- 1Renal Division, Heilongjiang Academy of Chinese Medicine Sciences, Harbin, China
- 2Shunyi Hospital, Beijing Hospital of Traditional Chinese Medicine, Beijing, China
- 3China-Japan Friendship Hospital, Beijing, China
Diabetic nephropathy (DN) is a common and serious micro-vascular complication of diabetes and a leading cause of end-stage renal disease globally. This disease primarily affects middle-aged and elderly individuals, especially those with a diabetes history of over 10 years and poor long-term blood glucose control. Small ubiquitin-related modifiers (SUMOs) are a group of reversible post-translational modifications of proteins that are widely expressed in eukaryotes. SUMO proteins intervene in the progression of DN by modulating various signaling cascades, such as Nrf2-mediated oxidative stress, NF-κB, TGF-β, and MAPK pathways. Recent advancements indicate that natural products regulating SUMOylation hold promise as targets for intervening in DN. In a previous article published in 2022, we reviewed the mechanisms by which SUMOylation intervenes in renal fibrosis and presented a summary of some natural products with therapeutic potential. Therefore, this paper will focus on DN. The aim of this review is to elucidate the mechanism of action of SUMOylation in DN and related natural products with therapeutic potential, thereby summarising the targets and candidate natural products for the treatment of DN through the modulation of SUMOylation, such as ginkgolic acid, ginkgolide B, resveratrol, astragaloside IV, etc., and highlighting that natural product-mediated modulation of SUMOylation is a potential therapeutic strategy for the treatment of DN as a potential therapeutic strategy.
1 Introduction
Diabetes represents a significant challenge to global health. This issue affects over 500 million individuals worldwide. The global prevalence of this condition is on a startling upward trajectory, with projections indicating a rise to 700 million by the year 2045 (Smith et al., 2022). Particularly, the elderly demographic faces a heightened risk of developing diabetes-related complications, including Diabetic Nephropathy (DN) (Lin et al., 2022). DN is a prevalent micro-vascular complication among individuals with diabetes and serves as a primary mechanism leading to end-stage renal disease (Shoeib et al., 2023). The pathological characteristics of DN encompass thickening of the glomerular basement membrane, mesangial matrix expansion, interstitial fibrosis in the renal tubules, and podocyte loss (Harlan et al., 2018; Wang H. et al., 2021). In individuals with diabetes, the lifetime prevalence of DN exceeds 50% (Pop-Busui et al., 2022). Factors such as hypertension (Skov et al., 2016), hyperglycemia (Wu et al., 2023), oxidative stress (Ma et al., 2021), advanced glycation end products (AGEs), and angiotensin II can induce the onset of DN by activating pathways like transforming growth factor β (TGF-β), nuclear factor kappa B (NF-κB), Nrf2-mediated oxidative stress, and mitogen-activated protein kinases (MAPK) (Hwang et al., 2019). To date, the treatment for DN has primarily focused on correcting the metabolic dysregulation and hemodynamic abnormalities in patients with diabetes. However, clinical studies have demonstrated that these therapeutic measures only partially alleviate the pathology of DN (Gross et al., 2005). Evidence suggests that standard treatments aimed at strict control of blood sugar and blood pressure are insufficient to halt the progression of DN to end stage renal disease (ESRD) (Arora and Singh, 2013). Consequently, the search for new therapeutic targets to prevent and treat DN has emerged as a critical challenge in this field.
SUMOylation is a reversible post-translational modification (PTM) process (Han et al., 2018), intricately linked with various cellular processes including nucleocytoplasmic transport (Melchior et al., 2003), transcriptional regulation (Muller et al., 2004), apoptosis (Choi et al., 2017), protein stability, stress response, and cell cycle progression (Eifler and Vertegaal, 2015). It has been demonstrated that SUMO can alter and influence the function of specific metabolic enzymes in the pathway, thereby regulating the entire metabolic pathway (Gareau and Lima, 2010). Previous studies have demonstrated that SUMO is associated with a number of diseases related to metabolic disorders, including Type I diabetes (Li et al., 2005; Wang and She, 2008), Type II diabetes (Dou et al., 2022), and diabetes-mediated cardiovascular diseases (Chang and Abe, 2016). The SUMOylation E2 conjugase UBC9 was first reported to be expressed in the kidneys in 2003 (Golebiowski et al., 2003). A growing body of research suggests that SUMOylation is associated with the progression of DN through the modulation of multiple signalling pathways (Gao et al., 2014). It is also linked to the damage process of podocyte (Li et al., 2019). Although some natural products have been proven to inhibit enzymes involved in the SUMOylation process, progress in developing more selective and potent inhibitors of SUMOylation remains limited (Brackett et al., 2020). In previous publications, we have reviewed the mechanisms involved in modulating SUMOylation for the treatment of renal fibrosis, as well as candidate natural products (Liu P. et al., 2022). This article connects the mechanisms of SUMOylation with natural products, exploring the therapeutic prospects of SUMOylation in the progression of DN and the candidate natural products. Figure 1 depicts the chemical structures of the pertinent natural compounds. Table 1 lists the targets associated with SUMOylation for these compounds.
2 SUMOylation
2.1 SUMO proteins
Small ubiquitin-related modifiers (SUMOs) are members of the ubiquitin-like protein family and can conjugate with a wide array of proteins. Predominantly localized in the nucleus, SUMOs are crucial for various cellular processes, including cell cycle progression, genome stability, and transcription (Vertegaal, 2022). Sumoylation of proteins can influence their stability and enzymatic activity, alter their localization, or mediate novel interactions with other proteins containing SUMO-interacting motifs (SIMs) (Geiss-Friedlander and Melchior, 2007). Research has shown that the PTM of proteins by SUMOs can regulate chromatin structure and function at multiple levels, influencing gene expression and maintaining genomic integrity through various mechanisms. Both SUMO ligases and deconjugating enzymes are key factors in modulating chromatin structure. Sumoylation plays a multifaceted role in regulating chromatin architecture, gene expression, and genomic stability. Part of its function lies in the diverse downstream effects that occur when SUMO is conjugated to different proteins (Cubenas-Potts and Matunis, 2013).
SUMOs, approximately 11 kDa in size and slightly larger than ubiquitin, can bind to target proteins as single or multiple monomers or in various polymeric forms. Like ubiquitin, SUMO can undergo self-modification, such as phosphorylation and/or acetylation. The SUMO conjugation mechanism involves three types of enzymes: the activating E1 enzyme, the conjugating E2 enzyme, and the E3 ligase. In addition to covalent attachment to substrates, SUMO can interact with other proteins through SIMs. Non-covalent protein-protein interactions between sumoylated proteins containing SIMs and SUMO readers can alter the subcellular localization of the sumoylated proteins, including facilitating SUMO-mediated phase separation. SIMs in SUMO-targeted ubiquitin ligases (STUbLs) enable binding to poly-sumoylated proteins, which are subsequently ubiquitinated and degraded, forming a SUMO-mediated protein turnover pathway. Additionally, when SUMO monomers effectively compete with ubiquitin for lysine residues on target proteins, they can prevent the degradation of these proteins (Vertegaal, 2022). The specificity of the SUMO pathway is achieved through redox regulation, acetylation, phosphorylation, or other post-translational modifications of SUMOylation and deSUMOylation enzymes.
SUMO-1 typically modifies substrates as a monomer; however, SUMO-2/3 can form poly-SUMO chains. Monomeric SUMO-1 or poly-SUMO chains can interact with other proteins via SIMs, thus providing a platform to enhance protein interactions. The consequences of SUMOylation include changes in cellular localization, protein activity, or protein stability. Moreover, SUMO can facilitate protein degradation by binding ubiquitin through STUbLs (Chang and Yeh, 2020). To date, five genes encoding SUMO paralogs, known as SUMO1, SUMO2, SUMO3, SUMO4, and SUMO5, have been identified in the human genome (Wu and Huang, 2023). SUMO-1 shares only 56% identity with SUMO-2 and -3. SUMO2 and SUMO3 are 95% homologous, collectively referred to as SUMO2/3 (Kim and Baek, 2009). Mice with a knockout of the SUMO1 gene can survive, thanks to compensatory mechanisms involving SUMO2 or SUMO3 (Wang et al., 2014a; Evdokimov et al., 2008). Under different physiological and pathological conditions, the conjugation of the same substrate protein with different SUMO isoforms can lead to distinct functional outcomes. For instance, the modification of Drp1 by SUMO1 and SUMO2/3 has opposing effects on mitochondrial apoptosis regulation. SUMO1-conjugated Drp1 significantly accelerates mitochondrial fission, ultimately promoting the apoptotic process. In contrast, SUMO2/3-conjugated Drp1 effectively delays mitochondrial fission and inhibits apoptosis (Sheng et al., 2021). Similarly, in the regulation of the stability and function of the circadian rhythm protein Period2 (PER2), SUMO2 modification promotes proteasomal degradation of PER2, whereas SUMO1 conjugation inhibits its degradation and enhances its function as a transcriptional repressor (Chen et al., 2021). Additionally, the poly-SUMO2/3 modification of mutant CFTR protein associated with cystic fibrosis promotes its degradation, while SUMO1 conjugation enhances CFTR stability (Gong et al., 2019). Generally, SUMO1 modification promotes the dissolution of aberrant proteins and inhibits their aggregation, thereby protecting cells, a function particularly significant in preventing protein misfolding-related diseases. On the other hand, SUMO2/3, in coordination with STUBL and the ubiquitin-proteasome system, facilitates substrate degradation, helping cells eliminate damaged or unnecessary proteins. This mechanism is especially important in response to oxidative stress, DNA damage, and other stress conditions (Wang and Matunis, 2023). Although SUMO1, SUMO2, and SUMO3 all play important roles in cellular regulation, current studies suggest that SUMO2/3 may be more critical in the progression of DN. Unlike SUMO1, SUMO2/3 is more readily induced under conditions of cellular stress, particularly in response to oxidative stress and inflammation, which are closely associated with the high-stress environment of DN. SUMO2/3 has the ability to form poly-SUMO chains, thereby influencing a broader network of proteins. This feature gives SUMO2/3 a unique advantage in regulating complex cellular functions such as cell proliferation, differentiation, and apoptosis, making it especially relevant in the context of DN progression. SUMO4 is most similar to SUMO2/3 but contains a unique proline residue (P90) at its C-terminus, which impedes its effective maturation (Owerbach et al., 2005). Under normal culture conditions, SUMO4 is rapidly degraded; however, during cellular stress, SUMO4 can mature through stress-induced endogenous hydrolases and covalently conjugate to its substrate proteins (Wei et al., 2008). Genetic studies have linked SUMO4 to both type 1 and type 2 diabetes, suggesting that the unbound form of SUMO4 may play a role (Guo et al., 2004; Lin et al., 2007), although this association remains controversial (Podolsky et al., 2009). Nonetheless, recent research by Nisha Sinha and colleagues, through gene polymorphism analysis in diabetic patients without nephropathy (DM) and those with DN, has demonstrated that the SUMO4 c.163 G> A polymorphism is associated with an increased susceptibility to DN in North Indian patients with type 2 diabetes (Sinha et al., 2016). A recent meta-analysis has confirmed that the SUMO4 (M55V) variant is associated with both type 1 and type 2 diabetes in Asian and European populations (Li et al., 2017). The newest member of the SUMO family, SUMO5, highly homologous to SUMO1, has been shown to regulate the dynamics of PML nuclear bodies when exogenously expressed in cells (Liang et al., 2016).
2.2 SUMOylation and deSUMOylation process
SUMOylation is a reversible PTM involving the covalent attachment of small ubiquitin-like modifier proteins to substrate proteins (Tokarz and Wozniak, 2021). The attachment of SUMO1-SUMO3 to proteins, known as SUMOylation, is mediated by a mechanism that involves the dimeric SUMO activating enzyme subunits 1 and 2 (SAE1-SAE2; also known as UBA2-AOS1), the E2 conjugating enzyme UBC9 (also referred to as UBE2I), and E3 SUMO ligases, whereas deSUMOylation is regulated by the sentrin/SUMO-specific protease (SENP) family (Kunz et al., 2018; Yeh, 2009). The first SUMO proteases described were the ubiquitin-like proteases 1 and 2 (Ulp1 and Ulp2) in yeast (Li and Hochstrasser, 1999; Li and Hochstrasser, 2000). The mammalian SENP family consists of six SUMO proteases (SENP1-3 and SENP5-7), which were identified based on their sequence similarity to the Ulp family. These enzymes are all cysteine proteases and contain a catalytic triad (Cys-His-Asp) within a conserved protease domain (Jia et al., 2019). Based on sequence homology, substrate specificity, and subcellular localization, these six SENPs can be categorized into three subfamilies (Kumar and Zhang, 2015). SENP1 and SENP2 form the first subfamily. SENP1 is primarily localized in the nucleus, where it is involved in the maturation of SUMO precursors and the deconjugation of SUMOylated proteins. SENP2 is found in both the nucleus and the nuclear envelope, regulating the SUMOylation of nuclear pore components and other nuclear proteins. Both SENP1 and SENP2 exhibit broad specificity for SUMO-1, SUMO-2, and SUMO-3 and are highly homologous to the yeast Ulp1 protease. By processing the precursors of SUMO-1, SUMO-2, and SUMO-3, SENP1 and SENP2 play a multifunctional role in the SUMOylation process, which is crucial for the regulation of gene expression, DNA repair, and cell cycle progression (Kunz et al., 2018). SENP3 and SENP5 belong to the second subfamily of SUMO proteases and are also located in the nucleus. They exhibit a preference for SUMO-2 and SUMO-3 over SUMO-1, thereby regulating the function and localization of SUMOylated proteins (Yun et al., 2008; Gong and Yeh, 2006; Di Bacco et al., 2006). SENP6 and SENP7 are members of the third subfamily, typically localized in the nucleus. Their catalytic domains contain four conserved loop insertions that are absent in other SENPs, which are believed to confer their SUMO subtype specificity and preference for cleaving SUMO2/3 chains (Claessens and Vertegaal, 2024). Subsequently, the SUMO proteases deSUMOylating isopeptidase 1 (DESI1), DESI2, and USPL1 were identified, which share little sequence homology with the Ulp/SENP family (Schulz et al., 2012; Shin et al., 2012). SUMO is conjugated to lysine residues on target proteins through an isopeptide bond, catalyzed by SUMO-specific activating (E1), conjugating (E2), and ligating (E3) enzymes (Chang and Yeh, 2020). In mammalian cells, SUMOs are translated as inactive precursors and are processed into their mature forms by specific neutral proteases known as SENPs. The maturation of SUMO involves the hydrolysis of a peptide bond at the C-terminus, thereby exposing a diglycine (GG) motif. This motif subsequently forms a thioester bond with the SUMO E1 enzyme (SAE1/UBA2) in an ATP-dependent manner. Subsequently, SUMO is transferred to the catalytic cysteine residue of the SUMO E2 enzyme UBC9 through a transesterification reaction. UBC9 then conjugates SUMO to a lysine residue on the substrate, either with the assistance of a SUMO-E3 ligase or independently (Claessens and Vertegaal, 2024). The complete process of SUMOylation and deSUMOylation is illustrated in Figure 2.
3 Mechanisms of SUMOylation intervention in DN
DN is a principal cause of End-Stage Renal Disease (ESRD) worldwide and is a major contributor to the morbidity and mortality among individuals with diabetes (Reutens and Atkins, 2011). The clinical intervention for DN primarily focuses on controlling risk factors such as hyperglycemia, dyslipidemia, hypertension, and proteinuria, aiming to alleviate symptoms and slow the progression of DN. However, these interventions have limited efficacy (Tang et al., 2021; Xiong and Zhou, 2019; Magee et al., 2017). SUMOylation has been found to be extensively involved in the pathogenesis of DN. Under normal physiological conditions, SUMOylation and deSUMOylation are maintained in dynamic balance, regulating critical cellular functions such as protein stability, transcriptional control, and DNA repair. However, in the context of DN, this balance is disrupted, leading to abnormal increases or decreases in SUMOylation levels. Modification of the NR5A2 protein, a key mediator in the transcriptional regulation of the calreticulin gene, by SUMO exacerbates renal fibrosis (Arvaniti et al., 2016). Furthermore, SUMO may play a protective role against the apoptosis of podocyte (Yang et al., 2019). These findings have been summarized in detail (Figure 3; Table 2).
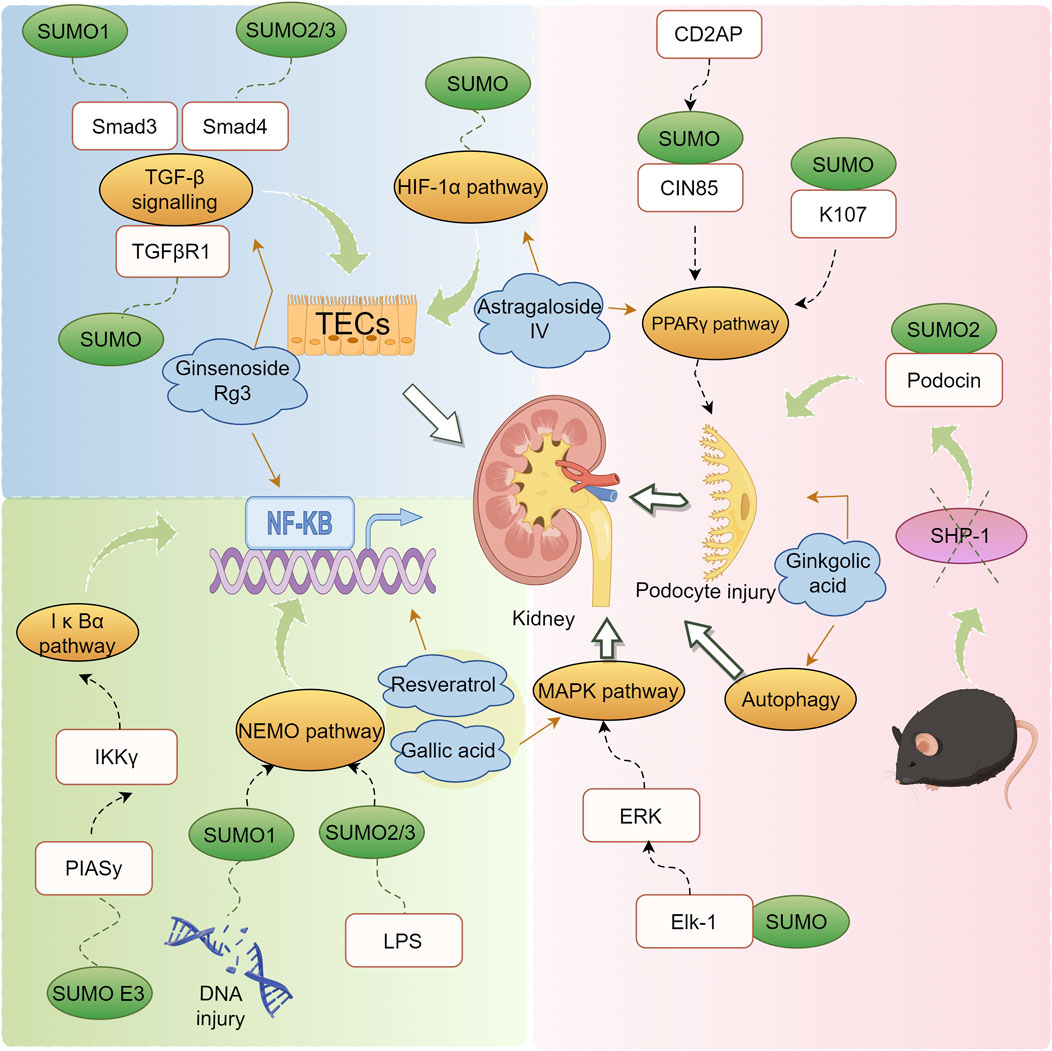
Figure 3. Mechanism of action of natural products in the treatment of DN through modulation of the SUMO pathway.
3.1 SUMOylation interferes with insulin secretion
The SUMO pathway is associated with the production and secretion of insulin (Kamynina and Stover, 2017). In a diabetic mouse model established through UBC9 deficiency, β-cells exhibited impaired antioxidant capacity, and Ubc9 deficiency resulted in reduced NRF2 activity and decreased expression of its downstream antioxidant genes, leading to Reactive Oxygen Species (ROS) accumulation and oxidative stress (He et al., 2018). SENP1 plays a crucial role in insulin secretion in type 2 diabetes (Ferdaoussi et al., 2015). SENP1 is essential for glucose-dependent insulin secretion. SUMOylation responds to intracellular Ca2+ elevation by directly and reversibly inhibiting β-cell exocytosis, thereby acutely regulating insulin secretion (Dai et al., 2011; Manning et al., 2012). NADPH equivalents and reduced glutathione activate SENP1 function, thereby increasing insulin secretion (Ferdaoussi et al., 2015). Conversely, over-expression of SENP1 reduces insulin secretion and impairs the handling of Ca2+-induced cell death (Hajmrle et al., 2014). Glucagon-like peptide-1 (GLP-1) is an incretin hormone secreted by intestinal L-cells, crucial for postprandial insulin secretion (Drucker et al., 1987). In mouse pancreatic β-cell lines and primary mouse β-cells, cAMP produced by GLP-1R stimulation was shown to be downregulated by SUMO. Therefore, SUMO modification of GLP-1R may be a contributing factor to the diminished incretin response (Rajan et al., 2012). Oxidative stress-mediated inactivation of SENP1 may be a critical step in impaired insulin secretion in type 2 diabetes (Shoeib et al., 2023; Attie, 2015). Studies indicate that SENP2 is involved in adipocyte differentiation (Liang et al., 2019) and adipogenesis (Chung et al., 2010), adipose tissue thermogenesis (Liu et al., 2021), and insulin sensitivity (Zheng et al., 2018). Expression of SENP2 in insulin-secreting cells is triggered by chronic high glucose stimulation. In vitro, the increase in SENP2 levels under high glucose conditions was more pronounced in the cytoplasm than in the nucleus (Jung et al., 2016). Experiments have shown that SENP2 regulates mitochondrial function and insulin secretion in pancreatic β-cells, with the regulation of DRP1 sumoylation-mediated phosphorylation being a potential mechanism (Nan et al., 2022). Endoplasmic reticulum protein 44 (ERp44) is closely related to glucose and lipid metabolism (Nyirimigabo et al., 2019). Interfering with ERp44 SUMOylation enhances ERp44 degradation and impairs ER retention by Ero1α, thereby ameliorating ER stress and maintaining adipose tissue homeostasis. Therefore, modulating ERp44 SUMOylation in adipocyte may be a viable strategy to combat obesity and insulin resistance (Xie et al., 2023). A synopsis of the preceding discussion is provided in Figure 4.
3.2 Improvement of the inflammatory response
Inflammation is closely related to the development and progression of DN (Deepa and Venkatraman, 2013). A significant body of research indicates that the activation of inflammatory signaling and the infiltration of inflammatory cells are crucial for the development of DN (Galkina and Ley, 2006). Increasing evidence suggests that SENPs play roles in modulating macrophage function and inflammation. For instance, Lao et al. (2018) reported that SENP3 enhances lipopolysaccharide (LPS) induced TLR4 signal transduction via the deSUMOylation of MKK7, and myeloid-specific deletion of SENP3 can alleviate LPS-induced endotoxic shock and acute lung injury (Chen et al., 2020). During high-fat diet (HFD)-induced obesity in mice, SENP3 expression in adipose tissue macrophages (ATMs) is significantly increased, while myeloid-specific SENP3 deficiency protects mice from HFD-induced obesity and systemic inflammation. This protective effect is partly mediated by alterations in YAP1 sumoylation. The YAP1 signaling pathway plays a role in regulating macrophage function and inflammation (Zhou et al., 2019; Liu M. et al., 2020). In the context of HFD-induced obesity in mice, SENP3 regulates the deSUMOylation of YAP1, while the absence of SENP3 can abolish the upregulation of YAP1 induced by IL-1β. Myeloid-specific deletion of SENP3 attenuates macrophage infiltration in adipose tissue and reduces serum inflammatory factor levels during diet and age-related obesity (Jiang et al., 2023).
3.2.1 NF-κB signaling
As a pivotal coordinator of inflammatory responses, NF-κB controls the expression of various inflammatory cytokines associated with the pathogenesis of DN (Perez-Morales et al., 2019; Wu et al., 2016). Some components of the NF-κB pathway can be modified by the SUMOylation process (Mabb and Miyamoto, 2007). NEMO can be considered a potential target for downregulating NF-κB activity, as NF-κB activation requires SUMO modification of NEMO before its accumulation in the nucleus (Hamdoun and Efferth, 2017). SUMO1 is conjugated by Ubc9 to K21 and K22 lysines of IκBα, preventing its ubiquitination and degradation, and further inhibiting the activation of NF-κB. Specifically, the NEMO kinase, a part of the IKK complex essential for NF-κB activation, is modified by SUMO1 in response to DNA damage, while exposure to lipopolysaccharide induces the modification of NEMO by SUMO2/3. This modification prevents the deubiquitinase CYLD from binding to NEMO, thereby enhancing the activation of the NF-κB essential modifier (IKK) (Liu X. et al., 2013). P100 can also activate the NF-κB pathway upon SUMOylation, with NEMO SUMOylation involving SUMO-1, mainly regulating the activation of NF-κB in genotoxic responses (Liu et al., 2016). PIAS proteins enhance the SUMOylation of tissue TG2, keeping TG2 stable and avoiding ubiquitin-mediated degradation, thereby sustaining oxidative stress and chronic inflammation in cells (Luciani et al., 2009). It has been elucidated that elevated glucose upregulates PIASy expression and that the SUMO E3 ligase PIASy mediates high-glucose-induced NF-κB inflammatory signalling, suggesting that PIASy is a potential therapeutic target for DN (Huang et al., 2017). Studies have found that high glucose stimulation enhances the expression of SUMO1 and SUMO2/3 in a dose- and time-dependent mannerand significantly reduces SUMOylation of IκBα, thereby activating NF-κB signalling (Huang et al., 2013). SUMO 4 binds to IκBα and negatively regulates NF-κB transcriptional activity (Guo et al., 2004).
3.2.2 TGF-β signaling
TGF-β is a multifunctional cytokine recognized, with Smad4 identified as a central channel in the TGF-β signaling mechanism (Wang L. et al., 2021). In the unilateral ureteral obstruction (UUO) mouse model, the absence of Smad3 significantly reduces renal fibrosis (Sato et al., 2003). Regarding DN, in streptozotocin (STZ)-induced DN models, mice lacking Smad3 were found to avoid renal fibrosis, including glomerular basement membrane (GBM) thickening and excessive extracellular matrix (ECM) production (Fujimoto et al., 2003), although the suppression of albuminuria was consistently observed (Wang et al., 2007). Studies have shown that Smad4 knockdown enhances pro-inflammatory NF-κB signalling while inactivating fibrotic Smad3 signalling (Meng et al., 2012). High glucose significantly increases the expression of SUMO1 and SUMO2/3 and promotes the conjugation of SUMO2/3 to Smad4 (Zhou et al., 2014). In addition to Smad4, Smad3 also plays an important role in coordinating TGF-β-mediated signalling processes. Studies have verified that PIASy, acting as a SUMOylation E3 ligase, intervenes in TGF-β signaling by binding to and SUMOylating Smad3, thus serving as a regulatory checkpoint (Derynck et al., 1998; Imoto et al., 2008). PIASy regulates TGF-β/Smad3-mediated signaling by stimulating the SUMOylation and nuclear export of Smad3. SUMOylation also inhibits the transcriptional activity of Smad4 (Gao et al., 2014; Long et al., 2004).
3.2.3 MAPK signaling
The Mitogen-Activated Protein Kinase (MAPK) pathway is a crucial conduit for transmitting external signals to internal cellular responses and is recognized as a prototypical pro-inflammatory signaling pathway. The over-activation of the MAPK pathway has been closely linked to numerous inflammatory diseases. Indeed, several MAPK inhibitors have undergone preclinical evaluation in a wide range of disease models, showing significant therapeutic potential (Yong et al., 2009). This pathway primarily participates in various cell functions by regulating the phosphorylation of its substrates, a process closely associated with SUMOylation (Guo et al., 2007). In conditions of chronic hyperglycemia, the MAPK pathway is activated, leading to localized inflammatory responses (Sakai et al., 2005). Recent research has revealed that the inhibition of the transcription factor Elk-1 due to SUMOylation can be counteracted by activating the ERK pathway (Besnard et al., 2011). Moreover, the SUMOylation of key proteins such as p53 and ERK5 has profound effects, influencing the apoptosis of endothelial cells under disturbed blood flow and regulating inflammatory processes (Heo et al., 2013).
3.3 The SUMOylation of podocyte apoptosis pathway
Clinical and experimental studies have shown that podocytopenia leads to proteinuria and/or glomerulosclerosis. And apoptosis is the main reason for the decrease in podocyte numbers (Asanuma, 2015; Nagata, 2016). Podocyte injury is an early critical event in the progression of DN, in which autophagy is an important factor (Xu et al., 2020; Deretic, 2021). This degradation process involves pathways such as mTOR, AMPK, and PI3K, playing a crucial role in inhibiting inflammation and kidney damage, thereby slowing the progression of DN (Zhang Z. et al., 2022).
Podocyte injury, including ultra-structural changes and reduced expression of slit diaphragm components like nephrin, podocin, and CD2AP, underlies many glomerular diseases. Nephrin is a SUMO-1 and SUMO-2/3 SUMOylated target protein and the presence of SUMOylation increases nephrin stability (Tossidou et al., 2014). CD2AP affects the post-translational structure of CIN85 in podocyte, enhancing its modification by SUMO-1, -2, and -3. Converting lysine 598 to arginine eliminates this modification and enhances the interaction between CIN85 and nephrin, indicating a newly discovered regulatory function of CD2AP (Tossidou et al., 2012). SENP6 deficiency enhances podocyte loss induced by high glucose (HG) through activation of the Notch1 signaling. Simultaneously, the lack of SENP6 promotes the production of EDN1 by regulating the transcription of EDN1 in podocyte, thereby exacerbating HG-induced glomerular endothelial cell injury and dysfunction. This suggests that the protective role of SENP6 may be related to its deSUMOylation function (Guo et al., 2023).
SHP-1 is a cell membrane protein tyrosine phosphatase expressed primarily in haematopoietic and epithelial cells. In the kidney, increased expression of SHP-1 in mouse and human podocytes exposed to HG leads to decreased insulin and nephrin action and podocyte dysfunction (Drapeau et al., 2013; Lizotte et al., 2016). Research has demonstrated that SHP-1 can decrease the PTM of podocin by SUMO2. In renal tissues of diabetic mice and patients, a reduction in SUMO2 and an increase in SUMO1 are observed. Upon deletion of SHP-1 in podocyte, the SUMOylation of structural proteins is restored, thereby maintaining their integrity and mitigating the progression of DN. Thus, targeting SHP-1 could represent a strategic approach to prevent podocyte injury caused by diabetes (Lizotte et al., 2023).
In kidney disease, p53 has been identified as a key factor in regulating podocyte apoptosis (Wada et al., 2005). Data indicate that SENP1 expression was low in normal podocyte and significantly increases in podocyte undergoing apoptosis and oxidative stress induced by puromycin aminonucleoside (PAN). The absence of SENP1 results in the accumulation of SUMOylated p53, which directly mediates increased apoptosis in podocytes treated with PAN (Wang et al., 2014b).
Renal tubular hypoxia is a major driving factor in DN proximal tubular pathology (Miyata and de Strihou, 2010). Hypoxia-inducible factor (HIF)-1 plays a crucial role under hypoxic conditions. There is increasing evidence that HIF-1α expression is elevated in renal proximal tubule cells under hyperglycaemic conditions (Bessho et al., 2019). Elevated levels of ROS may lead to renal cell damage, resulting in renal dysfunction (Liang et al., 2021). It was shown that HIF-1α attenuates high glucose-mediated associated renal tubular cell injury by promoting Parkin/PINK1-mediated mitosis (Yu et al., 2021). Hypoxia might promote the stability and activation of HIF-1α by increasing the expression of SENP1 in podocyte, thereby inducing survival and angiogenesis in Glomerular Endothelial Cells to combat hypoxia. The deSUMOylation of HIF-1α signaling protects glomerular endothelial cells from hypoxic injury (Wang L. et al., 2015). Over-expression of SUMO or SUMO E3 ligase can enhance the stability and transcriptional activity of HIF-1α in Human Lymphatic Endothelial Cells (Han et al., 2015).
Although the current study reveals beneficial effects of SENP1, it is important to consider the insights from other studies. For instance, Meinecke et al. demonstrated that elevated levels of SUMO acetylation in promyelocytic leukaemia proteins result in resistance to Fas-induced apoptosis (Meinecke et al., 2007). In a study conducted by Li et al. (2008) it was demonstrated that the deSUMOylation of HIPK1 by SENP1 in TNF-treated human umbilical vein endothelial cells resulted in the enhancement of ASK1-dependent apoptosis. These contradictory results are intriguing and, aside from the different types of stimuli and signaling pathways studied, might also relate to the different cell types investigated. Therefore, the relationship between SUMOylation and apoptosis warrants further exploration.
4 Natural products that combat DN by regulating SUMOylation
In the clinical treatment of DN, pharmacological interventions are being explored alongside natural products. Traditional drug therapies focus primarily on blood glucose control, blood pressure management, and inhibition of the renin-angiotensin-aldosterone system (RAAS). Antidiabetic medications like metformin and SGLT2 inhibitors have shown some degree of nephroprotective effects. Additionally, ACE inhibitors (ACEIs) and angiotensin II receptor blockers (ARBs) are effective in reducing proteinuria and slowing the progression of renal dysfunction (Hu et al., 2023a). However, these synthetic drugs are often accompanied by side effects, including ketoacidosis, weight gain, polyuria, liver damage, renal impairment, and cardiovascular complications (Roborel et al., 2020; Blahova et al., 2021; Kushwaha et al., 2020a). This has prompted further exploration of phytochemicals as alternative therapeutic approaches (Kushwaha et al., 2020b). Phytochemicals are considered potential natural modulators due to their ability to interact with multiple targets, slow disease progression, and their relatively low toxicity (Khatoon et al., 2022). Among these, natural compounds like polyphenols, flavonoids, and saponins possess antioxidant, anti-inflammatory, and antifibrotic properties, offering protective effects on the kidneys (Hu et al., 2023b). Research suggests that certain natural compounds and herbal extracts may exert therapeutic effects on DN through mechanisms such as SUMOylation, highlighting their potential as a treatment option for DN.
Ginkgo biloba is a traditional herbal medicine with thousands of years of clinical use history and stands as one of the most widely used botanical treatment products globally (Howes and Houghton, 2012). It is primarily utilized for treating conditions such as cough, asthma, and enuresis (Nash and Shah, 2015). Previously, researchers found that ginkgo biloba alleviated the worsening of proteinuria in patients with type 2 diabetes, suggesting that ginkgo biloba is a promising option for early protection of the kidneys in patients with DN (Howes and Houghton, 2012). Modern pharmacological research indicates that Ginkgo biloba possesses anti-oxidative, anti-inflammatory (Mohanta et al., 2014), neuroprotective (Zuo et al., 2017), and anti-platelet aggregation effects (Koch, 2005). Animal studies have confirmed Ginkgo biloba’s preventive and therapeutic effects on DN (Avula et al., 2015; Qiu et al., 2015; Tang et al., 2009). Ginkgolic acid (GA), an alkylphenol constituent found in Ginkgo biloba leaves and fruits (Isah, 2015), significantly reduces and reverses the modulation induced by oxidized low-density lipoprotein, indicating its anti-inflammatory action through the NF-κB pathway (Zhang and Yan, 2019). GA is a SUMO chemical inhibitor that binds directly to the E1 enzyme and inhibits the formation of the E1-SUMO intermediate, thereby effectively inhibiting the SUMO chemical pathway (Fukuda et al., 2009). Research has demonstrated that this compound can downregulate the expression of SUMO1 and SUMO2/3 in macrophages, an effect consistently replicated in both experimental and clinical models (Liu X. et al., 2022). The application of GA to inhibit SUMOylation or suppress SUMO1 expression can regulate the levels of SUMO1 and its conjugation with p53, promoting autophagy while inhibiting cell proliferation. These results reveal the ability of GA to control the multifaceted regulation of complex cellular processes (Tan et al., 2022). Beyond its cell regulatory functions, SUMOylation is also essential for the exchange on slit diaphragms, a critical component of the renal unit in kidney architecture. Treatment with the SUMOylation inhibitor GA reduces the expression of nephrin on podocyte membranes, highlighting the importance of SUMOylation in renal homeostasis and its potential as a therapeutic intervention target (Tossidou et al., 2014).
Research indicates that polyphenols hold promise for treating various chronic diseases, including diabetes and its complications, with minimal toxic effects observed in vitro and in animal models (Kabir et al., 2021). Resveratrol (RES) is a naturally occurring non-flavonoid polyphenolic compound derived mainly from natural plants such as grapes, cranberries, lingonberries and certain herbs (Nanjan and Betz, 2014). The pharmacological actions of RSV have been studied, including its anti-oxidative, anti-inflammatory, immunomodulatory, hepatoprotective, anticancer, and anti-atherosclerotic effects (Pandey and Rizvi, 2009; Prasad, 2012; Bo et al., 2013; Carrizzo et al., 2013; Carter et al., 2014; Wang Y. et al., 2015; Peiyuan et al., 2017). Studies have shown that RSV treatment has a protective effect on DN, with RSV treatment in animals with DN alleviating hyperglycemia, hyperlipidemia, and improving kidney structure integrity and renal function (Den Hartogh and Tsiani, 2019). Resveratrol effectively inhibits carcinogen-induced expression of cyclooxygenase-2 (COX-2). This process requires the phosphorylation of extracellular signal-regulated kinases 1 and 2 (ERK1/2) and the translocation of the enzyme to the nucleus by SUMO-1. COX-2 then accumulates in the nucleus, forming a complex with SUMO-1, which binds to phosphorylated p53, potentially influencing the carcinogenic process (Cheng et al., 2018). Furthermore, resveratrol can mediate the downregulation of SUMO1, inhibit the expression of Wnt5a, deactivate β-catenin, and prevent their entry into the cell nucleus. This molecular cascade attenuates the Wnt/β-catenin signaling pathway, thereby producing an anti-inflammatory effect (Wang et al., 2020). Experimental evidence suggests that resveratrol may be an effective agent for the prevention and inflammatory response of renal mesangial cells induced by LPS and the expression of fibronectin through the SphK1/S1P2/NF-κB signaling pathway, highlighting resveratrol’s potential role in combating renal fibrosis in addition to its anti-hyperglycemic properties (Gong et al., 2020).
Astragalus membranaceus, known as Huangqi, is the dried root of Astragalus and a commonly used herb in traditional Chinese medicine. Increasing research demonstrates its beneficial effects on various diseases through activating immune mechanisms, alleviating oxidative stress, and reducing inflammation (Cho and Leung, 2007; Ko et al., 2005; Qin et al., 2012). Astragaloside IV (AS-IV), a saponin extracted from Astragalus membranaceus Bunge, is one of the main pharmacologically active components of Astragalus (Fu et al., 2014). Recent studies report that AS-IV possesses extensive pharmacological activities both in vivo and in vitro, including anti-oxidative stress, anti-inflammatory, anti-diabetic, and renal protective effects (Zhou et al., 2017; Chen et al., 2018). Further research utilizing advanced Western blotting techniques has found that the sharp decline and delayed recovery of SUMO1 activity are responsible for the initial increase and subsequent decrease in HIF-1α concentration. Enhanced expression of SUMO1 stabilizes HIF-1α within the nucleus, effectively reducing the extent of vascular anomalies following hypoxia. Astragaloside IV (AS-IV) triggers sustained production of SUMO1 in vascular endothelial cells, consolidating the HIF-1α/VEGF axis and thus promoting angiogenesis under hypoxic conditions (Wang B. et al., 2021). Additionally, AS-IV seems to alleviate podocyte apoptosis by activating the PPARγ-Klotho-FoxO1 signaling pathway, offering therapeutic potential for DN (Xing et al., 2021). Peroxisome proliferator-activated receptor γ (PPARγ), a target of thiazolidinediones used to treat patients with type 2 diabetes mellitus (T2DM), plays a crucial role in enhancing insulin sensitivity, promoting adipogenesis and exerting anti-inflammatory effects (Sauer, 2015; Stechschulte et al., 2016; Rao et al., 2015). In cell-based studies, the SUMOylation of the N-terminal K107 on PPARγ has been shown to inhibit its transcriptional activity, suggesting that targeting this modification could be a strategic approach in drug therapy. This strategy aims to separate the beneficial insulin-sensitizing properties of PPARγ from its adverse effects on body weight (Katafuchi et al., 2018). Extensive research indicates that PTM of PPARγ regulate its transcriptional activity, with SUMOylation being a crucial negative regulatory mechanism (Ohshima et al., 2004). Studies have demonstrated that excessive SUMOylation of PPARγ, similar to the effects of HFD, induces endothelial insulin resistance (IR) and dysfunction by negatively regulating the eNOS-NO pathway. More importantly, it was discovered that excessive SUMOylation of PPARγ triggers an endogenous SUMOylation cascade, exacerbating endothelial IR and dysfunction (Yuan et al., 2019).
Gallic acid (GA) is a bio-active compound found, known for its antioxidant, anti-allergic, anti-cancer, anti-inflammatory, and anti-diabetic properties (Chen et al., 2009; D’Souza et al., 2014; Huang et al., 2012; Hidalgo et al., 2012; Ho et al., 2013; Habtemariam, 1997; Nabavi et al., 2013; Punithavathi et al., 2011; Liu K. Y. et al., 2013). In human diets, the primary sources of this compound are non-glycosylated esters of gallic acid (Wianowska and Olszowy-Tomczyk, 2023). Gallic acid is a potent phenolic substance involved in crucial deSUMOylation processes through SENP1, particularly targeting GATA2, NEMO, Pin1, SMAD4, and HIF-1α, thus having significant impacts on various diseases (Taghvaei et al., 2022). Studies have shown that gallic acid effectively inhibits the renal activation of p38 MAPK and nuclear NF-κB, ultimately alleviating renal dysfunction in diabetic rats induced by a combination of HFD and STZ through p38 MAPK-mediated pathways (Ahad et al., 2015). Toxicological investigations indicate that gallic acid exhibits minimal toxicity or adverse reactions across a range of animal experiments and clinical studies (Bai et al., 2021).
Ginsenosides, the primary active components extracted from the roots, stems, leaves, or fruits of ginseng, are extensively cultivated in Korea and Northeast China (Sui et al., 2023). Ginseng has been reported to have anti-diabetic properties due to its ability to induce insulin secretion, stimulate glucose uptake, inhibit intestinal glucose absorption, and reduce glycogen breakdown (Chen et al., 2012; Yuan et al., 2012). Ginsenoside Rg3 has been shown to effectively protect against hyperglycemia, obesity, and diabetes by preventing the death of pancreatic β-cell (Kim et al., 2016; Kim et al., 2009). In a supplementary study, an eight-week treatment with 20(R)-Rg3 not only lowered fasting blood glucose levels and harmful levels of advanced glycation end-products but also beneficially regulated insulin modulation, lipid profiles, oxidative markers, and overall kidney health. This was achieved by affecting the MAPK and NF-κB signaling cascades in DN mice, highlighting 20(R)-Rg3 as a promising therapeutic agent (Li et al., 2021). Additionally, the well-known ginsenoside Rg3 has been identified as up-regulating the phosphorylated RanBP2 (an E3 SUMO-protein ligase), thereby exerting inhibitory effects on the NF-κB signaling pathway and offering new therapeutic avenues for cancer treatment (Zou et al., 2018). This inhibitory effect may be related to the regulation of nucleocytoplasmic transport. The phosphorylation of RanBP2 might affect its interaction with Nup153, leading to the aggregation of these nucleoporins. This aggregation could prevent IκB from entering the nucleus, thereby prolonging its binding to the p65 subunit and inhibiting NF-κB activity (Liu Y. et al., 2020).
In addition to natural products, TAK-981 and ML-792 are small-molecule SUMO inhibitors with significant selectivity. Research has shown that the SUMOylation inhibitors TAK-981 and ML-792 stimulate cytotoxic NK cells, M1 macrophages, and CTLs, while also preventing the exhaustion of CD8+ T cells, thereby enhancing antitumor activity (Wang et al., 2024). The SUMO E1 inhibitor TAK-981 is currently undergoing clinical trials for cancer treatment (Langston et al., 2021). The discovery of TAK-981 opens up possibilities for novel immunotherapies, offering new opportunities for the treatment of diseases such as cancer and sepsis (Zhang H. et al., 2022; Youssef et al., 2023).
Recent research indicates that the activation of the Notch, Wnt, and Hedgehog (Hh) signaling pathways plays a crucial role in the regeneration of damaged organs. However, excessive stimulation of these pathways can lead to fibrosis development. Therefore, reducing the activity of Notch, Wnt, or Hh signaling may represent a new therapeutic strategy for DN (Edeling et al., 2016). The Wnt pathway, particularly the canonical Wnt pathway, is considered a key regulator in the progression of DN. In DN, the TGF-β and Wnt signaling pathways mutually activate and regulate each other, promoting tissue fibrosis and damage repair, which exacerbates disease progression (Wang H. et al., 2021). At the same time, glucocorticoid receptors (GR) in endothelial cells play a key role in regulating renal fibrosis by inhibiting the canonical Wnt signaling pathway (Srivastava et al., 2021). Research on DN has shown that the Notch signaling pathway significantly affects podocytes and tubular epithelial cells (Yang and Liu, 2022; Ma et al., 2022). Additionally, fibroblast growth factor receptor 1 (FGFR1) signaling is involved in regulating kidney damage. Studies suggest that FGFR1 promotes the fibrotic response of renal tubular epithelial cells in the context of hypertension and elevated angiotensin II, indicating that FGFR1 is a potential target for preserving kidney function and integrity (Xu et al., 2022). Sirtuin 3 (SIRT3), a major mitochondrial deacetylase, plays a crucial role in the production and detoxification of reactive ROS. Experimental results show that regulating the SIRT3 pathway can improve diabetes-induced kidney damage. Specific activation of SIRT3 reduces oxidative stress induced by diabetes, protecting podocytes and glomeruli from damage (Locatelli et al., 2020). These signaling pathways and their interactions offer potential targets for future therapeutic strategies in treating DN.
5 Conclusion
To conclude, the present investigation provides an integrated review of SUMOylation’s involvement in DN, alongside the medicinal actions of natural products that influence transcription factors and pivotal signaling molecules. Given the intricate nature of DN onset, which is characterized by an interplay of diverse factors and a spectrum of molecular dynamics, and is indicative of both systemic and renal-specific pathological conditions, efficacious therapeutic strategies are markedly lacking. The role of SUMOylation in DN is complex, as its effects depend on specific target proteins and pathological conditions. The application of SUMOylation regulation in DN requires further research and validation. Current studies indicate that SUMOylation levels are often elevated in DN, particularly in response to high glucose and oxidative stress. This process offers some benefits by enhancing the activity of antioxidant proteins, which temporarily protect the kidneys from further oxidative damage. However, excessive SUMOylation can also drive the activation of profibrotic and pro-inflammatory signaling pathways, such as TGF-β and NF-κB, exacerbating kidney fibrosis and chronic inflammation. Therefore, future research may need to focus on balancing SUMOylation regulation through precise therapeutic targeting. This approach would aim to prevent the excessive accumulation of SUMO modifications while preserving the necessary cellular stress responses.
Natural products are widespread and diverse in nature and may provide additional directions and options for the treatment of DN. The burgeoning research into natural products capable of modulating SUMOylation is poised to elucidate the role of SUMOylation in the progression of diabetic kidney ailments, spotlighting compounds like ginkgolic acid, ginkgolide B, astragaloside, resveratrol, gallic acid, and ginsenoside Rg3. It is important to note that the difference in specificity between natural products and modern synthetic inhibitors is significant, directly influencing their efficacy and safety in research and therapeutic applications. While the natural products mentioned in this paper can affect SUMOylation, they often exert their effects through multiple pathways that are not necessarily related to SUMO modification. In contrast, compounds like TAK981 are designed with high specificity, targeting the SUMOylation pathway by inhibiting the SUMO E1 enzyme, thereby blocking the SUMOylation process. This high specificity allows TAK981 to more precisely regulate SUMOylation. However, natural products, due to their broad availability and multi-target characteristics, can simultaneously influence multiple biological pathways, which is particularly advantageous for complex diseases. Moreover, they tend to have lower side effects and reduced risk of resistance, making them a crucial component in disease treatment. While investigations into natural products modulating SUMOylation are nascent, the pursuit of viable SUMOylation-based treatments is a considerable hurdle, necessitating extensive and detailed foundational studies.
Future research could focus on developing natural product-based drugs targeting SUMOylation pathways, with the aim of regulating specific SUMOylation processes to slow or prevent the progression of DN. Additionally, SUMOylation modulators could be combined with other DN treatments, such as SGLT2 inhibitors and RAAS inhibitors, to create multi-target therapeutic strategies. This approach could act on multiple pathological mechanisms simultaneously, improving treatment efficacy while reducing the side effects associated with monotherapies. In summary, regulating SUMOylation may open new avenues for DN treatment, especially by targeting various pathological processes to achieve more personalized and precise therapeutic interventions.
Author contributions
JW: Writing–original draft. RZ: Writing–original draft. CW: Writing–original draft. LW: Conceptualization, Writing–review and editing. PeL: Conceptualization, Supervision, Writing–review and editing. PiL: Conceptualization, Supervision, Writing–review and editing.
Funding
The author(s) declare that financial support was received for the research, authorship, and/or publication of this article. This study was supported by the National Natural Science Foundation of China (No. 82274489, 82174296, U23A20504), the Fifth Batch of National Training Program for Excellent Clinical Talents of Traditional Chinese Medicine [No.1 (2022) of People’s Education of Traditional Chinese Medicine], Beijing Natural Science Foundation (No.7232326).
Conflict of interest
The authors declare that the research was conducted in the absence of any commercial or financial relationships that could be construed as a potential conflict of interest.
Publisher’s note
All claims expressed in this article are solely those of the authors and do not necessarily represent those of their affiliated organizations, or those of the publisher, the editors and the reviewers. Any product that may be evaluated in this article, or claim that may be made by its manufacturer, is not guaranteed or endorsed by the publisher.
References
Ahad, A., Ahsan, H., Mujeeb, M., and Siddiqui, W. A. (2015). Gallic acid ameliorates renal functions by inhibiting the activation of p38 MAPK in experimentally induced type 2 diabetic rats and cultured rat proximal tubular epithelial cells. Chem. Biol. Interact. 240, 292–303. doi:10.1016/j.cbi.2015.08.026
Arora, M. K., and Singh, U. K. (2013). Molecular mechanisms in the pathogenesis of diabetic nephropathy: an update. Vasc. Pharmacol. 58 (4), 259–271. doi:10.1016/j.vph.2013.01.001
Arvaniti, E., Vakrakou, A., Kaltezioti, V., Stergiopoulos, A., Prakoura, N., Politis, P. K., et al. (2016). Nuclear receptor NR5A2 is involved in the calreticulin gene regulation during renal fibrosis. Biochim. Biophys. Acta 1862 (9), 1774–1785. doi:10.1016/j.bbadis.2016.06.013
Asanuma, K. (2015). The role of podocyte injury in chronic kidney disease. Gakkai Kaishi 38 (1), 26–36. doi:10.2177/jsci.38.26
Attie, A. D. (2015). How do reducing equivalents increase insulin secretion? J. Clin. Invest. 125 (10), 3754–3756. doi:10.1172/JCI84011
Avula, B., Sagi, S., Gafner, S., Upton, R., Wang, Y. H., Wang, M., et al. (2015). Identification of Ginkgo biloba supplements adulteration using high performance thin layer chromatography and ultra high performance liquid chromatography-diode array detector-quadrupole time of flight-mass spectrometry. Anal. Bioanal. Chem. 407 (25), 7733–7746. doi:10.1007/s00216-015-8938-1
Bai, J., Zhang, Y., Tang, C., Hou, Y., Ai, X., Chen, X., et al. (2021). Gallic acid: pharmacological activities and molecular mechanisms involved in inflammation-related diseases. Biomed. Pharmacother. 133, 110985. doi:10.1016/j.biopha.2020.110985
Besnard, A., Galan-Rodriguez, B., Vanhoutte, P., and Caboche, J. (2011). Elk-1 a transcription factor with multiple facets in the brain. Front. Neurosci. 5, 35. doi:10.3389/fnins.2011.00035
Bessho, R., Takiyama, Y., Takiyama, T., Kitsunai, H., Takeda, Y., Sakagami, H., et al. (2019). Hypoxia-inducible factor-1α is the therapeutic target of the SGLT2 inhibitor for diabetic nephropathy. Sci. Rep. 9 (1), 14754. doi:10.1038/s41598-019-51343-1
Blahova, J., Martiniakova, M., Babikova, M., Kovacova, V., Mondockova, V., and Omelka, R. (2021). Pharmaceutical drugs and natural therapeutic products for the treatment of type 2 diabetes mellitus. Pharm. (Basel) 14 (8), 806. doi:10.3390/ph14080806
Bo, S., Ciccone, G., Castiglione, A., Gambino, R., De Michieli, F., Villois, P., et al. (2013). Anti-inflammatory and antioxidant effects of resveratrol in healthy smokers a randomized, double-blind, placebo-controlled, cross-over trial. Curr. Med. Chem. 20 (10), 1323–1331. doi:10.2174/0929867311320100009
Brackett, C. M., García-Casas, A., Castillo-Lluva, S., and Blagg, B. S. J. (2020). Synthesis and evaluation of ginkgolic acid derivatives as SUMOylation inhibitors. ACS Med. Chem. Lett. 11 (11), 2221–2226. doi:10.1021/acsmedchemlett.0c00353
Carrizzo, A., Forte, M., Damato, A., Trimarco, V., Salzano, F., Bartolo, M., et al. (2013). Antioxidant effects of resveratrol in cardiovascular, cerebral and metabolic diseases. Food Chem. Toxicol. 61, 215–226. doi:10.1016/j.fct.2013.07.021
Carter, L. G., D'Orazio, J. A., and Pearson, K. J. (2014). Resveratrol and cancer: focus on in vivo evidence. Endocr. Relat. Cancer 21 (3), R209–R225. doi:10.1530/ERC-13-0171
Chang, E., and Abe, J. I. (2016). Kinase-SUMO networks in diabetes-mediated cardiovascular disease. Metabolism 65 (5), 623–633. doi:10.1016/j.metabol.2016.01.007
Chang, H. M., and Yeh, E. (2020). SUMO: from bench to bedside. Physiol. Rev. 100 (4), 1599–1619. doi:10.1152/physrev.00025.2019
Chen, F., Chen, Y., Kang, X., Zhou, Z., Zhang, Z., and Liu, D. (2012). Anti-apoptotic function and mechanism of ginseng saponins in Rattus pancreatic β-cells. Biol. Pharm. Bull. 35 (9), 1568–1573. doi:10.1248/bpb.b12-00461
Chen, H. M., Wu, Y. C., Chia, Y. C., Chang, F. R., Hsu, H. K., Hsieh, Y. C., et al. (2009). Gallic acid, a major component of Toona sinensis leaf extracts, contains a ROS-mediated anti-cancer activity in human prostate cancer cells. Cancer Lett. 286 (2), 161–171. doi:10.1016/j.canlet.2009.05.040
Chen, L. C., Hsieh, Y. L., Tan, G. Y. T., Kuo, T. Y., Chou, Y. C., Hsu, P. H., et al. (2021). Differential effects of SUMO1 and SUMO2 on circadian protein PER2 stability and function. Sci. Rep. 11 (1), 14431. doi:10.1038/s41598-021-93933-y
Chen, Q., Su, Y., Ju, Y., Ma, K., Li, W., and Li, W. (2018). Astragalosides IV protected the renal tubular epithelial cells from free fatty acids-induced injury by reducing oxidative stress and apoptosis. Biomed. Pharmacother. 108, 679–686. doi:10.1016/j.biopha.2018.09.049
Chen, X., Lao, Y., Yi, J., Yang, J., He, S., and Chen, Y. (2020). SENP3 in monocytes/macrophages up-regulates tissue factor and mediates lipopolysaccharide-induced acute lung injury by enhancing JNK phosphorylation. J. Cell. Mol. Med. 24 (10), 5454–5462. doi:10.1111/jcmm.15199
Cheng, T. M., Chin, Y. T., Ho, Y., Chen, Y. R., Yang, Y. N., Yang, Y. C., et al. (2018). Resveratrol induces sumoylated COX-2-dependent anti-proliferation in human prostate cancer LNCaP cells. Food Chem. Toxicol. 112, 67–75. doi:10.1016/j.fct.2017.12.011
Cho, W. C., and Leung, K. N. (2007). In vitro and in vivo anti-tumor effects of Astragalus membranaceus. Cancer Lett. 252 (1), 43–54. doi:10.1016/j.canlet.2006.12.001
Choi, S. G., Kim, H., Jeong, E. I., Lee, H. J., Park, S., Lee, S. Y., et al. (2017). SUMO-modified FADD recruits cytosolic Drp1 and caspase-10 to mitochondria for regulated necrosis. Mol. Cell. Biol. 37 (2), 002544–e316. doi:10.1128/MCB.00254-16
Chung, S. S., Ahn, B. Y., Kim, M., Choi, H. H., Park, H. S., Kang, S., et al. (2010). Control of adipogenesis by the SUMO-specific protease SENP2. Mol. Cell. Biol. 30 (9), 2135–2146. doi:10.1128/MCB.00852-09
Claessens, L. A., and Vertegaal, A. (2024). SUMO proteases: from cellular functions to disease. Trends Cell. Biol. doi:10.1016/j.tcb.2024.01.002
Cubenas-Potts, C., and Matunis, M. J. (2013). SUMO: a multifaceted modifier of chromatin structure and function. Dev. Cell. 24 (1), 1–12. doi:10.1016/j.devcel.2012.11.020
Dai, X. Q., Plummer, G., Casimir, M., Kang, Y., Hajmrle, C., Gaisano, H. Y., et al. (2011). SUMOylation regulates insulin exocytosis downstream of secretory granule docking in rodents and humans. Diabetes 60 (3), 838–847. doi:10.2337/db10-0440
Deepa, B., and Venkatraman, A. C. (2013). Effects of linalool on inflammation, matrix accumulation and podocyte loss in kidney of streptozotocin-induced diabetic rats. Toxicol. Mech. Methods 23 (4), 223–234. doi:10.3109/15376516.2012.743638
Den Hartogh, D. J., and Tsiani, E. (2019). Health benefits of resveratrol in kidney disease: evidence from in vitro and in vivo studies. Nutrients 11 (7), 1624. doi:10.3390/nu11071624
Deretic, V. (2021). Autophagy in inflammation, infection, and immunometabolism. Immunity 54 (3), 437–453. doi:10.1016/j.immuni.2021.01.018
Derynck, R., Zhang, Y., and Feng, X. H. (1998). Smads: transcriptional activators of TGF-beta responses. Cell. 95 (6), 737–740. doi:10.1016/s0092-8674(00)81696-7
Di Bacco, A., Ouyang, J., Lee, H. Y., Catic, A., Ploegh, H., and Gill, G. (2006). The SUMO-specific protease SENP5 is required for cell division. Mol. Cell. Biol. 26 (12), 4489–4498. doi:10.1128/MCB.02301-05
Dou, X., Zhou, W. Y., Ding, M., Ma, Y. J., Yang, Q. Q., Qian, S. W., et al. (2022). The protease SENP2 controls hepatic gluconeogenesis by regulating the SUMOylation of the fuel sensor AMPKα. J. Biol. Chem. 298 (2), 101544. doi:10.1016/j.jbc.2021.101544
Drapeau, N., Lizotte, F., Denhez, B., Guay, A., Kennedy, C. R., and Geraldes, P. (2013). Expression of SHP-1 induced by hyperglycemia prevents insulin actions in podocytes. Am. J. Physiol. Endocrinol. Metab. 304 (11), E1188–E1198. doi:10.1152/ajpendo.00560.2012
Drucker, D. J., Philippe, J., Mojsov, S., Chick, W. L., and Habener, J. F. (1987). Glucagon-like peptide I stimulates insulin gene expression and increases cyclic AMP levels in a rat islet cell line. Proc. Natl. Acad. Sci. U. S. A. 84 (10), 3434–3438. doi:10.1073/pnas.84.10.3434
D'Souza, J. J., D'souza, P. P., Fazal, F., Kumar, A., Bhat, H. P., and Baliga, M. S. (2014). Anti-diabetic effects of the Indian indigenous fruit Emblica officinalis Gaertn: active constituents and modes of action. Food Funct. 5 (4), 635–644. doi:10.1039/c3fo60366k
Edeling, M., Ragi, G., Huang, S., Pavenstädt, H., and Susztak, K. (2016). Developmental signalling pathways in renal fibrosis: the roles of Notch, Wnt and Hedgehog. Nat. Rev. Nephrol. 12 (7), 426–439. doi:10.1038/nrneph.2016.54
Eifler, K., and Vertegaal, A. C. (2015). Mapping the SUMOylated landscape. FEBS J. 282 (19), 3669–3680. doi:10.1111/febs.13378
Evdokimov, E., Sharma, P., Lockett, S. J., Lualdi, M., and Kuehn, M. R. (2008). Loss of SUMO1 in mice affects RanGAP1 localization and formation of PML nuclear bodies, but is not lethal as it can be compensated by SUMO2 or SUMO3. J. Cell. Sci. 121 (Pt 24), 4106–4113. doi:10.1242/jcs.038570
Ferdaoussi, M., Dai, X., Jensen, M. V., Wang, R., Peterson, B. S., Huang, C., et al. (2015). Isocitrate-to-SENP1 signaling amplifies insulin secretion and rescues dysfunctional β cells. J. Clin. Invest. 125 (10), 3847–3860. doi:10.1172/JCI82498
Fu, J., Wang, Z., Huang, L., Zheng, S., Wang, D., Chen, S., et al. (2014). Review of the botanical characteristics, phytochemistry, and pharmacology of Astragalus membranaceus (Huangqi). Phytother. Res. 28 (9), 1275–1283. doi:10.1002/ptr.5188
Fujimoto, M., Maezawa, Y., Yokote, K., Joh, K., Kobayashi, K., Kawamura, H., et al. (2003). Mice lacking Smad3 are protected against streptozotocin-induced diabetic glomerulopathy. Biochem. Biophys. Res. Commun. 305 (4), 1002–1007. doi:10.1016/s0006-291x(03)00885-4
Fukuda, I., Ito, A., Hirai, G., Nishimura, S., Kawasaki, H., Saitoh, H., et al. (2009). Ginkgolic acid inhibits protein SUMOylation by blocking formation of the E1-SUMO intermediate. Chem. Biol. 16 (2), 133–140. doi:10.1016/j.chembiol.2009.01.009
Galkina, E., and Ley, K. (2006). Leukocyte recruitment and vascular injury in diabetic nephropathy. J. Am. Soc. Nephrol. 17 (2), 368–377. doi:10.1681/ASN.2005080859
Gao, C., Huang, W., Kanasaki, K., and Xu, Y. (2014). The role of ubiquitination and sumoylation in diabetic nephropathy. Biomed. Res. Int. 2014, 160692. doi:10.1155/2014/160692
Gareau, J. R., and Lima, C. D. (2010). The SUMO pathway: emerging mechanisms that shape specificity, conjugation and recognition. Nat. Rev. Mol. Cell. Biol. 11 (12), 861–871. doi:10.1038/nrm3011
Geiss-Friedlander, R., and Melchior, F. (2007). Concepts in sumoylation: a decade on. Nat. Rev. Mol. Cell. Biol. 8 (12), 947–956. doi:10.1038/nrm2293
Golebiowski, F., Szulc, A., Sakowicz, M., Szutowicz, A., and Pawełczyk, T. (2003). Expression level of Ubc9 protein in rat tissues. Acta Biochim. Pol. 50 (4), 1065–1073.
Gong, L., and Yeh, E. T. (2006). Characterization of a family of nucleolar SUMO-specific proteases with preference for SUMO-2 or SUMO-3. J. Biol. Chem. 281 (23), 15869–15877. doi:10.1074/jbc.M511658200
Gong, W., Li, J., Chen, W., Feng, F., and Deng, Y. (2020). Resveratrol inhibits lipopolysaccharide-induced extracellular matrix accumulation and inflammation in rat glomerular mesangial cells by SphK1/S1P2/NF-κB pathway. Diabetes Metab. Syndr. Obes. 13, 4495–4505. doi:10.2147/DMSO.S278267
Gong, X., Liao, Y., Ahner, A., Larsen, M. B., Wang, X., Bertrand, C. A., et al. (2019). Different SUMO paralogues determine the fate of wild-type and mutant CFTRs: biogenesis versus degradation. Mol. Biol. Cell. 30 (1), 4–16. doi:10.1091/mbc.E18-04-0252
Gross, J. L., de Azevedo, M. J., Silveiro, S. P., Canani, L. H., Caramori, M. L., and Zelmanovitz, T. (2005). Diabetic nephropathy: diagnosis, prevention, and treatment. Diabetes Care 28 (1), 164–176. doi:10.2337/diacare.28.1.164
Guo, B., Yang, S. H., Witty, J., and Sharrocks, A. D. (2007). Signalling pathways and the regulation of SUMO modification. Biochem. Soc. Trans. 35 (Pt 6), 1414–1418. doi:10.1042/BST0351414
Guo, D., Li, M., Zhang, Y., Yang, P., Eckenrode, S., Hopkins, D., et al. (2004). A functional variant of SUMO4, a new I kappa B alpha modifier, is associated with type 1 diabetes. Nat. Genet. 36 (8), 837–841. doi:10.1038/ng1391
Guo, F., Song, Y., Wu, L., Zhao, Y., Ma, X., Wang, J., et al. (2023). SUMO specific peptidase 6 regulates the crosstalk between podocytes and glomerular endothelial cells in diabetic kidney disease. Biochim. Biophys. Acta Mol. Basis Dis. 1869 (5), 166685. doi:10.1016/j.bbadis.2023.166685
Habtemariam, S. (1997). Flavonoids as inhibitors or enhancers of the cytotoxicity of tumor necrosis factor-alpha in L-929 tumor cells. J. Nat. Prod. 60 (8), 775–778. doi:10.1021/np960581z
Hajmrle, C., Ferdaoussi, M., Plummer, G., Spigelman, A. F., Lai, K., Manning Fox, J. E., et al. (2014). SUMOylation protects against IL-1β-induced apoptosis in INS-1 832/13 cells and human islets. Am. J. Physiol. Endocrinol. Metab. 307 (8), E664–E673. doi:10.1152/ajpendo.00168.2014
Hamdoun, S., and Efferth, T. (2017). Ginkgolic acids inhibit migration in breast cancer cells by inhibition of NEMO sumoylation and NF-κB activity. Oncotarget 8 (21), 35103–35115. doi:10.18632/oncotarget.16626
Han, X., Wang, X. L., Li, Q., Dong, X. X., Zhang, J. S., and Yan, Q. C. (2015). HIF-1α SUMOylation affects the stability and transcriptional activity of HIF-1α in human lens epithelial cells. Graefes Arch. Clin. Exp. Ophthalmol. 253 (8), 1279–1290. doi:10.1007/s00417-015-2999-x
Han, Z. J., Feng, Y. H., Gu, B. H., Li, Y. M., and Chen, H. (2018). The post-translational modification, SUMOylation, and cancer (Review). Int. J. Oncol. 52 (4), 1081–1094. doi:10.3892/ijo.2018.4280
Harlan, S. M., Heinz-Taheny, K. M., Sullivan, J. M., Wei, T., Baker, H. E., Jaqua, D. L., et al. (2018). Progressive renal disease established by renin-coding adeno-associated virus-driven hypertension in diverse diabetic models. J. Am. Soc. Nephrol. 29 (2), 477–491. doi:10.1681/ASN.2017040385
He, X., Lai, Q., Chen, C., Li, N., Sun, F., Huang, W., et al. (2018). Both conditional ablation and overexpression of E2 SUMO-conjugating enzyme (UBC9) in mouse pancreatic beta cells result in impaired beta cell function. Diabetologia 61 (4), 881–895. doi:10.1007/s00125-017-4523-9
Heo, K. S., Chang, E., Le, N. T., Cushman, H., Yeh, E. T. H., Fujiwara, K., et al. (2013). De-SUMOylation enzyme of sentrin/SUMO-specific protease 2 regulates disturbed flow-induced SUMOylation of ERK5 and p53 that leads to endothelial dysfunction and atherosclerosis. Circ. Res. 112 (6), 911–923. doi:10.1161/CIRCRESAHA.111.300179
Hidalgo, M., Martin-Santamaria, S., Recio, I., Sanchez-Moreno, C., de Pascual-Teresa, B., Rimbach, G., et al. (2012). Potential anti-inflammatory, anti-adhesive, anti/estrogenic, and angiotensin-converting enzyme inhibitory activities of anthocyanins and their gut metabolites. Genes. Nutr. 7 (2), 295–306. doi:10.1007/s12263-011-0263-5
Ho, H. H., Chang, C. S., Ho, W. C., Liao, S. Y., Lin, W. L., and Wang, C. J. (2013). Gallic acid inhibits gastric cancer cells metastasis and invasive growth via increased expression of RhoB, downregulation of AKT/small GTPase signals and inhibition of NF-κB activity. Toxicol. Appl. Pharmacol. 266 (1), 76–85. doi:10.1016/j.taap.2012.10.019
Howes, M. J., and Houghton, P. J. (2012). Ethnobotanical treatment strategies against Alzheimer's disease. Curr. Alzheimer Res. 9 (1), 67–85. doi:10.2174/156720512799015046
Hu, Q., Chen, Y., Deng, X., Li, Y., Ma, X., Zeng, J., et al. (2023a). Diabetic nephropathy: focusing on pathological signals, clinical treatment, and dietary regulation. Biomed. Pharmacother. 159, 114252. doi:10.1016/j.biopha.2023.114252
Hu, Q., Jiang, L., Yan, Q., Zeng, J., Ma, X., and Zhao, Y. (2023b). A natural products solution to diabetic nephropathy therapy. Pharmacol. Ther. 241, 108314. doi:10.1016/j.pharmthera.2022.108314
Huang, H. L., Lin, C. C., Jeng, K. C. G., Yao, P. W., Chuang, L. T., Kuo, S. L., et al. (2012). Fresh green tea and gallic acid ameliorate oxidative stress in kainic acid-induced status epilepticus. J. Agric. Food Chem. 60 (9), 2328–2336. doi:10.1021/jf203709q
Huang, W., Liang, Y., Dong, J., Zhou, L., Gao, C., Jiang, C., et al. (2017). SUMO E3 ligase PIASy mediates high glucose-induced activation of NF-κB inflammatory signaling in rat mesangial cells. Mediat. Inflamm. 2017, 1685194. doi:10.1155/2017/1685194
Huang, W., Xu, L., Zhou, X., Gao, C., Yang, M., Chen, G., et al. (2013). High glucose induces activation of NF-κB inflammatory signaling through IκBα sumoylation in rat mesangial cells. Biochem. Biophys. Res. Commun. 438 (3), 568–574. doi:10.1016/j.bbrc.2013.07.065
Hwang, J. Y., Kan, W. C., Liu, Y. B., Chuang, L. Y., Guh, J. Y., Yang, Y. L., et al. (2019). Angiotensin-converting enzyme inhibitors attenuated advanced glycation end products-induced renal tubular hypertrophy via enhancing nitric oxide signaling. J. Cell. Physiol. 234 (10), 17473–17481. doi:10.1002/jcp.28369
Imoto, S., Ohbayashi, N., Ikeda, O., Kamitani, S., Muromoto, R., Sekine, Y., et al. (2008). Sumoylation of Smad3 stimulates its nuclear export during PIASy-mediated suppression of TGF-beta signaling. Biochem. Biophys. Res. Commun. 370 (2), 359–365. doi:10.1016/j.bbrc.2008.03.116
Isah, T. (2015). Rethinking Ginkgo biloba L.: medicinal uses and conservation. Pharmacogn. Rev. 9 (18), 140–148. doi:10.4103/0973-7847.162137
Jia, Y., Claessens, L. A., Vertegaal, A. C. O., and Ovaa, H. (2019). Chemical tools and biochemical assays for SUMO specific proteases (SENPs). ACS Chem. Biol. 14 (11), 2389–2395. doi:10.1021/acschembio.9b00402
Jiang, Y., Liang, M., Chen, L., Wang, J., Huang, Y., Huo, H., et al. (2023). Myeloid SENP3 deficiency protects mice from diet and age-induced obesity via regulation of YAP1 SUMOylation. Cell. Mol. Life Sci. 81 (1), 4. doi:10.1007/s00018-023-05050-w
Jung, H. S., Kang, Y. M., Park, H. S., Ahn, B. Y., Lee, H., Kim, M. J., et al. (2016). Senp2 expression was induced by chronic glucose stimulation in INS1 cells, and it was required for the associated induction of Ccnd1 and Mafa. Islets 8 (6), 207–216. doi:10.1080/19382014.2016.1235677
Kabir, M. T., Tabassum, N., Uddin, M. S., Aziz, F., Behl, T., Mathew, B., et al. (2021). Therapeutic potential of polyphenols in the management of diabetic neuropathy. Evid. Based Complement. Altern. Med. 2021, 9940169. doi:10.1155/2021/9940169
Kamynina, E., and Stover, P. J. (2017). The roles of SUMO in metabolic regulation. Adv. Exp. Med. Biol. 963, 143–168. doi:10.1007/978-3-319-50044-7_9
Katafuchi, T., Holland, W. L., Kollipara, R. K., Kittler, R., Mangelsdorf, D. J., and Kliewer, S. A. (2018). PPARγ-K107 SUMOylation regulates insulin sensitivity but not adiposity in mice. Proc. Natl. Acad. Sci. U. S. A. 115 (48), 12102–12111. doi:10.1073/pnas.1814522115
Khatoon, E., Banik, K., Harsha, C., Sailo, B. L., Thakur, K. K., Khwairakpam, A. D., et al. (2022). Phytochemicals in cancer cell chemosensitization: current knowledge and future perspectives. Semin. Cancer Biol. 80, 306–339. doi:10.1016/j.semcancer.2020.06.014
Kim, K. I., and Baek, S. H. (2009). Small ubiquitin-like modifiers in cellular malignancy and metastasis. Int. Rev. Cell. Mol. Biol. 273, 265–311. doi:10.1016/S1937-6448(08)01807-8
Kim, M., Ahn, B. Y., Lee, J. S., Chung, S. S., Lim, S., Park, S. G., et al. (2009). The ginsenoside Rg3 has a stimulatory effect on insulin signaling in L6 myotubes. Biochem. Biophys. Res. Commun. 389 (1), 70–73. doi:10.1016/j.bbrc.2009.08.088
Kim, Y. J., Park, S. M., Jung, H. S., Lee, E. J., Kim, T. K., Kim, T. N., et al. (2016). Ginsenoside Rg3 prevents INS-1 cell death from intermittent high glucose stress. Islets 8 (3), 57–64. doi:10.1080/19382014.2016.1161874
Ko, J. K., Lam, F. Y., and Cheung, A. P. (2005). Amelioration of experimental colitis by Astragalus membranaceus through anti-oxidation and inhibition of adhesion molecule synthesis. World J. Gastroenterol. 11 (37), 5787–5794. doi:10.3748/wjg.v11.i37.5787
Koch, E. (2005). Inhibition of platelet activating factor (PAF)-induced aggregation of human thrombocytes by ginkgolides: considerations on possible bleeding complications after oral intake of Ginkgo biloba extracts. Phytomedicine 12 (1-2), 10–16. doi:10.1016/j.phymed.2004.02.002
Kumar, A., and Zhang, K. Y. (2015). Advances in the development of SUMO specific protease (SENP) inhibitors. Comput. Struct. Biotechnol. J. 13, 204–211. doi:10.1016/j.csbj.2015.03.001
Kunz, K., Piller, T., and Muller, S. (2018). SUMO-specific proteases and isopeptidases of the SENP family at a glance. J. Cell. Sci. 131 (6), jcs211904. doi:10.1242/jcs.211904
Kushwaha, K., Sharma, S., and Gupta, J. (2020a). Metabolic memory and diabetic nephropathy: beneficial effects of natural epigenetic modifiers. Biochimie 170, 140–151. doi:10.1016/j.biochi.2020.01.007
Kushwaha, K., Sharma, S., and Gupta, J. (2020b). Metabolic memory and diabetic nephropathy: beneficial effects of natural epigenetic modifiers. Biochimie 170, 140–151. doi:10.1016/j.biochi.2020.01.007
Langston, S. P., Grossman, S., England, D., Afroze, R., Bence, N., Bowman, D., et al. (2021). Discovery of TAK-981, a first-in-class inhibitor of SUMO-activating enzyme for the treatment of cancer. J. Med. Chem. 64 (5), 2501–2520. doi:10.1021/acs.jmedchem.0c01491
Lao, Y., Yang, K., Wang, Z., Sun, X., Zou, Q., Yu, X., et al. (2018). DeSUMOylation of MKK7 kinase by the SUMO2/3 protease SENP3 potentiates lipopolysaccharide-induced inflammatory signaling in macrophages. J. Biol. Chem. 293 (11), 3965–3980. doi:10.1074/jbc.M117.816769
Li, M., Guo, D., Isales, C. M., Eizirik, D. L., Atkinson, M., She, J. X., et al. (2005). SUMO wrestling with type 1 diabetes. J. Mol. Med. Berl. 83 (7), 504–513. doi:10.1007/s00109-005-0645-5
Li, O., Ma, Q., Li, F., Cai, G. Y., Chen, X. M., and Hong, Q. (2019). Progress of small ubiquitin-related modifiers in kidney diseases. Chin. Med. J. Engl. 132 (4), 466–473. doi:10.1097/CM9.0000000000000094
Li, S. J., and Hochstrasser, M. (1999). A new protease required for cell-cycle progression in yeast. Nature 398 (6724), 246–251. doi:10.1038/18457
Li, S. J., and Hochstrasser, M. (2000). The yeast ULP2 (SMT4) gene encodes a novel protease specific for the ubiquitin-like Smt3 protein. Mol. Cell. Biol. 20 (7), 2367–2377. doi:10.1128/mcb.20.7.2367-2377.2000
Li, X., Luo, Y., Yu, L., Lin, Y., Luo, D., Zhang, H., et al. (2008). SENP1 mediates TNF-induced desumoylation and cytoplasmic translocation of HIPK1 to enhance ASK1-dependent apoptosis. Cell. Death Differ. 15 (4), 739–750. doi:10.1038/sj.cdd.4402303
Li, Y., Hou, J. G., Liu, Z., Gong, X. J., Hu, J. N., Wang, Y. P., et al. (2021). Alleviative effects of 20(R)-Rg3 on HFD/STZ-induced diabetic nephropathy via MAPK/NF-κB signaling pathways in C57BL/6 mice. J. Ethnopharmacol. 267, 113500. doi:10.1016/j.jep.2020.113500
Li, Y. Y., Wang, H., Yang, X. X., Geng, H. Y., Gong, G., Kim, H. J., et al. (2017). Small ubiquitin-like modifier 4 (SUMO4) gene M55V polymorphism and type 2 diabetes mellitus: a meta-analysis including 6,823 subjects. Front. Endocrinol. (Lausanne) 8, 303. doi:10.3389/fendo.2017.00303
Liang, Q., Zheng, Q., Zuo, Y., Chen, Y., Ma, J., Ni, P., et al. (2019). SENP2 suppresses necdin expression to promote Brown adipocyte differentiation. Cell. Rep. 28 (8), 2004–2011. doi:10.1016/j.celrep.2019.07.083
Liang, X., Su, Y., and Huo, Y. (2021). Forkhead box protein O1 (FoxO1)/SERPINB1 ameliorates ROS production in diabetic nephropathy. Food Sci. Nutr. 9 (1), 44–51. doi:10.1002/fsn3.1859
Liang, Y. C., Lee, C. C., Yao, Y. L., Lai, C. C., Schmitz, M. L., and Yang, W. M. (2016). SUMO5, a novel poly-SUMO isoform, regulates PML nuclear bodies. Sci. Rep. 6, 26509. doi:10.1038/srep26509
Lin, H. Y., Wang, C. L., Hsiao, P. J., Lu, Y. C., Chen, S. Y., Lin, K. D., et al. (2007). SUMO4 M55V variant is associated with diabetic nephropathy in type 2 diabetes. Diabetes 56 (4), 1177–1180. doi:10.2337/db06-1283
Lin, Q., Chen, O., Wise, J. P., Shi, H., Wintergerst, K. A., Cai, L., et al. (2022). FGF1ΔHBS delays the progression of diabetic nephropathy in late-stage type 2 diabetes mouse model by alleviating renal inflammation, fibrosis, and apoptosis. Biochim. Biophys. Acta Mol. Basis Dis. 1868 (8), 166414. doi:10.1016/j.bbadis.2022.166414
Liu, J., Tao, X., Zhang, J., Wang, P., Sha, M., Ma, Y., et al. (2016). Small ubiquitin-related modifier 1 is involved in hepatocellular carcinoma progression via mediating p65 nuclear translocation. Oncotarget 7 (16), 22206–22218. doi:10.18632/oncotarget.8066
Liu, K. Y., Hu, S., Chan, B. C. L., Wat, E. C. L., Lau, C. B. S., Hon, K. L., et al. (2013b). Anti-inflammatory and anti-allergic activities of Pentaherb formula, Moutan Cortex (Danpi) and gallic acid. Molecules 18 (3), 2483–2500. doi:10.3390/molecules18032483
Liu, M., Yan, M., Lv, H., Wang, B., Lv, X., Zhang, H., et al. (2020a). Macrophage K63-linked ubiquitination of YAP promotes its nuclear localization and exacerbates atherosclerosis. Cell. Rep. 32 (5), 107990. doi:10.1016/j.celrep.2020.107990
Liu, P., Zhang, J., Wang, Y., Wang, C., Qiu, X., and Chen, D. Q. (2022a). Natural products against renal fibrosis via modulation of SUMOylation. Front. Pharmacol. 13, 800810. doi:10.3389/fphar.2022.800810
Liu, X., Chen, L., Zhang, C., Dong, W., Liu, H., Xiao, Z., et al. (2022b). Ginkgolic acid promotes inflammation and macrophage apoptosis via SUMOylation and NF-κB pathways in sepsis. Front. Med. (Lausanne) 9, 1108882. doi:10.3389/fmed.2022.1108882
Liu, X., Chen, W., Wang, Q., Li, L., and Wang, C. (2013a). Negative regulation of TLR inflammatory signaling by the SUMO-deconjugating enzyme SENP6. PLoS Pathog. 9 (6), e1003480. doi:10.1371/journal.ppat.1003480
Liu, Y., Dou, X., Zhou, W. Y., Ding, M., Liu, L., Du, R. Q., et al. (2021). Hepatic small ubiquitin-related modifier (SUMO)-Specific protease 2 controls systemic metabolism through SUMOylation-dependent regulation of liver-adipose tissue crosstalk. Hepatology 74 (4), 1864–1883. doi:10.1002/hep.31881
Liu, Y., Trnka, M. J., Guan, S., Kwon, D., Kim, D. H., Chen, J. J., et al. (2020b). A novel mechanism for NF-κB-activation via IκB-aggregation: implications for hepatic mallory-denk-body induced inflammation. Mol. Cell. Proteomics 19 (12), 1968–1986. doi:10.1074/mcp.RA120.002316
Lizotte, F., Denhez, B., Guay, A., Gévry, N., Côté, A. M., and Geraldes, P. (2016). Persistent insulin resistance in podocytes caused by epigenetic changes of SHP-1 in diabetes. Diabetes 65 (12), 3705–3717. doi:10.2337/db16-0254
Lizotte, F., Rousseau, M., Denhez, B., Lévesque, D., Guay, A., Liu, H., et al. (2023). Deletion of protein tyrosine phosphatase SHP-1 restores SUMOylation of podocin and reverses the progression of diabetic kidney disease. Kidney Int. 104 (4), 787–802. doi:10.1016/j.kint.2023.06.038
Locatelli, M., Zoja, C., Zanchi, C., Corna, D., Villa, S., Bolognini, S., et al. (2020). Manipulating Sirtuin 3 pathway ameliorates renal damage in experimental diabetes. Sci. Rep. 10 (1), 8418. doi:10.1038/s41598-020-65423-0
Long, J., Wang, G., He, D., and Liu, F. (2004). Repression of Smad4 transcriptional activity by SUMO modification. Biochem. J. 379 (Pt 1), 23–29. doi:10.1042/BJ20031867
Luciani, A., Villella, V. R., Vasaturo, A., Giardino, I., Raia, V., Pettoello-Mantovani, M., et al. (2009). SUMOylation of tissue transglutaminase as link between oxidative stress and inflammation. J. Immunol. 183 (4), 2775–2784. doi:10.4049/jimmunol.0900993
Ma, L., Wu, F., Shao, Q., Chen, G., Xu, L., and Lu, F. (2021). Baicalin alleviates oxidative stress and inflammation in diabetic nephropathy via Nrf2 and MAPK signaling pathway. Drug Des. Devel Ther. 15, 3207–3221. doi:10.2147/DDDT.S319260
Ma, T., Li, X., Zhu, Y., Yu, S., Liu, T., Zhang, X., et al. (2022). Excessive activation of Notch signaling in macrophages promote kidney inflammation, fibrosis, and necroptosis. Front. Immunol. 13, 835879. doi:10.3389/fimmu.2022.835879
Mabb, A. M., and Miyamoto, S. (2007). SUMO and NF-kappaB ties. Cell. Mol. Life Sci. 64 (15), 1979–1996. doi:10.1007/s00018-007-7005-2
Magee, C., Grieve, D. J., Watson, C. J., and Brazil, D. P. (2017). Diabetic nephropathy: a tangled web to unweave. Cardiovasc Drugs Ther. 31 (5-6), 579–592. doi:10.1007/s10557-017-6755-9
Manning, F. J., Hajmrle, C., and Macdonald, P. E. (2012). Novel roles of SUMO in pancreatic β-cells: thinking outside the nucleus. Can. J. Physiol. Pharmacol. 90 (6), 765–770. doi:10.1139/y11-134
Meinecke, I., Cinski, A., Baier, A., Peters, M. A., Dankbar, B., Wille, A., et al. (2007). Modification of nuclear PML protein by SUMO-1 regulates Fas-induced apoptosis in rheumatoid arthritis synovial fibroblasts. Proc. Natl. Acad. Sci. U. S. A. 104 (12), 5073–5078. doi:10.1073/pnas.0608773104
Melchior, F., Schergaut, M., and Pichler, A. (2003). SUMO: ligases, isopeptidases and nuclear pores. Trends Biochem. Sci. 28 (11), 612–618. doi:10.1016/j.tibs.2003.09.002
Meng, X. M., Huang, X. R., Xiao, J., Chung, A. C. K., Qin, W., Chen, H. y., et al. (2012). Disruption of Smad4 impairs TGF-β/Smad3 and Smad7 transcriptional regulation during renal inflammation and fibrosis in vivo and in vitro. Kidney Int. 81 (3), 266–279. doi:10.1038/ki.2011.327
Miyata, T., and de Strihou, C. (2010). Diabetic nephropathy: a disorder of oxygen metabolism? Nat. Rev. Nephrol. 6 (2), 83–95. doi:10.1038/nrneph.2009.211
Mohanta, T. K., Tamboli, Y., and Zubaidha, P. K. (2014). Phytochemical and medicinal importance of Ginkgo biloba L. Nat. Prod. Res. 28 (10), 746–752. doi:10.1080/14786419.2013.879303
Muller, S., Ledl, A., and Schmidt, D. (2004). SUMO: a regulator of gene expression and genome integrity. Oncogene 23 (11), 1998–2008. doi:10.1038/sj.onc.1207415
Nabavi, S. M., Habtemariam, S., Nabavi, S. F., Sureda, A., Daglia, M., Moghaddam, A. H., et al. (2013). Protective effect of gallic acid isolated from Peltiphyllum peltatum against sodium fluoride-induced oxidative stress in rat's kidney. Mol. Cell. Biochem. 372 (1-2), 233–239. doi:10.1007/s11010-012-1464-y
Nagata, M. (2016). Podocyte injury and its consequences. Kidney Int. 89 (6), 1221–1230. doi:10.1016/j.kint.2016.01.012
Nan, J., Lee, J. S., Moon, J. H., Lee, S. A., Park, Y. J., Lee, D. S., et al. (2022). SENP2 regulates mitochondrial function and insulin secretion in pancreatic β cells. Exp. Mol. Med. 54 (1), 72–80. doi:10.1038/s12276-021-00723-7
Nanjan, M. J., and Betz, J. (2014). Resveratrol for the management of diabetes and its downstream pathologies. Eur. Endocrinol. 10 (1), 31–35. doi:10.17925/EE.2014.10.01.31
Nash, K. M., and Shah, Z. A. (2015). Current perspectives on the beneficial role of ginkgo biloba in neurological and cerebrovascular disorders. Integr. Med. Insights 10, 1–9. doi:10.4137/IMI.S25054
Nyirimigabo, E., Jin, M., Yang, Z., Wang, J., Zhai, K., Mao, Y., et al. (2019). The role of ERp44 in glucose and lipid metabolism. Arch. Biochem. Biophys. 671, 175–184. doi:10.1016/j.abb.2019.06.011
Ohshima, T., Koga, H., and Shimotohno, K. (2004). Transcriptional activity of peroxisome proliferator-activated receptor gamma is modulated by SUMO-1 modification. J. Biol. Chem. 279 (28), 29551–29557. doi:10.1074/jbc.M403866200
Owerbach, D., McKay, E. M., Yeh, E. T. H., Gabbay, K. H., and Bohren, K. M. (2005). A proline-90 residue unique to SUMO-4 prevents maturation and sumoylation. Biochem. Biophys. Res. Commun. 337 (2), 517–520. doi:10.1016/j.bbrc.2005.09.090
Pandey, K. B., and Rizvi, S. I. (2009). Plant polyphenols as dietary antioxidants in human health and disease. Oxid. Med. Cell. Longev. 2 (5), 270–278. doi:10.4161/oxim.2.5.9498
Peiyuan, H., Zhiping, H., Chengjun, S., Chunqing, W., Bingqing, L., and Imam, M. U. (2017). Resveratrol ameliorates experimental alcoholic liver disease by modulating oxidative stress. Evid. Based Complement. Altern. Med. 2017, 4287890. doi:10.1155/2017/4287890
Perez-Morales, R. E., Del Pino, M. D., Valdivielso, J. M., Ortiz, A., Mora-Fernández, C., and Navarro-González, J. F. (2019). Inflammation in diabetic kidney disease. Nephron 143 (1), 12–16. doi:10.1159/000493278
Podolsky, R., Prasad, L. M., and She, J. X.Type I Diabetes Genetics Consortium (2009). Analyses of multiple single-nucleotide polymorphisms in the SUMO4/IDDM5 region in affected sib-pair families with type I diabetes. Genes. Immun. 10 (Suppl. 1), S16–S20. doi:10.1038/gene.2009.86
Pop-Busui, R., Ang, L., Boulton, A., Feldman, E., Marcus, R., Mizokami-Stout, K., et al. (2022). Diagnosis and treatment of painful diabetic peripheral neuropathy. Arlingt. (VA) Am. Diabetes Assoc. 2022, 1–32. doi:10.2337/db2022-01
Prasad, K. (2012). Resveratrol, wine, and atherosclerosis. Int. J. Angiol. 21 (1), 7–18. doi:10.1055/s-0032-1306417
Punithavathi, V. R., Stanely Mainzen Prince, P., Kumar, M. R., and Selvakumari, C. J. (2011). Protective effects of gallic acid on hepatic lipid peroxide metabolism, glycoprotein components and lipids in streptozotocin-induced type II diabetic Wistar rats. J. Biochem. Mol. Toxicol. 25 (2), 68–76. doi:10.1002/jbt.20360
Qin, Q., Niu, J., Wang, Z., Xu, W., Qiao, Z., and Gu, Y. (2012). Astragalus membranaceus inhibits inflammation via phospho-P38 mitogen-activated protein kinase (MAPK) and nuclear factor (NF)-κB pathways in advanced glycation end product-stimulated macrophages. Int. J. Mol. Sci. 13 (7), 8379–8387. doi:10.3390/ijms13078379
Qiu, J. Y., Chen, X., Zheng, X. x., Jiang, X. l., Yang, D. z., Yu, Y. y., et al. (2015). Target cell extraction coupled with LC-MS/MS analysis for screening potential bioactive components in Ginkgo biloba extract with preventive effect against diabetic nephropathy. Biomed. Chromatogr. 29 (2), 226–232. doi:10.1002/bmc.3264
Rajan, S., Torres, J., Thompson, M. S., and Philipson, L. H. (2012). SUMO downregulates GLP-1-stimulated cAMP generation and insulin secretion. Am. J. Physiol. Endocrinol. Metab. 302 (6), E714–E723. doi:10.1152/ajpendo.00486.2011
Rao, A., Pandya, V., and Whaley-Connell, A. (2015). Obesity and insulin resistance in resistant hypertension: implications for the kidney. Adv. Chronic Kidney Dis. 22 (3), 211–217. doi:10.1053/j.ackd.2014.12.004
Reutens, A. T., and Atkins, R. C. (2011). Epidemiology of diabetic nephropathy. Contrib. Nephrol. 170, 1–7. doi:10.1159/000324934
Roborel, D. C. A., Pain, E., Boss, A., and Shaunik, A. (2020). Understanding reasons for treatment discontinuation, attitudes and education needs among People who discontinue type 2 diabetes treatment: results from an online patient survey in the USA and UK. Diabetes Ther. 11 (8), 1873–1881. doi:10.1007/s13300-020-00843-9
Sakai, N., Wada, T., Furuichi, K., Iwata, Y., Yoshimoto, K., Kitagawa, K., et al. (2005). Involvement of extracellular signal-regulated kinase and p38 in human diabetic nephropathy. Am. J. Kidney Dis. 45 (1), 54–65. doi:10.1053/j.ajkd.2004.08.039
Sato, M., Muragaki, Y., Saika, S., Roberts, A. B., and Ooshima, A. (2003). Targeted disruption of TGF-beta1/Smad3 signaling protects against renal tubulointerstitial fibrosis induced by unilateral ureteral obstruction. J. Clin. Invest. 112 (10), 1486–1494. doi:10.1172/JCI19270
Sauer, S. (2015). Ligands for the nuclear peroxisome proliferator-activated receptor gamma. Trends Pharmacol. Sci. 36 (10), 688–704. doi:10.1016/j.tips.2015.06.010
Schulz, S., Chachami, G., Kozaczkiewicz, L., Winter, U., Stankovic-Valentin, N., Haas, P., et al. (2012). Ubiquitin-specific protease-like 1 (USPL1) is a SUMO isopeptidase with essential, non-catalytic functions. EMBO Rep. 13 (10), 930–938. doi:10.1038/embor.2012.125
Sheng, Z., Zhu, J., Deng, Y. N., Gao, S., and Liang, S. (2021). SUMOylation modification-mediated cell death. Open Biol. 11 (7), 210050. doi:10.1098/rsob.210050
Shin, E. J., Shin, H. M., Nam, E., Kim, W. S., Kim, J. H., Oh, B. H., et al. (2012). DeSUMOylating isopeptidase: a second class of SUMO protease. EMBO Rep. 13 (4), 339–346. doi:10.1038/embor.2012.3
Shoeib, H. M., Keshk, W. A., Al-Ghazaly, G. M., Wagih, A. A., and El-Dardiry, S. A. (2023). Interplay between long non-coding RNA MALAT1 and pyroptosis in diabetic nephropathy patients. Gene 851, 146978. doi:10.1016/j.gene.2022.146978
Sinha, N., Yadav, A. K., Kumar, V., Dutta, P., Bhansali, A., and Jha, V. (2016). SUMO4 163 G>A variation is associated with kidney disease in Indian subjects with type 2 diabetes. Mol. Biol. Rep. 43 (5), 345–348. doi:10.1007/s11033-016-3979-x
Skov, J., Christiansen, J. S., and Poulsen, P. L. (2016). Hypertension and diabetic nephropathy. Endocr. Dev. 31, 97–107. doi:10.1159/000439393
Smith, S., Normahani, P., Lane, T., Hohenschurz-Schmidt, D., Oliver, N., and Davies, A. H. (2022). Prevention and management strategies for diabetic neuropathy. Life (Basel) 12 (8), 1185. doi:10.3390/life12081185
Srivastava, S. P., Zhou, H., Setia, O., Liu, B., Kanasaki, K., Koya, D., et al. (2021). Loss of endothelial glucocorticoid receptor accelerates diabetic nephropathy. Nat. Commun. 12 (1), 2368. doi:10.1038/s41467-021-22617-y
Stechschulte, L. A., Czernik, P. J., Rotter, Z. C., Tausif, F. N., Corzo, C. A., Marciano, D. P., et al. (2016). PPARG post-translational modifications regulate bone formation and bone resorption. EBioMedicine 10, 174–184. doi:10.1016/j.ebiom.2016.06.040
Sui, Z., Sui, D., Li, M., Yu, Q., Li, H., and Jiang, Y. (2023). Ginsenoside Rg3 has effects comparable to those of ginsenoside re on diabetic kidney disease prevention in db/db mice by regulating inflammation, fibrosis and PPARγ. Mol. Med. Rep. 27 (4), 84. doi:10.3892/mmr.2023.12971
Taghvaei, S., Sabouni, F., Minuchehr, Z., and Taghvaei, A. (2022). Identification of novel anti-cancer agents, applying in silico method for SENP1 protease inhibition. J. Biomol. Struct. Dyn. 40 (14), 6228–6242. doi:10.1080/07391102.2021.1880480
Tan, X., Liu, Y., Liu, D., Tang, X., Xia, M., Chen, G., et al. (2022). SUMO1 promotes mesangial cell proliferation through inhibiting autophagy in a cell model of IgA nephropathy. Front. Med. (Lausanne) 9, 834164. doi:10.3389/fmed.2022.834164
Tang, D., Zhang, Z., Gao, Y., Wei, Y., and Han, L. (2009). Protective effects of serum containing Ginkgo biloba extract on glomerulosclerosis in rat mesangial cells. J. Ethnopharmacol. 124 (1), 26–33. doi:10.1016/j.jep.2009.04.017
Tang, G., Li, S., Zhang, C., Chen, H., Wang, N., and Feng, Y. (2021). Clinical efficacies, underlying mechanisms and molecular targets of Chinese medicines for diabetic nephropathy treatment and management. Acta Pharm. Sin. B 11 (9), 2749–2767. doi:10.1016/j.apsb.2020.12.020
Tokarz, P., and Wozniak, K. (2021). SENP proteases as potential targets for cancer therapy. Cancers (Basel) 13 (9), 2059. doi:10.3390/cancers13092059
Tossidou, I., Himmelseher, E., Teng, B., Haller, H., and Schiffer, M. (2014). SUMOylation determines turnover and localization of nephrin at the plasma membrane. Kidney Int. 86 (6), 1161–1173. doi:10.1038/ki.2014.198
Tossidou, I., Niedenthal, R., Klaus, M., Teng, B., Worthmann, K., King, B. L., et al. (2012). CD2AP regulates SUMOylation of CIN85 in podocytes. Mol. Cell. Biol. 32 (6), 1068–1079. doi:10.1128/MCB.06106-11
Vertegaal, A. (2022). Signalling mechanisms and cellular functions of SUMO. Nat. Rev. Mol. Cell. Biol. 23 (11), 715–731. doi:10.1038/s41580-022-00500-y
Wada, T., Pippin, J. W., Marshall, C. B., Griffin, S. V., and Shankland, S. J. (2005). Dexamethasone prevents podocyte apoptosis induced by puromycin aminonucleoside: role of p53 and Bcl-2-related family proteins. J. Am. Soc. Nephrol. 16 (9), 2615–2625. doi:10.1681/ASN.2005020142
Wang, A., Ziyadeh, F. N., Lee, E. Y., Pyagay, P. E., Sung, S. H., Sheardown, S. A., et al. (2007). Interference with TGF-beta signaling by Smad3-knockout in mice limits diabetic glomerulosclerosis without affecting albuminuria. Am. J. Physiol. Ren. Physiol. 293 (5), F1657–F1665. doi:10.1152/ajprenal.00274.2007
Wang, B., Zhang, C., Chu, D., Ma, X., Yu, T., Liu, X., et al. (2021c). Astragaloside IV improves angiogenesis under hypoxic conditions by enhancing hypoxia‑inducible factor‑1α SUMOylation. Mol. Med. Rep. 23 (4), 244. doi:10.3892/mmr.2021.11883
Wang, C. Y., and She, J. X. (2008). SUMO4 and its role in type 1 diabetes pathogenesis. Diabetes Metab. Res. Rev. 24 (2), 93–102. doi:10.1002/dmrr.797
Wang, H., Zhang, R., Wu, X., Chen, Y., Ji, W., Wang, J., et al. (2021a). The Wnt signaling pathway in diabetic nephropathy. Front. Cell. Dev. Biol. 9, 701547. doi:10.3389/fcell.2021.701547
Wang, J., Zhang, Z., Fang, A., Wu, K., Chen, X., Wang, G., et al. (2020). Resveratrol attenuates inflammatory bowel disease in mice by regulating SUMO1. Biol. Pharm. Bull. 43 (3), 450–457. doi:10.1248/bpb.b19-00786
Wang, L., Wang, H. L., Liu, T. T., and Lan, H. Y. (2021b). TGF-beta as a master regulator of diabetic nephropathy. Int. J. Mol. Sci. 22 (15), 7881. doi:10.3390/ijms22157881
Wang, L., Wansleeben, C., Zhao, S., Miao, P., Paschen, W., and Yang, W. (2014a). SUMO2 is essential while SUMO3 is dispensable for mouse embryonic development. EMBO Rep. 15 (8), 878–885. doi:10.15252/embr.201438534
Wang, L., Zhang, T., Fang, M., Shen, N., Wang, D., Teng, J., et al. (2015a). Podocytes protect glomerular endothelial cells from hypoxic injury via deSUMOylation of HIF-1α signaling. Int. J. Biochem. Cell. Biol. 58, 17–27. doi:10.1016/j.biocel.2014.10.030
Wang, L., Zhu, J., Fang, M., Zhang, T., Xie, H., Wang, N., et al. (2014b). Inhibition of p53 deSUMOylation exacerbates puromycin aminonucleoside-induced apoptosis in podocytes. Int. J. Mol. Sci. 15 (11), 21314–21330. doi:10.3390/ijms151121314
Wang, W., and Matunis, M. J. (2023). Paralogue-specific roles of SUMO1 and SUMO2/3 in protein quality control and associated diseases. Cells 13 (1), 8. doi:10.3390/cells13010008
Wang, Y., Jiang, Y., Fan, X., Tan, H., Zeng, H., Wang, Y., et al. (2015b). Hepato-protective effect of resveratrol against acetaminophen-induced liver injury is associated with inhibition of CYP-mediated bioactivation and regulation of SIRT1-p53 signaling pathways. Toxicol. Lett. 236 (2), 82–89. doi:10.1016/j.toxlet.2015.05.001
Wang, Z., Pan, B., Su, L., Yu, H., Wu, X., Yao, Y., et al. (2024). SUMOylation inhibitors activate anti-tumor immunity by reshaping the immune microenvironment in a preclinical model of hepatocellular carcinoma. Cell. Oncol. (Dordr) 47 (2), 513–532. doi:10.1007/s13402-023-00880-z
Wei, W., Yang, P., Pang, J., Zhang, S., Wang, Y., Wang, M. H., et al. (2008). A stress-dependent SUMO4 sumoylation of its substrate proteins. Biochem. Biophys. Res. Commun. 375 (3), 454–459. doi:10.1016/j.bbrc.2008.08.028
Wianowska, D., and Olszowy-Tomczyk, M. (2023). A concise profile of gallic acid-from its natural sources through biological properties and chemical methods of determination. Molecules 28 (3), 1186. doi:10.3390/molecules28031186
Wu, T., Ding, L., Andoh, V., Zhang, J., and Chen, L. (2023). The mechanism of hyperglycemia-induced renal cell injury in diabetic nephropathy disease: an update. Life (Basel) 13 (2), 539. doi:10.3390/life13020539
Wu, W., and Huang, C. (2023). SUMOylation and DeSUMOylation: prospective therapeutic targets in cancer. Life Sci. 332, 122085. doi:10.1016/j.lfs.2023.122085
Wu, X. M., Gao, Y. B., Cui, F. Q., and Zhang, N. (2016). Exosomes from high glucose-treated glomerular endothelial cells activate mesangial cells to promote renal fibrosis. Biol. Open 5 (4), 484–491. doi:10.1242/bio.015990
Xie, H., Wang, Y. H., Liu, X., Gao, J., Yang, C., Huang, T., et al. (2023). SUMOylation of ERp44 enhances Ero1α ER retention contributing to the pathogenesis of obesity and insulin resistance. Metabolism 139, 155351. doi:10.1016/j.metabol.2022.155351
Xing, L., Fang, J., Zhu, B., Wang, L., Chen, J., Wang, Y., et al. (2021). Astragaloside IV protects against podocyte apoptosis by inhibiting oxidative stress via activating PPARγ-Klotho-FoxO1 axis in diabetic nephropathy. Life Sci. 269, 119068. doi:10.1016/j.lfs.2021.119068
Xiong, Y., and Zhou, L. (2019). The signaling of cellular senescence in diabetic nephropathy. Oxid. Med. Cell. Longev. 2019, 7495629. doi:10.1155/2019/7495629
Xu, J., Deng, Y., Wang, Y., Sun, X., Chen, S., and Fu, G. (2020). SPAG5-AS1 inhibited autophagy and aggravated apoptosis of podocytes via SPAG5/AKT/mTOR pathway. Cell. Prolif. 53 (2), e12738. doi:10.1111/cpr.12738
Xu, Z., Luo, W., Chen, L., Zhuang, Z., Yang, D., Qian, J., et al. (2022). Ang II (angiotensin II)-Induced FGFR1 (fibroblast growth factor receptor 1) activation in tubular epithelial cells promotes hypertensive kidney fibrosis and injury. Hypertension 79 (9), 2028–2041. doi:10.1161/HYPERTENSIONAHA.122.18657
Yang, J., and Liu, Z. (2022). Mechanistic pathogenesis of endothelial dysfunction in diabetic nephropathy and retinopathy. Front. Endocrinol. (Lausanne) 13, 816400. doi:10.3389/fendo.2022.816400
Yang, Z., Zhang, Y., and Sun, S. (2019). Deciphering the SUMO code in the kidney. J. Cell. Mol. Med. 23 (2), 711–719. doi:10.1111/jcmm.14021
Yeh, E. T. (2009). SUMOylation and De-SUMOylation: wrestling with life's processes. J. Biol. Chem. 284 (13), 8223–8227. doi:10.1074/jbc.R800050200
Yong, H. Y., Koh, M. S., and Moon, A. (2009). The p38 MAPK inhibitors for the treatment of inflammatory diseases and cancer. Expert Opin. Investig. Drugs 18 (12), 1893–1905. doi:10.1517/13543780903321490
Youssef, A., Mohammed, B. K., Prasad, A., Del Aguila, A., Bassi, G., Yang, W., et al. (2023). Splenic SUMO1 controls systemic inflammation in experimental sepsis. Front. Immunol. 14, 1200939. doi:10.3389/fimmu.2023.1200939
Yu, L., Wang, Y., Guo, Y. H., Wang, L., Yang, Z., Zhai, Z. H., et al. (2021). HIF-1α alleviates high-glucose-induced renal tubular cell injury by promoting Parkin/PINK1-mediated mitophagy. Front. Med. (Lausanne) 8, 803874. doi:10.3389/fmed.2021.803874
Yuan, H. D., Kim, J. T., Kim, S. H., and Chung, S. H. (2012). Ginseng and diabetes: the evidences from in vitro, animal and human studies. J. Ginseng Res. 36 (1), 27–39. doi:10.5142/jgr.2012.36.1.27
Yuan, W., Zhou, Y., Wang, M., Zeng, G., and Huang, Q. (2019). Negative regulation of eNOS-NO signaling by over-SUMOylation of PPARγ contributes to insulin resistance and dysfunction of vascular endothelium in rats. Vasc. Pharmacol. 122-123, 106597. doi:10.1016/j.vph.2019.106597
Yun, C., Wang, Y., Mukhopadhyay, D., Backlund, P., Kolli, N., Yergey, A., et al. (2008). Nucleolar protein B23/nucleophosmin regulates the vertebrate SUMO pathway through SENP3 and SENP5 proteases. J. Cell. Biol. 183 (4), 589–595. doi:10.1083/jcb.200807185
Zhang, H., Tomar, V. S., Li, J., Basavaraja, R., Yan, F., Gui, J., et al. (2022b). Protection of regulatory T cells from fragility and inactivation in the tumor microenvironment. Cancer Immunol. Res. 10 (12), 1490–1505. doi:10.1158/2326-6066.CIR-22-0295
Zhang, J., and Yan, J. (2019). Protective effect of ginkgolic acid in attenuating LDL induced inflammation human peripheral blood mononuclear cells via altering the NF-κB signaling pathway. Front. Pharmacol. 10, 1241. doi:10.3389/fphar.2019.01241
Zhang, Z., Sun, Y., Xue, J., Jin, D., Li, X., Zhao, D., et al. (2022a). The critical role of dysregulated autophagy in the progression of diabetic kidney disease. Front. Pharmacol. 13, 977410. doi:10.3389/fphar.2022.977410
Zheng, Q., Cao, Y., Chen, Y., Wang, J., Fan, Q., Huang, X., et al. (2018). Senp2 regulates adipose lipid storage by de-SUMOylation of Setdb1. J. Mol. Cell. Biol. 10 (3), 258–266. doi:10.1093/jmcb/mjx055
Zhou, X., Gao, C., Huang, W., Yang, M., Chen, G., Jiang, L., et al. (2014). High glucose induces sumoylation of Smad4 via SUMO2/3 in mesangial cells. Biomed. Res. Int. 2014, 782625. doi:10.1155/2014/782625
Zhou, X., Li, W., Wang, S., Zhang, P., Wang, Q., Xiao, J., et al. (2019). YAP aggravates inflammatory bowel disease by regulating M1/M2 macrophage polarization and gut microbial homeostasis. Cell. Rep. 27 (4), 1176–1189. doi:10.1016/j.celrep.2019.03.028
Zhou, X., Sun, X., Gong, X., Yang, Y., Chen, C., Shan, G., et al. (2017). Astragaloside IV from Astragalus membranaceus ameliorates renal interstitial fibrosis by inhibiting inflammation via TLR4/NF-кB in vivo and in vitro. Int. Immunopharmacol. 42, 18–24. doi:10.1016/j.intimp.2016.11.006
Zou, M., Wang, J., Gao, J., Han, H., and Fang, Y. (2018). Phosphoproteomic analysis of the antitumor effects of ginsenoside Rg3 in human breast cancer cells. Oncol. Lett. 15 (3), 2889–2898. doi:10.3892/ol.2017.7654
Keywords: diabetic nephropathy, sumoylation, natural products, ginkgolic acid, ginkgolide B, resveratrol, astragaloside IV
Citation: Wang J, Zhang R, Wu C, Wang L, Liu P and Li P (2024) Exploring potential targets for natural product therapy of DN: the role of SUMOylation. Front. Pharmacol. 15:1432724. doi: 10.3389/fphar.2024.1432724
Received: 14 May 2024; Accepted: 20 September 2024;
Published: 04 October 2024.
Edited by:
Swayam Prakash Srivastava, Weill Cornell Medical Center, NewYork-Presbyterian, United StatesReviewed by:
Jiaoyi Chen, University Health Network (UHN), CanadaDavid Ribet, Institut National de la Santé et de la Recherche Médicale (INSERM), France
Copyright © 2024 Wang, Zhang, Wu, Wang, Liu and Li. This is an open-access article distributed under the terms of the Creative Commons Attribution License (CC BY). The use, distribution or reproduction in other forums is permitted, provided the original author(s) and the copyright owner(s) are credited and that the original publication in this journal is cited, in accordance with accepted academic practice. No use, distribution or reproduction is permitted which does not comply with these terms.
*Correspondence: Lifan Wang, d2xmNDY0ODM3NEAxNjMuY29t; Peng Liu, ZHJsaXVwZW5nQHNpbmEuY24=; Ping Li, bHA4Njc1QDE2My5jb20=
†These authors have contributed equally to this work