- 1Center of Liver Diseases Division 3, Beijing Ditan Hospital, Capital Medical University, Beijing, China
- 2Division of Gastroenterology and Hepatology, BaoJi Central Hospital, Shaanxi, China
- 3Division of Gastroenterology and Hepatology, NYU Langone Health, New York University School of Medicine, New York, NY, United States
- 4Center of Liver Diseases, Peking University Ditan Teaching Hospital, Beijing, China
Alcohol-related liver disease (ALD) from excessive alcohol intake has a unique gut microbiota profile. The disease progression-free survival in ALD patients has been associated with the degree of gut dysbiosis. The vicious cycles between gut dysbiosis and the disease progression in ALD including: an increase of acetaldehyde production and bile acid secretion, impaired gut barrier, enrichment of circulating microbiota, toxicities of microbiota metabolites, a cascade of pro-inflammatory chemokines or cytokines, and augmentation in the generation of reactive oxygen species. The aforementioned pathophysiology process plays an important role in different disease stages with a spectrum of alcohol hepatitis, ALD cirrhosis, neurological dysfunction, and hepatocellular carcinoma. This review aims to illustrate the pathophysiology of gut microbiota and clarify the gut-brain crosstalk in ALD, which may provide the opportunity of identifying target points for future therapeutic intervention in ALD.
1 Introduction
Alcohol-related liver disease (ALD) has become an important public health problem with high incidence and mortality (Llopis et al., 2016; EASL Clinical Practice Guidelines: European Association for the Study of the Liver, 2018). It has a variety of clinical presentations with a spectrum from alcoholic hepatitis to liver cirrhosis. ALD has become a worldwide public health concern (approximately 4.2% of the population) and caused approximately 5.9% of all deaths every year (2018). However, 2.3 billion people between the ages of 15 and 59 were still active alcohol drinkers (Borrelli et al., 2022). The economic burden of ALD contributes significantly to the healthcare cost, which has been estimated at 125 billion Euros in the European Union and 249 billion dollars in the United States in 2010 (Axley et al., 2019).
The gut microbiome thrives in our alimentary tract in a symbiotic manner and affects the function of the gastrointestinal tract or liver, and vice versa (Gupta et al., 2021). In the last decade, lots of articles illustrated the pathophysiology of ALD and gut microbiota (Mutlu et al., 2012; Leclercq et al., 2014b; Bajaj et al., 2017; Brandl et al., 2018; Ciocan et al., 2018; Chu et al., 2020; Lang et al., 2020; Bajaj et al., 2021). To be specific, the gut microbiota contributed to individual susceptibility to ALD through the metabolic pathway, immune signaling pathway, and gut-brain axis (Queipo-Ortuño et al., 2012; Llopis et al., 2016; Bajaj, 2019). Moreover, alcohol dependence and liver dysfunction have a negative impact on the gut microbiota in ALD patients (Dubinkina et al., 2017). This current review mainly focused on the pathophysiology of gut microbiota and clarifies the gut-brain cross talk in ALD, which may provide the opportunity of identifying target points for future therapeutic intervention in ALD. We believe that the review will enhance our understanding of the topic and provide clear information for the potential therapeutic intervention through the targeting therapy of gut microbiota.
2 Literature search method
The literature search was performed on the PubMed database for studies published between 1/2010 to 6/2022 by using the search strategy shown in the Supplementary Material S1. The current review included data from randomized controlled trials, observational cohort studies, and animal studies. Studies were excluded: 1) All reviews including meta-analysis or the sample size too small in the ALD arm (n < 10); 2) Patients or animals co-infected with other liver diseases. 3) Not related to our topic. Based on the keyword search on the aforementioned, a total of 1398 articles were identified, and 69 articles were finally enrolled (Supplementary Material S2). The literature search was performed by 2 authors (LZ and YW). The disagreement on the study selections was arbitrated by the discussions with the corresponding author (HX and CP) and resolved with the group consensus.
3 Alcohol effects on gut pathophysiology and microbiota
Alcohol has a direct toxic effect on endoplasmic reticulum structure and function in hepatocytes, also resulting in intestinal stem cell dysregulation and long-lasting intestinal damage (Howarth et al., 2012; Lu et al., 2017). Chronic alcohol intake also enhances Tumor Necrosis Factor (TNF)-α expression in the jejunum of mice, human intestinal monocytes, and macrophages (Chen et al., 2015a). Elevated systemic TNF-α contributed to the activation of TNF receptor I on intestinal epithelial cells and phosphorylation of myosin light chain kinase to redistribute tight junction proteins and increases intestinal permeability (Chen et al., 2015a). Additionally, alcohol-induced gut leakiness from binging alcohol has been linked to the increase of cytochrome P450-2E1(CYP2E1), apoptosis of enterocytes, and production of nitration followed by ubiquitin-dependent proteolytic degradation of the junctional complex proteins (Abdelmegeed et al., 2013). Alcohol suppressed antimicrobial-regenerating islet-derived (REG)-3B lectins and REG3G gene and protein expression. REG3B and REG3G, as secreted C-type lectins mainly expressed in the intestinal epithelial and Paneth cells, had bactericidal activity against Gram-positive and Gram-negative bacteria respectively (Wang et al., 2016). Moreover, alcohol feeding of mice also disrupted the gut mucus layer and diminished mucosal thickness (Grander et al., 2018). Several studies suggest that intestinal aerobes and facultative anaerobes, like Ruminococcus, Prevotella, Collinsella, Staphylococcus, Corynebacterium, Escherichia, Streptococcus, were responsible for alcohol dehydrogenase-mediated ethanol oxidation under aerobic and even microaerobic conditions (Suen et al., 2011; Tsuruya et al., 2016). The accumulation of acetaldehyde further inhibited the expression of tight junction protein and promotes mucosa-associated microbiota translocated through the intestinal barrier to mesenteric lymph nodes and liver (Wang et al., 2016).
Alcohol can also affect gut bacteria profiles and features directly or change the composition of gut biofilms through bile acid synthesis indirectly. In the presence of alcohol, pathogenic bacteria like Acinetobacter baumannii enhanced its virulence significantly correlates with the acidification of bacterial cultures (Nwugo et al., 2012). Chronic alcohol abuse suppressed the bacterial genes involved in the biosynthesis of saturated long-chain fatty acids (LCFAs), inhibiting the proliferation of Lactobacilli which metabolizes saturated LCFA (Chen et al., 2015b). Primary bile acids such as cholic acid and chenodeoxycholic acid were synthesized through the Cholesterol 7-alpha-hydroxycholesterol (CYP7A1) classical pathway or the Cholesterol 27-hydroxycholesterol (CYP27A1) alternative pathway (Chiang, 2013). Alcohol upregulated the expression of CYP7A1 and CYP27A1 via the activation of hepatic cannabinoid receptor type 1 and suppressed fibroblast growth factor 15 gene expression to promote the synthesis of bile acids (Xie et al., 2013). Continued alcohol misuse also induced human fibroblast growth factor 19 gene expression in biliary epithelial cells and ductular cells, followed by total and conjugated bile acids increased significantly (Bajaj et al., 2017; Brandl et al., 2018). Bile acids exert a bacteriostatic effect, directly damaging DNA and destroying the bacterial membrane of bacterial. (Merritt and Donaldson, 2009; Kakiyama et al., 2013).
4 Microbiota in patients with Alcohol-related liver disease
Alcoholics with dysbiosis had a reduced bacterial diversity, decreased abundance of Bacteroidetes, and increased Proteobacteria (Mutlu et al., 2012). The changes of gut microbiotas have been observed at both of the phylum and family levels in patients with alcohol dependence syndrome (ADS) (Leclercq et al., 2014b; Dubinkina et al., 2017). At the phylum level, Bacteroidetes and Firmicutes of alcoholics decreased significantly, whereas Proteobacteria, Fusobacteria, and Actinobacteria increased. At the family level, there was a decrease in Ruminococcaceae significantly but an increase in both Lachnospiraceae and Enterobacteriaceae (Leclercq et al., 2014b; Dubinkina et al., 2017). Also observed that Prevotellaceae was enriched in alcohol-related cirrhosis (ALC) compared with controls and other etiologies cirrhotics (Chen et al., 2011). Moreover, Bajaj has summarized articles about alcoholic cirrhosis and microbiota composition or function in humans from 2012 to 2018 (Bajaj, 2019). There were 2 updated novel-related studies from 2018 to now. It is shown that ALD patients who had a lower fungal diversity with an overgrowth of Candida and higher serum anti-Saccharomyces cerevisiae antibodies had increased mortality (Lang et al., 2020).
Upregulation of systemic inflammation including inflammation in the oral cavity, gut, and liver is postulated to be a motivating factor of ALD (Acharya et al., 2017). The oral mucosa serves as the first line of defense due to the function of salivary immunoglobulins, agglutinins, histatins, and lysozyme. Enrolled 102 cirrhotics (38% alcohol-related) to analyze salivary and stool microbiomes. They observed that the lower salivary microbiota ratio (calculated by Lachnospiraceae + Ruminococcaceae + Clostridiales Incertae Sedis XIV/Streptococcaceae), the lower cirrhosis dysbiosis ratio (calculated by Lachnospiraceae + Ruminococcaceae + Clostridiales Incertae Sedis XIV + Veillonelllaceae/Enterobacteriaceae + Bacteroidaceae), indicates oral dysbiosis and gut dysbiosis respectively (Bajaj et al., 2015). Furthermore, patients marked with salivary dysbiosis confront a higher 90-day liver-related hospitalization rate than those not. The possible cause may be related to oral microbiota invading the gut under the changes in gut pH or bile acid dysregulation of ALD. Patients with ADS had significantly increased oral-oriented microbes like Lactobacillus salivarius, Veillonella parvula, and Streptococcus salivarius (Dubinkina et al., 2017). When applying periodontal therapy in alcohol-related cirrhotics, the model for end-stage liver disease (MELD) scores were improved as the results of alleviating oral-gut dysbiosis, and decreased endotoxin, lipopolysaccharide-binding protein (Bajaj et al., 2018).
5 Metabolites from microbiota in patients with Alcohol-related liver disease
Gut microbe-derived metabolites mainly included long-chain fatty acids (LCFAs), short-chain fatty acids (SCFAs), mucus, secondary bile acids, indole or phenol derivatives, and vitamin B (Table 1) (Fan and Pedersen, 2021). Methods like integrated analyses of the microbiome and linked metabolomes as well as microbiome-wide association studies can be used to profile the gut metabolome characteristic, including shotgun-based sequencing, various bioinformatic algorithms, strain-level profiling, and so on (Fan and Pedersen, 2021). Bioinformatics analyses such as the Kyoto Encyclopedia of Genes and Genomes (KEGG) analysis have been applied to identify potential mechanistic links between the gut microbiome and metabolome. KEGG is a database and helpful tool for understanding most of the known metabolic pathways based on sequence similarity to proteins with known functional characteristics. For ALD patients, seven KEGG pathways were significantly increased (Galactose metabolism Porphyrin, chlorophyll metabolism, ABC transporters, phosphotransferase system, fructose or mannose metabolism, glutathione metabolism, and biosynthesis of siderophore group non-ribosomal peptides) (Dubinkina et al., 2017). Besides, there were significantly lower fecal metabolites focused on bioenergetics (citrate, malate, or phosphate), amino acids (threonine, ornithine, or serine), and pyrimidine intermediates (ribosine, orotic acid, or hexonate) (Kakiyama et al., 2013; Bajaj et al., 2017; Brandl et al., 2018; Ciocan et al., 2018; Fan and Pedersen, 2021).
5.1 Bile acids involved in the disease progression
Gut microbiota played a key role in bile acid homeostasis. Several studies found that alcohol-related hepatitis or cirrhosis in patients with ALD had higher total fecal bile acids, total secondary bile acids, deoxycholic acid, lithocholic acid, and a higher concentration of serum conjugated deoxycholic acid (Kakiyama et al., 2014; Ciocan et al., 2018). As a choloylglycine hydrolase produced by gut microbiota, bile salt hydrolase (BSHs) can catalyze the hydrolysis of conjugated bile salts into deconjugated bile acids. As a result, the transformation of primary bile acids to secondary bile acids increased. BSHs were distributed in 591 intestinal bacterial strains within 117 genera in human microbiota, mainly distributed in the family Firmicutes and Proteobacteria, genus Bacillus, Staphylococcus, Bacteroides, Lactobacillus, Enterococcus, and Clostridium (Song et al., 2019). Gut microbiota, Serving as an important metabolic organ of the host (Chen et al., 2011), has significant effects on the metabolism of bile acids in patients with ALD. Bile acids positively correlate with the histological severity of liver inflammation via the activation of various nuclear receptors, such as the farnesoid X receptor (FXR) (Ciocan et al., 2018). In animal studies, under the same drinking state, the fecal chenodeoxycholic acid (CDCA) and ursodeoxycholic acid (UDCA) of mice were decreased with the progression from non-hepatitis to severe AH (sAH) (Llopis et al., 2016). Ursodeoxycholic acid (UDCA), a hydrophilic bile acid that is the hepatoprotective metabolite of CDCA could induce the production of class I alcohol dehydrogenase (ADH1) and accelerate the transformation and metabolism of ethanol. Alcohol dehydrogenase 1 (ADH1) gene polymorphism may be one of the reasons for the different susceptibility to ALD (Ehlers et al., 2012).
5.2 Other gut metabolites served as the protective factors in Alcohol-related liver disease
Some gut microbe-derived metabolites involving SCFAs, LCFAs, mucus, indole derivatives, and vitamin B had protective effects on the gut and liver (Miyamoto et al., 2019; Zheng et al., 2020). Lactobacillus was involved in arachidonic acid metabolism and can reverse arachidonic acid-mediated adipose inflammation and liver lipid accumulation, thus exerting protective effects on ALD liver steatosis (Miyamoto et al., 2019; Zheng et al., 2020). Moreover, arachidonic acid can be transformed into potent bioactive compounds like lipoxins, prostaglandin E1, and prostaglandin I2, exerting anti-inflammatory effects in ALD (Das, 2019). Butyrate, as the major fuel source for the colonocyte and one of three predominant SCFAs (acetate, propionate, and butyrate), has a protective effect on the gut barrier in preserving tight junction protein expression (Cresci et al., 2017; Roychowdhury et al., 2019). Thus, the butyrate-producing bacteria Faecalibacterium prausnitzii, a dominant member of the Clostridium leptum subgroup, possessed anti-inflammatory and mucosal protective properties to mitigate ethanol-induced gut-liver injury (Rios-Covian et al., 2015).
The mucus barrier integrity is the first line of the intestinal tract. Akkermansia muciniphila, a mucin-degrading anaerobic bacterium that resides in the gut mucus layer, can utilize mucins as carbon and nitrogen source to restore mucus thickening and control the host mucus turnover (Everard et al., 2013; Grander et al., 2018). Moreover, Gegus Roseburia isolated from the gut can recover gut mucus integrity through upregulation of the protein occludin and MUC2(Grander et al., 2018). Restoration of mucus barrier function decreased expression of pro-inflammatory cytokines and dissemination of endotoxins in ALD (Grander et al., 2018).
Diverse bacterial species have been reported to produce tryptophan catabolites (Hendrikx et al., 2019). Indole-3-acetic acid (IAA), as a tryptophan catabolite and a microbiota-derived ligand of the aryl hydrocarbon receptor (AHR) which regulates AhR-dependent IL22 and Reg3g expression, strengthened the integrity of intestinal mucosa and reduced alcoholic-related liver inflammation (Roager and Licht, 2018). IAA degradation by a common pathogenic pathogen of ALD, such as Acinetobacter baumannii, via the indole-3-pyruvate (IPyA) pathway, which primarily involves IAA catabolic sites, catechol-degrading genes, and phenylacetic acid degrading genes, may be linked to the significant reduction of IAA levels in feces of patients with alcoholic hepatitis (Shu et al., 2015; Lin et al., 2018). Indolepropionic acid (IPA) is another tryptophan catabolite produced by gut symbiont Clostridium sporogenes, a bacteria from the phylum Firmicutes. Clostridium sporogenes can generate aromatic amino acid (tryptophan, phenylalanine, and tyrosine) metabolites via aromatic amino acid aminotransferase reductive pathways (Dodd et al., 2017). IPA can regulate intestinal barrier function and decrease intestinal permeability mediated by the pregnane X receptor (Jennis et al., 2018). The vitamin B was able to regulate the methionine metabolic cycle in the liver and essential for the production of glutathione, the antioxidant against oxidative liver injury (Halsted, 2013). Some related species of Bifidobacterium and Lactobacillus (like Bifidobacterium longum and Lactobacillus reuteri), normally existing in the human gut, were involved in the denovo synthesis process of folate and vitamin B12. These species may have a vital role in the treatment of ALD (LeBlanc et al., 2013).
6 Gut microbiota and immune response
The appropriate immune response in the liver is dependent on the pattern recognition receptors expressed by liver sinusoidal endothelial cells, hepatocytes, Kupffer cells, dendritic cells, and liver-resident lymphocytes through the activation of receptors like Toll-like receptors (TLRs) (Jenne and Kubes, 2013). In ALD patients, gut microbiota can get across the intestinal barrier and circulate to the liver through the portal vein to interact with the liver (Bruellman and Llorente, 2021). As a result, a prolonged inflammatory immune response is activated, as shown in Figure 1.
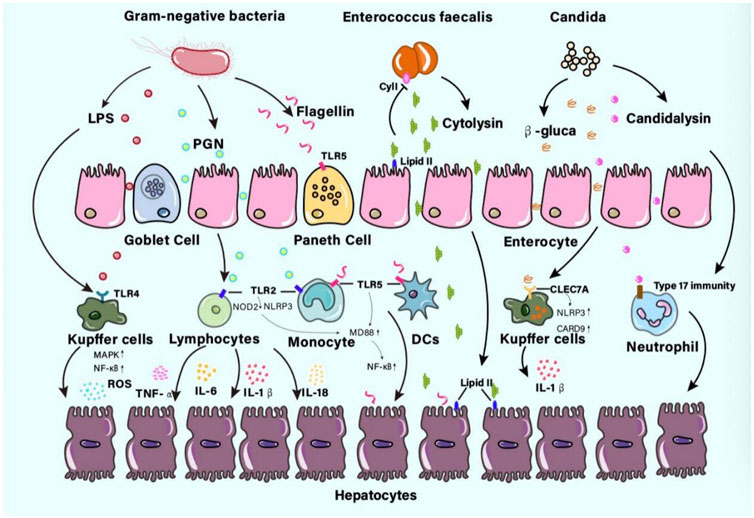
FIGURE 1. The pathogens or components interact with the liver in ALD patients Gut microbes can get across the intestinal barrier then interact with the liver. The pathogens or components like lipopolysaccharide (LPS), peptidoglycan (PGN), flagellin, cytolysin, β-glucan, or candidalysin, prolonged inflammatory immune response may contribute to fibrosis even cirrhosis. Abbreviations: LPS, lipopolysaccharide; PGN, peptidoglycan.
6.1 Mechanism of gut microbiota aggravating liver injury
Recently, the pathophysiology of several bacteria or their products triggering liver immune injury has been studied extensively. Gram-negative bacteria were surrounded by a thin PGN cell wall. Muramyl dipeptide, the minimal bioactive cytosolic structure of PGN, can not only stimulate TLR2 receptors on lymphocytes and monocytes but also interact with Nod-like receptor proteins NOD2 or NLRP3 (Leclercq et al., 2014a). The MyD88-dependent pathway in lymphocytes or monocytes was activated, which lead to the production of TNF-α, IL-6, IL-1β, and IL-18 (Schroder and Tschopp, 2010). In a recent study on alcohol-dependence patients, there were pieces of evidence that PGN may also cross the gut barrier and contribute to the high concentration of PGN in the plasma (Leclercq et al., 2014a). As an antigen from Gram-negative bacteria surface, the LPS can bind into the pattern-recognition receptors such as TLR4 of the Kupffer cells in the liver and trigger the production of proinflammatory mediators including TNFα (Mukherjee et al., 2014; Llopis et al., 2016). Furthermore, this can induce the activation of mitogen-activated protein kinase and nuclear transcription factor kappa B(NF-κB) pathway and lead to reactive oxygen species and persistent liver inflammation in ALD (Mukherjee et al., 2014; Llopis et al., 2016). As a main component of the bacterial flagellum, flagellin plays an important role in bacterial motility. In an animal study, liver injury was observed in mice because of increased levels of flagellin (Yoon et al., 2012). The injury was thought to be an immune injury triggered by the activation of TLR5 in the antimicrobial peptide-secreting paneth cells, which had the unique protein-binding TLR for the flagellin component in b- and g-Proteobacteria. The activation of TLR5 further initiated the MyD88-dependent signaling pathway and promoted the proinflammatory transcription factor NF-κB, leading to immune clearance against flagellated bacteria (Yang and Yan, 2017). Since rapid immune responses to flagellin have been observed in hepatocytes, epithelial cells, monocytes, and DCs, and increased Proteobacteria were found in ALD-related studies, this has led to the disease progression in patients with ALD (Chen et al., 2011; Engen et al., 2015; Leclercq et al., 2020). Cytolysin is a two-subunit exotoxin secreted by Enterococcus faecalis. Cytolysin may use lipid II as a docking molecule to lyse target cell membranes such as bacteria, erythrocytes, or eukaryotic cells. However, it can prevent self-lysis through the activity of the immunity factor CyII and the pheromone quorum signaling pathway (Van Tyne et al., 2013). A study showed that alcoholic hepatitis patients had increased fecal numbers of E. faecalis (Duan et al., 2019), and the presence of cytolysin-positive (cytolytic) E. faecalis correlated with severe liver damage and high mortality in patients with ALD (Duan et al., 2019).
There are three predominant commensal fungal species in the human gut, which include Candida, Saccharomyces cerevisiae, and Malassezia (Wang et al., 2014). Alcohol-dependent patients displayed decreased gut fungal diversity and overgrowth of Candida. β-glucan is a cell wall polysaccharide found in most fungi like Candida. As a result of candida overgrowth, a higher concentration of β-glucan circulated to the liver and induced hepatic inflammation via a receptor of the C-type lectin domain family 7 member A (CLEC7A) on Kupffer cells (Yang et al., 2017). More specifically, the CLEC7A signaling pathway activates NLR family pyrin domain-containing 3 and caspase recruitment domain family member 9, leading to the production of mature IL-1β. IL-1β secretion contributed to hepatocyte injury and the development of ALD (Dambuza and Brown, 2015). Candidalysin is a cytolytic peptide toxin secreted by Candida, and associated with liver disease severity and mortality in alcohol-related hepatitis (AH) patients via participation in neutrophil recruitment and Type 17 immunity (Naglik et al., 2019).
6.2 Mechanism of gut microbiota attenuating liver injury
In contrast, the attenuating effect on hepatic injury has been associated with several microbiota in the gut. Study showed that L. reuteri promoted the expression of the anti-inflammatory cytokine IL-10 by suppressing NF-κB signaling pathways (Hsu et al., 2017). They also decreased the expression levels of hepatic IL-1β, IL-6, and TNF-α leading to the reduction of necro-inflammation in hepatocytes (Hsu et al., 2017). Besides, Lactobacillus rhamnosus GG inhibited CYP2E1 expression, p38 MAP kinase phosphorylation, TLR4/TLR5, and NFκB activation, which upregulated Nrf2 protein levels and attenuated the production of proinflammatory TNFα in C57BL/6N mice of chronic liver injury from alcohol (Wang et al., 2013). In addition, the protective effects of L. rhamnosus GG in ALD were also documented. The mechanism was speculated as a process of up-regulating multiple inducers and stabilizers including intestinal trefoil factor for the mucus layer (Wang et al., 2013). Regarding the effects of A. muciniphila, the presence of the bacteria not only reduce IL-1β and TNF-α expression but also reduces infiltration of myeloperoxidase and neutrophils in ALD (Grander et al., 2018). In terms of restoring intestinal barrier function, it is worth noting that some butyrate-producing bacteria such as Roseburia intestinalis can possibly enhance the expression of IL-22 and REG3g through their flagellin binding with TLR5 and attenuated the hepatic expression of TNF-a and IL-1b as well as lipid transport factors (Seo et al., 2020), such as PPAR-g and CD36. Restoring intestinal barriers help ameliorate ALD (Seo et al., 2020). The protective effects were summarized in Figure 2. Flagellin is a double-edged sword that have some protective immune effects and the potential of inducing further liver injury, which depended on the bacterial density. Thus, caution should be taken when interpreting the study results or reviewing the contradictory data.
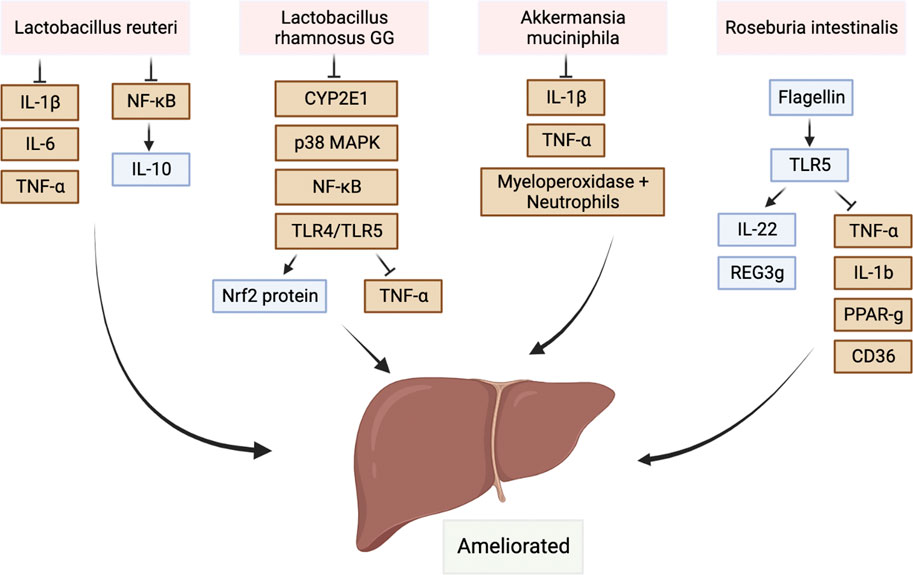
FIGURE 2. Protective commensal bacteria protect the liver by regulating the immune system. There were also some protective commensal bacteria such as Lactobacillus, Akkermansia muciniphila, and Roseburia can also protect the liver by regulating the immune system.Abbreviations: IL-1β, interleukin-1 beta; IL-6, interleukin-6; IL-10, interleukin-10; p38 MAPK, P38 mitogen-activated protein kinase; Nrf2 protein, nuclear factor erythroid 2-related factor 2 protein; REG3g, regenerating islet-derived protein 3 gamma; PPAR-g, Peroxisome proliferative activated receptor gamma; TLR, toll-like receptor; CD36, fatty acid transporter/CD36 molecule; NF-κB, nuclear transcription factor kappa B; CYP2E1, cytochrome P450-2E1.
7 Gut-brain axis in Alcohol-related liver disease
The changes in gut microbiota also influence the function of the brain. The gut microbiota communicates with the brain via the neuroendocrine or neuroimmune mechanisms, known as the gut-brain axis. Gut microbiota can produce neurotransmitters, precursors like 5-hydroxytryptamine(5-HT), dopamine, noradrenaline, L-glutamic acid, γ-and aminobutyric acid (GABA), which can regulate the function of the central nervous system (Seo et al., 2020). In addition, other metabolites of gut microbiota, such as acetate, may affect the levels of 5-HT or other neurotransmitters and therefore influence human behavior (Holzer et al., 2012). Recently, Qamar et al., 2019 used the Ingenuity Pathway Analysis Software to identify the alteration in neurotransmitters associated with alcohol consumption.
Several bacteria such as Streptococcus, Acinetobacter, Escherichia coli, and Clostridia can produce 5-HT directly or generate it via a bacteria-mediated tryptophan metabolism pathway (Williams et al., 2014; Zhang et al., 2021). The 5-HT levels were always significantly increased in ALD patients when compared with those in non-ALD patients (Williams et al., 2014; Zhang et al., 2021). The activation of 5-HT receptors promoted dopamine release in the reward circuitry and increased the risk of alcohol addiction (Enoch et al., 2011). In addition, central noradrenergic neurons can convert dopamine to norepinephrine (Enoch et al., 2011). Norepinephrine can affect the growth of Prevotella and other anaerobic bacteria as it can increase virulence gene expression and enhance iron acquisition in Clostridium (Boyanova, 2017). Furthermore, several bacteria strains have been reported to be able to produce dopamine and norepinephrine. They included E. coli, Proteus vulgaris, Staphylococcus aureus, and Bacillus subtilis (Strandwitz, 2018). Noradrenergic neurons also can be regulated by transmitters like glutamate and GABA. The GABA was produced through the α-decarboxylation of glutamate by the enzyme glutamate decarboxylase (Sarasa et al., 2020). Chronic alcohol exposure increased the extracellular glutamate concentration and suppressed GABA activity, leading to decrease glutamatergic synaptic transmission and further alcohol consumption. However, acute alcohol exposure resulted in the opposite effects (Alasmari et al., 2018). As GABA-producing bacteria and neuroactive bacteria, L. reuteri and L. rhamnosus, act on intrinsic primary afferent neurons in the mouse model (Mao et al., 2013; Pokusaeva et al., 2017; Tsai et al., 2020; Zheng et al., 2020), which transmit microbial messages to the brain via the vagus nerve. As a result, alcohol-induced hepatitis was alleviated.
The anxiety/depression-like behaviors in patients with chronic alcohol intake have been associated with the increase (Zhao et al., 2020) of pathogenic colonies like Actinobacteria and Adlercreutzia, which drive changes in inflammatory signaling and cytokine release, leading to GABA-1 receptor changes in the prefrontal cortex and the brain-derived neurotrophic factor/α1 subunit of γ-aminobutyric acid A (Kelly et al., 2016; Xu et al., 2019), and finally influenced people’s emotions. For example, at the withdrawal affect stage, microbiome-immune disruptions have been identified as potential mediators of front-limbic anomalies and derived emotional dysregulation in the alcohol addiction cycle (Gorky and Schwaber, 2016). On the one hand, the “leaky gut” allows inflammatory molecules to leak outside of the gut into the blood to generate peripheral inflammation, further activating microglia and causing a neuroinflammatory response (Skinner et al., 2009; Keita and Söderholm, 2010). On the other hand, such alterations may prime the vagus nerve to alter its neuroprotective afferent signals or promote vagal signals that are in some harmful way (Gorky and Schwaber, 2016; Carbia et al., 2021). With the brain primed by vagal afferent alterations, it may be more susceptible to influence from the peripheral inflammatory response that occurs in response to alcohol withdrawal (Figure 3 and Suplementary Material S3). Another common brain disorder among ALD patients is dementia which mostly occurs in women and may triple the risk of death (Bahorik et al., 2021). Gut microbiota dysbiosis such as the increase of Muribaculaceae and the decrease of Lactobacillus can lead to the peripheral accumulation of phenylalanine and isoleucine, which stimulates the proliferation of pro-inflammatory T helper 1 cells, contributing to dementia-associated neuroinflammation (Sahlman et al., 2016). Gut-resident Cyanobacteria-generated neurotoxins involving β-N-methylamino-l-alanine and saxitoxin may further aggravate neurodegenerative disease (Alkasir et al., 2017).
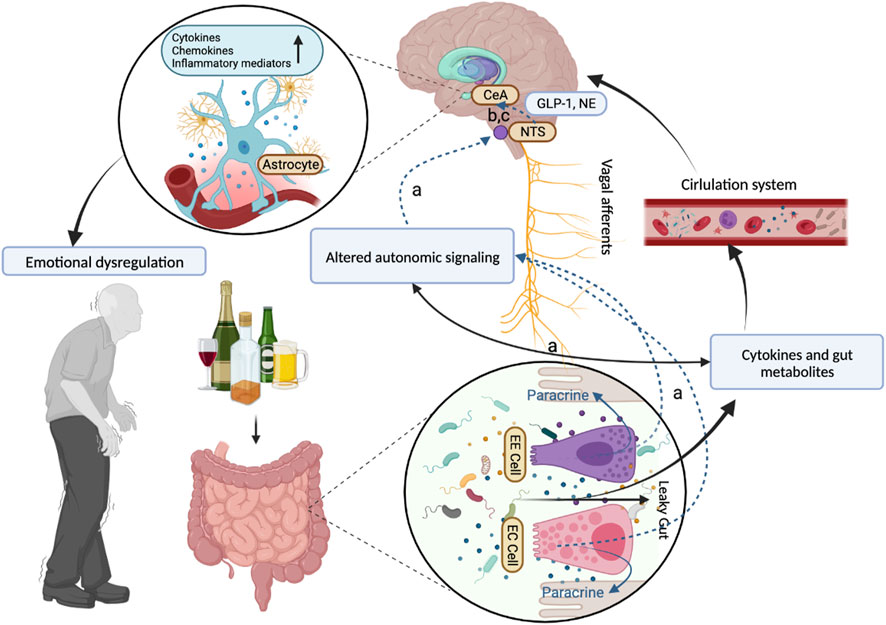
FIGURE 3. The role of the gut-brain cross talk in alcohol withdrawal reaction.The whole mechanism of associations between the gut-brain cross-talk and the mental and neurological abnormal manifestations in the alcohol withdrawal reaction. (A) The vagus afferent nerve senses change in relative bacterial abundance through two pathways: one route of communication may be through direct interactions between vagal afferent neurons and bacteria or their metabolic products. Another route includes the effects of paracrine signaling from enterochromaffin (EC) cells and from enteroendocrine (EE) cells that interact with the gut lumen causing secretion of factors that affect nearby cells in the gut wall. (B) The state of the gut microbiome is reported to the brain via vagal projections to the nucleus tractus solitarius (NTS) by changes in the activity of the vagus nerve and in the neurochemical phenotype of the vagal afferent neurons in the nodose ganglia and their targets in the NTS. The NTS in turn has viscerosensory projections to the central nucleus of the amygdala (CeA) that involves norepinephrine (NE). Neuroinflammation in regions like the central nucleus of the amygdala (CeA) and others can contribute to emotional dysregulation seen in withdrawal. (C) Neurons expressing glucagon-like peptide 1 (GLP-1) and glutamate have been described to participate in these NTS viscerosensory projections to the CeA. Abbreviations: EE Cell, enteroendocrine cells; EC Cell, enterochromaffin cells; NTS, nucleus tractus solitarius; CeA, central nucleus of the amygdala; GLP-1, glucagon-like peptide 1; NE, norepinephrine.
Hepatic encephalopathy (HE) is one of the end-stage manifestations of ALD, characterized by disturbances in mental status and neurological function. In cirrhosis, ammonia crossed the blood-brain barrier and led to astrocyte swelling and reactive oxygen species formation (Alsahhar and Rahimi, 2019). The increased Klebsiella, Proteus, and E. coli were related to the increased plasma levels of ammonia and endotoxin, contributing to the occurrence of hepatic encephalopathy in ALD (Wang et al., 2019). Moreover, the gut microbiota diversity was decreased during acute episodes of overt HE, and the relative abundances of Alistipes, Bacteroides, and Phascolarctobacterium were respectively associated with HE recurrence and overall survival during the subsequent 1-year follow-up (Sung et al., 2019).
8 Conclusion
The gut microbiota dysbiosis in ALD was initiated by oral-gut dysbiosis, followed by gut microbes’ colonization and circulating microbiota change. The factors that persistently aggravate disease progression in ALD patients have been explored in recent studies, which included Alcohol intake, gut microbiota change, gut metabolic dysregulation, activated inflammatory immune response, and neurological or behavioral deficits, giving a better understanding of the pathophysiology of gut dysbiosis in ALD and the pathway of the gut-brain cross talk. Further investigations into the pathogenic of dysbiosis and the mechanisms of certain protective bacteria in ALD may provide the therapeutic targeting points for the treatment of ALD and aid in selecting effective regimens with a favorable safety profile.
Author contributions
LZ: Writing–original draft. YW: Writing–original draft. CP: Investigation, Project administration, Supervision, Writing–review and editing. HX: Investigation, Project administration, Supervision, Writing–review and editing. All authors contributed to the article and approved the submitted version.
Funding
This work was supported by National Key R&D Program of China (2022YFC2304500); National Key R&D Program of China (2021YFC2301801); The Beijing Municipal of Science and Technology Major Project (20220383ky); Capital’s Funds for Health Improvement and Research of China (CFH 2020-1-2171). The authors declare financial support was received for the research, authorship, and/or publication of this article.
Conflict of interest
The authors declare that the research was conducted in the absence of any commercial or financial relationships that could be construed as a potential conflict of interest.
Publisher’s note
All claims expressed in this article are solely those of the authors and do not necessarily represent those of their affiliated organizations, or those of the publisher, the editors and the reviewers. Any product that may be evaluated in this article, or claim that may be made by its manufacturer, is not guaranteed or endorsed by the publisher.
Supplementary material
The Supplementary Material for this article can be found online at: https://www.frontiersin.org/articles/10.3389/fphar.2023.1258062/full#supplementary-material
Abbreviations
AH, Alcohol-related Hepatitis; AHR, Aryl Hydrocarbon Receptor; ALD, Alcohol-related Liver Disease; BSHs, Bile Salt Hydrolase; CDCA, Chenodeoxycholic Acid; CYP2E1, Cytochrome P450-2E1; FXR,farnesoid X receptor; GABA, γ-Aminobutyric Acid; HE, Hepatic Encephalopathy; IAA, Indole-3-acetic Acid; IPA, Indolepropionic Acid; KEGG, Kyoto Encyclopedia of Genes and Genomes; LCFAs, Long-Chain Fatty Acids; LPS, Lipopolysaccharide; NF-κB, Nuclear Transcription Factor Kappa B; PGN, Peptidoglycan; REG3B, Antimicrobial-regener; TNF, Tumor Necrosis Factor; 5HT, 5-Hydroxytryptamine.
References
Abdelmegeed, M. A., Banerjee, A., Jang, S., Yoo, S. H., Yun, J. W., Gonzalez, F. J., et al. (2013). CYP2E1 potentiates binge alcohol-induced gut leakiness, steatohepatitis, and apoptosis. Free Radic. Biol. Med. 65, 1238–1245. doi:10.1016/j.freeradbiomed.2013.09.009
Acharya, C., Sahingur, S. E., and Bajaj, J. S. (2017). Microbiota, cirrhosis, and the emerging oral-gut-liver axis. JCI Insight 2 (19), e94416. doi:10.1172/jci.insight.94416
Alasmari, F., Goodwani, S., Mccullumsmith, R. E., and Sari, Y. (2018). Role of glutamatergic system and mesocorticolimbic circuits in alcohol dependence. Prog. Neurobiol. 171, 32–49. doi:10.1016/j.pneurobio.2018.10.001
Alkasir, R., Li, J., Li, X., Jin, M., and Zhu, B. (2017). Human gut microbiota: The links with dementia development. Protein Cell. 8 (2), 90–102. doi:10.1007/s13238-016-0338-6
Alsahhar, J. S., and Rahimi, R. S. (2019). Updates on the pathophysiology and therapeutic targets for hepatic encephalopathy. Curr. Opin. Gastroenterol. 35 (3), 145–154. doi:10.1097/MOG.0000000000000527
Axley, P. D., Richardson, C. T., and Singal, A. K. (2019). Epidemiology of alcohol consumption and societal burden of alcoholism and alcoholic liver disease. Clin. Liver Dis. 23 (1), 39–50. doi:10.1016/j.cld.2018.09.011
Bahorik, A., Bobrow, K., Hoang, T., and Yaffe, K. (2021). Increased risk of dementia in older female US veterans with alcohol use disorder. Addiction 116 (8), 2049–2055. doi:10.1111/add.15416
Bajaj, J. S. (2019). Alcohol, liver disease and the gut microbiota. Nat. Rev. Gastroenterol. Hepatol. 16 (4), 235–246. doi:10.1038/s41575-018-0099-1
Bajaj, J. S., Betrapally, N. S., Hylemon, P. B., Heuman, D. M., Daita, K., White, M. B., et al. (2015). Salivary microbiota reflects changes in gut microbiota in cirrhosis with hepatic encephalopathy. Hepatology 62 (4), 1260–1271. doi:10.1002/hep.27819
Bajaj, J. S., Gavis, E. A., Fagan, A., Wade, J. B., Thacker, L. R., Fuchs, M., et al. (2021). A randomized clinical trial of fecal microbiota transplant for alcohol use disorder. Hepatology 73 (5), 1688–1700. doi:10.1002/hep.31496
Bajaj, J. S., Kakiyama, G., Zhao, D., Takei, H., Fagan, A., Hylemon, P., et al. (2017). Continued alcohol misuse in human cirrhosis is associated with an impaired gut-liver Axis. Alcohol Clin. Exp. Res. 41 (11), 1857–1865. doi:10.1111/acer.13498
Bajaj, J. S., Matin, P., White, M. B., Fagan, A., Deeb, J. G., Acharya, C., et al. (2018). Periodontal therapy favorably modulates the oral-gut-hepatic axis in cirrhosis. Am. J. Physiol. Gastrointest. Liver Physiol. 315 (5), G824–G837. doi:10.1152/ajpgi.00230.2018
Borrelli, I., Santoro, P. E., Gualano, M. R., Perrotta, A., Daniele, A., Amantea, C., et al. (2022). Alcohol consumption in the workplace: A comparison between European union countries' policies. Int. J. Environ. Res. Public Health 19 (24), 16964. doi:10.3390/ijerph192416964
Boyanova, L. (2017). Stress hormone epinephrine (adrenaline) and norepinephrine (noradrenaline) effects on the anaerobic bacteria. Anaerobe 44, 13–19. doi:10.1016/j.anaerobe.2017.01.003
Brandl, K., Hartmann, P., Jih, L. J., Pizzo, D. P., Argemi, J., Ventura-Cots, M., et al. (2018). Dysregulation of serum bile acids and FGF19 in alcoholic hepatitis. J. Hepatol. 69 (2), 396–405. doi:10.1016/j.jhep.2018.03.031
Bruellman, R., and Llorente, C. (2021). A perspective of intestinal immune-microbiome interactions in alcohol-associated liver disease. Int. J. Biol. Sci. 17 (1), 307–327. doi:10.7150/ijbs.53589
Carbia, C., Lannoy, S., Maurage, P., López-Caneda, E., O'riordan, K. J., Dinan, T. G., et al. (2021). A biological framework for emotional dysregulation in alcohol misuse: From gut to brain. Mol. Psychiatry 26 (4), 1098–1118. doi:10.1038/s41380-020-00970-6
Chen, P., Stärkel, P., Turner, J. R., Ho, S. B., and Schnabl, B. (2015a). Dysbiosis-induced intestinal inflammation activates tumor necrosis factor receptor I and mediates alcoholic liver disease in mice. Hepatology 61 (3), 883–894. doi:10.1002/hep.27489
Chen, P., Torralba, M., Tan, J., Embree, M., Zengler, K., Stärkel, P., et al. (2015b). Supplementation of saturated long-chain fatty acids maintains intestinal eubiosis and reduces ethanol-induced liver injury in mice. Gastroenterology 148 (1), 203–214. doi:10.1053/j.gastro.2014.09.014
Chen, Y., Yang, F., Lu, H., Wang, B., Chen, Y., Lei, D., et al. (2011). Characterization of fecal microbial communities in patients with liver cirrhosis. Hepatology 54 (2), 562–572. doi:10.1002/hep.24423
Chiang, J. Y. (2013). Bile acid metabolism and signaling. Compr. Physiol. 3 (3), 1191–1212. doi:10.1002/cphy.c120023
Chu, H., Duan, Y., Lang, S., Jiang, L., Wang, Y., Llorente, C., et al. (2020). The Candida albicans exotoxin candidalysin promotes alcohol-associated liver disease. J. Hepatol. 72 (3), 391–400. doi:10.1016/j.jhep.2019.09.029
Ciocan, D., Voican, C. S., Wrzosek, L., Hugot, C., Rainteau, D., Humbert, L., et al. (2018). Bile acid homeostasis and intestinal dysbiosis in alcoholic hepatitis. Aliment. Pharmacol. Ther. 48 (9), 961–974. doi:10.1111/apt.14949
Cresci, G. A., Glueck, B., Mcmullen, M. R., Xin, W., Allende, D., and Nagy, L. E. (2017). Prophylactic tributyrin treatment mitigates chronic-binge ethanol-induced intestinal barrier and liver injury. J. Gastroenterol. Hepatol. 32 (9), 1587–1597. doi:10.1111/jgh.13731
Dambuza, I. M., and Brown, G. D. (2015). C-Type lectins in immunity: Recent developments. Curr. Opin. Immunol. 32, 21–27. doi:10.1016/j.coi.2014.12.002
Das, U. N. (2019). Beneficial role of bioactive lipids in the pathobiology, prevention, and management of HBV, HCV and alcoholic hepatitis, nafld, and liver cirrhosis: A review. J. Adv. Res. 17, 17–29. doi:10.1016/j.jare.2018.12.006
Dodd, D., Spitzer, M. H., Van Treuren, W., Merrill, B. D., Hryckowian, A. J., Higginbottom, S. K., et al. (2017). A gut bacterial pathway metabolizes aromatic amino acids into nine circulating metabolites. Nature 551 (7682), 648–652. doi:10.1038/nature24661
Duan, Y., Llorente, C., Lang, S., Brandl, K., Chu, H., Jiang, L., et al. (2019). Bacteriophage targeting of gut bacterium attenuates alcoholic liver disease. Nature 575 (7783), 505–511. doi:10.1038/s41586-019-1742-x
Dubinkina, V. B., Tyakht, A. V., Odintsova, V. Y., Yarygin, K. S., Kovarsky, B. A., Pavlenko, A. V., et al. (2017). Links of gut microbiota composition with alcohol dependence syndrome and alcoholic liver disease. Microbiome 5 (1), 141. doi:10.1186/s40168-017-0359-2
EASL Clinical Practice Guidelines: European Association for the Study of the Liver (2018). EASL clinical Practice Guidelines: Management of alcohol-related liver disease. J. Hepatol. 69 (1), 154–181. doi:10.1016/j.jhep.2018.03.018
Ehlers, C. L., Liang, T., and Gizer, I. R. (2012). ADH and ALDH polymorphisms and alcohol dependence in Mexican and Native Americans. Am. J. Drug Alcohol Abuse 38 (5), 389–394. doi:10.3109/00952990.2012.694526
Engen, P. A., Green, S. J., Voigt, R. M., Forsyth, C. B., and Keshavarzian, A. (2015). The gastrointestinal microbiome: Alcohol effects on the composition of intestinal microbiota. Alcohol Res. 37 (2), 223–236.
Enoch, M. A., Gorodetsky, E., Hodgkinson, C., Roy, A., and Goldman, D. (2011). Functional genetic variants that increase synaptic serotonin and 5-HT3 receptor sensitivity predict alcohol and drug dependence. Mol. Psychiatry 16 (11), 1139–1146. doi:10.1038/mp.2010.94
Everard, A., Belzer, C., Geurts, L., Ouwerkerk, J. P., Druart, C., Bindels, L. B., et al. (2013). Cross-talk between Akkermansia muciniphila and intestinal epithelium controls diet-induced obesity. Proc. Natl. Acad. Sci. U. S. A. 110 (22), 9066–9071. doi:10.1073/pnas.1219451110
Fan, Y., and Pedersen, O. (2021). Gut microbiota in human metabolic health and disease. Nat. Rev. Microbiol. 19 (1), 55–71. doi:10.1038/s41579-020-0433-9
Gorky, J., and Schwaber, J. (2016). The role of the gut-brain axis in alcohol use disorders. Prog. Neuropsychopharmacol. Biol. Psychiatry 65, 234–241. doi:10.1016/j.pnpbp.2015.06.013
Grander, C., Adolph, T. E., Wieser, V., Lowe, P., Wrzosek, L., Gyongyosi, B., et al. (2018). Recovery of ethanol-induced Akkermansia muciniphila depletion ameliorates alcoholic liver disease. Gut 67 (5), 891–901. doi:10.1136/gutjnl-2016-313432
Gupta, H., Suk, K. T., and Kim, D. J. (2021). Gut microbiota at the intersection of alcohol, brain, and the liver. J. Clin. Med. 10 (3), 541. doi:10.3390/jcm10030541
Halsted, C. H. (2013). B-Vitamin dependent methionine metabolism and alcoholic liver disease. Clin. Chem. Lab. Med. 51 (3), 457–465. doi:10.1515/cclm-2012-0308
Hendrikx, T., Duan, Y., Wang, Y., Oh, J. H., Alexander, L. M., Huang, W., et al. (2019). Bacteria engineered to produce IL-22 in intestine induce expression of REG3G to reduce ethanol-induced liver disease in mice. Gut 68 (8), 1504–1515. doi:10.1136/gutjnl-2018-317232
Holzer, P., Reichmann, F., and Farzi, A. (2012). Neuropeptide Y, peptide YY and pancreatic polypeptide in the gut-brain axis. Neuropeptides 46 (6), 261–274. doi:10.1016/j.npep.2012.08.005
Howarth, D. L., Vacaru, A. M., Tsedensodnom, O., Mormone, E., Nieto, N., Costantini, L. M., et al. (2012). Alcohol disrupts endoplasmic reticulum function and protein secretion in hepatocytes. Alcohol Clin. Exp. Res. 36 (1), 14–23. doi:10.1111/j.1530-0277.2011.01602.x
Hsu, T. C., Huang, C. Y., Liu, C. H., Hsu, K. C., Chen, Y. H., and Tzang, B. S. (2017). Lactobacillus paracasei GMNL-32, Lactobacillus reuteri GMNL-89 and L. reuteri GMNL-263 ameliorate hepatic injuries in lupus-prone mice. Br. J. Nutr. 117 (8), 1066–1074. doi:10.1017/S0007114517001039
Jenne, C. N., and Kubes, P. (2013). Immune surveillance by the liver. Nat. Immunol. 14 (10), 996–1006. doi:10.1038/ni.2691
Jennis, M., Cavanaugh, C. R., Leo, G. C., Mabus, J. R., Lenhard, J., and Hornby, P. J. (2018). Microbiota-derived tryptophan indoles increase after gastric bypass surgery and reduce intestinal permeability in vitro and in vivo. Neurogastroenterol. Motil. 30 (2), e13178. doi:10.1111/nmo.13178
Kakiyama, G., Hylemon, P. B., Zhou, H., Pandak, W. M., Heuman, D. M., Kang, D. J., et al. (2014). Colonic inflammation and secondary bile acids in alcoholic cirrhosis. Am. J. Physiol. Gastrointest. Liver Physiol. 306 (11), G929–G937. doi:10.1152/ajpgi.00315.2013
Kakiyama, G., Pandak, W. M., Gillevet, P. M., Hylemon, P. B., Heuman, D. M., Daita, K., et al. (2013). Modulation of the fecal bile acid profile by gut microbiota in cirrhosis. J. Hepatol. 58 (5), 949–955. doi:10.1016/j.jhep.2013.01.003
Keita, A. V., and Söderholm, J. D. (2010). The intestinal barrier and its regulation by neuroimmune factors. Neurogastroenterol. Motil. 22 (7), 718–733. doi:10.1111/j.1365-2982.2010.01498.x
Kelly, J. R., Borre, Y., Patterson, E., El Aidy, S., Deane, J., Kennedy, P. J., et al. (2016). Transferring the blues: Depression-associated gut microbiota induces neurobehavioural changes in the rat. J. Psychiatr. Res. 82, 109–118. doi:10.1016/j.jpsychires.2016.07.019
Lang, S., Duan, Y., Liu, J., Torralba, M. G., Kuelbs, C., Ventura-Cots, M., et al. (2020). Intestinal fungal dysbiosis and systemic immune response to fungi in patients with alcoholic hepatitis. Hepatology 71 (2), 522–538. doi:10.1002/hep.30832
Leblanc, J. G., Milani, C., De Giori, G. S., Sesma, F., Van Sinderen, D., and Ventura, M. (2013). Bacteria as vitamin suppliers to their host: A gut microbiota perspective. Curr. Opin. Biotechnol. 24 (2), 160–168. doi:10.1016/j.copbio.2012.08.005
Leclercq, S., De Saeger, C., Delzenne, N., De Timary, P., and Stärkel, P. (2014a). Role of inflammatory pathways, blood mononuclear cells, and gut-derived bacterial products in alcohol dependence. Biol. Psychiatry 76 (9), 725–733. doi:10.1016/j.biopsych.2014.02.003
Leclercq, S., De Timary, P., and Stärkel, P. (2020). Targeting the gut microbiota to treat alcoholic liver diseases: Evidence and promises. Acta Gastroenterol. Belg 83 (4), 616–621.
Leclercq, S., Matamoros, S., Cani, P. D., Neyrinck, A. M., Jamar, F., Stärkel, P., et al. (2014b). Intestinal permeability, gut-bacterial dysbiosis, and behavioral markers of alcohol-dependence severity. Proc. Natl. Acad. Sci. U. S. A. 111 (42), E4485–E4493. doi:10.1073/pnas.1415174111
Lin, H. R., Shu, H. Y., and Lin, G. H. (2018). Biological roles of indole-3-acetic acid in Acinetobacter baumannii. Microbiol. Res. 216, 30–39. doi:10.1016/j.micres.2018.08.004
Llopis, M., Cassard, A. M., Wrzosek, L., Boschat, L., Bruneau, A., Ferrere, G., et al. (2016). Intestinal microbiota contributes to individual susceptibility to alcoholic liver disease. Gut 65 (5), 830–839. doi:10.1136/gutjnl-2015-310585
Lu, R., Voigt, R. M., Zhang, Y., Kato, I., Xia, Y., Forsyth, C. B., et al. (2017). Alcohol injury damages intestinal stem cells. Alcohol Clin. Exp. Res. 41 (4), 727–734. doi:10.1111/acer.13351
Mao, Y. K., Kasper, D. L., Wang, B., Forsythe, P., Bienenstock, J., and Kunze, W. A. (2013). Bacteroides fragilis polysaccharide A is necessary and sufficient for acute activation of intestinal sensory neurons. Nat. Commun. 4, 1465. doi:10.1038/ncomms2478
Merritt, M. E., and Donaldson, J. R. (2009). Effect of bile salts on the DNA and membrane integrity of enteric bacteria. J. Med. Microbiol. 58 (12), 1533–1541. doi:10.1099/jmm.0.014092-0
Miyamoto, J., Igarashi, M., Watanabe, K., Karaki, S. I., Mukouyama, H., Kishino, S., et al. (2019). Gut microbiota confers host resistance to obesity by metabolizing dietary polyunsaturated fatty acids. Nat. Commun. 10 (1), 4007. doi:10.1038/s41467-019-11978-0
Mukherjee, S., Zheng, H., Derebe, M. G., Callenberg, K. M., Partch, C. L., Rollins, D., et al. (2014). Antibacterial membrane attack by a pore-forming intestinal C-type lectin. Nature 505 (7481), 103–107. doi:10.1038/nature12729
Mutlu, E. A., Gillevet, P. M., Rangwala, H., Sikaroodi, M., Naqvi, A., Engen, P. A., et al. (2012). Colonic microbiome is altered in alcoholism. Am. J. Physiol. Gastrointest. Liver Physiol. 302 (9), G966–G978. doi:10.1152/ajpgi.00380.2011
Naglik, J. R., Gaffen, S. L., and Hube, B. (2019). Candidalysin: Discovery and function in Candida albicans infections. Curr. Opin. Microbiol. 52, 100–109. doi:10.1016/j.mib.2019.06.002
Nwugo, C. C., Arivett, B. A., Zimbler, D. L., Gaddy, J. A., Richards, A. M., and Actis, L. A. (2012). Effect of ethanol on differential protein production and expression of potential virulence functions in the opportunistic pathogen Acinetobacter baumannii. PLoS One 7 (12), e51936. doi:10.1371/journal.pone.0051936
Pokusaeva, K., Johnson, C., Luk, B., Uribe, G., Fu, Y., Oezguen, N., et al. (2017). GABA-producing Bifidobacterium dentium modulates visceral sensitivity in the intestine. Neurogastroenterol. Motil. 29 (1), e12904. doi:10.1111/nmo.12904
Qamar, N., Castano, D., Patt, C., Chu, T., Cottrell, J., and Chang, S. L. (2019). Meta-analysis of alcohol induced gut dysbiosis and the resulting behavioral impact. Behav. Brain Res. 376, 112196. doi:10.1016/j.bbr.2019.112196
Queipo-Ortuño, M. I., Boto-Ordóñez, M., Murri, M., Gomez-Zumaquero, J. M., Clemente-Postigo, M., Estruch, R., et al. (2012). Influence of red wine polyphenols and ethanol on the gut microbiota ecology and biochemical biomarkers. Am. J. Clin. Nutr. 95 (6), 1323–1334. doi:10.3945/ajcn.111.027847
Rios-Covian, D., Gueimonde, M., Duncan, S. H., Flint, H. J., and De Los Reyes-Gavilan, C. G. (2015). Enhanced butyrate formation by cross-feeding between Faecalibacterium prausnitzii and Bifidobacterium adolescentis. FEMS Microbiol. Lett. 362 (21), fnv176. doi:10.1093/femsle/fnv176
Roager, H. M., and Licht, T. R. (2018). Microbial tryptophan catabolites in health and disease. Nat. Commun. 9 (1), 3294. doi:10.1038/s41467-018-05470-4
Roychowdhury, S., Glueck, B., Han, Y., Mohammad, M. A., and Cresci, G. a. M. (2019). A designer synbiotic attenuates chronic-binge ethanol-induced gut-liver injury in mice. Nutrients 11 (1), 97. doi:10.3390/nu11010097
Sahlman, P., Nissinen, M., Pukkala, E., and Färkkilä, M. (2016). Incidence, survival and cause-specific mortality in alcoholic liver disease: A population-based cohort study. Scand. J. Gastroenterol. 51 (8), 961–966. doi:10.3109/00365521.2016.1157889
Sarasa, S. B., Mahendran, R., Muthusamy, G., Thankappan, B., Selta, D. R. F., and Angayarkanni, J. (2020). A brief review on the non-protein amino acid, gamma-amino butyric acid (GABA): Its production and role in microbes. Curr. Microbiol. 77 (4), 534–544. doi:10.1007/s00284-019-01839-w
Schroder, K., and Tschopp, J. (2010). The inflammasomes. Cell. 140 (6), 821–832. doi:10.1016/j.cell.2010.01.040
Seo, B., Jeon, K., Moon, S., Lee, K., Kim, W. K., Jeong, H., et al. (2020). Roseburia spp. abundance associates with alcohol consumption in humans and its administration ameliorates alcoholic fatty liver in mice. Cell. Host Microbe 27 (1), 25–40. doi:10.1016/j.chom.2019.11.001
Shu, H. Y., Lin, L. C., Lin, T. K., Chen, H. P., Yang, H. H., Peng, K. C., et al. (2015). Transcriptional regulation of the iac locus from Acinetobacter baumannii by the phytohormone indole-3-acetic acid. Ant. Van Leeuwenhoek 107 (5), 1237–1247. doi:10.1007/s10482-015-0417-3
Skinner, R. A., Gibson, R. M., Rothwell, N. J., Pinteaux, E., and Penny, J. I. (2009). Transport of interleukin-1 across cerebromicrovascular endothelial cells. Br. J. Pharmacol. 156 (7), 1115–1123. doi:10.1111/j.1476-5381.2008.00129.x
Song, Z., Cai, Y., Lao, X., Wang, X., Lin, X., Cui, Y., et al. (2019). Taxonomic profiling and populational patterns of bacterial bile salt hydrolase (BSH) genes based on worldwide human gut microbiome. Microbiome 7 (1), 9. doi:10.1186/s40168-019-0628-3
Strandwitz, P. (2018). Neurotransmitter modulation by the gut microbiota. Brain Res. 1693, 128–133. doi:10.1016/j.brainres.2018.03.015
Suen, G., Stevenson, D. M., Bruce, D. C., Chertkov, O., Copeland, A., Cheng, J. F., et al. (2011). Complete genome of the cellulolytic ruminal bacterium Ruminococcus albus 7. J. Bacteriol. 193 (19), 5574–5575. doi:10.1128/JB.05621-11
Sung, C. M., Lin, Y. F., Chen, K. F., Ke, H. M., Huang, H. Y., Gong, Y. N., et al. (2019). Predicting clinical outcomes of cirrhosis patients with hepatic encephalopathy from the fecal microbiome. Cell. Mol. Gastroenterol. Hepatol. 8 (2), 301–318. doi:10.1016/j.jcmgh.2019.04.008
Tsai, Y. S., Lin, S. W., Chen, Y. L., and Chen, C. C. (2020). Effect of probiotics Lactobacillus paracasei GKS6, L. plantarum GKM3, and L. rhamnosus GKLC1 on alleviating alcohol-induced alcoholic liver disease in a mouse model. Nutr. Res. Pract. 14 (4), 299–308. doi:10.4162/nrp.2020.14.4.299
Tsuruya, A., Kuwahara, A., Saito, Y., Yamaguchi, H., Tenma, N., Inai, M., et al. (2016). Major anaerobic bacteria responsible for the production of carcinogenic acetaldehyde from ethanol in the colon and rectum. Alcohol Alcohol 51 (4), 395–401. doi:10.1093/alcalc/agv135
Van Tyne, D., Martin, M. J., and Gilmore, M. S. (2013). Structure, function, and biology of the Enterococcus faecalis cytolysin. Toxins (Basel) 5 (5), 895–911. doi:10.3390/toxins5050895
Wang, L., Fouts, D. E., Stärkel, P., Hartmann, P., Chen, P., Llorente, C., et al. (2016). Intestinal REG3 lectins protect against alcoholic steatohepatitis by reducing mucosa-associated microbiota and preventing bacterial translocation. Cell. Host Microbe 19 (2), 227–239. doi:10.1016/j.chom.2016.01.003
Wang, X., Sun, G., Feng, T., Zhang, J., Huang, X., Wang, T., et al. (2019). Sodium oligomannate therapeutically remodels gut microbiota and suppresses gut bacterial amino acids-shaped neuroinflammation to inhibit Alzheimer's disease progression. Cell. Res. 29 (10), 787–803. doi:10.1038/s41422-019-0216-x
Wang, Y., Liu, Y., Kirpich, I., Ma, Z., Wang, C., Zhang, M., et al. (2013). Lactobacillus rhamnosus GG reduces hepatic TNFα production and inflammation in chronic alcohol-induced liver injury. J. Nutr. Biochem. 24 (9), 1609–1615. doi:10.1016/j.jnutbio.2013.02.001
Wang, Z. K., Yang, Y. S., Stefka, A. T., Sun, G., and Peng, L. H. (2014). Review article: Fungal microbiota and digestive diseases. Aliment. Pharmacol. Ther. 39 (8), 751–766. doi:10.1111/apt.12665
Williams, B. B., Van Benschoten, A. H., Cimermancic, P., Donia, M. S., Zimmermann, M., Taketani, M., et al. (2014). Discovery and characterization of gut microbiota decarboxylases that can produce the neurotransmitter tryptamine. Cell. Host Microbe 16 (4), 495–503. doi:10.1016/j.chom.2014.09.001
Xie, G., Zhong, W., Li, H., Li, Q., Qiu, Y., Zheng, X., et al. (2013). Alteration of bile acid metabolism in the rat induced by chronic ethanol consumption. Faseb J. 27 (9), 3583–3593. doi:10.1096/fj.13-231860
Xu, Z., Wang, C., Dong, X., Hu, T., Wang, L., Zhao, W., et al. (2019). Chronic alcohol exposure induced gut microbiota dysbiosis and its correlations with neuropsychic behaviors and brain BDNF/Gabra1 changes in mice. Biofactors 45 (2), 187–199. doi:10.1002/biof.1469
Yang, A. M., Inamine, T., Hochrath, K., Chen, P., Wang, L., Llorente, C., et al. (2017). Intestinal fungi contribute to development of alcoholic liver disease. J. Clin. Invest. 127 (7), 2829–2841. doi:10.1172/JCI90562
Yang, J., and Yan, H. (2017). TLR5: Beyond the recognition of flagellin. Cell. Mol. Immunol. 14 (12), 1017–1019. doi:10.1038/cmi.2017.122
Yoon, S. I., Kurnasov, O., Natarajan, V., Hong, M., Gudkov, A. V., Osterman, A. L., et al. (2012). Structural basis of TLR5-flagellin recognition and signaling. Science 335 (6070), 859–864. doi:10.1126/science.1215584
Zhang, J., Zhu, S., Ma, N., Johnston, L. J., Wu, C., and Ma, X. (2021). Metabolites of microbiota response to tryptophan and intestinal mucosal immunity: A therapeutic target to control intestinal inflammation. Med. Res. Rev. 41 (2), 1061–1088. doi:10.1002/med.21752
Zhao, W., Hu, Y., Li, C., Li, N., Zhu, S., Tan, X., et al. (2020). Transplantation of fecal microbiota from patients with alcoholism induces anxiety/depression behaviors and decreases brain mGluR1/PKC ε levels in mouse. Biofactors 46 (1), 38–54. doi:10.1002/biof.1567
Keywords: alcohol-related liver disease, alcohol-related cirrhosis, alcohol-related hepatitis, alcohol use disorder, gut dysbiosis
Citation: Zhu L, Wang Y, Pan CQ and Xing H (2023) Gut microbiota in alcohol-related liver disease: pathophysiology and gut-brain cross talk. Front. Pharmacol. 14:1258062. doi: 10.3389/fphar.2023.1258062
Received: 13 July 2023; Accepted: 27 July 2023;
Published: 04 August 2023.
Edited by:
Zhengsheng Zou, Fifth Medical Center of the PLA General Hospital, ChinaReviewed by:
Hui Yang, First Hospital of Shanxi Medical University, ChinaYan Wang, Peking University, China
Copyright © 2023 Zhu, Wang, Pan and Xing. This is an open-access article distributed under the terms of the Creative Commons Attribution License (CC BY). The use, distribution or reproduction in other forums is permitted, provided the original author(s) and the copyright owner(s) are credited and that the original publication in this journal is cited, in accordance with accepted academic practice. No use, distribution or reproduction is permitted which does not comply with these terms.
*Correspondence: Calvin Q. Pan, UGFuYzAxQG55dS5lZHU=; Huichun Xing, aGNoeGluZ0Bzb2h1LmNvbQ==, aGNoeGluZ0BjY211LmVkdS4gY29t