- 1The School of Clinical Medicine, Hunan University of Chinese Medicine, Changsha, Hunan, China
- 2Department of Neurology, Brain Hospital of Hunan Province, Changsha, Hunan, China
- 3School of Medicine, Changsha Social Work College, Changsha, Hunan, China
- 4Department of Neurology, Huitong People’s Hospital, Huitong, Hunan, China
Copper is an important mineral, and moderate copper is required to maintain physiological processes in nervous system including cerebral ischemia/reperfusion (I/R) injury. Over the past few decades, copper induced cell death, named cuprotosis, has attracted increasing attention. Several lines of evidence have confirmed cuprotosis exerts pivotal role in diverse of pathological processes, such as cancer, neurodegenerative diseases, and I/R injury. Therefore, an in-depth understanding of the interaction mechanism between copper-mediated cell death and I/R injury may reveal the significant alterations about cellular copper-mediated homeostasis in physiological and pathophysiological conditions, as well as therapeutic strategies deciphering copper-induced cell death in cerebral I/R injury.
Introduction
Copper is an essential trace nutrient required for various cellular functions, meanwhile, copper accumulation beyond cellular needs is toxic (Khalfaoui-Hassani et al., 2021). Copper exists in two states, Cu+ and Cu2+, in body. In bodily fluids, copper is predominantly in the form of Cu2+, while inside cells, primarily exists as Cu+ (Kucková et al., 2015). Under the action of oxidoreductases, a conversion between Cu+ and Cu2+ existed, which electron transfer occurs through the Fenton reaction, leading to the generation of ROS, including superoxide anion (O2−), nitric oxide (NO-), hydroxyl radical (OH), and hydrogen peroxide (H2O2) (Valko et al., 2005). ROS could oxidize and damage biological molecules, including proteins, nucleic acids, and lipids. Additionally, ROS could interfer with the synthesis of iron-sulfur clusters (Slezak et al., 2021). Copper, assimilated from the digestive tract, undergoes hepatic metabolism to form ceruloplasmin, the primary copper-containing protein in the bloodstream, extensively present in diverse bodily organs. Ceruloplasmin is responsible for transporting 95% of copper in the bloodstream, making it a reliable marker for assessing the body’s copper levels (Piacenza et al., 2021). The diagnosis of specific copper metabolism disorders involves evaluating internal copper levels through the measurement of ceruloplasmin (CP) content (Bandmann et al., 2015). When ceruloplasmin (CP) amounts to the surface of target cells, which interacts with its corresponding receptors to release copper. The released copper is then absorbed and utilized by the targeted cells. The binding and release of copper ions by CP allow for distinct distribution of copper in multiple tissues and organs (Liu Z. et al., 2022). However, copper not bound to ceruloplasmin could still be continuously absorbed by tissues in the blood. Ceruloplasmin could oxidize ferrous ions (Fe2+) to ferric ions (Fe3+), facilitating the transportation of iron in the plasma, which plays a crucial role in maintaining the iron homeostasis in living organisms (Raia et al., 2023). During the oxidation of ferrous iron to ferric iron, ceruloplasmin facilitates the transfer of ferric iron into transferrin, preventing oxidative stress damage caused by the Fenton reaction (Vasilyev, 2019). Therefore, ceruloplasmin possesses certain antioxidant capabilities, manifested through its oxidase activity towards low-valent metal ions and glutathione peroxidase. It also exhibits the ability to eliminate reactive oxygen species (ROS) (Liu Z. et al., 2022; Samygina et al., 2017).
Copper enters the cell through binding with a copper chaperone, enters the mitochondria. Within the mitochondria, COX17 forms a complex with Cu+, and subsequently, Cu+ could be transferred to SCO1 or COX11, which is involved in the synthesis of cytochrome c oxidase (CcO), the terminal component of the mitochondrial respiratory chain. CcO catalyzes the transfer of electrons from reduced cytochrome c to oxygen, facilitating the oxidative phosphorylation process (McCann C. et al., 2022; Horng et al., 2004; Banci et al., 2008). CCS [Copper Chaperone for superoxide dismutase (SOD)] serves as the copper companion for Superoxide Dismutase 1 (SOD1) and involves transferring copper ions to SOD1, thereby activating the active site of SOD1, which exerts a crucial regulatory role in the cellular redox balance (Boyd et al., 2020; Boulis and Donsante, 2023). In cellular cytoplasm, Metallothionein 1/2 (MT1/2) forms complexes with numerous copper ions, acting as a repository for copper. This interaction is potentially involved in regulating the equilibrium of copper ions within the cell (Tapiero et al., 2003; Ruttkay-Nedecky et al., 2013; Yu et al., 2022). Glutathione (GSH), featuring thiol groups, functions as an antioxidant within cells by directly engaging in enzymatic antioxidant reactions. This involvement manifests anti-oxidative and anti-apoptotic effects. Additionally, GSH may play a role in directly or indirectly regulating the cellular copper pool (Ulrich and Jakob, 2019; Ferguson and Bridge, 2019). Copper transport protein (ATP7A) is expressed in most tissues and organs, while ATP7B is primarily expressed in the liver. ATP7A and ATP7B are located in the trans-Golgi network (TGN), which supply copper to copper-dependent enzymes synthesized in the secretory pathway. The copper efflux rate is determined by the transport or fusion of vesicles containing ATP7A with the plasma membrane (Chun et al., 2017; Janardhanan et al., 2022; Chen et al., 2020) (Figure 1).
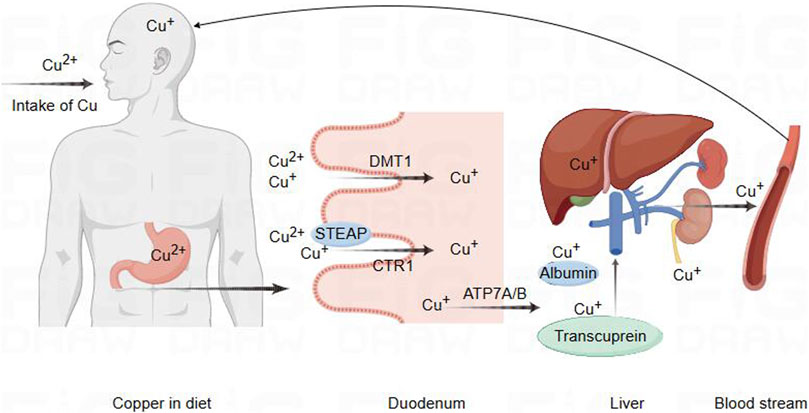
Figure 1. Schematic diagram of copper ion regulation in cells for cellular function. Copper metabolism and transport to the brain pathway. Copper in food is first reduced by STEAP, which promotes the absorption of copper by intestinal epithelial cells through the action of CTR1. In addition, DMT1 can also transport and absorb copper in certain situations. Subsequently, copper is transported to the portal vein through copper ATP7A, where it binds to plasma proteins such as albumin and copper transporter protein for transport to the liver. A small amount of copper is processed or transported from the liver and released into the bloodstream for circulation into the brain, while excess copper is excreted through bile.
Copper metabolism and internal balance
Copper metabolism
Copper is extensively distributed in the biological entities of the natural environment, and it is a crucial trace nutrient for human body. It plays a vital role in numerous redox reactions occurring within human cells, contributing to cellular processes (Chen et al., 2021; Chelyadina et al., 2023). As is well known, Cu is a static cofactor, serving as a catalytic or structural cofactor in the formation of enzymes and proteins, which might be buried and protected within its active sites of enzymes, participating in numerous vital biological activities. Cu is involved in various life processes by constituting enzymes such as cytochrome c oxidase (CCO), SOD, metallothionein (MT), cuproprotein (CP), and lysyl oxidase (LOX). Its participation extends to functions like energy metabolism, antioxidant stress response, vascular formation, and neurotransmitter synthesis (Chen et al., 2020; Tsang et al., 2021; Zhou et al., 2023). Internal copper metabolism is tightly regulated, and extreme deficiency or excess of copper disrupts the copper balance, leading to the onset of diseases and even posing a threat to life (Kirsipuu et al., 2020). Copper deficiency could influence the activity of SOD and the antioxidant capacity of other cellular components, such as iron, selenium, and glutathione (Altarelli et al., 2019; Trist et al., 2021). Copper deficiency may be associated with early pregnancy miscarriage, inflammatory responses, severe anemia, cardiovascular system issues, optic nerve function, and neuro-degenerative changes (Gurnari and Rogers, 2021; Hu et al., 2023; Cendrowska-Pinkosz et al., 2022; Wu et al., 2023). Similarly, copper accumulation could lead to abnormal oxidative metabolism, attacking to the structures and functions of biomolecules such as proteins, which could lead to the occurrence of diseases such as diabetes, neurological disorders, and cardiovascular dysfunctions (Bjørklund et al., 2020; Shi et al., 2023; Gaetke and Chow, 2003). Thus, copper homeostasis is regarded as crucial mechanism for maintaining the balance in the body. The absorption of dietary copper depends on the chemical form of copper and the interaction with dietary components. Daily intake below 0.8 mg/day results in a net loss of copper, while intake above 2.4 mg/day leads to a net increase (Bost et al., 2016). Studies indicates that excessive dietary copper intakes are associated with atherosclerosis, cardiovascular diseases, abdominal aortic calcification, neuronal and mixed neuronal-glial tumors, diabetes, and various neurodegenerative diseases (Yang et al., 2024; Li X. et al., 2023; Liu and Liang, 2024; Eljazzar et al., 2023; Zhang W. et al., 2023; Zhang Y. et al., 2023). In the mammalian digestive tract, copper in food is reduced from its divalent state by the metal-reducing enzyme (STEAP) to a monovalent state. Subsequently, it is absorbed by intestinal cells through the high-affinity copper transporter 1 (CTR1) (Li, 2020). Divalent metal transporter 1 (DMT1) could also transport copper under certain circumstances. The intestinal DMT1 is crucial for the absorption of iron but not essential for the absorption of copper or manganese in mice. A high-iron diet inhibited the intestinal transport of copper through DMT1 (Lin et al., 2015; Sánchez-González et al., 2022; Shawki et al., 2015). As previously reported, copper absorbed in the intestine is transported to the portal vein through ATP7A. In the portal vein, copper binds to plasma proteins such as albumin and transcuprein, facilitating transport to liver, which serves as the central control system for copper homeostasis (Alessi et al., 2021; Horn and Wittung-Stafshede, 2021). Most excess copper is excreted through the bile into the feces, with only a small amount excreted through the kidneys. Impairment of biliary copper excretion could lead to hepatic copper retention (Lenartowicz et al., 2015; Schilsky et al., 2023) (Figure 2).
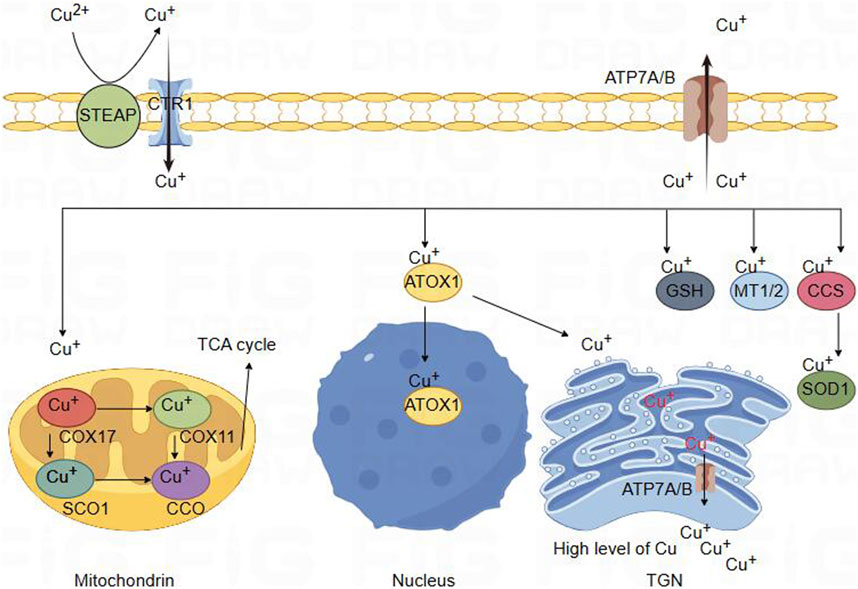
Figure 2. Schematic diagram of copper ion metabolism in body. Copper metabolism in brain cells. Divalent copper cannot be directly absorbed. It is first reduced by STEAP and enters cells through the high affinity of CTR1 for copper absorption on the plasma membrane. Copper binds to copper partners and enters mitochondria, where COX17 forms a complex with copper. Copper can then be transferred to SCO1 or COX11, which participate in the synthesis of CcO, the terminal component of the tricarboxylic acid cycle energy metabolism mitochondrial respiratory chain. Copper could bind with ATOXI into the nucleus and participate in gene expression regulation. It can also be transferred to the Golgi apparatus and released by binding with ATP7A/B, providing copper for copper dependent enzymes synthesized in the secretion pathway. In the cytoplasm, MT1/2 forms complexes with many copper ions, serving as a storage reservoir for copper. This interaction may be involved in regulating the balance of copper ions within cells. GSH can also directly or indirectly participate in regulating cellular copper pools. In addition, CCS acts as a copper partner of SOD1, involving the transfer of copper ions to SOD1. Finally, the efflux of copper is determined by the transport or fusion of ATP7A/B vesicles with the plasma membrane.
Cu protein in mammal metabolism
Ferredoxin (Fdx)
In mitochondrial matrix of eukaryotic cells, there is an unstable copper pool, which serves as the location for the assembly of mitochondrial iron-sulfur (Fe/S) clusters (Du et al., 2023). Ferredoxin (FDX) is a vital protein mediator in biological electron transfer reactions, which characterized by the presence of [2Fe-2S] or [4Fe-4S] clusters, allowing it to efficiently accept and release electrons, contributing to its active involvement in oxidation-reduction reactions (Nzuza et al., 2021). Disruption of copper homeostasis may negatively impact the formation of mitochondrial Fe/S clusters, which could lead to impaired iron-sulfur cluster biogenesis, affecting mitochondrial function. The impact extends beyond the respiratory chain, influencing various mitochondrial functions like citric acid cycle, heme biosynthesis, and sulfur-containing amino acid synthesis may be compromised (Ruiz et al., 2021; Cobine et al., 2021). FDX, as an electron donor, engages in various metabolic processes due to its versatile nature, which include participation in the synthesis of steroids, hemoglobin, vitamin D, and the biogenesis of Fe-S clusters in different organisms. Although Fdx1 and Fdx2 share significant functional similarities, they exhibit specific and distinct roles in different physiological pathways (Dreishpoon et al., 2023a; Braymer et al., 2021). Lipoic acid synthase (LIAS) generates lipoic acid cofactors, crucial components for tricarboxylic acid cycle (TCA) cycle enzymes like pyruvate dehydrogenase (PDH). FDX1 acts as an upstream regulator of LIAS, participating in the regulation of the PDH complex. DLAT is a component of the mitochondrial PDH. Loss of FDX1 resulted in the accumulation of pyruvate and α-ketoglutarate, impacting the TCA cycle (Joshi et al., 2023; Dreishpoon et al., 2023b). FDX1 is a crucial regulator of copper ionophore-induced cell death and serves as an upstream regulator of cellular protein lipidation. FDX1 directly binds to LIAS, facilitating its functional interaction with the lipoic acid carrier protein (glycine cleavage system protein H, GCSH), which direct interaction allows FDX1 to regulate protein lipoylation, bypassing indirect modulation of cellular Fe-S cluster biogenesis.
FDX1 promotes the formation of LIAS-GCSH bonds and activation of lipoic acid, which is crucial for cellular processes (Wang Z. et al., 2023; Ke et al., 2023; Schulz et al., 2023). The reductase encoded by FDX1 undergoes protein thioylation regulation during the reduction of Cu2+ to Cu+, promoting oligomerization of dihydrothioacyl S-acetyltransferase (DLAT). The regulatory mechanism subsequently induces protein toxicity stress, indicating the significant role of FDX1 in copper ion metabolism and cellular stress response (Joshi et al., 2023; Weiler et al., 2020; Li Y. et al., 2023). FDX1 knockdown results in a decrease in the lipoylation level of DLAT and DLST in thyroid cancer cells, which contributes to the alleviation of cell death induced by copper accumulation (Chen G. et al., 2023). FDX1 could regulate the activity of cytochrome c oxidase and mitochondrial respiration, and has multiple targets in vivo. FDX1 knockout led to a specific reduction in cytochrome c oxidase, influencing copper and heme A/a3 levels (Zulkifli et al., 2023).
Cytochrome c oxidase (COX)
Mitochondrial Cytochrome c Oxidase is located in the inner mitochondrial membrane and serves as a crucial enzyme in the respiratory chain. As the fourth central enzyme complex, also known as Complex IV, it receives electrons on cytochrome C molecules and transfers them to oxygen, generating water and releasing a substantial amount of energy (Blomberg, 2020; Ramzan et al., 2021; Brischigliaro and Zeviani, 2021). Copper serves as a necessary cofactor for COX, with three copper ions needed to construct the dual-core CuA and mononuclear CuB sites, ensuring the enzyme’s stability and activity (Maghool et al., 2019). The assembly of the CuA center necessitates the presence of copper chaperones SCO1, SCO2, and COA6. COA6 knockout in HEK293T cells resulted in decreased activities of both complex I and IV, accompanied by a reduction in mitochondrial membrane potential (Pacheu-Grau et al., 2020). The cellular COX is a complex comprising 13 subunits, and its functionality involves a highly coordinated process. The regulation of COX expression and function is essential for sustaining cellular respiration and energy production (Watson and McStay, 2020). The involvement of Cytochrome c oxidase subunit 5a (COX5a) is essential for preserving regular mitochondrial function.
Metabolism of copper ions in brain
Copper has been confirmed abundantly present in the brain, playing a crucial role in brain function and development. The subventricular zone (SVZ) in the lateral ventricles, containing a high amount of copper, is the largest neurogenic region in the adult brain and plays a crucial role in neurogenesis (Liu L. L. et al., 2022). Clinical studies confirmed that the severity of brain atrophy is associated with the functional and neurological impairments in patients with Wilson’s disease (WD). Brain volume serves as an indicator of neuro-degeneration induced by copper (Shang et al., 2023). Disruption of copper homeostasis may be a primary cause of various neurological disorders, particularly when elevated copper ion levels occur during brain I/R injury, leading to neuronal damage (Zhang et al., 2020; Chen X. et al., 2023). The potential mechanisms underlying the toxicity induced by the excessive accumulation of free copper within neural cells remain unclear, which are associated with oxidative stress attacks, cell apoptosis, copper death, and other factors (Chen X. et al., 2023; Goto et al., 2020; Gao et al., 2024). In 2022, Peter Tsvetkov, et al. first proposed a novel form of cell death induced by copper ions, which named “cuproptosis” (Kahlson and Dixon, 2022). Emerging evidence supports the facts that copper death is a novel form of cell death involved in regulating cerebral I/R injury. In a rat model of cerebral I/R injury, elevated copper ions and abnormal expression of copper death-related proteins were observed (Guo Q. et al., 2023). Thus, much progress has been made in understanding the pathophysiological mechanisms of copper death in cerebral I/R, it has attracted more and more attention worldwide. It is accepted that the mechanism of I/R is an important driving force for cerebral I/R injury. In this review, we intended to discuss the recent advances in the copper balance.
Endothelial cells within the blood-brain barrier acquire copper from the blood through CTR1 and transport it to the brain parenchyma via ATP7A. Copper homeostasis in the brain involves a complex interplay between uptake, distribution, utilization, and efflux mechanisms (Washington-Hughes et al., 2023; An et al., 2022). Copper ions traverse the blood-brain barrier to enter the brain parenchyma, initially encountering astrocytes. Astrocytes possess the capability to absorb, store, and release copper (Li B. et al., 2024). Copper is allocated to various brain cells according to the demands of different cell types. The copper concentration within astrocytes is the highest, influencing copper homeostasis in the brain. When astrocytes die due to brain injury or aging, copper may be released from astrocytes, potentially exerting adverse effects on neighboring brain cells (Górska et al., 2023; Bhattacharjee et al., 2020). The copper transporter protein CTR1 on the cytoplasmic membrane of brain nerve cells could efficiently uptake copper with high affinity (Wen et al., 2021).
Biological functions of copper in brain/and neuronal cells
Cu protein in brain metabolism
Copper is crucial for the normal functioning of the brain, participating in the synthesis of neurotransmitters such as dopamine and adrenaline. Copper distribution in the brain is uneven, with the highest concentration in the locus coeruleus region of brainstem neurons (Rihel, 2018; Scheiber et al., 2014). This region serves as a primary source of norepinephrine (NA) in CNS. The neurotransmitter NA plays a role in regulating wakefulness, sensory processing, attention, aversion, adaptive stress responses, as well as higher-order cognitive functions and memory (Ma et al., 2023; Kawahara et al., 2021). The copper content is higher in the substantianigra, and the decreased levels of copper in the substantia nigra of Parkinson’s disease (PD) brain further indicate the significant role of copper in this brain region (Bisaglia and Bubacco, 2020). Copper also plays a crucial role in synaptic transmission. In the synaptic cleft, copper could directly or indirectly modulate the activity of neurotransmitter receptors (NMDA, AMPA, GABAA, P2X receptors), influencing neural excitability. Neurotransmission could influence the transport and transmission of copper within neuronal cells (D’Ambrosi and Rossi, 2015; Wang et al., 2020). Copper in brain, besides participating in specific functions mentioned earlier, is also associated with general metabolism, which includes energy metabolism (cytochrome c oxidase, TCA cycle (FDX1)), antioxidant defense (superoxide dismutase containing zinc and copper, copper blue protein), iron metabolism (copper blue protein), neurotransmitter synthesis (dopamine-β-monooxygenase), and neuropeptide synthesis (peptide glycine-α-amidating enzyme) (Liu L. L. et al., 2022; Scheiber et al., 2014; Wang et al., 2020; Masaldan et al., 2018; Li et al., 2022). Neural cells require enzymes and proteins that depend on copper coordination to perform their normal functions. Additionally, some proteins rely on copper for metabolic regulation. Several important proteins expressed in brain and involved in these processes are described as following:
Ceruloplasmin (both soluble and GPI-anchored ceruloplasmin)
Genetic mutations in the CP gene result in aceruloplasminemia, a hereditary condition characterized by progressive degeneration of the retina and basal ganglia neurons (Bonaccorsi di Patti et al., 2021). Patients with WD undergo impaired copper excretion from the liver, leading to copper ion deposition in the brain and the manifestation of neurological symptoms, even after undergoing liver transplantation (Xu et al., 2022). Additionally, in a mouse model of permanent middle cerebral artery occlusion-induced cerebral ischemia, mice lacking CP exhibit an increased area of cerebral infarction, leading to more severe oxidative stress damage to lipids and proteins (Ryan et al., 2019).
COX
Overexpressing COX5a in the SAMP8 aging mouse model led to improvements in spatial recognition, memory, and hippocampal synaptic plasticity and also restored hippocampal CA1 dendrites, playing a crucial role in modulating age-related cognitive decline (Xiyang et al., 2020). In hypoxia-ischemia (HI) rat model, a manifestation of severe neurological dysfunction, cerebral infarction, cell apoptosis, and notable neuronal loss is concomitant with reduction in COX5a expression. Conversely, COX5a upregulation facilitated neuronal survival post-oxygen-glucose deprivation (OGD), decreasing apoptosis and notably increasing the length of neuronal dendrites (Jiang et al., 2020). In addition, COX6b1 overexpression alleviated I/R-induced hippocampal neuron damage in rat (Yang et al., 2019).
Fdx
In a rat MCAO model, a significant upregulation of FDX1 was observed, while interventions with DEX resulted in reduction in FDX1 expression, which alleviates cerebral infarction area and decreases copper-induced neuronal death. Furthermore, in PC12 cells, post-OGD/R treatment led to increased FDX1 expression, causing DLAT oligomerization and triggering copper-induced neuronal death (Wang D. et al., 2023).
SOD
The structure, function, and mutations of copper-zinc SOD are associated with neurodegenerative disorders, particularly amyotrophic lateral sclerosis (ALS) (Altobelli et al., 2020; Sanghai and Tranmer, 2021). The activation of immature SOD1 involves in the copper chaperone (Ccs1), which includes Ccs1 delivering copper and promoting the oxidation of the intramolecular disulfide bonds within SOD1, or activation through the entry of copper ions into the sulfur-containing intermediate at the binding site (Fetherolf et al., 2017). In rats subjected to cerebral I/R injury, overexpression of transgenic SOD1 alleviates oxidative stress injury, which exhibited protective effects on nerve cells (Chan et al., 1998). The key to combating cerebral I/R injury is enhancing the activity of endogenous antioxidant enzymes, with SOD1 capable of clearing excessive ROS production (Park et al., 2021). Therefore, external intervention to enhance SOD1 activity could be utilized in the development of protective agents against ischemic injury.
Dopamine β-hydroxylase (DBH)
DBH is a member of the copper-dependent monooxygenase family, located in the endoplasmic reticulum, which encoded by the DBH gene and catalyzed the conversion of dopamine to norepinephrine (Juárez-Cedillo et al., 2023). Functionally similar to DBM, and both may be different names for the same enzyme. Dietary copper deficiency in rats leads to a downregulation of dopamine beta-monooxygenase levels in brain tissues (Prohaska and Brokate, 2001). In patients with Menkes disease, the reduction of copper content led to an increased ratio of DBM deficiency in dopamine/norepinephrine, resulting in abnormal behavioral and psychological manifestations (Guthrie et al., 2020; Ohkubo et al., 2019). DBH is the enzyme responsible for producing norepinephrine (NE), while dopamine (DA) and NE are crucial neurotransmitters necessary for normal brain function, which are associated with various diseases such as hypertension, congestive heart failure, Alzheimer’s disease, PD, and Huntington’s disease (Gonzalez-Lopez and Vrana, 2020; Vendelboe et al., 2016).
Peptidylglycine α-amidating monooxygenase (PAM)
Peptide hormones are synthesized, stored, and released in the anterior pituitary. The activation of inactive peptide hormone precursors requires the post-translational processing enzyme PAM (Bonnemaison et al., 2016), which converts peptides with extended glycine to amidated products, involved two single-electron transfer steps catalyzed by copper ions at two specific sites. This process is involved in the biosynthesis of peptide hormones (Bäck et al., 2022; Merkler et al., 2022). PAM, an ascorbate- and copper-dependent membrane enzyme that enters secretory granules along with its soluble substrates. Biological studies elucidated the highly conserved mechanism for amidated peptide production and raised many questions about PAM trafficking and the effects of PAM on cytoskeletal organization and gene expression. With the identification of PAM, it showed that PAM was composed of two soluble catalytic cores, including PHMcc (the catalytic core of PHM) and PALcc (the catalytic core of PAL) and a protease-sensitive cytoplasmic domain. Copper ions bound at two sites separated by an 11 Å aqueous cleft participate in the two single electron transfer steps needed to generate a peptidyl-α-hydroxyglycine product (Grechnikova et al., 2020). As previously reported, PAM−/− mice could only survive until mid-pregnancy (Kumar et al., 2016). PAM (+/−) heterozygous mice exhibited anxiety-like behavior, alterations in temperature regulation, and increased susceptibility to seizures, which could be reversed by dietary copper supplementation, suggesting that physiological functions sensitive to PAM genetic constraints could be reversed by copper supplementation (Bousquet-Moore et al., 2010). In primary pituitary cells subjected to 4 h of hypoxia, hypoxia-inducible factor 1α (HIF-1α) exhibited an upregulation, which restricts the ability of PAM to generate amidated peptides, further impacting the enzyme’s activity (Rao et al., 2021).
The pathophysiological role of copper in cerebral I/R injury
Cerebral I/R injury and cuproptosis
Stroke is a severe neurological disease, which remains the second leading cause of death (GBD, 2019 Stroke Collaborators, 2021). Early identification, thrombolytic therapy, and emergency intervention for acute ischemic stroke could significantly reduce the incidence and mortality associated with strokes (Herpich and Rincon, 2020). The recovery reperfusion of acute ischemic stroke has a high degree of time dependence (Reziya et al., 2023). In clinical trials, it is challenging to grasp the time window for vascular reconstruction. When the recovery of cerebral blood flow exceeds the time window, it cannot improve the tissue state of reversible damage, and may even deteriorate irreversible sequelas, such as malignant edema and even bleeding (Magoufis et al., 2021). However, the molecular mechanisms remained not fully elucidated, which are associated with oxidative stress, apoptosis, ferroptosis, calcium overload, and copper death. Increasing evidence has showed that copper death participates in brain I/R injury. In cerebral I/R injury, ischemia or hypoxia causes decreases in mitochondrial ATP production, which restricts copper transportation, and results in the of copper accumulation (Cunnane et al., 2020). As previously reported, copper-targeted delivery alleviates neuronal damage in ischemic stroke (Huuskonen et al., 2017). During cerebral I/R, the intracellular copper ion uptake and excretion reached a homeostatic balance, which could be disrupted by mitochondrial respiratory attack and limitation in ATP production, potentially the accumulation of copper ions within the cell and cellular cuproptosis (Lin et al., 2021). Therefore, inhibiting neuronal cuproptosis could decrease the levels of copper ions inside nerve cells, mitigating neuronal injury during cerebral I/R.
Copper accumulation and cuproptosis
Reperfusion of blood supply in ischemic region of the brain after an ischemic stroke yields to irreversible cellular and biochemical consequences, including generation of ROS, expressions of inflammatory cytokines, and inflammation (Lim et al., 2021). Brain tissue is rich in polyunsaturated fatty acids, which is particularly susceptible to attack by ROS and undergoing lipid peroxidation. Intervention with polyunsaturated fatty acids also held potentials for improving neurological outcomes in neonatal hypoxic/ischemic encephalopathy (sHIE) (Martinat et al., 2021; Ng et al., 2022; Dyall et al., 2023). Cu2+ exposure reduced levels of glutathione (GSH) and antioxidant capacity while promoting lipid peroxidation in tissues. Some studies indicate that copper (Cu-HCF) nanocomposites catalyze the conversion of reduced glutathione (GSH) to oxidized glutathione within tumor cells, leading to the depletion of GSH (Li C. et al., 2024). The generation of mitochondrial ATP occurs through the process of oxidative phosphorylation (OXPHOS), where the OXPHOS system operates the following five enzyme complexes in the electron transport chain (ETC): Complex I (NADH: ubiquinone oxidoreductase), Complex II (succinate dehydrogenase, SDH), Dimeric Complex III2 (cytochrome bc1 oxidoreductase), and Complex IV (cytochrome c oxidase) (Vercellino and Sazanov, 2022). Due to the presence of two crucial copper sites essential for its functionality, respiratory Complex IV in mitochondria requires copper to sustain the functionality of cytochrome c oxidase (CcO). Therefore, its activity could be easily manipulated by either copper removal/chelation or copper overload, thus influencing the structural and functional aspects of the Electron Transport Chain (ETC) (Ruiz et al., 2021; Swaminathan and Gohil, 2022). Some studies indicate that prolonged exposure to copper in liver and muscles of the viviparous killifish leads to a reduction in energy production, which attribute to copper inhibiting anaerobic pathways and the mitochondrial respiratory chain (Abou Anni et al., 2019). Furthermore, in the liver of patients with Wilson’s disease (WD), copper overload could lead to mitochondrial injury, impairing energy metabolism (Mazi et al., 2020). In cerebral I/R injury, the level of copper ions increases. Cu+ directly binds to the sulfur-containing components in the mitochondrial TCA, leading to copper-induced reduction of Fe-S (iron-sulfur) proteins, which triggers protein toxicity stress, inducing copper-dependent cell death. Recent study indicates that Disulfiram (DSF), a widely used drug for controlling alcoholism, possesses anticancer activity by inducing apoptosis in a copper Cu-dependent manner (You et al., 2019). DSF/Cu could accelerate copper death in liver cancer cells (HCC), accompanied by GSH depletion and increased lipid peroxides (Zhang et al., 2024). Taken together, these findings suggest that sustained copper accumulation may induce oxidative stress damage, mitochondrial dysfunction, and the occurrence of cuproptosis in cerebral I/R injury (Figure 3).
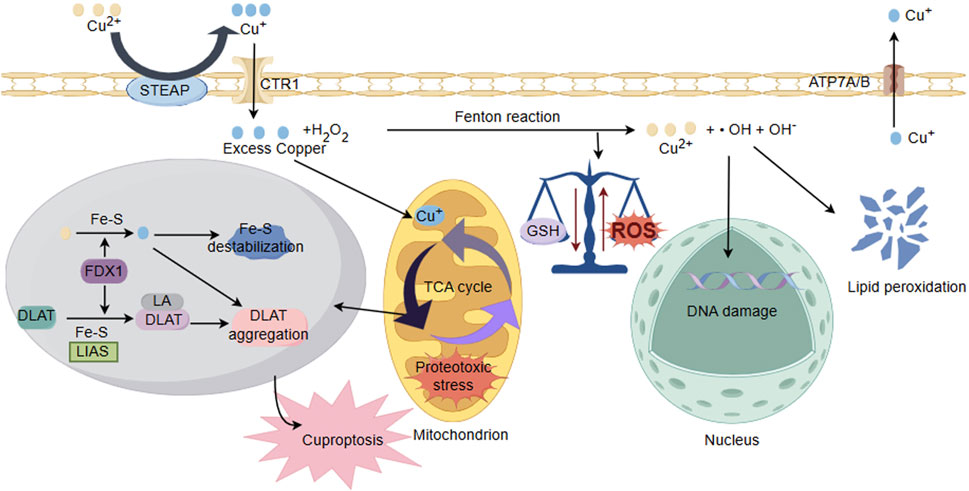
Figure 3. Schematic illustration of copper ion overload inducing neuronal cell injury. Under the action of cytoplasmic oxidoreductases, there is a conversion between Cu+ and Cu2+, and electron transfer occurs through the Fenton reaction, producing ROS. The accumulation of Cu2+ reduces GSH levels and antioxidant capacity, disrupting the balance of cellular redox states and damaging biomolecules including proteins, nucleic acids, and lipids. In addition, ROS could interfere with the synthesis of iron sulfur clusters. Cu binds to thiolated mitochondrial enzymes in the TCA cycle (such as DLAT), inducing the aggregation of these proteins. FDX1/LIAS is an upstream regulator of protein sulfhydrylation. FDX1 reduces Cu2+ to more toxic Cu+, leading to inhibition of Fe-S cluster protein synthesis, aggregation of mitochondrial proteins and loss of Fe-S clusters, blocking the TCA cycle of the tricarboxylic acid cycle. Collectively, these abnormal processes lead to protein toxicity stress and induce cell death.
Regulating the level of copper ion and inhibit cuproptosis in cerebral I/R
Clinical studies have found a significant increase in free copper ions in blood samples collected from patients with acute myocardial infarction and successful percutaneous coronary intervention (Hao et al., 2022). Similarly, there is an increase in copper ion levels in the kidney and small intestine models of mice with I/R injury (Akçil et al., 2000; Lee et al., 2019). In a rat cerebral I/R model, the copper ion content in the hippocampal CA1 region of the brain tissue significantly increased compared with the sham group (Shang et al., 2023). In another MCAO rat study, pretreatment with the copper chelator D-penicillamine significantly reduced cerebral infarct volume and copper ion levels which could be improved mitochondrial respiration and membrane potential, possibly exerting its effects by blocking the cuproptosis pathway mediated by FDX1 (Guo Qingduo et al., 2023). Therefore, reducing the increase in copper ion levels in tissues and organs can inhibit the occurrence of cuproptosis. By regulating the copper ion transport, storage, and output processes in neural cells, the copper ion levels in neuronal cells could be reduced, thereby inhibiting the occurrence of cuproptosis. Seeking targeted therapeutic strategies to alleviate I/R injury by specifically reducing cuproptosis is becoming urgent.
Regulating copper ion levels by blocking the entry of copper ions into CTR1
CTR, located at the plasma membrane and composed of two types of subunits including CTR1 and CTR2, especially which CTR1 belongs to the superfamily of membrane spanning transport proteins responsible for dietary copper homeostasis in mammalian cells (Schoeberl et al., 2022a). Under a normal physiological copper homeostasis, CTR1 is described to be mainly located at the plasma membrane. However, when the cells are medicated with a high amount of Cu, CTR1 is internalized to Early Endosomal Antigen (EEA1)- and Rab5-marked compartments by endocytosis. After removal of extracellular Cu, the transport protein recycles back to the plasma membrane via the slower recycling endosomes (Garmann et al., 2008; Mandal et al., 2020). The missense mutation of the CTR1 coding gene leads to copper deficiency in the central nervous system, thereby affecting the mitochondrial function of nerve cells (Batzios et al., 2022). The increase in copper content during the aging process of the human brain may be attributed to the dysfunction of the brain barrier CTR1, which may be a trigger for neurodegenerative diseases (Haywood and Vaillant, 2014). The increase of intracellular copper is parallel to the expression of CTR1 mRNA. Chakraborty K, et al. found that the differentiation of PC-12 cells (neurons) involves an increase in intracellular copper (Chakraborty et al., 2022). Copper ions must be transported into cells through the transporter protein CTR1, so it is possible to reduce intracellular copper ions by regulating CTR1.
Interference in copper and platinum uptake mediated by CTR1
CTR1 is responsible for copper transport through the N-terminal domain of its amino acid residues. Interestingly, CTR1 also affects platinum uptake in tumor cells (Wang X. et al., 2023). Cisplatin, as a competitor to hCtr1 mediated copper transport, leads to a decrease in cellular copper levels (Akerfeldt et al., 2017). Liang et al.'s study reported that cisplatin reduced copper uptake by at least 50% in ovarian cancer cells (Liang et al., 2014). In addition, Pt (II) drugs can also specifically affect Cu (I) homeostasis by interfering with the rapid exchange of Cu (I) between Atox1 and Cu ATPases (Lasorsa et al., 2019). Which indicated that interference existed between copper and platinum that are substrates for cellular uptake mediated by CTR1. Several in vitro studies have disclosed that platinum drugs disrupt cellular copper uptake. In the ovarian cancer cell lines A2780 and the cisplatin-resistant A2780cis cells, utilizing cisplatin treatment exhibited a significant decrease in copper content compared with untreated cells (Schoeberl et al., 2022b). Therefore, platinum based drugs may become a backup drug for intervening in elevated copper ion levels in the future, by reducing intracellular copper ion concentration and inhibiting copper induced cell death.
Factors influencing CTR1 expression
In the study of improving the anti-tumor efficacy of platinum-based drugs, upregulation of CTR1 expression can be achieved. NSCLC cells, through glucose restriction, upregulate ROS and induce CTR1 expression in AMPK. Similarly, mice fed a low carbohydrate ketogenic diet (glucose restriction) showed an increase in CTR1 expression in tumor tissue, significantly enhancing the efficacy of cisplatin (Zhang et al., 2022). Changes in the expression of CTR1 within cells can directly affect the transport of copper ions. Downregulation of CTR1 expression reduces copper ion uptake. In the investigation of anti-pancreatic cancer, Song, et al. used targeted CTR1 mRNA therapy to effectively inhibit the expression of CTR1 in PANC-1 cells, reduce copper intake, and delay the progress of pancreatic cancer (Song et al., 2021). In a rat brain I/R injury model, the expression of intracellular CTR1 is downregulated, the concentration of copper ions decreases, and copper induced neuronal death is alleviated, effectively protecting neural function (Strenkert et al., 2024).
However, there are many factors that affect the expression of CTR1, such as the regulation of gene transcription encoded by CTR1. Treatment of M21 melanoma cell lines with the Sp1 selective inhibitor Plicamycin for 24 h or knockout of the Sp1 gene resulted in a decrease in the expression of Sp1 and CTR1, as well as a decrease in intracellular copper ion levels and an increase in cell survival rate (Lin et al., 2014). In U2OS cells, SP1 can directly bind to the CTR1 promoter. Overexpression SP1 stimulates CTR1 expression, increases copper ions and inhibits nuclear translocation of (SP1), knockout of p53 promotes CTR1 expression and cisplatin uptake, while p53 overexpression inhibits CTR1 expression and cisplatin uptake (Yong et al., 2023). In VPS35 knockout in HeLa cells, the results showed a significant decrease in the abundance of CTR1 on the cell surface and a significant decrease in copper ion levels in the absence of reverse transcriptase function, indicating that reverse transcriptase affects the transport of copper by CTR1 (Curnock and Cullen, 2020). Carine White’s et al. found that RAW264.7 macrophages pre-exposed to hypoxic conditions showed a significant increase in copper ion uptake compared to normoxia (White et al., 2009). Experimental evidence points that the copper uptake of Caco-2 cells under hypoxia is five times higher than normoxia, and the increase in copper uptake in Caco-2 cells under hypoxic conditions is related to the increase in Ctr1mRNA expression (Pourvali et al., 2012). In addition, studies have found that the expression of CTR1 gene is regulated by hypoxia inducible factor (HIF) and Myc transcription (Feng et al., 2009; Porcu et al., 2018).
After the transcription of the CTR1 gene, the stability of its protein can be translated in order to perform its function. Jianping Guo, et al. found that Nedd4l can induce CTR1 ubiquitination and subsequently degrade CTR1 (Guo et al., 2021). Nedd4l can negatively regulate CTR1 copper signaling to regulate AKT kinase activity and reduce tumor occurrence. Based on CRISPR/Cas9, the upstream kinase of CTR1 was identified through transcriptome screening. It was found that AMPK can enhance the localization of CTR1 membrane, and phosphorylation and stabilization of CTR1 are present and play a role in the plasma membrane (Zhang X. et al., 2023). CTR1 is essential for the activation of the MAPK signaling pathway by ligands of three major receptor tyrosine kinases (RTKs): FGF, PDGF, and EGF (Tsai et al., 2012). In summary, during cerebral I/R, modulation of post-translational modifications of CTR1, such as ubiquitination and phosphorylation, could influence its function and inhibit neuronal uptake of copper ions.
Factors influencing CTR1 activity
X-ray diffraction shows that CTR1 adopts a homologous trimeric structure similar to Cu+ selective ion channels. Two layers of cysteine triad form a selective filter that coordinates two bound copper ions near the extracellular entrance (Ren et al., 2019). Changes in the activity of CTR1 in cells may interfere with intracellular copper ion levels. Initially, it was believed that, similar to other ion channels, its activity could be altered by affecting energy metabolism. However, studies have shown that both mammalian and yeast CTR families lack clear ATP binding domains, and CTR1 mediated copper uptake may not be energy dependent (Margaret et al., 2023). Moreover, Peter Tsvetkov et al.'s research show that mitochondrial respiration is involved in copper-induced cell death, on the contrary, disrupting ATP generation with mitochondrial uncoupling agents showed no effect on copper-induced cell death (Tsvetkov et al., 2022). However, changes in the extracellular environment may affect CTR1 activity. The N-terminal region of the human copper transporter protein Ctr1 exhibits pH and metal oxidation state-dependent multi-metal binding capabilities (Lee et al., 2002). Experimental findings on the impact of extracellular pH, Na, and K+ on copper absorption indicated a significant increase in copper accumulation in Hek5 cells at pH 5.5 and 5.7 compared to pH 7.5. Compared with low K buffer, incubation of Hek5 cells in high K buffer significantly increased copper accumulation (Ren et al., 2019). Collectively, these findings suggest that acidic or high potassium could activate CTR1 activity, once neuronal cells undergo anaerobic glycolysis, acidosis, and high potassium levels, changes in the cellular microenvironment might cause stimulation on CTR1 activity, which led to increased copper ion uptake and subsequent copper-induced neuronal cell death. Furthermore, emerging studies indicate that the endogenous retrovirus envelope glycoprotein Refrex1 could interact with CTR1, reducing its transport activity to regulate copper uptake (Nardella et al., 2022). These results indicated that altering CTR1 activity through changes in the extracellular environment and protein interactions represents a potential target against copper-induced cell death due to elevated copper ion levels.
Regulating copper ion levels by modulating the copper efflux pathway ATP7A/B
ATP7A/B is a Cu + transporter ATPase, which maintains copper homeostasis within cells and involves the transmembrane transfer of Cu + ions from the cytoplasm to receptor proteins, and requires ATP hydrolysis (Tury et al., 2023). Mutations in ATP7A and ATP7B (more specific to liver where it plays an important role to deliver Cu to apoceruloplasmin) are the basis for MD and WD, respectively. ATP7A facilitates the extrusion of copper through the cell membrane to maintain cellular copper homeostasis. The copper accumulation in motor neurons is the pathogenic mechanism associated with ATP7A in the hereditary motor neuropathy dHMN (Tadini-Buoninsegni and Smeazzetto, 2017). However, some results indicated that genetic mutations in the genes encoding copper efflux proteins ATP7A/B could lead to functional impairment, which might impair Cu+ efflux within cells, copper overload, and induction of copper related cell death. A study in a Wilson Disease (WD) mouse model demonstrated that a decrease in Fe-S proteins, protein sulfhydration, and expression of copper death-related proteins showed shared occurrence of copper-induced cell death mechanisms in genetic models (Dong et al., 2021). Hepatolenticular degeneration is a mutation in the ATP7B gene, where copper ions continue to accumulate in brain, liver, and other tissues. The intervention strategy for WD primarily depended on the utilization of copper ion chelators such as D-penicillamine and tetrathiomolybdate (TTM) (Litwin et al., 2019). Studies have revealed that heterogeneous nuclear ribonucleoprotein hnRNPA2/B1 regulates Cu + homeostasis by modulating the Cu(I)-transporting protein ATP7A (McCann Courtney J. et al., 2022). In Hela cells, hnRNPA2/B1 downregulation increases ATP7A mRNA and protein levels, significantly decreasing cellular copper levels (Natera-de Benito et al., 2021). In HPrEC cells, certain anticancer drugs, like flavonoids, disrupt the expression of the copper transport gene ATP7A, leading to impaired copper ion transport and subsequent cell death (McCann C. J. et al., 2022). Furthermore, in human neuroblastoma SH-SY5Y cells, NaHS significantly reduces the levels of ATP7A, promotes intracellular Cu + accumulation, which induce cellular toxicity (Goto et al., 2020). COMMD1 performs intracellular ATP7A/B stability chaperone function, and copper levels increase in cells lacking functional COMMD1 protein (Singla et al., 2021). Therefore, by regulating the expression of ATP7A/B, it is possible to influence the intracellular copper ion levels. In the study of cerebral I/R in rats, it was found that the expression of ATP7B was significantly downregulated compared to the control group, and the intracellular copper ion level was increased. Dexmedetomidine pretreatment in cerebral I/R causes increase in ATP7B strongly expressed in brain, reduce intracellular copper ions, and inhibit neuronal copper death (Guo Qingduo et al., 2023). These results indicate that overexpression of ATP7A/B in neuronal cells, increased excretion of copper ions, and inhibition of copper induced neuronal cell death, which provided significant implications for the treatment of cerebral I/R injury.
Future prospectives
Taken together, the primary treatment approach for stroke is early perfusion to alleviate ischemic damage. However, early reperfusion could result in I/R injury, necessitating urgent exploration of therapeutic strategies to mitigate these assaults. During cerebral I/R, there is an elevation in copper ion levels, abnormal expression of copper death-related proteins, and the occurrence of copper-induced cell death in neurons. Understanding the physiological metabolism of copper ions in the brain, reducing cerebral copper ion levels during I/R injury aims to alleviate copper-induced cell death, offering a novel avenue for treating cerebral ischemic injury. While current research on cuproptosis primarily focuses on tumor treatment and drug resistance mechanisms, lessons from various strategies to decrease intracellular copper ions in cancer research could be applied to inhibit cuproptosis, contributing to studies aimed at alleviating cerebral I/R injury. Recently, although substantial progress has been made in unraveling the mechanisms of cuproptosis during cerebral I/R, there are still no effective remedies available for reliving cerebral I/R injury. This requires further studies and novel approaches to facilitate the molecular features and excavate more rational strategies.
Author contributions
GP: Writing–original draft, Software, Writing–review and editing. GX: Formal Analysis, Data curation, Writing–review and editing. YH: Supervision, Funding acquisition, Conceptualization, Writing–review and editing. JT: Writing–review and editing, Validation, Supervision.
Funding
The author(s) declare that financial support was received for the research, authorship, and/or publication of this article. This study was partly funded by Hunan Natural Science Foundation (2023JJ60262).
Conflict of interest
The authors declare that the research was conducted in the absence of any commercial or financial relationships that could be construed as a potential conflict of interest.
The reviewer CS declared a shared affiliation with the author YH to the handling editor at the time of review.
Publisher’s note
All claims expressed in this article are solely those of the authors and do not necessarily represent those of their affiliated organizations, or those of the publisher, the editors and the reviewers. Any product that may be evaluated in this article, or claim that may be made by its manufacturer, is not guaranteed or endorsed by the publisher.
References
Abou Anni, I. S., Zebral, Y. D., Afonso, S. B., Moreno Abril, S. I., Lauer, M. M., and Bianchini, A. (2019). Life-time exposure to waterborne copper III: effects on the energy metabolism of the killifish Poecilia vivipara. Chemosphere 227, 580–588. doi:10.1016/j.chemosphere.2019.04.080
Akçil, E., Tuğ, T., and Döşeyen, Z. (2000). Antioxidant enzyme activities and trace element concentrations in ischemia-reperfusion. Biol. Trace Elem. Res. 76 (1), 13–17. doi:10.1385/BTER:76:1:13
Akerfeldt, M. C., Tran, C. M., Shen, C., Hambley, T. W., and New, E. J. (2017). Interactions of cisplatin and the copper transporter CTR1 in human colon cancer cells. J. Biol. Inorg. Chem. 22 (5), 765–774. doi:10.1007/s00775-017-1467-y
Alessi, K., Delima, M., Pfautsch, M., Shriver, M. E., and Parmar, M. S. (2021). Neurological type Wilson's disease: a case report. SN Compr. Clin. Med. 3 (9), 1946–1950. doi:10.1007/s42399-021-00960-x
Altarelli, M., Ben-Hamouda, N., Schneider, A., and Berger, M. M. (2019). Copper deficiency: causes, manifestations, and treatment. Nutr. Clin. Pract. 34 (4), 504–513. doi:10.1002/ncp.10328
Altobelli, G. G., Van Noorden, S., Balato, A., and Cimini, V. (2020). Copper/zinc superoxide dismutase in human skin: current knowledge. Front. Med. (Lausanne) 7, 183. doi:10.3389/fmed.2020.00183
An, Y., Li, S., Huang, X., Chen, X., Shan, H., and Zhang, M. (2022). The role of copper homeostasis in brain disease. Int. J. Mol. Sci. 23 (22), 13850. doi:10.3390/ijms232213850
Bäck, N., Mains, R. E., and Eipper, B. A. (2022). PAM: diverse roles in neuroendocrine cells, cardiomyocytes, and green algae. FEBS J. 289 (15), 4470–4496. doi:10.1111/febs.16049
Banci, L., Bertini, I., Ciofi-Baffoni, S., Hadjiloi, T., Martinelli, M., and Palumaa, P. (2008). Mitochondrial copper(I) transfer from Cox17 to Sco1 is coupled to electron transfer. Proc. Natl. Acad. Sci. U. S. A. 105 (19), 6803–6808. doi:10.1073/pnas.0800019105
Bandmann, O., Weiss, K. H., and Kaler, S. G. (2015). Wilson's disease and other neurological copper disorders. Lancet Neurol. 14 (1), 103–113. doi:10.1016/S1474-4422(14)70190-5
Batzios, S., Tal, G., DiStasio, A. T., Peng, Y., Charalambous, C., Nicolaides, P., et al. (2022). Newly identified disorder of copper metabolism caused by variants in CTR1, a high-affinity copper transporter. Hum. Mol. Genet. 31 (24), 4121–4130. doi:10.1093/hmg/ddac156
Bhattacharjee, A., Ghosh, S., Chatterji, A., and Chakraborty, K. (2020). Neuron-glia: understanding cellular copper homeostasis, its cross-talk and their contribution towards neurodegenerative diseases. Metallomics. 12 (12), 1897–1911. doi:10.1039/d0mt00168f
Bisaglia, M., and Bubacco, L. (2020). Copper ions and Parkinson's disease: why is homeostasis so relevant? Biomolecules 10 (2), 195. doi:10.3390/biom10020195
Bjørklund, G., Dadar, M., Pivina, L., Doşa, M. D., Semenova, Y., and Aaseth, J. (2020). The role of zinc and copper in insulin resistance and diabetes mellitus. Curr. Med. Chem. 27 (39), 6643–6657. doi:10.2174/0929867326666190902122155
Blomberg, M. R. A. (2020). The structure of the oxidized state of cytochrome c oxidase - experiments and theory compared. J. Inorg. Biochem. 206, 111020. doi:10.1016/j.jinorgbio.2020.111020
Bonaccorsi di Patti, M. C., Cutone, A., Nemčovič, M., Pakanová, Z., Baráth, P., and Musci, G. (2021). Production of recombinant human ceruloplasmin: improvements and perspectives. Int. J. Mol. Sci. 22 (15), 8228. doi:10.3390/ijms22158228
Bonnemaison, M. L., Duffy, M. E., Mains, R. E., Vogt, S., Eipper, B. A., and Ralle, M. (2016). Copper, zinc and calcium: imaging and quantification in anterior pituitary secretory granules. Metallomics 8 (9), 1012–1022. doi:10.1039/c6mt00079g
Bost, M., Houdart, S., Oberli, M., Kalonji, E., Huneau, J. F., and Margaritis, I. (2016). Dietary copper and human health: current evidence and unresolved issues. J. Trace Elem. Med. Biol. 35, 107–115. doi:10.1016/j.jtemb.2016.02.006
Boulis, N. M., and Donsante, A. (2023). A novel antibody to treat SOD1-related amyotrophic lateral sclerosis. Mol. Ther. Methods Clin. Dev. 29, 236–237. doi:10.1016/j.omtm.2023.03.011
Bousquet-Moore, D., Prohaska, J. R., Nillni, E. A., Czyzyk, T., Wetsel, W. C., Mains, R. E., et al. (2010). Interactions of peptide amidation and copper: novel biomarkers and mechanisms of neural dysfunction. Neurobiol. Dis. 37 (1), 130–140. doi:10.1016/j.nbd.2009.09.016
Boyd, S. D., Ullrich, M. S., Skopp, A., and Winkler, D. D. (2020). Copper sources for Sod1 activation. Antioxidants (Basel) 9 (6), 500. doi:10.3390/antiox9060500
Braymer, J. J., Freibert, S. A., Rakwalska-Bange, M., and Lill, R. (2021). Mechanistic concepts of iron-sulfur protein biogenesis in Biology. Biochim. Biophys. Acta Mol. Cell Res. 1868 (1), 118863. doi:10.1016/j.bbamcr.2020.118863
Brischigliaro, M., and Zeviani, M. (2021). Cytochrome c oxidase deficiency. Biochim. Biophys. Acta Bioenerg. 1862 (1), 148335. doi:10.1016/j.bbabio.2020.148335
Cendrowska-Pinkosz, M., Ostrowska-Lesko, M., Ognik, K., Krauze, M., Juskiewicz, J., Dabrowska, A., et al. (2022). Dietary copper deficiency leads to changes in gene expression indicating an increased demand for NADH in the prefrontal cortex of the rat's brain. Int. J. Mol. Sci. 23 (12), 6706. doi:10.3390/ijms23126706
Chakraborty, K., Kar, S., Rai, B., Bhagat, R., Naskar, N., Seth, P., et al. (2022). Copper dependent ERK1/2 phosphorylation is essential for the viability of neurons and not glia. Metallomics. 14 (4), mfac005. doi:10.1093/mtomcs/mfac005
Chan, P. H., Kawase, M., Murakami, K., Chen, S. F., Li, Y., Calagui, B., et al. (1998). Overexpression of SOD1 in transgenic rats protects vulnerable neurons against ischemic damage after global cerebral ischemia and reperfusion. J. Neurosci. 18 (20), 8292–8299. doi:10.1523/JNEUROSCI.18-20-08292.1998
Chelyadina, N. S., Kapranov, S. V., Popov, M. A., Smirnova, L. L., and Bobko, N. I. (2023). The mussel Mytilus galloprovincialis (Crimea, Black Sea) as a source of essential trace elements in human nutrition. Biol. Trace Elem. Res. 201 (11), 5415–5430. doi:10.1007/s12011-023-03607-1
Chen, C. H., Chou, Y. T., Yang, Y. W., and Lo, K. Y. (2021). High-dose copper activates p53-independent apoptosis through the induction of nucleolar stress in human cell lines. Apoptosis 26 (11-12), 612–627. doi:10.1007/s10495-021-01692-y
Chen, G., Zhang, J., Teng, W., Luo, Y., and Ji, X. (2023a). FDX1 inhibits thyroid cancer malignant progression by inducing cuprotosis. Heliyon 9 (8), e18655. doi:10.1016/j.heliyon.2023.e18655
Chen, J., Jiang, Y., Shi, H., Peng, Y., Fan, X., and Li, C. (2020). The molecular mechanisms of copper metabolism and its roles in human diseases. Pflugers Arch. 472 (10), 1415–1429. doi:10.1007/s00424-020-02412-2
Chen, X., Cai, Q., Liang, R., Zhang, D., Liu, X., Zhang, M., et al. (2023b). Copper homeostasis and copper-induced cell death in the pathogenesis of cardiovascular disease and therapeutic strategies. Cell Death Dis. 14 (2), 105. doi:10.1038/s41419-023-05639-w
Chun, H., Catterton, T., Kim, H., Lee, J., and Kim, B. E. (2017). Organ-specific regulation of ATP7A abundance is coordinated with systemic copper homeostasis. Sci. Rep. 7 (1), 12001. doi:10.1038/s41598-017-11961-z
Cobine, P. A., Moore, S. A., and Leary, S. C. (2021). Getting out what you put in: copper in mitochondria and its impacts on human disease. Biochim. Biophys. Acta Mol. Cell Res. 1868 (1), 118867. doi:10.1016/j.bbamcr.2020.118867
Cunnane, S. C., Trushina, E., Morland, C., Prigione, A., Casadesus, G., Andrews, Z. B., et al. (2020). Brain energy rescue: an emerging therapeutic concept for neurodegenerative disorders of ageing. Nat. Rev. Drug Discov. 19 (9), 609–633. doi:10.1038/s41573-020-0072-x
Curnock, R., and Cullen, P. J. (2020). Mammalian copper homeostasis requires retromer-dependent recycling of the high-affinity copper transporter 1. J. Cell Sci. 133 (16), jcs249201. doi:10.1242/jcs.249201
D’Ambrosi, N., and Rossi, L. (2015). Copper at synapse: release, binding and modulation of neurotransmission. Neurochem. Int. 90, 36–45. doi:10.1016/j.neuint.2015.07.006
Dong, J., Wang, X., Xu, C., Gao, M., Wang, S., Zhang, J., et al. (2021). Inhibiting NLRP3 inflammasome activation prevents copper-induced neuropathology in a murine model of Wilson's disease. Cell Death Dis. 12 (1), 87. doi:10.1038/s41419-021-03397-1
Dreishpoon, M. B., Bick, N. R., Petrova, B., Warui, D. M., Cameron, A., Booker, S. J., et al. (2023b). FDX1 regulates cellular protein lipoylation through direct binding to LIAS. J. Biol. Chem. 299 (9), 105046. doi:10.1016/j.jbc.2023.105046
Dreishpoon, M. B., Bick, N. R., Petrova, B., Warui, D. M., Cameron, A., Booker, S. J., et al. (2023a). FDX1 regulates cellular protein lipoylation through direct binding to LIAS. J. Biol. Chem. 299 (9), 105046. doi:10.1016/j.jbc.2023.105046
Du, J., Huang, Z., Li, Y., Ren, X., Zhou, C., Liu, R., et al. (2023). Copper exerts cytotoxicity through inhibition of iron-sulfur cluster biogenesis on ISCA1/ISCA2/ISCU assembly proteins. Free Radic. Biol. Med. 204, 359–373. doi:10.1016/j.freeradbiomed.2023.05.017
Dyall, S. C., Nessel, I., Sharpe, J. A., Yip, P. K., Michael-Titus, A. T., and Shah, D. K. (2023). Long-chain omega-3 polyunsaturated fatty acids are reduced in neonates with substantial brain injury undergoing therapeutic hypothermia after hypoxic-ischemic encephalopathy. Front. Neurol. 14, 1231743. doi:10.3389/fneur.2023.1231743
Eljazzar, S., Abu-Hijleh, H., Alkhatib, D., Sokary, S., Ismail, S., Al-Jayyousi, G. F., et al. (2023). The role of copper intake in the development and management of type 2 diabetes: a systematic review. Nutrients 15 (7), 1655. doi:10.3390/nu15071655
Feng, W., Ye, F., Xue, W., Zhou, Z., and Kang, Y. J. (2009). Copper regulation of hypoxia-inducible factor-1 activity. Mol. Pharmacol. 75 (1), 174–182. doi:10.1124/mol.108.051516
Ferguson, G. D., and Bridge, W. J. (2019). The glutathione system and the related thiol network in Caenorhabditis elegans. Redox Biol. 24, 101171. doi:10.1016/j.redox.2019.101171
Fetherolf, M. M., Boyd, S. D., Taylor, A. B., Kim, H. J., Wohlschlegel, J. A., Blackburn, N. J., et al. (2017). Copper-zinc superoxide dismutase is activated through a sulfenic acid intermediate at a copper ion entry site. J. Biol. Chem. 292 (29), 12025–12040. doi:10.1074/jbc.M117.775981
Gaetke, L. M., and Chow, C. K. (2003). Copper toxicity, oxidative stress, and antioxidant nutrients. Toxicology 189 (1-2), 147–163. doi:10.1016/s0300-483x(03)00159-8
Gao, S., Zhou, M., and Tang, Z. (2024). The Tao of copper metabolism: from physiology to pathology. Curr. Med. Chem. 31 (35), 5805–5817. doi:10.2174/0929867331666230915162405
Garmann, D., Warnecke, A., Kalayda, G. V., Kratz, F., and Jaehde, U. (2008). Cellular accumulation and cytotoxicity of macromolecular platinum complexes in cisplatin-resistant tumor cells. J. Control. Release. 131 (2), 100–106. doi:10.1016/j.jconrel.2008.07.017
GBD 2019 Stroke Collaborators (2021). Global, regional, and national burden of stroke and its risk factors, 1990-2019: a systematic analysis for the Global Burden of Disease Study 2019. Lancet Neurol. 20 (10), 795–820. doi:10.1016/S1474-4422(21)00252-0
Gonzalez-Lopez, E., and Vrana, K. E. (2020). Dopamine beta-hydroxylase and its genetic variants in human health and disease. J. Neurochem. 152 (2), 157–181. doi:10.1111/jnc.14893
Górska, A., Markiewicz-Gospodarek, A., Markiewicz, R., Chilimoniuk, Z., Borowski, B., Trubalski, M., et al. (2023). Distribution of iron, copper, zinc and cadmium in glia, their influence on glial cells and relationship with neurodegenerative diseases. Brain Sci. 13 (6), 911. doi:10.3390/brainsci13060911
Goto, N., Hara, H., Kondo, M., Yasuda, N., Kamiya, T., Okuda, K., et al. (2020). Hydrogen sulfide increases copper-dependent neurotoxicity via intracellular copper accumulation. Metallomics. 12 (6), 868–875. doi:10.1039/d0mt00015a
Grechnikova, M., Ženíšková, K., Malych, R., Mach, J., and Sutak, R. (2020). Copper detoxification machinery of the brain-eating amoeba Naegleria fowleri involves copper-translocating ATPase and the antioxidant system. Int. J. Parasitol. Drugs Drug Resist 14, 126–135. doi:10.1016/j.ijpddr.2020.10.001
Guo, J., Cheng, J., Zheng, N., Zhang, X., Dai, X., Zhang, L., et al. (2021). Copper promotes tumorigenesis by activating the PDK1-AKT oncogenic pathway in a copper transporter 1 dependent manner. Adv. Sci. (Weinh) 8 (18), e2004303. doi:10.1002/advs.202004303
Guo, Q., Ma, M., Yu, H., Han, Y., and Zhang, D. (2023a). Dexmedetomidine enables copper homeostasis in cerebral ischemia/reperfusion via ferredoxin 1. Ann. Med. 55 (1), 2209735. doi:10.1080/07853890.2023.2209735
Guo, Q., Ma, M., Yu, H., Han, Y., and Zhang, D. (2023b). Dexmedetomidine enables copper homeostasis in cerebral ischemia/reperfusion via ferredoxin 1. Ann. Med. 55 (1), 2209735. doi:10.1080/07853890.2023.2209735
Gurnari, C., and Rogers, H. J. (2021). Copper deficiency. N. Engl. J. Med. 385 (7), 640. doi:10.1056/NEJMicm2103532
Guthrie, L. M., Soma, S., Yuan, S., Silva, A., Zulkifli, M., Snavely, T. C., et al. (2020). Elesclomol alleviates Menkes pathology and mortality by escorting Cu to cuproenzymes in mice. Science 368 (6491), 620–625. doi:10.1126/science.aaz8899
Hao, P., Li, H., Zhou, L., Sun, H., Han, J., and Zhang, Z. (2022). Serum metal ion-induced cross-linking of photoelectrochemical peptides and circulating proteins for evaluating cardiac ischemia/reperfusion. ACS Sens. 7 (3), 775–783. doi:10.1021/acssensors.1c02305
Haywood, S., and Vaillant, C. (2014). Overexpression of copper transporter CTR1 in the brain barrier of North Ronaldsay sheep: implications for the study of neurodegenerative disease. J. Comp. Pathol. 150 (2-3), 216–224. doi:10.1016/j.jcpa.2013.09.002
Herpich, F., and Rincon, F. (2020). Management of acute ischemic stroke. Crit. Care Med. 48 (11), 1654–1663. doi:10.1097/CCM.0000000000004597
Horn, N., and Wittung-Stafshede, P. (2021). ATP7A-Regulated enzyme metalation and trafficking in the Menkes disease puzzle. Biomedicines 9 (4), 391. doi:10.3390/biomedicines9040391
Horng, Y. C., Cobine, P. A., Maxfield, A. B., Carr, H. S., and Winge, D. R. (2004). Specific copper transfer from the Cox17 metallochaperone to both Sco1 and Cox11 in the assembly of yeast cytochrome C oxidase. J. Biol. Chem. 279 (34), 35334–35340. doi:10.1074/jbc.M404747200
Hu, Y., Zhang, D., Zhang, Q., Yin, T., Jiang, T., He, S., et al. (2023). Serum Cu, Zn and IL-1β levels may predict fetal miscarriage risk after IVF cycles: a nested case-control study. Biol. Trace Elem. Res. 201 (12), 5561–5574. doi:10.1007/s12011-023-03621-3
Huuskonen, M. T., Tuo, Q. Z., Loppi, S., Dhungana, H., Korhonen, P., McInnes, L. E., et al. (2017). The copper bis(thiosemicarbazone) complex CuII(atsm) Is protective against cerebral ischemia through modulation of the inflammatory milieu. Neurotherapeutics 14 (2), 519–532. doi:10.1007/s13311-016-0504-9
Janardhanan, P., Somasundaran, A. K., Balakrishnan, A. J., and Pilankatta, R. (2022). Sensitization of cancer cells towards Cisplatin and Carboplatin by protein kinase D inhibitors through modulation of ATP7A/B (copper transport ATPases). Cancer Treat. Res. Commun. 32, 100613. doi:10.1016/j.ctarc.2022.100613
Jiang, Y., Bai, X., Li, T. T., Al-Hawwas, M., Jin, Y., Zou, Y., et al. (2020). COX5A over-expression protects cortical neurons from hypoxic ischemic injury in neonatal rats associated with TPI up-regulation. BMC Neurosci. 21 (1), 18. doi:10.1186/s12868-020-00565-5
Joshi, P. R., Sadre, S., Guo, X. A., McCoy, J. G., and Mootha, V. K. (2023). Lipoylation is dependent on the ferredoxin FDX1 and dispensable under hypoxia in human cells. J. Biol. Chem. 299 (9), 105075. doi:10.1016/j.jbc.2023.105075
Juárez-Cedillo, T., Martínez-Rodríguez, N., Fragoso, J. M., Islas-Pérez, V., López-Martínez, A., and Valle-Medina, A. (2023). Multifactor dimensionality reduction analysis to evaluate the association of dopamine beta-hydroxylase (dβh) polymorphisms with susceptibility to dementia (SADEM study). Mol. Neurobiol. 60 (8), 4731–4737. doi:10.1007/s12035-023-03367-y
Kahlson, M. A., and Dixon, S. J. (2022). Copper-induced cell death. Science 375 (6586), 1231–1232. doi:10.1126/science.abo3959
Kawahara, M., Tanaka, K. I., and Kato-Negishi, M. (2021). Copper as a collaborative partner of zinc-induced neurotoxicity in the pathogenesis of vascular dementia. Int. J. Mol. Sci. 22 (14), 7242. doi:10.3390/ijms22147242
Ke, D., Zhang, Z., Liu, J., Chen, P., Li, J., Sun, X., et al. (2023). Ferroptosis, necroptosis and cuproptosis: novel forms of regulated cell death in diabetic cardiomyopathy. Front. Cardiovasc Med. 10, 1135723. doi:10.3389/fcvm.2023.1135723
Khalfaoui-Hassani, B., Trasnea, P. I., Steimle, S., Koch, H. G., and Daldal, F. (2021). Cysteine mutants of the major facilitator superfamily-type transporter CcoA provide insight into copper import. mBio 12 (4), e0156721. doi:10.1128/mBio.01567-21
Kirsipuu, T., Zadorožnaja, A., Smirnova, J., Friedemann, M., Plitz, T., Tõugu, V., et al. (2020). Copper (II)-binding equilibria in human blood. Sci. Rep. 10 (1), 5686. doi:10.1038/s41598-020-62560-4
Kucková, L., Jomová, K., Švorcová, A., Valko, M., Segľa, P., Moncoľ, J., et al. (2015). Synthesis, crystal structure, spectroscopic properties and potential biological activities of salicylate‒neocuproine ternary copper(II) complexes. Molecules 20 (2), 2115–2137. doi:10.3390/molecules20022115
Kumar, D., Mains, R. E., and Eipper, B. A. (2016). 60 Years of POMC: from POMC and α-MSH to PAM, molecular oxygen, copper, and vitamin C. J. Mol. Endocrinol. 56 (4), T63–T76. doi:10.1530/JME-15-0266
Lasorsa, A., Nardella, M. I., Rosato, A., Mirabelli, V., Caliandro, R., Caliandro, R., et al. (2019). Mechanistic and structural basis for inhibition of copper trafficking by platinum anticancer drugs. J. Am. Chem. Soc. 141 (30), 12109–12120. doi:10.1021/jacs.9b05550
Lee, E. J., Kolb, J., Hwee, D. T., Malik, F. I., and Granzier, H. L. (2019). Functional characterization of the intact diaphragm in a nebulin-based nemaline myopathy (NM) model-effects of the fast skeletal muscle troponin activator tirasemtiv. Int. J. Mol. Sci. 20 (20), 5008. doi:10.3390/ijms20205008
Lee, J., Peña, M. M., Nose, Y., and Thiele, D. J. (2002). Biochemical characterization of the human copper transporter Ctr1. J. Biol. Chem. 277 (6), 4380–4387. doi:10.1074/jbc.M104728200
Lenartowicz, M., Krzeptowski, W., Lipiński, P., Grzmil, P., Starzyński, R., Pierzchała, O., et al. (2015). Mottled mice and non-mammalian models of Menkes disease. Front. Mol. Neurosci. 8, 72. doi:10.3389/fnmol.2015.00072
Li, B., Yu, W., and Verkhratsky, A. (2024a). Trace metals and astrocytes physiology and pathophysiology. Cell Calcium 118, 102843. doi:10.1016/j.ceca.2024.102843
Li, C., Jia, W., Guo, Z., Kang, Y., Zhou, C., Zhao, R., et al. (2024b). A copper-platinum nanoplatform for synergistic photothermal and chemodynamic tumor therapy via ROS outburst and GSH exhaustion. J. Mater Chem. B 12 (3), 800–813. doi:10.1039/d3tb02288a
Li, S. R., Bu, L. L., and Cai, L. (2022). Cuproptosis: lipoylated TCA cycle proteins-mediated novel cell death pathway. Signal Transduct. Target Ther. 7 (1), 158. doi:10.1038/s41392-022-01014-x
Li, X., Dehghan, M., Tse, L. A., Lang, X., Rangarajan, S., Liu, W., et al. (2023a). Associations of dietary copper intake with cardiovascular disease and mortality: findings from the Chinese Perspective Urban and Rural Epidemiology (PURE-China) Study. BMC Public Health 23 (1), 2525. doi:10.1186/s12889-023-17441-6
Li, Y. (2020). Copper homeostasis: emerging target for cancer treatment. IUBMB Life 72, 1900–1908. doi:10.1002/iub.2341
Li, Y., Du, Y., Zhou, Y., Chen, Q., Luo, Z., Ren, Y., et al. (2023b). Iron and copper: critical executioners of ferroptosis, cuproptosis and other forms of cell death. Cell Commun. Signal 21 (1), 327. doi:10.1186/s12964-023-01267-1
Liang, Z. D., Long, Y., Chen, H. H., Savaraj, N., and Kuo, M. T. (2014). Regulation of the high-affinity copper transporter (hCtr1) expression by cisplatin and heavy metals. J. Biol. Inorg. Chem. 19 (1), 17–27. doi:10.1007/s00775-013-1051-z
Lim, S., Kim, T. J., Kim, Y. J., Kim, C., Ko, S. B., and Kim, B. S. (2021). Senolytic therapy for cerebral ischemia-reperfusion injury. Int. J. Mol. Sci. 22 (21), 11967. doi:10.3390/ijms222111967
Lin, C., Zhang, Z., Wang, T., Chen, C., and James Kang, Y. (2015). Copper uptake by DMT1: a compensatory mechanism for CTR1 deficiency in human umbilical vein endothelial cells. Metallomics 7 (8), 1285–1289. doi:10.1039/c5mt00097a
Lin, M. C., Liu, C. C., Liao, C. S., and Ro, J. H. (2021). Neuroprotective effect of Quercetin during cerebral ischemic injury involves regulation of essential elements, transition metals, Cu/Zn ratio, and antioxidant activity. Molecules 26 (20), 6128. doi:10.3390/molecules26206128
Lin, X., Shang, X., Manorek, G., Fofana, M., and Stephen, B. H. (2014). Integrin αV modulates the cellular pharmacology of copper and cisplatin by regulating expression of the influx transporter CTR1. Oncoscience 1 (3), 185–195. doi:10.18632/oncoscience.22
Litwin, T., Dzieżyc, K., and Członkowska, A. (2019). Wilson disease-treatment perspectives. Ann. Transl. Med. 7 (Suppl. 2), S68. doi:10.21037/atm.2018.12.09
Liu, C., and Liang, D. (2024). High copper intake is associated with decreased likelihood of abdominal aortic calcification in middle-aged and older US adults. Biol. Trace Elem. Res. 202 (4), 1390–1400. doi:10.1007/s12011-023-03765-2
Liu, L. L., van Rijn, R. M., and Zheng, W. (2022b). Copper modulates adult neurogenesis in brain subventricular zone. Int. J. Mol. Sci. 23 (17), 9888. doi:10.3390/ijms23179888
Liu, Z., Wang, M., Zhang, C., Zhou, S., and Ji, G. (2022a). Molecular functions of ceruloplasmin in metabolic disease pathology. Diabetes Metab. Syndr. Obes. 15, 695–711. doi:10.2147/DMSO.S346648
Ma, H. T., Zhang, H. C., Zuo, Z. F., and Liu, Y. X. (2023). Heterogeneous organization of Locus coeruleus: an intrinsic mechanism for functional complexity. Physiol. Behav. 268, 114231. doi:10.1016/j.physbeh.2023.114231
Maghool, S., Cooray, N. D. G., Stroud, D. A., Aragão, D., Ryan, M. T., and Maher, M. J. (2019). Structural and functional characterization of the mitochondrial complex IV assembly factor Coa6. Life Sci. Alliance 2 (5), e201900458. doi:10.26508/lsa.201900458
Magoufis, G., Safouris, A., Raphaeli, G., Kargiotis, O., Psychogios, K., Krogias, C., et al. (2021). Acute reperfusion therapies for acute ischemic stroke patients with unknown time of symptom onset or in extended time windows: an individualized approach. Ther. Adv. Neurol. Disord. 14, 17562864211021182. doi:10.1177/17562864211021182
Mandal, T., Kar, S., Maji, S., Sen, S., and Gupta, A. (2020). Structural and functional diversity among the members of CTR, the membrane copper transporter family. J. Membr. Biol. 253 (5), 459–468. doi:10.1007/s00232-020-00139-w
Margaret, B. D., Bick, N. R., Petrova, B., Douglas, M. W., Cameron, A., Booker, S. J., et al. (2023). FDX1 regulates cellular protein lipoylation through direct binding to LIAS. J. Biol. Chem. 299 (9), 105046. doi:10.1016/j.jbc.2023.105046
Martinat, M., Rossitto, M., Di Miceli, M., and Layé, S. (2021). Perinatal dietary polyunsaturated fatty acids in brain development, role in neurodevelopmental disorders. Nutrients 13 (4), 1185. doi:10.3390/nu13041185
Masaldan, S., Clatworthy, S. A. S., Gamell, C., Smith, Z. M., Francis, P. S., Denoyer, D., et al. (2018). Copper accumulation in senescent cells: interplay between copper transporters and impaired autophagy. Redox Biol. 16, 322–331. doi:10.1016/j.redox.2018.03.007
Mazi, T. A., Shibata, N. M., and Medici, V. (2020). Lipid and energy metabolism in Wilson disease. Liver Res. 4 (1), 5–14. doi:10.1016/j.livres.2020.02.002
McCann, C., Quinteros, M., Adelugba, I., Morgada, M. N., Castelblanco, A. R., Davis, E. J., et al. (2022a). The mitochondrial Cu+ transporter PiC2 (SLC25A3) is a target of MTF1 and contributes to the development of skeletal muscle in vitro. Front. Mol. Biosci. 9, 1037941. doi:10.3389/fmolb.2022.1037941
McCann, C. J., Hasan, N. M., Padilla-Benavides, T., Roy, S., and Lutsenko, S. (2022b). Heterogeneous nuclear ribonucleoprotein hnRNPA2/B1 regulates the abundance of the copper-transporter ATP7A in an isoform-dependent manner. Front. Mol. Biosci. 9, 1067490. doi:10.3389/fmolb.2022.1067490
McCann, C. J., Hasan, N. M., Padilla-Benavides, T., Roy, S., and Lutsenko, S. (2022c). Heterogeneous nuclear ribonucleoprotein hnRNPA2/B1 regulates the abundance of the copper-transporter ATP7A in an isoform-dependent manner. Front. Mol. Biosci. 9, 1067490. doi:10.3389/fmolb.2022.1067490
Merkler, D. J., Hawley, A. J., Eipper, B. A., and Mains, R. E. (2022). Peptidylglycine α-amidating monooxygenase as a therapeutic target or biomarker for human diseases. Br. J. Pharmacol. 179 (13), 3306–3324. doi:10.1111/bph.15815
Nardella, M. I., Fortino, M., Barbanente, A., Natile, G., Pietropaolo, A., and Arnesano, F. (2022). Multinuclear metal-binding ability of the N-terminal region of human copper transporter Ctr1: dependence upon pH and metal oxidation state. Front. Mol. Biosci. 9, 897621. doi:10.3389/fmolb.2022.897621
Natera-de Benito, D., Sola, A., Sousa, P. R., Boronat, S., Expósito-Escudero, J., Carrera-García, L., et al. (2021). Copper toxicity associated with an ATP7A-related complex phenotype. Pediatr. Neurol. 119, 40–44. doi:10.1016/j.pediatrneurol.2021.03.005
Ng, S. C. W., Furman, R., Axelsen, P. H., and Shchepinov, M. S. (2022). Free radical chain reactions and polyunsaturated fatty acids in brain lipids. ACS Omega 7 (29), 25337–25345. doi:10.1021/acsomega.2c02285
Nzuza, N., Padayachee, T., Chen, W., Gront, D., Nelson, D. R., and Syed, K. (2021). Diversification of ferredoxins across living organisms. Curr. Issues Mol. Biol. 43 (3), 1374–1390. doi:10.3390/cimb43030098
Ohkubo, N., Aoto, M., Kon, K., and Mitsuda, N. (2019). Lack of zinc finger protein 521 upregulates dopamine β-hydroxylase expression in the mouse brain, leading to abnormal behavior. Life Sci. 231, 116559. doi:10.1016/j.lfs.2019.116559
Pacheu-Grau, D., Wasilewski, M., Oeljeklaus, S., Gibhardt, C. S., Aich, A., Chudenkova, M., et al. (2020). COA6 facilitates cytochrome c oxidase biogenesis as thiol-reductase for copper metallochaperones in mitochondria. J. Mol. Biol. 432 (7), 2067–2079. doi:10.1016/j.jmb.2020.01.036
Park, Y. E., Noh, Y., Kim, D. W., Lee, T.-K., Ahn, J. H., Kim, B., et al. (2021). Experimental pretreatment with YES-10®, a plant extract rich in scutellarin and chlorogenic acid, protects hippocampal neurons from ischemia/reperfusion injury via antioxidant role. Exp. Ther. Med. 21 (3), 183. doi:10.3892/etm.2021.9614
Piacenza, F., Giacconi, R., Costarelli, L., Basso, A., Bürkle, A., Moreno-Villanueva, M., et al. (2021). Age, sex, and BMI influence on copper, zinc, and their major serum carrier proteins in a large European population including nonagenarian offspring from MARK-AGE study. J. Gerontol. A Biol. Sci. Med. Sci. 76 (12), 2097–2106. doi:10.1093/gerona/glab134
Porcu, C., Antonucci, L., Barbaro, B., Illi, B., Nasi, S., Martini, M., et al. (2018). Copper/MYC/CTR1 interplay: a dangerous relationship in hepatocellular carcinoma. Oncotarget 9 (10), 9325–9343. doi:10.18632/oncotarget.24282
Pourvali, K., Matak, P., Latunde-Dada, G. O., Solomou, S., Mastrogiannaki, M., Peyssonnaux, C., et al. (2012). Basal expression of copper transporter 1 in intestinal epithelial cells is regulated by hypoxia-inducible factor 2α. FEBS Lett. 586 (16), 2423–2427. doi:10.1016/j.febslet.2012.05.058
Prohaska, J. R., and Brokate, B. (2001). Dietary copper deficiency alters protein levels of rat dopamine beta-monooxygenase and tyrosine monooxygenase. Exp. Biol. Med. (Maywood) 226 (3), 199–207. doi:10.1177/153537020122600307
Raia, S., Conti, A., Zanardi, A., Ferrini, B., Scotti, G. M., Gilberti, E., et al. (2023). Ceruloplasmin-deficient mice show dysregulation of lipid metabolism in liver and adipose tissue reduced by a protein replacement. Int. J. Mol. Sci. 24 (2), 1150. doi:10.3390/ijms24021150
Ramzan, R., Napiwotzki, J., Weber, P., Kadenbach, B., and Vogt, S. (2021). Cholate disrupts regulatory functions of cytochrome c oxidase. Cells 10 (7), 1579. doi:10.3390/cells10071579
Rao, V. K. S., Eipper, B. A., and Mains, R. E. (2021). Multiple roles for peptidylglycine α-amidating monooxygenase in the response to hypoxia. J. Cell Physiol. 236 (11), 7745–7758. doi:10.1002/jcp.30457
Ren, F., Logeman, B. L., Zhang, X., Liu, Y., Thiele, D. J., and Yuan, P. (2019). X-ray structures of the high-affinity copper transporter Ctr1. Nat. Commun. 10 (1), 1386. doi:10.1038/s41467-019-09376-7
Reziya, H., Sayifujiamali, K., Han, H. J., Wang, X. M., Nuerbiya, T., Nuerdong, D., et al. (2023). Real-time feedback on mobile application use for emergency management affects the door-to-needle time and functional outcomes in acute ischemic stroke. J. Stroke Cerebrovasc. Dis. 32 (4), 107055. doi:10.1016/j.jstrokecerebrovasdis.2023.107055
Rihel, J. (2018). Copper on the brain. Nat. Chem. Biol. 14 (7), 638–639. doi:10.1038/s41589-018-0089-1
Ruiz, L. M., Libedinsky, A., and Elorza, A. A. (2021). Role of copper on mitochondrial function and metabolism. Front. Mol. Biosci. 8, 711227. doi:10.3389/fmolb.2021.711227
Ruttkay-Nedecky, B., Nejdl, L., Gumulec, J., Zitka, O., Masarik, M., Eckschlager, T., et al. (2013). The role of metallothionein in oxidative stress. Int. J. Mol. Sci. 14 (3), 6044–6066. doi:10.3390/ijms14036044
Ryan, F., Zarruk, J. G., Lößlein, L., and David, S. (2019). Ceruloplasmin plays a neuroprotective role in cerebral ischemia. Front. Neurosci. 12, 988. doi:10.3389/fnins.2018.00988
Samygina, V. R., Sokolov, A. V., Bourenkov, G., Schneider, T. R., Anashkin, V. A., Kozlov, S. O., et al. (2017). Rat ceruloplasmin: a new labile copper binding site and zinc/copper mosaic. Metallomics 9 (12), 1828–1838. doi:10.1039/c7mt00157f
Sánchez-González, C., Moreno, L., Aranda, P., Montes-Bayón, M., Llopis, J., and Rivas-García, L. (2022). Effect of bis (maltolato) oxovanadium (IV) on zinc, copper, and manganese homeostasis and DMT1 mRNA expression in streptozotocin-induced hyperglycemic rats. Biol. (Basel). 11 (6), 814. doi:10.3390/biology11060814
Sanghai, N., and Tranmer, G. K. (2021). Hydrogen peroxide and amyotrophic lateral sclerosis: from biochemistry to pathophysiology. Antioxidants (Basel) 11 (1), 52. doi:10.3390/antiox11010052
Scheiber, I. F., Mercer, J. F., and Dringen, R. (2014). Metabolism and functions of copper in brain. Prog. Neurobiol. 116, 33–57. doi:10.1016/j.pneurobio.2014.01.002
Schilsky, M. L., Roberts, E. A., Bronstein, J. M., Dhawan, A., Hamilton, J. P., Rivard, A. M., et al. (2023). A multidisciplinary approach to the diagnosis and management of Wilson disease: executive summary of the 2022 practice guidance on Wilson disease from the American association for the study of liver diseases. Hepatology 77 (4), 1428–1455. doi:10.1002/hep.32805
Schoeberl, A., Gutmann, M., Theiner, S., Corte-Rodríguez, M., Braun, G., Vician, P., et al. (2022a). The copper transporter CTR1 and cisplatin accumulation at the single-cell level by LA-ICP-TOFMS. Front. Mol. Biosci. 9, 1055356. doi:10.3389/fmolb.2022.1055356
Schoeberl, A., Gutmann, M., Theiner, S., Corte-Rodríguez, M., Braun, G., Vician, P., et al. (2022b). The copper transporter CTR1 and cisplatin accumulation at the single-cell level by LA-ICP-TOFMS. Front. Mol. Biosci. 9, 1055356. doi:10.3389/fmolb.2022.1055356
Schulz, V., Basu, S., Freibert, S. A., Webert, H., Boss, L., Mühlenhoff, U., et al. (2023). Functional spectrum and specificity of mitochondrial ferredoxins FDX1 and FDX2. Nat. Chem. Biol. 19 (2), 206–217. doi:10.1038/s41589-022-01159-4
Shang, J., Jiao, J., Yan, M., Wang, J., Li, Q., Shabuerjiang, L., et al. (2023). Chrysin protects against cerebral ischemia-reperfusion injury in hippocampus via restraining oxidative stress and transition elements. Biomed. Pharmacother. 161, 114534. doi:10.1016/j.biopha.2023.114534
Shawki, A., Anthony, S. R., Nose, Y., Engevik, M. A., Niespodzany, E. J., Barrientos, T., et al. (2015). Intestinal DMT1 is critical for iron absorption in the mouse but is not required for the absorption of copper or manganese. Am. J. Physiol. Gastrointest. Liver Physiol. 309 (8), G635–G647. doi:10.1152/ajpgi.00160.2015
Shi, W., Zhang, H., Zhang, Y., Lu, L., Zhou, Q., Wang, Y., et al. (2023). Co-exposure to Fe, Zn, and Cu induced neuronal ferroptosis with associated lipid metabolism disorder via the ERK/cPLA2/AA pathway. Environ. Pollut. 336, 122438. doi:10.1016/j.envpol.2023.122438
Singla, A., Chen, Q., Suzuki, K., Song, J., Fedoseienko, A., Wijers, M., et al. (2021). Regulation of murine copper homeostasis by members of the COMMD protein family. Dis. Model Mech. 14 (1), dmm045963. doi:10.1242/dmm.045963
Slezak, J., Kura, B., LeBaron, T. W., Singal, P. K., Buday, J., and Barancik, M. (2021). Oxidative stress and pathways of molecular hydrogen effects in medicine. Curr. Pharm. Des. 27 (5), 610–625. doi:10.2174/1381612826666200821114016
Song, G., Dong, H., Ma, D., Wang, H., Ren, X., Qu, Y., et al. (2021). Tetrahedral framework nucleic acid delivered RNA therapeutics significantly attenuate pancreatic cancer progression via inhibition of CTR1-dependent copper absorption. ACS Appl. Mater Interfaces 13 (39), 46334–46342. doi:10.1021/acsami.1c13091
Strenkert, D., Schmollinger, S., Paruthiyil, S., Brown, B. C., Green, S., Shafer, C. M., et al. (2024). Distinct function of Chlamydomonas CTRA-CTR transporters in Cu assimilation and intracellular mobilization. Metallomics 16 (3), mfae013. doi:10.1093/mtomcs/mfae013
Swaminathan, A. B., and Gohil, V. M. (2022). The role of COA6 in the mitochondrial copper delivery pathway to cytochrome c oxidase. Biomolecules 12 (1), 125. doi:10.3390/biom12010125
Tadini-Buoninsegni, F., and Smeazzetto, S. (2017). Mechanisms of charge transfer in human copper ATPases ATP7A and ATP7B. IUBMB Life 69 (4), 218–225. doi:10.1002/iub.1603
Tapiero, H., Townsend, D. M., and Tew, K. D. (2003). Trace elements in human physiology and pathology. Copper. Copp. Biomed. Pharmacother. 57 (9), 386–398. doi:10.1016/s0753-3322(03)00012-x
Trist, B. G., Hilton, J. B., Hare, D. J., Crouch, P. J., and Double, K. L. (2021). Superoxide dismutase 1 in health and disease: how a frontline antioxidant becomes neurotoxic. Angew. Chem. Int. Ed. Engl. 60 (17), 9215–9246. doi:10.1002/anie.202000451
Tsai, C. Y., Finley, J. C., Ali, S. S., Patel, H. H., and Howell, S. B. (2012). Copper influx transporter 1 is required for FGF, PDGF and EGF-induced MAPK signaling. Biochem. Pharmacol. 84 (8), 1007–1013. doi:10.1016/j.bcp.2012.07.014
Tsang, T., Davis, C. I., and Brady, D. C. (2021). Copper biology. Curr. Biol. 31 (9), R421–R427. doi:10.1016/j.cub.2021.03.054
Tsvetkov, P., Coy, S., Petrova, B., Dreishpoon, M., Verma, A., Abdusamad, M., et al. (2022). Copper induces cell death by targeting lipoylated TCA cycle proteins. Science 375 (6586), 1254–1261. doi:10.1126/science.abf0529
Tury, S., Chauveau, L., Lecante, A., Courgnaud, V., and Battini, J. L. (2023). A co-opted endogenous retroviral envelope promotes cell survival by controlling CTR1-mediated copper transport and homeostasis. Cell Rep. 42 (9), 113065. doi:10.1016/j.celrep.2023.113065
Ulrich, K., and Jakob, U. (2019). The role of thiols in antioxidant systems. Free Radic. Biol. Med. 140, 14–27. doi:10.1016/j.freeradbiomed.2019.05.035
Valko, M., Morris, H., and Cronin, M. T. (2005). Metals, toxicity and oxidative stress. Curr. Med. Chem. 12 (10), 1161–1208. doi:10.2174/0929867053764635
Vasilyev, V. B. (2019). Looking for a partner: ceruloplasmin in protein-protein interactions. Biometals 32 (2), 195–210. doi:10.1007/s10534-019-00189-1
Vendelboe, T. V., Harris, P., Zhao, Y., Walter, T. S., Harlos, K., El Omari, K., et al. (2016). The crystal structure of human dopamine β-hydroxylase at 2.9 Å resolution. Sci. Adv. 2 (4), e1500980. doi:10.1126/sciadv.1500980
Vercellino, I., and Sazanov, L. A. (2022). The assembly, regulation and function of the mitochondrial respiratory chain. Nat. Rev. Mol. Cell Biol. 23 (2), 141–161. doi:10.1038/s41580-021-00415-0
Wang, D., Tian, Z., Zhang, P., Zhen, L., Meng, Q., Sun, B., et al. (2023b). The molecular mechanisms of cuproptosis and its relevance to cardiovascular disease. Biomed. Pharmacother. 163, 114830. doi:10.1016/j.biopha.2023.114830
Wang, L., Yin, Y. L., Liu, X. Z., Shen, P., Zheng, Y. G., Lan, X. R., et al. (2020). Current understanding of metal ions in the pathogenesis of Alzheimer's disease. Transl. Neurodegener. 9, 10. doi:10.1186/s40035-020-00189-z
Wang, X., Lou, Q., Fan, T., Zhang, Q., Yang, X., Liu, H., et al. (2023c). Copper transporter Ctr1 contributes to enhancement of the sensitivity of cisplatin in esophageal squamous cell carcinoma. Transl. Oncol. 29, 101626. doi:10.1016/j.tranon.2023.101626
Wang, Z., Jin, D., Zhou, S., Dong, N., Ji, Y., An, P., et al. (2023a). Regulatory roles of copper metabolism and cuproptosis in human cancers. Front. Oncol. 13, 1123420. doi:10.3389/fonc.2023.1123420
Washington-Hughes, C. L., Roy, S., Seneviratne, H. K., Karuppagounder, S. S., Morel, Y., Jones, J. W., et al. (2023). Atp7b-dependent choroid plexus dysfunction causes transient copper deficit and metabolic changes in the developing mouse brain. PLoS Genet. 19 (1), e1010558. doi:10.1371/journal.pgen.1010558
Watson, S. A., and McStay, G. P. (2020). Functions of cytochrome c oxidase assembly factors. Int. J. Mol. Sci. 21 (19), 7254. doi:10.3390/ijms21197254
Weiler, B. D., Brück, M. C., Kothe, I., Bill, E., Lill, R., and Mühlenhoff, U. (2020). Mitochondrial [4Fe-4S] protein assembly involves reductive [2Fe-2S] cluster fusion on ISCA1-ISCA2 by electron flow from ferredoxin FDX2. Proc. Natl. Acad. Sci. U. S. A. 117 (34), 20555–20565. doi:10.1073/pnas.2003982117
Wen, M. H., Xie, X., Huang, P. S., Yang, K., and Chen, T. Y. (2021). Crossroads between membrane trafficking machinery and copper homeostasis in the nerve system. Open Biol. 11 (12), 210128. doi:10.1098/rsob.210128
White, C., Kambe, T., Fulcher, Y. G., Sachdev, S. W., Bush, A. I., Fritsche, K., et al. (2009). Copper transport into the secretory pathway is regulated by oxygen in macrophages. J. Cell Sci. 122 (Pt 9), 1315–1321. doi:10.1242/jcs.043216
Wu, Y., Liu, C., and Dong, W. (2023). Adjunctive therapeutic effects of micronutrient supplementation in inflammatory bowel disease. Front. Immunol. 14, 1143123. doi:10.3389/fimmu.2023.1143123
Xiyang, Y. B., Liu, R., Wang, X. Y., Li, S., Zhao, Y., Lu, B. T., et al. (2020). COX5A plays a vital role in memory impairment associated with brain aging via the BDNF/ERK1/2 signaling pathway. Front. Aging Neurosci. 12, 215. doi:10.3389/fnagi.2020.00215
Xu, W. Q., Wang, R. M., Dong, Y., and Wu, Z. Y. (2022). Emerging neurological symptoms after liver transplantation: a 6-year follow-up of an adolescent patient with Wilson's disease. CNS Neurosci. Ther. 28 (5), 788–791. doi:10.1111/cns.13798
Yang, S., Li, Y., Zhou, L., Wang, X., Liu, L., and Wu, M. (2024). Copper homeostasis and cuproptosis in atherosclerosis: metabolism, mechanisms and potential therapeutic strategies. Cell Death Discov. 10 (1), 25. doi:10.1038/s41420-023-01796-1
Yang, S., Wu, P., Xiao, J., and Jiang, L. (2019). Overexpression of COX6B1 protects against I/R-induced neuronal injury in rat hippocampal neurons. Mol. Med. Rep. 19 (6), 4852–4862. doi:10.3892/mmr.2019.10144
Yong, L., Shi, Y., Wu, H. L., Dong, Q. Y., Guo, J., Hu, L. S., et al. (2023). p53 inhibits CTR1-mediated cisplatin absorption by suppressing SP1 nuclear translocation in osteosarcoma. Front. Oncol. 12, 1047194. doi:10.3389/fonc.2022.1047194
You, S. Y., Rui, W., Chen, S. T., Chen, H. C., Liu, X. W., Huang, J., et al. (2019). Process of immunogenic cell death caused by disulfiram as the anti-colorectal cancer candidate. Biochem. Biophys. Res. Commun. 513 (4), 891–897. doi:10.1016/j.bbrc.2019.03.192
Yu, Y., Wu, Q., Niu, M., Gou, L., Tan, L., Fu, C., et al. (2022). A core-shell liquid metal-Cu nanoparticle with glutathione consumption via an in-situ replacement strategy for tumor combination treatment of chemodynamic, microwave dynamic and microwave thermal therapy. Biomater. Sci. 10 (13), 3503–3513. doi:10.1039/d2bm00435f
Zhang, M., Li, W., Wang, Y., Wang, T., Ma, M., and Tian, C. (2020). Association between the change of serum copper and ischemic stroke: a systematic review and meta-analysis. J. Mol. Neurosci. 70 (3), 475–480. doi:10.1007/s12031-019-01441-6
Zhang, P., Li, B., Chen, Q., Wang, H., and Feng, Q. (2022). Glucose restriction induces ROS-AMPK-mediated CTR1 expression and increases cisplatin efficiency in NSCLC. Cancer Lett. 543, 215793. doi:10.1016/j.canlet.2022.215793
Zhang, P., Zhou, C., Ren, X., Jing, Q., Gao, Y., Yang, C., et al. (2024). Inhibiting the compensatory elevation of xCT collaborates with disulfiram/copper-induced GSH consumption for cascade ferroptosis and cuproptosis. Redox Biol. 69, 103007. doi:10.1016/j.redox.2023.103007
Zhang, W., He, Y., Kang, X., Wang, C., Chen, F., Kang, Z., et al. (2023a). Association between dietary minerals and glioma: a case-control study based on Chinese population. Front. Nutr. 10, 1118997. doi:10.3389/fnut.2023.1118997
Zhang, X., Jiang, Q., Su, Y., Bu, L., Sun, Z., Wu, X., et al. (2023c). AMPK phosphorylates and stabilises copper transporter 1 to synergise metformin and copper chelator for breast cancer therapy. Br. J. Cancer 128 (8), 1452–1465. doi:10.1038/s41416-022-02127-4
Zhang, Y., Zhou, Q., Lu, L., Su, Y., Shi, W., Zhang, H., et al. (2023b). Copper induces cognitive impairment in mice via modulation of cuproptosis and CREB signaling. Nutrients 15 (4), 972. doi:10.3390/nu15040972
Zhou, C., Yang, J., Liu, T., Jia, R., Yang, L., Sun, P., et al. (2023). Copper metabolism and hepatocellular carcinoma: current insights. Front. Oncol. 13, 1186659. doi:10.3389/fonc.2023.1186659
Keywords: copper ions, cerebral ischemia/reperfusion injury, cuproptosis, apoptosis, oxidative stress
Citation: Peng G, Huang Y, Xie G and Tang J (2024) Exploring Copper’s role in stroke: progress and treatment approaches. Front. Pharmacol. 15:1409317. doi: 10.3389/fphar.2024.1409317
Received: 29 March 2024; Accepted: 16 September 2024;
Published: 26 September 2024.
Edited by:
Viola B. Morris, Emory University, United StatesReviewed by:
François Canonne-Hergaux, Institut National de la Santé et de la Recherche Médicale (INSERM), FranceZhong-Bao Yang, The affiliated Changsha hospital of hunan normal university, China
Chong Song, Changsha Social Work College, China
Anna Vasilaki, University of Thessaly, Greece
Copyright © 2024 Peng, Huang, Xie and Tang. This is an open-access article distributed under the terms of the Creative Commons Attribution License (CC BY). The use, distribution or reproduction in other forums is permitted, provided the original author(s) and the copyright owner(s) are credited and that the original publication in this journal is cited, in accordance with accepted academic practice. No use, distribution or reproduction is permitted which does not comply with these terms.
*Correspondence: Yongpan Huang, eW9uZ3Bhbmh1YW5neXh5QDE2My5jb20=; Jiayu Tang, dGFuZ2ppYXl1MTk3OEAxNjMuY29t