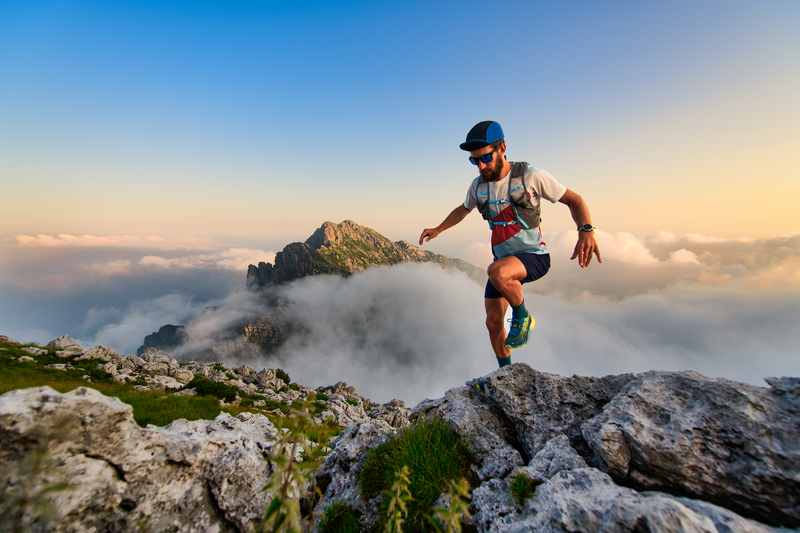
95% of researchers rate our articles as excellent or good
Learn more about the work of our research integrity team to safeguard the quality of each article we publish.
Find out more
ORIGINAL RESEARCH article
Front. Pharmacol. , 06 November 2023
Sec. Experimental Pharmacology and Drug Discovery
Volume 14 - 2023 | https://doi.org/10.3389/fphar.2023.1298085
Immune checkpoint molecules such as programmed death-1 (PD-1) and programmed death ligand-1 (PD-L1) have revolutionized the field of lung cancer treatment. As part of our study, we examined the role of these proteins in acute rejection in a mouse model of heterotopic tracheal transplantation. Recipient mice were untreated (Allo group) or treated with anti-PD-L1 (aPDL1 group) or PD-L1 Fc recombinant protein (PD-L1 Fc group). A further group of C57BL/6 mice received isografts (Iso group). The occlusion rate was significantly higher in the Allo group than in the Iso group (p = 0.0075), and also higher in the aPD-L1 group (p = 0.0066) and lower in the PD-L1 Fc group (p = 0.030) than in the Allo group. PD-L1 Fc recombinant protein treatment significantly decreased interleukin-6 and interferon-γ levels and reduced the CD4+/CD8+ T cell ratio, without increasing PD-1 and T-cell immunoglobulin mucin 3 expression in CD4+ T cells. These data suggest that PD-L1 Fc recombinant protein decreases the levels of inflammatory cytokines and the proportion of CD4+ T cells without exhaustion. The PD-L1-mediated immune checkpoint mechanism was associated with rejection in the murine tracheal transplant model, suggesting a potential novel target for immunotherapy in lung transplantation.
Survival rates in patients who have undergone lung transplantation are much lower than those in patients who receive other solid organ grafts (Gracon and Wilkes, 2014). The leading cause of death more than 1 year after lung transplantation is bronchiolitis obliterans syndrome (Yusen et al., 2015). A major form of chronic lung allograft dysfunction (Glanville et al., 2019), bronchiolitis obliterans syndrome has the most significant impact on the long-term survival of lung transplant patients (Verleden et al., 2014). Treatments for chronic lung allograft dysfunction are still limited and have not substantially improved patient prognosis.
Acute rejection is a serious complication in the early phase after lung transplantation, and is also an established risk factor for the development of chronic lung allograft dysfunction (Benzimra et al., 2017).
Programmed death 1 (PD-1) was first described by Ishida et al., in 1992 (Ishida et al., 1992) and is a negative regulator that modulates T-cell activation and homeostasis. Programmed death ligand-1 (PD-L1; also known as CD274 or B7H1) is a member of the B7 family of immuno-coinhibitory and costimulatory molecules and functions as an immune checkpoint via its interaction with its receptors PD-1 and CD80.
PD-1/PD-L1 interactions play a crucial suppressive role in the immune system, attenuating autoimmunity and promoting self-tolerance by preventing T-cell activation. PD-1/PD-L1 axis targeting has been reported in other conditions, such as autoimmune diabetes (Ben Nasr et al., 2017; Ben Nas et al., 2018; Ben Nasr et al., 2022) and coronavirus disease-2019 (Loretelli et al., 2021). Furthermore, the development of immune checkpoint inhibitors, such as anti-PD-1 or anti-PD-L1 inhibitors, has revolutionized the treatment of non-small cell lung cancer (Okazaki et al., 2013; Brahmer et al., 2015; Herbst et al., 2016; Rittmeyer et al., 2017).
Associations between PD-1/PD-L1 interactions and prolonged allograft survival have been reported in murine kidney transplantation (Peng et al., 2011; Jaworska et al., 2015), heterotopic heart transplantation (Ozkaynak et al., 2002; Tanaka et al., 2007; Yang et al., 2011), liver transplantation (Morita et al., 2010), and hematopoietic cell transplantation (Al-Chaqmaqchi et al., 2013; Cassa et al., 2018). However, there are few studies on lung transplantation (Takahashi et al., 2018), and immunological tolerance has not yet been achieved through targeting of PD-1.
In this study, we investigated the role of immune checkpoint molecules in acute rejection using a murine tracheal transplant model.
Specific pathogen-free male inbred C57BL/6 (H-2b) and BALB/c (H-2d) mice (CLEA Japan, Inc., Tokyo, Japan) were used for heterotopic tracheal transplantation. They were housed at the Biomedical Research Center at Chiba University School of Medicine (Chiba, Japan) in accordance with institutional guidelines. BALB/c (H-2d) and C57BL/6 (H-2b) mice were used as donors and C57BL/6 (H-2b) mice as recipients at 8–12 weeks of age (body weight, 24–32 g).
Heterotopic tracheal transplantation was carried out as previously described (Hua et al., 2010). The transplanted tracheas were harvested on day 14 post-surgery, along with serum. We established four groups. In the Allo group, BALB/c (H-2d) mice were used as donors and C57BL/6 (H-2b) mice as recipients (n = 5). In the aPD-L1 group, recipient mice were treated intraperitoneally on days −2, 0, and 2 with 250 μg mouse anti-PD-L1 (Bio X Cell, Lebanon, NH, USA) (n = 5). In the PD-L1 Fc group, recipient mice were treated intraperitoneally on days 1, 4, and 6 with 100 μg mouse PD-L1 Fc recombinant protein (PD-L1 Fc RP; Acro Biosystems, Newark, DE, USA) (n = 5). In the Iso group, C57BL/6 (H-2b) mice were used as donors and as recipients (n = 5) (Figure 1).
FIGURE 1. Study design. Tracheas from donor BALB/c (H-2d) mice were transplanted into major histocompatibility antigen-mismatched recipient C57BL/6 (H-2b) mice in the tracheal transplant model. Four groups (allografts (Allo), mouse anti-PD-L1 antibody (aPD-L1), PD-L1 Fc recombinant protein (PD-L1 Fc), and isografts (Iso) (n = 5)) were confirmed pathologically and analyzed. Rejection was evaluated by assessing pathologic occlusion rate, immunohistochemistry, and cytokine levels and cell surface markers by cytokine bead assay and flow cytometry, respectively. PD-L1, programmed death ligand-1.
This study was approved by the Institute for Animal Care at Chiba University (approval code: A2-015) and was performed in compliance with the Guide for the Care and Use of Laboratory Animals (National Institutes of Health publication 86–23, revised 1996). All surgical procedures were performed utilizing a sterile technique. No antibiotics were given to either donor or recipient mice. Induction of anesthesia of the donor mouse was initiated with 5% isoflurane. The mouse was then orotracheally intubated with a 20-gauge intravenous catheter and placed on a rodent ventilator, using 100% oxygen at a rate of 125 breaths/minute and approximately 0.5 mL tidal volume (2% of its body weight). The animals were maintained under general anesthesia with a mixture of isoflurane and oxygen. The donor and recipient mice were swabbed with 70% alcohol before incision. Buprenorphine (0.05–0.15 mg/kg) was administered immediately after surgery and every 8 h for 2–3 days post-surgery for analgesia, and all efforts were made to minimize suffering. Animals were monitored every day for signs of respiratory difficulty, weight loss, and the development of a moribund state. Animals were euthanized under anesthesia, and the transplanted grafts were harvested at that time. Euthanasia was performed by cervical dislocation under deep anesthesia induced by isoflurane or CO2.
Trachea grafts were harvested on day 14 post-surgery, fixed in glutaraldehyde, and paraffin embedded. Each trachea was sectioned and stained with hematoxylin/eosin and Masson’s trichrome to evaluate the presence of inflammatory cells and airway fibrosis and to diagnose acute rejection.
Luminal occlusion of the transplanted trachea was determined by measuring the area containing tissue inside the cartilage ring using ImageJ software (National Institutes of Health, Bethesda, MD, United States) (Reichenspurner et al., 1997; Schneider et al., 2012). The percentage of luminal occlusion was calculated as follows: (area within cartilage—area within residual lumen)/area within cartilage × 100%. The presence of mucus, produced by airway epithelial cells, in the lumen was not counted as occlusion. The histological changes in the respiratory epithelium were evaluated as the percentage of luminal circumference covered by ciliated epithelium (Sato et al., 2008).
Immunohistochemistry was performed with the following primary antibodies as per the manufacturer’s protocols: rabbit polyclonal anti-PD-1/CD279 antibody (catalog no. 18106-1-AP; ProteinTech Group, Rosemont, IL, USA) and polyclonal anti-PD-L1/CD274 antibody (catalog no. 17952-1-AP; ProteinTech Group).
Serum from individual representative recipient mice from each of the Iso (n = 5), Allo (n = 5), aPD-L1 (n = 5), and PD-L1 Fc (n = 5) groups was analyzed using the BD Cytometric Bead Array system (BD, Franklin Lakes, NJ, United States), as previously described (Morgan et al., 2004).
Single cell suspensions were obtained from fresh trachea graft tissue by enzymatic digestion using collagenase type III (6000 U/mL; Worthington Biochemical Corp., Lakewood, NJ, United States) and DNase (10 mg/mL; Merck Group, Darmstadt, Germany) for 30 min at 37°C. After counting, cells were incubated with antibodies for 30 min at 4°C and analyzed using a FACSCanto II flow cytometer (BD).
Single cell suspensions were stained with fluorochrome-labeled antibodies specific for CD44 (clone IM7), CD8a (clone 53–6.7), and CD4 (clone RM45) (all from eBioscience, Inc., San Diego, CA, United States), and PD-1 (clone RMT1-30), and T-cell immunoglobulin mucin 3 (Tim-3; clone RMT3-23) (from BioLegend, San Diego, CA, United States).
All data are presented as the mean ± standard error of the mean. When comparing two groups, data were analyzed using the Student’s t-test. All statistical analyses were performed using JMP software version 13.2 (SAS Institute, Cary, NC, United States). p-values <0.05 were considered statistically significant.
Trachea graft sections were stained with hematoxylin/eosin (Figures 2A–D) or Masson’s trichrome (Figures 2E–H). The airway was not obstructed by fibrotic tissue in the Iso group (Figures 2A,E). The Allo group showed airway occlusion with luminal cell infiltration and fibrotic tissue, which was deposition of mononuclear cells in the graft/denuded epithelium as evidence of acute rejection (Figures 2B,F). The airway occlusion was greater in the aPD-L1 group (Figures 2C,G) and less in the PD-L1 Fc group (Figures 2D,H) than in the Allo group.
FIGURE 2. Histological features of the trachea grafts. Representative images of hematoxylin/eosin-stained tissue sections (A–D) (original, 10× and 40x), and Masson’s trichrome-stained tissue sections (E–H) in the Allo (A and E), aPD-L1 (B and F), PD-L1 Fc (C and G), and Iso (D and H) groups (original, 10× and 40x). aPD-L1, anti-programmed death ligand-1; PD-L1, programmed death ligand-1. Black arrows indicate epithelium and red arrows indicate locations of cellular infiltration. Scale bar = 500 μm.
Trachea graft sections were also stained using antibodies against PD-1 (Figures 3A–D) and PD-L1 (Figures 3E–H). Immunohistochemistry revealed that luminal infiltrating cells were positive for PD-1 (Figures 3B,C,I,J) and PD-L1 (Figures 3F,G) in the Allo and aPD-L1 groups.
FIGURE 3. Immunohistochemical features of trachea grafts. Representative images of PD-1 immunohistochemical staining (A–D) and PD-L1 immunohistochemical staining (E–H) in the Allo (A and E), aPD-L1 (B and F), PD-L1 Fc (C and G), and Iso (D and H) groups. aPD-L1, anti-programmed death ligand-1; PD-1, programmed death-1; PD-L1, programmed death ligand-1. Scale bar = 500 μm.
The luminal occlusion rate was significantly higher in the Allo group than in the Iso group (58.24% ± 5.02% vs. 35.58% ± 2.17%, p = 0.0075), higher in the aPD-L1 group than in the Allo group (81.36% ± 8.77% vs. 58.24% ± 5.02%, p = 0.0066), and lower in the PD-L1 Fc group than in the Allo group (40.54% ± 1.76% vs. 58.24% ± 5.02%, p = 0.030) (Figure 4).
FIGURE 4. Pathological occlusion rate. The occlusion rate was significantly higher in the Allo group than in the Iso group (58.24% ± 5.02% vs. 35.58% ± 2.17%, p = 0.0075), higher in the aPD-L1 group than in the Allo group (81.36% ± 8.77% vs. 58.24% ± 5.02%, p = 0.0066), and lower in the PD-L1 Fc group than in the Allo group (40.54% ± 1.76% vs. 58.24% ± 5.02%, p = 0.0297) (n = 5). *p < 0.05, **p < 0.01. aPD-L1, anti-programmed death ligand-1; PD-L1, programmed death ligand-1.
Serum cytokine analysis revealed that interleukin-6 levels were significantly suppressed in the PD-L1 Fc group, whereas they increased in the aPD-L1 group (0.43 ± 0.12 pg/mL vs. 1.55 ± 0.52 pg/mL, p = 0.037) (Figure 5A). Interferon-γ levels were also suppressed in the PD-L1 Fc group (1.20 ± 0.06 pg/mL) and increased in the aPD-L1 group (1.34 ± 0.20 pg/mL), although not significantly compared with the Allo group (Figure 5B). In contrast, levels of interleukin-10, a cytokine with potent anti-inflammatory properties, were increased in the Allo group (2.87 ± 0.41 pg/mL) and the PD-L1 Fc group (2.79 ± 0.10 pg/mL) and suppressed in the aPD-L1 group (2.02 ± 0.53 pg/mL) (Figure 5C).
FIGURE 5. Serum cytokine levels. (A) IL-6 levels were significantly suppressed in the PD-L1 Fc group but increased in the aPD-L1 group (0.43 ± 0.12 pg/mL vs. 1.55 ± 0.52 pg/mL, p = 0.0366). (B) A similar trend was observed in IFN-γ in the four groups. (C) IL-10 levels were increased in the Allo (2.87 ± 0.41 pg/mL) and PD-L1 Fc (2.79 ± 0.10 pg/mL) groups and suppressed in the aPD-L1 group (2.02 ± 0.53 pg/mL) (n = 5). *p < 0.05. aPD-L1, anti-programmed death ligand-1; IFN-γ, interferon-γ; IL, interleukin; PD-L1, programmed death ligand-1.
In flow cytometric analyses of whole tracheal tissue, the CD4+/CD8+ ratio was lower in the PD-L1 Fc group (0.78 ± 0.25) than in the Allo group (4.74 ± 3.47) and the Iso group (3.33 ± 1.78) (Figure 6) (n = 3).
FIGURE 6. CD4+/CD8+ T cell ratio. In flow cytometric analyses of whole tracheal tissue, the CD4+/CD8+ ratio was lower in the PD-L1 Fc group (0.78 ± 0.25) than in the Allo group (4.74 ± 3.47) and the Iso group (3.33 ± 1.78) (n = 3). This ratio was increased in the Allo group compared with the Iso group. PD-L1, programmed death ligand-1.
CD44 is an activation marker that distinguishes memory and effector T cells from their naïve counterparts (Schumann et al., 2015). CD4+CD44+ T cells are antigen-stimulated memory T cells. Both PD-1 and Tim-3 are exhaustion markers and can function as negative regulators of T-cell responses. CD4+CD44+ T cells were analyzed for expression of the immune checkpoint molecules PD-1 and Tim-3. In this analysis, cells that coexpressed PD-1 and Tim-3 represented the most exhausted fraction.
The proportion of CD4+CD44+ T cells that were PD-1-/Tim-3-, PD-1+/Tim-3-, and PD-1+/Tim3+ was not significantly different in the PD-L1 Fc group (44.3% ± 9.8%, 17.6% ± 10.2%, and 2.4% ± 1.2%, respectively) compared to the Allo group (42.5% ± 7.7%, 10.6% ± 6.1%, and 8.7% ± 2.7%, respectively) (Figure 7).
FIGURE 7. Proportions of CD4+CD44+ T cells expressing PD-1 and Tim3. The proportion of CD4+CD44+ T cells that were PD-1-/Tim-3-, PD-1+/Tim-3-, and PD-1+/Tim3+ was not significantly different in the PD-L1 Fc group (44.3% ± 9.8%, 17.6% ± 10.2%, and 2.4% ± 1.2%, respectively) compared to the Allo group (42.5% ± 7.7%, 10.6% ± 6.1%, and 8.7% ± 2.7%, respectively) (n = 3). PD-1, programmed death-1; PD-L1, programmed death ligand-1; Tim-3, T-cell immunoglobulin mucin 3.
This study examined the role of PD-1/PD-L1 in acute rejection using a murine tracheal transplant model. The administration of PD-L1 Fc RP decreased inflammatory cytokine levels and inhibited CD4+ T-cell proliferation; allograft airway fibrosis was also suppressed. In cancer immunotherapy, PD-1/PD-L1 blockade activates the PD-1/PD-L1 axis, which serves as a mechanism for tumor evasion of host tumor antigen-specific T-cell immunity. This study was initiated to investigate the role of immune checkpoint molecules in lung transplantation and their potential therapeutic applications.
The main finding of the present study was that PD-1/PD-L1 was associated with transplant rejection in the tracheal transplant model. In our study, administration of PD-L1 Fc RP suppressed airway occlusion by luminal cell infiltration and fibrotic tissue, suggesting that acute rejection could be regulated by targeting this axis. These results were consistent with those described in other transplant models.
The present study is, to the best of our knowledge, the first to report the potential utility of PD-1/PD-L1 for the treatment of lung transplant rejection in a murine heterotopic tracheal transplant model. Takahashi et al. reported that PD-1 expression regulates the differentiation of CD8+ T cells within lung allografts (Takahashi et al., 2018), but the effect of PD-1 targeting therapy was not mentioned. The results of this study provide a first step toward the treatment of lung transplant rejection by targeting immune checkpoint molecules. T-cell immunoglobulin and ITIM domain is another immune checkpoint molecule, and it has been reported that administering T-cell immunoglobulin and ITIM domain-Fc recombinant protein suppressed the proliferation of T cells and interferon-γ production in a mouse model (Yu et al., 2009). Other immune checkpoint molecules, such as lymphocyte activation gene-3 or Tim-3, also modulate the immune system. Combined blockade of the Tim-3 and PD-1 pathways has been reported to be a promising strategy for tumor immunotherapy (Tian and Li, 2021). These immune checkpoint molecules may possibly be of use in transplant rejection therapy (McGrath and Najafian, 2012).
Another important finding of the present study is that PD-1/PD-L1 was associated with suppression of the inflammatory cytokines, interleukin-6 and interferon-γ, and a decrease in the proportion of CD4+ T cells without exhaustion. Treatment with PD-L1 Fc RP did not increase the expression of Tim-3 in CD4+CD44+ T cells. This result may provide insight into the mechanism of allograft rejection in lung transplantation and provide new options for the targeting of immune checkpoint inhibitors in tumor immunology. Further in vivo studies are required to establish the molecular mechanisms of immune checkpoints in therapeutic approaches, including their association with CD4+Foxp3+ T cells. Translational research on immune checkpoint molecules in lung cancer is currently being performed (Kamada et al., 2019), and there is potential for clinical applications of immune checkpoint molecules for lung allograft rejection in the future.
The current study has some limitations. First, this murine heterotopic tracheal transplant model is an acute rejection model with a major histocompatibility mismatch. Heterotopic tracheal transplant model is a high-throughput procedure useful for studying the cellular requirements for acute rejection models. Histological changes also make this model especially valuable for studying chronic rejection observed in the airways of human lungs (Lama et al., 2017). The tracheal transplant model has disadvantages including no ventilation of the transplanted trachea, inhibition of mucociliary clearance and retained secretions, and differences in the microenvironment. To analyze acute rejection more precisely, a murine orthotopic lung transplant model should be utilized. This model was developed by Okazaki et al., 2007, and a murine obliterative bronchiolitis orthotopic lung transplant model has also been established (Suzuki et al., 2012; Hata et al., 2020). Orthotopic lung transplant models are a closer reflection of the clinical situation than heterotopic lung transplant models and will therefore be essential to understand the mechanisms underlying the pathogenesis of rejection in lung transplantation.
Second, cellular rejection was mainly analyzed in this study of acute rejection; however, humoral rejection may also be involved. The association between antibody-mediated rejection and C4d staining analysis (Levine et al., 2016) was not investigated in this study and will be further explored in the future.
Finally, the PD-1/PD-L1 mechanism involved in acute rejection following lung transplantation remains unidentified. In the present study, PD-L1 Fc RP was found to upregulate PD-L1 levels; however, it has been reported that overexpression of PD-L1 in dendritic cells inhibits allogeneic lymphocyte activation in mice (Peng et al., 2011; Li et al., 2012). This mechanism suggests the possibility of donor-specific immune tolerance, which has been reported in other murine organ transplant models but has not been reported in the lung transplant model. This donor-specific treatment might be a potential therapeutic modality for rejection in lung transplantation.
In conclusion, the PD-L1-mediated immune checkpoint mechanism was associated with rejection, which suggests a potential novel target for immunotherapy in lung transplantation. PD-1/PD-L1 should be further explored as a potential therapeutic modality for rejection.
The raw data supporting the conclusion of this article will be made available by the authors, without undue reservation.
The animal study was approved by the Institute for Animal Care at Chiba University. The study was conducted in accordance with the local legislation and institutional requirements.
TK: Conceptualization, Data curation, Formal Analysis, Funding acquisition, Investigation, Methodology, Project administration, Visualization, Writing–original draft, Writing–review and editing. HS: Conceptualization, Data curation, Formal Analysis, Funding acquisition, Investigation, Methodology, Project administration, Supervision, Writing–original draft, Writing–review and editing. AH: Project administration, Writing–review and editing. HM: Project administration, Writing–review and editing. KT: Project administration, Writing–review and editing. YS: Project administration, Writing–review and editing. SM: Methodology, Project administration, Resources, Software, Supervision, Writing–review and editing. IY: Conceptualization, Data curation, Formal Analysis, Funding acquisition, Methodology, Project administration, Resources, Supervision, Writing–review and editing.
The author(s) declare financial support was received for the research, authorship, and/or publication of this article. This study was supported by grants from JSPS KAKENHI for TK (https://www.jsps.go.jp/j-grantsinaid/; Grant Number JP18K16411) and Chiba Foundation for Health Promotion and Disease Prevention for TK (Grant Number 4974-22). The funders had no role in study design, data collection and analysis, decision to publish, or preparation of the manuscript.
We thank Yumiko Sasaki for technical assistance and Masahiro Kiuchi and Toshinori Nakayama for the immunological analysis. We would like to thank Editage (www.editage.jp) for English language editing.
The authors declare that the research was conducted in the absence of any commercial or financial relationships that could be construed as a potential conflict of interest.
All claims expressed in this article are solely those of the authors and do not necessarily represent those of their affiliated organizations, or those of the publisher, the editors and the reviewers. Any product that may be evaluated in this article, or claim that may be made by its manufacturer, is not guaranteed or endorsed by the publisher.
PD-1, programmed death-1; PD-L1, programmed death ligand-1; RP, recombinant protein; Tim-3, T-cell immunoglobulin mucin 3.
Al-Chaqmaqchi, H., Sadeghi, B., Abedi-Valugerdi, M., Al-Hashmi, S., Fares, M., Kuiper, R., et al. (2013). The role of programmed cell death ligand-1 (PD-L1/CD274) in the development of graft versus host disease. PloS one 8, e60367. doi:10.1371/journal.pone.0060367
Ben Nasr, M., D'Addio, F., Malvandi, A. M., Faravelli, S., Castillo-Leon, E., Usuelli, V., et al. (2018). Prostaglandin E2 stimulates the expansion of regulatory hematopoietic stem and progenitor cells in type 1 diabetes. Front. Immunol. 9, 1387. doi:10.3389/fimmu.2018.01387
Ben Nasr, M., Robbins, D., Parone, P., Usuelli, V., Tacke, R., Seelam, A. J., et al. (2022). Pharmacologically enhanced regulatory hematopoietic stem cells revert experimental autoimmune diabetes and mitigate other autoimmune disorders. J. Immunol. 208 (7), 1554–1565. doi:10.4049/jimmunol.2100949
Ben Nasr, M., Tezza, S., D'Addio, F., Mameli, C., Usuelli, V., Maestroni, A., et al. (2017). PD-L1 genetic overexpression or pharmacological restoration in hematopoietic stem and progenitor cells reverses autoimmune diabetes. Sci. Transl. Med. 9 (416), eaam7543. doi:10.1126/scitranslmed.aam7543
Benzimra, M., Calligaro, G. L., and Glanville, A. R. (2017). Acute rejection. J. Thorac. Dis. 9, 5440–5457. doi:10.21037/jtd.2017.11.83
Brahmer, J., Reckamp, K. L., Baas, P., Crinò, L., Eberhardt, W. E. E., Poddubskaya, E., et al. (2015). Nivolumab versus docetaxel in advanced squamous-cell non-small-cell lung cancer. N. Engl. J. Med. 373, 123–135. doi:10.1056/NEJMoa1504627
Cassady, K., Martin, P. J., and Zeng, D. (2018). Regulation of GVHD and GVL activity via PD-L1 interaction with PD-1 and CD80. Front. Immunol. 9, 3061. doi:10.3389/fimmu.2018.03061
Glanville, A. R., Verleden, G. M., Todd, J. L., Benden, C., Calabrese, F., Gottlieb, J., et al. (2019). Chronic lung allograft dysfunction: definition and update of restrictive allograft syndrome-A consensus report from the Pulmonary Council of the ISHLT. J. Heart Lung Transpl. 38, 483–492. doi:10.1016/j.healun.2019.03.008
Gracon, A. S., and Wilkes, D. S. (2014). Lung transplantation: chronic allograft dysfunction and establishing immune tolerance. Hum. Immunol. 75, 887–894. doi:10.1016/j.humimm.2014.06.015
Hata, A., Suzuki, H., Nakajima, T., Fujiwara, T., Shiina, Y., Kaiho, T., et al. (2020). Differential gene analysis during the development of obliterative bronchiolitis in a murine orthotopic lung transplantation model: a comprehensive transcriptome-based analysis. PloS one 15, e0232884. doi:10.1371/journal.pone.0232884
Herbst, R. S., Baas, P., Kim, D.-W., Felip, E., Pérez-Gracia, J. L., Han, J. Y., et al. (2016). Pembrolizumab versus docetaxel for previously treated, PD-L1-positive, advanced non-small-cell lung cancer (KEYNOTE-010): a randomised controlled trial. Lancet 387, 1540–1550. doi:10.1016/S0140-6736(15)01281-7
Hua, X., Deuse, T., Tang-Quan, K. R., Robbins, R. C., Reichenspurner, H., and Schrepfer, S. (2010). Heterotopic and orthotopic tracheal transplantation in mice used as models to study the development of obliterative airway disease. J. Vis. Exp., 1437. doi:10.3791/1437
Ishida, Y., Agata, Y., Shibahara, K., and Honjo, T. (1992). Induced expression of PD-1, a novel member of the immunoglobulin gene superfamily, upon programmed cell death. Embo J. 11, 3887–3895. doi:10.1002/j.1460-2075.1992.tb05481.x
Jaworska, K., Ratajczak, J., Huang, L., Whalen, K., Yang, M., Stevens, B. K., et al. (2015). Both PD-1 ligands protect the kidney from ischemia reperfusion injury. J. Immunol. 194, 325–333. doi:10.4049/jimmunol.1400497
Kamada, T., Togashi, Y., Tay, C., Ha, D., Sasaki, A., Nakamura, Y., et al. (2019). PD-1(+) regulatory T cells amplified by PD-1 blockade promote hyperprogression of cancer. Proc. Natl. Acad. Sci. U. S. A. 116, 9999–10008. doi:10.1073/pnas.1822001116
Lama, V. N., Belperio, J. A., Christie, J. D., El-Chemaly, S., Fishbein, M. C., Gelman, A. E., et al. (2017). Models of lung transplant research: a consensus statement from the national heart, lung, and blood Institute workshop. JCI Insight 2 (9), e93121. doi:10.1172/jci.insight.93121
Levine, D. J., Glanville, A. R., Aboyoun, C., Belperio, J., Benden, C., Berry, G. J., et al. (2016). Antibody-mediated rejection of the lung: a consensus report of the international society for heart and lung transplantation. J. Heart Lung Transpl. 35, 397–406. doi:10.1016/j.healun.2016.01.1223
Li, W., Wang, X., Chen, R., Zhu, H., Chen, G., and Sun, X. (2012). Overexpression of programmed death ligand 1 in dendritic cells inhibits allogeneic lymphocyte activation in mice. J. Surg. Res. 176, e79–e87. doi:10.1016/j.jss.2011.12.009
Loretelli, C., Abdelsalam, A., D'Addio, F., Ben Nasr, M., Assi, E., Usuelli, V., et al. (2021). PD-1 blockade counteracts post-COVID-19 immune abnormalities and stimulates the anti-SARS-CoV-2 immune response. JCI Insight 6 (24), e146701. doi:10.1172/jci.insight.146701
McGrath, M. M., and Najafian, N. (2012). The role of coinhibitory signaling pathways in transplantation and tolerance. Front. Immunol. 3, 47. doi:10.3389/fimmu.2012.00047
Morgan, E., Varro, R., Sepulveda, H., Ember, J. A., Apgar, J., Wilson, J., et al. (2004). Cytometric bead array: a multiplexed assay platform with applications in various areas of biology. Clin. Immunol. 110, 252–266. doi:10.1016/j.clim.2003.11.017
Morita, M., Fujino, M., Jiang, G., Kitazawa, Y., Xie, L., Azuma, M., et al. (2010). PD-1/B7-H1 interaction contribute to the spontaneous acceptance of mouse liver allograft. Am. J. Transpl. 10, 40–46. doi:10.1111/j.1600-6143.2009.02859.x
Okazaki, M., Krupnick, A. S., Kornfeld, C. G., Lai, J. M., Ritter, J. H., Richardson, S. B., et al. (2007). A mouse model of orthotopic vascularized aerated lung transplantation. Am. J. Transpl. 7, 1672–1679. doi:10.1111/j.1600-6143.2007.01819.x
Okazaki, T., Chikuma, S., Iwai, Y., Fagarasan, S., and Honjo, T. (2013). A rheostat for immune responses: the unique properties of PD-1 and their advantages for clinical application. Nat. Immunol. 14, 1212–1218. doi:10.1038/ni.2762
Ozkaynak, E., Wang, L., Goodearl, A., McDonald, K., Qin, S., O'Keefe, T., et al. (2002). Programmed death-1 targeting can promote allograft survival. J. Immunol. 169, 6546–6553. doi:10.4049/jimmunol.169.11.6546
Peng, W., Ran, B., Ma, Y., Huang, X., Chang, Q., and Wang, X. (2011). Dendritic cells transfected with PD-L1 recombinant adenovirus induces T cell suppression and long-term acceptance of allograft transplantation. Cell. Immunol. 271, 73–77. doi:10.1016/j.cellimm.2011.06.007
Reichenspurner, H., Soni, V., Nitschke, M., Berry, G. J., Brazelton, T. R., Shorthouse, R., et al. (1997). Obliterative airway disease after heterotopic tracheal xenotransplantation: pathogenesis and prevention using new immunosuppressive agents. Transplantation 64, 373–383. doi:10.1097/00007890-199708150-00001
Rittmeyer, A., Barlesi, F., Waterkamp, D., Park, K., Ciardiello, F., von Pawel, J., et al. (2017). Atezolizumab versus docetaxel in patients with previously treated non-small-cell lung cancer (OAK): a phase 3, open-label, multicentre randomised controlled trial. Lancet 389, 255–265. doi:10.1016/S0140-6736(16)32517-X
Sato, M., Liu, M., Anraku, M., Ogura, T., D'Cruz, G., Alman, B. A., et al. (2008). Allograft airway fibrosis in the pulmonary milieu: a disorder of tissue remodeling. Am. J. Transpl. 8, 517–528. doi:10.1111/j.1600-6143.2007.02106.x
Schneider, C. A., Rasband, W. S., and Eliceiri, K. W. (2012). NIH Image to ImageJ: 25 years of image analysis. Nat. Methods 9, 671–675. doi:10.1038/nmeth.2089
Schumann, J., Stanko, K., Schliesser, U., Appelt, C., and Sawitzki, B. (2015). Differences in CD44 surface expression levels and function discriminates IL-17 and IFN-gamma producing helper T cells. PloS one 10, e0132479. doi:10.1371/journal.pone.0132479
Suzuki, H., Fan, L., and Wilkes, D. S. (2012). Development of obliterative bronchiolitis in a murine model of orthotopic lung transplantation. J. Vis. Exp., 3947. doi:10.3791/3947
Takahashi, T., Hsiao, H. M., Tanaka, S., Li, W., Higashikubo, R., Scozzi, D., et al. (2018). PD-1 expression on CD8(+) T cells regulates their differentiation within lung allografts and is critical for tolerance induction. Am. J. Transpl. 18, 216–225. doi:10.1111/ajt.14437
Tanaka, K., Albin, M. J., Yuan, X., Yamaura, K., Habicht, A., Murayama, T., et al. (2007). PDL1 is required for peripheral transplantation tolerance and protection from chronic allograft rejection. J. Immunol. 179, 5204–5210. doi:10.4049/jimmunol.179.8.5204
Tian, T., and Li, Z. (2021). Targeting tim-3 in cancer with resistance to PD-1/PD-L1 blockade. Front. Oncol. 11, 731175. doi:10.3389/fonc.2021.731175
Verleden, G. M., Raghu, G., Meyer, K. C., Glanville, A. R., and Corris, P. (2014). A new classification system for chronic lung allograft dysfunction. J. Heart Lung Transpl. 33, 127–133. doi:10.1016/j.healun.2013.10.022
Yang, J., Riella, L. V., Chock, S., Liu, T., Zhao, X., Yuan, X., et al. (2011). The novel costimulatory programmed death ligand 1/B7.1 pathway is functional in inhibiting alloimmune responses in vivo. J. Immunol. 187, 1113–1119. doi:10.4049/jimmunol.1100056
Yu, X., Harden, K., Gonzalez, L. C., Francesco, M., Chiang, E., Irving, B., et al. (2009). The surface protein TIGIT suppresses T cell activation by promoting the generation of mature immunoregulatory dendritic cells. Nat. Immunol. 10, 48–57. doi:10.1038/ni.1674
Yusen, R. D., Edwards, L. B., Kucheryavaya, A. Y., Benden, C., Dipchand, A. I., Goldfarb, S. B., et al. (2015). The registry of the international society for heart and lung transplantation: thirty-second official adult lung and heart-lung transplantation report--2015; focus theme: early graft failure. J. Heart Lung Transpl. 34, 1264–1277. doi:10.1016/j.healun.2015.08.014
Keywords: programmed death-1, programmed death ligand-1, lung transplantation, mouse model, immunotherapy
Citation: Kaiho T, Suzuki H, Hata A, Matsumoto H, Tanaka K, Sakairi Y, Motohashi S and Yoshino I (2023) Targeting PD-1/PD-L1 inhibits rejection in a heterotopic tracheal allograft model of lung transplantation. Front. Pharmacol. 14:1298085. doi: 10.3389/fphar.2023.1298085
Received: 21 September 2023; Accepted: 23 October 2023;
Published: 06 November 2023.
Edited by:
Mohamed Abdo Rizk, Mansoura University, EgyptReviewed by:
Mohammad Afzal Khan, University of Maryland, United StatesCopyright © 2023 Kaiho, Suzuki, Hata, Matsumoto, Tanaka, Sakairi, Motohashi and Yoshino. This is an open-access article distributed under the terms of the Creative Commons Attribution License (CC BY). The use, distribution or reproduction in other forums is permitted, provided the original author(s) and the copyright owner(s) are credited and that the original publication in this journal is cited, in accordance with accepted academic practice. No use, distribution or reproduction is permitted which does not comply with these terms.
*Correspondence: Hidemi Suzuki, aGlkZW1pc3V6dWtpZGVzdUB5YWhvby5jby5qcA==
†These authors have contributed equally to this work and share first authorship
Disclaimer: All claims expressed in this article are solely those of the authors and do not necessarily represent those of their affiliated organizations, or those of the publisher, the editors and the reviewers. Any product that may be evaluated in this article or claim that may be made by its manufacturer is not guaranteed or endorsed by the publisher.
Research integrity at Frontiers
Learn more about the work of our research integrity team to safeguard the quality of each article we publish.