- 1College of Life Sciences, Northwest University, Xi’an, Shaanxi, China
- 2Department of Nephrology, Xi’an Chang’an District Hospital, Xi’an, Shaanxi, China
- 3Beijing Key Lab for Immune-Mediated Inflammatory Diseases, Institute of Clinical Medical Sciences, China-Japan Friendship Hospital, Beijing, China
Pyruvate kinase M2 (PKM2), a rate limiting enzyme in glycolysis, is a cellular regulator that has received extensive attention and regards as a metabolic regulator of cellular metabolism and energy. Kidney is a highly metabolically active organ, and glycolysis is the important energy resource for kidney. The accumulated evidences indicates that the enzymatic activity of PKM2 is disturbed in kidney disease progression and treatment, especially diabetic kidney disease and acute kidney injury. Modulating PKM2 post-translational modification determines its enzymatic activity and nuclear translocation that serves as an important interventional approach to regulate PKM2. Emerging evidences show that PKM2 and its post-translational modification participate in kidney disease progression and treatment through modulating metabolism regulation, podocyte injury, fibroblast activation and proliferation, macrophage polarization, and T cell regulation. Interestingly, PKM2 activators (TEPP-46, DASA-58, mitapivat, and TP-1454) and PKM2 inhibitors (shikonin, alkannin, compound 3k and compound 3h) have exhibited potential therapeutic property in kidney disease, which indicates the pleiotropic effects of PKM2 in kidney. In the future, the deep investigation of PKM2 pleiotropic effects in kidney is urgently needed to determine the therapeutic effect of PKM2 activator/inhibitor to benefit patients. The information in this review highlights that PKM2 functions as a potential biomarker and therapeutic target for kidney diseases.
1 Introduction
Kidney is a highly metabolically active organ. The metabolic programing in the kidney is different in the distinct regions of kidney. The cortex has high rates of gluconeogenesis and fatty acid oxidation, while the medulla mainly relies on glycolysis, which indicates that oxygen requirement reduces gradually from cortex to medulla in kidney. In the cortex, proximal tubules are highly aerobic and mainly responsible for fluid reabsorption, while in the medulla, distal nephron segments have lower oxygen tension. Under physiological condition, the medulla papilla and distal convoluted tubules has high glycolytic activity (Tang and Wei, 2023), and glycolysis is also the major energy source of podocytes (Brinkkoetter et al., 2019). Even proximal tubular cells have low glycolytic activity, glycolysis plays an important role in maintaining phosphate homeostasis (Zhou et al., 2022; Zhou et al., 2023). Since emerging evidences demonstrated that glycolysis is significantly disturbed during kidney disease (Nakagawa et al., 2020; Gu et al., 2022; Ito et al., 2022), glycolysis is the potential therapeutic target for kidney disease.
Glycolysis is a major metabolic process that converts glucose into pyruvate with the production of adenosine triphosphate (ATP) and nicotinamide adenine dinucleotide. Glycolysis is mediated by a series of cellular enzymes, and hexokinase, phosphofructokinase and pyruvate kinase (PK) serves as key rate-limiting enzymes. The final product of glycolysis pyruvate is converted to lactic acid or acetyl-coenzyme A (CoA) for utilization in tricarboxylic acid cycle by mitochondria, which produces energy precursors for oxidative phosphorylation (Figure 1).
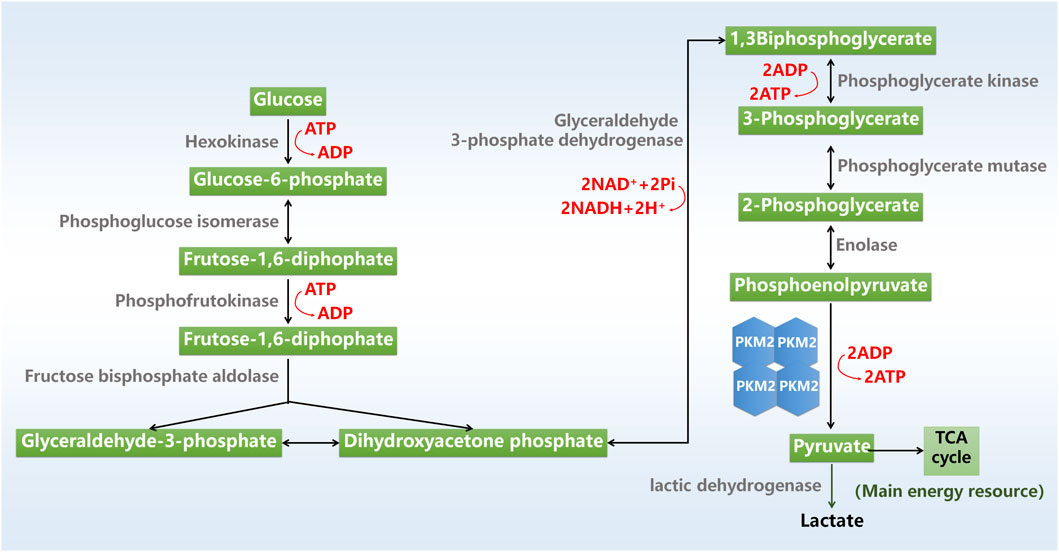
Figure 1. Glycolysis process. ADP, adenosine triphosphate; ATP, adenosine triphosphate; NAD, nicotinamide adenine dinucleotide; TCA cycle, tricarboxylic acid cycle.
PK mediates its substrate phosphoenolpyruvate (PEP) to pyruvate. PK has four different subtypes including L, R, M1, and M2 (Zhang et al., 2019). PKL isoforms are mainly distributed in liver, pancreas and kidney, and PKR is distributed in erythrocytes (Puckett et al., 2021). PKM1 are predominantly expressed in muscle, mature spermatozoa and central nervous system, while PKM2 is expressed in brain, kidney, lung and spleen (Alquraishi et al., 2019; Puckett et al., 2021). PKM2 is the dominant form of PK in kidney tissue (Alquraishi et al., 2019), and a lot of researches have revealed that regulating PKM2 affects kidney disease progression and treatment (Li et al., 2020a; Chen et al., 2023; Xie et al., 2023), highlighting the important role of PKM2 in kidney disease.
In this review, we describe some important research progresses of PKM2 in recent 5 years, from its structure and post-translational modifications to its role in kidney, and introduce the potential intervention effects of PKM2 agonists and antagonists. We further present clinical application of PKM2 in kidney disease, with the goal of highlighting the therapeutic potential of PKM2 in kidney disease. Finally, we discuss the major opportunities and obstacles of PKM2 in kidney disease to facilitate the clinical treatment.
2 PKM2 structure and its post-translational modifications
2.1 PKM2 structure
Human PKM2 protein consists of 531 amino acids that is subdivided as N (43aa), A (244aa), B (102aa) and C (142aa) domains according to their characteristic features (Figure 2A). The catalytic active site locates at the adjoining region between the A1 and B domains, while the intersubunit contact domain (ISCD) locates at the adjoining region between the A2 and C domains. Nuclear localization signal sequence and the binding site of the allosteric activator (FBP) locate at C domain. The A domain mediates the subunits interaction to form a dimer and functions as the core of monomer, while the formation of the tetramer form of PKM2 is assembled through the binding of two dimers’ C-subunits in ISCD.
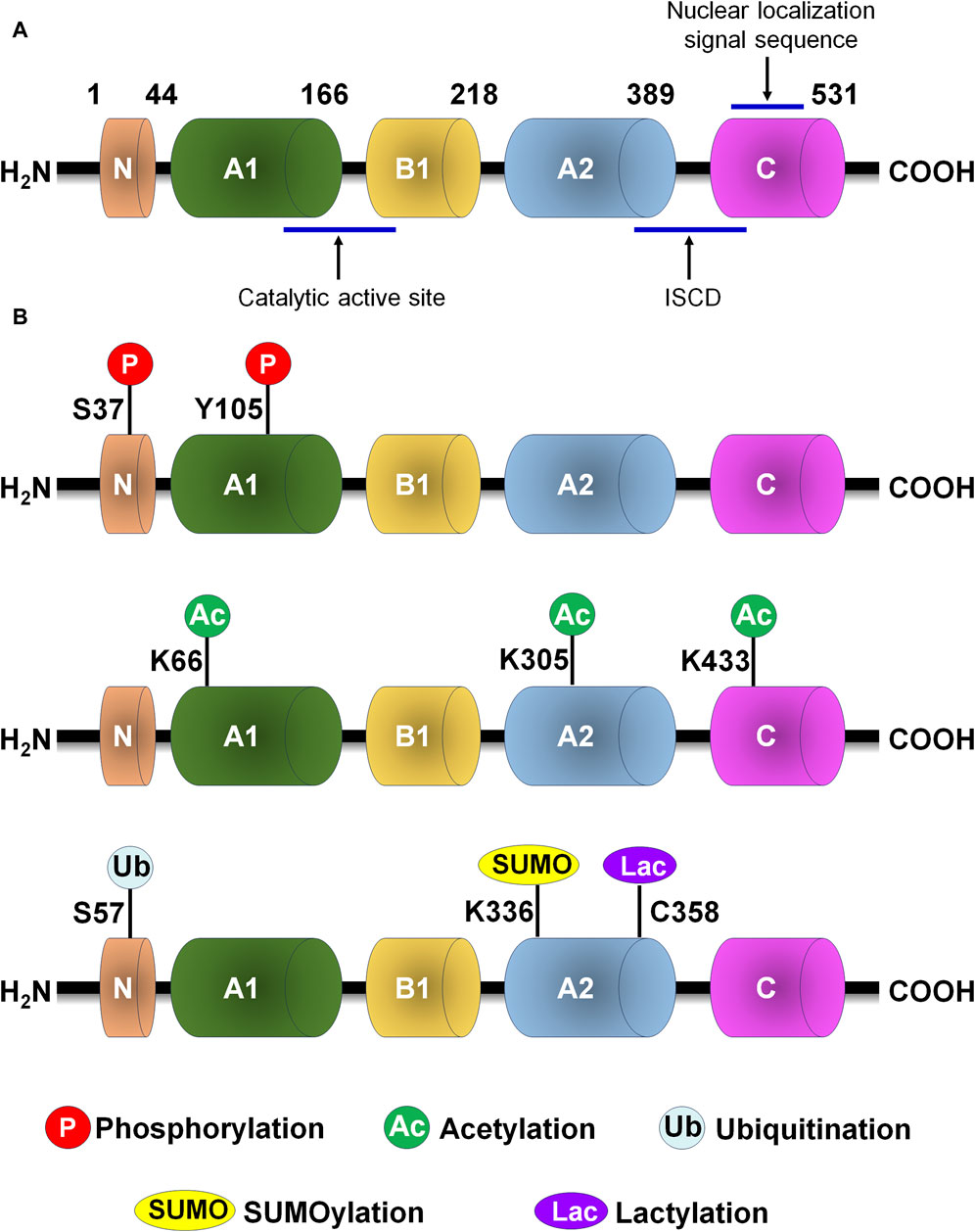
Figure 2. PKM2 protein structure and post-translational modification of PKM2. (A) PKM2 protein structure. (B) The major post-translational modification sites of PKM2, including phosphorylation, acetylation, ubiquitination, SUMOylation and lactylation. C, cysteine; ISCD, intersubunit contact domain; K, lysine; S, serine; Y, tyrosine.
The enzyme activity of PKM2 is affected by its oligomeric state. PKM2 has four different enzymatic states: an inactive monomer, a nearly inactive dimer, an inactive T state tetramer, and an active R state tetramer (Prakasam et al., 2018; Alquraishi et al., 2019). The tetrameric form has a high PEP affinity, while the dimeric form has a low affinity for PEP and is almost inactive under physiological conditions.
2.2 PKM2 post-translational modification
Numerous conserved post-translational modification sites exist in PKM2 protein, including phosphorylation, acetylation, methylation, SUMOylation and oxidation (Figure 2B; Table 1). Post-translational modification of PKM2 determines its structural and functional properties, including oligomeric state, catalytic activity, protein stability, binding of allosteric activators, conditional protein interaction, and subcellular localization, which influences disease progression and therapeutic effect.
The Tyr105 and Ser37 are the common phosphorylation site of PKM2 phosphorylation, which mainly control PKM2 active tetramer formation and nuclear translocation. CoA binds to PKM2 to block its Tyr105 phosphorylation and nuclear translocation, resulting in reduced glycolysis in Th17 cell. These data indicate that targeting Th17 cell metabolic reprograming via PKM2 functions as a potential therapeutic intervention for Th17 cell-associated autoimmune diseases (Chen et al., 2022). Annexin A5 has the ability to switch metabolic reprogramming from glycolysis to oxidative phosphorylation in activated macrophages by modulating PKM2 post-translational modification. Mechanistically, Annexin A5 directly interacts with PKM2 and further reduces Tyr105 phosphorylation to facilitate tetramer formation in macrophage polarization (Xu et al., 2020). The phosphorylation of PKM2 at Tyr105 promotes its dimer formation and translocation into the mitochondria after treatment with staurosporine or cisplatin in acute kidney injury (AKI). Mitochondrial PKM2 binds myosin heavy chain 9 to facilitate dynamin-related protein 1-mediated mitochondrial fragmentation, and the loss of PKM2 attenuates mitochondrial fragmentation and suppresses renal tubular injury and cell death (Xie et al., 2023). Immunoglobulin superfamily 11 suppresses PKM2 activity through promoting PKM2 phosphorylation at Tyr105 to modulate osteoclast differentiation. Modulating PKM2 activity is considered as a metabolic switch that is necessary for optimal osteoclast maturation (Kim et al., 2023). The phosphorylation of PKM2 at Tyr105 and Ser37 can be activated by an allosteric activator, TEPP-46, results in PKM2 tetramerization and inhibits its nuclear translocation to prevent glycolysis, which reduces CD4+ T cell pathogenicity and inhibits autoimmunity (Angiari et al., 2020). PKM2 phosphorylation involves in aggressive breast cancer cell phenotypes, and PKM2 phosphorylation at Ser37 functions as an effective therapeutic target for triple-negative breast cancer treatment (Apostolidi et al., 2021). Follistatin-like protein 1 (FSTL1), a secreted glycoprotein, could directly bound to PKM2 through its FK domain. FSTL1 facilitates PKM2 phosphorylation and nuclear translocation and inhibits PKM2 ubiquitination to enhance PKM2-dependent glycolysis and M1 polarization to promote liver fibrosis (Rao et al., 2022). In addition, succinate stimulates PKM2 dimerization that further translocate into the nucleus and mitochondria to accelerate fibroblast activation and apoptosis resistance in heart (Wang et al., 2023).
The acetylation of PKM2 occurs in Lys433, Lys305, and Lys66, and this process can be reversed by the deacetylase. JNK induces the acetylation of PKM2 at Lys433, resulting in PKM2 detetramerization and nuclear translocation during dendritic cell activation through modulating glycolysis and fatty acid synthesis (Jin et al., 2020). Testes-specific protease 50 (TSP50) maintains the low activity of PKM2 to control aerobic glycolysis in heaptocellular carcinoma (HCC) cells. Mechanistically, TSP50 enhances the acetylation of PKM2 at Lys433, while the acetyl-insensitive mutation of PKM2 K433R obviously counteracts TSP50-induced aerobic glycolysis (Gao et al., 2021). Phosphoserine aminotransferase 1 (PSAT1) accumulates nuclear PKM2 translocation to facilitate lung cancer cell migration, while the acetyl-mimetic mutant of PKM2 (K433Q) affected PSAT1-mediated cell migration (Biyik-Sit et al., 2021). Class IIa histone deacetylase HDAC7 mediates the deacetylation of PKM2 at Lys433 to aggravate its proinflammatory property (Das Gupta et al., 2020). Sirt3 mediates the deacetylation of PKM2 at Lys433 in A549 cells, which process significantly alleviates apoptosis against lung ischemia/reperfusion injury (Zhao et al., 2022). Phosphoglycerate dehydrogenase (PHGDH) increases the stability and activity of PKM2 through interacting with PKM2 to facilitate Lys433 acetylation and prevent Lys305 acetylation, which leading to PKM2 nuclear translocation and ultimately prevents premature senescence (Wu et al., 2023). A common environmental carcinogen, 1,4-benzoquinone, results in the acetylation of PKM2 at Lys66 that contributes to the upregulation of PKM2. However, the acetyltransferase general control non-derepressible 5 could reverse the acetylation of PKM2 at Lys66 (Zhang et al., 2022). Besides, Sirt2 mediates PKM2 deacetylation, which is the potential therapeutic approach for psoriasis therapy (Hao et al., 2021).
The SUMOylation often occurs in lysine residue in PKM2. Small ubiquitin-like modifier-activating (SUMO-activating) enzyme 1/ubiquitin like modifier activating enzyme 2 mediates the SUMOylation of PKM2 that facilitates PKM2 phosphorylation and nuclear translocation and reduces enzymatic activity (Wang et al., 2020). HCC-derived ectosomal PKM2 induced metabolic reprogramming in monocytes to accelerate HCC progression. In HCC cells, the SUMOylation of PKM2 facilitates its plasma membrane targeting and subsequent ectosomal excretion. Ectosomal PKM2 is clearly detected in the plasma of HCC patients and functions as a potential diagnostic marker for HCC (Hou et al., 2020). The SUMOylation of PKM2 at Lys270 induces PKM2 from tetrameric to dimeric formation, and nuclear translocation. The replacement of wild type PKM2 to a SUMOylation-deficient mutant (K270R) weakens PKM2 effect on RUNX1 in leukemia cells (Xia et al., 2021). The knockdown of small ubiquitin-like modifier 1 (SUMO1) causes the downregulation of PKM2 protein in A549 cells. SUMO1 directly mediates the SUMO1 modification of PKM2 at Lys336 that enhances glycolysis and cell proliferation (An et al., 2018). The activated guanosine triphosphate binding protein 4 (GTPBP4) promotes PKM2 SUMOylation via facilitating SUMO1 protein activation and functioning as a linker between SUMO1 and PKM2 protein, which process accelerates aerobic glycolysis in HCC (Zhou et al., 2022).
The ubiquitination and deubiquitination of PKM2 are reported in plenty of researches. DExD-box helicase 39B (DDX39B) directly interacts with PKM2 and stabilizes PKM2 by competitively suppressing STIP1 homology and U-box-containing protein 1 (STUB1)-mediated PKM2 ubiquitination and degradation. DDX39B accelerates the nuclear translocation of PKM2 to promote the activation of oncogenes and glycolysis-related genes, which process is independent of ERK1/2-mediated phosphorylation of PKM2 at Ser37 (Zhao et al., 2022). CNRIP1 overexpression activates Parkin (an E3 ubiquitin ligase), and triggers the protein degradation of PKM2 in intrahepatic cholangiocarcinoma cells (Chen et al., 2021). In addition, the deubiquitinating enzyme, herpesvirus-associated ubiquitin-specific protease (HAUSP), interacts with PKM2 at Ser57 to deubiquitinate PKM2, while HAUSP knockdown increases PKM2 ubiquitination (Choi et al., 2020). OTUB2, an OTU deubiquitinase, directly interacts with PKM2 and suppresses its ubiquitination via weakening the interaction of PKM2 and Parkin to increase PKM2 activity and glycolysis in colorectal cancer (Yu et al., 2022).
Additionally, the lactylation of PKM2 is found in macrophages, and Lys62 site is responsible for PKM2 lactylation to inhibit inflammatory metabolic adaptation. Lactate facilitates PKM2 lactylation that delays the transition from tetramer to dimer, and increase its enzymatic activity and suppress nuclear distribution (Wang et al., 2022). O-GlcNAc transferase mediates PKM2 O-GlcNAcylation to suppress PKM2 catalytic activity that increases aerobic glycolysis and tumor growth (Singh et al., 2020). The S-nitrosylation of PKM2 by JSD26 intraperitoneal injection protects against AKI in mice (Zhou et al., 2023). The modification at Cys358 by acrolein leads to PKM2 inactivation and aberrant glycolysis to promote renal fibrosis progression in high-fat diet-streptozotocin-induced diabetic kidney disease (DKD) mice. Treatment with acrolein scavengers (hydralazine and carnosine) obviously attenuates PKM2 activity and renal fibrosis (Kuo et al., 2023). Inhibiting neddylation modification by MLN4924 treatment induces tetramerization and activates PKM2 to increase glycolysis against cancer cell growth (Zhou et al., 2019). These data demonstrate that targeting post-translational modification of PKM2 is a promising therapeutic approach to treat renal and various diseases.
3 The role of PKM2 in kidney disease
3.1 Metabolism regulation
PKM2 plays a vital role in regulating the glycolytic reprogramming in multiple renal cells and various kidney diseases (Figure 3). In tubular epithelial cell, the phosphorylation of PKM2 at Tyr105 promotes its dimer formation and translocation into the mitochondria after treatment with staurosporine or cisplatin in AKI. Hsp90 triggers the activation of PKM2-Akt signaling pathway to exhibit antiapoptotic effect against heat-stress injury in AKI (Chen et al., 2020). Mitochondrial PKM2 binds myosin heavy chain 9 to facilitate dynamin-related protein 1-mediated mitochondrial fragmentation, and the loss of PKM2 attenuates mitochondrial fragmentation and suppresses renal tubular injury and cell death by cisplatin (Xie et al., 2023). PKM2 interacts with mitofusin 2 to enhance mitochondrial fusion and oxidative phosphorylation, and suppress glycolysis (Li et al., 2019). Metabolomic study reveals that in cisplatin-induced normal kidney tubular epithelial NRK-52E cells, the excretion of PKM2 is significantly elevated in the media (Kim et al., 2022). PKM2 serves as the direct target of miR-144-5p. The long non-coding RNA (lncRNA) Opa-interacting protein 5 antisense RNA 1 targets miR-144-5p/PKM2 axis to attenuate the apoptosis of renal epithelial cells induced by cisplatin (Chang et al., 2022). Additionally, the activation of ERK modulated glycolysis and resulted in the reduced reserve respiratory capacity during cisplatin treatment (Suman et al., 2024).
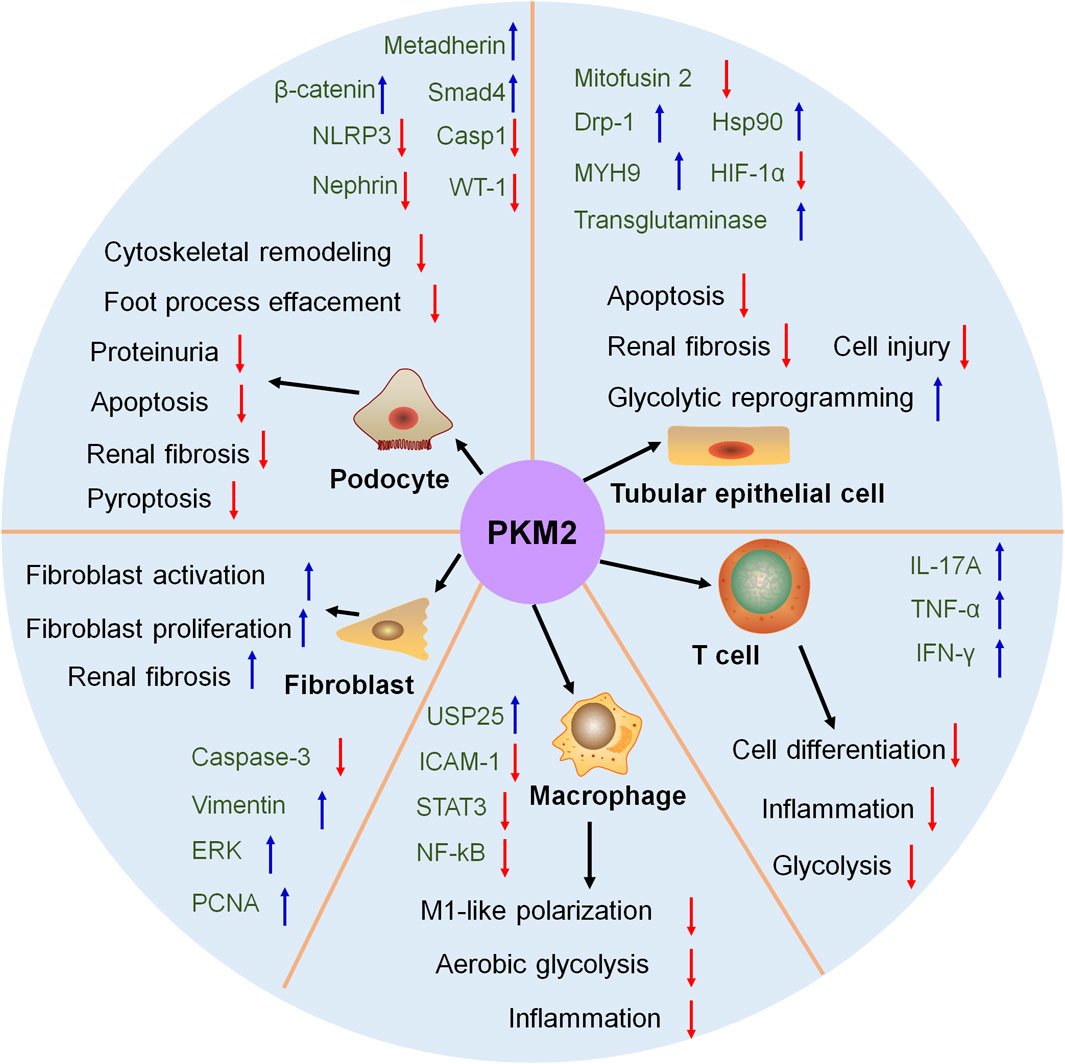
Figure 3. The role of PKM2 in kidney. In tubular epithelial cell, podocyte, fibroblast, macrophage and T cell, PKM2 participate in kidney disease and treatment. Drp-1, dynamin-related protein-1; ERK, extracellular regulated protein kinase; HIF-1α, hypoxia inducible factor-1; HSP90, heat shock protein 90; ICAM-1, intercellular cell adhesion molecule-1; IFN-γ, interferon gamma; IL-17A, interleukin-17A; MYH9, myosin heavy chain 9; NF-κB, nuclear factor kappa-B; NLPR3, NOD-like receptor thermal protein domain associated protein 3; PCNA, proliferating cell nuclear antigen; STAT3, signal transducer and activator of transcription 3; TNF-α, tumor necrosis factor-α; USP25, ubiquitin specific peptidase 25; WT-1, Wilm’s tumor-1.
PKM2 is significantly increased in glomeruli of patients with DKD and corelated with estimated glomerular filtration rate, which involves in preserving kidney function. The elevated PKM2 in circulation functions as biomarkers in DKD (Gordin et al., 2019). PKM2 protein is highly detected in the urine of DKD patients but not found in the urine of normal subjects. PKM2 is identified as the new biomarker for the early diagnosis of DKD (Park et al., 2023). DKD process is accompanied with Sirt3 suppression and PKM2 dimer formation. Sirt3 deficiency-induced abnormal glycolysis facilitates renal fibrosis (Srivastava et al., 2018). Sodium glucose cotransporter 2 inhibitor, empagliflozin, induces PKM2 dimer formation to normalize aberrant glycolysis, and eventually exhibits renal protection against DKD in proximal tubules (Li et al., 2020a). Besides, in the present of aminylation, tissue transglutaminase modulates glycolysis in normal and indoxyl sulfate-induced endothelial cell injury via activating PKM2 (Lin et al., 2023).
3.2 Podocyte injury
PKM2 is identified as a vital metabolic regulator for podocyte development (Figure 3). PKM2 is downregulated in podocytes from kidney biopsies of patients with DKD and hypertensive nephropathy (Luo et al., 2022; Chen et al., 2023). The injured glycolysis in podocytes enhances ornithine catabolism under diabetic conditions, and podocyte-specific loss of PKM2 aggravates ornithine catabolism to modulate cytoskeletal remodeling in podocytes in DKD (Luo et al., 2022). Podocyte-specific deletion of PKM2 in mice promotes angiotensin II-induced glomerular and podocyte injury with foot process effacement and proteinuria. Mechanistically, angiotensin II-induced glycolysis impairment aggravates an insufficient energy supply to the foot process and leads to podocyte injury (Chen et al., 2023). Podocyte-specific overexpression of PKM2 exhibits potent therapeutic effects on albumin/creatinine ratio, mesangial expansion, basement membrane thickness, and podocyte foot process effacement in streptozotocin-induced DKD mice (Fu et al., 2022). Podocyte-specific deletion of Smad4 alleviates DKD. Mechanistically, hyperglycaemia causes Smad4 localization to mitochondria in podocytes, and Smad4 directly binds to PKM2 and reduces the formation of active tetrameric form to decrease glycolysis (Li et al., 2020b).
In adriamycin-induced mice, podocyte-specific deletion of PKM2 exhibits limited energy metabolism that induces cell differentiation defects. Podocyte-specific knockout of PKM2 in mice worsens albuminuria and podocyte injury in adriamycin-induced mice (Yuan et al., 2020). Metadherin triggers the deaggregation of PKM2 tetramers and facilitates PKM2 monomers to enter the nucleus in podocyte to accelerate podocyte injury and proteinuria. Podocyte-specific knockout of metadherin attenuates proteinuria, podocyte injury and glomerulosclerosis after advanced oxidation protein products challenge or in adriamycin-induced mice (Chen et al., 2023).
In AKI, podocyte-specific deletion of PKM2 alleviates LPS-induced inflammation and apoptosis via the activation of β-catenin and the loss of Wilms' Tumor 1 and nephrin. These data reveals PKM2 as a promising therapeutic target for AKI (Alquraishi et al., 2022). The elevated PKM2 and increased glycolysis is detected during renal fibrosis. These contribute to hypoxic and acidic environment, and eventually suppress podocyte proliferation and differentiation and accelerate renal interstitial fibrosis. These results elicit that increased glycolysis-induced energy metabolism recodification influences podocyte number and function and aggravates fibrosis (Li et al., 2018).
PKM2 also participate in podocyte death. Podocyte-specific deletion of PKM2 contributes to glomerular and podocyte injury that involves in reduced glycolysis, cytoskeletal remodeling and podocyte apoptosis (Chen et al., 2023). The inhibition of dihydroxyacetone phosphate exhibits protective property on podocyte pyroptosis via downregulating PKM2 expression (Zhang et al., 2023).
3.3 Fibroblast activation and proliferation
Fibroblast-specific loss of PKM2 inhibits fibroblast proliferation and triggers tubular epithelial cell death during AKI process (Figure 3). PKM2-mediated fibroblast proliferation activates pro-survival signals to reduce tubular cell death (Ye et al., 2021). Overexpression of PKM2 triggers fibroblast activation and renal interstitial fibrosis that is accompanied by elevated glycometabolism, which elicits the important role of metabolic reprogramming in renal interstitial fibrosis (Yin et al., 2018). The knock-out of a multifunctional E3 ubiquitin-protein ligase, WWP2, facilitates myofibroblast proliferation and suppresses its activation. The loss of WWP2 sacrifices glycolysis and boosts mitochondrial respiration to activate fatty acid oxidation and the pentose phosphate pathway, which is the promising therapeutic target for renal fibrosis (Chen et al., 2024).
3.4 Macrophage polarization
Ubiquitin-specific protease 25 (USP25)-PKM2-aerobic glycolysis axis positively modulates M1-like polarization and accelerates ischemic AKI in mice, indicating potential therapeutic targets for AKI treatment (Figure 3). Mechanistically, USP25 controls aerobic glycolysis and lactate production during M1-like polarization via PKM2 (Yang et al., 2023). Besides, the regulation of PKM2 modulates inflammation by phosphorylating STAT3 and NF-κB in DKD that delays the differentiation of macrophages to M1 cells, and the downregulation of phosphorylated PKM2 is beneficial for DKD treatment (Li et al., 2020).
3.5 T cell regulation
Even few researches report the effect of PKM2 on T cell regulation in the kidney, the regulatory role of PKM2 on T cell regulation is confirmed in other tissues (Figure 3). PKM2 participates in T cell biology, and the upregulation, phosphorylation and nuclear accumulation of PKM2 is observed in CD4+ T cells. The activation of PKM2 by TEPP-46 treatment restricts T helper 17 and T helper 1 cells development and alleviates experimental autoimmune encephalomyelitis (Angiari et al., 2020). PRAK-Nrf2-mediated antioxidant signaling is a metabolic checkpoint that controls Th17 cell glycolysis and differentiation via restoring PKM2 phosphorylation to exhibit potent Th17 cell antitumor immunity (Zhao et al., 2023). Specific deletion of PKM2 in inflammatory hepatic CXCR3+ Th17 cells has ability to reverse inflammatory vigor and non-alcoholic fatty liver disease severity (Moreno-Fernandez et al., 2021). PKM2 is increased in infiltrated T lymphocytes of vascular lesions. Extracellular vesicles (EVs) from PKM2-activated T lymphocyte triggers abdominal aortic aneurysm progression through facilitating macrophage redox imbalance and migration (Dang et al., 2022). These data confirm the regulatory effect of PKM2 on T cell.
4 The activators and inhibitors of PKM2 in kidney disease
4.1 Activators
TEPP-46, a small-molecule PKM2 activator, leads to PKM2 tetramer formation through enhancing PKM2 subunit interactions and increasing PKM2 enzymatic activity (Table 2). Several studies reveal the protective role of TEPP-46 in DKD. The PKM2 activator TEPP-46 increases the glycolytic activity and lactate production in the kidney against DKD, suggesting targeting PKM2 as a promising therapeutic target for DKD treatment (Bertelsen et al., 2021). PKM2 activator TEPP-46 that promotes tetramerization enhances the interaction of endocytic trafficking through the versatile networks of Hsp70s and rewrites the crosstalk of EGFR signal transduction circuits and metabolic stress to promote resilience in DKD (Wang et al., 2022). PKM2 activation by TEPP-46 treatment improves tubular phenotype through suppressing the epithelial-mesenchymal transition program and normalizing aberrant glycolysis in high glucose-induced renal tubular epithelial cell and DKD model (Liu et al., 2021).
DASA-58 is able to induce PKM2 activation by promoting PKM2 tetramerization and reducing lactate secretion (Table 2). Pharmacological activation of PKM2 by DASA-58 partially counters glycolysis and inflammation during liver fibrosis process (Rao et al., 2022). DASA-58 treatment facilitates the proliferation of vascular resident endothelial progenitor cell and enhances PKM2 activity via promoting glycolysis and mitochondrial fusion (Ren et al., 2020). Besides, treatment with TEPP-46 or DASA-58 obviously hinders IL-17-producing T helper cell 17 development against multiple sclerosis (Seki et al., 2020). Even if few studies report the effect of DASA-58 in kidney disease, the above-mentioned data provide a promising prospect for DASA-58 in kidney disease treatment.
Mitapivat, also called AG-348, is the first-line oral small molecule allosteric activator of PK (Table 2). Several clinical trials have confirmed the beneficial role of mitapivat in pyruvate kinase deficiency (PKD) treatment, and mitapivat has been granted orphan drug designation by the FDA for PKD. A phase 3, randomized, placebo-controlled clinical trial demonstrates that mitapivat substantially elevates hemoglobin level, reduces hemolysis, and improves patient-reported outcomes in patients with PKD (Al-Samkari et al., 2022). Another multicentre, open-label, single-arm, phase 3 trial shows that mitapivat functions as a novel therapy and becomes first disease-modifying agent for PKD that alleviates transfusion burden in adult patients who accepts regular transfusions (Glenthøj et al., 2022). Besides, an open-label, multicentre, phase 2 study reveals that mitapivat also exhibits potent therapeutic effect against α-thalassaemia and β-thalassaemia, and haemoglobin concentration significantly increases after treatment (Kuo et al., 2022).
TP-1454, derived from SGI-9380, enhances the bind of two dimer PKM2 as a glue of monomer PKM2 (Xu et al., 2014). TP-1454 is a potent PKM2 activator and has enter clinical trials as the first oral PKM2 activator for advanced solid tumors treatment (Weagel et al., 2022). In addition, PKM2 is identified as a major celastrol-bound protein, and celastrol binds to the residue Cys31 and further alters the spatial conformation to reduce the enzyme activity of PKM2 in hepatic macrophages against non-alcoholic fatty liver disease (Fan et al., 2022).
PKM2 is also the therapeutic target of traditional medicine against kidney disease. Modified Hu-lu-ba-wan exhibits beneficial effect on DKD patients and animal model through facilitating mitochondrial dynamic homeostasis. The one of its components, berberine, has a high affinity with PKM2, and prevent apoptosis by enhancing PKM2-mediated mitochondrial dynamic homeostasis (Gong et al., 2023).
4.2 Inhibitors
Shikonin and alkannin, enantiomeric pair of pigments derived from Lithospermum erythrorhizon roots, are identified as potent and highly selective PKM2 inhibitor (Table 2). The protective role of shikonin has been confirmed in kidney diseases (Wei et al., 2019; Wu et al., 2021; Peng et al., 2022). The inhibition of PKM2 by shikonin notably suppresses the expression of HIF-1α and apoptosis-related factors such as BNIP3, Bax, and caspase-3, while the inhibition of PKM2 by shikonin significantly improves the histopathological symptoms of LPS-induced AKI. The inhibition of PKM2 by shikonin significantly ameliorates the histopathological symptoms in LPS-induced AKI mice and inhibits apoptosis via downregulating BNIP3, Bax, and caspase-3 (Wu et al., 2021). Shikonin inhibits renal oxidative stress, inflammatory and tubular epithelial cell apoptosis through regulating NOX4/PTEN/AKT pathway against sepsis-induced AKI rat model (Peng et al., 2022). The blockade of glycolysis by shikonin obviously attenuates renal fibrosis through modulating PKM2 in unilateral ureteral obstruction mice (Wei et al., 2019). Shikonin effectively controls mitochondrial membrane potential to exhibit renal protection in high glucose-induced NRK-52E cells (Tong et al., 2018). Shikonin suppresses DKD progression and gentamicin-induced renal injury through inhibiting renal oxidative stress and inflammation (Balaha et al., 2023; Zhu et al., 2023). In addition, PKM2 antagonist (shikonin) and its allosteric agent (TEPP-46) substantially attenuates liver fibrosis by modulating PKM2-mediated aerobic glycolysis (Zheng et al., 2020). As for alkannin, few studies investigate its renal protection, but alkannin exhibits potent therapeutic property on Alzheimer’s disease and anti-cancer treatment (Chang et al., 2020; Hosoi et al., 2023).
The selectivity of compound 3k is higher than shikonin for PKM2 (Gao et al., 2022). Compound 3k triggers PKM2 tetramer disruption to delay fibrosis progression in idiopathic pulmonary fibrosis (Gao et al., 2022). Treatment with compound 3k hinders glycolysis and reprograms metabolism against ovarian cancer (Park et al., 2021). Besides, comparing with compound 3k, compound 3h has a higher affinity with PKM2. Treatment with compound 3h hinders glycolytic pathways to attenuate apoptotic and autophagic cell death against prostate cancer cells (Jiang et al., 2022).
PKM2 severs as a potential therapeutic target for natural product and antisense against kidney diseases. Microcystin-RR directly binds to PKM2 and suppresses phosphorylated PKM2 at Lys105 to impair PKM2-HIF-1α pathway against chronic kidney disease (CKD) (Ren et al., 2022). The aquatic extract of Dendropanax morbifera alleviates PKM2 expression against DKD and renal fibrosis via ameliorating oxidative stress and inflammation (Sachan et al., 2020). Huangqi-Danshen decoction, a Chinese herbal preparation, attenuates PKM2 expression to reshape glucose metabolism profiles against adenine-induced CKD (Huang et al., 2023). Qian Yang Yu Yin granule suppresses PKM2 expression in hypertensive nephropathy rat model and modulates metabolic reprogramming via HIF-1α/PKM2 positive feedback loop (Qian et al., 2021). Tianshu capsule alleviates serum PKM2 expression to normalize energy metabolism in spontaneously hypertensive rat model (Gao et al., 2019). Besides, natural antisense lncRNA ARAP1 knock-down suppresses dimeric PKM2 expression and restores tetrameric PKM2 formation to normalize aberrant glycolysis and fibrosis in DKD models (Li et al., 2023).
5 Conclusion and perspectives
Glycolysis is the key energy resource for kidney, and PKM2 plays a vital role in glycolysis. PKM2 is not only the rate-limiting enzyme that mediates PEP to pyruvate, also functions as a co-transcription factor that triggers the upregulation of glycolysis-related genes including MYC, SLC2A1, LDHA and PDK1 (Li et al., 2023). PKM2 monomer has 531 amino acids and owns four domains (N, A, B and C). The inactive monomer, nearly inactive dimer, inactive T state tetramer, and active R state tetramer are four states of PKM2 protein. The post-translational modification of PKM2 modulates its enzymatic activity and nuclear localization. PKM2 phosphorylation at Tyr105 and Ser37 inhibits active tetramer formation and induces nuclear translocation, and the acetylation of PKM2 at Lys433, Lys305 and Lys66 also influences its enzymatic activity and nuclear translocation. The SUMOylation at Lys270 triggers PKM2 dimeric formation and nuclear translocation, while the SUMOylation at Lys336 enhances PKM2 enzymatic activity. The deubiquitination of PKM2 at Ser57 by HAUSP protects PKM2 from degradation. Besides, the lactylation and the modification at Cys358 by acrolein also affects PKM2 enzymatic activity to regulate glycolysis. These data indicates that the post-translational modification of PKM2 is responsible for PKM2 enzymatic activity and nuclear translocation that is the promising therapeutic target.
Emerging evidences show that the aberrant glycolysis contributes to kidney disease progression accompanying PKM2 dysfunction. PKM2 participates in kidney disease and treatment through modulating metabolism regulation, podocyte injury, fibroblast activation and proliferation, macrophage polarization, and T cell regulation. Notably, the expression and enzymatic activity of PKM2 is substantially disturbed in kidney and targeting PKM2 has been proved a promising therapeutic target, especially in DKD and AKI that is consistent with previous study (Rabelink and Carmeliet, 2018). Notably, DKD and AKI accompany with the accumulation of glycolytic intermediate products (Rabelink and Carmeliet, 2018). The obvious elevation of PKM2 is observed in glomeruli of patients with DKD (Gordin et al., 2019), and the knock-down of PKM2 in podocyte aggravates glomerular injury and albuminuria in DKD mice (Qi et al., 2017). In AKI, PKM2 severs as a novel biomarker for early detection (Cheon et al., 2016), and the deletion of PKM2 in several kidney cells is beneficial in AKI. The deletion of PKM2 in podocyte attenuates AKI, and the deletion of PKM2 in fibroblast triggers fibroblast activation and AKI progression. Besides, PKM2 also controls macrophage polarization to participate in AKI. The possible reason that PKM2 is more important in DKD and AKI than other kidney diseases is that DKD and AKI suffer aberrant glycolysis and energy metabolism more during their progression. These results reveal that targeting PKM2 is emerging as a promising therapeutic approach for DKD and AKI treatment.
Interestingly, both PKM2 activator TEPP-46 and PKM2 inhibitor shikonin exhibits protective effect on kidney diseases, suggesting the importance of PKM2 during kidney disease progression. Importantly, these results remind us that the deep investigation of PKM2 pleiotropic effects are urgent before the application in clinical. The novel positron emission tomography [18F]DASA-23 helps to determine PKM2 level in human to visualize intracranial malignancies that is a useful tool to explore of PKM2 pleiotropic effects in kidney (Beinat et al., 2020). Both the regulation of PKM2 post-translational modification and pharmacological modulation of PKM2 is the promising approach to control PKM2 activity and kidney disease progression. PKM2 activator (TEPP-46, DASA-58, mitapivat and TP-1454) and PKM2 inhibitor (shikonin, alkannin, compound 3k and compound 3h) have exhibited potential therapeutic property in kidney disease, which provides candidates for kidney disease in further pre-clinical and clinical investigation. Another limitation that hinders PKM2 as a therapeutic target is restricted clinical trials in kidney disease and other disease. Clinical trials demonstrates that PKM2 is the potential biomarker in inflammatory bowel disease and Crohn’s disease (Almousa et al., 2018), and elevated plasma PKM2 is associated with poorer prognosis of pancreatic and peri-ampullary cancer in clinical (Bandara et al., 2018). The increased PKM2 expression are more frequent in cirrhotic liver than non-cirrhotic liver, and is associated with poor survival rates in both cirrhotic liver and non-cirrhotic liver (Liu et al., 2017). Although the clinical and related renal studies referring PKM2 are limited, the promising potential of PKM2 has been verified, which provides the candidates for kidney disease treatment.
Overall, PKM2 is the promising therapeutic target for kidney disease. The deep investigation of PKM2 pleiotropic effects in kidney and clinical trial are urgently needed to verify the therapeutic effect of PKM2 activator/inhibitor to benefit patients.
Author contributions
D-QC: Funding acquisition, Writing-original draft, Writing-review and editing. JH: Writing-review and editing. HL: Writing-review and editing. KF: Writing-review and editing. PL: Writing-review and editing.
Funding
The author(s) declare that financial support was received for the research, authorship, and/or publication of this article. This study was supported by the National Natural Science Foundation of China (Grant No. 82104511), Young Elite Scientists Sponsorship Program by China Association for Science and Technology (Grant No. YESS20230162), Shaanxi Provincial Natural Science Basic Research Program (Grant No. 2024JC-YBQN-0875), Yantai School-Government Integration Development Project (2023XDRHXMXK08), and Joint Project by National Division and Provincial Bureau of TCM (GZY-KJS-SD-2023-052).
Conflict of interest
The authors declare that the research was conducted in the absence of any commercial or financial relationships that could be construed as a potential conflict of interest.
Publisher’s note
All claims expressed in this article are solely those of the authors and do not necessarily represent those of their affiliated organizations, or those of the publisher, the editors and the reviewers. Any product that may be evaluated in this article, or claim that may be made by its manufacturer, is not guaranteed or endorsed by the publisher.
References
Almousa, A. A., Morris, M., Fowler, S., Jones, J., and Alcorn, J. (2018). Elevation of serum pyruvate kinase M2 (PKM2) in IBD and its relationship to IBD indices. Clin. Biochem. 53, 19–24. doi:10.1016/j.clinbiochem.2017.12.007
Alquraishi, M., Chahed, S., Alani, D., Puckett, D. L., Dowker, P. D., Hubbard, K., et al. (2022). Podocyte specific deletion of PKM2 ameliorates LPS-induced podocyte injury through beta-catenin. Cell Commun. 20 (1), 76. doi:10.1186/s12964-022-00884-6
Alquraishi, M., Puckett, D. L., Alani, D. S., Humidat, A. S., Frankel, V. D., Donohoe, D. R., et al. (2019). Pyruvate kinase M2: a simple molecule with complex functions. Free Radic. Biol. Med. 143, 176–192. doi:10.1016/j.freeradbiomed.2019.08.007
Al-Samkari, H., Galactéros, F., Glenthøj, A., Rothman, J. A., Andres, O., Grace, R. F., et al. (2022). Mitapivat versus placebo for pyruvate kinase deficiency. N. Engl. J. Med. 386 (15), 1432–1442. doi:10.1056/NEJMoa2116634
An, S., Huang, L., Miao, P., Shi, L., Shen, M., Zhao, X., et al. (2018). Small ubiquitin-like modifier 1 modification of pyruvate kinase M2 promotes aerobic glycolysis and cell proliferation in A549 human lung cancer cells. Onco. Targets Ther. 11, 2097–2109. doi:10.2147/ott.S156918
Angiari, S., Runtsch, M. C., Sutton, C. E., Palsson-McDermott, E. M., Kelly, B., Rana, N., et al. (2020). Pharmacological activation of pyruvate kinase M2 Inhibits CD4(+) T cell pathogenicity and suppresses autoimmunity. Cell Metab. 31 (2), 391–405. doi:10.1016/j.cmet.2019.10.015
Apostolidi, M., Vathiotis, I. A., Muthusamy, V., Gaule, P., Gassaway, B. M., Rimm, D. L., et al. (2021). Targeting pyruvate kinase M2 Phosphorylation reverses aggressive cancer phenotypes. Cancer Res. 81 (16), 4346–4359. doi:10.1158/0008-5472.Can-20-4190
Balaha, M. F., Alamer, A. A., Eisa, A. A., and Aljohani, H. M. (2023). Shikonin alleviates gentamicin-induced renal injury in rats by targeting renal endocytosis, SIRT1/Nrf2/HO-1, TLR-4/NF-κB/MAPK, and PI3K/Akt cascades. Antibiotics 12 (5), 826. doi:10.3390/antibiotics12050826
Bandara, I. A., Baltatzis, M., Sanyal, S., and Siriwardena, A. K. (2018). Evaluation of tumor M2-pyruvate kinase (Tumor M2-PK) as a biomarker for pancreatic cancer. World J. Surg. Oncol. 16 (1), 56. doi:10.1186/s12957-018-1360-3
Beinat, C., Patel, C. B., Haywood, T., Shen, B., Naya, L., Gandhi, H., et al. (2020). Human biodistribution and radiation dosimetry of [(18)F]DASA-23, a PET probe targeting pyruvate kinase M2. Eur. J. Nucl. Med. Mol. Imaging 47 (9), 2123–2130. doi:10.1007/s00259-020-04687-0
Bertelsen, L. B., Hansen, E. S. S., Sadowski, T., Ruf, S., and Laustsen, C. (2021). Hyperpolarized pyruvate to measure the influence of PKM2 activation on glucose metabolism in the healthy kidney. NMR Biomed. 34 (11), e4583. doi:10.1002/nbm.4583
Biyik-Sit, R., Kruer, T., Dougherty, S., Bradley, J. A., Wilkey, D. W., Merchant, M. L., et al. (2021). Nuclear Pyruvate kinase M2 (PKM2) contributes to phosphoserine aminotransferase 1 (PSAT1)-mediated cell migration in EGFR-Activated lung cancer cells. Cancers 13 (16), 3938. doi:10.3390/cancers13163938
Brinkkoetter, P. T., Bork, T., Salou, S., Liang, W., Mizi, A., Özel, C., et al. (2019). Anaerobic glycolysis maintains the glomerular filtration barrier independent of mitochondrial metabolism and dynamics. Cell Rep. 27 (5), 1551–1566. doi:10.1016/j.celrep.2019.04.012
Chang, M., Wang, H., Niu, J., Song, Y., and Zou, Z. (2020). Alkannin-induced oxidative DNA damage synergizes with PARP inhibition to cause cancer-specific cytotoxicity. Front. Pharmacol. 11, 610205. doi:10.3389/fphar.2020.610205
Chang, S., Chang, M., Liu, G., Xu, D., Wang, H., Sun, R., et al. (2022). LncRNA OIP5-AS1 reduces renal epithelial cell apoptosis in cisplatin-induced AKI by regulating the miR-144-5p/PKM2 axis. Biomed. J. 45 (4), 642–653. doi:10.1016/j.bj.2021.07.005
Chen, B., Yang, B., Zhu, J., Wu, J., Sha, J., Sun, J., et al. (2020). Hsp90 relieves heat stress-induced damage in mouse kidneys: involvement of antiapoptotic PKM2-AKT and autophagic HIF-1α signaling. Int. J. Mol. Sci. 21 (5), 1646. doi:10.3390/ijms21051646
Chen, C., Zhang, W., Zhou, T., Liu, Q., Han, C., Huang, Z., et al. (2022). Vitamin B5 rewires Th17 cell metabolism via impeding PKM2 nuclear translocation. Cell Rep. 41 (9), 111741. doi:10.1016/j.celrep.2022.111741
Chen, D., Wu, H., Feng, X., Chen, Y., Lv, Z., Kota, V. G., et al. (2021). DNA methylation of cannabinoid receptor interacting protein 1 promotes pathogenesis of intrahepatic cholangiocarcinoma through suppressing parkin-dependent pyruvate kinase M2 ubiquitination. Hepatology 73 (5), 1816–1835. doi:10.1002/hep.31561
Chen, H., You, R., Guo, J., Zhou, W., Chew, G., Devapragash, N., et al. (2024). WWP2 regulates kidney fibrosis and the metabolic reprogramming of profibrotic myofibroblasts. J. Am. Soc. Nephrol. doi:10.1681/asn.0000000000000328
Chen, X., Xiao, J., Tao, D., Liang, Y., Chen, S., Shen, L., et al. (2023a). Metadherin orchestrates PKA and PKM2 to activate β-catenin signaling in podocytes during proteinuric chronic kidney disease. Transl. Res. 266, 68–83. doi:10.1016/j.trsl.2023.11.006
Chen, Y., Bai, X., Chen, J., Huang, M., Hong, Q., Ouyang, Q., et al. (2023b). Pyruvate kinase M2 regulates kidney fibrosis through pericyte glycolysis during the progression from acute kidney injury to chronic kidney disease. Cell Prolif. 57, e13548. doi:10.1111/cpr.13548
Chen, Z., Zhu, Z., Liang, W., Luo, Z., Hu, J., Feng, J., et al. (2023c). Reduction of anaerobic glycolysis contributes to angiotensin II-induced podocyte injury with foot process effacement. Kidney Int. 103 (4), 735–748. doi:10.1016/j.kint.2023.01.007
Cheon, J. H., Kim, S. Y., Son, J. Y., Kang, Y. R., An, J. H., Kwon, J. H., et al. (2016). Pyruvate kinase M2: a novel biomarker for the early detection of acute kidney injury. Toxicol. Re.s 32 (1), 47–56. doi:10.5487/tr.2016.32.1.047
Choi, H. S., Pei, C. Z., Park, J. H., Kim, S. Y., Song, S. Y., Shin, G. J., et al. (2020). Protein stability of pyruvate kinase isozyme M2 is mediated by HAUSP. Cancers 12 (6), 1548. doi:10.3390/cancers12061548
Dang, G., Li, T., Yang, D., Yang, G., Du, X., Yang, J., et al. (2022). T lymphocyte-derived extracellular vesicles aggravate abdominal aortic aneurysm by promoting macrophage lipid peroxidation and migration via pyruvate kinase muscle isozyme 2. Redox Biol. 50, 102257. doi:10.1016/j.redox.2022.102257
Das Gupta, K., Shakespear, M. R., Curson, J. E. B., Murthy, A. M. V., Iyer, A., Hodson, M. P., et al. (2020). Class IIa histone deacetylases drive toll-like receptor-inducible glycolysis and macrophage inflammatory responses via pyruvate kinase M2. Cell Rep. 30 (8), 2712–2728. doi:10.1016/j.celrep.2020.02.007
Fan, N., Zhang, X., Zhao, W., Zhao, J., Luo, D., Sun, Y., et al. (2022). Covalent inhibition of pyruvate kinase M2 reprograms metabolic and inflammatory pathways in hepatic macrophages against non-alcoholic fatty liver disease. Int. J. Biol. Sci. 18 (14), 5260–5275. doi:10.7150/ijbs.73890
Fu, J., Shinjo, T., Li, Q., St-Louis, R., Park, K., Yu, M. G., et al. (2022). Regeneration of glomerular metabolism and function by podocyte pyruvate kinase M2 in diabetic nephropathy. JCI Insight 7 (5), e155260. doi:10.1172/jci.insight.155260
Gao, F., Zhang, X., Wang, S., Zheng, L., Sun, Y., Wang, G., et al. (2021). TSP50 promotes the Warburg effect and hepatocyte proliferation via regulating PKM2 acetylation. Cell Death Dis. 12 (6), 517. doi:10.1038/s41419-021-03782-w
Gao, J., Wang, T., Wang, C., Wang, S., Wang, W., Ma, D., et al. (2019). Effects of Tianshu capsule on spontaneously hypertensive rats as revealed by (1)H-NMR-based metabolic profiling. Front. Pharmacol. 10, 989. doi:10.3389/fphar.2019.00989
Gao, S., Li, X., Jiang, Q., Liang, Q., Zhang, F., Li, S., et al. (2022). PKM2 promotes pulmonary fibrosis by stabilizing TGF-β1 receptor I and enhancing TGF-β1 signaling. Sci. Adv. 8 (38), eabo0987. doi:10.1126/sciadv.abo0987
Glenthøj, A., van Beers, E. J., Al-Samkari, H., Viprakasit, V., Kuo, K. H. M., Galactéros, F., et al. (2022). Mitapivat in adult patients with pyruvate kinase deficiency receiving regular transfusions (ACTIVATE-T): a multicentre, open-label, single-arm, phase 3 trial. Lancet Haematol. 9 (10), e724–e732. doi:10.1016/s2352-3026(22)00214-9
Gong, M., Guo, Y., Dong, H., Wu, F., He, Q., Gong, J., et al. (2023). Modified Hu-lu-ba-wan protects diabetic glomerular podocytes via promoting PKM2-mediated mitochondrial dynamic homeostasis. Phytomedicine 123, 155247. doi:10.1016/j.phymed.2023.155247
Gordin, D., Shah, H., Shinjo, T., St-Louis, R., Qi, W., Park, K., et al. (2019). Characterization of glycolytic enzymes and pyruvate kinase M2 in type 1 and 2 diabetic nephropathy. Diabetes Care 42 (7), 1263–1273. doi:10.2337/dc18-2585
Gu, M., Tan, M., Zhou, L., Sun, X., Lu, Q., Wang, M., et al. (2022). Protein phosphatase 2Acα modulates fatty acid oxidation and glycolysis to determine tubular cell fate and kidney injury. Kidney Int. 102 (2), 321–336. doi:10.1016/j.kint.2022.03.024
Hao, L., Park, J., Jang, H. Y., Bae, E. J., and Park, B. H. (2021). Inhibiting protein kinase activity of pyruvate kinase M2 by SIRT2 deacetylase attenuates psoriasis. J. Invest. Dermatol. 141 (2), 355–363.e6. doi:10.1016/j.jid.2020.06.024
Hosoi, T., Yazawa, K., Imada, M., Tawara, A., Tohda, C., Nomura, Y., et al. (2023). Alkannin attenuates Amyloid β aggregation and Alzheimer's disease pathology. Mol. Pharmacol. 103 (5), 266–273. doi:10.1124/molpharm.121.000468
Hou, P. P., Luo, L. J., Chen, H. Z., Chen, Q. T., Bian, X. L., Wu, S. F., et al. (2020). Ectosomal PKM2 promotes HCC by inducing macrophage differentiation and remodeling the tumor microenvironment. Mol. Cell 78 (6), 1192–1206. doi:10.1016/j.molcel.2020.05.004
Huang, X., Gao, L., Deng, R., Peng, Y., Wu, S., Lu, J., et al. (2023). Huangqi-Danshen decoction reshapes renal glucose metabolism profiles that delays chronic kidney disease progression. Biomed. Pharmacother. 164, 114989. doi:10.1016/j.biopha.2023.114989
Ito, M., Gurumani, M. Z., Merscher, S., and Fornoni, A. (2022). Glucose- and non-glucose-induced mitochondrial dysfunction in diabetic kidney disease. Biomolecules 12 (3), 351. doi:10.3390/biom12030351
Jiang, C., Zhao, X., Jeong, T., Kang, J. Y., Park, J. H., Kim, I. S., et al. (2022). Novel specific pyruvate kinase M2 inhibitor, compound 3h, induces apoptosis and autophagy through suppressing Akt/mTOR signaling pathway in LNCaP cells. Cancers 15 (1), 265. doi:10.3390/cancers15010265
Jin, X., Zhang, W., Wang, Y., Liu, J., Hao, F., Li, Y., et al. (2020). Pyruvate kinase M2 promotes the activation of dendritic cells by enhancing IL-12p35 expression. Cell Rep. 31 (8), 107690. doi:10.1016/j.celrep.2020.107690
Kim, H., Takegahara, N., and Choi, Y. (2023). IgSF11-mediated phosphorylation of pyruvate kinase M2 regulates osteoclast differentiation and prevents pathological bone loss. Bone Res. 11 (1), 17. doi:10.1038/s41413-023-00251-2
Kim, H. R., Park, J. H., Lee, S. H., Kwack, S. J., Lee, J., Kim, S., et al. (2022). Using intracellular metabolic profiling to identify novel biomarkers of cisplatin-induced acute kidney injury in NRK-52E cells. J. Toxicol. Environ. Health A 85 (1), 29–42. doi:10.1080/15287394.2021.1969305
Kuo, C. W., Chen, D. H., Tsai, M. T., Lin, C. C., Cheng, H. W., Tsay, Y. G., et al. (2023). Pyruvate kinase M2 modification by a lipid peroxidation byproduct acrolein contributes to kidney fibrosis. Front. Med. 10, 1151359. doi:10.3389/fmed.2023.1151359
Kuo, K. H. M., Layton, D. M., Lal, A., Al-Samkari, H., Bhatia, J., Kosinski, P. A., et al. (2022). Safety and efficacy of mitapivat, an oral pyruvate kinase activator, in adults with non-transfusion dependent α-thalassaemia or β-thalassaemia: an open-label, multicentre, phase 2 study. Lancet 400 (10351), 493–501. doi:10.1016/s0140-6736(22)01337-x
Li, J., Liu, H., Takagi, S., Nitta, K., Kitada, M., Srivastava, S. P., et al. (2020a). Renal protective effects of empagliflozin via inhibition of EMT and aberrant glycolysis in proximal tubules. JCI Insight 5 (6), e129034. doi:10.1172/jci.insight.129034
Li, J., Sun, Y. B. Y., Chen, W., Fan, J., Li, S., Qu, X., et al. (2020b). Smad4 promotes diabetic nephropathy by modulating glycolysis and OXPHOS. EMBO Rep. 21 (2), e48781. doi:10.15252/embr.201948781
Li, L., Tang, L., Yang, X., Chen, R., Zhang, Z., Leng, Y., et al. (2020c). Gene regulatory effect of pyruvate kinase M2 is involved in renal inflammation in type 2 diabetic nephropathy. Exp. Clin. Endocrinol. Diabetes 128 (9), 599–606. doi:10.1055/a-1069-7290
Li, M., Jia, F., Zhou, H., Di, J., and Yang, M. (2018). Elevated aerobic glycolysis in renal tubular epithelial cells influences the proliferation and differentiation of podocytes and promotes renal interstitial fibrosis. Eur. Rev. Med. Pharmacol. Sci. 22 (16), 5082–5090. doi:10.26355/eurrev_201808_15701
Li, T., Han, J., Jia, L., Hu, X., Chen, L., and Wang, Y. (2019). PKM2 coordinates glycolysis with mitochondrial fusion and oxidative phosphorylation. Protein Cell 10 (8), 583–594. doi:10.1007/s13238-019-0618-z
Li, X., Ma, T. K., Wang, M., Zhang, X. D., Liu, T. Y., Liu, Y., et al. (2023a). YY1-induced upregulation of LncRNA-ARAP1-AS2 and ARAP1 promotes diabetic kidney fibrosis via aberrant glycolysis associated with EGFR/PKM2/HIF-1α pathway. Front. Pharmacol. 14, 1069348. doi:10.3389/fphar.2023.1069348
Li, Y. J., Zhang, C., Martincuks, A., Herrmann, A., and Yu, H. (2023b). STAT proteins in cancer: orchestration of metabolism. Nat. Rev. Cancer 23 (3), 115–134. doi:10.1038/s41568-022-00537-3
Lin, C. J., Chiu, C. Y., Liao, E. C., Wu, C. J., Chung, C. H., Greenberg, C. S., et al. (2023). S-nitrosylation of tissue transglutaminase in modulating glycolysis, oxidative stress, and inflammatory responses in normal and indoxyl-sulfate-induced endothelial cells. Int. J. Mol. Sci. 24 (13), 10935. doi:10.3390/ijms241310935
Liu, H., Takagaki, Y., Kumagai, A., Kanasaki, K., and Koya, D. (2021). The PKM2 activator TEPP-46 suppresses kidney fibrosis via inhibition of the EMT program and aberrant glycolysis associated with suppression of HIF-1α accumulation. J. Diabetes Investig. 12 (5), 697–709. doi:10.1111/jdi.13478
Liu, Y., Wu, H., Mei, Y., Ding, X., Yang, X., Li, C., et al. (2017). Clinicopathological and prognostic significance of PKM2 protein expression in cirrhotic hepatocellular carcinoma and non-cirrhotic hepatocellular carcinoma. Sci. Rep. 7 (1), 15294. doi:10.1038/s41598-017-14813-y
Luo, Q., Liang, W., Zhang, Z., Zhu, Z., Chen, Z., Hu, J., et al. (2022). Compromised glycolysis contributes to foot process fusion of podocytes in diabetic kidney disease: role of ornithine catabolism. Metabolism 134, 155245. doi:10.1016/j.metabol.2022.155245
Moreno-Fernandez, M. E., Giles, D. A., Oates, J. R., Chan, C. C., Damen, M., Doll, J. R., et al. (2021). PKM2-dependent metabolic skewing of hepatic Th17 cells regulates pathogenesis of non-alcoholic fatty liver disease. Cell Metab. 33 (6), 1187–1204.e9. doi:10.1016/j.cmet.2021.04.018
Nakagawa, T., Johnson, R. J., Andres-Hernando, A., Roncal-Jimenez, C., Sanchez-Lozada, L. G., Tolan, D. R., et al. (2020). Fructose production and metabolism in the kidney. J. Am. Soc. Nephrol. 31 (5), 898–906. doi:10.1681/asn.2019101015
Park, J. H., Kundu, A., Lee, S. H., Jiang, C., Lee, S. H., Kim, Y. S., et al. (2021). Specific pyruvate kinase M2 inhibitor, compound 3K, induces autophagic cell death through disruption of the glycolysis pathway in ovarian cancer cells. Int. J. Biol. Sci. 17 (8), 1895–1908. doi:10.7150/ijbs.59855
Park, Y. S., Han, J. H., Park, J. H., Choi, J. S., Kim, S. H., and Kim, H. S. (2023). Pyruvate kinase M2: a new biomarker for the early detection of diabetes-induced nephropathy. Int. J. Mol. Sci. 24 (3), 2683. doi:10.3390/ijms24032683
Peng, Y., Li, Y., Li, H., and Yu, J. (2022). Shikonin attenuates kidney tubular epithelial cells apoptosis, oxidative stress, and inflammatory response through nicotinamide adenine dinucleotide phosphate oxidase 4/PTEN pathway in acute kidney injury of sepsis model. Drug Dev. Res. 83 (5), 1111–1124. doi:10.1002/ddr.21936
Prakasam, G., Iqbal, M. A., Bamezai, R. N. K., and Mazurek, S. (2018). Posttranslational modifications of pyruvate kinase M2: tweaks that benefit cancer. Front. Oncol. 8, 22. doi:10.3389/fonc.2018.00022
Puckett, D. L., Alquraishi, M., Chowanadisai, W., and Bettaieb, A. (2021). The role of PKM2 in metabolic reprogramming: insights into the regulatory roles of non-coding RNAs. Int. J. Mol. Sci. 22 (3), 1171. doi:10.3390/ijms22031171
Qi, W., Keenan, H. A., Li, Q., Ishikado, A., Kannt, A., Sadowski, T., et al. (2017). Pyruvate kinase M2 activation may protect against the progression of diabetic glomerular pathology and mitochondrial dysfunction. Nat. Med. 23 (6), 753–762. doi:10.1038/nm.4328
Qian, L., Ren, S., Xu, Z., Zheng, Y., Wu, L., Yang, Y., et al. (2021). Qian Yang Yu Yin granule improves renal injury of hypertension by regulating metabolic reprogramming mediated by HIF-1α/PKM2 positive feedback loop. Front. Pharmacol. 12, 667433. doi:10.3389/fphar.2021.667433
Rabelink, T. J., and Carmeliet, P. (2018). Renal metabolism in 2017: glycolytic adaptation and progression of kidney disease. Nat. Rev. Nephrol. 14 (2), 75–76. doi:10.1038/nrneph.2017.173
Rao, J., Wang, H., Ni, M., Wang, Z., Wang, Z., Wei, S., et al. (2022). FSTL1 promotes liver fibrosis by reprogramming macrophage function through modulating the intracellular function of PKM2. Gut 71 (12), 2539–2550. doi:10.1136/gutjnl-2021-325150
Ren, R., Guo, J., Shi, J., Tian, Y., Li, M., and Kang, H. (2020). PKM2 regulates angiogenesis of VR-EPCs through modulating glycolysis, mitochondrial fission, and fusion. J. Cell Physiol. 235 (9), 6204–6217. doi:10.1002/jcp.29549
Ren, Y., Wang, J., Guo, W., Chen, J., Wu, X., Gu, S., et al. (2022). Renoprotection of microcystin-RR in unilateral ureteral obstruction-induced renal fibrosis: targeting the PKM2-HIF-1α pathway. Front. Pharmacol. 13, 830312. doi:10.3389/fphar.2022.830312
Sachan, R., Kundu, A., Dey, P., Son, J. Y., Kim, K. S., Lee, D. E., et al. (2020). Dendropanax morbifera protects against renal fibrosis in streptozotocin-Induced diabetic rats. Antioxidants 9 (1), 84. doi:10.3390/antiox9010084
Seki, S. M., Posyniak, K., McCloud, R., Rosen, D. A., Fernández-Castañeda, A., Beiter, R. M., et al. (2020). Modulation of PKM activity affects the differentiation of T(H)17 cells. Sci. Signal. 13 (655), eaay9217. doi:10.1126/scisignal.aay9217
Singh, J. P., Qian, K., Lee, J. S., Zhou, J., Han, X., Zhang, B., et al. (2020). O-GlcNAcase targets pyruvate kinase M2 to regulate tumor growth. Oncogene 39 (3), 560–573. doi:10.1038/s41388-019-0975-3
Srivastava, S. P., Li, J., Kitada, M., Fujita, H., Yamada, Y., Goodwin, J. E., et al. (2018). SIRT3 deficiency leads to induction of abnormal glycolysis in diabetic kidney with fibrosis. Cell Death Dis. 9 (10), 997. doi:10.1038/s41419-018-1057-0
Suman, I., Šimić, L., Čanadi Jurešić, G., Buljević, S., Klepac, D., and Domitrović, R. (2024). The interplay of mitophagy, autophagy, and apoptosis in cisplatin-induced kidney injury: involvement of ERK signaling pathway. Cell Death Discov. 10 (1), 98. doi:10.1038/s41420-024-01872-0
Tang, W., and Wei, Q. (2023). The metabolic pathway regulation in kidney injury and repair. Front. Physiol. 14, 1344271. doi:10.3389/fphys.2023.1344271
Tong, Y., Chuan, J., Bai, L., Shi, J., Zhong, L., Duan, X., et al. (2018). The protective effect of shikonin on renal tubular epithelial cell injury induced by high glucose. Biomed. Pharmacother. 98, 701–708. doi:10.1016/j.biopha.2017.12.112
Wang, C., Xiao, Y., Lao, M., Wang, J., Xu, S., Li, R., et al. (2020). Increased SUMO-activating enzyme SAE1/UBA2 promotes glycolysis and pathogenic behavior of rheumatoid fibroblast-like synoviocytes. JCI Insight 5 (18), e135935. doi:10.1172/jci.insight.135935
Wang, J., Yang, P., Yu, T., Gao, M., Liu, D., Zhang, J., et al. (2022a). Lactylation of PKM2 suppresses inflammatory metabolic adaptation in pro-inflammatory macrophages. Int. J. Biol. Sci. 18 (16), 6210–6225. doi:10.7150/ijbs.75434
Wang, Z., Yang, S., Ping, Z., Li, Y., Jiang, T., Zheng, X., et al. (2023). Age-induced accumulation of succinate promotes cardiac fibrogenesis. Circ. Res. 134 (5), 1–23. doi:10.1161/circresaha.123.323651
Wang, Z., Yu, J., Hao, D., Liu, X., and Wang, X. (2022b). Transcriptomic signatures responding to PKM2 activator TEPP-46 in the hyperglycemic human renal proximal epithelial tubular cells. Front. Endocrinol. 13, 965379. doi:10.3389/fendo.2022.965379
Weagel, E. G., Foulks, J. M., Siddiqui, A., and Warner, S. L. (2022). Molecular glues: enhanced protein-protein interactions and cell proteome editing. Med. Chem. Res. 31 (7), 1068–1087. doi:10.1007/s00044-022-02882-2
Wei, Q., Su, J., Dong, G., Zhang, M., Huo, Y., and Dong, Z. (2019). Glycolysis inhibitors suppress renal interstitial fibrosis via divergent effects on fibroblasts and tubular cells. Am. J. Physiol. Ren. Physiol. 316 (6), F1162–f1172. doi:10.1152/ajprenal.00422.2018
Wu, J., Rong, S., Zhou, J., and Yuan, W. (2021). The role and mechanism of PKM2 in the development of LPS-induced acute kidney injury. Histol. Histopathol. 36 (8), 845–852. doi:10.14670/hh-18-343
Wu, Y., Tang, L., Huang, H., Yu, Q., Hu, B., Wang, G., et al. (2023). Phosphoglycerate dehydrogenase activates PKM2 to phosphorylate histone H3T11 and attenuate cellular senescence. Nat. Commun. 14 (1), 1323. doi:10.1038/s41467-023-37094-8
Xia, L., Jiang, Y., Zhang, X. H., Wang, X. R., Wei, R., Qin, K., et al. (2021). SUMOylation disassembles the tetrameric pyruvate kinase M2 to block myeloid differentiation of leukemia cells. Cell Death Dis. 12 (1), 101. doi:10.1038/s41419-021-03400-9
Xie, W., He, Q., Zhang, Y., Xu, X., Wen, P., Cao, H., et al. (2023). Pyruvate kinase M2 regulates mitochondrial homeostasis in cisplatin-induced acute kidney injury. Cell Death Dis. 14 (10), 663. doi:10.1038/s41419-023-06195-z
Xu, F., Guo, M., Huang, W., Feng, L., Zhu, J., Luo, K., et al. (2020). Annexin A5 regulates hepatic macrophage polarization via directly targeting PKM2 and ameliorates NASH. Redox Biol. 36, 101634. doi:10.1016/j.redox.2020.101634
Xu, Y., Liu, X. H., Saunders, M., Pearce, S., Foulks, J. M., Parnell, K. M., et al. (2014). Discovery of 3-(trifluoromethyl)-1H-pyrazole-5-carboxamide activators of the M2 isoform of pyruvate kinase (PKM2). Bioorg. Med. Chem. Lett. 24 (2), 515–519. doi:10.1016/j.bmcl.2013.12.028
Yang, Y., Zhan, X., Zhang, C., Shi, J., Wu, J., Deng, X., et al. (2023). USP25-PKM2-glycolysis axis contributes to ischemia reperfusion-induced acute kidney injury by promoting M1-like macrophage polarization and proinflammatory response. Clin. Immunol. 251, 109279. doi:10.1016/j.clim.2023.109279
Ye, Y., Xu, L., Ding, H., Wang, X., Luo, J., Zhang, Y., et al. (2021). Pyruvate kinase M2 mediates fibroblast proliferation to promote tubular epithelial cell survival in acute kidney injury. Faseb J. 35 (7), e21706. doi:10.1096/fj.202100040R
Yin, X. N., Wang, J., Cui, L. F., and Fan, W. X. (2018). Enhanced glycolysis in the process of renal fibrosis aggravated the development of chronic kidney disease. Eur. Rev. Med. Pharmacol. Sci. 22 (13), 4243–4251. doi:10.26355/eurrev_201807_15419
Yu, S., Zang, W., Qiu, Y., Liao, L., and Zheng, X. (2022). Deubiquitinase OTUB2 exacerbates the progression of colorectal cancer by promoting PKM2 activity and glycolysis. Oncogene 41 (1), 46–56. doi:10.1038/s41388-021-02071-2
Yuan, Q., Miao, J., Yang, Q., Fang, L., Fang, Y., Ding, H., et al. (2020). Role of pyruvate kinase M2-mediated metabolic reprogramming during podocyte differentiation. Cell Death Dis. 11 (5), 355. doi:10.1038/s41419-020-2481-5
Zhang, W., Guo, X., Ren, J., Chen, Y., Wang, J., and Gao, A. (2022). GCN5-mediated PKM2 acetylation participates in benzene-induced hematotoxicity through regulating glycolysis and inflammation via p-Stat3/IL17A axis. Environ. Pollut. 295, 118708. doi:10.1016/j.envpol.2021.118708
Zhang, Z., Deng, X., Liu, Y., Liu, Y., Sun, L., and Chen, F. (2019). PKM2, function and expression and regulation. Cell Biosci. 9, 52. doi:10.1186/s13578-019-0317-8
Zhang, Z., Hu, H., Luo, Q., Yang, K., Zou, Z., Shi, M., et al. (2023). Dihydroxyacetone phosphate accumulation leads to podocyte pyroptosis in diabetic kidney disease. J. Cell. Mol. Med. 28 (3), e18073. doi:10.1111/jcmm.18073
Zhao, G., Yuan, H., Li, Q., Zhang, J., Guo, Y., Feng, T., et al. (2022a). DDX39B drives colorectal cancer progression by promoting the stability and nuclear translocation of PKM2. Signal Transduct. Target Ther. 7 (1), 275. doi:10.1038/s41392-022-01096-7
Zhao, J., Wang, G., Han, K., Wang, Y., Wang, L., Gao, J., et al. (2022b). Mitochondrial PKM2 deacetylation by procyanidin B2-induced SIRT3 upregulation alleviates lung ischemia/reperfusion injury. Cell Death Dis. 13 (7), 594. doi:10.1038/s41419-022-05051-w
Zhao, Z., Wang, Y., Gao, Y., Ju, Y., Zhao, Y., Wu, Z., et al. (2023). The PRAK-NRF2 axis promotes the differentiation of Th17 cells by mediating the redox homeostasis and glycolysis. Proc. Natl. Acad. Sci. U. S. A. 120 (19), e2212613120. doi:10.1073/pnas.2212613120
Zheng, D., Jiang, Y., Qu, C., Yuan, H., Hu, K., He, L., et al. (2020). Pyruvate kinase M2 tetramerization protects against hepatic stellate cell activation and liver fibrosis. Am. J. Pathol. 190 (11), 2267–2281. doi:10.1016/j.ajpath.2020.08.002
Zhou, H. L., Hausladen, A., Anand, P., Rajavel, M., Stomberski, C. T., Zhang, R., et al. (2023a). Identification of a selective SCoR2 inhibitor that protects against acute kidney injury. J. Med. Chem. 66 (8), 5657–5668. doi:10.1021/acs.jmedchem.2c02089
Zhou, Q., Li, H., Li, Y., Tan, M., Fan, S., Cao, C., et al. (2019). Inhibiting neddylation modification alters mitochondrial morphology and reprograms energy metabolism in cancer cells. JCI Insight 4 (4), e121582. doi:10.1172/jci.insight.121582
Zhou, Q., Yin, Y., Yu, M., Gao, D., Sun, J., Yang, Z., et al. (2022a). GTPBP4 promotes hepatocellular carcinoma progression and metastasis via the PKM2 dependent glucose metabolism. Redox Biol. 56, 102458. doi:10.1016/j.redox.2022.102458
Zhou, W., Simic, P., and Rhee, E. P. (2022b). Fibroblast growth factor 23 regulation and acute kidney injury. Nephron 146 (3), 239–242. doi:10.1159/000517734
Zhou, W., Simic, P., Zhou, I. Y., Caravan, P., Vela Parada, X., Wen, D., et al. (2023b). Kidney glycolysis serves as a mammalian phosphate sensor that maintains phosphate homeostasis. J. Clin. Invest. 133 (8), e164610. doi:10.1172/jci164610
Keywords: pyruvate kinase M2, diabetic kidney disease, acute kidney injury, post-translational modification, glycolysis
Citation: Chen D-Q, Han J, Liu H, Feng K and Li P (2024) Targeting pyruvate kinase M2 for the treatment of kidney disease. Front. Pharmacol. 15:1376252. doi: 10.3389/fphar.2024.1376252
Received: 25 January 2024; Accepted: 05 April 2024;
Published: 07 June 2024.
Edited by:
Ya-Long Feng, Xianyang Normal University, ChinaReviewed by:
Bardia Askari, New York Institute of Technology, United StatesTian Li, Tianjin Medical University, China
Yong Zhang, Jianli County People’s Hospital, China
Copyright © 2024 Chen, Han, Liu, Feng and Li. This is an open-access article distributed under the terms of the Creative Commons Attribution License (CC BY). The use, distribution or reproduction in other forums is permitted, provided the original author(s) and the copyright owner(s) are credited and that the original publication in this journal is cited, in accordance with accepted academic practice. No use, distribution or reproduction is permitted which does not comply with these terms.
*Correspondence: Dan-Qian Chen, Y2hlbmRhbnFpYW4yMDEzQDE2My5jb20=; Ping Li, bHA4Njc1QDE2My5jb20=
†These authors have contributed equally to this work.