- 1College of Basic Medicine, Heilongjiang University of Chinese Medicine, Harbin, China
- 2The Second Hospital Affiliated Heilongjiang University of Traditional Chinese Medicine, Harbin, China
Objective: This study aims to elucidate the intervention effects of saponin components from Polygala tenuifolia Willd (Polygalaceae) on dementia, providing experimental evidence and new insights for the research and application of saponins in the field of dementia.
Materials and Methods: This review is based on a search of the PubMed, NCBI, and Google Scholar databases from their inception to 13 May 2024, using terms such as “P. tenuifolia,” “P. tenuifolia and saponins,” “toxicity,” “dementia,” “Alzheimer’s disease,” “Parkinson’s disease dementia,” and “vascular dementia.” The article summarizes the saponin components of P. tenuifolia, including tenuigenin, tenuifolin, polygalasaponins XXXII, and onjisaponin B, as well as the pathophysiological mechanisms of dementia. Importantly, it highlights the potential mechanisms by which the active components of P. tenuifolia prevent and treat diseases and relevant clinical studies.
Results: The saponin components of P. tenuifolia can reduce β-amyloid accumulation, exhibit antioxidant effects, regulate neurotransmitters, improve synaptic function, possess anti-inflammatory properties, inhibit neuronal apoptosis, and modulate autophagy. Therefore, P. tenuifolia may play a role in the prevention and treatment of dementia.
Conclusion: The saponin components of P. tenuifolia have shown certain therapeutic effects on dementia. They can prevent and treat dementia through various mechanisms.
1 Introduction
Dementia is a common disabling syndrome characterized primarily by the gradual or progressive loss of memory, executive abilities, and other cognitive functions (Galasko, 2013). As cognitive abilities deteriorate, approximately 90% of individuals with dementia exhibit psychological and behavioral abnormalities, including aggression, psychosis, agitation, and depression (Aarsland, 2020). The decline in cognitive function is typically attributed to neurodegenerative processes, such as the abnormal accumulation of proteins within brain cells and changes in the function of cellular components, which ultimately impair neuronal function and diminish cognitive and memory capacities (Ritchie and Lovestone, 2002). Recent research suggests that vascular aging contributes, at least in part, to the onset of dementia, a finding substantiated in various subtypes of the condition (Andersson and Stone, 2023; Raz et al., 2016). High-risk factors such as hypertension are significant contributors to vascular damage (Lyon et al., 2024). Autopsies indicate that over half of those aged 65 and older exhibit small vessel disease (Hainsworth et al., 2024), underscoring the importance of mitigating risk factors that contribute to cerebrovascular and cumulative brain damage as a strategy for preventing dementia. Dementia is classified into several subtypes, with Alzheimer’s disease (AD) and vascular dementia (VaD) being the most prevalent, accounting for approximately 60% and 20% of cases, respectively. Other forms include Lewy body dementia (about 10%), frontotemporal dementia (about 5%), and Parkinson’s disease dementia (about 2%) (Duong et al., 2017).
According to projections, the global dementia population in 2019 was approximately 55.2 million, with associated societal costs estimated at $1.313 trillion (Wimo et al., 2023). In the United States, the number of Alzheimer’s disease patients aged 65 and older stood at about 6.7 million in 2023, projected to increase to 13.8 million by 2060. The financial burden related to healthcare, long-term care, and end-of-life services is projected to reach $345 billion (Alzheimer’s disease facts and figures, 2023). A further forecast for 2023 estimates the global Alzheimer’s population at 416 million, representing roughly 22% of those aged 50 and over, with 315 million potentially in the preclinical stages of the disease (Gustavsson et al., 2023). Overall, dementia imposes a substantial socio-economic burden, particularly in low- and middle-income countries, where it is estimated that 61% of global dementia costs account for only 26% of total expenditures (Wimo et al., 2023). This disparity likely contributes to a lower quality of life for dementia patients in these regions. The uneven distribution and increasing prevalence of the condition significantly heighten the burden on families and societies (Arruda and Paun, 2017). Given the limited effectiveness of current treatments, there is an urgent need for innovative therapeutic strategies.
Polygala tenuifolia (PT) is extensively used in China, Japan, and South Korea as a component of traditional Asian medicine, boasting a long history of application (Jiang et al., 2021; Haraguchi et al., 2022). Ancient Chinese medical texts document PT’s efficacy in treating ailments such as insomnia, neurasthenia, excessive cough with phlegm, and palpitations (Jiang et al., 2021; Chen et al., 2023). In preclinical research, PT and its extracts have demonstrated neuroprotective properties, as well as potential for dementia prevention and antidepressant effects (Shin et al., 2018; Han et al., 2021). Traditional plant-based medicinal combinations containing PT, such as Kaixinsan (Fu et al., 2019), Sagacious Confucius’ Pillow Elixir (Hou et al., 2019), and Buyuan Congnao decoction (Chen et al., 2012a), have also exhibited pharmacological actions against AD. Regarding its chemical composition, PT contains saponins, sugars and glycosides, polygols, ketones, alkaloids, fatty oils, and resins (Tian et al., 2015; Ba et al., 2019; Luo et al., 2024), with several of these constituents confirmed to possess anti-dementia properties. Mechanistically, its actions encompass anti-inflammatory (Zhou et al., 2021), antioxidant (Tang et al., 2022), anti-apoptotic (Choi et al., 2011), anti-amyloid beta protein deposition (Zhao et al., 2015), and neurotransmitter regulation (Lee et al., 2004), providing a broad spectrum of interventions.
Among these, saponin compounds are one of the primary components of P. tenuifolia. Saponins are mainly distributed in terrestrial plants, with a diverse array of types and complex structures characterized by their triterpene or steroid aglycone and one or more sugar chains (Güçlü-Ustündağ and Mazza, 2007). Currently, all saponin compounds identified in PT are triterpenoid saponins. Studies have shown that these saponin compounds can penetrate the blood-brain barrier and have a prolonged retention time in the body, providing a material basis for their use in treating brain lesions. Some saponin extracts from PT have been proven to intervene in the progression of dementia through various mechanisms, including anti-inflammatory, antioxidant, anti-apoptotic, and autophagy regulation.
The aim of this review was to examine the pharmacological effects of the saponin active components in PT for the treatment of dementia.
2 Saponin compounds in Polygala tenuifolia
The saponin compounds of P. tenuifolia represent some of the most significant active ingredients in PT, all categorized as triterpenoid saponins with a fundamental structure of pentacyclic triterpenes, specifically of the oleanane-type (Liu et al., 2021). To date, numerous PT saponins have been identified, including Sibiricasaponins A-E (Song et al., 2013), onjisaponins (A, B, E, F, etc.) (Zeng et al., 2021), and tenuifoliside A-C (Son et al., 2022). Although extensive, pharmacological research has primarily concentrated on tenuigenin (senegenin), polygalasaponins XXXII, tenuifolin, and onjisaponin B (Figure 1). These saponin compounds have demonstrated extensive neuroprotective effects in the treatment of dementia, employing diverse mechanisms of intervention, and have shown particularly effective outcomes in managing AD and PDD. This review will provide a systematic summary of the action mechanisms of these compounds.
3 Plant localization and pharmacokinetics of saponin compounds
The saponin components of PT are predominantly found in the roots, with the concentration in the aboveground parts amounting to only about one-fifth to one-quarter of that in the roots, which is the primary rationale for utilizing the roots for extraction and medicinal purposes (Park et al., 2008; Vinh et al., 2020). Regarding age, there is an inverse relationship between the age of the plant and the concentration of total saponins and sapogenins; older roots contain lower levels of saponins, with annual roots offering the highest quality (Teng et al., 2009). However, the diameter of triennial roots is approximately 2–3 times that of annual roots. Consequently, triennial roots yield a higher biomass, aligning more closely with traditional harvesting practices (Teng et al., 2009).
In terms of pharmacokinetics, saponin compounds exhibit poor membrane permeability and generally demonstrate suboptimal absorption due to physicochemical properties such as a high molecular weight (>500 Da), substantial hydrogen bonding capability (>12), and significant polymer flexibility (>10) (Yu et al., 2012). Tenuigenin, an active ingredient in PT extract, was tested using Institute of Cancer Research (ICR) mice. The half-lives for oral and intravenous administration were recorded at 2.6 ± 0.6 h and 1.6 ± 0.4 h, respectively, with an oral bioavailability of 8.7% (Shen et al., 2022). In contrast, tenuifolin has an oral and intravenous half-life of 1.1 ± 0.2 h and 0.8 ± 0.2 h, respectively, with an oral bioavailability of 4.0% (Shen et al., 2022). When Sprague-Dawley (SD) rats were utilized as research subjects, tenuifolin achieved peak concentrations within 24 min, with terminal elimination half-lives of 4.8 ± 1.6 h and 2.0 ± 0.3 h for oral and intravenous injections, respectively, and an oral bioavailability of 2.0% (Wang et al., 2015). Another study revealed that in SD rats, tenuifolin reached peak concentration 46 min post oral administration, with half-lives of 1.80 ± 0.39 h and 1.41 ± 0.54 h for oral and intravenous injection, respectively, and a bioavailability of 0.83% ± 0.28% (Ma et al., 2014). In terms of tissue distribution, tenuifolin primarily accumulates in the liver and kidneys, with a small amount penetrating the blood-brain barrier and infiltrating brain tissues. The drug’s retention time in various organs does not exceed 12 h (Ma et al., 2014). In summary, PT’s saponin compounds have lower bioavailability but benefit from longer residence times in vivo and the ability to penetrate the blood-brain barrier, potentially providing the material basis for treating dementia with PT saponin compounds.
4 Pharmacological effects of saponin compounds
Accumulating evidence indicates that the saponin compounds in PT possess neuroprotective effects, and they are primarily utilized in treating AD within the spectrum of dementia. There is also limited evidence suggesting their potential for interventional in PDD and VaD subtypes. The focus of research on these compounds includes tenuigenin, tenuifolin, polygalasaponins XXXII, and onjisaponin B. Mechanistically, their actions encompass reducing β-amyloid (Aβ) accumulation, providing antioxidant effects, regulating neurotransmitters, enhancing synaptic function, offering anti-inflammatory benefits, preventing neuronal apoptosis, and modulating autophagy (Table 1; Figure 2).
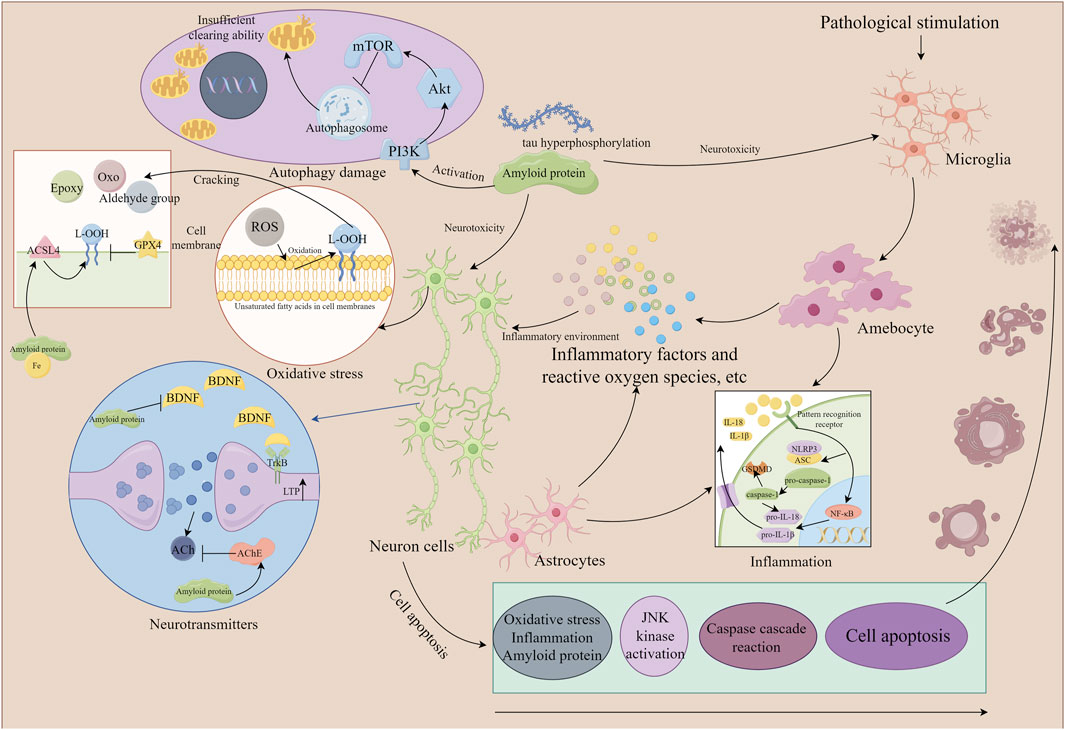
Figure 2. The mechanism of neuronal damage (the part that Polygala tenuifolia saponins can intervene in). By Figdraw. Note: ROS, reactive oxygen species; ACSL4, acyl-CoA synthetase long chain family member 4; GPX4, glutathione peroxidase 4; ACh, acetylcholine; JNK, c-Jun N-terminal kinase; NLRP3, recombinant NLR family, pyrin domain containing protein 3; IL, interleukin; NF-κB, nuclear factor-κB; mTOR, mammalian target of rapamycin; BDNF, brain-derived neurotrophic factor; ASC, apoptosis-associated speck-like protein containing a CARD; TrkB, tyrosine kinase receptor B; Akt, protein kinase B; ACSL4, acyl-CoA synthetase long-chain family member 4.
4.1 Improving learning and memory
Extracellular amyloid protein deposition and intracellular neurofibrillary tangles are classic pathological hallmarks of Alzheimer’s disease (Bergamino et al., 2022). An imbalance between the generation and degradation of Aβ protein leads to amyloid accumulation (Cai et al., 2023). Aβ protein synthesis is dependent on the β-secretase pathway (von Einem et al., 2010) and is regulated by γ-secretase, with dysregulation of γ-secretase resulting in increased Aβ production (Yang et al., 2021). The primary pathways for Aβ clearance involve glial cell clearance (McAlpine et al., 2021), autophagy (Luo et al., 2020), transport across the blood-brain barrier (Cai et al., 2018), and proteolytic degradation (Zheng et al., 2022). Factors such as an abnormal increase in Aβ protein or aging can impair these clearance mechanisms. Aβ proteins that are not cleared in a timely manner can form oligomers or dispersed amyloid deposits, activating microglia and astrocytes to create a pro-inflammatory environment (Phillips et al., 2014; Franco-Bocanegra et al., 2019), leading to oxidative stress. This stress promotes the excessive phosphorylation of tau proteins, forming neurofibrillary tangles, disrupting microtubule structural stability, causing depolymerization, and ultimately inducing neurotoxicity (Canudas et al., 2005).
Reducing the neuronal toxicity caused by excessive phosphorylation of Aβ and tau proteins is beneficial for ameliorating memory impairment. Studies have demonstrated that tenuigenin and tenuifolin can mitigate Aβ25-35-induced neuronal cytotoxicity, thereby enhancing neuronal survival rates (Liu et al., 2015). Furthermore, tenuigenin has been shown to increase the length of neural synapses (Jesky and Chen, 2016). It can also reduce tau protein hyperphosphorylation and oxidative stress levels in the brains of streptozotocin-induced AD rats, thereby intervening in cognitive dysfunction (Huang et al., 2018). Another therapeutic strategy for cognitive impairments involves increasing the number of new neurons and glial cells. Neural stem cells, capable of self-renewal (Andreotti et al., 2019), are located in the subventricular zone of the lateral ventricles and the subgranular zone of the dentate gyrus in humans or mice. These cells possess the potential to differentiate into neurons and astrocytes of the central nervous system. Tenuigenin promotes the proliferation of neural stem cells and induces their differentiation into neurons and astrocytes (Chen et al., 2012b). Both onjisaponin B and tenuifolin enhance the proliferation of neural stem cells, though tenuifolin lacks the capability to facilitate migration and differentiation (Wang et al., 2021), which are crucial for neurogenesis. In contrast, onjisaponin B promotes neural stem cell migration and induces their differentiation into astrocytes (Wang et al., 2021).
4.2 Reducing Aβ protein secretion
Excessive secretion of Aβ protein can damage central nervous system cells (Xia et al., 2024). Aβ protein is produced through the enzymatic cleavage of amyloid precursor protein (APP), which is encoded by the APP gene located on chromosome 21 (Maurya et al., 2023). APP is a transmembrane protein regulated by two cleavage pathways: the non-Aβ protein generation pathway (Zhao et al., 2022) and the Aβ protein generation pathway (Meng et al., 2023a). Dysregulation of the Aβ protein generation pathway is the primary cause of imbalances in Aβ protein secretion. The β-secretase enzyme is responsible for hydrolyzing and releasing the C-terminal transmembrane peptide segment, C99 (Capone et al., 2021), which is subsequently cleaved by the γ-secretase complex to release Aβ proteins of varying amino acid lengths (Devkota et al., 2024). The most commonly released forms of Aβ protein are Aβ1-40 and Aβ1-42, with Aβ1-42 being more pathogenic and associated with poorer prognosis (Wittrahm et al., 2023).
Inhibiting APP levels and secretase activity can effectively reduce Aβ protein expression and protect central nervous system cells. Tenuigenin reduces APP protein expression by suppressing APP mRNA levels in PC12 neural cells, thereby leading to a decrease in the levels of the hydrolysis product C99 (Jia et al., 2004). Further research has revealed that tenuifolin consistently inhibits both Aβ1-40 and Aβ1-42. However, it does not affect the γ-secretase-mediated cleavage of C99; instead, it reduces Aβ protein expression through the inhibition of β-secretase (Lv et al., 2009). Additionally, presenilin (PS) 1, the catalytic center of γ-secretase, is affected by mutations in the PS1 gene (PSEN1), which are a causative factor in early-onset familial Alzheimer’s disease. PSEN1 mutations increase the expression of β-secretase and γ-secretase, enhancing the sequential cleavage of APP proteins (Li et al., 2022). Onjisaponin B inhibits the interaction between PS1 and β-secretase in APP/PS1 mice, leading to a reduction in Aβ protein secretion, without affecting the activity of β-secretase and γ-secretase (Li et al., 2016).
4.3 Antioxidant effect
The imbalance between the production and clearance of reactive oxygen species (ROS) or free radicals leads to oxidative stress, as ROS are natural byproducts of biological oxygen metabolism (Palma et al., 2024). Under normal physiological conditions, ROS stimulate cell growth and rely on antioxidant enzymes to facilitate redox reactions that eliminate excess ROS (Helli et al., 2024). Pathologically, due to the brain’s high oxygen demand and damage to the blood-brain barrier, the transport of ROS and antioxidants both intra- and extracranially becomes challenging (Cornacchia et al., 2022), resulting in an accumulation of ROS within the brain. Furthermore, the neuronal membrane structure, which is rich in unsaturated fatty acids (Yammine et al., 2020), is prone to oxidation. This oxidation, when combined with ROS, forms lipid peroxides and contributes to oxidative damage in the brain. In dementia patients, oxidative stress is closely linked to pathological brain changes. Specifically, Aβ proteins induce neurotoxicity characterized by oxidative stress, oxidizing unsaturated fatty acids on cell membranes to produce lipid peroxides (Koenig and Meyerhoff, 2003), which lead to synaptic loss and ultimately neuron death. Aβ25-35 plays a significant catalytic role in this process (Smith and Klimov, 2019). In patients with AD and PDD, there is often an increase in lipid hydroperoxide (L-OOH) and malondialdehyde (MDA) activity, alongside decreased activity of antioxidant enzymes such as superoxide dismutase (SOD), catalase (CAT), and glutathione (GSH) (Chen et al., 2022; Atiq et al., 2023). Moreover, the brain’s rich content of metal ions, such as iron, facilitates oxidative stress and DNA damage through the Haber-Weiss reaction (Suma et al., 2020), and promotes the aggregation of α-synuclein, leading to the formation of Lewy bodies—a key pathological feature of PDD and Lewy body dementia (Wakabayashi et al., 2007). In summary, oxidative stress is an early event leading to cell death, which triggers brain lesions in dementia through programmed cell death processes, such as apoptosis and ferroptosis.
Tenuigenin can reduce the levels of ROS within neuronal cells (Chen et al., 2010). Under pathological conditions, one of the primary sources of ROS is NADPH oxidase, activated when cytokines stimulate cells (Schröder, 2019). Acting as an inhibitor of NADPH oxidase, tenuigenin significantly lowers the accumulation of ROS in PC12 cells (Zhu et al., 2016). Excessive ROS is counteracted by the antioxidant system, with heme oxygenase-1 (HO-1) serving as a crucial metabolic enzyme in responding to oxidative stress. HO-1 is positively regulated by the antioxidant regulatory factor NF-E2 related factor 2 (Nrf2). Upon cellular stimulation, Nrf2 dissociates from Kelch-like ECH-associated protein 1 (Keap1) and translocates from the cytoplasm to the nucleus to activate gene expression. Moreover, HO-1 enhances the expression of glutathione peroxidase (GSH-Px), SOD, and CAT, thus boosting antioxidant capacity (Ryter, 2021). When the antioxidant system fails to effectively suppress ROS, substantial amounts of lipid peroxides are produced, leading to the generation of toxic 4-hydroxynonenal (4-HNE) through non-enzymatic reactions. 4-HNE induces the secretion of Aβ protein, exacerbating cytotoxicity (Takagane et al., 2015). Studies have demonstrated that tenuigenin promotes the nuclear translocation of Nrf2 in neuronal cells, enhances HO-1 expression (Ren et al., 2022), and consequently upregulates the expression of the antioxidant enzyme GSH-Px, while downregulating MDA and 4-HNE levels (Huang et al., 2018). Nitric oxide (NO), another free radical, is positively regulated by inducible nitric oxide synthase (iNOS), which triggers lipid peroxidation by producing peroxynitrite anions (Wan et al., 2021). In microglia, tenuigenin acts as a direct scavenger of NO, although its inhibition of NO is not regulated by iNOS (Lu et al., 2017). Analogously, onjisaponin B reduces oxidative stress in aging rats by downregulating MDA accumulation and upregulating the production of GSH, GSH-Px, and SOD (Li et al., 2018). Tenuifolin elevates the expression of Nrf2 and HO-1 in SH-SY5Y cells, increasing levels of GSH, SOD, and CAT, and reducing MDA and ROS content. Additionally, it suppresses Aβ42 oligomer-induced increases in iNOS and NO in microglia (Chen and Jia, 2020). Polygalasaponins enhance SOD activity in the cerebral cortex and hippocampus, lower MDA levels, and inhibit Aβ25-35-induced lipid peroxidation and oxidative stress damage (Xu et al., 2011).
4.4 Improving synaptic plasticity
The synapse serves as a crucial functional unit of neuronal connectivity, consisting of the presynaptic membrane, synaptic cleft, and postsynaptic membrane. Information is transmitted across these components in sequence, making synaptic plasticity a vital element in facilitating learning and memory functions (Choquet and Triller, 2013). Synaptic plasticity typically manifests as long-term potentiation (LTP) and long-term inhibition, both crucial feedback mechanisms for its regulation. In dementia, LTP is commonly inhibited; thus, stimulating or extending LTP may aid in restoring learning and memory functions (Paoletti et al., 2013). In dementia subtypes such as AD and VaD, synapses display functional deficits, including reductions in synapse numbers, impaired dendritic spine morphology, and diminished neurotransmission capabilities (Piscopo et al., 2022; Che et al., 2023).
Enhancing synaptic morphological changes is beneficial for improving brain information transmission and treating cognitive impairments. Tenuigenin has been shown to improve learning and memory capabilities and offers protective effects on synaptic morphology (Cai et al., 2013). Neurotrophic factors can induce the expression of synaptic-related proteins, such as growth-associated protein-43 (GAP-43), synaptophysin (Syn), and postsynaptic density protein 95, all of which play roles in regulating synaptic plasticity and neurotransmitter release. Tenuigenin enhances the expression of GAP-43 and Syn in PC12 cells induced by Aβ25-35, increasing both neurogenesis and the number and length of synapses (Jesky and Chen, 2016). Additionally, tenuigenin affects other proteins involved in memory and synaptic plasticity regulation, reducing the expression of histone deacetylase two and hippocalcin in the rat hippocampus, promoting the phosphorylation of cyclic AMP-responsive element-binding protein and the N-methyl-D-aspartate receptor (NMDAR) 2B subunit, thereby enhancing long-term potentiation (LTP) and synaptic plasticity (Lin et al., 2018). Other compounds, like onjisaponin B, can elevate the expression of neurotrophic factors (Yabe et al., 2003). Tenuifolin directly impacts synaptic status (Koganezawa et al., 2021), increasing the total length and intersection numbers of dendrites in hippocampal CA1 neurons, notably affecting only female mice (Kong et al., 2024). Brain-derived neurotrophic factor (BDNF) is known to activate LTP and increase dendritic spine formation, thus potentially reversing LTP deficits. The tyrosine kinase receptor B (TrkB) serves as a receptor for BDNF, maintaining synaptic protein expression and neuronal survival (Moya-Alvarado et al., 2023). Tenuifolin promotes neuronal cell survival and synaptic protein expression through the BDNF/TrkB signaling pathway (Li et al., 2023). Aβ proteins can inhibit BDNF by reducing the expression of the cyclic AMP response element-binding protein (CREB), while CREB and BDNF-dependent increases promote dendritic growth in neuronal cell bodies. Polyalasaponin XXXII promotes the expression of CREB and BDNF, enhancing the phosphorylation of TrkB and activating downstream cascades, which strengthens synaptic transmission in the dentate gyrus and induces and maintains LTP, enhancing hippocampal synaptic plasticity (Xue et al., 2009; Zhou et al., 2016). NMDAR plays a critical role in regulating synaptic plasticity; under pathological conditions, damage to NMDAR promotes the splicing of APP into Aβ protein, which in turn damages neurons and reduces NMDAR expression (Shankar et al., 2008). Studies have shown that polygalasaponin can reverse the expression of NMDAR subtypes NMDAR1 and NMDAR2B in the hippocampus and cortex of SAMP8 mice, ameliorating cognitive impairments (Xu et al., 2016).
4.5 Regulating neurotransmitters
Neurotransmitters are synthesized by presynaptic neurons and released into the synaptic gaps to mediate information transmission between neurons. In dementia patients, neurotransmitter release is typically abnormal (Teleanu et al., 2022; Yang et al., 2023). Over a hundred types of neurotransmitters have been identified, and current research on the effects of saponins from Radix Polygalae primarily focuses on acetylcholine (ACh), 5-hydroxytryptamine (5-HT), norepinephrine (NE), and dopamine (DA). The cholinergic system, closely associated with memory and learning abilities, utilizes choline acetyltransferase (ChAT) as a specific marker for cholinergic neurons. ChAT catalyzes the formation of ACh from choline and acetyl CoA, which then triggers neural impulses in the synaptic gaps, subsequently broken down into choline and acetic acid by acetylcholinesterase (AChE) (Hampel et al., 2018). In dementia, factors such as the loss of cholinergic neurons or deposition of Aβ proteins lead to abnormally elevated levels of AChE (Allard and Hussain Shuler, 2023; Lazarova et al., 2024). Furthermore, 5-HT, DA, and NE, which are closely linked to cognitive functions, when diminished, impair cognitive abilities, manifesting as neuronal damage or metabolic dysfunctions in the brain (Kaneko et al., 2005).
Tenuigenin can enhance learning abilities by inhibiting AChE activity in the hippocampus (Huang et al., 2013) and enhancing field excitatory postsynaptic potentials, which improve synaptic transmission functions (Wei et al., 2015). Onjisaponin B has been shown to strengthen cholinergic neurons and to increase the expression of ChAT (Yabe et al., 2003). Research on tenuifolin indicates that it not only reduces cortical AChE activity but also elevates the expression levels of NE and DA in the hippocampus, although it does not affect the regulation of 5-HT (Zhang et al., 2008). Additionally, NE serves as an anti-inflammatory agent by regulating microglia, astrocytes, and pro-inflammatory factors, thereby reducing brain inflammation (O’Donnell et al., 2012), which underscores tenuifolin’s potential anti-inflammatory effects.
4.6 Anti-inflammatory effects
The inflammatory response is intricately linked to the onset and progression of dementia. At early stages of dementia, the brain exhibits high levels of inflammatory responses, primarily involving microglia and astrocytes (Ahmad et al., 2022). Mechanistically, the assembly of inflammasomes is one of the key drivers in the onset and persistence of brain inflammation. Pattern recognition receptors, such as Toll-like receptors, engage with pathogen-related molecular patterns or damage-related molecular patterns (Swanson et al., 2019), leading to the activation of NLR family pyrin domain containing protein 3 (NLRP3) and nuclear factor kappa-B (NF-κB). Upon NLRP3 activation, apoptosis-associated speck-like protein containing a CARD (ASC) and the precursor of pro-caspase-1 are recruited to form an inflammasome complex (Jiang et al., 2023b), subsequently triggering the self-cleavage of pro-caspase-1 to form active caspase-1 (Meng et al., 2023b). Besides activation through pattern recognition receptors, NF-κB is also regulated by pro-inflammatory cytokines such as tumor necrosis factor (TNF)-α and interleukin-1β (IL-1β) (Yu et al., 2020), leading to the transcription of inactive pro-IL-18 and pro-IL-1β (Wang et al., 2023b). Caspase-1 cleaves Gasdermin-D (GSDMD) into GSDMD-N and GSDMD-C, with GSDMD-N binding to the cell membrane to create pores (Meng et al., 2023b). Moreover, caspase-1 cleaves pro-IL-18 and pro-IL-1β into mature IL-18 and IL-1β (Qin and Zhao, 2023), which are released through these membrane pores to promote an inflammatory milieu. Additionally, pro-inflammatory cytokines TNF-α and IL-6 can be directly induced by NF-κB (Yu et al., 2020), further activating NF-κB and perpetuating the release of inflammatory mediators, thus sustaining the inflammatory cycle. The excessive expression of inflammatory factors not only damages neurons and disrupts glial cell function but also causes abnormal glial proliferation, intensifying neurodegenerative changes. Research suggests that certain inflammatory markers such as IL-6 and TNF-α may serve as biomarkers for diagnosing dementia (Darweesh et al., 2018; Custodero et al., 2022).
Microglia are the primary cells responsible for releasing inflammatory mediators in the brain. Under pathological conditions, they serve as a significant source of cytokines and neurotoxic substances. Tenuigenin inhibits lipopolysaccharide-induced microglial activation and the activation of NLRP3 inflammasomes (Fan et al., 2017). The substantia nigra, the principal nucleus responsible for dopamine synthesis, experiences delayed behavioral movements due to dopamine neuron deficiencies and dopamine depletion in the substantia nigra compacta (Kanan et al., 2024). Tenuigenin effectively prevents the degeneration of dopamine neurons and inhibits NLRP3 inflammasome activation in the substantia nigra (Fan et al., 2017). Additionally, Tenuigenin suppresses NF-κB activation in microglia, reducing the cleavage of pro-IL-1β (Li et al., 2017), and decreases the expression of TNF-α, IL-6, and IL-1β (Wang et al., 2017). Moreover, matrix metalloproteinases (MMP) 2 and MMP9, effector factors secreted by microglia, exhibit enhanced expression under the pathological conditions of dementia, promoting neuroinflammation and accelerating neuronal degeneration (Qiu et al., 2023). Studies have shown that tenuigenin reduces the protein expression of MMP2 and MMP9 in primary rat microglia, without affecting the mRNA expression of MMP2 (Lu et al., 2017). Onjisaponin B by inhibiting the NF-κB pathway in the hippocampus, lowers the expression of IL-6, IL-1β, and TNF-α, exerting anti-inflammatory effects (Li et al., 2018). Tenuifolin, by inhibiting the activation of Toll-like receptor 4 and NF-κB in mice with cognitive impairment (Wang et al., 2022), decreases the translation levels of NLRP3, ASC, and caspase-1, reduces inflammasome assembly, and consequently downregulates the expression of IL-6, IL-1β, and IL-18 (Jiang et al., 2023a). In patients with AD, microglia can be activated by Aβ protein, leading to morphological changes and the activation of nitric oxide synthase, which increases NO (Wu et al., 2024) and causes neuronal damage. Cyclooxygenase-2, positively correlated with the density of amyloid plaques, when overexpressed, can worsen cognitive impairments (McLarnon, 2023). Tenuifolin inhibits the Aβ42 oligomer-induced inflammatory response in microglia, reducing the release of TNF-α, IL-6, and IL-1β, and suppressing the expression of nitric oxide synthase and cyclooxygenase-2, thus protecting neuronal cells from inflammatory damage (Chen and Jia, 2020).
4.7 Anti neuronal apoptosis effect
Apoptosis, a programmed cell death mode, intersects with several dementia pathogenesis hypotheses, including oxidative stress, inflammation, and Aβ-induced cell damage, and represents one of their ultimate outcomes. This process contributes to neuronal loss, which is a pivotal cause of dementia (Liang et al., 2024). Apoptosis is modulated by multiple pathways; notably, saponin components from P. tenuifolia primarily inhibit neuronal apoptosis via the c-Jun N-terminal kinase (JNK) pathway, thereby enhancing memory and learning capabilities. Stress stimuli or inflammatory factors can activate JNK kinase, increasing mitochondrial membrane permeability and initiating a caspase cascade reaction (Yuan et al., 2023). The caspase protease family is broadly categorized into initiator caspases (such as caspase-8, 9, and 10) and effector caspases (caspase-3, 6, and 7). Once activated, initiator caspases further activate effector caspases, leading to DNA fragmentation, cytoskeletal and nuclear protein degradation, apoptotic body formation, and subsequent phagocytic uptake (Elmore, 2007). Additionally, JNK pathway activation can regulate the levels of B-cell lymphoma-2 (Bcl-2) family proteins, such as the prototypical anti-apoptotic protein Bcl-2 and the pro-apoptotic protein Bcl2-associated X protein (Bax), which are abnormally expressed in the brains of those with dementia (Rajesh and Kanneganti, 2022).
Recent studies have demonstrated that tenuigenin suppresses caspase-3 activation by downregulating the expression of JNK and phosphorylated JNK in neuronal cells (Chen et al., 2010), thus reversing the expression ratio of Bcl-2 to Bax (Ren et al., 2022). Rho guanine nucleotide dissociation inhibitor α (RhoGDIα), a RhoGDP dissociation inhibitor, participates in the processes of AD and VaD through RhoGTPase activity. Specifically, overexpression of RhoGDIα can reduce Tau hyperphosphorylation and inhibit neuronal apoptosis (Zhang et al., 2023). Tenuigenin elevates the expression of RhoGDIα, which decreases the apoptosis rate in neuronal cells, and this process may be mediated by the JNK pathway (Li et al., 2015). Aβ proteins disrupt intracellular Ca2+ distribution, increasing membrane permeability and Ca2+ permeability, leading to excessive calpain activation and oxidative stress-induced apoptosis. Tenuifolin inhibits neuronal apoptosis by modulating the calpain system (Li et al., 2023). Furthermore, tenuifolin protects against Aβ25-35-induced apoptosis and mitochondrial membrane potential loss in hippocampal neurons, downregulates the expression of caspase-3 and caspase-9, thereby reversing spatial learning and memory deficits in AD mice (Wang et al., 2019a).
4.8 Regulating cellular autophagy function
Cellular autophagy is a metabolic regulatory process that recycles and degrades damaged organelles, proteins, and other biomolecules, thereby supporting the growth and development of organisms (Wang et al., 2024). Abnormal regulation of autophagy is commonly observed in dementia patients. In the early stages of AD, there is evident impairment of autophagy, characterized by the earlier aggregation of autophagosomes than Aβ protein deposition, and their failure to be timely degraded by lysosomes (Zhang et al., 2021). As AD progresses, autophagy function significantly weakens, exemplified by the decreased expression of Beclin-1, which is crucial for the formation of autophagic precursors (Bieri et al., 2018). The phosphorylation of intracellular phosphatidylinositol-3-hydroxykinase (PI3K) produces phosphatidylinositol-3,4,5-triphosphate (PIP3), which accumulates on the cell membrane and activates downstream pathways by recruiting and binding proteins such as protein kinase B (Akt) and insulin receptor substrate (IRS) 1 (Zang et al., 2023). A key regulator of autophagy, the mammalian target of rapamycin (mTOR), when activated by Akt, suppresses autophagy by inhibiting the Unc-51 Like Autophagy Activating Kinase 1 (ULK1) complex, preventing the formation of autophagosomes (Kim et al., 2011). In summary, insufficient autophagy flux in the brain may lead to mitochondrial damage and Aβ protein deposition, ultimately impairing cognitive functions.
Aβ1-42 can activate the PI3K/Akt signaling pathway in neuronal cells, inhibit autophagy, and subsequently lead to neuronal cell damage. Tenuigenin treatment results in a dose-dependent downregulation of p-PI3K and p-Akt (Ren et al., 2022). Microtubule-associated protein 1A/1B-light chain 3 (LC3)-I is converted to LC3-II through lipid modification. LC3-II is a component of autophagosomes and correlates directly with autophagy flux (Wan et al., 2023). Tenuigenin enhances the expression of LC3-I/LC3-II. Additionally, mitochondrial autophagy is essential for cell survival as it maintains mitochondrial quality by clearing damaged or dysfunctional mitochondria. Under stress conditions, PTEN-induced putative kinase 1 (PINK1) phosphorylates the E3 ubiquitin ligase Parkin, promoting its localization on mitochondrial membranes. This ubiquitination degrades certain mitochondrial proteins and recruits LC3 proteins, which facilitates mitochondrial fusion and the formation of autophagosomes, thereby initiating the autophagy pathway (Eiyama and Okamoto, 2015). Research indicates that tenuigenin upregulates neuronal expression of PINK1 and Parkin, enhances Parkin’s mitochondrial localization, activates autophagy, and increases the rate of autophagic degradation of damaged mitochondria (Tian et al., 2022). Onjisaponin B enhances neuronal autophagy by upregulating the expression of LC3-II (Wu et al., 2015). Tenuifolin improves autophagy levels in neuronal cells induced by Aβ25-35 by increasing protein levels of Beclin-1 and LC3-II/I, activating AMP-activated protein kinase (AMPK) and ULK1 expression, and inhibiting mTOR activity to boost autophagy flux. Interestingly, this study also found that Aβ protein itself increases the expression of Beclin-1 and LC3-II/I, possibly reflecting the stress response of neuronal cells in early AD (Wang et al., 2019b).
4.9 Maintain iron steady state
Under physiological conditions, central nervous system cells require iron as a cofactor for redox reactions to generate energy. However, oxidative damage can occur when free iron levels increase or antioxidant systems are compromised, ultimately leading to cell ferroptosis (Deng et al., 2023). Elevated iron content has been observed in the brains of patients with several degenerative diseases. Lipid peroxidation acts as a trigger for ferroptosis in cells. Free polyunsaturated fatty acids undergo autooxidation to form L-OOH, and the epoxy, oxo-, or aldehyde groups produced upon L-OOH cleavage are highly toxic oxidative products (Yan et al., 2021). In this context, acyl-CoA synthetase long-chain family member 4 (ACSL4) serves as a critical checkpoint in ferroptosis, facilitating the disease process by catalyzing the formation of L-OOH from arachidonic acid (Tuo et al., 2022). In combating lipid oxidation products, glutathione peroxidase 4 (GPX4) plays a crucial role by detoxifying L-OOH in the membrane, converting toxic L-OOH into non-toxic lipid alcohols, thus significantly mitigating ferroptosis (Reichert et al., 2020).
Some residues of Aβ protein have the ability to bind iron, which upon binding promotes Aβ aggregation and induces neuronal cell death (Wang et al., 2023a). Research has demonstrated that tenuigenin effectively inhibits iron-induced ferroptosis in Aβ25-35 stimulated PC12 neurons, mitigating oxidative damage and reversing mitochondrial depolarization. Mechanistically, treatment with tenuigenin reduces the expression of ACSL4 and PEBP1 proteins while elevating GPX4 expression, thereby preventing L-OOH-induced neuronal damage (Zhang et al., 2022). Solute carrier family 7 member 11 (SLC7A11) can be induced under various stress conditions to uptake cysteine, leading to cysteine production and subsequent glutathione synthesis, which supports GPX4 in detoxifying L-OOH (Koppula et al., 2021). The co-administration of d-galactose with Aβ1-42 initiates the ferroptosis pathway in HT22 neuronal cells, significantly reducing the expression of L-OOH-associated proteins GPX4 and SLC7A11 and increasing ACSL4 expression. Treatment with tenuifolin reverses these protein expression anomalies and inhibits the ferroptosis process in HT22 cells (Li et al., 2023).
5 Toxic effects
PT exhibits inhibitory effects on gastrointestinal peristalsis, potentially leading to intestinal bloating or necrosis (Wen et al., 2015). Further research has demonstrated that onjisaponin B and tenuifolin can act as sub-hypnotic agents, extending the duration of pentobarbital-induced sleep in mice. Additionally, onjisaponin B stimulates gastrointestinal tension in rabbits, inducing irregular contractions of the isolated intestine at an 80 mg/L dosage. Notably, tenuigenin, onjisaponin B, and tenuifolin have all been shown to reduce the expression of prostaglandin E2 (PGE2) in mouse stomachs. PGE2 serves as a gastric mucosal protector, and its reduced expression can compromise this protective effect, leading to gastric damage (Wen et al., 2015). Moreover, polygalasaponins exhibit a synergistic effect with pentobarbital sodium, lengthening sleep duration and decreasing sleep latency (Yao et al., 2010). Regarding acute toxicity, polygalasaponins have a 0% lethal dose of 2.6 g/kg and a median lethal dose of 3.95 g/kg (Yao et al., 2010). In conclusion, the available evidence indicates that PT and its saponin extracts pose certain gastrointestinal toxicities. However, reports on acute, subacute, or long-term toxicity are lacking, making toxicity studies essential for further clinical research. Additionally, since PT saponin components are frequently used in the treatment and research of chronic diseases requiring prolonged medication, defining their safe usage range is crucial.
6 Clinical experimental research
According to current reports, there have been no independent clinical studies on PT saponin components. Memantine, an NMDA receptor antagonist, is utilized in treating moderate to severe AD patients (Tang et al., 2023). Research involving 152 AD patients has shown that a 12-week treatment with the combination of tenuigenin (10 mg/d), β-asarone (10 mg/d), and memantine significantly enhances the average Mini-Mental State Examination (MMSE) scores, markedly reduces the Clinical Dementia Rating Scale (CDR) and Activities of Daily Living (ADL) scores, and improves cognitive functions. This combination produces adverse reactions similar to those observed with memantine alone, including mild, transient hallucinations, headaches, nausea, and drowsiness. Additionally, a subgroup analysis identified male patients aged 60–74 with moderate AD as the most beneficial recipients of this treatment regimen (Chang and Teng, 2018). Another clinical study applied the same treatment protocol to 93 AD patients, with increased dosages of tenuigenin (20 mg/d) and β-asarone (20 mg/d) over a 12-week period, resulting in consistent outcomes regarding MMSE, CDR, ADL scores, and side effects (Dong et al., 2018). In conclusion, clinical trials suggest that tenuigenin may have therapeutic effects on AD. However, due to the complexity of the combination therapy, the independent pharmacological impact of tenuigenin requires further validation. Notably, the combination therapy increased symptoms such as drowsiness and nausea compared to using memantine alone (Chang and Teng, 2018), aligning with reports of tenuigenin’s toxicity. Therefore, the possibility that these adverse effects are attributable to tenuigenin cannot be excluded. Future research should intensify preclinical toxicity studies on PT saponin components.
7 Discussion
Dementia, as a neurodegenerative disease, exhibits a high incidence rate and a prolonged disease course, imposing substantial psychological and economic burdens on patients and their families (Ayhan et al., 2023). Despite numerous pathogenic hypotheses proposed for its various subtypes, effective medications for treating this condition remain undeveloped, and the pathogenic mechanisms and therapeutic approaches require further exploration. This article provides a comprehensive analysis of the pharmacological effects of the main saponin components of PT in the treatment of dementia, particularly in addressing AD and PDD. The therapeutic potential of PT’s saponins is demonstrated through various mechanisms, including anti-inflammatory, antioxidant, anti-apoptotic, anti-amyloid protein deposition, and neurotransmitter regulation activities. Notably, tenuigenin, onjisaponin B, and tenuifolin exhibit significant neuroprotective effects, contributing to enhanced cognitive function and neuroprotection.
The neuroprotective mechanisms of PT’s saponin components are diverse. Tenuigenin and tenuifolin have been shown to reduce Aβ accumulation, which is central to the pathogenesis of AD. By inhibiting β-secretase activity and modulating γ-secretase, these saponins reduce Aβ production and enhance its clearance through the regulation of microglial activity and autophagy pathways. Additionally, PT’s saponin components mitigate oxidative stress by enhancing the expression of SOD and CAT and by lowering MDA and ROS levels. In terms of neurotransmitter regulation and synaptic plasticity, several saponins from PT enhance cholinergic function by inhibiting AChE activity and increasing ACh levels, thereby improving synaptic plasticity and cognitive function. Moreover, these saponins upregulate the expression of synaptic proteins such as Syn and BDNF, which are crucial for maintaining synaptic integrity and promoting neurogenesis.
However, despite the promising pharmacological effects of PT’s saponin components, their bioavailability remains a significant challenge. Saponin compounds naturally possess characteristics such as high molecular weight, poor membrane permeability, and low oral bioavailability. For instance, tenuigenin and tenuifolin exhibit low oral bioavailability and rapid clearance from the body, necessitating the development of novel delivery systems to enhance their therapeutic efficacy. Clinically, a trial combining tenuigenin with memantine has shown potential benefits in improving cognitive function in AD patients. However, observed side effects, such as gastrointestinal discomfort and sedation, are unfavorable for long-term treatment regimens aimed at slowing disease progression. Therefore, comprehensive toxicological evaluations are required to ensure the safety of long-term use.
Future research should focus on enhancing the bioavailability of PT’s saponin compounds through advanced drug delivery systems such as nanoparticles or liposomes (Kumar and Nair, 2024). Additionally, extensive preclinical toxicity tests and clinical trials are needed to confirm the efficacy and safety of PT’s saponin compounds, whether used as monotherapy or in combination with existing dementia treatments.
8 Conclusion
In summary, PT’s saponin compounds show considerable promise in treating dementia through various neuroprotective mechanisms. However, challenges such as low bioavailability and potential side effects must be addressed through further research and clinical validation. The combination of PT’s saponin compounds with existing treatment regimens may offer a novel approach to slowing the progression of neurodegenerative diseases.
Author contributions
SL: Conceptualization, Data curation, Formal Analysis, Investigation, Methodology, Project administration, Resources, Software, Supervision, Validation, Visualization, Writing–original draft, Writing–review and editing. ZH: Data curation, Formal Analysis, Methodology, Project administration, Software, Supervision, Validation, Writing–review and editing. TY: Data curation, Formal Analysis, Methodology, Project administration, Supervision, Visualization, Writing–review and editing. XS: Data curation, Formal Analysis, Methodology, Supervision, Writing–review and editing. XH: Data curation, Formal Analysis, Methodology, Project administration, Supervision, Writing–review and editing. JC: Conceptualization, Data curation, Formal Analysis, Funding acquisition, Investigation, Methodology, Project administration, Resources, Software, Supervision, Validation, Visualization, Writing–review and editing.
Funding
The author(s) declare that financial support was received for the research, authorship, and/or publication of this article. This study was supported by the National Nature Science Foundation of China (Grant No. 82274395).
Acknowledgments
We acknowledge the invaluable support of the National Nature Science Foundation.
Conflict of interest
The authors declare that the research was conducted in the absence of any commercial or financial relationships that could be construed as a potential conflict of interest.
Publisher’s note
All claims expressed in this article are solely those of the authors and do not necessarily represent those of their affiliated organizations, or those of the publisher, the editors and the reviewers. Any product that may be evaluated in this article, or claim that may be made by its manufacturer, is not guaranteed or endorsed by the publisher.
References
Alzheimer’s disease facts and figures (2023). Alzheimer’s disease facts and figures. Alzheimer’s and Dementia 19, 1598–1695. doi:10.1002/alz.13016
Aarsland, D. (2020). Epidemiology and pathophysiology of dementia-related psychosis. J. Clin. Psychiatry 81, 27625. doi:10.4088/JCP.AD19038BR1C
Ahmad, M. A., Kareem, O., Khushtar, M., Akbar, M., Haque, M. R., Iqubal, A., et al. (2022). Neuroinflammation: A potential risk for dementia. International Journal of Molecular Sciences 23, 616. doi:10.3390/ijms23020616
Allard, S., and Hussain Shuler, M. G. (2023). Cholinergic reinforcement signaling is impaired by amyloidosis prior to its synaptic loss. J. Neurosci. 43, 6988–7005. doi:10.1523/JNEUROSCI.0967-23.2023
Andersson, M. J., and Stone, J. (2023). Best medicine for dementia: The life-long defense of the brain. J. Alzheimer’s dis. JAD 94, 51–66. doi:10.3233/JAD-230429
Andreotti, J. P., Silva, W. N., Costa, A. C., Picoli, C. C., Bitencourt, F. C. O., Coimbra-Campos, L. M. C., et al. (2019). Neural stem cell niche heterogeneity. Semin. Cell Dev. Biol. 95, 42–53. doi:10.1016/j.semcdb.2019.01.005
Arruda, E. H., and Paun, O. (2017). Dementia caregiver grief and bereavement: An integrative review. West. J. Nurs. Res. 39, 825–851. doi:10.1177/0193945916658881
Atiq, A., Lee, H. J., Khan, A., Kang, M. H., Rehman, I. U., Ahmad, R., et al. (2023). Vitamin E analog Trolox attenuates MPTP-induced parkinson’s disease in mice, mitigating oxidative stress, neuroinflammation, and motor impairment. International Journal of Molecular Sciences 24, 9942. doi:10.3390/ijms24129942
Ayhan, Y., Yoseph, S. A., and Miller, B. L. (2023). Management of psychiatric symptoms in dementia. Neurol. Clin. 41, 123–139. doi:10.1016/j.ncl.2022.05.001
Ba, Y., Wang, M., Zhang, K., Chen, Q., Wang, J., Lv, H., et al. (2019). Intestinal absorption profile of three polygala oligosaccharide esters in polygalae radix and the effects of other components in polygalae radix on their absorption. Evidence-Based Complementary and Alternative Medicine 2019, 1379531. doi:10.1155/2019/1379531
Bergamino, M., Schiavi, S., Daducci, A., Walsh, R. R., and Stokes, A. M. (2022). Analysis of brain structural connectivity networks and white matter integrity in patients with mild cognitive impairment. Front. Aging Neurosci. 14, 793991. doi:10.3389/fnagi.2022.793991
Bieri, G., Lucin, K. M., O’Brien, C. E., Zhang, H., Villeda, S. A., and Wyss-Coray, T. (2018). Proteolytic cleavage of beclin 1 exacerbates neurodegeneration. Mol. Neurodegener. 13, 68. doi:10.1186/s13024-018-0302-4
Cai, W., Wu, T., and Chen, N. (2023). The amyloid-beta clearance: From molecular targets to glial and neural cells. Biomolecules 13, 313. doi:10.3390/biom13020313
Cai, Z., Qiao, P.-F., Wan, C.-Q., Cai, M., Zhou, N.-K., and Li, Q. (2018). Role of blood-brain barrier in alzheimer’s disease. Journal of Alzheimer’s disease 63, 1223–1234. doi:10.3233/JAD-180098
Cai, Z.-L., Wang, C.-Y., Gu, X.-Y., Wang, N.-J., Wang, J.-J., Liu, W.-X., et al. (2013). Tenuigenin ameliorates learning and memory impairments induced by ovariectomy. Physiol. Behav. 118, 112–117. doi:10.1016/j.physbeh.2013.05.025
Canudas, A. M., Gutierrez-Cuesta, J., Rodríguez, M. I., Acuña-Castroviejo, D., Sureda, F. X., Camins, A., et al. (2005). Hyperphosphorylation of microtubule-associated protein tau in senescence-accelerated mouse (SAM). Mech. Ageing Dev 126, 1300–1304. doi:10.1016/j.mad.2005.07.008
Capone, R., Tiwari, A., Hadziselimovic, A., Peskova, Y., Hutchison, J. M., Sanders, C. R., et al. (2021). The C99 domain of the amyloid precursor protein resides in the disordered membrane phase. J. Biol. Chem. 296, 100652. doi:10.1016/j.jbc.2021.100652
Chang, W., and Teng, J. (2018). Combined application of tenuigenin and β-asarone improved the efficacy of memantine in treating moderate-to-severe Alzheimer's disease. Drug Design Development and Therapy 12, 455–462. doi:10.2147/DDDT.S155567
Che, P., Zhang, J., Yu, M., Tang, P., Wang, Y., Lin, A., et al. (2023). Dl-3-n-butylphthalide promotes synaptic plasticity by activating the akt/ERK signaling pathway and reduces the blood-brain barrier leakage by inhibiting the HIF-1α/MMP signaling pathway in vascular dementia model mice. CNS Neurosci. Ther. 29, 1392–1404. doi:10.1111/cns.14112
Chen, J., Zhang, M., Bai, H., Shi, P., Du, M., Zhang, S., et al. (2022). Overexpression of C9orf72 exacerbates Aβ25-35-induced oxidative stress and apoptosis in PC12 cells. Acta Neurobiol. Exp. (warsz.) 82, 77–87. doi:10.55782/ane-2022-007
Chen, M., Wang, J., and Ming, C. (2012a). Buyuan congnao decoction decreases hippocampal beta-amyloid expression in a rat model of alzheimer’s disease. Neural Regen. Res. 7, 664–668. doi:10.3969/j.issn.1673-5374.2012.09.004
Chen, Q., Jia, T., Wu, X., Chen, X., Wang, J., and Ba, Y. (2023). Polygalae radix oligosaccharide esters may relieve depressive-like behavior in rats with chronic unpredictable mild stress via modulation of gut microbiota. International Journal of Molecular Sciences 24, 13877. doi:10.3390/ijms241813877
Chen, S., and Jia, J. (2020). Tenuifolin Attenuates Amyloid-β42-Induced Neuroinflammation in Microglia Through the NF-κB Signaling Pathway. J. Alzheimer’s dis. JAD 76, 195–205. doi:10.3233/JAD-200077
Chen, Y., Huang, X., Chen, W., Wang, N., and Li, L. (2012b). Tenuigenin promotes proliferation and differentiation of hippocampal neural stem cells. Neurochem. Res. 37, 771–777. doi:10.1007/s11064-011-0671-3
Chen, Y.-J., Huang, X.-B., Li, Z.-X., Yin, L.-L., Chen, W.-Q., and Li, L. (2010). Tenuigenin protects cultured hippocampal neurons against methylglyoxal-induced neurotoxicity. Eur. J. Pharmacol. 645, 1–8. doi:10.1016/j.ejphar.2010.06.034
Choi, J. G., Kim, H. G., Kim, M. C., Yang, W. M., Huh, Y., Kim, S. Y., et al. (2011). Polygalae radix inhibits toxin-induced neuronal death in the parkinson’s disease models. J. Ethnopharmacol. 134, 414–421. doi:10.1016/j.jep.2010.12.030
Choquet, D., and Triller, A. (2013). The dynamic synapse. Neuron 80, 691–703. doi:10.1016/j.neuron.2013.10.013
Cornacchia, C., Marinelli, L., Di Rienzo, A., Dimmito, M. P., Serra, F., Di Biase, G., et al. (2022). Development of l-dopa-containing diketopiperazines as blood-brain barrier shuttle. Eur. J. Med. Chem. 243, 114746. doi:10.1016/j.ejmech.2022.114746
Custodero, C., Ciavarella, A., Panza, F., Gnocchi, D., Lenato, G. M., Lee, J., et al. (2022). Role of inflammatory markers in the diagnosis of vascular contributions to cognitive impairment and dementia: A systematic review and meta-analysis. GeroScience 44, 1373–1392. doi:10.1007/s11357-022-00556-w
Darweesh, S. K. L., Wolters, F. J., Ikram, M. A., de Wolf, F., Bos, D., and Hofman, A. (2018). Inflammatory markers and the risk of dementia and alzheimer’s disease: A meta-analysis. Alzheimer’s and Dementia 14, 1450–1459. doi:10.1016/j.jalz.2018.02.014
Deng, L., Mo, M.-Q., Zhong, J., Li, Z., Li, G., and Liang, Y. (2023). Iron overload induces islet β cell ferroptosis by activating ASK1/P-P38/CHOP signaling pathway. Peerj 11, e15206. doi:10.7717/peerj.15206
Devkota, S., Zhou, R., Nagarajan, V., Maesako, M., Do, H., Noorani, A., et al. (2024). Familial alzheimer mutations stabilize synaptotoxic γ-secretase-substrate complexes. Cell Rep 43, 113761. doi:10.1016/j.celrep.2024.113761
Dong, H., Wu, S., Hu, N., and Xing, G. (2018). Efficacy of tenuigenin and β-asarone as augmentations for memantine in the treatment of Alzheimer's disease. Neuroreport 29, 203–207. doi:10.1097/WNR.0000000000000952
Duong, S., Patel, T., and Chang, F. (2017). Dementia: what pharmacists need to know. Can. pharm. j. CPJ = Rev. pharm. du Can. RPC 150, 118–129. doi:10.1177/1715163517690745
Eiyama, A., and Okamoto, K. (2015). PINK1/parkin-mediated mitophagy in mammalian cells. Curr. Opin. Cell Biol. 33, 95–101. doi:10.1016/j.ceb.2015.01.002
Elmore, S. (2007). Apoptosis: A review of programmed cell death. Toxicol. Pathol. 35, 495–516. doi:10.1080/01926230701320337
Fan, Z., Liang, Z., Yang, H., Pan, Y., Zheng, Y., and Wang, X. (2017). Tenuigenin protects dopaminergic neurons from inflammation via suppressing NLRP3 inflammasome activation in microglia. J. Neuroinflamm. 14, 256. doi:10.1186/s12974-017-1036-x
Franco-Bocanegra, D. K., George, B., Lau, L. C., Holmes, C., Nicoll, J. A. R., and Boche, D. (2019). Microglial motility in Alzheimer's disease and after Aβ42 immunotherapy: a human post-mortem study. Acta Neuropathologica Communications 7, 174. doi:10.1186/s40478-019-0828-x
Fu, H., Xu, Z., Zhang, X., and Zheng, G.-Q. (2019). Kaixinsan, a well-known Chinese herbal prescription, for alzheimer’s disease and depression: A preclinical systematic review. Front. Neurosci. 13, 1421. doi:10.3389/fnins.2019.01421
Galasko, D. (2013). The diagnostic evaluation of a patient with dementia. Continuum : Lifelong Learning in Neurology 19, 397–410. doi:10.1212/01.CON.0000429176.37224.58
Güçlü-Ustündağ, O., and Mazza, G. (2007). Saponins: Properties, applications and processing. Crit. Rev. Food Sci. Nutr. 47, 231–258. doi:10.1080/10408390600698197
Gustavsson, A., Norton, N., Fast, T., Frölich, L., Georges, J., Holzapfel, D., et al. (2023). Global estimates on the number of persons across the alzheimer’s disease continuum. Alzheimer’s and Dementia 19, 658–670. doi:10.1002/alz.12694
Hainsworth, A. H., Markus, H. S., and Schneider, J. A. (2024). Cerebral small vessel disease, hypertension, and vascular contributions to cognitive impairment and dementia. Hypertension 81, 75–86. doi:10.1161/HYPERTENSIONAHA.123.19943
Hampel, H., Mesulam, M.-M., Cuello, A. C., Farlow, M. R., Giacobini, E., Grossberg, G. T., et al. (2018). The cholinergic system in the pathophysiology and treatment of alzheimer’s disease. Brain: J. Neurol. 141, 1917–1933. doi:10.1093/brain/awy132
Han, G., Choi, J., Cha, S.-Y., Kim, B. I., Kho, H. K., Jang, M.-J., et al. (2021). Effects of radix polygalae on cognitive decline and depression in estradiol depletion mouse model of menopause. Curr. Issues Mol. Biol. 43, 1669–1684. doi:10.3390/cimb43030118
Haraguchi, A., Saito, K., Tahara, Y., and Shibata, S. (2022). Polygalae radix shortens the circadian period through activation of the CaMKII pathway. Pharm. Biol. 60, 689–698. doi:10.1080/13880209.2022.2048863
Helli, B., Navabi, S. P., Hosseini, S. A., Sabahi, A., Khorsandi, L., Amirrajab, N., et al. (2024). The protective effects of syringic acid on bisphenol a-induced neurotoxicity possibly through AMPK/PGC-1α/Fndc5 and CREB/BDNF signaling pathways. Mol. Neurobiol. doi:10.1007/s12035-024-04048-0
Hou, Z., Li, F., Chen, J., Liu, Y., He, C., Wang, M., et al. (2019). Beneficial effects of sagacious confucius’ pillow elixir on cognitive function in senescence-accelerated P8 mice (SAMP8) via the NLRP3/caspase-1 pathway. Evidence-Based Complementary and Alternative Medicine 2019, 3097923. doi:10.1155/2019/3097923
Huang, J., Wang, C., Wang, X., Wu, B., Gu, X., Liu, W., et al. (2013). Tenuigenin treatment improves behavioral Y-maze learning by enhancing synaptic plasticity in mice. Behav. Brain Res. 246, 111–115. doi:10.1016/j.bbr.2013.03.001
Huang, X.-B., Chen, Y.-J., Chen, W.-Q., Wang, N.-Q., Wu, X.-L., and Liu, Y. (2018). Neuroprotective effects of tenuigenin on neurobehavior, oxidative stress, and tau hyperphosphorylation induced by intracerebroventricular streptozotocin in rats. Brain Circulation 4, 24–32. doi:10.4103/bc.bc_2_17
Jesky, R., and Chen, H. (2016). The neuritogenic and neuroprotective potential of senegenin against Aβ-induced neurotoxicity in PC 12 cells. BMC Complement. Altern. Med. 16, 26. doi:10.1186/s12906-016-1006-3
Jia, H., Jiang, Y., Ruan, Y., Zhang, Y., Ma, X., Zhang, J., et al. (2004). Tenuigenin treatment decreases secretion of the alzheimer’s disease amyloid beta-protein in cultured cells. Neurosci. Lett. 367, 123–128. doi:10.1016/j.neulet.2004.05.093
Jiang, N., Wei, S., Zhang, Y., He, W., Pei, H., Huang, H., et al. (2021). Protective effects and mechanism of radix polygalae against neurological diseases as well as effective substance. Front. Psychiatry 12, 688703. doi:10.3389/fpsyt.2021.688703
Jiang, N., Zhang, Y., Yao, C., Liu, Y., Chen, Y., Chen, F., et al. (2023a). Tenuifolin ameliorates the sleep deprivation-induced cognitive deficits. Phytotherapy research: PTR 37, 464–476. doi:10.1002/ptr.7627
Jiang, Q., Zhu, Z., and Mao, X. (2023b). Ubiquitination is a major modulator for the activation of inflammasomes and pyroptosis. Biochimica Et Biophysica Acta. Gene Regulatory Mechanisms 1866, 194955. doi:10.1016/j.bbagrm.2023.194955
Kanan, M. F., Sheehan, P. W., Haines, J. N., Gomez, P. G., Dhuler, A., Nadarajah, C. J., et al. (2024). Neuronal deletion of the circadian clock gene Bmal1 induces cell-autonomous dopaminergic neurodegeneration. JCI insight 9, e162771. doi:10.1172/jci.insight.162771
Kaneko, A., Cho, S., Hirai, K., Okabe, T., Iwasaki, K., Nanba, Y., et al. (2005). Hange-koboku-to, a kampo medicine, modulates cerebral levels of 5-HT (5-hydroxytryptamine), NA (noradrenaline) and DA (dopamine) in mice. Phytother. res. PTR 19, 491–495. doi:10.1002/ptr.1669
Kim, J., Kundu, M., Viollet, B., and Guan, K.-L. (2011). AMPK and mTOR regulate autophagy through direct phosphorylation of Ulk1. Nat. Cell Biol. 13, 132–141. doi:10.1038/ncb2152
Koenig, M. L., and Meyerhoff, J. L. (2003). In vitro neuroprotection against oxidative stress by pre-treatment with a combination of dihydrolipoic acid and phenyl-butyl nitrones. Neurotox. Res. 5, 265–272. doi:10.1007/BF03033384
Koganezawa, N., Sekino, Y., Kawakami, H., Fuchino, H., Kawahara, N., and Shirao, T. (2021). NMDA receptor-dependent and -independent effects of natural compounds and crude drugs on synaptic states as revealed by drebrin imaging analysis. Eur. J. Neurosci. 53, 3548–3560. doi:10.1111/ejn.15231
Kong, H., Han, Y.-Y., Yang, G.-L., Li, K., Yu, L., Xie, X.-K., et al. (2024). Tenuifolin improves learning and memory by regulating long-term potentiation and dendritic structure of hippocampal CA1 area in healthy female mice but not male mice. Behav. Brain Res. 466, 114974. doi:10.1016/j.bbr.2024.114974
Koppula, P., Zhuang, L., and Gan, B. (2021). Cystine transporter SLC7A11/xCT in cancer: Ferroptosis, nutrient dependency, and cancer therapy. Protein and Cell 12, 599–620. doi:10.1007/s13238-020-00789-5
Kumar, V., and Nair, S. C. (2024). Nano lipid carriers as a promising drug delivery carrier for neurodegenerative disorders - an overview of recent advances. Recent Pat. Biotechnol 18, 2–21. doi:10.2174/1872208317666230320164219
Lazarova, M., Tsvetanova, E., Georgieva, A., Stefanova, M., Uzunova, D., Denev, P., et al. (2024). Extracts of sideritis scardica and clinopodium vulgare alleviate cognitive impairments in scopolamine-induced rat dementia. Int. J. Mol. Sci. 25, 1840. doi:10.3390/ijms25031840
Lee, H. J., Ban, J. Y., Koh, S. B., Seong, N. S., Song, K. S., Bae, K. W., et al. (2004). Polygalae radix extract protects cultured rat granule cells against damage induced by NMDA. Am. J. Chin. Med. 32, 599–610. doi:10.1142/S0192415X04002235
Li, C., Gao, F., Qu, Y., Zhao, P., Wang, X., and Zhu, G. (2023). Tenuifolin in the prevention of alzheimer’s disease-like phenotypes: investigation of the mechanisms from the perspectives of calpain system, ferroptosis, and apoptosis. Phytother. res. PTR, 4621–4638. doi:10.1002/ptr.7930
Li, G., Yu, J., Zhang, L., Wang, Y., Wang, C., and Chen, Q. (2018). Onjisaponin B prevents cognitive impairment in a rat model of D-galactose-induced aging. Biomed. Pharmacother. 99, 113–120. doi:10.1016/j.biopha.2018.01.006
Li, H., Lin, S., Qin, T., Li, H., Ma, Z., and Ma, S. (2017). Senegenin exerts anti-depression effect in mice induced by chronic un-predictable mild stress via inhibition of NF-κB regulating NLRP3 signal pathway. Int. Immunopharmacol. 53, 24–32. doi:10.1016/j.intimp.2017.10.001
Li, N., Qiu, Y., Wang, H., Zhao, J., and Qing, H. (2022). PS1 affects the pathology of alzheimer’s disease by regulating BACE1 distribution in the ER and BACE1 maturation in the golgi apparatus. International Journal of Molecular Sciences 23, 16151. doi:10.3390/ijms232416151
Li, X., Cui, J., Yu, Y., Li, W., Hou, Y., Wang, X., et al. (2016). Traditional Chinese Nootropic Medicine Radix Polygalae and Its Active Constituent Onjisaponin B Reduce β-Amyloid Production and Improve Cognitive Impairments. PLoS One 11, e0151147. doi:10.1371/journal.pone.0151147
Li, X., Zhao, Y., Liu, P., Zhu, X., Chen, M., Wang, H., et al. (2015). Senegenin inhibits hypoxia/reoxygenation-induced neuronal apoptosis by upregulating RhoGDIα. Mol. Neurobiol. 52, 1561–1571. doi:10.1007/s12035-014-8948-6
Liang, S., Zhou, J., Yu, X., Lu, S., and Liu, R. (2024). Neuronal conversion from glia to replenish the lost neurons. Neural Regen. Res. 19, 1446–1453. doi:10.4103/1673-5374.386400
Lin, J., Wang, S., Feng, Y., Zhao, W., Zhao, W., Luo, F., et al. (2018). Propofol exposure during early gestation impairs learning and memory in rat offspring by inhibiting the acetylation of histone. J. Cell. Mol. Med. 22, 2600–2611. doi:10.1111/jcmm.13524
Liu, L., Feng, W.-H., Liu, X.-Q., Liang, Y.-H., Li, C., and Wang, Z.-M. (2021). research progress on polygalae radix. Zhongguo Zhong Yao Za Zhi 46, 5744–5759. doi:10.19540/j.cnki.cjcmm.20210518.601
Liu, Y., Li, Z., Hu, H., Xu, S., Chang, Q., Liao, Y., et al. (2015). Tenuifolin, a secondary saponin from hydrolysates of polygalasaponins, counteracts the neurotoxicity induced by Aβ25-35 peptides in vitro and in vivo. Pharmacology, Biochemistry, and Behavior 128, 14–22. doi:10.1016/j.pbb.2014.11.010
Lu, L., Li, X., Xu, P., Zheng, Y., and Wang, X. (2017). Tenuigenin down-regulates the release of nitric oxide, matrix metalloproteinase-9 and cytokines from lipopolysaccharide-stimulated microglia. Neurosci. Lett. 650, 82–88. doi:10.1016/j.neulet.2017.04.001
Luo, R., Su, L.-Y., Li, G., Yang, J., Liu, Q., Yang, L.-X., et al. (2020). Activation of PPARA-mediated autophagy reduces alzheimer disease-like pathology and cognitive decline in a murine model. Autophagy 16, 52–69. doi:10.1080/15548627.2019.1596488
Luo, Y., Hu, B., Ji, H., Jing, Y., Dang, X., Zhang, H., et al. (2024). Comprehensive evaluation of chemical constituents and antioxidant activity between crude and processed polygalae radix based on UPLC-Q-TOF-MS/MS combined with multivariate statistical analysis. Heliyon 10, e27622. doi:10.1016/j.heliyon.2024.e27622
Lv, J., Jia, H., Jiang, Y., Ruan, Y., Liu, Z., Yue, W., et al. (2009). Tenuifolin, an extract derived from tenuigenin, inhibits amyloid-beta secretion in vitro. Acta Physiol. (Oxf. Engl.) 196, 419–425. doi:10.1111/j.1748-1716.2009.01961.x
Lyon, M., Fullerton, J. L., Kennedy, S., and Work, L. M. (2024). Hypertension and dementia: Pathophysiology and potential utility of antihypertensives in reducing disease burden. Pharmacol. Ther. 253, 108575. doi:10.1016/j.pharmthera.2023.108575
Ma, B., Li, X., Li, J., Zhang, Q., Liu, Y., Yang, X., et al. (2014). Quantitative analysis of tenuifolin concentrations in rat plasma and tissue using LC⬜MS/MS: Application to pharmacokinetic and tissue distribution study. J. Pharm. Biomed. Anal. 88, 191–200. doi:10.1016/j.jpba.2013.07.012
Maurya, R., Bhattacharjee, G., Khambhati, K., Gohil, N., Singh, P., Mani, I., et al. (2023). Amyloid precursor protein in alzheimer’s disease. Prog. Mol. Biol. Transl. Sci. 196, 261–270. doi:10.1016/bs.pmbts.2022.09.006
McAlpine, C. S., Park, J., Griciuc, A., Kim, E., Choi, S. H., Iwamoto, Y., et al. (2021). Astrocytic interleukin-3 programs microglia and limits alzheimer’s disease. Nature 595, 701–706. doi:10.1038/s41586-021-03734-6
McLarnon, J. G. (2023). Glial-derived neuroinflammation induced with amyloid-beta-peptide plus fibrinogen injection in rat hippocampus. Curr. Alzheimer Res. 20, 515–522. doi:10.2174/1567205020666230912113501
Meng, H. W., Kim, J.-H., Kim, H. Y., Lee, A. Y., and Cho, E. J. (2023a). Paeoniflorin attenuates lipopolysaccharide-induced cognitive dysfunction by inhibition of amyloidogenesis in mice. International Journal of Molecular Sciences 24, 4838. doi:10.3390/ijms24054838
Meng, H., Zhou, J., Wang, M., Zheng, M., Xing, Y., and Wang, Y. (2023b). SARS-CoV-2 papain-like protease negatively regulates the NLRP3 inflammasome pathway and pyroptosis by reducing the oligomerization and ubiquitination of ASC. Microorganisms 11, 2799. doi:10.3390/microorganisms11112799
Moya-Alvarado, G., Tiburcio-Felix, R., Ibáñez, M. R., Aguirre-Soto, A. A., Guerra, M. V., Wu, C., et al. (2023). BDNF/TrkB signaling endosomes in axons coordinate CREB/mTOR activation and protein synthesis in the cell body to induce dendritic growth in cortical neurons. eLife 12, e77455. doi:10.7554/eLife.77455
O’Donnell, J., Zeppenfeld, D., McConnell, E., Pena, S., and Nedergaard, M. (2012). Norepinephrine: a neuromodulator that boosts the function of multiple cell types to optimize CNS performance. Neurochem. Res. 37, 2496–2512. doi:10.1007/s11064-012-0818-x
Palma, F. R., Gantner, B. N., Sakiyama, M. J., Kayzuka, C., Shukla, S., Lacchini, R., et al. (2024). ROS production by mitochondria: Function or dysfunction? Oncogene 43, 295–303. doi:10.1038/s41388-023-02907-z
Paoletti, P., Bellone, C., and Zhou, Q. (2013). NMDA receptor subunit diversity: impact on receptor properties, synaptic plasticity and disease. Nat. Rev. Neurosci. 14, 383–400. doi:10.1038/nrn3504
Park, H.-J., Lee, K., Heo, H., Lee, M., Kim, J. W., Whang, W. W., et al. (2008). Effects of polygala tenuifolia root extract on proliferation of neural stem cells in the hippocampal CA1 region. Phytotherapy Research 22, 1324–1329. doi:10.1002/ptr.2488
Phillips, E. C., Croft, C. L., Kurbatskaya, K., O’Neill, M. J., Hutton, M. L., Hanger, D. P., et al. (2014). Astrocytes and neuroinflammation in alzheimer’s disease. Biochem. Soc. Trans. 42, 1321–1325. doi:10.1042/BST20140155
Piscopo, P., Crestini, A., Carbone, E., Rivabene, R., Ancidoni, A., Lo Giudice, M., et al. (2022). A systematic review on drugs for synaptic plasticity in the treatment of dementia. Ageing Res. Rev. 81, 101726. doi:10.1016/j.arr.2022.101726
Qin, Y., and Zhao, W. (2023). Posttranslational modifications of NLRP3 and their regulatory roles in inflammasome activation. Eur. J. Immunol. 53, e2350382. doi:10.1002/eji.202350382
Qiu, L., Wang, Y., Wang, Y., Liu, F., Deng, S., Xue, W., et al. (2023). Ursolic acid ameliorated neuronal damage by restoring microglia-activated MMP/TIMP imbalance in vitro. Drug Design Development and Therapy 17, 2481–2493. doi:10.2147/DDDT.S411408
Rajesh, Y., and Kanneganti, T.-D. (2022). Innate immune cell death in neuroinflammation and alzheimer’s disease. Cells 11, 1885. doi:10.3390/cells11121885
Raz, L., Knoefel, J., and Bhaskar, K. (2016). The neuropathology and cerebrovascular mechanisms of dementia. Journal of Cerebral Blood Flow and Metabolism 36, 172–186. doi:10.1038/jcbfm.2015.164
Reichert, C. O., de Freitas, F. A., Sampaio-Silva, J., Rokita-Rosa, L., Barros, P. de L., Levy, D., et al. (2020). Ferroptosis mechanisms involved in neurodegenerative diseases. International Journal of Molecular Sciences 21, 8765. doi:10.3390/ijms21228765
Ren, X., Zhang, J., Zhao, Y., and Sun, L. (2022). Senegenin inhibits Aβ1-42-induced PC12 cells apoptosis and oxidative stress via activation of the PI3K/akt signaling pathway. Neuropsych. Dis. Treat. 18, 513–524. doi:10.2147/NDT.S346238
Ritchie, K., and Lovestone, S. (2002). The dementias. Lancet (London, England) 360, 1759–1766. doi:10.1016/S0140-6736(02)11667-9
Ryter, S. W. (2021). Heme oxgenase-1, a cardinal modulator of regulated cell death and inflammation. Cells 10, 515. doi:10.3390/cells10030515
Schröder, K. (2019). NADPH oxidase-derived reactive oxygen species: Dosis facit venenum. Exp. Physiol. 104, 447–452. doi:10.1113/EP087125
Shankar, G. M., Li, S., Mehta, T. H., Garcia-Munoz, A., Shepardson, N. E., Smith, I., et al. (2008). Amyloid-beta protein dimers isolated directly from alzheimer’s brains impair synaptic plasticity and memory. Nat. Med. 14, 837–842. doi:10.1038/nm1782
Shen, X., Dai, X., He, Y., Wen, C., and Zhang, Q. (2022). Determination of senegenin and tenuifolin in mouse blood by ultra-high performance liquid chromatography-tandem mass spectrometry and their pharmacokinetics. International Journal of Analytical Chemistry 2022, e3401355. doi:10.1155/2022/3401355
Shin, J.-Y., Shin, J.-W., Ha, S.-K., Kim, Y., Swanberg, K. M., Lee, S., et al. (2018). Radix polygalae extract attenuates PTSD-like symptoms in a mouse model of single prolonged stress and conditioned fear possibly by reversing BAG1. Exp. Neurobiol. 27, 200–209. doi:10.5607/en.2018.27.3.200
Smith, A. K., and Klimov, D. K. (2019). De novo aggregation of Alzheimer's Aβ25-35 peptides in a lipid bilayer. Sci. Rep. 9, 7161. doi:10.1038/s41598-019-43685-7
Son, S.-R., Yoon, Y.-S., Hong, J.-P., Kim, J.-M., Lee, K.-T., and Jang, D. S. (2022). Chemical constituents of the roots of polygala tenuifolia and their anti-inflammatory effects. Plants (Basel Switz.) 11, 3307. doi:10.3390/plants11233307
Song, Y.-L., Zeng, K.-W., Shi, T.-X., Jiang, Y., and Tu, P.-F. (2013). Sibiricasaponins a-E, five new triterpenoid saponins from the aerial parts of polygala sibirica L. Fitoterapia 84, 295–301. doi:10.1016/j.fitote.2012.12.017
Suma, P. R., Padmanabhan, R. A., Telukutla, S. R., Ravindran, R., Velikkakath, A. K. G., Dekiwadia, C. D., et al. (2020). Vanadium pentoxide nanoparticle mediated perturbations in cellular redox balance and the paradigm of autophagy to apoptosis. Free Radic. Biol. Med. 161, 198–211. doi:10.1016/j.freeradbiomed.2020.10.008
Swanson, K. V., Deng, M., and Ting, J. P.-Y. (2019). The NLRP3 inflammasome: Molecular activation and regulation to therapeutics. Nat. Rev. Immunol. 19, 477–489. doi:10.1038/s41577-019-0165-0
Takagane, K., Nojima, J., Mitsuhashi, H., Suo, S., Yanagihara, D., Takaiwa, F., et al. (2015). Aβ induces oxidative stress in senescence-accelerated (SAMP8) mice. Biosci. Biotechnol. Biochem. 79, 912–918. doi:10.1080/09168451.2014.1002449
Tang, B.-C., Wang, Y.-T., and Ren, J. (2023). Basic information about memantine and its treatment of alzheimer’s disease and other clinical applications. Ibrain 9, 340–348. doi:10.1002/ibra.12098
Tang, X., Zhao, Y., Liu, Y., Liu, Y., Liu, Y., Niu, F., et al. (2022). 3,6’-disinapoyl sucrose attenuates Aβ1-42 - induced neurotoxicity in caenorhabditis elegans by enhancing antioxidation and regulating autophagy. J. Cell. Mol. Med. 26, 1024–1033. doi:10.1111/jcmm.17153
Teleanu, R. I., Niculescu, A.-G., Roza, E., Vladâcenco, O., Grumezescu, A. M., and Teleanu, D. M. (2022). Neurotransmitters-key factors in neurological and neurodegenerative disorders of the central nervous system. Int. J. Mol. Sci. 23, 5954. doi:10.3390/ijms23115954
Teng, H., Fang, M., Cai, X., and Hu, Z. (2009). Localization and dynamic change of saponin in vegetative organs of polygala tenuifolia. J. Integr. Plant Biol. 51, 529–536. doi:10.1111/j.1744-7909.2009.00830.x
Tian, H., Xu, X., Zhang, F., Wang, Y., Guo, S., Qin, X., et al. (2015). Analysis of polygala tenuifolia transcriptome and description of secondary metabolite biosynthetic pathways by illumina sequencing. Int. J. Genomics 2015, 782635. doi:10.1155/2015/782635
Tian, Y., Qi, Y., Cai, H., Xu, M., and Zhang, Y. (2022). Senegenin alleviates Aβ1-42 induced cell damage through triggering mitophagy. J. Ethnopharmacol. 295, 115409. doi:10.1016/j.jep.2022.115409
Tuo, Q.-Z., Liu, Y., Xiang, Z., Yan, H.-F., Zou, T., Shu, Y., et al. (2022). Thrombin induces ACSL4-dependent ferroptosis during cerebral ischemia/reperfusion. Signal Transduction and Targeted Therapy 7, 59. doi:10.1038/s41392-022-00917-z
Vinh, L. B., Heo, M., Phong, N. V., Ali, I., Koh, Y. S., Kim, Y. H., et al. (2020). Bioactive compounds from polygala tenuifolia and their inhibitory effects on lipopolysaccharide-stimulated pro-inflammatory cytokine production in bone marrow-derived dendritic cells. Plants (Basel, Switzerland) 9, 1240. doi:10.3390/plants9091240
von Einem, B., Schwanzar, D., Rehn, F., Beyer, A.-S., Weber, P., Wagner, M., et al. (2010). The role of low-density receptor-related protein 1 (LRP1) as a competitive substrate of the amyloid precursor protein (APP) for BACE1. Exp. Neurol. 225, 85–93. doi:10.1016/j.expneurol.2010.05.017
Wakabayashi, K., Tanji, K., Mori, F., and Takahashi, H. (2007). The lewy body in parkinson’s disease: Molecules implicated in the formation and degradation of alpha-synuclein aggregates. Neuropathology 27, 494–506. doi:10.1111/j.1440-1789.2007.00803.x
Wan, W., Qian, C., Wang, Q., Li, J., Zhang, H., Wang, L., et al. (2023). STING directly recruits WIPI2 for autophagosome formation during STING-induced autophagy. Embo J 42, e112387. doi:10.15252/embj.2022112387
Wan, Y., Song, M., Xie, X., Chen, Z., Gao, Z., Wu, X., et al. (2021). BMSCs regulate astrocytes through TSG-6 to protect the blood-brain barrier after subarachnoid hemorrhage. Mediators Inflamm 2021, 5522291. doi:10.1155/2021/5522291
Wang, H., Huang, H., Jiang, N., Zhang, Y., Lv, J., and Liu, X. (2022). Tenuifolin ameliorates chronic restraint stress-induced cognitive impairment in C57BL/6J mice. Phytother. Res. 36, 1402–1412. doi:10.1002/ptr.7402
Wang, J., Fu, J., Zhao, Y., Liu, Q., Yan, X., and Su, J. (2023a). Iron and targeted iron therapy in alzheimer’s disease. International Journal of Molecular Sciences 24, 16353. doi:10.3390/ijms242216353
Wang, L., Jin, G. F., Yu, H. H., Lu, X. H., Zou, Z. H., Liang, J. Q., et al. (2019a). Protective effects of tenuifolin isolated from polygala tenuifolia willd roots on neuronal apoptosis and learning and memory deficits in mice with alzheimer’s disease. Food Funct 10, 7453–7460. doi:10.1039/C9FO00994A
Wang, L., Jin, G., Yu, H., Li, Q., and Yang, H. (2019b). Protective effect of tenuifolin against alzheimer’s disease. Neurosci. Lett. 705, 195–201. doi:10.1016/j.neulet.2019.04.045
Wang, L., Zhang, S., Yi, S., and Ho, M. S. (2024). A new regulator of autophagy initiation in glia. Autophagy 20, 207–209. doi:10.1080/15548627.2023.2251821
Wang, P., Tang, C.-T., Li, J., Huang, X., Jin, R., Yin, F., et al. (2023b). The E3 ubiquitin ligase RNF31 mediates the development of ulcerative colitis by regulating NLRP3 inflammasome activation. Int. Immunopharmacol. 125, 111194. doi:10.1016/j.intimp.2023.111194
Wang, Q., Xiao, B.-X., Pan, R.-L., Liu, X.-M., Liao, Y.-H., Feng, L., et al. (2015). An LC-MS/MS method for simultaneous determination of three polygala saponin hydrolysates in rat plasma and its application to a pharmacokinetic study. J. Ethnopharmacol. 169, 401–406. doi:10.1016/j.jep.2015.04.033
Wang, X., Li, M., Cao, Y., Wang, J., Zhang, H., Zhou, X., et al. (2017). Tenuigenin inhibits LPS-induced inflammatory responses in microglia via activating the Nrf2-mediated HO-1 signaling pathway. Eur. J. Pharmacol. 809, 196–202. doi:10.1016/j.ejphar.2017.05.004
Wang, X., Xiao, H., Wu, Y., Kong, L., Chen, J., Yang, J., et al. (2021). Active constituent of polygala tenuifolia attenuates cognitive deficits by rescuing hippocampal neurogenesis in APP/PS1 transgenic mice. BMC Complementary Medicine and Therapies 21, 267. doi:10.1186/s12906-021-03437-5
Wei, P.-J., Yao, L.-H., Dai, D., Huang, J.-N., Liu, W.-X., Xiao, P., et al. (2015). Tenuigenin enhances hippocampal schaffer collateral-CA1 synaptic transmission through modulating intracellular calcium. Phytomedicine 22, 807–812. doi:10.1016/j.phymed.2015.05.008
Wen, L., Xia, N., Tang, P., Hong, Y., Wang, Z., Liu, Y., et al. (2015). The gastrointestinal irritation of polygala saponins and its potential mechanism in vitro and in vivo. Biomed Res. Int. 2015, 918048. doi:10.1155/2015/918048
Wimo, A., Seeher, K., Cataldi, R., Cyhlarova, E., Dielemann, J. L., Frisell, O., et al. (2023). The worldwide costs of dementia in 2019. Alzheimer’s and Dementia 19, 2865–2873. doi:10.1002/alz.12901
Wittrahm, R., Takalo, M., Kuulasmaa, T., Mäkinen, P. M., Mäkinen, P., Končarević, S., et al. (2023). Protective alzheimer’s disease-associated APP A673T variant predominantly decreases sAPPβ levels in cerebrospinal fluid and 2D/3D cell culture models. Neurobiol. Dis. 182, 106140. doi:10.1016/j.nbd.2023.106140
Wu, A.-G., Wong, V. K.-W., Zeng, W., Liu, L., and Law, B. Y.-K. (2015). Identification of novel autophagic radix polygalae fraction by cell membrane chromatography and UHPLC-(Q)TOF-MS for degradation of neurodegenerative disease proteins. Sci. Rep. 5, 17199. doi:10.1038/srep17199
Wu, J., Meng, Q., Liu, D., Fan, A., Huang, J., and Lin, W. (2024). Targeted isolation of sorbicilinoids from a deep-sea derived fungus with anti-neuroinflammatory activities. Phytochemistry 219, 113976. doi:10.1016/j.phytochem.2024.113976
Xia, X., He, X., Zhao, T., Yang, J., Bi, Z., Fu, Q., et al. (2024). Inhibiting mtDNA-STING-NLRP3/IL-1β axis-mediated neutrophil infiltration protects neurons in alzheimer’s disease. Cell Prolif 57, e13529. doi:10.1111/cpr.13529
Xu, P., Xu, S.-P., Wang, K.-Z., Lu, C., Zhang, H.-X., Pan, R., et al. (2016). Cognitive-enhancing effects of hydrolysate of polygalasaponin in SAMP8 mice. J. Zhejiang Univ. Sci. B 17, 503–514. doi:10.1631/jzus.B1500321
Xu, S. P., Yang, Y. Y., Xue, D., Liu, J. X., Liu, X. M., Fan, T.-P., et al. (2011). Cognitive-enhancing effects of polygalasaponin hydrolysate in aβ(25-35)-induced amnesic mice. Evid.-Based Complement. Altern. Med 2011, 839720. doi:10.1155/2011/839720
Xue, W., Hu, J., Yuan, Y., Sun, J., Li, B., Zhang, D., et al. (2009). Polygalasaponin XXXII from polygala tenuifolia root improves hippocampal-dependent learning and memory. Acta Pharmacol. Sin. 30, 1211–1219. doi:10.1038/aps.2009.112
Yabe, T., Tuchida, H., Kiyohara, H., Takeda, T., and Yamada, H. (2003). Induction of NGF synthesis in astrocytes by onjisaponins of polygala tenuifolia, constituents of kampo (japanese herbal) medicine, ninjin-yoei-to. Phytomed. Int. J. Phytother. Phytopharm. 10, 106–114. doi:10.1078/094471103321659799
Yammine, A., Nury, T., Vejux, A., Latruffe, N., Vervandier-Fasseur, D., Samadi, M., et al. (2020). Prevention of 7-ketocholesterol-induced overproduction of reactive oxygen species, mitochondrial dysfunction and cell death with major nutrients (polyphenols, ω3 and ω9 unsaturated fatty acids) of the mediterranean diet on N2a neuronal cells. Molecules (Basel, Switzerland) 25, 2296. doi:10.3390/molecules25102296
Yan, H.-F., Zou, T., Tuo, Q.-Z., Xu, S., Li, H., Belaidi, A. A., et al. (2021). Ferroptosis: Mechanisms and links with diseases. Signal Transduction and Targeted Therapy 6, 49. doi:10.1038/s41392-020-00428-9
Yang, G., Zhou, R., Guo, X., Yan, C., Lei, J., and Shi, Y. (2021). Structural basis of γ-secretase inhibition and modulation by small molecule drugs. Cell 184, 521–533.e14. doi:10.1016/j.cell.2020.11.049
Yang, Z., Zou, Y., and Wang, L. (2023). Neurotransmitters in prevention and treatment of alzheimer’s disease. Int. J. Mol. Sci. 24, 3841. doi:10.3390/ijms24043841
Yao, Y., Jia, M., Wu, J.-G., Zhang, H., Sun, L.-N., Chen, W.-S., et al. (2010). Anxiolytic and sedative-hypnotic activities of polygalasaponins from polygala tenuifolia in mice. Pharm. Biol. 48, 801–807. doi:10.3109/13880200903280042
Yu, H., Lin, L., Zhang, Z., Zhang, H., and Hu, H. (2020). Targeting NF-κB pathway for the therapy of diseases: Mechanism and clinical study. Signal Transduction and Targeted Therapy 5, 209. doi:10.1038/s41392-020-00312-6
Yu, K., Chen, F., and Li, C. (2012). Absorption, disposition, and pharmacokinetics of saponins from Chinese medicinal herbs: What do we know and what do we need to know more? Curr. Drug Metab. 13, 577–598. doi:10.2174/1389200211209050577
Yuan, Y., Tan, H., Chen, H., Zhang, J., Shi, F., Wang, M., et al. (2023). Peroxiredoxin 1 alleviates oxygen-glucose deprivation/reoxygenation injury in N2a cells via suppressing the JNK/caspase-3 pathway. Iran. J. Basic Med. Sci. 26, 1305–1312. doi:10.22038/IJBMS.2023.71390.15528
Zang, L., Fu, D., Zhang, F., Li, N., and Ma, X. (2023). Tenuigenin activates the IRS1/akt/mTOR signaling by blocking PTPN1 to inhibit autophagy and improve locomotor recovery in spinal cord injury. J. Ethnopharmacol. 317, 116841. doi:10.1016/j.jep.2023.116841
Zeng, W., Wu, A. G., Zhou, X.-G., Khan, I., Zhang, R. L., Lo, H. H., et al. (2021). Saponins isolated from radix polygalae extent lifespan by modulating complement C3 and gut microbiota. Pharmacol. Res. 170, 105697. doi:10.1016/j.phrs.2021.105697
Zhang, H., Han, T., Zhang, L., Yu, C.-H., Wan, D.-G., Rahman, K., et al. (2008). Effects of tenuifolin extracted from radix polygalae on learning and memory: a behavioral and biochemical study on aged and amnesic mice. Phytomed 15, 587–594. doi:10.1016/j.phymed.2007.12.004
Zhang, H., Lu, F., Liu, P., Qiu, Z., Li, J., Wang, X., et al. (2023). A direct interaction between RhoGDIα/tau alleviates hyperphosphorylation of tau in alzheimer’s disease and vascular dementia. Journal of Neuroimmune Pharmacology 18, 58–71. doi:10.1007/s11481-021-10049-w
Zhang, H., Zhou, W., Li, J., Qiu, Z., Wang, X., Xu, H., et al. (2022). Senegenin rescues PC12 cells with oxidative damage through inhibition of ferroptosis. Mol. Neurobiol. 59, 6983–6992. doi:10.1007/s12035-022-03014-y
Zhang, Z., Yang, X., Song, Y.-Q., and Tu, J. (2021). Autophagy in alzheimer’s disease pathogenesis: Therapeutic potential and future perspectives. Ageing Res. Rev. 72, 101464. doi:10.1016/j.arr.2021.101464
Zhao, H., Wang, Z.-C., Wang, K.-F., and Chen, X.-Y. (2015). Aβ peptide secretion is reduced by Radix Polygalae-induced autophagy via activation of the AMPK/mTOR pathway. Mol. Med. Rep. 12, 2771–2776. doi:10.3892/mmr.2015.3781
Zhao, L., Yue, Z., Wang, Y., Wang, J., Ullah, I., Muhammad, F., et al. (2022). Autophagy activation by terminalia chebula retz. reduce aβ generation by shifting APP processing toward non-amyloidogenic pathway in APPswe transgenic SH-SY5Y cells. Phytomed. Int. J. Phytother. Phytopharm. 103, 154245. doi:10.1016/j.phymed.2022.154245
Zheng, Q., Song, B., Li, G., Cai, F., Wu, M., Zhao, Y., et al. (2022). USP25 inhibition ameliorates alzheimer’s pathology through the regulation of APP processing and aβ generation. J. Clin. Invest. 132, e152170. doi:10.1172/JCI152170
Zhou, H., Xue, W., Chu, S.-F., Wang, Z.-Z., Li, C.-J., Jiang, Y.-N., et al. (2016). Polygalasaponin XXXII, a triterpenoid saponin from polygalae radix, attenuates scopolamine-induced cognitive impairments in mice. Acta Pharmacol. Sin. 37, 1045–1053. doi:10.1038/aps.2016.17
Zhou, Y., Yan, M., Pan, R., Wang, Z., Tao, X., Li, C., et al. (2021). Radix polygalae extract exerts antidepressant effects in behavioral despair mice and chronic restraint stress-induced rats probably by promoting autophagy and inhibiting neuroinflammation. J. Ethnopharmacol. 265, 113317. doi:10.1016/j.jep.2020.113317
Keywords: triterpenoid saponins, cognitive functions, Alzheimer’s disease, tenuigenin, traditional Chinese medicine, neurodegenerative processes
Citation: Li S, Hou Z, Ye T, Song X, Hu X and Chen J (2024) Saponin components in Polygala tenuifolia as potential candidate drugs for treating dementia. Front. Pharmacol. 15:1431894. doi: 10.3389/fphar.2024.1431894
Received: 13 May 2024; Accepted: 21 June 2024;
Published: 10 July 2024.
Edited by:
Ana Hortência Fonsêca Castro, Universidade Federal de São João del-Rei, BrazilReviewed by:
Safaet Alam, Bangladesh Council of Scientific and Industrial Research (BCSIR), BangladeshSimony Mendonça, Federal University of Rio de Janeiro, Brazil
Copyright © 2024 Li, Hou, Ye, Song, Hu and Chen. This is an open-access article distributed under the terms of the Creative Commons Attribution License (CC BY). The use, distribution or reproduction in other forums is permitted, provided the original author(s) and the copyright owner(s) are credited and that the original publication in this journal is cited, in accordance with accepted academic practice. No use, distribution or reproduction is permitted which does not comply with these terms.
*Correspondence: Jing Chen, Y2hlbmppbmc2Mzg1QDE2My5jb20=