- 1Henan University of Chinese Medicine, Zhengzhou, China
- 2Collaborative Innovation Center of Research and Development on the Whole Industry Chain of Yu-Yao, Zhengzhou, China
- 3Jacobs School of Medicine and Biomedical Sciences, The State University of New York, University at Buffalo, Buffalo, NY, United States
- 4School of Community Health and Policy, Morgan State University, Baltimore, MD, United States
- 5The First Affiliated Hospital of Henan University of Chinese Medicine, Zhengzhou, China
The Vitamin D receptor (VDR) is a crucial nuclear receptor that plays a vital role in various physiological functions. To a larger extent, the genomic effects of VDR maintain general wellbeing, and its modulation holds implications for multiple diseases. Current evidence regarding using vitamin D or its synthetic analogs to treat non-communicable diseases is insufficient, though observational studies suggest potential benefits. Traditional Chinese medicines (TCMs) and bioactive compounds derived from natural sources have garnered increasing attention. Interestingly, TCM formulae and TCM-derived bioactive compounds have shown promise in modulating VDR activities. This review explores the intriguing potential of TCM and bioactive compounds in modulating VDR activity. We first emphasize the latest information on the genetic expression, function, and structure of VDR, providing a comprehensive understanding of this crucial receptor. Following this, we review several TCM formulae and herbs known to influence VDR alongside the mechanisms underpinning their action. Similarly, we also discuss TCM-based bioactive compounds that target VDR, offering insights into their roles and modes of action.
1 Introduction
Traditional Chinese Medicine (TCM) has been an integral part of the healthcare system in China and other parts of the world for over three millennia. Recent studies on TCM herbal formulations and bioactive compounds have led to cutting-edge research findings, resulting in significant advances in medical knowledge about the therapeutic potential of TCM formulations. These studies have shown that TCM formulations can treat diseases and disorders like osteoporosis, cancer, alopecia, etc (An et al., 2016; Li et al., 2017; Zhang et al., 2022a). This is so because most of these TCM formulations have a rich variety of bioactive compounds, such as alkaloids, flavonoids, terpenoids, and polysaccharides, which have been demonstrated to modulate molecular cascades of biological activities, including anti-inflammatory, antioxidant, and immunomodulatory effects (Bai et al., 2019; Fang et al., 2020; Dai et al., 2021; Lu et al., 2021; Su et al., 2021; Cao et al., 2022a; Cao et al., 2022b; Gao et al., 2022). Importantly, recent emerging works show that some TCM-based compounds regulate Vitamin D receptor (VDR) [also known as 1α,25-dihydroxy vitamin D receptor or nuclear receptor subfamily 1 group 1 member 1 (NR1l1)] activity, potentially offering new opportunities for developing novel therapies for VDR-dependent diseases (Hou et al., 2017; Xiong et al., 2022).
VDR was first cloned and expressed in 1988 by Andrew Baker and his colleagues. It is part of the nuclear receptor 1 superfamily. VDR senses the active form of vitamin D3 (calcitriol or 1α,25-dihydroxy vitamin D3) (Baker et al., 1988) and, in response, triggers diverse specific gene expressions which are vital in the development and metabolism of an organism (Rochel et al., 2000). VDR can be found in the nucleus, cytosol, or plasma membrane, but in general, it is mainly localized to the nucleus (Tamura et al., 2017). VDR is conserved among many organisms, including dogs, frogs, rats, etc. In worms like Caenorhabditis elegans, the nuclear hormone receptor DAF-12 is the closest homolog to vertebrate VDR (Aghayeva et al., 2021). Sequence analysis of the VDR gene reveals highly conserved DNA-binding and hydrophobic ligand-binding domains, which are typical of nuclear receptors (Poon et al., 2012). VDR is found to be widely expressed in numerous tissues (Norman, 2008; Fagerberg et al., 2014; Carlberg and Muñoz, 2022). In addition, VDR is also found in some tumor tissues like mammary tumors, leukemia, etc. However, VDR’s expression and function are tissue-specific, and its native agonist autoregulates VDR expression levels in-vivo and in cells (Zella et al., 2006).
VDR is linked to many non-communicable diseases like osteoporosis, alopecia, diabetes, heart disease, and cancer. Activation of VDR by its ligand initiates signaling through VDR-retinoid-X-receptor heterodimers, which later bind to specific vitamin D response elements on DNA, resulting in the transcription of target genes. Today, our understanding of the functions of VDR continues to evolve. VDR drives the expression of genes that participate in cellular homeostasis, calcium and phosphate regulation, bone formation, cell growth and differentiation, immunity, and many more (Pike and Meyer, 2010; Christakos et al., 2016). Consequently, VDR represents an attractive drug target for many cellular metabolic pathways. Therefore, efforts are underway to unlock the tremendous value of TCM formulations and compounds as regulators of VDR activity in many diseases (Antony et al., 2010; Matsuo et al., 2013; Maestro et al., 2016; Sawada et al., 2018).
To provide a comprehensive overview of the potential of TCM as a modulator of VDR activity, this article will first establish a foundation by discussing VDR expression, functions, structure, and activation mechanism. We will then explore the therapeutic potential of VDR as a target for diseases resulting from VDR dysregulation and mutations. The main focus of this review will be on recent advances that demonstrate the potential of TCM-based formulations/compounds in modulating VDR.
2 Vitamin D receptor: expression, function, and structure
The VDR gene, which encodes vitamin D receptor protein, is located on chromosome 12q13.11 in humans (Figure 1) and chromosome 15 in mice (Haussler et al., 2013). It consists of 9 exons and has multiple isoforms (Esteban et al., 2005; Zenata and Vrzal, 2017). Various polymorphic sites have been identified in the VDR gene, including VDR Apa1, Bsm1, Taq1, Fok1, etc. These polymorphisms can affect mRNA expression patterns, protein translation efficiency, and VDR transcriptional activity (Fleet and Schoch, 2010; Han et al., 2017; Li et al., 2021). VDR expression has been detected in various cell types, including those involved in mineral homeostasis and immune cells (da Silva Teixeira et al., 2020; Provvedini et al., 1983; Kadowaki and Norman, 1984). The regulation of VDR expression is influenced by factors such as the presence of natural agonist, cellular molecules, including parathyroid hormone and retinoic acid hormone, and tissue-specific regulatory sites (Crofts et al., 1998; Lee et al., 2015).
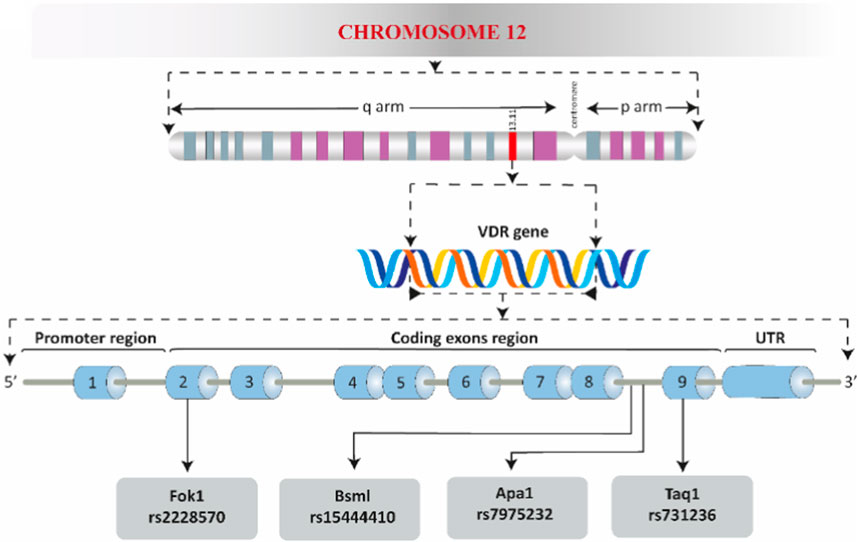
FIGURE 1. A schematic overview of the human VDR. The promoter region, coding exons region, the three prime untranslated region (UTR), and the well-known polymorphic sites are shown.
The functions and molecular activation of VDR have been extensively studied since the cloning of the full-length VDR in 1988 (Baker et al., 1988). VDR’s function at the cellular level is primarily dependent on ligand binding, with 1α,25-dihydroxy vitamin D3 being a key agonist. The binding of an agonist to VDR initiates a sequence of events, starting with the formation of a heterodimer with the retinoid-X-receptor (Carlberg, 2004). This complex then translocates from the cytosol to the nucleus, although the specifics of this process remain unclear. In the nucleus, the ligand-bound VDR-retinoid-X-receptor complex interacts with vitamin D response elements to initiate transcription, aided by various coactivators (Prüfer et al., 2000). Without an agonist, VDR can still enter the nucleus but is associated with transcriptional corepressors that prevent gene transcription (Prüfer and Barsony, 2002; Carlberg, 2004; Deeb et al., 2007; Peleg and Nguyen, 2010; Toropainen et al., 2010; Takeyama and Kato, 2011) (Figure 2). The biological functions of VDR are diverse and complex, primarily due to its varied cell-specific actions. One of the most established roles of VDR is in the regulation of mineral metabolism and bone health, where it is known to suppress bone resorption (Nakamichi et al., 2017). Studies have shown that the absence of VDR leads to impaired bone growth, and VDR influences the expression of genes involved in bone differentiation and mineralization (Mathieu et al., 2001; van de Peppel and van Leeuwen, 2014). However, the relationship between VDR polymorphisms and bone diseases in humans remains uncertain, with conflicting results in clinical studies (Hunter et al., 2000; Thakkinstian et al., 2004; Uitterlinden et al., 2006). Besides bone health, VDR also plays a significant role in immune cell activation, cell proliferation, and differentiation, highlighting its broad functional spectrum in various cellular contexts (Bakke and Sun, 2018; von Essen et al., 2010; Halfon et al., 2015).
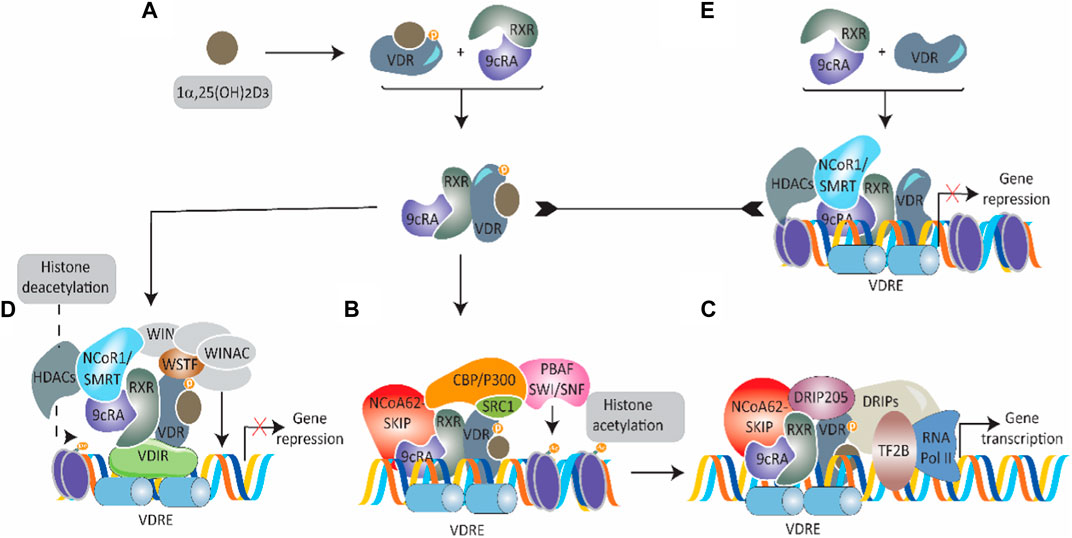
FIGURE 2. Overview of VDR transcriptional signaling. (A) A schematic representation of 1α,25-dihydroxy vitamin D3 [1α, 25 (OH)2D3]-induced activation of cytosolic VDR. On binding, VDR complexes with the retinoid-X-receptor coupled with 9-cis-retinoic acid (9cRA) and moves into the nucleus. (B) In the nucleus, the 1α, 25 (OH)2D3-VDR-RXR-9cRA complex binds to nuclear vitamin D response elements (nVDRE) and recruits several coactivators [nuclear coactivator—62 kDa-Ski-interacting protein (NCoA62-SKIP), steroid receptor coactivators (SRC1), CREB binding protein-p300 (CBP/p300), and SWI-2-related gene 1 associated factor (PBAF-SNF)] to promote histone acetylation and unwrap chromatin. (C) The mediator complex VDR-interacting proteins (DRIPs) are brought in by binding VDR-interacting protein 205. Transcription initiation is triggered by transcription factor 2B (TF2B) and RNA polymerase II. (D) In certain situations, transcriptional activation is prevented in 1α, 25 (OH)2D3 presence. This happens when 1α, 25 (OH)2D3-VDR-RXR-9cRA complex binds to a VDR-interacting repressor (VDIR) recognized by nVDRE, resulting in the dissociation of histone acetyltransferase (HAT) coactivators and the association of corepressors NCoR1/SMRT with histone deacetylase (HDAC) activity. The subsequent recruitment and interaction with the Williams syndrome transcription factor (WSTF) hinder gene transcription expression via the multifunctional ATP-dependent chromatin-remodeling complex (WINAC). (E) VDR that is not bound to 1α, 25 (OH)2D3 and is linked to RXR-9cRA moves into the nucleus and then binds to nVDRE. With the help of corepressors (NCoR1/SMRT) with histone deacetylase activity, transcription is inhibited.
The structural and biochemical features of VDR protein are multifaceted and crucial for its function. In human and mouse cells, the VDR consists of 427 and 423 amino acids, respectively, and is primarily located in the nucleus, although it can also be found in the cytoplasm and plasma membrane (Barsony and Prufer, 2002). VDR is characterized by its modular structure, which includes various domains such as A/B, C, D, E, and F (Figure 3), common to nuclear receptor 1 superfamily receptors (Wurtz et al., 1996; Rochel et al., 2000; Tocchini-Valentini et al., 2001). The A/B region at the N-terminus is relatively short, and the C domain is the highly conserved DNA binding domain featuring two “zinc finger” motifs. These motifs are critical for binding to DNA at vitamin D response elements, thereby facilitating VDR-induced transcription. The D domain, or flexible hinge, connects the DNA-binding and ligand-binding domains and plays a key role in nuclear translocation and in determining the orientation of the ligand-binding domain to DNA sequences (Michigami et al., 1999; Pike and Meyer, 2010; Nwachukwu and Nettles, 2012). The E domain is the ligand-binding portion, composed of ⍺-helices and β-sheets forming a hydrophobic pocket essential for binding ligands, coactivators, and corepressors (Mizwicki et al., 2007; Campbell et al., 2010; Wan et al., 2015; Sawada et al., 2018). This domain includes an activation function crucial for ligand-induced VDR activation and subsequent engagement with coactivators. The combined actions of the ligand-binding and DNA-binding domains influence VDR’s role in gene expression (Rochel et al., 2000; Orlov et al., 2012). Uniquely, VDR also features a F region, the function of which is not fully understood (Wurtz et al., 1996; Rochel et al., 2000; Tocchini-Valentini et al., 2001; Shaffer and Gewirth, 2004). Post-translational modifications, such as phosphorylation, SUMOylation, and potential ubiquitination, play significant roles in regulating VDR’s activity, localization, stability, and protein interactions (Arriagada et al., 2007; Zenata and Vrzal, 2017; Bakke et al., 2018). These modifications can also either enhance or suppress VDR’s transcriptional activities, exemplifying the complex regulation of VDR in cellular environments (Hsieh et al., 2004; Chi et al., 2009; Jena et al., 2012).
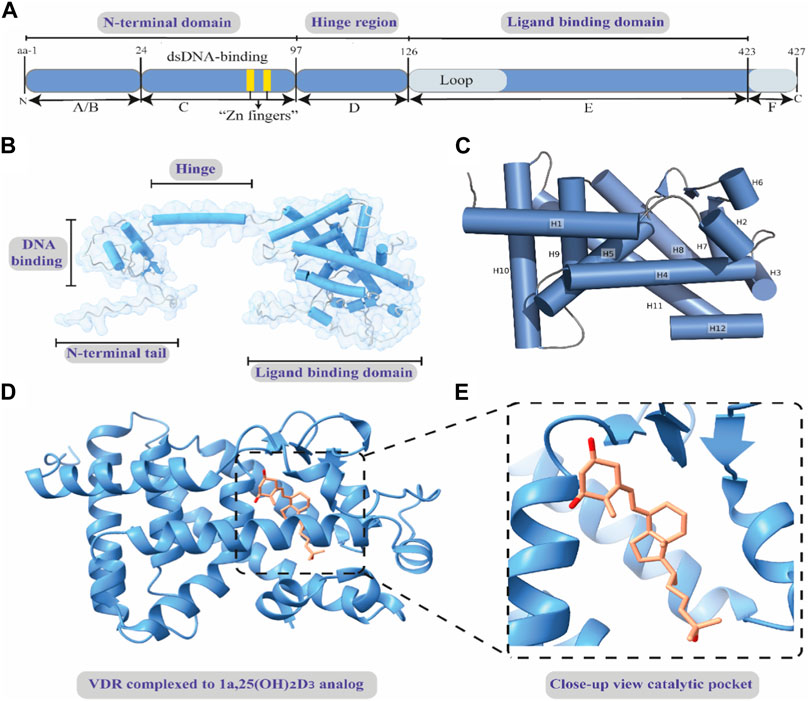
FIGURE 3. Basic structural features of human VDR. (A) Diagram of the functional domains of the human VDR. (B) The full-length 3-dimensional structure of apo-human VDR, showing the functional domains. (C) A close view of the crystal structure of the ligand-unbound ligand-binding domain of VDR. The α-helices are shown as cylindrical and numbered from H1-H12. (D) A cartoon representation of the ligand-binding domain of human VDR bound to 1α,25-dihydroxy vitamin D3 analog. (E) A magnified view of the binding site showing the binding mode of the inset ligand 1α,25-dihydroxy vitamin D3 analog. Full-length apo-human VDR (alpha fold model); Crystal structure of apo-human VDR ligand-binding domain (PDB ID: 3A78); and crystal structure of the nuclear receptor for vitamin D ligand binding domain bound to 1α,25-dihydroxy vitamin D3 analog (PDB ID: 1IE9).
3 VDR and human diseases
In recent decades, the therapeutic potential of VDR signaling in various diseases has been recognized (Figure 4). Modulating the VDR protein through genetic or drug-based strategies has improved our understanding of the many cellular processes controlled by VDR and how changes in VDR function can contribute to disease development or regression. VDR expression is present in most human body systems, and approximately 50–60 mutations in the VDR gene have been identified, most of which are found in the DNA-binding and ligand-binding domains, as well as other essential regions of the gene. These mutations have clinical implications and have been associated with severe hypocalcemic rickets, a condition linked to structural defects in bones in children (Hughes et al., 1988). One example of a VDR mutation is Gln152Xaa, which is found in the hinge region of the gene. This region is believed to be involved in the nuclear transportation of VDR or in supporting its association with specific response elements on DNA. The Gln152Xaa mutation is known to cause severe rickets associated with alopecia. This likely occurs because the mutation prevents VDR from carrying out its transcriptional activity to induce genes that control calcium and phosphate metabolism and hair growth signals. As a result, the body’s ability to maintain proper bone mineralization and hair growth signal is disrupted, leading to the development of severe rickets and total alopecia. Depending on the location of the mutations in the VDR gene, individuals with VDR mutation-induced rickets can be sub-grouped as having defects in hormone and DNA binding activity on VDR or restrains that prevent VDR nuclear localization. Most patients with VDR-dependent rickets condition have a range of signs and symptoms, including alopecia, hypocalcemia, hypophosphatemia, impaired bone formation, growth retardation, female infertility, uterine hypoplasia, impaired folliculogenesis, and hyperparathyroidism.
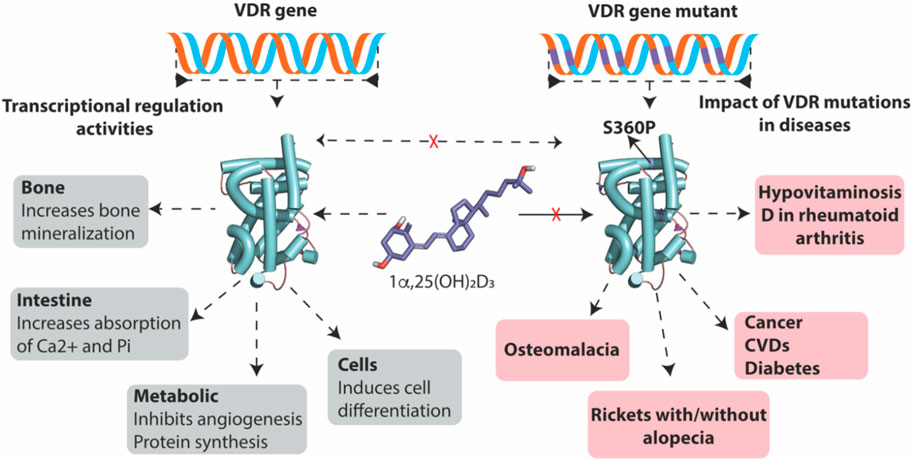
FIGURE 4. Disease mutants VDR but not wild-type VDR causes diseases. Wild-type VDR, upon binding to its ligand 1α,25-dihydroxy vitamin D3, plays a vital role in maintaining overall health by facilitating calcium and phosphate absorption and promoting bone mineralization. In addition, it also inhibits angiogenesis, promotes protein synthesis, and induces cell differentiation. In contrast, disease-associated mutants of VDR are resistant to 1α,25-dihydroxy vitamin D3, disrupting VDR signaling and leading to the development of various bone-related diseases and the progression of cancer, diabetes, and CVDs.
Another class of mutations of importance to our discussion here is missense mutations, point mutations in which a single nucleotide base change results in a codon that encodes for a different amino acid. Most missense mutations found in the VDR gene are associated with the rare disorder termed hereditary 1,25-dihydroxyvitamin D-resistant rickets or vitamin D-dependent rickets type IIA. It is worth noting that this type of rickets is different from vitamin D-dependent rickets type 1A—a disease caused by mutants in 25-hydroxyvitamin D3 1α-hydroxylase, the enzyme that catalyzes the synthesis of the active form (1α,25-dihydroxy vitamin D3) of vitamin D (Kitanaka et al., 1998). The first discovery of a missense mutation in the VDR gene was reported first in 1991 in Japanese rickets patients from heterozygous carrier parents (Saijo et al., 1991). Although it was not substantiated clearly on the exact missense mutant that was responsible for the vitamin D-dependent rickets type IIA, the authors’ analysis of the VDR gene from these patients strongly pinpointed that Arg47, located between the two zinc fingers, homozygous substitution is responsible for this disorder. However, subsequent advances in VDR gene analysis have confirmed this homozygous substitution and also further revealed unique homozygous missense substitutions such as Ser360Pro, Val346Met, Arg274Leu, and His305Gln in VDR gene in patients with vitamin D-dependent rickets type II (Tamura et al., 2017). In most cases, VDR transcriptional activity and ligand-binding affinity are lost entirely or partially impaired in all these missense mutants. For this reason, vitamin D-dependent rickets type II is resistant to 1α,25-dihydroxy vitamin D3 and its synthetic analogs treatment.
Due to space issues, we summarize three important VDR-dependent non-communicable diseases (Cancer, cardiometabolic disorders, and bone diseases) that are particularly important for our subsequent session discussion below.
3.1 Cancer and VDR
Cancer is one of the most therapeutically challenging diseases with extreme financial burdens on afflicted individuals. The link between VDR and cancer emanated from the “inconclusive” antineoplastic effects of the natural substrate 1α,25-dihydroxy vitamin D3. This led to the hypothesis that the VDR gene may act to repress tumorigenesis. It has also been observed that VDR is either highly expressed or downregulated in many histological types of cancers. For instance, in colon cancer, VDR mRNA is decreased (Zhang et al., 2020), while the opposite is true for basal cell carcinoma, squamous cell carcinoma, and breast, cervical, and ovarian cancers, suggesting a tissue-specific effect of VDR signaling. Of interest, although it remains unclear what drives the overexpression of VDR, the importance of overexpression of VDR in specific tumor types has been associated with better prognosis and prolonged survival in study participants. In fact, it is suggested that tumors with overexpression of VDR are more responsive to vitamin D supplementation. On the other hand, the low expression of VDR in host with colon cancers have been linked to colon cancer disease development and progression and a slight improvement in relapse-free survival at 5 years after vitamin D supplementation. Besides the tumor tissue-specific levels of VDR, several non-synonymous and synonymous single-nucleotide polymorphisms (Fok1, Bsml, Apa1, Tru91, and Taq1) in the VDR gene have been found. Most of them have been reported to be associated with either increased or decreased susceptibility to primary cancer and invasive and metastatic cancers of the prostate, breast, and head and neck. Also, data are available for their correlation with modifiable and unmodifiable risk factors of cancers. However, these associations are often based on small-sample sizes and do not account for ethnic differences and subgroup variations among study participants. Thus, light has not been adequately shed on the functional role of VDR signaling in cancers.
However, basic studies have tried to answer some of the questions relating to the role of VDR signaling in cancers by showing that VDR knockout results in increased susceptibility to chemicals-induced carcinogenesis. Thus, VDR signaling can potentially prevent tumorigenesis (Zinser et al., 2002; Zinser and Welsh, 2004; Zinser et al., 2005). For instance, hyperproliferation and enhanced mitotic activity in the descending colon were seen in laboratory-adapted Vdr−/− mice. To further examine how intestinal VDR contributes to the barrier function and protects against carcinogenesis, Zhang et al. utilized azoxymethane/dextran sulfate sodium-induced cancer model in intestinal VDR conditional knockout (VDR∆IEC) mice, 2D and 3D cell cultures, and human colon cancer samples. In a snapshot, the authors found that intestinal VDR helps keep the intestinal barrier structure and function and also acts as a tumor suppressor by regulating the JAK/STAT pathway in the intestine (Zhang et al., 2020). Another recent elegant study, which was aimed at investigating how VDR coordinates with other intestinal barrier structural integrity players like tight junctions to protect the host from colorectal cancer, found that intestinal epithelial VDR regulates cellular levels of tight junction protein claudin-5 to prevent inflammation and carcinogenesis in the colon. In addition, CLDN-5 gene was identified as a novel target of VDR. This is because when the VDR gene was deleted, a decrease in claudin-5 and an increase in tumor development were observed. In contrast, increased claudin-5 and protection against tumorigenesis in intestinal epithelial cells were found after enhancing VDR activity (Zhang et al., 2022b). In sum, VDR status and signaling may be related to cancer prevention or retrogression. However, many critical translational questions must be explored and answered on the cellular and molecular mechanisms by which VDR prevents the survival of cancer cells and inhibit angiogenesis.
3.2 Cardiometabolic diseases and VDR
Cardiovascular diseases and metabolic disorders, collectively known as cardiometabolic diseases, remain a leading cause of disease-related mortality worldwide. In an effort to understand the factors that contribute to the development and progression of these conditions, researchers have been studying the role of metabolic genes in cardiometabolic diseases. One area of interest is the link between cardiometabolic events and the seasons, as several observational studies have shown that cardiometabolic diseases tend to be more common during the winter months (Motiwala and Wang, 2012). These observations have spurred researchers to investigate the role of VDR signaling in cardiometabolic diseases such as diabetes, hypertension, and metabolic syndrome. A growing body of research using human and animal disease model samples has shown that VDR is a metabolic gene and that individuals with metabolic syndrome and vascular diseases tend to have downregulated VDR expression. This suggests that VDR may have an essential role in the development and progression of cardiometabolic diseases and that targeting VDR signaling may be a promising approach for preventing and treating these conditions (de Albuquerque Borborema et al., 2021). In support of this idea, it has been discovered that VDR plays a key role in regulating cellular processes that are essential to cardiovascular and metabolic health, such as cell proliferation and differentiation, metabolic homeostasis, tissue mineralization, protein synthesis and degradation, and ion transport for catalysis. In fact, most cardiovascular and metabolic cell types, including endothelial cells, adipocytes, hepatocytes, smooth muscle cells, immune cells, cardiomyocytes, and platelets, have been found to express VDR strongly (Somjen et al., 2005; Tishkoff et al., 2008).
Secondary bile acids, which are derived from gut microbial metabolism, are VDR ligands and regulators of VDR expression. Multiple gut microbiome analyses using 16s rRNA gene sequencing have shown that alterations in the gut microbiome composition can lead to cardiovascular and metabolic diseases. In fact, a genome-wide association and shotgun metagenomic analysis published in Nature Genetics found that the gut microbiome composition of VDR−/− mice differed significantly from that of control mice that express VDR. Additionally, VDR-mediated signaling was found to be critical in the gut-liver signaling axis and microbial co-metabolism (Wang et al., 2016), suggesting that VDR signaling can influence gene targets that are relevant to cardiometabolic health and that changes in VDR gene expression can lead to disruptions in the gut microbiome that can cause cardiometabolic diseases. Furthermore, several observational studies have suggested that VDR gene polymorphic alleles and genotypes are associated with an increased risk of cardiovascular and metabolic diseases in certain populations, such as pregnant women, offspring, and Asians (Apaydın et al., 2019; Mokhtar et al., 2019). For example, it has been found that individuals carrying the VDR SNPs Apa1, Taq1, and Bsm1 genotypes were more likely to be obese, while those with the Fok1 genotype were at an increased risk of heart failure and hypertension (Abouzid et al., 2021). These findings highlight the potential role of VDR in the development of cardiometabolic diseases and the need for further research to understand the mechanisms underlying this relationship.
Although this mini-review in its entirety seeks to focus on VDR, it is worth noting that several experimental pieces of evidence have shown that exposure 1α,25-dihydroxy vitamin D3 in cardiovascular and metabolic cell types, leads to a decrease in endothelial inflammation, improves flow-mediated dilation, reduces endothelium-dependent contraction, enhances nitric oxide release, improves tissue mineralization, decreases cholesterol uptake and foam cell formation, modulates immune cells differentiation, etc. (Norman and Powell, 2014). Besides, significant associations between low vitamin D intake and adverse endpoints of cardioembolic diseases have been found. It has been suggested that adequate supplementation with vitamin D or 1α,25-dihydroxy vitamin D3 has favorable outcomes on biochemical and metabolic parameters (Breslavsky et al., 2013), suggesting a therapeutic implication for VDR signaling. However, despite the asserted beneficial effect of VDR signaling on the cardiovascular system, other epidemiological studies, mainly priming VDR signaling via vitamin D supplementation, suggest a disease-causal role for VDR in the pathogenesis of cardiovascular and metabolic diseases (Kayaniyil et al., 2010). These deleterious effects of VDR signaling, perhaps, can be attributed to the high intake of vitamin D used in most of these studies. Altogether, the role of VDR signaling cannot be overlooked in cardiometabolic health, but several knowledge gaps, like the tissue-specific pattern effects of VDR and its relevance in a specific cardiometabolic disease, need to be addressed.
3.3 Bone disorders and VDR
Bone is widely recognized as the primary target of vitamin D, as numerous studies have shown that 1α,25-dihydroxy vitamin D3 supplementation can alleviate bone diseases such as rickets, osteomalacia, osteosclerosis, and osteoporosis. Osteoblasts, osteoclasts, chondrocytes, and osteocytes are important human bone cells that regulate bone formation, resorption, and mineralization. These cells express the VDR gene, and mice that lack this gene develop severe rickets and osteomalacia. These findings demonstrate the crucial role of the VDR-1α,25-dihydroxy vitamin D3 complex in maintaining healthy bones (Nakamichi et al., 2018). Besides, it is well established that VDR is the epicenter regulator of bone formation, resorption, mineralization, and metabolism. Mainly via the expression of genes that interact with other key cellular factors and other metabolic pathways that help to ensure the proper development and mineralization of bones (Luo et al., 2018). However, despite the known role of the VDR-1α,25-dihydroxy vitamin D3 complex in regulating bone mineralization, formation, and resorption, our understanding of these processes is still incomplete. This is because studies using VDR−/− genetic systems to investigate the functions of VDR in bone-specific cells have yielded conflicting results. For example, some studies using osteoblast lineage-specific VDR cKO mice and osteocyte-specific VDR cKO mice have reported significant abnormalities in bone mineralization and disruptions in bone formation and resorption processes in these mice, while others have found the opposite (Verlinden et al., 2019). The conflicting results of these studies may be due to differences in the specificity of the cell systems used to knockout VDR or the effectiveness of the Cre activity in the Cre transgenic mice that were often used to delete VDR gene. Therefore, further research is needed to clarify the role of VDR in regulating bone mineralization, formation, and resorption (Verlinden et al., 2019).
In elderly individuals, the expression of VDR and its target genes, which are crucial for cell differentiation, is decreased. This decrease in VDR expression is believed to be caused by well-known risk factors for diseases such as aging. As a result, the ability of the VDR-1α,25-dihydroxy vitamin D3 complex to regulate bone health may be impaired in the elderly, contributing to the increased risk of bone-related diseases (Jiang et al., 2020). This is consistent with the well-established idea that aging makes us more susceptible to diseases, which explains why older adults are at increased risk of developing metabolic bone diseases such as osteoporosis, rheumatoid arthritis, and osteomalacia. Notably, studies involving elderly patients have found that vitamin D intake can promote longevity by inducing VDR expression and enhancing metabolic bone processes that have declined due to aging. As with other diseases discussed earlier, the effect of VDR polymorphisms on the risk of bone diseases in specific ethnic populations has also been studied. A meta-analysis of almost 10,000 people recently found that ApaI, BsmI, and FokI polymorphisms, but not TaqI polymorphism, were associated with the metabolic bone disease osteoporosis. Further subgroup analysis focused on ethnicity revealed that ApaI, BsmI, and TaqI polymorphisms were significantly associated with the incidence of osteoporosis in Caucasians, while VDR polymorphisms BsmI and FokI were linked to an increased risk of osteoporosis among Asians. These findings highlight the importance of considering the role of VDR polymorphisms in the development of bone-related diseases in different ethnic populations (Jiang et al., 2022). In summary, while the exact mechanisms by which VDR-mediated transcription promotes osteogenesis and osteoblastic bone formation while also suppressing osteocyte senescence and osteoclastic bone resorption remain unclear, the significant role of VDR in bone formation and bone-related diseases is undeniable. Further research is needed to better understand the specific mechanisms by which VDR regulates these processes and to identify potential therapeutic strategies for maintaining healthy bones and treating bone-related diseases.
3.4 Kidney diseases and VDR
Kidney diseases, encompassing a range of pathologies such as IgA nephropathy, idiopathic nephrotic syndrome, renal cell carcinoma, diabetic nephropathy, and lupus nephritis, pose serious health challenges globally. Recent strides in understanding the role of the VDR in these diseases offer a promising therapeutic frontier. VDR activation, shown to protect renal function, operates through a multitude of mechanisms: it suppresses the renin-angiotensin system, reduces inflammation, inhibits fibrogenesis, enhances mitochondrial function, attenuates autoimmunity, and reduces renal cell apoptosis (Yang et al., 2018). Such multifaceted roles underscore VDR’s potential as a therapeutic target. For example, in the context of lupus nephritis, a notable condition marked by increased disease activity and severity, a correlation has been observed with decreased VDR expression in renal tissues (Huang et al., 2021). This finding is pivotal as it suggests that VDR not only plays a role in disease manifestation but also in its progression. VDR agonists have emerged as a beacon of hope in these scenarios, showing efficacy in reducing renal inflammation and restraining the nuclear translocation of inflammatory mediators (Sanchez-Niño et al., 2012).
Another particularly intriguing study in the realm of IgA nephropathy revealed differential expressions of VDR and the calcitriol-synthesizing enzyme, 1alpha-OHase (Arapović et al., 2021). Patients showed elevated VDR expression in distal tubular cells, contrasted with a marked decrease in 1alpha-OHase (Arapović et al., 2021). This disparity opens new avenues for understanding the disease’s molecular underpinnings and, possibly, its management. The role of VDR extends into the broader spectrum of chronic kidney disease, where its diminished levels and activity exacerbate conditions like secondary hyperparathyroidism and renal fibrosis (Bakdash et al., 2014; Barragan et al., 2015). This insight gains practical significance in experimental models of chronic renal failure (CRF), where VDR agonists like paricalcitol and calcitriol have demonstrated remarkable anti-fibrotic effects, offering a therapeutic window (Martínez-Arias et al., 2021). Moreover, the genetic landscape of VDR in kidney diseases has been illuminated by a comprehensive meta-analysis. This analysis identified specific VDR gene polymorphisms, such as BsmI, FokI, and TaqI, associated with an increased risk of renal diseases, including end-stage renal disease (Xu et al., 2022). These genetic insights not only deepen our understanding of the disease etiology but also pave the way for personalized medicine approaches in renal pathology.
The accumulating evidence highlights a significant yet complex relationship between VDR and kidney diseases. It urges the scientific community to delve deeper into this nexus, exploring how VDR modulation can be effectively harnessed in therapeutic strategies. This endeavor demands a multi-dimensional approach, integrating molecular biology, genetics, and clinical research to unravel the connection of VDR in renal health and disease. The ultimate goal is to translate these findings into clinically effective, patient-centric therapies, offering new hope in the battle against kidney diseases. As such, the path ahead is laden with opportunities for groundbreaking research and innovative therapeutic developments, beckoning researchers to explore this promising and largely uncharted research field.
4 Therapeutic applications of TCM and bioactive compounds in VDR-dependent cancer diseases
TCM has a history spanning over two thousand years, offering a unique perspective on health, disease, and healing that differs from Western medicine. TCM embraces a holistic approach, viewing the body as an interconnected system rather than a collection of individual parts. Its principles are rooted in the concept of balance and harmony, emphasizing the importance of the body’s ability to self-regulate and heal (Zou, 2016). In recent years, the relationship between TCM and modern science has garnered significant attention. On a yearly basis, several scientific investigations come out to demonstrate that, indeed, TCM formulae, herbs, and bioactive compounds exert their therapeutic outcomes by targeting several regulating several diseased biomolecules, hence improving health and alleviating disease (Liu et al., 2014; Zhou et al., 2021). Similarly, studies have revealed that TCM formulae, herbs, and compounds also possess the ability to influence the activity of the VDR (Kuai et al., 2020; Chen et al., 2021; Xiong et al., 2022). The discovery of VDR-modulating properties in TCM formulae components, herbs, and TCM compounds opens up new avenues to uncover novel therapeutic approaches for VDR-dependent human diseases, potentially offering safer and more effective treatment options.
4.1 VDR-modulating traditional Chinese medicine formulae and herbs: examples and mechanisms
TCM, with its diverse array of formulas and herbs, has been discovered to interact with and modulate VDR activity in ways that are crucial for the alleviation or improvement of VDR-dependent diseases (Xiong et al., 2022). TCM formulas and herbs have been used in numerous clinics to treat, for example, bone and skin disorders (Si et al., 2018; Wu et al., 2018; Wang et al., 2020). In our world today, particularly in the aging population, for instance, TCM has emerged as an economical alternative to well-known western medicines for bone discomfort and aging skin. This is so because, in various VDR-dependent diseases such as psoriasis, osteomalacia, joint sprains, osteoporosis, inflammatory disorders, and even broken bones, topical applications of TCM formulas or herbal remedies have proven beneficial (Li et al., 2017; Zhang et al., 2022a). For example, clinical studies using TCM for the management of musculoskeletal disorders, particularly bone fractures, osteoporosis, and rheumatoid arthritis, have reported some significant success (Li et al., 2007; He et al., 2008; Xu et al., 2023). In addition, it has been shown that some TCM natural products investigated in laboratory settings for the treatment of osteoporosis appear to have both anabolic and anticatabolic effects, enhancing osteogenesis and reducing unbalanced osteoclast activity, resulting in improved bone mineral density and biomechanical properties, and reduced bone microstructural degeneration (An et al., 2016). These impressive findings suggest that TCM formulas and herbs act at the molecular level to influence, for example, osteoporosis-dependent genes, particularly VDR, to promote these beneficial effects. As seen in Table 1, a comprehensive list of TCM formulas and herbs have been studied for their ability to modulate VDR activity. These TCM interventions range from single herbs to complex formulas, each providing unique insights into how VDR activity can be influenced to alleviate VDR-dependent diseases such as osteoporosis, cancer, psoriasis, diabetes, etc.
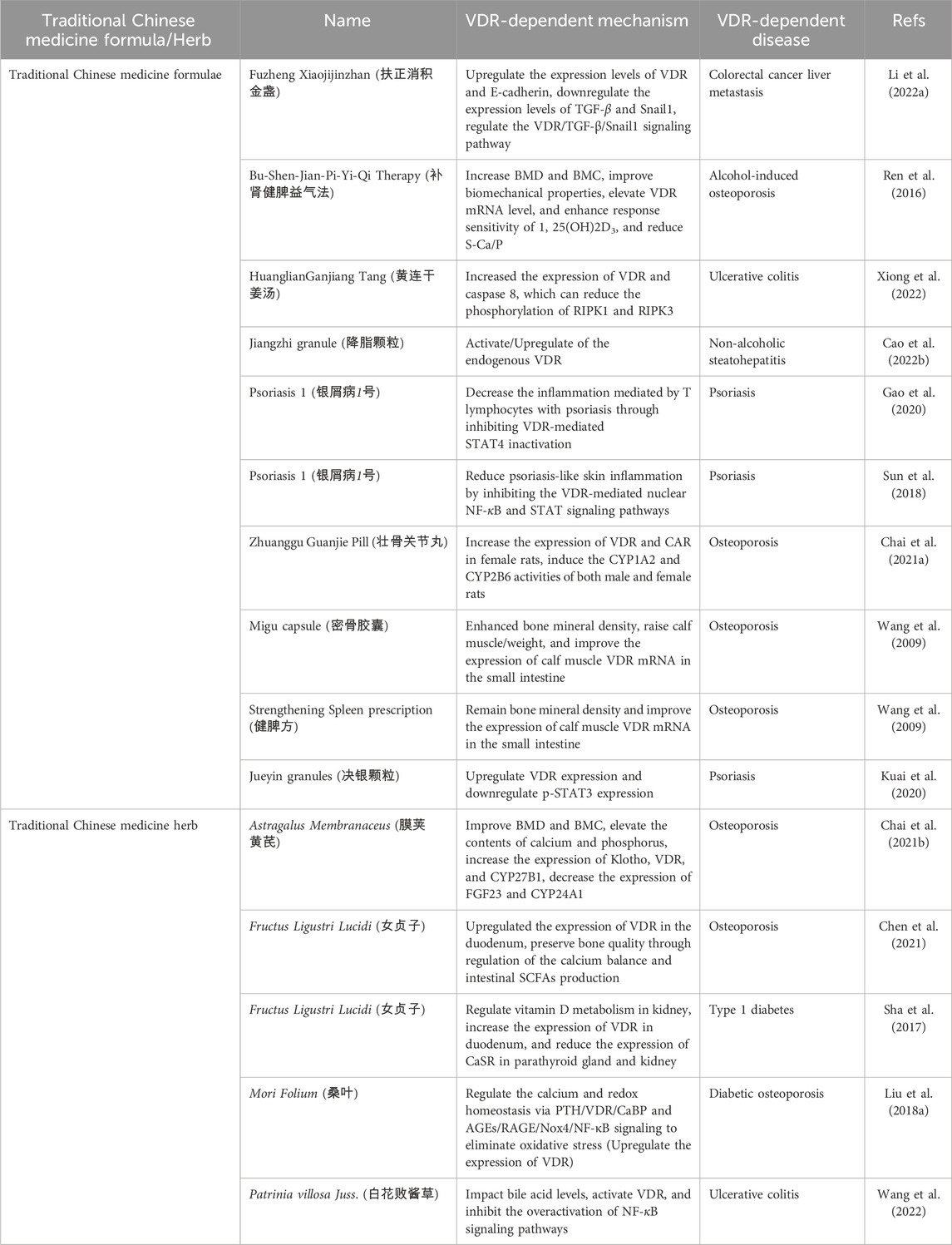
TABLE 1. Traditional Chinese medicine formulas and herbs target VDR activity to improve VDR-dependent diseases.
5 Natural bioactive compounds targeting VDR activity
The active natural metabolite of vitamin D3, 1α,25-dihydroxy vitamin D3, binds to the VDR-ligand binding domain, initiating physiological responses linked to VDR stimulation. This interaction has been discovered to have numerous positive effects on health and disease. Therefore, scientists have been striving to create chemical alterations that can produce active analogs of 1α,25-dihydroxy vitamin D3 with the desired pharmacokinetic and pharmacodynamic profiles, as well as minimal toxicity. In the past, these endeavors were based on “trial and error” or “educated guess” methods, but this changed in 2000 when the first X-ray crystal structure of the VDR-ligand binding domain bound to 1α,25-dihydroxy vitamin D3 was deciphered. This significant breakthrough allowed for a more targeted and effective approach to the development of 1α,25-dihydroxy vitamin D3 analogs (Christakos et al., 2016). Consequently, the crystal structure of the VDR-1α,25-dihydroxy vitamin D3 complex sparked a revolution in the rational design of second-generation 1α,25-dihydroxy vitamin D3 analogs.
To date, approximately 5,000 synthetic or semi-synthetic 1α,25-dihydroxy vitamin D3 analogs have been developed by pharmaceutical and academic research institutions. These analogs can be classified based on the chemical modifications made to 1α,25-dihydroxy vitamin D3 structural motifs, including A-ring analogs, Seco B-ring analogs, CD-ring analogs, and side chain analogs (Figure 5). Most of these analogs have been demonstrated to have reduced calcemic side effects and potent therapeutic effects that are similar to or better than those of the parent 1α,25-dihydroxy vitamin D3 compound in cell cultures, animal models, and several human diseases. In addition to the steroidal VDR modulators, nonsteroidal VDR modulators, including LY2108491, LY2109866, and LG190119, have also been reported. Their efficacy in diseases is currently limited to studies using laboratory-adapted animal species (Maestro et al., 2019). However, it is expected that more nonsteroidal compounds capable of modulating the activity of VDR will be discovered in the future, potentially offering promising therapeutic prospects for VDR-dependent human diseases.
5.1 TCM-based bioactive compounds and VDR activity
TCM-based compounds refer to the bioactive molecules derived from medicinal herbs and other source formulations used in TCM-based clinics. These compounds encompass a broad range of chemical substances belonging to the phytochemicals, such as alkaloids, flavonoids, saponins, polysaccharides, and terpenoids, among others. Examples of TCM-based compounds include artemisinin, which is extracted from the plant Artemisia annua and is used as a potent antimalarial drug, and berberine, which is found in several plants used in TCM and has been shown to have antibacterial, anti-inflammatory, neuroprotective and anti-diabetic effects (Wang et al., 2017; Feng et al., 2020). Other examples include ginsenosides from ginseng, which have a variety of therapeutic effects, and curcumin from turmeric, known for its anti-inflammatory and antioxidant properties via the radical scavenging activity (Hung et al., 2021). The therapeutic potential of TCM-based bioactive compounds is vast and continually being explored. Their diverse chemical scaffolds and biological activities make them an excellent source of novel drugs or therapeutic agents against numerous human diseases. Because, these bioactive compounds have been found to possess various pharmacological properties, including modulating cellular proteins or enzymes activity at the cellular and molecular levels (Zhang et al., 2019; Yang et al., 2020).
As discussed earlier, dysregulation or alterations in VDR activity have been implicated in a variety of diseases, including osteoporosis, autoimmune disorders, and certain types of cancer. Therefore, targeting VDR activity has therapeutic potential in the treatment of VDR-dependent diseases. Recent research indicates that certain TCM-based compounds can interact with and modulate VDR activity, presenting a promising avenue for the treatment of VDR-dependent diseases. For instance, some TCM-based bioactive compounds might enhance VDR gene expression or antagonize VDR receptor, thereby positively or negatively influencing the biological processes regulated by VDR and potentially ameliorating the symptoms or progression of VDR-dependent diseases like bone diseases, neurodegenerative diseases, etc. (Ding et al., 2021; Luo et al., 2022). Moreover, considering that low vitamin D levels and VDR Fokl variants are linked to sepsis susceptibility (Yang et al., 2022), a study on the TCM-based bioactive compound emodin demonstrated that emodin treatment can increase both mRNA and protein expression of VDR and its downstream molecules. It can also inhibit the expressions of TNF-α, IL-6, and MDA in serum and tissue and increase the levels of SOD and GSH. This regulation of the VDR/Nrf2/HO-1 signaling pathway can improve immune function and protect against sepsis-induced intestinal barrier damage (Shang et al., 2021).
Below, we delve deeper into the intriguing world of TCM-based bioactive compounds and their impact on VDR activity. We explore how these bioactive substances, rooted in centuries of traditional use, can offer novel solutions to improve the outcomes of VDR-dependent diseases. This discussion sheds light on the untapped potential of TCM-based bioactive compounds in addressing complex metabolic health challenges through the lens of VDR signaling and activity.
1. Astragalus polysaccharide: The Astragalus membranaceus plant, also known as Huang Qi, belongs to the family Fabaceae and is native to China. The roots of A. membranaceus are used in TCM for their immune-modulating, cardiotonic, hepatoprotective, and diuretic properties (Liu et al., 2018b). Astragalus polysaccharide is a high molecular weight heteropolysaccharide compound isolated from the A. membranaceus plant, which is commonly used in TCM. This polysaccharide is made up of various monosaccharides, including glucose, galactose, and arabinose, among others. It is a member of the polysaccharide class of phytochemicals.
The pharmacological activity of astragalus polysaccharide has been extensively studied. It has been shown to have antioxidant, anti-inflammatory, antiviral, and immunomodulatory properties (Li et al., 2022b). Additionally, Astragalus polysaccharide has shown immense potential in the treatment of various diseases, including cancer, diabetes, cardiovascular diseases, and liver diseases (Li et al., 2022b). Its efficacy extends not only to alleviating these conditions but also to combating sepsis, as revealed by a recent study (Li et al., 2022c). This study suggested that the upregulation of adrenocortical VDR activity and vitamin D levels might be the mechanism by which astragalus polysaccharide improves sepsis outcomes.
Using a septic rat model, the authors found that treatment with astragalus polysaccharide led to an upregulation of the vitamin D metabolic axis. This upregulation had a dual effect: it suppressed the expression of inflammatory cytokines while promoting the production of adrenal cortical proteins such as VDR. The latter is especially significant as these proteins play a crucial role in modulating the gut microbiota flora, specifically, those species that produce short-chain fatty acids. These fatty acids can directly or indirectly mitigate sepsis processes and renal damage induced by sepsis (Li et al., 2022c). This is consistent with a previous study that also show the protective effects of astragalus polysaccharide on sepsis-indued acute kidney injury (Sun et al., 2021). Importantly, the significance of these findings are underscored by the established correlation between vitamin D levels and disease severity, including mortality (Parekh et al., 2017). Patients with vitamin D deficiency have a higher probability of developing sepsis and acute renal failure compared to healthy individuals (Olejarova et al., 2019). Thus, the potential of astragalus polysaccharide to upregulate vitamin D activity makes it a promising target for improving sepsis outcomes.
However, the path to developing astragalus polysaccharide into a therapeutic drug is not without its challenges. A critical hurdle lies in the standardization of the plant material, given the complex phytochemical mixture found in A. membranaceus (Fu et al., 2014). Furthermore, the current lack of well-designed human clinical trials evaluating the efficacy and safety of astragalus polysaccharide presents another significant obstacle. Despite these challenges, promising results from preclinical studies advocate for more comprehensive human-based studies. This further investigation is vital to truly unlock and harness the full therapeutic potential of astragalus polysaccharide.
2. Bufalin: Is a cardiotonic digoxin-like steroid that is extracted from the skin and parotid venom glands of the Chinese toad species Bufo melanostictus and Bufo gargarizans, also known as Chan Su. This TCM material has been used for centuries as a painkiller and for the treatment of heart failure, cancer, and other diseases (Long et al., 2022). Bufalin is one of the major active components of Chan Su, alongside other bufadienolides such as bufalon, resibufagin, gambufotalin, bufataline, and cinobufotalin (Wei et al., 2019). Bufalin has a chemical structure consisting of a five-member lactone ring with a C-24 steroid backbone and four fused rings. It is a member of the cardiotonic steroid class of phytochemicals. Bufalin’s pharmacological activities have been widely studied, including anti-inflammatory, anti-angiogenic, and anti-viral activities, as well as effects on the cardiovascular and nervous systems (Tsai et al., 2012; Wei et al., 2019). Most importantly, bufalin has shown therapeutic potential in the treatment of various types of cancers, including liver cancer, pancreatic cancer, and breast cancer, because of its ability to act at the molecular level to arrest cell proliferation and induce several forms of cell death, including apoptosis and necroptosis (Soumoy et al., 2022).
Clinical studies have demonstrated that a deficiency in vitamin D is connected to an elevated risk of cardiovascular disease and cancer (see the above discussion). Therefore, vitamin D’s regulatory role extends beyond bone and mineral homeostasis to include cardiovascular and metabolic functions, suggesting a cooperative mechanism with cardiotonic steroids within the body. Bufalin has been proposed as a valuable tool for exploring VDR regulation by signals originating from the cell membrane and for understanding the pathophysiological mechanisms that link VDR to cardiovascular dysfunction and cancer (Nakano et al., 2005; Amano et al., 2009). Pre-clinical evidence on bufalin suggests that bufalin, potentially in combination with 1α,25-dihydroxy vitamin D3 or its synthetic analogs, could have therapeutic value in the treatment of myeloid leukemia (Amano et al., 2009). The authors found that bufalin treatment stabilized the nuclear expression of VDR and enhanced the expression of the VDR-target gene Cyp24A1 and cathelicidin antimicrobial peptide. They also found that bufalin effectively enhanced VDR activity induced by 1α,25-dihydroxy vitamin D3 (Nakano et al., 2005) and also expanded the levels of nuclear VDR expression by inhibiting its degradation. However, this effect was not observed in other cell types, such as THP-1 and U937 cells, suggesting that the effect of bufalin on nuclear VDR expression might be cell-type dependent. In addition to bufalin modulating the interaction of VDR and its cofactors, its treatment inhibited the proliferation of HL60 cells and enhanced their differentiation when induced by 1α,25-dihydroxy vitamin D3 (Nakano et al., 2005; Amano et al., 2009).
Despite bufalin’s therapeutic potential, there are challenges associated with its development into a drug. One challenge is its toxicity to normal cells and low solubility in aqueous solutions adds to the challenge. Nevertheless, preclinical studies have shown promising results, and further studies are warranted to unlock the full therapeutic potential of Bufalin.
3. Tanshinone IIA: Is a natural compound that is isolated from the root of Salvia miltiorrhiza, also known as Danshen, a traditional Chinese medicinal herb. The roots of Danshen have been used for centuries for the treatment of cardiovascular and cerebrovascular diseases and several other conditions (Li et al., 2018). Tanshinone IIA belongs to a group of phytochemicals known as diterpenoids, which are organic compounds with a diverse array of chemical structures. Tanshinone IIA contains a quinone substructure in its chemical structure, which gives it unique pharmacological properties (Ansari et al., 2021). A wealth of literature provides thorough reviews on the extensive range of pharmacological effects tanshinone IIA exerts on various bodily systems. These effects encompass anti-inflammatory, anti-tumor, anti-oxidative, and antimicrobial activities (Ansari et al., 2021; Bi et al., 2021; Subedi and Gaire, 2021). Moreover, preclinical studies have demonstrated the ability of tanshinone IIA to deter platelet aggregation and enhance blood circulation, thereby suggesting its potential utility in managing cardiovascular diseases. Such an outcome has proven beneficial in mitigating myocardial injury and reducing short-term cardiovascular events. Specifically, this has been observed in individuals with non-ST elevation acute coronary syndrome who underwent percutaneous coronary intervention and were administered sodium tanshinone IIA sulfonate (Mao et al., 2021). In addition, tanshinone IIA has been shown to have neuroprotective effects, which could make it useful in the treatment of neurodegenerative diseases like Alzheimer’s disease (Subedi and Gaire, 2021).
Years of research have revealed that tanshinone IIA may ameliorate diabetes and its associated complications, such as diabetic kidney diseases (Ansari et al., 2021). A recent study offered mechanistic insight into this process, demonstrating that high glucose hindered cell proliferation and spurred the transition of renal tubular epithelial cells to mesenchymal cells in HK-2 samples. Remarkably, the introduction of tanshinone IIA was able to reverse this high glucose-induced transition. It accomplished this by elevating the levels of VDR protein while concurrently reducing the levels of β-catenin and GSK-3b proteins, suggesting a potential inverse relationship between VDR and the Wnt/β-catenin pathway. This hypothesis was further validated by the team using VDR-siRNA transfection, where they found out that when cells were exposed to high glucose, the β-catenin pathway. However, upon the restoration of VDR levels through the introduction of tanshinone IIA, the inhibitory effect of VDR on the pathway was recovered, and the pathway’s activation was suppressed (Zeng and Bao, 2021). These findings indicate that tanshinone IIA may modulate VDR expression levels either directly or indirectly, which in turn may help mitigate cardiometabolic diseases, such as diabetic kidney diseases. However, the challenges of developing tanshinone IIA into a drug include its poor solubility in water and low bioavailability, which make it difficult to deliver effectively to the body.
4. Vitexin: Popularly referred to as ‘Mujingsu’ in Chinese, is a natural flavonoid compound that is found in a wide variety of Chinese medicinal plants. It is particularly abundant in the leaves of the Vitex negundo plant, also known as the Five-leaved Chaste Tree, which is a traditional Chinese medicinal herb used in the treatment of inflammation, infection, and other conditions (Liang et al., 2023). Vitexin has a chemical structure characterized by a flavone substructure, which belongs to the flavonoid family. It has been found to have a wide range of pharmacological activities, including anti-inflammatory, antioxidant, anti-tumor, and neuroprotective effects (Liu et al., 2018c; Zhao et al., 2021; Chen et al., 2022). Studies have also shown that vitexin has potential therapeutic effects for metabolic conditions such as liver injury and diabetes (Akter et al., 2021; Noor et al., 2022).
Several compelling studies suggest a potential role for vitexin in the treatment of osteoporosis, a disease characterized by weakened bones. For instance, reports indicate that vitexin inhibits RANKL-induced osteoclast formation, a process associated with bone resorption, and impedes lipopolysaccharide-induced osteolysis, thus preserving bone integrity (Jiang et al., 2019). Furthermore, vitexin has shown the ability to promote the proliferation of osteoblasts and increase calcium deposition in osteoblast-like cells (Lin et al., 2020). Such evidence points to vitexin’s potential to restore bone microarchitecture, particularly in osteoporosis. This potential was further demonstrated by a recent study that reported that vitexin’s positive impact on osteoblast biochemical indicators such as VEGFA, ALP, and Runx2 in the ovariectomized rat model (Liu et al., 2023a). Additionally, the authors found that vitexin appears to foster angiogenesis, the formation of new blood vessels, an essential process for bone health and regeneration. This dual role in promoting angiogenesis and osteogenesis suggests that vitexin could facilitate a process known as angiogenesis-dependent osteogenesis, which is crucial for bone health (Liu et al., 2023a).
Notably, vitexin was found to increase the expression of VDR in the femur tissue of osteoporosis rats (Liu et al., 2023a). VDR is known to be a significant target for osteoporosis treatment, and this increase in expression suggests that vitexin might exert its bone-protective effects through VDR modulation. Further supporting this idea, the authors show that vitexin directly binds to VDR with good affinity (Liu et al., 2023a). The VDR/eNOS signaling pathway is another critical player in angiogenesis and osteogenesis regulation, and vitexin seems to operate at least in part through this pathway. By activating the VDR/eNOS signaling, vitexin may promote bone angiogenesis, which in turn supports osteogenesis, thereby offering a potential strategy for osteoporosis treatment (Liu et al., 2023a). Therefore, vitexin’s apparent interaction with the VDR/eNOS signaling pathway and the subsequent effects on angiogenesis-dependent osteogenesis further solidifies vitexin’s potential as a novel therapeutic strategy for osteoporosis.
5. Asperuloside: Is a natural iridoid glucoside compound that is found in several plant species, including Asperula odorata, Galium odoratum, and Galium verum. These plants are commonly used in TCM and European folk medicine for treating various conditions, including inflammation, pain, and infection (Manzione et al., 2020). Characterized by its iridoid glycoside substructure, asperuloside is also considered a member of O-glycosyl compounds, which are defined by the presence of an O-glycosidic bond. Asperuloside is highly water-soluble and has a weakly acidic nature (Cha et al., 2020). Like other bioactive glucosides, asperuloside has been found to have a range of pharmacological activities, including anti-inflammatory, antioxidant, anti-obesity, and laxative effects (Cha et al., 2020; Nakamura et al., 2020). Studies have also shown that asperuloside has potential therapeutic effects for conditions such as cancer (Qi et al., 2023).
VDR signaling pathway has a crucial role in several physiological activities, such as bone development, anti-inflammatory response, and anti-tumor activities (Shang and Sun, 2017). Notably, a decrease in both the serum vitamin D levels and the expression of VDR in the colon has been observed in patients with colitis or colorectal cancer (Chen and Jin, 2022; Ma et al., 2023). In a study using a mouse model of colitis-associated cancer, treatment with asperuloside was found to significantly enhance the expression of VDR in the colon, leading to marked reductions in colitis symptoms and a decrease in both the quantity and size of tumors (Lu et al., 2022). This highlights the potential of asperuloside as a promising therapeutic agent for colorectal cancer. In-depth in-vitro investigations revealed that asperuloside not only increases VDR expression but also reduces Smad3 mRNA levels, thereby suggesting its potential to inhibit the development of the epithelial-mesenchymal transition via VDR regulation (Lu et al., 2022). Intriguingly, asperuloside was observed to enhance VDR signaling and suppress Smad3 mRNA in NF-κB knockout cells, implying that asperuloside may inhibit Smad3 mRNA through VDR signaling activation, independent of NF-kB signaling. This conclusion aligns with the observed reduction in the transformation from the epithelial phenotype to the more motile mesenchymal phenotype, along with a decrease in epithelial-mesenchymal transition markers (Lu et al., 2022). These findings underscore the potential of asperuloside to prevent colitis-associated cancer by hindering the development of EMT, a pivotal process in the disease’s progression. This beneficial effect is achieved through the regulation of the VDR/Smad3 pathway, further underscoring asperuloside’s potential in the fight against colitis-associated cancer.
6. Berberine: Berberine is an isoquinoline alkaloid that is found in many plant species, including Berberis aristata, Coptidis rhizoma, and Hydrastis canadensis. These plants are commonly used in TCM and Ayurvedic medicine for treating various conditions, including infections, inflammation, and metabolic disorders such as diabetes and biological aging (Wang et al., 2019). Berberine, in particular, is a popular natural compound that has been studied extensively and found to have a wide range of pharmacological activities, including but not limited to anti-inflammatory, antioxidant, anti-hyperglycemia, and antitumor effects (Wang et al., 2017). Study reports that have surfaced have demonstrated that berberine possesses potential therapeutic benefits for conditions mainly including cardiovascular disease and metabolic disorders. This is attributed to berberine’s capability to interact with key metabolic molecules, specifically AMPK, SIRT1, LDLR, PCSK9, and PTP1B (Feng et al., 2019). Berberine has shown promising effects in the context of alleviating irritable bowel syndrome (IBS) (Hou et al., 2019). VDR plays a crucial role in the homeostasis of the mucosal barrier by maintaining the integrity of junction complexes and promoting the healing capacity of the colonic epithelium. This is evident from studies showing that VDR-deficient mice are more prone to mucosal injury induced by dextran sulfate sodium (Kong et al., 2008). Also, the treatment with 1α,25-dihydroxy vitamin D3, an active form of Vitamin D, enhances tight junctions in Caco-2 monolayers, a model of human intestinal cells, by increasing junction protein expression and transepithelial electric resistance (Kong et al., 2008). This vitamin D-dependent effect on junction integrity is vital, as reduced VDR expression has been shown to decrease junction proteins and intestinal transepithelial electric resistance.
In the context of IBS, particularly the diarrhea-predominant form (IBS-D), vitamin D deficiency may compromise the mucosal barrier, leading to increased susceptibility to mucosal damage and worsening symptoms. In clinical studies, IBS-D patients receiving vitamin D supplementation experienced significant improvements in symptom severity, quality of life, hospital anxiety and depression, and visceral sensitivity index scores compared to a placebo group (Khalighi Sik et al., 2020). In a rat model of IBS-D, berberine improved symptoms in a dose-dependent manner. Importantly, berberine effectively promoted the activity of the vitamin D receptor response element promoter, enhancing the expression of tight junction proteins in colonic epithelial cells (Huang et al., 2023). The upregulated proteins included occludin and zonula occludens-1, crucial components of the intestinal mucosal barrier. This suggests that berberine can enhance the intestinal mucosal barrier function in IBS-D, primarily through promoting VDR activity. Importantly, it was found that berberine primarily targets the N-terminal region of the VDR to regulate VDR activity (Huang et al., 2023). These findings show that berberine represents a novel and potentially effective approach for treating IBS-D via targeting VDR.
7. Astragaloside: This is a natural bioactive compound that is extracted from the root of Astragalus membranaceus, a traditional Chinese herb commonly used in herbal formulations for various medicinal purposes (Yu et al., 2022). Astragaloside (3-O-β-D-xylopyranosyl-6-O-β-D-glucopyranosyl-cycloastragenol) has a complex chemical structure consisting of a triterpenoid backbone with a cycloartane structure, which belongs to the saponin family. Astragaloside has been found to have a range of pharmacological activities, including antioxidant, anti-inflammatory, and immunomodulatory effects (Zhou et al., 2023). Some meta-analyses focused on clinical and preclinical studies also indicate that astragaloside may offer potential treatment benefits for conditions including hepatic fibrosis, heart failure, neurological diseases, diabetes, and cancer (Costa et al., 2019; Xiao et al., 2020; Zhou et al., 2020; Han et al., 2021; Li et al., 2023). Further evidence is mounting to suggest that astragaloside’s anti-cancer properties are largely delivered through mechanisms that promote apoptosis and autophagy while simultaneously impeding cell growth, invasion, movement, and spread. In addition, astragaloside appears to modulate the tumor microenvironment, influencing factors like new blood vessel formation and both innate and adaptive immune responses (Zhou et al., 2023).
The use of laboratory-adapted Vdr KO animal models and cardiovascular tissue-specific Vdr null mice has revealed key insights into the role of vitamin D signaling in the cardiovascular system. Notably, mice lacking Vdr or Cyp27B1 exhibited elevated renin levels, leading to an increase in angiotensin II, which in turn resulted in hypertension and cardiac hypertrophy. Furthermore, aged normocalcemic Vdr null mice on a rescue diet developed a series of cardiovascular complications, including endothelial dysfunction, increased arterial stiffness, augmented aortic impedance, and compromised systolic and diastolic functions. These issues are believed to be mechanistically linked to the lack of Vdr signaling, which chronically reduces the bioavailability of nitric oxide (NO)—a potent vasodilator—through decreased expression of NO synthesizing enzyme (Christakos et al., 2016). In light of these findings, astragaloside has been found to counteract the effects of endothelin-1 (ET-1), which induces hypertrophy and apoptosis in cardiomyocytes. Specifically, the investigation revealed that astragaloside treatment led to a dose-dependent increase in VDR and CYP27B1 mRNA expression levels to improve cardiomyocyte injury. The evident link between astragaloside and the modulation of VDR signaling strengthens the case for the viability of VDR as a potential target for cardiovascular disease treatment (Yunzhi et al., 2017). This also emphasizes the need for further exploration into the mechanisms of astragaloside’s effects, as it shows great promise for the treatment of cardiovascular diseases.
8. Parthenolide: Is a natural compound that is found in many plants, including feverfew (Tanacetum parthenium), leaf vegetables, and herbs such as chamomile. Feverfew has been used for centuries in traditional medicine to treat various conditions, including migraines, arthritis, and menstrual pain (Pareek et al., 2011). The chemical structure of parthenolide contains a lactone moiety and is classified as a germacrene sesquiterpene lactone. Parthenolide has been found to have a range of pharmacological activities, including anti-inflammatory, anticancer, redox-modulation, and neuroprotective effects (Freund et al., 2020). Studies have also shown that parthenolide has potential therapeutic effects for conditions such as leukemia, Alzheimer’s disease, and migraines (Materazzi et al., 2013; Yang et al., 2017; Fan et al., 2023). In addition to these diseases, parthenolide has promising effects on diabetes and its related complications (Liu et al., 2023b). Mounting evidence also has established a close relationship between VDR signaling and diabetes, as well as diabetic nephropathy and insulin resistance. For example, high serum levels of 1α,25-dihydroxy vitamin D3 decrease the risk for diabetic nephropathy (Chen et al., 2023). Besides, increasing VDR levels may alleviate proteinuria, renal fibrosis and inflammation and prevent podocyte damage (Zhang et al., 2010; Wang et al., 2012).
Treatment with parthenolide resulted in improvements in glomerular mesangial expansion, glomerular hypertrophy, and albuminuria. In addition, parthenolide effectively ameliorated diabetic nephropathy-associated complications, including renal tubular basement membrane thickening, renal fibrosis, proteinuria excretion, and high blood sugar levels. In a cellular context, parthenolide significantly improved high glucose-induced apoptosis and inflammation in MPC5 cells, a podocyte cell line. It was also observed to increase the expression levels of matrix-related proteins such as nephrin and podocin, key elements in maintaining podocyte integrity (Yan et al., 2022).
Parthenolide has shown potential as a DNA methylation inhibitor—it was observed to inhibit DNA methyltransferase 1 (DNMT1) (Liu et al., 2009), a noteworthy effect considering DNMT1 was found to be upregulated in diabetic nephropathy mice and high glucose-induced MPC5 cells (Yan et al., 2022). This upregulation contributes to the hypermethylation of the VDR gene, which is significantly associated with lower VDR gene expression levels and vitamin D levels (Guan et al., 2022). Consequently, in high glucose-induced podocytes, VDR protein expression was significantly reduced, while the methylation level of its promoter was increased. Parthenolide demonstrated its ability to promote VDR protein expression while inhibiting the methylation level of the VDR promoter. Parthenolide’s promotion of VDR expression led to the upregulation of the AKT phosphorylation level in HG-induced podocytes, a process that was reversed by the addition of the AKT inhibitor MK2206(193). The multifaceted capabilities of parthenolide, from its influence on diabetic nephropathy-associated complications to its modulation of VDR expression, position it as a potential therapeutic agent in managing diabetic nephropathy.
6 Challenges, limitations, and future directions
The VDR plays a leading role in many physiological processes in various biological systems. As an intracellular receptor for vitamin D, VDR helps regulate the genomic effects essential for maintaining general wellbeing, pointing to another reason why avoiding vitamin D deficiency is valuable to overall health. In addition, its wide distribution in different cells and tissues explains the numerous therapeutic functions of VDR modulation in different systems, subsequently implicating its alteration in different body system diseases. This also highlights that more VDR-dependent genes may be discovered in the future. These genes could provide insights into and solidify our understanding of how VDR contributes to overall health or causes VDR-dependent diseases—a spectrum of conditions that are either directly influenced by the activity of the VDR protein or indirectly affected through its regulatory impact on various physiological processes.
To date, there is insufficient evidence to support whether using vitamin D or its synthetic analogs can help treat common but detrimental non-communicable diseases such as cancers, diabetes, heart and vascular diseases, and many more. However, numerous observational clinical studies have established the relationship between vitamin D supplementation and these non-communicable diseases, hinting that there is a need to use appropriate disease models to delve into how VDR activity can be modulated to help treat these diseases. New advances in methods and bioinformatic tools are also providing a better understanding of the structural interactions between VDR and its protein partners, which will help explain the selective effects of VDR in different target tissues and how the expression of VDR target proteins can be controlled. While most known VDR modulators are steroids, nonsteroidal modulators have also been identified with strong therapeutic effects without causing high calcium levels in the blood.
TCM have been historically recognized for their multi-target, multi-component therapeutic effects. Interestingly, recent investigations have highlighted the potential of various TCM-derived bioactive compounds in modulating VDR activities, signifying a promising avenue for therapeutic applications in VDR-dependent diseases. Nevertheless, the use of TCM in VDR modulation presents its own set of challenges and limitations. One significant challenge lies in the complexity of TCM, given that each preparation typically comprises multiple bioactive compounds, each of which may interact differently with the VDR. Therefore, identifying the specific component responsible for the therapeutic effect could be laborious and complex. Additionally, there are limitations concerning the quality control of TCM preparations, the quantification of their active ingredients, and the standardization of treatment protocols. The interindividual variability in response to TCM therapies also presents a significant challenge. These differences could be attributed to genetic factors, diet, gut microbiota, and other environmental influences that can significantly affect the absorption, distribution, metabolism, and excretion of TCM compounds.
Moreover, the underlying mechanisms of how TCM modulates VDR are still largely unexplored, which limits our understanding on the therapeutic outcomes. A better understanding of these mechanisms will allow the development of more effective therapeutic strategies for VDR-dependent diseases. On the bright side, the integration of modern scientific techniques with traditional medical knowledge offers promising future directions. For example, utilizing advanced bioinformatics and molecular biology tools has helped elucidate the specific interactions between TCM compounds and VDR. These approaches have also aided in the identification and characterization of new TCM-based VDR modulators, enabling the design of more effective therapeutic strategies. This suggests that in the coming years, the drug discovery field of VDR research will continue to grow and provide new insights into novel TCM-based nonsteroidal modulators of VDR that will help us better appreciate the implications of VDR signaling in health and disease. However, to better have this sufficient evidence, studies must push beyond the primary in-vitro activity assays to more complex structural-activity study designs that seek to offer atomic-level knowledge about the dynamics between VDR and its activity-supporting partners and these TCM compounds. Such future advances may provide a rationale understanding for addressing most of the mechanistic issues associated with how the genomic effects of VDR are stimulated in the presence of TCM interventions.
Author contributions
MY: Writing–original draft. PO: Writing–original draft. AA: Writing–review and editing. HY: Writing–review and editing.
Funding
The author(s) declare financial support was received for the research, authorship, and/or publication of this article. This study was supported by HSRP-DFCTCM-2023.
Conflict of interest
The authors declare that the research was conducted in the absence of any commercial or financial relationships that could be construed as a potential conflict of interest.
Publisher’s note
All claims expressed in this article are solely those of the authors and do not necessarily represent those of their affiliated organizations, or those of the publisher, the editors and the reviewers. Any product that may be evaluated in this article, or claim that may be made by its manufacturer, is not guaranteed or endorsed by the publisher.
References
Abouzid, M., Kruszyna, M., Burchardt, P., Kruszyna, Ł., Główka, F. K., and Karaźniewicz-Łada, M. (2021). Vitamin D receptor gene polymorphism and vitamin D status in population of patients with cardiovascular disease-A preliminary study. Nutrients 13 (9), 3117. doi:10.3390/nu13093117
Aghayeva, U., Bhattacharya, A., Sural, S., Jaeger, E., Churgin, M., Fang-Yen, C., et al. (2021). DAF-16/FoxO and DAF-12/VDR control cellular plasticity both cell-autonomously and via interorgan signaling. PLoS Biol. 19 (4), e3001204. doi:10.1371/journal.pbio.3001204
Akter, R., Afrose, A., Rahman, M. R., Chowdhury, R., Nirzhor, S. S. R., Khan, R. I., et al. (2021). A comprehensive analysis into the therapeutic application of natural products as SIRT6 modulators in Alzheimer’s disease, aging, cancer, inflammation, and diabetes. Int. J. Mol. Sci. 22 (8), 4180. doi:10.3390/ijms22084180
Amano, Y., Cho, Y., Matsunawa, M., Komiyama, K., and Makishima, M. (2009). Increased nuclear expression and transactivation of vitamin D receptor by the cardiotonic steroid bufalin in human myeloid leukemia cells. J. Steroid Biochem. Mol. Biol. 114 (3-5), 144–151. doi:10.1016/j.jsbmb.2009.01.022
An, J., Yang, H., Zhang, Q., Liu, C., Zhao, J., Zhang, L., et al. (2016). Natural products for treatment of osteoporosis: the effects and mechanisms on promoting osteoblast-mediated bone formation. Life Sci. 147, 46–58. doi:10.1016/j.lfs.2016.01.024
Ansari, M. A., Khan, F. B., Safdari, H. A., Almatroudi, A., Alzohairy, M. A., Safdari, M., et al. (2021). Prospective therapeutic potential of Tanshinone IIA: an updated overview. Pharmacol. Res. 164, 105364. doi:10.1016/j.phrs.2020.105364
Antony, P., Sigüeiro, R., Huet, T., Sato, Y., Ramalanjaona, N., Rodrigues, L. C., et al. (2010). Structure-function relationships and crystal structures of the vitamin D receptor bound 2 alpha-methyl-(20S,23S)- and 2 alpha-methyl-(20S,23R)-epoxymethano-1 alpha,25-dihydroxyvitamin D3. J. Med. Chem. 53 (3), 1159–1171. doi:10.1021/jm9014636
Apaydın, M., Beysel, S., Eyerci, N., Pinarli, F. A., Ulubay, M., Kizilgul, M., et al. (2019). The VDR gene FokI polymorphism is associated with gestational diabetes mellitus in Turkish women. BMC Med. Genet. 20 (1), 82. doi:10.1186/s12881-019-0820-0
Arapović, A., Vukojević, K., Glavina Durdov, M., Benzon, B., Šolić, I., Racetin, A., et al. (2021). Expression of renal vitamin D receptors and metabolizing enzymes in IgA nephropathy. Acta Histochem. 123 (5), 151740. doi:10.1016/j.acthis.2021.151740
Arriagada, G., Paredes, R., Olate, J., van Wijnen, A., Lian, J. B., Stein, G. S., et al. (2007). Phosphorylation at serine 208 of the 1alpha,25-dihydroxy Vitamin D3 receptor modulates the interaction with transcriptional coactivators. J. Steroid Biochem. Mol. Biol. 103 (3-5), 425–429. doi:10.1016/j.jsbmb.2006.12.021
Bai, J., Kwok, W. C., and Thiery, J. P. (2019). Traditional Chinese Medicine and regulatory roles on epithelial-mesenchymal transitions. Chin. Med. 14, 34. doi:10.1186/s13020-019-0257-6
Bakdash, G., van Capel, T. M., Mason, L. M., Kapsenberg, M. L., and de Jong, E. C. (2014). Vitamin D3 metabolite calcidiol primes human dendritic cells to promote the development of immunomodulatory IL-10-producing T cells. Vaccine 32 (47), 6294–6302. doi:10.1016/j.vaccine.2014.08.075
Baker, A. R., McDonnell, D. P., Hughes, M., Crisp, T. M., Mangelsdorf, D. J., Haussler, M. R., et al. (1988). Cloning and expression of full-length cDNA encoding human vitamin D receptor. Proc. Natl. Acad. Sci. U. S. A. 85 (10), 3294–3298. doi:10.1073/pnas.85.10.3294
Bakke, D., Chatterjee, I., Agrawal, A., Dai, Y., and Sun, J. (2018). Regulation of microbiota by vitamin D receptor: a nuclear weapon in metabolic diseases. Nucl. Recept. Res. 5, 101377. doi:10.11131/2018/101377
Bakke, D., and Sun, J. (2018). Ancient nuclear receptor VDR with new functions: microbiome and inflammation. Inflamm. Bowel Dis. 24 (6), 1149–1154. doi:10.1093/ibd/izy092
Barragan, M., Good, M., and Kolls, J. K. (2015). Regulation of dendritic cell function by vitamin D. Nutrients 7 (9), 8127–8151. doi:10.3390/nu7095383
Barsony, J., and Prufer, K. (2002). Vitamin D receptor and retinoid X receptor interactions in motion. Vitam. Horm. 65, 345–376. doi:10.1016/s0083-6729(02)65071-x
Bi, Z., Wang, Y., and Zhang, W. (2021). A comprehensive review of tanshinone IIA and its derivatives in fibrosis treatment. Biomed. Pharmacother. 137, 111404. doi:10.1016/j.biopha.2021.111404
Breslavsky, A., Frand, J., Matas, Z., Boaz, M., Barnea, Z., and Shargorodsky, M. (2013). Effect of high doses of vitamin D on arterial properties, adiponectin, leptin and glucose homeostasis in type 2 diabetic patients. Clin. Nutr. Edinb. Scotl. 32 (6), 970–975. doi:10.1016/j.clnu.2013.01.020
Campbell, F. C., Xu, H., El-Tanani, M., Crowe, P., and Bingham, V. (2010). The yin and yang of vitamin D receptor (VDR) signaling in neoplastic progression: operational networks and tissue-specific growth control. Biochem. Pharmacol. 79 (1), 1–9. doi:10.1016/j.bcp.2009.09.005
Cao, L., Xu, E., Zheng, R., Zhangchen, Z., Zhong, R., Huang, F., et al. (2022a). Traditional Chinese medicine Lingguizhugan decoction ameliorate HFD-induced hepatic-lipid deposition in mice by inhibiting STING-mediated inflammation in macrophages. Chin. Med. 17 (1), 7. doi:10.1186/s13020-021-00559-3
Cao, Y., Shu, X., Li, M., Yu, S., Li, C., Ji, G., et al. (2022b). Jiangzhi granule attenuates non-alcoholic steatohepatitis through modulating bile acid in mice fed high-fat vitamin D deficiency diet. Biomed. Pharmacother. 149, 112825. doi:10.1016/j.biopha.2022.112825
Carlberg, C. (2004). Ligand-mediated conformational changes of the VDR are required for gene transactivation. J. Steroid Biochem. Mol. Biol. 89-90, 227–232. doi:10.1016/j.jsbmb.2004.03.112
Carlberg, C., and Muñoz, A. (2022). An update on vitamin D signaling and cancer. Semin. Cancer Biol. 79, 217–230. doi:10.1016/j.semcancer.2020.05.018
Chai, Y., Pu, X., Wu, Y., Tian, X., Li, Q., Zeng, F., et al. (2021b). Inhibitory effect of Astragalus Membranaceus on osteoporosis in SAMP6 mice by regulating vitaminD/FGF23/Klotho signaling pathway. Bioengineered 12 (1), 4464–4474. doi:10.1080/21655979.2021.1946633
Chai, Y., Xu, Y., Xia, Z., Huang, X., Zhang, L., and Jiang, Z. (2021a). Study on the effects of Zhuanggu Guanjie Pill, a modern Chinese medicine formula, on the activities and mRNA expression of seven CYP isozymes in rats. J. Ethnopharmacol. 281, 114521. doi:10.1016/j.jep.2021.114521
Chan, Y., Ng, S. W., Xin Tan, J. Z., Gupta, G., Tambuwala, M. M., Bakshi, H. A., et al. (2020). Emerging therapeutic potential of the iridoid molecule, asperuloside: a snapshot of its underlying molecular mechanisms. Chem. Biol. Interact. 315, 108911. doi:10.1016/j.cbi.2019.108911
Chen, B., and Jin, L. (2022). Low serum level of 25-OH vitamin D relates to Th17 and treg changes in colorectal cancer patients. Immun. Inflamm. Dis. 10 (11), e723. doi:10.1002/iid3.723
Chen, B., Wei, J., Zhu, R., Zhang, H., Xia, B., Liu, Y., et al. (2021). Fructus Ligustri Lucidi aqueous extract promotes calcium balance and short-chain fatty acids production in ovariectomized rats. J. Ethnopharmacol. 279, 114348. doi:10.1016/j.jep.2021.114348
Chen, X., Wan, Z., Geng, T., Zhu, K., Li, R., Lu, Q., et al. (2023). Vitamin D status, vitamin D receptor polymorphisms, and risk of microvascular complications among individuals with type 2 diabetes: a prospective study. Diabetes Care 46 (2), 270–277. doi:10.2337/dc22-0513
Chen, Y., Yang, J., Huang, Z., Yin, B., Umar, T., Yang, C., et al. (2022). Vitexin mitigates Staphylococcus aureus-induced mastitis via regulation of ROS/ER stress/NF-κB/MAPK pathway. Oxid. Med. Cell Longev. 2022, 7977433. doi:10.1155/2022/7977433
Chi, Y., Hong, Y., Zong, H., Wang, Y., Zou, W., Yang, J., et al. (2009). CDK11p58 represses vitamin D receptor-mediated transcriptional activation through promoting its ubiquitin-proteasome degradation. Biochem. biophysical Res. Commun. 386 (3), 493–498. doi:10.1016/j.bbrc.2009.06.061
Christakos, S., Dhawan, P., Verstuyf, A., Verlinden, L., and Carmeliet, G. (2016). Vitamin D: metabolism, molecular mechanism of action, and pleiotropic effects. Physiol. Rev. 96 (1), 365–408. doi:10.1152/physrev.00014.2015
Costa, I. M., Lima, F. O. V., Fernandes, L. C. B., Norrara, B., Neta, F. I., Alves, R. D., et al. (2019). Astragaloside IV supplementation promotes A neuroprotective effect in experimental models of neurological disorders: a systematic review. Curr. Neuropharmacol. 17 (7), 648–665. doi:10.2174/1570159X16666180911123341
Crofts, L. A., Hancock, M. S., Morrison, N. A., and Eisman, J. A. (1998). Multiple promoters direct the tissue-specific expression of novel N-terminal variant human vitamin D receptor gene transcripts. Proc. Natl. Acad. Sci. U. S. A. 95 (18), 10529–10534. doi:10.1073/pnas.95.18.10529
Dai, X., Feng, J., Chen, Y., Huang, S., Shi, X., Liu, X., et al. (2021). Traditional Chinese Medicine in nonalcoholic fatty liver disease: molecular insights and therapeutic perspectives. Chin. Med. 16 (1), 68. doi:10.1186/s13020-021-00469-4
da Silva Teixeira, S., Harrison, K., Uzodike, M., Rajapakshe, K., Coarfa, C., He, Y., et al. (2020). Vitamin D actions in neurons require the PI3K pathway for both enhancing insulin signaling and rapid depolarizing effects. J. Steroid Biochem. Mol. Biol. 200, 105690. doi:10.1016/j.jsbmb.2020.105690
de Albuquerque Borborema, M. E., Oliveira, D. C., and de Azevedo Silva, J. (2021). Down regulation of VDR gene expression in metabolic syndrome and atherosclerosis’ patients: cause or consequence? Gene 771, 145341. doi:10.1016/j.gene.2020.145341
Deeb, K. K., Trump, D. L., and Johnson, C. S. (2007). Vitamin D signalling pathways in cancer: potential for anticancer therapeutics. Nat. Rev. Cancer 7 (9), 684–700. doi:10.1038/nrc2196
Ding, M. Y., Peng, Y., Li, F., Li, Z. Q., Wang, D., Zhou, G. C., et al. (2021). Andrographolide derivative as antagonist of vitamin D receptor to induce lipidation of microtubule associate protein 1 light chain 3 (LC3). Bioorg Med. Chem. 51, 116505. doi:10.1016/j.bmc.2021.116505
Esteban, L. M., Fong, C., Amr, D., Cock, T. A., Allison, S. J., Flanagan, J. L., et al. (2005). Promoter-cell-and ligand-specific transactivation responses of the VDRB1 isoform. Biochem. biophysical Res. Commun. 334 (1), 9–15. doi:10.1016/j.bbrc.2005.06.054
Fagerberg, L., Hallström, B. M., Oksvold, P., Kampf, C., Djureinovic, D., Odeberg, J., et al. (2014). Analysis of the human tissue-specific expression by genome-wide integration of transcriptomics and antibody-based proteomics. Mol. Cell Proteomics 13 (2), 397–406. doi:10.1074/mcp.M113.035600
Fan, M., Wang, C., Zhao, X., Jiang, Y., and Wang, C. (2023). Parthenolide alleviates microglia-mediated neuroinflammation via MAPK/TRIM31/NLRP3 signaling to ameliorate cognitive disorder. Int. Immunopharmacol. 120, 110287. doi:10.1016/j.intimp.2023.110287
Fang, Z., Tang, Y., Ying, J., Tang, C., and Wang, Q. (2020). Traditional Chinese medicine for anti-Alzheimer’s disease: berberine and evodiamine from Evodia rutaecarpa. Chin. Med. 15, 82. doi:10.1186/s13020-020-00359-1
Feng, X., Cao, S., Qiu, F., and Zhang, B. (2020). Traditional application and modern pharmacological research of Artemisia annua L. Pharmacol. Ther. 216, 107650. doi:10.1016/j.pharmthera.2020.107650
Feng, X., Sureda, A., Jafari, S., Memariani, Z., Tewari, D., Annunziata, G., et al. (2019). Berberine in cardiovascular and metabolic diseases: from mechanisms to therapeutics. Theranostics 9 (7), 1923–1951. doi:10.7150/thno.30787
Fleet, J. C., and Schoch, R. D. (2010). Molecular mechanisms for regulation of intestinal calcium absorption by vitamin D and other factors. Crit. Rev. Clin. Lab. Sci. 47 (4), 181–195. doi:10.3109/10408363.2010.536429
Freund, R. R. A., Gobrecht, P., Fischer, D., and Arndt, H. D. (2020). Advances in chemistry and bioactivity of parthenolide. Nat. Prod. Rep. 37 (4), 541–565. doi:10.1039/c9np00049f
Fu, J., Wang, Z., Huang, L., Zheng, S., Wang, D., Chen, S., et al. (2014). Review of the botanical characteristics, phytochemistry, and pharmacology of Astragalus membranaceus (Huangqi). Phytother. Res. 28 (9), 1275–1283. doi:10.1002/ptr.5188
Gao, Y., Sun, W., Cha, X., and Wang, H. (2020). ’Psoriasis 1’ reduces T-lymphocyte-mediated inflammation in patients with psoriasis by inhibiting vitamin D receptor-mediated STAT4 inactivation. Int. J. Mol. Med. 46 (4), 1538–1550. doi:10.3892/ijmm.2020.4695
Gao, Z. R., Feng, Y. Z., Zhao, Y. Q., Zhao, J., Zhou, Y. H., Ye, Q., et al. (2022). Traditional Chinese medicine promotes bone regeneration in bone tissue engineering. Chin. Med. 17 (1), 86. doi:10.1186/s13020-022-00640-5
Guan, H., Zhu, N., Tang, G., Du, Y., Wang, L., and Yuan, W. (2022). DNA methyltransferase 1 knockdown reverses PTEN and VDR by mediating demethylation of promoter and protects against renal injuries in hepatitis B virus-associated glomerulonephritis. Cell Biosci. 12 (1), 98. doi:10.1186/s13578-022-00835-1
Halfon, M., Phan, O., and Teta, D. (2015). Vitamin D: a review on its effects on muscle strength, the risk of fall, and frailty. Biomed. Res. Int. 2015, 953241. doi:10.1155/2015/953241
Han, F. F., Lv, Y. L., Gong, L. L., Liu, H., Wan, Z. R., and Liu, L. H. (2017). VDR Gene variation and insulin resistance related diseases. Lipids health Dis. 16 (1), 157. doi:10.1186/s12944-017-0477-7
Han, Z., Zhu, J., and Han, Z. (2021). Evaluation of astragaloside IV in hepatic fibrosis: a meta-analysis. Med. Baltim. 100 (13), e25105. doi:10.1097/MD.0000000000025105
Haussler, M. R., Whitfield, G. K., Kaneko, I., Haussler, C. A., Hsieh, D., Hsieh, J. C., et al. (2013). Molecular mechanisms of vitamin D action. Calcif. Tissue Int. 92 (2), 77–98. doi:10.1007/s00223-012-9619-0
He, Y., Lu, A., Lu, C., Zha, Y., Yan, X., Song, Y., et al. (2008). Symptom combinations assessed in traditional Chinese medicine and its predictive role in ACR20 efficacy response in rheumatoid arthritis. Am. J. Chin. Med. 36 (4), 675–683. doi:10.1142/S0192415X08006144
Hou, Q., Zhu, S., Zhang, C., Huang, Y., Guo, Y., Li, P., et al. (2019). Berberine improves intestinal epithelial tight junctions by upregulating A20 expression in IBS-D mice. Biomed. Pharmacother. 118, 109206. doi:10.1016/j.biopha.2019.109206
Hou, Q. K., Huang, Y. Q., Luo, Y. W., Wang, B., Liu, Y. M., Deng, R. D., et al. (2017). (+)-Cholesten-3-one induces osteogenic differentiation of bone marrow mesenchymal stem cells by activating vitamin D receptor. Exp. Ther. Med. 13 (5), 1841–1849. doi:10.3892/etm.2017.4200
Hsieh, J. C., Dang, H. T., Galligan, M. A., Whitfield, G. K., Haussler, C. A., Jurutka, P. W., et al. (2004). Phosphorylation of human vitamin D receptor serine-182 by PKA suppresses 1,25(OH)2D3-dependent transactivation. Biochem. biophysical Res. Commun. 324 (2), 801–809. doi:10.1016/j.bbrc.2004.09.139
Huang, J., An, Q., Ju, B. M., Zhang, J., Fan, P., He, L., et al. (2021). Role of vitamin D/VDR nuclear translocation in down-regulation of NF-κB/NLRP3/caspase-1 axis in lupus nephritis. Int. Immunopharmacol. 100, 108131. doi:10.1016/j.intimp.2021.108131
Huang, Y. Q., Liu, J. L., Chen, G. X., Shen, D. T., Zhu, W., Chen, X. L., et al. (2023). Berberine enhances intestinal mucosal barrier function by promoting vitamin D receptor activity. Chin. J. Integr. Med. 23, 3547. doi:10.1007/s11655-023-3547-x
Hughes, M. R., Malloy, P. J., Kieback, D. G., Kesterson, R. A., Pike, J. W., Feldman, D., et al. (1988). Point mutations in the human vitamin D receptor gene associated with hypocalcemic rickets. Science 242 (4886), 1702–1705. doi:10.1126/science.2849209
Hung, S. W., Zhang, R., Tan, Z., Chung, J. P. W., Zhang, T., and Wang, C. C. (2021). Pharmaceuticals targeting signaling pathways of endometriosis as potential new medical treatment: a review. Med. Res. Rev. 41 (4), 2489–2564. doi:10.1002/med.21802
Hunter, D., Major, P., Arden, N., Swaminathan, R., Andrew, T., MacGregor, A. J., et al. (2000). A randomized controlled trial of vitamin D supplementation on preventing postmenopausal bone loss and modifying bone metabolism using identical twin pairs. J. Bone Min. Res. 15 (11), 2276–2283. doi:10.1359/jbmr.2000.15.11.2276
Jena, S., Lee, W. P., Doherty, D., and Thompson, P. D. (2012). PIAS4 represses vitamin D receptor-mediated signaling and acts as an E3-SUMO ligase towards vitamin D receptor. J. Steroid Biochem. Mol. Biol. 132 (1-2), 24–31. doi:10.1016/j.jsbmb.2012.04.006
Jiang, J., Jia, Y., Lu, X., Zhang, T., Zhao, K., Fu, Z., et al. (2019). Vitexin suppresses RANKL-induced osteoclastogenesis and prevents lipopolysaccharide (LPS)-induced osteolysis. J. Cell Physiol. 234 (10), 17549–17560. doi:10.1002/jcp.28378
Jiang, L. L., Zhang, C., Zhang, Y., Ma, F., and Guan, Y. (2022). Associations between polymorphisms in VDR gene and the risk of osteoporosis: a meta-analysis. Arch. Physiol. Biochem. 128 (6), 1637–1644. doi:10.1080/13813455.2020.1787457
Jiang, Y., Liao, L., Li, J., Wang, L., and Xie, Z. (2020). Older age is associated with decreased levels of VDR, CYP27B1, and CYP24A1 and increased levels of PTH in human parathyroid glands. Int. J. Endocrinol. 2020, 7257913. doi:10.1155/2020/7257913
Kadowaki, S., and Norman, A. W. (1984). Dietary vitamin D is essential for normal insulin secretion from the perfused rat pancreas. J. Clin. Invest. 73 (3), 759–766. doi:10.1172/JCI111269
Kayaniyil, S., Vieth, R., Retnakaran, R., Knight, J. A., Qi, Y., Gerstein, H. C., et al. (2010). Association of vitamin D with insulin resistance and beta-cell dysfunction in subjects at risk for type 2 diabetes. Diabetes Care 33 (6), 1379–1381. doi:10.2337/dc09-2321
Khalighi Sikaroudi, M., Mokhtare, M., Shidfar, F., Janani, L., Faghihi Kashani, A., Masoodi, M., et al. (2020). Effects of vitamin D3 supplementation on clinical symptoms, quality of life, serum serotonin (5-hydroxytryptamine), 5-hydroxy-indole acetic acid, and ratio of 5-HIAA/5-HT in patients with diarrhea-predominant irritable bowel syndrome: a randomized clinical trial. Excli J. 19, 652–667. doi:10.17179/excli2020-2247
Kitanaka, S., Takeyama, K., Murayama, A., Sato, T., Okumura, K., Nogami, M., et al. (1998). Inactivating mutations in the 25-hydroxyvitamin D3 1alpha-hydroxylase gene in patients with pseudovitamin D-deficiency rickets. N. Engl. J. Med. 338 (10), 653–661. doi:10.1056/NEJM199803053381004
Kong, J., Zhang, Z., Musch, M. W., Ning, G., Sun, J., Hart, J., et al. (2008). Novel role of the vitamin D receptor in maintaining the integrity of the intestinal mucosal barrier. Am. J. Physiol. Gastrointest. Liver Physiol. 294 (1), G208–G216. doi:10.1152/ajpgi.00398.2007
Kuai, L., Song, J. K., Zhang, R. X., Xing, M., Luo, Y., Ru, Y., et al. (2020). Uncovering the mechanism of Jueyin granules in the treatment of psoriasis using network pharmacology. J. Ethnopharmacol. 262, 113214. doi:10.1016/j.jep.2020.113214
Lee, S. M., Meyer, M. B., Benkusky, N. A., O’Brien, C. A., and Pike, J. W. (2015). Mechanisms of enhancer-mediated hormonal control of vitamin D receptor gene expression in target cells. J. Biol. Chem. 290 (51), 30573–30586. doi:10.1074/jbc.M115.693614
Li, C. X., Liu, Y., Zhang, Y. Z., Li, J. C., and Lai, J. (2022b). Astragalus polysaccharide: a review of its immunomodulatory effect. Arch. Pharm. Res. 45 (6), 367–389. doi:10.1007/s12272-022-01393-3
Li, E. K., Tam, L. S., Wong, C. K., Li, W. C., Lam, C. W., Wachtel-Galor, S., et al. (2007). Safety and efficacy of Ganoderma lucidum (lingzhi) and San Miao San supplementation in patients with rheumatoid arthritis: a double-blind, randomized, placebo-controlled pilot trial. Arthritis Rheum. 57 (7), 1143–1150. doi:10.1002/art.22994
Li, H. M., Liu, Y., Zhang, R. J., Ding, J. Y., and Shen, C. L. (2021). Vitamin D receptor gene polymorphisms and osteoarthritis: a meta-analysis. Rheumatol. Oxf. 60 (2), 538–548. doi:10.1093/rheumatology/keaa644
Li, J., Zhao, J., Chai, Y., Li, W., Liu, X., and Chen, Y. (2022c). Astragalus polysaccharide protects sepsis model rats after cecum ligation and puncture. Front. Bioeng. Biotechnol. 10, 1020300. doi:10.3389/fbioe.2022.1020300
Li, N., Zhao, W., Xing, J., Liu, J., Zhang, G., Zhang, Y., et al. (2017). Chinese herbal Pulian ointment in treating psoriasis vulgaris of blood-heat syndrome: a multi-center, double-blind, randomized, placebo-controlled trial. BMC Complement. Altern. Med. 17 (1), 264. doi:10.1186/s12906-017-1631-5
Li, Q., Chen, J. X., Wu, Y., Lv, L. L., Ying, H. F., Zhu, W. H., et al. (2022a). The mechanism of FZXJJZ decoction suppresses colorectal liver metastasis via the VDR/TGF-β/Snail1 signaling pathways based on network pharmacology-TCGA data-transcriptomics analysis. J. Ethnopharmacol. 287, 114904. doi:10.1016/j.jep.2021.114904
Li, X. X., Li, D., Cui, X. Y., Zhou, K., Liu, J., Lu, J. J., et al. (2023). Astragaloside IV for heart failure: preclinical evidence and possible mechanisms, A systematic review and meta-analysis. Chin. J. Integr. Med. 29 (7), 626–633. doi:10.1007/s11655-023-3636-x
Li, Z. M., Xu, S. W., and Liu, P. Q. (2018). Salvia miltiorrhizaBurge (Danshen): a golden herbal medicine in cardiovascular therapeutics. Acta Pharmacol. Sin. 39 (5), 802–824. doi:10.1038/aps.2017.193
Liang, C., Jiang, Y., and Sun, L. (2023). Vitexin suppresses the proliferation, angiogenesis and stemness of endometrial cancer through the PI3K/AKT pathway. Pharm. Biol. 61 (1), 581–589. doi:10.1080/13880209.2023.2190774
Lin, Y. T., Mao, Y. W., Imtiyaz, Z., Chiou, W. F., and Lee, M. H. (2020). Comprehensive LC-MS/MS-based phytochemical perspectives and osteogenic effects of Uraria crinita. Food Funct. 11 (6), 5420–5431. doi:10.1039/d0fo00782j
Liu, C., Zhu, R., Liu, H., Li, L., Chen, B., Jia, Q., et al. (2018a). Aqueous extract of mori folium exerts bone protective effect through regulation of calcium and redox homeostasis via PTH/VDR/CaBP and AGEs/RAGE/Nox4/NF-κB signaling in diabetic rats. Front. Pharmacol. 9, 1239. doi:10.3389/fphar.2018.01239
Liu, D., Chen, L., Zhao, J., and Cui, K. (2018b). Cardioprotection activity and mechanism of Astragalus polysaccharide in vivo and in vitro. Int. J. Biol. Macromol. 111, 947–952. doi:10.1016/j.ijbiomac.2018.01.048
Liu, L., Feng, L., Gao, J., Hu, J., Li, A., Zhu, Y., et al. (2023b). Parthenolide targets NLRP3 to treat inflammasome-related diseases. Int. Immunopharmacol. 119, 110229. doi:10.1016/j.intimp.2023.110229
Liu, N., Wang, K. S., Qi, M., Zhou, Y. J., Zeng, G. Y., Tao, J., et al. (2018c). Vitexin compound 1, a novel extraction from a Chinese herb, suppresses melanoma cell growth through DNA damage by increasing ROS levels. J. Exp. Clin. Cancer Res. 37 (1), 269. doi:10.1186/s13046-018-0897-x
Liu, Y., Huang, Y., Zhao, C., Qin, X., Zhu, Q., Chen, S., et al. (2014). Salvia miltiorrhiza injection on pulmonary heart disease: a systematic review and meta-analysis. Am. J. Chin. Med. 42 (6), 1315–1331. doi:10.1142/S0192415X14500827
Liu, Y., Zhu, S., Liu, J., Chen, Y., Zhong, S., Lian, D., et al. (2023a). Vitexin regulates angiogenesis and osteogenesis in ovariectomy-induced osteoporosis of rats via the VDR/PI3K/AKT/eNOS signaling pathway. J. Agric. Food Chem. 71 (1), 546–556. doi:10.1021/acs.jafc.2c07005
Liu, Z., Liu, S., Xie, Z., Pavlovicz, R. E., Wu, J., Chen, P., et al. (2009). Modulation of DNA methylation by a sesquiterpene lactone parthenolide. J. Pharmacol. Exp. Ther. 329 (2), 505–514. doi:10.1124/jpet.108.147934
Long, L., Zhong, W., Guo, L., Ji, J., and Nie, H. (2022). Effect of bufalin-PLGA microspheres in the alleviation of neuropathic pain via the CCI model. Front. Pharmacol. 13, 910885. doi:10.3389/fphar.2022.910885
Lu, Y., Guan, T., Xu, S., Chen, Y. E., Shen, Q., Zhu, S., et al. (2022). Asperuloside inhibited epithelial-mesenchymal transition in colitis associated cancer via activation of vitamin D receptor. Phytomedicine 101, 154070. doi:10.1016/j.phymed.2022.154070
Lu, Y., Zhou, C., Zhu, M., Fu, Z., Shi, Y., Li, M., et al. (2021). Traditional Chinese medicine syndromes classification associates with tumor cell and microenvironment heterogeneity in colorectal cancer: a single cell RNA sequencing analysis. Chin. Med. 16 (1), 133. doi:10.1186/s13020-021-00547-7
Luo, J., Wang, N., Hua, L., Deng, F., Liu, D., Zhou, J., et al. (2022). The anti-sepsis effect of isocorydine screened from guizhou ethnic medicine is closely related to upregulation of vitamin D receptor expression and inhibition of NFκB p65 translocation into the nucleus. J. Inflamm. Res. 15, 5649–5664. doi:10.2147/JIR.S365191
Luo, W., Liu, L., Yang, L., Dong, Y., Liu, T., Wei, X., et al. (2018). The vitamin D receptor regulates miR-140-5p and targets the MAPK pathway in bone development. Metabolism 85, 139–150. doi:10.1016/j.metabol.2018.03.018
Ma, Y., Deng, L., Huangfu, Y., Zhou, Y., Wang, P., and Shen, L. (2023). Adequate vitamin D level associated with reduced risk of sporadic colorectal cancer. Front. Nutr. 10, 1024849. doi:10.3389/fnut.2023.1024849
Maestro, M. A., Molnár, F., and Carlberg, C. (2019). Vitamin D and its synthetic analogs. J. Med. Chem. 62 (15), 6854–6875. doi:10.1021/acs.jmedchem.9b00208
Maestro, M. A., Molnár, F., Mouriño, A., and Carlberg, C. (2016). Vitamin D receptor 2016: novel ligands and structural insights. Expert Opin. Ther. Pat. 26 (11), 1291–1306. doi:10.1080/13543776.2016.1216547
Manzione, M. G., Martorell, M., Sharopov, F., Bhat, N. G., Kumar, N. V. A., Fokou, P. V. T., et al. (2020). Phytochemical and pharmacological properties of asperuloside, a systematic review. Eur. J. Pharmacol. 883, 173344. doi:10.1016/j.ejphar.2020.173344
Mao, S., Wang, L., Zhao, X., Guo, L., Lin, Q., Wang, X., et al. (2021). Efficacy of sodium tanshinone IIA sulfonate in patients with non-ST elevation acute coronary syndrome undergoing percutaneous coronary intervention: results from a multicentre, controlled, randomized trial. Cardiovasc Drugs Ther. 35 (2), 321–329. doi:10.1007/s10557-020-07077-8
Martínez-Arias, L., Panizo, S., Alonso-Montes, C., Martín-Vírgala, J., Martín-Carro, B., Fernández-Villabrille, S., et al. (2021). Effects of calcitriol and paricalcitol on renal fibrosis in CKD. Nephrol. Dial. Transpl. 36 (5), 793–803. doi:10.1093/ndt/gfaa373
Materazzi, S., Benemei, S., Fusi, C., Gualdani, R., De Siena, G., Vastani, N., et al. (2013). Parthenolide inhibits nociception and neurogenic vasodilatation in the trigeminovascular system by targeting the TRPA1 channel. Pain 154 (12), 2750–2758. doi:10.1016/j.pain.2013.08.002
Mathieu, C., Van Etten, E., Gysemans, C., Decallonne, B., Kato, S., Laureys, J., et al. (2001). In vitro and in vivo analysis of the immune system of vitamin D receptor knockout mice. J. Bone Min. Res. 16 (11), 2057–2065. doi:10.1359/jbmr.2001.16.11.2057
Matsuo, M., Hasegawa, A., Takano, M., Saito, H., Kakuda, S., Chida, T., et al. (2013). Synthesis of 2α-heteroarylalkyl active vitamin d3 with therapeutic effect on enhancing bone mineral density in vivo. ACS Med. Chem. Lett. 4 (7), 671–674. doi:10.1021/ml400098w
Michigami, T., Suga, A., Yamazaki, M., Shimizu, C., Cai, G., Okada, S., et al. (1999). Identification of amino acid sequence in the hinge region of human vitamin D receptor that transfers a cytosolic protein to the nucleus. J. Biol. Chem. 274 (47), 33531–33538. doi:10.1074/jbc.274.47.33531
Mizwicki, M. T., Bula, C. M., Bishop, J. E., and Norman, A. W. (2007). New insights into Vitamin D sterol-VDR proteolysis, allostery, structure-function from the perspective of a conformational ensemble model. J. Steroid Biochem. Mol. Biol. 103 (3-5), 243–262. doi:10.1016/j.jsbmb.2006.12.004
Mokhtar, W. A., Fawzy, A., Allam, R. M., Amer, R. M., and Hamed, M. S. (2019). Maternal vitamin D level and vitamin D receptor gene polymorphism as a risk factor for congenital heart diseases in offspring; an Egyptian case-control study. Genes Dis. 6 (2), 193–200. doi:10.1016/j.gendis.2018.08.001
Motiwala, S. R., and Wang, T. J. (2012). Vitamin D and cardiovascular risk. Curr. Hypertens. Rep. 14 (3), 209–218. doi:10.1007/s11906-012-0262-y
Nakamichi, Y., Udagawa, N., Horibe, K., Mizoguchi, T., Yamamoto, Y., Nakamura, T., et al. (2017). VDR in osteoblast-lineage cells primarily mediates vitamin D treatment-induced increase in bone mass by suppressing bone resorption. J. Bone Min. Res. 32 (6), 1297–1308. doi:10.1002/jbmr.3096
Nakamichi, Y., Udagawa, N., Suda, T., and Takahashi, N. (2018). Mechanisms involved in bone resorption regulated by vitamin D. J. Steroid Biochem. Mol. Biol. 177, 70–76. doi:10.1016/j.jsbmb.2017.11.005
Nakamura, A., Yokoyama, Y., Tanaka, K., Benegiamo, G., Hirayama, A., Zhu, Q., et al. (2020). Asperuloside improves obesity and type 2 diabetes through modulation of gut microbiota and metabolic signaling. iScience 23 (9), 101522. doi:10.1016/j.isci.2020.101522
Nakano, H., Matsunawa, M., Yasui, A., Adachi, R., Kawana, K., Shimomura, I., et al. (2005). Enhancement of ligand-dependent vitamin D receptor transactivation by the cardiotonic steroid bufalin. Biochem. Pharmacol. 70 (10), 1479–1486. doi:10.1016/j.bcp.2005.08.012
Noor, K. K., Ijaz, M. U., Ehsan, N., Tahir, A., Yeni, D. K., Neamul Kabir Zihad, S. M., et al. (2022). Hepatoprotective role of vitexin against cadmium-induced liver damage in male rats: a biochemical, inflammatory, apoptotic and histopathological investigation. Biomed. Pharmacother. 150, 112934. doi:10.1016/j.biopha.2022.112934
Norman, A. W. (2008). From vitamin D to hormone D: fundamentals of the vitamin D endocrine system essential for good health. Am. J. Clin. Nutr. 88 (2), 491S–499s. doi:10.1093/ajcn/88.2.491S
Norman, P. E., and Powell, J. T. (2014). Vitamin D and cardiovascular disease. Circ. Res. 114 (2), 379–393. doi:10.1161/CIRCRESAHA.113.301241
Nwachukwu, J. C., and Nettles, K. W. (2012). The nuclear receptor signalling scaffold: insights from full-length structures. EMBO J. 31 (2), 251–253. doi:10.1038/emboj.2011.475
Olejarova, M., Dobisova, A., Suchankova, M., Tibenska, E., Szaboova, K., Koutun, J., et al. (2019). Vitamin D deficiency-a potential risk factor for sepsis development, correlation with inflammatory markers, SOFA score and higher early mortality risk in sepsis. Bratisl. Med. Journal/Bratislavske Lek. Listy. 120 (4), 284–290. doi:10.4149/BLL_2019_040
Orlov, I., Rochel, N., Moras, D., and Klaholz, B. P. (2012). Structure of the full human RXR/VDR nuclear receptor heterodimer complex with its DR3 target DNA. EMBO J. 31 (2), 291–300. doi:10.1038/emboj.2011.445
Pareek, A., Suthar, M., Rathore, G. S., and Bansal, V. (2011). Feverfew (Tanacetum parthenium L.): a systematic review. Pharmacogn. Rev. 5 (9), 103–110. doi:10.4103/0973-7847.79105
Parekh, D., Patel, J. M., Scott, A., Lax, S., Dancer, R. C., D’Souza, V., et al. (2017). Vitamin D deficiency in human and murine sepsis. Crit. Care Med. 45 (2), 282–289. doi:10.1097/CCM.0000000000002095
Peleg, S., and Nguyen, C. V. (2010). The importance of nuclear import in protection of the vitamin D receptor from polyubiquitination and proteasome-mediated degradation. J. Cell Biochem. 110 (4), 926–934. doi:10.1002/jcb.22606
Pike, J. W., and Meyer, M. B. (2010). The vitamin D receptor: new paradigms for the regulation of gene expression by 1,25-dihydroxyvitamin D(3). Endocrinol. Metab. Clin. North Am. 39 (2), 255–269. doi:10.1016/j.ecl.2010.02.007
Poon, A. H., Gong, L., Brasch-Andersen, C., Litonjua, A. A., Raby, B. A., Hamid, Q., et al. (2012). Very important pharmacogene summary for VDR. Pharmacogenet Genomics 22 (10), 758–763. doi:10.1097/FPC.0b013e328354455c
Provvedini, D. M., Tsoukas, C. D., Deftos, L. J., and Manolagas, S. C. (1983). 1,25-dihydroxyvitamin D3 receptors in human leukocytes. Science 221 (4616), 1181–1183. doi:10.1126/science.6310748
Prüfer, K., and Barsony, J. (2002). Retinoid X receptor dominates the nuclear import and export of the unliganded vitamin D receptor. Mol. Endocrinol. 16 (8), 1738–1751. doi:10.1210/me.2001-0345
Prüfer, K., Racz, A., Lin, G. C., and Barsony, J. (2000). Dimerization with retinoid X receptors promotes nuclear localization and subnuclear targeting of vitamin D receptors. J. Biol. Chem. 275 (52), 41114–41123. doi:10.1074/jbc.M003791200
Qi, Z. M., Wang, X., Liu, X., and Zhao, J. (2023). Asperuloside promotes apoptosis of cervical cancer cells through endoplasmic reticulum stress-mitochondrial pathway. Chin. J. Integr. Med. 30, 34–41. doi:10.1007/s11655-023-3695-z
Ren, S. J., Xing, G. L., Hu, N. W., Xu, W. M., Wang, Y. Q., Dong, Q. P., et al. (2016). Bu-shen-jian-pi-yi-qi therapy prevents alcohol-induced osteoporosis in rats. Am. J. Ther. 23 (5), e1135–e1142. doi:10.1097/MJT.0000000000000298
Rochel, N., Wurtz, J. M., Mitschler, A., Klaholz, B., and Moras, D. (2000). The crystal structure of the nuclear receptor for vitamin D bound to its natural ligand. Mol. Cell 5 (1), 173–179. doi:10.1016/s1097-2765(00)80413-x
Saijo, T., Ito, M., Takeda, E., Huq, A. H., Naito, E., Yokota, I., et al. (1991). A unique mutation in the vitamin D receptor gene in three Japanese patients with vitamin D-dependent rickets type II: utility of single-strand conformation polymorphism analysis for heterozygous carrier detection. Am. J. Hum. Genet. 49 (3), 668–673.
Sanchez-Niño, M. D., Bozic, M., Córdoba-Lanús, E., Valcheva, P., Gracia, O., Ibarz, M., et al. (2012). Beyond proteinuria: VDR activation reduces renal inflammation in experimental diabetic nephropathy. Am. J. Physiol. Ren. Physiol. 302 (6), F647–F657. doi:10.1152/ajprenal.00090.2011
Sawada, D., Kakuda, S., Takeuchi, A., Kawagoe, F., Takimoto-Kamimura, M., and Kittaka, A. (2018). Effects of 2-substitution on 14-epi-19-nortachysterol-mediated biological events: based on synthesis and X-ray co-crystallographic analysis with the human vitamin D receptor. Org. Biomol. Chem. 16 (14), 2448–2455. doi:10.1039/C8OB00158H
Sha, N. N., Zhao, Y. J., Zhao, D. F., Mok, D. K., Shi, Q., Wang, Y. J., et al. (2017). Effect of the water fraction isolated from Fructus Ligustri Lucidi extract on bone metabolism via antagonizing a calcium-sensing receptor in experimental type 1 diabetic rats. Food Funct. 8 (12), 4703–4712. doi:10.1039/c7fo01259d
Shaffer, P. L., and Gewirth, D. T. (2004). Structural analysis of RXR-VDR interactions on DR3 DNA. J. Steroid Biochem. Mol. Biol. 89-90 (1-5), 215–219. doi:10.1016/j.jsbmb.2004.03.084
Shang, L., Liu, Y., Li, J., Pan, G., Zhou, F., and Yang, S. (2021). Emodin protects sepsis associated damage to the intestinal mucosal barrier through the VDR/Nrf2/HO-1 pathway. Front. Pharmacol. 12, 724511. doi:10.3389/fphar.2021.724511
Shang, M., and Sun, J. (2017). Vitamin D/VDR, probiotics, and gastrointestinal diseases. Curr. Med. Chem. 24 (9), 876–887. doi:10.2174/0929867323666161202150008
Si, Y., Ma, Y., Guo, Y., Pan, Y., Zheng, S., Xu, G., et al. (2018). Efficacy and safety of Shaoyang Xibi decoction in patients with knee osteoarthritis: a multi-center, single-blind, randomized controlled trial. J. Tradit. Chin. Med. 38 (5), 733–739. doi:10.1016/s0254-6272(18)30912-9
Somjen, D., Weisman, Y., Kohen, F., Gayer, B., Limor, R., Sharon, O., et al. (2005). 25-hydroxyvitamin D3-1alpha-hydroxylase is expressed in human vascular smooth muscle cells and is upregulated by parathyroid hormone and estrogenic compounds. Circulation 111 (13), 1666–1671. doi:10.1161/01.CIR.0000160353.27927.70
Soumoy, L., Ghanem, G. E., Saussez, S., and Journe, F. (2022). Bufalin for an innovative therapeutic approach against cancer. Pharmacol. Res. 184, 106442. doi:10.1016/j.phrs.2022.106442
Su, Y., Qin, W., Wu, L., Yang, B., Wang, Q., Kuang, H., et al. (2021). A review of Chinese medicine for the treatment of psoriasis: principles, methods and analysis. Chin. Med. 16 (1), 138. doi:10.1186/s13020-021-00550-y
Subedi, L., and Gaire, B. P. (2021). Tanshinone IIA: a phytochemical as a promising drug candidate for neurodegenerative diseases. Pharmacol. Res. 169, 105661. doi:10.1016/j.phrs.2021.105661
Sun, J., Wei, S., Zhang, Y., and Li, J. (2021). Protective effects of Astragalus polysaccharide on sepsis-induced acute kidney injury. Anal. Cell Pathol. (Amst). 2021, 7178253. doi:10.1155/2021/7178253
Sun, W., Gao, Y., Yu, X., Yuan, Y., Yi, J., Zhang, Z., et al. (2018). ’Psoriasis 1’ reduces psoriasis‑like skin inflammation by inhibiting the VDR‑mediated nuclear NF‑κB and STAT signaling pathways. Mol. Med. Rep. 18 (3), 2733–2743. doi:10.3892/mmr.2018.9262
Takeyama, K., and Kato, S. (2011). The vitamin D3 1alpha-hydroxylase gene and its regulation by active vitamin D3. Biosci. Biotechnol. Biochem. 75 (2), 208–213. doi:10.1271/bbb.100684
Tamura, M., Ishizawa, M., Isojima, T., Özen, S., Oka, A., Makishima, M., et al. (2017). Functional analyses of a novel missense and other mutations of the vitamin D receptor in association with alopecia. Sci. Rep. 7 (1), 5102. doi:10.1038/s41598-017-05081-x
Thakkinstian, A., D’Este, C., Eisman, J., Nguyen, T., and Attia, J. (2004). Meta-analysis of molecular association studies: vitamin D receptor gene polymorphisms and BMD as a case study. J. Bone Min. Res. 19 (3), 419–428. doi:10.1359/JBMR.0301265
Tishkoff, D. X., Nibbelink, K. A., Holmberg, K. H., Dandu, L., and Simpson, R. U. (2008). Functional vitamin D receptor (VDR) in the t-tubules of cardiac myocytes: VDR knockout cardiomyocyte contractility. Endocrinology 149 (2), 558–564. doi:10.1210/en.2007-0805
Tocchini-Valentini, G., Rochel, N., Wurtz, J. M., Mitschler, A., and Moras, D. (2001). Crystal structures of the vitamin D receptor complexed to superagonist 20-epi ligands. Proc. Natl. Acad. Sci. U. S. A. 98 (10), 5491–5496. doi:10.1073/pnas.091018698
Toropainen, S., Väisänen, S., Heikkinen, S., and Carlberg, C. (2010). The down-regulation of the human MYC gene by the nuclear hormone 1alpha,25-dihydroxyvitamin D3 is associated with cycling of corepressors and histone deacetylases. J. Mol. Biol. 400 (3), 284–294. doi:10.1016/j.jmb.2010.05.031
Tsai, S. C., Yang, J. S., Peng, S. F., Lu, C. C., Chiang, J. H., Chung, J. G., et al. (2012). Bufalin increases sensitivity to AKT/mTOR-induced autophagic cell death in SK-HEP-1 human hepatocellular carcinoma cells. Int. J. Oncol. 41 (4), 1431–1442. doi:10.3892/ijo.2012.1579
Uitterlinden, A. G., Ralston, S. H., Brandi, M. L., Carey, A. H., Grinberg, D., Langdahl, B. L., et al. (2006). The association between common vitamin D receptor gene variations and osteoporosis: a participant-level meta-analysis. Ann. Intern Med. 145 (4), 255–264. doi:10.7326/0003-4819-145-4-200608150-00005
van de Peppel, J., and van Leeuwen, J. P. (2014). Vitamin D and gene networks in human osteoblasts. Front. Physiol. 5, 137. doi:10.3389/fphys.2014.00137
Verlinden, L., Janssens, I., Doms, S., Vanhevel, J., Carmeliet, G., and Verstuyf, A. (2019). Vdr expression in osteoclast precursors is not critical in bone homeostasis. J. Steroid Biochem. Mol. Biol. 195, 105478. doi:10.1016/j.jsbmb.2019.105478
von Essen, M. R., Kongsbak, M., Schjerling, P., Olgaard, K., Odum, N., and Geisler, C. (2010). Vitamin D controls T cell antigen receptor signaling and activation of human T cells. Nat. Immunol. 11 (4), 344–349. doi:10.1038/ni.1851
Wan, L. Y., Zhang, Y. Q., Chen, M. D., Du, Y. Q., Liu, C. B., and Wu, J. F. (2015). Relationship between structure and conformational change of the vitamin D receptor ligand binding domain in 1α,25-dihydroxyvitamin D3 signaling. Mol. (Basel, Switz. 20 (11), 20473–20486. doi:10.3390/molecules201119713
Wang, J., Thingholm, L. B., Skiecevičienė, J., Rausch, P., Kummen, M., Hov, J. R., et al. (2016). Genome-wide association analysis identifies variation in vitamin D receptor and other host factors influencing the gut microbiota. Nat. Genet. 48 (11), 1396–1406. doi:10.1038/ng.3695
Wang, J., Wang, L., Lou, G. H., Zeng, H. R., Hu, J., Huang, Q. W., et al. (2019). Coptidis Rhizoma: a comprehensive review of its traditional uses, botany, phytochemistry, pharmacology and toxicology. Pharm. Biol. 57 (1), 193–225. doi:10.1080/13880209.2019.1577466
Wang, J., Wang, X., Ma, X., Xu, B., Chen, L., Chen, C., et al. (2022). Therapeutic effect of Patrinia villosa on TNBS-induced ulcerative colitis via metabolism, vitamin D receptor and NF-κB signaling pathways. J. Ethnopharmacol. 288, 114989. doi:10.1016/j.jep.2022.114989
Wang, K., Feng, X., Chai, L., Cao, S., and Qiu, F. (2017). The metabolism of berberine and its contribution to the pharmacological effects. Drug Metab. Rev. 49 (2), 139–157. doi:10.1080/03602532.2017.1306544
Wang, L., Zhang, X. F., Zhang, X., Guo, D. Y., Duan, Y. W., Wang, Z. C., et al. (2020). Evaluation of the therapeutic effect of traditional Chinese medicine on osteoarthritis: a systematic review and meta-analysis. Pain Res. Manag. 2020, 5712187. doi:10.1155/2020/5712187
Wang, L. T., Zhao, Y. F., Wang, X., Xu, Y., Chen, Y. C., and Zhan, H. S. (2009). Influence of Migu capsule and strengthening spleen prescription on the expression of small intestine VDR mRNA in calf muscle on ovariectomized rats. Zhongguo Gu Shang 22 (5), 367–370.
Wang, Y., Deb, D. K., Zhang, Z., Sun, T., Liu, W., Yoon, D., et al. (2012). Vitamin D receptor signaling in podocytes protects against diabetic nephropathy. J. Am. Soc. Nephrol. 23 (12), 1977–1986. doi:10.1681/ASN.2012040383
Wei, W. L., Hou, J. J., Wang, X., Yu, Y., Li, H. J., Li, Z. W., et al. (2019). Venenum bufonis: an overview of its traditional use, natural product chemistry, pharmacology, pharmacokinetics and toxicology. J. Ethnopharmacol. 237, 215–235. doi:10.1016/j.jep.2019.03.042
Wu, M., Deng, Y., Li, S., Chen, Y., Guo, D., Jin, X., et al. (2018). The immunoregulatory effects of traditional Chinese medicine on psoriasis via its action on interleukin: advances and considerations. Am. J. Chin. Med. 46 (4), 739–750. doi:10.1142/S0192415X18500386
Wurtz, J. M., Bourguet, W., Renaud, J. P., Vivat, V., Chambon, P., Moras, D., et al. (1996). A canonical structure for the ligand-binding domain of nuclear receptors. Nat. Struct. Biol. 3 (1), 87–94. doi:10.1038/nsb0196-87
Xiao, Z., Jiang, Y., Wang, C. Q., Hu, S. S., Huang, X. R., Chen, X. F., et al. (2020). Clinical efficacy and safety of aidi injection combination with vinorelbine and cisplatin for advanced non-small-cell lung carcinoma: a systematic review and meta-analysis of 54 randomized controlled trials. Pharmacol. Res. 153, 104637. doi:10.1016/j.phrs.2020.104637
Xiong, X., Cheng, Z., Zhou, Y., Wu, F., Xie, L., Lawless, L., et al. (2022). HuanglianGanjiang Tang alleviates DSS-induced colitis in mice by inhibiting necroptosis through vitamin D receptor. J. Ethnopharmacol. 298, 115655. doi:10.1016/j.jep.2022.115655
Xu, J., Zhang, L., Xu, Y., Yu, J., Zhao, L., Deng, H., et al. (2023). Effectiveness of Yishen Tongbi decoction versus methotrexate in patients with active rheumatoid arthritis: a double-blind, randomized, controlled, non-inferiority trial. Phytomedicine 112, 154704. doi:10.1016/j.phymed.2023.154704
Xu, N., Yang, M., Liu, J., and Li, X. (2022). Association of vitamin D receptor genetic polymorphisms with nephrolithiasis and end-stage renal disease: a meta-analysis. Transpl. Proc. 54 (7), 1773–1780. doi:10.1016/j.transproceed.2022.04.022
Yang, J., Tian, S., Zhao, J., and Zhang, W. (2020). Exploring the mechanism of TCM formulae in the treatment of different types of coronary heart disease by network pharmacology and machining learning. Pharmacol. Res. 159, 105034. doi:10.1016/j.phrs.2020.105034
Yang, S., Li, A., Wang, J., Liu, J., Han, Y., Zhang, W., et al. (2018). Vitamin D receptor: a novel therapeutic target for kidney diseases. Curr. Med. Chem. 25 (27), 3256–3271. doi:10.2174/0929867325666180214122352
Yang, X., Ru, J., Li, Z., Jiang, X., and Fan, C. (2022). Lower vitamin D levels and VDR FokI variants are associated with susceptibility to sepsis: a hospital-based case-control study. Biomarkers 27 (2), 188–195. doi:10.1080/1354750X.2021.2024598
Yang, X., Zhang, Y., Yang, N., Yu, X., Gao, X., and Zhao, M. (2022). Parthenolide regulates DNMT1-mediated methylation of VDR promoter to relieve podocyte damage in mice with diabetic nephropathy. Food Sci. Technol. 42. doi:10.1590/fst.51221
Yang, Z., Kuang, B., Kang, N., Ding, Y., Ge, W., Lian, L., et al. (2017). Synthesis and anti-acute myeloid leukemia activity of C-14 modified parthenolide derivatives. Eur. J. Med. Chem. 127, 296–304. doi:10.1016/j.ejmech.2016.12.044
Yu, S., Peng, W., Qiu, F., and Zhang, G. (2022). Research progress of astragaloside IV in the treatment of atopic diseases. Biomed. Pharmacother. 156, 113989. doi:10.1016/j.biopha.2022.113989
Yunzhi, C., Jiaxu, C., Jie, G., Yihui, C., Wen, L., and Zhong, Q. (2017). Effect of astragaloside on vitamin D-receptor expression after endothelin-1-induced cardiomyocyte injury. Afr. J. Tradit. Complement. Altern. Med. 14 (4), 278–288. doi:10.21010/ajtcam.v14i4.31
Zella, L. A., Kim, S., Shevde, N. K., and Pike, J. W. (2006). Enhancers located within two introns of the vitamin D receptor gene mediate transcriptional autoregulation by 1,25-dihydroxyvitamin D3. Mol. Endocrinol. 20 (6), 1231–1247. doi:10.1210/me.2006-0015
Zenata, O., and Vrzal, R. (2017). Fine tuning of vitamin D receptor (VDR) activity by post-transcriptional and post-translational modifications. Oncotarget 8 (21), 35390–35402. doi:10.18632/oncotarget.15697
Zeng, J., and Bao, X. (2021). Tanshinone IIA attenuates high glucose-induced epithelial-to-mesenchymal transition in HK-2 cells through VDR/Wnt/β-catenin signaling pathway. Folia Histochem Cytobiol. 59 (4), 259–270. doi:10.5603/FHC.a2021.0025
Zhang, B. X., Wang, L., Wang, Q. X., Tu, Y. H., and Li, J. (2022a). Clinical study of modified Yiyi Baijiang Decoction on psoriasis vulgaris due to dampness-heat accumulation. Zhongguo Zhong Yao Za Zhi 47 (11), 3105–3110. doi:10.19540/j.cnki.cjcmm.20220222.501
Zhang, R., Zhu, X., Bai, H., and Ning, K. (2019). Network pharmacology databases for traditional Chinese medicine: review and assessment. Front. Pharmacol. 10, 123. doi:10.3389/fphar.2019.00123
Zhang, Y., Garrett, S., Carroll, R. E., Xia, Y., and Sun, J. (2022b). Vitamin D receptor upregulates tight junction protein claudin-5 against colitis-associated tumorigenesis. Mucosal Immunol. 15 (4), 683–697. doi:10.1038/s41385-022-00502-1
Zhang, Y., Kong, J., Deb, D. K., Chang, A., and Li, Y. C. (2010). Vitamin D receptor attenuates renal fibrosis by suppressing the renin-angiotensin system. J. Am. Soc. Nephrol. 21 (6), 966–973. doi:10.1681/ASN.2009080872
Zhang, Y. G., Lu, R., Wu, S., Chatterjee, I., Zhou, D., Xia, Y., et al. (2020). Vitamin D receptor protects against dysbiosis and tumorigenesis via the JAK/STAT pathway in intestine. Cell Mol. Gastroenterol. Hepatol. 10 (4), 729–746. doi:10.1016/j.jcmgh.2020.05.010
Zhao, C. R., Yang, F. F., Cui, Q., Wang, D., Zhou, Y., Li, Y. S., et al. (2021). Vitexin inhibits APEX1 to counteract the flow-induced endothelial inflammation. Proc. Natl. Acad. Sci. U. S. A. 118 (48), e2115158118. doi:10.1073/pnas.2115158118
Zhou, L., Li, M., Chai, Z., Zhang, J., Cao, K., Deng, L., et al. (2023). Anticancer effects and mechanisms of astragaloside-IV (Review). Oncol. Rep. 49 (1), 5. doi:10.3892/or.2022.8442
Zhou, W., Lai, X., Wang, X., Yao, X., Wang, W., and Li, S. (2021). Network pharmacology to explore the anti-inflammatory mechanism of Xuebijing in the treatment of sepsis. Phytomedicine 85, 153543. doi:10.1016/j.phymed.2021.153543
Zhou, X. T., Zou, J. J., Ao, C., Gong, D. Y., Chen, X., and Ma, Y. R. (2020). Renal protective effects of astragaloside IV, in diabetes mellitus kidney damage animal models: a systematic review, meta-analysis. Pharmacol. Res. 160, 105192. doi:10.1016/j.phrs.2020.105192
Zinser, G., Packman, K., and Welsh, J. (2002). Vitamin D(3) receptor ablation alters mammary gland morphogenesis. Development 129 (13), 3067–3076. doi:10.1242/dev.129.13.3067
Zinser, G. M., Suckow, M., and Welsh, J. (2005). Vitamin D receptor (VDR) ablation alters carcinogen-induced tumorigenesis in mammary gland, epidermis and lymphoid tissues. J. Steroid Biochem. Mol. Biol. 97 (1-2), 153–164. doi:10.1016/j.jsbmb.2005.06.024
Zinser, G. M., and Welsh, J. (2004). Vitamin D receptor status alters mammary gland morphology and tumorigenesis in MMTV-neu mice. Carcinogenesis 25 (12), 2361–2372. doi:10.1093/carcin/bgh271
Keywords: TCM, VDR, bioactive compounds, VDR-dependent diseases, 1α,25-dihydroxyvitamin D3
Citation: Yao M, Oduro PK, Akintibu AM and Yan H (2024) Modulation of the vitamin D receptor by traditional Chinese medicines and bioactive compounds: potential therapeutic applications in VDR-dependent diseases. Front. Pharmacol. 15:1298181. doi: 10.3389/fphar.2024.1298181
Received: 28 September 2023; Accepted: 08 January 2024;
Published: 22 January 2024.
Edited by:
Patrícia Mendonça Rijo, Lusofona University, PortugalCopyright © 2024 Yao, Oduro, Akintibu and Yan. This is an open-access article distributed under the terms of the Creative Commons Attribution License (CC BY). The use, distribution or reproduction in other forums is permitted, provided the original author(s) and the copyright owner(s) are credited and that the original publication in this journal is cited, in accordance with accepted academic practice. No use, distribution or reproduction is permitted which does not comply with these terms.
*Correspondence: Haifeng Yan, aGZ5YW4xMjZAMTI2LmNvbQ==
†These authors have contributed equally to this work and share first authorship