- Department of Biochemistry and Molecular Medicine, School of Medicine, University of California at Davis, Sacramento, CA, United States
ATP-binding cassette (ABC) transporters are transmembrane proteins expressed commonly in metabolic and excretory organs to control xenobiotic or endobiotic disposition and maintain their homeostasis. Changes in ABC transporter expression may directly affect the pharmacokinetics of relevant drugs involving absorption, distribution, metabolism, and excretion (ADME) processes. Indeed, overexpression of efflux ABC transporters in cancer cells or bacteria limits drug exposure and causes therapeutic failure that is known as multidrug resistance (MDR). With the discovery of functional noncoding microRNAs (miRNAs) produced from the genome, many miRNAs have been revealed to govern posttranscriptional gene regulation of ABC transporters, which shall improve our understanding of complex mechanism behind the overexpression of ABC transporters linked to MDR. In this article, we first overview the expression and localization of important ABC transporters in human tissues and their clinical importance regarding ADME as well as MDR. Further, we summarize miRNA-controlled posttranscriptional gene regulation of ABC transporters and effects on ADME and MDR. Additionally, we discuss the development and utilization of novel bioengineered miRNA agents to modulate ABC transporter gene expression and subsequent influence on cellular drug accumulation and chemosensitivity. Findings on posttranscriptional gene regulation of ABC transporters shall not only improve our understanding of mechanisms behind variable ADME but also provide insight into developing new means towards rational and more effective pharmacotherapies.
1 Introduction
ATP-binding cassette (ABC) transporters are a superfamily of transmembrane proteins which transport substrates by overcoming the concentration gradients across the membrane critical for cell functions (Robey et al., 2018; Wang et al., 2021). ABC transporters are expressed ubiquitously in human body while they are relatively more extensively distributed in metabolic organs such as small intestine and liver (Robey et al., 2018; Koehn, 2021). When ABC transporter domains recognize respective substrates, including xenobiotics (e.g., anticancer drugs and environmental agents) and endogenous compounds (e.g., nutrients and hormones), these transporters dispose such agents to maintain cell functions (Wang et al., 2021).
The clinical significance of ABC transporters has been well recognized. Since ABC transporters contribute to the homeostasis of some nutrients while exporting toxins, genetic mutations of ABC transporters might change transporter functions and be involved in certain disorders such as cystic fibrosis and retinal degeneration (Dean, 2005). On the other hand, the roles of ABC transporters in drug absorption, distribution, metabolism, and excretion (ADME) or pharmacokinetics (PK) are firmly established (Choi and Yu, 2014; Koehn, 2021), and overexpression of efflux ABC transporters in carcinoma cells confers multidrug resistance (MDR) (Choi and Yu, 2014; Bukowski et al., 2020; Koehn, 2021). Moreover, more than 90% of cancer patient deaths are caused by MDR during chemotherapy (Bukowski et al., 2020), in which the overexpression of efflux ABC transporters is considered to be one of the most important mechanisms (Choi and Yu, 2014; Fan et al., 2023). Therefore, understanding the factors in the control of ABC efflux transporter gene expression is important for identifying proper ways to overcome the MDR towards an improved therapy, in addition to the development of chemical inhibitors.
Studies have revealed the importance of several nuclear receptors, such as pregnane X receptor (PXR), constitutive androstane receptor (CAR), proliferator-activated receptor (PPAR) and aryl hydrocarbon receptor (AHR), in transcriptional gene expression of ABC transporters (Chen et al., 2012). For instance, PXR has been shown to regulate ABCB1 expression in human colon cancer LS174T cells through direct binding to the direct repeat separated by four base pairs (DR4) motif within the ABCB1 promoter region, and thus PXR ligand rifampin induces ABCB1 expression (Geick et al., 2001). As another example, 3-methylcholanthrene (3MC) upregulates ABCG2 expression in colon cancer LS174T cells via the activation of AHR which binds to the AHR response element 5 within ABCG2 promoter region and thus activate the transcription of ABCG2 (Tompkins et al., 2010).
Recent research has also revealed posttranscriptional gene regulation (PTGR) of ABC transporters by the genome-derived, small noncoding microRNAs (miRNAs or miRs) (Yu and Pan, 2012; Ingelman-Sundberg et al., 2013; Zhong and Leeder, 2013; Yu et al., 2016). By acting on the 3′-untranslated region (3′UTR) of target transcripts, miRNAs lead to the inhibition of mRNA translation or enhancement of mRNA degradation (Lai, 2002; Ambros, 2004). For example, hsa-miR-519c has been shown to target the 3′UTR of ABCG2 to modulate its protein outcomes in parental human colon cancer S1 cells whereas not in the drug-resistant S1MI80 cells, as the latter are comprised of shortened ABCG2 mRNA escaping miR-519c-controlled PTGR and leading to ABCG2 overexpression in the drug-resistant cells (To et al., 2008; To et al., 2009). In addition, hsa-miR-328 controls the PTGR of ABCG2 in the drug-resistant breast cancer cells to modulate mitoxantrone sensitivity (Pan et al., 2009). These findings not only offer insights into the presence of PTGR mechanisms behind variable ABC transporter expression levels and thus drug disposition capacities but also the development of new means to improve therapies.
Nevertheless, studies on miRNA-controlled PTGR have been limited to using vector or virus based expression materials or chemically synthesized miRNA agents (Yu et al., 2019; Traber and Yu, 2023). The former are not RNA but DNA molecules, while the latter are comprised of extensive artificial modifications differing from natural miRNAs. Therefore, novel recombinant technologies have been developed to achieve in vivo production of true biological miRNA agents, namely BERAs or BioRNAs (Li et al., 2014; Chen et al., 2015; Ho et al., 2018; Li et al., 2021; Tu et al., 2021; Traber et al., 2024). Recombinant miRNAs have been shown to be active and selectively regulate target gene expression, including many ABC transporters (Li et al., 2019; Yi et al., 2020), representing a new class of RNA molecules for basic research and showing potential as therapeutics (Ho et al., 2018; Cronin and Yu, 2023; Traber and Yu, 2023).
In this article, we first summarize the clinical importance of ABC transporters, specifically in ADME and MDR. Following a brief introduction of PTGR mechanism controlled by the genome-derived miRNAs, we overview the regulation of some important efflux ABC transporters by specific miRNAs and the consequent effects on drug transport and chemosensitivity. Further, we summarize the production and utilization of unparalleled recombinant miRNAs for the investigation of ABC transporter PTGR and discuss their potential applications.
2 Clinical importance of ABC transporters
2.1 Discovery and general properties of ABC transporters
Research on ABC transporters emerged following the discovery of a nutrient-transporting protein from membrane vesicles of Escherichia coli that was dependent on ATP hydrolysis (Berger and Heppel, 1974). Later, Juliano and Ling (1976) disclosed a 170-kD, surface membrane glycoprotein affecting colchicine permeation, namely P-glycoprotein (P-gp or ABCB1), which was responsible for drug resistance in the mammalian cells. Further genetic analyses of the mammalian and bacterial transporters revealed that their coding genes were highly conserved (Walker et al., 1982; Riordan et al., 1985). With valuable insights of human genome, many human ABC transporter genes were identified (Luciani et al., 1994; Allikmets et al., 1996; Klein et al., 1999; Dean et al., 2001; Olsen et al., 2001; Venter et al., 2001). A total of 48 ABC genes have been revealed to encode functional ABC transmembrane proteins in humans and classified into seven subfamilies, from ABCA to ABCG (Dean et al., 2001; Dean, 2005; Vasiliou et al., 2009; Alam and Locher, 2023).
Human ABC transporters are expressed in almost all organs (Figure 1), especially those metabolic tissues such as intestine, liver, and kidney to greater degrees. These transporters are also critical for the body homeostasis and physiology by translocating xenobiotics and endobiotics across cells, and defects of ABC transporters may contribute to certain types of metabolic diseases. For instance, ABCA1 is a membrane protein which maintains lipid homeostasis by effluxing phospholipids and cholesterol to the extracellular lipid-poor apolipoprotein (Dean et al., 2001; Vincent et al., 2019), and ABCA1 within adipose tissues contributes to the high-density lipoprotein (HDL) biogenesis in the body (Chung et al., 2011). Through analyzing the visceral and subcutaneous adipose tissue ABCA1 expression in both lean and obese individuals, Vincent et al. (2019) found that lower expression of ABCA1 was correlated with obesity and insulin resistance. Notably, the precise location of important ABC transporters (e.g., ABCB1, ABCC1-4, and ABCG2) in major organs relevant to ADME, including apical (cell faces to the lumen) and basolateral (faces to the extracellular fluid) sides of cells (Figure 2), have been well established for improved understanding of the directions of drug transport within the body.
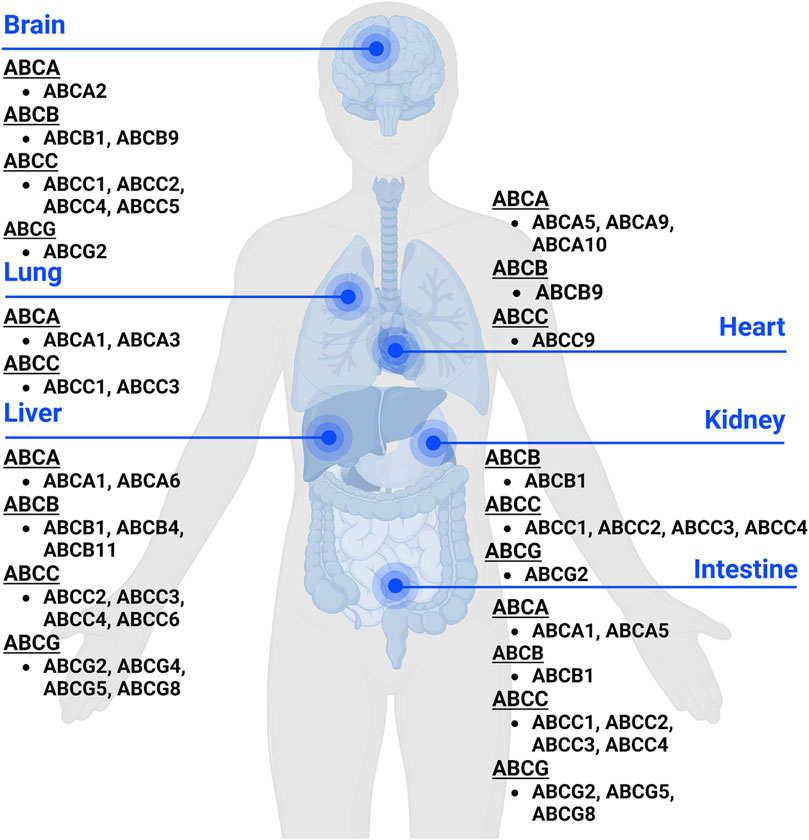
Figure 1. Expression of ABC transporters in human body. ABC transporters are mostly expressed in metabolic and excretory organs, such as liver, kidney, and intestine. This figure was created with BioRender.com.
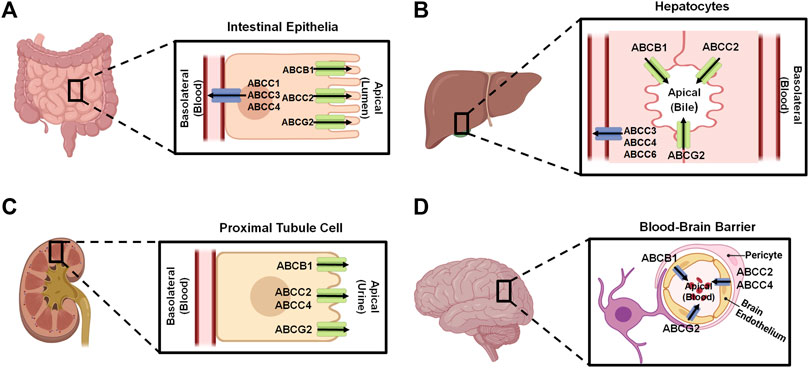
Figure 2. Localization of important ABC transporters in human intestine (A), liver (B), kidney (C), and brain (D). The apical side refers to the cell surface oriented towards the lumen, while the basolateral side facing the extracellular fluid, such as blood. ABC transporters located to the apical surfaces export substances from the tissues to the lumens, and transporters expressed at the basolateral surfaces facing the blood vessels substrate efflux from the tissues to the portal bloods. At the blood-brain barrier, transporters localized on the apical sides of brain endothelium cells facing the bloodstream transfer substrates into the blood. This figure was made by using BioRender.com.
An ABC transporter is usually comprised of two nucleotide-binding domains (NBDs) to which ATP is bound and two transmembrane domains (TMDs) that allow transporter protein to reside on cell membrane and interact with specific substrates (Dean et al., 2001; Choi and Yu, 2014; Fan et al., 2023). Indeed, the classification of the ABC transporters is based on the sequences of conserved NBDs consisting of characteristic motifs (Walker A and Walker B) and the ABC signature motif (Walker et al., 1982; Hyde et al., 1990; Dean et al., 2022). X-ray crystallography studies have showed a similar conformational change of ABCB1 transporters from non-human species upon interacting with poly-substrates (Jin et al., 2012; Esser et al., 2017). Most recent applications of novel cryo-electron microscopy (cryoEM) technology have provided further structures of ABC transporters (Kim and Chen, 2018; Nosol et al., 2020), including human ABCB1 in the outward-facing conformation as well as interactions with substrates and inhibitors. The latter shall facilitate the prediction of possible, transporter-based drug-drug interactions as well as the discovery and development of more effective inhibitors to overcome MDR.
2.2 Roles of ABC transporters in ADME
ABC transporters expressed in human body (Figures 1, 2) are known to directly affect drug absorption, distribution, and excretion as well as metabolism via interacting with metabolic enzymes. Among various factors affecting oral drug absorption from gastrointestinal tract to the bloodstream (Stillhart et al., 2020), transporters ABCB1, ABCC2, and ABCG2 localized on the apical sides of intestinal epithelial cells (Figure 2A) contribute to limiting oral absorption while ABCC1, ABCC3, and ABCC4 on the basolateral sides facilitate the absorption of substrate drugs. Digoxin, a cardiac glycoside used to enhance heart function, has been identified as one of many substrates of ABCB1 (Table 1), and the absorption and overall PK of digoxin may be determined by ABCB1 status (de Lannoy and Silverman, 1992; Fromm et al., 1999; Greiner et al., 1999). Indeed, ABCB1 inducer rifampin was shown to have significant impact on the PK of digoxin in healthy volunteers, associated with the increase in intestinal ABCB1 expression levels (Greiner et al., 1999). Besides clinical investigations, genetically modified mouse models are widely used to define the importance of ABC transporters in ADME/PK (Jiang et al., 2011; Durmus et al., 2015), including the role of ABCB1 in digoxin PK (Fromm et al., 1999). As another example, comparative studies with wild type, Abcc2-knockout, Abcb1a/1b-knockout, and Abcb1a/1b/Abcc2-knockout mice demonstrated the important role of Abcc2 and Abcb1 in paclitaxel PK, in particular, Abcc2 in hepatobiliary excretion (Figure 2B) over renal excretion (Figure 2C), as well as Abcb1 in intestinal absorption (Lagas et al., 2006).
ABC transporters may restrict the distribution of substrate drugs (Table 1) from the bloodstream to target organs, thereby the study of how ABC transporters impede drug penetration into the central nervous system (CNS) remains an important area in ADME/PK or drug delivery fields (Daood et al., 2008; Miller, 2015). ABCB1 and ABCG2 are two major efflux transporters expressed at the apical sides of brain endothelium cells (Figure 2D) which prevent substrates across the blood-brain barrier (BBB) and protect the brain from toxins or other xenobiotics including many therapeutic drugs (Dean et al., 2001; Daood et al., 2008). For instance, cyclin-dependent kinase inhibitors (CDKis) (Table 1) are a group of medications approved for the treatment of metastatic breast cancer, and it is important to understand their penetration into the brain (De Gooijer et al., 2015; Martínez-Chávez et al., 2019). In vitro studies revealed the CDKi ribociclib as a substrate for human ABCB1 whereas poorly transported by mouse abcg2 (Sorf et al., 2018; Martínez-Chávez et al., 2019). In vivo study with abcb1 and abcg2 knockout mice not only showed a 2.3-fold increase of ribociclib oral bioavailability but also 30-fold sharp elevation of brain-to-plasma (Kp,brain) ratio, supporting the role of abcb1 in brain distribution of ribociclib (Martínez-Chávez et al., 2019). Additionally, the coadministration of an abcb1 and abcg2 dual inhibitor (e.g., elacridar) resulted in a significant increase in ribociclib concentrations in the brain (Martínez-Chávez et al., 2019). Similarly, the other CDKi palbociclib exhibited higher brain distribution when abcb1 and abcg2 were knocked out in mice (De Gooijer et al., 2015).
Kidney is the primary excretory organ which eliminates drugs, metabolites, and toxins. Therefore, some efflux transporters (e.g., ABCC2, ABCC4, and ABCG2) located on the apical membranes of kidney proximal tubule cells (Figure 2C) may pump their substrates into the urine and contribute to their elimination (Masereeuw and Russel, 2012). Adefovir and tenofovir (Table 1), two antiviral drugs, were identified as ABCC4 substrates (Imaoka et al., 2007). Compared to wild-type mice, abcc4-knockout mice exhibited higher plasma drug concentrations and kidney accumulation following adefovir treatment. Additionally, the total clearance (CLtotal) of adefovir was found to be lower in the absence of abcc4, and tubular secretion clearance (CLrenal, kidney) of adefovir and tenofovir was also reduced over 50% in the knockout mice (Imaoka et al., 2007).
Clinical investigations have further revealed an altered expression of ABC transporters among patients with acute or chronic diseases and potential influence on drug ADME, efficacy or toxicity (Evers et al., 2018; Laddha et al., 2024). One study on hyperbilirubinemia and association with bile acid homeostasis and regulation among intensive care unit patients showed that protein levels of ABCC3 (Figure 2B) were strongly increased at the basolateral sides of patient liver biopsies (Vanwijngaerden et al., 2011). Another study with intestinal biopsy specimens from healthy volunteers as well as human immunodeficiency virus (HIV)-positive subjects with or without antiretroviral therapy revealed a significantly lower mRNA and protein levels of ABCC2 in antiretroviral therapy-naive subjects than the control group which was partially restored to baseline levels along with significant increase in ABCB1 expression in HIV-positive subjects following antiretroviral therapy (Kis et al., 2016). A very recent article provides a comprehensive review on the expression and function of hepatic transporters under fatty liver conditions, as well as subsequent effects on the PK properties of relevant drugs, including some clinical findings (Laddha et al., 2024).
2.3 Roles of ABC transporters in MDR
Besides their importance in controlling drug ADME/PK, the overexpression of efflux ABC transporters is a common mechanism behind MDR (Figure 3) (Hrycyna and Gottesman, 1998; Choi and Yu, 2014; Robey et al., 2018; Orelle et al., 2019) that leads to pharmacotherapy failure among patients with infections, cancers, or other diseases. Specific ABC transporters, including ABCB1, ABCC family, and ABCG2, have been shown to be overexpressed in human carcinoma cells to confer MDR, attributed to a lower intracellular drug exposure (Figure 3A) and manifested by low or lost chemosensitivity (Figure 3B). Among them, ABCB1 was highly expressed in almost all types of MDR cancers, such as adrenocortical, colon, breast, and kidney; and the contribution of ABCB1 to MDR has been proven for many drugs, including vincristine, doxorubicin (DOX), and 5-fluorouracil (5-FU) (Fojo et al., 1987) (Table 1). MDR appeared after the initial chemotherapy (e.g., vincristine) with the increase in ABCB1 levels during relapse and eventually led to mortality (Fojo et al., 1987). Another study also revealed much higher frequency or extent of ABCB1 expression among relapse acute nonlymphoblastic leukemia (ANLL) patients (60%) than initial ANLL patients (26%) (Han et al., 2001), indicating the involvement of efflux transporter ABCB1 in MDR.
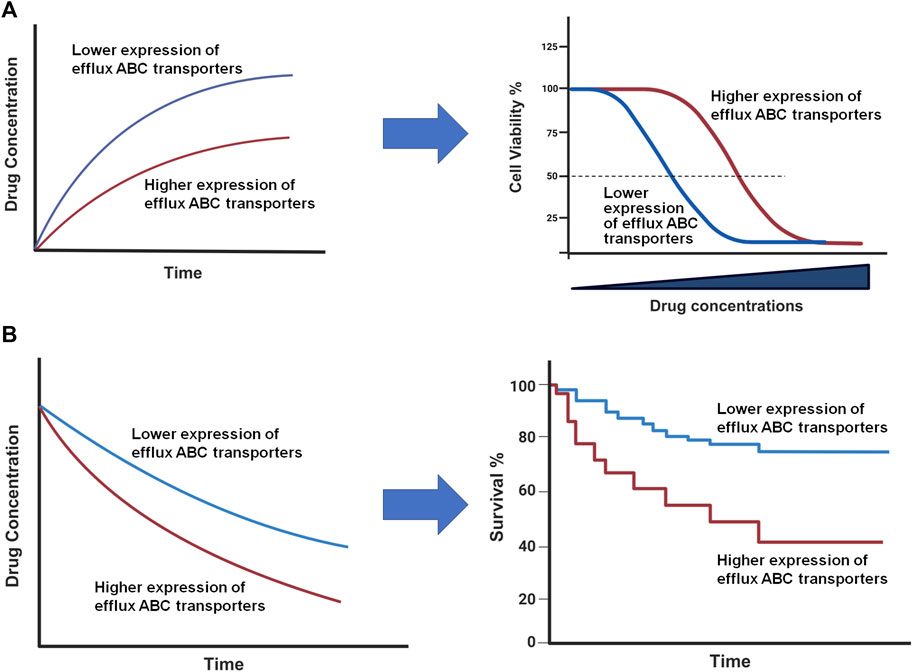
Figure 3. Impact of ABC transporters on drug exposure and therapeutic outcome in vitro (A) and in vivo (B). (A) Overexpression of efflux ABC transporters leads to a lower intracellular drug accumulation (left) and antiproliferation activity (right). (B) Drug concentrations are reduced in patient tumor tissues with higher ABC transporter expression (left), which may lead to a lower survival rate (right). This figure was created with BioRender.com.
ABCC family transporters were found to be highly expressed in lung cancers associated with MDR, including both small cell lung cancer (SCLC) and non-small cell lung cancer (NSCLC) (Young et al., 1999). Among them, ABCC3 was revealed to contribute greatly to the resistance to both DOX and vincristine (Table 1) during the treatment of lung cancer while other ABCC transporters could be involved in it. As another example, imatinib was identified as a substrate of ABCG2 (Table 1) (Burger et al., 2004) in treating leukemia, and ABCG2 was found to be upregulated in imatinib-resistant leukemia cells (Kaehler et al., 2017). Further, overexpression of ABCG2 was demonstrated to correlate with the resistance to many other drugs such as mitoxantrone (Rocchi et al., 2000), daunorubicin (Robey et al., 2003), DOX (Robey et al., 2003), and topotecan (Rocchi et al., 2000).
While overexpression of efflux ABC transporters confers MDR in carcinoma cells, it is also accompanied by many other cellular changes (Choi and Yu, 2014). For instance, MDR cells were revealed to exhibit a higher intracellular pH than parental cells (Boscoboinik et al., 1990), and the increased intracellular pH in MDR cells might decrease apoptosis as the caspase-dependent apoptosis was elevated under acidic conditions (Muriithi et al., 2020). Indeed, the reversal of MDR by small molecules were not necessarily correlated with their effects on intracellular pH (Boscoboinik et al., 1990). Furthermore, lipid compositions of plasma membranes were found to be altered in MDR cells, such as higher levels of sphingomyelin, phosphatidylinositol, cholesterol, cholesterol esters in MDR cell membranes (Peetla et al., 2013; Kopecka et al., 2020), which could contribute to the altered permeability of anticancer drugs (Mohammad et al., 2020; Szlasa et al., 2020).
As the impact of high-expressing efflux ABC transporters on the sensitivity of cells to respective substrate drugs, such as DOX (Fojo et al., 1987; Lemontt et al., 1988; Kim et al., 2015), has well demonstrated by preclinical studies (Figure 3A), clinical studies have also showed that high levels of ABC transporters contribute to the limited tissue and/or systemic drug exposure and subsequently poor therapeutic outcomes (Figure 3B) albeit there are some controversial reports (Tamaki et al., 2011). A recent study on the expression status of various ABC transporters in different tumors revealed that, while ABC transporter expression correlated with different stages of breast, kidney or lung tumors, a lower ABCB1 mRNA expression level was predictive of significantly longer survival of patients with ovarian or kidney cancer and thymoma (Kadioglu et al., 2020), supporting the clinical significance of efflux ABC transporters and needs for new means to achieve individualized pharmacotherapy.
One way to overcome MDR is to develop and co-administer with ABC transporter inhibitors (Robey et al., 2018), which rather has not found any success, given the complex roles of these transporters in ADME/PK (Choi and Yu, 2014). Understanding the mechanisms by which efflux transporters are overexpressed in MDR cells and organisms may offer clues for developing new strategies. Indeed, various factors such as gene duplication or multiplication as well as the changes in transcriptional regulation or signaling have been shown to contribute to the overexpression of efflux ABC transporters, and respective remedies may be explored and critically evaluated.
3 MicroRNAs in posttranscriptional gene regulation
MiRNAs are small noncoding RNAs generated from the genome, around 22 nt in length, which play important roles in PTGR in cells (Ambros, 2004). Lin-4 (Lee et al., 1993) and let-7 (Reinhart et al., 2000) are among the first functional miRNAs discovered in Caenorhabditis elegans, while let-7 is the first miRNA identified in humans (Pasquinelli et al., 2000). With more studies to the miRNAs, they have been found across many animal species which are highly conserved (Pasquinelli et al., 2000; Li et al., 2010; De Rie et al., 2017). Regulation of target genes by miRNAs loaded within the RNA-induced silencing complex (RISC) involve the inhibition of mRNA translation and enhancement of mRNA decay or degradation (Lee et al., 1993; Lai, 2002; Doench and Sharp, 2004). Through PTGR of targeted genes, miRNAs influence essentially all biological processes, including those important in maintaining metabolite homeostasis and cellular defenses (Ha and Kim, 2014). Furthermore, miRNAs hold promise as therapeutic targets or entities for the treatment of various human diseases (Yu et al., 2020; Yu and Tu, 2022; Traber and Yu, 2023, 2024).
3.1 MicroRNA biogenesis
Most miRNAs are transcribed from corresponding coding genes by RNA polymerase II (Pol II) (Lee et al., 2004) to primary miRNAs (pri-miRNAs) which are recognized and cleaved by micro-processor proteins, DROSHA and DGCR8, to generate short-hairpin, precursor miRNAs (pre-miRNAs) within the nucleus (Lee et al., 2003; Denli et al., 2004; Gregory et al., 2004; Wang et al., 2007) (Figure 4). After the pre-miRNA is translocated from nucleus to cytoplasm by Exportin-5 (XPO5) (Yi et al., 2003; Lund et al., 2004), it is further processed by the RISC-loading complex (RLC), consisting of the RNase III family protein DICER (Bernstein et al., 2001; Ketting et al., 2001), transactivation response element RNA-binding protein (TRBP) (Lee et al., 2003; Fareh et al., 2016), and Argonaute 2 (AGO2) protein (Liu et al., 2004; Chendrimada et al., 2005; MacRae et al., 2008), to miRNA duplex. Subsequently, the AGO protein unwinds the miRNA duplex and guides one strand (3p- or 5p-) into the RISC (Khvorova et al., 2003; Kwak and Tomari, 2012) to achieve RNA interference (RNAi) (Figure 4).
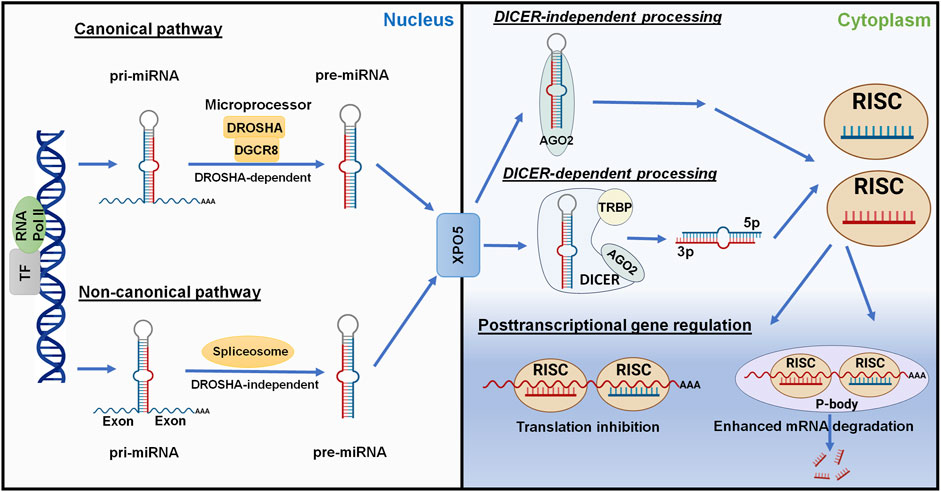
Figure 4. Posttranscriptional gene regulation controlled by the genome-derived miRNAs. The canonical and non-canonical pathways of miRNA biogenesis are dependent and independent upon DROSHA, respectively, to generate pre-miRNAs from longer pri-miRNAs transcribed from the genome within nucleus. Following Exportin-5 (XPO5)-mediated translocation, cytoplasmic pre-miRNAs are recognized and processed by DICER, TRBP, and AGO2 to the miRNA duplex. The AGO protein unwinds the miRNA duplex where either the 5p or 3p strand may be incorporated into the RISC. Some mature miRNAs are generated through DICER-independent processing as pre-miRNAs directly bind to AGO2. The mature miRNA usually acts on the 3′UTR of target mRNA, leading to translation inhibition or enhanced mRNA degradation. This figure was made by using BioRender.com and Microsoft Powerpoint.
Complementary to the canonical biogenesis pathway, the maturation of some miRNAs is independent on the micro-processor (DROSHA/DGCR8) or DICER to offer noncanonical miRNAs (Figure 4). The short-hairpin introns may be spliced and debranched to produce pre-miRNAs directly (Okamura et al., 2007; Ruby et al., 2007). After exported into the cytoplasm, noncanonical pre-miRNAs may follow the DICER-dependent maturation pathway (Okamura et al., 2007). This DROSHA-independent mirtron pathway is conserved for some miRNAs (e.g., miR-887) including mammals (Berezikov et al., 2007; Babiarz et al., 2011). On the other hand, some miRNAs (e.g., miR-451) is formed independent on DICER (Figure 4), despite that pre-miRNA production is DROSHA-dependent. AGO2 protein has been revealed as an alternative enzyme to directly cleave pre-miRNA (Cheloufi et al., 2010; Yang et al., 2010), which may involve subsequent trimming by poly(A)-specific ribonuclease (PARN) (Yoda et al., 2013; Kim et al., 2016), to generate functional miRNA strand for target silencing within the RISC (Figure 4). Interestingly, miR-451 generally partners with miR-144, relying on the transfer of micro-processor proteins from miR-144 to continue its further maturation (Yang et al., 2010; Shang et al., 2020).
3.2 Posttranscriptional gene regulation by microRNAs
Derived from either canonical or noncanonical pathway, functional miRNA activates the RISC to control target gene expression at the posttranscriptional level, namely PTGR. In particular, one strand (5p- or 3p-) recognizes target mRNA sequences through imperfect base pairing to induce translation inhibition or mRNA cleavage or degradation (Figure 4). The human genome contains four AGO proteins (AGO1-4), among them AGO2 plays a major role in PTGR (Liu et al., 2004; O’Carroll et al., 2007). The seed region at the 5′ of an miRNA may include 7–8 nt that are fully complementary to the miRNA response element within 3′UTR of target mRNA to effectively inhibit mRNA translation and/or degradation (Doench and Sharp, 2004). While some research indicated possible contribution of post-initiation inhibition to translation repression, other studies identified the interruption of mRNA translation at the initiation steps and involvement of specific initiation factors or components (see reviews Gu and Kay, 2010; Wilczynska and Bushell, 2015), highlighting the complexity in studying miRNA-mediated translation inhibition. Meanwhile, processing bodies (P-bodies) and cytoplasmic granules seem associated with mRNA decay and degradation (Sheth and Parker, 2003; Sen and Blau, 2005).
4 MicroRNA-controlled posttranscriptional gene regulation of ABC transporters
The miRNA mediated PTGR has been established as a critical regulatory mechanism behind target protein outcomes and the whole proteome critical for almost all cellular processes, including ABC transporters vital in ADME and MDR (Figure 5) (Yu, 2009; Yu and Pan, 2012; Haenisch et al., 2014; Yu et al., 2016). Many miRNAs have been shown to impact protein expression of specific ABC transporters through direct targeting of the transporter mRNAs or indirectly acting on their regulators. The reduction of efflux ABC transporter protein levels may subsequently alter the disposition of relevant substrates, such as antineoplastic drugs, and subsequently influence chemosensitivity (Table 2) (Figure 5).
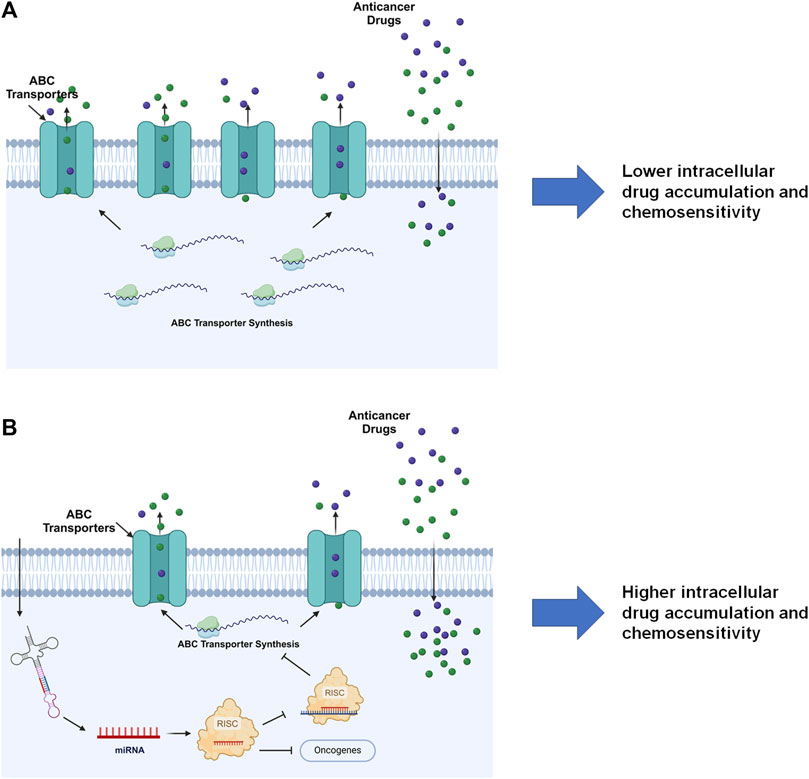
Figure 5. MiRNA-controlled posttranscriptional gene regulation of ABC transporters in drug disposition and chemosensitivity. (A) By escaping miRNA mediated PTGR, efflux ABC transporters are overexpressed in cells to pump out substrate drugs, exhibiting lower drug exposure and chemosensitivity. (B) Restoration of PTGR of efflux transporters, e.g., through the introduction of bioengineered miRNA molecules, reduces ABC transporter expression, enhances intracellular drug exposure, and increases cell sensitivity to the drugs. Note that miRNAs introduced into the cells may inhibit the expression of some oncogenes to exert anticancer effects. This figure was made by using BioRender.com.
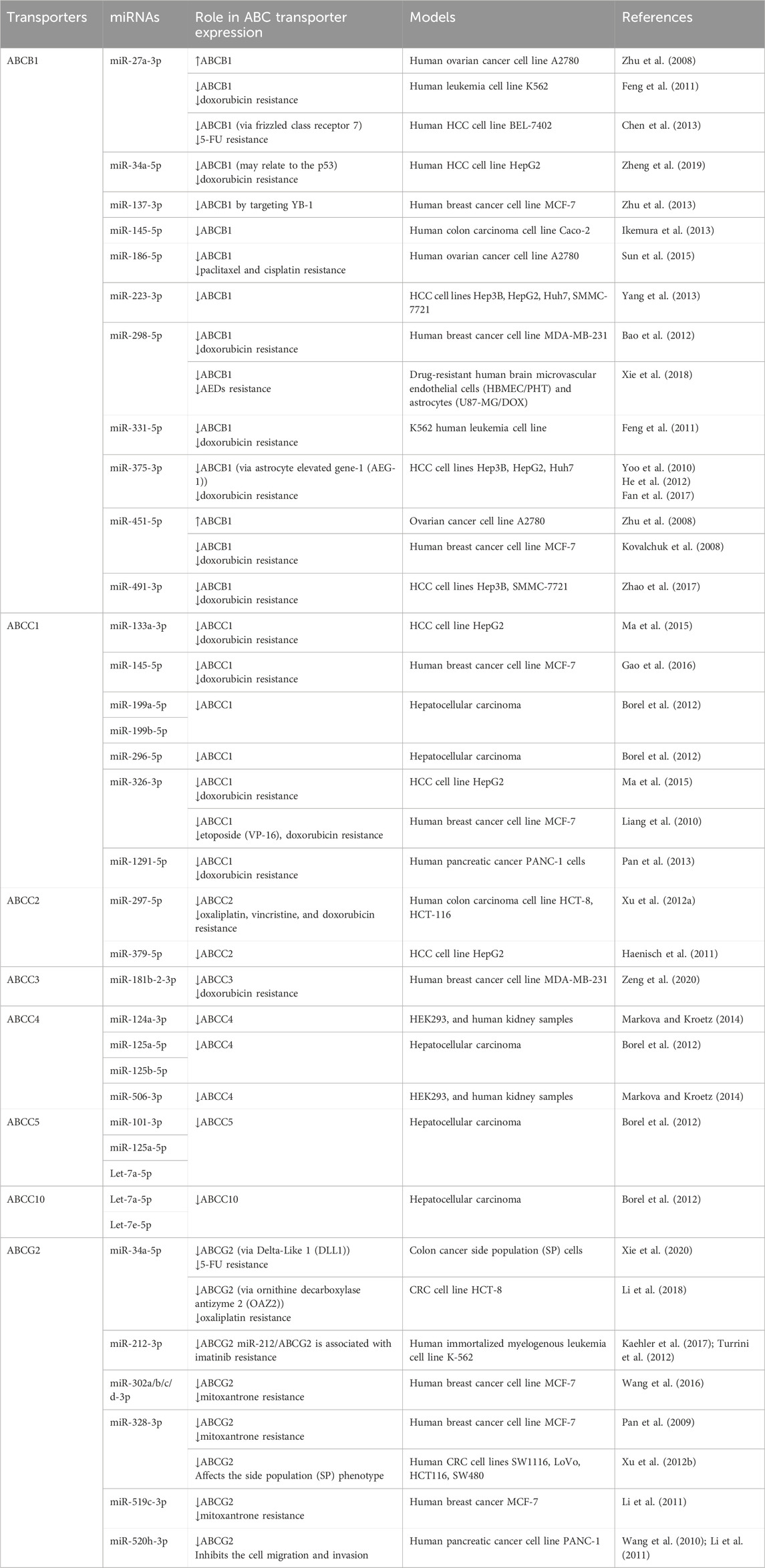
Table 2. Roles of specific miRNAs in the regulation of human ABC transporter gene expression and influence on drug disposition.
4.1 ABCB1
MiR-451 represents one of the first identified miRNAs involved in PTGR of ABCB1 (Kovalchuk et al., 2008) (Table 2). Deregulation of miR-451 was revealed in the DOX-resistant, human breast cancer cell line MCF-7/DOX, in which overexpression of ABCB1 contributes to DOX resistance. Luciferase reporter assay showed that miR-451 reduced ABCB1 3′UTR-luciferase activity, validating direct interaction between miR-451 and ABCB1 3′UTR. Further, transfection with miR-451 reduced the protein expression of ABCB1 in MCF-7/DOX cells and increased the sensitivity to DOX (Kovalchuk et al., 2008), suggesting the contribution of miR-451 mediated PTGR of efflux transporter ABCB1 behind MDR.
Likewise, decrease of miR-27a and miR-331-5p were found to be associated with DOX resistance in leukemia cells, and overexpression of these miRNAs increased the sensitivity of these cells to DOX (Feng et al., 2011) (Table 2). Luciferase reporter assay was also used to validate the miR-27a and miR-331-5p binding sites with ABCB1 3′UTR (Feng et al., 2011). In a separate study, miR-27a was showed to modulate ABCB1 expression in human hepatocellular carcinoma (HCC) cells through interference with upstream regulator, and downregulation of ABCB1 increased cell sensitivity to 5-FU (Chen et al., 2013). These findings suggest the potential role of miR-27a in reversing MDR by the reduction of efflux transporter expression. However, opposite effects were reported for miR-27a in human ovarian cancer A2780 cells where miR-27a mimics upregulated ABCB1 expression and increased chemoresistance (Zhu et al., 2008) (Table 2).
The adriamycin (ADM)-selected breast cancer MCF-7/ADM cell line exhibited higher levels of ABCB1 and Y-box binding protein-1 (YB-1), and transfection of miR-137 reduced both YB-1 and ABCB1 levels (Zhu et al., 2013) (Table 2). Further study indicated that miR-137 interfered with YB-1 mRNA, thereby inhibiting protein translation. Notably, YB-1 is a transcription factor that activates ABCB1 expression (Bargou et al., 1997), suggesting the contribution of YB-1 reduction to the suppression of ABCB1 expression in MCF-7/ADM cells by miR-137. Consequently, introduction of miR-137 elevated the sensitivity of MCF-7/ADM cells to anti-cancer drugs DOX, vincristine, and paclitaxel, as manifested by lower IC50 values (Zhu et al., 2013).
Recent study on HCC cells showed that resistance to DOX was correlated with overexpression of ABCB1, whereas miR-491-3p levels were reduced (Zhao et al., 2017) (Table 2). In line with the finding on the transcription factor Sp3 in transcriptional regulation of ABCB1 (Gromnicova et al., 2012), Zhao et al. (2017) demonstrated the actions of miR-491-3p on the 3′UTR of ABCB1 and Sp3 mRNAs that explained the suppression of ABCB1 expression and increase of cell sensitivity to DOX by miR-491-3p.
As ABCB1 transports many anti-epilepsy drugs (AEDs) across the blood-brain barrier (BBB) that may alter the exposure and efficacy of AEDs in patients with refractory epilepsy, another recent study investigated potential use of ABCB1 regulatory miRNA to reverse ABCB1-mediated MDR to AEDs (Xie et al., 2018). Lower levels of miR-298-5p were identified in drug-selected human brain microvascular endothelial cell lines (HBMEC/PHT) and astrocytes (U87-MG/DOX) cell lines, and forced expression of miR-298-5p reduced ABCB1 mRNA and protein levels and consequently, increased the intracellular accumulation of AEDs in drug-resistant HBMEC/PHT and U87-MG/DOX cells (Xie et al., 2018) (Table 2), illustrating the utility of miRNAs to overcome MDR in refractory epilepsy.
4.2 ABCC subfamily transporters
ABCC1 expression was found to be elevated in the etoposide-resistant breast cancer cell line MCF-7/VP, accompanied by a pronounced decline in miR-326 levels (Liang et al., 2010) (Table 2). Following the identification and validation of miR-326 binding site within ABCC1 3′UTR, transfection of MCF-7/VP cells with miR-326 was showed to reduce ABCC1 mRNA and protein levels as well as sensitivity to etoposide and DOX (Liang et al., 2010). In another study, miR-1291-5p was revealed to be derived from the small nucleolar RNA H/ACA box 34 (SNORA34) in human pancreatic carcinoma PANC-1 cells overexpressing ABCC1 (Pan et al., 2013). Control of ABCC1 protein outcomes by miR-1291 was verified by both gain- and loss-of-miR-1291 function approaches, besides the validation of miR-1291 response elements within ABCC1 3′UTR. As miR-1291 did not affect ABCC1 mRNA stability, PTGR of ABCC1 by miR-1291 is likely attributable to translation repression mechanism. In addition, downregulation of ABCC1 by miR-1291 elevated intracellular DOX accumulation and sensitized PANC-1 cells to DOX (Pan et al., 2013) (Table 2), illustrating the importance of miRNA-controlled PTGR in drug disposition and MDR.
In the multidrug-resistant, human colorectal carcinoma (CRC) cell line HCT-116/L-OHP overexpressing efflux transporter ABCC2, miR-297 level was found to be reduced (Xu K. et al., 2012) (Table 2). Reporter assay revealed that miR-297 suppressed ABCC2 3′UTR-luciferase activity. As miR-297 was effective to repress ABCC2 expression, overexpression of miR-297 in drug-resistant CRC cell lines sensitized the cells to anticancer drugs, including oxaliplatin, vincristine, and DOX, which was associated with an enhanced apoptosis of multidrug-resistant CRC cells compared to antineoplastic drug monotherapy (Xu K. et al., 2012).
One most recent study (Zeng et al., 2020) first showed that curcumol improved the sensitivity of drug-resistant, triple-negative breast cancer (TNBC) MDA-MB-231 cells to DOX in vitro and in vivo. That was related to the increase of miR-181b-2-3p levels which was found to target ABCC3 directly. Furthermore, curcumol activated a transcription factor, NFAT1, which could directly bind to the promoter region of miR-181b-2-3p to enhance its expression. Therefore, this NFAT1/miR-181b-2-3p/ABCC3 axis was likely involved in the sensitization of TNBC cells to DOX by curcumol (Table 2).
There are also many other reports on PTGR of human ABCC transporters by specific miRNAs, such as ABCC4 by miR-124 and -506 (Markova and Kroetz, 2014), as well as ABCC5 and ABCC10 by let-7 (Borel et al., 2012), as summarized in Table 2. Likewise, repression of ABCC transporter protein levels by the miRNAs could be translated into an altered drug exposure and response in the cells. Together, these findings not only demonstrate the involvement of miRNA-controlled PTGR in variations in efflux ABCC transporter expression and chemosensitivity but also provide insights into development of new remedies to overcome MDR (Choi and Yu, 2014; Yu et al., 2016).
4.3 ABCG2
Consisting of miR-519c binding sites within the 3′UTR, ABCG2 was showed to be repressed by miR-519c in the S1 colon cancer cells (To et al., 2008) (Table 2). However, the drug-resistant subline S1MI80 with truncated ABCG2 3′UTR lacking miR-519c binding sites escaped miR-519c mediated PTGR, providing new insights into ABCG2 overexpression conferring MDR in these cells (To et al., 2008). In the mitoxantrone-resistant breast cancer cell line MCF-7/MX100 overexpressing ABCG2 transporter, miR-328 was able to increase the sensitivity to mitoxantrone by decreasing the ABCG2 protein expression via complementary targeting the 3′UTR of ABCG2 mRNA (Pan et al., 2009). Further, miR-520h was found to modulate ABCG2 expression in PANC-1 cells and contribute to cancer cell migration and invasion (Wang et al., 2010). Another side-by-side study on the efficiency of miR-328, -519c, and -520h revealed that miR-328 and -519c were effective to repress ABCG2 protein levels in MCF-7/MX100 cells and thus, increase intracellular drug accumulation and chemosensitivity (Li et al., 2011). By contrast, miR-520h was found to be ineffective to regulate ABCG2 expression in MCF-7/MX100 cells in which miR-520h levels were unchanged, highlighting the importance to monitor miRNA levels in these studies.
Some recent studies demonstrated the regulation of ABCG2 by several other miRNAs, including miR-302a/b/c/d in mitoxantrone-resistant breast cancer cells (Wang et al., 2016) and miR-212 in imatinib-resistant leukemia cells (Kaehler et al., 2017) (Table 2). Both studies also showed the effectiveness of such miRNAs to sensitize drug-resistant cancer cells, supporting the roles of miRNA-controlled PTGR of the efflux ABC transporter as well as implications to drug disposition and MDR.
5 Recombinant RNAs to study ABC transporter posttranscriptional gene regulation
Recombinant DNA (rDNA) technology, which modifies genetic materials to produce specific products or introduce certain traits into living organisms, has been widely used for precise molecular, cellular, or systems studies on ADME genes (Cronin and Yu, 2023). The basis of rDNA technology is the manipulation of DNA sequences within host organisms using viable vectors or plasmids (Vieira and Messing, 1982). Application of rDNA technologies enables the production of gene products related to drug metabolism and disposition, such as drug-metabolizing enzymes (Dai et al., 1993; Crespi and Miller, 1999; Gonzalez, 2004) and efflux transporters (Galluccio et al., 2022; Cronin and Yu, 2023), which have greatly improved our understanding of roles of ADME genes in drug metabolism and disposition as well as possible influence on therapy.
While heterologous overexpression of RNAs proved to be challenging, novel technologies have been developed to achieve high yield production of recombinant miRNAs (named BERAs or BioRNAs) for PTGR studies, by using unique transfer RNA (tRNA) fused pre-miRNA carriers (Yu et al., 2019; Cronin and Yu, 2023; Traber and Yu, 2023). The tRNA fused pre-miRNA (e.g., pre-miR-34a) carriers allow a consistent, high-level and large-scale heterologous overexpression (e.g., >30% of total bacterial RNA) as well as high-yield production (e.g., tens milligrams from 1 L fermentation) of high-purity, target BioRNAs (e.g., >97% homogeneity, by HPLC; <3.0 EU/µg RNA) bearing target miRNAs, siRNAs, aptamers, or other forms of small RNAs (Chen et al., 2015; Ho et al., 2018; Li et al., 2021; Traber et al., 2024), in contrast to the tRNA scaffold (Ponchon et al., 2009) that commonly leads to the absence of heterologous expression of target RNAs or merely offers relatively a low yield (Li et al., 2014; Chen et al., 2015). Distinguished from conventional miRNA reagents synthesized in vitro through chemical or enzymatic reactions consisting of either extensive artificial modifications or none, BERAs or BioRNAs are similar as natural RNAs made and folded in vivo and being comprised of only a few essential posttranscriptional modifications (Li et al., 2015; Wang et al., 2015).
Recombinant BioRNA/miRNAs are precisely processed to target miRNAs in human cells to selectively regulate target gene expression, including many human ABC transporters (Table 3; Figure 5). For instance, recombinant miRNA let-7c-5p was successfully produced and introduced into human HCC Huh7 cells, leading to a sharp increase of let-7c-5p levels (Jilek et al., 2020). This resulted in a significant downregulation of ABCC5 protein levels in Huh7 cells, while ABCC4 and ABCG2 levels were not or minimally affected (Table 3). Additionally, coadministration of BioRNA/miR-7c-5p and 5-FU showed a strong synergism to inhibit HCC cell proliferation, partially attributable to the elevation in intracellular accumulation of 5-FU, a known substrate of ABCC5 transporter (Wijnholds et al., 2000; Pratt et al., 2005) (Table 1). As another example, Li et al. (2019) produced BERA/miR-328-3p that was revealed to control PTGR of ABCG2 transporter overexpressed to render mitoxantrone drug resistance in human breast cancer cells, namely MCF-7/MX100 cells (Pan et al., 2009; Li et al., 2011) (Table 3). After transfection with the BERA/miR-328-3p, the levels of miR-328-3p were greatly elevated in MCF-7/MX100 cells, leading to the reduction of ABCG2 and consequently, greater cellular exposure of and sensitivity to MX (Li et al., 2019). Rather, treatment of human placental BeWo cells with BERA/miR-328-3p did not alter intrinsic ABCG2 levels, intracellular MX accumulation, and chemosensitivity, indicating the difference in PTGR mechanism of ABCG2 between BeWo and drug resistant MCF-7/MX100 cells.
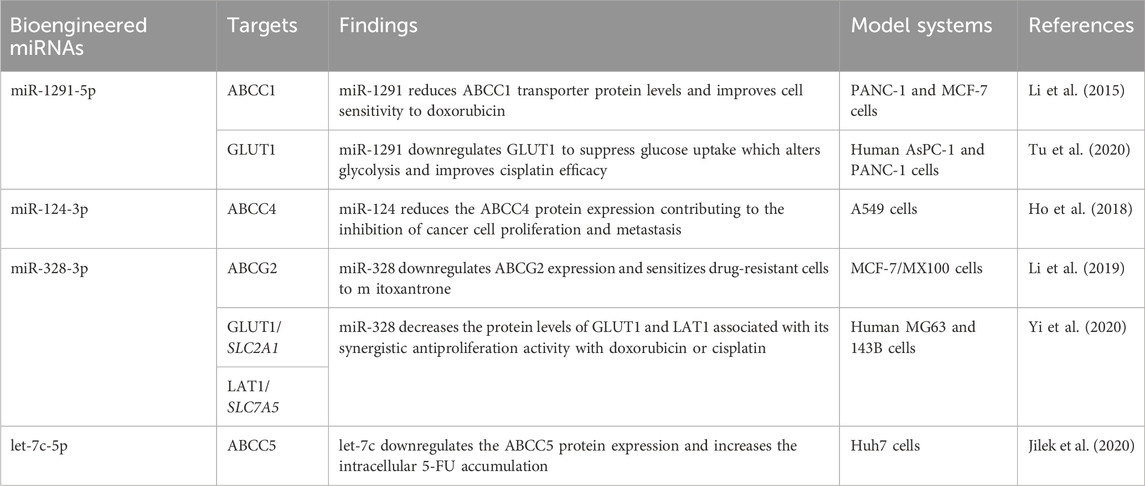
Table 3. Utilization of novel recombinant miRNA agents in studying transporter gene expression and impact on xenobiotic flux and multidrug resistance. GLUT1/SLC2A1, glucose transporter protein type 1, gene symbol solute carrier family two member 1; LAT1/SLC7A5, large neutral amino acid transporter 1, gene symbol solute carrier family seven member 5.
Besides efflux ABC transporter, bioengineered miRNAs have been utilized to investigate PTGR of some solute carrier transporters (Tu et al., 2020; Yi et al., 2020) and enzymes (Li et al., 2014; Li et al., 2019; Tu et al., 2020) in ADME (Table 3). The involvement of miR-27b-3p in PTGR of human cytochrome P450 3A4 (CYP3A4) was demonstrated not only by a tRNA fused pre-miR-27b (Li et al., 2014) but also the tRNA/pre-miR-34a carrier based miR-27b-3p (Li et al., 2019). Following the downregulation of CYP3A4 proteins by recombinant miR-27b-3p, cellular midazolam 1′-hydroxylation capacity was reduced, indicating the involvement of miR-27b-3p signaling in drug metabolism. Further, miR-1291-5p was selectively released from recombinant miR-1291 in human pancreatic cancer cells (Tu et al., 2020). The suppression of arginosuccinate synthase (ASS1) in L3.3 cells by miR-1291-5p altered arginine homeostasis and increased the sensitivity of cells to arginine deprivation therapy (Tu et al., 2020). On the other hand, the reduction of glucose transporter protein type 1 (GLUT1) by miR-1291-5p decreased glucose uptake and glycolysis capacity and improved cisplatin efficacy in human AsPC-1 and PANC-1 cells (Tu et al., 2020).
Research on PTGR in ADME with recombinant RNAi molecules has advanced our knowledge of complex mechanisms behind interindividual variations in ADME and offered clues to developing new therapies. Indeed, a total of 6 RNAi therapeutics have been approved by the United States Food and Drug Administration for the treatment of various disorders, such as patisiran for hereditary transthyretin-mediated amyloidosis and nedosiran for primary hyperoxaluria type 1, respectively (Yu et al., 2019; Yu et al., 2020; Traber and Yu, 2023, 2024). Many studies have demonstrated the efficacy and safety of recombinant miRNAs and siRNAs in the control of tumor growth in clinically relevant animal models (Ho et al., 2018; Jilek et al., 2019; Tu et al., 2019; Deng et al., 2021; Ning et al., 2022; Chen et al., 2023; Luo et al., 2024) that are amenable to clinical investigations.
6 Conclusion and perspectives
The ubiquitous expression of membrane ABC transporters in human body maintains normal biologic processes through preserving endobiotic homeostasis, protecting against environmental toxins, and limiting the exposure to other xenobiotics. ABC transporters localized among metabolic organs pose an important role in substrate drug absorption, distribution, excretion, and overall PK properties, which may be altered among patients with certain conditions. Intrinsic or acquired overexpression of ABC transporters in the organisms or diseased cells has been shown to confer MDR by reducing drug exposure, which remains a major challenge in treating infectious diseases and cancers. While targeting efflux ABC transporters is a potential strategy to overcome MDR, clinical trials on previous generations of inhibitors reveals just a limited benefit whereas some toxicity. Therefore, a thorough understanding of complex mechanisms underlying dysregulation of ABC transporters would offer new insights into developing other possible means to improve therapies.
With the discovery of functional miRNAs derived from the genome, many recent studies have demonstrated the contribution of specific miRNAs in PTGR of various ABC transporters by targeting respective transcripts. Coadministration of certain miRNAs is proven effective to sensitize drug-resistant cells or tumors to anticancer drugs through the reduction of efflux ABC transporter expression, which rather warrants more extensive studies and clinical investigations. Furthermore, novel, in vivo fermentation based RNA molecular bioengineering technologies have been developed to offer high-quality, recombinant miRNA molecules that are a unique addition to conventional miRNA agents synthesized in vitro chemically or enzymatically. Bioengineered miRNA molecules have been revealed functional to modulate the expression of ABC transporters and other ADME genes and subsequently alter cellular drug metabolism and transport capacities as well as chemosensitivities. Such recombinant miRNA and RNAi molecules represent a novel class of tools for ADME and broader general biomedical research while holding promise as therapeutics.
Author contributions
YW: Writing–review and editing, Writing–original draft, Visualization, Investigation, Formal Analysis, Data curation. M-JT: Writing–review and editing, Validation, Investigation. A-MY: Writing–review and editing, Supervision, Funding acquisition, Conceptualization.
Funding
The author(s) declare that financial support was received for the research, authorship, and/or publication of this article. This work is supported by the National Institute of General Medical Sciences (R35GM140835) and National Cancer Institute (R01CA225958 and R01CA253230), National Institutes of Health (NIH).
Conflict of interest
The authors declare that the research was conducted in the absence of any commercial or financial relationships that could be construed as a potential conflict of interest.
Publisher’s note
All claims expressed in this article are solely those of the authors and do not necessarily represent those of their affiliated organizations, or those of the publisher, the editors and the reviewers. Any product that may be evaluated in this article, or claim that may be made by its manufacturer, is not guaranteed or endorsed by the publisher.
References
Alam, A., and Locher, K. P. (2023). Structure and mechanism of human ABC transporters. Annu. Rev. Biophys. 52, 275–300. doi:10.1146/annurev-biophys-111622-091232
Allikmets, R., Gerrard, B., Hutchinson, A., and Dean, M. (1996). Characterization of the human ABC superfamily: isolation and mapping of 21 new genes using the expressed sequence tags database. Hum. Mol. Genet. 5, 1649–1655. doi:10.1093/hmg/5.10.1649
Babiarz, J. E., Hsu, R., Melton, C., Thomas, M., Ullian, E. M., and Blelloch, R. (2011). A role for noncanonical microRNAs in the mammalian brain revealed by phenotypic differences in Dgcr8 versus Dicer1 knockouts and small RNA sequencing. Rna 17, 1489–1501. doi:10.1261/rna.2442211
Bao, L., Hazari, S., Mehra, S., Kaushal, D., Moroz, K., and Dash, S. (2012). Increased expression of P-glycoprotein and doxorubicin chemoresistance of metastatic breast cancer is regulated by miR-298. Am. J. pathology 180, 2490–2503. doi:10.1016/j.ajpath.2012.02.024
Bargou, R. C., Jürchott, K., Wagener, C., Bergmann, S., Metzner, S., Bommert, K., et al. (1997). Nuclear localization and increased levels of transcription factor YB-1 in primary human breast cancers are associated with intrinsic MDR1 gene expression. Nat. Med. 3, 447–450. doi:10.1038/nm0497-447
Berezikov, E., Chung, W.-J., Willis, J., Cuppen, E., and Lai, E. C. (2007). Mammalian mirtron genes. Mol. Cell. 28, 328–336. doi:10.1016/j.molcel.2007.09.028
Berger, E. A., and Heppel, L. A. (1974). Different mechanisms of energy coupling for the shock-sensitive and shock-resistant amino acid permeases of Escherichia coli. J. Biol. Chem. 249, 7747–7755. doi:10.1016/s0021-9258(19)42031-0
Bernstein, E., Caudy, A. A., Hammond, S. M., and Hannon, G. J. (2001). Role for a bidentate ribonuclease in the initiation step of RNA interference. Nature 409, 363–366. doi:10.1038/35053110
Borel, F., Han, R., Visser, A., Petry, H., Van Deventer, S. J., Jansen, P. L., et al. (2012). Adenosine triphosphate-binding cassette transporter genes up-regulation in untreated hepatocellular carcinoma is mediated by cellular microRNAs. Hepatology 55, 821–832. doi:10.1002/hep.24682
Boscoboinik, D., Gupta, R., and Epand, R. (1990). Investigation of the relationship between altered intracellular pH and multidrug resistance in mammalian cells. Br. J. cancer 61, 568–572. doi:10.1038/bjc.1990.127
Bukowski, K., Kciuk, M., and Kontek, R. (2020). Mechanisms of multidrug resistance in cancer chemotherapy. Int. J. Mol. Sci. 21, 3233. doi:10.3390/ijms21093233
Burger, H., Van Tol, H., Boersma, A. W., Brok, M., Wiemer, E. A., Stoter, G., et al. (2004). Imatinib mesylate (STI571) is a substrate for the breast cancer resistance protein (BCRP)/ABCG2 drug pump. Blood 104, 2940–2942. doi:10.1182/blood-2004-04-1398
Cheloufi, S., Dos Santos, C. O., Chong, M. M., and Hannon, G. J. (2010). A dicer-independent miRNA biogenesis pathway that requires Ago catalysis. Nature 465, 584–589. doi:10.1038/nature09092
Chen, C., Scott, D., Hanson, E., Franco, J., Berryman, E., Volberg, M., et al. (2003). Impact of Mrp2 on the biliary excretion and intestinal absorption of furosemide, probenecid, and methotrexate using Eisai hyperbilirubinemic rats. Pharm. Res. 20, 31–37. doi:10.1023/a:1022238506509
Chen, J., Wang, Z., Gao, S., Wu, K., Bai, F., Zhang, Q., et al. (2021). Human drug efflux transporter ABCC5 confers acquired resistance to pemetrexed in breast cancer. Cancer Cell. Int. 21, 136. doi:10.1186/s12935-021-01842-x
Chen, Q.-X., Wang, W.-P., Zeng, S., Urayama, S., and Yu, A.-M. (2015). A general approach to high-yield biosynthesis of chimeric RNAs bearing various types of functional small RNAs for broad applications. Nucleic acids Res. 43, 3857–3869. doi:10.1093/nar/gkv228
Chen, Y., Agarwal, S., Shaik, N. M., Chen, C., Yang, Z., and Elmquist, W. F. (2009). P-glycoprotein and breast cancer resistance protein influence brain distribution of dasatinib. J. Pharmacol. Exp. Ther. 330, 956–963. doi:10.1124/jpet.109.154781
Chen, Y., Tang, Y., Guo, C., Wang, J., Boral, D., and Nie, D. (2012). Nuclear receptors in the multidrug resistance through the regulation of drug-metabolizing enzymes and drug transporters. Biochem. Pharmacol. 83, 1112–1126. doi:10.1016/j.bcp.2012.01.030
Chen, Y., Tu, M. J., Han, F., Liu, Z., Batra, N., Lara, P. N., et al. (2023). Use of recombinant microRNAs as antimetabolites to inhibit human non-small cell lung cancer. Acta Pharm. Sin. B 13, 4273–4290. doi:10.1016/j.apsb.2023.07.011
Chen, Z., Ma, T., Huang, C., Zhang, L., Lv, X., Xu, T., et al. (2013). MiR-27a modulates the MDR1/P-glycoprotein expression by inhibiting FZD7/β-catenin pathway in hepatocellular carcinoma cells. Cell. Signal. 25, 2693–2701. doi:10.1016/j.cellsig.2013.08.032
Chen, Z.-S., Lee, K., and Kruh, G. D. (2001). Transport of cyclic nucleotides and estradiol 17-beta-D-glucuronide by multidrug resistance protein 4. Resistance to 6-mercaptopurine and 6-thioguanine. J. Biol. Chem. 276, 33747–33754. doi:10.1074/jbc.M104833200
Chendrimada, T. P., Gregory, R. I., Kumaraswamy, E., Norman, J., Cooch, N., Nishikura, K., et al. (2005). TRBP recruits the Dicer complex to Ago2 for microRNA processing and gene silencing. Nature 436, 740–744. doi:10.1038/nature03868
Choi, Y. H., and Yu, A.-M. (2014). ABC transporters in multidrug resistance and pharmacokinetics, and strategies for drug development. Curr. Pharm. Des. 20, 793–807. doi:10.2174/138161282005140214165212
Chu, X.-Y., Suzuki, H., Ueda, K., Kato, Y., Akiyama, S.-I., and Sugiyama, Y. (1999). Active efflux of CPT-11 and its metabolites in human KB-derived cell lines. J. Pharmacol. Exp. Ther. 288, 735–741.
Chung, S., Sawyer, J. K., Gebre, A. K., Maeda, N., and Parks, J. S. (2011). Adipose tissue ATP binding cassette transporter A1 contributes to high-density lipoprotein biogenesis in vivo. Circulation 124, 1663–1672. doi:10.1161/CIRCULATIONAHA.111.025445
Cole, S., Bhardwaj, G., Gerlach, J., Mackie, J., Grant, C., Almquist, K., et al. (1992). Overexpression of a transporter gene in a multidrug-resistant human lung cancer cell line. Science 258, 1650–1654. doi:10.1126/science.1360704
Crespi, C. L., and Miller, V. P. (1999). The use of heterologously expressed drug metabolizing enzymes—state of the art and prospects for the future. Pharmacol. Ther. 84, 121–131. doi:10.1016/s0163-7258(99)00028-5
Cronin, J. M., and Yu, A.-M. (2023). Recombinant technologies facilitate drug metabolism, pharmacokinetics, and general biomedical research. Drug Metabolism Dispos. 51, 685–699. doi:10.1124/dmd.122.001008
Cui, Y., König, J., Buchholz, U., Spring, H., Leier, I., and Keppler, D. (1999). Drug resistance and ATP-dependent conjugate transport mediated by the apical multidrug resistance protein, MRP2, permanently expressed in human and canine cells. Mol. Pharmacol. 55, 929–937.
Dai, Y., Rashba-Step, J., and Cederbaum, A. I. (1993). Stable expression of human cytochrome P4502E1 in HepG2 cells: characterization of catalytic activities and production of reactive oxygen intermediates. Biochemistry 32, 6928–6937. doi:10.1021/bi00078a017
Daood, M., Tsai, C., Ahdab-Barmada, M., and Watchko, J. F. (2008). Abc transporter (P-Gp/Abcb1, Mrp1/Abcc1, Bcrp/Abcg2) expression in the developing human Cns. Neuropediatrics 39, 211–218. doi:10.1055/s-0028-1103272
Davey, R. A., Longhurst, T. J., Davey, M. W., Belov, L., Harvie, R. M., Hancox, D., et al. (1995). Drug resistance mechanisms and MRP expression in response to epirubicin treatment in a human leukaemia cell line. Leukemia Res. 19, 275–282. doi:10.1016/0145-2126(94)00159-8
Dean, M. (2005). The genetics of ATP-binding cassette transporters. Methods Enzym. 400, 409–429. doi:10.1016/S0076-6879(05)00024-8
Dean, M., Hamon, Y., and Chimini, G. (2001). The human ATP-binding cassette (ABC) transporter superfamily. J. lipid Res. 42, 1007–1017. doi:10.1016/s0022-2275(20)31588-1
Dean, M., Moitra, K., and Allikmets, R. (2022). The human ATP-binding cassette (ABC) transporter superfamily. Hum. Mutat. 43, 1162–1182. doi:10.1002/humu.24418
De Gooijer, M. C., Zhang, P., Thota, N., Mayayo-Peralta, I., Buil, L. C., Beijnen, J. H., et al. (2015). P-glycoprotein and breast cancer resistance protein restrict the brain penetration of the CDK4/6 inhibitor palbociclib. Investig. new drugs 33, 1012–1019. doi:10.1007/s10637-015-0266-y
De Lannoy, I. A., and Silverman, M. (1992). The MDR1 gene product, P-glycoprotein, mediates the transport of the cardiac glycoside, digoxin. Biochem. biophysical Res. Commun. 189, 551–557. doi:10.1016/0006-291x(92)91593-f
Deng, L., Petrek, H., Tu, M.-J., Batra, N., Yu, A.-X., and Yu, A.-M. (2021). Bioengineered miR-124-3p prodrug selectively alters the proteome of human carcinoma cells to control multiple cellular components and lung metastasis in vivo. Acta Pharm. Sin. B 11, 3950–3965. doi:10.1016/j.apsb.2021.07.027
Denli, A. M., Tops, B. B., Plasterk, R. H., Ketting, R. F., and Hannon, G. J. (2004). Processing of primary microRNAs by the Microprocessor complex. Nature 432, 231–235. doi:10.1038/nature03049
De Rie, D., Abugessaisa, I., Alam, T., Arner, E., Arner, P., Ashoor, H., et al. (2017). An integrated expression atlas of miRNAs and their promoters in human and mouse. Nat. Biotechnol. 35, 872–878. doi:10.1038/nbt.3947
Di Martino, M. T., Arbitrio, M., Leone, E., Guzzi, P. H., Saveria Rotundo, M., Ciliberto, D., et al. (2011). Single nucleotide polymorphisms of ABCC5 and ABCG1 transporter genes correlate to irinotecan-associated gastrointestinal toxicity in colorectal cancer patients: a DMET microarray profiling study. Cancer Biol. Ther. 12, 780–787. doi:10.4161/cbt.12.9.17781
Doench, J. G., and Sharp, P. A. (2004). Specificity of microRNA target selection in translational repression. Genes and Dev. 18, 504–511. doi:10.1101/gad.1184404
Durmus, S., Hendrikx, J. J., and Schinkel, A. H. (2015). Apical ABC transporters and cancer chemotherapeutic drug disposition. Adv. Cancer Res. 125, 1–41. doi:10.1016/bs.acr.2014.10.001
Esser, L., Zhou, F., Pluchino, K. M., Shiloach, J., Ma, J., Tang, W.-K., et al. (2017). Structures of the multidrug transporter P-glycoprotein reveal asymmetric ATP binding and the mechanism of polyspecificity. J. Biol. Chem. 292, 446–461. doi:10.1074/jbc.M116.755884
Evers, R., Piquette-Miller, M., Polli, J. W., Russel, F. G. M., Sprowl, J. A., Tohyama, K., et al. (2018). Disease-associated changes in drug transporters may impact the pharmacokinetics and/or toxicity of drugs: a white paper from the international transporter consortium. Clin. Pharmacol. Ther. 104, 900–915. doi:10.1002/cpt.1115
Evers, R., Zaman, G., Van Deemter, L., Jansen, H., Calafat, J., Oomen, L., et al. (1996). Basolateral localization and export activity of the human multidrug resistance-associated protein in polarized pig kidney cells. J. Clin. Investigation 97, 1211–1218. doi:10.1172/JCI118535
Fan, J., To, K. K. W., Chen, Z.-S., and Fu, L. (2023). ABC transporters affects tumor immune microenvironment to regulate cancer immunotherapy and multidrug resistance. Drug Resist. Updat. 66, 100905. doi:10.1016/j.drup.2022.100905
Fan, Y.-P., Liao, J.-Z., Lu, Y.-Q., Tian, D.-A., Ye, F., Zhao, P.-X., et al. (2017). MiR-375 and doxorubicin co-delivered by liposomes for combination therapy of hepatocellular carcinoma. Mol. Therapy-Nucleic Acids 7, 181–189. doi:10.1016/j.omtn.2017.03.010
Fareh, M., Yeom, K.-H., Haagsma, A. C., Chauhan, S., Heo, I., and Joo, C. (2016). TRBP ensures efficient Dicer processing of precursor microRNA in RNA-crowded environments. Nat. Commun. 7, 13694. doi:10.1038/ncomms13694
Feng, D. D., Zhang, H., Zhang, P., Zheng, Y. S., Zhang, X. J., Han, B. W., et al. (2011). Down-regulated miR-331–5p and miR-27a are associated with chemotherapy resistance and relapse in leukaemia. J. Cell. Mol. Med. 15, 2164–2175. doi:10.1111/j.1582-4934.2010.01213.x
Flens, M. J., Zaman, G., Van Der Valk, P., Izquierdo, M. A., Schroeijers, A. B., Scheffer, G. L., et al. (1996). Tissue distribution of the multidrug resistance protein. Am. J. Pathology 148, 1237–1247.
Fojo, A. T., Ueda, K., Slamon, D. J., Poplack, D., Gottesman, M., and Pastan, I. (1987). Expression of a multidrug-resistance gene in human tumors and tissues, Proc. Natl. Acad. Sci. U. S. A. 84, 265–269. doi:10.1073/pnas.84.1.265
Fromm, M. F., Kim, R. B., Stein, C. M., Wilkinson, G. R., and Roden, D. M. (1999). Inhibition of P-glycoprotein-mediated drug transport: a unifying mechanism to explain the interaction between digoxin and quinidine [seecomments]. Circulation 99, 552–557. doi:10.1161/01.cir.99.4.552
Fukuda, Y., Takenaka, K., Sparreboom, A., Cheepala, S. B., Wu, C.-P., Ekins, S., et al. (2013). Human immunodeficiency virus protease inhibitors interact with ATP binding cassette transporter 4/multidrug resistance protein 4: a basis for unanticipated enhanced cytotoxicity. Mol. Pharmacol. 84, 361–371. doi:10.1124/mol.113.086967
Galluccio, M., Scalise, M., Pappacoda, G., Scarpelli, M., Bonanomi, M., Gaglio, D., et al. (2022). Production of recombinant human xCT (SLC7A11) and reconstitution in proteoliposomes for functional studies. Front. Physiology 13, 993626. doi:10.3389/fphys.2022.993626
Gao, M., Miao, L., Liu, M., Li, C., Yu, C., Yan, H., et al. (2016). miR-145 sensitizes breast cancer to doxorubicin by targeting multidrug resistance-associated protein-1. Oncotarget 7, 59714–59726. doi:10.18632/oncotarget.10845
Geick, A., Eichelbaum, M., and Burk, O. (2001). Nuclear receptor response elements mediate induction of intestinal MDR1 by rifampin. J. Biol. Chem. 276, 14581–14587. doi:10.1074/jbc.M010173200
Gonzalez, F. J. (2004). Cytochrome P450 humanised mice. Hum. genomics 1, 300–306. doi:10.1186/1479-7364-1-4-300
Gregory, R. I., Yan, K.-P., Amuthan, G., Chendrimada, T., Doratotaj, B., Cooch, N., et al. (2004). The Microprocessor complex mediates the genesis of microRNAs. Nature 432, 235–240. doi:10.1038/nature03120
Greiner, B., Eichelbaum, M., Fritz, P., Kreichgauer, H.-P., Von Richter, O., Zundler, J., et al. (1999). The role of intestinal P-glycoprotein in the interaction of digoxin and rifampin. J. Clin. investigation 104, 147–153. doi:10.1172/JCI6663
Gromnicova, R., Romero, I., and Male, D. (2012). Transcriptional control of the multi-drug transporter ABCB1 by transcription factor Sp3 in different human tissues. PLoS One 7, e48189. doi:10.1371/journal.pone.0048189
Gu, S., and Kay, M. A. (2010). How do miRNAs mediate translational repression? Silence 1, 11. doi:10.1186/1758-907X-1-11
Ha, M., and Kim, V. N. (2014). Regulation of microRNA biogenesis. Nat. Rev. Mol. Cell. Biol. 15, 509–524. doi:10.1038/nrm3838
Haenisch, S., Laechelt, S., Bruckmueller, H., Werk, A., Noack, A., Bruhn, O., et al. (2011). Down-regulation of ATP-binding cassette C2 protein expression in HepG2 cells after rifampicin treatment is mediated by microRNA-379. Mol. Pharmacol. 80, 314–320. doi:10.1124/mol.110.070714
Haenisch, S., Werk, A. N., and Cascorbi, I. (2014). Micro RNA s and their relevance to ABC transporters. Br. J. Clin. Pharmacol. 77, 587–596. doi:10.1111/bcp.12251
Han, K., Kahng, J., Kim, M., Lim, J., Kim, Y., Cho, B., et al. (2001). Expression of functional markers in acute nonlymphoblastic leukemia. Acta Haematol. 104, 174–180. doi:10.1159/000046511
He, X., Chang, Y., Meng, F., Wang, M., Xie, Q., Tang, F., et al. (2012). MicroRNA-375 targets AEG-1 in hepatocellular carcinoma and suppresses liver cancer cell growth in vitro and in vivo. Oncogene 31, 3357–3369. doi:10.1038/onc.2011.500
Hegedűs, C., Özvegy-Laczka, C., Apati, A., Magocsi, M., Nemet, K., Őrfi, L., et al. (2009). Interaction of nilotinib, dasatinib and bosutinib with ABCB1 and ABCG2: implications for altered anti-cancer effects and pharmacological properties. Br. J. Pharmacol. 158, 1153–1164. doi:10.1111/j.1476-5381.2009.00383.x
Hegedűs, T., Őrfi, L., Seprődi, A., Váradi, A., Sarkadi, B., and Kéri, G. (2002). Interaction of tyrosine kinase inhibitors with the human multidrug transporter proteins, MDR1 and MRP1. Biochimica Biophysica Acta (BBA)-Molecular Basis Dis. 1587, 318–325. doi:10.1016/s0925-4439(02)00095-9
Ho, P. Y., Duan, Z., Batra, N., Jilek, J. L., Tu, M.-J., Qiu, J.-X., et al. (2018). Bioengineered noncoding RNAs selectively change cellular miRNome profiles for cancer therapy. J. Pharmacol. Exp. Ther. 365, 494–506. doi:10.1124/jpet.118.247775
Hopper-Borge, E., Chen, Z.-S., Shchaveleva, I., Belinsky, M. G., and Kruh, G. D. (2004). Analysis of the drug resistance profile of multidrug resistance protein 7 (ABCC10) resistance to docetaxel. Cancer Res. 64, 4927–4930. doi:10.1158/0008-5472.CAN-03-3111
Hrycyna, C. A., and Gottesman, M. M. (1998). Multidrug ABC transporters from bacteria to man: an emerging hypothesis for the universality of molecular mechanism and function. Drug Resist Updat 1, 81–83. doi:10.1016/s1368-7646(98)80019-8
Huisman, M. T., Smit, J. W., Crommentuyn, K. M., Zelcer, N., Wiltshire, H. R., Beijnen, J. H., et al. (2002). Multidrug resistance protein 2 (MRP2) transports HIV protease inhibitors, and transport can be enhanced by other drugs. Aids 16, 2295–2301. doi:10.1097/00002030-200211220-00009
Hyde, S., Emsley, P., Hartshorn, M., Mimmack, M. M., Gileadi, U., Pearce, S. R., et al. (1990). Structural model of ATP-binding proteins associated with cystic fibrosis, multidrug resistance and bacterial transport. Nature 346, 362–365. doi:10.1038/346362a0
Ikemura, K., Yamamoto, M., Miyazaki, S., Mizutani, H., Iwamoto, T., and Okuda, M. (2013). MicroRNA-145 post-transcriptionally regulates the expression and function of P-glycoprotein in intestinal epithelial cells. Mol. Pharmacol. 83, 399–405. doi:10.1124/mol.112.081844
Imaoka, T., Kusuhara, H., Adachi, M., Schuetz, J. D., Takeuchi, K., and Sugiyama, Y. (2007). Functional involvement of multidrug resistance-associated protein 4 (MRP4/ABCC4) in the renal elimination of the antiviral drugs adefovir and tenofovir. Mol. Pharmacol. 71, 619–627. doi:10.1124/mol.106.028233
Ingelman-Sundberg, M., Zhong, X.-B., Hankinson, O., Beedanagari, S., Yu, A.-M., Peng, L., et al. (2013). Potential role of epigenetic mechanisms in the regulation of drug metabolism and transport. Drug Metabolism Dispos. 41, 1725–1731. doi:10.1124/dmd.113.053157
Janneh, O., Jones, E., Chandler, B., Owen, A., and Khoo, S. H. (2007). Inhibition of P-glycoprotein and multidrug resistance-associated proteins modulates the intracellular concentration of lopinavir in cultured CD4 T cells and primary human lymphocytes. J. Antimicrob. Chemother. 60, 987–993. doi:10.1093/jac/dkm353
Jedlitschky, G., Hoffmann, U., and Kroemer, H. K. (2006). Structure and function of the MRP2 (ABCC2) protein and its role in drug disposition. Expert Opin. drug metabolism Toxicol. 2, 351–366. doi:10.1517/17425255.2.3.351
Jiang, X. L., Gonzalez, F. J., and Yu, A. M. (2011). Drug-metabolizing enzyme, transporter, and nuclear receptor genetically modified mouse models. Drug Metab. Rev. 43, 27–40. doi:10.3109/03602532.2010.512294
Jilek, J. L., Tu, M.-J., Zhang, C., and Yu, A.-M. (2020). Pharmacokinetic and pharmacodynamic factors contribute to synergism between Let-7c-5p and 5-fluorouracil in inhibiting hepatocellular carcinoma cell viability. Drug Metabolism Dispos. 48, 1257–1263. doi:10.1124/dmd.120.000207
Jilek, J. L., Zhang, Q.-Y., Tu, M.-J., Ho, P. Y., Duan, Z., Qiu, J.-X., et al. (2019). Bioengineered Let-7c inhibits orthotopic hepatocellular carcinoma and improves overall survival with minimal immunogenicity. Mol. Therapy-Nucleic Acids 14, 498–508. doi:10.1016/j.omtn.2019.01.007
Jin, M. S., Oldham, M. L., Zhang, Q., and Chen, J. (2012). Crystal structure of the multidrug transporter P-glycoprotein from Caenorhabditis elegans. Nature 490, 566–569. doi:10.1038/nature11448
Juliano, R. L., and Ling, V. (1976). A surface glycoprotein modulating drug permeability in Chinese hamster ovary cell mutants. Biochimica Biophysica Acta (BBA)-Biomembranes 455, 152–162. doi:10.1016/0005-2736(76)90160-7
Kadioglu, O., Saeed, M. E., Munder, M., Spuller, A., Greten, H. J., and Efferth, T. (2020). Effect of ABC transporter expression and mutational status on survival rates of cancer patients. Biomed. Pharmacother. 131, 110718. doi:10.1016/j.biopha.2020.110718
Kaehler, M., Ruemenapp, J., Gonnermann, D., Nagel, I., Bruhn, O., Haenisch, S., et al. (2017). MicroRNA-212/ABCG2-axis contributes to development of imatinib-resistance in leukemic cells. Oncotarget 8, 92018–92031. doi:10.18632/oncotarget.21272
Keppler, D., and König, J. (1997). Expression and localization of the conjugate export pump encoded by the MRP2 (cMRP/cMOAJ) gene in liver. FASEB J. 11, 509–515. doi:10.1096/fasebj.11.7.9212074
Ketting, R. F., Fischer, S. E., Bernstein, E., Sijen, T., Hannon, G. J., and Plasterk, R. H. (2001). Dicer functions in RNA interference and in synthesis of small RNA involved in developmental timing in C. elegans. Genes. and Dev. 15, 2654–2659. doi:10.1101/gad.927801
Khvorova, A., Reynolds, A., and Jayasena, S. D. (2003). Functional siRNAs and miRNAs exhibit strand bias. Cell. 115, 209–216. doi:10.1016/s0092-8674(03)00801-8
Kim, K. Y., Kim, S. H., Yu, S. N., Park, S. K., Choi, H. D., Yu, H. S., et al. (2015). Salinomycin enhances doxorubicin-induced cytotoxicity in multidrug resistant MCF-7/MDR human breast cancer cells via decreased efflux of doxorubicin. Mol. Med. Rep. 12, 1898–1904. doi:10.3892/mmr.2015.3633
Kim, Y., and Chen, J. (2018). Molecular structure of human P-glycoprotein in the ATP-bound, outward-facing conformation. Science 359, 915–919. doi:10.1126/science.aar7389
Kim, Y.-K., Kim, B., and Kim, V. N. (2016). Re-evaluation of the roles of DROSHA, Export in 5, and DICER in microRNA biogenesis, Proc. Natl. Acad. Sci. U. S. A. 113, E1881–E1889. doi:10.1073/pnas.1602532113
Kis, O., Sankaran-Walters, S., Hoque, M. T., Walmsley, S. L., Dandekar, S., and Bendayan, R. (2016). HIV-1 alters intestinal expression of drug transporters and metabolic enzymes: implications for antiretroviral drug disposition. Antimicrob. Agents Chemother. 60, 2771–2781. doi:10.1128/AAC.02278-15
Kitazaki, T., Oka, M., Nakamura, Y., Tsurutani, J., Doi, S., Yasunaga, M., et al. (2005). Gefitinib, an EGFR tyrosine kinase inhibitor, directly inhibits the function of P-glycoprotein in multidrug resistant cancer cells. Lung Cancer 49, 337–343. doi:10.1016/j.lungcan.2005.03.035
Klein, I., Sarkadi, B., and Váradi, A. (1999). An inventory of the human ABC proteins. Biochimica Biophysica Acta (BBA)-Biomembranes 1461, 237–262. doi:10.1016/s0005-2736(99)00161-3
Knauer, M. J., Urquhart, B. L., Meyer Zu Schwabedissen, H. E., Schwarz, U. I., Lemke, C. J., Leake, B. F., et al. (2010). Human skeletal muscle drug transporters determine local exposure and toxicity of statins. Circulation Res. 106, 297–306. doi:10.1161/CIRCRESAHA.109.203596
Koehn, L. M. (2021). “ABC transporters: an overview,” in The ADME encyclopedia: a comprehensive guide on biopharmacy and pharmacokinetics. Editor A. Talevi (Springer), 1–10.
Kool, M., Van Der Linden, M., De Haas, M., Scheffer, G. L., De Vree, J. M. L., Smith, A. J., et al. (1999). MRP3, an organic anion transporter able to transport anti-cancer drugs. Proc. Natl. Acad. Sci. 96, 6914–6919. doi:10.1073/pnas.96.12.6914
Kopecka, J., Trouillas, P., Gašparović, A. Č., Gazzano, E., Assaraf, Y. G., and Riganti, C. (2020). Phospholipids and cholesterol: inducers of cancer multidrug resistance and therapeutic targets. Drug Resist. Updat. 49, 100670. doi:10.1016/j.drup.2019.100670
Kovalchuk, O., Filkowski, J., Meservy, J., Ilnytskyy, Y., Tryndyak, V. P., Chekhun, V. F., et al. (2008). Involvement of microRNA-451 in resistance of the MCF-7 breast cancer cells to chemotherapeutic drug doxorubicin. Mol. Cancer Ther. 7, 2152–2159. doi:10.1158/1535-7163.MCT-08-0021
Kwak, P. B., and Tomari, Y. (2012). The N domain of Argonaute drives duplex unwinding during RISC assembly. Nat. Struct. Mol. Biol. 19, 145–151. doi:10.1038/nsmb.2232
Laddha, A. P., Dzielak, L., Lewis, C., Xue, R., and Manautou, J. E. (2024). Impact of non-alcoholic fatty liver disease (NAFLD) and non-alcoholic steatohepatitis (NASH) on the expression and function of hepatobiliary transporters: a comprehensive mechanistic review. Biochim. Biophys. Acta Mol. Basis Dis. 1870, 167037. doi:10.1016/j.bbadis.2024.167037
Lagas, J. S., Vlaming, M. L., Van Tellingen, O., Wagenaar, E., Jansen, R. S., Rosing, H., et al. (2006). Multidrug resistance protein 2 is an important determinant of paclitaxel pharmacokinetics. Clin. Cancer Res. 12, 6125–6132. doi:10.1158/1078-0432.CCR-06-1352
Lai, E. C. (2002). Micro RNAs are complementary to 3′ UTR sequence motifs that mediate negative post-transcriptional regulation. Nat. Genet. 30, 363–364. doi:10.1038/ng865
Lee, R. C., Feinbaum, R. L., and Ambros, V. (1993). The C. elegans heterochronic gene lin-4 encodes small RNAs with antisense complementarity to lin-14. Cell. 75, 843–854. doi:10.1016/0092-8674(93)90529-y
Lee, Y., Ahn, C., Han, J., Choi, H., Kim, J., Yim, J., et al. (2003). The nuclear RNase III Drosha initiates microRNA processing. Nature 425, 415–419. doi:10.1038/nature01957
Lee, Y., Kim, M., Han, J., Yeom, K. H., Lee, S., Baek, S. H., et al. (2004). MicroRNA genes are transcribed by RNA polymerase II. EMBO J. 23, 4051–4060. doi:10.1038/sj.emboj.7600385
Leggas, M., Adachi, M., Scheffer, G. L., Sun, D., Wielinga, P., Du, G., et al. (2004). Mrp4 confers resistance to topotecan and protects the brain from chemotherapy. Mol. Cell. Biol. 24, 7612–7621. doi:10.1128/MCB.24.17.7612-7621.2004
Lemontt, J. F., Azzaria, M., and Gros, P. (1988). Increased mdr gene expression and decreased drug accumulation in multidrug-resistant human melanoma cells. Cancer Res. 48, 6348–6353.
Lemos, C., Kathmann, I., Giovannetti, E., Calhau, C., Jansen, G., and Peters, G. (2009). Impact of cellular folate status and epidermal growth factor receptor expression on BCRP/ABCG2-mediated resistance to gefitinib and erlotinib. Br. J. Cancer 100, 1120–1127. doi:10.1038/sj.bjc.6604980
Li, M.-M., Addepalli, B., Tu, M.-J., Chen, Q.-X., Wang, W.-P., Limbach, P. A., et al. (2015). Chimeric microRNA-1291 biosynthesized efficiently in Escherichia coli is effective to reduce target gene expression in human carcinoma cells and improve chemosensitivity. Drug Metabolism Dispos. 43, 1129–1136. doi:10.1124/dmd.115.064493
Li, M.-M., Wang, W.-P., Wu, W.-J., Huang, M., and Yu, A.-M. (2014). Rapid production of novel pre-microRNA agent hsa-mir-27b in Escherichia coli using recombinant RNA technology for functional studies in mammalian cells. Drug Metabolism Dispos. 42, 1791–1795. doi:10.1124/dmd.114.060145
Li, P.-C., Tu, M.-J., Ho, P. Y., Batra, N., Tran, M. M., Qiu, J.-X., et al. (2021). In vivo fermentation production of humanized noncoding RNAs carrying payload miRNAs for targeted anticancer therapy. Theranostics 11, 4858–4871. doi:10.7150/thno.56596
Li, S.-C., Chan, W.-C., Hu, L.-Y., Lai, C.-H., Hsu, C.-N., and Lin, W.-C. (2010). Identification of homologous microRNAs in 56 animal genomes. Genomics 96, 1–9. doi:10.1016/j.ygeno.2010.03.009
Li, X., Pan, Y.-Z., Seigel, G. M., Hu, Z.-H., Huang, M., and Yu, A.-M. (2011). Breast cancer resistance protein BCRP/ABCG2 regulatory microRNAs (hsa-miR-328,-519c and-520h) and their differential expression in stem-like ABCG2+ cancer cells. Biochem. Pharmacol. 81, 783–792. doi:10.1016/j.bcp.2010.12.018
Li, X., Tian, Y., Tu, M.-J., Ho, P. Y., Batra, N., and Yu, A.-M. (2019). Bioengineered miR-27b-3p and miR-328-3p modulate drug metabolism and disposition via the regulation of target ADME gene expression. Acta Pharm. Sin. B 9, 639–647. doi:10.1016/j.apsb.2018.12.002
Li, Y., Gong, P., Hou, J.-X., Huang, W., Ma, X.-P., Wang, Y.-L., et al. (2018). miR-34a regulates multidrug resistance via positively modulating OAZ2 signaling in colon cancer cells. J. Immunol. Res. 2018, 7498514. doi:10.1155/2018/7498514
Liang, Z., Wu, H., Xia, J., Li, Y., Zhang, Y., Huang, K., et al. (2010). Involvement of miR-326 in chemotherapy resistance of breast cancer through modulating expression of multidrug resistance-associated protein 1. Biochem. Pharmacol. 79, 817–824. doi:10.1016/j.bcp.2009.10.017
Liu, J., Carmell, M. A., Rivas, F. V., Marsden, C. G., Thomson, J. M., Song, J.-J., et al. (2004). Argonaute2 is the catalytic engine of mammalian RNAi. Science 305, 1437–1441. doi:10.1126/science.1102513
Lorico, A., Rappa, G., Finch, R. A., Yang, D., Flavell, R. A., and Sartorelli, A. C. (1997). Disruption of the murine MRP (multidrug resistance protein) gene leads to increased sensitivity to etoposide (VP-16) and increased levels of glutathione. Cancer Res. 57, 5238–5242.
Luciani, M. F., Denizot, F., Savary, S., Mattei, M. G., and Chimini, G. (1994). Cloning of two novel ABC transporters mapping on human chromosome 9. Genomics 21, 150–159. doi:10.1006/geno.1994.1237
Lund, E., Guttinger, S., Calado, A., Dahlberg, J. E., and Kutay, U. (2004). Nuclear export of microRNA precursors. Science 303, 95–98. doi:10.1126/science.1090599
Luo, Z., Huang, Y., Batra, N., Chen, Y., Huang, H., Wang, Y., et al. (2024). Inhibition of iRhom1 by CD44-targeting nanocarrier for improved cancer immunochemotherapy. Nat. Commun. 15, 255. doi:10.1038/s41467-023-44572-6
Ma, J., Wang, T., Guo, R., Yang, X., Yin, J., Yu, J., et al. (2015). Involvement of miR-133a and miR-326 in ADM resistance of HepG2 through modulating expression of ABCC1. J. Drug Target. 23, 519–524. doi:10.3109/1061186X.2015.1015536
Macrae, I. J., Ma, E., Zhou, M., Robinson, C. V., and Doudna, J. A. (2008). In vitro reconstitution of the human RISC-loading complex, Proc. Natl. Acad. Sci. U. S. A. 105, 512–517. doi:10.1073/pnas.0710869105
Markova, S. M., and Kroetz, D. L. (2014). ABCC4 is regulated by microRNA-124a and microRNA-506. Biochem. Pharmacol. 87, 515–522. doi:10.1016/j.bcp.2013.10.017
Martínez-Chávez, A., Van Hoppe, S., Rosing, H., Lebre, M. C., Tibben, M., Beijnen, J. H., et al. (2019). P-glycoprotein limits ribociclib brain exposure and CYP3A4 restricts its oral bioavailability. Mol. Pharm. 16, 3842–3852. doi:10.1021/acs.molpharmaceut.9b00475
Masereeuw, R., and Russel, F. G. (2012). Regulatory pathways for ATP-binding cassette transport proteins in kidney proximal tubules. AAPS J. 14, 883–894. doi:10.1208/s12248-012-9404-z
Meaden, E., Hoggard, P., Newton, P., Tjia, J., Aldam, D., Cornforth, D., et al. (2002). P-glycoprotein and MRP1 expression and reduced ritonavir and saquinavir accumulation in HIV-infected individuals. J. Antimicrob. Chemother. 50, 583–588. doi:10.1093/jac/dkf161
Mechetner, E., Kyshtoobayeva, A., Zonis, S., Kim, H., Stroup, R., Garcia, R., et al. (1998). Levels of multidrug resistance (MDR1) P-glycoprotein expression by human breast cancer correlate with in vitro resistance to taxol and doxorubicin. Clin. cancer Res. official J. Am. Assoc. Cancer Res. 4, 389–398.
Meyers, M. B., Scotto, K. W., and Sirotnak, F. M. (1991). P-glycoprotein content and mediation of vincristine efflux: correlation with the level of differentiation in luminal epithelium of mouse small intestine. Cancer Commun. 3, 159–165. doi:10.3727/095535491820873335
Miller, D. S. (2015). Regulation of ABC transporters at the blood–brain barrier. Clin. Pharmacol. Ther. 97, 395–403. doi:10.1002/cpt.64
Mohammad, I. S., He, W., and Yin, L. (2020). Insight on multidrug resistance and nanomedicine approaches to overcome MDR. Crit. Reviews™ Ther. Drug Carr. Syst. 37, 473–509. doi:10.1615/CritRevTherDrugCarrierSyst.2020025052
Muriithi, W., Macharia, L. W., Heming, C. P., Echevarria, J. L., Nyachieo, A., Niemeyer Filho, P., et al. (2020). ABC transporters and the hallmarks of cancer: roles in cancer aggressiveness beyond multidrug resistance. Cancer Biol. Med. 17, 253–269. doi:10.20892/j.issn.2095-3941.2019.0284
Naruhashi, K., Tamai, I., Inoue, N., Muraoka, H., Sai, Y., Suzuki, N., et al. (2002). Involvement of multidrug resistance-associated protein 2 in intestinal secretion of grepafloxacin in rats. Antimicrob. agents Chemother. 46, 344–349. doi:10.1128/aac.46.2.344-349.2002
Ning, S., Liu, C., Lou, W., Yang, J. C., Lombard, A. P., D’abronzo, L. S., et al. (2022). Bioengineered BERA-Wnt5a siRNA targeting Wnt5a/FZD2 signaling suppresses advanced prostate cancer tumor growth and enhances enzalutamide treatment. Mol. Cancer Ther. 21, 1594–1607. doi:10.1158/1535-7163.MCT-22-0216
Norris, M. D., Smith, J., Tanabe, K., Tobin, P., Flemming, C., Scheffer, G. L., et al. (2005). Expression of multidrug transporter MRP4/ABCC4 is a marker of poor prognosis in neuroblastoma and confers resistance to irinotecan in vitro. Mol. cancer Ther. 4, 547–553. doi:10.1158/1535-7163.MCT-04-0161
Nosol, K., Romane, K., Irobalieva, R. N., Alam, A., Kowal, J., Fujita, N., et al. (2020). Cryo-EM structures reveal distinct mechanisms of inhibition of the human multidrug transporter ABCB1. Proc. Natl. Acad. Sci. 117, 26245–26253. doi:10.1073/pnas.2010264117
O’carroll, D., Mecklenbrauker, I., Das, P. P., Santana, A., Koenig, U., Enright, A. J., et al. (2007). A Slicer-independent role for Argonaute 2 in hematopoiesis and the microRNA pathway. Genes. and Dev. 21, 1999–2004. doi:10.1101/gad.1565607
Okamura, K., Hagen, J. W., Duan, H., Tyler, D. M., and Lai, E. C. (2007). The mirtron pathway generates microRNA-class regulatory RNAs in Drosophila. Cell. 130, 89–100. doi:10.1016/j.cell.2007.06.028
Olsen, A., Linton, L. M., Birren, B., Nusbaum, C., Zody, M. C., Baldwin, J., et al. (2001). Initial sequencing and analysis of the human genome. nature 409, 860–921. doi:10.1038/35057062
Orelle, C., Mathieu, K., and Jault, J. M. (2019). Multidrug ABC transporters in bacteria. Res. Microbiol. 170, 381–391. doi:10.1016/j.resmic.2019.06.001
Pan, Y.-Z., Morris, M. E., and Yu, A.-M. (2009). MicroRNA-328 negatively regulates the expression of breast cancer resistance protein (BCRP/ABCG2) in human cancer cells. Mol. Pharmacol. 75, 1374–1379. doi:10.1124/mol.108.054163
Pan, Y. Z., Zhou, A., Hu, Z., and Yu, A. M. (2013). Small nucleolar RNA-derived microRNA hsa-miR-1291 modulates cellular drug disposition through direct targeting of ABC transporter ABCC1. Drug Metab. Dispos. 41, 1744–1751. doi:10.1124/dmd.113.052092
Pasquinelli, A. E., Reinhart, B. J., Slack, F., Martindale, M. Q., Kuroda, M. I., Maller, B., et al. (2000). Conservation of the sequence and temporal expression of let-7 heterochronic regulatory RNA. Nature 408, 86–89. doi:10.1038/35040556
Peetla, C., Vijayaraghavalu, S., and Labhasetwar, V. (2013). Biophysics of cell membrane lipids in cancer drug resistance: implications for drug transport and drug delivery with nanoparticles. Adv. drug Deliv. Rev. 65, 1686–1698. doi:10.1016/j.addr.2013.09.004
Ponchon, L., Beauvais, G., Nonin-Lecomte, S., and Dardel, F. (2009). A generic protocol for the expression and purification of recombinant RNA in Escherichia coli using a tRNA scaffold. Nat. Protoc. 4, 947–959. doi:10.1038/nprot.2009.67
Pratt, S., Shepard, R. L., Kandasamy, R. A., Johnston, P. A., Perry Iii, W., and Dantzig, A. H. (2005). The multidrug resistance protein 5 (ABCC5) confers resistance to 5-fluorouracil and transports its monophosphorylated metabolites. Mol. cancer Ther. 4, 855–863. doi:10.1158/1535-7163.MCT-04-0291
Reinhart, B. J., Slack, F. J., Basson, M., Pasquinelli, A. E., Bettinger, J. C., Rougvie, A. E., et al. (2000). The 21-nucleotide let-7 RNA regulates developmental timing in Caenorhabditis elegans. nature 403, 901–906. doi:10.1038/35002607
Renes, J., De Vries, E. G., Nienhuis, E. F., Jansen, P. L., and Müller, M. (1999). ATP-and glutathione-dependent transport of chemotherapeutic drugs by the multidrug resistance protein MRP1. Br. J. Pharmacol. 126, 681–688. doi:10.1038/sj.bjp.0702360
Riordan, J. R., Deuchars, K., Kartner, N., Alon, N., Trent, J., and Ling, V. (1985). Amplification of P-glycoprotein genes in multidrug-resistant mammalian cell lines. nature 316, 817–819. doi:10.1038/316817a0
Rius, M., Nies, A. T., Hummel-Eisenbeiss, J., Jedlitschky, G., and Keppler, D. (2003). Cotransport of reduced glutathione with bile salts by MRP4 (ABCC4) localized to the basolateral hepatocyte membrane. Hepatology 38, 374–384. doi:10.1053/jhep.2003.50331
Robey, R., Honjo, Y., Morisaki, K., Nadjem, T., Runge, S., Risbood, M., et al. (2003). Mutations at amino-acid 482 in the ABCG2 gene affect substrate and antagonist specificity. Br. J. Cancer 89, 1971–1978. doi:10.1038/sj.bjc.6601370
Robey, R. W., Pluchino, K. M., Hall, M. D., Fojo, A. T., Bates, S. E., and Gottesman, M. M. (2018). Revisiting the role of ABC transporters in multidrug-resistant cancer. Nat. Rev. Cancer 18, 452–464. doi:10.1038/s41568-018-0005-8
Rocchi, E., Khodjakov, A., Volk, E. L., Yang, C.-H., Litman, T., Bates, S. E., et al. (2000). The product of the ABC half-transporter gene ABCG2 (BCRP/MXR/ABCP) is expressed in the plasma membrane. Biochem. biophysical Res. Commun. 271, 42–46. doi:10.1006/bbrc.2000.2590
Ruby, J. G., Jan, C. H., and Bartel, D. P. (2007). Intronic microRNA precursors that bypass Drosha processing. Nature 448, 83–86. doi:10.1038/nature05983
Sen, G. L., and Blau, H. M. (2005). Argonaute 2/RISC resides in sites of mammalian mRNA decay known as cytoplasmic bodies. Nat. Cell. Biol. 7, 633–636. doi:10.1038/ncb1265
Shang, R., Baek, S. C., Kim, K., Kim, B., Kim, V. N., and Lai, E. C. (2020). Genomic clustering facilitates nuclear processing of suboptimal pri-miRNA loci. Mol. Cell. 78, 303–316. doi:10.1016/j.molcel.2020.02.009
Shen, T., Kuang, Y.-H., Ashby Jr, C. R., Lei, Y., Chen, A., Zhou, Y., et al. (2009). Imatinib and nilotinib reverse multidrug resistance in cancer cells by inhibiting the efflux activity of the MRP7 (ABCC10). PLoS One 4, e7520. doi:10.1371/journal.pone.0007520
Sheth, U., and Parker, R. (2003). Decapping and decay of messenger RNA occur in cytoplasmic processing bodies. Science 300, 805–808. doi:10.1126/science.1082320
Shirakawa, K., Takara, K., Tanigawara, Y., Aoyama, N., Kasuga, M., Komada, F., et al. (1999). Interaction of docetaxel (“Taxotere”) with human P-glycoprotein. Jpn. J. cancer Res. 90, 1380–1386. doi:10.1111/j.1349-7006.1999.tb00723.x
Sorf, A., Hofman, J., Kučera, R., Staud, F., and Ceckova, M. (2018). Ribociclib shows potential for pharmacokinetic drug-drug interactions being a substrate of ABCB1 and potent inhibitor of ABCB1, ABCG2 and CYP450 isoforms in vitro. Biochem. Pharmacol. 154, 10–17. doi:10.1016/j.bcp.2018.04.013
Stillhart, C., Vučićević, K., Augustijns, P., Basit, A. W., Batchelor, H., Flanagan, T. R., et al. (2020). Impact of gastrointestinal physiology on drug absorption in special populations––An UNGAP review. Eur. J. Pharm. Sci. 147, 105280. doi:10.1016/j.ejps.2020.105280
Su, Y., Zhang, X., and Sinko, P. J. (2004). Human organic anion-transporting polypeptide OATP-A (SLC21A3) acts in concert with P-glycoprotein and multidrug resistance protein 2 in the vectorial transport of Saquinavir in Hep G2 cells. Mol. Pharm. 1, 49–56. doi:10.1021/mp0340136
Sun, K.-X., Jiao, J.-W., Chen, S., Liu, B.-L., and Zhao, Y. (2015). MicroRNA-186 induces sensitivity of ovarian cancer cells to paclitaxel and cisplatin by targeting ABCB1. J. Ovarian Res. 8, 80–87. doi:10.1186/s13048-015-0207-6
Szlasa, W., Zendran, I., Zalesińska, A., Tarek, M., and Kulbacka, J. (2020). Lipid composition of the cancer cell membrane. J. bioenergetics Biomembr. 52, 321–342. doi:10.1007/s10863-020-09846-4
Tamaki, A., Ierano, C., Szakacs, G., Robey, R. W., and Bates, S. E. (2011). The controversial role of ABC transporters in clinical oncology. Essays Biochem. 50, 209–232. doi:10.1042/bse0500209
Thiebaut, F., Tsuruo, T., Hamada, H., Gottesman, M. M., Pastan, I., and Willingham, M. C. (1987). Cellular localization of the multidrug-resistance gene product P-glycoprotein in normal human tissues. Proc. Natl. Acad. Sci. 84, 7735–7738. doi:10.1073/pnas.84.21.7735
Tiwari, A. K., Sodani, K., Wang, S.-R., Kuang, Y.-H., Ashby Jr, C. R., Chen, X., et al. (2009). Nilotinib (AMN107, Tasigna) reverses multidrug resistance by inhibiting the activity of the ABCB1/Pgp and ABCG2/BCRP/MXR transporters. Biochem. Pharmacol. 78, 153–161. doi:10.1016/j.bcp.2009.04.002
To, K. K., Robey, R. W., Knutsen, T., Zhan, Z., Ried, T., and Bates, S. E. (2009). Escape from hsa-miR-519c enables drug-resistant cells to maintain high expression of ABCG2. Mol. Cancer Ther. 8, 2959–2968. doi:10.1158/1535-7163.MCT-09-0292
To, K. K., Zhan, Z., Litman, T., and Bates, S. E. (2008). Regulation of ABCG2 expression at the 3′ untranslated region of its mRNA through modulation of transcript stability and protein translation by a putative microRNA in the S1 colon cancer cell line. Mol. Cell. Biol. 28, 5147–5161. doi:10.1128/MCB.00331-08
Tompkins, L. M., Li, H., Li, L., Lynch, C., Xie, Y., Nakanishi, T., et al. (2010). A novel xenobiotic responsive element regulated by aryl hydrocarbon receptor is involved in the induction of BCRP/ABCG2 in LS174T cells. Biochem. Pharmacol. 80, 1754–1761. doi:10.1016/j.bcp.2010.08.016
Traber, G. M., Yi, C., Batra, N., Tu, M.-J., and Yu, A.-M. (2024). Novel RNA molecular bioengineering technology efficiently produces functional miRNA agents. RNA 30, 680–694. in press. doi:10.1261/rna.079904.123
Traber, G. M., and Yu, A.-M. (2023). RNAi-based therapeutics and novel RNA bioengineering Technologies. J. Pharmacol. Exp. Ther. 384, 133–154. doi:10.1124/jpet.122.001234
Traber, G. M., and Yu, A.-M. (2024). The growing class of novel RNAi therapeutics. Mol. Pharmacol. 106, 13–20. in press. doi:10.1124/molpharm.124.000895
Tu, M.-J., Duan, Z., Liu, Z., Zhang, C., Bold, R. J., Gonzalez, F. J., et al. (2020). MicroRNA-1291-5p sensitizes pancreatic carcinoma cells to arginine deprivation and chemotherapy through the regulation of arginolysis and glycolysis. Mol. Pharmacol. 98, 686–694. doi:10.1124/molpharm.120.000130
Tu, M.-J., Ho, P. Y., Zhang, Q.-Y., Jian, C., Qiu, J.-X., Kim, E. J., et al. (2019). Bioengineered miRNA-1291 prodrug therapy in pancreatic cancer cells and patient-derived xenograft mouse models. Cancer Lett. 442, 82–90. doi:10.1016/j.canlet.2018.10.038
Tu, M.-J., Wright, H. K., Batra, N., and Yu, A.-M. (2021). “Expression and purification purification of tRNA/transfer RNA (tRNA) pre-miRNA-Based recombinant noncoding RNAs,” in RNA scaffolds: methods and protocols (Springer), 249–265.
Turrini, E., Haenisch, S., Laechelt, S., Diewock, T., Bruhn, O., and Cascorbi, I. (2012). MicroRNA profiling in K-562 cells under imatinib treatment: influence of miR-212 and miR-328 on ABCG2 expression. Pharmacogenetics genomics 22, 198–205. doi:10.1097/FPC.0b013e328350012b
Ueda, K., Cardarelli, C., Gottesman, M. M., and Pastan, I. (1987). Expression of a full-length cDNA for the human" MDR1" gene confers resistance to colchicine, doxorubicin, and vinblastine. Proc. Natl. Acad. Sci. 84, 3004–3008. doi:10.1073/pnas.84.9.3004
Van Aubel, R. A., Smeets, P. H., Peters, J. G., Bindels, R. J., and Russel, F. G. (2002). The MRP4/ABCC4 gene encodes a novel apical organic anion transporter in human kidney proximal tubules: putative efflux pump for urinary cAMP and cGMP. J. Am. Soc. Nephrol. 13, 595–603. doi:10.1681/ASN.V133595
Vanwijngaerden, Y. M., Wauters, J., Langouche, L., Vander Perre, S., Liddle, C., Coulter, S., et al. (2011). Critical illness evokes elevated circulating bile acids related to altered hepatic transporter and nuclear receptor expression. Hepatology 54, 1741–1752. doi:10.1002/hep.24582
Vasiliou, V., Vasiliou, K., and Nebert, D. W. (2009). Human ATP-binding cassette (ABC) transporter family. Hum. Genomics 3, 281–290. doi:10.1186/1479-7364-3-3-281
Venter, J. C., Adams, M. D., Myers, E. W., Li, P. W., Mural, R. J., Sutton, G. G., et al. (2001). The sequence of the human genome. Science 291, 1304–1351. doi:10.1126/science.1058040
Vieira, J., and Messing, J. (1982). The pUC plasmids, an M13mp7-derived system for insertion mutagenesis and sequencing with synthetic universal primers. Gene 19, 259–268. doi:10.1016/0378-1119(82)90015-4
Vincent, V., Thakkar, H., Aggarwal, S., Mridha, A. R., Ramakrishnan, L., and Singh, A. (2019). ATP-binding cassette transporter A1 (ABCA1) expression in adipose tissue and its modulation with insulin resistance in obesity. Diabetes, metabolic syndrome Obes. Targets Ther. 12, 275–284. doi:10.2147/DMSO.S186565
Walker, J. E., Saraste, M., Runswick, M. J., and Gay, N. J. (1982). Distantly related sequences in the alpha-and beta-subunits of ATP synthase, myosin, kinases and other ATP-requiring enzymes and a common nucleotide binding fold. EMBO J. 1, 945–951. doi:10.1002/j.1460-2075.1982.tb01276.x
Wang, F., Xue, X., Wei, J., An, Y., Yao, J., Cai, H., et al. (2010). hsa-miR-520h downregulates ABCG2 in pancreatic cancer cells to inhibit migration, invasion, and side populations. Br. J. cancer 103, 567–574. doi:10.1038/sj.bjc.6605724
Wang, J. Q., Wu, Z. X., Yang, Y., Teng, Q. X., Li, Y. D., Lei, Z. N., et al. (2021). ATP-binding cassette (ABC) transporters in cancer: a review of recent updates. J. Evidence-Based Med. 14, 232–256. doi:10.1111/jebm.12434
Wang, W.-P., Ho, P. Y., Chen, Q.-X., Addepalli, B., Limbach, P. A., Li, M.-M., et al. (2015). Bioengineering novel chimeric microRNA-34a for prodrug cancer therapy: high-yield expression and purification, and structural and functional characterization. J. Pharmacol. Exp. Ther. 354, 131–141. doi:10.1124/jpet.115.225631
Wang, Y., Medvid, R., Melton, C., Jaenisch, R., and Blelloch, R. (2007). DGCR8 is essential for microRNA biogenesis and silencing of embryonic stem cell self-renewal. Nat. Genet. 39, 380–385. doi:10.1038/ng1969
Wang, Y., Zhao, L., Xiao, Q., Jiang, L., He, M., Bai, X., et al. (2016). miR-302a/b/c/d cooperatively inhibit BCRP expression to increase drug sensitivity in breast cancer cells. Gynecol. Oncol. 141, 592–601. doi:10.1016/j.ygyno.2015.11.034
Wijnholds, J., Mol, C. A., Van Deemter, L., De Haas, M., Scheffer, G. L., Baas, F., et al. (2000). “Multidrug-resistance protein 5 is a multispecific organic anion transporter able to transport nucleotide analogs,” in Proceedings of the national academy of Sciences 97, 7476–7481.
Wilczynska, A., and Bushell, M. (2015). The complexity of miRNA-mediated repression. Cell. Death Differ. 22, 22–33. doi:10.1038/cdd.2014.112
Williams, G. C., Liu, A., Knipp, G., and Sinko, P. J. (2002). Direct evidence that saquinavir is transported by multidrug resistance-associated protein (MRP1) and canalicular multispecific organic anion transporter (MRP2). Antimicrob. agents Chemother. 46, 3456–3462. doi:10.1128/aac.46.11.3456-3462.2002
Xie, Y., Shao, Y., Deng, X., Wang, M., and Chen, Y. (2018). MicroRNA-298 reverses multidrug resistance to antiepileptic drugs by suppressing MDR1/P-gp expression in vitro. Front. Neurosci. 12, 602. doi:10.3389/fnins.2018.00602
Xie, Z.-Y., Wang, F.-F., Xiao, Z.-H., Liu, S.-F., Tang, S.-L., and Lai, Y.-L. (2020). Overexpressing microRNA-34a overcomes ABCG2-mediated drug resistance to 5-FU in side population cells from colon cancer via suppressing DLL1. J. Biochem. 167, 557–564. doi:10.1093/jb/mvaa012
Xu, K., Liang, X., Shen, K., Cui, D., Zheng, Y., Xu, J., et al. (2012a). miR-297 modulates multidrug resistance in human colorectal carcinoma by down-regulating MRP-2. Biochem. J. 446, 291–300. doi:10.1042/BJ20120386
Xu, X., Xu, Q., Tong, J., Zhu, M., Nie, F., Chen, X., et al. (2012b). MicroRNA expression profiling identifies miR-328 regulates cancer stem cell-like SP cells in colorectal cancer. Br. J. cancer 106, 1320–1330. doi:10.1038/bjc.2012.88
Yang, J.-S., Maurin, T., Robine, N., Rasmussen, K. D., Jeffrey, K. L., Chandwani, R., et al. (2010). “Conserved vertebrate mir-451 provides a platform for Dicer-independent, Ago2-mediated microRNA biogenesis,” in Proceedings of the national academy of Sciences 107, 15163–15168.
Yang, T., Zheng, Z.-M., Li, X.-N., Li, Z.-F., Wang, Y., Geng, Y.-F., et al. (2013). MiR-223 modulates multidrug resistance via downregulation of ABCB1 in hepatocellular carcinoma cells. Exp. Biol. Med. 238, 1024–1032. doi:10.1177/1535370213497321
Yi, R., Qin, Y., Macara, I. G., and Cullen, B. R. (2003). Exportin-5 mediates the nuclear export of pre-microRNAs and short hairpin RNAs. Genes. and Dev. 17, 3011–3016. doi:10.1101/gad.1158803
Yi, W., Tu, M.-J., Liu, Z., Zhang, C., Batra, N., Yu, A.-X., et al. (2020). Bioengineered miR-328-3p modulates GLUT1-mediated glucose uptake and metabolism to exert synergistic antiproliferative effects with chemotherapeutics. Acta Pharm. Sin. B 10, 159–170. doi:10.1016/j.apsb.2019.11.001
Yoda, M., Cifuentes, D., Izumi, N., Sakaguchi, Y., Suzuki, T., Giraldez, A. J., et al. (2013). Poly (A)-specific ribonuclease mediates 3′-end trimming of Argonaute2-cleaved precursor microRNAs. Cell. Rep. 5, 715–726. doi:10.1016/j.celrep.2013.09.029
Yoo, B. K., Chen, D., Su, Z.-Z., Gredler, R., Yoo, J., Shah, K., et al. (2010). Molecular mechanism of chemoresistance by astrocyte elevated gene-1. Cancer Res. 70, 3249–3258. doi:10.1158/0008-5472.CAN-09-4009
Young, L. C., Campling, B. G., Voskoglou-Nomikos, T., Cole, S. P., Deeley, R. G., and Gerlach, J. H. (1999). Expression of multidrug resistance protein-related genes in lung cancer: correlation with drug response. Clin. Cancer Res. 5, 673–680.
Yu, A.-M. (2009). Role of microRNAs in the regulation of drug metabolism and disposition. Expert Opin. drug metabolism Toxicol. 5, 1513–1528. doi:10.1517/17425250903307448
Yu, A.-M., Choi, Y. H., and Tu, M.-J. (2020). RNA drugs and RNA targets for small molecules: principles, progress, and challenges. Pharmacol. Rev. 72, 862–898. doi:10.1124/pr.120.019554
Yu, A.-M., Jian, C., Allan, H. Y., and Tu, M.-J. (2019). RNA therapy: are we using the right molecules? Pharmacol. Ther. 196, 91–104. doi:10.1016/j.pharmthera.2018.11.011
Yu, A.-M., and Pan, Y.-Z. (2012). Noncoding microRNAs: small RNAs play a big role in regulation of ADME? Acta Pharm. Sin. B 2, 93–101. doi:10.1016/j.apsb.2012.02.011
Yu, A.-M., Tian, Y., Tu, M.-J., Ho, P. Y., and Jilek, J. L. (2016). MicroRNA pharmacoepigenetics: posttranscriptional regulation mechanisms behind variable drug disposition and strategy to develop more effective therapy. Drug Metabolism Dispos. 44, 308–319. doi:10.1124/dmd.115.067470
Yu, A. M., and Tu, M. J. (2022). Deliver the promise: RNAs as a new class of molecular entities for therapy and vaccination. Pharmacol. Ther. 230, 107967. doi:10.1016/j.pharmthera.2021.107967
Zeng, C., Fan, D., Xu, Y., Li, X., Yuan, J., Yang, Q., et al. (2020). Curcumol enhances the sensitivity of doxorubicin in triple-negative breast cancer via regulating the miR-181b-2-3p-ABCC3 axis. Biochem. Pharmacol. 174, 113795. doi:10.1016/j.bcp.2020.113795
Zeng, H., Bain, L. J., Belinsky, M. G., and Kruh, G. D. (1999). Expression of multidrug resistance protein-3 (multispecific organic anion transporter-D) in human embryonic kidney 293 cells confers resistance to anticancer agents. Cancer Res. 59, 5964–5967.
Zeng, H., Chen, Z.-S., Belinsky, M. G., Rea, P. A., and Kruh, G. D. (2001). Transport of methotrexate (MTX) and folates by multidrug resistance protein (MRP) 3 and MRP1: effect of polyglutamylation on MTX transport. Cancer Res. 61, 7225–7232.
Zhang, Y., Schuetz, J. D., Elmquist, W. F., and Miller, D. W. (2004). Plasma membrane localization of multidrug resistance-associated protein homologs in brain capillary endothelial cells. J. Pharmacol. Exp. Ther. 311, 449–455. doi:10.1124/jpet.104.068528
Zhao, Y., Qi, X., Chen, J., Wei, W., Yu, C., Yan, H., et al. (2017). The miR-491-3p/Sp3/ABCB1 axis attenuates multidrug resistance of hepatocellular carcinoma. Cancer Lett. 408, 102–111. doi:10.1016/j.canlet.2017.08.027
Zheng, S.-Z., Sun, P., Wang, J.-P., Liu, Y., Gong, W., and Liu, J. (2019). MiR-34a overexpression enhances the inhibitory effect of doxorubicin on HepG2 cells. World J. gastroenterology 25, 2752–2762. doi:10.3748/wjg.v25.i22.2752
Zhong, X.-B., and Leeder, J. S. (2013). Epigenetic regulation of ADME-related genes: focus on drug metabolism and transport. Drug Metabolism Dispos. 41, 1721–1724. doi:10.1124/dmd.113.053942
Zhu, H., Wu, H., Liu, X., Evans, B. R., Medina, D. J., Liu, C.-G., et al. (2008). Role of MicroRNA miR-27a and miR-451 in the regulation of MDR1/P-glycoprotein expression in human cancer cells. Biochem. Pharmacol. 76, 582–588. doi:10.1016/j.bcp.2008.06.007
Keywords: ABC transporter, gene regulation, multidrug resistance, microRNA, ADME, pharmacokinetics, cancer, disease
Citation: Wang Y, Tu M-J and Yu A-M (2024) Efflux ABC transporters in drug disposition and their posttranscriptional gene regulation by microRNAs. Front. Pharmacol. 15:1423416. doi: 10.3389/fphar.2024.1423416
Received: 25 April 2024; Accepted: 11 July 2024;
Published: 24 July 2024.
Edited by:
Junmei Wang, University of Pittsburgh, United StatesReviewed by:
Rania Harati, University of Sharjah, United Arab EmiratesVeronica Aran, Instituto Estadual do Cérebro Paulo Niemeyer (IECPN), Brazil
Imran Shair Mohammad, City of Hope National Medical Center, United States
Copyright © 2024 Wang, Tu and Yu. This is an open-access article distributed under the terms of the Creative Commons Attribution License (CC BY). The use, distribution or reproduction in other forums is permitted, provided the original author(s) and the copyright owner(s) are credited and that the original publication in this journal is cited, in accordance with accepted academic practice. No use, distribution or reproduction is permitted which does not comply with these terms.
*Correspondence: Ai-Ming Yu, YWlteXVAdWNkYXZpcy5lZHU=