- 1Chongqing Key Laboratory of Neurobiology, Department of Teaching Experiment Center, College of Basic Medicine, Army Medical University, Chongqing, China
- 2Battalion, College of Basic Medicine, Army Medical University, Chongqing, China
Low selectivity and tumor drug resistance are the main hinderances to conventional radiotherapy and chemotherapy against tumor. Ion interference therapy is an innovative anti-tumor strategy that has been recently reported to induce metabolic disorders and inhibit proliferation of tumor cells by reordering bioactive ions within the tumor cells. Calcium cation (Ca2+) are indispensable for all physiological activities of cells. In particular, calcium overload, characterized by the abnormal intracellular Ca2+ accumulation, causes irreversible cell death. Consequently, calcium overload-based ion interference therapy has the potential to overcome resistance to traditional tumor treatment strategies and holds promise for clinical application. In this review, we 1) Summed up the current strategies employed in this therapy; 2) Described the outcome of tumor cell death resulting from this therapy; 3) Discussed its potential application in synergistic therapy with immunotherapy.
1 Introduction
Toxicity to normal tissues and drug resistance have long limited the application of traditional tumor therapies, such as radiotherapy, chemotherapy, and immunotherapy. Even worse, there continues to be a rapid increase in the number of tumor patients worldwide. According to the “Global Cancer Report 2020” issued by the World Health Organization (WHO), the estimated number of new cancer cases worldwide in 2020 is approximately 19.3 million, with around 10 million cancer-related deaths (Sung et al., 2021). The global burden of cancer is estimated to reach 28.4 million cases by 2040, an increase of 47% compared to 2020. Therefore, it is urgent to develop novel cancer therapies to alleviate patient pain and improve patient outcomes.
Ca2+, an essential intracellular second messenger, plays an irreplaceable role in different kinds of fundamental physiological processes including cell cycle, gene expression, intracellular transport and cell death (Giorgi et al., 2018). Considering the significance of Ca2+, there are various calcium regulatory mechanisms, including transmembrane transport, mitochondrial and endoplasmic reticulum (ER) buffering, maintaining the concentration and distribution of intracellular Ca2+ at a physiological level. Nevertheless, the artificial manipulation of calcium regulatory mechanisms can disturb intracellular calcium homeostasis and potentially induce calcium overload. Calcium overload could cause severe affects to cell function, which mediates irreversible damage and even death of cells. Recently, researchers have reported a strategy that might potentially lead to a novel anti-tumor therapy, called calcium overload-based ion interference therapy (Zhang et al., 2019; Bai et al., 2022).
Calcium overload is a prevalent pathophysiological mechanisms of cell death, resulting in obvious alterations in cell structure and function due to the abnormal accumulation of Ca2+(Petersen et al., 2021). A growing number of evidence suggests that calcium overload has an irreplaceable role in tumor therapy. Although modestly increased Ca2+ have been reported to promote tumor proliferation and metastasis (Cui et al., 2017), calcium overload could reverse the pro-proliferative effect to a pro-apoptotic effect, which significantly inhibits tumor proliferation and improves drug susceptibility (Peters et al., 2017; Wang et al., 2019; Patergnani et al., 2020).
Therefore, calcium overload-based ion interference is considered to be a prospective anti-tumor therapy with the characteristics of disrupting calcium homeostasis in tumor cells, interfering with physiological processes related to tumor proliferation and disrupting the normal structure and function of tumor cells (Zhang et al., 2019). In addition, this therapy has also been shown to induce or augment immune responses, resulting in enhanced anti-tumor immune effects when synergistically employed with immunotherapy. (Chen et al., 2018; Zheng et al., 2021a). We have briefly described the main content of this review in Figure 1. To be specific, this review will present several strategies for implementing this therapy and summarize different outcomes of cell death mediated by this therapy, ending with a discussion of the feasibility of combining this therapy with immunotherapy.
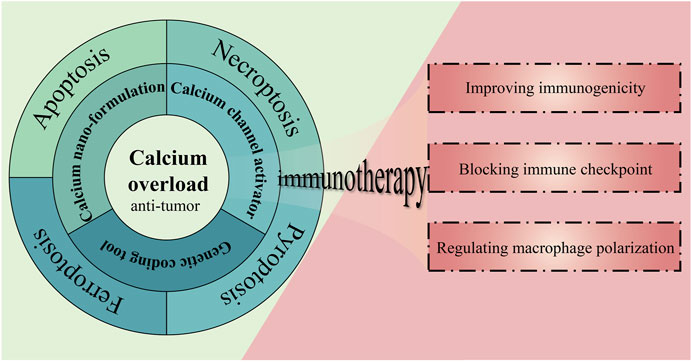
FIGURE 1. The strategies, outcomes, and prospects of calcium overload-based ion interference therapy in tumor treatment.
2 Incentive strategies of calcium overload-based ion interference therapy
2.1 The conventional calcium channel activators
Currently, calcium overload-based ion interference therapy primarily relies on conventional calcium channel activators. These activators can act on various types of intracellular calcium channels and result in a significant elevation in intracellular Ca2+. Here, we have summarized some conventional calcium channel activators with significant anti-tumor properties in Table 1. These conventional activators can disrupt intracellular calcium homeostasis and cause calcium overload through a variety of ways.
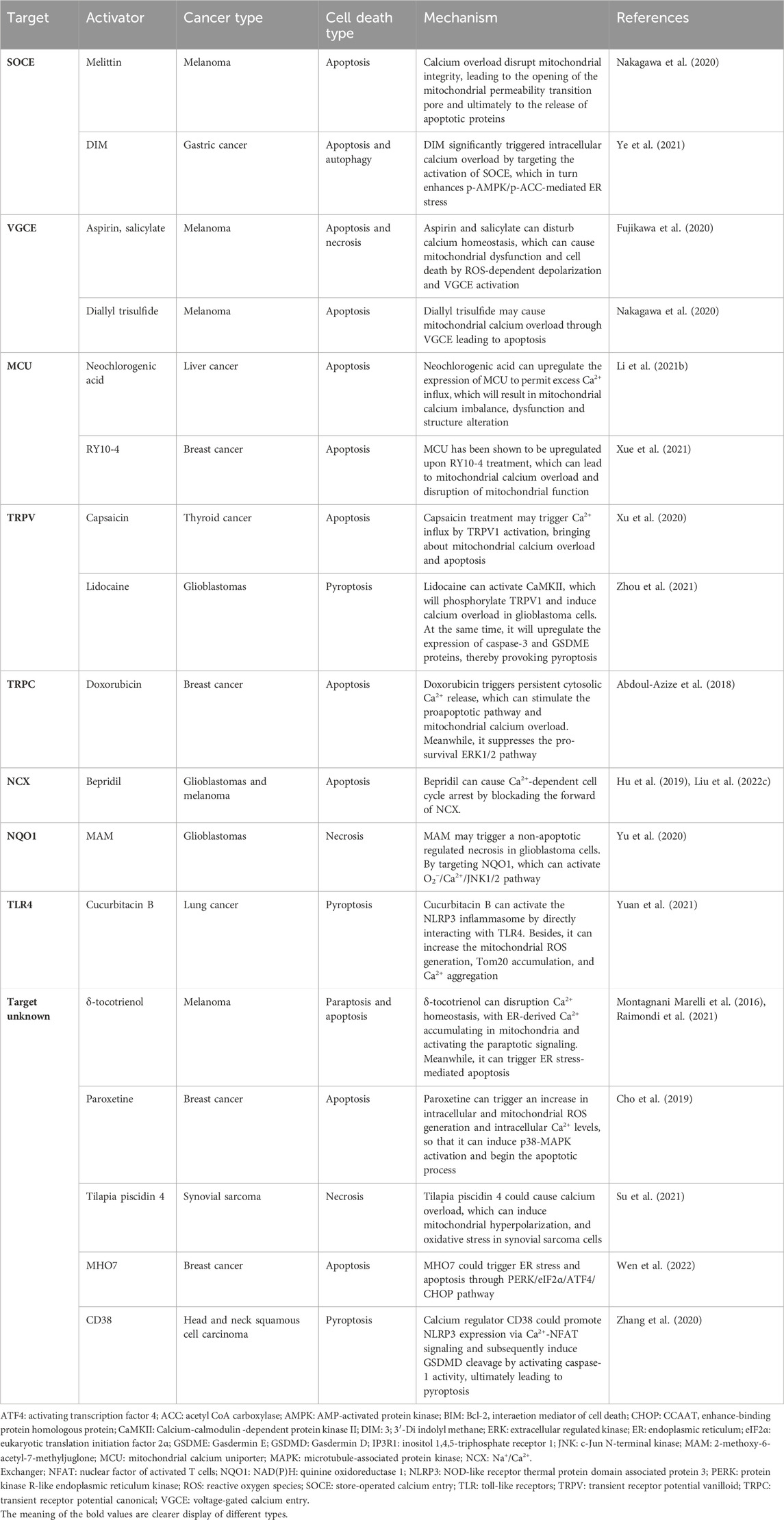
TABLE 1. The conventional calcium channel activators with the ability to induce calcium overload in tumor cells.
Firstly, with the continuous activation of the calcium channels located on the cell membrane, the intracellular Ca2+ accumulate abnormally so as to reach the level of calcium overload. Ye et al. found that 3, 3′-Diindolylmethane (DIM) could apparently inhibit proliferation and induce apoptosis as well as autophagy in two gastric cancer cell lines (Ye et al., 2021). Following DIM treatment, activation of stromal interaction molecule 1 (STIM1)-mediated store-operated Ca2+ entry (SOCE) could lead to sustained cytoplasmic calcium overload. Mechanistically, DIM activated SOCE, which apparently increase intracellular Ca2+ and subsequently led to the activation of p-AMPK/p-ACC expression and ER stress. Additionally, as well as promoting the inward flow of Ca2+, calcium overload could also be provoked by blocking the efflux of Ca2+. Hu et al. revealed that Bepridil could significantly elevate intracellular calcium concentrations and rapidly kill glioma cells by blocking the forward mode of Na+/Ca2+ exchanger (Hu et al., 2019). Furthermore, it has been confirmed that Bepridil wound not affect the growth of normal glial cells. In addition to affecting calcium channels on the cell membrane, certain activators can interact with calcium channels localized on organelles and release internal calcium stores. Neochlorogenic acid has been confirmed to upregulate the expression of mitochondrial calcium uniporter (MCU) which could permit excess Ca2+ influx (Li et al., 2021b). Analogously, when Yue et al. used the Sirtuin-1 inhibitor inauhzi to treat colorectal cancer (CRC), they demonstrated that inauhzi inhibited CRC by promoting acetylation of the MCU, which in turn enhanced mitochondrial Ca2+ uptake and caused mitochondria calcium overload (Sun et al., 2022). Thirdly, intracellular Ca2+ could be raised by indirectly affecting calcium channels. Ugur et al. found that digitalis toxin combined with microtubule-associated protein kinase (MAPK)/extracellular signal-regulated kinase inhibitors could increase intracellular calcium levels by lowering intracellular pH in certain melanoma cells. Then, it would transport extracellular Ca2+ into some organelles, resulting in calcium overload (Eskiocak et al., 2016).
2.2 Novel calcium nano-formulation
Novel calcium nano-formulations are increasingly favored by researchers in various aspects of tumor therapy for their low toxicity, excellent biocompatibility and ability to directly alter the distribution of intracellular Ca2+(Yao et al., 2022a; Bai et al., 2022). In Table 2, we have summarized various novel calcium nano-formulations that have displayed significant tumor-suppressive effects. For instance, Bu et al. designed and wet-chemically synthesized a class of ultra-small SH-CaO2 nanoparticles (Zhang et al., 2019). It was reported that transmitting the nanoparticles into tumor lesions could diminish calcium regulatory ability of the tumor and trigger a sustained cellular calcium overload effect. In this nano-system, the pH-sensitive CaO2 nanoparticles could slowly transform into Ca2+ and H2O2 in the mildly acidic tumor microenvironment (TME), concurrently triggering intracellular calcium overload and oxidative stress. However, introducing a large amount of exogenous Ca2+ would inevitably disrupt intracellular homeostasis and cause acute inflammatory reactions. It becomes an ideal anti-tumor strategy to mediate endogenous calcium overload in situ without introducing exogenous ions (Liu et al., 2020; Chu et al., 2021). Therefore, a nano-drug delivery system named UC-ZIF/BER was synthesized by Bu et al. Upon 980 nm near-infrared light stimulation, the release of nitric oxide from UC-ZIF/BER activated ryanodine receptors which rapidly increased intracellular Ca2+ concentration. At the same time, the calcium pump inhibitor berbamine specifically blocked Ca2+-ATPases on the cell membrane and thereby induced endogenous calcium overload (Chu et al., 2021). Moreover, calcium nano-formulations could combine with several efficient therapies, such as chemodynamical therapy, phototherapy, sonodynamic therapy. This strategy has shown greater anti-tumor effects and much broader application prospects. Ni et al. developed semiconductor polymer nanoparticles modified with capsaicin, which could cause intracellular calcium overload through near-infrared light stimulation without introducing additional Ca2+ (Schwartz et al., 2022). Specifically, under near-infrared light stimulation, it can release capsaicin to activate transient receptor potential vanilloid (TRPV1), leading to an influx of Ca2+ into cells. Moreover, singlet oxygen generated by nanoparticles can also induce phototherapy. Many ions such as iron, copper, and cobalt also have a significant effect on various physiological activities of cells. Therefore, the composite calcium nano-formulation based on multiple ions synergistically would theoretically exert more powerful anti-tumor ability. Yan et al. constructed a cascade nanocatalytic platform by curcumin and transferrin co-loaded CaO2 nanoparticles (Yin et al., 2021). This nanocatalytic platform could target to tumor and achieves highly efficient anti-tumor effects by interfering with the metabolic processes of calcium and iron ions. It was a great combination of ferroptosis, calcium overload therapy and chemotherapy.
2.3 Calcium channels-based gene coding tools
With the great development of gene editing technology, a series of gene encoding tools represented by optogenetic tools have been designed to achieve the precise regulation of various types of physiological processes within cells. Optogenetics is pioneered by the noted scientist Karl Deisseroth. It combines optical and genetic methods to accurately manipulate diverse physiological processes in specific cells of a living tissue (Tan et al., 2022). Recently, a large number of novel calcium channel-based optogenetic tools have been developed to manipulate calcium signaling pathway and influence the expression of related genes (Tan et al., 2022). For example, He et al. presented a light-controlled calcium channel named Opto-CRAC that can respond to 470 nm blue-light irradiation (He et al., 2015). It is composed of photosensitive protein LOV2 and stromal interaction molecule (STIM) 1 fragment, which achieves precise spatiotemporal control of Ca2+ release-activated Ca2+ (CRAC) channels through light stimulation. Moreover, it has been proven that Opto-CRAC could regulate immune responses and inhibit the proliferation of melanoma in mouse model. However, the calcium signaling induced by Opto-CRAC is dependent on the expression of ORAI proteins. The further application of Opto-CRAC in cells and tissues expressing ORAI at low levels is restricted. To overcome the limitation of Opto-CRAC, a novel light-controlled calcium channel called LOCa was developed by He et al. LOCa is a highly selective calcium channel precisely regulated and activated by blue light. It enables precise optical control over Ca2+ signals and hallmark Ca2+-dependent physiological responses (He et al., 2021). When the cells were exposed to a blue light the calcium ion gates opened. When the light was turned off, the gates closed. Furthermore, LOCa has been shown to be useful in delaying Alzheimer’s disease and inhibiting abnormal self-renewal of cancerous hematopoietic stem cells. However, it is regrettable that the application of light-controlled calcium channels, including Opto-CRAC and LOCa, in the field of tumor therapy is relatively rare and further exploration is still needed. Moreover, considering that the limited penetration of light may restrain its efficacy in deep tumors, some genetic coding tools that utilize chemical factors to manipulate calcium channels have been developed. Wang et al. reported a caffeine operated synthesis module (COSMO) that regulates calcium channels through caffeine, its metabolites (Wang et al., 2021). Even more impressively, it also can be activated by caffeinated beverages, including tea, coffee and energy drinks. Furthermore, how to target these tools to tumor cells in vivo is an urgent problem to be solved. One way to solve this problem is to bind these tools with tumor specific promoters. At the same time, according to the location of the patient’s tumor and systemic metastasis, we can choose different delivery methods, including systemic administration, intratumoral injection, etc. Moreover, we can combine optogenetic tools with upconversion nanoplates (Yu et al., 2019). Using upconversion nanomaterials as light sensors, near-infrared light with strong tissue penetration can be converted into blue light, and then optogenetic tools can be activated in situ. Meanwhile, we can reduce the side effects on normal tissues as much as possible by giving targeted light or chemicals. With the rapid development of nanomedicine, it is also possible to combine these tools with suitable nanocarriers. In addition, cells can not only perceive external light and chemical signals, but also respond to mechanical stress. Therefore, mechanical genetics is regarded as a new type of induction method (Song et al., 2022). It achieves non-invasive remote control of cells by converting mechanical stress into the control of cellular genetic. Robert B. et al. found that the mechano-transduction of pulsed focused ultrasound could cause DNA damage and superoxide formation in some tumor cells by calcium overload, further forming a proinflammatory TME (Rosenblatt et al., 2021). Similarly, Yue et al. used ultrasound combined with microbubbles to effectively activate the mechanical sensitive channel Piezo1. In that way, it caused the influx of Ca2+, resulted in calcium overload and ultimately induced apoptosis in pancreatic cancer cells (Song et al., 2022).
3 Different outcomes of calcium overload-based ion interference therapy
Calcium overload, characterized by the abnormal accumulation of Ca2+ in cytoplasm and mitochondria, leads to varying degrees of cell damage and even cell death through various mechanisms. In this process, different types of tumors and divergent induction conditions will induce assorted types of cell death.
3.1 Calcium overload and apoptosis
Apoptosis is the main type of cell death that induced by calcium overload, which is closely related to mitochondrial dysfunction and ER stress. We describe the role of mitochondrial dysfunction and ER stress in Figure 2. Notably, mitochondria are the core sites of calcium overload in tumor cells (Miwa et al., 2022). Triggering Ca2+-dependent mitochondrial dysfunction requires the accumulation of intracellular Ca2+ to a certain threshold and long-term maintenance at this level (Zhang et al., 2022). This process is closely related to the MCU located on the inner membrane of mitochondria (Marchi et al., 2020). Xue et al. used RY10-4 to upregulate the expression of MCU and enhanced mitochondrial calcium uptake, thereby inducing Ca2+-dependent mitochondrial dysfunction and inhibiting breast cancer. When the MCU is knocked out, the mitochondrial calcium uptake induced by several stimuli is completely inhibited (Zhang et al., 2022). Mechanistically, enhanced mitochondrial calcium uptake would lead to massive mitochondrial calcium accumulation and thus promote the occurrence of mitochondrial dysfunction by affecting mitochondrial metabolism and structure (Miwa et al., 2022). However, the role of MCU in cancer cell survival appears cell line-specific or drug-specific. Xiao et al. confirmed that MCU upregulation enhanced clone formation, migration, and mitochondrial activity of endometrial cancer cells (Xiao et al., 2023). Moreover, Yu et al. indicated that MCU silencing in MDA-MB-231 cells decreased migration and invasion in vitro and reduced lung metastasis in vivo (Yu et al., 2017).
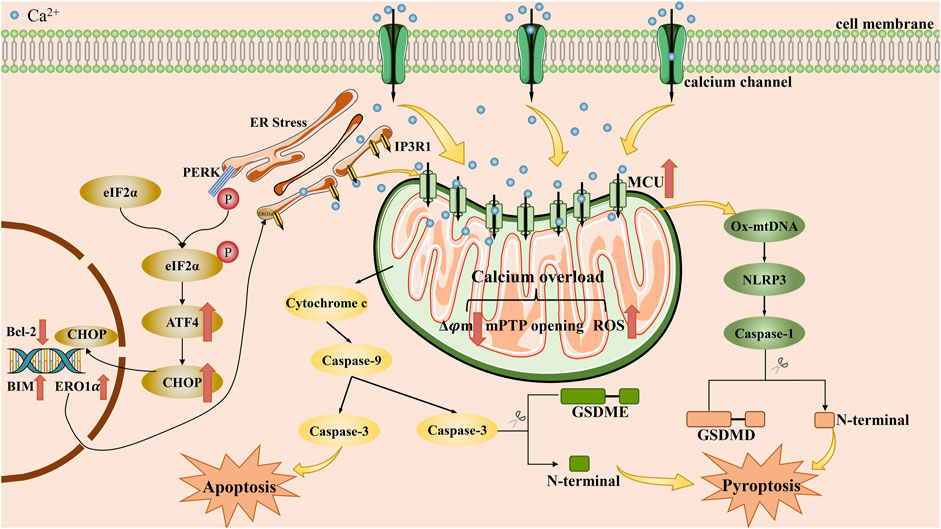
FIGURE 2. The main mechanisms by which calcium overload exerts its tumor suppressive effect by apoptosis and pyroptosis. Intracellular calcium overload, caused by the influx of exogenous calcium ions into the cytoplasm or the release of intracellular calcium stores, can induce apoptosis or pyroptosis in tumor cells, mainly through endoplasmic reticulum stress and mitochondrial dysfunction pathways. ATF4: activating transcription factor 4; BIM: Bcl-2 interaetion mediator of cell death; Bcl-2: B-cell lymphoma-2; CHOP: CCAAT enhance-binding protein homologous protein; eIF2α: eukaryotic translation initiation factor 2α; ERO1α: endoplasmic reticulum oxidoreductin-1α; ER: endoplasmic reticulum; GSDME: Gasdermin E; GSDMD: Gasdermin D; IP3R1: inositol 1,4,5-triphosphate receptor 1; MCU: mitochondrial calcium uniporter; mPTP: mitochondrial permeability transition pore; NLRP3: NOD-like receptor thermal protein domain associated protein 3; Ox-mtDNA: oxidized-mitochondrial DNA; PERK: protein kinase R-like endoplasmic reticulum kinase; ROS: reactive oxygen species.
However, it is undeniable that inducing the occurrence of calcium overload in some cancer cells is indeed an effective strategy. On the one hand, mitochondrial calcium overload can have an adverse effect on mitochondrial metabolism. The elevation of Ca2+ increases the activity of nicotinamide adenine dinucleotide oxidase and promotes the production of reactive oxygen species (ROS) by accelerating the tricarboxylic acid cycle and oxidative phosphorylation (Cui et al., 2019). Then, varieties of ROS inhibit tumor growth through sustained cell cycle arrest (Li et al., 2021a). During this process, many key protein phosphatases was inhibited in antioxidant pathways (Perillo et al., 2020; Cheung and Vousden, 2022). However, it is notable that sublethal levels of ROS do promote tumor progression (Sies and Jones, 2020). As Jing et al. found that the mitochondrial Ca2+ signaling pathway mediated by the MCU regulator 1 in hepatocellular carcinoma could promote epithelial-mesenchymal transition via activating the ROS/nuclear factor erythroid 2-related factor 2 (Nrf2)/Notch1 pathway by sublethal levels of ROS(Jin et al., 2019). On the other, calcium overload can be hazardous to the structure of mitochondria. Recent decade, researches have confirmed that mitochondria calcium overload can decrease mitochondrial membrane potential and open the mitochondrial permeability transition pore (MPTP), which will result in mitochondrial swelling and the rupture of the outer mitochondrial membrane (Zhang et al., 2022).
ER stress is another significant mechanism implicated in apoptosis triggered by calcium overload. The ER regulates the influx and efflux of Ca2+ mainly through ion channels on its membrane such as ryanodine receptor (RyR), inositol 1,4,5-triphosphate receptor (IP3R) and sarcoendoplasmic reticulum calcium transport ATPase (SERCA), ensuring proper calcium storage and adequate folding capacity (Zheng et al., 2022b). Once the calcium regulation mechanism of ER is disturbed, the ER calcium homeostasis would collapse, resulting in severe ER stress and crosstalk with mitochondrial dysfunction. According to Zhang et al., curcumin induced ER stress to inhibit thyroid cancer cells, while simultaneously initiating the mitochondrial apoptotic pathway by inhibiting the activity of SERCA2 and activating the calcium/calmodulin-dependent protein kinase (CaMK) II/c-Jun N-terminal kinase (JNK) pathway (Zhang et al., 2018). In parallel, the unfolded protein response (UPR) is also activated in reply to ER stress. Although the UPR might help reduce the load of unfolded proteins to maintain cell survival (Marciniak et al., 2022), persistent UPR caused by long-term severe ER stress could induce apoptosis through protein kinase R-like endoplasmic reticulum kinase (PERK)/eukaryotic translation initiation factor 2α (eIF2α)/activating transcription factor 4 (ATF4)/CCAAT enhance-binding protein homologous protein (CHOP) pathway (Hetz, 2012; Yu et al., 2021b; Coker-Gurkan et al., 2021). As a transcription factor, CHOP promotes mitochondrial dependent apoptosis by upregulating the expression of pro-apoptotic proteins comprise BAX, BAK and BIM. Meanwhile, it can downregulate the expression of anti-apoptotic proteins contain BCL-2, BCL-XL and MCL-1 (Zhou et al., 2022). Moreover, CHOP can also contribute to the death receptor pathway. For instance, CHOP can upregulate the expression of death receptor (DR) 4 or DR5, so that it can sensitize a variety of cancer cells to trail mediated apoptosis (Hu et al., 2018; Kim et al., 2019; Cao et al., 2020). In addition, Glutamine-rich protein 1 (QRICH1) has also been revealed as a key effector of the PERK/eIF2α/ATF4/CHOP pathway. QRICH1 controls proteostasis in ER by regulating protein translation and secretion, thereby determining cell fate at the end of the UPR(You et al., 2021). Recently, Recently, a research team from the University of Geneva unveiled a direct crosstalk between SOCE and UPR via inositol-requiring enzyme 1(IRE1), acting as key regulator of ER Ca2+ and proteostasis in T cells (Carreras-Sureda et al., 2023). Under ER stress, the IRE1-STIM1 axis may boost SOCE to preserve immune cell function. Maybe, the IRE1-STIM1 axis could be a significant pathway for cancer immunotherapy.
Additionally, there is a close contact between the mitochondrial membrane and the ER membrane, known as the mitochondrial associated endoplasmic reticulum membrane (MAM) (Loncke et al., 2021). One of the main functions of MAM is to mediate the flow of calcium ions between ER and mitochondria. Increasing Ca2+ transfer from ER to mitochondria is also an effective means of inducing calcium overload (Wu et al., 2023). Xie et al. confirmed that TAT-fused inositol 1,4,5-trisphosphate receptor-derived peptide targeting the BH4 domain of Bcl-2 increased cisplatin-induced Ca2+ flux from ER to mitochondria, thereby promoting apoptosis and enhancing cisplatin cytotoxicity in ovarian cancer cells (Xie et al., 2018). However, a large amount of research is still needed to confirm the targeted key factors in MAM. The cell line-specific and drug-specific also need to be explored.
3.2 Calcium overload and pyroptosis
Pyroptosis is a type of programmed cell death characterized by an inflammatory response, which is facilitated by the gasdermin family of proteins that disrupt the cell membrane. Its primary characteristics include cell swelling and the release of interleukin-1β. (Yu et al., 2021a). As research continues to deepen, more and more mechanisms of pyroptosis continue to be discovered, and at present, two main pathways and several alternative pathways have been recognized (Loveless et al., 2021). Among the classical pathways, pyroptosis is mainly mediated by gasdermin D and involves cysteinyl aspartate specific proteinase (caspase)-1 or caspase-4/5/11. One alternative pathway that has garnered significant attention is the caspase-3/gasdermin E pathway. Moreover, Ca2+ was found to regulate pyroptosis mainly by affecting activators of gasdermin proteins, including NOD-like receptor thermal protein domain associated protein 3 (NLRP3) inflammasome, caspase-3. Here, we describe the we describe the main mechanisms by which calcium overload leads to pyroptosis in Figure 2. Earlier studies have demonstrated that Ca2+ accumulation is tightly associated with the assembly and activation of NLRP3 inflammasome. It means that blocking intracellular calcium accumulation will arrest inflammasome activation (Murakami et al., 2012; Akbal et al., 2022). Geun Shik Lee et al. confirmed that hindering the release of Ca2+ from calcium stores will interrupt the activation of NLRP3 inflammasome (Lee et al., 2012). Subsequently, the activated NLRP3 inflammasome facilitates pyroptosis by promoting the cleavage of GSDMD protein through caspase-1. Additionally, activated NLRP3 inflammasome can accelerate Ca2+ accumulation, leading to the formation of positive feedback.
Pyroptosis can lead to membrane rupture, which is manifested by the formation of pores on the membrane the appearance of small pores on the cell membrane, so as to favor the influx of Ca2+. Yet NLRP3 alone can also promote the efflux of Ca2+ from the ER by regulating RyR2 and increase intracellular calcium levels (Kong et al., 2021). Yuan et al. demonstrated that during the process of cucurbitacin B inhibited non-small cell lung cancer, the release of Ca2+ from intracellular calcium stores and the occurrence of mitochondrial calcium overload are important for the toll-like receptors 4/NLRP3/gasdermin D pathway (Yuan et al., 2021). However, the specific mechanism by which accumulated Ca2+ promotes NLRP3 inflammasome activation requires further investigations. Nowadays, two possible molecular mechanisms have been identified: (1) Excessive Ca2+ transport from cytosol to mitochondria causes mitochondrial dysfunction. It would drive the release of oxidized-mitochondrial DNA into cytosol via MPTP and voltage-dependent anion channel, which has been proven that it is profit to the activation of NLRP3 inflammasome (Moossavi et al., 2018; Xian et al., 2022); (2) Ca2+ could directly contribute to the activation of NLRP3 inflammasome by promoting NLRP3 interaction with apoptosis-associated speck-like protein containing a CARD (Kong et al., 2021). More investigations are needed to be designed and further reveal the underlying mechanism by which Ca2+ promote the activation of NLRP3 inflammasome.
Beside regulating major pathways, Ca2+ also takes part in alternative pathways, particularly the caspase-3/gasdermin E pathway. Calcium overload could accelerate the assembly of a pyroptosome complex consisting of Apaf-1 and caspase-4 by inducing mitochondrial permeability transition. After the activation of a pyroptosome complex, caspase-3 and gasdermin E would be cleaved, thus activate gasdermin E-mediated pyroptosis (Xu et al., 2021). Similarly, a novel calcium nano-modulator constructed by Zhen et al. induced the occurrence of mitochondrial calcium overload with multiple effects, including GSDME-mediated pyroptosis (Zheng et al., 2022a).
3.3 Calcium overload and necroptosis
Necroptosis is a form of necrotic cell death mainly mediated by necrosome. The necrosome is a protein complex composed of receptor interacting serine/threonine protein (RIP) kinase 1, RIP kinase 3 and mixed lineage kinase region like protein (MLKL) (Yan et al., 2022). Calcium overload is recognized as be one of the key initiators of necroptosis (Faizan and Ahmad, 2021). The formation of necrosome can induce necroptosis by hastening the influx of Ca2+ to form calcium overload (Figure 3). An experiment on human colon cancer demonstrated that the formation of necrosome was followed by translocation to the plasma membrane (Cai et al., 2014). After that, it can facilitate a robust influx of Ca2+ mediated by transient receptor potential melastatin 7. Such an influx of Ca2+ may have a positive feedback effect and bring about the subsequent occurrence of necroptosis. Furthermore, it is reported that a large proportion of necrosome can also be found to be associated with ER and lead to ER stress (Liang et al., 2021).
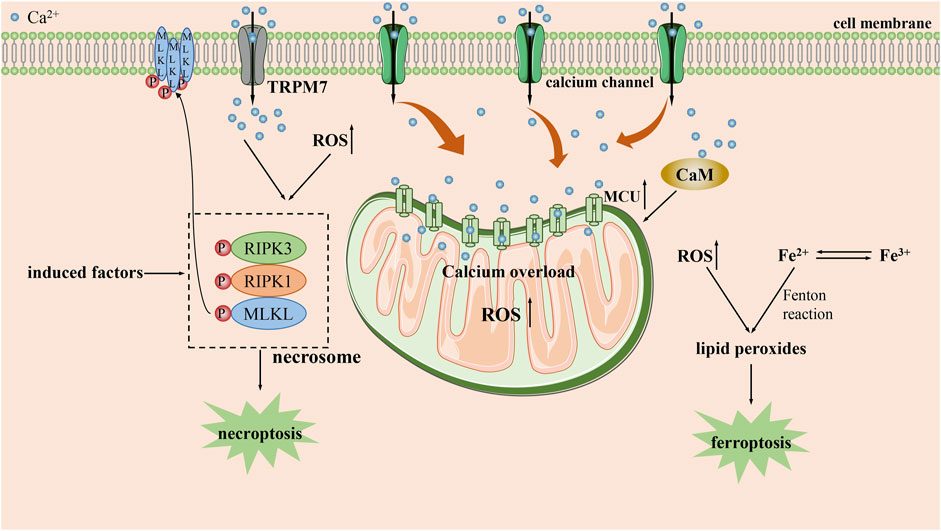
FIGURE 3. The main mechanisms by which calcium overload exerts its tumor suppressive effect by necroptosis and ferroptpsis. CaM: calcium/calmodulin-dependent protein; MCU: mitochondrial calcium uniporter; RIPK1: receptor interacting serine/threonine protein kinase 1; RIPK3: receptor interacting serine/threonine protein kinase 3; ROS: reactive oxygen species; TRPM7: transient receptor potential melastatin 7.
Moreover, calcium overload also contributes to the formation of necrosome. While using the antimicrobial peptide PFR to treat acute myeloid leukemia (AML), Yu et al. proved that PFR triggered increased mitochondrial ROS and thus accelerated the formation of necrosome through cytoplasmic calcium overload (Lv et al., 2019). Similarly, upon the treatment of neuroblastoma with HVJ-e, it was confirmed that continuously calcium overload in the cell lead to necrosome formation by activating CaMKⅡ-mediated phosphorylation of RIP1(Nomura et al., 2014). Additionally, calcium overload can also regulate necroptosis through alternative pathways. Poly (ADP-ribose) polymerase-1(PARP1) and AIF have been shown to be involved in calcium overload mediated necroptosis (Cabon et al., 2012). Similarly, Paul et al. authenticated that prolonged fumonisin B1 exposure in neuroblastoma can induce necroptosis by calcium overload (Paul et al., 2021). During this process, the accumulation of Ca2+ contributes to the induction of PARP1 activation, which results in an inhibitory effect via PARP1/JNK/AIF signaling pathway to induce necroptosis.
Ca2+ is also involved in regulating non canonical necroptosis. During the treatment of pancreatic cancer with adipoRon, Miho Akimoto et al. demonstrated that when adipoRon induced Ca2+-dependent mitochondrial dysfunction through calcium overload accompanying with the activation of RIP kinase 1 and extracellular signal regulated kinase 1/2, which led to non-canonical necroptosis (Akimoto et al., 2018).
3.4 Calcium overload and ferroptosis
Ferroptosis is an iron-dependent cell death characterized by the accumulation of lipid peroxides (Dixon et al., 2012; Stockwell et al., 2017). Surprisingly, previous studies have shown that there is crosstalk between iron and Ca2+ through ROS during ferroptosis. Large amounts of ROS generated by iron can act on the ER and stimulate calcium release from calcium stores, arousing a sustained increase in intracellular Ca2+ by activating IP3R, RyR and reducing the activity of SERCA, PMCA (Núñez and Hidalgo, 2019). The persistently increased Ca2+ that are transported into mitochondria will subsequently stimulate lipid peroxidation, contributing to a vicious cycle that exacerbates ferroptosis (Gleitze et al., 2021). However, the role of Ca2+ in ferroptosis is currently still contentious.
Some studies have argued that increased Ca2+ may have roles in delaying or inhibiting ferroptosis. Wang et al. discovered that CaMK kinase 2 activated by Ca2+ in melanoma could inhibit ferroptosis by strengthening the expression of anti-oxidant proteins such as heme oxygenase 1 and superoxide dismutase 2, which involved in Nrf2-dependent anti-oxidant mechanism (Wang et al., 2022). Similarly, Bing et al. demonstrated that the leakage of Ca2+ from the ER would be facilitated by enhancing the interaction of cell migration-inducing protein with IP3R in prostate cancer cells. Afterwards, Ca2+ could activate CaMKII, which further accelerates Nrf2 phosphorylation and nuclear localization, contributing to the uptake of cystine which counteract ferroptosis (Liu et al., 2022a). On the contrary, some studies also suggest that Ca2+ may also amplify the ferroptosis process (Figure 3). For example, Peng et al. used erastin to induce calcium-dependent ferroptosis in lung cancer cells and demonstrated that Ca2+/CaM signaling is a key mediator of ferroptosis mediated by erasin. Blocking this signaling would significantly rescue cell death by inhibiting ferroptosis (Chen et al., 2020). Analogously, some research have demonstrated that MCU-dependent mitochondrial calcium overload is integral for the induction of ferroptosis by long-term cold stimulation (Nakamura et al., 2021).
A recent study on ferroptosis could partly explain the contradictory influences of Ca2+(Pedrera et al., 2021). Early cytosolic Ca2+ increases could induce the activation of the endosomal sorting complex required for transport III-dependent membrane repair machinery and suspend ferroptosis. However, the progression of lipid peroxidation at the plasma membrane would not be interrupted. Furthermore, the paradoxical role of Ca2+ in ferroptosis may also be related to cell line heterogeneity and different stimuli.
4 The prospect of combining calcium overload-based ion interference therapy with immunotherapy
The discovery of immunotherapy has led to a better comprehension of tumors and significant advancements in tumor therapy. The regulation of intracellular Ca2+ is an indispensable part in various processes, including immune cell activation, the switching of phenotypes and TME enhancement (Vig and Kinet, 2009). For example, targeting elevated intracellular Ca2+ can effectively stimulate the proliferation of cytotoxic lymphocytes, ultimately enhancing their cytotoxic effect on tumor cells (Kim et al., 2017). What’s more, the activation of T lymphocyte-associated transcription factors such as nuclear factor of activated T cells, NF-κB, and JNK relies significantly on elevated levels of intracellular Ca2+(Trebak and Kinet, 2019; Meng et al., 2020). Consequently, the integration of calcium overload-based ion interference therapy with immunotherapy has the potential to substantially enhance the antitumor effect by regulating Ca2+. This is particularly true with the advancement of nanomedicine, which may propel the application of this therapy.
Lack of immunogenicity and insufficient activation of antitumor immune responses are key reasons contributing to the insensitivity of certain tumors to immunotherapy (Huang et al., 2021). Based on this, targeting improved immunogenicity to promote immunogenic cell death (ICD) has become a key aspect in immunotherapy. In this process, the role of multiple damage associated molecular patterns (DAMPs), including high mobility group box-1, calreticulin (CRT) and heat shock proteins, cannot be ignored (Galluzzi et al., 2017). Calcium overload has been confirmed to significantly promote the release of DAMPs to improve immunogenicity and efficiently enhance the anti-tumor immune effect (Zheng et al., 2021a). Zheng et al. developed a multifunctional calcium nano-formulation named PEGCaCUR, which significantly enhanced the release of DAMPs by inducing mitochondrial calcium overload. Subsequently, a significantly higher proportion of mature dendritic cells and activated T cells was observed, confirming the successful ICD elicitation (Zheng et al., 2021a). Furthermore, ER stress induced by calcium overload also contributes to the higher immunogenicity. The release of Ca2+ from the calcium store may also facilitate the exposure of CRT localized in the ER, contributing to better improve immunogenicity (Tufi et al., 2008). CRT exposure on the cell surface is known to deliver robust pro-phagocytic signals to myeloid cells (Guilbaud et al., 2023) For example, surface-exposed CRT has been shown to be fundamental for the uptake of dying cancer cells by dendritic cell precursors that can initiate adaptive tumor-targeting immune responses (Obeid et al., 2007). Dai et al. reported a novel ICD nanoinductor that enhanced CRT exposure by facilitating the release of Ca2+ from the store into the cytosol, which eventually triggered ICD(Dai et al., 2020). What’s more, severe ER stress in conjuction with oxidative stress will result in the release and exposure of more DAMPs.
With the exception of improving immunogenicity, targeting tumor associated macrophages (TAMs) is also an effective immunotherapy. TAMs mainly consist of M1 phenotype associated with anti-tumor activity and M2 phenotype associated with pro-tumor activity (Christofides et al., 2022). There are three strategies which can target TAMs to improve the TME: (1) directly clearing M2 type macrophages; (2) accurately inhibiting the recruitment of macrophages; (3) reprograming TAMs from M2 to M1 phenotype (Xiang et al., 2021). Numerous research have shown that the concentration of Ca2+ in TAMs might be closely related to the phenotype of TAM(Chen et al., 2018; Kang et al., 2018; Bi et al., 2020). For example, Chen et al. successfully switched TAMs from tumor-promoting M2 phenotype to tumor-inhibiting M1 phenotype with chloroquine (Chen et al., 2018). Mechanically, chloroquine can directly act on lysosomes, releasing Ca2+ stored in lysosomes. For one thing the increased cytoplasmic Ca2+ can activate p38 and NF-κB for polarizing TAMs to M1 phenotype, for another it can stimulate transcription factor EB for reprograming the metabolism of TAMs. Similarly, the use of near-infrared light to assist in regulating intracellular calcium levels also promotes macrophage polarization towards M1 phenotype by increasing intracellular Ca2+(Kang et al., 2018). Furthermore, ROS and nicotinamide adenine dinucleotide phosphate have also been considered as crucial players in the regulation of macrophage polarization (Mehla and Singh, 2019). During the suppression of AML progression by chenodeoxycholic acid, chenodeoxycholic acid could induce mitochondrial dysfunction via calcium overload and subsequently promote lipid peroxidation via the ROS/p38-MAPK/Diacylglycerol-O-Acyltransferase 1 pathway (Liu et al., 2022b). Besides, chenodeoxycholic acid has also been shown to inhibit M2 macrophage polarization, but the specific mechanism is unknown. Certainly, it can be speculated that the massive ROS and lipid peroxidation induced by calcium overload play a critical role in this process. However, further studies will be necessary for us to verify and reveal the specific mechanisms.
Immuno-checkpoint blocking therapy (ICB) has demonstrated great clinical application potential, but its clinical application response rate is often hindered by the tumor immune suppression microenvironment. Previous research have found that calcium signaling is bound up with the efficacy of ICB(Freitas et al., 2018). A nano-formulation named CaNP@CAD-PEG, constructed by An et al. not only takes advantages of the calcium overload to enhance immunogenicity and promote TAM reprogramming, but also achieves tumor cell specific programmed cell death ligand 1 silencing via a calcium activated DNAzyme, significantly boosting the antitumor immune effect (An et al., 2022). Apart from directly inhibiting the primary tumor by inducing the calcium overload, the nano-sonosensitizer called TiO2@CaP, activated by the acidic TME, could further combine with anti-programmed death 1 and bring about the inhibition of growth of distal tumors and lung metastasis of tumors (Tan et al., 2021).
However, it cannot be ignored that there are certain risks in combining calcium overload-based ion interference therapy with immunotherapy. It has been shown that cytotoxic T Lymphocytes have a bell-shaped Ca2+ dependence with an optimum for cancer cell elimination at rather low extracellular Ca2+ concentrations (23–625 μm) (Zhou et al., 2018). A decrease of extracellular Ca2+ concentrations or partial inhibition of ORAI1 activity by selective blockers in the TME could obviously inhibit cancer growth by simultaneously increasing cytotoxic T Lymphocytes and natural killer cell cytotoxicity and decreasing cancer cell proliferation. Therefore, the influx of Ca2+ has at least a dual role in the TME. We believe that the most important thing is to go for a balance that maximizes the elimination of tumors while protecting the stability of one’s immune system.
5 Conclusion
Calcium overload-based ion interference therapy caused various cellular damages by artificially inducing the occurrence of calcium overload and triggered different types of cell death through various mechanisms to suppress the proliferation and metastasis of tumor cells. This therapy has shown momentous tumor inhibitory effects and received increasing attention. However, there are still several issues that need to be addressed:
1) Although exciting results were obtained in some clinical trials, some calcium channel activators were also found to be extremely prone to trigger strong systemic toxic side effects, or need to be applied with doses higher than the current clinically safe dosage. At the same time, while utilizing novel calcium nano-formulations can localize calcium overload to tumor sites, further clinical research is needed to investigate the safety and efficacy of these novel calcium nano-formulations.
2) While calcium overload can inhibit tumors by inducing severe cell damage, it is nonnegligible that the increased Ca2+ could enhance tumor proliferation and decrease apoptosis before reaching the calcium overload level. Achieving a balance between cell death and proliferation is crucial to maximize the tumor-suppressive effects of calcium overload.
3) Recently, more and more ion interference therapy based on different metal ions have been widely developed and have shown great anti-tumor effects. Theoretically, combining multiple ion interference therapy would show more efficient anti-tumor effects. Therefore, further studies are needed to explore its influence on various normal tissues and organs throughout the body in order to ensure its biosafety.
4) Due to the heterogeneity between individuals and different kinds of tumors, a “one-size-fits-all” approach is not feasible. Developing individualized and precise treatment strategies is essential to optimize therapeutic outcomes.
In conclusion, the calcium overload-based ion interference therapy has a promising clinical application for its robust antitumor effect. As our understanding of the relationship between Ca2+ and tumors deepens, more effective therapies and technologies are expected to emerge, along with a better understanding of the mechanisms underlying this therapy to enhance therapeutic efficacy and facilitate clinical translation.
Author contributions
SL: Conceptualization, Writing–original draft, Writing–review and editing. RF: Conceptualization, Writing–original draft, Writing–review and editing. YW: Investigation, Writing–review and editing. KH: Investigation, Writing–review and editing. JX: Investigation, Writing–review and editing. HL: Conceptualization, Funding acquisition, Writing–original draft, Writing–review and editing.
Funding
The author(s) declare financial support was received for the research, authorship, and/or publication of this article. This work was supported by National Innovation and Entrepreneurship Training Project for University (China) (No. 202290031013), the Undergraduate Scientific Research Training Program of Army Medical University (No. 2022XBK03).
Conflict of interest
The authors declare that the research was conducted in the absence of any commercial or financial relationships that could be construed as a potential conflict of interest.
Publisher’s note
All claims expressed in this article are solely those of the authors and do not necessarily represent those of their affiliated organizations, or those of the publisher, the editors and the reviewers. Any product that may be evaluated in this article, or claim that may be made by its manufacturer, is not guaranteed or endorsed by the publisher.
References
Abdoul-Azize, S., Buquet, C., Li, H., Picquenot, J. M., and Vannier, J. P. (2018). Integration of Ca(2+) signaling regulates the breast tumor cell response to simvastatin and doxorubicin. Oncogene 37, 4979–4993. doi:10.1038/s41388-018-0329-6
Akbal, A., Dernst, A., Lovotti, M., Mangan, M. S. J., Mcmanus, R. M., and Latz, E. (2022). How location and cellular signaling combine to activate the NLRP3 inflammasome. Cell Mol. Immunol. 19, 1201–1214. doi:10.1038/s41423-022-00922-w
Akimoto, M., Maruyama, R., Kawabata, Y., Tajima, Y., and Takenaga, K. (2018). Antidiabetic adiponectin receptor agonist AdipoRon suppresses tumour growth of pancreatic cancer by inducing RIPK1/ERK-dependent necroptosis. Cell Death Dis. 9, 804. doi:10.1038/s41419-018-0851-z
An, J., Liu, M., Zhao, L., Lu, W., Wu, S., Zhang, K., et al. (2022). Boosting tumor immunotherapy by bioactive nanoparticles via Ca2+ interference mediated TME reprogramming and specific PD-L1 depletion. Adv. Funct. Mater. 32. doi:10.1002/adfm.202201275
An, J., Zhang, K., Wang, B., Wu, S., Wang, Y., Zhang, H., et al. (2020). Nanoenabled disruption of multiple barriers in antigen cross-presentation of dendritic cells via calcium interference for enhanced chemo-immunotherapy. ACS Nano 14, 7639–7650. doi:10.1021/acsnano.0c03881
Bai, S., Lan, Y., Fu, S., Cheng, H., Lu, Z., and Liu, G. (2022). Connecting calcium-based nanomaterials and cancer: from diagnosis to therapy. Nanomicro Lett. 14, 145. doi:10.1007/s40820-022-00894-6
Bi, S., Huang, W., Chen, S., Huang, C., Li, C., Guo, Z., et al. (2020). Cordyceps militaris polysaccharide converts immunosuppressive macrophages into M1-like phenotype and activates T lymphocytes by inhibiting the PD-L1/PD-1 axis between TAMs and T lymphocytes. Int. J. Biol. Macromol. 150, 261–280. doi:10.1016/j.ijbiomac.2020.02.050
Cabon, L., GaláN-Malo, P., Bouharrour, A., DelavalléE, L., Brunelle-Navas, M. N., Lorenzo, H. K., et al. (2012). BID regulates AIF-mediated caspase-independent necroptosis by promoting BAX activation. Cell Death Differ. 19, 245–256. doi:10.1038/cdd.2011.91
Cai, Z., Jitkaew, S., Zhao, J., Chiang, H. C., Choksi, S., Liu, J., et al. (2014). Plasma membrane translocation of trimerized MLKL protein is required for TNF-induced necroptosis. Nat. Cell Biol. 16, 55–65. doi:10.1038/ncb2883
Cao, Y., Kong, S., Xin, Y., Meng, Y., Shang, S., and Qi, Y. (2020). Lestaurtinib potentiates TRAIL-induced apoptosis in glioma via CHOP-dependent DR5 induction. J. Cell Mol. Med. 24, 7829–7840. doi:10.1111/jcmm.15415
Carreras-Sureda, A., Zhang, X., Laubry, L., Brunetti, J., Koenig, S., Wang, X., et al. (2023). The ER stress sensor IRE1 interacts with STIM1 to promote store-operated calcium entry, T cell activation, and muscular differentiation. Cell Rep. 42, 113540. doi:10.1016/j.celrep.2023.113540
Chang, M., Hou, Z., Jin, D., Zhou, J., Wang, M., Wang, M., et al. (2020). Colorectal tumor microenvironment-activated bio-decomposable and metabolizable Cu2 O@CaCO3 nanocomposites for synergistic oncotherapy. Adv. Mater 32, e2004647. doi:10.1002/adma.202004647
Chen, D., Xie, J., Fiskesund, R., Dong, W., Liang, X., Lv, J., et al. (2018). Chloroquine modulates antitumor immune response by resetting tumor-associated macrophages toward M1 phenotype. Nat. Commun. 9, 873. doi:10.1038/s41467-018-03225-9
Chen, F., Yang, B., Xu, L., Yang, J., and Li, J. (2021). A CaO(2) @tannic acid-Fe(III) nanoconjugate for enhanced chemodynamic tumor therapy. ChemMedChem 16, 2278–2286. doi:10.1002/cmdc.202100108
Cheng, X., Xu, Y., Zhang, Y., Jia, C., Wei, B., Hu, T., et al. (2021). Glucose-targeted hydroxyapatite/indocyanine green hybrid nanoparticles for collaborative tumor therapy. ACS Appl. Mater Interfaces 13, 37665–37679. doi:10.1021/acsami.1c09852
Chen, P., Wu, Q., Feng, J., Yan, L., Sun, Y., Liu, S., et al. (2020). Erianin, a novel dibenzyl compound in Dendrobium extract, inhibits lung cancer cell growth and migration via calcium/calmodulin-dependent ferroptosis. Signal Transduct. Target Ther. 5, 51. doi:10.1038/s41392-020-0149-3
Chen, Z., Deng, J., Cao, J., Wu, H., Feng, G., Zhang, R., et al. (2022). Nano-hydroxyapatite-evoked immune response synchronized with controllable immune adjuvant release for strengthening melanoma-specific growth inhibition. Acta Biomater. 145, 159–171. doi:10.1016/j.actbio.2022.04.002
Cheung, E. C., and Vousden, K. H. (2022). The role of ROS in tumour development and progression. Nat. Rev. Cancer 22, 280–297. doi:10.1038/s41568-021-00435-0
Cho, Y. W., Kim, E. J., Nyiramana, M. M., Shin, E. J., Jin, H., Ryu, J. H., et al. (2019). Paroxetine induces apoptosis of human breast cancer MCF-7 cells through Ca(2+)-and p38 MAP kinase-dependent ROS generation. Cancers (Basel) 11, 64. doi:10.3390/cancers11010064
Christofides, A., Strauss, L., Yeo, A., Cao, C., Charest, A., and Boussiotis, V. A. (2022). The complex role of tumor-infiltrating macrophages. Nat. Immunol. 23, 1148–1156. doi:10.1038/s41590-022-01267-2
Chu, X., Jiang, X. W., Liu, Y. Y., Zhai, S. J., Jiang, Y. Q., Chen, Y., et al. (2021). Nitric oxide modulating calcium store for Ca2+-initiated cancer therapy. Adv. Funct. Mater. 31, 12. doi:10.1002/adfm.202008507
Coker-Gurkan, A., Can, E., Sahin, S., Obakan-Yerlikaya, P., and Arisan, E. D. (2021). Atiprimod triggered apoptotic cell death via acting on PERK/eIF2α/ATF4/CHOP and STAT3/NF-ΚB axis in MDA-MB-231 and MDA-MB-468 breast cancer cells. Mol. Biol. Rep. 48, 5233–5247. doi:10.1007/s11033-021-06528-1
Cui, C., Merritt, R., Fu, L., and Pan, Z. (2017). Targeting calcium signaling in cancer therapy. Acta Pharm. Sin. B 7, 3–17. doi:10.1016/j.apsb.2016.11.001
Cui, C., Yang, J., Fu, L., Wang, M., and Wang, X. (2019). Progress in understanding mitochondrial calcium uniporter complex-mediated calcium signalling: a potential target for cancer treatment. Br. J. Pharmacol. 176, 1190–1205. doi:10.1111/bph.14632
Dai, Z., Tang, J., Gu, Z., Wang, Y., Yang, Y., Yang, Y., et al. (2020). Eliciting immunogenic cell death via a unitized nanoinducer. Nano Lett. 20, 6246–6254. doi:10.1021/acs.nanolett.0c00713
Dixon, S. J., Lemberg, K. M., Lamprecht, M. R., Skouta, R., Zaitsev, E. M., Gleason, C. E., et al. (2012). Ferroptosis: an iron-dependent form of nonapoptotic cell death. Cell 149, 1060–1072. doi:10.1016/j.cell.2012.03.042
Eskiocak, U., Ramesh, V., Gill, J. G., Zhao, Z., Yuan, S. W., Wang, M., et al. (2016). Synergistic effects of ion transporter and MAP kinase pathway inhibitors in melanoma. Nat. Commun. 7, 12336. doi:10.1038/ncomms12336
Faizan, M. I., and Ahmad, T. (2021). Altered mitochondrial calcium handling and cell death by necroptosis: an emerging paradigm. Mitochondrion 57, 47–62. doi:10.1016/j.mito.2020.12.004
Feng, X., Lin, T., Chen, D., Li, Z., Yang, Q., Tian, H., et al. (2023). Mitochondria-associated ER stress evokes immunogenic cell death through the ROS-PERK-eIF2α pathway under PTT/CDT combined therapy. Acta Biomater. 160, 211–224. doi:10.1016/j.actbio.2023.02.011
Freitas, C. M. T., Johnson, D. K., and Weber, K. S. (2018). T cell calcium signaling regulation by the Co-receptor CD5. Int. J. Mol. Sci. 19, 1295. doi:10.3390/ijms19051295
Fu, L.-H., Wan, Y., Li, C., Qi, C., He, T., Yang, C., et al. (2021a). Biodegradable calcium phosphate nanotheranostics with tumor-specific activatable cascade catalytic reactions-augmented photodynamic therapy. Adv. Funct. Mater. 31, 2009848. doi:10.1002/adfm.202009848
Fu, L. H., Wan, Y., Qi, C., He, J., Li, C., Yang, C., et al. (2021b). Nanocatalytic theranostics with glutathione depletion and enhanced reactive oxygen species generation for efficient cancer therapy. Adv. Mater 33, e2006892. doi:10.1002/adma.202006892
Fu, F., Wang, W., Wu, L., Wang, W., Huang, Z., Huang, Y., et al. (2023). Inhalable biomineralized liposomes for cyclic Ca(2+)-burst-centered endoplasmic reticulum stress enhanced lung cancer ferroptosis therapy. ACS Nano 17, 5486–5502. doi:10.1021/acsnano.2c10830
Fujikawa, I., Ando, T., Suzuki-Karasaki, M., Suzuki-Karasaki, M., Ochiai, T., and Suzuki-Karasaki, Y. (2020). Aspirin induces mitochondrial Ca(2+) remodeling in tumor cells via ROS‒depolarization‒voltage-gated Ca(2+) entry. Int. J. Mol. Sci. 21, 4771. doi:10.3390/ijms21134771
Galluzzi, L., Buqué, A., Kepp, O., Zitvogel, L., and Kroemer, G. (2017). Immunogenic cell death in cancer and infectious disease. Nat. Rev. Immunol. 17, 97–111. doi:10.1038/nri.2016.107
Giorgi, C., Danese, A., Missiroli, S., Patergnani, S., and Pinton, P. (2018). Calcium dynamics as a machine for decoding signals. Trends Cell Biol. 28, 258–273. doi:10.1016/j.tcb.2018.01.002
Gleitze, S., Paula-Lima, A., Nunez, M. T., and Hidalgo, C. (2021). The calcium-iron connection in ferroptosis-mediated neuronal death. Free Radic. Biol. Med. 175, 28–41. doi:10.1016/j.freeradbiomed.2021.08.231
Guan, Q., Zhou, L. L., Lv, F. H., Li, W. Y., Li, Y. A., and Dong, Y. B. (2020). A glycosylated covalent organic framework equipped with BODIPY and CaCO(3) for synergistic tumor therapy. Angew. Chem. Int. Ed. Engl. 59, 18042–18047. doi:10.1002/anie.202008055
Guilbaud, E., Kroemer, G., and Galluzzi, L. (2023). Calreticulin exposure orchestrates innate immunosurveillance. Cancer Cell 41, 1014–1016. doi:10.1016/j.ccell.2023.04.015
Guo, D., Dai, X., Liu, K., Liu, Y., Wu, J., Wang, K., et al. (2023). A self-reinforcing nanoplatform for highly effective synergistic targeted combinatary calcium-overload and photodynamic therapy of cancer. Adv. Healthc. Mater 12, e2202424. doi:10.1002/adhm.202202424
He, L., Wang, L., Zeng, H., Tan, P., Ma, G., Zheng, S., et al. (2021). Engineering of a bona fide light-operated calcium channel. Nat. Commun. 12, 164. doi:10.1038/s41467-020-20425-4
He, L., Zhang, Y., Ma, G., Tan, P., Li, Z., Zang, S., et al. (2015). Near-infrared photoactivatable control of Ca(2+) signaling and optogenetic immunomodulation. Elife 4, e10024. doi:10.7554/eLife.10024
Hetz, C. (2012). The unfolded protein response: controlling cell fate decisions under ER stress and beyond. Nat. Rev. Mol. Cell Biol. 13, 89–102. doi:10.1038/nrm3270
Hu, H. J., Wang, S. S., Wang, Y. X., Liu, Y., Feng, X. M., Shen, Y., et al. (2019). Blockade of the forward Na(+)/Ca(2+) exchanger suppresses the growth of glioblastoma cells through Ca(2+) -mediated cell death. Br. J. Pharmacol. 176, 2691–2707. doi:10.1111/bph.14692
Huang, C., Lin, B., Chen, C., Wang, H., Lin, X., Liu, J., et al. (2022). Synergistic reinforcing of immunogenic cell death and transforming tumor-associated macrophages via a multifunctional cascade bioreactor for optimizing cancer immunotherapy. Adv. Mater 34, e2207593. doi:10.1002/adma.202207593
Huang, J., Yang, B., Peng, Y., Huang, J., Wong, S. H. D., Bian, L., et al. (2021). Nanomedicine-boosting tumor immunogenicity for enhanced immunotherapy. Adv. Funct. Mater. 31. doi:10.1002/adfm.202011171
Hu, H., Tian, M., Ding, C., and Yu, S. (2018). The C/EBP homologous protein (CHOP) transcription factor functions in endoplasmic reticulum stress-induced apoptosis and microbial infection. Front. Immunol. 9, 3083. doi:10.3389/fimmu.2018.03083
Jin, M., Wang, J., Ji, X., Cao, H., Zhu, J., Chen, Y., et al. (2019). MCUR1 facilitates epithelial-mesenchymal transition and metastasis via the mitochondrial calcium dependent ROS/Nrf2/Notch pathway in hepatocellular carcinoma. J. Exp. Clin. Cancer Res. 38, 136. doi:10.1186/s13046-019-1135-x
Kang, H., Zhang, K., Wong, D. S. H., Han, F., Li, B., and Bian, L. (2018). Near-infrared light-controlled regulation of intracellular calcium to modulate macrophage polarization. Biomaterials 178, 681–696. doi:10.1016/j.biomaterials.2018.03.007
Kang, Y., Sun, W., Li, S., Li, M., Fan, J., Du, J., et al. (2019). Oligo hyaluronan-coated silica/hydroxyapatite degradable nanoparticles for targeted cancer treatment. Adv. Sci. (Weinh) 6, 1900716. doi:10.1002/advs.201900716
Kim, B. R., Park, S. H., Jeong, Y. A., Na, Y. J., Kim, J. L., Jo, M. J., et al. (2019). RUNX3 enhances TRAIL-induced apoptosis by upregulating DR5 in colorectal cancer. Oncogene 38, 3903–3918. doi:10.1038/s41388-019-0693-x
Kim, K. D., Bae, S., Capece, T., Nedelkovska, H., De Rubio, R. G., Smrcka, A. V., et al. (2017). Targeted calcium influx boosts cytotoxic T lymphocyte function in the tumour microenvironment. Nat. Commun. 8, 15365. doi:10.1038/ncomms15365
Kong, F., You, H., Zheng, K., Tang, R., and Zheng, C. (2021). The crosstalk between pattern-recognition receptor signaling and calcium signaling. Int. J. Biol. Macromol. 192, 745–756. doi:10.1016/j.ijbiomac.2021.10.014
Lee, G. S., Subramanian, N., Kim, A. I., Aksentijevich, I., Goldbach-Mansky, R., Sacks, D. B., et al. (2012). The calcium-sensing receptor regulates the NLRP3 inflammasome through Ca2+ and cAMP. Nature 492, 123–127. doi:10.1038/nature11588
Liang, W., Qi, W., Geng, Y., Wang, L., Zhao, J., Zhu, K., et al. (2021). Necroptosis activates UPR sensors without disrupting their binding with GRP78. Proc. Natl. Acad. Sci. U. S. A. 118, e2110476118. doi:10.1073/pnas.2110476118
Li, G., Fang, S., Shao, X., Li, Y., Tong, Q., Kong, B., et al. (2021a). Curcumin reverses NNMT-induced 5-fluorouracil resistance via increasing ROS and cell cycle arrest in colorectal cancer cells. Biomolecules 11, 1295. doi:10.3390/biom11091295
Liu, B., Li, X., Wang, D., Yu, Y., Lu, D., Chen, L., et al. (2022a). CEMIP promotes extracellular matrix-detached prostate cancer cell survival by inhibiting ferroptosis. Cancer Sci. 113, 2056–2070. doi:10.1111/cas.15356
Liu, J., Wei, Y., Jia, W., Can, C., Wang, R., Yang, X., et al. (2022b). Chenodeoxycholic acid suppresses AML progression through promoting lipid peroxidation via ROS/p38 MAPK/DGAT1 pathway and inhibiting M2 macrophage polarization. Redox Biol. 56, 102452. doi:10.1016/j.redox.2022.102452
Liu, Y., Zhang, M., and Bu, W. (2020). Bioactive nanomaterials for ion-interference therapy. View 1, e18. doi:10.1002/viw2.18
Liu, Z., Cheng, Q., Ma, X., and Song, M. (2022c). Suppressing effect of Na(+)/Ca(2+) exchanger (NCX) inhibitors on the growth of melanoma cells. Int. J. Mol. Sci. 23, 901. doi:10.3390/ijms23020901
Li, Y., Huang, C., and Xu, Y. (2022). Colon cancer exosome-derived biomimetic nanoplatform for curcumin-mediated sonodynamic therapy and calcium overload. Front. Bioeng. Biotechnol. 10, 1069676. doi:10.3389/fbioe.2022.1069676
Li, Y., Yu, X., Deng, L., Zhou, S., Wang, Y., Zheng, X., et al. (2021b). Neochlorogenic acid anchors MCU-based calcium overload for cancer therapy. Food Funct. 12, 11387–11398. doi:10.1039/d1fo01393a
Li, Y., Zhou, S., Song, H., Yu, T., Zheng, X., and Chu, Q. (2021c). CaCO(3) nanoparticles incorporated with KAE to enable amplified calcium overload cancer therapy. Biomaterials 277, 121080. doi:10.1016/j.biomaterials.2021.121080
Loncke, J., Kaasik, A., Bezprozvanny, I., Parys, J. B., Kerkhofs, M., and Bultynck, G. (2021). Balancing ER-mitochondrial Ca(2+) fluxes in Health and disease. Trends Cell Biol. 31, 598–612. doi:10.1016/j.tcb.2021.02.003
Loveless, R., Bloomquist, R., and Teng, Y. (2021). Pyroptosis at the forefront of anticancer immunity. J. Exp. Clin. Cancer Res. 40, 264. doi:10.1186/s13046-021-02065-8
Lv, Y., Shao, G., Zhang, Q., Wang, X., Meng, Y., Wang, L., et al. (2019). The antimicrobial peptide PFR induces necroptosis mediated by ER stress and elevated cytoplasmic calcium and mitochondrial ROS levels: cooperation with Ara-C to act against acute myeloid leukemia. Signal Transduct. Target Ther. 4, 38. doi:10.1038/s41392-019-0073-6
Marchi, S., Giorgi, C., Galluzzi, L., and Pinton, P. (2020). Ca(2+) fluxes and cancer. Mol. Cell 78, 1055–1069. doi:10.1016/j.molcel.2020.04.017
Marciniak, S. J., Chambers, J. E., and Ron, D. (2022). Pharmacological targeting of endoplasmic reticulum stress in disease. Nat. Rev. Drug Discov. 21, 115–140. doi:10.1038/s41573-021-00320-3
Mehla, K., and Singh, P. K. (2019). Metabolic regulation of macrophage polarization in cancer. Trends Cancer 5, 822–834. doi:10.1016/j.trecan.2019.10.007
Meng, X., Wu, X., Zheng, Y., Shang, K., Jing, R., Jiao, P., et al. (2020). Exploiting Ca(2+) signaling in T cells to advance cancer immunotherapy. Semin. Immunol. 49, 101434. doi:10.1016/j.smim.2020.101434
Miwa, S., Kashyap, S., Chini, E., and Von Zglinicki, T. (2022). Mitochondrial dysfunction in cell senescence and aging. J. Clin. Invest. 132, e158447. doi:10.1172/JCI158447
Montagnani Marelli, M., Marzagalli, M., Moretti, R. M., Beretta, G., Casati, L., Comitato, R., et al. (2016). Vitamin E δ-tocotrienol triggers endoplasmic reticulum stress-mediated apoptosis in human melanoma cells. Sci. Rep. 6, 30502. doi:10.1038/srep30502
Moossavi, M., Parsamanesh, N., Bahrami, A., Atkin, S. L., and Sahebkar, A. (2018). Role of the NLRP3 inflammasome in cancer. Mol. Cancer 17, 158. doi:10.1186/s12943-018-0900-3
Murakami, T., Ockinger, J., Yu, J., Byles, V., Mccoll, A., Hofer, A. M., et al. (2012). Critical role for calcium mobilization in activation of the NLRP3 inflammasome. Proc. Natl. Acad. Sci. U. S. A. 109, 11282–11287. doi:10.1073/pnas.1117765109
Nakagawa, C., Suzuki-Karasaki, M., Suzuki-Karasaki, M., Ochiai, T., and Suzuki-Karasaki, Y. (2020). The mitochondrial Ca(2+) overload via voltage-gated Ca(2+) entry contributes to an anti-melanoma effect of diallyl trisulfide. Int. J. Mol. Sci. 21, 491. doi:10.3390/ijms21020491
Nakamura, T., Ogawa, M., Kojima, K., Takayanagi, S., Ishihara, S., Hattori, K., et al. (2021). The mitochondrial Ca(2+) uptake regulator, MICU1, is involved in cold stress-induced ferroptosis. EMBO Rep. 22, e51532. doi:10.15252/embr.202051532
Nomura, M., Ueno, A., Saga, K., Fukuzawa, M., and Kaneda, Y. (2014). Accumulation of cytosolic calcium induces necroptotic cell death in human neuroblastoma. Cancer Res. 74, 1056–1066. doi:10.1158/0008-5472.CAN-13-1283
NúñEZ, M. T., and Hidalgo, C. (2019). Noxious iron-calcium connections in neurodegeneration. Front. Neurosci. 13, 48. doi:10.3389/fnins.2019.00048
Obeid, M., Tesniere, A., Ghiringhelli, F., Fimia, G. M., Apetoh, L., Perfettini, J. L., et al. (2007). Calreticulin exposure dictates the immunogenicity of cancer cell death. Nat. Med. 13, 54–61. doi:10.1038/nm1523
Patergnani, S., Danese, A., Bouhamida, E., Aguiari, G., Previati, M., Pinton, P., et al. (2020). Various aspects of calcium signaling in the regulation of apoptosis, autophagy, cell proliferation, and cancer. Int. J. Mol. Sci. 21, 8323. doi:10.3390/ijms21218323
Paul, S., Jakhar, R., Bhardwaj, M., Chauhan, A. K., and Kang, S. C. (2021). Fumonisin B1 induces poly (ADP-ribose) (PAR) polymer-mediated cell death (parthanatos) in neuroblastoma. Food Chem. Toxicol. 154, 112326. doi:10.1016/j.fct.2021.112326
Pedrera, L., Espiritu, R. A., Ros, U., Weber, J., Schmitt, A., Stroh, J., et al. (2021). Ferroptotic pores induce Ca(2+) fluxes and ESCRT-III activation to modulate cell death kinetics. Cell Death Differ. 28, 1644–1657. doi:10.1038/s41418-020-00691-x
Perillo, B., Di Donato, M., Pezone, A., Di Zazzo, E., Giovannelli, P., Galasso, G., et al. (2020). ROS in cancer therapy: the bright side of the moon. Exp. Mol. Med. 52, 192–203. doi:10.1038/s12276-020-0384-2
Peters, A. A., Jamaludin, S. Y. N., Yapa, K., Chalmers, S., Wiegmans, A. P., Lim, H. F., et al. (2017). Oncosis and apoptosis induction by activation of an overexpressed ion channel in breast cancer cells. Oncogene 36, 6490–6500. doi:10.1038/onc.2017.234
Petersen, O. H., Gerasimenko, J. V., Gerasimenko, O. V., Gryshchenko, O., and Peng, S. (2021). The roles of calcium and ATP in the physiology and pathology of the exocrine pancreas. Physiol. Rev. 101, 1691–1744. doi:10.1152/physrev.00003.2021
Qiu, M., Chen, J., Huang, X., Li, B., Zhang, S., Liu, P., et al. (2022). Engineering chemotherapeutic-augmented calcium phosphate nanoparticles for treatment of intraperitoneal disseminated ovarian cancer. ACS Appl. Mater Interfaces 14, 21954–21965. doi:10.1021/acsami.2c02552
Raimondi, M., Fontana, F., Marzagalli, M., Audano, M., Beretta, G., Procacci, P., et al. (2021). Ca(2+) overload- and ROS-associated mitochondrial dysfunction contributes to δ-tocotrienol-mediated paraptosis in melanoma cells. Apoptosis 26, 277–292. doi:10.1007/s10495-021-01668-y
Rosenblatt, R. B., Frank, J. A., and Burks, S. R. (2021). Cytosolic Ca(2+) transients during pulsed focused ultrasound generate reactive oxygen species and cause DNA damage in tumor cells. Theranostics 11, 602–613. doi:10.7150/thno.48353
Schwartz, A. V., Backlund, J. C., De Boer, I. H., Rubin, M. R., Barnie, A., Farrell, K., et al. (2022). Risk factors for lower bone mineral density in older adults with type 1 diabetes: a cross-sectional study. Lancet Diabetes Endocrinol. 10, 509–518. doi:10.1016/S2213-8587(22)00103-6
Sies, H., and Jones, D. P. (2020). Reactive oxygen species (ROS) as pleiotropic physiological signalling agents. Nat. Rev. Mol. Cell Biol. 21, 363–383. doi:10.1038/s41580-020-0230-3
Song, Y., Chen, J., Zhang, C., Xin, L., Li, Q., Liu, Y., et al. (2022). Mechanosensitive channel Piezo1 induces cell apoptosis in pancreatic cancer by ultrasound with microbubbles. iScience 25, 103733. doi:10.1016/j.isci.2022.103733
Stockwell, B. R., Friedmann Angeli, J. P., Bayir, H., Bush, A. I., Conrad, M., Dixon, S. J., et al. (2017). Ferroptosis: a regulated cell death nexus linking metabolism, redox biology, and disease. Cell 171, 273–285. doi:10.1016/j.cell.2017.09.021
Su, B. C., Hung, G. Y., Tu, Y. C., Yeh, W. C., Lin, M. C., and Chen, J. Y. (2021). Marine antimicrobial peptide TP4 exerts anticancer effects on human synovial sarcoma cells via calcium overload, reactive oxygen species production and mitochondrial hyperpolarization. Mar. Drugs 19, 93. doi:10.3390/md19020093
Sung, H., Ferlay, J., Siegel, R. L., Laversanne, M., Soerjomataram, I., Jemal, A., et al. (2021). Global cancer statistics 2020: GLOBOCAN estimates of incidence and mortality worldwide for 36 cancers in 185 countries. CA Cancer J. Clin. 71, 209–249. doi:10.3322/caac.21660
Sun, Q., Liu, B., Zhao, R., Feng, L., Wang, Z., Dong, S., et al. (2021). Calcium peroxide-based nanosystem with cancer microenvironment-activated capabilities for imaging guided combination therapy via mitochondrial Ca(2+) overload and chemotherapy. ACS Appl. Mater Interfaces 13, 44096–44107. doi:10.1021/acsami.1c13304
Sun, Y., Chen, Y., Ma, X., Yuan, Y., Liu, C., Kohn, J., et al. (2016). Mitochondria-targeted hydroxyapatite nanoparticles for selective growth inhibition of lung cancer in vitro and in vivo. ACS Appl. Mater Interfaces 8, 25680–25690. doi:10.1021/acsami.6b06094
Sun, Y., Yang, Y. M., Hu, Y. Y., Ouyang, L., Sun, Z. H., Yin, X. F., et al. (2022). Inhibition of nuclear deacetylase Sirtuin-1 induces mitochondrial acetylation and calcium overload leading to cell death. Redox Biol. 53, 102334. doi:10.1016/j.redox.2022.102334
Tan, P., He, L., Huang, Y., and Zhou, Y. (2022). Optophysiology: illuminating cell physiology with optogenetics. Physiol. Rev. 102, 1263–1325. doi:10.1152/physrev.00021.2021
Tan, X., Huang, J., Wang, Y., He, S., Jia, L., Zhu, Y., et al. (2021). Transformable nanosensitizer with tumor microenvironment-activated sonodynamic process and calcium release for enhanced cancer immunotherapy. Angew. Chem. Int. Ed. Engl. 60, 14051–14059. doi:10.1002/anie.202102703
Trebak, M., and Kinet, J. P. (2019). Calcium signalling in T cells. Nat. Rev. Immunol. 19, 154–169. doi:10.1038/s41577-018-0110-7
Tufi, R., Panaretakis, T., Bianchi, K., Criollo, A., Fazi, B., Di Sano, F., et al. (2008). Reduction of endoplasmic reticulum Ca2+ levels favors plasma membrane surface exposure of calreticulin. Cell Death Differ. 15, 274–282. doi:10.1038/sj.cdd.4402275
Vig, M., and Kinet, J. P. (2009). Calcium signaling in immune cells. Nat. Immunol. 10, 21–27. doi:10.1038/ni.f.220
Wang, S., Yi, X., Wu, Z., Guo, S., Dai, W., Wang, H., et al. (2022). CAMKK2 defines ferroptosis sensitivity of melanoma cells by regulating AMPKNRF2 pathway. J. Invest. Dermatol 142, 189–200 e8. doi:10.1016/j.jid.2021.05.025
Wang, T., He, L., Jing, J., Lan, T. H., Hong, T., Wang, F., et al. (2021). Caffeine-operated synthetic modules for chemogenetic control of protein activities by life style. Adv. Sci. (Weinh) 8, 2002148. doi:10.1002/advs.202002148
Wang, W., Yu, S., Huang, S., Deng, R., Ding, Y., Wu, Y., et al. (2019). A complex role for calcium signaling in colorectal cancer development and progression. Mol. Cancer Res. 17, 2145–2153. doi:10.1158/1541-7786.MCR-19-0429
Wen, H., Zhong, Y., Yin, Y., Qin, K., Yang, L., Li, D., et al. (2022). A marine-derived small molecule induces immunogenic cell death against triple-negative breast cancer through ER stress-CHOP pathway. Int. J. Biol. Sci. 18, 2898–2913. doi:10.7150/ijbs.70975
Wu, D., Zhu, Z. Q., Tang, H. X., Shi, Z. E., Kang, J., Liu, Q., et al. (2020). Efficacy-shaping nanomedicine by loading calcium peroxide into tumor microenvironment-responsive nanoparticles for the antitumor therapy of prostate cancer. Theranostics 10, 9808–9829. doi:10.7150/thno.43631
Wu, H., Chen, W., Chen, Z., Li, X., and Wang, M. (2023). Novel tumor therapy strategies targeting endoplasmic reticulum-mitochondria signal pathways. Ageing Res. Rev. 88, 101951. doi:10.1016/j.arr.2023.101951
Wu, H., Liu, S., Chen, S., Hua, Y., Li, X., Zeng, Q., et al. (2022). A selective reduction of osteosarcoma by mitochondrial apoptosis using hydroxyapatite nanoparticles. Int. J. Nanomedicine 17, 3691–3710. doi:10.2147/IJN.S375950
Wu, H., Li, Z., Tang, J., Yang, X., Zhou, Y., Guo, B., et al. (2019). The in vitro and in vivo anti-melanoma effects of hydroxyapatite nanoparticles: influences of material factors. Int. J. Nanomedicine 14, 1177–1191. doi:10.2147/IJN.S184792
Xiang, X., Wang, J., Lu, D., and Xu, X. (2021). Targeting tumor-associated macrophages to synergize tumor immunotherapy. Signal Transduct. Target Ther. 6, 75. doi:10.1038/s41392-021-00484-9
Xian, H., Watari, K., Sanchez-Lopez, E., Offenberger, J., Onyuru, J., Sampath, H., et al. (2022). Oxidized DNA fragments exit mitochondria via mPTP- and VDAC-dependent channels to activate NLRP3 inflammasome and interferon signaling. Immunity 55, 1370–1385.e8. doi:10.1016/j.immuni.2022.06.007
Xiao, H., Ma, L., Ding, J., Wang, H., Bi, X., Tan, F., et al. (2023). Mitochondrial calcium uniporter (MCU) that modulates mitochondrial calcium uptake and facilitates endometrial cancer progression through interaction with VDAC1. Curr. Cancer Drug Targets 24, 354–367. doi:10.2174/1568009624666230912095526
Xie, Q., Xu, Y., Gao, W., Zhang, Y., Su, J., Liu, Y., et al. (2018). TAT-fused IP3R-derived peptide enhances cisplatin sensitivity of ovarian cancer cells by increasing ER Ca2+ release. Int. J. Mol. Med. 41, 809–817. doi:10.3892/ijmm.2017.3260
Xue, P., Chen, Q., Ren, X., Liu, D., and Yang, X. (2021). A novel protoapigenone analog RY10-4 induces apoptosis of breast cancer cells by exacerbating mitochondrial Ca(2+) influx through mitochondrial calcium uniporter. Toxicol. Appl. Pharmacol. 433, 115776. doi:10.1016/j.taap.2021.115776
Xu, S., Cheng, X., Wu, L., Zheng, J., Wang, X., Wu, J., et al. (2020). Capsaicin induces mitochondrial dysfunction and apoptosis in anaplastic thyroid carcinoma cells via TRPV1-mediated mitochondrial calcium overload. Cell Signal 75, 109733. doi:10.1016/j.cellsig.2020.109733
Xu, W., Che, Y., Zhang, Q., Huang, H., Ding, C., Wang, Y., et al. (2021). Apaf-1 pyroptosome senses mitochondrial permeability transition. Cell Metab. 33, 424–436.e10. doi:10.1016/j.cmet.2020.11.018
Yan, J., Wan, P., Choksi, S., and Liu, Z. G. (2022). Necroptosis and tumor progression. Trends Cancer 8, 21–27. doi:10.1016/j.trecan.2021.09.003
Yao, J., Peng, H., Qiu, Y., Li, S., Xu, X., Wu, A., et al. (2022a). Nanoplatform-mediated calcium overload for cancer therapy. J. Mater Chem. B 10, 1508–1519. doi:10.1039/d1tb02721b
Yao, M., Han, W., Feng, L., Wei, Z., Liu, Y., Zhang, H., et al. (2022b). pH-programmed responsive nanoplatform for synergistic cancer therapy based on single atom catalysts. Eur. J. Med. Chem. 233, 114236. doi:10.1016/j.ejmech.2022.114236
Ye, Y., Li, X., Wang, Z., Ye, F., Xu, W., Lu, R., et al. (2021). 3,3'-Diindolylmethane induces gastric cancer cells death via STIM1 mediated store-operated calcium entry. Int. J. Biol. Sci. 17, 1217–1233. doi:10.7150/ijbs.56833
Yin, Y., Jiang, T., Hao, Y., Zhang, J., Li, W., Hao, Y., et al. (2021). Cascade catalytic nanoplatform based on ions interference strategy for calcium overload therapy and ferroptosis. Int. J. Pharm. 606, 120937. doi:10.1016/j.ijpharm.2021.120937
You, K., Wang, L., Chou, C. H., Liu, K., Nakata, T., Jaiswal, A., et al. (2021). QRICH1 dictates the outcome of ER stress through transcriptional control of proteostasis. Science 371, eabb6896. doi:10.1126/science.abb6896
Yu, X. X., Zhu, M. Y., Wang, J. R., Li, H., Hu, P., Qing, Y. J., et al. (2021b). LW-213 induces cell apoptosis in human cutaneous T-cell lymphomas by activating PERK-eIF2α-ATF4-CHOP axis. Acta Pharmacol. Sin. 42, 290–300. doi:10.1038/s41401-020-0466-7
Yuan, R., Zhao, W., Wang, Q. Q., He, J., Han, S., Gao, H., et al. (2021). Cucurbitacin B inhibits non-small cell lung cancer in vivo and in vitro by triggering TLR4/NLRP3/GSDMD-dependent pyroptosis. Pharmacol. Res. 170, 105748. doi:10.1016/j.phrs.2021.105748
Yu, C., Wang, Y., Peng, J., Shen, Q., Chen, M., Tang, W., et al. (2017). Mitochondrial calcium uniporter as a target of microRNA-340 and promoter of metastasis via enhancing the Warburg effect. Oncotarget 8, 83831–83844. doi:10.18632/oncotarget.19747
Yu, J., Zhong, B., Jin, L., Hou, Y., Ai, N., Ge, W., et al. (2020). 2-Methoxy-6-acetyl-7-methyljuglone (MAM) induced programmed necrosis in glioblastoma by targeting NAD(P)H: quinone oxidoreductase 1 (NQO1). Free Radic. Biol. Med. 152, 336–347. doi:10.1016/j.freeradbiomed.2020.03.026
Yu, N., Huang, L., Zhou, Y., Xue, T., Chen, Z., and Han, G. (2019). Near-infrared-light activatable nanoparticles for deep-tissue-penetrating wireless optogenetics. Adv. Healthc. Mater 8, e1801132. doi:10.1002/adhm.201801132
Yu, P., Zhang, X., Liu, N., Tang, L., Peng, C., and Chen, X. (2021a). Pyroptosis: mechanisms and diseases. Signal Transduct. Target Ther. 6, 128. doi:10.1038/s41392-021-00507-5
Zhang, M. J., Gao, W., Liu, S., Siu, S. P., Yin, M., Ng, J. C., et al. (2020). CD38 triggers inflammasome-mediated pyroptotic cell death in head and neck squamous cell carcinoma. Am. J. Cancer Res. 10, 2895–2908.
Zhang, L., Cheng, X., Xu, S., Bao, J., and Yu, H. (2018). Curcumin induces endoplasmic reticulum stress-associated apoptosis in human papillary thyroid carcinoma BCPAP cells via disruption of intracellular calcium homeostasis. Med. Baltim. 97, e11095. doi:10.1097/MD.0000000000011095
Zhang, L., Qi, J., Zhang, X., Zhao, X., An, P., Luo, Y., et al. (2022). The regulatory roles of mitochondrial calcium and the mitochondrial calcium uniporter in tumor cells. Int. J. Mol. Sci. 23, 6667. doi:10.3390/ijms23126667
Zhang, M., Song, R. X., Liu, Y. Y., Yi, Z. G., Meng, X. F., Zhang, J. W., et al. (2019). Calcium-overload-mediated tumor therapy by calcium peroxide nanoparticles. Chem 5, 2171–2182. doi:10.1016/j.chempr.2019.06.003
Zhao, Y., Bian, Y., Xiao, X., Liu, B., Ding, B., Cheng, Z., et al. (2022). Tumor microenvironment-responsive Cu/CaCO(3) -based nanoregulator for mitochondrial homeostasis disruption-enhanced chemodynamic/sonodynamic therapy. Small 18, e2204047. doi:10.1002/smll.202204047
Zheng, P., Ding, B., Jiang, Z., Xu, W., Li, G., Ding, J., et al. (2021a). Ultrasound-augmented mitochondrial calcium ion overload by calcium nanomodulator to induce immunogenic cell death. Nano Lett. 21, 2088–2093. doi:10.1021/acs.nanolett.0c04778
Zheng, P., Ding, B., Shi, R., Jiang, Z., Xu, W., Li, G., et al. (2021b). A multichannel Ca(2+) nanomodulator for multilevel mitochondrial destruction-mediated cancer therapy. Adv. Mater 33, e2007426. doi:10.1002/adma.202007426
Zheng, P., Ding, B., Zhu, G., Li, C., and Lin, J. (2022a). Biodegradable Ca(2+) nanomodulators activate pyroptosis through mitochondrial Ca(2+) overload for cancer immunotherapy. Angew. Chem. Int. Ed. Engl. 61, e202204904. doi:10.1002/anie.202204904
Zheng, S., Wang, X., Zhao, D., Liu, H., and Hu, Y. (2022b). Calcium homeostasis and cancer: insights from endoplasmic reticulum-centered organelle communications. Trends Cell Biol. 33, 312–323. doi:10.1016/j.tcb.2022.07.004
Zhou, B., Lin, Y., Chen, S., Cai, J., Luo, Z., Yu, S., et al. (2021). Activation of Ca(2+)/calmodulin-dependent protein kinase II (CaMKII) with lidocaine provokes pyroptosis of glioblastoma cells. Bull. Exp. Biol. Med. 171, 297–304. doi:10.1007/s10517-021-05216-1
Zhou, H., Wang, K., Wang, M., Zhao, W., Zhang, C., Cai, M., et al. (2022). ER-Phagy in the occurrence and development of cancer. Biomedicines 10, 707. doi:10.3390/biomedicines10030707
Zhou, X., Friedmann, K. S., Lyrmann, H., Zhou, Y., Schoppmeyer, R., KnöRCK, A., et al. (2018). A calcium optimum for cytotoxic T lymphocyte and natural killer cell cytotoxicity. J. Physiol. 596, 2681–2698. doi:10.1113/JP274964
Keywords: calcium overload, ion interference therapy, outcome, prospect, strategy, tumor treatment
Citation: Li S, Fan R, Wang Y, He K, Xu J and Li H (2024) Application of calcium overload-based ion interference therapy in tumor treatment: strategies, outcomes, and prospects. Front. Pharmacol. 15:1352377. doi: 10.3389/fphar.2024.1352377
Received: 08 December 2023; Accepted: 02 February 2024;
Published: 15 February 2024.
Edited by:
Jinwei Zhang, Chinese Academy of Sciences (CAS), ChinaReviewed by:
Barbara Niemeyer, Saarland University, GermanyDana-Lynn Koomoa-Lange, Western New England University, United States
Copyright © 2024 Li, Fan, Wang, He, Xu and Li. This is an open-access article distributed under the terms of the Creative Commons Attribution License (CC BY). The use, distribution or reproduction in other forums is permitted, provided the original author(s) and the copyright owner(s) are credited and that the original publication in this journal is cited, in accordance with accepted academic practice. No use, distribution or reproduction is permitted which does not comply with these terms.
*Correspondence: Hongli Li, bGlob25nbGlAdG1tdS5lZHUuY24=