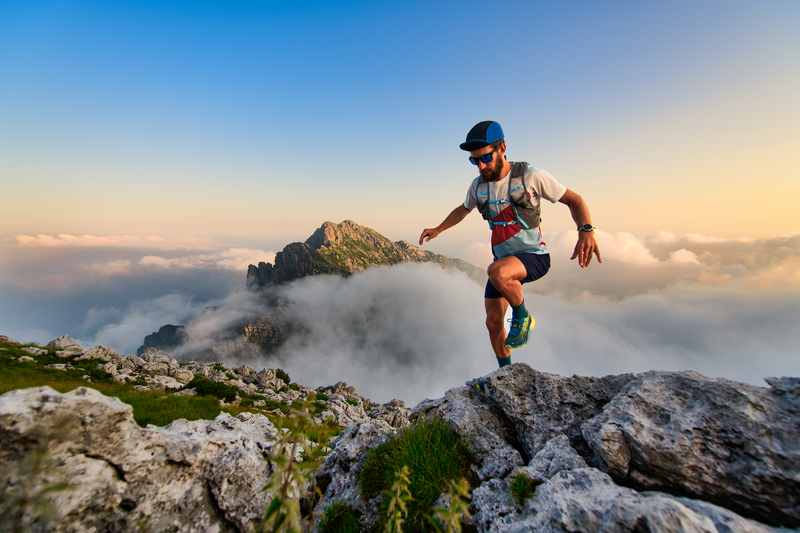
95% of researchers rate our articles as excellent or good
Learn more about the work of our research integrity team to safeguard the quality of each article we publish.
Find out more
ORIGINAL RESEARCH article
Front. Pharmacol. , 07 August 2024
Sec. Neuropharmacology
Volume 15 - 2024 | https://doi.org/10.3389/fphar.2024.1437445
Ischemic stroke poses a significant global health burden, with rapid revascularization treatments being crucial but often insufficient to mitigate ischemia-reperfusion (I/R) injury. Dexmedetomidine (DEX) has shown promise in reducing cerebral I/R injury, but its potential molecular mechanism, particularly its interaction with non-coding RNAs (ncRNAs), remains unclear. This study investigates DEX’s therapeutic effect and potential molecular mechanisms in reducing cerebral I/R injury. A transient middle cerebral artery obstruction (tMACO) model was established to simulate cerebral I/R injury in adult rats. DEX was administered pre-ischemia and post-reperfusion. RNA sequencing and bioinformatic analyses were performed on the ischemic cerebral cortex to identify differentially expressed non-coding RNAs (ncRNAs) and mRNAs. The sequencing results showed 6,494 differentially expressed (DE) mRNA and 2698 DE circRNA between the sham and tMCAO (I/R) groups. Additionally, 1809 DE lncRNA, 763 DE mRNA, and 2795 DE circRNA were identified between the I/R group and tMCAO + DEX (I/R + DEX) groups. Gene ontology (GO) analysis indicated significant enrichment in multicellular biogenesis, plasma membrane components, and protein binding. KEGG analysis further highlighted the potential mechanism of DEX action in reducing cerebral I/R injury, with hub genes involved in inflammatory pathways. This study demonstrates DEX’s efficacy in reducing cerebral I/R injury and offers insights into its brain-protective effects, especially in ischemic stroke. Further research is warranted to fully understand DEX’s neuroprotective mechanisms and its clinical applications.
Stroke remains a significant global health concern, ranking as the second leading cause of death and the third leading cause of long-term disability worldwide, with ischemic stroke constituting the majority of cases (Fan et al., 2023; Zhao Y. et al., 2023). Ischemic stroke, characterized by the obstruction of blood flow to the brain, represents approximately 70% of all stroke cases (Fan et al., 2023; Zhang R. et al., 2023). Currently, the most important treatment for acute ischemic stroke is rapid revascularization, including intravenous thrombolysis with tissue plasminogen activator (tPA) and mechanical thrombectomy, aimed at restoring blood flow to the affected brain tissue (Herpich and Rincon, 2020). Although the use of drugs and the development of thrombolytic technology can reduce nerve damage caused by ischemia, reperfusion following ischemia triggers a cascade of inflammatory reactions and oxidative stress, exacerbating tissue injury and neurological deficits, a phenomenon known as cerebral ischemia-reperfusion injury (IR) (Wu M. Y. et al., 2018). The reperfusion injury can lead to cell necrosis, apoptosis, and secondary neuron damage, further aggravating nerve function deficit (Wu M. Y. et al., 2018). Despite advancements in drug therapies and thrombolytic technologies to mitigate ischemic damage, there remain limited drugs for the effective treatment of cerebral IR injury (Paul and Candelario-Jalil, 2021), so there is still an urgent need to develop novel neuroprotective drugs to alleviate cerebral IR injury.
Dexmedetomidine (DEX), a highly selective α2 adrenergic receptor agonist, has garnered attention for its protective effect against IR injury in various organs, such as the heart, liver, lung, kidney, and brain (Zhao et al., 2020; Huang et al., 2021; Shi J. et al., 2021; Hu et al., 2022; Wang et al., 2022). In recent years, its neuroprotective properties have been increasingly recognized in diverse neurological conditions, including traumatic brain injury (TBI) (Wu J. et al., 2018; Li et al., 2020), subarachnoid hemorrhage (SAH) (Wei et al., 2022), and cerebral ischemia (Fang et al., 2021; Yin et al., 2021). The mechanism underlying the neuroprotective role of DEX involves the inhibition of inflammatory response, reducing apoptosis and autophagy, preservation of the blood-brain barrier integrity, and enhancing stable cell structure (Hu et al., 2022). Notably, DEX has been shown to modulate key signaling pathways implicated in IR, such as HIF-1α mediated autophagy inhibition (Wang et al., 2019) and NF-κB suppression (Wang et al., 2017). Furthermore, recent studies have demonstrated that DEX can reduce cerebral IR injury and play a brain-protective role by inhibiting copper inflow and iron death (Liu et al., 2022; Guo et al., 2023). However, the precise mechanism underlying the DEX neuroprotective effect in cerebral IR injury remains poorly understood and further research is still needed.
Non-coding RNAs (ncRNAs) have emerged as a pivotal regulator of gene expression and transcription regulation (Peschansky and Wahlestedt, 2014; Beermann et al., 2016). In recent years, more and more studies have shown that ncRNAs, particularly miRNAs and lncRNAs play an important role in the pathophysiology of I/R injury (Vasudeva et al., 2021; Yang et al., 2022; Xiaoqing et al., 2023). The brain is rich in ncRNAs, which regulate various central nervous system functions. Injuries such as stroke, traumatic encephalopathy, subarachnoid hemorrhage, and cerebral hemorrhage can lead to significant changes in the ncRNA expression profile. In the context of I/R injury, ncRNAs are involved in neurogenesis, angiogenesis, inflammation, and other functions, making them potential biomarkers for the diagnosis and prognosis of cerebral I/R injury (Bao et al., 2018; Wang et al., 2018; Sun et al., 2022). A variety of ncRNA, especially miRNA (such as miR-7-5p, miR-214-5p, miR-29a-3p, miR-381) and lncRNA (such as HOXA11-AS, SHNG16) have been identified as mediators in the regulation of cerebral I/R injury by DEX. These ncRNAs contribute to reducing cognitive dysfunction and improving neurodegeneration, thereby playing a neuroprotective role (Wang L. et al., 2020; Burlacu et al., 2022; Yan et al., 2023).
In this study, we aimed to investigate the impact of DEX on cerebral I/R injury using a transient middle cerebral artery occlusion (tMCAO) rats’ model. We evaluated its effects on infarct volume and neurological function and conducted whole-transcriptomic sequencing to elucidate the expression profiles of ncRNAs and mRNAs in the ischemic cortex. Bioinformatics analysis was employed to unravel the potential biological functions of differentially expressed genes and ncRNAs associated with stroke. This study provides novel insights into the pathogenesis of cerebral I/R injury and identifies potential therapeutic targets for the intervention.
Fifty-four male Sprague-Dawley rats, aged 7–8 weeks and weighing 180–200 g, were obtained from Henan Skobes Biotechnology Co., LTD. (China). The rats were housed in standard cages with controlled environmental conditions, including a temperature of 23°C ± 1°C, humidity maintained at 60% ± 5%, and a 12-h light/dark cycle. They had ad libitum access to food and water and underwent a 7-day acclimatization period before experimentation. All procedures were conducted in compliance with the National Institutes of Health Guidelines for the Care and Use of Laboratory Animals and were approved by the Zhengzhou University Animal Care and Use Committee.
tMCAO was used to induce focal cerebral IR injury following the previously described procedure (Yuan et al., 2017a). In brief, the rats fasted for 12 h before surgery and were then anesthetized with isoflurane (4% induction, 2.5% maintenance). First, the rats were fixed to cardboard in a supine position and the neck hair was removed using a hair removal cream. Disinfect the neck with a cotton ball containing 75% ethanol. Then, a small opening of about 2 cm is made in the midline of the neck with surgical scissors, and the right common carotid artery (CCA), external carotid artery (ECA), and internal carotid artery (ICA) are separated with ophthalmic bending forceps. After proximal ligation of the CCA and ECA, the surgical wire (Beijing Cinontect Co., Ltd., Beijing, China) was inserted into the ICA until the marked black spot reached the carotid artery bifurcation at a depth of approximately 18–20 mm, indicating occlusion of the middle cerebral artery (MCA), resulting in a brief cessation of blood flow, followed by cerebral infarction in the area provided by the MCA. The skin is sutured with 5–0 silk, then after 2 h of ischemia, the filaments are extracted to initiate the reperfusion process, and the neck skin is sutured. During the whole process, the rats’ body temperature was maintained at 37°C ± 0.5°C. In the sham operation group, only the skin was cut to separate blood vessels without inserting surgical wires.
The rats were randomly assigned to three groups: Sham group (Sham), tMCAO group (I/R), and tMCAO group treated with dexmedetomidine (50 μg/kg, i. p) (I/R + DEX). DEX was dissolved in saline at the concentration of 50 μg/kg and injected intraperitoneally 30 min before MCAO. The mice in sham and tMCAO groups without drug treatment were injected with the same volume of saline. Mice were re-anesthetized and sacrificed 24 h after tMCAO. The concentration of DEX used in the experiment was based on the concentration reported in previous studies (Yuan et al., 2017b; Zhao et al., 2022; Li et al., 2023a). Each group comprised three rats for sequencing, three rats for HE staining, six rats for 2,3,5-triphenyl tetrazolium chloride (TTC) staining, and six rats for reverse transcription polymerase chain reaction (RT-PCR).
Neurological tests were performed 24 h post-reperfusion using a modified scoring system developed by Longa et al. (1989). Neurological function was graded on a scale of 0–5 as follows: 0 = no deficits; 1 = failure to extend left forepaw fully; 2 = circling to the left; 3 = falling to the left; 4 = no spontaneous walking with a depressed level of consciousness; and 5 = dead. The higher the score, the more severe the injury. The mice with a neurological function score of longa was 0, meaning no nerve defect, death occurred within 24 h, and subarachnoid hemorrhage found after dissection were excluded from the study.
The whole brain of the rat was removed immediately after anesthesia, and the brain was placed in the refrigerator at −20°C for 15 min. Then the brain was placed in the brain tank, and the brain was cut into six consecutive 2 mm coronal slices. The slices were placed in 2% TTC (2,3, 5-triphenyl tetrazolium chloride) dye solution at 37°C and incubated for 15 min, during which the slices could be turned over to make them evenly stained. After staining was completed, the sections were placed in 4% paraformaldehyde-fixed overnight and photographed. ImageJ was used to calculate the infarct area, in which the non-infarct site was dyed red, while the infarct site was not stained and turned pale by researcher who were blinded to the study group. To exclude the effect of cerebral edema, the following calculation formula was used: (contralateral hemisphere area–ipsilateral non-ischemic hemisphere area)/contralateral hemisphere area × 100% (Lu et al., 2021).
The sections were deparaffinized first, followed by washes with various concentrations of ethanol and distilled water. Following the process of xylene translucency, the segments were sealed with neutral resin and stained with H&E. Lastly, a microscope was used to observe the alterations in the brain tissue’s pathological condition. Data was gathered and the necessary sections of the sample were examined.
Total RNA was extracted from cortical cerebral samples at the infarct site using an RNA mini kit (Qiagen, Germany) following the manufacturer’s instructions and RNA quality was examined by gel electrophoresis and with Qubit (Thermo, Waltham, MA, United States). Subsequently, sequencing libraries were generated using VAHTSTM Total RNA-seq (H/M/R) Library Prep Kit for Illumina Novaseq6000. The differential gene expression analysis included transcripts and ncRNAs. Raw sequences were quality-checked with trimming (Trimmomatic 0.32) before being mapped to the Musculus genome sequence (version GRCm38.72). Gene hit counts and RPKM were calculated using CLCbio software (CLC Genomics Workbench 7.0.2, CLC Genomics Server). Using bigwig files derived from bam files, the mapped reads were visualized in the UCSC browser.
Significant Differentially expressed (DE) mRNAs and ncRNAs were defined by utilizing a cutoff of P < 0.05 and fold change < 1.74 [log2 (±0.8)] for subsequent analyses. DE genes (DEGs) were further processed for GO annotations and KEGG pathways significant enrichment analysis. The functional connection between the DEGs from the two regions was analyzed and elucidated using the STRING (Search Tool for the Retrieval of Interacting Genes) version: 11.0 database. The STRING database version 12 (https://string-db.org/) was utilized for the protein-protein interaction (PPI) network and hub genes were screened according to the number of neighbors. Additionally, miRNA–lncRNA–mRNA networks and miRNA–circRNA–mRNA networks were established to elucidate the intricate regulatory interactions underlying cerebral IR injury.
The RNA sequence analysis results were verified through q-PCR. SteadyPure Quick RNA Extraction Kit (Accurate Biotechnology, Hunan, China) was used to extract total RNA, and then Evo M-MLV RT Mix Kit with gDNA Clean for qPCR (also from Accurate Biotechnology) was used to reverse-transcribe the RNA into cDNA for quantitative polymerase chain reaction. A 2 µL template was then amplified by PCR (Servicebio, Wuhan, China) using primers (Supplementary Table S1) in a reaction volume of 20 μL, including 250 nM of each primer (forward and reverse), 10 µL of SYBR Green Premix Pro Taq HS qPCR Kit (Rox Plus) (Accurate Biotechnology, Hunan, China), and 20 ng of cDNA. The reactions were performed using a 7,500 Fast Real-Time PCR Detection System (Applied Biosystems, United States) under the following conditions: 95°C for 30 s followed by 40 cycles of 95°C for 5 s and 60°C for 30 s. The ratios (I/R and I/R + DEX mRNA levels to Sham mRNA levels) were calculated using the Ct method (2−ΔΔCT) by normalizing all data to the housekeeping gene glyceraldehyde-3-phosphate dehydrogenase (GAPDH).
The data collected in this study were presented as mean ± SEM and obtained randomly. The data were statistically analyzed with a two-tailed, unpaired Student’s t-test and one-way and two-way ANOVA with repeated measures. The post hoc Tukey method was employed for pairwise comparisons when ANOVA showed significant differences. Statistically significant was considered when P < 0.05. GraphPad Prism 8.0 was used to analyze the data.
To assess the therapeutic efficacy of DEX in MCAO rats, neurological scores and TTC staining were performed 24 h after induction of cerebral ischemia and reperfusion. The neurological scores revealed a significant increase in deficits in the MCAO group compared to the sham group (p < 0.001). However, DEX treatment markedly improved the IR-induced neurological impairment (p < 0.01) when compared to the untreated MCAO group (Figure 1A).
Figure 1. (A) DEX improved neurological deficit scores in tMCAO mice. ***p < 0.001 vs. sham group; ##p < 0.01 vs. I/R group, n = 6. (B,C) DEX reduced infarct volume in I/R rats. ***p < 0.001 vs. sham group; ##p < 0.01 vs. I/R group, n = 6. (D) The representative images of H&E staining in Sham, I/R, and I/R + DEX groups.
Additionally, TTC staining was performed to assess the extent of brain damage caused by the ischemia and reperfusion. The results showed no evidence of infarction in the brain of sham rats, as demonstrated by the representative image of TTC staining (Figure 1B). In contrast, rats subjected to MCAO exhibited a substantial increase in infarct volume (24.00% ± 1.693%) compared to that in the Sham group (p < 0.0001) (Figure 1C). Remarkably, rats treated with DEX in conjunction with MCAO showed a significant reduction in infarct volume (16.83% ± 1.939%) compared to the untreated MCAO group (p < 0.005).
To investigate the effect of DEX on MCAO-induced rat neurons, HE staining showed no obvious pathological change in the sham brain tissue (Figure 1D). Rats subjected to MCAO exhibited the number of neuron cells decreased, the nucleolus was atrophic or disappeared, the cells showed vacuolar degeneration, inflammatory cell infiltration, and the number of denatured and necrotic neuron cells was large, suggesting severe neuron injury. Notably, in the DEX group, the neuronal damage and nerve cell necrosis decreased.
RNA-seq generated more than 769.3 M original reads (2.55 M in sham, 2.66 M IR group, 2.48 M IR + DEX group) on the Illumina Novaseq6000 sequencing platform. Overall, 380 M clean read lengths were obtained after removing the joint sequence and the low-quality sequence. The percentage of clean reads in each library varied from 98.41% to 99.12% in the raw data. A total of 3,251 new predictive lncRNA from the cerebral cortex were identified by screening using four analytical tools (PLEK, CPAT, CNCI, and CPC) (Supplementary Figure S1A). Our analysis further suggests that the 3,251 lncRNA were composed of 1790 (55.1%) long intergenic non-coding RNA (lncRNA), 185 (5.7%) antisense lncRNA, 1,214 (37.3%) intron lncRNA, and 62 (1.9%) bidirectional LncRNA (Supplementary Figure S1B).
Then, we analyzed DEG expression profiles in the IR group and IR + DEX group. About 7,267 and 518 significant genes were identified in 28,751 and 28,864 transcripts in the IR group and IR + DEX group, respectively, among which protein-coding RNA had the largest transcriptional changes (71.9%–72.08%), followed by known lncRNA (4.99%–5.19%). Predicted lncRNA (8.91%–9%) and other ncRNAs (13.92%–13.99%) (Supplementary Figures S1C, D). The distribution of transcript length and exons in mRNA and lncRNA is shown in Supplementary Figures S1E, F.
The expression of mRNA, lncRNA, and circRNA in the cerebral infarction area of rats was significantly changed after tMACO. Approximately 6,494 mRNA (4,110 upregulated and 2,384 downregulated), 1,809 lncRNA (513 upregulated and 1,296 downregulated), and 2,698 circRNA (1740 upregulated and 958 downregulated) were significantly altered in the I/R group as compared to sham group. In the case of the I/R + DEX group, approximately 763 mRNA (369 upregulated and 394 downregulated), 232 lncRNA (118 upregulated and 114 downregulated), and 2,795 circRNA (1,395 upregulated and 1,400 downregulated) were significantly altered as compared to I/R, as shown in Figures 2A–D.
Figure 2. Differentially expressed mRNA, lncRNA, and circRNA in I/R and DEX after tMCAO (A,B) Scatter diagrams and (C,D) Heatmaps of significantly differentially expressed mRNA, lncRNA, and circRNA in I/R and DEX from rats after tMCAO. n = 3 rats/group.
We conducted Gene Ontology (GO) analysis to explore the functional characteristics of differential expressed mRNA in the I/R and IR + DEX groups. The analysis revealed significant enrichment in biological processes (BPs), including the regulation of multicellular organismal processes, the biological development process of cells and systems, the immune regulation process, and the regulation of nociceptive stimuli. Notably, we observed Cellular components (CC) are enriched in cellular and neuronal projection, and the most robust molecular functions (MFs) are enriched in protein and receptor binding. Similarly, circRNA colocalization and co-expression genes analysis demonstrated analogous enrichment, while trans-lncRNA analysis highlighted involvement in cell and tissue genesis and regulation, particularly in neuronal projection, cell junction, cell projection, synapses, axons and dendrites (Figures 5A, B).
Furthermore, KEGG pathway analysis of DE RNA showed significant enrichment in pathways such as cytokine−cytokine receptor interaction, glutamatergic, synapse, cell adhesion molecules (CAMs), hematopoietic, cell lineage, chemokine signaling pathway and cAMP signaling pathway. DE circRNA analysis displayed enrichment in axon guidance, cAMP signaling pathway, glutamatergic synapse, phosphatidylinositol signaling system, ubiquitin-mediated proteolysis, long-term potentiation, morphine addiction, dopaminergic synapse, and endocytosis. Additionally, DE cis-lncRNA pathways included type I diabetes mellitus, epstein−Barr virus infection, graft−versus−host disease, and antigen processing and presentation, while DE trans-lncRNA pathways involved dopaminergic synapse, apoptosis, TNF signaling pathway and chemokine signaling pathway (Figures 3C, D).
Figure 3. Functional prediction of DE mRNAs, DE circRNAs, DE cis-lncRNAs, DE trans-lncRNAs in I/R (A) and I/R + DEX (B), by GO analyses. Analysis of the Gene Ontology database graphically displays the top 10 significant GO enrichment results with the candidate targeted genes in the biological process, molecular function, and cellular component in the I/R and I/R + DEX. Functional prediction of DE mRNAs, DE circRNAs, DE cis-lncRNAs, and DE trans-lncRNAs in I/R (C) and I/R + DEX (D), by KEGG analyses showed top 20 enrichments of KEGG pathways. The abscissa represents -log10 (p-value). The more significant the abscissa is, the more significant the pathway enrichment (p ≤ 0.05).
To identify the interaction between DE RNA in the I/R and the I/R + DEX group and predict their regulatory relationship, we constructed the ceRNA network diagram comprising the top 10 lncRNA-miRNA-mRNA and circRNA-miRNA-mRNA interactions based on correlation analysis. Through node analysis, we identified MSTRG.17966.6, MSTRG.288.3, MSTRG.288.2, MSTRG.2024.4, and specific loci like 9:67242046|67269326 (rno-Abi2_0020), and 15:48443441|48446043 (rno-intergenic_000444), which exhibited simultaneous interaction in both I/R and I/R + DEX groups with multiple miRNA and mRNA. These findings suggest the potential significance of these lncRNA/circRNA in the DEX treatment of cerebral I/R injury. Moreover, the establishment of co-expression networks serves as a foundation for further research exploration into the functional roles of ceRNAs and the lncRNA/circRNA-miRNA-mRNA axis in the pathophysiology of cerebral I/R injury. Notably, rno-miR-24-3p and rno-miR-6333 were found as key binding targets in the constructed lncRNA/circRNA-miRNA-mRNA axis. For instance, the MSTRG.288.3/rno-miR-6333/Grm1 axis, MSTRG.17966.6/rno-miR-24-3p/URGCP axis, and rno-Abi2_0020/rno-miR-24-3p/Plec axis are potential regulatory axes modulated by DEX to reduce cerebral I/R injury (Figure 4).
Figure 4. The top 10 lncRNAs and circRNAs and their target genes are displayed according to their respective network degrees of mRNA, lncRNA, and circRNAs. (A,C) ceRNA (lncRNA–miRNA–mRNA) network on I/R (A) and I/R + DEX (C). (B,D) ceRNA (circRNA–miRNA–mRNA) network on I/R (B) and I/R + DEX (D). Red represents upregulation, green represents downregulation, and blue represents unknown. Triangle: miRNA; Square: mRNA; Diamond: lncRNA or circRNA.
We employed Venn diagrams to show the genes whose expression was reversed after DEX injection. In the IR + DEX group, compared to IR alone, 204 mRNA, 53 lncRNA, and 902 circRNA were downregulated, while 163 mRNA, 55 lncRNA, and 183 circRNA were upregulated, suggesting their relevance to DEX-mediated neuroprotection (Figures 5A, B). Notably, analysis of 341 DE mRNA associated with stroke (259 upregulated, 82 downregulated) in the IR group (Supplementary Figure S2) revealed upregulation of genes like Ccl2, Cxcl1, C3, Serpine1, Adamts7, Csf3, Ptx3, MMP8, Lif, and Lcn2, and downregulation of Gdf10, Agtr2 and Shank3 (Figure 5C). GO and KEGG enrichment analysis underscored hypoxia, regulation of blood circulation, and wound healing among these genes. Subsequent analysis of 367 DE mRNA reversed by DEX treatment showed significant enrichment in inflammatory pathways such as leukocyte adhesion and immune complex formation (Figures 5D, E). Neuroinflammation and oxidative stress play an important role in I/R injury, and DEX has anti-inflammatory and anti-oxidative stress effects. Therefore, we used gene cards to characterize the above 367 DE mRNA. Gene characterization revealed associated with neuroinflammation (22 DE mRNA) and oxidative stress (16 DE mRNA). Among these, 10 DE mRNA are associated with both neuroinflammation and oxidative stress: Selp, Cxcl1, Fas, Sele, Esr1, Nod2, Mmp3, Tac1, Ttr, and Bdnf (Figures 5F, G). These genes participate in common biological processes such as leukocyte migration and response to external stimuli while exhibiting enrichment in cell components like perikaryon and the external side of the plasma membrane. Additionally, their molecular functions are enriched in signal receptors and chemokine binding. KEGG analysis identified the TNF signaling pathway and cytokine interaction as significantly enriched pathways, underscoring their role in cerebral I/R injury (Figure 5H). The STRING database was used for 10 significant differentially expressed mRNAs, which are identified as associated with both neuroinflammation and oxidative stress to establish the PPI network as shown in Figure 5I. Cxcl1 showed co-expression with Selp, Sele, and Mmp3, with co-expression scores were 0.657, 0.629, and 0.728, respectively. Ngfr showed co-expression with Tac1, Fas, and Esr, and co-expression scores were 0.533, 0.47, and 0.556 respectively. These findings implicate DEX in mitigating neuroinflammation and oxidative stress, offering promising therapeutic avenues for cerebral I/R injury.
Figure 5. (A) Venn diagrams indicate the downregulated mRNA, lncRNA, circRNA in I/R and upregulated in I/R + DEX, (B)Venn diagrams indicate the upregulated mRNA, lncRNA, circRNA in I/R and downregulated in I/R + DEX. n = 3 rats/group. (C) Heat map of the top 15 up- and downregulated genes of stroke in the I/R group, (D) and their GO and KEGG functional enrichment, (E) GO and KEGG functional enrichment analysis of reverse effect genes after DEX application, (F) Venn diagrams (G) Heat map (H) Functional enrichment analysis, and (I) PPI network analysis indicate the number of genes associated with both inflammation and oxidative stress. (J) Venn diagrams indicate the number of DEGs mapped to stroke, cognitive disorder, and sleep disorders and (K) the number of DEGs mapped to stroke, inflammation- and apoptosis-related genes in the I/R group.
DEGs associated with stroke, cognitive dysfunction, and sleep disorders after I/R injury were characterized using Gene Cards databases. Analysis of 6494 DE mRNA in the I/R group showed relevance to stroke, cognitive dysfunction, and sleep disorder, with 53 DEGs associated with stroke and cognitive dysfunction, 109 with sleep disorders, and 23 with all three conditions (Figure 5J). Further characterization via Venn diagram showed overlapping DEGs involved in neuroinflammation, apoptosis, and stroke. Specifically, 74 DEGs were associated with neuroinflammation, apoptosis, and stroke, while 138 DEGs were linked to stroke and neuroinflammation. Additionally, 89 DEGs were related to stroke and apoptosis, and 74 genes were associated with stroke, neuroinflammation, and apoptosis (Figure 5K). The proportion of these differential genes in stroke, neuroinflammation, apoptosis, cognitive dysfunction, and sleep disorders in the I/R group was 3.50%, 4.39%, 4.31%, 9.56%, and 3.59%, respectively (Supplementary Figure S2).
To verify the reliability of the sequencing results, we selected two mRNA (MMP3, Ntrk1), two circRNAs (Chd1, Robo2), and two lncRNAs (MSTRG.288.3, MSTRG.16732.1), we found that the results were consistent with sequencing results (Figures 6A–F).
Figure 6. Validations of DE mRNA, circRNAs, and lncRNAs, in I/R and I/R + DEX after tMCAO. The expression levels of mRNAs MMP3 and Ntrk1 (A,B), circRNA Chd1 and Robo2 (C,D), lncRNA MSTRG.288.3 and MSTRG.16732.1 (E,F). */#p < 0.05, **/##p < 0.01, ***/###p < 0.001, n = 6.
In this study, we established a clinically relevant model of I/R injury in rats and found that DEX significantly improved neurological impairment and reduced infarct volume. Non-treated rats exhibited increased infarct volumes and neurological deficits. Consistent with previous studies (Yuan et al., 2017a; Wang et al., 2019; Zhai et al., 2019), DEX reduced cerebral infarction size, improved motor dysfunction, and decreased neuronal damage. Notably, this study is the first to use full transcriptome sequencing and bioinformatic analysis to observe the expression profile of ncRNA in the cortex of infarct sites in rats after DEX treatment.
To predict gene functions of DE mRNA and ncRNA in I/R injury, we performed GO and KEGG enrichment analyses of these DEGs. The results highlighted significant roles in neuronal production and differentiation, response to stimulation, immune response, MAPK signaling pathway, cytokine-to-cytokine binding, cAMP signaling pathway, and TNF signaling pathway. These findings are consistent with previous reports (Dergunova et al., 2018). Many DEGs were associated with neuroinflammation and oxidative stress, key contributors to cerebral I/R injury (Wu et al., 2020). We constructed ceRNA networks for the top 10 DEGs, predicting targeted regulatory relationships and identifying potential therapeutic targets for I/R.
Increased oxygen supply after I/R leads to oxidative stress and increased release of inflammatory factors, triggering a cascade of reactions resulting in apoptosis, disruption of the blood-brain barrier (BBB), cerebral edema, and bleeding transformation (Jurcau and Simion, 2021). Anti-inflammatory as a potential therapeutic target for I/R injury has been studied for a long time (Tirandi et al., 2023). Various cytokines and signaling pathways, including NF-κB, MAPK, and PI3K-AKT, are implicated in cerebral I/R injury. Our study identified stroke-related molecules such as Ccl2 (Dimitrijevic et al., 2006; Stowe et al., 2012; Geng et al., 2022; Georgakis et al., 2022), Cxcl1 (Shi Y. et al., 2021), C3 (Mocco et al., 2006; Yang et al., 2013; Yang et al., 2021; Hou et al., 2022), Serpine1 (Pu et al., 2022; Palakurti et al., 2023), Adamts7 (Sharifi et al., 2023), Csf3 (Garcia-Bonilla et al., 2015), Ptx3 (Rodriguez-Grande et al., 2014; Rodriguez-Grande et al., 2015; Shindo et al., 2016; de Oliveira et al., 2019; Shindo et al., 2021), Mmp8 (Han et al., 2016; Ren et al., 2020), Lif (Cai et al., 2022), and Lcn2 (Jin et al., 2014; Zhao et al., 2019; Wang G. et al., 2020; Li et al., 2023b; Zhao R. Y. et al., 2023) were significantly upregulated after I/R, whereas Gdf10 (Li et al., 2015), Agtr2 (Iwai et al., 2004; Iwanami et al., 2011), Shank3 (Datta et al., 2011; Zhang et al., 2024) downregulated after I/R, aligning with existing literature.
DEX, a highly selective α2 adrenergic receptor agonist, has notable anti-inflammatory and anti-oxidative properties. By reducing cerebral blood flow and metabolic oxygen demand, DEX slightly lowers intracranial pressure. Its neuroprotective effects have been demonstrated in multiple brain injury models, primarily by reducing cerebral catecholamine release. Our high-throughput sequencing identified 367 genes whose expression was reversed by DEX treatment. GO and KEGG analyses identified these genes are involved in inflammatory and immune biological processes. We identified DEGs associated with both inflammation and oxidative stress, including Selp, Cxcl1, ESR1, NOD2, Fas, and BDNF, suggesting they play crucial roles in DEX’s neuroprotective mechanism. For instance, selectin (Selp, Sele), cell adhesion molecules, are upregulated following pro-inflammatory stimuli (Cappenberg et al., 2022) and promote thrombosis (Purdy et al., 2022). DEX inhibited the I/R-induced increase in Selp and Sele expression, potentially contributing to its protective effects. Cxcl1, neutrophil chemokine, is involved in post-ischemic inflammation and pain (Huang et al., 2023). DEX can reverse Cxcl increase, mitigating inflammation in various models (Shen et al., 2020; Zhang S. et al., 2023). ESR1, an estrogen receptor, mediates neuroprotection by reducing inflammation (Suzuki et al., 2009). DEX reversed the I/R-induced upregulation of ESR1, though its precise regulatory mechanism needs further research. NOD2, involved in I/R inflammatory response, exacerbates injury when activated (Liu et al., 2015). DEX’s modulation of NOD2’s role in I/R injury remains to be elucidated. Fas, an apoptotic receptor, increases in ischemic stroke and contributes to neuronal apoptosis (Chojnowski et al., 2023). DEX’s reduction of Fas expression supports its anti-apoptotic effects. Lastly, BDNF promotes neuronal survival and plasticity. DEX enhanced BDNF levels, supporting neuroprotection (Li et al., 2018; Chen et al., 2024).
Our study still had some limitations, first transcriptome analysis of differential genes was limited to a single time point, necessitating multi-time-point analyses. Second, our RNA-seq mainly tests the expression profiles of mRNA, lncRNA, and circRNA, but no miRNA. Further research is needed to validate whether these DEGs can serve as therapeutic targets.
To summarize, this study demonstrated that DEX significantly reduces I/R injury in a rat model by improving neurological outcomes and reducing infarct volumes. Through comprehensive transcriptome sequencing and bioinformatic analysis, we identified significant changes in the expression profiles of mRNAs and ncRNAs in the cortex of infarct sites. The study provides new insights into the molecular mechanisms of DEX’s protective effects against cerebral I/R injury, identifying several potential therapeutic targets. However, further research is needed to validate these findings and explore the therapeutic potential of these DEGs. These findings provide a basis for exploring DEX’s neuroprotective mechanisms and developing new therapies for I/R injury.
The data presented in the study are deposited in the https://www.ncbi.nlm.nih.gov/, accession GSE268634.
The animal study was approved by Animal Care and Use Committee of the Second Affiliated Hospital of Zhengzhou University. The study was conducted in accordance with the local legislation and institutional requirements.
ZZ: Conceptualization, Data curation, Formal Analysis, Investigation, Writing–original draft. AN: Conceptualization, Data curation, Formal Analysis, Investigation, Methodology, Project administration, Writing–original draft, Writing–review and editing. DL: Formal Analysis, Methodology, Writing–review and editing. SK: Writing–review and editing. QB: Project administration, Resources, Writing–review and editing. FY: Funding acquisition, Supervision, Writing–review and editing, Conceptualization.
The author(s) declare that financial support was received for the research, authorship, and/or publication of this article. This work was supported by the Science and Technology research project of Henan Province (NO:232102311118).
The authors declare that the research was conducted in the absence of any commercial or financial relationships that could be construed as a potential conflict of interest.
All claims expressed in this article are solely those of the authors and do not necessarily represent those of their affiliated organizations, or those of the publisher, the editors and the reviewers. Any product that may be evaluated in this article, or claim that may be made by its manufacturer, is not guaranteed or endorsed by the publisher.
The Supplementary Material for this article can be found online at: https://www.frontiersin.org/articles/10.3389/fphar.2024.1437445/full#supplementary-material
Bao, M. H., Szeto, V., Yang, B. B., Zhu, S. Z., Sun, H. S., and Feng, Z. P. (2018). Long non-coding RNAs in ischemic stroke. Cell. Death Dis. 9 (3), 281. doi:10.1038/s41419-018-0282-x
Beermann, J., Piccoli, M. T., Viereck, J., and Thum, T. (2016). Non-coding RNAs in development and disease: background, mechanisms, and therapeutic approaches. Physiol. Rev. 96 (4), 1297–1325. doi:10.1152/physrev.00041.2015
Burlacu, C. C., Neag, M. A., Mitre, A. O., Sirbu, A. C., Badulescu, A. V., and Buzoianu, A. D. (2022). The role of miRNAs in dexmedetomidine’s neuroprotective effects against brain disorders. Int. J. Mol. Sci. 23 (10), 5452. doi:10.3390/ijms23105452
Cai, W., Shi, L., Zhao, J., Xu, F., Dufort, C., Ye, Q., et al. (2022). Neuroprotection against ischemic stroke requires a specific class of early responder T cells in mice. J. Clin. Investig. 132 (15), e157678. doi:10.1172/JCI157678
Cappenberg, A., Kardell, M., and Zarbock, A. (2022). Selectin-mediated signaling-shedding light on the regulation of integrin activity in neutrophils. Cells 11 (8), 1310. doi:10.3390/cells11081310
Chen, X., Chen, A., Wei, J., Huang, Y., Deng, J., Chen, P., et al. (2024). Dexmedetomidine alleviates cognitive impairment by promoting hippocampal neurogenesis via BDNF/TrkB/CREB signaling pathway in hypoxic-ischemic neonatal rats. CNS Neurosci. Ther. 30 (1), e14486. doi:10.1111/cns.14486
Chojnowski, K., Opiełka, M., Gozdalski, J., Radziwon, J., Dańczyszyn, A., Aitken, A. V., et al. (2023). The role of arginine-vasopressin in stroke and the potential use of arginine-vasopressin type 1 receptor antagonists in stroke therapy: a narrative review. Int. J. Mol. Sci. 24 (3), 2119. doi:10.3390/ijms24032119
Datta, A., Jingru, Q., Khor, T. H., Heese, K., and Sze, S. K. (2011). Quantitative neuroproteomics of an in vivo rodent model of focal cerebral ischemia/reperfusion injury reveals a temporal regulation of novel pathophysiological molecular markers. J. Proteome Res. 10 (11), 5199–5213. doi:10.1021/pr200673y
de Oliveira, T. H. C., Souza, D. G., Teixeira, M. M., and Amaral, F. A. (2019). Tissue dependent role of PTX3 during ischemia-reperfusion injury. Front. Immunol. 10, 1461. doi:10.3389/fimmu.2019.01461
Dergunova, L. V., Filippenkov, I. B., Stavchansky, V. V., Denisova, A. E., Yuzhakov, V. V., Mozerov, S. A., et al. (2018). Genome-wide transcriptome analysis using RNA-Seq reveals a large number of differentially expressed genes in a transient MCAO rat model. BMC Genomics 19 (1), 655. doi:10.1186/s12864-018-5039-5
Dimitrijevic, O. B., Stamatovic, S. M., Keep, R. F., and Andjelkovic, A. V. (2006). Effects of the chemokine CCL2 on blood-brain barrier permeability during ischemia-reperfusion injury. J. Cereb. Blood Flow. Metab. 26 (6), 797–810. doi:10.1038/sj.jcbfm.9600229
Fan, J., Li, X., Yu, X., Liu, Z., Jiang, Y., Fang, Y., et al. (2023). Global burden, risk factor analysis, and prediction study of ischemic stroke, 1990-2030. Neurology 101 (2), e137–e150. doi:10.1212/WNL.0000000000207387
Fang, H., Li, H. F., Yan, J. Y., Yang, M., and Zhang, J. P. (2021). Dexmedetomidine-up-regulated microRNA-381 exerts anti-inflammatory effects in rats with cerebral ischaemic injury via the transcriptional factor IRF4. J. Cell. Mol. Med. 25 (4), 2098–2109. doi:10.1111/jcmm.16153
Garcia-Bonilla, L., Racchumi, G., Murphy, M., Anrather, J., and Iadecola, C. (2015). Endothelial CD36 contributes to postischemic brain injury by promoting neutrophil activation via CSF3. J. Neurosci. 35 (44), 14783–14793. doi:10.1523/JNEUROSCI.2980-15.2015
Geng, H., Chen, L., Tang, J., Chen, Y., and Wang, L. (2022). The role of CCL2/CCR2 Axis in cerebral ischemia-reperfusion injury and treatment: from animal experiments to clinical trials. Int. J. Mol. Sci. 23 (7), 3485. doi:10.3390/ijms23073485
Georgakis, M. K., Bernhagen, J., Heitman, L. H., Weber, C., and Dichgans, M. (2022). Targeting the CCL2-CCR2 axis for atheroprotection. Eur. Heart J. 43 (19), 1799–1808. doi:10.1093/eurheartj/ehac094
Guo, Q., Ma, M., Yu, H., Han, Y., and Zhang, D. (2023). Dexmedetomidine enables copper homeostasis in cerebral ischemia/reperfusion via ferredoxin 1. Ann. Med. 55 (1), 2209735. doi:10.1080/07853890.2023.2209735
Han, J. E., Lee, E. J., Moon, E., Ryu, J. H., Choi, J. W., and Kim, H. S. (2016). Matrix metalloproteinase-8 is a novel pathogenetic factor in focal cerebral ischemia. Mol. Neurobiol. 53 (1), 231–239. doi:10.1007/s12035-014-8996-y
Herpich, F., and Rincon, F. (2020). Management of acute ischemic stroke. Crit. Care Med. 48 (11), 1654–1663. doi:10.1097/CCM.0000000000004597
Hou, J. Y., Cao, G. Z., Tian, L. L., Zhou, R., Zhang, Y., Xu, H., et al. (2022). Integrated transcriptomics and metabolomics analysis reveals that C3 and C5 are vital targets of DuZhi Wan in protecting against cerebral ischemic injury. Biomed. Pharmacother. 155, 113703. doi:10.1016/j.biopha.2022.113703
Hu, Y., Zhou, H., Zhang, H., Sui, Y., Zhang, Z., Zou, Y., et al. (2022). The neuroprotective effect of dexmedetomidine and its mechanism. Front. Pharmacol. 13, 965661. doi:10.3389/fphar.2022.965661
Huang, X., Guo, M., Zhang, Y., Xie, J., Huang, R., Zuo, Z., et al. (2023). Microglial IL-1RA ameliorates brain injury after ischemic stroke by inhibiting astrocytic CXCL1-mediated neutrophil recruitment and microvessel occlusion. Glia 71 (7), 1607–1625. doi:10.1002/glia.24359
Huang, Y. Q., Wen, R. T., Li, X. T., Zhang, J., Yu, Z. Y., and Feng, Y. F. (2021). The protective effect of dexmedetomidine against ischemia-reperfusion injury after hepatectomy: a meta-analysis of randomized controlled trials. Front. Pharmacol. 12, 747911. doi:10.3389/fphar.2021.747911
Iwai, M., Liu, H. W., Chen, R., Ide, A., Okamoto, S., Hata, R., et al. (2004). Possible inhibition of focal cerebral ischemia by angiotensin II type 2 receptor stimulation. Circulation 110 (7), 843–848. doi:10.1161/01.CIR.0000138848.58269.80
Iwanami, J., Mogi, M., Tsukuda, K., Min, L. J., Sakata, A., Jing, F., et al. (2011). Effect of angiotensin II type 2 receptor deletion in hematopoietic cells on brain ischemia-reperfusion injury. Hypertension 58 (3), 404–409. doi:10.1161/HYPERTENSIONAHA.111.177873
Jin, M., Kim, J. H., Jang, E., Lee, Y. M., Soo Han, H., Woo, D. K., et al. (2014). Lipocalin-2 deficiency attenuates neuroinflammation and brain injury after transient middle cerebral artery occlusion in mice. J. Cereb. Blood Flow. Metab. 34 (8), 1306–1314. doi:10.1038/jcbfm.2014.83
Jurcau, A., and Simion, A. (2021). Neuroinflammation in cerebral ischemia and ischemia/reperfusion injuries: from pathophysiology to therapeutic strategies. Int. J. Mol. Sci. 23 (1), 14. doi:10.3390/ijms23010014
Li, H., Lu, C., Yao, W., Xu, L., Zhou, J., and Zheng, B. (2020). Dexmedetomidine inhibits inflammatory response and autophagy through the circLrp1b/miR-27a-3p/Dram2 pathway in a rat model of traumatic brain injury. Aging (Albany NY) 12 (21), 21687–21705. doi:10.18632/aging.103975
Li, J., Wang, K., Liu, M., He, J., Zhang, H., and Liu, H. (2023a). Dexmedetomidine alleviates cerebral ischemia-reperfusion injury via inhibiting autophagy through PI3K/Akt/mTOR pathway. J. Mol. Histology 54 (3), 173–181. doi:10.1007/s10735-023-10120-1
Li, J., Xu, P., Hong, Y., Xie, Y., Peng, M., Sun, R., et al. (2023b). Lipocalin-2-mediated astrocyte pyroptosis promotes neuroinflammatory injury via NLRP3 inflammasome activation in cerebral ischemia/reperfusion injury. J. Neuroinflammation 20 (1), 148. doi:10.1186/s12974-023-02819-5
Li, S., Nie, E. H., Yin, Y., Benowitz, L. I., Tung, S., Vinters, H. V., et al. (2015). GDF10 is a signal for axonal sprouting and functional recovery after stroke. Nat. Neurosci. 18 (12), 1737–1745. doi:10.1038/nn.4146
Li, Z. C., Jia, Y. P., Wang, Y., Qi, J. L., and Han, X. P. (2018). Effects of dexmedetomidine post-treatment on BDNF and VEGF expression following cerebral ischemia/reperfusion injury in rats. Mol. Med. Rep. 17 (4), 6033–6037. doi:10.3892/mmr.2018.8597
Liu, C., Li, Z., and Xi, H. (2022). Bioinformatics analysis and in vivo validation of ferroptosis-related genes in ischemic stroke. Front. Pharmacol. 13, 940260. doi:10.3389/fphar.2022.940260
Liu, H., Wei, X., Kong, L., Liu, X., Cheng, L., Yan, S., et al. (2015). NOD2 is involved in the inflammatory response after cerebral ischemia-reperfusion injury and triggers NADPH oxidase 2-derived reactive oxygen species. Int. J. Biol. Sci. 11 (5), 525–535. doi:10.7150/ijbs.10927
Longa, E. Z., Weinstein, P. R., Carlson, S., and Cummins, R. (1989). Reversible middle cerebral artery occlusion without craniectomy in rats. stroke 20 (1), 84–91. doi:10.1161/01.str.20.1.84
Lu, Y., Liu, Y., Zhou, J., Li, D., and Gao, W. (2021). Biosynthesis, total synthesis, structural modifications, bioactivity, and mechanism of action of the quinone-methide triterpenoid celastrol. Med. Res. Rev. 41 (2), 1022–1060. doi:10.1002/med.21751
Mocco, J., Mack, W. J., Ducruet, A. F., Sosunov, S. A., Sughrue, M. E., Hassid, B. G., et al. (2006). Complement component C3 mediates inflammatory injury following focal cerebral ischemia. Circ. Res. 99 (2), 209–217. doi:10.1161/01.RES.0000232544.90675.42
Palakurti, R., Biswas, N., Roy, S., Gnyawali, S. C., Sinha, M., Singh, K., et al. (2023). Inducible miR-1224 silences cerebrovascular Serpine1 and restores blood flow to the stroke-affected site of the brain. Mol. Ther. Nucleic Acids 31, 276–292. doi:10.1016/j.omtn.2022.12.019
Paul, S., and Candelario-Jalil, E. (2021). Emerging neuroprotective strategies for the treatment of ischemic stroke: an overview of clinical and preclinical studies. Exp. Neurol. 335, 113518. doi:10.1016/j.expneurol.2020.113518
Peschansky, V. J., and Wahlestedt, C. (2014). Non-coding RNAs as direct and indirect modulators of epigenetic regulation. Epigenetics 9 (1), 3–12. doi:10.4161/epi.27473
Pu, Z., Bao, X., Xia, S., Shao, P., and Xu, Y. (2022). Serpine1 regulates peripheral neutrophil recruitment and acts as potential target in ischemic stroke. J. Inflamm. Res. 15, 2649–2663. doi:10.2147/JIR.S361072
Purdy, M., Obi, A., Myers, D., and Wakefield, T. (2022). P- and E-selectin in venous thrombosis and non-venous pathologies. J. Thromb. Haemost. 20 (5), 1056–1066. doi:10.1111/jth.15689
Ren, Y., Gao, X. P., Liang, H., Zhang, H., and Hu, C. Y. (2020). LncRNA KCNQ1OT1 contributes to oxygen-glucose-deprivation/reoxygenation-induced injury via sponging miR-9 in cultured neurons to regulate MMP8. Exp. Mol. Pathol. 112, 104356. doi:10.1016/j.yexmp.2019.104356
Rodriguez-Grande, B., Swana, M., Nguyen, L., Englezou, P., Maysami, S., Allan, S. M., et al. (2014). The acute-phase protein PTX3 is an essential mediator of glial scar formation and resolution of brain edema after ischemic injury. J. Cereb. Blood Flow. Metab. 34 (3), 480–488. doi:10.1038/jcbfm.2013.224
Rodriguez-Grande, B., Varghese, L., Molina-Holgado, F., Rajkovic, O., Garlanda, C., Denes, A., et al. (2015). Pentraxin 3 mediates neurogenesis and angiogenesis after cerebral ischaemia. J. Neuroinflammation 12, 15. doi:10.1186/s12974-014-0227-y
Sharifi, M. A., Wierer, M., Dang, T. A., Milic, J., Moggio, A., Sachs, N., et al. (2023). ADAMTS-7 modulates atherosclerotic plaque formation by degradation of TIMP-1. Circ. Res. 133 (8), 674–686. doi:10.1161/CIRCRESAHA.123.322737
Shen, Q., Xu, G., Liu, J., Wang, L., Zhou, Y., Yu, Y., et al. (2020). Dexmedetomidine alleviates non-ventilation associated lung injury via modulating immunology phenotypes of macrophages. Life Sci. 259, 118249. doi:10.1016/j.lfs.2020.118249
Shi, J., Yu, T., Song, K., Du, S., He, S., Hu, X., et al. (2021a). Dexmedetomidine ameliorates endotoxin-induced acute lung injury in vivo and in vitro by preserving mitochondrial dynamic equilibrium through the HIF-1a/HO-1 signaling pathway. Redox Biol. 41, 101954. doi:10.1016/j.redox.2021.101954
Shi, Y., Yi, Z., Zhao, P., Xu, Y., and Pan, P. (2021b). MicroRNA-532-5p protects against cerebral ischemia-reperfusion injury by directly targeting CXCL1. Aging (Albany NY) 13 (8), 11528–11541. doi:10.18632/aging.202846
Shindo, A., Maki, T., Mandeville, E. T., Liang, A. C., Egawa, N., Itoh, K., et al. (2016). Astrocyte-derived pentraxin 3 supports blood-brain barrier integrity under acute phase of stroke. Stroke 47 (4), 1094–1100. doi:10.1161/STROKEAHA.115.012133
Shindo, A., Takase, H., Hamanaka, G., Chung, K. K., Mandeville, E. T., Egawa, N., et al. (2021). Biphasic roles of pentraxin 3 in cerebrovascular function after white matter stroke. CNS Neurosci. Ther. 27 (1), 60–70. doi:10.1111/cns.13510
Stowe, A. M., Wacker, B. K., Cravens, P. D., Perfater, J. L., Li, M. K., Hu, R., et al. (2012). CCL2 upregulation triggers hypoxic preconditioning-induced protection from stroke. J. Neuroinflammation 9, 33. doi:10.1186/1742-2094-9-33
Sun, P., Hamblin, M. H., and Yin, K. J. (2022). Non-coding RNAs in the regulation of blood-brain barrier functions in central nervous system disorders. Fluids Barriers CNS 19 (1), 27. doi:10.1186/s12987-022-00317-z
Suzuki, S., Brown, C. M., and Wise, P. M. (2009). Neuroprotective effects of estrogens following ischemic stroke. Front. Neuroendocrinol. 30 (2), 201–211. doi:10.1016/j.yfrne.2009.04.007
Tirandi, A., Sgura, C., Carbone, F., Montecucco, F., and Liberale, L. (2023). Inflammatory biomarkers of ischemic stroke. Intern Emerg. Med. 18 (3), 723–732. doi:10.1007/s11739-023-03201-2
Vasudeva, K., Dutta, A., and Munshi, A. (2021). Role of lncRNAs in the development of ischemic stroke and their therapeutic potential. Mol. Neurobiol. 58 (8), 3712–3728. doi:10.1007/s12035-021-02359-0
Wang, G., Weng, Y. C., Chiang, I. C., Huang, Y. T., Liao, Y. C., Chen, Y. C., et al. (2020b). Neutralization of lipocalin-2 diminishes stroke-reperfusion injury. Int. J. Mol. Sci. 21 (17), 6253. doi:10.3390/ijms21176253
Wang, L., Liu, H., Zhang, L., Wang, G., Zhang, M., and Yu, Y. (2017). Neuroprotection of dexmedetomidine against cerebral ischemia-reperfusion injury in rats: involved in inhibition of NF-κB and inflammation response. Biomol. Ther. Seoul. 25 (4), 383–389. doi:10.4062/biomolther.2015.180
Wang, L., Liu, W., Zhang, Y., Hu, Z., Guo, H., Lv, J., et al. (2020a). Dexmedetomidine had neuroprotective effects on hippocampal neuronal cells via targeting lncRNA SHNG16 mediated microRNA-10b-5p/BDNF axis. Mol. Cell. Biochem. 469 (1-2), 41–51. doi:10.1007/s11010-020-03726-6
Wang, S. W., Liu, Z., and Shi, Z. S. (2018). Non-coding RNA in acute ischemic stroke: mechanisms, biomarkers and therapeutic targets. Cell. Transpl. 27 (12), 1763–1777. doi:10.1177/0963689718806818
Wang, Y. Q., Tang, Y. F., Yang, M. K., and Huang, X. Z. (2019). Dexmedetomidine alleviates cerebral ischemia-reperfusion injury in rats via inhibition of hypoxia-inducible factor-1α. J. Cell. Biochem. 120 (5), 7834–7844. doi:10.1002/jcb.28058
Wang, Z., Yao, M., Jiang, L., Wang, L., Yang, Y., Wang, Q., et al. (2022). Dexmedetomidine attenuates myocardial ischemia/reperfusion-induced ferroptosis via AMPK/GSK-3β/Nrf2 axis. Biomed. Pharmacother. 154, 113572. doi:10.1016/j.biopha.2022.113572
Wei, B., Liu, W., Jin, L., Guo, S., Fan, H., Jin, F., et al. (2022). Dexmedetomidine inhibits gasdermin D-induced pyroptosis via the PI3K/AKT/GSK3β pathway to attenuate neuroinflammation in early brain injury after subarachnoid hemorrhage in rats. Front. Cell. Neurosci. 16, 899484. doi:10.3389/fncel.2022.899484
Wu, J., Vogel, T., Gao, X., Lin, B., Kulwin, C., and Chen, J. (2018b). Neuroprotective effect of dexmedetomidine in a murine model of traumatic brain injury. Sci. Rep. 8 (1), 4935. doi:10.1038/s41598-018-23003-3
Wu, L., Xiong, X., Wu, X., Ye, Y., Jian, Z., Zhi, Z., et al. (2020). Targeting oxidative stress and inflammation to prevent ischemia-reperfusion injury. Front. Mol. Neurosci. 13, 28. doi:10.3389/fnmol.2020.00028
Wu, M. Y., Yiang, G. T., Liao, W. T., Tsai, A. P. Y., Cheng, Y. L., Cheng, P. W., et al. (2018a). Current mechanistic concepts in ischemia and reperfusion injury. Cell. Physiol. Biochem. 46 (4), 1650–1667. doi:10.1159/000489241
Xiaoqing, S., Yinghua, C., and Xingxing, Y. (2023). The autophagy in ischemic stroke: a regulatory role of non-coding-RNAs. Cell. Signal 104, 110586. doi:10.1016/j.cellsig.2022.110586
Yan, F., Wang, P., Yang, X., and Wang, F. (2023). Long non-coding RNA HOXA11-AS regulates ischemic neuronal death by targeting miR-337-3p/YBX1 signaling pathway: protective effect of dexmedetomidine. Aging (Albany NY) 15 (7), 2797–2811. doi:10.18632/aging.204648
Yang, J., Ahn, H. N., Chang, M., Narasimhan, P., Chan, P. H., and Song, Y. S. (2013). Complement component 3 inhibition by an antioxidant is neuroprotective after cerebral ischemia and reperfusion in mice. J. Neurochem. 124 (4), 523–535. doi:10.1111/jnc.12111
Yang, K., Zeng, L., Ge, A., Wang, S., Zeng, J., Yuan, X., et al. (2022). A systematic review of the research progress of non-coding RNA in neuroinflammation and immune regulation in cerebral infarction/ischemia-reperfusion injury. Front. Immunol. 13, 930171. doi:10.3389/fimmu.2022.930171
Yang, P., Zhu, Z., Zang, Y., Bu, X., Xu, T., Zhong, C., et al. (2021). Increased serum complement C3 levels are associated with adverse clinical outcomes after ischemic stroke. Stroke 52 (3), 868–877. doi:10.1161/STROKEAHA.120.031715
Yin, J. W., Li, J., Ren, Y. M., Li, Y., Wang, R. X., Wang, S., et al. (2021). Dexmedetomidine and netrin-1 combination therapy inhibits endoplasmic reticulum stress by regulating the ERK5/mef2a pathway to attenuate cerebral ischemia injury. Front. Neurosci. 15, 641345. doi:10.3389/fnins.2021.641345
Yuan, F., Fu, H., Sun, K., Wu, S., and Dong, T. (2017a). Effect of dexmedetomidine on cerebral ischemia-reperfusion rats by activating mitochondrial ATP-sensitive potassium channel. Metab. Brain Dis. 32 (2), 539–546. doi:10.1007/s11011-016-9945-4
Yuan, F., Fu, H., Sun, K., Wu, S., and Dong, T. (2017b). Effect of dexmedetomidine on cerebral ischemia-reperfusion rats by activating mitochondrial ATP-sensitive potassium channel. Metab. Brain Dis. 32, 539–546. doi:10.1007/s11011-016-9945-4
Zhai, M., Liu, C., Li, Y., Zhang, P., Yu, Z., Zhu, H., et al. (2019). Dexmedetomidine inhibits neuronal apoptosis by inducing Sigma-1 receptor signaling in cerebral ischemia-reperfusion injury. Aging (Albany NY) 11 (21), 9556–9568. doi:10.18632/aging.102404
Zhang, H., Feng, Y., Si, Y., Lu, C., Wang, J., Wang, S., et al. (2024). Shank3 ameliorates neuronal injury after cerebral ischemia/reperfusion via inhibiting oxidative stress and inflammation. Redox Biol. 69, 102983. doi:10.1016/j.redox.2023.102983
Zhang, R., Liu, H., Pu, L., Zhao, T., Zhang, S., Han, K., et al. (2023a). Global burden of ischemic stroke in young adults in 204 countries and territories. Neurology 100 (4), e422–e434. doi:10.1212/WNL.0000000000201467
Zhang, S., Zhang, Y., Zheng, Y., Zhu, S., Sun, J., Deng, Y., et al. (2023b). Dexmedetomidine attenuates sleep deprivation-induced inhibition of hippocampal neurogenesis via VEGF-VEGFR2 signaling and inhibits neuroinflammation. Biomed. Pharmacother. 165, 115085. doi:10.1016/j.biopha.2023.115085
Zhao, N., Xu, X., Jiang, Y., Gao, J., Wang, F., Xu, X., et al. (2019). Lipocalin-2 may produce damaging effect after cerebral ischemia by inducing astrocytes classical activation. J. Neuroinflammation 16 (1), 168. doi:10.1186/s12974-019-1556-7
Zhao, R. Y., Wei, P. J., Sun, X., Zhang, D. H., He, Q. Y., Liu, J., et al. (2023b). Role of lipocalin 2 in stroke. Neurobiol. Dis. 179, 106044. doi:10.1016/j.nbd.2023.106044
Zhao, S., Wu, W., Lin, X., Shen, M., Yang, Z., Yu, S., et al. (2022). Protective effects of dexmedetomidine in vital organ injury: crucial roles of autophagy. Cell. Mol. Biol. Lett. 27 (1), 34. doi:10.1186/s11658-022-00335-7
Zhao, Y., Feng, X., Li, B., Sha, J., Wang, C., Yang, T., et al. (2020). Dexmedetomidine protects against lipopolysaccharide-induced acute kidney injury by enhancing autophagy through inhibition of the PI3K/AKT/mTOR pathway. Front. Pharmacol. 11, 128. doi:10.3389/fphar.2020.00128
Keywords: ischemia-reperfusion injury, dexmedetomidine, transcriptomics, non-coding RNAs, ischemic stroke
Citation: Zhang ZZ, Nasir A, Li D, Khan S, Bai Q and Yuan F (2024) Effect of dexmedetomidine on ncRNA and mRNA profiles of cerebral ischemia-reperfusion injury in transient middle cerebral artery occlusion rats model. Front. Pharmacol. 15:1437445. doi: 10.3389/fphar.2024.1437445
Received: 23 May 2024; Accepted: 18 July 2024;
Published: 07 August 2024.
Edited by:
Andleeb Khan, Integral University, IndiaReviewed by:
Rong Jin, Penn State Milton S. Hershey Medical Center, United StatesCopyright © 2024 Zhang, Nasir, Li, Khan, Bai and Yuan. This is an open-access article distributed under the terms of the Creative Commons Attribution License (CC BY). The use, distribution or reproduction in other forums is permitted, provided the original author(s) and the copyright owner(s) are credited and that the original publication in this journal is cited, in accordance with accepted academic practice. No use, distribution or reproduction is permitted which does not comply with these terms.
*Correspondence: Qian Bai, YmFpcWlhbkB6enUuZWR1LmNu; Feng Yuan, eXVhbmZlbmdmc0B6enUuZWR1LmNu
†These authors have contributed equally to this work
Disclaimer: All claims expressed in this article are solely those of the authors and do not necessarily represent those of their affiliated organizations, or those of the publisher, the editors and the reviewers. Any product that may be evaluated in this article or claim that may be made by its manufacturer is not guaranteed or endorsed by the publisher.
Research integrity at Frontiers
Learn more about the work of our research integrity team to safeguard the quality of each article we publish.