- 1Shifa College of Pharmaceutical Sciences, Shifa Tameer-e-Millat University, Islamabad, Pakistan
- 2Shifa College of Medicine, Shifa Tameer-e-Millat University, Islamabad, Pakistan
- 3Department of Chemistry, College of Science, United Arab Emirates University, Al Ain, United Arab Emirates
- 4Department of Clinical Psychology, College of Medicine and Health Sciences, United Arab Emirates University, Al Ain, United Arab Emirates
- 5Department of Biosciences, Shifa Tameer-e-Millat University, Islamabad, Pakistan
Malaria, transmitted through the bite of a Plasmodium-infected Anopheles mosquito, remains a significant global health concern. This review examines the complex life cycle of Plasmodium, emphasizing the role of humans and mosquitoes in its transmission and proliferation. Malarial parasites are transmitted as sporozoites to the human body by biting an infected female Anopheles mosquito. These sporozoites then invade liver cells, multiply, and release merozoites, which infect red blood cells, perpetuating the cycle. As this cycle continues, the affected person starts experiencing the clinical symptoms of the disease. The current treatments for malaria, including chloroquine, artemisinin-based combination therapy, and quinine, are discussed alongside the challenges of drug resistance and misdiagnosis. Although efforts have been made to develop a malarial vaccine, they have so far been unsuccessful. Additionally, the review explores the potential of medicinal plants as remedies for malaria, highlighting the efficacy of compounds derived from Artemisia annua, Cinchona species, and Helianthus annuus L., as well as exploration of plants and phytocompounds like cryptolepine, and isoliquiritigenin against drug-resistant Plasmodium species. Moreover, studies from Pakistan further highlight the diverse vegetal resources utilized in malaria treatment, emphasizing the need for further research into natural remedies. Despite the advantages of herbal medicines, including cost-effectiveness, and fewer side effects; their limitations must be taken into account, including variations in potency and potential drug interactions. The review concludes by advocating for a balanced approach to malaria treatment and prevention, emphasizing the importance of early detection, accurate diagnosis, and integrated efforts to combat the disease in the endemic regions.
1 Introduction
Malaria is one of the ancient diseases of humanity. Its historical records can be traced back as early as 2700 BC. By the early 1800s, malaria had become a global scourge, afflicting people across the world (Garcia, 2010). Despite scientific and medicinal advancements, malaria is still endemic in over 90 countries, and around 2 billion people are at risk of developing this infection annually (Finnigan, 2023). In the malaria hotspots, it is one of the leading causes of mortality, causing more than 1 million deaths worldwide (Pradines and Robert, 2019).
Malaria is a parasitic infection transmitted to humans by the Anopheles mosquito. When a mosquito bites a human, the parasite responsible for causing malaria is transmitted to the human body. Plasmodium exists in different forms, but only Plasmodium falciparum, Plasmodium vivax, Plasmodium ovale, and Plasmodium malariae are considered infectious. Among them, P. falciparum causes the most severe disease (Talapko et al., 2019). Malaria symptoms include headache, fever, myalgia, vomiting, and diarrhea. A severe infection can result in life-threatening conditions such as encephalopathy, high-grade fever, and gastroenteritis (Bartoloni and Zammarchi, 2012).
In endemic areas, malaria significantly impacts not only the health of individuals but also their socioeconomic profile. Due to poor living conditions and nutrition status, malaria disproportionately affects impoverished communities, exacerbating the challenges faced by vulnerable social groups (Suh, Kain, and Keystone, 2004). Malaria stands as one of humanity’s most fatal afflictions. The horrors unleashed by this ancient disease persist to the present day. Unfortunately, an effective and potent cure for the infection continues to elude us. The challenges span from complexities of early detection of malaria to obstacles to formulating a suitable remedial strategy, collectively presenting a formidable hurdle in its treatment.
Quinine was the first drug for malaria treatment (Weinke et al., 1992). However, with advancements in medicine, treatment options have also evolved. Currently, chloroquine, artemisinin, proguanil, primaquine, mefloquine, and sulfonamides are used to manage malaria (Gilles and Warrell, 1996). However, there is still no empirical drug for it, as these drugs are plagued by resistance and undesirable side effects (Castelli, Tomasoni, and Matteelli, 2012).
Furthermore, homology modeling and molecular dynamics simulations provide valuable insights into P. falciparum chloroquine resistance transporter (PfCRTs) and its interactions with chloroquine (Patel and Roy, 2021). In vitro assays reveal increased IC50 (a measure of potency) values for mefloquine, suggesting diminished parasite susceptibility. Moreover, the concurrent emergence of resistance to artesunate, coupled with mefloquine resistance, underscores the urgent requirement for alternative approaches to malaria treatment (Rogers et al., 2009b).
For centuries, natural products have been utilized in the treatment of various conditions. This is particularly true for malaria because many anti-malaria drugs are derived from plants, including quinine and artemisinin, which are crucial in malaria control (Kingston and Cassera, 2022). However, the reduced effectiveness of the old therapies due to various reasons including the emergence of resistance in the parasite necessitates the search for newer agents to treat malaria. This article aims to review natural remedies that can be used to treat malaria including the resistant strains while emphasizing the challenges faced by the empirical class of drugs.
2 Epidemiology
According to the World Health Organization’s annual report on malaria, there were more than 240 million malaria cases worldwide. From 2000 to 2021, around 2 billion people were estimated to have suffered from the infection. However, The number of total malaria cases globally increased in 2021, from 245 million in 2020 to 247 million in 2021 (WHO, 2023a; 2022). Most cases are concentrated in the African region (95%), with around 2% in the Southeast Asian region. Approximately 29 countries accounted for 96% of malarial deaths (Figures 1,2; Table 1).
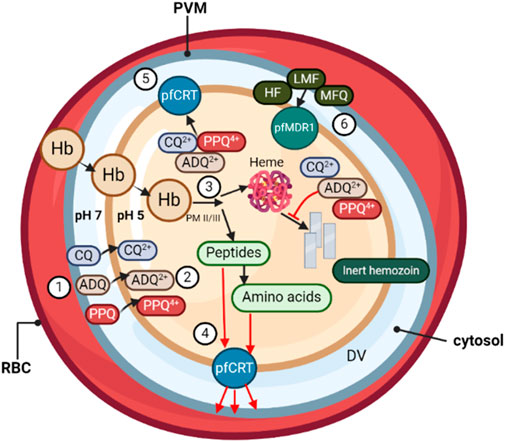
Figure 1. During its asexual blood stage, the P. falciparum parasite, surrounded by its parasitophorous vacuole membrane (PVM), develops within the host RBCs. (❶) Quinoline-based antimalarials, including chloroquine (CQ), amodiaquine (ADQ), and piperaquine (PPQ), concentrate from the parasitic cytosol (pH ∼7) into the digestive vacuole (DV) (pH ∼5.2). (❷) Once inside the DV, weak-base drugs are protonated, unable to passively diffuse out through the DV membrane. (❸) Protonated drug molecules bind to toxic heme by-products and grooves on hemozoin crystals in the DV, resulting in >1,000-fold drug accumulation due to pH trapping and heme-binding. (❹) The DV membrane protein PfCRT is believed to be involved in transporting peptides released from hemoglobin digestion into the parasite cytosol. (❺) In drug-resistant parasites, mutations in PfCRT enable the efflux of protonated drug molecules out of the DV, away from their heme target. (❻) DV membrane transporter PfMDR1 mutations influence parasite susceptibility by redirecting drugs such as halofantrine (HF), lumefantrine (LMF), and mefloquine (MFQ) into the DV, away from their primary site of action.
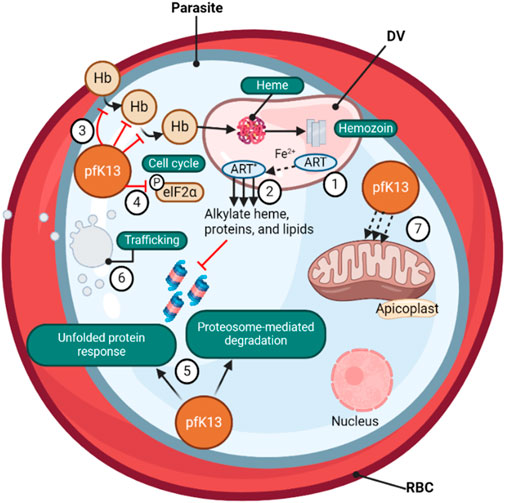
Figure 2. (❶) Artemisinin-based (ART) drugs are activated by cleavage of their endoperoxide by iron protoporphyrin IX (Fe2+-heme), a product of parasite-digested hemoglobin. (❷) The Fe2+-heme- ART carbon-centered radicals alkylate and damage a multitude of parasite proteins, heme, and lipids and inhibit proteasome-mediated protein degradation. PfK13 mutations, located primarily in the β-propeller kelch domain, confer ART resistance. (❸) The loss of PfK13 function provided by mutations has been shown to cause reduced endocytosis of host Hb and (❹) to extend the duration of ring-stage development, perhaps via PK4-mediated eIF2α phosphorylation, resulting in lowered levels of Hb catabolism and availability of Fe2+-heme as the drug activator, reducing ART activation (❺) PfK13 mutations activate the unfolded protein response, maintain proteasome-mediated degradation of polyubiquitinated proteins in the presence of ART, (❻) remove drugs and damaged proteins through an increase in PI3K-mediated vesicular trafficking. (❼) PfK13 may also help regulate mitochondrial physiology and maintain membrane potential during drug-induced ring-stage quiescence. The asterisk signifies the activated form of ART (Wicht, Mok, and Fidock, 2020).
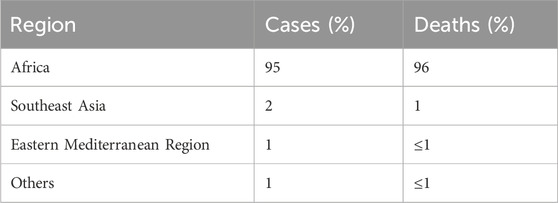
Table 1. Malaria cases and deaths by geographical region (WHO Annual Report, 2022).
A significant burden of malaria cases is shared by four countries, namely, Nigeria (27%), the Democratic Republic of Congo (12%), Uganda (5%), and Mozambique (4%) (Commission, 2023). Similarly, around 96% of deaths due to malaria occurred in four countries, i.e., Nigeria, the Democratic Republic of Congo, Niger, and the United Republic of Tanzania (WHO, 2023a). According to the WHO, more than 94% of malaria cases (233 million) and 95% of deaths occurred in the African region (Organization, 2023).
However, the epidemiology of malaria in India is distinct due to several features of the Plasmodium parasites, Anopheles vectors, ecoepidemiological aspects conducive to disease transmission, and susceptible humans living in rural and forested areas (Khan MuhammadImran et al, 2023). Notably, in 2021, India accounted for 79% of malaria cases and about 82.4% of all malaria deaths in the South-East Asia Region (Organization, 2022).
Lastly, a systematic review for finding out the prevalence of malaria (from 2001 to 2021) estimated pooled malaria prevalence in Pakistan to be 23.3%, with P. vivax, P. falciparum, and mixed infection rates of 79.13%, 16.29%, and 3.98%, respectively. Moreover, the analysis marked Karachi as the hotspot for malaria with a prevalence rate of 99.79% (Khan Nikhat et al., 2023). This data suggests that malaria is still endemic in most parts of the world. Although the infection rates have decreased over the years, it remains one of the most challenging health problems faced by the global south.
3 Pathophysiology of malaria
The life cycle of Plasmodium involves humans and mosquitoes, where the human body acts as a site for asexual reproduction, while mosquitoes are involved in the sexual stage of their lifecycle (Figure 3). Mosquitoes serve as vectors and transmit Plasmodium to humans, where it causes malaria (Giribaldi et al., 2015). Plasmodium is transmitted to humans by the female Anopheles mosquito. There are more than 450 species of Anopheles, but about 68 are involved in the transmission of the disease (Giribaldi et al., 2015).
Once it bites a human, the sporozoite form of the parasite is transmitted to the host.
The sporozoite form circulates via the bloodstream and resides in the liver, leading to malaria infection (Menard, 2005). The sporozoites undergo asexual reproduction, resulting in merozoites. This stage is categorized as the “liver stage” with no clinical symptoms. In the case of P. falciparum and P. malariae, the merozoites are released into the bloodstream, leading to the erythrogenic stage, where malaria infection symptoms start appearing. In the case of P. vivax and P. ovale, the parasite may enter a dormant hypnozoite stage, significantly increasing the risk of reinfection (Markus, 2011). As the merozoites invade RBCs, they rapidly grow and develop into ring-like forms called “trophozoites” that can be identified via Giemsa staining (Giribaldi et al., 2015).
4 Current treatments
The treatment and management of malaria depend upon accurate and timely diagnosis since its symptoms can differ significantly from one person to another (Bartoloni and Zammarchi, 2012). The clinical objectives of treating uncomplicated malaria are to cure the infection as rapidly as possible and to prevent progression to severe disease. “Cure” is defined as the elimination of all parasites from the body (WHO, 2023b). Current guidelines recommend that non-falciparum malaria should be treated with a 3-day course of oral chloroquine and oral analgesics (Chiodini et al., 2007). Primaquine is considered the most effective drug to eliminate P. vivax and P. ovale, even for the dormant hypnozoites in the liver (Wångdahl et al., 2022). Artemisinin-based combination therapy is used to treat uncomplicated P. falciparum malaria infection and the artemisinin-lumefantrine combination is a drug of choice for its treatment (McIntosh et al., 1996). Severe falciparum infection is treated with intravenous (IV) drugs until the patient can be switched to oral drugs. The treatment for severe malaria in adults and children is IV artesunate (Trampuz et al., 2003). The chemical structures of some of these drugs are provided in Figure 4.
Furthermore, treatment options for pregnant women and children afflicted with malaria are different and should be used with caution to avoid undesirable side effects (Whitty, Edmonds, and Mutabingwa, 2005). In addition, severe malaria infections in pediatrics should be treated with empirical broad-spectrum antibiotics. In case of uncomplicated infection, a combination of artemether-lumefantrine can be utilized as the first line of treatment (Chiodini et al., 2007). In the first trimester of pregnancy, uncomplicated malarial infection should be treated with quinine-clindamycin combination therapy. In the second and third trimesters of pregnancy, artemether-lumefantrine treats uncomplicated P. falciparum malaria (Whitty, Edmonds, and Mutabingwa, 2005). For severe malaria, in any trimester of pregnancy, artesunate is usually preferred over quinine. Severe malarial infection by P. falciparum in pregnancy can potentially result in stillbirth or early delivery, so it's advisable to start the treatment as early as possible, under the supervision of a specialist doctor (Nunes and Scherf, 2007).
Furthermore, in patients co-infected with HIV/AIDS and uncomplicated P. falciparum malaria, artesunate + sulfadoxine-pyrimethamine is not recommended if they are being treated with co-trimoxazole. Additionally, artesunate + amodiaquine is not recommended if they are being treated with efavirenz or zidovudine (WHO, 2023a; Obeagu et al., 2024). Another critical treatment case is malaria-tuberculosis co-infection. Severe drug interaction, for example, rifampicin-induced liver injury may be worsened by amodiaquine-containing artemisinin-based combination therapy, complicating the treatment plan (Adedeji et al., 2018). Currently, there is insufficient evidence to modify the existing dosing recommendations based on mg/kg body weight. However, due to the higher risk of recrudescent malaria infections in these patients, close monitoring is advised (World Health, 2023).
Moreover, the November 2022 update to treatment recommendations for malaria includes several key points. Firstly, it suggests the use of artesunate-pyronaridine as an artemisinin-based combination therapy option for treating uncomplicated P. falciparum malaria, despite low-certainty evidence. Additionally, pregnant women diagnosed with uncomplicated P. falciparum malaria during the first trimester are advised to undergo treatment with artemether-lumefantrine, again supported by a strong recommendation despite low certainty evidence. Additionally, to prevent relapse in cases of P. vivax or P. ovale malaria in children and adults (with specific exceptions), primaquine at a dosage of 0.5 mg/kg/day for 7 days was strongly recommended. Conversely, the update advises against using primaquine at a dosage of 1.0 mg/kg/day for 7 days to prevent relapse in P. vivax or P. ovale malaria, based on a conditional recommendation and very low-certainty evidence (Organization, 2023).
In addition to antipyretics, like acetaminophen, and anti-emetics, anti-seizure medications are also advised. Importantly, generalized seizures are more common in children with P. falciparum malaria than in those with malaria caused by other species. Usually, parenteral or rectal benzodiazepines or intramuscular paraldehyde are given. However, prophylactic use of anti-convulsants has no firm empirical foundation in otherwise uncomplicated malaria and therefore is not recommended (World Health, 2023).
5 Challenges associated with current treatments
One of the main challenges faced by clinicians in malaria treatment is drug resistance developed by Plasmodium. P. falciparum, responsible for severe malarial infection, has developed resistance to many drugs, including chloroquine, mefloquine, and quinine (Vestergaard and Ringwald, 2007). Chloroquine is considered the most cost-effective drug for malaria management, but recent data suggests that the treatment failure rate with chloroquine is more than 70% due to rapid drug-resistance development (Vestergaard and Ringwald, 2007). Amodiaquine, an essential part of the drug combinations used for treating malaria, is also facing the same threat.
Moreover, malaria misdiagnosis is another major challenge in combatting the epidemic. Accurate use of diagnostic procedures may not only lead to early infection treatment but also help select the correct antimalarial drug regimen against a particular pathogen (Shahbodaghi and Rathjen, 2022).
Vector control is one of the bigger challenges in the fight against malaria. In addition to outdoor transmission, growing levels of insecticide resistance in targeted vectors threaten the efficacy of long-lasting insecticidal nets and indoor residual spraying. These methods reduce malaria incidence but generally have little impact on malaria prevalence. Larvicidal treatments can be useful but are not recommended for rural areas. The research needed to improve the quality and delivery of mosquito vector control (Benelli and Beier, 2017). Due to the drug-drug interaction of antimalarial drugs, caution is always needed before prescribing. When treating malaria in someone who is also taking anti-tuberculosis medications, one must be careful to prevent harmful drug interactions. This is because the anti-tuberculosis drugs, such as rifampicin and isoniazid, can cause liver damage, and this risk could be heightened by using artemisinin-based combination therapy with amodiaquine to treat malaria, potentially leading to more severe outcomes (Adedeji et al., 2018).
6 Mechanisms of resistance
There is a plethora of factors behind anti-malaria drug resistance. The mechanisms of resistance to chloroquine in malaria parasites often involve mutations in the PfCRT gene, which reduce the drug accumulation within the parasite, diminishing its efficacy and facilitating parasite survival (Patel and Roy, 2021). In mosquitoes, exposure to chloroquine can lead to alterations in innate immunity, potentially affecting their ability to treat Plasmodium infections. Moreover, chloroquine disrupts normal apoptotic processes in mosquito midgut cells, potentially facilitating parasite survival and development within the mosquito host. These changes in parasite susceptibility and mosquito physiology can significantly influence malaria transmission dynamics and the proliferation of drug-resistant strains (Abrantes et al., 2008). The resistance mechanisms to mefloquine in P. falciparum involve mutations in the pfmdr1 gene, impacting drug transport and efficacy. Amplification of pfmdr1 correlates with heightened mefloquine resistance and treatment failure rates. Prolonged parasite clearance post-mefloquine treatment signifies potential resistance development. In vitro assays indicated elevated IC50 values for mefloquine, indicative of decreased parasite susceptibility. Furthermore, emerging resistance to artesunate, alongside mefloquine resistance, highlights the pressing need for alternative malaria treatment approaches (Rogers et al., 2009a).
Studies regarding molecular mechanisms involved in anti-malaria drug resistance found the involvement of variation in P. vivax multidrug resistance gene 1 (PvMDR1) (Reed et al., 2000; Chaorattanakawee et al., 2017; Li et al., 2020), P. vivax chloroquine resistance transporter (PvCRT) (Sá et al., 2019), P. vivax dihydrofolate reductase-thymidylate synthase (PvDHFR-TS), dihydropteroate synthase (PvDHPS) (Hawkins et al., 2007; Auburn et al., 2018; Auburn et al., 2019), and P. vivax multidrug resistance protein 1 (PvMRP1) (Bright et al., 2013; Flannery et al., 2015) in mediating drug resistance (Buyon, Elsworth, and Duraisingh, 2021). Furthermore, chemoprevention or chemoprophylaxis strategies may also inadvertently exacerbate the resistance problem. Malaria chemoprevention interventions do not always lead to favorable outcomes; counter-intuitively, they may increase the prevalence of resistance-associated genetic mutations (Plowe, 2022).
Drugs that are effective in adults cannot be used to treat malaria in pregnancy and children. This is another area of concern as many times, the same drugs are prescribed in all age groups leading to increased risk of adverse reactions and potentially life-threatening consequences during pregnancy (Phillips-Howard and Wood, 1996). Moreover (Acosta et al., 2020), assessed the relationship among drug dose, timing, and drug resistance in the malaria model. The analysis revealed that the higher dose administration may lead to high-level resistance. In addition, altering treatment timing can also influence the risk of resistance emergence. The study concluded that identifying the “right” time and dose was crucial for maximizing clinical goals (Acosta et al., 2020). In addition, an investigation focusing on the correlation between anti-malarial market characteristics and the emergence of arteminisin revealed that there was an urgent need for tight regulation to delay the emergence and spread of arteminisin (Guissou et al., 2023).
7 A glance at malaria vaccine-related advancements and development
About half of the world’s population is still at risk of contracting malaria, so making an effective malaria vaccine is the need of the hour, a goal that remains elusive for now. In 2021 the WHO approved the first malaria vaccine, RTS,S/AS01 vaccine (Mosquirix™), for widespread use. However, development continues on promising candidates such as R21, PfSPZ, and P. vivax vaccines (El-Moamly and El-Sweify, 2023).
R21/Matrix-M™ Malaria Vaccine is a product of collaboration between the University of Oxford and Novavax. By reaching 77% effectiveness in Phase 2 clinical trials, R21 demonstrated remarkable efficacy. Moreover, it is designed to be low-dose, accessible, and cost-effective, for use in children under 3 years of age (Aderinto et al., 2024). In addition to these developments, many researchers have emphasized the need for developing asexual blood-stage malaria vaccine development against Plasmodium (Takashima et al., 2024).
Thus far, two types of vaccines have been developed. One type, called subunit vaccines, like RTS,S, and R21, target a specific part of the parasite, but they have not been very effective at stimulating the immune system to protect against malaria for a long time (MacMillen et al., 2024). Another type of vaccine called whole-organism vaccines, such as Radiation Attenuated Sporozoites (RAS) exhibited better protection, but they are difficult and expensive to make since they need to be grown inside mosquitoes. Moreover, these vaccines require a series of IV doses administered over multiple clinic visits (Sissoko et al., 2022). To overcome this, researchers proposed a novel strategy called a “prime-and-trap” approach, involving using two different vaccines together. In vivo, the first vaccine (the priming dose) a single dose of a self-replicating RNA encoding full-length P. yoelii CS protein was delivered using advanced Nano carriers called LION™. The second vaccine (the trapping dose) consists of an attenuated malaria parasite, delivered as a whole-organism vaccine (WO RAS). Interestingly, an accelerated regimen, i.e., either 5-day or same-day immunization induced a strong immune response. Notably, mice vaccinated on the same day experienced a 2-day delay in the appearance of parasites in their blood and achieved 90% protection against a 3-week spz challenge. Moreover, this same-day regimen provided 70% protection against the 2-month spz challenge. This innovative approach may aid in developing vaccines that require fewer doses and provide long-lasting protection against malaria (MacMillen et al., 2024).
Furthermore, malaria transmission-blocking vaccines, which interrupt malaria transmission from one person to another, have also been tested in malaria-endemic areas of Africa. The study found that all the tested formulations were safe and well tolerated in healthy adults. Particularly, ProC6C-AlOH/Matrix-M vaccine elicited the highest levels of functional antibodies, requiring further investigation (Tiono et al., 2024).
8 Medicinal plants as remedies for malaria
Herbal medicines have many proven advantages over synthetic drugs, as the former is more cost-effective, has fewer side effects, and is more efficient (Mohammadi et al., 2020). Vegetal species have been used for centuries for different therapeutic purposes. Researchers from all over the world have identified numerous plant-based products that are effective in managing various diseases including malaria (Lemma et al., 2017; Hassan et al., 2021; Afzal et al., 2022; Nawaz, Ishtiaq, and Anwar, 2022). Derived from the plant named Artemisia annua, artemisinin has revolutionized the treatment of malaria (Phillipson, 1990). Similarly, various herbal medicines are derived from Cinchona species, with quinine being one of them (White, 1985). Febrifugine, another antimalarial drug, is derived from the plant Dichroea febrifuga Lour. (Farnsworth and Soejarto, 1991). Artemisinin and quinine contain bioactive compounds/phytochemicals, alkaloids and terpenoids that are effective for the treatment of malaria infection (Moyo et al., 2020). The structural formula for artemisinin, quinine, and febrifugine is shown in Figure 5. Here we describe some of the most important plants with promising anti-malarial activities.
8.1 Cryptolepis sanguinolenta (Lindl.) Schltr.
Cryptolepis sanguinolenta (Apocynaceae) leaves are used in the treatment of malaria. In 1996, cryptolepine was first isolated from the roots of C. sanguinolenta, and it inhibits DNA synthesis, which is the main reason for its antimalarial action (Osafo, Mensah, and Yeboah, 2017). In a study, both cryptolepine and isocryptolepine exhibited effective inhibition of P. falciparum in vitro, regardless of the strain’s resistance to chloroquine. Cryptolepine demonstrated slightly better performance in killing the parasite, with an IC50 ranging from 0.2 to 0.6 μM, compared to isocryptolepine’s (another alkaloid from C. sanguinolenta) IC50 of about 0.8 μM. The antimalarial activity of cryptolepine was also confirmed in-vivo rodent malarial parasites. The results showed that cryptolepine was effective against even the resistant varieties of Plasmodium parasites (Grellier et al., 1996; Table 3). This plant has been used in traditional African medicine to manage malaria, showing promising results (Mohammadi et al., 2020).
8.2 Artemisia annua L.
In a clinical study, patients with severe malaria who responded to neither Artemisinin combination Therapy (ACT) nor IV artesunate were treated with dried leaves of A. annua (Asteraceae). At the dose of 0.5g, twice daily for 5 days, the A. annua dried-leaf oral solution. Another study investigated A. annua anti-malaria potentials by subjecting its aqueous and hydroalcoholic extracts to in vitro and in vivo evaluations. Interestingly, the hydroalcoholic extract was found to be more active (IC50 = 3.27 ± 1.42 nM) than artemisinin (IC50 = 5.10 ± 1.89 nM) in vitro. However, the in vivo investigations using Plasmodium berghei NK 173 infected mice revealed that the aqueous extract of A. annua, with artemisinin content of 20 mg/kg, demonstrated comparable efficacy to pure artemisinin administered at a dosage of 140 mg/kg (Zime-Diawara et al., 2015). A series of clinical trials have proven the efficacy of these compounds, making these drugs one of the preferred choices of clinicians for eradicating Plasmodium parasites from the body (Chiodini et al., 2007). Information about several drugs derived from Artemisia species is provided in Table 2.
A study was conducted by (Elfawal et al., 2015) to demonstrate the efficacy of the whole A. annua plant, as a malaria therapy, is more potent than a comparable dose of pure artemisinin in a rodent malaria model. For this purpose, in the rodent malaria model, P. chabaudi was chosen due to its susceptibility to both whole-plant A. annua and artemisinin treatment. P. yoelii was selected as an artemisinin-resistant strain for challenge experiments. The study design consisted of five animal groups, 4 serving as treatment groups and 1 as control. Treatments included dried A. annua plant, and pure artemisinin, administered orally. The experiment utilized two doses of pure artemisinin and two doses of whole plant. The low dose of pure artemisinin was 24 mg/kg, while the high dose was 120 mg/kg. Similarly, the low dose of whole-plant A. annua corresponded to 24 mg of artemisinin per kilogram of body weight, and the high dose was 120 mg/kg. The results revealed that a stable resistance to the whole plant was achieved; three times slower than stable resistance to artemisinin. A. annua treatment was even more resilient than the double dose of artemisinin. This resilience was attributed to the potential evolutionary refinement of the plant’s secondary metabolic products into a redundant, multi-component defense system (Elfawal et al., 2015; Table 3).
8.3 Helianthus annuus L.
An Indonesian study investigated the in vivo and in vitro efficacy of H. annuus (Asteraceae). The ethanolic extracts from all parts of H. annuus were made separately. The root extract was more potent in vitro with IC50 values of 2.3 ± 1.4 μg/mL. In addition, leaf and flower extracts showed significant antimalarial activity with IC50 values of 4.3 ± 2.2 and 4.8 ± 0.0 μg/mL, respectively. In vivo studies in P. berghei-infected mice, treated with H. annuus root extract demonstrated the highest percentage inhibition, while leaf extract also showed significant inhibition (63.6 ± 8.0). Notably, the root extract in vivo curative assay showed a significant median effective (ED50) value of 10.6 ± 0.2 mg/kg. At 400 mg/kg dose, the group showed the highest prophylactic inhibition (79.2%) on day 3. Overall, the H. annuus extracts exhibited high activity against malaria (Ekasari et al., 2019; Table 4).
8.4 Cyperus articulates L.
A study focusing on the volatile oil obtained from rhizomes of the C. articulates (Cyperaceae) plant, commonly found in the Amazon region was carried out in Brazil. In this study, P. falciparum W2 (chloroquine-resistant) and 3D7 (chloroquine-sensitive) strains in erythrocytes were exposed to C. articulates volatile oils at different concentrations. The oil showed high activity against the two P. falciparum strains, with IC50 = 1.21 μg/mL for W2, and 2.30 μg/mL for 3D7. Furthermore, in vivo, antimalarial activity was tested in P. berghei-infected BALB/c mice. Treatment with C. articulates was a success in all 18 ACT-resistant cases (Daddy et al., 2017; Table 3).
The discovery of artemisinin has played a critical role in treating malaria, as it is not only active against various plasmodial forms, resistant to older drugs like chloroquine but also has fewer side effects. Its combination with other medications, such as lumefantrine, is crucial in combatting severe malarial infection (Marwa et al., 2022). With the development of different commercial forms of artemisinin-based drugs, there has been some improvement in the control over malaria endemics in the African and Mediterranean regions (Marwa et al., 2022). C. articulates volatile oil also significantly reduced parasitemia and anemia in animals treated with 100 and 200 mg/kg doses (Silva et al., 2019).
8.5 Hypoestes forskaleii (Vahl) R
Hypoestes forskalei (Acanthaceae) holds an eminent place in ethnopharmacology. In addition to its use as an anti-malaria, it is used as a cytotoxic, antimicrobial, larvicidal, antioxidant, antipyretic, antileismanial, and antitrypanosomal agent. To test H. forskalei anti-malaria effects, its leaf extracts were studied in both in vitro and in vivo settings. Notably, all the test doses of the crude extract and the fractions significantly reduced parasitemia and prolonged mean survival time (p < 0.001) as compared to their negative control groups. At 600 mg/kg dose of the crude extract during the 4-day suppressive test, maximum parasitemia suppression effect was observed (56%). At the same dose, the n-butanol, chloroform, and aqueous H. forskalei fractions revealed a percentage suppression of about 50, 38, and 19, respectively. Moreover, the n-butanol fraction, at a dose of 600 mg/kg, exhibited a significant curative effect (p < 0.001) in Rane’s test with a suppression of about 49% (Misganaw, Amare, and Mengistu, 2020; Table 4).
8.6 Angelica keiskei (Miq.) Koidz.
Chalcones is a renowned antimalarial phytocompound. A. keiskei (Apiaceae) is one of the plants that are rich in chalcone. A study aiming to determine the antimalarial activity of A. keiskei root extract by in vitro assay used the P. falciparum strain 3D7. The root extract, with an IC50 value of 16.091 μg/mL, exhibited an inhibitory effect as compared to chloroquine as a positive control with an IC50 value of 0.007 μg/mL. The study concluded that A. keiskei root extracts could be categorized as one of the antimalarial agents (Wardani, Wahid, and Rosa, 2020; Table 4).
8.7 Quercus infectoria G.Olivier
Similarly, a study conducted in Malaysia evaluated the anti-malaria potential of Quercus infectoria (Fagaceae). For this purpose, acetone, ethanol, methanol, and aqueous extracts were made from the Q. infectoria galls. Among these extracts, the acetone extract showed the highest antimalarial effects (IC50 = 5.85 ± 1.64 μg/mL), followed by the methanol extract (IC50 = 10.31 ± 1.90 μg/mL) against chloroquine-sensitive P. falciparum. Moreover, the study established the safety of these extracts, both acetone and methanol extracts were found to be non-toxic to the normal cell lines and statistically significant to artemisinin (p < 0.05) (Zin et al., 2020; Table 4).
8.8 Pogostemon cablin (Blanco) Benth
Another study conducted in Thailand found that the ethanol extract of Pogostemon cablin (Lamiaceae) carries the potential to become a more effective and safer anti-malarial agent. The efficacy and safety of the extract was tested by both in vitro and in vivo methods. P. cablin extract showed a significant IC50 of 24.49 ± 0.01 μg/mL against chloroquine-resistant P. falciparum K1. In comparison, the cytotoxic analyses revealed a nontoxic effect of the extract on Vero cells at a concentration of 80 μg/mL. In P. berghei-infected ICR mice, the ethanolic extract showed no toxic effect on mice at a dose of 2,000 mg/kg body weight. Notably, treatment with P. cablin extracts significantly suppressed parasitemia in mice by 38.41%, 45.12%, and 89.00% at doses of 200, 400, and 600 mg/kg body weight, respectively (Phuwajaroanpong et al., 2020; Table 3).
8.9 Kniphofia foliosa Hochst.
K. foliosa (Asphodelaceae) is indigenous to the Ethiopian highlands. Traditionally, its rhizomes are used for abdominal cramps, wound healing, as well as malaria management. To empirically establish K. foliosa as an anti-malaria agent, an investigation was carried out. In this study, two compounds (knipholone and dianellin) were isolated from the 80% methanolic extract of K. foliosa rhizomes. Upon evaluation by using Peters’ 4-day suppressive test against P. berghei in mice, the hydroalcoholic extract (400 mg/kg) and knipholone (200 mg/kg) demonstrated the highest activity with chemo suppression values of 61.52% and 60.16%, respectively. The dose-response plot analysis revealed the ED50 doses of knipholone and dianellin were 81.25 and 92.31 mg/kg, respectively. A molecular docking study revealed that knipholone had a strong binding affinity to the P. falciparum l-lactate dehydrogenase target (Alebachew et al., 2021; Table 4).
8.10 Physalis angulata L., Jatropha curcas L., Alstonia spectabilis R.Br.
To empirically understand the malaria treatment practices and evaluate the anti-plasmodial activity and phytochemicals of several plants used by the Tetun ethnic people in West Timor Indonesia, an experimental study was designed. In this study, ethanolic extracts from the whole P. angulate plant (family: Solanaceae), stem barks of A. spectabilis (family: Apocynaceae), and Jatropha curcas (family: Euphorbiaceae) were tested among others. Among the 11 plants studied in this investigation, P. angulata, J. curcas, and A. spectabilis extracts showed strong anti-plasmodial activity against the P. falciparum 3D7 strain in vitro, with IC50 values of 0.22, 0.22, and 1.23 μg/mL, respectively (Maximus et al., 2021) (Table 4).
8.11 Allium paradoxum (M.Bieb.) G.Don
A study aimed to examine the inhibitory effects of A. paradoxum (Amaryllidaceae) on P. falciparum and P. berghei. The highest efficacy of A. paradoxum extract was observed at 80 μg/mL in P. falciparum culture, resulting in 60.43% growth inhibition compared to control groups. The significantly highest parasite growth inhibition with 88.71% was seen in the mice infected with P. berghei when administered with 400 mg/kg extract compared to control groups. However, no significant changes in the liver and kidney cells were observed between the experimental and control groups (Elmi et al., 2021; Table 4).
8.12 Toddalia asiatica (L.) Lam., Rhamnus prinoides L'Hér., and Vernonia lasiopus O. Hoffm.
Extracts from various plant species were collected, authenticated, and processed using hot water extraction to mimic traditional methods. The activities of these extracts were tested against a chloroquine-resistant strain of P. berghei NK65 in mice. At the dose of 500 mg/kg, twice a day for 4 days, aqueous extract of V. lasiopus (root bark) and R. prinoides (leaves) showed remarkable suppression of parasitemia on day 4 post-infection (p.i.) with values of 51% and 54%, respectively. Moreover, the group treated with Toddalia asiatica (root bark) showed 100% survival up to day 15 p.i. Other extracts, including R. prinoides (leaves and root bark), showed prolonged survival with 40% of mice surviving beyond day 9 p.i. (Muregi et al., 2007; Table 3).
8.13 Maytenus senegalensis (Lam.) Exell, and Rhamnus staddo A.Rich.
In the same study by (Muregi et al., 2007), some plant extracts performed well when given in combination with chloroquine, particularly, extracts of M. senegalensis, R. staddo, T. asiatica, and V. lasiopus, in combination with chloroquine at a dose of 20 mg/kg, once a day for 2 days, and plant aqueous extracts at a dose of 500 mg/kg, twice a day for 4 days exhibited a remarkable suppression of parasitemia ranging from 51% to 66%. Survival analysis on day 14 p.i. showed that chloroquine in combination with certain plant extracts resulted in 40%–60% survival rates, with some mice surviving beyond day 14 p.i. Notably, combinations with R. prinoides (root bark) and V. lasiopus (root bark) showed 60% survival on day 14 p.i., with the last mice surviving beyond day 24 and day 30 p.i., respectively (Muregi et al., 2007; Table 3).
8.14 Citrullus colocynthis (L.) Schrad., Physalis alkekengi L., and Solanum nigrum L.
Drawing inspiration from Iranian traditional medicine, a study tested methanolic extracts from these plants against multi-drug resistant (K1) strains of P. falciparum. For the resistant strain, the study found that methanolic extracts of C. colocynthis exhibited promising in vitro anti-plasmodial activity, with an IC50 value of 6.9 μg/mL. Similarly, Solanum nigrum and P. alkekengi also showed activity against the resistant strain, with IC50 values of 18.67 and 13.08 μg/mL, respectively (Haddad et al., 2017; Table 3).
8.15 Glycyrrhiza glabra L.
In an in vivo investigation, the root extract of Glycyrrhiza glabra (Fabaceae) demonstrated anti-plasmodial potential. This activity was attributed to its phytomolecule: Isoliquiritigenin. Moreover, in-vivo antimalarial efficacy was evaluated through a 4-day suppression test in a mouse model. The phytocompound, in vitro, exhibited moderate anti-plasmodial activity against the multidrug-resistant strain (K1) of P. falciparum. Notably, the ethyl acetate extracts performed moderately against the resistant strain of P. falciparum (K1), with an IC50 value of 7.2 μg/mL. In vivo, mice infected with a chloroquine-resistant strain of P. yoelli nigeriensis were administered with the ethyl acetate extract at a dose of 500 mg/kg, which showed the highest inhibition of P. yoelii nigeriensis growth (82.73%), followed by doses of 250 mg/kg (64.40%) and 100 mg/kg (46.09%) compared to the control group. Also, the ethyl acetate extracts increased the mean survival time of the mice to 18.2 days, 13.5 days, and 16.5 days, respectively (Kumar et al., 2024; Table 3).
Interestingly, isoliquiritigenin was found to synergize with chloroquine and artemisinin against the multidrug-resistant strain (K1) of P. falciparum. The IC50 of chloroquine in the presence of isoliquiritigenin was found to drop from 0.132 to 0.013 μg/mL (10-fold). Importantly, the IC50 of isoliquiritigenin itself was found to drop from 5.2 to 1.90 μg/mL (up to 2.73-fold) in the presence of chloroquine. Similarly, with the isoliquiritigenin-artemisinin combination, it was found that the IC50 of artemisinin dropped from 3.9 to 1.75 ng/mL (2.22 times reduction). However, since all values fell within the range of >01 and ≤2, it indicated that the combination had an additive effect (Kumar et al., 2024; Table 3).
8.16 Cuscuta reflexa Roxb.
C. reflexa (Family: Convolvulaceae) has traditionally been used by the indigenous people of Odisha, India for the treatment of malaria. An in vitro evaluation was carried out to provide an empirical basis for this practice. In vitro, fractions of methanol extract from the shed-dried whole plant of C. reflexa exhibited promising effects (IC50 ranging from < 10.0 to 2.2 μg/mL) against P. falciparum R539T (artemisinin resistance strain) and RKL (chloroquine resistance strain) strains. Notably, no in vitro cytotoxicity was recorded. Moreover, C. reflexa fraction showed high in vivo parasite suppression, with a mean survival time similar to that of artesunate (19.3 vs 20.6 days) at a dose of 20 mg/kg in P. berghei ANKA-infected male Swiss-albino mice (Ojha et al., 2023; Table 3).
8.17 Caesalpinia bonducella (L.) Fleming
Another study explored the anti-malaria qualities of norcaesalpin D obtained from dichloromethane root extract of C. bonducella (Fabaceae). Additionally, crude extracts, fractions, and isolated compounds were evaluated for anti-plasmodial activity against chloroquine-resistant P. falciparum (Dd2, K1) and artemisinin-resistant P. falciparum strains. The results indicated the fractions from C. bonducella roots were found to be highly effective against K1, Dd2, and artemisinin-resistant parasites. Moreover, norcaesalpin D from C. bonducella root extract was active with IC50 of 0.98, 1.85, and 2.13 μg/mL against 3D7, Dd2, and IPC 4912-Mondolkiri parasites, respectively (Nondo et al., 2017; Table 3).
8.18 Momordica charantia L., and Diospyros monbuttensis Gürke
A Nigerian study tested the sensitivity of resistant P. falciparum species to plant extracts of M. charantia, D. monbuttensis, and M. lucida by using an in vitro micro-test (Mark III), based on assessing the inhibition of schizont maturation. Among the isolated parasites, all were sensitive to quinine, mefloquine, and artesunate; however, 51% of the isolates were resistant to chloroquine, 13% to amodiaquine, and 5% to sulphadoxine/pyrimethamine. The study found that the highest activity was obtained with an extract of D. monbuttensis with an IC50 of 3.2nM, while D. monbuttensis produced inhibitory activity with IC50 = 12.5 nM. However, the least satisfactory activity was obtained from M. lucida extract (IC50 = 25 nM) (Olasehinde et al., 2014; Table 3).
8.19 Costus afer Ker Gawl.
C. afer (Costaceae) was investigated for anti-malaria potentials in vitro activity by using its methanol stem extract and its residual aqueous fraction against chloroquine-resistant, and artemether-resistant P. falciparum strains. The extracts demonstrated significant and dose-dependent inhibitions of schizont growth in the resistant Plasmodium strains with IC50 values of 11.27 and 15.05, and 10.30 and 11.23 μg/mL against, chloroquine-resistant and artemether-resistant strains, respectively (Jimoh et al., 2019; Table 3).
8.20 Rubia cordifolia L.
R. cordifolia is part of Kenyan traditional medicine for malaria treatment; however, there is limited scientific evidence supporting this practice. To begin addressing this gap, a study aimed to assess the plant-extract efficacy, taken from the aerial part of R. cordifolia, against chloroquine-resistant (W2) P. falciparum strains. Major findings of the study included a significant inhibition of parasite growth, in a dose-dependent manner. At 50 μg/mL, inhibition was (84.07%, 77.94%, and 66.08%) for hexane, methanol, and water extracts, respectively, against the resistant strain. Hexane extract showed an IC50 0.551 μg/mL, while methanol extract gave encouraging results with an IC50 1.231 μg/mL. However, the aqueous extract showed moderate activity (IC50 = 5.348 μg/mL) (Jeremiah et al., 2023; Table 3).
9 Medicinal plants used for malaria treatment in Pakistan
The Himalayan region of Pakistan is renowned for its diverse herbal remedies the local communities use to treat various health problems. A study on these plants found that Asteraceae (11.9%) was the most cited vegetal family against malaria. Other plant families being used included Lamiaceae (5.9%), Solanaceae, Verbenaceae (4.7%), and Violaceae (3.5%). A study conducted by (Shah and Rahim, 2017), in the Soon Valley of Pakistan, indicated the use of more than fifty varieties for malaria treatment in the region. The researchers identified that anti-malarial properties of seven plant species were reported for the first time. These plant species included Withania coagulans (Stocks), Fagonia cretica L. (Zygophyllaceae), Carthamus oxyacanthus M.Bieb. (Asteraceae), Ehretia obtusifolia Hochst. ex A.DC. (Boraginaceae), Helianthus annuus L. (Asteraceae), Olea ferruginea (Aiton) Steud. (Oleaceae), and Vitex trifolia L. (Lamiaceae).
Another study focused on three local plants for their anti-malarial activity. The plant species analyzed included P. kurroa, C. bonducella, and A. absinthium. The investigators used cold alcohol, hot alcohol, and aqueous preparations of extracts obtained from the plants to ascertain their antimalarial activity. The study found that P. kurroa was more effective than the other two plants against malaria (Irshad, Mannan, and Mirza, 2011).
10 Discussion
One of the strengths of this review is its emphasis on the use of natural products for malaria treatment. Natural remedies for treating malaria have several advantages over mainstream drugs. First, natural products offer a vast array of chemical structures with diverse pharmacological properties (Dias, Urban, and Roessner, 2012). This chemical diversity provides a rich source of compounds with potential anti-malarial activity, allowing for the discovery of novel drugs or drug leads. This chemical diversity also helps in delaying the emergence of drug resistance. The prevention or at least delay of drug resistance may also be helped by the fact that many natural products exhibit activity against multiple stages of the malaria parasite’s life cycle, including the blood stages (schizonts), liver stages (hypnozoites), and transmission stages (gametocytes) (Roberts et al., 2017). This multifactorial action may help prevent the development of drug resistance and improve treatment outcomes. Natural products often contain multiple bioactive compounds that can act synergistically to enhance their anti-malarial activity. This synergism may result in greater efficacy and lower risk of drug resistance compared to single-compound therapies. Most natural products exhibit anti-malarial activity with minimal toxicity to human cells, making them potentially safer alternatives to synthetic drugs (Amelo, Nagpal, and Makonnen, 2014). This is particularly important in malaria-endemic regions where access to healthcare and monitoring for adverse drug reactions may be limited. This review not only focuses on the use of natural products for treating malaria but emphasizes that combining treatment with a hybrid approach of vector control methods with rigorous hygiene measures can be an effective strategy for combating malaria. Especially, the distribution of insecticide-treated bed nets and indoor residual spraying with long-lasting insecticides can significantly reduce mosquito populations and prevent malaria transmission indoors (Hellewell et al., 2021). Targeted application of larvicides to mosquito breeding sites, such as stagnant water bodies, can prevent the development of mosquito larvae and reduce adult mosquito populations (Lardeux et al., 2002). Implementing an integrated approach that combines multiple vector control methods, tailored to local epidemiological and ecological conditions, can enhance the effectiveness and sustainability of malaria control efforts. Establishing robust surveillance systems to monitor malaria transmission dynamics, vector populations, and insecticide resistance can inform evidence-based decision-making and guide the adaptation of control strategies over time.
11 Limitations of the herbal treatments
An important limitation of herbal medicine is the variation in the potency and effectiveness that may occur due to several factors, including geographic variations in malaria parasite strains, drug resistance patterns, and differences in local healthcare infrastructure and practices. For example, P. falciparum is the most prevalent and deadliest of the malaria parasite species, and resistance to anti-malarial drugs, such as chloroquine and sulfadoxine-pyrimethamine, has been widely reported in many regions, particularly in sub-Saharan Africa (Crider et al., 2022). National and international treatment guidelines for malaria may vary based on epidemiological data, drug resistance patterns, and healthcare resources. Treatment recommendations may be tailored to specific regions or populations to optimize treatment outcomes and minimize the risk of drug resistance. For example, ACTs are recommended as first-line treatment for uncomplicated P. falciparum malaria in most endemic regions, but the specific ACT regimen may vary based on local drug resistance patterns (Saito et al., 2023).
Another limitation of herbal treatments is the lack of standardized dosing for malaria which can have several implications, including variability in treatment efficacy, safety concerns, and challenges in comparing research findings across studies. Natural product treatments often contain complex mixtures of bioactive compounds, which may vary in concentration and composition depending on factors such as plant species, growing conditions, harvesting methods, and processing techniques (Santos-Zea et al., 2016). This variability makes it difficult to establish standardized dosing regimens across different preparations of the same natural product. Many natural products lack comprehensive pharmacokinetic data, including information on absorption, distribution, metabolism, and excretion (ADME) in humans. Without this data, it is challenging to determine optimal dosing regimens that achieve therapeutic levels of active ingredients while minimizing the risk of toxicity or suboptimal efficacy. Natural products may contain toxic compounds or interact with other medications, leading to adverse effects or drug interactions (Fugh-Berman, 2000). Without standardized dosing and rigorous safety evaluation, there is a risk of overdosing, under-dosing, or unintended adverse reactions, particularly in vulnerable populations such as children, pregnant women, or individuals with underlying health conditions.
The potential interactions of natural products such as for malaria with other medications are an important consideration, as they can impact treatment efficacy, safety, and patient outcomes, thus limiting their use (Fugh-Berman, 2000). While natural products are often perceived as safe, they contain bioactive compounds that may interact with conventional medications that may alter their pharmacokinetics or pharmacodynamics. Natural products may affect the ADME of other medications, leading to alterations in their blood levels and therapeutic effects. For example, certain natural products may inhibit or induce drug-metabolizing enzymes in the liver (e.g., cytochrome P450 enzymes), influencing the metabolism of co-administered medications and potentially leading to drug toxicity or treatment failure (Yang, Wang, and Zeng, 2002; Parvez and Rishi, 2019). Furthermore, natural products may have additive, synergistic, or antagonistic effects when combined with other medications, altering their pharmacological actions. For instance, some natural products may enhance the anticoagulant effects of blood-thinning medications or potentiate the sedative effects of central nervous system depressants. Similarly, St. John’s wort (Hypericum perforatum L.), a herbal remedy sometimes used for its antidepressant effects, could induce drug metabolizing enzymes and reduce the efficacy of medications, including certain anti-malarial drugs, oral contraceptives, and immunosuppressants (Yang, Wang, and Zeng, 2002).
12 Conclusion
Malaria remains a formidable global health challenge, causing significant morbidity and mortality, particularly in impoverished regions. Despite numerous diagnostic and medicinal breakthroughs, the Plasmodium resistance development to anti-malaria drugs will undo most of this progress. This threat has become an impetus behind the race for novel, effective, and affordable drugs and vaccines. Natural products derived from plants like A. annua, C. sanguinolenta, H. impetiginosus, P. kurroa, and A. hexapetalus are among the noteworthy sources. Interestingly, A. annua is the precursor of artemisinin, one of the most significant drugs considered a mainstay of treatment against malaria. Moreover, plants like V. lasiopus, R. prinoides, T. asiatica, C. colocynthis, and phytocompounds like isoliquiritigenin, and norcaesalpin D should be further studied for effects against drug-resistant Plasmodium species. However, while herbal medicines have advantages like cost-effectiveness and fewer side effects, they also have limitations. These limitations include variations in potency, lack of standardized dosing, and potential interactions with other medications. Therefore, either modern or herbal medicines should be used after conducting a cost-benefit analysis associated with the treatment plan. Only a balanced approach can have an effectual impact. In addition, an emphasis on early detection, accurate diagnosis, and integrated efforts for disease prevention is also crucial. Additionally, implementing stringent hygiene measures and promoting community awareness will also contribute to reducing the burden of malaria in endemic areas.
Author contributions
QA: Data curation, Formal Analysis, Investigation, Resources, Writing–original draft. NS: Data curation, Investigation, Methodology, Software, Writing–original draft, Writing–review and editing. NM: Data curation, Formal Analysis, Funding acquisition, Writing–review and editing. RN: Data curation, Formal Analysis, Funding acquisition, Writing–review and editing. FN: Data curation, Formal Analysis, Methodology, Project administration, Resources, Validation, Visualization, Writing–review and editing. SA: Formal Analysis, Methodology, Project administration, Resources, Supervision, Visualization, Writing–review and editing.
Funding
The author(s) declare financial support was received for the research, authorship, and/or publication of this article. The authors thank the United Arab Emirates University for financing this research under UPAR project G00003696, grant code 12S094.
Conflict of interest
The authors declare that the research was conducted in the absence of any commercial or financial relationships that could be construed as a potential conflict of interest.
Publisher’s note
All claims expressed in this article are solely those of the authors and do not necessarily represent those of their affiliated organizations, or those of the publisher, the editors and the reviewers. Any product that may be evaluated in this article, or claim that may be made by its manufacturer, is not guaranteed or endorsed by the publisher.
References
Abrantes, P., George, D., Grosso, A. R., Rosario, V. E. do, and Silveira, H. (2008). Chloroquine mediated modulation of Anopheles gambiae gene expression. PLoS One 3 (7), e2587. doi:10.1371/journal.pone.0002587
Acosta, M. M., Bram, J. T., Sim, D., and Read, A. F. (2020). Effect of drug dose and timing of treatment on the emergence of drug resistance in vivo in a malaria model. Evol. Med. Public Health 2020 (1), 196–210. doi:10.1093/emph/eoaa016
Adedeji, W. A., Balogun, T., Fehintola, F. A., and Morse, G. D. (2018). Drug-drug interactions of antimalarial drugs. Drug Interact. Infect. Dis. Antimicrob. Drug Interact., 503–514. doi:10.1007/978-3-319-72416-4_12
Aderinto, N., Olatunji, G., Kokori, E., Sikirullahi, S., Aboje, J. E., and Ojabo, R. E. (2024). A perspective on Oxford’s R21/Matrix-M™ malaria vaccine and the future of global eradication efforts. Malar. J. 23 (1), 16. doi:10.1186/s12936-024-04846-w
Afzal, H. S., Khan, W., Nawaz, S., Malik Utra, A., and Ahmad, T. (2022). Investigation of the diuretic potential of diosmetin, a flavonoid from citrus lemon in sprague-dawley rat model. Phytopharm. Commun. 2 (02), 115–126. doi:10.55627/ppc.002.02.0151
Alebachew, Y., Bisrat, D., Solomon, T., and Asres, K. (2021). In vivo anti-malarial activity of the hydroalcoholic extract of rhizomes of Kniphofia foliosa and its constituents. Malar. J. 20, 1–12.
Amelo, W., Nagpal, P., and Makonnen, E. (2014). Antiplasmodial activity of solvent fractions of methanolic root extract of Dodonaea angustifolia in Plasmodium berghei infected mice. BMC complementary Altern. Med. 14, 462–467. doi:10.1186/1472-6882-14-462
Auburn, S., Benavente, E. D., Miotto, O., Pearson, R. D., Amato, R., Grigg, M. J., et al. (2018). Genomic analysis of a pre-elimination Malaysian Plasmodium vivax population reveals selective pressures and changing transmission dynamics. Nat. Commun. 9 (1), 2585. doi:10.1038/s41467-018-04965-4
Auburn, S., Getachew, S., Pearson, R. D., Amato, R., Miotto, O., Trimarsanto, H., et al. (2019). Genomic analysis of Plasmodium vivax in southern Ethiopia reveals selective pressures in multiple parasite mechanisms. J. Infect. Dis. 220 (11), 1738–1749. doi:10.1093/infdis/jiz016
Bartoloni, A., and Zammarchi, L. (2012). Clinical aspects of uncomplicated and severe malaria. Mediterr. J. Hematol. Infect. Dis. 4 (1), e2012026. doi:10.4084/MJHID.2012.026
Benelli, G., and Beier, J. C. (2017). Current vector control challenges in the fight against malaria. Acta trop. 174, 91–96. doi:10.1016/j.actatropica.2017.06.028
Bright, A. T., Alenazi, T., Shokoples, S., Tarning, J., Paganotti, G. M., White, N. J., et al. (2013). Genetic analysis of primaquine tolerance in a patient with relapsing vivax malaria. Emerg. Infect. Dis. 19 (5), 802–805. doi:10.3201/eid1905.121852
Buyon, L. E., Elsworth, B., and Duraisingh, M. T. (2021). The molecular basis of antimalarial drug resistance in Plasmodium vivax. Int. J. Parasitol. Drugs Drug Resist. 16, 23–37. doi:10.1016/j.ijpddr.2021.04.002
Castelli, F., Tomasoni, L. R., and Matteelli, A. (2012). Advances in the treatment of malaria. Mediterr. J. Hematol. Infect. Dis. 4 (1), e2012064. doi:10.4084/MJHID.2012.064
Chaorattanakawee, S., Lon, C., Chann, S., Thay, K. H., Kong, N., You, Y., et al. (2017). Measuring ex vivo drug susceptibility in Plasmodium vivax isolates from Cambodia. Malar. J. 16, 392–413. doi:10.1186/s12936-017-2034-2
Chiodini, P. L., Lalloo, D. G., Shingadia, D., Pasvol, G., Whitty, C. J., Beeching, N. J., et al. HPA Advisory Committee on Malaria Prevention in UK Travellers (2007). UK malaria treatment guidelines. J. Infect. 54 (2), 111–121. doi:10.1016/j.jinf.2006.12.003
Commission, African Union (2023). 2023 Africa malaria progress report. Addis Ababa, Ethiopia: Commission, African Union.
Crider, K. S., Williams, J. L., Qi, Y. P., Gutman, J., Yeung, L. F., Mai, C. T., et al. (2022). Folic acid supplementation and malaria susceptibility and severity among people taking antifolate antimalarial drugs in endemic areas. Cochrane Database Syst. Rev. 2022 (2). doi:10.1002/14651858.CD014217
Daddy, N. B., Malemo Kalisya, L., Gisenya Bagire, P., Watt, R. L., Towler, M. J., and Weathers, P. J. (2017). Artemisia annua dried leaf tablets treated malaria resistant to ACT and i.v. artesunate: case reports. Phytomedicine 32, 37–40. doi:10.1016/j.phymed.2017.04.006
Dias, D. A., Urban, S., and Roessner, U. (2012). A historical overview of natural products in drug discovery. Metabolites 2 (2), 303–336. doi:10.3390/metabo2020303
Ekasari, W., Widya Pratiwi, D., Amanda, Z., Widyawaruyanti, A., Arwati, H., and Arwati, H. (2019). Various parts of Helianthus annuus plants as new sources of antimalarial drugs. Evidence-Based Complementary Altern. Med. 2019, 7390385. no. 2019. doi:10.1155/2019/7390385
Elfawal, M. A., Towler, M. J., Reich, N. G., Weathers, P. J., and Rich, S. M. (2015). Dried whole-plant Artemisia annua slows evolution of malaria drug resistance and overcomes resistance to artemisinin. Proc. Natl. Acad. Sci. 112 (3), 821–826. doi:10.1073/pnas.1413127112
Elmi, T., Hajialiani, F., Asadi, M. R., Sadeghi, S., Namazi, M. J., Tabatabaie, F., et al. (2021). Antimalarial effects of the hydroalcoholic extract of Allium paradoxum in vitro and in vivo. J. Parasit. Dis. 45 (4), 1055–1064. doi:10.1007/s12639-021-01359-0
El-Moamly, A. A., and El-Sweify, M. A. (2023). Malaria vaccines: the 60-year journey of hope and final success—lessons learned and future prospects. Trop. Med. Health 51 (1), 29. doi:10.1186/s41182-023-00516-w
Farnsworth, N. R., and Soejarto, D. D. (1991). Global importance of medicinal plants. conservation Med. plants 26 (26), 25–51.
Flannery, E. L., Wang, T., Akbari, A., Corey, V. C., Gunawan, F., Bright, A. T., et al. (2015). Next-generation sequencing of Plasmodium vivax patient samples shows evidence of direct evolution in drug-resistance genes. ACS Infect. Dis. 1 (8), 367–379. doi:10.1021/acsinfecdis.5b00049
Fugh-Berman, A. (2000). Herb-drug interactions. Lancet 355 (9198), 134–138. doi:10.1016/S0140-6736(99)06457-0
Garcia, L. S. (2010). Malaria. Clin. Laboratory Med. no. 30 (1), 93–129. doi:10.1016/j.cll.2009.10.001
Gilles, H. M., and Warrell, D. A. (1996). Bruce-Chwatt's essential malariology. Edward Arnold (Publisher) Ltd.
Giribaldi, G., D’Alessandro, S., Prato, M., and Basilico, N. (2015). Etiopathogenesis and pathophysiology of malaria. Hum. Mosquito Lysozymes Old Mol. New Approaches Against Malar., 1–18. doi:10.1007/978-3-319-09432-8_1
Grellier, P., Ramiaramanana, L., Millerioux, V., Deharo, E., Schrével, J., Frappier, F., et al. (1996). Antimalarial activity of cryptolepine and isocryptolepine, alkaloids isolated from Cryptolepis sanguinolenta. Phytotherapy Res. 10 (4), 317–321. doi:10.1002/(sici)1099-1573(199606)10:4<317::aid-ptr858>3.3.co;2-s
Guissou, R. M., Amaratunga, C., De Haan, F., Tou, F., Yeong Cheah, P., Yerbanga, R. S., et al. (2023). The impact of anti-malarial markets on artemisinin resistance: perspectives from Burkina Faso. Malar. J. 22 (1), 269. doi:10.1186/s12936-023-04705-0
Haddad, M. H. F., Mahbodfar, H., Zamani, Z., and Ali, R. (2017). Antimalarial evaluation of selected medicinal plant extracts used in Iranian traditional medicine. Iran. J. Basic Med. Sci. no. 20 (4), 415–422. doi:10.22038/IJBMS.2017.8583
Hassan, I., Haq, A.Ul, Shah, S. M., Akhtar, I., Begum, A., and Shaheen, S. (2021). Qualitative phytochemical screening and anti-inflammatory evaluation of aerial Part Extracts of medicago laciniata (L.) mill. Phytopharm. Commun. 1 (01), 09–16. doi:10.55627/ppc.001.01.0099
Hawkins, V. N., Joshi, H., Rungsihirunrat, K., Na-Bangchang, K., and Sibley, C. H. (2007). Antifolates can have a role in the treatment of Plasmodium vivax. Trends Parasitol. 23 (5), 213–222. doi:10.1016/j.pt.2007.03.002
Hellewell, J., Sherrard-Smith, E., Ogoma, S., and Churcher, T. S. (2021). Assessing the impact of low-technology emanators alongside long-lasting insecticidal nets to control malaria. Philosophical Trans. R. Soc. B 376 (1818), 20190817. doi:10.1098/rstb.2019.0817
Irshad, S., Mannan, A., and Mirza, B. (2011). Antimalarial activity of three Pakistani medicinal plants. Pak. J. Pharm. Sci. 24 (4), 589–591.
Jeremiah, M., Kamau, L., Nyambati, G., and Hastings, O. (2023). Rubia cordifolia L. Crude aerial Part Extracts show a high potential as antimalarials against chloroquine resistant plasmodium falciparum strains in vitro. Pharmacogn. Res. 15 (2), 288–296. doi:10.5530/pres.15.2.031
Jimoh, A. A., Maiha, B. B., Chindo, B. A., Ejiofor, J. I., Ehinmidu, J. O., Atang, D. A., et al. (2019). In vitro antiplasmodial activity of methanol stem extract of Costus afer Ker Gawl.(Costaceae) and its residual aqueous fraction against some drug-sensitive and drug-resistant Plasmodium falciparum strains. Trop. J. Nat. Prod. Res. 3 (5), 162–169. doi:10.26538/tjnpr/v3i5.3
Khan, M. I., Qureshi, H., Bae, S. J., Ali Khattak, A., Anwar, M. S., Ahmad, S., et al. (2023a). Malaria prevalence in Pakistan: a systematic review and meta-analysis (2006–2021). Heliyon 9, e15373. doi:10.1016/j.heliyon.2023.e15373
Khan, N., Awasthi, G., and Das, A. (2023b). How can the complex epidemiology of malaria in India impact its elimination? Trends Parasitol. 39, 432–444. doi:10.1016/j.pt.2023.03.006
Kingston, D. G. I., and Cassera, M. B. (2022). Antimalarial natural products. Berlin, Germany: Springer.
Kumar, S., Mina, P. R., Bhatt, D., Kumar, P., Singh, M., Shanker, K., et al. (2024). Isoliquiritigenin from licorice root: a multi-stage anti-malarial with synergistic impact on multidrug-resistant P. Falciparum. Pharmacol. Research-Modern Chin. Med. 10, 100396. doi:10.1016/j.prmcm.2024.100396
Lardeux, F., Rivière, F., Sechan, Y., and Loncke, S. (2002). Control of the Aedes vectors of the dengue viruses and Wuchereria bancrofti: the French Polynesian experience. Ann. Trop. Med. Parasitol. no. 96 (2), 105–116. doi:10.1179/000349802125002455
Lemma, M. T., Ali, M. A., Mohamed, T. E., Ngo, H. T., Tran, L.-H.Vu, Sang, To K., et al. (2017). Medicinal plants for in vitro antiplasmodial activities: a systematic review of literature. Parasitol. Int. 66 (6), 713–720. doi:10.1016/j.parint.2017.09.002
Li, J., Zhang, J., Li, Q., Hu, Y., Ruan, Y., Tao, Z., et al. (2020). Ex vivo susceptibilities of Plasmodium vivax isolates from the China-Myanmar border to antimalarial drugs and association with polymorphisms in Pvmdr1 and Pvcrt-o genes. PLOS Neglected Trop. Dis. 14 (6), e0008255. doi:10.1371/journal.pntd.0008255
MacMillen, Z., Hatzakis, K., Simpson, A., Shears, M. J., Watson, F., Erasmus, J. H., et al. (2024). Accelerated prime-and-trap vaccine regimen in mice using repRNA-based CSP malaria vaccine. npj Vaccines 9 (1), 12. doi:10.1038/s41541-023-00799-4
Markus, M. B. (2011). Malaria: origin of the term “hypnozoite”. J. Hist. Biol. 44, 781–786. doi:10.1007/s10739-010-9239-3
Marwa, K., Kapesa, A., Baraka, V., Konje, E., Kidenya, B., Jackson, M., et al. (2022). Therapeutic efficacy of artemether-lumefantrine, artesunate-amodiaquine and dihydroartemisinin-piperaquine in the treatment of uncomplicated Plasmodium falciparum malaria in Sub-Saharan Africa: a systematic review and meta-analysis. PloS one 17 (3), e0264339. doi:10.1371/journal.pone.0264339
Maximus, M. TAEK, Tukan, G. D., Prajogo, B. E. W., and Mangestuti, AGIL (2021). Antiplasmodial activity and phytochemical constituents of selected antimalarial plants used by native people in west timor Indonesia. Turkish J. Pharm. Sci. 18 (1), 80–90. doi:10.4274/tjps.galenos.2019.29000
McIntosh, H., and Olliaro, P.Cochrane Infectious Diseases Group (1996). Artemisinin derivatives for treating uncomplicated malaria. Cochrane database Syst. Rev. 2019 (5).
Misganaw, D., Amare, G. G., and Mengistu, G. (2020). Chemo suppressive and curative potential of Hypoestes forskalei against Plasmodium berghei: evidence for in vivo antimalarial activity. J. Exp. Pharmacol. 12, 313–323. doi:10.2147/JEP.S262026
Mohammadi, S., Jafari, B., Asgharian, P., Martorell, M., and Rad, J. S. (2020). Medicinal plants used in the treatment of Malaria: a key emphasis to Artemisia, Cinchona, Cryptolepis, and Tabebuia genera. Phytotherapy Res. 34 (7), 1556–1569. doi:10.1002/ptr.6628
Moyo, P., Mugumbate, G., Eloff, J. N., Louw, A. I., Maharaj, V. J., and Birkholtz, L.-M. (2020). Natural products: a potential source of malaria transmission blocking drugs? Pharmaceuticals 13 (9), 251. doi:10.3390/ph13090251
Muregi, F. W., Ishih, A., Suzuki, T., Kino, H., Amano, T., Mkoji, G. M., et al. (2007). In vivo antimalarial activity of aqueous extracts from Kenyan medicinal plants and their chloroquine (CQ) potentiation effects against a blood-induced CQ-resistant rodent parasite in mice. Phytotherapy Res. An Int. J. Devoted Pharmacol. Toxicol. Eval. Nat. Prod. Deriv. no. 21 (4), 337–343. doi:10.1002/ptr.2067
Nawaz, R., Ishtiaq, H., and Anwar, T. H. (2022). Cardioprotective effects of malus domestica (apple) may be mediated through antiplatelet, calcium channel blocking, and antioxidant properties. Phytopharm. Commun. 2 (01), 09–19. doi:10.55627/ppc.002.01.0048
Nondo, R. S. O., Julius Moshi, M., Erasto, P., Masimba, P. J., Machumi, F., Kidukuli, A. W., et al. (2017). Anti-plasmodial activity of Norcaesalpin D and extracts of four medicinal plants used traditionally for treatment of malaria. BMC complementary Altern. Med. 17, 167–168. doi:10.1186/s12906-017-1673-8
Nunes, M. C., and Scherf, A. (2007). Plasmodium falciparum during pregnancy: a puzzling parasite tissue adhesion tropism. Parasitology 134 (13), 1863–1869. doi:10.1017/S0031182007000133
Obeagu, E. I., Uzoma Obeagu, G., Ihuoma Ubosi, N., Ijeoma, C. U., and Eltayeb, M. A. T. (2024). Concurrent management of HIV and malaria: a comprehensive review of strategies to enhance quality of life. Medicine 103 (14), e37649. doi:10.1097/MD.0000000000037649
Ojha, S. B., Kumar Sah, R., Madan, E., Bansal, R., Roy, S., Singh, S., et al. (2023). Cuscuta reflexa possess potent inhibitory activity against human malaria parasite: an in vitro and in vivo study. Curr. Microbiol. 80 (5), 189. doi:10.1007/s00284-023-03289-x
Olasehinde, G. I., Ojurongbe, O., Adeyeba, A. O., Fagade, O. E., Valecha, N., Ayanda, I. O., et al. (2014). In vitro studies on the sensitivity pattern of Plasmodium falciparum to anti-malarial drugs and local herbal extracts. Malar. J. 13, 63–67. doi:10.1186/1475-2875-13-63
Organization, World Health (2023). WHO malaria policy advisory group (MPAG) meeting report. World Health Organization.
Osafo, N., Mensah, K. B., and Yeboah, O. K. (2017). Phytochemical and pharmacological review of cryptolepis sanguinolenta (lindl.) schlechter. Adv. Pharmacol. Pharm. Sci. 2017, 3026370. doi:10.1155/2017/3026370
Parvez, M. K., and Rishi, V. (2019). Herb-drug interactions and hepatotoxicity. Curr. drug Metab. 20 (4), 275–282. doi:10.2174/1389200220666190325141422
Patel, C., and Roy, D. (2021). A computational study of molecular mechanism of chloroquine resistance by chloroquine resistance transporter protein of plasmodium falciparum via molecular modeling and molecular simulations. Physchem 1 (3), 232–242. doi:10.3390/physchem1030017
Phillips-Howard, P. A., and Wood, D. (1996). The safety of antimalarial drugs in pregnancy. Drug Saf. 14 (3), 131–145. doi:10.2165/00002018-199614030-00001
Phillipson, J. D. (1990). “Possibilities of finding new products from plant cell cultures,” in Paper read at Progress in Plant Cellular and Molecular Biology: Proceedings of the VIIth International Congress on Plant Tissue and Cell Culture, Amsterdam, The Netherlands, June, 1990, 24–29.
Phuwajaroanpong, A., Chaniad, P., Horata, N., Muangchanburee, S., Kaewdana, K., and Punsawad, C. (2020). In vitro and in vivo antimalarial activities and toxicological assessment of Pogostemon cablin (Blanco) Benth. J. Evidence-Based Integr. Med. 25, 2515690X20978387. doi:10.1177/2515690X20978387
Plowe, C. V. (2022). Malaria chemoprevention and drug resistance: a review of the literature and policy implications. Malar. J. 21 (1), 104. doi:10.1186/s12936-022-04115-8
Pradines, B., and Robert, M.-G. (2019). Current situation of malaria in the world. La Rev. Du. Prat. 69 (2), 146–149.
Reed, M. B., Saliba, K. J., Caruana, S. R., Kirk, K., and Cowman, A. F. (2000). Pgh1 modulates sensitivity and resistance to multiple antimalarials in Plasmodium falciparum. Nature 403 (6772), 906–909. doi:10.1038/35002615
Roberts, B. F., Zheng, Y., Jacob, C., Lee, S., Lee, E., Ayong, L., et al. (2017). 4-Nitro styrylquinoline is an antimalarial inhibiting multiple stages of Plasmodium falciparum asexual life cycle. Int. J. Parasitol. Drugs Drug Resist. 7 (1), 120–129. doi:10.1016/j.ijpddr.2017.02.002
Rogers, W. O., Sem, R., Tero, T., Chim, P., Lim, P., Muth, S., et al. (2009a). Failure of artesunate-mefloquine combination therapy for uncomplicated Plasmodium falciparum malaria in southern Cambodia. Malar. J. 8 (1), 10–19. doi:10.1186/1475-2875-8-10
Rogers, W. O., Sem, R., Tero, T., Chim, P., Lim, P., Muth, S., et al. (2009b). Failure of artesunate-mefloquine combination therapy for uncomplicated Plasmodium falciparum malaria in southern Cambodia. Malar. J. 8, 10–19. doi:10.1186/1475-2875-8-10
Sá, J. M., Kaslow, S. R., Moraes Barros, R. R., Brazeau, N. F., Parobek, C. M., Tao, D., et al. (2019). Plasmodium vivax chloroquine resistance links to pvcrt transcription in a genetic cross. Nat. Commun. 10 (1), 4300. doi:10.1038/s41467-019-12256-9
Saito, M., Rose, M. G., Tinto, H., Rouamba, T., Mosha, D., Rulisa, S., et al. (2023). Pregnancy outcomes after first-trimester treatment with artemisinin derivatives versus non-artemisinin antimalarials: a systematic review and individual patient data meta-analysis. Lancet 401 (10371), 118–130. doi:10.1016/S0140-6736(22)01881-5
Santos-Zea, L., Pérez, A. M. R., Leal-Díaz, A. M., and Uribe, J. A. G. (2016). Variability in saponin content, cancer antiproliferative activity and physicochemical properties of concentrated agave sap. J. food Sci. 81 (8), H2069–H2075. doi:10.1111/1750-3841.13376
Shah, A., and Rahim, S. (2017). Ethnomedicinal uses of plants for the treatment of malaria in Soon Valley, Khushab, Pakistan. J. Ethnopharmacol. 200, 84–106. doi:10.1016/j.jep.2017.02.005
Shahbodaghi, S. D., and Rathjen, N. A. (2022). Malaria: prevention, diagnosis, and treatment. Am. Fam. physician 106 (3), 270–278.
Silva, N. C. da, Goncalves, S. F., Araújo, L. S. de, Kasper, A. A. M., Fonseca, A. L. da, Sartoratto, A., et al. (2019). In vitro and in vivo antimalarial activity of the volatile oil of Cyperus articulatus (Cyperaceae). Acta Amaz. 49, 334–342. doi:10.1590/1809-4392201804331
Sissoko, M. S., Healy, S. A., Katile, A., Zaidi, I., Hu, Z., Kamate, B., et al. (2022). Safety and efficacy of a three-dose regimen of Plasmodium falciparum sporozoite vaccine in adults during an intense malaria transmission season in Mali: a randomised, controlled phase 1 trial. Lancet Infect. Dis. 22 (3), 377–389. doi:10.1016/S1473-3099(21)00332-7
Suh, K. N., Kain, K. C., and Keystone, J. S. (2004). Malaria. Cmaj 170 (11), 1693–1702. doi:10.1503/cmaj.1030418
Takashima, E., Otsuki, H., Morita, M., Ito, D., Nagaoka, H., Yuguchi, T., et al. (2024). The need for novel asexual blood-stage malaria vaccine candidates for Plasmodium falciparum. Biomolecules 14 (1), 100. doi:10.3390/biom14010100
Talapko, J., Škrlec, I., Alebić, T., Jukić, M., and Včev, A. (2019). Malaria: the past and the present. Microorganisms 7 (6), 179. doi:10.3390/microorganisms7060179
Tiono, A. B., Plieskatt, J. L., Ouedraogo, A., Soulama, B. I., Miura, K., Bougouma, E. C., et al. (2024). A randomized first-in-human phase I trial of differentially adjuvanted Pfs48/45 malaria vaccines in Burkinabé adults. J. Clin. Investigation 134 (7), e175707. doi:10.1172/JCI175707
Trampuz, A., Jereb, M., Muzlovic, I., and Prabhu, R. M. (2003). Clinical review: severe malaria. Crit. care 7 (7), 315–323. doi:10.1186/cc2183
Vestergaard, L. S., and Ringwald, P. (2007). Responding to the challenge of antimalarial drug resistance by routine monitoring to update national malaria treatment policies. Defin. Defeating Intolerable Burd. Malar. III Prog. Perspect. Suppl. Volume 77 (6) Am. J. Trop. Med. Hyg. 77, 153–159. doi:10.4269/ajtmh.2007.77.153
Wångdahl, A., Sondén, K., Wyss, K., Stenström, C., Björklund, D., Zhang, J., et al. (2022). Relapse of Plasmodium vivax and Plasmodium ovale malaria with and without primaquine treatment in a nonendemic area. Clin. Infect. Dis. 74 (7), 1199–1207. doi:10.1093/cid/ciab610
Wardani, A. K., Wahid, A. R., and Rosa, N. S. (2020). In vitro antimalarial activity of ashitaba root extracts (Angelica keiskei k.). Res. J. Pharm. Technol. no. 13 (8), 3771–3776. doi:10.5958/0974-360x.2020.00667.8
Weinke, T., Held, T., Trautmann, M., Rögler, G., Mravak, S., Alexander, M., et al. (1992). Malaria therapy in 452 patients, with special reference to the use of quinine. J. Infect. 25 (2), 173–180. doi:10.1016/0163-4453(92)94012-m
White, N. J. (1985). Clinical pharmacokinetics of antimalarial drugs. Clin. Pharmacokinet. 10, 187–215. doi:10.2165/00003088-198510030-00001
Whitty, C. J. M., Edmonds, S., and Mutabingwa, T. K. (2005). Malaria in pregnancy. BJOG An Int. J. Obstetrics Gynaecol. 112 (9), 1189–1195. doi:10.1111/j.1471-0528.2005.00714.x
WHO (2022). Essential comprehensive up-to-date assessment of trends in global malaria control and elimination published by the World Health Organization. Geneva, Switzerland: World Health Organization.
WHO (2023a). WHO guidelines for malaria, 14 march 2023. Geneva, Switzerland: World Health Organization.
WHO (2023b). WHO malaria policy advisory group (MPAG) meeting report. Geneva, Switzerland: World Health Organization. 18–20 April 2023.
Wicht, K. J., Mok, S., and Fidock, D. A. (2020). Molecular mechanisms of drug resistance in plasmodium falciparum malaria. Annu. Rev. Microbiol. 74, 431–454. doi:10.1146/annurev-micro-020518-115546
World Health, Organization (2023). WHO guidelines for malaria, 14 March 2023. Geneva: World Health Organization.
Yang, X.-fen, Wang, N.-ping, and Zeng, F.-dian (2002). Effects of the active components of some Chinese herbs on drug metabolizing-enzymes. Zhongguo Zhong yao za zhi= Zhongguo zhongyao zazhi= China J. Chin. materia medica 27 (5), 325–328.
Zime-Diawara, H., Habib, G., Gbaguidi, F., Yemoa, A., Bero, J., Jansen, O., et al. (2015). The antimalarial action of aqueous and hydro alcoholic extracts of Artemisia annua L. cultivated in Benin: in vitro and in vivo studies. J. Chem. Pharm. Res. 7 (8), 817–823.
Keywords: malaria, natural products, Artemisia, Cinchona, drug resistance
Citation: Ain QT, Saleem N, Munawar N, Nawaz R, Naseer F and Ahmed S (2024) Quest for malaria management using natural remedies. Front. Pharmacol. 15:1359890. doi: 10.3389/fphar.2024.1359890
Received: 22 December 2023; Accepted: 29 May 2024;
Published: 26 June 2024.
Edited by:
Rajeev K. Singla, Sichuan University, ChinaReviewed by:
Vinod Kumar, GD Goenka University, IndiaMarcus Scotti, Federal University of Paraíba, Brazil
Michael P. Okoh, Sichuan University, China
Copyright © 2024 Ain, Saleem, Munawar, Nawaz, Naseer and Ahmed. This is an open-access article distributed under the terms of the Creative Commons Attribution License (CC BY). The use, distribution or reproduction in other forums is permitted, provided the original author(s) and the copyright owner(s) are credited and that the original publication in this journal is cited, in accordance with accepted academic practice. No use, distribution or reproduction is permitted which does not comply with these terms.
*Correspondence: Nayla Munawar, nmunawar@uaeu.ac.ae; Faiza Naseer, faiza.naseer@ymail.com