- 1Institute of Medical Technology, Peking University Health Science Center, Beijing, China
- 2Department of Pulmonary and Critical Care Medicine, Peking University Third Hospital, Beijing, China
- 3Department of Infectious Disease, Peking University Third Hospital, Beijing, China
- 4Center of Infectious Disease, Peking University Third Hospital, Beijing, China
Background: The rate of carbapenem-resistant Klebsiella pneumoniae (CRKP) infection has been increasing rapidly worldwide and, poses a significant risk to human health. Effective methods are urgently needed to address treatment failures related to antibiotic resistance. Recent research has reported that some drugs in combination with antibiotics have displayed synergistic killing of resistant bacteria. Here, we investigated whether glutathione (GSH) can synergize with meropenem, and enhance its effectiveness against CRKP.
Methods: Synergistic activity was assessed by checkerboard and time-killing assays. The mechanism of these combinations was assessed by total ROS and membrane permeability assays. The bacterial metabolites were assessed by LC‒MS/MS.
Results: The FICIs of GSH and meropenem were approximately 0.5 and the combined treatment with GSH and meropenem resulted in a more than 2log10 CFU/mL reduction in bacteria compared to the individual treatments. These findings indicated the synergistic effect of the two drugs. Moreover, the meropenem MIC of CRKP was reduced to less than 4 mg/L when combined with 6 mg/mL GSH, indicating that GSH could significantly reverse resistance to meropenem in bacteria. The production of ROS in bacteria was determined by flow cytometry. After adding GSH, the ROS in the GSH group and the combined group was significantly higher than that in the control and meropenem groups, but there was no significant difference between the combined and GSH groups. The metabolic disturbance caused by GSH alone and in combination with meropenem was significant intracellularly and extracellularly, especially in terms of glycerophospholipid metabolism, indicating that the synergistic effect of the combined use of GSH and meropenem was relevant to glycerophospholipid metabolism. In addition, we measured the cell membrane permeability. The cell membrane permeability of the combination group was significantly higher than that of the blank control or monotreatment groups. This confirmed that the GSH can serve as a meropenem enhancers by disturbing glycerophospholipid metabolism and increasing cell membrane permeability.
Conclusion: GSH and meropenem display a synergistic effect, wherein GSH increases the sensitivity of CRKP to meropenem. The synergy and susceptibility effects are thought to related to the increased membrane permeability resulting from the perturbations in glycerophospholipid metabolism, presenting a novel avenue for CRKP treatment.
1 Introduction
Klebsiella pneumoniae is an opportunistic Gram-negative pathogen that frequently causes nosocomial infections in humans and accounts for a significant proportion of hospital-acquired urinary tract infections, pneumonia, and bloodstream infections (Pendleton et al., 2013). In addition to its importance as a nosocomial pathogen, Klebsiella pneumoniae is notorious for its high frequency and diversity of antimicrobial resistance (AMR) genes. In recent years, there has been an increasing global incidence of K. pneumoniae strains with multidrug resistance traits. Carbapenems are considered the preferred antibiotics to treat health care-associated infections caused by ESBL and AmpC-producing Enterobacteriaceae, particularly Klebsiella pneumonia. However, in recent years, carbapenem-resistant Enterobacteriaceae (CRE) has been noted as one of the critical-priority bacteria by the WHO (Tacconelli et al., 2018; Zhen et al., 2021). In particular, carbapenem-resistant K. pneumonia (CRKP) accounted for a large proportion of CRE cases, according to a report from the China CRE Network (Zhang et al., 2018). In addition, CRKP usually exhibits multidrug resistance against most β-lactams, fluoroquinolones and aminoglycoside antibiotics, which complicates the selection of adequate treatments and contributes to overall mortality; this presents a challenge because antimicrobial treatment options remain very restricted.
As efforts to develop antimicrobial agents that can effectively kill MDR bacteria have not been successful thus far, there is an urgent need for drugs that can kill or prevent infection by these bacteria. Recent research has reported that some drugs in combination with antibiotics have displayed synergistic killing against resistant bacteria (Copp et al., 2020; Kim et al., 2020; Liu et al., 2021; Liu et al., 2022). Previous studies revealed that glutamine promoted antibiotic uptake to increase the killing of MDR bacteria, myo-inositol improved the host’s ability to eliminate balofloxacin-resistant Escherichia coli, and L-alanine metabolism conferred aminoglycoside resistance via Na+-NQR (Chen et al., 2015; Jiang et al., 2020; Zhao et al., 2021). These studies revealed the effect of the combination of nonantibacterial substances and antibiotics on the treatment of drug-resistant bacteria and provided a new direction for the treatment of drug-resistant bacteria.
Glutathione (GSH) is an antioxidant synthesized in most Gram-negative bacteria and a few Gram-positive bacteria (Smirnova and Oktyabrsky, 2005). In addition to its role in maintaining the particular reduction potential, GSH is also related to antibacterial activity and biofilm clearance (Klare et al., 2016). Das et al. reported that the growth and biofilm activity of all bacteria decreased by more than 50% under 30 mM exogenous GSH, whereas the effects on different bacteria were different (Das et al., 2019). In addition, exogenous GSH mediated ciprofloxacin protection in Escherichia coli by neutralizing antibiotic-induced oxidative stress, increasing the efflux of antibiotics, and promoting acid shock survival (Goswami and Narayana Rao, 2018). GSH-mediated protection has been observed when used with quinolones and aminoglycosides, such as ciprofloxacin, norfloxacin and spectinomycin (Goswami et al., 2006; Goswami and Narayana Rao, 2018). According to previous studies (Klare et al., 2016; Alharbe et al., 2017; Das et al., 2019), GSH differentially modulates the antibacterial activity of diverse antibiotics in various kinds of bacteria.
However, it is not known whether GSH can restore the activity of meropenem against CRKP. In this study, meropenem in combination with GSH was evaluated against clinical CRKP isolates. In addition, we explored whether the mechanism by which meropenem synergizes with GSH stems from elevated membrane permeability resulting from perturbations in glycerophospholipid metabolism.
2 Methods
2.1 Bacterial strains
This study included 30 CRKP strains isolated from the samples of 30 individual patients. These patients were enrolled from a retrospective cohort study in a tertiary care hospital. All specimens were obtained between February 2020 and February 2021. The isolates were confirmed by the combination of mass spectrometry and the Vitek 2 system (bioMérieux, Marcy-l’Étoile, France). The definition of CR was the resistance of the strains to meropenem or imipenem in antibiotic susceptibility testing (AST) (Magiorakos et al., 2012). The clinical information of these patients was collected, including age, sex, admitted department, isolation time, specimen type, and outcome in 30 days. The study was approved by the Peking University Third Hospital Medical Science Research Ethics Committee (M2021545).
2.2 Antibiotic susceptibility testing
The ASTs of these isolates was conducted by the Vitek 2 system and included the following agents: meropenem, imipenem, ceftazidime, cefepime, piperacillin/tazobactam, cefoperazone/sulbactam, levofloxacin, amikacin, minocycline, and trimethoprim/sulfamethoxazole. The AST results were interpreted according to the Clinical and Laboratory Standards Institute (CLSI) guidelines. Furthermore, the minimum inhibitory concentrations (MICs) of GSH and meropenem were determined by the microbroth dilution method as previously described (Timu et al., 2006). GSH and meropenem were prepared in CAMH broth. A final bacterial suspension of 5 × 105 CFU/mL was added to each well, and the bacteria were incubated with diluted broth for 18 h at 37°C. The MIC was the lowest concentration that inhibited the visible growth of the bacteria.
2.3 Checkerboard assays
The synergy between meropenem and GSH was tested using the checkerboard method (Flamm et al., 2019). Meropenem and GSH were prepared with twofold serial dilutions and mixed to create different combinations of concentrations. The concentrations employed in the checkerboard assays were established based on the MIC of each agent that was previously determined for each specific isolate. A final bacterial suspension of 5 × 105 CFU/mL was added to each well. After incubation for 18 h at 37°C, the fractional inhibitory concentration was calculated as the MIC of the combination divided by that of each compound alone. The sum of the fractional inhibitory concentrations of the two drugs was denoted as the fractional inhibitory concentration index (FICI). The results were interpreted as synergistic (FICI ≤0.5), additive (0.5 < FICI ≤ 1), or indifferent (FICI >1) (Hall et al., 1983; Antonelli et al., 2022).
2.4 Time-killing kinetics assays
A time-killing assay was performed to determine the synergy between GSH and meropenem as described by White et al. (1996) with few modifications. Briefly, overnight cultured bacteria were suspended and diluted to 1 × 106 CFU/mL in CAMH broth. Diluted bacteria were then incubated with GSH (6 mg/mL), meropenem (4 mg/L), and the combination. Aliquots (100 μL) were taken out at 0, 4, 8, 12 and 24 h postinoculation and serially diluted in PBS solution to determine viable counts. Diluted samples (100 μL) were uniformly plated on MH agar plates. After culturing at 37°C for 18 h, the total bacterial counts (CFU/mL) were recorded. Synergy was defined as a ≥2 log10 CFU/mL reduction at 24 h in a combination when compared to the CFU/mL of the most active individual drug (Gómara and Ramón-García, 2019).
2.5 Total ROS assay
2′,7′-dichlorofluorescein diacetate (DCFH-DA) dye was used to measure reactive oxygen species (ROS) levels in CRKP as previously described (Yu and Guo, 2022). In brief, each strain was grown overnight in MH agar at 37°C. A single colony of each strain was inoculated into 5 mL of LB medium for 6–8 h with shaking at 37°C and 200 rpm. The bacteria were collected by 4,000 × g centrifugation, washed with PBS twice, and resuspended to 0.5 MCF. Briefly, the suspension was treated with 4 mg/L meropenem, 6 mg/mL GSH or the combination at 37°C for 2 h. Then, bacteria collected by 4,000 × g centrifugation were washed twice with PBS, followed by the addition of DCFH-DA (10 μM) and incubation in the dark at 37°C for 30 min. After loading the dye, the probe was washed twice with PBS, and the fluorescence intensity was measured by flow cytometry within half an hour.
2.6 Bacterial metabolite extraction
Metabolite extraction was performed as described previously with a few modifications (Peng et al., 2015; Ye et al., 2021). Isolates cultured overnight were inoculated into LB broth at 37°C and incubated at 200 r/min for 6–8 h. The suspension was centrifuged and treated with 4 mg/L meropenem, 6 mg/mL GSH or the combination at 37°C for 4 h. And the bacteria cultured in broth for 4 h without any agent treatment served as a blank control group. The culture was diluted to the same concentration with fresh medium and centrifuged at 5,000 × g for 20 min at 4°C to separate bacteria from the supernatant. Bacteria were then washed twice in PBS at 4°C, and the supernatants were filtered through a 0.2 μm membrane filter. The bacteria and medium were both stored at −80°C until metabolites were extracted with cold methanol. When extracting, equivalent cells or medium were quenched using methanol (containing isotopically-labeled internal standard mixture) at −40°C and collected by centrifugation at 13,800 × g for 15 min. The resulting supernatant was transferred to a fresh glass vial for LC‒MS analysis.
2.7 LC‒MS/MS analysis and data preprocessing
A UHPLC system (Vanquish, Thermo Fisher Scientific) with a UPLC HSS T3 column coupled to an Orbitrap Exploris 120 mass spectrometer (Orbitrap MS, Thermo) was employed to perform LC‒MS/MS analysis. The mobile phase consisted of 5 mmol/L ammonium acetate, 5 mmol/L acetic acid in water (A) and acetonitrile (B). The information-dependent acquisition (IDA) mode was applied to acquire MS/MS spectra by acquisition software (Xcalibur, Thermo). The raw data were converted to the mzXML format by ProteoWizard and processed with XCMS (Sm et al., 2006) for peak detection, extraction, alignment, and integration. Then, an MS2 database (BiotreeDB) was applied for metabolite annotation. The cutoff for annotation was set at 0.3.
2.8 Membrane permeability assay
The treatment of bacteria for this assay was the same as the method for the ROS assay. The membrane permeability of the treated bacteria was measured based on the fluorescence intensity of propidium iodide (PI)-labeling. PI (10 μM) was added to the washed bacterial precipitate and the sample was incubated for 30 min at 37°C after shaking and mixing. Finally, the fluorescence intensity of each sample was detected by flow cytometry.
2.9 Statistical analysis
Statistical analysis was carried out by GraphPad Prism 8.3.5 and SIMCA V16.0.2. Except the data of metabolomic, all data were presented as the mean ± standard deviation. Student’s t-test or one-way ANOVA were used to determine the differences between groups and the differences were significant when p-value is less than 0.05 (*p < 0.05, **p < 0.01, ***p < 0.001). Metabolomic was analyzed by Student’s t-test, principal component analysis (PCA) and orthogonal projections to latent structures-discriminant analysis (OPLS-DA). The metabolites of the GSH, meropenem or the combination group were compared to the blank control group. The cutoff of the differentially expressed metabolites was based on the following two indicators: the p-value of Student’s t-test was less than 0.05, and the variable importance in the projection (VIP) of the OPLS-DA model was greater than 1. Taking Klebsiella pneumoniae subsp. pneumoniae MGH 78578 (serotype K52) as a reference, the screened differentially abundant metabolites were mapped using the KEGG pathway database (http://www.kegg.jp/kegg/pathway.html).
3 Results
3.1 Antimicrobial susceptibility and clinical characteristics of the Klebsiella pneumoniae isolates
The antimicrobial susceptibility results for each isolate are displayed in Table 1. All 30 isolates were resistant to meropenem (MIC, 32–128 mg/L), imipenem (MIC, ≥16 mg/L), cefepime (MIC, ≥32 mg/L), ceftazidime (MIC, 16 to ≥64 mg/L), cefoperazone-sulbactam (MIC, ≥64 mg/L), piperacillin-tazobactam (MIC, ≥128 mg/L), and levofloxacin (MIC, ≥8 mg/L). Most isolates were resistant to amikacin (73.3%; MIC, 2 to ≥16 mg/L). Approximately half of the 30 isolates were resistant to trimethoprim-sulfamethoxazole (56.7%). The lowest resistance rate was observed for minocycline (40.0%). According to the AST results, all isolates had CR phenotypes. These strains were mostly isolated from sputum specimens, primarily originating from the ICU, emergency department, and geriatric wards; these isolates were associated with a 50% mortality rate within 30 days for patients (Table 2).
3.2 Synergistic effect of glutathione and meropenem against CRKP
Most of the 30 isolates exhibited an MIC of 12 mg/mL for GSH (86.7%, Table 1). To precisely delineate the antibacterial efficacy of GSH, 8 strains were randomly selected, and the survival of clones in different concentrations of GSH were measured. Then, the minimum bactericidal concentration (MBC) was recorded. The results revealed that the isolates were not significantly affected by GSH in the range of 0–6 mg/mL (Figure 1A). However, as the concentration escalated from 6 to 9 mg/mL, there was a significant diminution in the surviving colony count. At 9 mg/mL, GSH effectively eradicated 99.9% of the bacteria, indicating an MBC of 9 mg/mL. Importantly, further augmentation of the GSH concentration led to a continued decrease in the bacterial survival rate. These results suggested that exogenous GSH inhibits CRKP growth.
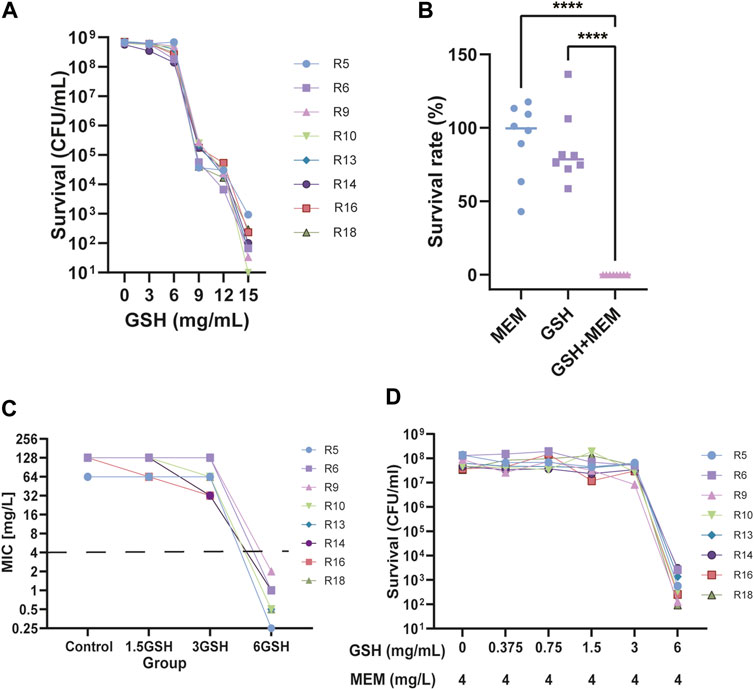
FIGURE 1. Effect of Glutathione (GSH) and meropenem on the growth of CRKP. (A) The number of surviving bacteria after using different concentrations of GSH for 24 h. (B) The survival rate of bacteria treatment with 4 mg/L meropenem (MEM), 6 mg/L GSH and in combination (GSH + MEM) after 24 h ****p < 0.0001. (C) The MIC of meropenem after adding different concentrations of GSH (1.5, 3, and 6 mg/mL). (D) The number of surviving bacteria after the use of 4 mg/L meropenem and different concentrations of GSH (0–6 mg/mL) for 24 h.
To determine whether there was synergy between GSH and meropenem, the FICI for GSH in combination with meropenem was measured. Among the majority of clinical isolates, specifically 86.7% of isolates, the FICI for the combination of GSH and meropenem approached 0.5, denoting an additive effect (Table 3). Conversely, the time-killing assay indicated that GSH or meropenem alone could not inhibit bacterial growth, while the combination of GSH and meropenem significantly antagonized the bacteria after 24 h of exposure, denoting a synergistic effect (Figure 1B). These data together suggest that GSH is synergistic with meropenem in vitro to fight against infections by CRKP.
3.3 Exogenous GSH prominently reverses meropenem resistance in CRKP
To further evaluate the potentiation, the effects of different concentrations of GSH on the sensitivity of meropenem were determined in these clinical isolates. The susceptibility of isolates to meropenem increased when the concentration of GSH was either 1.5 mg/mL or 3 mg/mL, with the maximum improvement reaching up to a 4-fold increase in susceptibility (Figure 1C). Nevertheless, not all isolates demonstrated augmented sensitivity at these particular concentrations. Importantly, the susceptibility of all isolates to meropenem significantly increased (≥32-fold) upon the introduction of 6 mg/mL GSH. This result implies that the potentiation of meropenem sensitivity by GSH was contingent on concentration. To further validate this result, the number of viable bacteria exposed to a 2-fold gradient concentration of GSH combined with 4 mg/L meropenem for 18–24 h was recorded. The results indicated that when combined with 4 mg/L meropenem, neither 1.5 mg/mL nor 3 mg/mL GSH affected the survival rate of bacteria (Figure 1D). Only when the GSH concentration was elevated to 6 mg/mL were the isolates growth significantly suppressed (≥99.9%). The decrease in viable bacteria occurred as early as 4 h after treatment and persisted through 24 h with the combination of 6 mg/mL GSH and 4 mg/L meropenem (Figure 1B; Figure 2).
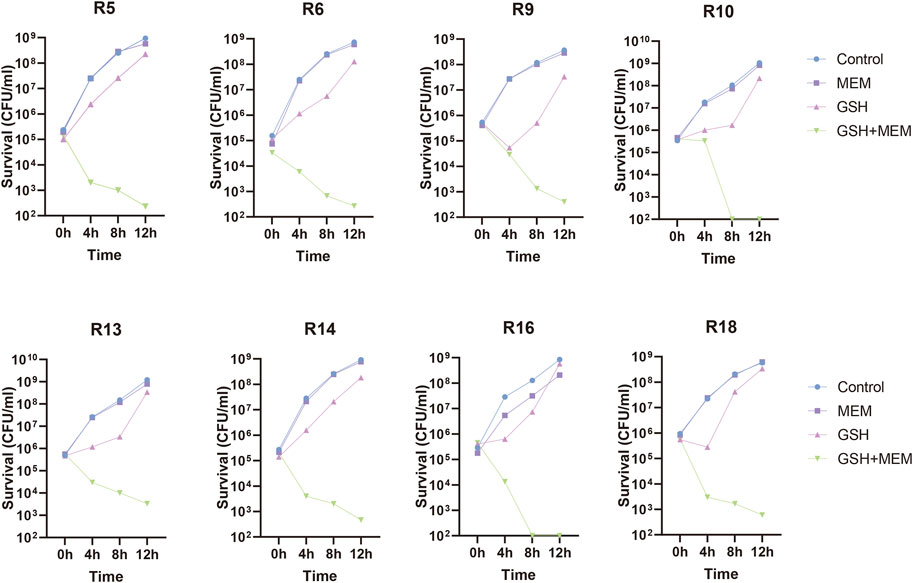
FIGURE 2. Time-killing curves of 8 CRKP treated with 4 mg/L meropenem (MEM), 6 mg/L GSH and in combination (GSH + MEM). Bacteria growing in blank culture medium as control group.
3.4 Exogenous GSH stimulates excess ROS production in CRKP
Recent studies have suggested that oxidative stress due to the increase in ROS caused by antibiotics is a common antimicrobial mechanism (Van Acker and Coenye, 2017; Hong et al., 2019). GSH is an antioxidant that helps maintain the optimum intracellular redox (Masip et al., 2006). The addition of exogenous GSH may cause the original redox homeostasis to be disrupted and increase the production of ROS, thus promoting sterilization. Therefore, we hypothesized that the antimicrobial effects of GSH might result from the overproduction of intracellular ROS. The results indicated that compared to the control group or the group treated with meropenem alone, the bacteria treated with GSH exhibited a significant increase in intracellular ROS levels after a 2 h treatment (Figure 3). Moreover, the combination GSH and meropenem treatment significantly increased the intracellular ROS levels. This result was consistent with the hypothesis that the addition of exogenous GSH promoted the production of ROS in K. pneumoniae. However, although there was a significant difference in bacterial survival between the GSH group and the combination group (Figure 1B), there was no significant difference in ROS production between the two groups, indicating that the bactericidal effect of the GSH-meropenem combination therapy was unlikely to be associated with the increase of ROS production.
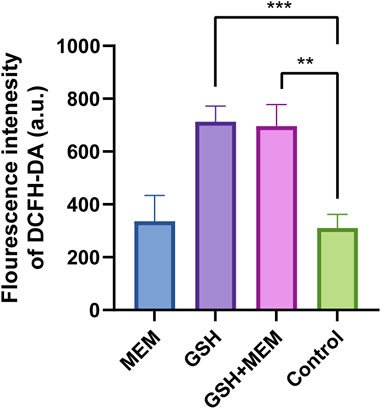
FIGURE 3. Fluorescence intensity of DCFH-DA in each group. MEM, meropenem 4 mg/L; GSH, glutathione 6 mg/mL; GSH + MEM, meropenem 4 mg/L and glutathione 6 mg/mL; Control, untreated. **p < 0.01; ***p < 0.001.
3.5 The combination of GSH and meropenem led to significant metabolic disruptions in CRKP
The resistance mechanisms of bacteria are associated with their metabolic state (Kok et al., 2022). To explore the potential mechanism by which GSH increases the sensitivity of CRKP to meropenem, the metabolic characteristics of strains were assessed. Strains in exponential growth were collected by centrifugation and resuspended in medium with a single drug or two drugs for 4 h, and strains resuspended in blank medium were used as controls. Eight biological replicates were used for each group. A total of 500 and 573 putatively identified metabolites were obtained in the extracellular and intracellular metabolomes, respectively (Supplementary Table 1 and 2). PCA was carried out to assess the quality of samples. All samples were located within Hotelling’s T-squared (95%) ellipse, and all QC samples were clustered together in the PCA score scatter plot (Supplementary Figure 1). OPLS-DA was applied to visualize group separation and identify metabolites with significantly different abundances. The GSH, meropenem or the combination group were clearly distinguished from the control group in the OPLS-DA score plot (Supplementary Figure 2). Significantly differential metabolites were screened, and the corresponding VIP and p-values for each metabolite can be found in Supplementary Materials 2 and 3. Meropenem alone induced minor metabolic changes, while GSH alone significantly changed the abundance of 94 and 59 extracellular and intracellular metabolites, respectively (Figure 4A). However, the results revealed that a total of 125 and 62 metabolites in the external and internal metabolome, respectively, had significant variations in the combination group (Figure 4). These results indicated that the antibacterial effect of the combination of GSH and meropenem may be due to profound changes in bacterial metabolism. Compared to the individual use of GSH, the combination with meropenem resulted in a greater number of differential metabolites in the external metabolome. Thus, the 125 significantly differential metabolites in the external metabolome were mapped to the KEGG database for further analysis to identify the most affected pathways.
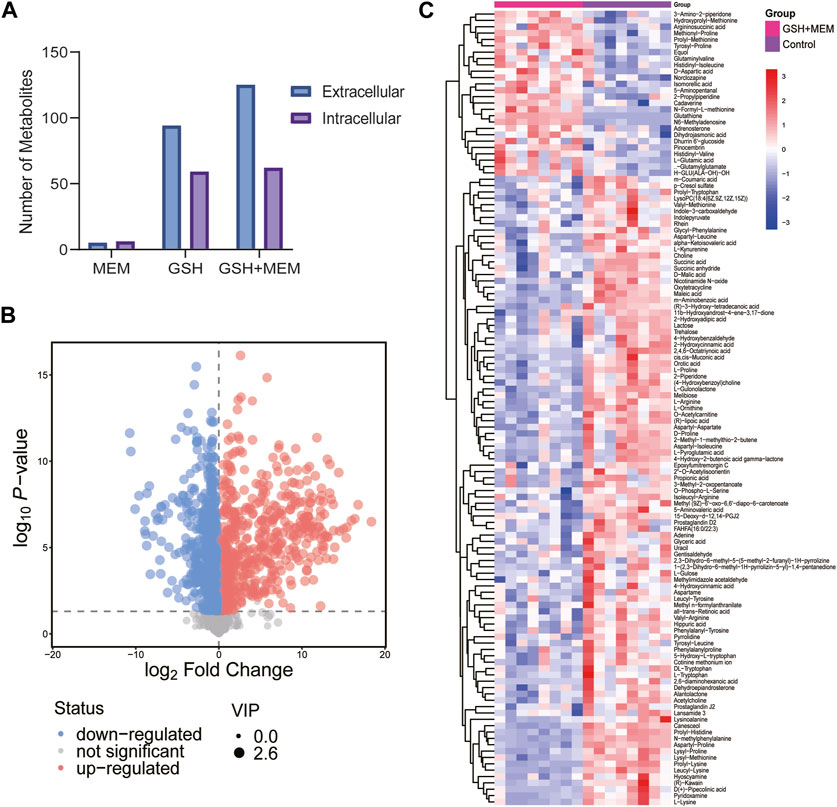
FIGURE 4. Metabolomics analysis results. (A) Number of different changed metabolites in each group. MEM, 4 mg/L meropenem; GSH, 6 mg/L glutathione; GSH + MEM, 6 mg/L glutathione and 4 mg/L meropenem. (B) Volcano plot of extracellular metabolites for the combination group vs. control group. (C) Clustered heat map profiles of the relative abundance for significantly affected extracellular metabolites in CRKP. Blue = significant decrease, red = significant increase. The metabolites with VIP>1 and p < 0.05 were considered as significantly changed metabolites.
3.6 KEGG pathway analysis of the significantly differential metabolites
According to the annotation results, the significantly differential metabolites were mainly involved in amino acid metabolism, carbohydrate metabolism, lipid metabolism, membrane transport and translation (Figure 5A). Then, the differential abundance scores (DA scores) were calculated, revealing that glycerophospholipid metabolism; glycine, serine and threonine metabolism; arginine and proline metabolism; ABC transporters; aminoacyl-tRNA biosynthesis; D-amino acid metabolism; and butanoate metabolism in the combination group were significantly inhibited (Figure 5B). In contrast, alanine, aspartate and glutamate metabolism, arginine biosynthesis and lysine degradation were promoted. KEGG analysis revealed the involvement of the differentially abundant metabolites in a spectrum of metabolic pathways, encompassing multiple amino acid metabolism pathways, membrane transport pathways and translation. More importantly, their substantial influence on glycerophospholipid metabolism, which was related to antibiotic sterilization, was noted (Dalebroux, 2017; Dias et al., 2018).
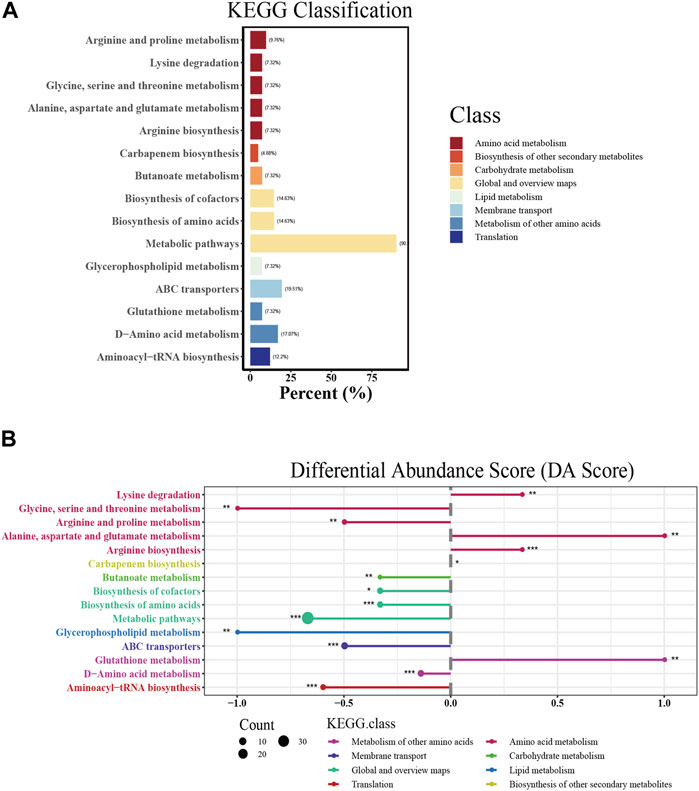
FIGURE 5. KEGG pathway analysis of significantly affected extracellular metabolites. (A) KEGG classification of each pathway. The abscissa represents the percentage of annotated metabolites in a certain pathway compared to all annotated metabolites. (B) Differential abundance score (DA score) of each pathway. The size of the dot indicates the number of significantly affected metabolites annotated in the pathway. DA score reflects the overall changes of all metabolites in the metabolic pathway. A score of 1 indicates an upward trend in the expression of all annotated metabolites in the pathway, while −1 indicates a downward trend in the expression of all annotated metabolites in the pathway. The length of the line segment represents the absolute value of DA score.
3.7 GSH disrupted glycerophospholipid metabolism and increased membrane permeability in CRKP
Glycerophospholipids constitute vital elements within the dual-membrane envelope of Gram-negative bacteria (Dalebroux, 2017). In this study, GSH and meropenem significantly interfered with the metabolism of glycerophospholipids (Figure 5B); thus, we performed a further analysis of the intracellular metabolome. In the internal metabolome, two types of phosphatidylserines (PSs) were identified. In both the GSH monotherapy and combined meropenem treatment groups, these two PSs exhibited significant reductions, while there were no substantial changes observed in the meropenem monotherapy group (Figure 6A). Phosphatidylethanolamine (PE) is the most abundant membrane phospholipid within prokaryotic cells, which originates from the decarboxylation of PS (Vance and Steenbergen, 2005). Noteworthy differences were observed in three PEs in this study. Notably, PE (2:0/16:1) exhibited a marked reduction in both the GSH and combination groups. Conversely, within the combination group, both PE (2:0/18:1) and PE (14:0/16:1) were substantially elevated. Meanwhile, a PE (2:0/18:1) increase was only discernible in the GSH group (Figure 6A). The decrease in PS and the increase in PE were more notable in the combination group than in the GSH group. The aminoacylation of phosphatidylglycerol (PG) constitutes a crucial mechanism that is employed by bacteria to withstand environmental stress (Slavetinsky et al., 2017). The results revealed a significant reduction in various PGs in both the GSH and combination groups. Particularly, the levels of PG (18:1/19:1) and PG (18:0/17:1) were reduced. Intriguingly, the GSH group exhibited a marginally greater increase in PG levels when compared to the combination group, which is different from the change in PS and PE.
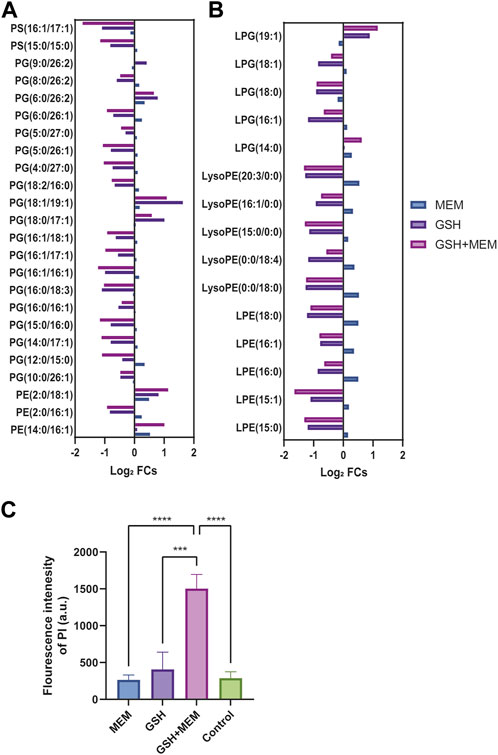
FIGURE 6. Glycerophospholipid metabolism and membrane permeability. (A) The log2 fold change of glycerophospholipids in each group. (B) The log2 fold change of lysophospholipids in each group. (C) The fluorescence intensity of PI in each group. Control, untreated; MEM, 4 mg/L meropenem; GSH, 6 mg/L glutathione; GSH + MEM, 6 mg/L glutathione and 4 mg/L meropenem. ***p < 0.001; ****p < 0.0001.
Lysophospholipids, which usually make a contribution of less than 1% to the formation of the bacterial envelope, can also be converted into PG, PE, and cardiolipin (Zheng et al., 2017). The results clearly demonstrated the contrasting effects of GSH or combined treatment versus meropenem on lysophosphatidylethanolamine (LPE) and lysophosphatidylglycerol (LPG). GSH and combined treatment primarily reduced their levels, while meropenem led to a slight increase (Figure 6B).
When considering that GSH combination with meropenem could disrupt glycerophospholipid metabolism, we further assessed its impact on the membrane permeability in CRKP. Consistent with previous studies, the fluorescence intensity of PI was used to label the membrane permeability of CRKP (Song et al., 2020). The results clearly showed that enhanced membrane permeability occurred solely when GSH was employed in conjunction with meropenem, whereas there was no such change when either GSH or meropenem was administered individually (Figure 6C).
4 Discussion
This study demonstrated that exogenous GSH had a synergistic effect with meropenem in vitro. Interestingly, this change mainly resulted from the increased sensitivity to meropenem rather than GSH activity. In addition, this study provides some indications of the underlying mechanisms. Interestingly, the metabolomics results showed that the synergistic effect was related to metabolic disorders, especially the substantial influence on glycerophospholipid metabolism, which significantly increased membrane permeability.
It has been reported that the mortality rate of MDR K. pneumoniae infection is higher than 40%–50% and these infections represent a major challenge in the field of infectious disease treatment (Xu et al., 2017). Appropriate treatment is essential for controlling MDR K. pneumoniae infection, especially in critically ill patients (Seo et al., 2020). However, due to the emergence of CRKP, treatment options are limited, so the choice of appropriate treatment has become a problem. Antibiotic combination therapy has been the most widely used during recent years. Despite resistance to carbapenem, some studies confirmed the effectiveness of meropenem-based regimens in the treatment of these infections (Daikos et al., 2014). Interestingly, some studies have revealed that the combination of non-antibiotic drugs and antibiotics that could confront MDR bacteria (Liu et al., 2019; Liu et al., 2021). Previous studies have shown that GSH alone mediated antibacterial effectiveness in E. coli, Pseudomonas aeruginosa and Acinetobacter baumannii with a dose-dependent manner (Goswami and Jawali, 2007; Klare et al., 2016; Alharbe et al., 2017; Das et al., 2019). Similar to previous studies, our research indicated that GSH has a bactericidal effect in K. pneumoniae. Furthermore, GSH and meropenem exhibited a synergistic effect. However, this study mainly focused on CRKP, the effect of the GSH-meropenem combination should be evaluated in a broader range of antibiotic-resistant bacteria. This result was similar to that of Goswami et al., which shows that GSH can be used as a synergist with ampicillin and penicillin (Goswami and Jawali, 2007). Additionally, we evaluated the ability of GSH to promote the sensitivity to meropenem. Surprisingly, at 6 mg/mL, GSH can reduce the MIC of meropenem to 2 mg/L, although the initial MIC of meropenem was greater than 64 mg/L. When 6 mg/mL GSH was used in combination with 4 mg/L meropenem, it can kill bacteria as early as 8 h after treatment. Previous studies have shown that 30 mM GSH could act as a biofilm disruptor in many bacterial species (Das et al., 2019). In our study, the effective concentration of GSH for the synergistic and sensitization effects was 6 mg/mL (20 mM), and the effective concentration when used alone was between 6 and 12 mg/mL (20–40 mM), similar to previous results.
GSH systems are a major thiol-dependent systems that provide promising antioxidant capacity targets for novel antibiotic development (Song et al., 2021). Recent studies have revealed that ebselen, auranofin and combining ebselen and silver exhibited a strong synergistic effect against MDR Gram-negative bacteria (Ren et al., 2020). The addition of exogenous GSH may disrupt the balance of the GSH system. GSH metabolism was analyzed in this study based on metabolic results and total ROS. The metabolic results of GSH alone or in combination with meropenem showed significant changes in the GSH metabolism pathway, and the total intracellular ROS increased. The change in the GSH pathway was consistent with the increase in ROS. However, the lack of a significant difference in ROS between the combination group and the GSH monotherapy group indicated that ROS may not have a significant correlation with this synergistic effect, and the specific mechanism needs further research. This results were similar to research on GSH-mediated augmentation of ampicillin and penicillin antibacterial activity against E. coli that showed that this effect was independent of its redox status outside the cell and the status of gamma glutamyl-transepeptidase (Goswami and Jawali, 2007).
Glycerophospholipid alterations can result from bacterial resistance and can also serve as the fundamental mechanism contributing to the synergy of drugs (Aye et al., 2020; Song et al., 2020). Song et al. reported that SLAP-S25 reverses the resistance of MDR Gram-negative pathogens by binding to both lipopolysaccharide and PG and triggering membrane damage to potentiate antibiotic efficacy (Song et al., 2020). In this study, we observed that the combined use of GSH and meropenem intensified the alterations in glycerophospholipid levels. This was confirmed by the increase in cell membrane permeability. Bacterial membrane damage has been reported boosting the action of antibiotics (Cai et al., 2023). These results suggest that the mechanism of synergy may involve multiple changes in glycerophospholipid levels, which led to an increase in membrane permeability. However, the specific mechanism needs further research. In addition, GSH in combination with meropenem also impacted the metabolism of multiple amino acids, which is related to the synthetic peptidoglycan skeleton (Tsai et al., 2016; Antonoplis et al., 2019; Charlier and Bervoets, 2019; Pereira et al., 2020; Miyamoto and Homma, 2021), indicating that the sensitizing effect of GSH on meropenem may be related to the inhibition of cell wall synthesis.
Although we investigated the synergistic effects of GSH and meropenem against CRKP in vitro, this study has certain limitations. One limitation is that further in vivo experiments are still needed. In addition, while the findings are promising, clinical validation in real-world patient populations is necessary to confirm the effectiveness and safety of the GSH-meropenem combination. However, we hope that future work will show that GSH-meropenem combination is a promising treatment candidate to improve the treatment of drug-resistant bacteria in the clinic.
5 Conclusion
In this study, the antibacterial effect of GSH was verified in CRKP isolated from the clinic, and the sensitizing effect of GSH on meropenem was verified. The possible mechanism of this sensitization was the increase in membrane permeability due to alterations to glycerophospholipids. The above results provide a new direction for the treatment of CRKP.
Data availability statement
The original contributions presented in the study are included in the article/Supplementary Material, further inquiries can be directed to the corresponding author.
Ethics statement
The studies involving humans were approved by the Peking University Third Hospital Medical Science Research Ethics Committee. The studies were conducted in accordance with the local legislation and institutional requirements. Written informed consent for participation was not required from the participants or the participants’ legal guardians/next of kin in accordance with the national legislation and institutional requirements.
Author contributions
JY: Methodology, Formal Analysis, Visualization, Writing–original draft, Writing–review and editing. CL: Resources, Formal Analysis, Methodology, Writing–original draft, Writing–review and editing. PY: Resources, Formal Analysis, Writing–original draft, Writing–review and editing. ZW: Formal Analysis, Methodology, Writing–review and editing. CD: Formal Analysis, Writing–review and editing. NS: Project administration, Formal Analysis, Funding acquisition, Validation, Supervision, Writing–review and editing.
Funding
The author(s) declare financial support was received for the research, authorship, and/or publication of this article. This work was supported by Key clinical project of Peking University Third Hospital (BYSYZD2022007), Clinical cohort project of Peking University Third Hospital (BYSYDL2019007) and National Natural Science Foundation of China (82200012).
Conflict of interest
The authors declare that the research was conducted in the absence of any commercial or financial relationships that could be construed as a potential conflict of interest.
Publisher’s note
All claims expressed in this article are solely those of the authors and do not necessarily represent those of their affiliated organizations, or those of the publisher, the editors and the reviewers. Any product that may be evaluated in this article, or claim that may be made by its manufacturer, is not guaranteed or endorsed by the publisher.
Supplementary material
The Supplementary Material for this article can be found online at: https://www.frontiersin.org/articles/10.3389/fphar.2023.1327230/full#supplementary-material
References
Alharbe, R., Almansour, A., and Kwon, D. H. (2017). Antibacterial activity of exogenous glutathione and its synergism on antibiotics sensitize carbapenem-associated multidrug resistant clinical isolates of Acinetobacter baumannii. Int. J. Med. Microbiol. 307, 409–414. doi:10.1016/j.ijmm.2017.07.009
Antonelli, A., Coppi, M., Tellapragada, C., Hasan, B., Maruri, A., Gijón, D., et al. (2022). Isothermal microcalorimetry vs checkerboard assay to evaluate in-vitro synergism of meropenem-amikacin and meropenem-colistin combinations against multi-drug-resistant Gram-negative pathogens. Int. J. Antimicrob. Agents 60, 106668. doi:10.1016/j.ijantimicag.2022.106668
Antonoplis, A., Zang, X., Wegner, T., Wender, P. A., and Cegelski, L. (2019). Vancomycin-arginine conjugate inhibits growth of carbapenem-resistant E. coli and targets cell-wall synthesis. ACS Chem. Biol. 14, 2065–2070. doi:10.1021/acschembio.9b00565
Aye, S. M., Galani, I., Han, M.-L., Karaiskos, I., Creek, D. J., Zhu, Y., et al. (2020). Lipid A profiling and metabolomics analysis of paired polymyxin-susceptible and -resistant MDR Klebsiella pneumoniae clinical isolates from the same patients before and after colistin treatment. J. Antimicrob. Chemother. 75, 2852–2863. doi:10.1093/jac/dkaa245
Cai, J., Shi, J., Chen, C., He, M., Wang, Z., and Liu, Y. (2023). Structural-activity relationship-inspired the discovery of saturated fatty acids as novel colistin enhancers. Adv. Sci. (Weinh). 10, e2302182. doi:10.1002/advs.202302182
Charlier, D., and Bervoets, I. (2019). Regulation of arginine biosynthesis, catabolism and transport in Escherichia coli. Amino Acids 51, 1103–1127. doi:10.1007/s00726-019-02757-8
Chen, X.-H., Zhang, B.-W., Li, H., and Peng, X.-X. (2015). Myo-inositol improves the host’s ability to eliminate balofloxacin-resistant Escherichia coli. Sci. Rep. 5, 10720. doi:10.1038/srep10720
Copp, J. N., Pletzer, D., Brown, A. S., Van der Heijden, J., Miton, C. M., Edgar, R. J., et al. (2020). Mechanistic understanding enables the rational design of salicylanilide combination therapies for gram-negative infections. mBio 11, 02068–e2120. doi:10.1128/mBio.02068-20
Daikos, G. L., Tsaousi, S., Tzouvelekis, L. S., Anyfantis, I., Psichogiou, M., Argyropoulou, A., et al. (2014). Carbapenemase-producing Klebsiella pneumoniae bloodstream infections: lowering mortality by antibiotic combination schemes and the role of carbapenems. Antimicrob. Agents Chemother. 58, 2322–2328. doi:10.1128/AAC.02166-13
Dalebroux, Z. D. (2017). Cues from the membrane: bacterial glycerophospholipids. J. Bacteriol. 199, 00136–e217. doi:10.1128/JB.00136-17
Das, T., Paino, D., Manoharan, A., Farrell, J., Whiteley, G., Kriel, F. H., et al. (2019). Conditions under which glutathione disrupts the biofilms and improves antibiotic efficacy of both ESKAPE and non-ESKAPE species. Front. Microbiol. 10, 2000. doi:10.3389/fmicb.2019.02000
Dias, C., Pais, J. P., Nunes, R., Blázquez-Sánchez, M.-T., Marquês, J. T., Almeida, A. F., et al. (2018). Sugar-based bactericides targeting phosphatidylethanolamine-enriched membranes. Nat. Commun. 9, 4857. doi:10.1038/s41467-018-06488-4
Flamm, R. K., Rhomberg, P. R., Lindley, J. M., Sweeney, K., Ellis-Grosse, E. J., and Shortridge, D. (2019). Evaluation of the bactericidal activity of fosfomycin in combination with selected antimicrobial comparison agents tested against gram-negative bacterial strains by using time-kill curves. Antimicrob. Agents Chemother. 63, 02549–e2618. doi:10.1128/AAC.02549-18
Gómara, M., and Ramón-García, S. (2019). The FICI paradigm: correcting flaws in antimicrobial in vitro synergy screens at their inception. Biochem. Pharmacol. 163, 299–307. doi:10.1016/j.bcp.2019.03.001
Goswami, M., and Jawali, N. (2007). Glutathione-mediated augmentation of b-lactam antibacterial activity against Escherichia coli. J. Antimicrob. Chemother. 5, 185. doi:10.1093/jac/dkm121
Goswami, M., Mangoli, S. H., and Jawali, N. (2006). Involvement of reactive oxygen species in the action of ciprofloxacin against Escherichia coli. Antimicrob. Agents Chemother. 50, 949–954. doi:10.1128/AAC.50.3.949-954.2006
Goswami, M., and Narayana Rao, AVSS (2018). Transcriptome profiling reveals interplay of multifaceted stress response in Escherichia coli on exposure to glutathione and ciprofloxacin. mSystems 3, e00001-18. doi:10.1128/msystems.00001-18
Hall, M. J., Middleton, R. F., and Westmacott, D. (1983). The fractional inhibitory concentration (FIC) index as a measure of synergy. J. Antimicrob. Chemother. 11, 427–433. doi:10.1093/jac/11.5.427
Hong, Y., Zeng, J., Wang, X., Drlica, K., and Zhao, X. (2019). Post-stress bacterial cell death mediated by reactive oxygen species. Proc. Natl. Acad. Sci. U. S. A. 116, 10064–10071. doi:10.1073/pnas.1901730116
Jiang, M., Kuang, S.-F., Lai, S.-S., Zhang, S., Yang, J., Peng, B., et al. (2020). Na+-NQR confers aminoglycoside resistance via the regulation of l-alanine metabolism. mBio 11, 02086–e2120. doi:10.1128/mBio.02086-20
Kim, S., Woo, J. H., Jun, S. H., Moon, D. C., Lim, S.-K., and Lee, J. C. (2020). Synergy between florfenicol and aminoglycosides against multidrug-resistant Escherichia coli isolates from livestock. Antibiot. (Basel) 9, 185. doi:10.3390/antibiotics9040185
Klare, W., Das, T., Ibugo, A., Buckle, E., Manefield, M., and Manos, J. (2016). Glutathione-disrupted biofilms of clinical Pseudomonas aeruginosa strains exhibit an enhanced antibiotic effect and a novel biofilm transcriptome. Antimicrob. Agents Chemother. 60, 4539–4551. doi:10.1128/AAC.02919-15
Kok, M., Maton, L., van der Peet, M., Hankemeier, T., and van Hasselt, J. G. C. (2022). Unraveling antimicrobial resistance using metabolomics. Drug Discov. Today 27, 1774–1783. doi:10.1016/j.drudis.2022.03.015
Liu, S., She, P., Li, Z., Li, Y., Li, L., Yang, Y., et al. (2022). Drug synergy discovery of tavaborole and aminoglycosides against Escherichia coli using high throughput screening. Amb. Express 12, 151. doi:10.1186/s13568-022-01488-6
Liu, Y., Li, R., Xiao, X., and Wang, Z. (2019). Antibiotic adjuvants: an alternative approach to overcome multi-drug resistant Gram-negative bacteria. Crit. Rev. Microbiol. 45, 301–314. doi:10.1080/1040841X.2019.1599813
Liu, Y., Tong, Z., Shi, J., Li, R., Upton, M., and Wang, Z. (2021). Drug repurposing for next-generation combination therapies against multidrug-resistant bacteria. Theranostics 11, 4910–4928. doi:10.7150/thno.56205
Magiorakos, A. P., Srinivasan, A., Carey, R. B., Carmeli, Y., Falagas, M. E., Giske, C. G., et al. (2012). Multidrug-resistant, extensively drug-resistant and pandrug-resistant bacteria: an international expert proposal for interim standard definitions for acquired resistance. Clin. Microbiol. Infect. 18, 268–281. doi:10.1111/j.1469-0691.2011.03570.x
Masip, L., Veeravalli, K., and Georgiou, G. (2006). The many faces of glutathione in bacteria. Antioxid. Redox Signal 8, 753–762. doi:10.1089/ars.2006.8.753
Miyamoto, T., and Homma, H. (2021). D-Amino acid metabolism in bacteria. J. Biochem. 170, 5–13. doi:10.1093/jb/mvab043
Pendleton, J. N., Gorman, S. P., and Gilmore, B. F. (2013). Clinical relevance of the ESKAPE pathogens. Expert Rev. Anti Infect. Ther. 11, 297–308. doi:10.1586/eri.13.12
Peng, B., Su, Y.-B., Li, H., Han, Y., Guo, C., Tian, Y.-M., et al. (2015). Exogenous alanine and/or glucose plus kanamycin kills antibiotic-resistant bacteria. Cell Metab. 21, 249–262. doi:10.1016/j.cmet.2015.01.008
Pereira, M. F., Rossi, C. C., da Silva, G. C., Rosa, J. N., and Bazzolli, D. M. S. (2020). Galleria mellonella as an infection model: an in-depth look at why it works and practical considerations for successful application. Pathog. Dis. 78, ftaa056. doi:10.1093/femspd/ftaa056
Ren, X., Zou, L., and Holmgren, A. (2020). Targeting bacterial antioxidant systems for antibiotics development. Curr. Med. Chem. 27, 1922–1939. doi:10.2174/0929867326666191007163654
Seo, H., Lee, S. C., Chung, H., Ra, S. H., Sung, H., Kim, M.-N., et al. (2020). Clinical and microbiological analysis of risk factors for mortality in patients with carbapenem-resistant Enterobacteriaceae bacteremia. Int. J. Antimicrob. Agents 56, 106126. doi:10.1016/j.ijantimicag.2020.106126
Slavetinsky, C., Kuhn, S., and Peschel, A. (2017). Bacterial aminoacyl phospholipids - biosynthesis and role in basic cellular processes and pathogenicity. Biochim. Biophys. Acta Mol. Cell Biol. Lipids 1862, 1310–1318. doi:10.1016/j.bbalip.2016.11.013
Smirnova, G. V., and Oktyabrsky, O. N. (2005). Glutathione in bacteria. Biochem. (Mosc) 70, 1199–1211. doi:10.1007/s10541-005-0248-3
Smith, C. A., Want, E. J., O’Maille, G., Abagyan, R., and Siuzdak, G. (2006). XCMS: processing mass spectrometry data for metabolite profiling using nonlinear peak alignment, matching, and identification. Anal. Chem. 78, 779–787. doi:10.1021/ac051437y
Song, M., Liu, Y., Huang, X., Ding, S., Wang, Y., Shen, J., et al. (2020). A broad-spectrum antibiotic adjuvant reverses multidrug-resistant Gram-negative pathogens. Nat. Microbiol. 5, 1040–1050. doi:10.1038/s41564-020-0723-z
Song, Y., Zhou, Z., Gu, J., Yang, J., and Deng, J. (2021). Reducing the periplasmic glutathione content makes Escherichia coli resistant to trimethoprim and other antimicrobial drugs. Microbiol. Spectr. 9, e0074321. doi:10.1128/Spectrum.00743-21
Tacconelli, E., Carrara, E., Savoldi, A., Harbarth, S., Mendelson, M., Monnet, D. L., et al. (2018). Discovery, research, and development of new antibiotics: the WHO priority list of antibiotic-resistant bacteria and tuberculosis. Lancet Infect. Dis. 18, 318–327. doi:10.1016/S1473-3099(17)30753-3
Timurkaynak, F., Can, F., Azap, O. K., Demirbilek, M., Arslan, H., and Karaman, S. O. (2006). In vitro activities of non-traditional antimicrobials alone or in combination against multidrug-resistant strains of Pseudomonas aeruginosa and Acinetobacter baumannii isolated from intensive care units. Int. J. Antimicrob. Agents 27, 224–228. doi:10.1016/j.ijantimicag.2005.10.012
Tsai, C. J.-Y., Loh, J. M. S., and Proft, T. (2016). Galleria mellonella infection models for the study of bacterial diseases and for antimicrobial drug testing. Virulence 7, 214–229. doi:10.1080/21505594.2015.1135289
Van Acker, H., and Coenye, T. (2017). The role of reactive oxygen species in antibiotic-mediated killing of bacteria. Trends Microbiol. 25, 456–466. doi:10.1016/j.tim.2016.12.008
Vance, J., and Steenbergen, R. (2005). Metabolism and functions of phosphatidylserine. Prog. Lipid Res. 44, 207–234. doi:10.1016/j.plipres.2005.05.001
White, R. L., Burgess, D. S., Manduru, M., and Bosso, J. A. (1996). Comparison of three different in vitro methods of detecting synergy: time-kill, checkerboard, and E test. Antimicrob. Agents Chemother. 40, 1914–1918. doi:10.1128/AAC.40.8.1914
Xu, L., Sun, X., and Ma, X. (2017). Systematic review and meta-analysis of mortality of patients infected with carbapenem-resistant Klebsiella pneumoniae. Ann. Clin. Microbiol. Antimicrob. 16, 18. doi:10.1186/s12941-017-0191-3
Ye, D., Li, X., Wang, C., Liu, S., Zhao, L., Du, J., et al. (2021). Improved sample preparation for untargeted metabolomics profiling of Escherichia coli. Microbiol. Spectr. 9, e0062521. doi:10.1128/Spectrum.00625-21
Yu, Z., and Guo, J. (2022). Non-caloric artificial sweeteners exhibit antimicrobial activity against bacteria and promote bacterial evolution of antibiotic tolerance. J. Hazard Mater 433, 128840. doi:10.1016/j.jhazmat.2022.128840
Zhang, Y., Wang, Q., Yin, Y., Chen, H., Jin, L., Gu, B., et al. (2018). Epidemiology of carbapenem-resistant Enterobacteriaceae infections: report from the China CRE Network. Antimicrob. Agents Chemother. 62, 01882–e1917. doi:10.1128/AAC.01882-17
Zhao, X.-L., Chen, Z.-G., Yang, T.-C., Jiang, M., Wang, J., Cheng, Z.-X., et al. (2021). Glutamine promotes antibiotic uptake to kill multidrug-resistant uropathogenic bacteria. Sci. Transl. Med. 13, eabj0716. doi:10.1126/scitranslmed.abj0716
Zhen, X., Stålsby Lundborg, C., Sun, X., Zhu, N., Gu, S., and Dong, H. (2021). Economic burden of antibiotic resistance in China: a national level estimate for inpatients. Antimicrob. Resist Infect. Control 10, 5. doi:10.1186/s13756-020-00872-w
Keywords: glutathione, synergy, meropenem, carbapenem-resistant Klebsiella pneumoniae, antimicrobial resistance, glycerophospholipid metabolism
Citation: Yi J, Liu C, Yang P, Wu Z-c, Du C-j and Shen N (2023) Exogenous glutathione reverses meropenem resistance in carbapenem-resistant Klebsiella pneumoniae. Front. Pharmacol. 14:1327230. doi: 10.3389/fphar.2023.1327230
Received: 24 October 2023; Accepted: 05 December 2023;
Published: 19 December 2023.
Edited by:
Amedeo De Nicolò, University of Turin, ItalyReviewed by:
Yuan Liu, Yangzhou University, ChinaVigyasa Singh, University of Arizona, United States
Copyright © 2023 Yi, Liu, Yang, Wu, Du and Shen. This is an open-access article distributed under the terms of the Creative Commons Attribution License (CC BY). The use, distribution or reproduction in other forums is permitted, provided the original author(s) and the copyright owner(s) are credited and that the original publication in this journal is cited, in accordance with accepted academic practice. No use, distribution or reproduction is permitted which does not comply with these terms.
*Correspondence: Ning Shen, c2hlbm5pbmdwdXRoQDE2My5jb20=