- 1Shemyakin-Ovchinnikov Institute of Bioorganic Chemistry, Russian Academy of Sciences, Moscow, Russia
- 2Engelhardt Institute of Molecular Biology, Russian Academy of Sciences, Moscow, Russia
- 3Moscow Institute of Physics and Technology (State University), Moscow, Russia
Oligoarginine peptides, known mostly for their cell-penetrating properties, are also inhibitors of the nicotinic acetylcholine receptors (nAChRs). Since octa-arginine (R8) inhibits α9α10 nAChR and suppresses neuropathic pain, we checked if other polycationic compounds containing amino and/or guanidino groups could be effective and tested the activity of the disulfide-fixed “cyclo”R8, a series of biogenic polyamines (putrescine, spermidine, and spermine), C-methylated spermine analogs, agmatine and its analogs, as well as acylpolyamine argiotoxin-636 from spider venom. Their inhibitory potency on muscle-type, α7 and α9α10 nAChRs was determined using radioligand analysis, electrophysiology, and calcium imaging. “Cyclo”R8 showed similar activity to that of R8 against α9α10 nAChR (IC50 ≈ 60 nM). Biogenic polyamines as well as agmatine and its analogs displayed low activity on muscle-type Torpedo californica, as well as α7 and α9α10 nAChRs, which increased with chain length, the most active being spermine and its C-methylated derivatives having IC50 of about 30 μM against muscle-type T. californica nAChR. Argiotoxin-636, which contains a polyamine backbone and terminal guanidino group, also weakly inhibited T. californica nAChR (IC50 ≈ 15 μM), but it revealed high potency against rat α9α10 nAChR (IC50 ≈ 200 nM). We conclude that oligoarginines and similar polycationic compounds effectively inhibiting α9α10 nAChR may serve as a basis for the development of analgesics to reduce neuropathic pain.
1 Introduction
Oligoarginine peptides as such or in polymeric vesicles are widely used for intracellular delivery of a wide variety of compounds [see reviews (Ruan et al., 2016; Xie et al., 2021)]. Positively charged oligo- and polyarginines exhibit numerous biological activities (Edwards et al., 2020). However, the molecular mechanisms of their action are poorly understood and there is almost no information on their binding to specific molecular targets. An exception is the interaction of oligoarginines containing from 8 to 18 arginine residues (R8 to R18) with N-methyl-D-aspartate (NMDA)-type glutamate receptors (MacDougall et al., 2017). These oligoarginines are considered as a new class of neuroprotective molecules for the treatment of a range of neurological disorders (Meloni et al., 2020).
It was found that oligoarginines containing from 6 to 16 arginine residues (R6 to R16) interact with both muscle and neuronal nicotinic acetylcholine receptors (nAChRs), while the potency of inhibition of a particular receptor subtype depends on the peptide length (Lebedev et al., 2019). Binding to nAChRs was studied not only for oligoarginines, but also for oligohistidines and oligolysines, and it was shown that oligoarginines of sufficient length are the most effective (Zhang et al., 2022).
It was established that octa-arginine R8 (Figure 1), which has a high affinity for α9α10 nAChR, reduces neuropathic pain with the same efficacy as α-conotoxin RgIA, a specific inhibitor of this receptor subtype (Dyachenko et al., 2022). In this regard, we decided to obtain a more metabolically stable derivative of R8. Cyclization is a standard method to increase the proteolytic resistance of pharmacologically active peptides, because the lack of free amino and carboxyl groups of N- and C-terminal amino acids makes cyclic peptides resistant towards blood exopeptidases (Costa et al., 2023). The structures of the oligoarginine peptides can be stabilized by various modes of cyclization to improve their stability and cell-penetrating properties (Bató et al., 2023). For example, on the basis of R8 its cyclic fluorescent analog has been prepared (Fang et al., 2022). Here, we stabilize the R8 conformation by attaching cysteine residues at its N- and C-termini and closing the disulfide bond between them (“cyclo”R8, Figure 1). We have recently used the same approach for the synthesis of the fragment of a three-finger protein, which preserved the structure of the central loop and displayed the activity of the parent protein in binding to muscle-type nAChR (Kryukova et al., 2019; Mineev et al., 2020). For “cyclo”R8, we compared its inhibitory potency with that of the linear R8 on the muscle-type, neuronal α7 and on α9α10 nAChRs normaly present on the cochlear cells and in the immune system [see review (Elgoyhen, 2023)].
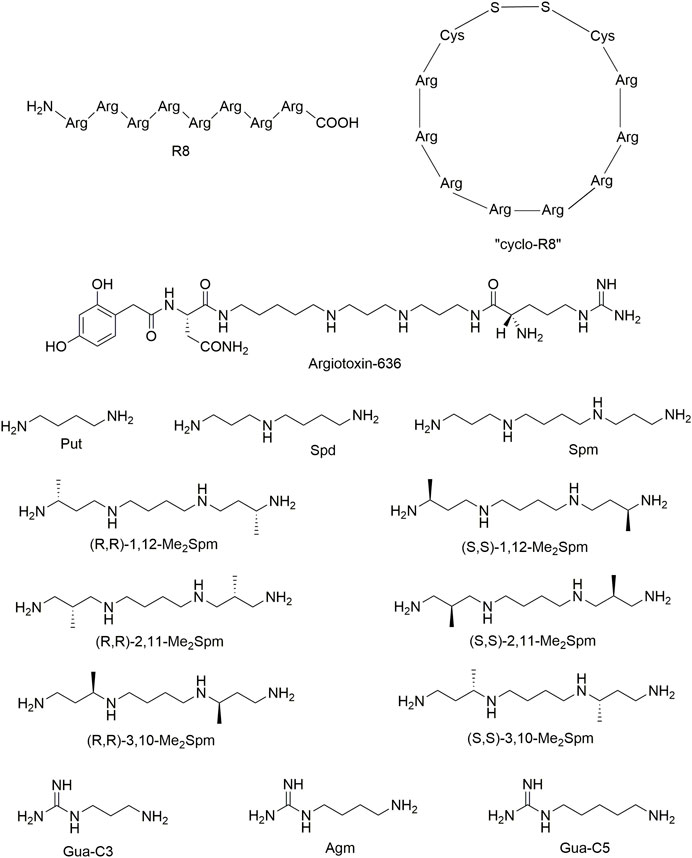
FIGURE 1. The chemical structures of tested polycationic compounds: octa-arginine R8 and “cyclo”R8, argiotoxin-636, putrescine (Put), spermidine (Spd), spermine (Spm), C-methylated spermine analogs (Me2Spm), agmatine (Agm) and its derivatives guanidine-C3 (Gua-C3) and guanidine-C5 (Gua-C5). The structures were drawn using ChemDraw Professional 18.0.
Since a number of positively charged oligopeptides inhibit nAChRs (Zhang et al., 2022), here we analyzed other low-molecular-mass polycationic compounds containing amino and guanidino groups. We started with biogenic polyamines putrescine (Put), spermidine (Spd), spermine (Spm), C-methylated Spm analogs, and agmatine (Agm) and its analogs (Figure 1). Being polycations under physiological pH, biogenic polyamines effectively interact with different negatively charged cellular targets, including nucleic acids, receptors and other proteins (Matta et al., 2021). Polyamines play an important role in synaptic transmission by conferring inward rectification to certain potassium channels, α-amino-3-hydroxy-5-methyl-4-isoxazolepropionate (AMPA)-type glutamate receptors and nAChRs (Haghighi and Cooper, 2000; Nichols and Lee, 2018; Twomey et al., 2018). Recently, it has been shown that polyamines selectively control the biogenesis of α7 or α4β2 nAChR receptors (Dhara et al., 2020). Analogs and derivatives of polyamines are widely used to investigate cellular functions of Spm and Spd (Holbert et al., 2022), while C-methylated analogs of polyamines are one of the research tools providing functionally active mimetics of Spm and Spd (for reviews see (Keinänen et al., 2014; Khomutov et al., 2019a)). Polyamines are accumulated in glial cells (astrocytes, oligodendrocytes, Müller and Bergmann glia), but not in neurons and this makes polyamine-reservoirs in brain/retina restricted to glial cells (Rieck et al., 2022; Ríos et al., 2023; Weiss et al., 2023). These cells express a variety of polyamine specific and nonselective organic cation transporters such as SLC22A-1,2,3 (Sala-Rabanal et al., 2013), SLC18B1 (Hiasa et al., 2014), and ATP13A2 (Qiao et al., 2016; Vrijsen et al., 2023) for polyamine uptake into the cytoplasm from any extracellular sources such as cerebral spinal fluid (Weiss et al., 2023). Since glial cells may take up di- and polyamine analogs and derivatives (Malpica-Nieves et al., 2021), these cells may also take up C-methylated analogs of Spm and Agm of this study.
Another polycationic compound of our choice is argiotoxin-636 (AgTx-636), also known as argiopin, purified from Argiope lobata spider venom. It is the first-in-class molecule belonging to the so-called acylpolyamines; AgTx-636 has a polyamine tail linked to an aromatic acyl head group and a terminal arginine residue (Figure 1) (Grishin et al., 1986; Antonov et al., 1987; Grishin et al., 1989). Binding of AgTx-636 to both AMPA and NMDA-type glutamate receptors was studied in detail (Davies et al., 1992; Twomey et al., 2018). In addition, it is known to inhibit weakly some neuronal nAChRs (Liu et al., 1997), but there are no data on its interaction with α9α10 nAChR. For a number of derivatives of another well-studied acylpolyamine philanthotoxin-433 (PTX-433), highly potent inhibition of α3β4 and α4β2 nAChRs was demonstrated (Kachel et al., 2019), but the action on α9α10 nAChR was not tested either.
In this study we tested the inhibitory potency of a number of polycationic compounds on α9α10 nAChR, a known molecular target to reduce neuropathic pain (Hone and McIntosh, 2023; Shelukhina et al., 2023), as well as on muscle and α7 nAChRs to check the possibility of undesirable side effects. Muscle relaxant activity mediated by controlled muscle nAChR inhibition (Shelukhina et al., 2018) may be profitable in complex analgesic therapy, but excessive affinity to muscle nAChR may lead to paralysis as in the case of the selective snake α-neurotoxins or α-conotoxins. Potentiation of α7 nAChR is beneficial in chronic pain, mostly by alleviating neuroinflammation (Zhou et al., 2022; Shelukhina et al., 2023), therefore, it is necessary to control its inhibition, which may lead to the opposite effect in vivo.
2 Methods
2.1 Synthesis of linear octa-arginine R8 and disulfide-stabilized “cyclo” R8
The peptides were prepared by solid-phase synthesis using Fmoc/t-butyl strategy on tritylchloride-polystyrene resin (Intavis, Tubingen, Germany). The disulfides were formed under conventional oxidation conditions: prolonged incubation on air in 50% aqueous acetonitrile at room temperature in the presence of N-ethyldiisopropylamine (pH 8.0). The peptides purity (>98%) was confirmed using reversed-phase high-performance liquid chromatography (RP-HPLC) and the respective monoisotopic molecular masses (MW (R8) = 1266.82 Da, MW (“cyclo”R8) = 1470.84 Da) were determined by matrix-assisted laser desorption/ionization mass-spectrometry (MALDI-MS) using Ultraflex I mass-spectrometer (Bruker Daltonik, Bremen, Germany). Peptide aliquots were prepared immediately after lyophylization by dissolving the dried compound in water of Milli-Q grade; the aliquots were stored at –20°C. Peptide concentration was determined by dissolving a weighted amount in a fixed volume of solution.
2.2 Synthesis of bis-methylated spermine analogs
(R,R)-2,13-Diamino-5,10-diazatetradecane and (S,S)-2,13-diamino-5,10-diazatetradecane tetrahydrochlorides, (R,R)-1,12-Me2Spm and (S,S)-1,12-Me2Spm were synthesized as described (Grigorenko et al., 2007).
(R,R)-1,12-Diamino-3,10-dimethyl-4,9-diazadodecane and (S,S)-1,12-diamino-3,10-dimethyl-4,9-diazadodecane tetrahydrochlorides, (R,R)-3,10-Me2Spm and (S,S)-3,10-Me2Spm were synthesized as described (Khomutov et al., 2020).
(R,R)-1,12-diamino-2,11-dimethyl-4,9-diazadodecane and (S,S)-1,12-diamino-2,11-dimethyl-4,9-diazadodecane tetrahydrochlorides, (R,R)-2,11-Me2Spm and (S,S)-2,11-Me2Spm were synthesized from (R)- and (S)-3-amino-2-methylpropanol-1 as described (Khomutov et al., 2019b).
2.3 AgTx-636 purification
AgTx-636 was isolated and purified from A. lobata venom as described (Grishin et al., 1989; Twomey et al., 2018). Briefly, the lyophilized venom was dissolved in water, mixed with ethanol, centrifuged, and the supernatant was separated by RP-HPLC. AgTx-636 was purified to homogeneity (>98%). AgTx-636 aliquots were freeze-dried; one was dissolved in MeCN–MeOH, and the concentration was determined by UV absorbance using the known extinction coefficient (ε280 = 4150). Other aliquots were dissolved in water or buffer solution to the desired concentrations.
2.4 Ca-imaging analysis of inhibition of α7 nAChR endogenously expressed in neuroblastoma SH-SY5Y cells
Human neuroblastoma SH-SY5Y cells were cultured in DMEM/F12 medium (Thermo Fisher Scientific, Waltham, MA, United States) supplemented with 10% fetal bovine serum (FBS) (PAA Laboratories, Pasching, Austria) in a CO2 incubator at 37°C and 5% CO2 atmosphere. After removing the growth medium, SH-SY5Y cells were loaded with Fluo-4 Direct Calcium Assay Kit (Thermo Fisher Scientific) for 30 min at 37°C and then were kept for 30 min at room temperature according to the manufacturer’s protocol. Then SH-SY5Y cells were preincubated with positive allosteric modulator of α7 nAChR, PNU120596 (10 μM, Tocris Bioscience, Bristol, United Kingdom), and inhibitors (R8 and “cyclo”R8, Agm, Spd, Spm, rac-2,11-Me2Spm, AgTx-636 and α-cobratoxin (CTX)) in a buffer containing 140 mM NaCl, 2 mM CaCl2, 2.8 mM KCl, 4 mM MgCl2, 20 mM HEPES, 10 mM glucose, pH 7.4 for 20 min before agonist PNU282987 (200 nM, Tocris Bioscience) addition. The Fluo-4 fluorescence was analyzed with the microplate reader Hidex Sence (Hidex, Turku, Finland) (excitaion/emission 485/535 nm). Data files were analyzed using Hidex Sence software and OriginPro 2015 software (OriginLab, Northampton, MA, United States).
2.5 Competition radioligand assay with 125I-labeled α-bungarotoxin
The binding capacity (IC50) of the low-molecular-mass compounds was evaluated by competition with 125I-labeled α-bungarotoxin (125I-αBgt) as described (Kryukova et al., 2019; Mineev et al., 2020). The suspensions of membranes from Torpedo californica ray electric organ or human α7 nAChR expressed in GH4C1 cells (1.25 nM or 0.4 nM αBgt binding sites, respectively) were incubated in 50 μL of binding buffer (20 mM Tris-HCl, pH 8.0, containing 1 mg/mL bovine serum albumin) for 90 min with various amounts of low-molecular-mass compounds. Then, 0.1–0.2 nM 125I-αBgt (500 Ci/mmol) was added and incubated for another 5 min. The T. californica membranes or cell suspensions were applied to glass GF/C filters (Whatman, Maidstone, United Kingdom) presoaked in 0.3% polyethylenimine. Filter washing, measurement of bound radioactivity and non-specific binding of 125I-αBgt were performed as in (Kryukova et al., 2019; Mineev et al., 2020).
2.6 Two-electrode voltage clamp analysis of rat and human α9α10 nAChR inhibition by polycationic compounds
Xenopus laevis frogs were fed twice a week and maintained according to supplier recommendations (https://www.enasco.com/page/xen_care). All experiments were carried out in strict accordance with the World Health Organization’s International Guiding Principles for Biomedical Research Involving Animals. The protocol (number 251/2018 26.02.18) was approved by the Institutional Animal Care and Use Committee based on the Institutional Policy on the Use of Laboratory Animals of the Shemyakin-Ovchinnikov Institute of Bioorganic Chemistry Russian Academy of Sciences.
Oocytes were removed from mature anesthetized X. laevis frogs by dissecting the abdomen and removing necessary amounts of ovarium. Stage V–VI X. laevis oocytes were defolliculated with collagenase type I (2 mg/mL, Life Technologies, Camarillo, CA, United States) at room temperature (21°C–24°C) for 2 h in Ca2+-free Barth’s solution composed of (in mM) 88 NaCl, 1.1 KCl, 2.4 NaHCO3, 0.8 MgSO4 and 15 HEPES, pH 7.6. Oocytes were injected with 9.2 ng of rat or human nAChR α9 and α10 cRNA (at a ratio of 1:1). Oocytes were incubated at 18°C for 2–4 days before electrophysiological recordings in Barth’s solution composed of (in mM) 88 NaCl, 1.1 KCl, 2.4 NaHCO3, 0.3 Ca(NO3)2, 0.4 CaCl2, 0.8 MgSO4 and 15 HEPES at pH 7.6, supplemented with 40 μg/mL gentamicin and 100 μg/mL ampicillin. Recordings were performed using a turbo TEC-03X amplifier (NPI Electronic, Tamm, Germany) and WinWCP recording software (University of Strathclyde, Glasgow, United Kingdom). The glass recording electrodes were filled with 3 M KCl and the electrode resistance was 0.1–0.5 MΩ. Membrane potential was clamped at −60 mV. Oocytes were briefly washed with Ba2+ Ringer’s solution composed of (in mM) 115 NaCl, 2.5 KCl, 1.8 BaCl2 and 10 HEPES, pH 7.2, followed by three applications of 500 μM acetylcholine (ACh). Washout with Ba2+ Ringer’s was performed for 5 min between ACh applications. Oocytes were preincubated with various concentrations of “cyclo”R8, rac-2,11-Me2Spm, Spm, and AgTx-636 for 1 min followed by their co-application with ACh. The peak current amplitudes of ACh-induced responses were measured before (ACh alone) and after the preincubation of oocytes with the inhibitors. The ratio between these two measurements was used to assess the activity of the tested compounds.
Rat α9 and α10 cDNAs was cloned in the pGEMHE vector; human α9 and α10 cDNAs were derived from the pT7TS vector. Plasmid pT7TS and pGEMHE constructs were linearized with XbaI and NheI (NEB, Ipswich, United States) restriction enzymes, respectively. mRNAs were transcribed in vitro using T7 or SP6 mMESSAGE mMACHINE High Yield Capped RNA Transcription Kit (Thermo Fisher Scientific). Transcribed mRNA was polyadenylated using the Poly-A-Tailing Kit (Thermo Fisher Scientific). The mRNAs were stored up to 6 months at −70°C.
2.7 Statistical analysis
Calcium imaging, radioligand analysis and electrophysiological data were statistically analyzed using OriginPro 7.5 software (Microcal, Northampton, MA, United States) and SigmaPlot 11.0 (Systat Software Inc., CA, United States). The data were first analyzed for normality using the Shapiro-Wilk test, then they were processed using one-way ANOVA test, Tukey’s test, or Student’s t-test, *p < 0.05.
3 Results
3.1 Inhibition of α7 and α9α10 nAChRs by “cyclo”R8
We prepared “cyclo”R8 (Figure 1) by solid-phase peptide synthesis and studied its activity in comparison with the parent R8 using electrophysiology and calcium imaging. We found that “cyclo”R8 showed high affinity to human α7 and α9α10 nAChRs; its inhibitory potency (IC50 = 13.3 ± 3.0 nM on α7 and 61.8 ± 16.0 nM on α9α10) was similar to that of R8 (IC50 = 14.5 ± 1.0 nM on α7 and 44 (95% CI = 26–75) nM on α9α10 (Lebedev et al., 2019); Figures 2A, B). Thus, the “cyclic” structure of R8 peptide, formed by the addition of two flanking cysteine residues and closing the disulfide bridge between them, did not affect significantly the pharmacological properties toward nAChRs.
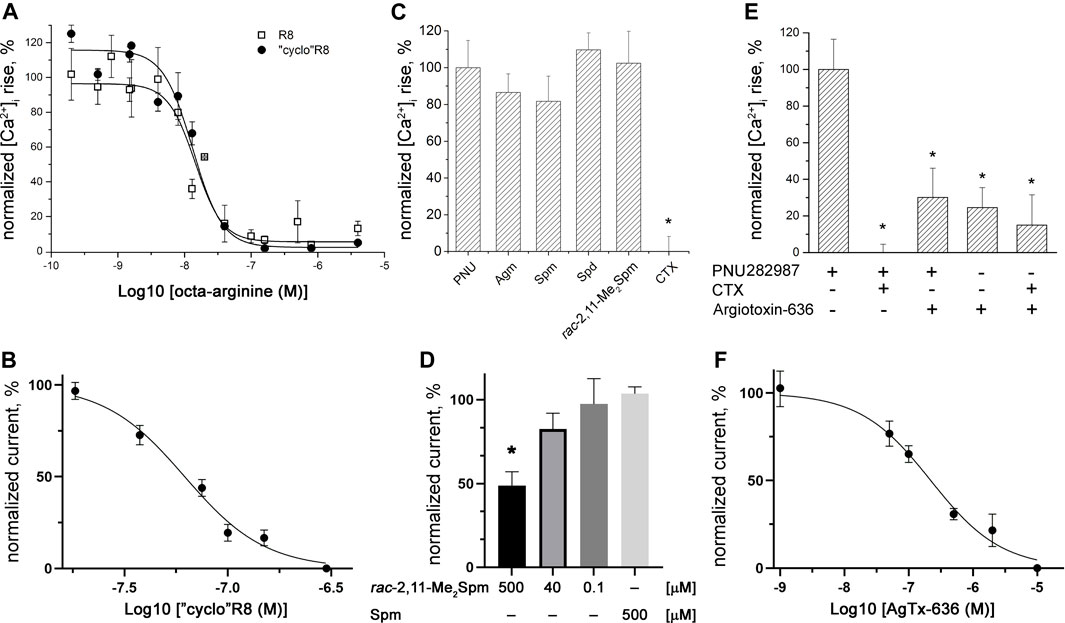
FIGURE 2. Inhibitory action of polycationic compounds toward α7 (upper panel) and α9α10 (lower panel) nAChRs. Calcium imaging of (A) R8 (IC50 = 14.5 ± 1.0 nM) and “cyclo”R8 (IC50 = 13.3 ± 3.0 nM), (C) 500 μM Agm, Spm, Spd, rac-2,11-Me2Spm, and 5 μM CTX, and (E) 10 μM AgTx-636 and 5 μM CTX inhibition of 200 nM PNU282987-evoked intracellular calcium concentration [Ca2+]i rise in SH-SY5Y neuroblastoma cells expressing human α7 nAChR. Electrophysiological recordings of (B) “cyclo”R8 (IC50 = 61.8 ± 16.0 nM), (D) rac-2,11-Me2Spm and Spm, and (F) AgTx-636 inhibition (IC50 = 220 ± 22 nM) of ACh (500 μM)-evoked currents in X. laevis oocytes expressing α9α10 nAChR. Each plot point represents data obtained from three independent experiments [(A, B, D, F) mean ± SEM; (C,E) mean ± SD, one-way ANOVA test, Tukey’s test, *p < 0.05].
3.2 Interaction of biogenic polyamines and their analogs with muscle-type, α7 and α9α10 nAChRs
Competitive radioligand analysis with 125I-αBgt was used to test the ability of all selected low-molecular-mass compounds containing amino or guanidino groups (Figure 1), to bind to muscle-type nAChR in T. californica membranes and to human neuronal α7 nAChR expressed in GH4C1 cells (Figure 3; Table 1). Binding capacity of Put, Spd, and Spm increased at longer chain of the polyamine. Spm was the most active at concentrations of 500 and 50 μM on both muscle-type T. californica nAChR (Figure 3A) and human neuronal α7 nAChR (Figure 3B). Binding capacity of Spd to muscle-type nAChR as well as α7 nAChR was more than five-fold lower than that of Spm, while Put was almost inactive. The replacement of the amino group with the guanidino group in some cases is known to enhance pharmacological activity of the compound. Thus, guanidine-containing analogs of amantadine (1-aminoadamantane) and memantine (3,5-dimethyl-1-aminoadamantane), i.e., 1-adamantylguanidine and 3,5-dimethyl-1-adamantylguanidine, have better therapeutic indices than memantine (Gmiro and Serdyuk, 2021). This is in line with the effects of Put and Agm on the muscle-type T. californica nAChR (Figure 3A). Moreover, the longest in chain guanidine-C5 (Gua-C5, Figure 1) was the most active among Agm analogs, although it failed to reach the effectiveness of Spm (Figure 3A). The binding capacity of the six diastereomers of bis-methylated Spm analogs (Figure 1) to muscle-type T. californica nAChR was almost identical, and similar to that of natural Spm (Figure 3C; Supplementary Figure S1; Table 1). The inhibition curves were plotted for two diastereomers of 1,12-Me2Spm and they were almost identical, but differed from that of Spm (Figure 3C), while their IC50 values were very similar (Table 1). Interestingly, the IC50 value for AgTx-636 (Figure 1), the central fragment of which is Spm-like 1,13-diamino-4,8-diazatridecane with N and N′-terminal substituents, was only two times lower compared to that of Spm (Table 1).
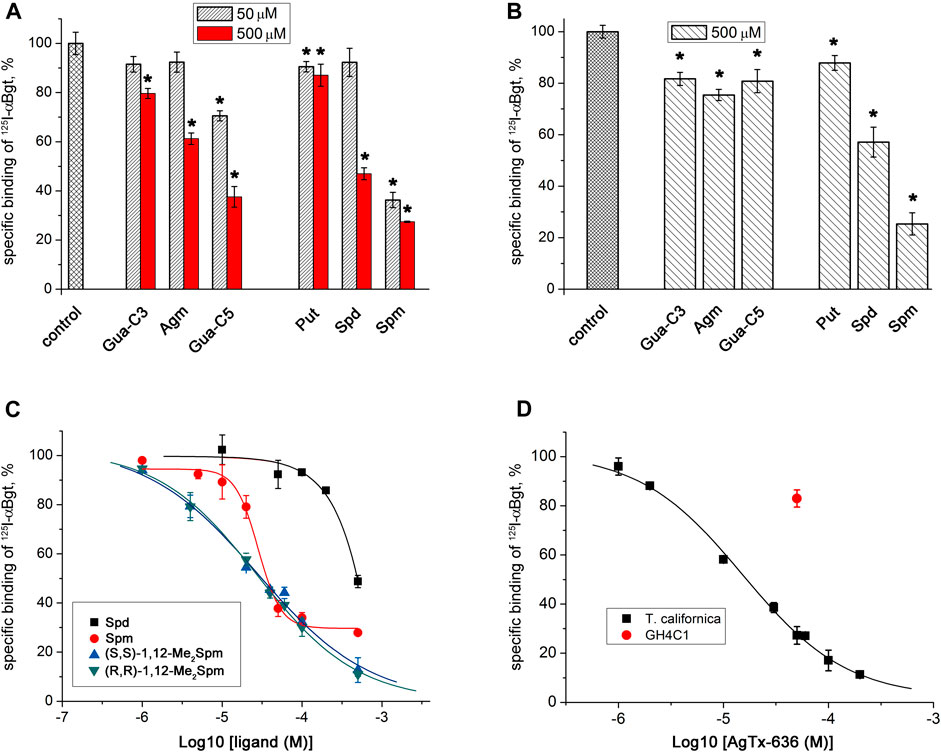
FIGURE 3. Inhibition of 125I-αBgt specific binding to nAChRs by natural polyamines, agmatine and its analogs, diastereomers of bis-methylated Spm, and AgTx-636. Bar presentation of the activity of the compounds against (A) T. californica nAChR and (B) human α7 nAChR in GH4C1 cells. (C) Dose-response curves of inhibition of 125I-αBgt binding to T. californica nAChR by Spd, Spm, and two diastereomers of 1,12-Me2Spm. (D) Radioligand assay of AgTx-636 competition with 125I-αBgt for binding to (■) T. californica nAChR (IC50 = 15.3 ± 1.3 μM) and (●) human α7 nAChR expressed in GH4C1 cells. Specific binding of 125I-αBgt in the absence of compounds was accepted as 100%. Each point is represented as the mean ± SEM of 2–4 independent experiments, Student’s t-test, *p < 0.05. IC50 values are presented in Table 1.
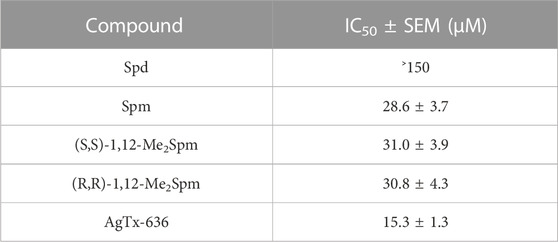
TABLE 1. Competition of Spd, Spm, diastereomers of 1,12-Me2Spm, and AgTx-636 with 125I-αBgt for binding to T. californica nAChR.
Since we observed that Spm, Spd, and Agm can orthosterically bind to human α7 nAChR (competing with 125I-αBgt), we checked their capability to inhibit this receptor subtype. The obtained calcium imaging results showed only a tendency of inhibition (around 20%) of PNU282987-evoked response by Spm and Agm (Figure 2C). Electrophysiological studies showed a very weak inhibition of rat α9α10 nAChR expressed in Xenopus oocytes by rac-2,11-Me2Spm (IC50 ≈ 500 μM, and no inhibition was observed for Spm at 500 μM (Figure 2D).
3.3 Interaction of argiotoxin-636 with muscle-type, α7 and α9α10 nAChRs
We studied the inhibitory activity of AgTx-636 toward both muscle-type and neuronal nAChRs, paying special attention to its interaction with α9α10 subtype. In competitive radioligand analysis we observed inhibition of 125I-αBgt binding to muscle-type T. californica nAChR (IC50 ≈ 15 μM, Table 1). In contrast, its interaction with human α7 nAChR expressed in GH4C1 cells was very weak. At a concentration of 50 μM, AgTx-636 inhibited ≤20% of 125I-αBgt binding to this nAChR subtype (Figure 3D).
According to calcium imaging data, at a concentration of 10 μM, AgTx-636 was able to inhibit the action of a specific α7 nAChR agonist PNU282987 (200 nM) by ≥ 70% (Figure 2E), while α-cobratoxin (CTX) at a lower concentration of 5 μM completely suppressed the activity of this receptor (Figures 2C, E). It is difficult to accurately determine the percentage of the inhibitory effect of AgTx-636, since this toxin alone caused a noticeable [Ca2+]i increase in human neuroblastoma SH-SY5Y cells [without α7 nAChR activation; Figure 2E, bar 4 (AgTx-636 “+”)]. This AgTx-636-evoked [Ca2+]i increase remains visible in the presence of PNU282987 or CTX (Figure 2E, bars 3 and 5). Therefore, in addition to binding to α7 nAChR, AgTx-636 also acts on some other target in SH-SY5Y cells. Such combination of inhibitory and activating effects of AgTx-636 is apparently due to its ability to bind to various molecular targets in neuroblastoma cells. The high-affinity targets of AgTx-636 and other acylpolyamines are calcium-permeable glutamate receptors, which are blocked in the nanomolar range (Hansen et al., 2021). At higher concentrations AgTx-636 and other acylpolyamines affect a range of targets including different ion channels and receptors (Vassilevski et al., 2009). Unfortunately, at this time it is not possible to figure out which target is affected in SH-SY5Y cells. Our electrophysiological study showed an effective inhibition of rat α9α10 nAChR expressed in Xenopus oocytes by AgTx-636 (IC50 = 220 ± 22 nM, Figure 2F).
4 Discussion
Here, we tested the inhibitory activity of the disulfide-fixed octa-arginine “cyclo”R8, a series of cationic amino and guanidino group-containing compounds including biogenic polyamines such as Agm, Put, Spd, and Spm, bis-methylated Spm analogs, and spider venom-derived toxin AgTx-636 against muscle-type, α7 and α9α10 nAChRs. Our attention was mostly focused on the inhibition of α9α10 nAChR, since it opens the possibility to develop new effective drugs reducing neuropathic pain (Hone and McIntosh, 2023; Shelukhina et al., 2023). In this connection testing the interaction of the polyamine-related compounds with α7 nAChR seemed necessary because activation of this receptor subtype is important in the cholinergic anti-inflammatory pathway (Zhou et al., 2022; Shelukhina et al., 2023) and its inhibition would limit possible application of polyamines as probable analgesics. As can be seen from Figure 2A, “cyclo”R8 inhibits α9α10 nAChR almost as effectively as R8 itself (Lebedev et al., 2019). There are also no considerable differences between these two compounds in their inhibition of α7 nAChR (Figure 2B). Thus, a new “cyclic” octa-arginine peptide R8, the N- and C-termini of which are connected by the added cysteine residues with the closed disulfide, preserves the high affinity of the original linear R8 peptide to α9α10 and α7 nAChRs. The resulting activity of “cyclo”R8 can be the basis for creating new compounds sharing such structure with a desired biological activity.
Biogenic polyamines Spd and Spm are essential, ubiquitous organic polycations present in all eukaryotic cells in μM–mM concentrations and vitally important for the differentiation, proliferation and normal functioning of cells (Miller-Fleming et al., 2015; Pegg, 2016). Disturbances of polyamine metabolism are associated with many diseases (Nakanishi and Cleveland, 2021), including malignant tumors, since cancer cells have an elevated level of polyamines (Casero et al., 2018). It is well known that polyamine level is a marker for different neurodegenerative diseases (Seidl et al., 1996; Lewandowski et al., 2010; Inoue et al., 2013; Vrijsen et al., 2023) and corresponding enzymes of polyamine metabolism are potential therapeutic targets. An example of this is the successful application of the combination of DFMO (difluoromethyl ornithine, a specific irreversible inhibitor of ornithine decarboxylase, the rate-limiting enzyme of polyamine biosynthesis) and a polyamine uptake inhibitor AMXT 1501 for the treatment of neuroblastoma (https://www.ccia.org.au/our-impact/a-new-treatment-for-neuroblastoma). Simultaneous blocking of the synthesis and uptake of polyamines with DFMO and AMXT 1501 effectively starves the tumor cells of polyamines, significantly reducing the growth of diffuse intrinsic pontine glioma cells (Khan et al., 2021).
The interaction of C-methylated analogs of Spm with enzymes of Spm catabolism and cancer cells may be modulated by moving the methyl group along the Spm backbone (Khomutov et al., 2019b). The introduction of methyl group(s) into the backbone of polyamine molecule leads to the appearance of a chiral center. This opens the possibility for the regulation of the biochemical properties of polyamine analogs by changing the configuration of thus formed chiral center as it was observed for the enzymes of polyamine metabolism and different cancer cells (Hyvönen et al., 2007; Hyvönen et al., 2011; Hyvönen et al., 2015; Keinänen et al., 2018). Respectively, we assumed that the efficiency of the interaction of C-methylated analogs of Spm with nAChRs might depend on the position of methyl group and/or stereo configuration of chiral centers.
Here, we checked the binding of naturally occurring polyamines, C-methylated analogs of Spm, as well as of agmatine and its analogs to the muscle-type nAChR of T. californica and to neuronal human α7 nAChR (Figure 3A, Figure 3B), the activity of some being tested on human α7 and rat α9α10 nAChRs (Figures 2C, D). In all cases a comparatively weak affinity was found. The activities of Spm and bis-methylated Spm analogs, including diastereomers, were similar toward muscle-type and neuronal α7 nAChRs, and only on α9α10 nAChR rac-2,11-Me2Spm was more potent than Spm, but still remained a very weak inhibitor (Figure 2D, IC50 of about 500 µM). This finding is of importance, since some of the C-methylated Spm derivatives are considered as potential drug candidates, so due to their low affinity there is no need to expect their undesirable effects on nAChRs. Interestingly, for both natural polyamines and agmatine analogs the binding capacity correlated with their chain length: the most active were the compounds with the longest polyamine chain, namely, Spm and diastereomers of Me2Spm.
We found that AgTx-636 inhibited αBgt binding to the muscle-type T. californica nAChR (IC50 ≈ 15 μM, Figure 3D; Table 1), its affinity being close to that of Spm and diastereomers of 1,12-Me2Spm (IC50 ≈ 30 μM, Figure 3C; Table 1). The similar IC50 values may indicate that in this case the polyamine backbone is responsible for the binding efficiency. AgTx-636 showed a very low affinity to the orthosteric ligand-binding site of α7 nAChR, competing only for ≤20% of 125I-αBgt binding sites in GH4C1 cells at 50 μM (Figure 3D). On the contrary, the calcium imaging experiments demonstrated a potent inhibitory action of AgTx-636 on human α7 nAChR in neuroblastoma SH-SY5Y cells (Figure 2E). Probably, in this case AgTx-636 acts as a pore blocker, similarly to its mode of action on glutamate receptors (Twomey et al., 2018). AgTx-636 also produced a weak [Ca2+]i increase independent of nAChR activation or inhibition, suggesting that there is an additional target for the toxin in these cells. The most important result is a comparatively high inhibitory activity of AgTx-636 against rat α9α10 nAChR (IC50 ≈ 200 nM) revealed by electrophysiological analysis (Figure 2F). Thus, in electrophysiological tests AgTx-636 has some advantages as compared to αBgt, which has a similarly high affinity to the muscle-type, α7 and α9α10 nAChRs.
In addition, it is well known that the receptors targeted by polyamine-related compounds, i.e., glutamate receptors and nAChRs, are widely expressed in glial cells. For example, astrocytes express α4* (Ma et al., 2023) and α7 nAChRs (Sharma and Vijayaraghavan, 2001; Fontana et al., 2023), which are involved in the pathogenesis of Alzheimer’s and other diseases. α9 subunit is expressed in glial type-II vestibular hair cells of the inner ear (Kong et al., 2006). Glial cells express functional AMPA and NMDA-type glutamate receptors (Burnashev et al., 1992; Lalo et al., 2006). We therefore anticipate that the compounds analyzed in this communication will be used to study not only neurons but also glia.
We conclude that oligoarginines and some low-molecular-mass polycationic compounds effectively inhibiting α9α10 nAChR may serve as a basis for the development of analgesics to reduce neuropathic pain.
Data availability statement
The raw data supporting the conclusions of this article will be made available by the authors, without undue reservation.
Ethics statement
The animal study was approved by Institutional Animal Care and Use Committee basing on the Institutional Policy on the Use of Laboratory Animals of the Shemyakin-Ovchinnikov Institute of Bioorganic Chemistry RAS (protocol number: 251/2018 26.02.18). The study was conducted in accordance with the local legislation and institutional requirements.
Author contributions
LO: Investigation, Writing–original draft. EK: Investigation, Writing–original draft. NE: Investigation, Writing–original draft. AS: Investigation, Writing–original draft. LE: Investigation, Writing–original draft. DD: Investigation, Writing–original draft. AK: Conceptualization, Supervision, Writing–original draft. DS: Investigation, Writing–original draft. AV: Funding acquisition, Investigation, Resources, Writing–original draft. MK: Investigation, Resources, Supervision, Writing–original draft. VT: Conceptualization, Resources, Supervision, Writing–original draft. IS: Data curation, Investigation, Supervision, Writing–original draft.
Funding
The author(s) declare financial support was received for the research, authorship, and/or publication of this article. This work was supported by the Russian Science Foundation (grant no. 22-14-00395).
Conflict of interest
The authors declare that the research was conducted in the absence of any commercial or financial relationships that could be construed as a potential conflict of interest.
Publisher’s note
All claims expressed in this article are solely those of the authors and do not necessarily represent those of their affiliated organizations, or those of the publisher, the editors and the reviewers. Any product that may be evaluated in this article, or claim that may be made by its manufacturer, is not guaranteed or endorsed by the publisher.
Supplementary material
The Supplementary Material for this article can be found online at: https://www.frontiersin.org/articles/10.3389/fphar.2023.1327603/full#supplementary-material
Abbreviations
nAChR, nicotinic acetylcholine receptor; R8, octa-arginine; CTX, α-cobratoxin; Spm, spermine; Spd, spermidine; Agm, agmatine; Me2Spm, C-methylated spermine; AgTx-636, argiotoxin-636; AMPA, α-amino-3-hydroxy-5-methyl-4-isoxazolepropionate; NMDA, N-methyl-D-aspartate; 125I-αBgt, 125I-α-bungarotoxin.
References
Antonov, S. M., Grishin, E. V., Magazanik, L. G., Shupliakov, O. V., Vesselkin, N. P., and Volkova, T. M. (1987). Argiopin blocks the glutamate responses and sensorimotor transmission in motoneurones of isolated frog spinal cord. Neurosci. Lett. 83, 179–184. doi:10.1016/0304-3940(87)90237-0
Bató, C., Szabó, I., and Bánóczi, Z. (2023). Enhancing cell penetration efficiency of cyclic oligoarginines using rigid scaffolds. Pharmaceutics 15, 1736. doi:10.3390/pharmaceutics15061736
Burnashev, N., Khodorova, A., Jonas, P., Helm, P. J., Wisden, W., Monyer, H., et al. (1992). Calcium-permeable AMPA-kainate receptors in fusiform cerebellar glial cells. Science 256, 1566–1570. doi:10.1126/science.1317970
Casero, R. A., Murray Stewart, T., and Pegg, A. E. (2018). Polyamine metabolism and cancer: treatments, challenges and opportunities. Nat. Rev. Cancer 18, 681–695. doi:10.1038/s41568-018-0050-3
Costa, L., Sousa, E., and Fernandes, C. (2023). Cyclic peptides in pipeline: what future for these great molecules? Pharmaceuticals 16, 996. doi:10.3390/ph16070996
Davies, M. S., Baganoff, M. P., Grishin, E. V., Lanthorn, T. H., Volkova, T. M., Watson, G. B., et al. (1992). Polyamine spider toxins are potent un-competitive antagonists of rat cortex excitatory amino acid receptors. Eur. J. Pharmacol. 227, 51–56. doi:10.1016/0922-4106(92)90141-h
Dhara, M., Matta, J. A., Lei, M., Knowland, D., Yu, H., Gu, S., et al. (2020). Polyamine regulation of ion channel assembly and implications for nicotinic acetylcholine receptor pharmacology. Nat. Commun. 11, 2799. doi:10.1038/s41467-020-16629-3
Dyachenko, I. A., Palikova, Y. A., Palikov, V. A., Korolkova, Y. V., Kazakov, V. A., Egorova, N. S., et al. (2022). α-Conotoxin RgIA and oligoarginine R8 in the mice model alleviate long-term oxaliplatin induced neuropathy. Biochimie 194, 127–136. doi:10.1016/j.biochi.2021.12.013
Edwards, A. B., Mastaglia, F. L., Knuckey, N. W., and Meloni, B. P. (2020). Neuroprotective cationic arginine-rich peptides (CARPs): an assessment of their clinical safety. Drug Saf. 43, 957–969. doi:10.1007/s40264-020-00962-z
Elgoyhen, A. B. (2023). The α9α10 acetylcholine receptor: a non-neuronal nicotinic receptor. Pharmacol. Res. 190, 106735. doi:10.1016/j.phrs.2023.106735
Fang, D., Wang, R., Yu, X., and Tian, Y. (2022). Construction of cyclic cell-penetrating peptides for enhanced penetration of biological barriers. J. Vis. Exp. 22, 64293. doi:10.3791/64293
Fontana, I. C., Kumar, A., and Nordberg, A. (2023). The role of astrocytic α7 nicotinic acetylcholine receptors in Alzheimer disease. Nat. Rev. Neurol. 19, 278–288. doi:10.1038/s41582-023-00792-4
Gmiro, V. E., and Serdyuk, S. E. (2021). Comparison of the pharmacological activity and safety of 1-adamantylguanidine and 3,5-dimethyl-1-adamantylguanidine to those of memantine. Pharm. Chem. J. 54, 1198–1204. doi:10.1007/s11094-021-02343-x
Grigorenko, N. A., Khomutov, A. R., Keinänen, T. A., Järvinen, A., Alhonen, L., Jänne, J., et al. (2007). Synthesis of novel optical isomers of α-methylpolyamines. Tetrahedron 63, 2257–2262. doi:10.1016/j.tet.2006.12.065
Grishin, E. V., Volkova, T. M., Arsen’ev, A. S., Reshetova, O. S., and Onoprienko, V. V. (1986). Structural-functional characteristics of argiopine--the ion channel blockers from the spider Argiope lobata venom. Bioorg Khim 12, 1121–1124.
Grishin, E. V., Volkova, T. M., and Arseniev, A. S. (1989). Isolation and structure analysis of components from venom of the spider Argiope lobata. Toxicon 27, 541–549. doi:10.1016/0041-0101(89)90115-3
Haghighi, A. P., and Cooper, E. (2000). A molecular link between inward rectification and calcium permeability of neuronal nicotinic acetylcholine alpha3beta4 and alpha4beta2 receptors. J. Neurosci. 20, 529–541. doi:10.1523/JNEUROSCI.20-02-00529.2000
Hansen, K. B., Wollmuth, L. P., Bowie, D., Furukawa, H., Menniti, F. S., Sobolevsky, A. I., et al. (2021). Structure, function, and pharmacology of glutamate receptor ion channels. Pharmacol. Rev. 73, 1469–1658. doi:10.1124/pharmrev.120.000131
Hiasa, M., Miyaji, T., Haruna, Y., Takeuchi, T., Harada, Y., Moriyama, S., et al. (2014). Identification of a mammalian vesicular polyamine transporter. Sci. Rep. 4, 6836. doi:10.1038/srep06836
Holbert, C. E., Cullen, M. T., Casero, R. A., and Stewart, T. M. (2022). Polyamines in cancer: integrating organismal metabolism and antitumour immunity. Nat. Rev. Cancer 22, 467–480. doi:10.1038/s41568-022-00473-2
Hone, A. J., and McIntosh, J. M. (2023). Nicotinic acetylcholine receptors: therapeutic targets for novel ligands to treat pain and inflammation. Pharmacol. Res. 190, 106715. doi:10.1016/j.phrs.2023.106715
Hyvönen, M. T., Keinänen, T. A., Cerrada-Gimenez, M., Sinervirta, R., Grigorenko, N., Khomutov, A. R., et al. (2007). Role of hypusinated eukaryotic translation initiation factor 5A in polyamine depletion-induced cytostasis. J. Biol. Chem. 282, 34700–34706. doi:10.1074/jbc.M704282200
Hyvönen, M. T., Keinänen, T. A., Khomutov, M., Simonian, A., Weisell, J., Kochetkov, S. N., et al. (2011). The use of novel C-methylated spermidine derivatives to investigate the regulation of polyamine metabolism. J. Med. Chem. 54, 4611–4618. doi:10.1021/jm200293r
Hyvönen, M. T., Khomutov, M., Petit, M., Weisell, J., Kochetkov, S. N., Alhonen, L., et al. (2015). Enantiomers of 3-methylspermidine selectively modulate deoxyhypusine synthesis and reveal important determinants for spermidine transport. ACS Chem. Biol. 10, 1417–1424. doi:10.1021/cb500938e
Inoue, K., Tsutsui, H., Akatsu, H., Hashizume, Y., Matsukawa, N., Yamamoto, T., et al. (2013). Metabolic profiling of Alzheimer’s disease brains. Sci. Rep. 3, 2364. doi:10.1038/srep02364
Kachel, H. S., Franzyk, H., and Mellor, I. R. (2019). Philanthotoxin analogues that selectively inhibit ganglionic nicotinic acetylcholine receptors with exceptional potency. J. Med. Chem. 62, 6214–6222. doi:10.1021/acs.jmedchem.9b00519
Keinänen, T. A., Grigorenko, N., Khomutov, A. R., Huang, Q., Uimari, A., Alhonen, L., et al. (2018). Controlling the regioselectivity and stereospecificity of FAD-dependent polyamine oxidases with the use of amine-attached guide molecules as conformational modulators. Biosci. Rep. 38, BSR20180527. doi:10.1042/BSR20180527
Keinänen, T. A., Hyvönen, M. T., Alhonen, L., Vepsäläinen, J., and Khomutov, A. R. (2014). Selective regulation of polyamine metabolism with methylated polyamine analogues. Amino Acids 46, 605–620. doi:10.1007/s00726-013-1587-9
Khan, A., Gamble, L. D., Upton, D. H., Ung, C., Yu, D. M. T., Ehteda, A., et al. (2021). Dual targeting of polyamine synthesis and uptake in diffuse intrinsic pontine gliomas. Nat. Commun. 12, 971. doi:10.1038/s41467-021-20896-z
Khomutov, M. A., Hyvönen, M. T., Salikhov, A. I., Chizhov, A. O., Ryzhov, I. M., Kochetkov, S. N., et al. (2020). Synthesis of (3R,10R)- and (3S,10S)-Diastereomers of 3,10-dimethylspermine. Russ. J. Bioorg Chem. 46, 1061–1066. doi:10.1134/S1068162020060126
Khomutov, M. A., Hyvönen, M. T., Simonian, A., Formanovsky, A. A., Mikhura, I. V., Chizhov, A. O., et al. (2019b). Unforeseen possibilities to investigate the regulation of polyamine metabolism revealed by novel C-methylated spermine derivatives. J. Med. Chem. 62, 11335–11347. doi:10.1021/acs.jmedchem.9b01666
Khomutov, M. A., Mikhura, I. V., Kochetkov, S. N., and Khomutov, A. R. (2019a). C-methylated analogs of spermine and spermidine: synthesis and biological activity. Russ. J. Bioorg Chem. 45, 463–487. doi:10.1134/S1068162019060207
Kong, W., Cheng, H., and van Cauwenberge, P. (2006). Expression of nicotinic acetylcholine receptor subunit alpha9 in type II vestibular hair cells of rats. Acta Pharmacol. Sin. 27, 1509–1514. doi:10.1111/j.1745-7254.2006.00423.x
Kryukova, E. V., Egorova, N. S., Kudryavtsev, D. S., Lebedev, D. S., Spirova, E. N., Zhmak, M. N., et al. (2019). From synthetic fragments of endogenous three-finger proteins to potential drugs. Front. Pharmacol. 10, 748. doi:10.3389/fphar.2019.00748
Lalo, U., Pankratov, Y., Kirchhoff, F., North, R. A., and Verkhratsky, A. (2006). NMDA receptors mediate neuron-to-glia signaling in mouse cortical astrocytes. J. Neurosci. 26, 2673–2683. doi:10.1523/JNEUROSCI.4689-05.2006
Lebedev, D. S., Kryukova, E. V., Ivanov, I. A., Egorova, N. S., Timofeev, N. D., Spirova, E. N., et al. (2019). Oligoarginine peptides, a new family of nicotinic acetylcholine receptor inhibitors. Mol. Pharmacol. 96, 664–673. doi:10.1124/mol.119.117713
Lewandowski, N. M., Ju, S., Verbitsky, M., Ross, B., Geddie, M. L., Rockenstein, E., et al. (2010). Polyamine pathway contributes to the pathogenesis of Parkinson disease. Proc. Natl. Acad. Sci. U. S. A. 107, 16970–16975. doi:10.1073/pnas.1011751107
Liu, M., Nakazawa, K., Inoue, K., and Ohno, Y. (1997). Potent and voltage-dependent block by philanthotoxin-343 of neuronal nicotinic receptor/channels in PC12 cells. Br. J. Pharmacol. 122, 379–385. doi:10.1038/sj.bjp.0701373
Ma, W., Si, T., Wang, Z., Wen, P., Zhu, Z., Liu, Q., et al. (2023). Astrocytic α4-containing nAChR signaling in the hippocampus governs the formation of temporal association memory. Cell Rep. 42, 112674. doi:10.1016/j.celrep.2023.112674
MacDougall, G., Anderton, R. S., Edwards, A. B., Knuckey, N. W., and Meloni, B. P. (2017). The neuroprotective peptide poly-arginine-12 (R12) reduces cell surface levels of NMDA NR2B receptor subunit in cortical neurons; investigation into the involvement of endocytic mechanisms. J. Mol. Neurosci. 61, 235–246. doi:10.1007/s12031-016-0861-1
Malpica-Nieves, C. J., Rivera, Y., Rivera-Aponte, D. E., Phanstiel, O., Veh, R. W., Eaton, M. J., et al. (2021). Uptake of biotinylated spermine in astrocytes: effect of Cx43 siRNA, HIV-tat protein and polyamine transport inhibitor on polyamine uptake. Biomolecules 11, 1187. doi:10.3390/biom11081187
Matta, J. A., Gu, S., Davini, W. B., and Bredt, D. S. (2021). Nicotinic acetylcholine receptor redux: discovery of accessories opens therapeutic vistas. Science 373, eabg6539. doi:10.1126/science.abg6539
Meloni, B. P., Mastaglia, F. L., and Knuckey, N. W. (2020). Cationic arginine-rich peptides (CARPs): a novel class of neuroprotective agents with a multimodal mechanism of action. Front. Neurol. 11, 108. doi:10.3389/fneur.2020.00108
Miller-Fleming, L., Olin-Sandoval, V., Campbell, K., and Ralser, M. (2015). Remaining mysteries of molecular biology: the role of polyamines in the cell. J. Mol. Biol. 427, 3389–3406. doi:10.1016/j.jmb.2015.06.020
Mineev, K. S., Kryukova, E. V., Kasheverov, I. E., Egorova, N. S., Zhmak, M. N., Ivanov, I. A., et al. (2020). Spatial structure and activity of synthetic fragments of Lynx1 and of nicotinic receptor loop C models. Biomolecules 11, 1. doi:10.3390/biom11010001
Nakanishi, S., and Cleveland, J. L. (2021). Polyamine homeostasis in development and disease. Med. Sci. (Basel) 9, 28. doi:10.3390/medsci9020028
Nichols, C. G., and Lee, S.-J. (2018). Polyamines and potassium channels: a 25-year romance. J. Biol. Chem. 293, 18779–18788. doi:10.1074/jbc.TM118.003344
Pegg, A. E. (2016). Functions of polyamines in mammals. J. Biol. Chem. 291, 14904–14912. doi:10.1074/jbc.R116.731661
Qiao, C., Yin, N., Gu, H.-Y., Zhu, J.-L., Ding, J.-H., Lu, M., et al. (2016). Atp13a2 deficiency aggravates astrocyte-mediated neuroinflammation via NLRP3 inflammasome activation. CNS Neurosci. Ther. 22, 451–460. doi:10.1111/cns.12514
Rieck, J., Skatchkov, S. N., Derst, C., Eaton, M. J., and Veh, R. W. (2022). Unique chemistry, intake, and metabolism of polyamines in the central nervous system (CNS) and its body. Biomolecules 12, 501. doi:10.3390/biom12040501
Ríos, D. S., Malpica-Nieves, C. J., Díaz-García, A., Eaton, M. J., and Skatchkov, S. N. (2023). Changes in the localization of polyamine spermidine in the rat retina with age. Biomedicines 11, 1008. doi:10.3390/biomedicines11041008
Ruan, R., Chen, M., Zou, L., Wei, P., Liu, J., Ding, W., et al. (2016). Recent advances in peptides for enhancing transdermal macromolecular drug delivery. Ther. Deliv. 7, 89–100. doi:10.4155/tde.15.94
Sala-Rabanal, M., Li, D. C., Dake, G. R., Kurata, H. T., Inyushin, M., Skatchkov, S. N., et al. (2013). Polyamine transport by the polyspecific organic cation transporters OCT1, OCT2, and OCT3. Mol. Pharm. 10, 1450–1458. doi:10.1021/mp400024d
Seidl, R., Beninati, S., Cairns, N., Singewald, N., Risser, D., Bavan, H., et al. (1996). Polyamines in frontal cortex of patients with Down syndrome and Alzheimer disease. Neurosci. Lett. 206, 193–195. doi:10.1016/s0304-3940(96)12451-4
Sharma, G., and Vijayaraghavan, S. (2001). Nicotinic cholinergic signaling in hippocampal astrocytes involves calcium-induced calcium release from intracellular stores. Proc. Natl. Acad. Sci. U. S. A. 98, 4148–4153. doi:10.1073/pnas.071540198
Shelukhina, I., Siniavin, A., Kasheverov, I., Ojomoko, L., Tsetlin, V., and Utkin, Y. (2023). α7-and α9-containing nicotinic acetylcholine receptors in the functioning of immune system and in pain. Int. J. Mol. Sci. 24, 6524. doi:10.3390/ijms24076524
Shelukhina, I. V., Zhmak, M. N., Lobanov, A. V., Ivanov, I. A., Garifulina, A. I., Kravchenko, I. N., et al. (2018). Azemiopsin, a selective peptide antagonist of muscle nicotinic acetylcholine receptor: preclinical evaluation as a local muscle relaxant. Toxins (Basel) 10, 34. doi:10.3390/toxins10010034
Twomey, E. C., Yelshanskaya, M. V., Vassilevski, A. A., and Sobolevsky, A. I. (2018). Mechanisms of channel block in calcium-permeable AMPA receptors. Neuron 99, 956–968. doi:10.1016/j.neuron.2018.07.027
Vassilevski, A. A., Kozlov, S. A., and Grishin, E. V. (2009). Molecular diversity of spider venom. Biochem. (Mosc) 74, 1505–1534. doi:10.1134/s0006297909130069
Vrijsen, S., Houdou, M., Cascalho, A., Eggermont, J., and Vangheluwe, P. (2023). Polyamines in Parkinson’s disease: balancing between neurotoxicity and neuroprotection. Annu. Rev. Biochem. 92, 435–464. doi:10.1146/annurev-biochem-071322-021330
Weiss, T., Bernard, R., Laube, G., Rieck, J., Eaton, M. J., Skatchkov, S. N., et al. (2023). As verified with the aid of biotinylated spermine, the brain cannot take up polyamines from the bloodstream leaving it solely dependent on local biosynthesis. Biomolecules 13, 1114. doi:10.3390/biom13071114
Xie, L., Liu, R., Chen, X., He, M., Zhang, Y., and Chen, S. (2021). Micelles based on lysine, histidine, or arginine: designing structures for enhanced drug delivery. Front. Bioeng. Biotechnol. 9, 744657. doi:10.3389/fbioe.2021.744657
Zhang, B., Ren, M., Yang, F., Li, R., Yu, L., Luo, A., et al. (2022). Oligo-basic amino acids, potential nicotinic acetylcholine receptor inhibitors. Biomed. Pharmacother. 152, 113215. doi:10.1016/j.biopha.2022.113215
Keywords: nicotinic acetylcholine receptor, oligoarginine, polyamines, acylpolyamine, radioligand analysis, electrophysiology, calcium imaging, argiopin
Citation: Ojomoko LO, Kryukova EV, Egorova NS, Salikhov AI, Epifanova LA, Denisova DA, Khomutov AR, Sukhov DA, Vassilevski AA, Khomutov MA, Tsetlin VI and Shelukhina IV (2023) Inhibition of nicotinic acetylcholine receptors by oligoarginine peptides and polyamine-related compounds. Front. Pharmacol. 14:1327603. doi: 10.3389/fphar.2023.1327603
Received: 25 October 2023; Accepted: 30 November 2023;
Published: 15 December 2023.
Edited by:
Murat Oz, Health Science Center, KuwaitReviewed by:
Arik J. Hone, The University of Utah, United StatesSerguei Skatchkov, Central University of the Caribbean, Puerto Rico
Copyright © 2023 Ojomoko, Kryukova, Egorova, Salikhov, Epifanova, Denisova, Khomutov, Sukhov, Vassilevski, Khomutov, Tsetlin and Shelukhina. This is an open-access article distributed under the terms of the Creative Commons Attribution License (CC BY). The use, distribution or reproduction in other forums is permitted, provided the original author(s) and the copyright owner(s) are credited and that the original publication in this journal is cited, in accordance with accepted academic practice. No use, distribution or reproduction is permitted which does not comply with these terms.
*Correspondence: Irina V. Shelukhina, c2hlbHVraGluYS5pdkB5YW5kZXgucnU=