- 1Key Laboratory of Pathobiology, Ministry of Education, Jilin University, Changchun, Jilin, China
- 2Department of Urology, The First Hospital of Jilin University, Changchun, Jilin, China
Maintaining the structural integrity of genomic chromosomal DNA is an essential role of cellular life and requires two important biological mechanisms: the DNA damage response (DDR) mechanism and telomere protection mechanism at chromosome ends. Because abnormalities in telomeres and cellular DDR regulation are strongly associated with human aging and cancer, there is a reciprocal regulation of telomeres and cellular DDR. Moreover, several drug treatments for DDR are currently available. This paper reviews the progress in research on the interaction between telomeres and cellular DNA damage repair pathways. The research on the crosstalk between telomere damage and DDR is important for improving the efficacy of tumor treatment. However, further studies are required to confirm this hypothesis.
1 Introduction
The maintenance of genomic integrity is a fundamental feature of cellular physiology (Maréchal and Zou, 2013). However, DNA damage occurs continuously in cells exposed to numerous extrinsic sources, including ionizing radiation, ultraviolet irradiation, and chemical exposure, as well as intrinsic sources, including metabolic responses, oxidative stress, and replication errors, that eventually result in single- or double-stranded DNA breaks (DSBs) (Basu et al., 2012; De Falco and De, 2021; Cheng et al., 2022). In normal cells, several DNA damage response (DDR) mechanism participate in maintaining cell viability and genomic stability. The primary types of DDR include homologous recombination (HR), base excision repair (BER), non-homologous end-joining (NHEJ), break-induced replication (BIR), mismatch repair (MMR), nucleotide excision repair (NER), direct repair (DR), and single-stranded annealing (SSA), which can facilitate pinpoint DNA restoration, identify DNA lesions, prevent cell division-related processes, and enhance aberrant apoptosis (Beard et al., 2019). Missing information in the DDR pathway can lead to mutations that result in genomic destabilization, thereby contributing to carcinoma formation.
DDR functions in two ways: by preventing cells from entering mitosis until repair is completed via activating DNA damage checkpoints and by coordinating and activating various repair pathways and inducing metabolic reprogramming. DNA-dependent protein kinase (DNA-PK) and capillary dilated ataxia mutated (ATM) and ATM and Rad3-related (ATR) kinases, which belong to the PI3K-related kinase (PIKK) family, play essential roles in this process. Among them, DNA-PK and ATM are primarily responsible for DSB repair, whereas ATR repairs the damage induced by DNA replication (Roos et al., 2016; Blackford and Jackson, 2017). Telomeres are nuclear protein complexes comprising TTAGGG repeats located at the ends of linear chromosomes. Under normal conditions, the telomere is covered with the shelterin protein complex, which comprises six proteins, including TRF1, TRF2, POT1, RAP1, TPP1, and TIN2 (de Lange, 2005). Their essential function is to protect chromosomal ends from recognition by free ends generated by DSB, which incorrectly activate DDR mechanisms that result in DNA degradation, end-to-end fusion, and genomic instability (Li et al., 2022). Therefore, telomeres are strongly associated with DDR.
Shelterin protein complexes are involved in distinguishing telomere ends from damaged DNA and can induce DDR. The TRF2 and POT1 proteins suppress ATM- and ATR-mediated DDR pathways, respectively, thereby avoiding the onset of the response. As cells divide, telomeres become progressively shorter, and when they reach a certain level of shortening, the ATM- and ATR-mediated DDR pathways are activated, which results in cell death or senescence. However, several studies have shown that proteins that are associated with DDR appear at telomeres and are directly or indirectly involved in telomere maintenance. Moreover, defects in DSB repair proteins, such as ATM, Ku, DNA-PKcs, RAD51, and MRN complexes, lead to the mismetabolism of telomeres. Gabriel Arantes Dos Santos et al. showed that upregulation of shelterin and CST (Cdc13/Ctc1, Stn1, Ten1) led to telomere lengthening and promoted invasion of prostate cancer cells (Dos Santos et al., 2024). Importantly, shelterin links to tumor immunity and predicts response to PD-1 blockade immune therapy (Luo et al., 2021). Therefore, functional telomeres interact with DDR proteins (Slijepcevic, 2006).
The activation of telomere maintenance mechanisms, including telomerase and the alternative lengthening of telomeres (ALTs), is essential for tumor cell growth, although its regulatory mechanisms are not completely understood (Kaul et al., 2021). ALT is a BIR-based mechanism that elongates telomeres in a subset of human cancer cells (Silva et al., 2021). Telomeres in ALT + cells are inherently unstable and prone to replicative stress and spontaneous DSB formation, which causes the repeated activation of DDR (Feretzaki et al., 2020). In ALT + cancer cells, telomeric repeats that contain long noncoding RNA (TERRA) interact with DNA repair proteins involved in several DNA repair pathways, including NER, DSB, and BER, indicating a strong link between DDR and telomere function (Guh et al., 2022). Therefore, telomeres in normal cells need to avoid DDR employment. In contrast, telomere replication and protection require the involvement of DDR-related proteins (Verdun and Karlseder, 2007). Thus, this review illuminates the relationship between telomeres and cellular DDR and provides clues on how to target telomere-associated DDR for cancer treatment.
2 Multiple DDRs and telomere-related DNA repairing
DNA damage, in the form of DNA base abnormalities or DSBs, may produce mutations in cells, promote malignant proliferation, and induce cancer. However, such mutations can be avoided if a precise DNA repair system can recognize and repair the damage before replication. In particular, DDR disruption occurs during cancer progression and can be used as a target for cancer treatment. Functional abnormalities in various proteins responsible for repair pathways can also contribute to the build-up of damage and, consequently, to the induction of cancer. Therefore, DNA repair is a key protective mechanism against malignant cell proliferation and cancer development (Nagel et al., 2017; Zimmermann et al., 2022).
Four primary types of DDR are present in eukaryotes: NER, BER, MMR, and double-strand break repair (DSBR). NER excises large segments of damaged DNA, BER repairs damage to individual bases, MMR is used to repair base mismatches, and DSBR includes two mechanisms (i.e., HR and NHEJ). NHEJ directly attaches to the fractured ends without a template, whereas HR requires complete sister chromatids as a repair pattern. DR is another DDR system that can repair certain forms of base damage without removing the bases (Maréchal and Zou, 2013; Caldecott, 2020; Bayley et al., 2022). BIR relies on homologous sequence templates for DNA synthesis and repair, particularly for repairing one-ended DSBs. SSA is a repair process that occurs when homologous sequences are present in the same direction at both ends of a DSB.
2.1 HR
HR is a multistep process that prevents genomic instability and maintains cellular homeostasis. After DSBs occur, BRCA1 promotes HR pathways. First, the MRN complex (mre11-rad50-nbs1) activates this pathway by coupling to DSB (Belan et al., 2022). The MRN complex acts synergistically with BRCA1 and CtIP endonucleases to mediate DNA end resection (Kishkevich et al., 2022). Furthermore, the MRN complex triggers ATM, which in turn initiates PALB2, BRCA1, and BRCA2 expressions (Murciano-Goroff et al., 2022; Peng et al., 2023). Subsequently, RAD51 loads onto the DNA damage site to form a nucleoprotein, which further invades sister chromosomes to search for orthologous DNA sequences that can be used as templates for novel DNA synthesis (Cleary et al., 2020; Murciano-Goroff et al., 2022).
By losing ATM in cancer cells, HR is undermined; therefore, when DNA is damaged, these cancer cells depend on the rest of the DDR pathway for repair (Kaminski et al., 2022). Cancers with specific HR defects can be treated with targeted agents that inhibit HR proteins.
In cancer cells, a mechanism of telomere lengthening via homologous targeted repair (i.e., ALT) is similar to HR and triggers DNA repair to maintain telomere length (Kaminski et al., 2022). TERRA is a transcription factor involved in telomere elongation. Chia et al. reported that several HR-related proteins, including RAD50, BRCA1, WRN, ATR, and WRNIP1, are potential TERRA-interacting proteins (Guh et al., 2022). TERRA initiates RAD51-dependent strand invasion (Feretzaki et al., 2020), whereas BRCA1 binds to and represses TERRA transcription (Vohhodina et al., 2021). A strong correlation exists between telomere function and the DNA damage response, particularly HR, which might be a potential therapeutic target for ALT cancer treatment.
2.2 NHEJ
NHEJ is a DDR pathway for the repair of DSBs and is activated by 53BP1, RIF1, and the shieldin complex. NHEJ includes classical non-homologous end-joining (cNHEJ), alternative non-homologous end-joining (alt-EJ), and MMEJ (Gómez-Cabello et al., 2022). cNHEJ utilizes nonspecific ligation to correct DNA breaks, resulting in error-prone repair that can occur at any time in the cell cycle (van de Kooij et al., 2022). cNHEJ is initiated via binding of the Ku70–Ku80 (also known as XRCC6–XRCC5) heterodimer to DSB ends (Luedeman et al., 2022). This event activates DNA-PK, which, in turn, activates a multi-protein complex of XRCC4, Artemis, and DNA ligase. Although the NHEJ mechanism is simpler than the HR mechanism, it can sometimes result in rearrangements, whereas the HR mechanism does not produce errors (Findlay et al., 2018). Alt-EJ primarily utilizes microhomologous fragments (2–25 bp) of the damaged region to generate an annealing reaction and remove nonhomologous ssDNA (Oanh et al., 2022). Alt-EJ is poly ADP-ribose polymerase 1 (PARP1)-dependent, and polymerase θ (Polθ) mediates break repair after MRN complex and PARP1-binding double-strand breaks. When HR is defective in tumors, it causes an enhanced reliance on alt-EJ. The correlation between the effect of alt-EJ on Polθ indicates that Polθ inhibitors are likely to be potent in HR-deficient tumors (Findlay et al., 2018; Scully et al., 2019; Shibata and Jeggo, 2020; Murciano-Goroff et al., 2022).
Ribes-Zamora et al. have determined the role of shelterin in suppressing the NHEJ function of Ku in human telomeres (Ribes-Zamora et al., 2013). Telomere fusion occurs when NHEJ acts on uncapped telomeres (Ueno, 2023). As the center of telomere maintenance and structure, the complex between TRF2 and Rap1 blocks NHEJ, and together with DNA-PK, inhibits telomere end-joining (Arat and Griffith, 2012). NHP2 is a component of the telomerase–holoenzyme complex. In telomere RNA subunit (hTR)-expressing ALT + cells, NHP2 is downregulated, and 53BP1 foci at telomeres are increased. The depletion of NHP2 in hTR-expressing cells rarely reduces the total 53BP1 level, but does decrease TIF reduction compared to NHP2 depletion in non-hTR-expressing cells (Raghunandan et al., 2021). Therefore, NHEJ factors are attractive targets for cancer therapies because of the reliance of tumor cell division on DNA repair mechanisms.
2.3 BIR
BIR primarily repairs single-ended DSBs similar to those resulting from telomere encroachment or replication fork crashes (Kockler et al., 2021). BIR was first reported in bacteriophage T4 and occurs in both mammalian cells and humans (Kreuzer, 2000). One study found that the overexpression of oncogenes activates BIR in human cells, resulting in chromosomal rearrangements (Elango et al., 2019). In ALT + cells, BIR is active during the G2 stage of the cell cycle and RAD52 is recruited to the replication stress site, a process that requires the two regulatory subunits of DNA polymerase δ, namely, POLD3 and POLD4 (Silva et al., 2019). ALT is a BIR that functions through both RAD52-and non-dependent processes. (Verma et al., 2019). In ALT cells, RAD52 is primarily responsible for D-loop formation and mediates the RAD52-dependent BIR process, whereas RAD51AP1 is primarily responsible for TERRA-mediated R-loop formation in telomeres, which promotes G4 formation. Subsequently, G4 promotes R-loop-to-D-loop conversion, which promotes ALT (Kaminski et al., 2022; Yadav et al., 2022). Moreover, BIR can trigger the SUMOylation of PIAS4-mediated TRF2, and the deprivation of PIAS4 renders APB devoid of repair proteins, which in turn compromises the synthesis of ALT telomeres (Zhang et al., 2021). Therefore, understanding the role of BIR in the treatment of ALT cancer cells is vital (Elango et al., 2017).
2.4 SSA
SSA is a double-stranded oligonucleotide with a 3′ overhang of three random nucleotides that can be efficiently ligated to the 3’ end of single-stranded DNA using T4 DNA ligase (Wu et al., 2018). Similar to the alt-EJ mechanism, SSA requires homologous DNA sites to catalyze DSB repair. However, SSA can occur over long stretches of DNA and result in large deletions that can cause intrachromosomal translocations. Mechanistically, SSA is inhibited by RAD51. Unlike alt-EJ, which requires PARP and Polθ, SSA requires RAD52 to influence the annealing of the homologous stretches of ssDNA (Scully et al., 2019; Vancevska et al., 2020; Subecz et al., 2021).
The mammalian ERCC1/XPF endonuclease plays an important role in DSB repair via SSA (Kim et al., 2020). TERRA triggers XPF localization to telomeres and results in FANCM deficiency, eventually leading to DSBs (Guh et al., 2022). SLX4, a coordinator of multiple DNA structure-specific endonucleases, plays important roles in several DNA repair pathways. The Slx4-Rad1 complex is required for the SSA pathway, in which the Mec1/Tel1-dependent phosphorylation of Slx4 is essential (Saito et al., 2009). SLX4 cooperates with XPF for interstrand DNA crosslink repair and is required for XPF-mediated DDR at ALT telomeres (Guh et al., 2022). Therefore, therapies targeting the SSA repair pathway may be useful for treating ALT cancer cells (Pfitzer et al., 2019).
2.5 BER and NER
BER is initiated by an impaired base and is substituted by de novo-synthesized DNA. Then, APE (depurine/depyrimidine nuclease) cleaves it to form a 3′OH end at the site of damage (Szymanski et al., 2022; Tang et al., 2022). Finally, DNA ligase and polymerase are used to bridge these gaps (Farag et al., 2022). The NER mechanism involves the removal of damaged DNA by the excision repair cross-complementary protein 1 (ERCCI), which is substituted with normal DNA replication (Elango et al., 2017; Scully et al., 2019). Defects in BER are correlated with premature aging, and BER genes are overexpressed in various cancers, such as POLβ, XRCC1, and APE1, thus indicating that BER is essential for genome maintenance (Somuncu et al., 2020).
XPF is an NER factor with nucleic acid endonuclease activity and is the most enriched TERRA-binding protein according to mass spectrum results (Guh et al., 2022). XPF can generate DSBs while promoting DDR in ALT telomeres (Guh et al., 2022). Yang et al. discovered that the principal mechanism of telomere replication may be linked to TFIIH, because as an NER element, TFIIH is an important factor in TRF1 and its absence results in several telomere replication phenotypes (Yang et al., 2022).
2.6 MMR
MMR restores the nucleotide sequence in DNA molecules with mismatched bases. When microsatellite instability (MSI) occurs in an organism, MMR proteins repair the errors. Normal functioning of the MMR protein repairs the MSI and maintains microsatellite stability; however, when the MMR protein is absent, MSI is not repaired, and it will gradually accumulate, thus resulting in high MSI. MSI is typically found in colorectal cancer but can also occur in gastric and endometrial cancers (Scully et al., 2019; Shibata and Jeggo, 2020; Ngo et al., 2021).
Recent literature suggests that the loss of MMR function may play a significant role in ALT activity in human cancer cell lines; however, this correlation has not been confirmed in human primary tumors. Furthermore, the loss of MMR function is associated with ALT, improved organism survival, and health in yeast and mice, thus supporting the role of the loss of the MMR pathway in promoting the development of ALT (Stundon et al., 2022). MMR is initiated by one of two heterodimers: MSH2/MSH6 (MutSα) and MSH2/MSH3 (MutSβ). MutSα binds to base–base mismatches or the insertion and deletion loops of 1–3 nt, whereas MutSβ binds to insertion and deletion loops containing up to 16 nt (Kunkel and Erie, 2015). A recent study revealed that MutSα restricts telomere extension via ALT-associated homology-directed repair in human cancer cells (Barroso-González et al., 2021). MutSβ precludes the aggregation of R-loops and telomeric G-quadruplex (G4) structures (Sakellariou et al., 2022). Additionally, SLX4 is associated with the proteins MSH2-MSH1 and TRF2 (Ouyang et al., 2015). These associations suggest a link between telomeres and MMR, thus offering potential therapeutic alternatives for cancer treatment.
3 Protective role of the shelterin complex in DDR
The shelterin complex at the telomere ends forms a protective T-loop, which alters the end of the chromosome similar to that of the recombinant D-loop, thus concealing the 3′one-stranded DNA overhanging ends and preventing the false activation of DDR. Most somatic cells have progressively shorter telomeres, but carcinomas can sustain telomere length by upregulating telomerase activity or using the ALT mechanism (Barnes et al., 2023). How does DDR at telomere ends in tumors protect cells from overproliferation and promote tumorigenesis? Shelterin inhibits multiple DDR pathways, and different shelterin subunits are involved in diverse reparative pathways and signaling. For instance, the absence of TRF2 triggers ATM signaling, which leads to telomere fusion. In contrast, in the absence of POT1, ATR signaling is activated at telomeres, but the ATM signaling pathway remains inhibited. HR inhibition results in telomeric sister chromatid exchange, which involves the presence of concurrent RAP1 and POT1 proteins in the telomere. TRF2 and another shelterin protein also inhibit the ALT–NHEJ pathway (Doksani and de Lange, 2014; Doksani and de Lange, 2016). The CST complex in mammals has been reported to boost telomere replication but has no direct role in inhibiting telomere DDR (Gu and Chang, 2013). Disruption of the DDR can promote cancer development and progression; thus, the destruction of DDR pathways in cancer cells could be used to treat cancer.
4 Damaged DNA-targeted therapies in cancer
4.1 Relevant treatments of telomere and drugs available
4.1.1 PARP inhibitors
The development of PARP inhibitors has resulted in synthetic lethality. The binding of PARP1 to single-stranded DNA breaks produced during BER forms the basis of a synthetic lethal interaction with HR defects (de Vos et al., 2012). Preclinical and clinical studies on PARP inhibitors have revealed additional mechanisms of their activity (Xue et al., 2022). PARP inhibitors trap the PARP enzyme at damaged DNA sites, thereby influencing the prevention of essential cellular processes, including DNA repair and transcription. However, in HR-deficient cells, the trapped PARP–DNA complex is lethal (Illuzzi et al., 2022). In HR-deficient cell lines, PARP inhibitors are currently available, including niraparib (Chi et al., 2023), talazoparib (Agarwal et al., 2023), rucaparib (Fizazi et al., 2023), olaparib (Robson et al., 2017), veliparib (Coleman et al., 2019), AZD5305 (78) and IMP4297(79). Clinical trials of these inhibitors have been approved and used for several cancers, including ovarian, breast, and pancreatic cancers. However, the acquired resistance to PARP inhibitors in clinical settings remains to be resolved (Vancevska et al., 2020; Vernì, 2022) (Table 1).
PARP inhibitors have been reported to cause TRF2 to decapitate telomeres, resulting in the stimulation of incorrect NHEJ repair in ALT-positive cancer cells. Loss-of-function ATRX and/or DAXX mutations have been found in ALT-positive cancer cells. Currently, no drugs related to ATRX are being investigated in clinical trials (Cavalcante et al., 2021; Qin et al., 2022).
4.1.2 Targeting ATM and ATR
As an apical DDR kinase, ATM regulates DSB repair in various cell types. Mechanistically, single-ended DSBs caused by PARP and topoisomerase one inhibitors require HR for accurate repair. The deletion of ATM signaling causes delayed end resection and repairs single-ended DSBs via NHEJ, resulting in irregular chromosome fusion and tumor cell death. ATM is considered a tumor suppressor and may lead to de novo tumor formation in various tissues when exposed to ATM inhibitors for prolonged periods (Pobiega et al., 2021). ATM defects or mutations are commonly found in solid tumors and B-cell lymphomas. Therefore, ensuring that the therapeutic benefits of ATM inhibition outweigh the therapeutic risks are important (Mukherjee et al., 2018; Scully et al., 2019; Subecz et al., 2021). A Phase I trial has already evaluated the ATM inhibitor AZD0156 as both a monotherapy and in combination with the PARP inhibitors irinotecan and olaparib, which is another cytotoxic agent (Davis et al., 2022; Qin et al., 2022; Wong et al., 2022).
During the S phase, ATR ensures accurate DNA replication by regulating the initiation of ignition and fork progression. ATR inhibitors can also increase replication fork arrest, induce chromosomal breakage, and cause cytotoxicity (Ouyang et al., 2015). The sensitivity of cancer cells to ATR inhibitors, which is caused by the overexpression of the oncogenic protein E1, is higher than that of other cell lines. Therefore, ATR inhibitors are often used to treat PARP inhibitor-resistant tumors (Kim et al., 2022). Cancer cells with BRCA1 mutations can overcome the toxicity of PARP inhibitors by loading DSB with BRCA1-independent RAD51, thereby overcoming drug resistance. ATR inhibitors block BRCA1-independent function and re-sensitize tumor cells to PARP inhibition in vitro. Preclinical data showed that berzosertib (also known as M6620 and VX-970) was the first ATR inhibitor to reveal that lung cancer cells were primarily sensitive to chemotherapeutic agents. The drug can result in the collapse of replication forks, such as cisplatin and gemcitabine (in vitro), and improve antitumor activity when combined with cisplatin (in vivo) (Perkhofer et al., 2021; Scheper et al., 2022; Telli et al., 2022; Viol et al., 2022; Takahashi et al., 2023). Other ATR inhibitors currently in clinical trials are as follows, for example, ceralasertib (AZD6738) (Vendetti et al., 2018; Biegała et al., 2023), elimusertib (BAY1895344) (Harold et al., 2023), tuvusertib (M1774) (Yap et al., 2024) and camonsertib (RP-3500) (Yap et al., 2023).
Replication stress occurs when damaged DNA impedes the progression of replication forks, leading to stagnation. If unrepaired, the stalled fork may deteriorate into a DSB, eventually facilitating the recruitment of DNA repair factors and engagement of HR mechanisms to lengthen telomeres. Therefore, ATM and ATR inhibitors are also used in cancer therapy to treat patients with ALT-positive cancers. The ATM inhibitor AZD0156 has shown selective toxicity in melanoma cells, neuroblastoma, and preclinical models of colorectal cancer (Davis et al., 2022; Qin et al., 2022; Wong et al., 2022; Yilmaz et al., 2023).
In contrast, other studies showed that ATM activation balances senescence, apoptosis, and autophagy. The G-quadruplex ligand 20A elicits global DNA damage and activates the ATM pathway in both cancer cells (HeLa) and xenograft mouse models (Beauvarlet et al., 2019). Other DDR-related inhibitors currently in clinical trials such as APE1 inhibitors (Fishel et al., 2019; Eads et al., 2021; Radin et al., 2024), DNA-PK inhibitors (Blackwell et al., 2015; Wen et al., 2015; Brown et al., 2018; Sweeney et al., 2022; Selvaraj et al., 2023; Samuels et al., 2024), CHK1/CHK2 inhibitors (Infante et al., 2017; van Harten et al., 2019; Konstantinopoulos et al., 2022; Park et al., 2022; Huffman et al., 2023), and WEE1 inhibitors (Huang et al., 2021; Fu et al., 2023; Schutte et al., 2023) are summarized in Table 1.
4.1.3 Immune related telomere targeted therapy strategies
Ilgen Mender et al. elucidated for the first time the mechanism and potential clinical translational value of 6-thio-dG, a nucleoside analogue targeting telomere damage, in activating host DNA-cyclic GMP- AMP synthase (cGAS)–stimulator of interferon genes (STING) pathway -dependent immune cells to inhibit tumor growth (Mender et al., 2020). 6-thio-2′-deoxyguanosine (6-thio-dG) is a telomerase substrate precursor nucleoside analog that has been validated as a telomere-targeting strategy. Mechanistically, 6-thio-dG induces persistent telomere dysfunction and sequentially activates the ATR pathway, followed by ATM activation in telomerase-positive cells (Sengupta et al., 2018). Notably, ATR activation decreases after activation with 6-thio-dG, indicating the dual effect of DDR on telomere-related cell death. Previous studies have shown that treatment with low-dose THIO followed by anti-PD-1/PD-L1 immunotherapy eliminated advanced tumors in a clinical precursor cell model and generated cancer cell-specific immune memory, thus enabling the immune system to retain its activity against cancer cells after treatment cessation (Kodym et al., 2009). 6-thio-dG induces a high antitumor activity in chemotherapy-resistant tumor cells and mouse models (Mender et al., 2018). This co-treatment strategy is expected to provide clinical benefits to patients with small cell lung and colorectal cancers, as well as hepatocellular carcinoma, who have had unsuccessful first-line therapies (George et al., 2020; Yu et al., 2021). Due to structural similarities, many analogues previously used to inhibit human immunodeficiency virus (HIV) reverse transcriptase have also been found to inhibit the hTERT catalytic site. Drugs currently being investigated in telomerase-positive cancers include azidothymidine (AZT) and 5-methylcarboxy-indolyl-2′-deoxyribonucleoside 5′- triphosphate (5- MeCITP) (Gomez et al., 2012; Hernandez-Sanchez et al., 2019). GV1001 is a peptide derived from the reverse transcriptase subunit of telomerase (hTERT) that has been developed as a vaccine against a variety of cancers. GV1001 interacts with heat shock proteins (HSPs) and penetrates cell membranes to localize in the cytoplasm (Kim et al., 2016). It has been shown in the literature that chemotherapeutic agents combined with the GV1001 vaccine enhance the immune response but do not improve the overall survival of pancreatic cancer patients (Middleton et al., 2014). The UV1 vaccine consists of three synthetic long peptides and is a peptide vaccine against telomerase (Gerada et al., 2020). The UV1 vaccine has been tested in prostate cancer (Lilleby et al., 2017)、lung cancer (Brunsvig et al., 2020)and malignant melanoma (Aamdal et al., 2021), either alone or in combination with checkpoint inhibitors. A phase II trial of the UV1 telomerase vaccine in combination with ibritumomab and nifedumab together in pleural mesothelioma is currently underway, and the results have shown that the addition of the vaccine is more effective (Haakensen et al., 2024). Vx-001 is the first antitumor vaccine based on optimized cryptic peptides, targeting tumor antigen TERT, and its functional peptide is hidden inside the protein (Vassilis et al., 2013). Phase I/II trials of Vx-001 in patients with non-small cell lung cancer, melanoma, breast cancer, and many other cancers have been completed (Athanasios et al., 2014). In clinical trials, this vaccine demonstrated high hTERT-specific immune responses, good anti-tumor efficacy, good tolerability and few side effects (Menez-Jamet et al., 2016). INVAC-1 is a DNA plasmid encoding a modified hTERT protein for patients with relapsed or refractory solid tumors (Calvet et al., 2014). Phase I clinical trials of INVAC1 found that the vaccine was well tolerated, triggered hTERT-specific CD4+ and CD8+ T-cell responses, and blocked cancer progression in the majority of patients with relapsed or refractory solid tumors (Teixeira et al., 2020).
4.2 Other applications of telomere-related treatment in cancer
Owing to the prevalence of telomerase-positive cancer in all cancer patients, targeted telomerase therapy is considered a potential approach for cancer treatment. Several promising candidates are currently being investigated in clinical trials and pre-clinical studies (Table 2).
Small-molecule telomerase reverse transcriptase (TERT) has been well studied and has achieved good practical prospects. BIBR1532 is a representative drug of this type that binds to TERT at the non-catalytic site and inhibits telomerase activity in a non-competitive manner (Liu B. et al., 2022). Its cytotoxicity is primarily caused by direct damage to the telomere structure, resulting in the loss of TRF2 binding, which induces telomere dysfunction, acts as a telomere end-to-end fusion, and increases p53 activation. The preclinical studies have shown that BIBR1532 is effective against several cancer cell lines, including breast cancer, fibrosarcoma, endometrial cancer, and leukemia (Qin and Guo, 2022; Al-Karmalawy et al., 2023). Due to the relative instability of epigallocatechin gallate (EGCG), improved TERT inhibitors synthesized from EGCG-related fractions have been developed (Wu et al., 2020). Among these, MST-312 has been confirmed to be involved in various types of cancers. In breast, lung, and colon cancers, MST-312 treatment significantly downregulates TERT expression, reduces telomerase activity, and results in telomere shortening. It can also lead to cell cycle arrest and apoptosis in cancer cells (Wu et al., 2020). However, MST-312 works only on cancer cells with short telomeres, according to the time required for telomere shortening to a critical length (90 d) (Fernandes et al., 2022). Nu-1 and erythromycin antibiotics are other TERT inhibitors that can directly bind to the TERT catalytic domain and block TERT transcription. However, these drugs have not been well studied and have only been investigated in early clinical research because of their low potency and slow onset of cytotoxicity (Ameri et al., 2019; Wu et al., 2020). G4 ligands inhibit telomerase binding to telomeric DNA, eventually inhibiting telomerase activity (Tiek et al., 2022). G4-stabilizing ligands include telomeric repressors, TMPyP4, RHPS4, pyridostatin and telomestatin (Teng et al., 2021; Alessandrini et al., 2022; Liu LY. et al., 2022; Iida et al., 2022). Owing to the high levels of G-rich DNA throughout the genome, particularly in the promoter regions of oncogenes, G4 ligands pose numerous risks when used as telomerase inhibitors and might have a significant off-target effect (Yan et al., 2021; Shankar et al., 2022; Tsai et al., 2022). Oligonucleotides can form stable double-stranded bodies with complementary DNA, thereby disrupting hTR function. Binding to the hTR sequence template effectively inhibits the catalytic action of telomeric repeat addition, thereby inhibiting telomerase activity. The representative drug in this category is imetelstat (GRN163L), which has been tested in different tumor models and is currently the only anti-telomerase oligonucleotide in clinical use (Djojosubroto et al., 2005; Fischer-Mertens et al., 2022).
Regarding ALT-positive cancer cells, the development of cancer drugs targeting ALT can be traced back to its upstream and downstream pathways, particularly the DDR. In ALT-positive cancer cells, PARP inhibitors can cause TRF2 to dissociate from telomeres, thereby stimulating the inappropriate repair of non-homologous end connections. ATM and ATR inhibitors can also be used for the treatment of ALT-positive cancers. AZD0156, an ATM inhibitor, has been used to treat ALT neuroblastomas and overcomes chemotherapy resistance (Koneru et al., 2021). As an inhibitor of the FANCM-BTR interaction (Wu et al., 2023), PIP-199 may be selectively toxic to ALT cancer cells (Lu et al., 2019), rendering it a potential therapeutic target. Tetra-Pt (bpy), a cisplatin derivative that targets telomeric G-quadruplexes, severely inhibits the growth of ALT cell xenograft tumors, indicating that it may be a novel oncotherapeutic agent for targeting ALT cancer cells (Zheng et al., 2017). In addition, bpy disrupts telomere maintenance in telomerase cancer cells, further elucidating the function of G-quadruplexes in the human genome (Shen et al., 2022). The potential of bpy as a chemotherapeutic target has been demonstrated in both ALT-positive and telomerase cancer cells.
The shelterin protein complex prevents chromosome ends from being recognised as DSB and activates the DDR (Tesmer et al., 2023). As cells divide repeatedly and telomeres continue to shorten, shelterin binding and telomere-loop (t-loop) formation are impaired, and this weakened protection leads to telomere dysfunction, cellular senescence or apoptosis (Shi et al., 2023). TRF2 overexpression has been reported to be present in a variety of malignant cancers, and its downregulation leads to cell death. Yin-da Qiu et al. showed that FKB04, a flavokawain B derivative, effectively inhibited TRF2 expression in hepatocellular carcinoma cells and also induced telomere shortening, increased the number of telomere-free ends, and led to the disruption of the T-loop structure (Qiu et al., 2024). These results suggest that TRF2 is a potential therapeutic target for hepatocellular carcinoma and indicate that FKB04 may be a selective small-molecule inhibitor of TRF2, which is expected to be used in the treatment of hepatocellular carcinoma. Mutations in TRF2 lead to changes in telomeric DNA topology, which initiates ATM-dependent DDR (Benarroch-Popivker et al., 2016). TRF1 and TRF2 form a homodimer that binds to double-stranded telomere DNA. TRF1 inhibits ATR signalling during S phase and otherwise induces a fragile telomere phenotype. TRF1 small molecule inhibitors (ETP-47228 and ETP-47037) inhibit TRF1 binding to DNA, induce DNA damage and inhibit lung cancer and glioblastoma progression, suggesting that TRF1 is a potential therapeutic target and that ETP-47228 and ETP-47037 small molecule inhibitors may be useful in treating lung cancer and glioblastoma (García-Beccaria et al., 2015; Bejarano et al., 2017). TRF2 also binds to RAP1 and inhibits the localisation of SLX4 and PARP1 to telomeres, thereby inhibiting NHEJ (Rai et al., 2016). It has been reported in the literature that Triazole-stapled peptides can block protein interactions between RAP1 and TRF2, thereby inhibiting HR (Ran et al., 2016). POT1 has been reported to co-localize with the ubiquitin-specific processing protease 7 (USP7) deubiquitinating enzyme within APB, which is capable of deubiquitinating and stabilising the POT1-targeted ubiquitin ligase, whereas testis-specific Y-coding-like protein 5 (TSPYL5) has recently been identified as a PML component and functions as a USP7 inhibitor (Episkopou et al., 2019). This suggests that TSPYL5 may be a therapeutic target for ALT-positive cancer types. The above suggests that targeting a reduction in DDR-related proteins on telomeres would reduce cancer progression. Although no reliable inhibitors are available for the above, their importance for the types of cancers in which ALT is used suggests that they should be considered in the development of future ALT-targeted therapies.
5 Conclusion and perspectives
Replicative immortality, a hallmark of cancer, is achieved by activating the telomere maintenance machinery (TMM), where the TMM consists of telomerase (85%–90% of tumors) and the telomere lengthening (ALT) pathway (10%–15% of tumors) (Hanahan, 2022). While telomerase inhibitors are considered promising anticancer agents, the reality is challenging; ALT cancer types are aggressive and have a poor prognosis, but no therapeutic options are currently available. Targeting telomere maintenance therefore represents an opportunity to treat the vast majority of cancer types. In this review, we identify the link between telomeres and DDR and the promising use of drugs targeting DDR therapy for the treatment of ALT cancers, and summarize recent advances in drugs targeting DDR, telomerase and ALT therapy.
Owing to the critical function of the DDR in cancer cells, balanced DNA damage and repair, particularly in the telomere region, is vital for cancer treatment. For example, the traditional antitumor drug, cisplatin, kills cancer cells via DNA crosstalk and induces DNA damage, which activates the ATM signaling pathway (Bian et al., 2023). Therefore, ATMi may be a potential combined drug to improve the efficacy of chemotherapy drugs. However, when considering the function of telomeres in cancer cells, the G-quadruplex ligands 20A and 6-thio-dG, which can activate ATM and/or ATR, were also confirmed to have effective antitumor activity. Therefore, the opposite effects of the same molecule should be considered in cancer treatment, which might be the key to resolving the low response rate to antitumor drugs (Figure 1).
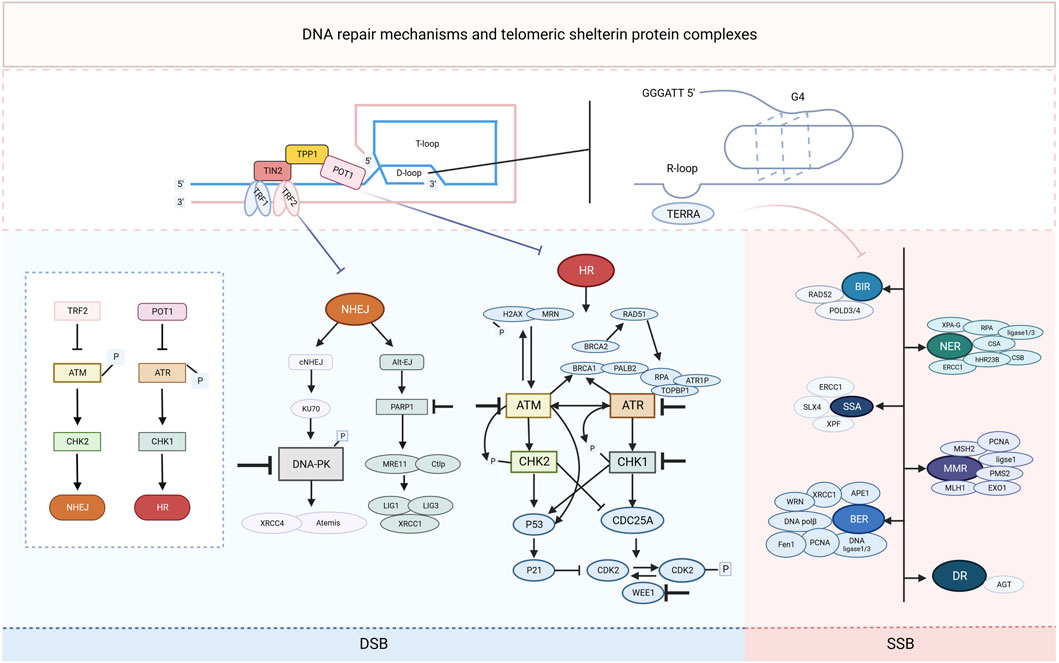
Figure 1. Schematic representation of DNA repair mechanisms and telomeric shelterin protein complexes. In repairing exogenous and endogenous DNA damage, cells use a range of DNA repair mechanisms, including single-strand break (SSB) and double-strand break (DSB) DNA repair pathways. SSB DNA repair mechanisms include direct repair (DR), nucleotide excision repair (NER), mismatch repair (MMR), break-induced replication (BIR), single-stranded annealing (SSA), and base excision repair (BER). The DSB response signaling is orchestrated by two kinases, ATR and ATM, which phosphorylate the substrate mainly in the G2 or G1 phases, respectively, and send signals to the cell cycle through CHK1 and CHK2. CHK2 signals cell cycle arrest and triggers both homologous recombination (HR) and non-homologous end-joining (NHEJ) DSB repair mechanisms. Each DNA repair pathway consists of a complex of signaling sources, transcription factors and effectors of the DNA repair restoration mechanism, some of the key players of which are highlighted in the figure. The telomeric shelterin protein complex inhibits the NHEJ and HR repair mechanisms, and the RNA it transcriptionally generates (TERRA) as well as the secondary structures it forms are also associated with some of the key molecules in the SSB repair mechanism. Inhibitors of these pathway components (denoted by a “T" bar) are currently in preclinical and clinical use as drugs targeting the DNA damage response.
Research on the development of drugs targeting telomeres or telomerases is ongoing. BIBR1532, MST-312, TMPyP4, RHPS4, and pyridostatin are currently undergoing preclinical research. The oligonucleotide, imetelstat, has been approved by the Food and Drug Administration for the treatment of recurrent or refractory myelofibrosis. Instead of telomerase, ALT activation in approximately 10%–15% of cancer should also be considered as a potential treatment target, particularly for DDR-related molecules.
The cGAS-STING signalling pathway is part of the innate immune system that senses both host and foreign cytosolic double-stranded DNA to initiate a type I inter feron response (Barber, 2015). Studies have shown an association between TMM and the cGAS-STING pathway, which contributes to cancer development (Ebata et al., 2022). Spontaneous immortalisation of non-malignant cells induced by TERT expression has been reported to trigger the cGAS-STING pathway, thereby altering their microenvironment to become tumour-friendly (Yang et al., 2017). ALT cancer cells have a unique feature of extrachromosomal telomere repeats (ECTR) in the cytoplasm. ECTR in normal cells activate the cGAS-STING pathway and promote immune responses leading to proliferative disorders, whereas ALT cells have a defective cGAS-STING pathway that escapes antiproliferative effects (Li et al., 2022). This suggests two major weaknesses of ALT cells. Firstly, ALT cells are able to evade ECTR-induced antiproliferative effects, but it may also lead to cells being susceptible to viral infection. Second, if the cGAS-STING pathway is active, it is potent in killing ALT cells. This suggests that testing the end product of this pathway, such as FDA-approved interferon beta (IFNβ), may be a therapeutic approach to inhibit the growth of ALT-positive cancer cells (Moglan et al., 2023). Therefore, research on the crosstalk between telomere damage and DDR is important for improving the efficacy of tumor treatment. However, further studies are required to confirm this hypothesis.
Author contributions
LG: Conceptualization, Methodology, Writing–original draft, Writing–review and editing. ML: Conceptualization, Methodology, Writing–original draft, Writing–review and editing. YZ: Investigation, Writing–review and editing. HZ: Supervision, Writing–review and editing. YW: Funding acquisition, Supervision, Writing–review and editing. Z-XX: Funding acquisition, Supervision, Writing–review and editing.
Funding
The author(s) declare financial support was received for the research, authorship, and/or publication of this article. This research was funded by grants from the National Natural Science Foundation of China (82020108024 and 81772924), International Cooperation project of the Department of Science and Technology of Jilin Province (No: 20210402005GH), Department of Finance of Jilin Province (Nos. JLSWSRCZX 2021–015), and Graduate Innovation Fund of Jilin University (101832020CX260). The funding body did not have any role in the design of the study and collection, analysis, interpretation of data, decision to publish, or preparation of the manuscript.
Conflict of interest
The authors declare that the research was conducted in the absence of any commercial or financial relationships that could be construed as a potential conflict of interest.
Publisher’s note
All claims expressed in this article are solely those of the authors and do not necessarily represent those of their affiliated organizations, or those of the publisher, the editors and the reviewers. Any product that may be evaluated in this article, or claim that may be made by its manufacturer, is not guaranteed or endorsed by the publisher.
References
Aamdal, E., Inderberg, E. M., Ellingsen, E. B., Rasch, W., Brunsvig, P. F., Aamdal, S., et al. (2021). Combining a universal telomerase based cancer vaccine with ipilimumab in patients with metastatic melanoma - five-year follow up of a phase I/IIa trial. Front. Immunol. 12, 663865. doi:10.3389/fimmu.2021.663865
Agarwal, N., Azad, A. A., Carles, J., Fay, A. P., Matsubara, N., Heinrich, D., et al. (2023). Talazoparib plus enzalutamide in men with first-line metastatic castration-resistant prostate cancer (TALAPRO-2): a randomised, placebo-controlled, phase 3 trial. Lancet 402 (10398), 291–303. doi:10.1016/S0140-6736(23)01055-3
Alessandrini, I., Percio, S., Naghshineh, E., Zuco, V., Stacchiotti, S., Gronchi, A., et al. (2022). Telomere as a therapeutic target in dedifferentiated liposarcoma. Cancers (Basel) 14 (11), 2624. doi:10.3390/cancers14112624
Al-Karmalawy, A. A., Nafie, M. S., Shaldam, M. A., Elmaaty, A. A., Antar, S. A., El-Hamaky, A. A., et al. (2023). Ligand-based design on the dog-bone-shaped BIBR1532 pharmacophoric features and synthesis of novel analogues as promising telomerase inhibitors with in vitro and in vivo evaluations. J. Med. Chem. 66 (1), 777–792. doi:10.1021/acs.jmedchem.2c01668
Ameri, Z., Ghiasi, S., Farsinejad, A., Hassanshahi, G., Ehsan, M., and Fatemi, A. (2019). Telomerase inhibitor MST-312 induces apoptosis of multiple myeloma cells and down-regulation of anti-apoptotic, proliferative and inflammatory genes. Life Sci. 228, 66–71. doi:10.1016/j.lfs.2019.04.060
Arat, N., and Griffith, J. D. (2012). Human Rap1 interacts directly with telomeric DNA and regulates TRF2 localization at the telomere. J. Biol. Chem. 287 (50), 41583–41594. doi:10.1074/jbc.M112.415984
Athanasios, K., Elisavet, P., Eleni Kyriaki, V., Despoina, A., Eleftheria Kleio, D., Dora, H., et al. (2014). A phase II trial evaluating the clinical and immunologic response of HLA-A2(+) non-small cell lung cancer patients vaccinated with an hTERT cryptic peptide. Lung Cancer 86 (1), 59–66. doi:10.1016/j.lungcan.2014.07.018
Barber, G. N. (2015). STING: infection, inflammation and cancer. Nat. Rev. Immunol. 15 (12), 760–770. doi:10.1038/nri3921
Barnes, R. P., Thosar, S. A., and Opresko, P. L. (2023). Telomere fragility and MiDAS: managing the gaps at the end of the road. Genes (Basel) 14 (2), 348. doi:10.3390/genes14020348
Barroso-González, J., García-Expósito, L., Galaviz, P., Lynskey, M. L., Allen, J. A. M., Hoang, S., et al. (2021). Anti-recombination function of MutSα restricts telomere extension by ALT-associated homology-directed repair. Cell Rep. 37 (10), 110088. doi:10.1016/j.celrep.2021.110088
Basu, B., Yap, T. A., Molife, L. R., and de Bono, J. S. (2012). Targeting the DNA damage response in oncology: past, present and future perspectives. Curr. Opin. Oncol. 24 (3), 316–324. doi:10.1097/CCO.0b013e32835280c6
Bayley, R., Borel, V., Moss, R. J., Sweatman, E., Ruis, P., Ormrod, A., et al. (2022). H3K4 methylation by SETD1A/BOD1L facilitates RIF1-dependent NHEJ. Mol. Cell 82 (10), 1924–1939.e10. doi:10.1016/j.molcel.2022.03.030
Beard, W. A., Horton, J. K., Prasad, R., and Wilson, S. H. (2019). Eukaryotic base excision repair: new approaches shine light on mechanism. Annu. Rev. Biochem. 88, 137–162. doi:10.1146/annurev-biochem-013118-111315
Beauvarlet, J., Bensadoun, P., Darbo, E., Labrunie, G., Rousseau, B., Richard, E., et al. (2019). Modulation of the ATM/autophagy pathway by a G-quadruplex ligand tips the balance between senescence and apoptosis in cancer cells. Nucleic Acids Res. 47 (6), 2739–2756. doi:10.1093/nar/gkz095
Bejarano, L., Schuhmacher, A. J., Méndez, M., Megías, D., Blanco-Aparicio, C., Martínez, S., et al. (2017). Inhibition of TRF1 telomere protein impairs tumor initiation and progression in glioblastoma mouse models and patient-derived xenografts. Cancer Cell 32 (5), 590–607. doi:10.1016/j.ccell.2017.10.006
Belan, O., Sebald, M., Adamowicz, M., Anand, R., Vancevska, A., Neves, J., et al. (2022). POLQ seals post-replicative ssDNA gaps to maintain genome stability in BRCA-deficient cancer cells. Mol. Cell 82 (24), 4664–4680.e9. doi:10.1016/j.molcel.2022.11.008
Benarroch-Popivker, D., Pisano, S., Mendez-Bermudez, A., Lototska, L., Kaur, P., Bauwens, S., et al. (2016). TRF2-Mediated control of telomere DNA topology as a mechanism for chromosome-end protection. Mol. Cell 61 (2), 274–286. doi:10.1016/j.molcel.2015.12.009
Bian, H., Gu, Y., Chen, C., Chen, J., Zhang, F., Xu, Z., et al. (2023). Silence of URI in gastric cancer cells promotes cisplatin-induced DNA damage and apoptosis. Am. J. Cancer Res. 13 (3), 936–949.
Biegała, Ł., Gajek, A., Szymczak-Pajor, I., Marczak, A., Śliwińska, A., and Rogalska, A. (2023). Targeted inhibition of the ATR/CHK1 pathway overcomes resistance to olaparib and dysregulates DNA damage response protein expression in BRCA2(MUT) ovarian cancer cells. Sci. Rep. 13 (1), 22659. doi:10.1038/s41598-023-50151-y
Blackford, A. N., and Jackson, S. P. (2017). ATM, ATR, and DNA-PK: the trinity at the heart of the DNA damage response. Mol. Cell 66 (6), 801–817. doi:10.1016/j.molcel.2017.05.015
Blackwell, K., Burris, H., Gomez, P., Lynn Henry, N., Isakoff, S., Campana, F., et al. (2015). Phase I/II dose-escalation study of PI3K inhibitors pilaralisib or voxtalisib in combination with letrozole in patients with hormone-receptor-positive and HER2-negative metastatic breast cancer refractory to a non-steroidal aromatase inhibitor. Breast Cancer Res. Treat. 154 (2), 287–297. doi:10.1007/s10549-015-3615-9
Brown, J. R., Hamadani, M., Hayslip, J., Janssens, A., Wagner-Johnston, N., Ottmann, O., et al. (2018). Voxtalisib (XL765) in patients with relapsed or refractory non-Hodgkin lymphoma or chronic lymphocytic leukaemia: an open-label, phase 2 trial. Lancet Haematol. 5 (4), e170–e180. doi:10.1016/S2352-3026(18)30030-9
Brunsvig, P. F., Guren, T. K., Nyakas, M., Steinfeldt-Reisse, C. H., Rasch, W., Kyte, J. A., et al. (2020). Long-term outcomes of a phase I study with UV1, a second generation telomerase based vaccine, in patients with advanced non-small cell lung cancer. Front. Immunol. 11, 572172. doi:10.3389/fimmu.2020.572172
Caldecott, K. W. (2020). Mammalian DNA base excision repair: dancing in the moonlight. DNA Repair (Amst) 93, 102921. doi:10.1016/j.dnarep.2020.102921
Calvet, C. Y., Thalmensi, J., Liard, C., Pliquet, E., Bestetti, T., Huet, T., et al. (2014). Optimization of a gene electrotransfer procedure for efficient intradermal immunization with an hTERT-based DNA vaccine in mice. Mol. Ther. Methods Clin. Dev. 1, 14045. doi:10.1038/mtm.2014.45
Cavalcante, S. G., Pereira, B. J. A., Lerario, A. M., Sola, P. R., Oba-Shinjo, S. M., and Marie, S. K. N. (2021). The chromatin remodeler complex ATRX-DAXX-H3.3 and telomere length in meningiomas. Clin. Neurol. Neurosurg. 210, 106962. doi:10.1016/j.clineuro.2021.106962
Cheng, B., Pan, W., Xing, Y., Xiao, Y., Chen, J., and Xu, Z. (2022). Recent advances in DDR (DNA damage response) inhibitors for cancer therapy. Eur. J. Med. Chem. 230, 114109. doi:10.1016/j.ejmech.2022.114109
Chi, K. N., Rathkopf, D., Smith, M. R., Efstathiou, E., Attard, G., Olmos, D., et al. (2023). Niraparib and abiraterone acetate for metastatic castration-resistant prostate cancer. J. Clin. Oncol. 41 (18), 3339–3351. doi:10.1200/JCO.22.01649
Cleary, J. M., Aguirre, A. J., Shapiro, G. I., and D'Andrea, A. D. (2020). Biomarker-guided development of DNA repair inhibitors. Mol. Cell 78 (6), 1070–1085. doi:10.1016/j.molcel.2020.04.035
Coleman, R. L., Fleming, G. F., Brady, M. F., Swisher, E. M., Steffensen, K. D., Friedlander, M., et al. (2019). Veliparib with first-line chemotherapy and as maintenance therapy in ovarian cancer. N. Engl. J. Med. 381 (25), 2403–2415. doi:10.1056/NEJMoa1909707
Davis, S. L., Hartman, S. J., Bagby, S. M., Schlaepfer, M., Yacob, B. W., Tse, T., et al. (2022). ATM kinase inhibitor AZD0156 in combination with irinotecan and 5-fluorouracil in preclinical models of colorectal cancer. BMC Cancer 22 (1), 1107. doi:10.1186/s12885-022-10084-7
De Falco, M., and De, F. M. (2021). Take a break to repair: a dip in the world of double-strand break repair mechanisms pointing the gaze on archaea. Int. J. Mol. Sci. 22 (24), 13296. doi:10.3390/ijms222413296
de Lange, T. (2005). Shelterin: the protein complex that shapes and safeguards human telomeres. Genes Dev. 19 (18), 2100–2110. doi:10.1101/gad.1346005
de Vos, A., van der Helm, J., Prins, M., and Kretzschmar, M. (2012). Determinants of persistent spread of HIV in HCV-infected populations of injecting drug users. Epidemics 4 (2), 57–67. doi:10.1016/j.epidem.2012.01.001
Djojosubroto, M. W., Chin, A. C., Go, N., Schaetzlein, S., Manns, M. P., Gryaznov, S., et al. (2005). Telomerase antagonists GRN163 and GRN163L inhibit tumor growth and increase chemosensitivity of human hepatoma. Hepatology 42 (5), 1127–1136. doi:10.1002/hep.20822
Doksani, Y., and de Lange, T. (2014). The role of double-strand break repair pathways at functional and dysfunctional telomeres. Cold Spring Harb. Perspect. Biol. 6 (12), a016576. doi:10.1101/cshperspect.a016576
Doksani, Y., and de Lange, T. (2016). Telomere-internal double-strand breaks are repaired by homologous recombination and PARP1/lig3-dependent end-joining. Cell Rep. 17 (6), 1646–1656. doi:10.1016/j.celrep.2016.10.008
Dos Santos, G. A., Viana, N. I., Pimenta, R., de Camargo, J. A., Guimaraes, V. R., Romão, P., et al. (2024). Upregulation of shelterin and CST genes and longer telomeres are associated with unfavorable prognostic characteristics in prostate cancer. Cancer Genet. 284-285, 20–29. doi:10.1016/j.cancergen.2024.03.006
Eads, J. R., Krishnamurthi, S. S., Saltzman, J., Bokar, J. A., Savvides, P., Meropol, N. J., et al. (2021). Phase I clinical trial of temozolomide and methoxyamine (TRC-102), an inhibitor of base excision repair, in patients with advanced solid tumors. Invest. New Drugs 39 (1), 142–151. doi:10.1007/s10637-020-00962-x
Ebata, H., Loo, T. M., and Takahashi, A. (2022). Telomere maintenance and the cGAS-STING pathway in cancer. Cells 11 (12), 1958. doi:10.3390/cells11121958
Elango, R., Osia, B., Harcy, V., Malc, E., Mieczkowski, P. A., Roberts, S. A., et al. (2019). Repair of base damage within break-induced replication intermediates promotes kataegis associated with chromosome rearrangements. Nucleic Acids Res. 47 (18), 9666–9684. doi:10.1093/nar/gkz651
Elango, R., Sheng, Z., Jackson, J., DeCata, J., Ibrahim, Y., Pham, N. T., et al. (2017). Break-induced replication promotes formation of lethal joint molecules dissolved by Srs2. Nat. Commun. 8 (1), 1790. doi:10.1038/s41467-017-01987-2
Episkopou, H., Diman, A., Claude, E., Viceconte, N., and Decottignies, A. (2019). TSPYL5 depletion induces specific death of ALT cells through USP7-dependent proteasomal degradation of POT1. Mol. Cell 75 (3), 469–482. doi:10.1016/j.molcel.2019.05.027
Farag, N., Ercolani, G., Del Grosso, E., and Ricci, F. (2022). DNA tile self-assembly guided by base excision repair enzymes. Angew. Chem. Int. Ed. Engl. 61 (34), e202208367. doi:10.1002/anie.202208367
Feretzaki, M., Pospisilova, M., Valador Fernandes, R., Lunardi, T., Krejci, L., and Lingner, J. (2020). RAD51-dependent recruitment of TERRA lncRNA to telomeres through R-loops. Nature 587 (7833), 303–308. doi:10.1038/s41586-020-2815-6
Fernandes, S. G., Gala, K., and Khattar, E. (2022). Telomerase inhibitor MST-312 and quercetin synergistically inhibit cancer cell proliferation by promoting DNA damage. Transl. Oncol. 27, 101569. doi:10.1016/j.tranon.2022.101569
Findlay, S., Heath, J., Luo, V. M., Malina, A., Morin, T., Coulombe, Y., et al. (2018). SHLD2/FAM35A co-operates with REV7 to coordinate DNA double-strand break repair pathway choice. EMBO J. 37 (18), e100158. doi:10.15252/embj.2018100158
Fischer-Mertens, J., Otte, F., Roderwieser, A., Rosswog, C., Kahlert, Y., Werr, L., et al. (2022). Telomerase-targeting compounds Imetelstat and 6-thio-dG act synergistically with chemotherapy in high-risk neuroblastoma models. Cell Oncol. (Dordr) 45 (5), 991–1003. doi:10.1007/s13402-022-00702-8
Fishel, M. L., Xia, H., McGeown, J., McIlwain, D. W., Elbanna, M., Craft, A. A., et al. (2019). Antitumor activity and mechanistic characterization of APE1/ref-1 inhibitors in bladder cancer. Mol. Cancer Ther. 18 (11), 1947–1960. doi:10.1158/1535-7163.MCT-18-1166
Fizazi, K., Piulats, J. M., Reaume, M. N., Ostler, P., McDermott, R., Gingerich, J. R., et al. (2023). Rucaparib or physician's choice in metastatic prostate cancer. N. Engl. J. Med. 388 (8), 719–732. doi:10.1056/NEJMoa2214676
Fu, S., Yao, S., Yuan, Y., Previs, R. A., Elias, A. D., Carvajal, R. D., et al. (2023). Multicenter phase II trial of the WEE1 inhibitor adavosertib in refractory solid tumors harboring CCNE1 amplification. J. Clin. Oncol. 41 (9), 1725–1734. doi:10.1200/JCO.22.00830
García-Beccaria, M., Martínez, P., Méndez-Pertuz, M., Martínez, S., Blanco-Aparicio, C., Cañamero, M., et al. (2015). Therapeutic inhibition of TRF1 impairs the growth of p53-deficient K-RasG12V-induced lung cancer by induction of telomeric DNA damage. EMBO Mol. Med. 7 (7), 930–949. doi:10.15252/emmm.201404497
George, S. L., Parmar, V., Lorenzi, F., Marshall, L. V., Jamin, Y., Poon, E., et al. (2020). Novel therapeutic strategies targeting telomere maintenance mechanisms in high-risk neuroblastoma. J. Exp. Clin. Cancer Res. 39 (1), 78. doi:10.1186/s13046-020-01582-2
Gerada, C., Campbell, T. M., Kennedy, J. J., McSharry, B. P., Steain, M., Slobedman, B., et al. (2020). Manipulation of the innate immune response by varicella zoster virus. Front. Immunol. 11, 1. doi:10.3389/fimmu.2020.00001
Gomez, D. E., Armando, R. G., and Alonso, D. F. (2012). AZT as a telomerase inhibitor. Front. Oncol. 2, 113. doi:10.3389/fonc.2012.00113
Gómez-Cabello, D., Pappas, G., Aguilar-Morante, D., Dinant, C., and Bartek, J. (2022). CtIP-dependent nascent RNA expression flanking DNA breaks guides the choice of DNA repair pathway. Nat. Commun. 13 (1), 5303. doi:10.1038/s41467-022-33027-z
Gu, P., and Chang, S. (2013). Functional characterization of human CTC1 mutations reveals novel mechanisms responsible for the pathogenesis of the telomere disease Coats plus. Aging Cell 12 (6), 1100–1109. doi:10.1111/acel.12139
Guh, C. Y., Shen, H. J., Chen, L. W., Chiu, P. C., Liao, I. H., Lo, C. C., et al. (2022). XPF activates break-induced telomere synthesis. Nat. Commun. 13 (1), 5781. doi:10.1038/s41467-022-33428-0
Haakensen, V. D., Öjlert, Å. K., Thunold, S., Farooqi, S., Nowak, A. K., Chin, W. L., et al. (2024). UV1 telomerase vaccine with ipilimumab and nivolumab as second line treatment for pleural mesothelioma - a phase II randomised trial. Eur. J. Cancer 202, 113973. doi:10.1016/j.ejca.2024.113973
Hanahan, D. (2022). Hallmarks of cancer: new dimensions. Cancer Discov. 12 (1), 31–46. doi:10.1158/2159-8290.CD-21-1059
Harold, J., Bellone, S., Manavella, D. D., Mutlu, L., McNamara, B., Hartwich, T. M. P., et al. (2023). Elimusertib (BAY1895344), a novel ATR inhibitor, demonstrates in vivo activity in ATRX mutated models of uterine leiomyosarcoma. Gynecol. Oncol. 168, 157–165. doi:10.1016/j.ygyno.2022.11.014
Hernandez-Sanchez, W., Huang, W., Plucinsky, B., Garcia-Vazquez, N., Robinson, N. J., Schiemann, W. P., et al. (2019). A non-natural nucleotide uses a specific pocket to selectively inhibit telomerase activity. PLoS Biol. 17 (4), e3000204. doi:10.1371/journal.pbio.3000204
Hu, X., Hsieh, C. Y., Zhang, Y., Liu, W., Xu, S., Cai, S. X., et al. (2023). Effect of a strong CYP3A4 inhibitor and inducer on the pharmacokinetics of senaparib (IMP4297) in healthy volunteers: a drug-drug interaction study. Br. J. Clin. Pharmacol. 89 (6), 1767–1779. doi:10.1111/bcp.15624
Huang, P. Q., Boren, B. C., Hegde, S. G., Liu, H., Unni, A. K., Abraham, S., et al. (2021). Discovery of ZN-c3, a highly potent and selective Wee1 inhibitor undergoing evaluation in clinical trials for the treatment of cancer. J. Med. Chem. 64 (17), 13004–13024. doi:10.1021/acs.jmedchem.1c01121
Huffman, B. M., Feng, H., Parmar, K., Wang, J., Kapner, K. S., Kochupurakkal, B., et al. (2023). A phase I expansion cohort study evaluating the safety and efficacy of the CHK1 inhibitor LY2880070 with low-dose gemcitabine in patients with metastatic pancreatic adenocarcinoma. Clin. Cancer Res. 29 (24), 5047–5056. doi:10.1158/1078-0432.CCR-23-2005
Iida, K., Suzuki, N., Sasaki, A., Ishida, S., and Arai, T. (2022). Development of a novel light-up probe for detection of G-quadruplexes in stress granules. Sci. Rep. 12 (1), 12892. doi:10.1038/s41598-022-17230-y
Illuzzi, G., Staniszewska, A., Gill, S., Pike, A., McWilliams, L., Critchlow, S., et al. (2022). Preclinical characterization of AZD5305, A next-generation, highly selective PARP1 inhibitor and trapper. Clin. cancer Res. 28 (21), 4724–4736. doi:10.1158/1078-0432.CCR-22-0301
Infante, J. R., Hollebecque, A., Postel-Vinay, S., Bauer, T. M., Blackwood, E. M., Evangelista, M., et al. (2017). Phase I study of GDC-0425, a checkpoint kinase 1 inhibitor, in combination with gemcitabine in patients with refractory solid tumors. Clin. Cancer Res. 23 (10), 2423–2432. doi:10.1158/1078-0432.CCR-16-1782
Kaminski, N., Wondisford, A. R., Kwon, Y., Lynskey, M. L., Bhargava, R., Barroso-González, J., et al. (2022). RAD51AP1 regulates ALT-HDR through chromatin-directed homeostasis of TERRA. Mol. Cell 82 (21), 4001–4017.e7. doi:10.1016/j.molcel.2022.09.025
Kaul, Z., Cheung, C. T. Y., Bhargava, P., Sari, A. N., Yu, Y., Huifu, H., et al. (2021). Functional characterization of miR-708 microRNA in telomerase positive and negative human cancer cells. Sci. Rep. 11 (1), 17052. doi:10.1038/s41598-021-96096-y
Kim, D. E., Dollé, M. E. T., Vermeij, W. P., Gyenis, A., Vogel, K., Hoeijmakers, J. H. J., et al. (2020). Deficiency in the DNA repair protein ERCC1 triggers a link between senescence and apoptosis in human fibroblasts and mouse skin. Aging Cell 19 (3), e13072. doi:10.1111/acel.13072
Kim, H., Seo, E. H., Lee, S. H., and Kim, B. J. (2016). The telomerase-derived anticancer peptide vaccine GV1001 as an extracellular heat shock protein-mediated cell-penetrating peptide. Int. J. Mol. Sci. 17 (12), 2054. doi:10.3390/ijms17122054
Kim, R., Kwon, M., An, M., Kim, S., Smith, S., Loembé, A., et al. (2022). Phase II study of ceralasertib (AZD6738) in combination with durvalumab in patients with advanced/metastatic melanoma who have failed prior anti-PD-1 therapy. Ann. Oncol. 33 (2), 193–203. doi:10.1016/j.annonc.2021.10.009
Kishkevich, A., Tamang, S., Nguyen, M. O., Oehler, J., Bulmaga, E., Andreadis, C., et al. (2022). Rad52's DNA annealing activity drives template switching associated with restarted DNA replication. Nat. Commun. 13 (1), 7293. doi:10.1038/s41467-022-35060-4
Kockler, Z. W., Osia, B., Lee, R., Musmaker, K., and Malkova, A. (2021). Repair of DNA breaks by break-induced replication. Annu. Rev. Biochem. 90, 165–191. doi:10.1146/annurev-biochem-081420-095551
Kodym, E., Kodym, R., Reis, A., Habib, A., Story, M., and Saha, D. (2009). The small-molecule CDK inhibitor, SNS-032, enhances cellular radiosensitivity in quiescent and hypoxic non-small cell lung cancer cells. Lung cancer (Amsterdam, Neth.) 66 (1), 37–47. doi:10.1016/j.lungcan.2008.12.026
Koneru, B., Farooqi, A., Nguyen, T. H., Chen, W. H., Hindle, A., Eslinger, C., et al. (2021). ALT neuroblastoma chemoresistance due to telomere dysfunction-induced ATM activation is reversible with ATM inhibitor AZD0156. Sci. Transl. Med. 13 (607), eabd5750. doi:10.1126/scitranslmed.abd5750
Konstantinopoulos, P. A., Lee, J. M., Gao, B., Miller, R., Lee, J. Y., Colombo, N., et al. (2022). A Phase 2 study of prexasertib (LY2606368) in platinum resistant or refractory recurrent ovarian cancer. Gynecol. Oncol. 167 (2), 213–225. doi:10.1016/j.ygyno.2022.09.019
Kreuzer, K. N. (2000). Recombination-dependent DNA replication in phage T4. Trends Biochem. Sci. 25 (4), 165–173. doi:10.1016/s0968-0004(00)01559-0
Kunkel, T. A., and Erie, D. A. (2015). Eukaryotic mismatch repair in relation to DNA replication. Annu. Rev. Genet. 49, 291–313. doi:10.1146/annurev-genet-112414-054722
Li, X., Li, X., Xie, C., Cai, S., Li, M., Jin, H., et al. (2022). cGAS guards against chromosome end-to-end fusions during mitosis and facilitates replicative senescence. Protein Cell 13 (1), 47–64. doi:10.1007/s13238-021-00879-y
Lilleby, W., Gaudernack, G., Brunsvig, P. F., Vlatkovic, L., Schulz, M., Mills, K., et al. (2017). Phase I/IIa clinical trial of a novel hTERT peptide vaccine in men with metastatic hormone-naive prostate cancer. Cancer Immunol. Immunother. 66 (7), 891–901. doi:10.1007/s00262-017-1994-y
Liu, B., He, Y., Wang, Y., Song, H., Zhou, Z., and Feigon, J. (2022a). Structure of active human telomerase with telomere shelterin protein TPP1. Nature 604 (7906), 578–583. doi:10.1038/s41586-022-04582-8
Liu, L. Y., Ma, T. Z., Zeng, Y. L., Liu, W., and Mao, Z. W. (2022b). Structural basis of pyridostatin and its derivatives specifically binding to G-quadruplexes. J. Am. Chem. Soc. 144 (26), 11878–11887. doi:10.1021/jacs.2c04775
Lu, R., O'Rourke, J. J., Sobinoff, A. P., Allen, J. A. M., Nelson, C. B., Tomlinson, C. G., et al. (2019). The FANCM-BLM-TOP3A-RMI complex suppresses alternative lengthening of telomeres (ALT). Nat. Commun. 10 (1), 2252. doi:10.1038/s41467-019-10180-6
Luedeman, M. E., Stroik, S., Feng, W., Luthman, A. J., Gupta, G. P., and Ramsden, D. A. (2022). Poly(ADP) ribose polymerase promotes DNA polymerase theta-mediated end joining by activation of end resection. Nat. Commun. 13 (1), 4547. doi:10.1038/s41467-022-32166-7
Luo, Z., Liu, W., Sun, P., Wang, F., and Feng, X. (2021). Pan-cancer analyses reveal regulation and clinical outcome association of the shelterin complex in cancer. Brief. Bioinform 22 (5), bbaa441. doi:10.1093/bib/bbaa441
Maréchal, A., and Zou, L. (2013). DNA damage sensing by the ATM and ATR kinases. Cold Spring Harb. Perspect. Biol. 5 (9), a012716. doi:10.1101/cshperspect.a012716
Mender, I., LaRanger, R., Luitel, K., Peyton, M., Girard, L., Lai, T., et al. (2018). Telomerase-mediated strategy for overcoming non-small cell lung cancer targeted therapy and chemotherapy resistance. Neoplasia (New York, NY) 20 (8), 826–837. doi:10.1016/j.neo.2018.06.002
Mender, I., Zhang, A., Ren, Z., Han, C., Deng, Y., Siteni, S., et al. (2020). Telomere stress potentiates STING-dependent anti-tumor immunity. Cancer Cell 38 (3), 400–411. doi:10.1016/j.ccell.2020.05.020
Menez-Jamet, J., Gallou, C., Rougeot, A., and Kosmatopoulos, K. (2016). Optimized tumor cryptic peptides: the basis for universal neo-antigen-like tumor vaccines. Ann. Transl. Med. 4 (14), 266. doi:10.21037/atm.2016.05.15
Middleton, G., Silcocks, P., Cox, T., Valle, J., Wadsley, J., Propper, D., et al. (2014). Gemcitabine and capecitabine with or without telomerase peptide vaccine GV1001 in patients with locally advanced or metastatic pancreatic cancer (TeloVac): an open-label, randomised, phase 3 trial. Lancet Oncol. 15 (8), 829–840. doi:10.1016/S1470-2045(14)70236-0
Moglan, A. M., Albaradie, O. A., Alsayegh, F. F., Alharbi, H. M., Samman, Y. M., Jalal, M. M., et al. (2023). Preclinical efficacy of oncolytic VSV-IFNβ in treating cancer: a systematic review. Front. Immunol. 14, 1085940. doi:10.3389/fimmu.2023.1085940
Mukherjee, J., Johannessen, T. C., Ohba, S., Chow, T. T., Jones, L., Pandita, A., et al. (2018). Mutant IDH1 cooperates with ATRX loss to drive the alternative lengthening of telomere phenotype in glioma. Cancer Res. 78 (11), 2966–2977. doi:10.1158/0008-5472.CAN-17-2269
Murciano-Goroff, Y., Schram, A., Rosen, E., Won, H., Gong, Y., Noronha, A., et al. (2022). Reversion mutations in germline BRCA1/2-mutant tumors reveal a BRCA-mediated phenotype in non-canonical histologies. Nat. Commun. 13 (1), 7182. doi:10.1038/s41467-022-34109-8
Nagel, Z. D., Kitange, G. J., Gupta, S. K., Joughin, B. A., Chaim, I. A., Mazzucato, P., et al. (2017). DNA repair capacity in multiple pathways predicts chemoresistance in glioblastoma multiforme. Cancer Res. 77 (1), 198–206. doi:10.1158/0008-5472.CAN-16-1151
Ngo, G. H. P., Grimstead, J. W., and Baird, D. M. (2021). UPF1 promotes the formation of R loops to stimulate DNA double-strand break repair. Nat. Commun. 12 (1), 3849. doi:10.1038/s41467-021-24201-w
Oanh, N. T. K., Lee, H. S., Kim, Y. H., Min, S., Park, Y. J., Heo, J., et al. (2022). Regulation of nuclear DNA damage response by mitochondrial morphofunctional pathway. Nucleic Acids Res. 50 (16), 9247–9259. doi:10.1093/nar/gkac690
Ouyang, J., Garner, E., Hallet, A., Nguyen, H. D., Rickman, K. A., Gill, G., et al. (2015). Noncovalent interactions with SUMO and ubiquitin orchestrate distinct functions of the SLX4 complex in genome maintenance. Mol. Cell 57 (1), 108–122. doi:10.1016/j.molcel.2014.11.015
Park, S. J., Chang, S. J., Suh, D. H., Kong, T. W., Song, H., Kim, T. H., et al. (2022). A phase IA dose-escalation study of PHI-101, a new checkpoint kinase 2 inhibitor, for platinum-resistant recurrent ovarian cancer. BMC Cancer 22 (1), 28. doi:10.1186/s12885-021-09138-z
Peng, X., Zhang, S., Wang, Y., Zhou, Z., Yu, Z., Zhong, Z., et al. (2023). Stellettin B sensitizes glioblastoma to DNA-damaging treatments by suppressing PI3K-mediated homologous recombination repair. Adv. Sci. (Weinh) 10 (3), e2205529. doi:10.1002/advs.202205529
Perkhofer, L., Gout, J., Roger, E., Kude de Almeida, F., Baptista Simões, C., Wiesmüller, L., et al. (2021). DNA damage repair as a target in pancreatic cancer: state-of-the-art and future perspectives. Gut 70 (3), 606–617. doi:10.1136/gutjnl-2019-319984
Pfitzer, L., Moser, C., Gegenfurtner, F., Arner, A., Foerster, F., Atzberger, C., et al. (2019). Targeting actin inhibits repair of doxorubicin-induced DNA damage: a novel therapeutic approach for combination therapy. Cell Death Dis. 10 (4), 302. doi:10.1038/s41419-019-1546-9
Pobiega, S., Alibert, O., and Marcand, S. (2021). A new assay capturing chromosome fusions shows a protection trade-off at telomeres and NHEJ vulnerability to low-density ionizing radiation. Nucleic Acids Res. 49 (12), 6817–6831. doi:10.1093/nar/gkab502
Qin, H., and Guo, Y. (2022). Targeting telomerase enhances cytotoxicity of salinomycin in cancer cells. ACS Omega 7 (34), 30565–30570. doi:10.1021/acsomega.2c04082
Qin, T., Mullan, B., Ravindran, R., Messinger, D., Siada, R., Cummings, J. R., et al. (2022). ATRX loss in glioma results in dysregulation of cell-cycle phase transition and ATM inhibitor radio-sensitization. Cell Rep. 38 (2), 110216. doi:10.1016/j.celrep.2021.110216
Qiu, Y. D., Yan, Q., Wang, Y., Ye, Y. F., Wang, Y., Wang, M. Y., et al. (2024). Discovery of a selective TRF2 inhibitor FKB04 induced telomere shortening and senescence in liver cancer cells. Acta Pharmacol. Sin. doi:10.1038/s41401-024-01243-6
Radin, D. P., Shifman, S., Outhwaite, I. R., Sharma, A., Bases, R., Seeliger, M. A., et al. (2024). Lucanthone, a potential PPT1 inhibitor, perturbs stemness, reduces tumor microtube formation, and slows the growth of temozolomide-resistant gliomas in vivo. J. Pharmacol. Exp. Ther. 389 (1), 51–60. doi:10.1124/jpet.123.002021
Raghunandan, M., Geelen, D., Majerova, E., and Decottignies, A. (2021). NHP2 downregulation counteracts hTR-mediated activation of the DNA damage response at ALT telomeres. Embo J. 40 (6), e106336. doi:10.15252/embj.2020106336
Rai, R., Chen, Y., Lei, M., and Chang, S. (2016). TRF2-RAP1 is required to protect telomeres from engaging in homologous recombination-mediated deletions and fusions. Nat. Commun. 7, 10881. doi:10.1038/ncomms10881
Ran, X., Liu, L., Yang, C. Y., Lu, J., Chen, Y., Lei, M., et al. (2016). Design of high-affinity stapled peptides to target the repressor activator protein 1 (RAP1)/Telomeric repeat-binding factor 2 (TRF2) protein-protein interaction in the shelterin complex. J. Med. Chem. 59 (1), 328–334. doi:10.1021/acs.jmedchem.5b01465
Ribes-Zamora, A., Indiviglio, S. M., Mihalek, I., Williams, C. L., and Bertuch, A. A. (2013). TRF2 interaction with Ku heterotetramerization interface gives insight into c-NHEJ prevention at human telomeres. Cell Rep. 5 (1), 194–206. doi:10.1016/j.celrep.2013.08.040
Robson, M., Im, S. A., Senkus, E., Xu, B., Domchek, S. M., Masuda, N., et al. (2017). Olaparib for metastatic breast cancer in patients with a germline BRCA mutation. N. Engl. J. Med. 377 (6), 523–533. doi:10.1056/NEJMoa1706450
Roos, W. P., Thomas, A. D., and Kaina, B. (2016). DNA damage and the balance between survival and death in cancer biology. Nat. Rev. Cancer 16 (1), 20–33. doi:10.1038/nrc.2015.2
Saito, T. T., Youds, J. L., Boulton, S. J., and Colaiácovo, M. P. (2009). Caenorhabditis elegans HIM-18/SLX-4 interacts with SLX-1 and XPF-1 and maintains genomic integrity in the germline by processing recombination intermediates. PLoS Genet. 5 (11), e1000735. doi:10.1371/journal.pgen.1000735
Sakellariou, D., Bak, S. T., Isik, E., Barroso, S. I., Porro, A., Aguilera, A., et al. (2022). MutSβ regulates G4-associated telomeric R-loops to maintain telomere integrity in ALT cancer cells. Cell Rep. 39 (1), 110602. doi:10.1016/j.celrep.2022.110602
Samuels, M., Falkenius, J., Bar-Ad, V., Dunst, J., van Triest, B., Yachnin, J., et al. (2024). A phase 1 study of the DNA-PK inhibitor peposertib in combination with radiation therapy with or without cisplatin in patients with advanced head and neck tumors. Int. J. Radiat. Oncol. Biol. Phys. 118 (3), 743–756. doi:10.1016/j.ijrobp.2023.09.024
Scheper, J., Hildebrand, L. S., Faulhaber, E. M., Deloch, L., Gaipl, U. S., Symank, J., et al. (2022). Tumor-specific radiosensitizing effect of the ATM inhibitor AZD0156 in melanoma cells with low toxicity to healthy fibroblasts. Strahlenther Onkol. 199, 1128–1139. doi:10.1007/s00066-022-02009-x
Schutte, T., Embaby, A., Steeghs, N., van der Mierden, S., van Driel, W., Rijlaarsdam, M., et al. (2023). Clinical development of WEE1 inhibitors in gynecological cancers: a systematic review. Cancer Treat. Rev. 115, 102531. doi:10.1016/j.ctrv.2023.102531
Scully, R., Panday, A., Elango, R., and Willis, N. A. (2019). DNA double-strand break repair-pathway choice in somatic mammalian cells. Nat. Rev. Mol. Cell Biol. 20 (11), 698–714. doi:10.1038/s41580-019-0152-0
Selvaraj, S., Feist, W. N., Viel, S., Vaidyanathan, S., Dudek, A. M., Gastou, M., et al. (2023). High-efficiency transgene integration by homology-directed repair in human primary cells using DNA-PKcs inhibition. Nat. Biotechnol. 42, 731–744. doi:10.1038/s41587-023-01888-4
Sengupta, S., Sobo, M., Lee, K., Senthil Kumar, S., White, A. R., Mender, I., et al. (2018). Induced telomere damage to treat telomerase expressing therapy-resistant pediatric brain tumors. Mol. Cancer Ther. 17 (7), 1504–1514. doi:10.1158/1535-7163.MCT-17-0792
Shankar, U., Mishra, S. K., Jain, N., Tawani, A., Yadav, P., and Kumar, A. (2022). Ni(+2) permease system of Helicobacter pylori contains highly conserved G-quadruplex motifs. Infect. Genet. Evol. 101, 105298. doi:10.1016/j.meegid.2022.105298
Shen, Z., Zheng, R., Yang, H., Xing, S., Jin, X., Yan, H., et al. (2022). G-quadruplex stabilizer Tetra-Pt(bpy) disrupts telomere maintenance and impairs FAK-mediated migration of telomerase-positive cells. Int. J. Biol. Macromol. 213, 858–870. doi:10.1016/j.ijbiomac.2022.06.015
Shi, J., Zhang, M., Zhang, L., Yu, X., Sun, L., Liu, J., et al. (2023). Shelterin dysfunction promotes CD4+ T cell senescence in behçet's disease. Rheumatol. Oxf., kead703. doi:10.1093/rheumatology/kead703
Shibata, A., and Jeggo, P. A. (2020). Canonical DNA non-homologous end-joining; capacity versus fidelity. Br. J. Radiol. 93 (1115), 20190966. doi:10.1259/bjr.20190966
Silva, B., Arora, R., Bione, S., and Azzalin, C. M. (2021). TERRA transcription destabilizes telomere integrity to initiate break-induced replication in human ALT cells. Nat. Commun. 12 (1), 3760. doi:10.1038/s41467-021-24097-6
Silva, B., Pentz, R., Figueira, A. M., Arora, R., Lee, Y. W., Hodson, C., et al. (2019). FANCM limits ALT activity by restricting telomeric replication stress induced by deregulated BLM and R-loops. Nat. Commun. 10 (1), 2253. doi:10.1038/s41467-019-10179-z
Slijepcevic, P. (2006). The role of DNA damage response proteins at telomeres-an "integrative" model. DNA Repair (Amst). 5 (11), 1299–1306. doi:10.1016/j.dnarep.2006.05.038
Somuncu, B., Keskin, S., Antmen, F. M., Saglican, Y., Ekmekcioglu, A., Ertuzun, T., et al. (2020). Non-muscle invasive bladder cancer tissues have increased base excision repair capacity. Sci. Rep. 10 (1), 16371. doi:10.1038/s41598-020-73370-z
Stundon, J. L., Ijaz, H., Gaonkar, K. S., Kaufman, R. S., Jin, R., Karras, A., et al. (2022). ALT in pediatric high-grade gliomas can occur without ATRX mutation and is enriched in patients with pathogenic germline MMR variants OGG1 in the kidney: beyond base excision repair. Neuro Oncol. 2022, 5774641.
Subecz, C., Sun, J. S., and Roger, L. (2021). Effect of DNA repair inhibitor AsiDNA on the incidence of telomere fusion in crisis. Hum. Mol. Genet. 30 (3-4), 172–181. doi:10.1093/hmg/ddab008
Sweeney, C. J., Percent, I. J., Babu, S., Cultrera, J. L., Mehlhaff, B. A., Goodman, O. B., et al. (2022). Phase ib/II study of enzalutamide with samotolisib (LY3023414) or placebo in patients with metastatic castration-resistant prostate cancer. Clin. Cancer Res. 28 (11), 2237–2247. doi:10.1158/1078-0432.CCR-21-2326
Szymanski, M. R., Karlowicz, A., Herrmann, G. K., Cen, Y., and Yin, Y. W. (2022). Human EXOG possesses strong AP hydrolysis activity: implication on mitochondrial DNA base excision repair. J. Am. Chem. Soc. 144 (51), 23543–23550. doi:10.1021/jacs.2c10558
Takahashi, N., Hao, Z., Villaruz, L. C., Zhang, J., Ruiz, J., Petty, W. J., et al. (2023). Berzosertib plus topotecan vs topotecan alone in patients with relapsed small cell lung cancer: a randomized clinical trial. JAMA Oncol. 9 (12), 1669–1677. doi:10.1001/jamaoncol.2023.4025
Tang, Q., Gulkis, M., McKenna, R., and Çağlayan, M. (2022). Structures of LIG1 that engage with mutagenic mismatches inserted by polβ in base excision repair. Nat. Commun. 13 (1), 3860. doi:10.1038/s41467-022-31585-w
Teixeira, L., Medioni, J., Garibal, J., Adotevi, O., Doucet, L., Durey, M. D., et al. (2020). A first-in-human phase I study of INVAC-1, an optimized human telomerase DNA vaccine in patients with advanced solid tumors. Clin. Cancer Res. 26 (3), 588–597. doi:10.1158/1078-0432.CCR-19-1614
Telli, M. L., Tolaney, S. M., Shapiro, G. I., Middleton, M., Lord, S. R., Arkenau, H. T., et al. (2022). Phase 1b study of berzosertib and cisplatin in patients with advanced triple-negative breast cancer. NPJ Breast Cancer 8 (1), 45. doi:10.1038/s41523-022-00406-0
Teng, F. Y., Jiang, Z. Z., Guo, M., Tan, X. Z., Chen, F., Xi, X. G., et al. (2021). G-quadruplex DNA: a novel target for drug design. Cell Mol. Life Sci. 78 (19-20), 6557–6583. doi:10.1007/s00018-021-03921-8
Tesmer, V. M., Brenner, K. A., and Nandakumar, J. (2023). Human POT1 protects the telomeric ds-ss DNA junction by capping the 5' end of the chromosome. Science 381 (6659), 771–778. doi:10.1126/science.adi2436
Tiek, D., Erdogdu, B., Razaghi, R., Jin, L., Sadowski, N., Alamillo-Ferrer, C., et al. (2022). Temozolomide-induced guanine mutations create exploitable vulnerabilities of guanine-rich DNA and RNA regions in drug-resistant gliomas. Sci. Adv. 8 (25), eabn3471. doi:10.1126/sciadv.abn3471
Tsai, R., Fang, K., Yang, P., Hsieh, Y., Chiang, I., Chen, Y., et al. (2022). TERRA regulates DNA G-quadruplex formation and ATRX recruitment to chromatin. Nucleic acids Res. 50 (21), 12217–12234. doi:10.1093/nar/gkac1114
Ueno, M. (2023). Exploring genetic interactions with telomere protection gene pot1 in fission yeast. Biomolecules 13 (2), 370. doi:10.3390/biom13020370
Vancevska, A., Ahmed, W., Pfeiffer, V., Feretzaki, M., Boulton, S. J., and Lingner, J. (2020). SMCHD1 promotes ATM-dependent DNA damage signaling and repair of uncapped telomeres. EMBO J. 39 (7), e102668. doi:10.15252/embj.2019102668
van de Kooij, B., Kruswick, A., van Attikum, H., and Yaffe, M. B. (2022). Multi-pathway DNA-repair reporters reveal competition between end-joining, single-strand annealing and homologous recombination at Cas9-induced DNA double-strand breaks. Nat. Commun. 13 (1), 5295. doi:10.1038/s41467-022-32743-w
van Harten, A. M., Buijze, M., van der Mast, R., Rooimans, M. A., Martens-de Kemp, S. R., Bachas, C., et al. (2019). Targeting the cell cycle in head and neck cancer by Chk1 inhibition: a novel concept of bimodal cell death. Oncogenesis 8 (7), 38. doi:10.1038/s41389-019-0147-x
Vassilis, G., Jean-Yves, D., David, K., Christian, M., Rafael, R., Antonio, R., et al. (2013). A multicenter randomized phase IIb efficacy study of Vx-001, a peptide-based cancer vaccine as maintenance treatment in advanced non-small-cell lung cancer: treatment rationale and protocol dynamics. Clin. Lung Cancer 14 (4), 461–465. doi:10.1016/j.cllc.2013.02.001
Vendetti, F. P., Karukonda, P., Clump, D. A., Teo, T., Lalonde, R., Nugent, K., et al. (2018). ATR kinase inhibitor AZD6738 potentiates CD8+ T cell-dependent antitumor activity following radiation. J. Clin. Invest. 128 (9), 3926–3940. doi:10.1172/JCI96519
Verdun, R. E., and Karlseder, J. (2007). Replication and protection of telomeres. Nature 447 (7147), 924–931. doi:10.1038/nature05976
Verma, P., Dilley, R. L., Zhang, T., Gyparaki, M. T., Li, Y., and Greenberg, R. A. (2019). RAD52 and SLX4 act nonepistatically to ensure telomere stability during alternative telomere lengthening. Genes Dev. 33 (3-4), 221–235. doi:10.1101/gad.319723.118
Vernì, F. (2022). DNA damage response (DDR) and DNA repair. Int. J. Mol. Sci. 23 (13), 7204. doi:10.3390/ijms23137204
Viol, F., Sipos, B., Fahl, M., Clauditz, T. S., Amin, T., Kriegs, M., et al. (2022). Novel preclinical gastroenteropancreatic neuroendocrine neoplasia models demonstrate the feasibility of mutation-based targeted therapy. Cell Oncol. (Dordr) 45 (6), 1401–1419. doi:10.1007/s13402-022-00727-z
Vohhodina, J., Goehring, L. J., Liu, B., Kong, Q., Botchkarev, V. V., Huynh, M., et al. (2021). BRCA1 binds TERRA RNA and suppresses R-Loop-based telomeric DNA damage. Nat. Commun. 12 (1), 3542. doi:10.1038/s41467-021-23716-6
Wen, P. Y., Omuro, A., Ahluwalia, M. S., Fathallah-Shaykh, H. M., Mohile, N., Lager, J. J., et al. (2015). Phase I dose-escalation study of the PI3K/mTOR inhibitor voxtalisib (SAR245409, XL765) plus temozolomide with or without radiotherapy in patients with high-grade glioma. Neuro Oncol. 17 (9), 1275–1283. doi:10.1093/neuonc/nov083
Wong, W. K., Guerra Liberal, F. D. C., and McMahon, S. J. (2022). DNA repair inhibitors potentiate fractionated radiotherapy more than single-dose radiotherapy in breast cancer cells. Cancers (Basel) 14 (15), 3794. doi:10.3390/cancers14153794
Wu, J., Dai, W., Wu, L., and Wang, J. (2018). SALP, a new single-stranded DNA library preparation method especially useful for the high-throughput characterization of chromatin openness states. BMC Genomics 19 (1), 143. doi:10.1186/s12864-018-4530-3
Wu, L., Fidan, K., Um, J. Y., and Ahn, K. S. (2020). Telomerase: key regulator of inflammation and cancer. Pharmacol. Res. 155, 104726. doi:10.1016/j.phrs.2020.104726
Wu, X., Krishna Sudhakar, H., Alcock, L. J., and Lau, Y. H. (2023). Mannich base PIP-199 is a chemically unstable pan-assay interference compound. J. Med. Chem. 66 (16), 11271–11281. doi:10.1021/acs.jmedchem.3c00674
Xue, H., Bhardwaj, A., Yin, Y., Fijen, C., Ephstein, A., Zhang, L., et al. (2022). A two-step mechanism governing PARP1-DNA retention by PARP inhibitors. Sci. Adv. 8 (36), eabq0414. doi:10.1126/sciadv.abq0414
Yadav, T., Zhang, J. M., Ouyang, J., Leung, W., Simoneau, A., and Zou, L. (2022). TERRA and RAD51AP1 promote alternative lengthening of telomeres through an R-to D-loop switch. Mol. Cell 82 (21), 3985–4000.e4. doi:10.1016/j.molcel.2022.09.026
Yan, C., Chang, Y., Gao, H., Zhang, Q., Peng, S., Wang, D., et al. (2021). G-quadruplex apurinic site-programmed chiral cyanine assemblies for specifically recognizing guanosine and guanine. Analyst 146 (19), 5866–5872. doi:10.1039/d1an01110c
Yang, H., Wang, H., Ren, J., Chen, Q., and Chen, Z. J. (2017). cGAS is essential for cellular senescence. Proc. Natl. Acad. Sci. U. S. A. 114 (23), E4612–e20. doi:10.1073/pnas.1705499114
Yang, Z., Sharma, K., and de Lange, T. (2022). TRF1 uses a noncanonical function of TFIIH to promote telomere replication. Genes Dev. 36 (17-18), 956–969. doi:10.1101/gad.349975.122
Yap, T. A., Fontana, E., Lee, E. K., Spigel, D. R., Højgaard, M., Lheureux, S., et al. (2023). Camonsertib in DNA damage response-deficient advanced solid tumors: phase 1 trial results. Nat. Med. 29 (6), 1400–1411. doi:10.1038/s41591-023-02399-0
Yap, T. A., Tolcher, A. W., Plummer, R., Mukker, J. K., Enderlin, M., Hicking, C., et al. (2024). First-in-Human study of the ataxia telangiectasia and rad3-related (ATR) inhibitor tuvusertib (M1774) as monotherapy in patients with solid tumors. Clin. Cancer Res. 30, 2057–2067. doi:10.1158/1078-0432.CCR-23-2409
Yilmaz, U., Kamer, D., Asik, A., Kara, H. G., Gündüz, C., and Kamer, S. (2023). The effect of the ATM inhibitor AZD0156 on the radiosensitivity of human breast cancer and lung fibroblast cells. J. Cancer Res. Ther. 19 (2), 203–207. doi:10.4103/jcrt.JCRT_1540_20
Yu, S., Wei, S., Savani, M., Lin, X., Du, K., Mender, I., et al. (2021). A modified nucleoside 6-thio-2'-deoxyguanosine exhibits antitumor activity in gliomas. Clin. Cancer Res. 27 (24), 6800–6814. doi:10.1158/1078-0432.CCR-21-0374
Zhang, J. M., Genois, M. M., Ouyang, J., Lan, L., and Zou, L. (2021). Alternative lengthening of telomeres is a self-perpetuating process in ALT-associated PML bodies. Mol. Cell 81 (5), 1027–1042.e4. doi:10.1016/j.molcel.2020.12.030
Zheng, J., Li, Z., and Min, W. (2022). Current status and future promise of next-generation poly (ADP-Ribose) polymerase 1-selective inhibitor AZD5305. Front. Pharmacol. 13, 979873. doi:10.3389/fphar.2022.979873
Zheng, X. H., Nie, X., Fang, Y., Zhang, Z., Xiao, Y., Mao, Z., et al. (2017). A cisplatin derivative tetra-Pt(bpy) as an oncotherapeutic agent for targeting ALT cancer. J. Natl. Cancer Inst. 109 (10). doi:10.1093/jnci/djx061
Keywords: alternative lengthening of telomeres, cancer, DNA damage response, telomerase inhibitor, telomere
Citation: Gu L, Liu M, Zhang Y, Zhou H, Wang Y and Xu Z-X (2024) Telomere-related DNA damage response pathways in cancer therapy: prospective targets. Front. Pharmacol. 15:1379166. doi: 10.3389/fphar.2024.1379166
Received: 30 January 2024; Accepted: 23 May 2024;
Published: 07 June 2024.
Edited by:
Junmin Zhang, Lanzhou University, ChinaReviewed by:
Marla Weetall, PTC Therapeutics, United StatesVenkataswarup Tiriveedhi, Tennessee State University, United States
Copyright © 2024 Gu, Liu, Zhang, Zhou, Wang and Xu. This is an open-access article distributed under the terms of the Creative Commons Attribution License (CC BY). The use, distribution or reproduction in other forums is permitted, provided the original author(s) and the copyright owner(s) are credited and that the original publication in this journal is cited, in accordance with accepted academic practice. No use, distribution or reproduction is permitted which does not comply with these terms.
*Correspondence: Honglan Zhou, aGx6aG91QGpsdS5lZHUuY24=; Yishu Wang, d2FuZ3lzQGpsdS5lZHUuZW4=; Zhi-Xiang Xu, emhpeGlhbmd4dUBqbHUuZWR1LmNu
†These authors have contributed equally to this work