- Institute of Mental Health Research, University of Ottawa, Ottawa, ON, Canada
Ketamine acts primarily by blocking the N-methyl-D-aspartate (NMDA) receptor at the phencyclidine site. The rapid antidepressant properties of ketamine were demonstrated in the clinic and several behavioral models of depression in rodents. We hypothesized that the normalization of abnormal activity of monoamine neurons in Wistar Kyoto (WKY) rats contributes to the rapid antidepressant effects of ketamine. A single administration of ketamine (10 mg/kg, i. p) or saline was administered to anesthetized WKY rats before in vivo electrophysiological recordings of dorsal raphe nucleus (DRN) serotonin (5-HT), locus coeruleus (LC) norepinephrine (NE) and ventral tegmental area (VTA) dopamine (DA) neuronal activity. Pyramidal neurons from the medial prefrontal cortex (mPFC) were also recorded before and after a ketamine injection. In the VTA, ketamine elicited a significant increase in the population activity of DA neurons. This enhancement was consistent with findings in other depression-like models in which such a decreased population activity was observed. In the LC, ketamine normalized increased NE neuron burst activity found in WKY rats. In the DRN, ketamine did not significantly reverse 5-HT neuronal activity in WKY rats, which is dampened compared to Wistar rats. Ketamine did not significantly alter the neuronal activity of mPFC pyramidal neurons. These findings demonstrate that ketamine normalized NE neuronal activity and enhanced DA neuronal activity in WKY rats, which may contribute to its rapid antidepressant effect.
Introduction
Clinical evidence demonstrates that a subanesthetic single dose of ketamine, a noncompetitive N-methyl-D-aspartate receptor (NMDAR) antagonist, induces a rapid antidepressant effect in treatment-resistant patients with major depressive disorder (MDD; McIntyre et al., 2021; Swainson et al., 2021). Preclinical studies have shown that ketamine increased the activation of α-amino-3-hydroxy-5-methyl-4-isoxazolepropionic acid receptor (AMPAR), brain-derived neurotrophic factor (BDNF) release and/or expression, activation of protein synthesis pathways (i.e., eEF2 kinase), increased expression of synaptic proteins (GluA1, PSD95, and synapsin), and increased synaptic number and function in the medial prefrontal cortex (mPFC; Duman et al., 2019; Hashimoto, 2019). These data indicate that the rapid antidepressant effect of ketamine is supported by increases in GluA1 and other synaptic proteins, in line with a reported enhancement in the formation and connectivity of new synapses. Importantly, studies also indicate that bidirectional interactions between glutamatergic pyramidal neurons in the PFC, and monoamine neurons in the dorsal raphe nucleus (DRN), locus coeruleus (LC), and ventral tegmental area (VTA) may contribute to the antidepressant response, including that of ketamine (Fukumoto et al., 2014; Nishitani et al., 2014; El Iskandrani et al., 2015; Fukumoto et al., 2016; Witkin et al., 2016; Hare et al., 2017; Lopez-Gil et al., 2019; Rincón-Cortés and Grace, 2020; Iro et al., 2021).
It was previously reported that reciprocal interactions between glutamate neurons in the PFC with serotonin (5-HT) neurons in the DRN play a fundamental role in the learned helplessness model of depression (Maier and Watkins, 2005). Indeed, the DRN gives rise to a majority of the 5-HT innervation of limbic and cortical brain regions, including the mPFC. On the other hand, the inactivation of mPFC at the time of stress exposure abolishes the influence of this structure in regulating stressor controllability (Amat et al., 2005), emphasizing the importance of this interaction in the deleterious effects of inescapable stress. Interestingly, microinjection of ketamine in the mPFC increases c-fos immunoreactivity in DRN 5-HT neurons (Fukumoto et al., 2016). In addition, the ketamine-induced reduction in the latency to feed in a novel environment was abolished by 5-HT depletion or by infusion of a 5- HT1A receptor antagonist into the mPFC (Fukumoto et al., 2014; Fukumoto et al., 2016).
LC norepinephrine (NE) neurons are regulated by a stimulatory glutamatergic efferent emanating from mPFC pyramidal neurons, which in turn are controlled by NE innervation from LC. NE exerts an inverted U-shaped influence on PFC physiology and cognition. Accordingly, low levels of NE are found during fatigue, while phasic release takes place under alert and non-stress conditions, and high levels are observed during exposure to uncontrollable stress (Hains and Arnsten, 2008; Arnsten, 2009). The activation of postsynaptic α2A-adrenoceptor by NE inhibits irrelevant and distracting sensory processing by acting on glutamate pyramidal neurons of the PFC (Arnsten, 1998), which is thought to be beneficial in MDD. Interestingly, ketamine induces an increase in the NE level in mPFC (Lorrain et al., 2003a) and enhances climbing in the forced swim test (FST) in rodents (Lopez-Gil et al., 2019), interpreted as an antidepressant-like effect.
Dopamine (DA) and glutamate interact in a bidirectional manner to regulate reward processing. Anhedonia, which is a cardinal feature of depression (Yang et al., 2014; Daniels et al., 2021), is regulated by DA activity in the mesocorticolimbic pathway (Düzel et al., 2010). The glutamatergic tone has been shown to modulate mesolimbic functioning through a polysynaptic circuit projecting from the PFC onto midbrain DA neurons. Indeed, inhibition of VTA projections to the mPFC increased social avoidance in animals subjected to chronic social defeat stress (Chaudhury et al., 2013). Furthermore, acute ketamine administration rescued decreased sucrose consumption, a measure of anhedonia, in mice and rats exposed to chronic mild stress (Li et al., 2011) and also reversed depressive-like behavior in the FST (Rincón-Cortés and Grace, 2017). Finally, acute ketamine injection leads to enhancement in DA levels in the nucleus accumbens and the PFC (Moghaddam et al., 1997).
Crosstalk between glutamate and monoamine pathways thus appears important in the antidepressant response and modulation of one system affect the others (Figure 1). Accordingly in the present study, the effects of a single administration of ketamine were examined on 5-HT, NE, DA, and mPFC neuronal activity in WKY, an animal model of depression.
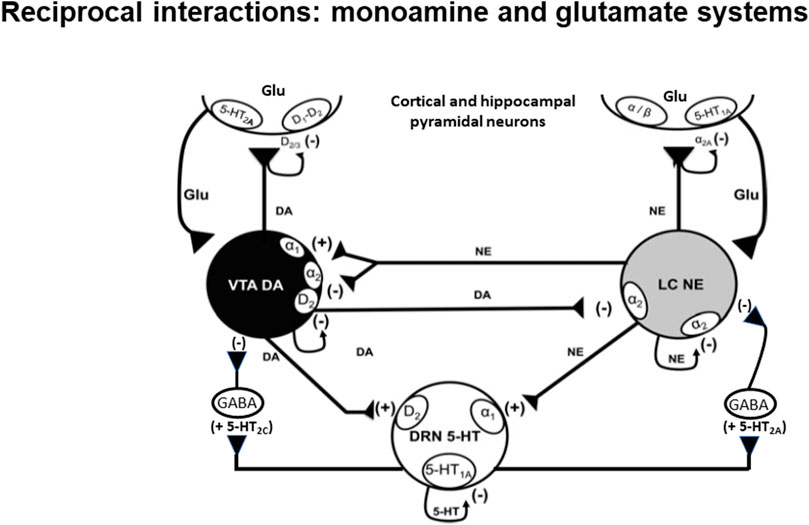
FIGURE 1. Schematic representation of the reciprocal interaction between glutamatergic pyramidal neurons and 5-HT, NE and DA neurons. It also depicts interplay between monoamine neurons (see Guiard et al., 2008). The nature of modulation of different neurotransmitters on classes of auto- and heteroreceptors is shown with (+) signs indicating a stimulatory effect and (−) signs indicating an inhibitory one.
Materials and methods
Animals
Adult male Wistar Kyoto (WKY) rats weighing approximately 280–350 g at the time of recording, were obtained from Charles River (St. Constant, Quebec, Canada). Animals were always kept under a constant temperature of 22°C in the facility and were housed in pairs under standard laboratory conditions (12:12-h light-dark cycle with ad libitum access to food and water). Rats were allowed 1 week to acclimatize to laboratory conditions. All animals were handled according to the guidelines of the Canadian Council on Animal Care (CCAC) and the local Animal Care Committee (University of Ottawa, Ottawa, Canada), which approved all protocols.
Drug administration
Ketamine hydrochloride (10 mg/kg; ERFA Canada Inc.) was diluted in 0.9% saline solution and administered intraperitoneally (i.p.). Control rats received a single saline (0.9%) i. p. injection. All injections were given 30 min before electrophysiological recordings. Recordings were carried out starting 30 min after ketamine injection until 120 min after. This is in accordance with clinical findings (Zarate et al., 2006; Phillips et al., 2019) and animal studies including behavioral, molecular and electrophysiological reports showing important changes related to the antidepressant-like effects of ketamine can take place very rapidly (Li et al, 2010; Beurel et al, 2011; Lindholm et al., 2012; El Iskandrani et al., 2015; Lopez-Gil et al., 2019).
In Vivo electrophysiological experiments
Rats were anesthetized using chloral hydrate (400 mg/kg i. p), and placed on a stereotaxic frame (David Kopf, CA, United States) with the skull positioned horizontally. Supplemental doses of chloral hydrate (100 mg/kg i. p) were given to maintain constant anesthesia to prevent any nociceptive response to the palpebral reflex or pinching of the hind paw (pedal withdrawal reflex). During electrophysiological recordings, rats were maintained at a constant body temperature of 37°C by a thermistor-controlled heating pad. A burr hole was drilled at the stereotaxic coordinates corresponding to the brain structure of interest. Electrode descents were carried out and neurons were identified and recorded for at least 2 min using the Spike2 program (Cambridge Electronic Design, Cambridge, United Kingdom).
Extracellular recordings of DRN 5-HT, LC NE, VTA DA, and mPFC pyramidal neurons were performed using single-barrel glass micropipettes (Stoelting, IL, United States) prepared using a pipette puller (Narishige, Japan) and filled with 2 M NaCl solution at an impedance range of 2–4 MΩ. Several electrode descents were carried out in each structure to record an optimal number of neurons (see detail below for VTA). The firing rate, number of neurons firing in bursts, percentage of spikes in bursts, and number of neurons per track were analyzed.
Recording of DRN 5-HT neurons
5-HT neurons were recorded using a single-barrel glass micropipette positioned 0.9–1.2 mm anterior to lambda, on the midline, and lowered into the DRN, encountered at a depth of 4.5–5.5 mm from the surface of the brain. The following criteria were used to identify presumptive 5-HT neurons: a slow (0.5–2.5 Hz), regular firing rate, long duration (2–5 milliseconds [ms]), bi- or triphasic extracellular waveforms (Vandermaelen and Aghajanian, 1983).
Recording of LC NE neurons
NE neurons were recorded using a single-barrel glass micropipette positioned at 1.1–1.2 mm posterior to lambda and 0.9–1.3 mm from the midline suture and at a depth of 4.5–6.0 mm from the surface of the brain. NE neurons were identified by their regular firing rate (0.5–5 Hz), a biphasic action potential of long duration (>2 ms), and a characteristic volley of spikes followed by a quiescent period in response to a nociceptive pinch of the contralateral hind paw (Marwaha and Aghajanian, 1982).
Recording of VTA DA neurons
VTA DA neurons were recorded using a single-barrel glass micropipette positioned at 3.1–3.3 mm and 0.7–1.0 mm lateral to the midline and lowered to a depth of 6.5–9 mm from the surface of the brain. The number of spontaneously active DA neurons found per track was determined by recording multiple tracks in a grid of 6–9 tracks per rat. Presumed DA neurons were identified based on the following criteria (Ungless and Grace, 2012): 1) Regular or irregular single spiking pattern that may include burst firing with a rate between 2 and 10 Hz; 2) biphasic or triphasic waveforms, with an initial positive deflection (usually notched) followed by a prominent negative phase, with a duration >1.1 ms from start to trough of the waveform; 3) long-duration action potentials (2.5–4 ms), and 4) low-pitch sound when monitored by an audio amplifier.
Recording of pyramidal neurons in the mPFC
Previous work has reported that pyramidal neurons in the mPFC are glutamatergic in nature (Santana et al., 2004). Putative mPFC pyramidal neurons were recorded by positioning a single-barrel glass micropipette according to the following coordinates (in mm from bregma): A-P, 3.2–3.4; M-L, 0.6–0.8; D-V, 2.5–5.5. Pyramidal neurons were identified according to the following electrophysiological properties: 1) A firing rate of 0.01–3 Hz, 2) a biphasic or triphasic action potential with irregular firing, and 3) a spike with a positive inflection duration greater than 0.36 ms and negative inflection duration greater than 1.08 ms to exclude any fast-spiking interneurons (Riga et al., 2017). In the acute experiments carried out in the mPFC, 9–23 pyramidal neurons were recorded in each rat to establish a mean baseline firing rate and a percentage of burst activity, then ketamine was injected. Subsequently, another 7–13 neurons were recorded in the same rat.
Burst analyses
The firing activity of neurons was analyzed using spike-sorting software (www.github.com/nno/burstidator/releases). For burst activity, the start of a burst was indicated by the occurrence of 2 spikes with interspike intervals (ISI) < 0.08 s for NE and DA neurons and <0.01 s for 5-HT neurons. Burst termination was defined as an ISI >0.16 s for NE and DA (Grace and Bunney, 1983) and ISI >0.01 s for 5-HT neurons (Hajós and Sharp, 1996). For mPFC pyramidal neurons, burst determination was based on the following criteria: a series of 2 or more spikes, with ISI <0.045 s for the initiation and >0.045 s for termination of burst (Laviolette et al., 2005).
Statistical analyses
All data are presented based on mean values ± standard error of the mean (SEM). Student’s t-tests were used to compare group means using two-tailed tests when the assumption of normality passed using the normality test Shapiro-Wilk. In cases where normality failed, the non-parametric Mann-Whitney test was utilized, which compared the median values of groups. In such cases, median values are indicated in the corresponding legend to figures. Statistical comparisons and graphing of data were conducted using SigmaPlot software 12.5 (Systat Software Inc., California, United States).
Results
Effect of ketamine on DRN 5-HT neuron activity in WKY rats
As illustrated in Figure 2, a single administration of ketamine (10 mg/kg, i. p.) before electrophysiological recordings, did not significantly alter the firing rate of 5-HT neurons (saline: 1.3 ± 0.2 Hz; ketamine 1.0 ± 0.1 Hz; Two-tailed t-test, t [12] = 1.4, p = 0.2; Figure 2A).
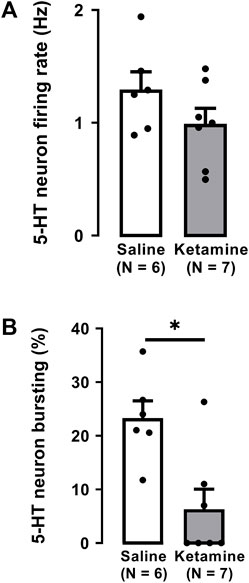
FIGURE 2. Effects of a single administration of ketamine (10 mg/kg, i. p.) on the firing rate (A) and burst activity (B) of 5-HT neurons in the DRN of WKY rats (number of rats = 7, number of neurons = 125) compared to WKY receiving saline (number of rats = 6, number of neurons = 139). Each dot represents an individual data point from one rat. The histograms show data as mean values ±SEM. Statistical significance is indicated where it applies, *p < 0.05.
A single administration of ketamine to WKY rats significantly decreased by 73% the number of neurons firing in burst mode when compared to saline administration (saline: 23% ± 3%; ketamine 6.3% ± 3.7%; Two-tailed t-test, t [11] = 3.4, p < 0.01; Figure 2B).
Effect of ketamine on LC NE neuron activity in WKY rats
There was no significant difference in the firing rate of NE neurons in WKY rats administered ketamine (3.0 ± 0.1 Hz) compared to WKY rats administered saline (2.7 ± 0.2 Hz; Two-tailed t-test, t [9] = 1.7, p = 0.1; Figure 3A).
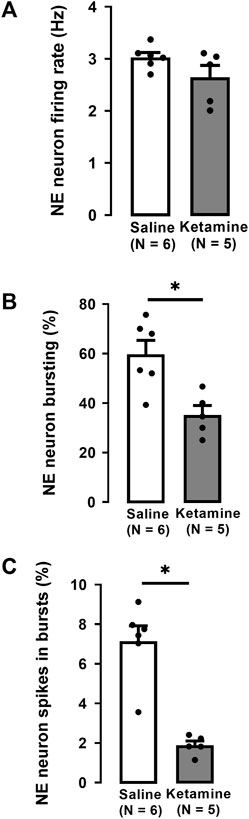
FIGURE 3. Effects of a single administration of ketamine (10 mg/kg, i. p.) on the firing rate (A), percentage of neurons with bursts (B), and the percentage of spikes in bursts (C) of NE neurons in the LC of WKY rats (number of rats = 5, number of neurons = 145) compared to WKY receiving saline (number of rats = 6, number of neurons = 175; median, saline: 7.6%, ketamine: 1.8%). Each dot represents an individual data point from one rat. The histograms show data as mean values ±SEM. Statistical significance is indicated where it applies, *p < 0.05.
A single administration of ketamine to WKY rats significantly decreased by 42% the number of NE neurons firing in burst mode compared to rats receiving saline (saline 60% ± 6%; ketamine 35% ± 4%; Two-tailed t-test, t [9] = 3.5, p < 0.01; Figure 3B). Similarly, the percentage of spikes in bursts was significantly decreased by 74% in WKY rats administered ketamine compared to saline (saline: 8% ± 0.7%; ketamine 2% ± 0.2%; Mann-Whitney U test, U = 0, p < 0.004; Figure 3C).
Effect of ketamine on VTA DA neuron activity in WKY rats
In the VTA of WKY rats, a single administration of ketamine caused no significant effect on the firing activity of DA neurons (saline: 3.7 ± 0.3 Hz; ketamine 3.2 ± 0.2 Hz; Two-tailed t-test, t [10] = 1.1, p = 0.3; Figure 4A).
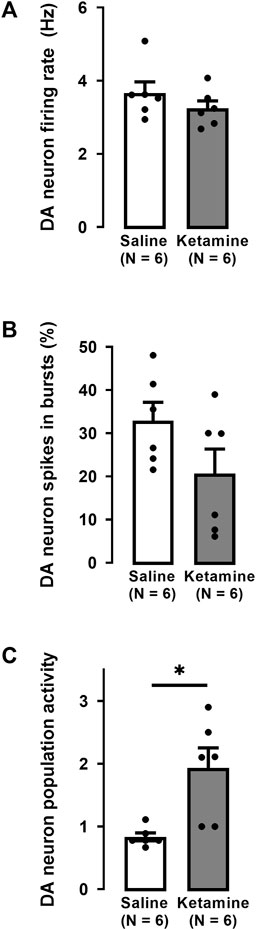
FIGURE 4. Effects of a single administration of ketamine (10 mg/kg, i. p.) on the firing rate (A), percentage of spikes in bursts (B), and population activity (C) of DA neurons in the VTA of WKY rats (number of rats = 6, number of neurons = 87) compared to WKY rats receiving saline (number of rats = 6, number of neurons = 45). Each dot represents an individual data point from one rat. The histograms show data as mean values ±SEM. Statistical significance is indicated where it applies, *p < 0.05.
The percentage of spikes occurring in bursts was not significantly altered by ketamine compared to saline administration (saline: 28% ± 4%; ketamine 21% ± 6%; Two-tailed t-test, t [10] = 1.0, p = 0.4; Figure 4B). However, there was a two-fold enhancement of the number of spontaneously active DA neurons (population activity) after ketamine compared to saline administration (saline: 0.8 ± 0.06; ketamine: 1.9 ± 0.3; Two-tailed t-test, t [10] = −3.4, p < 0.01; Figure 4C).
Effect of ketamine on mPFC pyramidal neuron activity in WKY rats
For each of the 5 WKY rats used in this series of experiments, a mean firing rate and a percentage of spikes in bursts of a population of mPFC pyramidal neurons was determined before and after the ketamine injection. When all data were pooled (Figure 5), there was no significant difference in the mean firing rate of neurons recorded before and after the ketamine injection (before: 2.1 ± 0.3 Hz, n = 53 versus 1.9 ± 0.3 Hz after ketamine, n = 60; 5 rats; Two-tailed t-test, t [4] = 1, p = 0.4; Figure 5A). The percentage of spikes in burst was also not altered following injection of ketamine (before: 56% ± 5% versus 56% ± 3% after ketamine; 5 rats; Two-tailed t-test, t [4] = −0.2, p = 0.9, Figure 5B).
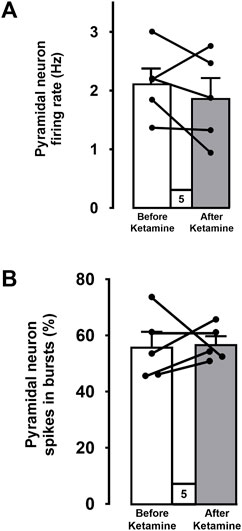
FIGURE 5. Mean firing rates (A) and percentages of spikes firing in bursts (B) of pooled data from 5 WKY rats before and after ketamine injections. Each dot represents an individual data point from one rat. Data points (before and after ketamine) from each rat are connected. The histograms show data as mean values ±SEM.
Discussion
Several lines of evidence show an involvement of 5-HT in the antidepressant-like effect of ketamine, including in the FST paradigm in which paracholoro-phenylanaline (PCPA) lowers the level of 5-HT and results in blunting of its effect on immobility in Sprague-Dawley (SD) rats (Gigliucci et al., 2013). In the current experiments, the mean firing rate of 5-HT neurons was similar in WKY rats that received ketamine compared to those that received saline. Considering the fact that 5-HT neuronal activity was decreased in WKY rats (Bruzos-Cidón et al., 2014; El Mansari et al., 2023), these results suggested that acute ketamine had no effect in restoring deficiency in the firing activity of DRN 5-HT neurons. Such a lack of effect of ketamine was not because 5-HT neurons activity was abnormally decreased in WKY rats, since even in naïve SD rats, where 5-HT neurons fire at control levels, acute injection of ketamine also did not alter the firing activity of 5-HT neurons (El Iskandrani et al., 2015). Such an absence of normalization of the firing of 5-HT neurons was also observed after administration of the selective 5-HT reuptake inhibitors (SSRIs) escitalopram and paroxetine, respectively, in heterozygous mice for 5-HT transporters (5-HTT+/−) and olfactory bulbectomized rats, that exhibit dampened firing activity of 5-HT neurons (Guiard et al., 2012; El Mansari et al., 2014). Nevertheless, it is possible to restore to normal levels, or even increase above baseline the firing activity of 5-HT neurons, using medications for MDD that act directly on monoamine receptors (Blier and El Mansari, 2013). For instance, the NE/DA releaser bupropion re-established normal firing in rats subjected to olfactory bulbectomy, which caused dampened firing activity (Dong and Blier, 2001; El Mansari et al., 2014). Normalization of 5-HT neuronal firing that is dampened following acute administration of an SSRI/selective norepinephrine reuptake inhibitor (SNRI) was, however, observed in SD rats that received an adjunct medication such as DA/5-HT modulators (Seager et al., 2004; Chernoloz et al., 2009; El Mansari et al., 2020). In addition, some antidepressant strategies for MDD such as vagus nerve stimulation, agomelatine, mirtazapine, bupropion, and pramipexole all enhance the firing activity of 5-HT neurons above their baseline in naïve SD rats (Blier and El Mansari, 2013). In these rats, the two latter drugs also increased 5-HT neuron burst activity (Chernoloz et al., 2009; Ghanbari et al., 2010), while this was significantly decreased by ketamine, here in WKY rats. This could not have been due to a direct action of ketamine on 5-HT neurons since the iontophoretic application of the NMDAR blocker, 2-APV, does not have any effect on their firing activity (Ferraro et al., 1997). It is conceivable that a dampening of the firing activity of 5-HT (including bursts) may have taken place indirectly through the activation of mPFC exerting an inhibition on 5-HT neurons (Celada et al., 2001). However, our present results showed that there was no increase in the firing activity of mPFC pyramidal neurons after ketamine injection and second, local blockade of NMDAR does not change the activity of these pyramidal neurons (Jodo et al., 2005). Nevertheless, the decrease in burst activity of 5-HT neurons by ketamine did not result in decreased levels of 5-HT in projection areas at least in SD rats. Microdialysis studies showed that 5-HT concentrations are enhanced in Wistar rat mPFC, but not the DRN. Although it was transient (1 h) after a systemic injection of 10 mg/kg of ketamine, 5-HT levels increased immediately after 25 mg/kg and lasted for at least 2 h (Lopez-Gil et al., 2012; Lopez-Gil et al., 2019). Indeed, non-competitive NMDAR antagonists selectively decrease GABA inhibition of pyramidal neurons resulting in their disinhibition and increase 5-HT levels in the mPFC (Adams and Moghaddam, 2001; Lopez-Gil et al., 2007). Altogether, these results suggest that the rapid antidepressant-like effect of ketamine is independent of the firing activity of 5-HT neurons, in line with results showing that the increase of c-fos-positive cells induced by NMDAR blockade was abolished by tetrodotoxin (TTX) in the mPFC, but not the DRN (Lopez-Gil et al., 2012).
Ketamine increases climbing in the FST, indicating the involvement of NE transmission in its antidepressant-like effect in Wistar rats (Lopez-Gil et al., 2019). In WKY rats, a previous electrophysiological study has also shown that the percentage of LC NE neurons displaying burst activity was significantly higher compared to Wistar rats by 41% (El Mansari et al., 2023). Interestingly, the present study showed in this same strain, that a single ketamine administration reduced by a similar proportion (42%) the number of NE neurons displaying burst activity and the percentage of spikes occurring in bursts as well. These observations taken together suggest that ketamine administration normalized maladaptive NE neuronal firing by decreasing the percentage of NE neurons exhibiting burst activity seen in WKY rats. This effect of ketamine could be mediated through direct blockade of NMDAR or via an increase in AMPAR activation following NMDAR blockade on GABA neurons (Miller et al., 2016; Widman and McMahon, 2018). It is unlikely that ketamine acts directly by blocking the NMDAR because it was shown that the selective NMDAR antagonist AP5 does not affect spontaneous NE firing activity (Charlety et al., 1993), although local and intracerebroventricular administration of the non-specific excitatory amino acid antagonist kynurenic acid decreases NE neurons burst activity (Tung et al., 1989; Saunier et al., 1993). An increase in AMPA throughput secondary to the blockade of NMDAR by ketamine is also not a likely explanation since AMPA does not affect the burst firing of NE neurons, albeit it enhances their mean firing activity (Olpe et al., 1989). The latter increase also cannot be due to the blockade of NMDARs in the mPFC because this would rather increase NE neuronal discharge (Jodo et al., 1998). Therefore, how ketamine decreases burst activity in WKY rats remains to be elucidated.
In SD rats, a single ketamine injection induced an increase in the firing and burst activity of NE neurons (El Iskandrani et al., 2015). The opposite effects of ketamine observed in WKY rats herein, versus SD rats, may be attributable to a difference in basal NE transmission between the two strains. Such differences include lower levels of NE and desensitized α2-adrenoceptors on the cell body in the LC and terminals in the hippocampus of WKY rats (Scholl et al., 2010; Jedema et al., 2008; Bruzos-Cidón et al., 2014; El Mansari et al., 2023). Whether an increase of NE neuronal activity as in WKY rats is a maladaptation or rather constitutes a beneficial adaptation induced by drugs with antidepressant-like action in SD rats remains to be elucidated. Nevertheless, similar increased phasic NE transmission is also manifested in behavioral and neuropsychological functions of patients experiencing anxiety, particularly those with post-traumatic stress syndrome (Hains and Arnsten, 2008; Arnsten, 2009). These manifestations seem to be mimicked by an enhanced phasic burst activity as in WKY rats (El Mansari et al., 2023; Bruzos-Cidón et al., 2014). On the other hand, an enhanced NE transmission through an increase in firing and/or burst activity, as well as tonic activation of α2-adrenoceptors, was also reported using several antidepressant strategies modulating 5-HT, NE, and DA systems in naïve SD rats (Blier and El Mansari, 2013). It was hypothesized that increasing tonic NE function within their basal firing range consequently dampens excessive phasic firing through activation of the inhibitory α2-adrenergic autoreceptors (see Montoya et al., 2016). The present results suggest that ketamine decreased the burst activity of NE neurons while their tonic firing remained at basal levels in WKY rats. In SD naïve rats, however, the firing activity of NE neurons was dampened following acute and long-term administration of medications acting directly on the NE system, including the NE reuptake inhibitors reboxetine and desipramine (Lacroix et al., 1991; Szabo and Blier, 2001a). It has been postulated that the antidepressant response induced by these medications is rather based on a tonic increase in NE in projection areas, such as the frontal cortex and hippocampus, due to a desensitization of terminal α2-adrenoceptors (Szabo and Blier, 2001b; Invernizzi et al., 2001). Regardless of NE neuronal firing pattern, microdialysis studies in SD rats have shown that acute ketamine (12.5–25 mg/kg) increased, for at least 2 h, NE levels in the mPFC and the ventral hippocampus (Lorrain et al., 2003a; Lopez-Gil et al., 2019). This is congruent with an increase in climbing in the FST (Lopez-Gil et al., 2019) and thus indicates a net augmentation in NE transmission (Detke et al., 1995). Altogether, these data imply that ketamine normalized phasic NE neuronal firing in WKY rats, and increased tonic NE transmission found in SD rats, which may underlie at least in part the rapid antidepressant action of ketamine. A similar augmentation in NE transmission is also achieved with standard antidepressant treatments, but only after chronic administration (El Mansari et al., 2020).
Previous studies in SD rats documented increased population activity of DA neurons by acute administration of ketamine, while firing and burst activity was unchanged (El Iskandrani et al., 2015; Witkin et al., 2016). A similar increase was also observed herein, utilizing the same regimen in WKY rats, where the firing properties of DA neurons were previously shown not to be different from those of Wistar rats (El Mansari et al., 2023). Since 5-HT and NE neurons exert an inhibitory effect on DA neurons activity (Figure 1; Guiard et al., 2008), it is possible that decreased neuronal activity of 5-HT and NE in WKY rats that received ketamine resulted in an increase in DA neurons activity, albeit the effect on population activity remains to be elucidated. Population activity of DA neurons, however, is regulated by the ventral subiculum where a local infusion of NMDA elicits an increase in this parameter (Floresco et al., 2003). Hence, it is unlikely that the increase in DA neuron population activity observed herein is due to the direct blockade of NMDAR per se because this effect induces rather a decrease in the number of DA neurons recorded per track (Floresco et al., 2003). It is possible, however, that the observed ketamine-induced increase is instead due to the promotion of AMPAR-mediated glutamatergic neurotransmission, as previously hypothesized (Miller et al., 2016; Widman and McMahon, 2018). Indeed, previous results had shown an increase in the firing of DA neurons after the local application of AMPA in the VTA, although the effect on burst was not assessed (Tong et al., 1996; Zhang et al., 1997). In learned helpless rats, another model of depression, showing dampened population activity of VTA DA neurons, ketamine rescued this deficit and also increased the basal number of spontaneously active DA neurons (Belujon and Grace, 2014). A similar restoration of dampened population activity of DA neurons was also reported in male and female rats subjected to chronic mild stress (Rincón-Cortés and Grace, 2017). Altogether, these results show that ketamine can increase DA neuronal activity, particularly population activity, which produces a significant enhancement in DA concentration in the nucleus accumbens (Floresco et al., 2003; Lisman and Grace, 2005), and modulates lower reward expectation (i.e., anhedonia; Markovic et al., 2021). Consistent with this interpretation, acute ketamine administration (10–25 mg/kg) increased DA levels, for at least 1 h, in the mPFC, although in naïve SD rats (Lorrain et al., 2003b; Lindefors et al.,1997; Moghaddam et al., 1997). As with ketamine, classical medications indicated for MDD administered alone or in combination with DA/5-HT modulators (i.e., aripiprazole, cariprazine) either increased above basal level or normalized dampened DA neurons firing/bursts/population activity, only following repeated but not acute regimens (Chernoloz et al., 2009; El Mansari et al., 2020). Thus, it is probable that an increase in DA transmission contributes significantly to the rapid antidepressant effect of ketamine.
Previous work has demonstrated that local injection of ketamine into the mPFC is sufficient to reproduce rapid antidepressant-like action in rats (Fuchikami et al., 2015). Furthermore, optogenetic stimulation of the infralimbic part of the PFC induces a rapid antidepressant-like effect, as measured in behavior and synaptogenesis (Fuchikami et al., 2015), indicating an important contribution of mPFC neuronal activity. Indeed, disinhibited activity of pyramidal neurons following a selective reduction of GABAergic inhibition is considered a possible mechanism of action of ketamine (Miller et al., 2016; Widman and McMahon, 2018). This would be consistent with an increased efflux of glutamate within the mPFC by ketamine (Moghaddam et al., 1997; Lopez-Gil et al., 2007), resulting in an enhancement of the firing rate of these glutamatergic neurons. Although the local infusion of ketamine enhanced c-fos immunolabeling in the infralimbic part of the PFC, the present study showed no change in the mean firing and burst activity of mPFC pyramidal neurons after acute ketamine administration. Our results are also inconsistent with a previous study showing that cumulative injections of ketamine (1.25–20 mg/kg, i. v.) inhibited the firing activity of pyramidal neurons in the PFC (Shen et al., 2018). Such a discrepancy may stem from the fact the latter study assessed the effect of ketamine injection on single neurons in each rat, while the current study assessed the mean firing of a population of neurons before and after i. p. ketamine, which may reflect more the average change in such a neuronal population. Moreover, in the majority of a population of pyramidal neurons, their mean firing was enhanced following the blockade of NMDARs using MK-801, another NMDAR antagonist (Homayoun and Moghaddam, 2007). PCP administered systemically resulted in half of PFC pyramidal neurons increasing their firing activity, while the rest either decreased or showed no variation (Kargieman et al., 2007). The current approach sampling the firing activity of PFC pyramidal neurons before and after ketamine injection did not reveal the crucial role of this brain structure in the antidepressant-like effect of ketamine. However, the action of ketamine and especially regarding a subpopulation of mPFC pyramidal neurons projecting to monoamines neurons remains to be further elucidated.
As illustrated in Table 1, the present study showed that in the DRN, the acute injection of ketamine did not change the firing activity of 5-HT neurons in WKY rats, where activity is below normal, although previously it did increase 5-HT levels in the mPFC and the hippocampus. In the LC, acute ketamine administration reversed abnormally higher burst activity of NE neurons in WKY rats. In the VTA, ketamine enhanced population activity, which is similar to the effects of several medications used to treat MDD. Ketamine promptly normalized or enhanced monoamine neurotransmissions, indicating that such alterations can contribute, at least in part, to its rapid antidepressant-like effects.

TABLE 1. Summary of effects of a single administration of ketamine (10 mg/kg) in WKY rats on the firing and burst activity (% spikes in bursts) of DRN 5-HT, LC NE and mPFC glutamatergic (Glu) pyramidal neurons. For the VTA, DA neurons population activity was also included. The upward and downward arrows represent, respectively, a significant increase or decrease compared to saline administered WKY rats. The circle with a line through represents no significant change.
Study limitations
In the current studies, experiments in both vehicle- and ketamine-treated rats were carried out under chloral hydrate anesthesia, to ensure a similar basal condition in both groups. The assumption then is that any difference between these two groups would be attributable to effects induced by ketamine. Furthermore, several lines of evidence show that ketamine effects are more likely in opposite direction to chloral hydrate. On the one hand, chloral hydrate through its metabolite trichloroethanol enhances GABAA-mediated transmission, which is responsible for its anesthetic effects (Lovinger et al., 1993; Krasowski and Harrison, 2000). In addition, its potency to enhance this GABA effect appears to be greater than to depress excitatory transmission (Lovinger et al., 1993). Moreover, chloral hydrate inhibits the AMPA response by 50% (Carlà and Moroni, 1992; Peoples and Weight, 1998; Fischer et al., 2000). On the other hand, ketamine induces a disinhibition of GABA neurons through a blockade of NMDA receptors, which results in an increase in AMPA throughput (Li et al., 2010; Miller et al., 2016; Widman and McMahon, 2018). Therefore, it is possible that effects obtained after ketamine administration herein are even more likely to be under-estimated because of the opposing action of chloral hydrate on the impact of ketamine.
Although these findings are limited to male rats, clinical studies have shown no difference in the effects of a single ketamine infusion in women and men with treatment-resistant depression, even when menopause status is considered (Freeman et al., 2019; Phillips et al., 2019).
Data availability statement
The raw data supporting the conclusion of this article will be made available by the authors, without undue reservation.
Ethics statement
The animal study was approved by the Canadian Council on Animal Care (CCAC) and the local Animal Care Committee (University of Ottawa, Ottawa, Canada). The study was conducted in accordance with the local legislation and institutional requirements.
Author contributions
SD: Investigation, Writing–original draft, Writing–review and editing. ME: Conceptualization, Investigation, Writing–original draft, Writing–review and editing. RH: Investigation. PB: Conceptualization, Funding acquisition, Writing–review and editing.
Funding
The authors declare financial support was received for the research, authorship, and/or publication of this article. This research was funded by the Canadian Institutes of Health Research (grant PJT-169078). SD received a fellowship from Janssen Canada.
Conflict of interest
The authors declare that the research was conducted in the absence of any commercial or financial relationships that could be construed as a potential conflict of interest.
Publisher’s note
All claims expressed in this article are solely those of the authors and do not necessarily represent those of their affiliated organizations, or those of the publisher, the editors and the reviewers. Any product that may be evaluated in this article, or claim that may be made by its manufacturer, is not guaranteed or endorsed by the publisher.
Supplementary material
The Supplementary Material for this article can be found online at: https://www.frontiersin.org/articles/10.3389/fphar.2023.1276309/full#supplementary-material
References
Adams, B. W., and Moghaddam, B. (2001). Effect of clozapine, haloperidol, or M100907 on phencyclidine-activated glutamate efflux in the prefrontal cortex. Biol. Psychiatry 50, 750–757. doi:10.1016/s0006-3223(01)01195-7
Amat, J., Baratta, M. V., Paul, E., Bland, S. T., Watkins, L. R., and Maier, S. F. (2005). Medial prefrontal cortex determines how stressor controllability affects behavior and dorsal raphe nucleus. Nat. Neurosci. 8, 365–371. doi:10.1038/NN1399
Arnsten, A. F. T. (1998). The biology of being frazzled. Science 280, 1711–1712. doi:10.1126/SCIENCE.280.5370.1711
Arnsten, A. F. T. (2009). Stress signalling pathways that impair prefrontal cortex structure and function. Nat. Rev. Neurosci. 10, 410–422. doi:10.1038/NRN2648
Belujon, P., and Grace, A. A. (2014). Restoring mood balance in depression: ketamine reverses deficit in dopamine-dependent synaptic plasticity. Biol. Psychiatry 76, 927–936. doi:10.1016/j.biopsych.2014.04.014
Beurel, E., Song, L., and Jope, R. S. (2011). Inhibition of glycogen synthase kinase-3 is necessary for the rapid antidepressant effect of ketamine in mice. Mol. Psychiatry 16, 1068–1070. doi:10.1038/mp.2011.47
Blier, P., and El Mansari, M. (2013). Serotonin and beyond: therapeutics for major depression. Philos. Trans. R. Soc. Lond B Biol. Sci. 368 (1615), 20120536. doi:10.1098/RSTB.2012.0536
Bruzos-Cidón, C., Miguelez, C., Rodríguez, J. J., Gutiérrez-Lanza, R., Ugedo, L., and Torrecilla, M. (2014). Altered neuronal activity and differential sensitivity to acute antidepressants of locus coeruleus and dorsal raphe nucleus in Wistar Kyoto rats: a comparative study with Sprague Dawley and Wistar rats. Eur. Neuropsychopharmacol. 24, 1112–1122. doi:10.1016/J.EURONEURO.2014.02.007
Carlà, V., and Moroni, F. (1992). General anaesthetics inhibit the responses induced by glutamate receptor agonists in the mouse cortex. Neurosci. Lett. 146, 21–24. doi:10.1016/0304-3940(92)90162-z
Celada, P., Victoria Puig, M., Casanovas, J. M., Guillazo, G., and Artigas, F. (2001). Control of dorsal raphe serotonergic neurons by the medial prefrontal cortex: involvement of serotonin-1A, GABAA, and glutamate receptors. J. Neurosci. 21, 9917–9929. doi:10.1523/JNEUROSCI.21-24-09917.2001
Charléty, P. J., Chergui, K., Akaoka, H., Saunier, F., Buda, M., Aston-Jones, G., et al. (1993). Serotonin differentially modulates responses mediated by specific excitatory amino acid receptors in the rat locus coeruleus. Eur. J. Neurosci. 5, 1024–1028. doi:10.1111/j.1460-9568.1993.tb00954.x
Chaudhury, D., Walsh, J. J., Friedman, A. K., Juarez, B., Ku, S. M., Koo, J. W., et al. (2013). Rapid regulation of depression-related behaviours by control of midbrain dopamine neurons. Nature 493, 532–536. doi:10.1038/nature11713
Chernoloz, O., El Mansari, M., and Blier, P. (2009). Electrophysiological studies in the rat brain on the basis for aripiprazole augmentation of antidepressants in major depressive disorder. Psychopharmacol. Berl. 206, 335–344. doi:10.1007/S00213-009-1611-7
Daniels, S., Lemaire, D., Lapointe, T., Limebeer, C., Parker, L., and Leri, F. (2021). Effects of inescapable stress on responses to social incentive stimuli and modulation by escitalopram. Psychopharmacol. Berl. 238, 3239–3247. doi:10.1007/s00213-021-05940-6
Detke, M. J., Rickels, M., and Lucki, I. (1995). Active behaviors in the rat forced swimming test differentially produced by serotonergic and noradrenergic antidepressants. Psychopharmacol. (Berl.) 121, 66–72. doi:10.1007/BF02245592
Dong, J., and Blier, P. (2001). Modification of norepinephrine and serotonin, but not dopamine, neuron firing by sustained bupropion treatment. Psychopharmacol. Berl. 155, 52–57. doi:10.1007/s002130000665
Duman, R. S., Sanacora, G., and Krystal, J. H. (2019). Altered connectivity in depression: GABA and glutamate neurotransmitter deficits and reversal by novel treatments. Neuron 102, 75–90. doi:10.1016/J.NEURON.2019.03.013
Düzel, E., Bunzeck, N., Guitart-Masip, M., and Düzel, S. (2010). Novelty-related motivation of anticipation and exploration by dopamine (NOMAD): implications for healthy aging. Neurosci. Biobehav Rev. 34, 660–669. doi:10.1016/J.NEUBIOREV.2009.08.006
El Iskandrani, K. S., Oosterhof, C. A., El Mansari, M., and Blier, P. (2015). Impact of subanesthetic doses of ketamine on AMPA-mediated responses in rats: an in vivo electrophysiological study on monoaminergic and glutamatergic neurons. J. Psychopharmacol. 29, 792–801. doi:10.1177/0269881115573809
El Mansari, M., Manta, S., Oosterhof, C., El Iskandrani, K. S., Chenu, F., Shim, S., et al. (2014). Restoration of serotonin neuronal firing following long-term administration of bupropion but not paroxetine in olfactory bulbectomized rats. Int. J. Neuropsychopharmacol. 18, pyu050–8. doi:10.1093/ijnp/pyu050
El Mansari, M., Ebrahimzadeh, M., Hamati, R., Iro, C. M., Farkas, B., Kiss, B., et al. (2020). Long-term administration of cariprazine increases locus coeruleus noradrenergic neurons activity and serotonin1A receptor neurotransmission in the hippocampus. J. Psychopharmacol. 34, 1143–1154. doi:10.1177/0269881120936891
El Mansari, M., Hamoudeh, R., Daniels, S., and Blier, P. (2023). Wistar Kyoto rats exhibit decreased serotonin neuronal firing and increased norepinephrine burst activity but dampened hippocampal α2-adrenoceptor sensitivity. J. Psychopharmacol. (in press).
Ferraro, G., Montalbano, M. E., Sardo, P., and La Grutta, V. (1997). Lateral habenula and hippocampus: a complex interaction raphe cells-mediated. J. Neural. Transm. 104, 615–631. doi:10.1007/BF01291880
Fischer, W., Allgaier, C., and Illes, P. (2000). Inhibition by chloral hydrate and trichloroethanol of AMPA-induced Ca(2+) influx in rat cultured cortical neurones. Eur. J. Pharmacol. 394, 41–45. doi:10.1016/s0014-2999(00)00160-6
Floresco, S. B., West, A. R., Ash, B., Moorel, H., and Grace, A. A. (2003). Afferent modulation of dopamine neuron firing differentially regulates tonic and phasic dopamine transmission. Nat. Neurosci. 6, 968–973. doi:10.1038/NN1103
Freeman, M. P., Papakostas, G. I., Hoeppner, B., Mazzone, E., Judge, H., Cusin, C., et al. (2019). Sex differences in response to ketamine as a rapidly acting intervention for treatment resistant depression. J. Psychiatr. Res. 110, 166–171. doi:10.1016/j.jpsychires.2019.01.010
Fuchikami, M., Thomas, A., Liu, R., Wohleb, E. S., Land, B. B., DiLeone, R. J., et al. (2015). Optogenetic stimulation of infralimbic PFC reproduces ketamine’s rapid and sustained antidepressant actions. Proc. Natl. Acad. Sci. U. S. A. 112, 8106–8111. doi:10.1073/PNAS.1414728112
Fukumoto, K., Iijima, M., and Chaki, S. (2014). Serotonin-1A receptor stimulation mediates effects of a metabotropic glutamate 2/3 receptor antagonist, 2S-2-amino-2- (1S, 2S-2-carboxycycloprop-1-yl)- 3-(xanth-9-yl)propanoic acid (LY341495), and an N-methyl-D-aspartate receptor antagonist, ketamine, in the novelty-suppressed feeding test. Psychopharmacol. Berl. 231, 2291–2298. doi:10.1007/S00213-013-3378-0
Fukumoto, K., Iijima, M., and Chaki, S. (2016). The antidepressant effects of an mGlu2/3 receptor antagonist and ketamine require AMPA receptor stimulation in the mPFC and subsequent activation of the 5-HT neurons in the DRN. Neuropsychopharmacology 41, 1046–1056. doi:10.1038/npp.2015.233
Ghanbari, R., El Mansari, M., and Blier, P. (2010). Electrophysiological effects of the co-administration of escitalopram and bupropion on rat serotonin and norepinephrine neurons. J. Psychopharmacol. 24, 39–50. doi:10.1177/0269881108095714
Gigliucci, V., O’Dowd, G., Casey, S., Egan, D., Gibney, S., and Harkin, A. (2013). Ketamine elicits sustained antidepressant-like activity via a serotonin-dependent mechanism. Psychopharmacol. Berl. 228, 157–166. doi:10.1007/S00213-013-3024-x
Grace, A. A., and Bunney, B. S. (1983). Intracellular and extracellular electrophysiology of nigral dopaminergic neurons-1. Identification and characterization. Neurosci 10, 301–315. doi:10.1016/0306-4522(83)90135-5
Guiard, B. P., El Mansari, M., Merali, Z., and Blier, P. (2008). Functional interactions between dopamine, serotonin and norepinephrine neurons: an in-vivo electrophysiological study in rats with monoaminergic lesions. Int. J. Neuropsychopharmacol. 11, 625–639. doi:10.1017/S1461145707008383
Guiard, B. P., Mansari, M.El, Murphy, D. L., and Blier, P. (2012). Altered response to the selective serotonin reuptake inhibitor escitalopram in mice heterozygous for the serotonin transporter: an electrophysiological and neurochemical study. J. Neuropsychopharmacol. 15, 349–361. doi:10.1017/S1461145711000484
Hains, A. B., and Arnsten, A. F. T. (2008). Molecular mechanisms of stress-induced prefrontal cortical impairment: implications for mental illness. Learn Mem. 15, 551–564. doi:10.1101/LM.921708
Hajós, M., and Sharp, T. (1996). Burst-firing activity of presumed 5-HT neurones of the rat dorsal raphe nucleus: electrophysiological analysis by antidromic stimulation. Brain Res. 740, 162–168. doi:10.1016/S0006-8993(96)00869-4
Hare, B. D., Ghosal, S., and Duman, R. S. (2017). Rapid acting antidepressants in chronic stress models: molecular and cellular mechanisms. Chr Stress 1, 2470547017697317. doi:10.1177/2470547017697317
Hashimoto, K. (2019). Rapid-acting antidepressant ketamine, its metabolites and other candidates: a historical overview and future perspective. Psychiatry Clin. Neurosci. 73, 613–627. doi:10.1111/PCN.12902
Homayoun, H., and Moghaddam, B. (2007). NMDA receptor hypofunction produces opposite effects on prefrontal cortex interneurons and pyramidal neurons. J. Neurosci. 27, 11496–11500. doi:10.1523/JNEUROSCI.2213-07.2007
Invernizzi, R. W., Parini, S., Sacchetti, G., Fracasso, C., Caccia, S., Annoni, K., et al. (2001). Chronic treatment with reboxetine by osmotic pumps facilitates its effect on extracellular noradrenaline and may desensitize alpha(2)-adrenoceptors in the prefrontal cortex. Br. J. Pharmacol. 132, 183–188. doi:10.1038/sj.bjp.0703821
Iro, C. M., Hamati, R., El Mansari, M., and Blier, P. (2021). Repeated but not single administration of ketamine prolongs increases of the firing activity of norepinephrine and dopamine neurons. Neuropsychopharmacology 24, 570–579. doi:10.1093/ijnp/pyab010
Jedema, H. P., Gold, S. J., Gonzalez-Burgos, G., Sved, A. F., Tobe, B. J., Wensel, T. G., et al. (2008). Chronic cold exposure increases RGS7 expression and decreases alpha(2)-autoreceptor-mediated inhibition of noradrenergic locus coeruleus neurons. Eur. J. Neurosci. 27, 2433–2443. doi:10.1111/J.1460-9568.2008.06208.X
Jodo, E., Chiang, C., and Aston-Jones, G. (1998). Potent excitatory influence of prefrontal cortex activity on noradrenergic locus coeruleus neurons. Neurosci 83, 63–79. doi:10.1016/S0306-4522(97)00372-2
Jodo, E., Suzuki, Y., Katayama, T., Hoshino, K. Y., Takeuchi, S., Niwa, S. I., et al. (2005). Activation of medial prefrontal cortex by phencyclidine is mediated via a hippocampo-prefrontal pathway. Cereb. Cortex 15, 663–669. doi:10.1093/CERCOR/BHH168
Kargieman, L., Santana, N., Mengod, G., Celada, P., and Artigas, F. (2007). Antipsychotic drugs reverse the disruption in prefrontal cortex function produced by NMDA receptor blockade with phencyclidine. Proc. Natl. Acad. Sci. U. S. A. 104, 14843–14848. doi:10.1073/PNAS.0704848104
Krasowski, M. D., and Harrison, N. L. (2000). The actions of ether, alcohol and alkane general anaesthetics on GABAA and glycine receptors and the effects of TM2 and TM3 mutations. Br. J. Pharmacol. 129, 731–743. doi:10.1038/sj.bjp.0703087
Lacroix, D., Blier, P., Curet, O., and De Montigny, C. (1991). Effects of long-term desipramine administration on noradrenergic neurotransmission: electrophysiological studies in the rat brain. J. Pharmacol. Exp. Ther. 257, 1081–1090. Available at: https://pubmed.ncbi.nlm.nih.gov/1646320/.
Laviolette, S. R., Lipski, W. J., and Grace, A. A. (2005). A subpopulation of neurons in the medial prefrontal cortex encodes emotional learning with burst and frequency codes through a dopamine D4 receptor-dependent basolateral amygdala input. J. Neurosci. 25, 6066–6075. doi:10.1523/JNEUROSCI.1168-05.2005
Li, N., Lee, B., Liu, R. J., Banasr, M., Dwyer, J. M., Iwata, M., et al. (2010). mTOR-dependent synapse formation underlies the rapid antidepressant effects of NMDA antagonists. Science 329, 959–964. doi:10.1126/SCIENCE.1190287
Li, N., Liu, R. J., Dwyer, J. M., Banasr, M., Lee, B., Son, H., et al. (2011). Glutamate N-methyl-D-aspartate receptor antagonists rapidly reverse behavioral and synaptic deficits caused by chronic stress exposure. Biol. Psychiatry 69, 754–761. doi:10.1016/J.BIOPSYCH.2010.12.015
Lindefors, N., Barati, S., and O’Connor, W. T. (1997). Differential effects of single and repeated ketamine administration on dopamine, serotonin and GABA transmission in rat medial prefrontal cortex. Brain Res. 759, 205–212. doi:10.1016/S0006-8993(97)00255-2
Lindholm, J. S. O., Autio, H., Vesa, L., Antila, H., Lindemann, L., Hoener, M. C., et al. (2012). The antidepressant-like effects of glutamatergic drugs ketamine and AMPA receptor potentiator LY 451646 are preserved in bdnf⁺/⁻ heterozygous null mice. Neuropharmacology 62, 391–397. doi:10.1016/j.neuropharm.2011.08.015
Lisman, J. E., and Grace, A. A. (2005). The hippocampal-VTA loop: controlling the entry of information into long-term memory. Neuron 46, 703–713. doi:10.1016/J.NEURON.2005.05.002
López-Gil, X., Babot, Z., Amargós-Bosch, M., Suñol, C., Artigas, F., and Adell, A. (2007). Clozapine and haloperidol differently suppress the MK-801-increased glutamatergic and serotonergic transmission in the medial prefrontal cortex of the rat. Neuropsychopharmacology 32, 2087–2097. doi:10.1038/SJ.NPP.1301356
López-Gil, X., Jiménez-Sánchez, L., Romón, T., Campa, L., Artigas, F., and Adell, A. (2012). Importance of inter-hemispheric prefrontal connection in the effects of non-competitive NMDA receptor antagonists. Int. J. Neuropsychopharmacol. 15, 945–956. doi:10.1017/S1461145711001064
López-Gil, X., Jiménez-Sánchez, L., Campa, L., Castro, E., Frago, C., and Adell, A. (2019). Role of serotonin and noradrenaline in the rapid antidepressant action of ketamine. ACS Chem. Neurosci. 10, 3318–3326. doi:10.1021/acschemneuro.9b00288
Lorrain, D. S., Schaffhauser, H., Campbell, U. C., Baccei, C. S., Correa, L. D., Rowe, B., et al. (2003a). Group II mGlu receptor activation suppresses norepinephrine release in the ventral hippocampus and locomotor responses to acute ketamine challenge. Neuropsychopharmacology 28, 1622–1632. doi:10.1038/sj.npp.1300238
Lorrain, D. S., Baccei, C. S., Bristow, L. J., Anderson, J. J., and Varney, M. A. (2003b). Effects of ketamine and N-methyl-D-aspartate on glutamate and dopamine release in the rat prefrontal cortex: modulation by a group II selective metabotropic glutamate receptor agonist LY379268. Neuroscience 117, 697–706. doi:10.1016/S0306-4522(02)00652-8
Lovinger, D. M., Zimmerman, S. A., Levitin, M., Jones, M. V., and Harrison, N. L. (1993). Trichloroethanol potentiates synaptic transmission mediated by gamma-aminobutyric acid A receptors in hippocampal neurons. J. Pharmacol. Exp. Ther. 264, 1097–1103. PMID: 8383736.
Maier, S. F., and Watkins, L. R. (2005). Stressor controllability and learned helplessness: the roles of the dorsal raphe nucleus, serotonin, and corticotropin-releasing factor. Neurosci. Biobehav Rev. 29, 829–841. doi:10.1016/J.NEUBIOREV.2005.03.021
Markovic, T., Pedersen, C. E., Massaly, N., Vachez, Y. M., Ruyle, B., Murphy, C. A., et al. (2021). Pain induces adaptations in ventral tegmental area dopamine neurons to drive anhedonia-like behavior. Nat. Neurosci. 24, 1601–1613. doi:10.1038/S41593-021-00924-3
Marwaha, J., and Aghajanian, G. K. (1982). Relative potencies of alpha-1 and alpha-2 antagonists in the locus ceruleus, dorsal raphe and dorsal lateral geniculate nuclei: an electrophysiological study. J. Pharmacol. Exp. Ther. 222, 287–293.
McIntyre, R. S., Rosenblat, J. D., Nemeroff, C. B., Sanacora, G., Murrough, J. W., Berk, M., et al. (2021). Synthesizing the evidence for ketamine and esketamine in treatment-resistant depression: an international expert opinion on the available evidence and implementation. Am. J. Psychiatry 178, 383–399. doi:10.1176/APPI.AJP.2020.20081251
Miller, O. H., Moran, J. T., and Hall, B. J. (2016). Two cellular hypotheses explaining the initiation of ketamine’s antidepressant actions: direct inhibition and disinhibition. Neuropharmacology 100, 17–26. doi:10.1016/J.NEUROPHARM.2015.07.028
Moghaddam, B., Adams, B., Verma, A., and Daly, D. (1997). Activation of glutamatergic neurotransmission by ketamine: a novel step in the pathway from NMDA receptor blockade to dopaminergic and cognitive disruptions associated with the prefrontal cortex. J. Neurosci. 17, 2921–2927. doi:10.1523/JNEUROSCI.17-08-02921.1997
Montoya, A., Bruins, R., Katzman, M. A., and Blier, P. (2016). The noradrenergic paradox: implications in the management of depression and anxiety. Neuropsychiatr. Dis. Treat. 12, 541–557. doi:10.2147/NDT.S91311
Nishitani, N., Nagayasu, K., Asaoka, N., Yamashiro, M., Shirakawa, H., Nakagawa, T., et al. (2014). Raphe AMPA receptors and nicotinic acetylcholine receptors mediate ketamine-induced serotonin release in the rat prefrontal cortex. Int. J. Neuropsychopharmacol. 17, 1321–1326. doi:10.1017/S1461145714000649
Olpe, H. R., Steinmann, M. W., Brugger, F., and Pozza, M. F. (1989). Excitatory amino acid receptors in rat locus coeruleus. An extracellular in vitro study. Naunyn Schmiedeb. Arch. Pharmacol. 339, 312–314. doi:10.1007/BF00173584
Peoples, R. W., and Weight, F. F. (1998). Inhibition of excitatory amino acid-activated currents by trichloroethanol and trifluoroethanol in mouse hippocampal neurones. Br. J. Pharmacol. 124, 1159–1164. doi:10.1038/sj.bjp.0701949
Phillips, J. L., Norris, S., Talbot, J., Birmingham, M., Hatchard, T., Ortiz, A., et al. (2019). Single, repeated, and maintenance ketamine infusions for treatment-resistant depression: a randomized controlled trial. Am. J. Psychiatry 176, 401–409. doi:10.1176/appi.ajp.2018.18070834
Riga, M. S., Teruel-Martí, V., Sánchez, C., Celada, P., and Artigas, F. (2017). Subchronic vortioxetine treatment -but not escitalopram-enhances pyramidal neuron activity in the rat prefrontal cortex. Neuropharmacology 113, 148–155. doi:10.1016/j.neuropharm.2016.09.024
Rincón-Cortés, M., and Grace, A. A. (2017). Sex-dependent effects of stress on immobility behavior and VTA dopamine neuron activity: modulation by ketamine. Int. J. Neuropsychopharmacol. 20, 823–832. doi:10.1093/ijnp/pyx048
Rincón-Cortés, M., and Grace, A. A. (2020). Antidepressant effects of ketamine on depression-related phenotypes and dopamine dysfunction in rodent models of stress. Beh Br. Res. 379, 112367. doi:10.1016/j.bbr.2019.112367
Santana, N., Bortolozzi, A., Serrats, J., Mengod, G., and Artigas, F. (2004). Expression of serotonin1A and serotonin2A receptors in pyramidal and GABAergic neurons of the rat prefrontal cortex. Cereb. Cortex 14, 1100–1109. doi:10.1093/cercor/bhh070
Saunier, C. F., Akaoka, H., De la Chapelle, B., Charlety, P. J., Chergui, K., Chouvet, G., et al. (1993). Activation of brain noradrenergic neurons during recovery from halothane anesthesia. Persistence of phasic activation after clonidine. Anesthesiology 79, 1072–1082. doi:10.1097/00000542-199311000-00026
Scholl, J. L., Renner, K. J., Forster, G. L., and Tejani-Butt, S. (2010). Central monoamine levels differ between rat strains used in studies of depressive behavior. Brain Res. 1355, 41–51. doi:10.1016/J.BRAINRES.2010.08.003
Seager, M. A., Huff, K. D., Barth, V. N., Phebus, L. A., and Rasmussen, K. (2004). Fluoxetine administration potentiates the effect of olanzapine on locus coeruleus neuronal activity. Biol. Psychiatry 55, 1103–1109. doi:10.1016/j.biopsych.2004.02.012
Shen, G., Han, F., and Shi, W. X. (2018). Effects of low doses of ketamine on pyramidal neurons in rat prefrontal cortex. Neuroscience 384, 178–187. doi:10.1016/J.NEUROSCIENCE.2018.05.037
Swainson, J., McGirr, A., Blier, P., Brietzke, E., Richard-Devantoy, S., Ravindran, N., et al. (2021). The Canadian network for mood and anxiety treatments (CANMAT) task force recommendations for the use of racemic ketamine in adults with major depressive disorder: recommandations du groupe de travail du réseau canadien pour les traitements de L'humeur et de L'anxiété (canmat) concernant L'utilisation de La kétamine racémique chez les adultes souffrant de trouble dépressif majeur. Can. J. Psychiatry 66, 113–125. doi:10.1177/0706743720970860
Szabo, S. T., and Blier, P. (2001a). Effect of the selective noradrenergic reuptake inhibitor reboxetine on the firing activity of noradrenaline and serotonin neurons. Eur. J. Neurosci. 13, 2077–2087. doi:10.1046/j.0953-816X.2001.01583.x
Szabo, S. T., and Blier, P. (2001b). Effects of the selective norepinephrine reuptake inhibitor reboxetine on norepinephrine and serotonin transmission in the rat hippocampus. Neuropsychopharmacology 25, 845–857. doi:10.1016/S0893-133X(01)00284-6
Tong, Z. Y., Overton, P. G., and Clark, D. (1996). Antagonism of NMDA receptors but not AMPA/kainate receptors blocks bursting in dopaminergic neurons induced by electrical stimulation of the prefrontal cortex. J. Neural Transm. 103, 889–904. doi:10.1007/BF01291780
Tung, C.-S., Ugedo, L., Grenhoff, J., Engberg, G., and Svensson, T. H. (1989). Peripheral induction of burst firing in locus coeruleus neurons by nicotine mediated via excitatory amino acids. Synapse 4, 313–318. doi:10.1002/SYN.890040407
Ungless, M. A., and Grace, A. A. (2012). Are you or aren’t you? Challenges associated with physiologically identifying dopamine neurons. Trends Neurosci. 35, 422–430. doi:10.1016/J.TINS.2012.02.003
Vandermaelen, C. P., and Aghajanian, G. K. (1983). Electrophysiological and pharmacological characterization of serotonergic dorsal raphe neurons recorded extracellularly and intracellularly in rat brain slices. Brain Res. 289, 109–119. doi:10.1016/0006-8993(83)90011-2
Widman, A. J., and McMahon, L. L. (2018). Disinhibition of CA1 pyramidal cells by low-dose ketamine and other antagonists with rapid antidepressant efficacy. Proc. Natl. Acad. Sci. U. S. A. 115, E3007-E3016–E3016. doi:10.1073/pnas.1718883115
Witkin, J. M., Monn, J. A., Schoepp, D. D., Li, X., Overshiner, C., Mitchell, S. N., et al. (2016). The rapidly acting antidepressant ketamine and the mGlu2/3 receptor antagonist LY341495 rapidly engage dopaminergic mood circuits. J. Pharmacol. Exp. Ther. 358, 71–82. doi:10.1124/JPET.116.233627
Yang, X., Huang, J., Zhu, C. Y., Wang, Y. F., Cheung, E. F. C., Chan, R. C. K., et al. (2014). Motivational deficits in effort-based decision making in individuals with subsyndromal depression, first-episode and remitted depression patients. Psychiatry Res. 220, 874–882. doi:10.1016/j.psychres.2014.08.056
Zarate, C. A., Singh, J. B., Carlson, P. J., Brutsche, N. E., Ameli, R., Luckenbaugh, D. A., et al. (2006). A randomized trial of an N-methyl-D-aspartate antagonist in treatment-resistant major depression. Arch. Gen. Psychiatry 63, 856–864. doi:10.1001/archpsyc.63.8.856
Keywords: Wistar Kyoto, ketamine, serotonin, norepinephrine, dopamine, electrophysiology, depression
Citation: Daniels S, El Mansari M, Hamoudeh R and Blier P (2023) Ketamine promptly normalizes excess norepinephrine and enhances dopamine neuronal activity in Wistar Kyoto rats. Front. Pharmacol. 14:1276309. doi: 10.3389/fphar.2023.1276309
Received: 11 August 2023; Accepted: 06 October 2023;
Published: 31 October 2023.
Edited by:
Daniela Jezova, Slovak Academy of Sciences (SAS), SlovakiaReviewed by:
Bruno Pierre Guiard, Université de Toulouse, FranceEliyahu Dremencov, Slovak Academy of Sciences, Slovakia
Copyright © 2023 Daniels, El Mansari, Hamoudeh and Blier. This is an open-access article distributed under the terms of the Creative Commons Attribution License (CC BY). The use, distribution or reproduction in other forums is permitted, provided the original author(s) and the copyright owner(s) are credited and that the original publication in this journal is cited, in accordance with accepted academic practice. No use, distribution or reproduction is permitted which does not comply with these terms.
*Correspondence: Mostafa El Mansari, bW9zdGFmYS5lbG1hbnNhcmlAdGhlcm95YWwuY2E=