- 1Guangzhou Key Laboratory of Basic and Applied Research of Oral Regenerative Medicine, Department of Pharmacy, Guangdong Engineering Research Center of Oral Restoration and Reconstruction, Affiliated Stomatology Hospital of Guangzhou Medical University, Guangzhou, China
- 2Department of Gastrointestinal Surgery, The Second Affiliated Hospital of Dalian Medical University, Dalian, China
- 3Affiliated Foshan Maternity and Child Healthcare Hospital, Southern Medical University, Foshan, China
- 4School of Pharmaceutical Sciences, Southern Medical University, Guangzhou, China
The human gut microbiota is a complex ecosystem regulating the host’s environmental interaction. The same functional food or drug may have varying bioavailability and distinct effects on different individuals. Drugs such as antibiotics can alter the intestinal flora, thus affecting health. However, the relationship between intestinal flora and non-antibiotic drugs is bidirectional: it is not only affected by drugs; nevertheless, it can alter the drug structure through enzymes and change the bioavailability, biological activity, or toxicity of drugs to improve their efficacy and safety. This review summarizes the roles and mechanisms of antibiotics, antihypertensive drugs, nonsteroidal anti-inflammatory drugs, lipid-lowering drugs, hypoglycemic drugs, virus-associated therapies, metabolites, and dietary in modulating the colorectal cancer gut microbiota. It provides a reference for future antitumor therapy targeting intestinal microorganisms.
1 Introduction
There are over 193 million cases of colorectal cancer (CRC) diagnosed each year in the world, despite the rising use of colonoscopies (Siegel et al., 2023). Approximately 50%–60% of incident cases of CRC can be attributed to modifiable risk factors such as smoking, drinking a lot of alcohol, being overweight, being obese, being inactive, eating much red and processed meat, and eating little whole grains or fiber (Li et al., 2022). A growing body of evidence indicates that intestinal microbiomes are influenced by the environment and contribute to disease (Sepich-Poore et al., 2021; Xia et al., 2023a; Xia et al., 2023b). It has been found that patients with CRC have different intestinal microbiomes compared to healthy individuals. Moreover, it has been shown that gut microbes change during colorectal carcinogenesis assisting in identifying individuals at risk (Hagan et al., 2019). People have learned a great deal about the composition of the human gut microbiota in the last decade, but the complex temporally spatial interplay between gut microbes and humans remains an ongoing mystery.
It is well-known that the gut microbiota can be shifted into alternative stable states or quasi-stable states by antibiotics, which may become more resilient to external influences (Hao et al., 2020; Reyman et al., 2022). However, a bidirectional relationship exists between intestinal flora and non-antibiotic drugs. Despite its impact on drugs, it can also alter the structure of drugs via enzymes, enhance the bioavailability and biological activity of drugs, or reduce their toxicity (de Gunzburg et al., 2018; Willmann et al., 2019). There have also been new changes in the treatment of tumors (Ye et al., 2023). In this review, we summarize the roles and mechanisms of antibiotics, antihypertensive drugs, nonsteroidal anti-inflammatory drugs, lipid-lowering drugs, hypoglycemic drugs, virus-associated therapies, metabolites, and dietary in modulating the CRC gut microbiota. It provides evidence that intestinal microorganisms could be used as targets for future antitumor therapies.
2 Drug repurposing and microbiota in colorectal cancer
Drug repurposing involves using existing drugs to treat diseases not included in the original indication. Based on the side effects of the current chemotherapeutic agents, the repurposing of noncancer drugs in the prevention or treatment of CRC has the advantage of a high safety level and fewer side effects. Currently, the relationship between the gut microbiome and CRC is gradually being elucidated (Karpiński et al., 2022), and we have summarized the anticancer activity of some traditional drugs and attempted to analyze their association with the gut microbiome during treatment.
2.1 Antihypertensive drugs
Hypertension is the most prevalent chronic cardiovascular disease globally and is closely associated with the gut microbiota. Human global gut microbiota diversity is associated with hypertension (Silveira-Nunes et al., 2020; Wang et al., 2021). The gut microbiota is high in Proteobacteria and Actinobacteria at a low level; at the genus level, Klebsiella, Clostridium, Streptococcus, Parabacteroides, Eggerthella, and Salmonella are more prevalent (Yan et al., 2017). The proportion of butyrate decreased (Wang et al., 2021). Short-chain fatty acids (SCFA), including butyrate, regulate the activity of G protein-coupled receptors and these metabolites have immunomodulatory functions. They also contribute to blood pressure homeostasis and are implicated in the pathogenesis of hypertension (Katsi et al., 2019; Duttaroy, 2021). Meanwhile, trimethylamine, trimethylamine-producing bacteria, and trimethylamine-N-oxide have been linked to hypertension in several pathways (Zhang et al., 2021).
In animal studies, the ratio of Firmicutes to Bacteroidetes in the hypertensive model rats was increased (Santisteban et al., 2017; Li et al., 2021). Prehypertension and hypertension are associated with the risk of multiple cancers, including CRC (Seretis et al., 2019; Lee et al., 2020). Both elevated systolic and diastolic blood pressure and stage 2 hypertension were positively related to the CRC risk, and metabolic syndrome was associated with an increased risk of early-onset CRC, including hypertension (Chen et al., 2021; Kaneko et al., 2021).
Drugs for hypertension include angiotensin I-converting enzyme inhibitors (ACEI), angiotensin II receptor blockers (ARB), and β-blockers. Angiotensin II differential blood pressure was regulated by gut microbiota metabolites. Mice lacking gut microbiota are protected from angiotensin II-induced arterial hypertension, vascular dysfunction, and hypertension-induced end-organ damage (Karbach et al., 2016). ACEI, ARB, and β -blockers significantly affect gut microbiota (Zhernakova et al., 2016; Jackson et al., 2018). Among them, telmisartan induces specific gut microbiota characteristics to mediate its anti-obesity effect; irbesartan attenuates pulmonary arterial pressure in the high-altitude pulmonary hypertension model rats by increasing the abundance of Lactobacillaceae and Lachnospiraceae in the gut and reducing the abundance of Prevotellaceae and Desulfovibrionaceae; captopril exerts its sustained antihypertensive effect by mediating captopril-reactive bacteria (including Parabacteroides, Mucispirillum, and Allobaculum) (Figure 1), and re-balancing of the brain-gut axis; Antihypertensive peptides and the α-lactalbumin hydrolysates under 3 kDa can restore the diversity of the intestinal microbiota, induce SCFA, and relieve hypertension-associated gut dysbiosis (Yang et al., 2019; Beckmann et al., 2021; Nijiati et al., 2021; Xie et al., 2022).
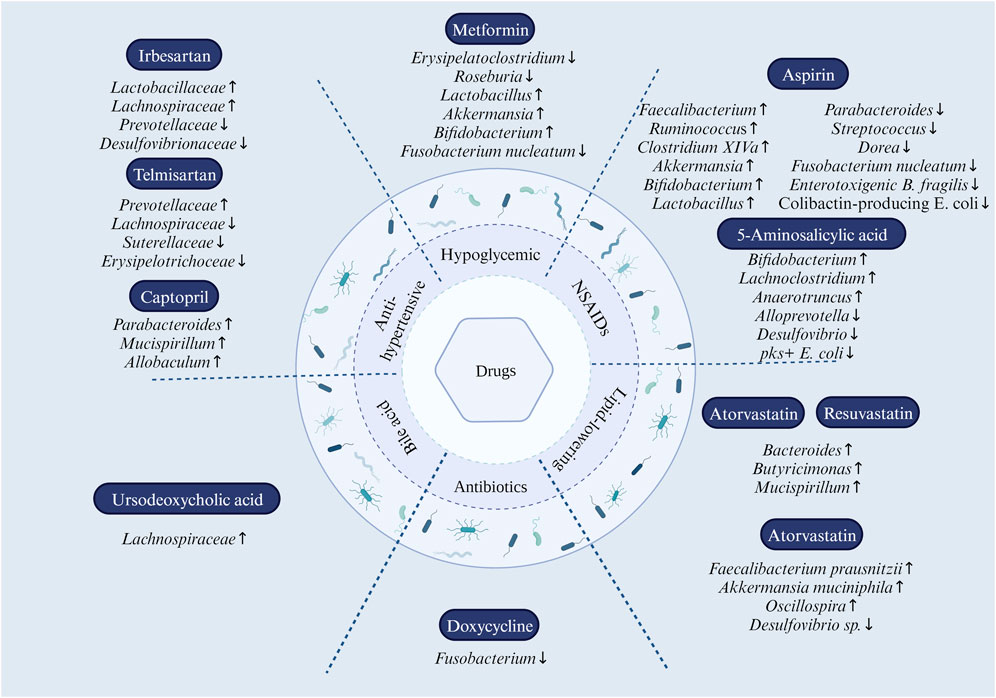
FIGURE 1. Effect of drug reuse on intestinal microbiota. In antihypertensive drugs, Prevotellaceae were elevated, and Lachnospiraceae, Suterellaceae, and Erysipelotrichoceae were decreased by the use of telmisartan; irbesartan upregulates the abundance of Lactobacillaceae and Lachnospiraceae and downregulates the abundance of Prevotellaceae and Desulfovibrionaceae; captopril modulates the abundance of Parabacteroides, Mucispirillum, and Allobaculum. In NSAIDs, aspirin increases the abundance of Faecalibacterium, Ruminococcus, Clostridium XIVa, Akkermansia, Bifidobacterium, and Lactobacillus; while reducing the abundance of Parabacteroides, Streptococcus, Dorea, Fusobacterium nucleatum, enterotoxigenic B. fragilis, and colibactin-producing E. coli. 5-Aminosalicylic acid modulates intestinal flora dysbiosis by increasing the abundance of Bifidobacterium, Lachnoclostridium, and Anaerotruncus and decreasing the abundance of Alloprevotella and Desulfovibrio. In statins, atorvastatin and rosuvastatin upregulated the abundance of Bacteroides, Butyricimonas, and Mucispirillum; atorvastatin helps to reshape the dysbiosis of the gut microbiota by increasing the abundance of anti-inflammatory bacteria such as Faecalibacterium prausnitzii, Akkermansia muciniphila, and the genus Oscillospira, and decreasing the abundance of pro-inflammatory bacteria Desulfovibrio sp. Metformin treatment altered the composition of the gut microbiota by increasing the relative abundance of Lactobacillus and Akkermansia species while reducing Erysipelatoclostridium. At the genus level, Bifidobacterium increased and Fusobacterium nucleatum decreased. Doxycycline eliminated Fusobacterium spp. Members of the Lachnospiraceae Family (Phylum Firmicutes, Class Clostridia) were significantly increased in the gut microbial community of the ursodeoxycholic acid-treated group.
These hypertensive drugs also have a preventive or therapeutic effect on CRC. Some studies demonstrated that the application of ACEI and ARB might reduce CRC incidence, polyp formation, and metastasis and prevent the development of CRC via a mechanism involving inhibition of angiotensin-converting enzyme activity, angiotensin Ⅱ synthesis, and the epidermal growth factor receptor expression (Kedika et al., 2011; Makar et al., 2014; Childers, 2015; Asgharzadeh et al., 2018; Nakamura et al., 2018;Cheung et al., 2020a). ARB did not change the number of cluster of differentiation 11 b + bone marrow cells in tumors; nonetheless, it significantly reduced the T cell inhibition ability while reducing the production of various immunosuppressive factors. ARB also decreased the cancer-related fibroblasts, chemokine ligand 12, and nitric oxide synthase 2 expressions, indicating that the renin-angiotensin-system is involved in the production of immunosuppressive cells initiated by bone marrow cells and fibroblasts tumor microenvironment (Nakamura et al., 2018). ACEI/ARB and β-blockers are associated with improved survival, tumor progression, and decreased hospitalization in patients with advanced CRC (Engineer et al., 2013). The β-blocker nebivolol co-inhibited complex I and adenosine triphosphate synthase activity, precisely hindering oxidative phosphorylation of cancer cells (Nuevo-Tapioles et al., 2020). Antihypertensive medications regulate intestinal flora and maintain a healthy brain-gut axis. Angiotensin II regulates blood pressure through gut microbiota metabolites, thereby involving intestinal flora participation in the renin-angiotensin-system and the formation of the suppressive tumor microenvironment.
2.2 Nonsteroidal anti-inflammatory drugs
Nonsteroidal anti-inflammatory drugs (NSAIDs) can reduce the incidence and recurrence of advanced colorectal adenomas and CRC, and numerous randomized, controlled, and double-blind clinical trials are undergoing to evaluate NSAIDs in cancer chemoprevention (Wang et al., 2018; Cheung et al., 2020b; Chen et al., 2021; Chudy-Onwugaje et al., 2021; Maniewska and Jeżewska, 2021). NSAIDs play an anti-inflammatory role by inhibiting the cyclooxygenase enzyme, prostaglandin E2 pathway, and cyclooxygenase-independent pathway. The inflammatory response and accessory inflammation, which is an intermediate state between chronic inflammation and basal homeostasis, significantly impacted CRC progression and p53 to maintain homeostasis (Ghanghas et al., 2016). Upon loss of p53, para-inflammation loses its tumor-suppressive properties and becomes tumor-promoting (Lasry et al., 2016). Phosphatidylinositol-4,5-bisphosphate 3-kinase catalytic subunit alpha mutations are present in approximately 15% to 20% of CRC. Upregulation of phosphatidylinositol 3-kinase increases cyclooxygenase-2 activity, promotes prostaglandin E2 synthesis, and inhibits apoptosis in colon cancer cells (Amitay et al., 2019). NSAIDs can also inhibit the mechanistic target of rapamycin kinase signaling to induce autophagy, inhibit tumor cell viability via p53-dependent autophagy, and increase chemotherapeutic drug radiosensitivity and cytotoxicity (Yu et al., 2018). Patients using NSAIDs have distinct gastrointestinal microbiome profiles compared to those not using NSAIDs. The abundance of Prevotella spp., Bacteroides spp., family Ruminococaceae, and Barnesiella spp. can discriminate aspirin users from no medication; celecoxib and ibuprofen users showed enrichment of Acidaminococcaceae and Enterobacteriaceae in the gut (Rogers and Aronoff, 2016).
Aspirin has potent chemopreventive activity in CRC and regular use of aspirin significantly reduces the incidence of CRC (Bosetti et al., 2020). The incidence of CRC is significantly reduced in people with long-term aspirin use for more than 10 years (Zhang et al., 2021). Low-dose aspirin and flexible sigmoidoscopy are equally effective in reducing the incidence and mortality of CRC (Emilsson et al., 2017). Kane, A.M. et al. used the B-Raf proto-oncogene mutation model study and found that aspirin treatment could significantly reduce the incidence of metastatic disease (Kane et al., 2021). The 10-year overall survival was significantly prolonged with aspirin in patients with wild-type phosphatidylinositol-4,5-bisphosphate 3-kinase catalytic subunit alpha and mutated KRAS tumors (Gebauer et al., 2021). The chemopreventive effect of aspirin in CRC might be related to various mechanisms, including inhibition of cyclooxygenase, cyclin-dependent kinase, β-catenin phosphorylation, mechanistic target of rapamycin kinase, MYC, cyclin A2, nuclear factor of kappa light polypeptide gene enhancer in B cells, and Wnt signaling pathways, activation of adenosine monophosphate kinase, induction of polyamine catabolism and deoxyribonucleic acid mismatch repair proteins, and acetylation of p53, glucose-6-phosphate dehydrogenase, and other proteins (Sankaranarayanan et al., 2020).
It has been found that aspirin can regulate the tumor microenvironment via gut microbes, induce autophagy, reduce the inflammatory response induced by necrosis, and inhibit tumor development (Frouws et al., 2017). Aspirin alters the gut microbiome and modifies the bacterial taxa in the gut, thereby protecting against CRC (Chan et al., 2012). Specifically, aspirin downregulated flora positively associated with CRC risk, including Parabacteroides and Streptococcus, while up-regulating flora negatively associated with CRC, including Faecalibacterium and Ruminococcus (Figure 1). In double-blind, randomized, placebo-controlled trials, aspirin users have a higher abundance of Ruminococcaceae and Clostridium XIVa and a lower abundance of Parabacteroides and Dorea. In addition, aspirin increased the abundance of the anti-inflammatory bacterium Akkermansia more than the placebo (Figure 1) (Prizment et al., 2020; Brennan et al., 2021). Likewise, the inhibitory effect of aspirin on the notorious Fusobacterium nucleatum, enterotoxigenic Bacteroides fragilis, and colibactin-producing Escherichia coli is noteworthy for its ability to diminish F. nucleatum on CRC promotion (Figure 1). In mice treated with azomethane and dextran sulfate, probiotic bacteria, including Bifidobacterium pseudolongum and Faecalibacterium rodentium, were enriched in aspirin-treated mice; a similar effect also occurs for Bifidobacterium and Lactobacillus genera, including B. pseudolongum, Bifidobacterium breve, Bifidobacterium animalis, Lactobacillus reuteri, Lactobacillus gasseri, and Lactobacillus johnsonii in aspirin-treated APCmin/+ mice (Figure 1) (Zhao et al., 2020).
Celecoxib is a Food and Drug Administration-approved drug used for colorectal polyps and prevents high-risk adenoma recurrence (Lynch et al., 2010; Thompson et al., 2016). In a 5-year clinical trial designed to prevent sporadic adenomatous polyps in colon, the incidence of new adenoma was substantially lower in the celecoxib group than in the placebo group. Celecoxib reduced the luminal microbiome and metabolome associated with intestinal stem cell proliferation, inhibited c-Met, and exerted anti-CRC effects (Jendrossek, 2013; Lin et al., 2019). The ability of celecoxib to chemoprevent CRC is mediated by the gut microbiota and microbe-derived metabolites (Ferrara et al., 2022). In hepatocellular carcinoma, celecoxib enhances the anti-tumor function of immune cells by up-regulating the abundance of Bacteroides Acidifaciens, Odoribacter Laneus, and Odoribacter splanchnicus (Pan et al., 2023).
5-Aminosalicylic acid has also been found to exert anti-inflammatory effects by modulating the intestinal flora. 5-Aminosalicylic acid modulates intestinal flora dysbiosis by increasing the abundance of Bifidobacterium, Lachnoclostridium, and Anaerotruncus and decreasing the abundance of Alloprevotella and Desulfovibrio (Figure 1). 5-Aminosalicylic acid is an important metabolite of aspirin and has been found to exert anti-inflammatory effects by modulating intestinal flora. abundance to modulate intestinal dysbiosis and ultimately ameliorate dextran sulfate sodium-induced colitis (Huang et al., 2022; Wada et al., 2023). In addition, 5-aminosalicylic acid may also exert a preventive effect against CRC by inhibiting pks + E. coli (Figure 1) (Tang-Fichaux et al., 2021). Naproxen may influence the production of trimethylamine and trimethylamine-N-oxide by altering the type of choline-utilizing anaerobic bacteria implicated with CRC progression (Rogers and Aronoff, 2016).
Besides, intestinal flora also impacts the drug use of NSAIDs. The bioavailability of aspirin is related to its chemopreventive effect on CRC, however, the bioavailability of oral drugs, including aspirin, is related to intestinal flora (Zhang et al., 2021). Lysinibacillus sphaericus weakens the chemopreventive effect of aspirin by degrading it (Zhao et al., 2020). Not coincidentally, enzymes produced by the intestinal flora reduced the efficacy of 5-aminosalicylic acid in the treatment of inflammatory bowel disease (Mehta et al., 2023). Therefore, these findings reveal the complex interactions between aspirin, intestinal flora, and CRC, and provide additional mechanisms and references to follow for the use of aspirin in the treatment of CRC.
2.3 Lipid-lowering drugs
High serum triglyceride levels and high serum cholesterol levels are positively associated with the incidence of CRC (Yang et al., 2022). Targeting cholesterol biosynthesis, which contributes to CRC cell growth and liver metastasis, is a promising therapy for CRC (Zhang et al., 2021). As lipid-lowering agents, statins are among the chemopreventive agents for CRC and play an important role in the treatment and prognosis of CRC. A population-based case-control study found statins to be moderately chemoprotective against CRC (Rodríguez-Miguel et al., 2022). After undergoing a colonoscopy, statin users were found to have a lower risk of CRC (Cheung et al., 2019). Furthermore, atorvastatin eliminated microadenomas in tumor-free mice (Chang et al., 2018). Statins also inhibit the proliferation of CRC cells and promote apoptosis (Palko-Łabuz et al., 2019). Statins may be considered as targeted therapy for CRC (Shailes et al., 2022). Meanwhile, in the treatment, statins can enhance the anticancer activity of the chemotherapeutic drug oxaliplatin, enhance the sensitivity of radiotherapy for CRC and synergize the antitumor effect of the targeted drug regorafenib, which may arise from the weakening of stemness and drug resistance of CRC cells by statins (Karagkounis et al., 2018; Gao et al., 2021; Gonçalves et al., 2021; Tsubaki et al., 2023; Yuan et al., 2023). Pravastatin, a metabolite of intestinal flora, can promote interleukin (IL)-13 release from type Ⅱ innate lymphocytes through IL-33 signaling, which has been shown to promote self-renewal of pluripotent intestinal stem cells at the base of intestinal crypts (Zhu et al., 2019; Deng et al., 2021). In postoperative patients, statin use has been associated with lower short- and long-term mortality (Pourlotfi et al., 2021a; Pourlotfi et al., 2021b).
The gut microbiota is an important part of the pharmacological action of statins. Statin therapy is negatively associated with obesity-related microbiota dysbiosis (Vieira-Silva et al., 2020). Statins modulate gut microbiota dysbiosis due to hyperglycemia and hyperlipidemia, and atorvastatin and rosuvastatin upregulated the abundance of Bacteroides, Butyricimonas, and Mucispirillum, and notably, fecal transplants of gut microbiota altered by rosuvastatin still exerted ameliorative effects on hyperglycemia (Figure 1) (Kim et al., 2019). Atorvastatin helps to reshape the dysbiosis of the gut microbiota induced by hyperlipidemia, which includes reversing the ratio between Firmicutes to Bacteroidetes, increasing the abundance of anti-inflammatory bacteria such as Faecalibacterium prausnitzii, Akkermansia muciniphila, and genus Oscillospira, and decrease the abundance of pro-inflammatory bacteria Desulfovibrio sp. (Figure 1) (Wang L. et al., 2021). There is no doubt that the same microbiota regulated by statins are associated with CRC. An interesting perspective is that the gut microbiota may already be a common target for statins against atherosclerosis and tumors (Wu et al., 2021). The study found that intestinal flora is crucial for preventing or treating CRC with statins. Statins may affect the gut microbiota, with a high proportion of Bacteroides, a low proportion of Faecalibacterium, and a low microbial cell density in the gut of patients not receiving statin therapy compared with patients taking statins. Statins can enhance the number of Anaerostipes hadrus and Bifidobacterium longum subsp and their ability to produce butyrate Statins also increase the abundance of Bifidobacterium, Anaerobes, Broucella, and B. longum subsp (Figure 1) (Vieira-Silva et al., 2020; Hu et al., 2021). Statins are equally involved in the metabolism of the gut microbiota. The application of atorvastatin enhances the availability of tryptophan in the gut, which in turn leads to an increase in L. reuteri, which then inhibits colorectal carcinogenesis by metabolizing tryptophan to indole-3-lactic acid. This process ultimately contributes to the chemopreventive effects of atorvastatin (Figure 1) (Han et al., 2023).
2.4 Hypoglycemic drugs
Diabetes mellitus is associated with an increased risk of CRC, and their association is related to the family history of CRC (Ali Khan et al., 2020a; Ali Khan et al., 2020b; Amadou et al., 2021; Hu et al., 2021). High insulin levels occurring in the pre-diabetic phase act as a major driver of the positive association of type 2 diabetes with CRC (Murphy et al., 2022). The duration of obesity reflects the level and duration of hyperinsulinemia, patients with long-term obesity had an increased risk of CRC compared to patients with no history of obesity (Peeters et al., 2015). Stratification based on the cumulative period of obesity revealed that patients with long-term obesity had an increased risk of CRC compared to patients with no history of obesity. Furthermore, insulin and insulin like growth factor 1 (IGF1) are ligands for the IGF1 receptor, and their binding induces autophosphorylation and conformational changes in the cytoplasmic tyrosine domain to stimulate a signaling cascade, including primarily the phosphatidylinositol 3′-kinase/protein kinase B and mitogen-activated protein kinases pathways that are closely associated with protein synthesis, survival, and proliferation (Yu et al., 2022).
Certain hypoglycemic drugs can prevent and treat CRC. Metformin has a potential role in the chemoprevention of CRC, and low-dose metformin reduces the incidence and number of metachronous adenoma or polyps after polypectomy (Higurashi et al., 2016); Metformin, as neoadjuvant therapy, can reduce the adverse effects of diabetes and improve the prognosis of patients with diabetes and CRC in conjunction with 5-fluorouracil (Sang et al., 2020). The mechanisms include metformin targeting the mechanistic target of rapamycin kinase via the adenosine monophosphate-activated protein kinase and insulin/insulin-like growth factor pathway, and inducing apoptosis and autophagy through oxidative stress, inflammation, and metabolic homeostasis (Mallik and Chowdhury, 2018).
Studies have shown that metformin inhibits tumor progression by modifying the gut microbiome. Transplantation of metformin-treated mice’s feces into mice with metastatic tumors revealed increased SCFA and decreased expression of tumor cholesterol metabolism genes in tumor-bearing mice (Broadfield et al., 2022). Metformin can increase Firmicutes and reduce Fusobacteria and Bacteroidetes at the phylum level. At the genus level, there was an increased Bifidobacterium and decreased Fusobacterium nucleatum (Figure 1) (Huang et al., 2020; Huang et al., 2020). Metformin altered the composition of gut flora associated with CRC, including Bacteroides, Streptococcus, Achromobacter, Alistipes, and Fusobacterium. Importantly, metformin inhibited the growth of Fusobacterium in vitro and was shown to inhibit Fusobacterium in APC Min/+ mice (Figure 1) (Huang et al., 2020).
The complex mechanism of metformin action maybe 1) metformin is metabolized in the gut, reacts with intestinal microbes, alters the composition and abundance of intestinal flora, and causes a cascade related to intestinal flora, such as regulating inflammation, innate immunity, and adaptive immunity. 2) metformin alters gut metabolomics, including some substances that can weaken CRC such as SCFA (Vallianou et al., 2019). 3) complex interactions between metformin, gut microbiota, and the growth hormone/IGF-1 axis. Metformin can affect IGF-1 levels (Khan et al., 2023). Similarly, the intestinal flora can regulate IGF-1 through the growth hormone/IGF-1 axis. Microbial metabolites, including SCFA, also regulate the release of growth hormones in cancer (Yan and Charles, 2018). The gut microbiota produces SCFA and other microbial metabolites that act on the liver and adipose tissue to induce IGF-1 production (Matsushita et al., 2021). IGF-1 production is associated with CRC risk and promotes inflammation-related tumorigenesis (Youssif et al., 2018; Murphy et al., 2020).
2.5 Antibiotics
Antibiotics have contradictory effects on intestinal microflora. First, prolonged misuse of antibiotics, generation, and drug resistance severely disrupt the microbial ecosystem and increase the risk of CRC. Antibiotic use was associated with an increased risk of colorectal polyps, and gut dysbiosis was implicated in the early phases of colorectal carcinogenesis, according to a case-control study conducted in Sweden. Tetracycline and quinolone antibiotics are strongly linked to an elevated risk of colon and rectal polyps (Song et al., 2021). Second, chronic inflammation induced by antibiotics can cause gene mutation in colon epithelial cells and aberrant deoxyribonucleic acid methylation modifications, contributing to CRC development. In the azoxymethane/dextran sodium sulfate mouse model, antibiotics that modulate the gut microbiota can reduce colonic inflammation and inhibit colonic tumorigenesis. The study revealed that when tumor-bearing mice were treated with the Fusobacterium-resistant antibiotic erythromycin, neither the tumor volume nor the abundance of Clostridium bacteria in the tumor tissue changed; however, when the tumor-bearing mice were treated with the Fusobacterium-sensitive antibiotic metronidazole, both the tumor volume and the abundance of Fusobacterium in the tumor tissue decreased (Mihai et al., 2021). This indicates that antibiotics regulate Fusobacterium and inhibit CRC tumor cell proliferation. We demonstrated that the anticancer activity of doxycycline is mainly because the drug can significantly affect the diversity of Bifidobacterium populations, eliminating Fusobacterium without any effect on other intestinal bacterial populations (Figure 1) (Elvers et al., 2020).
The relationships between gut microbiota, inflammation, and tumors are complicated. Antibiotics are a double-edged sword against intestinal flora. Antibiotics can interfere with normal intestinal flora and promote tumor occurrence; they can also inhibit pathogenic intestinal bacteria, reduce inflammation and inhibit tumor occurrence. Therefore, the effect on gut microbes should be considered when investigating antitumoral antibiotics.
2.6 Virus-related therapy
Viruses are part of the gut microbiota and significantly affect the occurrence and progression of CRC. Compared to healthy control, CRC patients showed higher viral diversity in the gut. Enterovirus and disease stage-specific changes and prognosis were also associated with CRC patients’ prognosis (Nakatsu et al., 2018). Epstein-Barr virus, human cytomegalovirus, human papillomavirus, and other deoxyribonucleic acid viruses replicate within infected cells, target p53, pRb, and p21, and disrupt the cell cycle. Therefore, it can produce an anti-CRC effect by interfering with the virus. Zidovudine is a nucleoside antiretroviral drug inhibiting CRC cell proliferation (Fang et al., 2017; Sherif et al., 2021). Ganciclovir inhibits the NLR family pyrin domain containing 3 activations and reduces the irinotecan-induced intestinal toxicity (Huang et al., 2020). The combination of zidovudine and veavirren completely blocked tumorigenesis in tumor-bearing mice (Schneider et al., 2021). Nelfinavir is a human immunodeficiency virus protease inhibitor and fractionated radiotherapy for locally advanced rectal cancer (Hill et al., 2016). Chemokine receptors C-X-C motif chemokine receptor 4 and C-X-C motif chemokine receptor 7 are involved in CRC progression, and HIV drugs targeting C-X-C motif chemokine receptor 4 may inhibit CRC (Goïta and (Goïta and Guenot, 2022).
Studies have shown that double-stranded DNA viruses in the gut are mainly phages (Hannigan et al., 2018; Lin and Lin, 2019). The abundance of phages was significantly higher in azoxymethane-induced colorectal tumors. It disrupts the intestinal microbiota balance and induces a specific immune response that exacerbates colitis through the toll-like receptor 9 and interferon-gamma pathways. Because antibiotics are often difficult to regulate the intestinal flora precisely and can interfere with normal intestinal flora, bacteria will develop gradual antibiotic resistance. Thus, antitumor therapies targeting phages have distinct advantages. Bakuradze et al. found that adding phage VA7 to enterotoxin-producing Bacteroides fragilis-infected CRC cells significantly decreased bacterial counts and IL-8 levels in the VA7-treated group compared to untreated infected cells (Bakuradze et al., 2021). Bacteriophage FNU 1 can decompose, lyse F. nucleatum biofilms, and play an antitumor role (Kabwe et al., 2019). Bacteriophage EFA 1 upregulates reactive oxygen species in the culture system and inhibits HCT116 CRC cell growth (Kabwe et al., 2021).
It is possible to obtain phage nanoparticles with increased antibacterial activity through phage display technology by selecting phages with a high affinity for the target and combining them with inorganic nanomaterials having elevated antibacterial activity. For instance, the incorporation of silver nanoparticles into the phage surface resulted in precise clearance of Fusobacterium, blocked the expansion of bone marrow-derived suppressor cells in the tumor microenvironment, enhanced the host immune response, and prolonged the survival of CRC mice (Dong et al., 2020). Oral administration of phage nanoparticles containing irinotecan did not affect the number of blood cell levels, immunoglobulin and histamine levels, and liver and kidney function of tumor-bearing animals indicated that the safety of phage nanoparticles was good (Zheng et al., 2019). Phages can carry different fragments and have different effects on the tumor microenvironment. It was found that bacteriophage M13, which targets carcinoembryonic antigen, can specifically bind to the carcinoembryonic antigen in tumor cells, activate antitumor immunity, and substantially inhibit tumor growth in CRC tumor-bearing mice (Murgas et al., 2018).
Oncolytic viruses can target cancer cells, release viral particles, cytokines, and their contents in the tumor cells, induce local inflammation inside the cells, and play an antitumor role. The virus uses its protein sequence to stimulate the immune system through pathogen-associated molecular patterns (Cook and Chauhan, 2020). Taking the talimogene laherparepvec system as an example, the investigators knocked out genes related to neurovirulence and antiviral evasion in VEC, a herpes simplex virus, and lead-in the single chain variable fragment of monoclonal antibody to increased talimogene laherparepvec’s target (Turkington et al., 2020). Studies have shown that the unmodified reovirus Pelareorep can also treat CRC (Goel et al., 2020). Oncolytic virotherapy can alter the tumor microenvironment and improve the efficacy of anti-programmed cell death protein 1 therapy (Ribas et al., 2017).
3 Metabolites, dietary, and gut microbiota in colorectal cancer
3.1 Metabolites
Bile acids are mainly synthesized by cholesterol in hepatocytes. Bile acids synthesized by hepatocytes are primary bile acids. Bile acids have bidirectional regulation effects on the body. At physiological concentrations, secondary bile acids have immune regulation and anti-inflammatory effects on the body, inhibiting intestinal inflammatory disease progression. High levels of secondary bile acids in the blood, bile, and feces increase the risk of cholesterol stones, damage the intestinal epithelium, and induce excessive proliferation of undifferentiated cells, leading to a premalignant state.
The metabolites of certain gut microorganisms contain secondary bile acids and SCFA, which play a role in cell proliferation. These microbial metabolites can promote colonic cell proliferation at low concentrations and inhibit cell proliferation at high concentrations. Bile acids, although synthesized in the liver, have direct or indirect antibacterial effects, thereby modulating the composition of the microbiota, which in turn regulates the size and composition of the bile acid pool (Liu et al., 2020). Patients with liver diseases such as fatty liver, fibrosis, cirrhosis, and hepatocellular carcinoma frequently exhibited intestinal dysbiosis characterized by a significant increase in aerobic and pro-inflammatory bacteria such as Enterobacter, Enterococcus, and Clostridium, which can accelerate production from secondary bile acids. Cholalic acid-fed mice, with a significant decrease in Firmicutes, the primary SCFA producer, from the gut. Chic acid-induced micro-dysbiosis impaired intestinal barrier function and induced low-grade intestinal inflammation, activating the transcription pathway’s IL-6 signal transducer and activator and promoting tumor progression (Wang et al., 2019). Increased bile acid levels induced by a high-fat diet promoted an increase in 7 α-dehydroxylated bacteria and increased secondary bile acids with tumor-promoting activity in the colon, particularly deoxycholic acid (Ocvirk and O'Keefe, 2017). Studies have shown that members of the Lachnospiraceae Family (Phylum Firmicutes, Class Clostridia) were significantly increased in the gut microbial community of the ursodeoxycholic acid-treated group (Figure 1). Bile acids bind to the receptor to regulate organisms' physiological response (Winston et al., 2021). Ursodeoxycholic acid can activate farnesoid X-activated receptor and Takeda G-protein coupled receptor 5 receptors and regulate the host’s innate immune response (Winston et al., 2020). Obercholic acid, a novel farnesoid X-activated receptor agonist, combined with the β-catenin inhibitor nitazoxanide, can inhibit CRC progression (Yu et al., 2021).
3.2 Dietary
The species and abundance of gut flora varied between CRC and healthy individuals. The intestinal flora is closely linked to food intake. Young women with high consumption of sugary drinks were substantially associated with increased publication rates of colorectal adenomas (particularly rectal adenomas) (Joh et al., 2021). Mice fed a high-fructose diet exhibited increased intestinal permeability, a lower proportion of Bacteroidetes in the gut microbiota, and a significantly increased proportion of Proteobacteria susceptible to colorectal tumors; diet feeding with fructose removal and supplementation could reverse tumor progression (Do et al., 2018; Nishiguchi et al., 2021). Starch-rich diet can increase the sulfur mucin content in the intestine and have a protective effect on the intestinal mucosa (Gabrielli and Tomassoni, 2018). The gut microbiota can ferment dietary fiber to produce SCFA, such as acetate, propionate, and butyrate, essential for maintaining intestinal homeostasis and intestinal epithelial cell health (Wu et al., 2018).
Regarding protein, the intake of red meat and industrially processed meat raises the risk of CRC in people (Farvid et al., 2021). In a pooled analysis of data from extensively prospective studies of Japanese men and women, meat subtype and sex were associated with CRC risk, and increased beef consumption was associated with an increased risk of CRC (women) and distal CRC (men) (Islam et al., 2019). Heme iron is a crucial component of red meat, and dietary heme influences the microbiota composition and its diversity, thus leading to dysbiosis. A heme diet reduced the gut flora α-diversity and altered the gut microbiota composition, with reduced Firmicutes and increased Proteobacteria (Seiwert et al., 2020).
A high-fat diet will increase the dietary fat in the intestine, and the intestinal dietary fat can further regulate the intestinal flora by screening fatty acid degradation genes and replacing the compound medium with the fat medium, significantly reducing the overall abundance of Clostridium (Budu et al., 2022). Microdysbiosis can activate the monocyte chemoattractant protein-1/C-C motif chemokine receptor 2 axis, promote the recruitment and polarization of M2 tumor-associated macrophages, and reduce SCFA content, leading to intestinal tumor cell proliferation (Martin-Gallausiaux et al., 2021). A high-fat diet increases the bile acid levels in the body, stimulates secondary bile acids levels with tumor-promoting activity in the colon, activates the bile acids-farnesoid X-activated receptor axis, stimulates the colon, and increases Wnt family member 2 B expression in fibroblast cells, thus promoting the formation of tumor immunosuppressive microenvironment (Zeng et al., 2019).
Furthermore, trace elements in the diet, such as iron, selenium, magnesium, and calcium, can regulate intestinal microbes and affect the occurrence or progression of CRC. Iron-deficiency anemia is a common complication of CRC. Studies have shown that in patients treated with oral iron, their extratemporal microbiome is rich in Bacteriaceae and Bacteroides. In contrast, the intratumoral microbiome is rich in Nocardiaceae, Intrasporangiaceae, and Brevibacteriaceae families and Prevotella 9, Nocardioides, Kocuria, Brevibacterium, Veillonella, and Catenibacterium genera (Phipps et al., 2021). Populations with a high in vivo selenium status have a lower risk of CRC. Selenoproteins can affect various signaling pathways related to the pathogenesis of CRC, and play antitumor effects (Peters et al., 2018). Studies demonstrated that selenium can modulate the intestinal flora and that the intestinal flora can also influence the absorption of selenium. Intestinal flora can produce selenomethionine from metabolizing biological selenium compounds (Peters and Takata, 2008; Takahashi et al., 2017). Magnesium intake was associated with the risk of colorectal adenoma and CRC. Studies showed that high magnesium intake may reduce the incidence of CRC in women (Larsson et al., 2005). During short-term magnesium deficiency, the content of Bifidobacteria and Lactobacilli in the intestine is low, leading to increased intestinal permeability and inflammation. Mice that were fed a regular magnesium-containing diet showed no signs of inflammation in the intestine (Pachikian et al., 2010). Calcium is an effective chemopreventive agent for colorectal adenoma (Huang et al., 2020; Emami et al., 2022). Calcium consumption can reduce the risk of death in CRC patients (Yang et al., 2019). Studies have shown that a low calcium diet can lead to intestinal microbiota dysbiosis, and high calcium supplement can restore the ecological imbalance of intestinal flora, increase the abundance of L. reuteri, Lactobacillus plantarum, Firmicutes, Lactobacillus bulgaricus, Streptococcus thermophilus, and Lactobacillus, and play an antitumor role (Gomes et al., 2015; Hua et al., 2019).
4 Conclusion
This paper summarized the complex interactions between antitumor agents and the gut microbiota in CRC (Figure 1). Clinicians and clinical pharmacists must realize that antineoplastic drugs affect the types and abundance of microorganisms in the gut microbiota; gut microbes can also change the effects of drugs, leading to impaired health outcomes. Simultaneously, the discipline of pharmaceutical microbiology is emerging, and clinical trials in this area have already underway. Understanding how the microbiota metabolizes drugs or improves the efficacy of anticancer therapy will significantly impact the clinical practice of drugs and generate novel ideas for regulating the intestinal microbiota and enhancing the efficacy of drug therapy.
Author contributions
JW: Conceptualization, Investigation, Writing–original draft. CoX: Writing–original draft. CL: Writing–review and editing. QZ: Writing–review and editing, Conceptualization. ChX: Conceptualization, Supervision, Writing–review and editing.
Funding
The author(s) declare financial support was received for the research, authorship, and/or publication of this article. This work was supported by grants from the Science and Technology Bureau of Foshan (No. FS0AA-KJ819-4901-0082). We use biorender (https://biorender.com/) to create our figures.
Conflict of interest
The authors declare that the research was conducted in the absence of any commercial or financial relationships that could be construed as a potential conflict of interest.
Publisher’s note
All claims expressed in this article are solely those of the authors and do not necessarily represent those of their affiliated organizations, or those of the publisher, the editors and the reviewers. Any product that may be evaluated in this article, or claim that may be made by its manufacturer, is not guaranteed or endorsed by the publisher.
Abbreviations
ACEI, angiotensin I-converting enzyme inhibitors; ARB, angiotensin II receptor blockers; CRC, colorectal cancer; IGF1, Insulin like growth factor 1; IL, interleukin; NSAIDs, nonsteroidal anti-inflammatory drugs; SCFA, short-chain fatty acids.
References
Ali Khan, U., Fallah, M., Sundquist, K., Sundquist, J., Brenner, H., and Kharazmi, E. (2020a). Risk of colorectal cancer in patients with diabetes mellitus: A Swedish nationwide cohort study. PLoS Med. 17, e1003431. doi:10.1371/journal.pmed.1003431
Ali Khan, U., Fallah, M., Tian, Y., Sundquist, K., Sundquist, J., Brenner, H., et al. (2020b). Personal history of diabetes as important as family history of colorectal cancer for risk of colorectal cancer: A nationwide cohort study. Am. J. Gastroenterol. 115, 1103–1109. doi:10.14309/ajg.0000000000000669
Amadou, A., Freisling, H., Jenab, M., Tsilidis, K. K., Trichopoulou, A., Boffetta, P., et al. (2021). Prevalent diabetes and risk of total, colorectal, prostate and breast cancers in an ageing population: meta-analysis of individual participant data from cohorts of the CHANCES consortium. Br. J. Cancer 124, 1882–1890. doi:10.1038/s41416-021-01347-4
Amitay, E. L., Carr, P. R., Jansen, L., Walter, V., Roth, W., Herpel, E., et al. (2019). Association of aspirin and nonsteroidal anti-inflammatory drugs with colorectal cancer risk by molecular subtypes. J. Natl. Cancer Inst. 111, 475–483. doi:10.1093/jnci/djy170
Asgharzadeh, F., Hassanian, S. M., Ferns, G. A., Khazaei, M., and Hasanzadeh, M. (2018). The therapeutic potential of angiotensin-converting enzyme and angiotensin receptor inhibitors in the treatment of colorectal cancer: rational strategies and recent progress. Curr. Pharm. Des. 24, 4652–4658. doi:10.2174/1381612825666190111145140
Bakuradze, N., Merabishvili, M., Makalatia, K., Kakabadze, E., Grdzelishvili, N., Wagemans, J., et al. (2021). In vitro evaluation of the therapeutic potential of phage VA7 against enterotoxigenic Bacteroides fragilis infection. Viruses 13, 2044. doi:10.3390/v13102044
Beckmann, L., Künstner, A., Freschi, M. L., Huber, G., Stölting, I., Ibrahim, S. M., et al. (2021). Telmisartan induces a specific gut microbiota signature which may mediate its antiobesity effect. Pharmacol. Res. 170, 105724. doi:10.1016/j.phrs.2021.105724
Bosetti, C., Santucci, C., Gallus, S., Martinetti, M., and La Vecchia, C. (2020). Aspirin and the risk of colorectal and other digestive tract cancers: an updated meta-analysis through 2019. Ann. Oncol. 31, 558–568. doi:10.1016/j.annonc.2020.02.012
Brennan, C. A., Nakatsu, G., Gallini Comeau, C. A., Drew, D. A., Glickman, J. N., Schoen, R. E., et al. (2021). Aspirin modulation of the colorectal cancer-associated microbe Fusobacterium nucleatum. mBio 12, e00547–e00521. doi:10.1128/mBio.00547-21
Broadfield, L. A., Saigal, A., Szamosi, J. C., Hammill, J. A., Bezverbnaya, K., Wang, D., et al. (2022). Metformin-induced reductions in tumor growth involves modulation of the gut microbiome. Mol. Metab. 61, 101498. doi:10.1016/j.molmet.2022.101498
Budu, O., Banciu, C., Pinzaru, I., Sarău, C., Lighezan, D., Șoica, C., et al. (2022). A combination of two probiotics, Lactobacillus sporogenes and Clostridium butyricum, inhibits colon cancer development: an in vitro study. Microorganisms 10, 1692. doi:10.3390/microorganisms10091692
Chan, A. T., Arber, N., Burn, J., Chia, W. K., Elwood, P., Hull, M. A., et al. (2012). Aspirin in the chemoprevention of colorectal neoplasia: an overview. Cancer Prev. Res. Phila. Pa) 5, 164–178. doi:10.1158/1940-6207.CAPR-11-0391
Chang, W. L., Jackson, C., Riel, S., Cooper, H. S., Devarajan, K., Hensley, H. H., et al. (2018). Differential preventive activity of sulindac and atorvastatin in Apc(+/Min-FCCC)mice with or without colorectal adenomas. Gut 67, 1290–1298. doi:10.1136/gutjnl-2017-313942
Chen, H., Zheng, X., Zong, X., Li, Z., Li, N., Hur, J., et al. (2021a). Metabolic syndrome, metabolic comorbid conditions and risk of early-onset colorectal cancer. Gut 70, 1147–1154. doi:10.1136/gutjnl-2020-321661
Chen, X., Guo, F., Hoffmeister, M., Chang-Claude, J., and Brenner, H. (2021b). Non-steroidal anti-inflammatory drugs, polygenic risk score and colorectal cancer risk. Aliment. Pharmacol. Ther. 54, 167–175. doi:10.1111/apt.16438
Cheung, K. S., Chan, E. W., Seto, W. K., Wong, I., and Leung, W. K. (2020a). ACE (Angiotensin-Converting enzyme) inhibitors/angiotensin receptor blockers are associated with lower colorectal cancer risk: A territory-wide study with propensity score analysis. Hypertension 76, 968–975. doi:10.1161/HYPERTENSIONAHA.120.15317
Cheung, K. S., Chen, L., Chan, E. W., Seto, W. K., Wong, I., and Leung, W. K. (2020b). Nonsteroidal anti-inflammatory drugs but not aspirin are associated with a lower risk of post-colonoscopy colorectal cancer. Aliment. Pharmacol. Ther. 51, 899–908. doi:10.1111/apt.15693
Cheung, K. S., Chen, L., Chan, E. W., Seto, W. K., Wong, I., and Leung, W. K. (2019). Statins reduce the progression of non-advanced adenomas to colorectal cancer: A postcolonoscopy study in 187 897 patients. Gut 68, 1979–1985. doi:10.1136/gutjnl-2018-317714
Childers, W. K. (2015). Interactions of the renin-angiotensin system in colorectal cancer and metastasis. Int. J. Colorectal Dis. 30, 749–752. doi:10.1007/s00384-014-2118-1
Chudy-Onwugaje, K., Huang, W. Y., Su, L. J., Purdue, M. P., Johnson, C. C., Wang, L., et al. (2021). Aspirin, ibuprofen, and reduced risk of advanced colorectal adenoma incidence and recurrence and colorectal cancer in the PLCO Cancer Screening Trial. Cancer 127, 3145–3155. doi:10.1002/cncr.33623
Cook, M., and Chauhan, A. (2020). Clinical application of oncolytic viruses: A systematic review. Int. J. Mol. Sci. 21, 7505. doi:10.3390/ijms21207505
de Gunzburg, J., Ghozlane, A., Ducher, A., Le Chatelier, E., Duval, X., Ruppé, E., et al. (2018). Protection of the human gut microbiome from antibiotics. J. Infect. Dis. 217, 628–636. doi:10.1093/infdis/jix604
Deng, F., Hu, J. J., Yang, X., Sun, Q. S., Lin, Z. B., Zhao, B. C., et al. (2021). Gut microbial metabolite pravastatin attenuates intestinal ischemia/reperfusion injury through promoting IL-13 release from type II innate lymphoid cells via IL-33/ST2 signaling. Front. Immunol. 12, 704836. doi:10.3389/fimmu.2021.704836
Do, M. H., Lee, E., Oh, M. J., Kim, Y., and Park, H. Y. (2018). High-glucose or -fructose diet cause changes of the gut microbiota and metabolic disorders in mice without body weight change. Nutrients 10, 761. doi:10.3390/nu10060761
Dong, X., Pan, P., Zheng, D. W., Bao, P., Zeng, X., and Zhang, X. Z. (2020). Bioinorganic hybrid bacteriophage for modulation of intestinal microbiota to remodel tumor-immune microenvironment against colorectal cancer. Sci. Adv. 6, eaba1590. doi:10.1126/sciadv.aba1590
Duttaroy, A. K. (2021). Role of gut microbiota and their metabolites on atherosclerosis, hypertension and human blood platelet function: A review. Nutrients 13, 144. doi:10.3390/nu13010144
Elvers, K. T., Wilson, V. J., Hammond, A., Duncan, L., Huntley, A. L., Hay, A. D., et al. (2020). Antibiotic-induced changes in the human gut microbiota for the most commonly prescribed antibiotics in primary care in the UK: A systematic review. BMJ Open 10, e035677. doi:10.1136/bmjopen-2019-035677
Emami, M. H., Salehi, M., Hassanzadeh Keshteli, A., Mansourian, M., Mohammadzadeh, S., and Maghool, F. (2022). Calcium and dairy products in the chemoprevention of colorectal adenomas: A systematic review and meta-analysis. Crit. Rev. Food Sci. Nutr. 62, 7168–7183. doi:10.1080/10408398.2021.1911927
Emilsson, L., Holme, Ø., Bretthauer, M., Cook, N. R., Buring, J. E., Løberg, M., et al. (2017). Systematic review with meta-analysis: the comparative effectiveness of aspirin vs. screening for colorectal cancer prevention. Aliment. Pharmacol. Ther. 45, 193–204. doi:10.1111/apt.13857
Engineer, D. R., Burney, B. O., Hayes, T. G., and Garcia, J. M. (2013). Exposure to ACEI/ARB and β-blockers is associated with improved survival and decreased tumor progression and hospitalizations in patients with advanced colon cancer. Transl. Oncol. 6, 539–545. doi:10.1593/tlo.13346
Fang, X., Hu, T., Yin, H., Yang, J., Tang, W., Hu, S., et al. (2017). Differences in telomerase activity and the effects of AZT in aneuploid and euploid cells in colon cancer. Int. J. Oncol. 51, 525–532. doi:10.3892/ijo.2017.4043
Farvid, M. S., Sidahmed, E., Spence, N. D., Mante Angua, K., Rosner, B. A., and Barnett, J. B. (2021). Consumption of red meat and processed meat and cancer incidence: A systematic review and meta-analysis of prospective studies. Eur. J. Epidemiol. 36, 937–951. doi:10.1007/s10654-021-00741-9
Ferrara, C. R., Bai, J., McNally, E. M., Putzel, G. G., Zhou, X. K., Wang, H., et al. (2022). Microbes contribute to chemopreventive efficacy, intestinal tumorigenesis, and the metabolome. Cancer Prev. Res. Phila. Pa) 15, 803–814. doi:10.1158/1940-6207.CAPR-22-0244
Frouws, M. A., Bastiaannet, E., Langley, R. E., Chia, W. K., van Herk-Sukel, M. P., Lemmens, V. E., et al. (2017). Effect of low-dose aspirin use on survival of patients with gastrointestinal malignancies; an observational study. Br. J. Cancer 116, 405–413. doi:10.1038/bjc.2016.425
Gabrielli, M. G., and Tomassoni, D. (2018). Starch-enriched diet modulates the glucidic profile in the rat colonic mucosa. Eur. J. Nutr. 57, 1109–1121. doi:10.1007/s00394-017-1393-3
Gao, S., Soares, F., Wang, S., Wong, C. C., Chen, H., Yang, Z., et al. (2021). CRISPR screens identify cholesterol biosynthesis as a therapeutic target on stemness and drug resistance of colon cancer. Oncogene 40, 6601–6613. doi:10.1038/s41388-021-01882-7
Gebauer, L., Nist, A., Mernberger, M., Stiewe, T., Moll, R., Stabla, K., et al. (2021). Superior overall survival in patients with colorectal cancer, regular aspirin use, and combined wild-type PIK3CA and KRAS-mutated tumors. Cancers (Basel) 13, 4959. doi:10.3390/cancers13194959
Ghanghas, P., Jain, S., Rana, C., and Sanyal, S. N. (2016). Chemopreventive action of non-steroidal anti-inflammatory drugs on the inflammatory pathways in colon cancer. Biomed. Pharmacother. 78, 239–247. doi:10.1016/j.biopha.2016.01.024
Goel, S., Ocean, A. J., Parakrama, R. Y., Ghalib, M. H., Chaudhary, I., Shah, U., et al. (2020). Elucidation of Pelareorep pharmacodynamics in A phase I trial in patients with KRAS-mutated colorectal cancer. Mol. Cancer Ther. 19, 1148–1156. doi:10.1158/1535-7163.MCT-19-1117
Goïta, A. A., and Guenot, D. (2022). Colorectal cancer: the contribution of CXCL12 and its receptors CXCR4 and CXCR7. Cancers (Basel) 14, 1810. doi:10.3390/cancers14071810
Gomes, J. M., Costa, J. A., and Alfenas, R. C. (2015). Could the beneficial effects of dietary calcium on obesity and diabetes control be mediated by changes in intestinal microbiota and integrity. Br. J. Nutr. 114, 1756–1765. doi:10.1017/S0007114515003608
Gonçalves, A. C., Richiardone, E., Jorge, J., Polónia, B., Xavier, C., Salaroglio, I. C., et al. (2021). Impact of cancer metabolism on therapy resistance - clinical implications. Drug resist. updat. 59, 100797. doi:10.1016/j.drup.2021.100797
Hagan, T., Cortese, M., Rouphael, N., Boudreau, C., Linde, C., Maddur, M. S., et al. (2019). Antibiotics-driven gut microbiome perturbation alters immunity to vaccines in humans. Cell. 178, 1313–1328. doi:10.1016/j.cell.2019.08.010
Han, J. X., Tao, Z. H., Wang, J. L., Zhang, L., Yu, C. Y., Kang, Z. R., et al. (2023). Microbiota-derived tryptophan catabolites mediate the chemopreventive effects of statins on colorectal cancer. Nat. Microbiol. 8, 919–933. doi:10.1038/s41564-023-01363-5
Hannigan, G. D., Duhaime, M. B., Ruffin, M. T. 4th, Koumpouras, C. C., and Schloss, P. D. (2018). Diagnostic potential and interactive dynamics of the colorectal cancer virome. mBio 9, e02248–e02218. doi:10.1128/mBio.02248-18
Hao, W. Z., Li, X. J., Zhang, P. W., and Chen, J. X. (2020). A review of antibiotics, depression, and the gut microbiome. Psychiatry Res. 284, 112691. doi:10.1016/j.psychres.2019.112691
Higurashi, T., Hosono, K., Takahashi, H., Komiya, Y., Umezawa, S., Sakai, E., et al. (2016). Metformin for chemoprevention of metachronous colorectal adenoma or polyps in post-polypectomy patients without diabetes: A multicentre double-blind, placebo-controlled, randomised phase 3 trial. Lancet Oncol. 17, 475–483. doi:10.1016/S1470-2045(15)00565-3
Hill, E. J., Roberts, C., Franklin, J. M., Enescu, M., West, N., MacGregor, T. P., et al. (2016). Clinical trial of oral nelfinavir before and during radiation therapy for advanced rectal cancer. Clin. Cancer Res. 22, 1922–1931. doi:10.1158/1078-0432.CCR-15-1489
Hu, X., Li, H., Zhao, X., Zhou, R., Liu, H., Sun, Y., et al. (2021a). Multi-omics study reveals that statin therapy is associated with restoration of gut microbiota homeostasis and improvement in outcomes in patients with acute coronary syndrome. Theranostics 11, 5778–5793. doi:10.7150/thno.55946
Hu, Y., Zhang, X., Ma, Y., Yuan, C., Wang, M., Wu, K., et al. (2021b). Incident type 2 diabetes duration and cancer risk: A prospective study in two us cohorts. J. Natl. Cancer Inst. 113, 381–389. doi:10.1093/jnci/djaa141
Hua, P., Xiong, Y., Yu, Z., Liu, B., and Zhao, L. (2019). Effect of chlorella pyrenoidosa protein hydrolysate-calcium chelate on calcium absorption metabolism and gut microbiota composition in low-calcium diet-fed rats. Mar. Drugs 17, 348. doi:10.3390/md17060348
Huang, D., Lei, S., Wu, Y., Weng, M., Zhou, Y., Xu, J., et al. (2020a). Additively protective effects of vitamin D and calcium against colorectal adenoma incidence, malignant transformation and progression: A systematic review and meta-analysis. Clin. Nutr. Edinb. Scotl. 39, 2525–2538. doi:10.1016/j.clnu.2019.11.012
Huang, H., Wang, X., Zhang, X., Zhang, G., Jinbo, M., Wang, H., et al. (2020b). Ganciclovir reduces irinotecan-induced intestinal toxicity by inhibiting NLRP3 activation. Cancer Chemother. Pharmacol. 85, 195–204. doi:10.1007/s00280-019-03996-y
Huang, L., Zheng, J., Sun, G., Yang, H., Sun, X., Yao, X., et al. (2022). 5-Aminosalicylic acid ameliorates dextran sulfate sodium-induced colitis in mice by modulating gut microbiota and bile acid metabolism. Cell. Mol. Life Sci. 79, 460. doi:10.1007/s00018-022-04471-3
Huang, Q. Y., Yao, F., Zhou, C. R., Huang, X. Y., Wang, Q., Long, H., et al. (2020c). Role of gut microbiome in regulating the effectiveness of metformin in reducing colorectal cancer in type 2 diabetes. World J. Clin. Cases 8, 6213–6228. doi:10.12998/wjcc.v8.i24.6213
Huang, X., Hong, X., Wang, J., Sun, T., Yu, T., Yu, Y., et al. (2020d). Metformin elicits antitumour effect by modulation of the gut microbiota and rescues Fusobacterium nucleatum-induced colorectal tumourigenesis. EBioMedicine 61, 103037. doi:10.1016/j.ebiom.2020.103037
Islam, Z., Akter, S., Kashino, I., Mizoue, T., Sawada, N., Mori, N., et al. (2019). Meat subtypes and colorectal cancer risk: A pooled analysis of 6 cohort studies in Japan. Cancer Sci. 110, 3603–3614. doi:10.1111/cas.14188
Jackson, M. A., Verdi, S., Maxan, M. E., Shin, C. M., Zierer, J., Bowyer, R., et al. (2018). Gut microbiota associations with common diseases and prescription medications in a population-based cohort. Nat. Commun. 9, 2655. doi:10.1038/s41467-018-05184-7
Jendrossek, V. (2013). Targeting apoptosis pathways by Celecoxib in cancer. Cancer Lett. 332, 313–324. doi:10.1016/j.canlet.2011.01.012
Joh, H. K., Lee, D. H., Hur, J., Nimptsch, K., Chang, Y., Joung, H., et al. (2021). Simple sugar and sugar-sweetened beverage intake during adolescence and risk of colorectal cancer precursors. Gastroenterology 161, 128–142.e20. doi:10.1053/j.gastro.2021.03.028
Kabwe, M., Brown, T. L., Dashper, S., Speirs, L., Ku, H., Petrovski, S., et al. (2019). Genomic, morphological and functional characterisation of novel bacteriophage FNU1 capable of disrupting Fusobacterium nucleatum biofilms. Sci. Rep. 9, 9107. doi:10.1038/s41598-019-45549-6
Kabwe, M., Meehan-Andrews, T., Ku, H., Petrovski, S., Batinovic, S., Chan, H. T., et al. (2021). Lytic bacteriophage EFA1 modulates HCT116 colon cancer cell growth and upregulates ROS production in an Enterococcus faecalis Co-culture system. Front. Microbiol. 12, 650849. doi:10.3389/fmicb.2021.650849
Kane, A. M., Liu, C., Fennell, L. J., McKeone, D. M., Bond, C. E., Pollock, P. M., et al. (2021). Aspirin reduces the incidence of metastasis in a pre-clinical study of Braf mutant serrated colorectal neoplasia. Br. J. Cancer 124, 1820–1827. doi:10.1038/s41416-021-01339-4
Kaneko, H., Yano, Y., Itoh, H., Morita, K., Kiriyama, H., Kamon, T., et al. (2021). Untreated hypertension and subsequent incidence of colorectal cancer: analysis of a nationwide epidemiological database. J. Am. Heart Assoc. 10, e022479. doi:10.1161/JAHA.121.022479
Karagkounis, G., DeVecchio, J., Ferrandon, S., and Kalady, M. F. (2018). Simvastatin enhances radiation sensitivity of colorectal cancer cells. Surg. Endosc. 32, 1533–1539. doi:10.1007/s00464-017-5841-1
Karbach, S. H., Schönfelder, T., Brandão, I., Wilms, E., Hörmann, N., Jäckel, S., et al. (2016). Gut microbiota promote angiotensin II-induced arterial hypertension and vascular dysfunction. J. Am. Heart Assoc. 5, e003698. doi:10.1161/JAHA.116.003698
Karpiński, T. M., Ożarowski, M., and Stasiewicz, M. (2022). Carcinogenic microbiota and its role in colorectal cancer development. Semin. Cancer Biol. 86, 420–430. doi:10.1016/j.semcancer.2022.01.004
Katsi, V., Didagelos, M., Skevofilax, S., Armenis, I., Kartalis, A., Vlachopoulos, C., et al. (2019). GUT microbiome-GUT dysbiosis-arterial hypertension: new horizons. Curr. Hypertens. Rev. 15, 40–46. doi:10.2174/1573402114666180613080439
Kedika, R., Patel, M., Pena Sahdala, H. N., Mahgoub, A., Cipher, D., and Siddiqui, A. A. (2011). Long-term use of angiotensin converting enzyme inhibitors is associated with decreased incidence of advanced adenomatous colon polyps. J. Clin. Gastroenterol. 45, e12–e16. doi:10.1097/MCG.0b013e3181ea1044
Khan, J., Pernicova, I., Nisar, K., and Korbonits, M. (2023). Mechanisms of ageing: growth hormone, dietary restriction, and metformin. Lancet Diabetes Endocrinol. 11, 261–281. doi:10.1016/S2213-8587(23)00001-3
Kim, J., Lee, H., An, J., Song, Y., Lee, C. K., Kim, K., et al. (2019). Alterations in gut microbiota by statin therapy and possible intermediate effects on hyperglycemia and hyperlipidemia. Front. Microbiol. 10, 1947. doi:10.3389/fmicb.2019.01947
Larsson, S. C., Bergkvist, L., and Wolk, A. (2005). Magnesium intake in relation to risk of colorectal cancer in women. JAMA 293, 86–89. doi:10.1001/jama.293.1.86
Lasry, A., Zinger, A., and Ben-Neriah, Y. (2016). Inflammatory networks underlying colorectal cancer. Nat. Immunol. 17, 230–240. doi:10.1038/ni.3384
Lee, S. H., Lee, H. A., Lee, S. S., Kim, S. E., Shim, K. N., Jung, H. K., et al. (2020). Clinical impact of pre-hypertension on the risk of cancer in male and female subjects. Sci. Rep. 10, 9974. doi:10.1038/s41598-020-66653-y
Li, L., Zhong, S. J., Hu, S. Y., Cheng, B., Qiu, H., and Hu, Z. X. (2021). Changes of gut microbiome composition and metabolites associated with hypertensive heart failure rats. BMC Microbiol. 21, 141. doi:10.1186/s12866-021-02202-5
Li, S., Miller-Wilson, L. A., Guo, H., Hoover, M., and Fisher, D. A. (2022). Incident colorectal cancer screening and associated healthcare resource utilization and Medicare cost among Medicare beneficiaries aged 66-75 years in 2016-2018. BMC Health Serv. Res. 22, 1228. doi:10.1186/s12913-022-08617-8
Lin, D. M., and Lin, H. C. (2019). A theoretical model of temperate phages as mediators of gut microbiome dysbiosis. F1000Research 8, F1000. doi:10.12688/f1000research.18480.1
Lin, Y. M., Lu, C. C., Hsiang, Y. P., Pi, S. C., Chen, C. I., Cheng, K. C., et al. (2019). c-Met inhibition is required for the celecoxib-attenuated stemness property of human colorectal cancer cells. J. Cell. Physiol. 234, 10336–10344. doi:10.1002/jcp.27701
Liu, T., Song, X., Khan, S., Li, Y., Guo, Z., Li, C., et al. (2020). The gut microbiota at the intersection of bile acids and intestinal carcinogenesis: an old story, yet mesmerizing. Int. J. Cancer 146, 1780–1790. doi:10.1002/ijc.32563
Lynch, P. M., Ayers, G. D., Hawk, E., Richmond, E., Eagle, C., Woloj, M., et al. (2010). The safety and efficacy of celecoxib in children with familial adenomatous polyposis. Am. J. Gastroenterol. 105, 1437–1443. doi:10.1038/ajg.2009.758
Makar, G. A., Holmes, J. H., and Yang, Y. X. (2014). Angiotensin-converting enzyme inhibitor therapy and colorectal cancer risk. J. Natl. Cancer Inst. 106, djt374. doi:10.1093/jnci/djt374
Mallik, R., and Chowdhury, T. A. (2018). Metformin in cancer. Diabetes Res. Clin. Pract. 143, 409–419. doi:10.1016/j.diabres.2018.05.023
Maniewska, J., and Jeżewska, D. (2021). Non-Steroidal anti-inflammatory drugs in colorectal cancer chemoprevention. Cancers (Basel) 13, 594. doi:10.3390/cancers13040594
Martin-Gallausiaux, C., Marinelli, L., Blottière, H. M., Larraufie, P., and Lapaque, N. (2021). Scfa: mechanisms and functional importance in the gut. Proc. Nutr. Soc. 80, 37–49. doi:10.1017/S0029665120006916
Matsushita, M., Fujita, K., Hayashi, T., Kayama, H., Motooka, D., Hase, H., et al. (2021). Gut microbiota-derived short-chain fatty acids promote prostate cancer growth via IGF1 signaling. Cancer Res. 81, 4014–4026. doi:10.1158/0008-5472.CAN-20-4090
Mehta, R. S., Mayers, J. R., Zhang, Y., Bhosle, A., Glasser, N. R., Nguyen, L. H., et al. (2023). Gut microbial metabolism of 5-ASA diminishes its clinical efficacy in inflammatory bowel disease. Nat. Med. 29, 700–709. doi:10.1038/s41591-023-02217-7
Mihai, M. M., Ion, A., Giurcăneanu, C., Nițipir, C., Popa, A. M., Chifiriuc, M. C., et al. (2021). The impact of long-term antibiotic therapy of cutaneous adverse reactions to EGFR inhibitors in colorectal cancer patients. J. Clin. Med. 10, 3219. doi:10.3390/jcm10153219
Murgas, P., Bustamante, N., Araya, N., Cruz-Gómez, S., Durán, E., Gaete, D., et al. (2018). A filamentous bacteriophage targeted to carcinoembryonic antigen induces tumor regression in mouse models of colorectal cancer. Cancer Immunol. Immunother. 67, 183–193. doi:10.1007/s00262-017-2076-x
Murphy, N., Carreras-Torres, R., Song, M., Chan, A. T., Martin, R. M., Papadimitriou, N., et al. (2020). Circulating levels of insulin-like growth factor 1 and insulin-like growth factor binding protein 3 associate with risk of colorectal cancer based on serologic and mendelian randomization analyses. Gastroenterology 158, 1300–1312. doi:10.1053/j.gastro.2019.12.020
Murphy, N., Song, M., Papadimitriou, N., Carreras-Torres, R., Langenberg, C., Martin, R. M., et al. (2022). Associations between glycemic traits and colorectal cancer: A mendelian randomization analysis. J. Natl. Cancer Inst. 114, 740–752. doi:10.1093/jnci/djac011
Nakamura, K., Yaguchi, T., Ohmura, G., Kobayashi, A., Kawamura, N., Iwata, T., et al. (2018). Involvement of local renin-angiotensin system in immunosuppression of tumor microenvironment. Cancer Sci. 109, 54–64. doi:10.1111/cas.13423
Nakatsu, G., Zhou, H., Wu, W., Wong, S. H., Coker, O. O., Dai, Z., et al. (2018). Alterations in enteric virome are associated with colorectal cancer and survival outcomes. Gastroenterology 155, 529–541. doi:10.1053/j.gastro.2018.04.018
Nijiati, Y., Maimaitiyiming, D., Yang, T., Li, H., and Aikemu, A. (2021). Research on the improvement of oxidative stress in rats with high-altitude pulmonary hypertension through the participation of irbesartan in regulating intestinal flora. Eur. Rev. Med. Pharmacol. Sci. 25, 4540–4553. doi:10.26355/eurrev_202107_26247
Nishiguchi, R., Basu, S., Staab, H. A., Ito, N., Zhou, X. K., Wang, H., et al. (2021). Dietary interventions to prevent high-fructose diet-associated worsening of colitis and colitis-associated tumorigenesis in mice. Carcinogenesis 42, 842–852. doi:10.1093/carcin/bgab007
Nuevo-Tapioles, C., Santacatterina, F., Stamatakis, K., Núñez de Arenas, C., Gómez de Cedrón, M., Formentini, L., et al. (2020). Coordinate β-adrenergic inhibition of mitochondrial activity and angiogenesis arrest tumor growth. Nat. Commun. 11, 3606. doi:10.1038/s41467-020-17384-1
Ocvirk, S., and O'Keefe, S. J. (2017). Influence of bile acids on colorectal cancer risk: potential mechanisms mediated by diet - gut microbiota interactions. Curr. Nutr. Rep. 6, 315–322. doi:10.1007/s13668-017-0219-5
Pachikian, B. D., Neyrinck, A. M., Deldicque, L., De Backer, F. C., Catry, E., Dewulf, E. M., et al. (2010). Changes in intestinal bifidobacteria levels are associated with the inflammatory response in magnesium-deficient mice. J. Nutr. 140, 509–514. doi:10.3945/jn.109.117374
Palko-Łabuz, A., Środa-Pomianek, K., Wesołowska, O., Kostrzewa-Susłow, E., Uryga, A., and Michalak, K. (2019). MDR reversal and pro-apoptotic effects of statins and statins combined with flavonoids in colon cancer cells. Biomed. Pharmacother. 109, 1511–1522. doi:10.1016/j.biopha.2018.10.169
Pan, B., Chen, Z., Zhang, X., Wang, Z., Yao, Y., Wu, X., et al. (2023). 2,5-dimethylcelecoxib alleviated NK and T-cell exhaustion in hepatocellular carcinoma via the gastrointestinal microbiota-AMPK-mTOR axis. J. Immunother. Cancer 11, e006817. doi:10.1136/jitc-2023-006817
Peeters, P. J., Bazelier, M. T., Leufkens, H. G., de Vries, F., and De Bruin, M. L. (2015). The risk of colorectal cancer in patients with type 2 diabetes: associations with treatment stage and obesity. Diabetes Care 38, 495–502. doi:10.2337/dc14-1175
Peters, K. M., Carlson, B. A., Gladyshev, V. N., and Tsuji, P. A. (2018). Selenoproteins in colon cancer. Free Radic. Biol. Med. 127, 14–25. doi:10.1016/j.freeradbiomed.2018.05.075
Peters, U., and Takata, Y. (2008). Selenium and the prevention of prostate and colorectal cancer. Mol. Nutr. Food Res. 52, 1261–1272. doi:10.1002/mnfr.200800103
Phipps, O., Al-Hassi, H. O., Quraishi, M. N., Dickson, E. A., Segal, J., Steed, H., et al. (2021). Oral and intravenous iron therapy differentially alter the on- and off-tumor microbiota in anemic colorectal cancer patients. Cancers (Basel) 13, 1341. doi:10.3390/cancers13061341
Pourlotfi, A., Ahl, R., Sjolin, G., Forssten, M. P., Bass, G. A., Cao, Y., et al. (2021a). Statin therapy and postoperative short-term mortality after rectal cancer surgery. Colorectal Dis. 23, 875–881. doi:10.1111/codi.15481
Pourlotfi, A., Bass, G. A., Ahl Hulme, R., Forssten, M. P., Sjolin, G., Cao, Y., et al. (2021b). Statin use and long-term mortality after rectal cancer surgery. Cancers (Basel) 13, 4288. doi:10.3390/cancers13174288
Prizment, A. E., Staley, C., Onyeaghala, G. C., Vivek, S., Thyagarajan, B., Straka, R. J., et al. (2020). Randomised clinical study: oral aspirin 325 mg daily vs placebo alters gut microbial composition and bacterial taxa associated with colorectal cancer risk. Aliment. Pharmacol. Ther. 52, 976–987. doi:10.1111/apt.16013
Reyman, M., van Houten, M. A., Watson, R. L., Chu, M., Arp, K., de Waal, W. J., et al. (2022). Effects of early-life antibiotics on the developing infant gut microbiome and resistome: A randomized trial. Nat. Commun. 13, 893. doi:10.1038/s41467-022-28525-z
Ribas, A., Dummer, R., Puzanov, I., VanderWalde, A., Andtbacka, R., Michielin, O., et al. (2017). Oncolytic virotherapy promotes intratumoral T cell infiltration and improves anti-PD-1 immunotherapy. Cell. 170, 1109–1119. doi:10.1016/j.cell.2017.08.027
Rodríguez-Miguel, A., Fernández-Antón, E., Barreira-Hernández, D., García-Rodríguez, L. A., Gil, M., García-Lledó, A., et al. (2022). Statins and colorectal cancer risk: A population-based case-control study and synthesis of the epidemiological evidence. J. Clin. Med. 11, 1528. doi:10.3390/jcm11061528
Rogers, M., and Aronoff, D. M. (2016). The influence of non-steroidal anti-inflammatory drugs on the gut microbiome. Clin. Microbiol. Infect. 22, 178.e1–178. doi:10.1016/j.cmi.2015.10.003
Sang, J., Tang, R., Yang, M., and Sun, Q. (2020). Metformin inhibited proliferation and metastasis of colorectal cancer and presented a synergistic effect on 5-FU. Biomed. Res. Int. 2020, 9312149. doi:10.1155/2020/9312149
Sankaranarayanan, R., Kumar, D. R., Altinoz, M. A., and Bhat, G. J. (2020). Mechanisms of colorectal cancer prevention by aspirin-A literature review and perspective on the role of COX-dependent and -independent pathways. Int. J. Mol. Sci. 21, 9018. doi:10.3390/ijms21239018
Santisteban, M. M., Qi, Y., Zubcevic, J., Kim, S., Yang, T., Shenoy, V., et al. (2017). Hypertension-linked pathophysiological alterations in the gut. Circ. Res. 120, 312–323. doi:10.1161/CIRCRESAHA.116.309006
Schneider, M. A., Buzdin, A. A., Weber, A., Clavien, P. A., and Borger, P. (2021). Combination of antiretroviral drugs zidovudine and efavirenz impairs tumor growths in a mouse model of cancer. Viruses 13, 2396. doi:10.3390/v13122396
Seiwert, N., Heylmann, D., Hasselwander, S., and Fahrer, J. (2020). Mechanism of colorectal carcinogenesis triggered by heme iron from red meat. Biochim. Biophys. Acta Rev. Cancer 1873, 188334. doi:10.1016/j.bbcan.2019.188334
Sepich-Poore, G. D., Zitvogel, L., Straussman, R., Hasty, J., Wargo, J. A., and Knight, R. (2021). The microbiome and human cancer. Science 371, eabc4552. doi:10.1126/science.abc4552
Seretis, A., Cividini, S., Markozannes, G., Tseretopoulou, X., Lopez, D. S., Ntzani, E. E., et al. (2019). Association between blood pressure and risk of cancer development: A systematic review and meta-analysis of observational studies. Sci. Rep. 9, 8565. doi:10.1038/s41598-019-45014-4
Shailes, H., Tse, W. Y., Freitas, M. O., Silver, A., and Martin, S. A. (2022). Statin treatment as a targeted therapy for APC-mutated colorectal cancer. Front. Oncol. 12, 880552. doi:10.3389/fonc.2022.880552
Sherif, D. A., Makled, M. N., and Suddek, G. M. (2021). The HIV reverse transcriptase Inhibitor Tenofovir suppressed DMH/HFD-induced colorectal cancer in Wistar rats. Fundam. Clin. Pharmacol. 35, 940–954. doi:10.1111/fcp.12679
Siegel, R. L., Miller, K. D., Wagle, N. S., and Jemal, A. (2023). Cancer statistics, 2023. CA Cancer J. Clin. 73, 17–48. doi:10.3322/caac.21763
Silveira-Nunes, G., Durso, D. F., Cunha, E., Maioli, T. U., and Vieira, A. T. (2020). Hypertension is associated with intestinal microbiota dysbiosis and inflammation in a Brazilian population. Front. Pharmacol. 11, 258. doi:10.3389/fphar.2020.00258
Song, M., Nguyen, L. H., Emilsson, L., Chan, A. T., and Ludvigsson, J. F. (2021). Antibiotic use associated with risk of colorectal polyps in a nationwide study. Clin. Gastroenterol. Hepatol. 19, 1426–1435.e6. doi:10.1016/j.cgh.2020.05.036
Takahashi, K., Suzuki, N., and Ogra, Y. (2017). Bioavailability comparison of nine bioselenocompounds in vitro and in vivo. Int. J. Mol. Sci. 18, 506. doi:10.3390/ijms18030506
Tang-Fichaux, M., Branchu, P., Nougayrède, J. P., and Oswald, E. (2021). Tackling the threat of cancer due to pathobionts producing colibactin: is mesalamine the magic bullet. Toxins (Basel) 13, 897. doi:10.3390/toxins13120897
Thompson, P. A., Ashbeck, E. L., Roe, D. J., Fales, L., Buckmeier, J., Wang, F., et al. (2016). Celecoxib for the prevention of colorectal adenomas: results of a suspended randomized controlled trial. J. Natl. Cancer Inst. 108, djw151. doi:10.1093/jnci/djw151
Tsubaki, M., Takeda, T., Matsuda, T., Kishimoto, K., Takefuji, H., Taniwaki, Y., et al. (2023). Statins enhances antitumor effect of oxaliplatin in KRAS-mutated colorectal cancer cells and inhibits oxaliplatin-induced neuropathy. Cancer Cell. Int. 23, 73. doi:10.1186/s12935-023-02884-z
Turkington, C., Varadan, A. C., Grenier, S. F., and Grasis, J. A. (2020). The viral janus: viruses as aetiological agents and treatment options in colorectal cancer. Front. Cell. Infect. Microbiol. 10, 601573. doi:10.3389/fcimb.2020.601573
Vallianou, N. G., Stratigou, T., and Tsagarakis, S. (2019). Metformin and gut microbiota: their interactions and their impact on diabetes. Horm. (Athens, Greece) 18, 141–144. doi:10.1007/s42000-019-00093-w
Vieira-Silva, S., Falony, G., Belda, E., Nielsen, T., Aron-Wisnewsky, J., Chakaroun, R., et al. (2020). Statin therapy is associated with lower prevalence of gut microbiota dysbiosis. Nature 581, 310–315. doi:10.1038/s41586-020-2269-x
Wada, H., Miyoshi, J., Kuronuma, S., Nishinarita, Y., Oguri, N., Hibi, N., et al. (2023). 5-Aminosalicylic acid alters the gut microbiota and altered microbiota transmitted vertically to offspring have protective effects against colitis. Sci. Rep. 13, 12241. doi:10.1038/s41598-023-39491-x
Wang, L., Zhou, W., Guo, M., Hua, Y., Zhou, B., Li, X., et al. (2021a). The gut microbiota is associated with clinical response to statin treatment in patients with coronary artery disease. Atherosclerosis 325, 16–23. doi:10.1016/j.atherosclerosis.2021.03.007
Wang, S., Dong, W., Liu, L., Xu, M., Wang, Y., Liu, T., et al. (2019). Interplay between bile acids and the gut microbiota promotes intestinal carcinogenesis. Mol. Carcinog. 58, 1155–1167. doi:10.1002/mc.22999
Wang, X., Chan, A. T., Slattery, M. L., Chang-Claude, J., Potter, J. D., Gallinger, S., et al. (2018). Influence of smoking, body mass index, and other factors on the preventive effect of nonsteroidal anti-inflammatory drugs on colorectal cancer risk. Cancer Res. 78, 4790–4799. doi:10.1158/0008-5472.CAN-18-0326
Wang, Y., Wang, H., Howard, A. G., Tsilimigras, M., Avery, C. L., Meyer, K. A., et al. (2021b). Gut microbiota and host plasma metabolites in association with blood pressure in Chinese adults. Hypertension 77, 706–717. doi:10.1161/HYPERTENSIONAHA.120.16154
Willmann, M., Vehreschild, M., Biehl, L. M., Vogel, W., Dörfel, D., Hamprecht, A., et al. (2019). Distinct impact of antibiotics on the gut microbiome and resistome: A longitudinal multicenter cohort study. BMC Biol. 17, 76. doi:10.1186/s12915-019-0692-y
Winston, J. A., Rivera, A., Cai, J., Patterson, A. D., and Theriot, C. M. (2021). Secondary bile acid ursodeoxycholic acid alters weight, the gut microbiota, and the bile acid pool in conventional mice. PLoS ONE 16, e0246161. doi:10.1371/journal.pone.0246161
Winston, J. A., Rivera, A. J., Cai, J., Thanissery, R., Montgomery, S. A., Patterson, A. D., et al. (2020). Ursodeoxycholic acid (UDCA) mitigates the host inflammatory response during clostridioides difficile infection by altering gut bile acids. Infect. Immun. 88, e00045–e00020. doi:10.1128/IAI.00045-20
Wu, W. K., Ivanova, E. A., and Orekhov, A. N. (2021). Gut microbiome: A possible common therapeutic target for treatment of atherosclerosis and cancer. Semin. Cancer Biol. 70, 85–97. doi:10.1016/j.semcancer.2020.06.017
Wu, X., Wu, Y., He, L., Wu, L., Wang, X., and Liu, Z. (2018). Effects of the intestinal microbial metabolite butyrate on the development of colorectal cancer. J. Cancer 9, 2510–2517. doi:10.7150/jca.25324
Xia, C., Su, J., Liu, C., Mai, Z., Yin, S., Yang, C., et al. (2023a). Human microbiomes in cancer development and therapy. MedComm 4, e221. doi:10.1002/mco2.221
Xia, C., Yin, S., To, K., and Fu, L. (2023b). CD39/CD73/A2AR pathway and cancer immunotherapy. Mol. Cancer 22, 44. doi:10.1186/s12943-023-01733-x
Xie, D., Shen, Y., Su, E., Du, L., Xie, J., and Wei, D. (2022). The effects of angiotensin I-converting enzyme inhibitory peptide VGINYW and the hydrolysate of α-lactalbumin on blood pressure, oxidative stress and gut microbiota of spontaneously hypertensive rats. Food Funct. 13, 2743–2755. doi:10.1039/d1fo03570c
Yan, J., and Charles, J. F. (2018). Gut microbiota and IGF-1. Calcif. Tissue Int. 102, 406–414. doi:10.1007/s00223-018-0395-3
Yan, Q., Gu, Y., Li, X., Yang, W., Jia, L., Chen, C., et al. (2017). Alterations of the gut microbiome in hypertension. Front. Cell. Infect. Microbiol. 7, 381. doi:10.3389/fcimb.2017.00381
Yang, T., Aquino, V., Lobaton, G. O., Li, H., Colon-Perez, L., Goel, R., et al. (2019a). Sustained captopril-induced reduction in blood pressure is associated with alterations in gut-brain Axis in the spontaneously hypertensive rat. J. Am. Heart Assoc. 8, e010721. doi:10.1161/JAHA.118.010721
Yang, W., Ma, Y., Smith-Warner, S., Song, M., Wu, K., Wang, M., et al. (2019b). Calcium intake and survival after colorectal cancer diagnosis. Clin. Cancer Res. 25, 1980–1988. doi:10.1158/1078-0432.CCR-18-2965
Yang, Z., Tang, H., Lu, S., Sun, X., and Rao, B. (2022). Relationship between serum lipid level and colorectal cancer: A systemic review and meta-analysis. BMJ Open 12, e052373. doi:10.1136/bmjopen-2021-052373
Ye, F., Dewanjee, S., Li, Y., Jha, N. K., Chen, Z. S., Kumar, A., et al. (2023). Advancements in clinical aspects of targeted therapy and immunotherapy in breast cancer. Mol. Cancer 22, 105. doi:10.1186/s12943-023-01805-y
Youssif, C., Cubillos-Rojas, M., Comalada, M., Llonch, E., Perna, C., Djouder, N., et al. (2018). Myeloid p38α signaling promotes intestinal IGF-1 production and inflammation-associated tumorigenesis. EMBO Mol. Med. 10, e8403. doi:10.15252/emmm.201708403
Yu, C., Li, W. B., Liu, J. B., Lu, J. W., and Feng, J. F. (2018). Autophagy: novel applications of nonsteroidal anti-inflammatory drugs for primary cancer. Cancer Med. 7, 471–484. doi:10.1002/cam4.1287
Yu, G. H., Li, S. F., Wei, R., and Jiang, Z. (2022). Diabetes and colorectal cancer risk: clinical and therapeutic implications. J. Diabetes Res. 2022, 1747326. doi:10.1155/2022/1747326
Yu, J., Yang, K., Zheng, J., Zhao, W., and Sun, X. (2021). Synergistic tumor inhibition of colon cancer cells by nitazoxanide and obeticholic acid, a farnesoid X receptor ligand. Cancer Gene Ther. 28, 590–601. doi:10.1038/s41417-020-00239-8
Yuan, T., Wu, R., Wang, W., Liu, Y., Kong, W., Yang, B., et al. (2023). Synergistic antitumor activity of regorafenib and rosuvastatin in colorectal cancer. Front. Pharmacol. 14, 1136114. doi:10.3389/fphar.2023.1136114
Zeng, H., Umar, S., Rust, B., Lazarova, D., and Bordonaro, M. (2019). Secondary bile acids and short chain fatty acids in the colon: A focus on colonic microbiome, cell proliferation, inflammation, and cancer. Int. J. Mol. Sci. 20, 1214. doi:10.3390/ijms20051214
Zhang, K. L., Zhu, W. W., Wang, S. H., Gao, C., Pan, J. J., Du, Z. G., et al. (2021a). Organ-specific cholesterol metabolic aberration fuels liver metastasis of colorectal cancer. Theranostics 11, 6560–6572. doi:10.7150/thno.55609
Zhang, W. Q., Wang, Y. J., Zhang, A., Ding, Y. J., Zhang, X. N., Jia, Q. J., et al. (2021b). TMA/TMAO in hypertension: novel horizons and potential therapies. J. Cardiovasc Transl. Res. 14, 1117–1124. doi:10.1007/s12265-021-10115-x
Zhang, X., Han, Y., Huang, W., Jin, M., and Gao, Z. (2021c). The influence of the gut microbiota on the bioavailability of oral drugs. Acta Pharm. Sin. B 11, 1789–1812. doi:10.1016/j.apsb.2020.09.013
Zhang, Y., Chan, A. T., Meyerhardt, J. A., and Giovannucci, E. L. (2021d). Timing of aspirin use in colorectal cancer chemoprevention: A prospective cohort study. J. Natl. Cancer Inst. 113, 841–851. doi:10.1093/jnci/djab009
Zhao, R., Coker, O. O., Wu, J., Zhou, Y., Zhao, L., Nakatsu, G., et al. (2020). Aspirin reduces colorectal tumor development in mice and gut microbes reduce its bioavailability and chemopreventive effects. Gastroenterology 159, 969–983. doi:10.1053/j.gastro.2020.05.004
Zheng, D. W., Dong, X., Pan, P., Chen, K. W., Fan, J. X., Cheng, S. X., et al. (2019). Phage-guided modulation of the gut microbiota of mouse models of colorectal cancer augments their responses to chemotherapy. Nat. Biomed. Eng. 3, 717–728. doi:10.1038/s41551-019-0423-2
Zhernakova, A., Kurilshikov, A., Bonder, M. J., Tigchelaar, E. F., Schirmer, M., Vatanen, T., et al. (2016). Population-based metagenomics analysis reveals markers for gut microbiome composition and diversity. Science 352, 565–569. doi:10.1126/science.aad3369
Keywords: gut microbiota, drugs, interactions, colorectal cancer, mechanism of action
Citation: Wu J, Xia C, Liu C, Zhang Q and Xia C (2023) The role of gut microbiota and drug interactions in the development of colorectal cancer. Front. Pharmacol. 14:1265136. doi: 10.3389/fphar.2023.1265136
Received: 22 July 2023; Accepted: 14 August 2023;
Published: 23 August 2023.
Edited by:
Guoxin Huang, Macau University of Science and Technology, Macao SAR, ChinaReviewed by:
Qing Li, The Chinese University of Hong Kong, ChinaHailin Tang, Sun Yat-sen University Cancer Center (SYSUCC), China
Lipeng Xu, Jinan University, China
Copyright © 2023 Wu, Xia, Liu, Zhang and Xia. This is an open-access article distributed under the terms of the Creative Commons Attribution License (CC BY). The use, distribution or reproduction in other forums is permitted, provided the original author(s) and the copyright owner(s) are credited and that the original publication in this journal is cited, in accordance with accepted academic practice. No use, distribution or reproduction is permitted which does not comply with these terms.
*Correspondence: Qianshi Zhang, emhhbmdxaWFuc2hpMTk4N0BxcS5jb20=; Chenglai Xia, eGlhY2hlbmdsYWlAc211LmVkdS5jbg==
†These authors have contributed equally to this work