- Cancer Center, The First Hospital of Jilin University, Changchun, Jilin, China
Ferroptosis, a recently identified form of non-apoptotic cell death, is distinguished by its dependence on iron-triggered lipid peroxidation and accumulation of iron. It has been linked to various disorders, including the development of tumours. Interestingly, ferroptosis appears to exhibit a dual role in the context of tumour growth. This article provides a thorough exploration of the inherent ambivalence within ferroptosis, encompassing both its facilitation and inhibition of tumorous proliferation. It examines potential therapeutic targets associated with ferroptosis, the susceptibility of cancerous cells to ferroptosis, strategies to enhance the efficacy of existing cancer treatments, the interaction between ferroptosis and the immune response to tumours, and the fundamental mechanisms governing ferroptosis-induced tumour progression. A comprehensive understanding of how ferroptosis contributes to tumour biology and the strategic management of its dual nature are crucial for maximizing its therapeutic potential.
1 Introduction
Cell death plays a crucial role in maintaining tissue balance and controlling the unregulated growth of tumour cells (Fuchs and Steller, 2011). However, tumour cells have evolved mechanisms to evade cell death regulation, promoting unchecked cell replication. Ferroptosis, a unique form of non-apoptotic cell death characterised by lipid peroxidation and unstable iron buildup, differs in morphology, physiology, and biochemistry from classical programmed cell death (Dixon et al., 2012; Friedmann Angeli et al., 2014; Stockwell et al., 2017; Hassannia et al., 2019). An increasing body of evidence implicates ferroptosis in the development of various diseases, including the onset and progression of tumours (Tang et al., 2021).
Currently, ferroptosis has emerged as a significant focus in oncology research. Most studies suggest a beneficial role in restraining tumour growth through interactions between ferroptosis and tumours (Table 1), highlighting its potential as a therapeutic target in oncology. Tumour cells can bypass ferroptosis to promote their own growth by employing defense mechanisms, such as activating System Xc-, boosting glutathione peroxidase 4 (GPX4) activity, and altering glutathione (GSH) metabolism (Dixon et al., 2012). Disrupting or eliminating these mechanisms can trigger ferroptosis and hinder tumour expansion. Additionally, regulating lipid metabolism and iron metabolism pathways can induce ferroptosis, thereby inhibiting tumour growth (Martinez-Outschoorn et al., 2017; Wolpaw and Dang, 2018; Sang et al., 2019; Zou et al., 2020; Lei et al., 2022). Ferroptosis inducers presents a promising approach to curbing tumour growth. Furthermore, combining ferroptosis with chemotherapy, radiotherapy, targeted therapy, or immunotherapy shows potential to enhance antitumour effectiveness and overcome drug resistance (Yamaguchi et al., 2013; Yu et al., 2015; Wang et al., 2019a; Ye et al., 2020) (Table 3). Consequently, ferroptosis holds the potential to reshape tumour treatment strategies and improve clinical outcomes.
However, it’s important to note that ferroptosis can also have a negative impact on promoting tumour growth (Table 2). Through various pathways, such as ferroptosis metabolic pathways (Dai et al., 2020), inflammation-related pathways (Tang et al., 2021; Li and Li, 2020), antigen presentation process (Legrand et al., 2019), and the modulation of immune cell function (Wang et al., 2020; Luo et al., 2021), ferroptosis has been identified as a promoter of tumour growth. This article offers a comprehensive review of ferroptosis’s dual role in both promoting and inhibiting tumours, laying a theoretical foundation for further research into ferroptosis in tumour treatment. A thorough understanding of this duality allows for maximizing the clinical effectiveness of ferroptosis-based treatments while minimizing potential adverse effects.
2 The mechanism of ferroptosis
Ferroptosis is an iron-dependent type of programmed cell death caused by excessive polyunsaturated fatty acids (PUFAs). PUFAs are essential components of cell membrane phospholipid layers, significantly influencing membrane structure, fluidity, and permeability (Luo et al., 2021). The main mechanism behind ferroptosis is that when the balance between cellular oxidation and the antioxidant system is disrupted (Kuang et al., 2020), PUFAs in the cell membrane undergo oxidation, forming hydroxyl radicals catalyzed by Fe2+ or ester oxygenase (Figure 1). This process creates lipid peroxides, leading to cellular ferroptosis (Yang and Stockwell, 2016; Yang et al., 2016; Stockwell et al., 2017). The products of lipid peroxidation in cell membranes act as a source of reactive oxygen species (ROS), triggering increased cellular oxidative stress that damages DNA, proteins, or lipids, ultimately resulting in cellular ferroptosis (Trachootham et al., 2009; Reczek and Chandel, 2018).
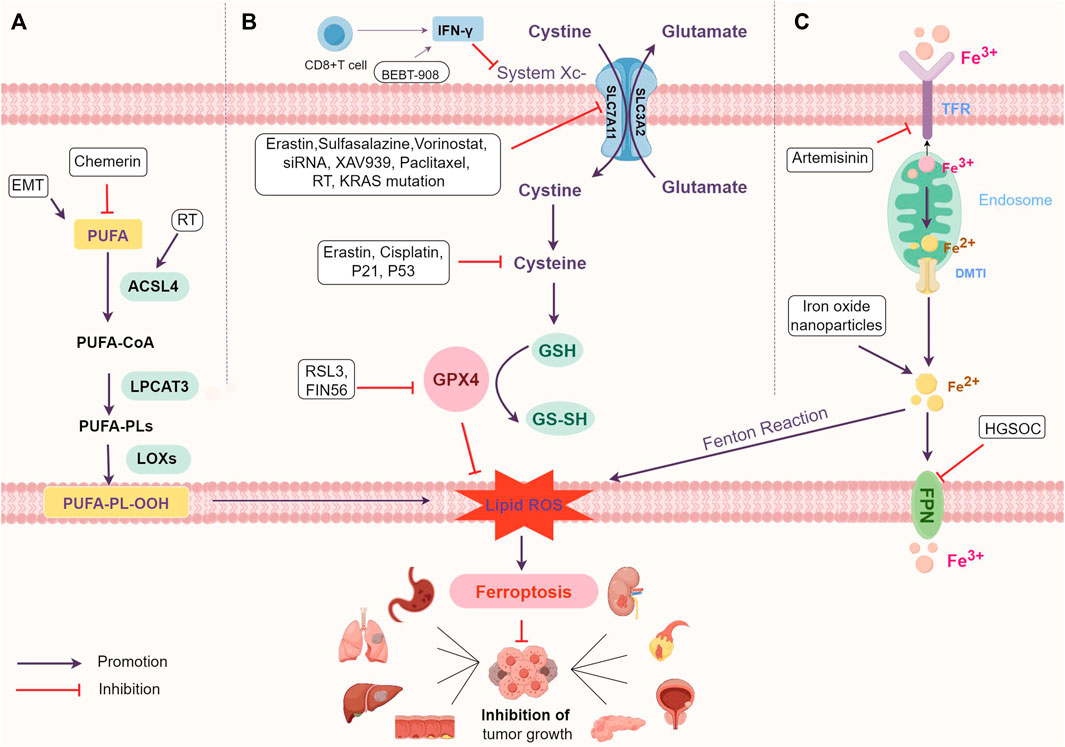
FIGURE 1. The mechanism of ferroptosis and main regulatory mechanism. (A) Regulate lipid metabolism pathways. (B) Interfere with amino acid metabolism pathways. (C) Influence iron metabolism pathways. Abbreviations: PUFAs, polyunsaturated fatty acids; PUFA-PLs, PUFA-containing phospholipids; RT, radiation therapy; ACSL4, Acyl-coenzyme A synthetase long chain family member 4; LPCAT3, Lysophosphatidylcholine acyltransferase 3; LOXs, lipoxygenase; EMT, epithelial-mesenchymal transition; ROS, reactive oxygen species; IFN-γ, interferon-γ; GSH, glutathione; GPX4, glutathione Peroxidase 4; TFR, transferrin; DMT1, divalent metal ion transporter protein-1; FPN, ferroportin; HGSOC, high-grade serous ovarian cancer.
3 Inhibitory effect of ferroptosis on tumour growth
Ferroptosis can inhibit tumour growth. The inhibitory effect of ferroptosis on tumour growth is discussed in terms of regulating lipid metabolism, amino acid metabolism, and iron metabolism. It has been reported that inhibition of ferroptosis can also promote tumour growth.
3.1 Inhibition of ferroptosis through regulating lipid metabolism thereby promoting tumour growth
Lipid metabolism is closely related to ferroptosis. Lipid peroxidation is a free radical-driven reaction that primarily affects unsaturated fatty acids in cell membranes (Tang et al., 2021). Acyl-coenzyme A synthetase long chain family member 4 (ACSL4) and Lysophosphatidylcholine acyltransferase 3 (LPCAT3) are key regulators of PUFA-PLs synthesis. Phospholipase A2 (PLA2) cleaves PUFAs into free PUFAs and lysophospholipids (Tang et al., 2021). ACSL4 catalyzes the attachment of free PUFAs to coenzyme A to generate PUFA-CoAs, which are re-esterified and incorporated into phospholipids (PLs) by LPCAT3 to form PUFA-containing phospholipids (PUFA-PLs) (Lei et al., 2022; Doll et al., 2017; Dixon et al., 2015). Due to the presence of a bis-allylic moieties of PUFAs, PUFA-PLs are especially susceptible to peroxidation (Conrad and Pratt, 2019).
The downregulation of PUFAs in tumour cells is associated with ferroptosis evasion and the promotion of tumour growth (Lei et al., 2022) (Figure 1; Table 1). For instance, in renal cell carcinoma (RCC), reducing peroxidized PUFAs through the adipokine chemerin allows tumour cells to avoid ferroptosis and supports RCC growth (Tan et al., 2021). KRAS mutations in lung cancer also increase the expression of Acyl-coenzyme A synthetase long chain family member 3 (ACSL3) to reprogram lipid metabolism, promote Monounsaturated fatty acids-phospholipids (MUFA-PL) biosynthesis and ferroptosis resistance, and facilitate lung cancer progression (Friedmann Angeli et al., 2014; Padanad et al., 2016).
In human tumour cell lines, cells in a mesenchymal-like state show selective susceptibility to ferroptosis (Sang et al., 2019). Research indicates that mesenchymal tumour cells exhibit higher enzyme activity, promoting PUFAs synthesis and lipid peroxide production, ultimately leading to ferroptosis occurrence (Viswanathan et al., 2017; Xu et al., 2019) (Figure 1). Specific overexpression of elongation of very long-chain fatty acid protein 5 (ELOVL5) and fatty acid desaturase 1 (FADS1) in mesenchymal gastric cancer cells, both involved in PUFAs synthesis, makes cancer cells particularly susceptible to ferroptosis (Lee et al., 2020) (Table 1).
3.2 Escaping ferroptosis by interfering with the antioxidant system and affecting amino acid metabolism contributes to tumour growth
Ferroptosis is associated with disruption of the antioxidant system and amino acid metabolism (Figure 1). GSH-GPX4 is involved in the intracellular antioxidant system and is a key factor influencing the onset of ferroptosis. GPX4, the only member of the GPX protein family capable of converting phospholipid hydroperoxides into phosphatidyl alcohols, prevents lipid peroxidation, thus restraining ferroptosis and supporting tumour growth (Ursini et al., 1982; Brigelius-Flohé and Maiorino, 2013; Seibt et al., 2019; Brigelius-Flohé and Flohé, 2020). GSH, a co-factor for GPX4, is synthesized from glycine, glutamate, and cysteine, with cysteine being the rate-limiting precursor (Forman et al., 2009; Koppula et al., 2018; Friedmann Angeli et al., 2019).
Cysteine/glutathione antiporter, also known as System Xc-, is an important intracellular antioxidant element. System Xc-is a transmembrane protein, consisting of SLC7A11 and SLC3A2, responsible for the exchange of extracellular cystine with intracellular glutamate (Bannai, 1986; Conrad and Sato, 2012). SLC7A11 mediates cystine/glutamate antotransporter protein activity and SLC3A2 maintains SLC7A11 protein stability (Bannai, 1986; Sato et al., 1999; Conrad and Sato, 2012; Koppula et al., 2018). Therefore, inhibition of System Xc—leads to an imbalance of the antioxidant system thereby causing ferroptosis.
The SLC7A11-GSH-GPX4 system plays a crucial role as the main defense against ferroptosis in tumours (Dixon et al., 2012; Friedmann Angeli et al., 2014; Stockwell et al., 2017; Hassannia et al., 2019). GPX4 is a central control factor of ferroptosis, and intracellular GSH content directly affects GPX4 activity (Maiorino et al., 2018). Ferroptosis inducers have demonstrated efficacy in tumour cells by directly binding to and inhibiting GPX4 (Table 1). The ferroptosis activator RSL3, an inhibitor of the antioxidant system, directly inactivates GPX4 and inhibits tumour growth in a xenograft mouse model of BJeLR cell origin (Fuchs and Steller, 2011). FIN56, induces ferroptosis in HT1080 cells by depleting GPX4 protein as well as activating farnesyl-diphosphate farnesyltransferase 1 (FDFT1/SQS) to block coenzyme Q10 production (Shimada et al., 2016). Kras/TP53-driven pancreatic tumours induce ferroptosis and inhibit tumour growth by depleting cystine or cysteine through cyst (e) inase (Badgley et al., 2020). Sulfasalazine inhibits System Xc- and diminishes cellular glutathione, leading to the excessive buildup of lipid peroxides in tumour cells, inducing ferroptosis. This demonstrates an antitumour effect in pancreatic cancer (Lo et al., 2010).
In pancreatic ductal adenocarcinomas (PDACs), overexpressing SLC7A11 inhibits ferroptosis by increasing cystine uptake and GSH production, promoting tumour growth (Badgley et al., 2020). In patients with KRAS-mutant lung adenocarcinoma (LUAD), SLC7A11 mediates cystine uptake, decreases ROS production and thus promotes lung adenocarcinoma proliferation and migration (Hu et al., 2020; Liu et al., 2020; Chen et al., 2021; Lou et al., 2021). These suggest that SLC7A11 overexpression is positively associated with tumour progression. In contrast, downregulation of SLC7A11 gene expression by siRNA induced ROS accumulation, promoted ferroptosis and inhibited A549 cell proliferation (Huang et al., 2018). XAV939 induces ferroptosis and inhibits non-small cell lung cancer (NSCLC) by downregulating SLC7A11 through long non-coding RNA (lncRNA) (Yu et al., 2019).
3.3 Inhibiting tumour growth by affecting iron metabolism pathways to induce ferroptosis
Iron metabolism is a necessary process for ferroptosis. Iron overload induces ferroptosis through the Fenton reaction, which generates a large number of hydroxyl radicals and triggers a strong oxidative stress response that produces a large number of ROS (Conrad and Pratt, 2019). Transferrin (TFR) and divalent metal ion transporter protein-1 (DMT1) take up extracellular iron, and ferroportin (FPN) transfers intracellular iron to the outside of the cell (Figure 1). These proteins collaborate to maintain intracellular iron homeostasis (Seiler et al., 2008; Mandal et al., 2010). Iron is also essential for participation in lipid peroxidation, and lipoxygenase (LOXs) and cytochrome P450 oxidoreductase (PORs) require iron for catalysis (Jiang et al., 2021a).
Tumour cells show an increased demand for iron and display heightened oxidative metabolic processes compared to non-malignant cells (Martinez-Outschoorn et al., 2017; Wolpaw and Dang, 2018; Zou et al., 2020). The level of intracellular iron impacts sensitivity to ferroptosis. Elevated intracellular iron in tumour cells leads to higher production of ROS and lipid metabolites, aiding ferroptosis development (Table 1; Figure 1). Tumours abundant in iron, like hepatocellular carcinoma (HCC) and breast cancer, or those rich in ROS like lung cancer, along with tumours with increased iron use and overload, demonstrate heightened sensitivity to ferroptosis (Ma et al., 2016).
Iron oxide nanoparticles, breaking down within the acidic tumour cell environment, release intracellular iron, leading to increased iron and ROS production, ultimately inducing ferroptosis and hindering tumour growth (Ma et al., 2017). Artemisinin prompts lung cancer cells to absorb and release substantial iron amounts, heightening their susceptibility to ferroptosis (Chen et al., 2020). In high-grade serous ovarian cancer (HGSOC), elevated iron intake and reduced expression of the iron efflux pump FPN result in excessive intracellular iron, further promoting ferroptosis onset (Basuli et al., 2017).
Thus, adjusting iron levels—enhancing iron intake, reducing storage, and restricting iron release—holds potential to promote ferroptosis and impede tumour growth.
4 Promotional effect of ferroptosis on tumour growth
Ferroptosis promotes tumour growth and progression by regulating metabolic pathways, triggering inflammation-associated immunosuppression, and cancer cells dying from ferroptosis to compromise antitumour immune responses (Figure 2; Table 2).
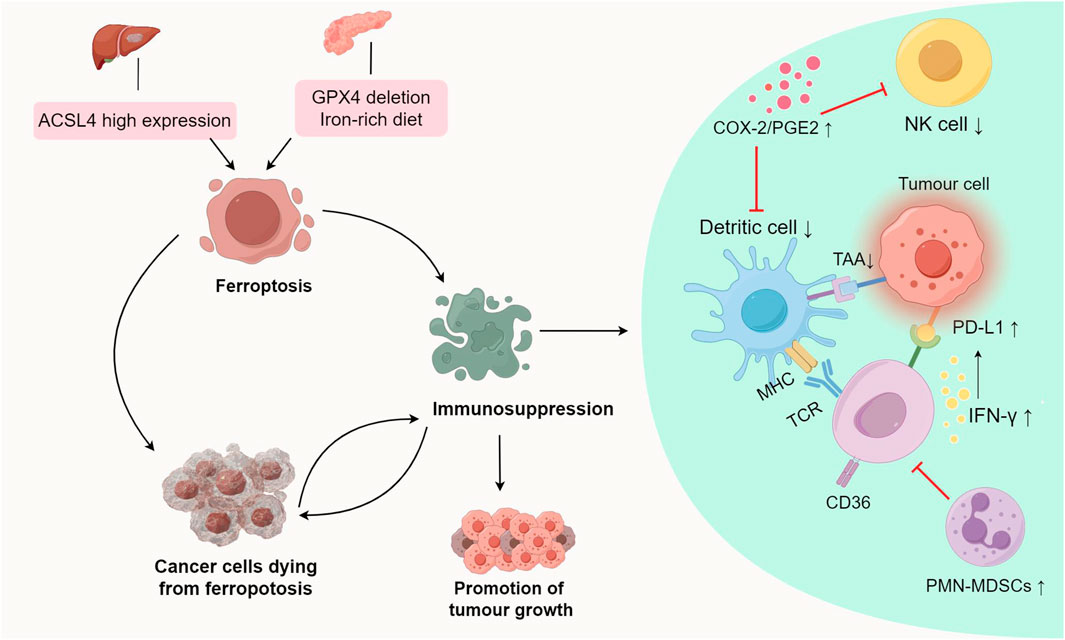
FIGURE 2. Promotional effect of ferroptosis on tumour growth.ACSL4-dependent ferroptosis promotes hepatocellular carcinoma development. A diet rich in iron or GPX4 depletion can induce ferroptosis, thereby promoting the development of pancreatic tumours. Ferroptosis inhibits antitumour immunity by triggering inflammation-related pathways, and influencing antigen presentation processes and the production of immunosuppressive molecules, thereby promoting tumour growth. Abbreviations: ACSL4, Acyl-coenzyme A synthetase long chain family member 4; GPX4, glutathione Peroxidase 4; PGE2, Prostaglandin E2; COX-2, cyclooxygenase-2; TAAs, tumour-associated antigens; IFN-γ, interferon-γ; PD-L1, Programmed cell death ligand 1; MHC, Major Histocompatibility Complex; PMN-MDSCs, polymorphonuclear myeloid derived suppressor cells.
4.1 Ferroptosis promotes pancreatic tumour and hepatocellular carcinoma development and progression by regulating metabolic pathways
ACSL4, an enzyme involved in synthesizing phospholipids from PUFAs plays a crucial role in ferroptosis (Dixon et al., 2015; Yuan et al., 2016). In HCC, ACSL4 expression surpasses that in normal liver tissue, and hepatocyte ferroptosis relies on ACSL4, suggesting its involvement in HCC development (Ndiaye et al., 2020) (Figure 2). In a mature toxic injury model, intervention in ACSL4-dependent ferroptosis notably suppressed HCC progression, likely due to reduced fibrosis in the absence of ACSL4 (Tsurusaki et al., 2019; Li et al., 2020a; Luo et al., 2020; Qi et al., 2020) (Table 2).
Studies suggest that an iron-rich diet or Gpx4 depletion can induce ferroptosis in tumour cells, releasing 8-hydroxy-2′-deoxyguanosine (8-OHdG). This activates the TMEM173/STING-dependent DNA sensor pathway, thereby promoting pancreatic tumour development (Dai et al., 2020; Cooke et al., 2003) (Table 2). TMEM173 regulates inflammation and immune responses, associated with macrophage activation and migration triggered by 8-OHdG (Barber, 2015; Motwani et al., 2019). Tumour-associated macrophages (TAMs) influence early stages of pancreatic tumour formation, activating KRAS-driven PDACs (Mielgo and Schmid, 2013; Zhu et al., 2017). GPX4 deletion or a high-iron diet increases acinar-to-duct metaplasia, ductal lesions, stromal reactions, metastasis, as well as expression of Ki67 and a ferroptosis marker (prostaglandin-endoperoxide synthase 2, PTGS2) in the pancreas (Yang et al., 2014). These studies collectively suggest that ferroptosis contributes to promoting tumour growth.
4.2 Ferroptosis inhibits antitumour immunity by triggering inflammation-related pathways, thereby promoting tumour growth
4.2.1 COX-2/PGE2 pathway
PTGS2, also known as cyclooxygenase-2 (COX-2), is a marker of ferroptosis (Yang et al., 2014). Lipopolysaccharide (LPS) induces lipid peroxidation and PTGS2 expression, which activates ferroptosis (Li et al., 2020b). Ferroptosis detrimentally influences tumour growth by specifically enhancing the COX-2/PGE2 pathway, leading to inflammation-associated immunosuppression (Tang et al., 2021; Li and Li, 2020; Yang et al., 2014) (Figure 2; Table 2). Prostaglandin E2 (PGE2), an inflammatory and immunosuppressive agent, undermines immune control mediated by conventional type 1 dendritic cells (cDC1s) and allows tumour cells to evade the immune system, resulting in immunotherapy resistance (Goodwin and Ceuppens, 1983; Wang and DuBois, 2015). Further studies reveal that PGE2 limits the infiltration of cDC1s into the tumour site by suppressing the chemokines CCL5 and XCL1, secreted by NK cells. Apart from impacting NK cell activity, PGE2 directly hampers cDC1s by reducing levels of tumour-recruited chemokine receptors (Böttcher et al., 2018; Zhang et al., 2022).
4.2.2 IFN-γ-related immune resistance
Ferroptosis can induce immune response through ferroptotic tumour cells, exposing danger-associated molecular patterns (DAMPs) and releasing tumour-associated antigens (TAAs), which stimulate T cells to secrete Interferon-γ (IFN-γ) (Tang et al., 2019; Zhang et al., 2019). Though IFN-γ secretion by T cells induced by ferroptosis effectively eliminates tumour cells, it can also elevate programmed death ligand-1 (PD-L1) levels, triggering IFN-γ-related adaptive immune resistance (Dorand et al., 2016; Li et al., 2021) (Figure 2; Table 2). This phenomenon affects the antitumour efficiency of immune cells, contributing to tumour progression. PD-L1, an immunosuppressive molecule, is overexpressed in various cancers like gastric, kidney, pancreatic, bladder cancers, among others, and is linked to poor clinical prognosis (Ohigashi et al., 2005; Hamanishi et al., 2007; Nakanishi et al., 2007; Hou et al., 2014; Wang et al., 2016). Binding of PD-L1 to PD-1 depletes effector T cells, allowing tumour cells to evade the immune response, ultimately promoting tumour growth.
4.2.3 CD36-mediated ferroptosis
The study noted notably elevated fatty acid levels in tumour tissues compared to normal skin or spleen tissues (Zhang et al., 2018). CD36, involved in DCs’ antigen presentation function, emerged as a T cell function regulator. The study showed that within the tumour microenvironment, CD8 (+) T cells uptake fatty acids via CD36, impairing their antitumour functionality by inducing ferroptosis and reducing cytotoxic cytokine production (Wang et al., 2020; Su et al., 2020) (Table 2). Effective blocking of CD36-mediated ferroptosis restored CD8 (+) T cells’ antitumour activity. Moreover, inhibiting CD36-mediated ferroptosis alongside immunotherapy notably enhanced the antitumour effects of CD8 (+) T cells (Wang et al., 2020).
4.3 Cancer cells dying from ferroptosis affects the antigen presentation process and produces immunosuppressive effects, thus compromising antitumour immune responses
Immunogenicity refers to antigens’ ability to provoke an immune response, involving immune effector molecule production, activation, proliferation, and differentiation (Aaes and Vandenabeele, 2021). Immunogenic cell death occurs when tumour-associated antigens (TAAs) are processed and presented on the surfaces of tumour cells and dendritic cells (DCs) (Legrand et al., 2019; Yatim et al., 2017). Research suggests that ferroptosis may possess immunomodulatory traits influencing neighbouring tumour cells’ response to immunogenic death (Blüml et al., 2005; Blüml et al., 2009). Observations indicate that cancer cells undergoing ferroptosis impede TAA processing and presentation. Co-culturing ferroptotic cancer cells with DCs revealed that these “initial” iron-depleted cells hinder DCs maturation, phagocytosis, and antigen-cross-presentation (Wiernicki et al., 2022) (Figure 2; Table 2). Consequently, ferroptosis negatively impacts antigen-presenting cells, influencing adaptive immune responses and antitumour immunity.
Pathologically activated neutrophils, termed polymorphonuclear myeloid-derived suppressor cells (PMN-MDSCs), negatively impact the regulation of antitumour immunity (Condamine et al., 2015; Wang et al., 2019b; Ostrand-Rosenberg et al., 2020). Their presence in cancer patients correlates with poor prognoses in immunotherapy (Zhou et al., 2018). Studies indicate that genes linked to PMN-MDSCs are enriched in the ferroptosis pathway, suggesting their susceptibility to ferroptosis (Zhang et al., 2020). Observations reveal that PMN-MDSCs can undergo ferroptosis within the tumour microenvironment. While ferroptosis reduces PMN-MDSC numbers, cancer cells undergoing ferroptosis release immunosuppressive molecules hindering T cells, reducing the effectiveness of antitumour therapy (Kim et al., 2022) (Table 2). Conversely, inhibiting ferroptosis decreases immunosuppressive activity, significantly impeding tumour growth. In immune-active mice, ferroptosis inhibition abolishes PMN-MDSCs’ suppressive activity on T cells and synergizes with immune checkpoint inhibitors to halt tumour progression (Kim et al., 2022). Hence, targeting ferroptosis in PMN-MDSCs holds promise as a future therapeutic avenue.
5 The role of ferroptosis in different cancer therapies, such as chemotherapy, immunotherapy, radiotherapy and reversal of tumour resistance
Currently, numerous studies corroborate the synergistic effect of ferroptosis combination therapy in bolstering the efficacy of antitumour treatment. However, evidence supporting the inhibitory effect of combination regimens remains relatively scarce. We’ve compiled studies showcasing the potential of combined ferroptosis therapy to heighten antitumour efficacy and surmount drug resistance (Table 3). Further pertinent research is warranted to build upon this foundation.
5.1 Ferroptosis combination with chemotherapy
Combining chemotherapeutic agents with ferroptosis inducers amplifies anticancer effects, as these drugs themselves can induce ferroptosis in tumour cells. For instance, the ferroptosis inducer erastin notably enhances cisplatin’s efficacy across various tumour types by antagonizing system Xc- or GPX4 functions (Yamaguchi et al., 2013; Yu et al., 2015). Studies illustrate that erastin combined with cisplatin impedes ovarian cancer progression via a ROS-mediated mechanism, augmenting cisplatin’s antitumour impact (Cheng et al., 2021) (Table 3). Moreover, cisplatin lowers GSH levels in tumour cells, compromising GPX4 function and triggering ferroptosis in NSCLC cells (Guo et al., 2018). Paclitaxel (PTX) reduces SLC7A11 expression, promoting ferroptosis and retarding colorectal carcinoma cell growth (Lv et al., 2017). The synergy of PTX with RSL3 induces ferroptosis in mutant p53 hypopharyngeal squamous carcinoma (Ye et al., 2019) (Figure 1; Table 3).
5.2 Ferroptosis combination with radiation therapy
Radiation therapy (RT) has been linked to inducing ferroptosis in tumours through diverse pathways (Lei et al., 2020), such as ROS production, GSH depletion, ACSL4 upregulation, and SLC7A11 inhibition (Azzam et al., 2012; Lei et al., 2020) (Figure 1). RT generates surplus ROS and triggers ACSL4 expression by breaking down cellular water, leading to PUFA peroxidation and ferroptosis (Ye et al., 2020). Additionally, RT can promote ferroptosis by suppressing SLC7A11 expression, thereby enhancing radiosensitivity (Lang et al., 2019). Ferroptosis inducers synergize with RT in tumour treatment. For instance, erastin and salazopyridine enhance NSCLC sensitivity to RT (Lei et al., 2020) (Figure 1; Table 3).
5.3 Ferroptosis combination with immunotherapy
The immune system wields significant influence over both tumour development and treatment. Recent research highlights ferroptosis as a factor impeding tumour growth by modulating the immune response (Wang et al., 2019a). Combining immune checkpoint inhibitors with ferroptosis inducers enhances immunotherapy efficacy (Jiang et al., 2021b). Ferroptosis, by recruiting and activating immune cells within the tumour environment, serves as a foundation for using ferroptosis inducers to augment immunotherapy. Ferroptotic tumour cells release DAMPs and trigger Major Histocompatibility Complex (MHC) class I molecule expression, activating T cells and macrophages (Wen et al., 2019). In the tumour microenvironment, CD8 (+) T cells produce IFN-γ, downregulating SLC7A11 expression, reducing cystine uptake, fostering lipid peroxide accumulation, and inducing ferroptosis in Melanoma and ovarian cancer cells (Wang et al., 2019a). The ferroptosis inducer BEBT-908 triggers ferroptosis, elevates MHC class I molecule expression, and activates the IFN-γ signalling pathway in Colorectal cancer (CRC), human diffuse large B-cell lymphoma, and lung cancer, bolstering the body’s immune response and exerting antitumour effects (Fan et al., 2021) (Figure 1; Table 3).
5.4 Ferroptosis reverses resistance to tumour therapy
Tumour drug resistance presents a formidable treatment challenge, often reinforced by tumour cells suppressing ferroptosis (Lu et al., 2017). Survival of drug-resistant cells often hinges on GPX4 (Hangauer et al., 2017). Inducing ferroptosis can reverse resistance to conventional chemotherapy, targeted therapy, and immunotherapy. In colon cancer, Lipocalin 2 (LCN2) overexpression leads to resistance to 5-fluorouracil. Targeted inhibition of LCN2 by anti-LCN2 monoclonal antibody (3D12B2) increases intracellular iron levels, decreases GPX4 expression, and induces ferroptosis in tumor cells, thereby overcoming resistance (Chaudhary et al., 2021) (Table 3). EGFR-mutated lung cancer cells, facing acquired drug resistance, exhibit heightened sensitivity to ferroptosis inducers. Vorinostat triggers ferroptosis in resistant cells by inhibiting System Xc- and SLC7A11 expression (Zhang et al., 2021) (Table 3). These findings underscore ferroptosis’ therapeutic potential in combatting drug resistance.
6 Future perspective and conclusion
We have summarized multiple studies delving into the interplay between ferroptosis and tumour growth, aiming to grasp their relationship comprehensively and provide insights for targeted therapeutic strategies. It’s vital to decipher how to counteract ferroptosis’ role in promoting tumour growth while harnessing its therapeutic potential. One study proposed RCH NPs, a self-amplifying nanomedicine, aiming to optimize therapeutic efficacy in tumours by addressing ferroptosis’ dual nature. RCH NPs displayed robust ferroptotic damage and bolstered the immune response, enhancing ferroptosis’ positive effects in inhibiting tumour growth. They also mitigated inflammation-linked immunosuppression and IFN-γ-associated adaptive immune resistance, countering ferroptosis’ negative impact on immunotherapy (Zhang et al., 2022). More research is anticipated to design effective therapies that balance ferroptosis’ dual effects on tumour growth.
Yet, ongoing research on ferroptosis remains in its early stages, leaving unanswered queries. Most studies investigating ferroptosis and its tumour association have relied on cellular and animal models, lacking validated clinical evidence. For instance, though targeting GPX4, a crucial component of the ferroptosis defense system, might theoretically restrain tumour growth, GPX4 is essential for life, with studies suggesting its loss heightens mortality rates in mice (Yant et al., 2003). Hence, it's crucial to ascertain the potential harm to normal tissue due to GPX4 inhibitors. Despite numerous potential targets linked to tumours and ferroptosis, it’s unclear which of these findings can transition into clinical investigations.
Furthermore, there’s a lack of research examining the effectiveness and safety of drugs intended to target ferroptosis. For instance, inhibiting SLC7A11 has shown potential in triggering tumour ferroptosis and reversing tumour resistance without observable impact on the development and survival of mice (Sato et al., 2005). However, this treatment may not be effective for tumours not reliant on the System Xc-. Ferroptosis is linked to the onset and progression of various diseases, extending beyond cancer to include degenerative conditions (Stockwell et al., 2017). Therefore, it’s crucial to develop tailored therapies inducing ferroptosis in tumours while avoiding systemic adverse reactions. Combination therapies involving ferroptosis inducers and RT have shown safety in preclinical studies, but there are indications that ferroptosis might also contribute to radiation-induced damage in normal tissues (Su et al., 2022). Hence, further research is necessary to understand the impact of ferroptosis inducers on normal tissues and identify the patient population most likely to benefit from these treatments.
Finally, there’s a notable absence of biomarkers available for assessing ferroptosis within the human body. Identifying suitable biomarkers would significantly aid in in vivo studies and clinical monitoring. Discovering predictive biomarkers capable of forecasting a tumour’s response to ferroptosis-inducing therapies is crucial for categorizing tumour patients and guiding subsequent antitumour interventions involving ferroptosis induction.
Author contributions
XZ: Conceptualization, Visualization, Writing–original draft, Writing–review and editing. XL: Conceptualization, Writing–original draft. YX: Conceptualization, Supervision, Writing–review and editing.
Funding
The authors declare that no financial support was received for the research, authorship, and/or publication of this article.
Conflict of interest
The authors declare that the research was conducted in the absence of any commercial or financial relationships that could be construed as a potential conflict of interest.
Publisher’s note
All claims expressed in this article are solely those of the authors and do not necessarily represent those of their affiliated organizations, or those of the publisher, the editors and the reviewers. Any product that may be evaluated in this article, or claim that may be made by its manufacturer, is not guaranteed or endorsed by the publisher.
References
Aaes, T. L., and Vandenabeele, P. (2021). The intrinsic immunogenic properties of cancer cell lines, immunogenic cell death, and how these influence host antitumor immune responses. Cell Death Differ. 28 (3), 843–860. doi:10.1038/s41418-020-00658-y
Azzam, E. I., Jay-Gerin, J.-P., and Pain, D. (2012). Ionizing radiation-induced metabolic oxidative stress and prolonged cell injury. Cancer Lett. 327 (1-2), 48–60. doi:10.1016/j.canlet.2011.12.012
Badgley, M. A., Kremer, D. M., Maurer, H. C., DelGiorno, K. E., Lee, H.-J., Purohit, V., et al. (2020). Cysteine depletion induces pancreatic tumor ferroptosis in mice. Sci. (New York, N.Y.) 368 (6486), 85–89. doi:10.1126/science.aaw9872
Bannai, S. (1986). Exchange of cystine and glutamate across plasma membrane of human fibroblasts. J. Biol. Chem. 261 (5), 2256–2263. doi:10.1016/s0021-9258(17)35926-4
Barber, G. N. (2015). STING: infection, inflammation and cancer. Nat. Rev. Immunol. 15 (12), 760–770. doi:10.1038/nri3921
Basuli, D., Tesfay, L., Deng, Z., Paul, B., Yamamoto, Y., Ning, G., et al. (2017). Iron addiction: a novel therapeutic target in ovarian cancer. Oncogene 36 (29), 4089–4099. doi:10.1038/onc.2017.11
Blüml, S., Kirchberger, S., Bochkov, V. N., Krönke, G., Stuhlmeier, K., Majdic, O., et al. (2005). Oxidized phospholipids negatively regulate dendritic cell maturation induced by TLRs and CD40. J. Immunol. 175 (1), 501–508. doi:10.4049/jimmunol.175.1.501
Blüml, S., Zupkovitz, G., Kirchberger, S., Seyerl, M., Bochkov, V. N., Stuhlmeier, K., et al. (2009). Epigenetic regulation of dendritic cell differentiation and function by oxidized phospholipids. Blood 114 (27), 5481–5489. doi:10.1182/blood-2008-11-191429
Böttcher, J. P., Bonavita, E., Chakravarty, P., Blees, H., Cabeza-Cabrerizo, M., Sammicheli, S., et al. (2018). NK cells stimulate recruitment of cDC1 into the tumor microenvironment promoting cancer immune control. Cell 172 (5), 1022–1037. doi:10.1016/j.cell.2018.01.004
Brigelius-Flohé, R., and Flohé, L. (2020). Regulatory phenomena in the glutathione peroxidase superfamily. Antioxidants redox Signal. 33 (7), 498–516. doi:10.1089/ars.2019.7905
Brigelius-Flohé, R., and Maiorino, M. (2013). Glutathione peroxidases. Biochim. Biophys. Acta 1830 (5), 3289–3303. doi:10.1016/j.bbagen.2012.11.020
Chaudhary, N., Choudhary, B. S., Shah, S. G., Khapare, N., Dwivedi, N., Gaikwad, A., et al. (2021). Lipocalin 2 expression promotes tumor progression and therapy resistance by inhibiting ferroptosis in colorectal cancer. Int. J. Cancer 149 (7), 1495–1511. doi:10.1002/ijc.33711
Chen, G. Q., Benthani, F. A., Wu, J., Liang, D., Bian, Z. X., and Jiang, X. (2020). Artemisinin compounds sensitize cancer cells to ferroptosis by regulating iron homeostasis. Cell Death Differ. 27 (1), 242–254. doi:10.1038/s41418-019-0352-3
Chen, M., Jiang, Y., and Sun, Y. (2021). KDM4A-mediated histone demethylation of SLC7A11 inhibits cell ferroptosis in osteosarcoma. Biochem. Biophys. Res. Commun. 550, 77–83. doi:10.1016/j.bbrc.2021.02.137
Cheng, Q., Bao, L., Li, M., Chang, K., and Yi, X. (2021). Erastin synergizes with cisplatin via ferroptosis to inhibit ovarian cancer growth in vitro and in vivo. J. Obstetrics Gynaecol. Res. 47 (7), 2481–2491. doi:10.1111/jog.14779
Chu, B., Kon, N., Chen, D., Li, T., Liu, T., Jiang, L., et al. (2019). ALOX12 is required for p53-mediated tumour suppression through a distinct ferroptosis pathway. Nat. Cell Biol. 21 (5), 579–591. doi:10.1038/s41556-019-0305-6
Condamine, T., Mastio, J., and Gabrilovich, D. I. (2015). Transcriptional regulation of myeloid-derived suppressor cells. J. Leukoc. Biol. 98 (6), 913–922. doi:10.1189/jlb.4RI0515-204R
Conrad, M., and Pratt, D. A. (2019). The chemical basis of ferroptosis. Nat. Chem. Biol. 15 (12), 1137–1147. doi:10.1038/s41589-019-0408-1
Conrad, M., and Sato, H. (2012). The oxidative stress-inducible cystine/glutamate antiporter, system x (c) (-): cystine supplier and beyond. Amino Acids 42 (1), 231–246. doi:10.1007/s00726-011-0867-5
Cooke, M. S., Evans, M. D., Dizdaroglu, M., and Lunec, J. (2003). Oxidative DNA damage: mechanisms, mutation, and disease. FASEB J. Official Publ. Fed. Am. Soc. Exp. Biol. 17 (10), 1195–1214. doi:10.1096/fj.02-0752rev
Dai, E., Han, L., Liu, J., Xie, Y., Zeh, H. J., Kang, R., et al. (2020). Ferroptotic damage promotes pancreatic tumorigenesis through a TMEM173/STING-dependent DNA sensor pathway. Nat. Commun. 11 (1), 6339. doi:10.1038/s41467-020-20154-8
Dixon, S. J., Lemberg, K. M., Lamprecht, M. R., Skouta, R., Zaitsev, E. M., Gleason, C. E., et al. (2012). Ferroptosis: an iron-dependent form of nonapoptotic cell death. Cell 149 (5), 1060–1072. doi:10.1016/j.cell.2012.03.042
Dixon, S. J., Winter, G. E., Musavi, L. S., Lee, E. D., Snijder, B., Rebsamen, M., et al. (2015). Human haploid cell genetics reveals roles for lipid metabolism genes in nonapoptotic cell death. ACS Chem. Biol. 10 (7), 1604–1609. doi:10.1021/acschembio.5b00245
Doll, S., Proneth, B., Tyurina, Y. Y., Panzilius, E., Kobayashi, S., Ingold, I., et al. (2017). ACSL4 dictates ferroptosis sensitivity by shaping cellular lipid composition. Nat. Chem. Biol. 13 (1), 91–98. doi:10.1038/nchembio.2239
Dorand, R. D., Nthale, J., Myers, J. T., Barkauskas, D. S., Avril, S., Chirieleison, S. M., et al. (2016). Cdk5 disruption attenuates tumor PD-L1 expression and promotes antitumor immunity. Sci. (New York, N.Y.) 353 (6297), 399–403. doi:10.1126/science.aae0477
Fan, F., Liu, P., Bao, R., Chen, J., Zhou, M., Mo, Z., et al. (2021). A dual PI3K/HDAC inhibitor induces immunogenic ferroptosis to potentiate cancer immune checkpoint therapy. Cancer Res. 81 (24), 6233–6245. doi:10.1158/0008-5472.CAN-21-1547
Forman, H. J., Zhang, H., and Rinna, A. (2009). Glutathione: overview of its protective roles, measurement, and biosynthesis. Mol. Asp. Med. 30 (1-2), 1–12. doi:10.1016/j.mam.2008.08.006
Friedmann Angeli, J. P., Krysko, D. V., and Conrad, M. (2019). Ferroptosis at the crossroads of cancer-acquired drug resistance and immune evasion. Nat. Rev. Cancer 19 (7), 405–414. doi:10.1038/s41568-019-0149-1
Friedmann Angeli, J. P., Schneider, M., Proneth, B., Tyurina, Y. Y., Tyurin, V. A., Hammond, V. J., et al. (2014). Inactivation of the ferroptosis regulator Gpx4 triggers acute renal failure in mice. Nat. Cell Biol. 16 (12), 1180–1191. doi:10.1038/ncb3064
Fuchs, Y., and Steller, H. (2011). Programmed cell death in animal development and disease. Cell 147 (4), 742–758. doi:10.1016/j.cell.2011.10.033
Gaschler, M. M., and Stockwell, B. R. (2017). Lipid peroxidation in cell death. Biochem. Biophys. Res. Commun. 482 (3), 419–425. doi:10.1016/j.bbrc.2016.10.086
Goodwin, J. S., and Ceuppens, J. (1983). Regulation of the immune response by prostaglandins. J. Clin. Immunol. 3 (4), 295–315. doi:10.1007/BF00915791
Guo, J., Xu, B., Han, Q., Zhou, H., Xia, Y., Gong, C., et al. (2018). Ferroptosis: a novel anti-tumor action for cisplatin. Cancer Res. Treat. 50 (2), 445–460. doi:10.4143/crt.2016.572
Hamanishi, J., Mandai, M., Iwasaki, M., Okazaki, T., Tanaka, Y., Yamaguchi, K., et al. (2007). Programmed cell death 1 ligand 1 and tumor-infiltrating CD8+ T lymphocytes are prognostic factors of human ovarian cancer. Proc. Natl. Acad. Sci. U. S. A. 104 (9), 3360–3365. doi:10.1073/pnas.0611533104
Hangauer, M. J., Viswanathan, V. S., Ryan, M. J., Bole, D., Eaton, J. K., Matov, A., et al. (2017). Drug-tolerant persister cancer cells are vulnerable to GPX4 inhibition. Nature 551 (7679), 247–250. doi:10.1038/nature24297
Hassannia, B., Vandenabeele, P., and Vanden Berghe, T. (2019). Targeting ferroptosis to iron out cancer. Cancer Cell 35 (6), 830–849. doi:10.1016/j.ccell.2019.04.002
Hou, J., Yu, Z., Xiang, R., Li, C., Wang, L., Chen, S., et al. (2014). Correlation between infiltration of FOXP3+ regulatory T cells and expression of B7-H1 in the tumor tissues of gastric cancer. Exp. Mol. Pathol. 96 (3), 284–291. doi:10.1016/j.yexmp.2014.03.005
Hu, K., Li, K., Lv, J., Feng, J., Chen, J., Wu, H., et al. (2020). Suppression of the SLC7A11/glutathione axis causes synthetic lethality in KRAS-mutant lung adenocarcinoma. J. Clin. Invest. 130 (4), 1752–1766. doi:10.1172/jci124049
Huang, C., Yang, M., Deng, J., Li, P., Su, W., and Jiang, R. (2018). Upregulation and activation of p53 by erastin-induced reactive oxygen species contribute to cytotoxic and cytostatic effects in A549 lung cancer cells. Oncol. Rep. 40 (4), 2363–2370. doi:10.3892/or.2018.6585
Jiang, X., Stockwell, B. R., and Conrad, M. (2021a). Ferroptosis: mechanisms, biology and role in disease. Nat. Rev. Mol. Cell Biol. 22 (4), 266–282. doi:10.1038/s41580-020-00324-8
Jiang, Z., Lim, S.-O., Yan, M., Hsu, J. L., Yao, J., Wei, Y., et al. (2021b). TYRO3 induces anti-PD-1/PD-L1 therapy resistance by limiting innate immunity and tumoral ferroptosis. J. Clin. Investigation 131 (8), e139434. doi:10.1172/JCI139434
Kim, R., Hashimoto, A., Markosyan, N., Tyurin, V. A., Tyurina, Y. Y., Kar, G., et al. (2022). Ferroptosis of tumour neutrophils causes immune suppression in cancer. Nature 612 (7939), 338–346. doi:10.1038/s41586-022-05443-0
Koppula, P., Zhang, Y., Zhuang, L., and Gan, B. (2018). Amino acid transporter SLC7A11/xCT at the crossroads of regulating redox homeostasis and nutrient dependency of cancer. Cancer Commun. Lond. Engl. 38 (1), 12. doi:10.1186/s40880-018-0288-x
Kuang, F., Liu, J., Tang, D., and Kang, R. (2020). Oxidative damage and antioxidant defense in ferroptosis. Front. Cell Dev. Biol. 8, 586578. doi:10.3389/fcell.2020.586578
Lang, X., Green, M. D., Wang, W., Yu, J., Choi, J. E., Jiang, L., et al. (2019). Radiotherapy and immunotherapy promote tumoral lipid oxidation and ferroptosis via synergistic repression of SLC7A11. Cancer Discov. 9 (12), 1673–1685. doi:10.1158/2159-8290.CD-19-0338
Lee, J. Y., Nam, M., Son, H. Y., Hyun, K., Jang, S. Y., Kim, J. W., et al. (2020). Polyunsaturated fatty acid biosynthesis pathway determines ferroptosis sensitivity in gastric cancer. Proc. Natl. Acad. Sci. U. S. A. 117 (51), 32433–32442. doi:10.1073/pnas.2006828117
Legrand, A. J., Konstantinou, M., Goode, E. F., and Meier, P. (2019). The diversification of cell death and immunity: memento mori. Mol. Cell 76 (2), 232–242. doi:10.1016/j.molcel.2019.09.006
Lei, G., Zhang, Y., Koppula, P., Liu, X., Zhang, J., Lin, S. H., et al. (2020). The role of ferroptosis in ionizing radiation-induced cell death and tumor suppression. Cell Res. 30 (2), 146–162. doi:10.1038/s41422-019-0263-3
Lei, G., Zhuang, L., and Gan, B. (2022). Targeting ferroptosis as a vulnerability in cancer. Nat. Rev. Cancer 22 (7), 381–396. doi:10.1038/s41568-022-00459-0
Li, D., and Li, Y. (2020). The interaction between ferroptosis and lipid metabolism in cancer. Signal Transduct. Target. Ther. 5 (1), 108. doi:10.1038/s41392-020-00216-5
Li, N., Wang, W., Zhou, H., Wu, Q., Duan, M., Liu, C., et al. (2020b). Ferritinophagy-mediated ferroptosis is involved in sepsis-induced cardiac injury. Free Radic. Biol. Med. 160, 303–318. doi:10.1016/j.freeradbiomed.2020.08.009
Li, Q., Zhou, Y., He, W., Ren, X., Zhang, M., Jiang, Y., et al. (2021). Platelet-armored nanoplatform to harmonize janus-faced IFN-γ against tumor recurrence and metastasis. J. Control. Release Official J. Control. Release Soc. 338, 33–45. doi:10.1016/j.jconrel.2021.08.020
Li, X., Wang, T.-X., Huang, X., Li, Y., Sun, T., Zang, S., et al. (2020a). Targeting ferroptosis alleviates methionine-choline deficient (MCD)-diet induced NASH by suppressing liver lipotoxicity. Liver Int. Official J. Int. Assoc. Study Liver 40 (6), 1378–1394. doi:10.1111/liv.14428
Liu, Y., Fan, X., Zhao, Z., and Shan, X. (2020). LncRNA slc7a11-AS1 contributes to lung cancer progression through facilitating TRAIP expression by inhibiting miR-4775. Onco Targets Ther. 13, 6295–6302. doi:10.2147/ott.S253082
Lo, M., Ling, V., Low, C., Wang, Y. Z., and Gout, P. W. (2010). Potential use of the anti-inflammatory drug, sulfasalazine, for targeted therapy of pancreatic cancer. Curr. Oncol. 17 (3), 9–16. doi:10.3747/co.v17i3.485
Lou, J. S., Zhao, L. P., Huang, Z. H., Chen, X. Y., Xu, J. T., Tai, W. C., et al. (2021). Ginkgetin derived from Ginkgo biloba leaves enhances the therapeutic effect of cisplatin via ferroptosis-mediated disruption of the Nrf2/HO-1 axis in EGFR wild-type non-small-cell lung cancer. Phytomedicine 80, 153370. doi:10.1016/j.phymed.2020.153370
Lu, B., Chen, X. B., Ying, M. D., He, Q. J., Cao, J., and Yang, B. (2017). The role of ferroptosis in cancer development and treatment response. Front. Pharmacol. 8, 992. doi:10.3389/fphar.2017.00992
Luo, L., Wang, H., Tian, W., Zeng, J., Huang, Y., and Luo, H. (2021). Targeting ferroptosis for cancer therapy: iron metabolism and anticancer immunity. Am. J. Cancer Res. 11 (11), 5508–5525.
Luo, Y., Chen, H., Liu, H., Jia, W., Yan, J., Ding, W., et al. (2020). Protective effects of ferroptosis inhibition on high fat diet-induced liver and renal injury in mice. Int. J. Clin. Exp. Pathol. 13 (8), 2041–2049.
Lv, C., Qu, H., Zhu, W., Xu, K., Xu, A., Jia, B., et al. (2017). Low-dose paclitaxel inhibits tumor cell growth by regulating glutaminolysis in colorectal carcinoma cells. Front. Pharmacol. 8, 244. doi:10.3389/fphar.2017.00244
Ma, P. a., Xiao, H., Yu, C., Liu, J., Cheng, Z., Song, H., et al. (2017). Enhanced cisplatin chemotherapy by iron oxide nanocarrier-mediated generation of highly toxic reactive oxygen species. Nano Lett. 17 (2), 928–937. doi:10.1021/acs.nanolett.6b04269
Ma, S., Henson, E. S., Chen, Y., and Gibson, S. B. (2016). Ferroptosis is induced following siramesine and lapatinib treatment of breast cancer cells. Cell Death Dis. 7 (7), e2307. doi:10.1038/cddis.2016.208
Maiorino, M., Conrad, M., and Ursini, F. (2018). GPx4, lipid peroxidation, and cell death: discoveries, rediscoveries, and open issues. Antioxidants redox Signal. 29 (1), 61–74. doi:10.1089/ars.2017.7115
Mandal, P. K., Seiler, A., Perisic, T., Kölle, P., Banjac Canak, A., Förster, H., et al. (2010). System x(c)- and thioredoxin reductase 1 cooperatively rescue glutathione deficiency. J. Biol. Chem. 285 (29), 22244–22253. doi:10.1074/jbc.M110.121327
Martinez-Outschoorn, U. E., Peiris-Pagés, M., Pestell, R. G., Sotgia, F., and Lisanti, M. P. (2017). Cancer metabolism: a therapeutic perspective. Nat. Rev. Clin. Oncol. 14 (1), 11–31. doi:10.1038/nrclinonc.2016.60
Mielgo, A., and Schmid, M. C. (2013). Impact of tumour associated macrophages in pancreatic cancer. BMB Rep. 46 (3), 131–138. doi:10.5483/bmbrep.2013.46.3.036
Motwani, M., Pesiridis, S., and Fitzgerald, K. A. (2019). DNA sensing by the cGAS-STING pathway in health and disease. Nat. Rev. Genet. 20 (11), 657–674. doi:10.1038/s41576-019-0151-1
Nakamura, E., Sato, M., Yang, H., Miyagawa, F., Harasaki, M., Tomita, K., et al. (1999). 4F2 (CD98) heavy chain is associated covalently with an amino acid transporter and controls intracellular trafficking and membrane topology of 4F2 heterodimer. J. Biol. Chem. 274 (5), 3009–3016. doi:10.1074/jbc.274.5.3009
Nakanishi, J., Wada, Y., Matsumoto, K., Azuma, M., Kikuchi, K., and Ueda, S. (2007). Overexpression of B7-H1 (PD-L1) significantly associates with tumor grade and postoperative prognosis in human urothelial cancers. Cancer Immunol. Immunother. 56 (8), 1173–1182. doi:10.1007/s00262-006-0266-z
Ndiaye, H., Liu, J. Y., Hall, A., Minogue, S., Morgan, M. Y., and Waugh, M. G. (2020). Immunohistochemical staining reveals differential expression of ACSL3 and ACSL4 in hepatocellular carcinoma and hepatic gastrointestinal metastases. Biosci. Rep. 40 (4). doi:10.1042/BSR20200219
Ohigashi, Y., Sho, M., Yamada, Y., Tsurui, Y., Hamada, K., Ikeda, N., et al. (2005). Clinical significance of programmed death-1 ligand-1 and programmed death-1 ligand-2 expression in human esophageal cancer. Clin. Cancer Res. Official J. Am. Assoc. Cancer Res. 11 (8), 2947–2953. doi:10.1158/1078-0432.CCR-04-1469
Ostrand-Rosenberg, S., Beury, D. W., Parker, K. H., and Horn, L. A. (2020). Survival of the fittest: how myeloid-derived suppressor cells survive in the inhospitable tumor microenvironment. Cancer Immunol. Immunother. 69 (2), 215–221. doi:10.1007/s00262-019-02388-8
Padanad, M. S., Konstantinidou, G., Venkateswaran, N., Melegari, M., Rindhe, S., Mitsche, M., et al. (2016). Fatty acid oxidation mediated by acyl-CoA synthetase long chain 3 is required for mutant KRAS lung tumorigenesis. Cell Rep. 16 (6), 1614–1628. doi:10.1016/j.celrep.2016.07.009
Qi, J., Kim, J.-W., Zhou, Z., Lim, C.-W., and Kim, B. (2020). Ferroptosis affects the progression of nonalcoholic steatohepatitis via the modulation of lipid peroxidation-mediated cell death in mice. Am. J. Pathology 190 (1), 68–81. doi:10.1016/j.ajpath.2019.09.011
Reczek, C. R., and Chandel, N. S. (2018). ROS promotes cancer cell survival through calcium signaling. Cancer Cell 33 (6), 949–951. doi:10.1016/j.ccell.2018.05.010
Sang, M., Luo, R., Bai, Y., Dou, J., Zhang, Z., Liu, F., et al. (2019). Mitochondrial membrane anchored photosensitive nano-device for lipid hydroperoxides burst and inducing ferroptosis to surmount therapy-resistant cancer. Theranostics 9 (21), 6209–6223. doi:10.7150/thno.36283
Sato, H., Shiiya, A., Kimata, M., Maebara, K., Tamba, M., Sakakura, Y., et al. (2005). Redox imbalance in cystine/glutamate transporter-deficient mice. J. Biol. Chem. 280 (45), 37423–37429. doi:10.1074/jbc.M506439200
Sato, H., Tamba, M., Ishii, T., and Bannai, S. (1999). Cloning and expression of a plasma membrane cystine/glutamate exchange transporter composed of two distinct proteins. J. Biol. Chem. 274 (17), 11455–11458. doi:10.1074/jbc.274.17.11455
Seibt, T. M., Proneth, B., and Conrad, M. (2019). Role of GPX4 in ferroptosis and its pharmacological implication. Free Radic. Biol. Med. 133, 144–152. doi:10.1016/j.freeradbiomed.2018.09.014
Seiler, A., Schneider, M., Förster, H., Roth, S., Wirth, E. K., Culmsee, C., et al. (2008). Glutathione peroxidase 4 senses and translates oxidative stress into 12/15-lipoxygenase dependent- and AIF-mediated cell death. Cell Metab. 8 (3), 237–248. doi:10.1016/j.cmet.2008.07.005
Shimada, K., Skouta, R., Kaplan, A., Yang, W. S., Hayano, M., Dixon, S. J., et al. (2016). Global survey of cell death mechanisms reveals metabolic regulation of ferroptosis. Nat. Chem. Biol. 12 (7), 497–503. doi:10.1038/nchembio.2079
Stockwell, B. R., Friedmann Angeli, J. P., Bayir, H., Bush, A. I., Conrad, M., Dixon, S. J., et al. (2017). Ferroptosis: a regulated cell death nexus linking metabolism, redox biology, and disease. Cell 171 (2), 273–285. doi:10.1016/j.cell.2017.09.021
Su, J., Bian, C., Zheng, Z., Wang, H., Meng, L., Xin, Y., et al. (2022). Cooperation effects of radiation and ferroptosis on tumor suppression and radiation injury. Front. Cell Dev. Biol. 10, 951116. doi:10.3389/fcell.2022.951116
Su, P., Wang, Q., Bi, E., Ma, X., Liu, L., Yang, M., et al. (2020). Enhanced lipid accumulation and metabolism are required for the differentiation and activation of tumor-associated macrophages. Cancer Res. 80 (7), 1438–1450. doi:10.1158/0008-5472.CAN-19-2994
Tan, S. K., Mahmud, I., Fontanesi, F., Puchowicz, M., Neumann, C. K. A., Griswold, A. J., et al. (2021). Obesity-dependent adipokine chemerin suppresses fatty acid oxidation to confer ferroptosis resistance. Cancer Discov. 11 (8), 2072–2093. doi:10.1158/2159-8290.CD-20-1453
Tang, D., Chen, X., Kang, R., and Kroemer, G. (2021). Ferroptosis: molecular mechanisms and health implications. Cell Res. 31 (2), 107–125. doi:10.1038/s41422-020-00441-1
Tang, D., Kang, R., Berghe, T. V., Vandenabeele, P., and Kroemer, G. (2019). The molecular machinery of regulated cell death. Cell Res. 29 (5), 347–364. doi:10.1038/s41422-019-0164-5
Trachootham, D., Alexandre, J., and Huang, P. (2009). Targeting cancer cells by ROS-mediated mechanisms: a radical therapeutic approach? Nat. Rev. Drug Discov. 8 (7), 579–591. doi:10.1038/nrd2803
Tsurusaki, S., Tsuchiya, Y., Koumura, T., Nakasone, M., Sakamoto, T., Matsuoka, M., et al. (2019). Hepatic ferroptosis plays an important role as the trigger for initiating inflammation in nonalcoholic steatohepatitis. Cell Death Dis. 10 (6), 449. doi:10.1038/s41419-019-1678-y
Ursini, F., Maiorino, M., Valente, M., Ferri, L., and Gregolin, C. (1982). Purification from pig liver of a protein which protects liposomes and biomembranes from peroxidative degradation and exhibits glutathione peroxidase activity on phosphatidylcholine hydroperoxides. Biochim. Biophys. Acta 710 (2), 197–211. doi:10.1016/0005-2760(82)90150-3
Viswanathan, V. S., Ryan, M. J., Dhruv, H. D., Gill, S., Eichhoff, O. M., Seashore-Ludlow, B., et al. (2017). Dependency of a therapy-resistant state of cancer cells on a lipid peroxidase pathway. Nature 547 (7664), 453–457. doi:10.1038/nature23007
Wang, D., and DuBois, R. N. (2015). Immunosuppression associated with chronic inflammation in the tumor microenvironment. Carcinogenesis 36 (10), 1085–1093. doi:10.1093/carcin/bgv123
Wang, H., Franco, F., Tsui, Y.-C., Xie, X., Trefny, M. P., Zappasodi, R., et al. (2020). CD36-mediated metabolic adaptation supports regulatory T cell survival and function in tumors. Nat. Immunol. 21 (3), 298–308. doi:10.1038/s41590-019-0589-5
Wang, W., Green, M., Choi, J. E., Gijón, M., Kennedy, P. D., Johnson, J. K., et al. (2019a). CD8+ T cells regulate tumour ferroptosis during cancer immunotherapy. Nature 569 (7755), 270–274. doi:10.1038/s41586-019-1170-y
Wang, W., Xia, X., Mao, L., and Wang, S. (2019b). The CCAAT/Enhancer-Binding protein family: its roles in MDSC expansion and function. Front. Immunol. 10, 1804. doi:10.3389/fimmu.2019.01804
Wang, X., Teng, F., Kong, L., and Yu, J. (2016). PD-L1 expression in human cancers and its association with clinical outcomes. Onco Targets Ther. 9, 5023–5039. doi:10.2147/OTT.S105862
Wen, Q., Liu, J., Kang, R., Zhou, B., and Tang, D. (2019). The release and activity of HMGB1 in ferroptosis. Biochem. Biophys. Res. Commun. 510 (2), 278–283. doi:10.1016/j.bbrc.2019.01.090
Wiernicki, B., Maschalidi, S., Pinney, J., Adjemian, S., Vanden Berghe, T., Ravichandran, K. S., et al. (2022). Cancer cells dying from ferroptosis impede dendritic cell-mediated anti-tumor immunity. Nat. Commun. 13 (1), 3676. doi:10.1038/s41467-022-31218-2
Wolpaw, A. J., and Dang, C. V. (2018). Exploiting metabolic vulnerabilities of cancer with precision and accuracy. Trends Cell Biol. 28 (3), 201–212. doi:10.1016/j.tcb.2017.11.006
Xu, T., Ding, W., Ji, X., Ao, X., Liu, Y., Yu, W., et al. (2019). Molecular mechanisms of ferroptosis and its role in cancer therapy. J. Cell. Mol. Med. 23 (8), 4900–4912. doi:10.1111/jcmm.14511
Yamaguchi, H., Hsu, J. L., Chen, C.-T., Wang, Y.-N., Hsu, M.-C., Chang, S.-S., et al. (2013). Caspase-independent cell death is involved in the negative effect of EGF receptor inhibitors on cisplatin in non-small cell lung cancer cells. Clin. Cancer Res. Official J. Am. Assoc. Cancer Res. 19 (4), 845–854. doi:10.1158/1078-0432.CCR-12-2621
Yang, W. S., Kim, K. J., Gaschler, M. M., Patel, M., Shchepinov, M. S., and Stockwell, B. R. (2016). Peroxidation of polyunsaturated fatty acids by lipoxygenases drives ferroptosis. Proc. Natl. Acad. Sci. U. S. A. 113 (34), E4966–E4975. doi:10.1073/pnas.1603244113
Yang, W. S., SriRamaratnam, R., Welsch, M. E., Shimada, K., Skouta, R., Viswanathan, V. S., et al. (2014). Regulation of ferroptotic cancer cell death by GPX4. Cell 156 (1-2), 317–331. doi:10.1016/j.cell.2013.12.010
Yang, W. S., and Stockwell, B. R. (2016). Ferroptosis: death by lipid peroxidation. Trends Cell Biol. 26 (3), 165–176. doi:10.1016/j.tcb.2015.10.014
Yant, L. J., Ran, Q., Rao, L., Van Remmen, H., Shibatani, T., Belter, J. G., et al. (2003). The selenoprotein GPX4 is essential for mouse development and protects from radiation and oxidative damage insults. Free Radic. Biol. Med. 34 (4), 496–502. doi:10.1016/s0891-5849(02)01360-6
Yatim, N., Cullen, S., and Albert, M. L. (2017). Dying cells actively regulate adaptive immune responses. Nat. Rev. Immunol. 17 (4), 262–275. doi:10.1038/nri.2017.9
Ye, J., Jiang, X., Dong, Z., Hu, S., and Xiao, M. (2019). Low-concentration PTX and RSL3 inhibits tumor cell growth synergistically by inducing ferroptosis in mutant p53 hypopharyngeal squamous carcinoma. Cancer Manag. Res. 11, 9783–9792. doi:10.2147/CMAR.S217944
Ye, L. F., Chaudhary, K. R., Zandkarimi, F., Harken, A. D., Kinslow, C. J., Upadhyayula, P. S., et al. (2020). Radiation-induced lipid peroxidation triggers ferroptosis and synergizes with ferroptosis inducers. ACS Chem. Biol. 15 (2), 469–484. doi:10.1021/acschembio.9b00939
Yu, H., Han, Z., Xu, Z., An, C., Xu, L., and Xin, H. (2019). RNA sequencing uncovers the key long non-coding RNAs and potential molecular mechanism contributing to XAV939-mediated inhibition of non-small cell lung cancer. Oncol. Lett. 17 (6), 4994–5004. doi:10.3892/ol.2019.10191
Yu, Y., Xie, Y., Cao, L., Yang, L., Yang, M., Lotze, M. T., et al. (2015). The ferroptosis inducer erastin enhances sensitivity of acute myeloid leukemia cells to chemotherapeutic agents. Mol. Cell. Oncol. 2 (4), e1054549. doi:10.1080/23723556.2015.1054549
Yuan, H., Li, X., Zhang, X., Kang, R., and Tang, D. (2016). Identification of ACSL4 as a biomarker and contributor of ferroptosis. Biochem. Biophys. Res. Commun. 478 (3), 1338–1343. doi:10.1016/j.bbrc.2016.08.124
Zhang, F., Li, F., Lu, G. H., Nie, W., Zhang, L., Lv, Y., et al. (2019). Engineering magnetosomes for ferroptosis/immunomodulation synergism in cancer. ACS Nano 13 (5), 5662–5673. doi:10.1021/acsnano.9b00892
Zhang, M., Di Martino, J. S., Bowman, R. L., Campbell, N. R., Baksh, S. C., Simon-Vermot, T., et al. (2018). Adipocyte-derived lipids mediate melanoma progression via FATP proteins. Cancer Discov. 8 (8), 1006–1025. doi:10.1158/2159-8290.CD-17-1371
Zhang, M., Qin, X., Zhao, Z., Du, Q., Li, Q., Jiang, Y., et al. (2022). A self-amplifying nanodrug to manipulate the Janus-faced nature of ferroptosis for tumor therapy. Nanoscale Horizons 7 (2), 198–210. doi:10.1039/d1nh00506e
Zhang, T., Sun, B., Zhong, C., Xu, K., Wang, Z., Hofman, P., et al. (2021). Targeting histone deacetylase enhances the therapeutic effect of Erastin-induced ferroptosis in EGFR-activating mutant lung adenocarcinoma. Transl. Lung Cancer Res. 10 (4), 1857–1872. doi:10.21037/tlcr-21-303
Zhang, Y., Song, J., Zhao, Z., Yang, M., Chen, M., Liu, C., et al. (2020). Single-cell transcriptome analysis reveals tumor immune microenvironment heterogenicity and granulocytes enrichment in colorectal cancer liver metastases. Cancer Lett. 470, 84–94. doi:10.1016/j.canlet.2019.10.016
Zhou, J., Nefedova, Y., Lei, A., and Gabrilovich, D. (2018). Neutrophils and PMN-MDSC: their biological role and interaction with stromal cells. Semin. Immunol. 35, 19–28. doi:10.1016/j.smim.2017.12.004
Zhu, Y., Herndon, J. M., Sojka, D. K., Kim, K.-W., Knolhoff, B. L., Zuo, C., et al. (2017). Tissue-resident macrophages in pancreatic ductal adenocarcinoma originate from embryonic hematopoiesis and promote tumor progression. Immunity 47 (2), 323–338. doi:10.1016/j.immuni.2017.07.014
Zou, Y., Henry, W. S., Ricq, E. L., Graham, E. T., Phadnis, V. V., Maretich, P., et al. (2020). Plasticity of ether lipids promotes ferroptosis susceptibility and evasion. Nature 585 (7826), 603–608. doi:10.1038/s41586-020-2732-8
Glossary
Keywords: ferroptosis, tumour, iron, metabolism, antitumour therapy
Citation: Zhao X, Li X and Xu Y (2024) Ferroptosis: a dual-edged sword in tumour growth. Front. Pharmacol. 14:1330910. doi: 10.3389/fphar.2023.1330910
Received: 31 October 2023; Accepted: 27 December 2023;
Published: 11 January 2024.
Edited by:
Elisa Giovannetti, VU Medical Center, NetherlandsReviewed by:
Chunming Cheng, University of Oklahoma, United StatesMahrou Vahabi, VU Medical Center, Netherlands
Copyright © 2024 Zhao, Li and Xu. This is an open-access article distributed under the terms of the Creative Commons Attribution License (CC BY). The use, distribution or reproduction in other forums is permitted, provided the original author(s) and the copyright owner(s) are credited and that the original publication in this journal is cited, in accordance with accepted academic practice. No use, distribution or reproduction is permitted which does not comply with these terms.
*Correspondence: Yinghui Xu, xuyinghui@jlu.edu.cn