- 1Department of Obstetrics and Gynecology, The Second Affiliated Hospital, Hengyang Medical School, University of South China, Hengyang, Hunan, China
- 2Department of Assisted Reproductive Centre, The affiliated Zhuzhou hospital Xiangya medical college, Central South University, Zhuzhou, Hunan, China
Malignant tumors are among the most important causes of death worldwide. The pathogenesis of a malignant tumor is complex and has not been fully elucidated. Studies have shown that such pathogenesis is related to abnormal cell cycle progression. The expression levels of cyclins, cyclin-dependent kinases (CDKs), and CDK inhibitors as well as functions of the cell cycle checkpoints determine whether the cell cycle progression is smooth. Cell-cycle-targeting drugs have the advantages of high specificity, low toxicity, low side effects, and low drug resistance. Identifying drugs that target the cell cycle and applying them in clinical treatments are expected to promote chemotherapeutic developments against malignant tumors. This article aims to review drugs targeted against the cell cycle and their action mechanisms.
1 Introduction
The cell cycle refers to a series of events within a cell that cause it to divide into two new daughter cells. The typical cell cycle is divided into four phases, namely, G1, S, G2, and M phases. The function of the G1 phase is to prepare for the S phase and synthesize large amounts of RNA and proteins. The S phase mainly involves DNA replication. Small amounts of RNA and proteins are synthesized in the G2 phase. Finally, the cells undergo karyokinesis and cytokinesis in the M phase. In the process of cell life, various factors (environmental factors, self-factors, etc.) can easily affect the integrity of the genetic material of the cell (Barnum and O’Connell, 2014; Li et al., 2022). Cells have developed a series of regulatory mechanisms to ensure continuous cell division and accurate replication of the cellular genetic material known as the cell cycle regulatory system.
The cell cycle consists of three important nodes that we refer to as the cell cycle checkpoints. The first is the G1/S checkpoint between the G1 and S phases and is also called as the restriction point. The second is the G2/M checkpoint located between the G2 and M phases and is also called as the DNA damage checkpoint. The third is the mitotic spindle assembly checkpoint (SAC) (Khan and Wang, 2022). The cell cycle can function properly only through these checkpoints (Poon, 2016), and the three most critical types of proteins involved in regulating the cell cycle are the cyclins, cyclin-dependent kinases (CDKs), and CDK inhibitor proteins (Khan and Wang, 2022). Cyclins bind to the CDKs to form complexes that drive the cell cycle (Gao et al., 2020). Different complexes can act on different phases of the cell cycle. For example, the cyclinD–CDK4/6 and cyclinE–CDK2 complexes act on the G1 phase; the cyclinA–CDK2 complex acts on the S phase; the cyclinB–CDK1 complex acts on the G2 and M phases (Pan et al., 2023).
Malignant tumors are among the most important causes of death worldwide, and their incidence is increasing annually. In the 21st century, cancer is expected to become the leading cause of death in every country and imposes an enormous burden (Lin et al., 2021). The treatments for malignant tumors include surgery, radiotherapy, and chemotherapy; however, patients can relapse easily after treatment, and the mortality rate is high, which is a major problem for not only clinicians but also researchers. Studies have shown that the pathogenesis of a malignant tumor is related to abnormal cell cycle progression (Liu et al., 2022), and the search for targeted drugs acting on the cell cycle is expected to provide new avenues for the treatment of malignant tumors.
Herein, our main purpose is to explore the relationships between malignant tumors and cell cycle regulation as well as between the cell cycle and targeted drugs (Table 1); we also review these relationships to provide references and evidence for subsequent research on malignant tumors.
2 Differences between normal and malignant cells
In normal cells, the progression and termination of the cell cycle are determined by the cell cycle regulatory system (Khan and Wang, 2022). Once a given cell cycle ends, the commencement of the next cell cycle is dependent upon the needs of the body. Unlike normal cells, malignant tumor cells are relatively autonomous. Owing to the instability of the genome, regulated growth of the malignant tumor cells is disrupted, cell replication is dysregulated by the body, and cell apoptosis is hindered (Hanahan and Weinberg, 2011), which manifests as a cell cycle disorder. Studies have shown that cell cycle disorders in malignant tumors are associated with the cell cycle checkpoints. Malignant tumor cells can overcome the limitations of these checkpoints (Suski et al., 2021) by activating various signaling pathways and altering the expression levels of the intracellular proteins (Li et al., 2024; Zhang et al., 2024; Zhou et al., 2024), eventually leading to imbalances in cell cycle regulation.
3 Changes in the cyclin–CDK complexes during different cell cycle phases
3.1 G1 phase
The cell cycle ends when the last cell completes mitosis. Then, if the cell needs to undergo another round of mitosis, the new daughter cells will enter the G1 phase and start a new cell cycle. In the G1 phase, the cells synthesize proteins (such as the protein replication complex (pre-RC)), RNA, ribosomes, and other substances in preparation for DNA synthesis in the next phase (Bandura and Calvi, 2002). The cyclinD–CDK4/6 complex plays an important role in this phase; CDK4/6 binds to cyclinD to form a complex, which further activates CDK4/6 to continue the cell cycle. The cyclinD–CDK4/6 complex binds to members of the retinoblastoma (RB) protein family and phosphorylates them. The phosphorylated RB proteins stimulate downstream signaling pathways to release E2F transcription factors and activate the E2F-responsive genes. The E2F-mediated gene expression generates cyclinE, which interacts with CDK2 to form the cyclinE–CDK2 complex that phosphorylates RB, further activating the E2F genes and promoting the progression of the cell division cycle (Wang, 2022; Hamilton and Infante, 2016). The cyclinD–CDK4/6 and cyclinE–CDK2 complexes phosphorylate the RB proteins sequentially to release the restriction of the G1/S checkpoint (Liu et al., 2022); the cells then enter the S phase after passing the G1/S checkpoint.
3.2 S phase
In the S phase, the E2F-mediated gene expression stimulates the synthesis of a large number of proteins, and the generated cyclinA and CDK2 form the cyclinA–CDK2 complex (Hume et al., 2020). Moreover, the cyclinE–CDK2 and cyclinA–CDK2 complexes further activate CDK, eventually activating pre-RC and initiating DNA replication. The cells in the S phase complete DNA replication and then enter the G2 phase (Bandura and Calvi, 2002).
3.3 G2 phase
During the G2 phase, proteins and other substances are synthesized, and the cells grow in preparation for the next stage of mitosis (Wang, 2022). In the G2 phase, CDK1 interacts with cyclinA to form the cyclinA–CDK1 complex, which plays an irreplaceable role in the cell cycle (Santaguida and Nepveu, 2005). The cyclinA–CDK1 complex activates and stabilizes the cyclinB–CDK1 complex, steadily increasing the activity of the cyclinB–CDK1 complex and advancing the cell cycle to the next stage until mitosis is completed (Wang, 2022). The G2/M phase checkpoint is responsible for detecting DNA damage in the cells; DNA damage that occurs during DNA replication needs to be repaired before the cells enter the next stage to prevent errors in the genetic material (Khan and Wang, 2022).
3.4 M phase
The M phase is divided into mitotic and cytoplasmic divisions; here, mitosis is further divided into prophase, prometaphase, metaphase, anaphase, and telophase. Prophase is characterized by intracellular chromatin/chromosome aggregation, centrosome separation, and nuclear membrane rupture (Figure 1); once the chromosomes in the cell are condensed, the centrosomes separate and move toward the poles of the cell, following which the nuclear membrane ruptures. During the prometaphase, the spindle microtubules attach to the centromere of the chromosomal centromere. During metaphase, the centromere microtubules pull the chromosomes and align them along the equatorial plate to ensure accurate chromosomal separation. During this stage, SAC confirms that the chromosomes are in the proper positions along the equatorial plate, in addition to ensuring that the chromosomes are properly connected to the spindles (Poon, 2016) and that the microtubules are pulling in the correct direction. After passing the SAC, the cell cycle continues and enters the mitotic anaphase. During anaphase, the microtubules pull the chromatids toward each pole. During telophase, the chromosomes uncoil to become chromatin, and the nuclear membrane is formed again. Eventually, the cell membrane contracts inward, dividing the cytoplasm equally between the two cells and forming the two new daughter cells with identical genetic material (Wang, 2022). The cyclinB–CDK1 complex has an irreplaceable role in mitosis, and its activation is believed to trigger mitosis. The cyclinB–CDK1 complex is activated during prophase, degraded in the middle stage, and dephosphorylated in the late stage, until it drops below the threshold and the cell exits mitosis (Gavet and Pines, 2010).
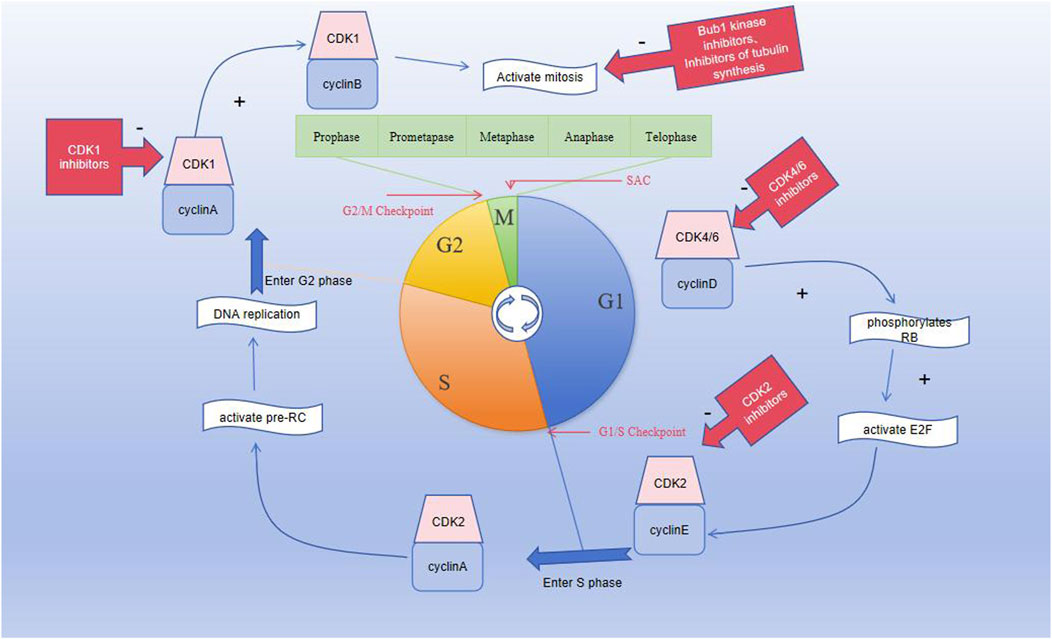
Figure 1. Graph showing the division of the cell cycle into G1, S, G2, and M phases; the M phase is further divided into prophase, prometaphase, metaphase, anaphase, and telophase. Three important checkpoints exist in the cell cycle, namely, the G1/S, G2/M, and spindle assembly checkpoints.
4 DNA replication and damage repair during the cell cycle
Although DNA replication has high fidelity, the cells are affected by various damaging factors at all times, making DNA damage inevitable. The DNA-damaging factors can be divided into endogenous and exogenous factors. The endogenous factors include errors in base pairing during DNA replication, instability in the DNA structure, and reactive oxygen species produced by metabolism, among others. The exogenous factors can be roughly divided into biological, chemical, and physical factors. Biological factors mainly include viruses that can reverse transcribe directly to affect the DNA or its metabolites, damaging the integrity of the DNA. The common physical factors include electromagnetic radiation like X-rays, gamma rays, and ultraviolet rays. The chemical factors include free radicals, base analogs, and alkylating agents (Carusillo and Mussolino, 2020). DNA damage activates the DNA damage repair pathway in the body, and the intracellular checkpoint proteins are activated to provide the necessary time for DNA repair, following which the cell cycle continues. If the DNA damage exceeds the limit of repair, the cell will activate the apoptotic pathway and eventually undergo cell death. If the mutated DNA is not repaired and cell replication is not halted, the damaged genetic material will be passed on to the next generation of daughter cells, which will eventually develop into malignant cells. Cancer is believed to result from mutations in cellular DNA through mutations occurring in only a few genes (Basu, 2018).
During the replication of malignant cells, complete DNA replication depends on normal progression of the DNA replication forks. The arresting or deceleration of replication fork progression is called as replication stress, which is an important cause of instability of the genetic material in malignant tumor cells. Damaged DNA not only affects the progression of the DNA replication forks but also causes replication stress (da Costa et al., 2023). However, malignant tumor cells do not die easily from DNA damage because they have special damage repair mechanisms. Malignant tumor cells can repair damaged DNA replication forks through break-induced replication (BIR), thereby ensuring the integrity of the genetic material (Costantino et al., 2014). Understanding the specific DNA repair mechanisms of malignant tumors is thus helpful for identifying the causes of the difficulty with curing malignant tumors and chemotherapeutic resistance, providing new ideas for the development of targeted drugs in the future.
5 Cell cycle checkpoints and target drugs
5.1 G1/S checkpoint and target drugs
The G1/S checkpoint is an important cell cycle checkpoint that can detect DNA integrity. It prevents DNA damage from being replicated and determines whether the cells can easily enter the S phase. Ataxia telangiectasia mutation (ATM) is a type of DNA damage sensor, and the ATM-Chk2-p53 pathway is activated when DNA damage occurs. Activated ATM regulates the activities of CHK2 and P53, thereby affecting DNA repair and cell cycle progression. ATM phosphorylates CHK2, which then inhibits CDC25A dephosphorylation and ultimately inhibits CDK2, leading to cell cycle disruption (Figure 2). Research shows that ATM is lost in gastrointestinal, respiratory, and lymphatic malignancies, suggesting that the absence of ATM is associated with the development of malignant tumors (Smith et al., 2020). Moreover, studies have shown that the cyclinD–CDK4/6 complex is an important part of the cellular transition from G1 to S phases and that the expressions of the cyclinD–CDK4/6 complex change in various tumors, showing abnormal elevation (Hamilton and Infante, 2016; Chen et al., 2003). Blocking the signaling pathway downstream of cyclinD–CDK4/6 prevents RB protein phosphorylation, thereby preventing cells from entering the S phase and undergoing subsequent tumorigenesis (Hume et al., 2020). In conclusion, the ATM-Chk2-P53 pathway, CDK2, and CDK4/6 may constitute breakthroughs for which we can develop targeted drugs against the proteins; this is expected to improve the prognosis of patients with malignant tumors.
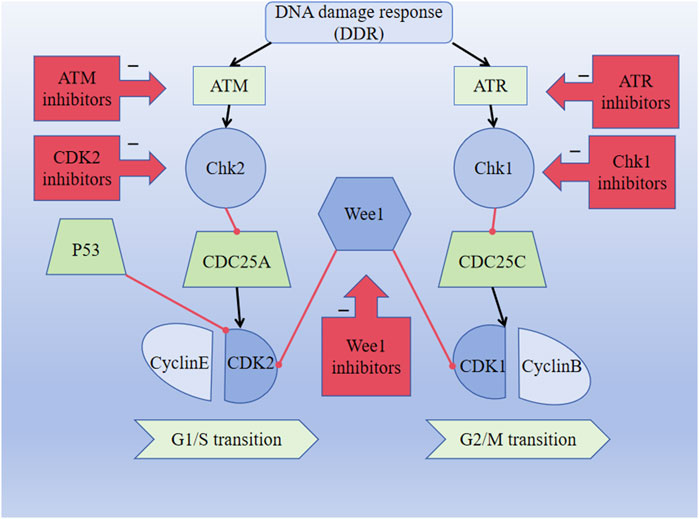
Figure 2. DNA damage response (DDR) pathway activates the ATM-CHK2-P53 signaling pathway and inhibits CDK2 expression, thereby affecting the G1/S transition. DDR pathway activates the ATR-CHK1-Wee1 signaling pathway and inhibits CDK1 expression, thus affecting the G2/M transition. The black lines indicate activation, and the red lines indicate inhibition.
ATM inhibitors: Clinical trials of the ATM inhibitors, including M3541, AZD0156, and AZD1390, are underway. In phase I clinical trials, M3541 adjuvant radiotherapy has been used to treat solid tumors; AZD0156 combined with olaparib, irinotecan, fluorouracil, and folinic acid has been used to treat advanced solid tumors; AZD1390 combined with radiotherapy has been used to treat brain tumors (Smith et al., 2020).
CDK2 inhibitors: Indisulam (E7070) is a type of CDK2 inhibitor that inhibits the activation of cyclinE–CDK2 and the cell cycle, causing G1/S arrest. Ziva Pogacar et al. reported that indisulam combined with the CDK4/6 inhibitor palbociclib could cause senescence or death of lung cancer cells (Pogacar et al., 2022).
CDK4/6 inhibitors: At present, three CDK4/6 inhibitors are in clinical use or trials: abemaciclib (LY2835219), palbociclib (PD-0332991), and ribociclib (LEE011) (Hamilton and Infante, 2016). Palbociclib is currently used to treat perimenopausal or premenopausal patients with breast cancer and is relatively effective against hormone-receptor-positive (HR+) breast cancer cells. In patients with partial mantle cell lymphoma (MCL), palbociclib has been found to be clinically beneficial. Ribociclib can be used to treat tumors with abnormal activities of the cyclinD–CDK complex and downstream pathways; some examples include neurological tumors, fat sarcomas, breast cancer, melanoma, and squamous cell carcinoma of the head and neck, with a certain safety profile. Abemaciclib can be used to treat postmenopausal women with breast cancer, with a clinical benefit rate of up to 72% for patients with HR+/HER2 breast cancers (Gao et al., 2020).
5.2 G2/M checkpoint and target agents
The G2/M checkpoint is responsible for monitoring DNA damage during cell cycle progression. The DNA damage response (DDR) is crucial for sustaining the integrity of the genetic material, and activation of the DDR pathway is closely coordinated with cell cycle arrest to prevent transmission of DNA damage to the next generation. Ataxia telangiectasia and Rad3 related (ATR) is a DNA damage sensor associated with phosphorylation and dephosphorylation that inactivates the CDKs, resulting in cessation of the cellular replication process. ATR is activated when the DNA is damaged by external or self-harmful factors. At this point, ATR fully activates the cell cycle checkpoint kinase 1 (CHK1) through serine phosphorylation. The overexpression of Wee1 has been observed in malignant tumor cells, including hepatic cell carcinomas, breast cancers, glioblastoma, respiratory tumors, and gastrointestinal tumors. The upregulation of the ATR-Chk1-Wee1 pathway in tumors may indicate poor prognosis, and the ATR-Chk1-Wee1 pathway is likely to be an attractive focus for the treatment of malignancies (Smith et al., 2020). Moreover, CHK1 inhibits the dephosphorylation functions of the CDC25 family of phosphatases and eventually inhibits CDK1, preventing cell entry into mitosis and thereby blocking the activity of the cyclinB–CDK1 complex (Figure 2) (Smith et al., 2010). Dysregulation of CDK1 expression is associated with the development of various malignant tumors and affects the survival probabilities of patients with different tumor types. Drugs targeting the ATR-Chk1-Wee1 pathway and CDK1 may thus be key strategies for the treatment of malignant tumors (Wang et al., 2023).
ATR inhibitors: Clinical trials of ATR inhibitors, including M6620 (VX-970), AZD6738, BAY1895344, and M4344 (VX-803), are underway. In phase I clinical trials, M6620 combined with radiotherapy was used to treat non-small cell lung carcinoma (NSCLC), small cell lung carcinoma (SCLC), and neural endocrine cancers that metastasized intracranially. M6620 combined with gemcitabine, cisplatin, carboplatin, paclitaxel, irinotecan, and other drugs have been used to treat late-stage solid tumors. M6620 combined with radiation and chemotherapy (cisplatin + capecitabine) has been used to treat esophageal cancer. AZD6738 monotherapy has been used for head and neck squamous cell carcinoma, chronic lymphocytic leukemia (CLL), prolymphocytic leukemia (PLL), B-cell lymphomas, chronic myeloid leukemia (CML), or myelodysplastic syndrome (MDS); AZD6738 combined with acalabrutinib has been prescribed for the treatment of non-Hodgkin lymphoma (NHL); AZD6738 combined with gemcitabine has been used in the treatment of late-stage solid tumors; AZD6738 with paclitaxel has been used to treat metastatic tumors for which standard chemotherapy has failed. BAY1895344 single therapy has been utilized for the treatment of late-stage solid tumors and lymphomas. M4344 (VX-803) is available as a standalone agent or is combination with cisplatin, carboplatin, and gemcitabine as a therapy for late-stage solid tumors. In phase I/II clinical trials, AZD6738 combined with carboplatin, olaparib, or durvalumab was used to treat NSCLC, breast cancer, squamous cell carcinoma of the head and neck, and gastrointestinal malignancies; AZD6738 was combined with acalcitoninib to treat refractory CLL. In phase II trials, M6620 combined with avelumab and carboplatin have been shown to treat primary peritoneal cancer and some malignant tumors of the female reproductive system, such as fallopian tube cancer and ovarian cancer; M6620 combined with cisplatin and gemcitabine has been used to treat metastatic urothelial carcinoma; M6620 combined with carboplatin/docetaxel has been used to treat prostate cancer; M6620 combined with gemcitabine has been used to treat recurrent ovarian cancer, primary peritoneal cancer, and fallopian tube cancer; M6620 combined with Irinotecan has been used to treat gastric or gastroesophageal junction cancers and metastatic gastric cancer. AZD6738 alone has been used to treat triple-negative mammary cancer (TNBC); AZD6738 combined with olaparib has been used in the treatment of nephrocellular carcinoma, urinary tract epithelial carcinoma, pancreatic carcinoma, recurrent ovarian carcinoma, SCLC, and carcinoma of the prostate, among others; AZD6738 and durvalumab combination has been used in the treatment of NSCLC, gastric cancer, and melanoma.
CHK1 inhibitors: At present, CHK1 inhibitors like MK-8776 (SCH900776) and LY2606368 (prexasertib) are used in clinical research. In phase I clinical trials, MK-8776 alone or in combination with cytarabine was used to treat acute leukemia; MK-8776 in combination with hydroxyurea was used to treat late-stage solid tumors. LY2606368 (prexasertib) alone or in combination with gemcitabine/pemetrexed or ralimetanib/olaparib/PD-L1 inhibitor was used to treat solid tumors; LY2606368 combined with cisplatin, cisplatin, cetuximab, or radiotherapy was used to treat head and neck cancers; LY2606368 and cytarabine has been used to treat myeloid leukemia. In phase I/II clinical trials, LY2606368 alone or in combination with gemcitabine was used to treat pancreatic cancer; LY2606368 combined with cisplatin or pemetrexed was used to treat NSCLC. MK-8776 as a single agent or in combination with cytarabine has been used to treat acute myeloid leukemia. In phase II clinical trials, LY2606368 monotherapy was used to treat SCLC, platinum-resistant ovarian cancer, some solid tumors, breast cancer, and prostate cancer; LY2606368 combined with pemetrexed has been used to treat NSCLC (Smith et al., 2020). SRA737 is an FDA-approved CHK1 inhibitor. Sen et al. (2019) reported that SRA737 and gemcitabine (LDG) could be combined to treat SCLC and other cancers (Sen et al., 2019).
Wee1 inhibitors: The Wee1 inhibitor AZD1775 (MK-1775) is currently in clinical trial. In phase I clinical trials, AZD1775 monotherapy has been used to treat ovarian cancer, SCLC, and solid tumors. AZD1775/paclitaxel and carboplatin/orapanitan/gemcitabine/cisplatin combinations have been used to treat solid tumors; AZD1775 combined with irinotecan has been used to treat metastatic colorectal cancer. In phase II clinical trials, AZD1775 monotherapy or in combination with carboplatin/taxol has been used to treat SCLC; AZD1775 and other combinations have been used to treat NSCLC; AZD1775 combined with cisplatin has been used for the treatment of TNBC; the combination of AZD1775 and cytarabine has been used to treat advanced acute myeloid leukemia or MDS (Smith et al., 2020).
CDK1 inhibitors: Ro-3306 and CGP74514A are selective CDK1 inhibitors, and the elimination of CDK1 phosphorylation causes cell cycle arrest at the G2/M checkpoint (Ly et al., 2015). Chen et al. (2023) reported that RO-3306 could significantly reduce the multiplication, mobility, and invasiveness of TNBC while increasing the susceptibility of cancer cells to cisplatin and paclitaxel (Chen et al., 2023). Huang et al. (2023) investigated both an ovarian cancer cell line and a high-grade serous ovarian cancer in a genetically engineered mouse model and reported that CDK1 inhibition plays an antitumor role (Huang et al., 2023). Yang et al. (2021) reported that the combination of CGP74514A (CGP) and a broad-spectrum CDK inhibitor flavopiridol (pull pingdu) can synergistically inhibit acute myeloid leukemia cell proliferation and induce apoptosis (Yang et al., 2021).
5.3 SAC and target drugs
The SAC is an important mechanism for safeguarding mitotic fidelity to ensure the accuracy of karyotype numbers in meristematic cells. SAC impairment can cause destabilization of the chromosomes and also tumor development (Hosea et al., 2024). The major SAC components include BUB1, BUBR1, MAD2, CENP-E, and CDC20, among others. Mutations of the SAC components are associated with tumor progression, suggesting that the SAC components may be potential therapeutic targets for the treatment of malignant tumors (Bolanos-Garcia, 2009). BAY1816032 is a selective BUB1 kinase inhibitor, and Siemeister et al. (2019) reported that BAY1816032 can increase tumor cell sensitivity to paclitaxel, ATR inhibitors, and PARP inhibitors. In addition, the combination of BAY1816032 and paclitaxel has synergistic or additive antiproliferative effects during the treatment of malignancies, including cervical cancers, TNBC, NSCLC, prostate cancer, and intracranial malignant tumor cells (Siemeister et al., 2019). Huang et al. (2021) reported that BAY1816032 can significantly reduce the multiplication, aggressiveness, and migration of osteosarcoma cells and could be a novel therapeutic target for osteosarcoma (Huang et al., 2021). Indibulin is an inhibitor of tubulin synthesis that can activate the spindle assembly checkpoint proteins MAD2 and BUBR1 to halt the cell cycle. Kapoor et al. (2018a) observed that indibulin has favorable anticancer activity and less neural toxicity in both preclinical animal models and phase I clinical trials of carcinogenic chemistry; derivatives of indibulin have strong antiproliferative effects on different types of tumor cells, such as head and neck tumors, NSCLC, gastric cancer, and breast cancer (Kapoor et al., 2018a). Li et al. (2019) showed that the CDC20-MAD2 complex could prevent apoptosis by preventing the early biodegradation of cyclin B1; M2I-1 is an MAD2 inhibitor that interferes with the interactions between CDC20 and MAD2, thereby increasing the susceptibility of cancer cells to antiangiogenic drugs such as paclitaxel (Li et al., 2019). GSK923295 is a specific CENP-E inhibitor that causes chromosomal dislocation and interrupts mitotic progression (Qian et al., 2010). Chung et al. (2012) demonstrated that GSK923295 exhibits in vitro antitumor activity against several solid tumor cell lines and hematological malignant cell lines; it also exhibits in vivo antitumor activity against numerous solid tumor xenograft models, with very few grade 3 or 4 adverse reactions (Chung et al., 2012).
6 Traditional chemotherapeutic drugs
Chemotherapy is an important approach to treating malignant tumors. Based on the different mechanisms of operation, commonly used chemotherapy drugs can be classified into the categories listed below.
6.1 Drugs affecting DNA biosynthesis
Methotrexate: Methotrexate mainly affects the S phase of the cell cycle and DNA synthesis, thereby inhibiting the growth and proliferation of malignant tumor cells. Methotrexate is a dihydrofolate reductase inhibitor that prevents the conversion of dihydrofolate to tetrahydrofolate, ultimately reducing the synthesis of deoxythymidine acid and leading to impaired DNA synthesis (Olsen, 1991). Methotrexate is commonly used to treat acute leukemia and choriocarcinoma, and the common adverse reactions are myelosuppression, liver damage, and kidney damage, among others.
Fluorouracil: Fluorouracil mainly acts on the S phase of the cell cycle and can affect DNA synthesis in malignant tumor cells to inhibit the growth of malignant tumors. Fluorouracil is converted to fluorouracil deoxynucleotides in the cells, thereby inhibiting deoxythymidylate synthase and preventing the conversion of deoxyuridine acid to deoxythymidylate to interfere with DNA synthesis. Fluorouracil is mainly used to treat malignant tumors of the digestive system (such as colorectal cancer), breast cancer, and head and neck cancers. The common adverse reactions of this drug are bone marrow suppression and gastrointestinal damage (Longley et al., 2003).
Cytarabine: Cytarabine mainly targets the S phase of the cell cycle, affecting DNA synthesis and interfering with DNA replication to kill the malignant tumor cells. Cytarabine is a DNA polymerase inhibitor; after it enters the body, it produces metabolites such as cytarabine triphosphate, resulting in abnormal functions of DNA polymerase and interfering with DNA synthesis and replication to inhibit the proliferation of malignant tumor cells (Faruqi and Tadi, 2023). Cytarabine is commonly used to treat acute leukemia, and its common adverse reactions are myelosuppression and gastrointestinal reactions (Baker et al., 1991).
6.2 Drugs affecting DNA structure and functions
Cyclophosphamide: Cyclophosphamide is a cell cycle non-specific drug that acts by disrupting the structure of the cellular DNA. After cyclophosphamide enters the body, it produces phosphoramide nitrogen mustard, which can undergo alkylation reactions with the DNA in cells, cause DNA breakage, and destroy the normal structure and functions of DNA. Cyclophosphamide is commonly used to treat multiple myeloma, leukemia, and solid tumors. The common adverse reactions of this drug are bone marrow suppression, nausea, and vomiting (Emadi et al., 2009).
Platinum-based drugs: Cisplatin is a cell cycle non-specific drug. After entering the body, cisplatin binds to the double strand of the DNA and forms intrastrand/interstrand crosslinks, which can prevent DNA replication or break the DNA strand. Cisplatin is commonly used to treat testicular cancer and ovarian cancer. The common adverse reactions of these drugs include gastrointestinal reactions, bone marrow suppression, and otonephrotoxicity (Dasari and Tchounwou, 2014).
6.3 Drugs affecting RNA and protein syntheses
Adriamycin: Adriamycin acts on cells in all phases of the cell cycle, but cells in the S phase are more sensitive to it. Adriamycin is an anthracycline antibiotic that can bind tightly to DNA, affecting not only DNA replication but also RNA transcription and synthesis. Adriamycin is commonly used to treat acute leukemia, breast cancer, and ovarian cancer. Its common adverse reactions are cardiotoxicity, bone marrow suppression, and gastrointestinal reactions (Tsukagoshi, 1988).
Vincristine: Vincristine mainly targets the M phase of the cell cycle and affects cell cycle progression by altering spindle filament formation. When vincristine binds to tubulin, it inhibits microtubule polymerization, blocks the generation of spindle filaments, and eventually leads to cell cycle arrest. Vincristine is commonly used to treat acute leukemia and lymphoma. Its common adverse reactions are neurotoxicity, bone marrow suppression, and gastrointestinal reactions.
Taxanes: Paclitaxel mainly acts on the M phase of the cell cycle and can affect the normal functions of the spindles. When paclitaxel enters the cell, it promotes tubulin polymerization and inhibits its depolymerization, thus causing a loss of function of the spindle and ultimately blocking cell mitosis to inhibit tumor cell growth. Paclitaxel is commonly used to treat ovarian and breast cancers. Its common adverse reactions are myelosuppression, neurotoxicity, and anaphylaxis (Alqahtani et al., 2019).
6.4 Other drugs
Minichromosome maintenance (MCM) complex inhibitors have also been developed. The MCM complex is closely linked to DNA duplication, and its dysfunction can lead to the development of malignant tumors. The inhibition of MCM or its downstream signaling pathway is expected to be a therapeutic target for treating malignant tumors (Li et al., 2021; Cao et al., 2020; Tang et al., 2023; Zeng et al., 2021).
7 Cell-cycle-targeting drugs reduce chemotherapeutic resistance
With the development of medicine and pharmacology, various chemotherapeutic drugs have been developed and applied clinically, but drug resistance or even multidrug resistance (MDR) can occur in patients during chemotherapy, whose mechanisms are not fully clear. At present, the possible resistance mechanisms listed below are considered.
7.1 Mutations in cell cycle regulatory genes
Mutations in the cell cycle regulatory genes may lead to cell cycle disorders, which potentially affect the efficacies of chemotherapeutic drugs and lead to drug resistance. For example, P53 is a protein that regulates the cell cycle and plays an important role in various malignant tumors. Frequent P53 mutations can eliminate the inhibitory effects on tumor cells and increase the DNA repair functions of malignant tumor cells such that the killing effects of chemotherapeutic drugs on DNA are reduced, leading to chemotherapeutic drug resistance (Alvarado-Ortiz et al., 2021).
7.2 Abnormal expressions of cyclins or altered functions of cell cycle checkpoints
The efficacy of a chemotherapeutic drug is closely related to the cell cycle of the malignant tumor cells, and interfering with the cell cycle affects the efficacy of the drug. The progression of the cell cycle is related to the expression levels of cyclins and functions of the cell cycle checkpoints. Malignant tumor cells can interfere with cellular processes by regulating the expressions of cyclins or altering the functions of the cell cycle checkpoints, thereby affecting the efficacies of the chemotherapeutic drugs and leading to resistance. For example, cyclinD expression affects the sensitivity of multiple myeloma cells to chemotherapeutic agents (Bustany et al., 2016). The overexpression of cyclinA is significantly associated with resistance to first-line platinum-based chemotherapy in ovarian cancer cells (Cybulski et al., 2015). The activities of cell cycle checkpoint kinases and functions of these checkpoints are altered in lung cancer cells, thereby interfering with cell cycle progression and leading to chemotherapeutic resistance (Ke et al., 2021).
7.3 Activation of antiapoptotic mechanisms
In the occurrence and development of malignant tumors, loss of control of the apoptotic signals and even activation of the antiapoptotic mechanisms can occur in tumor cells, which lead to failure of chemotherapy drugs that induce apoptosis (Mohammad et al., 2015). For example, mutations in the CHEK2 gene activate the P53 apoptotic pathway and induce apoptosis in TNMC cells, leading to chemotherapeutic resistance (Luo et al., 2018).
Cell-cycle-targeting drugs have advantages over traditional chemotherapeutic drugs. In particular, targeted drugs can act on target organs with high specificity and have fewer toxic side effects, which can improve the survival rates of patients (Lee et al., 2018). In contrast to traditional chemotherapy drugs, cell-cycle-targeting drugs have certain advantages in overcoming MDR. On the one hand, given the precise actions of cell-cycle-targeting drugs, the genes of the tumor cells do not readily mutate, thereby reducing the occurrence of drug resistance. On the other hand, cell-cycle-targeting drugs act on cell cycle checkpoints and related pathways, do not directly damage the DNA of the malignant tumor cells, reduce the repair of DNA damage by tumor cells, and reduce the occurrence of chemotherapeutic resistance (Wu et al., 2014). Studies have shown that combination therapy not only reduces the toxic side effects of chemotherapeutic drugs on normal cells but also minimizes drug resistance (Mokhtari et al., 2017; Yue et al., 2021). Moreover, eradicating malignant tumor cells as much as possible and shrinking the tumor can reduce the occurrence of drug resistance (Chatterjee and Bivona, 2019). For example, in the treatment of SCLC, the combination of Wee1 inhibitors can ensure efficacy while reducing the side effects of chemotherapy (Meijer et al., 2022). In NSCLC, the use of a Wee1 inhibitor can increase the sensitivity of the tumor cells to sorafenib (Caiola et al., 2018). Ma et al. (2017) reported that the combination of the CDK4/6 inhibitor palbociclib and anastrozole can inhibit the proliferation of tumor cells and reduce drug resistance in patients with ER+/HER2- breast cancers (Ma et al., 2017).
8 Conclusions and perspectives
In this review, we summarize the pathological changes to the cell cycle in malignant tumors and the mechanisms of cell-cycle-targeting drugs. The action mechanisms of malignant tumors are very complex and are have not been fully elucidated thus far; these mechanisms are usually characterized by cell proliferation and are not subject to regulation or cell cycle disorders. Studies have shown that cell cycle progression, cell-cycle-related protein (cyclin, CDK, and CDK inhibitor) expressions, and activation of relevant proteins indicate that malignant tumors are potential therapeutic targets. In recent years, major advancements have been achieved in research on cell-cycle-targeting drugs, and some drugs such as the CDK4/6 inhibitors have been licensed for the clinical treatment of malignant tumors. Moreover, the combination of cell-cycle-targeting drugs and traditional chemotherapeutic drugs can significantly increase the therapeutic effects. However, methods to ensure the efficacy and safety of the drugs and resistance to subsequent treatment are still major problems that must be solved.
Therefore, future research efforts need to be focused on elucidating the pathogenesis of malignant tumors and developing cell-cycle-targeting drugs to formulate novel treatment options with increased scientific and clinical value while providing new hope for the treatment of malignant tumors in the future.
Author contributions
GS: writing–original draft and writing–review and editing. JL: writing–original draft and writing–review and editing. XT: writing–review and editing. JZ (4th author): writing–review and editing. YZ: writing–review and editing. XZ: writing–review and editing. JbZ: writing–review and editing. JZ (8th author): writing–review and editing. LC: writing–review and editing. QZ: writing–review and editing. YL: writing–review and editing.
Funding
The authors declare that no financial support was received for the research, authorship, and/or publication of this article.
Conflict of interest
The authors declare that the research was conducted in the absence of any commercial or financial relationships that could be construed as a potential conflict of interest.
Publisher’s note
All claims expressed in this article are solely those of the authors and do not necessarily represent those of their affiliated organizations or those of the publisher, editors, and reviewers. Any product that may be evaluated in this article or claim that may be made by its manufacturer is not guaranteed or endorsed by the publisher.
References
Alqahtani, F. Y., Aleanizy, F. S., El Tahir, E., Alkahtani, H. M., and AlQuadeib, B. T. (2019). Paclitaxel. Relat. Methodol. 44, 205–238. doi:10.1016/bs.podrm.2018.11.001
Alvarado-Ortiz, E., de la Cruz-López, K. G., Becerril-Rico, J., Sarabia-Sánchez, M. A., Ortiz-Sánchez, E., and García-Carrancá, A. (2021). Mutant p53 gain-of-function: role in cancer development, progression, and therapeutic approaches. Front. Cell Dev. Biol. 8, 607670. Published 2021 Feb 11. doi:10.3389/fcell.2020.607670
Baker, W. J., Royer, G. L., and Weiss, R. B. (1991). Cytarabine and neurologic toxicity. J. Clin. Oncol. 9 (4), 679–693. doi:10.1200/JCO.1991.9.4.679
Bandura, J. L., and Calvi, B. R. (2002). Duplication of the genome in normal and cancer cell cycles. Cancer Biol. Ther. 1 (1), 8–13. doi:10.4161/cbt.1.1.31
Barnum, K. J., and O’Connell, M. J. (2014). Cell cycle regulation by checkpoints. Methods Mol. Biol. 1170, 29–40. doi:10.1007/978-1-4939-0888-2_2
Basu, A. K. (2018). DNA damage, mutagenesis and cancer. Int. J. Mol. Sci. 19 (4), 970. Published 2018 Mar 23. doi:10.3390/ijms19040970
Bolanos-Garcia, V. M. (2009). Assessment of the mitotic spindle assembly checkpoint (SAC) as the target of anticancer therapies. Curr. Cancer Drug Targets 9 (2), 131–141. doi:10.2174/156800909787580980
Bridges, K. A., Hirai, H., Buser, C. A., Brooks, C., Liu, H., Buchholz, T. A., et al. (2011). MK-1775, a novel Wee1 kinase inhibitor, radiosensitizes p53-defective human tumor cells. Clin. Cancer Res. 17 (17), 5638–5648. Epub 2011 Jul 28. doi:10.1158/1078-0432.CCR-11-0650
Bustany, S., Bourgeais, J., Tchakarska, G., Body, S., Hérault, O., Gouilleux, F., et al. (2016). Cyclin D1 unbalances the redox status controlling cell adhesion, migration, and drug resistance in myeloma cells. Oncotarget 7 (29), 45214–45224. doi:10.18632/oncotarget.9901
Caiola, E., Frapolli, R., Tomanelli, M., Valerio, R., Iezzi, A., Garassino, M. C., et al. (2018). Wee1 inhibitor MK1775 sensitizes KRAS mutated NSCLC cells to sorafenib. Sci. Rep. 8 (1), 948. Published 2018 Jan 17. doi:10.1038/s41598-017-18900-y
Cao, T., Yi, S. J., Wang, L. X., Zhao, J. X., Xiao, J., Xie, N., et al. (2020). Identification of the DNA replication regulator MCM complex expression and prognostic significance in hepatic carcinoma. Biomed. Res. Int. 2020, 3574261. Published 2020 Sep 9. doi:10.1155/2020/3574261
Carusillo, A., and Mussolino, C. (2020). DNA damage: from threat to treatment. Cells 9 (7), 1665. Published 2020 Jul 10. doi:10.3390/cells9071665
Chatterjee, N., and Bivona, T. G. (2019). Polytherapy and targeted cancer drug resistance. Trends Cancer 5 (3), 170–182. doi:10.1016/j.trecan.2019.02.003
Chen, J., Li, M., Liu, Y., Guan, T., Yang, X., Wen, Y., et al. (2023). PIN1 and CDK1 cooperatively govern pVHL stability and suppressive functions. Cell Death Differ. 30 (4), 1082–1095. doi:10.1038/s41418-023-01128-x
Chen, Q., Lin, J., Jinno, S., and Okayama, H. (2003). Overexpression of Cdk6-cyclin D3 highly sensitizes cells to physical and chemical transformation. Oncogene 22 (7), 992–1001. doi:10.1038/sj.onc.1206193
Chung, V., Heath, E. I., Schelman, W. R., Johnson, B. M., Kirby, L. C., Lynch, K. M., et al. (2012). First-time-in-human study of GSK923295, a novel antimitotic inhibitor of centromere-associated protein E (CENP-E), in patients with refractory cancer. Cancer Chemother. Pharmacol. 69 (3), 733–741. doi:10.1007/s00280-011-1756-z
Costantino, L., Sotiriou, S. K., Rantala, J. K., Magin, S., Mladenov, E., Helleday, T., et al. (2014). Break-induced replication repair of damaged forks induces genomic duplications in human cells. Science 343 (6166), 88–91. doi:10.1126/science.1243211
Cybulski, M., Jarosz, B., Nowakowski, A., Jeleniewicz, W., Kutarska, E., Bednarek, W., et al. (2015). Cyclin A correlates with YB1, progression and resistance to chemotherapy in human epithelial ovarian cancer. Anticancer Res. 35 (3), 1715–1721.
da Costa, AABA, Chowdhury, D., Shapiro, G. I., D'Andrea, A. D., and Konstantinopoulos, P. A. (2023). Targeting replication stress in cancer therapy. Nat. Rev. Drug Discov. 22 (1), 38–58. doi:10.1038/s41573-022-00558-5
Dasari, S., and Tchounwou, P. B. (2014). Cisplatin in cancer therapy: molecular mechanisms of action. Eur. J. Pharmacol. 740, 364–378. doi:10.1016/j.ejphar.2014.07.025
Durant, S. T., Zheng, L., Wang, Y., Chen, K., Zhang, L., Zhang, T., et al. (2018). The brain-penetrant clinical ATM inhibitor AZD1390 radiosensitizes and improves survival of preclinical brain tumor models. Sci. Adv. 4 (6), eaat1719. doi:10.1126/sciadv.aat1719
Emadi, A., Jones, R. J., and Brodsky, R. A. (2009). Cyclophosphamide and cancer: golden anniversary. Nat. Rev. Clin. Oncol. 6 (11), 638–647. doi:10.1038/nrclinonc.2009.146
Faruqi, A., and Tadi, P. (2023). “Cytarabine,” in StatPearls. Treasure island (FL) (Treasure Island (FL): StatPearls Publishing).
Fokas, E., Prevo, R., Pollard, J. R., Reaper, P. M., Charlton, P. A., Cornelissen, B., et al. (2012). Targeting ATR in vivo using the novel inhibitor VE-822 results in selective sensitization of pancreatic tumors to radiation. Cell Death Dis. 3, e441. doi:10.1038/cddis.2012.181
Fry, D. W., Harvey, P. J., Keller, P. R., Elliott, W. L., Meade, M., Trachet, E., et al. (2004). Specific inhibition of cyclin-dependent kinase 4/6 by PD 0332991 and associated antitumor activity in human tumor xenografts. Mol. Cancer Ther. 3 (11), 1427–1438. doi:10.1158/1535-7163.1427.3.11
Gao, X., Leone, G. W., and Wang, H. (2020). Cyclin D-CDK4/6 functions in cancer. Adv. Cancer Res. 148, 147–169. doi:10.1016/bs.acr.2020.02.002
Gavet, O., and Pines, J. (2010). Progressive activation of CyclinB1-Cdk1 coordinates entry to mitosis. Dev. Cell 18 (4), 533–543. doi:10.1016/j.devcel.2010.02.013
Gelbert, L. M., Cai, S., Lin, X., Sanchez-Martinez, C., Del Prado, M., Lallena, M. J., et al. (2014). Preclinical characterization of the CDK4/6 inhibitor LY2835219: in-vivo cell cycle-dependent/independent anti-tumor activities alone/in combination with gemcitabine. Invest New Drugs 32 (5), 825–837. doi:10.1007/s10637-014-0120-7
Guzi, T. J., Paruch, K., Dwyer, M. P., Labroli, M., Shanahan, F., Davis, N., et al. (2011). Targeting the replication checkpoint using SCH 900776, a potent and functionally selective CHK1 inhibitor identified via high content screening. Mol. Cancer Ther. 10 (4), 591–602. doi:10.1158/1535-7163.MCT-10-0928
Hamilton, E., and Infante, J. R. (2016). Targeting CDK4/6 in patients with cancer. Cancer Treat. Rev. 45, 129–138. doi:10.1016/j.ctrv.2016.03.002
Hanahan, D., and Weinberg, R. A. (2011). Hallmarks of cancer: the next generation. Cell 144 (5), 646–674. doi:10.1016/j.cell.2011.02.013
Hosea, R., Duan, W., Meliala, I. T. S., Li, W., Wei, M., Hillary, S., et al. (2024). YY2/BUB3 Axis promotes SAC hyperactivation and inhibits colorectal cancer progression via regulating chromosomal instability. Adv. Sci. (Weinh) 11, e2308690. Published online April 29. doi:10.1002/advs.202308690
Huang, Y., Fan, Y., Zhao, Z., Zhang, X., Tucker, K., Staley, A., et al. (2023). Inhibition of CDK1 by RO-3306 exhibits anti-tumorigenic effects in ovarian cancer cells and a transgenic mouse model of ovarian cancer. Int. J. Mol. Sci. 24 (15), 12375. Published 2023 Aug 3. doi:10.3390/ijms241512375
Huang, Z., Wang, S., Wei, H., Chen, H., Shen, R., Lin, R., et al. (2021). Inhibition of BUB1 suppresses tumorigenesis of osteosarcoma via blocking of PI3K/Akt and ERK pathways. J. Cell Mol. Med. 25 (17), 8442–8453. doi:10.1111/jcmm.16805
Hume, S., Dianov, G. L., and Ramadan, K. (2020). A unified model for the G1/S cell cycle transition. Nucleic Acids Res. 48 (22), 12483–12501. doi:10.1093/nar/gkaa1002
Imidazo 4,5-c quinolin-2-one compounds and their use in treating cancer. (2019). US-10189834-B2. Issued 2019.
Kapoor, S., Srivastava, S., and Panda, D. (2018a). Indibulin dampens microtubule dynamics and produces synergistic antiproliferative effect with vinblastine in MCF-7 cells: implications in cancer chemotherapy. Sci. Rep. 8 (1), 12363. Published 2018 Aug 17. doi:10.1038/s41598-018-30376-y
Kapoor, S., Srivastava, S., and Panda, D. (2018b). Indibulin dampens microtubule dynamics and produces synergistic antiproliferative effect with vinblastine in MCF-7 cells: implications in cancer chemotherapy. Sci. Rep. 8 (1), 12363. doi:10.1038/s41598-018-30376-y
Ke, Z., Liang, A., and Liu, Y. (2021). Zhongguo Fei Ai Za Zhi 24 (4), 265–270. doi:10.3779/j.issn.1009-3419.2021.101.09
Khan, M. G. M., and Wang, Y. (2022). Cell cycle-related clinical applications. Methods Mol. Biol. 2579, 35–46. doi:10.1007/978-1-0716-2736-5_3
King, C., Diaz, H. B., McNeely, S., Barnard, D., Dempsey, J., Blosser, W., et al. (2015). LY2606368 causes replication catastrophe and antitumor effects through CHK1-dependent mechanisms. Mol. Cancer Ther. 14 (9), 2004–2013. doi:10.1158/1535-7163.MCT-14-1037
Lee, Y. T., Tan, Y. J., and Oon, C. E. (2018). Molecular targeted therapy: treating cancer with specificity. Eur. J. Pharmacol. 834, 188–196. doi:10.1016/j.ejphar.2018.07.034
Li, J., Dang, N., Martinez-Lopez, N., Jowsey, P. A., Huang, D., Lightowlers, R. N., et al. (2019). M2I-1 disrupts the in vivo interaction between CDC20 and MAD2 and increases the sensitivities of cancer cell lines to anti-mitotic drugs via MCL-1s. Cell Div. 14, 5. Published 2019 Jun 15. doi:10.1186/s13008-019-0049-5
Li, S., Wang, L., Wang, Y., Zhang, C., Hong, Z., and Han, Z. (2022). The synthetic lethality of targeting cell cycle checkpoints and PARPs in cancer treatment. J. Hematol. Oncol. 15 (1), 147. doi:10.1186/s13045-022-01360-x
Li, Y., Zou, J., Zhang, Q., Quan, F., Cao, L., Zhang, X., et al. (2021). Systemic analysis of the DNA replication regulator MCM complex in ovarian cancer and its prognostic value. Front. Oncol. 11, 681261. Published 2021 Jun 9. doi:10.3389/fonc.2021.681261
Li, Y. K., Gao, A. B., Zeng, T., Liu, D., Zhang, Q. F., Ran, X. M., et al. (2024). ANGPTL4 accelerates ovarian serous cystadenocarcinoma carcinogenesis and angiogenesis in the tumor microenvironment by activating the JAK2/STAT3 pathway and interacting with ESM1. J. Transl. Med. 22 (1), 46. doi:10.1186/s12967-023-04819-8
Lin, L., Li, Z., Yan, L., Liu, Y., Yang, H., and Li, H. (2021). Global, regional, and national cancer incidence and death for 29 cancer groups in 2019 and trends analysis of the global cancer burden, 1990-2019. J. Hematol. Oncol. 14 (1), 197. doi:10.1186/s13045-021-01213-z
Liu, J., Peng, Y., and Wei, W. (2022). Cell cycle on the crossroad of tumorigenesis and cancer therapy. Trends Cell Biol. 32 (1), 30–44. doi:10.1016/j.tcb.2021.07.001
Longley, D. B., Harkin, D. P., and Johnston, P. G. (2003). 5-fluorouracil: mechanisms of action and clinical strategies. Nat. Rev. Cancer 3 (5), 330–338. doi:10.1038/nrc1074
Luo, L., Gao, W., Wang, J., Wang, D., Peng, X., Jia, Z., et al. (2018). Study on the mechanism of cell cycle checkpoint kinase 2 (CHEK2) gene dysfunction in chemotherapeutic drug resistance of triple negative breast cancer cells. Med. Sci. Monit. 24, 3176–3183. Published 2018 May 15. doi:10.12659/MSM.907256
Ly, T., Endo, A., and Lamond, A. I. (2015). Proteomic analysis of the response to cell cycle arrests in human myeloid leukemia cells. Elife 4, e04534. Published 2015 Jan 2. doi:10.7554/eLife.04534
Ma, C. X., Gao, F., Luo, J., Northfelt, D. W., Goetz, M., Forero, A., et al. (2017). NeoPalAna: neoadjuvant palbociclib, a cyclin-dependent kinase 4/6 inhibitor, and anastrozole for clinical stage 2 or 3 estrogen receptor-positive breast cancer. Clin. Cancer Res. 23 (15), 4055–4065. doi:10.1158/1078-0432.CCR-16-3206
Meijer, J. J., Leonetti, A., Airò, G., Tiseo, M., Rolfo, C., Giovannetti, E., et al. (2022). Small cell lung cancer: novel treatments beyond immunotherapy. Semin. Cancer Biol. 86 (Pt 2), 376–385. doi:10.1016/j.semcancer.2022.05.004
Mohammad, R. M., Muqbil, I., Lowe, L., Yedjou, C., Hsu, H. Y., Lin, L. T., et al. (2015). Broad targeting of resistance to apoptosis in cancer. Semin. Cancer Biol. 35 (Suppl. l(0), S78-S103–S103. doi:10.1016/j.semcancer.2015.03.001
Mokhtari, R. B., Homayouni, T. S., Baluch, N., Morgatskaya, E., Kumar, S., Das, B., et al. (2017). Combination therapy in combating cancer. Oncotarget 8 (23), 38022–38043. doi:10.18632/oncotarget.16723
Olsen, E. A. (1991). The pharmacology of methotrexate. J. Am. Acad. Dermatol 25 (2 Pt 1), 306–318. doi:10.1016/0190-9622(91)70199-c
Osborne, J. D., Matthews, T. P., McHardy, T., Proisy, N., Cheung, K. M. J., Lainchbury, M., et al. (2016). Multiparameter lead optimization to give an oral checkpoint kinase 1 (CHK1) inhibitor clinical candidate: (R)-5-((4-((Morpholin-2-ylmethyl)amino)-5-(trifluoromethyl)pyridin-2-yl)amino)pyrazine-2-carbonitrile (CCT245737). J. Med. Chem. 59 (11), 5221–5237. doi:10.1021/acs.jmedchem.5b01938
Ozawa, Y., Sugi, N. H., Nagasu, T., Owa, T., Watanabe, T., Koyanagi, N., et al. (2001). E7070, a novel sulphonamide agent with potent antitumour activity in vitro and in vivo. Eur. J. Cancer 37 (17), 2275–2282. doi:10.1016/s0959-8049(01)00275-1
Pan, J., Shang, F., Ma, R., Rong, Y., and Zhang, Y. (2023). Sheng Wu Gong Cheng Xue Bao 39 (4), 1525–1547. doi:10.13345/j.cjb.220478
Pogacar, Z., Johnson, J. L., Krenning, L., De Conti, G., Jochems, F., Lieftink, C., et al. (2022). Indisulam synergizes with palbociclib to induce senescence through inhibition of CDK2 kinase activity. PLoS One 17 (9), e0273182. Published 2022 Sep 6. doi:10.1371/journal.pone.0273182
Poon, R. Y. (2016). Cell cycle control: a system of interlinking oscillators. Methods Mol. Biol. 1342, 3–19. doi:10.1007/978-1-4939-2957-3_1
Qian, X., McDonald, A., Zhou, H. J., Adams, N. D., Parrish, C. A., Duffy, K. J., et al. (2010). Discovery of the first potent and selective inhibitor of centromere-associated protein E: GSK923295. ACS Med. Chem. Lett. 1 (1), 30–34. Published 2010 Jan 19. doi:10.1021/ml900018m
Santaguida, M., and Nepveu, A. (2005). Differential regulation of CDP/Cux p110 by cyclin A/Cdk2 and cyclin A/Cdk1. J. Biol. Chem. 280 (38), 32712–32721. doi:10.1074/jbc.M505417200
Sen, T., Della Corte, C. M., Milutinovic, S., Cardnell, R. J., Diao, L., Ramkumar, K., et al. (2019). Combination treatment of the oral CHK1 inhibitor, SRA737, and low-dose gemcitabine enhances the effect of programmed death ligand 1 blockade by modulating the immune microenvironment in SCLC. J. Thorac. Oncol. 14 (12), 2152–2163. doi:10.1016/j.jtho.2019.08.009
Siemeister, G., Mengel, A., Bone, W., Schröder, J., Zitzmann-Kolbe, S., Briem, H., et al. (2017). Abstract 287: BAY 1816032, a novel BUB1 kinase inhibitor with potent antitumor activity. Cancer Res. 77 (13 Suppl. l), 287. doi:10.1158/1538-7445.AM2017-287
Siemeister, G., Mengel, A., Fernández-Montalván, A. E., Bone, W., Schröder, J., Zitzmann-Kolbe, S., et al. (2019). Inhibition of BUB1 kinase by BAY 1816032 sensitizes tumor cells toward taxanes, ATR, and PARP inhibitors in vitro and in vivo. Clin. Cancer Res. 25 (4), 1404–1414. doi:10.1158/1078-0432.CCR-18-0628
Smith, H. L., Southgate, H., Tweddle, D. A., and Curtin, N. J. (2020). DNA damage checkpoint kinases in cancer. Expert Rev. Mol. Med. 22, e2. Published 2020 Jun 8. doi:10.1017/erm.2020.3
Smith, J., Tho, L. M., Xu, N., and Gillespie, D. A. (2010). The ATM-Chk2 and ATR-Chk1 pathways in DNA damage signaling and cancer. Adv. Cancer Res. 108, 73–112. doi:10.1016/B978-0-12-380888-2.00003-0
Suski, J. M., Braun, M., Strmiska, V., and Sicinski, P. (2021). Targeting cell-cycle machinery in cancer. Cancer Cell 39 (6), 759–778. doi:10.1016/j.ccell.2021.03.010
Tang, M., Chen, J., Zeng, T., Ye, D. M., Li, Y. K., Zou, J., et al. (2023). Systemic analysis of the DNA replication regulator origin recognition complex in lung adenocarcinomas identifies prognostic and expression significance. Cancer Med. 12 (4), 5035–5054. doi:10.1002/cam4.5238
Ulrich, T. L., Lefranc, J., Wengner, A., Wortmann, L., Schick, H., Briem, H., et al. (2017). Abstract 983: identification of potent, highly selective and orally available ATR inhibitor BAY 1895344 with favorable PK properties and promising efficacy in monotherapy and combination in preclinical tumor models. Cancer Res. 77 (Issue 13 Suppl. ment), 983. doi:10.1158/1538-7445.am2017-983
VanArsdale, T., Boshoff, C., Arndt, K. T., and Abraham, R. T. (2015). Molecular pathways: targeting the cyclin D-CDK4/6 Axis for cancer treatment. Clin. Cancer Res. 21 (13), 2905–2910. doi:10.1158/1078-0432.CCR-14-0816
Vassilev, L. T., Tovar, C., Chen, S., Knezevic, D., Zhao, X., Sun, H., et al. (2006). Selective small-molecule inhibitor reveals critical mitotic functions of human CDK1. Proc. Natl. Acad. Sci. U. S. A. 103 (28), 10660–10665. doi:10.1073/pnas.0600447103
Vendetti, F. P., Lau, A., Schamus, S., Conrads, T. P., O’Connor, M. J., and Bakkenist, C. J. The orally active and bioavailable ATR kinase inhibitor AZD6738 potentiates the anti-tumor effects of CDDP to resolve ATM-deficient non-small cell lung cancer in vivo.
Wang, Q., Bode, A. M., and Zhang, T. (2023). Targeting CDK1 in cancer: mechanisms and implications. NPJ Precis. Oncol. 7 (1), 58. Published 2023 Jun 13. doi:10.1038/s41698-023-00407-7
Wang, Z. (2022). Cell cycle progression and synchronization: an overview. Methods Mol. Biol. 2579, 3–23. doi:10.1007/978-1-0716-2736-5_1
Wood, K. W., Lad, L., Luo, L., Qian, X., Knight, S. D., Nevins, N., et al. (2010). Antitumor activity of an allosteric inhibitor of centromere-associated protein-E. Proc. Natl. Acad. Sci. U. S. A. 107 (13), 5839–5844. doi:10.1073/pnas.0915068107
Wu, Q., Yang, Z., Nie, Y., Shi, Y., and Fan, D. (2014). Multi-drug resistance in cancer chemotherapeutics: mechanisms and lab approaches. Cancer Lett. 347 (2), 159–166. doi:10.1016/j.canlet.2014.03.013
Yang, Y., Dai, Y., Yang, X., Wu, S., and Wang, Y. (2021). DNMT3A mutation-induced CDK1 overexpression promotes leukemogenesis by modulating the interaction between EZH2 and DNMT3A. Biomolecules 11 (6), 781. Published 2021 May 22. doi:10.3390/biom11060781
Yue, S., Li, Y., Chen, X., Wang, J., Li, M., Chen, Y., et al. (2021). FGFR-TKI resistance in cancer: current status and perspectives. J. Hematol. Oncol. 14 (1), 23. Published 2021 Feb 10. doi:10.1186/s13045-021-01040-2
Zeng, T., Guan, Y., Li, Y. K., Wu, Q., Tang, X. J., Zeng, X., et al. (2021). The DNA replication regulator MCM6: an emerging cancer biomarker and target. Clin. Chim. Acta 517, 92–98. doi:10.1016/j.cca.2021.02.005
Zenke, F. T., Zimmermann, A., Dahmen, H., Elenbaas, B., and Blaukat, A. (2019). “Antitumor activity of M4344, a potent and selective ATR inhibitor, in monotherapy and combination therapy,” in Proceedings: AACR Annual Meeting 2019; March 29–April 3, 2019 (Atlanta, GA). doi:10.1158/1538-7445.AM2019-369
Zhang, J., Ouyang, F., Gao, A., Zeng, T., Li, M., Li, H., et al. (2024). ESM1 enhances fatty acid synthesis and vascular mimicry in ovarian cancer by utilizing the PKM2-dependent warburg effect within the hypoxic tumor microenvironment. Mol. Cancer 23 (1), 94. doi:10.1186/s12943-024-02009-8
Keywords: malignant tumor, cell cycle, cell cycle checkpoint, cyclin, cyclin-dependent kinase, chemotherapeutic drug
Citation: Song G, Liu J, Tang X, Zhong J, Zeng Y, Zhang X, Zhou J, Zhou J, Cao L, Zhang Q and Li Y (2024) Cell cycle checkpoint revolution: targeted therapies in the fight against malignant tumors. Front. Pharmacol. 15:1459057. doi: 10.3389/fphar.2024.1459057
Received: 03 July 2024; Accepted: 16 September 2024;
Published: 11 October 2024.
Edited by:
Hailin Tang, Sun Yat-sen University Cancer Center (SYSUCC), ChinaReviewed by:
Jinjun Wu, Guangzhou University of Chinese Medicine, ChinaXiaoning Gan, Guangzhou First People’s Hospital, China
Copyright © 2024 Song, Liu, Tang, Zhong, Zeng, Zhang, Zhou, Zhou, Cao, Zhang and Li. This is an open-access article distributed under the terms of the Creative Commons Attribution License (CC BY). The use, distribution or reproduction in other forums is permitted, provided the original author(s) and the copyright owner(s) are credited and that the original publication in this journal is cited, in accordance with accepted academic practice. No use, distribution or reproduction is permitted which does not comply with these terms.
*Correspondence: Qunfeng Zhang, eGlhb2ZlbmcyOUAxNjMuY29t; Yukun Li, eXVrdW5fbGlAZm94bWFpbC5jb20=; eXVrdW5fbGlAY3N1LmVkdS5jbg==
†These authors have contributed equally to this work