- 1Department of Hematology, The Affiliated Huaian No. 1 People’s Hospital of Nanjing Medical University, Huai’an, China
- 2Northern Jiangsu Institute of Clinical Medicine, Nanjing Medical University, Nanjing, China
- 3Department of Hematology, The Huaian Clinical College of Xuzhou Medical University, Huai’an, China
Diffuse large B-cell lymphoma (DLBCL) is the most common subtype of B-cell non-Hodgkin’s lymphoma (NHL), up to 30%–40% of patients will relapse and 10%–15% of patients have primary refractory disease, so exploring new treatment options is necessary. Ferroptosis is a non-apoptotic cell death mode discovered in recent years. Its occurrence pathway plays an essential impact on the therapeutic effect of tumors. Numerous studies have shown that modulating critical factors in the ferroptosis pathway can influence the growth of tumor cells in hematological malignancies including DLBCL. This review highlights recent advances in ferroptosis-related genes (FRGs), including STAT3, Nrf2, and ZEB1, and focuses on the clinical potential of ferroptosis inducers such as IKE, α-KG, DMF, and APR-246, which are currently being explored in clinical studies for their therapeutic effects in DLBCL. Correlational studies provide a novel idea for the research and treatment of ferroptosis in DLBCL and other hematological malignancies and lay a solid foundation for future studies.
1 Introduction
Diffuse large B-cell lymphoma (DLBCL) is the most common subtype of B-cell non-Hodgkin’s lymphoma (NHL), accounting for 30%–40% of all newly diagnosed NHL cases worldwide (Al-Hamadani et al., 2015). According to the gene expression profile, DLBCL mainly has two biological molecular forms: germinal center B-cell-like (GCB) and activated B-cell-like (ABC) (Alizadeh et al., 2000). As of 2024, the standard treatment for DLBCL remains R-CHOP and Pola-R-CHP chemotherapy (R: rituximab, C: cyclophosphamide, H: doxorubicin, O: vincristine, P: prednisone), although this method is safe and effective, up to 30%–40% of patients will relapse and 10%–15% of patients have primary refractory disease (Tavakkoli and Barta, 2023).
The concept of ferroptosis was proposed by Dixon et al. (2012) when they observed that ferrostatin-1 (Fer-1) could specifically counteract cell death induced by RAS-selective lethal (RSL) compounds. Unlike classical cell death modes such as apoptosis, necrosis, autophagy, and pyroptosis, ferroptosis is a non-apoptotic, programmed cell death mode that is iron-dependent and mediated by multiple small molecules. Recent work has identified some FRGs that were mainly involved in iron and lipid metabolism. Abnormal iron metabolism and lipid peroxidation lead to an imbalance between the generation and degradation of reactive oxygen species (ROS), which can cause oxidative stress reactions (Pizzino et al., 2017). In normal cells, ROS is mainly produced by the electron transport chain and exists at low and fixed levels (Herb et al., 2021). Excessive ROS could cause cells to undergo ferroptosis and accompany a series of changes in cell morphology, such as outer membrane rupture, nuclear integrity, reduction of mitochondrial ridges, and membrane condensation (Cao and Dixon, 2016).
Recent studies have found that the loss of histone deubiquitinase MYSM1 can induce ferroptosis and affect the function of hematopoietic stem cell, providing a new clinical approach for treating complex blood diseases (Zhao et al., 2023). Regrading the role of ferroptosis in DLBCL mainly focused on ferroptosis-related regulatory factors. By regulating these factors, the susceptibility of tumor cells to ferroptosis would be changed accordingly.
2 Ferroptosis regulation and its relevance in DLBCL
Ferroptosis is a form of regulated cell death driven by the accumulation of lipid peroxides to toxic levels, which leads to cellular damage and death. The key regulators include iron metabolism proteins and lipid metabolic enzymes like ACSL4, which promote the incorporation of polyunsaturated fatty acids (PUFAs) into membranes, making them prone to peroxidation. Unlike apoptosis or necrosis, ferroptosis is primarily characterized by iron-dependent oxidative stress. The process involves the failure of cellular antioxidant defenses, particularly the depletion of GSH and inhibition of the enzyme GPX4, which normally prevents lipid peroxidation (Figure 1) (Zhang et al., 2021a). Recent studies have highlighted the influence of epigenetic and post-translational modifications on ferroptosis regulation (Wang et al., 2023a). Non-coding RNAs (e.g., miRNAs, lncRNAs, and circRNAs) modulate ferroptosis by targeting key genes like GPX4 and SLC7A11, thereby affecting cancer cell sensitivity to chemotherapy (Wang et al., 2024). Emerging evidence suggests that the mechanisms underlying DLBCL development and progression are intimately linked to the regulation of ferroptosis. The precise processes and components underlying the mechanisms will be elaborated in the subsequent subsections.
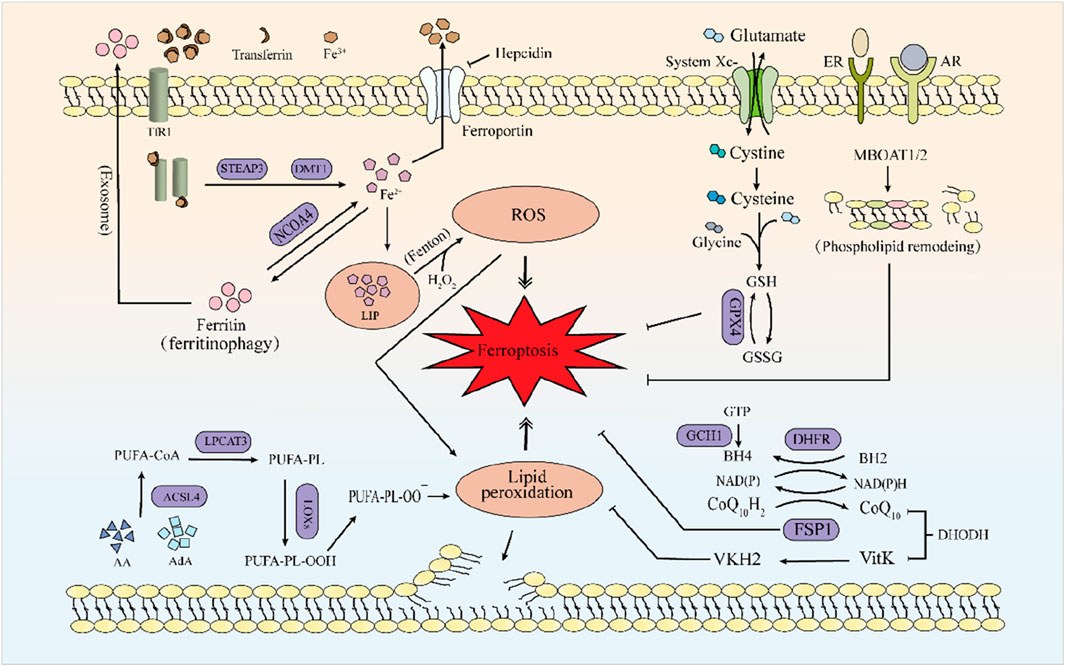
Figure 1. The mechanism of ferroptosis. Iron metabolism primarily involves the Fenton reaction, accumulating excessive Fe2+, leading to increased ROS and triggering ferroptosis. Lipids primarily composed of AA and AdA undergo lipid peroxidation catalyzed by a series of enzymes (ACSL4, LPCAT3), ultimately leading to ferroptosis. GPX4, the FSP1-CoQ10-NAD(P)H system and MBOAT1/2-mediated phospholipid remodeling can all suppress the occurrence of ferroptosis. Among these, the generation of GPX4 requires the xc-system. FSP1 can mediate the reduction of VKH2 and maintain VKH2 as an RTA to inhibit ferroptosis.
2.1 Iron metabolism
Iron deficiency or iron overload can have specific adverse effects on the body (Andrews, 2000), so iron metabolism plays a vital role in the body’s homeostasis. Hepcidin, the main regulatory factor of iron homeostasis, which can induce the degradation of iron transporter protein (FPN, SLC40A1, initially also known as Ireg-1 or MTP-1), inhibiting intestinal iron absorption (Coffey and Ganz, 2017; Camaschella et al., 2020). In the case of cellular iron deficiency, divalent metal transporter 1 (DMT1, also known as DCT1 in the early stage) is upregulated (Gunshin et al., 1997). Furthermore, iron regulatory protein 2 removes iron–sulfur clusters and binds to the iron regulatory elements of target mRNA, stimulating the translation of transferrin receptor 1 (TfR1) and DMT1 and inhibiting the expression of ferritin and FPN (Anderson and Vulpe, 2009; Rouault, 2013; Hentze et al., 2010; Anderson et al., 2012).
Heme is partially degraded into Fe2+ under heme oxygenase-1, and Fe2+ enters intestinal cells after binding to heme carrier protein 1 (Choby and Skaar, 2016; Otterbein et al., 2003; Shayeghi et al., 2005). Ferritin is the principal storage form of Fe2+, and the surface arginine of ferritin heavy chain 1 can be re-generated into Fe2+ under the mediation of nuclear receptor coactivator 4 (NCOA4) (Mancias et al., 2015) a process known as ferritinophagy. Excess Fe2+ can form a labile iron pool (LIP) and induce ferroptosis through the Fenton reaction. FPN is the only known mammalian iron exporter to transport intracellular Fe2+ to the blood. Ceruloplasmin induces the conversion of Fe2+ to Fe3+ and promotes the binding of Fe3+ to transferrin, accelerating the mobilization of iron stores and the turnover of plasma iron (Abboud and Haile, 2000; McKie et al., 2000; Qian and Ke, 2001; Lin et al., 2024). Fe3+, transferrin, and TfR1 form a complex and enter the cell. The complex disintegrates under the mediation of prostate transmembrane epithelial antigen 3 (STEAP3), releasing Fe2+, which then enters the cytoplasm through the action of DMT1 (Fleming et al., 1997; Ohgami et al., 2005). Incorporation of iron into heme occurs within the mitochondria, where free cytoplasmic iron is utilized for the synthesis of heme and iron–sulfur clusters. Iron–sulfur clusters, as essential components of the electron transport chain complexes, facilitate electron transfer. However, electron leakage at complex I and III can lead to the generation of ROS, and excessive ROS levels may result in cellular damage or death (Read et al., 2021).
The accumulation of ROS and intracellular labile iron can enhance the sensitivity of DLBCL tumor cells to ferroptosis (Huang et al., 2023), while ROS-induced oxidative stress can activate the NF-κB pathway, which is somewhat related to the pathogenesis of DLBCL (Lingappan, 2018; Compagno et al., 2009).
2.2 Lipid metabolism
Arachidonic acid (AA) or its metabolite adrenic acid (AdA) is a typical free PUFA. PUFA and CoA form fatty acyl-CoA esters under the action of long-chain family member 4 of acyl-CoA synthetase (ACSL4), and fatty acyl-CoA esters form PUFA-PE with lysophosphatidylcholine acyltransferase 3 (LPCAT3) and phosphatidylethanolamine (PE) (Doll et al., 2017). PUFA-PE generates lipid hydroperoxides under the catalysis of lipoxygenase (LOX), especially 15-LOX. It is worth noting that LOX may require phosphatidylethanolamine binding protein 1 (PEBP1) to induce lipid peroxidation on the membrane (Wenzel et al., 2017). Vitamin E and tocopherol could inhibit LOX to prevent ferroptosis (Kagan et al., 2017). In addition, other oxygenases, such as NADPH oxidase (NOX) and cytochrome P450 oxidoreductase (Manea and Simionescu, 2012; Ai et al., 2021), can also cause lipid peroxidation. Free PUFA alone cannot induce ferroptosis because cells have a powerful ferroptosis defence mechanism, including glutathione peroxidase 4 (GPX4) (Gan, 2022).
Monounsaturated fatty acids (MUFA) may inhibit lipid peroxidation and ferroptosis by competing with PUFA in incorporate into phospholipids, reducing the number of PUFA-phospholipid associations. However, the lipid peroxidation reaction requires the presence of the diene-propyl portion, which exists in PUFA but not in MUFA (Magtanong et al., 2019). Therefore, MUFA itself does not induce ferroptosis. The combined action of PUFA and IFN-γ can enhance the effect of ferroptosis in tumor cells (Gan, 2022). The mechanism may be related to the increased sensitivity of tumor cells to ferroptosis by IFN-γ by inhibiting solute carrier family 7 member 11 (SLC7A11) expression (Liao et al., 2022).
Research has found that the intake of PUFAs is inversely associated with the risk of NHL, with this association being particularly prominent in DLBCL (Charbonneau et al., 2013). The lipid metabolism changes are one of the significant pathogenic factors in DLBCL (Caro et al., 2012). The latest research reveals that the immune response mechanism in DLBCL is correlated with the lipid metabolism risk model (Zhang et al., 2024).
2.3 The system Xc-/GSH/GPX4 axis
Organism activity is a dynamic equilibrium process, and the system Xc−/GSH/GPX4 axis is an important antioxidant system for ferroptosis (Li et al., 2022). Most ferroptosis inducers, such as Erastin and RSL3, act on this axis (Yang et al., 2014). The system Xc− is a chloride-dependent and sodium-dependent reverse heterodimer of cysteine and glutamate located on the plasma membrane, composed of a light chain (xCT or SLC7A11) and a heavy chain 4F2hc (CD98hc or SLC3A2) (Parker et al., 2021). Among them, SLC7A11 can be regulated by critical oncogenic transcription factors, including mutant p53 (Jiang et al., 2015), Nrf2 (Sun et al., 2016), ATF4 (Chen et al., 2017), and others. The cysteine absorbed by system Xc− is reduced to cysteine by thioredoxin reductase 1 (TrxR1) and then used for GSH biosynthesis (Mandal et al., 2010; Conrad and Sato, 2012). Since cysteine is the rate-limiting substrate for GSH biosynthesis and GSH is the primary antioxidant in mammalian cells, blocking the levels of cysteine and GSH in cells can directly affect the activity of GPX4 (Ursini and Maiorino, 2020). GPX4 protects cells from lipid peroxidation by converting lipid hydroperoxides (PLOOH) into the corresponding alcohols (Brigelius-Flohé and Maiorino, 2013) and plays a crucial role in regulating ferroptosis.
Lymphoid hematological diseases relatively lack glutathione peroxidase compared to normal cells, making DLBCL cells more susceptible to ferroptosis induced by system Xc-inhibitors (Iglehart et al., 1977). Since 2018, researchers have found that DLBCL cells are susceptible to ferroptosis regulated by GPX4 (Yang et al., 2014; Kinowaki et al., 2018).
2.4 The FSP1-CoQ10-NAD(P)H axis
Ferroptosis suppressor protein 1 (FSP1), formerly known as apoptosis-inducing factor mitochondria 2, reduces ubiquinone (CoQ10) in a way that does not depend on GSH and GPX4, captures free radicals by producing ubiquinol, induces lipid peroxidation, and prevents lipid peroxidation and related ferroptosis by directly or indirectly recycling α-tocopherol as a radical trapping antioxidant (RTA) to clear lipid radicals in the membrane. At the same time, FSP1 uses NAD(P)H to catalyze the regeneration of CoQ10 (Doll et al., 2019). As a ubiquinone reductase located on the outer surface of the mitochondrial inner membrane, dihydroorotate dehydrogenase (DHODH) is also a ubiquinone reductase. The research found that DHODH can inhibit ferroptosis by reducing CoQ10 and vitamin K (Mao et al., 2021). However, Mishima et al. (2023) proposed through experimental research that DHODH inhibitors only show significant sensitivity to ferroptosis when FSP1 is effectively inhibited, and their effect on ferroptosis is minimal. Therefore, the role of DHODH in ferroptosis is still debatable. Similar to the mechanism of action of DHODH, FSP1 can mediate the reduction of vitamin K to hydroquinone (VKH2) and maintain VKH2 as an RTA to inhibit ferroptosis and improve warfarin toxicity (Mishima et al., 2022), providing a new clinical approach for patients who cannot clot due to the use of warfarin to treat thrombosis.
2.5 Additional anti-ferroptosis pathways
Through CRISPR whole-genome screening, a new ferroptosis control mechanism has been identified that does not depend on GPX4 or FSP1. One is guanosine triphosphate cyclohydrolase 1, and its metabolite tetrahydrobiopterin (BH4), which mainly inhibits ferroptosis by selectively preventing phospholipid consumption, and BH4 itself is also an effective RTA (Kraft et al., 2020). Dihydrofolate reductase (DHFR) is involved in the recycling of BH4. Blocking DHFR can synergize with GPX4 inhibitors to induce ferroptosis (Soula et al., 2020). The other is sex hormone signaling, which inhibits cancer cell ferroptosis through MBOAT1/2-mediated PL remodeling (Liang et al., 2023).
3 Development of a ferroptosis-related gene risk scoring model for DLBCL
Up of now, the most commonly used methods for predicting the prognosis of DLBCL still include the use of the International Prognostic Index (IPI), revised IPI, and the National Comprehensive Cancer Network (NCCN-IPI). However, with the discovery that the prognosis of DLBCL patients with GPX4 positive and 8-hydroxydeoxyguanosine negative is significantly poorer in terms of OS (p = 0.0170) and PFS (p = 0.0005) (Kinowaki et al., 2018), researchers have begun to pay attention to the prognostic role of FRGs in DLBCL patients. The following study suggests that a risk-scoring model based on FRGs can be used for personalized risk assessment and prognosis prediction in DLBCL patients.
Chen et al. (2021a) used the Gene Expression Omnibus to obtain mRNA expression and matched clinical data of DLBCL patients established a personalized risk assessment model based on FRGs for DLBCL patients. However, whether these FRGs are associated with survival remains to be elusived. Subsequent studies have found that the risk-scoring model can predict the OS of DLBCL patients, with the high-risk group having lower OS. Among them, the DCA calibration curve and results have demonstrated the excellent performance of this method (Wang et al., 2023b). In addition, the model could be used for the prediction of resistance of ABC-DLBCL cells to ibrutinib treatment (Weng et al., 2022); Immune infiltration is commonly observed in the tumor cells of DLBCL (Chang et al., 2007). Concurrently, risk scores exhibit a significant correlation with immune infiltration (Xiong et al., 2022); STEAP3 plays a crucial role in the Tumor Immune Microenvironment of DLBCL (Chen et al., 2023a); the expression levels of FRGs (CAPG, HAMP, NOX4, and SLC1A5) are associated with dendritic cells, which may be significantly associated with DLBCL (Wu et al., 2023a). Previous studies have shown that dendritic cells are pivotal in anti-tumor responses (Scheuerpflug et al., 2021). In a study of tumor cells from 48 DLBCL patients, it was found that in 21% of patients, there was an increased number of CD1a+ dendritic cells at the tumor margins, and this was significantly correlated with a favorable prognosis (p = 0.015) (Chang et al., 2007).
Based on the relevant risk scoring models mentioned above, we have sorted out and summarized three genes that are closely related to ferroptosis. These genes are promising as new targets for DLBCL treatment and provide a strong candidate direction for future treatment strategies.
3.1 Signal transducer and activator of transcription 3
STAT3, as the core transcription factor in the JAK/STAT signaling pathway, was first discovered by the scientific community in 1993. This transcription factor plays a crucial role in the vital activities of cells, and can regulate key processes such as cell proliferation, survival, differentiation, migration, and immune response (Wegenka et al., 1993; O'Shea et al., 2013). The search results indicate that STAT3 plays a critical role in regulating ferroptosis in various cancer types and other diseases (Lin et al., 2024; Li and Liu, 2023; Zhang et al., 2022a).
The four splicing variants of STAT3 (α, β, △S-α, and △S-β) can all be found in DLBCL tumor cells (Turton et al., 2015). The IL-6 and IL-10 produced by ABC-DLBCL cells can lead to constitutive activation of STAT3 through autocrine action (Lam et al., 2008). This activation is an indispensable step for the survival and proliferation of ABC- DLBCL cells (Ding et al., 2008). In ABC-DLBCL patients treated with R-CHOP, STAT3 activation is closely related to poorer survival rates (Huang et al., 2013). Further studies have shown that the expression level of pSTAT3 can predict the OS and PFS of newly diagnosed DLBCL patients, suggesting its importance as a prognostic indicator (Ok et al., 2014). In mouse models, directly inhibiting STAT3 with short hairpin RNA can significantly suppress the growth of ABC-DLBCL, further confirming the critical role of STAT3 in the development of DLBCL (Scuto et al., 2011). In addition, ruxolitinib, while inhibiting STAT3 activity, also acts synergistically with lenalidomide to effectively inhibit the growth of ABC-DLBCL cells both in vitro and in xenograft mouse models, providing us with a new therapeutic approach (Lu et al., 2018). AZD9150, an antisense oligonucleotide targeting STAT3 mRNA, has been tested in a clinical trial involving relapsed or refractory DLBCL patients. The results demonstrated clinical benefits, including both complete and partial remissions (Shiah et al., 2021). Thus, targeting STAT3 for the development of ferroptosis-inducing drugs presents a promising research direction for treating DLBCL patients.
3.2 The nuclear factor erythroid 2-related factor 2
Nrf2 is a basic leucine zipper transcription factor that was first reported by Moi et al. (1994). The upregulation of Nrf2 expression can effectively inhibit the occurrence of ferroptosis, and this view has been confirmed by research (Fan et al., 2017). Conversely, when the expression level of Nrf2 decreases, the sensitivity of cancer cells to pro-ferroptosis drugs is significantly enhanced (Roh et al., 2017). The activities of glutamate-cysteine ligase, glutathione synthetase, and SLC7A11, closely related to glutathione synthesis and metabolism, are closely related to the expression level of Nrf2 in the cells, collectively maintaining the stability of the intracellular environment (Yang et al., 2005; Chan and Kwong, 2000; Habib et al., 2015; Ye et al., 2014).
The expression of Nrf2 in DLBCL tissues is significantly higher than that in reactive lymph node hyperplasia tissues, and its expression level is significantly correlated with IPI and Ann-Arbor clinical stage (p < 0.05) (Yi et al., 2018). Furthermore, studies have shown that the upregulation of Nrf2 expression may be related to the development of bortezomib resistance in mantle cell lymphoma (Chen et al., 2013). Further observation found that low expression of Nrf2 in the nucleus, high expression of Nrf2 in the cytoplasm, low expression of Nrf1 in the nucleus, and low expression of Keap1 in the cytoplasm are all closely related to the poor prognosis of high-risk DLBCL patients (Kari et al., 2019).
Recent studies have investigated the use of zotatifin, a clinical-stage protein synthesis inhibitor, to disrupt Nrf2’s protective role in DLBCL. When combined with ferroptosis inducers, such as IKE, zotatifin enhances the sensitivity of DLBCL cells to ferroptosis, demonstrating increased anti-cancer activity (Hsu et al., 2025; Manara et al., 2024). Thus, targeting Nrf2 in combination with ferroptosis inducers or other therapies holds potential as a novel treatment strategy for DLBCL.
3.3 Zinc finger E-box binding homeobox 1
ZEB1, a well-established gene associated with ferroptosis, plays a dual role in modulating this form of cell death. Depending on the specific regulatory mechanisms involved, ZEB1 can either promote or inhibit ferroptosis, highlighting its complex involvement in this process (Wu et al., 2023b; Wang et al., 2022). ZEB1 transcriptionally activates small nucleolar RNA host gene 14 and programmed death ligand 1, significantly promoting the immune evasion mechanism of DLBCL cells, thereby exacerbating the progression of the disease (Zhao et al., 2019). Furthermore, ZEB1 is considered one of the key genes closely associated with the poor clinical presentation and outcomes of DLBCL, and its aberrant expression often indicates a poorer prognosis (Lemma et al., 2013). These findings provide important clues for us to deeply understand the pathogenesis of DLBCL and develop new treatment strategies.
4 Therapeutic strategies targeting ferroptosis in DLBCL
Ferroptosis inducers in solid and hematologic tumors were explored extensively, as shown in Table 1 (Liu et al., 2023a). Moreover, therapeutic strategies focusing on ferroptosis induction—such as inhibiting GPX4, enhancing iron metabolism, or disrupting antioxidant defenses—have shown promise in overcoming chemoresistance (Wang et al., 2023c). However, challenges like tumor heterogeneity and the complex tumor microenvironment need to be addressed. Following the understanding of the role of the ferroptosis in the pathogenesis of DLBCL (Figure 2), several ferroptosis inducers have been explored as potential ferroptosis inducers in preclinical studies for therapeutic intervention in DLBCL (Figure 3). Additionally, ferroptosis may contribute to the efficacy of R-CHOP therapy, commonly used for DLBCL. R-CHOP-induced oxidative stress and metabolic disruptions can sensitize cancer cells to ferroptosis (Hertzberg, 2022; Vitolo and Chiappella, 2023; Zhou and Busino, 2023). The development of ferroptosis-inducing therapies remains in its early stages. While preclinical findings provide valuable insights, substantial further research is required to translate these findings into clinical practice.
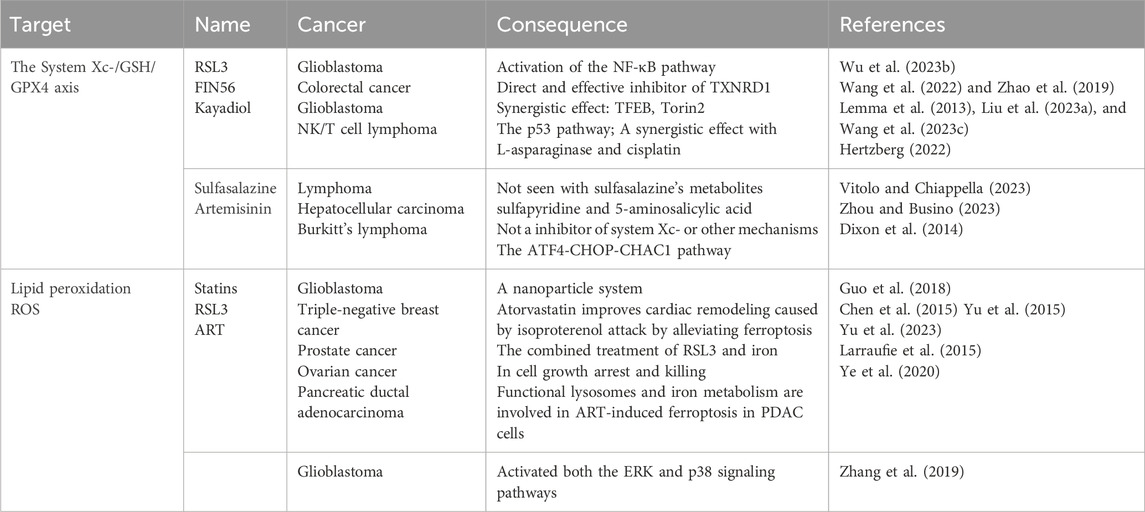
Table 1. Research progress and controversial points of ferroptosis-related drugs in solid and hematologic tumors.
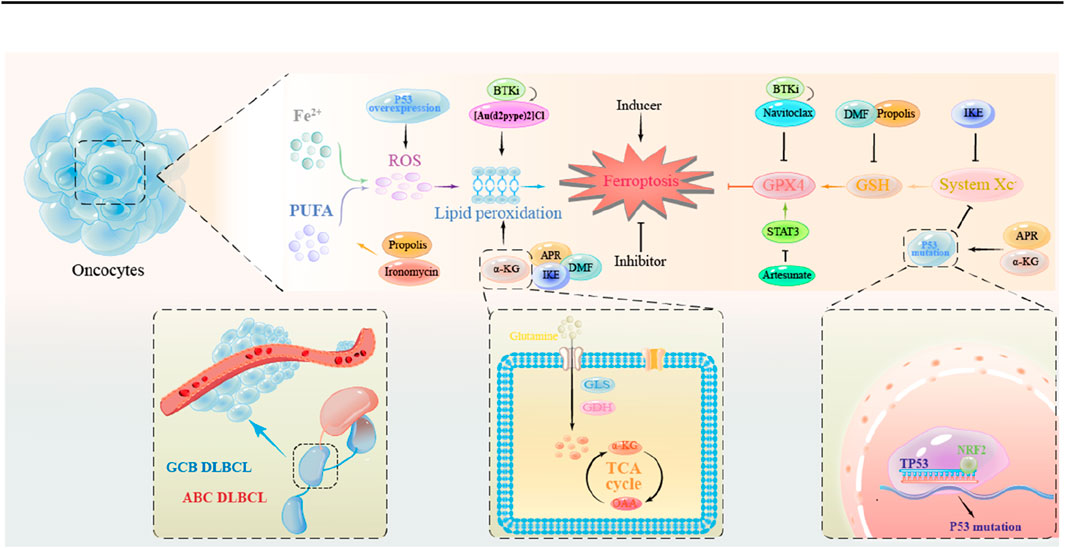
Figure 3. The mechanism of action of ferroptosis inducers in DLBCL tumor cells. Promotion of lipid peroxidation: APR, DMF, IKE, α-KG; Reduction of GSH synthesis: DMF; Inhibition of the System Xc-: IKE; Inhibition of the System Xc-through Mutant p53: APR, α-KG.
4.1 imidazole ketone erastin
Erastin, as a well-studied inducer of ferroptosis, has demonstrated efficacy in preclinical models. However, its clinical application is currently limited due to potential toxic side effects and the need for further optimization to enhance therapeutic effects. Future studies are required to address these challenges and to evaluate its safety and efficacy in clinical settings.
Erastin inhibits tumor cell growth by inhibiting the system Xc- (Dixon et al., 2014). Meanwhile, Erastin can increase the sensitivity of lung cancer cells to cisplatin (Guo et al., 2018), the sensitivity of glioblastoma cells to temozolomide (Chen et al., 2015), and the sensitivity of AML cells to anthracyclines (Yu et al., 2015). A recent experimental study found that EZH2 inhibitor can induce simultaneous upregulation of GPX4 and TfR-1, leading to resistance of DLBCL cells to EZH2 inhibitor. However, the combination of EZH2 inhibitor and erastin can effectively overcome the drug resistance of DLBCL (Yu et al., 2023).
Recently, it has been found that IKE, as an improved analogue of erastin, is more than 100 times more potent than erastin (Larraufie et al., 2015), it effectively inhibits system Xc-at low concentrations (Ye et al., 2020), making it a promising candidate for further investigation. However, its efficacy and safety profile remain to be validated in clinical trials. Zhang et al. (2019) found in a mouse DLBCL xenograft model that IKE, by inhibiting system Xc-, depleting GSH, inducing lipidomic changes through non-Fenton reaction-mediated lipid peroxidation, promotes ferroptosis, inhibits tumor cell growth. At the same time, the research used PEG-PLGA nanoparticles as carriers to combine IKE for DLBCL treatment, further increasing the therapeutic window of IKE, and concluded that the combination of IKE and other therapies may serve as a potential treatment for DLBCL.
While the preclinical data for IKE is promising, its translation into clinical practice is still in its early stages. Future research should focus on conducting rigorous clinical trials to assess its safety, efficacy, and optimal therapeutic combinations, which are crucial for determining its role in treating DLBCL.
4.2 APR-246
Wild-type p53 coordinates the transcription of various genes to respond to cellular stress, reduce DNA damage, and induce cell cycle arrest, senescence, or apoptosis through differential activation of target genes (Liu and Chen, 2006), preventing the replication of damaged DNA (Lim et al., 2007). Mature p53 signifies the active and stabilized state of p53 in response to cellular stressors. TP53 gene mutations impair the activity of wild-type p53, which is essentially a stress-responsive transcription factor that binds to DNA in tetrameric form (El-Deiry et al., 1992). Cells sensitive to p53-induced apoptosis produce ROS when p53 is overexpressed, cells insensitive to this mechanism cannot generate ROS (THOMAS et al., 1996). Previous studies have shown that TP53 mutations are an adverse factor for the prognosis of DLBCL, especially in GCB DLBCL (Qin et al., 2020). Accumulated mutant TP53, by binding to the main antioxidant transcription factor NRF2, inhibits the expression of SLC7A11, affects glutathione synthesis, and significantly reduces GPX4, making mutant p53 tumors more susceptible to oxidative damage (Liu et al., 2017). A recent study found that in cells with NRF2 knockout, the expression of HERC2 and VAMP8 is relatively low, ferritin and NCOA4 are increased, and low ferritin accumulates in autophagosomes, ultimately controlling iron homeostasis and increasing sensitivity to ferroptosis (Anandhan et al., 2023).
APR-246 is a small molecule that can reactivate mutant p53 in cancer cells (Lambert et al., 2009), and antagonize the thioredoxin reductase system, directly affecting the cellular redox state (Peng et al., 2013). Hong et al. (2022) found through in vitro and in vivo experiments that TP53 exon 7 mutations are associated with poorer overall survival (OS), TP53 exon 5 and 6 mutations are associated with poorer progression-free survival (PFS), and DLBCL with TP53 mutations is more susceptible to the effects of APR-246. APR-246 induces the accumulation of excess ROS, leading to ferroptosis and inhibiting tumor cell growth. Additionally, APR-246 has no significant toxicity in mice (Hong et al., 2022). The team also pointed out that in DLBCL with TP53 exon 7 missense mutations, APR-246 can induce ferritin autophagy, further triggering the Fenton reaction, increasing ROS levels, and ultimately leading to ferroptosis.
While APR-246 has shown promising preclinical results, including synergy with system Xc-antagonists in mutant p53 tumor cells, its clinical translation remains in its early stages (Liu et al., 2017). Although this compound has demonstrated efficacy in ovarian cancer models, including reducing resistance to chemotherapeutic agents like cisplatin and doxorubicin (Mohell et al., 2015), no clinical trials have yet evaluated its safety and efficacy specifically in DLBCL.
The identification of SLC7A11 as a predictive biomarker provides a rational basis for future clinical investigation into the therapeutic potential of APR-246 in DLBCL treatment. However, rigorous clinical trials are required to validate these findings, optimize dosing strategies, and assess potential toxicities before this compound can be considered a viable therapeutic option.
4.3 α-Ketoglutarate
In recent years, in vitro and in vivo studies have indicated that α-KG has anti-cancer effects and may serve as a potential anti-cancer drug (Abla et al., 2020). α-KG is mainly derived from glutamine and generates energy in the citric acid cycle within the cell.
Glutamine, as an essential metabolic fuel, can meet the high demand of rapidly proliferating cells for ATP, biosynthetic precursors, and reducing agents (DeBerardinis et al., 2008), with cancer cells exhibiting a greater demand for glutamine than normal cells (Yuneva et al., 2007). With the assistance of the transport system, extracellular glutamine crosses the plasma membrane and is converted to α-KG by the combined action of glutaminase and glutamate dehydrogenase. In turn, α-KG can be converted to glutamine by glutamine synthetase or to CO2 through the tricarboxylic acid cycle to provide energy for the cell (Xiao et al., 2016). Accumulated α-KG is reduced by malate dehydrogenase 1 in an acidic environment and converted to 2-hydroxyglutarate, which increases ROS levels and can induce GSDMC-dependent pyroptosis through caspase-8 activated by death receptor 6 (Zhang et al., 2021b).
Hong et al. (2022) conducted a non-targeted metabolomic analysis of serum samples from 60 newly diagnosed DLBCL patients and found that glutamine metabolism is upregulated in primary DLBCL patients and is associated with poor outcomes.
Their preclinical experiments demonstrated that α-KG induces ferroptosis by increasing ROS, promoting lipid peroxidation, and DNA oxidation damage-mediated TP53 expression, further inhibiting DLBCL tumor growth. Notably, DM-αKG treatment, a modified form of α-KG, effectively inhibited DLBCL tumor growth in mouse models, especially in cells with double hits (Cai et al., 2023). It is important to highlight that these findings are derived from preclinical models and require rigorous validation through clinical trials to confirm their translational potential.
Furthermore, it is worth noting that the α-ketoglutarate dehydrogenase complex (α-KGDC) is responsible for converting α-KG to succinyl-CoA and can generate ROS, promoting ferroptosis (Vatrinet et al., 2017), warrants additional investigation as a possible target for therapeutic intervention.
4.4 Dimethyl fumarate
DMF is a clinically approved drug that can impair the NF-κB/STAT3 signalling pathway in adult T-cell leukemia/lymphoma cells (MAETA et al., 2022). NF-κB and STAT3 are two transcription factors that act synergistically and play crucial roles in both inflammation and cancer (Grivennikov and Karin, 2010). STAT3 belongs to the signal transducer and activator of transcription (STAT) family of transcription factors, and it is mediated by phosphorylation of a critical tyrosine residue (Tyr 705), which induces STAT3 dimerization through phosphorylated tyrosine-SH2 domain interactions (Yang et al., 2007). Once dimerized, STAT transcription factors enter the nucleus and activate various target genes.
In preclinical models, DMF has been highlighted as a potential therapeutic agent for GCB-DLBCLs. Schmitt et al. (2021) proposed electrophiles as a new class of ferroptosis inducers, and unlike BSO and erastin, DMF induces lipid peroxidation and effectively and rapidly depletes GSH by directly inducing succinylation of cysteine residues, thereby promoting ferroptosis. Their research data suggests that the sensitivity of GCB- DLBCL cell lines to DMF-induced ferroptosis is related to their high expression of 5-LOX. Additionally, in ABC-DLBCL cells dependent on NF-κB and STAT3 survival signals, DMF can effectively inhibit the IKK complex’s and Janus kinase’s activity (Schmitt et al., 2021).
This research team found that BRD4, as a member of the BET protein family, can control the expression of FSP1 and SLC7A11, thereby protecting GCB-DLBCL cells from ferroptosis. Chromatin immunoprecipitation sequencing further confirmed this finding. The team proposed that the use of DMF in combination with other drugs that impair the cell’s detoxification of lipid peroxides may be a powerful and attractive option for the future treatment of DLBCLs (Schmitt et al., 2023). Additionally, the BRD4 inhibitor JQ1 can induce ferroptosis through ferritinophagy or by regulating the expression of iron-related genes (Sui et al., 2019). The development of combination therapies that exploit ferroptosis mechanisms represents a promising direction for future research and clinical translation.
4.5 Ironomycin
To some extent, Ferroptosis can be considered a form of lysosomal cell death (Gao et al., 2018). Lysosomal membrane permeabilization (LMP) is the process by which lysosomal membrane rupture leads to the release of tissue proteases and other hydrolases from the lysosomal lumen into the cytoplasm (Boya and Kroemer, 2008), and lysosomal exocytosis can reduce LMP, reducing LMP damage to normal cells (Zhong et al., 2023). Ironomycin is a derivative of salinomycin that can sequester iron in lysosomes, leading to ROS generation and ultimately causing ferroptosis (Mai et al., 2017; Versini et al., 2020).
Iron metabolism gene expression profiles in DLBCL patients has been conducted, and an iron score was established to differentiate the prognosis of DLBCL patients. Ironomycin at nanomolar concentrations can induce growth inhibition, ferroptosis, and autophagy in DLBCL cells, significantly reducing the median survival of primary DLBCL cells and has no significant toxicity to non-tumor cells (Devin et al., 2022). It is worth mentioning that significant synergistic effects were observed when ironomycin combined with doxorubicin, BH3 mimetics, and Bruton’s tyrosine kinase (BTK) inhibitors in mantle cell lymphoma (Ovejero-Merino et al., 2022). These data suggest that ironomycin is an attractive treatment strategy for DLBCL, especially in high-risk patients defined by iron score.
These findings suggest that ironomycin has potential therapeutic value in DLBCL, particularly for high-risk patients identified by the iron score. However, these observations are primarily derived from preclinical models, necessitating rigorous clinical trials to confirm ironomycin’s safety, efficacy, and combinatorial potential in DLBCL treatment.
4.6 BTK inhibitors
BTK is crucial in regulating the proliferation, survival, and function of B cells and plasma cells, making it a promising therapeutic target for various B-cell malignancies (Alu et al., 2022). Research suggests that BTK inhibitors may regulate ferroptosis in lymphoma and other cancers, although further studies are needed to understand the role of BTK in ferroptosis fully. BTK inhibitors have shown significant antitumor activity in various types of B cell malignancies, and their use has created new possibilities for chemotherapy-free management of these conditions.
4.6.1 Ibrutinib and [Au(d2pype)2]Cl
The B cell receptor (BCR) signaling pathway can activate downstream pathways involved in cell proliferation and differentiation, including NF-κB (Pontoriero et al., 2019), ERK/MAPK (Shukla et al., 2016) and AKT (Longo et al., 2008). Bruton’s tyrosine kinase (BTK) is a crucial protein that links BCR activity to downstream pathways (Mohamed et al., 2009). By analyzing the RNA-seq dataset from TCGA, studies have indicated that the expression levels of the thioredoxin (Trx) system and the BCR signaling pathway are higher in DLBCL patient samples than in healthy samples. Knockdown of TrxR can reduce BTK mRNA and protein expression. The combination of an inhibitor of TrxR ([Au(d2pype)2]Cl) and a BTK inhibitor can activate apoptosis and ferroptosis pathways in SUDHL4 cells (Wang et al., 2023d). The combined targeted therapy of BTK and TrxR inhibitors may be an effective treatment strategy for DLBCL.
4.6.2 Zanubrutinib and navitoclax
Double-hit lymphoma (DHL) is an aggressive subset of DLBCL with a poor prognosis and no satisfactory treatment options (Riedell and Smith, 2018). Zanubrutinib and navitoclax (a BCL-2 inhibitor) synergistically downregulate NRF2 and HMOX1 while inactivating GPX4, triggering ferroptosis and inhibiting the growth of DHL tumor cells. A comprehensive computational approach from single-cell to bulk analysis and in vitro and in vivo validation in DHL cell lines has demonstrated that ablating BTK enhances DHL cell sensitivity to navitoclax, inhibiting tumor cell proliferation (Setiawan et al., 2023).
4.7 Other potential ferroptosis inducers
4.7.1 Artesunate
Artesunate (ATS) may induce apoptosis, regulate autophagy, and induce ferroptosis in DLBCL cells by disrupting the STAT3 signaling pathway (Chen et al., 202b). Based on this, the latest discovery shows that ATS has a synergistic effect with Sorafenib (Chen et al., 2023b).
4.7.2 Propolis
It was found that propolis from poplar buds may inhibit the proliferation of DLBCL SU-DHL-2 cells through the ferroptosis pathway, accelerate cell death, downregulate serine/threonine-protein kinase PLK1, and affect apoptosis (Liu et al., 2023b).
5 Conclusion
Recent studies have shown that ferroptosis is essential in occurrence, development and the treatment of hematological malignancies (Zhou et al., 2022). Ferroptosis is critical in inhibiting tumor development and enhancing treatment efficacy (Zhang et al., 2022b). Leukemia and lymphoma are the most common hematological malignancies, and their main treatment methods include chemotherapy and stem cell transplantation. Although stem cell transplantation has improved significantly in recent years (Sakurai et al., 2023; Martin-Rufino et al., 2023), there are still certain limitations. At the same time, the remission rate of chemotherapy is low, and there has been no substantial progress in recent years. Therefore, exploring novel therapeutic approaches that are beneficial to patients remains necessary. With the continuous understanding and improvement of the mechanism of ferroptosis, inducing ferroptosis in DLBCL through different pathways is becoming a promising new option. It has been verified in animal model and in vitro experiments. Inducing ferroptosis in DLBCL cells, overcoming drug resistance, and combination therapy are potential applications of ferroptosis in DLBCL treatment.
Therefore, utilizing the ferroptosis mechanism and its inducers to inhibit DLBCL tumor cell growth may be a promising research direction. Ferroptosis inducers can not only enhance the efficacy of chemotherapy by inducing the death of cancer cells with low sensitivity to chemotherapy (Chen et al., 2023c) but also be used in combination with other treatments such as radiotherapy, immunotherapy, and nanotherapy to inhibit tumor cell growth further and improve efficacy. In addition to the known classic ferroptosis-related mechanisms, researchers have also found preliminary conclusions, including the use of ranolazine as an FAO inhibitor to promote ferroptosis (Sekine et al., 2022) and the regulation of low-density lipoprotein receptor-mediated PI3K/AKT pathway to promote ferroptosis in DLBCL tumor cells by progesterone and progestin receptor 3 (Song et al., 2023). However, as ferroptosis is a double-edged sword (Xu et al., 2021), researchers need to further study it to maximize its advantages and minimize its side effect. Although some clinical trials have tested the role of ferroptosis inducers in DLBCL treatment, most of the evidence is obtained from animal models, so more research is still needed to reveal its potential mechanisms and evaluate the safety and actual efficacy of human-induced ferroptosis therapy.
Author contributions
YW: Writing–original draft. ZH: Writing–original draft. XD: Writing–original draft. YY: Writing–original draft. QC: Writing–original draft. YS: Writing–original draft. YD: Writing–original draft. QZ: Writing–original draft. LY: Writing–review and editing. CW: Writing–review and editing.
Funding
The author(s) declare that no financial support was received for the research, authorship, and/or publication of this article.
Conflict of interest
The authors declare that the research was conducted in the absence of any commercial or financial relationships that could be construed as a potential conflict of interest.
Publisher’s note
All claims expressed in this article are solely those of the authors and do not necessarily represent those of their affiliated organizations, or those of the publisher, the editors and the reviewers. Any product that may be evaluated in this article, or claim that may be made by its manufacturer, is not guaranteed or endorsed by the publisher.
References
Abboud, S., and Haile, D. J. (2000). A novel mammalian iron-regulated protein involved in intracellular iron metabolism. J. Biol. Chem. 275, 19906–19912. doi:10.1074/jbc.M000713200
Abla, H., Sollazzo, M., Gasparre, G., Iommarini, L., and Porcelli, A. M. (2020). The multifaceted contribution of α-ketoglutarate to tumor progression: an opportunity to exploit? Semin. Cell Dev. Biol. 98, 26–33. doi:10.1016/j.semcdb.2019.05.031
Ai, Y., Yan, B., and Wang, X. (2021). The oxidoreductases POR and CYB5R1 catalyze lipid peroxidation to execute ferroptosis. Mol. Cell Oncol. 8, 1881393. doi:10.1080/23723556.2021.1881393
Al-Hamadani, M., Habermann, T. M., Cerhan, J. R., Macon, W. R., Maurer, M. J., and Go, R. S. (2015). Non-Hodgkin lymphoma subtype distribution, geodemographic patterns, and survival in the US: a longitudinal analysis of the National Cancer Data Base from 1998 to 2011. Am. J. Hematol. 90, 790–795. doi:10.1002/ajh.24086
Alizadeh, A. A., Eisen, M. B., Davis, R. E., Ma, C., Lossos, I. S., Rosenwald, A., et al. (2000). Distinct types of diffuse large B-cell lymphoma identified by gene expression profiling. Nature 403, 503–511. doi:10.1038/35000501
Alu, A., Lei, H., Han, X., Wei, Y., and Wei, X. (2022). BTK inhibitors in the treatment of hematological malignancies and inflammatory diseases: mechanisms and clinical studies. J. Hematol. Oncol. 15, 138. doi:10.1186/s13045-022-01353-w
Anandhan, A., Dodson, M., Shakya, A., Chen, J., Liu, P., Wei, Y., et al. (2023). NRF2 controls iron homeostasis and ferroptosis through HERC2 and VAMP8. Sci. Adv. 9, eade9585. doi:10.1126/sciadv.ade9585
Anderson, C. P., Shen, M., Eisenstein, R. S., and Leibold, E. A. (2012). Mammalian iron metabolism and its control by iron regulatory proteins. Biochimica Biophysica Acta (Bba) - Mol. Cell Res. 1823, 1468–1483. doi:10.1016/j.bbamcr.2012.05.010
Anderson, G. J., and Vulpe, C. D. (2009). Mammalian iron transport. Cell Mol. Life Sci. 66, 3241–3261. doi:10.1007/s00018-009-0051-1
Andrews, N. C. (2000). Iron metabolism: iron deficiency and iron overload. Annu. Rev. Genomics Hum. Genet. 1, 75–98. doi:10.1146/annurev.genom.1.1.75
Boya, P., and Kroemer, G. (2008). Lysosomal membrane permeabilization in cell death. Oncogene 27, 6434–6451. doi:10.1038/onc.2008.310
Brigelius-Flohé, R., and Maiorino, M. (2013). Glutathione peroxidases. Biochim. Biophys. Acta 1830, 3289–3303. doi:10.1016/j.bbagen.2012.11.020
Cai, Y., Lv, L., Lu, T., Ding, M., Yu, Z., Chen, X., et al. (2023). α-KG inhibits tumor growth of diffuse large B-cell lymphoma by inducing ROS and TP53-mediated ferroptosis. Cell Death Discov. 9, 182. doi:10.1038/s41420-023-01475-1
Camaschella, C., Nai, A., and Silvestri, L. (2020). Iron metabolism and iron disorders revisited in the hepcidin era. Haematol. (Roma) 105, 260–272. doi:10.3324/haematol.2019.232124
Cao, J. Y., and Dixon, S. J. (2016). Mechanisms of ferroptosis. Cell Mol. Life Sci. 73, 2195–2209. doi:10.1007/s00018-016-2194-1
Caro, P., Kishan, A. U., Norberg, E., Stanley, I. A., Chapuy, B., Ficarro, S. B., et al. (2012). Metabolic signatures uncover distinct targets in molecular subsets of diffuse large B cell lymphoma. Cancer Cell 22, 547–560. doi:10.1016/j.ccr.2012.08.014
Chan, J. Y., and Kwong, M. (2000). Impaired expression of glutathione synthetic enzyme genes in mice with targeted deletion of the Nrf2 basic-leucine zipper protein. Biochim. Biophys. Acta 1517, 19–26. doi:10.1016/s0167-4781(00)00238-4
Chang, K. C., Huang, G. C., Jones, D., and Lin, Y. H. (2007). Distribution patterns of dendritic cells and T cells in diffuse large B-cell lymphomas correlate with prognoses. Clin. Cancer Res. 13, 6666–6672. doi:10.1158/1078-0432.CCR-07-0504
Charbonneau, B., O'Connor, H. M., Wang, A. H., Liebow, M., Thompson, C. A., Fredericksen, Z. S., et al. (2013). Trans fatty acid intake is associated with increased risk and n3 fatty acid intake with reduced risk of non-hodgkin lymphoma. J. Nutr. 143, 672–681. doi:10.3945/jn.112.168658
Chen, D., Fan, Z., Rauh, M., Buchfelder, M., Eyupoglu, I. Y., and Savaskan, N. (2017). ATF4 promotes angiogenesis and neuronal cell death and confers ferroptosis in a xCT-dependent manner. Oncogene 36, 5593–5608. doi:10.1038/onc.2017.146
Chen, H., He, Y., Pan, T., Zeng, R., Li, Y., Chen, S., et al. (2021a). Ferroptosis-related gene signature: a new method for personalized risk assessment in patients with diffuse large B-cell lymphoma. Pharmgenomics Pers. Med. 14, 609–619. doi:10.2147/PGPM.S309846
Chen, L., Li, X., Liu, L., Yu, B., Xue, Y., and Liu, Y. (2015). Erastin sensitizes glioblastoma cells to temozolomide by restraining xCT and cystathionine-gamma-lyase function. Oncol. Rep. 33, 1465–1474. doi:10.3892/or.2015.3712
Chen, X., Hu, S., Han, Y., Cai, Y., Lu, T., Hu, X., et al. (2023a). Ferroptosis-related STEAP3 acts as predictor and regulator in diffuse large B cell lymphoma through immune infiltration. Clin. Exp. Med. 23, 2601–2617. doi:10.1007/s10238-023-00996-4
Chen, Y., Tao, H., Wang, F., Wu, P., Gao, J., Zhang, X., et al. (2023b). Artesunate synergistically promotes sorafenib-induced apoptosis and ferroptosis in non-Hodgkin lymphoma cells through inhibition of the STAT3 pathway. Oncol. Rep. 50, 147. doi:10.3892/or.2023.8584
Chen, Y., Wang, F., Wu, P., Gong, S., Gao, J., Tao, H., et al. (2021b). Artesunate induces apoptosis, autophagy and ferroptosis in diffuse large B cell lymphoma cells by impairing STAT3 signaling. Cell Signal 88, 110167. doi:10.1016/j.cellsig.2021.110167
Chen, Z., Pittman, E. F., Romaguera, J., Fayad, L., Wang, M., Neelapu, S. S., et al. (2013). Nuclear translocation of B-cell-specific transcription factor, BACH2, modulates ROS mediated cytotoxic responses in mantle cell lymphoma. Plos One 8, e69126. doi:10.1371/journal.pone.0069126
Chen, Z., Wang, W., Abdul Razak, S. R., Han, T., Ahmad, N. H., and Li, X. (2023c). Ferroptosis as a potential target for cancer therapy. Cell Death Dis. 14, 460. doi:10.1038/s41419-023-05930-w
Choby, J. E., and Skaar, E. P. (2016). Heme synthesis and acquisition in bacterial pathogens. J. Mol. Biol. 428, 3408–3428. doi:10.1016/j.jmb.2016.03.018
Coffey, R., and Ganz, T. (2017). Iron homeostasis: an anthropocentric perspective. J. Biol. Chem. 292, 12727–12734. doi:10.1074/jbc.R117.781823
Compagno, M., Lim, W. K., Grunn, A., Nandula, S. V., Brahmachary, M., Shen, Q., et al. (2009). Mutations of multiple genes cause deregulation of NF-kappaB in diffuse large B-cell lymphoma. Nature 459, 717–721. doi:10.1038/nature07968
Conrad, M., and Sato, H. (2012). The oxidative stress-inducible cystine/glutamate antiporter, system x (c) (-): cystine supplier and beyond. Amino Acids 42, 231–246. doi:10.1007/s00726-011-0867-5
DeBerardinis, R. J., Sayed, N., Ditsworth, D., and Thompson, C. B. (2008). Brick by brick: metabolism and tumor cell growth. Curr. Opin. Genet. Dev. 18, 54–61. doi:10.1016/j.gde.2008.02.003
Devin, J., Cañeque, T., Lin, Y. L., Mondoulet, L., Veyrune, J. L., Abouladze, M., et al. (2022). Targeting cellular iron homeostasis with ironomycin in diffuse large B-cell lymphoma. Cancer Res. 82, 998–1012. doi:10.1158/0008-5472.CAN-21-0218
Ding, B. B., Yu, J. J., Yu, R. Y., Mendez, L. M., Shaknovich, R., Zhang, Y., et al. (2008). Constitutively activated STAT3 promotes cell proliferation and survival in the activated B-cell subtype of diffuse large B-cell lymphomas. Blood 111, 1515–1523. doi:10.1182/blood-2007-04-087734
Dixon, S. J., Lemberg, K. M., Lamprecht, M. R., Skouta, R., Zaitsev, E. M., Gleason, C. E., et al. (2012). Ferroptosis: an iron-dependent form of nonapoptotic cell death. Cell 149, 1060–1072. doi:10.1016/j.cell.2012.03.042
Dixon, S. J., Patel, D. N., Welsch, M., Skouta, R., Lee, E. D., Hayano, M., et al. (2014). Pharmacological inhibition of cystine-glutamate exchange induces endoplasmic reticulum stress and ferroptosis. Elife 3, e02523. doi:10.7554/eLife.02523
Doll, S., Freitas, F. P., Shah, R., Aldrovandi, M., Da, S. M., Ingold, I., et al. (2019). FSP1 is a glutathione-independent ferroptosis suppressor. Nature 575, 693–698. doi:10.1038/s41586-019-1707-0
Doll, S., Proneth, B., Tyurina, Y. Y., Panzilius, E., Kobayashi, S., Ingold, I., et al. (2017). ACSL4 dictates ferroptosis sensitivity by shaping cellular lipid composition. Nat. Chem. Biol. 13, 91–98. doi:10.1038/nchembio.2239
El-Deiry, W. S., Kern, S. E., Pietenpol, J. A., Kinzler, K. W., and Vogelstein, B. (1992). Definition of a consensus binding site for p53. Nat. Genet. 1, 45–49. doi:10.1038/ng0492-45
Fan, Z., Wirth, A. K., Chen, D., Wruck, C. J., Rauh, M., Buchfelder, M., et al. (2017). Nrf2-Keap1 pathway promotes cell proliferation and diminishes ferroptosis. Oncogenesis 6, e371. doi:10.1038/oncsis.2017.65
Fleming, M. D., Trenor, C. R., Su, M. A., Foernzler, D., Beier, D. R., Dietrich, W. F., et al. (1997). Microcytic anaemia mice have a mutation in Nramp2, a candidate iron transporter gene. Nat. Genet. 16, 383–386. doi:10.1038/ng0897-383
Gan, B. (2022). ACSL4, PUFA, and ferroptosis: new arsenal in anti-tumor immunity. Signal Transduct. Target Ther. 7, 128. doi:10.1038/s41392-022-01004-z
Gao, H., Bai, Y., Jia, Y., Zhao, Y., Kang, R., Tang, D., et al. (2018). Ferroptosis is a lysosomal cell death process. Biochem. Biophys. Res. Commun. 503, 1550–1556. doi:10.1016/j.bbrc.2018.07.078
Grivennikov, S. I., and Karin, M. (2010). Dangerous liaisons: STAT3 and NF-kappaB collaboration and crosstalk in cancer. Cytokine Growth Factor Rev. 21, 11–19. doi:10.1016/j.cytogfr.2009.11.005
Gunshin, H., Mackenzie, B., Berger, U. V., Gunshin, Y., Romero, M. F., Boron, W. F., et al. (1997). Cloning and characterization of a mammalian proton-coupled metal-ion transporter. Nature 388, 482–488. doi:10.1038/41343
Guo, J., Xu, B., Han, Q., Zhou, H., Xia, Y., Gong, C., et al. (2018). Ferroptosis: a novel anti-tumor action for cisplatin. Cancer Res. Treat. 50, 445–460. doi:10.4143/crt.2016.572
Habib, E., Linher-Melville, K., Lin, H. X., and Singh, G. (2015). Expression of xCT and activity of system xc(-) are regulated by NRF2 in human breast cancer cells in response to oxidative stress. Redox Biol. 5, 33–42. doi:10.1016/j.redox.2015.03.003
Hentze, M. W., Muckenthaler, M. U., Galy, B., and Camaschella, C. (2010). Two to tango: regulation of Mammalian iron metabolism. Cell 142, 24–38. doi:10.1016/j.cell.2010.06.028
Herb, M., Gluschko, A., and Schramm, M. (2021). Reactive oxygen species: not omnipresent but important in many locations. Front. Cell Dev. Biol. 9, 716406. doi:10.3389/fcell.2021.716406
Hertzberg, M. R. (2022). R-CHOP in DLBCL: priming for success. Blood 139, 1121–1122. doi:10.1182/blood.2021013620
Hong, Y., Ren, T., Wang, X., Liu, X., Fei, Y., Meng, S., et al. (2022). APR-246 triggers ferritinophagy and ferroptosis of diffuse large B-cell lymphoma cells with distinct TP53 mutations. Leukemia 36, 2269–2280. doi:10.1038/s41375-022-01634-w
Hsu, C. M., Kao, S. Y., Yen, C. H., Hsiao, C. E., Cho, S. F., Wang, H. C., et al. (2025). Biomarker potential of nuclear Nrf2 activation in the ABC subtype of diffuse large B-cell lymphoma. Oncol. Lett. 29, 30. doi:10.3892/ol.2024.14776
Huang, Q. T., Hu, Q. Q., Wen, Z. F., and Li, Y. L. (2023). Iron oxide nanoparticles inhibit tumor growth by ferroptosis in diffuse large B-cell lymphoma. Am. J. Cancer Res. 13, 498–508.
Huang, X., Meng, B., Iqbal, J., Ding, B. B., Perry, A. M., Cao, W., et al. (2013). Activation of the STAT3 signaling pathway is associated with poor survival in diffuse large B-cell lymphoma treated with R-CHOP. J. Clin. Oncol. 31, 4520–4528. doi:10.1200/JCO.2012.45.6004
Iglehart, J. K., York, R. M., Modest, A. P., Lazarus, H., and Livingston, D. M. (1977). Cystine requirement of continuous human lymphoid cell lines of normal and leukemic origin. J. Biol. Chem. 252, 7184–7191. doi:10.1016/s0021-9258(19)66953-x
Jiang, L., Kon, N., Li, T., Wang, S. J., Su, T., Hibshoosh, H., et al. (2015). Ferroptosis as a p53-mediated activity during tumour suppression. Nature 520, 57–62. doi:10.1038/nature14344
Kagan, V. E., Mao, G., Qu, F., Angeli, J. P. F., Doll, S., Croix, C. S., et al. (2017). Oxidized arachidonic and adrenic PEs navigate cells to ferroptosis. Nat. Chem. Biol. 13, 81–90. doi:10.1038/nchembio.2238
Kari, E., Teppo, H. R., Haapasaari, K. M., Kuusisto, M., Lemma, A., Karihtala, P., et al. (2019). Nuclear factor erythroid 2-related factors 1 and 2 are able to define the worst prognosis group among high-risk diffuse large B cell lymphomas treated with R-CHOEP. J. Clin. Pathol. 72, 316–321. doi:10.1136/jclinpath-2018-205584
Kinowaki, Y., Kurata, M., Ishibashi, S., Ikeda, M., Tatsuzawa, A., Yamamoto, M., et al. (2018). Glutathione peroxidase 4 overexpression inhibits ROS-induced cell death in diffuse large B-cell lymphoma. Lab. Invest 98, 609–619. doi:10.1038/s41374-017-0008-1
Kraft, V., Bezjian, C. T., Pfeiffer, S., Ringelstetter, L., Müller, C., Zandkarimi, F., et al. (2020). GTP cyclohydrolase 1/tetrahydrobiopterin counteract ferroptosis through lipid remodeling. Acs Cent. Sci. 6, 41–53. doi:10.1021/acscentsci.9b01063
Lam, L. T., Wright, G., Davis, R. E., Lenz, G., Farinha, P., Dang, L., et al. (2008). Cooperative signaling through the signal transducer and activator of transcription 3 and nuclear factor-{kappa}B pathways in subtypes of diffuse large B-cell lymphoma. Blood 111, 3701–3713. doi:10.1182/blood-2007-09-111948
Lambert, J. M. R., Gorzov, P., Veprintsev, D. B., Söderqvist, M., Segerbäck, D., Bergman, J., et al. (2009). PRIMA-1 reactivates mutant p53 by covalent binding to the core domain. Cancer Cell 15, 376–388. doi:10.1016/j.ccr.2009.03.003
Larraufie, M., Yang, W. S., Jiang, E., Thomas, A. G., Slusher, B. S., and Stockwell, B. R. (2015). Incorporation of metabolically stable ketones into a small molecule probe to increase potency and water solubility. Bioorg Med. Chem. Lett. 25, 4787–4792. doi:10.1016/j.bmcl.2015.07.018
Lemma, S., Karihtala, P., Haapasaari, K. M., Jantunen, E., Soini, Y., Bloigu, R., et al. (2013). Biological roles and prognostic values of the epithelial-mesenchymal transition-mediating transcription factors Twist, ZEB1 and Slug in diffuse large B-cell lymphoma. Histopathology 62, 326–333. doi:10.1111/his.12000
Li, F., Long, H., Zhou, Z., Luo, H., Xu, S., and Gao, L. (2022). System Xc−/GSH/GPX4 axis: an important antioxidant system for the ferroptosis in drug-resistant solid tumor therapy. Front. Pharmacol. 13, 910292. doi:10.3389/fphar.2022.910292
Li, X., and Liu, J. (2023). FANCD2 inhibits ferroptosis by regulating the JAK2/STAT3 pathway in osteosarcoma. Bmc Cancer 23, 179. doi:10.1186/s12885-023-10626-7
Liang, D., Feng, Y., Zandkarimi, F., Wang, H., Zhang, Z., Kim, J., et al. (2023). Ferroptosis surveillance independent of GPX4 and differentially regulated by sex hormones. Cell 186, 2748–2764.e22. doi:10.1016/j.cell.2023.05.003
Liao, P., Wang, W., Wang, W., Kryczek, I., Li, X., Bian, Y., et al. (2022). CD8(+) T cells and fatty acids orchestrate tumor ferroptosis and immunity via ACSL4. Cancer Cell 40, 365–378.e6. doi:10.1016/j.ccell.2022.02.003
Lim, Y. P., Lim, T. T., Chan, Y. L., Song, A. C., Yeo, B. H., Vojtesek, B., et al. (2007). The p53 knowledgebase: an integrated information resource for p53 research. Oncogene 26, 1517–1521. doi:10.1038/sj.onc.1209952
Lin, S., Yan, J., Wang, W., and Luo, L. (2024). STAT3-Mediated ferroptosis is involved in sepsis-associated acute respiratory distress syndrome. Inflammation 47, 1204–1219. doi:10.1007/s10753-024-01970-2
Lingappan, K. (2018). NF-κB in oxidative stress. Curr. Opin. Toxicol. 7, 81–86. doi:10.1016/j.cotox.2017.11.002
Liu, D. S., Duong, C. P., Haupt, S., Montgomery, K. G., House, C. M., Azar, W. J., et al. (2017). Inhibiting the system xC−/glutathione axis selectively targets cancers with mutant-p53 accumulation. Nat. Commun. 8, 14844. doi:10.1038/ncomms14844
Liu, G., and Chen, X. (2006). Regulation of the p53 transcriptional activity. J. Cell Biochem. 97, 448–458. doi:10.1002/jcb.20700
Liu, X., Tian, Y., Yang, A., Zhang, C., Miao, X., and Yang, W. (2023b). Antitumor effects of poplar propolis on DLBCL SU-DHL-2 cells. Foods 12, 283. doi:10.3390/foods12020283
Liu, Y., Du, Z., Huang, J., Li, T., Zhang, J., Li, Y., et al. (2023a). Ferroptosis in hematological malignant tumors. Front. Oncol. 13, 1127526. doi:10.3389/fonc.2023.1127526
Longo, P. G., Laurenti, L., Gobessi, S., Sica, S., Leone, G., and Efremov, D. G. (2008). The Akt/Mcl-1 pathway plays a prominent role in mediating antiapoptotic signals downstream of the B-cell receptor in chronic lymphocytic leukemia B cells. Blood 111, 846–855. doi:10.1182/blood-2007-05-089037
Lu, L., Zhu, F., Zhang, M., Li, Y., Drennan, A. C., Kimpara, S., et al. (2018). Gene regulation and suppression of type I interferon signaling by STAT3 in diffuse large B cell lymphoma. Proc. Natl. Acad. Sci. U. S. A. 115, E498-E505–E505. doi:10.1073/pnas.1715118115
Maeta, T., Sato, T., Asano, K., and Ito, S. (2022). Dimethyl fumarate induces apoptosisvia inhibiting NF-κB and STAT3 signaling in adult T-cell leukemia/lymphoma cells. Anticancer Res. 42, 2301–2309. doi:10.21873/anticanres.15709
Magtanong, L., Ko, P., To, M., Cao, J. Y., Forcina, G. C., Tarangelo, A., et al. (2019). Exogenous monounsaturated fatty acids promote a ferroptosis-resistant cell state. Cell Chem. Biol. 26, 420–432. doi:10.1016/j.chembiol.2018.11.016
Mai, T. T., Hamaï, A., Hienzsch, A., Cañeque, T., Müller, S., Wicinski, J., et al. (2017). Salinomycin kills cancer stem cells by sequestering iron in lysosomes. Nat. Chem. 9, 1025–1033. doi:10.1038/nchem.2778
Manara, P., Newsam, A. D., Saralamma, V. V., Russo, M. V., Martinez, A. B., Fattakhov, N., et al. (2024). NRF2 translation block by inhibition of cap-dependent initiation sensitizes lymphoma cells to ferroptosis and CAR-T immunotherapy. Biorxiv, 2024.09.09.612133–2029. doi:10.1101/2024.09.09.612133
Mancias, J. D., Pontano, V. L., Nissim, S., Biancur, D. E., Kim, A. J., Wang, X., et al. (2015). Ferritinophagy via NCOA4 is required for erythropoiesis and is regulated by iron dependent HERC2-mediated proteolysis. Elife 4, e10308. doi:10.7554/eLife.10308
Mandal, P. K., Seiler, A., Perisic, T., Kölle, P., Banjac Canak, A., Förster, H., et al. (2010). System xc− and thioredoxin reductase 1 cooperatively rescue glutathione deficiency. J. Biol. Chem. 285, 22244–22253. doi:10.1074/jbc.M110.121327
Manea, A., and Simionescu, M. (2012). Nox enzymes and oxidative stress in atherosclerosis. Front. Biosci. Sch. Ed. 4, 651–670. doi:10.2741/s291
Mao, C., Liu, X., Zhang, Y., Lei, G., Yan, Y., Lee, H., et al. (2021). DHODH-mediated ferroptosis defence is a targetable vulnerability in cancer. Nature 593, 586–590. doi:10.1038/s41586-021-03539-7
Martin-Rufino, J. D., Castano, N., Pang, M., Grody, E. I., Joubran, S., Caulier, A., et al. (2023). Massively parallel base editing to map variant effects in human hematopoiesis. Cell 186, 2456–2474.e24. doi:10.1016/j.cell.2023.03.035
McKie, A. T., Marciani, P., Rolfs, A., Brennan, K., Wehr, K., Barrow, D., et al. (2000). A novel duodenal iron-regulated transporter, IREG1, implicated in the basolateral transfer of iron to the circulation. Mol. Cell 5, 299–309. doi:10.1016/s1097-2765(00)80425-6
Mishima, E., Ito, J., Wu, Z., Nakamura, T., Wahida, A., Doll, S., et al. (2022). A non-canonical vitamin K cycle is a potent ferroptosis suppressor. Nature 608, 778–783. doi:10.1038/s41586-022-05022-3
Mishima, E., Nakamura, T., Zheng, J., Zhang, W., Mourão, A., Sennhenn, P., et al. (2023). DHODH inhibitors sensitize to ferroptosis by FSP1 inhibition. Nature 619, E9–E18. doi:10.1038/s41586-023-06269-0
Mohamed, A. J., Yu, L., Bäckesjö, C. M., Vargas, L., Faryal, R., Aints, A., et al. (2009). Bruton's tyrosine kinase (Btk): function, regulation, and transformation with special emphasis on the PH domain. Immunol. Rev. 228, 58–73. doi:10.1111/j.1600-065X.2008.00741.x
Mohell, N., Alfredsson, J., Fransson, Å., Uustalu, M., Byström, S., Gullbo, J., et al. (2015). APR-246 overcomes resistance to cisplatin and doxorubicin in ovarian cancer cells. Cell Death Dis. 6, e1794. doi:10.1038/cddis.2015.143
Moi, P., Chan, K., Asunis, I., Cao, A., and Kan, Y. W. (1994). Isolation of NF-E2-related factor 2 (Nrf2), a NF-E2-like basic leucine zipper transcriptional activator that binds to the tandem NF-E2/AP1 repeat of the beta-globin locus control region. Proc. Natl. Acad. Sci. U. S. A. 91, 9926–9930. doi:10.1073/pnas.91.21.9926
Ohgami, R. S., Campagna, D. R., Greer, E. L., Antiochos, B., McDonald, A., Chen, J., et al. (2005). Identification of a ferrireductase required for efficient transferrin-dependent iron uptake in erythroid cells. Nat. Genet. 37, 1264–1269. doi:10.1038/ng1658
Ok, C. Y., Chen, J., Xu-Monette, Z. Y., Tzankov, A., Manyam, G. C., Li, L., et al. (2014). Clinical implications of phosphorylated STAT3 expression in de novo diffuse large B-cell lymphoma. Clin. Cancer Res. 20, 5113–5123. doi:10.1158/1078-0432.CCR-14-0683
O'Shea, J. J., Holland, S. M., and Staudt, L. M. (2013). JAKs and STATs in immunity, immunodeficiency, and cancer. N. Engl. J. Med. 368, 161–170. doi:10.1056/NEJMra1202117
Otterbein, L. E., Soares, M. P., Yamashita, K., and Bach, F. H. (2003). Heme oxygenase-1: unleashing the protective properties of heme. Trends Immunol. 24, 449–455. doi:10.1016/s1471-4906(03)00181-9
Ovejero-Merino, S., Alibert, L., Devin, J., Cañeque, T., Karmous Gadacha, O., Abouladze, M., et al. (2022). Ironomycin induces mantle cell lymphoma cell death by targeting iron metabolism addiction. Blood 140, 6439–6440. doi:10.1182/blood-2022-164644
Parker, J. L., Deme, J. C., Kolokouris, D., Kuteyi, G., Biggin, P. C., Lea, S. M., et al. (2021). Molecular basis for redox control by the human cystine/glutamate antiporter system xc<sup/>. Nat. Commun. 12, 7147. doi:10.1038/s41467-021-27414-1
Peng, X., Zhang, M., Conserva, F., Hosny, G., Selivanova, G., Bykov, V. J. N., et al. (2013). APR-246/PRIMA-1MET inhibits thioredoxin reductase 1 and converts the enzyme to a dedicated NADPH oxidase. Cell Death Dis. 4, e881. doi:10.1038/cddis.2013.417
Pizzino, G., Irrera, N., Cucinotta, M., Pallio, G., Mannino, F., Arcoraci, V., et al. (2017). Oxidative stress: harms and benefits for human health. Oxid. Med. Cell Longev. 2017, 8416763–8416813. doi:10.1155/2017/8416763
Pontoriero, M., Fiume, G., Vecchio, E., de Laurentiis, A., Albano, F., Iaccino, E., et al. (2019). Activation of NF-κB in B cell receptor signaling through Bruton's tyrosine kinase-dependent phosphorylation of IκB-α. J. Mol. Med. Berl. 97, 675–690. doi:10.1007/s00109-019-01777-x
Qian, Z. M., and Ke, Y. (2001). Rethinking the role of ceruloplasmin in brain iron metabolism. Brain Res. Rev. 35, 287–294. doi:10.1016/s0165-0173(01)00056-x
Qin, Y., Jiang, S., Liu, P., Yang, J., Yang, S., He, X., et al. (2020). Characteristics and management of TP53-mutated diffuse large B-cell lymphoma patients. Cancer Manag. Res. 12, 11515–11522. doi:10.2147/CMAR.S269624
Read, A. D., Bentley, R. E., Archer, S. L., and Dunham-Snary, K. J. (2021). Mitochondrial iron-sulfur clusters: structure, function, and an emerging role in vascular biology. Redox Biol. 47, 102164. doi:10.1016/j.redox.2021.102164
Riedell, P. A., and Smith, S. M. (2018). Double hit and double expressors in lymphoma: definition and treatment. Cancer 124, 4622–4632. doi:10.1002/cncr.31646
Roh, J. L., Kim, E. H., Jang, H., and Shin, D. (2017). Nrf2 inhibition reverses the resistance of cisplatin-resistant head and neck cancer cells to artesunate-induced ferroptosis. Redox Biol. 11, 254–262. doi:10.1016/j.redox.2016.12.010
Rouault, T. A. (2013). Iron metabolism in the CNS: implications for neurodegenerative diseases. Nat. Rev. Neurosci. 14, 551–564. doi:10.1038/nrn3453
Sakurai, M., Ishitsuka, K., Ito, R., Wilkinson, A. C., Kimura, T., Mizutani, E., et al. (2023). Chemically defined cytokine-free expansion of human haematopoietic stem cells. Nature 615, 127–133. doi:10.1038/s41586-023-05739-9
Scheuerpflug, A., Ahmetlić, F., Bauer, V., Riedel, T., Röcken, M., and Mocikat, R. (2021). The role of dendritic cells for therapy of B-cell lymphoma with immune checkpoint inhibitors. Cancer Immunol. Immunother. 70, 1343–1350. doi:10.1007/s00262-020-02767-6
Schmitt, A., Grimm, M., Kreienkamp, N., Junge, H., Labisch, J., Schuhknecht, L., et al. (2023). BRD4 inhibition sensitizes diffuse large B-cell lymphoma cells to ferroptosis. Blood 142, 1143–1155. doi:10.1182/blood.2022019274
Schmitt, A., Xu, W., Bucher, P., Grimm, M., Konantz, M., Horn, H., et al. (2021). Dimethyl fumarate induces ferroptosis and impairs NF-κB/STAT3 signaling in DLBCL. Blood 138, 871–884. doi:10.1182/blood.2020009404
Scuto, A., Kujawski, M., Kowolik, C., Krymskaya, L., Wang, L., Weiss, L. M., et al. (2011). STAT3 inhibition is a therapeutic strategy for ABC-like diffuse large B-cell lymphoma. Cancer Res. 71, 3182–3188. doi:10.1158/0008-5472.CAN-10-2380
Sekine, Y., Yamamoto, K., Kurata, M., Honda, A., Onishi, I., Kinowaki, Y., et al. (2022). HADHB, a fatty acid beta-oxidation enzyme, is a potential prognostic predictor in malignant lymphoma. Pathology 54, 286–293. doi:10.1016/j.pathol.2021.06.119
Setiawan, S. A., Liu, W. Z., Weng, P. W., Lee, C. H., Yadav, V. K., Hardianti, M. S., et al. (2023). Synergistic disruption of BTK and BCL-2 causes apoptosis while inducing ferroptosis in double-hit lymphoma. Eur. J. Pharmacol. 943, 175526. doi:10.1016/j.ejphar.2023.175526
Shayeghi, M., Latunde-Dada, G. O., Oakhill, J. S., Laftah, A. H., Takeuchi, K., Halliday, N., et al. (2005). Identification of an intestinal heme transporter. Cell 122, 789–801. doi:10.1016/j.cell.2005.06.025
Shiah, J. V., Grandis, J. R., and Johnson, D. E. (2021). Targeting STAT3 with proteolysis targeting chimeras and next-generation antisense oligonucleotides. Mol. Cancer Ther. 20, 219–228. doi:10.1158/1535-7163.MCT-20-0599
Shukla, A., Rai, K., Shukla, V., Chaturvedi, N. K., Bociek, R. G., Pirruccello, S. J., et al. (2016). Sprouty 2: a novel attenuator of B-cell receptor and MAPK-Erk signaling in CLL. Blood 127, 2310–2321. doi:10.1182/blood-2015-09-669317
Song, X., Zhang, W., Yu, N., and Zhong, X. (2023). PAQR3 facilitates the ferroptosis of diffuse large B-cell lymphoma via the regulation of LDLR-mediated PI3K/AKT pathway. Hematol. Oncol. 42, e3219. doi:10.1002/hon.3219
Soula, M., Weber, R. A., Zilka, O., Alwaseem, H., La, K., Yen, F., et al. (2020). Metabolic determinants of cancer cell sensitivity to canonical ferroptosis inducers. Nat. Chem. Biol. 16, 1351–1360. doi:10.1038/s41589-020-0613-y
Sui, S., Zhang, J., Xu, S., Wang, Q., Wang, P., and Pang, D. (2019). Ferritinophagy is required for the induction of ferroptosis by the bromodomain protein BRD4 inhibitor (+)-JQ1 in cancer cells. Cell Death Dis. 10, 331. doi:10.1038/s41419-019-1564-7
Sun, X., Ou, Z., Chen, R., Niu, X., Chen, D., Kang, R., et al. (2016). Activation of the p62-Keap1-NRF2 pathway protects against ferroptosis in hepatocellular carcinoma cells. Hepatology 63, 173–184. doi:10.1002/hep.28251
Tavakkoli, M., and Barta, S. K. (2023). 2024 Update: advances in the risk stratification and management of large B-cell lymphoma. Am. J. Hematol. 98, 1791–1805. doi:10.1002/ajh.27075
Thomas, M., Johnson, ZYVJ, Ferrans, V. J., Lowenstein, R. A., and Finkel, T. (1996). Reactive oxygen species are downstream mediators ofp53-dependent apoptosis. Med. Sci. 93, 11848–11852. doi:10.1073/pnas.93.21.11848
Turton, K. B., Annis, D. S., Rui, L., Esnault, S., and Mosher, D. F. (2015). Ratios of four STAT3 splice variants in human eosinophils and diffuse large B cell lymphoma cells. Plos One 10, e0127243. doi:10.1371/journal.pone.0127243
Ursini, F., and Maiorino, M. (2020). Lipid peroxidation and ferroptosis: the role of GSH and GPx4. Free Radic. Biol. Med. 152, 175–185. doi:10.1016/j.freeradbiomed.2020.02.027
Vatrinet, R., Leone, G., De Luise, M., Girolimetti, G., Vidone, M., Gasparre, G., et al. (2017). The α-ketoglutarate dehydrogenase complex in cancer metabolic plasticity. Cancer Metab. 5, 3. doi:10.1186/s40170-017-0165-0
Versini, A., Colombeau, L., Hienzsch, A., Gaillet, C., Retailleau, P., Debieu, S., et al. (2020). Salinomycin derivatives kill breast cancer stem cells by lysosomal iron targeting. Chemistry 26, 7416–7424. doi:10.1002/chem.202000335
Vitolo, U., and Chiappella, A. (2023). A new frontier for R-CHOP: is two better than one? Blood 142, 1331–1332. doi:10.1182/blood.2023021521
Wang, H., Fleishman, J. S., Cheng, S., Wang, W., Wu, F., Wang, Y., et al. (2024). Epigenetic modification of ferroptosis by non-coding RNAs in cancer drug resistance. Mol. Cancer 23, 177. doi:10.1186/s12943-024-02088-7
Wang, J., Peng, H., Zhang, G., and Yan, W. (2023b). Identification of a novel model based on ferroptosis-related genes for predicting the prognosis of diffuse large B-cell lymphomas. Hematology 28, 2198862. doi:10.1080/16078454.2023.2198862
Wang, S., Clapper, E., Tonissen, K. F., and Di Trapani, G. (2023d). Co-targeting of BTK and TrxR as a therapeutic approach to the treatment of lymphoma. Antioxidants (Basel) 12, 529. doi:10.3390/antiox12020529
Wang, X., Liu, M., Chu, Y., Liu, Y., Cao, X., Zhang, H., et al. (2022). O-GlcNAcylation of ZEB1 facilitated mesenchymal pancreatic cancer cell ferroptosis. Int. J. Biol. Sci. 18, 4135–4150. doi:10.7150/ijbs.71520
Wang, Y., Hu, J., Wu, S., Fleishman, J. S., Li, Y., Xu, Y., et al. (2023a). Targeting epigenetic and posttranslational modifications regulating ferroptosis for the treatment of diseases. Signal Transduct. Target Ther. 8, 449. doi:10.1038/s41392-023-01720-0
Wang, Y., Wu, X., Ren, Z., Li, Y., Zou, W., Chen, J., et al. (2023c). Overcoming cancer chemotherapy resistance by the induction of ferroptosis. Drug Resist Updat 66, 100916. doi:10.1016/j.drup.2022.100916
Wegenka, U. M., Buschmann, J., Lütticken, C., Heinrich, P. C., and Horn, F. (1993). Acute-phase response factor, a nuclear factor binding to acute-phase response elements, is rapidly activated by interleukin-6 at the posttranslational level. Mol. Cell Biol. 13, 276–288. doi:10.1128/mcb.13.1.276
Weng, J., Chen, L., Liu, H., Yang, X. P., and Huang, L. (2022). Ferroptosis markers predict the survival, immune infiltration, and ibrutinib resistance of diffuse large B cell lymphoma. Inflammation 45, 1146–1161. doi:10.1007/s10753-021-01609-6
Wenzel, S. E., Tyurina, Y. Y., Zhao, J., St, C. C., Dar, H. H., Mao, G., et al. (2017). PEBP1 wardens ferroptosis by enabling lipoxygenase generation of lipid death signals. Cell 171, 628–641. doi:10.1016/j.cell.2017.09.044
Wu, C., Hou, X., Li, S., and Luo, S. (2023b). Long noncoding RNA ZEB1-AS1 attenuates ferroptosis of gastric cancer cells through modulating miR-429/BGN axis. J. Biochem. Mol. Toxicol. 37, e23381. doi:10.1002/jbt.23381
Wu, H., Zhang, J., Fu, L., Wu, R., Gu, Z., Yin, C., et al. (2023a). Identification and development of a 4-gene ferroptosis signature predicting overall survival for diffuse large B-cell lymphoma. Technol. Cancer Res. Treat. 22, 15330338221147772. doi:10.1177/15330338221147772
Xiao, D., Zeng, L., Yao, K., Kong, X., Wu, G., and Yin, Y. (2016). The glutamine-alpha-ketoglutarate (AKG) metabolism and its nutritional implications. Amino Acids 48, 2067–2080. doi:10.1007/s00726-016-2254-8
Xiong, D., Li, M., and Zeng, C. (2022). Construction and validation of a risk scoring model for diffuse large B-cell lymphoma based on ferroptosis-related genes and its association with immune infiltration. Transl. Oncol. 16, 101314. doi:10.1016/j.tranon.2021.101314
Xu, C., Liu, Z., and Xiao, J. (2021). Ferroptosis: a double-edged sword in gastrointestinal disease. Int. J. Mol. Sci. 22, 12403. doi:10.3390/ijms222212403
Yang, H., Magilnick, N., Lee, C., Kalmaz, D., Ou, X., Chan, J. Y., et al. (2005). Nrf1 and Nrf2 regulate rat glutamate-cysteine ligase catalytic subunit transcription indirectly via NF-kappaB and AP-1. Mol. Cell Biol. 25, 5933–5946. doi:10.1128/MCB.25.14.5933-5946.2005
Yang, J., Liao, X., Agarwal, M. K., Barnes, L., Auron, P. E., and Stark, G. R. (2007). Unphosphorylated STAT3 accumulates in response to IL-6 and activates transcription by binding to NFkappaB. Genes Dev. 21, 1396–1408. doi:10.1101/gad.1553707
Yang, W. S., SriRamaratnam, R., Welsch, M. E., Shimada, K., Skouta, R., Viswanathan, V. S., et al. (2014). Regulation of ferroptotic cancer cell death by GPX4. Cell 156, 317–331. doi:10.1016/j.cell.2013.12.010
Ye, L. F., Chaudhary, K. R., Zandkarimi, F., Harken, A. D., Kinslow, C. J., Upadhyayula, P. S., et al. (2020). Radiation-induced lipid peroxidation triggers ferroptosis and synergizes with ferroptosis inducers. Acs Chem. Biol. 15, 469–484. doi:10.1021/acschembio.9b00939
Ye, P., Mimura, J., Okada, T., Sato, H., Liu, T., Maruyama, A., et al. (2014). Nrf2-and ATF4-dependent upregulation of xCT modulates the sensitivity of T24 bladder carcinoma cells to proteasome inhibition. Mol. Cell Biol. 34, 3421–3434. doi:10.1128/MCB.00221-14
Yi, X., Zhao, Y., Xue, L., Zhang, J., Qiao, Y., Jin, Q., et al. (2018). Expression of Keap1 and Nrf2 in diffuse large B-cell lymphoma and its clinical significance. Exp. Ther. Med. 16, 573–578. doi:10.3892/etm.2018.6208
Yu, L., Wang, Y. F., Xiao, J., Shen, Q. Q., Chi, S. S., Gao, Y. L., et al. (2023). Dysregulation of iron homeostasis by TfR-1 renders EZH2 wild type diffuse large B-cell lymphoma resistance to EZH2 inhibition. Acta Pharmacol. Sin. 44, 2113–2124. doi:10.1038/s41401-023-01097-4
Yu, Y., Xie, Y., Cao, L., Yang, L., Yang, M., Lotze, M. T., et al. (2015). The ferroptosis inducer erastin enhances sensitivity of acute myeloid leukemia cells to chemotherapeutic agents. Mol. Cell Oncol. 2, e1054549. doi:10.1080/23723556.2015.1054549
Yuneva, M., Zamboni, N., Oefner, P., Sachidanandam, R., and Lazebnik, Y. (2007). Deficiency in glutamine but not glucose induces MYC-dependent apoptosis in human cells. J. Cell Biol. 178, 93–105. doi:10.1083/jcb.200703099
Zhang, C., Liu, X., Jin, S., Chen, Y., and Guo, R. (2022b). Ferroptosis in cancer therapy: a novel approach to reversing drug resistance. Mol. Cancer 21, 47. doi:10.1186/s12943-022-01530-y
Zhang, J., Du, J., Kong, N., Zhang, G., Liu, M., and Liu, C. (2021a). Mechanisms and pharmacological applications of ferroptosis: a narrative review. Ann. Transl. Med. 9, 1503. doi:10.21037/atm-21-1595
Zhang, J. Y., Zhou, B., Sun, R. Y., Ai, Y. L., Cheng, K., Li, F. N., et al. (2021b). The metabolite α-KG induces GSDMC-dependent pyroptosis through death receptor 6-activated caspase-8. Cell Res. 31, 980–997. doi:10.1038/s41422-021-00506-9
Zhang, W., Gong, M., Zhang, W., Mo, J., Zhang, S., Zhu, Z., et al. (2022a). Thiostrepton induces ferroptosis in pancreatic cancer cells through STAT3/GPX4 signalling. Cell Death Dis. 13, 630. doi:10.1038/s41419-022-05082-3
Zhang, Y., Tan, H., Daniels, J. D., Zandkarimi, F., Liu, H., Brown, L. M., et al. (2019). Imidazole ketone erastin induces ferroptosis and slows tumor growth in a mouse lymphoma model. Cell Chem. Biol. 26, 623–633. doi:10.1016/j.chembiol.2019.01.008
Zhang, Z., Zhao, C., Yang, S., Lu, W., and Shi, J. (2024). A novel lipid metabolism-based risk model associated with immunosuppressive mechanisms in diffuse large B-cell lymphoma. Lipids Health Dis. 23, 20. doi:10.1186/s12944-024-02017-z
Zhao, J., Jia, Y., Mahmut, D., Deik, A. A., Jeanfavre, S., Clish, C. B., et al. (2023). Human hematopoietic stem cell vulnerability to ferroptosis. Cell 186, 732–747.e16. doi:10.1016/j.cell.2023.01.020
Zhao, L., Liu, Y., Zhang, J., Liu, Y., and Qi, Q. (2019). LncRNA SNHG14/miR-5590-3p/ZEB1 positive feedback loop promoted diffuse large B cell lymphoma progression and immune evasion through regulating PD-1/PD-L1 checkpoint. Cell Death Dis. 10, 731. doi:10.1038/s41419-019-1886-5
Zhong, D., Wang, R., Zhang, H., Wang, M., Zhang, X., and Chen, H. (2023). Induction of lysosomal exocytosis and biogenesis via TRPML1 activation for the treatment of uranium-induced nephrotoxicity. Nat. Commun. 14, 3997. doi:10.1038/s41467-023-39716-7
Zhou, N., and Busino, L. (2023). Targeting epigenetics and ferroptosis in DLBCL. Blood 142, 1108–1109. doi:10.1182/blood.2023021310
Zhou, Q., Li, T., Qin, Q., Huang, X., and Wang, Y. (2022). Ferroptosis in lymphoma: emerging mechanisms and a novel therapeutic approach. Front. Genet. 13, 1039951. doi:10.3389/fgene.2022.1039951
Glossary
α-KG alpha-ketoglutarate
AA arachidonic acid
ABC activated B-cell-like
ACSL4 long-chain family member 4 of acyl-CoA synthetase
AdA adrenic acid
ATS Artesunate
BCR B cell receptor
BH4 tetrahydrobiopterin
BTK bruton’s tyrosine kinase
CoQ10 ubiquinone
DHFR Dihydrofolate reductase
DHL double-hit lymphoma
DHODH dihydroorotate dehydrogenase
DLBCL diffuse large B-cell lymphoma
DMF dimethyl fumarate
FPN iron transporter protein
FRGs ferroptosis-Related Genes
FSP1 ferroptosis suppressor protein 1
FTH1 ferritin heavy chain 1
GCB germinal center B-cell-like
GPX4 glutathione peroxidase 4
IKE imidazole ketone erastin
LIP labile iron pool
LMP lysosomal membrane permeabilization
LOX lipoxygenase
LPCAT3 lysophosphatidylcholine acyltransferase 3
MUFA monounsaturated fatty acids
NOX NADPH oxidase
NCOA4 nuclear receptor coactivator 4
NHL non-Hodgkin’s lymphoma
NKTCL NK/T cell lymphoma
NRF2 The nuclear factor erythroid 2-related factor 2
ROS reactive oxygen species
RSL RAS-selective lethal
RTA radical trapping antioxidant
OS overall survival
PE phosphatidylethanolamine
PFS progression-free survival
PLOOH lipid hydroperoxides
PUFA polyunsaturated fatty acid
SLC7A11 solute carrier family 7 member 11
STAT signal transducer and activator of transcription
STEAP3 prostate transmembrane epithelial antigen 3
Tf transferrin
TfR1 transferrin receptor 1
TrxR thioredoxin reductase
VKH2 vitamin K to hydroquinone
ZEB1 Zinc finger E-box binding homeobox 1
Keywords: ferroptosis, STAT3, Nfr2, ZEB1, IKE, α-KG, DMF, APR-246
Citation: Wang Y, He Z, Dong X, Yao Y, Chen Q, Shi Y, Deng Y, Zhang Q, Yu L and Wang C (2025) Regulation and therapy: the role of ferroptosis in DLBCL. Front. Pharmacol. 15:1458412. doi: 10.3389/fphar.2024.1458412
Received: 02 July 2024; Accepted: 09 December 2024;
Published: 06 January 2025.
Edited by:
Apurva Srivastava, National Cancer Institute at Frederick (NIH), United StatesReviewed by:
Hongquan Wang, Peking University Aerospace School of Clinical Medicine, ChinaArchita Venugopal Menon, Beam Therapeutics, United States
Copyright © 2025 Wang, He, Dong, Yao, Chen, Shi, Deng, Zhang, Yu and Wang. This is an open-access article distributed under the terms of the Creative Commons Attribution License (CC BY). The use, distribution or reproduction in other forums is permitted, provided the original author(s) and the copyright owner(s) are credited and that the original publication in this journal is cited, in accordance with accepted academic practice. No use, distribution or reproduction is permitted which does not comply with these terms.
*Correspondence: Liang Yu, eXVsaWFuZ2hhQDE2My5jb20=; Chunling Wang, d2NsNjUwNkAxNjMuY29t
†These authors have contributed equally to this work and share first authorship