- 1Hospital of Chengdu University of Traditional Chinese Medicine, Chengdu, Sichuan, China
- 2TCM Regulating Metabolic Diseases Key Laboratory of Sichuan Province, Chengdu, Sichuan, China
- 3Department of Endocrinology, Hospital of Chengdu University of Traditional Chinese Medicine, Chengdu, Sichuan, China
- 4Affiliated Hospital of Jiangxi University of Chinese Medicine, Nanchang, Jiangxi, China
Fibrosis is a public health issue of great concern characterized by the excessive deposition of extracellular matrix, leading to the destruction of parenchymal tissue and organ dysfunction that places a heavy burden on the global healthcare system due to its high incidence, disability, and mortality. Salvianolic acid B (SalB) has positively affected various human diseases, including fibrosis. In this review, we concentrate on the anti-fibrotic effects of SalB from a molecular perspective while providing information on the safety, adverse effects, and drug interactions of SalB. Additionally, we discuss the innovative SalB formulations, which give some references for further investigation and therapeutic use of SalB’s anti-fibrotic qualities. Even with the encouraging preclinical data, additional research is required before relevant clinical trials can be conducted. Therefore, we conclude with recommendations for future studies. It is hoped that this review will provide comprehensive new perspectives on future research and product development related to SalB treatment of fibrosis and promote the efficient development of this field.
1 Introduction
Fibrosis is characterized by the destruction of fibrous connective tissue, activation and proliferation of fibroblasts, elevated collagen fiber secretion, and excessive deposition of extracellular matrix (ECM) (Zhang and Zhang, 2020). Activation of myofibroblasts, which produce ECM as effector cells in fibrotic illnesses, is a notable feature of these conditions (Wynn and Ramalingam, 2012; Walraven and Hinz, 2018). According to reports, the stimulation and maintenance of the myofibroblast phenotype are dependent on the transforming growth factor-β (TGF-β)/Smad, wingless/Integrated (Wnt), and yes-associated protein 1 (YAP)/transcriptional coactivator with PDZ-binding motif (TAZ) signaling pathways (Figure 1) (Piersma et al., 2015; Ricard-Blum and Miele, 2020). Notably, excessive deposition of ECM within organs leads to the destruction or replacement of parenchymal tissue, irreversible scarring, and organ dysfunction or failure, such as heart failure, chronic renal failure, and chronic pancreatitis (King et al., 2011; Weiskirchen et al., 2019). Currently, Nidanib and Pirfenidone are the only approved drugs for treating idiopathic pulmonary fibrosis (IPF). However, they carry the risk of gastrointestinal and dermatological adverse reactions and cardiovascular events (Henderson et al., 2020). Furthermore, there is currently no specific treatment strategy for other tissue fibrosis (Rosenbloom et al., 2017). Therefore, it is extremely urgent to investigate efficient and safe anti-fibrotic therapeutic approaches.
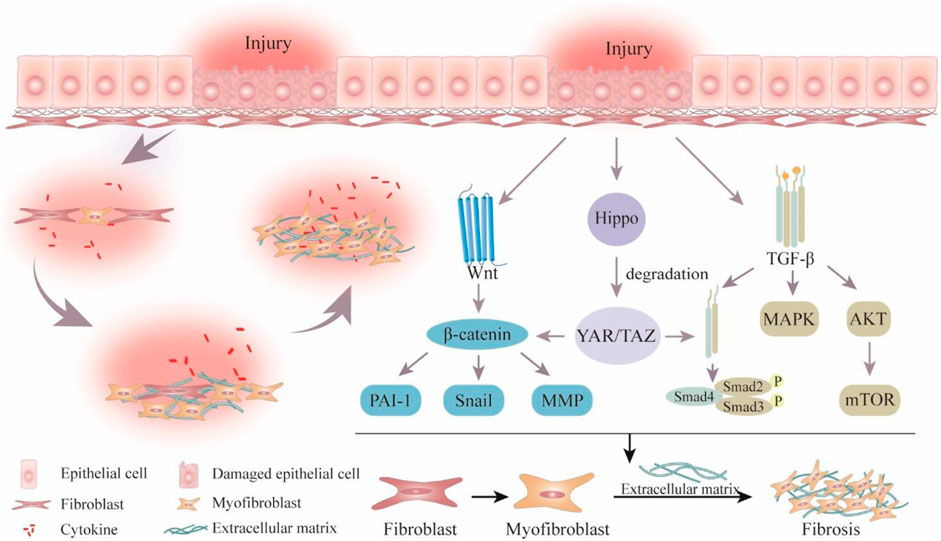
Figure 1. Schematic overview of pathological process and signaling pathways related to fibrosis. Wingless/Integrated (Wnt), plasminogen activator inhibitor-1 (PAI-1), matrix metalloproteinase (MMP), Yes-associated protein 1 (YAP), transcriptional coactivator with PDZ-binding motif (TAZ), transforming growth factor-β (TGF-β), mitogen-activated protein kinase (MAPK), the protein kinase (AKT), mechanistic target of rapamycin (mTOR).
Natural products are uniquely suited to treat fibrotic disorders because of their high level of safety and precise efficacy (Wang Y. et al., 2024; Deng et al., 2024; Liu et al., 2024; Xing et al., 2024). It not only adjusts immune function but also lessens side effects from other medications, slows the growth of fibrosis, and greatly improves the quality of life for patients. Danshen, the dried root of rhizome of Salvia miltiorrhiza Burge, a perennial herb in the genus Salvia, family Labiatae, was originally recorded in Shennong Bencao Jing. The main bioactive monomeric component of the hydrophilic compounds of Danshen is salvianolic acid B(SalB) (Figure 2A), which is produced through the condensation of one molecule of caffeic acid and three molecules of salvianic acid A (Liu et al., 2007). Modern pharmacological research has found that SalB has anti-oxidant, anti-apoptotic, anti-inflammatory, and anti-fibrotic effects (Guo and Wang, 2022; Liu et al., 2023a; Yan et al., 2023; Zhou et al., 2023). Moreover, it exerts protective effects on various organs and tissues, such as the heart, liver, kidney, lung, skin, etc. (Figure 2B) (Cao et al., 2012; Jia et al., 2019). Consequently, it has drawn a lot of attention. Numerous studies have clarified SalB’s potential therapeutic effectiveness in a wide range of pathological conditions, including but not limited to myocardial infarction, membranous nephropathy, ischemic brain injury, retinal defects, intervertebral disc degeneration, diabetes mellitus, sepsis, and various other ailments (Su et al., 2020; Chen et al., 2022a; Zhang F. X. et al., 2022; Hu et al., 2022; Yan M. et al., 2023; Wang et al., 2023).
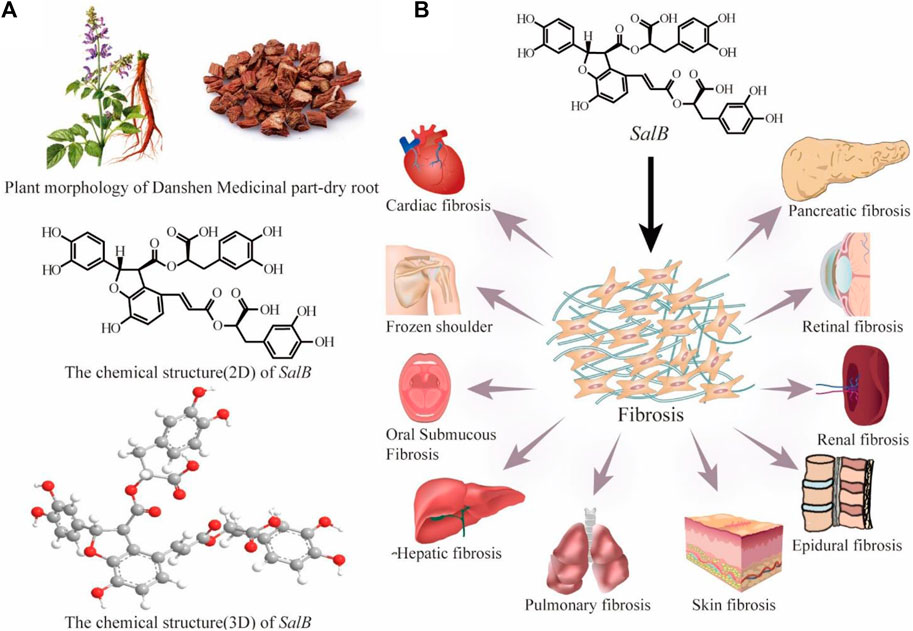
Figure 2. Plant morphology and medical part-dry root of Danshen and the chemical structure (2D and 3D) of SalB (A); SalB is effective for numerous fibrosis diseases (B).
Despite extensive confirmation of SalB’s anti-fibrotic pharmacological action, no comprehensive literature has been produced to summarize this effect and related mechanisms. Therefore, Studies related to the anti-fibrotic effects of SalB published in the last 23 years were identified through major scientific databases (PubMed, Web of Science, Embase, Google Scholar). Additional articles were identified through citation tracking or by visiting journal websites. Keywords used during the search included salvianolic acid B, fibrosis, fibrotic disease, anti-fibrotic effects, pharmacological effects, pharmacological mechanism, safety, drug interactions, combination therapy, and new dosage.
2 Anti-fibrotic effect of SalB
2.1 Hepatic fibrosis
Hepatic fibrosis (HF) is a common pathological feature seen in many etiologies of chronic liver disease and is an intermediate stage in the disease’s progression (Tacke and Trautwein, 2015). It is caused by excessive deposition of type I and type III collagen-rich ECM in the liver, resulting in the formation of fibrous scars. (Tacke and Trautwein, 2015; Zhao et al., 2016). Inhibiting hepatic fibrogenesis is crucial for successful prevention and therapy methods against chronic liver illnesses, as numerous studies have shown the possibility of reversing liver injury at different stages of fibrosis (Lee et al., 2015). Pro-fibrotic factors secreted by hepatic stellate cells (HSC) aid in the creation of collagen by bone marrow-derived fibroblasts and myofibroblasts, which in turn contributes to the development and progression of HF (Trautwein et al., 2015; Zhang C. Y. et al., 2016). It is imperative to inhibit the proliferation, activation, and migration of HSC for effective HF prevention (Higashi et al., 2017).
2.1.1 TGF-β
TGF-β is the most effective cytokine for sustaining HF due to its pro-fibrotic effects, and it is also an essential inducer of ECM formation (Tsuchida and Friedman, 2017). SalB has demonstrated its effectiveness in reducing diethyl nitrosamine-induced HF via altering the TGF-β/Smad and mitogen-activated protein kinase (MAPK) signaling pathways (Wu et al., 2019). Additionally, SalB downregulates the expression of the fibrosis gene plasminogen activator inhibitor-1 (PAI-1) and enhances protein levels of α-smooth muscle actin (α-SMA) and collagen type I (CoI), both indicative markers associated with HF. Furthermore, enhancer factor 2 (EF2) acts as a downstream effector within the TGF-β1 pathway and plays an essential role in HSC activation and the progression of fibrosis. Zhang et al. found that SalB exerts an anti-fibrotic effect by antagonizing TGF-β1-induced activation of myocyte enhancer factor 2 (MEF2) at protein and RNA levels (Zhang et al., 2019). Additionally, TGF-β1 promotes fibrosis by enhancing autophagic flux through increasing cellular autophagosomes, thus reducing the level of autophagy in fibrotic tissues, which may be a potential target for anti-fibrotic therapy (Weiskirchen and Tacke, 2019; Ye et al., 2020). Potent anti-fibrotic agent SalB suppressed autophagosome formation and autophagic flux in HSC by down-regulating the extracellular regulated protein kinases (ERK), c-Jun N-terminal kinase (JNK), and p38-MAPK pathways, leading to decreased expression of light chain 3β II, autophagy-related gene 5 (Atg5), α-SMA, and CoI (Jiang et al., 2022). To sum up, SalB might be a useful and potent TGF-β antagonist to postpone the development of HF.
2.1.2 PDGF
Platelet-derived growth factor (PDGF) is a significant pathway to promote HF (Roehlen et al., 2020). Fibroblasts produce PDGF in response to stimulation, which causes active fibroblasts to develop into myofibroblasts, which express the PDGF receptor (PDGFR). The activation of the PDGF/PDGFR pathway drives the proliferation and migration of HSC and promotes ECM deposition, all of which advance the course of fibrosis (Klinkhammer et al., 2018). Liu et al. employed molecular docking and ion resonance biosensors to illustrate the strong binding affinity between PDGFR-β and SalB, and SalB relieves HF by inhibiting the PDGFR-β signaling pathway and inducing HSC apoptosis, and inflammatory reaction of HSC (Liu et al., 2023b). This suggests that Sal B exerts anti-fibrotic effects by directly targeting the PDGFRβ signaling cascade.
2.1.3 Hedgehog
The hedgehog (Hh) pathway is thought to be connected with the level of fibrosis and has the capacity to activate HSC (Choi et al., 2009; Bar-Gal et al., 2012). Epithelial-mesenchymal transition (EMT) is a key process in which epithelial cells undergo phenotypic changes from an epithelial state to a mesenchymal state (Zhao et al., 2016). SalB effectively suppresses EMT in activated HSC by inducing microRNA (miR)-152 via the Hh signaling pathway, which in turn causes methyltransferase1 to be downregulated and Patched1 to be demethylated (Yu et al., 2015). Furthermore, the importance of EMT stimulated by Hh in the pathophysiology of HF has been shown in an increasing number of studies (Song et al., 2019; Zhang et al., 2022).
2.1.4 Wnt
HF is dependent on the Wnt signaling system, which coordinates complex cell signaling networks, promotes HSC proliferative activation, and interacts cooperatively with other pro-fibrotic factors (Wang J. N. et al., 2018; Wang et al., 2024). Yu et al. discovered that SalB inhibited the Wnt/β-catenin signaling pathway, downregulated the production of miR-17-5p, and reduced the expression of α-SMA and ECM to limit the activation of HSC (Yu et al., 2016). Moreover, the specific mechanism of HF delay following SalB treatment is due to upregulated LincRNA-p21’s inhibition of HSC proliferation through the Wnt/β-catenin pathway (Yu et al., 2017). These findings identify the Wnt signaling pathway as potentially important for therapeutic targets in HF.
2.1.5 NF-κB
The inflammatory response is mostly regulated by nuclear factor-κ-gene binding (NF-κB), a transcriptional regulator that is activated in chronic liver disorders and that promotes the production and secretion of pro-inflammatory cytokines (Karin and Ben-Neriah, 2000). Furthermore, the main ways that NF-κB contributes to HF are through controlling hepatocellular damage, altering inflammatory signaling pathways, and controlling fibrotic responses in HSC (Luedde and Schwabe, 2011). Wang et al.‘s study showed that SalB attenuated HF in mice by inhibiting the proliferation and activation of HSC by regulating the miR-6499-3p/LncRNA-ROR-mediated NF-κB signaling pathway (Wang et al., 2022). Additionally, SalB exhibited a dose-dependent reduction in the activation of nucleolus NF-κB, accompanied by an increase in cytoplasmic NF-κB levels (Wang et al., 2012). Thus, the regulation of the NF-κB pathway is one of the effective pathways of anti-HF (Basso et al., 2021; Ciceu et al., 2021).
2.1.6 FGF
The fibroblast growth factor (FGF) family is involved in cellular proliferation and differentiation, angiogenesis, wound healing, and tissue regeneration (Degirolamo et al., 2016). FGF19 is an endocrine gastrointestinal hormone that regulates the metabolism of bile acids and possesses anti-fibrotic properties (Hirschfield et al., 2019). Several studies have reported that the FGF19/FGF Receptor4 (FGFR4) signaling pathway exerts an anti-fibrotic effect primarily through the inhibition of proliferative activation in HSC (Zhou et al., 2017; Gadaleta et al., 2018; Hirschfield et al., 2019). SalB exhibits the ability to upregulate the FGF19/FGFR4 pathway, which was disrupted by lipopolysaccharide (LPS) treatment, with the result that HSC activation and proliferation are inhibited (Tian et al., 2021). Given these functions, the endocrine FGF has therapeutic potential for inhibiting HSC.
2.1.7 UGCG
The development of HF is closely associated with the overexpression of UDP-glucose ceramide glucosyltransferase (UGCG) in several chronic liver illnesses (Li et al., 2021). SalB considerably inhibits the progression of HF by inhibiting collagen deposition and HSC activation (Li et al., 2023). This is accomplished by the inhibition of UGCG by SalB, the additional reduction of immune cell infiltration brought on by carbon tetrachloride, the downregulation of α-SMA and CoI, and the suppression of phosphorylated histone, a hallmark of hepatic DNA damage.
Based on the above studies, we can find that SalB exhibits anti-HF properties by impeding HSC proliferation and activation as well as suppressing collagen accumulation through diverse mechanisms, including TGF, Wnt, PDGF, Hh, etc. (Figure 3). Since HF is a result of multiple variables, even though the role of HSC in HF progression is obvious, is SalB useful in treating other pathogenic factors? Furthermore, all of the latest research confirms the efficacy of SalB on a single target in the HF process; more investigation is required to determine how several pathways interact.
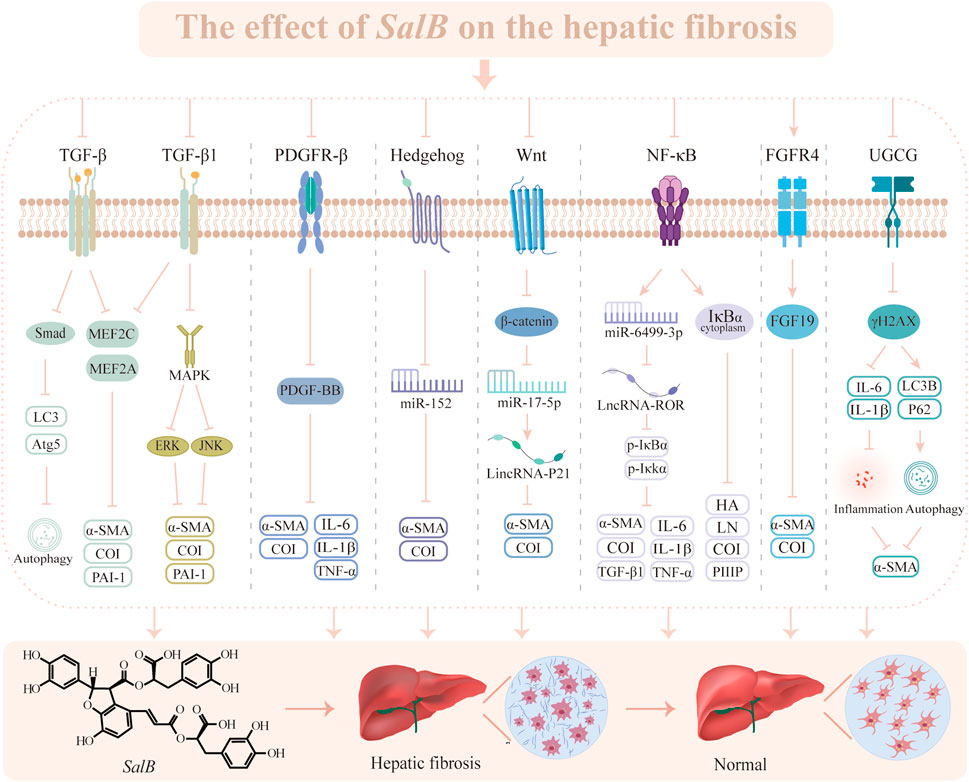
Figure 3. The effect of SalB on the hepatic fibrosis. Transforming growth factor-β (TGF-β), microtubule-associated protein light chain 3 (LC3), autophagy-related gene 5 (Atg5), myocyte enhancer factor 2 (MEF2), α-smooth muscle actin (α-SMA), collagen type I (CoI), plasminogen activator inhibitor-1 (PAI-1), mitogen-activated protein kinase (MAPK), extracellular regulated protein kinases (ERK), c-Jun N-terminal kinase (JNK), platelet-derived growth factor receptor-β (PDGFR-β), platelet-derived growth factor subunit B (PDGF-BB), interleukin-6 (IL-6), interleukin-1β (IL-1β), tumor necrosis factor α (TNF-α), microRNA (miR), wingless/Integrated (Wnt), nuclear factor-κ-gene binding (NF-κB), inhibitor of nuclear factor-κBα (IκBα), inhibiting kappa B kinase α (IκKα), hyaluronic acid (HA), laminin (LN), procollagen III peptide (PIIIP), fibroblast growth factor receptor 4 (FGFR4), fibroblast growth factor 19 (FGF19), UDP-glucose ceramide glucosyltransferase (UGCG), phosphorylation of Histone H2A Family Member X (γH2AX).
2.2 Pulmonary fibrosis
Pulmonary fibrosis (PF) is an aberrant ECM deposition and excessive fibroblast accumulation that causes a chronic, progressive alveolar illness (Strieter and Mehrad, 2009). Lung epithelial cells are damaged under the influence of various stimuli, which trigger activation of the fibrotic pathway and subsequent collagen deposition. Consequently, excessive mesenchymal stromal cells and ECM replace normal lung tissue, leading to the structural destruction of the alveoli, dyspnea as a result of diminished lung compliance, and ultimately respiratory failure or death (Glass et al., 2020; Wu et al., 2021; Lu et al., 2022).
2.2.1 TGF-β
TGF-β is the most potent pro-fibrotic mediator, inducing ECM, EMT, and pro-fibrotic mediators’ production by promoting the binding of Smad2/3 to Smad4, and driving myofibroblast differentiation to promote the PF process (Hu et al., 2018; Kramer and Clancy, 2018). Research has elucidated that patients with PF can exhibit elevated expression of TGF-β in their fibroblasts and airway epithelium (Xu et al., 2016). TGF-β, which plays a vital role in the development of PF, can stimulate airway smooth muscle hyperresponsiveness, goblet cell hyperplasia, and increased mucin secretion through Smad signaling (Ouyang et al., 2010; Ojiaku et al., 2018). Liu et al. interfered with the bleomycin (BLM)-induced PF model with SalB. The mechanism of SalB in relieving PF involves inhibiting the TGF-β pathway, including alleviating inflammatory injury, reducing disruption of alveolar architecture and collagen deposition, and inhibiting myofibroblast differentiation and EMT (Liu et al., 2016). SalB is a key subtype of TGF-β1 that serves an essential part in PF pathogenesis. It can attenuate CoI, α-SMA, and endogenous TGF-β1 production and inflammatory processes while inhibiting TGF-β1-induced proliferation, differentiation, and fibroblast-to-myofibroblast transformation in PF (Zhang et al., 2014; Jiang et al., 2020). Thus, targeting the TGF-β signaling pathway has emerged as an efficient therapeutic strategy for PF.
2.2.2 PAR1
Protease-activated receptor 1 (PAR1) promotion of PF is attributed to various pathways that promote mitosis and angiogenesis, modulate pulmonary vascular permeability, and stimulate fibroblast migration (Kaneider et al., 2007; Tressel et al., 2011). By down-regulating PAR1, SalB inhibits coagulation factors, activates the fibrinolytic system, dissolves excessive fibrin deposition, preserves the structural integrity of pulmonary tissues, and suppresses fibrous tissue proliferation (Zhang et al., 2021). Therefore, PAR1 may be a promising target for the prevention and treatment of PF.
2.2.3 Nrf2
Compared to other organs, the lung is exposed to higher oxygen tension and is the main organ in direct contact with inhaled oxidants. When fibrotic lesions appear in lung tissue, the body’s antioxidants may not be sufficient to counteract the significant amounts of oxidants that result from an imbalance between oxidation and anti-oxidation (Amara et al., 2010; Gao et al., 2024). In the meantime, the activated inflammatory factors trigger the production of substantial quantities of pro-fibrotic cytokines, which exacerbate oxidative stress in pulmonary tissue and intensify fibrosis (Obayashi et al., 2000; Kliment and Oury, 2010). SalB decreases malondialdehyde (MDA), myeloperoxidase, and reactive oxygen species (ROS) levels and increases superoxide dismutase activity, moreover, it inhibits inflammatory factors and activates the nuclear factor erythroid 2-related factor 2 (Nrf2) pathway to suppress myofibroblast transdifferentiating (Liu et al., 2018; Lu et al., 2022). Furthermore, SalB attenuated alveolar wall congestion, inflammatory cell infiltration, and emphysema in PF rat lung tissues while exerting notable anti-fibrotic effects. The results showed that Nrf2 was absent in PF areas, while the SalB treatment could increase the expression of Nrf2 in lung tissues.
2.2.4 MAPK and NF-κB
Chronic inflammation is a feature of PF, which is closely associated with tissue damage resulting from the fibrotic response (Paola et al., 2011). Increased microvascular permeability brought on by ROS causes inflammatory cells to migrate and exacerbates the inflammatory process (Hecquet and Malik, 2009). MAPK and NF-κB are involved in chronic inflammation and oxidative stress-induced hyperpermeability of endothelial cells. BLM encourages the release of ROS, enhances endothelial cell permeability, and mediates apoptosis. Subsequent investigations have shown that SalB protects endothelial cells from oxidative stress injury by up-regulating the expression of the tight junction gene, reducing the permeability of injured endothelial cells, and counteracting the increase in ROS caused by BLM through the MAPK and NF-κB signaling pathways (Liu et al., 2018). SalB exhibits multiple features of anti-oxidant, inflammation inhibition, and immune modulation, which are indispensable for the treatment of PF (Ho and Hong, 2011).
In summary, one of S. miltiorrhiza’s most physiologically active ingredients, SalB, modulates TGF-β, PAR1, and oxidative stress to produce anti-fibrotic actions (Figure 4). But PF is a complex process that leads to the loss of structural lung tissue. EMT and circulating fibroblasts play a major role in controlling fibrosis, and their biological characteristics will be important to take into account when developing new treatment targets in the future to slow the progression of PF through SalB.
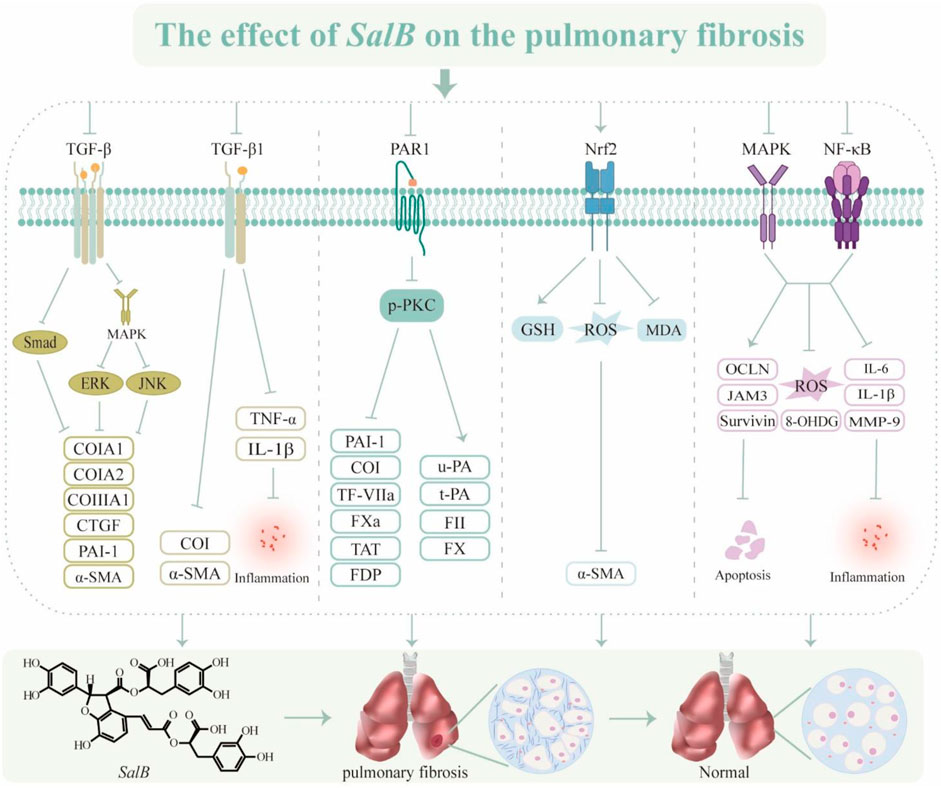
Figure 4. The effect of SalB on the pulmonary fibrosis. Transforming growth factor-β (TGF-β), mitogen-activated protein kinase (MAPK), extracellular regulated protein kinases (ERK), c-Jun N-terminal kinase (JNK), collagen type I (CoI), collagen type III (CoIII), connective tissue growth factor (CTGF), plasminogen activator inhibitor-1 (PAI-1), α-smooth muscle actin (α-SMA), tumor necrosis factor α (TNF-α), interleukin-1β (IL-1β), protease-activated receptor 1 (PAR1), phospho-protein kinase C (p-PKC), tissue factor (TF)/coagulation factor VII (TF-VIIa), activated coagulation factor X (FXa), thrombin-antithrombin complex (TAT), fibrinogen degradation product (FDP), urokinase type plasminogen activator (u-PA), tissue type plasminogen activator (t-PA), coagulation factor II (FII), coagulation factor X (FX), nuclear factor erythroid 2-related factor 2 (Nrf2), glutathione (GSH), reactive oxygen species (ROS), malondialdehyde (MDA), mitogen-activated protein kinase (MAPK), nuclear factor-κ-gene binding (NF-κB), occludin (OCLN), recombinant junctional adhesion molecule 3 (JAM3), 8-Hydroxy-2′-deoxyguanine (8-OHDG), interleukin-6 (IL-6), matrix metalloproteinase-9 (MMP-9).
2.3 Cardiac fibrosis
Cardiac fibrosis (CF) is cardiac interstitial remodeling caused by excessive proliferative activation of fibroblasts and excessive deposition of secreted collagen matrix (Yang et al., 2024). Regardless of ejection fraction, CF is an essential event in the transition of cardiac function from compensatory to decompensated period and represents one of the significant factors contributing to mortality in patients with heart failure (Kanagala et al., 2019). The formation of this phenomenon is closely associated with the dysregulation of NF-κB, matrix metalloproteinase (MMP), fibronectin (FN), α-SMA, and connective tissue growth factor (CTGF), as well as the modulation of the renin-angiotensin-aldosterone system (RAAS) (Oatmen et al., 2020).
2.3.1 NF-κB
NF-κB can participate in the occurrence and development of pathological cardiac remodeling by regulating the synthesis and release of ECM, cytokines, and chemokines (Wei et al., 2013; Zhang et al., 2016). Increasing evidence substantiates that NF-κB regulates the expression of vital genes in CF by intricate positive and negative feedback mechanisms, facilitating myofibroblast differentiation and promoting the fibrotic process (Li et al., 2020; Gong et al., 2021). Abnormal proliferation and differentiation of cardiac fibroblasts (CFB) can promote CF, and SalB inhibits Ang II-induced CFB activation and proliferation, as well as the fibrotic process by suppressing the expression of NF-κB and pro-fibrotic factors and ECM accumulation (Fan and Guan, 2016; Wang et al., 2018). More importantly, SalB also exhibits cardioprotective effects by protecting against LPS-induced cardiomyocyte injury through the Toll-like receptor 4 (TLR4)/NF-κB/Tumor Necrosis Factor α (TNF-α) pathway (Wang et al., 2011). In general, CF prevention and treatment techniques place a high priority on inhibiting the NF-κB signaling pathway via SalB’s effects (Wei et al., 2013).
2.3.2 TRIM8
The tripartite motif-containing protein (TRIM) family has been acknowledged as an indispensable regulator in the process of myocardial injury (Yin et al., 2016). Studies have shown that heart tissue with strong TRIM32 expression further controls cystic fibrosis (Chen et al., 2016). Additionally, TRIM72 has been found to decrease fibroblast proliferation to promote fibrosis, while the knockdown of TRIM8 has been clarified to reduce myocardial ischemia-reperfusion injury (Zhao and Lei, 2016; Dang et al., 2020). By down-regulating TRIM8 expression, SalB effectively prevents oxygen radical generation and cardiomyocyte apoptosis via the TRIM8/recombinant glutathione peroxidase 1 (GPX1) signaling pathway both in vivo and in vitro (Lu et al., 2022). This elucidates a potential mechanism underlying SalB-mediated myocardial protection.
2.3.3 MMP-9
The MMP-9 maintains the dynamic microenvironmental homeostasis of the ECM, involving collagen degradation associated with cardiovascular remodeling (Qin et al., 2010; Radosinska et al., 2017). In the context of myocardial infarction, MMP-9 prevents angiogenesis and exacerbates CF(Wang et al., 2021). During ventricular remodeling, MMP-9 activation upregulates fibrotic signaling and facilitates CF (Frangogiannis et al., 2002). SalB suppresses MMP-9 activity, diminishes the ratio of CoI/III, enhances cardiac contractility, and mitigates fibrosis (Jiang et al., 2010; Nandi et al., 2020). It is a competitive inhibitor of MMP-9 that guards against structural damage to the ventricles.
2.3.4 AMPK
Adenosine monophosphate-regulated protein kinase (AMPK), a receptor of cellular energy status expressed in cardiomyocytes, is an essential part of CF (Harada et al., 2015). Research has illustrated that promoting the AMPK cascade can prevent cardiac hypertrophy, repair cardiac function, and impede the progression of fibrosis (Yun et al., 2014; Samanta et al., 2020). Ang II can stimulate collagen production and secretion, as well as fibroblast proliferation (Leask, 2015). SalB attenuates Ang II-induced ECM deposition via the forkhead box protein O1/miR-148a-3p axis and significantly reduces the levels of CoIA1, CoIII A1, α-SMA, CTGF, and ROS (Liu et al., 2023). By encouraging AMPK phosphorylation, SalB may counteract Ang II’s pro-fibrotic action on CF.
2.3.5 IGFBP3
Insulin-like growth factor binding protein 3 (IGFBP3) has been evaluated to regulate cellular proliferation and migration and decrease activation of cardiac fibroblasts through epigenetic mechanisms (Ding et al., 2023). Besides, IGFBP3 miRNA and protein levels increase in CF. SalB inhibits the expression of IGFBP3, triggering the vascular endothelial growth factor receptor 2 (VEGFR2)/vascular endothelial growth factor A (VEGFA) pathway to enhance ventricular remodeling, angiogenesis, and collagen deposition linked to hyperglycemia, ultimately protecting cardiac function (Li et al., 2020).
Natural products have multitargeted actions that show promise in treating CF. SalB has the potential to serve as a complementary therapeutic agent for CF and exert an anti-fibrotic effect by modulating the formation of these substances (Figure 5). Even though their therapeutic effects have been demonstrated to be significant, the mechanisms of action are still unclear. Therefore, more research is required to understand the unique pathophysiology of CF and the synergistic process of SalB’s multi-point adjustments.
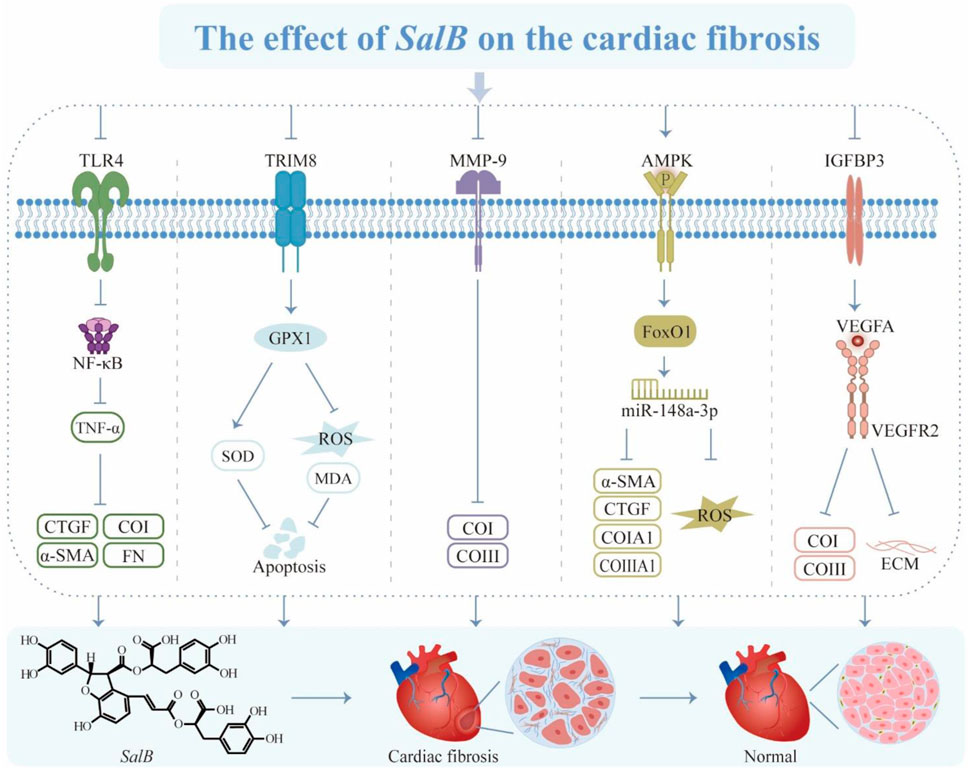
Figure 5. The effect of SalB on the cardiac fibrosis. Toll-like receptor 4 (TLR4), nuclear factor-κ-gene binding (NF-κB), tumor necrosis factor α (TNF-α), connective tissue growth factor (CTGF), α-smooth muscle actin (α-SMA), collagen type I (CoI), fibronectin (FN), tripartite motif 8 (TRIM8), glutathione peroxidase 1 (GPX1), superoxide dismutase (SOD), reactive oxygen species (ROS), malondialdehyde (MDA), matrix metalloproteinase-9 (MMP-9), collagen type III (CoIII), adenosine monophosphate-regulated protein kinase (AMPK), forkhead box protein 1 (FoxO1), connective tissue growth factor (CTGF), insulin-like growth factor binding protein 3 (IGFBP3), vascular endothelial growth factor A (VEGFA), vascular endothelial growth factor receptor 2 (VEGFR2), extracellular matrix (ECM).
2.4 Renal fibrosis
Fibrous scar tissue generated by renal fibrosis (RF) replaces functional tissue, which impairs the regenerative ability of the kidneys and deteriorates renal function and tissue structure (Liu et al., 2018; Lin et al., 2023). RF is a prevalent feature observed in renal injury and the decline of renal function caused by various etiologies, serving as a common pathway for the progression of diverse chronic kidney diseases (Boor and Floege, 2012; Kramann et al., 2013). To avoid or delay the onset of chronic renal disease and improve the prognosis, it is imperative to target and block RF.
2.4.1 TGF-β
TGF-β directly affects intrinsic renal cells, promoting cellular proliferation and triggering podocyte clearance and apoptosis of RTEC (López-Hernández and López-Novoa, 2012; Meng et al., 2015). Wang et al. found that SalB alleviated tubular fibrosis by inhibiting Smad2/3 phosphorylation as well as MMP-2 and MMP-9 activity via TGF-β1/Smad (Wang et al., 2010). SalB reverses TGF-β1-induced EMT, suppresses α-SMA expression, and facilitates the restoration of tubular epithelial structure to mitigate RF in vitro (Pan et al., 2011). A direct target of miRs is the TGF-β type II receptor, which regulates TGF-β signaling. SalB therapy increases miR-106b expression and inhibits TGF-β type II receptor expression to reduce TGF-β1-induced EMT (Tang et al., 2014). Despite the current dearth of efficacious medications for the treatment of RF, above numerous studies have manifested that targeting the TGF-β cascade represents an efficacious therapeutic approach for this condition.
2.4.2 HPSE
Heparinase (HPSE) is an endonucleating β-D-glucuronidase that interacts with TGF-β to modulate the remodeling and degradation of the ECM, and the release of various cytokines (Ilan et al., 2006; Masola et al., 2014). The HPSE protein is expressed in RTEC and actively participates in the TGF-β-induced EMT in renal tubular cells (Masola et al., 2015). The integrity of the filtration barrier and the operation of glomerular filtration depends on this regulating mechanism (Masola et al., 2012; Rabelink et al., 2017). Tubular epithelial fibroblast transdifferentiation (TEMT) has been proposed as a viable therapeutic option to decrease RF since it stimulates the expression of several fibro-cytokines, which represent a critical mechanism in the development of RF (Li et al., 2004; Liu, 2010). SalB mitigates TEMT and has renal protective effects by down-regulating the expression of FGF2/TGF-β1/α-SMA via the HPSE/Syndecan-l axis (Hu et al., 2020).
2.4.3 PDGF
PDGF is a profibrotic mediator that induces fibroblast activation and proliferation and promotes ECM contraction (Bonner, 2004). Numerous pro-fibrotic mediators, such as TGF-β, TNF-α, and FGF, exhibit PDGF-associated pro-fibrotic activity (Chaudhary et al., 2007). At locations where renal interstitial fibrosis occurs, there is an upregulation of PDGF-C expression, which stimulates PDGFRα to further the fibrotic process (Eitner et al., 2008; Folestad et al., 2018; Li et al., 2021). The efficacy of SalB in reducing renal inflammation and CTGF production, improving renal function, and inhibiting the progression of RF through the PDGF-C/PDGFRα pathway has been well-documented (Yao et al., 2022). In consequence, targeting PDGF with SalB presents a promising and effective new strategy for treating RF.
2.4.4 PTEN/AKT
Phosphatase Tensin Homolog (PTEN) dephosphorylates protein kinase B (AKT), which inhibits pro-fibrotic signaling pathways and epithelial cell transdifferentiating; furthermore, RF is attenuated through the activation of AKT (Lan and Du, 2015; An et al., 2022). The administration of SalB has been shown to enhance renal function and prevent fibrosis by inhibiting Zeste gene enhancer homolog-2 (EZH2) and H3 lysine 27 trimethylation and down-regulating FN and α-SMA expression through the PTEN/AKT pathway (Lin et al., 2023).
2.4.5 NLRP3
Nucleotide-binding oligomerization domain-like pyrin domain-containing protein 3 (NLRP3) is becoming increasingly implicated in the regulation of RF, as evidenced by the significant upregulation of its expression in renal fibrotic tissue (Ke et al., 2018; Wu et al., 2018; Mulay, 2019; Zhang and Wang, 2019). Moreover, NLRP3 influences RTEC apoptosis through mitochondrial connections in addition to promoting the advancement of the disease by inducing an inflammatory reaction (Qi and Yang, 2018). The effect of enhancing RTEC viability and mitigating cellular damage and apoptosis of SalB by modulating the TLR4/NF-κB/NLRP3 pathway to increase mitochondrial membrane potential levels (Zhang and Wang, 2019). Further, activation of the NLRP3 cascade promotes RF through RTEC pyroptosis (Hutton et al., 2016; Miao et al., 2019). To lessen renal injury, SalB enhances the nuclear accumulation of Nrf2 and suppresses NLRP3 activation, oxidative stress, and Caspase-1/Gasdermin D-mediated cellular pyroptosis via the Nrf2/NLRP3 pathway (Pang et al., 2020). According to the information above, SalB suppression of NLRP3 regulates RF and may be a useful target for treating RF.
2.4.6 PI3K/AKT and Sirtuin1
The autophagy-lysosomal pathway is responsible for maintaining the metabolic balance of the ECM by sequestering FN within the cytosol and activating lysosomes for degradation in fibrosis (Guo et al., 2020). Autophagy stimulation can decrease synthesis, accelerate ECM degradation, and alleviate RF (Guo et al., 2020). SalB controls the important autophagy-mediating factor miR-145-5p to safeguard renal function. The mechanism is activating cellular autophagy, reducing cell proliferation, inflammation, and immune deposition via the phosphatidylinositol-3-kinase (PI3K)/AKT pathway (Chen et al., 2022a). SalB can also ameliorate autophagy and upregulate Sirtuin1, which can both lessen renal pathological damage and improve renal insufficiency (Pan et al., 2011; He et al., 2020). Additionally, it inhibits the expression of fibrogenic factors. Consequently, SalB as an autophagy mediator can protect renal function and ameliorate RF injury.
2.4.7 Apoptosis
While the suppression of apoptosis ameliorates renal interstitial fibrotic lesions, the degree of apoptosis of RTEC corresponds with the grade of fibrosis, the index of renal interstitial damage, and the degree of renal function impairment (Docherty et al., 2006; Teteris et al., 2007). SalB therapy reduced ROS levels and lessened cellular damage brought on by endoplasmic reticulum stress (ERS) activation. It also suppressed the production of proteins linked to apoptosis, such as p-JNK, C/EBP homologous protein, and BCL2-associated X/B-cell lymphoma-2 (Dong et al., 2021). Intracellular free fatty acid-mediated lipotoxicity induces apoptosis in RTEC and contributes to fibrosis. This lipotoxicity is attenuated by SalB, which reduces apoptosis and tubular injury by inhibiting the activation of ERS and apoptotic markers (Mai et al., 2020).
SalB affects the fibrosis of renal tubular epithelial cells (RTEC) by inhibiting various cytokines (PTEN, Ang II, TGF-β, EZH2, NF-κB, PDGF-C, CTGF) and pathways (cellular pyroptosis, autophagy, and apoptosis) (Figure 6). However, the pathophysiology of renal fibrosis is still poorly known, and renal fibrosis involves the dynamic process of various renal cells and inflammatory cells. Clinical research is lacking from the small number of cellular and animal tests that have been done so far, and several of the signaling pathways remain unclear.
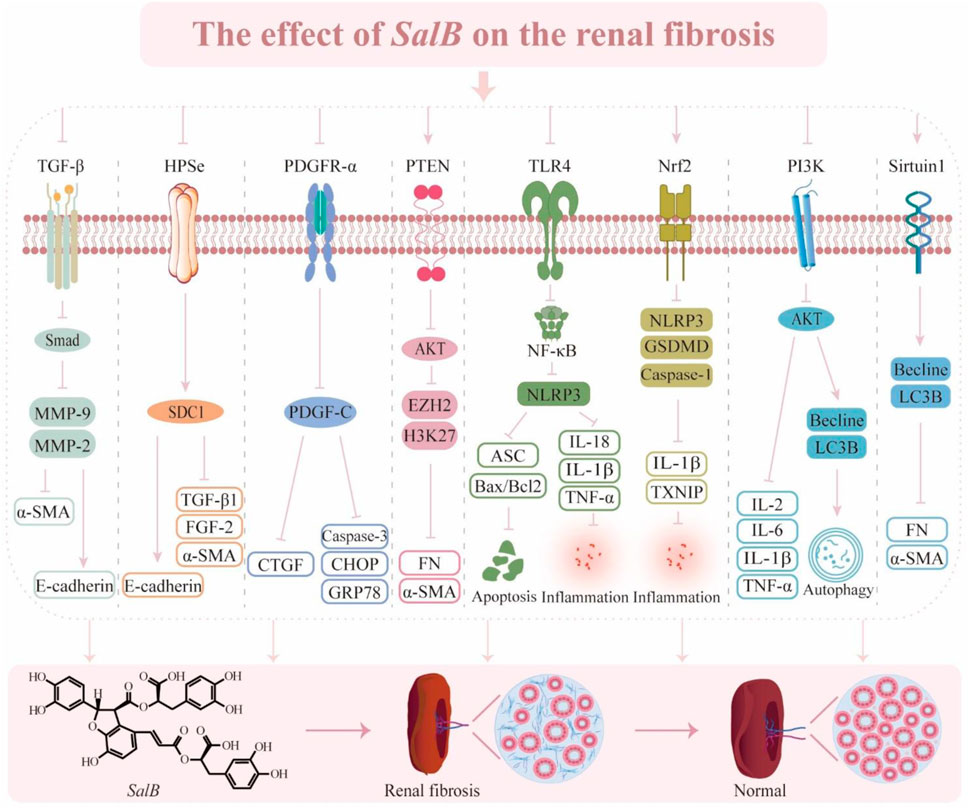
Figure 6. The effect of SalB on the renal fibrosis. Transforming growth factor-β (TGF-β), matrix metalloproteinase-9 (MMP-9), matrix metalloproteinase-2 (MMP-2), α-smooth muscle actin (α-SMA), heparinase (HPSe), syndecan-1(SDC1), fibroblast growth factor2 (FGF 2), platelet-derived growth factor receptor α (PDGFR-α), platelet-derived growth factor C (PDGF-C), connective tissue growth factor (CTGF), C/EBP homologous protein (CHOP), glucose regulated protein 78 (GRP78), phosphatase tensin homolog (PTEN), protein kinase B (AKT), enhancer of zeste homolog-2 (E2H2), histone H3 lysine 27 (H3K27), fibronectin (FN), toll-like receptor 4 (TLR4), nuclear factor-κ-gene binding (NF-κB), nucleotide-binding oligomerization domain-like pyrin domain containing protein 3 (NLRP3), apoptosis-associated speck-like protein containing a CARD (ASC), bcl-2 assaciated x protein (Bax), b-cell lymphoma-2 (Bcl2), interleukin-18 (IL-18), interleukin-1β (IL-1β), tumor necrosis factor α (TNF-α), nuclear factor erythroid 2-related factor 2 (Nrf2), gasdermin D (GSDMD), hioredoxin-interacting protein (TXNIP), phosphatidylinositol 3-kinase (PI3K), interleukin-2 (IL-2), interleukin-6 (IL-6), microtubule-associated protein light chain 3B (LC3B).
2.5 Skin fibrosis
Hypertrophic scar is a prevalent yet inadequately resolved fibrotic dermatological condition, presenting as elevated, erythematous, indurated, and non-elastic plaques (Finnerty et al., 2016). They are among the most common side effects that arise when cutaneous damage heals. Targeting its downstream effector CD36, the SalB intervention lowers reticular fibroblast numbers and fibrogenic factors, which in turn inhibit cell proliferation and the production of skin scars (Griffin et al., 2021).
The pathogenesis of systemic sclerosis (SSc) is associated with endothelial damage, fibroproliferative vasculopathy, and fibroblast dysfunction (Taflinski et al., 2014; Garrett et al., 2017). TGF-β is a highly potent inducer of cell proliferation and collagen synthesis (Kim et al., 2015). SalB decreases skin thickness and collagen deposition by regulating the TGF-β/Smad and MAPK/ERK pathways, while down-regulating levels of CTGF, FN1, PAI-1, and α-SMA (Liu et al., 2019).
2.6 Epidural fibrosis
Epidural fibrosis (EF) is formed by excessive deposition of scar tissue in the epidural space following lumbar laminectomy (Zhang et al., 2013). Postoperative back and lower extremity pain is caused by the proliferation and adherence of scar tissue to the dura mater, as well as fibrosis around and enclosing the nerve roots (Fritsch et al., 1996; Ross et al., 1996). EF is therefore one of the main causes of the syndrome known as “failed lumbar spine surgery.” Inhibition of vascular regeneration and over-proliferation of fibroblasts is a promising strategy for reducing EF (Zhang et al., 2015). SalB inhibits the angiogenic factor VEGF, which mitigates fibroblast proliferation and blood vessel infiltration, reduces tissue adhesion, and prevents epidural scarring (Chen et al., 2016).
2.7 Frozen shoulder
Current treatments for arthrofibrosis are limited in terms of efficacy and diversity (Blessing et al., 2019). Fibro-proliferative tissue fibrosis is regarded as the primary pathological process underlying frozen shoulder (FS) (Challoumas et al., 2020). SalB has been found to have anti-inflammatory and anti-fibrotic effects in the treatment of FS. SalB inhibits the CD36-mediated PI3K/AKT pathway, downregulates the expression of fibrosis-associated molecules such as CoI, CoⅢ, FN, and α-SMA, and blocks the pathologic fibrotic process in vitro and in vivo (Yan et al., 2023).
2.8 Retinal fibrosis
Retinal fibrosis is a vascularized disease with excessive deposition of large numbers of immune cells, myofibroblasts, and ECM (Little et al., 2018; Tenbrock et al., 2022). A sustained low-grade inflammatory response drives the formation of fibrosis-associated lesions, facilitating the release of pro-fibrotic factors and the deposition of fibrotic tissue. In addition, pro-angiogenic astrocytes and microglia also contribute to the development of fibrotic lesions by producing VEGF and FN (Uemura et al., 2006). SalB attenuated the activation and proliferation of retinal microglia and astrocytes induced by amyloid β-protein deposition, along with the release of inflammatory factors through the β-Secretase one pathway (Wang et al., 2023). This increased dark-adapted and light-adapted amplitudes in mice restored the structure and function of the retina, and was associated with the reduction of ganglion cell apoptosis, attenuation of neuronal damage caused by oxidative stress, and restoration of mitochondrial function (Tian et al., 2008; He et al., 2018). According to other research, SalB protects retinal pigment epithelial cells from oxidative stress damage and H2O2-induced apoptosis while also restoring cell viability (Liu et al., 2016). These effects are mainly attributed to the activation of Nrf2.
2.9 Pancreatic fibrosis
Pancreatic fibrosis is a prominent morphological alteration observed in chronic pancreatitis, which is a multifaceted pathological process driven by the activation of pancreatic stellate cells (PSC) and distinguished by enhanced fibroblast proliferation and deposition of ECM (Singh et al., 2019; Beyer et al., 2020). Therefore, impeding the activation and proliferation of PSC is considered an approach for anti-pancreatic fibrosis therapy. SalB attenuates fibrotic damage, inhibits PSC activation and proliferation, and lowers the concentration of MDA in pancreatic tissues, all of which mitigate pathological harm (Lu et al., 2009).
2.10 Oral submucous fibrosis
Oral submucous fibrosis (OSF) is a chronic inflammatory disease identified by aberrant collagen deposition and progressive fibrosis of the subepithelial connective tissue in the oral submucosa (Utsunomiya et al., 2005). OSF is also a disorder of collagen metabolism caused by a disturbed balance of ECM synthesis and degradation. SalB inhibits the transcription of pro-collagen genes CoI and CoⅢ, and the expression of CTGF, IL-1β, and IL-6 by modulating the AKT, MAPK/ERK, and TGF-β/Smad signaling pathways (Dai et al., 2015a). It has notable anti-fibrotic action and decreases collagen deposition as well.
The abovementioned studies validate the great potential of SalB in fibrosis of skin, pancreas, oral mucosa, and other tissues (Figure 7). However, further research is required to fully understand the role of SalB in these tissues to produce relevant and appropriate data for therapeutic trials and the interpretation of findings.
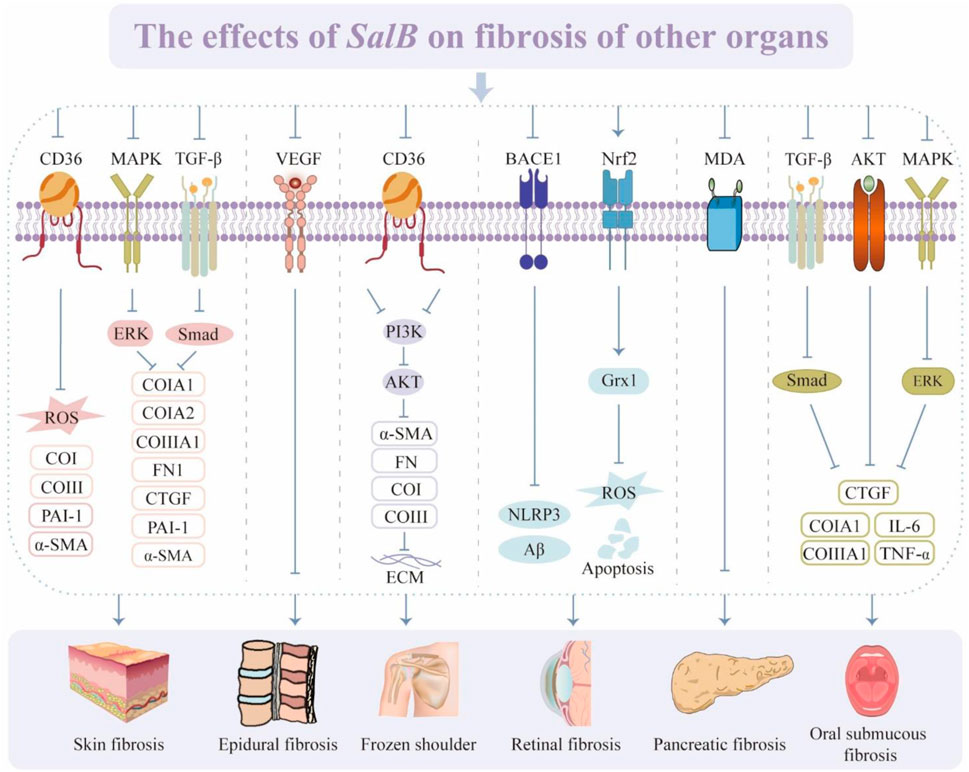
Figure 7. The effects of SalB on fibrosis of other organs. Recombinant Cluster of Differentiation 36 (CD36), reactive oxygen species (ROS), collagen type I (CoI), collagen type III (CoIII), plasminogen activator inhibitor-1 (PAI-1), α-smooth muscle actin (α-SMA), mitogen-activated protein kinase (MAPK), transforming growth factor-β (TGF-β), extracellular regulated protein kinases (ERK), fibronectin (FN), connective tissue growth factor (CTGF), vascular endothelial growth factor (VEGF), phosphatidylinositol 3-kinase (PI3K), protein kinase B (AKT), extracellular matrix (ECM), recombinant beta-site APP cleaving enzyme 1 (BACE1), nucleotide-binding oligomerization domain-like pyrin domain containing protein 3 (NLRP3), nuclear factor erythroid 2-related factor 2 (Nrf2), glutaredoxin 1 (Grx1), malondialdehyde (MDA), interleukin-6 (IL-6), tumor necrosis factor α (TNF-α).
3 Safety and adverse effects
SalB has many biological benefits, such as anti-inflammatory, anti-oxidant, anti-fibrotic, and enhanced perfusion. It is extensively used in phenolic acids. Thus, the safety and adverse drug reactions of SalB have gradually become the focus of researchers’ attention. Compared to the equivalent doses of caffeic acid and ferulic acid, SalB does not induce the production of ROS or oxidative stress in the walls of small veins, preventing an imbalance in oxidative and anti-oxidant mechanisms (Du et al., 2017). Furthermore, a concentration of SalB of less than 300 mg/kg won't harm pregnant rats; at 100 mg/kg, SalB won't harm developing embryos or cause genotoxicity. SalB was tested for toxicity at a high dose of 750 mg/kg via the tail vein, and the findings point to SalB’s safety (He et al., 2023). According to another study, nebulized inhalation of SalB as a dry powder inhalation formulation demonstrated satisfactory mobility and no mortality in rat models. No significant side effects were noted in a randomized, double-blind clinical trial of SalB at single ascending doses and multiple ascending doses (Cheng et al., 2023). These findings suggest a favorable safety profile for SalB. In addition, the rate of fibrosis stage reversal was 36.67%, the rate of inflammation remission was 40.0%, and the serum fibrosis markers were significantly improved after 6 months of continuous use of SalB tablets (30 mg/tablet, 2 tablets once, three times a day) in the treatment of patients with hepatic fibrosis combined with consolidated hepatitis B. Furthermore, all members of the SalB group displayed normal test results and no adverse responses, indicating a high level of safety (Liu et al., 2002). The aforementioned research has established the safety of SalB in comparison to other synthetic medicines. To determine the optimal supplemental dosage and duration for treating fibrosis in different organs, as well as the possibility of hepatorenal toxicity when used over an extended period of time, more preclinical research is still required to support the data, and clinical studies are needed to produce high-caliber evidence-based research.
4 Drug interactions
Drug interaction (DI) between SalB and several other medications has been reported. For instance, the co-administration of tanshinone and polyphenol extract contributed to an increase in the area under the plasma concentration-time curve and a decrease in the total plasma clearance of SalB (Guo et al., 2008). When aspirin is taken together, SalB has a synergistic effect that inhibits CD62p, a molecular marker of platelet activation and extends SalB’s half-life by preventing the liver metabolizing enzyme catechol-O-methyltransferase (COMT) from being active (Cao et al., 2021). SalB also raises plasma levodopa levels after extended usage and prevents levodopa from being methylated via COMT. The activity of SalB was further enhanced in combination with ferulic acid (Chen et al., 2022). Nonetheless, the majority of the recent research on SalB’s DI focuses on synergistic potentiation rather than minimizing harmful side effects. Additionally, little is known about how SalB interacts with anti-fibrotic medications. Consequently, further studies are required to clarify these possible connections.
5 Different dosage forms of SalB
The absolute bioavailability of SalB is only 3.9% after oral administration of 50 mg/kg SalB to rats (Zhou et al., 2009). Another study found that dogs fed 180 mg/kg SalB orally had an AUC of 1680 ± 670 ng/mL h and a bioavailability of only 1.07% ± 0.43% (Gao et al., 2009). The main reasons for this include the enterohepatic circulation phenomena, hepatic first-pass effect, and inadequate permeability (Zhang et al., 2004a; 2004b; Wu et al., 2006; Zhou et al., 2009; Li et al., 2019). This limits SalB’s effectiveness and absorption in the gastrointestinal system and makes it less useful for clinical use. Researchers have manipulated SalB into several formulations to maximize its medicinal efficacy and increase its bioavailability. SalB was transformed into a lyophilized powder and dissolved in sodium chloride injection for intravenous infusion. Pharmacokinetic analysis revealed that there was no discernible accumulation of SalB in the body as the maximum plasma concentration and area under the curve (AUC) rose proportionately with increasing doses (Bi et al., 2016). Pulmonary administration via dry powder inhaler circumvents first-pass effects and diminishes enzymatic degradation reactions (Newman, 2017). The drug concentration in the lungs was elevated by the dry powder inhalation formulation of SalB, which had an AUC (0–1) that was almost 2099.12 times higher than that of intravenous treatment (Jiang et al., 2021). Loading SalB into a solid self-micro emulsifying drug delivery system for lipid delivery prepared by coagulating the liquid excipient into a powder (Bi et al., 2016). Higher bioavailability is made possible by this technique, which also guarantees a more effective medication delivery mechanism. Nanotechnology has made a major impact on the advancement of medicine, as nano-encapsulated natural products have better safety, stability, and efficacy. Compared to previous formulations, SalB-loaded PEGylated liposomes at an active dose of 100 mg/kg showed higher than 4-fold plasma concentrations (Isacchi et al., 2011). SalB’s bioavailability can be significantly increased by using different dosage forms. However, less research has been done on fibrosis treatment. Anti-fibrotic drug development at the nexus of biology, pharmacy, and materials science may take an important turn in this direction in the future.
6 Discussion
Natural products are crucial sources of modern drug discovery and play a pivotal role in disease prevention and treatment. With the advancements in molecular biology and modern chromatographic separation techniques and the mechanisms of the fibrotic process constantly being elucidated, an increasing number of natural anti-fibrotic products are being discovered. SalB is considered a promising treatment in the field of combating fibrotic diseases. The details of its anti-fibrotic effects on multiple tissues and organs through activation of different signaling pathways and modulation of multiple targets are shown in Table 1. While significant progress has been achieved in current research on the pathogenesis of fibrosis, there are several challenges to translating this research into clinical practice.
Adequacy of resources is a challenge for drugs derived from natural products. The problem of natural product resources can be greatly solved by synthesizing bioactive compounds having pharmacological properties using either biotic or abiotic approaches. Nevertheless, SalB extraction from the natural product S. miltiorrhiza is the main method to obtain SalB. However, according to some research, SalB can be produced artificially using biotechnological means: rosmarinic acid, the precursor compound for SalB, can be made into salvianolic acid by creating a full biometabolic pathway for it in a strain of Saccharomyces cerevisiae. This can be achieved by using endogenous enzymes in the yeast chassis cells in conjunction with an exogenous biosynthetic pathway (Di et al., 2013; Mateljak et al., 2017). While it is possible to produce SalB through a technological method, more research is necessary to determine whether the molecules produced through this process have the same pharmacological effects as SalB derived from natural sources.
One more problem that must be resolved before SalB can be used in the clinic is bioavailability. Due to SalB’s restricted oral bioavailability, attempts are now being made in research to find substitute formulations. Research is now being done on SalB’s lyophilized powder, dry powder inhaler, and lipid carrier alternatives. However, little is known about its use in fibrotic disorders. The effectiveness of SalB in treating fibrotic diseases has been confirmed. To enhance efficacy and improve bioavailability, future research endeavors ought to concentrate on the preparation of SalB into diverse dosage forms that are customized to the unique features of the disease.
The comprehension of fibrotic molecules is improved through modeling (Ricard-Blum and Miele, 2020). It is essential to create predictive animal models, primary human tissue culture systems, and virtualized medications based on particular genetic profiles of fibrotic patients due to the developments of modern technology, such as single-cell multi-omics. Before a medicine enters clinical trials, its effect on particular molecular disease phenotypes can be predicted.
One significant barrier to the development of anti-fibrotic medications is the lack of suitable biomarkers for identifying disease-specific traits (Wynn and Ramalingam, 2012). Therefore, methods for quickly, non-invasively, and precisely determining the anti-fibrotic efficacy of SalB are essential for its clinical application. Normal repair processes require the production of FN, the release of inflammatory agents, and the deposition of ECM during fibrosis. To avoid off-target toxicity, more research is needed to ascertain whether SalB can target key fibrotic components upstream and downstream and whether its use affects the normal repair process in any way. In addition, the majority of recent research on SalB’s anti-fibrotic action focuses mostly on one target or pathway. On the other hand, little is known about the ways in which various targets and pathways interact. Thus, in order to more thoroughly understand the overall regulation of SalB, we can conduct studies on the regulatory mechanism of the intricate network of interacting targets using techniques including single-cell sequencing, proteomics, transcriptomics, and metabolomics.
Few comprehensive clinical investigations have been conducted on the safety and side effects of SalB, despite multiple preclinical studies showing the drug’s anti-fibrotic efficacy in a variety of organs (Ma et al., 2017; Cheng et al., 2023). There is an urgent need to address these issues through large-scale clinical studies. Furthermore, selecting subjects for clinical trials is difficult due to the diversity of clinical conditions and the slow course of fibrosis. The development of successful anti-fibrotic therapeutics depends on defining reliable and valid predictors of the course of fibrotic disease and planning clinical studies with distinct clinical outcomes to typify patients prior to trial enrollment (Henderson et al., 2020).
7 Conclusion
SalB has garnered interest due to its diverse array of anti-fibrotic properties. Several studies have been done on SalB’s impact on fibrosis in both cells and animals. SalB may be able to mitigate the fibrotic process by modifying multiple aspects of immune cells, inflammatory factors, oxidative stress, ERS, and pyroptosis to lessen the release of fibrogenic factors, ECM over deposition, and fibroblast proliferation. It is reasonable to conclude that SalB may be a pleiotropic molecule exerting anti-fibrotic effects against numerous signaling cascades after carefully reviewing the studies on SalB’s effects on fibrosis. There are some limitations since the current study is a review that focuses on SalB’s involvement in fibrosis in various organs. Firstly, as the study is a review of the research that has been done, it falls short in terms of offering clinical evidence supporting SalB’s use in the treatment of fibrosis. Second, this review aims to shed light on the scope and efficacy of SalB in treating fibrotic diseases by describing and summarizing SalB’s effects on fibrosis in various organ tissues. It is necessary to reevaluate from a different angle whether SalB’s mechanism of action is similar for different fibrotic disorders or whether similar therapeutic targets are present. Lastly, the evidence that can be offered for this study is limited because there are not enough studies on SalB for the therapy of fibrosis in other organs, such as the skin, pancreas, retina, etc. All of these issues require further in-depth research, discussion, and augmentation in the future.
In conclusion, additional excellent, advanced basic and clinical research is required to fully comprehend how SalB affects fibrosis, to enhance SalB’s therapeutic benefits, particularly with regard to organ damage carried on by fibrosis, and to fulfill the intended purpose of targeted therapy.
Author contributions
QL: Writing–original draft, Writing–review and editing. XL: Visualization, Writing–review and editing. XP: Writing–original draft, Writing–review and editing. TL: Supervision, Writing–review and editing. YS: Supervision, Writing–review and editing. XX: Writing–review and editing. HX: Writing–review and editing. HG: Writing–review and editing. ZC: Supervision, Writing–review and editing. CX: Conceptualization, Supervision, Writing–review and editing.
Funding
The author(s) declare that financial support was received for the research, authorship, and/or publication of this article. This work is supported by the innovation team and talents cultivation program of National Administration of Traditional Chinese Medicine (ZYYCXTD-C-202209), study on the effect of Shenqi Compound series on cardiovascular benefit in diabetes mellitus based on macrovascular protective effect, Sichuan Provincial Department of Science and Technology (2022ZDZX0022), and the Talent Support Project of the National Administration of Traditional Chinese Medicine: Qihuang Scholar (National Chinese Medicine Letter 2022 No. 6).
Conflict of interest
The authors declare that the research was conducted in the absence of any commercial or financial relationships that could be construed as a potential conflict of interest.
The reviewer DL declared a shared parent affiliation with the authors to the handling editor at the time of review.
Publisher’s note
All claims expressed in this article are solely those of the authors and do not necessarily represent those of their affiliated organizations, or those of the publisher, the editors and the reviewers. Any product that may be evaluated in this article, or claim that may be made by its manufacturer, is not guaranteed or endorsed by the publisher.
References
Amara, N., Goven, D., Prost, F., Muloway, R., Crestani, B., and Boczkowski, J. (2010). NOX4/NADPH oxidase expression is increased in pulmonary fibroblasts from patients with idiopathic pulmonary fibrosis and mediates TGFbeta1-induced fibroblast differentiation into myofibroblasts. Thorax 65, 733–738. doi:10.1136/thx.2009.113456
An, C., Jiao, B., Du, H., Tran, M., Zhou, D., and Wang, Y. (2022). Myeloid PTEN deficiency aggravates renal inflammation and fibrosis in angiotensin II-induced hypertension. J. Cell. Physiol. 237, 983–991. doi:10.1002/jcp.30574
Bar-Gal, G. K., Kim, M. J., Klein, A., Shin, D. H., Oh, C. S., Kim, J. W., et al. (2012). Tracing hepatitis B virus to the 16th century in a Korean mummy. Hepatology 56, 1671–1680. doi:10.1002/hep.25852
Basso, B. de S., Haute, G. V., Ortega-Ribera, M., Luft, C., Antunes, G. L., Bastos, M. S., et al. (2021). Methoxyeugenol deactivates hepatic stellate cells and attenuates liver fibrosis and inflammation through a PPAR-ɣ and NF-kB mechanism. J. Ethnopharmacol. 280, 114433. doi:10.1016/j.jep.2021.114433
Beyer, G., Habtezion, A., Werner, J., Lerch, M. M., and Mayerle, J. (2020). Chronic pancreatitis. Lancet 396, 499–512. doi:10.1016/S0140-6736(20)31318-0
Bi, X., Liu, X., Di, L., and Zu, Q. (2016). Improved oral bioavailability using a solid self-microemulsifying drug delivery system containing a multicomponent mixture extracted from salvia miltiorrhiza. Molecules 21, 456. doi:10.3390/molecules21040456
Blessing, W. A., Okajima, S. M., Cubria, M. B., Villa-Camacho, J. C., Perez-Viloria, M., Williamson, P. M., et al. (2019). Intraarticular injection of relaxin-2 alleviates shoulder arthrofibrosis. Proc. Natl. Acad. Sci. U. S. A. 116, 12183–12192. doi:10.1073/pnas.1900355116
Bonner, J. C. (2004). Regulation of PDGF and its receptors in fibrotic diseases. Cytokine Growth Factor Rev. 15, 255–273. doi:10.1016/j.cytogfr.2004.03.006
Boor, P., and Floege, J. (2012). The renal (myo-)fibroblast: a heterogeneous group of cells. Nephrol. Dial. Transpl. 27, 3027–3036. doi:10.1093/ndt/gfs296
Cao, W., Guo, X., Zheng, H., Li, D., Jia, G., and Wang, J. (2012). Current progress of research on pharmacologic actions of salvianolic acid B. Chin. J. Integr. Med. 18, 316–320. doi:10.1007/s11655-012-1052-8
Cao, W., Yang, Q., Zhang, W., Xu, Y., Wang, S., Wu, Y., et al. (2021). Drug-drug interactions between salvianolate injection and aspirin based on their metabolic enzymes. Biomed. Pharmacother. 135, 111203. doi:10.1016/j.biopha.2020.111203
Challoumas, D., Biddle, M., McLean, M., and Millar, N. L. (2020). Comparison of treatments for frozen shoulder: a systematic review and meta-analysis. JAMA Netw. Open 3, e2029581. doi:10.1001/jamanetworkopen.2020.29581
Chaudhary, N. I., Roth, G. J., Hilberg, F., Müller-Quernheim, J., Prasse, A., Zissel, G., et al. (2007). Inhibition of PDGF, VEGF and FGF signalling attenuates fibrosis. Eur. Respir. J. 29, 976–985. doi:10.1183/09031936.00152106
Chen, F., Wang, C., Sun, J., Wang, J., Wang, L., and Li, J. (2016a). Salvianolic acid B reduced the formation of epidural fibrosis in an experimental rat model. J. Orthop. Surg. Res. 11, 141. doi:10.1186/s13018-016-0475-x
Chen, J., Hu, Q., Luo, Y., Luo, L., Lin, H., Chen, D., et al. (2022a). Salvianolic acid B attenuates membranous nephropathy by activating renal autophagy via microRNA-145-5p/phosphatidylinositol 3-kinase/AKT pathway. Bioengineered 13, 13956–13969. doi:10.1080/21655979.2022.2083822
Chen, J., Wang, Y., Wang, S., Zhao, X., Zhao, L., and Wang, Y. (2022b). Salvianolic acid B and ferulic acid synergistically promote angiogenesis in HUVECs and zebrafish via regulating VEGF signaling. J. Ethnopharmacol. 283, 114667. doi:10.1016/j.jep.2021.114667
Chen, L., Huang, J., Ji, Y., Zhang, X., Wang, P., Deng, K., et al. (2016b). Tripartite motif 32 prevents pathological cardiac hypertrophy. Clin. Sci. (Lond) 130, 813–828. doi:10.1042/CS20150619
Cheng, J., Long, J., Zhang, J., Han, L., Hu, Y., Liu, J., et al. (2023). Safety, tolerance, and pharmacokinetics of salvianolic acid B in healthy Chinese volunteers: a randomized, double-blind, placebo-controlled phase 1 clinical trial. Front. Pharmacol. 14, 1146309. doi:10.3389/fphar.2023.1146309
Choi, S. S., Omenetti, A., Witek, R. P., Moylan, C. A., Syn, W. K., Jung, Y., et al. (2009). Hedgehog pathway activation and epithelial-to-mesenchymal transitions during myofibroblastic transformation of rat hepatic cells in culture and cirrhosis. Am. J. Physiol. Gastrointest. Liver Physiol. 297, G1093–G1106. doi:10.1152/ajpgi.00292.2009
Ciceu, A., Balta, C., Herman, H., Gharbia, S., Ignat, S. R., Dinescu, S., et al. (2021). Complexation with random methyl-β-cyclodextrin and (2-hidroxypropyl)-β-cyclodextrin enhances in vivo anti-fibrotic and anti-inflammatory effects of chrysin via the inhibition of NF-κB and TGF-β1/smad signaling pathways and modulation of hepatic pro/anti-fibrotic miRNA. Int. J. Mol. Sci. 22, 1869. doi:10.3390/ijms22041869
Dai, J. P., Zhu, D. X., Sheng, J. T., Chen, X. X., Li, W. Z., Wang, G. F., et al. (2015a). Inhibition of tanshinone iia, salvianolic acid A and salvianolic acid B on areca nut extract-induced oral submucous fibrosis in vitro. Molecules 20, 6794–6807. doi:10.3390/molecules20046794
Dai, J. P., Zhu, D. X., Sheng, J. T., Chen, X. X., Li, W. Z., Wang, G. F., et al. (2015b). Inhibition of Tanshinone IIA, salvianolic acid A and salvianolic acid B on Areca nut extract-induced oral submucous fibrosis in vitro. Molecules 20, 6794–6807. doi:10.3390/molecules20046794
Dang, X., Qin, Y., Gu, C., Sun, J., Zhang, R., and Peng, Z. (2020). Knockdown of tripartite motif 8 protects H9C2 cells against hypoxia/reoxygenation-induced injury through the activation of PI3K/akt signaling pathway. Cell. Transpl. 29, 963689720949247. doi:10.1177/0963689720949247
Degirolamo, C., Sabbà, C., and Moschetta, A. (2016). Therapeutic potential of the endocrine fibroblast growth factors FGF19, FGF21 and FGF23. Nat. Rev. Drug Discov. 15, 51–69. doi:10.1038/nrd.2015.9
Deng, L., Ouyang, B., Tang, W., Wang, N., Yang, F., Shi, H., et al. (2024). Icariside II modulates pulmonary fibrosis via PI3K/Akt/β-catenin pathway inhibition of M2 macrophage program. Phytomedicine 130, 155687. doi:10.1016/j.phymed.2024.155687
Di, P., Zhang, L., Chen, J., Tan, H., Xiao, Y., Dong, X., et al. (2013). 13 C tracer reveals phenolic acids biosynthesis in hairy root cultures of Salvia miltiorrhiza. ACS Chem. Biol. 8, 1537–1548. doi:10.1021/cb3006962
Ding, J. F., Sun, H., Song, K., Zhou, Y., Tu, B., Shi, K. H., et al. (2023). IGFBP3 epigenetic promotion induced by METTL3 boosts cardiac fibroblast activation and fibrosis. Eur. J. Pharmacol. 942, 175494. doi:10.1016/j.ejphar.2023.175494
Docherty, N. G., O’Sullivan, O. E., Healy, D. A., Fitzpatrick, J. M., and Watson, R. W. G. (2006). Evidence that inhibition of tubular cell apoptosis protects against renal damage and development of fibrosis following ureteric obstruction. Am. J. Physiol. Ren. Physiol. 290, F4–F13. doi:10.1152/ajprenal.00045.2005
Dong, S. J., Gao, X. Y., Pei, M. X., Luo, T., Fan, D., Chen, Y. L., et al. (2021). Effects and mechanism of salvianolic acid B on the injury of human renal tubular epithelial cells induced by iopromide. Front. Pharmacol. 12, 761908. doi:10.3389/fphar.2021.761908
Du, W. Y., Xiao, Y., Yao, J. J., Hao, Z., and Zhao, Y. B. (2017). Involvement of NADPH oxidase in high-dose phenolic acid-induced pro-oxidant activity on rat mesenteric venules. Exp. Ther. Med. 13, 17–22. doi:10.3892/etm.2016.3923
Eitner, F., Bücher, E., Roeyen, C. V., Kunter, U., Rong, S., Seikrit, C., et al. (2008). PDGF-C is a proinflammatory cytokine that mediates renal interstitial fibrosis. J. Am. Soc. Nephrol. 19, 281–289. doi:10.1681/ASN.2007030290
Fan, Z., and Guan, J. (2016). Antifibrotic therapies to control cardiac fibrosis. Biomater. Res. 20, 13. doi:10.1186/s40824-016-0060-8
Finnerty, C. C., Jeschke, M. G., Branski, L. K., Barret, J. P., Dziewulski, P., and Herndon, D. N. (2016). Hypertrophic scarring: the greatest unmet challenge after burn injury. Lancet London, Engl. 388, 1427–1436. doi:10.1016/S0140-6736(16)31406-4
Folestad, E., Kunath, A., and Wågsäter, D. (2018). PDGF-C and PDGF-D signaling in vascular diseases and animal models. Mol. Asp. Med. 62, 1–11. doi:10.1016/j.mam.2018.01.005
Frangogiannis, N. G., Smith, C. W., and Entman, M. L. (2002). The inflammatory response in myocardial infarction. Cardiovasc Res. 53, 31–47. doi:10.1016/s0008-6363(01)00434-5
Fritsch, E. W., Heisel, J., and Rupp, S. (1996). The failed back surgery syndrome: reasons, intraoperative findings, and long-term results: a report of 182 operative treatments. Spine (Phila Pa 1976) 21, 626–633. doi:10.1097/00007632-199603010-00017
Gadaleta, R. M., Scialpi, N., Peres, C., Cariello, M., Ko, B., Luo, J., et al. (2018). Suppression of hepatic bile acid synthesis by a non-tumorigenic FGF19 analogue protects mice from fibrosis and hepatocarcinogenesis. Sci. Rep. 8, 17210. doi:10.1038/s41598-018-35496-z
Gao, D. Y., Han, L. M., Zhang, L. H., Fang, X. L., and Wang, J. X. (2009). Bioavailability of salvianolic acid B and effect on blood viscosities after oral administration of salvianolic acids in beagle dogs. Archives pharmacal Res. 32, 773–779. doi:10.1007/s12272-009-1517-2
Gao, Y., Liu, M. F., Li, Y., Liu, X., Cao, Y. J., Long, Q. F., et al. (2024). Mesenchymal stem cells-extracellular vesicles alleviate pulmonary fibrosis by regulating immunomodulators. World J. Stem Cells 16, 670–689. doi:10.4252/wjsc.v16.i6.670
Garrett, S. M., Baker Frost, D., and Feghali-Bostwick, C. (2017). The mighty fibroblast and its utility in scleroderma research. J. Scleroderma Relat. Disord. 2, 69–134. doi:10.5301/jsrd.5000240
Glass, D. S., Grossfeld, D., Renna, H. A., Agarwala, P., Spiegler, P., Kasselman, L. J., et al. (2020). Idiopathic pulmonary fibrosis: molecular mechanisms and potential treatment approaches. Respir. Investig. 58, 320–335. doi:10.1016/j.resinv.2020.04.002
Gong, Z., Ye, Q., Wu, J. W., Zhou, J. L., Kong, X. Y., and Ma, L. K. (2021). UCHL1 inhibition attenuates cardiac fibrosis via modulation of nuclear factor-κB signaling in fibroblasts. Eur. J. Pharmacol. 900, 174045. doi:10.1016/j.ejphar.2021.174045
Griffin, M. F., Borrelli, M. R., Garcia, J. T., Januszyk, M., King, M., Lerbs, T., et al. (2021). JUN promotes hypertrophic skin scarring via CD36 in preclinical in vitro and in vivo models. Sci. Transl. Med. 13, eabb3312. doi:10.1126/scitranslmed.abb3312
Guo, L., Tan, K., Luo, Q., and Bai, X. (2020). Dihydromyricetin promotes autophagy and attenuates renal interstitial fibrosis by regulating miR-155-5p/PTEN signaling in diabetic nephropathy. Bosn. J. Basic Med. Sci. 20, 372–380. doi:10.17305/bjbms.2019.4410
Guo, S. S., and Wang, Z. G. (2022). Salvianolic acid B from Salvia miltiorrhiza bunge: a potential antitumor agent. Front. Pharmacol. 13, 1042745. doi:10.3389/fphar.2022.1042745
Guo, Z. J., Zhang, Y., Tang, X., Li, H., and Sun, Q. S. (2008). Pharmacokinetic interaction between tanshinones and polyphenolic extracts of salvia miltinorrhiza BUNGE after intravenous administration in rats. Biol. Pharm. Bull. 31, 1469–1474. doi:10.1248/bpb.31.1469
Harada, M., Tadevosyan, A., Qi, X., Xiao, J., Liu, T., Voigt, N., et al. (2015). Atrial fibrillation activates AMP-dependent protein kinase and its regulation of cellular calcium handling: potential role in metabolic adaptation and prevention of progression. J. Am. Coll. Cardiol. 66, 47–58. doi:10.1016/j.jacc.2015.04.056
He, G., Chen, G., Liu, W., Ye, D., Liu, X., Liang, X., et al. (2023). Salvianolic acid B: a review of pharmacological effects, safety, combination therapy, new dosage forms, and novel drug delivery routes. Pharmaceutics 15, 2235. doi:10.3390/pharmaceutics15092235
He, Y., Jia, K., Li, L., Wang, Q., Zhang, S., Du, J., et al. (2018). Salvianolic acid B attenuates mitochondrial stress against Aβ toxicity in primary cultured mouse neurons. Biochem. Biophys. Res. Commun. 498, 1066–1072. doi:10.1016/j.bbrc.2018.03.119
He, Y., Lu, R., Wu, J., Pang, Y., Li, J., Chen, J., et al. (2020). Salvianolic acid B attenuates epithelial-mesenchymal transition in renal fibrosis rats through activating Sirt1-mediated autophagy. Biomed. Pharmacother. 128, 110241. doi:10.1016/j.biopha.2020.110241
Hecquet, C. M., and Malik, A. B. (2009). Role of H(2)O(2)-activated TRPM2 calcium channel in oxidant-induced endothelial injury. Thromb. Haemost. 101, 619–625. doi:10.1160/th08-10-0641
Henderson, N. C., Rieder, F., and Wynn, T. A. (2020). Fibrosis: from mechanisms to medicines. Nature 587, 555–566. doi:10.1038/s41586-020-2938-9
Higashi, T., Friedman, S. L., and Hoshida, Y. (2017). Hepatic stellate cells as key target in liver fibrosis. Adv. Drug Deliv. Rev. 121, 27–42. doi:10.1016/j.addr.2017.05.007
Hirschfield, G. M., Chazouillères, O., Drenth, J. P., Thorburn, D., Harrison, S. A., Landis, C. S., et al. (2019). Effect of NGM282, an FGF19 analogue, in primary sclerosing cholangitis: a multicenter, randomized, double-blind, placebo-controlled phase II trial. J. Hepatol. 70, 483–493. doi:10.1016/j.jhep.2018.10.035
Ho, J. H. C., and Hong, C. Y. (2011). Salvianolic acids: small compounds with multiple mechanisms for cardiovascular protection. J. Biomed. Sci. 18, 30. doi:10.1186/1423-0127-18-30
Hu, H. H., Chen, D. Q., Wang, Y. N., Feng, Y. L., Cao, G., Vaziri, N. D., et al. (2018). New insights into TGF-β/Smad signaling in tissue fibrosis. Chem. Biol. Interact. 292, 76–83. doi:10.1016/j.cbi.2018.07.008
Hu, J., Li, C., Jin, S., Ye, Y., Fang, Y., Xu, P., et al. (2022). Salvianolic acid B combined with bone marrow mesenchymal stem cells piggybacked on HAMA hydrogel re-transplantation improves intervertebral disc degeneration. Front. Bioeng. Biotechnol. 10, 950625. doi:10.3389/fbioe.2022.950625
Hu, Y., Wang, M., Pan, Y., Li, Q., and Xu, L. (2020). Salvianolic acid B attenuates renal interstitial fibrosis by regulating the HPSE/SDC1 axis. Mol. Med. Rep. 22, 1325–1334. doi:10.3892/mmr.2020.11229
Hutton, H. L., Ooi, J. D., Holdsworth, S. R., and Kitching, A. R. (2016). The NLRP3 inflammasome in kidney disease and autoimmunity. Nephrol. Carlt. 21, 736–744. doi:10.1111/nep.12785
Ilan, N., Elkin, M., and Vlodavsky, I. (2006). Regulation, function and clinical significance of heparanase in cancer metastasis and angiogenesis. Int. J. Biochem. Cell. Biol. 38, 2018–2039. doi:10.1016/j.biocel.2006.06.004
Isacchi, B., Fabbri, V., Galeotti, N., Bergonzi, M. C., Karioti, A., Ghelardini, C., et al. (2011). Salvianolic acid B and its liposomal formulations: anti-hyperalgesic activity in the treatment of neuropathic pain. Eur. J. Pharm. Sci. 44, 552–558. doi:10.1016/j.ejps.2011.09.019
Jia, Q., Zhu, R., Tian, Y., Chen, B., Li, R., Li, L., et al. (2019). Salvia miltiorrhiza in diabetes: a review of its pharmacology, phytochemistry, and safety. Phytomedicine 58, 152871. doi:10.1016/j.phymed.2019.152871
Jiang, B., Chen, J., Xu, L., Gao, Z., Deng, Y., Wang, Y., et al. (2010). Salvianolic acid B functioned as a competitive inhibitor of matrix metalloproteinase-9 and efficiently prevented cardiac remodeling. BMC Pharmacol. 10, 10. doi:10.1186/1471-2210-10-10
Jiang, L., Li, Y., Yu, J., Wang, J., Ju, J., and Dai, J. (2021). A dry powder inhalable formulation of salvianolic acids for the treatment of pulmonary fibrosis: safety, lung deposition, and pharmacokinetic study. Drug Deliv. Transl. Res. 11, 1958–1968. doi:10.1007/s13346-020-00857-7
Jiang, L., Wang, J., Ju, J., and Dai, J. (2020). Salvianolic acid B and sodium tanshinone II A sulfonate prevent pulmonary fibrosis through anti-inflammatory and anti-fibrotic process. Eur. J. Pharmacol. 883, 173352. doi:10.1016/j.ejphar.2020.173352
Jiang, N., Zhang, J., Ping, J., and Xu, L. (2022). Salvianolic acid B inhibits autophagy and activation of hepatic stellate cells induced by TGF-β1 by downregulating the MAPK pathway. Front. Pharmacol. 13, 938856. doi:10.3389/fphar.2022.938856
Kanagala, P., Cheng, A. S. H., Singh, A., Khan, J. N., Gulsin, G. S., Patel, P., et al. (2019). Relationship between focal and diffuse fibrosis assessed by CMR and clinical outcomes in heart failure with preserved ejection fraction. JACC Cardiovasc Imaging 12, 2291–2301. doi:10.1016/j.jcmg.2018.11.031
Kaneider, N. C., Leger, A. J., Agarwal, A., Nguyen, N., Perides, G., Derian, C., et al. (2007). “Role reversal” for the receptor PAR1 in sepsis-induced vascular damage. Nat. Immunol. 8, 1303–1312. doi:10.1038/ni1525
Karin, M., and Ben-Neriah, Y. (2000). Phosphorylation meets ubiquitination: the control of NF-[kappa]B activity. Annu. Rev. Immunol. 18, 621–663. doi:10.1146/annurev.immunol.18.1.621
Ke, B., Shen, W., Fang, X., and Wu, Q. (2018). The NLPR3 inflammasome and obesity-related kidney disease. J. Cell. Mol. Med. 22, 16–24. doi:10.1111/jcmm.13333
Kim, Y. M., Huh, J. S., Lim, Y., and Cho, M. (2015). Soy isoflavone glycitin (4'-hydroxy-6-methoxyisoflavone-7-D-glucoside) promotes human dermal fibroblast cell proliferation and migration via TGF-β signaling. Phytother. Res. 29, 757–769. doi:10.1002/ptr.5313
King, T. E., Pardo, A., and Selman, M. (2011). Idiopathic pulmonary fibrosis. Lancet 378, 1949–1961. doi:10.1016/S0140-6736(11)60052-4
Kliment, C. R., and Oury, T. D. (2010). Oxidative stress, extracellular matrix targets, and idiopathic pulmonary fibrosis. Free Radic. Biol. Med. 49, 707–717. doi:10.1016/j.freeradbiomed.2010.04.036
Klinkhammer, B. M., Floege, J., and Boor, P. (2018). PDGF in organ fibrosis. Mol. Asp. Med. 62, 44–62. doi:10.1016/j.mam.2017.11.008
Kramann, R., Dirocco, D. P., Maarouf, O. H., and Humphreys, B. D. (2013). Matrix producing cells in chronic kidney disease: origin, regulation, and activation. Curr. Pathobiol. Rep. 1, 301–311. doi:10.1007/s40139-013-0026-7
Kramer, E. L., and Clancy, J. P. (2018). TGFβ as a therapeutic target in cystic fibrosis. Expert Opin. Ther. Targets 22, 177–189. doi:10.1080/14728222.2018.1406922
Lan, A., and Du, J. (2015). Potential role of Akt signaling in chronic kidney disease. Nephrol. Dial. Transpl. 30, 385–394. doi:10.1093/ndt/gfu196
Leask, A. (2015). Getting to the heart of the matter: new insights into cardiac fibrosis. Circ. Res. 116, 1269–1276. doi:10.1161/CIRCRESAHA.116.305381
Lee, Y. A., Wallace, M. C., and Friedman, S. L. (2015). Pathobiology of liver fibrosis: a translational success story. Gut 64, 830–841. doi:10.1136/gutjnl-2014-306842
Li, C., Liu, B., Wang, Z., Xie, F., Qiao, W., Cheng, J., et al. (2020a). Salvianolic acid B improves myocardial function in diabetic cardiomyopathy by suppressing IGFBP3. J. Mol. Cell. Cardiol. 139, 98–112. doi:10.1016/j.yjmcc.2020.01.009
Li, H., Cao, X., Liu, Y., Liu, T., Wang, M., Ren, X., et al. (2019). Establishment of modified biopharmaceutics classification system absorption model for oral Traditional Chinese Medicine (Sanye Tablet). J. Ethnopharmacol. 244, 112148. doi:10.1016/j.jep.2019.112148
Li, J. H., Wang, W., Huang, X. R., Oldfield, M., Schmidt, A. M., Cooper, M. E., et al. (2004). Advanced glycation end products induce tubular epithelial-myofibroblast transition through the RAGE-ERK1/2 MAP kinase signaling pathway. Am. J. Pathol. 164, 1389–1397. doi:10.1016/S0002-9440(10)63225-7
Li, Q., Ming, Y., Jia, H., and Wang, G. (2021a). Poricoic acid A suppresses TGF-β1-induced renal fibrosis and proliferation via the PDGF-C, Smad3 and MAPK pathways. Exp. Ther. Med. 21, 289. doi:10.3892/etm.2021.9720
Li, Y., Feng, L., Li, G., An, J., Zhang, S., Li, J., et al. (2020b). Resveratrol prevents ISO-induced myocardial remodeling associated with regulating polarization of macrophages through VEGF-B/AMPK/NF-kB pathway. Int. Immunopharmacol. 84, 106508. doi:10.1016/j.intimp.2020.106508
Li, Z., Chiang, Y. P., He, M., Worgall, T. S., Zhou, H., and Jiang, X. C. (2021b). Liver sphingomyelin synthase 1 deficiency causes steatosis, steatohepatitis, fibrosis, and tumorigenesis: an effect of glucosylceramide accumulation. iScience 24, 103449. doi:10.1016/j.isci.2021.103449
Li, Z., Jiang, L., Ni, J., Xu, Y., Liu, F., Liu, W., et al. (2023). Salvianolic acid B suppresses hepatic fibrosis by inhibiting ceramide glucosyltransferase in hepatic stellate cells. Acta Pharmacol. Sin. 44, 1191–1205. doi:10.1038/s41401-022-01044-9
Lin, P., Qiu, F., Wu, M., Xu, L., Huang, D., Wang, C., et al. (2023). Salvianolic acid B attenuates tubulointerstitial fibrosis by inhibiting EZH2 to regulate the PTEN/Akt pathway. Pharm. Biol. 61, 23–29. doi:10.1080/13880209.2022.2148169
Little, K., Ma, J. H., Yang, N., Chen, M., and Xu, H. (2018). Myofibroblasts in macular fibrosis secondary to neovascular age-related macular degeneration - the potential sources and molecular cues for their recruitment and activation. EBioMedicine 38, 283–291. doi:10.1016/j.ebiom.2018.11.029
Liu, B. C., Tang, T. T., Lv, L. L., and Lan, H. Y. (2018a). Renal tubule injury: a driving force toward chronic kidney disease. Kidney Int. 93, 568–579. doi:10.1016/j.kint.2017.09.033
Liu, F., Li, S., Chen, P., Gu, Y., Wang, S., Wang, L., et al. (2023a). Salvianolic acid B inhibits hepatic stellate cell activation and liver fibrosis by targeting PDGFRβ. Int. Immunopharmacol. 122, 110550. doi:10.1016/j.intimp.2023.110550
Liu, F., Li, S., Chen, P., Gu, Y., Wang, S., Wang, L., et al. (2023b). Salvianolic acid B inhibits hepatic stellate cell activation and liver fibrosis by targeting PDGFRβ. Int. Immunopharmacol. 122, 110550. doi:10.1016/j.intimp.2023.110550
Liu, F., Yao, Y., Guo, C., Dai, P., Huang, J., Peng, P., et al. (2024). Trichodelphinine A alleviates pulmonary fibrosis by inhibiting collagen synthesis via NOX4-ARG1/TGF-β signaling pathway. Phytomedicine 132, 155755. doi:10.1016/j.phymed.2024.155755
Liu, J., Sun, Q., Sun, X., Wang, Q., Zou, G., Wang, D., et al. (2023c). Therapeutic effects of salvianolic acid B on angiotensin II–induced atrial fibrosis by regulating atrium metabolism via targeting AMPK/FoxO1/miR-148a-3p Axis. J. Cardiovasc. Trans. Res. 16, 341–357. doi:10.1007/s12265-022-10303-3
Liu, M., Li, Y. G., Zhang, F., Yang, L., Chou, G. X., Wang, Z. T., et al. (2007). Chromatographic fingerprinting analysis of Danshen root (Salvia miltiorrhiza Radix et Rhizoma) and its preparations using high performance liquid chromatography with diode array detection and electrospray mass spectrometry (HPLC-DAD-ESI/MS). J. Sep. Sci. 30, 2256–2267. doi:10.1002/jssc.200700149
Liu, M., Xu, H., Zhang, L., Zhang, C., Yang, L., Ma, E., et al. (2018b). Salvianolic acid B inhibits myofibroblast transdifferentiation in experimental pulmonary fibrosis via the up-regulation of Nrf2. Biochem. Biophysical Res. Commun. 495, 325–331. doi:10.1016/j.bbrc.2017.11.014
Liu, P., Hu, Y. Y., Liu, C., Zhu, D. Y., Xue, H. M., Xu, Z. Q., et al. (2002). Clinical observation of salvianolic acid B in treatment of liver fibrosis in chronic hepatitis B. World J. Gastroenterol. 8, 679–685. doi:10.3748/wjg.v8.i4.679
Liu, Q., Chu, H., Ma, Y., Wu, T., Qian, F., Ren, X., et al. (2016a). Salvianolic acid B attenuates experimental pulmonary fibrosis through inhibition of the TGF-β signaling pathway. Sci. Rep. 6, 27610. doi:10.1038/srep27610
Liu, Q., Lu, J., Lin, J., Tang, Y., Pu, W., Shi, X., et al. (2019). Salvianolic acid B attenuates experimental skin fibrosis of systemic sclerosis. Biomed. Pharmacother. 110, 546–553. doi:10.1016/j.biopha.2018.12.016
Liu, Q., Shi, X., Tang, L., Xu, W., Jiang, S., Ding, W., et al. (2018c). Salvianolic acid B attenuates experimental pulmonary inflammation by protecting endothelial cells against oxidative stress injury. Eur. J. Pharmacol. 840, 9–19. doi:10.1016/j.ejphar.2018.09.030
Liu, X., Xavier, C., Jann, J., and Wu, H. (2016b). Salvianolic acid B (sal B) protects retinal pigment epithelial cells from oxidative stress-induced cell death by activating glutaredoxin 1 (Grx1). Int. J. Mol. Sci. 17, 1835. doi:10.3390/ijms17111835
Liu, Y. (2010). New insights into epithelial-mesenchymal transition in kidney fibrosis. J. Am. Soc. Nephrol. 21, 212–222. doi:10.1681/ASN.2008121226
López-Hernández, F. J., and López-Novoa, J. M. (2012). Role of TGF-β in chronic kidney disease: an integration of tubular, glomerular and vascular effects. Cell. Tissue Res. 347, 141–154. doi:10.1007/s00441-011-1275-6
Lu, B., Li, J., Gui, M., Yao, L., Fan, M., Zhou, X., et al. (2022a). Salvianolic acid B inhibits myocardial I/R-induced ROS generation and cell apoptosis by regulating the TRIM8/GPX1 pathway. Pharm. Biol. 60, 1458–1468. doi:10.1080/13880209.2022.2096644
Lu, P., Li, J., Liu, C., Yang, J., Peng, H., Xue, Z., et al. (2022b). Salvianolic acid B dry powder inhaler for the treatment of idiopathic pulmonary fibrosis. Asian J. Pharm. Sci. 17, 447–461. doi:10.1016/j.ajps.2022.04.004
Lu, X. L., Dong, X. Y., Fu, Y. B., Cai, J. T., Du, Q., Si, J. M., et al. (2009). Protective effect of salvianolic acid B on chronic pancreatitis induced by trinitrobenzene sulfonic acid solution in rats. Pancreas 38, 71–77. doi:10.1097/MPA.0b013e3181855d0d
Luedde, T., and Schwabe, R. F. (2011). NF-κB in the liver--linking injury, fibrosis and hepatocellular carcinoma. Nat. Rev. Gastroenterol. Hepatol. 8, 108–118. doi:10.1038/nrgastro.2010.213
Ma, G., Zhang, Y., Chen, W., Tang, Z., Xin, X., Yang, P., et al. (2017). Inhibition of human ugt1a1-mediated bilirubin glucuronidation by polyphenolic acids impact safety of popular salvianolic acid A/B-containing drugs and herbal products. Mol. Pharm. 14, 2952–2966. doi:10.1021/acs.molpharmaceut.7b00365
Mai, X., Yin, X., Chen, P., and Zhang, M. (2020). Salvianolic acid B protects against fatty acid-induced renal tubular injury via inhibition of endoplasmic reticulum stress. Front. Pharmacol. 11, 574229. doi:10.3389/fphar.2020.574229
Masola, V., Gambaro, G., Tibaldi, E., Brunati, A. M., Gastaldello, A., D’Angelo, A., et al. (2012). Heparanase and syndecan-1 interplay orchestrates fibroblast growth factor-2-induced epithelial-mesenchymal transition in renal tubular cells. J. Biol. Chem. 287, 1478–1488. doi:10.1074/jbc.M111.279836
Masola, V., Zaza, G., Onisto, M., Lupo, A., and Gambaro, G. (2015). Impact of heparanase on renal fibrosis. J. Transl. Med. 13, 181. doi:10.1186/s12967-015-0538-5
Masola, V., Zaza, G., Secchi, M. F., Gambaro, G., Lupo, A., and Onisto, M. (2014). Heparanase is a key player in renal fibrosis by regulating TGF-β expression and activity. Biochim. Biophys. Acta 1843, 2122–2128. doi:10.1016/j.bbamcr.2014.06.005
Mateljak, I., Tron, T., and Alcalde, M. (2017). Evolved α-factor prepro-leaders for directed laccase evolution in Saccharomyces cerevisiae. Microb. Biotechnol. 10, 1830–1836. doi:10.1111/1751-7915.12838
Meng, X. M., Tang, P. M. K., Li, J., and Lan, H. Y. (2015). TGF-β/Smad signaling in renal fibrosis. Front. Physiol. 6, 82. doi:10.3389/fphys.2015.00082
Miao, N. J., Xie, H. Y., Xu, D., Yin, J. Y., Wang, Y. Z., Wang, B., et al. (2019). Caspase-11 promotes renal fibrosis by stimulating IL-1β maturation via activating caspase-1. Acta Pharmacol. Sin. 40, 790–800. doi:10.1038/s41401-018-0177-5
Mulay, S. R. (2019). Multifactorial functions of the inflammasome component NLRP3 in pathogenesis of chronic kidney diseases. Kidney Int. 96, 58–66. doi:10.1016/j.kint.2019.01.014
Nandi, S. S., Katsurada, K., Sharma, N. M., Anderson, D. R., Mahata, S. K., and Patel, K. P. (2020). MMP9 inhibition increases autophagic flux in chronic heart failure. Am. J. Physiol. Heart Circ. Physiol. 319, H1414-H1437–H1437. doi:10.1152/ajpheart.00032.2020
Newman, S. P. (2017). Drug delivery to the lungs: challenges and opportunities. Ther. Deliv. 8, 647–661. doi:10.4155/tde-2017-0037
Oatmen, K. E., Cull, E., and Spinale, F. G. (2020). Heart failure as interstitial cancer: emergence of a malignant fibroblast phenotype. Nat. Rev. Cardiol. 17, 523–531. doi:10.1038/s41569-019-0286-y
Obayashi, Y., Fujita, J., Nishiyama, T., Yoshinouchi, T., Kamei, T., Yamadori, I., et al. (2000). Role of carbohydrate antigens sialyl Lewis (a) (CA19-9) in bronchoalveolar lavage in patients with pulmonary fibrosis. Respiration 67, 146–152. doi:10.1159/000029502
Ojiaku, C. A., Cao, G., Zhu, W., Yoo, E. J., Shumyatcher, M., Himes, B. E., et al. (2018). TGF-β1 evokes human airway smooth muscle cell shortening and hyperresponsiveness via Smad3. Am. J. Respir. Cell. Mol. Biol. 58, 575–584. doi:10.1165/rcmb.2017-0247OC
Ouyang, Y., Miyata, M., Hatsushika, K., Ohnuma, Y., Katoh, R., Ogawa, H., et al. (2010). TGF-beta signaling may play a role in the development of goblet cell hyperplasia in a mouse model of allergic rhinitis. Allergol. Int. 59, 313–319. doi:10.2332/allergolint.10-SC-0172
Pan, R. H., Xie, F. yan, Chen, H. M., Xu, L. Z., Wu, X. C., Xu, L. L., et al. (2011). Salvianolic acid B reverses the epithelial-to-mesenchymal transition of HK-2 cells that is induced by transforming growth factor-β. Archives pharmacal Res. 34, 477–483. doi:10.1007/s12272-011-0317-7
Pang, Y., Zhang, P. C., Lu, R. R., Li, H. L., Li, J. C., Fu, H. X., et al. (2020). Andrade-oliveira salvianolic acid B modulates caspase-1-mediated pyroptosis in renal ischemia-reperfusion injury via Nrf2 pathway. Front. Pharmacol. 11, 541426. doi:10.3389/fphar.2020.541426
Paola, R. D., Talero, E., Galuppo, M., Mazzon, E., Bramanti, P., Motilva, V., et al. (2011). Adrenomedullin in inflammatory process associated with experimental pulmonary fibrosis. Respir. Res. 12, 41. doi:10.1186/1465-9921-12-41
Pei, M. X., Dong, S. J., Gao, X. Y., Luo, T., Fan, D., Jin, J. F., et al. (2022). Salvianolic acid B attenuates iopromide-induced renal tubular epithelial cell injury by inhibiting the TLR4/NF-κB/NLRP3 signaling pathway. Evidence-Based Complementary Altern. Med. 2022, 8400496. doi:10.1155/2022/8400496
Piersma, B., Bank, R. A., and Boersema, M. (2015). Signaling in fibrosis: TGF-β, WNT, and YAP/TAZ converge. Front. Med. (Lausanne) 2, 59. doi:10.3389/fmed.2015.00059
Qi, R., and Yang, C. (2018). Renal tubular epithelial cells: the neglected mediator of tubulointerstitial fibrosis after injury. Cell. Death Dis. 9, 1126. doi:10.1038/s41419-018-1157-x
Qin, Y., Ye, P., He, J., Sheng, L., Wang, L., and Du, J. (2010). Simvastatin inhibited cardiac hypertrophy and fibrosis in apolipoprotein E-deficient mice fed a “Western-style diet” by increasing PPAR α and γ expression and reducing TC, MMP-9, and Cat S levels. Acta Pharmacol. Sin. 31, 1350–1358. doi:10.1038/aps.2010.109
Rabelink, T. J., Berg, B. M. van den, Garsen, M., Wang, G., Elkin, M., and Vlag, J. van der (2017). Heparanase: roles in cell survival, extracellular matrix remodelling and the development of kidney disease. Nat. Rev. Nephrol. 13, 201–212. doi:10.1038/nrneph.2017.6
Radosinska, J., Barancik, M., and Vrbjar, N. (2017). Heart failure and role of circulating MMP-2 and MMP-9. Panminerva Med. 59, 241–253. doi:10.23736/S0031-0808.17.03321-3
Ricard-Blum, S., and Miele, A. E. (2020). Omic approaches to decipher the molecular mechanisms of fibrosis, and design new anti-fibrotic strategies. Semin. Cell. Dev. Biol. 101, 161–169. doi:10.1016/j.semcdb.2019.12.009
Roehlen, N., Crouchet, E., and Baumert, T. F. (2020). Liver fibrosis: mechanistic concepts and therapeutic perspectives. Cells 9, 875. doi:10.3390/cells9040875
Rosenbloom, J., Macarak, E., Piera-Velazquez, S., and Jimenez, S. A. (2017). Human fibrotic diseases: current challenges in fibrosis research. Methods Mol. Biol. 1627, 1–23. doi:10.1007/978-1-4939-7113-8_1
Ross, J. S., Robertson, J. T., Frederickson, R. C., Petrie, J. L., Obuchowski, N., Modic, M. T., et al. (1996). Association between peridural scar and recurrent radicular pain after lumbar discectomy: magnetic resonance evaluation. Neurosurgery 38, 855–863. ; discussion 861-863. doi:10.1227/00006123-199604000-00053
Samanta, J., Mondal, A., Saha, S., Chakraborty, S., and Sengupta, A. (2020). Oleic acid protects from arsenic-induced cardiac hypertrophy via AMPK/FoxO/NFATc3 pathway. Cardiovasc Toxicol. 20, 261–280. doi:10.1007/s12012-019-09550-9
Singh, V. K., Yadav, D., and Garg, P. K. (2019). Diagnosis and management of chronic pancreatitis: a review. JAMA 322, 2422–2434. doi:10.1001/jama.2019.19411
Song, L., Chen, T. Y., Zhao, X. J., Xu, Q., Jiao, R. Q., Li, J. M., et al. (2019). Pterostilbene prevents hepatocyte epithelial-mesenchymal transition in fructose-induced liver fibrosis through suppressing miR-34a/Sirt1/p53 and TGF-β1/Smads signalling. Br. J. Pharmacol. 176, 1619–1634. doi:10.1111/bph.14573
Strieter, R. M., and Mehrad, B. (2009). New mechanisms of pulmonary fibrosis. Chest 136, 1364–1370. doi:10.1378/chest.09-0510
Su, H., Ma, Z., Guo, A., Wu, H., and Yang, X. (2020). Salvianolic acid B protects against sepsis-induced liver injury via activation of SIRT1/PGC-1α signaling. Exp. Ther. Med. 20, 2675–2683. doi:10.3892/etm.2020.9020
Tacke, F., and Trautwein, C. (2015). Mechanisms of liver fibrosis resolution. J. hepatology 63, 1038–1039. doi:10.1016/j.jhep.2015.03.039
Taflinski, L., Demir, E., Kauczok, J., Fuchs, P. C., Born, M., Suschek, C. V., et al. (2014). Blue light inhibits transforming growth factor-β1-induced myofibroblast differentiation of human dermal fibroblasts. Exp. Dermatol. 23, 240–246. doi:10.1111/exd.12353
Tang, Q., Zhong, H., Xie, F., Xie, J., Chen, H., and Yao, G. (2014). Expression of miR-106b-25 induced by salvianolic acid B inhibits epithelial-to-mesenchymal transition in HK-2 cells. Eur. J. Pharmacol. 741, 97–103. doi:10.1016/j.ejphar.2014.07.051
Tenbrock, L., Wolf, J., Boneva, S., Schlecht, A., Agostini, H., Wieghofer, P., et al. (2022). Subretinal fibrosis in neovascular age-related macular degeneration: current concepts, therapeutic avenues, and future perspectives. Cell. Tissue Res. 387, 361–375. doi:10.1007/s00441-021-03514-8
Teteris, S. A., Menahem, S. A., Perry, G., Maguire, J. A., Dowling, J. P., Langham, R. G., et al. (2007). Dysregulated growth factor gene expression is associated with tubulointerstitial apoptosis and renal dysfunction. Kidney Int. 71, 1044–1053. doi:10.1038/sj.ki.5002176
Tian, L. L., Wang, X. J., Sun, Y. N., Li, C. R., Xing, Y. L., Zhao, H. B., et al. (2008). Salvianolic acid B, an antioxidant from Salvia miltiorrhiza, prevents 6-hydroxydopamine induced apoptosis in SH-SY5Y cells. Int. J. Biochem. Cell. Biol. 40, 409–422. doi:10.1016/j.biocel.2007.08.005
Tian, S., Chen, M., Wang, B., Han, Y., Shang, H., and Chen, J. (2021). Salvianolic acid B blocks hepatic stellate cell activation via FGF19/FGFR4 signaling. Ann. Hepatol. 20, 100259. doi:10.1016/j.aohep.2020.07.013
Trautwein, C., Friedman, S. L., Schuppan, D., and Pinzani, M. (2015). Hepatic fibrosis: concept to treatment. J. hepatology 62, S15–S24. doi:10.1016/j.jhep.2015.02.039
Tressel, S. L., Kaneider, N. C., Kasuda, S., Foley, C., Koukos, G., Austin, K., et al. (2011). A matrix metalloprotease-PAR1 system regulates vascular integrity, systemic inflammation and death in sepsis. EMBO Mol. Med. 3, 370–384. doi:10.1002/emmm.201100145
Tsuchida, T., and Friedman, S. L. (2017). Mechanisms of hepatic stellate cell activation. Nat. Rev. Gastroenterol. Hepatol. 14, 397–411. doi:10.1038/nrgastro.2017.38
Uemura, A., Kusuhara, S., Wiegand, S. J., Yu, R. T., and Nishikawa, S. (2006). Tlx acts as a proangiogenic switch by regulating extracellular assembly of fibronectin matrices in retinal astrocytes. J. Clin. Investig. 116, 369–377. doi:10.1172/JCI25964
Utsunomiya, H., Tilakaratne, W. M., Oshiro, K., Maruyama, S., Suzuki, M., Ida-Yonemochi, H., et al. (2005). Extracellular matrix remodeling in oral submucous fibrosis: its stage-specific modes revealed by immunohistochemistry and in situ hybridization. J. Oral Pathol. Med. 34, 498–507. doi:10.1111/j.1600-0714.2005.00339.x
Walraven, M., and Hinz, B. (2018). Therapeutic approaches to control tissue repair and fibrosis: extracellular matrix as a game changer. Matrix Biol. 71–72, 205–224. doi:10.1016/j.matbio.2018.02.020
Wang, C., Luo, H., Xu, Y., Tao, L., Chang, C., and Shen, X. (2018a). Salvianolic acid B-alleviated angiotensin II induces cardiac fibrosis by suppressing NF-κB pathway in vitro. Med. Sci. Monit. 24, 7654–7664. doi:10.12659/MSM.908936
Wang, F., Chen, L., Kong, D., Zhang, X., Xia, S., Liang, B., et al. (2024a). Canonical Wnt signaling promotes HSC glycolysis and liver fibrosis through an LDH-A/HIF-1α transcriptional complex. Hepatology 79, 606–623. doi:10.1097/HEP.0000000000000569
Wang, J., Zhang, Y., Guo, L., Wu, G., and Liu, R. (2011). Salvianolic acid B inhibits the TLR4-NFκB-TNFα pathway and attenuates neonatal rat cardiomyocyte injury induced by lipopolysaccharide. Chin. J. Integr. Med. 17, 775–779. doi:10.1007/s11655-011-0877-x
Wang, J. N., Li, L., Li, L. Y., Yan, Q., Li, J., and Xu, T. (2018b). Emerging role and therapeutic implication of Wnt signaling pathways in liver fibrosis. Gene 674, 57–69. doi:10.1016/j.gene.2018.06.053
Wang, M. D., Zhang, S., Liu, X. Y., Wang, P. P., Zhu, Y. F., Zhu, J. R., et al. (2023). Salvianolic acid B ameliorates retinal deficits in an early-stage Alzheimer’s disease mouse model through downregulating BACE1 and Aβ generation. Acta Pharmacol. Sin. 44, 2151–2168. doi:10.1038/s41401-023-01125-3
Wang, Q. L., Tao, Y. Y., Yuan, J. L., Shen, L., and Liu, C. H. (2010). Salvianolic acid B prevents epithelial-to-mesenchymal transition through the TGF-β1 signal transduction pathway in vivo and in vitro.
Wang, R., Li, S., Chen, P., Yue, X., Wang, S., Gu, Y., et al. (2022). Salvianolic acid B suppresses hepatic stellate cell activation and liver fibrosis by inhibiting the NF-κB signaling pathway via miR-6499-3p/LncRNA-ROR. Phytomedicine 107, 154435. doi:10.1016/j.phymed.2022.154435
Wang, R., Yu, X. Y., Guo, Z. Y., Wang, Y. J., Wu, Y., and Yuan, Y. F. (2012). Inhibitory effects of salvianolic acid B on CCl4-induced hepatic fibrosis through regulating NF-κB/IκBα signaling. J. Ethnopharmacol. 144, 592–598. doi:10.1016/j.jep.2012.09.048
Wang, X., Ma, J., Zhang, S., Li, Z., Hong, Z., Jiang, L., et al. (2021). G protein-coupled estrogen receptor 30 reduces transverse aortic constriction-induced myocardial fibrosis in aged female mice by inhibiting the ERK1/2 -MMP-9 signaling pathway. Front. Pharmacol. 12, 731609. doi:10.3389/fphar.2021.731609
Wang, Y., Han, Y., Shang, S., Xiao, J., Tao, L., Peng, Z., et al. (2024b). Kokusaginine attenuates renal fibrosis by inhibiting the PI3K/AKT signaling pathway. Biomed. Pharmacother. = Biomedecine Pharmacother. 175, 116695. doi:10.1016/j.biopha.2024.116695
Wei, C., Kim, I. K., Kumar, S., Jayasinghe, S., Hong, N., Castoldi, G., et al. (2013). NF-κB mediated miR-26a regulation in cardiac fibrosis. J. Cell. Physiology 228, 1433–1442. doi:10.1002/jcp.24296
Weiskirchen, R., and Tacke, F. (2019). Relevance of autophagy in parenchymal and non-parenchymal liver cells for health and disease. Cells 8, 16. doi:10.3390/cells8010016
Weiskirchen, R., Weiskirchen, S., and Tacke, F. (2019). Organ and tissue fibrosis: molecular signals, cellular mechanisms and translational implications. Mol. Asp. Med. 65, 2–15. doi:10.1016/j.mam.2018.06.003
Wu, C., Chen, W., Ding, H., Li, D., Wen, G., Zhang, C., et al. (2019). Salvianolic acid B exerts anti-liver fibrosis effects via inhibition of MAPK-mediated phospho-Smad2/3 at linker regions in vivo and in vitro. Life Sci. 239, 116881. doi:10.1016/j.lfs.2019.116881
Wu, H., Yu, Y., Huang, H., Hu, Y., Fu, S., Wang, Z., et al. (2021). Progressive pulmonary fibrosis is caused by elevated mechanical tension on alveolar stem cells. Cell. 184, 845–846. doi:10.1016/j.cell.2021.01.020
Wu, M., Han, W., Song, S., Du, Y., Liu, C., Chen, N., et al. (2018). NLRP3 deficiency ameliorates renal inflammation and fibrosis in diabetic mice. Mol. Cell. Endocrinol. 478, 115–125. doi:10.1016/j.mce.2018.08.002
Wu, Y. T., Chen, Y. F., Hsieh, Y. J., Jaw, I., Shiao, M. S., and Tsai, T. H. (2006). Bioavailability of salvianolic acid B in conscious and freely moving rats. Int. J. Pharm. 326, 25–31. doi:10.1016/j.ijpharm.2006.07.003
Wynn, T. A., and Ramalingam, T. R. (2012). Mechanisms of fibrosis: therapeutic translation for fibrotic disease. Nat. Med. 18, 1028–1040. doi:10.1038/nm.2807
Xing, Z., Yang, C., Feng, Y., He, J., Peng, C., and Li, D. (2024). Understanding aconite’s anti-fibrotic effects in cardiac fibrosis. Phytomedicine 122, 155112. doi:10.1016/j.phymed.2023.155112
Xu, Y., Mizuno, T., Sridharan, A., Du, Y., Guo, M., Tang, J., et al. (2016). Single-cell RNA sequencing identifies diverse roles of epithelial cells in idiopathic pulmonary fibrosis. JCI Insight 1, e90558. doi:10.1172/jci.insight.90558
Yan, M., Li, Z., Dai, S., Li, S., and Yu, P. (2023a). The potential effect of salvianolic acid B against rat ischemic brain injury in combination with mesenchymal stem cells. J. Chem. Neuroanat. 133, 102338. doi:10.1016/j.jchemneu.2023.102338
Yan, Y., Zhou, M., Meng, K., Zhou, C. H., Jia, X. Y., Li, X. H., et al. (2023b). Salvianolic acid B attenuates inflammation and prevent pathologic fibrosis by inhibiting CD36-mediated activation of the PI3K-Akt signaling pathway in frozen shoulder. Front. Pharmacol. 14, 1230174. doi:10.3389/fphar.2023.1230174
Yang, B., Qiao, Y., Yan, D., and Meng, Q. (2024). Targeting interactions between fibroblasts and macrophages to treat cardiac fibrosis. Cells 13, 764. doi:10.3390/cells13090764
Yao, L., Zhao, R., He, S., Feng, Q., Qiao, Y., Wang, P., et al. (2022). Effects of salvianolic acid A and salvianolic acid B in renal interstitial fibrosis via PDGF-C/PDGFR-α signaling pathway. Phytomedicine 106, 154414. doi:10.1016/j.phymed.2022.154414
Ye, H. L., Zhang, J. W., Chen, X. Z., Wu, P. B., Chen, L., and Zhang, G. (2020). Ursodeoxycholic acid alleviates experimental liver fibrosis involving inhibition of autophagy. Life Sci. 242, 117175. doi:10.1016/j.lfs.2019.117175
Yin, Y., Zhong, J., Li, S. W., Li, J. Z., Zhou, M., Chen, Y., et al. (2016). TRIM11, a direct target of miR-24-3p, promotes cell proliferation and inhibits apoptosis in colon cancer. Oncotarget 7, 86755–86765. doi:10.18632/oncotarget.13550
Yu, F., Guo, Y., Chen, B., Shi, L., Dong, P., Zhou, M., et al. (2017). LincRNA-p21 inhibits the wnt/β-catenin pathway in activated hepatic stellate cells via sponging MicroRNA-17-5p. Cell. Physiol. Biochem. 41, 1970–1980. doi:10.1159/000472410
Yu, F., Lu, Z., Chen, B., Wu, X., Dong, P., and Zheng, J. (2015). Salvianolic acid B-induced microRNA-152 inhibits liver fibrosis by attenuating DNMT1-mediated Patched1 methylation. J. Cell. Mol. Med. 19, 2617–2632. doi:10.1111/jcmm.12655
Yu, F., Lu, Z., Huang, K., Wang, X., Xu, Z., Chen, B., et al. (2016). MicroRNA-17-5p-activated Wnt/β-catenin pathway contributes to the progression of liver fibrosis. Oncotarget 7, 81–93. doi:10.18632/oncotarget.6447
Yun, H., Park, S., Kim, M. J., Yang, W. K., Im, D. U., Yang, K. R., et al. (2014). AMP-activated protein kinase mediates the antioxidant effects of resveratrol through regulation of the transcription factor FoxO1. FEBS J. 281, 4421–4438. doi:10.1111/febs.12949
Zhang, C., Feng, S., Hu, N., Bryukhovetskiy, A. S., and Chekhonin, V. P. (2015). Letter to the editor regarding: “Evaluation of topical application and systemic administration of rosuvastatin in preventing epidural fibrosis in rats” by Bora Gürer et al. Spine J. 15, 1165–1166. doi:10.1016/j.spinee.2015.01.009
Zhang, C., Kong, X., Zhou, H., Liu, C., Zhao, X., Zhou, X., et al. (2013). An experimental novel study: angelica sinensis prevents epidural fibrosis in laminectomy rats via downregulation of hydroxyproline, IL-6, and TGF- β 1. Evid. Based Complement. Altern. Med. 2013, 291814. doi:10.1155/2013/291814
Zhang, C. Y., Yuan, W. G., He, P., Lei, J. H., and Wang, C. X. (2016a). Liver fibrosis and hepatic stellate cells: etiology, pathological hallmarks and therapeutic targets. World J. Gastroenterol. 22, 10512–10522. doi:10.3748/wjg.v22.i48.10512
Zhang, F. X., Cui, S. S., Yuan, Y. L. L., Li, C., and Li, R. M. (2022a). Dissection of the potential anti-diabetes mechanism of salvianolic acid B by metabolite profiling and network pharmacology. Rapid Commun. Mass Spectrom. 36, e9205. doi:10.1002/rcm.9205
Zhang, H., and Wang, Z. (2019). Effect and regulation of the NLRP3 inflammasome during renal fibrosis. Front. Cell. Dev. Biol. 7, 379. doi:10.3389/fcell.2019.00379
Zhang, M., Cao, S. R., Zhang, R., Jin, J. L., and Zhu, Y. F. (2014). The inhibitory effect of salvianolic acid B on TGF-β1-induced proliferation and differentiation in lung fibroblasts. Exp. Lung Res. 40, 172–185. doi:10.3109/01902148.2014.895070
Zhang, M., and Zhang, S. (2020). T cells in fibrosis and fibrotic diseases. Front. Immunol. 11, 1142. doi:10.3389/fimmu.2020.01142
Zhang, T., Liu, M., Gao, Y., Li, H., Song, L., Hou, H., et al. (2021). Salvianolic acid B inhalation solution enhances antifibrotic and anticoagulant effects in a rat model of pulmonary fibrosis. Biomed. Pharmacother. 138, 111475. doi:10.1016/j.biopha.2021.111475
Zhang, W., Ping, J., Zhou, Y., Chen, G., and Xu, L. (2019). Salvianolic acid B inhibits activation of human primary hepatic stellate cells through downregulation of the myocyte enhancer factor 2 signaling pathway. Front. Pharmacol. 10, 322. doi:10.3389/fphar.2019.00322
Zhang, X. L., Zhang, X. Y., Ge, X. Q., and Liu, M. X. (2022b). Mangiferin prevents hepatocyte epithelial-mesenchymal transition in liver fibrosis via targeting HSP27-mediated JAK2/STAT3 and TGF-β1/Smad pathway. Phytother. Res. 36, 4167–4182. doi:10.1002/ptr.7549
Zhang, Y., Akao, T., Nakamura, N., Duan, C. L., Hattori, M., Yang, X. W., et al. (2004a). Extremely low bioavailability of magnesium lithospermate B, an active component from Salvia miltiorrhiza, in rat. Planta Med. 70, 138–142. doi:10.1055/s-2004-815490
Zhang, Y., Akao, T., Nakamura, N., Hattori, M., Yang, X. W., Duan, C. L., et al. (2004b). Magnesium lithospermate B is excreted rapidly into rat bile mostly as methylated metabolites, which are potent antioxidants. Drug Metab. Dispos. 32, 752–757. doi:10.1124/dmd.32.7.752
Zhang, Y., Zhang, L., Zhang, Y., Xu, J. J., Sun, L. L., and Li, S.-Z. (2016b). The protective role of liquiritin in high fructose-induced myocardial fibrosis via inhibiting NF-κB and MAPK signaling pathway. Biomed. Pharmacother. 84, 1337–1349. doi:10.1016/j.biopha.2016.10.036
Zhao, J., and Lei, H. (2016). Tripartite motif protein 72 regulates the proliferation and migration of rat cardiac fibroblasts via the transforming growth factor-β signaling pathway. Cardiology 134, 340–346. doi:10.1159/000443703
Zhao, Y. L., Zhu, R. T., and Sun, Y. L. (2016). Epithelial-mesenchymal transition in liver fibrosis. Biomed. Rep. 4, 269–274. doi:10.3892/br.2016.578
Zhou, L., Chow, M. S. S., and Zuo, Z. (2009). Effect of sodium caprate on the oral absorptions of danshensu and salvianolic acid B. Int. J. Pharm. 379, 109–118. doi:10.1016/j.ijpharm.2009.06.016
Zhou, M., Learned, R. M., Rossi, S. J., DePaoli, A. M., Tian, H., and Ling, L. (2017). Engineered FGF19 eliminates bile acid toxicity and lipotoxicity leading to resolution of steatohepatitis and fibrosis in mice. Hepatol. Commun. 1, 1024–1042. doi:10.1002/hep4.1108
Zhou, S., Gao, X., Chen, C., Zhang, J., Zhang, Y., Zhang, L., et al. (2023). Porcine cardiac blood - salvia miltiorrhiza root alleviates cerebral ischemia reperfusion injury by inhibiting oxidative stress induced apoptosis through PI3K/AKT/Bcl-2/Bax signaling pathway. J. Ethnopharmacol. 316, 116698. doi:10.1016/j.jep.2023.116698
Keywords: Salvianolic acid B, fibrosis, natural product, pharmacological mechanism, treatment
Citation: Liang Q, Liu X, Peng X, Luo T, Su Y, Xu X, Xie H, Gao H, Chen Z and Xie C (2024) Salvianolic acid B in fibrosis treatment: a comprehensive review. Front. Pharmacol. 15:1442181. doi: 10.3389/fphar.2024.1442181
Received: 01 June 2024; Accepted: 16 July 2024;
Published: 30 July 2024.
Edited by:
Javier Echeverria, University of Santiago, ChileReviewed by:
Feng Zhang, Nanjing University of Chinese Medicine, ChinaDan Li, Chengdu University of Traditional Chinese Medicine, China
Liang Shan, Anhui Medical University, China
Copyright © 2024 Liang, Liu, Peng, Luo, Su, Xu, Xie, Gao, Chen and Xie. This is an open-access article distributed under the terms of the Creative Commons Attribution License (CC BY). The use, distribution or reproduction in other forums is permitted, provided the original author(s) and the copyright owner(s) are credited and that the original publication in this journal is cited, in accordance with accepted academic practice. No use, distribution or reproduction is permitted which does not comply with these terms.
*Correspondence: Zhengtao Chen, NzYwODM5NzcyQHFxLmNvbQ==; Chunguang Xie, eGllY2dAY2R1dGNtLmVkdS5jbg==
†These authors have contributed equally to this work and share first authorship