- Institute of General Pathology and Pathophysiology, Moscow, Russia
γ-Aminobutyric acid type A receptors (GABAARs) are members of the pentameric ligand-gated ion channel (pLGIC) family, which are widespread throughout the invertebrate and vertebrate central nervous system. GABAARs are engaged in short-term changes of the neuronal concentrations of chloride (Cl−) and bicarbonate (HCO3−) ions by their passive permeability through the ion channel pore. GABAARs are regulated by various structurally diverse phenolic substances ranging from simple phenols to complex polyphenols. The wide chemical and structural variability of phenols suggest similar and different binding sites on GABAARs, allowing them to manifest themselves as activators, inhibitors, or allosteric ligands of GABAAR function. Interest in phenols is associated with their great potential for GABAAR modulation, but also with their subsequent negative or positive role in neurological and psychiatric disorders. This review focuses on the GABAergic deficit hypotheses during neurological and psychiatric disorders induced by various phenols. We summarize the structure–activity relationship of general phenol groups concerning their differential roles in the manifestation of neuropsychiatric symptoms. We describe and analyze the role of GABAAR subunits in manifesting various neuropathologies and the molecular mechanisms underlying their modulation by phenols. Finally, we discuss how phenol drugs can modulate GABAAR activity via desensitization and resensitization. We also demonstrate a novel pharmacological approach to treat neuropsychiatric disorders via regulation of receptor phosphorylation/dephosphorylation.
1 Introduction
Pentameric ligand-gated ion channels (pLGICs), also known as Cys-loop receptors, are the main conduits of chemical neurotransmission in the central nervous system (CNS) (Calimet et al., 2013; Hu et al., 2020). Ion flow via channel gating induces short-term and decremental fluctuations in the transmembrane potential (TMP), allowing dynamic change and control of neuronal excitation and inhibition (E:I) balance in the brain (Gielen and Corringer, 2018). pLGICs have a conserved cylindrical structure in which five subunits are arranged around a central axis (Howard, 2021). Each subunit has a large hydrophilic extracellular domain, which contains a mediator-binding site; four transmembrane domains (TMDs), where TMD2 shapes the ion pore; an intracellular domain; and a short extracellular C-terminal region (Jaiteh et al., 2016; Lara et al., 2020). A dysfunction in pLGICs and subsequent E:I imbalance has been hypothesized to be a key molecular events responsible for neuropsychiatric disorders (Sauguet et al., 2015). Therefore, therapeutic approaches targeting the recovery of the E:I balance are associated with regulating pLGICs.
As members of the pLGIC family, GABAARs undergo conformational change upon binding of ligand opening a channel that provides passive permeability for Cl−, thereby hyperpolarizing/inhibiting the TMP and reducing neuronal excitability in the adult brain (Ghit et al., 2021). Under certain circumstances (e.g., strong activation), there is also an outflux of HCO3− via the receptor channel, resulting in the depolarization/excitation of the TMP (Staley and Proctor, 1999; Kaila et al., 2014). GABAARs have a pentameric structure formed from 19 identified subunits: α1–6, β1–3, γ1–3, δ, ϵ, π, θ, and ρ1–3 (Zhu et al., 2018). Although the presence of various subunits provides diversity, the most common subunit combinations in the vertebrate brain have tri-heteromeric compositions (2α:2β:1γ), arranged in the following order: γ–β–α–β–α (Sigel and Steinmann, 2012; Borghese et al., 2021). The combination, localization, and functional properties of GABAAR subunits are key determining factors in neuronal circuits and the genesis of neurological and psychiatric disorders. Specifically, the α(1–3)βγ2 subtypes are mainly located in the synapses and mediate the phasic current (Figure 1), whereas α(4–6)βγ2 or αβε receptor isoforms are partially localized outside the synapse and therefore contribute to both phasic and tonic inhibition (Feng and Forman, 2018; Sallard et al., 2021). On the contrary, αβ or αβδ GABAAR combinations are located extrasynaptically and are involved only in the tonic inhibition of neurons (Sieghart and Savić, 2018). GABAARs are targets for the action of various drugs, including benzodiazepines, alcohol, barbiturates, neurosteroids, or anesthetics; such pharmacological diversity is determined by the presence of separate binding sites in the structures of the subunits (Sieghart and Sperk, 2002; Olsen and Sieghart, 2009; Sieghart, 2015; Masiulis et al., 2019; Edwards and Preuss, 2022). Many of these drugs act as allosteric modulators of the gating process and stabilize the open state of the receptor pore, potentiating or activating the ionic currents by changing the desensitization processes (with or without orthosteric ligand binding) (Gielen and Corringer, 2018; Kang et al., 2020). Currently, phenols of various origins have attracted special attention because they have great potential for positively modulating GABAARs and treating diverse diseases, comprising epilepsy, insomnia, anxiety/depression, Parkinson’s disorder, autism, or schizophrenia (Yamaura et al., 2016; Hu et al., 2020). Although analyses of the structure and function of GABAARs (Phulera et al., 2018; Zhu et al., 2018) and their binding with various drugs have expanded our understanding of their pharmacological profiles (Sieghart, 2015; Puthenkalam et al., 2016; Engin et al., 2018; Liu et al., 2018; Masiulis et al., 2019), the molecular events via which phenols interact with specific sites on GABAARs remain poorly understood, although models are beginning to emerge.
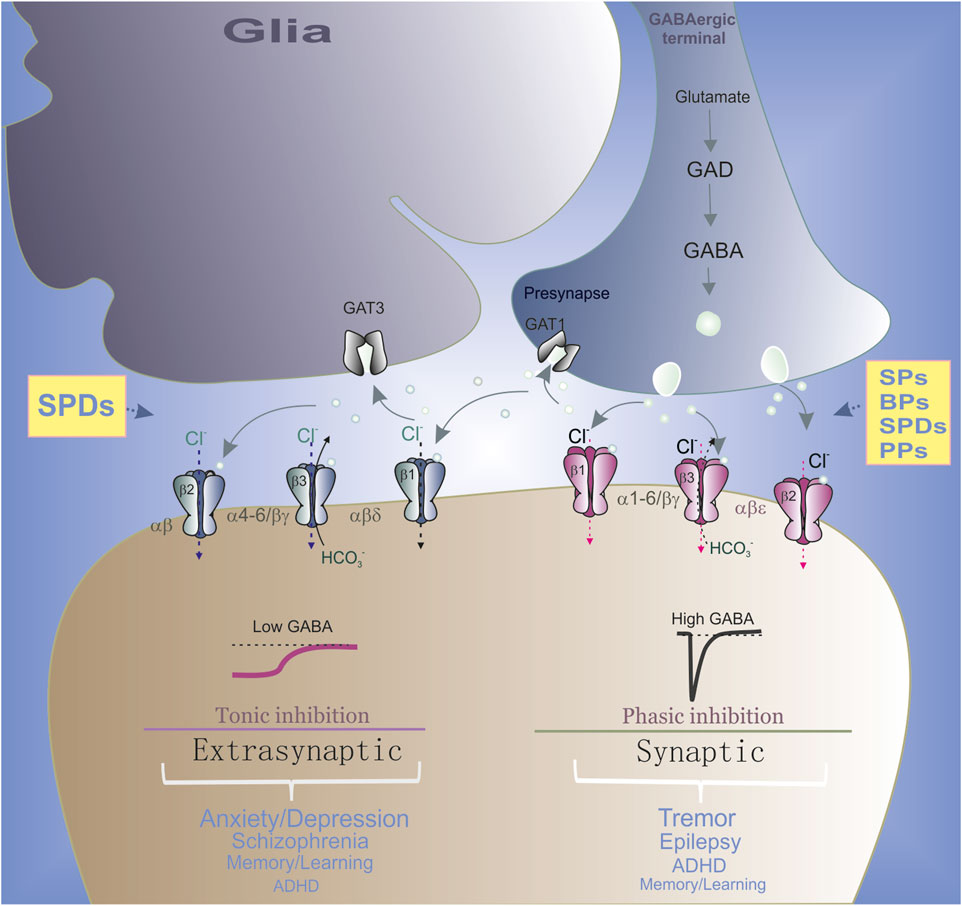
FIGURE 1. Role of synaptic and extrasynaptic GABAAR subtypes in the neurological and psychiatric disorders. In GABAergic terminals, GABA is synthesized from glutamate via glutamic acid decarboxylase (GAD) and then is released from presynaptic neuronal vesicles into the synaptic cleft (circle). GABA reuptake is carried out by GABA transporters (GAT) in both neurons and glia. Specifically, α(1–3)βγ2 subtypes are mainly located in the synapses and mediated the phasic current. Whereas α(4–6)βγ2 or αβε receptor ensembles are partially localized outside the synapse and therefore contributing to both phasic and tonic inhibition. Synaptic GABAAR ensembles preferably are involved in the neurological disorders and extrasynaptic subtypes preferably are involved in the neuropsychiatric disorders. All phenols regulate the synaptic GABAAR ensembles and SPDs also modulate extrasynaptic subtypes.
Phenols display a great variety of structures, ranging from simple phenols (SPs) and their derivatives (SPDs) to bisphenols (BPs) and complex natural polyphenols (PPs) (Figure 2). Natural phenolic compounds’ features and roles in GABAAR regulation have been extensively discussed in recent reviews (Cichon et al., 2020; Rahman et al., 2021; Ríos et al., 2022). However, the chemical and structural variability of phenols suggests the presence of various binding sites on GABAARs, allowing them to be activators, blockers, or allosteric ligands of GABAAR activity (Al Mamari, 2021). The presence of several opposing characteristics determines interest in SPDs and PPs as independent modulators of GABAARs function: their potential pharmacological use as anesthetic, anxiolytic, anticonvulsant, sedative, or muscle relaxant drugs (Sauguet et al., 2015) and their ability to exert a neuropathological effect upon accumulation at high concentrations, prompting the search for the optimal phenol structure with the least negative effect (Mohammadi et al., 2001). Moreover, the interest in natural phenols is associated with the need to find new active chemicals different from other pharmacological drugs, avoiding several undesirable effects, such as tolerance, abstinence, dependence, and memory disorders. The ability of SPs and BPs to cause neuropsychiatric disorders such as head twitching/tremors and seizures (Figure 2) (Spencer et al., 2007; Menzikov and Morozov, 2019), attention-deficit/hyperactivity disorder (ADHD), depression, anxiety, and schizophrenia (Bowman et al., 2015; Perera et al., 2016) require a clarification of the molecular mechanisms underlying the appearance of these disorders (Detyniecki, 2022). Literature evidence supports the potential action of various phenols as positive modulators of GABAARs, which favors their use in developing new therapeutic drugs (Lasoń and Leśkiewicz, 2013; Cordeiro et al., 2022). However, additional investigation is needed to determine the minimal structural complexity needed for phenolic compounds to activate GABAARs.
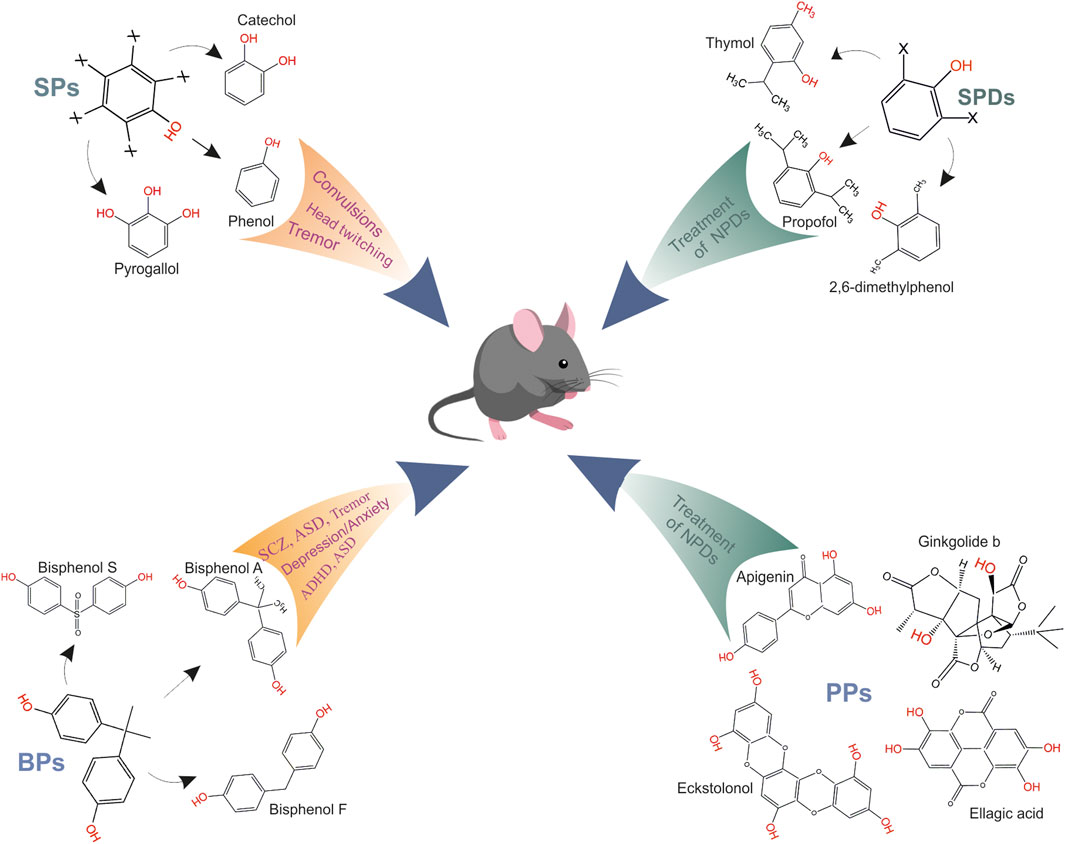
FIGURE 2. Role of various phenol groups in appearance of neuropsychiatric disorders. SPs mediate head twitching, tremor and epileptic seizures (sceleton structure of SPs, where x is possible presence of a hydroxyl group and representatives of group are phenol, catechol or pyrogallol); BPs mediate the tremor, anxiety/depression, ADHD, schizophrenia and memory/learning, ASD (sceleton structure of BPs and representatives of group are bisphenol A, bisphenol S or bisphenol F); SPDs are general anesthetics and use for treatment of neuropsychiatric disorders (sceleton structure of SPDs, where x is possible presence of a substituting group and representatives of group are propofol, thymol or 2,6-dimethylphenol); PPs have neuroprotective effects and were used for treatment of neuropsychiatric disorders, and representatives of group are apigenin, ginkgolide b, eckstolonol, ellagic acid.
This review focuses on the GABAAergic deficit hypotheses during neuropsychiatric disorders induced by various phenols and the molecular mechanisms underlying their interaction with GABAARs. We analyzed the structures of various phenols and how they induce different neuropsychiatric manifestations, from negative (by SPs or BPs) to positive effects in the CNS (by SPDs and PPs). We also highlighted and discussed the role of phenols in the modulation of GABAARs by analyzing their extra- and intracellular binding sites. Next, we analyzed the role of GABAAR subunits in phenol-induced neuropsychiatric disorders and the molecular mechanisms underlying their modulatory effects. Finally, based on current literature, we discussed how phenols can regulate GABAAR activity via processes of the desensitization and resensitization. Overall, this review demonstrated novel pharmacological approaches when using phenolic substances to treat diseases via regulation of the receptor phosphorylation/dephosphorylation.
2 Phenols and neuropsychiatric disorders
Phenols are a class of aromatic chemicals characterized by a hydroxyl (-OH) group attached directly to a carbon atom of a benzene ring (Al Mamari, 2021). Phenols are among the largest and most widely distributed group of aromatic hydrocarbons. Phenols can be divided into subgroups according to their structural and biological characteristics since they can produce both beneficial and harmful effects on living systems depending on the phenols’ structural features and doses (Figure 3). Indeed, despite the wide use of phenol compounds in various industries, their biological significance is usually considered in the context of their effects on the environment and on human health (Li, 2017; Tufarelli et al., 2017). Human and animal health, especially, is adversely impacted through lifetime exposures to environmental stressors, such as chemicals present in the water, air, and food. Crucial sources of environmental pollution are phenolic compounds (Vermerris and Nicholson, 2008). Moreover, many studies have demonstrated that phenols cross the blood-brain barrier (BBB) and can exert positive or negative health effects on the brain (Pletz et al., 2016; Velásquez-Jiménez et al., 2021). For example, when phenols of various origins enter the bodies of animals and humans, these compounds can have undesirable and beneficial neuropsychiatric consequences (Tufarelli et al., 2017). Although, based on their neurotoxicological features, phenols are essentially distinct from each other, there are not only visible differences but also similarities in their influence on the CNS (Giri et al., 2016; Ismail and Mahboub, 2016).
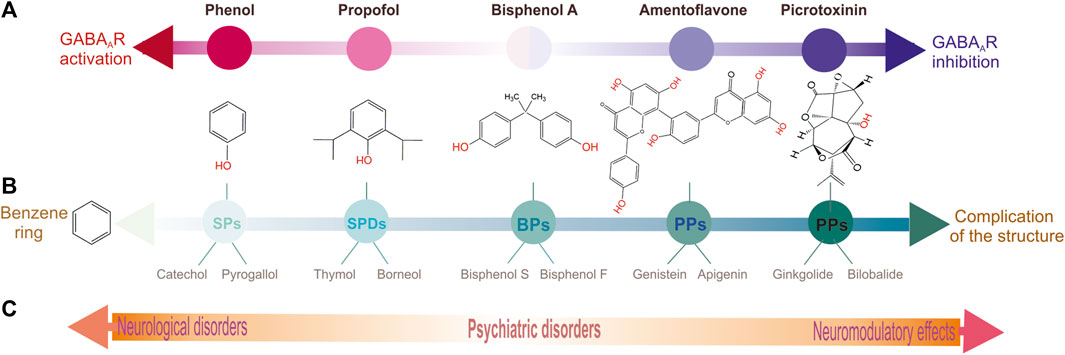
FIGURE 3. Schematic representation distributing of various phenol groups by their biological and structural peculiarities. (A) Chemicals distribution in according to their type of action on GABAARs: left is activation, right is inhibition and in the middle is intermediate state. (B) Chemicals distribution in according to complexity of their structure. (C) Chemicals distribution in according to their the role in the neuropsychiatric sequelae.
2.1 Simple phenols
Simple phenols (SPs) are molecules with one or several -OH groups attached to a benzene ring, which is their basic skeleton (Figure 2). The simplest representative compound of the SP class is phenol (C6H6O), also known as benzenol or carbolic acid, which contains a single hydroxyl group. Within the SP group, catechol, resorcinol, pyrogallol, and phloroglucinol contain either two or three hydroxyl groups (Figure 3). SPs can either be natural or artificial compounds; phenol is found in coat tar, while pyrogallol, resorcinol, catechol, and phloroglucinol are found in plants. SPs are ubiquitous chemicals used in manufacturing fragrances, pharmaceutical drugs, and flavor enhancers and are essential for producing polycarbonates, epoxies, bakelite, nylon, detergents, herbicides, and fur dyes. However, the main use of phenol, which accounts for two-thirds of its production, involves its conversion to precursors that are used in plastics. Environmental exposure to phenols occurs in various ways via industrial processes, such as during pyrolysis and in chemical effluents. Many SPs are corrosive to the eyes, skin, and respiratory tract, and long-term and/or repeated exposure to these substances may harm the liver and kidneys. SPs may also cause harmful effects on the CNS and heart, resulting in arrhythmia, seizures, and coma. Phenol-induced neurological disorders include consecutive manifestations of several neurological symptoms, such as head twitching, tremors, epileptic seizures, coma, and even death (Hodson, 1985; Rice et al., 1997; Todorović, 2003; Giri et al., 2016; Menzikov and Morozov, 2019).
Tremors are neurodegenerative movement disorders (Gironell, 2014). Tremor-related pathologies are classified according to their characteristics, and their clinical manifestations include motor symptoms (including bradykinesia, rigidity, postural instability, and/or resting tremors) and/or non-motor symptoms (Błaszczyk, 2016). Phenol-induced effects manifest primarily as head twitching and muscular tremors followed by the spreading of such manifestations to other body parts (Spencer et al., 2007). The magnitude of head twitching/tremors depends on the compound dose, and the maximum effect occurs 5–10 min after exposure (Menzikov and Morozov, 2019; Itoh, 1995; Windus-Podehl et al., 1983).
Epilepsy comprises a group of neurological disorders with neurobiological, cognitive, and psychological symptoms characterized by repetitive epileptic seizures with a range of etiologies and comorbidities (Hirose, 2014; Kaila et al., 2014; Fei et al., 2020). Epilepsy is mostly considered an impairment of the excitation: inhibition (E:I) balance due to alterations in synaptic neurotransmission. Phenol-induced tremors are accompanied by severe seizures which are dependent on the phenol dose (Windus-Podehl et al., 1983; Itoh, 1995). GABAAergic ligands (phenobarbital or picrotoxin) have been shown to restore phenol-mediated behavioral changes (Menzikov and Morozov, 2019).
2.2 Simple phenol derivatives
Simple phenol derivatives (SPDs) are simple phenols on which one or more hydroxyl groups are substituted with methyl, amino, or halogen groups as shown in Figure 2) (Al Mamari, 2021). Propofol and thymol, which are general anesthetics, are well-known as representative SPDs. Propofol induces and maintains general anesthesia and sedation in human adults and is administered via injection into a vein. It takes approximately 2 min to reach its maximum effect and typically lasts 5–10 min. This drug may cause a decrease in the processes associated with consciousness and memory and can also induce euphoria, hallucinations, and disinhibition. Propofol is also used to treat epileptic disorders if other drugs are ineffective (Meyer et al., 2006).
The use of SPDs is limited because of the severe neuroexcitatory symptoms that occur at high concentrations of these compounds that accumulate in the brain. Propofol-induced convulsions are related to the suppression of inhibitory circuits in the CNS (Islander and Vinge, 2000; Pantelakis et al., 2021). SPDs can cause excitatory phenomena, such as epileptiform movement or seizures (Moore et al., 2021). Moreover, among the various SPDs studied, only OH-substituted naphthalenes were active in inducing myoclonic convulsions in rodents, indicating that the phenolic hydroxyl group is an essential requirement for inducing these neurological manifestations (Haeseler and Leuwer, 2002).
2.3 Bisphenols
Bisphenols (BPs) are a large group of compounds that contain two phenolic rings linked by a bridge that is formed by carbon and other chemical structures (Figure 2). BP compounds are derivatives of bisphenols for which the capital letter after that acronym is used to express the reactant atom/component within its chemical structure. For example, the A in bisphenol A (BPA) stands for acetone, the S in bisphenol S (BPS) denotes sulfur trioxide, and the F in bisphenol F (BPF) stands for formaldehyde. Other letters representing other compounds are also used as shown in Figure 3 (Qiu et al., 2019). BPs are widely produced synthetic chemicals and are typically used to manufacture polycarbonate plastics, including dental composites, bottles, and sealants (Yoo et al., 2020). BPs are also used to add strength and resilience to materials and are found in many products, such as toys, water supply pipes, and medical tubing (Brown, 2009; Li et al., 2023). BPs are ubiquitous within the environment and have become a health threat due to their neurotoxicological effects on animals and humans. Many studies on the biological and neurotoxicological actions of BPs on the CNS have focused on their estrogenic action as an endocrine disrupter (Choi et al., 2007; Braun et al., 2009; Marques et al., 2021; Rebolledo-Solleiro et al., 2021).
BPA is a contaminant of utmost concern because it is an endocrine disruptor that may also affect neurodevelopment in children (Brown, 2009). The primary route for introducing BPA in humans is via the consumption of food products that have been in contact with materials containing this chemical. BPA is a major component of epoxy and polycarbonate resins, which are widely used as ingredients in protective coatings on food containers and as adhesives used in packaging products. Low doses of BPA induce cancer, an increase in body weight, adverse effects on the male reproductive tract, and long-term changes in brain structure and function. Neurocognitive effects, such as hyperactivity, aggression, and impaired learning have also been demonstrated in animals treated with BPA. Although other BP compounds have been used to replace BPA, evidence that such replacement compounds show similar toxicity to BPA has been reported (Naderi et al., 2022). However, a comprehensive assessment of these replacement compounds still needs to be done.
Invertebrate and vertebrate animal models have shown that exposure to BPA can adversely affect multiple aspects of neuronal development, including neural stem cell proliferation, differentiation, and synaptic plasticity. Investigations on animal models have revealed that BPA treatment considerably concurrently affects behavioral endophenotypes, including changes in locomotor activity, induction of anxiety-like behavior, and learning/memory deficits (Stump et al., 2010; Bowman et al., 2015; Mornagui et al., 2019; Welch and Mulligan, 2022).
Tremor − Some reports demonstrate that BPA exposure may affect the development of Parkinson’s disorder (Landolfi et al., 2017; Li et al., 2023). Administration of BPs led to a worsening of tremors in mice via NRF2/HO-1 signaling (D’Amico et al., 2022). BPA (≤1 mM) was found to induce oxidative stress and impair mitochondrial and cellular metabolic activity in the heads of flies (Drosophila melanogaster), causing the development of a Parkinson ’s-like disease (Musachio et al., 2020). In addition, high concentrations of BPA produced changes in the expression of tremor marker proteins in the zebrafish brain (Olsvik et al., 2019).
ADHD is a childhood neuropsychiatric disorder characterized by behavioral deviations, including hyperactivity, attention deficit, and impulsivity (Kwon et al., 2017). However, despite the availability of knowledge regarding the diverse mechanisms of ADHD, its pathogenesis is not fully understood, so correct early diagnosis and provision of accurate clinical management are challenging (Kessi et al., 2022). Recent papers have speculated a strong correlation between BPA treatment and behavioral/cognitive dysfunction in children with ADHD (Tewar et al., 2016; Rochester et al., 2018; Yoo et al., 2020; Subramani et al., 2023). Life-course analysis revealed that a diet containing BPA led to an increase in energy expenditure in mice and was associated with hyperactive and lean phenotypes (Anderson et al., 2013). In another study, BPA administration (0.01–1 μM) led to larval hyperactivity or learning deficits in adult zebrafish (Saili et al., 2012). Interestingly, exposure to β-estradiol (0.1 μM) also led to larval hyperactivity. In a recent paper, it was demonstrated that treating D. melanogaster embryos with BPA (1 mM) induced pathological developmental changes in behavior, such as incompatibility in social interactions and hyperactivity, which manifest as an enhancement of locomotion in open field tests and aggression episodes (Musachio et al., 2021).
Anxiety is characterized by worry, apprehension, and specific somatic, neurocognitive, and behavioral manifestations (Hu et al., 2023). Depression includes emotional symptoms, such as persistent anhedonia, sadness, reduced interest of the person in their environment, and psychomotor changes (Xing et al., 2012). Several studies have suggested that gestational exposure to BPs may lead to neurobehavioral problems during childhood and impaired socio-cognitive development and associated mechanisms. In a study by Perera et al. (2016), BPA administration was coupled with symptoms of anxiety and depression more frequently in boys than in girls aged 10–12 years. Many studies have also shown that long-term application of BPA and its phenolic analogs induces various sex-specific anxiety and depression symptoms in rodents (Xu et al., 2015; Xu et al., 2012; Hong et al., 2013; Kumar and Thakur, 2017; Wiersielis et al., 2020). Specifically, mice exposed to low doses of BPA in their drinking water during the pre- and post-natal periods showed anxiety-related behaviors (Sasaki et al., 2023). In another study, BPA administration elevated anxiety behaviors in F0 rats (Fan et al., 2018). Paternal exposure of F1 rats also led to an increase in anxiety behaviors in F1 females and aggravated depressive behaviors in both sexes. A recent study revealed that administration with low concentrations of BPA and BPS in early life caused an elevation in anxiety-like behavior in zebrafish (Naderi et al., 2022). In contrast, exposure to higher concentrations of BPA resulted in social deficits and impaired object recognition memory. Additionally, co-exposure to an aromatase inhibitor antagonized the BPA- and BPS-induced effects on anxiety levels and social behaviors in zebrafish larvae. Co-application with an estrogen receptor antagonist restored normal recognition memory. The authors speculated that BPA and BPS affect social and cognitive functions via different mechanisms.
Schizophrenia is a disorder with an unknown etiopathogenesis. It manifests as disturbed behavior characterized by delusions, hallucinations, abnormal mental functions, disorganized thinking, and impaired cognitive function. Although several hypotheses have been proposed, the etiology of schizophrenia remains unclear. Accumulating data demonstrate that native endocrine disrupters are associated with this disease (Bowman et al., 2015). Moreover, the pathology of schizophrenia has been associated with impairments in mitochondrial function, energy expenditure, and oxidative stress (Chiapponi et al., 2016).
Memory and learning refer to how an individual acquires, encodes, stores, and retrieves information (Rebolledo-Solleiro et al., 2021). The hippocampus is one of the main structures involved in various cognitive functions, including memory and learning. Several studies have reported that BPA could influence learning and memory, which sometimes appear to be sex-dependent. For example, male rats that received high concentrations of BPA during adolescence showed deficient spatial memory and anxiety in adulthood (Bowman et al., 2015; Wu et al., 2020; Hu et al., 2023). In contrast, other studies report that rats exposed to BPA (25 μg/kg/day) had altered spatial learning as determined using a Morris water maze; however, this observation was only noted in females (Hass et al., 2016). However, some authors have not found significant differences in spatial learning between male and female deer mice (Jašarević et al., 2013). It was also reported that mice showed decreased alternation behavior in a Y-maze after treatment with BPA at doses of 100 and 500 mg/kg/day (Rebolledo-Solleiro et al., 2021). These results indicated working memory impairment. BPA-treated mice also showed a decrease in novel object recognition as indicated by a reduction in central locomotion and frequency in the central zone (Tian et al., 2010). Mice of both sexes that received BPA (0.5–5,000 μg/kg/day) presented increased anxiety, impaired spatial memory, and reduced dendritic spine density in the CA1 region of the hippocampus and medial prefrontal cortex (Xingyi et al., 2013; Zhang et al., 2019; Zhang et al., 2020). Moreover, low-dose maternal BPA application caused significant impairment in the learning/memory capabilities of F1 male mice but not in the F2 generation. Wang et al. reported adverse effects of BPA on the CNS, especially learning and memory (Wang et al., 2014). BPA has been shown to cause adverse effects on the synaptic structure of pregnant Sprague-Dawley rats, resulting in a widened synaptic cleft, thinned postsynaptic density, and unclear synaptic surface. In addition, environmental exposure to BPA may impair childhood behavior and learning development. For example, urinary BPA levels are negatively coupled to the learning quotient based on the Learning Disability Evaluation Scale (LDES) as described in several studies (Hong et al., 2013).
Autism is characterized by several behavioral features, including social deficits, impaired communication, and repetitive behaviors with sex-specific manifestations (Stein et al., 2015). Pre-natal exposure to high BPA concentrations is thought to elevate the risk of developing autism. Recent studies have identified candidate autism-related genes responsible for the sex-specific prenatal action of BPA (Thongkorn et al., 2023). Molecular docking analysis of BPA and autism-related transcription factors revealed targets for BPs (Kanlayaprasit et al., 2021). In addition, it was found that prenatal BPA administration caused an elevation in neurite length, the number of primary neurites, and the number of neurite branches but diminished the size of the hippocampal cell body in both sexes. Several epidemiological studies demonstrated the association between prenatal BPA application and autism (Kanlayaprasit et al., 2021); however, other studies did not find any association between prenatal BPA application and neuropathology (Hansen et al., 2021).
2.4 Polyphenols
Polyphenols (PPs) are a class of compounds with a polyphenol structure and one or more -OH groups attached directly to several benzene rings (Figure 2). Natural phenols include two types of compounds: 1) flavonoids and 2) non-flavonoids (Balland et al., 2022; Ríos et al., 2022). Flavonoids consist of flavones, flavonols, flavonones, isoflavones, and anthocyanins, which differ via their hydrogenation status and the identity of their heterocyclic substitutions (Figure 3). Other PPs include phenolic acids, terpenes (mono-, di-, tri-, and sesquiterpenoids), stilbenes, phlorotannins, and lignans.
Natural polyphenols are secondary metabolites ubiquitous throughout the plant kingdom. PPs are formed in variable amounts depending on the taxonomic group (family or species) and plant parts, such as roots, stems, flowers, and fruits. In plants, these chemicals play a key role in many vital physiological processes, such as lignification, pigmentation of flowers and fruits, and pollination, and also act as growth factors. They also govern plant responses to environmental stress, such as protection from excess ultraviolet radiation, nutrient deficiency, and drought stress, and act as chemical defenses against herbivores, insects, and microbial pathogens. Natural phenolics are important constituents of many edible and medicinal plants and are widely used in the food industry as flavorings, antioxidants, and antibacterial agents. Indeed, PPs have attracted significant attention due to their wide presence in our daily diet and, more importantly, their antioxidant properties (Rahman et al., 2021; Karioti et al., 2016). Although the relationships between food phenolics and health are not yet fully understood, human epidemiological studies demonstrate that consuming food rich in polyphenols might be beneficial, especially for age-related disorders, such as cardiovascular and neurodegenerative diseases. While various studies have investigated extracts and essential oils as potential sources of active agents in animals and humans, other authors studied their possible pharmacological potential in silico. For example, flavonoids have antimicrobial, hepatoprotective, cardioprotective, anti-inflammatory, neuroprotective, antiviral, and anticancer properties (Rahman et al., 2021). Of all tested compounds, phenolics were of greatest interest, especially flavonoids and certain tannins. At present, convincing evidence that the mechanisms by which flavonoids exert their pharmacological effects are not simply due to their redox properties but their capability to bind directly to target proteins or peptides that regulate different cellular functions exists (Karioti et al., 2016).
Natural phenols can be beneficial to health because they cause a reduction in the risk of developing neurological and psychiatric diseases (Vermerris and Nicholson, 2008; Szwajgier et al., 2017). Many authors have reported the neuroprotective role of various phenolic acids in controlling epilepsy and ameliorating anxiety and depression, imbalance after traumatic brain injury, hyperinsulinemia-induced memory impairment, and Parkinson’s disorder (Szwajgier et al., 2017; Fasipe et al., 2020; Cordeiro et al., 2022; De Vries et al., 2022; Dong and Huang, 2022). Ellagic acid has antioxidant, anti-inflammatory, and neuroprotective properties (Ríos et al., 2022). Several recent reviews have discussed the antidepressant effects of compounds, such as amentoflavone, apigenin, chlorogenic acid, curcumin, and ferulic acid (Pathak et al., 2013; Wang et al., 2022; Winiarska-Mieczan et al., 2023). In another study, Nigella sativa oil produced a positive motor coordinative effect on phenol-mediated essential tremors in a mouse model (Folarin et al., 2020). Recent studies have also suggested using plant-derived polyphenolic compounds for antiepileptic treatment. In particular, extracts from Urtica dioica Linn. root or Lactuca serriola (Asteraceae) can cause antiepileptic effects in pentylenetetrazole-induced seizure models (Loshali et al., 2021). Among flavonoids, chrysin, resveratrol, baicalein, quercetin, and rutin produced significant anti-seizure activity or mild sedative and anxiolytic effects (Ullah et al., 2022; Karioti et al., 2016). Thus, flavonoids can prevent neuronal excitability, suggesting their potential use as an adjunctive therapy for treating epilepsy. However, although natural phenols possess diverse biological properties, the molecular mechanisms underlying their effects on the CNS remain unknown.
3 Phenols and GABAARs
Although the neurotoxic actions of aromatic hydrocarbons on animals and humans manifest themselves at the organismic, cellular, and molecular levels, they primarily affect the CNS. Therefore, understanding where and how phenol and phenol derivatives interact with subcellular brain structures remains relevant at the present time. Moreover, substances with a wide range of action on the organism can have additive and/or neutralizing influences on each other (Hellström et al., 2016). The research concerning the brain structures that are specific and highly sensitive to a wide row of phenolic compounds is important for obtaining a better understanding of their neuropsychiatric mechanisms action. Many studies have confirmed that their neurotoxicity effects are associated with modulating pentameric ligand-gated ion channels (pLGIC) activity. Specifically, data demonstrate that phenolic compounds selectively interact with GABAARs and prove to be modulators by inducing negative or positive effects on the CNS (Figure 3).
3.1 Simple phenols
Early studies demonstrated heterogeneous data regarding the mechanisms underlying phenol-induced NDs. For example, the effects of catechols on neuromodulator uptake in rat brain slices showed that low catechol concentrations (10 μM) had little effect on the release of acetylcholine, noradrenaline, or GABA but produced an increase in aspartate release (Minchin and Pearson, 1981). In contrast, a high catechol concentration (100 μM) led to an inhibition of acetylcholine and GABA release. Ducis et al. have shown that phenol affects the peripheral benzodiazepine receptors on astrocytes (Ducis et al., 1990). However, it is likely that the effects were directed at a translocator protein (TSPO), also known as a peripheral benzodiazepine receptor, which is a transmembrane protein located on the outer mitochondria membrane in the glial cells of the brain (Lee et al., 2020). Several authors have shown that 10 mM phenol activates oocyte-expressed GABAAR-mediated Cl− currents by displacing water as shown in Figure 4A (Brosnan and Pham, 2018). Both in vitro and in vivo studies recently demonstrated that phenol has a biphasic action on the Cl−-ATPase activity of β3-containing GABAARs isolated from rat and fish brains with receptor activation at low concentrations and inhibition at high concentrations (Menzikov, 2018; Menzikov and Morozov, 2019). Moreover, at low doses (<100 μM), phenol was found to inhibit Cl−/HCO3− ATPase function and activate Cl− transport via GABAARs that had been purified from the rat brain (Figure 3).
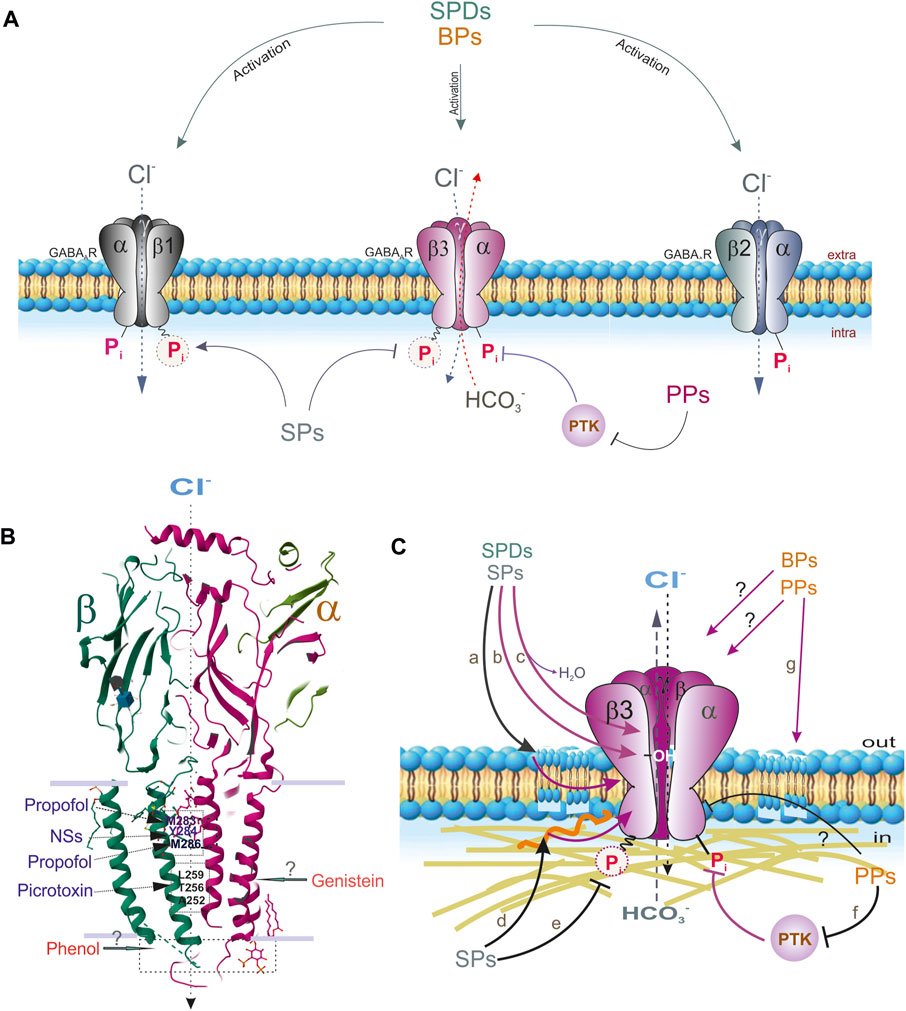
FIGURE 4. Extra- and intracellular effects of different phenols on the GABAAR subtypes containing β1, β2 or β3 subunits. (A) SPDs interact with all receptor subtypes. SPs interact with β3 and β1-containing GABAAR subtypes via binding with ATP-hydrolyzing site. PPs directly inhibit GABAAR function or via PTK way. (B) Sites binding of propofol, picrotoxin or NSs with GABAAR β and α subunit (Protein Data Bank, 4COF). Sites binding of phenol or genistein are not known. (C) The suspected molecular mechanisms interactions of SPs or SPDs on the GABAARs: a) and g) via membrane lipids modification; b) via H-bond formation with OH-groups; c) via displacing of water; d) via cytoskeleton disturbance; e) via changes of the formation of high-energetic acyl-phosphate bond; f) via the processes of phosphorylation by PTKs (or other protein kinases) or direct inhibition of the receptor function.
3.2 Simple phenol derivatives
Primary alkylphenol-injectable anesthetics are potent drugs with a high affinity to recombinant GABAARs; at high doses, these anesthetics may also directly potentiate receptors as shown in Figure 4A (Weir et al., 2017; Borghese et al., 2021). General anesthetics can induce different changes in the GABAAR αβγ and αβδ complexes in either their activation or desensitization states (Feng and Forman, 2018). Structure–activity studies using recombinant α1β2γ2 GABAARs addressing the direct agonist effects of SPDs revealed that only chemicals with both unsubstituted phenolic hydroxyls attached directly to the benzene ring or a methyl or isopropyl group inserted in the ortho position relative to the -OH group induced inward Cl− currents (Mohammadi et al., 2001). In addition, thymol (one isopropyl group at the ortho-position) was slightly more potent than 2,6-dimethylphenol; however, propofol possesses the highest potency for GABAARs.
The propofol binding sites on the GABAAR have been identified via site-directed mutagenesis (Shin et al., 2018), substituted cysteine modification protection (Forman and Miller, 2016), or photo-affinity labeling (Olsen and Li, 2011; Jayakar et al., 2014). Specifically, results of modeling, photolabeling, and/or functional studies demonstrated two propofol binding domains on GABAARs (Krasowski et al., 1998; Krasowski et al., 2001). These domains are defined based on either the β(M283) or β(M286) residues at the β+α interface in the transmembrane domain (TMD) and the β(Y143) residue close to the β-surface in the junction between the extracellular domain (ECD) and TMD (Figure 4B) (Shin et al., 2018; Borghese et al., 2021). Although it has been found that the β subunits are important for SPD-mediated regulation of GABAARs, subtypes containing β3 subunits are the primary targets (Jurd et al., 2003; Eaton et al., 2015; Kreuzer et al., 2020). In mutant β3(N265M) mice, the action of propofol via β3-containing GABAARs was severely restricted. It has also been speculated that the propofol binding sites are preferably located at the αβ+/α−γ interface and the α+/β− or α+/γ− TMD interfaces in the α1β3γ2 isoform (Bali and Akabas, 2004; Maldifassi et al., 2016). In addition, photolabeling of β3 homomeric and α1β3 receptors also suggested that propofol is in contact with β3H267 (Yip et al., 2013). However, the results of a mutational analysis do not support the idea that β3H267 is a propofol binding site (Stern and Forman, 2016; Feng and Forman, 2018).
3.3 Bisphenols
The first study reporting the action of BPA on GABAARs was conducted on recombinant α1β1 isoforms expressed in Xenopus oocytes as shown in Figure 4) (Aoshima et al., 2001). Depending on its concentration, BPA has dual action on GABAARs; ≤100 μM BPA causes an increase in GABA-mediated Cl− currents while >100 μM BPA causes a decrease. This action was found to be induced by the direct binding of BPA to GABAARs in an estrogen-independent manner (Figure 4A). Other authors investigated the action of BPA on GABA-induced Cl− currents (IGABA) in isolated rat CA3 pyramidal neurons (Choi et al., 2007). In those studies, <10 μM BPA potentiated the GABA-mediated IGABA peak, while diazepam (or ethanol) led to a great reduction in the BPA-mediated activation of IGABA. However, >30 μM BPA caused suppression of the peak IGABA mediated by GABA and accelerated the desensitization state. BPA (>30 μM) inhibited the steady-state GABA-mediated IGABA) in a noncompetitive manner. Perinatal administration of BPA at low doses produced an inhibition of GABAAR-mediated Cl− currents in neurons of the amygdala, leading to abnormal cortical-amygdala synaptic transmission and inducing neurobehavioral alterations (Zhou et al., 2011); however, the long-term behavioral effects of developmental BPA exposure can be reversed (Soriano et al., 2016).
According to data from various studies, BPA-mediated memory/learning disorders may be caused by altering GABAAR α1 subunit expression and distribution in the CA1 region of the hippocampus and prefrontal cortex. Specifically, BPA administration caused a considerable reduction in the density of GABAAR α1 subunits in the prefrontal cortex and hippocampus (Taherianfard and Taci, 2015). The distribution of GABAARs was denser in BPA-exposed rats that were subject to learning than in non-learning rats. Recent studies have reported sex-specific effects of long-term BPA administration on the level of α2 subunit expression in the hippocampus with increased levels in females and decreased levels in males (Xu et al., 2015). These data concerning behavioral alterations prompted the authors to suggest that long-term exposure to BPA affects anxiety- and depression-like behaviors in adult mice, which are mediated by changes in the expression levels of the GABAAR α2 subunit in the hippocampus.
The binding sites of BP on the GABAAR have not yet been identified, but they may be close to the sites for neurosteroid binding, which are distinct from sites at which other phenols bind (Figure 4B). Endogenous steroids display GABAAR-mediated neuroactive effects, including anesthesia, analgesia, and sedation. Although the exact location of the neurosteroid-binding sites has yet to be determined, many residues in the TMDs (αS240, αQ241, αN407, αY410, αT236, and βY284) have been shown to affect neurosteroid activity (Li et al., 2009). The modulatory and activation sites of neurosteroids are located at the TMDs of the α subunit and β+/α–interfaces, respectively (Miller et al., 2017). In particular, photolabeling of β3 homomeric receptors identified F301 in β3-M3 as a possible neurosteroid binding site (Chen et al., 2012).
3.4 Polyphenols
Complex polyphenols, such as flavonoids, terpenoids, and polyacetylenic alcohols, affect the GABA system (Hanrahan et al., 2011; Ríos et al., 2022). Such compounds can manifest as agonists (such as thymol), inhibitors (such as picrotoxinin, bilobalide, or ginkgolide), or allosteric modulators of GABAAR activity (Figure 3). Moreover, glycosylation reduces the binding of natural phenols to GABAARs (Wang et al., 2002). Flavonoids can also interact with flumazenil-sensitive or -insensitive GABAARs (Hanrahan et al., 2015). Several studies have shown that some isoflavones (such as genistein or tyrphostin) are protein tyrosine kinase (PTK) inhibitors that can directly block GABAAR function or act in a kinase-dependent manner to block receptor function (Dunne et al., 1998; Huang and Hsu, 1999). Earlier studies have shown that GABAAR-mediated Cl− currents are directly depressed by genistein or tyrphostin in neuronal and recombinant GABAARs (Moss et al., 1995; Jassar et al., 1997; Wan et al., 1997; Castel et al., 2000). The flavone, hispidulin, was found to potently activate the α6β2γ2 GABAAR isoform and led to a reduction in susceptibility to seizures (Kavvadias et al., 2004). These data indicate that flavonoids can be considered GABAergic agents (Figure 4A). Although the role of flavonoids in GABAAR regulation has received attention (Hanrahan et al., 2011; Ríos et al., 2022), the molecular mechanisms underlying their direct actions on GABAARs remain poorly understood.
Many flavonoids (including isoflavones and flavones) possess modulatory actions on the benzodiazepine-binding site of GABAARs (Hanrahan et al., 2015). However, the compounds within this family show potential actions at more than one additional binding site on GABAARs (Figure 4B). Notably, benzodiazepine-sensitive GABAAR subtypes comprise two α and two β subunits in addition to one γ subunit (Fritschy, 2008). GABAARs containing α4, α6, γ2, and, to a lesser extent, δ, subunits, can potently bind many benzodiazepine ligands (Olsen and Sieghart, 2009; Ghit et al., 2021). Mutations that convert histidine to arginine (α1H101R, α2H101R, α3H126R, and α5H105R) of the β2γ2 subtype of GABAARs eliminated diazepam activity, whereas reverse mutations elicited diazepam responses (Benson et al., 1998).
4 GABAARs and neuropsychiatric disorders
Although data concerning the role of GABAergic receptors in different areas (including cortex, hippocampus, hypothalamus, amygdala or spinal cord) of the brain are limited, evidence that the same GABAAR subtypes which are expressed in various neuronal populations can modulate the excitability and neuronal synchronization. Accumulated data in the literature indicate that disturbance of GABAAergic signaling is a root cause for the appearance of neuropsychiatric disorders (Chiapponi et al., 2016; Van Nuland et al., 2020). To confirm the interaction of phenols with GABAARs in the development of neurobiological consequences, we considered the role of these receptors in the manifestation of neurological and psychiatric diseases. In this chapter, we investigated correlations between deficiencies in specific GABAAR subunits and the occurrence of NPDs.
4.1 Tremor
Although it is believed that bradykinesia results from a reduction in dopaminergic neurons (Calon et al., 2003), research done over the last two decades revealed that the major pathophysiological paradigm underlying tremors is the GABAAR hypothesis (Boecker et al., 2010; Paris-Robidas et al., 2012; van Nuland et al., 2020). In addition, the reciprocity between dopamine and GABA in the basal ganglia has been recognized (Hoerbelt et al., 2015). Recently, it was shown that dopamine without agonists can directly regulate recombinant GABAARs by interacting with the β3 subunit. The etiology of essential tremors involves the abnormal firing of Purkinje cells, which receive excitatory inputs from granule cells in the cerebellum (Nietz et al., 2020). A previous study showed that the Purkinje cell-specific knockout of the GABAAR α1 subunit eliminated all GABAAR-mediated inhibition in Purkinje cells while retaining the GABAAR-mediated inhibition of intact cerebellar molecular layer interneurons. The selective depletion of the GABAAR α1 subunit from Purkinje cells did not induce deficiencies in the accelerating rotarod test or decreased survival rates. However, an essential tremor-like phenotype has been observed in animals with a global knockout of the GABAAR α1 subunit (Nietz et al., 2020). This finding is similar to the essential tremors observed in patients. In contrast, results from recent studies provide important new clues into tremor suppression mechanisms initiated by the enhancement of GABA-driven inhibition in pathways controlled by the α2/3 GABAAR subunits but not the α1 subunit (Kosmowska et al., 2023). In addition, GABAAR α6 subunit-selective drugs were found to cause a substantial reduction in tremors and restoration of physical wellbeing in a mouse model (Huang et al., 2021).
4.2 Epileptic seizures
The GABAAR β3 subunit is highly expressed in immature and adult brains, specifically in circuits involved in seizure generation and epileptic seizures (Hörtnagl et al., 2013). Moreover, changes in the physiological and biochemical properties of β3-containing GABAAR assemblies in the brains of patients with epilepsy (Menzikov et al., 2021). Several studies have reported that GABRB3 mutations with juvenile myoclonic epilepsy, childhood absence epilepsy, and/or other syndromes and that reduced GABAAR function causes an E: I imbalance (Gurba et al., 2012; Janve et al., 2016). Animal models of epilepsy have demonstrated obvious alterations in the expression and rearrangement of GABAAR subunits in the hippocampus and the para-hippocampal areas, including downregulation of the α5 and δ subunits and upregulation of the α4 subunit (Sperk et al., 2021). Findings addressing the increased expression of the α4 subunit in patients with temporal lobe epilepsy are similar to those observed in rodent models (Schipper et al., 2016).
4.3 ADHD
Although the etiology and pathophysiology of ADHD remain unclear, some data support the interaction between genetic and environmental factors (Wang et al., 2012; Sun et al., 2018). In particular, Kwon and coauthors have shown an association between GABAB3 gene polymorphisms and ADHD in children (Kwon et al., 2017). Rodents with a deficiency in the β3 subunit exhibit thalamic disinhibition, seizures associated with learning/memory deficits, hyperactivity, poor motor skills in repetitive tasks, and a disturbed rest–activity cycle (DeLorey et al., 1998).
4.4 Anxiety/depression
Accumulating data suggest that the major cause of depressive disorder is GABAergic dysfunction (Luscher et al., 2011; Nuss, 2015). Specifically, it was previously established that cortical GABAARs decreased in patients with depressive pathologies (Fogaça and Duman, 2019). In addition, abundant evidence that the expression of various GABAAR subunit transcripts is altered in depression in animal models has been reported. For example, partial GABAAR deficits in mice (heterozygous γ2 or α2 subunits) induced depression-like behavior (Möhler, 2012) and found that GABRA1 gene expression is subject to epigenetic control (Luscher et al., 2011). In addition, the behavioral deficit was restored by chronic antidepressant treatment in γ2 mice (Luscher et al., 2011). Analysis of the frontopolar cortex of suicide victims who had suffered from various forms of depressive disorders revealed reductions in the abundance of α1, α3, α4, and δ subunit mRNAs (Oquendo et al., 2014). A comparison of the brains of suicide victims with or without depression showed elevated mRNA expression levels of the α5, γ2, and δ subunits in the dorsolateral prefrontal cortex (Merali et al., 2004). However, some studies suggest that variations in the gamma subunits, GABRA2, GABRA3, GABRA6, and GABRG2, do not play a key role in the susceptibility to anxiety spectrum disorders (Pham et al., 2009). Recent anatomical and electrophysiological data indicate that α6-containing GABAARs in cerebellar granule cells can be specific targets for treating NPDs as described in several studies (Sieghart et al., 2022).
4.5 Schizophrenia
A deficit in GABAAR signaling is the current hypothesis underlying the manifestation of schizophrenia (Chiapponi et al., 2016). A decrease in the mRNA expression of the GABAAR α1, γ2, α4, α5, and δ subunits in the dorsolateral prefrontal cortex (DLPFC) of patients with schizophrenia was found (Gill and Grace, 2014; Mueller et al., 2015; Marques et al., 2021). Changes in the expression of the GABAAR α2, β1, and ε subunits in the lateral cerebellum were found in patients with schizophrenia, depression, and bipolar disorder (Fatemi et al., 2013; Fatemi et al., 2017). Meanwhile, several reports about an increase in the mRNA expression of the GABAAR α1 and α5 subunits and no change in the expression of the α4 receptor subunit in schizophrenic brains of humans (Maldonado-Avilés et al., 2009; de Jonge et al., 2017). Several studies on schizophrenic brains showed a decrease in high-mannose N-glycan levels in GABA-associated proteins specific to different GABAAR subunits (α1, α4, and β1–3), increased levels of high-mannose N-glycans in the β1 subunit, decreased levels of high-mannose N-glycans on the α1 subunit, and alterations in the total N-glycan content of the β2 subunits (Mueller et al., 2014; Williams et al., 2020).
4.6 Memory/learning
The GABAAR α5 subunit preferably localizes in the hippocampus of the mature brain. It is related to learning and memory and was discovered through the targeted disruption of the α5 gene in mice (Collinson et al., 2002) in which it was found that α5−/− mice demonstrated elevated spatial learning performance. In contrast, no changes in performance were observed in non-hippocampal-dependent learning and anxiety tasks. In addition, α5−/− mice showed a decrease in the amplitude of inhibitory postsynaptic currents (IPSCs) and an increase in the paired-pulse facilitation of field excitatory post-synaptic potentials (EPSP) in the CA1 region of the hippocampus. Wiltgen et al. showed an increase in the expression of the α1 subunit in the lateral nucleus of the amygdala (Wiltgen et al., 2009). Administering an α1 subunit antagonist into the lateral amygdala caused a selective impairment in auditory learning. Mice with a selective knockout of the α1 subunit in excitatory cells did not exhibit enhanced learning.
In the hippocampus, GABAergic tonic currents are tightly associated with memory and play an essential role in cognition. In the extra-synapses, the distribution density of α5-containing GABAAR subtypes is relatively high in the hippocampus, which can be considered an amnesia-like mechanism during anesthesia (Zhao et al., 2019). Sedation refers to a decrease in the arousal level as indicated by longer response times, decreased motor activity, and slurred speech. In animal models, sedation is characterized by reduced motor activity and arousal (Rudolph and Antkowiak, 2004).
4.7 Autism
Several studies concluded that an impairment in the E: I balance is the main reason for autism development, which was confirmed in several mouse models of autism (Paine et al., 2020). Mendez and coauthors conducted a positron-emission tomography (PET) imaging study using the radioactive ligand [11C]-Ro15–4513 VT to trace levels of the GABAAR α5 subunit in autism (Mendez et al., 2013). Their results showed a reduction in the expression of the GABAAR α5 subunit in two limbic areas of the brains (amygdala and nucleus accumbens) of autistic patients. In contrast, a recent study showed that disturbance in the GABAergic system in autism mouse models and patients with autism was not associated with alterations in the number of GABAARs between healthy and diseased individuals (Horder et al., 2018), and based on a meta-analysis, which also failed to show a link between changes in the GABAAR subunits (β3, α5, and α3) and autism in children (Mahdavi et al., 2018).
5 Molecular mechanisms underlying the action of phenols on GABAARs
The capability of phenols to interact with different target structures and cause a broad range of effects can be attributed largely to their amphiphilic character. Indeed, an oxygen atom normally forms δ bonds with other atoms (as an example, with hydrogen atoms in H2O molecule). When one hydrogen atom is missing in a water molecule, the formation of the -OH group, which is responsible for various physical and chemical features of various compounds, including phenols, is prone to electrophilic substitution reactions due to its rich electron density. In particular, these reactions are initiated by reactions of electron-deficient groups with the negatively charged oxygen atom or by reaction of electron-rich groups to the positively charged atoms (C or H bonds) whereas the availability of the hydrophobic planar benzene ring in phenolic molecules is responsible for π-binding (π-stacking) and other non-covalent interactions. Such structural peculiarities allow phenols to induce positive and/or negative effects on various target proteins, including enzymes, receptors, and lipid structures, which subsequently modulates their properties (Tungmunnithum et al., 2018; Lin et al., 2021). Thus, the action of phenols on GABAAR function could result from their specific binding with their target subunits, nonspecific interactions with surrounding membrane lipids, or perturbation of the cytoskeleton in the receptor environment (Figure 4C).
5.1 Via hydrogen-bond formation
Hydrogen bonds are important in potentiating diverse cellular functions, including protein–ligand interactions (Chen et al., 2016). Some authors demonstrated that tyrosine H-bonds largely contribute to protein stability and are considered the strongest conventional H-bonds (Pace et al., 2001; Scheiner et al., 2002). Compounds containing -OH groups in their structure interact with receptors via H-bond formation (Starovoytov et al., 2014). H-bonds usually occur between the phenolic OH-group and the protein–peptide bonds; the strongest of all is the conventional H-bonds, namely, OH‧‧O and OH‧‧N (Scheiner et al., 2002). SPs and SPDs have been shown to interact with GABAARs partly via the formation of H-bonds between their -OH groups and various amino acid residues (Figure 4C). Specifically, H-bonds form between the −OH groups of phenol compounds and amino acid residues within the binding cavity of the GABAAR β3 subunit (Krasowski et al., 2001). In addition, a structure analysis suggests a direct interaction between propofol and the phenol residue of tyrosine in the receptor channel (Leon et al., 2012). The strengths of H-bonds at interaction with aromatic amino acids have followed this trend–Trp > His > Tyr ∼ Phe. In other studies, the formation of H-bonds between phenols and amino acid residues was used as an introductory model for biological systems because of their structural similarities to tyrosine, a para-substituted phenol (Fedor and Toda, 2014). However, the molecular mechanism and the intensity by which H-bonds regulate molecular interactions of phenol with GABAARs remain unclear because the H-bonding continuously competes with bulk water (Utas et al., 2006).
5.2 Via water displacement
Some authors suggest that phenol might modulate rat recombinant α1β2γ2s GABAARs by replacing H2O from one or more low-affinity amphipathic binding sites, resulting in a conformational rearrangement that increases anion conductance as shown in Figure 4C (Brosnan and Pham, 2016; Brosnan and Pham, 2018). It was previously suggested that the presence of amphipathic receptor sites normally occupied by H2O molecules is associated with dissociation constants inversely related to the cut-off solubility value of phenols, which is between 0.10 and 0.46 mM. Weakly soluble phenol compounds cannot reach concentrations sufficient to compete with H2O for binding-site access and, therefore, fail to modulate GABAARs (Brosnan and Pham, 2018).
5.3 Via membrane lipid modification
Various phenols can modify the properties of membranes, including their fluidity, thickness, or lateral structure, with several conformational changes in membrane proteins (Tsuchiya and Mizogami, 2014). Several studies used various phenols, model lipids, and measurement methods to collect detailed information on phenol–lipid interactions (Figure 4C). In particular, it was shown that various phenol compounds at concentrations of 1–10 μM interacted with the model or neuronal membranes to raise their fluidity in the order of potency: propofol > thymol > isothymol > guaiacol > phenol > eugenol, which is consistent with the order of their activity (Tsuchiya and Mizogami, 2020). In addition, these chemicals caused a reduction in membrane lipid peroxidation at potencies correlating with their membrane activities. Miguel et al. (2019) used an equilibrium molecular dynamics simulation approach and showed that applying SPDs in bilayers affected lipid acyl chains in carbons near the interface, but their influence is negligible at the center of the bilayer. Some authors who use nuclear magnetic resonance (1H-NMR) spectroscopy showed that GABAergic phenol derivatives can be incorporated into phospholipid vesicles, specifically within the region between polar groups (choline molecules), glycerol, and the first atoms of the acyl chains (Reiner et al., 2013). Furthermore, inserting these chemicals into membranes leads to a reduction in the repulsion between the phospholipid head groups and a reduction in the overall mobility of the hydrocarbon chains. Natural polyphenols can also interact with the membrane and penetrate lipid bilayers depending on the structure, concentration, and composition of the membrane lipids (Bonarska-Kujawa et al., 2011; Karonen, 2022).
5.4 Via cytoskeletal modification
It is known that pLGICs interact with signaling and cytoskeletal proteins (Sheng and Pak, 2000; Björnström and Eintrei, 2003). Phenol compounds can induce reorganization of the actin cytoskeleton in neurons into ring structures (Figure 4C). For example, the interaction between propofol and actin triggers a dose-dependent internalization of GABAAR β2 subunits (Oscarsson et al., 2007); such an increase in internal GABAA β2 subunit content is closely related to actin polymerization.
Using fluorescence-labeled actin in cultured rat neurons, several authors evaluated the percentage of actin rings induced by propofol or GABA in combination with Rho, Rho kinase (ROCK), phosphoinositide 3-kinase (PI3K), or protein tyrosine kinase (PTK) inhibitors (Björnström et al., 2008). In contrast to GABA, propofol induces transcellular actin ring organization that is dependent on the influx of extracellular calcium, which can be blocked by ROCK, PI3-kinase, or tyrosine kinase inhibitors. Thus, propofol utilizes Rho/ROCK to facilitate actin translocation from the cytoskeleton to the plasma membrane, while actin ring organization depends on an interaction site close to the agonist site on GABAAR. GABA did not mediate actin ring formation, indicating that this effect was propofol-specific.
5.5 Via kinase regulation
GABAAR phosphorylation governs numerous processes, including channel activity regulation, control of receptor trafficking, effects on receptor-interacting proteins, and altering sensitivity to various drugs (Balduzzi et al., 2002). Cyclic-AMP-, Ca2+/phospholipid-, Ca2+/calmodulin-, and cGMP-dependent kinases catalyze the transfer of γ-phosphate from ATP to a serine or threonine residue, which typically causes a reduction in GABAAR function (Nakamura et al., 2015). In recent decades, studies have demonstrated the importance of PTKs in regulating the properties of GABAARs (Moss et al., 1995). Tyrosine residue phosphorylation by PTKs (Src family) causes an increase in receptor activity (Jassar et al., 1997). The tyrosine residues of the β3 subunit (Tyr365 and Tyr367) and serine residue of the γ2 subunit (Ser343) are substrates of PTKs as shown in Figure 3C (Wan et al., 1997). In cultured frog pituitary melanotrophs, extracellular application of the PTK blocker, genistein, can cause a bell-shaped modification of the whole-cell GABAAR-mediated Cl− currents (Castel et al., 2000). In particular, low concentrations of genistein (<0.1 μM) induced receptor activation, whereas high concentrations (>50 μM) caused a reversible reduction in the GABAAR-mediated Cl− current. In addition, administration of recombinant PTK pp60c-src (inside-out configuration) inhibited GABAAR-mediated Cl− currents; this effect was reversed by genistein (Figures 4A–C). Immunoblotting revealed that genistein markedly inhibited the tyrosine phosphorylation of GABAAR β2/β3 subunits. In addition, propofol caused an increase in the intracellular calcium levels ([Ca2+]i) of primary neurons cultured from newborn rats when exposed to neurons cultured in a Ca2+-free medium (Björnström et al., 2002). This increase in [Ca2+]i declined when the cells were preincubated with the PTK blocker, herbimycin A. Propofol treatment caused an increase in tyrosine phosphorylation of the GABAAR β subunits. Thus, PTKs participate in these propofol-mediated biological effects by inducing calcium release from intracellular stores and modulating the GABAAR β subunits (Björnström et al., 2002). In addition, polyphenols isolated from the Chinese mangrove plant can inhibit GABAARs via individual kinases (IC50 = 2–4 μg) as described in several studies (Shi et al., 2010).
5.6 Via modification of receptor desensitization
GABAARs quickly open their transmembrane pores upon neuromodulator binding thus enabling anions to flow passively into neurons via the plasma membrane (Ghit et al., 2021). However, GABAARs undergo desensitization following activation, which provides incremental entry into a long-term closed state refractory to excessive or repeated activation (Figure 5) (Field et al., 2021). The desensitization process causes a decrease in Cl− currents and facilitates GABA entry into a bound state with GABAARs (Bai et al., 1999). Some studies addressing desensitization have focused on changes in the conformation of GABAARs following significant activation by activators or allosteric modulators (Kang et al., 2020). In addition, functional and structural data support a “dual-gate” model in which the TMD of pLGICs contains both an activation gate, located at the top of the channel, and a deactivation gate, located at the intracellular end of the channel as shown in Figure 5 (Gielen and Corringer, 2018; Kaczor et al., 2021). Recent structural studies have shown conformational changes between the mediator-bound open state and the desensitized state that occurs at the “internal face” of the receptor (Rovšnik et al., 2021). Such alterations also include phosphorylation of GABAAR subunits by protein kinases, their expression, clustering, and pharmacology.
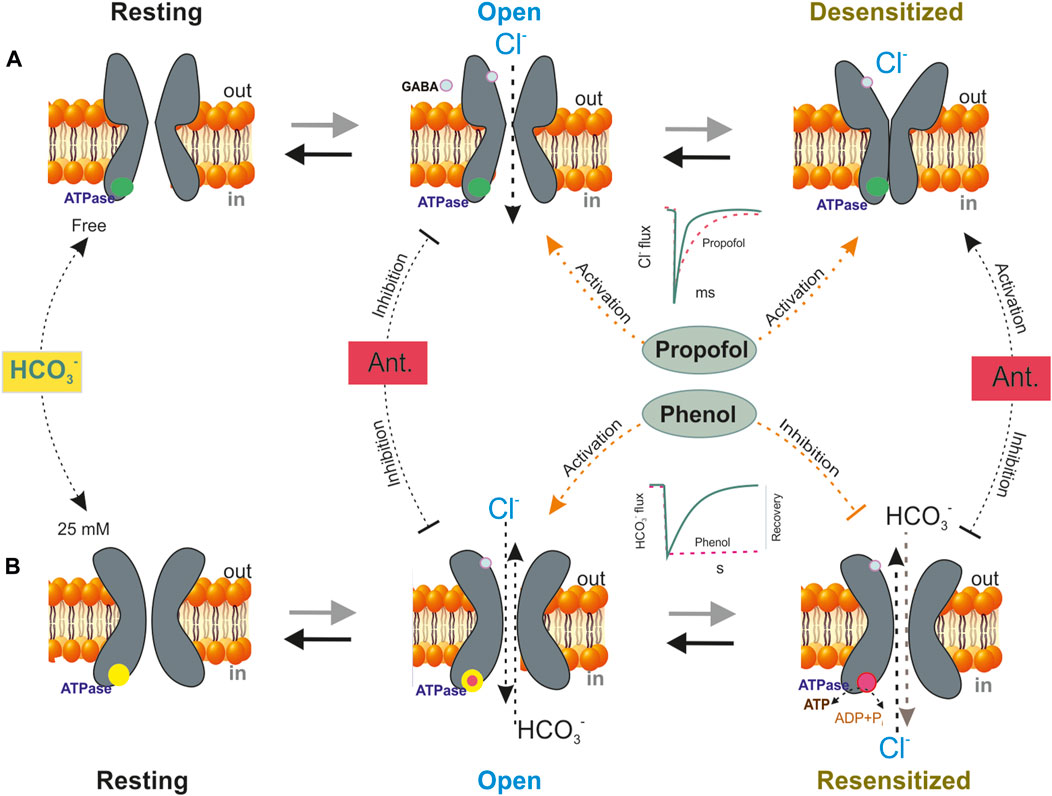
FIGURE 5. A schematic model showing four basic conformation states that depict the GABAAR function–a resting, an open, a desensitization or resensitization states. (A) In an HCO3− free medium, GABA (green circles) exposure shifts the equilibrium from resting to open state and on repeat (or prolongation) mediator application is observed the GABAAR desensitization. Propofol influences the GABA-mediated response by stabilizing different states, including resting, desensitized conformations and controlling their transitions. The blue circles are the ATPase in the none active form. The most noticeable effect of propofol on the responses to short exposures to mediators is that it prolongs deactivation. (B) In the presence of physiological concentration of HCO3− (25 mM), GABA changes the conformation of channel and shifted the equilibrium from resting state to open state. Bicarbonate determines the phenol action on the GABAAR. Phenol activates the open state and inhibits the resensitization state of the receptor − ([Cl−]i/[HCO3−]i recovery. The GABAAR-coupled ATPase only possesses low sensitivity (>1 mM) to phenol effect if it is activated only Cl− or HCO3−, whereas the Cl−, HCO3− ATPase is inhibited by low concentrations of phenols (<1 mM). It was shown that the transition from the desensitization state to resensitization is coupled with a fall in [ATP]i, which is carried out via ATPase performance. The red circles are the ATPase in the active form. Antagonists (Ant.–picrotoxin or bicuculline) inhibit the open state but activate the desensitization state.
Although sufficient structural and pharmacological data are present, our understanding of the effects of different phenols on receptor desensitization is formed mainly by studies conducted using propofol (Borghese et al., 2021) or neurosteroides (Miller et al., 2017). The most noticeable effect of propofol on the responses to short exposures to mediators is that it causes prolongation of deactivation. Specifically, an earlier study noted that propofol prolongs desensitization as evidenced by changes over the time course of the GABA responses (Orser et al., 1994). In addition, propofol influences the GABA-mediated response by stabilizing different states, including the resting and desensitized conformations in addition to controlling the transition between these states (Figure 5). Such channel activation and changes of desensitization may result from propofol binding near the GABA recognition site. Several structural studies have shown at least five certain receptor states, namely, three nonconducting states (resting, diazepam-bound, and potentiating propofol-bound) and two conducting-desensitized states (activating propofol-bound and mediator-bound states) as described in several studies (Williams and Akabas, 2002; Shin et al., 2018).
5.7 Via modification of receptor resensitization
Regulating GABAAR desensitization is an essential process that is controlled at the signaling pathway and receptor levels; however, modulating receptor function at the level of the energy processes plays a key role in understanding withdrawal in neurological and psychiatric disorders (Rezin et al., 2009). Mitochondrial dysfunction is a key factor in epilepsy, Parkinson’s disorder, schizophrenia, and other synaptic and extra-synaptic pathologies (De Simone et al., 2023). However, the relationship between GABAAR activity, impaired energy balance, and manifestations of NPDs remains poorly understood.
Early research has shown that exposure to SPs (such as catechol and pyrogallol) induces convulsive activity accompanied by a rapid decline in neuronal ATP levels ([ATP]i) in the brains of mice (Angel et al., 1969; Angel and Rogers, 1972). Other studies showed that neuronal [ATP]i led to a decrease in energy production (Pi/min/g), which is elevated abruptly during seizures and recovered after phenobarbital treatment (Stanley and Mantz, 1971; Winn, 1980; Walton et al., 1998). However, these studies reported that the reduction in [ATP]i was partly due to mitochondrial dysfunction that occurred during the seizure state; the main reason behind the reduction in [ATP]i during neurological disorders is still elusive (Walton et al., 1998). Furthermore, many reports have demonstrated that even due to the effects of various ligands (including GABA), the reduction in [ATP]i is the main reason underlying the reduction in GABAAR functional activity, which ultimately manifests as neuronal excitation (Sallard et al., 2021). However, the molecular events determining the decline in ATP levels during the GABAAR run-down phenomenon and desensitization state remain unknown (Figure 5).
Many literature reports show that GABAAR desensitization is tightly associated with its slow deactivation, namely, so-called process of resensitization (Hinkle and Macdonald, 2003; Kang et al., 2020). Although some kinases play a role in the desensitization and retardation of GABAAR deactivation, the main players involved in the resensitization process have not been fully established. Recently, it was reported that the β3 and β1 subunits possess a phenol-regulated ATPase, which is localized in the ICD (Menzikov, 2018; Menzikov et al., 2021) and that resensitization by the enzyme facilitates the replenishment of the neuronal concentrations of chloride ([Cl−]i) and bicarbonate ([HCO3−]i) after GABAAR activity and desensitization (Menzikov et al., 2021; Menzikov et al., 2022). A recent finding showed that the Cl− ATPase activities belonging to the β1 and the β3 subunits have Cl−, HCO3−ATPase activity, which determines differences in their sensitivity to phenol (Menzikov et al., 2023). Furthermore, this enzyme is within the scope of GABAAR performance as Cl−ATPase during the first week of postnatal rodent development and then as a Cl−, HCO3−ATPase. The GABAAR-coupled ATPase only possesses low sensitivity (>1 mM) to the phenol effect if it is activated only by Cl− or HCO3−, whereas the Cl−, HCO3− ATPase is inhibited by low concentrations of phenols (<300 μM). It was shown that the transition from the desensitization state to resensitization is coupled with a fall in [ATP]i, which is carried out via ATPase performance (Figure 5). The critical role of ATPase during the resensitization process and the resulting conformational changes in GABAARs were confirmed using a thiol alkylating agent and a mutant receptor (Menzikov et al., 2022). Phenol modulates the formation of a phosphoprotein (high-energy phosphate bond) and the rate of ATP-consuming Cl− transport by the ATPase (Menzikov and Morozov, 2019). Moreover, it was established that phenol interacts with the GABAAR β3 subunit by binding with the ATP-hydrolyzing site of ICD a finding that directly confirms the interaction of phenol with GABAARs.
6 Phenols and ionic plasticity
Neuronal/synaptic plasticity concerns structural and functional alterations that take place in different parts of the brain over a range of timescales and adapt their function in response to specific stimuli. Ionic plasticity is directly associated with the modulation of the functional expression and properties of ionic channels. Neuronal chloride ([Cl−]i) and bicarbonate ([HCO3−]i) ion concentrations are pivotal parameters that control the E: I balance, and their effects depend on specialization and the level of neuronal development. Ionic plasticity of GABAergic signaling refers to the modulation of neuronal functions via changes in the driving force (DFGABA) of the underlying anionic currents via short- and long-term mechanisms that can lead to significant changes (EGABA) (Raimondo et al., 2017; Sallard et al., 2021). Specifically, GABAAR dynamics is the result of the short-term changes in the neuronal Cl− and HCO3− gradients that control the nature and strength of GABAAR-mediated currents (EGABA), whereas activity-dependent changes in the trafficking, kinetics, and function of [Cl−]i/[HCO3−]i regulating systems (cation-chloride cotransporters, carbonic anhydrases, and Na+, K+ATPase) can result in long-term shifts in the DFGABA. For example, in mature neurons, the [Cl−]i is low, and GABAAR activation triggers Cl− influx into neurons, which results in the hyperpolarization of the TMP. Under certain conditions (such as the massive action of ligands or spinal cord lesions), the [Cl−]i can reach high values, close to the [Cl−]i in immature neurons (Fritschy, 2008). In this state, GABA can shift the TMP from hyperpolarization toward depolarization and even excitation, which depends not only on Cl− influx but also on HCO3− efflux via the channel (Fujiwara-Tsukamoto et al., 2003; Kaila et al., 2014). Several reports showed that changing the [Cl−]i is essential for desensitization (Ghit et al., 2021), whereas changing the [HCO3−]i is essential for receptor resensitization (Menzikov et al., 2022).
Interneuronal GABAA receptors inside and outside of synapses are required for normal brain function, including plasticity, learning/memory, and neuronal networks. Phenols interact with GABAAR subunits (preferably β subunits) and act as activators (such as SPs and SPDs) or inhibitors (PPs) depending on the complexity of their structure (Figure 3). A comparative analysis of various chemicals belonging to various phenolic groups demonstrated similarities and differences in their capabilities to regulate GABAARs and subsequently cause neuropsychiatric disorders. A certain pattern can be observed between the structure of phenolic substances and their effects on receptors (activation or inhibition); the more complex the structure of the substance, the more likely they are to elicit inhibitory effects (Figure 3). Other literature studies also demonstrate that the effect of many phenols (including natural compounds) on the CNS exhibits a concentration dependence; at low concentrations, it activates, and at high concentrations, it inhibits receptor function (Borghese et al., 2021). In addition, in vivo and in vitro studies have shown that administering low phenol concentrations caused an increase in GABAAR-coupled ATPase function and induced mild manifestations of the disease in animals, whereas the effect of high concentrations of phenols on receptor activity may represent another side of their influence on GABAARs; higher phenol concentrations inhibit ATPase activity but mediate the development of severe disorders. Thus, phenols activate the passive permeability of anions via GABAAR channels into neurons (Brosnan and Pham, 2018; Menzikov and Morozov, 2019) but inhibit the GABAAR-coupled ATPase activity, which recovers anion homeostasis in neurons that can be associated not only with different sites of binding but and modification of non-covalent interactions (firstly, H-bonds) as shown in Figure 6. Moreover, non-covalent interactions between phenols and amino acid residues are quite labile and especially dependent on the pH value or ionic strength (Schefer et al., 2021). Such a double role of phenols in the modulation of the GABAAR dynamic is prone to disrupt the short- and long-term alterations of ionic plasticity and, as a result, impact neuronal network activity and neuropsychiatric sequelae. As noted in previous chapters, phenol compounds with varying potency can impair GABAAR desensitization/resensitization and cause a collapse of neuronal Cl− and HCO3− gradients that ultimately cause neurobiological sequelae (Lillis et al., 2012; Hudson and Grau, 2022). A substantial development of phenol-induced neurological symptoms and the concurrent recovery of ATPase activity after administering GABAAergic drugs or phosphorylation blockers indicate that GABAAR/ATPase subtypes are the target of phenol action and can be used to investigate the effects of other phenols.
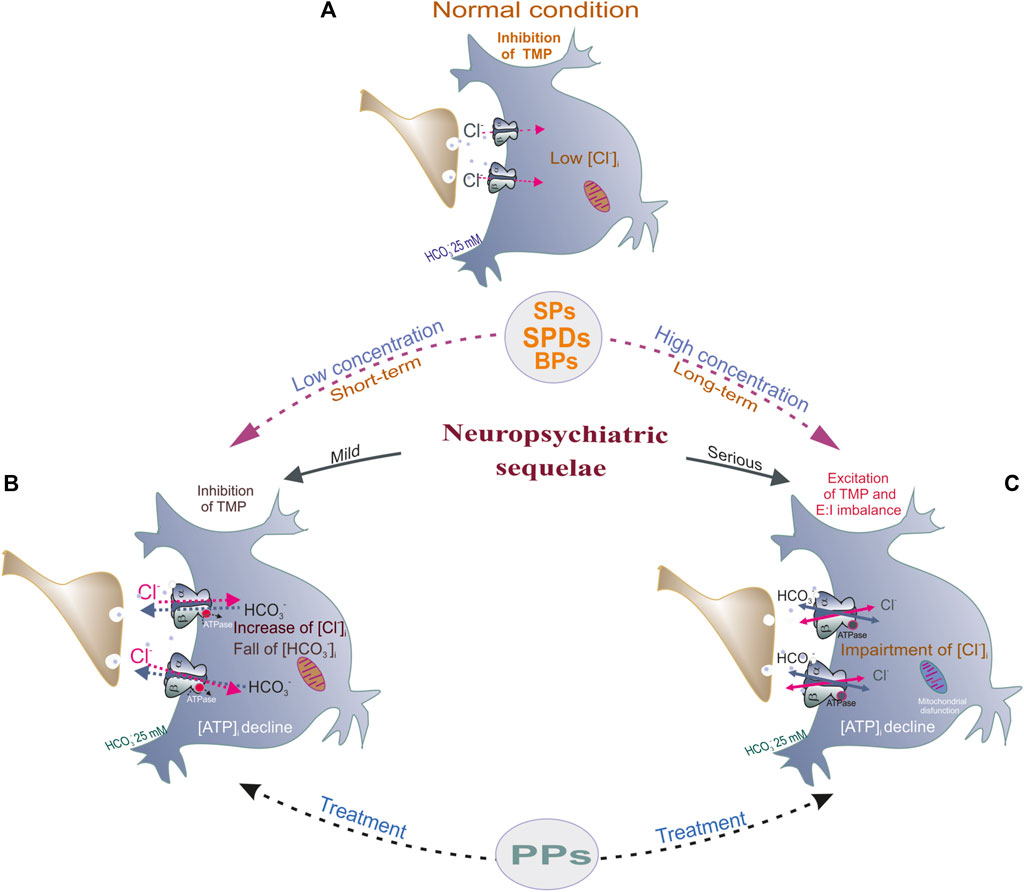
FIGURE 6. Potential mechanism underlying phenol-mediated changes of E:I balance by modulation of GABAARs function. (A) In normal condition, in mature neurons, the [Cl−]i is low, and GABAAR activation by GABA (green circles) triggers a fast Cl− influx and subsequent hyperpolarization/inhibition of the TMP. (B) Low concentration (or short-term of phenols (SPs, SPDs or BPs) exposure induced an essential increase of Cl− influx and HCO3− outflux via receptor pore and as a result, the increase of hyperpolarization of the TMP. Here, phenol compounds increase the GABAAR-coupled Cl−, HCO3−ATPase activity, the decline of [ATP]i, and what accompanies by mild neuropsychiatric pathologies (head twitching, tremor). (C) High concentration (or long-term) of phenols application induced impaired of synchronicity of multidirectional Cl− and HCO3− fluxes via receptor pore, ATPase inhibition and as a result, the depolarization of TMP, E:I imbalance (collapse). These pathologies are characterized by the decline of [ATP]i, mitochondrial dysfunction and accompanies by serious neuropsychiatric manifestation.
7 Conclusion
The first reports regarding the role of phenols in neuropsychiatric consequences and the establishment of the possible role of GABAARs in their manifestation have primarily focused on phenol derivatives. In this review, we examined the roles of phenols with different structures and neurobiological sequelae in GABAAR regulation. Due to the diversity and complexity of the structures of phenolic substances, they have different modes of action on GABAARs and elicit various diseases. We showed the similarities and differences between various phenol groups and how they can induce neurobiological changes ranging from negative action (e.g., SPs or BPs) to positive manifestations (e.g., PPs) in the CNS. The neuropsychiatric sequelae after exposure of GABAARs to phenols are confirmed by a number of studies, suggesting the dominant role of this type of pLGICs in the development of these diseases. In particular, simple phenols elevate GABAAR functional activity, whereas many natural polyphenols most often causes an inhibitory effect on receptor activity, albeit at varying efficiencies; however, mechanisms of action for most remain unclear. As shown above, the complex structures of natural chemicals imply the presence of diverse mechanisms regarding their interaction with GABAAR subunits, making it difficult to establish their molecular mechanisms of effect. However, although natural polyphenols are of particular interest, probably when establishing molecular causes in the development of neuropsychiatric disorders consideration primarily of simple phenol action on the GABAARs was preferable.
Indeed, the first step toward achieving effective GABAAR regulation using complex phenols (including natural phenolics) to treat the different neurobiological sequelae is to elucidate the specific sites that interact with simple phenols and the molecular events leading to positive conformational changes in their corresponding receptor subtypes. However, although our understanding of the interaction between phenol derivatives and GABAARs continues to increase, the molecular mechanisms underlying the interaction of the GABAARs to simple phenols (and primarily to phenol) remain largely elusive. In addition, crystal/cryo-EM and site-directed mutagenesis may not always identify the functional, critical, and complex phenols binding sites, as various compounds may interact with similar or distinct sites and elicit various functional consequences. Therefore, determining the mechanistic basis of phenol-GABAAR interactions at the molecular level is essential for elucidating the conformational rearrangements in the channel and could pave the way for the design of drugs with improved activity and target specificity.
One unique feature of GABAARs is their adoption of a phenol-dependent conformation. Recent data suggest that phenols mainly target the β subunit (primarily the β3 subunit) of GABAARs (Menzikov et al., 2021). Comparative analysis showed that phenols have different effects on the mediator-mediated responses by stabilizing or inhibiting different receptor conformations/states depending on the presence of bicarbonate. Although the exact molecular mechanism of phenol-receptor interactions remains unresolved, H-bond formation between the hydroxyl group of the phenol and the amino acid residues of the target subunits is likely; however, as shown above, other interactions are also possible.
The construction of phenol-specific structures (Leon et al., 2012) that target the GABAAR subunits is required to fully understand the molecular nature of the interaction of compounds with the GABAA receptor. Using these model structures, along with a combination of functional (e.g., electrophysiology, fluorimetry) and structural (e.g., cryo-EM, MS, or NMR) studies, should eventually provide detailed insight into the mechanisms underlying phenol-GABAAR interactions. This will also allow us a better understanding of the pharmacological effects of other phenols that might modulate GABAAR conformation, which could result in a generate chemical templates for developing clinically important drugs. Finally, a mechanistic understanding of the interactions of phenol with the GABAAR-coupled ATPase during resensitization may have clinical significance for treating neurobiological sequelae. Indeed, this ATPase plays an essential role in GABAAR resensitization and the elimination of neuropsychiatric symptoms. However, discovering the mechanism whereby phenols modulate ATPase activity requires further detailed structural analyses the interactions of phenol with the GABAARs and specific enzyme inhibitors.
Author contributions
SAM: Conceptualization, Writing–original draft. DZ: Formal Analysis, Validation, Writing–review and editing. AM: Formal Analysis, Validation, Writing–review and editing. SGM: Project administration, Resouces, Writing–review and editing. AK: Project administration, Resources, Writing–review and editing.
Funding
The author(s) declare that no financial support was received for the research, authorship, and/or publication of this article.
Acknowledgments
The authors want to thank Olga Menzikova for the help in preparing the figures.
Conflict of interest
The authors declare that the research was conducted in the absence of any commercial or financial relationships that could be construed as a potential conflict of interest.
Publisher’s note
All claims expressed in this article are solely those of the authors and do not necessarily represent those of their affiliated organizations, or those of the publisher, the editors and the reviewers. Any product that may be evaluated in this article, or claim that may be made by its manufacturer, is not guaranteed or endorsed by the publisher.
References
Al Mamari, H. H. (2021). “Phenolic compounds: classification, chemistry, and updated techniques of analysis and synthesis,” in Phenolic Compounds—Chemistry, Synthesis, Diversity, Non-Conventional Industrial, Pharmaceutical and Therapeutic Applications. (London: IntechOpen). doi:10.5772/intechopen.98958
Anderson, O. S., Peterson, K. E., Sanchez, B. N., Zhang, Z., Mancuso, P., and Dolinoy, D. C. (2013). Perinatal bisphenol A exposure promotes hyperactivity, lean body composition, and hormonal responses across the murine life course. FASEB J. 27 (4), 1784–1792. doi:10.1096/fj.12-223545
Angel, A., Lemon, R. N., Rogers, K. J., and Banks, P. (1969). The effect of polyhydroxyphenols on brain ATP in the mouse. Exp. Brain Res. 7, 250–257. doi:10.1007/BF00239032
Angel, A., and Rogers, K. J. (1972). An analysis of the convulsant activity of substituted benzenes in the mouse. Toxicol. Appl. Pharmacol. 21, 214–219. doi:10.1016/0041-008x(72)90064-6
Aoshima, H., Hossain, S. J., Imamura, H., and Shingai, R. (2001). Effects of bisphenol A and its derivatives on the response of GABA(A) receptors expressed in Xenopus oocytes. Biosci. Biotechnol. Biochem. 65, 2070–2077. doi:10.1271/bbb.65.2070
Bai, D., Pennefather, P. S., MacDonald, J. F., and Orser, B. A. (1999). The general anesthetic propofol slows deactivation and desensitization of GABA(A) receptors. J. Neurosci. 19 (24), 10635–10646. doi:10.1523/JNEUROSCI.19-24-10635.1999
Balduzzi, R., Cupello, A., and Robello, M. (2002). Modulation of the expression of GABA(A) receptors in rat cerebellar granule cells by protein tyrosine kinases and protein kinase C. Biochim. Biophys. Acta 1564, 263–270. doi:10.1016/s0005-2736(02)00460-1
Bali, M., and Akabas, M. H. (2004). Defining the propofol binding site location on the GABAA receptor. Mol. Pharmacol. 65 (1), 68–76. doi:10.1124/mol.65.1.68
Balland, E., Lafenetre, P., and Vauzour, D. (2022). Editorial: polyphenols' action on the brain. Front. Neurosci. 21 (16), 947761. doi:10.3389/fnins.2022.947761
Benson, J. A., Löw, K., Keist, R., Mohler, H., and Rudolph, U. (1998). Pharmacology of recombinant gamma-aminobutyric acidA receptors rendered diazepam-insensitive by point-mutated alpha-subunits. FEBS Lett. 431 (3), 400–404. doi:10.1016/s0014-5793(98)00803-5
Björnström, K., and Eintrei, C. (2003). The difference between sleep and anaesthesia is in the intracellular signal: propofol and GABA use different subtypes of the GABA(A) receptor beta subunit and vary in their interaction with actin. Acta Anaesthesiol. Scand. 47 (2), 157–164. doi:10.1034/j.1399-6576.2003.00007.x
Björnström, K., Sjölander, A., Schippert, A., and Eintrei, C. (2002). A tyrosine kinase regulates propofol-induced modulation of the beta-subunit of the GABA(A) receptor and release of intracellular calcium in cortical rat neurones. Acta Physiol. Scand. 175 (3), 227–235. doi:10.1046/j.1365-201X.2002.00991.x
Björnström, K., Turina, D., Loverock, A., Lundgren, S., Wijkman, M., Lindroth, M., et al. (2008). Characterisation of the signal transduction cascade caused by propofol in rat neurons: from the GABA(A) receptor to the cytoskeleton. J. Physiol. Pharmacol. 59 (3), 617–632.
Błaszczyk, J. W. (2016). Parkinson’s disease and neurodegeneration: GABA-collapse hypothesis. Front. Neurosci. 10, 269. doi:10.3389/fnins.2016.00269
Boecker, H., Weindl, A., Brooks, D. J., Ceballos-Baumann, A. O., Liedtke, C., Miederer, M., et al. (2010). GABAergic dysfunction in essential tremor: an 11C-flumazenil PET study. J. Nucl. Med. 51 (7), 1030–1035. doi:10.2967/jnumed.109.074120
Bonarska-Kujawa, D., Pruchnik, H., Oszmiański, J., Sarapuk, J., and Kleszczyńska, H. (2011). Changes caused by fruit extracts in the lipid phase of biological and model membranes. Food Biophys. 6 (1), 58–67. doi:10.1007/s11483-010-9175-y
Borghese, C. M., Wang, H. L., McHardy, S. F., Messing, R. O., Trudell, J. R., Harris, R. A., et al. (2021). Modulation of α1β3γ2 GABAA receptors expressed in X. laevis oocytes using a propofol photoswitch tethered to the transmembrane helix. Proc. Natl. Acad. Sci. U.S.A. 118 (8), e2008178118. doi:10.1073/pnas.2008178118
Bowman, R. E., Luine, V., Diaz Weinstein, S., Khandaker, H., DeWolf, S., et al. (2015). Bisphenol-A exposure during adolescence leads to enduring alterations in cognition and dendritic spine density in adult male and female rats. Horm. Behav. 69, 89–97. doi:10.1016/j.yhbeh.2014.12.007
Braun, J. M., Yolton, K., Dietrich, K. N., Hornung, R., Ye, X., Calafat, A. M., et al. (2009). Prenatal bisphenol A exposure and early childhood behavior. Environ. Health Perspect. 117 (12), 1945–1952. doi:10.1289/ehp.0900979
Brosnan, R. J., and Pham, T. L. (2016). GABAA receptor modulation by phenyl ring compounds is associated with a water solubility cut-off value. Pharmacology 98, 13–19. doi:10.1159/000444935
Brosnan, R. J., and Pham, T. L. (2018). Anesthetic-sensitive ion channel modulation is associated with a molar water solubility cut-off. BMC Pharmacol. Toxicol. 19 (1), 57. doi:10.1186/s40360-018-0244-z
Brown, J. S. (2009). Effects of bisphenol-A and other endocrine disruptors compared with abnormalities of schizophrenia: an endocrine-disruption theory of schizophrenia. Schizophr. Bull. 35 (1), 256–278. doi:10.1093/schbul/sbm147
Calimet, N., Simoes, M., Changeux, J. P., Karplus, M., Taly, A., and Cecchini, M. (2013). A gating mechanism of pentameric ligand-gated ion channels. Proc. Natl. Acad. Sci. U. S. A. 110 (42), E3987–E3996. doi:10.1073/pnas.1313785110
Calon, F., Morissette, M., Rajput, A. H., Hornykiewicz, O., Bédard, P. J., and Di Paolo, T. (2003). Changes of GABA receptors and dopamine turnover in the postmortem brains of parkinsonians with levodopa-induced motor complications. Mov. Disord. 18, 241–253. doi:10.1002/mds.10343
Castel, H., Louiset, E., Anouar, Y., Le Foll, F., Cazin, L., and Vaudry, H. (2000). Regulation of GABAA receptor by protein tyrosine kinases in frog pituitary melanotrophs. J. Neuroendocrinol. 12 (1), 41–52. doi:10.1046/j.1365-2826.2000.00417.x
Chen, D., Oezguen, N., Urvil, P., Ferguson, C., Dann, S. M., and Savidge, T. C. (2016). Regulation of protein-ligand binding affinity by hydrogen bond pairing. Sci. Adv. 2 (3), e1501240. doi:10.1126/sciadv.1501240
Chen, Z. W., Manion, B., Townsend, R. R., Reichert, D. E., Covey, D. F., Steinbach, J. H., et al. (2012). Neurosteroid analog photolabeling of a site in the third transmembrane domain of the β3 subunit of the GABA(A) receptor. Mol. Pharmacol. 82 (3), 408–419. doi:10.1124/mol.112.078410
Chiapponi, C., Piras, F., Piras, F., Caltagirone, C., and Spalletta, G. (2016). GABA system in schizophrenia and mood disorders: a mini review on third-generation imaging studies. Front. Psychiatry 7, 61. doi:10.3389/fpsyt.2016.00061
Choi, I. S., Cho, J. H., Park, E. J., Park, J. W., Kim, S. H., Lee, M. G., et al. (2007). Multiple effects of bisphenol A, an endocrine disrupter, on GABA(A) receptors in acutely dissociated rat CA3 pyramidal neurons. Neurosci. Res. 59 (1), 8–17. doi:10.1016/j.neures.2007.05.003
Cichon, N., Saluk-Bijak, J., Gorniak, L., Przyslo, L., and Bijak, M. (2020). Flavonoids as a natural enhancer of neuroplasticity-an overview of the mechanism of neurorestorative action. Antioxidants 9 (11), 1035. doi:10.3390/antiox9111035
Collinson, N., Kuenzi, F. M., Jarolimek, W., Maubach, K. A., Cothliff, R., Sur, C., et al. (2002). Enhanced learning and memory and altered GABAergic synaptic transmission in mice lacking the alpha 5 subunit of the GABAA receptor. J. Neurosci. 22 (13), 5572–5580. doi:10.1523/JNEUROSCI.22-13-05572.2002
Cordeiro, M. L. D. S., Martins, V. G. Q. A., Silva, A. P. D., Rocha, H. A. O., Rachetti, V. P. S., and Scortecci, K. C. (2022). Phenolic acids as antidepressant agents. Nutrients 14 (20), 4309. doi:10.3390/nu14204309
D'Amico, R., Gugliandolo, E., Siracusa, R., Cordaro, M., Genovese, T., Peritore, A. F., et al. (2022). Toxic exposure to endocrine disruptors worsens Parkinson's disease progression through NRF2/HO-1 alteration. Biomedicines 10 (5), 1073. doi:10.3390/biomedicines10051073
De Jonge, J. C., Vinkers, C. H., Hulshoff Pol, H. E., and Marsman, A. (2017). GABAergic mechanisms in schizophrenia: linking postmortem and in vivo studies. Front. Psychiatry 8, 118. doi:10.3389/fpsyt.2017.00118
DeLorey, T. M., Handforth, A., Anagnostaras, S. G., Homanics, G. E., Minassian, B. A., Asatourian, A., et al. (1998). Mice lacking the beta3 subunit of the GABAA receptor have the epilepsy phenotype and many of the behavioral characteristics of Angelman syndrome. J. Neurosci. 18 (20), 8505–8514. doi:10.1523/JNEUROSCI.18-20-08505.1998
De Simone, G., Mazza, B., Vellucci, L., Barone, A., Ciccarelli, M., and de Bartolomeis, A. (2023). Schizophrenia synaptic pathology and antipsychotic treatment in the framework of oxidative and mitochondrial dysfunction: translational highlights for the clinics and treatment. Antioxidants (Basel) 12 (4), 975. doi:10.3390/antiox12040975
Detyniecki, K. (2022). Do psychotropic drugs cause epileptic seizures? A review of the available evidence. Curr. Top. Behav. Neurosci. 55, 267–279. doi:10.1007/7854_2021_226
de Vries, K., Medawar, E., Korosi, A., and Witte, A. V. (2022). The effect of polyphenols on working and episodic memory in non-pathological and pathological aging: a systematic review and meta-analysis. Front. Nutr. 8, 720756. doi:10.3389/fnut.2021.720756
Dong, X., and Huang, R. (2022). Ferulic acid: an extraordinarily neuroprotective phenolic acid with anti-depressive properties. Phytomedicine 105, 154355. doi:10.1016/j.phymed.2022.154355
Ducis, I., Norenberg, L. O., and Norenberg, M. D. (1990). Effect of phenol and sodium octanoate on the astrocyte benzodiazepine receptor. Brain Res. 514 (2), 349–351. doi:10.1016/0006-8993(90)91431-f
Dunne, E. L., Moss, S. J., and Smart, T. G. (1998). Inhibition of GABAA receptor function by tyrosine kinase inhibitors and their inactive analogues. Mol. Cell Neurosci. 12 (4-5), 300–310. doi:10.1006/mcne.1998.0717
Eaton, M. M., Cao, L. Q., Chen, Z., Franks, N. P., Evers, A. S., and Akk, G. (2015). Mutational analysis of the putative high-affinity propofol binding site in human β3 homomeric GABAA receptors. Mol. Pharmacol. 88 (4), 736–745. doi:10.1124/mol.115.100347
Edwards, Z., and Preuss, C. V. (2022). “GABA receptor positive allosteric modulators,” in StatPearls (Treasure Island (FL): StatPearls Publishing).
Engin, E., Benham, R. S., and Rudolph, U. (2018). An emerging circuit pharmacology of GABAA receptors. Trends Pharmacol. Sci. 39 (8), 710–732. doi:10.1016/j.tips.2018.04.003
Fan, Y., Tian, C., Liu, Q., Zhen, X., Zhang, H., Zhou, L., et al. (2018). Preconception paternal bisphenol A exposure induces sex-specific anxiety and depression behaviors in adult rats. PLoS One 13 (2), e0192434. doi:10.1371/journal.pone.0192434
Fasipe, O. J., Agede, O. A., and Enikuomehin, A. C. (2020). Announcing the novel class of GABA-A receptor selective positive allosteric modulator antidepressants. Future Sci. OA 7 (2), FSO654. doi:10.2144/fsoa-2020-0108
Fatemi, S. H., Folsom, T. D., Rooney, R. J., and Thuras, P. D. (2013). Expression of GABAA α2-β1- and ε-receptors are altered significantly in the lateral cerebellum of subjects with schizophrenia, major depression and bipolar disorder. Transl. Psychiatry 3 (9), e303. doi:10.1038/tp.2013.64
Fatemi, S. H., Folsom, T. D., and Thuras, P. D. (2017). GABAA and GABAB receptor dysregulation in superior frontal cortex of subjects with schizophrenia and bipolar disorder. Synapse 71 (7). doi:10.1002/syn.21973
Fedor, A. M., and Toda, M. J. (2014). Investigating hydrogen bonding in phenol using infrared spectroscopy and computational chemistry. J. Chem. Educ. 91 (12), 2191–2194. doi:10.1021/ed500563w
Fei, Y., Shi, R., Song, Z., and Wu, J. (2020). Metabolic control of epilepsy: a promising therapeutic target for epilepsy. Front. Neurol. 11, 592514. doi:10.3389/fneur.2020.592514
Feng, H. J., and Forman, S. A. (2018). Comparison of αβδ and αβγ GABAA receptors: allosteric modulation and identification of subunit arrangement by site-selective general anesthetics. Pharmacol. Res. 133, 289–300. doi:10.1016/j.phrs.2017.12.031
Field, M., Dorovykh, V., Thomas, P., and Smart, T. G. (2021). Physiological role for GABAA receptor desensitization in the induction of long-term potentiation at inhibitory synapses. Nat. Commun. 12, 2112. doi:10.1038/s41467-021-22420-9
Fogaça, M. V., and Duman, R. S. (2019). Cortical GABAergic dysfunction in stress and depression: new insights for therapeutic interventions. Front. Cell. Neurosci. 13, 87. doi:10.3389/fncel.2019.00087
Folarin, R. O., Surajudeen, O. B., Omotosho, E. O., Surajudeen, O. B., Oyeleye, O. D. O., and Shallie, P. (2020). Motor co-ordinative roles of Nigella sativa oil in mice models of phenol-induced essential tremor. Ann. Health Res. 61, 85–99. doi:10.30442/ahr.0601-10-70
Forman, S. A., and Miller, K. W. (2016). Mapping general anesthetic sites in heteromeric γ-aminobutyric acid type A receptors reveals a potential for targeting receptor subtypes. Anesth. Analg. 123 (5), 1263–1273. doi:10.1213/ANE.0000000000001368
Fritschy, J. M. (2008). Epilepsy, E/I balance and GABA(A) receptor plasticity. Front. Mol. Neurosci. 1, 5. doi:10.3389/neuro.02.005.2008
Fujiwara-Tsukamoto, Y., Isomura, Y., Nambu, A., and Takada, M. (2003). Excitatory GABA input directly drives seizure-like rhythmic synchronization in mature hippocampal CA1 pyramidal cells. Neuroscience 119, 265–275. doi:10.1016/s0306-4522(03)00102-7
Ghit, A., Assal, D., Al-Shami, A. S., and Hussein, D. E. E. (2021). GABAA receptors: structure, function, pharmacology, and related disorders. J. Genet. Eng. Biotechnol. 19 (1), 123. doi:10.1186/s43141-021-00224-0
Gielen, M., and Corringer, P. J. (2018). The dual-gate model for pentameric ligand-gated ion channels activation and desensitization. J. Physiol. 596 (10), 1873–1902. doi:10.1113/JP275100
Gill, K. M., and Grace, A. A. (2014). The role of α5 GABAA receptor agonists in the treatment of cognitive deficits in schizophrenia. Curr. Pharm. 20 (31), 5069–5076. doi:10.2174/1381612819666131216114612
Giri, P. P., Sinha, R., Sikka, S., and Meur, S. (2016). Acute carbolic acid poisoning: a report of four cases. Indian J. Crit. Care Med. 20 (11), 668–670. doi:10.4103/0972-5229.194014
Gironell, A. (2014). The GABA hypothesis in essential tremor: lights and shadows. Tremor Other Hyperkinet. Mov. (NY) 4, 254. doi:10.7916/D8SF2T9C
Gurba, K. N., Hernandez, C. C., Hu, N., and Macdonald, R. L. (2012). GABRB3 mutation, G32R, associated with childhood absence epilepsy alters α1β3γ2L γ-aminobutyric acid type A (GABAA) receptor expression and channel gating. J. Biol. Chem. 287 (15), 12083–12097. doi:10.1074/jbc.M111.332528
Haeseler, G., and Leuwer, M. (2002). Interaction of phenol derivatives with ion channels. Eur. J. Anaesthesiol. 19 (1), 1–8. doi:10.1017/s0265021502000017
Hanrahan, J. R., Chebib, M., and Johnston, G. A. (2011). Flavonoid modulation of GABA(A) receptors. Br. J. Pharmacol. 163 (2), 234–245. doi:10.1111/j.1476-5381.2011.01228.x
Hanrahan, J. R., Chebib, M., and Johnston, G. A. (2015). Interactions of flavonoids with ionotropic GABA receptors. Adv. Pharmacol. 72, 189–200. doi:10.1016/bs.apha.2014.10.007
Hansen, J. B., Bilenberg, N., Timmermann, C. A. G., Jensen, R. C., Frederiksen, H., Andersson, A. M., et al. (2021). Prenatal exposure to bisphenol A and autistic- and ADHD-related symptoms in children aged 2 and5 years from the Odense Child Cohort. Environ. Health 20 (1), 24. doi:10.1186/s12940-021-00709-y
Hass, U., Christiansen, S., Boberg, J., Rasmussen, M. G., Mandrup, K., and Axelstad, M. (2016). Low-dose effect of developmental bisphenol A exposure on sperm count and behaviour in rats. Andrology 4 (4), 594–607. doi:10.1111/andr.12176
Hellström, G., Klaminder, J., Finn, F., Persson, L., Alanärä, A., Jonsson, M., et al. (2016). GABAergic anxiolytic drug in water increases migration behaviour in salmon. Nat. Commun. 7, 13460. doi:10.1038/ncomms13460
Hinkle, D. J., and Macdonald, R. L. (2003). Beta subunit phosphorylation selectively increases fast desensitization and prolongs deactivation of alpha1beta1gamma2L and alpha1beta3gamma2L GABA(A) receptor currents. J. Neurosci. 23, 11698–11710. doi:10.1523/JNEUROSCI.23-37-11698.2003
Hirose, S. (2014). Mutant GABA(A) receptor subunits in genetic (idiopathic) epilepsy. Prog. Brain Res. 213, 55–85. doi:10.1016/B978-0-444-63326-2.00003-X
Hodson, P. V. (1985). A comparison of the acute toxicity of chemicals to fish, rats and mice. J. Appl. Toxicol. 5, 220–226. doi:10.1002/jat.2550050403
Hoerbelt, P., Lindsley, T. A., and Fleck, M. W. (2015). Dopamine directly modulates GABAA receptors. J. Neurosci. 35, 3525–3536. doi:10.1523/JNEUROSCI.4390-14.2015
Hong, S. B., Hong, Y. C., Kim, J. W., Park, E. J., Shin, M. S., Kim, B. N., et al. (2013). Bisphenol A in relation to behavior and learning of school-age children. J. Child. Psychol. Psychiatry 54 (8), 890–899. doi:10.1111/jcpp.12050
Horder, J., Andersson, M., Mendez, M. A., Singh, N., Tangen, Ä., Lundberg, J., et al. (2018). GABAA receptor availability is not altered in adults with autism spectrum disorder or in mouse models. Sci. Transl. Med. 10 (461), eaam8434. doi:10.1126/scitranslmed.aam8434
Hörtnagl, H., Tasan, R. O., Wieselthaler, A., Kirchmair, E., Sieghart, W., and Sperk, G. (2013). Patterns of mRNA and protein expression for 12 GABAA receptor subunits in the mouse brain. Neuroscience 236, 345–372. doi:10.1016/j.neuroscience.2013.01.008
Howard, R. J. (2021). Elephants in the dark: insights and incongruities in pentameric ligand-gated ion channel models. J. Mol. Biol. 433 (17), 167128. doi:10.1016/j.jmb.2021.167128
Hu, H., Howard, R. J., Bastolla, U., Lindahl, E., and Delarue, M. (2020). Structural basis for allosteric transitions of a multidomain pentameric ligand-gated ion channel. Proc. Natl. Acad. Sci. U. S. A. 117 (24), 13437–13446. doi:10.1073/pnas.1922701117
Hu, L., Mei, H., Feng, H., Huang, Y., Cai, X., Xiang, F., et al. (2023). Exposure to bisphenols, parabens and phthalates during pregnancy and postpartum anxiety and depression symptoms: evidence from women with twin pregnancies. Environ. Res. 221, 115248. doi:10.1016/j.envres.2023.115248
Huang, C. C., and Hsu, K. S. (1999). Protein tyrosine kinase is required for the induction of long-term potentiation in the rat hippocampus. J. Physiol. 520, 783–796. doi:10.1111/j.1469-7793.1999.00783.x
Huang, Y., Lee, M. T., Sieghart, W., Knutson, D. E., Wimmer, L. R., Sharmin, D., et al. (2021). Positive modulation of cerebellar α6 GABAA receptors for treating essential tremor: a proof-of-concept study in harmaline-treated mice. BioRxiv. doi:10.1101/2021.04.19.440397
Hudson, K. E., and Grau, J. W. (2022). Ionic plasticity: common mechanistic underpinnings of pathology in spinal cord injury and the brain. Cells 11 (18), 2910. doi:10.3390/cells11182910
Islander, G., and Vinge, E. (2000). Severe neuroexcitatory symptoms after anaesthesia - with focus on propofol anaesthesia. Acta Anaesthesiol. Scand. 44 (2), 144–149. doi:10.1034/j.1399–6576.2000.440203.x
Ismail, H. T., and Mahboub, H. H. (2016). Effect of acute exposure to nonylphenol on biochemical, hormonal, and hematological parameters and muscle tissues residues of Nile tilapia; Oreochromis niloticus. Vet. World 9 (6), 616–625. doi:10.14202/vetworld.2016.616-625
Itoh, M. (1995). The role of brain acetylcholine in phenol-induced tremor in mice. Arch. Oral Biol. 40, 365–372. doi:10.1016/0003-9969(94)00191-d
Jaiteh, M., Taly, A., and Hénin, J. (2016). Evolution of pentameric ligand-gated ion channels: pro-loop receptors. PLoS One 11 (3), e0151934. doi:10.1371/journal.pone.0151934
Janve, V. S., Hernandez, C. C., Verdier, K. M., Hu, N., and Macdonald, R. L. (2016). Epileptic encephalopathy de novo GABRB mutations impair γ-aminobutyric acid type A receptor function. Ann. Neurol. 79, 806–825. doi:10.1002/ana.24631
Jašarević, E., Williams, S. A., Vandas, G. M., Ellersieck, M. R., Liao, C., Kannan, K., et al. (2013). Sex and dose-dependent effects of developmental exposure to bisphenol A on anxiety and spatial learning in deer mice (Peromyscus maniculatus bairdii) offspring. Horm. Behav. 63 (1), 180–189. doi:10.1016/j.yhbeh.2012.09.009
Jassar, B. S., Ostashewski, P. M., and Jhamandas, J. H. (1997). GABAA receptor modulation by protein tyrosine kinase in the rat diagonal band of Broca. Brain Res. 775 (1-2), 127–133. doi:10.1016/s0006-8993(97)00892-5
Jayakar, S. S., Zhou, X., Chiara, D. C., Dostalova, Z., Savechenkov, P. Y., Bruzik, K. S., et al. (2014). Multiple propofol-binding sites in a γ-aminobutyric acid type A receptor (GABAAR) identified using a photoreactive propofol analog. J. Biol. Chem. 289 (40), 27456–27468. doi:10.1074/jbc.M114.581728
Jurd, R., Arras, M., Lambert, S., Drexler, B., Siegwart, R., Crestani, F., et al. (2003). General anesthetic actions in vivo strongly attenuated by a point mutation in the GABA(A) receptor beta3 subunit. FASEB J. 17 (2), 250–252. doi:10.1096/fj.02-0611fje
Kaczor, P. T., Wolska, A. D., and Mozrzymas, J. W. (2021). α1 subunit histidine 55 at the interface between extracellular and transmem-brane domains affects preactivation and desensitization of the GABAA receptor. ACS Chem. Neurosci. 12, 562–572. doi:10.1021/acschemneuro.0c00781
Kaila, K., Ruusuvuori, E., Seja, P., Voipio, J., and Puskarjov, M. (2014). GABA actions and ionic plasticity in epilepsy. Curr. Opin. Neurobiol. 26, 34–41. doi:10.1016/j.conb.2013.11.004
Kang, Y., Saito, M., and Toyoda, H. (2020). Molecular and regulatory mechanisms of desensitization and resensitization of GABAA receptors with a special reference to propofol/barbiturate. Int. J. Mol. Sci. 21 (2), 563. doi:10.3390/ijms21020563
Kanlayaprasit, S., Thongkorn, S., Panjabud, P., Jindatip, D., Hu, V. W., Kikkawa, T., et al. (2021). Autism-Related transcription factors underlying the sex-specific effects of prenatal bisphenol A exposure on transcriptome-interactome profiles in the offspring prefrontal cortex. Int. J. Mol. Sci. 22 (24), 13201. doi:10.3390/ijms222413201
Karioti, A., Carta, F., and Supuran, C. T. (2016). Phenols and polyphenols as carbonic anhydrase inhibitors. Molecules 21 (12), 1649. doi:10.3390/molecules21121649
Karonen, M. (2022). Insights into polyphenol-lipid interactions: chemical methods, molecular aspects and their effects on membrane structures. Plants (Basel) 11 (14), 1809. doi:10.3390/plants11141809
Kavvadias, D., Sand, P., Youdim, K. A., Qaiser, M. Z., Rice-Evans, C., Baur, R., et al. (2004). The flavone hispidulin, a benzodiazepine receptor ligand with positive allosteric properties, traverses the blood-brain barrier and exhibits anticonvulsive effects. Br. J. Pharmacol. 142 (5), 811–820. doi:10.1038/sj.bjp.0705828
Kessi, M., Duan, H., Xiong, J., Chen, B., He, F., Yang, L., et al. (2022). Attention-deficit/hyperactive disorder updates. Front. Mol. Neurosci. 15, 925049. doi:10.3389/fnmol.2022.925049
Kosmowska, B., Paleczna, M., Biała, D., Kadłuczka, J., Wardas, J., Witkin, J. M., et al. (2023). GABA-A alpha 2/3 but not alpha 1 receptor subunit ligand inhibits harmaline and pimozide-induced tremor in rats. Biomolecules 13 (2), 197. doi:10.3390/biom13020197
Krasowski, M. D., Koltchine, V. V., Rick, C. E., Ye, Q., Finn, S. E., and Harrison, N. L. (1998). Propofol and other intravenous anesthetics have sites of action on the gamma-aminobutyric acid type A receptor distinct from that for isoflurane. Mol. Pharmacol. 53 (3), 530–538. doi:10.1124/mol.53.3.530
Krasowski, M. D., Nishikawa, K., Nikolaeva, N., Lin, A., and Harrison, N. L. (2001). Methionine 286 in transmembrane domain 3 of the GABAA receptor beta subunit controls a binding cavity for propofol and other alkylphenol general anesthetics. Neuropharmacology 41 (8), 952–964. doi:10.1016/s0028-3908(01)00141-1
Kreuzer, M., Butovas, S., García, P. S., Schneider, G., Schwarz, C., Rudolph, U., et al. (2020). Propofol affects cortico-hippocampal interactions via β3 subunit-containing GABAA receptors. Int. J. Mol. Sci. 21 (16), 5844. doi:10.3390/ijms21165844
Kumar, D., and Thakur, M. K. (2017). Anxiety like behavior due to perinatal exposure to Bisphenol-A is associated with decrease in excitatory to inhibitory synaptic density of male mouse brain. Toxicology 378, 107–113. doi:10.1016/j.tox.2017.01.010
Kwon, H. J., Kim, W., and Lim, M. H. (2017). Association between GABA3 gene polymorphisms and attention deficit hyperactivity disorder in Korean children. Psychiatry Investig. 14 (5), 693–697. doi:10.4306/pi.2017.14.5.693
Landolfi, A., Troisi, J., Savanelli, M. C., Vitale, C., Barone, P., and Amboni, M. (2017). Bisphenol A glucuronidation in patients with Parkinson's disease. Neurotoxicology 63, 90–96. doi:10.1016/j.neuro.2017.09.008
Lara, C. O., Burgos, C. F., Moraga-Cid, G., Carrasco, M. A., and Yévenes, G. E. (2020). Pentameric ligand-gated ion channels as pharmacological targets against chronic pain. Front. Pharmacol. 11, 167. doi:10.3389/fphar.2020.00167
Lasoń, W., and Leśkiewicz, M. (2013). Effect of plant polyphenols on seizures – animal studies. J. Epileptol. 21, 79–87. doi:10.1515/joepi-2015-0007
Lee, Y., Park, Y., Nam, H., Lee, J. W., and Yu, S. W. (2020). Translocator protein (TSPO): the new story of the old protein in neuroinflammation. BMB Rep. 53 (1), 20–27. doi:10.5483/BMBRep.2020.53.1.273
Leon, I., Millán, J., Cocinero, E. J., Lesarri, A., Castañoa, F., and Fernández, J. A. (2012). Mimicking anaesthetic-receptor interaction: a combined spectroscopic and computational study of propofol···phenol. Phys. Chem. Chem. Phys. 14, 8956–8963. doi:10.1039/c2cp40656j
Li, A. M. (2017). Ecological determinants of health: food and environment on human health. Environ. Sci. Pollut. Res. Int. 24 (10), 9002–9015. doi:10.1007/s11356-015-5707-9
Li, C., Sang, C., Zhang, S., Zhang, S., and Gao, H. (2023). Effects of bisphenol A and bisphenol analogs on the nervous system. Chin. Med. J. Engl. 136 (3), 295–304. doi:10.1097/CM9.0000000000002170
Li, P., Bandyopadhyaya, A. K., Covey, D. F., Steinbach, J. H., and Akk, G. (2009). Hydrogen bonding between the 17beta-substituent of a neurosteroid and the GABA(A) receptor is not obligatory for channel potentiation. Br. J. Pharmacol. 158 (5), 1322–1329. doi:10.1111/j.1476-5381.2009.00390.x
Lillis, K. P., Kramer, M. A., Mertz, J., Staley, K. J., and White, J. A. (2012). Pyramidal cells accumulate chloride at seizure onset. Neurobiol. Dis. 47 (3), 358–366. doi:10.1016/j.nbd.2012.05.016
Lin, K., Li, Y., Toit, E. D., Wendt, L., and Sun, J. (2021). Effects of polyphenol supplementations on improving depression, anxiety, and quality of life in patients with depression. Front. Psychiatry. 12, 765485. doi:10.3389/fpsyt.2021.765485
Liu, S., Xu, L., Guan, F., Liu, Y. T., Cui, Y., Zhang, Q., et al. (2018). Cryo-EM structure of the human α5β3 GABAA receptor. Cell Res. 28 (9), 958–961. doi:10.1038/s41422-018-0077-8
Loshali, A., Joshi, B. C., Sundriyal, A., and Uniyal, S. (2021). Antiepileptic effects of antioxidant potent extract from Urtica dioica Linn. root on pentylenetetrazole and maximal electroshock induced seizure models. Heliyon 7 (2), e06195. doi:10.1016/j.heliyon.2021.e06195
Luscher, B., Shen, Q., and Sahir, N. (2011). The GABAergic deficit hypothesis of major depressive disorder. Mol. Psychiatry. 16 (4), 383–406. doi:10.1038/mp.2010.120
Mahdavi, M., Kheirollahi, M., Riahi, R., Khorvash, F., Khorrami, M., and Mirsafaie, M. (2018). Meta-analysis of the association between GABA receptor polymorphisms and autism spectrum disorder (ASD). J. Mol. Neurosci. 65 (1), 1–9. doi:10.1007/s12031-018-1073-7
Maldifassi, M. C., Baur, R., and Sigel, E. (2016). Functional sites involved in modulation of the GABAA receptor channel by the intravenous anesthetics propofol, etomidate and pentobarbital. Neuropharmacology 105, 207–214. doi:10.1016/j.neuropharm.2016.01.003
Maldonado-Avilés, J. G., Curley, A. A., Hashimoto, T., Morrow, A. L., Ramsey, A. J., O'Donnell, P., et al. (2009). Altered markers of tonic inhibition in the dorsolateral prefrontal cortex of subjects with schizophrenia. Am. J. Psychiatry 166 (4), 450–459. doi:10.1176/appi.ajp.2008.08101484
Marques, T. R., Ashok, A. H., Angelescu, I., Borgan, F., Myers, J., Lingford-Hughes, A., et al. (2021). GABA-A receptor differences in schizophrenia: a positron emission tomography study using [11C]Ro154513. Mol. Psychiatry 26, 2616–2625. doi:10.1038/s41380-020-0711-y
Masiulis, S., Desai, R., Uchański, T., Martin, I. S., Laverty, D., Karia, D., et al. (2019). GABAA receptor signalling mechanisms revealed by structural pharmacology. Nature 565 (7740), 454–459. doi:10.1038/s41586-018-0832-5
Mendez, M. A., Horder, J., Myers, J., Coghlan, S., Stokes, P., Erritzoe, D., et al. (2013). The brain GABA-benzodiazepine receptor alpha-5 subtype in autism spectrum disorder: a pilot [(11)C]Ro15-4513 positron emission tomography study. Neuropharmacology 68, 195–201. doi:10.1016/j.neuropharm.2012.04.008
Menzikov, S. A. (2018). Effect of phenol on the GABAAR-coupled Cl-/HCO3--ATPase from fish brain: an in vitro approach on the enzyme function. Toxicol. Vitro 46, 129–136. doi:10.1016/j.tiv.2017.09.027
Menzikov, S. A., and Morozov, S. G. (2019). Involvement of brain GABAAR-coupled Cl−/HCO3−-ATPase in phenol-induced the head-twitching and tremor responses in rats. Neurotoxicology 71, 122–131. doi:10.1016/j.neuro.2018.12.007
Menzikov, S. A., Morozov, S. G., and Kubatiev, A. A. (2021). Intricacies of GABAA receptor function: the critical role of the β3 subunit in norm and pathology. Int. J. Mol. Sci. 22, 1457. doi:10.3390/ijms22031457
Menzikov, S. A., Zaichenko, D. M., Moskovtsev, A. A., Morozov, S. G., and Kubatiev, A. A. (2022). Physiological role of ATPase for GABAA receptor resensitization. Int. J. Mol. Sci. 23, 5320. doi:10.3390/ijms23105320
Menzikov, S. A., Zaichenko, D. M., Moskovtsev, A. A., Morozov, S. G., and Kubatiev, A. A. (2023). Zinc inhibits the GABAAR/ATPase during postnatal rat development: the role of cysteine residue. Int. J. Mol. Sci. 24 (3), 2764. doi:10.3390/ijms24032764
Merali, Z., Du, L., Hrdina, P., Palkovits, M., Faludi, G., Poulter, M. O., et al. (2004). Dysregulation in the suicide brain: mRNA expression of corticotropin-releasing hormone receptors and GABA(A) receptor subunits in frontal cortical brain region. J. Neurosci. 24 (6), 1478–1485. doi:10.1523/JNEUROSCI.4734-03.2004
Meyer, S., Shamdeen, M. G., Kegel, B., Mencke, T., Gottschling, S., Gortner, L., et al. (2006). Effect of propofol on seizure-like phenomena and electroencephalographic activity in children with epilepsy vs children with learning difficulties. Anaesthesia 61 (11), 1040–1047. doi:10.1111/j.1365-2044.2006.04782.x
Miguel, V., Villarreal, M. A., and García, D. A. (2019). Effects of gabergic phenols on the dynamic and structure of lipid bilayers: a molecular dynamic simulation approach. PLoS One 14 (6), e0218042. doi:10.1371/journal.pone.0218042
Miller, P. S., Scott, S., Masiulis, S., De Colibus, L., Pardon, E., Steyaert, J., et al. (2017). Structural basis for GABAA receptor potentiation by neurosteroids. Nat. Struct. Mol. Biol. 24 (11), 986–992. doi:10.1038/nsmb.3484
Minchin, M. C., and Pearson, G. (1981). The effect of the convulsant agent, catechol, on neurotransmitter uptake and release in rat brain slices. Br. J. Pharmacol. 74 (3), 715–721. doi:10.1111/j.1476-5381.1981.tb10483.x
Mohammadi, B., Haeseler, G., Leuwer, M., Dengler, R., Krampfl, K., and Bufler, J. (2001). Structural requirements of phenol derivatives for direct activation of chloride currents via GABA(A) receptors. Eur. J. Pharmacol. 421, 85–91. doi:10.1016/s0014-2999(01)01033-0
Möhler, H. (2012). The GABA system in anxiety and depression and its therapeutic potential. Neuropharmacology 62 (1), 42–53. doi:10.1016/j.neuropharm.2011.08.040
Moore, J. L., Carvalho, D. Z., St Louis, E. K., and Bazil, C. (2021). Sleep and epilepsy: a focused review of pathophysiology, clinical syndromes, Co-morbidities, and therapy. Neurotherapeutics 18 (1), 170–180. doi:10.1007/s13311-021-01021-w
Mornagui, B., Rezg, R., Repond, C., and Pellerin, L. (2019). Effects of bisphenol S, a major substitute of bisphenol A, on neurobehavioral responses and cerebral monocarboxylate transporters expression in mice. Food Chem. Toxicol. 132, 110670. doi:10.1016/j.fct.2019.110670
Moss, S. J., Gorrie, G. H., Amato, A., and Smart, T. G. (1995). Modulation of GABAA receptors by tyrosine phosphorylation. Nature 377 (6547), 344–348. doi:10.1038/377344a0
Mueller, T. M., Haroutunian, V., and Meador-Woodruff, J. H. (2014). N-Glycosylation of GABAA receptor subunits is altered in Schizophrenia. Neuropsychopharmacology 39 (3), 528–537. doi:10.1038/npp.2013.190
Mueller, T. M., Remedies, C. E., Haroutunian, V., and Meador-Woodruff, J. H. (2015). Abnormal subcellular localization of GABAA receptor subunits in schizophrenia brain. Transl. Psychiatry 5 (8), e612. doi:10.1038/tp.2015.102
Musachio, E. A. S., Araujo, S. M., Bortolotto, V. C., de Freitas Couto, S., Dahleh, M. M. M., Poetini, M. R., et al. (2020). Bisphenol A exposure is involved in the development of Parkinson like disease in Drosophila melanogaster. Food. Chem. Toxicol. 137, 111128. doi:10.1016/j.fct.2020.111128
Musachio, E. A. S., de Freitas Couto, S., Poetini, M. R., Bortolotto, V. C., Dahleh, M. M. M., Janner, D. E., et al. (2021). Bisphenol A exposure during the embryonic period: insights into dopamine relationship and behavioral disorders in Drosophila melanogaster. Food Chem. Toxicol. 157, 112526. doi:10.1016/j.fct.2021.112526
Naderi, M., Puar, P., JavadiEsfahani, R., and Kwong, R. W. M. (2022). Early developmental exposure to bisphenol A and bisphenol S disrupts socio-cognitive function, isotocin equilibrium, and excitation-inhibition balance in developing zebrafish. Neurotoxicology 88, 144–154. doi:10.1016/j.neuro.2021.11.009
Nakamura, Y., Darnieder, L. M., Deeb, T. Z., and Moss, S. J. (2015). Regulation of GABAARs by phosphorylation. Adv. Pharmacol. 72, 97–146. doi:10.1016/bs.apha.2014.11.008
Nietz, A., Krook-Magnuson, C., Gutierrez, H., Klein, J., Sauve, C., Hoff, I., et al. (2020). Selective loss of the GABAAα1 subunit from Purkinje cells is sufficient to induce a tremor phenotype. J. Neurophysiol. 124 (4), 1183–1197. doi:10.1152/jn.00100.2020
Nuss, P. (2015). Anxiety disorders and GABA neurotransmission: a disturbance of modulation. Neuropsychiatr. Dis. Treat. 11, 165–175. doi:10.2147/NDT.S58841
Olsen, R. W., and Li, G.-D. (2011). GABA(A) receptors as molecular targets of general anesthetics: identification of binding sites provides clues to allosteric modulation. Can. J. Anaesth. 58, 206–215. doi:10.1007/s12630-010-9429-7
Olsen, R. W., and Sieghart, W. (2009). GABA A receptors: subtypes provide diversity of function and pharmacology. Neuropharmacology 56, 141–148. doi:10.1016/j.neuropharm.2008.07.045
Olsvik, P. A., Whatmore, P., Penglase, S. J., Skjærven, K. H., Anglès d'Auriac, M., and Ellingsen, S. (2019). Associations between behavioral effects of bisphenol A and dna methylation in zebrafish embryos. Front. Genet. 10, 184. doi:10.3389/fgene.2019.00184
Oquendo, M. A., Sullivan, G. M., Sudol, K., Baca-Garcia, E., Stanley, B. H., Sublette, M. E., et al. (2014). Toward a biosignature for suicide. Am. J. Psychiatry. 171 (12), 1259–1277. doi:10.1176/appi.ajp.2014.14020194
Orser, B. A., Wang, L. Y., Pennefather, P. S., and MacDonald, J. F. (1994). Propofol modulates activation and desensitization of GABAA receptors in cultured murine hippocampal neurons. J. Neurosci. 14 (12), 7747–7760. doi:10.1523/JNEUROSCI.14-12-07747.1994
Oscarsson, A., Juhas, M., Sjölander, A., and Eintrei, C. (2007). The effect of propofol on actin, ERK-1/2 and GABAA receptor content in neurones. Acta Anaesthesiol. Scand. 51 (9), 1184–1189. doi:10.1111/j.1399-6576.2007.01388.x
Pace, C. N., Horn, G., Hebert, E. J., Bechert, J., Shaw, K., Urbanikova, L., et al. (2001). Tyrosine hydrogen bonds make a large contribution to protein stability. J. Mol. Biol. 312 (2), 393–404. doi:10.1006/jmbi.2001.4956
Paine, T. A., Chang, S., and Poyle, R. (2020). Contribution of GABAA receptor subunits to attention and social behavior. Behav. Brain Res. 378, 112261. doi:10.1016/j.bbr.2019.112261
Pantelakis, L., Alvarez, V., Gex, G., and Godio, M. (2021). Severe neuroexcitatory reaction: a rare and underrecognized life-threatening complication of propofol-induced anesthesia. Neurohospitalist 11 (1), 49–53. doi:10.1177/1941874420929536
Paris-Robidas, S., Brochu, E., Sintes, M., Emond, V., Bousquet, M., Vandal, M., et al. (2012). Defective dentate nucleus GABA receptors in essential tremor. Brain 135, 105–116. doi:10.1093/brain/awr301
Pathak, L., Agrawal, Y., and Dhir, A. (2013). Natural polyphenols in the management of major depression. Expert. Opin. Investig. Drugs 22 (7), 863–880. doi:10.1517/13543784.2013.794783
Perera, F., Nolte, E. L. R., Wang, Y., Margolis, A. E., Calafat, A. M., Wang, S., et al. (2016). Bisphenol A exposure and symptoms of anxiety and depression among inner city children at 10-12 years of age. Environ. Res. 151, 195–202. doi:10.1016/j.envres.2016.07.028
Pham, X., Sun, C., Chen, X., van den Oord, E. J., Neale, M. C., Kendler, K. S., et al. (2009). Association study between GABA receptor genes and anxiety spectrum disorders. Depress Anxiety 26 (11), 998–1003. doi:10.1002/da.20628
Phulera, S., Zhu, H., Yu, J., Claxton, D. P., Yoder, N., Yoshioka, C., et al. (2018). Cryo-EM structure of the benzodiazepine-sensitive α1β1γ2S tri-heteromeric GABAA receptor in complex with GABA. Elife 7, e39383. doi:10.7554/eLife.39383
Pletz, J., Sánchez-Bayo, F., and Tennekes, H. A. (2016). Dose-response analysis indicating time-dependent neurotoxicity caused by organic and inorganic mercury-Implications for toxic effects in the developing brain. Toxicology 347–349, 1–5. doi:10.1016/j.tox.2016.02.006
Puthenkalam, R., Hieckel, M., Simeone, X., Suwattanasophon, C., Feldbauer, R. V., Ecker, G. F., et al. (2016). Structural studies of GABAA receptor binding sites: which experimental structure tells us what? Front. Mol. Neurosci. 9, 44. doi:10.3389/fnmol.2016.00044
Qiu, W., Zhan, H., Hu, J., Zhang, T., Xu, H., Wong, M., et al. (2019). The occurrence, potential toxicity, and toxicity mechanism of bisphenol S, a substitute of bisphenol A: a critical review of recent progress. Ecotoxicol. Environ. Saf. 173, 192–202. doi:10.1016/j.ecoenv.2019.01.114
Rahman, M. M., Rahaman, M. S., Islam, M. R., Rahman, F., Mithi, F. M., Alqahtani, T., et al. (2021). Role of phenolic compounds in human disease: current knowledge and future prospects. Molecules 27 (1), 233. doi:10.3390/molecules27010233
Raimondo, J. V., Richards, B. A., and Woodin, M. A. (2017). Neuronal chloride and excitability-the big impact of small changes. Curr. Opin. Neurobiol. 43, 35–42. doi:10.1016/j.conb.2016.11.012
Rebolledo-Solleiro, D., Castillo Flores, L. Y., and Solleiro-Villavicencio, H. (2021). Impact of BPA on behavior, neurodevelopment and neurodegeneration. Front. Biosci. (Landmark Ed. 26 (2), 363–400. doi:10.2741/4898
Reiner, G. N., Fraceto, L. F., Paula, E., Perillo, M. A., and García, D. A. (2013). Effects of gabaergic phenols on phospholipid bilayers as evaluated by 1H-NMR. J. Biomaterials Nanobiotechnology 4, 28–34. doi:10.4236/jbnb.2013.43a004
Rezin, G. T., Amboni, G., Zugno, A. I., Quevedo, J., and Streck, E. L. (2009). Mitochondrial dysfunction and psychiatric disorders. Neurochem. Res. 34 (6), 1021–1029. doi:10.1007/s11064-008-9865-8
Rice, P. J., Drewes, C. D., Klubertanz, T. M., Bradbury, S. P., and Coats, J. R. (1997). Acute toxicity and behavioral effects of chlorpyrifos, permethrin, phenol, strychnine, and 2,4-dinitrophenol to 30-day-old Japanese medaka (Oryzias latipes). Environ. Toxicol. Chem. 16, 696–704. doi:10.1897/1551-5028(1997)016<0696:atabeo>2.3.co;2
Ríos, J. L., Schinella, G. R., and Moragrega, I. (2022). Phenolics as GABAA receptor ligands: an updated review. Molecules 27 (6), 1770. doi:10.3390/molecules27061770
Rochester, J. R., Bolden, A. L., and Kwiatkowski, C. F. (2018). Prenatal exposure to bisphenol A and hyperactivity in children: a systematic review and meta-analysis. Environ. Int. 114, 343–356. doi:10.1016/j.envint.2017.12.028
Rovšnik, U., Zhuang, Y., Forsberg, B. O., Carroni, M., Yvonnesdotter, L., Howard, R. J., et al. (2021). Dynamic closed states of a ligand-gated ion channel captured by cryo-EM and simulations. Life Sci. Alliance 4 (8), e202101011. doi:10.26508/lsa.202101011
Rudolph, U., and Antkowiak, B. (2004). Molecular and neuronal substrates for general anaesthetics. Nat. Rev. Neurosci. 5 (9), 709–720. doi:10.1038/nrn1496
Saili, K. S., Corvi, M. M., Weber, D. N., Patel, A. U., Das, S. R., Przybyla, J., et al. (2012). Neurodevelopmental low-dose bisphenol A exposure leads to early life-stage hyperactivity and learning deficits in adult zebrafish. Toxicology 291 (1-3), 83–92. doi:10.1016/j.tox.2011.11.001
Sallard, E., Letourneur, D., and Legendre, P. (2021). Electrophysiology of ionotropic GABA receptors. Cell Mol. Life Sci. 78 (13), 5341–5370. doi:10.1007/s00018-021-03846-2
Sasaki, T., Saito, H., Furukawa, Y., Tominaga, T., Kitajima, S., Kanno, J., et al. (2023). Exposure to bisphenol A or its phenolic analogs during early life induces different types of anxiety-like behaviors after maturity in male mice. J. Toxicol. Sci. 48 (4), 211–219. doi:10.2131/jts.48.211
Sauguet, L., Shahsavar, A., and Delarue, M. (2015). Crystallographic studies of pharmacological sites in pentameric ligand-gated ion channels. Biochim. Biophys. Acta 1850 (3), 511–523. doi:10.1016/j.bbagen.2014.05.007
Schefer, S., Oest, M., and Rohn, S. (2021). Interactions between phenolic acids, proteins, and carbohydrates-influence on dough and bread properties. Foods 10 (11), 2798. doi:10.3390/foods10112798
Scheiner, S., Kar, T., and Pattanayak, J. (2002). Comparison of various types of hydrogen bonds involving aromatic amino acids. J. Am. Chem. Soc. 124 (44), 13257–13264. doi:10.1021/ja027200q
Schipper, S., Aalbers, M. W., Rijkers, K., Swijsen, A., Rigo, J. M., Hoogland, G., et al. (2016). GABAA receptors as potential target for the treatment of temporal lobe epilepsy. Mol. Neurobiol. 53 (8), 5252–5265. doi:10.1007/s12035-015-9423-8
Sheng, M., and Pak, D. T. (2000). Ligand-gated ion channel interactions with cytoskeletal and signaling proteins. Annu. Rev. Physiol. 62, 755–778. doi:10.1146/annurev.physiol.62.1.755
Shi, C., Xu, M. J., Bayer, M., Deng, Z. W., Kubbutat, M. H., Wätjen, W., et al. (2010). Phenolic compounds and their anti-oxidative properties and protein kinase inhibition from the Chinese mangrove plant Laguncularia racemosa. Phytochemistry 71 (4), 435–442. doi:10.1016/j.phytochem.2009.11.008
Shin, D. J., Germann, A. L., Johnson, A. D., Forman, S. A., Steinbach, J. H., and Akk, G. (2018). Propofol is an allosteric agonist with multiple binding sites on concatemeric ternary GABAA receptors. Mol. Pharmacol. 93 (2), 178–189. doi:10.1124/mol.117.110403
Sieghart, W. (2015). Allosteric modulation of GABAA receptors via multiple drug-binding sites. Adv. Pharmacol. 72, 53–96. doi:10.1016/bs.apha.2014.10.002
Sieghart, W., Chiou, L. C., Ernst, M., Fabjan, J. M., Savić, M., and Lee, M. T. (2022). α6-Containing GABAA receptors: functional roles and therapeutic potentials. Pharmacol. Rev. 74 (1), 238–270. doi:10.1124/pharmrev.121.000293
Sieghart, W., and Savić, M. M. (2018). International union of basic and clinical pharmacology. CVI: GABAA receptor subtype- and function-selective ligands: key issues in translation to humans. Pharmacol. Rev. 70 (4), 836–878. doi:10.1124/pr.117.014449
Sieghart, W., and Sperk, G. (2002). Subunit composition, distribution and function of GABA-A receptor subtypes. Curr. Top. Med. Chem. 2, 795–816. doi:10.2174/1568026023393507
Sigel, E., and Steinmann, M. E. (2012). Structure, function, and modulation of GABA(A) receptors. J. Biol. Chem. 287 (48), 40224–40231. doi:10.1074/jbc.R112.386664
Soriano, S., Ripoll, C., Alonso-Magdalena, P., Fuentes, E., Quesada, I., Nadal, A., et al. (2016). Effects of Bisphenol A on ion channels: experimental evidence and molecular mechanisms. Steroids 111, 12–20. doi:10.1016/j.steroids.2016.02.020
Spencer, P. J., Gollapudi, B. B., and Waechter, J. M. (2007). Induction of micronuclei by phenol in the mouse bone marrow: I. Association with chemically induced hypothermia. Toxicol. Sci. 97, 120–127. doi:10.1093/toxsci/kfm032
Sperk, G., Pirker, S., Gasser, E., Wieselthaler, A., Bukovac, A., Kuchukhidze, G., et al. (2021). Increased expression of GABAA receptor subunits associated with tonic inhibition in patients with temporal lobe epilepsy. Brain Commun. 3 (4), fcab239. doi:10.1093/braincomms/fcab239
Staley, K. J., and Proctor, W. R. (1999). Modulation of mammalian dendritic GABA(A) receptor function by the kinetics of Cl- and HCO3- transport. J. Physiol. 519 (3), 693–712. doi:10.1111/j.1469-7793.1999.0693n.x
Stanley, R., and Mantz, N. M. (1971). Brain energy reserve levels at the onset of convulsions in hypoxic mice. Life Sci. 10, 1901–1907.
Starovoytov, O. N., Liu, Y., Tan, L., and Yang, S. (2014). Effects of the hydroxyl group on phenyl based ligand/ERRγ protein binding. Chem. Res. Toxicol. 27 (8), 1371–1379. doi:10.1021/tx500082r
Stein, T. P., Schluter, M. D., Steer, R. A., Guo, L., and Ming, X. (2015). Bisphenol A exposure in children with autism spectrum disorders. Autism Res. 8 (3), 272–283. doi:10.1002/aur.1444
Stern, A. T., and Forman, S. A. (2016). A cysteine substitution probes β3H267 interactions with propofol and other potent anesthetics in α1β3γ2L γ-aminobutyric acid type A receptors. Anesthesiology 124 (1), 89–100. doi:10.1097/ALN.0000000000000934
Stump, D. G., Beck, M. J., Radovsky, A., Garman, R. H., Freshwater, L. L., Sheets, L. P., et al. (2010). Developmental neurotoxicity study of dietary bisphenol A in Sprague-Dawley rats. Toxicol. Sci. 115 (1), 167–182. doi:10.1093/toxsci/kfq025
Subramani, B., Chang, C. H., Fang, T. Y., Yu, C.-J., Du, J.-C., Chiou, H.-C., et al. (2023). The association of bisphenol A and parabens exposure and oxidative stress in attention-deficit/hyperactivity disorder in children and adolescents. Expo. Health. doi:10.1007/s12403-023-00561-0
Sun, H., Chen, Y., Huang, Q., Lui, S., Huang, X., Shi, Y., et al. (2018). Psychoradiologic utility of MR imaging for diagnosis of attention deficit hyperactivity disorder: a radiomics analysis. Radiology 287, 620–630. doi:10.1148/radiol.2017170226
Szwajgier, D., Borowiec, K., and Pustelniak, K. (2017). The neuroprotective effects of phenolic acids: molecular mechanism of action. Nutrients 9 (5), 477. doi:10.3390/nu9050477
Taherianfard, M., and Taci, A. A. (2015). Effects of bisphenol A and learning on the distribution of GABAAα1 receptors in the rat Hippocampus and prefrontal cortex. Neurophysiology 47, 23–29. doi:10.1007/s11062-015-9492-3
Tewar, S., Auinger, P., Braun, J. M., Lanphear, B., Yolton, K., Epstein, J. N., et al. (2016). Association of Bisphenol A exposure and Attention-Deficit/Hyperactivity Disorder in a national sample of U.S. children. Environ. Res. 150, 112–118. doi:10.1016/j.envres.2016.05.040
Thongkorn, S., Kanlayaprasit, S., Kasitipradit, K., Lertpeerapan, P., Panjabud, P., Hu, V. W., et al. (2023). Investigation of autism-related transcription factors underlying sex differences in the effects of bisphenol A on transcriptome profiles and synaptogenesis in the offspring hippocampus. Biol. Sex. Differ. 14 (1), 8. doi:10.1186/s13293-023-00496-w
Tian, Y. H., Baek, J. H., Lee, S. Y., and Jang, C. G. (2010). Prenatal and postnatal exposure to bisphenol a induces anxiolytic behaviors and cognitive deficits in mice. Synapse 64 (6), 432–439. doi:10.1002/syn.20746
Tsuchiya, H., and Mizogami, M. (2014). Comparative interactions of anesthetic alkylphenols with lipid membranes. Open J. Anesthesiol. 4, 308–317. doi:10.4236/ojanes.2014.412044
Tsuchiya, H., and Mizogami, M. (2020). Interaction of drugs with lipid raft membrane domains as a possible target. Drug Target Insights 14, 34–47. doi:10.33393/dti.2020.2185
Tufarelli, V., Casalino, E., D'Alessandro, A. G., and Laudadio, V. (2017). Dietary phenolic compounds: biochemistry, metabolism and significance in animal and human health. Curr. Drug Metab. 18 (10), 905–913. doi:10.2174/1389200218666170925124004
Tungmunnithum, D., Thongboonyou, A., Pholboon, A., and Yangsabai, A. (2018). Flavonoids and other phenolic compounds from medicinal plants for pharmaceutical and medical aspects: an overview. Med. (Basel) 5 (3), 93. doi:10.3390/medicines5030093
Ullah, M. I., Anwar, R., Kamran, S., Gul, B., Elhady, S. S., and Youssef, F. S. (2022). Evaluation of the anxiolytic and anti-epileptogenic potential of Lactuca serriola seed using pentylenetetrazol-induced kindling in mice and metabolic profiling of its bioactive extract. Antioxidants (Basel) 11 (11), 2232. doi:10.3390/antiox11112232
Utas, J. E., Kritikos, M., Sandström, D., and Akermark, B. (2006). Water as a hydrogen bonding bridge between a phenol and imidazole. A simple model for water binding in enzymes. Biochim. Biophys. Acta 1757 (12), 1592–1596. doi:10.1016/j.bbabio.2006.08.005
van Nuland, A. J. M., den Ouden, H. E. M., Zach, H., Dirkx, M. F. M., van Asten, J. J. A., Scheenen, T. W. J., et al. (2020). GABAergic changes in the thalamocortical circuit in Parkinson’s disease. Hum. Brain Mapp. 41, 1017–1029. doi:10.1002/hbm.24857
Velásquez-Jiménez, D., Corella-Salazar, D. A., Zuñiga-Martínez, B. S., Domínguez-Avila, J. A., Montiel-Herrera, M., Salazar-López, N. J., et al. (2021). Phenolic compounds that cross the blood-brain barrier exert positive health effects as central nervous system antioxidants. Food Funct. 12 (21), 10356–10369. doi:10.1039/d1fo02017j
Vermerris, W., and Nicholson, R. (2008). “Phenolic compounds and their effects on human health,” in Phenolic compound biochemistry (Dordrecht: Springer), 235–255. doi:10.1007/978-1-4020-5164-7_7
Walton, N. Y., Nagy, A. K., and Treiman, D. M. (1998). Altered residual ATP content in rat brain cortex subcellular fractions following status epilepticus induced by lithium and pilocarpine. J. Mol. Neurosci. 11, 233–242. doi:10.1385/JMN:11:3:233
Wan, Q., Man, H. Y., Braunton, J., Wang, W., Salter, M. W., Becker, L., et al. (1997). Modulation of GABAA receptor function by tyrosine phosphorylation of beta subunits. J. Neurosci. 17 (13), 5062–5069. doi:10.1523/JNEUROSCI.17-13-05062.1997
Wang, C., Niu, R., Zhu, Y., Han, H., Luo, G., Zhou, B., et al. (2014). Changes in memory and synaptic plasticity induced in male rats after maternal exposure to bisphenol A. Toxicology 322, 51–60. doi:10.1016/j.tox.2014.05.001
Wang, G., Clark, C. G., and Rodgers, F. G. (2002). Detection in Escherichia coli of the genes encoding the major virulence factors, the genes defining the O157:H7 serotype, and components of the type 2 Shiga toxin family by multiplex PCR. J. Clin. Microbiol. 40 (10), 3613–3619. doi:10.1128/JCM.40.10.3613-3619.2002
Wang, G., Ma, Y., Wang, S., Ren, G., and Guo, H. (2012). Association of dopaminergic/GABAergic genes with attention deficit hyperactivity disorder in children. Mol. Med. Rep. 6, 1093–1098. doi:10.3892/mmr.2012.1028
Wang, X., Yu, J., and Zhang, X. (2022). Dietary polyphenols as prospective natural-compound depression treatment from the perspective of intestinal microbiota regulation. Molecules 27 (21), 7637. doi:10.3390/molecules27217637
Weir, C. J., Mitchell, S. J., and Lambert, J. J. (2017). Role of GABAA receptor subtypes in the behavioural effects of intravenous general anaesthetics. Br. J. Anaesth. 119 (1), 167–175. doi:10.1093/bja/aex369
Welch, C., and Mulligan, K. (2022). Does bisphenol A confer risk of neurodevelopmental disorders? What we have learned from developmental neurotoxicity studies in animal models. Int. J. Mol. Sci. 23 (5), 2894. doi:10.3390/ijms23052894
Wiersielis, K. R., Samuels, B. A., and Roepke, T. A. (2020). Perinatal exposure to bisphenol A at the intersection of stress, anxiety, and depression. Neurotoxicol. Teratol. 79, 106884. doi:10.1016/j.ntt.2020.106884
Williams, D. B., and Akabas, M. H. (2002). Structural evidence that propofol stabilizes different GABA(A) receptor states at potentiating and activating concentrations. J. Neurosci. 22 (17), 7417–7424. doi:10.1523/JNEUROSCI.22-17-07417.2002
Williams, S. E., Mealer, R. G., Scolnick, E. M., Smoller, J. W., and Cummings, R. D. (2020). Aberrant glycosylation in schizophrenia: a review of 25 years of post-mortem brain studies. Mol. Psychiatry. 25 (12), 3198–3207. doi:10.1038/s41380-020-0761-1
Wiltgen, B. J., Godsil, B. P., Peng, Z., Saab, F., June, H. L., Linn, M. L., et al. (2009). The alpha1 subunit of the GABA(A) receptor modulates fear learning and plasticity in the lateral amygdala. Front. Behav. Neurosci. 3, 37. doi:10.3389/neuro.08.037.2009
Windus-Podehl, G., Lyftogt, C., Zieve, L., and Brunner, G. (1983). Encephalopathic effect of phenol in rats. J. Lab. Clin. Med. 101, 586–592.
Winiarska-Mieczan, A., Kwiecień, M., Jachimowicz-Rogowska, K., Donaldson, J., Tomaszewska, E., and Baranowska-Wójcik, E. (2023). Anti-inflammatory, antioxidant, and neuroprotective effects of polyphenols-polyphenols as an element of diet therapy in depressive disorders. Int. J. Mol. Sci. 24 (3), 2258. doi:10.3390/ijms24032258
Winn, H. R., Welsh, J. E., Rubio, R., and Berne, R. M. (1980). Changes in brain adenosine during bicuculline-induced seizures in rats Effects of hypoxia and altered systemic blood pressure. Circ. Res. 47, 568–577. doi:10.1161/01.res.47.4.568
Wu, D., Wu, F., Lin, R., Meng, Y., Wei, W., Sun, Q., et al. (2020). Impairment of learning and memory induced by perinatal exposure to BPA is associated with ERα-mediated alterations of synaptic plasticity and PKC/ERK/CREB signaling pathway in offspring rats. Brain Res. Bull. 161, 43–54. doi:10.1016/j.brainresbull.2020.04.023
Xing, H., Xiao-Hong, X., Yu-Jie, Y., Ling-Dan, X., Zhen-Lu, W., Qin, Z., et al. (2012). Early life exposure to bisphenol A affects anxiety and depression behaviors in mice and its molecular mechanism. Acta Psychol. Sin. 44 (9), 1167–1179. doi:10.3724/sp.j.1041.2012.01167
Xingyi, L., Xiaohong, X., Qin, Z., Guangxia, Z., Jialin, J. I., Fangni, D., et al. (2013). The effects of environmental endocrine disrupter bisphenol A on learning-memory and synaptic structure of adult mice. Acta Psychol. Sin. 45 (9), 981–992. doi:10.3724/sp.j.1041.2013.00981
Xu, X., Dong, F., Yang, Y., Wang, Y., Wang, R., and Shen, X. (2015). Sex-specific effects of long-term exposure to bisphenol-A on anxiety- and depression-like behaviors in adult mice. Chemosphere 120, 258–266. doi:10.1016/j.chemosphere.2014.07.021
Xu, X., Hong, X., Xie, L., Li, T., Yang, Y., Zhang, Q., et al. (2012). Gestational and lactational exposure to bisphenol-A affects anxiety- and depression-like behaviors in mice. Horm. Behav. 62 (4), 480–490. doi:10.1016/j.yhbeh.2012.08.005
Yamaura, K., Kiyonaka, S., Numata, T., Inoue, R., and Hamachi, I. (2016). Discovery of allosteric modulators for GABAA receptors by ligand-directed chemistry. Nat. Chem. Biol. 12 (10), 822–830. doi:10.1038/nchembio.2150
Yip, G. M., Chen, Z. W., Edge, C. J., Smith, E. H., Dickinson, R., Hohenester, E., et al. (2013). A propofol binding site on mammalian GABAA receptors identified by photolabeling. Nat. Chem. Biol. 9 (11), 715–720. doi:10.1038/nchembio.1340
Yoo, S. J., Joo, H., Kim, D., Lim, M. H., Kim, E., Ha, M., et al. (2020). Associations between exposure to bisphenol A and behavioral and cognitive function in children with attention-deficit/hyperactivity disorder: a case-control study. Clin. Psychopharmacol. Neurosci. 18 (2), 261–269. doi:10.9758/cpn.2020.18.2.261
Zhang, H., Kuang, H., Luo, Y., Liu, S., Meng, L., Pang, Q., et al. (2019). Low-dose bisphenol A exposure impairs learning and memory ability with alterations of neuromorphology and neurotransmitters in rats. Sci. Total Environ. 697, 134036. doi:10.1016/j.scitotenv.2019.134036
Zhang, H., Wang, Z., Meng, L., Kuang, H., Liu, J., Lv, X., et al. (2020). Maternal exposure to environmental bisphenol A impairs the neurons in hippocampus across generations. Toxicology 432, 152393. doi:10.1016/j.tox.2020.152393
Zhao, Z. F., Du, L., Gao, T., Bao, L., Luo, Y., Yin, Y. Q., et al. (2019). Inhibition of α5 GABAA receptors has preventive but not therapeutic effects on isoflurane-induced memory impairment in aged rats. Neural Regen. Res. 14 (6), 1029–1036. doi:10.4103/1673-5374.250621
Zhou, R., Bai, Y., Yang, R., Zhu, Y., Chi, X., Li, L., et al. (2011). Abnormal synaptic plasticity in basolateral amygdala may account for hyperactivity and attention-deficit in male rat exposed perinatally to low-dose bisphenol-A. Neuropharmacology 60 (5), 789–798. doi:10.1016/j.neuropharm.2011.01.031
Keywords: GABAA receptors, phenols, structures, neuropsychiatric manifestations, molecular mechanisms, ionic plasticity
Citation: Menzikov SA, Zaichenko DM, Moskovtsev AA, Morozov SG and Kubatiev AA (2024) Phenols and GABAA receptors: from structure and molecular mechanisms action to neuropsychiatric sequelae. Front. Pharmacol. 15:1272534. doi: 10.3389/fphar.2024.1272534
Received: 04 August 2023; Accepted: 03 January 2024;
Published: 18 January 2024.
Edited by:
César Mattei, Université d'Angers, FranceReviewed by:
Giulia Puja, University of Modena and Reggio Emilia, ItalyRochelle Marie Hines, University of Nevada, Las Vegas, United States
Copyright © 2024 Menzikov, Zaichenko, Moskovtsev, Morozov and Kubatiev. This is an open-access article distributed under the terms of the Creative Commons Attribution License (CC BY). The use, distribution or reproduction in other forums is permitted, provided the original author(s) and the copyright owner(s) are credited and that the original publication in this journal is cited, in accordance with accepted academic practice. No use, distribution or reproduction is permitted which does not comply with these terms.
*Correspondence: Sergey A. Menzikov, cy5hLm1lbnppa292QGdtYWlsLmNvbQ==