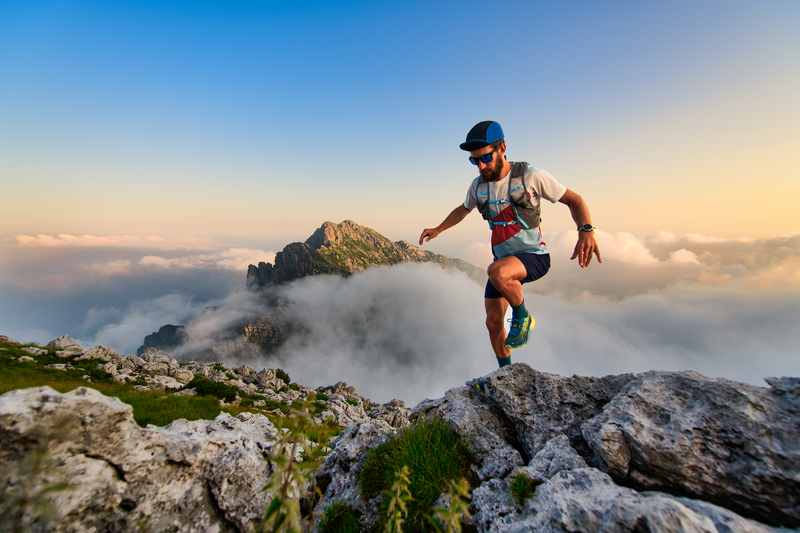
95% of researchers rate our articles as excellent or good
Learn more about the work of our research integrity team to safeguard the quality of each article we publish.
Find out more
REVIEW article
Front. Pharmacol. , 23 July 2024
Sec. Ethnopharmacology
Volume 15 - 2024 | https://doi.org/10.3389/fphar.2024.1399598
The liver, a complex parenchymal organ, possesses a distinctive microcirculatory system crucial for its physiological functions. An intricate interplay exists between hepatic microcirculatory disturbance and the manifestation of pathological features in diverse liver diseases. This review updates the main characteristics of hepatic microcirculatory disturbance, including hepatic sinusoidal capillarization, narrowing of sinusoidal space, portal hypertension, and pathological angiogenesis, as well as their formation mechanisms. It also summarized the detection methods for hepatic microcirculation. Simultaneously, we have also reviewed the characteristics of microcirculatory disturbance in diverse liver diseases such as acute liver failure, hepatic ischemia-reperfusion injury, viral hepatitis, non-alcoholic fatty liver disease, hepatic fibrosis, hepatic cirrhosis, and hepatocellular carcinoma. Finally, this review also summarizes the advancement in hepatic microcirculation attributed to traditional Chinese medicine (TCM) and its active metabolites, providing novel insights into the application of TCM in treating liver diseases.
The liver is a crucial metabolic organ within the human body, serving various physiological functions, including macronutrient metabolism, blood volume regulation, blood sugar regulation, immune system support, endocrine control of growth signaling pathways, lipid and cholesterol homeostasis, and the breakdown of xenobiotic metabolites (Trefts et al., 2017; Zhang et al., 2019). The liver’s microcirculatory system comprises structures such as the hepatic artery, portal vein, hepatic sinusoids, and central vein. It features a unique dual blood supply system, with blood sourced from terminal branches of the portal vein and hepatic artery flow into the hepatic sinusoids, accompanied by the hepatic bile duct and lymphatic vessels, ultimately flowing into the central vein (Burchill et al., 2019; Torres Rojas and Lorente, 2023). Hepatic microcirculation, the crucial role in the overall physiology and function of the whole organism, supplies oxygen and nutrients to the substantial tissues and clears toxicants and foreign bodies from the bloodstream. However, various factors such as emotional stress (Li et al., 2013), medication (Wang et al., 2020), alcohol (Han et al., 2023) and virus (Orabueze et al., 2024) can disrupt the complex microcirculation in the liver, leading to liver ischemia, hypoxia, and metabolic disturbance, which in turn leads to diseases such as acute liver failure (ALF) (Gurakar et al., 2024), non-alcoholic fatty liver disease (NAFLD) (Pan et al., 2021), alcoholic liver disease (ALD) (Han et al., 2023), viral hepatitis (Kao et al., 2021), hepatic cirrhosis (Davies et al., 2017), and hepatocellular carcinoma (HCC) (Rumgay et al., 2022). Therefore, improving hepatic microcirculation has become a promising way to prevent and treat liver diseases.
At present, western medical approaches in treating hepatic microcirculatory disturbance include drug therapy and surgical treatment, yet all these methods possess certain limitations and side effects. In terms of drug therapy, vasoactive modulators or anticoagulants are the first choice to treat hepatic microcirculation, such as β Receptor blockers, rivaroxaban, and aspirin, which can cause symptoms like dizziness, hypotension, and gastrointestinal bleeding (Guixé-Muntet et al., 2020; Zhang et al., 2022). Surgical treatment, such as interventional therapy based on catheters, like transjugular intrahepatic portosystemic shunt (Jin and Zhang, 2024), can improve liver blood flow and alleviate portal hypertension but also come with risks like bleeding, infection, and embolism. Surgical removal (Pan et al., 2024) or transplantation of the liver (Lieber et al., 2024) could eliminate diseased tissue or restore liver function. Nevertheless, these surgical procedures carry significant risks, including trauma, bleeding, infection, and rejection. Hence, implementing secure and potent strategies to ameliorate hepatic microcirculation is critical for preventing and treating liver diseases. Traditional Chinese medicine (TCM) possesses multi-metabolites, multi-targets, and multi-pathways characteristics that comprehensive regulation and personalized treatment of patients, with significant advantages in regulating hepatic microcirculatory disturbance (Han et al., 2017). In this review, we aim to summarize the pathological characteristics and detection methods of hepatic microcirculatory disturbance, the characteristics of hepatic microcirculatory disturbance in diverse liver diseases as well as the potential therapeutic effects of TCM on treating diverse liver diseases by modulating hepatic microcirculatory disturbance, providing reference and inspiration for clinical and scientific research.
The hepatic microcirculation is a highly complex and coordinated system that involves the synergistic effect of various cell types, such as hepatic stellate cells (HSCs), liver sinusoidal endothelial cells (LSECs), and Kupffer cells (KCs) (Gracia-Sancho et al., 2019). Its main goal is to maintain the homeostasis of liver metabolism and immune function (Figures 1A, B). However, when hepatic microcirculation experiences disruption, it can develop characteristics such as hepatic sinusoidal capillarization (Zhang et al., 2022), narrowing of sinusoidal space (Mitten et al., 2023), portal hypertension (Iwakiri and Trebicka, 2021), and pathological angiogenesis (Li et al., 2023). Therefore, a deeper understanding of these characteristics can help reveal the complexity of hepatic microcirculation and provide crucial insights into the pathogenesis of liver diseases.
Figure 1. Structures and characteristics of normal hepatic microcirculation and hepatic microcirculatory disturbance. (A) Schematic diagram of the liver composed of hepatic lobules. (B) Normal hepatic sinusoidal microcirculation. (C) characteristics of hepatic microcirculatory disturbance. (1) Hepatic sinusoidal capillarization, (2) narrowing of sinusoidal space, (3) portal hypertension, and (4) pathological angiogenesis. This figure is created with biorender.com.
The hepatic sinusoid is a particular type of capillary located between adjacent liver plates, which is the histological basis for normal material exchange between blood and liver cells. Its inner wall has a layer of LSECs, the liver’s highest proportion of non-parenchymal cells (Gracia-Sancho et al., 2021). The normal morphology and function of the hepatic sinusoid play a crucial role in maintaining liver physiological function. Prolonged damage may result in hepatic sinusoidal capillarization, marked by the loss of fenestrae in LSECs and the formation of an endothelial basement membrane (Zheng et al., 2020) (Figure 1C-1). The phenomenon of hepatic sinusoidal capillarization occurs in a variety of liver diseases, including hepatic fibrosis (Zhang et al., 2022), cirrhosis, and HCC (Fu et al., 2023). Therefore, an in-depth understanding of hepatic sinusoidal capillarization is crucial for understanding liver disease pathogenesis.
Fenestrae are distinctive structures in LSECs, with diameters ranging from 50 to 100 nm, creating relatively wide intercellular gaps that allow passive transport of solutes, large molecules, and particles between the hepatic sinusoidal lumen and hepatocytes (Shetty et al., 2018). Various factors, including drugs, hormones, diseases, and aging, can impact the diameter, porosity, and frequency of fenestrations in LSECs (Szafranska et al., 2021). Studies have shown that activated KCs can secrete various signaling molecules such as reactive oxygen species (ROS), nitric oxide synthase (NOS), platelet-derived growth factor (PDGF), transforming growth factor (TGF)-β, monocyte chemoattractant protein-1 (MCP-1), tumor necrosis factor-α (TNF-α), and interleukins (ILs) to induce the activation of HSCs (Yang et al., 2009; Yoshizawa et al., 2022; Blas-García and Apostolova, 2023). Furthermore, the release of ROS, vascular endothelial growth factor (VEGF), lipid peroxide, TGF-β, PDGF and ILs from hepatocytes has also reported to induce the activation of HSCs. Subsequently, HSCs are converted into myofibroblasts, producing collagen and extracellular matrix (ECM), leading to a decrease or disappearance of fenestrae in LSECs (Blas-García and Apostolova, 2023). Thrombospondin-1 (TSP-1) secreted by activated HSCs play a pivotal role in regulating the contractile force and tension of LSECs through cytoskeletal signaling pathways, ultimately resulting in the closure and disappearance of fenestrae (Venkatraman and Tucker-Kellogg, 2013). Moreover, CD47-mediated downregulation of the endothelial nitric oxide synthase-nitric oxide (eNOS-NO) signaling pathway inhibits nitric oxide (NO) levels and induces contraction of LSECs, thereby leading to disappearance of fenestrae (Koch et al., 2021; Bian et al., 2022). Leukocyte cell-derived chemotaxin 2 (LECT2) bind to the tyrosine kinase receptor (Tie1) on the surface of LSECs, affecting the quantity and size of fenestrae (Du and Wang, 2022). During the early stages of hepatic sinusoidal capillarization, activated phagocytic cells migrate into the hepatic sinusoids, causing narrowing or obstruction of the sinusoids and reducing the number of fenestrae in LSECs, as well as decreasing fenestrae diameter (Cai et al., 2021). Furthermore, hepatocytes in iron-overloaded mice exhibit high expression of nerve growth factor (NGF), which actively induces the closure of fenestrae in LSECs by interacting with the NGF receptor Tropomyosin receptor kinase A (TrkA) (Addo et al., 2015).
A basement membrane between endothelial cells and hepatocytes is absent in normal liver tissue, providing high permeability to LSECs. The formation of basement membrane may result from HSCs activation and ECM deposition during the liver injury (Ortiz et al., 2021). Laminin and collage Type IV, the main components of the basement membrane, are deposited in the space of Disse. Nidogen-1 is mainly deposited in the parenchymal area around the portal ducts, and perlecan is mainly distributed in the basement membrane of the portal bile ducts and vascular structures and the hepatic sinusoidal wall. Other minor basement membrane molecules, such as Col XVIII, can be found in the space of Disse (Mak and Mei, 2017).
Research has demonstrated that numerous medications actively regulate hepatic sinusoidal capillarization to exert hepatoprotective effects. For example, Hunt et al. (2019) found that NO donors, including sildenafil, amlodipine, and nitroglycerin, can increase the porosity of LSECs. Through the eNOS and AMP-activated protein kinase pathways, metformin sulfate may improve insulin resistance, increase the porosity of LSECs fenestrae in both young and elderly animals, and slow down the aging process (Hunt et al., 2020).
Under normal circumstances, the diameter of hepatic sinusoids remains relatively stable, ensuring sufficient blood flow through the liver to maintain normal physiological function. However, when the sinusoidal space narrows, this passage becomes restricted, impeding blood flow (Mitten et al., 2023). Narrowing of sinusoidal space is a common pathological feature observed in viral hepatitis (Maini and Peppa, 2013), NAFLD (Mitten et al., 2023), and cirrhosis (Shenoda and Boselli, 2019). Research suggests that fibrosis regulation and contractile factors play a critical role in narrowing of sinusoidal space (Figure 1C-2). Activated HSCs release the ECM, leading to fibrosis and scar formation, and they mechanically compress the hepatic sinusoids, narrowing of sinusoidal space (Shenoda and Boselli, 2019). Vasoconstrictors, such as norepinephrine, angiotensin II (Ang II), and endothelin, can also cause constriction of the vessels surrounding the hepatic sinusoids and the small branches of the hepatic veins, thereby reducing the diameter of the hepatic sinusoids (Shenoda and Boselli, 2019; Rajapaksha et al., 2021).
Research on drug formulations for widening the hepatic sinusoidal spaces still needs to be completed. The primary solution is to address the root causes of hepatic sinusoidal space constriction, such as vascular obstruction. For instance, sildenafil can reduce platelet aggregation markers CD41 and P-selectin, mitigate LSECs dysfunction and endothelial barrier damage associated with sinusoidal obstruction syndrome, thereby improving blood hypercoagulability and contributing to alleviating hepatic sinusoidal stenosis (Mansour. et al., 2021).
Portal hypertension is an essential manifestation of microcirculation disturbance in diseases such as NAFLD (Baffy, 2018), hepatic fibrosis (Torres Rojas and Lorente, 2023), cirrhosis (Iwakiri and Trebicka, 2021), and is also one of the main complications of chronic liver disease. The primary cause of portal hypertension is a pathological increase in intrahepatic vascular resistance (Gracia-Sancho et al., 2019). The activation of HSCs and the function of LSECs directly contribute to the formation of portal vein hypertension (Figure 1C-3). Research has found that impaired function of LSECs results in decreased of NO synthesis, disappearance of fenestrae, increase synthesis of microvilli, adhesion molecule and basement membrane, resulting in a decrease in hepatic sinusoidal permeability, changes in hemodynamics and vascular tension, ultimately leading to the development of portal hypertension (Schierwagen et al., 2020; Iwakiri and Trebicka, 2021). Additionally, portal hypertension is also related to HSCs contraction, which is mainly due to factors such as endothelin and catecholamines acting on G protein-coupled receptors (GPCRs) or the RhoA/Rho kinase pathway, inhibiting myosin light chain phosphatase (MLCP) to further constrict HSCs, thus leading to hepatic sinusoidal stenosis and inducing portal hypertension (Iwakiri and Trebicka, 2021).
Nowadays, research has demonstrated that some medications, including tofogliflozin, possess the effects to suppressed Ac-HSC-stimulated capillarization and vasoconstriction in LSECs by enhancing the antioxidant capacity, as well as to inhibit the capillaries LSEC-stimulated contractive, profibrogenic and proliferative activities of Ac-HSCs, thus prevention portal hypertension (Asada et al., 2024).
Angiogenesis is the dynamic process of generating new blood vessels from existing vessels, and this microcirculatory disturbance mainly occurs in HCC (Fu et al., 2023). There are two primary modalities: budding and invagination. Either way, it requires the formation of a lumen in the nascent vessel and stabilization of the nascent vessel by structures such as the basement membrane, smooth muscle cells, and peripheral nerves (Thabut and Shah, 2010). Evidence suggests that LSECs and HSCs are crucial to hepatic angiogenesis (Figure 1C-4). HSCs can directly wrap around newly formed blood vessels, providing stability and durability to prevent the collapse or degradation of blood vessels composed by LSECs. Conversely, HSCs can release pro-angiogenic substances like VEGF and angiopoietin-1, which activate LSECs to create a favorable sinusoidal environment for angiogenesis. Moreover, LSECs can also release NO, TGF-β, and PDGF to encourage HSCs migration toward neovascularization (Thabut and Shah, 2010).
Several drugs have demonstrated the ability to inhibit abnormal neovascularization. Sorafenib, for instance, reduces angiogenesis by inhibiting hypoxia-inducible factor-1α (HIF-1α) and VEGF protein expression (Liu et al., 2012). By interfering with the vascular supply, lidocaine can prevent neovascularization (Suzuki et al., 2020).
The microstructure and blood flow influence the liver’s overall function. Any abnormalities in the liver’s structure or blood flow can directly impact its blood supply and oxygen delivery, impairing its function. Consequently, employing specific methods to examine the microstructure and blood flow of the liver holds excellent significance in gaining insights into the liver’s pathophysiological processes.
Pathological examination is a crucial detection technique that permits the observation of alterations in tissue or cell morphology, structure, and function. It facilitates the evaluation of the extent and mechanisms of hepatic microcirculatory changes by examining the steady state of the liver’s microstructure. H&E staining (Cardiff et al., 2014) is one of the crucial methods for diagnosing tissue diseases, as well as simple staining techniques by using two dyes, hematoxylin and eosin, to label the nuclei and cytoplasm of liver tissue. Using this technique, researchers could observe the structure of liver lobules, including hepatocytes, central veins, portal veins, and hepatic artery branches (Boyd et al., 2020). However, H&E staining only focuses on cellular morphological features without providing information regarding intracellular molecular expression and function. Furthermore, it cannot differentiate between components such as proteins, nucleic acids, or polysaccharides within tissues. Additionally, it is unable to depict cellular organelle structures and to be proceed with the in vivo observation.
The immunofluorescence technique utilizes fluorescently labeled antibodies as probes to detect the expression of specific antigens within liver tissue or cells, followed by scanning and imaging using fluorescence microscopy or confocal imaging. This method enables us to observe the localization of HSCs, hepatocytes, bile duct epithelial cells, KCs, LSECs, or proteins and to understand the patterns of cellular morphological changes (He et al., 2023). Immunofluorescence technology offers the advantage of detecting expression of target molecules with strong specificity and achieving multi-color fluorescence staining to detect the position and interrelationships of target molecules. However, it typically cannot be carried out directly in living animals or cells, and fluorescent dyes may gradually quench due to prolonged exposure, thus limiting observation time and continuity.
FISH utilizes the principle of complementary base pairing to hybridize fluorescently labeled probes with target DNA following denaturation and renaturation, enabling direct visualization of the target DNA’s location using fluorescence microscopy, confocal microscopy or other equipment. The location of the periportal vein regions, central vein regions, or cells and the status of hepatic sinusoid damage (Ben-Moshe et al., 2022) could be observed by using FISH to label specific target genes in hepatic microcirculation. FISH offers high resolution and strong specificity, achieving visualization of the distribution and expression of target genes. However, this method entails complex sample processing to maintain tissue structural integrity and stability. Additionally, it may lack sufficient sensitivity for detecting genes with low expression levels.
While H&E staining provides clear insight into the macroscopic morphology and structure of the liver, it falls short of revealing its nanoscale structure. To address these limitations, researchers utilize SEM to observe the liver microstructure. SEM operates on the principle of using electron beams to scan the sample’s surface, generating high-resolution images. Within liver tissue, SEM enables the observation of various microstructures, including hepatocytes, endothelial cells, KCs, leukocytes, fenestrae, filopodia, and collagen fibers. These observations could directly reflect microscopic spatial morphological changes within the liver (Nafady et al., 2017). However, it is essential to note that SEM imposes stringent requirements on sample preparation. Damage to tissue samples must be meticulously avoided during fixation, dehydration, drying, gold-plating, and other processes, as any such damage can significantly impact microstructure observations. Additionally, SEM is restricted to capturing two-dimensional surface information of the sample and cannot provide a clear view of organelle structures or three-dimensional arrangements.
It is essential to explore the changes in subcellular structures within cells. TEM employs electron beams to penetrate samples, generating high-resolution, high-contrast images. It allows for a detailed examination of the ultra-microstructure of the liver, including mitochondria, cell nuclei, rough endoplasmic reticulum, microvilli, Disse space, lysosomes, vesicles, and collagen deposition (Nafady et al., 2017). Nonetheless, TEM presents challenges in sample preparation, risking potential damage. Prepared samples must endure vacuum conditions and exposure to high-energy electron beams. Additionally, TEM is limited to observing thin tissue sections, lacking the ability to detect the tissue’s three-dimensional structure.
Color Doppler ultrasound is a better way to visualize the shape and function of blood vessels and blood flow characteristics (Zhang and Han, 2021). The principle of color Doppler ultrasound relies on the Doppler effect, which means that when ultrasound encounters moving erythrocytes, the frequency will change, reflecting the direction, speed, and distribution of blood flow. Color Doppler ultrasound codes blood flow in different directions and speeds with different colors. Then, it superimposes them on the two-dimensional image to form a color Doppler ultrasound blood flow image. This technique could help to identify vessels, assesses blood flow as well as detect the hemodynamic characteristics of the hepatic artery, portal vein, and hepatic vein (Tanaka, 2020). It can visually display the vascular structure and hemodynamic characteristics in liver, which is of great value in diagnosing liver lesions. However, color Doppler ultrasound still possesses certain limitations in detecting deep tissues.
Researchers have developed a laser speckle imaging system with a higher spatial resolution to capture more detailed and comprehensive blood flow information (Li et al., 2021). Exposing tissue containing flowing blood cells to laser irradiation generates random interference speckle patterns, which underlie the principle behind this technology. These speckle patterns change with variations in blood flow. Analyzing temporal and spatial changes in speckle patterns enables the acquisition of information on blood flow velocity and distribution. This breakthrough makes it possible to visualize blood flow conditions in real-time, particularly in liver diseases like NAFLD (Pan et al., 2021). Remarkably, however, the laser speckle imaging system is limited to detecting blood flow at a depth of 1 mm below the tissue surface.
Whereas the laser speckle imaging system offers benefits like non-contact, non-invasive, and rapid imaging, it suffers from low resolution in deep tissues, making it challenging to distinguish individual blood vessels. In contrast, two-photon imaging employs two low-energy infrared photons to achieve deep three-dimensional imaging in living tissues, with depths of up to 250–500 μM or exceeding 1 mm. Researchers can utilize this instrument to observe the inner diameter of liver sinusoids and calculate the blood flow velocity in the sinusoids based on distance-time images (Fan et al., 2019). An advantage lies in using near-infrared light, which causes less damage to biological tissues, allowing for long-term, high-resolution functional imaging of living tissues. Nevertheless, this technology presents challenges, including demanding design requirements for fluorescent probes, high equipment costs, and the need for objective quantitative analysis methods and standardized evaluation criteria, which require further research and validation to address these limitations.
SMI technology is a cutting-edge hepatic microcirculation detection method with the advantages of non-invasive, radiation-free and no contrast agent requirement. It employs intelligent filtering to isolate very low-speed (min. 0.8 cm/s) blood flow signals from tiny vessels (diameter >0.1 mm) in liver lesions. This technology can help to identify benign/malignant liver lesions, assess tumor metastasis, and gauge liver function and perfusion (He et al., 2017). However, the main drawback of SMI is its limit ability to quantify blood flow velocity, which cannot provide parameters such as blood flow velocity, direction, and resistance index. There is still a need for clinical validation and standardized assessment methods. Further research and verification are required to establish the diagnostic efficacy and clinical significance.
Single-cell sequencing is a genomics approach that relies on comprehensive, high-throughput genomic analysis of individual cells through RNA amplification technology. It detects gene expression patterns and transcriptome features of individual cells, thereby annotating multiple cell types and providing insight into the diversity of cellular states. For example, researchers achieved to obtain transcriptional profiles of 20 discrete cell populations, including hepatocytes, endothelial cells, cholangiocytes, HSCs, B-cells, T-cells and NK cells by single-cell sequencing. This comprehensive analysis delineates the characteristics of the resident cells in the liver and provides a detailed map of the immune microenvironment of the human liver (MacParland et al., 2018). Analyzing the gene expression of different cell types in the hepatic sinusoidal microcirculation enables the identification of changes in the expression patterns of specific genes and cellular subpopulations associated with abnormal microcirculatory function. This capability aids in uncovering the underlying biological mechanisms of microcirculatory disturbance, providing an essential basis for diagnosing and treating related diseases. Despite the advancements in single-cell sequencing technology, challenges persist in processing and analyzing large datasets. Furthermore, it still needed to elucidate global transcriptional differences across lobular units in physical space.
Spatial transcriptomics is a genomic approach based on detecting gene expression and spatial location information on tissue sections through the combination of spatial localization technology and high-throughput RNA sequencing to reveal the distribution of cell types in tissues and the interactions between adjacent cells. Researchers have conducted studies to spatially annotate the hepatic sinusoids at the level of the liver lobules using spatial transcriptomics to classify the hepatic sinusoids into periportal, intermediate, and pericentral venous zones and to compare the overall transcriptional differences in the hepatic lobular axis (Hildebrandt et al., 2021). This approach can provide a crucial spatial analysis tool for revealing the pathophysiological mechanisms of microcirculatory disturbance and help to develop precise therapeutic strategies for related diseases. However, it still needs to be continuously improved in sample processing and imaging resolution to enhance the efficiency and accuracy of its application in the study of hepatic sinusoidal microcirculation.
Beyond these methods, various approaches can be used for observing and evaluating hepatic microcirculation, such as immunoelectron microscopy (Yokomori et al., 2015), perfusion-weighted MRI (Ding et al., 2021), computed tomography (CT) angiography (Kim J. S. et al., 2022), hepatic arteriography (Murata et al., 2014), inverted intravital microscope (Mu et al., 2018), laser Doppler flowmetry (Papagiouvanni et al., 2022), CT perfusion imaging (Brehmer et al., 2018), contrast-enhanced Ultrasound (CEUS) (Pang et al., 2018) (Table 1). Each has unique advantages and disadvantages. In practice, the choice of detection method should align with specific needs to obtain precise and comprehensive information about hepatic microcirculation.
The liver’s normal function relies on the integrity and stability of hepatic microcirculation. Nonetheless, various factors such as viral infections, alcohol consumption, hypoxia, and ischemia can disrupt hepatic microcirculation, leading to conditions such as acute liver failure, viral hepatitis, alcoholic liver disease, and hepatocellular carcinoma. This section will summarize the characteristics of microcirculatory disturbance in various liver diseases.
ALF refers to a large amount of hepatocyte necrosis, apoptosis, and severe liver dysfunction occurring within a short period in the absence of underlying liver disease, characterized by severe coagulation dysfunction and encephalopathy. Liver transplantation is the ultimate curative option for ALF. Although in some cases, spontaneous regeneration is possible if the patient is managed conservatively in intensive care units (Ribaud et al., 2022). Microcirculatory disturbance in ALF manifests through various abnormalities, including HSCs and KCs activation, fibrin deposition, and thrombosis (Figure 2A). Firstly, study has elucidated the pivotal role of HSCs in ALF, in which HSCs relays inflammation signaling from sinusoids to parenchyma via the secretion of inflammatory cytokines. Conversely, HSCs aid in liver regeneration by releasing growth factors and maintaining hepatocyte attachment and liver tissue architecture via ECM production (Li et al., 2019). Additionally, study also have revealed that activated KCs contribute to ALF by secreting chemokines that recruit monocytes, neutrophils, and cytokines, thus exacerbating inflammation and sensitize hepatocytes to apoptosis. Neutrophils and monocyte-derived macrophages also could secrete cytokines and pro-angiogenic factors (Krenkel et al., 2014; Kolodziejczyk et al., 2020). In ALF, damage to LSECs, characterized by degeneration, necrosis, and detachment, triggers fibrin deposition in liver sinusoids and platelet aggregation, contributing to intravascular coagulation (Hirata et al., 1989).
Figure 2. Microcirculatory disturbance in liver diseases. (A) Shows microcirculatory disturbance in acute liver failure; (B) Shows microcirculatory disturbance in hepatic ischemia-reperfusion injury; (C) Shows microcirculatory disturbance in viral hepatitis, (D) Shows microcirculatory disturbance in non-alcoholic fatty liver disease; (E) Shows microcirculatory disturbance in alcoholic liver injury; (F) Shows microcirculatory disturbance in hepatic fibrosis and cirrhosis, and (G) Represents microcirculatory disturbance in hepatocellular carcinoma. This figure is created with biorender.
HIRI is a pathological condition characterized by hepatocyte damage and inflammation triggered by transient ischemia and subsequent restoration of blood flow, commonly occurring during surgical procedures or transplantation (Nakamura et al., 2019). Recent investigation has demonstrated that the administration of prostaglandin (PG)E1 can ameliorate microcirculation dysfunction in hepatic I/R syndrome by expanding blood vessels and enhancing perfusion status (Mouratidou et al., 2023). The primary manifestations of hepatic microcirculatory disturbance in HIRI encompass abnormal hemodynamics, hepatocyte necrosis and apoptosis, activation of KCs and HSCs (Figure 2B). During HIRI, notable sinusoidal congestion occurs, accompanied by edema, deformation, necrosis and apoptosis of KCs, LSECs and hepatocytes. Additionally, hepatocytes may display cytoplasmic vacuolization, while LSECs may exhibit plasma membrane rupture, nuclear membrane vacuolization and cell morphological changes, collectively contributing to the narrowing of sinusoidal space (Peralta et al., 2013; Sheng et al., 2015; Mu et al., 2018). In terms of inflammation, upregulation of nuclear factor-κB (NF-κB) p65 acetylation occurs, along with KCs and neutrophils releasing inflammatory mediators and ROS. This cascade promotes neutrophil recruitment and adhesion to sinusoids, disrupting LSECs and microvascular integrity (Peralta et al., 2013; Tao et al., 2014; Cannistrà et al., 2016). Activated HSCs release cytokines, rho-associated kinase, endothelin-1 (ET-1) and matrix metalloproteinases (MMPs), thereby stimulating the recruitment of CD4+ T cells to the injury site and increasing the quantity and activity of inflammatory factors (Peng et al., 2022). Under the stimulation of pro-inflammatory cytokines, HSCs are activated and differentiate into myofibroblasts, thereby inducing fibrosis through ECM deposition (Liu et al., 2019). Researchers have observed a significantly reduction in the number and proportion of hepatocytes following HIRI injury, with the pericentral venous zone exhibiting heightened sensitive to HIRI injury by using spatial transcriptomics. Furthermore, enrichment of endothelial cells, epithelial cells and HSCs was observed in the periportal zone, revealing pericentral zone-specific injury-related change in differentially expressed genes, cellular composition and functional pathways following HIRI injury (Xin et al., 2023).
Viral hepatitis refers to liver inflammation caused by different types of viruses, such as hepatitis A virus (HAV), hepatitis B virus (HBV) and hepatitis C virus (HCV). Initially, interferon therapy was utilized following the identification of viral hepatitis. However, due to its limited efficacy and significant side effects, treatment strategies later transitioned towards antiviral medications (Bush et al., 2023). The pathogenesis of hepatic microcirculatory disturbance in viral hepatitis involves coagulation dysfunction, capillarization and HSCs activation (Figure 2C). Studies has found that HBV and HCV primarily attack hepatocytes, where they replicate and release extensively (Maini and Peppa, 2013; Klenerman and Ramamurthy, 2015). HBV infection can cause narrowing of sinusoidal space and the decreased of blood flow (Maini and Peppa, 2013). In advanced stages of the HBV infection, HSCs activation is induced through endoplasmic reticulum stress and ferroptosis pathways, ultimately leading to fibrosis (Kuo et al., 2020). Similarly, During HCV infection, elevated thrombin levels, along with the presence of numerous thrombi in the portal vein, lead to HSCs activation and hepatic fibrosis progression (González-Reimers et al., 2016). In the early stages of infection with mouse hepatitis virus type 3, microthrombi form occurs in the portal vein and periportal sinusoids, resulting in obstruction of sinusoidal blood flow. Subsequently, during the mid-stage of viral infection, hepatocyte swelling ensues, causing altered blood flow patterns from damaged to undamaged areas. Finally, in the late stage of viral infection, extensive thrombus formation and hepatocyte death occur (Levy et al., 1983).
NAFLD is characterized by the excessive accumulation of fat in the liver, in the absence of alcohol abuse or other clear causes. Despite its prevalence, there are currently no approved pharmacological interventions for NAFLD (Pereira et al., 2022). Notably, simvastatin has shown to improve microcirculatory function in NAFLD by mitigating oxidative and advanced lipoxidation end product–receptors of advanced glycation end products (ALE-RAGE) stress, while also ameliorating steatosis, fibrosis and inflammatory markers (Pereira et al., 2022). Microcirculatory disturbances in NAFLD are typified by the activation of HSCs and KCs, along with the narrowing of sinusoidal space and diminished blood flow (Figure 2D). Studies have indicated that a high-fat diet can lead to structural and functional alterations in LSECs, activation of HSCs, enhanced release of NO by KCs, as well as increased adhesion of macrophages and monocytes within the hepatic sinusoids, exacerbating oxidative stress. Additionally, it induces hepatocyte enlargement and deposition of ECM into the Disse space, thereby inducing liver microcirculatory damage and a 42% reduction in hepatic blood flow (Pereira et al., 2017; Nasiri-Ansari et al., 2022). Moreover, excessive triglycerides (TG) accumulation can also induce swelling and apoptosis of hepatocyte, and platelet aggregation, further contributing to the narrowing of sinusoidal space, and reduced blood flow (Fan et al., 2019; Miao et al., 2024). High-fat diets also elevate liver vascular tension and perfusion while impairing endothelial dilation response to acetylcholine (Ach), thereby disrupting normal blood flow dynamics (Pasarín et al., 2012). As NAFLD progresses, disappearance of LSECs fenestrae, formation of the basement membrane forms and extensive ECM deposition occurs in the Disse space, ultimately leading to fibrosis (Baffy, 2018).
ALD is a liver disorder caused by prolonged and excessive alcohol consumption. While modest alcohol intake can appropriately increase liver blood flow, acute alcohol consumption can cause hepatic microvascular dysfunction, exacerbating gut I/R-induced hepatic microvascular dysfunction and subsequent liver injury (Israel and Orrego, 1987; Horie and Ishii, 2001). Although glucocorticoids and hepatic protectants have received FDA approval for ALD treatment, ongoing debate surrounds their efficacy (Mai et al., 2022). Microcirculatory disturbance in ALD is associated with sinusoidal capillarization, and inflammation (Figure 2E). Researchers have found that hepatocyte enlargement in ALD leads to sinusoidal vessel compression, disrupting erythrocyte circulation within liver sinusoids and impeding hepatic microcirculation (Mak et al., 2022). Ethanol consumption can also modulate the composition of the intestinal microbiota, facilitating the translocation of gut-derived lipopolysaccharide (LPS) and other bacterial products into the portal vein. Subsequent activation of toll-like receptor (TLR) 4 signaling in KCs incites liver inflammation (Inokuchi et al., 2011). Moreover, ethanol intake reduces the number and diameter of fenestrations in LSECs, induces marginal contraction of LSECs, and activates HSCs, leading to increased synthesis of collagen fiber bundles and resultant sinusoidal capillarization (Mak et al., 2022).
Hepatic fibrosis represents an abnormal wound healing response in the liver caused by long-term liver damage from diverse etiologies. Currently, drugs for treating hepatic fibrosis exit limited efficacy and lack clinical and commercial validation. Although several medications, including Selonsertib, Simtuzumab, and GR-MD-02, are undergoing clinical trials for hepatic fibrosis treatment, none have garnered approval (Zhang et al., 2024). Microcirculatory disturbance in hepatic fibrosis mainly manifests as increased immune response, HSCs activation, capillarization and reduced blood flow (Figure 2F). In fibrotic conditions, hepatocytes undergo ballooning degeneration accompanied by degenerative necrosis (Fu et al., 2021). Neutrophils play a pivotal role in the activation of HSCs by generating ROS and myeloperoxidase (MPO). Subsequently, activated HSCs, in turn, secrete cytokines such as granulocyte-macrophage colony-stimulating factor (GM-CSF) and IL-15, thereby promoting neutrophil activation. Additionally, activated HSCs release neutrophil chemotactic factors, recruiting more neutrophils, forming a positive feedback loop that promotes the development of hepatic fibrosis (Tang et al., 2021). KCs contribute to the activation of HSCs and the survival of myofibroblasts by secrete TGF-β1 and PDGF (Krenkel and Tacke, 2017). KCs also release inflammatory factors like IL-1β and chemokines which activate HSCs and recruit other immune cells (Roehlen et al., 2020). An in vitro study indicates that LPS can activate liver TLR4 signaling pathway, transform hematopoietic stem cells into fibroblasts, and produce inflammatory factors like NF-κB to activate HSCs (Liang et al., 2016). Additionally, multiple signaling pathways, including Src/extracellular regulated protein kinases/drosophila mothers against decapentaplegic homolog 3 (Src/ERK/Smad3) (Huang Y. et al., 2023), Sirt1/Notch (Sun et al., 2022), ferroptosis (Huang et al., 2022), and phosphatase and tensin homolog (Geng et al., 2020) are all involved in the activation of HSCs and hepatic fibrosis. Deposition of collagen and other ECM proteins culminates basement membrane and loss of fenestrae, resulting in the capillarization of LSECs (Lafoz et al., 2020; Ma et al., 2021). Imbalances in vasoactive substances like ET-1, AngⅡ, norepinephrine, NO, carbon monoxide, thromboxane A2 (TXA2), PGI2, etc., increase intrahepatic resistance, thereby reducing hepatic sinusoidal blood flow (Huang Q. et al., 2023; Fu et al., 2023; Velez et al., 2024). Furthermore, VEGF and its receptors VEGFR1 and VEGFR2 promote abnormal angiogenesis in hepatic fibrosis (Wang et al., 2021).
Cirrhosis is the later stage of fibrosis. It characterized by portal hypertension leading to gastroesophageal varices and bleeding , which is the primary cause of mortality in cirrhotic patients (Gunarathne et al., 2020). Currently, effective treatments for preventing cirrhosis are lacking, thus the focus is primarily on managing liver diseases and their associated complications (Mendez-Guerrero et al., 2024). Microcirculatory disturbance in hepatic cirrhosis manifest through various abnormalities including abnormal neovascular proliferation, vascular occlusion, HSCs activation, capillarization and thrombosis (Figure 2F). Studies have shown an increase in the number of abnormal hepatic sinusoidal vessels in cirrhotic conditions (Thabut and Shah, 2010). Elevated coagulation factor VIII and decreased anticoagulation factor protein C result in portal and hepatic vein occlusion (Tripodi, 2015). Furthermore, activated KCs in the liver secrete TGF-β and inflammatory factors, contributing to reduced fenestrae in LSECs (Yang et al., 2009). Impaired LSECs function also affects substance exchange within liver sinusoids, leading to increased secretion of vasoconstrictive factors like ET-1, Ang II, PGH2, TXA2, and decreased secretion of vasodilatory factor NO. This imbalance induces pericellular contraction and thrombus formation, subsequently elevated intrahepatic pressure (Bosch et al., 2015; Gracia-Sancho et al., 2019; Gunarathne et al., 2020). Moreover, aberrant LSECs’ function reduces sinusoidal permeability, leading to hepatic ischemia, which further stimulates HSCs to secrete collagen, thereby exacerbating fibrosis (Thabut and Shah, 2010; Greuter and Shah, 2016; McConnell and Iwakiri, 2018). Recent advancements utilizing single-cell sequencing have identified a novel subpopulation of scar-associated TREM2+CD9+ macrophages with pro-fibrotic effects in cirrhotic disease. Additionally, researchers have defined novel ACKR1+ and PLVAP+ endothelial cells that expand in cirrhosis, are topographically scar-restricted, and enhance leucocyte transmigration (Ramachandran et al., 2019).
HCC primarily evolves from chronic viral hepatitis, cirrhosis, prolonged alcohol abuse and other liver diseases. Mainstream medicine employs various strategies for HCC treatment including surgical resection, liver transplantation, radiofrequency, chemotherapy and targeted molecular therapy (Kim et al., 2022). Microcirculatory disturbance in HCC involves not only capillarization and activation of KCs but also neovascularization and the formation of platelet-tumor cell aggregates (Figure 2G). In the development of HCC, tumor suppressor mechanisms are inhibited, leading to an increased risk of carcinogenesis via DNA mutations. Chronic hepatitis-induced hepatic sinusoidal inflammation and ROS contribute to increased DNA damage and proliferation of cancer cells (Gibert-Ramos et al., 2021). During the cirrhotic stage, the accumulation of matrix cells promotes the proliferation of HCC cells (Baglieri et al., 2019). Simultaneously, HSCs produce growth differentiation factor 15 (GDF15) through autophagy-dependent pathways, further promoting the proliferation of HCC cells (Myojin et al., 2021). Extensive fibrosis and capillarization inducing hypoxia in liver sinusoids, enable KCs to release various chemokines and cytokines. Through the HIF-1 pathway, they can secrete PDGF-β, VEGF, angiopoietin-1 (ANG-1), and MCP-1, thereby impairing the function of CD8+ T cells and dampening their anti-tumor effects, ultimately promoting tumor growth and metastasis (Liu et al., 2011; Gibert-Ramos et al., 2021). As the tumor progresses, tumor and surrounding cells secrete VEGF, fibroblast growth factor (FGF), PDGF and other angiogenic factors. These factors stimulate the proliferation, migration and differentiation of endothelial cell, thereby forming new capillaries and creating a microenvironment favorable for tumor cell proliferation (Li, 2016). Midkine (MK) can modulate NF-κB and promote the expression of VEGF, Ang-2, and Tie2 by activating integrin α4 (Itgα4) through autocrine signaling, inducing pathological angiogenesis (Fu et al., 2023). Tumor cells shed from the primary focus into the bloodstream can induce platelets to aggregate on their surfaces, forming platelet-tumor cell aggregates. This process helps tumor cells evade immune system attacks and shear force damage (Kanikarla Marie et al., 2021). Simultaneously, platelet aggregates can carry tumor cells to other organs, facilitating their adhesion to blood vessel walls and promoting tumor metastasis (Kanikarla Marie et al., 2021).
TCM formulas and their active metabolites play potential roles in protecting liver cells and promoting repair and regeneration. They possess the ability to regulate qi and blood, invigorate blood circulation, and remove stasis. These properties have demonstrated significant efficacy in managing hepatic microcirculatory disturbance by increasing blood flow, reducing thrombosis, and inhibiting abnormal angiogenesis and capillarization. Consequently, TCM formulas have exhibited remarkable therapeutic effects in various liver diseases such as ALF, HIRI, NAFLD, hepatic fibrosis, and HCC.
Research has demonstrated the potential of TCM formulas and their active metabolites in regulating hepatic microcirculatory disturbance by reducing thrombosis and increasing blood flow. For example, Shen-Ling-Bai-Zhu-San, a formula traditionally used to tonify the spleen and eliminate dampness, has been shown to improve organelle morphology, reduce hepatocyte necrosis, and alleviate lipid droplet accumulation in hepatocytes of NAFLD. Additionally, liver perfusion measured by a moorFLPI-2 blood flow imager showed significant improvement, possibly regulated by serum adiponectin (Tang et al., 2020; Pan et al., 2021). Active metabolites also play a significant role to these effects. Caffeic acid 1), extracted from Salvia miltiorrhiza Bge. [Lamiaceae; Salviae miltiorrhizae radix et rhizoma], was found to restore hepatic sinusoidal perfusion and erythrocyte velocity in HIRI mice using an inverted intravital microscope and Laser-Doppler Perfusion Imager. It was also reported to reduce leukocyte adhesion, blood cell count, liver lobule distortion, hepatic sinusoidal disturbance, congestion, hepatocellular vacuolization, and necrosis (Mu et al., 2015; Mu et al., 2018). Similarly, plumbagin 2), an active metabolite of Plumbago zeylanica L. [Plumbaginaceae; Plumbago radix et folium], has shown promise in reducing liver thrombosis, inflammatory cell infiltration, and macrophage recruitment in mice with ALF (Wang et al., 2016). Berberine 3) from Coptis chinensis Franch. [Ranunculaceae; Coptidis rhizoma] effectively alleviated HIRI symptoms such as hepatic lobular edema, hemorrhage, deformation and necrosis, inhibited neutrophil inhibited neutrophil infiltration and hepatocyte apoptosis (Sheng et al., 2015). Furthermore, acteoside 4), extracted from Lantana camara L. [Verbenaceae; Lantana radix, folium and flos], was reported to reverse the senescent fate of LSECs, restore sinusoidal networks, as well as ameliorate sinusoidal congestion, vacuolization, hepatocyte necrosis and oxidative stress by targeting the HMGB1-TLR3/4-IRF1 signaling pathway, thus providing protection against HIRI and offering the potential for new therapeutic developments (Jia et al., 2023).
Furthermore, TCM formulas and their active metabolites offer potential in improving hepatic microcirculatory disturbance by regulating abnormal angiogenesis. For instance, formulas like Si-Ni-San, aimed at soothing the liver and resolving stagnation, have been shown to inhibit angiogenesis in hepatic fibrosis tissues, reverse the activation of HSCs, reduce ECM accumulation, and alleviate hepatic fibrosis (Wang et al., 2021). Similarly, Xia-Yu-Xue decoction exerts its anti-angiogenic effects by decreasing the activities of MMPs (MMP-2 and MMP-9), inhibiting HSC activation, and damaging the integrity of new vessels, thus improving hepatic fibrosis (Du et al., 2011). Moreover, Xue-Fu-Zhu-Yu decoction demonstrates inhibitory effects on angiogenesis, hypoxia alleviation, and protective effect on LSECs function, thereby improving hepatic fibrosis (Zhou et al., 2014). The Tao Ren-Hong Hua herb pair can inhibit pathological hepatic angiogenesis, inflammation and fibrosis induced by carbon tetrachloride (CCL4) in chronic liver disease (Xi et al., 2016). Da-Huang-Zhe-Chong pill, which focuses on dispelling pathogenic factors, breaking blood stasis, and promoting blood circulation, can reduce pathological angiogenesis in HCC by inhibiting the MK/Itgα4 signaling pathway (Fu et al., 2023). Yi-Guan-Jian decoction can inhibit liver angiogenesis in cirrhotic mice treated with CCl4 by inhibiting the HIF-1α/VEGF signaling pathway (Zhou et al., 2015). Furthermore, gene Ontology analysis found that Jie-Du-Hua-Yu granule protects against liver failure by negatively regulating angiogenesis, fibrinolysis, and cell shape (Qiu et al., 2019). Yu-Ping-Feng-San attenuates the activation of the thymic stromal lymphopoietin-signal transducer and activator of transcription 3 (TSLP-STAT3) signaling pathway by inhibiting the immune-related factor TSLP, thereby inhibiting the formation of hepatic microvessels and exerting an anti-HCC effect (Yuan et al., 2019). Jie-du recipe may inhibit hypoxia-induced angiogenesis by suppressing IL-8/HIF-1α/phosphatidylinositol-3-kinase (P13k) and mitogen-activated protein kinase (MAPK)/ERK pathways after transcatheter arterial chemoembolization in HCC patients (Lin et al., 2021). Active metabolites such as Amarogentin 5), extracted from Swertia davidii Franch. [Gentianaceae; Swertia davidii Franch herba] can inhibit cancer cell angiogenesis by affecting stemness and the p53-dependent VEGFA/Dll4/Notch1 signaling pathway, thus preventing the malignant transformation of liver cancer cells (Zhang et al., 2020). Levistilide A 6), an active metabolite of Angelica sinensis (Oliv.) Diels. [Apiaceae; Angelicae sinensis radix], can inhibit hepatic fibrosis through anti-angiogenesis by alleviating sinusoid capillarization via the VEGF signaling pathway (Zhao et al., 2017). Hydroxysafflor Yellow A 7) from Carthamus tinctorius L. [Asteraceae; Carthami flos] has the potential to significantly suppress tumor growth by inhibiting the secretion of angiogenesis factors, such as VEGF-A and basic FGF, as well as VEGFR1 (Yang et al., 2015). Additionally, it can also suppress angiogenesis in HCC by regulating the p38 MAPK signaling pathway (Zhang et al., 2019). Oroxylin A 8), an active metabolite of Cutellaria baicalensis Georgi. [Lamiaceae; Scutellariae baicalensis radix], can inhibit hypoxia-induced nuclear translocation of YAP, which may influence the accumulation of HIF-1α and subsequently decrease the transcription of downstream target genes, including VEGF-A and Ang-2, thereby exerting anti-angiogenic activity (Zhang et al., 2018).
In addition, certain active metabolites have been reported to regulate hepatic microcirculatory disturbance by addressing sinusoidal capillarization. For instance, Curcumol 9), an extract of Curcuma longa L. [Zingiberaceae; Curcumae longae rhizoma], has shown to restore microcirculation and improve sinusoidal capillarization in hepatic fibrosis (Zheng et al., 2020).
In summary, TCM formulas (Table 2) and their active metabolites (Table 3; Figure 3) demonstrate promise to improve hepatic microcirculation in diverse liver diseases. They maintain the stable structure of the liver antrum and enhance blood circulation by promoting blood flow and removing blood stasis, thus preventing diseases such as fibrosis, cirrhosis, and HCC. As safe and effective drugs, TCM offers a valuable adjunctive treatment option for patients with liver diseases.
Table 2. Chinese medicinal formulae with potential activity in regulating hepatic microcirculatory disturbance.
Table 3. Active metabolites with potential activity in regulating hepatic microcirculatory disturbance.
Figure 3. The molecular structure of metabolites with activity to improve hepatic microcirculatory disturbance. The numbers within parentheses correspond to the numbers in the main text and table. These chemical structures were plotted using ChemBioDraw Ultra 14.0.
This review provides updated insights into the pathogenic mechanisms underlying hepatic microcirculatory disturbance and the associated detection techniques. It also summarizes the characteristics of hepatic microcirculatory disturbance in various liver diseases and elucidates the regulatory effects of TCM. Therefore, hepatic microcirculatory disturbance plays a crucial role in the pathogenesis of liver diseases and may become an effective approach for the future treatment of liver diseases.
Although significant strides have been made in understanding the mechanisms underlying hepatic microcirculatory disturbance, numerous issues still need to be resolved. Firstly, the detection techniques for hepatic microcirculation cover histopathology, microcirculation detection, and advanced genomic technologies. While these techniques play an important role in detecting hepatic microcirculation, the diversity of detection methods may lead to a lack of standardization, complicating research results. Additionally, it remains unclear whether there are specific pathological changes in hepatic microcirculatory disturbance caused by different etiologies, which requires further research to uncover characteristic markers. Secondly, although various treatment methods are available, their efficacy and safety remain uncertain, especially in long-term management and individualized treatment. More clinical trials and research data are needed to guide clinical practice. Lastly, early diagnosis and prevention of hepatic microcirculatory disturbance are pressing issues, but effective early screening tools and strategies are currently lacking. Therefore, developing new early diagnostic techniques and preventive measures will have a profound impact on improving the quality of life for patients with liver diseases.
Hepatic microcirculatory disturbance is an essential factor leading to the occurrence, development, and worsening of liver disease. It is also the key to preventing and treating liver disease with TCM. TCM emphasizes holistic treatment and syndrome differentiation. It is often used as an alternative or complementary therapy, and combined with Western medicine to maximize the therapeutic effects. TCM shows advantages in treating hepatic microcirculatory disturbance due to its multi-metabolites, multi-targets methods that regulate hepatic hemodynamics and maintain microcirculatory homeostasis. Nevertheless, the application of TCM in treating hepatic microcirculatory disturbance faces several challenges and limitations. Firstly, the complexity of its metabolites poses difficulties in isolating and verifying active metabolites. Secondly, the small sample sizes of TCM clinical trials and lake strict control groups of have led to a lack of clinical evidence. Furthermore, the placebo effect and patient expectancy can also potentially skew the outcomes of some studies. Given the variability in TCM formulas and dosages, efforts to standardized treatment protocols for hepatic microcirculatory disturbance are crucial. To address cultural and regulatory challenges in TCM clinical trials for hepatic microcirculatory disturbance, it’s essential to enhance international cooperation and communication to overcome cultural differences. Additionally, working closely with regulatory agencies and adhering to laws and regulations will improve clinical trials compliance.
In summary, although significant progress has been made in the study of hepatic microcirculatory disturbance, many unknown areas still require further exploration. Future research should employ multidisciplinary collaboration and innovative technologies such as genomics, proteomics, and metabolomics to comprehensively elucidate the molecular mechanisms of hepatic microcirculatory disturbance. Additionally, the use of emerging research tools, such as organoid models (Panwar et al., 2021) to simulate the microenvironment of the human liver will facilitate the pathophysiological research of hepatic microcirculatory disturbance as well as the study of the efficacy and mechanisms of TCM formula and active metabolites, potentially addressing the limitations of traditional clinical research. In addition, utilizing liver-targeted drug delivery systems, such as passive and active targeted drug delivery systems, as well as the physicochemical strategies for targeted drug delivery, can ensure the precise delivery of TCM to the liver (Ma et al., 2019). Finally, exploring new therapeutic strategies, such as gene therapy and cell therapy, promote the development of TCM in treating hepatic microcirculatory disturbance, and enhance the prognosis and quality of life for patients.
PL: Conceptualization, Investigation, Writing–original draft. W-LL: Writing–review and editing, Investigation. R-TH: Investigation, Writing–review and editing. X-XC: Writing–review and editing. D-HZ: Writing–review and editing. HK: Supervision, Writing–review and editing. Y-FL: Funding acquisition, Supervision, Writing–review and editing. Y-HX: Funding acquisition, Supervision, Writing–review and editing. S-HO: Conceptualization, Funding acquisition, Supervision, Writing–review and editing. R-RH: Conceptualization, Funding acquisition, Supervision, Writing–review and editing.
The author(s) declare that financial support was received for the research, authorship, and/or publication of this article. This work was supported by the National Natural Science Foundation of China (T2341004, 82321004, 82174054, 82125038), Guangdong Basic and Applied Basic Research Foundation (2021B1515120023, 2023B1515040016, 2020A1515110596), Guangzhou Basic and Applied Basic Research Foundation (2024A04J4093), Guangdong-Hong Kong-Macao Universities Joint Laboratory for the Internationalization of Traditional Chinese Medicine (2023LSYS002), and Guangzhou Key Laboratory of Traditional Chinese Medicine & Disease Susceptibility (2024A03J090), the GDUPS (2019), Innovation Team Project of Guangdong Provincial Department of Education (2020KCXTD003), Science and Technology Development Fund of Macau (0055/2019/AMJ).
Figures in this review were created using the BioRender platform (https://www.biorender.com/). Thank you for providing exquisite source material.
The authors declare that the research was conducted in the absence of any commercial or financial relationships that could be construed as a potential conflict of interest.
The author(s) declared that they were an editorial board member of Frontiers, at the time of submission. This had no impact on the peer review process and the final decision.
All claims expressed in this article are solely those of the authors and do not necessarily represent those of their affiliated organizations, or those of the publisher, the editors and the reviewers. Any product that may be evaluated in this article, or claim that may be made by its manufacturer, is not guaranteed or endorsed by the publisher.
Addo, L., Tanaka, H., Yamamoto, M., Toki, Y., Ito, S., Ikuta, K., et al. (2015). Hepatic nerve growth factor induced by iron overload triggers defenestration in liver sinusoidal endothelial cells. Biochim. Biophys. Acta 1852 (1), 175–183. doi:10.1016/j.bbadis.2014.11.014
Asada, S., Kaji, K., Nishimura, N., Koizumi, A., Matsuda, T., Tanaka, M., et al. (2024). Tofogliflozin delays portal hypertension and hepatic fibrosis by inhibiting sinusoidal capillarization in cirrhotic rats. Cells 13 (6), 538. doi:10.3390/cells13060538
Baffy, G. (2018). Origins of portal hypertension in nonalcoholic fatty liver disease. Dig. Dis. Sci. 63 (3), 563–576. doi:10.1007/s10620-017-4903-5
Baglieri, J., Brenner, D. A., and Kisseleva, T. (2019). The role of fibrosis and liver-associated fibroblasts in the pathogenesis of hepatocellular carcinoma. Int. J. Mol. Sci. 20 (7), 1723. doi:10.3390/ijms20071723
Ben-Moshe, S., Veg, T., Manco, R., Dan, S., Papinutti, D., Lifshitz, A., et al. (2022). The spatiotemporal program of zonal liver regeneration following acute injury. Cell Stem Cell 29 (6), 973–989.e10. doi:10.1016/j.stem.2022.04.008
Bian, H. T., Shen, Y. W., Zhou, Y. D., Nagle, D. G., Guan, Y. Y., Zhang, W. D., et al. (2022). CD47: beyond an immune checkpoint in cancer treatment. Biochim. Biophys. Acta Rev. Cancer 1877 (5), 188771. doi:10.1016/j.bbcan.2022.188771
Blas-García, A., and Apostolova, N. (2023). Novel therapeutic approaches to liver fibrosis based on targeting oxidative stress. Antioxidants (Basel) 12 (8), 1567. doi:10.3390/antiox12081567
Bosch, J., Groszmann, R. J., and Shah, V. H. (2015). Evolution in the understanding of the pathophysiological basis of portal hypertension: how changes in paradigm are leading to successful new treatments. J. Hepatol. 62 (1 Suppl. l), S121–S130. doi:10.1016/j.jhep.2015.01.003
Boyd, A., Cain, O., Chauhan, A., and Webb, G. J. (2020). Medical liver biopsy: background, indications, procedure and histopathology. Frontline Gastroenterol. 11 (1), 40–47. doi:10.1136/flgastro-2018-101139
Brehmer, K., Brismar, T. B., Morsbach, F., Svensson, A., Stål, P., Tzortzakakis, A., et al. (2018). Triple arterial phase CT of the liver with radiation dose equivalent to that of single arterial phase CT: initial experience. Radiology 289 (1), 111–118. doi:10.1148/radiol.2018172875
Burchill, M. A., Goldberg, A. R., and Tamburini, B. A. J. (2019). Emerging roles for lymphatics in chronic liver disease. Front. Physiol. 10, 1579. doi:10.3389/fphys.2019.01579
Bush, A. M., Deegan, C., and Gleeson, T. (2023). Viral hepatitis C-related care in active duty and reserve service members: a retrospective review. Mil. Med. 188 (1-2), e267–e269. doi:10.1093/milmed/usab340
Cai, J., Hu, M., Chen, Z., and Ling, Z. (2021). The roles and mechanisms of hypoxia in liver fibrosis. J. Transl. Med. 19 (1), 186. doi:10.1186/s12967-021-02854-x
Cannistrà, M., Ruggiero, M., Zullo, A., Gallelli, G., Serafini, S., Maria, M., et al. (2016). Hepatic ischemia reperfusion injury: a systematic review of literature and the role of current drugs and biomarkers. Int. J. Surg. 33 (Suppl. 1), S57–S70. doi:10.1016/j.ijsu.2016.05.050
Cardiff, R. D., Miller, C. H., and Munn, R. J. (2014). Manual hematoxylin and eosin staining of mouse tissue sections. Cold Spring Harb. Protoc. 2014 (6), 655–658. doi:10.1101/pdb.prot073411
Davies, T., Wythe, S., O'Beirne, J., Martin, D., and Gilbert-Kawai, E. (2017). Review article: the role of the microcirculation in liver cirrhosis. Aliment. Pharmacol. Ther. 46 (9), 825–835. doi:10.1111/apt.14279
Ding, K., Liu, M., Wei, X., Huang, R., Chen, J., Lu, S., et al. (2021). Comparison of MR-PWI quantitative and semi-quantitative parameters for the evaluation of liver fibrosis. BMC Med. Imaging 21 (1), 8. doi:10.1186/s12880-020-00539-3
Du, J. X., Liu, P., Sun, M. Y., Tao, Q., Zhang, L. J., Chen, G. F., et al. (2011). Chinese herbal medicine Xiayuxue Decoction inhibits liver angiogenesis in rats with carbon tetrachloride-induced liver fibrosis. Zhong Xi Yi Jie He Xue Bao 9 (8), 878–887. doi:10.3736/jcim20110810
Du, W., and Wang, L. (2022). The crosstalk between liver sinusoidal endothelial cells and hepatic microenvironment in NASH related liver fibrosis. Front. Immunol. 13, 936196. doi:10.3389/fimmu.2022.936196
Fan, J., Chen, C. J., Wang, Y. C., Quan, W., Wang, J. W., and Zhang, W. G. (2019). Hemodynamic changes in hepatic sinusoids of hepatic steatosis mice. World J. Gastroenterol. 25 (11), 1355–1365. doi:10.3748/wjg.v25.i11.1355
Fu, C., Zhang, Y., Xi, W. J., Xu, K., Meng, F., Ma, T., et al. (2023). Dahuang Zhechong pill attenuates hepatic sinusoidal capillarization in liver cirrhosis and hepatocellular carcinoma rat model via the MK/integrin signaling pathway. J. Ethnopharmacol. 308, 116191. doi:10.1016/j.jep.2023.116191
Fu, Y., Xiao, Z., Tian, X., Liu, W., Xu, Z., Yang, T., et al. (2021). The novel Chinese medicine JY5 formula alleviates hepatic fibrosis by inhibiting the notch signaling pathway. Front. Pharmacol. 12, 671152. doi:10.3389/fphar.2021.671152
Geng, W., Zhou, G., Zhao, B., Xiao, Q., Li, C., Fan, S., et al. (2020). Liquiritigenin suppresses the activation of hepatic stellate cells via targeting miR-181b/PTEN axis. Phytomedicine 66, 153108. doi:10.1016/j.phymed.2019.153108
Gibert-Ramos, A., Sanfeliu-Redondo, D., Aristu-Zabalza, P., Martínez-Alcocer, A., Gracia-Sancho, J., Guixé-Muntet, S., et al. (2021). The hepatic sinusoid in chronic liver disease: the optimal milieu for cancer. Cancers (Basel) 13 (22), 5719. doi:10.3390/cancers13225719
González-Reimers, E., Quintero-Platt, G., Martín-González, C., Pérez-Hernández, O., Romero-Acevedo, L., and Santolaria-Fernández, F. (2016). Thrombin activation and liver inflammation in advanced hepatitis C virus infection. World J. Gastroenterol. 22 (18), 4427–4437. doi:10.3748/wjg.v22.i18.4427
Gracia-Sancho, J., Caparrós, E., Fernández-Iglesias, A., and Francés, R. (2021). Role of liver sinusoidal endothelial cells in liver diseases. Nat. Rev. Gastroenterol. Hepatol. 18 (6), 411–431. doi:10.1038/s41575-020-00411-3
Gracia-Sancho, J., Marrone, G., and Fernández-Iglesias, A. (2019). Hepatic microcirculation and mechanisms of portal hypertension. Nat. Rev. Gastroenterol. Hepatol. 16 (4), 221–234. doi:10.1038/s41575-018-0097-3
Greuter, T., and Shah, V. H. (2016). Hepatic sinusoids in liver injury, inflammation, and fibrosis: new pathophysiological insights. J. Gastroenterol. 51 (6), 511–519. doi:10.1007/s00535-016-1190-4
Guixé-Muntet, S., Zhu, C. P., Xie, W. F., and Gracia-Sancho, J. (2020). Novel therapeutics for portal hypertension and fibrosis in chronic liver disease. Pharmacol. Ther. 215, 107626. doi:10.1016/j.pharmthera.2020.107626
Gunarathne, L. S., Rajapaksha, H., Shackel, N., Angus, P. W., and Herath, C. B. (2020). Cirrhotic portal hypertension: from pathophysiology to novel therapeutics. World J. Gastroenterol. 26 (40), 6111–6140. doi:10.3748/wjg.v26.i40.6111
Gurakar, A., Conde Amiel, I., Ozturk, N. B., Artru, F., Selzner, N., Psoter, K. J., et al. (2024). An international, multicenter, survey-based analysis of practice and management of acute liver failure. Liver Transpl. doi:10.1097/lvt.0000000000000402
Han, J. Y., Li, Q., Ma, Z. Z., and Fan, J. Y. (2017). Effects and mechanisms of compound Chinese medicine and major ingredients on microcirculatory dysfunction and organ injury induced by ischemia/reperfusion. Pharmacol. Ther. 177, 146–173. doi:10.1016/j.pharmthera.2017.03.005
Han, S. C., Huang, R. P., Zhang, Q. Y., Yan, C. Y., Li, X. Y., Li, Y. F., et al. (2023). Antialcohol and hepatoprotective effects of tamarind shell extract on ethanol-induced damage to HepG2 cells and animal models. Foods 12 (5), 1078. doi:10.3390/foods12051078
He, M. N., Lv, K., Jiang, Y. X., and Jiang, T. A. (2017). Application of superb microvascular imaging in focal liver lesions. World J. Gastroenterol. 23 (43), 7765–7775. doi:10.3748/wjg.v23.i43.7765
He, Y. F., Cheng, K., Zhong, Z. T., Hou, X. L., An, C. Z., Chen, W., et al. (2023). Simultaneous labeling and multicolor fluorescence imaging of multiple immune cells on liver frozen section by polychromatic quantum dots below freezing points. J. Colloid Interface Sci. 636, 42–54. doi:10.1016/j.jcis.2022.12.165
Hildebrandt, F., Andersson, A., Saarenpää, S., Larsson, L., Van Hul, N., Kanatani, S., et al. (2021). Spatial Transcriptomics to define transcriptional patterns of zonation and structural components in the mouse liver. Nat. Commun. 12 (1), 7046. doi:10.1038/s41467-021-27354-w
Hirata, K., Ogata, I., Ohta, Y., and Fujiwara, K. (1989). Hepatic sinusoidal cell destruction in the development of intravascular coagulation in acute liver failure of rats. J. Pathol. 158 (2), 157–165. doi:10.1002/path.1711580211
Horie, Y., and Ishii, H. (2001). Effect of alcohol on organ microcirculation: its relation to hepatic, pancreatic and gastrointestinal diseases due to alcohol. Nihon Arukoru Yakubutsu Igakkai Zasshi 36 (5), 471–485.
Huang, Q., Su, J., Xu, J., Yu, H., Jin, X., Wang, Y., et al. (2023a). Beneficial effects of Panax notoginseng (Burkill) F. H. Chen flower saponins in rats with metabolic hypertension by inhibiting the activation of the renin-angiotensin-aldosterone system through complement 3. BMC Complement. Med. Ther. 23 (1), 13. doi:10.1186/s12906-022-03828-2
Huang, S., Wang, Y., Xie, S., Lai, Y., Mo, C., Zeng, T., et al. (2022). Isoliquiritigenin alleviates liver fibrosis through caveolin-1-mediated hepatic stellate cells ferroptosis in zebrafish and mice. Phytomedicine 101, 154117. doi:10.1016/j.phymed.2022.154117
Huang, Y., Wang, Z. L., He, Y., Ye, L. M., Guo, W. Q., and Zhang, J. J. (2023b). Jiawei Taohe Chengqi Decoction attenuates hepatic fibrosis by preventing activation of HSCs through regulating Src/ERK/Smad3 signal pathway. J. Ethnopharmacol. 305, 116059. doi:10.1016/j.jep.2022.116059
Hunt, N. J., Lockwood, G. P., Kang, S. W. S., Pulpitel, T., Clark, X., Mao, H., et al. (2020). The effects of metformin on age-related changes in the liver sinusoidal endothelial cell. J. Gerontol. A Biol. Sci. Med. Sci. 75 (2), 278–285. doi:10.1093/gerona/glz153
Hunt, N. J., Lockwood, G. P., Warren, A., Mao, H., McCourt, P. A. G., Le Couteur, D. G., et al. (2019). Manipulating fenestrations in young and old liver sinusoidal endothelial cells. Am. J. Physiol. Gastrointest. Liver Physiol. 316 (1), G144–g154. doi:10.1152/ajpgi.00179.2018
Inokuchi, S., Tsukamoto, H., Park, E., Liu, Z. X., Brenner, D. A., and Seki, E. (2011). Toll-like receptor 4 mediates alcohol-induced steatohepatitis through bone marrow-derived and endogenous liver cells in mice. Alcohol Clin. Exp. Res. 35 (8), 1509–1518. doi:10.1111/j.1530-0277.2011.01487.x
Israel, Y., and Orrego, H. (1987). Hypermetabolic state, hepatocyte expansion, and liver blood flow: an interaction triad in alcoholic liver injury. Ann. N. Y. Acad. Sci. 492, 303–323. doi:10.1111/j.1749-6632.1987.tb48683.x
Iwakiri, Y., and Trebicka, J. (2021). Portal hypertension in cirrhosis: pathophysiological mechanisms and therapy. JHEP Rep. 3 (4), 100316. doi:10.1016/j.jhepr.2021.100316
Jia, K., Zhang, Y., Luo, R., Liu, R., Li, Y., Wu, J., et al. (2023). Acteoside ameliorates hepatic ischemia-reperfusion injury via reversing the senescent fate of liver sinusoidal endothelial cells and restoring compromised sinusoidal networks. Int. J. Biol. Sci. 19 (15), 4967–4988. doi:10.7150/ijbs.87332
Jin, Y. N., and Zhang, W. (2024). Transjugular intrahepatic portosystemic shunt: a promising therapy for recompensation in cirrhotic patients. World J. Gastroenterol. 30 (16), 2285–2286. doi:10.3748/wjg.v30.i16.2285
Kanikarla Marie, P., Fowlkes, N. W., Afshar-Kharghan, V., Martch, S. L., Sorokin, A., Shen, J. P., et al. (2021). The provocative roles of platelets in liver disease and cancer. Front. Oncol. 11, 643815. doi:10.3389/fonc.2021.643815
Kao, C., Surjit, M., and Ranjith-Kumar, C. T. (2021). Editorial: viral hepatitis: pathophysiology, prevention, and control. Front. Cell Infect. Microbiol. 11, 633580. doi:10.3389/fcimb.2021.633580
Kim, D. B., Lee, D. K., Cheon, C., Ribeiro, R., and Kim, B. (2022a). Natural products for liver cancer treatment: from traditional medicine to modern drug discovery. Nutrients 14 (20), 4252. doi:10.3390/nu14204252
Kim, J. S., Kim, D. W., Kim, K. W., Song, G. W., and Lee, S. G. (2022b). Improving the specificity of CT angiography for the diagnosis of hepatic artery occlusion after liver transplantation in suspected patients with Doppler ultrasound abnormalities. Korean J. Radiol. 23 (1), 52–59. doi:10.3348/kjr.2021.0266
Klenerman, P., and Ramamurthy, N. (2015). Liver sinusoidal endothelial cells: an antiviral "defendothelium. Gastroenterology 148 (2), 288–291. doi:10.1053/j.gastro.2014.12.010
Koch, P. S., Lee, K. H., Goerdt, S., and Augustin, H. G. (2021). Angiodiversity and organotypic functions of sinusoidal endothelial cells. Angiogenesis 24 (2), 289–310. doi:10.1007/s10456-021-09780-y
Kolodziejczyk, A. A., Federici, S., Zmora, N., Mohapatra, G., Dori-Bachash, M., Hornstein, S., et al. (2020). Acute liver failure is regulated by MYC- and microbiome-dependent programs. Nat. Med. 26 (12), 1899–1911. doi:10.1038/s41591-020-1102-2
Krenkel, O., Mossanen, J. C., and Tacke, F. (2014). Immune mechanisms in acetaminophen-induced acute liver failure. Hepatobiliary Surg. Nutr. 3 (6), 331–343. doi:10.3978/j.issn.2304-3881.2014.11.01
Krenkel, O., and Tacke, F. (2017). Liver macrophages in tissue homeostasis and disease. Nat. Rev. Immunol. 17 (5), 306–321. doi:10.1038/nri.2017.11
Kuo, C. Y., Chiu, V., Hsieh, P. C., Huang, C. Y., Huang, S. J., Tzeng, I. S., et al. (2020). Chrysophanol attenuates hepatitis B virus X protein-induced hepatic stellate cell fibrosis by regulating endoplasmic reticulum stress and ferroptosis. J. Pharmacol. Sci. 144 (3), 172–182. doi:10.1016/j.jphs.2020.07.014
Lafoz, E., Ruart, M., Anton, A., Oncins, A., and Hernández-Gea, V. (2020). The endothelium as a driver of liver fibrosis and regeneration. Cells 9 (4), 929. doi:10.3390/cells9040929
Levy, G. A., MacPhee, P. J., Fung, L. S., Fisher, M. M., and Rappaport, A. M. (1983). The effect of mouse hepatitis virus infection on the microcirculation of the liver. Hepatology 3 (6), 964–973. doi:10.1002/hep.1840030614
Li, D. Y., Xia, Q., Yu, T. T., Zhu, J. T., and Zhu, D. (2021). Transmissive-detected laser speckle contrast imaging for blood flow monitoring in thick tissue: from Monte Carlo simulation to experimental demonstration. Light Sci. Appl. 10 (1), 241. doi:10.1038/s41377-021-00682-8
Li, H. M. (2016). Microcirculation of liver cancer, microenvironment of liver regeneration, and the strategy of Chinese medicine. Chin. J. Integr. Med. 22 (3), 163–167. doi:10.1007/s11655-016-2460-y
Li, J., Zhao, Y. R., and Tian, Z. (2019). Roles of hepatic stellate cells in acute liver failure: from the perspective of inflammation and fibrosis. World J. Hepatol. 11 (5), 412–420. doi:10.4254/wjh.v11.i5.412
Li, W. X., Li, Y. F., Zhai, Y. J., Chen, W. M., Kurihara, H., and He, R. R. (2013). Theacrine, a purine alkaloid obtained from Camellia assamica var. kucha, attenuates restraint stress-provoked liver damage in mice. J. Agric. Food Chem. 61 (26), 6328–6335. doi:10.1021/jf400982c
Li, Z., Zhu, J., and Ouyang, H. (2023). Research progress of traditional Chinese medicine in improving hepatic fibrosis based on inhibiting pathological angiogenesis. Front. Pharmacol. 14, 1303012. doi:10.3389/fphar.2023.1303012
Liang, L., Yang, X., Yu, Y., Li, X., Wu, Y., Shi, R., et al. (2016). Babao Dan attenuates hepatic fibrosis by inhibiting hepatic stellate cells activation and proliferation via TLR4 signaling pathway. Oncotarget 7 (50), 82554–82566. doi:10.18632/oncotarget.12783
Lieber, S. R., Jones, A. R., Jiang, Y., Gowda, P., Patel, M., Lippe, B., et al. (2024). Psychiatric diagnoses are common after liver transplantation and associated with increased healthcare utilization and patient financial burden. Liver Transpl. doi:10.1097/lvt.0000000000000390
Lin, W., Wang, H., Zhong, M., Yu, S., Zhao, S., Liang, S., et al. (2021). Effect and molecular mechanisms of jiedu recipe on hypoxia-induced angiogenesis after transcatheter arterial chemoembolization in hepatocellular carcinoma. Evid. Based Complement. Altern. Med. 2021, 6529376. doi:10.1155/2021/6529376
Liu, L. P., Ho, R. L., Chen, G. G., and Lai, P. B. (2012). Sorafenib inhibits hypoxia-inducible factor-1α synthesis: implications for antiangiogenic activity in hepatocellular carcinoma. Clin. Cancer Res. 18 (20), 5662–5671. doi:10.1158/1078-0432.Ccr-12-0552
Liu, Q., Zhang, A., Xu, W., and Dong, J. (2011). A new view of the roles of blood flow dynamics and Kupffer cell in intra-hepatic metastasis of hepatocellular carcinoma. Med. Hypotheses 77 (1), 87–90. doi:10.1016/j.mehy.2011.03.033
Liu, Y., Lu, T., Zhang, C., Xu, J., Xue, Z., Busuttil, R. W., et al. (2019). Activation of YAP attenuates hepatic damage and fibrosis in liver ischemia-reperfusion injury. J. Hepatol. 71 (4), 719–730. doi:10.1016/j.jhep.2019.05.029
Ma, H., Liu, X., Zhang, M., and Niu, J. (2021). Liver sinusoidal endothelial cells are implicated in multiple fibrotic mechanisms. Mol. Biol. Rep. 48 (3), 2803–2815. doi:10.1007/s11033-021-06269-1
Ma, Z., Zhang, B., Fan, Y., Wang, M., Kebebe, D., Li, J., et al. (2019). Traditional Chinese medicine combined with hepatic targeted drug delivery systems: a new strategy for the treatment of liver diseases. Biomed. Pharmacother. 117, 109128. doi:10.1016/j.biopha.2019.109128
MacParland, S. A., Liu, J. C., Ma, X. Z., Innes, B. T., Bartczak, A. M., Gage, B. K., et al. (2018). Single cell RNA sequencing of human liver reveals distinct intrahepatic macrophage populations. Nat. Commun. 9 (1), 4383. doi:10.1038/s41467-018-06318-7
Mai, B., Han, L., Zhong, J., Shu, J., Cao, Z., Fang, J., et al. (2022). Rhoifolin alleviates alcoholic liver disease in vivo and in vitro via inhibition of the TLR4/NF-κB signaling pathway. Front. Pharmacol. 13, 878898. doi:10.3389/fphar.2022.878898
Maini, M. K., and Peppa, D. (2013). NK cells: a double-edged sword in chronic hepatitis B virus infection. Front. Immunol. 4, 57. doi:10.3389/fimmu.2013.00057
Mak, K. M., Kee, D., and Shin, D. W. (2022). Alcohol-associated capillarization of sinusoids: a critique since the discovery by Schaffner and Popper in 1963. Anat. Rec. Hob. 305 (7), 1592–1610. doi:10.1002/ar.24829
Mak, K. M., and Mei, R. (2017). Basement membrane type IV collagen and laminin: an overview of their biology and value as fibrosis biomarkers of liver disease. Anat. Rec. Hob. 300 (8), 1371–1390. doi:10.1002/ar.23567
Mansour, M., Gad, A. M., Zaky, H. S., and Nayira A, A. B. (2021). Repurposing of sildenafil as hepatoprotective in sinusoidal obstruction syndrome: amendment of endothelial cell damage and inhibition of platelet aggregation. Azhar Int. J. Pharm. Med. Sci. 1 (3), 21–31. doi:10.21608/aijpms.2021.206681
McConnell, M., and Iwakiri, Y. (2018). Biology of portal hypertension. Hepatol. Int. 12 (Suppl. 1), 11–23. doi:10.1007/s12072-017-9826-x
Mendez-Guerrero, O., Carranza-Carrasco, A., Chi-Cervera, L. A., Torre, A., and Navarro-Alvarez, N. (2024). Optimizing nutrition in hepatic cirrhosis: a comprehensive assessment and care approach. World J. Gastroenterol. 30 (10), 1313–1328. doi:10.3748/wjg.v30.i10.1313
Miao, Z., Wang, W., Miao, Z., Cao, Q., and Xu, S. (2024). Role of Selenoprotein W in participating in the progression of non-alcoholic fatty liver disease. Redox Biol. 71, 103114. doi:10.1016/j.redox.2024.103114
Mitten, E. K., Portincasa, P., and Baffy, G. (2023). Portal hypertension in nonalcoholic fatty liver disease: challenges and paradigms. J. Clin. Transl. Hepatol. 11 (5), 1201–1211. doi:10.14218/jcth.2023.00029
Mouratidou, C., Pavlidis, E. T., Katsanos, G., Kotoulas, S. C., Mouloudi, E., Tsoulfas, G., et al. (2023). Hepatic ischemia-reperfusion syndrome and its effect on the cardiovascular system: the role of treprostinil, a synthetic prostacyclin analog. World J. Gastrointest. Surg. 15 (9), 1858–1870. doi:10.4240/wjgs.v15.i9.1858
Mu, H. N., Li, Q., Fan, J. Y., Pan, C. S., Liu, Y. Y., Yan, L., et al. (2018). Caffeic acid attenuates rat liver injury after transplantation involving PDIA3-dependent regulation of NADPH oxidase. Free Radic. Biol. Med. 129, 202–214. doi:10.1016/j.freeradbiomed.2018.09.009
Mu, H. N., Li, Q., Pan, C. S., Liu, Y. Y., Yan, L., Hu, B. H., et al. (2015). Caffeic acid attenuates rat liver reperfusion injury through sirtuin 3-dependent regulation of mitochondrial respiratory chain. Free Radic. Biol. Med. 85, 237–249. doi:10.1016/j.freeradbiomed.2015.04.033
Murata, S., Jeppsson, B., Lunderquist, A., and Ivancev, K. (2014). Hemodynamics in rat liver tumor model during retrograde-outflow isolated hepatic perfusion with aspiration from the portal vein: angiography and in vivo microscopy. Acta Radiol. 55 (6), 737–744. doi:10.1177/0284185113505258
Myojin, Y., Hikita, H., Sugiyama, M., Sasaki, Y., Fukumoto, K., Sakane, S., et al. (2021). Hepatic stellate cells in hepatocellular carcinoma promote tumor growth via growth differentiation factor 15 production. Gastroenterology 160 (5), 1741–1754.e16. doi:10.1053/j.gastro.2020.12.015
Nafady, A. M., Ahmed, O. B., and Ghafeer, H. H. (2017). Scanning and transmission electron microscopy of the cells forming the hepatic sinusoidal wall of rat in acetaminophen and Escherichia coli endotoxin-induced hepatotoxicity. J. Microsc. Ultrastruct. 5 (1), 21–27. doi:10.1016/j.jmau.2016.04.003
Nakamura, K., Kageyama, S., and Kupiec-Weglinski, J. W. (2019). The evolving role of neutrophils in liver transplant ischemia-reperfusion injury. Curr. Transpl. Rep. 6 (1), 78–89. doi:10.1007/s40472-019-0230-4
Nasiri-Ansari, N., Androutsakos, T., Flessa, C. M., Kyrou, I., Siasos, G., Randeva, H. S., et al. (2022). Endothelial cell dysfunction and nonalcoholic fatty liver disease (NAFLD): a concise review. Cells 11 (16), 2511. doi:10.3390/cells11162511
Orabueze, I. N., Ike, A. C., Aniche, O. M., Nwafia, I. N., and Ebede, S. O. (2024). Hepatitis B virus infection among illegal drug users in Enugu State, Nigeria: prevalence, immune status, and related risk factors. BMC Public Health 24 (1), 1203. doi:10.1186/s12889-024-18675-8
Ortiz, C., Schierwagen, R., Schaefer, L., Klein, S., Trepat, X., and Trebicka, J. (2021). Extracellular matrix remodeling in chronic liver disease. Curr. Tissue Microenviron. Rep. 2 (3), 41–52. doi:10.1007/s43152-021-00030-3
Pan, M. X., Zheng, C. Y., Deng, Y. J., Tang, K. R., Nie, H., Xie, J. Q., et al. (2021). Hepatic protective effects of Shenling Baizhu powder, a herbal compound, against inflammatory damage via TLR4/NLRP3 signalling pathway in rats with nonalcoholic fatty liver disease. J. Integr. Med. 19 (5), 428–438. doi:10.1016/j.joim.2021.07.004
Pan, Y., Zheng, Z., Zhang, X., Liu, S., Zhuansun, S., Gong, S., et al. (2024). Hybrid bioactive hydrogel promotes liver regeneration through the activation of kupffer cells and ECM remodeling after partial hepatectomy. Adv. Healthc. Mater, e2303828. doi:10.1002/adhm.202303828
Pang, E. H. T., Chan, A., Ho, S. G., and Harris, A. C. (2018). Contrast-enhanced ultrasound of the liver: optimizing technique and clinical applications. AJR Am. J. Roentgenol. 210 (2), 320–332. doi:10.2214/ajr.17.17843
Panwar, A., Das, P., and Tan, L. P. (2021). 3D hepatic organoid-based advancements in LIVER tissue engineering. Bioeng. (Basel) 8 (11), 185. doi:10.3390/bioengineering8110185
Papagiouvanni, I., Sarafidis, P., Theodorakopoulou, M. P., Sinakos, E., and Goulis, I. (2022). Endothelial and microvascular function in liver cirrhosis: an old concept that needs re-evaluation? Ann. Gastroenterol. 35 (5), 471–482. doi:10.20524/aog.2022.0734
Pasarín, M., La Mura, V., Gracia-Sancho, J., García-Calderó, H., Rodríguez-Vilarrupla, A., García-Pagán, J. C., et al. (2012). Sinusoidal endothelial dysfunction precedes inflammation and fibrosis in a model of NAFLD. PLoS One 7 (4), e32785. doi:10.1371/journal.pone.0032785
Peng, Y., Yin, Q., Yuan, M., Chen, L., Shen, X., Xie, W., et al. (2022). Role of hepatic stellate cells in liver ischemia-reperfusion injury. Front. Immunol. 13, 891868. doi:10.3389/fimmu.2022.891868
Peralta, C., Jiménez-Castro, M. B., and Gracia-Sancho, J. (2013). Hepatic ischemia and reperfusion injury: effects on the liver sinusoidal milieu. J. Hepatol. 59 (5), 1094–1106. doi:10.1016/j.jhep.2013.06.017
Pereira, E., Araujo, B. P., Rodrigues, K. L., Silvares, R. R., Martins, C. S. M., Flores, E. E. I., et al. (2022). Simvastatin improves microcirculatory function in nonalcoholic fatty liver disease and downregulates oxidative and ALE-RAGE stress. Nutrients 14 (3), 716. doi:10.3390/nu14030716
Pereira, E., Silvares, R. R., Flores, E. E. I., Rodrigues, K. L., Ramos, I. P., da Silva, I. J., et al. (2017). Hepatic microvascular dysfunction and increased advanced glycation end products are components of non-alcoholic fatty liver disease. PLoS One 12 (6), e0179654. doi:10.1371/journal.pone.0179654
Qiu, H., Mao, D., Tang, N., Long, F., Zhang, R., Wang, M., et al. (2019). The underlying mechanisms of Jie-Du-Hua-Yu granule for protecting rat liver failure. Drug Des. Devel Ther. 13, 589–600. doi:10.2147/dddt.S180969
Rajapaksha, I. G., Gunarathne, L. S., Angus, P. W., and Herath, C. B. (2021). Update on new aspects of the renin-angiotensin system in hepatic fibrosis and portal hypertension: implications for novel therapeutic options. J. Clin. Med. 10 (4), 702. doi:10.3390/jcm10040702
Ramachandran, P., Dobie, R., Wilson-Kanamori, J. R., Dora, E. F., Henderson, B. E. P., Luu, N. T., et al. (2019). Resolving the fibrotic niche of human liver cirrhosis at single-cell level. Nature 575 (7783), 512–518. doi:10.1038/s41586-019-1631-3
Ribaud, J., McLernon, S., and Auzinger, G. (2022). Targeted temperature management in acute liver failure: a systematic review. Nurs. Crit. Care 27 (6), 784–795. doi:10.1111/nicc.12524
Roehlen, N., Crouchet, E., and Baumert, T. F. (2020). Liver fibrosis: mechanistic concepts and therapeutic perspectives. Cells 9 (4), 875. doi:10.3390/cells9040875
Rumgay, H., Arnold, M., Ferlay, J., Lesi, O., Cabasag, C. J., Vignat, J., et al. (2022). Global burden of primary liver cancer in 2020 and predictions to 2040. J. Hepatol. 77 (6), 1598–1606. doi:10.1016/j.jhep.2022.08.021
Schierwagen, R., Dietrich, P., Klein, S., Uschner, F. E., Ortiz, C., Tyc, O., et al. (2020). β-Arrestin2 is increased in liver fibrosis in humans and rodents. Proc. Natl. Acad. Sci. U. S. A. 117 (44), 27082–27084. doi:10.1073/pnas.2014337117
Sheng, M., Zhou, Y., Yu, W., Weng, Y., Xu, R., and Du, H. (2015). Protective effect of Berberine pretreatment in hepatic ischemia/reperfusion injury of rat. Transpl. Proc. 47 (2), 275–282. doi:10.1016/j.transproceed.2015.01.010
Shenoda, B., and Boselli, J. (2019). Vascular syndromes in liver cirrhosis. Clin. J. Gastroenterol. 12 (5), 387–397. doi:10.1007/s12328-019-00956-0
Shetty, S., Lalor, P. F., and Adams, D. H. (2018). Liver sinusoidal endothelial cells - gatekeepers of hepatic immunity. Nat. Rev. Gastroenterol. Hepatol. 15 (9), 555–567. doi:10.1038/s41575-018-0020-y
Sun, S., Huan, S., Li, Z., Yao, Y., Su, Y., Xia, S., et al. (2022). Curcumol alleviates liver fibrosis by inducing endoplasmic reticulum stress-mediated necroptosis of hepatic stellate cells through Sirt1/NICD pathway. PeerJ 10, e13376. doi:10.7717/peerj.13376
Suzuki, S., Mori, A., Fukui, A., Ema, Y., and Nishiwaki, K. (2020). Lidocaine inhibits vascular endothelial growth factor-A-induced angiogenesis. J. Anesth. 34 (6), 857–864. doi:10.1007/s00540-020-02830-7
Szafranska, K., Kruse, L. D., Holte, C. F., McCourt, P., and Zapotoczny, B. (2021). The wHole story about fenestrations in LSEC. Front. Physiol. 12, 735573. doi:10.3389/fphys.2021.735573
Tanaka, H. (2020). Current role of ultrasound in the diagnosis of hepatocellular carcinoma. J. Med. Ultrason. 47 (2), 239–255. doi:10.1007/s10396-020-01012-y
Tang, J., Yan, Z., Feng, Q., Yu, L., and Wang, H. (2021). The roles of neutrophils in the pathogenesis of liver diseases. Front. Immunol. 12, 625472. doi:10.3389/fimmu.2021.625472
Tang, K., Deng, Y., Zheng, C., Nie, H., Pan, M., Chen, R., et al. (2020). Prevention of nonalcoholic hepatic steatosis by shenling baizhu powder: involvement of adiponectin-induced inhibition of hepatic SREBP-1c. Oxid. Med. Cell Longev. 2020, 9701285. doi:10.1155/2020/9701285
Tao, T., Chen, F., Bo, L., Xie, Q., Yi, W., Zou, Y., et al. (2014). Ginsenoside Rg1 protects mouse liver against ischemia-reperfusion injury through anti-inflammatory and anti-apoptosis properties. J. Surg. Res. 191 (1), 231–238. doi:10.1016/j.jss.2014.03.067
Thabut, D., and Shah, V. (2010). Intrahepatic angiogenesis and sinusoidal remodeling in chronic liver disease: new targets for the treatment of portal hypertension? J. Hepatol. 53 (5), 976–980. doi:10.1016/j.jhep.2010.07.004
Torres Rojas, A. M., and Lorente, S. (2023). Liver fibrosis emulation: impact of the vascular fibrotic alterations on hemodynamics. Comput. Biol. Med. 166, 107563. doi:10.1016/j.compbiomed.2023.107563
Trefts, E., Gannon, M., and Wasserman, D. H. (2017). The liver. Curr. Biol. 27 (21), R1147–r1151. doi:10.1016/j.cub.2017.09.019
Tripodi, A. (2015). Hemostasis abnormalities in cirrhosis. Curr. Opin. Hematol. 22 (5), 406–412. doi:10.1097/moh.0000000000000164
Velez, J. C. Q., Latt, N., and Rodby, R. A. (2024). Pathophysiology of hepatorenal syndrome. Adv. Kidney Dis. Health 31 (2), 87–99. doi:10.1053/j.akdh.2024.01.002
Venkatraman, L., and Tucker-Kellogg, L. (2013). The CD47-binding peptide of thrombospondin-1 induces defenestration of liver sinusoidal endothelial cells. Liver Int. 33 (9), 1386–1397. doi:10.1111/liv.12231
Wang, H., Zhang, H., Zhang, Y., Wang, D., Cheng, X., Yang, F., et al. (2016). Plumbagin protects liver against fulminant hepatic failure and chronic liver fibrosis via inhibiting inflammation and collagen production. Oncotarget 7 (50), 82864–82875. doi:10.18632/oncotarget.12655
Wang, M., Liu, C. Y., Wang, T., Yu, H. M., Ouyang, S. H., Wu, Y. P., et al. (2020). (+)-Clausenamide protects against drug-induced liver injury by inhibiting hepatocyte ferroptosis. Cell Death Dis. 11 (9), 781. doi:10.1038/s41419-020-02961-5
Wang, S., Tang, C., Zhao, H., Shen, P., Lin, C., Zhu, Y., et al. (2021). Network pharmacological analysis and experimental validation of the mechanisms of action of Si-Ni-san against liver fibrosis. Front. Pharmacol. 12, 656115. doi:10.3389/fphar.2021.656115
Xi, S., Yue, L., Shi, M., Peng, Y., Xu, Y., Wang, X., et al. (2016). The effects of taoren-honghua herb pair on pathological microvessel and angiogenesis-associated signaling pathway in mice model of CCl4-induced chronic liver disease. Evid. Based Complement. Altern. Med. 2016, 2974256. doi:10.1155/2016/2974256
Xin, J., Yang, T., Wu, X., Wu, Y., Liu, Y., Liu, X., et al. (2023). Spatial transcriptomics analysis of zone-dependent hepatic ischemia-reperfusion injury murine model. Commun. Biol. 6 (1), 194. doi:10.1038/s42003-023-04564-0
Yang, F., Li, J., Zhu, J., Wang, D., Chen, S., and Bai, X. (2015). Hydroxysafflor yellow A inhibits angiogenesis of hepatocellular carcinoma via blocking ERK/MAPK and NF-κB signaling pathway in H22 tumor-bearing mice. Eur. J. Pharmacol. 754, 105–114. doi:10.1016/j.ejphar.2015.02.015
Yang, Z. X., Han, D. W., Xing, Y. Y., Liu, H. R., and Yan, Z. (2009). Pathogenesis of hepatic sinusoidal capillarization in hepatic cirrhosis. World Chin. J. Dig. 17 (30), 3101–3108. doi:10.11569/wcjd.v17.i30.3101
Yokomori, H., Ando, W., Yoshimura, K., Yamazaki, H., Takahashi, Y., and Oda, M. (2015). Increases in endothelial caveolin-1 and cavins correlate with cirrhosis progression. Micron 76, 52–61. doi:10.1016/j.micron.2015.03.009
Yoshizawa, M., Sugimoto, M., Tanaka, M., Sakai, Y., and Nishikawa, M. (2022). Computational simulation of liver fibrosis dynamics. Sci. Rep. 12 (1), 14112. doi:10.1038/s41598-022-18123-w
Yuan, Q., Yao, F., Zhou, L., Liang, G., Song, X., Jiang, G., et al. (2019). Yu ping feng san exert anti-angiogenesis effects through the inhibition of TSLP-STAT3 signaling pathways in hepatocellular carcinoma. Evid. Based Complement. Altern. Med. 2019, 1947156. doi:10.1155/2019/1947156
Zhang, C., Bian, M., Chen, X., Jin, H., Zhao, S., Yang, X., et al. (2018). Oroxylin A prevents angiogenesis of LSECs in liver fibrosis via inhibition of YAP/HIF-1α signaling. J. Cell Biochem. 119 (2), 2258–2268. doi:10.1002/jcb.26388
Zhang, C., and Han, D. (2021). The value of color Doppler ultrasound in the diagnosis of upper gastrointestinal hemorrhage in patients with liver cirrhosis. Panminerva Med. 63 (4), 570–571. doi:10.23736/s0031-0808.20.04071-9
Zhang, J., Li, J., Song, H., Xiong, Y., Liu, D., and Bai, X. (2019a). Hydroxysafflor yellow A suppresses angiogenesis of hepatocellular carcinoma through inhibition of p38 MAPK phosphorylation. Biomed. Pharmacother. 109, 806–814. doi:10.1016/j.biopha.2018.09.086
Zhang, L., Tao, M., Zhang, H., Zhang, S., Hou, X., Zong, C., et al. (2024). Lipopolysaccharide modification enhances the inhibitory effect of clodronate liposomes on hepatic fibrosis by depletion of macrophages and hepatic stellate cells. Chem. Biol. Interact. 395, 111015. doi:10.1016/j.cbi.2024.111015
Zhang, L. F., Wang, X. H., Zhang, C. L., Lee, J., Duan, B. W., Xing, L., et al. (2022a). Sequential nano-penetrators of capillarized liver sinusoids and extracellular matrix barriers for liver fibrosis therapy. ACS Nano 16 (9), 14029–14042. doi:10.1021/acsnano.2c03858
Zhang, Q., Ding, Q., Yan, S., and Yue, Q. Y. (2022b). Fatal adverse events of rivaroxaban combined with aspirin: an analysis using data from VigiBase. Eur. J. Clin. Pharmacol. 78 (9), 1521–1526. doi:10.1007/s00228-022-03357-4
Zhang, W. Q., Zhao, T. T., Gui, D. K., Gao, C. L., Gu, J. L., Gan, W. J., et al. (2019b). Sodium butyrate improves liver glycogen metabolism in type 2 diabetes mellitus. J. Agric. Food Chem. 67 (27), 7694–7705. doi:10.1021/acs.jafc.9b02083
Zhang, Y., Zhang, Y., Wang, J., and Gu, H. (2020). Amarogentin inhibits liver cancer cell angiogenesis after insufficient radiofrequency ablation via affecting stemness and the p53-dependent VEGFA/Dll4/Notch1 pathway. Biomed. Res. Int. 2020, 5391058. doi:10.1155/2020/5391058
Zhao, Z. M., Liu, H. L., Sun, X., Guo, T., Shen, L., Tao, Y. Y., et al. (2017). Levistilide A inhibits angiogenesis in liver fibrosis via vascular endothelial growth factor signaling pathway. Exp. Biol. Med. (Maywood) 242 (9), 974–985. doi:10.1177/1535370217701005
Zheng, Y., Wang, J., Wang, J., Xie, H., and Zhao, T. (2020). Effect of Curcumol on the fenestrae of liver sinusoidal endothelial cells based on NF-κB signaling pathway. Evid. Based Complement. Altern. Med. 2020, 8590638. doi:10.1155/2020/8590638
Zhou, Y. N., Mu, Y. P., Fu, W. W., Ning, B. B., Du, G. L., Chen, J. M., et al. (2015). Yiguanjian decoction and its ingredients inhibit angiogenesis in carbon tetrachloride-induced cirrhosis mice. BMC Complement. Altern. Med. 15, 342. doi:10.1186/s12906-015-0862-6
Zhou, Y. N., Sun, M. Y., Mu, Y. P., Yang, T., Ning, B. B., Ren, S., et al. (2014). Xuefuzhuyu decoction inhibition of angiogenesis attenuates liver fibrosis induced by CCl₄ in mice. J. Ethnopharmacol. 153 (3), 659–666. doi:10.1016/j.jep.2014.03.019
Keywords: hepatic microcirculatory disturbance, liver disease, pathogenesis, traditional Chinese medicine, active metabolite
Citation: Liu P, Liang W-L, Huang R-T, Chen X-X, Zou D-H, Kurihara H, Li Y-F, Xu Y-H, Ouyang S-H and He R-R (2024) Hepatic microcirculatory disturbance in liver diseases: intervention with traditional Chinese medicine. Front. Pharmacol. 15:1399598. doi: 10.3389/fphar.2024.1399598
Received: 13 March 2024; Accepted: 25 June 2024;
Published: 23 July 2024.
Edited by:
Somasundaram Arumugam, National Institute of Pharmaceutical Education and Research, Kolkata, IndiaReviewed by:
Priyanka Banerjee, Texas A&M Health Science Center, United StatesCopyright © 2024 Liu, Liang, Huang, Chen, Zou, Kurihara, Li, Xu, Ouyang and He. This is an open-access article distributed under the terms of the Creative Commons Attribution License (CC BY). The use, distribution or reproduction in other forums is permitted, provided the original author(s) and the copyright owner(s) are credited and that the original publication in this journal is cited, in accordance with accepted academic practice. No use, distribution or reproduction is permitted which does not comply with these terms.
*Correspondence: You-Hua Xu, eWh4dUBtdXN0LmVkdS5tbw==; Shu-Hua Ouyang, c2hvdXlhbmdAam51LmVkdS5jbg==; Rong-Rong He, cm9uZ3JvbmdoZUBqbnUuZWR1LmNu
†These authors have contributed equally to this work
Disclaimer: All claims expressed in this article are solely those of the authors and do not necessarily represent those of their affiliated organizations, or those of the publisher, the editors and the reviewers. Any product that may be evaluated in this article or claim that may be made by its manufacturer is not guaranteed or endorsed by the publisher.
Research integrity at Frontiers
Learn more about the work of our research integrity team to safeguard the quality of each article we publish.