- 1Department of Oral and Maxillofacial Surgery, Hospital of Stomatology, Jilin University, Changchun, China
- 2Jilin Provincial Key Laboratory of Tooth Development and Bone Remodeling, Jilin University, Changchun, China
Natural polyphenols may have a role in counteracting oxidative stress, which is associated with aging and several bone-related diseases. Chlorogenic acid (CGA) is a naturally occurring polyphenolic compound formed by the esterification of caffeic and quininic acids with osteogenic, antioxidant, and anti-inflammatory properties. This review discusses the potential of CGA to enhance osteogenesis by increasing the osteogenic capacity of mesenchymal stem cells (MSCs), osteoblast survival, proliferation, differentiation, and mineralization, as well as its ability to attenuate osteoclastogenesis by enhancing osteoclast apoptosis and impeding osteoclast regeneration. CGA can be involved in bone remodeling by acting directly on pro-osteoclasts/osteoblasts or indirectly on osteoclasts by activating the nuclear factor kB (RANK)/RANK ligand (RANKL)/acting osteoprotegerin (OPG) system. Finally, we provide perspectives for using CGA to treat bone diseases.
1 Introduction
Bones are a very specialized and dynamic organ that undergoes constant regeneration. Bone generation begins in fetal life, and regeneration continues after skeletal maturation (Siddiqui and Partridge, 2016). Bone homeostasis is maintained by osteoclastic bone resorption and osteoblastic bone formation within the basic multicellular unit, both of which are well coordinated in time and space to maintain bone integrity (Borciani et al., 2020; Kim et al., 2020). The dysregulation of bone homeostasis is associated with the development of jaw disorders (Freeman et al., 2021). MSCs can differentiate into multiple cell types, including adipocytes, myocytes, chondrocytes, and osteoblasts, under the influence of regulatory transcription factors. Osteoblasts produce osteoid by synthesizing and secreting type I collagen, which promotes their mineralization (Katsimbri, 2017; Na et al., 2021). Osteoclasts develop from hematopoietic stem cells in the bone marrow, mature in response to differentiation factors, such as macrophage colony-stimulating factor and receptor-activated nuclear factor kappa B (RANKL), and have the ability to absorb bone matrix. Mediators that inhibit osteoclast differentiation and their ability to resorb bone can lead to increased bone mass. Therefore, understanding the mechanisms of osteoclast bone resorption associated with jaw disorders is important (Yahara et al., 2020; Freeman et al., 2021; Udagawa et al., 2021).
With the development of herbal medicine, natural drug extracts have played increasingly important drug roles due to their efficiency and safety. Some researchers found that CGA could promote osteoblast activity and inhibit osteoclast activity (Kawabata et al., 2018; Wu et al., 2023). CGA is a polyphenolic compound formed by the esterification of caffeic acid and quininic acid and is known as 5-O-caffeoylquinic acid (Stefanello et al., 2019; Lu et al., 2020). (Figure 1)
CGA is not only the main active ingredient in many traditional Chinese medicines but also widely found in a variety of plant tissues and foods, such as coffee, beans, potatoes, plums, and honeysuckle. Previous reports showed that CGA exerts many different pharmacological effects, such as antioxidant (Khochapong et al., 2021), antiviral, anti-inflammatory, antibacterial (Munteanu and Apetrei, 2021), antithrombotic, and antitumor activity (Ye et al., 2020). (Figure 2) Clinical trials have also reported the beneficial effects of CGA on diabetes (Zhao et al., 2020; Mansour et al., 2021), hyperlipidemia (Ontawong et al., 2019; Ye et al., 2022), renal diseases (Zeng et al., 2022), and neurological disorders (Heitman and Ingram, 2017). (Table 1)
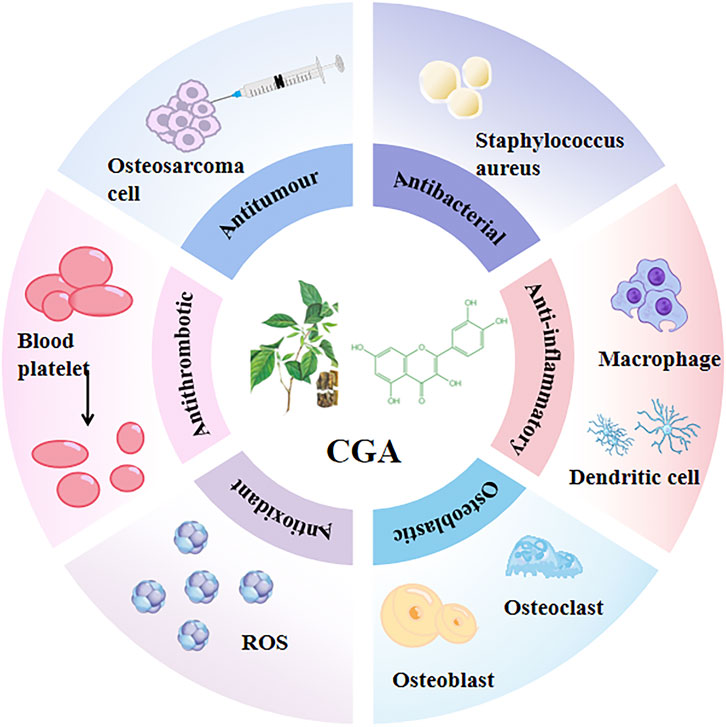
Figure 2. Pharmacological effects of CGA CGA has a variety of different pharmacological effects, such as antioxidant activity (Khochapong et al., 2021), antiviral, anti-inflammatory, antibacterial (Munteanu and Apetrei, 2021), antithrombotic, and antitumor activities (Ye et al., 2020).
CGA bioavailability is influenced by many factors, including oral and gastrointestinal conditions, hepatic-mediated metabolic processes (phase I and II metabolism), and gut and microbial flora (Alqahtani et al., 2021). In the oral cavity, CGA is scarcely metabolized but can interact with the oral microbiota, affecting its composition and metabolism, potentially playing a positive role in the prevention and treatment of oral diseases (Takahama et al., 2007; Barbour et al., 2022). A small fraction of CGA is absorbed intact in the stomach and small intestine, while the majority is metabolized into caffeic acid and quinic acid. These undergo further methylation, sulfation, and glucuronidation under the control of specific enzymes. Upon entering the liver, caffeic acid is metabolized to ferulic acid and isoferulic acid, while quinic acid is converted to gallic acid, which is further degraded to p-hydroxybenzoic acid and syringic acid. About two-thirds of CGA, in the form of CA and QA, enter the cecum, where it is hydrolyzed to p-coumaric acid, 3-(3-hydroxyphenyl) propionic acid, or 3-(3-hydroxyphenyl) acetic acid by the gut microbiota (such as Escherichia coli, Bifidobacterium, Lactobacillus, and Enterococcus) (Olthof et al., 2001; Lu et al., 2020). (Figure 3) Tomas-Barberan et al. suggested that the bioavailability of CGA metabolites largely depends on the composition and activity of the microbial community. By comparing the CGA-biotransforming capabilities of different human colonic microbiota, researchers found that diverse microbial communities act on CGA through different metabolic pathways, ultimately producing 3-(3-hydroxyphenyl) propionic acid (HPPA). Only a minority of individuals can further convert HPPA to phenylpropionic acid. Finally, the metabolites are absorbed and undergo phase II metabolism (conjugation with glucuronic acid, sulfate, methyl, or glycine) or other metabolic processes, such as hydrogenation, dehydrogenation, and α- or β-oxidation (Tomas-Barberan et al., 2014). Ultimately, 37 metabolites of CGA have been identified in human blood, urine, and feces (Nabavi et al., 2017; Lu et al., 2020; Liu et al., 2022). (Figure 4) This review discusses the potential mechanisms of CGA to regenerate bone tissue by promoting osteoblast activity and inhibiting osteoclast activity. Finally, we provide perspectives on the treatment of bone diseases with CGA.
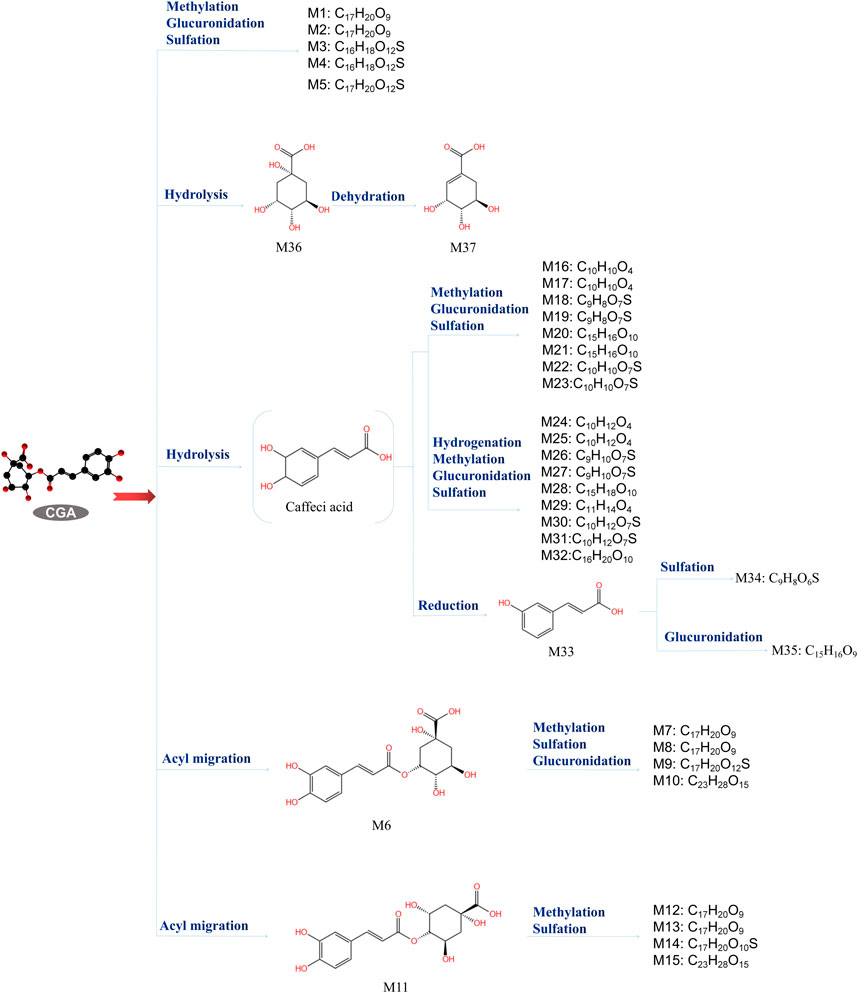
Figure 3. Chlorogenic acid metabolites (Zeng et al., 2022). The metabolic process of CGA and the structure and molecular formula of 37 metabolites.
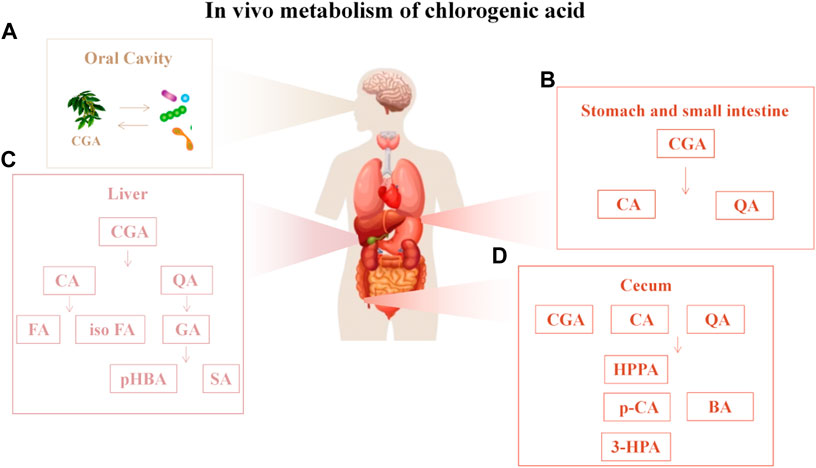
Figure 4. In vivo metabolism of chlorogenic acid (A) CGA is scarcely metabolized but can interact with the oral microbiota; (B) In the stomach and small intestine, chlorogenic acid (CGA) is metabolized into caffeic acid and quinic acid; (C) Upon entering the liver, caffeic acid is metabolized into ferulic acid and isoferulic acid, while quinic acid is converted into gallic acid, which is further degraded into p-hydroxybenzoic acid and syringic acid; (D) After entering the cecum in the form of CA and QA, CA is hydrolyzed into p-coumaric acid, 3-HPA or HPPA, and QA is hydrolyzed into BA. Abbreviations: CA, caffeic acid; QA, quinic acid; FA, ferulic acid; isoFA, isoferulic acid; GA, gallic acid; pHBA, 4-Hydroxybenzoic acid; SA, Gallic acid; HPPA, hydroxyphenyl propionic acid; p-CA, p-Coumaric acid; 3-HPA, 3-hydroxyphenyl propionic acid; BA, Benzoic acid.
2 Chlorogenic acid promotes osteoblasts
2.1 CGA enhances the proliferation and differentiation of osteoblasts
CGA has been shown to promote osteogenic proliferation and differentiation in vivo, thereby increasing bone mineralization and improving bone strength (Kawabata et al., 2018; Léotoing et al., 2016; Léotoing et al., 2016). These effects may be related to the amplification of tumor necrosis factor-α (TNF-α)-stimulated interleukin (IL-6) synthesis in osteoblasts by CGA (Yamamoto et al., 2015; Kozawa et al., 1997). Osteoblasts are derived from MSCs in the bone marrow, and differentiation is regulated by the bone morphogenetic protein (BMP) and wingless-associated integration site (WNT) pathways, which, in turn, increase cytoplasmic cAMP levels and stimulate DNA and collagen synthesis (Zhao et al., 2018; Ponzetti and Rucci, 2021).
2.2 The antioxidant and anti-apoptotic effects of CGA
Osteoblast precursors are recruited from the bone marrow to the bone surface by molecules, such as transforming growth factor β (TGF-β) and IGF-14. The first step in differentiation is the activation of runt-related transcription factor 2 (RUNX2), osterix and distal Drosophila 5. At the molecular level, RUNX2 is the main transcription factor regulating osteoblast function, while loss-of-function mutations in nuclear factor E2 p45-related factor 2 (Nrf2) reduce bone mass and load-driven anabolic responses (Dirckx et al., 2019; Komori, 2011). The role of Nrf2 in osteoblast differentiation may be related to intracellular reactive oxygen species (ROS) levels, which are elevated in Nrf2-deficient stromal cells, thereby causing oxidative stress and inhibiting osteoblast differentiation (Han et al., 2019; Park et al., 2014). Runx2 also promotes the proliferation of osteoblast progenitors and drives their commitment to the osteoblast lineage by directly regulating the expression of genes involved in hedgehog, fibroblast growth factor (Fgf), Wnt, and parathyroid hormone-like hormone (Pthlh) signaling pathways, as well as distal-less homeobox 5(Dlx5). In addition, smad ubiquitination regulatory factors (Smurfs) negatively modulate the TGF-β/BMP signaling pathway by promoting the ubiquitination and subsequent degradation of key signaling components (Kushioka et al., 2020; Komori, 2022). CGA inhibits apoptosis by reversing increased intracellular ROS production, H2O2 concentrations, and mitochondrial superoxide overproduction. The decreased expression of RUNX2, a transcription factor important for osteoblast differentiation, led to decreases in the expression of the important extracellular matrix protein osteocalcin. CGA extracts alleviated glucocorticoid-induced increases in bone resorption markers and decreased osteogenic markers by upregulating RANKL/OPG and RUNX2 signaling mRNA expression and protein levels (Naveed et al., 2018; Mo et al., 2019; Cai et al., 2021; Xiao et al., 2020). Zhou et al. found that Shp2 is a major cytoplasmic tyrosine phosphatase and that CGA promotes osteoblast proliferation and osteoclast differentiation in Bone marrow mesenchymal stem cells via the Shp2/PI3K/Akt/cyclinD1 pathway (Zhou et al., 2016). Upregulation of cellular proliferation by CGA was blocked by inhibiting Akt or Shp2. Recently, Bax and Bcl-2 were found to be two important genes regulating apoptosis. Bax overexpression accelerates apoptosis, whereas Bcl-2 overexpression inhibits apoptosis. High doses of CGA can prevent decreases in Bcl-2 and increases in Bax during apoptosis, thus inhibiting apoptosis (Zhang and Hu, 2016).
2.3 The benefits of CGA on the skeletal system (related to hormones)
One study found that CGA not only ameliorated ovariectomy-induced decreases in bone mineral density, but also upregulated osteocalcin and deoxypyridine, and stimulated alkaline phosphatase activity in primary osteoblasts. In addition, it prevented decreases in osteoblast viability after H2O2 treatment and reduced the rate of apoptosis and Caspase-3 activity. CGA activates Akt phosphorylation in osteoblasts and protects osteoblasts from oxidative damage via the PI3K/Akt-mediated Nrf2/HO-1 pathway (Han et al., 2019; Han et al., 2017; Nugraha et al., 2023). CGA was reported to reverse the dexamethasone-induced downregulation of p21 and promote the expression of nuclear Nrf2 and total Nrf2, as well as their downstream target protein heme oxygenase-1 (HO-1). These results suggest that the protective effects of CGA in osteoblasts are closely related to the activation of the PI3K/Akt-Nrf2/HO-1 and p21-Nrf2/HO-1 anti-oxidative stress signaling pathways, providing experimental evidence for the cytoprotective effects of CGA (Han et al., 2019; Han et al., 2017). Zhou et al. suggested that CGA might inhibit bone resorption in a manner similar to that of phytoestrogens, i.e., by competing with estrogen for estrogen receptors (ERs). In rodents and humans, ERs exist in both α and β isoforms. The Erβ isoforms are more abundant, and CGA has a higher affinity for ERβ. Studies have shown that CGA has a direct stimulatory effect on the proliferation and differentiation of cultured rat osteoblast precursors (Dirckx et al., 2019; Innocenti et al., 2007; Yang and Wan, 2019). At the cellular level, high doses of CGA at a concentration of 20 mg/kg enhanced bone density in the femoral head and femoral neck after ischemia. CGA can improve the proliferative capacity of osteoblasts, accelerate the transition of osteoblasts from the G1 phase to the S phase, and enhance mitosis and osteoblast regeneration, thus effectively improving hormone-induced necrosis (Zhang and Hu, 2016).
2.4 Derivatives of CGA and their osteogenic effects
Karadeniz et al. found that 3, 5-dicaffeoyl-epi-quinic acid (DCEQA), a derivative of CGA, enhanced osteoblast differentiation by stimulating Wnt/BMP signaling. It upregulated the expression of osteogenic markers alkaline phosphatase, osteocalcin, Runx2, BMP2, and Wnt10a (Karadeniz et al., 2020). Min et al. found that the Hspa1a/Fgfr2/Gadd45a/Tgfb3/Hspa1b gene was localized to the MAPK pathway using Kyoto Encyclopedia of Genes and Genomes analysis. Previous studies reported that CGA might affect the expression of apoptosis-related genes that are part of the oxidative stress and p38 MAP-dependent pathways (Min et al., 2018). (Figure 5) A clinical study conducted by Ferrantelli et al. demonstrated that daily consumption of 100 g of fresh lettuce with high concentrations of polyphenols, including CGA and CA, for a duration of 12 days resulted in the regulation of bone metabolism and improved phosphate absorption rate in participants. Consequently, natural polyphenols exert a positive influence on bone health (Ferrantelli et al., 2023).
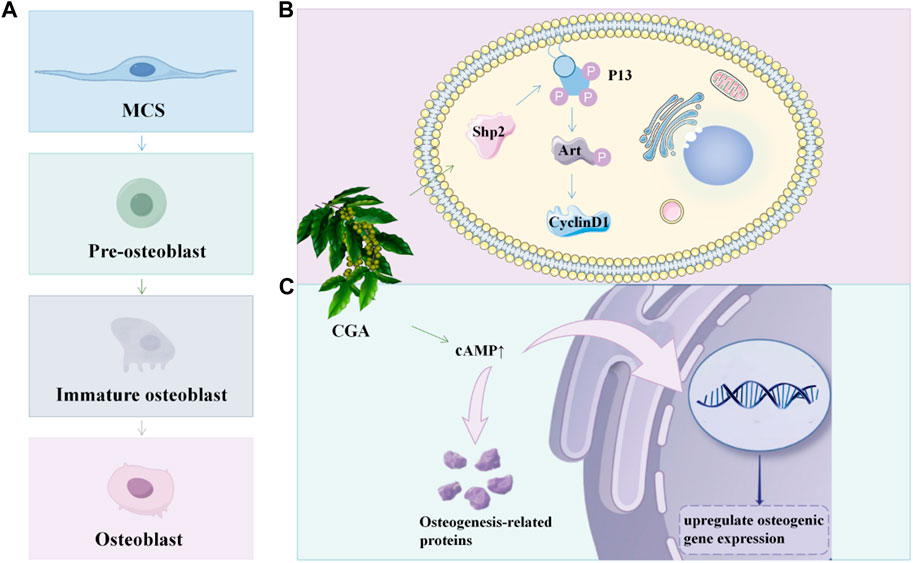
Figure 5. CGA promotes osteoblast proliferation and differentiation (A) The developmental process of osteoblasts; (B) CGA promote osteoblast proliferation in BMSC via the Shp2/PI3K/Akt/cyclin D1 pathway; (C) CGA could upregulate osteogenic gene expression and osteogenic protein synthesis by promoting cAMP expression.
However, some scholars remain skeptical of osteogenic promotion by CGA. Sakai suggested that the enhancement of PGF2α-induced Osteoprotegerin mRNA expression levels may not be attributed to CGA but to Epigallocatechin Gallate (Sakai et al., 2017). Folwarczna and colleagues also found that low doses of caffeic acid had detrimental effects on the skeletal system, while high doses of caffeic acid and CGA increased tibial mineralization and improved the mechanical properties of the femoral shaft (Folwarczna et al., 2015). In summary, CGA positively influences the proliferation, differentiation, and apoptosis of osteoblasts through various mechanisms, thereby helping to maintain skeletal health. These findings provide experimental evidence and a theoretical basis for applying CGA to treat skeletal diseases, offering potential new drug candidates or therapeutic strategies for the prevention and treatment of related conditions. However, further research is needed to explore the specific mechanisms of action of CGA in vivo, as well as its safety and efficacy in different populations and disease models.
3 Chlorogenic acid inhibits osteoclasts
3.1 The interaction between osteoclasts and osteoblasts
Osteoclasts are derived from mononuclear hematopoietic marrow lineage cells and are attracted to the bloodstream and then to the bone surface by factors such as sphinxin-1 phosphate. Multinucleated bone-resorbing cells are formed by the uptake of chemokines and other factors. The interaction between osteoblasts and osteoclasts is crucial for maintaining site-specific bone homeostasis and regulating bone remodeling. Both small extracellular vesicles and apoptotic vesicles from mature osteoblasts were shown to express membrane-bound RANK, which is a ligand for osteoblastic RANKL-activated RUNX2 expression. Faqeer et al. identified a new method of communication between osteoclasts and osteoblasts through proteomics analysis. Specifically, Secreted phosphoprotein one from osteoclasts activates TGFβ1/SMAD signaling in MSCs, promoting their osteogenic differentiation (Faqeer et al., 2023).
3.2 The regulatory role of CGA on osteoclasts
3.2.1 CGA regulated the RANKL signaling pathway
In recent years, CGA has been reported to reduce osteoclast differentiation and bone resorption. It was also shown to increase tibial mineralization and improve bone strength of the femoral backbone in in vivo experiments, which may be related to its regulation of the RANKL signaling pathway (Kuroyanagi et al., 2017; Chen et al., 2021; Ho et al., 2024). RANKL can bind to the RANK receptor on the surface of osteoblasts, thereby activating a number of major intracellular signaling pathways, including nuclear factor-κB (NF-κB), JNK, ERK, and p38 MAPK. NF-κβ is translocated to the nucleus through IκB kinase (IKK) phosphorylation and degradation. Recently, CGA was recently discovered to block RANKL-mediated IκB-α phosphorylation and degradation, hence decreasing NF-κB activation. In addition, RANKL-induced MAPK kinase activation further leads to the activation of the NFATc1 factor. NFATc1 is thought to be the master transcription factor regulating the terminal differentiation of osteoblasts (Boyce, 2013; Kwak et al., 2013; Ono et al., 2020; Ono and Nakashima, 2018). CGA showed an inhibitory effect on osteoclast formation by downregulating the expression of NFATc1 and also regulated the expression of the osteoclast-specific genes OSCAR and TRAP. This effectively prevented RANKL-induced osteoclast production and osteolytic bone destruction in vivo (Kwak et al., 2013; Ono et al., 2020; Ono and Nakashima, 2018; Tang et al., 2006; Lee S. H. et al., 2017; Xu et al., 2022). The RANKL signaling pathway plays an important role in bone resorption, and factors affecting osteoclasts all act directly or indirectly through this pathway. Osteoclasts secrete OPG, which acts as a decoy receptor to bind to RANK ligands and prevents them from binding to RANK on osteoclasts, thereby inhibiting bone resorption (Faqeer et al., 2023; Simonet et al., 1997). Osteoclast development may be regulated indirectly through the expression of OPG and RANKL in osteoblasts. The binding of RANKL to receptors is induced by low levels of ROS and multiple growth factors and cytokines (Lee et al., 2005; Ashtar et al., 2020). Hence, Increased ROS may play an important role as a secondary messenger in the RANKL-mediated osteoclast differentiation signaling pathway.
3.2.2 CGA regulates V-ATPase and autophagy
Emerging evidence suggests that bone resorption requires osteoclasts to possess the capability to generate protons, as the dissolution of the bone matrix by alkaline salts and the digestion of the bone matrix by acid phosphatases secreted by osteoclasts necessitate an acidic pH. The ruffled border of osteoclasts contains vacuolar H + -adenosine triphosphatase (V-ATPase), which hydrolyzes ATP to produce protons that are then translocated to the extracellular environment. The interaction of V-ATPase with TNF receptor-associated factor 6 (TRAF6), which is recruited by RANK, leads to its activation. Lee et al. found that CGA, while not significantly affecting the expression of TRAF6 or V-ATPase, blocked the association between V-ATPase and TRAF6 to some extentt (Rousselle and Heymann, 2002; Lee S. H. et al., 2017; Yang Y. et al., 2022). (Figure 6) A recent study found that the processes of endocytosis, secretion, and translocation, which occur at the fold boundary, may be related to cellular autophagy. The loss of lysosome-microtubule connections impairs bone resorption and lysosomal distribution in osteoblasts. The formation of fold boundaries requires several autophagy-associated proteins (Atg) to act in concert with microtubule-associated protein light chain three for actin ring formation, tissue proteinase K release, and bone resorption in osteoclasts (Pantoom et al., 2021; Fujiwara et al., 2016; Hiura et al., 2022; Wang et al., 2011). Caffeic acid, which is structurally similar to CGA, was found to effectively inhibit the formation of the osteoclast differentiation factor NF-κB ligand (receptor activator of RANKL). It directly inhibits osteoclast differentiation by inhibiting NF-κB activity and downregulating c-Fos and NFATc1. Dried plums rich in caffeic acid and CGA induced reductions in osteoclast surface areas, inhibited intrinsic osteoclast activity (reduced ctsk expression), and decreased serum C-terminal cross-linking telopeptide levels (a marker of bone resorption) (Ha et al., 2009; Shahnazari et al., 2016).
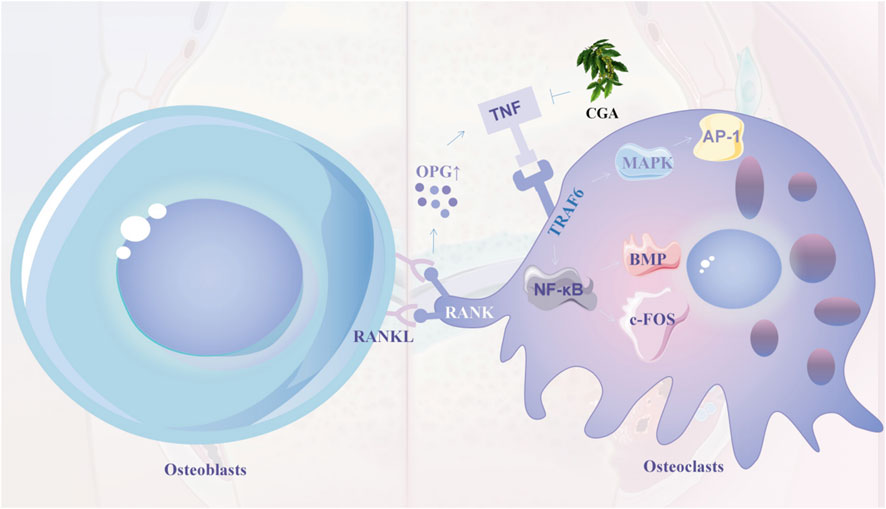
Figure 6. OPG is upregulated after RANKL and RANK junction, thereby recruiting TRAF6 molecules and activating MAPK and NF-κB pathways, thereby promoting the expression of AP-1, BMP and c-FOS (early signals in endochondral bone formation). Chlorogenic acid inhibits osteoclast formation and differentiation by inhibiting TRAF6 and MAPK and NF-κB signalling pathways in osteoblasts. 7.
Overall, CGA can inhibit osteoclasts by regulating the RANKL signaling pathway, V-ATPase, and autophagy. Therefore, CGA can be used to provide new strategies and methods for the prevention and treatment of bone-related diseases.
4 Chlorogenic acid in clinical applications
4.1 Applications in the oral cavity
Periodontitis is a common oral disease affected by dental plaque. Xia et al. found that CGA modulates the PI3K/AKT and NF-κB/MAPK signaling pathways targeting key genes (AKT, MAPK1, MAPK14, NF-κB, TNF, IL-2, and IL-1B). CGA treatment also inhibited Porphyromonas gingivalis (PG)-lipopolysaccharide (LPS)-induced increases in IL-1β and IL-18 in gingival fibroblasts. It also inhibited the expression of CysLT1R, HO-1, NLRP3, ASC, cysteinogen-1, active cysteine-1, and other proteins associated with LPS induction. In conclusion, CGA inhibited oxidative stress by promoting the nuclear translocation of Nrf2, and increased mitochondrial membrane potential, thereby decreasing the protease activity of PG, reducing alveolar bone loss, and inhibiting the development of inflammation (Kwon et al., 2021; Xia et al., 2023; Scannapieco and Gershovich, 2020; Huang et al., 2022; Tsou et al., 2019). Some authors found that CGA treatment significantly inhibited the production of inflammatory mediators and the expression of inducible nitric oxide synthase (iNOS) and cyclooxygenase-2 (COX-2) proteins mediated by PG in a dose-dependent manner by the Griess reaction, enzyme-linked immunosorbent assay (ELISA), and Western blotting analysis. Treatment of cells with the indicated concentrations of CGA and PG-LPS (1 μg/mL) at 37°C for 4 h revealed that CGA treatment inhibited the activation of the Toll-like receptor 4/myeloid differentiation primary response gene 88 (MyD88) and NF-κB in LPS-PG-stimulated HGFs (Human gingival fibroblasts). CGA treatment also inhibited LPS-PG-induced ERK and Akt phosphorylation but did not affect JNK or p38. The inhibitory effect of CGA on PG was investigated by turbidity tests and plate counts. The minimum inhibitory concentration of CGA was found to be 4 mg/mL, and the minimum bactericidal concentration was found to be 16 mg/mL (Naveed et al., 2018; Tsou et al., 2019; Park and Yoon, 2022; Marchesan et al., 2018; Liang and Kitts, 2015; Rashidi et al., 2022; Arfian et al., 2019). A concentration of 4 mg/mL CGA reduced cysteine protease activity by more than 40%. Hu et al. treated human dental pulp stem cells with CGA for 72 h. Cell proliferation in the 100 μg/mL group was significantly increased compared to the 0.1, 10 μg/mL, and 1 mg/mL groups. Frizzled-elated protein (FRZB), a member of the secreted frizzled-related family proteins, plays an important role in the osteogenic differentiation of MSCs as a regulator of Wnt signaling. RNA sequencing and real-time quantitative polymerase chain reaction analyses showed that CGA treatment increased the production of FRZB and pyruvate dehydrogenase kinase 4(PDK4) osteogenic gene expression and suppressed that of asperulins and cytokine-like 1. Western blot analysis revealed that in addition to FRZB, CGA treatment decrease total β-linked protein activity and increased total calcium/calmodulin-dependent kinase II, phosphorylated CamKII, and phosphorylated cAMP response element-binding protein (Hu et al., 2021; Yagi et al., 2022; Corr and Lane, 2007; Thysen et al., 2016). CGA-PLGA was recently synthesized by loading CGA onto poly (D,L-propylene-co-ethyleneglycolate) (PLGA) and modifying it with polyvinylpyrrolidone, resulting in the sustained release of CGA, which not only effectively removed ROS but also inhibited the cellular overexpression of pro-inflammatory cytokines. CGA-PLGA remained in gingival tissue for more than 24 h after local injection in a mouse model, thus effectively inhibiting alveolar bone resorption and stopping the progression of periodontitis (Li et al., 2022). In conclusion, CGA alleviates inflammation and oxidative stress induced by PG by modulating signaling pathways and gene expression, thereby reducing alveolar bone loss. CGA also inhibits bacterial growth and biofilm formation. The development of CGA nanocarriers has facilitated the sustained release of CGA in gingival tissue, suppressing disease progression and offering a novel strategy for treating periodontitis. Palaniraj et al. developed an antimicrobial CGA-loaded porous nanogel based on a calcium phosphate-chitosan nanogel. The base nanostructure was formed by the ionic gelation of calcium phosphate nanoparticles as a cross-linking agent, and its porous structure enabled controlled drug release. The negative charge on the phosphate ion and the positive charge on chitosan neutralize bacterial growth. Chitosan and CGA can disrupt bacterial cell membranes and penetrate the cells, thus eliminating biofilm formation. Future nanogel designs could promote the differentiation of human dental pulp MSCs (Palaniraj et al., 2019). He et al. addressed the problem of massive defects and associated infections in clinical bone implants by loading a CGA drug/graft peptide (bone-forming peptide, BFP) hydrogel system onto the surface of sulfonated polyetheretherketone (SPEEK). Sodium alginate was used as the drug carrier, and the BFP peptide was grafted onto the sodium alginate surface by further modification. The results showed that the SPEEK-CGA-grafted peptide hydrogel system was more active than CGA in terms of cell adhesion, proliferation, and ALP activity, thus providing an alternative approach for bone repair (Chahardoli et al., 2023; He et al., 2019). (Figure 7)
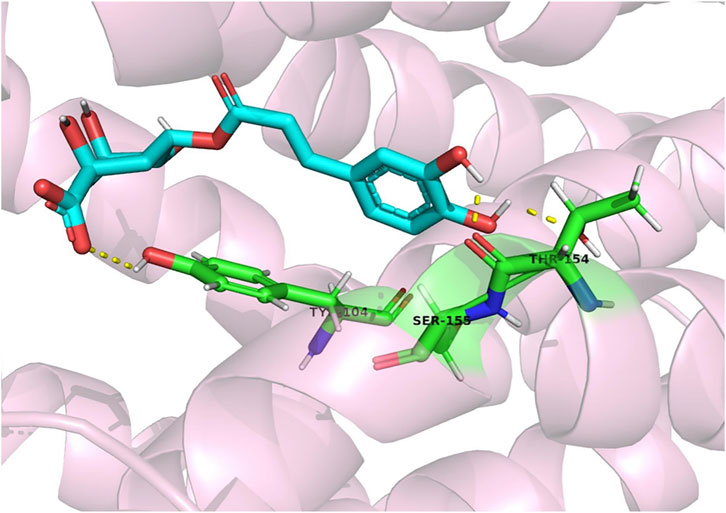
Figure 7. CGA forms a molecular docking with CysLT1R, which in turn reduces lipopolysaccharide-induced inflammation in gingival fibroblasts by affecting the CysLT1R/Nrf2/NLRP3 signalling pathway (Huang et al., 2022).
4.2 Applications in other diseases
CGA, renowned for its notable antioxidant and anti-inflammatory properties, mitigates oxidative stress and inflammatory responses in chondrocytes through diverse mechanisms. CGA suppresses the proliferation of osteosarcoma cells and demonstrates potential in treating osteoarthritis and rheumatoid arthritis. CGA also regulates the immune system by reducing the expression of pro-inflammatory cytokines and modulates the balance between bone resorption and formation in osteoporosis. Nanocarriers and hydrogel systems loaded with CGA offer novel therapeutic strategies for treating related skeletal diseases (Yang et al., 2023). The antioxidant potential of CGA has been suggested as it can prevent oxidative stress by activating multiple signaling pathways in chondrocytes. First, CGA can inhibit the STAT3 snail pathway, thereby reducing cell viability and decreasing the expression of the proliferating cell nuclear antigen (PCNA) in osteosarcoma cells. CGA has also been shown to have anticancer effects in several types of tumors, including osteosarcoma. Researchers reported changes in the expression of the signal transducer and activator of transcription 3 (STAT3)/snail pathway by Western blotting analysis to argue the toxicity of CGA on osteosarcoma cells and explore its potential mechanism. Zhang et al. found that CGA dose-dependently decreased cell viability and PCNA expression in osteosarcoma cells. At the same time, CGA increased apoptosis and cysteine-3/7 activity in osteosarcoma cells in a concentration-dependent manner. Si-TAT3 inhibited the STAT3/snail pathway to retard the growth of osteosarcoma cells and induce apoptosis. Studies have shown that the induction of apoptosis is the main way in which CGA affects the cell cycle and inhibits cell growth. On this basis, the cooperative effects of CGA and adriamycin were investigated in U2OS and MG-63 human OS cells. In cells treated with adriamycin, the concomitant administration of CGA further reduced cell viability and growth and potentially promoted cell death by inducing apoptosis (Zhang et al., 2019; Sapio et al., 2020; Salzillo et al., 2021; Xu et al., 2023). Yang and colleagues reported the preparation of AuNR@CA nanohybrids from CGA and gold nanorods. These nanohybrids triggered apoptosis and inhibited the growth of osteosarcoma. Additionally, under controlled mild near-infrared irradiation, the nanohybrids stimulated cellular osteogenic differentiation by upregulating Hsp47 and Hsp70 genes and promoting the expression of heat shock proteins (Yang et al., 2023).
Osteoarthritis (OA) is a progressive disease of cartilage damage. The pro-inflammatory cytokine IL-1β plays a key role in the progression of OA by inducing inflammation and upregulating the expression of matrix metalloproteinases (MMPs), a major mediator of bone degradation, leading to cartilage matrix degradation. IL-1β also induces the production of the inflammatory mediators nitric oxide (NO) and prostaglandin E2 (PGE). Chen et al. studied the IL-1β-induced expression levels of MMP-1, MMP-3, MMP-13, and tissue inhibitor of metalloproteinase-1 (TIMP-1) in rabbit chondrocytes using quantitative real-time fluorescence PCR and ELISA, and found that CGA inhibited MMP mRNA and protein expression and increased TIMP-1 expression at the mRNA and protein levels. CGA inhibited IL-1β-induced NF-κB activation and degradation of the κB inhibitor (IκB)-α. Other investigators examined the expression levels of iNOS, PGE2, COX-2, collagen II, MMP13, NF-κB, and inhibitor-κB α by Western blot analysis. The results showed that CGA prevented IL-1β-induced increases in iNOS/NO, IL-6, MMP-13, and COX-2/PGE2 production, and the IL-1β-mediated downregulation of collagen II. The data also suggest that CGA inhibits IL-1β-induced inflammatory responses associated with the NF-κB signaling pathway. Taken together, CGA has potential value for treating OA (Huh et al., 2012; Zheng et al., 2023; Chen and Wu, 2014; Liu et al., 2017; Zada et al., 2021; Blaney Davidson et al., 2007). Additionally, it downregulates the expression of pro-inflammatory cytokines, such as TNF-α, IL-6, interferon-gamma (IFN-γ), and MMP-associated proteins. CGA was found to prevent the inflammatory responses induced by IL-1β, which are associated with the NF-κB signaling pathway. This suggests that CGA may have potential value in treating OA (Singh et al., 2023).
Rheumatoid arthritis (RA) is a systemic inflammatory disease, and B-cell activating factor (BAFF), a member of the TNF) family, was recently found to play a key role in the pathogenesis and progression of RA (Smolen et al., 2016). CGA was found to reduce the DNA binding activity of NF-κB to the BAFF promoter region and inhibit BAFF via the NF-κB pathway in TNF-α-stimulated MH7A cells, thereby significantly attenuating the progression of arthritis. At a dosage of 40 mg/L, CGA effectively controlled the total CD3 cell counts and significantly inhibited the binding of CD80/86 to T-cell receptor. The interaction of CD80 or CD86 with the T-cell receptor promotes T-cell responses, enhancing the expansion of antigen-specific T cells. Thus, it can be hypothesized that CGA reduces inflammation by inhibiting T cells, possibly by inhibiting CD80 and CD86 molecules (Fu et al., 2019; Chauhan et al., 2012). The effect of CGA on CD4 T-cell-specific Th1/Th2 cytokines was examined. CGA significantly inhibited Th1 cytokines but elevated Th2 cytokines in a dose-dependent manner. The administration of ASEDS, an aqueous seed extract of Astragalus membranaceus rich in CGA, markedly improved immune cell counts, immunoglobulin synthesis, high-density lipoproteins concentrations, and antioxidant status in treated rats (p < 0.05). A therapeutic effect on arthritic caused by systemic lupus erythematosus (SLE) was also seen. In an SLE model, 10-week-old female MRL/lpr mice were treated with 40 mg/kg CGA daily for 12 weeks by intraperitoneal injection. Scholars observed that CGA significantly reduced joint swelling in the MRL/lpr mice. Pathological examination showed arthritic cell infiltration and cartilage erosion in the model group, and a slight relief of joint lesions was observed in the CGA group. Therefore, CGA is also gradually being used to treat RA (Joshua et al., 2022; Lee et al., 2018). Lee et al. examined the effect of CGA on septic arthritis caused by Candida albicans and found a significant reduction in edema (Lee et al., 2008).
Osteoporosis is a systemic skeletal disease characterized by reduced bone mineral density and is particularly prevalent among the elderly and women. Postmenopausal osteoporosis is the most common form in women, with approximately 50% experiencing of women at least one fracture after menopause. This condition is typically caused by an imbalance between excessive bone resorption and insufficient bone formation. Estrogen deficiency further impairs the function of osteoblasts, exacerbating bone loss and intensifying the imbalance between bone absorption and formation (Cui et al., 2024; Cui et al., 2022a; Cui et al., 2022b).
Osteoporosis is characterized by reduced bone mass, deteriorating microarchitecture, and fragility fractures, which are often seen in older women. The anti-osteoporotic activity of Artemisia capillaris was investigated using an ethanolic extract of capillary salt (ACHE) containing CGA, caffeic acid, gibberellin, isoquercitrin, isochlorogenic acid A, and hyoscovone. ACHE, particularly CGA, reduced osteoclast differentiation and bone resorption. CGA downregulated the interaction of V-ATPase with TRAF6, partly mediating the blockade of bone resorption by capillaries (Lee et al., 2016; Lee S. K. et al., 2017; Lane et al., 2000).
Finally, CGA/BFP hydrogel systems loaded onto SPEEK surfaces demonstrated excellent antimicrobial activity, offering a potential treatment for defects and associated infections in clinical bone implants (Salzillo et al., 2021; Nandhini et al., 2023). (Table 2)
5 Discussion
The current review has several limitations. Firstly, the optimal concentration of CGA for osteogenesis must be investigated, and specific mechanisms by which CGA inhibits osteogenesis at low concentrations while promoting it at high concentrations must be elucidated to lay the groundwork for the future development of CGA-related drugs to enhance bone formation. Secondly, the inconsistency in the dosages and models used in current experimental research poses a challenge in reaching consistent conclusions. Lastly, few studies have investigated the effects of CGA on osteoclasts and osteoarthritis, and clinical trials assessing the safety and efficacy of CGA in diverse populations and disease models are lacking.
6 Conclusion
The increasing number of bone-related diseases has helped them to become a pressing concern, especially for the aging population facing challenges associated with such disorders. Oral-related bone issues diminish the quality of life and impose significant financial burdens. Chronic bone illnesses can progress over time, potentially resulting in fractures and disabilities (Chen et al., 2019; Aspray and Hill, 2019; Lorentzon and Cummings, 2015; Parsons et al., 2018; Black and Rosen, 2016; Schwendicke, 2020; Nazir, 2017). Currently, the primary clinical treatment for osteoporosis in Western medicine involves bone resorption-inhibiting drugs, which often have extended treatment cycles and substantial adverse effects. These issues make it challenging for patients to adhere to the treatments over extended periods, resulting in suboptimal outcomes (Prestwood et al., 1995; Miller, 2016; Lei et al., 2023).
In contrast, CGA controls cellular signaling pathways influencing osteoblast and osteoclast development, promotes bone production, prevents bone resorption, and exhibits potent osteogenic characteristics. In recent years, research on senescence and bone diseases has gradually increased. Ambrosi et al. reported that senescent cells tended to accumulate in bone and trigger chronic inflammation by releasing secreted phenotypic factors associated with senescence. Several signaling pathways, including Hedgehog, Notch, Wnt/β-linker protein, TGF/BMP, and fibroblast growth factor, are involved in regulating cellular senescence in the bone and bone marrow microenvironments (He et al., 2023; Ambrosi et al., 2021). CGA has been shown to inhibit endothelial senescence both in vivo and in vitro by regulating the Nrf2/HO-1 pathway (Hada et al., 2020). CGA activates the FOXO transcription factors DAF-16, HSF-1, SKN-1, and HIF-1 and prolongs the lifespan of Hidradenitis elegans nematodes through DAF-16 in the insulin/IGF-1 signaling pathway. Therefore, CGA is expected to regulate bone regeneration and treat bone-related diseases through senescence-related pathways (Zheng et al., 2017).
Although few clinical trials have focused on CGA, the existing studies show promising results for the potential of CGS to protect bones. Research outcomes in mice suggest that CGA has the ability to protect various macromolecules against oxidative damage. As a significant source of polyphenols in the human diet, CGA is considered a promising candidate for the treatment of bone-related conditions. Further studies should delve into how CGA interacts with biological processes and its potential application in preventing or treating bone deformities.
The available safety evidence suggests that CGA should be relatively safe for use in enhancing bone health. However, recent studies have indicated that CGA can act as an allergen in certain individuals, potentially triggering allergic reactions such as asthma and dermatitis (Lin et al., 2013). Therefore, dose-specific clinical trials are needed to determine the safety of its long-term use before it can be clinically applied. In recent years, with the advancement of biomedicine, topical drug delivery systems have gained popularity in clinical settings due to their low side effects, high concentrations in target tissues, and low systemic uptake (Zhao et al., 2022). Rui et al. found that CGA-chitosan complex significantly enhanced metal ion-chelating activity, total antioxidant capacity, scavenging activity, and lipid peroxidation (Rui et al., 2017). CGA also exhibited excellent antibacterial activity against both Gram-negative and Gram-positive bacteria (Yang X. et al., 2022). Determining the effective dose of CGA to promote osteogenesis will be crucial for future clinical trials and investments. In the dental field, CGA is anticipated to be used to treat periodontitis and peri-implantitis (Zhou et al., 2016; Sari et al., 2023)..
Author contributions
JS: Conceptualization, Formal Analysis, Funding acquisition, Software, Writing–original draft. SZ: Supervision, Visualization, Writing–original draft. JZ: Investigation, Project administration, Supervision, Writing–original draft. XW: Conceptualization, Data curation, Formal Analysis, Writing–original draft. ZW: Funding acquisition, Project administration, Resources, Writing–review and editing. BH: Funding acquisition, Project administration, Resources, Writing–review and editing.
Funding
The author(s) declare that financial support was received for the research, authorship, and/or publication of this article. This research was funded by Natural Science Fund of China, grant number 82301026; Jilin Province Science and Technology Development plan project, grant number 20230204079YY.
Conflict of interest
The authors declare that the research was conducted in the absence of any commercial or financial relationships that could be construed as a potential conflict of interest.
Publisher’s note
All claims expressed in this article are solely those of the authors and do not necessarily represent those of their affiliated organizations, or those of the publisher, the editors and the reviewers. Any product that may be evaluated in this article, or claim that may be made by its manufacturer, is not guaranteed or endorsed by the publisher.
References
Alqahtani, M. S., Kazi, M., Alsenaidy, M. A., and Ahmad, M. Z. (2021). Advances in oral drug delivery. Front. Pharmacol. 12, 618411. doi:10.3389/fphar.2021.618411
Ambrosi, T. H., Marecic, O., McArdle, A., Sinha, R., Gulati, G. S., Tong, X., et al. (2021). Aged skeletal stem cells generate an inflammatory degenerative niche. Nature 597, 256–262. doi:10.1038/s41586-021-03795-7
Arfian, N., Wahyudi, D. A. P., Zulfatina, I. B., Citta, A. N., Anggorowati, N., Multazam, A., et al. (2019). Chlorogenic acid attenuates kidney ischemic/reperfusion injury via reducing inflammation, tubular injury, and myofibroblast formation. Biomed. Res. Int. 2019, 5423703. doi:10.1155/2019/5423703
Ashtar, M., Tenshin, H., Teramachi, J., Bat-Erdene, A., Hiasa, M., Oda, A., et al. (2020). The roles of ROS generation in RANKL-induced osteoclastogenesis: suppressive effects of febuxostat. Cancers (Basel) 12, 929. doi:10.3390/cancers12040929
Aspray, T. J., and Hill, T. R. (2019). Osteoporosis and the ageing skeleton. Subcell. Biochem. 91, 453–476. doi:10.1007/978-981-13-3681-2_16
Bagdas, D., Gul, Z., Meade, J. A., Cam, B., Cinkilic, N., and Gurun, M. S. (2020). Pharmacologic overview of chlorogenic acid and its metabolites in chronic pain and inflammation. Curr. Neuropharmacol. 18, 216–228. doi:10.2174/1570159X17666191021111809
Barbour, A., Elebyary, O., Fine, N., Oveisi, M., and Glogauer, M. (2022). Metabolites of the oral microbiome: important mediators of multikingdom interactions. FEMS Microbiol. Rev. 46, fuab039. doi:10.1093/femsre/fuab039
Bisht, A., Dickens, M., Rutherfurd-Markwick, K., Thota, R., Mutukumira, A. N., and Singh, H. (2020). Chlorogenic acid potentiates the anti-inflammatory activity of curcumin in LPS-stimulated THP-1 cells. Nutrients 12, 2706. doi:10.3390/nu12092706
Black, D. M., and Rosen, C. J. (2016). Clinical practice. Postmenopausal osteoporosis. N. Engl. J. Med. 374, 254–262. doi:10.1056/NEJMcp1513724
Blaney Davidson, E. N., van der Kraan, P. M., and van den Berg, W. B. (2007). TGF-beta and osteoarthritis. Osteoarthr. Cartil. 15, 597–604. doi:10.1016/j.joca.2007.02.005
Borciani, G., Montalbano, G., Baldini, N., Cerqueni, G., Vitale-Brovarone, C., and Ciapetti, G. (2020). Co-culture systems of osteoblasts and osteoclasts: simulating in vitro bone remodeling in regenerative approaches. Acta Biomater. 108, 22–45. doi:10.1016/j.actbio.2020.03.043
Boyce, B. F. (2013). Advances in the regulation of osteoclasts and osteoclast functions. J. Dent. Res. 92, 860–867. doi:10.1177/0022034513500306
Cai, J., Qin, H., and Yu, G. (2021). Effect of periostin silencing on Runx2, RANKL and OPG expression in osteoblasts. J. Orofac. Orthop. 82, 82–91. doi:10.1007/s00056-020-00253-3
Chahardoli, F., Pourmoslemi, S., Soleimani Asl, S., Tamri, P., and Haddadi, R. (2023). Preparation of polyvinyl alcohol hydrogel containing chlorogenic acid microspheres and its evaluation for use in skin wound healing. J. Biomater. Appl. 37, 1667–1675. doi:10.1177/08853282221150845
Chauhan, P. S., Satti, N. K., Sharma, P., Sharma, V. K., Suri, K. A., and Bani, S. (2012). Differential effects of chlorogenic acid on various immunological parameters relevant to rheumatoid arthritis. Phytother. Res. 26, 1156–1165. doi:10.1002/ptr.3684
Chen, C. H., Elsalmawy, A. H., Ish-Shalom, S., Lim, S. J., Al-Ali, N. S., Cunha-Borges, J. L., et al. (2019). Study description and baseline characteristics of the population enrolled in a multinational, observational study of teriparatide in postmenopausal women with osteoporosis: the Asia and Latin America Fracture Observational Study (ALAFOS). Curr. Med. Res. Opin. 35, 1041–1049. doi:10.1080/03007995.2018.1552576
Chen, W. P., and Wu, L. D. (2014). Chlorogenic acid suppresses interleukin-1β-induced inflammatory mediators in human chondrocytes. Int. J. Clin. Exp. Pathol. 7, 8797–8801.
Chen, X., Zhu, X., Wei, A., Chen, F., Gao, Q., Lu, K., et al. (2021). Nrf2 epigenetic derepression induced by running exercise protects against osteoporosis. Bone Res. 9, 15. doi:10.1038/s41413-020-00128-8
Corr, M., and Lane, N. E. (2007). FRZB: a bone and joint connection. Arthritis Rheum. 56, 3881–3883. doi:10.1002/art.23139
Cui, Y., Guo, Y., Kong, L., Shi, J., Liu, P., Li, R., et al. (2022a). A bone-targeted engineered exosome platform delivering siRNA to treat osteoporosis. Bioact. Mater 10, 207–221. doi:10.1016/j.bioactmat.2021.09.015
Cui, Y., Li, Z., Guo, Y., Qi, X., Yang, Y., Jia, X., et al. (2022b). Bioinspired nanovesicles convert the skeletal endothelium-associated secretory phenotype to treat osteoporosis. ACS Nano 16, 11076–11091. doi:10.1021/acsnano.2c03781
Cui, Y., Lv, B., Li, Z., Ma, C., Gui, Z., Geng, Y., et al. (2024). Bone-Targeted biomimetic nanogels Re-establish osteoblast/osteoclast balance to treat postmenopausal osteoporosis. Small 20, e2303494. doi:10.1002/smll.202303494
Dirckx, N., Moorer, M. C., Clemens, T. L., and Riddle, R. C. (2019). The role of osteoblasts in energy homeostasis. Nat. Rev. Endocrinol. 15, 651–665. doi:10.1038/s41574-019-0246-y
dos Santos, M. D., Almeida, M. C., Lopes, N. P., and de Souza, G. E. (2006). Evaluation of the anti-inflammatory, analgesic and antipyretic activities of the natural polyphenol chlorogenic acid. Biol. Pharm. Bull. 29, 2236–2240. doi:10.1248/bpb.29.2236
Faqeer, A., Wang, M., Alam, G., Padhiar, A. A., Zheng, D., Luo, Z., et al. (2023). Cleaved SPP1-rich extracellular vesicles from osteoclasts promote bone regeneration via TGFβ1/SMAD3 signaling. Biomaterials 303, 122367. doi:10.1016/j.biomaterials.2023.122367
Ferrantelli, V., Vasto, S., Alongi, A., Sabatino, L., Baldassano, D., Caldarella, R., et al. (2023). Boosting plant food polyphenol concentration by saline eustress as supplement strategies for the prevention of metabolic syndrome: an example of randomized interventional trial in the adult population. Front. Nutr. 22, 1288064. doi:10.3389/fnut.2023.1288064
Folwarczna, J., Pytlik, M., Zych, M., Cegieła, U., Nowinska, B., Kaczmarczyk-Sedlak, I., et al. (2015). Effects of caffeic and chlorogenic acids on the rat skeletal system. Eur. Rev. Med. Pharmacol. Sci. 19, 682–693.
Freeman, F. E., Burdis, R., and Kelly, D. J. (2021). Printing new bones: from print-and-implant devices to bioprinted bone organ precursors. Trends Mol. Med. 27, 700–711. doi:10.1016/j.molmed.2021.05.001
Fu, X., Lyu, X., Liu, H., Zhong, D., Xu, Z., He, F., et al. (2019). Chlorogenic acid inhibits BAFF expression in collagen-induced arthritis and human synoviocyte MH7A cells by modulating the activation of the NF-κB signaling pathway. J. Immunol. Res. 2019, 8042097. doi:10.1155/2019/8042097
Fujiwara, T., Ye, S., Castro-Gomes, T., Winchell, C. G., Andrews, N. W., Voth, D. E., et al. (2016). PLEKHM1/DEF8/RAB7 complex regulates lysosome positioning and bone homeostasis. JCI Insight 1, e86330. doi:10.1172/jci.insight.86330
Ha, J., Choi, H. S., Lee, Y., Lee, Z. H., and Kim, H. H. (2009). Caffeic acid phenethyl ester inhibits osteoclastogenesis by suppressing NF kappaB and downregulating NFATc1 and c-Fos. Int. Immunopharmacol. 9, 774–780. doi:10.1016/j.intimp.2009.03.001
Hada, Y., Uchida, H. A., Otaka, N., Onishi, Y., Okamoto, S., Nishiwaki, M., et al. (2020). The protective effect of chlorogenic acid on vascular senescence via the Nrf2/HO-1 pathway. Int. J. Mol. Sci. 21, 4527. doi:10.3390/ijms21124527
Han, D., Chen, W., Gu, X., Shan, R., Zou, J., Liu, G., et al. (2017). Cytoprotective effect of chlorogenic acid against hydrogen peroxide-induced oxidative stress in MC3T3-E1 cells through PI3K/Akt-mediated Nrf2/HO-1 signaling pathway. Oncotarget 8, 14680–14692. doi:10.18632/oncotarget.14747
Han, D., Gu, X., Gao, J., Wang, Z., Liu, G., Barkema, H. W., et al. (2019). Chlorogenic acid promotes the Nrf2/HO-1 anti-oxidative pathway by activating p21(Waf1/Cip1) to resist dexamethasone-induced apoptosis in osteoblastic cells. Free Radic. Biol. Med. 137, 1–12. doi:10.1016/j.freeradbiomed.2019.04.014
He, X., Deng, Y., Yu, Y., Lyu, H., and Liao, L. (2019). Drug-loaded/grafted peptide-modified porous PEEK to promote bone tissue repair and eliminate bacteria. Colloids Surf. B Biointerfaces 181, 767–777. doi:10.1016/j.colsurfb.2019.06.038
He, X., Hu, W., Zhang, Y., Chen, M., Ding, Y., Yang, H., et al. (2023). Cellular senescence in skeletal disease: mechanisms and treatment. Cell Mol. Biol. Lett. 28, 88. doi:10.1186/s11658-023-00501-5
Heitman, E., and Ingram, D. K. (2017). Cognitive and neuroprotective effects of chlorogenic acid. Nutr. Neurosci. 20, 32–39. doi:10.1179/1476830514Y.0000000146
Hiura, F., Kawabata, Y., Aoki, T., Mizokami, A., and Jimi, E. (2022). Inhibition of the ATG4-LC3 pathway suppressed osteoclast maturation. Biochem. Biophys. Res. Commun. 632, 40–47. doi:10.1016/j.bbrc.2022.09.065
Ho, C. Y., Tang, C. H., Ho, T. L., Wang, W. L., and Yao, C. H. (2024). Chlorogenic acid prevents ovariectomized-induced bone loss by facilitating osteoblast functions and suppressing osteoclast formation. Aging (Albany NY) 16, 4832–4840. doi:10.18632/aging.205635
Hu, X., Wang, L., He, Y., Wei, M., Yan, H., and Zhu, H. (2021). Chlorogenic acid promotes osteogenic differentiation of human dental pulp stem cells through Wnt signaling. Stem Cells Dev. 30, 641–650. doi:10.1089/scd.2020.0193
Huang, S., Wang, L. L., Xue, N. N., Li, C., Guo, H. H., Ren, T. K., et al. (2019). Chlorogenic acid effectively treats cancers through induction of cancer cell differentiation. Theranostics 9, 6745–6763. doi:10.7150/thno.34674
Huang, X., Liu, Y., Shen, H., Fu, T., Guo, Y., and Qiu, S. (2022). Chlorogenic acid attenuates inflammation in LPS-induced Human gingival fibroblasts via CysLT1R/Nrf2/NLRP3 signaling. Int. Immunopharmacol. 107, 108706. doi:10.1016/j.intimp.2022.108706
Huh, J. E., Seo, B. K., Baek, Y. H., Lee, S., Lee, J. D., Choi, D. Y., et al. (2012). Standardized butanol fraction of WIN-34B suppresses cartilage destruction via inhibited production of matrix metalloproteinase and inflammatory mediator in osteoarthritis human cartilage explants culture and chondrocytes. BMC Complement. Altern. Med. 12, 256. doi:10.1186/1472-6882-12-256
Innocenti, G., Vegeto, E., Dall'Acqua, S., Ciana, P., Giorgetti, M., Agradi, E., et al. (2007). In vitro estrogenic activity of Achillea millefolium L. Phytomedicine 14, 147–152. doi:10.1016/j.phymed.2006.05.005
Joshua, P. E., Yahaya, J., Ekpo, D. E., Ogidigo, J. O., Odiba, A. S., Asomadu, R. O., et al. (2022). Modulation of immunological responses by aqueous extract of Datura stramonium L. seeds on cyclophosphamide-induced immunosuppression in Wistar rats. BMC Immunol. 23, 50. doi:10.1186/s12865-022-00519-y
Karadeniz, F., Oh, J. H., Lee, J. I., Seo, Y., and Kong, C. S. (2020). 3,5-dicaffeoyl-epi-quinic acid from Atriplex gmelinii enhances the osteoblast differentiation of bone marrow-derived human mesenchymal stromal cells via WnT/BMP signaling and suppresses adipocyte differentiation via AMPK activation. Phytomedicine 71, 153225. doi:10.1016/j.phymed.2020.153225
Katsimbri, P. (2017). The biology of normal bone remodelling. Eur. J. Cancer Care (Engl) 26, e12740. doi:10.1111/ecc.12740
Kawabata, T., Otsuka, T., Fujita, K., Sakai, G., Matsushima-Nishiwaki, R., Kozawa, O., et al. (2018). (-)-Epigallocatechin gallate but not chlorogenic acid suppresses EGF-stimulated migration of osteoblasts via attenuation of p38 MAPK activity. Int. J. Mol. Med. 42, 3149–3156. doi:10.3892/ijmm.2018.3884
Khochapong, W., Ketnawa, S., Ogawa, Y., and Punbusayakul, N. (2021). Effect of in vitro digestion on bioactive compounds, antioxidant and antimicrobial activities of coffee (Coffea arabica L.) pulp aqueous extract. Food Chem. 348, 129094. doi:10.1016/j.foodchem.2021.129094
Kim, J. M., Lin, C., Stavre, Z., Greenblatt, M. B., and Shim, J. H. (2020). Osteoblast-osteoclast communication and bone homeostasis. Cells 9, 2073. doi:10.3390/cells9092073
Komori, T. (2011). Signaling networks in RUNX2-dependent bone development. J. Cell Biochem. 112, 750–755. doi:10.1002/jcb.22994
Komori, T. (2022). Whole aspect of Runx2 functions in skeletal development. Int. J. Mol. Sci. 23, 5776. doi:10.3390/ijms23105776
Kozawa, O., Suzuki, A., Kaida, T., Tokuda, H., and Uematsu, T. (1997). Tumor necrosis factor-alpha autoregulates interleukin-6 synthesis via activation of protein kinase C. Function of sphingosine 1-phosphate and phosphatidylcholine-specific phospholipase C. J. Biol. Chem. 272, 25099–25104. doi:10.1074/jbc.272.40.25099
Kuroyanagi, G., Tokuda, H., Yamamoto, N., Kainuma, S., Fujita, K., Ohguchi, R., et al. (2017). (-)-Epigallocatechin gallate synergistically potentiates prostaglandin E(2)-stimulated osteoprotegerin synthesis in osteoblasts. Prostagl. Other Lipid Mediat 128-129, 27–33. doi:10.1016/j.prostaglandins.2017.02.001
Kushioka, J., Kaito, T., Okada, R., Ishiguro, H., Bal, Z., Kodama, J., et al. (2020). A novel negative regulatory mechanism of Smurf2 in BMP/Smad signaling in bone. Bone Res. 8, 41. doi:10.1038/s41413-020-00115-z
Kwak, S. C., Lee, C., Kim, J. Y., Oh, H. M., So, H. S., Lee, M. S., et al. (2013). Chlorogenic acid inhibits osteoclast differentiation and bone resorption by down-regulation of receptor activator of nuclear factor kappa-B ligand-induced nuclear factor of activated T cells c1 expression. Biol. Pharm. Bull. 36, 1779–1786. doi:10.1248/bpb.b13-00430
Kwon, T., Lamster, I. B., and Levin, L. (2021). Current concepts in the management of periodontitis. Int. Dent. J. 71, 462–476. doi:10.1111/idj.12630
Lane, J. M., Russell, L., and Khan, S. N. (2000). Osteoporosis. Clin. Orthop. Relat. Res. 372, 139–150. doi:10.1097/00003086-200003000-00016
Le, Y. J., He, L. Y., Li, S., Xiong, C. J., Lu, C. H., and Yang, X. Y. (2022). Chlorogenic acid exerts antibacterial effects by affecting lipid metabolism and scavenging ROS in Streptococcus pyogenes. FEMS Microbiol. Lett. 369, fnac061. doi:10.1093/femsle/fnac061
Lee, C. J., Shim, K. S., and Ma, J. Y. (2016). Artemisia capillaris alleviates bone loss by stimulating osteoblast mineralization and suppressing osteoclast differentiation and bone resorption. Am. J. Chin. Med. 44, 1675–1691. doi:10.1142/S0192415X16500944
Lee, J. H., Park, J. H., Kim, Y. S., and Han, Y. (2008). Chlorogenic acid, a polyphenolic compound, treats mice with septic arthritis caused by Candida albicans. Int. Immunopharmacol. 8, 1681–1685. doi:10.1016/j.intimp.2008.08.002
Lee, N. K., Choi, Y. G., Baik, J. Y., Han, S. Y., Jeong, D. W., Bae, Y. S., et al. (2005). A crucial role for reactive oxygen species in RANKL-induced osteoclast differentiation. Blood 106, 852–859. doi:10.1182/blood-2004-09-3662
Lee, S. A., Moon, S. M., Han, S. H., Hwang, E. J., Park, B. R., Kim, J. S., et al. (2018). Chondroprotective effects of aqueous extract of Anthriscus sylvestris leaves on osteoarthritis in vitro and in vivo through MAPKs and NF-κB signaling inhibition. Biomed. Pharmacother. 103, 1202–1211. doi:10.1016/j.biopha.2018.04.183
Lee, S. H., Lee, J. Y., Kwon, Y. I., and Jang, H. D. (2017a). Anti-osteoclastic activity of Artemisia capillaris thunb. Extract depends upon attenuation of osteoclast differentiation and bone resorption-associated acidification due to chlorogenic acid, hyperoside, and scoparone. Int. J. Mol. Sci. 18, 322. doi:10.3390/ijms18020322
Lee, S. K., Kim, H., Park, J., Kim, H. J., Kim, K. R., Son, S. H., et al. (2017b). Artemisia annua extract prevents ovariectomy-induced bone loss by blocking receptor activator of nuclear factor kappa-B ligand-induced differentiation of osteoclasts. Sci. Rep. 7, 17332. doi:10.1038/s41598-017-17427-6
Lei, C., Song, J. H., Li, S., Zhu, Y. N., Liu, M. Y., Wan, M. C., et al. (2023). Advances in materials-based therapeutic strategies against osteoporosis. Biomaterials 296, 122066. doi:10.1016/j.biomaterials.2023.122066
Léotoing, L., Wauquier, F., Davicco, M. J., Lebecque, P., Gaudout, D., Rey, S., et al. (2016). The phenolic acids of Agen prunes (dried plums) or Agen prune juice concentrates do not account for the protective action on bone in a rat model of postmenopausal osteoporosis. Nutr. Res. 36, 161–173. doi:10.1016/j.nutres.2015.10.002
Li, H., Xu, J., Hu, J. F., Hu, Q. Y., Fang, X., Sun, Z. J., et al. (2022). Sustained release of chlorogenic acid-loaded nanomicelles alleviates bone loss in mouse periodontitis. Biomater. Sci. 10, 5583–5595. doi:10.1039/d2bm01099b
Liang, N., and Kitts, D. D. (2015). Role of chlorogenic acids in controlling oxidative and inflammatory stress conditions. Nutrients 8, 16. doi:10.3390/nu8010016
Lin, M., Gong, W., Chen, Q., Sun, L., Wang, Y., and Fan, X. (2013). Evaluation of the potential sensitization of chlorogenic Acid: a meta-analysis. Evid. Based Complement. Altern. Med. 2013, 208467. doi:10.1155/2013/208467
Liu, C. C., Zhang, Y., Dai, B. L., Ma, Y. J., Zhang, Q., Wang, Y., et al. (2017). Chlorogenic acid prevents inflammatory responses in IL‑1β‑stimulated human SW‑1353 chondrocytes, a model for osteoarthritis. Mol. Med. Rep. 16, 1369–1375. doi:10.3892/mmr.2017.6698
Liu, W., Li, W., Zhang, P., Gong, X., Tu, P., Tang, L., et al. (2022). Quality structural annotation for the metabolites of chlorogenic acid in rat. Food Chem. 379, 132134. doi:10.1016/j.foodchem.2022.132134
Lorentzon, M., and Cummings, S. R. (2015). Osteoporosis: the evolution of a diagnosis. J. Intern Med. 277, 650–661. doi:10.1111/joim.12369
Lou, Z., Wang, H., Zhu, S., Ma, C., and Wang, Z. (2011). Antibacterial activity and mechanism of action of chlorogenic acid. J. Food Sci. 76, M398–M403. doi:10.1111/j.1750-3841.2011.02213.x
Lu, H., Tian, Z., Cui, Y., Liu, Z., and Ma, X. (2020). Chlorogenic acid: a comprehensive review of the dietary sources, processing effects, bioavailability, beneficial properties, mechanisms of action, and future directions. Compr. Rev. Food Sci. Food Saf. 19, 3130–3158. doi:10.1111/1541-4337.12620
Luo, J., He, W., Li, X., Ji, X., and Liu, J. (2021). Anti-acne vulgaris effects of chlorogenic acid by anti-inflammatory activity and lipogenesis inhibition. Exp. Dermatol 30, 865–871. doi:10.1111/exd.14277
Mansour, A., Mohajeri-Tehrani, M. R., Samadi, M., Qorbani, M., Merat, S., Adibi, H., et al. (2021). Effects of supplementation with main coffee components including caffeine and/or chlorogenic acid on hepatic, metabolic, and inflammatory indices in patients with non-alcoholic fatty liver disease and type 2 diabetes: a randomized, double-blind, placebo-controlled, clinical trial. Nutr. J. 20, 35. doi:10.1186/s12937-021-00694-5
Marchesan, J., Girnary, M. S., Jing, L., Miao, M. Z., Zhang, S., Sun, L., et al. (2018). An experimental murine model to study periodontitis. Nat. Protoc. 13, 2247–2267. doi:10.1038/s41596-018-0035-4
Miller, P. D. (2016). Management of severe osteoporosis. Expert Opin. Pharmacother. 17, 473–488. doi:10.1517/14656566.2016.1124856
Min, J., Yuan, Z., Zhang, Q., Lin, S., Wang, K., and Luo, J. (2018). Analysis of anti-osteoporosis function of chlorogenic acid by gene microarray profiling in ovariectomy rat model. Biosci. Rep. 38. doi:10.1042/BSR20180775
Mo, H., Zhang, N., Li, H., Li, F., and Pu, R. (2019). Beneficial effects of Cuscuta chinensis extract on glucocorticoid-induced osteoporosis through modulation of RANKL/OPG signals. Braz J. Med. Biol. Res. 52, e8754. doi:10.1590/1414-431X20198754
Munteanu, I. G., and Apetrei, C. (2021). A review on electrochemical sensors and biosensors used in chlorogenic acid electroanalysis. Int. J. Mol. Sci. 22, 13138. doi:10.3390/ijms222313138
Na, W., Kang, M. K., Park, S. H., Kim, D. Y., Oh, S. Y., Oh, M. S., et al. (2021). Aesculetin accelerates osteoblast differentiation and matrix-vesicle-mediated mineralization. Int. J. Mol. Sci. 22, 12391. doi:10.3390/ijms222212391
Nabavi, S. F., Tejada, S., Setzer, W. N., Gortzi, O., Sureda, A., Braidy, N., et al. (2017). Chlorogenic acid and mental diseases: from chemistry to medicine. Curr. Neuropharmacol. 15, 471–479. doi:10.2174/1570159X14666160325120625
Nandhini, P., Gupta, P. K., Mahapatra, A. K., Das, A. P., Agarwal, S. M., Mickymaray, S., et al. (2023). In-Silico molecular screening of natural compounds as a potential therapeutic inhibitor for Methicillin-resistant Staphylococcus aureus inhibition. Chem. Biol. Interact. 374, 110383. doi:10.1016/j.cbi.2023.110383
Naveed, M., Hejazi, V., Abbas, M., Kamboh, A. A., Khan, G. J., Shumzaid, M., et al. (2018). Chlorogenic acid (CGA): a pharmacological review and call for further research. Biomed. Pharmacother. 97, 67–74. doi:10.1016/j.biopha.2017.10.064
Nazir, M. A. (2017). Prevalence of periodontal disease, its association with systemic diseases and prevention. Int. J. Health Sci. (Qassim) 11, 72–80.
Nugraha, A. P., Ardani, I., Sitalaksmi, R. M., Ramadhani, N. F., Rachmayanti, D., Kumala, D., et al. (2023). Anti-Peri-implantitis bacteria's ability of robusta green coffee bean (coffea canephora) ethanol extract: an in silico and in vitro study. Eur. J. Dent. 17, 649–662. doi:10.1055/s-0042-1750803
Olthof, M. R., Hollman, P. C., and Katan, M. B. (2001). Chlorogenic acid and caffeic acid are absorbed in humans. J. Nutr. 131, 66–71. doi:10.1093/jn/131.1.66
Ono, T., Hayashi, M., Sasaki, F., and Nakashima, T. (2020). RANKL biology: bone metabolism, the immune system, and beyond. Inflamm. Regen. 40, 2. doi:10.1186/s41232-019-0111-3
Ono, T., and Nakashima, T. (2018). Recent advances in osteoclast biology. Histochem Cell Biol. 149, 325–341. doi:10.1007/s00418-018-1636-2
Ontawong, A., Duangjai, A., Muanprasat, C., Pasachan, T., Pongchaidecha, A., Amornlerdpison, D., et al. (2019). Lipid-lowering effects of Coffea arabica pulp aqueous extract in Caco-2 cells and hypercholesterolemic rats. Phytomedicine 52, 187–197. doi:10.1016/j.phymed.2018.06.021
Palaniraj, S., Murugesan, R., and Narayan, S. (2019). Chlorogenic acid-loaded calcium phosphate chitosan nanogel as biofilm degradative materials. Int. J. Biochem. Cell Biol. 114, 105566. doi:10.1016/j.biocel.2019.105566
Pantoom, S., Konstantinidis, G., Voss, S., Han, H., Hofnagel, O., Li, Z., et al. (2021). RAB33B recruits the ATG16L1 complex to the phagophore via a noncanonical RAB binding protein. Autophagy 17, 2290–2304. doi:10.1080/15548627.2020.1822629
Park, C. K., Lee, Y., Kim, K. H., Lee, Z. H., Joo, M., and Kim, H. H. (2014). Nrf2 is a novel regulator of bone acquisition. Bone 63, 36–46. doi:10.1016/j.bone.2014.01.025
Park, C. M., and Yoon, H. S. (2022). Chlorogenic acid as a positive regulator in LPS-PG-induced inflammation via TLR4/MyD88-mediated NF-κB and PI3K/MAPK signaling cascades in human gingival fibroblasts. Mediat. Inflamm. 2022, 2127642. doi:10.1155/2022/2127642
Parsons, N., Griffin, X. L., Achten, J., Chesser, T. J., Lamb, S. E., and Costa, M. L. (2018). Modelling and estimation of health-related quality of life after hip fracture: a re-analysis of data from a prospective cohort study. Bone Jt. Res. 7, 1–5. doi:10.1302/2046-3758.71.BJR-2017-0199
Ponzetti, M., and Rucci, N. (2021). Osteoblast differentiation and signaling: established concepts and emerging topics. Int. J. Mol. Sci. 22, 6651. doi:10.3390/ijms22136651
Prestwood, K. M., Pilbeam, C. C., and Raisz, L. G. (1995). Treatment of osteoporosis. Annu. Rev. Med. 46, 249–256. doi:10.1146/annurev.med.46.1.249
Rashidi, R., Rezaee, R., Shakeri, A., Hayes, A. W., and Karimi, G. (2022). A review of the protective effects of chlorogenic acid against different chemicals. J. Food Biochem. 46, e14254. doi:10.1111/jfbc.14254
Rousselle, A. V., and Heymann, D. (2002). Osteoclastic acidification pathways during bone resorption. Bone 30, 533–540. doi:10.1016/s8756-3282(02)00672-5
Rui, L., Xie, M., Hu, B., Zhou, L., Saeeduddin, M., and Zeng, X. (2017). Enhanced solubility and antioxidant activity of chlorogenic acid-chitosan conjugates due to the conjugation of chitosan with chlorogenic acid. Carbohydr. Polym. 170, 206–216. doi:10.1016/j.carbpol.2017.04.076
Sakai, G., Otsuka, T., Fujita, K., Kainuma, S., Kuroyanagi, G., Kawabata, T., et al. (2017). Amplification by (-)-epigallocatechin gallate of prostaglandin F2α-stimulated synthesis of osteoprotegerin in osteoblasts. Mol. Med. Rep. 16, 6376–6381. doi:10.3892/mmr.2017.7354
Salzillo, A., Ragone, A., Spina, A., Naviglio, S., and Sapio, L. (2021). Chlorogenic acid enhances doxorubicin-mediated cytotoxic effect in osteosarcoma cells. Int. J. Mol. Sci. 22, 8586. doi:10.3390/ijms22168586
Santana-Gálvez, J., Cisneros-Zevallos, L., and Jacobo-Velázquez, D. A. (2017). Chlorogenic acid: recent advances on its dual role as a food additive and a nutraceutical against metabolic syndrome. Molecules 22, 358. doi:10.3390/molecules22030358
Sapio, L., Salzillo, A., Illiano, M., Ragone, A., Spina, A., Chiosi, E., et al. (2020). Chlorogenic acid activates ERK1/2 and inhibits proliferation of osteosarcoma cells. J. Cell Physiol. 235, 3741–3752. doi:10.1002/jcp.29269
Sari, D. S., Pujiastuti, P., Fatmawati, D. W. A., Mardiyana, M. A., Wulandari, A. T., and Arina, Y. M. D. (2023). Inhibiting the growth of periopathogenic bacteria and accelerating bone repair processes by using robusta coffee bean extract. Saudi Dent. J. 35, 322–329. doi:10.1016/j.sdentj.2023.03.007
Scannapieco, F. A., and Gershovich, E. (2020). The prevention of periodontal disease-An overview. Periodontol 84, 9–13. doi:10.1111/prd.12330
Schwendicke, F. (2020). Digital dentistry: advances and challenges. J. Clin. Med. 9, 4005. doi:10.3390/jcm9124005
Shahabadi, N., Akbari, A., Karampour, F., Falsafi, M., and Zendehcheshm, S. (2023). In vitro cytotoxicity, antibacterial activity and HSA and ct-DNA interaction studies of chlorogenic acid loaded on γ-Fe(2)O(3)@SiO(2) as new nanoparticles. J. Biomol. Struct. Dyn. 41, 2300–2320. doi:10.1080/07391102.2022.2030799
Shahnazari, M., Turner, R. T., Iwaniec, U. T., Wronski, T. J., Li, M., Ferruzzi, M. G., et al. (2016). Dietary dried plum increases bone mass, suppresses proinflammatory cytokines and promotes attainment of peak bone mass in male mice. J. Nutr. Biochem. 34, 73–82. doi:10.1016/j.jnutbio.2016.04.007
Siddiqui, J. A., and Partridge, N. C. (2016). Physiological bone remodeling: systemic regulation and growth factor involvement. Physiol. (Bethesda) 31, 233–245. doi:10.1152/physiol.00061.2014
Simonet, W. S., Lacey, D. L., Dunstan, C. R., Kelley, M., Chang, M. S., Lüthy, R., et al. (1997). Osteoprotegerin: a novel secreted protein involved in the regulation of bone density. Cell 89, 309–319. doi:10.1016/s0092-8674(00)80209-3
Singh, A. K., Singla, R. K., and Pandey, A. K. (2023). Chlorogenic acid: a dietary phenolic acid with promising pharmacotherapeutic potential. Curr. Med. Chem. 30, 3905–3926. doi:10.2174/0929867329666220816154634
Smolen, J. S., Aletaha, D., and McInnes, I. B. (2016). Rheumatoid arthritis. Lancet 388, 2023–2038. doi:10.1016/S0140-6736(16)30173-8
Stefanello, N., Spanevello, R. M., Passamonti, S., Porciúncula, L., Bonan, C. D., Olabiyi, A. A., et al. (2019). Coffee, caffeine, chlorogenic acid, and the purinergic system. Food Chem. Toxicol. 123, 298–313. doi:10.1016/j.fct.2018.10.005
Takahama, U., Ryu, K., and Hirota, S. (2007). Chlorogenic acid in coffee can prevent the formation of dinitrogen trioxide by scavenging nitrogen dioxide generated in the human oral cavity. J. Agric. Food Chem. 55, 9251–9258. doi:10.1021/jf071700r
Tang, Q. Y., Kukita, T., Ushijima, Y., Kukita, A., Nagata, K., Sandra, F., et al. (2006). Regulation of osteoclastogenesis by Simon extracts composed of caffeic acid and related compounds: successful suppression of bone destruction accompanied with adjuvant-induced arthritis in rats. Histochem Cell Biol. 125, 215–225. doi:10.1007/s00418-005-0062-4
Thysen, S., Cailotto, F., and Lories, R. (2016). Osteogenesis induced by frizzled-related protein (FRZB) is linked to the netrin-like domain. Lab. Invest. 96, 570–580. doi:10.1038/labinvest.2016.38
Tomas-Barberan, F., García-Villalba, R., Quartieri, A., Raimondi, S., Amaretti, A., Leonardi, A., et al. (2014). In vitro transformation of chlorogenic acid by human gut microbiota. Mol. Nutr. Food Res. 58, 1122–1131. doi:10.1002/mnfr.201300441
Tsou, S. H., Hu, S. W., Yang, J. J., Yan, M., and Lin, Y. Y. (2019). Potential oral health care agent from coffee against virulence factor of periodontitis. Nutrients 11, 2235. doi:10.3390/nu11092235
Udagawa, N., Koide, M., Nakamura, M., Nakamichi, Y., Yamashita, T., Uehara, S., et al. (2021). Osteoclast differentiation by RANKL and OPG signaling pathways. J. Bone Min. Metab. 39, 19–26. doi:10.1007/s00774-020-01162-6
Wang, K., Niu, J., Kim, H., and Kolattukudy, P. E. (2011). Osteoclast precursor differentiation by MCPIP via oxidative stress, endoplasmic reticulum stress, and autophagy. J. Mol. Cell Biol. 3, 360–368. doi:10.1093/jmcb/mjr021
Wang, X., Liu, J., Xie, Z., Rao, J., Xu, G., Huang, K., et al. (2019). Chlorogenic acid inhibits proliferation and induces apoptosis in A498 human kidney cancer cells via inactivating PI3K/Akt/mTOR signalling pathway. J. Pharm. Pharmacol. 71, 1100–1109. doi:10.1111/jphp.13095
Wu, T., Zhou, Q., Hong, G., Bai, Z., Bian, J., Xie, H., et al. (2023). A chlorogenic acid-chitosan complex bifunctional coating for improving osteogenesis differentiation and bactericidal properties of zirconia implants. Colloids Surf. B Biointerfaces 230, 113484. doi:10.1016/j.colsurfb.2023.113484
Xia, Z., Li, Q., and Tang, Z. (2023). Network pharmacology, molecular docking, and experimental pharmacology explored Ermiao wan protected against periodontitis via the PI3K/AKT and NF-κB/MAPK signal pathways. J. Ethnopharmacol. 303, 115900. doi:10.1016/j.jep.2022.115900
Xiao, L., Zhang, H., Wang, Y., Li, J., Yang, G., Wang, L., et al. (2020). Dysregulation of the ghrelin/RANKL/OPG pathway in bone mass is related to AIS osteopenia. Bone 134, 115291. doi:10.1016/j.bone.2020.115291
Xu, W., Li, Y., Zhang, X., Chen, L., Wang, S., Wang, Y., et al. (2023). A novel approach for assessing osteoporosis utilizing DXA, HU and VBQ. Biomed. Technol. 5, 102–108. doi:10.1016/j.bmt.2023.08.001
Xu, Q., Cao, Z., Xu, J., Dai, M., Zhang, B., Lai, Q., et al. (2022). Effects and mechanisms of natural plant active compounds for the treatment of osteoclast-mediated bone destructive diseases. J. Drug Target 30, 394–412. doi:10.1080/1061186X.2021.2013488
Yagi, H., Takahata, Y., Murakami, T., Nakaminami, Y., Hagino, H., Yamamoto, S., et al. (2022). Transcriptional regulation of FRZB in chondrocytes by osterix and Msx2. J. Bone Min. Metab. 40, 723–734. doi:10.1007/s00774-022-01345-3
Yahara, Y., Barrientos, T., Tang, Y. J., Puviindran, V., Nadesan, P., Zhang, H., et al. (2020). Erythromyeloid progenitors give rise to a population of osteoclasts that contribute to bone homeostasis and repair. Nat. Cell Biol. 22, 49–59. doi:10.1038/s41556-019-0437-8
Yamamoto, N., Tokuda, H., Kuroyanagi, G., Kainuma, S., Ohguchi, R., Fujita, K., et al. (2015). Amplification by (-)-epigallocatechin gallate and chlorogenic acid of TNF-α-stimulated interleukin-6 synthesis in osteoblasts. Int. J. Mol. Med. 36, 1707–1712. doi:10.3892/ijmm.2015.2381
Yang, D., and Wan, Y. (2019). Molecular determinants for the polarization of macrophage and osteoclast. Semin. Immunopathol. 41, 551–563. doi:10.1007/s00281-019-00754-3
Yang, S., Wang, F., Han, H., Santos, H. A., Zhang, Y., and Zhang, H. (2023). Fabricated technology of biomedical micro-nano hydrogel. Biomedical Technol. 2, 31–48. doi:10.1016/j.bmt.2022.11.012
Yang, X., Lan, W., and Xie, J. (2022b). Antimicrobial and anti-biofilm activities of chlorogenic acid grafted chitosan against Staphylococcus aureus. Microb. Pathog. 173, 105748. doi:10.1016/j.micpath.2022.105748
Yang, Y., Wei, Q., An, R., Zhang, H. M., Shen, J. Y., Qin, X. Y., et al. (2022a). Anti-osteoporosis effect of Semen Cuscutae in ovariectomized mice through inhibition of bone resorption by osteoclasts. J. Ethnopharmacol. 285, 114834. doi:10.1016/j.jep.2021.114834
Yang, Y. Y., Zheng, Y., Liu, J. J., Chang, Z. P., Wang, Y. H., Shao, Y. Y., et al. (2023). Natural chlorogenic acid planted nanohybrids with steerable hyperthermia for osteosarcoma suppression and bone regeneration. Adv. Healthc. Mater 12, e2300325. doi:10.1002/adhm.202300325
Ye, J., Yang, Y., Jin, J., Ji, M., Gao, Y., Feng, Y., et al. (2020). Targeted delivery of chlorogenic acid by mannosylated liposomes to effectively promote the polarization of TAMs for the treatment of glioblastoma. Bioact. Mater 5, 694–708. doi:10.1016/j.bioactmat.2020.05.001
Ye, X., Li, J., Gao, Z., Wang, D., Wang, H., and Wu, J. (2022). Chlorogenic acid inhibits lipid deposition by regulating the enterohepatic FXR-FGF15 pathway. Biomed. Res. Int. 2022, 4919153. doi:10.1155/2022/4919153
Zada, S., Pham, T. M., Hwang, J. S., Ahmed, M., Lai, T. H., Elashkar, O., et al. (2021). Chlorogenic acid protects human chondrocyte C28/I2 cells from oxidative stress-induced cell death through activation of autophagy. Life Sci. 285, 119968. doi:10.1016/j.lfs.2021.119968
Zeng, L., Xiang, R., Fu, C., Qu, Z., and Liu, C. (2022). The Regulatory effect of chlorogenic acid on gut-brain function and its mechanism: a systematic review. Biomed. Pharmacother. 149, 112831. doi:10.1016/j.biopha.2022.112831
Zhang, F., Yin, G., Han, X., Jiang, X., and Bao, Z. (2019). Chlorogenic acid inhibits osteosarcoma carcinogenesis via suppressing the STAT3/Snail pathway. J. Cell Biochem. 120, 10342–10350. doi:10.1002/jcb.28318
Zhang, M., and Hu, X. (2016). Mechanism of chlorogenic acid treatment on femoral head necrosis and its protection of osteoblasts. Biomed. Rep. 5, 57–62. doi:10.3892/br.2016.679
Zhao, P., Xiao, L., Peng, J., Qian, Y. Q., and Huang, C. C. (2018). Exosomes derived from bone marrow mesenchymal stem cells improve osteoporosis through promoting osteoblast proliferation via MAPK pathway. Eur. Rev. Med. Pharmacol. Sci. 22, 3962–3970. doi:10.26355/eurrev_201806_15280
Zhao, X., Yang, Y., Yu, J., Ding, R., Pei, D., Zhang, Y., et al. (2022). Injectable hydrogels with high drug loading through B-N coordination and ROS-triggered drug release for efficient treatment of chronic periodontitis in diabetic rats. Biomaterials 24, 121387. doi:10.1016/j.biomaterials.2022.121387
Zhao, X. Q., Guo, S., Lu, Y. Y., Hua, Y., Zhang, F., Yan, H., et al. (2020). Lycium barbarum L. leaves ameliorate type 2 diabetes in rats by modulating metabolic profiles and gut microbiota composition. Biomed. Pharmacother. 121, 109559. doi:10.1016/j.biopha.2019.109559
Zheng, S. Q., Huang, X. B., Xing, T. K., Ding, A. J., Wu, G. S., and Luo, H. R. (2017). Chlorogenic acid extends the lifespan of Caenorhabditis elegans via insulin/IGF-1 signaling pathway. J. Gerontol. A Biol. Sci. Med. Sci. 72, 464–472. doi:10.1093/gerona/glw105
Zheng, W., Zhou, T., Zhang, Y., Ding, J., Xie, J., Wang, S., et al. (2023). Simplified α(2)-macroglobulin as a TNF-α inhibitor for inflammation alleviation in osteoarthritis and myocardial infarction therapy. Biomaterials 301, 122247. doi:10.1016/j.biomaterials.2023.122247
Keywords: chlorogenic acid, osteoblast, osteoclast, osteoporosis, periodontitis
Citation: Shen J, Zhang S, Zhang J, Wei X, Wang Z and Han B (2024) Osteogenic mechanism of chlorogenic acid and its application in clinical practice. Front. Pharmacol. 15:1396354. doi: 10.3389/fphar.2024.1396354
Received: 05 March 2024; Accepted: 06 May 2024;
Published: 30 May 2024.
Edited by:
Chen Chen, Nanjing Medical University, ChinaReviewed by:
Hidestugu Kohzaki, Shumei University, JapanZiyi Wang, Johns Hopkins University, United States
Yongzhi Cui, Shanghai Jiao Tong University, China
Copyright © 2024 Shen, Zhang, Zhang, Wei, Wang and Han. This is an open-access article distributed under the terms of the Creative Commons Attribution License (CC BY). The use, distribution or reproduction in other forums is permitted, provided the original author(s) and the copyright owner(s) are credited and that the original publication in this journal is cited, in accordance with accepted academic practice. No use, distribution or reproduction is permitted which does not comply with these terms.
*Correspondence: Zilin Wang, V1pMMDcxNDM2QGhvdG1haWwuY29t; Bing Han, aGJpbmdAamx1LmVkdS5jbg==