- 1Department of Botany and Microbiology, Faculty of Science, Cairo University, Giza, Egypt
- 2Department of Microbial Chemistry, Biotechnology Research Institute, National Research Centre, Dokki, Cairo, Egypt
- 3Department of Clinical Microbiology and Immunology, Faculty of Medicine, King Abdulaziz University, Jeddah, Saudi Arabia
- 4Department of Clinical Laboratories-Diagnostic Immunology Division, King Abdulaziz University Hospital, Jeddah, Saudi Arabia
- 5Department of Pharmacology, Faculty of Medicine, Benha University, Benha, Egypt
- 6Department of Microbiology, Medicine Program, Batterjee Medical College, Jeddah, Saudi Arabia
- 7Inpatient Pharmacy, Mansoura University Hospitals, Mansoura, Egypt
- 8Department of Animal Histology and Anatomy, School of Veterinary Medicine, Badr University in Cairo (BUC), Badr City, Egypt
- 9Department of Anatomy and Embryology, Faculty of Veterinary Medicine, University of Sadat City, Sadat City, Egypt
- 10Department of Biology and Plant Protection, Faculty of Agriculture, University of Life Sciences “King Michael I” from Timișoara, Timișoara, Romania
- 11Department of Pharmacology and Toxicology, Faculty of Pharmacy, Egyptian Russian University, Cairo, Egypt
- 12Department of Medical Biochemistry, Faculty of Medicine, Mansoura University, Mansoura, Egypt
- 13Department of Basic Medical Sciences, College of Medicine, AlMaarefa University, Riyadh, Saudi Arabia
- 14Department of Forensic Medicine and Toxicology, Faculty of Veterinary Medicine, Benha University, Toukh, Egypt
Antibiotics represent a frequently employed therapeutic modality for the management of bacterial infections across diverse domains, including human health, agriculture, livestock breeding, and fish farming. The efficacy of antibiotics relies on four distinct mechanisms of action, which are discussed in detail in this review, along with accompanying diagrammatic illustrations. Despite their effectiveness, antibiotic resistance has emerged as a significant challenge to treating bacterial infections. Bacteria have developed defense mechanisms against antibiotics, rendering them ineffective. This review delves into the specific mechanisms that bacteria have developed to resist antibiotics, with the help of diagrammatic illustrations. Antibiotic resistance can spread among bacteria through various routes, resulting in previously susceptible bacteria becoming antibiotic-resistant. Multiple factors contribute to the worsening crisis of antibiotic resistance, including human misuse of antibiotics. This review also emphasizes alternative solutions proposed to mitigate the exacerbation of antibiotic resistance.
1 Introduction
Microbiology originated the term “antibiotic” from the French words “antibiose” and “antibiotique,” as defined by Vuillemin in the late 19th century to delineate substances that exert detrimental effects on living organisms, particularly microorganisms (Laskin, Bennett, and Gadd, 2002). Subsequently, in 1947, Selman A. Waksman provided an all-encompassing definition of antibiotics as chemical compounds produced by microorganisms that possess the capacity to impede the growth and induce the demise of bacteria and other microorganisms (Waksman, 1947; Kaur Sodhi and Singh, 2022). Antibiotics have found widespread application across various sectors, encompassing human health, agriculture, livestock breeding, and fish farms (Okocha, Olatoye, and Adedeji, 2018; Kaur Sodhi and Singh, 2022). The mechanisms underlying the efficacy of antibiotics involve four distinct modes of action (Kaur Sodhi and Singh, 2022), including inhibition of DNA replication (Fàbrega et al., 2009), protein biosynthesis (Tenson, Lovmar, and Ehrenberg, 2003), cell wall biosynthesis (Cho, Uehara, and Bernhardt, 2014), and folic acid metabolism (Saverus 2019). However, the emergence of antibiotic resistance has escalated into a critical global issue (Sarkar et al., 2021; Shree et al., 2023).
This review article comprehensively discusses the multifaceted factors contributing to the propagation of antibiotic resistance, alongside providing potential strategies for mitigating this problem. Furthermore, innovative solutions to combat antibiotic resistance have been uncovered by scientists, such as the utilization of nanoantibiotics, and antibiotic adjuvants, the discovery of novel antibiotics, and the exploration of alternatives to antibiotics, including bacteriophages and botanicals. In addition to exploring the aforementioned topics, this review article delves into the utilization of antibiotics in diverse sectors, the controversies encircling their use, the mechanisms by which antibiotics act, the emergence of antibiotic resistance, the mechanisms governing antibiotic resistance in bacteria, the dissemination of resistance among bacterial populations, and the pivotal factors that contribute to the escalation of resistance. Moreover, this article highlights the accomplishments achieved thus far and presents solutions that scientists have uncovered to combat the global crisis of antibiotic resistance.
2 Antibiotics use
Antibiotics are utilized in a variety of sectors, including agriculture, aquaculture, animal husbandry, and human health (Okocha et al., 2018; Kaur Sodhi and Singh, 2022) (Table 1). These substances are used to treat bacterial infections in humans, animals, and crops, thereby preventing crop loss from bacterial diseases (Svircev, Roach, and Castle, 2018; Kaur Sodhi and Singh, 2022). Additionally, antibiotics are widely used as growth-promoting agents in animal husbandry (Sriram et al., 2021; Kaur Sodhi and Singh, 2022). The utilization of antibiotics in livestock is classified into three categories by scientists (Gonzalez Ronquillo and Angeles Hernandez, 2017): therapeutic agents, prophylactic agents, and growth promoters. Therapeutic agents are administered in high doses to infected animals to treat illnesses (Schwarz, Kehrenberg, and Walsh, 2001), while prophylactic agents are given in sub-therapeutic doses through feed or drinking water to prevent disease when there are no evident symptoms of infection. Antibiotics are periodically administered to animals throughout their life cycle (Greene and Pisano 2012). Growth promoters are used to improve an animal’s growth rate and production, and a tiny quantity of antibiotics is regularly administered through its feed (Wierup, 2001). In aquaculture, antimicrobial substances are used to treat fish infections. Fish are given antibiotics by incorporating them into specially formulated feed, and they mostly excrete them into the environment (Dawood, Koshio, and Esteban, 2018). It is worth noting that approximately 75% of the antibiotics provided to fish are excreted into the water (Benbrook, 2002).
3 Mechanisms of action of antibiotics
Not all antibiotics have the exact mechanism of action; thus, scientists classify antibiotics according to their mechanism of action and chemical structure (Kaur Sodhi and Singh, 2022) into four mechanisms described as follows:
3.1 Antibiotics inhibit DNA replication
Binary fission is a type of cell division used by bacteria that produces two daughter cells (Bhattacharyya, 2012). Before that can happen, however, bacteria must create exact duplicates of their circular DNA. DNA replication is the procedure used to duplicate DNA (Gilbert, 2001). A DNA double helix strand is split into two single strands by the enzyme DNA helicase to begin this process. The enzyme DNA polymerase then produces new DNA Strands that are complementary to the old ones (Li and Araki, 2013). Positive DNA helical twists build up as a result of DNA helicase and DNA polymerase activity. If not eliminated, these positive helical twists stop DNA replication from continuing. The removal of the positive superhelical twists is carried out by the enzyme DNA gyrase, also known as topoisomerase II, allowing DNA replication to continue (Bush, Evans-roberts, and Maxwell, 2015). A crucial bacterial enzyme called DNA gyrase is made up of 2A and 2B subunits. This enzyme also plays a crucial role in the transcription of several genes and the start of DNA replication. Once the new two daughter DNA molecules have been created, they eventually connect and are interlinked. In order to divide the bacterial cell into two new daughter bacterial cells, the enzyme topoisomerase IV (related to DNA gyrase) enables the separation of the two connected DNA molecules (Nagaraja et al., 2017) (Figure 1).
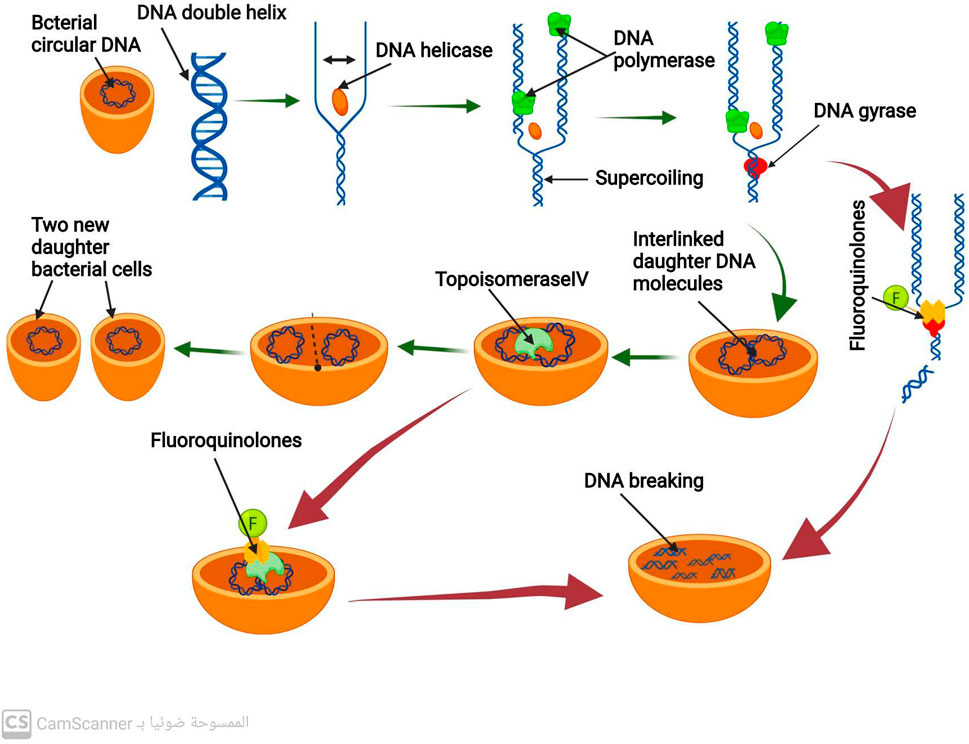
FIGURE 1. Mechanism of DNA replication process and mechanism of action of antibiotics that inhibit DNA replication.
3.2 Fluoroquinolones antibiotics
By inhibiting the activity of DNA gyrase and topoisomerase IV, fluoroquinolone antibiotics prevent the synthesis of bacterial DNA (Fàbrega et al., 2009; Shree et al., 2023). These antibiotics have a particular affinity for binding to the complex formed by DNA gyrase and DNA (Pham, Ziora, and Blaskovich, 2019). Such binding destabilizes the enzyme-DNA complex, causing DNA cleavage and ultimately leading to bacterial cell death (Malik, Zhao, and Drlica, 2006) (Figure 1). Fluoroquinolones primarily target DNA gyrase, which is the reason for their effectiveness against most gram-negative bacteria (Blondeau, 2004).
In contrast, in most gram-positive bacteria, fluoroquinolones target topoisomerase IV as their primary mechanism (Blondeau, 2004). However, they also act as a secondary target for DNA gyrase. This results in the binding of fluoroquinolones to DNA and the complex formed by topoisomerase IV, which causes disruption of the separation of the two daughter DNA molecules and eventually leads to DNA breakage (Malik et al., 2006).
3.3 Antibiotics inhibit protein biosynthesis
Like all other living things, bacteria have DNA, which contains the genetic information for every protein they need to survive. This includes the protein needed for metabolism regulation, growth, repair, and reproduction (Wu, 2009). Additionally, it encodes for mRNA, rRNA, and tRNA, three types of RNA that are essential for carrying out protein synthesis (Wu, 2009).
The unwinding and separation of the DNA molecule at a region that codes for the necessary protein to be produced is the first step in the process of protein biosynthesis. The transcription, the process of making mRNA, uses only one strand of DNA as a scaffold. When the mRNA strand is finished, it separates from the DNA template and then is connected to a ribosome. The 50 s and 30 s ribosomal subunits make up the bacterial ribosome. Following the joining of these two subunits along the mRNA strand, the synthesis of the polypeptide chain starts. Up until it encounters the signal along the mRNA to stop, the ribosome keeps adding amino acids to the lengthening polypeptide chain. The full polypeptide chain is generated at this point (Johansson, Lovmar, and Ehrenberg, 2008) (Figure 2). Therefore, targeting the ribosomal 30 or 50 subunits is necessary for antibiotics to inhibit protein synthesis (Scott Champney, 2008).
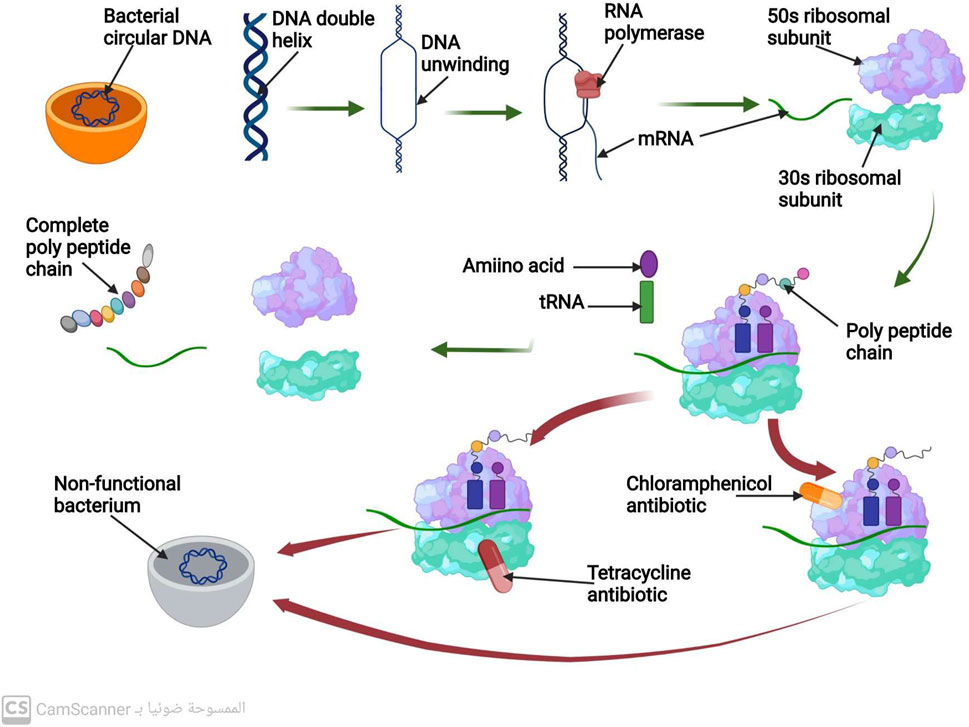
FIGURE 2. Mechanism of protein biosynthesis process and mechanism of action of antibiotics that inhibit protein biosynthesis.
3.3.1 Antibiotics inhibit protein biosynthesis by targeting the ribosomal 305 subunits
3.3.1.1 Aminoglycosides antibiotics
Antibiotics known as aminoglycosides function through a specific mechanism. These antibiotics are positively charged, which attracts the negatively charged outer membrane of bacteria, causing the membrane to develop large pores (Delcour, 2009). The aminoglycosides are then able to enter the bacterial cell through these pores. Additionally, aminoglycosides are able to pass through the bacterial cytoplasmic membrane by utilizing the energy of active bacterial transport (Jana and Deb, 2006). The target of aminoglycosides is the 16s rRNA of the 30s, which they bind to via hydrogen bonds. This binding inhibits protein biosynthesis before it can be completed (Vicens and Westhof, 2003).
Although aminoglycosides are effective against many types of bacteria, they have low efficacy against anaerobic bacteria, as these bacteria require oxygen for active transport pathways to function (Kohanski, Dwyer, and Collins, 2010). However, when combined with an antibiotic that inhibits cell wall synthesis, aminoglycosides have been found to have a greater ability to penetrate bacterial cells at low doses (Wang et al., 2022) (Figure 2).
3.3.1.2 Tetracycline antibiotics
This class of antibiotics targets the highly conserved sequence of the 16S rRNA present in the ribosomal 30S subunit. Tetracycline, for instance, functions by hindering the binding of tRNA to the A-site of the ribosome, which ultimately impedes the process of protein synthesis (Brodersen et al., 2000).
3.3.2 Antibiotics inhibit protein biosynthesis by targeting the ribosomal 50s subunit
3.3.2.1 Macrolides antibiotics
Macrolide antibiotics bind to the 50S subunit of the ribosome, thereby preventing the synthesis of polypeptide chains and inhibiting protein production (Tenson et al., 2003) (Figure 2).
3.3.2.2 Chloramphenicol antibiotics
Chloramphenicol antibiotics inhibit peptidyl transferase, an enzyme located on the 50S ribosomal subunit that is necessary for protein synthesis. This inhibition prevents t-RNA from connecting to the ribosomal A site, leading to the inhibition of protein synthesis (Syroegin et al., 2022) (Figure 2).
3.3.2.3 Oxazolidinone antibiotics
Oxazolidinone antibiotics prevent the synthesis of the initiation complex by binding to the 50S subunit of the ribosome, thereby preventing the production of proteins (Foti et al., 2021) (Figure 2).
3.4 Antibiotics inhibit cell wall synthesis
Most bacteria are composed of a cell membrane enclosed in a cell wall, while some bacteria also have an extra outer layer. The bacterial cell wall serves two purposes: to keep the bacteria in their distinctive shape and to stop them from bursting when osmosis is used to introduce fluid into the cell (Gupta and Gupta, 2021). The peptidoglycan is the most significant part of the cell wall. N-acetyl muramic acid (NAM) alternates with N-acetyl Glucosamine (NAG), and the two are connected by chains of amino acids to form the polymer known as peptidoglycan (Meroueh et al., 2006). There are several steps in the peptidoglycan synthesis process, which ultimately results in bacterial cell wall formation. A precursor to peptidoglycan is created by combining N-acetyl glucosamine (NAG) with N-acetyl muramic acid. The cell wall acceptors in the periplasm receive this peptidoglycan precursor after it has been delivered across the membrane. The peptidoglycan precursors attach to cell wall receptors in the periplasm and go through a lot of cross-linking (Liu and Breukink, 2016). Trans peptidase and carboxy peptidase enzymes are the two main enzymes in cross-linking. A cell wall eventually develops from many peptidoglycan layers that are all cross-linked (Liu and Breukink, 2016). Gram-positive bacteria may have a thicker cell wall than gram-negative bacteria because they may contain more layers of peptidoglycan (Figure 3) (Pasquina-Lemonche et al., 2020).
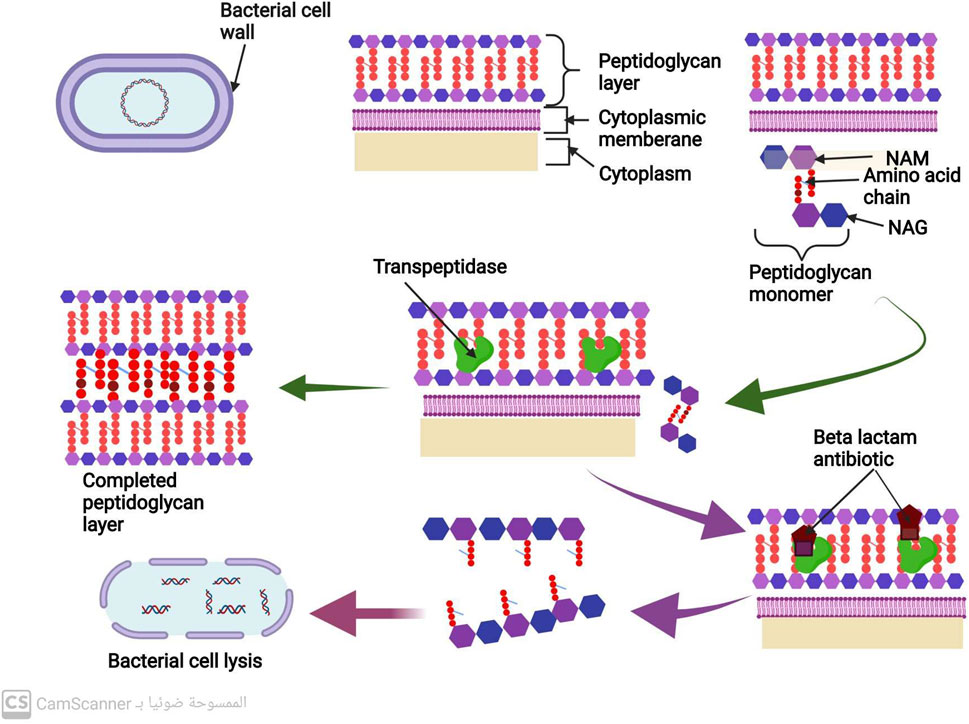
FIGURE 3. Mechanism of cell wall synthesis and Mechanism of action of antibiotics that inhibit cell wall synthesis.
The peptidoglycan is the most significant part of the bacterial cell wall. Transpeptidase and carboxypeptidase are the two primary enzymes in cross-linking during cell wall biosynthesis (Liu and Breukink, 2016) (Figure 3).
3.4.1 Betalactams antibiotics
All penicillins and cephalosporins with the beta-lactam ring chemical structure are included in this group (Fernandes, Amador, and Prudêncio, 2013). This distinctive structure allows them to attach to peptidoglycan cross-linking enzymes, such as transpeptidase and carboxypeptidase, ultimately inhibiting bacterial cell wall synthesis and preventing cross-linking (Cho et al., 2014). This inhibition of cell wall manufacturing leads to the destruction of the bacterial cell, as shown in Figure 3 (Cho et al., 2014).
3.4.2 Glycopeptides antibiotics
These antibiotics form non-covalent bonds with the terminal carbohydrates, which prevents the cross-linking of peptidoglycan precursors. This process ultimately leads to the degradation of bacterial cell walls, resulting in the destruction and elimination of bacterial cells (Kang and Park, 2015).
3.5 Antibiotics inhibit folic acid metabolism
These antibiotics are designed to selectively inhibit a key enzyme involved in the pathway for folic acid metabolism. Sulfonamide antibiotics target dihydropteroate synthase, an enzyme in the metabolic pathway. On the other hand, trimethoprim antibiotics target dihydrofolate reductase, another enzyme in the same pathway (Capasso and Supuran, 2014) (Figure 4).
4 Emergence of antibiotic resistance
Dr. Tedros Adhanom Ghebreyesus, CEO of the World Health Organization (WHO), has warned that the global epidemic of antibiotic resistance poses a significant threat to a century of progress in healthcare and the achievement of sustainable development goals (Sarkar et al., 2021). According to current predictions, it is expected that in a quarter of a century, almost 100% of bacteria will be resistant to most antibiotics used in medicine (Kaur Sodhi and Singh, 2022; Murray et al., 2022). Experts also predict that the number of deaths due to antimicrobial resistance may rise to 10 million by the middle of the twenty-first century, up from the current figure of over 700,000 per year (Romandini et al., 2021).
In response to this alarming situation, the WHO proposed the ranking of bacteria with the highest trend in resistance in 2017. This list includes Acinobacter baumannii, Pseudomonas aeruginosa, Enterobacteriaceae, Enterococcus faecium, Helicobacter pylori, Salmonella species, Campylobacter species, Staphylococcus aureus, and Nisseria gonorrhoeae. These microbe species are highly resistant to multiple classes of antibiotic treatments, making them less susceptible to antibiotic therapy (Biondo, 2023).
5 Mechanisms of antibiotic resistance
Bacteria employ three primary mechanisms to counteract the effects of antibiotics (Zhou et al., 2015). These mechanisms are outlined below:
5.1 Bacteria prevent antibiotic accumulation in their cells
5.1.1 Through limiting the entrance of drugs into bacterial cells
Gram-negative bacteria have porin channels in their outer membrane (Kang and Park, 2015). These channels act as gatekeepers, allowing only certain antibiotics like B-lactams and quinolones to enter the bacterial cell. Therefore, the reduced number of bacterial porins can hinder the entry of these antibiotics into the cell, leading to increased resistance to these drugs (Darby et al., 2023) (Figure 5).
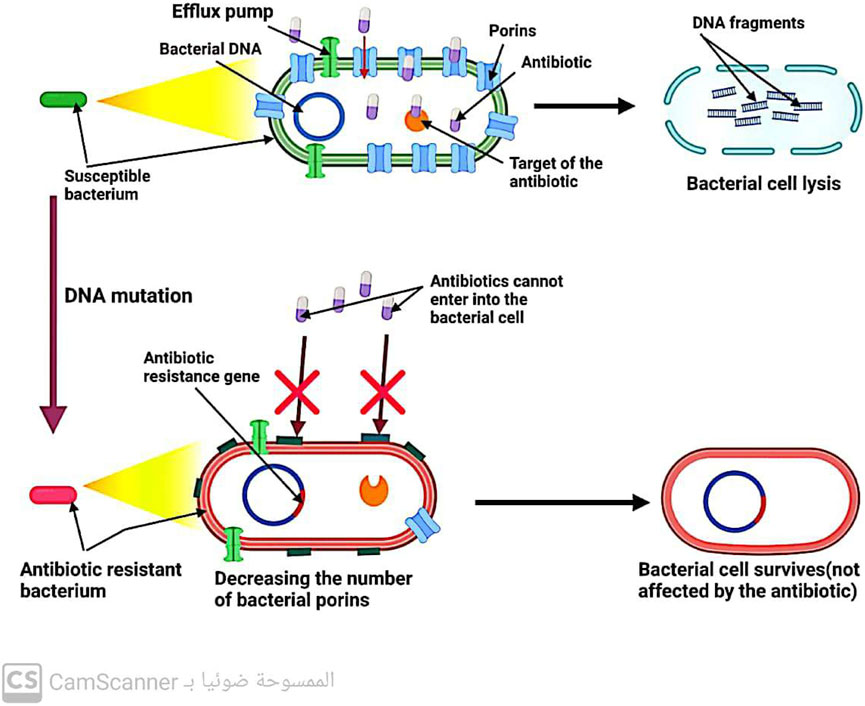
FIGURE 5. Mechanism of antibiotic resistance by decreasing antibiotic entry into the bacterial cell.
5.1.2 Increasing the rate at which antibiotics leave bacterial cells
Efflux pumps, located in the cytoplasmic membrane of bacteria, play a crucial role in maintaining the balance of solutes within bacterial cells. However, these pumps also contribute to antibiotic resistance by removing drugs from bacterial cells before they can reach their intended targets (Džidić, Šušković, and Kos, 2008) (Figure 6). Notably, efflux systems have been found to confer resistance (Kaur Sodhi and Singh, 2022; Shree et al., 2023; Sodhi et al., 2023). to all antibiotic classes except for polymyxin (Fernández-Billón et al., 2023). Increasing our understanding of the mechanisms underlying efflux systems may provide new strategies for combating antibiotic resistance.
5.2 Bacteria modify the target molecule of antibiotics
Antibiotics are designed to target specific molecules, but even the slightest alteration can prevent their binding, leading to the emergence of antibiotic resistance (Kang and Park, 2015; Shree et al., 2023).
5.2.1 Modifications to the ribosomal 30s or 50s subunits
One-way bacteria can develop resistance to drugs that affect protein production is by modifying their ribosomal 30S or 50S subunits (Kaur Sodhi and Singh, 2022; Sodhi et al., 2023) (Figure 7). This type of resistance is observed with antibiotics such as aminoglycosides, tetracycline, macrolides, chloramphenicol, lincosamides, and streptogramin (Tenover, 2006; Fernández-Billón et al., 2023).
5.2.2 Changes in penicillin-binding protein (PBP)
Penicillin-binding proteins (PBPs) are enzymes known as transpeptidases, which play a vital role in cross-linking peptidoglycan precursors during the biosynthesis of bacterial cell walls. As these enzymes are the primary targets of β-lactam antibiotics, any changes in their structure or function can lead to bacterial resistance to these drugs (Kaur Sodhi and Singh, 2022; Sodhi et al., 2023) (Figure 8).
5.2.3 Changes in DNA gyrase and topoisomerase enzymes
DNA replication involves the enzymes DNA gyrase and topoisomerase (Hirsch and Klostermeier, 2021). Quinolone antibiotics specifically target these two enzymes, which is why modifications in their structure can lead to bacterial resistance against quinolones (Fàbrega et al., 2009) (Figure 9).
5.2.4 Changes in D-alanyl-D-alanine
The peptidoglycan precursors contain a dipeptide residue known as D-alanyl-D-alanine, which plays a crucial role in cell wall formation (Peschel et al., 2000). Alterations to this D-alanyl-D-alanine residue can lead to bacterial resistance to antibiotics that target it (Džidić et al., 2008).
5.2.5 Protection of ribosome
Tetracycline antibiotics are known to target the ribosomal 30S subunit, but the ribosome has defense mechanisms that can resist their action (Kang and Park, 2015).
5.2.6 Alteration in RNA polymerase enzyme importing resistance to rifampicin antibiotics
Rifampicin is an antibiotic commonly used to treat bacterial infections. It works by inhibiting the RNA synthesis process in bacteria, specifically by binding to the beta-subunit of the DNA-dependent RNA polymerase enzyme (Hasan et al., 2021). This binding prevents the enzyme from effectively transcribing DNA into RNA, leading to the inhibition of bacterial growth and ultimately causing cell death.
However, bacteria can develop resistance to rifampicin through alterations in the RNA polymerase enzyme. Mutations in the rpoB gene, which encodes the beta-subunit of RNA polymerase, can confer resistance to rifampicin (Patel et al., 2023). These mutations can affect the binding affinity between rifampicin and the RNA polymerase enzyme, reducing the ability of the antibiotic to inhibit RNA synthesis.
The alterations in the RNA polymerase enzyme that result in rifampicin resistance can have several consequences. One of the effects is the alteration of the levels of peptidoglycan precursors, which are essential components of the bacterial cell wall. Changes in the levels of these precursors can impact the integrity and stability of the cell wall, potentially affecting the susceptibility of bacteria to other antibiotics, such as beta-lactams (Patel et al., 2023).
It is important to note that rifampicin resistance can arise through various mechanisms, and alterations in the RNA polymerase enzyme are just one of them. Other mechanisms include the acquisition of resistance genes through horizontal gene transfer and the overexpression of efflux pumps that can actively remove rifampicin from the bacterial cell (Hasan et al., 2021).
5.3 Bacteria inactivate the antibiotic by enzymes
Three key enzymes are responsible for antibiotic inactivation (Figure 10). These enzymes include the following:
5.3.1 Beta-lactamases enzymes
These enzymes produced by bacteria have the ability to break down all B-lactam antibiotics that are bonded with ester and amide. This leads to the development of resistance in bacteria that can produce beta-lactamase enzymes toward beta-lactam antibiotics (Fernández-Billón et al., 2023).
5.3.2 Aminoglycoside modifying enzymes (AGES)
Enzymes are known to play a crucial role in antibiotic resistance. Specifically, enzymes such as aminoglycoside-modifying enzymes (AMEs) have been found to prevent the attachment of aminoglycoside antibiotics to their ribosomal target (Strateva and Yordanov 2009). These enzymes are present in various bacterial strains, including E. faecalis, S. aureus, and S. pneumoniae. In addition to their role in preventing antibiotic attachment, these enzymes also aid in conferring resistance to aminoglycosides and fluoroquinolones (Kang and Park, 2015). Thus, the presence of AMEs in bacterial strains is a major concern in the field of antibiotic resistance, as it poses a significant challenge to the effectiveness of these antibiotics in treating bacterial infections.
5.3.3 Chloramphenicol-acetyl-transferases enzymes
Enzymes known as chloramphenicol-acetyltransferases modify the antibiotic chloramphenicol by acetylating its hydroxyl group, resulting in an altered form of the antibiotic that is unable to bind to its ribosomal target. Consequently, bacteria possessing the chloramphenicol-acetyltransferase enzyme are resistant to chloramphenicol antibiotics, rendering them ineffective (Varela et al., 2021).
6 Spread of antibiotic resistance among bacteria
When a microorganism is able to survive or grow in an antibiotic concentration that would normally inhibit or kill other organisms of the same species, it is considered resistant (Kester and Fortune, 2014; Kaur Sodhi and Singh, 2022). In clinical practice, the terms “susceptible” and “resistant” are often used to describe the likelihood of successful treatment with antibiotics (Sabtu, Enoch, and Brown, 2015). Resistance is more likely to occur when a patient is unable to attain the concentration of antibiotic necessary to inhibit or kill the bacteria (Ordway et al., 2003). Microorganisms can either inherently possess resistance to an antibiotic or acquire it after exposure (Premlatha, 2019). The development of resistance can occur through gene mutations or direct transfer of resistance genes (Sodhi et al., 2023), which can be carried on plasmids (mobile genetic elements) (Kaur Sodhi and Singh, 2022) and transmitted through conjugation (Shree et al., 2023), or through the direct transfer of naked DNA through transformation (Shree et al., 2023) (Figure 11) or the transfer of similar DNA through bacteriophages (Kaur Sodhi and Singh, 2022; Shree et al., 2023), a process known as transduction. Even among bacteria of different species, genetic material, including antibiotic-resistance genes, can spread rapidly (Džidić et al., 2008). It was reported that heavy metals (Sodhi et al., 2023) and biofilm formation (Shree et al., 2023) increase the spread of antibiotic resistance among bacteria.
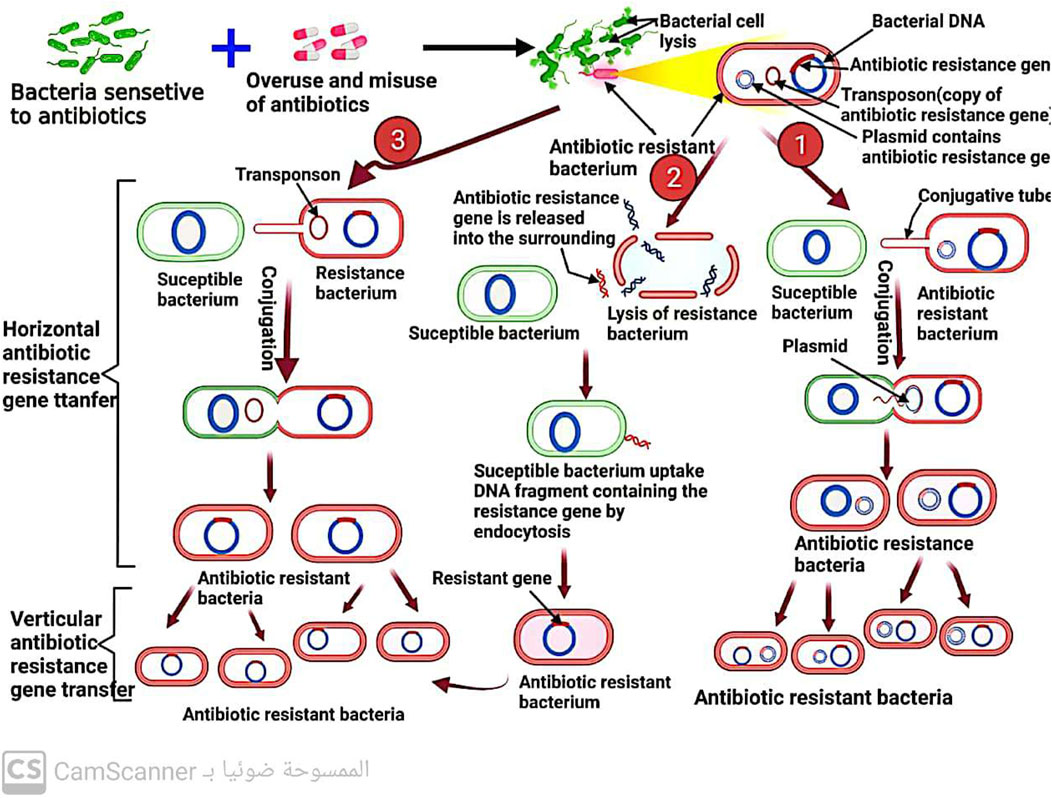
FIGURE 11. Mechanism of antibiotic resistance spread among bacteria by conjugation and transformation.
Different resistant bacteria can travel through various means, allowing them to spread and potentially cause infections in different settings. While the specific mechanisms of travel may vary depending on the bacteria and the environment, there are several common routes through which resistant bacteria can spread. For example:
1. Person-to-person transmission: Resistant bacteria can be transmitted directly from one person to another through close contact. This can occur through physical contact, such as touching or shaking hands with an infected person, or through respiratory droplets when an infected person coughs or sneezes (Ohmagari, 2014).
2. Contaminated surfaces: Resistant bacteria can survive on surfaces for extended periods. When a person touches a contaminated surface, such as doorknobs, countertops, or medical equipment, they can transfer the bacteria to their hands. If they then touch their face or mouth, the bacteria can enter their body and potentially cause an infection (Ohmagari, 2014).
3. Healthcare settings: Hospitals and healthcare facilities can be hotspots for the spread of resistant bacteria. Factors such as poor hygiene practices, inadequate infection control measures, and the close proximity of patients can contribute to the transmission of bacteria. Healthcare workers can inadvertently spread resistant bacteria from one patient to another if proper hand hygiene and infection control protocols are not followed (Clements et al., 2008; Tacconelli et al., 2014).
4. Animal-to-human transmission: Resistant bacteria can also be transmitted from animals to humans. This can occur through direct contact with infected animals or through the consumption of contaminated food products, such as meat or dairy products. Farm animals, in particular, can harbor resistant bacteria due to the use of antibiotics in agriculture (Garcia-Graells et al., 2012).
5. Travel and international spread: Resistant bacteria can be carried across borders through international travel. People who are infected or colonized with resistant bacteria can unknowingly spread them to other countries. This can contribute to the global dissemination of resistant strains and make it more challenging to control their spread (Ohmagari, 2014).
It is important to note that the specific mechanisms of travel and transmission can vary depending on the bacteria and the setting. Additionally, the spread of resistant bacteria can be influenced by factors such as poor hygiene, inadequate sanitation, and suboptimal infection control practices (Tacconelli et al., 2014).
7 Factors affecting the resistance of antibiotics
The emergence of antibiotic resistance is accelerated by the under, over, or improper use of antibiotics (Anwar, Iqbal, and Saleem, 2020; Kaur Sodhi and Singh, 2022; Shree et al., 2023; Sodhi et al., 2023). The indiscriminate use of antibiotics, which promotes antibiotic resistance, is caused by a variety of factors, including patients’ noncompliance with prescribed treatment and demand, irrational use of antibiotics by prescribers in human medicine, drug advertising, dispensing doctors, antibiotic use in agriculture, poor antibiotic quality, inadequate surveillance, and susceptibility testing. Despite their knowledge of a patient’s diagnosis, doctors and prescribers can be heavily influenced by patient demand, which can contribute to antimicrobial and antibiotic resistance (Acharya and Wilson, 2019).
Patients may discontinue their treatment once they begin to feel better, forget to take their prescriptions, or only purchase a portion of the medication. In such cases, increased physician-patient interaction is often necessary to ensure proper compliance with treatment (Carlet et al., 2012). Additionally, antibiotics are readily available in pharmacies without a prescription, which further contributes to their misuse by patients (Darj, Newaz, and Zaman, 2019; Machowska and Lundborg, 2019).
The pharmaceutical industry also plays a role in promoting antibiotic misuse through advertising. For example, some advertisements claim that certain antibiotics, such as Ciprofloxacin, are the best option for at-risk patients. In the past, advertisements in the Philippines encouraged the use of lincomycin for pharyngitis/tonsillitis and clindamycin for upper respiratory tract infections, despite the fact that these conditions are often caused by viral infections that do not require antibiotics (Ladd, 2005; Tanday, 2016). Overall, inappropriate antibiotic use is a multifaceted issue that requires cooperation between healthcare providers, patients, and the pharmaceutical industry to address.
Medical professionals have a significant impact on the development of antibiotic resistance in bacteria. It is common for doctors to prescribe broad-spectrum antibiotics when narrow-spectrum ones would be more appropriate (Mincey and Parkulo, 2001; Om et al., 2016). The prescribing patterns of doctors can vary, and studies have shown that 30%–60% of patients receive more antibiotics than necessary. Additionally, incorrect prescriptions and recommendations from untrained medical professionals pose a significant risk. A related study found that private practitioners often prescribe unnecessary medications (Thakolkaran et al., 2017).
Hospitals and clinics are significant contributors to the development of microbial resistance to antibiotics (Shree et al., 2023). Inadequate infection control protocols, such as failure to wash hands or change gloves regularly, have been identified as factors contributing to this problem (Weinstein, 2001). Another issue is the use of poor-quality antibiotics. This problem persists due to the lack of quality compliance and monitoring, leading to the use of expired and counterfeit antibiotics (Ayukekbong, Ntemgwa, and Atabe, 2017). Inappropriate use of antibiotics in animals is also a concern. Some antibiotics are administered to animals to boost their growth and prevent sickness (Manishimwe, Nishimwe, and Ojok, 2017). However, the surveillance and susceptibility testing of antibiotics are insufficient (Tebano et al., 2020) (Table 2).
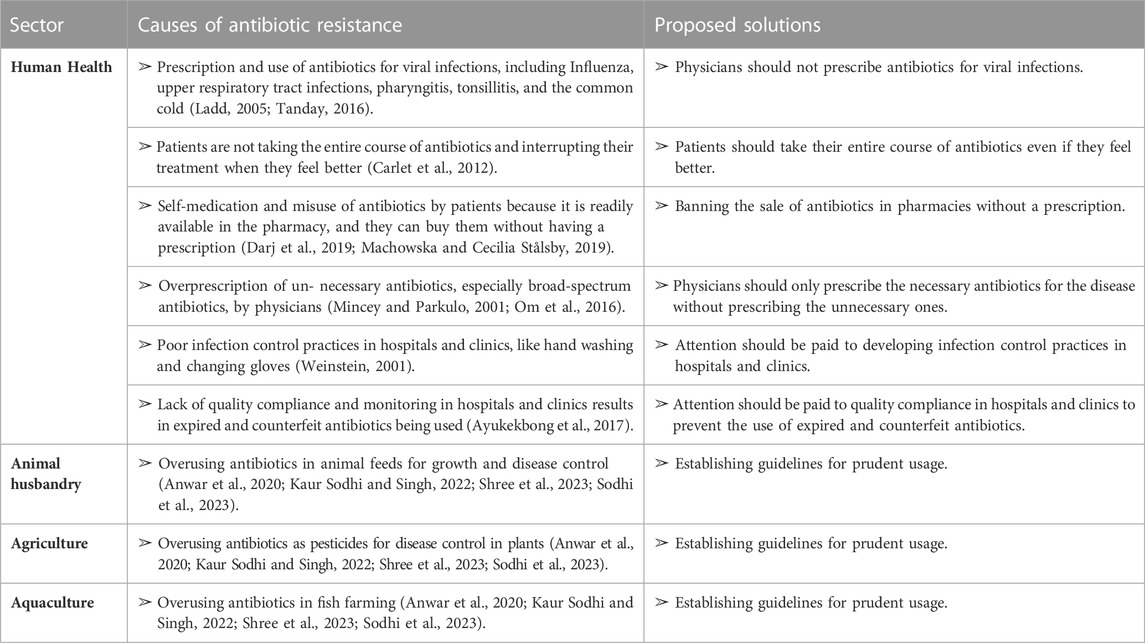
TABLE 2. Factors causing antibiotic resistance in different sectors and some proposed solutions to overcome these factors.
8 Alternative approaches to combat antibiotic resistance
We are now in grave danger because of the global antibiotic resistance crisis, which is constantly increasing. Therefore, we should focus on finding solutions to fight this resistance crisis. There are several approaches to fight this crisis which we discuss in this review article as follows:
8.1 Discovery of new antibiotics
In 2015, researchers discovered a new antibiotic called teixobactin, which demonstrated bactericidal activity against S. aureus, Clostridium difficile, and Bacillus anthracis (Piddock, 2015). On 20 February 2020, researchers published an article in Cell titled “A Deep Learning Approach to Antibiotic Discovery.” Using artificial intelligence, they discovered a new antibiotic called halicin, which showed bactericidal activity against a broad spectrum of pathogenic and resistant bacteria (Stokes et al., 2020).
On 26 September 2022, researchers published an article in Nature Microbiology titled “Computational identification of a systemic antibiotic for Gram-negative Bacteria.” Using computational screening, they discovered a new antibiotic called dynobactin, which demonstrated potent bactericidal activity against dangerous Gram-negative bacteria resistant to other antibiotics (Miller et al., 2022).
The dysfunctional R&D market for detecting new antibiotics refers to the challenges and limitations faced in the research and development of new antibiotics. These challenges include economic, regulatory, and scientific barriers that hinder the discovery and development of effective antibiotics to combat bacterial infections. Some of the key issues in the dysfunctional R&D market for detecting new antibiotics include:
8.1.1 A-economic challenges
Limited financial incentives: The high cost and low profitability of developing new antibiotics have led to a lack of investment from pharmaceutical companies (Clardy, Fischbach, and Walsh, 2006).
Long development timelines: The lengthy and expensive process of developing new antibiotics makes it less attractive for companies to invest in this area (Clardy et al., 2006).
8.1.2 B-regulatory challenges
Stringent regulatory requirements: The regulatory approval process for new antibiotics is complex and time-consuming, leading to delays in bringing new drugs to market (McDevitt and Rosenberg, 2001).
Limited guidance on clinical trial design: There is a lack of clear guidelines for conducting clinical trials for antibiotics, which can further hinder the development process (McDevitt and Rosenberg, 2001).
8.1.3 C-scientific challenges
Antibiotic resistance: The rise of antibiotic-resistant bacteria poses a significant challenge in the development of new antibiotics (McDevitt and Rosenberg, 2001).
Limited understanding of bacterial biology: Despite advances in genomics and other technologies, there is still much to learn about the biology of bacteria and their mechanisms of resistance (McDevitt and Rosenberg, 2001).
8.2 Antibiotic adjuvants
Antibiotic adjuvants are compounds that do not directly kill bacteria but instead enhance an antibiotic’s effectiveness by inhibiting resistance mechanisms. For example, beta-lactamase inhibitors are small-molecule antibiotic adjuvants. Beta-lactamase inhibitors, when combined with beta-lactam antibiotics, have been used successfully for over 30 years to treat Gram-positive and Gram-negative infections. Their use has been extensively documented (Melander and Melander, 2017).
8.3 Nano antibiotics
Nanoscale antibiotics, which consist of pure antibiotic molecules between 1 and 100 nm in size or antibiotic molecules physically attached to nanoparticles, represent one beneficial use of nanotechnology (Soares et al., 2018; Singh and Sodhi, 2023). By reengineering antibiotics at the nanoscale, this new antimicrobial approach revives the arsenal of available medications by making them effective against various clinically important microorganisms. Unlike their bulk chemical counterparts, nanoantibiotics have different physicochemical properties and increased potency (Mamun et al., 2021). Because nanoscale drug delivery systems can transport and bind to intracellular targets, reducing bacterial growth and metabolism and ultimately causing cell death, a medication delivered via or incorporated into nanoparticles at the same dose has significantly greater inhibitory effects on bacterial growth (Jijie et al., 2017; Singh and Sodhi, 2023).
8.4 Botanicals
Plants produce secondary metabolites, including alkaloids, flavonoids, phenolics, quinones, tannins, coumarins, terpenes, lectins, and saponins. These secondary metabolites exhibit antimicrobial activity against various microorganisms (Gupta and Birdi, 2017).
8.5 Bacteriophages
According to the National Institute of Health (NIH, 2014), bacteriophages are innovative elements that could combat microbial resistance (Shree et al., 2023; NIAID’s Antibacterial Resistance Program, 2014). Numerous studies applied bacteriophages on humans and animals to treat various bacterial diseases and showed positive, effective results. These bacterial pathogens include Shigella dysenteriae (Chanishvili, 2012), Vibrio cholera (Chanishvili, 2012), P. aeruginosa (Watanabe et al., 2007), C. difficile (Meader et al., 2013), Vancomycin-resistant E. faecium (Biswas et al., 2002), β-lactamase-producing E. coli (Wang et al., 2006), imipenem resistant P. aeruginosa (Wang et al., 2006), Acinetobacter baumannii (Yuan et al., 2019), E. coli (Pouillot et al., 2012), MDR-S. aureus (Fish et al., 2016), unclassified bacterial dysentery (Chanishvili and Sharp, 2008), S. typhi (Kutateladze and Adamia, 2008), and antibiotic-resistant P. aeruginosa (Wright et al., 2009).
9 Conclusion
This review article has discussed various aspects related to antibiotics, their mechanisms of action, the problem of antibiotic resistance, and potential solutions to combat resistance. Antibiotics have diverse applications in different fields but their use remains controversial owing to resistance issues. Antibiotics act through four primary mechanisms to kill or inhibit the growth of bacteria. However, bacteria have evolved mechanisms to become resistant to antibiotics, diminishing the effectiveness of these drugs. Given the limited capabilities of traditional antibiotics due to resistance, we discussed several promising alternative approaches, including the discovery of new antibiotics, the use of antibiotic adjuvants and nanoparticle-based antibiotics, botanicals, and bacteriophages. Although developing new antibiotics is challenging, a combination of complementary strategies such as nanoantibiotics, adjuvants, botanicals, and phage therapy could help address the resistance crisis.
Author contributions
EH: Conceptualization, Data curation, Formal Analysis, Investigation, Project administration, Resources, Validation, Visualization, Writing–original draft, Writing–review and editing. MF: Conceptualization, Data curation, Methodology, Software, Validation, Writing–original draft. MA-R: Data curation, Formal Analysis, Investigation, Software, Validation, Visualization, Writing–original draft. AB: Data curation, Formal Analysis, Software, Validation, Visualization, Writing–original draft. NN: Data curation, Formal Analysis, Methodology, Software, Validation, Writing–original draft. MA: Data curation, Formal Analysis, Investigation, Methodology, Software, Validation, Writing–original draft. RO: Data curation, Formal Analysis, Funding acquisition, Methodology, Software, Validation, Writing–original draft, Writing–review and editing. LF: Data curation, Formal Analysis, Methodology, Resources, Software, Validation, Writing–original draft, Writing–review and editing. AAt: Data curation, Formal Analysis, Methodology, Software, Validation, Writing–original draft. ME-N: Data curation, Formal Analysis, Investigation, Methodology, Software, Validation, Writing–original draft. AAb: Conceptualization, Software, Supervision, Validation, Visualization, Writing–original draft, Writing–review and editing.
Funding
The author(s) declare financial support was received for the research, authorship, and/or publication of this article. This paper was supported from the project 6PFE of the University of Life Sciences “King Mihai I” from Timisoara and Research Institute for Biosecurity and Bioengineering from Timisoara.
Conflict of interest
The authors declare that the research was conducted in the absence of any commercial or financial relationships that could be construed as a potential conflict of interest.
Publisher’s note
All claims expressed in this article are solely those of the authors and do not necessarily represent those of their affiliated organizations, or those of the publisher, the editors and the reviewers. Any product that may be evaluated in this article, or claim that may be made by its manufacturer, is not guaranteed or endorsed by the publisher.
References
Acharya, K. P., and Trevor Wilson, R. (2019). Antimicrobial resistance in Nepal. Front. Med. 6, 105–109. May. doi:10.3389/fmed.2019.00105
Anwar, M., Iqbal, Q., and Saleem, F. (2020). Improper disposal of unused antibiotics: an often overlooked driver of antimicrobial resistance. Expert Rev. Anti-Infective Ther. 18 (8), 697–699. doi:10.1080/14787210.2020.1754797
Ayukekbong, J. A., Ntemgwa, M., and Atabe, A. N. (2017). The threat of antimicrobial resistance in developing countries: causes and control strategies. Antimicrob. Resist. Infect. Control 6 (1), 47–48. doi:10.1186/s13756-017-0208-x
Benbrook, C. M. (2002). Antibiotic drug use in US aquaculture. United States: Institute for Agriculture and Trade Policy Report February, 1–18.
Biondo, C. (2023). Bacterial antibiotic resistance: the most critical pathogens. Pathogens 12 (1), 116–214. doi:10.3390/pathogens12010116
Biswas, B., Sankar, A., Paul, W., Paul, B., Trostel, A. N., Powell, B., et al. (2002). Erratum: bacteriophage therapy rescues mice bacteremic from a clinical isolate of vancomycin-resistant Enterococcus faecium (infection and immunity (2002) 70:1 (204-210)). Infect. Immun. 70 (3), 1664. doi:10.1128/iai.70.3.1664-1664.2002
Blondeau, J. M. (2004). Fluoroquinolones: mechanism of action, classification, and development of resistance. Surv. Ophthalmol. 49, S73–S78. 2 SUPPL. 2. doi:10.1016/j.survophthal.2004.01.005
Brodersen, D. E., Clemons, W. M., Carter, A. P., Morgan-Warren, R. J., Wimberly, B. T., and Ramakrishnan, V. (2000). The structural basis for the action of the antibiotics tetracycline, pactamycin, and hygromycin B, on the 30S ribosomal subunit. Cell 103 (7), 1143–1154. doi:10.1016/S0092-8674(00)00216-6
Bush, N. G., Evans-roberts, K., and Anthony, M. (2015). DNA topoisomerases. EcoSal Plus 6 (2), 1–34. doi:10.1128/ecosalplus.ESP-0010-2014
Capasso, C., and Supuran, C. T. (2014). Sulfa and trimethoprim-like drugs-antimetabolites acting as carbonic anhydrase, dihydropteroate synthase and dihydrofolate reductase inhibitors. J. Enzyme Inhibition Med. Chem. 29 (3), 379–387. doi:10.3109/14756366.2013.787422
Carlet, J., Jarlier, V., Harbarth, S., Voss, A., Goossens, H., and Pittet, D. (2012). Ready for a World without antibiotics? The pensières antibiotic resistance call to action. Antimicrob. Resist. Infect. Control 1, 11–13. doi:10.1186/2047-2994-1-11
Chanishvili, N. (2012). Phage therapy-history from twort and d’Herelle through soviet experience to current approaches. Vol. 83. 1. Amsterdam, Netherlands: Elsevier Inc.
Chanishvili, N., and Sharp, R. (2008). Bacteriophage therapy: experience from the eliava Institute, Georgia. Microbiol. Aust. 29 (2), 96. doi:10.1071/ma08096
Cho, H., Uehara, T., and Bernhardt, T. G. (2014). Beta-lactam antibiotics induce a lethal malfunctioning of the bacterial cell wall synthesis machinery. Cell 159 (6), 1300–1311. doi:10.1016/j.cell.2014.11.017
Clardy, J., Fischbach, M. A., and Walsh, C. T. (2006). New antibiotics from bacterial natural products. Nat. Biotechnol. 24 (12), 1541–1550. doi:10.1038/nbt1266
Clements, A., Halton, K., Graves, N., Pettitt, A., Morton, A., Looke, D., et al. (2008). Overcrowding and understaffing in modern health-care systems: key determinants in meticillin-resistant Staphylococcus aureus transmission. Lancet Infect. Dis. 8 (7), 427–434. doi:10.1016/S1473-3099(08)70151-8
Darby, E. M., Trampari, E., Siasat, P., Solsona Gaya, M., Alav, I., Webber, M. A., et al. (2023). Molecular mechanisms of antibiotic resistance revisited. Nat. Rev. Microbiol. 21 (5), 280–295. doi:10.1038/s41579-022-00820-y
Darj, E., Md Shah, N., and Zaman, M. H. (2019). Pharmacists’ perception of their challenges at work, focusing on antimicrobial resistance: a qualitative study from Bangladesh. Glob. Health Action 12, 1735126. sup1. doi:10.1080/16549716.2020.1735126
Dawood, M. A. O., Koshio, S., and Ángeles Esteban., M. (2018). Beneficial roles of feed additives as immunostimulants in aquaculture: a review. Rev. Aquac. 10 (4), 950–974. doi:10.1111/raq.12209
Delcour, A. H. (2009). Outer membrane permeability and antibiotic resistance. Biochimica Biophysica Acta - Proteins Proteomics 1794 (5), 808–816. doi:10.1016/j.bbapap.2008.11.005
Džidić, S., Šušković, J., and Kos, B. (2008). Antibiotic resistance mechanisms in bacteria: biochemical and genetic aspects. Food Technol. Biotechnol. 46 (1), 11–21.
Fàbrega, A., Madurga, S., Giralt, E., and Vila, J. (2009). Mechanism of action of and resistance to quinolones. Microb. Biotechnol. 2 (1), 40–61. doi:10.1111/j.1751-7915.2008.00063.x
Fernandes, R., Amador, P., and Prudêncio, C. (2013). β-Lactams: chemical structure, mode of action and mechanisms of resistance. Rev. Res. Med. Microbiol. 24 (1), 7–17. doi:10.1097/MRM.0b013e3283587727
Fernandez, B., María, A. E., Llambías, C., Jordana-Lluch, E., Oliver, A., and Macià, M. D. (2023). Mechanisms of antibiotic resistance in Pseudomonas aeruginosa biofilms. Biofilm 5, 100129. Figure 1. doi:10.1016/j.bioflm.2023.100129
Fish, R., Kutter, E., Wheat, G., Blasdel, B., Kutateladze, M., and Kuhl, S. (2016). Saint peter, hospital family, and medicine residency. England: Fish2016.
Foti, C., Piperno, A., Scala, A., and Giuffrè, O. (2021). Oxazolidinone antibiotics: chemical, biological and analytical aspects. Molecules 26 (14), 4280. doi:10.3390/molecules26144280
Garcia-Graells, C., Antoine, J., Larsen, J., Catry, B., Skov, R., and Denis, O. (2012). Livestock veterinarians at high risk of acquiring methicillin-resistant Staphylococcus aureus ST398. Epidemiol. Infect. 140 (3), 383–389. doi:10.1017/S0950268811002263
Gilbert, D. M. (2001). Making sense of eukaryotic DNA replication origins. Science 294 (5540), 96–100. doi:10.1126/science.1061724
Gonzalez Ronquillo, M., and Juan Carlos Angeles, H. (2017). Antibiotic and synthetic growth promoters in animal diets: review of impact and analytical methods. Food control. 72, 255–267. doi:10.1016/j.foodcont.2016.03.001
Gupta, P. D., and Birdi, T. J. (2017). Development of botanicals to combat antibiotic resistance. J. Ayurveda Integr. Med. 8 (4), 266–275. doi:10.1016/j.jaim.2017.05.004
Gupta, R., and Gupta, N. (2021). Fundamentals of bacterial physiology and metabolism. Singapore: Springer.
Hasan, C. M., Dutta, D., and Nguyen, A. N. T. (2021). Revisiting antibiotic resistance: mechanistic foundations to evolutionary outlook Antibiotics 11, 40. doi:10.3390/antibiotics11010040
Hirsch, J., and Klostermeier, D. (2021). What makes a type IIA topoisomerase a gyrase or a topo IV? Nucleic Acids Res. 49 (11), 6027–6042. doi:10.1093/nar/gkab270
Jana, S., and Deb, J. K. (2006). Molecular understanding of aminoglycoside action and resistance. Appl. Microbiol. Biotechnol. 70 (2), 140–150. doi:10.1007/s00253-005-0279-0
Jijie, R., Barras, A., Teodorescu, F., Boukherroub, R., and Szunerits, S. (2017). Advancements on the molecular design of nanoantibiotics: current level of development and future challenges. Mol. Syst. Des. Eng. 2 (4), 349–369. doi:10.1039/c7me00048k
Johansson, M., Lovmar, M., and Ehrenberg, M. (2008). Rate and accuracy of bacterial protein synthesis revisited. Curr. Opin. Microbiol. 11 (2), 141–147. doi:10.1016/j.mib.2008.02.015
Kang, H. K., and Park, Y. (2015). Glycopeptide antibiotics: structure and mechanisms of action. J. Bacteriol. Virology 45 (2), 67–78. doi:10.4167/jbv.2015.45.2.67
Kaur Sodhi, K., and Singh, C. K. (2022). Recent development in the sustainable remediation of antibiotics: a review. Total Environ. Res. Themes 3–4, 100008. May. doi:10.1016/j.totert.2022.100008
Kester, J. C., and Fortune, S. M. (2014). Persisters and beyond: mechanisms of phenotypic drug resistance and drug tolerance in bacteria. Crit. Rev. Biochem. Mol. Biol. 49 (2), 91–101. doi:10.3109/10409238.2013.869543
Kohanski, M. A., Dwyer, D. J., and Collins, J. J. (2010). How antibiotics kill bacteria: from targets to networks. Nat. Rev. Microbiol. 8 (6), 423–435. doi:10.1038/nrmicro2333
Kutateladze, M., and Adamia, R. (2008). Phage therapy experience at the eliava Institute. Med. Maladies Infect. 38 (8), 426–430. doi:10.1016/j.medmal.2008.06.023
Ladd, E. (2005). The use of antibiotics for viral upper respiratory tract infections: an Analysis of nurse practitioner and physician prescribing practices in ambulatory care, 1997-2001. J. Am. Acad. Nurse Pract. 17 (10), 416–424. doi:10.1111/j.1745-7599.2005.00072.x
Li, Y., and Araki, H. (2013). Loading and activation of DNA replicative helicases: the key step of initiation of DNA replication. Genes Cells 18 (4), 266–277. doi:10.1111/gtc.12040
Liu, Y., and Breukink, E. (2016). The membrane steps of bacterial cell wall synthesis as antibiotic targets. Antibiotics 5 (3), 28. doi:10.3390/antibiotics5030028
Machowska, A., and Cecilia Stålsby, L. (2019). Drivers of irrational use of antibiotics in europe. Int. J. Environ. Res. Public Health 16 (1), 27. doi:10.3390/ijerph16010027
Malik, M., Zhao, X., and Drlica, K. (2006). Lethal fragmentation of bacterial chromosomes mediated by DNA gyrase and quinolones. Mol. Microbiol. 61 (3), 810–825. doi:10.1111/j.1365-2958.2006.05275.x
Mamun, M. M., Sorinolu, A. J., Munir, M., and Eric, P. V. (2021). Nanoantibiotics: functions and properties at the nanoscale to combat antibiotic resistance. Front. Chem. 9, 687660–687723. May. doi:10.3389/fchem.2021.687660
Manishimwe, R., Nishimwe, K., and Lonzy, O. (2017). Assessment of antibiotic use in farm animals in Rwanda. Trop. Animal Health Prod. 49 (6), 1101–1106. doi:10.1007/s11250-017-1290-z
McDevitt, D., and Rosenberg, M. (2001). Exploiting genomics to discover new antibiotics. Trends Microbiol. 9 (12), 611–617. doi:10.1016/S0966-842X(01)02235-1
Meader, E., Mayer, M. J., Steverding, D., Carding, S. R., and Narbad, A. (2013). Evaluation of bacteriophage therapy to control Clostridium difficile and toxin production in an invitro human colon model system. Anaerobe 22, 25–30. doi:10.1016/j.anaerobe.2013.05.001
Melander, R. J., and Melander., C. (2017). The challenge of overcoming antibiotic resistance: an adjuvant approach? ACS Infect. Dis. 3 (8), 559–563. doi:10.1021/acsinfecdis.7b00071
Meroueh, S. O., Bencze, K. Z., Hesek, D., Lee, M., Jed, F. F., Timothy, L. S., et al. (2006). Three-dimensional structure of the bacterial cell wall peptidoglycan. Proc. Natl. Acad. Sci. U. S. A. 103 (12), 4404–4409. doi:10.1073/pnas.0510182103
Miller, R. D., Iinishi, A., Curtis, T. D., Lariviere, P. J., Liang, L., Son, S., et al. (2022). Computational identification of a systemic antibiotic for gram-negative bacteria. Nat. Microbiol. 7 (10), 1661–1672. doi:10.1038/s41564-022-01227-4
Mincey, B. A., and Parkulo, M. A. (2001). Antibiotic prescribing practices in a TeachingClinic: comparison of resident and staff physicians. South. Med. J. 94 (4), 365–369. doi:10.1097/00007611-200194040-00001
Murray, C. J. L., Kevin, S. I., Sharara, F., Swetschinski, L., Aguilar, G. R., Gray, A., et al. (2022). Global burden of bacterial antimicrobial resistance in 2019: a systematic Analysis. Lancet 399 (10325), 629–655. doi:10.1016/S0140-6736(21)02724-0
Nagaraja, V., Godbole, A. A., Henderson, S. R., and Anthony, M. (2017). DNA topoisomerase I and DNA gyrase as targets for TB therapy. Drug Discov. Today 22 (3), 510–518. doi:10.1016/j.drudis.2016.11.006
NIAID’s Antibacterial Resistance Program (2014). NIAID’s antibacterial resistance Program: current status and future directions. 13.
Okocha, R. C., Isaac, O. O., and Olufemi, B. A. (2018). Food safety impacts of antimicrobial use and their residues in aquaculture. Public Health Rev. 39 (1), 1–22. doi:10.1186/s40985-018-0099-2
Om, C., Frances Daily, , Vlieghe, E., McLaughlin, J. C., and McLaws, M. L. (2016). ‘If it’s a broad spectrum, it can shoot better’: inappropriate antibiotic prescribing in Cambodia. Antimicrob. Resist. Infect. Control 5 (1), 58–8. doi:10.1186/s13756-016-0159-7
Ordway, D., Viveiros, M., Leandro, C., Bettencourt, R., Almeida, J., Martins, M., et al. (2003). Clinical concentrations of thioridazine kill intracellular multidrug-resistant Mycobacterium tuberculosis. Antimicrob. Agents Chemother. 47 (3), 917–922. doi:10.1128/AAC.47.3.917-922.2003
Pasquina-Lemonche, L., Burns, J., Turner, R. D., Kumar, S., Tank, R., Mullin, N., et al. (2020). The architecture of the gram-positive bacterial cell wall. Nature 582 (7811), 294–297. doi:10.1038/s41586-020-2236-6
Peschel, A., Vuong, C., Otto, M., and Gotz, F. (2000). The D-alanine residues of Staphylococcus aureus teichoic acids alter the susceptibility to Vancomycin and the activity of autolytic enzymes. Antimicrob. Agents Chemother. 44 (10), 2845–2847. doi:10.1128/AAC.44.10.2845-2847.2000
Patel, Y., Soni, V., Rhee, K. Y., and Helmann, J. D. (2023). Mutations in rpoB that confer rifampicin resistance can alter levels of peptidoglycan precursors and affect β-lactam susceptibility. Mbio 14 (2), e03168–22.
Pham, T. D. M., Ziora, Z. M., and Blaskovich, M. A. T. (2019). Quinolone antibiotics. MedChemComm 10 (10), 1719–1739. doi:10.1039/c9md00120d
Piddock, L. J. V. (2015). Teixobactin, the first of a new class of antibiotics discovered by ichip technology? J. Antimicrob. Chemother. 70 (10), 2679–2680. doi:10.1093/jac/dkv175
Pouillot, F., Chomton, M., Blois, H., Courroux, C., Julien, N., Bidet, P., et al. (2012). Efficacy of bacteriophage therapy in experimental sepsis and meningitis caused by a clone O25b: H4-st131 Escherichia coli strain producing CTX-M-15. Antimicrob. Agents Chemother. 56 (7), 3568–3575. doi:10.1128/AAC.06330-11
Premlatha, M. (2019). Microbial resistance to antibiotics. Bact. Adapt. Co-Resistance 4, 61–80. December. doi:10.1007/978-981-13-8503-2_4
Romandini, A., Pani, A., Andrea Schenardi, P., De Giacomo, C., Scaglione, F., and Scaglione, F. (2021). Antibiotic resistance in pediatric infections: global emerging threats, predicting the near future. Antibiotics 10 (4), 393–412. doi:10.3390/antibiotics10040393
Sabtu, N., Enoch, D. A., and Brown, N. M. (2015). Antibiotic resistance: what, why, where, when and how? Br. Med. Bull. 116 (1), 105–113. doi:10.1093/bmb/ldv041
Sala, F. (1972). Structure and function of bacterial flagella. Bolletino Di Zool. 39 (2), 111–118. doi:10.1080/11250007209430052
Sarkar, R., Kaushik, P. B., Champak, D., Palash, J. S., and Dutta, S. (2021). Bacteriophage therapy to combat antibiotic resistance: a brief review. ∼ 389 ∼ Pharma Innovation J. 10 (5), 389–394.
Schwarz, S., Kehrenberg, C., and Walsh, T. R. (2001). Use of antimicrobial agents in veterinary medicine and food animal production. Int. J. Antimicrob. Agents 17 (6), 431–437. doi:10.1016/S0924-8579(01)00297-7
Scott Champney, W. (2008). The other target for ribosomal antibiotics: inhibition of bacterial ribosomal subunit formation. Infect. Disord. - Drug Targets 6 (4), 377–390. doi:10.2174/187152606779025842
Shree, P., Singh, C. K., Kaur Sodhi, K., Surya, J. N., and Singh, D. K. (2023). Biofilms: understanding the structure and contribution towards bacterial resistance in antibiotics. Med. Microecology 16, 100084. October 2022. doi:10.1016/j.medmic.2023.100084
Singh, C. K., and Kaur Sodhi, K. (2023). The emerging significance of nanomedicine-based approaches to fighting COVID-19 variants of concern: a perspective on the nanotechnology’s role in COVID-19 diagnosis and treatment. Front. Nanotechnol. 4, 1–13. January. doi:10.3389/fnano.2022.1084033
Soares, S., Sousa, J., Pais, A., and Vitorino, C. (2018). Nanomedicine: principles, properties, and regulatory issues. Front. Chem. 6, 360–415. AUG. doi:10.3389/fchem.2018.00360
Sodhi, K. K., Singh, C. K., Kumar, M., and Singh, D. K. (2023). Whole-genome sequencing of alcaligenes sp. Strain MMA: insight into the antibiotic and heavy metal resistant genes. Front. Pharmacol. 14, 1144561–1144611. May. doi:10.3389/fphar.2023.1144561
Sriram, A., Kalanxhi, E., Kapoor, G., Craig, J., Balasubramanian, R., Sehr Brar, , et al. (2021). State World ’ s Antibiotics 8 (2), 30–34.
Stokes, J. M., Yang, K., Swanson, K., Jin, W., Cubillos-Ruiz, A., Donghia, N. M., et al. (2020). A Deep learning approach to antibiotic discovery. Cell 180 (4), 688–702. doi:10.1016/j.cell.2020.01.021
Strateva, T., and Yordanov, D. (2009). Pseudomonas aeruginosa - a phenomenon of bacterial resistance. J. Med. Microbiol. 58 (9), 1133–1148. doi:10.1099/jmm.0.009142-0
Svircev, A., Roach, D., and Castle, A. (2018). Framing the future with bacteriophages in agriculture. Viruses 10 (5), 218–313. doi:10.3390/v10050218
Syroegin, E. A., Flemmich, L., Klepacki, D., Vazquez-Laslop, N., Micura, R., and Polikanov, Y. S. (2022). Structural basis for the context-specific action of the classic peptidyl transferase inhibitor chloramphenicol. Nat. Struct. Mol. Biol. 29 (2), 152–161. doi:10.1038/s41594-022-00720-y
Tacconelli, E., Cataldo, M. A., Dancer, S. J., De Angelis, G., Falcone, M., Frank, U., et al. (2014). ESCMID guidelines for the management of the infection control measures to reduce transmission of multidrug-resistant gram-negative bacteria in hospitalized patients. Clin. Microbiol. Infect. 20, 1–55. doi:10.1111/1469-0691.12427
Tanday, S. (2016). Resisting the use of antibiotics for viral infections. Lancet. Respir. Med. 4 (3), 179. doi:10.1016/S2213-2600(16)00060-6
Tebano, G., Mouelhi, Y., Zanichelli, V., Charmillon, A., Fougnot, S., Lozniewski, A., et al. (2020). Selective reporting of antibiotic susceptibility testing results: a promising antibiotic stewardship tool. Expert Rev. Anti-Infective Ther. 18 (3), 251–262. doi:10.1080/14787210.2020.1715795
Tenover, F. C. (2006). Mechanisms of antimicrobial resistance in bacteria. Am. J. Med. 119, S3–S10. 6 SUPPL. 1. doi:10.1016/j.amjmed.2006.03.011
Tenson, T., Lovmar, M., and Ehrenberg, M. (2003). The mechanism of action of macrolides, lincosamides and streptogramin B reveals the nascent peptide exit path in the ribosome. J. Mol. Biol. 330 (5), 1005–1014. doi:10.1016/S0022-2836(03)00662-4
Thakolkaran, N., Shetty, A. V., D′Souza, N. D. R., and Shetty, A. K. (2017). Antibiotic prescribing knowledge, attitudes, and practice among physicians in teaching Hospitals in south India. J. Fam. Med. Prim. Care 6 (3), 526–532. doi:10.4103/2249-4863.222057
Varela, M. F., Stephen, J., Lekshmi, M., Ojha, M., Wenzel, N., Sanford, L. M., et al. (2021). Bacterial resistance to antimicrobial agents. Antibiotics 10, 593. doi:10.3390/antibiotics10050593
Vicens, Q., and Westhof, E. (2003). RNA as a drug target: the case of aminoglycosides. ChemBioChem 4 (10), 1018–1023. doi:10.1002/cbic.200300684
Waksman, S. A. (1947). What is an antibiotic or an antibiotic substance? Mycologia 39 (5), 565–569. doi:10.1080/00275514.1947.12017635
Wang, J., Hu, B., Xu, M., Yan, Q., Liu, S., Zhu, X., et al. (2006). Use of bacteriophage in the treatment of experimental animal bacteremia from imipenem-resistant Pseudomonas aeruginosa. Int. J. Mol. Med. 17 (2), 309–317. doi:10.3892/ijmm.17.2.309
Wang, N., Luo, J., Deng, F., Huang, Y., and Zhou, H. (2022). Antibiotic combination therapy: a strategy to overcome bacterial resistance to aminoglycoside antibiotics. Front. Pharmacol. 13, 839808–839815. February. doi:10.3389/fphar.2022.839808
Watanabe, R., Matsumoto, T., Go, S., Ishii, Y., Tateda, K., Sumiyama, Y., et al. (2007). Efficacy of bacteriophage therapy against gut-derived sepsis caused by Pseudomonas aeruginosa in mice. Antimicrob. Agents Chemother. 51 (2), 446–452. doi:10.1128/AAC.00635-06
Weinstein, R. A. (2001). Controlling antimicrobial resistance in Hospitals: infection control and use of antibiotics. Emerg. Infect. Dis. 7 (2), 188–192. doi:10.3201/eid0702.010206
Wierup, M. (2001). The Swedish experience of the 1986 Year ban of antimicrobial growth promoters, with special reference to animal health, disease prevention, productivity, and usage of antimicrobials. Microb. Drug Resist. 7 (2), 183–190. doi:10.1089/10766290152045066
Wright, A., Hawkins, C. H., Änggård, E. E., and Harper, D. R. (2009). A controlled clinical trial of a therapeutic bacteriophage preparation in chronic otitis due to antibiotic-resistant Pseudomonas aeruginosa; A preliminary report of efficacy. Clin. Otolaryngol. 34 (4), 349–357. doi:10.1111/j.1749-4486.2009.01973.x
Wu, G. (2009). Amino acids: metabolism, functions, and nutrition. Amino Acids 37 (1), 1–17. doi:10.1007/s00726-009-0269-0
Yuan, Y., Wang, L., Li, X., Tan, D., Cong, C., and Xu, Y. (2019). Efficacy of a phage cocktail in controlling phage resistance development in multidrug resistant acinetobacter baumannii. Virus Res. 272 (June), 197734. doi:10.1016/j.virusres.2019.197734
Keywords: antibiotics, antimicrobial, bacteria, antibiotic resistance, disease spreading
Citation: Halawa EM, Fadel M, Al-Rabia MW, Behairy A, Nouh NA, Abdo M, Olga R, Fericean L, Atwa AM, El-Nablaway M and Abdeen A (2024) Antibiotic action and resistance: updated review of mechanisms, spread, influencing factors, and alternative approaches for combating resistance. Front. Pharmacol. 14:1305294. doi: 10.3389/fphar.2023.1305294
Received: 01 October 2023; Accepted: 18 December 2023;
Published: 12 January 2024.
Edited by:
Zul Kamal, Shaheed Benazir Bhutto University, PakistanReviewed by:
Manigandan Venkatesan, The University of Texas Health Science Center at San Antonio, United StatesHans Gersbach, ETH Zurich, Switzerland
Copyright © 2024 Halawa, Fadel, Al-Rabia, Behairy, Nouh, Abdo, Olga, Fericean, Atwa, El-Nablaway and Abdeen. This is an open-access article distributed under the terms of the Creative Commons Attribution License (CC BY). The use, distribution or reproduction in other forums is permitted, provided the original author(s) and the copyright owner(s) are credited and that the original publication in this journal is cited, in accordance with accepted academic practice. No use, distribution or reproduction is permitted which does not comply with these terms.
*Correspondence: Esraa M. Halawa, ZXNyYWFoYWxhd2FAZ3N0ZC5zY2kuY3UuZWR1LmVn; Rada Olga, b2xnYV9yYWRhQHVzdnQucm8=; Ahmed Abdeen, YWhtZWQuYWJkZWVuQGZ2dG0uYnUuZWR1LmVn