- 1School of Pharmacy, Nantong University, Nantong, Jiangsu, China
- 2School of Public Health, Nantong University, Nantong, Jiangsu, China
Vitamins are dietary components necessary for cellular metabolic balance, especially redox homeostasis; deficient or excessive supply may give rise to symptoms of psychiatric disorders. Exploring the nutritional and metabolic pathways of vitamins could contribute to uncovering the underlying pathogenesis of ferroptosis-associated diseases. This mini-review aims to provide insights into vitamins closely linked to the regulation of ferroptosis from the perspective of cellular reactive oxygen species biology. The mainstream reprogramming mechanisms of ferroptosis are overviewed, focusing on unique biological processes of iron metabolism, lipid metabolism, and amino acid metabolism. Moreover, recent breakthroughs in therapeutic interventions targeting ferroptosis via fully utilizing vitamin-based pharmacological tools were overviewed, covering vitamins (B, C, E, and K). Finally, mechanism insight related to vitamin-associated nutrient signaling was provided, highlighting the pharmacological benefits of metabolically reprogramming ferroptosis-associated diseases.
Introduction
Cell death can occur accidentally or controllably to maintain biological homeostasis by the removal of unnecessary cells and cell debris. In particular, regulated cell death controlled by interconnected signaling pathways is linked with tumorigenesis, apparently different from accidental cell death (Tang et al., 2019; Tong et al., 2022). Since its discovery in 1973, clinical oncology investigation has been devoted to promoting the development of therapeutics that are efficient in apoptosis induction (Kerr et al., 1972; Peng et al., 2022). In-depth studies of this lethal subroutine revealed the general characteristics of cancers that are resistant to apoptosis, and induction of other regulated routes involving necroptosis (Gong et al., 2019), autophagy (White, 2015), and pyroptosis (Yu et al., 2021), and so on. Over the past decades, regulated cell death pathways have been extensively studied in the field of cancer treatment, especially for newly emerging reactive oxygen species (ROS) nanomedicine that is amenable to the therapeutic intervention of ferroptosis execution. ROS-producing nanoparticles function by infiltrating cancerous tissues and exploiting the altered redox balance prevalent in these cells. Once activated, they intensify intracellular oxidative stress beyond the threshold cancer cells can manage, leading to uncontrolled lipid oxidation and membrane damage-hallmark of ferroptosis.
Up to 2012, ferroptosis featured phospholipid peroxidation driven by an iron-dependent manner, displaying significant differences at morphological, biochemically, and genetic levels (Dixon et al., 2012; Tang et al., 2020). Morphologically, unrepaired cellular damage spreads in a wave-like pattern, characterized by cytoplasmic and organelle ruptures, chromatin condensation, and a rise in autophagosomes (Riegman et al., 2020). Conceptually, ferroptosis was advanced to describe the result of iron accumulation and lipid peroxides (LPO) from the perspective of biochemistry (Liang et al., 2022). At present, GSH depletion and GPX4 inhibition play a crucial role in the initiation of ferroptosis, and other free radical-trapping targets involved ferroptosis suppressor protein 1 (FSP1) and GTP cyclohydrolase 1 (GCH1) (Jiang et al., 2021). To date, the underlying molecular mechanisms related to ferroptotic cell death have been extensively studied for therapeutic purposes.
Intriguingly, recent preclinical evidence suggests that certain malignancies are prone to chemoresistance and metastasis but are exquisitely vulnerable to ferroptosis (Liang et al., 2019; Mou et al., 2019; Lei et al., 2022). Consequently, modulating ferroptosis via metabolic interventions has garnered significant attention (Wu et al., 2020; Koppula et al., 2021). In this mini-review, we first summarized the key metabolic reprogramming of ferroptotic cell death, covering unique biological processes and pathophysiological characteristics in terms of iron metabolism, lipid metabolism, and amino acid metabolism. Further, we delve into the nutritional vitamins harnessed to govern ferroptosis, emphasizing their potential in tumor suppression and immune surveillance, underscoring the therapeutic promise of metabolic reprogramming in ferroptosis-linked diseases. Finally, we also outlined the current understanding of induction/inhibition mechanisms and conceptualized promising strategies that contribute to ROS biology for collaborative ferroptosis regulation.
Nutrient vitamins assosiated ferroptosis regulation
The downstream regulatory network of ferroptotic cell death is driven by the disequilibrium of redox hemostasis, owing to the inactivation of reducing enzymes or lipid oxidation products. As depicted in Figure 1, canonical nutrient vitamins actively participate in metabolic reprogramming mechanisms governing ferroptosis were outlined, including iron metabolism (Rizzollo et al., 2021), lipid metabolism (Snaebjornsson et al., 2020), and amino acid metabolism (Sies, 2021). Overall, vitamin B2 has been proven to be efficient in promoting ROS production assisted by laser or ultrasound irradiation, while B12 functions via methionine cycle to promote ferroptosis execution. In contrast, vitamin C may interfere in iron homeostasis, vitamin E act as endogenous antioxidants participate in neutralizing excessive free radicals to prevent oxidative damage of PUFA. Natural vitamin K is commonly recognized for its role as a potent inhibitor of ferroptosis, whereas synthetic variants can facilitate this process instead.
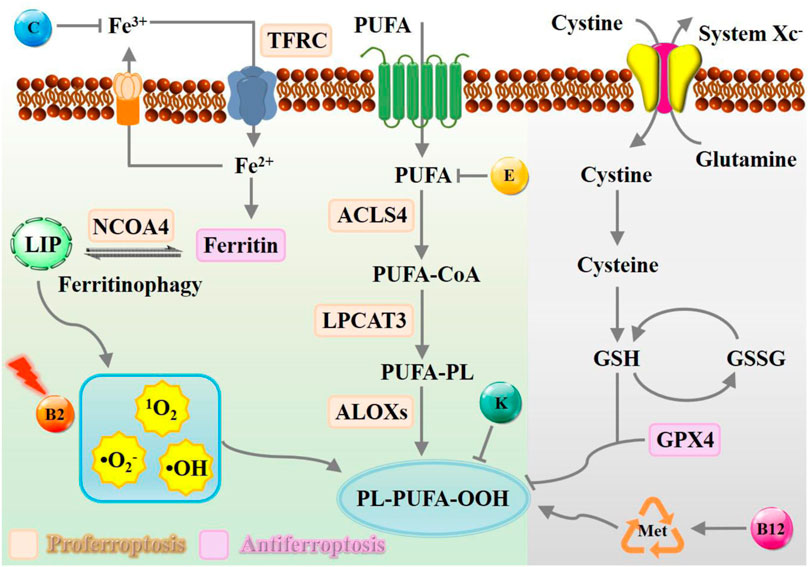
Figure 1. Schematic representation of metabolic processes of ferroptosis modulated by nutrient vitamins. The modulation of the ferroptosis process can be facilitated by the presence of vitamin B, C, E, and K, which exert a multifaceted influence towards iron deposition, ROS accumulation, and radical-trapping. Abbreviation: TFRC, transferrin receptor; NCOA4, nuclear receptor coactivator 4; LIP, labile iron pool; PUFA, polyunsaturated fatty acid; ACSL4, acyl-CoA synthetase long-chain family member 4; LPCAT3, lysophosphatidylcholine acyltransferase 3; LOX, lipoxygenase.
Iron metabolism
Cancer cells exhibited strong iron dependency to support their rapid proliferation, which is usually accompanied by upregulated transferrin and downregulated ferroportin, thus can be harnessed to therapeutic interventions of ferroptosis-associated diseases (Bao et al., 2020; Bao et al., 2021). Excessive intracellular iron is accompanied by highly oxidative stress that can propagate LPO accumulation (Zhang S. et al., 2022; Zhu et al., 2022). Thus, sensitizing cancer cells to oxidative damage and ferroptosis can be orchestrated by modulating iron uptake, storage, utilization, and export (Hassannia et al., 2019). Generally, cellular transport systems function well to preserve iron homeostasis (Andrews and Schmidt, 2007; Bogdan et al., 2016). In brief, iron can be internalized by circulating glycoprotein transferrin and discharged by ferroportin (Zhang C. et al., 2020). The intracellular labile iron pool (LIP) maintains a delicate balance normally and can be elevated by transferrin-mediated uptake or autophagic degradation (Lin et al., 2020). Inhibition of nuclear receptor coactivator 4 (NCOA4) mediated ferritinophagic flux or abnormal expression of iron metabolism proteins iron-responsive element binding protein 2 can dramatically decrease the vulnerability to ferroptosis (Fang et al., 2021; Zhou H. et al., 2023). Especially, vitamin C may interference iron metabolism by enhancing iron absorption. In the biological system, iron is an essential micronutrient element that can be engaged in hydroxyl radicals (•OH) formation through the Haber-Weiss and Fenton reaction, relying on the cycle between the ferrous (Fe2+) and ferric (Fe3+) states (Wang et al., 2020). Vitamin C helps convert poorly absorbed Fe3+ into its more absorbable Fe2+. Additionally, vitamin C acts as an antioxidant, protecting against oxidative stress that are associated with iron metabolism, thereby maintaining iron homeostasis and overall metabolic health.
Lipid metabolism
Lipid peroxidation is one of the canonical processes catalyzed by spontaneous autoxidation or endogenous enzymes that directly contribute to promoting ferroptosis (Yang and Stockwell, 2016; von Krusenstiern et al., 2023). At present, the widely recognized pathway is excessive peroxidation of polyunsaturated fatty acid (PUFA), comprising arachidonic acid and adrenic acid (Bazinet and Layé, 2014; Kagan et al., 2016). PUFAs, especially long-chain ones like ω-3 and ω-6 LCPUFAs, readily oxidize due to their numerous double bonds, rendering them susceptible to free radical assault. Vitamin E, an effective fat-loving antioxidant, acts as a membrane shield, with heightened importance in PUFA-dense areas. It directly neutralizes free radicals, halting their damaging cascade to preserve PUFAs. Teaming up with trace elements like selenium, it enhances the body’s antioxidant shield, defending both PUFAs and other cellular elements against oxidative stress. Additionally, vitamin E regulates metabolic interactions with PUFAs, impacting the progression of ferroptosis.
Generally, the peroxidation process of PUFA-containing phospholipids can be divided into three steps. Firstly, acyl-CoA synthetase long-chain family member 4 (ACSL4) converts the PUFA into acylated form (Gan, 2022). Then, lysophosphatidylcholine acyltransferase 3 (LPCAT3) participates in the process of esterifying acyl Co-A derivatives, incorporating PUFAs into phospholipid membranes (Hashidate-Yoshida et al., 2015). Finally, peroxidation occurs to form lipid hydroperoxide under the catalysis of lipoxygenase (LOX) (Stoyanovsky et al., 2019). Excessive oxidation of PUFA-containing phospholipids might disrupt the integrity of biological membranes, eventually decomposing into reactive toxic aldehydes (such as hydroxynonenal or malondialdehydes) facilitating ferroptosis execution (Dyall et al., 2022). Vitamin K1 has also emerged as a potent blocker of ferroptosis, a controlled cellular death mechanism linked to lipid oxidation. Research from Kolbrink et al. (Kolbrink et al., 2022) demonstrated its effectiveness in stopping ferroptosis in cellular models, reversing cell death and suggesting its potential in treating issues arising from oxygen deprivation and restoration cycles, like tissue injury. These discoveries emphasize vitamin K’s pivotal role, via unconventional metabolic pathways, in regulating ferroptosis-related diseases and its promise as a target for innovative therapies. Besides, the antioxidant mechanism of nutrient vitamins involves FSP1-triggered reduction, sustaining its radical-trapping properties to interrupt ferroptosis pathways.
Amino acid metabolism
In the biological system, extracellular glutamate and cystine are transported into the cell by amino acid transporter receptors system Xc−, while inhibiting the function of system Xc− can decrease the intracellular cysteine and GSH content (Koppula et al., 2021; Xiong et al., 2021). The cysteine required for GSH synthesis is generally obtained by the transsulfuration pathway of sulfur transfer from homocysteine to cysteine (Stockwell et al., 2020; Lee and Roh, 2022). GPX4 can protect cells from ferroptosis by reducing the lethal PUFA-containing phospholipid hydroperoxides (PL-OOH) into phospholipid alcohols (PL-OH) (Yang et al., 2014). Overall, the existing intracellular GSH/Xc−/GPX4 antioxidant defense system is of great importance in maintaining cellular redox homeostasis.
Moreover, the methionine-synthase cycle has been proven to be relevant to metabolic process of ROS generation, in which vitamin B12 plays a crucial role as a cofactor. It primarily functions to regenerate methionine, an essential amino acid, from homocysteine. The methionine cycle, involving SBP-1/SREBP1 and lipogenesis, is crucial for how early-life B12 levels influence later health outcomes. Research indicates that a lack of vitamin B12 early in life can result in elevated fat levels and toxic lipid accumulation, notably long-chain polyunsaturated fatty acids (LC-PUFAs), during adulthood, which triggers ferroptosis in reproductive cells (Qin et al., 2022). From the perspective of these signaling pathways, amino acid metabolism is crucial for the regulation of intracellular redox balance, especially amino acid-related metabolic processes, which could significantly affect the therapeutic effect of ferroptosis.
Metabolic regulation of ferroptosis with vitamins
Multiple nutrients including exogenous fatty acids and amino acids have been reviewed to regulate redox homeostasis and ferroptosis execution (Koppula et al., 2018; Qi et al., 2021). However, the underlying mechanism of vitamins and ferroptosis and corresponding nutrient signaling related to ferroptosis regulation is still limited. Vitamins are dietary components that have functioned for therapeutic applications over the past decades (Saghiri et al., 2017; Di Tano et al., 2020; Hou et al., 2020). In this section, vitamins B, C, E, and K are essential for regulating cellular homeostasis that directly linked to ferroptotic cell death has been overviewed.
Vitamin B
The vitamin B family generally serves as enzyme cofactors carrying out essential cellular functions, such as energy metabolism, DNA synthesis, and phospholipids metabolism (Lindschinger et al., 2019). B-group vitamins are water-soluble; the metabolic half-life is only a few hours and must be supplemented daily in most cases (Tardy et al., 2020). Existing evidence indicates that insufficient or excessive supply of B vitamins may elicit oxidative stress and modulate the expression of numerous pathogenic genes (Kennedy, 2016; Peterson et al., 2020). As a proof-of-concept, assembled Vitamin B2 nanocrystals have been exploited to favor the massive production of ROS under blue light irradiation taking advantage of optical waveguiding properties (Fei et al., 2019). Inspired by these interesting properties, Zhou et al. subtly reported the strategy by combining Vitamin B2 and trivalent iron in a self-assembly nanostructure (VFNC) for multiple ferroptosis regulation (Figure 2A; Zhou M. et al., 2023). This vitamin B2-ferric nanocomplex exhibited appropriate size and favorable biocompatibility. Activated by ultrasound, VB2 could contribute to producing a significant quantity of lethal 1O2 to inhibit cell proliferation. Simultaneously, the disassembled nanocomplex will release Fe3+ ion, which could generate •OH through the Fenton reaction and destroy the System Xc−-GSH-GPX4 antioxidant defense system. The downregulated GPX4 and SLC7A11 protein and more LPO accumulation suggested the effective facilitation of metformin to VFNC-based ferroptosis therapy (Figures 2B,C). The synergistic sonodynamic/ferroptotic therapy and the coactivation effect of metformin could exert a superadditive anti-tumor effect by completely disintegrating the redox homeostasis and antioxidant defense system of triple-negative breast cancer. Overall, this coordination-driven self-assembly system provides a general strategy for nutritional vitamins in clinical applications.
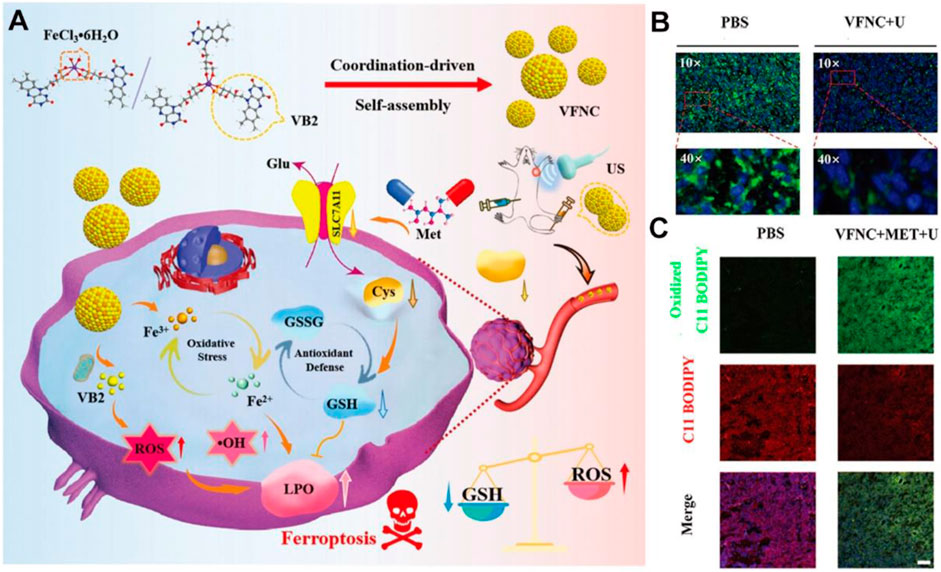
Figure 2. Schematic illustration of VFNC-based ferroptosis therapy towards triple-negative breast cancer. (A) Schematic of the preparation process and therapeutic mechanism of the VFNC nanoplatform. (B) Immunofluorescence staining of GPX4. (C) CLSM images of LPO accumulation. Adapted with permission from Zhou M. et al. (2023). Copyright (2023) John Wiley & Sons, Inc.
Recently, Qin et al. established the impact of early vitamin B12 insufficiency in ferroptosis induction (Figure 3A; Qin et al., 2022). Vitamin B12 sufficiency has no adverse impact on germ cells; however, early vitamin B12 deficiency leads to ferroptosis in germ cells and causes obesity and infertility. Mechanistically, vitamin B12 deficiency directly affects the methionine cycle-SBP-1/sterol regulatory element-binding protein-1 (SREBP1)-adipogenesis axis, resulting in the accumulation of polyunsaturated fatty acids and heightened peroxidation levels, which was significantly suppressed by the addition of B12 supplementation (Figures 3B,C). Overall, this study highlights the relationship between vitamin B12 deficiency and ferroptosis in early life, providing a potential target for the late-life treatment of the disease in adulthood.
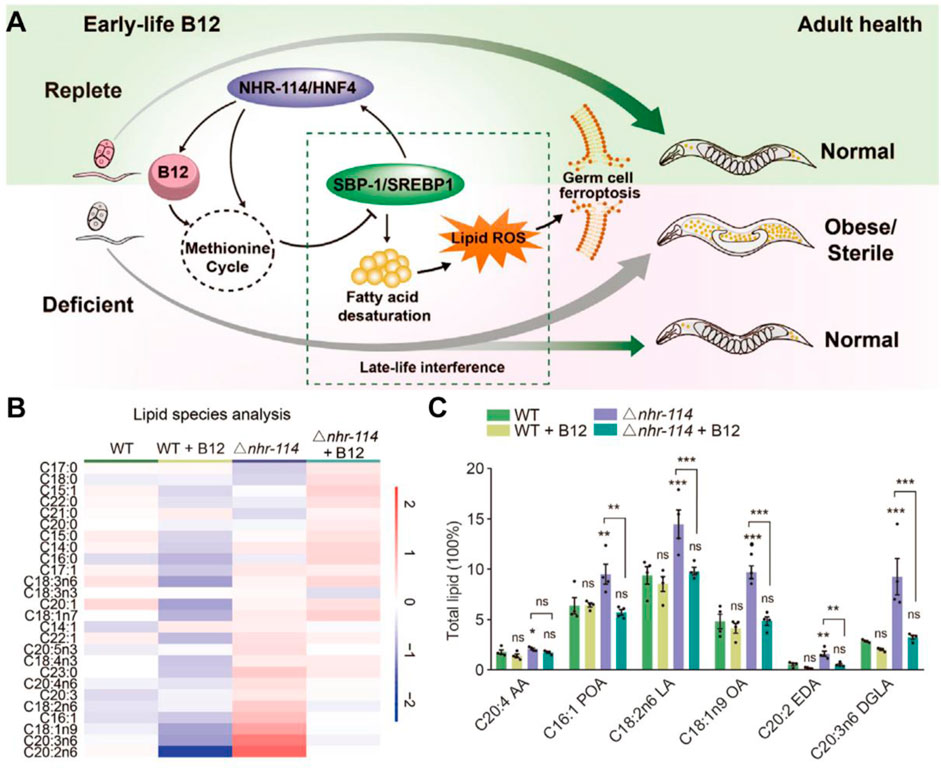
Figure 3. (A) Mechanism of action of early-life B12 in programming of adult health. (B,C) Heatmap and quantification of lipid species with maternal B12 supplementation. Reproduced with permission (Qin et al., 2022). Copyright 2022, Cell Press.
Vitamin C
In biological systems, vitamin C exists in different redox forms, including ascorbate, ascorbate radical, dehydroascorbic acid (DHA), 2,3-l-diketoglutonate (2,3-DKG), oxalic acid, and so on. Specifically, the ascorbyl radical is generated upon donating electrons to ferric iron, leading to the formation of superoxide anion (•O2−) and H2O2, while ascorbate in pharmacologic concentration can contribute to the suspended formation of •OH (Szarka et al., 2021).
In addition, vitamin C can affect iron metabolism by inhibiting the degradation of ferritin, increasing the synthesis of ferritin, and thus promoting iron absorption (Wang X. et al., 2021). This cellular death pathway shows similar characteristics to erastin-mediated ferroptosis (Liu et al., 2022). Although vitamin C as an anticancer therapeutic is still up for debate, ongoing recent clinical trials are investigating the combination of pharmacological ascorbate with concurrent radiochemotherapy. Various studies have demonstrated the enhanced therapeutic effect of employing vitamin C to treat troublesome non-small cell lung cancer (NSCLC) (Ou et al., 2020). Recently, a nanocatalytic sensitizer strategy with vitamin C involved has been proposed for treating metastatic NSCLC (Figure 4A; Wang et al., 2022). In this nanotherapy platform, the rational encapsulation of vitamin C and ferrous iron within the liposome enabled the reversible redox cycle. The ferrous ions can interact with hydrogen peroxide, yielding highly reactive •OH radicals, inducing lipid peroxidation (LPO) and ultimately triggering ferroptosis. As shown in Figure 4B, cells underwent oxidative stress and formed vacuolated mitochondria after drug administration. In addition, vitamin C is oxidized to produce DHA, which consumes intracellular GSH, resulting in decreased GPX4 expression (Figure 4C). Moreover, this effective nanocatalytic platform can simultaneously solve the problem of resistance to the kinase inhibitor oxitinib in metastasis NSCLC.
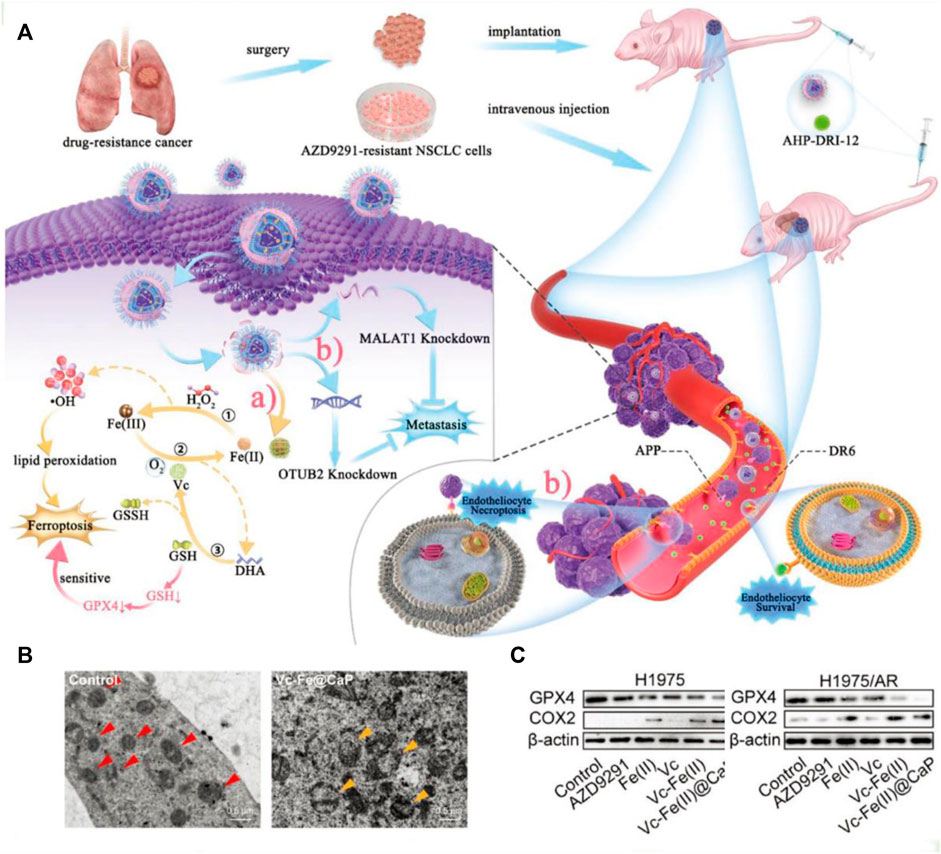
Figure 4. (A) Schematic illustration of the tumoricidal effect of combination therapy of VF/S/A@CaP and AHP-DRI-12. (B) The cryo-electron microscope images of H1975/AR cells from the control group or Vc–Fe(II)@CaP group. The red triangle serves as a marker for healthy mitochondria, whereas the yellow triangle signifies vacuolated mitochondria that have sustained severe oxidative damage. (C) The expression levels of GPX4 and COX2 in both H1975 and H1975/AR cells have been assayed. Reproduced with permission (Wang et al., 2022). Copyright (2022), John Wiley & Sons, Inc.
It is a widely recognized fact that mitochondria serve as the primary organelles responsible for iron metabolism within cells. Consequently, the development of mitochondria-targeting ferroptosis platforms has demonstrated promising antitumor effects (Gan et al., 2023). Nevertheless, recent studies have revealed that the rational design of lysosome-targeting systems capable of inducing ferroptosis may yield significant outcomes. These findings broaden our understanding of the complex mechanisms involved in ferroptosis and suggest novel therapeutic strategies for targeting this process. For instance, Chen et al. successfully synthesized a lysosome-targeting ferroptosis nanoplatform (Figure 5; Chen et al., 2022). The synthesis process and chemical structures of the components of VC@N3AMcLAVs are thoroughly outlined in Figures 5A,B. Notably, the incorporation of morpholine enabled the nanotherapeutic platform to specifically target lysosomes. Once inside the lysosome, the nanosystem interacts with excess GSH leading to the reduction of lipoic acid and oxidation of vitamin C. Additionally, the oxygen present in the lysosome is converted to hydrogen peroxide. Furthermore, this efficient redox cycle facilitates the conversion of ferric ions to ferrous ions through the degradation of ferritin within the lysosome, further enhancing the antitumor effects of ferroptosis. The produced excess hydrogen peroxide will interact with the ferrous ions to produce highly oxidizing •OH. Due to the depletion of GSH and deficiency of catalase, large numbers of ROS will destroy the redox homeostasis of lysosomes and result in the complete breakdown of the lysosomes membrane, inducing the release of proteolytic enzymes and other substances into the cytosol. This lysosomal membrane permeabilization will cause irreversible damage to tumor cells, resulting in efficient ferroptosis therapy (Figure 5C). The system successfully uses the interaction between the external vitamin C and the intracellular GSH to cause the irreversible death of tumor cells, which provides a new option for effective anticancer therapy. This design offers a promising approach for precision delivery and enhanced antitumor effects through ferroptosis induction in lysosomes.
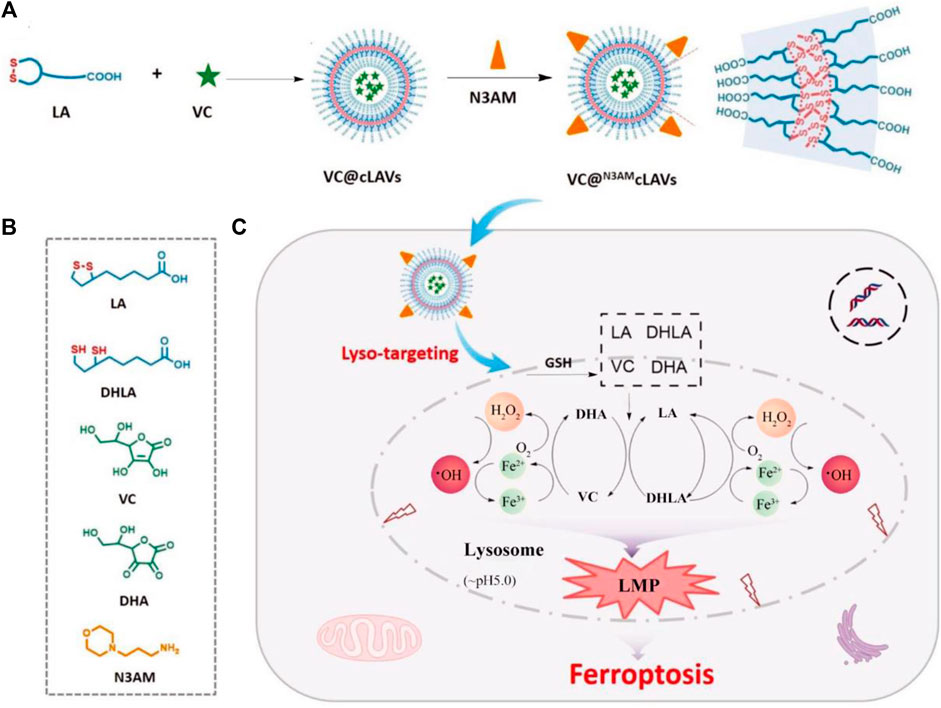
Figure 5. The schematic illustration outlines the process of enhancing ROS-mediated lysosomal membrane permeabilization for cancer ferroptosis therapy. (A) The design methods of VC@N3AMcLAVs. (B) The various molecular structures from the composition of VC@N3AMcLAVs. (C) Lysosome-targeting process of VC@N3AMcLAVs and the specific mechanism to promote ROS-mediated lysosomal membrane permeabilization for efficient ferroptosis therapy. Adapted with permission from Chen et al. (2022). Copyright (2023) John Wiley & Sons, Inc.
Recently, vitamin C at pharmaceutical dosage has been demonstrated to be efficient in strengthening the antineoplastic effect by eliciting host immune systems (Ngo et al., 2019). In particular, high-dose vitamin C treatment exhibits great synergy in potentiating the efficacy of immune checkpoint therapy (Magrì et al., 2020). Exploring vitamin C-mediated immunomodulation, Yu et al. proposed hybrid core-shell vesicles (HCSVs) to augment ferroptosis-based therapy by an exogenous circularly polarized magnetic field, as well as responsive magnetic resonance imaging capability (Yu et al., 2020). The HCSVs were constructed by encapsulating ascorbic acid within poly lactic-co-glycolic acid, and the iron oxide nanocubes were embedded into the outer layer. Under the external magnetic field, the vesicle structure is destroyed along with the release of ascorbic acid to interact with magnetic iron oxide. Ascorbic acid can oxidize ferric ions to produce ferrous ions, which could induce subsequent Fenton chemical reaction processes. Subsequently, the Fenton reaction leads to ferroptosis-mediated cancer inhibition by excessive LPO accumulation. Concurrently, the elevated oxidative stress could induce the activation of dendritic cells and infiltration of cytotoxic T lymphocytes to exert an immunomodulatory effect. During the reduction of magnetic iron oxide by ascorbic acid, the iron-based magnetic resonance imaging signal provides an efficient strategy for visualization of the Fenton reaction.
Vitamin E
As an endogenous radical-trapping antioxidant, vitamin E is a general term for tocopherol substances exhibiting multiple health benefits. Vitamin E comprises different forms of tocopherol and tocotrienol generally functions by the electron-transport coupled enzymatic mechanisms to prevent oxidative damage (Peh et al., 2016; Blaner et al., 2021). For ferroptosis execution, vitamin E actively binds to the PUFA substrate to favor the protective effects against LPO propagation (Hu et al., 2021). Recently, Zhang et al. revealed the anti-ferroptotic defense of nutrient vitamin E for chronic neurological diseases (Zhang X. et al., 2022). Mechanistically, vitamin E exerts neuroprotective effects largely by inhibiting LOX-15, and vitamin E pretreatment can reduce neuronal damage in pentylenetetrazol-induced epileptic rats. In addition, tetrahydrobiopterin (BH4) has been screened out to protect lipids from autoxidation in synergy with vitamin E (Soula et al., 2020). Metabolism-focused screens demonstrated that BH4 biosynthesis is essential for GPX4 inhibition, and can regenerate from its oxidative forms upon dihydrofolate reductase (DHFR), maintaining sustained anti-ferroptotic function. According to the autoxidations of STY-BODIPY, BH4 had similar ferroptosis-inhibitory effects in comparison to α-tocopherol (α-TOH). In the liposomal system, the combination of BH4 and α-TOH yielded superior anti-ferroptosis defenses, partially because of the scavenging of α-TOH-derived radicals.
Vitamin K
Vitamin K is a general term referring to a group of structurally related compounds that possess a common naphthoquinone ring. These compounds encompass both natural and synthetic forms, including phylloquinone (known as vitamin K1) found primarily in plants, menaquinones (vitamin K2) found in animal products, and synthetic analogs such as vitamin K3 and menaquinone-4 (MK4) (Welsh et al., 2022). The primary biological role of vitamin K is serving as a cofactor for the γ-carboxylation process, which is crucial in preventing excessive hemorrhaging and osteoporosis. This cofactor function is vital for maintaining bone health and blood coagulation (Wallin and Hutson, 2004; Shearer and Okano, 2018). Recently, vitamin K1 as the predominant dietary form has been investigated as a potential ferroptosis suppressor (Kolbrink et al., 2022). In 2022, Kolbrink et al. proved that vitamin K1 can participate in ferroptosis inhibitory systems, providing pharmacological control of ischemia-reperfusion injury (Kolbrink et al., 2022). In the NIH3T3 cells, the frequency of cell death in the RSL3 group and RSL3 + vitamin K1 group was observed to be 80.8% and 2.2%, respectively. Both vitamin K1 (10 μM) and ferrostatin-1 (1 μM) could remarkably counteract the cytotoxicity by inhibiting RSL3-induced ferroptosis at the cellular level. As evidenced by the phosphatidylserine accessibility, vitamin K1 can also reverse ferroptotic cell death by inhibition of FSP1 and mitochondrially dihydroorotate dehydrogenase (DHODH). For renal ischemia-reperfusion, vitamin K1 had a protective effect on acute kidney injury by inhibiting tubular necrosis and ACSL4 expression. Overall, vitamin K1 demonstrated great therapeutic potential for many diseases associated with ferroptosis by targeting multiple inhibitory pathways. Apart from vitamin K1, variant synthetic menadione has recently been proven to rescue cells from ferroptosis by GPX4 deletion (Mishima et al., 2022). As depicted in Figure 6A, vitamin K elicits ferroptotic inhibitory function that largely depends on FSP1-mediated reduction. In the canonical vitamin K cycle, vitamin K is typically reduced to vitamin K hydroquinone (VKH2), which is generally recognized as the radical-trapping antioxidant (Figure 6A). However, VKH2 is easily oxidized to vitamin K epoxide and then converted to vitamin K quinone losing antioxidant activity, this process is also known as warfarin poisoning. Intriguingly, FSP1 was able to form a direct cycle from vitamin K to VKH2, maintaining lipid radical-trapping activity to attenuate ferroptosis (Figure 6B). In pfa1 cells, MK4 is the most efficacious form of vitamin K derivatives in preventing ferroptosis induced by GPX4 deletion or knockdown (Figure 6C). The significantly reduced release percentage of lactate dehydrogenase further indicated the protective effect of three forms of vitamin K derivative (PK/MK4/Menad) against cell death (Figure 6D). In particular, the presence of MK4 was able to successfully prevent HT-1080 cells from undergoing pathologic changes associated with ferroptosis induced by RSL3 (Figure 6E). Lipid peroxidation is a key process in ferroptosis, where the accumulation of lipid peroxides leads to membrane damage and ultimately cell death. By reducing the level of lipid peroxide, the three forms of vitamin K can mitigate the progression of ferroptosis (Figure 6F). This finding suggests that vitamin K may act as an efficient antioxidant or regulate cellular processes that lead to lipid peroxidation, thereby protecting cells from ferroptosis. Overall, vitamin K metabolism is thought to play a critical role in ferroptosis-associated disease, acting as a natural active ingredient in anti-ferroptotic therapy through the non-classical vitamin K cycle.
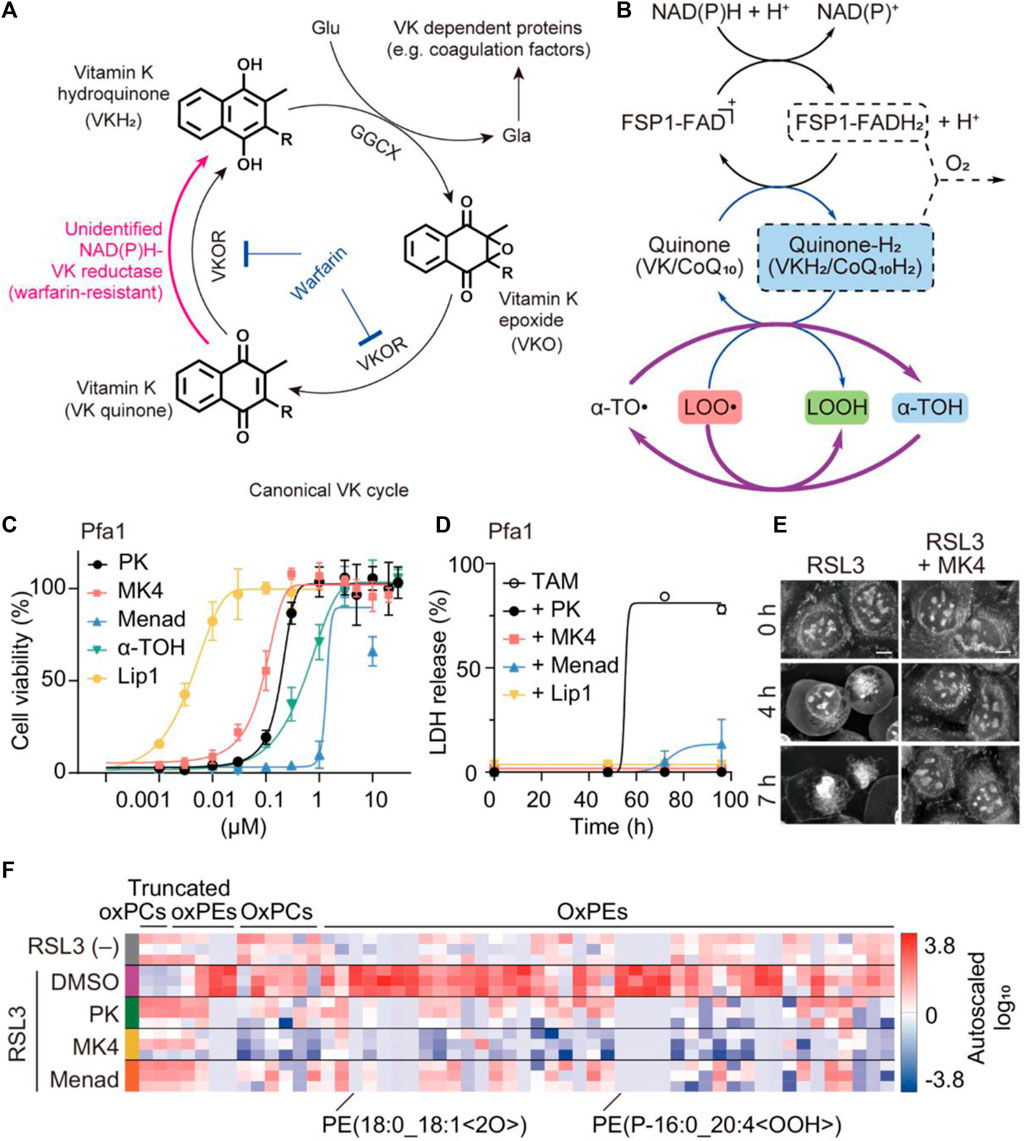
Figure 6. The anti-ferroptotic mechanism of non-canonical vitamin K cycle. (A) Mechanism of vitamin K supplying VKH2 to GGCX via the alternative vitamin K reduction pathway. (B) The possible recycling pathway of α-tocopherol via the CoQ10/VK-hydroquinone transformation process. (C) The cell viability and (D) lactate dehydrogenase (LDH) release from GPX4-KO mouse embryonic fibroblasts (Pfa1 cells) treated with different conditions (three forms of vitamin K and liproxstatin-1 (Lip1) as a ferroptosis inhibitor). (E) The cellular morphology images of RSL3-treated HT-1080 cells were observed irrespective of whether MK4 was present. (F) Proportional levels of oxidized phospholipids are visualized through heatmaps during an 8-h exposure to RSL3 in Pfa1 cells (Mishima et al., 2022). Copyright (2021) Springer Nature.
Furthermore, the synthetic variant menadione (vitamin K3) is known to be a notable anti-inflammatory drug, which can exacerbate ROS generation to achieve therapeutic effects against bacteria or viruses at appropriate wavelengths (Wang R. et al., 2021). Given that, vitamin K3 and its derivatives have been employed in the field of ROS-based nanomedicine aiming at achieving improved therapeutic index (Yang et al., 2018; Wellington et al., 2020; Chauhan et al., 2023). Utilizing iron ion therapy, nanoscale coordination polymers known as Fe-NQA have been suggested as a potential tumor treatment method, capable of initiating the Fenton reaction while simultaneously suppressing antioxidant activity (Figure 7; Zhang Z. et al., 2020). The Fe-NQA was prepared by one-step coordination self-assembly of Fe3+ and 6-[2-(3-methyl)naphthoquinolyl]hexanoic acid (NQA). The NQA can not only facilitate semiquinone radical formation but also inhibit GPX4 activation by binding cysteine or selenocysteine residues. After internalization, Fe-NQA disassembles in a pH-responsive manner to form a sustained cycle favoring the Fenton reaction by catalyzed cytochrome P450 reductase. Additionally, Fe-NQA sustains GPX4 inactivation, and this cellular death can be reversed by ferroptosis inhibitors. The staining process employed FerroOrange and BODIPY-C11 probes, revealing that the level of lipid peroxide (LPO) in CT26 cells incubated with Fe-NQA nanoparticles was notably elevated compared to the group treated with normal saline (Figure 7B). Taken together, Fe-NQA can synergistically initiate ferroptosis execution, enabling efficient tumor regression, metastasis prevention, and radiotherapy sensitization.
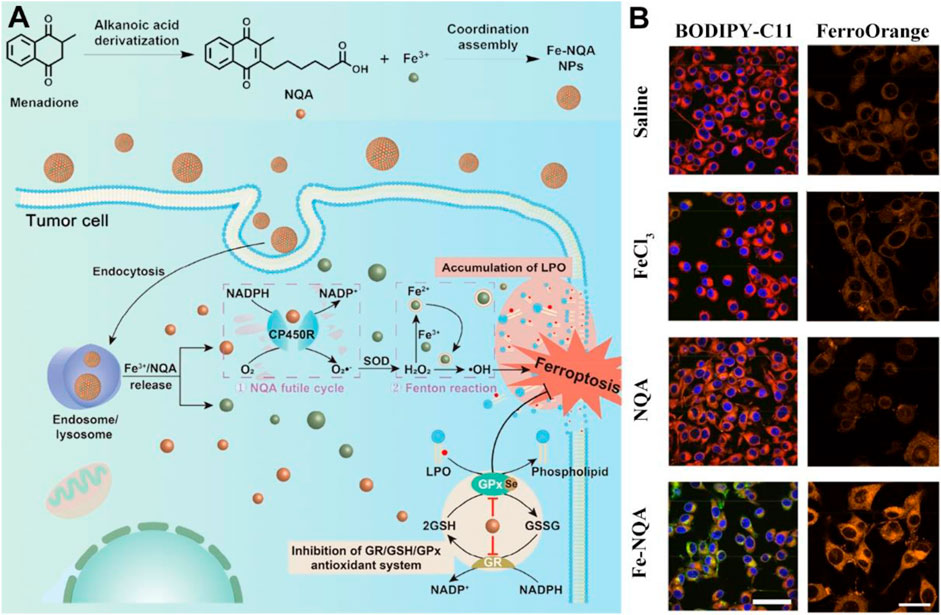
Figure 7. The preparation and action mechanism of Fe-NQA for augmenting tumor ferroptotic therapy. (A) Schematic illustration of Fe-NQA NPs induced ferroptotic therapy. (B) The LSCM images of LPO and Fe2+ detected in CT26 cells after incubation of cells with saline, FeCl3, NQA, and Fe-NQA NPs (scale bar: 50 μm). Adapted with permission from Zhang Z. et al. (2020). Copyright (2020) Springer Nature.
Conclusion and perspectives
Ferroptosis has achieved unprecedented outcomes since coined in 2012, and therapeutic intervention has been demonstrated to be tightly linked to neurodegeneration, viral infection, and cardiovascular disease. Especially for malignant tumors, proferroptotic stimulation has sufficiently indicated significant impacts on the inhibition, development, and metastasis of tumor cells. Existing research has sufficiently indicated ferroptosis inducers or inhibitors, and compartmentalized by regulatory networks involves iron, amino acids, and lipids metabolism. Elucidating these metabolism networks may be critical for therapeutic benefit in various ferroptosis-associated pathophysiological contexts. However, the complicated ferroptosis-based crosstalk between multiple nutrients and metabolic regulation of cellular homeostasis is still limited, especially in the field of vitamin-based essential nutrients.
The administration of nutritional vitamin supplements could potentially serve as an effective approach to modulate sublethal ferroptosis-associated disorders. To date, essential vitamins enabling the regulation of redox homeostasis and LPO generally depend on perturbing ROS hemostasis. However, the current understanding of ROS biology is orchestrated by complex molecular pathways comprising different cell death modalities. Conversely, the interplay between the mechanism of ferroptotic cell death and its associated factors with nutrient signaling remains relatively unexplored.
Furthermore, metabolism regulation of iron availability, cystine deprivation, and ROS biology has yielded insight into the nutrient vitamins in remodeled biological hemostasis. However, interconnected metabolism pathways are highly complex, which is also referred to as metabolic plasticity. The modulation of ferroptosis may involve various endogenous substrates, and vitamins could potentially play a role in other forms of controlled cell death. Nevertheless, the intricate interactions and transitions between ferroptosis and other cell death mechanisms remain elusive.
Finally, the pathophysiological signals of ferroptotic cell death must be unambiguously elucidated stem from the fact that these effectors are directly related to the therapeutic potential of ferroptosis-associated disease. Stratifying ferroptosis sensitivity in patients holds promise for conferring significant health benefits in clinical practice. Current oxidative lipidomics is largely time-consuming, while other reliable molecular imaging methodologies are relatively not enough to favor real-time monitoring of this regulated cell death modality. There is an urgent need for advanced techniques and reagents capable of monitoring treatment processes, in order to rigorously assess the pathological significance of ferroptosis.
Author contributions
JW: Investigation, Writing–original draft, Writing–review and editing. YS: Investigation, Writing–original draft, Writing–review and editing. MaZ: Investigation, Writing–original draft, Writing–review and editing. MC: Formal Analysis, Investigation, Methodology, Writing–original draft. SJ: Formal Analysis, Investigation, Methodology, Writing–original draft. XL: Formal Analysis, Investigation, Methodology, Writing–original draft. MeZ: Formal Analysis, Investigation, Methodology, Writing–original draft. RX: Writing–original draft, Writing–review and editing. XZ: Funding acquisition, Writing–original draft, Writing–review and editing. WW: Writing–original draft, Writing–review and editing.
Funding
The author(s) declare that financial support was received for the research, authorship, and/or publication of this article. This work was supported by the National Natural Science Foundation of China (52103169), Jiangsu Provincial Association for Science and Technology Youth Science and Technology Talent Support Project (TJ-2023-006), the Large Instruments Open Foundation of Nantong University (KFJN2372), Undergraduate Innovation and Entrepreneurship Training Program of Jiangsu Province (202310304155Y), and the College Students’ Innovation and Entrepreneurship Training Program of Nantong University (Grant no. 2022239) are gratefully acknowledged.
Conflict of interest
The authors declare that the research was conducted in the absence of any commercial or financial relationships that could be construed as a potential conflict of interest.
Publisher’s note
All claims expressed in this article are solely those of the authors and do not necessarily represent those of their affiliated organizations, or those of the publisher, the editors and the reviewers. Any product that may be evaluated in this article, or claim that may be made by its manufacturer, is not guaranteed or endorsed by the publisher.
References
Andrews, N. C., and Schmidt, P. J. (2007). Iron homeostasis. Annu. Rev. Physiol. 69, 69–85. doi:10.1146/annurev.physiol.69.031905.164337
Bao, W. D., Pang, P., Zhou, X. T., Hu, F., Xiong, W., Chen, K., et al. (2021). Loss of ferroportin induces memory impairment by promoting ferroptosis in Alzheimer's disease. Cell Death Differ. 28, 1548–1562. doi:10.1038/s41418-020-00685-9
Bao, W. D., Zhou, X. T., Zhou, L. T., Wang, F., Yin, X., Lu, Y., et al. (2020). Targeting miR-124/Ferroportin signaling ameliorated neuronal cell death through inhibiting apoptosis and ferroptosis in aged intracerebral hemorrhage murine model. Aging Cell 19, e13235. doi:10.1111/acel.13235
Bazinet, R. P., and Layé, S. (2014). Polyunsaturated fatty acids and their metabolites in brain function and disease. Nat. Rev. Neurosci. 15, 771–785. doi:10.1038/nrn3820
Blaner, W. S., Shmarakov, I. O., and Traber, M. G. (2021). Vitamin A and vitamin E: will the real antioxidant please stand up? Annu. Rev. Nutr. 41, 105–131. doi:10.1146/annurev-nutr-082018-124228
Bogdan, A. R., Miyazawa, M., Hashimoto, K., and Tsuji, Y. (2016). Regulators of iron homeostasis: new players in metabolism, cell death, and disease. Trends biochem. Sci. 41, 274–286. doi:10.1016/j.tibs.2015.11.012
Chauhan, A., Anjaly, K., Saini, A., Kumar, R., Kuanr, B. K., and Sharma, D. (2023). Vitamin k3-loaded magnetic nanoparticle-mediated synergistic magnetothermodynamic therapy evokes massive ROS and immune modulation for augmented antitumor potential. ACS Appl. Mater Interfaces 15, 27515–27532. doi:10.1021/acsami.3c01702
Chen, Y., Yang, Z., Wang, S., Ma, Q., Li, L., Wu, X., et al. (2022). Boosting ROS-mediated lysosomal membrane permeabilization for cancer ferroptosis therapy. Adv. Healthc. Mater. 12, e2202150. doi:10.1002/adhm.202202150
Di Tano, M., Raucci, F., Vernieri, C., Caffa, I., Buono, R., Fanti, M., et al. (2020). Synergistic effect of fasting-mimicking diet and vitamin C against KRAS mutated cancers. Nat. Commun. 11, 2332. doi:10.1038/s41467-020-16243-3
Dixon, S. j., Lemberg, K. m., Lamprecht, M. r., Skouta, R., Zaitsev, E. m., Gleason, C. e., et al. (2012). Ferroptosis: an iron-dependent form of nonapoptotic cell death. Cell 149, 1060–1072. doi:10.1016/j.cell.2012.03.042
Dyall, S. C., Balas, L., Bazan, N. G., Brenna, J. T., Chiang, N., Da Costa Souza, F., et al. (2022). Polyunsaturated fatty acids and fatty acid-derived lipid mediators: recent advances in the understanding of their biosynthesis, structures, and functions. Prog. Lipid Res. 86, 101165. doi:10.1016/j.plipres.2022.101165
Fang, Y., Chen, X., Tan, Q., Zhou, H., Xu, J., and Gu, Q. (2021). Inhibiting ferroptosis through disrupting the NCOA4–FTH1 interaction: a new mechanism of action. ACS Cent. Sci. 7, 980–989. doi:10.1021/acscentsci.0c01592
Fei, J., Dai, L., Gao, F., Zhao, J., and Li, J. (2019). Assembled vitamin B2 nanocrystals with optical waveguiding and photosensitizing properties for potential biomedical application. Angew. Chem. Int. Ed. Engl. 58, 7254–7258. doi:10.1002/anie.201900124
Gan, B. (2022). ACSL4, PUFA, and ferroptosis: new arsenal in anti-tumor immunity. Signal Transduct. Target Ther. 7, 128. doi:10.1038/s41392-022-01004-z
Gan, H., Huang, X., Luo, X., Li, J., Mo, B., Cheng, L., et al. (2023). A mitochondria-targeted ferroptosis inducer activated by glutathione-responsive imaging and depletion for triple negative breast cancer theranostics. Adv. Healthc. Mater 12, e2300220. doi:10.1002/adhm.202300220
Gong, Y., Fan, Z., Luo, G., Yang, C., Huang, Q., Fan, K., et al. (2019). The role of necroptosis in cancer biology and therapy. Mol. Cancer 18, 100. doi:10.1186/s12943-019-1029-8
Hashidate-Yoshida, T., Harayama, T., Hishikawa, D., Morimoto, R., Hamano, F., Tokuoka, S. M., et al. (2015). Fatty acid remodeling by LPCAT3 enriches arachidonate in phospholipid membranes and regulates triglyceride transport. Elife 4, e06328. doi:10.7554/eLife.06328
Hassannia, B., Vandenabeele, P., and Vanden Berghe, T. (2019). Targeting ferroptosis to iron out cancer. Cancer Cell 35, 830–849. doi:10.1016/j.ccell.2019.04.002
Hou, X., Zhang, X., Zhao, W., Zeng, C., Deng, B., Mccomb, D. W., et al. (2020). Vitamin lipid nanoparticles enable adoptive macrophage transfer for the treatment of multidrug-resistant bacterial sepsis. Nat. Nanotechnol. 15, 41–46. doi:10.1038/s41565-019-0600-1
Hu, Q., Zhang, Y., Lou, H., Ou, Z., Liu, J., Duan, W., et al. (2021). GPX4 and vitamin E cooperatively protect hematopoietic stem and progenitor cells from lipid peroxidation and ferroptosis. Cell Death Dis. 12, 706. doi:10.1038/s41419-021-04008-9
Jiang, X., Stockwell, B. R., and Conrad, M. (2021). Ferroptosis: mechanisms, biology and role in disease. Nat. Rev. Mol. Cell Biol. 22, 266–282. doi:10.1038/s41580-020-00324-8
Kagan, V. E., Mao, G., Qu, F., Angeli, J. P. F., Doll, S., Croix, C. S., et al. (2016). Oxidized arachidonic and adrenic PEs navigate cells to ferroptosis. Nat. Chem. Biol. 13, 81–90. doi:10.1038/nchembio.2238
Kennedy, D. (2016). B vitamins and the brain: mechanisms, dose and efficacy-A review. Nutrients 8, 68. doi:10.3390/nu8020068
Kerr, J. F. R., Wyllie, A. H., and Currie, A. R. (1972). Apoptosis: a basic biological phenomenon with wideranging implications in tissue kinetics. Br. J. Cancer 26, 239–257. doi:10.1038/bjc.1972.33
Kolbrink, B., Von Samson-Himmelstjerna, F. A., Messtorff, M. L., Riebeling, T., Nische, R., Schmitz, J., et al. (2022). Vitamin K1 inhibits ferroptosis and counteracts a detrimental effect of phenprocoumon in experimental acute kidney injury. Cell Mol. Life Sci. 79, 387. doi:10.1007/s00018-022-04416-w
Koppula, P., Zhang, Y., Zhuang, L., and Gan, B. (2018). Amino acid transporter SLC7A11/xCT at the crossroads of regulating redox homeostasis and nutrient dependency of cancer. Cancer Commun. 38, 12. doi:10.1186/s40880-018-0288-x
Koppula, P., Zhuang, L., and Gan, B. (2021). Cystine transporter SLC7A11/xCT in cancer: ferroptosis, nutrient dependency, and cancer therapy. Protein & Cell 12, 599–620. doi:10.1007/s13238-020-00789-5
Lee, J., and Roh, J.-L. (2022). SLC7A11 as a gateway of metabolic perturbation and ferroptosis vulnerability in cancer. Antioxidants 11, 2444. doi:10.3390/antiox11122444
Lei, G., Zhuang, L., and Gan, B. (2022). Targeting ferroptosis as a vulnerability in cancer. Nat. Rev. Cancer 22, 381–396. doi:10.1038/s41568-022-00459-0
Liang, C., Zhang, X., Yang, M., and Dong, X. (2019). Recent progress in ferroptosis inducers for cancer therapy. Adv. Mater 31, e1904197. doi:10.1002/adma.201904197
Liang, D., Minikes, A. M., and Jiang, X. (2022). Ferroptosis at the intersection of lipid metabolism and cellular signaling. Mol. Cell 82, 2215–2227. doi:10.1016/j.molcel.2022.03.022
Lin, L., Wang, S., Deng, H., Yang, W., Rao, L., Tian, R., et al. (2020). Endogenous labile iron pool-mediated free radical generation for cancer chemodynamic therapy. J. Am. Chem. Soc. 142, 15320–15330. doi:10.1021/jacs.0c05604
Lindschinger, M., Tatzber, F., Schimetta, W., Schmid, I., Lindschinger, B., Cvirn, G., et al. (2019). A randomized pilot trial to evaluate the bioavailability of natural versus synthetic vitamin B complexes in healthy humans and their effects on homocysteine, oxidative stress, and antioxidant levels. Oxid. Med. Cell Longev. 2019, 6082613. doi:10.1155/2019/6082613
Liu, Y., Huang, P., Li, Z., Xu, C., Wang, H., Jia, B., et al. (2022). Vitamin C sensitizes pancreatic cancer cells to erastin-induced ferroptosis by activating the AMPK/Nrf2/HMOX1 pathway. Oxid. Med. Cell Longev. 2022, 5361241. doi:10.1155/2022/5361241
Magrì, A., Germano, G., Lorenzato, A., Lamba, S., Chilà, R., Montone, M., et al. (2020). High-dose vitamin C enhances cancer immunotherapy. Sci. Transl. Med. 12, eaay8707. doi:10.1126/scitranslmed.aay8707
Mishima, E., Ito, J., Wu, Z., Nakamura, T., Wahida, A., Doll, S., et al. (2022). A non-canonical vitamin K cycle is a potent ferroptosis suppressor. Nature 608, 778–783. doi:10.1038/s41586-022-05022-3
Mou, Y., Wang, J., Wu, J., He, D., Zhang, C., Duan, C., et al. (2019). Ferroptosis, a new form of cell death: opportunities and challenges in cancer. J. Hematol. Oncol. 12, 34. doi:10.1186/s13045-019-0720-y
Ngo, B., Van Riper, J. M., Cantley, L. C., and Yun, J. (2019). Targeting cancer vulnerabilities with high-dose vitamin C. Nat. Rev. Cancer 19, 271–282. doi:10.1038/s41568-019-0135-7
Ou, J., Zhu, X., Chen, P., Du, Y., Lu, Y., Peng, X., et al. (2020). A randomized phase II trial of best supportive care with or without hyperthermia and vitamin C for heavily pretreated, advanced, refractory non-small-cell lung cancer. J. Adv. Res. 24, 175–182. doi:10.1016/j.jare.2020.03.004
Peh, H. Y., Tan, W. S., Liao, W., and Wong, W. S. (2016). Vitamin E therapy beyond cancer: tocopherol versus tocotrienol. Pharmacol. Ther. 162, 152–169. doi:10.1016/j.pharmthera.2015.12.003
Peng, F., Liao, M., Qin, R., Zhu, S., Peng, C., Fu, L., et al. (2022). Regulated cell death (RCD) in cancer: key pathways and targeted therapies. Signal Transduct. Target. Ther. 7, 286. doi:10.1038/s41392-022-01110-y
Peterson, C. T., Rodionov, D. A., Osterman, A. L., and Peterson, S. N. (2020). B vitamins and their role in immune regulation and cancer. Nutrients 12, 3380. doi:10.3390/nu12113380
Qi, Y., Zhang, X., Wu, Z., Tian, M., Chen, F., Guan, W., et al. (2021). Ferroptosis regulation by nutrient signalling. Nutr. Res. Rev. 35, 282–294. doi:10.1017/S0954422421000226
Qin, S., Wang, Y., Li, L., Liu, J., Xiao, C., Duan, D., et al. (2022). Early-life vitamin B12 orchestrates lipid peroxidation to ensure reproductive success via SBP-1/SREBP1 in Caenorhabditis elegans. Cell Rep. 40, 111381. doi:10.1016/j.celrep.2022.111381
Riegman, M., Sagie, L., Galed, C., Levin, T., Steinberg, N., Dixon, S. J., et al. (2020). Ferroptosis occurs through an osmotic mechanism and propagates independently of cell rupture. Nat. Cell Biol. 22, 1042–1048. doi:10.1038/s41556-020-0565-1
Rizzollo, F., More, S., Vangheluwe, P., and Agostinis, P. (2021). The lysosome as a master regulator of iron metabolism. Trends Biochem. Sci. 46, 960–975. doi:10.1016/j.tibs.2021.07.003
Saghiri, M. A., Asatourian, A., Ershadifar, S., Moghadam, M. M., and Sheibani, N. (2017). Vitamins and regulation of angiogenesis: [A, B1, B2, B3, B6, B9, B12, C, D, E, K]. J. Funct. Foods 38, 180–196. doi:10.1016/j.jff.2017.09.005
Shearer, M. J., and Okano, T. (2018). Key pathways and regulators of vitamin K function and intermediary metabolism. Annu. Rev. Nutr. 38, 127–151. doi:10.1146/annurev-nutr-082117-051741
Sies, H. (2021). Oxidative eustress: on constant alert for redox homeostasis. Redox Biol. 41, 101867. doi:10.1016/j.redox.2021.101867
Snaebjornsson, M. T., Janaki-Raman, S., and Schulze, A. (2020). Greasing the wheels of the cancer machine: the role of lipid metabolism in cancer. Cell Metab. 31, 62–76. doi:10.1016/j.cmet.2019.11.010
Soula, M., Weber, R. A., Zilka, O., Alwaseem, H., La, K., Yen, F., et al. (2020). Metabolic determinants of cancer cell sensitivity to canonical ferroptosis inducers. Nat. Chem. Biol. 16, 1351–1360. doi:10.1038/s41589-020-0613-y
Stockwell, B. R., Jiang, X., and Gu, W. (2020). Emerging mechanisms and disease relevance of ferroptosis. Trends Cell Biol. 30, 478–490. doi:10.1016/j.tcb.2020.02.009
Stoyanovsky, D. A., Tyurina, Y. Y., Shrivastava, I., Bahar, I., Tyurin, V. A., Protchenko, O., et al. (2019). Iron catalysis of lipid peroxidation in ferroptosis: regulated enzymatic or random free radical reaction? Free Radic. Biol. Med. 133, 153–161. doi:10.1016/j.freeradbiomed.2018.09.008
Szarka, A., Kapuy, O., Lőrincz, T., and Bánhegyi, G. (2021). Vitamin C and cell death. Antioxid. Redox Signal. 34, 831–844. doi:10.1089/ars.2019.7897
Tang, D., Chen, X., Kang, R., and Kroemer, G. (2020). Ferroptosis: molecular mechanisms and health implications. Cell Res. 31, 107–125. doi:10.1038/s41422-020-00441-1
Tang, D., Kang, R., Berghe, T. V., Vandenabeele, P., and Kroemer, G. (2019). The molecular machinery of regulated cell death. Cell Res. 29, 347–364. doi:10.1038/s41422-019-0164-5
Tardy, A. L., Pouteau, E., Marquez, D., Yilmaz, C., and Scholey, A. (2020). Vitamins and minerals for energy, fatigue and cognition: a narrative review of the biochemical and clinical evidence. Nutrients 12, 228. doi:10.3390/nu12010228
Tong, X., Tang, R., Xiao, M., Xu, J., Wang, W., Zhang, B., et al. (2022). Targeting cell death pathways for cancer therapy: recent developments in necroptosis, pyroptosis, ferroptosis, and cuproptosis research. J. Hematol. Oncol. 15, 174. doi:10.1186/s13045-022-01392-3
Von Krusenstiern, A. N., Robson, R. N., Qian, N., Qiu, B., Hu, F., Reznik, E., et al. (2023). Identification of essential sites of lipid peroxidation in ferroptosis. Nat. Chem. Biol. 19, 719–730. doi:10.1038/s41589-022-01249-3
Wallin, R., and Hutson, S. M. (2004). Warfarin and the vitamin K-dependent gamma-carboxylation system. Trends Mol. Med. 10, 299–302. doi:10.1016/j.molmed.2004.05.003
Wang, L., Fu, H., Song, L., Wu, Z., Yu, J., Guo, Q., et al. (2022). Overcoming AZD9291 resistance and metastasis of NSCLC via ferroptosis and multitarget interference by nanocatalytic sensitizer plus AHP-DRI-12. Small 19, e2204133. doi:10.1002/smll.202204133
Wang, R., Hu, Q., Wang, H., Zhu, G., Wang, M., Zhang, Q., et al. (2021a). Identification of Vitamin K3 and its analogues as covalent inhibitors of SARS-CoV-2 3CL(pro). Int. J. Biol. Macromol. 183, 182–192. doi:10.1016/j.ijbiomac.2021.04.129
Wang, W., Jin, Y., Xu, Z., Liu, X., Bajwa, S. Z., Khan, W. S., et al. (2020). Stimuli-activatable nanomedicines for chemodynamic therapy of cancer. Wiley Interdiscip. Rev. Nanomed Nanobiotechnol 12, e1614. doi:10.1002/wnan.1614
Wang, X., Xu, S., Zhang, L., Cheng, X., Yu, H., Bao, J., et al. (2021b). Vitamin C induces ferroptosis in anaplastic thyroid cancer cells by ferritinophagy activation. Biochem. Biophys. Res. Commun. 551, 46–53. doi:10.1016/j.bbrc.2021.02.126
Wellington, K. W., Hlatshwayo, V., Kolesnikova, N. I., Saha, S. T., Kaur, M., and Motadi, L. R. (2020). Anticancer activities of vitamin K3 analogues. Invest. New Drugs 38, 378–391. doi:10.1007/s10637-019-00855-8
Welsh, J., Bak, M. J., and Narvaez, C. J. (2022). New insights into vitamin K biology with relevance to cancer. Trends Mol. Med. 28, 864–881. doi:10.1016/j.molmed.2022.07.002
White, E. (2015). The role for autophagy in cancer. J. Clin. Invest. 125, 42–46. doi:10.1172/JCI73941
Wu, Y., Zhang, S., Gong, X., Tam, S., Xiao, D., Liu, S., et al. (2020). The epigenetic regulators and metabolic changes in ferroptosis-associated cancer progression. Mol. Cancer 19, 39. doi:10.1186/s12943-020-01157-x
Xiong, Y., Xiao, C., Li, Z., and Yang, X. (2021). Engineering nanomedicine for glutathione depletion-augmented cancer therapy. Chem. Soc. Rev. 50, 6013–6041. doi:10.1039/D0CS00718H
Yang, G. G., Zhang, H., Zhang, D. Y., Cao, Q., Yang, J., Ji, L. N., et al. (2018). Cancer-specific chemotherapeutic strategy based on the vitamin K3 mediated ROS regenerative feedback and visualized drug release in vivo. Biomaterials 185, 73–85. doi:10.1016/j.biomaterials.2018.08.065
Yang, W. s., Sriramaratnam, R., Welsch, M. e., Shimada, K., Skouta, R., Viswanathan, V. s., et al. (2014). Regulation of ferroptotic cancer cell death by GPX4. Cell 156, 317–331. doi:10.1016/j.cell.2013.12.010
Yang, W. S., and Stockwell, B. R. (2016). Ferroptosis: death by lipid peroxidation. Trends Cell Biol. 26, 165–176. doi:10.1016/j.tcb.2015.10.014
Yu, B., Choi, B., Li, W., and Kim, D.-H. (2020). Magnetic field boosted ferroptosis-like cell death and responsive MRI using hybrid vesicles for cancer immunotherapy. Nat. Commun. 11, 3637. doi:10.1038/s41467-020-17380-5
Yu, P., Zhang, X., Liu, N., Tang, L., Peng, C., and Chen, X. (2021). Pyroptosis: mechanisms and diseases. Signal Transduct. Target. Ther. 6, 128. doi:10.1038/s41392-021-00507-5
Zhang, C., Liu, Z., Zhang, Y., Ma, L., Song, E., and Song, Y. (2020a). Iron free" zinc oxide nanoparticles with ion-leaking properties disrupt intracellular ROS and iron homeostasis to induce ferroptosis. Cell Death Dis. 11, 183. doi:10.1038/s41419-020-2384-5
Zhang, S., Xin, W., Anderson, G. J., Li, R., Gao, L., Chen, S., et al. (2022a). Double-edge sword roles of iron in driving energy production versus instigating ferroptosis. Cell Death Dis. 13, 40. doi:10.1038/s41419-021-04490-1
Zhang, X., Wu, S., Guo, C., Guo, K., Hu, Z., Peng, J., et al. (2022b). Vitamin E exerts neuroprotective effects in pentylenetetrazole kindling epilepsy via suppression of ferroptosis. Neurochem. Res. 47, 739–747. doi:10.1007/s11064-021-03483-y
Zhang, Z., Ding, Y., Li, J., Wang, L., Xin, X., Yan, J., et al. (2020b). Versatile iron-vitamin K3 derivative-based nanoscale coordination polymer augments tumor ferroptotic therapy. Nano Res. 14, 2398–2409. doi:10.1007/s12274-020-3241-7
Zhou, H., Chen, J., Fan, M., Cai, H., Dong, Y., Qiu, Y., et al. (2023a). KLF14 regulates the growth of hepatocellular carcinoma cells via its modulation of iron homeostasis through the repression of iron-responsive element-binding protein 2. J. Exp. Clin. Cancer Res. 42, 5. doi:10.1186/s13046-022-02562-4
Zhou, M., Yuan, M., Jin, Y., Zhou, Q., Yu, Y., Li, J., et al. (2023b). Vitamin B2-based ferroptosis promoter for sono-enhanced nanocatalytic therapy of triple-negative breast cancer. Adv. Funct. Mater. 33. doi:10.1002/adfm.202303899
Keywords: ferroptosis, vitamins, nutrient signaling, metabolic regulation, reactive oxygen species
Citation: Wu J, Shi Y, Zhou M, Chen M, Ji S, Liu X, Zhou M, Xia R, Zheng X and Wang W (2024) Nutrient vitamins enabled metabolic regulation of ferroptosis via reactive oxygen species biology. Front. Pharmacol. 15:1434088. doi: 10.3389/fphar.2024.1434088
Received: 17 May 2024; Accepted: 01 July 2024;
Published: 18 July 2024.
Edited by:
Wagdy Mohamed Eldehna, Kafrelsheikh University, EgyptReviewed by:
Alessandra Fiore, University of Verona, ItalyHongbao Fang, Nanjing Normal University, China
Copyright © 2024 Wu, Shi, Zhou, Chen, Ji, Liu, Zhou, Xia, Zheng and Wang. This is an open-access article distributed under the terms of the Creative Commons Attribution License (CC BY). The use, distribution or reproduction in other forums is permitted, provided the original author(s) and the copyright owner(s) are credited and that the original publication in this journal is cited, in accordance with accepted academic practice. No use, distribution or reproduction is permitted which does not comply with these terms.
*Correspondence: Rui Xia, eHJhQG50dS5lZHUuY24=; Xiaohua Zheng, eGlhb2h1YXpAbnR1LmVkdS5jbg==; Weiqi Wang, d3dxMTk5MEBudHUuZWR1LmNu
†These authors have contributed equally to this work