- 1The First Clinical Institute, Zunyi Medical University, Zunyi, Guizhou, China
- 2Office of Drug Clinical Trial Institution, The Affiliated Hospital of Zunyi Medical University, Zunyi, China
- 3Department of General Surgery, The Affiliated Hospital of Zunyi Medical University, Zunyi, Guizhou, China
- 4Department of Oncology, The Second Affiliated Hospital of Zunyi Medical University, Zunyi, China
- 5Key Laboratory of Basic Pharmacology of Ministry of Education and Joint International Research Laboratory of Ethnomedicine of Ministry of Education, Zunyi Medical University, Zunyi, China
Ferroptosis represents a distinct form of cell death that is not associated with necrosis, autophagy, apoptosis, or pyroptosis. It is characterised by intracellular iron-dependent lipid peroxidation. The current literature indicates that a number of botanical drugs and isolated metabolites can modulate ferroptosis, thereby exerting inhibitory effects on lung cancer cells or animal models. The aim of this review is to elucidate the mechanisms through which botanical drugs and isolated metabolites regulate ferroptosis in the context of lung cancer, thereby providing potential insights into lung cancer treatment. It is crucial to highlight that these preclinical findings should not be interpreted as evidence that these treatments can be immediately translated into clinical applications. In the future, we will continue to study the pharmacology, pharmacokinetics and toxicology of these drugs, as well as evaluating their efficacy and safety in clinical trials, with the aim of providing new approaches to the development of new agents for the treatment of lung cancer.
1 Introduction
Recent statistics indicate that lung cancer is the leading cause of cancer-related mortality on a global scale. The disease encompasses both non-small cell lung cancer (NSCLC) and small cell lung cancer (SCLC). NSCLC represents the most prevalent type, accounting for approximately 86% of cases (Barta et al., 2019). Despite recent advances in the treatment of lung cancer, challenges remain due to factors such as drug dose-limiting toxicity (Cannon et al., 2013), drug resistance (Lin and Shaw, 2016; Wang et al., 2024). It is therefore imperative to explore and develop new drugs with enhanced efficacy and reduced toxicity.
Ferroptosis, a newly identified cell death pathway distinct from necrosis, autophagy, apoptosis, along with pyroptosis, relies on the buildup of ROS induced by iron-mediated lipid peroxidation (Yin et al., 2022). It presents cellular, molecular, and genetic features that set it apart from other cell death forms. Recent studies have indicated that the induction of ferroptosis may represent a potential mechanism for tumour cell death (Friedmann Angeli et al., 2019; Elgendy et al., 2020; Bell et al., 2024; Lei et al., 2024). Consequently, these findings have become a focal point for researchers engaged in the investigation of anti-tumour therapies. Botanical drugs and isolated metabolites have progressively become a burgeoning area of interest in anti-tumor drug research (Xing et al., 2023; Zhang et al., 2024), supported by recent in-depth studies highlighting their diverse anti-tumor activities (Zhang et al., 2017b; Jiang H. et al., 2021). Unprecedented strides have been made in studying ferroptosis induction by botanical drugs and isolated metabolites in lung cancer cells (Chen et al., 2020; Zhang R. et al., 2022). This review provides an overview of the ongoing research progress concerning established botanical drugs and isolated metabolites targeting the ferroptosis pathway in lung cancer.
2 Role of botanical drugs and isolated metabolites in regulating ferroptosis in lung cancer
These peroxides adversely impact cell membrane integrity, ultimately inducing ferroptosis. This distinctive type of cell death, reliant on iron-mediated phospholipid peroxidation (Wang et al., 2023), is intricately regulated by various cellular metabolic processes involving redox balance, iron homeostasis, mitochondrial function, as well as the metabolism of amino acids, lipids, and sugars, along with multiple associated signaling pathways relating to disease progression (Jiang X. et al., 2021; Zhou et al., 2024) (Table 1).
2.1 System Xc−-GSH-GPX4
The system Xc− comprises transmembrane transport proteins, including SLC7A11 and SLC3A2, located on phospholipid bilayers (Conrad and Sato, 2012; Lewerenz et al., 2013). System Xc− is the predominant aminoacid antiporter, importing L-cystine in exchange for glutamate via the transporter subunit SLC7A11 (Koppula et al., 2021a). Cystine is converted to cysteine intracellularly through a NADPH-consuming reduction reaction and can then be used to synthesize GSH, an important cellular cofactor for antioxidant systems (Yang et al., 2014; Jyotsana et al., 2022). GSH plays a critical role in protecting cells from oxidative damage and the toxicity of xenobiotic electrophiles, and maintaining redox homeostasis (Forman et al., 2009). The main function of GPX4 is to use GSH as a co-factor to resist lipid peroxidation, thereby protecting the integrity of the membrane (Xie et al., 2023). GPX4 moonlights as structural protein and antioxidase that powerfully inhibits lipid oxidation. It is considered as a key regulator of ferroptosis, which takes role in metabolism of lipids and amine acids (Liu et al., 2023). The role of GPX4 as the main regulator in the ferroptotic process is based on its unique function to reduce complex hydroperoxides including phospholipid hydroperoxides and cholesterol hydroperoxides to their corresponding counterparts, thereby interrupting the lipid peroxidation chain reaction (Seibt et al., 2019). Deficiency in GSH leads to GPX4 dysfunction and the substantial accumulation of lipid ROS, thereby initiating ferroptosis (Ursini et al., 1985; Yang et al., 2014). An imbalance in the Xc−-GSH-GPX4 pathway affects GPX4 homeostasis and ferroptosis activity (Figure 1).
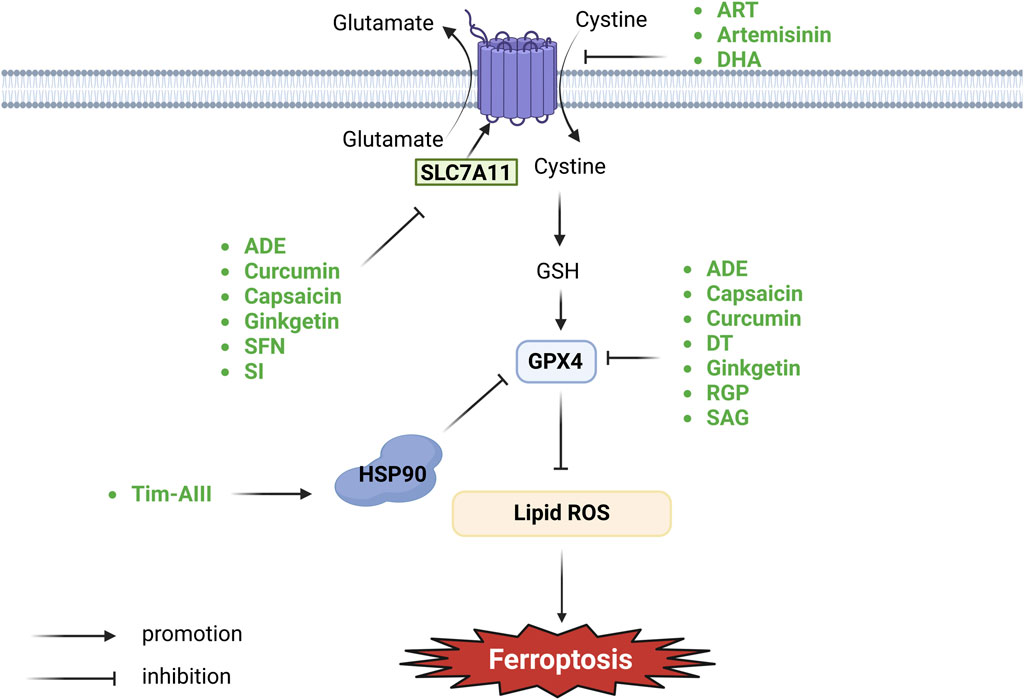
Figure 1. Botanical drugs and isolated metabolites through system Xc−-GSH-GPX4 pathway regulate ferroptosis in lung cancer (SLC7A11, solute carrier family seven member 11; HSP90, heat shock protein 90; GSH, glutathione; GPX4, glutathione peroxidase 4).
2.1.1 Salvia miltiorrhiza Bunge [Lamiaceae; Salviae miltiorrhizae radix et rhizoma]
Dihydroisotanshinone I (DT) is extracted from the dried roots of Salvia miltiorrhiza Bunge. GPX4 is responsible for breaking down small molecule peroxides and complex lipid peroxides in a GSH-dependent manner, thereby safeguarding cells from ferroptosis (Seibt et al., 2019). In instances of GPX4 inactivation or GSH depletion, accumulated fatty acids and hydroperoxides undergo catalysis to form lipid peroxyl radicals in the Fenton reaction mediated by iron, ultimately resulting in cellular ferroptosis. Wu et al. (2021) found that DT inhibited GPX4 expression and subsequently induced ferroptosis through lipid peroxidation, displaying an inhibitory effect on the growth of A549, H460, and other lung cancer cell lines. DT can induce apoptosis and ferroptosis in A549 and H460 cells.
2.1.2 Panax ginseng C. A. Mey. [Araliaceae; red asian ginseng radix et rhizoma]
Red ginseng, known scientifically as Panax ginseng C. A. Meyer, is extensively used in traditional Asian herbal medicine and is gaining popularity in Western countries (Helms, 2004). Ginseng polysaccharide (GP) is among the active metabolites of red ginseng. Recent findings indicate that red ginseng polysaccharide (RGP) shows potential as a immune-stimulating modifier and may hold significant value in the treatment of tumors (Zhou et al., 2014). Additionally, elevated levels of red ginseng acidic polysaccharide (RGAP) have a notable correlation with heightened immune system activity, indicating its role in activating immune activity (Youn et al., 2020). Zhai et al. (2022) discovered that RGP was observed to exert a significant inhibitory effect on cell proliferation and promote GPX4 downregulation-induced ferroptosis induction in A549 cells. These findings suggest that RGP may have potential applications in cancer treatment.
2.1.3 Anemarrhena asphodeloides Bunge [Asparagaceae; Anemarrhena asphodeloides Bunge dry radix et rhizome]
Timosaponin AⅢ (Tim-AⅢ), a steroid saponin, serves as the primary active metabolite of Anemarrhena asphodeloides Bunge (Zhou et al., 2023). HSP90, a chaperone protein, holds a critical role in essential cellular processes and regulatory pathways such as apoptosis, cell cycle control, protein folding and degradation, cell signaling, and cell viability (Hoter et al., 2018). Zhou et al., (2023) observed that Tim-AⅢ targeted GPX4 degradation and promoted GPX4 ubiquitination by binding and complexing with HSP90, resulting in ROS and iron accumulation, malondialdehyde production, and GSH depletion, ultimately inducing ferroptosis in NSCLC cells. Tim-AIII triggers cell death, inhibits cell proliferation, and promotes cell cycle arrest at G2/M phase via induction of ferroptosis in NSCLC cell lines.
2.1.4 Sanguinaria canadensis L. [Papaveraceae; Sanguinaria canadensis radix et rhizome]
Sanguinarine (SAG), a natural benzophenanthridine alkaloid derived from the root of Sanguinaria canadensis L., exhibits promising anticancer activity. Xu et al. (2022) discovered that SAG increased Fe2+ concentration, ROS levels, and malondialdehyde content while reducing GSH content. Additionally, SAG lowered the protein stability of GPX4 through E3 ligase STUB1-mediated ubiquitination and degradation of endogenous GPX4. This process inhibited the growth and metastasis of NSCLC by promoting ferroptosis.
2.1.5 Capsicum annuum L. [Solanaceae]
Capsaicin (trans-8-methyl-N-vanillyl-6-nonanamide, C18H27NO3) is the primary metabolite of Capsicum annuum L. (Bley et al., 2012) and has been reported to possess various functions such as antioxidant, anti-inflammatory, cardiovascular disease prevention, and gastrointestinal mucosal protection (Luo et al., 2011). Furthermore, several previous studies have demonstrated the anti-cancer effects of capsaicin on various malignant tumors, including NSCLC, liver cancer, and prostate cancer (Huang et al., 2009; Chakraborty et al., 2014; Venier et al., 2015). Capsaicin exerts anti-tumor effects by inhibiting cancer cell proliferation, inducing cell cycle arrest, inhibiting tumor angiogenesis, and promoting cancer autophagy (Chakraborty et al., 2014; Zheng et al., 2015; Islam et al., 2021). SLC7A11 is believed to play a crucial role in regulating ferroptosis, where its suppression initiates ferroptosis, resulting in a significant reduction in the proliferation of malignant cells (Daher et al., 2019; Lim et al., 2019). Liu (Liu X.-Y. et al., 2022) revealed that capsaicin exerted an anti-proliferative effect on A549 cells as well as NCI-H23 cells through SLC7A11/GPX4 signaling, ultimately resulting in ferroptosis. These data suggest that capsaicin inhibits the proliferation of A549 and NCI-H23 cells by inducing ferroptosis.
2.1.6 Ginkgo biloba L. [Ginkgoaceae; Ginkgo biloba plant dried leaves]
Ginkgetini is derived from Ginkgo biloba L. Lou et al. (2021) discovered that Ginkgetin mediated ferroptosis in NSCLC by increasing iron concentration, promoting lipid peroxidation, inhibiting SLC7A11 and GPX4 expression, and reducing the GSH/GSSG ratio. Ginkgetin trigger non-apoptotic cell death or disrupts the redox homeostasis in A549 by inducing ferroptosis.
2.1.7 Brassicaceae burnett
Sulforaphane (SFN), an isothiocyanate abundant in Brassicaceae, particularly in broccoli and broccoli sprouts, exhibits a wide array of anticancer properties (Clarke et al., 2008). Iida et al. (2021) found that SFN-induced cell death was mediated via ferroptosis and inhibition of SLC7A11 mRNA and protein expression levels in SCLC cells, leading to reduced GSH and increased lipid ROS levels. Following the addition of SFN to the cell culture, cell growth was significantly inhibited, and cell death was shown in SCLC and multidrug-resistant H69AR cells.
2.1.8 Artemisia annua L. [Compositae]
Dihydroartemisinin (DHA) is a derivative of Artemisia annua L. The PRIM2 is located on human chromosome 6p11.1-p12 and encodes a 58 kDa protein containing a 4Fe-4S cofactor that forms a heterodimeric DNA primase with PRIM1, a small subunit of DNA primase. This protein, in conjunction with the p49 subunit, forms the heterodimeric DNA primase enzyme. DNA primase is crucial for initiating DNA replication and synthesizing Okazaki fragments during the synthesis of lagging strand (Shiratori et al., 1995; Yatsula et al., 2006). The β-catenin signaling pathway is crucial in lung cancer carcinogenesis, particularly regarding the downregulation of both SLC7A11 and β-catenin expression in cells associated with PRIM2 loss. Yuan et al. (2020) discovered that DHA inhibited the expression of PRIM2, leading to the downregulation of SLC7A11 and β-catenin, key regulators of ferroptosis in lung cancer cells. This resulted in decreased GSH, increased ROS and malondialdehyde, ultimately inhibiting proliferation, clone formation, and inducing ferroptosis in A549 cells.
2.2 Lipid peroxidation
The sensitivity of ferroptosis is closely related to lipid metabolism and directly affects the biosynthesis and storage of lipid peroxidation (Yang and Stockwell, 2016). ACSL4 and LPCAT3 play pivotal roles in PUFA-PL synthesis (Dixon et al., 2015; Doll et al., 2017; Kagan et al., 2017). ACSL4 is one of a number of fatty acid activating enzymes functioning by esterifying CoA to free fatty acids in an ATP dependent manner (Seibt et al., 2019). ACSL4 is responsible for shaping the cellular lipidome by acting as an important node that determines sensitivity versus resistance to this form of cell death. ACSL4-dependent modulation of phospholipids, specifically that of PE, is a critical determinant of sensitivity to ferroptosis (Doll et al., 2017; Kagan et al., 2017). ACSL4 catalyses the ligation of free PUFAs, such as arachidonic acids and adrenic acids, with CoA to generate PUFA-CoAs, which are subsequently re-esterified and incorporated into PLs by LPCAT3 to form PUFA-PLs (Doll et al., 2017; Kagan et al., 2017). PUFA-PLs are particularly susceptible to peroxidation under the catalysis of ROS produced by the Fenton reaction initiated by iron, mitochondria or NOX, ultimately generating lipid peroxides (Dixon et al., 2012; Yang and Stockwell, 2016; Conrad and Pratt, 2019). ACC-catalysed carboxylation of acetyl-CoA generates malonyl-CoA, which is required for the synthesis of some PUFAs and therefore for ferroptosis (Dixon et al., 2015; Shimada et al., 2016; Lee et al., 2020b; Li et al., 2020). Inactivation of ACSL4, LPCAT3, or ACC blocks or attenuates ferroptosis (Doll et al., 2017; Lee et al., 2020a). The enzymatic reactions mediated by ALOX or Cytochrome P450 oxidoreductase are also involved in facilitating lipid peroxides (Yang et al., 2016; Wenzel et al., 2017; Zou et al., 2020; Koppula et al., 2021b; Yan et al., 2021). A great diversity of aldehydes are formed when lipid hydroperoxides break down in biological systems. Some of these aldehydes such as 4-hydroxynonenal, 4-hydroxyhexenal, and malonaldehyde are highly reactive and may be considered as second toxic messengers which will cause the cross-linking polymerization of life macromolecules such as proteins and nucleic acids, and affect the activities of mitochondrial respiratory chain complexes and key enzymes in mitochondria, resulting in cell death (Esterbauer et al., 1991) (Figure 3).
2.2.1 Curcuma longa L. [Zingiberaceae; Curcuma longa radix et rhizome]
Curcumin, a yellow polyphenolic metabolite commonly found in Curcuma longa L., exhibits anticancer properties through various mechanisms, including the inhibition of tumor proliferation, invasion, and metastasis, as well as the regulation of apoptosis and autophagy (Tomeh et al., 2019). Increasing evidence suggests that ACSL4 is a critical factor for ferroptosis sensitivity (Doll et al., 2017; Kenny et al., 2019). ACSL4 catalyzes the reaction of PUFAs with CoA to generate PUFA-CoA derivatives, which contribute to esterification into PUFA-PL. Subsequently, LPCAT3 specifically inserts acyl groups into lysophospholipids to synthesize PUFA-PL. Quantitative lipidomics analysis has revealed that PUFA-PL containing arachidonic acid or adrenaline acid is critical and is oxidized to PL-PUFA-OOH via the Fenton reaction, thereby driving ferroptosis. Tang et al. (2021) discovered that Curcumin could upregulate the protein levels of ACSL4 in tumor tissues and significantly downregulated SLC7A11 and GPX4 protein levels. It also found that Curcumin induced ferroptosis in NSCLC by activating autophagy. Moreover, Curcumin has been observed to deplete GSH and increase iron content in NSCLC cells. Consequently, tumor cell proliferation was inhibited and ferroptosis was promoted. As a result, curcumin significantly suppressed the proliferation of tumor cells and promoted the death of tumor cells.
2.3 Iron metabolism
Maintaining iron homeostasis is vital for sustaining physiological cellular functions. However, excessive iron not only induces the peroxidation of lipids through the mediation of the Fenton reaction, but also acts as an essential cofactor for enzymes that participate in lipid peroxidation (such as ALOX and POR) (Gaschler and Stockwell, 2017; Conrad and Pratt, 2019). The metabolism of irons is related with several stages encompassing absorption, storage, utilization, and efflux, rendering it a complex process. Imbalanced regulation of these iron metabolism processes can promote or inhibit ferroptosis. Typically, Fe3+ is internalized and carried to endosomes by means of the transferrin receptor (Kawabata, 2019). Within this process, the six-transmembrane epithelial antigen of the prostate three changes Fe3+ into Fe2+ (Shi et al., 2023). Subsequently, divalent metal transporters facilitate the discharge of Fe2+ from endosomes into the cytoplasmic labile iron pool, serving as a source for the Fenton reaction (Yanatori and Kishi, 2019). Excess intracellular iron is typically sequestered within the ferritin protein, which consists of two subunits: FTH1 and FTL (Yang and Stockwell, 2008). NCOA4-mediated phagocytosis of ferritin promotes the autophagic degradation of ferritin, leading to increased intracellular iron levels during periods of iron deficiency, ultimately driving the process of ferroptosis (Hou et al., 2016). Ferroportin, the sole recognized mammalian protein accountable for iron release, facilitates the transfer of cytoplasmic Fe2+ into the bloodstream (Billesbølle et al., 2020). In addition, it is reported that silencing iron response element binding protein 2 can increase the content of iron in cells through transferrin receptor, and eventually induce ferroptosis (Dixon et al., 2012; Reed and Pellecchia, 2012). Furthermore, Fe2+ can exit cells through exosomes or undergo re-oxidation to Fe3+ via ferric oxidases like ceruloplasmin or hephaestin (Torti and Torti, 2013; Brown et al., 2019) (Figure 2).
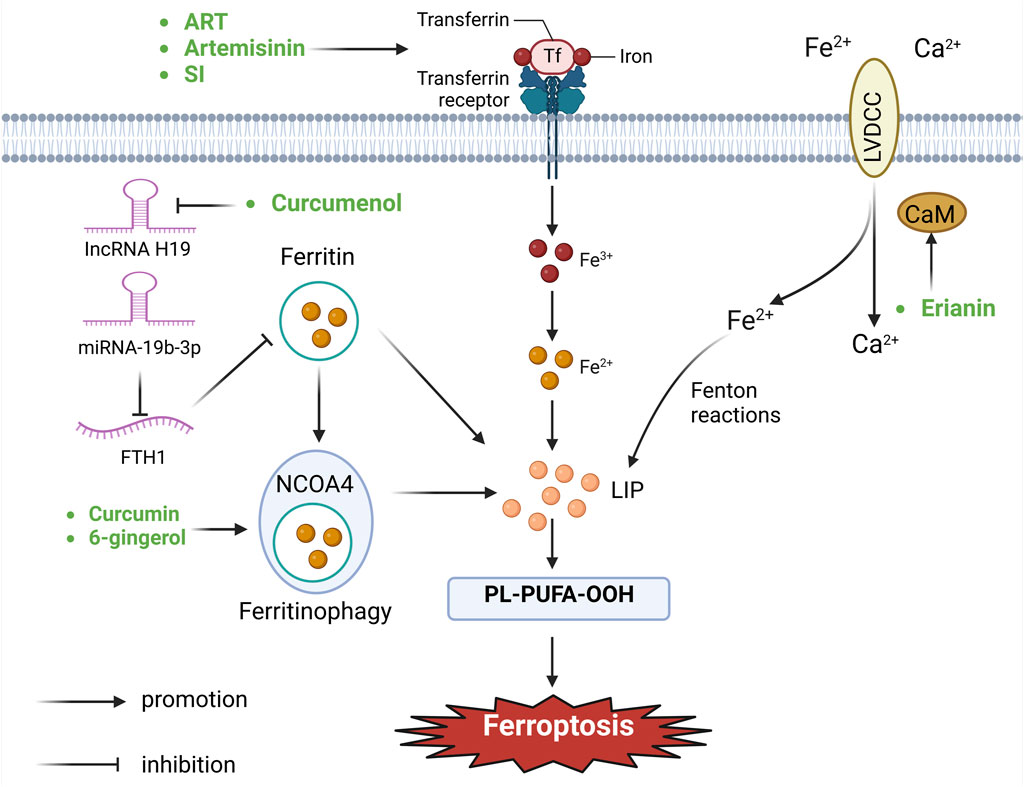
Figure 2. Botanical drugs and isolated metabolites through iron metabolism pathway regulate ferroptosis in lung cancer (CaM, calmodulin; FTH1, ferritin heavy chain 12; NCOA4, nuclear receptor coactivator 4; LVDCC, L-type voltage-dependent Ca2+ channels; LIP, lipid).
2.3.1 Dendrobium chrysotoxum Lindl. [Orchidaceae;Dendrobium chrysotoxum radix et rhizome]
Erianin is a natural product isolated from Dendrobium chrysotoxum Lindl. CAM, a key intracellular Ca2+ binding protein, regulates L-type voltage-dependent Ca2+ channels, which are involved in both Ca2+ transportation and iron uptake (Zühlke et al., 1999; Oudit et al., 2003). Elevated Ca2+ uptake leads to ROS production and heightened levels of ferric iron ions. According to Chen (Chen et al., 2020), treatment of lung cancer cells H460 and H1299 with Erianin activated the Ca2+/CAM signaling pathway. CAM increased cellular Ca2+ uptake by regulating L-type voltage-dependent Ca2+ channels, resulting in elevated ROS production and increased Fe2+ levels, ultimately inducing ferroptosis in lung cancer cells.
2.3.2 Brassica oleracea L. [Brassicaceae]
Sinapine (SI), an alkaloid obtained from Brassica oleracea L. and cruciferous plant species, possesses antioxidant (Boulghobra et al., 2020), neuroprotective (Pohl et al., 2019), and anti-inflammatory properties (Bhinu et al., 2009). Shao et al. (2022) revealed that SI induced ferroptosis in NSCLC through upregulation of transferrin/transferrin receptors and downregulation of SLC7A11. SI selectively inhibited NSCLC cell proliferation and growth in vivo.
2.3.3 Artemisia annua L. [Compositae]
Artemisinin, the primary active metabolite in Artemisia annua L., has been found by Liu et al. (2022c) to upregulate transferrin receptor mRNA levels and suppress cystine/glutamate transporter expression, thereby promoting ferroptosis in NSCLC cells. Among the anti-insect drugs, the derivatives of Artemisia annua L., artesunate (ART), a well-known anti-malarial drug, have been shown to possess selective anti-cancer properties. Transferrin receptor imports extracellular iron into cells, playing a catalytic role in promoting ferroptosis (Lu et al., 2021). ART have been found by Zhang et al. (2021) to induce ferroptosis in A549 cells by upregulating transferrin receptor and downregulating system Xc−. The results indicated that ART inhibited cell viability in a dose-dependent manner in NSCLC cells.
2.3.4 Zingiber officinale Roscoe [Zingiberaceae; Zingiber officinale radix et rhizome]
6-Gingerol is a naturally occurring phenol Zingiber officinale Roscoe, which has been demonstrated to exhibit anti-inflammatory, anti-tumor and antioxidant bioactivities (Zhang et al., 2017a; Koch et al., 2017; de Lima et al., 2018). Deubiquitination of USP14 inhibits autophagy, while ferritin promotes ferroptosis, and autophagy has the ability to regulate ferroptosis through the degradation of ferritin (Mancias et al., 2014; Hou et al., 2016; Xu et al., 2016). Tsai et al. (2020) observed that 6-gingerol decreased the expression of USP14, leading to an increase in the number of autophagosomes and ROS levels, along with an elevation in ferritin concentration. This heightened vulnerability of A549 cells led to their susceptibility to ferroptosis and inhibited cell proliferation of lung cancer.
2.3.5 Curcuma wenyujin Y. H. Chen and C. Ling [Zingiberaceae]
Curcumenol, an active metabolite of Curcuma wenyujin Y. H. Chen and C. Ling, has been demonstrated to exert antitumor potential in a number of cancer types. Overexpression of lncRNA H19 significantly increased the expression levels of negative regulators of ferroptosis, namely Nrf2, GPX4, FTH1, and SLC7A11. Zhang et al. (2022) demonstrated that Curcumenol could induce ferroptosis in lung cancer cells through the lncRNA H19/miR-19b-3p/FTH1 axis. The expression of lncRNA H19 decreased in cells after treatment with Curcumenol. IncRNA H19 regulated FTH1 levels by targeting miR-19b-3p. Curcumenol significantly increased the expression levels of HMOX-1 and transferrin but decreased the expression levels of GPX4, SLC40A1, SLC7A11, FTH1, Nrf2, and glutaminase in lung cancer cells, resulting in elevated ROS levels and decreased GSH levels, ultimately inducing ferroptosis in lung cancer cells. Curcumenol dramatically inhibited the growth of xenograft tumors as well as induced cell death and suppressed cell proliferation in H1299 and H460 cells.
2.4 Other mechanisms
Apart from the aforementioned major regulatory mechanisms, ferroptosis is also governed by additional mechanisms (Lei et al., 2022), such as the DHODH–CoQH2 system, the GCH1-BH4 system, the Mitochondrial metabolism and so on (Figure 3).
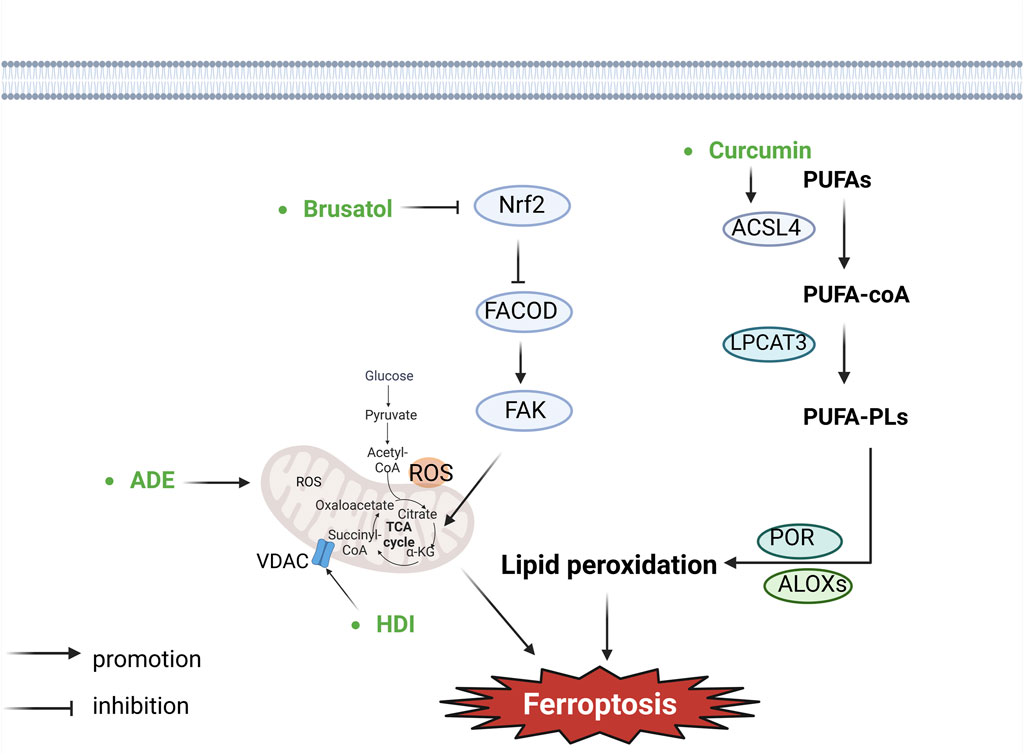
Figure 3. Botanical drugs and isolated metabolites through lipid peroxidation and other pathways regulate ferroptosis in lung cancer (ACSL4, acyl-CoA synthetase long-chain member 4; LPCAT3, lysophosphatidyl-choline acyltransferase 3; POR, cytochrome P450 reductase; ALOXs, arachidonate lipoxyge-nases; PUFAs, polyunsaturated fatty acids; PUFA-PLs, polyunsaturated fatty acid phospholipids; ROS, reactive oxygen species; VDAC, voltage-dependent anion channel; FAK, focal adhesion kinase; FACOD, focadhesin).
A newly identified GPX4-independent mitochondria-localized ferroptosis defense system, the DHODH–CoQH2 system can compensate for GPX4 loss and detoxify mitochondrial lipid peroxides (Mao et al., 2021). DHODH is an enzyme involved in pyrimidine synthesis that can reduce CoQ to CoQH2 in the inner mitochondrial membrane (Lei et al., 2022). When GPX4 is acutely inactivated, the flux through DHODH is significantly increased, resulting in enhanced CoQH2 generation that neutralises lipid peroxidation and prevents ferroptosis in mitochondria (Mao et al., 2021).
Recent studies revealed that the GCH1-BH4 system is another critical inhibitor of ferroptosis (Kraft et al., 2020; Soula et al., 2020). GCH1 mediates the rate-limiting reaction generating the endogenous metabolite BH4, and BH4 is a cofactor of aromatic amino acid hydroxylases and other enzymes (Thöny et al., 2000). BH4 is another radical-trapping antioxidant capable of trapping lipid peroxyl radicals (Soula et al., 2020). GCH1-mediated BH4 synthesis reprograms lipid metabolism and inhibits ferroptosis by selectively preventing two polyunsaturated fatty acyl tails from depleting PLs (Kraft et al., 2020).
The mitochondrion is the major organelle to produce ROS (Murphy, 2009), in which electron leakage from electron transport chain complexes I and III generates superoxides. And it is subsequently converted to hydrogen peroxide (H2O2) through superoxide dismutase mediated dismutation (Murphy, 2009). H2O2 can react with ferrous ion (Fe2+) to generate hydroxyl radicals (•OH), which then abstract the bis-allylic hydrogen in PUFAs to generate PUFA radicals (PUFA•) (Murphy, 2009; Zheng and Conrad, 2020). Moreover, electron transport and proton pumping in mitochondria are important for ATP production (Friedman and Nunnari, 2014; Vasan et al., 2020), which also promotes ferroptosis (Lee et al., 2020a; Li et al., 2020). Finally, mitochondria also have a biosynthetic role in cellular metabolism which contributes to ferroptosis. The underlying mechanisms of the TCA cycle in regulating ferroptosis likely relate to their function in supporting electron transport and fatty acid biosynthesis. The mitochondrion houses the TCA cycle and various anaplerotic reactions that replenish the TCA cycle, such as glutaminolysis (Friedman and Nunnari, 2014), which may drive ferroptosis by promoting ROS, ATP, and/or PUFA-PL generation (Heldt and Piechulla, 2011; Gao et al., 2015; Gao et al., 2019). Therefore, current studies suggest that the diverse roles of mitochondria in bioenergetic, biosynthetic, and ROS regulation contribute to its pro-ferroptosis function (Gan, 2021).
2.4.1 Andrographis paniculata (Burm.f.) Wall. ex Nees. [Acanthaceae]
Andrographolide (ADE) is a diterpenoid lactone isolated from Andrographis paniculata (Burm.f.). Mitochondria can control the intracellular intake of iron, influencing its availability, which plays a crucial role in ferroptosis. Li (Jiaqi et al., 2023) found that ADE could induce mitochondrial dysfunction, evidenced by elevated levels of mitochondrial ROS release, depolarization of the mitochondrial membrane potential, and decreased mitochondrial ATP. Additionally, it suppressed the expression of ferroptosis-related proteins, GPX4 and SLC7A11. The study validated that ADE could restrain proliferation and metastases of NSCLC cells (H460 and H1650) and mouse lung cancer cells (Lewis) through induction of ferroptosis via potentiating mitochondrial dysfunction.
2.4.2 Brucea javanica (L.) Merr [Simaroubaceae]
Brusatol, a triterpene lactone metabolite primarily derived from the Brucea javanica (L.) Merr (Zhao et al., 2014; Yu et al., 2020), has been observed to interact with Nrf2, a key regulator of cellular antioxidant responses, redox homeostasis, as well as metabolic homeostasis. The downstream targets of Nrf2 encompass crucial genes such as HMOX1, GPX4, along with SLC7A11 (Ishii et al., 2000; Osburn et al., 2006; Kerins and Ooi, 2018; Dodson et al., 2019), all of which play pivotal roles in inhibiting lipid peroxidation and the initiation of ferroptosis. Notably, Nrf2 exerts negative transcriptional regulation over the FOCAD gene, which is essential for modulating FAK activity. In instances where Nrf2 is inhibited, heightened FOCAD expression suppresses colony formation, migration, and invasive capacity of cancer cells (Brockschmidt et al., 2012; Brand et al., 2020). Furthermore, it has been established that the tricarboxylic acid cycle and the activity of the mitochondrial electron transport chain are indispensable for the production of lipid ROS in ferroptosis induced by cysteine deprivation. Liu et al. (2020) have provided evidence that Brusatol, functioning as an Nrf2 inhibitor, demonstrated inhibitory effects in human NSCLC by instigating ferroptosis through modulation of the FOCAD-FAK signaling pathway. The upregulation of FOCAD facilitated the activation of FAK. Moreover, Brusatol effectively managed NSCLC by augmenting the tricarboxylic acid cycle as well as Complex I activity within the mitochondrial electron transport chain, consequently enhancing the susceptibility of NSCLC cells to ferroptosis induced by cysteine deprivation. Brusatol suppresses colony formation, migration, and invasive capacity of cancer cells.
2.4.3 Hedyotis diffusa Willd. [Rubiaceae; Oldenlandia diffusa (Willd.) Roxb.]
Hedyotis diffusa Willd. [Rubiaceae; Oldenlandia diffusa (Willd.) Roxb.] (HDW) is the dried whole botanical drug of Hedyotis diffusa, belonging to the Rubiaceae family. Its antitumor active metabolites include Asperuloside, Quercetin, and β-sitosterol (Han et al., 2020). The VDAC is a channel protein situated in the outer membrane of the mitochondria, facilitating the movement of ions and metabolites between the cytoplasm and mitochondria. Activating VDAC facilitates the release of substantial intramitochondrial ROS, subsequently increasing intracellular levels of ROS and promoting ferroptosis (Maldonado et al., 2013; DeHart et al., 2018; Lipper et al., 2019). Bcl2/Bcl-xl inhibits the activation of VDAC2/3, exerting an inhibitory effect on cell death. Conversely, Bax/Bak promotes the activation of VDAC2/3 channels, as well as the release of cytochrome C and ROS, thereby promoting ferroptosis (Tsujimoto and Shimizu, 2000). Huang (Huang et al., 2022) observed that hedyotis diffusa injection (HDI) activated VDAC2/3 channels by inhibiting Bcl2 and promoting Bax, leading to the release of significant amounts of intramitochondrial ROS. This elevation in intracellular ROS levels induced ferroptosis in lung adenocarcinoma cells. Additionally, HDI-induced ferroptosis in lung adenocarcinoma cells was found to be independent of the GPX4 and PUFA-PLS pathways. In vitro experiments showed that HDI could inhibit the viability of lung adenocarcinoma cells (H23, A549 and H460 cells) and induce ferroptosis. In addition, this study investigated only HDI, without assessing the role of monomers; therefore, the effective monomeric components for an in-depth study of the mechanism underlying ferroptosis to be isolated.
3 Botanical drugs and isolated metabolites in lung cancer clinical trials
The process of clinical trials is imperative for the approval and subsequent introduction of drugs into the market. Only when the efficacy and safety of a drug are established through such trials can it be considered for clinical use. Several botanical drugs and isolated metabolites are currently undergoing clinical trials for the treatment of lung cancer.
3.1 Artesunate [Compositae]
To assess the effectiveness and safety of chemotherapy using artesunate in conjunction with the NP regimen (a vinorelbine and cisplatin chemotherapy regimen) for advanced lung cancer, 120 patients with advanced NSCLC were allocated into a chemotherapy group (control group, n = 60) as well as an Artemisinin combined chemotherapy group (experimental group, n = 60) in random. The control group underwent NP regimen, comprising 25 mg/m2 vinorelbine once daily via intravenous injection on the 1st and 8th day along with 25 mg/m2 cisplatin once daily via intravenous drip from the 2nd to 4th day. The experimental group received the standard NP therapy along with 120 mg Artesunate once daily via intravenous injection from the 1st to the 8th day, for 8 days. No less than two 21-day cycles of treatment were achieved. The primary endpoints examined included the short-term survival rate, DCR, TTP, MST, as well as 1-year survival rate; meanwhile, the toxicity and safety were also evaluated. The results demonstrated that the incorporation of Artesunate alongside NP led to the enhanced short-term survival rates along with the prolonged TTP among patients with advanced NSCLC. These findings suggest that Artesunate has an inhibitory effect on NSCLC (Zhang et al., 2008).
Upon reviewing Clinical Trials (https://clinicaltrials.gov/), it was noted that C. longa L. (NCT02321293) have been registered in clinical trials for lung cancer. Although these treatments remain in the research phase, the preliminary results appear promising (Table 2).

Table 2. Clinical trial in selected botanical drugs and isolated metabolites regulate ferroptosis in lung cancer.
4 Discussion and conclusion
The induction of ferroptosis in lung cancer cells by botanical drugs and isolated metabolites represents a burgeoning and promising area of oncological pharmacology. Our review of the literature has highlighted that various natural compounds demonstrate a capacity to induce ferroptosis through diverse mechanisms. By using lung cancer cell lines and animal models, we can assess whether ferroptosis inducers can reduce tumor growth and improve survival rates. This is crucial for determining the potential of these agents and justifying further development.
Despite promising preclinical data, the current research landscape is fraught with several limitations and challenges. A primary concern is the scarcity of comprehensive in vivo studies and clinical trials. Most current evidence is derived from in vitro studies using lung cancer cell lines, which, while informative, do not fully replicate the complexity of tumor biology within an organism. Furthermore, the pharmacokinetics, bioavailability, and potential toxicity of these botanical compounds in humans are inadequately understood. The absence of standardized protocols for extraction, purification, and dosing further complicates the translation of in vitro findings into clinical practice. Moreover, the mechanisms by which these compounds induce ferroptosis remain incompletely understood. Although key pathways and targets have been identified, the intricate network of interactions and regulatory mechanisms remains poorly elucidated. This gap in knowledge hinders future clinical applications.
It is recommended that future studies investigate the potential synergistic effects of botanical drugs in conjunction with existing therapies, such as chemotherapy, immunotherapy and targeted therapy. Furthermore, the establishment of standardised protocols for the extraction, purification and characterisation of botanical drugs is essential for ensuring the reproducibility and comparability of research findings. To ensure the consistency, safety, and efficacy of these compounds, rigorous quality control measures are imperative. Following successful preclinical evaluations, clinical trials are crucial to determine the therapeutic potential, optimal dosing regimens, and safety in human patients. As the old adage goes, “It is always dark before the dawn.” Despite the lengthy and challenging road ahead, we are optimistic that botanical drugs-induced ferroptosis may pave the way for innovative new strategies in the treatment of lung cancer.
Author contributions
XT: Formal Analysis, Investigation, Visualization, Writing–original draft. KF: Conceptualization, Data curation, Investigation, Writing–original draft. XH: Conceptualization, Visualization, Writing–original draft. HZ: Investigation, Visualization, Writing–original draft. NS: Software, Visualization, Writing–original draft. JL: Data curation, Investigation, Writing–original draft. YB: Investigation, Software, Writing–original draft. SH: Formal Analysis, Project administration, Writing–review and editing. JL: Conceptualization, Methodology, Project administration, Supervision, Writing–review and editing.
Funding
The author(s) declare that financial support was received for the research, authorship, and/or publication of this article. This study was financially supported by National Natural Science Foundation of China (Nos 82204692 and 81960513), the Natural Science Foundation of Guizhou Provincial Scientific and Technology Department Grant [(2022)626], the Science and Technology Innovation Talent Team Training Program of Zunyi [No. 2023 (4)], the College Student Innovation and Entrepreneurship Training Program of Zunyi Medical University (No. ZYDC202202044).
Acknowledgments
Figures in the article were drawn using BioRender.com.
Conflict of interest
The authors declare that the research was conducted in the absence of any commercial or financial relationships that could be construed as a potential conflict of interest.
Publisher’s note
All claims expressed in this article are solely those of the authors and do not necessarily represent those of their affiliated organizations, or those of the publisher, the editors and the reviewers. Any product that may be evaluated in this article, or claim that may be made by its manufacturer, is not guaranteed or endorsed by the publisher.
Supplementary material
The Supplementary Material for this article can be found online at: https://www.frontiersin.org/articles/10.3389/fphar.2024.1430561/full#supplementary-material
References
Barta, J. A., Powell, C. A., and Wisnivesky, J. P. (2019). Global epidemiology of lung cancer. Ann. Glob. Health 85, 8. doi:10.5334/aogh.2419
Bell, H. N., Stockwell, B. R., and Zou, W. (2024). Ironing out the role of ferroptosis in immunity. Immunity 57, 941–956. doi:10.1016/j.immuni.2024.03.019
Bhinu, V. S., Schäfer, U. A., Li, R., Huang, J., and Hannoufa, A. (2009). Targeted modulation of sinapine biosynthesis pathway for seed quality improvement in Brassica napus. Transgenic Res. 18, 31–44. doi:10.1007/s11248-008-9194-3
Billesbølle, C. B., Azumaya, C. M., Kretsch, R. C., Powers, A. S., Gonen, S., Schneider, S., et al. (2020). Structure of hepcidin-bound ferroportin reveals iron homeostatic mechanisms. Nature 586, 807–811. doi:10.1038/s41586-020-2668-z
Bley, K., Boorman, G., Mohammad, B., McKenzie, D., and Babbar, S. (2012). A comprehensive review of the carcinogenic and anticarcinogenic potential of capsaicin. Toxicol. Pathol. 40, 847–873. doi:10.1177/0192623312444471
Boulghobra, D., Grillet, P.-E., Laguerre, M., Tenon, M., Fauconnier, J., Fança-Berthon, P., et al. (2020). Sinapine, but not sinapic acid, counteracts mitochondrial oxidative stress in cardiomyocytes. Redox Biol. 34, 101554. doi:10.1016/j.redox.2020.101554
Brand, F., Förster, A., Christians, A., Bucher, M., Thomé, C. M., Raab, M. S., et al. (2020). FOCAD loss impacts microtubule assembly, G2/M progression and patient survival in astrocytic gliomas. Acta Neuropathol. 139, 175–192. doi:10.1007/s00401-019-02067-z
Brockschmidt, A., Trost, D., Peterziel, H., Zimmermann, K., Ehrler, M., Grassmann, H., et al. (2012). KIAA1797/FOCAD encodes a novel focal adhesion protein with tumour suppressor function in gliomas. Brain 135, 1027–1041. doi:10.1093/brain/aws045
Brown, C. W., Amante, J. J., Chhoy, P., Elaimy, A. L., Liu, H., Zhu, L. J., et al. (2019). Prominin2 drives ferroptosis resistance by stimulating iron export. Dev. Cell 51, 575–586.e4. doi:10.1016/j.devcel.2019.10.007
Cannon, D. M., Mehta, M. P., Adkison, J. B., Khuntia, D., Traynor, A. M., Tomé, W. A., et al. (2013). Dose-limiting toxicity after hypofractionated dose-escalated radiotherapy in non–small-cell lung cancer. J. Clin. Oncol. 31, 4343–4348. doi:10.1200/jco.2013.51.5353
Chakraborty, S., Adhikary, A., Mazumdar, M., Mukherjee, S., Bhattacharjee, P., Guha, D., et al. (2014). Capsaicin-induced activation of p53-SMAR1 auto-regulatory loop down-regulates VEGF in non-small cell lung cancer to restrain angiogenesis. PLoS One 9, e99743. doi:10.1371/journal.pone.0099743
Chen, P., Wu, Q., Feng, J., Yan, L., Sun, Y., Liu, S., et al. (2020). Erianin, a novel dibenzyl compound in Dendrobium extract, inhibits lung cancer cell growth and migration via calcium/calmodulin-dependent ferroptosis. Signal Transduct. Target Ther. 5, 51. doi:10.1038/s41392-020-0149-3
Clarke, J. D., Dashwood, R. H., and Ho, E. (2008). Multi-targeted prevention of cancer by sulforaphane. Cancer Lett. 269, 291–304. doi:10.1016/j.canlet.2008.04.018
Conrad, M., and Pratt, D. A. (2019). The chemical basis of ferroptosis. Nat. Chem. Biol. 15, 1137–1147. doi:10.1038/s41589-019-0408-1
Conrad, M., and Sato, H. (2012). The oxidative stress-inducible cystine/glutamate antiporter, system x (c) (-): cystine supplier and beyond. Amino Acids 42, 231–246. doi:10.1007/s00726-011-0867-5
Daher, B., Parks, S. K., Durivault, J., Cormerais, Y., Baidarjad, H., Tambutte, E., et al. (2019). Genetic ablation of the cystine transporter xCT in PDAC cells inhibits mTORC1, growth, survival, and tumor formation via nutrient and oxidative stresses. Cancer Res. 79, 3877–3890. doi:10.1158/0008-5472.Can-18-3855
DeHart, D. N., Fang, D., Heslop, K., Li, L., Lemasters, J. J., and Maldonado, E. N. (2018). Opening of voltage dependent anion channels promotes reactive oxygen species generation, mitochondrial dysfunction and cell death in cancer cells. Biochem. Pharmacol. 148, 155–162. doi:10.1016/j.bcp.2017.12.022
de Lima, R. M. T., Dos Reis, A. C., de Menezes, A. P. M., Santos, J. V. O., Filho, J., Ferreira, J. R. O., et al. (2018). Protective and therapeutic potential of ginger (Zingiber officinale) extract and [6]-gingerol in cancer: a comprehensive review. Phytother. Res. 32, 1885–1907. doi:10.1002/ptr.6134
Dixon, S. J., Lemberg, K. M., Lamprecht, M. R., Skouta, R., Zaitsev, E. M., Gleason, C. E., et al. (2012). Ferroptosis: an iron-dependent form of nonapoptotic cell death. Cell 149, 1060–1072. doi:10.1016/j.cell.2012.03.042
Dixon, S. J., Winter, G. E., Musavi, L. S., Lee, E. D., Snijder, B., Rebsamen, M., et al. (2015). Human haploid cell genetics reveals roles for lipid metabolism genes in nonapoptotic cell death. ACS Chem. Biol. 10, 1604–1609. doi:10.1021/acschembio.5b00245
Dodson, M., Castro-Portuguez, R., and Zhang, D. D. (2019). NRF2 plays a critical role in mitigating lipid peroxidation and ferroptosis. Redox Biol. 23, 101107. doi:10.1016/j.redox.2019.101107
Doll, S., Proneth, B., Tyurina, Y. Y., Panzilius, E., Kobayashi, S., Ingold, I., et al. (2017). ACSL4 dictates ferroptosis sensitivity by shaping cellular lipid composition. Nat. Chem. Biol. 13, 91–98. doi:10.1038/nchembio.2239
Elgendy, S. M., Alyammahi, S. K., Alhamad, D. W., Abdin, S. M., and Omar, H. A. (2020). Ferroptosis: an emerging approach for targeting cancer stem cells and drug resistance. Crit. Rev. Oncol. Hematol. 155, 103095. doi:10.1016/j.critrevonc.2020.103095
Esterbauer, H., Schaur, R. J., and Zollner, H. (1991). Chemistry and biochemistry of 4-hydroxynonenal, malonaldehyde and related aldehydes. Free Radic. Biol. Med. 11, 81–128. doi:10.1016/0891-5849(91)90192-6
Forman, H. J., Zhang, H., and Rinna, A. (2009). Glutathione: overview of its protective roles, measurement, and biosynthesis. Mol. Asp. Med. 30, 1–12. doi:10.1016/j.mam.2008.08.006
Friedman, J. R., and Nunnari, J. (2014). Mitochondrial form and function. Nature 505, 335–343. doi:10.1038/nature12985
Friedmann Angeli, J. P., Krysko, D. V., and Conrad, M. (2019). Ferroptosis at the crossroads of cancer-acquired drug resistance and immune evasion. Nat. Rev. Cancer 19, 405–414. doi:10.1038/s41568-019-0149-1
Gan, B. (2021). Mitochondrial regulation of ferroptosis. J. Cell Biol. 220, e202105043. doi:10.1083/jcb.202105043
Gao, M., Monian, P., Quadri, N., Ramasamy, R., and Jiang, X. (2015). Glutaminolysis and transferrin regulate ferroptosis. Mol. Cell 59, 298–308. doi:10.1016/j.molcel.2015.06.011
Gao, M., Yi, J., Zhu, J., Minikes, A. M., Monian, P., Thompson, C. B., et al. (2019). Role of mitochondria in ferroptosis. Mol. Cell 73, 354–363.e3. doi:10.1016/j.molcel.2018.10.042
Gaschler, M. M., and Stockwell, B. R. (2017). Lipid peroxidation in cell death. Biochem. Biophys. Res. Commun. 482, 419–425. doi:10.1016/j.bbrc.2016.10.086
Han, X., Zhang, X., Wang, Q., Wang, L., and Yu, S. (2020). Antitumor potential of Hedyotis diffusa Willd: a systematic review of bioactive constituents and underlying molecular mechanisms. Biomed. Pharmacother. 130, 110735. doi:10.1016/j.biopha.2020.110735
Heldt, H.-W., and Piechulla, B. (2011). “'15 - lipids are membrane constituents and function as carbon stores,” in Plant biochemistry. Editors H.-W. Heldt, and B. Piechulla Fourth Edition (San Diego: Academic Press). doi:10.1016/B978-0-12-384986-1.00015-6
Hoter, A., El-Sabban, M., and Naim, H. (2018). The HSP90 family: structure, regulation, function, and implications in health and disease. Int. J. Mol. Sci. 19, 2560. doi:10.3390/ijms19092560
Hou, W., Xie, Y., Song, X., Sun, X., Lotze, M. T., Zeh Iii, H. J., et al. (2016). Autophagy promotes ferroptosis by degradation of ferritin. Autophagy 12, 1425–1428. doi:10.1080/15548627.2016.1187366
Huang, F., Pang, J., Xu, L., Niu, W., Zhang, Y., Li, S., et al. (2022). Hedyotis diffusa injection induces ferroptosis via the Bax/Bcl2/VDAC2/3 axis in lung adenocarcinoma. Phytomedicine 104, 154319. doi:10.1016/j.phymed.2022.154319
Huang, S. P., Chen, J. C., Wu, C. C., Chen, C. T., Tang, N. Y., Ho, Y. T., et al. (2009). Capsaicin-induced apoptosis in human hepatoma HepG2 cells. Anticancer Res. 29, 165–174. doi:10.1186/1423-0127-17-35
Iida, Y., Okamoto-Κatsuyama, M., Maruoka, S., Mizumura, K., Shimizu, T., Shikano, S., et al. (2021). Effective ferroptotic small-cell lung cancer cell death from SLC7A11 inhibition by sulforaphane. Oncol. Lett. 21, 71. doi:10.3892/ol.2020.12332
Ishii, T., Itoh, K., Takahashi, S., Sato, H., Yanagawa, T., Katoh, Y., et al. (2000). Transcription factor Nrf2 coordinately regulates a group of oxidative stress-inducible genes in macrophages. J. Biol. Chem. 275, 16023–16029. doi:10.1074/jbc.275.21.16023
Islam, A., Hsieh, P. F., Liu, P. F., Chou, J. C., Liao, J. W., Hsieh, M. K., et al. (2021). Capsaicin exerts therapeutic effects by targeting tNOX-SIRT1 axis and augmenting ROS-dependent autophagy in melanoma cancer cells. Am. J. Cancer Res. 11, 4199–4219.
Jiang, H., Li, M., Du, K., Ma, C., Cheng, Y., Wang, S., et al. (2021a). Traditional Chinese medicine for adjuvant treatment of breast cancer: taohong siwu decoction. Chin. Med. 16, 129. doi:10.1186/s13020-021-00539-7
Jiang, X., Stockwell, B. R., and Conrad, M. (2021b). Ferroptosis: mechanisms, biology and role in disease. Nat. Rev. Mol. Cell Biol. 22, 266–282. doi:10.1038/s41580-020-00324-8
Jiaqi, L., Siqing, H., qin, W., di, Z., bei, Z., and jialin, Y. (2023). Andrographolide promoted ferroptosis to repress the development of non-small cell lung cancer through activation of the mitochondrial dysfunction. Phytomedicine 109, 154601. doi:10.1016/j.phymed.2022.154601
Jyotsana, N., Ta, K. T., and DelGiorno, K. E. (2022). The role of cystine/glutamate antiporter slc7a11/xCT in the pathophysiology of cancer. Front. Oncol. 12, 858462. doi:10.3389/fonc.2022.858462
Kagan, V. E., Mao, G., Qu, F., Angeli, J. P., Doll, S., Croix, C. S., et al. (2017). Oxidized arachidonic and adrenic PEs navigate cells to ferroptosis. Nat. Chem. Biol. 13, 81–90. doi:10.1038/nchembio.2238
Kawabata, H. (2019). Transferrin and transferrin receptors update. Free Radic. Biol. Med. 133, 46–54. doi:10.1016/j.freeradbiomed.2018.06.037
Kenny, E. M., Fidan, E., Yang, Q., Anthonymuthu, T. S., New, L. A., Meyer, E. A., et al. (2019). Ferroptosis contributes to neuronal death and functional outcome after traumatic brain injury. Crit. Care Med. 47, 410–418. doi:10.1097/ccm.0000000000003555
Kerins, M. J., and Ooi, A. (2018). The roles of NRF2 in modulating cellular iron homeostasis. Antioxid. Redox Signal 29, 1756–1773. doi:10.1089/ars.2017.7176
Koch, W., Kukula-Koch, W., Marzec, Z., Kasperek, E., Wyszogrodzka-Koma, L., Szwerc, W., et al. (2017). Application of chromatographic and spectroscopic methods towards the quality assessment of ginger (zingiber officinale) rhizomes from ecological plantations. Int. J. Mol. Sci. 18, 452. doi:10.3390/ijms18020452
Koppula, P., Zhuang, L., and Gan, B. (2021a). Cystine transporter SLC7A11/xCT in cancer: ferroptosis, nutrient dependency, and cancer therapy. Protein Cell 12, 599–620. doi:10.1007/s13238-020-00789-5
Koppula, P., Zhuang, L., and Gan, B. (2021b). Cytochrome P450 reductase (POR) as a ferroptosis fuel. Protein Cell 12, 675–679. doi:10.1007/s13238-021-00823-0
Kraft, V. A. N., Bezjian, C. T., Pfeiffer, S., Ringelstetter, L., Müller, C., Zandkarimi, F., et al. (2020). GTP cyclohydrolase 1/tetrahydrobiopterin counteract ferroptosis through lipid remodeling. ACS Cent. Sci. 6, 41–53. doi:10.1021/acscentsci.9b01063
Lee, H., Zandkarimi, F., Zhang, Y., Meena, J. K., Kim, J., Zhuang, L., et al. (2020a). Energy-stress-mediated AMPK activation inhibits ferroptosis. Nat. Cell Biol. 22, 225–234. doi:10.1038/s41556-020-0461-8
Lee, H., Zhuang, L., and Gan, B. (2020b). Energy stress inhibits ferroptosis via AMPK. Mol. Cell Oncol. 7, 1761242. doi:10.1080/23723556.2020.1761242
Lei, G., Zhuang, L., and Gan, B. (2022). Targeting ferroptosis as a vulnerability in cancer. Nat. Rev. Cancer 22, 381–396. doi:10.1038/s41568-022-00459-0
Lei, G., Zhuang, L., and Gan, B. (2024). The roles of ferroptosis in cancer: tumor suppression, tumor microenvironment, and therapeutic interventions. Cancer Cell 42, 513–534. doi:10.1016/j.ccell.2024.03.011
Lewerenz, J., Hewett, S. J., Huang, Y., Lambros, M., Gout, P. W., Kalivas, P. W., et al. (2013). The cystine/glutamate antiporter system x(c)(-) in health and disease: from molecular mechanisms to novel therapeutic opportunities. Antioxid. Redox Signal 18, 522–555. doi:10.1089/ars.2011.4391
Li, C., Dong, X., Du, W., Shi, X., Chen, K., Zhang, W., et al. (2020). LKB1-AMPK axis negatively regulates ferroptosis by inhibiting fatty acid synthesis. Signal Transduct. Target Ther. 5, 187. doi:10.1038/s41392-020-00297-2
Lim, J. K. M., Delaidelli, A., Minaker, S. W., Zhang, H.-F., Colovic, M., Yang, H., et al. (2019). Cystine/glutamate antiporter xCT (SLC7A11) facilitates oncogenic RAS transformation by preserving intracellular redox balance. Proc. Natl. Acad. Sci. U. S. A. 116, 9433–9442. doi:10.1073/pnas.1821323116
Lin, J. J., and Shaw, A. T. (2016). Resisting resistance: targeted therapies in lung cancer. Trends Cancer 2, 350–364. doi:10.1016/j.trecan.2016.05.010
Lipper, C. H., Stofleth, J. T., Bai, F., Sohn, Y. S., Roy, S., Mittler, R., et al. (2019). Redox-dependent gating of VDAC by mitoNEET. Proc. Natl. Acad. Sci. U. S. A. 116, 19924–19929. doi:10.1073/pnas.1908271116
Liu, J., Jiang, G., He, P., Du, X., Hu, Z., and Li, F. (2022c). Mechanism of ferroptosis in traditional Chinese medicine for clinical treatment: a review. Front. Pharmacol. 13, 1108836. doi:10.3389/fphar.2022.1108836
Liu, P., Wu, D., Duan, J., Xiao, H., Zhou, Y., Zhao, L., et al. (2020). NRF2 regulates the sensitivity of human NSCLC cells to cystine deprivation-induced ferroptosis via FOCAD-FAK signaling pathway. Redox Biol. 37, 101702. doi:10.1016/j.redox.2020.101702
Liu, X.-Y., Wei, D.-G., and Li, R.-S. (2022). Capsaicin induces ferroptosis of NSCLC by regulating SLC7A11/GPX4 signaling in vitro. Sci. Rep. 12, 11996. doi:10.1038/s41598-022-16372-3
Liu, Y., Wan, Y., Jiang, Y., Zhang, L., and Cheng, W. (2023). GPX4: the hub of lipid oxidation, ferroptosis, disease and treatment. Biochim. Biophys. Acta Rev. Cancer 1878, 188890. doi:10.1016/j.bbcan.2023.188890
Lou, J.-S., Zhao, L.-P., Huang, Z.-H., Chen, X.-Y., Xu, J.-T., Tai, W. C.-S., et al. (2021). Ginkgetin derived from Ginkgo biloba leaves enhances the therapeutic effect of cisplatin via ferroptosis-mediated disruption of the Nrf2/HO-1 axis in EGFR wild-type non-small-cell lung cancer. Phytomedicine 80, 153370. doi:10.1016/j.phymed.2020.153370
Lu, Y., Yang, Q., Su, Y., Ji, Y., Li, G., Yang, X., et al. (2021). MYCN mediates TFRC-dependent ferroptosis and reveals vulnerabilities in neuroblastoma. Cell Death Dis. 12, 511. doi:10.1038/s41419-021-03790-w
Luo, X. J., Peng, J., and Li, Y. J. (2011). Recent advances in the study on capsaicinoids and capsinoids. Eur. J. Pharmacol. 650, 1–7. doi:10.1016/j.ejphar.2010.09.074
Maldonado, E. N., Sheldon, K. L., DeHart, D. N., Patnaik, J., Manevich, Y., Townsend, D. M., et al. (2013). Voltage-dependent anion channels modulate mitochondrial metabolism in cancer cells: regulation by free tubulin and erastin. J. Biol. Chem. 288, 11920–11929. doi:10.1074/jbc.M112.433847
Mancias, J. D., Wang, X., Gygi, S. P., Harper, J. W., and Kimmelman, A. C. (2014). Quantitative proteomics identifies NCOA4 as the cargo receptor mediating ferritinophagy. Nature 509, 105–109. doi:10.1038/nature13148
Mao, C., Liu, X., Zhang, Y., Lei, G., Yan, Y., Lee, H., et al. (2021). DHODH-mediated ferroptosis defence is a targetable vulnerability in cancer. Nature 593, 586–590. doi:10.1038/s41586-021-03539-7
Murphy, M. P. (2009). How mitochondria produce reactive oxygen species. Biochem. J. 417, 1–13. doi:10.1042/bj20081386
Osburn, W. O., Wakabayashi, N., Misra, V., Nilles, T., Biswal, S., Trush, M. A., et al. (2006). Nrf2 regulates an adaptive response protecting against oxidative damage following diquat-mediated formation of superoxide anion. Arch. Biochem. Biophys. 454, 7–15. doi:10.1016/j.abb.2006.08.005
Oudit, G. Y., Sun, H., Trivieri, M. G., Koch, S. E., Dawood, F., Ackerley, C., et al. (2003). L-type Ca2+ channels provide a major pathway for iron entry into cardiomyocytes in iron-overload cardiomyopathy. Nat. Med. 9, 1187–1194. doi:10.1038/nm920
Pohl, F., Teixeira-Castro, A., Costa, M. D., Lindsay, V., Fiúza-Fernandes, J., Goua, M., et al. (2019). GST-4-Dependent suppression of neurodegeneration in C. elegans models of Parkinson's and machado-joseph disease by rapeseed pomace extract supplementation. Front. Neurosci. 13, 1091. doi:10.3389/fnins.2019.01091
Reed, J. C., and Pellecchia, M. (2012). Ironing out cell death mechanisms. Cell 149, 963–965. doi:10.1016/j.cell.2012.05.009
Seibt, T. M., Proneth, B., and Conrad, M. (2019). Role of GPX4 in ferroptosis and its pharmacological implication. Free Radic. Biol. Med. 133, 144–152. doi:10.1016/j.freeradbiomed.2018.09.014
Shao, M., Jiang, Q., Shen, C., Liu, Z., and Qiu, L. (2022). Sinapine induced ferroptosis in non-small cell lung cancer cells by upregulating transferrin/transferrin receptor and downregulating SLC7A11. Gene 827, 146460. doi:10.1016/j.gene.2022.146460
Shi, Y., Shi, X., Zhao, M., Chang, M., Ma, S., and Zhang, Y. (2023). Ferroptosis: a new mechanism of traditional Chinese medicine compounds for treating acute kidney injury. Biomed. Pharmacother. 163, 114849. doi:10.1016/j.biopha.2023.114849
Shimada, K., Skouta, R., Kaplan, A., Yang, W. S., Hayano, M., Dixon, S. J., et al. (2016). Global survey of cell death mechanisms reveals metabolic regulation of ferroptosis. Nat. Chem. Biol. 12, 497–503. doi:10.1038/nchembio.2079
Shiratori, A., Okumura, K., Nogami, M., Taguchi, H., Onozaki, T., Inoue, T., et al. (1995). Assignment of the 49-kDa (PRIM1) and 58-kDa (PRIM2A and PRIM2B) subunit genes of the human DNA primase to chromosome bands 1q44 and 6p11.1-p12. Genomics 28, 350–353. doi:10.1006/geno.1995.1155
Soula, M., Weber, R. A., Zilka, O., Alwaseem, H., La, K., Yen, F., et al. (2020). Metabolic determinants of cancer cell sensitivity to canonical ferroptosis inducers. Nat. Chem. Biol. 16, 1351–1360. doi:10.1038/s41589-020-0613-y
Tang, X., Ding, H., Liang, M., Chen, X., Yan, Y., Wan, N., et al. (2021). Curcumin induces ferroptosis in non-small-cell lung cancer via activating autophagy. Thorac. Cancer 12, 1219–1230. doi:10.1111/1759-7714.13904
Thöny, B., Auerbach, G., and Blau, N. (2000). Tetrahydrobiopterin biosynthesis, regeneration and functions. Biochem. J. 347 (Pt 1), 1–16. doi:10.1042/bj3470001
Tomeh, M. A., Hadianamrei, R., and Zhao, X. (2019). A review of curcumin and its derivatives as anticancer agents. Int. J. Mol. Sci. 20, 1033. doi:10.3390/ijms20051033
Torti, S. V., and Torti, F. M. (2013). Iron and cancer: more ore to be mined. Nat. Rev. Cancer 13, 342–355. doi:10.1038/nrc3495
Tsai, Y., Xia, C., and Sun, Z. (2020). The inhibitory effect of 6-gingerol on ubiquitin-specific peptidase 14 enhances autophagy-dependent ferroptosis and anti-tumor in vivo and in vitro. Front. Pharmacol. 11, 598555. doi:10.3389/fphar.2020.598555
Tsujimoto, Y., and Shimizu, S. (2000). VDAC regulation by the Bcl-2 family of proteins. Cell Death Differ. 7, 1174–1181. doi:10.1038/sj.cdd.4400780
Ursini, F., Maiorino, M., and Gregolin, C. (1985). The selenoenzyme phospholipid hydroperoxide glutathione peroxidase. Biochim. Biophys. Acta 839, 62–70. doi:10.1016/0304-4165(85)90182-5
Vasan, K., Werner, M., and Chandel, N. S. (2020). Mitochondrial metabolism as a target for cancer therapy. Cell Metab. 32, 341–352. doi:10.1016/j.cmet.2020.06.019
Venier, N. A., Yamamoto, T., Sugar, L. M., Adomat, H., Fleshner, N. E., Klotz, L. H., et al. (2015). Capsaicin reduces the metastatic burden in the transgenic adenocarcinoma of the mouse prostate model. Prostate 75, 1300–1311. doi:10.1002/pros.23013
Wang, Y., Hu, J., Fleishman, J. S., Li, Y., Ren, Z., Wang, J., et al. (2024). Inducing ferroptosis by traditional medicines: a novel approach to reverse chemoresistance in lung cancer. Front. Pharmacol. 15, 1290183. doi:10.3389/fphar.2024.1290183
Wang, Y., Hu, J., Wu, S., Fleishman, J. S., Li, Y., Xu, Y., et al. (2023). Targeting epigenetic and posttranslational modifications regulating ferroptosis for the treatment of diseases. Signal Transduct. Target Ther. 8, 449. doi:10.1038/s41392-023-01720-0
Wenzel, S. E., Tyurina, Y. Y., Zhao, J., St Croix, C. M., Dar, H. H., Mao, G., et al. (2017). PEBP1 wardens ferroptosis by enabling lipoxygenase generation of lipid death signals. Cell 171, 628–641.e26. doi:10.1016/j.cell.2017.09.044
Wu, C.-Y., Yang, Y.-H., Lin, Y.-S., Chang, G.-H., Tsai, M.-S., Hsu, C.-M., et al. (2021). Dihydroisotanshinone I induced ferroptosis and apoptosis of lung cancer cells. Biomed. Pharmacother. 139, 111585. doi:10.1016/j.biopha.2021.111585
Xie, Y., Kang, R., Klionsky, D. J., and Tang, D. (2023). GPX4 in cell death, autophagy, and disease. Autophagy 19, 2621–2638. doi:10.1080/15548627.2023.2218764
Xing, N., Du, Q., Guo, S., Xiang, G., Zhang, Y., Meng, X., et al. (2023). Ferroptosis in lung cancer: a novel pathway regulating cell death and a promising target for drug therapy. Cell Death Discov. 9, 110. doi:10.1038/s41420-023-01407-z
Xu, D., Shan, B., Sun, H., Xiao, J., Zhu, K., Xie, X., et al. (2016). USP14 regulates autophagy by suppressing K63 ubiquitination of Beclin 1. Genes Dev. 30, 1718–1730. doi:10.1101/gad.285122.116
Xu, R., Wu, J., Luo, Y., Wang, Y., Tian, J., Teng, W., et al. (2022). Sanguinarine represses the growth and metastasis of non-small cell lung cancer by facilitating ferroptosis. Curr. Pharm. Des. 28, 760–768. doi:10.2174/1381612828666220217124542
Yan, B., Ai, Y., Sun, Q., Ma, Y., Cao, Y., Wang, J., et al. (2021). Membrane damage during ferroptosis is caused by oxidation of phospholipids catalyzed by the oxidoreductases POR and CYB5R1. Mol. Cell. 81, 355–369.e10. doi:10.1016/j.molcel.2020.11.024
Yanatori, I., and Kishi, F. (2019). DMT1 and iron transport. Free Radic. Biol. Med. 133, 55–63. doi:10.1016/j.freeradbiomed.2018.07.020
Yang, W. S., Kim, K. J., Gaschler, M. M., Patel, M., Shchepinov, M. S., and Stockwell, B. R. (2016). Peroxidation of polyunsaturated fatty acids by lipoxygenases drives ferroptosis. Proc. Natl. Acad. Sci. U. S. A. 113, E4966–E4975. doi:10.1073/pnas.1603244113
Yang, W. S., SriRamaratnam, R., Welsch, M. E., Shimada, K., Skouta, R., Viswanathan, V. S., et al. (2014). Regulation of ferroptotic cancer cell death by GPX4. Cell 156, 317–331. doi:10.1016/j.cell.2013.12.010
Yang, W. S., and Stockwell, B. R. (2008). Synthetic lethal screening identifies compounds activating iron-dependent, nonapoptotic cell death in oncogenic-RAS-harboring cancer cells. Chem. Biol. 15, 234–245. doi:10.1016/j.chembiol.2008.02.010
Yang, W. S., and Stockwell, B. R. (2016). Ferroptosis: death by lipid peroxidation. Trends Cell Biol. 26, 165–176. doi:10.1016/j.tcb.2015.10.014
Yatsula, B., Galvao, C., McCrann, M., and Perkins, A. S. (2006). Assessment of F-MuLV-induced tumorigenesis reveals new candidate tumor genes including Pecam1, St7, and Prim2. Leukemia 20, 162–165. doi:10.1038/sj.leu.2404034
Yin, L., Liu, P., Jin, Y., Ning, Z., Yang, Y., and Gao, H. (2022). Ferroptosis-related small-molecule compounds in cancer therapy: strategies and applications. Eur. J. Med. Chem. 244, 114861. doi:10.1016/j.ejmech.2022.114861
Youn, S. H., Lee, S. M., Han, C.-K., In, G., Park, C.-K., and Hyun, S. H. (2020). Immune activity of polysaccharide fractions isolated from Korean red ginseng. Molecules 25, 3569. doi:10.3390/molecules25163569
Yu, X.-Q., Shang, X.-Y., Huang, X.-X., Yao, G.-D., and Song, S.-J. (2020). Brusatol: a potential anti-tumor quassinoid from Brucea javanica. Chin. Herb. Med. 12, 359–366. doi:10.1016/j.chmed.2020.05.007
Yuan, B., Liao, F., Shi, Z. Z., Ren, Y., Deng, X. L., Yang, T. T., et al. (2020). Dihydroartemisinin inhibits the proliferation, colony formation and induces ferroptosis of lung cancer cells by inhibiting PRIM2/slc7a11 Axis. Onco Targets Ther. 13, 10829–10840. doi:10.2147/OTT.S248492
Zhai, F. G., Liang, Q. C., Wu, Y. Y., Liu, J. Q., and Liu, J. W. (2022). Red ginseng polysaccharide exhibits anticancer activity through GPX4 downregulation-induced ferroptosis. Pharm. Biol. 60, 909–914. doi:10.1080/13880209.2022.2066139
Zhang, F., Ma, N., Gao, Y. F., Sun, L. L., and Zhang, J. G. (2017a). Therapeutic effects of 6-gingerol, 8-gingerol, and 10-gingerol on dextran sulfate sodium-induced acute ulcerative colitis in rats. Phytother. Res. 31, 1427–1432. doi:10.1002/ptr.5871
Zhang, Q., Xia, Y., Wang, F., Yang, D., and Liang, Z. (2024). Induction of ferroptosis by natural products in non-small cell lung cancer: a comprehensive systematic review. Front. Pharmacol. 15, 1385565. doi:10.3389/fphar.2024.1385565
Zhang, Q., Yi, H., Yao, H., Lu, L., He, G., Wu, M., et al. (2021). Artemisinin derivatives inhibit non-small cell lung cancer cells through induction of ROS-dependent apoptosis/ferroptosis. J. Cancer 12, 4075–4085. doi:10.7150/jca.57054
Zhang, R., Pan, T., Xiang, Y., Zhang, M., Xie, H., Liang, Z., et al. (2022). Curcumenol triggered ferroptosis in lung cancer cells via lncRNA H19/miR-19b-3p/FTH1 axis. Bioact. Mater 13, 23–36. doi:10.1016/j.bioactmat.2021.11.013
Zhang, Y., Liang, Y., and He, C. (2017b). Anticancer activities and mechanisms of heat-clearing and detoxicating traditional Chinese herbal medicine. Chin. Med. 12, 20. doi:10.1186/s13020-017-0140-2
Zhang, Z. Y., Yu, S. Q., Miao, L. Y., Huang, X. Y., Zhang, X. P., Zhu, Y. P., et al. (2008). Artesunate combined with vinorelbine plus cisplatin in treatment of advanced non-small cell lung cancer: a randomized controlled trial. Zhong Xi Yi Jie He Xue Bao 6, 134–138. doi:10.3736/jcim20080206
Zhao, L., Li, C., Zhang, Y., Wen, Q., and Ren, D. (2014). Phytochemical and biological activities of an anticancer plant medicine: Brucea javanica. Anticancer Agents Med. Chem. 14, 440–458. doi:10.2174/18715206113136660336
Zheng, J., and Conrad, M. (2020). The metabolic underpinnings of ferroptosis. Cell Metab. 32, 920–937. doi:10.1016/j.cmet.2020.10.011
Zheng, L., Chen, J., Ma, Z., Liu, W., Yang, F., Yang, Z., et al. (2015). Capsaicin causes inactivation and degradation of the androgen receptor by inducing the restoration of miR-449a in prostate cancer. Oncol. Rep. 34, 1027–1034. doi:10.3892/or.2015.4055
Zhou, C., Yu, T., Zhu, R., Lu, J., Ouyang, X., Zhang, Z., et al. (2023). Timosaponin AIII promotes non-small-cell lung cancer ferroptosis through targeting and facilitating HSP90 mediated GPX4 ubiquitination and degradation. Int. J. Biol. Sci. 19, 1471–1489. doi:10.7150/ijbs.77979
Zhou, Q., Meng, Y., Li, D., Yao, L., Le, J., Liu, Y., et al. (2024). Ferroptosis in cancer: from molecular mechanisms to therapeutic strategies. Signal Transduct. Target Ther. 9, 55. doi:10.1038/s41392-024-01769-5
Zhou, X., Shi, H., Jiang, G., Zhou, Y., and Xu, J. (2014). Antitumor activities of ginseng polysaccharide in C57BL/6 mice with Lewis lung carcinoma. Tumor Biol. 35, 12561–12566. doi:10.1007/s13277-014-2576-7
Zou, Y., Li, H., Graham, E. T., Deik, A. A., Eaton, J. K., Wang, W., et al. (2020). Cytochrome P450 oxidoreductase contributes to phospholipid peroxidation in ferroptosis. Nat. Chem. Biol. 16, 302–309. doi:10.1038/s41589-020-0472-6
Zühlke, R. D., Pitt, G. S., Deisseroth, K., Tsien, R. W., and Reuter, H. (1999). Calmodulin supports both inactivation and facilitation of L-type calcium channels. Nature 399, 159–162. doi:10.1038/20200
Glossary
Keywords: ferroptosis, lung cancer, botanical drugs, pharmacology, preclinical study
Citation: Tian X, Fu K, Huang X, Zou H, Shi N, Li J, Bao Y, He S and Lv J (2024) Ferroptosis in the adjuvant treatment of lung cancer-the potential of selected botanical drugs and isolated metabolites. Front. Pharmacol. 15:1430561. doi: 10.3389/fphar.2024.1430561
Received: 10 May 2024; Accepted: 26 July 2024;
Published: 13 August 2024.
Edited by:
Michael Heinrich, University College London, United KingdomReviewed by:
Feng Zhang, Nanjing University of Chinese Medicine, ChinaHongquan Wang, Peking University Aerospace School of Clinical Medicine, China
Copyright © 2024 Tian, Fu, Huang, Zou, Shi, Li, Bao, He and Lv. This is an open-access article distributed under the terms of the Creative Commons Attribution License (CC BY). The use, distribution or reproduction in other forums is permitted, provided the original author(s) and the copyright owner(s) are credited and that the original publication in this journal is cited, in accordance with accepted academic practice. No use, distribution or reproduction is permitted which does not comply with these terms.
*Correspondence: Sisi He, c2lzaWhlMTIxOUAxNjMuY29t; Junyuan Lv, anVueXVhbmx2QHptdS5lZHUuY24=
†These authors have contributed equally to this work