- 1Radiation Oncology Key Laboratory of Sichuan Province, Department of Experimental Research, Sichuan Clinical Research Center for Cancer, Sichuan Cancer Center, Sichuan Cancer Hospital and Institute, Affiliated Cancer Hospital of University of Electronic Science and Technology of China, Chengdu, China
- 2College of Basic Medicine, Chengdu University of Traditional Chinese Medicine, Chengdu, China
Mitochondria are critical for cellular energetic metabolism, intracellular signaling orchestration and programmed death regulation. Therefore, mitochondrial dysfunction is associated with various pathogeneses. The maintenance of mitochondrial homeostasis and functional recovery after injury are coordinated by mitochondrial biogenesis, dynamics and autophagy, which are collectively referred to as mitochondrial quality control. There is increasing evidence that mitochondria are important targets for melatonin to exert protective effects under pathological conditions. Melatonin, an evolutionarily conserved tryptophan metabolite, can be synthesized, transported and metabolized in mitochondria. In this review, we summarize the important role of melatonin in the damaged mitochondria elimination and mitochondrial energy supply recovery by regulating mitochondrial quality control, which may provide new strategies for clinical treatment of mitochondria-related diseases.
1 Introduction
Mitochondria are important organelles that not only regulate cellular energy synthesis but also determine cell fate by coordinating multiple intracellular signaling pathways (Ito and Ito, 2016; Chakrabarty and Chandel, 2021; Diehl et al., 2023). Mitochondria are dynamically changing and their quality is regulated through complex interacting processes, among which mitochondrial biogenesis, dynamics, and mitophagy are the key factors. These mitochondrial regulations are known as mitochondrial quality control (MQC). Moreover, MQC is associated with a variety of diseases, such as aging (Okatani et al., 2002; Zhang et al., 2023), cancers (de Almeida Chuffa et al., 2019; Reiter et al., 2020a; Zhang et al., 2023), cardiomyopathy (Cai et al., 2022; Zhou et al., 2023), intestinal inflammation (Motilva et al., 2011; Ma et al., 2020), ischemia/reperfusion injury (Chen et al., 2016; Bai et al., 2023), liver disease (Mauriz et al., 2007; Solís-Muñoz et al., 2011; Zhou et al., 2018a), and neurodegenerative diseases (Jauhari et al., 2020; Hossain et al., 2021; Austad et al., 2022; Xu et al., 2023; Han et al., 2023). Therefore, revealing the molecular mechanisms of MQC dysregulated may provide new prospects for therapeutic drug development in related diseases.
Interestingly, more increasing studies are showing that mitochondrial biogenesis, dynamics and autophagy are tightly regulated by melatonin, which is an evolutionarily conserved tryptophan metabolite (Tan et al., 2016a; Reiter et al., 2017; Roohbakhsh et al., 2018; Mehrzadi et al., 2021; Wu et al., 2021). Melatonin, N-acetyl-5-methoxytryptamine, is a natural endogenous hormone. In mammals, melatonin is produced in many organs such as the pineal, small intestine, retina, brain, liver, thymus, kidney, skin, and other tissues (Minich et al., 2022). In addition, melatonin is involved in the regulation of biological rhythms, free radical scavenging, anti-aging, anti-cancer, immunity and other aspects (Minich et al., 2022).
Mechanistically, melatonin modulates mitochondrial metabolism, promotes mitochondrial fusion and maintains mitochondrial oxidative stress by altering autophagy to protect against mitochondrial injury. Importantly, these protective functions of mitochondria exhibit an evolutionarily conserved pattern. Furthermore, melatonin can not only be taken up from the circulation and is produced and metabolized in mitochondria (Tan et al., 2013). An increasing body of evidence suggests that melatonin may play an important role in MQC regulation (Reiter et al., 2021; Wu et al., 2021; Reiter et al., 2022). However, the regulatory roles played by melatonin in MQC have not been fully clarified. Here, we review recent available studies and propose the possible regulatory mechanism by which melatonin maintains the homeostasis of mitochondria.
2 Melatonin synthesis and degradation
2.1 Melatonin synthesis
Tryptophan can be converted into melatonin through four enzymatic steps in pineal, retinal, intestine and other tissues (Zhao et al., 2019). Briefly, L-tryptophan is initially hydroxylated by tryptophan hydroxylase (TPH) to form 5-hydroxytryptophan (5-HTP) (Tan et al., 2016b), which is decarboxylated to produce 5-hydroxytryptophan (5-HT, serotonin) via aromatic amino acid decarboxylase (AADC) (Tan et al., 2015). Subsequently, serotonin is acetylated to produce N-acetylserotonin under the catalysis of arylalkylamine-N-acetyltransferase (AANAT). Finally, N-acetylserotonin is methylated to form 5-methoxytryptanmine (melatonin) via hydroxyindole-O-methyltransferase (HIOMT) (Figure 1A) (Tan et al., 2015; Zhao et al., 2019; Ma et al., 2020; Wu, 2021a).
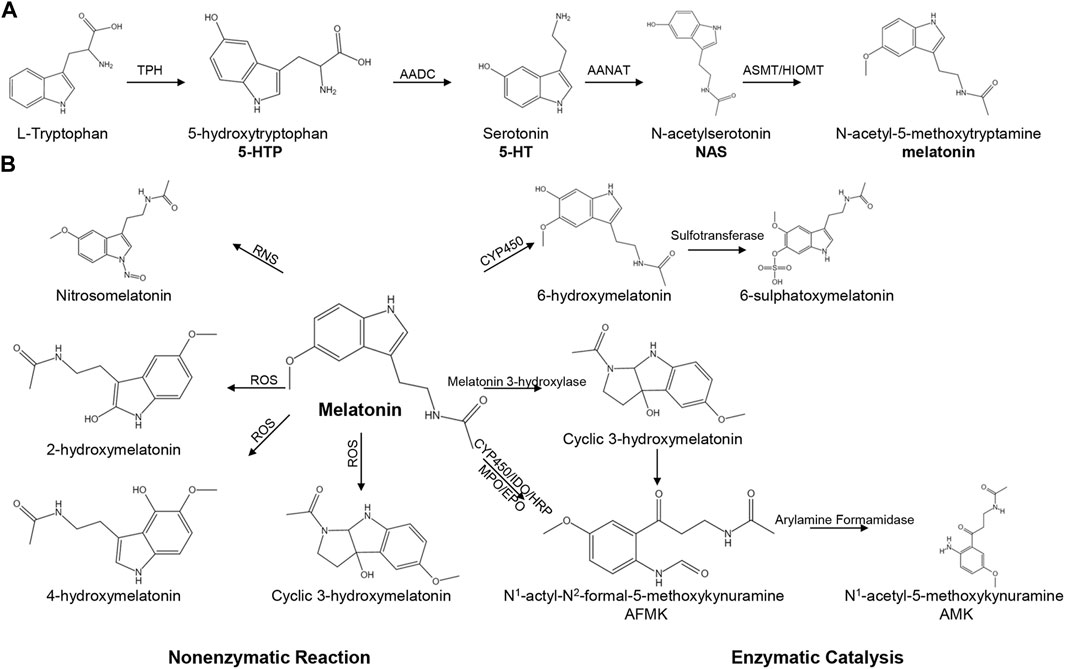
FIGURE 1. The pathways of melatonin biosynthesis and degradation. (A) The pathway of melatonin biosynthesis. (B) Melatonin degradation can be classified into non-enzymatic and enzymatic catalysis.
TPH is the initial enzyme in the biosynthesis of melatonin and has two isoforms in mammals: TPH-1 and TPH-2 (Waløen et al., 2017). TPH-1 is predominantly expressed in endothelial cells of the gastrointestinal tract (Yadav et al., 2010), and other non-neural cell types, such as kidney (Chen et al., 2019), skin (Nowak et al., 2012; Duerschmied et al., 2013), pineal gland (Rath et al., 2016) and pituitary gland (Waløen et al., 2017). In contrast, TPH-2 is expressed in serotonergic neurons in brainstem raphe nuclei (Walther et al., 2003), the orbitofrontal cortex (Booij et al., 2012) enteric nerves (Sia et al., 2013; Waløen et al., 2017; Kulikova and Kulikov, 2019). HIOMT, as known as acetylserotonin methyltransferase (ASMT), is the catalytic enzyme in the final step in melatonin or 5-MTP biosynthesis. HIOMT has been reported to be expressed in the small intestine or colon in many species, such as humans (Chojnacki et al., 2013; Chojnacki et al., 2018), rats (Al-Ghoul et al., 2010) and sheep (Zhao et al., 2019). The expression of HIOMT was significantly increased in ulcerative colitis and lymphocytic colitis patients compared with that in healthy subjects (Chojnacki et al., 2013; Chojnacki et al., 2018). Human HIOMT is encoded by a single gene that encodes three possible isoforms: P46597-1, P46597-2 and P46597-3 (Botros et al., 2013). Among them, P46597-1 is the major isoform and the only isoform, which can synthesize melatonin. Isoform P46597-2, a truncated HIOMT, can catalyse 5-MTP synthesis (Wu, 2021b). However, P46597-3 shows no ASMT activity (Botros et al., 2013).
2.2 Degradation of melatonin
Melatonin can be metabolized through non-enzymatic reactions and enzymatic catalysis (Figure 1B). During the non-enzymatic process, melatonin interacts with reactive nitrogen species (RNS) to generate nitrosomelatonin under nitrosomelatonin action (Tan et al., 2007). Moreover, melatonin can react with hydroxyl radicals (.OH) to generate 2-hydroxymelatonin, 4-hydroxymelatonin and cyclic 3-hydroxymelatonin (Tan et al., 2007). The enzymatic catalysis of melatonin involves the cytochrome C-based pathway and the classic kynurenine pathway. The cytochrome C-based pathway is the primary metabolic pathway for melatonin, and melatonin can be catalysed to form 6-hydroxymelatonin and 6-sulphatoxymelatonin (Ma et al., 2005). In bacteria, melatonin is metabolized to form cyclic 3-hydroxymelatonin via melatonin 3-hydroxylase (Hardeland, 2017). In an inflammatory environment, melatonin is degraded through the kynurenine pathway to form N1-actyl-N2-formal-5-methoxykynuramine (AFMK) and N1-acetyl-5-methoxykynuramine (AMK) (Hammerle and Surawicz, 2008; Hardeland, 2017; Ma et al., 2020).
2.3 Uptake, synthesis and degradation of melatonin by mitochondria
Melatonin is an amphiphilic molecule which can easily interact with the phospholipid bilayers and directly cross the cell membrane (Costa et al., 1995). In addition, melatonin can also be transported into cells via binding to the membrane receptors such as melatonin receptor 1 A (MTNR1A) (Liu et al., 2016; Mayo et al., 2017), melatonin receptor 1 B (MTNR1B) (Liu et al., 2016; Mayo et al., 2017), glucose transporters (GLUT) (Hevia et al., 2015; Hevia et al., 2017; Mayo et al., 2017; Pal et al., 2023), and proton-driven oligopeptide transporter 1/2 (PEPT1/2) (Wang et al., 2011). Quinone reductase two also acts as a target of melatonin to regulate downstream signaling pathways (Nosjean et al., 2000; Boutin, 2016). In the cytoplasm, melatonin can directly bind to nuclear receptors (retinoid-related orphan nuclear hormone receptor family) (Carlberg and Wiesenberg, 1995; Karasek et al., 2003; Ma et al., 2021). Besides nucleus, melatonin also exhibits regulatory effects by modulating endoplasmic reticulum (ER) stress (Martínez-Campa et al., 2006; Wu et al., 2016; Xue et al., 2017; Fang et al., 2018; Shi et al., 2018; Lee et al., 2019; Fan et al., 2020; Mahalanobish et al., 2020; Zhang et al., 2020; Guan et al., 2023). Additionally, melatonin can be transported into mitochondria through PEPT1/2 (Huo et al., 2017). Furthermore, Xin Wang et al. found that the melatonin receptor 1 A stretched across the membrane of mitochondria in mouse brains (Wang et al., 2011). Administration of melatonin to pinealectomized rats increased the concentration of melatonin in mitochondria (Tan et al., 2013). However, the melatonin level in mitochondria was not continually increased with increases in the dose administration (Tan et al., 2013). These results suggest that mitochondria may take up melatonin to maintain their function after pinealectomy.
Mitochondria may directly synthesize and degrade melatonin. First, pinealocytes contain plenty of mitochondria, and the morphology of mitochondria changes depending on the circadian clock (Bucana et al., 1974; Calvo and Boya, 1984; Tan et al., 2016a). The mitochondrial volume in pinealocytes is significantly increased in darkness, which is the peak for the synthesis of melatonin (Calvo and Boya, 1984; Tan et al., 2016a). Second, the concentration of melatonin in mitochondria has been reported approximately 100 times higher than that in mice plasma (Martín et al., 2000a). In addition, pinealectomy would not significantly decrease the melatonin level in the cerebral cortex or liver mitochondria compared (Venegas et al., 2012). Finally, and the most importantly, AANAT, the rate-limiting enzyme in the synthetic process of melatonin, resides in the mitochondria of pinealocytes and oocytes (Kerényi et al., 1979; Sakaguchi et al., 2013; Tan et al., 2013; Coelho et al., 2015). Under the stimulation of tryptophan, mitochondria derived from oocytes produce more melatonin (Sakaguchi et al., 2013; Coelho et al., 2015). Moreover, melatonin can be converted into AFMK by cytochrome P450 in the liver mitochondria of rats (Semak et al., 2008). Taken together, these studies indicate that melatonin can be transported, synthesized, and metabolized in mitochondria, which implies that melatonin may play an important role in mitochondrial homeostasis.
3 Melatonin regulates mitochondrial homeostasis
3.1 The function of melatonin in mitochondrial biogenesis
Melatonin can regulate mitochondrial biogenesis by influencing metabolism and the redox state (Figure 2). First, melatonin regulates the mitochondrial concentration of acetyl-CoA to alter mitochondrial metabolism. Melatonin regulates pyruvate or fatty acid metabolism to increase the concentration of acetyl-CoA in mitochondria. For instance, melatonin increases the activity of pyruvate kinase M1/2 (PKM) to regulate glycolysis and ultimately affects the content of acetyl-CoA in mitochondria (Vakhitova et al., 2019). Moreover, melatonin activates pyruvate dehydrogenase kinase 4 (PDK4) to regulate acetyl-CoA content (Ghareghani et al., 2019). In addition, melatonin can promote fatty acid metabolism by directly enhancing β-oxidation or increasing the transfer of fatty acid-derived acetyl-CoA into mitochondria (Kato et al., 2015; Liu et al., 2020). In addition, melatonin synthesis can consume acetyl-CoA derived from glucose and fatty acid metabolism as required by AANAT. Taken together, these studies indicate that melatonin increases or decreases acetyl-CoA content in mitochondria to regulate mitochondrial metabolism.
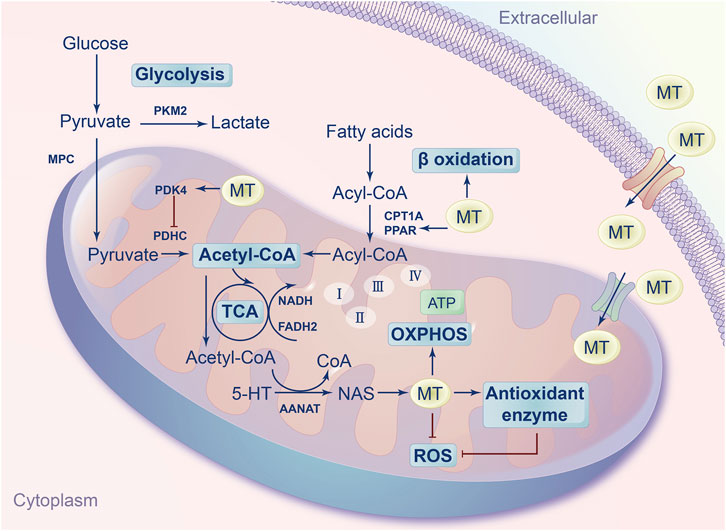
FIGURE 2. Overview of melatonin on mitochondrial metabolism and redox state. Melatonin can not only be taken from the blood into the mitochondria, but also can be synthesized in the mitochondria. Melatonin can regulate mitochondrial metabolism and redox state by regulating the concentration of acetyl-CoA, electron-transport chain, oxidative phosphorylation, reactive oxygen species, antioxidant enzymes.
Second, melatonin can enhance the activity of the electron-transport chain (ETC) and oxidative phosphorylation (OXPHOS) to regulate mitochondrial metabolism. For instance, melatonin enhanced the activities of mitochondrial complexes I and IV to protect mitochondria from ruthenium-induced injury (Martín et al., 2002). Moreover, melatonin enhanced OXPHOS and promoted adenosine triphosphate (ATP) synthesis in rat brain and liver mitochondria (Martín et al., 2000b). In addition, some studies have found that melatonin drove the switch from cytosolic glycolysis to mitochondrial OXPHOS in cancer cells (Bilska et al., 2021; Chen et al., 2021; Guerra-Librero et al., 2021). Moreover, melatonin can regulate the membrane potential of mitochondria and decrease excessive calcium levels to enhance ETC activity to increase ATP production (Xu et al., 2016).
Third, melatonin regulates the cellular redox state to influence mitochondrial metabolism. Melatonin exhibits superior antioxidant ability. Melatonin, as a major scavenger of reactive oxygen species (ROS), may play a pivotal role in protecting mitochondria from ROS-induced injury (Socaciu et al., 2020). In contrast to other antioxidants, melatonin is an amphiphilic molecule, enabling it to be distributed in aqueous or lyophobic medium (Minich et al., 2022). These specific characteristics make melatonin a broad-spectrum antioxidant. Moreover, melatonin directly scavenges various ROS, such as superoxide anions, hydroxyl radicals and hydrogen peroxide, via cascade reactions (Tan et al., 2007). In addition, melatonin increases the activity of antioxidant enzymes to eliminate ROS. Specifically, melatonin can upregulate the expression of superoxide dismutase (MnSOD), glutathione peroxidase (GSH-Px) and catalase (CAT) to prevent cell stress and injury (Fischer et al., 2013).
3.2 Melatonin and mitochondrial dynamics
In addition to mitochondrial biogenesis, melatonin inhibits fission and promotes fusion to affect mitochondrial dynamics, which contributes to the damaged mitochondria elimination and mitochondrial energy supply recovery (Figure 3). On the one hand, melatonin increases mitochondrial fusion-related genes such as mitofusin-1 (Mfn1), mitofusin-2 (Mfn2) and optic atrophy1 (Opa1) to promote mitochondrial fusion (Singhanat et al., 2021). Mechanistically, melatonin decreases calcium accumulation and eliminates extensive ROS production to regulate mitochondrial fusion. Notably, melatonin enhanced the fusion of mitochondria by activating adenosine monophosphate activated protein kinase (AMPK) to stabilize Opa1 in myocardial ischemia/reperfusion injury (Zhang et al., 2019). In addition, studies have found that melatonin activated the Yap-Hippo pathway to increase Opa1-related fusion (Ma and Dong, 2019).
Furthermore, melatonin prevents mitochondrial fission by regulating fission-related genes such as dynamin-related protein 1 (Drp1) and Fission 1 (Fis1). For instance, in cardiac ischaemia/reperfusion induced injury, melatonin decreased the ratio of p-Drp1ser616/Drp1 to inhibit mitochondrial fission. Moreover, melatonin reversed methamphetamine-induced mitochondrial fission in neuroblastoma by downregulating Fis1 expression and Drp1 mitochondrial translocation (Parameyong et al., 2013; Parameyong et al., 2015). In addition, Shangcheng Xu et al. found that melatonin blocked the translocation of Drp1 from the cytoplasm to mitochondria and eliminated excess cytosolic calcium to reduce cadmium-induced neurotoxicity (Xu et al., 2016). Mechanistically, melatonin alleviated cardiac dysfunction induced by diabetes by upregulating SIRT1-PGC1α to inhibit the expression of Drp1 (Ding et al., 2018). In addition, melatonin also activates SIRT1- PGC1α to attenuate colon injury (Yao et al., 2023). Moreover, melatonin decreased excessive Ca2+, eliminated Ca2+-induced mROS and stabilized cardiolipin to prevent mitochondrial fission and swelling during oxidative injury (Peng et al., 2012). Additionally, melatonin may activate AMPK/SERCA2a to inhibit Ca2+ overload and thus decrease calcium-dependent xanthine oxidase and ROS levels, ultimately resulting in dephosphorylation at Ser616 inhibiting migration on the surface of mitochondria and ultimately inhibiting mitochondrial fission in LPS-induced human umbilical vein endothelial cell injury (Cui et al., 2018).
3.3 Melatonin regulates mitochondrial oxidative stress through autophagy
As a highly conserved mechanism, autophagy degrades misfolding proteins or damaged organelles, to fulfill the recycling of amino acids and lipids (Xu et al., 2023; Liu et al., 2023). Insufficient autophagy leads to incomplete removal of damaged organelles, which exacerbates cell or tissue injury (Lin et al., 2023). However, uncontrolled and exacerbated autophagy causes excessive degradation of cellular components, which finally induces cell death and contributes to the development of diseases (Xu X. et al., 2023; Liu et al., 2023). Therefore, the balance of appropriate autophagy is critical to maintain cellular and tissue homeostasis, and autophagy plays differential roles in different steps of disease.
Autophagy plays an important role in cellular oxidative stress. As the major ROS generating organelles, mitochondria can also maintain the homeostasis of cellular oxidative stress via modulating autophagy (Scherz-Shouval and ElazarROS, 2007). ROS, as a signaling molecule, can enhance or attenuate autophagy through regulating AMPK. ROS can activate AMPK to enhance autophagy by increasing the ratio of AMP:ATP or enhancing the activity of LKB1, which is an upstream kinase of AMPK (Park et al., 2006; Fitzwalter et al., 2018). Under some circumstance, ROS can also inhibit autophagy via enhancing the activity of AKT, which is a negative regulator of AMPK (Jiang et al., 2021). Therefore, the regulation of ROS or oxidative stress on autophagy may vary depending on different circumstances (Agostini et al., 2023). Meanwhile, mitochondria are one of the main target organelles of melatonin. So that, melatonin might orchestrate autophagy to response mitochondrial oxidative stress under physiological and pathological conditions (Figure 4).
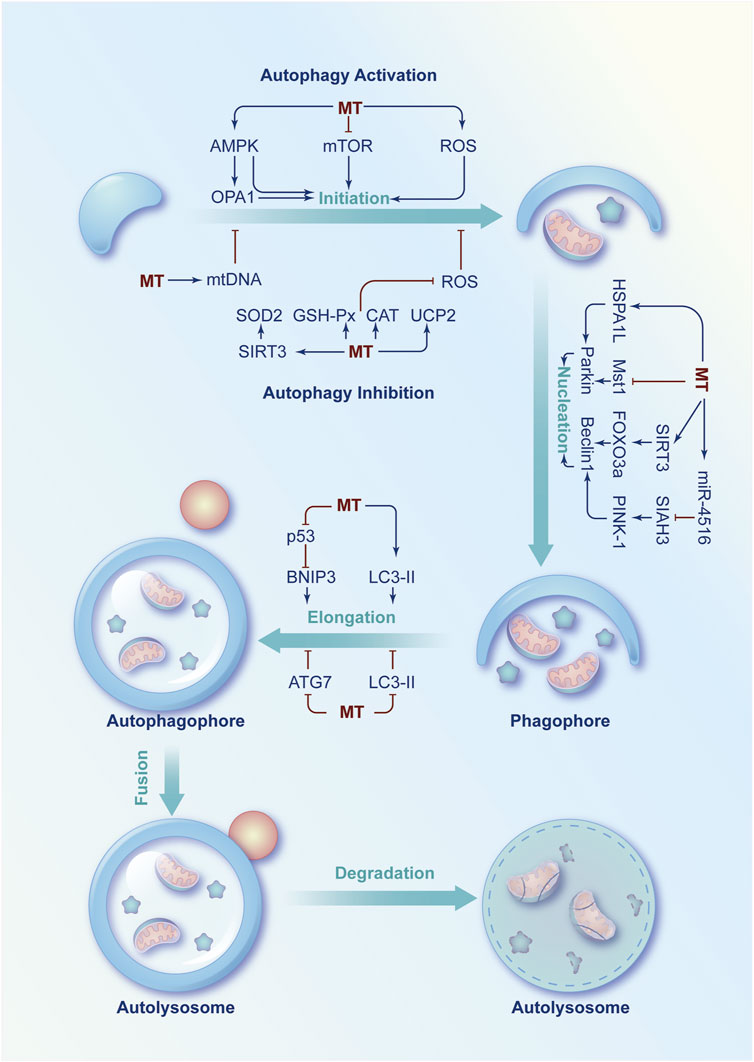
FIGURE 4. Schematic diagram of the regulatory role of melatonin in autophagy imbalance. Under physiological or pathological conditions, several signaling molecules are involved in the regulation of autophagy at the initiation, nucleation and phagophore elongation phases. Melatonin can modulate these molecules to increase or decrease autophagy rate to exert a protective effect under pathological conditions.
On the one hand, melatonin enhances autophagy to protect mitochondria from oxidative damage at the initiation, nucleation and phagophore elongation phases of autophagy. First, melatonin activates AMPK to regulate autophagy under different pathological conditions, such as myocardial ischemia-reperfusion injury (Zhang et al., 2019), doxorubicin-induced cardiotoxicity (Liu et al., 2018), PBDE-47 neurotoxicity (Dong et al., 2023), bone loss (McCarty et al., 2022), and lipopolysaccharide-induced blood-brain barrier injury (Wang et al., 2017). In addition, melatonin can also initiate autophagy by inhibiting the AKT/mTOR activation to enhance the therapeutic effect of rapamycin on head and neck cancer (Shen et al., 2018) or prevent hypertrophic scar (Dong et al., 2023). Moreover, melatonin combined with rapamycin and NLRP3-selective inhibitor can reduce cadmium-induced bone defects by downregulating ROS/NLRP3 signaling pathway (Hu et al., 2023). Then, melatonin modulates Beclin1 and Parkin to promote nucleation (X et al., 2023). Specifically, melatonin activates Parkin via upregulation of 70-kDa heat shock protein 1L (HSPA1L) to suppress the senescence of mesenchymal stem cells (Lee et al., 2020). Melatonin also inhibits mammalian Ste20-like kinase 1 (Mst1) to protect against diabetic cardiomyopathy (Zhang et al., 2017). Moreover, melatonin can enhance autophagy via regulating the SIRT3/FOXO3a signalling pathway to improve intervertebral disc degeneration (Chen et al., 2019). In addition, melatonin can activate the miR-4516/SIAH3/PINK-1 signalling pathway to attenuate renal fibrosis (Yoon et al., 2021). Finally, melatonin promotes the elongation of the autophagosome by relieving the repressive effect of p53 on BNIP3 (Zhou et al., 2018b) and enhancing the level of LC3-Ⅱ (Jeong et al., 2012).
On the other hand, melatonin could also inhibit autophagy to keep the mitochondrial redox state in balance in some cases. First, melatonin inhibits the initiation of autophagy by increasing mitochondrial DNA copy number or decreasing ROS levels and improve mitochondrial damage of non-alcoholic fatty liver disease or LPS-induced cardiomyopathy (Feng et al., 2013; Wang et al., 2023). In addition, melatonin might inhibit autophagy in cadmium-induced liver injury by increasing the expression and activity of silent information regulator 3 (SIRT3) to inhibit the acetylation of SOD2 and enhance the clearance ability of mitochondrial ROS (Pi et al., 2015). Moreover, melatonin can inhibit autophagy after osteoporosis by upregulating miR-224-5p to downregulate SIRT3 and AMPK (Chen and Dai, 2023). Furthermore, melatonin could elevate mitochondrial uncoupling protein 2 (UCP2) to decrease the production of mROS and inhibit autophagy in LPS-induced cardiomyopathy (Pan et al., 2018). Melatonin exerts neuroprotective effects by increasing AKT, mTOR and Unc-51 like autophagy activating kinase 1 (ULK1) to inhibit autophagy (Xiong et al., 2023). In addition, melatonin is capable to enhance the activity of SOD, CAT and GSH-Px to inhibit the formation of autophagosomes in oxidative stress-induced damaged goat spermatogonial stem cells or deoxynivalenol induced cell damage (Feng et al., 2020; Xu et al., 2023). Second, melatonin can inhibit elongation to prevent autophagy by inhibiting autophagy-related protein 7 (ATG7) activity or decreasing LC3-Ⅱ levels in kainic acid or arsenite-induced neurotoxicity (Chang et al., 2012; Teng et al., 2015).
Taken together, autophagy has a bidirectional regulatory role in cell survival or death, depending on the severity of cellular oxidative damage (Liu et al., 2023; Piletic et al., 2023). Melatonin has been proven to have a strong antioxidant effect. An intimate relationship between melatonin and autophagy has been found in various pathologies. Melatonin may modulate autophagy by regulating mitochondria and oxidative stress (Wu et al., 2021). However, the specific role of melatonin in the regulation of autophagic processes has not been extensively investigated in each pathology.
4 Conclusion and future prospects
In this paper, we discussed the regulatory effects of melatonin on mitochondrial quality control. Mechanistically, melatonin reprograms cellular metabolism by orchestrating the concentration of acetyl-CoA, OXPHOS levels, cellular redox state in mitochondria. Melatonin helps restore the damaged mitochondrial energy supply by regulating mitochondrial dynamics. Melatonin not only enhances mitochondrial fusion, but also has a dual role in regulating cellular autophagy. In other words, melatonin either enhances or attenuates autophagy depending on specific conditions. Under conditions of insufficient autophagy, melatonin can remove excess ROS and damaged mitochondria by upregulating autophagy. On the contrary, melatonin can down-regulate autophagy and increase cell death caused by excessive autophagy. In summary, melatonin, as a broad-spectrum antioxidant and modulator of mitochondrial activity, appears to be a promising approach for the treatment of many MQC-related injuries or diseases.
However, more basic and clinical trials are necessary to further validate the exact therapeutic effect of melatonin on MQC-related diseases (Boga et al., 2019; Reiter et al., 2020b; Melhuish Beaupre et al., 2021; Wu et al., 2021; Boc et al., 2022). For example, multiple signaling pathway are involved in aging (Martín Giménez et al., 2022; Yan et al., 2022), but the safety and efficacy of melatonin used as antioxidant therapy for clinical treatment of aging-related diseases still need further exploration (Yan et al., 2022). In addition, cutaneous cells can synthesize melatonin fight against UV-induced cutaneous damage (Slominski et al., 2008; Slominski et al., 2018; Boc et al., 2022). Is it possible to use melatonin topically to prevent the aging of skin? Why does melatonin differentially impact autophagy in different stages of aging (Hardeland, 2012), cancer (Reiter et al., 2021; Zhang et al., 2023; Li et al., 2023; Qin et al., 2023), degenerative disease (Feybesse et al., 2023; Litwiniuk et al., 2023) and ischemia/reperfusion injury (Loh and Reiter, 2021)? How does melatonin enhance or attenuate autophagy according to different stimulations or cell types? (Boga et al., 2019; Wu et al., 2021). In addition, many discoveries of melatonin are made on animals, how can they be successfully translated into MQC-related diseases in humans? The answers to these questions would not only allow us to further understand the relationship between melatonin mediated MQC and disease, but also provide more critical clues and evidence for the development of promising agents.
Author contributions
XL: Writing–review and editing, Writing–original draft. ZX: Writing–original draft. LH: Writing–original draft. YH: Investigation, Writing–original draft. ST: Investigation, Writing–original draft. LX: Writing–original draft. DL: Writing–review and editing.
Funding
The author(s) declare financial support was received for the research, authorship, and/or publication of this article. This research was financially supported by National Natural Science Foundation of China (81874255), Science and Technology Department of Sichuan Province (2021YJ0194, 2023JDRC0019, 2022ZYD0091) and Health Commission of Sichuan Province (21ZD008, 2023-802), Cadres Health Scientific Project of Sichuan Province (2021-803) and Sichuan Cancer Hospital Research Program (YB2023015, YBR2019003, YB2021023).
Conflict of interest
The authors declare that the research was conducted in the absence of any commercial or financial relationships that could be construed as a potential conflict of interest.
Publisher’s note
All claims expressed in this article are solely those of the authors and do not necessarily represent those of their affiliated organizations, or those of the publisher, the editors and the reviewers. Any product that may be evaluated in this article, or claim that may be made by its manufacturer, is not guaranteed or endorsed by the publisher.
References
Agostini, F., Bisaglia, M., and Plotegher, N. (2023). Linking ROS levels to autophagy: the key role of AMPK. Antioxidants (Basel, Switz. 12 (7), 1406. doi:10.3390/antiox12071406
Al-Ghoul, W. M., Abu-Shaqra, S., Park, B. G., and Fazal, N. (2010). Melatonin plays a protective role in postburn rodent gut pathophysiology. Int. J. Biol. Sci. 6 (3), 282–293. doi:10.7150/ijbs.6.282
Austad, S. N., Ballinger, S., Buford, T. W., Carter, C. S., Smith, D. L., Darley-Usmar, V., et al. (2022). Targeting whole body metabolism and mitochondrial bioenergetics in the drug development for Alzheimer's disease. Acta Pharm. Sin. B 12 (2), 511–531. doi:10.1016/j.apsb.2021.06.014
Bai, Y., Wu, J., Yang, Z., Wang, X., Zhang, D., and Ma, J. (2023). Mitochondrial quality control in cardiac ischemia/reperfusion injury: new insights into mechanisms and implications. Cell Biol. Toxicol. 39 (1), 33–51. doi:10.1007/s10565-022-09716-2
Bilska, B., Schedel, F., Piotrowska, A., Stefan, J., Zmijewski, M., Pyza, E., et al. (2021). Mitochondrial function is controlled by melatonin and its metabolites in vitro in human melanoma cells. J. pineal Res. 70 (3), e12728. doi:10.1111/jpi.12728
Bocheva, G., Slominski, R. M., Janjetovic, Z., Kim, T. K., Böhm, M., Steinbrink, K., et al. (2022). Protective role of melatonin and its metabolites in skin aging. Int. J. Mol. Sci. 23 (3), 1238. doi:10.3390/ijms23031238
Boga, J. A., Caballero, B., Potes, Y., Perez-Martinez, Z., Reiter, R. J., Vega-Naredo, I., et al. (2019). Therapeutic potential of melatonin related to its role as an autophagy regulator: a review. J. pineal Res. 66 (1), e12534. doi:10.1111/jpi.12534
Booij, L., Turecki, G., Leyton, M., Gravel, P., Lopez De Lara, C., Diksic, M., et al. (2012). Tryptophan hydroxylase(2) gene polymorphisms predict brain serotonin synthesis in the orbitofrontal cortex in humans. Mol. psychiatry 17 (8), 809–817. doi:10.1038/mp.2011.79
Botros, H. G., Legrand, P., Pagan, C., Bondet, V., Weber, P., Ben-Abdallah, M., et al. (2013). Crystal structure and functional mapping of human ASMT, the last enzyme of the melatonin synthesis pathway. J. pineal Res. 54 (1), 46–57. doi:10.1111/j.1600-079X.2012.01020.x
Boutin, J. A. (2016). Quinone reductase 2 as a promising target of melatonin therapeutic actions. Expert Opin. Ther. targets 20 (3), 303–317. doi:10.1517/14728222.2016.1091882
Bucana, C. D., Nadakavukaren, M. J., and Frehn, J. L. (1974). Novel features of hamster pinealocyte ultrastructure. Tissue & Cell 6 (1), 85–93. doi:10.1016/0040-8166(74)90024-x
Cai, C., Wu, F., He, J., Zhang, Y., Shi, N., Peng, X., et al. (2022). Mitochondrial quality control in diabetic cardiomyopathy: from molecular mechanisms to therapeutic strategies. Int. J. Biol. Sci. 18 (14), 5276–5290. doi:10.7150/ijbs.75402
Calvo, J., and Boya, J. (1984). Ultrastructure of the pineal gland in the adult rat. J. Anat. 138 (Pt 3), 405–409.
Carlberg, C., and Wiesenberg, I. (1995). The orphan receptor family RZR/ROR, melatonin and 5-lipoxygenase: an unexpected relationship. J. pineal Res. 18 (4), 171–178. doi:10.1111/j.1600-079x.1995.tb00157.x
Chakrabarty, R. P., and Chandel, N. S. (2021). Mitochondria as signaling organelles control mammalian stem cell fate. Cell Stem Cell 28 (3), 394–408. doi:10.1016/j.stem.2021.02.011
Chang, C. F., Huang, H. J., Lee, H. C., Hung, K. C., Wu, R. T., and Lin, A. M. (2012). Melatonin attenuates kainic acid-induced neurotoxicity in mouse hippocampus via inhibition of autophagy and α-synuclein aggregation. J. pineal Res. 52 (3), 312–321. doi:10.1111/j.1600-079X.2011.00945.x
Chen, D. Q., Cao, G., Chen, H., Argyopoulos, C. P., Yu, H., Su, W., et al. (2019a). Identification of serum metabolites associating with chronic kidney disease progression and anti-fibrotic effect of 5-methoxytryptophan. Nat. Commun. 10 (1), 1476. doi:10.1038/s41467-019-09329-0
Chen, H. H., Chen, Y. T., Yang, C. C., Chen, K. H., Sung, P. H., Chiang, H. J., et al. (2016). Melatonin pretreatment enhances the therapeutic effects of exogenous mitochondria against hepatic ischemia-reperfusion injury in rats through suppression of mitochondrial permeability transition. J. pineal Res. 61 (1), 52–68. doi:10.1111/jpi.12326
Chen, S., and Dai, M. (2023). The miR-224-5p/SIRT3/AMPK/mTOR axis is involved in the melatonin-mediated inhibition of glucocorticoid-induced osteoporosis by activating autophagy. Hum. Cell 36, 1965–1977. doi:10.1007/s13577-023-00929-z
Chen, X., Hao, B., Li, D., Reiter, R. J., Bai, Y., Abay, B., et al. (2021). Melatonin inhibits lung cancer development by reversing the Warburg effect via stimulating the SIRT3/PDH axis. J. pineal Res. 71, e12755. doi:10.1111/jpi.12755
Chen, Y., Wu, Y., Shi, H., Wang, J., Zheng, Z., Chen, J., et al. (2019b). Melatonin ameliorates intervertebral disc degeneration via the potential mechanisms of mitophagy induction and apoptosis inhibition. J. Cell. Mol. Med. 23 (3), 2136–2148. doi:10.1111/jcmm.14125
Chojnacki, C., Błasiak, J., Fichna, J., Chojnacki, J., and Popławski, T. (2018). Evaluation of melatonin secretion and metabolism exponents in patients with ulcerative and lymphocytic colitis. Mol. (Basel, Switz. 23 (2), 272. doi:10.3390/molecules23020272
Chojnacki, C., Wiśniewska-Jarosińska, M., Kulig, G., Majsterek, I., Reiter, R. J., and Chojnacki, J. (2013). Evaluation of enterochromaffin cells and melatonin secretion exponents in ulcerative colitis. World J. Gastroenterol. 19 (23), 3602–3607. doi:10.3748/wjg.v19.i23.3602
Coelho, L. A., Peres, R., Amaral, F. G., Reiter, R. J., and Cipolla-Neto, J. (2015). Daily differential expression of melatonin-related genes and clock genes in rat cumulus-oocyte complex: changes after pinealectomy. J. pineal Res. 58 (4), 490–499. doi:10.1111/jpi.12234
Costa, E. J., Lopes, R. H., and Lamy-Freund, M. T. (1995). Permeability of pure lipid bilayers to melatonin. J. pineal Res. 19 (3), 123–126. doi:10.1111/j.1600-079x.1995.tb00180.x
Cui, J., Li, Z., Zhuang, S., Qi, S., Li, L., Zhou, J., et al. (2018). Melatonin alleviates inflammation-induced apoptosis in human umbilical vein endothelial cells via suppression of Ca(2+)-XO-ROS-Drp1-mitochondrial fission axis by activation of AMPK/SERCA2a pathway. Cell stress & chaperones 23 (2), 281–293. doi:10.1007/s12192-017-0841-6
de Almeida Chuffa, L. G., Seiva, F. R. F., Cucielo, M. S., Silveira, H. S., Reiter, R. J., and Lupi, L. A. (2019). Mitochondrial functions and melatonin: a tour of the reproductive cancers. Cell. Mol. life Sci. CMLS 76 (5), 837–863. doi:10.1007/s00018-018-2963-0
Diehl, F. F., Sapp, K. M., and Vander Heiden, M. G. (2023). The bidirectional relationship between metabolism and cell cycle control. Trends Cell Biol. doi:10.1016/j.tcb.2023.05.012
Ding, M., Feng, N., Tang, D., Feng, J., Li, Z., Jia, M., et al. (2018). Melatonin prevents Drp1-mediated mitochondrial fission in diabetic hearts through SIRT1-PGC1α pathway. J. pineal Res. 65 (2), e12491. doi:10.1111/jpi.12491
Dong, L., Sun, Q., Qiu, H., Yang, K., Xiao, B., Xia, T., et al. (2023a). Melatonin protects against developmental PBDE-47 neurotoxicity by targeting the AMPK/mitophagy axis. J. pineal Res. 75 (1), e12871. doi:10.1111/jpi.12871
Dong, Y., Cao, X., Huang, J., Hu, Z., Chen, C., Chen, M., et al. (2023b). Melatonin inhibits fibroblast cell functions and hypertrophic scar formation by enhancing autophagy through the MT2 receptor-inhibited PI3K/Akt/mTOR signaling. Biochimica biophysica acta Mol. basis Dis. 1870 (1), 166887. doi:10.1016/j.bbadis.2023.166887
Duerschmied, D., Suidan, G. L., Demers, M., Herr, N., Carbo, C., Brill, A., et al. (2013). Platelet serotonin promotes the recruitment of neutrophils to sites of acute inflammation in mice. Blood 121 (6), 1008–1015. doi:10.1182/blood-2012-06-437392
Fan, C., Feng, J., Tang, C., Zhang, Z., Feng, Y., Duan, W., et al. (2020). Melatonin suppresses ER stress-dependent proapoptotic effects via AMPK in bone mesenchymal stem cells during mitochondrial oxidative damage. Stem Cell Res. Ther. 11 (1), 442. doi:10.1186/s13287-020-01948-5
Fang, J., Yan, Y., Teng, X., Wen, X., Li, N., Peng, S., et al. (2018). Melatonin prevents senescence of canine adipose-derived mesenchymal stem cells through activating NRF2 and inhibiting ER stress. Aging 10 (10), 2954–2972. doi:10.18632/aging.101602
Feng, T. Y., Li, Q., Ren, F., Xi, H. M., Lv, D. L., Li, Y., et al. (2020). Melatonin protects goat spermatogonial stem cells against oxidative damage during cryopreservation by improving antioxidant capacity and inhibiting mitochondrial apoptosis pathway. Oxidative Med. Cell. Longev. 2020, 5954635. doi:10.1155/2020/5954635
Feng, Y. M., Jia, Y. F., Su, L. Y., Wang, D., Lv, L., Xu, L., et al. (2013). Decreased mitochondrial DNA copy number in the hippocampus and peripheral blood during opiate addiction is mediated by autophagy and can be salvaged by melatonin. Autophagy 9 (9), 1395–1406. doi:10.4161/auto.25468
Feybesse, C., Chokron, S., and Tordjman, S. (2023). Melatonin in neurodevelopmental disorders: a critical literature review. Antioxidants (Basel, Switz. 12 (11), 2017. doi:10.3390/antiox12112017
Fischer, T. W., Kleszczyński, K., Hardkop, L. H., Kruse, N., and Zillikens, D. (2013). Melatonin enhances antioxidative enzyme gene expression (CAT, GPx, SOD), prevents their UVR-induced depletion, and protects against the formation of DNA damage (8-hydroxy-2'-deoxyguanosine) in ex vivo human skin. J. pineal Res. 54 (3), 303–312. doi:10.1111/jpi.12018
Fitzwalter, B. E., Towers, C. G., Sullivan, K. D., Andrysik, Z., Hoh, M., Ludwig, M., et al. (2018). Autophagy inhibition mediates apoptosis sensitization in cancer therapy by relieving FOXO3a turnover. Dev. Cell 44 (5), 555–565. doi:10.1016/j.devcel.2018.02.014
Ghareghani, M., Scavo, L., Jand, Y., Farhadi, N., Sadeghi, H., Ghanbari, A., et al. (2019). Melatonin therapy modulates cerebral metabolism and enhances remyelination by increasing PDK4 in a mouse model of multiple sclerosis. Front. Pharmacol. 10, 147. doi:10.3389/fphar.2019.00147
Guan, Q., Wang, Z., Hu, K., Cao, J., Dong, Y., and Chen, Y. (2023). Melatonin ameliorates hepatic ferroptosis in NAFLD by inhibiting ER stress via the MT2/cAMP/PKA/IRE1 signaling pathway. Int. J. Biol. Sci. 19 (12), 3937–3950. doi:10.7150/ijbs.85883
Guerra-Librero, A., Fernandez-Gil, B. I., Florido, J., Martinez-Ruiz, L., Rodríguez-Santana, C., Shen, Y. Q., et al. (2021). Melatonin targets metabolism in head and neck cancer cells by regulating mitochondrial structure and function. Antioxidants (Basel, Switz. 10 (4), 603. doi:10.3390/antiox10040603
Hammerle, C. W., and Surawicz, C. M. (2008). Updates on treatment of irritable bowel syndrome. World J. Gastroenterol. 14 (17), 2639–2649. doi:10.3748/wjg.14.2639
Han, Y., Liu, D., Cheng, Y., Ji, Q., Liu, M., Zhang, B., et al. (2023). Maintenance of mitochondrial homeostasis for Alzheimer's disease: strategies and challenges. Redox Biol. 63, 102734. doi:10.1016/j.redox.2023.102734
Hardeland, R. (2012). Melatonin in aging and disease -multiple consequences of reduced secretion, options and limits of treatment. Aging Dis. 3 (2), 194–225.
Hardeland, R. (2017). Taxon- and site-specific melatonin catabolism. Mol. (Basel, Switz. 22 (11), 2015. doi:10.3390/molecules22112015
Hevia, D., Gonzalez-Menendez, P., Fernandez-Fernandez, M., Cueto, S., Rodriguez-Gonzalez, P., Garcia-Alonso, J. I., et al. (2017). Melatonin decreases glucose metabolism in prostate cancer cells: a (13)C stable isotope-resolved metabolomic study. Int. J. Mol. Sci. 18 (8), 1620. doi:10.3390/ijms18081620
Hevia, D., González-Menéndez, P., Quiros-González, I., Miar, A., Rodríguez-García, A., Tan, D. X., et al. (2015). Melatonin uptake through glucose transporters: a new target for melatonin inhibition of cancer. J. pineal Res. 58 (2), 234–250. doi:10.1111/jpi.12210
Hossain, M. F., Wang, N., Chen, R., Li, S., Roy, J., Uddin, M. G., et al. (2021). Exploring the multifunctional role of melatonin in regulating autophagy and sleep to mitigate Alzheimer's disease neuropathology. Ageing Res. Rev. 67, 101304. doi:10.1016/j.arr.2021.101304
Hu, R., Luo, H., Ji, Y., Wang, Z., Zheng, P., Ouyang, H., et al. (2023). Activation of NLRP3 signaling contributes to cadmium-induced bone defects, associated with autophagic flux obstruction. Sci. total Environ. 893, 164787. doi:10.1016/j.scitotenv.2023.164787
Huo, X., Wang, C., Yu, Z., Peng, Y., Wang, S., Feng, S., et al. (2017). Human transporters, PEPT1/2, facilitate melatonin transportation into mitochondria of cancer cells: an implication of the therapeutic potential. J. pineal Res. 62 (4), e12390. doi:10.1111/jpi.12390
Ito, K., and Ito, K. (2016). Metabolism and the control of cell fate decisions and stem cell renewal. Annu. Rev. Cell Dev. Biol. 32, 399–409. doi:10.1146/annurev-cellbio-111315-125134
Jauhari, A., Baranov, S. V., Suofu, Y., Kim, J., Singh, T., Yablonska, S., et al. (2020). Melatonin inhibits cytosolic mitochondrial DNA-induced neuroinflammatory signaling in accelerated aging and neurodegeneration. J. Clin. investigation 130 (6), 3124–3136. doi:10.1172/JCI135026
Jeong, J. K., Moon, M. H., Lee, Y. J., Seol, J. W., and Park, S. Y. (2012). Melatonin-induced autophagy protects against human prion protein-mediated neurotoxicity. J. pineal Res. 53 (2), 138–146. doi:10.1111/j.1600-079X.2012.00980.x
Jiang, P., Ren, L., Zhi, L., Yu, Z., Lv, F., Xu, F., et al. (2021). Negative regulation of AMPK signaling by high glucose via E3 ubiquitin ligase MG53. Mol. Cell 81 (3), 629–637.e5. doi:10.1016/j.molcel.2020.12.008
Karasek, M., Gruszka, A., Lawnicka, H., Kunert-Radek, J., and Pawlikowski, M. (2003). Melatonin inhibits growth of diethylstilbestrol-induced prolactin-secreting pituitary tumor in vitro: possible involvement of nuclear RZR/ROR receptors. J. pineal Res. 34 (4), 294–296. doi:10.1034/j.1600-079x.2003.00046.x
Kato, H., Tanaka, G., Masuda, S., Ogasawara, J., Sakurai, T., Kizaki, T., et al. (2015). Melatonin promotes adipogenesis and mitochondrial biogenesis in 3T3-L1 preadipocytes. J. pineal Res. 59 (2), 267–275. doi:10.1111/jpi.12259
Kerényi, N. A., Balogh, I., Somogyi, E., and Sótonyi, P. (1979). Cytochemical investigation of acetyl-serotonin-transferase activity in the pineal gland. Cell. Mol. Biol. Incl. cyto-enzymology 25 (4), 259–262.
Kulikova, E. A., and Kulikov, A. V. (2019). Tryptophan hydroxylase 2 as a therapeutic target for psychiatric disorders: focus on animal models. Expert Opin. Ther. targets 23 (8), 655–667. doi:10.1080/14728222.2019.1634691
Lee, J. H., Yoon, Y. M., Han, Y. S., Jung, S. K., and Lee, S. H. (2019). Melatonin protects mesenchymal stem cells from autophagy-mediated death under ischaemic ER-stress conditions by increasing prion protein expression. Cell Prolif. 52 (2), e12545. doi:10.1111/cpr.12545
Lee, J. H., Yoon, Y. M., Song, K. H., Noh, H., and Lee, S. H. (2020). Melatonin suppresses senescence-derived mitochondrial dysfunction in mesenchymal stem cells via the HSPA1L-mitophagy pathway. Aging Cell 19 (3), e13111. doi:10.1111/acel.13111
Li, D., Peng, X., He, G., Liu, J., Li, X., Lin, W., et al. (2023). Crosstalk between autophagy and CSCs: molecular mechanisms and translational implications. Cell death Dis. 14 (7), 409. doi:10.1038/s41419-023-05929-3
Lin, Z., Long, F., Kang, R., Klionsky, D. J., Yang, M., and Tang, D. (2023). The lipid basis of cell death and autophagy. Autophagy, 1–20. doi:10.1080/15548627.2023.2259732
Litwiniuk, A., Juszczak, G. R., Stankiewicz, A. M., and Urbańska, K. (2023). The role of glial autophagy in Alzheimer's disease. Mol. psychiatry, 101917. doi:10.1038/s41380-023-02242-5
Liu, D., Ma, Z., Di, S., Yang, Y., Yang, J., Xu, L., et al. (2018). AMPK/PGC1α activation by melatonin attenuates acute doxorubicin cardiotoxicity via alleviating mitochondrial oxidative damage and apoptosis. Free Radic. Biol. Med. 129, 59–72. doi:10.1016/j.freeradbiomed.2018.08.032
Liu, J., Clough, S. J., Hutchinson, A. J., Adamah-Biassi, E. B., Popovska-Gorevski, M., and Dubocovich, M. L. (2016). MT1 and MT2 melatonin receptors: a therapeutic perspective. Annu. Rev. Pharmacol. Toxicol. 56, 361–383. doi:10.1146/annurev-pharmtox-010814-124742
Liu, S., Yao, S., Yang, H., Liu, S., and Wang, Y. (2023). Autophagy: regulator of cell death. Cell death Dis. 14 (10), 648. doi:10.1038/s41419-023-06154-8
Liu, W., Zhang, Y., Chen, Q., Liu, S., Xu, W., Shang, W., et al. (2020). Melatonin alleviates glucose and lipid metabolism disorders in Guinea pigs caused by different artificial light rhythms. J. diabetes Res. 2020, 4927403. doi:10.1155/2020/4927403
Loh, D., and Reiter, R. J. (2021). Melatonin: regulation of biomolecular condensates in neurodegenerative disorders. Antioxidants (Basel, Switz. 10 (9), 1483. doi:10.3390/antiox10091483
Ma, H., Kang, J., Fan, W., He, H., and Huang, F. (2021). ROR: nuclear receptor for melatonin or not? Mol. (Basel, Switz. 26 (9), 2693. doi:10.3390/molecules26092693
Ma, N., Zhang, J., Reiter, R. J., and Ma, X. (2020). Melatonin mediates mucosal immune cells, microbial metabolism, and rhythm crosstalk: a therapeutic target to reduce intestinal inflammation. Med. Res. Rev. 40 (2), 606–632. doi:10.1002/med.21628
Ma, S., and Dong, Z. (2019). Melatonin attenuates cardiac reperfusion stress by improving OPA1-related mitochondrial fusion in a yap-hippo pathway-dependent manner. J. Cardiovasc. Pharmacol. 73 (1), 27–39. doi:10.1097/FJC.0000000000000626
Ma, X., Idle, J. R., Krausz, K. W., and Gonzalez, F. J. (2005). Metabolism of melatonin by human cytochromes p450. Drug metabolism Dispos. Biol. fate Chem. 33 (4), 489–494. doi:10.1124/dmd.104.002410
Mahalanobish, S., Dutta, S., Saha, S., and Sil, P. C. (2020). Melatonin induced suppression of ER stress and mitochondrial dysfunction inhibited NLRP3 inflammasome activation in COPD mice. Food Chem. Toxicol. Int. J. Publ. Br. Industrial Biol. Res. Assoc. 144, 111588. doi:10.1016/j.fct.2020.111588
Martín, M., Macías, M., Escames, G., León, J., and Acuña-Castroviejo, D. (2000a). Melatonin but not vitamins C and E maintains glutathione homeostasis in t-butyl hydroperoxide-induced mitochondrial oxidative stress. FASEB J. official Publ. Fed. Am. Soc. Exp. Biol. 14 (12), 1677–1679. doi:10.1096/fj.99-0865fje
Martín, M., Macías, M., Escames, G., Reiter, R. J., Agapito, M. T., Ortiz, G. G., et al. (2000b). Melatonin-induced increased activity of the respiratory chain complexes I and IV can prevent mitochondrial damage induced by ruthenium red in vivo. J. pineal Res. 28 (4), 242–248. doi:10.1034/j.1600-079x.2000.280407.x
Martín, M., Macías, M., León, J., Escames, G., Khaldy, H., and Acuña-Castroviejo, D. (2002). Melatonin increases the activity of the oxidative phosphorylation enzymes and the production of ATP in rat brain and liver mitochondria. Int. J. Biochem. Cell Biol. 34 (4), 348–357. doi:10.1016/s1357-2725(01)00138-8
Martínez-Campa, C., Alonso-González, C., Mediavilla, M. D., Cos, S., González, A., Ramos, S., et al. (2006). Melatonin inhibits both ER alpha activation and breast cancer cell proliferation induced by a metalloestrogen, cadmium. J. pineal Res. 40 (4), 291–296. doi:10.1111/j.1600-079X.2006.00315.x
Martín Giménez, V. M., de Las Heras, N., Lahera, V., Tresguerres, J. A. F., Reiter, R. J., and Manucha, W. (2022). Melatonin as an anti-aging therapy for age-related cardiovascular and neurodegenerative diseases. Front. aging Neurosci. 14, 888292. doi:10.3389/fnagi.2022.888292
Mauriz, J. L., Molpeceres, V., García-Mediavilla, M. V., González, P., Barrio, J. P., and González-Gallego, J. (2007). Melatonin prevents oxidative stress and changes in antioxidant enzyme expression and activity in the liver of aging rats. J. pineal Res. 42 (3), 222–230. doi:10.1111/j.1600-079X.2006.00409.x
Mayo, J. C., Sainz, R. M., González-Menéndez, P., Hevia, D., and Cernuda-Cernuda, R. (2017). Melatonin transport into mitochondria. Cell. Mol. life Sci. CMLS 74 (21), 3927–3940. doi:10.1007/s00018-017-2616-8
McCarty, M. F., Lewis Lujan, L., and Iloki Assanga, S. (2022). Targeting Sirt1, AMPK, Nrf2, CK2, and soluble guanylate cyclase with nutraceuticals: a practical strategy for preserving bone mass. Int. J. Mol. Sci. 23 (9), 4776. doi:10.3390/ijms23094776
Mehrzadi, S., Karimi, M. Y., Fatemi, A., Reiter, R. J., and Hosseinzadeh, A. (2021). SARS-CoV-2 and other coronaviruses negatively influence mitochondrial quality control: beneficial effects of melatonin. Pharmacol. Ther. 224, 107825. doi:10.1016/j.pharmthera.2021.107825
Melhuish Beaupre, L. M., Brown, G. M., Gonçalves, V. F., and Kennedy, J. L. (2021). Melatonin's neuroprotective role in mitochondria and its potential as a biomarker in aging, cognition and psychiatric disorders. Transl. psychiatry 11 (1), 339. doi:10.1038/s41398-021-01464-x
Minich, D. M., Henning, M., Darley, C., Fahoum, M., Schuler, C. B., and Frame, J. (2022). Is melatonin the "next vitamin D"? a review of emerging science, clinical uses, safety, and dietary supplements. Nutrients 14 3934, doi:10.3390/nu14193934(19)
Motilva, V., García-Mauriño, S., Talero, E., and Illanes, M. (2011). New paradigms in chronic intestinal inflammation and colon cancer: role of melatonin. J. pineal Res. 51 (1), 44–60. doi:10.1111/j.1600-079X.2011.00915.x
Nosjean, O., Ferro, M., Coge, F., Beauverger, P., Henlin, J. M., Lefoulon, F., et al. (2000). Identification of the melatonin-binding site MT3 as the quinone reductase 2. J. Biol. Chem. 275 (40), 31311–31317. doi:10.1074/jbc.M005141200
Nowak, E. C., de Vries, V. C., Wasiuk, A., Ahonen, C., Bennett, K. A., Le Mercier, I., et al. (2012). Tryptophan hydroxylase-1 regulates immune tolerance and inflammation. J. Exp. Med. 209 (11), 2127–2135. doi:10.1084/jem.20120408
Okatani, Y., Wakatsuki, A., and Reiter, R. J. (2002). Melatonin protects hepatic mitochondrial respiratory chain activity in senescence-accelerated mice. J. pineal Res. 32 (3), 143–148. doi:10.1034/j.1600-079x.2002.1o106.x
Pal, S., Sahu, A., Verma, R., and Haldar, C. (2023). BPS-induced ovarian dysfunction: protective actions of melatonin via modulation of SIRT-1/Nrf2/NFĸB and IR/PI3K/pAkt/GLUT-4 expressions in adult golden hamster. J. pineal Res. 75 (1), e12869. doi:10.1111/jpi.12869
Pan, P., Zhang, H., Su, L., Wang, X., and Liu, D. (2018). Melatonin balance the autophagy and apoptosis by regulating UCP2 in the LPS-induced cardiomyopathy. Mol. (Basel, Switz. 23 (3), 675. doi:10.3390/molecules23030675
Parameyong, A., Charngkaew, K., Govitrapong, P., and Chetsawang, B. (2013). Melatonin attenuates methamphetamine-induced disturbances in mitochondrial dynamics and degeneration in neuroblastoma SH-SY5Y cells. J. pineal Res. 55 (3), 313–323. doi:10.1111/jpi.12078
Parameyong, A., Govitrapong, P., and Chetsawang, B. (2015). Melatonin attenuates the mitochondrial translocation of mitochondrial fission proteins and Bax, cytosolic calcium overload and cell death in methamphetamine-induced toxicity in neuroblastoma SH-SY5Y cells. Mitochondrion 24, 1–8. doi:10.1016/j.mito.2015.07.004
Park, I. J., Hwang, J. T., Kim, Y. M., Ha, J., and Park, O. J. (2006). Differential modulation of AMPK signaling pathways by low or high levels of exogenous reactive oxygen species in colon cancer cells. Ann. N. Y. Acad. Sci. 1091, 102–109. doi:10.1196/annals.1378.059
Peng, T. I., Hsiao, C. W., Reiter, R. J., Tanaka, M., Lai, Y. K., and Jou, M. J. (2012). mtDNA T8993G mutation-induced mitochondrial complex V inhibition augments cardiolipin-dependent alterations in mitochondrial dynamics during oxidative, Ca(2+), and lipid insults in NARP cybrids: a potential therapeutic target for melatonin. J. pineal Res. 52 (1), 93–106. doi:10.1111/j.1600-079X.2011.00923.x
Pi, H., Xu, S., Reiter, R. J., Guo, P., Zhang, L., Li, Y., et al. (2015). SIRT3-SOD2-mROS-dependent autophagy in cadmium-induced hepatotoxicity and salvage by melatonin. Autophagy 11 (7), 1037–1051. doi:10.1080/15548627.2015.1052208
Piletic, K., Alsaleh, G., and Simon, A. K. (2023). Autophagy orchestrates the crosstalk between cells and organs. EMBO Rep. 24 (9), e57289. doi:10.15252/embr.202357289
Qin, Y., Ashrafizadeh, M., Mongiardini, V., Grimaldi, B., Crea, F., Rietdorf, K., et al. (2023). Autophagy and cancer drug resistance in dialogue: pre-clinical and clinical evidence. Cancer Lett. 570, 216307. doi:10.1016/j.canlet.2023.216307
Rath, M. F., Coon, S. L., Amaral, F. G., Weller, J. L., Møller, M., and Klein, D. C. (2016). Melatonin synthesis: acetylserotonin O-methyltransferase (ASMT) is strongly expressed in a subpopulation of pinealocytes in the male rat pineal gland. Endocrinology 157 (5), 2028–2040. doi:10.1210/en.2015-1888
Reiter, R. J., Ma, Q., and Sharma, R. (2020b). Melatonin in mitochondria: mitigating clear and present dangers. Physiol. (Bethesda, Md) 35 (2), 86–95. doi:10.1152/physiol.00034.2019
Reiter, R. J., Rosales-Corral, S., Tan, D. X., Jou, M. J., Galano, A., and Xu, B. (2017). Melatonin as a mitochondria-targeted antioxidant: one of evolution's best ideas. Cell. Mol. life Sci. CMLS 74 (21), 3863–3881. doi:10.1007/s00018-017-2609-7
Reiter, R. J., Sharma, R., Ma, Q., Rorsales-Corral, S., and de Almeida Chuffa, L. G. (2020a). Melatonin inhibits Warburg-dependent cancer by redirecting glucose oxidation to the mitochondria: a mechanistic hypothesis. Cell. Mol. life Sci. CMLS 77 (13), 2527–2542. doi:10.1007/s00018-019-03438-1
Reiter, R. J., Sharma, R., Rosales-Corral, S., de Campos Zuccari Dap, , and de Almeida Chuffa, L. G. (2022). Melatonin: a mitochondrial resident with a diverse skill set. Life Sci. 301, 120612. doi:10.1016/j.lfs.2022.120612
Reiter, R. J., Sharma, R., Rosales-Corral, S., Manucha, W., Chuffa, L. G. A., and Zuccari, D. (2021). Melatonin and pathological cell interactions: mitochondrial glucose processing in cancer cells. Int. J. Mol. Sci. 22 (22), 12494. doi:10.3390/ijms222212494
Roohbakhsh, A., Shamsizadeh, A., Hayes, A. W., Reiter, R. J., and Karimi, G. (2018). Melatonin as an endogenous regulator of diseases: the role of autophagy. Pharmacol. Res. 133, 265–276. doi:10.1016/j.phrs.2018.01.022
Sakaguchi, K., Itoh, M. T., Takahashi, N., Tarumi, W., and Ishizuka, B. (2013). The rat oocyte synthesises melatonin. Reproduction, Fertil. Dev. 25 (4), 674–682. doi:10.1071/RD12091
Scherz-Shouval, R., and ElazarROS, Z. (2007). ROS, mitochondria and the regulation of autophagy. Trends Cell Biol. 17 (9), 422–427. doi:10.1016/j.tcb.2007.07.009
Semak, I., Korik, E., Antonova, M., Wortsman, J., and Slominski, A. (2008). Metabolism of melatonin by cytochrome P450s in rat liver mitochondria and microsomes. J. pineal Res. 45 (4), 515–523. doi:10.1111/j.1600-079X.2008.00630.x
Shen, Y. Q., Guerra-Librero, A., Fernandez-Gil, B. I., Florido, J., García-López, S., Martinez-Ruiz, L., et al. (2018). Combination of melatonin and rapamycin for head and neck cancer therapy: suppression of AKT/mTOR pathway activation, and activation of mitophagy and apoptosis via mitochondrial function regulation. J. pineal Res. 64 (3). doi:10.1111/jpi.12461
Shi, C., Zeng, J., Li, Z., Chen, Q., Hang, W., Xia, L., et al. (2018). Melatonin mitigates kainic acid-induced neuronal tau hyperphosphorylation and memory deficits through alleviating ER stress. Front. Mol. Neurosci. 11, 5. doi:10.3389/fnmol.2018.00005
Sia, T. C., Flack, N., Robinson, L., Kyloh, M., Nicholas, S. J., Brookes, S. J., et al. (2013). Is serotonin in enteric nerves required for distension-evoked peristalsis and propulsion of content in Guinea-pig distal colon? Neuroscience 240, 325–335. doi:10.1016/j.neuroscience.2013.02.061
Singhanat, K., Apaijai, N., Jaiwongkam, T., Kerdphoo, S., Chattipakorn, S. C., and Chattipakorn, N. (2021). Melatonin as a therapy in cardiac ischemia-reperfusion injury: potential mechanisms by which MT2 activation mediates cardioprotection. J. Adv. Res. 29, 33–44. doi:10.1016/j.jare.2020.09.007
Slominski, A., Tobin, D. J., Zmijewski, M. A., Wortsman, J., and Paus, R. (2008). Melatonin in the skin: synthesis, metabolism and functions. Trends Endocrinol. metabolism TEM 19 (1), 17–24. doi:10.1016/j.tem.2007.10.007
Slominski, A. T., Hardeland, R., Zmijewski, M. A., Slominski, R. M., Reiter, R. J., and Paus, R. (2018). Melatonin: a cutaneous perspective on its production, metabolism, and functions. J. investigative dermatology 138 (3), 490–499. doi:10.1016/j.jid.2017.10.025
Socaciu, A. I., Ionuţ, R., Socaciu, M. A., Ungur, A. P., Bârsan, M., Chiorean, A., et al. (2020). Melatonin, an ubiquitous metabolic regulator: functions, mechanisms and effects on circadian disruption and degenerative diseases. Rev. Endocr. metabolic Disord. 21 (4), 465–478. doi:10.1007/s11154-020-09570-9
Solís-Muñoz, P., Solís-Herruzo, J. A., Fernández-Moreira, D., Gómez-Izquierdo, E., García-Consuegra, I., Muñoz-Yagüe, T., et al. (2011). Melatonin improves mitochondrial respiratory chain activity and liver morphology in ob/ob mice. J. pineal Res. 51 (1), 113–123. doi:10.1111/j.1600-079X.2011.00868.x
Tan, D. X., Hardeland, R., Back, K., Manchester, L. C., Alatorre-Jimenez, M. A., and Reiter, R. J. (2016b). On the significance of an alternate pathway of melatonin synthesis via 5-methoxytryptamine: comparisons across species. J. pineal Res. 61 (1), 27–40. doi:10.1111/jpi.12336
Tan, D. X., Manchester, L. C., Esteban-Zubero, E., Zhou, Z., and Reiter, R. J. (2015). Melatonin as a potent and inducible endogenous antioxidant: synthesis and metabolism. Mol. (Basel, Switz. 20 (10), 18886–18906. doi:10.3390/molecules201018886
Tan, D. X., Manchester, L. C., Liu, X., Rosales-Corral, S. A., Acuna-Castroviejo, D., and Reiter, R. J. (2013). Mitochondria and chloroplasts as the original sites of melatonin synthesis: a hypothesis related to melatonin's primary function and evolution in eukaryotes. J. pineal Res. 54 (2), 127–138. doi:10.1111/jpi.12026
Tan, D. X., Manchester, L. C., Qin, L., and Reiter, R. J. (2016a). Melatonin: a mitochondrial targeting molecule involving mitochondrial protection and dynamics. Int. J. Mol. Sci. 17 (12), 2124. doi:10.3390/ijms17122124
Tan, D. X., Manchester, L. C., Terron, M. P., Flores, L. J., and Reiter, R. J. (2007). One molecule, many derivatives: a never-ending interaction of melatonin with reactive oxygen and nitrogen species? J. pineal Res. 42 (1), 28–42. doi:10.1111/j.1600-079X.2006.00407.x
Teng, Y. C., Tai, Y. I., Huang, H. J., and Lin, A. M. (2015). Melatonin ameliorates arsenite-induced neurotoxicity: involvement of autophagy and mitochondria. Mol. Neurobiol. 52 (2), 1015–1022. doi:10.1007/s12035-015-9250-y
Vakhitova, Y. V., Kuzmina, U. S., Voronin, M. V., Zainullina, L. F., and Seredenin, S. B. (2019). Effect of fabomotizole on brain gene expression in MR rats in the open field test. Doklady Biochem. biophysics 488 (1), 313–315. doi:10.1134/S1607672919050090
Venegas, C., García, J. A., Escames, G., Ortiz, F., López, A., Doerrier, C., et al. (2012). Extrapineal melatonin: analysis of its subcellular distribution and daily fluctuations. J. pineal Res. 52 (2), 217–227. doi:10.1111/j.1600-079X.2011.00931.x
Waløen, K., Kleppe, R., Martinez, A., and Haavik, J. (2017). Tyrosine and tryptophan hydroxylases as therapeutic targets in human disease. Expert Opin. Ther. targets 21 (2), 167–180. doi:10.1080/14728222.2017.1272581
Walther, D. J., Peter, J. U., Bashammakh, S., Hörtnagl, H., Voits, M., Fink, H., et al. (2003). Synthesis of serotonin by a second tryptophan hydroxylase isoform. Science 299 (5603), 76. doi:10.1126/science.1078197
Wang, L., Wang, C., Li, X., Tao, Z., Zhu, W., Su, Y., et al. (2023). Melatonin and erastin emerge synergistic anti-tumor effects on oral squamous cell carcinoma by inducing apoptosis, ferroptosis, and inhibiting autophagy through promoting ROS. Cell. Mol. Biol. Lett. 28 (1), 36. doi:10.1186/s11658-023-00449-6
Wang, X., Sirianni, A., Pei, Z., Cormier, K., Smith, K., Jiang, J., et al. (2011). The melatonin MT1 receptor axis modulates mutant Huntingtin-mediated toxicity. J. Neurosci. official J. Soc. Neurosci. 31 (41), 14496–14507. doi:10.1523/JNEUROSCI.3059-11.2011
Wang, X., Xue, G. X., Liu, W. C., Shu, H., Wang, M., Sun, Y., et al. (2017). Melatonin alleviates lipopolysaccharide-compromised integrity of blood-brain barrier through activating AMP-activated protein kinase in old mice. Aging Cell 16 (2), 414–421. doi:10.1111/acel.12572
Wu, J., Bai, Y., Wang, Y., and Ma, J. (2021). Melatonin and regulation of autophagy: mechanisms and therapeutic implications. Pharmacol. Res. 163, 105279. doi:10.1016/j.phrs.2020.105279
Wu, K. K. (2021a). Control of mesenchymal stromal cell senescence by tryptophan metabolites. Int. J. Mol. Sci. 22 (2), 697. doi:10.3390/ijms22020697
Wu, K. K. (2021b). Cytoguardin: a tryptophan metabolite against cancer growth and metastasis. Int. J. Mol. Sci. 22 (9), 4490. doi:10.3390/ijms22094490
Wu, S. M., Lin, W. Y., Shen, C. C., Pan, H. C., Keh-Bin, W., Chen, Y. C., et al. (2016). Melatonin set out to ER stress signaling thwarts epithelial mesenchymal transition and peritoneal dissemination via calpain-mediated C/EBPβ and NFκB cleavage. J. pineal Res. 60 (2), 142–154. doi:10.1111/jpi.12295
Xiong, L., Liu, S., Liu, C., Guo, T., Huang, Z., and Li, L. (2023). The protective effects of melatonin in high glucose environment by alleviating autophagy and apoptosis on primary cortical neurons. Mol. Cell. Biochem. 478 (7), 1415–1425. doi:10.1007/s11010-022-04596-w
Xu, G., Dong, Y., Wang, Z., Ding, H., Wang, J., Zhao, J., et al. (2023). Melatonin attenuates oxidative stress-induced apoptosis of bovine ovarian granulosa cells by promoting mitophagy via SIRT1/FoxO1 signaling pathway. Int. J. Mol. Sci. 24 (16), 12854. doi:10.3390/ijms241612854
Xu, H., Liu, Y. Y., Li, L. S., and Liu, Y. S. (2023a). Sirtuins at the crossroads between mitochondrial quality control and neurodegenerative diseases: structure, regulation, modifications, and modulators. Aging Dis. 14 (3), 794–824. doi:10.14336/AD.2022.1123
Xu, S., Pi, H., Zhang, L., Zhang, N., Li, Y., Zhang, H., et al. (2016). Melatonin prevents abnormal mitochondrial dynamics resulting from the neurotoxicity of cadmium by blocking calcium-dependent translocation of Drp1 to the mitochondria. J. pineal Res. 60 (3), 291–302. doi:10.1111/jpi.12310
Xu, X., Wang, J., Xia, Y., Yin, Y., Zhu, T., Chen, F., et al. (2023b). Autophagy, a double-edged sword for oral tissue regeneration. J. Adv. Res. doi:10.1016/j.jare.2023.06.010
Xu, Y., Xie, Y., Wu, Z., Wang, H., Chen, Z., Wang, J., et al. (2023c). Protective effects of melatonin on deoxynivalenol-induced oxidative stress and autophagy in IPEC-J2 cells. Food Chem. Toxicol. Int. J. Publ. Br. Industrial Biol. Res. Assoc. 177, 113803. doi:10.1016/j.fct.2023.113803
Xue, F., Shi, C., Chen, Q., Hang, W., Xia, L., Wu, Y., et al. (2017). Melatonin mediates protective effects against kainic acid-induced neuronal death through safeguarding ER stress and mitochondrial disturbance. Front. Mol. Neurosci. 10, 49. doi:10.3389/fnmol.2017.00049
Yadav, V. K., Balaji, S., Suresh, P. S., Liu, X. S., Lu, X., Li, Z., et al. (2010). Pharmacological inhibition of gut-derived serotonin synthesis is a potential bone anabolic treatment for osteoporosis. Nat. Med. 16 (3), 308–312. doi:10.1038/nm.2098
Yan, F., Zhao, Q., Li, Y., Zheng, Z., Kong, X., Shu, C., et al. (2022). The role of oxidative stress in ovarian aging: a review. J. ovarian Res. 15 (1), 100. doi:10.1186/s13048-022-01032-x
Yao, Y., Chen, T., Wu, H., Yang, N., and Xu, S. (2023). Melatonin attenuates bisphenol A-induced colon injury by dual targeting mitochondrial dynamics and Nrf2 antioxidant system via activation of SIRT1/PGC-1α signaling pathway. Free Radic. Biol. Med. 195, 13–22. doi:10.1016/j.freeradbiomed.2022.12.081
Yoon, Y. M., Go, G., Yoon, S., Lim, J. H., Lee, G., Lee, J. H., et al. (2021). Melatonin treatment improves renal fibrosis via miR-4516/SIAH3/PINK1 Axis. Cells 10 (7), 1682. doi:10.3390/cells10071682
Zhang, C., Gao, X., Li, M., Yu, X., Huang, F., Wang, Y., et al. (2023a). The role of mitochondrial quality surveillance in skin aging: focus on mitochondrial dynamics, biogenesis and mitophagy. Ageing Res. Rev. 87, 101917. doi:10.1016/j.arr.2023.101917
Zhang, J., Qiao, W., and Luo, Y. (2023b). Mitochondrial quality control proteases and their modulation for cancer therapy. Med. Res. Rev. 43 (2), 399–436. doi:10.1002/med.21929
Zhang, J., Wang, L., Xie, W., Hu, S., Zhou, H., Zhu, P., et al. (2020). Melatonin attenuates ER stress and mitochondrial damage in septic cardiomyopathy: a new mechanism involving BAP31 upregulation and MAPK-ERK pathway. J. Cell. physiology 235 (3), 2847–2856. doi:10.1002/jcp.29190
Zhang, M., Lin, J., Wang, S., Cheng, Z., Hu, J., Wang, T., et al. (2017). Melatonin protects against diabetic cardiomyopathy through Mst1/Sirt3 signaling. J. pineal Res. 63 (2), e12418. doi:10.1111/jpi.12418
Zhang, Y., Wang, Y., Xu, J., Tian, F., Hu, S., Chen, Y., et al. (2019). Melatonin attenuates myocardial ischemia-reperfusion injury via improving mitochondrial fusion/mitophagy and activating the AMPK-OPA1 signaling pathways. J. pineal Res. 66 (2), e12542. doi:10.1111/jpi.12542
Zhang, Z., Zhao, Y., Wang, Y., Zhao, Y., and Guo, J. (2023c). Autophagy/ferroptosis in colorectal cancer: carcinogenic view and nanoparticle-mediated cell death regulation. Environ. Res. 238 (Pt 2), 117006. doi:10.1016/j.envres.2023.117006
Zhao, D., Yu, Y., Shen, Y., Liu, Q., Zhao, Z., Sharma, R., et al. (2019a). Melatonin synthesis and function: evolutionary history in animals and plants. Front. Endocrinol. 10, 249. doi:10.3389/fendo.2019.00249
Zhao, F., Ma, C., Zhao, G., Wang, G., Li, X., and Yang, K. (2019b). Rumen-protected 5-hydroxytryptophan improves sheep melatonin synthesis in the pineal gland and intestinal tract. Med. Sci. Monit. Int. Med. J. Exp. Clin. Res. 25, 3605–3616. doi:10.12659/MSM.915909
Zhou, H., Du, W., Li, Y., Shi, C., Hu, N., Ma, S., et al. (2018a). Effects of melatonin on fatty liver disease: the role of NR4A1/DNA-PKcs/p53 pathway, mitochondrial fission, and mitophagy. J. pineal Res. 64 (1). doi:10.1111/jpi.12450
Zhou, H., Du, W., Li, Y., Shi, C., Hu, N., Ma, S., et al. (2018b). Effects of melatonin on fatty liver disease: the role of NR4A1/DNA-PKcs/p53 pathway, mitochondrial fission, and mitophagy. J. pineal Res. 64 (1), e12450. doi:10.1111/jpi.12450
Zhou, Y., Suo, W., Zhang, X., Liang, J., Zhao, W., Wang, Y., et al. (2023). Targeting mitochondrial quality control for diabetic cardiomyopathy: therapeutic potential of hypoglycemic drugs. Biomed. Pharmacother. = Biomedecine Pharmacother. 168, 115669. doi:10.1016/j.biopha.2023.115669
Glossary
Keywords: autophagy, melatonin, mitochondrial dynamics, mitochondria-related diseases, oxidative phosphorylation
Citation: Lei X, Xu Z, Huang L, Huang Y, Tu S, Xu L and Liu D (2024) The potential influence of melatonin on mitochondrial quality control: a review. Front. Pharmacol. 14:1332567. doi: 10.3389/fphar.2023.1332567
Received: 03 November 2023; Accepted: 31 December 2023;
Published: 11 January 2024.
Edited by:
Ganji Purnachandra Nagaraju, University of Alabama at Birmingham, United StatesReviewed by:
Ruichao Yue, University of North Carolina at Greensboro, United StatesLourdes Franco, University of Extremadura, Spain
Copyright © 2024 Lei, Xu, Huang, Huang, Tu, Xu and Liu. This is an open-access article distributed under the terms of the Creative Commons Attribution License (CC BY). The use, distribution or reproduction in other forums is permitted, provided the original author(s) and the copyright owner(s) are credited and that the original publication in this journal is cited, in accordance with accepted academic practice. No use, distribution or reproduction is permitted which does not comply with these terms.
*Correspondence: Dengqun Liu, ZGVuZ3F1bmxpdUB1ZXN0Yy5lZHUuY24=