- 1Traditional Chinese Medicine Integrated Department of Nephrology, The First Affiliated Hospital of Zhengzhou University, Zhengzhou, China
- 2Institute of Nephrology, Zhengzhou University, Zhengzhou, China
- 3Henan Province Research Center for Kidney Disease, Zhengzhou, China
- 4Key Laboratory of Precision Diagnosis and Treatment for Chronic Kidney Disease in Henan Province, Zhengzhou, China
The global prevalence of diabetes mellitus (DM) has led to widespread multi-system damage, especially in cardiovascular and renal functions, heightening morbidity and mortality. Emerging antidiabetic drugs sodium-glucose cotransporter 2 inhibitors (SGLT2i), glucagon-like peptide-1 receptor agonists (GLP-1RAs), and dipeptidyl peptidase-4 inhibitors (DPP-4i) have demonstrated efficacy in preserving cardiac and renal function, both in type 2 diabetic and non-diabetic individuals. To understand the exact impact of these drugs on cardiorenal protection and underlying mechanisms, we conducted a comprehensive review of recent large-scale clinical trials and basic research focusing on SGLT2i, GLP-1RAs, and DPP-4i. Accumulating evidence highlights the diverse mechanisms including glucose-dependent and independent pathways, and revealing their potential cardiorenal protection in diabetic and non-diabetic cardiorenal disease. This review provides critical insights into the cardiorenal protective effects of SGLT2i, GLP-1RAs, and DPP-4i and underscores the importance of these medications in mitigating the progression of cardiovascular and renal complications, and their broader clinical implications beyond glycemic management.
1 Introduction
Diabetes mellitus (DM) is the most common metabolic disorder worldwide. It is reported that the prevalence of diabetes will increase to 12.2% (789.2 million) by 2045 (Sun et al., 2022). Type 2 diabetes mellitus (T2DM) is particularly prone to a range of complications, including macrovascular disease (cardiovascular and cerebrovascular disease), which is mainly characterized by atherosclerosis of large blood vessels, as well as microvascular disease (diabetic retinopathy and diabetic kidney disease), which often manifests as microvascular endothelial dysfunction and microthrombosis (Li et al., 2023; Mauricio et al., 2023). The driving factors for cardiovascular complications in diabetic patients including glucotoxicity, lipotoxicity, and hypertension (Kenny and Abel, 2019). Meanwhile, diabetic kidney disease (DKD) is the most common cause of death among microvascular complications of diabetes and is closely associated with cardiovascular outcomes (Blazek and Bakris, 2023). Currently, there has been a paradigm shift in the management of diabetes and its complications, with a focus on not only controlling blood glucose levels but also addressing the associated cardiovascular and renal risks.
In recent years, new classes of anti-diabetic medications such as sodium-glucose cotransporter 2 inhibitors (SGLT2i), glucagon-like peptide-1 receptor agonists (GLP-1RAs), and dipeptidyl peptidase-4 inhibitors (DPP-4i), have shown efficacy in reducing cardiovascular events, slowing the progression of DKD, and improving overall cardiovascular and renal health in diabetic patients (Yin et al., 2022; Guo et al., 2023; Klen and Dolžan, 2023; Panico et al., 2023). More importantly, these medications exhibit cardiorenal protective effects beyond glycemic control and have the potential to ameliorate non-diabetic cardiovascular and renal diseases.
Research have shown that these three classes of drugs can reduce oxidative stress and inflammation through various mechanisms, including reducing cell damage caused by advanced glycation end products, improving mitochondrial function, and inhibiting the production of reactive oxygen species. This suggests that these medications may have therapeutic potential beyond lowering glucose levels (Andreadi et al., 2023; Balogh et al., 2023). Understanding the mechanisms of these drugs is crucial for developing targeted therapies and improving the quality of life for millions of individuals affected by diabetes-related complications.
2 Emerging antidiabetic drugs
2.1 SGLT2i
Sodium-glucose cotransporter 2 (SGLT2) is located in the proximal tubules of the kidney and is responsible for reabsorbing 80%–90% of urine glucose. Studies have shown that SGLT2 expression is upregulated in the tubular tissues of T2DM and type 1 diabetes mellitus (T1DM) patients (Rahmoune et al., 2005). SGLT2i reduce glucose reabsorption by inhibiting this protein. Interestingly, this effect is independent of insulin secretion and β-cell function, largely reducing the burden on β-cells and the risk of hypoglycemia (Abdul-Ghani et al., 2013). The reduced glucose reabsorption also results in less fluid retention and better control of overweight and hypertension, which often accompany T2DM. Over the past decade, SGLT2i have become a hot topic in scientific and clinical research and a breakthrough in the field of new hypoglycemic agents because of their unique therapeutic effect on diabetes. Representative drugs include empagliflozin, canagliflozin, dapagliflozin, sotagliflozin, ertugliflozin, etc.
2.2 GLP-1RAs
Glucagon-like peptide-1 (GLP-1) is an incretin hormone that is secreted in large amounts by L cells located in the intestinal crypt when the intestine is stimulated by nutrients (Müller et al., 2019). The action of GLP-1 depends on the location of its receptors. GLP-1 receptors belong to the G protein-coupled receptors family and are widely distributed in various tissues of the body. In pancreatic α cells, GLP-1 can reduce the secretion of glucagon, while in β cells, it can increase the secretion of insulin, improve the body’s insulin sensitivity, and even promote the proliferation of β cells (Graaf et al., 2016). What’s more, GLP-1, which is located in the brain, suppresses appetite and reduces food intake, leading to weight loss, which is as important as glycemic control in patients with T2DM (Baggio and Drucker, 2014). GLP-1RAs are a new class of antidiabetic drugs that were first approved for the treatment of diabetes in 2005. The representative drugs are exenatide, dulaglutide, liraglutide, and semaglutide.
2.3 DPP-4i
Dipeptidyl peptidase-4 (DPP-4) is an enzyme that can rapidly cleave GLP-1, which is a hormone with a very short half-life (Tschöp et al., 2023). DPP-4i can effectively prolong the half-life of GLP-1, increase insulin in the body, and reduce blood glucose over a long period (Capuano et al., 2013). The representative drugs are sitagliptin, linagliptin, and saxagliptin.
3 Cardiorenal protection of SGLT2i, GLP-1RAs, and DPP-4i
The American Diabetes Association (ADA) recommends that SGLT2i and GLP-1RAs be used in combination with metformin as first-line initial therapy in patients at high risk of heart failure (HF), atherosclerotic cardiovascular disease (ASCVD), and chronic kidney disease (CKD). In patients with T2DM, GLP-1RAs are even more effective than insulin in some cases. For patients with established ASCVD, SGLT2i and GLP-1RAs can be used as additional agents alone, independent of metformin (Committee ADAPP, 2022b). Compared with the former two agents, DPP-4i are slightly inferior, and studies on cardiac and renal outcomes are limited. DPP-4i can be considered in patients with GLP-1RAs intolerance. Furthermore, the ADA also states that SGLT2i should be administered as early as possible in patients with stage CKD2 or worse, regardless of blood glucose. GLP-1RAs are principally used to delay cardiovascular disease, which may also delay CKD progression (Committee ADAPP, 2022a).
Similarly, the 2022 Kidney Disease: Improving Global Outcomes (KDIGO) guidelines also recommend SGLT2i therapy in patients with T2DM and CKD. Long-acting GLP-1RAs are recommended when ideal glycemic targets are not achieved with the combination of metformin and SGLT2i. Notably, when GLP-1RAs combined with insulin or sulfonylureas, reduced dose of these drugs is recommended to avoid hypoglycemia. Some DPP-4i, such as saxagliptin and sitagliptin, are accessible to patients with an estimated glomerular filtration rate (eGFR) of less than 30 mL/min/1.73 m2 or who are receiving dialysis, and offer a viable alternative for individuals who are not utilizing GLP-1RAs (de Boer et al., 2022).
In general, SGLT2i and GLP-1RAs have significant beneficial effects on renal and cardiac outcomes, while the cardiorenal protective effect of DPP-4i needs to be further explored and clarified. We reviewed the real-world clinical data and the literature on potential mechanisms to gain insight into the pleiotropic effects of emerging antidiabetic agents.
3.1 Cardiovascular protection
3.1.1 SGLT2i
3.1.1.1 Clinical trial
SGLT2i have demonstrated superiority over placebo in most cardiovascular outcome trials. In 2019, the DAPA-HF trial, which recruited 4744 patients with HF and reduced ejection fraction, indicated that once-daily dapagliflozin (10 mg) lowered the risk of composite cardiovascular outcomes when compared to placebo (HR, 0.74 [95% CI, 0.65 to 0.85]; p < 0.001) (Table 1) (McMurray et al., 2019). Furthermore, in a 2022 trial, dapagliflozin exhibited significant cardioprotective effects (DELIVER) on patients with HF with mild reduced ejection fraction or preserved left ventricular ejection fraction (>40%) (HR, 0.82 [95% CI, 0.73 to 0.92]; p < 0.001) (Solomon et al., 2022). These results are consistent with those of the empagliflozin and ertugliflozin outcomes trials in HF with a preserved ejection fraction (EMPEROR-Preserved trial, MK-8835-004 trial) (Cannon et al., 2020; Anker et al., 2021). However, the protective effect of SGLT2i appeared to vary based on gender; a study from Australia indicates that older men with baseline HF benefit more from SGLT2i than women (subdistribution HR, 0.78 [95% CI, 0.66 to 0.93] for men; subdistribution HR, 0.99 [95% CI, 0.77 to 1.28] for women). On the contrary, SGLT2i were observed to improve the outcomes of women with baseline ASCVD (subdistribution HR, 0.98 [95% CI, 0.74 to 0.73] for men; subdistribution HR, 0.36 [95% CI, 0.18 to 0.71] for women) (Sharma et al., 2023). In another study, the effect of canagliflozin on cardiovascular events did not differ by age or sex (HR, 0.71 [95% CI, 0.54 to 0.95] for women; HR, 0.69 [95% CI, 0.56 to 0.84] for men; p = 0.8 for interaction) (Yi et al., 2023). The disparate findings of these two reports are intriguing. The absence of beneficial effects of SGLT2i in women with baseline HF may, in part, be attributed to the limited number of women in this specific subgroup. Notably, given the age-related increase in cardiovascular disease (CVD) risk in both genders, particularly among post-menopausal women (Zhao et al., 2018), further investigations focusing on sex differences and involving a substantial number of patients are warranted to ascertain the potential sex-specific benefits of SGLT2i and elucidate the mechanisms involved. In addition, SGLT2i also have a significant advantage in acute HF, suggesting a lower risk of hospitalization (Park et al., 2023). Sotagliflozin has been shown to significantly reduce cardiovascular death-related events in T2DM patients with recent worsening HF (HR, 0.67 [95% CI, 0.52 to 0.85]; p < 0.001) (SOLOIST-WHF trial) (Bhatt et al., 2021). Empagliflozin was also associated with a greater reduction in the rate of worsening HF events (HR, 1.36 [95% CI, 1.09 to 1.68]; p = 0.0054) (EMPULSE trial) (Voors et al., 2022).
3.1.1.2 Basic research
SGLT2i exhibit superior efficacy in improving cardiovascular diseases, regardless of the presence of diabetes. In vivo experiments revealed that dapagliflozin reduced interleukin (IL)-1β expression and can downregulate the activity of [Na+] and [Ca2+]-related ion channels to alleviate mitochondrial reactive oxygen species, thereby improving angiotensin Ⅱ (Ang Ⅱ)-induced diabetic cardiomyopathy in db/db mice (Table 3) (Arow et al., 2020). Consistently, an in vivo study focusing on diabetic cardiomyopathy in T1DM rats revealed that dapagliflozin markedly reduced oxidative stress (Rosa et al., 2022). Notably, SGLT2i can restore and maintain sinus rhythm after ablation of atrial fibrillation in T2DM patients (Abu-Qaoud et al., 2023). Interestingly, empagliflozin was found to block the binding of CpG islands in the promoter regions of nuclear factor kappa-B (NF-κB) and superoxide dismutase 2 (SOD2) to ten-eleven translocation (TET2) in cardiomyocytes under high glucose conditions, preventing gene demethylation and alleviating myocardial injury (Scisciola et al., 2023).
In addition to their protective effect against diabetes-induced cardiomyopathy, SGLT2i also have a beneficial effect on other cardiovascular diseases, including ASCVD, which is primarily caused by hypertension-induced inflammation. SGLT2 receptor expression is present in macrophages, which are major players in the inflammatory response. Adenosine 5′-monophosphate-activated protein kinase (AMPK) is a key energy regulator that inhibits the pro-inflammatory effects of macrophages (Packer, 2020a; Jansen et al., 2020). In an atherosclerosis model characterized by a high-fat diet, empagliflozin inhibited NF-κB expression in plaque and reduced the viability of macrophages in Apoe−/− mice. Most importantly, it was able to restore p-AMPK expression in macrophages (Fu et al., 2022). Heme oxygenase-1 (HO-1) can protect the cardiovascular system by increasing the bioavailability of NO in endothelial cells. Canagliflozin can increase the expression of HO-1 in endothelial cells and attenuate the adhesion of monocytes to endothelial cells (Peyton et al., 2022). Vascular calcification is a common pathological process in ASCVD. Chen et al. demonstrated for the first time that canagliflozin could reduce vascular smooth muscle cells (VSMCs) calcification by down-regulating the expression of NOD-like receptor thermal protein domain associated protein 3 (NLRP3) (Chen et al., 2023). There are many ion channels in cardiomyocytes, such as Na+/H+ exchanger 1 (NHE1) and Na+/Ca2+ exchanger (NCX). Pathological conditions that lead to excessive activation or inhibition of ion channels significantly impact the systolic and diastolic movements of the heart. Various hormones (Ang Ⅱ, aldosterone) released during HF or myocardial ischemia (MI) can activate NHE1, inhibit NCX, and lead to intracellular calcium overload, which in turn activates NHE1 and exacerbates calcium overload (Figure 1A) (Kim et al., 2017). Studies have shown that dapagliflozin, empagliflozin, and canagliflozin can inhibit NHE1 to improve endothelial permeability induced by mechanical stretch (Li X. et al., 2021). These studies confirmed that SGLT2i can protect both static and dynamic endothelial cell function (Juni et al., 2021).
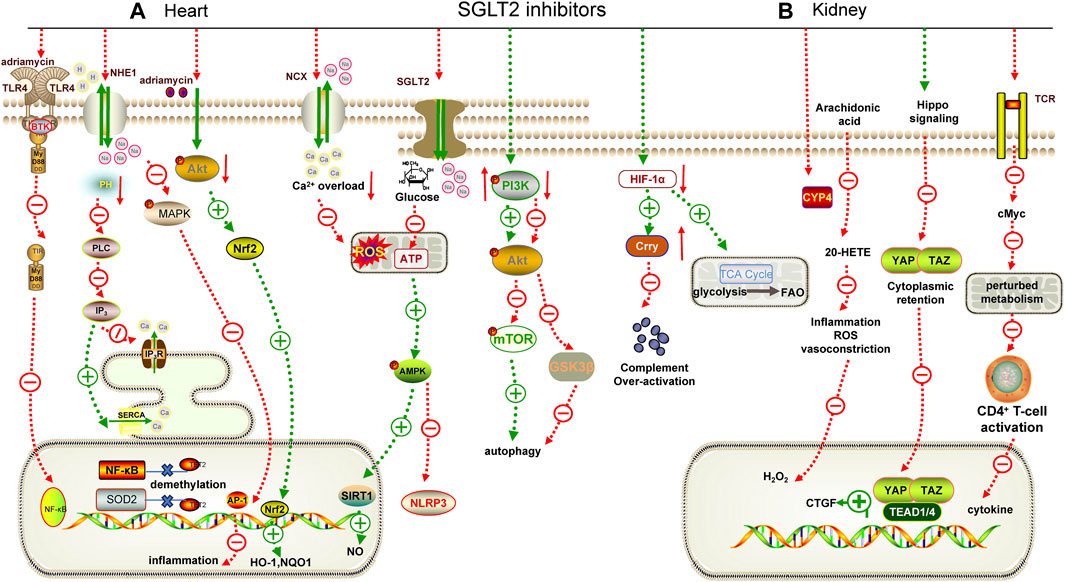
FIGURE 1. Mechanisms underlying the cardiorenal effects of SGLT2 inhibitors. (A) for heart; (B) for kidney. AMPK: adenosine monophosphate-activated protein kinase, TLR4: Toll-like receptor 4, MyD88: myeloid differentiation factor 88, NF-κB: nuclear factor-κB, PLC: phospholipase C, IP3: inositol 1,4,5-triphosphate, IP3R: inositol 1,4,5-triphosphate receptor, SERCA: sarcoplasmic reticulum Ca2+-ATPase, MAPK: mitogen-acticated protein kinase, AP-1: activator protein-1, Nrf2: nuclear factor erythroid2-related factor 2, SIRT1: sirtuin 1, PI3K: phosphatidylinositol 3-kinase, mTOR: mammalian target of rapamycin, GSK3β: glycogen synthase kinase 3β, HIF-1α: hypoxia-inducible factor 1-alpha, TCR: T cell receptor, CTGF: connective tissue growth factor, YAP: yes associated protein 1, TAZ: transcriptional coactivator PDZ-binding motif, RTK: receptor tyrosine kinase, NOQ1: NADH dehydrogenase quinone 1, SOD2: superoxide dismutase 2.
Notably, dapagliflozin also inhibits the mitogen-acticated protein kinase/activator protein-1 (MAPK/AP-1) pathway in an NHE1-dependent way to alleviate obesity-induced myocardial inflammation (Lin et al., 2022). Similarly, empagliflozin can improve myocardial injury in obese mice by regulating the AMPK/mammalian target of rapamycin (mTOR) pathway to maintain redox balance (Sun et al., 2020). In addition, empagliflozin also inhibited the overstimulated autophagy in cardiomyocytes by inhibiting the AMPK/glycogen synthase kinase 3β (GSK3β) pathway and NHE1 (Chung et al., 2023; Madonna et al., 2023). These findings highlight the multiple mechanisms by which SGLT2i contribute to the reduction of obesity-related myocardial complications.
SGLT2i also offer promising insights into preventing cardiac toxicity associated with antineoplastic agents. For example, dapagliflozin alleviated adriamycin-induced myocardial injury by inhibiting the phosphoinositide 3-kinase (PI3K)/protein kinase B (PKB)/nuclear factor erythroid 2-related factor 2 (Nrf2) pathway (Hsieh et al., 2022). Empagliflozin could significantly enhance the adriamycin-induced reduction of cardiomyocyte viability and inhibit the expression of NLRP3 and myeloid differentiation factor 88 (MyD88) (Quagliariello et al., 2021). Furthermore, empagliflozin was found to ameliorate sunitinib- and trastuzumab-induced cardiovascular complications by regulating the AMPK/mTOR pathway and ferroptosis (Ren et al., 2021; Min et al., 2023).
In conclusion, the cardiovascular protective effects of SGLT2i in relation to diabetes have been extensively explored. Additionally, SGLT2i have also shown multiple protective mechanisms in animal models of ASCVD, and it has been applied for the treatment of HF (Heidenreich et al., 2022). However, there is still new potential for clinical translation. SGLT2i have demonstrated remarkable advantages in obesity-related, antibiotic-induced, and antineoplastic drug-induced cardiotoxicity (Ren et al., 2021; Min et al., 2023). It is worth noting that although canagliflozin can exhibit anti-inflammatory effects in endothelial cells, recent reports have raised concerns about its specific impact on endothelial cells, which may elevate the risk of amputation (Peyton et al., 2022). Therefore, establishing SGLT2i as routine therapy for diseases beyond diabetes still has a way to go.
3.1.2 GLP-1RAs
3.1.2.1 Clinical trial
Although the previous ELIXA trial did not show superiority of lixisenatide in reducing the rate of major adverse cardiovascular events (MACE) (Table 1) (Pfeffer et al., 2015; Husain et al., 2019), GLP-1RAs has been gradually shown its advantage in improving cardiovascular outcomes in recent trials. Cardiovascular mortality among patients with T2DM and high cardiovascular risk was found to be lower with liraglutide than with placebo (HR, 0.87 [95% CI, 0.78 to 0.97]; p = 0.01) (LEADER) (Marso et al., 2016a). The SUSTAIN-6 trial, which involved 3,297 patients with T2DM and high cardiovascular risk, found that twice-weekly semaglutide significantly reduced the incidence of MACE (HR, 0.74 [95% CI, 0.58 to 0.95]; p = 0.02) (Marso et al., 2016b). In the Harmony Outcomes trial, the rate of MACE in T2DM patients with the addition of albiglutide (30–50 mg once-weekly) was lower than the placebo group (HR, 0.78 [95% CI, 0.68 to 0.90]; p = 0.0006) (Hernandez et al., 2018). Lastly, the REWIND trial revealed a reduction in MACE with once-weekly dulaglutide in T2DM patients (HR, 0.88 [95% CI, 0.79 to 0.99]; p = 0.026) (Gerstein et al., 2019).
3.1.2.2 Basic research
Extensive basic studies overwhelmingly support the observed beneficial effects of GLP-1RAs in clinical trials. Diabetes is often accompanied by lipid metabolism disorders, causing mitochondrial dysfunction. Studies have shown that liraglutide can inhibit the diacylglycerol/protein kinase C (DAG/PKC) pathway by activating AMPK, and upregulate Sirtuin 1 (SIRT1) to inactivate acetyl-CoA carboxylase phosphorylation, thereby reducing lipid-overloaded cardiomyocyte injury in streptozotocin-induced diabetic rats (Figure 2A) (Table 3)(Inoue et al., 2015). Similarly, liraglutide also increased adiponectin secretion and restored peroxisome proliferator-activated receptor gamma coactivator-1α (PGC-1α) expression by upregulating AMPK, which ameliorated IL-1β-induced mitochondrial damage in HL-1 cells (Zhang et al., 2020). Dulaglutide reduced the expression of NLRP3, IL-1β, and endoplasmic reticulum stress-related proteins induced by high glucose in human umbilical vein endothelial cells (HUVECs) via upregulating SIRT1 (Luo et al., 2019). Moreover, liraglutide enhanced the angiogenic potential of CD34 hematopoietic stem cells under high glucose conditions by activating the protective PI3K/PKB pathway and stimulating mitochondrial respiration (Sforza et al., 2022). Exenatide protects against high glucose-induced myocardial injury by inhibiting the NF-κB pathway and reducing the expression of tumor necrosis factor α (TNF-α) and monocyte chemotactic protein-1 (MCP-1) (Fu et al., 2020).
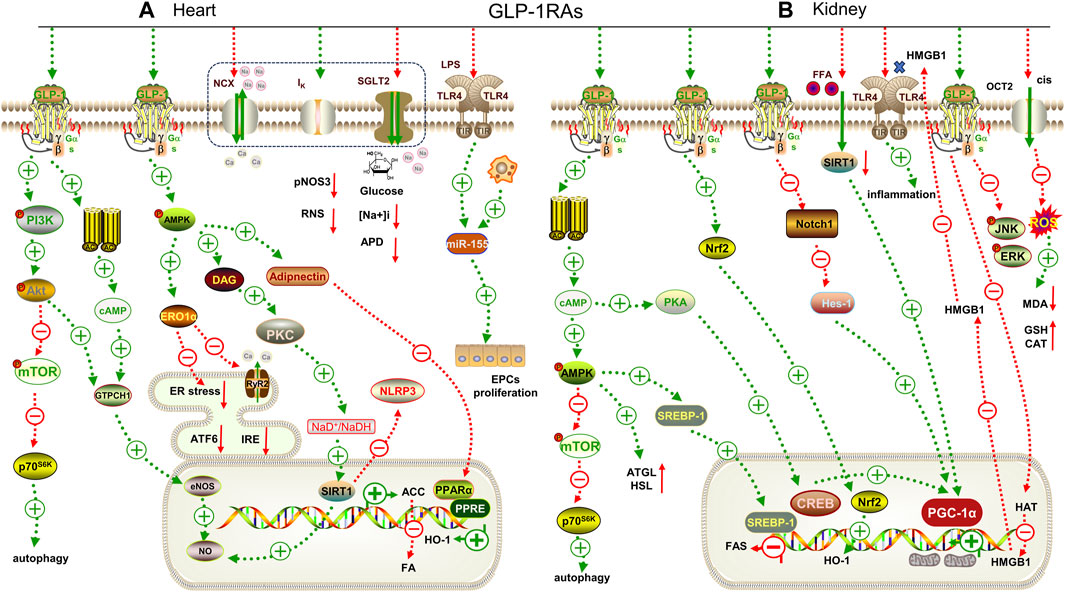
FIGURE 2. Mechanisms underlying the cardiorenal effects of GLP-1RAs. (A) for heart; (B) for kidney. GTPCH1: GTPcyclohydrolase Ⅰ, eNOS: endothelial nitric oxide synthase, ERO1α: endoplasmic oxidoreductin 1 like protein, ATF6: activating transcription factor 6, IRE: inositol-requiring enzyme, RyR2: ryanodine receptor 2, DAG: diacylglycerol, PPARα: peroxisome proliferator-activated receptor α, ATGL: adipose triglyceride lipase, HSL: hormone-sensitive lipase, SREBP-1: sterol regulatory element binding protein-1, FAS: fatty acid synthase, CREB: cAMP-response element binding protein, PGC-1α: peroxisome proliferator-activated receptor gamma coactivator-1alpha, Notch1: Notch homolog 1, Hes-1: hairy and enhancer of split-1, MDA: malondialdehyde. GSH: glutathione. pNOS3: phospho-endothelial nitric oxide, RNS: nitrogen species, APD: action potential duration, HMGB1: high mobility group protein 1, CAT: catalase, HAT: histone acrtyltransferases, LPS: lipopolysaccharide, ACC: acetyl CoA carboxylase.
GLP-1RAs exhibit similar potential to SGLT2i in improving ASCVD. Liraglutide has been shown to attenuate plaque formation in Apoe−/− mice by inducing cell cycle arrest in VSMCs in an AMPK-dependent or AMPK-independent manner (Jojima et al., 2017; Koshibu et al., 2019). Moreover, in the same mouse model, liraglutide was able to induce plaque regression by modulating bone marrow-derived macrophages to convert to anti-inflammatory phenotypes in the established plaque (Bruen et al., 2019).
GLP-1RAs have also demonstrated their potential in improving various other types of heart disease, including obesity-related, senile, and inflammatory heart disease, as well as improving the function of donor hearts after isolation. For example, liraglutide alleviates vascular inflammation in obesity by upregulating pAMPK expression and promoting Nrf2 nuclear translocation (Liu et al., 2023). It has also been shown to restore autophagy by inhibiting the mTOR/phosphoprotein 70 ribosomal protein S6 kinase (p70S6K) pathway caused by abdominal aortic coarctation (Zheng et al., 2020), regulate iNCX, delayed after potassium channel (Ik) and ryanodine receptor 2 (RyR2) channels in the myocardium, and restore mitochondrial membrane depolarization to protect the aged heart (Durak and Turan, 2023). Recent studies have also shown that acute administration of exenatide can increase NO to maintain good diastolic function after reperfusion in isolated hearts (Kadowaki et al., 2023). Furthermore, semaglutide can reduce lipopolysaccharides (LPS)-induced miR-155 secretion from macrophage exosomes to protect endothelial progenitor cell function (Pan et al., 2023). These findings highlight the multifaceted beneficial effects of GLP-1RAs on the cardiovascular system.
Currently, the superiority of GLP-1RAs in cardiovascular protection is primarily focused on diabetes-related conditions, as demonstrated above. However, recent findings have revealed that apart from their hypoglycemic effects, GLP-1RAs also have therapeutic efficacy in weight loss (Campbell et al., 2023). Basic research show their potential anti-inflammatory effects in obesity-related heart disease and advantages in ASCVD and aged animal models (Withaar et al., 2023). Despite the lack of clinical evidence, these findings suggest that GLP-1RAs may be used as a second or third-line therapy for non-diabetes-related cardiovascular diseases in the future.
3.1.3 DPP-4i
3.1.3.1 Clinical trial
Most of the cardiovascular outcome trials of DPP-4i have demonstrated cardiovascular safety rather than superiority. The EXAMINE trial, which included 5380 T2DM patients with a recent acute coronary event, showed that the rate of MACE with the addition of alogliptin was not superior to that of placebo (HR, 0.96 [upper boundary of the one-sided repeated CI, 1.16]; p < 0.001 for non-inferiority) (Table 1) (White et al., 2013). In the SAVOR-TIMI 53 trial, saxagliptin did not increase the rate of MACE in the elderly and very elderly patients (HR, 1.00 [95% CI, 0.89 to 1.12]; p = 0.99), but saxagliptin was surprisingly associated with an increased risk for HF hospitalization, thus its use requires detailed evaluation (HR, 1.27 [95% CI, 1.07 to 1.51]; p = 0.007) (Scirica et al., 2013; Leiter et al., 2015). The TECOS trial showed that sitagliptin did not increase the rate of MACE and hospitalizations for HF in patients with T2DM, even in high-risk patients (HR, 0.98 [95% CI, 0.88 to 1.09]; p = 0.65) (Green et al., 2015; McGuire et al., 2016). Similarly, sitagliptin was not superior to placebo in terms of efficacy in T2DM patients with ASCVD (Nauck et al., 2019). The CARMELINA trial indicated that linagliptin added to usual treatment resulted in a non-inferior risk of MACE in T2DM patients with high cardiovascular risk (HR, 1.02 [95% CI, 0.89 to 1.17]; p = 0.74) (Rosenstock et al., 2019). However, a trial from Thailand showed that linagliptin was superior in reducing 10-year cardiovascular risk score in patients with a baseline risk greater than 20%, with enhanced outcomes in older patients (Poonchuay et al., 2022).
3.1.3.2 Basic research
While clinical trials have not demonstrated significant advantages of DPP-4i, basic research has revealed their cardioprotective effect. The normal diastolic and systolic functions of the heart depend on the energy provided by a large number of mitochondria and fatty acid oxidation in cardiomyocytes. Evogliptin (an oral hypoglycemic drug approved for the treatment of T2DM in South Korea in 2015) can restore the expression of the mitochondrial-synthesis-related pathway, PGC-1α/Nrf2/mitochondrial transcription factor A (TFAM), to promote normal mitochondrial synthesis (Gureev et al., 2019) and inhibit the expression of lipid transmembrane transporters (fatty acid binding protein 3, FABP3) (Zhang et al., 2015) and synthetic proteins (Forkhead box protein O1, FOXO1; peroxisome proliferator activated receptor γ, PPARγ; diacylglycerol o-acyltransferase 1, DGAT1) (Kyriazis et al., 2021) to block the over-activated lipid pathway in db/db mice (Figure 3A) (Pham et al., 2023). Further studies have shown that sitagliptin combined with insulin can improve diabetic cardiomyopathy by reducing the expression of inflammatory factors to a greater extent (Table 3) (Wadie et al., 2022).
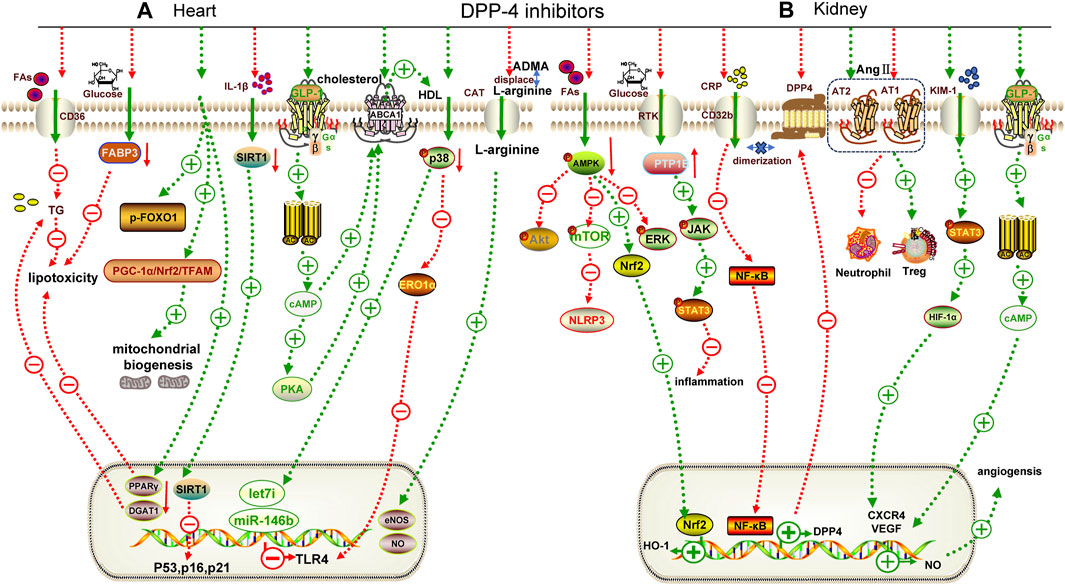
FIGURE 3. Mechanisms underlying the cardiorenal effects of DPP-4 inhibitors. (A) for heart; (B) for kidney. TG: triglyceride, FABP3: fatty acid binding protein 3, p-FOXO1: phospho-Forkhead box protein O1, ABCA1: adenosine triphosphate transporter A1, PTP1B: protein tyrosine phosphatase, CRP: C-reactive protein, KIM-1: kidney injury molecule 1, CXCR4: chemokine receptor 4, AT1: angiotensin receptor 1, AT2: angiotensin receptor 2, ERK: extracellular regulated protein kinase, DGAT1: diacylgycerol acyltransferase 1, CAT: cationic amino acid transporters, HDL: high density lipoprotein, TFAM: mitochondrial transcription factor A, eNOS: endothelial nitric oxide synthase, DGAT1: diacylglycerol o-acyltransferase 1, PPARγ: peroxisome proliferator activated receptor γ, JAK: janus tyrosine kinase, STAT3: signal transducer and activator of transcription 3.
In addition, DPP-4i have shown significant advantages in non-diabetic cardiovascular diseases, particularly ASCVD. Trelagliptin inhibits IL-1β-induced MCP-1 expression in human aortic endothelial cells by inhibiting the NF-κB pathway, preventing monocyte infiltration during atherosclerosis (Meng et al., 2020). Alogliptin has been shown to reduce IL-1β-induced inflammatory cytokine expression in VSMCs by restoring SIRT1 expression and downregulating senescence-related markers (p16, p21 and p53) to prevent premature smooth muscle cell senescence and enhance plaque progression (Zhao et al., 2021). Reverse cholesterol transport is an important mechanism for improving ASCVD, which is mainly mediated by high-density lipoprotein (HDL)-associated cyclic adenosine monophosphate (cAMP) and can activate adenosine triphosphate transporter A1 (ABCA1) to promote HDL formation. Sitagliptin increases intracellular cAMP levels by indirectly activating GLP-1R and up-regulating ABCA1 expression, thereby promoting reverse cholesterol transport in macrophages and reducing foam cell generation (Komatsu et al., 2023). In an MI model of db/db mice, linagliptin upregulated the expression of microRNAs (miR-146b and Let-7i) in cardiomyocytes by reducing p38 phosphorylation, thereby inhibiting toll-like receptor 4 (TLR4) upregulation (Birnbaum et al., 2019).
DPP-4i also offer benefits in improving metabolic syndrome. Plasma asymmetrical dimethylarginine (ADMA) is increased in fructose-induced metabolic syndrome, which can inhibit NO by replacing L-arginine, the substrate of NO synthase, and aggravate endothelial dysfunction. Sitagliptin reduces endothelial dysfunction by increasing the activity of dimethylarginine dimethylaminohydrolase 1 (DDAH1) (an enzyme that degrades ADMA) in the kidney to degrade ADMA, thereby increasing plasma NO levels (Wójcicka et al., 2023).
Although published clinical trials have not demonstrated the superior cardiovascular benefits of DPP-4i in diabetic population, extensive basic researches have highlighted its protective effects on the cardiovascular system in animal models of metabolic syndrome, ASCVD, and MI. These findings emphasize the necessity for further exploration into the potential clinical application of DPP-4i.
3.2 Renal protection
3.2.1 SGLT2i
3.2.1.1 Clinical trial
SGLT2i have demonstrated significant advantages in kidney- and cardiovascular system-related clinical trials. Dapagliflozin has been shown to reduce HF hospitalization rates in T2DM patients (DECLARE-TIMI 58) (HR, 0.83 [95% CI, 0.73 to 0.95]; p = 0.005) (Wiviott et al., 2019). In the DAPA-CKD trial, dapagliflozin exhibited superior efficacy in mitigating sustained eGFR decline of at least 50% in both diabetic and non-diabetic patients with CKD (Table 2) (HR, 0.61 [95% CI, 0.51 to 0.72]; p < 0.001) (Heerspink et al., 2020). The analysis of the DAPA-CKD trial also demonstrated the superior benefits of dapagliflozin in reducing albuminuria and improving eGFR in T2DM patients (Heerspink et al., 2021; Jongs et al., 2021). In addition, the analysis of the DELIVER trial (Solomon et al., 2022) also showed that dapagliflozin significantly slowed the decline in eGFR from the baseline (difference in eGFR decline from baseline was 0.5, [95% CI, 0.1–0.9 mL/min/1.73 m2 per year]; p = 0.01) (Mc Causland et al., 2023). However, the failure of dapagliflozin to improve GFR in the DIAMOND trial, which focused on patients with non-T2DM CKD (difference in mean proteinuria change from baseline was 0.9%, [95% CI, −16.6 to 22.1]; p = 0.93), suggests that the specific effects of dapagliflozin on GFR need to be further investigated (Cherney et al., 2020). In the CREDENCE trial, canagliflozin significantly reduced the rates of end-stage renal disease (ESRD) and doubled serum creatinine (HR, 0.70 [95% CI, 0.59 to 0.82]; p = 0.00001) (Perkovic et al., 2019). Lastly, empagliflozin (10 mg/day) was significantly superior to placebo in slowing kidney disease in the EMPA-KIDNEY trial (HR, 0.72 [95% CI, 0.6 to 0.82]; p < 0.001) (Herrington et al., 2023).
3.2.1.2 Basic research
Promising results have been obtained from basic research on the role of SGLT2i in alleviating kidney injury. High glucose stimulation induces heightened energy consumption in HK-2 cells, leading to a decrease in the intracellular adenosine-diphosphate/adenosine-triphosphate (ADP/ATP) ratio. This disruption affects the AMPK/mTOR pathway, resulting in reduced autophagy and inhibited energy production (Figure 1B) (Xiao et al., 2011; Packer, 2020b). Dapagliflozin may reverse this process (Xu et al., 2021). Cytochrome P4 (CYP4) is highly expressed in diabetic kidney and can metabolize arachidonic acid into 20-hydroxy-eicosapentaenoic acid (20-HETE), which promotes the formation of superoxide. Dapagliflozin can reduce the inflammation of DKD by targeting the CYP4/20-HETE pathway (Dia et al., 2023). In addition, dapagliflozin could appropriately restore fatty acid metabolism to improve the activation of hypoxia-inducible factor-1α (HIF-1α) and metabolite accumulation caused by mitochondrial tricarboxylic acid (TCA) cycle over-activation under DKD, suggesting that SGLT2i could prevent tubular cell metabolic shift and associate with inflammation (Ke et al., 2022). The Hippo-yes associated protein 1/transcriptional coactivator (YAP/TAZ) pathway plays an important role in fibrosis. Dapagliflozin can inhibit the nuclear translocation of YAP/TAZ, thereby reducing the transcription of its downstream pro-fibrotic target genes connective tissue growth factor (CTGF) to improve DKD fibrosis (Table 3) (Feng et al., 2023). Empagliflozin can also delay DKD fibrosis by preventing reprogramming of serine-threonine metabolism (Lu et al., 2022).
In recent years, the role of SGLT2i in mediating immune response has attracted great attention. Canagliflozin has been shown to inhibit CD4+T cell activation and reduce cancer myelocytomatosis oncogene (cMyc) to prevent metabolic reprogramming and immune inflammation (Jenkins et al., 2023). Consistently, a study by Zhao et al. revealed that empagliflozin can inhibit the over-activated SGLT2 in lupus kidney glomeruli, prevent the activation of mechanistic target of rapamycin complex 1 (mTORC1), and delay glomerular injury in lupus kidney (Zhao et al., 2023). Also, the expression of complement receptor type 1-related protein y (Crry), a key complement regulator, was upregulated by dapagliflozin, inhibiting HIF-1α accumulation under high glucose to alleviate immune inflammatory injury in db/db mice (Chang et al., 2021).
SGLT2i have a significant protective effect on DKD. Immune-related nephropathy is identified as a major contributor to CKD, and several basic studies have confirmed the positive role of SGLT2i in regulating the immune system. As a result, Säemann et al. advocate for the inclusion of patients with autoimmune diseases in large-scale renal outcome trials (Säemann and Kronbichler, 2022). Currently, relevant clinical trials have confirmed the acceptable safety profile of SGLT2i in the treatment of lupus nephritis, but further evaluation is needed to assess its efficacy (Wang et al., 2022).
3.2.2 GLP-1RAs
3.2.2.1 Clinical trial
Most clinical trials of GLP-1RAs have been post hoc and prespecified analyses, highlighting their role in reducing eGFR and urine albumin creatine ratio (UACR). A prespecified analysis of renal outcomes in the LEADER trial showed a significant improvement in macroalbuminuria with liraglutide (HR, 0.78 [95% CI, 0.67 to 0.92]; p = 0.003) (Table 2) (Mann et al., 2017). In a post hoc analysis of the (SUSTAIN1-7) trial, semaglutide has a significant effect on reducing UACR but decreasing eGFR only in an early stage in T2DM patients with established CKD (Mann et al., 2020). Similarly, in the pooled analysis of SUSTAIN 6 and LEADER, both liraglutide and semaglutide reduced albuminuria by 24% over 2 years (95% CI, 20%–27%; p < 0.001), with a greater delay in the continuous decline of eGFR at an eGFR of 30–60 mL/min/1.73 m2 (Shaman et al., 2022). In addition, a pooled analysis of the SUSTAIN 6 and PIONEER 6 trials showed that although the improvement in eGFR slope was not significant in subgroups, semaglutide still reduced the eGFR slope in an overall population analysis (Tuttle et al., 2023). Of note, in a post hoc analysis of the STEP1-3 trial in obese patients, a higher dose (2.4 mg) of once-weekly semaglutide reduced UACR by 20.6%, while there was no difference between semaglutide and placebo in the eGFR slope at week 68 (Heerspink et al., 2023). A direct, specific trial is underway to assess whether semaglutide can delay DKD (FLOW) in older patients who have had T2DM for nearly two decades, which will provide novel insights into the long-term renal effects of GLP-1RAs (Rossing et al., 2023).
3.2.2.2 Basic research
Similar to SGLT2i, GLP-1RAs have shown hopeful results in delaying the progression of kidney disease, regardless of diabetes status. Nrf2 expression was significantly upregulated by liraglutide, activating the AMPK/mTOR pathway and thereby alleviating DKD (Figure 2B) (Table 3) (Yang et al., 2020). Further, Nrf2 can regulate the disorder of lipid metabolism through the AMPK pathway to reduce ectopic lipid deposition in renal tubules in DKD (Su et al., 2020). Notably, co-administration of exenatide and adipose-derived mesenchymal stem cells (ADMSCs) significantly improved the renal function of DKD (Habib et al., 2021).
Additionally, GLP-1RAs may have therapeutic promise in renal injury caused by hypertension, obesity or ischemia reperfusion. Studies have shown that liraglutide can reduce blood pressure by increasing the expression of endothelial nitric oxide synthase (eNOS) and vascular endothelial growth factor (VEGF), thereby improving the vasoconstriction of intrarenal arterioles. It can also reduce the infiltration of macrophages into renal vascular endothelial cells and alleviate renal vascular inflammation in obese rats induced by a high-salt diet (Sukumaran et al., 2019). Exenatide can stabilize mitochondrial membrane potential and reduce palmitate-induced reactive oxygen species production in HK-2 cells through the upregulation of SIRT1 (Wang et al., 2021). High mobility group box 1 protein (HMGB1) is a damage-associated molecular pattern, which is released from the nucleus to the cytoplasm during renal ischemia and then binds to its receptors, such as TLR-4, to promote the inflammatory cascade. Liraglutide can downregulate the expression of HMGB1 receptors and prevent acetylation of HMGB1 by increasing histone acrtyltransferases (HAT) activity, thereby reducing neutrophil infiltration and delaying renal ischemia-reperfusion injury in vivo and in vitro (Li Y. et al., 2021).
GLP-1RAs have also been shown to have a positive role in reducing the nephrotoxic effects of antibiotics and antitumor drugs. Liraglutide can mediate mitochondrial biogenesis by regulating the protein kinase A/cyclic-AMP response binding protein (PKA/CREB) and notch homolog 1/hairy and enhancer of split-1 (Notch/Hes-1) pathways and up-regulating the expression of PGC-1α to activate Nrf2, thereby improving the nephrotoxicity induced by glucocorticoids (Elkhoely, 2023). Cisplatin, a common and effective chemotherapeutic agent, often causes irreversible acute kidney injury (AKI). Organic cations transporter 2 (OCT2) is located on the basement membrane of renal tubules and is responsible for the absorption of cisplatin. The MAPK pathway plays a pivotal role in cisplatin-induced AKI. Liraglutide can reduce renal injury by inhibiting the expression of OCT2 and c-Jun N-terminal kinase/extracellular regulated protein kinase (JNK/ERK), thereby restoring the oxidative/antioxidant balance (Sharaf et al., 2023). Additionally, liraglutide also inhibited the release of HMGB1 to reduce cisplatin-induced apoptosis in HK-2 cells (Xu et al., 2023).
These basic studies demonstrate that GLP-1RAs offer significant protection against obesity-related kidney disease, in addition to their benefits in improving DKD. Exenatide is even more effective than simvastatin in treating obesity-induced tubular epithelial cell lipotoxicity (Wang et al., 2021). The fact that GLP-1RAs has also become a second-line therapy for CKD expands its clinical benefits range besides weight loss (Navaneethan et al., 2023). Moreover, it has been reported that liraglutide, either alone or in combination with rabeprazole, can protect against cisplatin-induced nephrotoxicity (Sharaf et al., 2023), highlighting the potential of GLP-1RAs for further validation in clinical trials investigating nephrotoxicity associated with antineoplastic drugs.
3.2.3 DPP-4i
3.2.3.1 Clinical trial
The outcomes of clinical trials assessing the impact of DPP-4i on renal outcomes remain controversial. Initial findings indicated the potential benefits of DPP-4i in ameliorating DKD. In a retrospective analysis of four clinical datasets concerning linagliptin, it was observed that treatment with linagliptin led to a significant reduction in UACR after 12–24 weeks (Groop et al., 2013). Other studies showed that linagliptin reduced the probability of first adverse kidney events (HR, 0.84 [95% CI, 0.72–0.97]; p = 0.02) and new-onset albuminuria (HR, 0.82 [95% CI, 0.69–0.98]; p = 0.03) (Cooper et al., 2015). In the GUARD study, gemigliptin improved microalbuminuria (decrease in UACR was −41.9 mg/g creatinine; p = 0.03) and macroalbuminuria (decrease in UACR was −528.9 mg/g creatinine; p < 0.001) in both the short-term 12-week observation and the 40-week extension study (Table 2) (Yoon et al., 2017; Han et al., 2018). Analysis of the SAVOR-TIMI 53 trial found that saxagliptin improved UACR in patients with renal insufficiency (the difference in UACR change was −19.3 mg/g; p = 0.033) (Mosenzon et al., 2017). In the CARMELINA trial, linagliptin had a significant advantage in reducing UACR in T2DM patients with or without nephrotic range proteinuria (reduction of UACR ≥50%; HR, 1.15 [95% CI, 1.07 to 1.25] from baseline) (Wanner et al., 2021). In line with the CARMELINA trial, the EXAM trial showed that alogliptin may benefit patients with eGFR ≥60 mL/min/1.73 m2 (HR, 0.81 [95% CI, 0.65 to 0.99] for eGFR ≥60 mL/min/1.73 m2; HR, 1.2 [95% CI, 0.95 to 1.53] for eGFR <60 mL/min/1.73 m2) (Ferreira et al., 2020). However, there is contrary evidence to the above results. In the TECOS trial, sitagliptin did not significantly improve CKD progression, regardless of the baseline eGFR level (Cornel et al., 2016). The secondary analysis of CARMELINA also proved that linagliptin was not significantly different from placebo in improving renal outcomes (Perkovic et al., 2020). Postprandial glomerular hyperfiltration may be one of the renal risk factors in diabetic patients. Compared with glimepiride, linagliptin does not improve postprandial hemodynamics, and may even moderately induce postprandial glomerular hyperfiltration (Muskiet et al., 2020; Muskiet et al., 2022).
3.2.3.2 Basic research
Inconsistent with clinical trials, preclinical data have unequivocally demonstrated the beneficial effects of DPP-4i in alleviating DKD. High glucose activates the C-reactive protein (CRP)/FcγRIIb (CD32b)/NF-κB pathway, which enriches DPP-4 and forms a dimer with CD32b to maintain its expression, thereby forming an inflammatory cycle and aggravating the injury. Linagliptin can block this cycle (Tang et al., 2021). Omarigliptin can improve high glucose-induced glomerular endothelial cell inflammation by activating the AMPK/mTOR pathway and negatively regulating the NLRP3 inflammasome (Figure 3B) (Li L. et al., 2021). Protein tyrosine phosphatase 1B (PTP1B) participates in the inflammatory response by negatively regulating the janus tyrosine kinase/signal transducer and activator of transcription (JAK/STAT) pathway. Sitagliptin reduces renal inflammation in streptozotocin-induced rats by inhibiting PTP1B (Table 3) (Al-Qabbaa et al., 2023). Linagliptin alleviate renal fibrosis in streptozotocin-induced mice by increasing the expression of microRNA 29. Upregulation of microRNA 29 directly inhibited the expression of fibrosis genes (Kanasaki et al., 2014).
DPP-4i may also alleviate renal dysfunction induced by AngII. The expression of Ang Ⅱ receptor 2 (AT2R), which can antagonize Ang Ⅱ receptor 1 (AT1R)-mediated inflammatory responses, was upregulated by linagliptin to alleviate Ang Ⅱ-induced renal fibrosis (Bai et al., 2020). Additionally, saxagliptin can mediate innate and adaptive immune inflammation, inhibit the activity of pro-inflammatory cells (CD8+T cells, neutrophils), and convert them into anti-inflammatory cells (M2 macrophages and Treg cells) to reduce Ang Ⅱ-induced hypertensive nephropathy (Nistala et al., 2021). In addition, saxagliptin can also activate multiple pathways, such as GLP-1/cAMP/VEGF, kidney injury molecule-1 (KIM-1)/STAT3/HIF-1α/VEGF/eNOS, to increase the expression of NO and repair damaged blood vessels caused by inflammation after renal ischemia/reperfusion (Kamel et al., 2019).
Additionally, DPP-4i play a significant role in improving antibiotic-induced nephrotoxicity and nephritis. Saxagliptin can reduce the expression of malondialdehyde and increase the expression of glutathione to regulate the disorder of renal inflammation and oxidative stress caused by gentamicin (Helal et al., 2018). Interestingly, linagliptin also accelerated glomerular crescentic degeneration in anti-glomerular basement membrane (GBM) nephritis (Mayer et al., 2021).
Basic research are still ongoing to explore the potential benefits and mechanisms of DPP-4i in improving DKD. Additionally, DPP-4i can improve hypertensive nephropathy through immune mechanisms independent of blood pressure reduction (Nistala et al., 2021), and promote the regression of crescents in anti-GBM nephritis, thereby providing a clinical translation point for their future use in immune system diseases.
4 Conclusion
Patients with T2DM often suffer from adverse cardiovascular and renal outcomes. Accumulating evidence suggest that SGLT2i and GLP-1RAs have cardiorenal protective effects including glucose-dependent and independent pathways. They not only protect against heart and kidney diseases through classical anti-inflammatory, anti-oxidative stress, and anti-fibrosis pathways but are also implicated in non-classical epigenetics, mitochondrial energy metabolism, and immune complement pathways. They have also demonstrated positive effects on immune diseases and cardiovascular and renal toxicity caused by antineoplastic drugs and antibiotics. Although basic research indicate the beneficial effects of DPP-4i, most clinical studies have only demonstrated their non-inferiority, underscoring the necessity for further exploration. Therefore, more direct and larger clinical trials (involving a larger proportion of CVD/CKD patients without diabetes) are needed to assess this drug.
By exploring the cardiorenal protective effects of drugs, we can identify common mechanisms that contribute to cardiorenal injury in various diseases. These findings will establish a theoretical and experimental basis for developing novel clinical drugs. Additionally, a drug that can effectively treat both heart and kidney diseases has significant practical implications, including reducing the medication burden on patients, lowering adverse reactions, enhancing patient compliance, and alleviating financial strain and so on. Therefore, further research should investigate new mechanistic pathways to explore the effectiveness of second-generation anti-glucose drugs.
Author contributions
W-JF: Writing–original draft. J-LH: Writing–original draft. Z-HM: Writing–review and editing. S-KP: Writing–review and editing. D-WL: Writing–review and editing. Z-SL: Conceptualization, Writing–review and editing. PW: Conceptualization, Funding acquisition, Supervision, Writing–review and editing. Z-XG: Conceptualization, Funding acquisition, Supervision, Writing–review and editing.
Funding
The author(s) declare financial support was received for the research, authorship, and/or publication of this article. This work was supported by the Natural Science Foundation of Henan Province (222300420089 to PW), National Natural Science Foundation of China (32371170 to Z-XG, 31971065 to PW, and 82300323 to J-LH), and Scientific Research and Innovation Team of the First Affiliated Hospital of Zhengzhou University (QNCXTD2023006 to PW).
Acknowledgments
The authors would like to thank Editage for professional editing.
Conflict of interest
The authors declare that the research was conducted in the absence of any commercial or financial relationships that could be construed as a potential conflict of interest.
Publisher’s note
All claims expressed in this article are solely those of the authors and do not necessarily represent those of their affiliated organizations, or those of the publisher, the editors and the reviewers. Any product that may be evaluated in this article, or claim that may be made by its manufacturer, is not guaranteed or endorsed by the publisher.
References
Abdul-Ghani, M. A., DeFronzo, R. A., and Norton, L. (2013). Novel hypothesis to explain why SGLT2 inhibitors inhibit only 30-50% of filtered glucose load in humans. Diabetes 62 (10), 3324–3328. doi:10.2337/db13-0604
Abu-Qaoud, M. R., Kumar, A., Tarun, T., Abraham, S., Ahmad, J., Khadke, S., et al. (2023). Impact of SGLT2 inhibitors on AF recurrence after catheter ablation in Patients with type 2 diabetes. JACC Clin. Electrophysiol. 9 (10), 2109–2118. doi:10.1016/j.jacep.2023.06.008
Al-Qabbaa, S. M., Qaboli, S. I., Alshammari, T. K., Alamin, M. A., Alrajeh, H. M., Almuthnabi, L. A., et al. (2023). Sitagliptin mitigates diabetic nephropathy in a rat model of streptozotocin-induced type 2 diabetes: possible role of PTP1B/JAK-STAT pathway. Int. J. Mol. Sci. 24 (7), 6532. doi:10.3390/ijms24076532
Andreadi, A., Muscoli, S., Tajmir, R., Meloni, M., Muscoli, C., Ilari, S., et al. (2023). Recent pharmacological options in type 2 diabetes and synergic mechanism in cardiovascular disease. Int. J. Mol. Sci. 24 (2), 1646. doi:10.3390/ijms24021646
Anker, S. D., Butler, J., Filippatos, G., Ferreira, J. P., Bocchi, E., Böhm, M., et al. (2021). Empagliflozin in heart failure with a preserved ejection fraction. N. Engl. J. Med. 385 (16), 1451–1461. doi:10.1056/NEJMoa2107038
Arow, M., Waldman, M., Yadin, D., Nudelman, V., Shainberg, A., Abraham, N. G., et al. (2020). Sodium-glucose cotransporter 2 inhibitor Dapagliflozin attenuates diabetic cardiomyopathy. Cardiovasc. Diabetol. 19 (1), 7. doi:10.1186/s12933-019-0980-4
Baggio, L. L., and Drucker, D. J. (2014). Glucagon-like peptide-1 receptors in the brain: controlling food intake and body weight. J. Clin. investigation 124 (10), 4223–4226. doi:10.1172/jci78371
Bai, F., Zhang, L. H., Zhang, W. W., Zheng, R. H., Eskew, J. R., Bennett, J., et al. (2020). Conservation of glucagon like peptide-1 level with liraglutide and linagilptin protects the kidney against angiotensin II-induced tissue fibrosis in rats. Eur. J. Pharmacol. 867, 172844. doi:10.1016/j.ejphar.2019.172844
Balogh, D. B., Wagner, L. J., and Fekete, A. (2023). An overview of the cardioprotective effects of novel antidiabetic classes: focus on inflammation, oxidative stress, and fibrosis. Int. J. Mol. Sci. 24 (9), 7789. doi:10.3390/ijms24097789
Bhatt, D. L., Szarek, M., Steg, P. G., Cannon, C. P., Leiter, L. A., McGuire, D. K., et al. (2021). Sotagliflozin in patients with diabetes and recent worsening heart failure. N. Engl. J. Med. 384 (2), 117–128. doi:10.1056/NEJMoa2030183
Birnbaum, Y., Tran, D., Bajaj, M., and Ye, Y. (2019). DPP-4 inhibition by linagliptin prevents cardiac dysfunction and inflammation by targeting the Nlrp3/ASC inflammasome. Basic Res. Cardiol. 114 (5), 35. doi:10.1007/s00395-019-0743-0
Blazek, O., and Bakris, G. L. (2023). Slowing the progression of diabetic kidney disease. Cells 12 (15), 1975. doi:10.3390/cells12151975
Bruen, R., Curley, S., Kajani, S., Lynch, G., O'Reilly, M. E., Dillon, E. T., et al. (2019). Liraglutide attenuates preestablished atherosclerosis in apolipoprotein E-deficient mice via regulation of immune cell phenotypes and proinflammatory mediators. J. Pharmacol. Exp. Ther. 370 (3), 447–458. doi:10.1124/jpet.119.258343
Campbell, J. E., Müller, T. D., Finan, B., DiMarchi, R. D., Tschöp, M. H., and D'Alessio, D. A. (2023). GIPR/GLP-1R dual agonist therapies for diabetes and weight loss-chemistry, physiology, and clinical applications. Cell metab. 35 (9), 1519–1529. doi:10.1016/j.cmet.2023.07.010
Cannon, C. P., Pratley, R., Dagogo-Jack, S., Mancuso, J., Huyck, S., Masiukiewicz, U., et al. (2020). Cardiovascular outcomes with ertugliflozin in type 2 diabetes. N. Engl. J. Med. 383 (15), 1425–1435. doi:10.1056/NEJMoa2004967
Capuano, A., Sportiello, L., Maiorino, M. I., Rossi, F., Giugliano, D., and Esposito, K. (2013). Dipeptidyl peptidase-4 inhibitors in type 2 diabetes therapy--focus on alogliptin. Drug Des. Dev. Ther. 7, 989–1001. doi:10.2147/dddt.S37647
Chang, D. Y., Li, X. Q., Chen, M., and Zhao, M. H. (2021). Dapagliflozin ameliorates diabetic kidney disease via upregulating crry and alleviating complement over-activation in db/db mice. Front. Pharmacol. 12, 729334. doi:10.3389/fphar.2021.729334
Chen, A., Lan, Z., Li, L., Xie, L., Liu, X., Yang, X., et al. (2023). Sodium-glucose cotransporter 2 inhibitor canagliflozin alleviates vascular calcification through suppression of nucleotide-binding domain, leucine-rich-containing family, pyrin domain-containing-3 inflammasome. Cardiovasc. Res. 119 (13), 2368–2381. doi:10.1093/cvr/cvad119
Cherney, D. Z. I., Dekkers, C. C. J., Barbour, S. J., Cattran, D., Abdul Gafor, A. H., Greasley, P. J., et al. (2020). Effects of the SGLT2 inhibitor dapagliflozin on proteinuria in non-diabetic patients with chronic kidney disease (DIAMOND): a randomised, double-blind, crossover trial. lancet Diabetes and Endocrinol. 8 (7), 582–593. doi:10.1016/s2213-8587(20)30162-5
Chung, C. C., Lin, Y. K., Chen, Y. C., Kao, Y. H., Yeh, Y. H., Trang, N. N., et al. (2023). Empagliflozin suppressed cardiac fibrogenesis through sodium-hydrogen exchanger inhibition and modulation of the calcium homeostasis. Cardiovasc. Diabetol. 22 (1), 27. doi:10.1186/s12933-023-01756-0
Committee ADAPP (2022a). 11. Chronic kidney disease and risk management: standards of medical care in diabetes-2022. Diabetes care 45 (Suppl. 1), S175–s184. doi:10.2337/dc22-S011
Committee ADAPP (2022b). 9. Pharmacologic approaches to glycemic treatment: standards of medical care in diabetes-2022. Diabetes care 45 (Suppl. 1), S125–s143. doi:10.2337/dc22-S009
Cooper, M. E., Perkovic, V., McGill, J. B., Groop, P. H., Wanner, C., Rosenstock, J., et al. (2015). Kidney disease end points in a pooled analysis of individual patient-level data from a large clinical trials program of the dipeptidyl peptidase 4 inhibitor linagliptin in type 2 diabetes. Am. J. kidney Dis. 66 (3), 441–449. doi:10.1053/j.ajkd.2015.03.024
Cornel, J. H., Bakris, G. L., Stevens, S. R., Alvarsson, M., Bax, W. A., Chuang, L. M., et al. (2016). Effect of sitagliptin on kidney function and respective cardiovascular outcomes in type 2 diabetes: outcomes from TECOS. Diabetes care 39 (12), 2304–2310. doi:10.2337/dc16-1415
de Boer, I. H., Khunti, K., Sadusky, T., Tuttle, K. R., Neumiller, J. J., Rhee, C. M., et al. (2022). Diabetes management in chronic kidney disease: a consensus report by the American diabetes association (ADA) and kidney disease: improving global outcomes (KDIGO). Diabetes care 45 (12), 3075–3090. doi:10.2337/dci22-0027
Dia, B., Alkhansa, S., Njeim, R., Al Moussawi, S., Farhat, T., Haddad, A., et al. (2023). SGLT2 inhibitor-dapagliflozin attenuates diabetes-induced renal injury by regulating inflammation through a CYP4A/20-HETE signaling mechanism. Pharmaceutics 15 (3), 965. doi:10.3390/pharmaceutics15030965
Durak, A., and Turan, B. (2023). Liraglutide provides cardioprotection through the recovery of mitochondrial dysfunction and oxidative stress in aging hearts. J. physiology Biochem. 79 (2), 297–311. doi:10.1007/s13105-022-00939-9
Elkhoely, A. (2023). Liraglutide ameliorates gentamicin-induced acute kidney injury in rats via PGC-1α-mediated mitochondrial biogenesis: involvement of PKA/CREB and Notch/Hes-1 signaling pathways. Int. Immunopharmacol. 114, 109578. doi:10.1016/j.intimp.2022.109578
Feng, L., Chen, Y., Li, N., Yang, X., Zhou, L., Li, H., et al. (2023). Dapagliflozin delays renal fibrosis in diabetic kidney disease by inhibiting YAP/TAZ activation. Life Sci. 322, 121671. doi:10.1016/j.lfs.2023.121671
Ferreira, J. P., Mehta, C., Sharma, A., Nissen, S. E., Rossignol, P., and Zannad, F. (2020). Alogliptin after acute coronary syndrome in patients with type 2 diabetes: a renal function stratified analysis of the EXAMINE trial. BMC Med. 18 (1), 165. doi:10.1186/s12916-020-01616-8
Fu, J., Xu, H., Wu, F., Tu, Q., Dong, X., Xie, H., et al. (2022). Empagliflozin inhibits macrophage inflammation through AMPK signaling pathway and plays an anti-atherosclerosis role. Int. J. Cardiol. 367, 56–62. doi:10.1016/j.ijcard.2022.07.048
Fu, Z., Mui, D., Zhu, H., and Zhang, Y. (2020). Exenatide inhibits NF-κB and attenuates ER stress in diabetic cardiomyocyte models. Aging 12 (9), 8640–8651. doi:10.18632/aging.103181
Gerstein, H. C., Colhoun, H. M., Dagenais, G. R., Diaz, R., Lakshmanan, M., Pais, P., et al. (2019). Dulaglutide and cardiovascular outcomes in type 2 diabetes (REWIND): a double-blind, randomised placebo-controlled trial. Lancet 394 (10193), 121–130. doi:10.1016/s0140-6736(19)31149-3
Gerstein, H. C., Sattar, N., Rosenstock, J., Ramasundarahettige, C., Pratley, R., Lopes, R. D., et al. (2021). Cardiovascular and renal outcomes with efpeglenatide in type 2 diabetes. N. Engl. J. Med. 385 (10), 896–907. doi:10.1056/NEJMoa2108269
Graaf, C., Donnelly, D., Wootten, D., Lau, J., Sexton, P. M., Miller, L. J., et al. (2016). Glucagon-like peptide-1 and its class B G protein-coupled receptors: a long march to therapeutic successes. Pharmacol. Rev. 68 (4), 954–1013. doi:10.1124/pr.115.011395
Green, J. B., Bethel, M. A., Armstrong, P. W., Buse, J. B., Engel, S. S., Garg, J., et al. (2015). Effect of sitagliptin on cardiovascular outcomes in type 2 diabetes. N. Engl. J. Med. 373 (3), 232–242. doi:10.1056/NEJMoa1501352
Groop, P. H., Cooper, M. E., Perkovic, V., Emser, A., Woerle, H. J., and von Eynatten, M. (2013). Linagliptin lowers albuminuria on top of recommended standard treatment in patients with type 2 diabetes and renal dysfunction. Diabetes care 36 (11), 3460–3468. doi:10.2337/dc13-0323
Guo, X., Sang, C., Tang, R., Jiang, C., Li, S., Liu, N., et al. (2023). Effects of glucagon-like peptide-1 receptor agonists on major coronary events in patients with type 2 diabetes. Diabetes, Obes. metabolism 25 (Suppl. 1), 53–63. doi:10.1111/dom.15043
Gureev, A. P., Shaforostova, E. A., and Popov, V. N. (2019). Regulation of mitochondrial biogenesis as a way for active longevity: interaction between the Nrf2 and PGC-1α signaling pathways. Front. Genet. 10, 435. doi:10.3389/fgene.2019.00435
Habib, H. A., Heeba, G. H., and Khalifa, M. M. A. (2021). Effect of combined therapy of mesenchymal stem cells with GLP-1 receptor agonist, exenatide, on early-onset nephropathy induced in diabetic rats. Eur. J. Pharmacol. 892, 173721. doi:10.1016/j.ejphar.2020.173721
Han, S. Y., Yoon, S. A., Han, B. G., Kim, S. G., Jo, Y. I., Jeong, K. H., et al. (2018). Comparative efficacy and safety of gemigliptin versus linagliptin in type 2 diabetes patients with renal impairment: a 40-week extension of the GUARD randomized study. Diabetes, Obes. metabolism 20 (2), 292–300. doi:10.1111/dom.13059
Heerspink, H. J. L., Apperloo, E., Davies, M., Dicker, D., Kandler, K., Rosenstock, J., et al. (2023). Effects of semaglutide on albuminuria and kidney function in people with overweight or obesity with or without type 2 diabetes: exploratory analysis from the STEP 1, 2, and 3 trials. Diabetes care 46 (4), 801–810. doi:10.2337/dc22-1889
Heerspink, H. J. L., Jongs, N., Chertow, G. M., Langkilde, A. M., McMurray, J. J. V., Correa-Rotter, R., et al. (2021). Effect of dapagliflozin on the rate of decline in kidney function in patients with chronic kidney disease with and without type 2 diabetes: a prespecified analysis from the DAPA-CKD trial. lancet Diabetes and Endocrinol. 9 (11), 743–754. doi:10.1016/s2213-8587(21)00242-4
Heerspink, H. J. L., Stefánsson, B. V., Correa-Rotter, R., Chertow, G. M., Greene, T., Hou, F. F., et al. (2020). Dapagliflozin in patients with chronic kidney disease. N. Engl. J. Med. 383 (15), 1436–1446. doi:10.1056/NEJMoa2024816
Heidenreich, P. A., Bozkurt, B., Aguilar, D., Allen, L. A., Byun, J. J., Colvin, M. M., et al. (2022). 2022 AHA/ACC/HFSA guideline for the management of heart failure: a report of the American college of cardiology/American heart association joint committee on clinical practice guidelines. Circulation 145 (18), e895–e1032. doi:10.1161/CIR.0000000000001063
Helal, M. G., Zaki, M., and Said, E. (2018). Nephroprotective effect of saxagliptin against gentamicin-induced nephrotoxicity, emphasis on anti-oxidant, anti-inflammatory and anti-apoptic effects. Life Sci. 208, 64–71. doi:10.1016/j.lfs.2018.07.021
Hernandez, A. F., Green, J. B., Janmohamed, S., D'Agostino, R. B., Granger, C. B., Jones, N. P., et al. (2018). Albiglutide and cardiovascular outcomes in patients with type 2 diabetes and cardiovascular disease (Harmony Outcomes): a double-blind, randomised placebo-controlled trial. Lancet 392 (10157), 1519–1529. doi:10.1016/s0140-6736(18)32261-x
Herrington, W. G., Staplin, N., Wanner, C., Green, J. B., Hauske, S. J., Emberson, J. R., et al. (2023). Empagliflozin in patients with chronic kidney disease. N. Engl. J. Med. 388 (2), 117–127. doi:10.1056/NEJMoa2204233
Holman, R. R., Bethel, M. A., Mentz, R. J., Thompson, V. P., Lokhnygina, Y., Buse, J. B., et al. (2017). Effects of once-weekly exenatide on cardiovascular outcomes in type 2 diabetes. N. Engl. J. Med. 377 (13), 1228–1239. doi:10.1056/NEJMoa1612917
Hsieh, P. L., Chu, P. M., Cheng, H. C., Huang, Y. T., Chou, W. C., Tsai, K. L., et al. (2022). Dapagliflozin mitigates doxorubicin-caused myocardium damage by regulating AKT-mediated oxidative stress, cardiac remodeling, and inflammation. Int. J. Mol. Sci. 23 (17), 10146. doi:10.3390/ijms231710146
Husain, M., Birkenfeld, A. L., Donsmark, M., Dungan, K., Eliaschewitz, F. G., Franco, D. R., et al. (2019). Oral semaglutide and cardiovascular outcomes in patients with type 2 diabetes. N. Engl. J. Med. 381 (9), 841–851. doi:10.1056/NEJMoa1901118
Inoue, T., Inoguchi, T., Sonoda, N., Hendarto, H., Makimura, H., Sasaki, S., et al. (2015). GLP-1 analog liraglutide protects against cardiac steatosis, oxidative stress and apoptosis in streptozotocin-induced diabetic rats. Atherosclerosis 240 (1), 250–259. doi:10.1016/j.atherosclerosis.2015.03.026
Jansen, T., Kvandová, M., Daiber, A., Stamm, P., Frenis, K., Schulz, E., et al. (2020). The AMP-activated protein kinase plays a role in antioxidant defense and regulation of vascular inflammation. Antioxidants 9 (6), 525. doi:10.3390/antiox9060525
Jenkins, B. J., Blagih, J., Ponce-Garcia, F. M., Canavan, M., Gudgeon, N., Eastham, S., et al. (2023). Canagliflozin impairs T cell effector function via metabolic suppression in autoimmunity. Cell metab. 35 (7), 1132–1146.e9. doi:10.1016/j.cmet.2023.05.001
Jojima, T., Uchida, K., Akimoto, K., Tomotsune, T., Yanagi, K., Iijima, T., et al. (2017). Liraglutide, a GLP-1 receptor agonist, inhibits vascular smooth muscle cell proliferation by enhancing AMP-activated protein kinase and cell cycle regulation, and delays atherosclerosis in ApoE deficient mice. Atherosclerosis 261, 44–51. doi:10.1016/j.atherosclerosis.2017.04.001
Jongs, N., Greene, T., Chertow, G. M., McMurray, J. J. V., Langkilde, A. M., Correa-Rotter, R., et al. (2021). Effect of dapagliflozin on urinary albumin excretion in patients with chronic kidney disease with and without type 2 diabetes: a prespecified analysis from the DAPA-CKD trial. lancet Diabetes and Endocrinol. 9 (11), 755–766. doi:10.1016/s2213-8587(21)00243-6
Juni, R. P., Al-Shama, R., Kuster, D. W. D., van der Velden, J., Hamer, H. M., Vervloet, M. G., et al. (2021). Empagliflozin restores chronic kidney disease-induced impairment of endothelial regulation of cardiomyocyte relaxation and contraction. Kidney Int. 99 (5), 1088–1101. doi:10.1016/j.kint.2020.12.013
Kadowaki, S., Siraj, M. A., Chen, W., Wang, J., Parker, M., Nagy, A., et al. (2023). Cardioprotective actions of a glucagon-like peptide-1 receptor agonist on hearts donated after circulatory death. J. Am. Heart Assoc. 12 (3), e027163. doi:10.1161/jaha.122.027163
Kamel, N. M., Abd El Fattah, M. A., El-Abhar, H. S., and Abdallah, D. M. (2019). Novel repair mechanisms in a renal ischaemia/reperfusion model: subsequent saxagliptin treatment modulates the pro-angiogenic GLP-1/cAMP/VEGF, ANP/eNOS/NO, SDF-1α/CXCR4, and Kim-1/STAT3/HIF-1α/VEGF/eNOS pathways. Eur. J. Pharmacol. 861, 172620. doi:10.1016/j.ejphar.2019.172620
Kanasaki, K., Shi, S., Kanasaki, M., He, J., Nagai, T., Nakamura, Y., et al. (2014). Linagliptin-mediated DPP-4 inhibition ameliorates kidney fibrosis in streptozotocin-induced diabetic mice by inhibiting endothelial-to-mesenchymal transition in a therapeutic regimen. Diabetes 63 (6), 2120–2131. doi:10.2337/db13-1029
Ke, Q., Shi, C., Lv, Y., Wang, L., Luo, J., Jiang, L., et al. (2022). SGLT2 inhibitor counteracts NLRP3 inflammasome via tubular metabolite itaconate in fibrosis kidney. FASEB J. 36 (1), e22078. doi:10.1096/fj.202100909RR
Kenny, H. C., and Abel, E. D. (2019). Heart failure in type 2 diabetes mellitus. Circulation Res. 124 (1), 121–141. doi:10.1161/circresaha.118.311371
Kim, Y. M., Kim, S. J., Tatsunami, R., Yamamura, H., Fukai, T., and Ushio-Fukai, M. (2017). ROS-induced ROS release orchestrated by Nox4, Nox2, and mitochondria in VEGF signaling and angiogenesis. Am. J. physiology Cell physiology 312 (6), C749–C764. doi:10.1152/ajpcell.00346.2016
Klen, J., and Dolžan, V. (2023). SGLT2 inhibitors in the treatment of diabetic kidney disease: more than just glucose regulation. Pharmaceutics 15 (7), 1995. doi:10.3390/pharmaceutics15071995
Komatsu, T., Abe, S., Nakashima, S., Sasaki, K., Higaki, Y., Saku, K., et al. (2023). Dipeptidyl peptidase-4 inhibitor sitagliptin phosphate accelerates cellular cholesterol efflux in THP-1 cells. Biomolecules 13 (2), 228. doi:10.3390/biom13020228
Koshibu, M., Mori, Y., Saito, T., Kushima, H., Hiromura, M., Terasaki, M., et al. (2019). Antiatherogenic effects of liraglutide in hyperglycemic apolipoprotein E-null mice via AMP-activated protein kinase-independent mechanisms. Am. J. physiology Endocrinol. metabolism 316 (5), E895–E907. doi:10.1152/ajpendo.00511.2018
Kyriazis, I. D., Hoffman, M., Gaignebet, L., Lucchese, A. M., Markopoulou, E., Palioura, D., et al. (2021). KLF5 is induced by FOXO1 and causes oxidative stress and diabetic cardiomyopathy. Circulation Res. 128 (3), 335–357. doi:10.1161/circresaha.120.316738
Leiter, L. A., Teoh, H., Braunwald, E., Mosenzon, O., Cahn, A., Kumar, K. M., et al. (2015). Efficacy and safety of saxagliptin in older participants in the SAVOR-TIMI 53 trial. Diabetes care 38 (6), 1145–1153. doi:10.2337/dc14-2868
Li, L., Qian, K., Sun, Y., Zhao, Y., Zhou, Y., Xue, Y., et al. (2021b). Omarigliptin ameliorated high glucose-induced nucleotide oligomerization domain-like receptor protein 3 (NLRP3) inflammasome activation through activating adenosine monophosphate-activated protein kinase α (AMPKα) in renal glomerular endothelial cells. Bioengineered 12 (1), 4805–4815. doi:10.1080/21655979.2021.1957748
Li, X., Römer, G., Kerindongo, R. P., Hermanides, J., Albrecht, M., Hollmann, M. W., et al. (2021). Sodium glucose Co-transporter 2 inhibitors ameliorate endothelium barrier dysfunction induced by cyclic stretch through inhibition of reactive oxygen species. Int. J. Mol. Sci. 22 (11), 6044. doi:10.3390/ijms22116044
Li, Y., Liu, Y., Liu, S., Gao, M., Wang, W., Chen, K., et al. (2023). Diabetic vascular diseases: molecular mechanisms and therapeutic strategies. Signal Transduct. Target. Ther. 8 (1), 152. doi:10.1038/s41392-023-01400-z
Li, Y., Xu, B., Yang, J., Wang, L., Tan, X., Hu, X., et al. (2021a). Liraglutide protects against lethal renal ischemia-reperfusion injury by inhibiting high-mobility group box 1 nuclear-cytoplasmic translocation and release. Pharmacol. Res. 173, 105867. doi:10.1016/j.phrs.2021.105867
Lin, K., Yang, N., Luo, W., Qian, J. F., Zhu, W. W., Ye, S. J., et al. (2022). Direct cardio-protection of Dapagliflozin against obesity-related cardiomyopathy via NHE1/MAPK signaling. Acta Pharmacol. Sin. 43 (10), 2624–2635. doi:10.1038/s41401-022-00885-8
Liu, J., Aylor, K. W., and Liu, Z. (2023). Liraglutide and exercise synergistically attenuate vascular inflammation and enhance metabolic insulin action in early diet-induced obesity. Diabetes 72 (7), 918–931. doi:10.2337/db22-0745
Lu, Y. P., Zhang, Z. Y., Wu, H. W., Fang, L. J., Hu, B., Tang, C., et al. (2022). SGLT2 inhibitors improve kidney function and morphology by regulating renal metabolic reprogramming in mice with diabetic kidney disease. J. Transl. Med. 20 (1), 420. doi:10.1186/s12967-022-03629-8
Luo, X., Hu, Y., He, S., Ye, Q., Lv, Z., Liu, J., et al. (2019). Dulaglutide inhibits high glucose-induced endothelial dysfunction and NLRP3 inflammasome activation. Archives Biochem. biophysics 671, 203–209. doi:10.1016/j.abb.2019.07.008
Madonna, R., Moscato, S., Cufaro, M. C., Pieragostino, D., Mattii, L., Del Boccio, P., et al. (2023). Empagliflozin inhibits excessive autophagy through the AMPK/GSK3β signalling pathway in diabetic cardiomyopathy. Cardiovasc. Res. 119 (5), 1175–1189. doi:10.1093/cvr/cvad009
Mann, J. F. E., Hansen, T., Idorn, T., Leiter, L. A., Marso, S. P., Rossing, P., et al. (2020). Effects of once-weekly subcutaneous semaglutide on kidney function and safety in patients with type 2 diabetes: a post-hoc analysis of the SUSTAIN 1-7 randomised controlled trials. lancet Diabetes and Endocrinol. 8 (11), 880–893. doi:10.1016/s2213-8587(20)30313-2
Mann, J. F. E., Ørsted, D. D., Brown-Frandsen, K., Marso, S. P., Poulter, N. R., Rasmussen, S., et al. (2017). Liraglutide and renal outcomes in type 2 diabetes. N. Engl. J. Med. 377 (9), 839–848. doi:10.1056/NEJMoa1616011
Marso, S. P., Bain, S. C., Consoli, A., Eliaschewitz, F. G., Jódar, E., Leiter, L. A., et al. (2016b). Semaglutide and cardiovascular outcomes in patients with type 2 diabetes. N. Engl. J. Med. 375 (19), 1834–1844. doi:10.1056/NEJMoa1607141
Marso, S. P., Daniels, G. H., Brown-Frandsen, K., Kristensen, P., Mann, J. F., Nauck, M. A., et al. (2016a). Liraglutide and cardiovascular outcomes in type 2 diabetes. N. Engl. J. Med. 375 (4), 311–322. doi:10.1056/NEJMoa1603827
Mauricio, D., Gratacòs, M., and Franch-Nadal, J. (2023). Diabetic microvascular disease in non-classical beds: the hidden impact beyond the retina, the kidney, and the peripheral nerves. Cardiovasc. Diabetol. 22 (1), 314. doi:10.1186/s12933-023-02056-3
Mayer, A. L., Scheitacker, I., Ebert, N., Klein, T., Amann, K., and Daniel, C. (2021). The dipeptidyl peptidase 4 inhibitor linagliptin ameliorates renal injury and accelerated resolution in a rat model of crescentic nephritis. Br. J. Pharmacol. 178 (4), 878–895. doi:10.1111/bph.15320
Mc Causland, F. R., Claggett, B. L., Vaduganathan, M., Desai, A. S., Jhund, P., de Boer, R. A., et al. (2023). Dapagliflozin and kidney outcomes in patients with heart failure with mildly reduced or preserved ejection fraction: a prespecified analysis of the DELIVER randomized clinical trial. JAMA Cardiol. 8 (1), 56–65. doi:10.1001/jamacardio.2022.4210
McGuire, D. K., Van de Werf, F., Armstrong, P. W., Standl, E., Koglin, J., Green, J. B., et al. (2016). Association between sitagliptin use and heart failure hospitalization and related outcomes in type 2 diabetes mellitus: secondary analysis of a randomized clinical trial. JAMA Cardiol. 1 (2), 126–135. doi:10.1001/jamacardio.2016.0103
McMurray, J. J. V., Solomon, S. D., Inzucchi, S. E., Køber, L., Kosiborod, M. N., Martinez, F. A., et al. (2019). Dapagliflozin in patients with heart failure and reduced ejection fraction. N. Engl. J. Med. 381 (21), 1995–2008. doi:10.1056/NEJMoa1911303
Meng, J., Zhang, W., Wang, C., Xiong, S., Wang, Q., Li, H., et al. (2020). The dipeptidyl peptidase (DPP)-4 inhibitor trelagliptin inhibits IL-1β-induced endothelial inflammation and monocytes attachment. Int. Immunopharmacol. 89 (Pt B), 106996. doi:10.1016/j.intimp.2020.106996
Min, J., Wu, L., Liu, Y., Song, G., Deng, Q., Jin, W., et al. (2023). Empagliflozin attenuates trastuzumab-induced cardiotoxicity through suppression of DNA damage and ferroptosis. Life Sci. 312, 121207. doi:10.1016/j.lfs.2022.121207
Mosenzon, O., Leibowitz, G., Bhatt, D. L., Cahn, A., Hirshberg, B., Wei, C., et al. (2017). Effect of saxagliptin on renal outcomes in the SAVOR-TIMI 53 trial. Diabetes care 40 (1), 69–76. doi:10.2337/dc16-0621
Müller, T. D., Finan, B., Bloom, S. R., D'Alessio, D., Drucker, D. J., Flatt, P. R., et al. (2019). Glucagon-like peptide 1 (GLP-1). Mol. Metab. 30, 72–130. doi:10.1016/j.molmet.2019.09.010
Muskiet, M. H. A., Tonneijck, L., Smits, M. M., Kramer, M. H. H., Ouwens, D. M., Hartmann, B., et al. (2020). Effects of DPP-4 inhibitor linagliptin versus sulfonylurea glimepiride as add-on to metformin on renal physiology in overweight patients with type 2 diabetes (renalis): a randomized, double-blind trial. Diabetes care 43 (11), 2889–2893. doi:10.2337/dc20-0902
Muskiet, M. H. A., Tonneijck, L., Smits, M. M., Kramer, M. H. H., Ouwens, D. M., Hartmann, B., et al. (2022). Postprandial renal haemodynamic effects of the dipeptidyl peptidase-4 inhibitor linagliptin versus the sulphonylurea glimepiride in adults with type 2 diabetes (RENALIS): a predefined substudy of a randomized, double-blind trial. Diabetes, Obes. metabolism 24 (1), 115–124. doi:10.1111/dom.14557
Nauck, M. A., McGuire, D. K., Pieper, K. S., Lokhnygina, Y., Strandberg, T. E., Riefflin, A., et al. (2019). Sitagliptin does not reduce the risk of cardiovascular death or hospitalization for heart failure following myocardial infarction in patients with diabetes: observations from TECOS. Cardiovasc. Diabetol. 18 (1), 116. doi:10.1186/s12933-019-0921-2
Navaneethan, S. D., Zoungas, S., Caramori, M. L., Chan, J. C. N., Heerspink, H. J. L., Hurst, C., et al. (2023). Diabetes management in chronic kidney disease: synopsis of the 2020 KDIGO clinical practice guideline. Ann. Intern Med. 176 (3), 385–394. doi:10.7326/M20-5938
Nistala, R., Meuth, A. I., Smith, C., An, J., Habibi, J., Hayden, M. R., et al. (2021). DPP4 inhibition mitigates ANG II-mediated kidney immune activation and injury in male mice. Am. J. physiology Ren. physiology 320 (3), F505–f517. doi:10.1152/ajprenal.00565.2020
Packer, M. (2020a). SGLT2 inhibitors produce cardiorenal benefits by promoting adaptive cellular reprogramming to induce a state of fasting mimicry: a paradigm shift in understanding their mechanism of action. Diabetes care 43 (3), 508–511. doi:10.2337/dci19-0074
Packer, M. (2020b). Interplay of adenosine monophosphate-activated protein kinase/sirtuin-1 activation and sodium influx inhibition mediates the renal benefits of sodium-glucose co-transporter-2 inhibitors in type 2 diabetes: a novel conceptual framework. Diabetes, Obes. metabolism 22 (5), 734–742. doi:10.1111/dom.13961
Pan, X., Yang, L., Wang, S., Liu, Y., Yue, L., and Chen, S. (2023). Semaglutide alleviates inflammation-Induced endothelial progenitor cells injury by inhibiting MiR-155 expression in macrophage exosomes. Int. Immunopharmacol. 119, 110196. doi:10.1016/j.intimp.2023.110196
Panico, C., Bonora, B., Camera, A., Chilelli, N. C., Prato, G. D., Favacchio, G., et al. (2023). Pathophysiological basis of the cardiological benefits of SGLT-2 inhibitors: a narrative review. Cardiovasc. Diabetol. 22 (1), 164. doi:10.1186/s12933-023-01855-y
Park, S., Jeong, H. E., Lee, H., You, S. C., and Shin, J. Y. (2023). Association of sodium-glucose cotransporter 2 inhibitors with post-discharge outcomes in patients with acute heart failure with type 2 diabetes: a cohort study. Cardiovasc. Diabetol. 22 (1), 191. doi:10.1186/s12933-023-01896-3
Perkovic, V., Jardine, M. J., Neal, B., Bompoint, S., Heerspink, H. J. L., Charytan, D. M., et al. (2019). Canagliflozin and renal outcomes in type 2 diabetes and nephropathy. N. Engl. J. Med. 380 (24), 2295–2306. doi:10.1056/NEJMoa1811744
Perkovic, V., Toto, R., Cooper, M. E., Mann, J. F. E., Rosenstock, J., McGuire, D. K., et al. (2020). Effects of linagliptin on cardiovascular and kidney outcomes in people with normal and reduced kidney function: secondary analysis of the CARMELINA randomized trial. Diabetes care 43 (8), 1803–1812. doi:10.2337/dc20-0279
Peyton, K. J., Behnammanesh, G., Durante, G. L., and Durante, W. (2022). Canagliflozin inhibits human endothelial cell inflammation through the induction of heme oxygenase-1. Int. J. Mol. Sci. 23 (15), 8777. doi:10.3390/ijms23158777
Pfeffer, M. A., Claggett, B., Diaz, R., Dickstein, K., Gerstein, H. C., Køber, L. V., et al. (2015). Lixisenatide in patients with type 2 diabetes and acute coronary syndrome. N. Engl. J. Med. 373 (23), 2247–2257. doi:10.1056/NEJMoa1509225
Pham, T. K., Nguyen, T. H. T., Yi, J. M., Kim, G. S., Yun, H. R., Kim, H. K., et al. (2023). Evogliptin, a DPP-4 inhibitor, prevents diabetic cardiomyopathy by alleviating cardiac lipotoxicity in db/db mice. Exp. Mol. Med. 55 (4), 767–778. doi:10.1038/s12276-023-00958-6
Poonchuay, N., Wattana, K., and Uitrakul, S. (2022). Efficacy of linagliptin on cardiovascular risk and cardiometabolic parameters in Thai patients with type 2 diabetes mellitus: a real-world observational study. Diabetes and metabolic syndrome 16 (5), 102498. doi:10.1016/j.dsx.2022.102498
Quagliariello, V., De Laurentiis, M., Rea, D., Barbieri, A., Monti, M. G., Carbone, A., et al. (2021). The SGLT-2 inhibitor empagliflozin improves myocardial strain, reduces cardiac fibrosis and pro-inflammatory cytokines in non-diabetic mice treated with doxorubicin. Cardiovasc. Diabetol. 20 (1), 150. doi:10.1186/s12933-021-01346-y
Rahmoune, H., Thompson, P. W., Ward, J. M., Smith, C. D., Hong, G., and Brown, J. (2005). Glucose transporters in human renal proximal tubular cells isolated from the urine of patients with non-insulin-dependent diabetes. Diabetes 54 (12), 3427–3434. doi:10.2337/diabetes.54.12.3427
Ren, C., Sun, K., Zhang, Y., Hu, Y., Hu, B., Zhao, J., et al. (2021). Sodium-glucose CoTransporter-2 inhibitor empagliflozin ameliorates sunitinib-induced cardiac dysfunction via regulation of AMPK-mTOR signaling pathway-mediated autophagy. Front. Pharmacol. 12, 664181. doi:10.3389/fphar.2021.664181
Rosa, C. M., Campos, D. H. S., Reyes, D. R. A., Damatto, F. C., Kurosaki, L. Y., Pagan, L. U., et al. (2022). Effects of the SGLT2 inhibition on cardiac remodeling in streptozotocin-induced diabetic rats, a model of type 1 diabetes mellitus. Antioxidants 11 (5), 982. doi:10.3390/antiox11050982
Rosenstock, J., Perkovic, V., Johansen, O. E., Cooper, M. E., Kahn, S. E., Marx, N., et al. (2019). Effect of linagliptin vs placebo on major cardiovascular events in adults with type 2 diabetes and high cardiovascular and renal risk: the CARMELINA randomized clinical trial. Jama 321 (1), 69–79. doi:10.1001/jama.2018.18269
Rossing, P., Baeres, F. M. M., Bakris, G., Bosch-Traberg, H., Gislum, M., Gough, S. C. L., et al. (2023). The rationale, design and baseline data of FLOW, a kidney outcomes trial with once-weekly semaglutide in people with type 2 diabetes and chronic kidney disease. Nephrol. Dial. Transplant. 38 (9), 2041–2051. doi:10.1093/ndt/gfad009
Säemann, M., and Kronbichler, A. (2022). Call for action in ANCA-associated vasculitis and lupus nephritis: promises and challenges of SGLT-2 inhibitors. Ann. rheumatic Dis. 81 (5), 614–617. doi:10.1136/annrheumdis-2021-221474
Scirica, B. M., Bhatt, D. L., Braunwald, E., Steg, P. G., Davidson, J., Hirshberg, B., et al. (2013). Saxagliptin and cardiovascular outcomes in patients with type 2 diabetes mellitus. N. Engl. J. Med. 369 (14), 1317–1326. doi:10.1056/NEJMoa1307684
Scisciola, L., Taktaz, F., Fontanella, R. A., Pesapane, A., Surina, , Cataldo, V., et al. (2023). Targeting high glucose-induced epigenetic modifications at cardiac level: the role of SGLT2 and SGLT2 inhibitors. Cardiovasc. Diabetol. 22 (1), 24. doi:10.1186/s12933-023-01754-2
Sforza, A., Vigorelli, V., Rurali, E., Perrucci, G. L., Gambini, E., Arici, M., et al. (2022). Liraglutide preserves CD34(+) stem cells from dysfunction Induced by high glucose exposure. Cardiovasc. Diabetol. 21 (1), 51. doi:10.1186/s12933-022-01486-9
Shaman, A. M., Bain, S. C., Bakris, G. L., Buse, J. B., Idorn, T., Mahaffey, K. W., et al. (2022). Effect of the glucagon-like peptide-1 receptor agonists semaglutide and liraglutide on kidney outcomes in patients with type 2 diabetes: pooled analysis of SUSTAIN 6 and LEADER. Circulation 145 (8), 575–585. doi:10.1161/circulationaha.121.055459
Sharaf, G., E M, E. M., and El-Sayed, E. K. (2023). Augmented nephroprotective effect of liraglutide and rabeprazole via inhibition of OCT2 transporter in cisplatin-induced nephrotoxicity in rats. Life Sci. 321, 121609. doi:10.1016/j.lfs.2023.121609
Sharma, A., Wood, S., Bell, J. S., De Blasio, M. J., Ilomäki, J., and Ritchie, R. H. (2023). Sex differences in risk of cardiovascular events and mortality with sodium glucose co-transporter-2 inhibitors versus glucagon-like peptide 1 receptor agonists in Australians with type 2 diabetes: a population-based cohort study. Lancet regional health West. Pac. 33, 100692. doi:10.1016/j.lanwpc.2023.100692
Solomon, S. D., McMurray, J. J. V., Claggett, B., de Boer, R. A., DeMets, D., Hernandez, A. F., et al. (2022). Dapagliflozin in heart failure with mildly reduced or preserved ejection fraction. N. Engl. J. Med. 387 (12), 1089–1098. doi:10.1056/NEJMoa2206286
Su, K., Yi, B., Yao, B. Q., Xia, T., Yang, Y. F., Zhang, Z. H., et al. (2020). Liraglutide attenuates renal tubular ectopic lipid deposition in rats with diabetic nephropathy by inhibiting lipid synthesis and promoting lipolysis. Pharmacol. Res. 156, 104778. doi:10.1016/j.phrs.2020.104778
Sukumaran, V., Tsuchimochi, H., Sonobe, T., Shirai, M., and Pearson, J. T. (2019). Liraglutide improves renal endothelial function in obese zucker rats on a high-salt diet. J. Pharmacol. Exp. Ther. 369 (3), 375–388. doi:10.1124/jpet.118.254821
Sun, H., Saeedi, P., Karuranga, S., Pinkepank, M., Ogurtsova, K., Duncan, B. B., et al. (2022). IDF Diabetes Atlas: global, regional and country-level diabetes prevalence estimates for 2021 and projections for 2045. Diabetes Res. Clin. Pract. 183, 109119. doi:10.1016/j.diabres.2021.109119
Sun, X., Han, F., Lu, Q., Li, X., Ren, D., Zhang, J., et al. (2020). Empagliflozin ameliorates obesity-related cardiac dysfunction by regulating sestrin2-mediated AMPK-mTOR signaling and redox homeostasis in high-fat diet-induced obese mice. Diabetes 69 (6), 1292–1305. doi:10.2337/db19-0991
Tang, P. M., Zhang, Y. Y., Hung, J. S., Chung, J. Y., Huang, X. R., To, K. F., et al. (2021). DPP4/CD32b/NF-κB circuit: a novel druggable target for inhibiting CRP-driven diabetic nephropathy. Mol. Ther. J. Am. Soc. Gene Ther. 29 (1), 365–375. doi:10.1016/j.ymthe.2020.08.017
Tschöp, M., Nogueiras, R., and Ahrén, B. (2023). Gut hormone-based pharmacology: novel formulations and future possibilities for metabolic disease therapy. Diabetologia 66 (10), 1796–1808. doi:10.1007/s00125-023-05929-0
Tuttle, K. R., Bosch-Traberg, H., Cherney, D. Z. I., Hadjadj, S., Lawson, J., Mosenzon, O., et al. (2023). Post hoc analysis of SUSTAIN 6 and PIONEER 6 trials suggests that people with type 2 diabetes at high cardiovascular risk treated with semaglutide experience more stable kidney function compared with placebo. Kidney Int. 103 (4), 772–781. doi:10.1016/j.kint.2022.12.028
Voors, A. A., Angermann, C. E., Teerlink, J. R., Collins, S. P., Kosiborod, M., Biegus, J., et al. (2022). The SGLT2 inhibitor empagliflozin in patients hospitalized for acute heart failure: a multinational randomized trial. Nat. Med. 28 (3), 568–574. doi:10.1038/s41591-021-01659-1
Wadie, W., Ahmed, G. S., Shafik, A. N., and El-Sayed, M. (2022). Effects of insulin and sitagliptin on early cardiac dysfunction in diabetic rats. Life Sci. 299, 120542. doi:10.1016/j.lfs.2022.120542
Wang, H., Li, T., Sun, F., Liu, Z., Zhang, D., Teng, X., et al. (2022). Safety and efficacy of the SGLT2 inhibitor dapagliflozin in patients with systemic lupus erythematosus: a phase I/II trial. RMD open 8 (2), e002686. doi:10.1136/rmdopen-2022-002686
Wang, Y., He, W., Wei, W., Mei, X., Yang, M., and Wang, Y. (2021). Exenatide attenuates obesity-induced mitochondrial dysfunction by activating SIRT1 in renal tubular cells. Front. Endocrinol. 12, 622737. doi:10.3389/fendo.2021.622737
Wanner, C., Cooper, M. E., Johansen, O. E., Toto, R., Rosenstock, J., McGuire, D. K., et al. (2021). Effect of linagliptin versus placebo on cardiovascular and kidney outcomes in nephrotic-range proteinuria and type 2 diabetes: the CARMELINA randomized controlled trial. Clin. kidney J. 14 (1), 226–236. doi:10.1093/ckj/sfaa225
White, W. B., Cannon, C. P., Heller, S. R., Nissen, S. E., Bergenstal, R. M., Bakris, G. L., et al. (2013). Alogliptin after acute coronary syndrome in patients with type 2 diabetes. N. Engl. J. Med. 369 (14), 1327–1335. doi:10.1056/NEJMoa1305889
Withaar, C., Meems, L. M. G., Nollet, E. E., Schouten, E. M., Schroeder, M. A., Knudsen, L. B., et al. (2023). The cardioprotective effects of semaglutide exceed those of dietary weight loss in mice with HFpEF. JACC Basic Transl. Sci. 8 (10), 1298–1314. doi:10.1016/j.jacbts.2023.05.012
Wiviott, S. D., Raz, I., Bonaca, M. P., Mosenzon, O., Kato, E. T., Cahn, A., et al. (2019). Dapagliflozin and cardiovascular outcomes in type 2 diabetes. N. Engl. J. Med. 380 (4), 347–357. doi:10.1056/NEJMoa1812389
Wójcicka, G., Pradiuch, A., Fornal, E., Stachniuk, A., Korolczuk, A., Marzec-Kotarska, B., et al. (2023). The effect of exenatide (a GLP-1 analogue) and sitagliptin (a DPP-4 inhibitor) on asymmetric dimethylarginine (ADMA) metabolism and selected biomarkers of cardiac fibrosis in rats with fructose-induced metabolic syndrome. Biochem. Pharmacol. 214, 115637. doi:10.1016/j.bcp.2023.115637
Xiao, B., Sanders, M. J., Underwood, E., Heath, R., Mayer, F. V., Carmena, D., et al. (2011). Structure of mammalian AMPK and its regulation by ADP. Nature 472 (7342), 230–233. doi:10.1038/nature09932
Xie, Y., Bowe, B., Xian, H., Loux, T., McGill, J. B., and Al-Aly, Z. (2023). Comparative effectiveness of SGLT2 inhibitors, GLP-1 receptor agonists, DPP-4 inhibitors, and sulfonylureas on risk of major adverse cardiovascular events: emulation of a randomised target trial using electronic health records. lancet Diabetes and Endocrinol. 11 (9), 644–656. doi:10.1016/s2213-8587(23)00171-7
Xu, C., Lu, C., Wang, Z., Hu, X., Li, S., Xie, Y., et al. (2023). Liraglutide abrogates nephrotoxic effects of chemotherapies. Pharmacol. Res. 189, 106680. doi:10.1016/j.phrs.2023.106680
Xu, J., Kitada, M., Ogura, Y., Liu, H., and Koya, D. (2021). Dapagliflozin restores impaired autophagy and suppresses inflammation in high glucose-treated HK-2 cells. Cells 10 (6), 1457. doi:10.3390/cells10061457
Yang, S., Lin, C., Zhuo, X., Wang, J., Rao, S., Xu, W., et al. (2020). Glucagon-like peptide-1 alleviates diabetic kidney disease through activation of autophagy by regulating AMP-activated protein kinase-mammalian target of rapamycin pathway. Am. J. physiology Endocrinol. metabolism 319 (6), E1019–E1030. doi:10.1152/ajpendo.00195.2019
Yi, T. W., Smyth, B., Di Tanna, G. L., Arnott, C., Cardoza, K., Kang, A., et al. (2023). Kidney and cardiovascular effects of canagliflozin according to age and sex: a post hoc analysis of the CREDENCE randomized clinical trial. Am. J. kidney Dis. 82 (1), 84–96.e1. doi:10.1053/j.ajkd.2022.12.015
Yin, R., Xu, Y., Wang, X., Yang, L., and Zhao, D. (2022). Role of dipeptidyl peptidase 4 inhibitors in antidiabetic treatment. Mol. 27 (10), 3055. doi:10.3390/molecules27103055
Yoon, S. A., Han, B. G., Kim, S. G., Han, S. Y., Jo, Y. I., Jeong, K. H., et al. (2017). Efficacy, safety and albuminuria-reducing effect of gemigliptin in Korean type 2 diabetes patients with moderate to severe renal impairment: a 12-week, double-blind randomized study (the GUARD Study). Diabetes, Obes. metabolism 19 (4), 590–598. doi:10.1111/dom.12863
Zhang, L., Tian, J., Diao, S., Zhang, G., Xiao, M., and Chang, D. (2020). GLP-1 receptor agonist liraglutide protects cardiomyocytes from IL-1β-induced metabolic disturbance and mitochondrial dysfunction. Chemico-biological Interact. 332, 109252. doi:10.1016/j.cbi.2020.109252
Zhang, Y., Bao, M., Dai, M., Wang, X., He, W., Tan, T., et al. (2015). Cardiospecific CD36 suppression by lentivirus-mediated RNA interference prevents cardiac hypertrophy and systolic dysfunction in high-fat-diet induced obese mice. Cardiovasc. Diabetol. 14, 69. doi:10.1186/s12933-015-0234-z
Zhao, D., Guallar, E., Ouyang, P., Subramanya, V., Vaidya, D., Ndumele, C. E., et al. (2018). Endogenous sex hormones and incident cardiovascular disease in post-menopausal women. J. Am. Coll. Cardiol. 71 (22), 2555–2566. doi:10.1016/j.jacc.2018.01.083
Zhao, J., He, X., Zuo, M., Li, X., and Sun, Z. (2021). Anagliptin prevented interleukin 1β (IL-1β)-induced cellular senescence in vascular smooth muscle cells through increasing the expression of sirtuin1 (SIRT1). Bioengineered 12 (1), 3968–3977. doi:10.1080/21655979.2021.1948289
Zhao, X. Y., Li, S. S., He, Y. X., Yan, L. J., Lv, F., Liang, Q. M., et al. (2023). SGLT2 inhibitors alleviated podocyte damage in lupus nephritis by decreasing inflammation and enhancing autophagy. Ann. rheumatic Dis. 82 (10), 1328–1340. doi:10.1136/ard-2023-224242
Zheng, R. H., Zhang, W. W., Ji, Y. N., Bai, X. J., Yan, C. P., Wang, J., et al. (2020). Exogenous supplement of glucagon like peptide-1 protects the heart against aortic banding induced myocardial fibrosis and dysfunction through inhibiting mTOR/p70S6K signaling and promoting autophagy. Eur. J. Pharmacol. 883, 173318. doi:10.1016/j.ejphar.2020.173318
Keywords: diabetes mellitus, cardiorenal protection, SGLT2 inhibitors, GLP-1 receptor agonists, DPP-4 inhibitors
Citation: Fu W-J, Huo J-L, Mao Z-H, Pan S-K, Liu D-W, Liu Z-S, Wu P and Gao Z-X (2024) Emerging role of antidiabetic drugs in cardiorenal protection. Front. Pharmacol. 15:1349069. doi: 10.3389/fphar.2024.1349069
Received: 06 December 2023; Accepted: 26 January 2024;
Published: 06 February 2024.
Edited by:
Maria Consiglia Trotta, University of Campania Luigi Vanvitelli, ItalyReviewed by:
Aikaterini Andreadi, University of Rome Tor Vergata, ItalyMaria Antonietta Riemma, University of Campania Luigi Vanvitelli, Italy
Copyright © 2024 Fu, Huo, Mao, Pan, Liu, Liu, Wu and Gao. This is an open-access article distributed under the terms of the Creative Commons Attribution License (CC BY). The use, distribution or reproduction in other forums is permitted, provided the original author(s) and the copyright owner(s) are credited and that the original publication in this journal is cited, in accordance with accepted academic practice. No use, distribution or reproduction is permitted which does not comply with these terms.
*Correspondence: Zhong-Xiuzi Gao, Z2Fvemhvbmd4aXV6aUB6enUuZWR1LmNu; Peng Wu, d3VwZW5nY2dAenp1LmVkdS5jbg==; Zhang-Suo Liu, emhhbmdzdW9saXVAenp1LmVkdS5jbg==
†These authors have contributed equally to this work