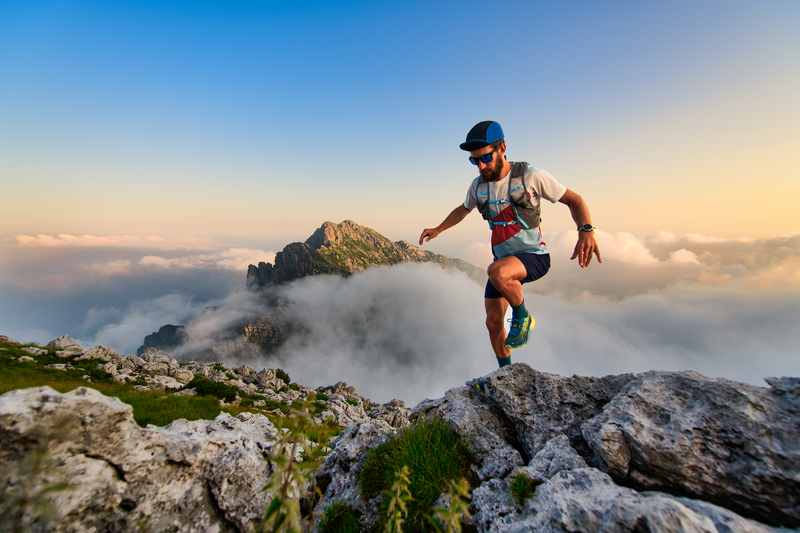
94% of researchers rate our articles as excellent or good
Learn more about the work of our research integrity team to safeguard the quality of each article we publish.
Find out more
SYSTEMATIC REVIEW article
Front. Pharmacol. , 29 January 2024
Sec. Ethnopharmacology
Volume 15 - 2024 | https://doi.org/10.3389/fphar.2024.1315584
This article is part of the Research Topic Preventing and Treating Liver Diseases: Medicinal and Food Plants, their metabolites as potential options View all 17 articles
Background: Matrine, an alkaloid derived from the dried roots of Sophora flavescens Aiton, has been utilized for the treatment of liver diseases, but its potential hepatotoxicity raises concerns. However, the precise condition and mechanism of action of matrine on the liver remain inconclusive. Therefore, the objective of this systematic review and meta-analysis is to comprehensively evaluate both the hepatoprotective and hepatotoxic effects of matrine and provide therapeutic guidance based on the findings.
Methods: The meta-analysis systematically searched relevant preclinical literature up to May 2023 from eight databases, including PubMed, Web of Science, Cochrane Library, Embase, China National Knowledge Infrastructure, WanFang Med Online, China Science and Technology Journal Database, and China Biomedical Literature Service System. The CAMARADES system assessed the quality and bias of the evidence. Statistical analysis was conducted using STATA, which included the use of 3D maps and radar charts to display the effects of matrine dosage and frequency on hepatoprotection and hepatotoxicity.
Results: After a thorough screening, 24 studies involving 657 rodents were selected for inclusion. The results demonstrate that matrine has bidirectional effects on ALT and AST levels, and it also regulates SOD, MDA, serum TG, serum TC, IL-6, TNF-α, and CAT levels. Based on our comprehensive three-dimensional analysis, the optimal bidirectional effective dosage of matrine ranges from 10 to 69.1 mg/kg. However, at a dose of 20–30 mg/kg/d for 0.02–0.86 weeks, it demonstrated high liver protection and low toxicity. The molecular docking analysis revealed the interaction between MT and SERCA as well as SREBP-SCAP complexes. Matrine could alter Ca2+ homeostasis in liver injury via multiple pathways, including the SREBP1c/SCAP, Notch/RBP-J/HES1, IκK/NF-κB, and Cul3/Rbx1/Keap1/Nrf2.
Conclusion: Matrine has bidirectional effects on the liver at doses ranging from 10 to 69.1 mg/kg by influencing Ca2+ homeostasis in the cytoplasm, endoplasmic reticulum, Golgi apparatus, and mitochondria.
Systematic review registration: https://inplasy.com/, identifier INPLASY202340114
Liver injury (LI) is a prevalent liver disease and a significant global health concern due to its high mortality rates. The European Association for the Study of the Liver (EASL) has reported that liver disease ranks 11th as the leading cause of death worldwide, accounting for 4% of all deaths. In 2023, Devarbhavi et al. estimated over two million fatalities annually due to liver disease (Devarbhavi et al., 2023). As a preliminary stage of liver disease, LI can be caused by various factors such as alcohol, infection, immunity, and drug-induced toxicity (Younossi et al., 2023). The severity of LI can range from mild inflammation to more severe conditions like liver cirrhosis, liver failure and even death. Symptoms of LI consist of abnormalities in liver function test abnormalities, fever, nausea, vomiting, jaundice, and right epigastric pain (Knight, 2005). Hepatocellular damage, fibrosis, and inflammatory infiltration are key pathological features of LI. Anti-viral drugs, liver protective agents, and immunosuppressive drugs are the mainstream drugs used to treat LI. While corticosteroids, pioglitazone, cholestyramine, and other medications are commonly prescribed to treat different types of LI (Devarbhavi et al., 2023), their hepatotoxicity can lead to drug-induced liver injury (DILI) in clinical settings. Therefore, exploring more effective and safer alternatives for LI is necessary.
The dried roots of Sophora flavescens Aiton (well-documented in The Plant List www.theplantlist.org), a Traditional Chinese Medicine (TCM) herb, commonly known as KuShen, was initially discovered for its therapeutic properties in the ancient text Shen Nong Ben Cao Jing. For thousands of years, the dried roots of S. flavescens Aiton has been widely used to treat various digestive disorders, such as dysentery, bloody stools, jaundice, and especially liver diseases (Chinese Pharmacopoeia Commission, 2020). Kushen Decoction and Longdan Kushen Decoction are the representative TCM prescriptions that incorporate the dried roots of S. flavescens Aiton for treating liver disease. Contemporary pharmacological studies have revealed that extracts of the dried roots of S. flavescens Aiton have hepatoprotective effects due to their anti-inflammatory and antiviral properties (He et al., 2015).
Matrine ((1R,2R,9S,17S)-7,13-diazatetracyclo [7.7.1.02,7.013,17]heptadecan-6-one; C15H24N2O; MW = 248.36) is an alkaloid extracted from the dried roots of S. flavescens Aiton and can be dissolved in various solvents such as water, ethanol, benzene, etc .,(Sun et al., 2022). As an active ingredient of the dried roots of S. flavescens Aiton, the total content of matrine and oxymatrine should not be less than 1.2% according to the Pharmacopoeia of the People’s Republic of China (Chinese Pharmacopoeia Commission, 2020). Numerous studies have shown that MT has anti-inflammatory, anti-viral, anti-tumor, and immune-suppressive abilities (Zhou et al., 2014; Wang et al., 2018; Peng et al., 2020; Chu et al., 2021; Jing et al., 2021). MT has been reported to regulate liver protective function, hepatic regeneration, and alleviate LI through several signaling pathways, such as TGF-β/Smad, NF-κB, Wnt/β-catenin, Notch/Jagged1/recombination signal binding protein for immunoglobulin kappa J (RBP-Jκ, RBP-J)/hairy and enhancer of split-1 (HES1) (Yu H. B. et al., 2011; Yu et al., 2014; Yang et al., 2016; Yin et al., 2018). Due to its extensive pharmacological effects, MT is often used as an injection in clinical practice for hepatitis B, tumors, and immune diseases (Liu and Zhang, 2021). In the clinical pharmacokinetic study of MT, serum MT concentrations ranged from 1 to 6 ug/mL after a large-dose intravenous infusion (6 mg/kg) (Zhang et al., 2009). In rats, the maximum blood concentration of MT was found to reach 2,412 ± 362 ng/mL and 94.6 ± 38.6 ng/mL after intravenous or oral administration of MT at a dose of 2 mg/kg (Yang et al., 2010). However, in recent years, several studies have demonstrated that MT can lead to DILI, reproductive toxicity, and neurotoxicity (Wang et al., 2017). MT has been demonstrated to induce hepatotoxicity through inhibiting the Nrf2 pathway and stimulating the reactive oxygen species (ROS)-mediated mitochondrial apoptosis pathway (You et al., 2019). Nevertheless, the mechanisms of MT in liver protection and hepatotoxicity are continually being improved and elucidated (Figure 1).
FIGURE 1. Chemical structure of Matrine. (PubChem Identifier: CID 91466, URL: https://pubchem.ncbi.nlm.nih.gov/compound/91466#section=2D-Structure).
Calcium ion (Ca2+), a multifunctional intracellular messenger, affects cellular metabolism, energy generation, and intracellular homeostasis under physiological conditions. Extracellular stress stimulation on the cell membrane could enhance Ca2+ influx, and increased cytoplasmic Ca2+ would be transported into the Endoplasmic reticulum (ER) lumen and stored via cross specific Ca2+ ion channels, such as sarcoendoplasmic reticulum calcium transport ATPase (SERCA) (Periasamy and Kalyanasundaram, 2007; Chemaly et al., 2018). Loss of Ca2+ homeostasis and irregular Ca2+ channels on the cell membrane, ER, and mitochondria might cause ER stress and modify the mitochondrial membrane potential, raising total ROS in hepatocytes (Kaufman and Malhotra, 2014; Zeeshan et al., 2016). Based on the current literature, distinct MT concentration gradients can influence diverse SERCA responses on the ER, regulate mitochondrial activity, and balance intracellular Ca2+ levels to alleviate or promote hepatocyte stress (Gao et al., 2019).
Previous studies have demonstrated that MT exhibits both hepatoprotective effects and the potential to cause liver damage, yet the underlying mechanisms remain unclear. Therefore, the objective of this study is to conduct a systematic review and meta-analysis to investigate the impact of MT on LI and elucidate the dynamic processes through which MT leads to liver protection and hepatotoxicity. Additionally, this study aims to explore the role of Ca2+ in these processes, offering innovative insights into the mechanisms involved.
The meta-analysis followed the PRISMA 2020 guidelines and has been submitted to the International Platform of Registered Systematic Review and Meta-analysis Protocols (INPLASY) database (https://inplasy.com/). The registration number for this submission is INPLASY202340114.
The retrieved databases included four English databases and four Chinese databases according to the five articles (Ju et al., 2018; Xiong et al., 2019; Liu et al., 2021; Luo et al., 2021; Zheng et al., 2021). The four English databases were: PubMed, Web of science, Cochrane library, Embase. And the four Chinese databases: China National Knowledge Infrastructure, WanFang Med Online, China Science and Technology Journal Database, and China Biomedical Literature Service System. The literature search in this study encompassed all pertinent literature up until May 2023.
The search terms were “Matrine,” “liver injury,” “hepatoprotection,” and “hepatotoxicity”. (Figure 2 and Supplementary Table S1).
Considering the difference between hepatotoxicity and hepatoprotection of MT, this article has formulated appropriate included and excluded criteria to address these dual effects.
Research of hepatoprotection needs to meet the following requirements: 1) Subjects: the study involved rats or mice as the population. 2) Control(C) group and intervention(I) group: each study included at least 1 LI group as the C group and 1 MT group as the I group. 3) The I groups were comprised of LI models and received MT monotherapy exclusively. The C groups consisted of LI models that either received no treatment or received non-functional intervention. 4) The indicators of the studies should encompass AST, ALT, MDA, SOD, serum TG, serum TC, IL-6, CAT, and TNF-α, either in their entirety or partially. 5) The quality evaluation results were above 5 points.
Research of hepatotoxicity needs to meet the following requirements: 1) Subjects: the study involved rats or mice as the population. 2) C group and I group: each study included at least 1 LI group as the C group and 1 MT group as the I group. 3). The I groups were comprised of normal animals and received MT monotherapy exclusively. The C groups consisted of normal animals that either received no treatment or received non-functional intervention. 4) The indicators of the studies should encompass AST and ALT, either in their entirety or partially. 5) The quality evaluation results were above 5 points.
Excluded criteria for the research of hepatoprotection: 1) LI rats or mice were not selected as animal subjects for hepatoprotection studies. 2) The experiments did not form controls. 3) The I group did not receive MT monotherapy. The C group used functional drugs (including Western medicines, TCMs and integrative medicines) as interventions, and could not provide specific outcome indices. 4) The common indicators of LI were not included in the study. 5) Quality evaluation results below 5 points.
Excluded criteria for the research of hepatotoxicity: 1) Normal rats or mice were not selected as subjects for hepatotoxicity studies. 2) The experiments did not form controls. 3) The I group did not receive MT monotherapy. The C group used functional drugs (including Western medicines, TCMs and integrative medicines) as interventions, and could not provide specific outcome indices. 4) The common indicators of LI were not included in the study. 5) Quality evaluation results below 5 points.
Two researchers extracted the following data from the included articles: 1) The first author’s name and publication year; 2) Basic animal characteristics: including the number, species (mice or rats), strain, sex, and weight; 3) Modeling details: including the method of modeling and criteria for successful modeling; 4) Specific intervention methods: including the drug used, dosage, and frequency of administration; 5) Outcome measures. (Table 1).
Regarding the preset indicators, we recorded only the highest dose group in the gradient dosages. For the experiments that observed data from multiple time points, only the last were recorded. We collected the experimental data by Universal Desktop Ruler and calculated the mean and standard deviation (SD) of the continuous variables. Results of the measurements were displayed in graphics rather than digital text.
The CAMARADES (Collaborative Approach to Meta-Analysis and Review of Animal Data from Experimental Studies) 10-point scoring scale, an internationally recognized criteria published in 2004, is utilized to assess and calculate a methodological quality score (Macleod et al., 2004). Two researchers made an independently assessment of the methodological quality of the surveys. The quality measures were changed in accordance with the study’s specificity. When there was a disagreement in the evaluation, the correspondence author came to an agreement or used arbitration. The specific methods were also provided in Figure 3.
To visualize the dose-time-effect relationship for hepatoprotection and hepatotoxicity of MT, this study unified the time units of all experiments into weeks (W), as well as constructed 3D maps and radar charts. In addition, the regulatory mechanism of MT role in the literature is summarized.
Statistical analysis of indicators in this study was conducted using STATA 16.0 software. When the results are statistically significant, the p-value should be less than 0.05 (p < 0.05). Results were quantified using the standardized mean differences (SMD) and accompanying 95% confidence intervals (95% CI). The I-squared (I2) test was used to assess the degree of heterogeneity and consistency between research (random-effects model [I2>50%] or fixed-effects model [I2 ≤ 50%]). Results were deemed to exhibit significant heterogeneity when I2 exceeded 50%. Investigators conducted subgroup analyses for animal species (rat, mouse), dose administered (low (L)≤25 mg/kg, 25<medium (M)≤50 mg/kg, high (H)>50 mg/kg), and time of administration (<4w, ≥4w) in order to identify the source of heterogeneity. To establish whether the findings were trustworthy enough to draw inferences, sensitivity analysis was done.
The compounds and ligands were obtained from the PubChem database (https://pubchem.ncbi.nlm.nih.gov) and RCSB Protein Data Bank database (https://www.rcsb.org/structure). Molecular docking was performed using AutoDockTools 1.5.6 and AutoDock Vina 4.2. Here is a brief summary of the docking process.
1) The structure of MT was downloaded from the PubChem database. It was then converted into a 3D structure using ChemDraw software to minimize the structural energy. The 3D structure was calculated using AutoDockTools 1.5.6 software and saved as a pdbqt file. 2) The ligands were obtained from the RCSB protein bank. They were imported into PyMOL, dehydrated, hydrogenated, and prepared for ligand separation. The docking grid box was constructed in AutoDockTools 1.5.6 at the active site for each target protein and saved in pdbqt format. 3) AutoDock Vina 1.1.2 was used for molecular docking of the potential targets and active compounds, as well as to evaluate free binding energies. 4) PyMOL 2.6 and Discovery Studio 2019 were utilized for visualizing and analyzing interactions.
By using keywords, 1,032 articles in all could be found (334 articles from the four English databases and 698 articles from the four Chinese databases). After eliminating 676 duplicate articles, the researchers further examined the rest 356 articles. Depending upon the inclusion and exclusion criteria, the researchers excluded 264 articles after reviewing the titles and abstracts. And 34 articles on MT reviews, conference reports, abstracts or editorials and web pharmacology were subsequently eliminated from consideration. The remaining 32 articles were excluded after reviewing the full text. This meta-analysis eventually comprised 24 publications, 16 of which were in Chinese (Li et al., 2005; Liu et al., 2008; Song et al., 2009; Zhou et al., 2009; Gao et al., 2013; Tang et al., 2013; Wu et al., 2014; Liang et al., 2015; Zhao, 2015; Zhu et al., 2015; Li et al., 2016; Guo et al., 2017; Bai et al., 2018; Yuan and Yang, 2020; Chang et al., 2021; Du et al., 2021) and others were in English (Shi et al., 2013; Yang et al., 2013; Zhang et al., 2013; Gao et al., 2018; Gu et al., 2019; Khan et al., 2019; Liu et al., 2020; Rao et al., 2022). Figure 2 illustrates a flowchart of the study selection process.
A modified 10-item CAMARADES checklist was used to assess the methodological quality of the included publications. Peer-reviewed articles were among the criteria; temperature management; construction of appropriate rodent models according to the study objectives; experimental animals were randomly assigned to treatment or control groups; blinded assessment of outcomes; explicit presentation of the use of anaesthetics without significant intrinsic neuroprotective activity; sample size calculations; compliance with animal welfare policies; and avoidance of potential conflicts of interest. All 24 articles used appropriate rodent models and reasonable groupings, all clearly reported sample sizes for each group and competing interests, and all were published in peer-reviewed publications. However, only 6 articles explicitly reported the use of anaesthetics with no apparent intrinsic neuroprotective activity, 4 did not mention temperature control in the experiments, 5 did not mention animal welfare policies, and no studies assessed outcomes blinded. The included articles’ overall quality ratings ranged from 6 to 9. Two of the 24 articles received a score of 6 (8.33%), six received a score of 7 (25.00%), ten received a score of 8 (41.67%), and six received a score of 9 (25.00%). The methodological quality of each selected article is demonstrated in Figure 3.
The 24 papers had enough information to conduct a meta-analysis. These trials involved a total of 657 rodents, 330 of which were divided into the treatment group and the others were control group (Table 1).
Based on their biological traits, the animals used in the included researches were roughly categorized. The creatures were categorized into seven groups based on their species: 8.52% (56/657) Kunming mice, 6.09% (40/657) ICR mice, 11.11% (73/657) C57BL/6 mice, 4.57% (30/657) BALB/c mice, 54.49% (358/657) Sprague Dawley Rats, 8.52% (56/657) Wistar rats and 6.70% (44/657) NIH mice. 63.01% (414/657) of rodents were rats, and 36.99% (243/657) of rodents were mice. The percentage of female and male rodents was 9.13% (60/657) and 87.21% (573/657) respectively regarding sex categorization, while 3.65% (24/657) of the rodents’ sexes were unknown. Furthermore, according to the quality assessment scores, 25.00% (6/24) had 9 points, 41.67 (10/24) had 8 points, 25.00% (6/24) had 7 points, and 8.33% (2/24) had 6 points. Regarding the intervention time of MT, all experiments were divided into two subgroups: 76.10% (500/657) <4W groups and 23.90% (157/657) ≥4W groups. And the dosage of each experiment was divided into three groups: 24.96% (164/657) L-dosage groups, 23.14% (152/657) M-dosage group and 51.90% (341/657) H-dosage group (Supplementary Figure S1).
Across the studies, the weight of the animals included in the analysis varied from 17.7 g to 250 g, with a total number of examinations ranging from 10 to 184. The daily dosage of MT administered ranged from 2.8 mg/kg to 160 mg/kg, and the frequency of administration varied from a single dose to a maximum of 90 days.
The levels of ALT, AST, TNF-α and SOD which were the primary outcomes were assessed after MT therapy as well as the levels of MDA, IL-6, serum TG, serum TC and CAT were also changed by MT (Supplementary Tables S2, S3). Liver tissues from animals with LI exhibited significant inflammatory cell infiltration, hepatocyte swelling, vacuolar degeneration, and hepatocellular necrosis, as evidenced by H&E staining. The pathogenic alterations were significantly improved with MT treatment at dosage of 1.4–100 mg/kg, but the most effective dosage was the medium (25–50 mg/kg/d).
Because there was considerable heterogeneity (I2 > 50%), we performed a random-effects analysis. The findings revealed that the ALT levels were significantly reduced in the MT groups compared to the LI model groups (n = 532; 95% CI [−4.34, −2.50]; SMD = |−3.42| > 1; I2 = 90.30%; p < 0.0001) (Figure 4).
FIGURE 4. Forest plot (effect size and 95% CI) summarising the effects of MT on ALT (A1–C1) and AST (A2–C2) levels in hepatoprotection. (A) Rat and mice subgroups; (B) L, M and H dosage subgroups; (C) < 4 weeks and ≥4 weeks of administration subgroups.
The random-effect analysis was used for further analysis as the significant heterogeneity (I2 > 50%). The random-effect analysis revealed that the AST levels between the MT and LI model groups were significantly different. The levels of AST were shown to be reduced by MT (n = 448; 95% CI [-4.74, −2.78]; SMD = |−3.76| > 1; I2 = 87.50%; p < 0.0001) (Figure 4).
Significant heterogeneity (I2 > 50%) was observed, and a random-effects analysis was conducted. The results indicated that the amounts of SOD protein in the MT groups were substantially greater than in the LI model groups (n = 120; 95% CI [2.66,5.33]; SMD = |4.00| > 1; I2 = 75.60%; p < 0.0001) (Figure 5).
FIGURE 5. Forest plot (effect size and 95% CI) summarising the effects of MT on SOD (A1–C1) and MDA (A2–C2) levels in hepatoprotection. (A) Rat and mice subgroups; (B) L, M and H dosage subgroups; (C) < 4 weeks and ≥4 weeks of administration subgroups.
The MDA levels in the mammalian models in the included study varied according to random-effects analyses. MDA levels in the MT groups were considerably lower than in the model groups (n = 130; 95% CI [-3.59, −1.81]; SMD = |-2.70| > 1; I2 = 64.40%; p < 0.0001) (Figure 5).
The random-effects analysis showed that there were differences in serum TG levels between the MT and LI model groups. The MT groups had significantly lower levels of serum TG compared to the LI model group (n = 100; 95% CI [-2.70, −0.67]; SMD = |-1.68| > 1; I2 = 77.00%; p = 0.001) (Supplementary Figure S2).
In accordance with the random-effects analysis, the animal models in the included research had different serum TC levels. The MT groups had considerably lower serum TC levels than the model groups (n = 88; 95% CI [-2.59, −0.78]; SMD = |-1.69| > 1; I2 = 68.60%; p < 0.0001) (Supplementary Figure S2).
A random-effects analysis found notable IL-6 levels discrepancies between the MT and LI model groups. When compared to the LI model groups, IL-6 levels were substantially lower in the MT groups (n = 254; 95% CI [-4.87, −2.67]; SMD = |-3.77| > 1; I2 = 66.80%; p < 0.0001) (Supplementary Figure S3).
Because there was considerable heterogeneity (I2 > 50%), we performed a random-effects analysis for additional research. Regarding the random-effect analysis, the MT and LI model groups showed significantly different levels of TNF-α. TNF-α levels was observed to be reduced by MT (n = 326; 95% CI [-5.50, −1.95]; SMD = |-3.72| > 1; I2 = 94.60%; p < 0.0001) (Supplementary Figure S3).
The animal models in the included studies showed several differences between the MT and LI model groups in CAT levels, according to random-effect analysis. The MT groups had significantly higher levels of CAT than model groups (n = 72; 95% CI [2.23,3.62]; SMD = |2.93| > 1; I2 = 0.00%; p < 0.0001) (Supplementary Figure S3).
In comparison to the LI model groups, the levels of ALT were found to be significantly reduced in the MT groups. MT was effective in both rats (n = 394; 95% CI [-4.96, −2.44]; SMD = |–3.70| > 1; I2 = 92.20%; p < 0.0001) and mice (n = 138; 95% CI [-4.42, −1.67]; SMD = |–3.05| > 1; I2 = 85.90%; p < 0.0001) (Figure 4A1). The ALT levels were found to reduced most by MT in the M-dosage subgroups (n = 112; 95% CI [-8.90,-2.86]; SMD = |–5.88| > 1; I2 = 93.60%; p < 0.0001) than H-dosage (n = 256; 95% CI [-3.85,-0.81]; SMD = |–2.33| > 1; I2 = 92.30%; p = 0.003) and L-dosage subgroups (n = 164; 95% CI [-4.32,-1.77]; SMD = |–3.05| > 1; I2 = 85.80%; p < 0.0001) (Figure 4B1). Furthermore, it worked in both ‘≥4W’ subgroups (n = 96; 95% CI [-1.77, −0.51]; SMD = |–1.14| > 1; I2 = 47.50%; p < 0.0001) and ‘<4W’ subgroups (n = 436; 95% CI [-5.47, −3.29]; SMD = |–4.38| > 1; I2 = 88.00%; p < 0.0001), but the lower levels were in the ‘<4W’ subgroups (Figure 4C1).
Compared with the LI model group, the levels of AST in MT groups were significantly lower. MT reduced substantially the AST levels in both rats’ subgroups (n = 346; 95% CI [-4.75, −2.60]; SMD = |–3.68| > 1; I2 = 85.80%; p < 0.0001) and mice subgroups (n = 102; 95% CI [-6.84, −2.16]; SMD = |–4.50| > 1; I2 = 89.80%; p < 0.0001) (Figure 4A2). MT had the most significant effect in the M-dosage subgroups (n = 96; 95% CI [-9.62,-2.74]; SMD = |–6.18| > 1; I2 = 91.30%; p < 0.0001) than the other two subgroups (H-dosage subgroups: n = 256; 95% CI [-3.52,-1.51]; SMD = |–2.52| > 1; I2 = 80.70%; p < 0.0001) (L-dosage subgroups: n = 96; 95% CI [-6.93,-2.08]; SMD = |-4.51| > 1; I2 = 90.60%; p < 0.0001) (Figure 4B2). The levels of AST were decreased in the both two time-subgroups, but the lower groups were ‘<4W’ subgroups (‘≥4W’ subgroups: n = 80; 95% CI [-2.32,-0.88]; SMD = |-1.60| > 1; I2 = 45.30%; p < 0.0001) (‘<4W’ subgroups: n = 368; 95% CI [-5.89,-3.53]; SMD = |-4.71| > 1; I2 = 83.50%; p < 0.0001) (Figure 4C2).
The amount of SOD was significantly greater in the groups treated with MT than in the LI model groups. The levels of SOD were increased by MT in both rats subgroups (n = 72; 95% CI [3.30,7.51]; SMD = |5.41| > 1; I2 = 73.70%; p < 0.0001) and mice subgroups (n = 48; 95% CI [1.79,3.38]; SMD = |2.58| > 1; I2 = 0.00%; p < 0.0001) (Figure 5A1). The levels of SOD of M-dosage subgroups (n = 36; 95% CI [2.85,10.54]; SMD = |6.70| > 1; I2 = 67.40%; p = 0.001) were the highest by MT than H-dosage subgroups (n = 56; 95% CI [1.57,5.93]; SMD = |3.75| > 1; I2 = 81.80%; p = 0.001) and L-dosage subgroups (n = 28; 95% CI [1.66,3.82]; SMD = |2.74| > 1; I2 = 0.00%; p < 0.0001) (Figure 5B1). Furthermore, it worked in both ‘≥4W’ subgroups (n = 60; 95% CI [2.77,6.70]; SMD = |4.73| > 1; I2 = 71.70%; p < 0.0001) and ‘<4W’ subgroups (n = 60; 95% CI [1.72,4.92]; SMD = |3.32| > 1; I2 = 70.60%; p < 0.0001), but the higher levels were in the ‘<4W’ subgroups (Figure 5C1).
The MDA levels in the MT groups were lower than those in the LI model groups. The MDA levels were decreased by MT in both rats subgroups (n = 72; 95% CI [-3.10, −1.84]; SMD = |-2.47| > 1; I2 = 0.00%; p < 0.0001) and mice subgroups (n = 58; 95% CI [-5.82, −1.23]; SMD = |-3.52| > 1; I2 = 83.60%; p = 0.003) (Figure 5A2). MT reduced the MDA levels most in the M-dosage subgroups (n = 46; 95% CI [-2.55,-0.02]; SMD = |-4.02| > 1; I2 = 81.50%; p = 0.006) among the time subgroups (H-dosage subgroups: n = 56; 95% CI [-3.05,-1.65]; SMD = |–2.35| > 1; I2 = 0.00%; p < 0.0001) (L-dosage subgroups: n = 28; 95% CI [-5.66,0.24]; SMD = |-4.51| > 1; I2 = 85.40%; p = 0.071) (Figure 5B2). Moreover, it substantially lowered MDA levels in both ‘≥4W’ subgroups (n = 60; 95% CI [-3.14, −1.76]; SMD = |-2.45| > 1; I2 = 0.00%; p < 0.0001) and ‘<4W’ subgroups (n = 70; 95% CI [-4.82, −1.42]; SMD = |-3.12| > 1; I2 = 78.20%; p < 0.0001) (Figure 5C2).
The sensitivity of ALT, AST, SOD, and MDA levels in detecting LI in mouse models did not differ significantly. To identify publication bias, we used the |t|-value and conducted Egger’s test. The |t|-values of these four factors did not indicate any publication bias in LI research (Supplementary Figures S4, S5).
ALT and AST levels were examined as significant main indicators of toxic effects of MT on liver in five investigations. All the five investigations showed that MT can significantly increase ALT and AST levels. According to the results, MT may increase hepatotoxicity by influencing ALT and AST levels (Supplementary Table S4). H&E staining of normal animal liver tissues revealed significant hepatotoxicity with inflammatory cell infiltration, cell edema, cytoplasmic loosening and vacuolar degeneration of cytoplasm. Significant pathogenic alterations occurred with the intervention of MT at 10–69.1 mg/kg, but the most toxic dosage was the 30–62.5 mg/kg/d.
Because of the considerable heterogeneity (I2 > 50%), the random-effects analysis was utilized for further investigation. The random-effects analysis revealed that the ALT levels between the MT and control groups were significantly different. The level of ALT was shown to be elevated by MT (n = 105; 95% CI [0.79, 3.02]; SMD = |1.91| > 1; I2 = 81.30%; p < 0.0001) (Figure 6A).
FIGURE 6. Forest plot (effect size and 95% CI) summarising the effects of MT on hepatotoxicity. (A) ALT levels; (B) AST levels.
The random-effect analysis revealed that the AST levels between the MT and control groups were significantly different. The level of AST was shown to be elevated by MT (n = 105; 95% CI [0.75, 3.68]; SMD = |2.21| > 1; I2 = 87.90%; p < 0.0001) (Figure 6B).
To achieve effective treatment for a disease, it is crucial to not only use the appropriate medications but also carefully consider the dosage and duration of drug administration. The three key elements in clinical treatment are identifying the most suitable medication, determining the ideal dosage, and establishing the optimal timing. In this study, we utilized three-dimensional mappings and radar charts to analyze the treatment duration and dosage in each research, aiming to identify the optimal length of treatment and dosage for MT that would yield the most effective results. Figures 7, 8 displayed 3D maps and radar charts corresponding to the four key indications.
FIGURE 7. 3D maps of dose–time–effect relationship. (A) ALT levels; (B) AST levels; (C) SOD levels; (D) MDA levels.
FIGURE 8. Radar charts of dose–time–effect relationship. (A) ALT levels; (B) AST levels; (C) SOD levels; (D) MDA levels.
The ALT and AST levels can be effectively reduced by MT in LI models at a dose of 1.4 mg/kg/d to 100 mg/kg/d, if all other conditions (except the dose of MT) are suitable. However, if the MT dose is less than 1.4 mg/kg/d or greater than 100 mg/kg/d, these effects are not observed. To ascertain the precise MT dosage that is effective, additional research are necessary. Considering the treatment period, 3D maps and radar charts indicate that MT effectively decrease ALT and AST levels at 0.02 W-4 W, but is unsuccessful in reducing these levels over 4.29 W. However, if the treatment period was 0.02W–0.86W at a medium dose (25–50 mg/kg/d), MT reduced ALT and AST levels more effectively than at a low dose (0–25 mg/kg/d) or a high dose (>50 mg/kg/d). Further study needs to be performed to determine the specific effective dose and administration of MT for a treatment duration of more than 4.29 W (Figures 7A,B; Figures 8A,B).
The levels of ALT and AST are increase by MT in normal models at a dosage of 10 mg/kg/d to 69.1 mg/kg/d, if all other conditions (except the dose of MT) are suitable. However, if the MT dose is less than 10 mg/kg/d or greater than 100 mg/kg/d, the toxicity of MT is not observed. Considering the treatment period, 3D maps and radar charts indicate that MT effectively increase ALT and AST levels at 1W-2 W or 8.57W-12.86W, but is unknown at 2W-8.57W. Additional investigation needs to be conducted to study the specific toxic dose and administration of MT in vivo (Figures 7A,B; Figures 8A,B).
According to the 3D maps and radar charts, the SOD levels in the MT groups were higher than those in the LI model groups at a dose of 1.4 mg/kg/d to 100 mg/kg/d. At 1W-4.29W, MT was found to increase the amounts of SOD in the MT groups. MT, in contrast with MDA, can lower MDA levels at a dosage of 0.7 mg/kg/d to 100 mg/kg/d and a duration of 0.14 W–4.29 W (Figures 7C,D; Figures 8C,D).
The hypothesized bilateral impacts of MT on LI are extensive and complicated. The identified signalling transduction pathways, namely, SERCA, SREBP1c/SCAP, Notch/RBP-J/HES1, IκK/NF-κB, Cul3/Rbx1/Keap1/Nrf2, and Bcl-2/ROS/Bax/caspase-9/caspase-3 have been evaluated in Supplementary Tables S5–S6.
To validate the potential mechanisms of action of MT, we utilized molecular docking to assess the binding affinity between MT and key targets. The molecular docking analysis demonstrated the interaction of MT with SERCA and SREBP-SCAP complexes, and the thermodynamic data was analyzed. The estimated free energy of −7.8 kcal/mol suggests that MT interacts with Phe256, Phe834, Ile829, Ile765, Tyr837, Val769, Val263, and Met83 on the SERCA protein. Additionally, with an estimated free energy of −6.8 kcal/mol, MT exhibits significant interactions with Glu605, Leu647, Pro649, Trp690, Ala646, Ala602, Val688, Val603, and Ile645 on the SREBP-SCAP complexes. These interactions between MT and the targets involve beneficial patterns of hydrogen bonds and hydrophobic interactions. The compound–target interactions were visualized using PyMoL 2.6 and Discovery Studio 2019 (Figure 9).
FIGURE 9. Docking patterns of MT and key targets. (A) MT binding to SERCA protein; (B) MT binding to SREBP-SCAP complexes.
According to our meta-analysis, consisting of 24 published studies with 657 rodent models, MT provides information on liver protection and hepatotoxicity. We analyzed a range of indicators, such as TNF-α, IL-6, serum TG, serum TC, SOD, MDA, CAT, ALT, and AST, to establish the biological efficacy and diverse dosages of MT for treating and managing LI. Furthermore, by utilizing molecular docking techniques, we confirmed the interaction of MT with SERCA and SREBP-SCAP complexes, while also summarizing the mechanisms of MT as described in relevant literature. These findings aimed to gain a better understanding of the potential protective and harmful signaling pathways linked to the included indicators of MT on the LI (Figure 10).
FIGURE 10. A graphical representation illustrates multiple molecular processes of matrine protection and toxicity in liver injury by modifying SERCA, SREBP1c/SCAP, Notch/RBP-J/HES1, IK/NF-B, Cul3/Rbx1/Keap1/Nrf2, and Bcl-2//Bax/caspase 9/caspase 3 signalling pathways. Matrine probably plays a significant role in Ca2+ homeostasis regulation within the endoplasmic reticulum, Golgi apparatus, and mitochondria. Matrine could additionally influence the expression of three essential metabolically related genes, including DHCR7, NSDHL, and HMGCS1. Please check the abbreviation list.
The comprehensive meta-analyses indicated that MT can protect from hepatotoxicity in animal models, and this protective effect is associated with variations in TNF-α, IL-6, serum TG, serum TC, MDA, ALT, AST, SOD, and CAT. Several signal transduction pathways are responsible for MT-induced alterations in these important indications of LI.
Sterol regulatory element-binding protein-1c (SREBP1c), a transcription factor which is generated from ER, might have a critical function in the regulation of lipogenesis and be activated by different nutrient states in the liver (Sekiya et al., 2008). SREBPs and SREBP cleavage activating protein (SCAP) interact to form a complex on the endoplasmic reticulum membrane. When cells in mammals lack cholesterol, SREBP-SCAP complexes assemble complexes in coat protein II (COPII) vesicles, facilitating transportation from the ER to the Golgi apparatus. To release the bHLH-Zip domains, SREBPs would be proteolyzed at the Golgi by site-1 and site-2 proteases (S1P and S2P) (Horton et al., 2002; Shimano and Sato, 2017; Lee et al., 2020). The translocated bHLH domain of SREBP1c interacts with the sterol regulatory element (SRE) in the nucleus, regulating the transcription of downstream lipid homeostasis genes such as fatty acid synthase (Fas), acetyl-CoA carboxylase (Acc), and stearoyl-CoA desaturase-1 (Scd1). SREBP1c also enhances the synthesis and accumulation of triacylglycerol (TG) in hepatocytes (Eberle et al., 2004). Previous studies have shown that the mechanistic target of rapamycin complex 1 mediates the nucleocytoplasmic transport of SREBP-1 and SREBP-2 (Peterson et al., 2011). A few findings indicate SREBP1c/2 may interact with NF-κB to modulate inflammation and cholesterol stability (Fowler et al., 2022; Guo et al., 2017). NF-κB enhances SCAP protein expression and promotes the activity of the SCAP-SREBP complex, which causes an inflammatory response and the accumulation of cholesterol (Lee et al., 2020; Li et al., 2013). In an animal model of nonalcoholic fatty liver disease (NAFLD) and nonalcoholic steatohepatitis (NASH) induced by a high-fat diet (HFD) or methionine-choline deficit (MCD), SREBP1c, Fas, Acc, ALT, AST, TNF-α, IL-6, and serum TC/TG levels are elevated. However, MT can reduce the levels of SREBP1c, Fas, Acc, ALT, AST, TNF-α, and IL-6 in hepatocytes of HFD and MCD mice by decreasing SREBP1c expression (Rinella et al., 2008; Gao et al., 2018). Modulation of SREBP1c may be positively correlated with the production of liver damage indicators, and MT may have a potential impact on hepatic injury biomarkers (Yang et al., 2022).
Ca2+ is a second messenger that is required for cellular homeostasis through mTORC1, calmodulin, mitochondrial nitric oxide synthase, citric acid cycle (TCA cycle, Kreb cycle) and electron transport chain (ECT) and associated with ROS generation (Wan et al., 1989; O-Uchi et al., 2014; Jin et al., 2016; Tang et al., 2017; Diaz-Garcia et al., 2021; Stork et al., 2022). Extracellular stress stimuli such as CCL4, hepatic ischemia-reperfusion injury (HIRI), alcohol, and so on would increase Ca2+ transport from the extracellular area to the cytosol and mitochondria during the development of hepatic damage. The activation of Kupffer cells by hepatic I/R injuries is probably produced by the stimulation of store-operated Ca2+ channels (SOC), which increases Ca2+ influx into the cells and exacerbates the I/R-induced Kupffer cell injury (Pan et al., 2012). In rat liver, CCl4 might increase the expression and distribution of acid-sensing ion channel 1 (ASIC-1) (Pan et al., 2012). Consistent consumption of alcohol improves Ca2+-mediated mitochondrial permeability transition pore opening and raises cyclophilin D levels within the liver (King et al., 2010).
A steady Ca2+ concentration gradient across the cell membrane is maintained by eukaryotic cells (∼100 nM within the cytoplasm and ∼1 mM extracellular milieu) (Bagur and Hajnoczky, 2017). The ER and mitochondria play essential roles in the storage, transport, and upkeep of Ca2+ within the cell. Ca2+ dysregulation in ER and mitochondria is associated with LI, including chronic viral hepatitis, alcoholic liver disease, and nonalcoholic fatty liver disease (Li et al., 2007; Mantena et al., 2008; King et al., 2010; Xiao et al., 2017). The connection between ER stress and lipid metabolism is linked to intracellular Ca2+ homeostasis in the liver. Recent accumulating investigations have connected Ca2+ concentration disruption to ER stress, proving to be a significant risk factor during the progression of NAFLD to NASH, leading to increased inositol-requiring enzyme 1α(IRE1α), activating transcription factor 6α (ATF6α), phosphor-plasmic reticulum kinase (p-PERK), the 78 kDa glucose-regulated protein (GRP78), and C/EBP homologous protein (CHOP) expression (Bartlett et al., 2014; Park and Lee, 2014; Rieusset, 2017; Gao et al., 2018). Inactive GRP78, an ER chaperone protein, binds to three transmembrane unfolded protein response (UPR) stress sensors under physiological conditions: IRE1, ATF6α, and PERK. When unfolding proteins assemble within the ER lumen, raising ER stress, GRP78 dissociates from these UPRs to capture the unfolding proteins and activate the UPR stress sensors.
ATF6 is transported from the ER to the Golgi apparatus once it has been separated from GRP78, where it can be cleaved by S1P and S2P. An activated form of ATF6α might migrate to the nucleus and activate downstream target genes related to X-box-binding protein 1 (XBP1) and CHOP. ATF6α signaling pathways may be able to alleviate ER stress. A serine-threonine kinase domain and an endoribonuclease domain have been identified in IRE1. The active IRE1 endonuclease activity could remove the introns of XBP1 mRNA to generate spliced XBP1 (sXBP1) mRNA. The sXBP1 protein functions as a transcription factor, translocating into the nucleus to stimulate the production of ER chaperones and the HSP40 family member P58 IPK gene. Activated PERK might cause dimerization and autophosphorylation of the kinase, allowing it to phosphorylate eukaryotic initiation factor 2 (eIF2). Phosphorylated eIF2 could block new protein translation while minimizing ER stress, hence assisting cell survival via transcription factor 4 (ATF4) activation. ATF4 could cate to the nucleus and stimulates the production of the survival gene and the apoptotic cell death gene CHOP (Harding et al., 2003; Szegezdi et al., 2006).
Ca2+ is transported across the plasma membrane, the endoplasmic reticulum, and the mitochondria through Ca2+ channels. Prior studies have demonstrated that the sarco/endoplasmic reticulum Ca2+-ATPase (SERCA) pump, a member of the P-type ATPase family of ion channels, transports intracellular Ca2+ from the cytosol to the ER and maintains Ca2+ homeostasis between the cytoplasm and ER lumen. Diminished SERCA activity could increase cytosolic Ca2+ level, ER stress, and apoptosis in NALFD, whereas increased SERCA activity would reverse the process (Zhang et al., 2014; Lai et al., 2017). Lai et al. revealed that suppressing protein kinase C delta (PKCδ) could increase SERCA activity, thereby reducing ER stress (Lai et al., 2017). Meanwhile, Gao et al. demonstrated that exposing PA-induced L02 cells to low (200 μM) and medium (400 μM) doses of MT enhanced SERCA activity and facilitated Ca2+ influx from the cytosol into the ER lumen (Gao et al., 2018). These findings suggest that changes in SERCA function may contribute to the development of LI and provide a potential therapeutic target for various hepatic disorders.
Previous research found that inhibiting Notch signaling using RBP-J deletion or a Notch inhibitor worsened hepatic I/R damage, demonstrated by impaired liver function and increased hepatocyte apoptosis (Yu H. C. et al., 2011; Yue et al., 2018). The intracellular transmembrane domain (NICD) of the Notch receptor is released through the catalytic action of an integral membrane protein γ-secretase complex. NICD might enter the nucleus and bind the DNA-binding protein RBP-J, allowing Notch target genes such as HES1 to be transcribed. Earlier research proposed that MT may diminish HES1 mRNA levels by downregulating RBP-Jκ mRNA expression to safeguard liver function and regeneration (Yang et al., 2013). The current study reveals that MT may promote hepatic progenitor cell development by obstructing the Notch/Jagged1/HES1 signaling pathway in vivo (Yang et al., 2016). By reducing SERCA activity and increasing ER stress in T-ALL cells, unmatured Notch signaling transduction pathways would be activated, contributing to apoptosis (De Ford et al., 2016). We hypothesize that MT can suppress the Notch/RBP-J/HES1 signaling cascade by promoting SERCA activity and minimize apoptosis in LI; however, more investigations are required.
The nuclear factor kappa B (NF-κB) is a recognized transcription factor in pro-inflammatory pathways. NF-κB could potentially have an impact on controlling the processes of cell proliferation, differentiation, and cell death (Gerondakis et al., 2006; Khandelwal et al., 2011; Mitchell et al., 2016). TNF-α and IL-1, pro-inflammatory cytokines, may mediate NF-κB signaling transduction pathways and encourage downstream target gene expression. NF-κB activation will trigger the transcription and translation of COX-2, IL-6, MCP-1, TNF-α, and IL-1. The IκK complex, which is composed of α and β subunits, is necessary for NF-κB pathway activation via phosphorylation and ubiquitination. Previous literature has indicated that the activity of IκK and NF-κB is linked to various chronic liver injuries, including steatohepatitis, hepatocellular carcinoma, alcoholic liver disease, NAFLD, viral hepatitis, and biliary liver disease. Present results demonstrated that IκKα would interact with NICD directly to maintain the nuclear factor-kappa B (NF-κB) activity in the T-ALL cells model (Vacca et al., 2006), and we proposed that MT therapeutic effect on Ca2+ ion channel SERCA might regulate the process of inflammation and apoptosis in the LI through NICD/NF-κB interaction.
Under normal state, nuclear factor erythroid 2-related factor 2 (Nrf2), as a protective molecule, could have a crucial function in preventing oxidation in the liver. In a physiological condition, Nrf2 might attach to the kelch-like ECH-associated protein 1 (Keap1), which is an adaptor to the E3 ubiquitin ligase complex Cullin3 (Cul3)/ring box protein 1 (Rbx1), and subsequently be ubiquitinated and suppressed by Cul3 in the cytoplasm. Recently research showed oxidative stress, the main pathologic feature of most liver diseases, could modify Keap1 and inhibit Nrf2 ubiquitination (Kobayashi et al., 2006; Saito et al., 2016). Nrf2 accumulation in the cytoplasm would translocate into the nucleus and bind to antioxidant-responsive elements (ARE) to transcript anti-oxidative and anti-inflammation genes expression involving superoxide dismutase (SOD), glutathione-S transferase (GST), glutathione peroxidase (GPx), catalase (CAT), heme oxygenase-1 (HO-1), quinone oxidoreductase-1 (NQO-1), and cyclooxygenase-2 (COX-2) (Prestera et al., 1995; Raghunath et al., 2018; Bardallo et al., 2022). Additionally, active Nrf2 may inhibit NF-κBp65 phosphorylation and reduce NF-κBp65 translocation to the nucleus in animal models to minimize inflammation and apoptosis. The PERK may collaborate with Nrf2 to improve cell survival after exposure to ER stress (Cullinan et al., 2003; Dai et al., 2018). We identified that MT could enhance the protective impact against hepatic damage via Nrf2 moving to the nucleus and activate downstream transcription of genes including CAT, SOD, and HO-1 in HFD-induced liver injury mouse models (Zhang et al., 2013).
As with the ER, mitochondria can potentially play an essential role in regulating Ca2+ homeostasis under physiological conditions. Specifically, Ca2+ could be transported to the outer membrane of mitochondria (OMM) through the voltage-dependent anion channels (VDACs) in the hepatocytes, and VDCAs would be regulated by a series of proteins, including inositol 1,4,5-trisphosphate receptors (IP3Rs), ryanodine receptor (RyR), glucose-regulated protein 75 (GRP75), and sigma-1 receptor (S1R), to transfer Ca2+ into the intermembranous space (IMS). Elevated Ca2+ levels in the IMS could lead the mitochondrial Ca2+ uniporter (MCU) on the inner mitochondrial membrane (IMM) to interact with the mitochondrial Ca2+ uptake 1/2 (MICU1/2) and promote Ca2+ influx to the matrix (Hirata et al., 2002; Csordas et al., 2013; Williams et al., 2015; Shoshan-Barmatz et al., 2018). Furthermore, previous research has suggested that matrix Ca2+ can influence the cycle of TCA and the process of oxidative phosphorylation for ATP production. Interestingly, the required ROS might be created simultaneously in aerobic metabolism to maintain microdomain cell signaling (Bertero and Maack, 2018).
Gao et al. observed that excessive amounts of MT (800 μM) elevated SREBP1c, Fas, and Acc expression in PA-induced L02 cells. In comparison to low and medium dosages of MT, overdosage treatment results in the opposite effect. High-MT treatment causes toxicity and ultimately loss of protective capacity in the PA-induced L02 cell line (Gao et al., 2018). Furthermore, at low and medium levels, MT might have a therapeutic function of active SERCA to increase Ca2+ ion influx to the ER in response to stress, but excessive MT would have a negative influence on this reaction. MT given in high doses inhibits SERCA activity, limiting Ca2+ transport from the cytosol to the ER lumen and increasing ER stress. Nonetheless, increased cytosolic Ca2+ may be transported across the mitochondria via VDACs, leading to a surge in Ca2+ accumulation within the IMS (Rapizzi et al., 2002; Shoshan-Barmatz et al., 2018). Ca2+ accumulation in the IMS stimulates the MCU to transport Ca2+ into the matrix. This process accelerates the metabolic rate of the TCA cycle and oxidative phosphorylation, resulting in an increase in mitochondrial ROS and apoptosis (Traaseth et al., 2004; Mallilankaraman et al., 2012; Csordas et al., 2013).
Overdosage of MT induces hepatotoxicity in animal models, and high-level MT may promote hepatocytes to produce higher ROS, increased HO-1, and the pro-apoptotic protein BAX while inhibiting the anti-apoptotic protein Bcl-2 synthesis. According to current research, mitochondrial ROS would activate NF-κB, improve the production of inflammatory cytokines, and prevent Nrf2 degradation, hence increasing HO-1 expression (Wang et al., 2015; Lingappan, 2018; Kasai et al., 2020; Liu et al., 2020; Li et al., 2021). The present literature has demonstrated that raising cytosolic Ca2+ concentration could also activate the NF-κB through elevating Ca2+/Calmodulin-Dependent Protein Kinase II (CaMKII) activity to phosphorylate and degrade IκK in the neurons (Snow and Albensi, 2016). Additionally, Rao et al. have discovered that MT-induced hepatotoxicity in the mice model suppresses three genes connected to steroid synthesis and metabolic processes in LI. These genes include 7-dehydrocholesterol reductase (DHCR7), NAD-(P)-dependent steroid dehydrogenase-like (NSDHL), and 3-hydroxy-3-methylglutaryl-coenzyme A synthase 1 (HMGCS1). However, the detailed mechanism is still to be further researched in the future (Rao et al., 2022).
The distinctive and dose-dependent effects of MT have been utilized to investigate various mechanisms of liver protection and hepatotoxicity. The suggested dosage, according to our data, is between 30–62. 5 mg/kg/d, which can be harmful to rodent animal models. When given at a dosage of 20 mg/kg/d from 0.02W to 0.86W, MT demonstrated significant liver protection with no hepatotoxicity. Finally, our findings show that a dosage of 20–30 mg/kg/d of 0.02–0.86 W has a considerable liver-protective effect with low hepatotoxicity. A dosage of more than 30–62.5 mg/kg/d of MT therapy, on the other hand, caused liver damage in animal models. Through the activation of SERCA, SREBP-SCAP complexes, and MT, the pharmacological actions of MT can produce both liver protection and damage. These complexes are responsible for linking the IRE1, ATF6, and PERK proteins, all of which play important roles in regulating ER stress. The interaction of MT with SERCA and SREBP-SCAP complexes was demonstrated utilizing molecular docking, and the thermodynamic data was analyzed. With an estimated free energy of −7.8 kcal/mol, the molecule MT interacts with Phe256, Phe834, Ile829, Ile765, Tyr837, Val769, Val263, and Met83 on the SERCA protein. With an estimated free energy of −6.8 kcal/mol, MT interacts substantially with the Glu605, Leu647, Pro649, Trp690, Ala646, Ala602, Val688, Val603, and Ile645 on the SREBP-SCAP complexes. SERCA and SREBP-SCAP complexes exhibit beneficial patterns of hydrogen bond and hydrophobic interactions.
This meta-analysis adhered to the PRISMA standards (http://prisma-statement.org/), despite several limitations. 1) As only four English and four Chinese databases were utilized for article inclusion, selective bias was inevitable. In addition, we have not been able to compile all the relevant literature. 2) The heterogeneity of various studies could not be unified because of instrument index measurement error, different units of indicators, different experimental methods, etc. 3) Even though articles with quality scores of less than 5 points were disregarded, there may be heterogeneity in the results due to differences in the quality of the included articles. 4) The absence of a standardized method for animal intervention, drug dosage, treatment regimens, and model species across studies was another factor that might have caused the high heterogeneity. The reliability of MT’s results in treating LI or causing hepatotoxicity was confirmed by the sensitivity analysis, Egger’s test, and subgroup analysis. 5) Although the primary pharmacological mechanisms of MT in terms of liver protection and hepatotoxicity have been summarized, not all mechanisms could be summarized due to the complex pathogenic mechanisms involved. 6) The reliability of MT for hepatotoxicity may be lower than for hepatoprotection because only 5 articles on this condition were included. Future research should be conducted to investigate the hepatotoxicity of MT. 7) For ethical reasons, there is a paucity of literature on the toxicological effects of MT in the human body. Therefore, we only included studies using animal models. It is important to conduct relevant clinical trials to confirm the efficacy and reliability of MT in the clinical management of hepatoprotection and hepatotoxicity. 8) We validated the binding of MT to key proteins using molecular docking, but experiments were still needed to prove it.
Although this meta-analysis has several limitations, the findings may provide new strategies for clinical medication and drug development.
In summary, our study revealed that within the dose range of 10–69.1 mg/kg and time range of 1–2 weeks, MT could have a bilateral impact on liver damage. However, at a dose of 20–30 mg/kg/d for 0.02–0.86 weeks, it demonstrated high protection and low toxicity on the liver. Molecular docking analysis indicated that MT interacts with SERCA and SREBP-SCAP complexes. These interactions involve beneficial patterns of hydrogen bonds and hydrophobic interactions. By activating SERCA, a Ca2+ ion channel on the ER, MT could play a crucial role in regulating Ca2+ homeostasis in damaged hepatocytes. This helps maintain the balance among the cytoplasm, ER, Golgi apparatus, and mitochondria. Our findings suggest that MT doses ranging from 1.4 mg/kg/d to 100 mg/kg/d may have a preventive and therapeutic effect on LI by modulating the expression of biomarkers such as TNF-α, IL-6, serum TG, serum TC, SOD, MDA, CAT, ALT, and AST. Additionally, signaling pathways such as SREBP1c/SCAP, Notch/RBP-J/HES1, IκK/NF-κB, and Cul3/Rbx1/Keap1/Nrf2 are likely involved in the protective process. It is interesting to note that many of these signaling pathways directly or indirectly interact with Ca2+ homeostasis. However, in normal hepatocytes, a high dosage of MT can suppress SERCA activity, leading to an adverse impact on Ca2+ homeostasis. This, in turn, can cause hepatotoxicity and promote apoptosis through the reduction of Bcl-2 and activation of the Ros/Bax/caspase 9/caspase 3 pathway. Elevated MT levels can also modulate the expression of various metabolic indicators, including AST, ALT, DHCR7, NSDHL, and HMGCS1. Further investigation is required to fully understand how MT influences the expression of these genes.
The original contributions presented in the study are included in the article/Supplementary Material, further inquiries can be directed to the corresponding authors.
WF: Conceptualization, Data curation, Methodology, Visualization, Writing–original draft. T-CK: Conceptualization, Data curation, Formal Analysis, Methodology, Visualization, Writing–original draft. JJ: Conceptualization, Data curation, Formal Analysis, Methodology, Visualization, Writing–original draft. XiZ: Data curation, Visualization, Writing–original draft. SC: Data curation, Visualization, Writing–original draft. JZ: Conceptualization, Writing–review and editing. YC: Conceptualization, Writing–review and editing. XM: Conceptualization, Writing–review and editing.
The author(s) declare financial support was received for the research, authorship, and/or publication of this article. This work was supported by Sichuan Science and Technology Program (2023NSFSC0687), Xinglin Scholar Research Promotion Project of Chengdu University of TCM (grant nos. QJRC2022028 and QJJJ2022010), Major scientific research problems and key topics of medical technology problems of China Medical Education Association (2022KTZ016) and “The Hundred Talents Program” of the Hospital of the Chengdu University of Traditional Chinese Medicine (grant no. 22- B09).
This paper has been greatly improved by the advice of the reviewers and the authors of all references. The authors wish to thank the reviewers and the authors of all references.
The authors declare that the research was conducted in the absence of any commercial or financial relationships that could be construed as a potential conflict of interest.
All claims expressed in this article are solely those of the authors and do not necessarily represent those of their affiliated organizations, or those of the publisher, the editors and the reviewers. Any product that may be evaluated in this article, or claim that may be made by its manufacturer, is not guaranteed or endorsed by the publisher.
The Supplementary Material for this article can be found online at: https://www.frontiersin.org/articles/10.3389/fphar.2024.1315584/full#supplementary-material
Bagur, R., and Hajnoczky, G. (2017). Intracellular Ca(2+) sensing: its role in calcium homeostasis and signaling. Mol. Cell 66 (6), 780–788. doi:10.1016/j.molcel.2017.05.028
Bai, N., Wang, D., Ouyang, X., Zhou, L., and Wang, Z. (2018). Inhibitory effect of matrine against hepatic ischemia-reperfusion injury in rats and its mechanis. Chin. J. General Surg. 27, 81–86. doi:10.3978/j.issn.1005-6947.2018.01.013
Bardallo, R. G., Panisello-Rosello, A., Sanchez-Nuno, S., Alva, N., Rosello-Catafau, J., and Carbonell, T. (2022). Nrf2 and oxidative stress in liver ischemia/reperfusion injury. FEBS J. 289 (18), 5463–5479. doi:10.1111/febs.16336
Bartlett, P. J., Gaspers, L. D., Pierobon, N., and Thomas, A. P. (2014). Calcium-dependent regulation of glucose homeostasis in the liver. Cell Calcium 55 (6), 306–316. doi:10.1016/j.ceca.2014.02.007
Bertero, E., and Maack, C. (2018). Calcium signaling and reactive oxygen species in mitochondria. Circ. Res. 122 (10), 1460–1478. doi:10.1161/CIRCRESAHA.118.310082
Chang, L., Wang, S., Dou, L., Jia, M., Wang, T., and Xu, B. (2021). Protective mechanism of matrine against liver injury induced by acetaminophen in mice. China J. Mod. Med. 31, 58–63. doi:10.3969/j.issn.1005-8982.2021.19.011
Chemaly, E. R., Troncone, L., and Lebeche, D. (2018). SERCA control of cell death and survival. Cell Calcium 69, 46–61. doi:10.1016/j.ceca.2017.07.001
Chinese Pharmacopoeia Commission (2020). The Pharmacopoeia of the people’s Republic of China, 2020. ed. Part I. Beijing, China: China Medical Science Press, 211.
Chu, Y., Jing, Y., Zhao, X., Wang, M., Zhang, M., Ma, R., et al. (2021). Modulation of the HMGB1/TLR4/NF-κB signaling pathway in the CNS by matrine in experimental autoimmune encephalomyelitis. J. Neuroimmunol. 352, 577480. doi:10.1016/j.jneuroim.2021.577480
Csordas, G., Golenar, T., Seifert, E. L., Kamer, K. J., Sancak, Y., Perocchi, F., et al. (2013). MICU1 controls both the threshold and cooperative activation of the mitochondrial Ca²⁺ uniporter. Cell Metab. 17 (6), 976–987. doi:10.1016/j.cmet.2013.04.020
Cullinan, S. B., Zhang, D., Hannink, M., Arvisais, E., Kaufman, R. J., and Diehl, J. A. (2003). Nrf2 is a direct PERK substrate and effector of PERK-dependent cell survival. Mol. Cell Biol. 23 (20), 7198–7209. doi:10.1128/MCB.23.20.7198-7209.2003
Dai, Y., Zhang, H., Zhang, J., and Yan, M. (2018). Isoquercetin attenuates oxidative stress and neuronal apoptosis after ischemia/reperfusion injury via Nrf2-mediated inhibition of the NOX4/ROS/NF-κB pathway. Chem. Biol. Interact. 284, 32–40. doi:10.1016/j.cbi.2018.02.017
De Ford, C., Heidersdorf, B., Haun, F., Murillo, R., Friedrich, T., Borner, C., et al. (2016). The clerodane diterpene casearin J induces apoptosis of T-ALL cells through SERCA inhibition, oxidative stress, and interference with Notch1 signaling. Cell Death Dis. 7 (1), e2070. doi:10.1038/cddis.2015.413
Devarbhavi, H., Asrani, S. K., Arab, J. P., Nartey, Y. A., Pose, E., and Kamath, P. S. (2023). Global burden of liver disease: 2023 update. J. Hepatol. 79 (2), 516–537. doi:10.1016/j.jhep.2023.03.017
Diaz-Garcia, C. M., Meyer, D. J., Nathwani, N., Rahman, M., Martinez-Francois, J. R., and Yellen, G. (2021). The distinct roles of calcium in rapid control of neuronal glycolysis and the tricarboxylic acid cycle. Elife 10, e64821. doi:10.7554/eLife.64821
Du, M., Xu, B., Xiang, R., Guo, D., Fan, Y., Shi, X., et al. (2021). The protective effect of Matrine injection on acute alcoholic liver injury in mice. China J. Mod. Med. 31, 13–18. doi:10.3969/j.issn.1005-8982.2021.24.003
Eberle, D., Hegarty, B., Bossard, P., Ferre, P., and Foufelle, F. (2004). SREBP transcription factors: master regulators of lipid homeostasis. Biochimie 86 (11), 839–848. doi:10.1016/j.biochi.2004.09.018
Fowler, J. W. M., Zhang, R., Tao, B., Boutagy, N. E., and Sessa, W. C. (2022). Inflammatory stress signaling via NF-kB alters accessible cholesterol to upregulate SREBP2 transcriptional activity in endothelial cells. Elife 86 (11). doi:10.7554/eLife.79529
Gao, X., Guo, S., Zhang, S., Liu, A., Shi, L., and Zhang, Y. (2018). Matrine attenuates endoplasmic reticulum stress and mitochondrion dysfunction in nonalcoholic fatty liver disease by regulating SERCA pathway. J. Transl. Med. 16 (1), 319. doi:10.1186/s12967-018-1685-2
Gao, X., Guo, S., Zhang, S., Liu, A., Shi, L., and Zhang, Y. (2019). Correction to: matrine attenuates endoplasmic reticulum stress and mitochondrion dysfunction in nonalcoholic fatty liver disease by regulating SERCA pathway. J. Transl. Med. 17 (1), 277. doi:10.1186/s12967-019-2020-2
Gao, Y., Zheng, P., Yan, L., and Dai, G. (2013). Preliminary mechanism study of matrine on chronic alcohol-induced hepatic injury in rats. Chin. Pharmacol. Bull. 29, 1012–1016. doi:10.3969/j.issn.1001-1978.2013.07.028
Gerondakis, S., Grumont, R., Gugasyan, R., Wong, L., Isomura, I., Ho, W., et al. (2006). Unravelling the complexities of the NF-kappaB signalling pathway using mouse knockout and transgenic models. Oncogene 25 (51), 6781–6799. doi:10.1038/sj.onc.1209944
Gu, Y., Lu, J., Sun, W., Jin, R., Ohira, T., Zhang, Z., et al. (2019). Oxymatrine and its metabolite matrine contribute to the hepatotoxicity induced by radix Sophorae tonkinensis in mice. Exp. Ther. Med. 17 (4), 2519–2528. doi:10.3892/etm.2019.7237
Guo, S., Zhang, S., Wei, H., Shi, L., Hu, N., Dang, X., et al. (2017). Protective effect and mechanism of matrine combined with glycyrrhizic acid in the treatment of chronic liver injury induced by carbon tetrachloride. China Pharm. 20, 1153–1158.
Harding, H. P., Zhang, Y., Zeng, H., Novoa, I., Lu, P. D., Calfon, M., et al. (2003). An integrated stress response regulates amino acid metabolism and resistance to oxidative stress. Mol. Cell 11 (3), 619–633. doi:10.1016/s1097-2765(03)00105-9
He, X., Fang, J., Huang, L., Wang, J., and Huang, X. (2015). Sophora flavescens Ait.: traditional usage, phytochemistry and pharmacology of an important traditional Chinese medicine. J. Ethnopharmacol. 172, 10–29. doi:10.1016/j.jep.2015.06.010
Hirata, K., Pusl, T., O'Neill, A. F., Dranoff, J. A., and Nathanson, M. H. (2002). The type II inositol 1,4,5-trisphosphate receptor can trigger Ca2+ waves in rat hepatocytes. Gastroenterology 122 (4), 1088–1100. doi:10.1053/gast.2002.32363
Horton, J. D., Goldstein, J. L., and Brown, M. S. (2002). SREBPs: activators of the complete program of cholesterol and fatty acid synthesis in the liver. J. Clin. Investigation 109 (9), 1125–1131. doi:10.1172/JCI15593
Jin, Y., Bai, Y., Ni, H., Qiang, L., Ye, L., Shan, Y., et al. (2016). Activation of autophagy through calcium-dependent AMPK/mTOR and PKCθ pathway causes activation of rat hepatic stellate cells under hypoxic stress. FEBS Lett. 590 (5), 672–682. doi:10.1002/1873-3468.12090
Jing, Y., Ma, R., Chu, Y., Dou, M., Wang, M., Li, X., et al. (2021). Matrine treatment induced an A2 astrocyte phenotype and protected the blood-brain barrier in CNS autoimmunity. J. Chem. Neuroanat. 117, 102004. doi:10.1016/j.jchemneu.2021.102004
Ju, J., Li, J., Lin, Q., and Xu, H. (2018). Efficacy and safety of berberine for dyslipidaemias: a systematic review and meta-analysis of randomized clinical trials. Phytomedicine 50, 25–34. doi:10.1016/j.phymed.2018.09.212
Kasai, S., Shimizu, S., Tatara, Y., Mimura, J., and Itoh, K. (2020). Regulation of Nrf2 by mitochondrial reactive oxygen species in physiology and pathology. Biomolecules 10 (2), 320. doi:10.3390/biom10020320
Kaufman, R. J., and Malhotra, J. D. (2014). Calcium trafficking integrates endoplasmic reticulum function with mitochondrial bioenergetics. Biochim. Biophys. Acta 1843 (10), 2233–2239. doi:10.1016/j.bbamcr.2014.03.022
Khan, A., Shal, B., Naveed, M., Shah, F. A., Atiq, A., Khan, N. U., et al. (2019). Matrine ameliorates anxiety and depression-like behaviour by targeting hyperammonemia-induced neuroinflammation and oxidative stress in CCl4 model of liver injury. Neurotoxicology 72, 38–50. doi:10.1016/j.neuro.2019.02.002
Khandelwal, N., Simpson, J., Taylor, G., Rafique, S., Whitehouse, A., Hiscox, J., et al. (2011). Nucleolar NF-κB/RelA mediates apoptosis by causing cytoplasmic relocalization of nucleophosmin. Cell Death Differ. 18 (12), 1889–1903. doi:10.1038/cdd.2011.79
King, A. L., Swain, T. M., Dickinson, D. A., Lesort, M. J., and Bailey, S. M. (2010). Chronic ethanol consumption enhances sensitivity to Ca(2+)-mediated opening of the mitochondrial permeability transition pore and increases cyclophilin D in liver. Am. J. Physiol. Gastrointest. Liver Physiol. 299 (4), G954–G966. doi:10.1152/ajpgi.00246.2010
Knight, J. A. (2005). Liver function tests: their role in the diagnosis of hepatobiliary diseases. J. Infus. Nurs. 28 (2), 108–117. doi:10.1097/00129804-200503000-00004
Kobayashi, A., Kang, M. I., Watai, Y., Tong, K. I., Shibata, T., Uchida, K., et al. (2006). Oxidative and electrophilic stresses activate Nrf2 through inhibition of ubiquitination activity of Keap1. Mol. Cell Biol. 26 (1), 221–229. doi:10.1128/MCB.26.1.221-229.2006
Lai, S., Li, Y., Kuang, Y., Cui, H., Yang, Y., Sun, W., et al. (2017). PKCδ silencing alleviates saturated fatty acid induced ER stress by enhancing SERCA activity. Biosci. Rep. 37 (6). doi:10.1042/BSR20170869
Lee, S. H., Lee, J. H., and Im, S. S. (2020). The cellular function of SCAP in metabolic signaling. Exp. Mol. Med. 52 (5), 724–729. doi:10.1038/s12276-020-0430-0
Li, L. C., Varghese, Z., Moorhead, J. F., Lee, C. T., Chen, J. B., and Ruan, X. Z. (2013). Cross-talk between TLR4-MyD88-NF-κB and SCAP-SREBP2 pathways mediates macrophage foam cell formation. Am J. Physiol. Heart Circ. Physiol. 304 (6), H874–H884. doi:10.1152/ajpheart.00096.2012
Li, C., Liu, L., Mo, C., and Huang, L. (2005). Effects of Matrine on release of interferon and pathological changes in concanavalin A-induced liver injury in mice. World Chin. J. Dig. 13, 640–643.
Li, D., Hong, X., Zhao, F., Ci, X., and Zhang, S. (2021). Targeting Nrf2 may reverse the drug resistance in ovarian cancer. Cancer Cell Int. 21 (1), 116. doi:10.1186/s12935-021-01822-1
Li, X., Cheng, G., and Zhou, F. (2016). The effects of matrine on blood lipids and antioxidant capacity in rats with chronic alcoholic liver injury. Chin. J. Geriatrics 36, 1838–1839. doi:10.3969/j.issn.1005-9202.2016.08.021
Li, Y., Boehning, D. F., Qian, T., Popov, V. L., and Weinman, S. A. (2007). Hepatitis C virus core protein increases mitochondrial ROS production by stimulation of Ca2+ uniporter activity. FASEB J. 21 (10), 2474–2485. doi:10.1096/fj.06-7345com
Liang, P., Yuan, T., Gu, L., and Lu, H. (2015). Study of hepatotoxicity and neural behavioral changes of Sophora flavescens and matrine in mice. Chin. J. Mod. Appl. Pharm. 32, 1444–1448. doi:10.13748/j.cnki.issn1007-7693.2015.12.008
Lingappan, K. (2018). NF-κB in oxidative stress. Curr. Opin. Toxicol. 7, 81–86. doi:10.1016/j.cotox.2017.11.002
Liu, H., Qiu, Y., Mao, L., Zhu, X., and Ding, Y. (2008). Protective effect of matrine against ischemia and reperfusion injury in rat partial liver transplantation. World Chin. J. Dig. 16, 1617–1621. doi:10.11569/wcjd.v16.i15.1617
Liu, J., Zhao, Y., Xia, J., and Qiu, M. (2020). Matrine induces toxicity in mouse liver cells through an ROS-dependent mechanism. Res. Vet. Sci. 132, 308–311. doi:10.1016/j.rvsc.2020.07.006
Liu, M., Pu, Y., Gu, J., He, Q., Liu, Y., Zeng, Y., et al. (2021). Evaluation of Zhilong Huoxue Tongyu capsule in the treatment of acute cerebral infarction: a systematic review and meta-analysis of randomized controlled trials. Phytomedicine 86, 153566. doi:10.1016/j.phymed.2021.153566
Liu, Q., and Zhang, X. S. (2021). Analysis of clinical application literature on matrine injection. Chin. Jof Clin. Ration. Drug Use 14, 12A. doi:10.15887/j.cnki.13-1389/r.2021.34.072
Luo, X., Ni, X., Lin, J., Zhang, Y., Wu, L., Huang, D., et al. (2021). The add-on effect of Chinese herbal medicine on COVID-19: a systematic review and meta-analysis. Phytomedicine 85, 153282. doi:10.1016/j.phymed.2020.153282
Macleod, M. R., O'Collins, T., Howells, D. W., and Donnan, G. A. (2004). Pooling of animal experimental data reveals influence of study design and publication bias. Stroke 35 (5), 1203–1208. doi:10.1161/01.Str.0000125719.25853.20
Mallilankaraman, K., Doonan, P., Cardenas, C., Chandramoorthy, H. C., Muller, M., Miller, R., et al. (2012). MICU1 is an essential gatekeeper for MCU-mediated mitochondrial Ca(2+) uptake that regulates cell survival. Cell 151 (3), 630–644. doi:10.1016/j.cell.2012.10.011
Mantena, S. K., King, A. L., Andringa, K. K., Eccleston, H. B., and Bailey, S. M. (2008). Mitochondrial dysfunction and oxidative stress in the pathogenesis of alcohol- and obesity-induced fatty liver diseases. Free Radic. Biol. Med. 44 (7), 1259–1272. doi:10.1016/j.freeradbiomed.2007.12.029
Mitchell, S., Vargas, J., and Hoffmann, A. (2016). Signaling via the NFκB system. Wiley Interdiscip. Rev. Syst. Biol. Med. 8 (3), 227–241. doi:10.1002/wsbm.1331
O-Uchi, J., Ryu, S. Y., Jhun, B. S., Hurst, S., and Sheu, S. S. (2014). Mitochondrial ion channels/transporters as sensors and regulators of cellular redox signaling. Antioxid. Redox Signal 21 (6), 987–1006. doi:10.1089/ars.2013.5681
Pan, L. J., Zhang, Z. C., Zhang, Z. Y., Wang, W. J., Xu, Y., and Zhang, Z. M. (2012). Effects and mechanisms of store-operated calcium channel blockade on hepatic ischemia-reperfusion injury in rats. World J. Gastroenterol. 18 (4), 356–367. doi:10.3748/wjg.v18.i4.356
Park, H. W., and Lee, J. H. (2014). Calcium channel blockers as potential therapeutics for obesity-associated autophagy defects and fatty liver pathologies. Autophagy 10 (12), 2385–2386. doi:10.4161/15548627.2014.984268
Peng, W., Xu, Y., Han, D., Feng, F., Wang, Z., Gu, C., et al. (2020). Potential mechanism underlying the effect of matrine on COVID-19 patients revealed through network pharmacological approaches and molecular docking analysis. Arch. Physiol. Biochem. 129, 253–260. doi:10.1080/13813455.2020.1817944
Periasamy, M., and Kalyanasundaram, A. (2007). SERCA pump isoforms: their role in calcium transport and disease. Muscle Nerve 35 (4), 430–442. doi:10.1002/mus.20745
Peterson, T. R., Sengupta, S. S., Harris, T. E., Carmack, A. E., Kang, S. A., Balderas, E., et al. (2011). mTOR complex 1 regulates lipin 1 localization to control the SREBP pathway. Cell 146 (3), 408–420. doi:10.1016/j.cell.2011.06.034
Prestera, T., Talalay, P., Alam, J., Ahn, Y. I., Lee, P. J., and Choi, A. M. (1995). Parallel induction of heme oxygenase-1 and chemoprotective phase 2 enzymes by electrophiles and antioxidants: regulation by upstream antioxidant-responsive elements (ARE). Mol. Med. 1, 827–837. doi:10.1007/bf03401897
Raghunath, A., Sundarraj, K., Nagarajan, R., Arfuso, F., Bian, J., Kumar, A. P., et al. (2018). Antioxidant response elements: Discovery, classes, regulation and potential applications. Redox Biol. 17, 297–314. doi:10.1016/j.redox.2018.05.002
Rao, S. W., Duan, Y. Y., Zhao, D. S., Liu, C. J., Xu, S. H., Liang, D., et al. (2022). Integrative analysis of transcriptomic and metabolomic data for identification of pathways related to matrine-induced hepatotoxicity. Chem. Res. Toxicol. 35 (12), 2271–2284. doi:10.1021/acs.chemrestox.2c00264
Rapizzi, E., Pinton, P., Szabadkai, G., Wieckowski, M. R., Vandecasteele, G., Baird, G., et al. (2002). Recombinant expression of the voltage-dependent anion channel enhances the transfer of Ca2+ microdomains to mitochondria. J. Cell Biol. 159 (4), 613–624. doi:10.1083/jcb.200205091
Rieusset, J. (2017). Endoplasmic reticulum-mitochondria calcium signaling in hepatic metabolic diseases. Biochim. Biophys. Acta Mol. Cell Res. 1864 (6), 865–876. doi:10.1016/j.bbamcr.2017.01.001
Rinella, M. E., Elias, M. S., Smolak, R. R., Fu, T., Borensztajn, J., and Green, R. M. (2008). Mechanisms of hepatic steatosis in mice fed a lipogenic methionine choline-deficient diet. J. Lipid Res. 49 (5), 1068–1076. doi:10.1194/jlr.M800042-JLR200
Saito, R., Suzuki, T., Hiramoto, K., Asami, S., Naganuma, E., Suda, H., et al. (2016). Characterizations of three major cysteine sensors of Keap1 in stress response. Mol. Cell Biol. 36 (2), 271–284. doi:10.1128/MCB.00868-15
Sekiya, M., Hiraishi, A., Touyama, M., and Sakamoto, K. (2008). Oxidative stress induced lipid accumulation via SREBP1c activation in HepG2 cells. Biochem. Biophys. Res. Commun. 375 (4), 602–607. doi:10.1016/j.bbrc.2008.08.068
Shi, D., Zhang, J., Qiu, L., Li, J., Hu, Z., and Zhang, J. (2013). Matrine inhibits infiltration of the inflammatory gr1(hi) monocyte subset in injured mouse liver through inhibition of monocyte chemoattractant protein-1. Evid. Based Complement. Altern. Med. 2013, 580673. doi:10.1155/2013/580673
Shimano, H., and Sato, R. (2017). SREBP-regulated lipid metabolism: convergent physiology - divergent pathophysiology. Nat. Rev. Endocrinol. 13 (12), 710–730. doi:10.1038/nrendo.2017.91
Shoshan-Barmatz, V., Krelin, Y., and Shteinfer-Kuzmine, A. (2018). VDAC1 functions in Ca(2+) homeostasis and cell life and death in health and disease. Cell Calcium 69, 81–100. doi:10.1016/j.ceca.2017.06.007
Snow, W. M., and Albensi, B. C. (2016). Neuronal gene targets of NF-κB and their dysregulation in alzheimer's disease. Front. Mol. Neurosci. 9, 118. doi:10.3389/fnmol.2016.00118
Song, B., Han, C., and Zhang, H. (2009). Toxicity of three Sophora flavescens ait alkaloids to mice. Acta Bot. Boreali-Occidentalia Sin. 29, 818–823.
Stork, B. A., Dean, A., Ortiz, A. R., Saha, P., Putluri, N., Planas-Silva, M. D., et al. (2022). Calcium/calmodulin-dependent protein kinase kinase 2 regulates hepatic fuel metabolism. Mol. Metab. 62, 101513. doi:10.1016/j.molmet.2022.101513
Sun, X. Y., Jia, L. Y., Rong, Z., Zhou, X., Cao, L. Q., Li, A. H., et al. (2022). Research advances on matrine. Front. Chem. 10, 867318. doi:10.3389/fchem.2022.867318
Szegezdi, E., Logue, S. E., Gorman, A. M., and Samali, A. (2006). Mediators of endoplasmic reticulum stress-induced apoptosis. EMBO Rep. 7 (9), 880–885. doi:10.1038/sj.embor.7400779
Tang, B., Ai, Z., and Yao, S. (2013). Effects of Matrine on the expression of COX-2 and iNOS in rats with non-alcoholic fatty liver disease. Chin. J. Clin. Electron. Ed. 7, 2011–2015. doi:10.3877/cma.j.issn.1674-0785.2013.05.038
Tang, B. D., Xia, X., Lv, X. F., Yu, B. X., Yuan, J. N., Mai, X. Y., et al. (2017). Inhibition of Orai1-mediated Ca(2+) entry enhances chemosensitivity of HepG2 hepatocarcinoma cells to 5-fluorouracil. J. Cell Mol. Med. 21 (5), 904–915. doi:10.1111/jcmm.13029
Traaseth, N., Elfering, S., Solien, J., Haynes, V., and Giulivi, C. (2004). Role of calcium signaling in the activation of mitochondrial nitric oxide synthase and citric acid cycle. Biochim. Biophys. Acta 1658 (1-2), 64–71. doi:10.1016/j.bbabio.2004.04.015
Vacca, A., Felli, M. P., Palermo, R., Di Mario, G., Calce, A., Di Giovine, M., et al. (2006). Notch3 and pre-TCR interaction unveils distinct NF-kappaB pathways in T-cell development and leukemia. Embo j. 25 (5), 1000–1008. doi:10.1038/sj.emboj.7600996
Wan, B., LaNoue, K. F., Cheung, J. Y., and Scaduto, R. C. (1989). Regulation of citric acid cycle by calcium. J. Biol. Chem. 264 (23), 13430–13439. doi:10.1016/s0021-9258(18)80015-1
Wang, F., Yang, J. L., Yu, K. K., Xu, M., Xu, Y. Z., Chen, L., et al. (2015). Activation of the NF-κB pathway as a mechanism of alcohol enhanced progression and metastasis of human hepatocellular carcinoma. Mol. Cancer 14 (1), 10. doi:10.1186/s12943-014-0274-0
Wang, L., Lu, J., Sun, W., Gu, Y., Zhang, C., Jin, R., et al. (2017). Hepatotoxicity induced by radix Sophorae tonkinensis in mice and increased serum cholinesterase as a potential supplemental biomarker for liver injury. Exp. Toxicol. Pathol. 69 (4), 193–202. doi:10.1016/j.etp.2017.01.003
Wang, Y., Zhang, S., Liu, J., Fang, B., Yao, J., and Cheng, B. (2018). Matrine inhibits the invasive and migratory properties of human hepatocellular carcinoma by regulating epithelial-mesenchymal transition. Mol. Med. Rep. 18 (1), 911–919. doi:10.3892/mmr.2018.9023
Williams, G. S., Boyman, L., and Lederer, W. J. (2015). Mitochondrial calcium and the regulation of metabolism in the heart. J. Mol. Cell Cardiol. 78, 35–45. doi:10.1016/j.yjmcc.2014.10.019
Wu, Y., Wang, Y., and Ma, X. (2014). Protective effects of matrine on acute ethanol-induced liver injury in mice. Asia-Pacific Tradit. Med. 10, 6–9.
Xiao, F., Zhang, J., Zhang, C., and An, W. (2017). Hepatic stimulator substance inhibits calcium overflow through the mitochondria-associated membrane compartment during nonalcoholic steatohepatitis. Lab. Invest. 97 (3), 289–301. doi:10.1038/labinvest.2016.139
Xiong, X., Wang, P., Duan, L., Liu, W., Chu, F., Li, S., et al. (2019). Efficacy and safety of Chinese herbal medicine Xiao Yao San in hypertension: a systematic review and meta-analysis. Phytomedicine 61, 152849. doi:10.1016/j.phymed.2019.152849
Yang, Z., Yu, G. L., Zhu, X., Peng, T. H., and Lv, Y. C. (2022). Critical roles of FTO-mediated mRNA m6A demethylation in regulating adipogenesis and lipid metabolism: Implications in lipid metabolic disorders. Genes Dis. 9 (1), 51–61. doi:10.1016/j.gendis.2021.01.005
Yang, Z., Gao, S., Yin, T., Kulkarni, K. H., Teng, Y., You, M., et al. (2010). Biopharmaceutical and pharmacokinetic characterization of matrine as determined by a sensitive and robust UPLC-MS/MS method. J. Pharm. Biomed. Anal. 51, 1120–1127. doi:10.1016/j.jpba.2009.11.020
Yang, Z., Wang, L., and Wang, X. (2016). Matrine induces the hepatic differentiation of WB-F344 rat hepatic progenitor cells and inhibits Jagged 1/HES1 signaling. Mol. Med. Rep. 14 (4), 3841–3847. doi:10.3892/mmr.2016.5668
Yang, Z. Y., Wang, L., Hou, Y. X., and Wang, X. B. (2013). Effects of matrine on oval cell‑mediated liver regeneration and expression of RBP‑Jκ and HES1. Mol. Med. Rep. 7 (5), 1533–1538. doi:10.3892/mmr.2013.1398
Yin, H., Que, R., Liu, C., Ji, W., Sun, B., Lin, X., et al. (2018). Survivin-targeted drug screening platform identifies a matrine derivative WM-127 as a potential therapeutics against hepatocellular carcinoma. Cancer Lett. 425, 54–64. doi:10.1016/j.canlet.2018.03.044
You, L., Yang, C., Du, Y., Liu, Y., Chen, G., Sai, N., et al. (2019). Matrine exerts hepatotoxic effects via the ROS-dependent mitochondrial apoptosis pathway and inhibition of nrf2-mediated antioxidant response. Oxid. Med. Cell Longev. 2019, 1045345. doi:10.1155/2019/1045345
Younossi, Z. M., Wong, G., Anstee, Q. M., and Henry, L. (2023). The global burden of liver disease. Clin. Gastroenterol. Hepatol. 21 (8), 1978–1991. doi:10.1016/j.cgh.2023.04.015
Yu, H. B., Zhang, H. F., Li, D. Y., Zhang, X., Xue, H. Z., and Zhao, S. H. (2011a). Matrine inhibits matrix metalloproteinase-9 expression and invasion of human hepatocellular carcinoma cells. J. Asian Nat. Prod. Res. 13 (3), 242–250. doi:10.1080/10286020.2010.551641
Yu, H. C., Qin, H. Y., He, F., Wang, L., Fu, W., Liu, D., et al. (2011b). Canonical notch pathway protects hepatocytes from ischemia/reperfusion injury in mice by repressing reactive oxygen species production through JAK2/STAT3 signaling. Hepatology 54 (3), 979–988. doi:10.1002/hep.24469
Yu, J. L., Li, J. H., Chengz, R. G., Ma, Y. M., Wang, X. J., and Liu, J. C. (2014). Effect of matrine on transforming growth factor β1 and hepatocyte growth factor in rat liver fibrosis model. Asian Pac J. Trop. Med. 7 (5), 390–393. doi:10.1016/s1995-7645(14)60062-6
Yuan, F., and Yang, Z. (2020). Influence of matrine on liver function and MAPK signaling pathway after hepatic ischemic reperfusion injury in rats. J. Guangdong Pharm. Univ. 36, 657–661. doi:10.16809/j.cnki.2096-3653.2020052003
Yue, Z.-S., Ruan, B., Duan, J.-L., Han, H., and Wang, L. (2018). The role of the Notch signaling pathway in liver injury and repair. J. Bio-X Res. 1 (2), 95–104. doi:10.1097/jbr.0000000000000014
Zeeshan, H. M., Lee, G. H., Kim, H. R., and Chae, H. J. (2016). Endoplasmic reticulum stress and associated ROS. Int. J. Mol. Sci. 17 (3), 327. doi:10.3390/ijms17030327
Zhang, H. F., Shi, L. J., Song, G. Y., Cai, Z. G., Wang, C., and An, R. J. (2013). Protective effects of matrine against progression of high-fructose diet-induced steatohepatitis by enhancing antioxidant and anti-inflammatory defences involving Nrf2 translocation. Food Chem. Toxicol. 55, 70–77. doi:10.1016/j.fct.2012.12.043
Zhang, J., Li, Y., Jiang, S., Yu, H., and An, W. (2014). Enhanced endoplasmic reticulum SERCA activity by overexpression of hepatic stimulator substance gene prevents hepatic cells from ER stress-induced apoptosis. Am. J. Physiol. Cell Physiol. 306 (3), C279–C290. doi:10.1152/ajpcell.00117.2013
Zhang, X. L., Xu, H. R., Chen, W. L., Chu, N. N., Li, X. N., Liu, G. Y., et al. (2009). Matrine determination and pharmacokinetics in human plasma using LC/MS/MS. J. Chromatogr. B Anal. Technol. Biomed. Life Sci. 877 (27), 3253–3256. doi:10.1016/j.jchromb.2009.08.026
Zhao, Y. (2015). Effects of Matrine on inflfl ammatory factor in rats with liver injury of carbon tetrachloride. Clin. J. Traditional Chin. Med. 27, 727–728. doi:10.16448/j.cjtcm.2015.0275
Zheng, Y., Ding, Q., Wei, Y., Gou, X., Tian, J., Li, M., et al. (2021). Effect of traditional Chinese medicine on gut microbiota in adults with type 2 diabetes: a systematic review and meta-analysis. Phytomedicine 88, 153455. doi:10.1016/j.phymed.2020.153455
Zhou, M., Li, Q., and Su, J. (2009). Effects of matrine on the expression of fas and fasL in liver tissue of mice injured by immune reaction. China Trop. Med. 9, 1445–1447.
Zhou, Y., Wu, Y., Deng, L., Chen, L., Zhao, D., Lv, L., et al. (2014). The alkaloid matrine of the root of Sophora flavescens prevents arrhythmogenic effect of ouabain. Phytomedicine 21 (7), 931–935. doi:10.1016/j.phymed.2014.02.008
Zhu, J., Zhu, J., Tang, F., Feng, Y., Zhou, Q., Jin, Q., et al. (2015). Influence of matrine on liver functions and TNF-α level following liver ischemic reperfusion injury in rats. Chin. J. Clin. Res. 28, 1147–1150. doi:10.13429/j.cnki.cjcr.2015.09.007
Keywords: matrine, hepatotoxicity, hepatoprotection, liver injury, meta-analysis
Citation: Feng W, Kao T-c, Jiang J, Zeng X, Chen S, Zeng J, Chen Y and Ma X (2024) The dynamic equilibrium between the protective and toxic effects of matrine in the development of liver injury: a systematic review and meta-analysis. Front. Pharmacol. 15:1315584. doi: 10.3389/fphar.2024.1315584
Received: 10 October 2023; Accepted: 08 January 2024;
Published: 29 January 2024.
Edited by:
Rongrui Wei, Jiangxi University of Traditional Chinese Medicine, ChinaReviewed by:
Feng Zhang, Nanjing University of Chinese Medicine, ChinaCopyright © 2024 Feng, Kao, Jiang, Zeng, Chen, Zeng, Chen and Ma. This is an open-access article distributed under the terms of the Creative Commons Attribution License (CC BY). The use, distribution or reproduction in other forums is permitted, provided the original author(s) and the copyright owner(s) are credited and that the original publication in this journal is cited, in accordance with accepted academic practice. No use, distribution or reproduction is permitted which does not comply with these terms.
*Correspondence: Jinhao Zeng, emVuZ2ppbmhhb0BjZHV0Y20uZWR1LmNu; Yu Chen, NzM1NDA1NjYxQHFxLmNvbQ==; Xiao Ma, dG9ieW1heGlhb0BjZHV0Y20uZWR1LmNu
†These authors have contributed equally to this work and share first authorship
Disclaimer: All claims expressed in this article are solely those of the authors and do not necessarily represent those of their affiliated organizations, or those of the publisher, the editors and the reviewers. Any product that may be evaluated in this article or claim that may be made by its manufacturer is not guaranteed or endorsed by the publisher.
Research integrity at Frontiers
Learn more about the work of our research integrity team to safeguard the quality of each article we publish.