- Département de Pharmacologie et Physiologie, Université de Montréal, Montréal, QC, Canada
Quetiapine is a second-generation atypical antipsychotic drug that has been commonly prescribed for the treatment of schizophrenia, major depressive disorder (depression), and other psychological disorders. Targeted inhibition of hyperpolarization-activated cyclic-nucleotide gated (HCN) channels, which generate Ih, may provide effective resistance against schizophrenia and depression. We investigated if HCN channels could contribute to the therapeutic effect of quetiapine, and its major active metabolite norquetiapine. Two-electrode voltage clamp recordings were used to assess the effects of quetiapine and its active metabolites 7-hydroxyquetiapine and norquetiapine on currents from HCN1 channels expressed in Xenopus laevis oocytes. Norquetiapine, but not quetiapine nor 7-hydroxyquetiapine, has an inhibitory effect on HCN1 channels. Norquetiapine selectively inhibited HCN1 currents by shifting the voltage-dependence of activation to more hyperpolarized potentials in a concentration-dependent manner with an IC50 of 13.9 ± 0.8 μM for HCN1 and slowing channel opening, without changing the kinetics of closing. Inhibition by norquetiapine primarily occurs from in the closed state. Norquetiapine inhibition is not sensitive to the external potassium concentration, and therefore, likely does not block the pore. Norquetiapine inhibition also does not dependent on the cyclic-nucleotide binding domain. Norquetiapine also inhibited HCN4 channels with reduced efficacy than HCN1 and had no effect on HCN2 channels. Therefore, HCN channels are key targets of norquetiapine, the primary active metabolite of quetiapine. These data help to explain the therapeutic mechanisms by which quetiapine aids in the treatment of anxiety, major depressive disorder, bipolar disorder, and schizophrenia, and may represent a novel structure for future drug design of HCN inhibitors.
Introduction
Hyperpolarization-activated cyclic-nucleotide gated (HCN) channels are the molecular correlate of Ih, and are widely expressed in the central and peripheral nervous systems. All four isoforms (HCN1-4) are expressed in the brain (Ludwig et al., 1998; Moosmang et al., 2001; Pape, 1996; Santoro et al., 1997; Santoro et al., 1998) where they play a role in setting the resting membrane potential, modulating dendritic integration of synaptic inputs, reducing neuronal input resistance, neuronal pacemaking, and establishing action potential threshold (Pape, 1996). HCN channels are important for learning and memory, pain sensation, sour taste sensation, and vision (Chaplan et al., 2003; Knop et al., 2008; Nolan et al., 2004; Nolan et al., 2003; Paspalas et al., 2013; Stevens et al., 2001). HCN1−/− mice show impaired motor learning but enhanced spatial learning and memory (Nolan et al., 2004; Nolan et al., 2003) and enhanced resistance to depression (Lewis et al., 2011; Huang et al., 2009). HCN1 expression increases in the CA1 region of the dorsal hippocampus in a chronic unpredictable stress rat model. Notably, shRNA knockdown of HCN1 reduces the stress response in this model (Kim et al., 2018). Targeted viral knockdown HCN1 in the CA1 hippocampal region also enhanced mobility in the Porsolt swim test (Kim et al., 2012). Similarly, genetic ablation of Trip8b, an auxiliary protein that regulates HCN1 and HCN2 expression, also increases resistance to depression (Lewis et al., 2011). Furthermore, altered HCN-cAMP signaling in prefrontal cortex networks also appears to contribute to the working memory deficits in schizophrenia and stress (Paspalas et al., 2013; Arnsten, 2011; Gamo et al., 2015), while mutations in SHANK3 linked to schizophrenia (Gauthier et al., 2010; Guilmatre et al., 2014) may induce an HCN channelopathy (Yi et al., 2016). Furthermore, polymorphisms in HCN4 channels were associated with mood disorders and/or obsessive compulsive disorder (Szabo et al., 2011).
Quetiapine fumarate (Seroquel®) (QTP) (Figure 1) is a second-generation atypical antipsychotic drug that has been commonly prescribed for the treatment of schizophrenia (Small et al., 1997; Dev and Raniwalla, 2000), acute bipolar mania (Janicak and Rado, 2012), insomnia (Lin et al., 2023), major depressive disorder (depression) (Ravindran et al., 2022), anxiety (Ravindran et al., 2022; Crapanzano et al., 2021; Bandelow et al., 2010), Post-traumatic stress disorder (Crapanzano et al., 2023) and other psychological disorders (Saller and Salama, 1993). Like other atypical antipsychotics, QTP is structurally similar to clozapine and acts as an antagonist to serotonin, dopamine, histamine, and adrenergic receptors (Saller and Salama, 1993; Burns, 2001). QTP is primarily metabolized by hepatic cytochrome P450 3A4 (Dev and Raniwalla, 2000), with norquetiapine (NQTP) and 7-hydroxyquetiapine (7-OH QTP) as its major active metabolites (Figure 1). NQTP exhibits pharmacological activity that differs from QTP (Bakken et al., 2012; DeVane and Nemeroff, 2001) and also exhibits antidepressant activity (Jensen et al., 2008; Lopez-Munoz and Alamo, 2013). In fact, NQTP shares structural similarities with several antidepressants including amoxapine and desipramine, and its physicochemical properties confer greater potential for its use as an antidepressant agent (Lopez-Munoz and Alamo, 2013; Kim et al., 2016). Indeed, the effect of QTP in major depressive disorder is probably mediated, at least in part, by NQTP, which selectively inhibits norepinephrine transporter reuptake (Bandelow et al., 2010; Lopez-Munoz and Alamo, 2013).
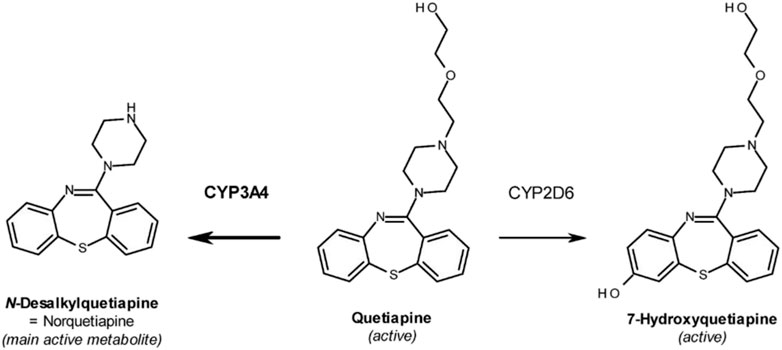
Figure 1. Chemical structures of Quetiapine (QTP) and its active metabolites Norquetiapine (NQTP) and 7-hydroxyquetiapine (7-OH QTP).
In addition to antagonizing serotonin, dopamine, histamine, and adrenergic receptors, and inhibiting norepinephrine transporter reuptake, QTP and NQTP block the hERG (human-Ether-a-go-go-Related Gene) potassium channel (Kongsamut et al., 2002; Lee et al., 2018), and the sodium channel, Nav1.5 (Kim et al., 2020). Given the role of HCN channels in major depressive disorders, anxiety, and schizophrenia, examining the effects of antipsychotic drugs on this current is worthwhile. In the present study, we investigated the inhibitory mechanisms of norquetiapine on the HCN channels expressed in Xenopus laevis oocytes.
Methods
Cloning, oocyte isolation and channel expression
cDNA coding for the mouse HCN1 gene and mHCN1-CX5 containing a stop codon at residue F472 (denoted as HCN1-ΔCNBD in this article) was sub-cloned into the expression vector pGH19, while mouse HCN2 was sub-cloned into the pGEM vector. hHCN4 was expressed in pcDNA3.1. All clones were verified by PCR sequencing of the complete ORF. cDNA was linearized using NheI (New England Biolabs) for mHCN1, SphI for mHCN2, or XbaI for hHCN4. To obtain RNA, ∼1.0 μg of linearized cDNA was used for in vitro transcription synthesis using the mMESSAGE mMACHINE™ T7 Transcription kit (Thermo Fisher Scientific, Life Technologies, United States).
All experiments were preformed using unfertilized Xenopus oocytes, extracted from anaesthetized female X. laevis. Once extracted, oocytes were injected with 4.6 ng of HCN RNA using a Drummond Nanoject II injector (Drummond Scientific Company). Prior to injection oocytes were subject to a controlled temperature of 17°C–19°C and placed in vials containing Barth antibiotic solution (mM): 90 NaCl, 3 KCl, 0.82 MgSO4.7H2O, 0.41 CaCl2.2H2O, 0.33 Ca(NO3)2.4H2O and 5 HEPES supplemented with 100 U/mL of penicillin-streptomycin and 10 mg/mL of kanamycin stock (10 mg/mL). Post injection cells were incubated in Barth antibiotic serum solution supplemented with ∼5% horse serum. Cells were expressed and ready to be used in electrophysiological recordings 1–3 days post injection. The number of cells recorded for each experimental group (n) are presented. Data for each group were collected from oocytes harvested on at least 3 separate occasions. Data subjected to statistical analysis had an n of at least five for each group.
Electrophysiological recordings
Electrophysiological studies were conducted using the two-electrode voltage clamp (TEVC) technique. Borosilicate rapid fill microelectrode pipettes (FHC Inc., United States) were filled with filtered 1 M KCL solution. Macroscopic currents were recorded from oocytes expressing full-length HCN1, HCN2, HCN4, or HCN1-ΔCNBD in a bath solution containing (in mM): 5 KCl, 84 NaCl 15 HEPES, 0.4 CaCl2, and 0.8 MgCl2, pH = 7.4 using OC-725C amplifier (Warner Instruments, United States) and digitized using a Digidata 1322 A (Molecular Devices, Sunnyvale, CA, United States). High [K+] recordings contained 30 mM KCl, and 59 mM NaCl instead. Quetiapine (QTP), 7-hydroxyquetiapine (7-OH QTP) or norquetiapine (NQTP) (Toronto Research Chemicals, Toronto, ON, Canada) were dissolved in DMSO to make 100 mM stock solutions that were stored at −20°C. On the day of the experiments, the stock solutions were diluted in extracellular solution to the final desired concentrations. All data were acquired using the software Clampex 10.5 at a sampling rate of 5 KHz with a filter of 1 kHz. HCN1 activation was assessed by 1.7 s test-steps between −130 and −30 mV (ΔV = +10 mV) from a VH = −30 mV, followed by a 1.5 s step to −130 mV. Deactivation was assessed by applying a 1.75 sec pre-pulse to −130 mV, followed by test pulses from +50 to −70 mV (ΔV = −10 mV). Since HCN2 channels activate slower and at more negative potentials, the protocol was altered to 2 s test-steps between −160 and −20 mV (ΔV = +10 mV) from a VH = −30 mV, followed by a 1.5 s step to −160 mV. Similarly, HCN4 were activate by 3 s test-steps between −160 to −20 mV (ΔV = +10 mV) from a VH = −30 mV, followed by a 1 s step to −160 mV. In all recordings, cells were held at the holding potential for an inter-pulse time of 27 s to allow the channels to fully close between sweeps. Control recordings (0 µM) were performed 2 min after impaling the cells, to allow stabilization of currents, and then QTP, 7-OH QTP or NQTP were added to the bath solution for at the defined concentration for 7.5 min (15 or 30 min in some cases as indicated) to enable pair-wise experiments. Experiments were also performed with equimolar quantities of DMSO used to solvate the drugs to their listed concentrations were used as additional controls. All compounds were tested for up to 30 min to ensure any effects or lack thereof were not time dependent. All recordings were conducted at room temperature (20–23°C).
Open-state inhibition was assessed using a constant pulse to −130 mV and adding 30 µM NQTP once HCN1 currents reached steady-state. Closed state inhibition was assessed using a repetitive pulse protocol with 2s pulses to −130 mV every 30 s from a holding potential (VH) of −10 mV. Inhibition by 30 µM NQTP was compared when repetitive pulses were continuously applied during NQTP addition, or cells were held at VH for 7.5 min.
Data analysis and statistics
All recordings were analyzed offline using the Clampfit (Molecular Devices) software. Data was analyzed and plotted using Origin 8.0 software (Northampton, MA, United States). Current-voltage relationships were analyzed using built in software in pClamp, taking each respective voltage to an inquired current. The I-V relationship was fit with the Boltzman I-V equation:
Activation and deactivation kinetics were determined with mono- or bi-exponential fits of test pulses after the initial lag period, as shown. Steady-state activation curves were fit with the Boltzmann equation:
where Vm corresponds to the test pulse, V1/2 is the midpoint of activation and k is the inverse slope factor. Concentration dependences of the drug-induced shift in V1/2 (ΔV1/2) were fit with the Hill equation:
where nH is the Hill co-efficient. Data are presented as means (±) standard error of the total number of cells (N). Statistical significance for I-V curves were determined measured using two-way ANOVA with Tukey HSD post hoc analysis. V1/2’s of steady-state dependencies were determined for each recording and pooled for a given treatment then analyzed by pairwise student t-test. A P value <0.05 was considered as statistically significant.
Results
Inhibition of HCN1 channel by norquetiapine but not quetiapine
Since 30 µM quetiapine (QTP) induces ∼80% inhibition of hERG channels (Lee et al., 2018) and 50% inhibition of Nav1.5 channels (Kim et al., 2020), we examined the effects of 30 µM QTP on HCN1 channels expressed in X. laevis oocytes using TEVC. At this concentration, in paired experiments, we observed no significant changes in the current-voltage relationship, steady-state voltage-dependence, nor gating kinetics of HCN1 (Figure 2, Tables 1, 2) using Equations 1, 2. No effect of QTP on HCN1 is observable, even if the incubation period is extended from 15 min to 30 min (Figure 2).
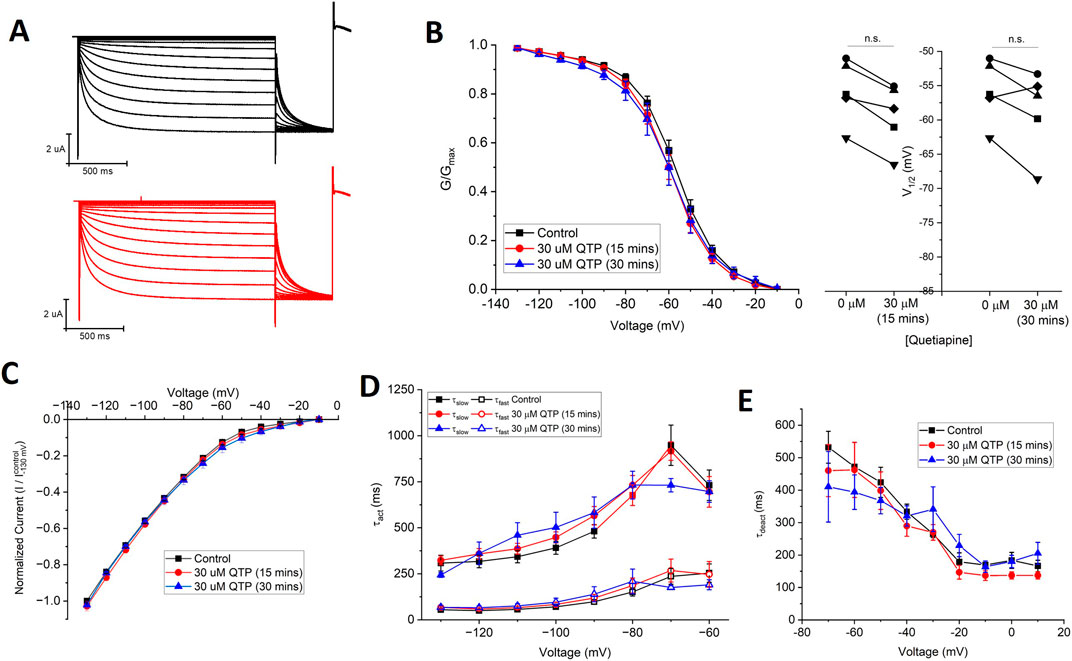
Figure 2. Quetiapine (QTP) does not regulate HCN1 channels. (A) Representative traces from a paired activation experiment following the addition of 30 µM QTP to oocytes expressing full-length HCN1. 30 μM QTP does not affect (B) the voltage-dependence of activation (V1/2 of each cell is shown on the right) (n = 5, P = 0.11) (C) the current-voltage (I-V) relationship (n = 5, P = 0.35) (D) activation kinetics (n = 5, P = 0.56) or (E) deactivation kinetics (n = 5, P = 0.72) of HCN1 channels.
Since NQTP also exhibits antidepressant activity (Jensen et al., 2008; Lopez-Munoz and Alamo, 2013) and a pharmacological activity that differs from QTP (Bakken et al., 2012; DeVane and Nemeroff, 2001), we also examined the effects of 30 µM NQTP on HCN1 channels (Figure 3). NQTP reduces HCN1 currents (Figure 3E), with a −11.8 ± 0.5 mV hyperpolarizing shift in the voltage-dependence of activation (Figure 3B) and slowing the kinetics of activation (Figure 3C). Deactivation kinetics are unchanged with NQTP treatment (Figure 3D). Examination of the concentration dependence of NQTP on the shift in voltage-dependence of activation (ΔV1/2) using Equation 3 indicates an IC50 of 13.9 ± 0.8 μM with a maximum ΔV1/2 of −15.4 ± 1.2 mV and a Hill co-efficient of 4.2 ± 0.1 (Figure 3F). Contrary to the effects of NQTP on HCN1 function, 7-OH QTP had no observable effects on HCN1 current, voltage-dependence, or gating kinetics (Figure 4) for incubation periods between 10 and 30 min.
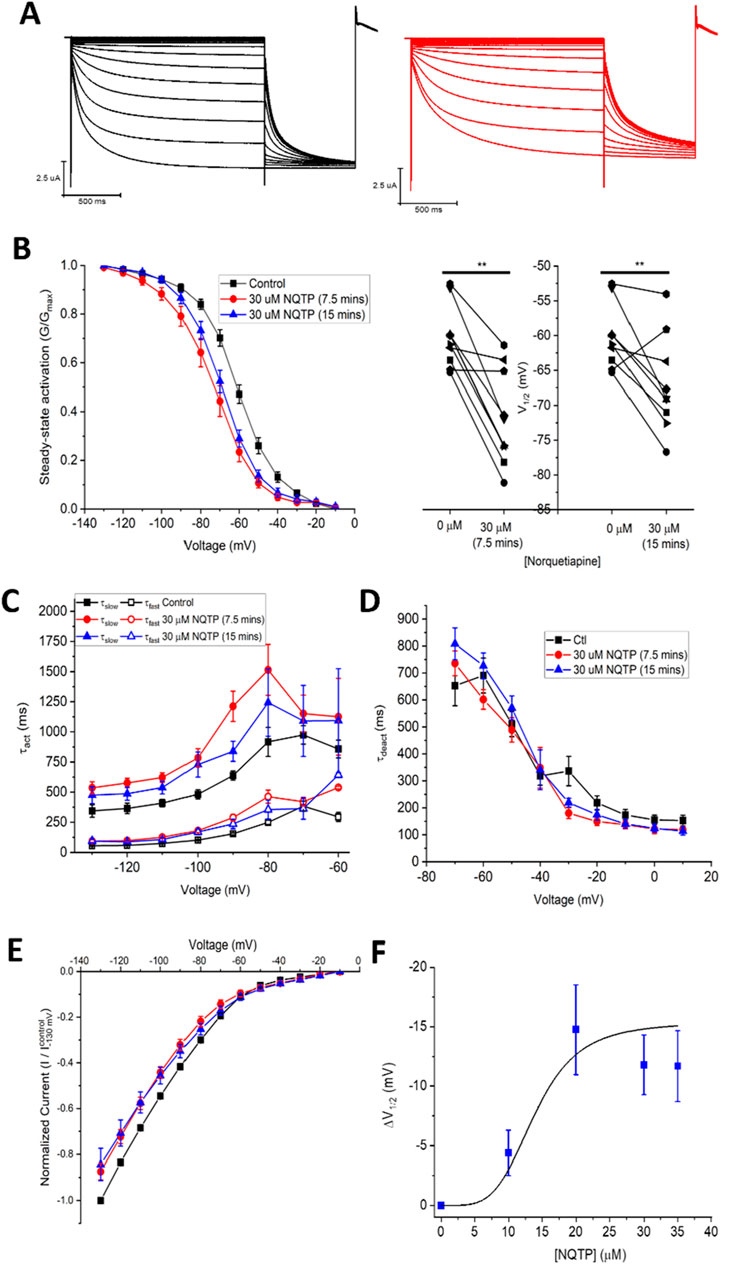
Figure 3. Norquetiapine (NQTP) inhibits HCN1 channels. (A) Representative traces from a paired activation experiment (control in black) following the addition of 30 µM NQTP (red traces) to oocytes expressing full-length HCN1. (B) NQTP induces a hyperpolarizing shift in the steady-state voltage-dependence of activation (P < 0.05 for V1/2 for 7.5 and 15 min compared to control). (C) Activation time constants (τfast and τslow) are greater in the presence of NQTP (P < 0.05). (D) Deactivation time constants (τdeact) are unchanged in presence of NQTP. (n = 6; P = 0.23). (E) Current-voltage (I-V) relationship in presence of NQTP normalized to maximal current (IControl (−130 mV)). (n = 9; P < 0.05 for 7.5 and 15 min compared to control). (F) Concentration dependence of ΔV1/2 fit with a Hill Equation 3 indicates NQTP inhibits HCN1 channels with an IC50 of 13.9 ± 0.8 μM, a maximum ΔV1/2 of −15.4 ± 1.2 mV and a Hill co-efficient of 4.2 ± 0.1.
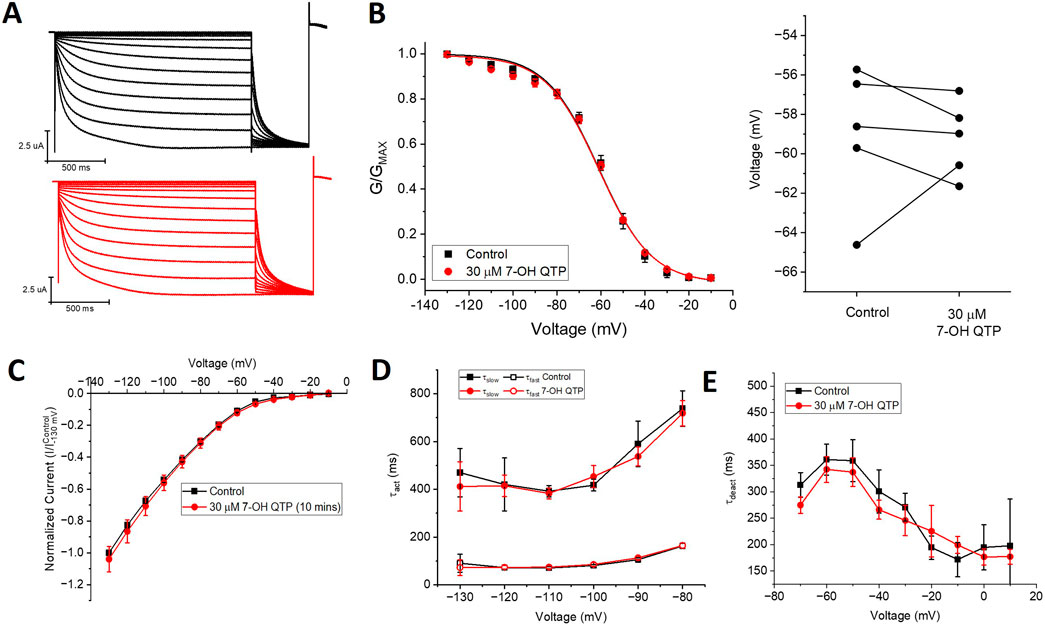
Figure 4. 7-hydroxyquetiapine (7-OH QTP) does not regulate HCN1 channels. (A) Representative traces from a paired activation experiment (control in black) following the addition of 30 µM 7-OH QTP (red traces) to oocytes expressing full-length HCN1. 30 μM 7-OH QTP does not affect (B) the voltage-dependence of activation (V1/2 of each cell is shown on the right) (n = 5, P = 0.64) (C), the current-voltage (I-V) relationship (n = 5, p = 0.47), (D) activation kinetics (n = 5, p = 0.88), or (E) deactivation kinetics (n = 6, P = 0.35) of HCN1 channels.
Comparison of inhibition of HCN isoforms by 30 µM norquetiapine
To determine if NQTP inhibition is specific to HCN1 channels, we assessed if 30 µM NQTP can also inhibit HCN2 or HCN4 channels. Pair-wised assessment of NQTP on HCN2 leads to a −3.2 ± 1.7 mV change in the voltage-dependence of activation, however, this did not reach statistical significance (P = 0.09). Thus, no significant changes in HCN2 function (I-V relationship, voltage-dependence of activation, or gating kinetics) are observed following incubation with NQTP at concentrations between 10 μM and 30 µM (Figure 5). To ensure the lack of an effect was due to equilibration within the membrane, we extended the period of incubation from 7.5 min to 15 min, however, still no effect is observable. On the other hand, NQTP inhibited HCN4 channels by inducing a −6.5 ± 2.1 mV hyperpolarizing shift in voltage-dependent activation and slowing the rate of activation (Figure 6). Thus, while HCN4 can be inhibited by NQTP, HCN1 channels are inhibited with higher efficacy than HCN2 and HCN4 at the concentrations tested. Notably, in our hands Xenopus oocytes do not tolerate the application of >35 µM NQTP and thus a concentration dependence for HCN4 could not be reliably collected.
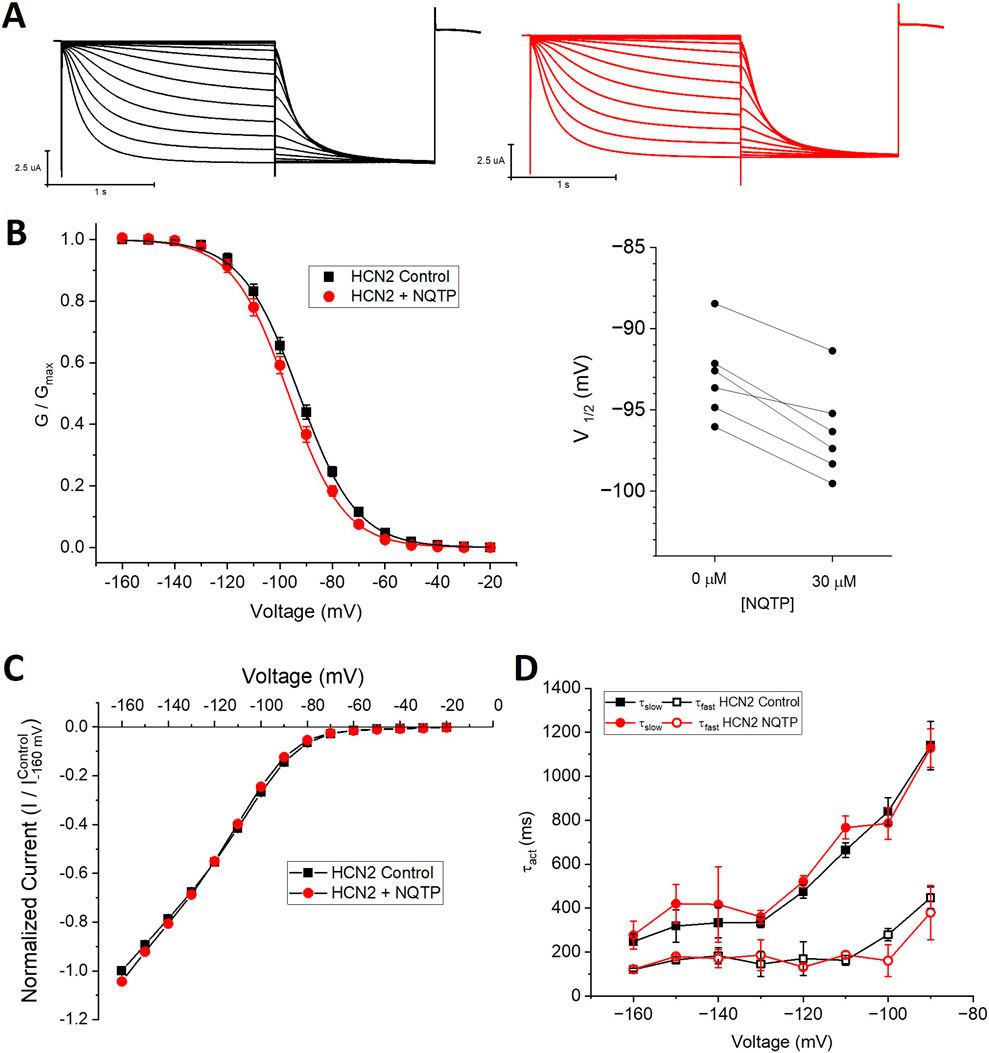
Figure 5. Norquetiapine (NQTP) does not inhibit HCN2 channels. (A) Representative traces from a paired activation experiment (control in black) following the addition of 30 µM NQTP (red traces) to oocytes expressing full-length HCN1. Unlike what we observe for HCN1 channels, 30 µM NQTP does not alter (B) the voltage-dependence of activation (V1/2 of each cell is shown on the right) (n = 6, P = 0.09), (C) the current-voltage (I-V) relationship (n = 6, p = 0.79) nor (D) the activation kinetics (n = 6, p = 0.65).
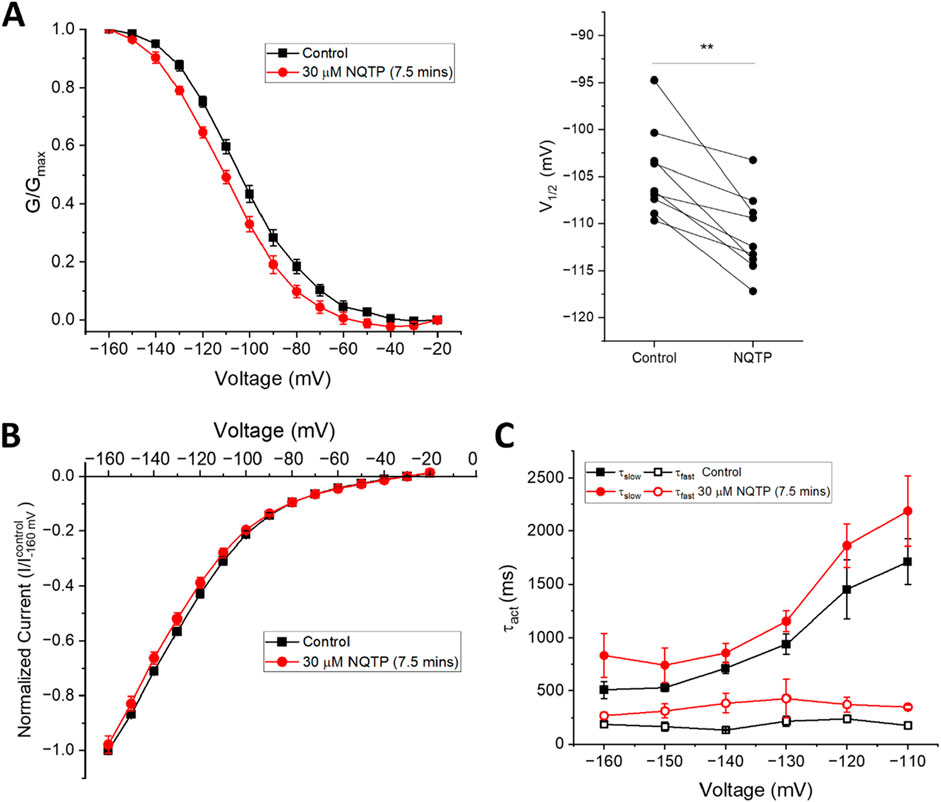
Figure 6. Norquetiapine (NQTP) inhibits HCN4 channels. (A) NQTP induces a hyperpolarizing shift in the steady-state voltage-dependence of activation (p < 0.05 for V1/2) (V1/2 of each cell is shown on the right) (n = 9, p = 0.001). (B) Current-voltage (I-V) relationship in presence of NQTP normalized to maximal current (IControl (-160 mV)). (n = 9; p = 0.63 for 7.5 min compared to control). (C) Activation time constants (τfast and τslow) are greater in the presence of NQTP (P < 0.05).
Mechanistic characterization of norquetiapine modulation of HCN1 channels
To assess if NQTP inhibits HCN function from the open state we applied a prolonged activation step to −130 mV, to fully activate the channels, and applied 30 µM NQTP at steady-state (Figure 7A). We observe a current ratio (INQTP/Icontrol) of 0.95 ± 0.01 following this protocol. Thus, NQTP has minimal effects on HCN channels in the open state.
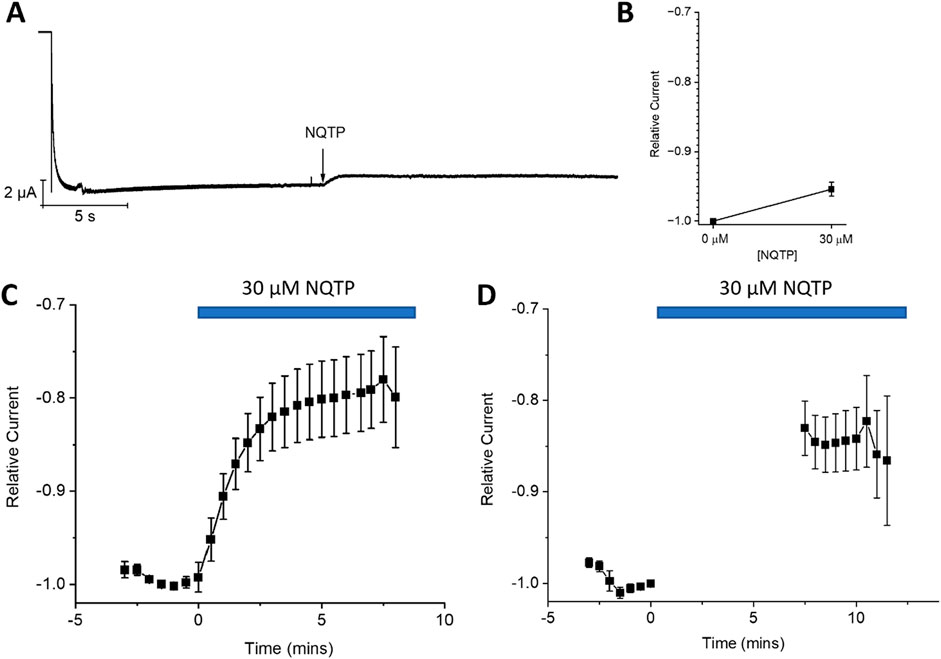
Figure 7. State-dependence of norquetiapine (NQTP) inhibition of HCN1 channels. (A) Open-state block was assessed by a prolonged activation step to −130 mV and applying 30 µM NQTP at steady-state. (B) Relative current (INQTP/Icontrol) to be 0.95 ± 0.01 following NQTP treatment (n = 5; P < 0.05). (C) Closed-state block was assessed using a repetitive −130 mV/+30 mV pulse protocol every 30 s 30 μM NQTP was applied after the stabilization of HCN1 currents, and resulted in a decrease of current by 20.4% ± 1.1% (n = 7). (D) When the repetitive protocol is interrupted and cells are held at VH = −10 mV for 7.5 min during the application of NQTP the amount of inhibition that is induced is 15.8% ± 0.7% (n = 9) indicating NQTP is a closed state blocker of HCN1.
We also examined if NQTP inhibition involves interactions in the closed state. We performed a repetitive pulse protocol in which channels were opened at −130 mV for 2 s then closed at +30 mV every 30 s. 30 μM NQTP was applied after the stabilization of HCN1 currents and resulted in a decrease of current by 20.4% ± 1.1% (Figure 7C). This is similar to the amount of inhibition that is induced when the repetitive protocol is interrupted and cells are held at VH = −10 mV for 7.5 min during the application of NQTP (15.8% ± 0.7%) (Figure 7B). Under this condition, cells are predominately closed. Therefore, HCN1 channels can be inhibited by NQTP from the closed state.
To determine if NQTP acts on HCN1 channels through interactions with the CNBD, we examined the effects on HCN1 channels lacking this domain (HCN1ΔCNBD) (Figure 8). Similarly to full-length HCN1 channels, 30 µM NQTP induces an approximate 10% reduction in the I-V relationship of HCN1ΔCNBD compared to control (Figure 8B). Additionally, 30 µM NQTP shifts the V1/2 of activation to more hyperpolarized potentials by −11.8 ± 1.2 mV (Figure 8C). Thus, inhibition of HCN1 channels by NQTP is not dependent on the CNBD.
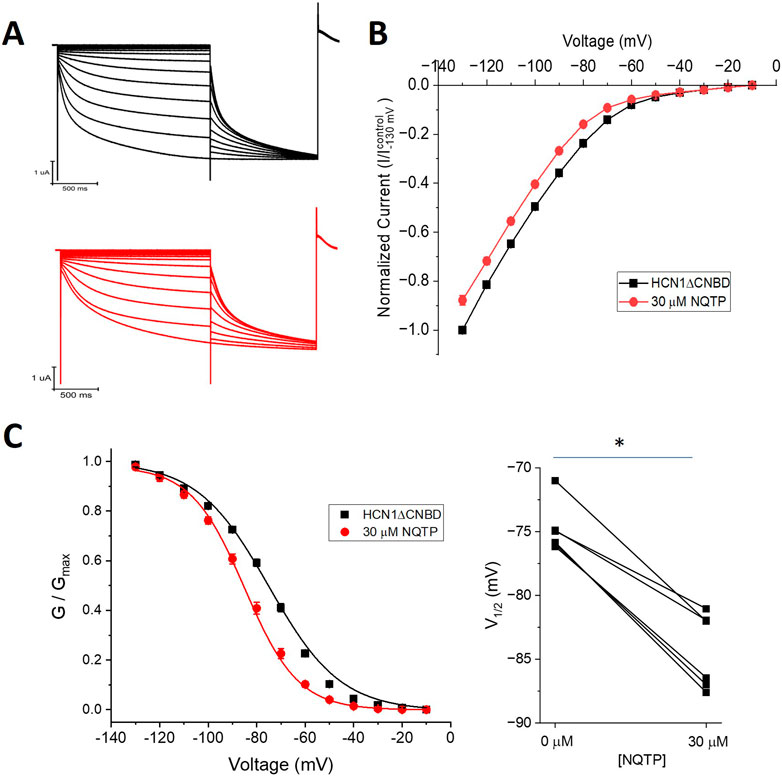
Figure 8. Norquetiapine (NQTP) inhibition of HCN1 channels does not depend on the CNBD. (A) Representative traces from a paired activation experiment (control in black) following the addition of 30 µM NQTP (red traces) to oocytes expressing HCN1ΔCNBD. (B) Current-voltage (I-V) relationship in presence of NQTP normalized to maximal current (Icontrol (-130 mV)). (n = 6; P < 0.05). (C) NQTP induces a hyperpolarizing shift in the steady-state voltage-dependence of activation (P < 0.05).
If NQTP inhibits HCN channels by binding in the pore region, similarly to ivabradine, ZD7288, clonidine, lidocaine, and other inhibitors (Cheng et al., 2007; Tanguay et al., 2019), we would anticipate that NQTP inhibition would depend on the extracellular K+ concentration ([K+]o). Specifically, we would anticipate that increasing [K+]o would reduce the effect of NQTP on HCN1. Instead, we observe that increasing [K+]o from 5 mM to 30 mM (by replacing the equivalent amount of extracellular Na+) had no effect on NQTP inhibition of HCN1 currents (Figure 9). 30 μM NQTP continues to reduce the I-V relationship (Figure 9B), and induces a hyperpolarizing shift in the V1/2 of activation by −9.5 ± 0.4 mV (Figure 8C) and slowed activation of HCN1 in 30 mM [K+]o. Thus, these data suggest that NQTP inhibition occurs via a different mechanism than many other known inhibitors of HCN channels which block the pore-domain and are sensitive to [K+]o.
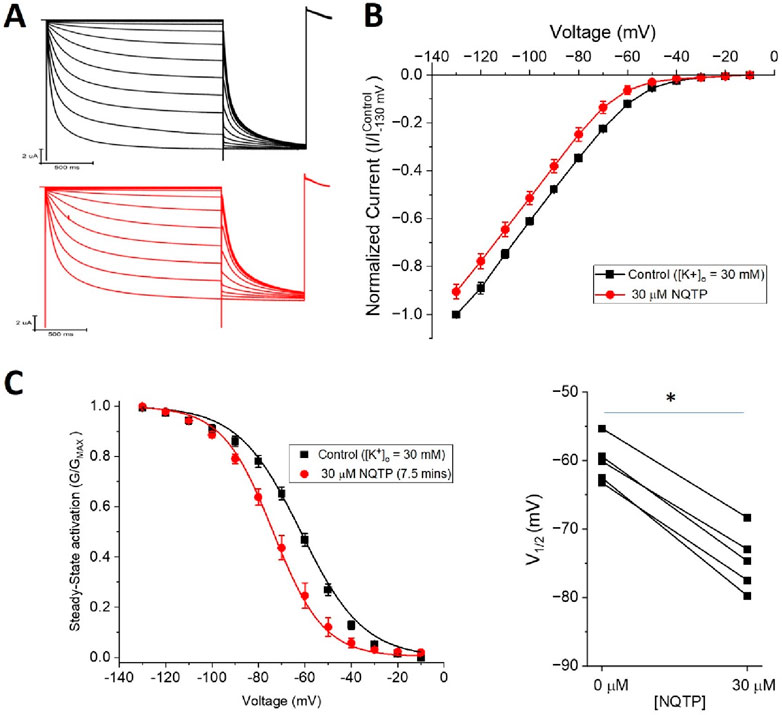
Figure 9. Norquetiapine (NQTP) inhibition of HCN1 channels is not affected by increasing [K+]o. (A) Representative traces from a paired activation experiment following the addition of 30 µM NQTP (red traces) to oocytes expressing full-length HCN1 bathed in high external potassium (control in black). (B) Current-voltage (I-V) relationship in presence of NQTP normalized to maximal current (Icontrol (-130 mV)). (n = 6; P < 0.05). (C) NQTP induces a hyperpolarizing shift in the steady-state voltage-dependence of activation (P < 0.05 for V1/2).
Discussion
Antipsychotics are a class of psychotropic medication primarily used to manage psychosis (including delusions, hallucinations, paranoia or disordered thought), principally in schizophrenia and bipolar disorder. All antipsychotics block dopamine D2 receptors, however, “atypical” antipsychotics (AAPs) bind less avidly to D2 receptors, leading to fewer extrapyramidal side effects at appropriate doses. AAPs also antagonize serotonin receptors, mainly 5HT2A. Consequently, AAPs are being used more often in recent years to treat anxiety, MDD, PTSD and other disorders. Quetiapine (QTP), a second-generation AAP commonly prescribed for the treatment of schizophrenia (Small et al., 1997; Dev and Raniwalla, 2000) and acute bipolar mania (Janicak and Rado, 2012), is now also used to treat insomnia (Lin et al., 2023), MDD (Ravindran et al., 2022), anxiety (Ravindran et al., 2022; Crapanzano et al., 2021; Bandelow et al., 2010), and PTSD (Crapanzano et al., 2023). Like other AAPs, QTP is structurally similar to clozapine and acts as an antagonist to serotonin, dopamine, histamine, and adrenergic receptors (Saller and Salama, 1993; Burns, 2001). QTP is primarily metabolized by hepatic cytochrome P450 3A4 (Dev and Raniwalla, 2000), with norquetiapine (NQTP) as its major active metabolite (Figure 1). NQTP exhibits pharmacological activity that differs from QTP (Bakken et al., 2012; DeVane and Nemeroff, 2001) and also exhibits antidepressant activity (Jensen et al., 2008; Lopez-Munoz and Alamo, 2013). In fact, NQTP shares structural similarities with several antidepressants including amoxapine and desipramine, and its physicochemical properties confer greater potential for its use as an antidepressant agent (Lopez-Munoz and Alamo, 2013; Kim et al., 2016). Indeed, the effect of QTP in major depressive disorder is probably mediated, at least in part, by NQTP, which selectively inhibits norepinephrine transporter reuptake (Bandelow et al., 2010; Lopez-Munoz and Alamo, 2013). Given the role of HCN channels in MDD, anxiety, and schizophrenia, we hypothesize that QTP and its major metabolites NQTP and 7-OH may work effectively in part via inhibition of HCN function.
Here we demonstrate that norquetiapine, but not quetiapine, inhibits HCN1 channels by shifting the voltage-dependence to hyperpolarized potentials and slowing channel opening. NQTP inhibited HCN1 channels with an IC50 of 13.9 ± 0.8 μM. This is similar to the ranges in which QTP and NQTP were found to inhibit other ion channels. Specifically, QTP and NQTP were found to block hERG current with a half-maximal inhibitory concentration of 8.3 and 10.8 μM, respectively (Lee et al., 2018). Nav1.5 currents were also shown to be inhibited by QTP and NQTP with IC50 of 30 and 6 μM respectively (Kim et al., 2020). Notably, HCN1 currents are still inhibited, with an approximate −3 to −7 mV shift in activation at these concentrations (Figure 3F). It is speculated that the inhibition of cardiac sodium channels by these drugs can reduce the risk of cardiotoxicity induced by the inhibition of hERG current. By comparison, inhibition of HCN channels by other molecules including ivabradine, ZD7288, clonidine, lidocaine, ketamine, and carvedilol also act within a similar concentration range (Cheng et al., 2007; BoSmith et al., 1993; Bucchi et al., 2006; Cao et al., 2018; Putrenko et al., 2017; Xing et al., 2017). Therefore, inhibition of HCN channels by NQTP occurs within the physiological range, and likely contributes to its therapeutic role.
It is not uncommon that for a number of drugs acting on a given target, the EC50/IC50 determined in oocyte experiments differ from EC50/IC50 determined in mammalian cells, however, the underlying effects remain. For example, the IC50 of hERG channel block by the antiarrhythmic drug BRL-32872 is ∼240 nM in Xenopus oocytes, but ∼20 nM for mammalian HEK293 cells (Thomas et al., 2001). Acehytisine blocks HCN4 channels expressed in Xenopus oocytes with an IC50 of ∼65 μM, but ∼10 µM in native If-channels of the rabbit SAN (Fan et al., 2012). Furthermore, the IC50 of ketamine inhibition of HCN1 expressed in HEK293 cells is ∼8 μm for V1/2 and ∼16 μm for current amplitude (Chen et al., 2009), but 67 μM in Xenopus oocytes (Xing et al., 2017). Thus, it is possible, or even likely, that NQTP effects HCN1 and HCN4 channels at even lower concentrations in mammalian cells.
Inhibition of HCN1 channels has been suggested as a key therapeutic target for depression. HCN1−/− mice show impaired motor learning but enhanced spatial learning and memory (Nolan et al., 2004; Nolan et al., 2003) and enhanced resistance to depression (Lewis et al., 2011; Huang et al., 2009). Targeted knockdown of HCN1 by shRNA in the CA1 hippocampal region enhances mobility in the Porsolt swim test, a behavioural model for anti-depressant effects (Kim et al., 2012). Furthermore, HCN1 expression increases in the CA1 region of the dorsal hippocampus of chronic unpredictable stress rats, while stress responses are reduced upon HCN1 shRNA knockdown (Kim et al., 2018). Additionally, Trip8b knockout mice are also more resistant to depression (Lewis et al., 2011). Recently, the benzisoxazole derivative Org 34,167, which has been patented for the treatment of depression and progressed to Phase I trials, was shown to be a broad-spectrum brain penetrant inhibitor of HCN channels, and resulted in reduced marble burying and increased the time spent mobile in the Porsolt swim and tail suspension tests in both male and female mice, suggesting reduced depressive-like behaviour (Pinares-Garcia et al., 2023). Thus, the inhibition of HCN1 channels by norquetiapine could provide a therapeutic benefit and contribute to the molecular mechanism of its anti-depressant action. Furthermore, the ability for HCN1 channels to be inhibited by NQTP but not QTP or 7-OH QTP may also provide a platform from which to develop even more specific negative gating modulators of HCN channels.
NQTP selectivity for HCN1 over HCN2 and HCN4 may also be an important factor in its therapeutic role. Intriguingly, the role of HCN2 and HCN4 in major depressive disorder is less straightforward than HCN1. HCN2−/− mice spend more time mobile in the tail suspension test (Lewis et al., 2011), suggesting a reduction in HCN2 has antidepressant effects. However, the expression of HCN2 is reduced in cholinergic interneurons in the nucleus accumbens of mice subjected to chronic stress, while HCN2 overexpression rescues the depressive phenotypes (Cheng et al., 2019). Similarly, HCN2 overexpression in dopamine neurons of the ventral tegmental area is effective in reversing the depressive phenotypes caused by chronic mild unpredictable stress in mice (Zhong et al., 2018). These data suggest that unlike what was observed for HCN1, stimulation of HCN2 is more favourable for the treatment of depression than HCN2 inhibition. Similarly, while HCN4 knockdown in the hippocampus suggested an increase in anxiety-like activity (Gunther et al., 2019), brain-specific HCN4 knockdown had a subtle anxiolytic effect (Kharouf et al., 2020). Thus, further supporting a role for NQTP inhibition of HCN1, and possibly HCN4, as part of the therapeutic mechanism of action of NQTP. However, since HCN4 channels also play a critical role in cardiac rhythmicity, examinations into the bradycardic effects of future NQTP-based treatments should also be considered.
HCN channels are blocked by a number of inhibitors, with ivabradine arguably the best characterized. Ivabradine does not demonstrate isoform specificity between HCN1-4 channels (Bucchi et al., 2006; Stieber et al., 2006). Ivabradine blocks the open state of HCN4 when the channels are opened by hyperpolarization, with enhanced binding upon frequent changes in the direction of ion flow (Bucchi et al., 2006; Bois et al., 1996; Bucchi et al., 2002) However, HCN1 channels can also be inhibited from the closed state (Bucchi et al., 2006). ZD7288 is also an open-state blocker of HCN channels (Cheng et al., 2007; Benetos et al., 1999; Shin et al., 2001; Wu et al., 2012) that induces at −15 mV shift in voltage-dependent Ih activation and reduces maximal activity by more than 50% (BoSmith et al., 1993). Lidocaine, bupivacaine and mepivacaine blockade of HCN channels also occurs from the inside of the cell (Putrenko et al., 2017). These inhibitors may bind in the open pore interacting with residues C358, A383, Y386, A387, V390 (HCN1 numbering) with residues C358, Y386, and A387 lining a hydrophobic groove within the pore cavity that may conformationally restrict the smaller ligands (Tanguay et al., 2019). Notably, these inhibitors are sensitive to extracellular potassium concentrations. Thus, it does not appear that NQTP inhibition of HCN1 follows the same pore-binding mechanism as these inhibitors.
On the other hand, other HCN inhibitors identified appear to act primarily as negative gating modulators of HCNs, rather than blockers of the ion conduction pathway. Niflumic acid may interact with the outer voltage-sensor domain (Cheng and Sanguinetti, 2009) While the binding site of carvedilol is not yet resolved, it is a closed-state inhibitor of HCN channels that induces a hyperpolarizing shift in the voltage-dependence of activation, but interacts with the channels at a site distinct from the pore-binding site of ivabradine or ZD7288 (Cao et al., 2018). There is also no evidence that ketamine, which inhibits HCN1 channels with a −20 mV shift in voltage-dependence and a reduction in activation kinetics at 25 μM, but not HCN2 and HCN4 at that concentration, could act as a pore inhibitor (Xing et al., 2017). Our data indicates that NQTP acts to inhibit HCN1 more like a negative gating modulator, rather than a pore blocker, since it modulates the voltage-dependence and gating kinetics of activation, is a closed-state blocker, and insensitive to external potassium concentration.
Our findings that NQTP is a selective inhibitor of HCN1 channels contributes to the understanding of the mode of action of quetiapine for the treatment of neuropsychiatric disorders such as anxiety, major mood disorder, and others. Our results may assist in the development of improved therapeutics based on this molecular scaffold.
Data availability statement
The raw data supporting the conclusions of this article will be made available by the authors, without undue reservation.
Ethics statement
The animal study was approved by the Comité de déontologie de l’expérimentation sur les animaux (CDEA). The study was conducted in accordance with the local legislation and institutional requirements.
Author contributions
AJ: Data curation, Formal Analysis, Methodology, Validation, Visualization, Writing–original draft, Writing–review and editing. ND’A: Conceptualization, Formal Analysis, Funding acquisition, Project administration, Resources, Supervision, Visualization, Writing–original draft, Writing–review and editing.
Funding
The author(s) declare that financial support was received for the research, authorship, and/or publication of this article. This work was supported by a Discovery Grant (RGPIN-2019-00373) from the National Science and Engineering Research Council (NSERC) and a Project Grant from the Canadian Institutes of Health Research (CIHR) (FRN 173388) awarded to ND’A.
Acknowledgments
mHCN1 in pGH19 and mHCN2 in pGEM were kindly provided by Dr. William Zagotta (University of Washington, Seattle, Washington) and mHCN1ΔCNBD (mHCN1-CX5) in pGH19 was kindly provided by Dr. Bina Santoro (Columbia University, New York).
Conflict of interest
The authors declare that the research was conducted in the absence of any commercial or financial relationships that could be construed as a potential conflict of interest.
Publisher’s note
All claims expressed in this article are solely those of the authors and do not necessarily represent those of their affiliated organizations, or those of the publisher, the editors and the reviewers. Any product that may be evaluated in this article, or claim that may be made by its manufacturer, is not guaranteed or endorsed by the publisher.
Abbreviations
MMD, major mood disorder; HCN, hyperpolarization-activated cyclic-nucleotide gated; CNBD, cyclic-nucleotide binding domain; WT, wild-type; FL, full-length; QTP quetiapine; NQTP, norquetiapine.
References
Arnsten, A. F. (2011). Prefrontal cortical network connections: key site of vulnerability in stress and schizophrenia. Int. J. Dev. Neurosci. 29, 215–223. doi:10.1016/j.ijdevneu.2011.02.006
Bakken, G. V., Molden, E., Knutsen, K., Lunder, N., and Hermann, M. (2012). Metabolism of the active metabolite of quetiapine, N-desalkylquetiapine in vitro. Drug Metab. Dispos. 40, 1778–1784. doi:10.1124/dmd.112.045237
Bandelow, B., Chouinard, G., Bobes, J., Ahokas, A., Eggens, I., Liu, S., et al. (2010). Extended-release quetiapine fumarate (quetiapine XR): a once-daily monotherapy effective in generalized anxiety disorder. Data from a randomized, double-blind, placebo- and active-controlled study. Int. J. Neuropsychopharmacol. 13, 305–320. doi:10.1017/S1461145709990423
Benetos, A., Rudnichi, A., Thomas, F., Safar, M., and Guize, L. (1999). Influence of heart rate on mortality in a French population: role of age, gender, and blood pressure. Hypertension 33, 44–52. doi:10.1161/01.hyp.33.1.44
Bois, P., Bescond, J., Renaudon, B., and Lenfant, J. (1996). Mode of action of bradycardic agent, S 16257, on ionic currents of rabbit sinoatrial node cells. Br. J. Pharmacol. 118, 1051–1057. doi:10.1111/j.1476-5381.1996.tb15505.x
BoSmith, R. E., Briggs, I., and Sturgess, N. C. (1993). Inhibitory actions of ZENECA ZD7288 on whole-cell hyperpolarization activated inward current (If) in Guinea-pig dissociated sinoatrial node cells. Br. J. Pharmacol. 110, 343–349. doi:10.1111/j.1476-5381.1993.tb13815.x
Bucchi, A., Baruscotti, M., and DiFrancesco, D. (2002). Current-dependent block of rabbit sino-atrial node I(f) channels by ivabradine. J. Gen. Physiol. 120, 1–13. doi:10.1085/jgp.20028593
Bucchi, A., Tognati, A., Milanesi, R., Baruscotti, M., and DiFrancesco, D. (2006). Properties of ivabradine-induced block of HCN1 and HCN4 pacemaker channels. J. Physiol. 572, 335–346. doi:10.1113/jphysiol.2005.100776
Burns, M. J. (2001). The pharmacology and toxicology of atypical antipsychotic agents. J. Toxicol. Clin. Toxicol. 39, 1–14. doi:10.1081/clt-100102873
Cao, Y., Chen, S., Liang, Y., Wu, T., Pang, J., Liu, S., et al. (2018). Inhibition of hyperpolarization-activated cyclic nucleotide-gated channels by β-blocker carvedilol. Br. J. Pharmacol. 175, 3963–3975. doi:10.1111/bph.14469
Chaplan, S. R., Guo, H. Q., Lee, D. H., Luo, L., Liu, C., Kuei, C., et al. (2003). Neuronal hyperpolarization-activated pacemaker channels drive neuropathic pain. J. Neurosci. 23, 1169–1178. doi:10.1523/JNEUROSCI.23-04-01169.2003
Chen, X., Shu, S., and Bayliss, D. A. (2009). HCN1 channel subunits are a molecular substrate for hypnotic actions of ketamine. J. Neurosci. 29, 600–609. doi:10.1523/JNEUROSCI.3481-08.2009
Cheng, L., Kinard, K., Rajamani, R., and Sanguinetti, M. C. (2007). Molecular mapping of the binding site for a blocker of hyperpolarization-activated, cyclic nucleotide-modulated pacemaker channels. J. Pharmacol. Exp. Ther. 322, 931–939. doi:10.1124/jpet.107.121467
Cheng, L., and Sanguinetti, M. C. (2009). Niflumic acid alters gating of HCN2 pacemaker channels by interaction with the outer region of S4 voltage sensing domains. Mol. Pharmacol. 75, 1210–1221. doi:10.1124/mol.108.054437
Cheng, J., Umschweif, G., Leung, J., Sagi, Y., and Greengard, P. (2019). HCN2 channels in cholinergic interneurons of nucleus accumbens shell regulate depressive behaviors. Neuron. 101, 662–672. doi:10.1016/j.neuron.2018.12.018
Crapanzano, C., Damiani, S., Casolaro, I., and Amendola, C. (2023). Quetiapine treatment for post-traumatic stress disorder: a systematic review of the literature. Clin. Psychopharmacol. Neurosci. 21, 49–56. doi:10.9758/cpn.2023.21.1.49
Crapanzano, C., Damiani, S., and Guiot, C. (2021). Quetiapine in the anxiety dimension of mood disorders: a systematic review of the literature to support clinical practice. J. Clin. Psychopharmacol. 41, 436–449. doi:10.1097/JCP.0000000000001420
Dev, V., and Raniwalla, J. (2000). Quetiapine: a review of its safety in the management of schizophrenia. Drug Saf. 23, 295–307. doi:10.2165/00002018-200023040-00003
DeVane, C. L., and Nemeroff, C. B. (2001). Clinical pharmacokinetics of quetiapine: an atypical antipsychotic. Clin. Pharmacokinet. 40, 509–522. doi:10.2165/00003088-200140070-00003
Fan, X., Chen, Y., Xing, J., Wu, P., Chen, H., Yang, J., et al. (2012). Blocking effects of acehytisine on pacemaker currents (I(f)) in sinoatrial node cells and human HCN4 channels expressed in Xenopus laevis oocytes. J. Ethnopharmacol. 139, 42–51. doi:10.1016/j.jep.2011.10.039
Gamo, N. J., Lur, G., Higley, M. J., Wang, M., Paspalas, C. D., Vijayraghavan, S., et al. (2015). Stress impairs prefrontal cortical function via D1 dopamine receptor interactions with hyperpolarization-activated cyclic nucleotide-gated channels. Biol. Psychiatry 78, 860–870. doi:10.1016/j.biopsych.2015.01.009
Gauthier, J., Champagne, N., Lafreniere, R. G., Xiong, L., Spiegelman, D., Brustein, E., et al. (2010). De novo mutations in the gene encoding the synaptic scaffolding protein SHANK3 in patients ascertained for schizophrenia. Proc. Natl. Acad. Sci. U. S. A. 107, 7863–7868. doi:10.1073/pnas.0906232107
Guilmatre, A., Huguet, G., Delorme, R., and Bourgeron, T. (2014). The emerging role of SHANK genes in neuropsychiatric disorders. Dev. Neurobiol. 74, 113–122. doi:10.1002/dneu.22128
Gunther, A., Luczak, V., Gruteser, N., Abel, T., and Baumann, A. (2019). HCN4 knockdown in dorsal hippocampus promotes anxiety-like behavior in mice. Genes Brain Behav. 18, e12550. doi:10.1111/gbb.12550
Huang, Z., Walker, M. C., and Shah, M. M. (2009). Loss of dendritic HCN1 subunits enhances cortical excitability and epileptogenesis. J. Neurosci. 29, 10979–10988. doi:10.1523/JNEUROSCI.1531-09.2009
Janicak, P. G., and Rado, J. T. (2012). Quetiapine for the treatment of acute bipolar mania, mixed episodes and maintenance therapy. Expert Opin. Pharmacother. 13, 1645–1652. doi:10.1517/14656566.2012.681377
Jensen, N. H., Rodriguiz, R. M., Caron, M. G., Wetsel, W. C., Rothman, R. B., and Roth, B. L. (2008). N-desalkylquetiapine, a potent norepinephrine reuptake inhibitor and partial 5-HT1A agonist, as a putative mediator of quetiapine's antidepressant activity. Neuropsychopharmacology 33, 2303–2312. doi:10.1038/sj.npp.1301646
Kharouf, Q., Phillips, A. M., Bleakley, L. E., Morrisroe, E., Oyrer, J., Jia, L., et al. (2020). The hyperpolarization-activated cyclic nucleotide-gated 4 channel as a potential anti-seizure drug target. Br. J. Pharmacol. 177, 3712–3729. doi:10.1111/bph.15088
Kim, C. S., Brager, D. H., and Johnston, D. (2018). Perisomatic changes in h-channels regulate depressive behaviors following chronic unpredictable stress. Mol. Psychiatry 23, 892–903. doi:10.1038/mp.2017.28
Kim, C. S., Chang, P. Y., and Johnston, D. (2012). Enhancement of dorsal hippocampal activity by knockdown of HCN1 channels leads to anxiolytic- and antidepressant-like behaviors. Neuron 75, 503–516. doi:10.1016/j.neuron.2012.05.027
Kim, D. H., Park, K. S., Park, S. H., Hahn, S. J., and Choi, J. S. (2020). Norquetiapine blocks the human cardiac sodium channel Na(v)1.5 in a state-dependent manner. Eur. J. Pharmacol. 885, 173532. doi:10.1016/j.ejphar.2020.173532
Kim, D. W., Weon, K. Y., Hong, E. P., Chung, E. K., and Lee, K. T. (2016). Comparative physicochemical and pharmacokinetic properties of quetiapine and its active metabolite norquetiapine. Chem. Pharm. Bull. (Tokyo) 64, 1546–1554. doi:10.1248/cpb.c16-00223
Knop, G. C., Seeliger, M. W., Thiel, F., Mataruga, A., Kaupp, U. B., Friedburg, C., et al. (2008). Light responses in the mouse retina are prolonged upon targeted deletion of the HCN1 channel gene. Eur. J. Neurosci. 28, 2221–2230. doi:10.1111/j.1460-9568.2008.06512.x
Kongsamut, S., Kang, J., Chen, X. L., Roehr, J., and Rampe, D. (2002). A comparison of the receptor binding and HERG channel affinities for a series of antipsychotic drugs. Eur. J. Pharmacol. 450, 37–41. doi:10.1016/s0014-2999(02)02074-5
Lee, H. J., Choi, J. S., Choi, B. H., and Hahn, S. J. (2018). Effects of norquetiapine, the active metabolite of quetiapine, on cloned hERG potassium channels. Neurosci. Lett. 664, 66–73. doi:10.1016/j.neulet.2017.11.029
Lewis, A. S., Vaidya, S. P., Blaiss, C. A., Liu, Z., Stoub, T. R., Brager, D. H., et al. (2011). Deletion of the hyperpolarization-activated cyclic nucleotide-gated channel auxiliary subunit TRIP8b impairs hippocampal Ih localization and function and promotes antidepressant behavior in mice. J. Neurosci. 31, 7424–7440. doi:10.1523/JNEUROSCI.0936-11.2011
Lin, C. Y., Chiang, C. H., Tseng, M. M., Tam, K. W., and Loh, E. W. (2023). Effects of quetiapine on sleep: a systematic review and meta-analysis of clinical trials. Eur. Neuropsychopharmacol. 67, 22–36. doi:10.1016/j.euroneuro.2022.11.008
Lopez-Munoz, F., and Alamo, C. (2013). Active metabolites as antidepressant drugs: the role of norquetiapine in the mechanism of action of quetiapine in the treatment of mood disorders. Front. Psychiatry 4, 102. doi:10.3389/fpsyt.2013.00102
Ludwig, A., Zong, X., Jeglitsch, M., Hofmann, F., and Biel, M. (1998). A family of hyperpolarization-activated mammalian cation channels. Nature 393, 587–591. doi:10.1038/31255
Moosmang, S., Stieber, J., Zong, X., Biel, M., Hofmann, F., and Ludwig, A. (2001). Cellular expression and functional characterization of four hyperpolarization-activated pacemaker channels in cardiac and neuronal tissues. Eur. J. Biochem./FEBS 268, 1646–1652. doi:10.1046/j.1432-1327.2001.02036.x
Nolan, M. F., Malleret, G., Dudman, J. T., Buhl, D. L., Santoro, B., Gibbs, E., et al. (2004). A behavioral role for dendritic integration: HCN1 channels constrain spatial memory and plasticity at inputs to distal dendrites of CA1 pyramidal neurons. Cell 119, 719–732. doi:10.1016/j.cell.2004.11.020
Nolan, M. F., Malleret, G., Lee, K. H., Gibbs, E., Dudman, J. T., Santoro, B., et al. (2003). The hyperpolarization-activated HCN1 channel is important for motor learning and neuronal integration by cerebellar Purkinje cells. Cell 115, 551–564. doi:10.1016/s0092-8674(03)00884-5
Pape, H. C. (1996). Queer current and pacemaker: the hyperpolarization-activated cation current in neurons. Annu. Rev. Physiol. 58, 299–327. doi:10.1146/annurev.ph.58.030196.001503
Paspalas, C. D., Wang, M., and Arnsten, A. F. (2013). Constellation of HCN channels and cAMP regulating proteins in dendritic spines of the primate prefrontal cortex: potential substrate for working memory deficits in schizophrenia. Cereb. Cortex 23, 1643–1654. doi:10.1093/cercor/bhs152
Pinares-Garcia, P., Spyrou, J., McKenzie, C. E., Forster, I. C., Soh, M. S., Mohamed Syazwan, E., et al. (2023). Antidepressant-like activity of a brain penetrant HCN channel inhibitor in mice. Front. Pharmacol. 14, 1159527. doi:10.3389/fphar.2023.1159527
Putrenko, I., Yip, R., Schwarz, S. K. W., and Accili, E. A. (2017). Cation and voltage dependence of lidocaine inhibition of the hyperpolarization-activated cyclic nucleotide-gated HCN1 channel. Sci. Rep. 7, 1281. doi:10.1038/s41598-017-01253-x
Ravindran, N., McKay, M., Paric, A., Johnson, S., Chandrasena, R., Abraham, G., et al. (2022). Randomized, placebo-controlled effectiveness study of quetiapine XR in comorbid depressive and anxiety disorders. J. Clin. Psychiatry 83, 21m14096. doi:10.4088/JCP.21m14096
Saller, C. F., and Salama, A. I. (1993). Seroquel: biochemical profile of a potential atypical antipsychotic. Psychopharmacol. Berl. 112, 285–292. doi:10.1007/BF02244923
Santoro, B., Grant, S. G., Bartsch, D., and Kandel, E. R. (1997). Interactive cloning with the SH3 domain of N-src identifies a new brain specific ion channel protein, with homology to eag and cyclic nucleotide-gated channels. Proc. Natl. Acad. Sci. U. S. A. 94, 14815–14820. doi:10.1073/pnas.94.26.14815
Santoro, B., Liu, D. T., Yao, H., Bartsch, D., Kandel, E. R., Siegelbaum, S. A., et al. (1998). Identification of a gene encoding a hyperpolarization-activated pacemaker channel of brain. Cell 93, 717–729. doi:10.1016/s0092-8674(00)81434-8
Shin, K. S., Rothberg, B. S., and Yellen, G. (2001). Blocker state dependence and trapping in hyperpolarization-activated cation channels: evidence for an intracellular activation gate. J. Gen. Physiol. 117, 91–101. doi:10.1085/jgp.117.2.91
Small, J. G., Hirsch, S. R., Arvanitis, L. A., Miller, B. G., and Link, C. G. (1997). Quetiapine in patients with schizophrenia. A high- and low-dose double-blind comparison with placebo. Seroquel Study Group. Arch. Gen. Psychiatry 54, 549–557. doi:10.1001/archpsyc.1997.01830180067009
Stevens, D. R., Seifert, R., Bufe, B., Muller, F., Kremmer, E., Gauss, R., et al. (2001). Hyperpolarization-activated channels HCN1 and HCN4 mediate responses to sour stimuli. Nature 413, 631–635. doi:10.1038/35098087
Stieber, J., Wieland, K., Stockl, G., Ludwig, A., and Hofmann, F. (2006). Bradycardic and proarrhythmic properties of sinus node inhibitors. Mol. Pharmacol. 69, 1328–1337. doi:10.1124/mol.105.020701
Szabo, Z., Bacskai, T., Deak, A., Matesz, K., Veress, G., and Sziklai, I. (2011). Dendrodendritic connections between the cochlear efferent neurons in Guinea pig. Neurosci. Lett. 504, 195–198. doi:10.1016/j.neulet.2011.09.012
Tanguay, J., Callahan, K. M., and D'Avanzo, N. (2019). Characterization of drug binding within the HCN1 channel pore. Sci. Rep. 9, 465. doi:10.1038/s41598-018-37116-2
Thomas, D., Wendt-Nordahl, G., Rockl, K., Ficker, E., Brown, A. M., and Kiehn, J. (2001). High-affinity blockade of human ether-a-go-go-related gene human cardiac potassium channels by the novel antiarrhythmic drug BRL-32872. J. Pharmacol. Exp. Ther. 297, 753–761.
Wu, S., Gao, W., Xie, C., Xu, X., Vorvis, C., Marni, F., et al. (2012). Inner activation gate in S6 contributes to the state-dependent binding of cAMP in full-length HCN2 channel. J. Gen. Physiol. 140, 29–39. doi:10.1085/jgp.201110749
Xing, J., Zhang, C., Jiang, W., Hao, J., Liu, Z., Luo, A., et al. (2017). The inhibitory effects of ketamine on human hyperpolarization-activated cyclic nucleotide-gated channels and action potential in rabbit sinoatrial node. Pharmacology 99, 226–235. doi:10.1159/000452975
Yi, F., Danko, T., Botelho, S. C., Patzke, C., Pak, C., Wernig, M., et al. (2016). Autism-associated SHANK3 haploinsufficiency causes Ih channelopathy in human neurons. Science 352, aaf2669. doi:10.1126/science.aaf2669
Keywords: HCN channel, norquetiapine, quetiapine, depression, major mood disorder
Citation: Jean Jacques A and D’Avanzo N (2024) Inhibition of HCN1 currents by norquetiapine, an active metabolite of the atypical anti-psychotic drug quetiapine. Front. Pharmacol. 15:1445509. doi: 10.3389/fphar.2024.1445509
Received: 07 June 2024; Accepted: 23 September 2024;
Published: 07 October 2024.
Edited by:
Christian Legros, Université d’Angers, FranceReviewed by:
Maria Novella Romanelli, University of Florence, ItalyAndrea Saponaro, University of Milan, Italy
Copyright © 2024 Jean Jacques and D’Avanzo. This is an open-access article distributed under the terms of the Creative Commons Attribution License (CC BY). The use, distribution or reproduction in other forums is permitted, provided the original author(s) and the copyright owner(s) are credited and that the original publication in this journal is cited, in accordance with accepted academic practice. No use, distribution or reproduction is permitted which does not comply with these terms.
*Correspondence: Nazzareno D’Avanzo, bmF6emFyZW5vLmQuYXZhbnpvQHVtb250cmVhbC5jYQ==