- 1Institute of Life Sciences, China Medical University, Shenyang, China
- 2The Sixth Affiliated Hospital of South China University of Technology, Foshan, Guangdong, China
- 3Department of Urology, The Fourth Affiliated Hospital of China Medical University, Shenyang, Liaoning, China
- 4Liaoning Key Laboratory of Bladder Disease Gene Research, Institute of Health Science, China Medical University, Shenyang, China
Background: The beneficial effects of fibroblast growth factor 21 (FGF21) and sodium butyrate (NaB) on protection against cholestasis-induced liver fibrosis are not well known. This study aimed to explore the effects of FGF21 and NaB on bile duct ligation (BDL)-induced liver fibrosis.
Methods: Wild-type (WT) and FGF21 knockout (KO) mice received BDL surgery for 14 days. Liver fibrosis was assessed by Masson’s staining for fibrosis marker expressions at the mRNA or protein levels. Adenovirus-mediated FGF21 overexpression in the WT mice was assessed against BDL damage. BDL surgeries were performed in WT and FGF21 KO mice that were administered either phosphate-buffered saline or NaB. The effects of NaB on the energy metabolism and gut microbiota were assessed using stable metabolism detection and 16S rRNA gene sequencing.
Results: BDL-induced liver fibrosis in the WT mice was accompanied by high induction of FGF21. Compared to the WT mice, the FGF21 KO mice showed more severe liver fibrosis induced by BDL. FGF21 overexpression protected against BDL-induced liver fibrosis, as proved by the decreasing α-SMA at both the mRNA and protein levels. NaB administration enhanced the glucose and energy metabolisms as well as remodeled the gut microbiota. NaB alleviated BDL-induced liver fibrosis in the WT mice but aggravated the same in FGF21 KO mice.
Conclusion: FGF21 plays a key role in alleviating cholestasis-induced liver damage and fibrosis. NaB has beneficial effects on cholestasis in an FGF21-dependent manner. NaB administration can thus be a novel nutritional therapy for treating cholestasis via boosting FGF21 signaling and regulating the gut microbiota.
Introduction
Cholestasis is a condition characterized by obstruction of bile flow within or outside the liver owing to progressive pathological states, which lead to chronic cholestatic liver diseases; this prevents the bile acids (BAs) from flowing into the small intestine and instead flow into the blood reversely (Penz-Osterreicher et al., 2011; Abshagen et al., 2020). The accumulated BAs in the liver and systemic circulation may then cause damage to the liver and other organs, thereby inducing complications such as liver injury, renal failure, osteoporosis/osteomalacia, portal hypertension, and cholangiocarcinoma that require close follow-up and specific interventions (Jansen et al., 2017). Furthermore, the excess hepatic BAs may cause liver fibrosis and progress to cirrhosis, which in turn could increase mortality as well as affect the quality of life and impose economic burden due to comorbidities associated with liver failure (Liu et al., 2020). Although significant progress has been made in understanding the pathogenesis of cholestasis, the specific mechanisms remain unclear and therapeutic drugs are rare.
Fibroblast growth factor 21 (FGF21) is a stress-induced hormone that exerts beneficial effects in adaptive responses to diverse physiological or pathological stressors, such as starvation, nutrient excess, and mitochondrial stress (Liu et al., 2015; Fisher and Maratos-Flier, 2016; Geng et al., 2020). FGF21 is primarily expressed in the liver and plays an important role in obesity as well as related metabolic diseases. The systemic deficiency of FGF21 has been shown to aggravate liver steatosis and fibrosis in methionine and choline deficient L-amino acid diet (MCD)-fed mice (Fisher et al., 2014), and FGF21 analogues could ameliorate liver injury and fibrosis through multiple mechanisms (Meng et al., 2021; Puengel et al., 2022). In particular, another study revealed that FGF21 can be regulated by the farnesoid X receptor (FXR), which is a well-known nuclear receptor maintaining the homeostasis of BAs, and that the FXR ligand obeticholic acid (OCA) can alleviate liver steatosis as well as fibrosis induced by a western diet by boosting the liver FGF21 signals (Hu et al., 2020). Although these data indicate that FGF21 could act as a therapeutic target in the treatment of liver failure, the beneficial effects of FGF21 have not been assessed under cholestasis conditions (Hu et al., 2020).
Recently, nutritional management was suggested as a therapeutic strategy for cholestatic liver disease (Kloosterman et al., 2022). Nutritional therapy has been demonstrated to play critical roles in multiple chronic liver diseases (Du et al., 2022; Shah and Barritt, 2022; Zhang et al., 2023). Sodium butyrate (NaB) is a well-known short-chain fatty acid (SCFA) with antioxidant capacity and has gained increasing attention for its vast beneficial effects, including modulation of hepatic antioxidation and anti-inflammation (Sun et al., 2018; Tayyeb et al., 2020). Previous works, including ours, indicate that the beneficial effects of NaB on boosting FGF21 signal could be a potential strategy in the treatment of cholestasis-induced liver damage and fibrosis (Li et al., 2012; Erickson and Moreau, 2016; Sheng et al., 2017). In addition, a recent study indicated that NaB administration could regulate the metabolism of liver BAs by modulating the gut microbiota (Ye et al., 2021). However, it remains unknown whether NaB could alleviate cholestasis-induced liver injury and fibrosis; further, it should be identified whether FGF21 is involved in the beneficial effects of NaB.
The present study aimed to examine the beneficial effects of FGF21 and NaB in cholestatic liver disease. We demonstrate that FGF21 could ameliorate cholestasis-induced liver injury and fibrosis and that NaB administration could prevent the progression of bile duct ligation (BDL)-induced liver fibrosis by boosting FGF21 signals and enhancing the host energy metabolism along with modulating the gut microbiota. We also show that the beneficial effects of NaB on cholestasis are dependent on FGF21.
Materials and methods
Animal study
All animal protocols in this work were approved by the Institutional Animal Care and Use Committee of China Medical University (CMUXN2022088). Eight-week old male C57BL/6 wild-type (WT) and FGF21 knockout (KO) mice were housed in a specific pathogen-free environment at 20–22°C with 12-h light–dark cycles along with free access to food and water.
BDL is a well-documented technique for inducing cholestasis in animals and was adopted in this study along with procedures described previously (Tag et al., 2015). In brief, the common bile duct was exposed and ligated twice under isoflurane inhalation (1.5 vol%) anesthesia; further, the BDL was not performed for the sham operation mice. For the FGF21 overexpression experiments, the mice were administered Ad-NC (empty control) and Ad-FGF21 (FGF21-overexpressing adenovirus) via tail-vein injection at a dose of 1 × 109 PFU per mouse 3 days before the BDL surgery. The mice were also administered NaB (500 mg/kg bodyweight) daily by oral gavage for 4 weeks before BDL and maintained on NaB administration till euthanization after BDL. The mice were euthanized 14 days after BDL, and their serum, liver, bile, and cecum were collected and stored at −80°C for further analyses.
Energy metabolism measurement
For the metabolic studies, the mice were housed individually in metabolic cages (Promethion, Sable Systems) and monitored for 24 h after NaB administration for 6 weeks while being allowed free access to food and water. Their food consumption, oxygen consumption, carbon dioxide production, energy expenditure, and respiratory exchange ratio were analyzed by CalR, as described previously (Mina et al., 2018).
Glucose and insulin tolerance analyses
Glucose tolerance test (GTT) and insulin tolerance test (ITT) were performed as described previously (Geng et al., 2022). Briefly, mice that were fasted overnight were intraperitoneally injected with D-glucose (2 g/kg bodyweight), and the ITT was conducted by injecting insulin (1 U/kg bodyweight) after 6 h of fasting. The blood glucose levels were measured at various time points, as specified in each experiment, using a blood glucose meter.
Biochemical analysis
The serum alanine aminotransferase (ALT), aspartate transaminase (AST), total bilirubin (TBIL), and total bile acid (TBA) amounts were quantified separately according to manufacturer instructions (Nanjing Jiancheng, China).
Histological and microscopy analyses
The liver tissues were first fixed in 4% paraformaldehyde and were then embedded in paraffin before being cut into 4-μm-thick slices. Hematoxylin and eosin (H&E) and Masson’s trichrome staining were performed according to standard protocols (Chang et al., 2022). The images of the stained tissues were acquired with a Leica microscope under magnification.
Gene expression analysis
Liver RNA was isolated using TRIzol (Invitrogen) and reverse transcribed to cDNA (TaKaRa). Real-time quantitative polymerase chain reaction (RT-qPCR) was then performed on a BIO-RAD real-time PCR system using SYBR Green PCR Master Premix (TaKaRa). The mRNA levels were then normalized against the levels of Gapdh expressions.
Western blot analysis
The liver tissues were homogenized on ice in RIPA buffer containing protease inhibitors, phosphatase inhibitors, and EDTA. After centrifugation, the proteins were quantified using a BCA protein assay kit. Equal amounts of the proteins were separated using SDS-PAGE gels and transferred onto polyvinylidene fluoride membranes. After blocking with 5% non-fat milk, the membranes were incubated overnight with primary antibodies at 4°C. The primary antibodies used were TGF-β (ab92486, Abcam), α-SMA (23,779,489, Millipore) Cytokeratin19 (60187-1-lg, Proteintech), and secondary horseradish-peroxidase-conjugated goat anti-rabbit or anti-mouse IgG antibodies (Zhongshan Golden Bridge Biotechnology, China) that were incubated at room temperature for 1 h. After washing in Tris-Buffered Saline and Tween 20 (TBST), the enhanced chemiluminescence (ECL) reagent (Advansta, USA) and Tanon 5200 (Shanghai Tianneng Technology, China) were used to analyze the bands.
Fecal microbiota 16S rRNA gene sequencing and analysis
The total genome DNA of the cecum content was extracted using hexadecyltrimethylammonium bromide (CTAB), as described previously (Zhao et al., 2022). The V4 hypervariable regions of the bacterial 16S rRNA genes were amplified using specific primers 515F (GTGCCAGCMGCCGCGGTAA) and 806R (GGACTACHVGGGTWTCTAAT). These libraries were sequenced on an Illumina HiSeq platform (Novogene, China) and 250-bp paired-end reads were generated using FLASH (version 1.2.7). The sequencing data were analyzed with QIIME V.2.0. pipeline (Hall and Beiko, 2018), and the Silva 16S rRNA gene reference database was used to classify the Amplicon Sequence Variant (ASV) taxonomically (Quast et al., 2013; Liu et al., 2016). The alpha and beta diversities were analyzed using the R package Vegan, as reported previously (Wang et al., 2018; Liu et al., 2022). Moreover, the predicted functions of the intestinal microbiota were analyzed using PICRUST2 (Douglas et al., 2020).
Statistical analysis
The data were expressed as means ± standard errors of mean (SEMs). Statistical analyses were carried out using two-tailed unpaired Student’s t-tests for two-group comparisons or one-way analysis of variance (ANOVA) for three or more group comparisons using GraphPad Prism; p-values below 0.05 were considered to be statistically significant.
Results
FGF21 expression was altered in cholestasis-induced liver fibrosis
The BDL surgery induced mild liver fibrosis, as shown in Masson’s staining (Figure 1A), accompanied by increased serum TBA and TBIL levels in the mice (Figure 1B, C). As expected, BDL surgery highly induced FGF21 at the mRNA and protein levels (Figure 1D, E). Additionally, we analyzed the single-cell sequencing data of primary sclerosing cholangitis (PSC) patients (shown in Supplementary Figure S1A) and found significant changes in FGF21 expression, indicating the potential of FGF21 as a therapeutic target in the treatment of cholestasis. Compared to the sham operations, the liver fibrosis markers (Tgf-β and α-Sma) were severely changed at the mRNA levels (Figure 1D–F) after BDL at 14 days. The protein levels of TGF-β, α-SMA, and bile duct hyperplasia marker CK19 were all evaluated in the surgical group and compared with the sham group (Figure 1G).
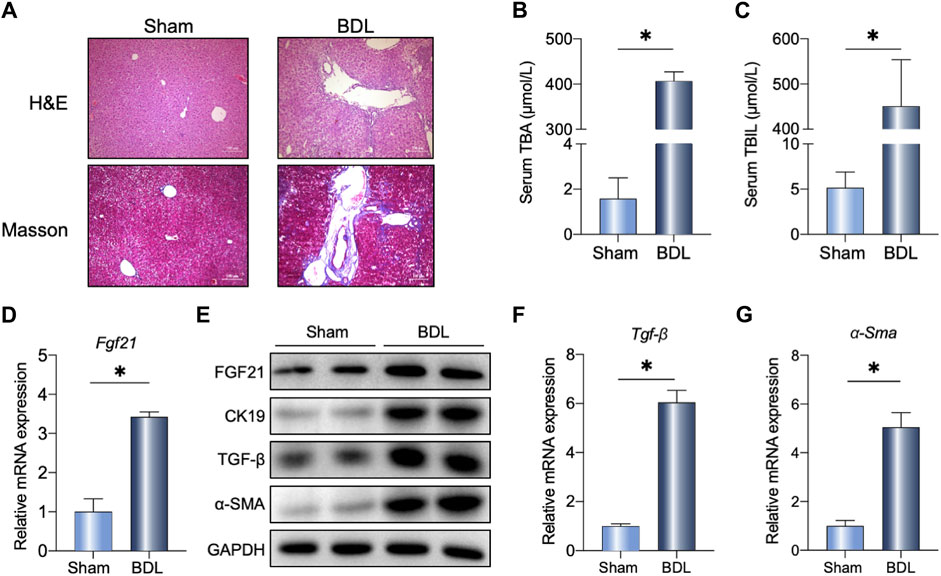
Figure 1. Liver FGF21 induced by bile duct ligation (BDL). (A) Representative images of H&E and Masson staining in wild-type (WT) mice 14 days after sham operation or BDL. (B, C) Serum total bile acids (TBAs) and total bilirubin (TBIL) in the indicated groups (n = 5). (D–F) Relative mRNA expression levels of Fgf21, Tgf-β, and α-Sma in the liver of mice 14 days after either sham operation or BDL. (G) Liver protein levels in the two groups. The data were statistically analyzed using unpaired t-test *p < 0.05.
Loss of FGF21 aggravated BDL-induced liver fibrosis
To determine the physiological role of FGF21 in cholestasis, FGF21 KO mice were subjected to BDL surgery. As shown in Figure 2B, C, increased liver and spleen organ indexes were observed in the FGF21 KO mice after BDL (KO-BDL) compared to the WT mice with BDL (WT-BDL). Histological analyses of the livers showed more necrosis and fibrosis in the KO-BDL mice (Figure 2D). Further, the mRNA levels of α-Sma and Timp1 were increased in the KO-BDL mice (Figure 2E, F); compared to the WT-BDL mice, the protein levels of CK19 and α-SMA were also consistently higher in the KO-BDL mice (Figure 2G).
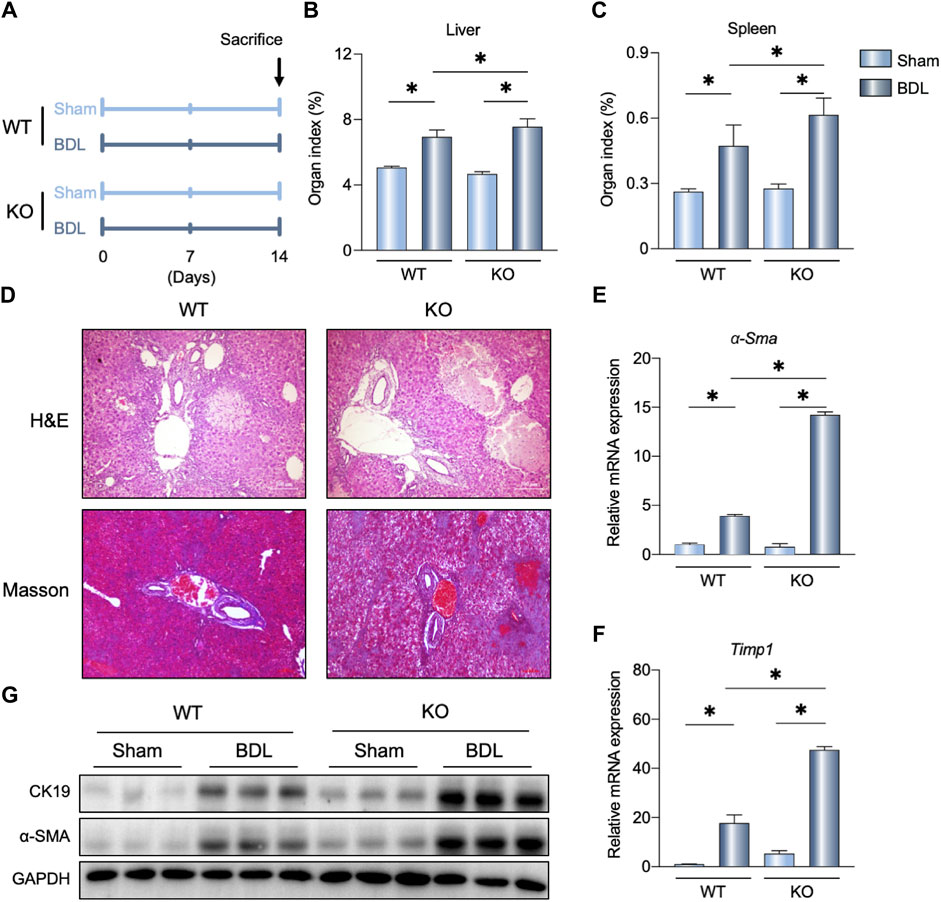
Figure 2. FGF21 knockout (KO) aggravates BDL-induced liver fibrosis. (A) Experimental outline (n = 5). (B, C) Liver and spleen organ indexes in the indicated groups. (D) Representative images of H&E and Masson staining in the WT and FGF21 KO mice 14 days after sham operation or BDL. (E, F) Relative mRNA expression levels of α-Sma and Timp1 in the liver of WT and FGF21 KO mice 14 days after either sham operation or BDL. (G) Liver protein levels in the four groups. The data were statistically analyzed using ANOVA and Tukey’s multiple comparisons test *p < 0.05.
FGF21 overexpression improved BDL-induced liver fibrosis
To confirm the potential therapeutic effects of FGF21 on cholestasis, we administered FGF21 to the WT-BDL mice (Figure 3A; Supplementary Figure S1B, C). As shown in Figure 3B, C, FGF21 overexpression reduced the liver and spleen organ indexes; interestingly, the volume of gallbladder bile also increased in the Ad-FGF21-BDL mice compared to the Ad-NC-BDL mice (Figure 3D–F). Furthermore, histological analysis showed that FGF21 overexpression alleviated BDL-induced liver injury and fibrosis (Figure 3G). The mRNA levels of Tgf-β and α-Sma also decreased in the Ad-FGF21-BDL mice than the Ad-NC-BDL mice (Figure 3H, I), and the protein levels of CK19 also decreased after FGF21 overexpression (Figure 3J).
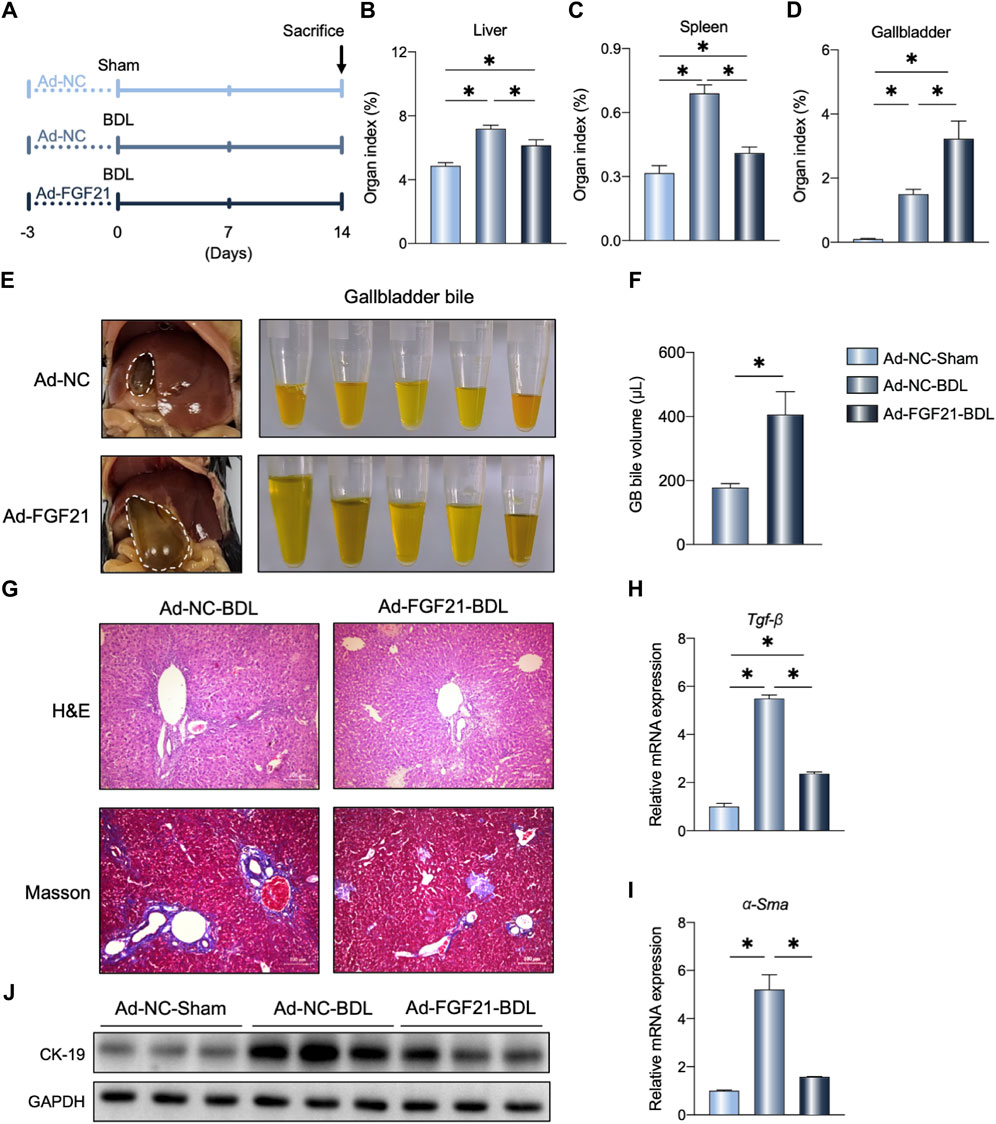
Figure 3. FGF21 overexpression in the WT mice protects against BDL-induced liver fibrosis. (A) Experimental outline (n = 5). (B–D) Liver, spleen, and gallbladder organ indexes in the indicated groups. (E, F) Representative photographs and volume of the gallbladder bile 14 days after BDL. (G) Representative images of H&E and Masson staining in the three groups. (H, I) Relative mRNA expression levels of Tgf-β and α-Sma in the liver of the indicated groups. (J) Liver protein levels in the three groups. The data were statistically analyzed using ANOVA and Tukey’s multiple comparisons test *p < 0.05.
NaB supplementation regulated liver FGF21 expression and gut microbiota composition
To assess the beneficial effects of NaB, the WT mice were administered NaB for 6 weeks (Figure 4A). As shown in Figure 4B, the hepatic FGF21 protein levels increased after NaB administration; although the ability for glucose metabolism did not change, insulin sensitivity improved significantly after NaB administration (Figures 4C–F). We also analyzed the alterations to the gut microbiota after NaB supplementation. As shown in Figure 4H, the phylum Firmicutes decreased after NaB administration but Bacteroidota were increased. At the genus level, Muribaculaceae and Muribaculum were evaluated in the groups with and without NaB supplementation and found to be significantly distinguishable upon Linear discriminant analysis Effect Size (LEfSe) analysis (Figure 4I, J, K); however, Lachnospiraceae and Lactobacillus decreased after NaB administration (Figure 4I).
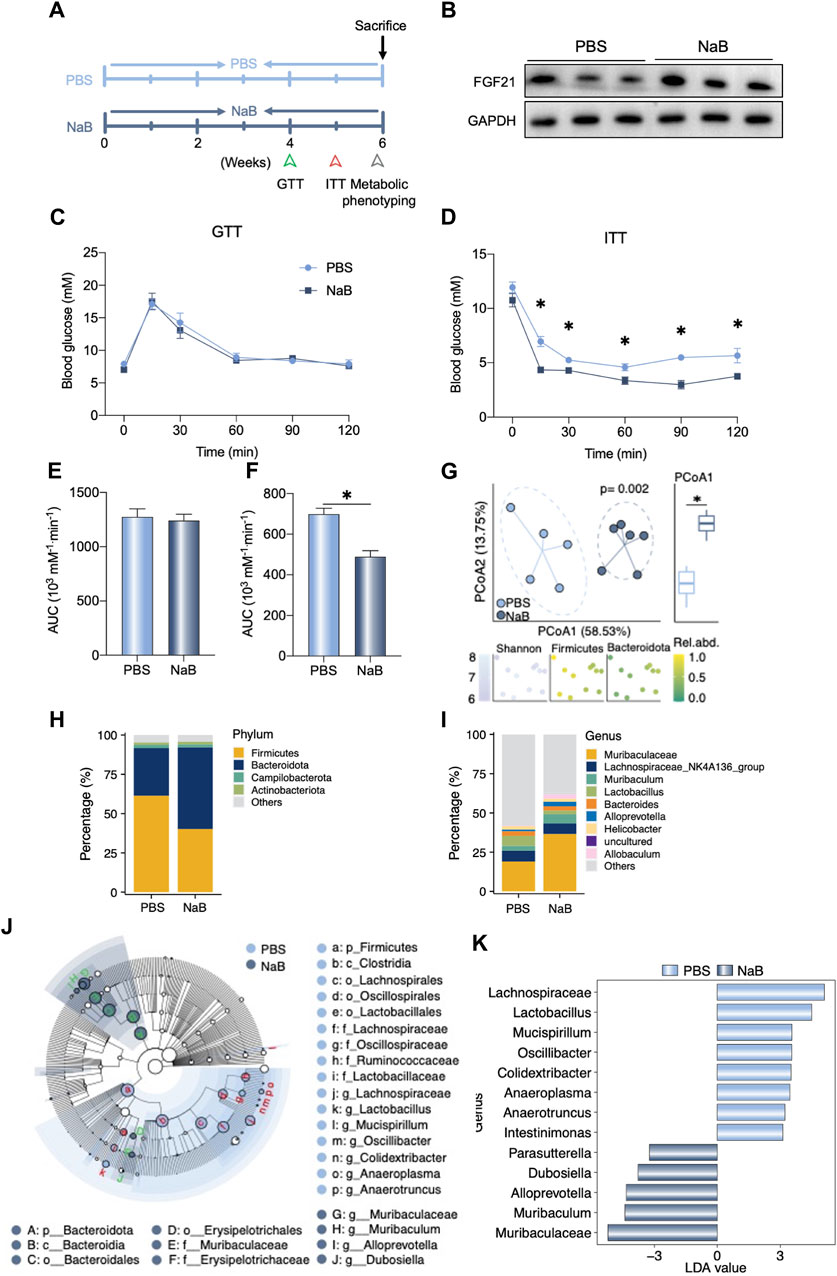
Figure 4. NaB supplementation regulates liver FGF21 expression and gut microbiota composition. (A) Experimental outline (n = 5–6). (B) Liver FGF21 protein levels in mice after receiving phosphate-buffered saline (PBS) or NaB for 6 weeks. (C, D) Glucose tolerance test (GTT) and insulin tolerance test (ITT) were performed in the two groups of mice. (E, F) Area under the curve (AUC) of the GTT and ITT data are quantified. (G) Beta diversity of the gut microbiota visualized by PCoA based on the Bray–Curtis distance matrix at the genera level. The plot color of the bottom graphic indicates the Shannon index, and the relative abundances of phylum Firmicutes and Bacteroidetes. In the PCoA1 of Principal Co-ordinates Analysis (PCoA), there was a significant difference between the two groups. (H, I) Relative abundances of the gut bacteria at the phylum and genera levels, respectively. (J) Cladograms generated on the basis of Linear discriminant analysis Effect Size (LEfSe) analysis indicating the differences in the fecal bacterial taxa. (K) Linear discriminant analysis (LDA) values of the LEfSe analysis between the two groups. Only LDA >3 genera are shown. The data were statistically analyzed using unpaired t-test*p < 0.05.
NaB enhanced basic metabolic capacity
To determine the metabolic effects of NaB on mice, fully automated metabolic cages were prepared to monitor the metabolic capacities of the mice. As shown in Figure 5A, B, NaB administration significantly increased food intake during the light cycle. However, oxygen consumption and carbon dioxide production rates of the mice increased after NaB administration (Figure 5C–F). The full-day energy expenditure was also evaluated in the mice in the NaB group (Figure 5G, H). The light-cycle respiratory exchange ratios of the NaB group mice were higher than those of the mice in the phosphate-buffered saline (PBS) group but decreased during the night cycle (Figure 5I). The elevated metabolic effects of NaB in the basic condition imply that NaB could promote host resistance after BDL. The enrichment of the Alloprevotella genus was negatively correlated with the area under the curve (AUC) of the ITT after NaB administration (Figure 5J); however, Muribaculaceae were positively correlated with food intake during the light cycle (Figure 5J). Moreover, enrichment of Oscillibacter and Lachnospiraceae genus in the PBS group were negatively associated with full-day oxygen consumption and carbon dioxide production during the light cycle, respectively (Figure 5J).
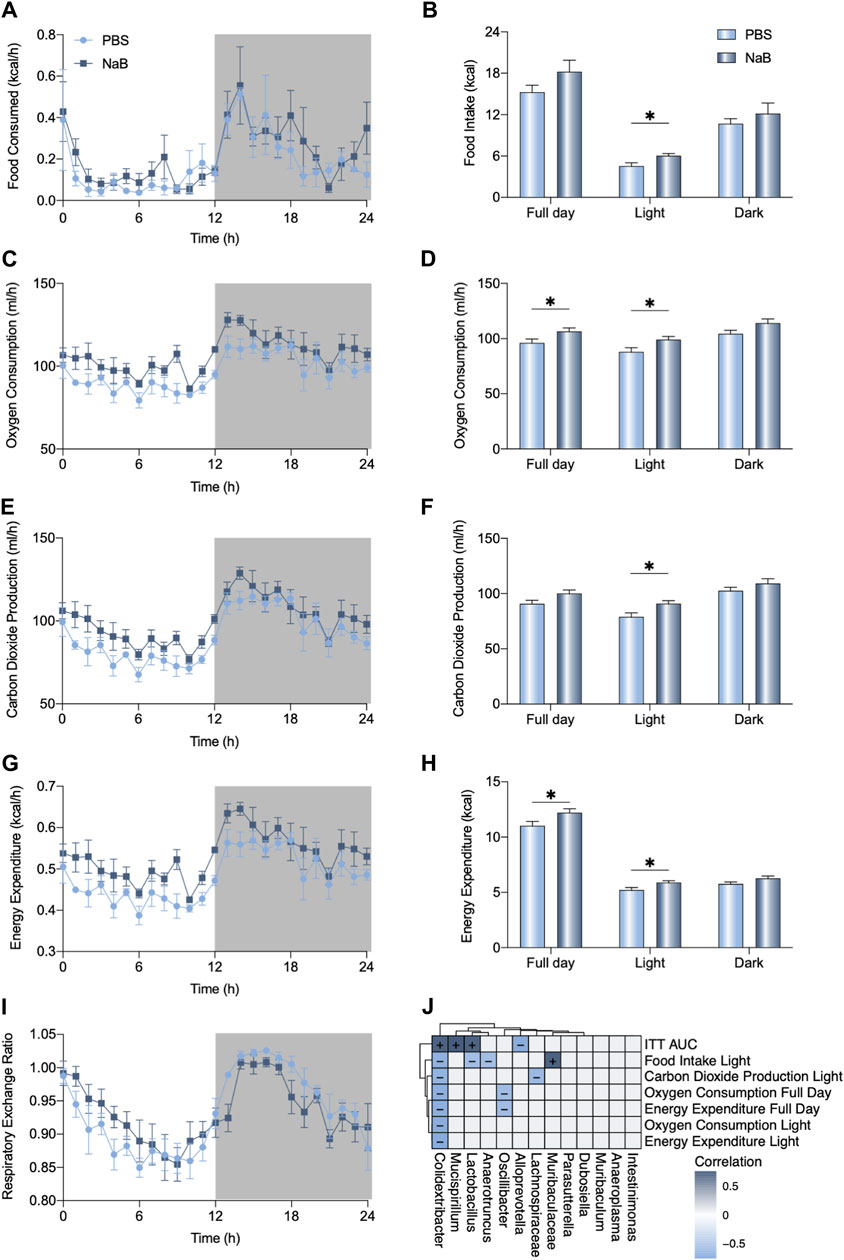
Figure 5. NaB promotes carbohydrate oxidation. (A, B) Food consumption, (C, D) oxygen consumption, (E, F) carbon dioxide production, (G, H) energy expenditure, and (I) respiratory exchange ratio of each group of mice (n = 5–6) monitored using fully automated metabolic cages during a 24-h cycle (07:00–07:00). (J) Correlations between the altered metabolic index with differences in genera (+: positive, -: negative). The data were statistically analyzed using unpaired t-test *p < 0.05.
NaB alleviated BDL-induced liver fibrosis
We primed the mice with NaB for 4 weeks before BDL surgery (Figure 6A). As shown in Figure 6B, these NaB-primed mice showed decreased liver portal area injury and fibrosis after BDL surgery based on histological staining (Figure 6B). Further, the hepatic protein levels of CK19 and α-SMA also decreased in the NaB-primed mice after BDL surgery (Figure 6C).
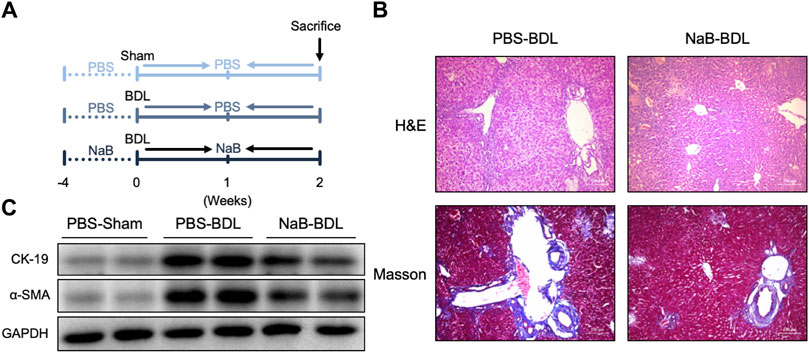
Figure 6. NaB alleviates BDL-induced liver fibrosis in WT mice. (A) Experimental outline (n = 5–6). (B) Representative images of H&E and Masson staining in the three groups. (C) Liver protein levels in the indicated groups.
NaB administration regulated gut microbiota in an FGF21-dependent manner
We analyzed the gut microbiota alterations in the WT and FGF21 KO mice that received either sham or BDL operations after NaB administration (Figure 7A). As shown in Figure 7B, there were significant differences among the six groups with regard to gut microbiota beta diversity. Specifically, the Proteobacteria phylum was enriched in the FGF21 KO mice that received BDL surgery and NaB administration (Figure 7C). At the genus level, NaB supplementation uniquely increased the Mycoplasma in the WT-BDL mice rather than KO-BDL mice (Figure 7D). However, NaB administration elevated the abundances of genus Alistipes, Lachnospiraceae_UGG-001, Oscillibacter, and Ralstonia in the KO-BDL group (Figure 7E). Peptidoglycan biosynthesis and glycolysis/gluconeogenesis capabilities were also uniquely elevated after NaB administration in the WT-BDL mice rather than the KO-BDL mice (Figure 7F). The predicted gut microbiota functions of fatty acid biosynthesis and thiamine metabolism in the KO-NaB-BDL group were higher those of the WT-NaB-BDL and KO-PBS-BDL groups (Figure 7F).
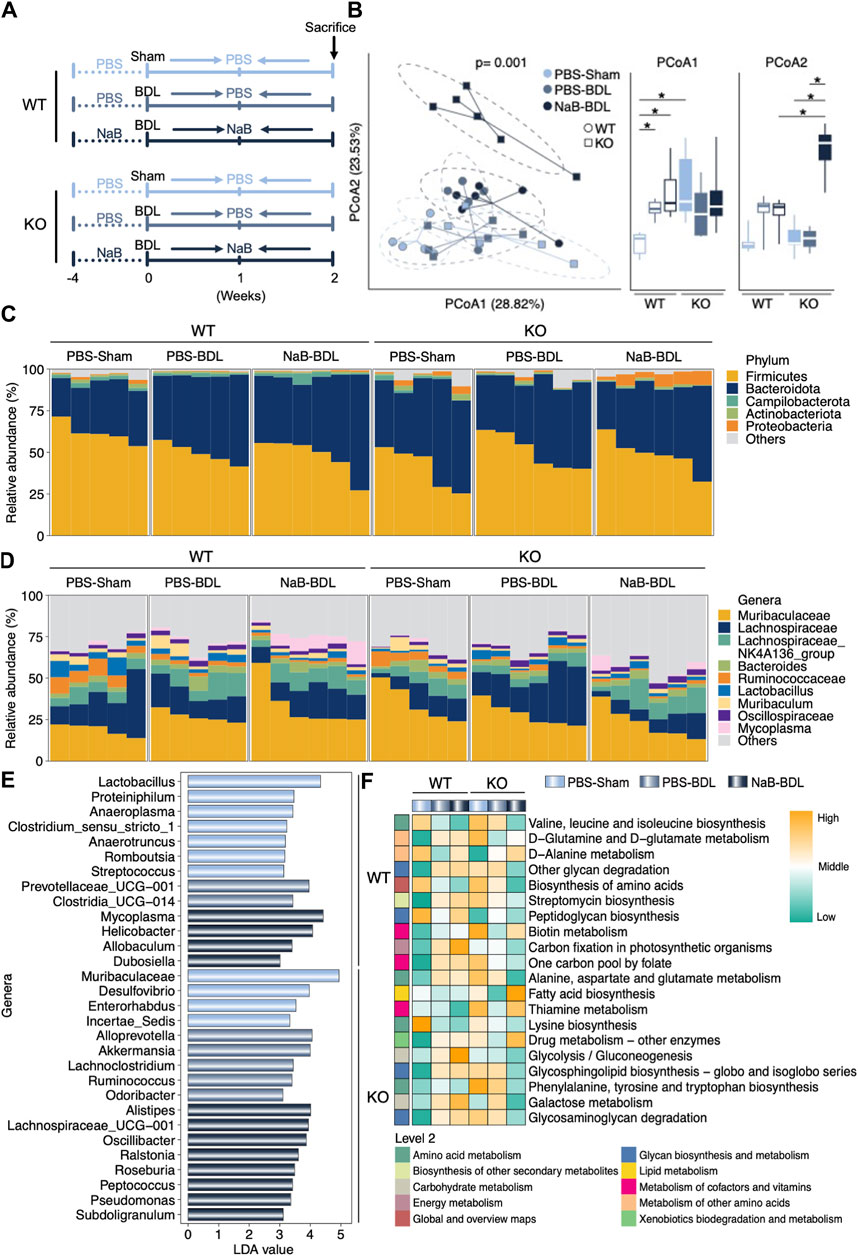
Figure 7. NaB regulates clear differences in the gut microbiota compositions in the WT and FGF21 KO mice that received either sham operation or BDL. (A) Experimental outline (n = 5–6). (B) Beta diversity of the gut microbiota visualized by PCoA based on the Bray–Curtis distance matrix at the genera level. Both PCoA1 and PCoA2 of PCoA show significant differences among the groups. (C, D) Relative abundances of the gut bacteria at the phylum and genera levels, respectively. (E) LDA values of the LEfSe analysis for the six groups. Only LDA >3 genera are shown. (F) Predicted functions in the indicated groups based on the gut microbiota. The data were statistically analyzed using unpaired t-test*p < 0.05.
NaB alleviated BDL-induced liver fibrosis dependent on FGF21
Higher serum ALT and AST levels were observed in the KO-NaB-BDL mice (Figure 8A, B); NaB administration alleviated BDL-induced liver injury and fibrosis in the WT mice rather than the FGF21 KO mice (Figure 8C). In the KO mice, NaB administration increased the serum TBAs concentration compared to the WT-NaB-BDL and KO-PBS-NaB groups (Figure 8D). The protein levels of hepatic α-SMA, PCAN, and HDCA1 in the KO-NaB-BDL group mice were consistently higher than those in the WT-NaB-BDL and KO-PBS-NaB groups (Figure 8E).
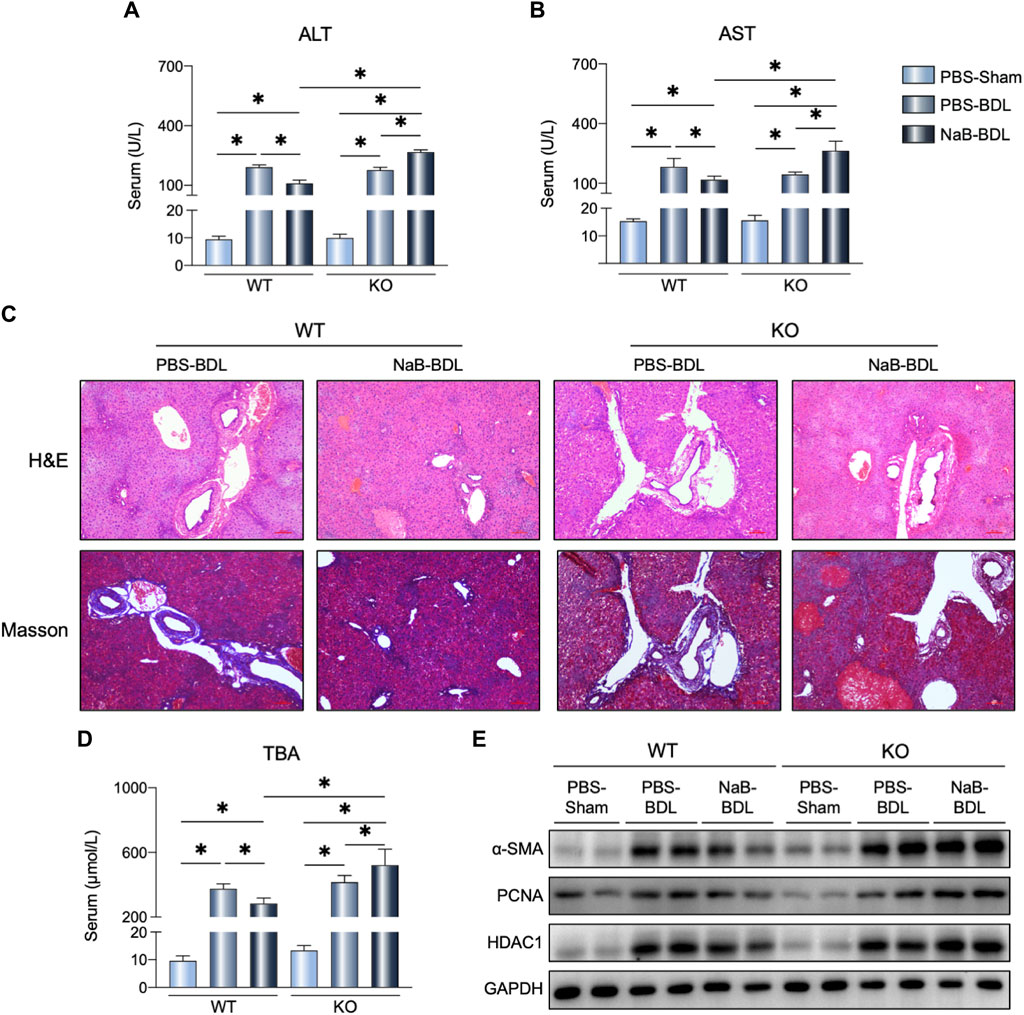
Figure 8. NaB alleviates BDL-induced liver fibrosis by FGF21 expression. (A, B) Serum ALT and AST levels in the six groups (n = 5–6). (C) Representative images of H&E and Masson staining in the indicated groups. (D) Serum TBAs in the six groups. (E) Liver protein levels in the indicated groups. The data were statistically analyzed using ANOVA and Tukey’s multiple comparisons test *p < 0.05.
Discussion
The physiological and pathological roles of FGF21 have been reported for multiple chronic and acute liver diseases (Guo et al., 2016; Choi et al., 2023). As a stress-induced hormone, the potential of FGF21 to act as a therapeutic target in the treatment of liver failure has been studied, but the beneficial effects of FGF21 on cholestasis is barely known. We found that liver FGF21 expression level was significantly higher in BDL-induced obstructive cholestasis in mice. This highly induced FGF21 could be a response to the overflow of BAs in the liver, thus preventing excess stress and protecting against liver injury (Cyphert et al., 2012). Moreover, mice with FGF21 deficiency showed more aggravated liver fibrosis and bile duct proliferation in BDL-induced cholestasis for the first time. NaB administration could highly induce hepatic FGF21 to protect against BDL-induced cholestasis, and this beneficial effect of NaB was FGF21-dependent. Together, our results indicate that FGF21 could act as a therapeutic target and that NaB could be an alternative approach for boosting FGF21 signaling in the prevention and treatment of cholestasis.
A previous study reported that FGF21 could ameliorate hepatic fibrosis through multiple mechanisms (Meng et al., 2021). In the obstructive cholestasis model, the excess accumulation of BAs is the principal cause of fibrosis; here, FGF21 could act as a negative regulator of BA synthesis in suppressing the plasma TBAs (Chen et al., 2018). Interestingly, our results revealed that FGF21 overexpression not only reduced liver fibrosis and bile duct proliferation but also expanded the gallbladder volume. However, limited studies are available on the connection between FGF21 and gallbladder function. These findings suggested that FGF21 may decrease liver cholestasis by increasing the expansion ability of the gallbladder, resulting in further alleviation of fibrosis induced by excess BA accumulation.
Owing to the protective effects of FGF21 on cholestasis-induced liver fibrosis, we further analyzed the beneficial effects of NaB, a well-known gut-microbiota-derived SCFA, to induce FGF21 expression in the liver through activation of PPARα (Kundu et al., 2019). As expected, NaB administration not only highly induced the expression of liver FGF21 but also enhanced insulin sensitivity. These data are consistent with the findings of previous studies that FGF21 could modulate glucose homeostasis (Jimenez et al., 2018; Szczepanska and Gietka-Czernel, 2022). Moreover, NaB could increase the Bacteroidota phylum in the gut microbiota, which has been identified to enhance the host energy metabolism through metabolization of the fibers in the food, consequently increasing the circulating SCFAs (Trompette et al., 2014). NaB is also known to facilitate the Alloprevotella genera, which are positively correlated with insulin sensitivity; a previous study showed that Alloprevotella could balance blood glucose levels (Ge et al., 2023). Moreover, NaB administration promotes energy expenditure and the respiratory exchange ratio; accordingly, a previous study showed that NaB supplementation increases the oxidation of fatty acids and carbohydrates (Li et al., 2018). Importantly, NaB inhibits Colidextribacter, which is negatively associated with energy expenditure. These findings suggest that NaB pretreatment enhances the energy metabolism of the mice by boosting FGF21 and regulating the gut microbiota.
As noted above, NaB could enhance the host metabolic ability and restore the intestinal microbiota. Therefore, it was not surprising that NaB administration alleviated BDL-induced liver damage. Considering the strong FGF21 induction capability of NaB, we speculated that the beneficial effects of NaB were dependent on the FGF21 signal. As expected, NaB administration in the FGF21-deficient mice receiving BDL operation significantly induced Proteobacteria phylum proportion as it particularly accounts for many pathogens (Jiang et al., 2017). More importantly, NaB treatment aggravated BDL-indued liver injury and fibrosis in the FGF21 KO mice. Numerous studies have demonstrated that NaB has beneficial effects for multiple metabolic diseases (Sun et al., 2018). However, in some cases, the overproduction of SCFAs can become unfavorable to the host (Serino, 2019); for example, increased propionate levels in the feces are related to increased risk of type 2 diabetes (Sanna et al., 2019). Importantly, our results indicate that NaB has detrimental effects on cholestasis mice with FGF21 deficiency. Thus, we speculate that higher levels of circuiting NaB could induce liver FGF21 expression, which in return could enhance the capability of NaB oxidation. However, in the FGF21-deficient mice, NaB administration did not facilitate NaB clearance via FGF21, consequently resulting in a high plasma concentration. As described previously, the higher plasma SCFA concentrations could be potentially harmful (van der Beek et al., 2015); therefore, the host FGF21 genetic expression should be taken into consideration before NaB application for disease treatment. More importantly, the short half-life of FGF21 poses challenges to its translational potential in clinical settings (Hu et al., 2020). Our results thus indicate that NaB administration could be an alternative approach to boosting FGF21 signaling and regulating gut microbiota in the treatment of cholestasis.
Although the above findings are encouraging, there are some limitations to our study. First, FGF21 overexpression alleviates BDL-induced liver fibrosis and bile duct proliferation accompanied by gallbladder volume expansion. To explore whether FGF21 alleviated BDL-induced liver damage depending on the gallbladder, a cholecystectomy should be considered in future research. Second, given that cholestasis is often caused by BA transportation and resorption dysbiosis, the BA pool and related gene expressions in treatment with FGF21 or NaB need to be studied further. Lastly, fecal microbiota transplantation from NaB-supplemented mice to BDL mice may be a possible strategy to confirm the beneficial effects of NaB on cholestasis through modulation of gut microbiota, even as this study shows that NaB administration could alleviate cholestasis via increasing FGF21 expression and regulating gut microbiota.
In conclusion, the present study shows that FGF21 could protect the host against BDL-induced liver injury and fibrosis and that NaB administration can enhance host metabolic capability by inducing liver FGF21 expression and restoring the gut microbiota. Importantly, this study identifies that NaB could alleviate BDL-induced liver damage and fibrosis in an FGF21-dependent manner. These comprehensive findings suggest that NaB administration can be a novel nutritional therapy for treating cholestasis by boosting FGF21 signaling and regulating the gut microbiota.
Data availability statement
The data presented in the study are deposited in the NCBI repository, accession number PRJNA1127873.
Ethics statement
The animal study was approved by the Institutional Animal Care and Use Committee of China Medical University. The study was conducted in accordance with all local legislations and institutional requirements.
Author contributions
JY: Data curation, Formal analysis, Writing–original draft, Writing–review and editing. LC: Data curation, Formal analysis, Methodology, Writing–original draft, Writing–review and editing. S-SZ: Writing–original draft, Writing–review and editing. CD: Writing–original draft, Writing–review and editing. Y-ZF: Writing–original draft, Writing–review and editing. H-XL: Conceptualization, Funding acquisition, Resources, Writing–original draft, Writing–review and editing. YL: Writing–original draft, Writing–review and editing. Y-ZL: Writing–original draft, Writing–review and editing.
Funding
The author(s) declare that financial support was received for the research, authorship, and/or publication of this article. This work was supported by the Liaoning Provincial Department of Education (grant no. LJKZ0758), Department of Science & Technology of Liaoning Province (grant no. 2022-MS-01), and China Medical University Innovation Team (grant no. 2400022020).
Conflict of interest
The authors declare that the research was conducted in the absence of any commercial or financial relationships that could be construed as a potential conflict of interest.
The author(s) declared that they were an editorial board member of Frontiers at the time of submission. This had no impact on the peer review process and the final decision.
Publisher’s note
All claims expressed in this article are solely those of the authors and do not necessarily represent those of their affiliated organizations or those of the publisher, editors, and reviewers. Any product that may be evaluated in this article or claim that may be made by its manufacturer is not guaranteed or endorsed by the publisher.
Supplementary material
The Supplementary Material for this article can be found online at: https://www.frontiersin.org/articles/10.3389/fphar.2024.1422770/full#supplementary-material
Abbreviations
ALT, serum alanine aminotransferase; AST, aspartate transaminase; BDL, bile duct ligation; BA, bile acid; FGF21, fibroblast growth factor 21; FXR, farnesoid X receptor; MCD, methionine and choline deficient L-amino acid diet; NaB, sodium butyrate; OCA, obeticholic acid; SCFA, short-chain fatty acid.
References
Abshagen, K., Hartmann, A., Gruner, L., Liebig, M., and Vollmar, B. (2020). Limited potential of resolvin D1 in treatment of cholestatic liver fibrosis. Hepatobiliary Surg. Nutr. 9 (5), 587–596. doi:10.21037/hbsn.2019.08.07
Chang, M. Y., Wang, H. H., Chen, L. H., Gao, J., Hung, S. Y., Chiou, Y. Y., et al. (2022). A mice model of chlorhexidine gluconate-induced peritoneal damage. J. Vis. Exp. 182. doi:10.3791/63903
Chen, M. M., Hale, C., Stanislaus, S., Xu, J., and Veniant, M. M. (2018). FGF21 acts as a negative regulator of bile acid synthesis. J. Endocrinol. 237 (2), 139–152. doi:10.1530/JOE-17-0727
Choi, M., Schneeberger, M., Fan, W., Bugde, A., Gautron, L., Vale, K., et al. (2023). FGF21 counteracts alcohol intoxication by activating the noradrenergic nervous system. Cell Metab. 35 (3), 429–437.e5. doi:10.1016/j.cmet.2023.02.005
Cyphert, H. A., Ge, X., Kohan, A. B., Salati, L. M., Zhang, Y., and Hillgartner, F. B. (2012). Activation of the farnesoid X receptor induces hepatic expression and secretion of fibroblast growth factor 21. J. Biol. Chem. 287 (30), 25123–25138. doi:10.1074/jbc.M112.375907
Douglas, G. M., Maffei, V. J., Zaneveld, J. R., Yurgel, S. N., Brown, J. R., Taylor, C. M., et al. (2020). PICRUSt2 for prediction of metagenome functions. Nat. Biotechnol. 38 (6), 685–688. doi:10.1038/s41587-020-0548-6
Du, C., Liu, W. J., Yang, J., Zhao, S. S., and Liu, H. X. (2022). The role of branched-chain amino acids and branched-chain α-keto acid dehydrogenase kinase in metabolic disorders. Front. Nutr. 9, 932670. doi:10.3389/fnut.2022.932670
Erickson, A., and Moreau, R. (2016). The regulation of FGF21 gene expression by metabolic factors and nutrients. Horm. Mol. Biol. Clin. Investig. 30 (1). doi:10.1515/hmbci-2016-0016
Fisher, F. M., Chui, P. C., Nasser, I. A., Popov, Y., Cunniff, J. C., Lundasen, T., et al. (2014). Fibroblast growth factor 21 limits lipotoxicity by promoting hepatic fatty acid activation in mice on methionine and choline-deficient diets. Gastroenterology 147 (5), 1073–1083. doi:10.1053/j.gastro.2014.07.044
Fisher, F. M., and Maratos-Flier, E. (2016). Understanding the physiology of FGF21. Annu. Rev. Physiol. 78, 223–241. doi:10.1146/annurev-physiol-021115-105339
Ge, X., He, X., Liu, J., Zeng, F., Chen, L., Xu, W., et al. (2023). Amelioration of type 2 diabetes by the novel 6, 8-guanidyl luteolin quinone-chromium coordination via biochemical mechanisms and gut microbiota interaction. J. Adv. Res. 46, 173–188. doi:10.1016/j.jare.2022.06.003
Geng, L., Lam, K. S. L., and Xu, A. (2020). The therapeutic potential of FGF21 in metabolic diseases: from bench to clinic. Nat. Rev. Endocrinol. 16 (11), 654–667. doi:10.1038/s41574-020-0386-0
Geng, L., Liao, B., Jin, L., Yu, J., Zhao, X., Zhao, Y., et al. (2022). β-Klotho promotes glycolysis and glucose-stimulated insulin secretion via GP130. Nat. Metab. 4 (5), 608–626. doi:10.1038/s42255-022-00572-2
Guo, Q., Shen, Z., Yu, H., Lu, G., Yu, Y., Liu, X., et al. (2016). Carnosic acid protects against acetaminophen-induced hepatotoxicity by potentiating Nrf2-mediated antioxidant capacity in mice. Korean J. Physiol. Pharmacol. 20 (1), 15–23. doi:10.4196/kjpp.2016.20.1.15
Hall, M., and Beiko, R. G. (2018). 16S rRNA gene analysis with QIIME2. Methods Mol. Biol. 1849, 113–129. doi:10.1007/978-1-4939-8728-3_8
Hu, Y., Liu, H. X., Jena, P. K., Sheng, L., Ali, M. R., and Wan, Y. Y. (2020). miR-22 inhibition reduces hepatic steatosis via FGF21 and FGFR1 induction. JHEP Rep. 2 (2), 100093. doi:10.1016/j.jhepr.2020.100093
Jansen, P. L., Ghallab, A., Vartak, N., Reif, R., Schaap, F. G., Hampe, J., et al. (2017). The ascending pathophysiology of cholestatic liver disease. Hepatology 65 (2), 722–738. doi:10.1002/hep.28965
Jiang, X., Ellabaan, M. M. H., Charusanti, P., Munck, C., Blin, K., Tong, Y., et al. (2017). Dissemination of antibiotic resistance genes from antibiotic producers to pathogens. Nat. Commun. 8, 15784. doi:10.1038/ncomms15784
Jimenez, V., Jambrina, C., Casana, E., Sacristan, V., Munoz, S., Darriba, S., et al. (2018). FGF21 gene therapy as treatment for obesity and insulin resistance. EMBO Mol. Med. 10 (8), e8791. doi:10.15252/emmm.201708791
Kloosterman, E., Dijkstra, T., and Verkade, H. J. (2022). 3.9 nutritional management in cholestatic liver disease. World Rev. Nutr. Diet. 124, 277–284. doi:10.1159/000516985
Kundu, P., Lee, H. U., Garcia-Perez, I., Tay, E. X. Y., Kim, H., Faylon, L. E., et al. (2019). Neurogenesis and prolongevity signaling in young germ-free mice transplanted with the gut microbiota of old mice. Sci. Transl. Med. 11 (518), eaau4760. doi:10.1126/scitranslmed.aau4760
Li, H., Gao, Z., Zhang, J., Ye, X., Xu, A., Ye, J., et al. (2012). Sodium butyrate stimulates expression of fibroblast growth factor 21 in liver by inhibition of histone deacetylase 3. Diabetes 61 (4), 797–806. doi:10.2337/db11-0846
Li, Z., Yi, C. X., Katiraei, S., Kooijman, S., Zhou, E., Chung, C. K., et al. (2018). Butyrate reduces appetite and activates brown adipose tissue via the gut-brain neural circuit. Gut 67 (7), 1269–1279. doi:10.1136/gutjnl-2017-314050
Liu, H. X., Hu, Y., French, S. W., Gonzalez, F. J., and Wan, Y. J. (2015). Forced expression of fibroblast growth factor 21 reverses the sustained impairment of liver regeneration in hPPARα(PAC) mice due to dysregulated bile acid synthesis. Oncotarget 6 (12), 9686–9700. doi:10.18632/oncotarget.3531
Liu, H. X., Rocha, C. S., Dandekar, S., and Wan, Y. J. (2016). Functional analysis of the relationship between intestinal microbiota and the expression of hepatic genes and pathways during the course of liver regeneration. J. Hepatol. 64 (3), 641–650. doi:10.1016/j.jhep.2015.09.022
Liu, Y., Chen, K., Li, F., Gu, Z., Liu, Q., He, L., et al. (2020). Probiotic Lactobacillus rhamnosus GG prevents liver fibrosis through inhibiting hepatic bile acid synthesis and enhancing bile acid excretion in mice. Hepatology 71 (6), 2050–2066. doi:10.1002/hep.30975
Liu, Y., Chen, L., Liu, L., Zhao, S. S., You, J. Q., Zhao, X. J., et al. (2022). Interplay between dietary intake, gut microbiota, and metabolic profile in obese adolescents: sex-dependent differential patterns. Clin. Nutr. 41 (12), 2706–2719. doi:10.1016/j.clnu.2022.10.009
Meng, F., Khoso, M. H., Kang, K., He, Q., Cao, Y., Jiang, X., et al. (2021). FGF21 ameliorates hepatic fibrosis by multiple mechanisms. Mol. Biol. Rep. 48 (11), 7153–7163. doi:10.1007/s11033-021-06707-0
Mina, A. I., LeClair, R. A., LeClair, K. B., Cohen, D. E., Lantier, L., and Banks, A. S. (2018). CalR: a web-based analysis tool for indirect calorimetry experiments. Cell Metab. 28 (4), 656–666. doi:10.1016/j.cmet.2018.06.019
Penz-Osterreicher, M., Osterreicher, C. H., and Trauner, M. (2011). Fibrosis in autoimmune and cholestatic liver disease. Best. Pract. Res. Clin. Gastroenterol. 25 (2), 245–258. doi:10.1016/j.bpg.2011.02.001
Puengel, T., Lefere, S., Hundertmark, J., Kohlhepp, M., Penners, C., Van de Velde, F., et al. (2022). Combined therapy with a CCR2/CCR5 antagonist and FGF21 analogue synergizes in ameliorating steatohepatitis and fibrosis. Int. J. Mol. Sci. 23 (12), 6696. doi:10.3390/ijms23126696
Quast, C., Pruesse, E., Yilmaz, P., Gerken, J., Schweer, T., Yarza, P., et al. (2013). The SILVA ribosomal RNA gene database project: improved data processing and web-based tools. Nucleic Acids Res. 41 (Database issue), D590–D596. doi:10.1093/nar/gks1219
Sanna, S., van Zuydam, N. R., Mahajan, A., Kurilshikov, A., Vich Vila, A., Vosa, U., et al. (2019). Causal relationships among the gut microbiome, short-chain fatty acids and metabolic diseases. Nat. Genet. 51 (4), 600–605. doi:10.1038/s41588-019-0350-x
Serino, M. (2019). SCFAs - the thin microbial metabolic line between good and bad. Nat. Rev. Endocrinol. 15 (6), 318–319. doi:10.1038/s41574-019-0205-7
Shah, N. D., and Barritt, A. S. (2022). Barritt ASt: nutrition as therapy in liver disease. Clin. Ther. 44 (5), 682–696. doi:10.1016/j.clinthera.2022.04.012
Sheng, L., Jena, P. K., Hu, Y., Liu, H. X., Nagar, N., Kalanetra, K. M., et al. (2017). Hepatic inflammation caused by dysregulated bile acid synthesis is reversible by butyrate supplementation. J. Pathol. 243 (4), 431–441. doi:10.1002/path.4983
Sun, B., Jia, Y., Hong, J., Sun, Q., Gao, S., Hu, Y., et al. (2018). Sodium butyrate ameliorates high-fat-diet-induced non-alcoholic fatty liver disease through peroxisome proliferator-activated receptor α-mediated activation of β oxidation and suppression of inflammation. J. Agric. Food Chem. 66 (29), 7633–7642. doi:10.1021/acs.jafc.8b01189
Szczepanska, E., and Gietka-Czernel, M. (2022). FGF21: a novel regulator of glucose and lipid metabolism and whole-body energy balance. Horm. Metab. Res. 54 (4), 203–211. doi:10.1055/a-1778-4159
Tag, C. G., Sauer-Lehnen, S., Weiskirchen, S., Borkham-Kamphorst, E., Tolba, R. H., Tacke, F., et al. (2015). Bile duct ligation in mice: induction of inflammatory liver injury and fibrosis by obstructive cholestasis. J. Vis. Exp. 96, 52438. doi:10.3791/52438
Tayyeb, J. Z., Popeijus, H. E., Mensink, R. P., Konings, M., Mokhtar, F. B. A., and Plat, J. (2020). Short-chain fatty acids (except hexanoic acid) lower NF-kB transactivation, which rescues inflammation-induced decreased apolipoprotein A-I transcription in HepG2 cells. Int. J. Mol. Sci. 21 (14), 5088. doi:10.3390/ijms21145088
Trompette, A., Gollwitzer, E. S., Yadava, K., Sichelstiel, A. K., Sprenger, N., Ngom-Bru, C., et al. (2014). Gut microbiota metabolism of dietary fiber influences allergic airway disease and hematopoiesis. Nat. Med. 20 (2), 159–166. doi:10.1038/nm.3444
van der Beek, C. M., Bloemen, J. G., van den Broek, M. A., Lenaerts, K., Venema, K., Buurman, W. A., et al. (2015). Hepatic uptake of rectally administered butyrate prevents an increase in systemic butyrate concentrations in humans. J. Nutr. 145 (9), 2019–2024. doi:10.3945/jn.115.211193
Wang, J., Zheng, J., Shi, W., Du, N., Xu, X., Zhang, Y., et al. (2018). Dysbiosis of maternal and neonatal microbiota associated with gestational diabetes mellitus. Gut 67 (9), 1614–1625. doi:10.1136/gutjnl-2018-315988
Ye, X., Shen, S., Xu, Z., Zhuang, Q., Xu, J., Wang, J., et al. (2021). Sodium butyrate alleviates cholesterol gallstones by regulating bile acid metabolism. Eur. J. Pharmacol. 908, 174341. doi:10.1016/j.ejphar.2021.174341
Zhang, X. L., Chen, L., Yang, J., Zhao, S. S., Jin, S., Ao, N., et al. (2023). Vitamin D alleviates non-alcoholic fatty liver disease via restoring gut microbiota and metabolism. Front. Microbiol. 14, 1117644. doi:10.3389/fmicb.2023.1117644
Keywords: FGF21, cholestasis, liver fibrosis, sodium butyrate, gut microbiota
Citation: Yang J, Chen L, Zhao S-S, Du C, Fan Y-Z, Liu H-X, Li Y and Li Y-Z (2024) FGF21-dependent alleviation of cholestasis-induced liver fibrosis by sodium butyrate. Front. Pharmacol. 15:1422770. doi: 10.3389/fphar.2024.1422770
Received: 24 April 2024; Accepted: 28 May 2024;
Published: 08 July 2024.
Edited by:
Yang Xie, Brigham and Women’s Hospital, Harvard Medical School, United StatesReviewed by:
Jingyuan Wang, University of Pittsburgh, United StatesXin Tong, Merck Sharp & Dohme Corp, United States
Copyright © 2024 Yang, Chen, Zhao, Du, Fan, Liu, Li and Li. This is an open-access article distributed under the terms of the Creative Commons Attribution License (CC BY). The use, distribution or reproduction in other forums is permitted, provided the original author(s) and the copyright owner(s) are credited and that the original publication in this journal is cited, in accordance with accepted academic practice. No use, distribution or reproduction is permitted which does not comply with these terms.
*Correspondence: Yong-Zhi Li, bGl5b25nemhpMjMyNEBob3RtYWlsLmNvbQ==; Yongchun Li, c3BseWNAMTI2LmNvbQ==; Hui-Xin Liu, bGl1aHhAY211LmVkdS5jbg==
†These authors have contributed equally to this work and share first authorship