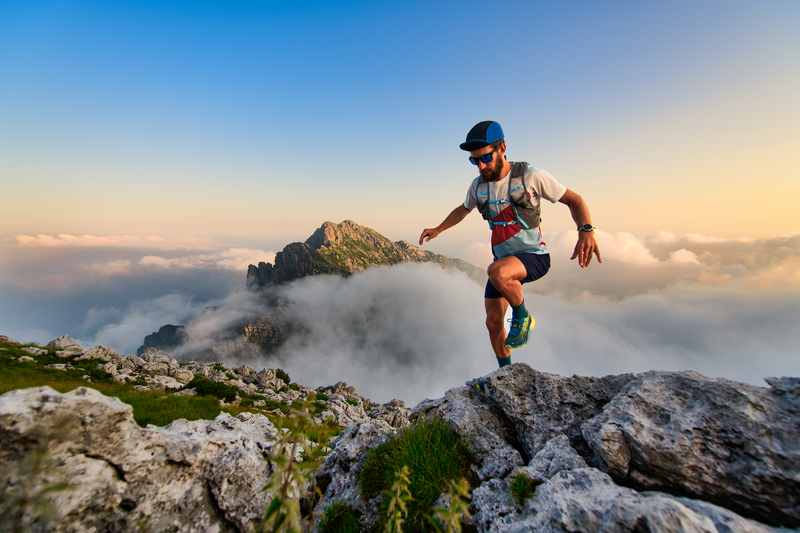
94% of researchers rate our articles as excellent or good
Learn more about the work of our research integrity team to safeguard the quality of each article we publish.
Find out more
REVIEW article
Front. Pharmacol. , 25 July 2024
Sec. Neuropharmacology
Volume 15 - 2024 | https://doi.org/10.3389/fphar.2024.1435133
Depression is a chronic mental disorder characterized by persistent low mood and loss of interest. Treatments for depression are varied but may not be sufficient cure. Drug-based treatment regimens have drawbacks such as slow onset of action, low bioavailability, and drug side effects. Nanocarrier Drug Delivery Systems (NDDS) has received increasing attention for brain drug delivery since it assists the drug through the blood-brain barrier and improves bioavailability, which may be beneficial for treating depression. Due to the particle size and physicochemical properties of nanocarriers, it presents a promise to improve the stability and solubility of antidepressants, thereby enhancing the drug concentration. Moreover, ligand-modified nanocarriers can be taken as a target direct medicines release system and reduce drug side effects. The purpose of the present review is to provide an up-to-date understanding of the Nanocarrier drug delivery system and relevant antidepressants in different routes of ingestion, to lay a foundation for the treatment of patients with depression.
Depression is a common psychosomatic disorder that features chronic emotional sadness and lack of energy. Unlike ordinary emotional sadness, depression is defined by persistent low mood and absence of pleasure for at least 2 weeks clinically, accompanied by typical symptoms such as a change in appetite, insomnia or drowsiness, low self-esteem, and difficulty concentrating (Malhi and Mann, 2018; McCarron et al., 2021). The above symptoms lasting for 4–6 months are considered significant depression, and someone even has suicidal tendencies (Marwaha et al., 2023). World Health Organization statistics in 2023 reported that 280 million people worldwide were suffering from depression, and the number of people with depression increased even more dramatically during COVID-19 (Woody et al., 2017; Tang et al., 2024).
The causes of depression are related to a variety of factors, including family genetics, neurotransmitter imbalances, environmental stressors, and individual personality traits. Treatment options for depression involve the use of antidepressants, psychological counseling, and physical therapy. Although psychological interventions and social support are essential, medication is necessary for moderate or severe depression (Davidson, 2010). To prevent recurrent episodes of depression, most patients need to take antidepressants regularly for 6–12 months and even two consecutive years in patients over 50 years of age. However, some currently approved antidepressant drugs offer various side effects like restricted penetration, high toxicity, and low bioavailability (Mutingwende et al., 2021; Patel et al., 2022), and medications are ineffective in 1/3 of patients with major depression (Tao et al., 2024). Furthermore, Some antidepressants are susceptible to destruction by gastrointestinal enzymes and may not reach the site of action intact, resulting in reduced antidepressant efficacy (Buckman et al., 2018; Mutingwende et al., 2021; Monroe and Harkness, 2022). Nano-based drug delivery systems can be employed to overcome these limitations.
Nanocarriers drug delivery systems have been extensively developed to transport medicines through the blood-brain barrier (BBB) due to their various advantageous features (Sharma et al., 2019). Nanocarriers can improve the solubility of drugs to enhance their stability. Nanoformulations can be designed to release drugs under specific conditions, achieving sustained drug release, decreasing dosing frequency, and reducing toxic side effects (Agrawal et al., 2018; Sharma and Dang, 2020). Additionally, drugs loaded on nanocarriers are protected from enzymatic destruction, further enhancing the effect of antidepressants on the brain. This review targets common nanocarriers’ nature and function, considering the possible safety issues of NDDS and the challenges of clinical applications.
The BBB is a selectively permeable physiological barrier between peripheral blood and brain tissue (Stamp et al., 2023). While useful for protecting the brain from harmful substances, it also limits drug entry. Depression is a neurological disorder of an imbalance of monoamine neurotransmitters, and several antidepressants have been developed based on this principle. How to get drugs across the BBB to the brain has been a focus of research in the treatment of depression. There are several strategies for delivering most types of drugs to the brain (Table 1). Either the drug is modified or encapsulated in a suitable carrier for transportation, or the BBB is modified to adapt it to drug passage. It is also possible to bypass the BBB to achieve this goal. In this regard, one can distinguish these four approaches in the following.
The BBB is mainly composed of brain microvascular endothelial cells and their intercellular tight junctions, intact basement membranes, pericytes, and astrocytes, of which the endothelium is the main structure. BBB permeability increases by opening tight junctions or inhibiting efflux systems to accommodate drug passage.
Tight junctions are located between brain endothelial cells and are formed by ZO, Claudin, and Occludin proteins on endothelial cell membranes (Pandit et al., 2020). Tight junctions are a significant component in maintaining permeability and tissue homeostasis and constitute the physical barrier of the BBB (Wu et al., 2023). It can be artificially induced to open by interfering with the BBB through biological, chemical, and physical stimuli. Hypertonic solutions such as arabinose, mannitol, and urea infused into the internal carotid artery increase BBB permeability, enhance drug delivery, and return to normal osmolality in about 8 h (Sharma et al., 2023). Histamine, bradykinin, oleic acid, and other organisms or chemicals can also open the BBB briefly by injection. Moreover, the transportation of drugs to the brain can be modulated by physical disruption approaches based on optical, electrical, magnetic, and mechanical stimulation (Stamp et al., 2023). For example, focused ultrasound (FUS), a non-invasive technique that briefly opens the BBB in combination with microbubbles, has been used for brain delivery of antibodies, nano-agents, and growth factors (Wang et al., 2022). It is considered an effective way to diagnose and treat brain diseases.
Efflux transport is the process by which various metabolites, toxic substances, neurotransmitters, and drugs are transported beyond the BBB through the efflux system. The efflux system consists of efflux transporters on the cell membranes or the BBB. They transport substances from the brain, eliminating wastes and preventing harmful substances enter. ATP-binding cassette (ABC) transporters, such as P-gp, BCRP, and MRP, are ubiquitously found in almost all organisms (Li et al., 2021). Thus, regulating the expression of efflux transporters on the brain microvascular endothelial cell membrane promotes the drugs across BBB.
P-gp is an important efflux protein that belongs to the ABC transporter family and is expressed in high abundance on the BBB. It regulates the uptake of endogenous and exogenous molecules and the clearance of waste products. Intentional modulation of P-gp activity has been studied for enhancing drug delivery to the brain and does show a bright prospect in animal models (Chai et al., 2022). Elkhayat et al. (2017) conducted a randomized double-blind trial recruiting drug-resistant epilepsy patients to compare the therapeutic effects of anti-epileptic drugs (AEDs) in combination with verapamil (P-gp inhibitor) and placebo. The results showed an improvement in the frequency of attacks and symptoms in patients taking verapamil. Verapamil increases the concentration of AEDs within the brain by inhibiting their efflux. Natural components derived from plants such as alkaloids, coumarins, flavonoids, and terpenoids have been found to inhibit ATPase activity and also serve as P-gp inhibitors (Mollazadeh et al., 2018).
However, there are certain research challenges associated with enhancing drug delivery through modulating efflux transporters. It is essential to know the corresponding efflux transporters for drugs and possess appropriate inhibitors, and typically more than one efflux system is involved in the drug efflux process (Banks, 2016).
Another way to enhance brain delivery of drugs may alter the physicochemical properties of a drug by modifying it, which in turn improves the transmembrane transport of the drug. Lipolysis may achieve the right lipophilicity for the drug to cross the BBB, and either insufficient or too much lipolysis will affect the penetration rate of the drug. In addition, prodrugs can be synthesized to overcome the low bioavailability of medications due to gastrointestinal digestion. A prodrug is a compound obtained by modification of a drug that is inactive or less active in vitro but is transformed in vivo to release activity and exert medicinal effects. Inatani et al. (2023) demonstrated TP0473292 as an ideal oral prodrug by animal studies. TP0473292 is an adamantane carboxylic acid ester prodrug, which can be used as a novel antidepressant that enhances metabotropic glutamate 2/3 receptor antagonist oral bioavailability.
In addition to the standard oral and IV administration of drugs, drugs can be delivered directly to the brain by bypassing the BBB. For example, intracerebroventricular injection administration allows the drug to go directly to the brain to work, but this is an invasive route that can cause intracranial infections. In addition, intranasal administration bypasses the BBB to reach the central nervous system (CNS) via the mucous membranes of the olfactory region, which is non-invasive and improves drug distribution in the brain. In 2019, esketamine intranasal formulation was approved by the FDA for treatment-resistant depression (Cristea and Naudet, 2019).
Viral vectors are tools that utilize a modified virus to deliver genetic material to a host. The virus carrying the target gene is delivered to the host cell to alter the host cell’s gene by utilizing the high efficiency of viral transfection. Common viral vectors have been widely used in vaccine development and gene therapy, including lentiviruses, adenoviruses, and adeno-associated viruses (AAV) (Ewer et al., 2016; Milone and O’Doherty, 2018). In recent years, viral vector-mediated genes have opened up more possibilities for treating neurological diseases. Viral vectors have shown rosy therapeutic efficacy in studies for treating Parkinson’s, Alzheimer’s disease, and brain tumors, and the lack of suitable animal prediction models and species differences have hindered the transition of viral vectors from the laboratory to clinical applications (Choudhury et al., 2017; Lundstrom, 2023). AAV9, the AAV serotype, has been extensively researched for its capacity to penetrate the BBB to transduce astrocytes (Liu et al., 2021). While gene therapy has demonstrated potential in animal and cell investigations for CNS diseases, the majority of clinical trials have yet to be successful enough. Finding new approaches to increase the efficacy of gene therapy for CNS disorders is still crucial (Liu et al., 2021).
Viral vector-based gene therapy medications have been approved for clinical, but more viral vectors are still in the preclinical and laboratory stages (Shirley et al., 2020). The obstacles to its development are mainly the possibility of causing host immune responses or genetic mutations, and the safety assessment of vectors with high replication capacity is even more important (Lundstrom, 2023). Currently, viral and non-viral vectors are the main methods for gene delivery. Non-viral vectors, such as liposomes, micelles, polymeric substances, and various nanoparticles, are frequently utilized (Mantz and Pannier, 2019). Nanocarriers increase drug loading and gene therapy efficacy and exhibit superior biosafety and targeting properties compared to viral vectors (Pan et al., 2021).
Successive invaginations of the cell membrane form multivesicular vesicles fuse with the cell membrane and are released extracellularly to form exosomes (Pegtel and Gould, 2019). They contain many cellular components such as proteins, DNA, RNA, and cell surface proteins. They can transmit these signaling molecules to other cells, thus affecting the biological behaviors and functions of the recipient cells. Hence, exosomes are regarded as a new cell-to-cell information transfer system (Zhang and Yu, 2019; Kalluri and LeBleu, 2020).
Tavakolizadeh et al. (2018) indicated that miRNAs and exosomes were novel biomarkers for the diagnosis and treatment of depression. Li et al. (2020) demonstrated exosomes from NK cells carrying miRNA-270 alleviated mouse depressive symptoms. Fluorescently labeled exosomes were injected by tail vein, and fluorescent signals were detected in the brains of mice after 12–24 h. ELISA analysis showed a reduction in pro-inflammatory cytokines associated with depression. The results displayed that NK cell-derived exosomes alleviated depressive symptoms in mice by reducing depression-associated inflammatory factors (Li et al., 2020). Fan et al. (2022) also demonstrated that microglia-derived exosomes enriched in miR-146a-5p induced downregulation of factors that inhibit excitatory neurons (predominantly KLF4) and alleviated the depressant drug phenotype in a rat model of depression.
Exosomes are natural products secreted by cells and are, therefore, biocompatible and have low immunogenicity. However, there are still many challenges to the clinical application of exosomes as drug-delivery vehicles. It is difficult to produce and purify, and their drug-carrying capacity may be limited, restricting the large-scale application of exosomes in the clinic (Mondal et al., 2023).
Unlike viral vectors and exosomes, nanocarriers are synthetic materials with significant drug delivery advantages and unique physicochemical properties. The major advantage of NDDS is its ability to increase the bioavailability of drugs (Khizar et al., 2023). Nanocarriers protect drug molecules from enzymatic damage and ensure their intact entry into the bloodstream, thus improving drug stability (Liu et al., 2023). Moreover, control over the drug release rate can be obtained by modifying the composition and architecture of the nanocarriers. It prolongs the antidepressants’ beneficial effects on the body, hence lowering the frequency of their administration. Nanocarrier systems can also be designed to enhance targeted delivery by designing specific surface antibodies or ligands (Miao et al., 2023). This means that antidepressants can act directly on particular lesions in the brain, increasing efficacy while reducing toxicity to normal tissue.
Currently, traditional formulations used to treat depression have some drawbacks such as limited penetration, frequent dosing, and side effects. It is a barrier to antidepressants reaching the brain through traditional formulations. Nano-formulations are expected to overcome this limitations and might be an effective treatment for depression (Table 2).
A suitable CNS drug delivery system should have the capability to target the BBB and reach the therapeutic area. The Enhanced Permeation and Retention (EPR) effect serves as a fundamental mechanism for nanocarrier targeting strategies. The EPR effect refers to the fact that during tumor growth, the vascular structure of the tumor region is more sparse compared to normal tissue, leading to increased permeability of the tissue (Cheng et al., 2021). This pathological change is considered beneficial as it enhances the accumulation of nanoparticles within the tumor area, leading to the formation of elevated concentrations. However, there are some unpredictable elements to this passive targeting (Furtado et al., 2018). If the vasculature is not sufficiently sparse, the nanocarriers may struggle to accumulate effectively at the desired site. Additionally, this delivery modality is also affected by multiple factors such as high intertumoral fluid pressure, poor blood flow, and other factors.
Coupling specific targeting molecules on the surface of nanocarriers, these ligands bind to specific receptors or markers on the surface of diseased tissues or cells to obtain better specificity (Cheng et al., 2021). Research has demonstrated that the p11 gene is involved in the modulation of brain serotonin levels and has been studied for applications in gene therapy for depression (Gałecka et al., 2022). Brain microvascular endothelium is rich in insulin-like growth factor II (IGF-II) receptors, and Gandhi et al. utilized IGF-II monoclonal antibodies coupled to liposomes to enhance targeted delivery to the p11 gene (Gandhi et al., 2019). The analysis of SD-PAGE and Caumas Brilliant Blue staining indicated that IGF-II bound to the surface of liposomes well. They utilized fluorescence-emitting plasmid DNA instead of p11 cDNA to visualize the localization of the targeting agent within rat brain tissue. This indicated that the specific formulation displayed increased fluorescence and uptake of the formulation at every stage in comparison to the non-targeted formulation (Gandhi et al., 2019). IGF-II coupled with liposomal may be considered a novel gene therapy for depression. Ge et al. prepared ketamine (KA) loaded nanopreparations with the bifunctional peptide (Ang-2-Con-G, AC) as a targeting factor (Ge et al., 2023). A peptide (Ang-2) specifically binds to relevant proteins in the BBB to improve penetration of the BBB. Another peptide (Con-G) is directed towards the transport of KA to hippocampal and prefrontal cortical areas. Morris water maze test and the Novel Object Recognition test showed that KA could be directed to accumulate in the treated area, resulting in the recovery of cognitive functions.
Ligand-modified nanoparticles are widely explored for brain delivery. Furthermore, the characteristics of nanomaterials, such as size, shape, chemical composition, surface charge, and other factors, also play a crucial role in the overall efficacy of nanocarrier. Through purposeful design, nanomaterials may transport drugs to the target site efficiently (Table 3) (Cheng et al., 2021; Li et al., 2021).
Table 3. A summary of implications of parameter design of nanocarriers for achieving brain-targeted delivery.
Nanocarriers are mainly classified into organic nanocarriers and inorganic nanocarriers (Patel et al., 2022). Organic nanocarriers typically consist of polymers, lipid materials, proteins, and other organic substances, such as liposomes, nylon, nanocapsules, and micelles. Inorganic nanocarriers are composed of inorganic materials like metal oxides, and magnetic nanomaterials etc. (Figure 1). The nanocarriers were categorized into polymer-based carriers, lipid-based carriers, and metallic carriers according to the constituent materials, which are applied to the study of antidepressant therapy.
Figure 1. Schematic representation of various types of nanocarriers used commonly for nano-drug delivery systems. (A) Organic nanocarriers: liposom, solid lipid nanoparticle, polymeric nanoparticle, micelles, dendrimer, nanogels; (B) Inorganic nanoparticle carrier: metallic nanocarriers and magnetic nanoparticle.
Liposomes are spherical drug delivery vehicles ranging from 25–1,000 nm in diameter, composed primarily of natural or synthetic phospholipids (Li et al., 2019). In the aqueous environment, the hydrophilic head is exposed to the inner and outer aqueous phases, and the hydrophobic tail aggregates to form closed vesicles with a stable bilayer structure (Has and Sunthar, 2020). Liposomes’ unique structure and composition determine that they can encapsulate hydrophilic drugs and transport lipophilic drugs (Fan et al., 2021; Guimarães et al., 2021). Liposomes have been used as drug carriers in therapeutic studies for malaria, cancer, breast cancer, and other diseases due to their targeting, controlled release, decreased drug toxicity, and enhanced drug stability (Almeida et al., 2020; Memvanga and Nkanga, 2021; Forouhari et al., 2022).
Dandekar et al. (2018) loaded xenon (Xe) into liposomes for mouse models of drug-administered depression to prove the antidepressant effects of Xe. The stimulatory effects of Xe-liposomes on locomotion in mice were conducted by spontaneous locomotion monitoring and forced swimming tests in rats. Behavioral studies in the mouse models of depression have shown that rats spend less time swimming and standing still and are much more active. Additionally, a group led by Nodari prepared liposomes loaded with Vortioxetine hydrobromide (VXT-Ls) to improve the stability of the refractory antidepressant Vortioxetine hydrobromide (VXT) stability and reduce drug-free toxicity (Nodari et al., 2022). This showed that the cell survival rate in the VXT-Ls group was significantly higher than in the VXT group at the same drug concentration. Studies have indicated liposomes with amphiphilic properties are positive carriers for transporting hydrophilic and hydrophobic drugs.
The transition of liposomes from the laboratory to large-scale production is a complex process in which much work remains. The stability of liposomes is a crucial factor in the safe transportation of encapsulated drugs in vivo to exert their therapeutic effects and a significant challenge in the production process of liposomal formulations (Guimarães et al., 2021). Therefore, there is also a need for more rapid assays to provide safety assurance for liposome characterization and quality (Shah et al., 2020).
Solid lipid nanoparticles (SLN) are discs or elliptical spherical particles composed of solid lipids, water, and surfactants at room temperature, and the drug may be attached to the surface of the carrier or encapsulated in a core (Scioli Montoto et al., 2020). SLN has the advantages of enhanced drug penetration, targeted delivery, loading of amphiphilic drugs, and protection from hepatic clearance for safe transportation and improved drug efficacy (Mishra et al., 2018). SLN prepared by various techniques (e.g., cold or hot homogenization, microemulsion method, ultrasound or high-speed homogenization, etc.) has been widely investigated for applications in vaccines, anticancer, antimicrobial, and gene delivery (Rajpoot, 2019).
Khan et al. (2021) demonstrated the antidepressant effects of diosgenin in a mouse animal model. Its low water solubility and low bioavailability affect its efficacy as an antidepressant. Diosgenin-loaded SLN was used to assess its antidepressant potential through a variety of behavioral tests, and the results indicated that SLN-encapsulated diosgenin had a positive effect in alleviating depressive behaviors. Thus, SLN allows improved bioavailability and penetration of drugs. The tricyclic antidepressant Amoxapine (AMX) is difficult to dissolve in water as well as to overcome first-pass metabolism, which affects its use as an antidepressant. Pawar et al. (2023) prepared solid liposome-encapsulated amoxapine (AMX-SLNs) by using a single emulsification method to enhance its antidepressant effect. Plasma and brain tissue AMX concentrations were determined using high-performance liquid chromatography (HPLC) and LC-MS/MS methods. Results confirmed a 1.6-fold increase in bioavailability and a 5.8-fold increase in brain drug concentration of AMX-SLN compared to oral AMX. Results indicated that SLN is an effective transport vehicle for increasing the distribution concentration of insoluble drugs in the brain.
Due to the instability of SLN, possible aggregation during storage, and limited drug loading capacity, nanostructured lipid carriers were developed to overcome these problems. Nanostructured lipid carriers (NLC) are regarded as an upgraded version of SLNs, containing more solid lipids than compared to liquid lipids, allowing for increased capacity for drug incorporation. Compared with SLN, NLC has better stability, controlled-release capability, and higher drug-loading capacity (Viegas et al., 2023).
Gul et al. (2022) designed Agomelatine-loaded nanostructured lipid carriers (AGM-NLC) to study the antidepressant effect of Agomelatine (AGM), a novel antidepressant encapsulated by NLC. The drug’s ability to act as an antidepressant was evaluated by forced swimming and tail-hanging tests, revealing that mice in the AGM-NLC group exhibited significantly decreased resting times compared to the control group. The pathogenesis of depression is linked to neurological impairment and inflammation. Therefore, they also evaluated the damage of AGM-NLC on brain neurons in mice, focusing on the effects of the drug on the levels of the inflammatory mediators TNF-α and COX-2. The frontal cortex of the mouse brain was examined under a microscope using Ni-staining, and AGM-NLC effectively maintained the quantity and morphological traits of the remaining neurons. Moreover, immunohistochemistry revealed decreased levels of TNF-α and COX-2 expression (Gul et al., 2022). These findings indicated that the administration of AGM may decrease in vivo toxicity and enhance the antidepressant properties when combined with NLC.
Dendritic macromolecules are specialized circular structures with many potential active sites and hyperbranched interactions (Shcharbin et al., 2017). Drugs could be encapsulated in the interior of dendritic macromolecules or affixed to their surfaces by hydrophobic forces or covalent binding, depending on the stability of the drug molecule (Dening et al., 2016; Patel et al., 2022). The unique structure and surface functional groups of dendritic macromolecules provide benefits for drug delivery, including biocompatibility, prolonged circulation time, and targeted delivery (Mutingwende et al., 2021). Polyamide-amine (PAMAM) is a tree-like macromolecule widely utilized in biomedical fields (Wilczewska et al., 2012). Tripathi et al. addressed the limitations associated with curcumin, including its instability, high metabolism, and poor absorption, by developing a novel delivery platform utilizing generation 4 poly-amido-amine (G4 PAMAM) as a carrier to enhance the bioavailability of curcumin (Tripathi et al., 2020). They conducted force swimming and tail-hanging tests with a stress-induced mouse model. Plasma curcumin levels were analyzed by reverse high-performance liquid chromatography to assess the pharmacokinetic parameters in vivo. The results showed an increase in peak concentration (Cmax), a delay in reaching maximum concentration, and a decrease in the mouse resting time in water. This suggests that G4 PAMAM exhibits a slow-release property that enhances efficacy and diminishes curcumin metabolism (Tripathi et al., 2020).
Polymeric micelles are nanostructures composed of amphiphilic block polymers (Nishiyama and Kataoka, 2006). When the surfactant concentration reaches the critical micelle concentration, the amphiphilic molecular polymer self-assembles in aqueous solution to form an ordered arrangement of thermodynamically stable lipophilic core and hydrophilic outer shell (Owen et al., 2012; Cagel et al., 2017). Polymeric micelles are known for their small size, structural stability, and minimal toxicity, and they also regulate the release of hydrophobic drugs and enhance drug availability and duration in the body (Kataoka et al., 2001; Rub et al., 2013). The highly functional core and shell allow them to play a vital role in drug delivery systems. There is an interaction between the hydrophobic nucleus and the drug, which enables the drug to be retained in the lipophilic nucleus, controlling the slow release of the drug from the micelle (Lin et al., 2003; Zhang and Zhuo, 2005; Hwang et al., 2020). Polymer micelles typically have a particle size ranging from 10 to 200 nm, making them difficult to recognize and capture by the endothelial reticular system in blood circulation. This characteristic enables the drug-loaded polymer micelles to remain stable and extend the time circulating in the bloodstream (Croy and Kwon, 2006; Lu and Park, 2013). The hydrophilic shell reduces the binding of serum proteins to the complement system and avoids the loss of the encapsulated drug during the body’s circulation (Xiao et al., 2011). Therefore, hydrophilic blocks should be added to the design of polymer micelles to avoid polymer adsorption by plasma proteins or removal from the body by complement activation (Hwang et al., 2020). Polyethylene glycol has been the most commonly used hydrophilic polymer in polymer micelles. It also showed that polyethylene glycol is permeable to mucus, which helps nanomedicines to cross the mucosal barrier effectively (Maisel et al., 2016).
Abourehab et al. (2018) analyzed the distribution concentration of polymer micelles loaded with dapoxetine (DPX-PM) in the brain. Results showed that DPX-PM reached its maximum drug concentration in the brain after 1.5 h of oral administration, and the drug concentration was 1.2 times that of ordinary DPX tablets. This illustrates that polymeric micelles may not only overcome the aggressive physical environment of the gastrointestinal tract but also prolong the duration of the drug’s residence in the brain (Abourehab et al., 2018). Moreover, El-Helaly and Rashad (2024) investigated the antidepressant activity of Mirtazapine (MTZ) through in vitro solubility and coefficient of dissolution studies, as well as drug content determination in vivo. The non-ionic nature of polymeric micelles reduces electrostatic repulsion between drug molecules, forming a hydrophilic outer shell around the nucleus. It greatly improves the solubility and stability of drug-loaded micelles. This finding indicates that polymeric micelles could enhance the oral bioavailability and solubility of drugs like MTZ (El-Helaly and Rashad, 2024).
Poly(lactic-co-glycolic acid) (PLGA) is a copolymer formed by the random polymerization of lactic acid and hydroxyacetic acid (Furtado et al., 2018). Parenteral administration of PLGA decreases the frequency of dosing, improves patient compliance, reduces dosage, and mitigates systemic side effects (Su et al., 2021). The FDA has approved PLGA for use as a parenteral transport vehicle in drug delivery systems (Singh et al., 2021).
Cayero-Otero et al. (2019) employed venlafaxine (VLF) as an antidepressant drug model encapsulated within PLGA nanomaterials (VLF-PLGA NPs) to achieve controlled release of VLF, thereby augmenting therapeutic levels of venlafaxine in the bloodstream. In vivo, biodistribution studies demonstrated that PLGA could cross the BBB to deliver the drug to the brain to improve bioavailability. Duloxetine hydrochloride (DXH) is a psychotropic drug for the treatment of major depressive disorder. Its poor water solubility and extensive metabolism necessitate frequent dosing to compensate for reduced bioavailability. Singh et al. prepared DLX-loaded PLGA (DLX-PLGA) using an emulsion solvent evaporation technique to enhance its antidepressant activity (Singh et al., 2021). They employed HPLC to analyze DLX levels present in blood and brain specimens, revealing that concentrations were higher than those of commercially available drugs in blood and brain. Moreover, the forced swimming test showed that the administration of DLX-PLGA notably reduced the immobility duration of the mice. Studies have shown that intramuscular injection of DLX-loaded polymer nanoparticles is a viable strategy to reduce the frequency of dosing and may help promote patient compliance for the effective management of major depressive disorder.
Chitosan is a biopolymer with good biocompatibility and biodegradability (Silant’ev et al., 2023). Its degradation components are non-toxic, do not re-accumulate within the body, and are non-antigenic, increasing its biomedical utility in the CNS due to its potential ability to cross the BBB (Silant’ev et al., 2023).
Tong et al. (2017) prepared PLGA-chitosan nanoparticles loaded with desvenlafaxine (DVF PLGA-CN) through solvent-emulsion evaporation technique and evaluated the antidepressant effectiveness of desvenlafaxine in a male rat depression model. DVF PLGA-CN nanoparticles significantly enhanced the levels of serotonin, norepinephrine, and dopamine within the brain when compared with depressed controls. Biochemical results showed that DVF PLGA-CN nanoparticles effectively alleviated depressive symptoms in rats. Salem et al. (2023) prepared and optimized DXH-loaded PLGA-chitosan nanoparticles (DXH-PLGA-CS-NPs) to improve the bioavailability of DXH. The sucrose preference test and forced swimming test indicated increased mobility and sucrose consumption in rats after the administration of DXH-PLGA-CS-NPs. This suggests that DXH-PLGA-CS-NPs may be a safe, stable, and efficient method for delivering drugs to address depression.
In another study, Lima et al. (2019) evaluated changes in Swiss mice exposed to different concentrations of chitosan-coated zein nanoparticles (ZNP-CS) for a short period. They assessed cognitive and behavioral changes in the mice through experiments such as behavioral tests like mazes and target recognition and motor performance assessments. Results showed that mice were sedentary for a longer time in the hanging tail test, that ZNP-CS induced depressive-like behaviors, and that exposure to ZNP-CS may lead to neurotoxic effects. This lays the foundation for the biosafety assessment of chitosan in the field of drug delivery.
Metallic nanoparticles have the advantages of the therapeutic efficacy of drugs by enabling targeted delivery and mitigating multidrug resistance. They are widely employed as vehicles for delivering a range of therapeutic agents such as antibodies, nucleic acids, chemotherapeutic drugs, etc. (Chandrakala et al., 2022). Metallic nanocarriers enhance the aqueous solubility of hydrophobic pharmaceutical compounds, prolong the systemic circulation of drugs in the bloodstream, and impede or prevent the rapid renal excretion of drugs (Chandrakala et al., 2022). In the field of drug delivery, common metal nanocarriers are gold, silver, iron oxide, and zinc oxide nanoparticles (Rathnam et al., 2024).
Saeidienik et al. (2018) studied the effect of iron oxide nanoparticles on lipopolysaccharide-induced depressive behavior in rats. Rats were exposed to the forced swim test (FST) and the open field test (OFT), with groups receiving iron nanoparticle treatment showing an increase in swimming duration and a decrease in the duration of immobilization. Results indicated the beneficial affect of administering iron nanoparticles in reducing depressive symptoms, which may be attributed to the influence of iron oxide nanoparticles on neurotransmitters and inflammatory factors associated with depression. Khadrawy et al. (2021) investigated the antidepressant effects of curcumin-coated iron oxide nanoparticles (Cur-IONs) using a rat model of rifampicin-induced depression. Lipid peroxidation, nitric oxide levels, monoamine oxidase activity, and monoamine neurotransmitters were assessed in the brain tissue of rats. Results showed that Cur-IONs may modulate depressive symptoms by increasing the synthesis of monoamine neurotransmitters and preventing their degradation.
Similarly, Fahmy et al. (2024) augmented the therapeutic efficacy for depression by synergistically incorporating curcumin with zinc oxide nanoparticles, leading to improved pharmacokinetic properties of curcumin. The study reported that exposure to zinc oxide nanoparticles (ZnO NPs) may reduce monoamine neurotransmitters in the brain (Jeyhoonabadi et al., 2022). Jeyhoonabadi et al. (2022) identified the protective impact of the novel psychotherapeutic medication betaine against ZnO NPs-induced toxicity in mice. Behavioral tests such as the FST showed that betaine mitigated depression-like behavior in mice. Furthermore, the histopathological in the hippocampus of mice indicated that the majority of cells in the group treated with betaine and ZnO NPs exhibited preserved morphology and dimensions.
Antidepressants currently approved for marketing by the FDA to alleviate depressive symptoms by modulating monoamine neurotransmitters and are mainly categorized as follows (Table 4).
Nearly half of the patients with significant depression still do not respond to first-line antidepressants. Also, there are side effects of conventional antidepressants and interactions between drugs or drugs and food, which has prompted scientists to start exploring more antidepressants. Several natural, plant-derived active ingredients are beginning to be studied for the treatment of depression, which may have fewer side effects and show better biocompatibility than synthetic drugs.
Many natural ingredients apply to antidepressant research, such as icariin, ginsenoside, chrysin, etc. However, its ability to cross the BBB is limited, and efficacy through oral delivery is inadequate. Li et al. (2022) explored the antidepressant mechanism of ginsenoside Rg1, confirming that ginsenoside Rg1 down-regulates the expression of GAS5 to attenuate microglia activation and alleviate depressive-like behavior. Yu et al. (2023) designed a drug-carrying platform consisting of Borneol and graphene to wrap ginsenoside Rg1 (BO-GO-Rg1). Behavioral tests in rats utilizing Borneol (BO) to enhance BBB permeability demonstrated that the compound BO-GO-Rg1 alleviated symptoms of anxiety and depression in rats 1-week following administration of BO-GO-Rg1 (Yu et al., 2023). Moreover, C-glycosylated flavonoids have been found to have antidepressant activity in the passion flower leaves, which grow mainly in Brazil. Alves et al. (2020) prepared nanoparticle-loaded passion flower leaf extract by nanoprecipitation to enhance its pharmacological activity. Results indicated that the extract exhibited low virulence, and high biocompatibility, and its antidepressant effect was similar to oral nortriptyline (Alves et al., 2020). Hypericin, an extract of Hypericum perforatum, is efficacious in improving mild and moderate depressive states. Waqar et al. (2023) evaluated the antidepressant activity of chrysin using nanoemulsion as a carrier for transnasal delivery. Tan et al. (2024) improved the poor water solubility, low bioavailability, and poor permeability of chrysin by using black phosphorus nanosheets as a carrier, significantly alleviating depressive symptoms. Besides, icariin primarily used for male reproduction, has shown potential therapeutic and preventive effects in the nervous system and can be used as an anti-inflammatory agent for depression, etc. (Jin et al., 2019; Luo et al., 2022). The emergence of natural antidepressants and the utilization of nano-drug delivery systems present encouraging prospects for the management of depression.
Oral, intranasal, and injection are the extensively studied routes for utilizing nanocarriers in depression treatment. There is an overview of the various methods for delivering nanocarriers (Figure 2).
Figure 2. Graphical overview of the routes toward nanocarrier-mediated administration. (A) Injections: nanopreparations are administered via intramuscular, subcutaneous, intravenous, and intradermal. (B) Nano-based eye drop: enhanced ability to penetrate the ocular barrier and prolonged drug residence time at the ocular surface. (C) Intranasal transport route of therapeutic nanomaterials from the nasal cavity to the brain through nasal mucosa, trigeminal nerve and respiratory epithelium. Intranasal drugs may be transported to the olfactory bulb by paracellular or transcellular transport along olfactory neurons or across the nasal epithelium. Within the nasal respiratory epithelial region, the drug would travel via the trigeminal nerve to the CNS, or traverse the respiratory epithelium to access the bloodstream prior to entering the CNS. (D) Oral: nanocarrier-encapsulated drug protects the drug from destruction by digestive enzymes and improves drug absorption through the intestinal epithelium. (E) Transdermal: the interaction between nanocarriers and skin is influenced by regulating the lipophilicity, size, shape, and surface charge of the nanocarriers, which improves the skin penetration efficiency of the nanocarriers.
Oral administration is the ideal route of administration for antidepressants because it is non-invasive, convenient, and low-cost. Nevertheless, oral medications undergo metabolism by the gastrointestinal wall or liver, which can diminish absorption rates and consequently reduce their effectiveness (Sato et al., 2023). Besides, the complex physiology of the BBB limits drug absorption. A variety of nanocarriers have been developed for oral antidepressants to improve the neuroavailability of drugs in the brain.
The curcumin extract (TUR) has been shown to have good antidepressant activity in various animal studies, and low oral solubility and malabsorption limit its clinical use. Sheng et al. designed an orally administered TUR nanoemulsion (TUR-NE) using PEG-400 and Tween 80 as surfactants to alleviate this problem (Sheng et al., 2023). The developed TUR-NE has a 5-fold increase in peak plasma concentration compared to the generic drug. Furthermore, in the model of Chronic Unpredictable Mild Stress (CUMS), tissue sections from the kidney and heart in the fluoxetine hydrochloride group were stained with mild lesions, whereas no lesions were evident in the TUR-NE group. The results showed that the nanoemulsions improve the water-solubility of the drug, which is more easily absorbed by the gastrointestinal tract and enhances the pharmacokinetics of the orally administered drug.
El-Helaly and Rashad (2024) established a polymeric micelle-based delivery platform to improve the oral bioavailability of mirtazapine. Pharmacokinetics showed that mirtazapine-loaded polymeric micelles (MTZ-PM) reached the maximum drug concentration faster than commercial mirtazapine tablets. This is because polymeric micelles improve the solubility and stability of the drug in the gastrointestinal tract and are more readily absorbed by the intestinal cells with the assistance of surfactants. They utilized the adsorption of Aerosil 200 to make the MTZ-PM liquid formulation into a dry powder, which was then mixed with a proportional surfactant and compressed into tablets. This provides the pharmaceutical industry with new ideas for making polymer micelles into oral tablets with high stability and bioavailability. The development of NDDS has undoubtedly opened up more possibilities for oral drug delivery for the treatment of depression.
Injectable administration delivers the drug directly into the circulation and avoids first-pass metabolism thus improving the efficacy of antidepressants. KA has been shown favorable antidepressant effect in treatment-resistant depression and is commonly delivered through intravenous infusion (Le Nedelec et al., 2018). Le Nedelec et al. (2018) compared plasma drug concentrations following the administration of KA through various routes in rats, including subcutaneous (SC), intramuscular (IM), intravenous infusion (IVI), and intravenous bolus (IVB) methods. Liquid chromatography-mass spectrometry measurements indicated that administration by the IBV route produced significantly elevated Cmax levels in comparison to alternative administration routes. Del Sant et al. (2020) monitored changes in blood pressure and heart rate in patients with treatment-resistant depression after subcutaneous injections of esketamine. It was found that there was minimal variation in blood pressure after repeated subcutaneous injections of esketamine and was effective in patients with comorbidities such as hypertension and diabetes mellitus. A randomized, double-blind trial designed by Loo et al. (2023) aimed to evaluate the antidepressant effectiveness and safety of repeated subcutaneous administrations of KA. This aligns with the findings of Del Sant et al.’s experimental study, suggesting that subcutaneous administration may offer a more straightforward and efficient approach to drug delivery.
Intranasal administration can be used for local, systemic, and even central nervous system administration (Keller et al., 2022). At the nasal cavity, drug molecules might bypass the BBB and be absorbed from the nasal mucosa into the brain via the trigeminal and olfactory pathways (Erdő et al., 2018). Compared to oral or intravenous administration, intranasal administration is non-invasive, fast-acting, has no first-pass metabolism and reduces side effects. Xu et al. (2020) designed a complex system loaded with Icariin to improve the antidepressant activity of Icariin and observed its antidepressant effect by nasal administration. Results suggested that the drug could be released continuously for 36 h, and fluorescently labeled icariin nanogel was detected in the brains of mice after 5 min of intranasal administration and reached the maximum fluorescence intensity within 30 min. In the classic CUMS animal model of depression, nasal administration shortened forced swimming and tail suspension times compared to oral administration. This suggests that nasal administration allows the drug to reach the brain quickly and accumulate, increasing the antidepressant activity of the drug (Xu et al., 2020). Intranasal administration has been approved primarily for treating localized systemic diseases, and its use in CNS disorders has attracted widespread interest. The clinical trials show the advantages of transnasal administration of esketamine in relieving depressive symptoms and preventing relapse (Daly et al., 2019; Popova et al., 2019; An et al., 2021).
In recent years, the application of nanoparticles has included biological imaging, targeted drug delivery, cancer vaccine delivery, mediated photothermal therapy, photodynamic therapy, etc. (Denkova et al., 2018; Zhao et al., 2022). In addition, nanomaterials have applications in brain tumors, epilepsy, Parkinson’s, Alzheimer’s, and other central system diseases (Islam et al., 2020). Numerous patents have been filed for nanoparticle drug delivery technologies for treating and diagnosing neurological disorders (Muheem et al., 2021).
As mentioned above, the nanocarriers can enter the body to achieve therapeutic effects by ingestion, inhalation, injection, and skin absorption. Oral administration might be the most commonly used route, and nanocarriers have shown an excellent future for oral drug delivery. However, studies have reported that the intestine is also an essential part of the immune system, maintaining the balance of intestinal flora and resisting oral pathogens. Although oral nanoformulation can improve the bioavailability of drugs, it may also cause an immune response (Ojer et al., 2015). Hort et al. (2021) encapsulated the drug within nanoemulsions (NE) and administered them to rats via oral and intraperitoneal injections of 200, 400, or 800 mg/kg of body weight. Results exhibited that oral ingestion of NE did not induce alterations in biochemical parameters in rats, whereas intraperitoneal injection at 800 mg/kg resulted in an inflammatory reaction. This finding indicates that the oral intake of nanoemulsions is safe, whereas elevated doses of parenteral administration may lead to adverse toxic responses (Hort et al., 2021). In addition, other studies have reported that nanoparticles can quickly accumulate in the brain after passing through the BBB, enter nerve cells, and produce neurotoxicity. Neurotoxic effects can directly affect the structure and function of the nervous system, thus affecting the function of the BBB (Teleanu et al., 2018). It is necessary to consider nanocarriers’ toxicity, metabolism, side effects, and other factors before delivering nanoformulations to the body through nano-drug delivery systems.
Toxicity assessment of nanoparticles is performed mainly in vivo and in vitro. In vitro techniques are commonly used to detect cytotoxicity, reactive oxygen species levels, immunotoxicity, carcinogenicity, hepatocytotoxicity, and genotoxicity by fluorescent probes, MTT, ELISA, and Tepan blue dye. The investigators simulated the performance of nanocarriers in the human body through animal models to ensure the safety of nanocarriers in clinical applications. The toxicity of nanoparticles is related to various factors such as route of administration, ingested dose, surface modification, particle size, shape, polydispersity coefficient, and others (Teleanu et al., 2018; Azarnezhad et al., 2020). Despite nanoparticles being extensively evaluated in laboratory and animal studies during the preclinical stage, their potential effects on the human body remain uncertain. To transfer nanoparticles from the laboratory to the clinic, it is necessary to master the interactions of nanoparticles with various tissue cells and blood components in the healthy or diseased state of the human body. This requires a complex and rigorous standard system to comprehensively evaluate nanoparticles from synthesis and characterization to drug delivery. Furthermore, the implementation of nanomedicine in clinical settings requires the consideration of intellectual property rights, governmental standardization, and market regulation, posing considerable challenges for both the pharmaceutical industry and regulatory bodies (Muheem et al., 2021). Ahmad et al. pointed out that nanotoxicology impacts the development and commercializing of nanocarriers, which requires the guidance of scientific teams and professional analysis (Ahmad et al., 2022).
NDDS provides essential opportunities for depression treatment. Currently used antidepressants have the disadvantages of high toxicity, poor permeability, and low bioavailability. NDDS encapsulates antidepressant drugs in nanoscale carriers, enabling precise release and targeting delivery in vivo, thereby improving therapeutic efficacy and patients’ quality of life. Although NDDS have shown excellent capability in treating depression, their clinical application still faces several challenges: 1) Toxicity studies are incomplete 2) Inadequate characterization techniques 3) Lack of standardized norms and regulations.
Much remains to be done to address the above issues. Firstly, The behavior of the nanocarriers in vivo such as circulation time, tissue distribution, and clearance rate needs to be determined. Also, the current commonly used toxicity evaluation methods need to be more accurate, and new toxicity evaluation strategies based on nanomaterials’ specificity in conjunction with nanotoxicology and neurology need to be designed (Szabat-Iriaka and Le Borgne, 2021). Secondly, there needs to be a transparent characterization scheme for the physicochemical properties of nanocarriers, which might be accountable for their letdown effect in late clinical trial stages. Interdepartmental collaboration between the National Cancer Institute (NCI), the National Institute of Standards and Technology (NIST), and the FDA established the Nanotechnology Characterization Laboratory (NCL) to improve the success of Phase II Human Trials. To adapt to the development of nanomedicine, NCL also needs to expand its popularity and communicate and collaborate with specialized pharmaceutical companies to understand the advances in nanomedicine preparation (Muheem et al., 2021). Finally, due to the specificity and complexity of nanotechnology, the traditional regulatory approach to drugs may not be able to fully cover nanomedicine, which will require multi-sectoral joint efforts to develop new regulations and regulatory measures (Mohapatra et al., 2024). The study of NDDS encompasses several academic disciplines, including but not limited to materials science, biology, pharmacology, etc. Strengthening interdisciplinary collaboration might promote the research and development of NDDS. Moreover, it is important to consistently exploring novel methodologies and technological domains, such as the integration of artificial intelligence and nanotechnology, as well as improving the design, material selection, and fabrication processes of nanocarriers.
Nanocarrier systems improve efficacy and reduce side effects by increasing the bioavailability of the drug, enabling targeted delivery and controlled release, thus sheding light on the novel solution through depression treatment may be developed.
XF: Writing–original draft, Formal Analysis, Data curation. PJ: Data curation, Writing–original draft, Visualization. DZ: Project administration, Writing–review and editing, Supervision, Funding acquisition, Conceptualization.
The author(s) declare that financial support was received for the research, authorship, and/or publication of this article. This work was supported by the University of Electronic Science and Technology of China (ZYGX2021YGLH217), Department of Science and Technology of Sichuan Province (2023YFS0311), and Science & Technology Bureau of Chengdu (YF05-00060-SN).
The authors declare that the research was conducted in the absence of any commercial or financial relationships that could be construed as a potential conflict of interest.
All claims expressed in this article are solely those of the authors and do not necessarily represent those of their affiliated organizations, or those of the publisher, the editors and the reviewers. Any product that may be evaluated in this article, or claim that may be made by its manufacturer, is not guaranteed or endorsed by the publisher.
Abourehab, M. A., Ahmed, O. A., Balata, G. F., and Almalki, W. H. (2018). Self-assembled biodegradable polymeric micelles to improve dapoxetine delivery across the blood-brain barrier. Int. J. Nanomedicine 13, 3679–3687. doi:10.2147/ijn.S168148
Agrawal, M., Saraf, S., Saraf, S., Antimisiaris, S. G., Hamano, N., Li, S. D., et al. (2018). Recent advancements in the field of nanotechnology for the delivery of anti-Alzheimer drug in the brain region. Expert Opin. Drug Deliv. 15 (6), 589–617. doi:10.1080/17425247.2018.1471058
Ahmad, A., Imran, M., and Sharma, N. (2022). Precision nanotoxicology in drug development: current trends and challenges in safety and toxicity implications of customized multifunctional nanocarriers for drug-delivery applications. Pharmaceutics 14 (11), 2463. doi:10.3390/pharmaceutics14112463
Almeida, B., Nag, O. K., Rogers, K. E., and Delehanty, J. B. (2020). Recent progress in bioconjugation strategies for liposome-mediated drug delivery. Molecules 25 (23), 5672. doi:10.3390/molecules25235672
Alves, J. S. F., Silva, A., da Silva, R. M., Tiago, P. R. F., de Carvalho, T. G., de Araújo Júnior, R. F., et al. (2020). In vivo antidepressant effect of Passiflora edulis f. flavicarpa into cationic nanoparticles: improving bioactivity and safety. Pharmaceutics 12 (4), 383. doi:10.3390/pharmaceutics12040383
An, D., Wei, C., Wang, J., and Wu, A. (2021). Intranasal ketamine for depression in adults: a systematic review and meta-analysis of randomized, double-blind, placebo-controlled trials. Front. Psychol. 12, 648691. doi:10.3389/fpsyg.2021.648691
Azarmi, M., Maleki, H., Nikkam, N., and Malekinejad, H. (2020). Transcellular brain drug delivery: a review on recent advancements. Int. J. Pharm. 586, 119582. doi:10.1016/j.ijpharm.2020.119582
Azarnezhad, A., Samadian, H., Jaymand, M., Sobhani, M., and Ahmadi, A. (2020). Toxicological profile of lipid-based nanostructures: are they considered as completely safe nanocarriers? Crit. Rev. Toxicol. 50 (2), 148–176. doi:10.1080/10408444.2020.1719974
Banks, W. A. (2016). From blood-brain barrier to blood-brain interface: new opportunities for CNS drug delivery. Nat. Rev. Drug Discov. 15 (4), 275–292. doi:10.1038/nrd.2015.21
Buckman, J. E. J., Underwood, A., Clarke, K., Saunders, R., Hollon, S. D., Fearon, P., et al. (2018). Risk factors for relapse and recurrence of depression in adults and how they operate: a four-phase systematic review and meta-synthesis. Clin. Psychol. Rev. 64, 13–38. doi:10.1016/j.cpr.2018.07.005
Cagel, M., Tesan, F. C., Bernabeu, E., Salgueiro, M. J., Zubillaga, M. B., Moretton, M. A., et al. (2017). Polymeric mixed micelles as nanomedicines: achievements and perspectives. Eur. J. Pharm. Biopharm. 113, 211–228. doi:10.1016/j.ejpb.2016.12.019
Cayero-Otero, M. D., Gomes, M. J., Martins, C., Álvarez-Fuentes, J., Fernández-Arévalo, M., Sarmento, B., et al. (2019). In vivo biodistribution of venlafaxine-PLGA nanoparticles for brain delivery: plain vs. functionalized nanoparticles. Expert Opin. Drug Deliv. 16 (12), 1413–1427. doi:10.1080/17425247.2019.1690452
Chai, A. B., Callaghan, R., and Gelissen, I. C. (2022). Regulation of P-glycoprotein in the brain. Int. J. Mol. Sci. 23 (23), 14667. doi:10.3390/ijms232314667
Chandrakala, V., Aruna, V., and Angajala, G. (2022). Review on metal nanoparticles as nanocarriers: current challenges and perspectives in drug delivery systems. Emergent Mater 5 (6), 1593–1615. doi:10.1007/s42247-021-00335-x
Cheng, Z., Li, M., Dey, R., and Chen, Y. (2021). Nanomaterials for cancer therapy: current progress and perspectives. J. Hematol. Oncol. 14 (1), 85. doi:10.1186/s13045-021-01096-0
Choudhury, S. R., Hudry, E., Maguire, C. A., Sena-Esteves, M., Breakefield, X. O., and Grandi, P. (2017). Viral vectors for therapy of neurologic diseases. Neuropharmacology 120, 63–80. doi:10.1016/j.neuropharm.2016.02.013
Cristea, I. A., and Naudet, F. (2019). US Food and Drug Administration approval of esketamine and brexanolone. Lancet Psychiatry 6 (12), 975–977. doi:10.1016/s2215-0366(19)30292-5
Croy, S. R., and Kwon, G. S. (2006). Polymeric micelles for drug delivery. Curr. Pharm. Des. 12 (36), 4669–4684. doi:10.2174/138161206779026245
Dadfar, S. M., Roemhild, K., Drude, N. I., von Stillfried, S., Knüchel, R., Kiessling, F., et al. (2019). Iron oxide nanoparticles: diagnostic, therapeutic and theranostic applications. Adv. Drug Deliv. Rev. 138, 302–325. doi:10.1016/j.addr.2019.01.005
Daly, E. J., Trivedi, M. H., Janik, A., Li, H., Zhang, Y., Li, X., et al. (2019). Efficacy of esketamine nasal spray plus oral antidepressant treatment for relapse prevention in patients with treatment-resistant depression: a randomized clinical trial. JAMA Psychiatry 76 (9), 893–903. doi:10.1001/jamapsychiatry.2019.1189
Dandekar, M. P., Peng, T., McPherson, D. D., Quevedo, J., Soares, J. C., and Huang, S. L. (2018). Intravenous infusion of xenon-containing liposomes generates rapid antidepressant-like effects. Prog. Neuropsychopharmacol. Biol. Psychiatry 86, 140–149. doi:10.1016/j.pnpbp.2018.03.011
Davidson, J. R. (2010). Major depressive disorder treatment guidelines in America and Europe. J. Clin. Psychiatry 71 (Suppl. E1), e04. doi:10.4088/JCP.9058se1c.04gry
Del Sant, L. C., Sarin, L. M., Magalhães, E. J. M., Lucchese, A. C., Tuena, M. A., Nakahira, C., et al. (2020). Effects of subcutaneous esketamine on blood pressure and heart rate in treatment-resistant depression. J. Psychopharmacol. 34 (10), 1155–1162. doi:10.1177/0269881120922955
Dening, T. J., Rao, S., Thomas, N., and Prestidge, C. A. (2016). Oral nanomedicine approaches for the treatment of psychiatric illnesses. J. Control Release 223, 137–156. doi:10.1016/j.jconrel.2015.12.047
Denkova, A. G., de Kruijff, R. M., and Serra-Crespo, P. (2018). Nanocarrier-mediated photochemotherapy and photoradiotherapy. Adv. Healthc. Mater 7 (8), e1701211. doi:10.1002/adhm.201701211
Ding, Q., Li, H., Tian, X., Shen, Z., Wang, X., Mo, F., et al. (2016). Zinc and imipramine reverse the depression-like behavior in mice induced by chronic restraint stress. J. Affect Disord. 197, 100–106. doi:10.1016/j.jad.2016.03.017
Dong, X. (2018). Current strategies for brain drug delivery. Theranostics 8 (6), 1481–1493. doi:10.7150/thno.21254
Dri, D. A., Marianecci, C., Carafa, M., Gaucci, E., and Gramaglia, D. (2021). Surfactants, nanomedicines and nanocarriers: a critical evaluation on clinical trials. Pharmaceutics 13 (3), 381. doi:10.3390/pharmaceutics13030381
El-Helaly, S. N., and Rashad, A. A. (2024). Mirtazapine loaded polymeric micelles for rapid release tablet: a novel formulation-in vitro and in vivo studies. Drug Deliv. Transl. Res. doi:10.1007/s13346-024-01525-w
Elkhayat, H. A., Aly, R. H., Elagouza, I. A., El-Kabarity, R. H., and Galal, Y. I. (2017). Role of P-glycoprotein inhibitors in children with drug-resistant epilepsy. Acta Neurol. Scand. 136 (6), 639–644. doi:10.1111/ane.12778
Erdő, F., Bors, L. A., Farkas, D., Bajza, Á., and Gizurarson, S. (2018). Evaluation of intranasal delivery route of drug administration for brain targeting. Brain Res. Bull. 143, 155–170. doi:10.1016/j.brainresbull.2018.10.009
Ewer, K. J., Lambe, T., Rollier, C. S., Spencer, A. J., Hill, A. V., and Dorrell, L. (2016). Viral vectors as vaccine platforms: from immunogenicity to impact. Curr. Opin. Immunol. 41, 47–54. doi:10.1016/j.coi.2016.05.014
Fahmy, H. M., Aboalasaad, F. A., Mohamed, A. S., Elhusseiny, F. A., Khadrawy, Y. A., and Elmekawy, A. (2024). Evaluation of the therapeutic effect of curcumin-conjugated zinc oxide nanoparticles on reserpine-induced depression in wistar rats. Biol. Trace Elem. Res. 202 (6), 2630–2644. doi:10.1007/s12011-023-03849-z
Fan, C., Li, Y., Lan, T., Wang, W., Long, Y., and Yu, S. Y. (2022). Microglia secrete miR-146a-5p-containing exosomes to regulate neurogenesis in depression. Mol. Ther. 30 (3), 1300–1314. doi:10.1016/j.ymthe.2021.11.006
Fan, Y., Marioli, M., and Zhang, K. (2021). Analytical characterization of liposomes and other lipid nanoparticles for drug delivery. J. Pharm. Biomed. Anal. 192, 113642. doi:10.1016/j.jpba.2020.113642
Forouhari, S., Beygi, Z., Mansoori, Z., Hajsharifi, S., Heshmatnia, F., and Gheibihayat, S. M. (2022). Liposomes: ideal drug delivery systems in breast cancer. Biotechnol. Appl. Biochem. 69 (5), 1867–1884. doi:10.1002/bab.2253
Furtado, D., Björnmalm, M., Ayton, S., Bush, A. I., Kempe, K., and Caruso, F. (2018). Overcoming the blood-brain barrier: the role of nanomaterials in treating neurological diseases. Adv. Mater 30 (46), e1801362. doi:10.1002/adma.201801362
Furukawa, T. A., Cipriani, A., Cowen, P. J., Leucht, S., Egger, M., and Salanti, G. (2019). Optimal dose of selective serotonin reuptake inhibitors, venlafaxine, and mirtazapine in major depression: a systematic review and dose-response meta-analysis. Lancet Psychiatry 6 (7), 601–609. doi:10.1016/s2215-0366(19)30217-2
Gałecka, M., Bliźniewska-Kowalska, K., Gałecki, P., Szemraj, J., and Orzechowska, A. (2022). Expression of p11 in patients with depression. J. Clin. Med. 11 (19), 5743. doi:10.3390/jcm11195743
Gandhi, M., Bhatt, P., Chauhan, G., Gupta, S., Misra, A., and Mashru, R. (2019). IGF-II-Conjugated nanocarrier for brain-targeted delivery of p11 gene for depression. AAPS PharmSciTech 20 (2), 50. doi:10.1208/s12249-018-1206-x
Gardner, L. A., and Levin, M. C. (2015). Importance of apolipoprotein A-I in multiple sclerosis. Front. Pharmacol. 6, 278. doi:10.3389/fphar.2015.00278
Ge, J., Tan, R., Gao, Q., Li, R., Xu, P., Song, H., et al. (2023). A multifunctional nanocarrier system for highly efficient and targeted delivery of ketamine to NMDAR sites for improved treatment of depression. Adv. Healthc. Mater 12 (21), e2300154. doi:10.1002/adhm.202300154
Guimarães, D., Cavaco-Paulo, A., and Nogueira, E. (2021). Design of liposomes as drug delivery system for therapeutic applications. Int. J. Pharm. 601, 120571. doi:10.1016/j.ijpharm.2021.120571
Gul, M., Shah, F. A., Sahar, N. U., Malik, I., Din, F. U., Khan, S. A., et al. (2022). Formulation optimization, in vitro and in vivo evaluation of agomelatine-loaded nanostructured lipid carriers for augmented antidepressant effects. Colloids Surf. B Biointerfaces 216, 112537. doi:10.1016/j.colsurfb.2022.112537
Has, C., and Sunthar, P. (2020). A comprehensive review on recent preparation techniques of liposomes. J. Liposome Res. 30 (4), 336–365. doi:10.1080/08982104.2019.1668010
Hort, M. A., Alves, B. D. S., Ramires Júnior, O. V., Falkembach, M. C., Araújo, G. M. S., Fernandes, C. L. F., et al. (2021). In vivo toxicity evaluation of nanoemulsions for drug delivery. Drug Chem. Toxicol. 44 (6), 585–594. doi:10.1080/01480545.2019.1659806
Huang, D., He, B., and Mi, P. (2019). Calcium phosphate nanocarriers for drug delivery to tumors: imaging, therapy and theranostics. Biomater. Sci. 7 (10), 3942–3960. doi:10.1039/c9bm00831d
Hwang, D., Ramsey, J. D., and Kabanov, A. V. (2020). Polymeric micelles for the delivery of poorly soluble drugs: from nanoformulation to clinical approval. Adv. Drug Deliv. Rev. 156, 80–118. doi:10.1016/j.addr.2020.09.009
Inatani, S., Ochi, M., Kinoshita, K., Yamaguchi, J. I., and Endo, H. (2023). Preclinical metabolism and disposition of TP0473292, a novel oral prodrug of the potent metabotropic glutamate 2/3 receptor antagonist TP0178894 for the treatment of depression. Drug Metab. Dispos. 51 (5), 572–582. doi:10.1124/dmd.122.001116
Islam, S. U., Shehzad, A., Ahmed, M. B., and Lee, Y. S. (2020). Intranasal delivery of nanoformulations: a potential way of treatment for neurological disorders. Molecules 25 (8), 1929. doi:10.3390/molecules25081929
Jain, A., and Jain, S. K. (2015). Ligand-appended BBB-targeted nanocarriers (LABTNs). Crit. Rev. Ther. Drug Carr. Syst. 32 (2), 149–180. doi:10.1615/critrevtherdrugcarriersyst.2015010903
Jannini, T. B., Lorenzo, G. D., Bianciardi, E., Niolu, C., Toscano, M., Ciocca, G., et al. (2022). Off-label uses of selective serotonin reuptake inhibitors (SSRIs). Curr. Neuropharmacol. 20 (4), 693–712. doi:10.2174/1570159x19666210517150418
Jeyhoonabadi, M., Alimoahmmadi, S., Hassanpour, S., and Hashemnia, M. (2022). Betaine ameliorates depressive-like behaviors in zinc oxide nanoparticles exposed mice. Biol. Trace Elem. Res. 200 (11), 4771–4781. doi:10.1007/s12011-021-03068-4
Jin, J., Wang, H., Hua, X., Chen, D., Huang, C., and Chen, Z. (2019). An outline for the pharmacological effect of icariin in the nervous system. Eur. J. Pharmacol. 842, 20–32. doi:10.1016/j.ejphar.2018.10.006
Kalbouneh, H. M., Toubasi, A. A., Albustanji, F. H., Obaid, Y. Y., and Al-Harasis, L. M. (2022). Safety and efficacy of SSRIs in improving poststroke recovery: a systematic review and meta-analysis. J. Am. Heart Assoc. 11 (13), e025868. doi:10.1161/jaha.122.025868
Kalluri, R., and LeBleu, V. S. (2020). The biology, function, and biomedical applications of exosomes. Science 367 (6478), eaau6977. doi:10.1126/science.aau6977
Kamel, M. S., Munds, R. A., and Verma, M. S. (2023). The quest for immunity: exploring human herpesviruses as vaccine vectors. Int. J. Mol. Sci. 24 (22), 16112. doi:10.3390/ijms242216112
Kataoka, K., Harada, A., and Nagasaki, Y. (2001). Block copolymer micelles for drug delivery: design, characterization and biological significance. Adv. Drug Deliv. Rev. 47 (1), 113–131. doi:10.1016/s0169-409x(00)00124-1
Keller, L. A., Merkel, O., and Popp, A. (2022). Intranasal drug delivery: opportunities and toxicologic challenges during drug development. Drug Deliv. Transl. Res. 12 (4), 735–757. doi:10.1007/s13346-020-00891-5
Khadrawy, Y. A., Hosny, E. N., Magdy, M., and Mohammed, H. S. (2021). Antidepressant effects of curcumin-coated iron oxide nanoparticles in a rat model of depression. Eur. J. Pharmacol. 908, 174384. doi:10.1016/j.ejphar.2021.174384
Khan, H., Nazir, S., Farooq, R. K., Khan, I. N., and Javed, A. (2021). Fabrication and assessment of diosgenin encapsulated stearic acid solid lipid nanoparticles for its anticancer and antidepressant effects using in vitro and in vivo models. Front. Neurosci. 15, 806713. doi:10.3389/fnins.2021.806713
Khizar, S., Alrushaid, N., Alam Khan, F., Zine, N., Jaffrezic-Renault, N., Errachid, A., et al. (2023). Nanocarriers based novel and effective drug delivery system. Int. J. Pharm. 632, 122570. doi:10.1016/j.ijpharm.2022.122570
Le Nedelec, M., Glue, P., Winter, H., Goulton, C., and Medlicott, N. J. (2018). The effect of route of administration on the enantioselective pharmacokinetics of ketamine and norketamine in rats. J. Psychopharmacol. 32 (10), 1127–1132. doi:10.1177/0269881118780013
Li, D., Wang, Y., Jin, X., Hu, D., Xia, C., Xu, H., et al. (2020). NK cell-derived exosomes carry miR-207 and alleviate depression-like symptoms in mice. J. Neuroinflammation 17 (1), 126. doi:10.1186/s12974-020-01787-4
Li, J., Gao, W., Zhao, Z., Li, Y., Yang, L., Wei, W., et al. (2022). Ginsenoside Rg1 reduced microglial activation and mitochondrial dysfunction to alleviate depression-like behaviour via the GAS5/EZH2/SOCS3/NRF2 Axis. Mol. Neurobiol. 59 (5), 2855–2873. doi:10.1007/s12035-022-02740-7
Li, J., Zheng, M., Shimoni, O., Banks, W. A., Bush, A. I., Gamble, J. R., et al. (2021). Development of novel therapeutics targeting the blood-brain barrier: from barrier to carrier. Adv. Sci. (Weinh) 8 (16), e2101090. doi:10.1002/advs.202101090
Li, M., Du, C., Guo, N., Teng, Y., Meng, X., Sun, H., et al. (2019). Composition design and medical application of liposomes. Eur. J. Med. Chem. 164, 640–653. doi:10.1016/j.ejmech.2019.01.007
Liang, Y., Duan, L., Lu, J., and Xia, J. (2021). Engineering exosomes for targeted drug delivery. Theranostics 11 (7), 3183–3195. doi:10.7150/thno.52570
Lima, V. S., Guimarães, A. T. B., da Costa Araújo, A. P., Estrela, F. N., da Silva, I. C., de Melo, N. F. S., et al. (2019). Depression, anxiety-like behavior, and memory impairment in mice exposed to chitosan-coated zein nanoparticles. Environ. Sci. Pollut. Res. Int. 26 (11), 10641–10650. doi:10.1007/s11356-019-04536-0
Lin, W. J., Juang, L. W., and Lin, C. C. (2003). Stability and release performance of a series of pegylated copolymeric micelles. Pharm. Res. 20 (4), 668–673. doi:10.1023/a:1023215320026
Liu, D., Zhu, M., Zhang, Y., and Diao, Y. (2021). Crossing the blood-brain barrier with AAV vectors. Metab. Brain Dis. 36 (1), 45–52. doi:10.1007/s11011-020-00630-2
Liu, Q., Zou, J., Chen, Z., He, W., and Wu, W. (2023). Current research trends of nanomedicines. Acta Pharm. Sin. B 13 (11), 4391–4416. doi:10.1016/j.apsb.2023.05.018
Loo, C., Glozier, N., Barton, D., Baune, B. T., Mills, N. T., Fitzgerald, P., et al. (2023). Efficacy and safety of a 4-week course of repeated subcutaneous ketamine injections for treatment-resistant depression (KADS study): randomised double-blind active-controlled trial. Br. J. Psychiatry 223 (6), 533–541. doi:10.1192/bjp.2023.79
Lu, Y., and Park, K. (2013). Polymeric micelles and alternative nanonized delivery vehicles for poorly soluble drugs. Int. J. Pharm. 453 (1), 198–214. doi:10.1016/j.ijpharm.2012.08.042
Lundstrom, K. (2023). Viral vectors in gene therapy: where do we stand in 2023? Viruses 15 (3), 698. doi:10.3390/v15030698
Luo, Z., Dong, J., and Wu, J. (2022). Impact of Icariin and its derivatives on inflammatory diseases and relevant signaling pathways. Int. Immunopharmacol. 108, 108861. doi:10.1016/j.intimp.2022.108861
Maisel, K., Reddy, M., Xu, Q., Chattopadhyay, S., Cone, R., Ensign, L. M., et al. (2016). Nanoparticles coated with high molecular weight PEG penetrate mucus and provide uniform vaginal and colorectal distribution in vivo. Nanomedicine (Lond) 11 (11), 1337–1343. doi:10.2217/nnm-2016-0047
Malhi, G. S., and Mann, J. J. (2018). Depression. Lancet 392 (10161), 2299–2312. doi:10.1016/s0140-6736(18)31948-2
Mantz, A., and Pannier, A. K. (2019). Biomaterial substrate modifications that influence cell-material interactions to prime cellular responses to nonviral gene delivery. Exp. Biol. Med. (Maywood) 244 (2), 100–113. doi:10.1177/1535370218821060
Marwaha, S., Palmer, E., Suppes, T., Cons, E., Young, A. H., and Upthegrove, R. (2023). Novel and emerging treatments for major depression. Lancet 401 (10371), 141–153. doi:10.1016/s0140-6736(22)02080-3
McCarron, R. M., Shapiro, B., Rawles, J., and Luo, J. (2021). Depression. Ann. Intern Med. 174 (5), Itc65–itc80. doi:10.7326/aitc202105180
McClure, E. W., and Daniels, R. N. (2021). Classics in chemical neuroscience: amitriptyline. ACS Chem. Neurosci. 12 (3), 354–362. doi:10.1021/acschemneuro.0c00467
Memvanga, P. B., and Nkanga, C. I. (2021). Liposomes for malaria management: the evolution from 1980 to 2020. Malar. J. 20 (1), 327. doi:10.1186/s12936-021-03858-0
Miao, Y. B., Zhao, W., Renchi, G., Gong, Y., and Shi, Y. (2023). Customizing delivery nano-vehicles for precise brain tumor therapy. J. Nanobiotechnology 21 (1), 32. doi:10.1186/s12951-023-01775-9
Milone, M. C., and O'Doherty, U. (2018). Clinical use of lentiviral vectors. Leukemia 32 (7), 1529–1541. doi:10.1038/s41375-018-0106-0
Mishra, V., Bansal, K. K., Verma, A., Yadav, N., Thakur, S., Sudhakar, K., et al. (2018). Solid lipid nanoparticles: emerging colloidal nano drug delivery systems. Pharmaceutics 10 (4), 191. doi:10.3390/pharmaceutics10040191
Mohapatra, P., Gopikrishnan, M., Doss, C. G., and Chandrasekaran, N. (2024). How precise are nanomedicines in overcoming the blood-brain barrier? A comprehensive review of the literature. Int. J. Nanomedicine 19, 2441–2467. doi:10.2147/ijn.S442520
Mollazadeh, S., Sahebkar, A., Hadizadeh, F., Behravan, J., and Arabzadeh, S. (2018). Structural and functional aspects of P-glycoprotein and its inhibitors. Life Sci. 214, 118–123. doi:10.1016/j.lfs.2018.10.048
Mondal, J., Pillarisetti, S., Junnuthula, V., Saha, M., Hwang, S. R., Park, I. K., et al. (2023). Hybrid exosomes, exosome-like nanovesicles and engineered exosomes for therapeutic applications. J. Control Release 353, 1127–1149. doi:10.1016/j.jconrel.2022.12.027
Monroe, S. M., and Harkness, K. L. (2022). Major depression and its recurrences: life course matters. Annu. Rev. Clin. Psychol. 18, 329–357. doi:10.1146/annurev-clinpsy-072220-021440
Muheem, A., Jahangir, M. A., Jaiswal, C. P., Jafar, M., Ahmad, M. Z., Ahmad, J., et al. (2021). Recent patents, regulatory issues, and toxicity of nanoparticles in neuronal disorders. Curr. Drug Metab. 22 (4), 263–279. doi:10.2174/1389200221999201210213036
Mutingwende, F. P., Kondiah, P. P. D., Ubanako, P., Marimuthu, T., and Choonara, Y. E. (2021). Advances in nano-enabled platforms for the treatment of depression. Polym. (Basel) 13 (9), 1431. doi:10.3390/polym13091431
Naidu, S. A. G., Wallace, T. C., Davies, K. J. A., and Naidu, A. S. (2023). Lactoferrin for mental health: neuro-redox regulation and neuroprotective effects across the blood-brain barrier with special reference to neuro-COVID-19. J. Diet. Suppl. 20 (2), 218–253. doi:10.1080/19390211.2021.1922567
Nishiyama, N., and Kataoka, K. (2006). Current state, achievements, and future prospects of polymeric micelles as nanocarriers for drug and gene delivery. Pharmacol. Ther. 112 (3), 630–648. doi:10.1016/j.pharmthera.2006.05.006
Nodari, C. H., De Quadros, N. D., Chiarentin, R., Da Silva, F. P., Morisso, F. D. P., Charão, M. F., et al. (2022). Vortioxetine liposomes as a novel alternative to improve drug stability under stress conditions: toxicity studies and evaluation of antidepressant-like effect. Pharmacol. Rep. 74 (5), 969–981. doi:10.1007/s43440-022-00412-w
Nowak, M., Brown, T. D., Graham, A., Helgeson, M. E., and Mitragotri, S. (2020). Size, shape, and flexibility influence nanoparticle transport across brain endothelium under flow. Bioeng. Transl. Med. 5 (2), e10153. doi:10.1002/btm2.10153
Ojer, P., Iglesias, T., Azqueta, A., Irache, J. M., and López de Cerain, A. (2015). Toxicity evaluation of nanocarriers for the oral delivery of macromolecular drugs. Eur. J. Pharm. Biopharm. 97 (Pt A), 206–217. doi:10.1016/j.ejpb.2015.10.005
Owen, S. C., Chan, D. P. Y., and Shoichet, M. S. (2012). Polymeric micelle stability. Nano Today 7 (1), 53–65. doi:10.1016/j.nantod.2012.01.002
Pan, X., Veroniaina, H., Su, N., Sha, K., Jiang, F., Wu, Z., et al. (2021). Applications and developments of gene therapy drug delivery systems for genetic diseases. Asian J. Pharm. Sci. 16 (6), 687–703. doi:10.1016/j.ajps.2021.05.003
Pandit, R., Chen, L., and Götz, J. (2020). The blood-brain barrier: physiology and strategies for drug delivery. Adv. Drug Deliv. Rev. 165-166, 1–14. doi:10.1016/j.addr.2019.11.009
Patel, R. B., Rao, H. R., Thakkar, D. V., and Patel, M. R. (2022). Comprehending the potential of metallic, lipid, and polymer-based nanocarriers for treatment and management of depression. Neurochem. Int. 153, 105259. doi:10.1016/j.neuint.2021.105259
Pawar, S. D., Gawali, K., Kulhari, H., Murty, U. S., and Kumar, P. (2023). Amoxapine-loaded solid lipid nanoparticles with superior preclinical pharmacokinetics for better brain delivery: LC-MS/MS and GC-MS analysis. ACS Chem. Neurosci. doi:10.1021/acschemneuro.2c00673
Pegtel, D. M., and Gould, S. J. (2019). Exosomes. Annu. Rev. Biochem. 88, 487–514. doi:10.1146/annurev-biochem-013118-111902
Popova, V., Daly, E. J., Trivedi, M., Cooper, K., Lane, R., Lim, P., et al. (2019). Efficacy and safety of flexibly dosed esketamine nasal spray combined with a newly initiated oral antidepressant in treatment-resistant depression: a randomized double-blind active-controlled study. Am. J. Psychiatry 176 (6), 428–438. doi:10.1176/appi.ajp.2019.19020172
Rajpoot, K. (2019). Solid lipid nanoparticles: a promising nanomaterial in drug delivery. Curr. Pharm. Des. 25 (37), 3943–3959. doi:10.2174/1381612825666190903155321
Rathnam, S. S., Deepak, T., Sahoo, B. N., Meena, T., Singh, Y., and Joshi, A. (2024). Metallic nanocarriers for therapeutic peptides: emerging solutions addressing the delivery challenges in brain ailments. J. Pharmacol. Exp. Ther. 388 (1), 39–53. doi:10.1124/jpet.123.001689
Ricken, R., Ulrich, S., Schlattmann, P., and Adli, M. (2017). Tranylcypromine in mind (Part II): review of clinical pharmacology and meta-analysis of controlled studies in depression. Eur. Neuropsychopharmacol. 27 (8), 714–731. doi:10.1016/j.euroneuro.2017.04.003
Rothmore, J. (2020). Antidepressant-induced sexual dysfunction. Med. J. Aust. 212 (7), 329–334. doi:10.5694/mja2.50522
Rub, M. A., Asiri, A. M., Azum, N., Khan, A., Aslam, A., Khan, P., et al. (2013). Amphiphilic antidepressant drug amitriptyline hydrochloride under the influence of ionic and nonionic hydrotropes; micellization and phase separation. J. Industrial Eng. Chem. 19 (6), 1774–1780. doi:10.1016/j.jiec.2013.02.019
Saeidienik, F., Shahraki, M. R., Fanaei, H., and Badini, F. (2018). The effects of iron oxide nanoparticles administration on depression symptoms induced by LPS in male wistar rats. Basic Clin. Neurosci. 9 (3), 209–216. doi:10.29252/nirp.Bcn.9.3.209
Salem, H. F., Ali, A. A., Rabea, Y. K., Abo El-Ela, F. I., and Khallaf, R. A. (2023). Optimization and appraisal of chitosan-grafted PLGA nanoparticles for boosting pharmacokinetic and pharmacodynamic effect of duloxetine HCl using box-benkhen design. J. Pharm. Sci. 112 (2), 544–561. doi:10.1016/j.xphs.2022.08.034
Sanganahalli, B. G., Joshi, P. G., and Joshi, N. B. (2000). Differential effects of tricyclic antidepressant drugs on membrane dynamics--a fluorescence spectroscopic study. Life Sci. 68 (1), 81–90. doi:10.1016/s0024-3205(00)00918-8
Sato, H., Yamada, K., Miyake, M., and Onoue, S. (2023). Recent advancements in the development of nanocarriers for mucosal drug delivery systems to control oral absorption. Pharmaceutics 15 (12), 2708. doi:10.3390/pharmaceutics15122708
Scioli Montoto, S., Muraca, G., and Ruiz, M. E. (2020). Solid lipid nanoparticles for drug delivery: pharmacological and biopharmaceutical aspects. Front. Mol. Biosci. 7, 587997. doi:10.3389/fmolb.2020.587997
Shah, S., Dhawan, V., Holm, R., Nagarsenker, M. S., and Perrie, Y. (2020). Liposomes: advancements and innovation in the manufacturing process. Adv. Drug Deliv. Rev. 154-155, 102–122. doi:10.1016/j.addr.2020.07.002
Sharma, A., Fernandes, D. C., Reis, R. L., Gołubczyk, D., Neumann, S., Lukomska, B., et al. (2023). Cutting-edge advances in modeling the blood-brain barrier and tools for its reversible permeabilization for enhanced drug delivery into the brain. Cell Biosci. 13 (1), 137. doi:10.1186/s13578-023-01079-3
Sharma, G., Sharma, A. R., Lee, S. S., Bhattacharya, M., Nam, J. S., and Chakraborty, C. (2019). Advances in nanocarriers enabled brain targeted drug delivery across blood brain barrier. Int. J. Pharm. 559, 360–372. doi:10.1016/j.ijpharm.2019.01.056
Sharma, S., and Dang, S. (2020). Neuropsychological disorders and their nanocarriers. Curr. Pharm. Des. 26 (19), 2247–2256. doi:10.2174/1381612826666200224111241
Shcharbin, D., Shcharbina, N., Dzmitruk, V., Pedziwiatr-Werbicka, E., Ionov, M., Mignani, S., et al. (2017). Dendrimer-protein interactions versus dendrimer-based nanomedicine. Colloids Surf. B Biointerfaces 152, 414–422. doi:10.1016/j.colsurfb.2017.01.041
Sheng, L., Wei, Y., Pi, C., Cheng, J., Su, Z., Wang, Y., et al. (2023). Preparation and evaluation of curcumin derivatives nanoemulsion based on turmeric extract and its antidepressant effect. Int. J. Nanomedicine 18, 7965–7983. doi:10.2147/ijn.S430769
Shirley, J. L., de Jong, Y. P., Terhorst, C., and Herzog, R. W. (2020). Immune responses to viral gene therapy vectors. Mol. Ther. 28 (3), 709–722. doi:10.1016/j.ymthe.2020.01.001
Silant’ev, V. E., Shmelev, M. E., Belousov, A. S., Patlay, A. A., Shatilov, R. A., Farniev, V. M., et al. (2023). How to develop drug delivery system based on carbohydrate nanoparticles targeted to brain tumors. Polym. (Basel) 15 (11), 2516. doi:10.3390/polym15112516
Singh, G., Sarwal, A., Sharma, S., Prasad, P., Kuhad, A., and Ali, W. (2021). Polymer-based prolonged-release nanoformulation of duloxetine: fabrication, characterization and neuropharmacological assessments. Drug Dev. Ind. Pharm. 47 (1), 12–21. doi:10.1080/03639045.2020.1851240
Song, Y. H., De, R., and Lee, K. T. (2023). Emerging strategies to fabricate polymeric nanocarriers for enhanced drug delivery across blood-brain barrier: an overview. Adv. Colloid Interface Sci. 320, 103008. doi:10.1016/j.cis.2023.103008
Stamp, M. E. M., Halwes, M., Nisbet, D., and Collins, D. J. (2023). Breaking barriers: exploring mechanisms behind opening the blood-brain barrier. Fluids Barriers CNS 20 (1), 87. doi:10.1186/s12987-023-00489-2
Su, Y., Zhang, B., Sun, R., Liu, W., Zhu, Q., Zhang, X., et al. (2021). PLGA-based biodegradable microspheres in drug delivery: recent advances in research and application. Drug Deliv. 28 (1), 1397–1418. doi:10.1080/10717544.2021.1938756
Szabat-Iriaka, B., and Le Borgne, M. (2021). Brain safety concerns of nanomedicines: the need for a specific regulatory framework. Drug Discov. Today 26 (11), 2502–2507. doi:10.1016/j.drudis.2021.06.011
Tábi, T., Vécsei, L., Youdim, M. B., Riederer, P., and Szökő, É. (2020). Selegiline: a molecule with innovative potential. J. Neural Transm. (Vienna) 127 (5), 831–842. doi:10.1007/s00702-019-02082-0
Tan, H., Cao, K., Zhao, Y., Zhong, J., Deng, D., Pan, B., et al. (2024). Brain-targeted black phosphorus-based nanotherapeutic platform for enhanced hypericin delivery in depression. Small, e2310608. doi:10.1002/smll.202310608
Tang, J., Chen, L. L., Zhang, H., Wei, P., and Miao, F. (2024). Effects of exercise therapy on anxiety and depression in patients with COVID-19: a systematic review and meta-analysis. Front. Public Health 12, 1330521. doi:10.3389/fpubh.2024.1330521
Tao, Y., Liang, Q., Zhang, F., Guo, S., Fan, L., and Zhao, F. (2024). Efficacy of non-invasive brain stimulation combined with antidepressant medications for depression: a systematic review and meta-analysis of randomized controlled trials. Syst. Rev. 13 (1), 92. doi:10.1186/s13643-024-02480-w
Tavakolizadeh, J., Roshanaei, K., Salmaninejad, A., Yari, R., Nahand, J. S., Sarkarizi, H. K., et al. (2018). MicroRNAs and exosomes in depression: potential diagnostic biomarkers. J. Cell Biochem. 119 (5), 3783–3797. doi:10.1002/jcb.26599
Teixeira, M. I., Lopes, C. M., Amaral, M. H., and Costa, P. C. (2023). Surface-modified lipid nanocarriers for crossing the blood-brain barrier (BBB): a current overview of active targeting in brain diseases. Colloids Surf. B Biointerfaces 221, 112999. doi:10.1016/j.colsurfb.2022.112999
Teleanu, D. M., Chircov, C., Grumezescu, A. M., Volceanov, A., and Teleanu, R. I. (2018). Impact of nanoparticles on brain health: an up to date overview. J. Clin. Med. 7 (12), 490. doi:10.3390/jcm7120490
Tenchov, R., Sasso, J. M., Wang, X., Liaw, W. S., Chen, C. A., and Zhou, Q. A. (2022). Exosomes─Nature's lipid nanoparticles, a rising star in drug delivery and diagnostics. ACS Nano 16 (11), 17802–17846. doi:10.1021/acsnano.2c08774
Tong, G. F., Qin, N., and Sun, L. W. (2017). Development and evaluation of Desvenlafaxine loaded PLGA-chitosan nanoparticles for brain delivery. Saudi Pharm. J. 25 (6), 844–851. doi:10.1016/j.jsps.2016.12.003
Tripathi, P. K., Gupta, S., Rai, S., Shrivatava, A., Tripathi, S., Singh, S., et al. (2020). Curcumin loaded poly (amidoamine) dendrimer-plamitic acid core-shell nanoparticles as anti-stress therapeutics. Drug Dev. Ind. Pharm. 46 (3), 412–426. doi:10.1080/03639045.2020.1724132
Unnisa, A., Greig, N. H., and Kamal, M. A. (2023). Nanotechnology: a promising targeted drug delivery system for brain tumours and Alzheimer's disease. Curr. Med. Chem. 30 (3), 255–270. doi:10.2174/0929867329666220328125206
Viegas, C., Patrício, A. B., Prata, J. M., Nadhman, A., Chintamaneni, P. K., and Fonte, P. (2023). Solid lipid nanoparticles vs. Nanostructured lipid carriers: a comparative review. Pharmaceutics 15 (6), 1593. doi:10.3390/pharmaceutics15061593
Wang, J., Li, Z., Pan, M., Fiaz, M., Hao, Y., Yan, Y., et al. (2022). Ultrasound-mediated blood-brain barrier opening: an effective drug delivery system for theranostics of brain diseases. Adv. Drug Deliv. Rev. 190, 114539. doi:10.1016/j.addr.2022.114539
Waqar, M. A., Zaman, M., Hameed, H., Jamshaid, M., Irfan, A., Shazly, G. A., et al. (2023). Formulation, characterization, and evaluation of β-cyclodextrin functionalized hypericin loaded nanocarriers. ACS Omega 8 (41), 38191–38203. doi:10.1021/acsomega.3c04444
Wilczewska, A. Z., Niemirowicz, K., Markiewicz, K. H., and Car, H. (2012). Nanoparticles as drug delivery systems. Pharmacol. Rep. 64 (5), 1020–1037. doi:10.1016/s1734-1140(12)70901-5
Woody, C. A., Ferrari, A. J., Siskind, D. J., Whiteford, H. A., and Harris, M. G. (2017). A systematic review and meta-regression of the prevalence and incidence of perinatal depression. J. Affect Disord. 219, 86–92. doi:10.1016/j.jad.2017.05.003
Wu, D., Chen, Q., Chen, X., Han, F., Chen, Z., and Wang, Y. (2023). The blood-brain barrier: structure, regulation, and drug delivery. Signal Transduct. Target Ther. 8 (1), 217. doi:10.1038/s41392-023-01481-w
Xiao, K., Li, Y., Luo, J., Lee, J. S., Xiao, W., Gonik, A. M., et al. (2011). The effect of surface charge on in vivo biodistribution of PEG-oligocholic acid based micellar nanoparticles. Biomaterials 32 (13), 3435–3446. doi:10.1016/j.biomaterials.2011.01.021
Xiong, Y., Xu, Z., and Li, Z. (2019). Polydopamine-based nanocarriers for photosensitizer delivery. Front. Chem. 7, 471. doi:10.3389/fchem.2019.00471
Xu, D., Lu, Y. R., Kou, N., Hu, M. J., Wang, Q. S., and Cui, Y. L. (2020). Intranasal delivery of icariin via a nanogel-thermoresponsive hydrogel compound system to improve its antidepressant-like activity. Int. J. Pharm. 586, 119550. doi:10.1016/j.ijpharm.2020.119550
Yu, S., Wang, X., Lv, L., Liu, T., and Guan, Q. (2023). Borneol-modified PEGylated graphene oxide as a nanocarrier for brain-targeted delivery of ginsenoside Rg1 against depression. Int. J. Pharm. 643, 123284. doi:10.1016/j.ijpharm.2023.123284
Zakharova, L. Y., Pashirova, T. N., Doktorovova, S., Fernandes, A. R., Sanchez-Lopez, E., Silva, A. M., et al. (2019). Cationic surfactants: self-assembly, structure-activity correlation and their biological applications. Int. J. Mol. Sci. 20 (22), 5534. doi:10.3390/ijms20225534
Zhang, L., and Yu, D. (2019). Exosomes in cancer development, metastasis, and immunity. Biochim. Biophys. Acta Rev. Cancer 1871 (2), 455–468. doi:10.1016/j.bbcan.2019.04.004
Zhang, Q. L., Fu, B. M., and Zhang, Z. J. (2017). Borneol, a novel agent that improves central nervous system drug delivery by enhancing blood-brain barrier permeability. Drug Deliv. 24 (1), 1037–1044. doi:10.1080/10717544.2017.1346002
Zhang, Y., and Zhuo, R. X. (2005). Synthesis and in vitro drug release behavior of amphiphilic triblock copolymer nanoparticles based on poly (ethylene glycol) and polycaprolactone. Biomaterials 26 (33), 6736–6742. doi:10.1016/j.biomaterials.2005.03.045
Zhao, X., Zhao, R., and Nie, G. (2022). Nanocarriers based on bacterial membrane materials for cancer vaccine delivery. Nat. Protoc. 17 (10), 2240–2274. doi:10.1038/s41596-022-00713-7
Zorkina, Y., Abramova, O., Ushakova, V., Morozova, A., Zubkov, E., Valikhov, M., et al. (2020). Nano carrier drug delivery systems for the treatment of neuropsychiatric disorders: advantages and limitations. Molecules 25 (22), 5294. doi:10.3390/molecules25225294
Keywords: depression, drug delivery system, nanocarriers, blood-brain barrier, antidepressants
Citation: Feng X, Jia P and Zhang D (2024) Nanocarrier drug delivery system: promising platform for targeted depression therapy. Front. Pharmacol. 15:1435133. doi: 10.3389/fphar.2024.1435133
Received: 19 May 2024; Accepted: 11 July 2024;
Published: 25 July 2024.
Edited by:
Zongchao Han, University of North Carolina at Chapel Hill, United StatesReviewed by:
Rania Harati, University of Sharjah, United Arab EmiratesCopyright © 2024 Feng, Jia and Zhang. This is an open-access article distributed under the terms of the Creative Commons Attribution License (CC BY). The use, distribution or reproduction in other forums is permitted, provided the original author(s) and the copyright owner(s) are credited and that the original publication in this journal is cited, in accordance with accepted academic practice. No use, distribution or reproduction is permitted which does not comply with these terms.
*Correspondence: Dingding Zhang, emhhbmdkZDI1QDEyNi5jb20=
†These authors have contributed equally to this work
Disclaimer: All claims expressed in this article are solely those of the authors and do not necessarily represent those of their affiliated organizations, or those of the publisher, the editors and the reviewers. Any product that may be evaluated in this article or claim that may be made by its manufacturer is not guaranteed or endorsed by the publisher.
Research integrity at Frontiers
Learn more about the work of our research integrity team to safeguard the quality of each article we publish.