- 1Shenzhen Baoan District Hospital of Traditional Chinese Medicine, Shenzhen, China
- 2Henan Hospital of Integrated Chinese and Western Medicine, Zhengzhou, China
The P2X7 receptor (P2X7R), an ATP-gated ion channel, has emerged as a crucial player in neuroinflammation and a promising therapeutic target for neurodegenerative disorders. This review explores the current understanding of P2X7R’s structure, activation, and physiological roles, focusing on its expression and function in microglial cells. The article examines the receptor’s involvement in calcium signaling, microglial activation, and polarization, as well as its role in the pathogenesis of Alzheimer’s disease, Parkinson’s disease, multiple sclerosis, and amyotrophic lateral sclerosis. The review highlights the complex nature of P2X7R signaling, discussing its potential neuroprotective and neurotoxic effects depending on the disease stage and context. It also addresses the development of P2X7R antagonists and their progress in clinical trials, identifying key research gaps and future perspectives for P2X7R-targeted therapy development. By providing a comprehensive overview of the current state of knowledge and future directions, this review serves as a valuable resource for researchers and clinicians interested in exploring the therapeutic potential of targeting P2X7R for the treatment of neurodegenerative disorders.
1 Introduction
The P2X7 receptor (P2X7R), an ATP-gated ion channel belonging to the P2X family, has recently emerged as a potential key player in the pathogenesis of various neurodegenerative disorders (Zheng et al., 2024). This receptor is predominantly expressed in immune cells, such as microglia, and regulates a wide range of cellular processes, including cell activation, calcium signaling, and cytokine release (Tewari et al., 2024). As a result, the P2X7R has been identified as a crucial mediator of neuroinflammation and immune responses within the central nervous system (CNS).
Growing evidence points to the P2X7R’s involvement in the onset and progression of neurodegenerative diseases, such as Alzheimer’s disease (AD) (Beltran-Lobo et al., 2023; Huang et al., 2024), Parkinson’s disease (PD) (Ren et al., 2021) and multiple sclerosis (MS) (Nobili et al., 2022), underscoring its potential as a therapeutic target. However, to fully harness the therapeutic potential of the P2X7R, a thorough understanding of its function, signaling pathways, and role in neurodegenerative disorders is crucial.
This review aims to provide a comprehensive overview of the current knowledge surrounding the P2X7R, with a focus on its expression in microglia, its role in calcium signaling, and its involvement in neurodegenerative disorders. We will explore recent advances in the development of P2X7R antagonists and their potential therapeutic applications, as well as discuss the challenges and future directions in P2X7R-targeted therapy research. The subsequent sections will delve into the P2X7R’s influence on microglial cell function through calcium signaling modulation, its role in neuroprotection and neurodegenerative disorders, and the current state of P2X7R antagonist research. Finally, we will synthesize the existing knowledge, identify research gaps and challenges, and provide future perspectives for the development of P2X7R-targeted therapies.
2 P2X7R
2.1 Unique characteristics of P2X7Rs
P2X7 receptors (P2X7Rs) exhibit unique properties that set them apart from other P2X receptors, making them an attractive target for research in various cellular contexts (Burnstock, 2017a). Activation of P2X7Rs necessitates higher ATP concentrations (−100 μM) compared to other P2X receptors (Surprenant et al., 1996). Upon activation, the P2X7R forms a non-selective cation channel, allowing the permeation of both monovalent (e.g., Na+ and K+) and divalent cations (e.g., Ca2+ and Mg2+) (North and Surprenant, 2000). Sustained activation can lead to the development of large, non-selective pores permeable to molecules up to 900 Da, a characteristic not observed in other P2X receptors (Pelegrin and Surprenant, 2006). The mechanism of P2X7R-mediated pore formation has been a subject of intense research and debate. Initially, it was thought that prolonged P2X7R activation led to the dilation of the receptor’s intrinsic channel, allowing the passage of larger molecules (Khakh and North, 2012). However, recent evidence suggests that the large pore formation may involve the recruitment and activation of additional proteins, such as pannexin-1 (Panx1) (Gulbransen et al., 2012). The P2X7R-Panx1 complex has been implicated in the release of ATP, glutamate, and other metabolites, as well as in the uptake of large molecules, such as fluorescent dyes (Gulbransen et al., 2012). However, the exact role of Panx1 in P2X7R-mediated pore formation remains controversial, as some studies have reported Panx1-independent mechanisms (Alberto et al., 2013). Moreover, recent structural studies have provided new insights into the potential mechanisms of P2X7R pore formation. The cryo-EM structure of the full-length rat P2X7R revealed a unique C-terminal domain that undergoes significant rearrangements upon ATP binding, potentially contributing to pore formation (McCarthy et al., 2019). Additionally, the identification of a cytoplasmic ballast domain and a C-cys anchor in the P2X7R structure suggests a role for these regions in the regulation of pore formation and channel gating (McCarthy et al., 2019). This distinctive feature is implicated in various physiological and pathological processes, such as the secretion of pro-inflammatory cytokines like interleukin-1β (IL-1β) and the induction of cell death (Di Virgilio et al., 2017).
2.2 Structure of P2X7Rs
P2X7Rs are trimeric ion channels expressed in various cell types throughout the body, particularly in macrophages and microglia (Collo et al., 1997). Each subunit is composed of 595 amino acids in mammals, forming a trimeric structure stabilized by hydrophobic and electrostatic interactions within its transmembrane domains and extracellular loop (Jiang et al., 2013; Karasawa and Kawate, 2016). Each subunit features two main structural domains: a cysteine-rich extracellular domain and the C- and N-termini, which enhance the channel’s functionality (Oken et al., 2022). The receptor includes extracellular, transmembrane, and cytoplasmic components (Tassetto et al., 2021). The ectodomain of P2X7R contains three ATP-binding sites and exhibits a chalice-like shape formed by interactions at various points (Jiang et al., 2013). Ligand-binding sites are created through interactions among the three extracellular domains of the subunits, which collectively comprise 282 amino acids (Di Virgilio et al., 2017; Jiang et al., 2021). Recent advancements in cryo-electron microscopy have shed light on the intricate structure of the full-length mammalian P2X7 receptor, revealing a more constricted channel in its apo state compared to the hP2X3 receptor, which effectively shields the orthosteric binding pocket from solvents and ligands (McCarthy et al., 2019). The transmembrane region of P2X7R consists of two domains: TM1 (amino acids 26–46) and TM2 (amino acids 330–349). TM1 harbors the ATP-binding pocket (Lara et al., 2020), while TM2 contains crucial pore-lining residues, such as S342 and Y343, which play a role in channel gating (Hansen et al., 1997; Pippel et al., 2017).
The cytoplasmic region of P2X7R is characterized by distinctive elements, including the C-cys anchor and the cytoplasmic ballast. The C-cys anchor serves as a link between the transmembrane domain and the cytoplasmic cap, preventing receptor desensitization through multiple palmitoylated residues (McCarthy et al., 2019). The cytoplasmic ballast, on the other hand, features a novel fold that incorporates a dinuclear zinc ion complex and a high-affinity guanosine nucleotide-binding site, which likely facilitates the connection between the receptor and intracellular signaling proteins (Adinolfi et al., 2010). The N- and C-termini play crucial roles in receptor expression and function (Costa-Junior et al., 2011). The N-terminus, which begins approximately 26 amino acids earlier than other P2X receptors, contains a protein kinase C (PKC) phosphorylation consensus site and plays a role in regulating calcium flow, extracellular signal-regulated kinase activation, and P2X7R gating (Allsopp and Evans, 2011). The C-terminal tail, the most extensive among all P2XRs (amino acids 356–595), contributes to the stabilization of macropore opening and features a cysteine-rich area with palmitoylated residues that form a binding site for GDP/GTP and two zinc ions (Durner et al., 2023). The initial portion of the C-terminal tail contains a cysteine-rich region with palmitoylation on residues C362, C363, C374, C377, and S360, which function as hinges. Furthermore, the tail possesses regions that share homology with tumor necrosis factor receptor 1 and the serum LPS-binding protein, highlighting its multifaceted role in receptor function (Denlinger et al., 2001) (Figure 1).
2.3 Activation of P2X7R
The activation of P2X7R is a complex process that involves the interplay of both extracellular and intracellular factors (Figure 2). Extracellular ATP serves as the primary ligand for P2X7R activation, with half maximal effective concentrations that vary across different species (Li et al., 2005; Bradley et al., 2011; He et al., 2017). Atomic modeling has revealed specific amino acid residues from two adjacent subunits that are crucial for ATP binding (Jiang et al., 2011; Hattori and Gouaux, 2012; Mansoor et al., 2016), while functional studies have highlighted the importance of surrounding residues in receptor activation (Stokes et al., 2006; Young et al., 2007; Adriouch et al., 2008; Liu et al., 2008; Martínez-Cuesta et al., 2020; De Salis et al., 2022). In addition to ATP, synthetic analogues such as BzATP and adenosine 5′-(γ-thio)-triphosphate can also activate P2X7Rs, albeit with varying potencies across species (Donnelly-Roberts et al., 2009). Divalent cations, including Ca2+ and Mg2+, act as allosteric inhibitors, modulating the activation of P2X7R (Roger et al., 2008). Intracellular regulation also plays a significant role in the activation of P2X7R. Protein-protein interactions, such as those with the 14-3-3 protein family, influence receptor trafficking and localization (Roger et al., 2008; Kopp et al., 2019). Post-translational modifications, including glycosylation, palmitoylation, and ubiquitination, alter the biophysical properties and stability of P2X7R, ultimately affecting its function and signaling (Oken et al., 2022; Li et al., 2023). Several compounds, such as.
LL-37, tenidap, polymyxin B, clemastine, ivermectin, and ginsenosides, have been identified as positive modulators of P2X7R activation (Elssner et al., 2004; Ferrari et al., 2006; Tomasinsig et al., 2008; Sanz et al., 2009; Nörenberg et al., 2011; 2012; Helliwell et al., 2015; Sivcev et al., 2019; Cui et al., 2023). LPS can modulate P2X7R activation through several distinct pathways. In murine cells, LPS stimulation of caspase-11 results in the cleavage of the ATP release channel pannexin-1, leading to an increase in extracellular ATP levels and subsequent P2X7R activation (Yang et al., 2015). Furthermore, intracellular LPS may reduce the threshold for P2X7R activation, possibly by interacting with an LPS-binding domain and inducing conformational changes that enhance the receptor’s sensitivity to ATP (Denlinger et al., 2001; Yang et al., 2015). However, further research is needed to provide direct evidence supporting this proposed mechanism. Moreover, Panx1 facilitates the release of ATP, which in turn activates P2X7R, resulting in caspase-3/7-mediated apoptosis and elevated IL-6 expression (Loureiro et al., 2022; Rusiecka et al., 2022). In summary, the activation of P2X7Rs is a multifaceted process influenced by various factors, including ligand binding, allosteric modulation, protein-protein interactions, post-translational modifications, and intracellular regulation.
2.4 Physiological roles of P2X7Rs
P2X7R participates in a wide array of physiological and pathophysiological processes, encompassing immune responses, inflammation, pain, and cell death (Ahn et al., 2023; Fan et al., 2024; Zou et al., 2024). In microglial cells, P2X7R activation leads to the release of pro-inflammatory cytokines, such as IL-1β and IL-18, through the activation of the NLRP3 inflammasome and caspase-1 (Xie et al., 2024), thereby contributing to the initiation and propagation of inflammatory responses in neurodegenerative diseases. Additionally, P2X7R plays a crucial role in regulating cell proliferation, differentiation, and migration (Di Virgilio et al., 2017). In certain cell types, such as osteoblasts and microglia, P2X7R activation can stimulate growth through increased intracellular calcium levels and the activation of downstream signaling pathways like MAPK and PI3K/Akt (Tóthová et al., 2021) (Figure 3). In contrast, P2X7R activation can inhibit proliferation in other cell types, such as lymphocytes, by triggering cell cycle arrest and apoptosis (Roger et al., 2008). P2X7R has been implicated in the differentiation of various cell types, including the promotion of osteoclastogenesis in bone remodeling (Gartland et al., 2012; Xu et al., 2023), modulation of neuronal differentiation in the CNS (Delarasse et al., 2009), and the glia proliferation in spinal cord (Stefanova et al., 2024). Moreover, P2X7R activation plays a crucial role in regulating the chemotactic response of immune cells, such as neutrophils, monocytes, and microglia, which is essential for immune surveillance and the resolution of inflammation (Chen et al., 2006; De Marchi et al., 2016). In conclusion, the P2X7R is a unique member of the P2X family with distinct properties and functions. Its complex structure and diverse physiological roles make it an attractive target for research in various cellular contexts.
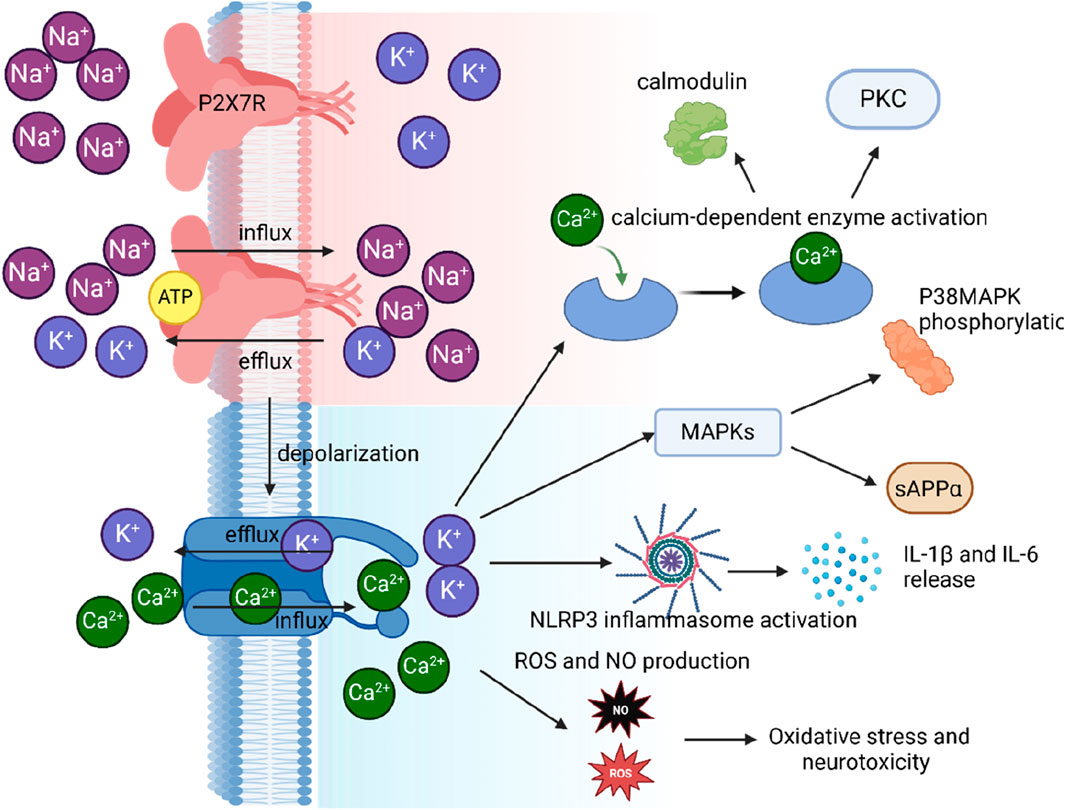
Figure 3. Illustration of P2X7R activation and its impact on ion flux, depolarization, and downstream signaling in microglial cells.
3 The role of P2X7 receptor in calcium signaling and homeostasis in microglial cells
P2X7R plays a crucial role in modulating intracellular calcium levels, which in turn regulates a wide array of microglial physiological functions. This section will delve into the intricate interactions between the P2X7R and calcium channels, and how these interactions shape microglial behavior.
Upon activation by extracellular ATP, the P2X7R forms a cation-selective channel that permits the influx of Na+ and Ca2+ ions. While the rapid influx of Ca2+ through the P2X7R was once considered a critical event, with the receptor undergoing pore dilation under certain conditions (Di Virgilio et al., 2017), recent findings have challenged this traditional understanding. Peverini et al. questioned the concept of pore dilation, suggesting it might be an artifact of earlier research methodologies (Peverini et al., 2018). They emphasized the significance of lipid composition in the cell membrane, with lipids such as sphingomyelin and phosphatidylglycerol facilitating the passage of large cations through the P2X7R, while cholesterol hindered this process. Di Virgilio et al. unveiled the structural dynamics of the P2X7R, demonstrating that the N-terminus and transmembrane domains are crucial for the gating and dilation of the receptor, contrasting with the previous notion that the binding of three ATP molecules to the receptor was necessary for its activation (Karasawa and Kawate, 2016; Di Virgilio et al., 2018). Intracellular ions also contribute to the modulation of P2X7R activity, with intracellular magnesium ions inhibiting pore dilation (Harkat et al., 2017) and extracellular divalent cations influencing the conformational plasticity in the selectivity filter of the P2X7 pore (Khakh and North, 2006; Wang et al., 2020a), rather than altering the sensitivity of the P2X7R to ATP as previously thought.
Transient exposure to high concentrations of extracellular ATP (≥1 mM) or the more potent ATP analog, BzATP, elicits inward currents in human microglia with characteristics consistent with a P2X7R-mediated response. These currents are non-selective for cations, partially carried by Ca2+, enhanced during prolonged exposure to agonists, and inhibited by P2X7R antagonists (Janks et al., 2018). At the resting membrane potential of around −40 mV in rodent microglia (Nörenberg et al., 1994; Madry et al., 2018), eATP activation of P2X7Rs causes Na+ and Ca2+ to rush into the cell while K+ exits, resulting in membrane depolarization (Figure 3). This inward Ca2+ current increases the concentration of free intracellular Ca2+ ([Ca2+]i), triggering various cellular processes such as cell cycle progression (Bianco et al., 2006), TNF-α release (Hide et al., 2000), activation of the transcription factor NFAT(Ferrari et al., 1999), and disruption of the cytoskeleton (Mackenzie et al., 2005). The outward K+ current decreases intracellular K+ ([K+]i), activating the NLRP3 inflammasome and promoting the maturation and release of proinflammatory cytokines IL-1β and IL-18 (Di Virgilio, 2007) (Figure 3). While the inflammasome activates when [K+]i drops below 90 mM (Pétrilli et al., 2007), recent evidence suggests that the P2X7R is not the primary K+ efflux pathway (Swanson et al., 2019). Instead, the two-pore K+ channels, THIK-1 and TWIK-2, are responsible in microglia (Drinkall et al., 2022; Ossola et al., 2023)and macrophages (Di et al., 2018), respectively. Despite this, eATP promotes the recruitment and colocalization of microglial P2X7Rs and NLRP3 to discrete sites in the subplasmalemmal cytoplasm, suggesting that the inflammasome is positioned close enough to directly sense the P2X7R-mediated local drop in [K+]i (Franceschini et al., 2015).
Another mechanism by which the P2X7R can regulate intracellular Ca2+ levels in microglial cells is through the modulation of store-operated calcium entry (SOCE). SOCE is a major mechanism of calcium influx in non-excitable cells, and is activated upon the depletion of intracellular Ca2+ stores,
The diagram shows ATP binding to the P2X7R, which results in the influx of Na+ and Ca2+ ions and the efflux of K+ ions. This ion flux causes depolarization of the cell membrane, which subsequently leads to an increase in intracellular calcium levels. The elevated intracellular calcium triggers three distinct signaling pathways: 1) activation of calcium-dependent enzymes, including calmodulin and PKC; 2) activation of the NLRP3 inflammasome, leading to the release of IL-1β and IL-18; and 3) production of ROS and NO, contributing to oxidative stress and neurotoxicity. primarily from the endoplasmic reticulum (ER) (Kodakandla et al., 2023). SOCE is mediated by the interaction between the ER calcium sensor STIM1 and the plasma membrane calcium channel Orai1, which allows the influx of Ca2+ ions into the cell (Kodakandla et al., 2023). This P2X7R-induced SOCE activation can further amplify the calcium signaling in microglial cells, modulating various physiological functions such as cytokine production, phagocytosis, and migration (Kettenmann et al., 2013). Moreover, P2X7R-mediated calcium signaling can activate the mitochondrial apoptotic pathway, involving the release of cytochrome c and the activation of caspases, ultimately leading to cell death (Pelegrin and Surprenant, 2007).
The P2X7R can also modulate intracellular calcium homeostasis in microglial cells through its interaction with various calcium transporters and pumps. For example, the P2X7R activation can affect the activity of the plasma membrane Ca2+-ATPase (PMCA) and the sarco/endoplasmic reticulum Ca2+-ATPase (SERCA), which are responsible for maintaining calcium homeostasis by pumping Ca2+ ions out of the cytoplasm and into the ER, respectively (Recourt et al., 2020). It has been suggested that the P2X7R-mediated Ca2+ influx can lead to the activation of PMCA and SERCA, thereby restoring intracellular Ca2+ levels to their resting state and protecting the cell from Ca2+ overload and subsequent cell death (Di Virgilio et al., 2017). However, sustained activation of the P2X7R or exposure to high concentrations of ATP can lead to calcium overload and the induction of apoptosis (Pelegrin and Surprenant, 2006).
In conclusion, the P2X7R plays a central role in the regulation of calcium signaling and homeostasis in microglial cells, with profound implications for their physiological functions. The modulation of intracellular calcium levels by the P2X7R can influence various aspects of microglial cell function, including cytokine production, migration, phagocytosis, and cell survival. Given the importance of calcium signaling in the regulation of microglial functions, targeting the P2X7R and its interactions with calcium channels may provide novel therapeutic strategies for the treatment of neuroinflammatory and neurodegenerative disorders.
4 P2X7R in microglial activation and polarization
Microglia exhibit diverse activation states that extend beyond the conventionally defined M1 and M2 phenotypes (Gao et al., 2023). M1 microglia produce pro-inflammatory cytokines (TNF-α, IL-1β, IL-6) and express inducible nitric oxide synthase (iNOS), while M2 microglia promote tissue repair and anti-inflammatory responses by secreting IL-10, IL-4, and TGF-β. Transcriptomic analyses have revealed intermediate phenotypes, highlighting the complexity of microglial activation (Masuda et al., 2020). These cells dynamically interact with pro-inflammatory and phagocytic functions (Long et al., 2024). P2X7R critically regulates microglial activation, and its overexpression can increase membrane permeability, contribute to microgliosis, trigger inflammatory factor release, and induce neuronal damage (Zhang et al., 2020). Without an external ATP agonist, P2X7R enhances phagocytosis via interactions involving extracellular residues 306–320 and intracellular regions associated with non-muscle myosin heavy chain IIA (NMMHC IIA) and the cytoskeleton (Gu et al., 2009; 2010; 2011). The receptor’s closed state influences this phagocytic capacity, which can be disrupted by cytochalasin D or a monoclonal antibody targeting the extracellular P2X7R domain (Gu et al., 2011). P2X7R synthesis and surface expression are essential for human monocytic cell phagocytosis, and siRNA-mediated disruption impairs this function (Gu et al., 2011).
Transient extracellular ATP level increases cause P2X7R to transition from a scavenger receptor, promoting autophagy and driving microglia towards a mixed M1/M2 activation state (Gu and Wiley, 2018). The M1/M2 balance is crucial for microglia’s transformation into disease-associated microglia (DAM) in neurodegenerative diseases (Keren-Shaul et al., 2017). Activated P2X7R releases neurotransmitters into the CNS extracellular space (Illes et al., 2020) and could serve as a molecular target for PET imaging of M1 microglia (Lee et al., 2002; Parvathenani et al., 2003; Sanz et al., 2012). Upon activation, P2X7R can release neurotransmitters into the extracellular space in the CNS(Sperlágh et al., 2002). Studies also showed that P2X7R could serve as a potential molecular target for positron emission tomography (PET) imaging of M1 microglia (Territo et al., 2017; Tronel et al., 2017). P2X7R inhibition promotes the M2 phenotype, reducing neuroinflammation and improving functional recovery in a mouse spinal cord injury model (Chen et al., 2013). As such, P2X7R antagonists show potential in mitigating various neurodegenerative conditions (Beamer et al., 2017; Fabbrizio et al., 2017; Wang et al., 2017; Domercq and Matute, 2019; Illes et al., 2019). P2X7R antagonism effectively reduces neuroinflammation, plaque deposition, and cognitive deficits in AD animal models (Martin et al., 2019; Verkhratsky et al., 2019). Furthermore, P2X7R blockade limits microglial migration and activation, thereby reducing neurodegeneration in a PD mouse model (Alves et al., 2014).
Pathological persistent ATP stimulation activates P2X7R, triggering cellular responses that lead to NLRP3 inflammasome-mediated caspase-1 activation and pro-inflammatory cytokine secretion (Muñoz-Planillo et al., 2013; Illes et al., 2019) primarily stimulated by decreased intracellular potassium levels, highlighting P2X7R’s intricate ion regulatory role (Di Virgilio et al., 2017; 2018; Madry et al., 2018). P2X7R activation can also release other pro-inflammatory cytokines (IL-6, TNF-α), further exacerbating inflammation (Shieh et al., 2014).
In conclusion, P2X7R plays a pivotal role in coordinating pro-inflammatory and phagocytic responses, influencing microglial activation state diversity. Moreover, P2X7R is implicated in DAM state progression, particularly in neurodegenerative conditions like AD, by modulating the M1/M2 microglia activation balance (Figure 4).
This diagram illustrates the key role of P2X7R in shaping microglial polarization states and its influence on immune responses. Under normal conditions without redundant ATP, P2X7R is unactivated and induces phagocytic activity, resulting in minimal inflammation and predominance of the M2 state. However, transient ATP stimulation alters this balance: P2X7R not only drives phagocytosis but also gets activated by ATP, promoting inflammatory responses, thereby leading to a balance between M1 and M2 states (DAM). In a pathological condition, chronic ATP binding and activation of P2X7R shifts the cell primarily towards a pro-inflammatory and phagocytosis-suppressed phenotype, characteristic of the M1 state.
Considering the importance of P2X7R in microglial function and neuroinflammation, a deeper understanding of the molecular mechanisms governing its regulation and interaction with microglial activation states could pave the way for the development of targeted therapies. For instance, identifying the specific signaling pathways that mediate P2X7R-induced M1/M2 microglia polarization could enable the design of pharmacological agents that modulate these processes to prevent or slow down the progression of neurodegenerative diseases. Overall, the P2X7R emerges as a key player in microglial activation and function, with significant implications for the onset and progression of neurodegenerative diseases. As such, P2X7R presents an attractive target for the development of novel therapeutic strategies aimed at modulating neuroinflammation and ameliorating the symptoms of these debilitating conditions.
5 P2X7R related signaling pathway
5.1 MAPK
MAPKs, a family of serine/threonine kinases (p38MAPK, ERK, JNK), are pivotal regulators of gene expression, cellular differentiation, stress response, and cell survival or death in response to extracellular stimuli (Kim and Choi, 2010; Plotnikov et al., 2011). P2X7R activation is linked to MAPK phosphorylation, leading to various cellular outcomes. LPS-induced inflammation in microglia is mediated by p38, JNK, and ERK pathway activation, causing pro-inflammatory cytokine secretion (Lowe et al., 2012). BBG, a P2X7R inhibitor, attenuates this neuroinflammatory response by preventing MAPK phosphorylation and reducing cytokine production, an effect potentiated by MAPK inhibitors (Wang et al., 2020b). JNK and ERK contribute to TNF mRNA production, while p38 regulates its transport from the nucleus to the cytoplasm and stimulates its release from microglial cells in a P2X7R-dependent manner (Hide, 2003; Suzuki et al., 2004). The activation of JNK and p38 downstream of P2X7R involves SRC family kinases and appears independent of Ca2+ influx (Hide, 2003), although this relationship remains controversial (Jandy et al., 2022). Increased P2X7R expression in microglia and enhanced p38MAPK phosphorylation are associated with dopaminergic neuron loss in the nigrostriatal pathway in LPS-induced PD models (Herrera et al., 2000; Wang et al., 2017; Brown, 2019). BBG-mediated P2X7R inhibition reduces microglial activation and prevents p38MAPK-driven neurodegeneration (Wang et al., 2017). However, the effectiveness of P2X7R inhibition varies in different chemical PD models (Hracskó et al., 2011; Carmo et al., 2014), possibly due to differences in nigral damage extent or antagonist treatment duration.
Besides its role in neuroinflammation, P2X7R activation also modulates APP processing through MAPK-dependent mechanisms (Figure 3). P2X7R stimulation leads to the phosphorylation of ADAMs (ADAM9, −10, −17), facilitating the non-amyloidogenic a-processing of APP(Camden et al., 2005; Surprenant and North, 2009). An ADAM-independent process for APP a-processing has also been identified, involving Erk1/2 and JNK pathways, resulting in increased sAPPα release while suppressing sAPPβ and Aβ peptide production (Ma et al., 2005; Delarasse et al., 2011). Moreover, P2X7R signaling triggers ERM protein phosphorylation, which interact with P2X7R at the plasma membrane and promote APP processing and sAPPa shedding (Darmellah et al., 2012). P2X7R activation differentially modulates AKT and ERK pathways in a cell-type-specific manner. In rat cortical astrocytes, P2X7 activation induces AKT phosphorylation (Jacques-Silva et al., 2004), while in microglial cells, BzATP stimulation results in ERK and AKT dephosphorylation (Bian et al., 2013; He et al., 2017). Notably, P2X7R activation is not associated with IL-1 family cytokine release in this context (He et al., 2017).
In summary, the diverse roles of P2X7R in regulating MAPK signaling pathways underscore its significance in modulating neuroinflammation, neurodegenerative processes, and APP processing. Targeting P2X7R and its downstream effectors may offer novel therapeutic approaches for neurological disorders associated with aberrant MAPK activation. However, further studies are required to elucidate the complex interplay between P2X7R, MAPKs, and their cell-type-specific effects in the central nervous system.
5.2 ROS
Oxidative stress, characterized by elevated ROS levels and diminished antioxidant capacity, plays a crucial role in the development of neurodegenerative diseases (Orfali et al., 2024). Inflammation and metabolic stress disrupt mitochondrial function, which compromises energy production, leads to ROS accumulation, and causes neuronal death, accelerating disease progression (Millichap et al., 2021). A significant contributor to progressive neuronal death in these conditions is the inflammatory response due to microglial activation (Qin et al., 2023). This inflammatory response, along with P2X7R-mediated Ca2+ influx and mitochondrial dysfunction, exacerbates ATP-induced neurodegeneration and oxidative stress (Nishida et al., 2012; Jiang et al., 2015; Zelentsova et al., 2022). During ischemic and hypoxic conditions, mitochondria become a major ROS source by generating superoxide at the respiratory chain’s origin (Zelentsova et al., 2022). This superoxide can oxidatively modify and damage macromolecules within the neuronal plasma membrane (Subramaniam and Chesselet, 2013). The activation of P2X7R by α-synuclein decreases mitochondrial membrane potential, increases mitochondrial ROS production, and triggers the intrinsic apoptotic pathway, leading to mitochondrial dysfunction and cell death (Figure 3) (Wu and Bratton, 2013; Jiang et al., 2015; Wilkaniec et al., 2020). This process results in reduced energy production and promotes cell death through the activation of pro-apoptotic proteins (Wilkaniec et al., 2020).
NOX2, is a significant ROS producer in microglia, generating ROS as a byproduct of cellular metabolism and playing roles in both intracellular and extracellular signaling (Munoz et al., 2017). While extracellular ROS are harmful to neurons, intracellular ROS serve as signaling molecules that activate p38 and ERK1/2 in microglia, prompting the production of pro-inflammatory and neurotoxic cytokines (Zheng et al., 2024). Intriguingly, the absence of NOX2 in SOD1-G93A mice enhances disease progression and survival, suggesting a complex role for NOX2 in disease dynamics (Apolloni et al., 2013b). P2X7R stimulation by BzATP in SOD1-G93A microglia activates Rac1, a critical Rho GTPase family member, which in turn activates NOX1 and NOX2, leading to increased ROS production (Apolloni et al., 2013b; Munoz et al., 2017). Notably, P2X7R-mediated NOX2 activation and subsequent ROS generation in microglia are entirely dependent on Rac1 activation (Apolloni et al., 2013b). Furthermore, ATP stimulation of P2X7R in ALS microglia increases ERK1/2 phosphorylation, with a noted interdependence between the NOX2 and ERK1/2 pathways contributing to ROS overproduction (Lenertz et al., 2009; Apolloni et al., 2013b).
In spinal cord astrocytes, P2X7R activation results in ROS production and IL-6 release, partly mediated by NADPH oxidase (Munoz et al., 2020). In EOC13 cells, P2X7 activation leads to ROS formation and subsequent cell death (Hewinson and Mackenzie, 2007; Di Virgilio et al., 2009) through a mechanism independent of Ca2+ influx and K+ efflux (Bartlett et al., 2013), whereas in primary rat microglia, P2X7-induced ROS formation is dependent on Ca2+ influx (Kim et al., 2007).
5.3 NLRP3 inflammasome
The link between inflammation and neurodegenerative diseases is intricate, revealing a complex interplay that requires further study. Pro-inflammatory cytokines are widely associated with various neurodegenerative disorders (Allan and Rothwell, 2001), but their exact role in disease pathogenesis remains unclear. Although inflammation has been shown to contribute to disease progression in animal models, it is not conclusively identified as the primary initiator of neurodegeneration (Glass et al., 2010).
At the core of this inflammatory process is the NLRP3 inflammasome, a protein complex that includes the sensor protein NLRP3, the adaptor ASC, and the effector caspase-1 (Xu et al., 2020). Activation of P2X7R in microglia leads to the release of IL-1β and IL-18, driven by NLRP3 inflammasome activation and subsequent caspase-1 activation (Xie et al., 2024) (Figure 3). This activation occurs in two phases: initiation and activation (Bai et al., 2021). During the initiation phase, pattern recognition receptors (such as Toll-like receptors) detect PAMPs, DAMPs, or environmental stress, or NF-κB is activated by TNF-α, leading to increased expression of NLRP3, pro-IL-1β, and pro-IL-18 (Toma et al., 2010; Saha et al., 2020). ROS are crucial in this process, facilitating NF-κB activation and upregulation of inflammasome components (Zhang et al., 2018). The activation phase involves the assembly of NLRP3 and ASC with pro-caspase-1, converting it into active caspase-1. This activated enzyme cleaves pro-IL-1β and pro-IL-18, releasing mature cytokines that mediate inflammation (Stephenson et al., 2018). A variety of stimuli, including ATP, Nigericin, and alum, can trigger this inflammasome activation (Stephenson et al., 2018). Interestingly, in the mouse brain, microglia uniquely form functional NLRP3 inflammasomes, secrete IL-1β, and release IL-18 and IL-1α, while astrocytes do not exhibit this capability (Gustin et al., 2015). This specialization underlines the critical role of microglia in neuroinflammation and their involvement in neurodegenerative disease progression.
6 Antagonists targeting P2X7R
The P2X7R antagonists has made substantial advancements, although only a few compounds have entered clinical trials, predominantly for peripheral disorders (Stock et al., 2012; Chitnis and Weiner, 2017). The limited clinical progress is often due to challenges in understanding the human P2X7R (hP2X7R) structure and achieving drug-like properties suitable for central nervous system (CNS) targeting, such as optimal lipophilicity, solubility, and brain tissue half-life (Stock et al., 2012; Chitnis and Weiner, 2017). Initial development efforts involved nucleotide analogs with broad-spectrum antipurinergic activity, like oATP, which mitigates proinflammatory signaling through mechanisms independent of P2 receptors (Beigi et al., 2003; Huo et al., 2018). One notable antagonist, BBG, has been widely utilized in both in vitro and in vivo studies despite its selectivity and blood-brain barrier (BBB) permeability limitations (Gargett and Wiley, 1997; Keystone et al., 2012; Bin Dayel et al., 2019). The quest to develop P2X7R antagonists for CNS conditions has intensified, leading to the discovery of novel derivatives with improved pharmacological properties (Guile et al., 2009; Lee et al., 2009). High-throughput screening has identified promising chemical scaffolds, such as berberine alkaloid analogs and chiral pyrazolodiazepinones, which show effective hP2X7R blockade (Lee et al., 2009; Park et al., 2015). Pyrimidine-2,4-dione derivatives, including compound 1 (Figure 5), have demonstrated superior inhibition compared to earlier compounds like KN-62 (Chambers et al., 2010). Cyclic pyroglutamic amides, such as compound 2 (Figure 5), have shown enhanced P2X7R blockade and better metabolic stability (Abberley et al., 2010), while imidazole moieties like unsubstituted imidazolone 4 have been developed to increase half-life (Recourt et al., 2020).
The search for effective P2X7R antagonists has also explored other structures, including o-chlorobenzamide derivatives like compound 3 (Figure 5), which exhibited moderate inhibitory activity (Honore et al., 2006) (Figure 5). Cyanoguanidines such as A-804598 (Figure 5) have shown high potency and favorable pharmacokinetic (PK) profiles (Able et al., 2011). Adamantane derivatives and triazolopiperidine scaffolds have also been investigated, with compounds like JNJ-54175446 demonstrating promising PK properties and tolerability (Able et al., 2011; Savall et al., 2015; Swanson et al., 2016; Wilkinson et al., 2017). A new family of triazolopiperidines was developed, with JNJ-55308942 emerging as a candidate for clinical trials (Chrovian et al., 2018). Various heterocyclic derivatives were also explored by linking o-chlorobenzamide to quinoline (Chen et al., 2010; Letavic et al., 2013). Another ligand, JNJ-47965567, reduced amphetamine-induced locomotion sensitization but showed less efficacy in neuropathic pain and depression models (Bhattacharya et al., 2013).
Several P2X7R antagonists have reached clinical trials for conditions such as depression, rheumatoid arthritis (RA), and inflammatory bowel disease, but none have achieved market approval (Keystone et al., 2012; Ali et al., 2013; Eser et al., 2015). AstraZeneca’s AZD9056 and Pfizer’s CE-224535, developed for RA and osteoarthritis, were discontinued in phase II trials due to lack of efficacy (Keystone et al., 2012; Eser et al., 2015). Similarly, GlaxoSmithKline’s GSK-1482160, targeting chronic inflammatory pain, failed to achieve therapeutic benefits in phase I within the safe dose range (Ali et al., 2013).
Despite these setbacks, the ongoing development of P2X7R antagonists underscores the receptor’s potential as a therapeutic target for neuroinflammation and peripheral inflammatory disorders (Sperlágh and Illes, 2014; Zheng et al., 2017; Meyer et al., 2020). The focus has increasingly shifted towards compounds that can effectively penetrate the BBB, as seen with JNJ-54175446 and JNJ-55308942, offering hope for more targeted treatments for neuroinflammatory diseases (Bhattacharya et al., 2018; Recourt et al., 2020).
The pursuit of effective P2X7R antagonists for neuroinflammatory conditions represents a significant step forward in the development of novel therapies for these debilitating disorders. As our understanding of the role of P2X7R in neuroinflammation continues to expand, it is anticipated that more targeted and efficacious compounds will emerge, offering hope for patients suffering from these conditions. However, the path to successful drug development is not without challenges, as evidenced by the discontinuation of several P2X7R antagonists in clinical trials.
Despite the setbacks, the ongoing clinical development of several promising P2X7R antagonists highlights the potential of targeting this receptor for the treatment of various neuroinflammatory and peripheral inflammatory disorders. The following section will provide an in-depth look at the clinical progress and challenges associated with these P2X7R antagonists.
6.1 JNJ-54175446
JNJ-54175446 (Figure 5), a highly selective and potent P2X7R antagonist, marks a crucial advance in the development of brain-penetrant drugs for neuroinflammationJNJ-54175446 (Letavic et al., 2017). This compound exhibits impressive activity across different species with PIC50 values between 7.8 and 8.81. It boasts favorable physicochemical properties and efficient central nervous system partitioning. JNJ-54175446 demonstrates robust in vivo target binding following oral administration, with preclinical pharmacological studies showing a dose-dependent inhibition of IL-1β release in the rat hippocampus (Letavic et al., 2017). The liver microsome stability across species and its exceptional pharmacokinetic profile in rats, dogs, and non-human primates—characterized by low clearance rates (Cl = 2.9, 0.9, and 4.4 mL/min/kg, respectively), suitable intravenous half-lives (T1/2 = 8.6, 32, and 6.9 h, respectively), and high oral bioavailability (%F = 96, 164, and 157, respectively)—underscore its potential (Letavic et al., 2017). Preclinical success led to the advancement of JNJ-54175446 into clinical trials. In single-ascending dose studies with healthy volunteers, the compound was well-tolerated across doses ranging from 0.5 mg to 600 mg (Timmers et al., 2018). A subsequent clinical study assessed the drug’s efficacy in 312 subjects, including 69 patients with depression, who were administered daily doses of 50–150 mg. In this study, healthy participants were given 20 mg of D-amphetamine to evaluate mood responses, while depressed patients underwent total sleep deprivation. JNJ-54175446 significantly mitigated the mood effects of D-amphetamine in healthy subjects and attenuated mood elevation following sleep deprivation in depressed individuals (Lee et al., 2023). Further evaluation in a double-blind, placebo-controlled trial involved multiple doses of JNJ-54175446. The compound was well-tolerated across all tested doses and effectively reduced the locomotor effects of D-amphetamine, while enhancing mood-lifting effects at doses of 100 mg and higher (Recourt et al., 2020). The promising results from both preclinical and clinical studies suggest that targeting P2X7R with brain-penetrant antagonists like JNJ-54175446 could offer new avenues for treating neuroinflammatory conditions. Continued research and development in this area are essential as we deepen our understanding of neuroinflammation and the role of P2X7R in its progression, aiming to provide effective, targeted therapies for patients afflicted by these debilitating disorders.
6.2 JNJ-55308942
JNJ-55308942 (Figure 5), the second compound advanced into clinical trials, exhibited significant potency and selectivity across both human and rat models, with IC50 values of 10 nM for hP2X7R and 15 nM for rP2X7R (Chrovian et al., 2018). Unlike its predecessor JNJ-54175446, JNJ-55308942 demonstrated enhanced solubility and metabolic stability due to the substitution of isonicotinamides for benzamides, which also contributed to reduced CYP2C19 inhibition (Chrovian et al., 2018). This compound remained stable in rat and human liver microsomes and showed no significant hERG toxicity, with an IC50 exceeding 19 μM for various CYP450 isoforms.
Pharmacokinetic assessments indicated that JNJ-55308942 had poor to moderate clearance rates (Cl = 3.7, 0.8, and 2.6 mL/min/kg) and acceptable intravenous half-lives (T1/2 = 5.6, 21.2, and 6.0 h) in rats, dogs, and monkeys, respectively [178]. The compound also exhibited high exposure (AUC24h = 17,549, 23,262, and 16,262 (ng/mL)·h) and favorable oral bioavailability in these species (%F = 81, 98, and 97), suggesting efficient absorption [178]. Intravenous to oral dose ratios were 1/5 for rats, 0.25/1.25 for dogs, and 0.5/2.5 for monkeys. Estimated human pharmacokinetics predicted a clearance of 0.8 mL/min/kg and a volume of distribution at steady state (Vss) of 1.1 L/kg, with a projected half-life (T1/2) of 16 h.
Preclinical pharmacodynamic studies using an in vivo microdialysis assay demonstrated that oral administration of 10 mg/kg JNJ-55308942 significantly inhibited Bz-ATP-induced IL-1β release in the rat hippocampus at 1 and 5 h (Chrovian et al., 2018). In the Bacillus Calmette-Guerin (BCG)-induced depression model, the compound reversed deficits in sucrose preference and social interaction at a dose of 30 mg/kg (p.o.), indicating its efficacy in this model of depression (Bhattacharya et al., 2018). Additionally, in a rat chronic stress model, JNJ-55308942 reversed stress-induced deficits in sucrose intake, with autoradiography confirming increased P2X7R occupancy in the brain following treatment (Bhattacharya et al., 2018). Phase I clinical trials of JNJ-55308942 (NCT03151486) were conducted to assess its safety, tolerability, and pharmacokinetics in healthy male and female subjects. Although detailed results have not been disclosed, these trials are crucial for understanding the clinical potential of JNJ-55308942 (Chrovian et al., 2018).
6.3 SGM-1019
SGM-1019, an orally bioavailable small molecule P2X7R antagonist developed by Evotec, has shown potential in treating nonalcoholic steatohepatitis (NASH) (Dibba et al., 2018). With a Ki value of 7.6 nM for human P2X7R, SGM-1019 exhibited low efficacy in lower species, prompting relevant preclinical studies in non-human primate models (Dibba et al., 2018). These studies revealed that SGM-1019 significantly improved liver histology in CCL4-induced hepatic fibrosis models by decreasing stellate cell activation and alpha smooth muscle actin expression, reducing liver pathology, and improving overall histological scores (Baeza-Raja et al., 2020). Additionally, non-human primates treated with SGM-1019 demonstrated reduced hepatic stellate cell activation, decreased collagen deposition, and downregulation of fibrosis-related genes, along with lower serum alanine aminotransferase levels (Baeza-Raja et al., 2020). The promising preclinical outcomes in non-human primate models suggest that P2X7R antagonists like SGM-1019 have considerable antifibrotic potential in vivo. These findings laid the groundwork for exploring their therapeutic effects in clinical settings. A randomized, double-blind, placebo-controlled clinical trial (NCT03676231) involving 100 NASH patients was initiated to assess the preliminary safety, tolerance, pharmacokinetics, and efficacy of SGM-1019. The study involved twice-daily oral administration of the compound. However, the trial was prematurely terminated due to safety concerns (Dibba et al., 2018). Despite the early termination of the clinical trial, the preclinical evidence supporting the antifibrotic effects of P2X7R antagonists remains compelling. SGM-1019s ability to reduce liver pathology, improve histological scores, and modulate key fibrotic processes such as stellate cell activation and collagen deposition underscores its potential as a novel therapeutic agent for NASH (Baeza-Raja et al., 2020). This experience underscores the complexities of translating preclinical success into clinical efficacy and serves as a valuable learning opportunity for future drug development efforts.
6.4 GSK-1482160
GSK-1482160 (Figure 5), a pyroglutamic acid amide P2X7R antagonist, displays distinct affinities between human and rat receptors, with a higher affinity for human P2X7R (hP2X7R pIC50 = 8.5) compared to rat P2X7R (rP2X7R pIC50 = 6.5) (Abdi et al., 2010). The compound exhibits stability in liver microsome metabolism, showing clearance rates below 0.5 mL/min/g in both human and rat liver microsomes. Additionally, it does not significantly inhibit various CYP450 enzyme subtypes at concentrations up to 100 μM(Abdi et al., 2010).
Pharmacokinetic evaluations in rats demonstrated that intravenous administration of GSK-1482160 at 1 mg/kg resulted in low plasma clearance (9 mL/min/kg) and a half-life of 1.5 h. Oral administration at 3 mg/kg showed rapid absorption (Tmax = 1 h), an AUC of 73 min kg/L, and an oral bioavailability of 65% (Abdi et al., 2010). Efficacy studies revealed that GSK-1482160 effectively reversed mechanical allodynia in chronic joint pain and chronic constriction injury (CCI) models of neuropathic pain on the first day of treatment at 20 mg/kg, maintaining this effect throughout the treatment period (Abdi et al., 2010).
In clinical trials involving healthy volunteers, GSK-1482160 was tested for its pharmacokinetics and pharmacodynamics across doses ranging from 0.3 to 1,000 mg. It was well-tolerated and exhibited a good safety profile. However, pharmacokinetic/pharmacodynamic (PK/PD) modeling suggested that the compound could not achieve the pharmacological inhibition required for a full P2X7R mechanism of action (>90% IL-1β release inhibition) while maintaining a safe margin relative to the steady-state no-observed-adverse-effect level (NOAEL) (Ali et al., 2013). As a result, GSK-1482160 was deemed unsuitable for treating rheumatoid arthritis or other inflammatory diseases.
Despite its limitations as a therapeutic agent, GSK-1482160 has been successfully repurposed as a radioactive tracer for P2X7R imaging. When labeled with 11C or 18F, it serves as a novel radioligand due to its good central nervous system permeability, strong selectivity, and affinity for P2X7R (Han et al., 2017; Huang et al., 2022). This repurposing underscores the versatility of GSK-1482160 and the importance of considering species-specific differences in receptor affinity when translating preclinical findings to human applications.
6.5 CE-224535
CE-224535 (Figure 5), an azazyrimidine-like hP2X7R antagonist developed by Pfizer, shows strong activity against the receptor with Yo-Pro IC50 of 4 nM and IL-1β IC50 of 1.4 nM, but its efficacy in rodent models is limited, resulting in a lack of useful published data [196]. Pharmacokinetic studies across various animal species revealed significant differences. In rats, oral administration of CE-224535 (5 mg/kg) led to a plasma clearance rate of 11 mL/min/kg and a half-life of 2.4 h, but the oral bioavailability was low (%F = 2.6) [196]. Conversely, in monkeys and dogs, the half-life was shorter (T1/2 = 0.46 and 0.77 h, respectively), but oral bioavailability was significantly higher (%F = 59 and 22, respectively) (Duplantier et al., 2011). Daily administration of CE-224535 at 500 mg/kg (p.o.) for 4 days did not result in toxicity in rats, and the compound showed no significant effect on hERG at 1 μM or on major human CYP enzymes at 30 μM (Duplantier et al., 2011). These findings led to a 4-week phase I clinical trial (NCT00446784) to evaluate its safety and tolerability in 20 RA subjects already receiving methotrexate. However, results were not posted. This was followed by a 2-week, randomized, double-blind, placebo- and positive-controlled phase II trial (NCT00418782) to assess its analgesic and anti-inflammatory efficacy in osteoarthritis (OA) knee pain. This trial was terminated due to lack of efficacy, reflecting the challenges in translating potent in vitro activity into effective clinical outcomes (Stock et al., 2012). Subsequent efforts included a phase IIA study where CE-224535, administered at 500 mg twice daily for 12 weeks, failed to show efficacy in RA patients who did not respond adequately to methotrexate (Stock et al., 2012). The compound’s low efficacy in rodent models and suboptimal pharmacokinetic properties in some species might have contributed to its clinical shortcomings.
The development trajectory of CE-224535 underscores the difficulties of achieving therapeutic success with P2X7R antagonists, despite strong receptor affinity in vitro. The lack of significant clinical benefits in patients with advanced RA or those unresponsive to methotrexate suggests that targeting P2X7R alone may not suffice for effective treatment. This emphasizes the necessity of further research to understand the role of P2X7R within the context of existing treatment regimens and disease progression.
6.6 AZD9056
AZD9056 (Figure 5), a methyleneamide hP2X7R antagonist with an adamantane functional group, exhibits dose-dependent inhibition of BzATP-induced IL-1β and IL-18 release from human peripheral blood monocytes (IL-1β IC50 = 10–13 nM)(McInnes et al., 2014). Given its lack of rodent activity, its analogue AZ11657312 was tested in a rat streptococcal cell wall (SCW) arthritis model. AZ11657312, at doses of 30 and 60 mg/kg, reduced synovitis, sub-lining inflammation, chondronecrosis, and sub-chondral bone resorption in granulocytic precursors, suggesting potential as a novel RA treatment (McInnes et al., 2014).
In phase I trials, AZD9056 was well-tolerated in healthy participants, with a daily oral dose of 400 mg for up to 6 months, showing a good safety profile (Snell, 2014). The compound advanced to phase II clinical trials for RA, where phase IIa results indicated a reduction in swollen and tender joint counts compared to placebo. However, there was no significant effect on the acute phase response (Keystone et al., 2012). In a subsequent phase IIb study, AZD9056 failed to show any clinically or statistically significant efficacy in RA patients despite being well-tolerated [166]. The compound was also evaluated in a 28-day phase IIa trial for Crohn’s disease (CD). Here, the Crohn’s Disease Activity Index (CDAI) decreased from a mean of 311 to 242 in the AZD9056 group, compared to a decrease from 262 to 239 in the placebo group (P = 0.049). Despite these results, the study showed no changes in inflammatory biomarkers (C-reactive protein and fecal calprotectin), and the development for the CD indication was halted due to this discrepancy.AZD9056 was further assessed in a 4-week study for chronic obstructive pulmonary disease (COPD), where patients received 400 mg orally once daily. This study did not demonstrate any clinical benefits of the compound (Genetzakis et al., 2022).
The journey of AZD9056 underscores the challenges in translating preclinical efficacy into clinical success. Despite its potent inhibition of pro-inflammatory cytokines in human cells and promising results in animal models using its analogue, the compound did not achieve significant clinical efficacy in RA and CD patients (Keystone et al., 2012; McInnes et al., 2014). The discrepancy between clinical symptom improvement and the lack of biomarker changes in CD raises questions about the true impact on disease pathology (Eser et al., 2015). Additionally, the failure to show benefit in COPD patients highlights the complexity of P2X7R targeting across different diseases and underscores the necessity of a thorough understanding of its role in disease-specific contexts (Snell, 2014).
7 Involvement in neurodegeneration disorders
7.1 Alzheimer’s disease
AD is marked by the presence of neuronal fibrillary tangles and senile plaques, with hyperphosphorylated tau protein forming the tangles inside neurons and extracellular amyloid-beta (Aβ) peptides contributing to senile plaques (Martin et al., 2019).The production of Aβ involves cleavage of amyloid precursor protein (APP) by α-, β-, and γ-secretases, with P2X7R potentially influencing this process (Allinson et al., 2003). Specifically, P2X7R activation stimulates ADAM9, ADAM10, and ADAM17, which possess α-secretase activity and promote the non-amyloidogenic processing of APP(Camden et al., 2005; Surprenant and North, 2009). Additionally, P2X7R activates an alternative α-cleavage pathway independent of ADAMs, enhancing sAPPα release while reducing sAPPβ and Aβ peptide production (Delarasse et al., 2011). This P2X7R-mediated sAPPα release has been observed in various cell types, and P2X7R inhibition can prevent this effect.
Emerging evidence supports the role of P2X7R inhibition in restoring hippocampal synaptic integrity and plasticity, reducing Aβ pathology, and rescuing memory deficits in APPPS1 mice, without involving the sAPPα pathway, IL-1β treatment, microglial activation, or phagocytosis (Bhattacharya and Biber, 2016). Extracellular Aβ peptides (Sanz et al., 2009), released from damaged neurons and glial cells, activate P2X7R, which is upregulated during microgliocytosis and significant cognitive and motor impairment (Mucke et al., 2000). Elevated P2X7R expression correlates with AD progression, promoting microglia migration towards plaques and inhibiting phagocytosis (Martínez-Frailes et al., 2019). In familial AD (FAD), Aβ aggregation occurs before P2X7R expression in microglia, as demonstrated in the P2X7R-EGFP/J20 transgenic mouse model (Grad et al., 2017). Furthermore, P2X7R is implicated in the production of Aβ-induced chemokines and the recruitment of CD8+ T cells in the brain (Cartier et al., 2005). These chemokines, overexpressed in response to Aβ peptides in vitro and in AD models, lead to inflammation, immune cell recruitment, and neurodegeneration (Jaerve and Müller, 2012).Astrocytes are increasingly recognized for their roles in synaptic transmission and protein clearance pathways in AD, affecting both excitatory and inhibitory neurotransmission (Sung and Jimenez-Sanchez, 2020; Andersen et al., 2022). Dysregulation of calcium homeostasis by Aβ in astrocytes can impair neurotransmission modulation, leading to neuronal hyperactivity through mechanisms such as TRPA1 activation in APP/PS1 mice (Haughey and Mattson, 2003; Chow et al., 2010; Paumier et al., 2022).
P2X7R is a key modulator of astrocyte function in AD, influencing neurotransmission, synaptic dysfunction, oxidative stress, and neuroinflammation (Fellin et al., 2006; Lee et al., 2011; Diaz-Hernandez et al., 2012; Ficker et al., 2014; Martin et al., 2019; Ruan et al., 2020; Carvalho et al., 2021; Di Lauro et al., 2022). Recent studies suggest astrocytes produce L-serine, which is transported to neurons for D-serine production, critical for NMDA receptor-mediated synaptic plasticity. Impaired glucose metabolism in prodromal AD decreases L-serine synthesis, affecting cognitive function (Le Douce et al., 2020). Astrocytic expression of glutamate transporters, particularly EAAT2, near Aβ plaques remains debated (Hefendehl et al., 2016; Kobayashi et al., 2018). In APP/PS1 mice, lower EAAT2 levels around plaques lead to extrasynaptic glutamate accumulation and neuronal hyperactivity (Hefendehl et al., 2016), though human AD brain analyses suggest astrocytic resilience (Kobayashi et al., 2018).
Astrocytes also internalize modified tau proteins through mechanisms involving heparan sulfate proteoglycans (HSPGs) and LRP1, with uptake efficiency varying based on tau modifications (Martini-Stoica et al., 2018; Perea et al., 2019; Puangmalai et al., 2020; Rauch et al., 2020; Reid et al., 2020; Wang and Ye, 2021). Tau internalization and release might be mediated by HSPGs, and P2X7R regulates HSPG expression in other tissues, suggesting potential parallels in astrocytes (Mankus et al., 2012; Holmes et al., 2013; Merezhko et al., 2018). The role of P2X7R in regulating astrocytic HSPGs and tau clearance warrants investigation, particularly as astrocytes play a role in protein clearance in AD. P2X7R-induced regulation of astrocytic autophagy may limit the spread of pathological proteins in AD and tauopathies (Takenouchi et al., 2009b; 2009a; Kim et al., 2018; Lee and Kim, 2020).
Genetic deletion or pharmacological inhibition of P2X7R has demonstrated benefits in AD and frontotemporal dementia (FTD) mouse models, improving cognitive, behavioral, and synaptic deficits (Diaz-Hernandez et al., 2012; Martin et al., 2019; Ruan et al., 2020; Carvalho et al., 2021; Di Lauro et al., 2022). Astrocytic P2X7R may contribute to elevated glutamate levels near plaques through Ca2+-dependent mechanisms (Fellin et al., 2006; Ficker et al., 2014), highlighting the need for further research on its specific impact on astrocytic functions in AD. The therapeutic potential of targeting P2X7R in AD lies in its ability to modulate synapse-related and protein clearance functions of astrocytes, presenting a promising avenue for developing effective interventions for neurodegenerative disorders (Kim et al., 2011; 2018; Ryu et al., 2011; Lee and Kim, 2020).
7.2 Parkinson’s disease
PD, the second most common neurodegenerative disorder globally, affects over 6 million individuals and is a leading cause of neurological disability (GBD, 2016 Neurology Collaborators, 2019). Characterized by motor symptoms such as bradykinesia, rigidity, tremor, and postural instability, PD is pathologically defined by α-synuclein-positive inclusions (Lewy bodies and Lewy neurites) and the degeneration of dopaminergic neurons in the substantia nigra pars compacta and striatal projections (Sorrentino and Giasson, 2020; Tan et al., 2020). Although most cases are idiopathic, a minority have genetic origins (Tufekci et al., 2011).
Immune system dysfunction has emerged as a critical factor in PD’s susceptibility and progression (Tan et al., 2020). Postmortem analyses have revealed increased reactive microglia and phagocytic activity in the brains of PD patients (Pasqualetti et al., 2015). Elevated levels of pro-inflammatory cytokines such as IL-1β and TNF-α in the cerebrospinal fluid of these patients further support this (McGeer et al., 1988; Mogi et al., 1994; Blum-Degen et al., 1995). These immune responses, once considered secondary, are now seen as potential primary contributors to the disease (Maatouk et al., 2019).
Microglial activation, driven by α-synuclein, plays a pivotal role in PD neuroinflammation. Alpha-synuclein binds to microglial NOX2, activating the NOX complex and inducing oxidative stress in vivo (Wang et al., 2016), and directly activates P2X7R without the need for exogenous ATP (Wilkaniec et al., 2017). P2X7R involvement in α-synuclein-mediated microglial activation leads to ATP and ROS release, damaging dopaminergic neurons and contributing to PD pathology (Jiang et al., 2015; Wilkinson et al., 2017; Dos-Santos-Pereira et al., 2018). This process also involves the release of excitotoxic glutamate from activated microglia, further exacerbating neuronal damage through ATP/glutamate secretion and ROS, which contribute to the destruction of dopaminergic neurons and PD development (Sperlágh et al., 2002; Simon et al., 2020).
Animal studies have shown that P2X7R antagonism by Brilliant Blue G (BBG) can alleviate PD symptoms and protect dopaminergic neurons (Ferrazoli et al., 2017; Kumar et al., 2017; Crabbé et al., 2019; Oliveira-Giacomelli et al., 2019). For instance, in an LPS-induced PD model in rats, BBG treatment reversed the reduction of tyrosine hydroxylase-immunoreactive (TH-ir) neurons in the substantia nigra by inhibiting the P38MAPK pathway, highlighting the neuroprotective effects of P2X7R antagonism (Wang et al., 2017). Increased P2X7R expression on microglia, leading to dopaminergic neuron loss, was observed following LPS injection into the substantia nigra (Wang et al., 2017). BBG treatment reduced both microglial activation and dopaminergic neuron loss, indicating that P2X7R contributes to the synthesis of pro-inflammatory cytokines involved in neuronal death (Dutta et al., 2008). In rat models with 6-OHDA-induced nigrostriatal damage, BBG treatment decreased apomorphine-induced rotational behavior, suggesting a reduction in motor deficits associated with PD (Ferrazoli et al., 2017). Further studies suggest that P2X7R-mediated neuronal swelling and necrosis might be related to substantia nigra striatal dysfunction in PD. Exposure of SN4741 neuronal cells, derived from transgenic mouse embryos, to high ATP concentrations led to rapid cell volume increase and subsequent necrotic changes such as nuclear swelling, DNA leakage, and cytoplasmic vacuole formation (Jun et al., 2007).
Recent evidence implicates P2X7Rs in PD pathogenesis, particularly regarding microglial activation, neuroinflammation, and dopaminergic neuron loss. ATP binding to P2X7R activates microglia and recruits peripheral immune cells through complex pathways, including calcium influx, NLRP3 inflammasome activation, cell death induction, tissue damage, and further ATP release (Illes et al., 2020). This ATP/P2X7R/apoptosis axis creates a positive feedback loop, perpetuating the neurodegenerative process in PD (Illes et al., 2020).
In conclusion, targeting P2X7R offers a promising therapeutic strategy for PD. Future research should focus on the precise mechanisms of P2X7R-mediated neurotoxicity and the potential of P2X7R modulators as new therapeutic agents for PD [244–262].
7.3 Multiple sclerosis
MS is a chronic neurological condition marked by autoimmune-driven demyelination and axonal damage in CNS (Dendrou et al., 2015; Domercq et al., 2019; Domercq and Matute, 2019). The role of P2X7R in MS has garnered attention for their contribution to disease progression through diverse mechanisms (Burnstock, 2017b; Domercq et al., 2019; Domercq and Matute, 2019). In the relapsing-remitting form of MS, autoreactive T and B cells infiltrate the CNS, intensifying the inflammatory response (Mahad et al., 2015). As MS progresses, the focus shifts from peripheral immune cells to CNS-resident cells like microglia and astrocytes, which continue to sustain inflammation (Dendrou et al., 2015; Mahad et al., 2015; Domercq et al., 2019; Domercq and Matute, 2019). Notably, microglia expressing P2X7R are found in greater numbers in post-mortem spinal cord samples from MS patients than in non-MS controls (Yiangou et al., 2006; Amadio et al., 2017).
P2X7R upregulation is evident in activated microglia during the early stages of experimental autoimmune encephalomyelitis (EAE), an animal model of MS(Grygorowicz et al., 2018). Treatment with the P2X7R antagonist BBG in EAE models alleviates symptoms and reduces pro-inflammatory cytokine levels, including IL-1β, IL-6, and TNF-α(Sharp et al., 2008). In astrocytes, P2X7R expression also increases during EAE, and BBG administration lessens astrogliosis and neurological symptoms (Grygorowicz et al., 2016). Furthermore, Panx-1 knockout mice, which exhibit reduced ATP release, experience delayed EAE onset and lower mortality rates, underscoring the link between diminished P2X7R signaling and improved outcomes (Lutz et al., 2013).
In addition to influencing immune responses, P2X7R activation directly affects oligodendrocytes—the cells responsible for myelin formation in the CNS. Activation of P2X7R leads to significant Ca2+ influx, potentially causing oligodendrocyte death and exacerbating demyelination (Matute et al., 2007). Increased P2X7R expression in oligodendrocytes is observed in optic nerve samples from MS patients compared to controls (Chen and Brosnan, 2006). P2X7R is also detected on the abluminal surface of brain microvessels, impacting BBB integrity during MS. BBG treatment in EAE rats helps preserve BBB integrity and mitigates clinical symptoms (Sharp et al., 2008; Grygorowicz et al., 2018).Genetic studies reveal that single nucleotide polymorphisms (SNPs) in the P2X7R gene are associated with MS risk. The loss-of-function SNP rs28360457 (Arg307Gln) is linked to a reduced risk of MS, while the gain-of-function SNP rs17525809 (Val76Ala) is associated with an increased risk (Miller et al., 2011; Oyanguren-Desez et al., 2011; Gu et al., 2015).
7.4 Amyotrophic lateral sclerosis
ALS is a fatal neurodegenerative disorder characterized by the progressive loss of motor neurons in the brainstem and spinal cord, which control voluntary movement (Ghasemi and Brown, 2018; Cieślak et al., 2019). Sporadic cases usually manifest around age 60, while inherited forms appear around age 50, with survival typically ranging from two to 4 years due to respiratory failure (Ly et al., 2020). ALS pathogenesis involves a complex interplay of mechanisms, including oxidative stress, neuroinflammation, mitochondrial dysfunction, protein misfolding, trophic growth factor deficiencies, impaired axonal conduction, and blood-brain barrier disruption (Cieślak et al., 2019).
The SOD1-G93A transgenic mouse model, which overexpresses a mutant form of superoxide dismutase 1 (SOD1), is commonly used to study ALS and displays clinical symptoms at 70–140 days of age (Ly et al., 2020). P2X7R activation plays a critical role in ALS by driving chronic microglial activation and contributing to motor neuron death (Ruiz-Ruiz et al., 2020). Postmortem spinal cord samples from ALS patients show increased P2X7R immunoreactivity, primarily in microglia/macrophages within affected regions (Yiangou et al., 2006). In vitro, stimulation of P2X7R in SOD1-G93A mouse-derived spinal cord microglia with Bz-ATP increases TNF-α and cyclooxygenase-2 levels, inducing toxicity in neuronal cell lines, an effect that BBG antagonism can mitigate (D’Ambrosi et al., 2009). Furthermore, Bz-ATP enhances NADPH oxidase-2 activity in SOD1-G93A microglia, generating reactive oxygen species (ROS), which is preventable through P2X7R knockout or BBG treatment (Apolloni et al., 2013b). SOD1-G93A astrocytes also contribute to P2X7R-mediated ROS production (Gandelman et al., 2010). Co-cultures of motoneurons with SOD1-G93A astrocytes show motoneuron death that can be prevented by using MnTBAB, BBG, or apyrase to degrade ATP (Gandelman et al., 2010).
Aggregated and soluble SOD1-G93A activate the NLRP3 inflammasome in primary mouse microglia, leading to IL-1β release, whereas NLRP3-deficient microglia do not show this response, indicating a specific role for NLRP3 in ALS neurotoxicity (Deora et al., 2020). Interestingly, short-term P2X7R activation in SOD1-G93A mouse microglia initiates autophagy and increases anti-inflammatory biomarkers (M2 microglia), whereas prolonged activation disrupts autophagic flux, shifting microglia towards a pro-inflammatory phenotype (M1 microglia) (Fabbrizio et al., 2017). Conflicting evidence exists regarding the role of P2X7R in ALS progression. Ablation of P2X7R in SOD1-G93A mice accelerates clinical onset and worsens disease progression, leading to increased astrogliosis, microgliosis, motoneuron loss, and pro-inflammatory markers (Apolloni et al., 2013a). Conversely, BBG treatment in the late pre-symptomatic phase enhances motor neuron survival, reduces microgliosis in the lumbar spinal cord, and lowers inflammatory markers such as NADPH-2 oxidase, IL-1β, and BDNF (Apolloni et al., 2014). This suggests that P2X7R activation might be beneficial in early disease stages but harmful later on. However, other studies report only mild effects with P2X7R antagonism in female SOD1-G93A mice using BBG, and no effect with the highly selective P2X7R antagonist JNJ-47965567, indicating the influence of factors such as antagonist type, dosage, administration method, disease phase, and mouse gender on treatment efficacy (Bartlett et al., 2017; Sluyter et al., 2017; Ly et al., 2020).
In summary, P2X7R plays a complex and multifaceted role in ALS pathogenesis. Its activation in spinal cord microglia and astrocytes leads to neuroinflammation and motor neuron death through mechanisms involving the NLRP3 inflammasome, ROS, and ATP production. The contrasting effects of P2X7R activation at different disease stages complicate its therapeutic potential. Further research should focus on understanding how P2X7R contributes to ALS pathology and refining the use of P2X7R modulators for effective treatment of ALS.
8 Research gaps and future perspectives for P2X7R-targeted therapy
The P2X7 receptor has emerged as a promising therapeutic target for various neurodegenerative disorders, including AD, PD, MS, and ALS. Despite the growing body of evidence supporting the involvement of P2X7R in the pathogenesis of these diseases and its potential as a therapeutic target, several research gaps and challenges need to be addressed to fully harness the therapeutic potential of P2X7R-targeted therapies.
One of the primary challenges is the complex and multifaceted role of P2X7R in neuroinflammation and neurodegeneration. While P2X7R activation is generally associated with pro-inflammatory responses and neurotoxicity, some studies have suggested that brief P2X7R stimulation may have neuroprotective effects, particularly in the early stages of disease progression. This dual function of P2X7R highlights the need for a more nuanced understanding of its role in different disease contexts and stages. Future research should focus on elucidating the precise mechanisms underlying the beneficial and detrimental effects of P2X7R activation, as well as identifying the optimal therapeutic window for P2X7R-targeted interventions.
Another important aspect is the characterization of P2X7R expression in different cell types within the central nervous system, particularly in neurons. While P2X7Rs are predominantly expressed in glial cells, such as microglia and astrocytes, their presence in neurons remains a topic of ongoing debate (Illes et al., 2017). Some studies have reported the expression of functional P2X7Rs in neurons, suggesting their involvement in neurotransmission, synaptic plasticity, and neuronal survival (Armstrong et al., 2002; Miras-Portugal et al., 2003). However, other studies have questioned the specificity of the antibodies and techniques used to detect neuronal P2X7Rs, leading to conflicting results (Anderson and Nedergaard, 2006; Illes et al., 2017). To resolve this controversy, future research should focus on developing highly specific tools for detecting P2X7R expression in neurons, such as novel antibodies, reporter mouse lines, or in situ hybridization techniques (Kaczmarek-Hajek et al., 2018). Additionally, investigating the functional role of neuronal P2X7Rs in different brain regions and under various physiological and pathological conditions will provide valuable insights into their potential involvement in neurodegenerative processes. Elucidating the cell-type specific expression and function of P2X7Rs will contribute to a better understanding of their therapeutic potential and guide the development of targeted interventions for neurodegenerative diseases.
To address these challenges, a comprehensive understanding of the molecular mechanisms underlying P2X7R signaling is crucial. While the P2X7R has been implicated in various cellular processes, including cell activation, calcium signaling, and cytokine release, the intricate molecular mechanisms underlying these effects remain elusive. Employing systems-level approaches, such as omics technologies and computational modeling, could shed light on these mechanisms and facilitate the development of more selective and effective P2X7R-targeted therapeutics.
Moreover, clarifying the context-specific role of P2X7R in different cell types and disease stages is essential. The P2X7R is predominantly expressed in immune cells like microglia and is also found in astrocytes. However, it is important to note that there is an ongoing debate regarding the existence of P2X7R in neurons (Illes et al., 2017; Miras-Portugal et al., 2017; Kaczmarek-Hajek et al., 2018). While some studies have reported the presence of P2X7R in neurons, others have questioned the specificity of the antibodies and techniques used to detect neuronal P2X7R expression. As a result, the extent of P2X7R expression and function in neurons remains a topic of active investigation. Nonetheless, a more nuanced understanding of the spatiotemporal dynamics of P2X7R expression and function across glial cell types and disease stages will help to determine its overall contribution to neurodegenerative disorders and inform the development of targeted therapies.
Another important aspect is the characterization of the functional diversity of P2X7R isoforms. Numerous P2X7R isoforms have been identified, yet their functional significance and involvement in disease progression remain poorly understood. Systematic functional analyses of distinct P2X7R isoforms could reveal novel therapeutic targets and provide insight into disease-specific mechanisms.
The development of brain-penetrant P2X7R antagonists remains a significant hurdle in the pursuit of effective therapies for neurodegenerative disorders. While some compounds, such as JNJ-54175446 and JNJ-55308942, have demonstrated improved brain penetrance and promising results in preclinical studies, further research is needed to optimize their pharmacokinetic and pharmacodynamic properties and assess their safety and efficacy in clinical trials. Moreover, efforts are needed to develop more selective and potent P2X7R modulators that can effectively penetrate the BBB and exhibit favorable pharmacological properties.
Addressing the translational gap in P2X7R-targeted therapies is another critical aspect. While preclinical studies have shown encouraging results, more extensive clinical trials are required to validate the long-term safety and efficacy of P2X7R-targeted therapies. Rigorous assessment of potential off-target effects, optimal dosing, treatment duration, and patient stratification strategies will be critical to the successful translation of these therapies to the clinic.
Finally, given the complex and multifactorial nature of neurodegenerative disorders, P2X7R-targeted therapies may need to be combined with other therapeutic approaches to achieve maximal clinical benefit. Systematic identification of synergistic combinations of P2X7R-targeted therapies with other treatments, such as immunomodulatory, neuroprotective, or neurorestorative agents, will be essential for optimizing their therapeutic potential. Exploring alternative strategies for modulating P2X7R function, such as targeting downstream effectors of P2X7R signaling or investigating the potential of gene therapy or RNA-based therapeutics, may also offer new avenues for therapeutic development.
In conclusion, while P2X7R-targeted therapy holds great promise for the treatment of neurodegenerative disorders, significant research gaps and challenges remain. Addressing these issues will require a concerted effort from the scientific community, involving a combination of basic research, preclinical studies, and well-designed clinical trials. By deciphering the complex molecular mechanisms of P2X7R signaling, clarifying its context-specific role in different cell types and disease stages, characterizing the functional diversity of isoforms, overcoming pharmacological challenges, addressing the translational gap, and unraveling the potential of combination therapies, we may pave the way for the development of more effective and targeted interventions for these devastating diseases. Future perspectives for P2X7R-targeted therapy development should focus on these critical aspects to maximize the therapeutic potential of this promising target.
Author contributions
XL: Investigation, Writing–original draft. YL: Writing–original draft. LH: Writing–original draft. YK: Writing–original draft. XW: Writing–original draft. XM: Writing–original draft. BZ: Writing–review and editing. JL: Writing–review and editing.
Funding
The author(s) declare that financial support was received for the research, authorship, and/or publication of this article. High-quality development project of public hospitals in Baoan District, Shenzhen. Nos YNXM2024016, YNXM2024062, and YNXM2024017.
Conflict of interest
The authors declare that the research was conducted in the absence of any commercial or financial relationships that could be construed as a potential conflict of interest.
Publisher’s note
All claims expressed in this article are solely those of the authors and do not necessarily represent those of their affiliated organizations, or those of the publisher, the editors and the reviewers. Any product that may be evaluated in this article, or claim that may be made by its manufacturer, is not guaranteed or endorsed by the publisher.
References
Abberley, L., Bebius, A., Beswick, P. J., Billinton, A., Collis, K. L., Dean, D. K., et al. (2010). Identification of 2-oxo-N-(phenylmethyl)-4-imidazolidinecarboxamide antagonists of the P2X(7) receptor. Bioorg. Med. Chem. Lett. 20, 6370–6374. doi:10.1016/j.bmcl.2010.09.101
Abdi, M. H., Beswick, P. J., Billinton, A., Chambers, L. J., Charlton, A., Collins, S. D., et al. (2010). Discovery and structure-activity relationships of a series of pyroglutamic acid amide antagonists of the P2X7 receptor. Bioorg. Med. Chem. Lett. 20, 5080–5084. doi:10.1016/j.bmcl.2010.07.033
Able, S. L., Fish, R. L., Bye, H., Booth, L., Logan, Y. R., Nathaniel, C., et al. (2011). Receptor localization, native tissue binding and ex vivo occupancy for centrally penetrant P2X7 antagonists in the rat. Br. J. Pharmacol. 162, 405–414. doi:10.1111/j.1476-5381.2010.01025.x
Adinolfi, E., Cirillo, M., Woltersdorf, R., Falzoni, S., Chiozzi, P., Pellegatti, P., et al. (2010). Trophic activity of a naturally occurring truncated isoform of the P2X7 receptor. FASEB J. Off. Publ. Fed. Am. Soc. Exp. Biol. 24, 3393–3404. doi:10.1096/fj.09-153601
Adriouch, S., Bannas, P., Schwarz, N., Fliegert, R., Guse, A. H., Seman, M., et al. (2008). ADP-ribosylation at R125 gates the P2X7 ion channel by presenting a covalent ligand to its nucleotide binding site. FASEB J. Off. Publ. Fed. Am. Soc. Exp. Biol. 22, 861–869. doi:10.1096/fj.07-9294com
Ahn, Y. H., Tang, Y., and Illes, P. (2023). The neuroinflammatory astrocytic P2X7 receptor: Alzheimer’s disease, ischemic brain injury, and epileptic state. Expert Opin. Ther. Targets 27, 763–778. doi:10.1080/14728222.2023.2258281
Alberto, A. V. P., Faria, R. X., Couto, C. G. C., Ferreira, L. G. B., Souza, C. A. M., Teixeira, P. C. N., et al. (2013). Is pannexin the pore associated with the P2X7 receptor? Naunyn. Schmiedeb. Arch. Pharmacol. 386, 775–787. doi:10.1007/s00210-013-0868-x
Ali, Z., Laurijssens, B., Ostenfeld, T., McHugh, S., Stylianou, A., Scott-Stevens, P., et al. (2013). Pharmacokinetic and pharmacodynamic profiling of a P2X7 receptor allosteric modulator GSK1482160 in healthy human subjects. Br. J. Clin. Pharmacol. 75, 197–207. doi:10.1111/j.1365-2125.2012.04320.x
Allan, S. M., and Rothwell, N. J. (2001). Cytokines and acute neurodegeneration. Nat. Rev. Neurosci. 2, 734–744. doi:10.1038/35094583
Allinson, T. M. J., Parkin, E. T., Turner, A. J., and Hooper, N. M. (2003). ADAMs family members as amyloid precursor protein alpha-secretases. J. Neurosci. Res. 74, 342–352. doi:10.1002/jnr.10737
Allsopp, R. C., and Evans, R. J. (2011). The intracellular amino terminus plays a dominant role in desensitization of ATP-gated P2X receptor ion channels. J. Biol. Chem. 286, 44691–44701. doi:10.1074/jbc.M111.303917
Alves, L. A., de Melo Reis, R. A., de Souza, C. A. M., de Freitas, M. S., Teixeira, P. C. N., Neto Moreira Ferreira, D., et al. (2014). The P2X7 receptor: shifting from a low-to a high-conductance channel - an enigmatic phenomenon? Biochim. Biophys. Acta 1838, 2578–2587. doi:10.1016/j.bbamem.2014.05.015
Amadio, S., Parisi, C., Piras, E., Fabbrizio, P., Apolloni, S., Montilli, C., et al. (2017). Modulation of P2X7 receptor during inflammation in multiple sclerosis. Front. Immunol. 8, 1529. doi:10.3389/fimmu.2017.01529
Andersen, J. V., Schousboe, A., and Verkhratsky, A. (2022). Astrocyte energy and neurotransmitter metabolism in Alzheimer’s disease: integration of the glutamate/GABA-glutamine cycle. Prog. Neurobiol. 217, 102331. doi:10.1016/j.pneurobio.2022.102331
Anderson, C. M., and Nedergaard, M. (2006). Emerging challenges of assigning P2X7 receptor function and immunoreactivity in neurons. Trends Neurosci. 29, 257–262. doi:10.1016/j.tins.2006.03.003
Apolloni, S., Amadio, S., Montilli, C., Volonté, C., and D’Ambrosi, N. (2013a). Ablation of P2X7 receptor exacerbates gliosis and motoneuron death in the SOD1-G93A mouse model of amyotrophic lateral sclerosis. Hum. Mol. Genet. 22, 4102–4116. doi:10.1093/hmg/ddt259
Apolloni, S., Amadio, S., Parisi, C., Matteucci, A., Potenza, R. L., Armida, M., et al. (2014). Spinal cord pathology is ameliorated by P2X7 antagonism in a SOD1-mutant mouse model of amyotrophic lateral sclerosis. Dis. Model. Mech. 7, 1101–1109. doi:10.1242/dmm.017038
Apolloni, S., Parisi, C., Pesaresi, M. G., Rossi, S., Carrì, M. T., Cozzolino, M., et al. (2013b). The NADPH oxidase pathway is dysregulated by the P2X7 receptor in the SOD1-G93A microglia model of amyotrophic lateral sclerosis. J. Immunol. 190, 5187–5195. doi:10.4049/jimmunol.1203262
Armstrong, J. N., Brust, T. B., Lewis, R. G., and MacVicar, B. A. (2002). Activation of presynaptic P2X7-like receptors depresses mossy fiber-CA3 synaptic transmission through p38 mitogen-activated protein kinase. J. Neurosci. Off. J. Soc. Neurosci. 22, 5938–5945. doi:10.1523/JNEUROSCI.22-14-05938.2002
Baeza-Raja, B., Goodyear, A., Liu, X., Lam, K., Yamamoto, L., Li, Y., et al. (2020). Pharmacological inhibition of P2RX7 ameliorates liver injury by reducing inflammation and fibrosis. PLoS One 15, e0234038. doi:10.1371/journal.pone.0234038
Bai, R., Lang, Y., Shao, J., Deng, Y., Refuhati, R., and Cui, L. (2021). The role of NLRP3 inflammasome in cerebrovascular diseases pathology and possible therapeutic targets. ASN Neuro 13, 17590914211018100. doi:10.1177/17590914211018100
Bartlett, R., Sluyter, V., Watson, D., Sluyter, R., and Yerbury, J. J. (2017). P2X7 antagonism using Brilliant Blue G reduces body weight loss and prolongs survival in female SOD1(G93A) amyotrophic lateral sclerosis mice. PeerJ 5, e3064. doi:10.7717/peerj.3064
Bartlett, R., Yerbury, J. J., and Sluyter, R. (2013). P2X7 receptor activation induces reactive oxygen species formation and cell death in murine EOC13 microglia. Mediat. Inflamm. 2013, 271813. doi:10.1155/2013/271813
Beamer, E., Fischer, W., and Engel, T. (2017). The ATP-gated P2X7 receptor as a target for the treatment of drug-resistant epilepsy. Front. Neurosci. 11, 21. doi:10.3389/fnins.2017.00021
Beigi, R. D., Kertesy, S. B., Aquilina, G., and Dubyak, G. R. (2003). Oxidized ATP (oATP) attenuates proinflammatory signaling via P2 receptor-independent mechanisms. Br. J. Pharmacol. 140, 507–519. doi:10.1038/sj.bjp.0705470
Beltran-Lobo, P., Reid, M. J., Jimenez-Sanchez, M., Verkhratsky, A., Perez-Nievas, B. G., and Noble, W. (2023). Astrocyte adaptation in Alzheimer’s disease: a focus on astrocytic P2X7R. Essays Biochem. 67, 119–130. doi:10.1042/EBC20220079
Bhattacharya, A., and Biber, K. (2016). The microglial ATP-gated ion channel P2X7 as a CNS drug target. Glia 64, 1772–1787. doi:10.1002/glia.23001
Bhattacharya, A., Lord, B., Grigoleit, J.-S., He, Y., Fraser, I., Campbell, S. N., et al. (2018). Neuropsychopharmacology of JNJ-55308942: evaluation of a clinical candidate targeting P2X7 ion channels in animal models of neuroinflammation and anhedonia. Neuropsychopharmacol. 43, 2586–2596. doi:10.1038/s41386-018-0141-6
Bhattacharya, A., Wang, Q., Ao, H., Shoblock, J. R., Lord, B., Aluisio, L., et al. (2013). Pharmacological characterization of a novel centrally permeable P2X7 receptor antagonist: JNJ-47965567. Br. J. Pharmacol. 170, 624–640. doi:10.1111/bph.12314
Bian, S., Sun, X., Bai, A., Zhang, C., Li, L., Enjyoji, K., et al. (2013). P2X7 integrates PI3K/AKT and AMPK-PRAS40-mTOR signaling pathways to mediate tumor cell death. PLoS One 8, e60184. doi:10.1371/journal.pone.0060184
Bianco, F., Ceruti, S., Colombo, A., Fumagalli, M., Ferrari, D., Pizzirani, C., et al. (2006). A role for P2X7 in microglial proliferation. J. Neurochem. 99, 745–758. doi:10.1111/j.1471-4159.2006.04101.x
Bin Dayel, A., Evans, R. J., and Schmid, R. (2019). Mapping the site of action of human P2X7 receptor antagonists AZ11645373, brilliant blue G, KN-62, calmidazolium, and ZINC58368839 to the intersubunit allosteric pocket. Mol. Pharmacol. 96, 355–363. doi:10.1124/mol.119.116715
Blum-Degen, D., Müller, T., Kuhn, W., Gerlach, M., Przuntek, H., and Riederer, P. (1995). Interleukin-1 beta and interleukin-6 are elevated in the cerebrospinal fluid of Alzheimer’s and de novo Parkinson’s disease patients. Neurosci. Lett. 202, 17–20. doi:10.1016/0304-3940(95)12192-7
Bradley, H. J., Baldwin, J. M., Goli, G. R., Johnson, B., Zou, J., Sivaprasadarao, A., et al. (2011). Residues 155 and 348 contribute to the determination of P2X7 receptor function via distinct mechanisms revealed by single-nucleotide polymorphisms. J. Biol. Chem. 286, 8176–8187. doi:10.1074/jbc.M110.211284
Brown, G. C. (2019). The endotoxin hypothesis of neurodegeneration. J. Neuroinflammation 16, 180. doi:10.1186/s12974-019-1564-7
Burnstock, G. (2017a). Purinergic signalling: therapeutic developments. Front. Pharmacol. 8, 661. doi:10.3389/fphar.2017.00661
Burnstock, G. (2017b). Purinergic signalling and neurological diseases: an update. CNS Neurol. Disord. Drug Targets 16, 257–265. doi:10.2174/1871527315666160922104848
Camden, J. M., Schrader, A. M., Camden, R. E., González, F. A., Erb, L., Seye, C. I., et al. (2005). P2Y2 nucleotide receptors enhance alpha-secretase-dependent amyloid precursor protein processing. J. Biol. Chem. 280, 18696–18702. doi:10.1074/jbc.M500219200
Carmo, M. R. S., Menezes, A. P. F., Nunes, A. C. L., Pliássova, A., Rolo, A. P., Palmeira, C. M., et al. (2014). The P2X7 receptor antagonist Brilliant Blue G attenuates contralateral rotations in a rat model of Parkinsonism through a combined control of synaptotoxicity, neurotoxicity and gliosis. Neuropharmacology 81, 142–152. doi:10.1016/j.neuropharm.2014.01.045
Cartier, L., Hartley, O., Dubois-Dauphin, M., and Krause, K.-H. (2005). Chemokine receptors in the central nervous system: role in brain inflammation and neurodegenerative diseases. Brain Res. Brain Res. Rev. 48, 16–42. doi:10.1016/j.brainresrev.2004.07.021
Carvalho, K., Martin, E., Ces, A., Sarrazin, N., Lagouge-Roussey, P., Nous, C., et al. (2021). P2X7-deficiency improves plasticity and cognitive abilities in a mouse model of Tauopathy. Prog. Neurobiol. 206, 102139. doi:10.1016/j.pneurobio.2021.102139
Chambers, L. J., Stevens, A. J., Moses, A. P., Michel, A. D., Walter, D. S., Davies, D. J., et al. (2010). Synthesis and structure-activity relationships of a series of (1H-pyrazol-4-yl)acetamide antagonists of the P2X7 receptor. Bioorg. Med. Chem. Lett. 20, 3161–3164. doi:10.1016/j.bmcl.2010.03.096
Chen, L., and Brosnan, C. F. (2006). Exacerbation of experimental autoimmune encephalomyelitis in P2X7R-/- mice: evidence for loss of apoptotic activity in lymphocytes. J. Immunol. 176, 3115–3126. doi:10.4049/jimmunol.176.5.3115
Chen, S., Ma, Q., Krafft, P. R., Hu, Q., Rolland, W., Sherchan, P., et al. (2013). P2X7R/cryopyrin inflammasome axis inhibition reduces neuroinflammation after SAH. Neurobiol. Dis. 58, 296–307. doi:10.1016/j.nbd.2013.06.011
Chen, X., Pierce, B., Naing, W., Grapperhaus, M. L., and Phillion, D. P. (2010). Discovery of 2-chloro-N-((4,4-difluoro-1-hydroxycyclohexyl)methyl)-5-(5-fluoropyrimidin-2-yl)benzamide as a potent and CNS penetrable P2X7 receptor antagonist. Bioorg. Med. Chem. Lett. 20, 3107–3111. doi:10.1016/j.bmcl.2010.03.094
Chen, Y., Corriden, R., Inoue, Y., Yip, L., Hashiguchi, N., Zinkernagel, A., et al. (2006). ATP release guides neutrophil chemotaxis via P2Y2 and A3 receptors. Science 314, 1792–1795. doi:10.1126/science.1132559
Chitnis, T., and Weiner, H. L. (2017). CNS inflammation and neurodegeneration. J. Clin. Invest. 127, 3577–3587. doi:10.1172/JCI90609
Chow, S.-K., Yu, D., Macdonald, C. L., Buibas, M., and Silva, G. A. (2010). Amyloid β-peptide directly induces spontaneous calcium transients, delayed intercellular calcium waves and gliosis in rat cortical astrocytes. ASN Neuro 2, e00026. doi:10.1042/AN20090035
Chrovian, C. C., Soyode-Johnson, A., Peterson, A. A., Gelin, C. F., Deng, X., Dvorak, C. A., et al. (2018). A dipolar cycloaddition reaction to access 6-Methyl-4,5,6,7-tetrahydro-1H-[1,2,3]triazolo[4,5-c]pyridines enables the discovery synthesis and preclinical profiling of a P2X7 antagonist clinical candidate. J. Med. Chem. 61, 207–223. doi:10.1021/acs.jmedchem.7b01279
Cieślak, M., Roszek, K., and Wujak, M. (2019). Purinergic implication in amyotrophic lateral sclerosis-from pathological mechanisms to therapeutic perspectives. Purinergic Signal 15, 1–15. doi:10.1007/s11302-018-9633-4
Collo, G., Neidhart, S., Kawashima, E., Kosco-Vilbois, M., North, R. A., and Buell, G. (1997). Tissue distribution of the P2X7 receptor. Neuropharmacology 36, 1277–1283. doi:10.1016/s0028-3908(97)00140-8
Costa-Junior, H. M., Sarmento Vieira, F., and Coutinho-Silva, R. (2011). C terminus of the P2X7 receptor: treasure hunting. Purinergic Signal 7, 7–19. doi:10.1007/s11302-011-9215-1
Crabbé, M., Van der Perren, A., Bollaerts, I., Kounelis, S., Baekelandt, V., Bormans, G., et al. (2019). Increased P2X7 receptor binding is associated with neuroinflammation in acute but not chronic rodent models for Parkinson’s disease. Front. Neurosci. 13, 799. doi:10.3389/fnins.2019.00799
Cui, L., Tan, Y.-J., Xu, S.-Q., Qin, B.-F., Xiu, M.-X., Zhang, X., et al. (2023). Ginsenoside Rd, a natural production for attenuating fibrogenesis and inflammation in hepatic fibrosis by regulating the ERRα-mediated P2X7r pathway. Food Funct. 14, 5606–5619. doi:10.1039/d3fo01315d
D’Ambrosi, N., Finocchi, P., Apolloni, S., Cozzolino, M., Ferri, A., Padovano, V., et al. (2009). The proinflammatory action of microglial P2 receptors is enhanced in SOD1 models for amyotrophic lateral sclerosis. J. Immunol. 183, 4648–4656. doi:10.4049/jimmunol.0901212
Darmellah, A., Rayah, A., Auger, R., Cuif, M.-H., Prigent, M., Arpin, M., et al. (2012). Ezrin/radixin/moesin are required for the purinergic P2X7 receptor (P2X7R)-dependent processing of the amyloid precursor protein. J. Biol. Chem. 287, 34583–34595. doi:10.1074/jbc.M112.400010
Delarasse, C., Auger, R., Gonnord, P., Fontaine, B., and Kanellopoulos, J. M. (2011). The purinergic receptor P2X7 triggers alpha-secretase-dependent processing of the amyloid precursor protein. J. Biol. Chem. 286, 2596–2606. doi:10.1074/jbc.M110.200618
Delarasse, C., Gonnord, P., Galante, M., Auger, R., Daniel, H., Motta, I., et al. (2009). Neural progenitor cell death is induced by extracellular ATP via ligation of P2X7 receptor. J. Neurochem. 109, 846–857. doi:10.1111/j.1471-4159.2009.06008.x
De Marchi, E., Orioli, E., Dal Ben, D., and Adinolfi, E. (2016). P2X7 receptor as a therapeutic target. Adv. Protein Chem. Struct. Biol. 104, 39–79. doi:10.1016/bs.apcsb.2015.11.004
Dendrou, C. A., Fugger, L., and Friese, M. A. (2015). Immunopathology of multiple sclerosis. Nat. Rev. Immunol. 15, 545–558. doi:10.1038/nri3871
Denlinger, L. C., Fisette, P. L., Sommer, J. A., Watters, J. J., Prabhu, U., Dubyak, G. R., et al. (2001). Cutting edge: the nucleotide receptor P2X7 contains multiple protein- and lipid-interaction motifs including a potential binding site for bacterial lipopolysaccharide. J. Immunol. 167, 1871–1876. doi:10.4049/jimmunol.167.4.1871
Deora, V., Lee, J. D., Albornoz, E. A., McAlary, L., Jagaraj, C. J., Robertson, A. A. B., et al. (2020). The microglial NLRP3 inflammasome is activated by amyotrophic lateral sclerosis proteins. Glia 68, 407–421. doi:10.1002/glia.23728
De Salis, S. K. F., Li, L., Chen, Z., Lam, K. W., Skarratt, K. K., Balle, T., et al. (2022). Alternatively spliced isoforms of the P2X7 receptor: structure, function and disease associations. Int. J. Mol. Sci. 23, 8174. doi:10.3390/ijms23158174
Di, A., Xiong, S., Ye, Z., Malireddi, R. K. S., Kometani, S., Zhong, M., et al. (2018). The TWIK2 potassium efflux channel in macrophages mediates NLRP3 inflammasome-induced inflammation. Immunity 49, 56–65. doi:10.1016/j.immuni.2018.04.032
Diaz-Hernandez, J. I., Gomez-Villafuertes, R., León-Otegui, M., Hontecillas-Prieto, L., Del Puerto, A., Trejo, J. L., et al. (2012). In vivo P2X7 inhibition reduces amyloid plaques in Alzheimer's disease through GSK3β and secretases. Neurobiol. Aging 33, 1816–1828. doi:10.1016/j.neurobiolaging.2011.09.040
Dibba, P., Li, A. A., Perumpail, B. J., John, N., Sallam, S., Shah, N. D., et al. (2018). Emerging therapeutic targets and experimental drugs for the treatment of NAFLD. Dis. 6, 83. doi:10.3390/diseases6030083
Di Lauro, C., Bianchi, C., Sebastián-Serrano, Á., Soria-Tobar, L., Alvarez-Castelao, B., Nicke, A., et al. (2022). P2X7 receptor blockade reduces tau induced toxicity, therapeutic implications in tauopathies. Prog. Neurobiol. 208, 102173. doi:10.1016/j.pneurobio.2021.102173
Di Virgilio, F. (2007). Liaisons dangereuses: P2X(7) and the inflammasome. Trends Pharmacol. Sci. 28, 465–472. doi:10.1016/j.tips.2007.07.002
Di Virgilio, F., Dal Ben, D., Sarti, A. C., Giuliani, A. L., and Falzoni, S. (2017). The P2X7 receptor in infection and inflammation. Immunity 47, 15–31. doi:10.1016/j.immuni.2017.06.020
Di Virgilio, F., Ferrari, D., and Adinolfi, E. (2009). P2X(7): a growth-promoting receptor-implications for cancer. Purinergic Signal 5, 251–256. doi:10.1007/s11302-009-9145-3
Di Virgilio, F., Schmalzing, G., and Markwardt, F. (2018). The elusive P2X7 macropore. Trends Cell Biol. 28, 392–404. doi:10.1016/j.tcb.2018.01.005
Domercq, M., and Matute, C. (2019). Targeting P2X4 and P2X7 receptors in multiple sclerosis. Curr. Opin. Pharmacol. 47, 119–125. doi:10.1016/j.coph.2019.03.010
Domercq, M., Zabala, A., and Matute, C. (2019). Purinergic receptors in multiple sclerosis pathogenesis. Brain Res. Bull. 151, 38–45. doi:10.1016/j.brainresbull.2018.11.018
Donnelly-Roberts, D. L., Namovic, M. T., Han, P., and Jarvis, M. F. (2009). Mammalian P2X7 receptor pharmacology: comparison of recombinant mouse, rat and human P2X7 receptors. Br. J. Pharmacol. 157, 1203–1214. doi:10.1111/j.1476-5381.2009.00233.x
Dos-Santos-Pereira, M., Acuña, L., Hamadat, S., Rocca, J., González-Lizárraga, F., Chehín, R., et al. (2018). Microglial glutamate release evoked by α-synuclein aggregates is prevented by dopamine. Glia 66, 2353–2365. doi:10.1002/glia.23472
Drinkall, S., Lawrence, C. B., Ossola, B., Russell, S., Bender, C., Brice, N. B., et al. (2022). The two pore potassium channel THIK-1 regulates NLRP3 inflammasome activation. Glia 70, 1301–1316. doi:10.1002/glia.24174
Duplantier, A. J., Dombroski, M. A., Subramanyam, C., Beaulieu, A. M., Chang, S.-P., Gabel, C. A., et al. (2011). Optimization of the physicochemical and pharmacokinetic attributes in a 6-azauracil series of P2X7 receptor antagonists leading to the discovery of the clinical candidate CE-224,535. Bioorg. Med. Chem. Lett. 21, 3708–3711. doi:10.1016/j.bmcl.2011.04.077
Durner, A., Durner, E., and Nicke, A. (2023). Improved ANAP incorporation and VCF analysis reveal details of P2X7 current facilitation and a limited conformational interplay between ATP binding and the intracellular ballast domain. Elife 12, e82479. doi:10.7554/eLife.82479
Dutta, G., Zhang, P., and Liu, B. (2008). The lipopolysaccharide Parkinson’s disease animal model: mechanistic studies and drug discovery. Fundam. Clin. Pharmacol. 22, 453–464. doi:10.1111/j.1472-8206.2008.00616.x
Elssner, A., Duncan, M., Gavrilin, M., and Wewers, M. D. (2004). A novel P2X7 receptor activator, the human cathelicidin-derived peptide LL37, induces IL-1 beta processing and release. J. Immunol. 172, 4987–4994. doi:10.4049/jimmunol.172.8.4987
Eser, A., Colombel, J.-F., Rutgeerts, P., Vermeire, S., Vogelsang, H., Braddock, M., et al. (2015). Safety and efficacy of an oral inhibitor of the purinergic receptor P2X7 in adult patients with moderately to severely active Crohn’s disease: a randomized placebo-controlled, double-blind, phase IIa study. Inflamm. Bowel Dis. 21, 2247–2253. doi:10.1097/MIB.0000000000000514
Fabbrizio, P., Amadio, S., Apolloni, S., and Volonté, C. (2017). P2X7 receptor activation modulates autophagy in SOD1-g93a mouse microglia. Front. Cell. Neurosci. 11, 249. doi:10.3389/fncel.2017.00249
Fan, Z., Wang, K., Zhao, X., and Sun, X. (2024). P2X7 receptor: a receptor closely linked with sepsis-associated encephalopathy. Open life Sci. 19, 20220775. doi:10.1515/biol-2022-0775
Fellin, T., Pozzan, T., and Carmignoto, G. (2006). Purinergic receptors mediate two distinct glutamate release pathways in hippocampal astrocytes. J. Biol. Chem. 281, 4274–4284. doi:10.1074/jbc.M510679200
Ferrari, D., Pizzirani, C., Adinolfi, E., Lemoli, R. M., Curti, A., Idzko, M., et al. (2006). The P2X7 receptor: a key player in IL-1 processing and release. J. Immunol. 176, 3877–3883. doi:10.4049/jimmunol.176.7.3877
Ferrari, D., Stroh, C., and Schulze-Osthoff, K. (1999). P2X7/P2Z purinoreceptor-mediated activation of transcription factor NFAT in microglial cells. J. Biol. Chem. 274, 13205–13210. doi:10.1074/jbc.274.19.13205
Ferrazoli, E. G., de Souza, H. D. N., Nascimento, I. C., Oliveira-Giacomelli, Á., Schwindt, T. T., Britto, L. R., et al. (2017). Brilliant blue G, but not fenofibrate, treatment reverts hemiparkinsonian behavior and restores dopamine levels in an animal model of Parkinson’s disease. Cell Transpl. 26, 669–677. doi:10.3727/096368917X695227
Ficker, C., Rozmer, K., Kató, E., Andó, R. D., Schumann, L., Krügel, U., et al. (2014). Astrocyte-neuron interaction in the substantia gelatinosa of the spinal cord dorsal horn via P2X7 receptor-mediated release of glutamate and reactive oxygen species. Glia 62, 1671–1686. doi:10.1002/glia.22707
Franceschini, A., Capece, M., Chiozzi, P., Falzoni, S., Sanz, J. M., Sarti, A. C., et al. (2015). The P2X7 receptor directly interacts with the NLRP3 inflammasome scaffold protein. FASEB J. Off. Publ. Fed. Am. Soc. Exp. Biol. 29, 2450–2461. doi:10.1096/fj.14-268714
Gandelman, M., Peluffo, H., Beckman, J. S., Cassina, P., and Barbeito, L. (2010). Extracellular ATP and the P2X7 receptor in astrocyte-mediated motor neuron death: implications for amyotrophic lateral sclerosis. J. Neuroinflammation 7, 33. doi:10.1186/1742-2094-7-33
Gao, C., Jiang, J., Tan, Y., and Chen, S. (2023). Microglia in neurodegenerative diseases: mechanism and potential therapeutic targets. Signal Transduct. Target Ther. 8, 359. doi:10.1038/s41392-023-01588-0
Gargett, C. E., and Wiley, J. S. (1997). The isoquinoline derivative KN-62 a potent antagonist of the P2Z-receptor of human lymphocytes. Br. J. Pharmacol. 120, 1483–1490. doi:10.1038/sj.bjp.0701081
Gartland, A., Skarratt, K. K., Hocking, L. J., Parsons, C., Stokes, L., Jørgensen, N. R., et al. (2012). Polymorphisms in the P2X7 receptor gene are associated with low lumbar spine bone mineral density and accelerated bone loss in post-menopausal women. Eur. J. Hum. Genet. 20, 559–564. doi:10.1038/ejhg.2011.245
GBD 2016 Neurology Collaborators (2019). Global, regional, and national burden of neurological disorders, 1990-2016: a systematic analysis for the Global Burden of Disease Study 2016. Lancet. Neurol. 18, 459–480. doi:10.1016/S1474-4422(18)30499-X
Genetzakis, E., Gilchrist, J., Kassiou, M., and Figtree, G. A. (2022). Development and clinical translation of P2X7 receptor antagonists: a potential therapeutic target in coronary artery disease? Pharmacol. Ther. 237, 108228. doi:10.1016/j.pharmthera.2022.108228
Ghasemi, M., and Brown, R. H. J. (2018). Genetics of amyotrophic lateral sclerosis. Cold Spring Harb. Perspect. Med. 8, a024125. doi:10.1101/cshperspect.a024125
Glass, C. K., Saijo, K., Winner, B., Marchetto, M. C., and Gage, F. H. (2010). Mechanisms underlying inflammation in neurodegeneration. Cell 140, 918–934. doi:10.1016/j.cell.2010.02.016
Grad, L. I., Rouleau, G. A., Ravits, J., and Cashman, N. R. (2017). Clinical spectrum of amyotrophic lateral sclerosis (ALS). Cold Spring Harb. Perspect. Med. 7, a024117. doi:10.1101/cshperspect.a024117
Grygorowicz, T., Dąbrowska-Bouta, B., and Strużyńska, L. (2018). Administration of an antagonist of P2X7 receptor to EAE rats prevents a decrease of expression of claudin-5 in cerebral capillaries. Purinergic Signal 14, 385–393. doi:10.1007/s11302-018-9620-9
Grygorowicz, T., Wełniak-Kamińska, M., and Strużyńska, L. (2016). Early P2X7R-related astrogliosis in autoimmune encephalomyelitis. Mol. Cell. Neurosci. 74, 1–9. doi:10.1016/j.mcn.2016.02.003
Gu, B. J., Field, J., Dutertre, S., Ou, A., Kilpatrick, T. J., Lechner-Scott, J., et al. (2015). A rare P2X7 variant Arg307Gln with absent pore formation function protects against neuroinflammation in multiple sclerosis. Hum. Mol. Genet. 24, 5644–5654. doi:10.1093/hmg/ddv278
Gu, B. J., Rathsam, C., Stokes, L., McGeachie, A. B., and Wiley, J. S. (2009). Extracellular ATP dissociates nonmuscle myosin from P2X(7) complex: this dissociation regulates P2X(7) pore formation. Am. J. Physiol. Cell Physiol. 297, C430–C439. doi:10.1152/ajpcell.00079.2009
Gu, B. J., Saunders, B. M., Jursik, C., and Wiley, J. S. (2010). The P2X7-nonmuscle myosin membrane complex regulates phagocytosis of nonopsonized particles and bacteria by a pathway attenuated by extracellular ATP. Blood 115, 1621–1631. doi:10.1182/blood-2009-11-251744
Gu, B. J., Saunders, B. M., Petrou, S., and Wiley, J. S. (2011). P2X(7) is a scavenger receptor for apoptotic cells in the absence of its ligand, extracellular ATP. J. Immunol. 187, 2365–2375. doi:10.4049/jimmunol.1101178
Gu, B. J., and Wiley, J. S. (2018). P2X7 as a scavenger receptor for innate phagocytosis in the brain. Br. J. Pharmacol. 175, 4195–4208. doi:10.1111/bph.14470
Guile, S. D., Alcaraz, L., Birkinshaw, T. N., Bowers, K. C., Ebden, M. R., Furber, M., et al. (2009). Antagonists of the P2X(7) receptor. From lead identification to drug development. J. Med. Chem. 52, 3123–3141. doi:10.1021/jm801528x
Gulbransen, B. D., Bashashati, M., Hirota, S. A., Gui, X., Roberts, J. A., MacDonald, J. A., et al. (2012). Activation of neuronal P2X7 receptor-pannexin-1 mediates death of enteric neurons during colitis. Nat. Med. 18, 600–604. doi:10.1038/nm.2679
Gustin, A., Kirchmeyer, M., Koncina, E., Felten, P., Losciuto, S., Heurtaux, T., et al. (2015). NLRP3 inflammasome is expressed and functional in mouse brain microglia but not in astrocytes. PLoS One 10, e0130624. doi:10.1371/journal.pone.0130624
Han, J., Liu, H., Liu, C., Jin, H., Perlmutter, J. S., Egan, T. M., et al. (2017). Pharmacologic characterizations of a P2X7 receptor-specific radioligand, [11C]GSK1482160 for neuroinflammatory response. Nucl. Med. Commun. 38, 372–382. doi:10.1097/MNM.0000000000000660
Hansen, M. A., Barden, J. A., Balcar, V. J., Keay, K. A., and Bennett, M. R. (1997). Structural motif and characteristics of the extracellular domain of P2X receptors. Biochem. Biophys. Res. Commun. 236, 670–675. doi:10.1006/bbrc.1997.6815
Harkat, M., Peverini, L., Cerdan, A. H., Dunning, K., Beudez, J., Martz, A., et al. (2017). On the permeation of large organic cations through the pore of ATP-gated P2X receptors. Proc. Natl. Acad. Sci. U. S. A. 114, E3786–E3795. doi:10.1073/pnas.1701379114
Hattori, M., and Gouaux, E. (2012). Molecular mechanism of ATP binding and ion channel activation in P2X receptors. Nature 485, 207–212. doi:10.1038/nature11010
Haughey, N. J., and Mattson, M. P. (2003). Alzheimer’s amyloid beta-peptide enhances ATP/gap junction-mediated calcium-wave propagation in astrocytes. Neuromolecular Med. 3, 173–180. doi:10.1385/NMM:3:3:173
He, Y., Taylor, N., Fourgeaud, L., and Bhattacharya, A. (2017). The role of microglial P2X7: modulation of cell death and cytokine release. J. Neuroinflammation 14, 135. doi:10.1186/s12974-017-0904-8
Hefendehl, J. K., LeDue, J., Ko, R. W. Y., Mahler, J., Murphy, T. H., and MacVicar, B. A. (2016). Mapping synaptic glutamate transporter dysfunction in vivo to regions surrounding Aβ plaques by iGluSnFR two-photon imaging. Nat. Commun. 7, 13441. doi:10.1038/ncomms13441
Helliwell, R. M., ShioukHuey, C. O., Dhuna, K., Molero, J. C., Ye, J.-M., Xue, C. C., et al. (2015). Selected ginsenosides of the protopanaxdiol series are novel positive allosteric modulators of P2X7 receptors. Br. J. Pharmacol. 172, 3326–3340. doi:10.1111/bph.13123
Herrera, A. J., Castaño, A., Venero, J. L., Cano, J., and Machado, A. (2000). The single intranigral injection of LPS as a new model for studying the selective effects of inflammatory reactions on dopaminergic system. Neurobiol. Dis. 7, 429–447. doi:10.1006/nbdi.2000.0289
Hewinson, J., and Mackenzie, A. B. (2007). P2X(7) receptor-mediated reactive oxygen and nitrogen species formation: from receptor to generators. Biochem. Soc. Trans. 35, 1168–1170. doi:10.1042/BST0351168
Hide, I. (2003). Mechanism of production and release of tumor necrosis factor implicated in inflammatory diseases. Nihon Yakurigaku Zasshi 121, 163–173. doi:10.1254/fpj.121.163
Hide, I., Tanaka, M., Inoue, A., Nakajima, K., Kohsaka, S., Inoue, K., et al. (2000). Extracellular ATP triggers tumor necrosis factor-alpha release from rat microglia. J. Neurochem. 75, 965–972. doi:10.1046/j.1471-4159.2000.0750965.x
Holmes, B. B., DeVos, S. L., Kfoury, N., Li, M., Jacks, R., Yanamandra, K., et al. (2013). Heparan sulfate proteoglycans mediate internalization and propagation of specific proteopathic seeds. Proc. Natl. Acad. Sci. U. S. A. 110, E3138–E3147. doi:10.1073/pnas.1301440110
Honore, P., Donnelly-Roberts, D., Namovic, M. T., Hsieh, G., Zhu, C. Z., Mikusa, J. P., et al. (2006). A-740003 [N-(1-{[(cyanoimino)(5-quinolinylamino) methyl]amino}-2,2-dimethylpropyl)-2-(3,4-dimethoxyphenyl)acetamide], a novel and selective P2X7 receptor antagonist, dose-dependently reduces neuropathic pain in the rat. J. Pharmacol. Exp. Ther. 319, 1376–1385. doi:10.1124/jpet.106.111559
Hracskó, Z., Baranyi, M., Csölle, C., Gölöncsér, F., Madarász, E., Kittel, A., et al. (2011). Lack of neuroprotection in the absence of P2X7 receptors in toxin-induced animal models of Parkinson’s disease. Mol. Neurodegener. 6, 28. doi:10.1186/1750-1326-6-28
Huang, G., Lu, X., Qiu, Y., Bi, L., Ye, P., Yang, M., et al. (2022). Hetero-aryl bromide precursor fluorine-18 radiosynthesis and preclinical evaluation of a novel positron emission tomography (PET) tracer [(18)F]GSK1482160. Bioorg. Med. Chem. 73, 116996. doi:10.1016/j.bmc.2022.116996
Huang, Q., Ying, J., Yu, W., Dong, Y., Xiong, H., Zhang, Y., et al. (2024). P2X7 receptor: an emerging target in alzheimer’s disease. Mol. Neurobiol. 61, 2866–2880. doi:10.1007/s12035-023-03699-9
Huo, H., Fryatt, A. G., Farmer, L. K., Schmid, R., and Evans, R. J. (2018). Mapping the binding site of the P2X receptor antagonist PPADS reveals the importance of orthosteric site charge and the cysteine-rich head region. J. Biol. Chem. 293, 12820–12831. doi:10.1074/jbc.RA118.003737
Illes, P., Khan, T. M., and Rubini, P. (2017). Neuronal P2X7 receptors revisited: do they really exist? J. Neurosci. Off. J. Soc. Neurosci. 37, 7049–7062. doi:10.1523/JNEUROSCI.3103-16.2017
Illes, P., Rubini, P., Huang, L., and Tang, Y. (2019). The P2X7 receptor: a new therapeutic target in Alzheimer’s disease. Expert Opin. Ther. Targets 23, 165–176. doi:10.1080/14728222.2019.1575811
Illes, P., Rubini, P., Ulrich, H., Zhao, Y., and Tang, Y. (2020). Regulation of microglial functions by purinergic mechanisms in the healthy and diseased CNS. Cells 9, 1108. doi:10.3390/cells9051108
Jacques-Silva, M. C., Rodnight, R., Lenz, G., Liao, Z., Kong, Q., Tran, M., et al. (2004). P2X7 receptors stimulate AKT phosphorylation in astrocytes. Br. J. Pharmacol. 141, 1106–1117. doi:10.1038/sj.bjp.0705685
Jaerve, A., and Müller, H. W. (2012). Chemokines in CNS injury and repair. Cell Tissue Res. 349, 229–248. doi:10.1007/s00441-012-1427-3
Jandy, M., Noor, A., Nelson, P., Dennys, C. N., Karabinas, I. M., Pestoni, J. C., et al. (2022). Peroxynitrite nitration of Tyr 56 in Hsp90 induces PC12 cell death through P2X7R-dependent PTEN activation. Redox Biol. 50, 102247. doi:10.1016/j.redox.2022.102247
Janks, L., Sharma, C. V. R., and Egan, T. M. (2018). A central role for P2X7 receptors in human microglia. J. Neuroinflammation 15, 325. doi:10.1186/s12974-018-1353-8
Jiang, L.-H., Baldwin, J. M., Roger, S., and Baldwin, S. A. (2013). Insights into the molecular mechanisms underlying mammalian P2X7 receptor functions and contributions in diseases, revealed by structural modeling and single nucleotide polymorphisms. Front. Pharmacol. 4, 55. doi:10.3389/fphar.2013.00055
Jiang, L. H., Caseley, E. A., Muench, S. P., and Roger, S. (2021). Structural basis for the functional properties of the P2X7 receptor for extracellular ATP. Purinergic Signal 17, 331–344. doi:10.1007/s11302-021-09790-x
Jiang, R., Lemoine, D., Martz, A., Taly, A., Gonin, S., Prado de Carvalho, L., et al. (2011). Agonist trapped in ATP-binding sites of the P2X2 receptor. Proc. Natl. Acad. Sci. U. S. A. 108, 9066–9071. doi:10.1073/pnas.1102170108
Jiang, T., Hoekstra, J., Heng, X., Kang, W., Ding, J., Liu, J., et al. (2015). P2X7 receptor is critical in α-synuclein--mediated microglial NADPH oxidase activation. Neurobiol. Aging 36, 2304–2318. doi:10.1016/j.neurobiolaging.2015.03.015
Jun, D.-J., Kim, J., Jung, S.-Y., Song, R., Noh, J.-H., Park, Y.-S., et al. (2007). Extracellular ATP mediates necrotic cell swelling in SN4741 dopaminergic neurons through P2X7 receptors. J. Biol. Chem. 282, 37350–37358. doi:10.1074/jbc.M707915200
Kaczmarek-Hajek, K., Zhang, J., Kopp, R., Grosche, A., Rissiek, B., Saul, A., et al. (2018). Re-evaluation of neuronal P2X7 expression using novel mouse models and a P2X7-specific nanobody. Elife 7, e36217. doi:10.7554/eLife.36217
Karasawa, A., and Kawate, T. (2016). Structural basis for subtype-specific inhibition of the P2X7 receptor. Elife 5, e22153. doi:10.7554/eLife.22153
Keren-Shaul, H., Spinrad, A., Weiner, A., Matcovitch-Natan, O., Dvir-Szternfeld, R., Ulland, T. K., et al. (2017). A unique microglia type associated with restricting development of alzheimer’s disease. Cell 169, 1276–1290. doi:10.1016/j.cell.2017.05.018
Kettenmann, H., Kirchhoff, F., and Verkhratsky, A. (2013). Microglia: new roles for the synaptic stripper. Neuron 77, 10–18. doi:10.1016/j.neuron.2012.12.023
Keystone, E. C., Wang, M. M., Layton, M., Hollis, S., and McInnes, I. B.D1520C00001 Study Team (2012). Clinical evaluation of the efficacy of the P2X7 purinergic receptor antagonist AZD9056 on the signs and symptoms of rheumatoid arthritis in patients with active disease despite treatment with methotrexate or sulphasalazine. Ann. Rheum. Dis. 71, 1630–1635. doi:10.1136/annrheumdis-2011-143578
Khakh, B. S., and North, R. A. (2006). P2X receptors as cell-surface ATP sensors in health and disease. Nature 442, 527–532. doi:10.1038/nature04886
Khakh, B. S., and North, R. A. (2012). Neuromodulation by extracellular ATP and P2X receptors in the CNS. Neuron 76, 51–69. doi:10.1016/j.neuron.2012.09.024
Kim, E. K., and Choi, E.-J. (2010). Pathological roles of MAPK signaling pathways in human diseases. Biochim. Biophys. Acta 1802, 396–405. doi:10.1016/j.bbadis.2009.12.009
Kim, J.-E., Ko, A.-R., Hyun, H.-W., Min, S.-J., and Kang, T.-C. (2018). P2RX7-MAPK1/2-SP1 axis inhibits MTOR independent HSPB1-mediated astroglial autophagy. Cell Death Dis. 9, 546. doi:10.1038/s41419-018-0586-x
Kim, J.-E., Ryu, H. J., Yeo, S.-I., and Kang, T.-C. (2011). P2X7 receptor differentially modulates astroglial apoptosis and clasmatodendrosis in the rat brain following status epilepticus. Hippocampus 21, 1318–1333. doi:10.1002/hipo.20850
Kim, S. Y., Moon, J. H., Lee, H. G., Kim, S. U., and Lee, Y. B. (2007). ATP released from beta-amyloid-stimulated microglia induces reactive oxygen species production in an autocrine fashion. Exp. Mol. Med. 39, 820–827. doi:10.1038/emm.2007.89
Kobayashi, E., Nakano, M., Kubota, K., Himuro, N., Mizoguchi, S., Chikenji, T., et al. (2018). Activated forms of astrocytes with higher GLT-1 expression are associated with cognitive normal subjects with Alzheimer pathology in human brain. Sci. Rep. 8, 1712. doi:10.1038/s41598-018-19442-7
Kodakandla, G., Akimzhanov, A. M., and Boehning, D. (2023). Regulatory mechanisms controlling store-operated calcium entry. ArXiv 14, 1330259. doi:10.3389/fphys.2023.1330259
Kopp, R., Krautloher, A., Ramírez-Fernández, A., and Nicke, A. (2019). P2X7 interactions and signaling - making head or tail of it. Front. Mol. Neurosci. 12, 183. doi:10.3389/fnmol.2019.00183
Kumar, S., Mishra, A., and Krishnamurthy, S. (2017). Purinergic antagonism prevents mitochondrial dysfunction and behavioral deficits associated with dopaminergic toxicity induced by 6-OHDA in rats. Neurochem. Res. 42, 3414–3430. doi:10.1007/s11064-017-2383-9
Lara, R., Adinolfi, E., Harwood, C. A., Philpott, M., Barden, J. A., Di Virgilio, F., et al. (2020). P2X7 in cancer: from molecular mechanisms to therapeutics. Front. Pharmacol. 11, 793. doi:10.3389/fphar.2020.00793
Le Douce, J., Maugard, M., Veran, J., Matos, M., Jégo, P., Vigneron, P.-A., et al. (2020). Impairment of glycolysis-derived l-serine production in astrocytes contributes to cognitive deficits in alzheimer’s disease. Cell Metab. 31, 503–517. doi:10.1016/j.cmet.2020.02.004
Lee, D.-S., and Kim, J.-E. (2020). P2 × 7 receptor inhibits astroglial autophagy via regulating FAK- and PHLPP1/2-mediated AKT-S473 phosphorylation following kainic acid-induced seizures. Int. J. Mol. Sci. 21, 6476. doi:10.3390/ijms21186476
Lee, G. E., Lee, H.-S., Lee, S. D., Kim, J.-H., Kim, W.-K., and Kim, Y.-C. (2009). Synthesis and structure-activity relationships of novel, substituted 5,6-dihydrodibenzo[a,g]quinolizinium P2X7 antagonists. Bioorg. Med. Chem. Lett. 19, 954–958. doi:10.1016/j.bmcl.2008.11.088
Lee, H. G., Won, S. M., Gwag, B. J., and Lee, Y. B. (2011). Microglial P2X₇ receptor expression is accompanied by neuronal damage in the cerebral cortex of the APPswe/PS1dE9 mouse model of Alzheimer’s disease. Exp. Mol. Med. 43, 7–14. doi:10.3858/emm.2011.43.1.001
Lee, J., Chan, S. L., and Mattson, M. P. (2002). Adverse effect of a presenilin-1 mutation in microglia results in enhanced nitric oxide and inflammatory cytokine responses to immune challenge in the brain. Neuromolecular Med. 2, 29–45. doi:10.1385/NMM:2:1:29
Lee, S., Ha, H., Jang, J., and Byun, Y. (2023). Recent advances in the development of antidepressants targeting the purinergic P2X7 receptor. Curr. Med. Chem. 30, 164–177. doi:10.2174/0929867329666220629141418
Lenertz, L. Y., Gavala, M. L., Hill, L. M., and Bertics, P. J. (2009). Cell signaling via the P2X(7) nucleotide receptor: linkage to ROS production, gene transcription, and receptor trafficking. Purinergic Signal 5, 175–187. doi:10.1007/s11302-009-9133-7
Letavic, M. A., Lord, B., Bischoff, F., Hawryluk, N. A., Pieters, S., Rech, J. C., et al. (2013). Synthesis and pharmacological characterization of two novel, brain penetrating P2X7 antagonists. ACS Med. Chem. Lett. 4, 419–422. doi:10.1021/ml400040v
Letavic, M. A., Savall, B. M., Allison, B. D., Aluisio, L., Andres, J. I., De Angelis, M., et al. (2017). 4-Methyl-6,7-dihydro-4H-triazolo[4,5-c]pyridine-Based P2X7 receptor antagonists: optimization of pharmacokinetic properties leading to the identification of a clinical candidate. J. Med. Chem. 60, 4559–4572. doi:10.1021/acs.jmedchem.7b00408
Li, J., Liu, D., Ke, H. Z., Duncan, R. L., and Turner, C. H. (2005). The P2X7 nucleotide receptor mediates skeletal mechanotransduction. J. Biol. Chem. 280, 42952–42959. doi:10.1074/jbc.M506415200
Li, Y., Zhang, R., and Hei, H. (2023). Advances in post-translational modifications of proteins and cancer immunotherapy. Front. Immunol. 14, 1229397. doi:10.3389/fimmu.2023.1229397
Liu, X., Surprenant, A., Mao, H.-J., Roger, S., Xia, R., Bradley, H., et al. (2008). Identification of key residues coordinating functional inhibition of P2X7 receptors by zinc and copper. Mol. Pharmacol. 73, 252–259. doi:10.1124/mol.107.039651
Long, Y., Li, X.-Q., Deng, J., Ye, Q.-B., Li, D., Ma, Y., et al. (2024). Modulating the polarization phenotype of microglia - a valuable strategy for central nervous system diseases. Ageing Res. Rev. 93, 102160. doi:10.1016/j.arr.2023.102160
Loureiro, A. V., Moura-Neto, L. I., Martins, C. S., Silva, P. I. M., Lopes, M. B. S., Leitão, R. F. C., et al. (2022). Role of Pannexin-1-P2X7R signaling on cell death and pro-inflammatory mediator expression induced by Clostridioides difficile toxins in enteric glia. Front. Immunol. 13, 956340. doi:10.3389/fimmu.2022.956340
Lowe, J., Cha, H., Lee, M.-O., Mazur, S. J., Appella, E., and Fornace, A. J. J. (2012). Regulation of the Wip1 phosphatase and its effects on the stress response. Front. Biosci. (Landmark Ed) 17, 1480–1498. doi:10.2741/3999
Lutz, S. E., González-Fernández, E., Ventura, J. C. C., Pérez-Samartín, A., Tarassishin, L., Negoro, H., et al. (2013). Contribution of pannexin1 to experimental autoimmune encephalomyelitis. PLoS One 8, e66657. doi:10.1371/journal.pone.0066657
Ly, D., Dongol, A., Cuthbertson, P., Guy, T. V., Geraghty, N. J., Sophocleous, R. A., et al. (2020). The P2X7 receptor antagonist JNJ-47965567 administered thrice weekly from disease onset does not alter progression of amyotrophic lateral sclerosis in SOD1(G93A) mice. Purinergic Signal 16, 109–122. doi:10.1007/s11302-020-09692-4
Ma, G., Chen, S., Wang, X., Ba, M., Yang, H., and Lu, G. (2005). Short-term interleukin-1(beta) increases the release of secreted APP(alpha) via MEK1/2-dependent and JNK-dependent alpha-secretase cleavage in neuroglioma U251 cells. J. Neurosci. Res. 80, 683–692. doi:10.1002/jnr.20515
Maatouk, L., Yi, C., Carrillo-de Sauvage, M.-A., Compagnion, A.-C., Hunot, S., Ezan, P., et al. (2019). Glucocorticoid receptor in astrocytes regulates midbrain dopamine neurodegeneration through connexin hemichannel activity. Cell Death Differ. 26, 580–596. doi:10.1038/s41418-018-0150-3
Mackenzie, A. B., Young, M. T., Adinolfi, E., and Surprenant, A. (2005). Pseudoapoptosis induced by brief activation of ATP-gated P2X7 receptors. J. Biol. Chem. 280, 33968–33976. doi:10.1074/jbc.M502705200
Madry, C., Kyrargyri, V., Arancibia-Cárcamo, I. L., Jolivet, R., Kohsaka, S., Bryan, R. M., et al. (2018). Microglial ramification, surveillance, and interleukin-1β release are regulated by the two-pore domain K+ channel THIK-1. Neuron 97, 299–312. doi:10.1016/j.neuron.2017.12.002
Mahad, D. H., Trapp, B. D., and Lassmann, H. (2015). Pathological mechanisms in progressive multiple sclerosis. Lancet. Neurol. 14, 183–193. doi:10.1016/S1474-4422(14)70256-X
Mankus, C., Chi, C., Rich, C., Ren, R., and Trinkaus-Randall, V. (2012). The P2X(7) receptor regulates proteoglycan expression in the corneal stroma. Mol. Vis. 18, 128–138.
Mansoor, S. E., Lü, W., Oosterheert, W., Shekhar, M., Tajkhorshid, E., and Gouaux, E. (2016). X-ray structures define human P2X(3) receptor gating cycle and antagonist action. Nature 538, 66–71. doi:10.1038/nature19367
Martin, E., Amar, M., Dalle, C., Youssef, I., Boucher, C., Le Duigou, C., et al. (2019). New role of P2X7 receptor in an Alzheimer’s disease mouse model. Mol. Psychiatry 24, 108–125. doi:10.1038/s41380-018-0108-3
Martínez-Cuesta, M. Á., Blanch-Ruiz, M. A., Ortega-Luna, R., Sánchez-López, A., and Álvarez, Á. (2020). Structural and functional basis for understanding the biological significance of P2X7 receptor. Int. J. Mol. Sci. 21, 8454. doi:10.3390/ijms21228454
Martínez-Frailes, C., Di Lauro, C., Bianchi, C., de Diego-García, L., Sebastián-Serrano, Á., Boscá, L., et al. (2019). Amyloid peptide induced neuroinflammation increases the P2X7 receptor expression in microglial cells, impacting on its functionality. Front. Cell. Neurosci. 13, 143. doi:10.3389/fncel.2019.00143
Martini-Stoica, H., Cole, A. L., Swartzlander, D. B., Chen, F., Wan, Y.-W., Bajaj, L., et al. (2018). TFEB enhances astroglial uptake of extracellular tau species and reduces tau spreading. J. Exp. Med. 215, 2355–2377. doi:10.1084/jem.20172158
Masuda, T., Sankowski, R., Staszewski, O., and Prinz, M. (2020). Microglia heterogeneity in the single-cell era. Cell Rep. 30, 1271–1281. doi:10.1016/j.celrep.2020.01.010
Matute, C., Torre, I., Pérez-Cerdá, F., Pérez-Samartín, A., Alberdi, E., Etxebarria, E., et al. (2007). P2X(7) receptor blockade prevents ATP excitotoxicity in oligodendrocytes and ameliorates experimental autoimmune encephalomyelitis. J. Neurosci. Off. J. Soc. Neurosci. 27, 9525–9533. doi:10.1523/JNEUROSCI.0579-07.2007
McCarthy, A. E., Yoshioka, C., and Mansoor, S. E. (2019). Full-length P2X(7) structures reveal how palmitoylation prevents channel desensitization. Cell 179, 659–670. doi:10.1016/j.cell.2019.09.017
McGeer, P. L., Itagaki, S., Boyes, B. E., and McGeer, E. G. (1988). Reactive microglia are positive for HLA-DR in the substantia nigra of Parkinson’s and Alzheimer’s disease brains. Neurology 38, 1285–1291. doi:10.1212/wnl.38.8.1285
McInnes, I. B., Cruwys, S., Bowers, K., and Braddock, M. (2014). Targeting the P2X7 receptor in rheumatoid arthritis: biological rationale for P2X7 antagonism. Clin. Exp. Rheumatol. 32, 878–882.
Merezhko, M., Brunello, C. A., Yan, X., Vihinen, H., Jokitalo, E., Uronen, R.-L., et al. (2018). Secretion of tau via an unconventional non-vesicular mechanism. Cell Rep. 25, 2027–2035. doi:10.1016/j.celrep.2018.10.078
Meyer, J. H., Cervenka, S., Kim, M.-J., Kreisl, W. C., Henter, I. D., and Innis, R. B. (2020). Neuroinflammation in psychiatric disorders: PET imaging and promising new targets. lancet. Psychiatry 7, 1064–1074. doi:10.1016/S2215-0366(20)30255-8
Miller, C. M., Boulter, N. R., Fuller, S. J., Zakrzewski, A. M., Lees, M. P., Saunders, B. M., et al. (2011). The role of the P2X₇ receptor in infectious diseases. PLoS Pathog. 7, e1002212. doi:10.1371/journal.ppat.1002212
Millichap, L. E., Damiani, E., Tiano, L., and Hargreaves, I. P. (2021). Targetable pathways for alleviating mitochondrial dysfunction in neurodegeneration of metabolic and non-metabolic diseases. Int. J. Mol. Sci. 22, 11444. doi:10.3390/ijms222111444
Miras-Portugal, M. T., Díaz-Hernández, M., Giráldez, L., Hervás, C., Gómez-Villafuertes, R., Sen, R. P., et al. (2003). P2X7 receptors in rat brain: presence in synaptic terminals and granule cells. Neurochem. Res. 28, 1597–1605. doi:10.1023/a:1025690913206
Miras-Portugal, M. T., Sebastián-Serrano, Á., de Diego García, L., and Díaz-Hernández, M. (2017). Neuronal P2X7 receptor: involvement in neuronal physiology and pathology. J. Neurosci. Off. J. Soc. Neurosci. 37, 7063–7072. doi:10.1523/JNEUROSCI.3104-16.2017
Mogi, M., Harada, M., Riederer, P., Narabayashi, H., Fujita, K., and Nagatsu, T. (1994). Tumor necrosis factor-alpha (TNF-alpha) increases both in the brain and in the cerebrospinal fluid from parkinsonian patients. Neurosci. Lett. 165, 208–210. doi:10.1016/0304-3940(94)90746-3
Mucke, L., Masliah, E., Yu, G. Q., Mallory, M., Rockenstein, E. M., Tatsuno, G., et al. (2000). High-level neuronal expression of abeta 1-42 in wild-type human amyloid protein precursor transgenic mice: synaptotoxicity without plaque formation. J. Neurosci. Off. J. Soc. Neurosci. 20, 4050–4058. doi:10.1523/JNEUROSCI.20-11-04050.2000
Munoz, F. M., Gao, R., Tian, Y., Henstenburg, B. A., Barrett, J. E., and Hu, H. (2017). Neuronal P2X7 receptor-induced reactive oxygen species production contributes to nociceptive behavior in mice. Sci. Rep. 7, 3539. doi:10.1038/s41598-017-03813-7
Munoz, F. M., Patel, P. A., Gao, X., Mei, Y., Xia, J., Gilels, S., et al. (2020). Reactive oxygen species play a role in P2X7 receptor-mediated IL-6 production in spinal astrocytes. Purinergic Signal 16, 97–107. doi:10.1007/s11302-020-09691-5
Muñoz-Planillo, R., Kuffa, P., Martínez-Colón, G., Smith, B. L., Rajendiran, T. M., and Núñez, G. (2013). K+ efflux is the common trigger of NLRP3 inflammasome activation by bacterial toxins and particulate matter. Immunity 38, 1142–1153. doi:10.1016/j.immuni.2013.05.016
Nishida, K., Nakatani, T., Ohishi, A., Okuda, H., Higashi, Y., Matsuo, T., et al. (2012). Mitochondrial dysfunction is involved in P2X7 receptor-mediated neuronal cell death. J. Neurochem. 122, 1118–1128. doi:10.1111/j.1471-4159.2012.07868.x
Nobili, P., Shen, W., Milicevic, K., Bogdanovic Pristov, J., Audinat, E., and Nikolic, L. (2022). Therapeutic potential of astrocyte purinergic signalling in epilepsy and multiple sclerosis. Front. Pharmacol. 13, 900337. doi:10.3389/fphar.2022.900337
Nörenberg, W., Gebicke-Haerter, P. J., and Illes, P. (1994). Voltage-dependent potassium channels in activated rat microglia. J. Physiol. 475, 15–32. doi:10.1113/jphysiol.1994.sp020046
Nörenberg, W., Hempel, C., Urban, N., Sobottka, H., Illes, P., and Schaefer, M. (2011). Clemastine potentiates the human P2X7 receptor by sensitizing it to lower ATP concentrations. J. Biol. Chem. 286, 11067–11081. doi:10.1074/jbc.M110.198879
Nörenberg, W., Sobottka, H., Hempel, C., Plötz, T., Fischer, W., Schmalzing, G., et al. (2012). Positive allosteric modulation by ivermectin of human but not murine P2X7 receptors. Br. J. Pharmacol. 167, 48–66. doi:10.1111/j.1476-5381.2012.01987.x
North, R. A., and Surprenant, A. (2000). Pharmacology of cloned P2X receptors. Annu. Rev. Pharmacol. Toxicol. 40, 563–580. doi:10.1146/annurev.pharmtox.40.1.563
Oken, A. C., Krishnamurthy, I., Savage, J. C., Lisi, N. E., Godsey, M. H., and Mansoor, S. E. (2022). Molecular pharmacology of P2X receptors: exploring druggable domains revealed by structural biology. Front. Pharmacol. 13, 925880. doi:10.3389/fphar.2022.925880
Oliveira-Giacomelli, Á., M Albino, C., de Souza, H. D. N., Corrêa-Velloso, J., de Jesus Santos, A. P., Baranova, J., et al. (2019). P2Y6 and P2X7 receptor antagonism exerts neuroprotective/neuroregenerative effects in an animal model of Parkinson’s disease. Front. Cell. Neurosci. 13, 476. doi:10.3389/fncel.2019.00476
Orfali, R., Alwatban, A. Z., Orfali, R. S., Lau, L., Chea, N., Alotaibi, A. M., et al. (2024). Oxidative stress and ion channels in neurodegenerative diseases. Front. Physiol. 15, 1320086. doi:10.3389/fphys.2024.1320086
Ossola, B., Rifat, A., Rowland, A., Hunter, H., Drinkall, S., Bender, C., et al. (2023). Characterisation of C101248: a novel selective THIK-1 channel inhibitor for the modulation of microglial NLRP3-inflammasome. Neuropharmacology 224, 109330. doi:10.1016/j.neuropharm.2022.109330
Oyanguren-Desez, O., Rodríguez-Antigüedad, A., Villoslada, P., Domercq, M., Alberdi, E., and Matute, C. (2011). Gain-of-function of P2X7 receptor gene variants in multiple sclerosis. Cell Calcium 50, 468–472. doi:10.1016/j.ceca.2011.08.002
Park, J.-H., Lee, G.-E., Lee, S.-D., Ko, H., and Kim, Y.-C. (2015). Structure-activity relationship studies of pyrimidine-2,4-dione derivatives as potent P2X7 receptor antagonists. Eur. J. Med. Chem. 106, 180–193. doi:10.1016/j.ejmech.2015.10.036
Parvathenani, L. K., Tertyshnikova, S., Greco, C. R., Roberts, S. B., Robertson, B., and Posmantur, R. (2003). P2X7 mediates superoxide production in primary microglia and is up-regulated in a transgenic mouse model of Alzheimer’s disease. J. Biol. Chem. 278, 13309–13317. doi:10.1074/jbc.M209478200
Pasqualetti, G., Brooks, D. J., and Edison, P. (2015). The role of neuroinflammation in dementias. Curr. Neurol. Neurosci. Rep. 15, 17. doi:10.1007/s11910-015-0531-7
Paumier, A., Boisseau, S., Jacquier-Sarlin, M., Pernet-Gallay, K., Buisson, A., and Albrieux, M. (2022). Astrocyte-neuron interplay is critical for Alzheimer’s disease pathogenesis and is rescued by TRPA1 channel blockade. Brain 145, 388–405. doi:10.1093/brain/awab281
Pelegrin, P., and Surprenant, A. (2006). Pannexin-1 mediates large pore formation and interleukin-1beta release by the ATP-gated P2X7 receptor. EMBO J. 25, 5071–5082. doi:10.1038/sj.emboj.7601378
Pelegrin, P., and Surprenant, A. (2007). Pannexin-1 couples to maitotoxin- and nigericin-induced interleukin-1beta release through a dye uptake-independent pathway. J. Biol. Chem. 282, 2386–2394. doi:10.1074/jbc.M610351200
Perea, J. R., López, E., Díez-Ballesteros, J. C., Ávila, J., Hernández, F., and Bolós, M. (2019). Extracellular monomeric tau is internalized by astrocytes. Front. Neurosci. 13, 442. doi:10.3389/fnins.2019.00442
Pétrilli, V., Papin, S., Dostert, C., Mayor, A., Martinon, F., and Tschopp, J. (2007). Activation of the NALP3 inflammasome is triggered by low intracellular potassium concentration. Cell Death Differ. 14, 1583–1589. doi:10.1038/sj.cdd.4402195
Peverini, L., Beudez, J., Dunning, K., Chataigneau, T., and Grutter, T. (2018). New insights into permeation of large cations through ATP-gated P2X receptors. Front. Mol. Neurosci. 11, 265. doi:10.3389/fnmol.2018.00265
Pippel, A., Stolz, M., Woltersdorf, R., Kless, A., Schmalzing, G., and Markwardt, F. (2017). Localization of the gate and selectivity filter of the full-length P2X7 receptor. Proc. Natl. Acad. Sci. U. S. A. 114, E2156–E2165. doi:10.1073/pnas.1610414114
Plotnikov, A., Zehorai, E., Procaccia, S., and Seger, R. (2011). The MAPK cascades: signaling components, nuclear roles and mechanisms of nuclear translocation. Biochim. Biophys. Acta 1813, 1619–1633. doi:10.1016/j.bbamcr.2010.12.012
Puangmalai, N., Bhatt, N., Montalbano, M., Sengupta, U., Gaikwad, S., Ventura, F., et al. (2020). Internalization mechanisms of brain-derived tau oligomers from patients with Alzheimer’s disease, progressive supranuclear palsy and dementia with Lewy bodies. Cell Death Dis. 11, 314. doi:10.1038/s41419-020-2503-3
Qin, J., Ma, Z., Chen, X., and Shu, S. (2023). Microglia activation in central nervous system disorders: a review of recent mechanistic investigations and development efforts. Front. Neurol. 14, 1103416. doi:10.3389/fneur.2023.1103416
Rauch, J. N., Luna, G., Guzman, E., Audouard, M., Challis, C., Sibih, Y. E., et al. (2020). LRP1 is a master regulator of tau uptake and spread. Nature 580, 381–385. doi:10.1038/s41586-020-2156-5
Recourt, K., van der Aart, J., Jacobs, G., de Kam, M., Drevets, W., van Nueten, L., et al. (2020). Characterisation of the pharmacodynamic effects of the P2X7 receptor antagonist JNJ-54175446 using an oral dexamphetamine challenge model in healthy males in a randomised, double-blind, placebo-controlled, multiple ascending dose trial. J. Psychopharmacol. 34, 1030–1042. doi:10.1177/0269881120914206
Reid, M. J., Beltran-Lobo, P., Johnson, L., Perez-Nievas, B. G., and Noble, W. (2020). Astrocytes in tauopathies. Front. Neurol. 11, 572850. doi:10.3389/fneur.2020.572850
Ren, C., Li, L.-X., Dong, A.-Q., Zhang, Y.-T., Hu, H., Mao, C.-J., et al. (2021). Depression induced by chronic unpredictable mild stress increases susceptibility to Parkinson’s disease in mice via neuroinflammation mediated by P2X7 receptor. ACS Chem. Neurosci. 12, 1262–1272. doi:10.1021/acschemneuro.1c00095
Roger, S., Pelegrin, P., and Surprenant, A. (2008). Facilitation of P2X7 receptor currents and membrane blebbing via constitutive and dynamic calmodulin binding. J. Neurosci. Off. J. Soc. Neurosci. 28, 6393–6401. doi:10.1523/JNEUROSCI.0696-08.2008
Ruan, Z., Delpech, J.-C., Venkatesan Kalavai, S., Van Enoo, A. A., Hu, J., Ikezu, S., et al. (2020). P2RX7 inhibitor suppresses exosome secretion and disease phenotype in P301S tau transgenic mice. Mol. Neurodegener. 15, 47. doi:10.1186/s13024-020-00396-2
Ruiz-Ruiz, C., García-Magro, N., Negredo, P., Avendaño, C., Bhattacharya, A., Ceusters, M., et al. (2020). Chronic administration of P2X7 receptor antagonist JNJ-47965567 delays disease onset and progression, and improves motor performance in ALS SOD1(G93A) female mice. Dis. Model. Mech. 13, dmm045732. doi:10.1242/dmm.045732
Rusiecka, O. M., Tournier, M., Molica, F., and Kwak, B. R. (2022). Pannexin1 channels—a potential therapeutic target in inflammation. Front. Cell Dev. Biol. 10, 1020826. doi:10.3389/fcell.2022.1020826
Ryu, H. J., Kim, J.-E., Yeo, S.-I., and Kang, T.-C. (2011). p65/RelA-Ser529 NF-κB subunit phosphorylation induces autophagic astroglial death (Clasmatodendrosis) following status epilepticus. Cell. Mol. Neurobiol. 31, 1071–1078. doi:10.1007/s10571-011-9706-1
Saha, S., Buttari, B., Panieri, E., Profumo, E., and Saso, L. (2020). An overview of Nrf2 signaling pathway and its role in inflammation. Molecules 25, 5474. doi:10.3390/molecules25225474
Sanz, J. M., Chiozzi, P., Colaianna, M., Zotti, M., Ferrari, D., Trabace, L., et al. (2012). Nimodipine inhibits IL-1β release stimulated by amyloid β from microglia. Br. J. Pharmacol. 167, 1702–1711. doi:10.1111/j.1476-5381.2012.02112.x
Sanz, J. M., Chiozzi, P., Ferrari, D., Colaianna, M., Idzko, M., Falzoni, S., et al. (2009). Activation of microglia by amyloid {beta} requires P2X7 receptor expression. J. Immunol. 182, 4378–4385. doi:10.4049/jimmunol.0803612
Savall, B. M., Wu, D., De Angelis, M., Carruthers, N. I., Ao, H., Wang, Q., et al. (2015). Synthesis, SAR, and pharmacological characterization of brain penetrant P2X7 receptor antagonists. ACS Med. Chem. Lett. 6, 671–676. doi:10.1021/acsmedchemlett.5b00089
Sharp, A. J., Polak, P. E., Simonini, V., Lin, S. X., Richardson, J. C., Bongarzone, E. R., et al. (2008). P2x7 deficiency suppresses development of experimental autoimmune encephalomyelitis. J. Neuroinflammation 5, 33. doi:10.1186/1742-2094-5-33
Shieh, C.-H., Heinrich, A., Serchov, T., van Calker, D., and Biber, K. (2014). P2X7-dependent, but differentially regulated release of IL-6, CCL2, and TNF-α in cultured mouse microglia. Glia 62, 592–607. doi:10.1002/glia.22628
Simon, D. K., Tanner, C. M., and Brundin, P. (2020). Parkinson disease epidemiology, pathology, genetics, and pathophysiology. Clin. Geriatr. Med. 36, 1–12. doi:10.1016/j.cger.2019.08.002
Sivcev, S., Slavikova, B., Rupert, M., Ivetic, M., Nekardova, M., Kudova, E., et al. (2019). Synthetic testosterone derivatives modulate rat P2X2 and P2X4 receptor channel gating. J. Neurochem. 150, 28–43. doi:10.1111/jnc.14718
Sluyter, R., Bartlett, R., Ly, D., and Yerbury, J. J. (2017). P2X7 receptor antagonism in amyotrophic lateral sclerosis. Neural Regen. Res. 12, 749–750. doi:10.4103/1673-5374.206643
Snell, N. J. C. (2014). Discontinued drug projects in the respiratory therapeutic area during 2012. Expert Opin. Investig. Drugs 23, 411–415. doi:10.1517/13543784.2014.873785
Sorrentino, Z. A., and Giasson, B. I. (2020). The emerging role of α-synuclein truncation in aggregation and disease. J. Biol. Chem. 295, 10224–10244. doi:10.1074/jbc.REV120.011743
Sperlágh, B., and Illes, P. (2014). P2X7 receptor: an emerging target in central nervous system diseases. Trends Pharmacol. Sci. 35, 537–547. doi:10.1016/j.tips.2014.08.002
Sperlágh, B., Köfalvi, A., Deuchars, J., Atkinson, L., Milligan, C. J., Buckley, N. J., et al. (2002). Involvement of P2X7 receptors in the regulation of neurotransmitter release in the rat hippocampus. J. Neurochem. 81, 1196–1211. doi:10.1046/j.1471-4159.2002.00920.x
Stefanova, E. E., Dychiao, J. V. T., Chinn, M. C., Borhani, M., and Scott, A. L. (2024). P2X7 regulates ependymo-radial glial cell proliferation in adult Danio rerio following spinal cord injury. Biol. Open 13, bio060270. doi:10.1242/bio.060270
Stephenson, J., Nutma, E., van der Valk, P., and Amor, S. (2018). Inflammation in CNS neurodegenerative diseases. Immunology 154, 204–219. doi:10.1111/imm.12922
Stock, T. C., Bloom, B. J., Wei, N., Ishaq, S., Park, W., Wang, X., et al. (2012). Efficacy and safety of CE-224,535, an antagonist of P2X7 receptor, in treatment of patients with rheumatoid arthritis inadequately controlled by methotrexate. J. Rheumatol. 39, 720–727. doi:10.3899/jrheum.110874
Stokes, L., Jiang, L.-H., Alcaraz, L., Bent, J., Bowers, K., Fagura, M., et al. (2006). Characterization of a selective and potent antagonist of human P2X(7) receptors, AZ11645373. Br. J. Pharmacol. 149, 880–887. doi:10.1038/sj.bjp.0706933
Subramaniam, S. R., and Chesselet, M.-F. (2013). Mitochondrial dysfunction and oxidative stress in Parkinson’s disease. Prog. Neurobiol. 106–107, 17–32. doi:10.1016/j.pneurobio.2013.04.004
Sung, K., and Jimenez-Sanchez, M. (2020). Autophagy in astrocytes and its implications in neurodegeneration. J. Mol. Biol. 432, 2605–2621. doi:10.1016/j.jmb.2019.12.041
Surprenant, A., and North, R. A. (2009). Signaling at purinergic P2X receptors. Annu. Rev. Physiol. 71, 333–359. doi:10.1146/annurev.physiol.70.113006.100630
Surprenant, A., Rassendren, F., Kawashima, E., North, R. A., and Buell, G. (1996). The cytolytic P2Z receptor for extracellular ATP identified as a P2X receptor (P2X7). Science 272, 735–738. doi:10.1126/science.272.5262.735
Suzuki, T., Hide, I., Ido, K., Kohsaka, S., Inoue, K., and Nakata, Y. (2004). Production and release of neuroprotective tumor necrosis factor by P2X7 receptor-activated microglia. J. Neurosci. Off. J. Soc. Neurosci. 24, 1–7. doi:10.1523/JNEUROSCI.3792-03.2004
Swanson, D. M., Savall, B. M., Coe, K. J., Schoetens, F., Koudriakova, T., Skaptason, J., et al. (2016). Identification of (R)-(2-Chloro-3-(trifluoromethyl)phenyl)(1-(5-fluoropyridin-2-yl)-4-methyl-6,7-dihydro-1H-imidazo[4,5-c]pyridin-5(4H)-yl)methanone (JNJ 54166060), a Small Molecule Antagonist of the P2X7 receptor. J. Med. Chem. 59, 8535–8548. doi:10.1021/acs.jmedchem.6b00989
Swanson, K. V., Deng, M., and Ting, J. P.-Y. (2019). The NLRP3 inflammasome: molecular activation and regulation to therapeutics. Nat. Rev. Immunol. 19, 477–489. doi:10.1038/s41577-019-0165-0
Takenouchi, T., Fujita, M., Sugama, S., Kitani, H., and Hashimoto, M. (2009a). The role of the P2X7 receptor signaling pathway for the release of autolysosomes in microglial cells. Autophagy 5, 723–724. doi:10.4161/auto.5.5.8478
Takenouchi, T., Nakai, M., Iwamaru, Y., Sugama, S., Tsukimoto, M., Fujita, M., et al. (2009b). The activation of P2X7 receptor impairs lysosomal functions and stimulates the release of autophagolysosomes in microglial cells. J. Immunol. 182, 2051–2062. doi:10.4049/jimmunol.0802577
Tan, E.-K., Chao, Y.-X., West, A., Chan, L.-L., Poewe, W., and Jankovic, J. (2020). Parkinson disease and the immune system - associations, mechanisms and therapeutics. Nat. Rev. Neurol. 16, 303–318. doi:10.1038/s41582-020-0344-4
Tassetto, M., Scialdone, A., Solini, A., and Di Virgilio, F. (2021). The P2X7 receptor: a promising pharmacological target in diabetic retinopathy. Int. J. Mol. Sci. 22, 7110. doi:10.3390/ijms22137110
Territo, P. R., Meyer, J. A., Peters, J. S., Riley, A. A., McCarthy, B. P., Gao, M., et al. (2017). Characterization of (11)C-GSK1482160 for targeting the P2X7 receptor as a biomarker for neuroinflammation. J. Nucl. Med. 58, 458–465. doi:10.2967/jnumed.116.181354
Tewari, M., Michalski, S., and Egan, T. M. (2024). Modulation of microglial function by ATP-gated P2X7 receptors: studies in rat, mice and human. Cells 13, 161. doi:10.3390/cells13020161
Timmers, M., Ravenstijn, P., Xi, L., Triana-Baltzer, G., Furey, M., Van Hemelryck, S., et al. (2018). Clinical pharmacokinetics, pharmacodynamics, safety, and tolerability of JNJ-54175446, a brain permeable P2X7 antagonist, in a randomised single-ascending dose study in healthy participants. J. Psychopharmacol. 32, 1341–1350. doi:10.1177/0269881118800067
Toma, C., Higa, N., Koizumi, Y., Nakasone, N., Ogura, Y., McCoy, A. J., et al. (2010). Pathogenic Vibrio activate NLRP3 inflammasome via cytotoxins and TLR/nucleotide-binding oligomerization domain-mediated NF-kappa B signaling. J. Immunol. 184, 5287–5297. doi:10.4049/jimmunol.0903536
Tomasinsig, L., Pizzirani, C., Skerlavaj, B., Pellegatti, P., Gulinelli, S., Tossi, A., et al. (2008). The human cathelicidin LL-37 modulates the activities of the P2X7 receptor in a structure-dependent manner. J. Biol. Chem. 283, 30471–30481. doi:10.1074/jbc.M802185200
Tóthová, Z., Šemeláková, M., Solárová, Z., Tomc, J., Debeljak, N., and Solár, P. (2021). The role of PI3K/AKT and MAPK signaling pathways in erythropoietin signalization. Int. J. Mol. Sci. 22, 7682. doi:10.3390/ijms22147682
Tronel, C., Largeau, B., Santiago Ribeiro, M. J., Guilloteau, D., Dupont, A.-C., and Arlicot, N. (2017). Molecular targets for PET imaging of activated microglia: the current situation and future expectations. Int. J. Mol. Sci. 18, 802. doi:10.3390/ijms18040802
Tufekci, K. U., Genc, S., and Genc, K. (2011). The endotoxin-induced neuroinflammation model of Parkinson’s disease. Park. Dis. 2011, 487450. doi:10.4061/2011/487450
Verkhratsky, A., Rodrigues, J. J., Pivoriunas, A., Zorec, R., and Semyanov, A. (2019). Astroglial atrophy in Alzheimer’s disease. Pflugers Arch. 471, 1247–1261. doi:10.1007/s00424-019-02310-2
Wang, P., and Ye, Y. (2021). Filamentous recombinant human Tau activates primary astrocytes via an integrin receptor complex. Nat. Commun. 12, 95. doi:10.1038/s41467-020-20322-w
Wang, S., Chu, C.-H., Guo, M., Jiang, L., Nie, H., Zhang, W., et al. (2016). Identification of a specific α-synuclein peptide (α-Syn 29-40) capable of eliciting microglial superoxide production to damage dopaminergic neurons. J. Neuroinflammation 13, 158. doi:10.1186/s12974-016-0606-7
Wang, W., Hu, D., Feng, Y., Wu, C., Song, Y., Liu, W., et al. (2020a). Paxillin mediates ATP-induced activation of P2X7 receptor and NLRP3 inflammasome. BMC Biol. 18, 182. doi:10.1186/s12915-020-00918-w
Wang, W., Huang, F., Jiang, W., Wang, W., and Xiang, J. (2020b). Brilliant blue G attenuates neuro-inflammation via regulating MAPKs and NF-κB signaling pathways in lipopolysaccharide-induced BV2 microglia cells. Exp. Ther. Med. 20, 116. doi:10.3892/etm.2020.9244
Wang, X.-H., Xie, X., Luo, X.-G., Shang, H., and He, Z.-Y. (2017). Inhibiting purinergic P2X7 receptors with the antagonist brilliant blue G is neuroprotective in an intranigral lipopolysaccharide animal model of Parkinson’s disease. Mol. Med. Rep. 15, 768–776. doi:10.3892/mmr.2016.6070
Wilkaniec, A., Cieślik, M., Murawska, E., Babiec, L., Gąssowska-Dobrowolska, M., Pałasz, E., et al. (2020). P2X7 receptor is involved in mitochondrial dysfunction induced by extracellular alpha synuclein in neuroblastoma SH-SY5Y cells. Int. J. Mol. Sci. 21, 3959. doi:10.3390/ijms21113959
Wilkaniec, A., Gąssowska, M., Czapski, G. A., Cieślik, M., Sulkowski, G., and Adamczyk, A. (2017). P2X7 receptor-pannexin 1 interaction mediates extracellular alpha-synuclein-induced ATP release in neuroblastoma SH-SY5Y cells. Purinergic Signal 13, 347–361. doi:10.1007/s11302-017-9567-2
Wilkinson, S. M., Barron, M. L., O’Brien-Brown, J., Janssen, B., Stokes, L., Werry, E. L., et al. (2017). Pharmacological evaluation of novel bioisosteres of an adamantanyl benzamide P2X(7) receptor antagonist. ACS Chem. Neurosci. 8, 2374–2380. doi:10.1021/acschemneuro.7b00272
Wu, C.-C., and Bratton, S. B. (2013). Regulation of the intrinsic apoptosis pathway by reactive oxygen species. Antioxid. Redox Signal. 19, 546–558. doi:10.1089/ars.2012.4905
Xie, Y., Han, R., Li, Y., Li, W., Zhang, S., Wu, Y., et al. (2024). P2X7 receptor antagonists modulate experimental autoimmune neuritis via regulation of NLRP3 inflammasome activation and Th17 and Th1 cell differentiation. J. Neuroinflammation 21, 73. doi:10.1186/s12974-024-03057-z
Xu, X.-Y., He, X.-T., Wang, J., Li, X., Xia, Y., Tan, Y.-Z., et al. (2023). Retraction Note: role of the P2X7 receptor in inflammation-mediated changes in the osteogenesis of periodontal ligament stem cells. Cell Death Dis. 14, 847. doi:10.1038/s41419-023-06390-y
Xu, Z., Chen, Z.-M., Wu, X., Zhang, L., Cao, Y., and Zhou, P. (2020). Distinct molecular mechanisms underlying potassium efflux for NLRP3 inflammasome activation. Front. Immunol. 11, 609441. doi:10.3389/fimmu.2020.609441
Yang, D., He, Y., Muñoz-Planillo, R., Liu, Q., and Núñez, G. (2015). Caspase-11 requires the pannexin-1 channel and the purinergic P2X7 pore to mediate pyroptosis and endotoxic shock. Immunity 43, 923–932. doi:10.1016/j.immuni.2015.10.009
Yiangou, Y., Facer, P., Durrenberger, P., Chessell, I. P., Naylor, A., Bountra, C., et al. (2006). COX-2, CB2 and P2X7-immunoreactivities are increased in activated microglial cells/macrophages of multiple sclerosis and amyotrophic lateral sclerosis spinal cord. BMC Neurol. 6, 12. doi:10.1186/1471-2377-6-12
Young, M. T., Pelegrin, P., and Surprenant, A. (2007). Amino acid residues in the P2X7 receptor that mediate differential sensitivity to ATP and BzATP. Mol. Pharmacol. 71, 92–100. doi:10.1124/mol.106.030163
Zelentsova, A. S., Deykin, A. V., Soldatov, V. O., Ulezko, A. A., Borisova, A. Y., Belyaeva, V. S., et al. (2022). P2X7 receptor and purinergic signaling: orchestrating mitochondrial dysfunction in neurodegenerative diseases. eNeuro 9, ENEURO.0092. doi:10.1523/ENEURO.0092-22.2022
Zhang, W.-J., Zhu, Z.-M., and Liu, Z.-X. (2020). The role and pharmacological properties of the P2X7 receptor in neuropathic pain. Brain Res. Bull. 155, 19–28. doi:10.1016/j.brainresbull.2019.11.006
Zhang, X., Fu, Y., Li, H., Shen, L., Chang, Q., Pan, L., et al. (2018). H3 relaxin inhibits the collagen synthesis via ROS- and P2X7R-mediated NLRP3 inflammasome activation in cardiac fibroblasts under high glucose. J. Cell. Mol. Med. 22, 1816–1825. doi:10.1111/jcmm.13464
Zheng, B., Lai, R., Li, J., and Zuo, Z. (2017). Critical role of P2X7 receptors in the neuroinflammation and cognitive dysfunction after surgery. Brain. Behav. Immun. 61, 365–374. doi:10.1016/j.bbi.2017.01.005
Zheng, H., Liu, Q., Zhou, S., Luo, H., and Zhang, W. (2024). Role and therapeutic targets of P2X7 receptors in neurodegenerative diseases. Front. Immunol. 15, 1345625. doi:10.3389/fimmu.2024.1345625
Zou, Y.-T., Li, J.-Y., Chai, J.-Y., Hu, Y.-S., Zhang, W.-J., and Zhang, Q. (2024). The impact of the P2X7 receptor on the tumor immune microenvironment and its effects on tumor progression. Biochem. Biophys. Res. Commun. 707, 149513. doi:10.1016/j.bbrc.2024.149513
Glossary
Keywords: P2X7R, neuroinflammation, neurodegeneration, microglia, ATP
Citation: Liu X, Li Y, Huang L, Kuang Y, Wu X, Ma X, Zhao B and Lan J (2024) Unlocking the therapeutic potential of P2X7 receptor: a comprehensive review of its role in neurodegenerative disorders. Front. Pharmacol. 15:1450704. doi: 10.3389/fphar.2024.1450704
Received: 18 June 2024; Accepted: 19 July 2024;
Published: 30 July 2024.
Edited by:
Michel Vignes, Université de Montpellier, FranceReviewed by:
Mariachiara Zuccarini, University of Studies G d’Annunzio Chieti and Pescara, ItalyIvar Von Kügelgen, University of Bonn, Germany
Copyright © 2024 Liu, Li, Huang, Kuang, Wu, Ma, Zhao and Lan. This is an open-access article distributed under the terms of the Creative Commons Attribution License (CC BY). The use, distribution or reproduction in other forums is permitted, provided the original author(s) and the copyright owner(s) are credited and that the original publication in this journal is cited, in accordance with accepted academic practice. No use, distribution or reproduction is permitted which does not comply with these terms.
*Correspondence: Jiao Lan, bGFuamlhb0BzemJhenl5Lm5ldA==; Beibei Zhao, emhhb2JlaWJlaUBzemJhenl5Lm5ldA==
†These authors share first authorship