- Department of Gastroenterology, General Hospital of the Chinese People’s Liberation Army, Beijing, China
Pancreatic diseases such as pancreatitis and pancreatic cancer represent significant health challenges characterized by high mortality rates and limited survival durations. Autophagy, a crucial cellular catabolic process, has emerged as a focal point in understanding various pathological conditions, spanning inflammation-related disorders to malignant neoplasms. This comprehensive review aims to elucidate the biological intricacies of autophagy and its pivotal roles within two extensively researched pancreatic diseases, namely pancreatitis and pancreatic cancer, drawing upon recent scholarly contributions. The discussion will delve into the nuanced mechanisms underlying autophagy’s involvement in these diseases, shedding light on its potential as a therapeutic target. Furthermore, the review will explore cutting-edge therapeutic interventions leveraging autophagy regulation for managing pancreatitis and pancreatic cancer. Through this analysis, we endeavor to offer novel insights into the pathophysiology of pancreatic disorders and contribute to the development of innovative therapeutic modalities in this challenging clinical domain.
Introduction
The pancreas plays a pivotal role as a secretory organ within the human body, comprising two distinct components: the exocrine and endocrine divisions (Czako et al., 2009; Das et al., 2014; Zhou and Melton, 2018). The exocrine segment encompasses acinar and ductal structures responsible for secreting pancreatic juice, a fluid containing vital enzymes such as trypsinogen, lipase, and amylase (Czako et al., 2009; Das et al., 2014; Zhou and Melton, 2018). This pancreatic fluid, essential for the digestion of proteins, fats, and sugars, is conveyed into the duodenum via the pancreatic duct network (Czako et al., 2009; Das et al., 2014; Zhou and Melton, 2018).
Comprising primarily pancreatic acinar and ductal cells (Wang et al., 2019; Huang et al., 2021; Grimont et al., 2022), any impairment or malfunction in these cellular components may precipitate pancreatic disorders, including pancreatitis and pancreatic cancer. However, due to the intricate nature of pancreatic pathology, the development of optimal therapeutic approaches remains imperative to combat such diseases effectively.
Autophagy, recognized as a critical metabolic process (Xu and Wan, 2023), facilitates the degradation and recycling of long-lived proteins, misfolded proteins, and redundant organelles during stress conditions, predominantly reliant on lysosomal activity (Debnath et al., 2023; Jain et al., 2023; Samare-Najaf et al., 2023). Initially documented in the mid-1950s, autophagy has since been implicated in the pathogenesis and progression of diverse disease states (Kumaresan et al., 2022; Yim et al., 2022; de Abreu et al., 2023). Notably, in the context of pancreatic disorders, extensive research has elucidated autophagy’s regulatory roles in the initiation and progression of pancreatitis and pancreatic cancer (Chen et al., 2024b; Cui et al., 2024; Liu et al., 2024b; Zhang et al., 2024). Contemporary investigations suggest that targeting autophagy holds promise as a therapeutic avenue for managing pancreatic disorders (Liu et al., 2023; Ashrafizadeh et al., 2024; Elango et al., 2024).
Building upon this foundation, this paper aims to comprehensively review and discuss the latest literature pertaining to the role of autophagy in pancreatitis and pancreatic cancer. Through this exploration, we endeavor to offer novel insights into the treatment of pancreatic disorders by leveraging the potential of autophagy modulation.
Biological features of autophagy
Autophagy represents a fundamental catabolic cellular process responsible for the degradation of protein aggregates and damaged organelles into metabolic components via lysosomal recycling, thereby maintaining cellular homeostasis and vitality (Miller and Thorburn, 2021; Liu et al., 2024a). This process is ubiquitously present in virtually all cell types and evolutionarily conserved from yeast to mammals (Feng et al., 2024; Proikas-Cezanne and Thumm, 2024; Riaz et al., 2024). Autophagy manifests in three primary forms based on cargo delivery and physiological function: macroautophagy, microautophagy, and chaperone-mediated autophagy (Kuchitsu and Taguchi, 2023; Yamamoto and Matsui, 2024). Macroautophagy, the most extensively studied form, entails the sequestration of degradable materials within double-membraned autophagosomes, subsequently fusing with lysosomes for degradation (Al-Kuraishy et al., 2024; Lewerissa et al., 2024). Microautophagy, in contrast, involves the non-selective engulfment of cytoplasmic materials through lysosomal/vacuolar membrane invagination (Alvarez-Guerra et al., 2024; Sakai and Oku, 2024). Chaperone-mediated autophagy is a selective process reliant on chaperone-mediated recognition of substrate proteins with specific motifs and lysosomal chaperones (Dong et al., 2021; Wang et al., 2023c). Since macroautophagy is, so far, the best-studied autophagy process, this review will mainly discuss the roles and mechanisms of macroautophagy in pancreatic disorders including pancreatitis and pancreatic cancer (hereafter referred to as “autophagy”).
The induction of autophagy comprises two essential steps, as elucidated in our prior reviews (Figure 1) (Wang et al., 2018; Shao et al., 2021; Shao et al., 2022a). Initially, substrate materials like aggregated proteins are enveloped by cup-shaped photophores with lipid bilayer membranes, leading to their sequestration within double-membrane autophagosomes. Subsequently, autophagosomes shed “coat proteins (LC3-II)” from their surfaces and merge with lysosomes to form functional autolysosomes. Over 30 autophagy-related genes (Atgs) are implicated in these processes. Furthermore, two pivotal signaling pathways regulate autophagy: the Class I PI3K-mammalian target of rapamycin (mTOR) pathway acts as an inhibitory regulator via mTOR complex 1 (mTORC1) stimulation (Borsa et al., 2024; Huang et al., 2024), while the Class III PI3K-Beclin-1 complex mediates an inductive pathway (Liu et al., 2021; Yan et al., 2023).
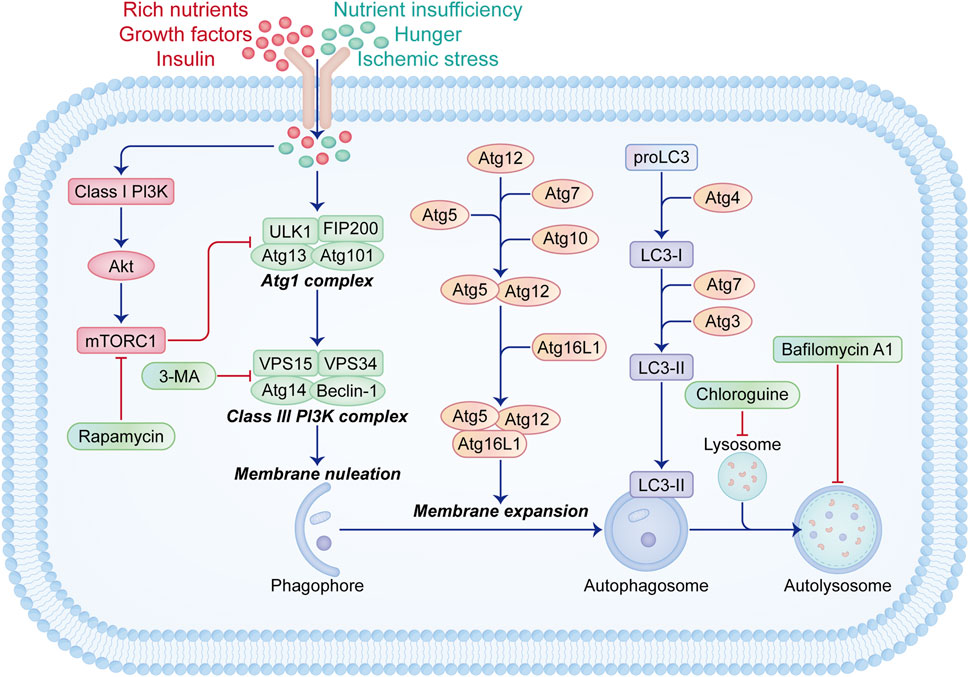
Figure 1. Schematic illustration of signaling pathways related to the initiation and regulation of autophagy. Autophagy is activated during nutrient deprivation, starvation, or ischemic stress conditions. The initiation of autophagy involves various groups of Atg proteins. The Atg1 complex, consisting of ULK1, FIP200, Atg13, and Atg101, initiates the assembly of the Class III PI3K complex containing Beclin-1, Atg14, VSP15, and VSP34, which promotes membrane nucleation and phagophore formation. Subsequent membrane expansion and fusion mediated by Atg5-Atg12-Atg16L1 and LC3-II lead to autophagosome formation, followed by fusion with lysosomes to form functional autophagy units. Under conditions of ample nutrients, growth factors, and insulin, the Class I PI3K-Akt-mTORC1 signaling pathway is activated, inhibiting the Atg1 complex formation. Rapamycin acts as an autophagy inducer by inhibiting mTORC1, while 3-MA, bafilomycin A1, and chloroquine block autophagy through distinct mechanisms.
In recent years, extensive research has explored the role of autophagy in various disease contexts, including cardiovascular disorders like myocardial infarction (Grazide et al., 2024; Li et al., 2024b) and atherosclerosis (Shao et al., 2023; Xu et al., 2024b), neurodegenerative conditions such as multiple sclerosis (Ke et al., 2017; Shao et al., 2017), metabolic diseases like diabetes (Yang et al., 2024; Yasasilka and Lee, 2024) and obesity (Behrooz et al., 2024; Li et al., 2024a), inflammatory and immune-related disorders such as pancreatitis (Wang et al., 2023a; Fu et al., 2024), inflammatory bowel disease (Shao et al., 2019; Shao et al., 2022b), and arthritis (Wang et al., 2024), as well as malignant tumors (Chen et al., 2024a; Mathur et al., 2024; Yu et al., 2024). While autophagy’s anti-inflammatory and immune-suppressive effects are well-documented, it has also been associated with cellular death termed “autophagic cellular death” in specific circumstances (Lin et al., 2024; Sakil et al., 2024; Xu et al., 2024a). These complexities highlight the intricate roles of autophagy in disease pathogenesis. Subsequently, this paper will delve into the detailed discussion of autophagy’s involvement in pancreatic disorders, including pancreatitis and pancreatic cancer.
Autophagy in pancreatic diseases
Autophagy in pancreatitis
The role of autophagy in pancreatitis
Pancreatitis, characterized by trypsin-induced self-digestion of the pancreas, manifests with edema, congestion, bleeding, or necrosis (Liu et al., 2019; Pesei et al., 2022). Clinical symptoms include abdominal pain, bloating, nausea, vomiting, and fever (Nguyen et al., 2023). This disease encompasses acute and chronic forms, with acute pancreatitis being a significant cause of gastrointestinal-related hospital admissions. While most cases of acute pancreatitis are mild to moderate, up to 30% of severe cases with persistent multi-organ dysfunction can be fatal (Lilja et al., 2008; Gukovskaya et al., 2017; Yoshimi et al., 2022). Chronic pancreatitis may arise from repeated subclinical or clinically evident episodes of acute pancreatitis, or develop independently (Lilja et al., 2008; Gukovskaya et al., 2017; Yoshimi et al., 2022). Contributing factors include genetic predisposition, gallstones, and alcohol misuse (Gukovskaya et al., 2017). The prevailing understanding posits acinar cell injury as the initial trigger, leading to parenchymal necrosis and inflammation, central to the disease’s pathology (Yu et al., 2023; Zaman and Gorelick, 2024). In addition, certain vacuole-associated proteins, including LC3 protein, lysosomal-associated membrane protein (LAMP)-1, and LAMP-2 in autophagic vacuoles, have been revealed to contribute greatly to the modulation of pancreatitis (Gukovsky et al., 2012; Mareninova et al., 2020). Besides, cholecystokinin (CCK), a major gastrointestinal hormone that plays an important role in stimulation of pancreatic secretion, has been revealed in high plasma level in patients with acute pancreatitis (Otsuki, 2000). Treatment of CCK-8 and CCK-1 receptor antagonist significantly alleviate pancreatitis through attenuating acinar necrosis in pancreas (Jia et al., 2015; Grupp et al., 2020). Emerging evidence suggests that systemic complications in acute pancreatitis stem from dysregulated immune system activation (Kessler et al., 2020).
We searched in PubMed and other digital sources with the keywords of “autophagy” and “pancreatitis” and more than 2400 studies have been retrieved. According to those previous findings, impaired autophagy plays a pivotal role in the pathogenesis and progression of pancreatitis. Studies have shown a heightened basal level of autophagy in mouse exocrine pancreas (Mizushima et al., 2004). In pancreatitis patients, increased levels of LC3-II and p62 in acinar cells alongside decreased LAMPs levels, indicative of impaired lysosomal function, have been observed (Mareninova et al., 2015; Mareninova et al., 2020; Yang et al., 2022). Autophagic vacuole accumulation, notably large autolysosomes, during pancreatitis points to inefficient lysosomal degradation (Mareninova et al., 2009; Gukovskaya and Gukovsky, 2012). Genetic modifications of core autophagy-related proteins like Atg5, Atg7, or LAMP2 in the pancreas lead to severe acinar cell degeneration, exocrine pancreas atrophy, fibrosis, inflammation, and cellular damage (Antonucci et al., 2015; Mareninova et al., 2015), highlighting autophagy impairment’s role in exacerbating pancreatitis severity. As recently reviewed by Tsomidis et al. (Tsomidis et al., 2024), autophagy plays an important role in pancreatitis through the connection of many mechanisms including endoplasmic reticulum (ER) stress, immunity, cell death process, and inflammation.
It is worth noting that the overload of calcium ion, an important secondary messenger, contributes to the pathogenesis and progression of pancreatitis through the regulation of impaired autophagy (Booth et al., 2011; Ono et al., 2023). Calcium ions have been revealed to regulate pancreatic secretion, acidic stores in pancreatic acinar cells, and lysosomal pH value, thus maintaining pancreatic homeostasis (Maleth and Hegyi, 2014; Petersen et al., 2021). It was recently revealed that the disturbance of calcium iron overload in pancreatic acinar cells via microRNA-26a significantly suppressed the severity of acute pancreatitis (Du et al., 2022). Such effect was mediated by the inhibition of impaired autophagy as well as inflammatory reaction and endoplasmic reticulum (ER) stress (Du et al., 2022).
Several signaling pathways regulate autophagy in pancreatitis. The STING/TBK1/IRF3 pathway modulates autophagy levels in cerulein-treated acinar cells (Song et al., 2021). The microRNA-30b-5p/CAMKII pathway contributes to impaired autophagy promotion in pancreatitis facilitated by Atg7 (Ji et al., 2022). The AMPK/mTOR pathway, a classic autophagy regulator, induces autophagy in pancreatitis (Ji et al., 2016). Furthermore, autophagy interacts with ferroptosis and inflammation mechanisms, exacerbating acute pancreatitis via impaired autophagy-induced ferroptosis (Yang et al., 2023) and strengthening the intestinal mucosal barrier during severe acute pancreatitis by attenuating oxidative stress (Huang et al., 2018). Crosstalk between autophagy and NF-κB-mediated inflammation is also noted in pancreatitis (Yang et al., 2012).
Pharmacological application of autophagy in pancreatitis
Numerous agents have demonstrated efficacy in mitigating pancreatitis by leveraging autophagy regulation (shown in Table 1). Rapamycin, a well-studied autophagy inducer, has shown considerable promise in ameliorating the pathogenesis and progression of pancreatitis. Mei et al. (Mei et al., 2020) reported rapamycin’s ability to alleviate hypertriglyceridemia-related acute pancreatitis by restoring autophagy flux and inhibiting endoplasmic reticulum stress, achieved through mTORC1/S6K1 signaling pathway inhibition. Müller et al. (Muller et al., 2012) demonstrated rapamycin’s potential to mitigate severe acute pancreatitis severity, possibly by early suppression of helper T cells. Additionally, rapamycin administration has been shown to mitigate oxidative stress and prevent apoptotic cell death in experimental chronic pancreatitis (Ozturk et al., 2015).
Several natural compounds have also exhibited efficacy in pancreatitis alleviation. Xanthohumol, derived from hops, attenuates severe acute pancreatitis by suppressing oxidative stress and restoring impaired autophagy via AKT/mTOR signaling pathway inhibition (Huangfu et al., 2023). Picroside II, from Picrorhiza scrophulariiflora Pennell, has shown efficacy in severe acute pancreatitis alleviation by enhancing antioxidant and anti-inflammatory activities through NF-κB-dependent autophagy modulation (Piao et al., 2017).
Other agents have been identified for their pancreatitis alleviation potential. Activation of α7 nicotinic acetylcholine receptor (α7nAChR), integral to the cholinergic anti-inflammatory pathway, protects against acute pancreatitis by enhancing transcription factor EB (TFEB)-regulated autophagy (Li et al., 2020). Additionally, β1 syntrophin, a critical regulator of actin cytoskeleton, shields against cerulein-induced acute pancreatitis by facilitating autophagy initiation in pancreatic acinar cells (Ye et al., 2019). Several microRNAs, including microRNA-20b-5p, microRNA-148a, microRNA-551b-5p, and microRNA-141, have been implicated in pancreatitis regulation through autophagy modulation (Zhu et al., 2016; Tang et al., 2021; Wei et al., 2024).
Autophagy in pancreatic cancer
The role of autophagy in pancreatic cancer
Pancreatic cancer stands out as one of the deadliest malignancies primarily due to delayed diagnosis, high metastatic potential, and aggressive local spread (Gorgulu et al., 2020). Despite the availability of various treatment modalities such as surgical resection, radiation therapy, immunotherapy, and chemotherapy, the median survival for pancreatic cancer patients remains a mere 6–9 months with a dismal 5-year survival rate of 9% (Rawla et al., 2019). Among pancreatic cancer subtypes, pancreatic ductal adenocarcinoma (PDAC) prevails as the most common, constituting approximately 3% of all cancers (Jiang et al., 2024). Noteworthy epidemiological risk factors for PDAC encompass smoking, excessive alcohol consumption, obesity, sedentary lifestyle, inadequate consumption of fruits and vegetables, and high-fat intake (Asahina et al., 2020; Li et al., 2021). These factors serve as significant extrinsic contributors to tumorigenesis, while gene mutations, particularly in Kras (Kras protooncogene, GTPase), Tp53 (tumor protein p53), Smad4 (Smad family member 4), and Brca2 (Brca2 DNA repair associated), represent intrinsic triggers for pancreatic tumorigenesis (Li et al., 2021; de Jesus et al., 2023; Gurreri et al., 2023). Besides, several vacuole-associated proteins, such as LC3B and vacuole membrane protein 1 (VMP1), play an important role in pancreatic cancer (Lima et al., 2023; Renna et al., 2023). The interplay of intrinsic and extrinsic factors disrupts cellular homeostasis, profoundly influencing tumorigenesis in both cell-autonomous and non-cell-autonomous manners.
We searched in PubMed and other digital sources with the keywords of “autophagy” and “pancreatic cancer” and more than 1300 studies have been retrieved. According to those previous findings, autophagy, a pivotal process in maintaining cellular homeostasis, plays a dual and intricate role in the pathogenesis and progression of pancreatic cancer, influenced by factors such as tumor stage, duration of exposure, and mode of autophagy modulation. Studies have shown significant upregulation of key autophagy genes like beclin-1 and LC3 in both mRNA and protein levels in tumor tissues compared to normal tissue (Cui et al., 2019). Elevated expression of these genes correlates with advanced TNM stages, lymphatic metastasis, and poor patient outcomes (Cui et al., 2019). PDAC cells exhibit heightened autophagy and lysosomal activity by uncoupling microphthalmia/transcription factor E (MiT/TFE) family transcription factors from mTOR signaling (Settembre et al., 2011; Ko et al., 2013; Perera et al., 2015). Additionally, PDAC cells effectively maintain basal autophagy levels by dephosphorylating ULK1 via PTPA/protein phosphatase 2 phosphatase activator (PP2A) (Wong et al., 2015).
Various signaling pathways related to cellular proliferation, apoptosis, and metabolism are implicated in autophagy’s impact on pancreatic cancer. For instance, concurrent inhibition of MAPK/ERK and IGF1R pathways enhances sensitivity to autophagy inhibitors in PDAC (Stalnecker et al., 2022a). Similarly, targeting IGF1R and ERK together enhances the efficacy of autophagy inhibitors (Stalnecker et al., 2022b). The microRNA454-FAM83A-TSPAN1 axis has been identified as a crucial regulator of pancreatic cancer cell proliferation through autophagy modulation (Zhou et al., 2021). Moreover, the intricate interplay between autophagy and inflammation further complicates the understanding of pancreatic cancer pathogenesis. Conditional knockout of Atg7 in pancreatic epithelial cells leads to acinar cell degeneration and inflammation, highlighting the multifaceted role of autophagy in pancreatic cancer progression (Antonucci et al., 2015; Zhou et al., 2017).
For the role of autophagy in tumor resistance to antineoplastic agents, it has been revealed that autophagy is highly involved in regulation of the long noncoding RNA PVT1-mediated gemcitabine resistance of pancreatic cancer via activating Wnt/β-catenin signaling pathway (Zhou et al., 2020). In addition, cancer-associated fibroblast autophagy was shown to contribute to pancreatic cancer resistance to immune surveillance and caner immunotherapy through modulating CD274/PDL1 levels (Zhang et al., 2024). Those findings indicate the close connection between autophagy and tumor resistance to antineoplastic therapies.
Pharmacological application of autophagy in pancreatic cancer
Numerous agents have demonstrated effectiveness in treating pancreatic cancer by modulating autophagy (shown in Table 2). Classic autophagy inhibitors, such as bafilomycin A1, have shown promise in lowering pHi, increasing thermosensitivity, and enhancing heat-induced growth delay in pancreatic cancer cells through vacuolar type H+ATPase inhibition and apoptosis induction (Ohta et al., 1998; Hayashi et al., 2006). Chloroquine (CQ) and hydroxychloroquine (HCQ), widely used autophagy inhibitors, have also been effective in mitigating pancreatic cancer growth by triggering autophagy-mediated inflammation, impacting cell survival via signaling pathways like CXCL12/CXCR4, ERK/STAT3, and RAGE/STATs (Balic et al., 2014; Chen et al., 2021a; McCubrey et al., 2023). Several clinical trials are available for CQ and/or HCQ with or without the combination of other classic chemotherapeutics in the treatment of pancreatic cancer (Samaras et al., 2017; Zeh et al., 2020).
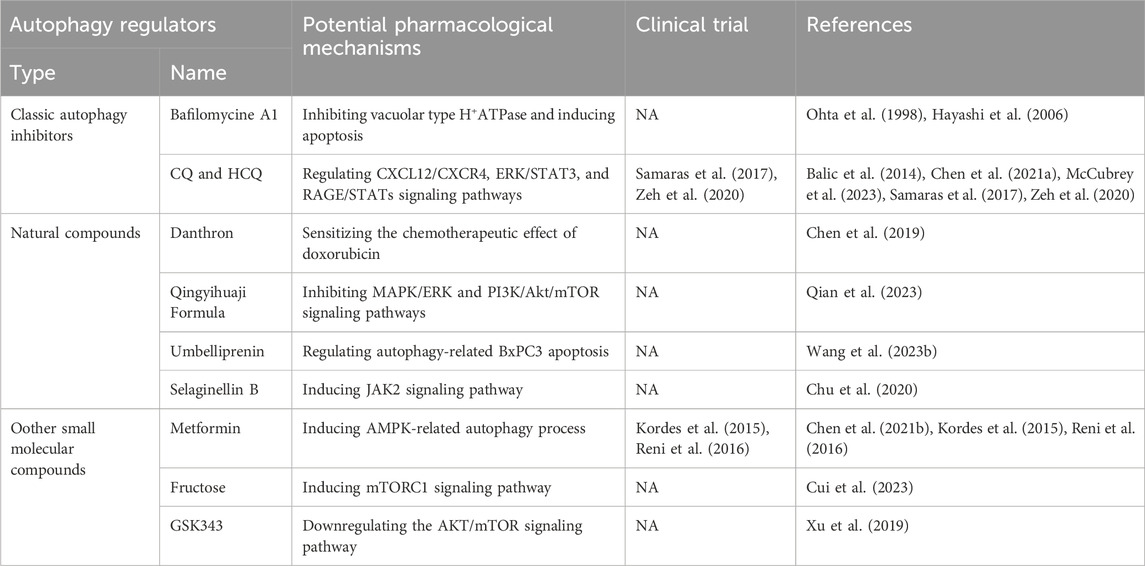
Table 2. Potential pharmacological mechanisms of autophagy regulators in pancreatic cancer treatment.
Natural compounds have also emerged as effective tools in pancreatic cancer alleviation through autophagy modulation. Danthron, from Rheum palmatum L., sensitizes pancreatic cancer cells to doxorubicin chemotherapy by suppressing autophagy (Chen et al., 2019). Qingyihuaji Formula, a traditional Chinese medicine, alleviates pancreatic cancer by modulating autophagy via MAPK/ERK and PI3K/Akt/mTOR pathways (Qian et al., 2023). Umbelliprenin from Artemisia absinthium L. inhibits tumor growth and induces apoptosis in pancreatic cancer cells through autophagy regulation (Wang et al., 2023b). Selaginellin B from Selaginella tamariscina targets Janus kinase 2 (JAK2) to treat pancreatic cancer via autophagy modulation (Chu et al., 2020).
Furthermore, small molecular compounds have exhibited anti-tumor effects in pancreatic cancer. Metformin combined with pitavastatin shows promise as a chemotherapeutic agent by inducing AMPK-related autophagy (Chen et al., 2021b). Several clinical trials are available to demonstrate the therapeutic effect of metformin on pancreatic cancer (Kordes et al., 2015; Reni et al., 2016). Fructose-induced mTORC1 activation promotes pancreatic cancer progression by inhibiting autophagy, highlighting potential strategies for targeting metabolism in PDAC treatment (Cui et al., 2023). GSK343, an EZH2 inhibitor, demonstrates anti-tumor effects by inducing autophagy and downregulating AKT/mTOR signaling in pancreatic cancer cells (Xu et al., 2019).
Conclusion
Recent research has shed light on autophagy’s involvement in the pathogenesis and progression of two extensively studied pancreatic disorders, namely pancreatitis and pancreatic cancer. Furthermore, we have witnessed the effectiveness of several autophagy-related therapies in mitigating these conditions. As discussed above, various agents leveraging autophagy have shown efficacy in treating both pancreatitis and pancreatic cancer. These agents encompass natural compounds as well as classic autophagy modulators, whether inducers or inhibitors. However, despite extensive investigations into the mechanisms of pancreatic diseases, the precise molecular pathways remain elusive, hindering the development of innovative treatments. Moreover, given the intricate impact of autophagy on pancreatitis and pancreatic cancer, further research is imperative to translate autophagy-based therapeutic strategies into clinical practice effectively.
Author contributions
W-GZ: Funding acquisition, Writing–original draft. Q-ZW: Writing–original draft, Data curation, Resources. B-ZS: Funding acquisition, Writing–review and editing.
Funding
The author(s) declare that financial support was received for the research, authorship, and/or publication of this article. This work was supported by grants from Beijing Natural Science Foundation Program (No.7244297), Chinese PLA General Hospital Young Independent Innovation Science Fund (No.22QNFC072), and National Natural Science Foundation of China (No. 82204483).
Conflict of interest
The authors declare that the research was conducted in the absence of any commercial or financial relationships that could be construed as a potential conflict of interest.
Publisher’s note
All claims expressed in this article are solely those of the authors and do not necessarily represent those of their affiliated organizations, or those of the publisher, the editors and the reviewers. Any product that may be evaluated in this article, or claim that may be made by its manufacturer, is not guaranteed or endorsed by the publisher.
References
Al-Kuraishy, H. M., Jabir, M. S., Al-Gareeb, A. I., Saad, H. M., Batiha, G. E., and Klionsky, D. J. (2024). The beneficial role of autophagy in multiple sclerosis: yes or No? Autophagy 20 (2), 259–274. doi:10.1080/15548627.2023.2259281
Alvarez-Guerra, I., Block, E., Broeskamp, F., Gabrijelcic, S., Infant, T., de Ory, A., et al. (2024). LDO proteins and Vac8 form a vacuole-lipid droplet contact site to enable starvation-induced lipophagy in yeast. Dev. Cell 59 (6), 759–775.e5. doi:10.1016/j.devcel.2024.01.014
Antonucci, L., Fagman, J. B., Kim, J. Y., Todoric, J., Gukovsky, I., Mackey, M., et al. (2015). Basal autophagy maintains pancreatic acinar cell homeostasis and protein synthesis and prevents ER stress. Proc. Natl. Acad. Sci. U. S. A. 112 (45), E6166–E6174. doi:10.1073/pnas.1519384112
Asahina, K., Balog, S., Hwang, E., Moon, E., Wan, E., Skrypek, K., et al. (2020). Moderate alcohol intake promotes pancreatic ductal adenocarcinoma development in mice expressing oncogenic Kras. Am. J. Physiol. Gastrointest. Liver Physiol. 318 (2), G265-G276–G276. doi:10.1152/ajpgi.00218.2019
Ashrafizadeh, M., Luo, K., Zhang, W., Reza Aref, A., and Zhang, X. (2024). Acquired and intrinsic gemcitabine resistance in pancreatic cancer therapy: environmental factors, molecular profile and drug/nanotherapeutic approaches. Environ. Res. 240 (Pt 2), 117443. doi:10.1016/j.envres.2023.117443
Balic, A., Sorensen, M. D., Trabulo, S. M., Sainz, B., Cioffi, M., Vieira, C. R., et al. (2014). Chloroquine targets pancreatic cancer stem cells via inhibition of CXCR4 and hedgehog signaling. Mol. Cancer Ther. 13 (7), 1758–1771. doi:10.1158/1535-7163.MCT-13-0948
Behrooz, A. B., Cordani, M., Fiore, A., Donadelli, M., Gordon, J. W., Klionsky, D. J., et al. (2024). The obesity-autophagy-cancer axis: mechanistic insights and therapeutic perspectives. Semin. Cancer Biol. 99, 24–44. doi:10.1016/j.semcancer.2024.01.003
Booth, D. M., Murphy, J. A., Mukherjee, R., Awais, M., Neoptolemos, J. P., Gerasimenko, O. V., et al. (2011). Reactive oxygen species induced by bile acid induce apoptosis and protect against necrosis in pancreatic acinar cells. Gastroenterology 140 (7), 2116–2125. doi:10.1053/j.gastro.2011.02.054
Borsa, M., Obba, S., Richter, F. C., Zhang, H., Riffelmacher, T., Carrelha, J., et al. (2024). Autophagy preserves hematopoietic stem cells by restraining MTORC1-mediated cellular anabolism. Autophagy 20 (1), 45–57. doi:10.1080/15548627.2023.2247310
Chen, G., Gao, C., Jiang, S., Cai, Q., Li, R., Sun, Q., et al. (2024a). Fusobacterium nucleatum outer membrane vesicles activate autophagy to promote oral cancer metastasis. J. Adv. Res. 56, 167–179. doi:10.1016/j.jare.2023.04.002
Chen, H., Zhao, C., He, R., Zhou, M., Liu, Y., Guo, X., et al. (2019). Danthron suppresses autophagy and sensitizes pancreatic cancer cells to doxorubicin. Toxicol Vitro 54, 345–353. doi:10.1016/j.tiv.2018.10.019
Chen, R. J., Lyu, Y. J., Chen, Y. Y., Lee, Y. C., Pan, M. H., Ho, Y. S., et al. (2021a). Chloroquine potentiates the anticancer effect of pterostilbene on pancreatic cancer by inhibiting autophagy and downregulating the RAGE/STAT3 pathway. Molecules 26 (21), 6741. doi:10.3390/molecules26216741
Chen, X., Tsvetkov, A. S., Shen, H. M., Isidoro, C., Ktistakis, N. T., Linkermann, A., et al. (2024b). International consensus guidelines for the definition, detection, and interpretation of autophagy-dependent ferroptosis. Autophagy 20, 1213–1246. doi:10.1080/15548627.2024.2319901
Chen, Y. H., Huang, Y. C., Yang, S. F., Yen, H. H., Tsai, H. D., Hsieh, M. C., et al. (2021b). Pitavastatin and metformin synergistically activate apoptosis and autophagy in pancreatic cancer cells. Environ. Toxicol. 36 (8), 1491–1503. doi:10.1002/tox.23146
Chu, P., Wang, S., Zhu, X., Yang, Y., Li, H., Tesfaldet, T., et al. (2020). Selaginellin B induces apoptosis and autophagy in pancreatic cancer cells via the JAK2/STAT3 signaling pathway. Am. J. Transl. Res. 12 (11), 7127–7143.
Cui, L., Wang, X., Zhao, X., Kong, C., Li, Z., Liu, Y., et al. (2019). The autophagy-related genes Beclin1 and LC3 in the prognosis of pancreatic cancer. Int. J. Clin. Exp. Pathol. 12 (8), 2989–2996.
Cui, Q., Liu, H. C., Liu, W. M., Ma, F., Lv, Y., Ma, J. C., et al. (2024). Milk fat globule epidermal growth factor 8 alleviates liver injury in severe acute pancreatitis by restoring autophagy flux and inhibiting ferroptosis in hepatocytes. World J. Gastroenterol. 30 (7), 728–741. doi:10.3748/wjg.v30.i7.728
Cui, Y., Tian, J., Wang, Z., Guo, H., Zhang, H., Wang, Z., et al. (2023). Fructose-induced mTORC1 activation promotes pancreatic cancer progression through inhibition of autophagy. Cancer Res. 83 (24), 4063–4079. doi:10.1158/0008-5472.CAN-23-0464
Czako, L., Hegyi, P., Rakonczay, Z., Wittmann, T., and Otsuki, M. (2009). Interactions between the endocrine and exocrine pancreas and their clinical relevance. Pancreatology 9 (4), 351–359. doi:10.1159/000181169
Das, S. L., Kennedy, J. I., Murphy, R., Phillips, A. R., Windsor, J. A., and Petrov, M. S. (2014). Relationship between the exocrine and endocrine pancreas after acute pancreatitis. World J. Gastroenterol. 20 (45), 17196–17205. doi:10.3748/wjg.v20.i45.17196
de Abreu, M. S., Demin, K. A., Kotova, M. M., Mirzaei, F., Shariff, S., Kantawala, B., et al. (2023). Developing novel experimental models of m-TORopathic epilepsy and related neuropathologies: translational insights from zebrafish. Int. J. Mol. Sci. 24 (2), 1530. doi:10.3390/ijms24021530
Debnath, J., Gammoh, N., and Ryan, K. M. (2023). Autophagy and autophagy-related pathways in cancer. Nat. Rev. Mol. Cell Biol. 24 (8), 560–575. doi:10.1038/s41580-023-00585-z
de Jesus, V. H. F., Mathias-Machado, M. C., de Farias, J. P. F., Aruquipa, M. P. S., Jacome, A. A., and Peixoto, R. D. (2023). Targeting KRAS in pancreatic ductal adenocarcinoma: the long road to cure. Cancers (Basel) 15 (20), 5015. doi:10.3390/cancers15205015
Dong, S., Wang, Q., Kao, Y. R., Diaz, A., Tasset, I., Kaushik, S., et al. (2021). Chaperone-mediated autophagy sustains haematopoietic stem-cell function. Nature 591 (7848), 117–123. doi:10.1038/s41586-020-03129-z
Du, W., Liu, G., Shi, N., Tang, D., Ferdek, P. E., Jakubowska, M. A., et al. (2022). A microRNA checkpoint for Ca(2+) signaling and overload in acute pancreatitis. Mol. Ther. 30 (4), 1754–1774. doi:10.1016/j.ymthe.2022.01.033
Elango, A., Nesam, V. D., Sukumar, P., Lawrence, I., and Radhakrishnan, A. (2024). Postbiotic butyrate: role and its effects for being a potential drug and biomarker to pancreatic cancer. Arch. Microbiol. 206 (4), 156. doi:10.1007/s00203-024-03914-8
Feng, Y., Chen, Y., Wu, X., Chen, J., Zhou, Q., Liu, B., et al. (2024). Interplay of energy metabolism and autophagy. Autophagy 20 (1), 4–14. doi:10.1080/15548627.2023.2247300
Fu, X., Xiu, Z., Xu, Q., Yue, R., and Xu, H. (2024). Interleukin-22 alleviates caerulein-induced acute pancreatitis by activating AKT/mTOR pathway. Dig. Dis. Sci. 69, 1691–1700. doi:10.1007/s10620-024-08360-6
Gorgulu, K., Diakopoulos, K. N., Kaya-Aksoy, E., Ciecielski, K. J., Ai, J., Lesina, M., et al. (2020). The role of autophagy in pancreatic cancer: from bench to the dark bedside. Cells 9 (4), 1063. doi:10.3390/cells9041063
Grazide, M. H., Ruidavets, J. B., Martinet, W., Elbaz, M., and Vindis, C. (2024). Association of circulating autophagy proteins ATG5 and Beclin 1 with acute myocardial infarction in a case-control study. Cardiology 149, 217–224. doi:10.1159/000537816
Grimont, A., Leach, S. D., and Chandwani, R. (2022). Uncertain beginnings: acinar and ductal cell plasticity in the development of pancreatic cancer. Cell Mol. Gastroenterol. Hepatol. 13 (2), 369–382. doi:10.1016/j.jcmgh.2021.07.014
Grupp, K., Bonk, S., Poppe, A., Wodack, K., Reeh, M., Gocht, A., et al. (2020). Cholecystokinin-8 treatment reduces acinar necrosis and edema of pigs with induced pancreatitis. Asian J. Surg. 43 (1), 272–277. doi:10.1016/j.asjsur.2019.05.002
Gukovskaya, A. S., and Gukovsky, I. (2012). Autophagy and pancreatitis. Am. J. Physiol. Gastrointest. Liver Physiol. 303 (9), G993-G1003–G1003. doi:10.1152/ajpgi.00122.2012
Gukovskaya, A. S., Gukovsky, I., Algul, H., and Habtezion, A. (2017). Autophagy, inflammation, and immune dysfunction in the pathogenesis of pancreatitis. Gastroenterology 153 (5), 1212–1226. doi:10.1053/j.gastro.2017.08.071
Gukovsky, I., Pandol, S. J., Mareninova, O. A., Shalbueva, N., Jia, W., and Gukovskaya, A. S. (2012). Impaired autophagy and organellar dysfunction in pancreatitis. J. Gastroenterol. Hepatol. 27, 27–32. doi:10.1111/j.1440-1746.2011.07004.x
Gurreri, E., Genovese, G., Perelli, L., Agostini, A., Piro, G., Carbone, C., et al. (2023). KRAS-dependency in pancreatic ductal adenocarcinoma: mechanisms of escaping in resistance to KRAS inhibitors and perspectives of therapy. Int. J. Mol. Sci. 24 (11), 9313. doi:10.3390/ijms24119313
Hayashi, Y., Katayama, K., Togawa, T., Kimura, T., and Yamaguchi, A. (2006). Effects of bafilomycin A1, a vacuolar type H+ ATPase inhibitor, on the thermosensitivity of a human pancreatic cancer cell line. Int. J. Hyperth. 22 (4), 275–285. doi:10.1080/02656730600708049
Huang, L., Desai, R., Conrad, D. N., Leite, N. C., Akshinthala, D., Lim, C. M., et al. (2021). Commitment and oncogene-induced plasticity of human stem cell-derived pancreatic acinar and ductal organoids. Cell Stem Cell 28 (6), 1090–1104.e6. doi:10.1016/j.stem.2021.03.022
Huang, L., Jiang, Y., Sun, Z., Gao, Z., Wang, J., and Zhang, D. (2018). Autophagy strengthens intestinal mucosal barrier by attenuating oxidative stress in severe acute pancreatitis. Dig. Dis. Sci. 63 (4), 910–919. doi:10.1007/s10620-018-4962-2
Huang, P., Dong, R. Y., Wang, P., Xu, M., Sun, X., and Dong, X. P. (2024). MCOLN/TRPML channels in the regulation of MTORC1 and autophagy. Autophagy 20, 1203–1204. doi:10.1080/15548627.2023.2300922
Huangfu, Y., Yu, X., Wan, C., Zhu, Y., Wei, Z., Li, F., et al. (2023). Xanthohumol alleviates oxidative stress and impaired autophagy in experimental severe acute pancreatitis through inhibition of AKT/mTOR. Front. Pharmacol. 14, 1105726. doi:10.3389/fphar.2023.1105726
Jain, V., Singh, M. P., and Amaravadi, R. K. (2023). Recent advances in targeting autophagy in cancer. Trends Pharmacol. Sci. 44 (5), 290–302. doi:10.1016/j.tips.2023.02.003
Ji, L., Li, L., Qu, F., Zhang, G., Wang, Y., Bai, X., et al. (2016). Hydrogen sulphide exacerbates acute pancreatitis by over-activating autophagy via AMPK/mTOR pathway. J. Cell Mol. Med. 20 (12), 2349–2361. doi:10.1111/jcmm.12928
Ji, L., Wang, Z. H., Zhang, Y. H., Zhou, Y., Tang, D. S., Yan, C. S., et al. (2022). ATG7-enhanced impaired autophagy exacerbates acute pancreatitis by promoting regulated necrosis via the miR-30b-5p/CAMKII pathway. Cell Death Dis. 13 (3), 211. doi:10.1038/s41419-022-04657-4
Jia, D., Yamamoto, M., and Otsuki, M. (2015). Effect of endogenous cholecystokinin on the course of acute pancreatitis in rats. World J. Gastroenterol. 21 (25), 7742–7753. doi:10.3748/wjg.v21.i25.7742
Jiang, L., Qin, J., Dai, Y., Zhao, S., Zhan, Q., Cui, P., et al. (2024). Prospective observational study on biomarkers of response in pancreatic ductal adenocarcinoma. Nat. Med. 30 (3), 749–761. doi:10.1038/s41591-023-02790-x
Ke, P., Shao, B. Z., Xu, Z. Q., Chen, X. W., Wei, W., and Liu, C. (2017). Activating α7 nicotinic acetylcholine receptor inhibits NLRP3 inflammasome through regulation of β-arrestin-1. CNS Neurosci. Ther. 23 (11), 875–884. doi:10.1111/cns.12758
Kessler, A., Weksler-Zangen, S., and Ilan, Y. (2020). Role of the immune system and the circadian rhythm in the pathogenesis of chronic pancreatitis: establishing a personalized signature for improving the effect of immunotherapies for chronic pancreatitis. Pancreas 49 (8), 1024–1032. doi:10.1097/MPA.0000000000001626
Ko, Y. H., Cho, Y. S., Won, H. S., Jeon, E. K., An, H. J., Hong, S. U., et al. (2013). Prognostic significance of autophagy-related protein expression in resected pancreatic ductal adenocarcinoma. Pancreas 42 (5), 829–835. doi:10.1097/MPA.0b013e318279d0dc
Kordes, S., Pollak, M. N., Zwinderman, A. H., Mathot, R. A., Weterman, M. J., Beeker, A., et al. (2015). Metformin in patients with advanced pancreatic cancer: a double-blind, randomised, placebo-controlled phase 2 trial. Lancet Oncol. 16 (7), 839–847. doi:10.1016/S1470-2045(15)00027-3
Kuchitsu, Y., and Taguchi, T. (2023). Lysosomal microautophagy: an emerging dimension in mammalian autophagy. Trends Cell Biol. 34, 606–616. doi:10.1016/j.tcb.2023.11.005
Kumaresan, V., Wang, J., Zhang, W., Zhang, Y., Xu, D., and Zhang, G. (2022). Coxiella burnetii virulent phase I and avirulent phase II variants differentially manipulate autophagy pathway in neutrophils. Infect. Immun. 90 (3), e0053421. doi:10.1128/IAI.00534-21
Lewerissa, E. I., Nadif Kasri, N., and Linda, K. (2024). Epigenetic regulation of autophagy-related genes: implications for neurodevelopmental disorders. Autophagy 20 (1), 15–28. doi:10.1080/15548627.2023.2250217
Li, B., Wu, J., Bao, J., Han, X., Shen, S., Ye, X., et al. (2020). Activation of α7nACh receptor protects against acute pancreatitis through enhancing TFEB-regulated autophagy. Biochim. Biophys. Acta Mol. Basis Dis. 1866 (12), 165971. doi:10.1016/j.bbadis.2020.165971
Li, C., Zhang, J., Dionigi, G., Liang, N., Guan, H., and Sun, H. (2024a). Adiponectin inhibits the progression of obesity-associated papillary thyroid carcinoma through autophagy. Endocrinology 165 (5), bqae030. doi:10.1210/endocr/bqae030
Li, J., Chen, X., Kang, R., Zeh, H., Klionsky, D. J., and Tang, D. (2021). Regulation and function of autophagy in pancreatic cancer. Autophagy 17 (11), 3275–3296. doi:10.1080/15548627.2020.1847462
Li, N., Xia, N., He, J., Liu, M., Gu, M., Lu, Y., et al. (2024b). Amphiregulin improves ventricular remodeling after myocardial infarction by modulating autophagy and apoptosis. FASEB J. 38 (4), e23488. doi:10.1096/fj.202302385R
Lilja, H. E., Leppaniemi, A., and Kemppainen, E. (2008). Utilization of intensive care unit resources in severe acute pancreatitis. JOP 9 (2), 179–184.
Lima, K., de Miranda, L. B. L., Del Milagro Bernabe Garnique, A., de Almeida, B. O., do Nascimento, M. C., Alcantara, G. A. S., et al. (2023). The multikinase inhibitor AD80 induces mitotic catastrophe and autophagy in pancreatic cancer cells. Cancers (Basel) 15 (15), 3866. doi:10.3390/cancers15153866
Lin, Z., Long, F., Kang, R., Klionsky, D. J., Yang, M., and Tang, D. (2024). The lipid basis of cell death and autophagy. Autophagy 20 (3), 469–488. doi:10.1080/15548627.2023.2259732
Liu, J., Liu, Y., Wang, Y., Li, C., Xie, Y., Klionsky, D. J., et al. (2023). TMEM164 is a new determinant of autophagy-dependent ferroptosis. Autophagy 19 (3), 945–956. doi:10.1080/15548627.2022.2111635
Liu, J., Wu, Y., Meng, S., Xu, P., Li, S., Li, Y., et al. (2024a). Selective autophagy in cancer: mechanisms, therapeutic implications, and future perspectives. Mol. Cancer 23 (1), 22. doi:10.1186/s12943-024-01934-y
Liu, M., Zhao, Y., Hu, D., Huang, X., Xiong, H., Qi, K., et al. (2019). Clinical and histologic characterization of Co-infection with astrovirus and goose parvovirus in goslings. Avian Dis. 63 (4), 731–736. doi:10.1637/aviandiseases-D-19-00110
Liu, X., Li, D., Ma, T., Luo, X., Peng, Y., Wang, T., et al. (2024b). Autophagy inhibition improves the targeted radionuclide therapy efficacy of (131)I-FAP-2286 in pancreatic cancer xenografts. J. Transl. Med. 22 (1), 156. doi:10.1186/s12967-024-04958-6
Liu, Y., Song, A., Wu, H., Sun, Y., and Dai, M. (2021). Paeonol inhibits apoptosis of vascular smooth muscle cells via up-regulation of autophagy by activating class III PI3K/Beclin-1 signaling pathway. Life Sci. 264, 118714. doi:10.1016/j.lfs.2020.118714
Maleth, J., and Hegyi, P. (2014). Calcium signaling in pancreatic ductal epithelial cells: an old friend and a nasty enemy. Cell Calcium 55 (6), 337–345. doi:10.1016/j.ceca.2014.02.004
Mareninova, O. A., Hermann, K., French, S. W., O'Konski, M. S., Pandol, S. J., Webster, P., et al. (2009). Impaired autophagic flux mediates acinar cell vacuole formation and trypsinogen activation in rodent models of acute pancreatitis. J. Clin. Invest 119 (11), 3340–3355. doi:10.1172/JCI38674
Mareninova, O. A., Jia, W., Gretler, S. R., Holthaus, C. L., Thomas, D. D. H., Pimienta, M., et al. (2020). Transgenic expression of GFP-LC3 perturbs autophagy in exocrine pancreas and acute pancreatitis responses in mice. Autophagy 16 (11), 2084–2097. doi:10.1080/15548627.2020.1715047
Mareninova, O. A., Sendler, M., Malla, S. R., Yakubov, I., French, S. W., Tokhtaeva, E., et al. (2015). Lysosome associated membrane proteins maintain pancreatic acinar cell homeostasis: LAMP-2 deficient mice develop pancreatitis. Cell Mol. Gastroenterol. Hepatol. 1 (6), 678–694. doi:10.1016/j.jcmgh.2015.07.006
Mathur, A., Ritu, , Chandra, P., and Das, A. (2024). Autophagy: a necessary evil in cancer and inflammation. 3 Biotech. 14 (3), 87. doi:10.1007/s13205-023-03864-w
McCubrey, J. A., Abrams, S. L., Follo, M. Y., Manzoli, L., Ratti, S., Martelli, A. M., et al. (2023). Effects of chloroquine and hydroxychloroquine on the sensitivity of pancreatic cancer cells to targeted therapies. Adv. Biol. Regul. 87, 100917. doi:10.1016/j.jbior.2022.100917
Mei, Q., Zeng, Y., Huang, C., Zheng, J., Guo, Y., Fan, J., et al. (2020). Rapamycin alleviates hypertriglyceridemia-related acute pancreatitis via restoring autophagy flux and inhibiting endoplasmic reticulum stress. Inflammation 43 (4), 1510–1523. doi:10.1007/s10753-020-01228-7
Miller, D. R., and Thorburn, A. (2021). Autophagy and organelle homeostasis in cancer. Dev. Cell 56 (7), 906–918. doi:10.1016/j.devcel.2021.02.010
Mizushima, N., Yamamoto, A., Matsui, M., Yoshimori, T., and Ohsumi, Y. (2004). In vivo analysis of autophagy in response to nutrient starvation using transgenic mice expressing a fluorescent autophagosome marker. Mol. Biol. Cell 15 (3), 1101–1111. doi:10.1091/mbc.e03-09-0704
Muller, C. A., Belyaev, O., Burr, W., Munding, J., McArthur, N., Bergmann, U., et al. (2012). Effects of FTY720 and rapamycin on inflammation in taurocholate-induced acute pancreatitis in the rat. Pancreas 41 (7), 1086–1091. doi:10.1097/MPA.0b013e3182496fd7
Nguyen, D. C., Nguyen, N. A., Dinh, Q. K., Le, D. T., Vu, T. B., and Hoang, V. T. (2023). Effect of plasma exchange treatment in patients with hypertriglyceridemia-induced acute pancreatitis. Med. Kaunas. 59 (5), 864. doi:10.3390/medicina59050864
Ohta, T., Arakawa, H., Futagami, F., Fushida, S., Kitagawa, H., Kayahara, M., et al. (1998). Bafilomycin A1 induces apoptosis in the human pancreatic cancer cell line Capan-1. J. Pathol. 185 (3), 324–330. doi:10.1002/(SICI)1096-9896(199807)185:3<324::AID-PATH72>3.0.CO;2-9
Ono, N., Horikoshi, J., Izawa, T., Nishiyama, K., Tanaka, M., Kuwamura, M., et al. (2023). L-arginine-induced pancreatitis aggravated by inhibiting Na(+)/Ca(2+) exchanger 1. J. Vet. Med. Sci. 85 (6), 657–666. doi:10.1292/jvms.22-0569
Otsuki, M. (2000). Pathophysiological role of cholecystokinin in humans. J. Gastroenterol. Hepatol. 15 (Suppl. l), D71–D83. doi:10.1046/j.1440-1746.2000.02178.x
Ozturk, K., Tasci, I., Yasar, M., Akay, C., Alcigir, M., Vural, S., et al. (2015). Effects of rapamycin treatment on pancreatic fibrosis, cellular apoptosis and oxidative stress in experimental chronic pancreatitis model. Acta Gastroenterol. Belg 78 (1), 3–7.
Perera, R. M., Stoykova, S., Nicolay, B. N., Ross, K. N., Fitamant, J., Boukhali, M., et al. (2015). Transcriptional control of autophagy-lysosome function drives pancreatic cancer metabolism. Nature 524 (7565), 361–365. doi:10.1038/nature14587
Pesei, Z. G., Jancso, Z., Demcsak, A., Nemeth, B. C., Vajda, S., and Sahin-Toth, M. (2022). Preclinical testing of dabigatran in trypsin-dependent pancreatitis. JCI Insight 7 (21), e161145. doi:10.1172/jci.insight.161145
Petersen, O. H., Gerasimenko, J. V., Gerasimenko, O. V., Gryshchenko, O., and Peng, S. (2021). The roles of calcium and ATP in the physiology and pathology of the exocrine pancreas. Physiol. Rev. 101 (4), 1691–1744. doi:10.1152/physrev.00003.2021
Piao, X., Liu, B., Guo, L., Meng, F., and Gao, L. (2017). Picroside II shows protective functions for severe acute pancreatitis in rats by preventing NF-κB-Dependent autophagy. Oxid. Med. Cell Longev. 2017, 7085709. doi:10.1155/2017/7085709
Proikas-Cezanne, T., and Thumm, M. (2024). Autophagy-from yeast to humans: thirty years of molecular autophagy. FEBS Lett. 598 (1), 3–6. doi:10.1002/1873-3468.14796
Qian, X., Bi, Q. Y., Wang, Z. N., Han, F., Liu, L. M., Song, L. B., et al. (2023). Qingyihuaji Formula promotes apoptosis and autophagy through inhibition of MAPK/ERK and PI3K/Akt/mTOR signaling pathway on pancreatic cancer in vivo and in vitro. J. Ethnopharmacol. 307, 116198. doi:10.1016/j.jep.2023.116198
Rawla, P., Sunkara, T., and Gaduputi, V. (2019). Epidemiology of pancreatic cancer: global trends, etiology and risk factors. World J. Oncol. 10 (1), 10–27. doi:10.14740/wjon1166
Reni, M., Dugnani, E., Cereda, S., Belli, C., Balzano, G., Nicoletti, R., et al. (2016). (Ir)relevance of metformin treatment in patients with metastatic pancreatic cancer: an open-label, randomized phase II trial. Clin. Cancer Res. 22 (5), 1076–1085. doi:10.1158/1078-0432.CCR-15-1722
Renna, F. J., Enrique Steinberg, J. H., Gonzalez, C. D., Manifava, M., Tadic, M. S., Orquera, T., et al. (2023). Ubiquitination is a novel post-translational modification of VMP1 in autophagy of human tumor cells. Int. J. Mol. Sci. 24 (16), 12981. doi:10.3390/ijms241612981
Riaz, M., Sultana, R., Ahmad, J., Mehmood, A., Sattar, S., Hammad Tanveer, M., et al. (2024). Autophagy related genes mediated mitophagy in yeast, mammals and higher plants. Cell Mol. Biol. (Noisy-le-grand) 70 (1), 1–11. doi:10.14715/cmb/2024.70.1.1
Sakai, Y., and Oku, M. (2024). ATG and ESCRT control multiple modes of microautophagy. FEBS Lett. 598 (1), 48–58. doi:10.1002/1873-3468.14760
Sakil, M. A., Mukae, K., Bao, J., Sadhu, A., Roni, M. S., Inoue-Aono, Y., et al. (2024). Autophagy promotes cell death induced by hydrogen peroxide in physcomitrium patens. Plant Cell Physiol. 65 (2), 269–281. doi:10.1093/pcp/pcad149
Samaras, P., Tusup, M., Nguyen-Kim, T. D. L., Seifert, B., Bachmann, H., von Moos, R., et al. (2017). Phase I study of a chloroquine-gemcitabine combination in patients with metastatic or unresectable pancreatic cancer. Cancer Chemother. Pharmacol. 80 (5), 1005–1012. doi:10.1007/s00280-017-3446-y
Samare-Najaf, M., Neisy, A., Samareh, A., Moghadam, D., Jamali, N., Zarei, R., et al. (2023). The constructive and destructive impact of autophagy on both genders' reproducibility, a comprehensive review. Autophagy 19 (12), 3033–3061. doi:10.1080/15548627.2023.2238577
Settembre, C., Di Malta, C., Polito, V. A., Garcia Arencibia, M., Vetrini, F., Erdin, S., et al. (2011). TFEB links autophagy to lysosomal biogenesis. Science 332 (6036), 1429–1433. doi:10.1126/science.1204592
Shao, B. Z., Chai, N. L., Yao, Y., Li, J. P., Law, H. K. W., and Linghu, E. Q. (2022a). Autophagy in gastrointestinal cancers. Front. Oncol. 12, 975758. doi:10.3389/fonc.2022.975758
Shao, B. Z., Ke, P., Xu, Z. Q., Wei, W., Cheng, M. H., Han, B. Z., et al. (2017). Autophagy plays an important role in anti-inflammatory mechanisms stimulated by Alpha7 nicotinic acetylcholine receptor. Front. Immunol. 8, 553. doi:10.3389/fimmu.2017.00553
Shao, B. Z., Liu, M. Z., Zhu, D. N., Yan, H., Ke, P., Wei, W., et al. (2023). Depletion of β-arrestin-1 in macrophages enhances atherosclerosis in ApoE-/- mice. Int. Immunopharmacol. 125 (Pt A), 111085. doi:10.1016/j.intimp.2023.111085
Shao, B. Z., Wang, P., and Bai, Y. (2022b). Editorial: autophagy in inflammation related diseases. Front. Pharmacol. 13, 912487. doi:10.3389/fphar.2022.912487
Shao, B. Z., Wang, S. L., Fang, J., Li, Z. S., Bai, Y., and Wu, K. (2019). Alpha7 nicotinic acetylcholine receptor alleviates inflammatory bowel disease through induction of AMPK-mTOR-p70S6K-mediated autophagy. Inflammation 42 (5), 1666–1679. doi:10.1007/s10753-019-01027-9
Shao, B. Z., Yao, Y., Zhai, J. S., Zhu, J. H., Li, J. P., and Wu, K. (2021). The role of autophagy in inflammatory bowel disease. Front. Physiol. 12, 621132. doi:10.3389/fphys.2021.621132
Song, Y., Zhang, Z., Yu, Z., Xia, G., Wang, Y., Wang, L., et al. (2021). Wip1 aggravates the cerulein-induced cell autophagy and inflammatory injury by targeting STING/TBK1/IRF3 in acute pancreatitis. Inflammation 44 (3), 1175–1183. doi:10.1007/s10753-021-01412-3
Stalnecker, C. A., Coleman, M. F., and Bryant, K. L. (2022a). Susceptibility to autophagy inhibition is enhanced by dual IGF1R and MAPK/ERK inhibition in pancreatic cancer. Autophagy 18 (7), 1737–1739. doi:10.1080/15548627.2022.2042782
Stalnecker, C. A., Grover, K. R., Edwards, A. C., Coleman, M. F., Yang, R., DeLiberty, J. M., et al. (2022b). Concurrent inhibition of IGF1R and ERK increases pancreatic cancer sensitivity to autophagy inhibitors. Cancer Res. 82 (4), 586–598. doi:10.1158/0008-5472.CAN-21-1443
Tang, G. X., Yang, M. S., Xiang, K. M., Yang, B. C., Liu, Z. L., and Zhao, S. P. (2021). MiR-20b-5p modulates inflammation, apoptosis and angiogenesis in severe acute pancreatitis through autophagy by targeting AKT3. Autoimmunity 54 (7), 460–470. doi:10.1080/08916934.2021.1953484
Tsomidis, I., Voumvouraki, A., and Kouroumalis, E. (2024). The pathogenesis of pancreatitis and the role of autophagy. Gastroenterol. insights 15 (2), 303–341. doi:10.3390/gastroent15020022
Wang, D., Han, S., Lv, G., Hu, Y., Zhuo, W., Zeng, Z., et al. (2023a). Pancreatic acinar cells-derived sphingosine-1-phosphate contributes to fibrosis of chronic pancreatitis via inducing autophagy and activation of pancreatic stellate cells. Gastroenterology 165 (6), 1488–1504.e20. doi:10.1053/j.gastro.2023.08.029
Wang, H., Liu, Y., Wang, Y., Xu, T., Xia, G., and Huang, X. (2023b). Umbelliprenin induces autophagy and apoptosis while inhibits cancer cell stemness in pancreatic cancer cells. Cancer Med. 12 (14), 15277–15288. doi:10.1002/cam4.6170
Wang, L., Cai, J., Zhao, X., Ma, L., Zeng, P., Zhou, L., et al. (2023c). Palmitoylation prevents sustained inflammation by limiting NLRP3 inflammasome activation through chaperone-mediated autophagy. Mol. Cell 83 (2), 281–297.e10. doi:10.1016/j.molcel.2022.12.002
Wang, L., Xie, D., and Wei, D. (2019). Pancreatic acinar-to-ductal metaplasia and pancreatic cancer. Methods Mol. Biol. 1882, 299–308. doi:10.1007/978-1-4939-8879-2_26
Wang, P., Shao, B. Z., Deng, Z., Chen, S., Yue, Z., and Miao, C. Y. (2018). Autophagy in ischemic stroke. Prog. Neurobiol. 163-164, 98–117. doi:10.1016/j.pneurobio.2018.01.001
Wang, X. H., Shen, C. P., Wang, T. T., Huang, Y., Jin, Y., Zhou, M. Y., et al. (2024). Shikonin suppresses rheumatoid arthritis by inducing apoptosis and autophagy via modulation of the AMPK/mTOR/ULK-1 signaling pathway. Phytomedicine 128, 155512. doi:10.1016/j.phymed.2024.155512
Wei, H., Zhao, H., Cheng, D., Zhu, Z., Xia, Z., Lu, D., et al. (2024). miR-148a and miR-551b-5p regulate inflammatory responses via regulating autophagy in acute pancreatitis. Int. Immunopharmacol. 127, 111438. doi:10.1016/j.intimp.2023.111438
Wong, P. M., Feng, Y., Wang, J., Shi, R., and Jiang, X. (2015). Regulation of autophagy by coordinated action of mTORC1 and protein phosphatase 2A. Nat. Commun. 6, 8048. doi:10.1038/ncomms9048
Xu, H., Cui, H., Weng, S., Zhang, Y., Wang, L., Xing, Z., et al. (2024a). Crosstalk of cell death pathways unveils an autophagy-related gene AOC3 as a critical prognostic marker in colorectal cancer. Commun. Biol. 7 (1), 296. doi:10.1038/s42003-024-05980-6
Xu, H., Fu, J., Tu, Q., Shuai, Q., Chen, Y., Wu, F., et al. (2024b). The SGLT2 inhibitor empagliflozin attenuates atherosclerosis progression by inducing autophagy. J. Physiol. Biochem. 80 (1), 27–39. doi:10.1007/s13105-023-00974-0
Xu, H., Zhang, L., Qian, X., Zhou, X., Yan, Y., Zhou, J., et al. (2019). GSK343 induces autophagy and downregulates the AKT/mTOR signaling pathway in pancreatic cancer cells. Exp. Ther. Med. 18 (4), 2608–2616. doi:10.3892/etm.2019.7845
Xu, Y., and Wan, W. (2023). Acetylation in the regulation of autophagy. Autophagy 19 (2), 379–387. doi:10.1080/15548627.2022.2062112
Yamamoto, H., and Matsui, T. (2024). Molecular mechanisms of macroautophagy, microautophagy, and chaperone-mediated autophagy. J. Nippon. Med. Sch. 91 (1), 2–9. doi:10.1272/jnms.JNMS.2024_91-102
Yan, R., Gao, W., Chen, W., Liu, Y., Shen, L., Dai, Y., et al. (2023). rTFPI protects cardiomyocytes from hypoxia/reoxygenation injury through inhibiting autophagy and the class III PI3K/Beclin-1 pathway. Cell Biochem. Biophys. 81 (1), 97–104. doi:10.1007/s12013-022-01113-0
Yang, L., Ye, F., Liu, J., Klionsky, D. J., Tang, D., and Kang, R. (2023). Extracellular SQSTM1 exacerbates acute pancreatitis by activating autophagy-dependent ferroptosis. Autophagy 19 (6), 1733–1744. doi:10.1080/15548627.2022.2152209
Yang, S., Bing, M., Chen, F., Sun, Y., Chen, H., and Chen, W. (2012). Autophagy regulation by the nuclear factor κB signal axis in acute pancreatitis. Pancreas 41 (3), 367–373. doi:10.1097/MPA.0b013e31822a9b05
Yang, X., Chen, J., Wang, J., Ma, S., Feng, W., Wu, Z., et al. (2022). Very-low-density lipoprotein receptor-enhanced lipid metabolism in pancreatic stellate cells promotes pancreatic fibrosis. Immunity 55 (7), 1185–1199.e8. doi:10.1016/j.immuni.2022.06.001
Yang, X., Ding, W., Chen, Z., Lai, K., and Liu, Y. (2024). The role of autophagy in insulin resistance and glucolipid metabolism and potential use of autophagy modulating natural products in the treatment of type 2 diabetes mellitus. Diabetes Metab. Res. Rev. 40 (1), e3762. doi:10.1002/dmrr.3762
Yasasilka, X. R., and Lee, M. S. (2024). Role of β-cell autophagy in β-cell physiology and the development of diabetes. J. Diabetes Investig. 15, 656–668. doi:10.1111/jdi.14184
Ye, R., Onodera, T., Blanchard, P. G., Kusminski, C. M., Esser, V., Brekken, R. A., et al. (2019). β1 syntrophin supports autophagy initiation and protects against cerulein-induced acute pancreatitis. Am. J. Pathol. 189 (4), 813–825. doi:10.1016/j.ajpath.2019.01.002
Yim, W. W., Kurikawa, Y., and Mizushima, N. (2022). An exploratory text analysis of the autophagy research field. Autophagy 18 (7), 1648–1661. doi:10.1080/15548627.2021.1995151
Yoshimi, A., Ishikawa, K., Niemeyer, C., and Grunert, S. C. (2022). Pearson syndrome: a multisystem mitochondrial disease with bone marrow failure. Orphanet J. Rare Dis. 17 (1), 379. doi:10.1186/s13023-022-02538-9
Yu, T., Rui, L., Jiumei, Z., Ziwei, L., and Ying, H. (2024). Advances in the study of autophagy in breast cancer. Breast Cancer 31 (2), 195–204. doi:10.1007/s12282-023-01541-7
Yu, X., Wang, M., and Kong, Q. (2023). Viral pancreatitis: research advances and mechanisms. Front. Microbiol. 14, 1326837. doi:10.3389/fmicb.2023.1326837
Zaman, S., and Gorelick, F. (2024). Acute pancreatitis: pathogenesis and emerging therapies. J. Pancreatol. 7 (1), 10–20. doi:10.1097/JP9.0000000000000168
Zeh, H. J., Bahary, N., Boone, B. A., Singhi, A. D., Miller-Ocuin, J. L., Normolle, D. P., et al. (2020). A randomized phase II preoperative study of autophagy inhibition with high-dose hydroxychloroquine and gemcitabine/nab-paclitaxel in pancreatic cancer patients. Clin. Cancer Res. 26 (13), 3126–3134. doi:10.1158/1078-0432.CCR-19-4042
Zhang, X., Lao, M., Yang, H., Sun, K., Dong, Y., He, L., et al. (2024). Targeting cancer-associated fibroblast autophagy renders pancreatic cancer eradicable with immunochemotherapy by inhibiting adaptive immune resistance. Autophagy 20 (6), 1314–1334. doi:10.1080/15548627.2023.2300913
Zhou, C., Liang, Y., Zhou, L., Yan, Y., Liu, N., Zhang, R., et al. (2021). TSPAN1 promotes autophagy flux and mediates cooperation between WNT-CTNNB1 signaling and autophagy via the MIR454-FAM83A-TSPAN1 axis in pancreatic cancer. Autophagy 17 (10), 3175–3195. doi:10.1080/15548627.2020.1826689
Zhou, C., Yi, C., Yi, Y., Qin, W., Yan, Y., Dong, X., et al. (2020). LncRNA PVT1 promotes gemcitabine resistance of pancreatic cancer via activating Wnt/β-catenin and autophagy pathway through modulating the miR-619-5p/Pygo2 and miR-619-5p/ATG14 axes. Mol. Cancer 19 (1), 118. doi:10.1186/s12943-020-01237-y
Zhou, Q., and Melton, D. A. (2018). Pancreas regeneration. Nature 557 (7705), 351–358. doi:10.1038/s41586-018-0088-0
Zhou, X., Xie, L., Xia, L., Bergmann, F., Buchler, M. W., Kroemer, G., et al. (2017). RIP3 attenuates the pancreatic damage induced by deletion of ATG7. Cell Death Dis. 8 (7), e2918. doi:10.1038/cddis.2017.313
Keywords: pancreatic disease, autophagy, pancreatitis, pancreatic cancer, inflammation
Citation: Zhang W-G, Wu Q-Z and Shao B-Z (2024) The role of autophagy in pancreatic diseases. Front. Pharmacol. 15:1444657. doi: 10.3389/fphar.2024.1444657
Received: 11 June 2024; Accepted: 20 September 2024;
Published: 30 September 2024.
Edited by:
Maria Ines Vaccaro, University of Buenos Aires, ArgentinaReviewed by:
Monika Anna Jakubowska, Jagiellonian University, PolandClaudio Daniel Gonzalez, Norberto Quirno Medical Education and Clinical Research Center (CEMIC), Argentina
Copyright © 2024 Zhang, Wu and Shao. This is an open-access article distributed under the terms of the Creative Commons Attribution License (CC BY). The use, distribution or reproduction in other forums is permitted, provided the original author(s) and the copyright owner(s) are credited and that the original publication in this journal is cited, in accordance with accepted academic practice. No use, distribution or reproduction is permitted which does not comply with these terms.
*Correspondence: Bo-Zong Shao, c2hhb2Jvem9uZ0AxMjYuY29t
†These authors have contributed equally to this work