- 1College of Life Science and Technology, Jinan University, Guangzhou, China
- 2Guangzhou ZhiYi Biotechnology Co. Ltd., Guangzhou, China
Bacteroides fragilis (B. fragilis) is a Gram-negative, obligate anaerobic, commensal bacterium residing in the human gut and holds therapeutic potential for ulcerative colitis (UC). Previous studies have indicated that capsular polysaccharide A (PSA) of B. fragilis is a crucial component for its effectiveness, possessing various biological activities such as anti-inflammatory, anti-tumor, and immune-modulating effects. We previously isolated and characterized the B. fragilis strain ZY-312 from the feces of a healthy breastfed infant, and extracted its PSA, named TP2. In this study, we explored the impact of TP2 on colonic inflammation and delved into its potential mechanisms. Initially, we used 2,4,6-trinitrobenzenesulfonic acid (TNBS) to induce colitis in rats and found that TP2 treatment significantly ameliorated TNBS-induced weight loss, increased clinical scores, extensive ulcers, and intestinal epithelial damage in UC rats. Further analysis revealed that TP2 effectively restored the intestinal barrier integrity in UC rats by regulating the expression of Muc-2, tight junction proteins (ZO-1, occludin, claudin-1, and claudin-2), as well as apoptosis-related proteins Bcl-2, BAX, and Cleaved-Caspase-3. Additionally, TP2 suppressed the expression of pro-inflammatory cytokines TNF-α, IL-1β, IL-6, and IL23, while promoting the secretion of anti-inflammatory cytokines IL-10 and IL-22, thereby inhibiting the occurrence of inflammation. TP2 also downregulated the phosphorylation levels of AKT and PI3K, effectively inhibiting the abnormal activation of the PI3K/AKT signaling pathway. More interestingly, 16S rRNA sequencing results showed that TP2 restored the ecological imbalance of the rat intestinal microbiota, with an increase in beneficial bacteria such as Lactobacillus and Limosilactobacillus observed in the treatment group. In conclusion, TP2 through the regulation of intestinal barrier-related cells and proteins, inhibition of apoptosis, modulation of inflammation-related cytokine levels, and control of abnormal activation of the PI3K/AKT signaling pathway, restores intestinal barrier integrity. Additionally, by reshaping the ecological imbalance of the gut microbiota, TP2 ultimately alleviates ulcerative colitis in rats.
1 Introduction
Ulcerative colitis (UC) is a type of inflammatory bowel disease (IBD), characterized by chronic and recurrent inflammation and ulceration of the colon and rectum walls, often associated with a certain proportion of colorectal cancer (Gros and Kaplan, 2023). The pathogenesis of UC is not fully elucidated, but increasing evidence suggests that the interaction between intestinal microbiota, mucosal barrier, and inflammatory responses plays a crucial role in the course of UC (Yan et al., 2019). Dysbiosis of the intestinal microbiota leads to rapid growth of harmful bacteria, invading the intestinal epithelial cells and causing damage to the intestinal mucosal barrier (Shen et al., 2018). This intestinal barrier damage results in the invasion of pathogens and antigens, triggering abnormal activation of the PI3K/AKT signaling pathway and inducing the upregulation of a series of pro-inflammatory factors such as TNF-α, IL-1β, IL-6, and IL-23 (Jiang et al., 2019; Peng et al., 2021; Zheng et al., 2022). The excessive expression of these pro-inflammatory cytokines promotes the infiltration of macrophages and neutrophils into the mucosa, exacerbating the inflammatory response and causing further damage to the intestinal mucosa (Neurath, 2014). Therefore, promoting intestinal barrier function, reshaping dysbiosis of the intestinal microbiota, and inhibiting the abnormal activation of the PI3K/AKT signaling pathway are important strategies for treating UC. Currently, the mainstream therapeutic drugs for UC include 5-aminosalicylic acid, glucocorticoids, immunosuppressants, and monoclonal antibodies. However, these drugs primarily offer symptomatic relief and fall short in providing long-term alleviation from intestinal inflammation in UC patients, often accompanied by various side effects. For instance, 5-aminosalicylic acid may lead to allergies, nausea, vomiting, etc. (Akobeng et al., 2016); glucocorticoids are prone to induce metabolic disorders and osteoporosis, weakening the immune system (Ford et al., 2011); antibody drugs often trigger immune responses in the body, leading to the production of neutralizing antibodies, rendering them ineffective (Baert et al., 2003). Therefore, there is an urgent need to develop new, safe, and effective drugs for the treatment of UC.
Bacteroides fragilis is a Gram-negative, rod-shaped, obligately anaerobic bacterium that acts as a commensal resident within the human colon, playing a role in maintaining the host’s health. Previously, we isolated a non-toxic strain of Bacteroides fragilis, ZY-312, from the feces of a healthy infant (Deng et al., 2016; Wang et al., 2017; Xu et al., 2018). This strain has shown therapeutic potential for various intestinal diseases, including antibiotic-associated diarrhea, Clostridium difficile infection, and ulcerative colitis (Li et al., 2017; Zhang et al., 2018; Deng et al., 2019; Zhou et al., 2022; Zhang et al., 2023). A substantial portion of the B. fragilis genome is dedicated to the synthesis of capsular polysaccharides, and the biological activity of B. fragilis is closely related to its highly complex and dynamic capsular structure (Comstock and Kasper, 2006; Eribo et al., 2022). The capsular polysaccharide of B. fragilis is the first recognized symbiotic factor that regulates the development of the host immune system, reversing functional defects in germ-free animals (Erturk-Hasdemir and Kasper, 2018). Studies by Mazmanian et al. demonstrated that capsular polysaccharides are crucial for B. fragilis to repair colitis. Mice colonized with wild-type B. fragilis or orally gavaged with PSA could avoid Helicobacter pylori-induced colitis, while mice colonized with a mutant strain that does not produce PSA could not escape the development of colitis (Mazmanian et al., 2008). Furthermore, PSA has been proven effective in treating various conditions such as hepatitis (Pagliuca et al., 2016), meningitis (Wang et al., 2014), melanoma (Vétizou et al., 2015), colorectal cancer (Sittipo et al., 2018), pneumonia (Johnson et al., 2018), and asthma (Johnson et al., 2015). In summary, PSA plays a vital role in maintaining host health and exhibits strong pharmaceutical potential.
We isolated and purified PSA from ZY-312 with an average relative molecular mass of 70 kDa, and named it TP2. Our previous research preliminarily confirmed that TP2 has therapeutic efficacy for UC similar to PSA reported in other literature, and it was observed that TP2 exerts its anti-inflammatory effects in vivo in an undegraded form (Zheng et al., 2020). However, the specific mechanism of how TP2 alleviates UC in vivo in its undegraded form is still not fully understood. This study aims to further validate the anti-inflammatory effects of TP2 in TNBS-induced rats colitis model and explore its potential anti-inflammatory mechanisms. The research focuses on assessing the impact of TP2 on intestinal barrier function and the intestinal microbiota ecosystem. Experimental results indicate that TP2 significantly alleviates TNBS-induced colitis in rats. Its mechanism of action includes the restoration of intestinal barrier function, inhibition of cell apoptosis, modulation of the expression of inflammatory factors, reshaping the intestinal microbiota, and inhibition of abnormal activation of the PI3K/AKT signaling pathway. This study provides a potential new therapy for treating UC and lays the foundation for further research into the anti-inflammatory mechanisms of TP2.
2 Materials and methods
2.1 Chemicals and reagents
Capsular polysaccharide TP2 was provided by Guangzhou Zhiyi Biotechnology Co., Ltd. (Guangzhou, China), with a total polysaccharide content exceeding 98%, protein content below 1%, and nucleic acid content less than 0.5%. Following preparation, it is stored in a freezer at −20°C. 2, 4, 6-trinitro-Benzenesulfonicacid (TNBS) was purchased from Sigma-Aldrich (St. Louis, United States), and prednisolone was obtained from SinePharm (Shanghai, China). Antibodies for Claudin-1 and Muc-2 were purchased from Abcam Biotechnology (Cambridge, United Kingdom), Claudin-1, PI3K, AKT, and GAPDH from Proteintech (Chicago, IL, United States), p-PI3K(Tyr458) and p-AKT (Ser473) from CST (Danvers, MA, United States), ZO-1 and Claudin-2 from Affinity Biosciences (Jiangsu, China), and Cleaved-Caspase-3, Bcl-2, BAX from Biodragon (Beijing, China). TNF-α, INF-γ, IL6, IL10, IL-17A, IL22 enzyme-linked immunosorbent assay (ELISA) kits were purchased from MultiSciences (Lianke) Biotechnology (Hangzhou, China), and the IL23 kit was obtained from JianglaiBiology (Shanghai, China). RIPA Lysis Buffer and Enhanced BCA Protein Assay Kit were purchased from Beyotime (Shanghai, China). All other reagents used in the experiments were of analytical grade.
2.2 Animals
Male Wistar rats (120 g ± 10 g) were procured from Guangzhou Ruige Biological Technology Co. Ltd (Guangzhou, China) with license number SCXK (Yue) 2023–0,059. The rats were accommodated in the animal facility of Guangzhou Ruige Biological Technology Co. Ltd., at a temperature of 21°C–24°C with a 12 h light/dark cycle. The animals were housed in IVC cages, with five rats per cage, and provided with unrestricted access to water from water bottles, along with ad libitum access to food. All animals were adapted to the new surroundings for at least 5 days before use in experiments. The animal use protocol listed below has been reviewed and approved by the Institutional Animal Care and Use Committee (IACUC) of Ruige Biotechnology (Number: 20,230,701–001).
2.3 Establishment of the UC model and TP2 treatment
A total of 60 male Wistar rats, six-week-old, were used in this study and were randomly allocated into the following groups: normal control group (NC), model control group (MC), 9 mg/kg prednisolone group (PD), 1 mg/kg TP2 group (LTP2), 2 mg/kg TP2 group (MTP2), and 4 mg/kg TP2 group (HTP2), each comprising 10 rats. To establish a colitis rat model, TNBS powder dissolved in 30% ethanol was used at a concentration of 60 mg/mL. This concentration has been shown to effectively induce colitis in previous studies (Motavallian-Naeini et al., 2012). Rats were fasted for 40 h before the experiment, during which they received subcutaneous injections of 5% glucose saline (10 mL/kg, once a day). On the first day of the experiment, fasting rats were anesthetized with intraperitoneal injection of 1% pentobarbital sodium at a dose of 40 mg/kg. The model control group, the prednisolone group, and the TP2 administration group used a disposable rectal administration tube inserted into the left colic flexure (approximately 8 cm from the anus) to induce colitis with TNBS enema (0.5 mL/piece). The normal control group received 0.5 mL of normal saline. To prevent enema fluid reflux, the animals’ heads were lowered for 15 min after enema, and they were kept in the Trendelenburg position until they woke up. On the first day post-modeling, the TP2 administration group and the prednisolone group received their respective drugs, while the blank group and the model group were instilled with the same volume of purified water for 7 days. Daily records were maintained for animal weights, and fecal status was scored. On day 8, animals were euthanized by cervical dislocation, and the colon was swiftly removed and washed with 0.9% saline. Measurements included the ulceration area, weight, and colon length (from the ileocecal junction to the anal margin) to calculate the weight/length (W/L) ratio.
2.4 Evaluating disease activity index (DAI) and scoring for the colon
DAI score is the important indicator to assess the pathological degree of colitis. According to Ho Pan Sham et al.’ report (Sham et al., 2018), the rat DAI scores were evaluated though the following formula: DAI = (weight loss percentage score + stool score + rectal bleed score)/3 (Table 1).
2.5 HE and PAS staining and analysis
To evaluate histopathological changes in the colon, both hematoxylin and eosin (HE) staining and Periodic Acid-Schiff (PAS) staining were conducted. Rat small intestinal tissue was fixed in 4% paraformaldehyde for 24 h, embedded in paraffin, and cut into 4 μm-thick slices for HE staining. Colonic mucosal damage was observed under a light microscope, and histopathological evaluation was based on the severity of inflammation, crypt loss, and mucosal damage, as outlined in Table 2 (Rahmani et al., 2020). PAS staining was performed following the manufacturer’s instructions to assess the structure of colonic goblet cells.
2.6 Western blot analyses
The colon tissues were cut into small pieces and lysed in ice-cold RIPA lysis buffer with a protease and phosphatase inhibitor cocktail. Subsequently, The tissue was lysed by an ultrasonic cell disruption apparatus. The protein concentration was determined using a BCA kit and then heated with loading buffer. Protein samples were separated by 10% gel electrophoresis and transferred to PVDF membranes. After blocking with 5% skim milk for 1 h at room temperature, the membranes were washed with Tris-Buffered Saline with Tween 20 (TBST) three times and then incubated with primary antibodies (1:1,000) overnight at 4°C refrigerator. After incubation with secondary antibodies (1:2000) for 1 h at room temperature, the membranes were washed with TBST three times, and the proteins were detected by ECL using ChemiDoc Imaging System (Biorad). GAPDH was used as an internal reference.
2.7 Measurement of cytokine level in the colon by ELISA
Cytokine concentrations in colon tissue were quantified using ELISA. Colon tissue was homogenized in a PBS solution, centrifuged at 3,500 rpm for 20 min at 4°C, and the supernatant was collected and stored at −80°C for further analysis. Levels of cytokines (IL-1β, IL-6, IL-10, IL-17A, IL-22, IL-23, TNF-α, IFN-γ) were determined using ELISA kits following the manufacturer’s protocol.
2.8 Stool DNA extraction and 16s RNA pyrosequencing
The Rat feces were collected, and total DNA was extracted using the QIAmp DNA microbiome kit (Qiagen). The 16S rRNA gene (V3-V4 region) was sequenced using the Illumina MiSeq system. The effective tags were clustered into operational taxonomic units (OTUs) with a similarity of ≥97% using Usearch software (version 11.0.667). Chimeric sequences and singleton OTUs (with only one read) were removed, after which the remaining sequences were sorted into each sample based on the OTUs. The tag sequence with the highest abundance was selected as a representative sequence within each cluster. Bacterial and fungal OTU representative sequences were classified taxonomically by blasting against the RDP Database and UNITE fungal ITS Database, respectively. All alpha diversity indices (including Chao1,Simpson, and Shannon indices) was calculated with Mothur software (version 1.30) and beta diversity were analyzed by R vegan package (version 2.5–6). Difference comparison was used to identify features with significantly different abundances between groups using LefSe (version 1.1.0). PICRUSt (v1.1.4) was utilized to predict functional profiles based on Kyoto Encyclopedia of Genes and Genomes (KEGG).
2.9 Statistical analysis
All data are presented as mean ± SEM. Statistical data analysis and graph creation were performed using GraphPad Prism10 software (Ver. 8.2.0). For each figure, one-way ANOVA was used to evaluate the statistical significance of differences among group means. If significant differences were detected by ANOVA, Tukey’s post hoc test was applied to perform pairwise comparisons between each group’s mean and the means of all other groups. Significance levels were defined as follows: p-value < 0.05 is considered as significant, p < 0.01 as very significant, p < 0.001 as highly significant, p < 0.0001 as extremely significant and p > 0.05 as not significant (NS), respectively.
3 Result
3.1 TP2 administration ameliorates the symptoms of TNBS-Induced colitis
UC symptoms were assessed based on factors such as body weight, DAI, and colon-related characteristics. In comparison to the NC group, the final body weight of rats in all modeled groups significantly decreased, while the DAI scores in the MC group rats significantly increased. Rats in the LTP2, MTP2, HTP2 and PD groups exhibited a significant decrease in DAI scores compared to the MC group (p < 0.001) (Figures 1B, C). Additionally, we evaluated colon tissue ulcer area, colon length, and colon weight/colon length (CW/CL). The ulcer area in the MC group was significantly larger than in the NC group (p < 0.0001), whereas the LTP2, MTP2, HTP2 and PD groups showed a significant reduction in ulcer area compared to the MC group (p < 0.01, p < 0.01, p < 0.05 and p < 0.01, respectively) (Figures 1D, F). Colon length in the MC group was significantly shorter than in the NC group (Fig. F), while the LTP2 and HTP2 groups exhibited significantly longer colon lengths than the MC group. The CW/CL in the LTP2, MTP2, HTP2 and PD groups was significantly lower than in the MC group (p < 0.0001, p < 0.0001, p < 0.01 and p < 0.001, respectively) (Figure 1E). Thus, TP2 alleviates the symptoms of TNBS-induced colitis, indicating its potential as a therapeutic agent for UC.
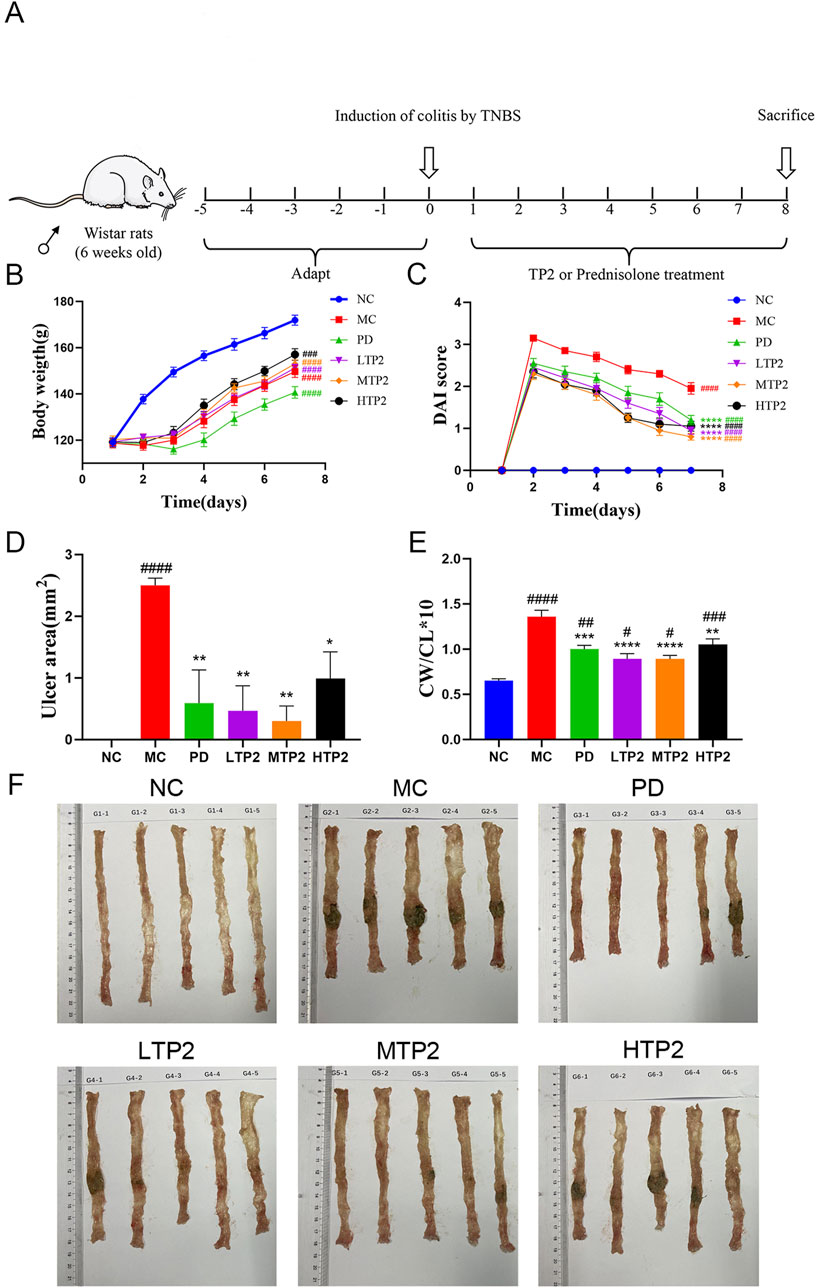
Figure 1. Experimental design and TP2 alleviate symptoms of Colitis. (A) The treatment regime of TP2 (B) Body weight (C) DAI score (D) Ulcer area (E) Colon weight/Colon length (F) Representative colon from each treatment group. Results reflected as mean ± SEM. ###p < 0.001, ####p < 0.0001 comparison to NC group. *p < 0.05, **p < 0.01, ***p < 0.001, ****p < 0.0001 comparison to MC group.
3.2 TP2 alleviates histological changes of colon
We histologically assessed the function of TP2 inrestoring intestinal epithelial integrity by HE staining. In the NC group, the intestinal wall exhibited normal tissue architecture with intact mucosal structure, clear crypt architecture, and evenly distributed glands. The MC group showed the disappearance of normal colonic wall structure, accompanied by partial cell necrosis. Crypt cells, goblet cells, and the majority of glands were largely absent, with a noticeable infiltration of inflammatory cells. In comparison to the MC group, the LTP2, MTP2 and PD groups displayed a mostly intact colonic structure with fewer inflammatory lesions. There was a slight loss of crypt cells and goblet cells (Figure 2A). These results were corroborated by histological score analysis. The MC group had significantly higher scores than the NC group, indicating severe colonic damage induced by TNBS-induced ulcerative colitis. Both the LTP2, MTP2 HTP2 and PD groups had significantly lower scores than the MC group (p < 0.01, p < 0.001, p < 0.05 and p < 0.01, respectively), indicating TP2 significantly reduced histopathological damage (Figure 2B).
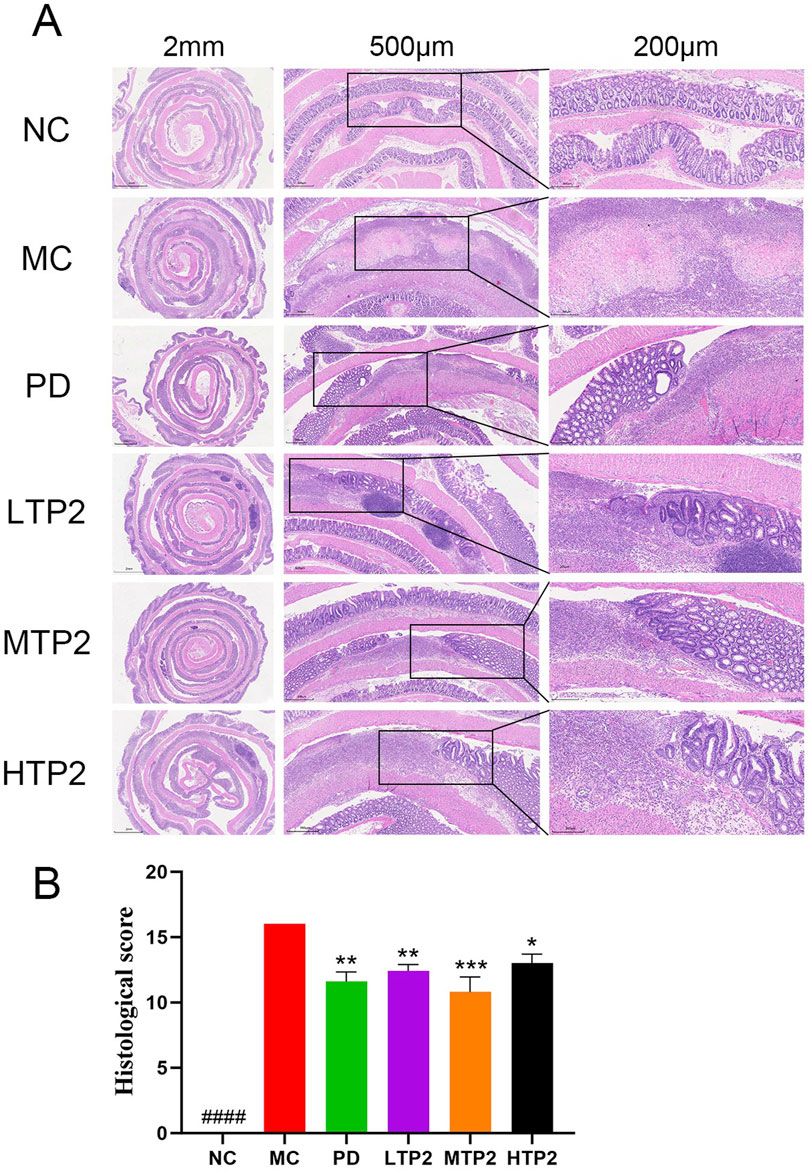
Figure 2. TP2 ameliorated colonic pathological damage in UC rats. (A) HE staining of colon tissue sections (B) Histopathological scores. ####p < 0.0001 comparison to NC group. *p < 0.05, **p < 0.01, ***p < 0.001, comparison to MC group. All data are presented as mean ± SEM (n = 5 rats per group).
3.3 TP2 protectes the integrity of barrier damage in rat
The intestinal mucosal barrier serves as the host’s first line of defense, protecting the intestinal epithelium from invasion (Pelaseyed et al., 2014). Structural weakening of the colonic mucosal barrier promotes the development of ulcerative colitis (van der Post et al., 2019). We utilized PAS staining and Western blot analysis to evaluate the protective effects of TP2 on the intestinal mucosal barrier. The results indicate severe damage to goblet cells in the MC group, with a significant decrease in Muc-2 expression. Treatment with TP2 and PD significantly alleviated the TNBS-induced reduction in goblet cells and Muc-2 secretion (Figures 3A, B). Considering that the MTP2 group demonstrated optimal protective efficacy in clinical symptoms, HE staining, and PAS staining in colitis rats, we selected the MTP2 group for further analysis in subsequent mechanistic investigations (In the following discussions, TP2 will be used to represent this group).
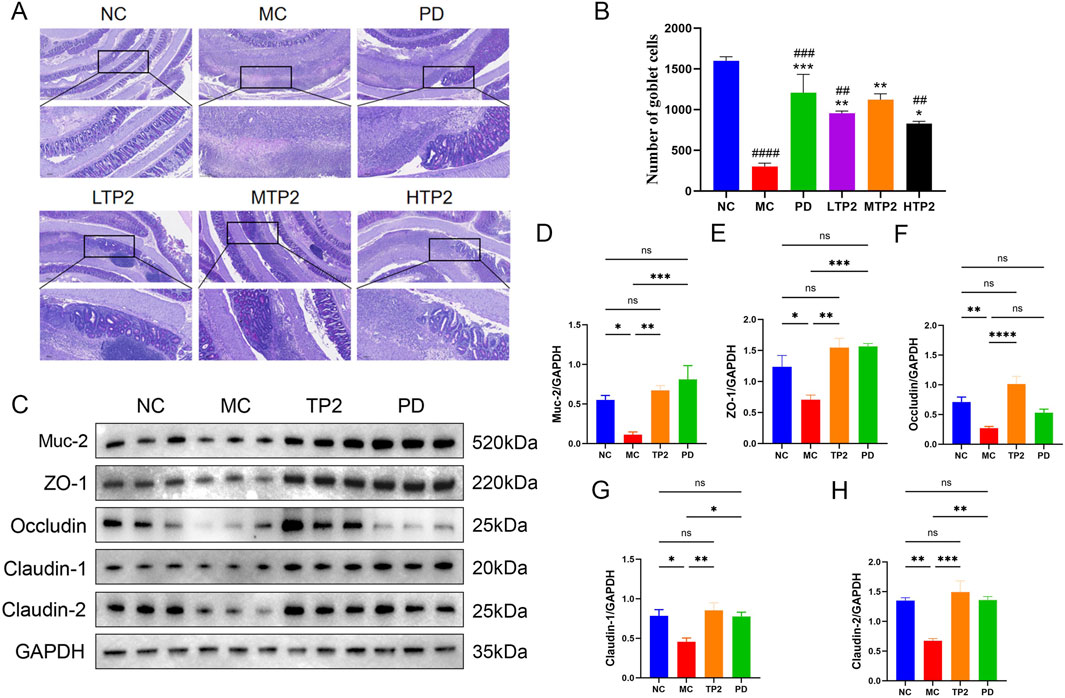
Figure 3. Therapeutic effects of TP2 on goblet cells and the intestinal barrier. (A) Representative PAS staining images (B) Quantification of goblet cells based on PAS staining. The relative protein expression of (C) Western blotting analysis of Muc-2, ZO-1, Occludin, Claudin-1 and claudin-2 in Colon tissue between groups (D) Muc-2 (E)ZO-1, (F) Occludin, (G) Claudin-1 and (H) Claudin-2 were normalized to GAPDH. ##p < 0.01, ###p < 0.001, ####p < 0.0001 comparison to NC group. *p < 0.05, **p < 0.01, ***p < 0.001 comparison to MC group. All data are presented as mean ± SEM (n = 5 rats per group).
Furthermore, intestinal tight junction proteins are crucial components of the intestinal barrier, preventing the leakage of harmful substances from the intestinal lumen (Buckley and Turner, 2018; Chelakkot et al., 2018). To investigate the protective effect of TP2 on TNBS-induced tight junction disruption, we measured the expression of ZO-1, Occludin, Claudin-1, and Claudin-2. The results showed a significant decrease in the expression of ZO-1, Occludin, Claudin-1, and Claudin-2 in the MC group compared to the NC group. TP2 and PD treatment, however, upregulated the expression levels of these proteins (Figures 3C–H). These findings suggest that TP2 restores the damaged intestinal barrier function by protecting goblet cells, upregulating Muc-2 expression, and enhancing the expression of tight junction proteins.
3.4 TP2 suppressed apoptosis in colon tissues
To assess apoptosis in colonic tissues, we investigated several proteins associated with apoptosis. Firstly, the proto-oncoproteins Bcl-2 and Bax were detected. As shown in Figure 4A, the expression of the anti-apoptotic protein Bcl-2 was downregulated, while the pro-apoptotic protein Bax was upregulated, resulting in a marked reduction in the Bcl-2/Bax ratio in the colonic tissues of TNBS-induced UC rats (p < 0.05). Additionally, Cleaved-Caspase-3 was selected to evaluate the effect of TP2 on TNBS-induced apoptosis. Under the challenge of TNBS, the expression of Cleaved-Caspase-3 significantly increased (p < 0.0001). By contrast, TP2 and PD treatment remarkably abrogated the downregulation of the Bcl-2/Bax ratio (p < 0.01, p < 0.05) (Figure 4B) and reduced Cleaved-Caspase-3 expression (p < 0.001, p < 0.001) (Figure 4C). In summary, TP2 suppressed apoptosis in colon tissues in TNBS-induced UC rats.
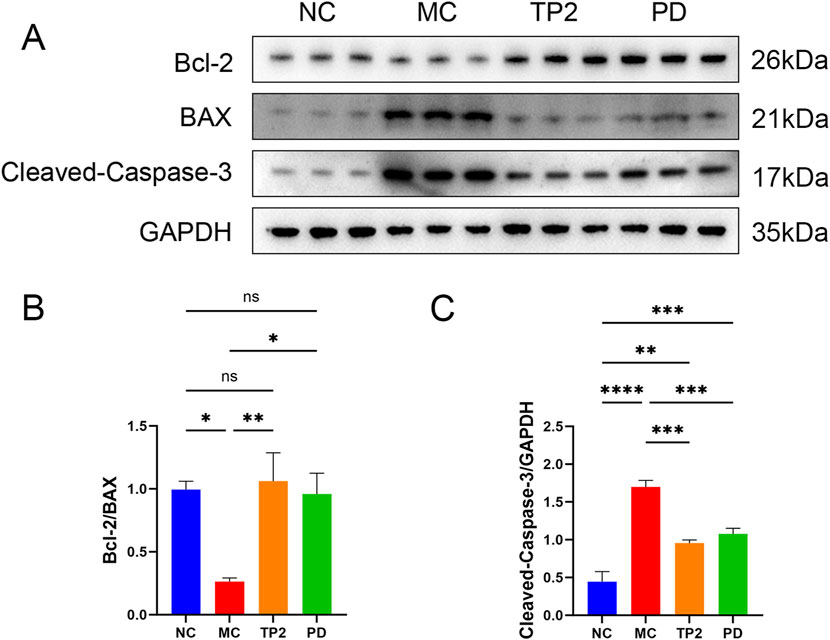
Figure 4. TP2 suppressed apoptosis in colon tissues (A) Western blotting analysis of Bcl-2, BAX and Cleaved-Caspase-3 in Colonic tissues (B) The relative protein expression levels of Bcl-2/BAX (C) The relative protein expression levels of Cleaved-Caspase-3/GAPDH. *p < 0.05, **p < 0.01, ***p < 0.001, ****p < 0.0001 and ns p > 0.05. All data are presented as mean ± SEM (n = 5 rats per group).
3.5 TP2 regulated cytokines and inflammatory factors in colon tissue
To gain a deeper understanding of the mechanism by which TP2 alleviates colitis, we utilized ELISA technology to assess typical pro-inflammatory and anti-inflammatory cytokines in rats’ colonic tissues, including TNF-α, IFN-γ, IL-1β, IL-6, IL-10, IL-17A, IL-22, and IL-23. As depicted in Figure 5, the MC group showed significantly increased levels of TNF-α (p < 0.05), IFN-γ (p < 0.05), IL-1β (p < 0.01), IL-6 (p < 0.01), and IL-23 (p < 0.01), indicating serious inflammation in UC rats. In comparison to the MC group, TP2 significantly enhanced the expression of IL-10 (p < 0.0001)and IL-22 (p < 0.05) in the colonic tissues of colitis-induced rats. Simultaneously, it reduced the levels of TNF-α (p < 0.055), IL-1β (p < 0.05), IL-6 (p < 0.05) and IL-23 (p < 0.01), while TP2 did not exhibit a significant impact on IFN-γ and IL-17 A. These research findings suggest that TP2 can promote the expression of anti-inflammatory cytokines and inhibit the secretion of pro-inflammatory cytokines, thereby mitigating colitis.
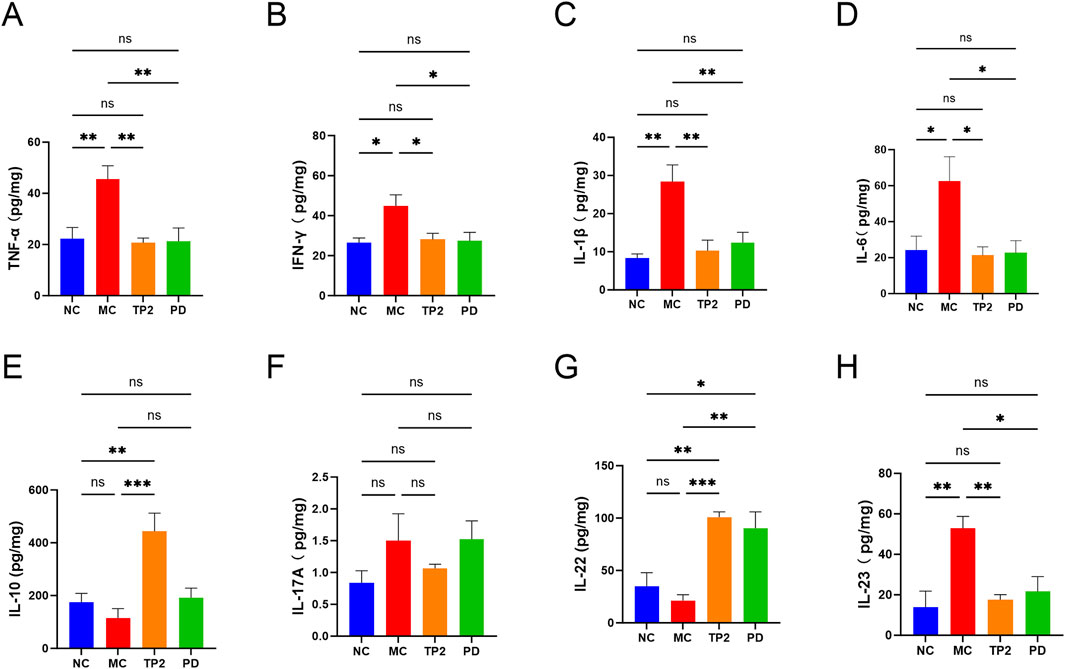
Figure 5. Effects of TP2 on inflammatory cytokines in colonic tissues. (A) TNF-α, (B) INF-γ, (C)IL-1β, (D) IL-6, (E) IL-10, (F) IL-17A, (G) IL-22 and (H) IL-23. *p < 0.05, **p < 0.01, ***p < 0.001 and ns p > 0.05. All data are presented as mean ± SEM (n = 5 rats per group).
3.6 TP2 inhibited PI3K/AKT signaling pathway in colonic tissues
Immunological dysregulation stands as a pivotal pathogenic factor in UC, manifested through an aberrant balance in the expression of pro-inflammatory and anti-inflammatory cytokines. The classical PI3K/AKT signaling pathway plays a crucial role in modulating diverse cellular processes, encompassing proliferation, differentiation, apoptosis, and inflammation-related responses. Numerous investigations underscore the interconnectedness of cytokine expression and closely associated proteins with the PI3K/AKT signaling pathway in the progression of UC (Huang et al., 2011; Chen et al., 2017; Li et al., 2022a). Consequently, we conducted Western blot analysis to scrutinize the influence of TP2 on the PI3K/AKT signaling pathway. The data revealed no discernible disparities in the total expression levels of PI3K and AKT across the experimental groups. Nevertheless, a noteworthy elevation in the expression levels of p-PI3K and p-AKT was observed in the MC group, signifying the induction of PI3K/AKT signaling pathway activation by TNBS (Figure 6A). Remarkably, treatment with TP2 and prednisolone effectively reversed this observed trend (Figures 6B, C). This aligns with the established regulatory effects of TP2 on inflammation, tight junction proteins, and cellular apoptosis. In summary, our research findings substantiate the proposition that TP2 mitigates TNBS-induced colonic injury by impeding the activation of the PI3K/AKT pathway.
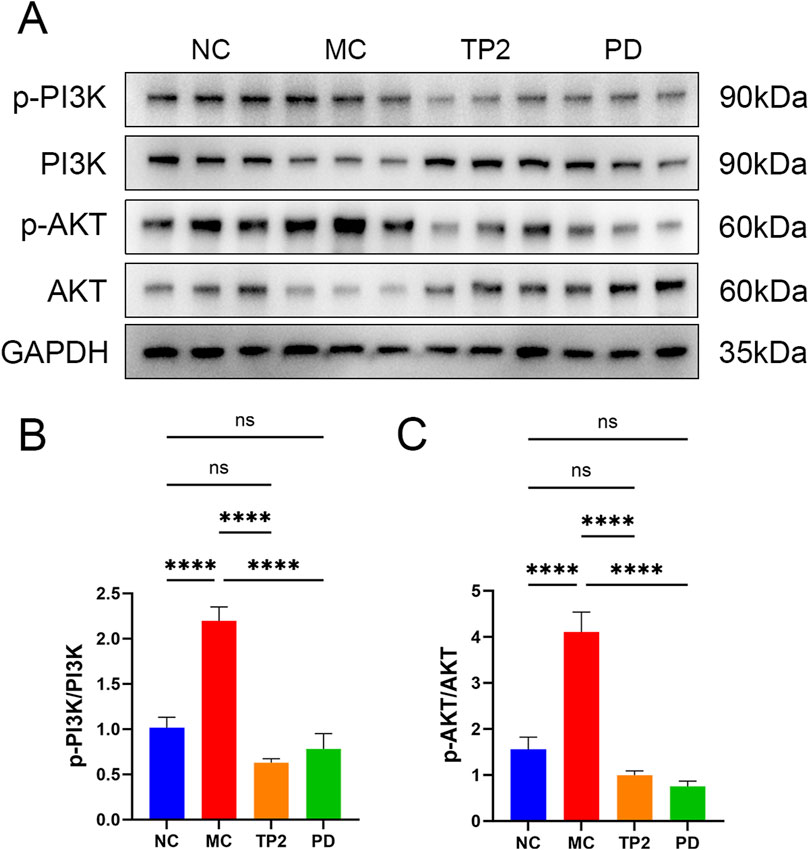
Figure 6. TP2 ameliorates TNBS-induced colitis relying on PI3K/AKT signaling pathway. (A) Protein levels of phosphor-PI3K, total PI3K, phosphor-AKT and total PI3K in colonic tissues were assessed by Western blotting (B) Representative protein levels of p-PI3K/PI3K (C) Representative protein levels of p-AKT/AKT. ****p < 0.0001 and ns p > 0.05. All data are presented as mean ± SEM (n = 5 rats per group).
3.7 TP2 reinstate gut microbiota dysbiosis
To investigate the changes in the structure and function of rat intestinal flora after drug administration, we performed 16S rRNA gene amplicon sequencing. Alpha diversity analysis at the OTU level showed that multiple indices (ACE, Chao, Shannon, Simpson) differed significantly in different groups (Figures 7A–E), the results of Principal Co-ordinates Analysis (PCoA) at the OTU level showed the same trend (Figure 7G), in which the MC group showed a significant separation compared to the NC group, and the TP2 group showed clustering with the PD and NC groups. The mean relative abundance at the genus level showed that TNBS reduced the abundance of major genera such as Lactobacillus, Limosilactobacillus, Ligilactobacillus and Akkermansia compared to the NC group, with the opposite trend in the TP2 and PD groups relative to the MC group (Figures 7E, F). These results suggest that TP2 treatment restored TNBS-induced disruption of UC intestinal microbiota. In addition, Functional prediction analysis of KEGG for bacteria and archaea was performed using PICRUSt (Figure 7G), Functional abundance heatmap showed that the abundance of probable phosphoglycerate mutase [EC:5.4.2.12], protein-tyrosine phosphatase [EC:3.1.3.48], probable phosphoglycerate mutase [EC:5.4.2.12] and L-lactate dehydrogenase [EC:1.1.1.27] was different in MC group and NC group, however, the abundance was similar in TP2 group and PD group and NC group. This may predict an important role for these KEGG ontologys (KOs) in the pathogenesis of inflammatory bowel disease.
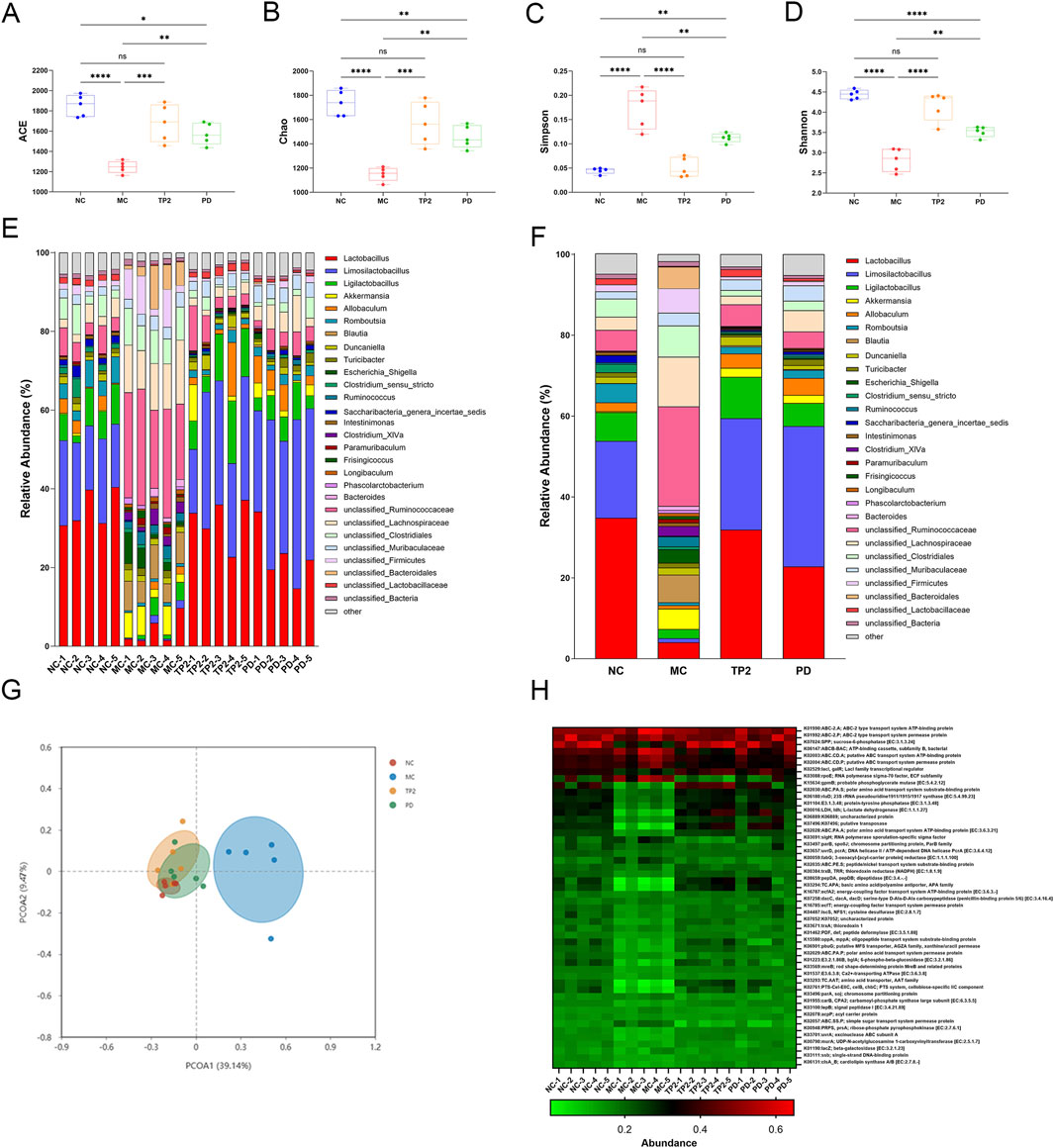
Figure 7. Effects of TP2 on intestinal microbiota in TNBS-induced rtas. (A) ACE (B) Chao (C) Simpsom (D) Shannon (E) Relative abundances of microbial genera in each sample (F) Relative abundances of microbial genera in each group (G) PCoA analysis (H) Relative abundances of microbial genera in each group (H) Microbial community functions against KEGG database between each group predicted by PICRUSt. *p < 0.05, **p < 0.01, ***p < 0.001, ****p < 0.0001 and ns p > 0.05. Values are mean ± SEM (n = 5 rats per group).
4 Discussion
The TNBS-induced model is widely recognized for its efficacy in replicating the pathological features of UC (Morris et al., 1989; Zhu et al., 2019; Bilsborough et al., 2021). Extensive research has consistently shown that TNBS can reliably induce a UC-like condition, characterized by inflammation predominantly affecting the mucosal and submucosal layers. This includes the formation of ulcers, crypt abscesses, goblet cell depletion, and significant inflammatory cell infiltration closely resembling the clinical and histopathological presentation of human UC (Silva et al., 2019). In this experiment, UC was induced in rats by administering a TNBS/ethanol mixture solution through the rectum. On the 2nd day of modeling, rats in the model group exhibited typical physiological symptoms of colitis, including weight loss, diarrhea, sticky stools with blood, and even anal bleeding. On the 8th day during dissection, the colon tissues of rats in the model group were significantly shortened, with large areas of ulcers observed. HE staining revealed the disappearance of colonic crypt structures, severe inflammation, and partial tissue necrosis. PAS staining and Western blotting demonstrated a significant decrease in the number of colonic goblet cells and mucin expression. These findings confirm the successful establishment of the UC rat model.
TP2, derived from the B. fragilis strain ZY-312, is a capsular polysaccharide. Previous studies have provided preliminary evidence of TP2’s efficacy in alleviating UC. However, the precise mechanism by which TP2 exerts its therapeutic effects in colitis remains unclear. This study aimed to further validate the therapeutic potential of TP2 in a rat model of TNBS-induced ulcerative colitis and explore the underlying mechanisms, including its impact on intestinal barrier function, microbial composition, and inflammation. The experimental results confirmed a significant alleviation of TNBS-induced colitis in rats following TP2 treatment, indicating a robust therapeutic effect. Figure 8 illustrates the comprehensive mechanisms by which TP2 mitigates TNBS-induced ulcerative colitis, demonstrating its effects across various systems including the gut microbiota, mucus layer, epithelial cells, and intracellular signaling pathways. TP2 intervention effectively ameliorated clinical manifestations such as weight loss, increased DAI score, large-area ulcers, and damage to the intestinal epithelium in UC rats. Further analyses demonstrated that TP2 contributed to the restoration of the rat intestinal barrier’s integrity, suppression of intestinal inflammatory responses, and inhibition of apoptosis in intestinal epithelial cells. Notably, TP2 played a crucial role in reshaping the gut microbiota. Additionally, TP2 significantly downregulated the expression of the PI3K/AKT signaling pathway, showcasing excellent anti-inflammatory properties.
Intestinal epithelial cells, along with the mucous layer, form the physiological barrier of the intestine—a critical defense against the invasion of toxic substances (Kotla et al., 2022). The intestinal mucous layer isolates bacteria from epithelial cells, safeguarding them against bacterial invasion and exposure to toxic substances (Hansson, 2012). Goblet cells and intestinal tight junction proteins are essential components of the intestinal barrier. Goblet cells, as specialized epithelial cells, are responsible for producing and secreting mucus, forming the epithelial scaffold of the mucous layer (Gonzalez-Perez et al., 2021; Kang et al., 2022). Tight junction proteins serve as the tightest adhesion between epithelial cells and endothelial cells, selectively allowing ions and small molecules from the intercellular space to pass through (Günzel and Fromm, 2012). Key tight junction proteins, including ZO-1, Occludin, Claudin-1, and Claudin-2, play a role in influencing the development of intestinal inflammation through the regulation of mucosal homeostasis and intestinal permeability (Ulluwishewa et al., 2011; Buckley and Turner, 2018; Chelakkot et al., 2018). Our research findings indicate that rats displayed typical colitis symptoms after TNBS induction. The MC group exhibited a significant loss of goblet cells and reduced expression of tight junction proteins, suggesting that TNBS induction impaired the colonic barrier function in rats. When the colonic barrier function is compromised, intestinal permeability increases, enabling harmful bacteria and excessive toxic substances to enter the colon, leading to inflammation. Following TP2 treatment, there was a significant increase in the number of goblet cells in colonic tissues. Moreover, the expression levels of Muc-2 and tight junction proteins ZO-1, Occludin, Claudin-1, and Claudin-2 were markedly upregulated. These findings suggest that TP2 protects the integrity of the intestinal barrier by augmenting the number of goblet cells and enhancing the expression of tight junction proteins. This action inhibits the entry of pathogenic factors into colonic tissues, thereby alleviating inflammation.
Research also indicates a close association between the disruption of tight junctions in intestinal tissues and inflammatory responses (Luissint et al., 2016). Under inflammatory conditions, cytokine-mediated dysfunction of tight junction barrier function promotes the development of intestinal diseases (Lee, 2015). Elevated levels of pro-inflammatory cytokines, such as TNF-α, IL-1β, IL-6, and IL-23 in colonic tissues, further exacerbate colonic damage and recruit a large number of inflammatory cells into the inflamed tissue (McGuckin et al., 2009). These cytokines are also associated with complications of colitis, including cell apoptosis, rectal bleeding, and the formation of colitis-associated cancer (Neurath, 2014). We observed a significant increase in the expression of pro-inflammatory cytokines such as TNF-α, IL-1β, IL-6, and IL-23 in the MC group compared to the NC group, while TP2 treatment inhibited the expression of these cytokines. In contrast to the aforementioned cytokines, anti-inflammatory cytokines such as IL-10 and IL-22 can restore intestinal barrier defects by enhancing intestinal cell permeability and promoting the expression of tight junction protein Claudin-2, respectively (Sun et al., 2008; Keir et al., 2020). In this study, we found that TP2 significantly promoted the secretion of anti-inflammatory cytokines IL-10 and IL-22. These research results suggest a close correlation between TP2 alleviating colonic damage and suppressing inflammation.
Besides the impact of inflammatory factors on the integrity of intestinal tissues, abnormal apoptosis of intestinal epithelial cells can also disrupt the integrity and barrier function of the intestinal mucosa (Blander, 2016; Subramanian et al., 2020). Previous studies found a significant increase in the apoptosis rate of intestinal epithelial cells in UC patients (Garcia-Carbonell et al., 2019). Inhibiting apoptosis of intestinal epithelial cells is crucial for improving the impaired intestinal barrier function in UC patients. Bcl-2 and Bax, key members of the mitochondrial apoptosis pathway, determine the balance between cell proliferation and apoptosis (Adams and Cory, 1998). Also, Caspase-3 and its cleavage product Cleaved-Caspase-3 play a crucial regulatory role in cell apoptosis (Riedl and Shi, 2004). The PI3K/AKT pathway, an important signaling pathway associated with apoptosis, regulates extracellular signals, influencing downstream effector molecules such as Bcl-2, Bax, and Caspase-3, thereby impacting inflammation, cell apoptosis, and the proliferation and differentiation of immune cells (Cantley, 2002; Chen et al., 2017; Jalil et al., 2023). Abnormal activation of the PI3K/AKT pathway has been found to lead to immune system imbalance and is one of the pathogenic mechanisms of UC (Nunes-Santos et al., 2019). Inhibiting the PI3K/AKT signaling pathway has significant benefits for alleviating UC (Park et al., 2014; Li et al., 2022a; Zhou et al., 2023) and is considered a promising target for treating UC in humans (Tokuhira et al., 2015). In this study, we observed that TP2 inhibited the activation of the PI3K/AKT signaling pathway by reducing the expression levels of p-PI3K and p-AKT.
In recent years, increasing evidence suggests that the dysregulation of the gut microbiota plays a crucial role in the pathological process of colitis (Lane et al., 2017). The interaction between the gut microbial community and the host is essential for maintaining the normal function of the intestinal epithelial barrier, regulating host immune responses, and ensuring the physiological health of the host (Heiman and Greenway, 2016). Disruption of the gut microbial community can trigger host immune reactions, activate inflammatory signaling pathways, and consequently lead to the occurrence of colitis (Park et al., 2017). Differences in the gut microbiota between UC patients and healthy individuals mainly include reduced microbial diversity and diminished abundance of specific bacteria (Miyoshi and Chang, 2017). 16S rRNA sequencing results indicate that TNBS induction results in a significant decrease in the abundance and diversity of the gut microbial community compared to normal rats, and TP2 treatment significantly improves this condition. PCoA analysis shows a distinct separation between the MC group and TP2 group rats, and the TP2 group tends to cluster with the NC group, indicating that TP2 treatment modifies the TNBS-induced changes in the gut microbiota. At the genus level, TNBS induction decreases the abundance of Lactobacillus, Limosilactobacillus, Ligilactobacillus, and Akkermansia, while increasing the abundance of unclassified_Ruminococcaceae and unclassified_Lachnospiraceae. Prior research has indicated that Lactobacillus and Limosilactobacillus can enhance the intestinal mucosal barrier by promoting the expression of intestinal tight junction proteins through interaction with host intestinal epithelial cells. Additionally, they regulate the host immune system by modulating inflammatory factors and immune cells (He et al., 2022; Luo et al., 2023). Ligilactobacillus has been found to contribute to nutrient absorption and the production of short-chain fatty acids (Li et al., 2022b). Akkermansia has been found to be negatively correlated with several pro-inflammatory cytokine levels and positively correlated with the intestinal tight junction protein ZO-1 (Ye et al., 2018). These results suggest that TP2 may alleviate colitis in rats by reshaping the structure and composition of the intestinal microbiota, influencing the physiological functions of the host.
5 Conclusion
This study confirmed the therapeutic effect of the capsular polysaccharide A of Bacteroides Fragilis TP2 on TNBS-induced UC in Wistar rat models. Furthermore, it revealed that TP2 restores intestinal barrier integrity by regulating barrier-related cells and proteins, inhibiting cell apoptosis, modulating levels of inflammation-related cytokines, and controlling the abnormal activation of the PI3K/AKT signaling pathway. Additionally, TP2 alleviates rat UC by reshaping the ecological imbalance of the intestinal microbiota.
Data availability statement
The processed 16S rRNA data has is deposited in the NCBI database, BioProject accession number PRJNA1189676; available at: https://www.ncbi.nlm.nih.gov/bioproject/PRJNA1189676. Further inquiries can be directed to the corresponding authors.
Ethics statement
The animal study was approved by Institutional Animal Care and Use Committee (IACUC) of Ruige Biotechnology. The study was conducted in accordance with the local legislation and institutional requirements.
Author contributions
YZ: Validation, Software, Investigation, Data curation, Conceptualization, Writing–review and editing, Writing–original draft. XC: Writing–review and editing, Supervision, Project administration, Investigation, Conceptualization. ZZ: Writing–original draft, Validation, Data curation, Formal Analysis. LZ: Writing–review and editing, Software, Data curation. GK: Writing–review and editing, Methodology. PL: Writing–review and editing, Supervision, Funding acquisition. CL: Writing–original draft, Project administration. YF: Writing–original draft, Investigation. ZL: Writing–original draft, Validation. KZ: Writing–original draft, Validation. QX: Writing–review and editing, Supervision, Resources, Investigation, Formal Analysis, Data curation. YL: Writing–review and editing, Supervision, Resources, Investigation, Funding acquisition, Formal Analysis.
Funding
The author(s) declare that financial support was received for the research, authorship, and/or publication of this article. This research was funded by the Guangzhou Science and Technology Program, Key Research and Development Plan (NO. 2024B03J1282).
Conflict of interest
Authors XC, GK, PL, CL, and YL were employed by Guangzhou ZhiYi Biotechnology Co. Ltd.
The remaining authors declare that the research was conducted in the absence of any commercial or financial relationships that could be construed as a potential conflict of interest.
Publisher’s note
All claims expressed in this article are solely those of the authors and do not necessarily represent those of their affiliated organizations, or those of the publisher, the editors and the reviewers. Any product that may be evaluated in this article, or claim that may be made by its manufacturer, is not guaranteed or endorsed by the publisher.
Supplementary material
The Supplementary Material for this article can be found online at: https://www.frontiersin.org/articles/10.3389/fphar.2024.1402465/full#supplementary-material
References
Adams, J. M., and Cory, S. (1998). The Bcl-2 protein family: arbiters of cell survival. Science 281, 1322–1326. doi:10.1126/science.281.5381.1322
Akobeng, A. K., Zhang, D., Gordon, M., and Macdonald, J. K. (2016). Oral 5-aminosalicylic acid for maintenance of medically-induced remission in Crohn's disease. Cochrane database Syst. Rev. 9, CD003715. doi:10.1002/14651858.CD003715.pub3
Baert, F., Noman, M., Vermeire, S., Van Assche, G., D'Haens, G., Carbonez, A., et al. (2003). Influence of immunogenicity on the long-term efficacy of infliximab in Crohn's disease. N. Engl. J. Med. 348, 601–608. doi:10.1056/NEJMoa020888
Bilsborough, J., Fiorino, M. F., and Henkle, B. W. (2021). Select animal models of colitis and their value in predicting clinical efficacy of biological therapies in ulcerative colitis. Expert Opin. Drug Discov. 16, 567–577. doi:10.1080/17460441.2021.1851185
Blander, J. M. (2016). Death in the intestinal epithelium—basic biology and implications for inflammatory bowel disease. FEBS J. 283, 2720–2730. doi:10.1111/febs.13771
Buckley, A., and Turner, J. R. (2018). Cell biology of tight junction barrier regulation and mucosal disease. Cold Spring Harb. Perspect. Biol. 10, a029314. doi:10.1101/cshperspect.a029314
Cantley, L. C. (2002). The phosphoinositide 3-kinase pathway. Science 296, 1655–1657. doi:10.1126/science.296.5573.1655
Chelakkot, C., Ghim, J., and Ryu, S. H. (2018). Mechanisms regulating intestinal barrier integrity and its pathological implications. Exp. and Mol. Med. 50, 103–109. doi:10.1038/s12276-018-0126-x
Chen, Q., Duan, X., Fan, H., Xu, M., Tang, Q., Zhang, L., et al. (2017). Oxymatrine protects against DSS-induced colitis via inhibiting the PI3K/AKT signaling pathway. Int. Immunopharmacol. 53, 149–157. doi:10.1016/j.intimp.2017.10.025
Comstock, L. E., and Kasper, D. L. (2006). Bacterial glycans: key mediators of diverse host immune responses. Cell 126, 847–850. doi:10.1016/j.cell.2006.08.021
Deng, H., Li, Z., Tan, Y., Guo, Z., Liu, Y., Wang, Y., et al. (2016). A novel strain of Bacteroides fragilis enhances phagocytosis and polarises M1 macrophages. Sci. Rep. 6, 29401. doi:10.1038/srep29401
Deng, H., Yang, S., Zhang, Y., Qian, K., Zhang, Z., Liu, Y., et al. (2019). Corrigendum: Bacteroides fragilis prevents Clostridium difficile infection in a mouse model by restoring gut barrier and microbiome regulation. Front. Microbiol. 10, 601. doi:10.3389/fmicb.2019.00601
Eribo, O. A., Du Plessis, N., and Chegou, N. N. (2022). The intestinal commensal, Bacteroides fragilis, modulates host responses to viral infection and therapy: lessons for exploration during mycobacterium tuberculosis infection. Infect. Immun. 90, e0032121. doi:10.1128/IAI.00321-21
Erturk-Hasdemir, D., and Kasper, D. L. (2018). Finding a needle in a haystack: Bacteroides fragilis polysaccharide A as the archetypical symbiosis factor. Ann. N. Y. Acad. Sci. 1417, 116–129. doi:10.1111/nyas.13660
Ford, A. C., Bernstein, C. N., Khan, K. J., Abreu, M. T., Marshall, J. K., Talley, N. J., et al. (2011). Glucocorticosteroid therapy in inflammatory bowel disease: systematic review and meta-analysis. Official J. Am. Coll. Gastroenterology| ACG 106, 590–599. doi:10.1038/ajg.2011.70
Garcia-Carbonell, R., Yao, S.-J., Das, S., and Guma, M. (2019). Dysregulation of intestinal epithelial cell RIPK pathways promotes chronic inflammation in the IBD gut. Front. Immunol. 10, 1094. doi:10.3389/fimmu.2019.01094
Gonzalez-Perez, V., Martinez-Espinosa, P. L., Sala-Rabanal, M., Bharadwaj, N., Xia, X.-M., Chen, A. C., et al. (2021). Goblet cell LRRC26 regulates BK channel activation and protects against colitis in mice. Proc. Natl. Acad. Sci. U. S. A. 118, e2019149118. doi:10.1073/pnas.2019149118
Gros, B., and Kaplan, G. G. (2023). Ulcerative colitis in adults: a review. Jama 330, 951–965. doi:10.1001/jama.2023.15389
GüNZEL, D., and Fromm, M. (2012). Claudins and other tight junction proteins. Compr. Physiol. 2, 1819–1852. doi:10.1002/cphy.c110045
Hansson, G. C. (2012). Role of mucus layers in gut infection and inflammation. Curr. Opin. Microbiol. 15, 57–62. doi:10.1016/j.mib.2011.11.002
He, X.-Q., Liu, D., Liu, H.-Y., Wu, D.-T., Li, H.-B., Zhang, X.-S., et al. (2022). Prevention of ulcerative colitis in mice by sweet tea (Lithocarpus litseifolius) via the regulation of gut microbiota and butyric-acid-mediated anti-inflammatory signaling. Nutrients 14, 2208. doi:10.3390/nu14112208
Heiman, M. L., and Greenway, F. L. (2016). A healthy gastrointestinal microbiome is dependent on dietary diversity. Mol. Metab. 5, 317–320. doi:10.1016/j.molmet.2016.02.005
Huang, X. L., Xu, J., Zhang, X. H., Qiu, B. Y., Peng, L., Zhang, M., et al. (2011). PI3K/Akt signaling pathway is involved in the pathogenesis of ulcerative colitis. Inflamm. Res. 60, 727–734. doi:10.1007/s00011-011-0325-6
Jalil, A. T., Hassan, N. F., Abdulameer, S. J., Farhan, Z. M., Suleiman, A. A., Al-Azzawi, A. K., et al. (2023). Phosphatidylinositol 3-kinase signaling pathway and inflammatory bowel disease: current status and future prospects. Fundam. and Clin. Pharmacol. 37, 910–917. doi:10.1111/fcp.12894
Jiang, W., Han, Y.-P., Hu, M., Bao, X.-Q., Yan, Y., and Chen, G. (2019). A study on regulatory mechanism of miR-223 in ulcerative colitis through PI3K/Akt-mTOR signaling pathway. Eur. Rev. Med. and Pharmacol. Sci. 23, 4865–4872. doi:10.26355/eurrev_201906_18074
Johnson, J. L., Jones, M. B., and Cobb, B. A. (2015). Bacterial capsular polysaccharide prevents the onset of asthma through T-cell activation. Glycobiology 25, 368–375. doi:10.1093/glycob/cwu117
Johnson, J. L., Jones, M. B., and Cobb, B. A. (2018). Polysaccharide-experienced effector T cells induce IL-10 in FoxP3+ regulatory T cells to prevent pulmonary inflammation. Glycobiology 28, 50–58. doi:10.1093/glycob/cwx093
Kang, Y., Park, H., Choe, B.-H., and Kang, B. (2022). The role and function of mucins and its relationship to inflammatory bowel disease. Front. Med. 9, 848344. doi:10.3389/fmed.2022.848344
Keir, M. E., Yi, T., Lu, T. T., and Ghilardi, N. (2020). The role of IL-22 in intestinal health and disease. J. Exp. Med. 217, e20192195. doi:10.1084/jem.20192195
Kotla, N. G., Isa, I. L. M., Rasala, S., Demir, S., Singh, R., Baby, B. V., et al. (2022). Modulation of gut barrier functions in ulcerative colitis by hyaluronic acid system. Adv. Sci. 9, 2103189. doi:10.1002/advs.202103189
Lane, E. R., Zisman, T. L., and Suskind, D. L. (2017). The microbiota in inflammatory bowel disease: current and therapeutic insights. J. Inflamm. Res. 10, 63–73. doi:10.2147/JIR.S116088
Lee, S. H. (2015). Intestinal permeability regulation by tight junction: implication on inflammatory bowel diseases. Intestinal Res. 13, 11–18. doi:10.5217/ir.2015.13.1.11
Li, C., Wang, L., Zhao, J., Wei, Y., Zhai, S., Tan, M., et al. (2022a). Lonicera rupicola Hook. f. et Thoms flavonoids ameliorated dysregulated inflammatory responses, intestinal barrier, and gut microbiome in ulcerative colitis via PI3K/AKT pathway. Phytomedicine 104, 154284. doi:10.1016/j.phymed.2022.154284
Li, Z., Deng, H., Zhou, Y., Tan, Y., Wang, X., Han, Y., et al. (2017). Bioluminescence imaging to track Bacteroides fragilis inhibition of Vibrio parahaemolyticus infection in mice. Front. Cell. Infect. Microbiol. 7, 170. doi:10.3389/fcimb.2017.00170
Li, Z., Zhang, S., Xu, L., Fang, X., Wan, Y., Yu, D., et al. (2022b). A tetrapeptide from maize combined with probiotics exerted strong anti-inflammatory effects and modulated gut microbiota in DSS-induced colitis mice. Food and Funct. 13, 12602–12618. doi:10.1039/d2fo02678c
Luissint, A.-C., Parkos, C. A., and Nusrat, A. (2016). Inflammation and the intestinal barrier: leukocyte–epithelial cell interactions, cell junction remodeling, and mucosal repair. Gastroenterology 151, 616–632. doi:10.1053/j.gastro.2016.07.008
Luo, Z., Chen, A., Xie, A., Liu, X., Jiang, S., and Yu, R. (2023). Limosilactobacillus reuteri in immunomodulation: molecular mechanisms and potential applications. Front. Immunol. 14, 1228754. doi:10.3389/fimmu.2023.1228754
Mazmanian, S. K., Round, J. L., and Kasper, D. L. (2008). A microbial symbiosis factor prevents intestinal inflammatory disease. Nature 453, 620–625. doi:10.1038/nature07008
Mcguckin, M. A., Eri, R., Simms, L. A., Florin, T. H., and Radford-Smith, G. (2009). Intestinal barrier dysfunction in inflammatory bowel diseases. Inflamm. bowel Dis. 15, 100–113. doi:10.1002/ibd.20539
Miyoshi, J., and Chang, E. B. (2017). The gut microbiota and inflammatory bowel diseases. Transl. Res. 179, 38–48. doi:10.1016/j.trsl.2016.06.002
Morris, G. P., Beck, P. L., Herridge, M. S., Depew, W. T., Szewczuk, M. R., and Wallace, J. L. (1989). Hapten-induced model of chronic inflammation and ulceration in the rat colon. Gastroenterology 96, 795–803. doi:10.1016/s0016-5085(89)80079-4
Motavallian-Naeini, A., Andalib, S., Rabbani, M., Mahzouni, P., Afsharipour, M., and Minaiyan, M. (2012). Validation and optimization of experimental colitis induction in rats using 2, 4, 6-trinitrobenzene sulfonic acid. Res. Pharm. Sci. 7, 159–169.
Neurath, M. F. (2014). Cytokines in inflammatory bowel disease. Nat. Rev. Immunol. 14, 329–342. doi:10.1038/nri3661
Nunes-Santos, C. J., Uzel, G., and Rosenzweig, S. D. (2019). PI3K pathway defects leading to immunodeficiency and immune dysregulation. J. Allergy Clin. Immunol. 143, 1676–1687. doi:10.1016/j.jaci.2019.03.017
Pagliuca, C., Cicatiello, A. G., Colicchio, R., Greco, A., Cerciello, R., Auletta, L., et al. (2016). Novel approach for evaluation of Bacteroides fragilis protective role against Bartonella henselae liver damage in immunocompromised murine model. Front. Microbiol. 7, 1750. doi:10.3389/fmicb.2016.01750
Park, J. H., Peyrin-Biroulet, L., Eisenhut, M., and Shin, J. I. (2017). IBD immunopathogenesis: a comprehensive review of inflammatory molecules. Autoimmun. Rev. 16, 416–426. doi:10.1016/j.autrev.2017.02.013
Park, S., Regmi, S. C., Park, S.-Y., Lee, E. K., Chang, J.-H., Ku, S. K., et al. (2014). Protective effect of 7-O-succinyl macrolactin A against intestinal inflammation is mediated through PI3-kinase/Akt/mTOR and NF-κB signaling pathways. Eur. J. Pharmacol. 735, 184–192. doi:10.1016/j.ejphar.2014.04.024
Pelaseyed, T., BergströM, J. H., Gustafsson, J. K., Ermund, A., Birchenough, G. M., SchüTTE, A., et al. (2014). The mucus and mucins of the goblet cells and enterocytes provide the first defense line of the gastrointestinal tract and interact with the immune system. Immunol. Rev. 260, 8–20. doi:10.1111/imr.12182
Peng, K.-Y., Gu, J.-F., Su, S.-L., Zhu, Y., Guo, J.-M., Qian, D.-W., et al. (2021). Salvia miltiorrhiza stems and leaves total phenolic acids combination with tanshinone protect against DSS-induced ulcerative colitis through inhibiting TLR4/PI3K/AKT/mTOR signaling pathway in mice. J. Ethnopharmacol. 264, 113052. doi:10.1016/j.jep.2020.113052
Rahmani, F., Asgharzadeh, F., Avan, A., Barneh, F., Parizadeh, M. R., Ferns, G. A., et al. (2020). Rigosertib potently protects against colitis-associated intestinal fibrosis and inflammation by regulating PI3K/AKT and NF-κB signaling pathways. Life Sci. 249, 117470. doi:10.1016/j.lfs.2020.117470
Riedl, S. J., and Shi, Y. (2004). Molecular mechanisms of caspase regulation during apoptosis. Nat. Rev. Mol. cell Biol. 5, 897–907. doi:10.1038/nrm1496
Sham, H. P., Bazett, M., Bosiljcic, M., Yang, H., Luk, B., Law, H. T., et al. (2018). Immune stimulation using a gut microbe-based immunotherapy reduces disease pathology and improves barrier function in ulcerative colitis. Front. Immunol. 9, 2211. doi:10.3389/fimmu.2018.02211
Shen, Z.-H., Zhu, C.-X., Quan, Y.-S., Yang, Z.-Y., Wu, S., Luo, W.-W., et al. (2018). Relationship between intestinal microbiota and ulcerative colitis: mechanisms and clinical application of probiotics and fecal microbiota transplantation. World J. gastroenterology 24, 5–14. doi:10.3748/wjg.v24.i1.5
Silva, I., Pinto, R., and Mateus, V. (2019). Preclinical study in vivo for new pharmacological approaches in inflammatory bowel disease: a systematic review of chronic model of TNBS-induced colitis. J. Clin. Med. 8, 1574. doi:10.3390/jcm8101574
Sittipo, P., Lobionda, S., Choi, K., Sari, I. N., Kwon, H. Y., and Lee, Y. K. (2018). Toll-like receptor 2-mediated suppression of colorectal cancer pathogenesis by polysaccharide A from Bacteroides fragilis. Front. Microbiol. 9, 1588. doi:10.3389/fmicb.2018.01588
Subramanian, S., Geng, H., and Tan, X.-D. (2020). Cell death of intestinal epithelial cells in intestinal diseases. Sheng li xue bao: Acta Physiol. Sin. 72, 308–324.
Sun, X., Yang, H., Nose, K., Nose, S., Haxhija, E. Q., Koga, H., et al. (2008). Decline in intestinal mucosal IL-10 expression and decreased intestinal barrier function in a mouse model of total parenteral nutrition. Am. J. Physiology-Gastrointestinal Liver Physiology 294, G139–G147. doi:10.1152/ajpgi.00386.2007
Tokuhira, N., Kitagishi, Y., Suzuki, M., Minami, A., Nakanishi, A., Ono, Y., et al. (2015). PI3K/AKT/PTEN pathway as a target for Crohn's disease therapy (Review). Int. J. Mol. Med. 35, 10–16. doi:10.3892/ijmm.2014.1981
Ulluwishewa, D., Anderson, R. C., Mcnabb, W. C., Moughan, P. J., Wells, J. M., and Roy, N. C. (2011). Regulation of tight junction permeability by intestinal bacteria and dietary components. J. Nutr. 141, 769–776. doi:10.3945/jn.110.135657
Van Der Post, S., Jabbar, K. S., Birchenough, G., Arike, L., Akhtar, N., Sjovall, H., et al. (2019). Structural weakening of the colonic mucus barrier is an early event in ulcerative colitis pathogenesis. Gut 68, 2142–2151. doi:10.1136/gutjnl-2018-317571
VéTIZOU, M., Pitt, J. M., DaillèRE, R., Lepage, P., Waldschmitt, N., Flament, C., et al. (2015). Anticancer immunotherapy by CTLA-4 blockade relies on the gut microbiota. Science 350, 1079–1084. doi:10.1126/science.aad1329
Wang, Y., Begum-Haque, S., Telesford, K. M., Ochoa-RepáRAZ, J., Christy, M., Kasper, E. J., et al. (2014). A commensal bacterial product elicits and modulates migratory capacity of CD39+ CD4 T regulatory subsets in the suppression of neuroinflammation. Gut microbes 5, 552–561. doi:10.4161/gmic.29797
Wang, Y., Deng, H., Li, Z., Tan, Y., Han, Y., Wang, X., et al. (2017). Safety evaluation of a novel strain of Bacteroides fragilis. Front. Microbiol. 8, 435. doi:10.3389/fmicb.2017.00435
Xu, W., Su, P., Zheng, L., Fan, H., Wang, Y., Liu, Y., et al. (2018). In vivo imaging of a novel strain of Bacteroides fragilis via metabolic labeling. Front. Microbiol. 9, 2298. doi:10.3389/fmicb.2018.02298
Yan, S., Yang, B., Zhao, J., Zhao, J., Stanton, C., Ross, R. P., et al. (2019). A ropy exopolysaccharide producing strain Bifidobacterium longum subsp. longum YS108R alleviates DSS-induced colitis by maintenance of the mucosal barrier and gut microbiota modulation. Food and Funct. 10, 1595–1608. doi:10.1039/c9fo00014c
Ye, J., Lv, L., Wu, W., Li, Y., Shi, D., Fang, D., et al. (2018). Butyrate protects mice against methionine–choline-deficient diet-induced non-alcoholic steatohepatitis by improving gut barrier function, attenuating inflammation and reducing endotoxin levels. Front. Microbiol. 9, 1967. doi:10.3389/fmicb.2018.01967
Zhang, W., Zhou, Q., Liu, H., Xu, J., Huang, R., Shen, B., et al. (2023). Bacteroides fragilis strain ZY-312 facilitates colonic mucosa regeneration in colitis via motivating STAT3 signaling pathway induced by IL-22 from ILC3 secretion. Front. Immunol. 14, 1156762. doi:10.3389/fimmu.2023.1156762
Zhang, W., Zhu, B., Xu, J., Liu, Y., Qiu, E., Li, Z., et al. (2018). Bacteroides fragilis protects against antibiotic-associated diarrhea in rats by modulating intestinal defenses. Front. Immunol. 9, 1040. doi:10.3389/fimmu.2018.01040
Zheng, L., Luo, M., Kuang, G., Liu, Y., Liang, D., Huang, H., et al. (2020). Capsular polysaccharide from Bacteroides fragilis protects against ulcerative colitis in an undegraded form. Front. Pharmacol. 11, 570476. doi:10.3389/fphar.2020.570476
Zheng, Y., Liang, C., Li, Z., Chen, J., Chen, Z., Jiang, Y., et al. (2022). Study on the mechanism of Huangqi Decoction on rats with ulcerative colitis of damp-heat type base on mtDNA, TLR4, p-PI3K, p-Akt protein expression and microbiota. J. Ethnopharmacol. 295, 115356. doi:10.1016/j.jep.2022.115356
Zhou, M., Zhi, J., Zhi, J., Xiong, Z., Wu, F., Lu, Y., et al. (2023). Polysaccharide from Strongylocentrotus nudus eggs regulates intestinal epithelial autophagy through CD36/PI3K-Akt pathway to ameliorate inflammatory bowel disease. Int. J. Biol. Macromol. 244, 125373. doi:10.1016/j.ijbiomac.2023.125373
Zhou, Q., Shen, B., Huang, R., Liu, H., Zhang, W., Song, M., et al. (2022). Bacteroides fragilis strain ZY-312 promotes intestinal barrier integrity via upregulating the STAT3 pathway in a radiation-induced intestinal injury mouse model. Front. Nutr. 9, 1063699. doi:10.3389/fnut.2022.1063699
Keywords: Bacteroides fragilis, capsular polysaccharide A, ulcerative colitis, intestinal barrier function, intestinal microbiota
Citation: Zhong Y, Chang X, Zhao Z, Zheng L, Kuang G, Li P, Liu C, Fan Y, Liang Z, Zhuang K, Xie Q and Liu Y (2024) Bacteroides fragilis capsular polysaccharide A ameliorates ulcerative colitis in rat by recovering intestinal barrier integrity and restoring gut microbiota. Front. Pharmacol. 15:1402465. doi: 10.3389/fphar.2024.1402465
Received: 17 March 2024; Accepted: 02 October 2024;
Published: 24 December 2024.
Edited by:
Barbara Romano, University of Naples Federico II, ItalyReviewed by:
Vanessa D'Antongiovanni, University of Pisa, ItalyLong Wu, University of Maryland, United States
Copyright © 2024 Zhong, Chang, Zhao, Zheng, Kuang, Li, Liu, Fan, Liang, Zhuang, Xie and Liu. This is an open-access article distributed under the terms of the Creative Commons Attribution License (CC BY). The use, distribution or reproduction in other forums is permitted, provided the original author(s) and the copyright owner(s) are credited and that the original publication in this journal is cited, in accordance with accepted academic practice. No use, distribution or reproduction is permitted which does not comply with these terms.
*Correspondence: Yijia Zhong, enlqOTkwOEBxcS5jb20=; Qiuling Xie, Mjk0NTQyMzY5QHFxLmNvbQ==; Yangyang Liu, bGl1eWFuZ3lhbmdAenlwaGFybS5jb20uY24=