- Department of Pharmacy, The First People’s Hospital of Changzhou/The Third Affiliated Hospital of Soochow University, Changzhou, Jiangsu, China
Voriconazole is a broad-spectrum triazole antifungal agent. A number of studies have revealed that the impact of C-reactive protein (CRP) on voriconazole pharmacokinetics was associated with the CYP2C19 phenotype. However, the combined effects of CYP2C19 genetic polymorphisms and inflammation on voriconazole pharmacokinetics have not been considered in previous population pharmacokinetic (PPK) studies, especially in the Chinese population. This study aimed to analyze the impact of inflammation on the pharmacokinetics of voriconazole in patients with different CYP2C19 genotypes and optimize the dosage of administration. Data were obtained retrospectively from adult patients aged ≥16 years who received voriconazole for invasive fungal infections from October 2020 to June 2023. Plasma voriconazole levels were measured via high-performance liquid chromatography coupled with tandem mass spectrometry (HPLC-MS/MS). CYP2C19 genotyping was performed using the fluorescence in situ hybridization method. A PPK model was developed using the nonlinear mixed-effect model (NONMEM). The final model was validated using bootstrap, visual predictive check (VPC), and normalized prediction distribution error (NPDE). The Monte Carlo simulation was applied to evaluate and optimize the dosing regimens. A total of 232 voriconazole steady-state trough concentrations from 167 patients were included. A one-compartment model with first order and elimination adequately described the data. The typical clearance (CL) and the volume of distribution (V) of voriconazole were 3.83 L/h and 134 L, respectively. The bioavailability was 96.5%. Covariate analysis indicated that the CL of voriconazole was substantially influenced by age, albumin, gender, CRP, and CYP2C19 genetic variations. The V of voriconazole was significantly associated with body weight. An increase in the CRP concentration significantly decreased voriconazole CL in patients with the CYP2C19 normal metabolizer (NM) and intermediate metabolizer (IM), but it had no significant effect on patients with the CYP2C19 poor metabolizer (PM). The Monte Carlo simulation based on CRP levels indicated that patients with high CRP concentrations required a decreased dose to attain the therapeutic trough concentration and avoid adverse drug reactions in NM and IM patients. These results indicate that CRP affects the pharmacokinetics of voriconazole and is associated with the CYP2C19 phenotype. Clinicians dosing voriconazole should consider the patient’s CRP level, especially in CYP2C19 NMs and IMs.
Introduction
Voriconazole is a broad-spectrum triazole antifungal agent that is extensively used in the treatment or prevention of invasive fungal disease caused by pathogens of Candida and Aspergillus species, as well as some emerging pathogens such as Scedosporium apiospermum and Paecilomyces lilacinus (Dolton and McLachlan, 2014). Voriconazole is rapidly absorbed after oral administration and exhibits nonlinear pharmacokinetics with disproportionate increases in plasma concentrations with increasing doses (Denning et al., 2002). Large inter-individual and intra-individual variations in concentrations have been observed in patients with invasive fungal disease treated according to the recommended dosage regimen (Wang et al., 2014). Numerous parameters, including liver diseases, inflammation, CYP2C19 gene polymorphisms, and drug–drug interactions, have been found to partly contribute to the variability (Veringa et al., 2017; Yan et al., 2018; Tang et al., 2019; Zhang et al., 2021). Therapeutic drug monitoring for voriconazole is recommended for optimizing outcomes and reducing toxicity in clinical practice (Park et al., 2012). However, therapeutic drug monitoring can only adjust the dose after starting treatment and reaching a steady state. Identifying the factors that contribute to the high variability in voriconazole pharmacokinetics is important for adjusting the appropriate dosage with the least delay possible.
Voriconazole is metabolized by the cytochrome P450 enzymes, and CYP2C19 is the main metabolic enzyme. CYP2C19 exhibits high genetic polymorphism, with the CYP2C19*2, CYP2C19*3, and CYP2C19*17 mutations being major factors, leading to variability in the metabolism (Li-Wan-Po et al., 2010; Lee et al., 2012). Based on the Clinical Pharmacogenetics Implementation Consortium (CPIC) Guidelines for CYP2C19 and voriconazole therapy (Moriyama et al., 2017), the patients could be classified as ultrarapid metabolizers (UMs, CYP2C19*17/*17), rapid metabolizers (RMs, CYP2C19*1/*17), normal metabolizers (NMs, CYP2C19*1/*1), intermediate metabolizers (IMs, CYP2C19*1/*2, CYP2C19*1/*3, and CYP2C19*2/*17), and poor metabolizers (PMs, CYP2C19*2/*2, CYP2C19*2/*3, and CYP2C19*3/*3). Several studies have shown that the CYP2C19 gene polymorphism is associated with the pharmacokinetics, efficacy, and safety of voriconazole (Abidi et al., 2015; Favela-Mendoza et al., 2015; Ho et al., 2017; Li et al., 2017). It has been found that CYP2C19 genotype-guided voriconazole dosing may improve the efficacy and safety of voriconazole (Hicks et al., 2020; Patel et al., 2020).
Inflammation is part of a complex biological response to harmful stimuli, such as pathogens, damaged cells, or irritants (Shah and Smith, 2015). In vivo and in vitro studies have shown that inflammation may induce the downregulation of CYP450 expression, which decreases the metabolism of the drug, resulting in increased drug concentration (Shah and Smith, 2015; Encalada et al., 2016; Stanke-Labesque et al., 2020). In recent years, numerous studies have investigated the relationship between inflammation and voriconazole exposure (Li et al., 2022). The results revealed that the inflammatory status, represented by C-reactive protein (CRP) and procalcitonin (PCT), can significantly affect the concentration of voriconazole (van Wanrooy et al., 2014; Encalada et al., 2015; Veringa et al., 2017; Cheng et al., 2020; Luo et al., 2021). Invasive fungal infections are often associated with inflammation, which increases the risk of voriconazole overexposure. Therefore, frequent monitoring of voriconazole trough concentrations is recommended during and following inflammation. Moreover, a meta-analysis revealed that the effect of inflammation appeared to be less important for patients with loss-of-function polymorphisms in CYP2C19 (Bolcato et al., 2021). However, in contrast to the findings of the meta-analysis, Vreugdenhil et al. (2018) argued that the impact of inflammation in patients who use voriconazole could be even greater in poor metabolizers.
Population pharmacokinetics (PPK) provides a quantitative analysis of the variables affecting the pharmacokinetic parameters and is a common method for individualized drug administration. Numerous PPK studies on voriconazole have been conducted in recent years (Shi et al., 2019). The most commonly identified covariates were body weight, the CYP2C19 genotype, liver function, CRP concentration, and concomitant medications. However, the combined effects of CYP2C19 genetic polymorphisms and inflammation on voriconazole pharmacokinetics have not been considered in previous PPK studies.
Therefore, we aimed to further quantify the impact of inflammation on the pharmacokinetics of voriconazole in patients with different CYP2C19 genotypes. A PPK model was constructed to identify the factors that significantly impact the pharmacokinetic parameters and provide dose optimization for the clinical rational use of voriconazole.
Materials and methods
Patients
This was a retrospective study performed at the Third Affiliated Hospital of Soochow University from March 2020 to August 2023. Inpatients aged ≥18 years with either a proven, probable, or possible diagnosis of invasive fungal infections treated with voriconazole and who underwent therapeutic drug monitoring were included. The exclusion criteria were as follows: 1) the absence of clinical data and 2) the combination of other antifungal agents or medications that significantly affect the pharmacokinetics of voriconazole. Demographic characteristics and clinical data were accurately extracted from the electronic medical records of each patient, including voriconazole treatment and therapeutic drug monitoring data and other factors that potentially influence the voriconazole trough concentration, such as CRP, albumin (ALB), alanine aminotransferase (ALT), aspartate transaminase (AST), total bilirubin (TBIL), hemoglobin (HB), platelet count (PLT), serum creatinine (Scr), and uric acid (UA), which were measured within the same day. The study was conducted in accordance with the guidelines of the Declaration of Helsinki and was approved by the Ethics Committee of the Third Affiliated Hospital of Soochow University (No. 2023-038).
Blood sampling and analytical assays
For the patients receiving the loading dose, the initial plasma sample was collected on the third day of treatment. For the patients without a loading dose, the plasma samples were collected after 5 days of treatment. All samples were obtained within 30 min before the next administration. Blood samples were centrifuged within 6 h and stored at −80°C; plasma was used to detect the concentration of voriconazole, and white blood cells were used for CYP2C19 identification. Plasma voriconazole concentration was measured using high-performance liquid chromatography coupled with tandem mass spectrometry. The plasma samples (50 μL) were precipitated with methanol-containing isotope internal standards. The chromatographic separation was performed on a Kinetex C18 Column (3 mm × 100 mm, 2.6 μm) using a mobile phase of 0.1% formic acid–water (containing 5 mmol·L–1 of the ammonium acetate solution) and 0.1% formic acid–methanol at a flow rate of 0.6 mL·min–1. The injection volume was 5 μL, and the analysis time was 5 min. The detection of the analytes was performed via electrospray ionization in the positive mode by multiple reaction monitoring. The plasma concentration of voriconazole was linear over the range of 0.1–20 μg·mL–1. The intra- and inter-day precisions were 1.92% and 4.60%, respectively.
Genotyping and phenotype assignment
CYP2C19 genotyping was performed by the fluorescence in situ hybridization method by using a fluorescence detector (Xi’an Tianlong Science and Technology Co., Ltd.). According to nomenclature by CPIC, the CYP2C19 phenotypes were classified into five categories: UMs (CYP2C19*17/*17), RMs (CYP2C19*1/*17), NMs (CYP2C19*1/*1), IMs (CYP2C19*1/*2, CYP2C19*1/*3, and CYP2C19*2/*17), and PMs (CYP2C19*2/*2, CYP2C19*2/*3, and CYP2C19*3/*3).
Population pharmacokinetic modeling
PPK analysis was conducted using the nonlinear mixed-effects model (NONMEM) program version 7.3 (ICON Development Solutions). The first-order conditional estimation method with interaction (FOCE-I) was selected to estimate the model. Graphical and statistical analyses were performed using R (version 2.15.1). A one-compartment model with first-order absorption and linear/non-linear elimination was used to analyze the pharmacokinetics of voriconazole following oral or intravenous administration. Given the small proportion (less than 5%) of the concentrations below the quantification limit (BQL), data were analyzed using the M1 methodology (discarding the BQL observations) (Xu et al., 2011). As the retrospective dataset consisted solely of trough concentrations and there were no data from the absorption phase, ka was fixed at 1.1 h–1, as previously reported by Pascual et al. (2012). The inter-individual variability in PK parameters was described using an exponential model:
where
where
Covariate model exploration was conducted after the selection of the basic model. Age, gender, body weight, the CYP2C19 phenotype, co-administered medications, liver and renal function indicators, and complete blood count were used as possible covariates to determine whether they explained the PK variability of voriconazole among patients. Pairwise correlations between all variables were assessed prior to covariate analysis, and highly collinear variables (correlation coefficient >0.5) would not be simultaneously incorporated into the final model. Continuous covariates were centered at their medians and explored using linear, power function, and exponential models. The categorical covariates were evaluated using the power model. The final model was built using stepwise covariate modeling with forward inclusion followed by a backward elimination method. It was considered significant when the inclusion of a covariate decreased the objective function value (OFV) by at least 3.84 (p < 0.05) and increased the OFV by at least 6.63 (p < 0.01) in the backward elimination.
Model validation
Goodness-of-fit plots were constructed to evaluate the adequacy of fitting. A total of 1,000 bootstrap replicates were used to assess the robustness and stability of the final model. All the parameters (median values and 95% confidence intervals) obtained from the bootstrap were compared with the final model parameter estimates. Furthermore, the normalized prediction distribution error (NPDE) and visual predictive check (VPC) were performed to evaluate the predictive performance of the final model.
Dosage regimen simulations
The Monte Carlo simulation was performed for each dosage regimen based on the final model. The target trough concentration range was defined as 0.5–5.0 μg/mL, which was recommended in the Chinese Practice Guidelines for the individualized medication of voriconazole (Chen et al., 2018). The probability of target attainment for the target trough concentration range was calculated for each of the different dosing regimens.
Results
Patient characteristics
One hundred and sixty-seven patients with a total of 232 voriconazole trough concentrations were included in the study. The demographics and clinical information of the patients are summarized in Table 1. Fifty-seven patients received an intravenous loading dose of 600 mg or 400 mg twice daily, with a subsequent intravenous maintenance dose of 300 or 200 mg twice daily; four patients received an oral loading dose of 400 mg or 300 mg twice daily and an oral maintenance dose of 200 mg twice daily; and one hundred and six patients were administered intravenously or orally at a dose of 200 mg twice daily without a loading dose. Oral preparations were taken 1 h before or after the meal. The rate of intravenous infusion was less than 3 mg/kg per hour. During subsequent treatment, the physician would adjust the dose according to the voriconazole concentrations. There was an extensive variation in the voriconazole trough concentration, with a median concentration of 4.45 μg/mL and a range of 0.25–16.75 μg/ml; 59.1% (137/232) of the trough concentration values were within the range of 0.5–5.0 μg/mL. Among the patients, there were 66 NM patients, 72 IM patients, and 29 PM patients. No UM or RM patients were included in the study. A total of 117 (70.1%) patients received a co-administration of proton pump inhibitors, and 43 (25.7%) patients received a co-administration of glucocorticoids.
Population pharmacokinetic analysis
A one-compartment model with first-order absorption and elimination was found to be the best base model due to the lowest OFV and the best goodness-of-fit plots. The model was parameterized using ka, clearance (CL), volume of distribution (V), and bioavailability (F). The assignment of inter-individual variability on F did not improve the data description and resulted in a large shrinkage (90.63%). As a result, inter-individual variabilities were detected for CL and V. The population pharmacokinetic parameters related to the base model are listed in Table 2.
The stepwise covariate modeling procedure revealed that CL was significantly affected by CRP (ΔOFV = 26.47), the CYP2C19 phenotype (ΔOFV = 21.26), albumin (ΔOFV = 20.14), gender (ΔOFV = 18.64), and age (ΔOFV = 16.31). Body weight (ΔOFV = 11.51) was found to significantly affect V. An increase in CRP levels significantly decreased the voriconazole CL in CYP2C19 NM and IM patients. However, the inhibitory effect of CRP appeared to be less important in CYP2C19 PM patients. Specifically, when CRP was tested in all CYP2C19 phenotype patients, the fitted exponential factors were −0.215, −0.109, and −0.0172 in CYP2C19 NM, IM, and PM patients, respectively. On the basis of these results, as the CRP concentration increased from 1 to 100 mg·L−1, the CL decreased by 34%, 19%, and 0.03% in CYP2C19 NM, IM, and PM patients, respectively. Considering that CRP has little effect on PM patients, this factor was not included in the model. Moreover, the same fitting exponential factor was used to fit the model in both CYP2C19 NM and IM patients. There was no significant increase in the OFV (ΔOFV = 0.994). Hence, for CYP2C19 NM and IM patients, the same fitting exponential factor of CRP was used in the final model. The final model included the following equations:
Model validation
Goodness-of-fit plots from the final model illustrated that both the predicted concentration (PRED) and the individual predicted concentration (IPRED) corresponded well with the observed value (DV). Most of the conditional weighted residuals were located between ±2 (Figure 1).
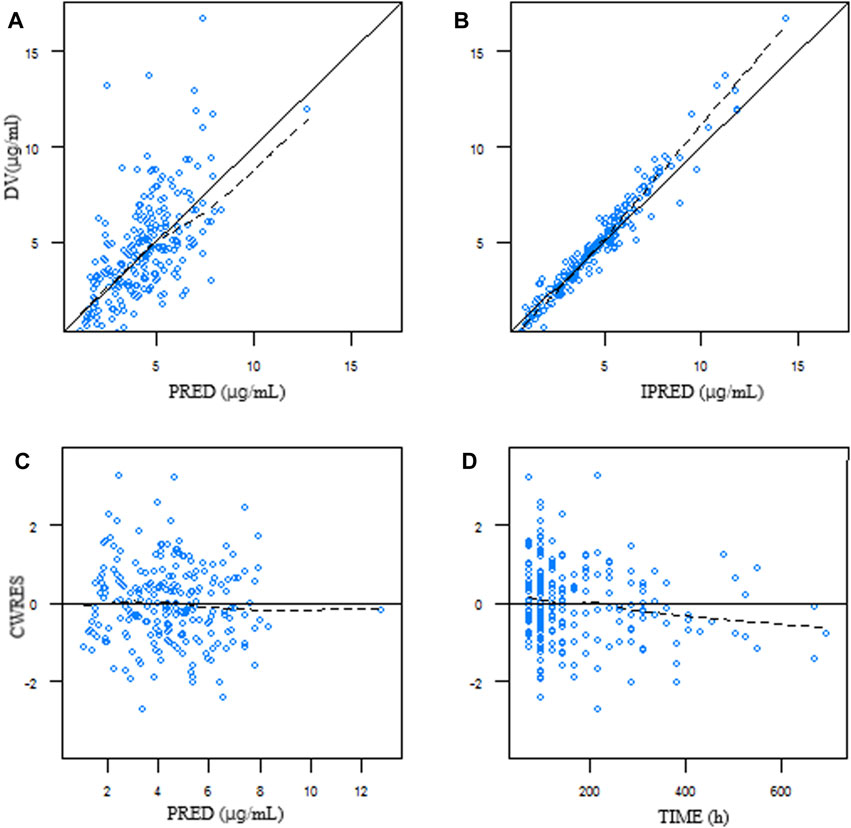
Figure 1. Goodness-of-fit plots of the final model. (A) DV plotted against PRED. (B) DV plotted against IPRED. (C) Correlation of CWRES with PRED. (D) Correlation of CWRES with the time after dosing. The line represents the line of identity. DV, observed concentration; PRED, predicted concentration; IPRED, individual predicted concentration; CWRES, conditional weighted residuals.
The bootstrap (n = 1,000) results are summarized in Table 2. The results showed that 898 out of 1,000 runs converged successfully. The estimated values of the final model were similar to the median values from the bootstrap results and fell within 95% confidence intervals, suggesting good accuracy and stability of the final model.
The NPDE result is presented in Figure 2. The mean and variance of the final model are 0.0257 and 1.06, respectively. The values of the t-test, Fisher test, and Shapiro–Wilk test of normality and global-adjusted p-value were 0.705, 0.490, 0.198, and 0.595, respectively. The results indicate that the final model has good predictive performance. The VPC plots displayed in Figure 3 showed that the model performed well in predicting the concentration of voriconazole for both oral and intravenous administration.
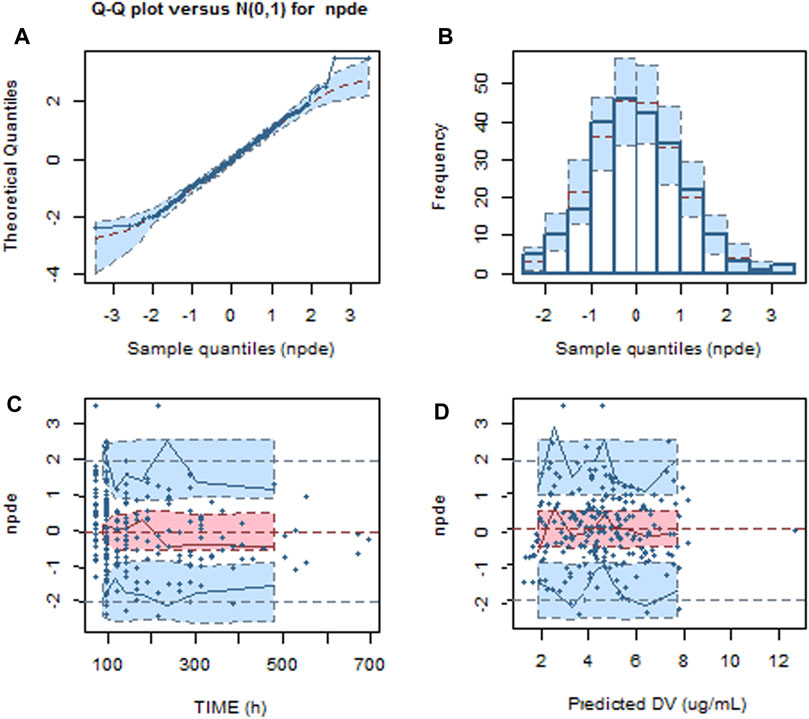
Figure 2. Normalized predictive distribution error (NPDE) of the final model. (A) Q–Q plot of the NPDE. (B) Histogram of the NPDE. (C) NPDE versus time after dosing. (D) NPDE versus population predicted concentration.
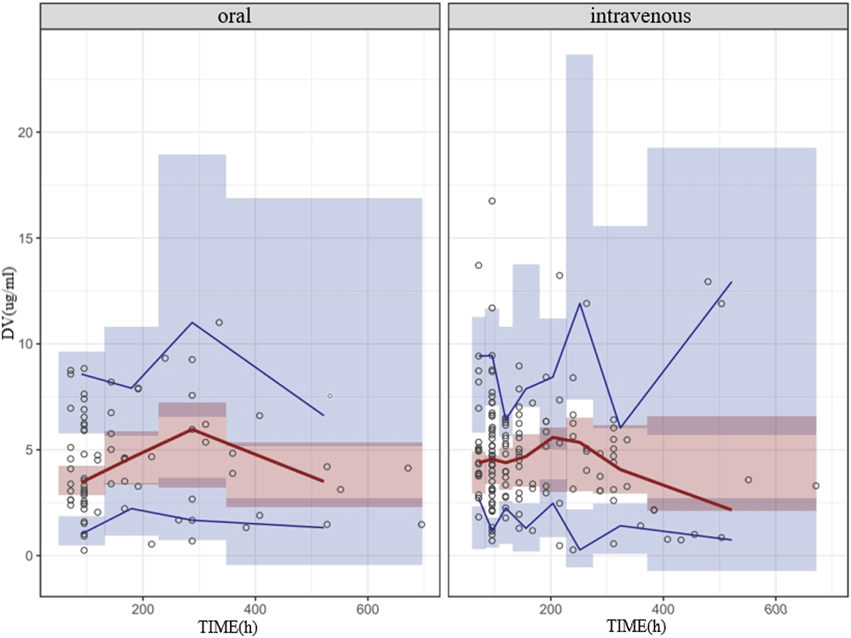
Figure 3. VPC of the final model for oral and intravenous administration of voriconazole. The hollow dots represent the observed data. Solid lines represent the 5th, 50th, and 95th percentiles of the observed data. Shaded areas represent the 95% confidence interval of the 5th, 50th, and 95th percentiles of the simulated data.
Simulation
Steady-state trough concentrations of voriconazole were simulated based on the final model using the dosage regimens of 50, 75, 100, 150, 200, and 250 mg by intravenous infusion twice daily in typical patients (a 71 year-old-man with a body weight of 65 kg and an albumin concentration of 34.8 g/L) with various CRP and CYP2C19 genotypes. The simulation trough concentrations are shown in Figures 4, 5. For example, in the group with CRP levels less than 10 mg/L, the 200 mg twice daily dosage regimen yielded therapeutic trough concentrations (0.5∼5.0 μg/mL) in 79.40% and 58.70% of CYP2C19 NM and IM patients, respectively, whereas the percentages that reached the toxic range were 20.10% and 41.10%, respectively. However, in the group with CRP levels more than 200 mg/L, the percentage of trough concentrations that achieved the therapeutic range (0.5∼5.0 μg/mL) was 26.65% and 14.06% in CYP2C19 NM and IM patients, respectively, whereas the percentages that reached the toxic range were 73.34% and 85.94%, respectively.
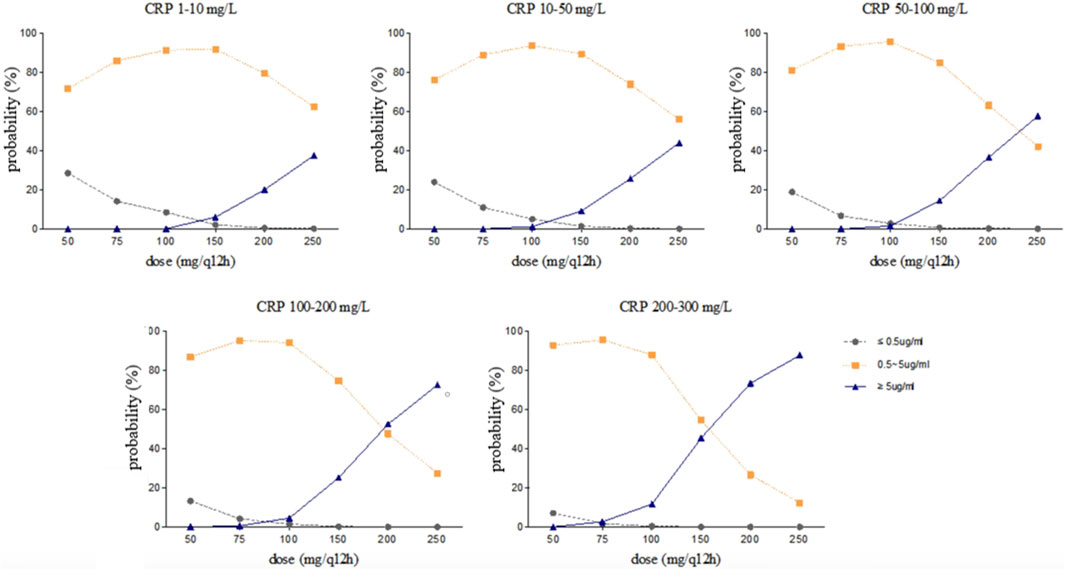
Figure 4. Simulated trough concentration stratified by CRP with the dosage regimens of 50, 75, 100, 150, 200, and 250 mg intravenous infusion twice daily in CYP2C19 NM patients.
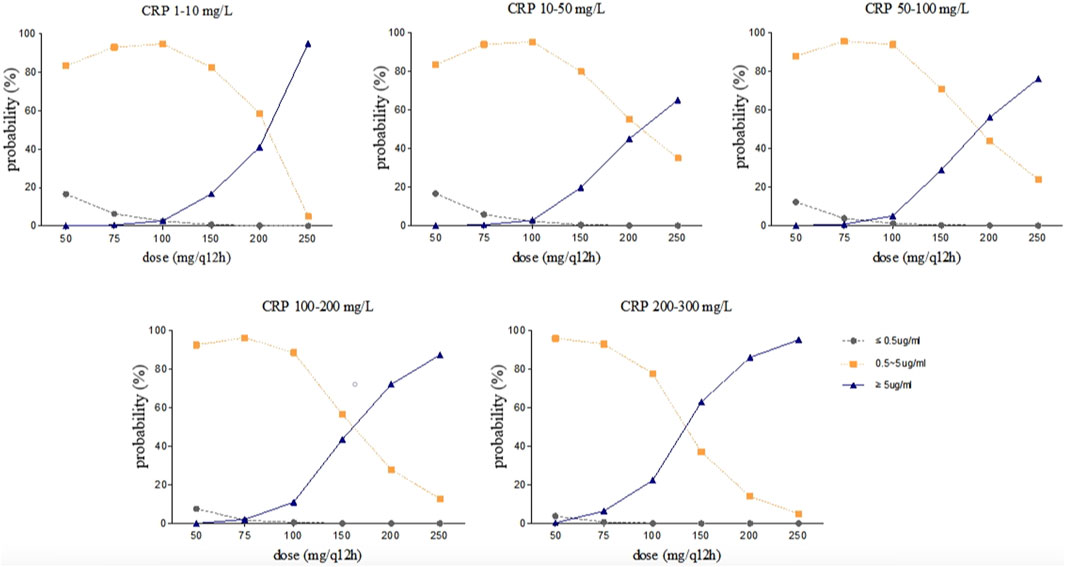
Figure 5. Simulated trough concentration stratified by CRP with the dosage regimens of 50, 75, 100, 150, 200, and 250 mg intravenous infusion twice daily in CYP2C19 IM patients.
Discussion
This retrospective study developed a population pharmacokinetic model of voriconazole in Chinese patients. To the authors’ knowledge, this is the first model-based study that focused on describing the influence of inflammation on the pharmacokinetics of voriconazole in relation to the CYP2C19 genotype. A one-compartment linear elimination model with first-order absorption best described the data. Potential covariates affecting the voriconazole plasma concentration were obtained based on the model. The typical population values for CL, V, and F were 3.83 L/h, 134 L, and 96.5%, respectively. This is aligned with previously reported models (Chen et al., 2015; Lin et al., 2018; Ren et al., 2019; Jiang et al., 2022). The shrinkage values of CL and V are 16.2% and 65.7%, respectively. The large shrinkage of V may be due to the absence of the data in the distribution phase.
The impact of inflammation on voriconazole exposure is substantial. Severe infections and inflammation reactions commonly appeared in invasive fungal infection patients (Boglione-Kerrien et al., 2023). CRP is an acute-phase protein produced by hepatocytes and triggered by pro-inflammatory cytokines such as IL-6, which can reflect the severity of inflammation. As the CRP concentration is widely used as a marker for inflammation and is measured frequently in daily practice, CRP was chosen as a covariate in our model. In our study, the CRP concentration had a significant effect on voriconazole pharmacokinetics. These findings are comparable to those of previously reported studies, which show that CYP450 isoenzymes are decreased at the transcriptional levels during inflammation, leading to reduced metabolism of voriconazole (van Wanrooy et al., 2014; Encalada et al., 2015; Vreugdenhil et al., 2018; Li et al., 2022). Increases of 10, 50, 100, and 200 mg/L in CRP concentrations were associated with a decrease in the CL of voriconazole by 3, 15, 34, and 61%, respectively. This covariate is of the same order of magnitude as that found in a Chinese study (Jiang et al., 2022).
Moreover, our data confirmed that the impact of CRP on voriconazole pharmacokinetics was associated with the CYP2C19 phenotypes. An increase in the CRP concentration significantly decreased the voriconazole CL in patients with CYP2C19 NM and IM, but there were no significant effects on patients with CYP2C19 PM. These results were consistent with those of a previous meta-analysis study, which demonstrated a smaller effect of inflammation for patients with decreased metabolic capacity than those with normal or elevated metabolic capacity (Bolcato et al., 2021). Although the results of the study by Chen et al. (2022), conducted in immunocompromised children <18 years of age, were inconsistent with our findings, their study showed that the impact of CRP on the trough concentration of voriconazole was modulated by the CYP2C19 phenotype. The extent of the increase in the voriconazole trough concentration was greater in the IM group than in the PM and NM groups. The difference may be explained by age. Moreover, Gautier-Veyret et al. (2017) showed a greater effect of inflammation on voriconazole metabolism in ultrafast metabolizers. The conflicting results could be explained by the genetic factors that integrated both CYP2C19 and CYP3A polymorphisms in their study. In addition, a higher frequency of PM of CYP2C19 was observed in the Asian population than those in the Caucasian population (Shimizu et al., 2003). Hence, ethnicity differences cannot be ignored.
Seventy percent of patients received a combination of proton pump inhibitors. Proton pump inhibitors are metabolized by CYP2C19, CYP3A4, and CYP2C9, and they competitively inhibit the metabolism of voriconazole. The degree of influence varies with the type of proton pump inhibitors (Yan et al., 2018). In this study, no significant effect of proton pump inhibitors on voriconazole pharmacokinetics was observed. This may be related to the fact that most of the patients included in this study used rabeprazole or pantoprazole, while a few patients received omeprazole. However, the concentration of voriconazole should be closely monitored when combined with proton pump inhibitors.
The covariate age was shown to have a significant effect on the CL of voriconazole. In our study, the median age of the patients was 71 years, and a majority of the patients were elderly (aged ≥ 65). The association between the CL of voriconazole and age is consistent with the fact that voriconazole is metabolized by drug-metabolizing enzymes and with the known negative relationship between age and enzyme functional activity (Shi et al., 2019). This finding is similar to that of the study conducted by Wang et al. (2014) Therefore, it is especially necessary to monitor the voriconazole concentration in elderly patients.
Gender, albumin, and body weight were commonly identified variables in previous analyses (Lin et al., 2018; Ren et al., 2019; Allegra et al., 2020). In the present study, the CL of voriconazole was greater in women than in men, which is consistent with the results of Allegra et al. (2020). This phenomenon could be explained by differences in sexual hormones and body fat percentage (Allegra et al., 2020). The covariate albumin was shown to have a significant effect on the CL of voriconazole. Several studies have suggested that albumin is associated with the concentration of voriconazole (Vanstraelen et al., 2014; Khan-Asa et al., 2020; Takahashi et al., 2022). Therefore, toxic reactions should be closely monitored in patients with low albumin levels. Moreover, V, instead of CL, was increased with body weight. Hence, the dosage of voriconazole adjusted based on body weight in adults may not be feasible; instead, using ideal body weight or adjusted body weight might be more appropriate, according to a previous study (Koselke et al., 2012).
One of the main limitations to this study is its retrospective design. Only trough concentrations were obtained, which made it difficult to estimate the pharmacokinetic parameters in the absorption and distribution phases. In addition, there might be some deviation in the results due to the limited sample size. Furthermore, genetic polymorphisms of CYP3A4, ABCB1, and FMO3 that might influence voriconazole pharmacokinetics were not analyzed. Finally, the determination of the major metabolite voriconazole N-oxide may be useful in evaluating the variability in voriconazole concentration. Future studies with larger sample sizes and more comprehensive patient data are recommended to further investigate the outcomes.
Conclusion
The population pharmacokinetic model developed in this study well described the plasma concentration of voriconazole. Dosing recommendations for voriconazole require considering the CRP level and CYP2C19 phenotypes. When administering voriconazole in CYP2C19 NM and IM patients with inflammation, close monitoring of the plasma concentration and proper adjustment of the dosage are recommended to maintain the voriconazole level within the therapeutic range and improve clinical outcomes.
Data availability statement
The original contributions presented in the study are included in the article/supplementary material; further inquiries can be directed to the corresponding author.
Ethics statement
The studies involving humans were approved by the Third Affiliated Hospital of Soochow University. The studies were conducted in accordance with the local legislation and institutional requirements. The participants provided their written informed consent to participate in this study.
Author contributions
JL: writing–original draft, writing–review and editing, conceptualization, methodology, and software. XY: data curation, formal analysis, and writing–original draft. LD: data curation, formal analysis, and writing–review and editing. YJ: data curation and writing–review and editing. SZ: data curation and writing–review and editing. NH: conceptualization, methodology, validation, writing–original draft, and writing–review and editing.
Funding
The authors are grateful for the support provided by Changzhou Science and Technology Program (CJ20229020 and CM20223005) and Young Talent Development Plan of Changzhou Health Commission (CZQM2020019).
Conflict of interest
The authors declare that the research was conducted in the absence of any commercial or financial relationships that could be construed as a potential conflict of interest.
Publisher’s note
All claims expressed in this article are solely those of the authors and do not necessarily represent those of their affiliated organizations, or those of the publisher, the editors, and the reviewers. Any product that may be evaluated in this article, or claim that may be made by its manufacturer, is not guaranteed or endorsed by the publisher.
References
Abidi, M. Z., D'Souza, A., Kuppalli, K., Ledeboer, N., and Hari, P. (2015). CYP2C19*17 genetic polymorphism--an uncommon cause of voriconazole treatment failure. Diagn. Micr. Infec. Dis. 83, 46–48. doi:10.1016/j.diagmicrobio.2015.05.002
Allegra, S., De Francia, S., De Nicolo, A., Cusato, J., Avataneo, V., Manca, A., et al. (2020). Effect of gender and age on voriconazole trough concentrations in Italian adult patients. Eur. J. Drug Metab. Ph. 45, 405–412. doi:10.1007/s13318-019-00603-6
Boglione-Kerrien, C., Zerrouki, S., Le Bot, A., Camus, C., Marchand, T., Bellissant, E., et al. (2023). Can we predict the influence of inflammation on voriconazole exposure? An overview. J. Antimicrob. Chemoth. 78, 2630–2636. doi:10.1093/jac/dkad293
Bolcato, L., Khouri, C., Veringa, A., Alffenaar, J., Yamada, T., Naito, T., et al. (2021). Combined impact of inflammation and pharmacogenomic variants on voriconazole trough concentrations: a meta-analysis of individual data. J. Clin. Med. 10, 2089. doi:10.3390/jcm10102089
Chen, J., Wu, Y., He, Y., Feng, X., Ren, Y., and Liu, S. (2022). Combined effect of CYP2C19 genetic polymorphisms and C-reactive protein on voriconazole exposure and dosing in immunocompromised children. Front. Pediatr. 10, 846411. doi:10.3389/fped.2022.846411
Chen, K., Zhang, X., Ke, X., Du, G., Yang, K., and Zhai, S. (2018). Individualized medication of voriconazole: a practice guideline of the division of therapeutic drug monitoring, Chinese pharmacological society. Ther. Drug Monit. 40, 663–674. doi:10.1097/FTD.0000000000000561
Chen, W., Xie, H., Liang, F., Meng, D., Rui, J., Yin, X., et al. (2015). Population pharmacokinetics in China: the dynamics of intravenous voriconazole in critically ill patients with pulmonary disease. Biol. Pharm. Bull. 38, 996–1004. doi:10.1248/bpb.b14-00768
Cheng, L., Xiang, R., Liu, F., Li, Y., Chen, H., Yao, P., et al. (2020). Therapeutic drug monitoring and safety of voriconazole in elderly patients. Int. Immunopharmacol. 78, 106078. doi:10.1016/j.intimp.2019.106078
Denning, D. W., Ribaud, P., Milpied, N., Caillot, D., Herbrecht, R., Thiel, E., et al. (2002). Efficacy and safety of voriconazole in the treatment of acute invasive aspergillosis. Clin. Infect. Dis. 34, 563–571. doi:10.1086/324620
Dolton, M. J., and McLachlan, A. J. (2014). Voriconazole pharmacokinetics and exposure-response relationships: assessing the links between exposure, efficacy and toxicity. Int. J. Antimicrob. Ag. 44, 183–193. doi:10.1016/j.ijantimicag.2014.05.019
Encalada, V. M., Span, L. F., van den Heuvel, E. R., Groothuis, G. M., and Alffenaar, J. W. (2015). Influence of inflammation on voriconazole metabolism. Antimicrob. Agents Ch. 59, 2942–2943. doi:10.1128/AAC.04789-14
Encalada, V. M., van Wanrooy, M. J., Span, L. F., Rodgers, M. G., van den Heuvel, E. R., Uges, D. R., et al. (2016). Longitudinal analysis of the effect of inflammation on voriconazole trough concentrations. Antimicrob. Agents Ch. 60, 2727–2731. doi:10.1128/AAC.02830-15
Favela-Mendoza, A. F., Martinez-Cortes, G., Hernandez-Zaragoza, M., Salazar-Flores, J., Munoz-Valle, J. F., Martinez-Sevilla, V. M., et al. (2015). Genetic variability of CYP2C19 in a Mexican population: contribution to the knowledge of the inheritance pattern of CYP2C19*17 to develop the ultrarapid metabolizer phenotype. J. Genet. 94, 3–7. doi:10.1007/s12041-015-0477-1
Gautier-Veyret, E., Bailly, S., Fonrose, X., Tonini, J., Chevalier, S., Thiebaut-Bertrand, A., et al. (2017). Pharmacogenetics may influence the impact of inflammation on voriconazole trough concentrations. Pharmacogenomics 18, 1119–1123. doi:10.2217/pgs-2017-0054
Hicks, J. K., Quilitz, R. E., Komrokji, R. S., Kubal, T. E., Lancet, J. E., Pasikhova, Y., et al. (2020). Prospective CYP2C19-guided voriconazole prophylaxis in patients with neutropenic acute myeloid leukemia reduces the incidence of subtherapeutic antifungal plasma concentrations. Clin. Pharmacol. Ther. 107, 563–570. doi:10.1002/cpt.1641
Ho, S., Rawlins, M., Ingram, P., and Boan, P. (2017). Voriconazole-induced hyponatraemia associated with homozygous CYP2C19*2 genotype. J. Chemother. 29, 325–326. doi:10.1080/1120009X.2017.1323395
Jiang, Z., Wei, Y., Huang, W., Li, B., Zhou, S., Liao, L., et al. (2022). Population pharmacokinetics of voriconazole and initial dosage optimization in patients with talaromycosis. Front. Pharmacol. 13, 982981. doi:10.3389/fphar.2022.982981
Khan-Asa, B., Punyawudho, B., Singkham, N., Chaivichacharn, P., Karoopongse, E., Montakantikul, P., et al. (2020). Impact of albumin and omeprazole on steady-state population pharmacokinetics of voriconazole and development of a voriconazole dosing optimization model in Thai patients with hematologic diseases. Antibiotics-Basel 9, 574. doi:10.3390/antibiotics9090574
Koselke, E., Kraft, S., Smith, J., and Nagel, J. (2012). Evaluation of the effect of obesity on voriconazole serum concentrations. J. Antimicrob. Chemoth. 67, 2957–2962. doi:10.1093/jac/dks312
Lee, S., Kim, B. H., Nam, W. S., Yoon, S. H., Cho, J. Y., Shin, S. G., et al. (2012). Effect of CYP2C19 polymorphism on the pharmacokinetics of voriconazole after single and multiple doses in healthy volunteers. J. Clin. Pharmacol. 52, 195–203. doi:10.1177/0091270010395510
Li, X., Lai, F., Jiang, Z., Li, M., Chen, Z., Cheng, J., et al. (2022). Effects of inflammation on voriconazole levels: a systematic review. Brit. J. Clin. Pharm. 88, 5166–5182. doi:10.1111/bcp.15495
Li, Z. W., Peng, F. H., Yan, M., Liang, W., Liu, X. L., Wu, Y. Q., et al. (2017). Impact of CYP2C19 genotype and liver function on voriconazole pharmacokinetics in renal transplant recipients. Ther. Drug Monit. 39, 422–428. doi:10.1097/FTD.0000000000000425
Lin, X. B., Li, Z. W., Yan, M., Zhang, B. K., Liang, W., Wang, F., et al. (2018). Population pharmacokinetics of voriconazole and CYP2C19 polymorphisms for optimizing dosing regimens in renal transplant recipients. Brit. J. Clin. Pharm. 84, 1587–1597. doi:10.1111/bcp.13595
Li-Wan-Po, A., Girard, T., Farndon, P., Cooley, C., and Lithgow, J. (2010). Pharmacogenetics of CYP2C19: functional and clinical implications of a new variant CYP2C19*17. . Brit. J. Clin. Pharm. 69, 222–230. doi:10.1111/j.1365-2125.2009.03578.x
Luo, X., Li, T., Hu, L., Liu, S., Zhao, H., Zhang, J., et al. (2021). Differential effects of C-reactive protein levels on voriconazole metabolism at three age groups in allogeneic hematopoietic cell transplant recipients. J. Chemother. 33, 95–105. doi:10.1080/1120009X.2020.1765604
Moriyama, B., Obeng, A. O., Barbarino, J., Penzak, S. R., Henning, S. A., Scott, S. A., et al. (2017). Clinical Pharmacogenetics implementation Consortium (CPIC) Guidelines for CYP2C19 and voriconazole therapy. Clin. Pharmacol. Ther. 102, 45–51. doi:10.1002/cpt.583
Park, W. B., Kim, N. H., Kim, K. H., Lee, S. H., Nam, W. S., Yoon, S. H., et al. (2012). The effect of therapeutic drug monitoring on safety and efficacy of voriconazole in invasive fungal infections: a randomized controlled trial. Clin. Infect. Dis. 55, 1080–1087. doi:10.1093/cid/cis599
Pascual, A., Csajka, C., Buclin, T., Bolay, S., Bille, J., Calandra, T., et al. (2012). Challenging recommended oral and intravenous voriconazole doses for improved efficacy and safety: population pharmacokinetics-based analysis of adult patients with invasive fungal infections. Clin. Infect. Dis. 55, 381–390. doi:10.1093/cid/cis437
Patel, J. N., Hamadeh, I. S., Robinson, M., Shahid, Z., Symanowski, J., Steuerwald, N., et al. (2020). Evaluation of CYP2C19 genotype-guided voriconazole prophylaxis after allogeneic hematopoietic cell transplant. Clin. Pharmacol. Ther. 107, 571–579. doi:10.1002/cpt.1642
Ren, Q. X., Li, X. G., Mu, J. S., Bi, J. F., Du, C. H., Wang, Y. H., et al. (2019). Population pharmacokinetics of voriconazole and optimization of dosage regimens based on Monte Carlo simulation in patients with liver cirrhosis. J. Pharm. Sci.-Us 108, 3923–3931. doi:10.1016/j.xphs.2019.09.019
Shah, R. R., and Smith, R. L. (2015). Inflammation-induced phenoconversion of polymorphic drug metabolizing enzymes: hypothesis with implications for personalized medicine. Drug Metab. Dispos. 43, 400–410. doi:10.1124/dmd.114.061093
Shi, C., Xiao, Y., Mao, Y., Wu, J., and Lin, N. (2019). Voriconazole: a review of population pharmacokinetic analyses. Clin. Pharmacokinet. 58, 687–703. doi:10.1007/s40262-019-00735-7
Shimizu, T., Ochiai, H., Asell, F., Shimizu, H., Saitoh, R., Hama, Y., et al. (2003). Bioinformatics research on inter-racial difference in drug metabolism I. Analysis on frequencies of mutant alleles and poor metabolizers on CYP2D6 and CYP2C19. Drug Metab. Pharmacok 18, 48–70. doi:10.2133/dmpk.18.48
Stanke-Labesque, F., Gautier-Veyret, E., Chhun, S., and Guilhaumou, R.French Society of Pharmacology and Therapeutics (2020). Inflammation is a major regulator of drug metabolizing enzymes and transporters: consequences for the personalization of drug treatment. Pharmacol. Ther. 215, 107627. doi:10.1016/j.pharmthera.2020.107627
Takahashi, T., Jaber, M. M., Smith, A. R., Jacobson, P. A., Fisher, J., and Kirstein, M. N. (2022). Predictive value of C-reactive protein and albumin for temporal within-individual pharmacokinetic variability of voriconazole in pediatric patients undergoing hematopoietic cell transplantation. J. Clin. Pharmacol. 62, 855–862. doi:10.1002/jcph.2024
Tang, D., Song, B. L., Yan, M., Zou, J. J., Zhang, M., Zhou, H. Y., et al. (2019). Identifying factors affecting the pharmacokinetics of voriconazole in patients with liver dysfunction: a population pharmacokinetic approach. Basic Clin. Pharmacol. 125, 34–43. doi:10.1111/bcpt.13208
Vanstraelen, K., Wauters, J., Vercammen, I., de Loor, H., Maertens, J., Lagrou, K., et al. (2014). Impact of hypoalbuminemia on voriconazole pharmacokinetics in critically ill adult patients. Antimicrob. Agents Ch. 58, 6782–6789. doi:10.1128/AAC.03641-14
van Wanrooy, M. J., Span, L. F., Rodgers, M. G., van den Heuvel, E. R., Uges, D. R., van der Werf, T. S., et al. (2014). Inflammation is associated with voriconazole trough concentrations. Antimicrob. Agents Ch. 58, 7098–7101. doi:10.1128/AAC.03820-14
Veringa, A., Ter Avest, M., Span, L. F., van den Heuvel, E. R., Touw, D. J., Zijlstra, J. G., et al. (2017). Voriconazole metabolism is influenced by severe inflammation: a prospective study. J. Antimicrob. Chemoth. 72, 261–267. doi:10.1093/jac/dkw349
Vreugdenhil, B., van der Velden, W., Feuth, T., Kox, M., Pickkers, P., van de Veerdonk, F. L., et al. (2018). Moderate correlation between systemic IL-6 responses and CRP with trough concentrations of voriconazole. Brit. J. Clin. Pharm. 84, 1980–1988. doi:10.1111/bcp.13627
Wang, T., Chen, S., Sun, J., Cai, J., Cheng, X., Dong, H., et al. (2014). Identification of factors influencing the pharmacokinetics of voriconazole and the optimization of dosage regimens based on Monte Carlo simulation in patients with invasive fungal infections. J. Antimicrob. Chemoth. 69, 463–470. doi:10.1093/jac/dkt369
Xu, X. S., Dunne, A., Kimko, H., Nandy, P., and Vermeulen, A. (2011). Impact of low percentage of data below the quantification limit on parameter estimates of pharmacokinetic models. J. Pharmacokinet. Phar. 38, 423–432. doi:10.1007/s10928-011-9201-9
Yan, M., Wu, Z. F., Tang, D., Wang, F., Xiao, Y. W., Xu, P., et al. (2018). The impact of proton pump inhibitors on the pharmacokinetics of voriconazole in vitro and in vivo. Biomed. Pharmacother. 108, 60–64. doi:10.1016/j.biopha.2018.08.121
Keywords: voriconazole, C-reactive protein, CYP2C19 genetic polymorphisms, population pharmacokinetics, nonlinear mixed-effects model
Citation: Ling J, Yang X, Dong L, Jiang Y, Zou S and Hu N (2024) Influence of C-reactive protein on the pharmacokinetics of voriconazole in relation to the CYP2C19 genotype: a population pharmacokinetics analysis. Front. Pharmacol. 15:1455721. doi: 10.3389/fphar.2024.1455721
Received: 27 June 2024; Accepted: 07 August 2024;
Published: 20 August 2024.
Edited by:
Momir Mikov, University of Novi Sad, SerbiaReviewed by:
Ziran Li, University of California, San Francisco, United StatesMuhammad Usman, University of Veterinary and Animal Sciences, Pakistan
Copyright © 2024 Ling, Yang, Dong, Jiang, Zou and Hu. This is an open-access article distributed under the terms of the Creative Commons Attribution License (CC BY). The use, distribution or reproduction in other forums is permitted, provided the original author(s) and the copyright owner(s) are credited and that the original publication in this journal is cited, in accordance with accepted academic practice. No use, distribution or reproduction is permitted which does not comply with these terms.
*Correspondence: Nan Hu, aG5fMzI0QDE2My5jb20=
†These authors have contributed equally to this work