- 1Department of Biochemistry and General Chemistry, Medical College of the University of Rzeszów, Rzeszów, Poland
- 2Students English Division Science Club, Medical College of the University of Rzeszów, Rzeszów, Poland
- 3Center for Innovative Research in Medical and Natural Sciences, Medical College of the University of Rzeszów, Rzeszów, Poland
- 4Department of Photomedicine and Physical Chemistry, Medical College of the University of Rzeszów, Rzeszów, Poland
Brain tumors, including glioblastoma multiforme, are currently a cause of suffering and death of tens of thousands of people worldwide. Despite advances in clinical treatment, the average patient survival time from the moment of diagnosis of glioblastoma multiforme and application of standard treatment methods such as surgical resection, radio- and chemotherapy, is less than 4 years. The continuing development of new therapeutic methods for targeting and treating brain tumors may extend life and provide greater comfort to patients. One such developing therapeutic method is photodynamic therapy. Photodynamic therapy is a progressive method of therapy used in dermatology, dentistry, ophthalmology, and has found use as an antimicrobial agent. It has also found wide application in photodiagnosis. Photodynamic therapy requires the presence of three necessary components: a clinically approved photosensitizer, oxygen and light. This paper is a review of selected literature from Pubmed and Scopus scientific databases in the field of photodynamic therapy in brain tumors with an emphasis on glioblastoma treatment.
1 Introduction
Malignant tumors of the brain and central nervous system (CNS) are a serious threat to health and cause the death of tens of thousands of people every year around the world (Ostrom et al., 2021). According to Ostrom et al., 83,029 deaths due to malignant brain tumors and other CNS tumors were recorded in 2014–2018 alone (Ostrom et al., 2021). In 2020, according to World Health Organization (WHO) reports, there were 308,102 new cases and 251,329 deaths due to malignant brain tumors, of which more than 50% of cases occur in Asia. In Poland in 2020, 4,413 new diagnoses were made (Raporty WHO, 2023). According to Fan et al., in 2019 alone, 347,992 cases of brain and CNS tumors were registered worldwide. This represents an increase of 94.35% compared to the period between the years 1990–2019 (Fan et al., 2022).
The most frequently diagnosed malignancy within the CNS is glioblastoma multiforme (GBM) which accounts for about 70% of all intracranial tumors with malignancy grade IV according to WHO classifications. It is estimated that in 80% of cases, tumor recurrence occurs after tumor resection, which makes the prognosis poor, despite the development of surgical techniques, as well as adjuvant radio- and chemotherapy, which are standard therapeutic procedures. The most common surgical procedures include: biopsy (i.e., taking a piece of tissue and analyzing it under a microscope), neurosurgical resection, partial or complete resection. The most commonly used radiotherapy is stereotactic radiotherapy, i.e., external radiotherapy. Depending on the type of dose application, we distinguish stereotactic radiosurgery (single dose) or fractionated stereotactic radiotherapy (several doses) (Fuentes et al., 2018). The application of a specific method depends to a large extent on the location of the tumor, the size and extent of the lesions. Other radiotherapy techniques for the treatment of brain tumors are: intensity modulated radiotherapy technique, volumetric modulated arc therapy. All these techniques enable the application of higher radioactive doses compared to conventional radiotherapy (Scaringi et al., 2018). Proton therapy, on the other hand, uses proton radiation to destroy dangerous lesions. In turn, in chemotherapy, the most commonly administered drugs are: tucatinib, adotrastuzumab emtansine, trastuzumab deruxtekan and neratinib, which show intracranial efficacy. The development of new therapeutic drugs and innovative strategies are still being developed (Newton, 2000; Quirk et al., 2015; Bartusik-Aebisher et al., 2022; Corti et al., 2022). Due to the infiltration of GBM into adjacent tissues, complete resection is difficult or even impossible due to the need to remove an appropriate margin of brain tissue, which may result in serious neurological consequences for the patient. On the other hand, traditional treatment involving irradiation and the use of chemotherapeutics carries many side effects, including the risk of damage to healthy tissues resulting from their low precision (Mahmoudi et al., 2019). For these reasons, the method of photodynamic therapy (PDT) is increasingly considered as an alternative.
The main principle of operation of PDT is based on the effect of combining photosensitizer molecules with cancer cells and their activation by excitation with laser light of the appropriate wavelength. The excited photosensitizer converts molecular oxygen to the singlet state as a result of the intersystem transition. This active form of oxygen generates a large amount of reactive oxygen species (ROS) (Bartusik-Aebisher et al., 2022). The generated reactive forms cause the oxidation of biological macromolecules (including proteins, fatty acids and DNA), consequently leading to damage to cellular organelles, i.e., lysosomes, nucleus, mitochondria, leading the cell to the path of programmed cell death (Xu et al., 2022). Figure 1 shows mechanism of PDT.
Photodynamic therapy is a progressive method of therapy used in dermatology, dentistry, ophthalmology and brain cancer. In dermatology, PDT is used in various types of skin diseases. Examples include: actinic keratosis (Mazur and Reich, 2023), acne vulgaris (Wang et al., 2023), port-wine stains (Kang et al., 2023) or more or less advanced skin cancers. In the treatment of dermatological diseases, the most often uses a cream with a component of one of the photosensitizers. On the other hand, in the treatment of brain tumors, the photosensitizer is administered systemically or orally (Hsia et al., 2023). The type of light source is adapted to the applied photosensitizer. Both in dermatological cases and brain tumors, the wavelength is in the range of 500–680 nm. The form of laser light delivery in dermatology takes place locally and centrally in the lesion area. However, in the case of brain tumors, a fiber optic diffuser, optical fiber, cavitation balloon, i.e., interstitial application, are used. Therapeutic effectiveness in the treatment of brain tumors depends on the forms of light application as well as on the geometry of the light. LED diodes are used more and more often, which prevent the tissue from heating up under the influence of the laser. In dermatological cases, the irradiation time varies from a few minutes to several minutes. According to Li et al., who conducted a systematic review of PDT in the treatment of rosacea, most studies showed satisfactory treatment effects (Li et al., 2022). In the case of brain tumor therapy, the effectiveness varies. According to Jamali et al., the number of killed human glioblastoma cells was higher after exposure to the blue LED compared to the red one (Jamali et al., 2018). Many studies and experiments have been developed to analyze the effectiveness of PDT in dentistry. PDT in dentistry is used in such diseases as: halitosis, biofilm, caries (Mazur et al., 2022). The average effectiveness of PDT in the treatment of oral lesions is 90%. Methylene Blue is a frequently used photosensitizer in dentistry and the light sources used have a wavelength in the range of 600–630 nm. The laser is applied directly to the changes or inserted into the periodontal pocket (Gholami et al., 2023) In turn, in ophthalmology, PDT is used to treat diseases such as: keratitis (de Paiva et al., 2022), treatment of choroidal melanoma (Kawczyk-Krupka et al., 2013) or hemangioma (Kumar et al., 2022). In ophthalmology, the most commonly used photosensitizers are: riboflavin, methylene blue and verteporfin (de Paiva et al., 2022). The most commonly used light source is ultraviolet and light-emitting diodes. There are numerous studies confirming the effectiveness of PDT in eye diseases. In the case of choroidal melanoma (studies led by Soucek and Cihelkova, PDT resulted in complete tumor regression (Soucek and Cihelkova, 2006). According to Kawczyk-krupka et al., Clinical trials on the application of PDT in ophthalmology should still be conducted (Kawczyk-Krupka et al., 2013).
In PDT photosensitizer (PS) accumulates in cancer cells prior to irradiation causing the production of toxic reactive oxygen species (ROS) that destroy abnormal tissue. Tumor destruction occurs in three primary ways: direct killing of the tumor cell, damage to the tumor vasculature, and activation of the body’s immune response (Dolmans et al., 2003). The effect of photodynamic therapy is affected by the modification of any of the PDT components (photosensitizer, visible light, reactive oxygen species), phenotypic variability of cancer cells and variability of the tumor environment. Different combinations of photosensitizers with appropriate beams of light produce different results. An ideal photosensitizer should absorb light in the red or far red wavelength, and must also be able to penetrate the blood-brain barrier. It should be highly selective towards tumor tissue, low-toxic and quickly eliminated from the body (Tetard et al., 2014). The effectiveness of the therapy is directly proportional to the amount of singlet oxygen produced after the use of the appropriate wavelength of light (Stylli and Andrew, 2006).
The aim of this study was to review the literature on targeted methods of treating brain tumors (primarily glioblastoma multiforme), with particular emphasis on the currently developed photodynamic therapy (taking into account the mechanism of its action, the impact of individual components) and immunotherapy.
2 Materials and methods
A search focused on the use photodynamic therapy and other targeted methods in the treatment of a brain tumor was conducted on Pubmed, and Scopus from 1990 to 2023. The search term included the phrase: “photodynamic therapy in brain cancer”, “targeted methods in the treatment of a brain tumor”. The authors of this review worked on the basis of an agreed scheme, selecting articles based on their title, language, abstract, and access. This review was conducted based on the Preferred Reporting Items for Systematic Reviews and Meta-Analyses (PRISMA) guidelines (Page et al., 2021). Full-text and accessible articles were reviewed.
In the selection of articles, inclusion and exclusion criteria were used (Figure 2).
Inclusion.
• all types of brain cancer
• qualifying both in vivo and in vitro studies
Exclusion.
• articles in a language other than English or Polish
• articles from before 1990
• no clearly defined effect of PDT on brain tumor cells/tissues
3 Results and discussion
3.1 Malignant neoplasms of the CNS
Central nervous system tumors include primary tumors (benign and malignant) and metastatic tumors located within the spinal cord and brain. Primary tumors of this system have characteristic features, such as no detectable pre-invasive or in situ features, no metastasis outside the CNS, possibility of dissemination through the cerebrospinal fluid (especially anaplastic tumors), and the location of the tumor has a key impact on prognosis. In children, tumors most often arise in the posterior cranial fossa, while in adults, the supratentorial. According to the WHO classification, CNS tumors can be divided into primary glioblastomas, peripheral nerve tumors, embryonic tumors, meningeal tumors, cardiovascular lymphomas, germline tumors, and metastatic tumors. Gliomas, classified on the basis of their similarity to various types of glial cells, include astrocytomas (including grade IV glioblastoma multiforme), oligodendrogliomas, and ependymomas. Poor prognosis is attributed to embryonic neoplasms, all of which are grade IV, especially medulloblastoma located in the cerebellum and occurring mainly in children. On the other hand, meningiomas are usually benign tumors and usually appear in adults (Fujisawa et al., 2000; Holland, 2000; Maher et al., 2006; Furnari et al., 2007; Kanu et al., 2009; Wirsching et al., 2016; Shah and Kochar, 2018; DeCordova et al., 2020; Ma et al., 2021; Martin et al., 2021). Despite medical progress, the average survival time of patients from the moment of diagnosis of GBM using standard treatment methods, such as surgical resection, radio- and chemotherapy, is less than 15 months (The median survival depends largely on the MGMT methylation state of the GBM and 15 months is the worst-case scenario) (Awad and Sloan, 2014), and in combination with temozolomide it ranges from 31.4 months to 48.1 months (DeCordova et al., 2020; Lazaridis et al., 2022). Factors contributing to poor prognosis include late diagnosis of advanced cancer, diffuse and invasive infiltration, pseudonecrosis, microvascular proliferation and resistance to conventional treatment, as well as heterogeneity of tumor cells and tumor microenvironment. Glioblastoma multiforme is usually located in the frontal or temporal lobes, rarely in the brainstem, cerebellum or spinal cord. In approximately 13% of cases, GBM occurs as multifocal or multicentric masses (more than two lesions, including the meninges), may form distant lesions or occur in a diffuse form (Khandwala et al., 2021). About 90% of cases of GBM are primary tumors, developing de novo, mainly in patients over 45 years of age. The remaining 10% develops within 5–10 years from a lower-grade malignancy (secondary GBM) and occurs mainly in patients under 45 years of age (Fujisawa et al., 2000; Holland, 2000; Maher et al., 2006; Furnari et al., 2007; Kanu et al., 2009; DeCordova et al., 2020). The symptoms of the disease depend on the area of the brain affected by the tumor. These include: headaches and dizziness, nausea, vomiting, impaired cognitive functions, confusion, speech disorders (the most common symptoms), convulsions, persistent weakness and fatigue, numbness, loss of vision, impaired executive functions, mood disorders, changes in behavior and even personality or memory disorders (Chaichana et al., 2009; Alexander and Cloughesy, 2017; Dajani et al., 2022). According to the WHO (Louis et al., 2021) classification updated in 2016, GBM is divided into the following molecular subtypes (division due to the presence or absence of mutations in the isocitrate dehydrogenase gene IDH): I- wild type (without mutations) - about 90% of cases, it is primary or de novo and common in patients >55 years of age; II- GBM with IDH mutation (approximately 10% of cases), includes secondary GBM in patients with a history of previous low-grade glioma and often occurs in younger patients; this type has a better prognosis than type I; III- GBM, not otherwise specified (NOS) - a diagnosis intended for tumors for which a full IDH analysis cannot be performed (Batash et al., 2017). Primary GBM markers include epidermal growth factor receptor (EGFR) amplification, PTEN gene mutations (phosphatase and tensin homolog deleted on chromosome 10) and telomerase promoter with reverse transcriptase activity (TERT). Secondary GBM show mutations in the isocitrate dehydrogenase 1 and 2 (IDH1/2) gene, the p53 protein and the ATRX gene (Parsons et al., 2008; Rohle et al., 2013; Schumacher et al., 2014; Bush et al., 2017). Figure 3 shows estimated total number of cases and deaths due to brain and CNS tumors in 2020 in various regions of the world.
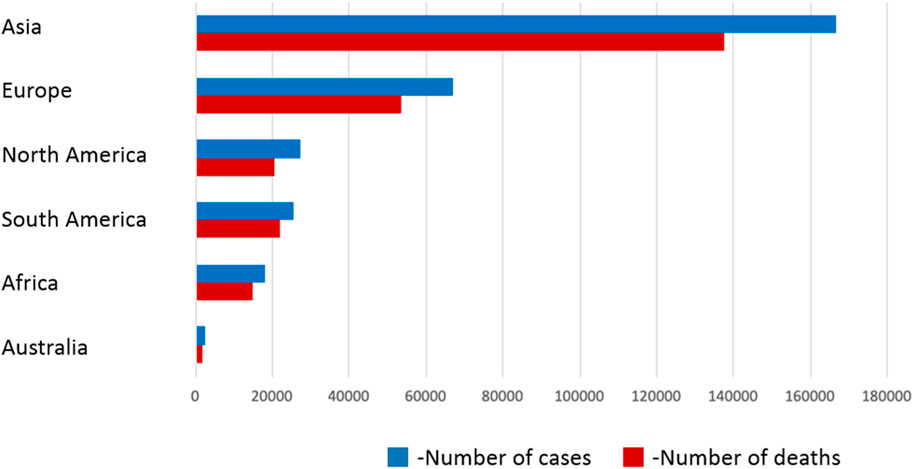
FIGURE 3. Estimated total number of cases and deaths due to brain and CNS tumors in 2020 in various regions of the world. Source: WHO Reports (Louis et al., 2021)
3.2 The use of photodynamic therapy in the treatment of brain tumors (GBM) and factors affecting the effect of therapy
In PDT irreversible destruction of neoplastic tissue can occur in several ways (de Paula et al., 2017). The first is direct cell death via apoptosis, necrotic cell death or autophagy, with apoptosis being the predominantly preferred mechanism (DUBEY et al., 2019). Apoptosis is characterized by nuclear fragmentation, chromatin condensation and formation of apoptotic bodies, while necrotic cell death is characterized by destruction of organelles, disruption of the plasma membrane and induction of an immune response (DUBEY et al., 2019). The second mechanism is the antivascular effect. Activation of photosensitizers leads to the destruction of endothelial cells, which in turn leads to the formation of thrombogenic sites, thus initiating a cascade of reactions leading to vessel closure or damage, leading to the death of cancer cells by depriving them of oxygen and nutrients (Velazquez, 2007). The third mechanism is the activation of the immune response against cancer cells through acute inflammatory processes and the release of cytokines into the tumor, which results in the influx of macrophages and leukocytes that can contribute to tumor destruction and stimulate the immune system to recognize and eliminate cancer cells (de Paula et al., 2017). These mechanisms are interrelated, and the predominance of one pathway over others depends on the parameters used in therapy as well as the disease state and health of the patient (Mroz et al., 2004).
Singlet oxygen has a short diffusion distance and short lifetime, which means that its activity is limited only to local tumor tissues without adversely affecting healthy tissues. According to Semyachkina-Glushkovskaya et al. and Ibarra et al., GBM cells are much more sensitive to the PDT method compared to other cancer cells (Semyachkina-Glushkovskaya et al., 2022a; Ibarra et al., 2021). Nevertheless, the effectiveness of photosensitizers is still not satisfactory, mainly due to their limitations.
3.2.1 Other factors affecting the effect of therapy
The effectiveness of photodynamic therapy is also influenced by the conditions of the tumor environment. The efficiency of production and use of free oxygen radicals is often reduced by deteriorating hypoxic conditions in the tumor. Oxygen concentration in brain tissue is normally 5%–15%, while in gliomas it may reach 0.1% in a necrotic tumor (Ihata et al., 2022).
The use of analgesics such as phenytoin (PHY) in vitro seems to affect the effective-ness of photodynamic therapy. Studies using this drug in 5-ALA therapy indicate a de-crease in PpIX synthesis. However, the effectiveness of PDT does not seem to change (Hefti et al., 2012).
In addition, photodynamic therapy of glioblastoma is also affected by ambient tem-perature. The mere use of a laser in PDT increases the temperature of the tissue, however, the simultaneous exposure of the in vitro culture to hyperthermia (HT) results in a synergistic effect of both therapies. The degree of synergism increases with increasing temperature (Hirschberg et al., 2004).
Proteins associated with oxidative stress (superoxide dismutase, catalase, NO syn-thase), as well as glutathione and related substances (glutathione peroxidase, glutathione S-transferase, omega-1 glutathione transferase, glutathione synthase) and hemoxygen-ase-1 (HO -1), apurin/apyrimidine endonuclease 1/redox factor-1 (APE1/Ref-1) and others affect the resistance of cells to PDT (Shinoda et al., 2021).
Müller et al., research from 2020 proved the different susceptibility of glioblastoma multiforme cells (U251MG line) to PDT depending on the expression of the transporter for protoporphyrin IX - ABCG2. High expression of this receptor reduces the accumulation of the photosensitizer, and then a higher dose of light is required for the appropriate effect of phototherapy. However, in order to restore the appropriate susceptibility of the cell population, an appropriate antagonist for ABCG2 can be used, e.g., non-toxic KO143, gefitinib or lapatinib (Sun et al., 2013; Zhao et al., 2013; Müller et al., 2020; Mansi et al., 2022).
Equally important is the expression of mRNA of the ferrochelatase (FECH) gene, which is an enzyme that catalyzes the conversion of protoporphyrin IX to heme. Silencing FECH results in a marked inhibition of growth and induction of apoptosis by PDT in glioblastoma cells (Teng et al., 2011).
In addition, prior administration of certain substances may result in increased cell death after photodynamic therapy. Such substances include, among others, calcitriol, arsenic trioxide, methadone, motexafin gadolinium (MGd), Shikonin (Madsen et al., 2009; Chen et al., 2014; Werner et al., 2022).
The main advantage of this method is its high precision—cancer tissue or tumor vasculature containing PS can be selectively irradiated sparing healthy tissue (Eljamel, 2008; Eljamel, 2010). Another issue related to PDT is the possibility of using 5-aminolevulinic acid (5-ALA) for intraoperative imaging during brain tumor resection. This compound, localized in cancer cells after administration, enzymatically forms protoporphyrin IV which fluoresces under exposure to blue light making it easier for surgeons to detect residual cancer tissue precisely for minimizing cancer recurrence (Eljamel, 2010). Delivery of PS and other therapeutic drugs to the CNS may be hindered by the need to overcome the blood-brain barrier (BBB) - a physical obstacle for the transport of substances between the blood and the cerebral spinal fluid (CSF), created by closely adhering capillary endothelial cells (Eljamel, 2008; Eljamel, 2010). It has been discovered that PDT can be used to open the BBB (for more effective delivery of drugs), and also to enhance lymphatic drainage and purify the CNS of unnecessary substances (Semyachkina-Glushkovskaya et al., 2022b). Currently, various methods of transporting medicinal substances to the tumor are being developed, of which the use of nanoparticles as carriers seems promising. They reach the tumor microenvironment (TME) by combining with its components (including immune cells) and are able to modify them, including stimulating the body’s immune-anti-cancer reactions. Nanoparticles accumulate in the tumor due to leaky vasculature and damaged lymphatic drainage, which significantly increases the concentration of drugs in the diseased tissue and reduces side effects (Casas, 2020; Yang et al., 2020). Currently, much research is focused on the development of targeted GBM immunotherapy, aimed at “reprogramming” the immune system to fight cancer. First of all, the use of immune checkpoint inhibitors (ICIs) and T lymphocytes with chimeric antigen receptors (CARs), which have been effective in the treatment of other cancers, is being considered (Yu and Quail, 2021). Other developed methods of targeted treatment of brain tumors include, among others, vaccine and gene therapy, which consists in introducing modified genes into tumor cells in order to destroy them (Eljamel, 2008).
3.3 Efficiency and advantage of PDT in the treatment of glioblastoma - examples from the literature
Photodynamic therapy was first approved in 1993 in Canada using the photosensitizer Photofrin for the treatment of bladder cancer (Dolmans et al., 2003). Since then, many papers have been published confirming the advantages of PDT in the treatment of gliomas and showing its advantage over standard surgical therapy. Examples are shown below.
Observations showed that patients who underwent surgery combined with photodynamic therapy showed a longer survival period than those who underwent surgery alone (Bartusik-Aebisher et al., 2022). A 2011 study reported 73 male patients with GBM. They received standard therapy (ST), ST + PDT or ST + PDT + IORT (intraoperative radiotherapy). The mean survival of patients treated with PDT was significantly longer than those treated with ST alone (62.9 weeks vs. 20.6 weeks). IORT alone did not make a significant difference in survival (Lyons et al., 2012). Another study showed that after using PTD with 5-ALA in a group of 10 patients with unresectable recurrent gliomas, the median survival from 6 to 8 months increased to about 15 (Beck et al., 2007). Similarly, another study (Stummer et al., 2006) showed that intraoperative PDT with 5-aminolevulinic acid allowed for more accurate glioblastoma resection and increased the number of patients with 6-month recurrence-free survival from 21.1% to 41%. Similar conclusions were included in the study (Eljamel et al., 2008), where it was indicated that intraoperative use of 5-ALA to facilitate GBM resection and repeated use of PTD allowed to extend the average survival in the study group from 24.6 to 52.8 weeks and the average progression-free survival tumor from 4.8 to 8.6 months. A study (Stummer et al., 2008) describes a case of a patient with GBM, previously treated with surgery, radiotherapy and chemotherapy, who developed a neoplastic lesion in the insula, resistant to secondary treatment. PDT with 5-ALA was applied and the lesion disappeared after 24 h. At the time of writing the paper by the researchers, the patient has still not relapsed after 56 months of therapy, which is an impressive result. In a study (Stylli et al., 2005), the effect of PDT with the use of HpD on the survival of patients with anaplastic astrocytoma (AA) and GBM after surgical removal of the tumor was tested. For newly diagnosed GBM, the median survival from diagnosis was 14.3 months, and after treatment with PDT, 25% of patients with this glioma survived for more than 36 months. In the case of recurrent GBM, the median survival from the time of surgery was 13.5 months, after PDT treatment, the survival rate of 41% of patients was more than 36 months. These examples and many others testify to the progress and increase in the effectiveness of GBM treatment through the use of photodynamic therapy. Lietke et al., in turn, performed interstitial PDT in combination with 5-ALA in patients with recurrent malignant glioma. Median age of patients 49.4 years. The eligibility criterion was the presence of glioblastoma recurrence. The maximum lesion size was not to exceed 3 cm. A diode laser (wavelength 635 nm) was used as the light source. The applied photosensitizer was 5-ALA at a dose of 20 mg/kg of body weight. The duration of the procedure was 60 min. The therapeutic effect was analyzed by magnetic resonance imaging with contrast. One of the main findings of the study was that interstitial PDT (iPDT) appears to be associated with beneficial treatment outcomes even in heavily treated malignant glioma relapses (Lietke et al., 2021).
3.4 Photosensitizers - 5-aminolevulinic acid, protoporphyrin IX, photofrin
5-aminolevulinic acid (ALA) is an organic chemical compound from the group of keto acids and amino acids, which is a derivative of levulinic acid. It is a precursor to the synthesis of porphyrins, including heme. In a several-stage process, ALA is converted to protoporphyrin IX (PpIX) - an endogenous photosensitizer accumulating in cancer cells (therefore, 5-ALA and its derivatives are considered prodrugs in PDT) (Mahmoudi et al., 2019). Protoporphyrin IV is a photoactive compound, which is used during tumor imaging during surgery - after exposure to blue-violet light, it is possible to observe the fluorescence of tumor cells in the red light wavelength range. Activation of PpIX by red light combined with oxygen produces singlet oxygen and causes cell death by apoptosis and necrosis. In this case, the use of red light for irradiation is more beneficial due to better penetration into tissues at a wavelength of 632 nm (Eljamel et al., 2008). Accumulation of protoporphyrin in tumor tissues results from, among others, due to the fact that their cells are characterized by lower expression of ferrocelatase - an enzyme converting PpIX into heme (Yang et al., 2015). In addition, it was found that by affecting the expression of enzymes involved in the synthesis of PpIX, such as porphobilinogen synthase (PBGS) and porphobilinogen deaminase (PBGD), tissue susceptibility to PDT can be regulated (Yang et al., 2015). The fluorescent and photosensitizing properties of 5-ALA/PpIX are particularly useful in the treatment of HGG (high-grade gliomas) by both FGS (Fluorescence-guided surgery) with blue light activation and PDT with red light activation (Mahmoudi et al., 2019). The first method (5-ALA in FGS for HGG) was approved by the European Union, while the FDA recognized 5-ALA as the first ever fluorescent agent for intraoperative visualization of brain tumors, enabling more effective resection (Mahmoudi et al., 2019). Another common photosensitizer is Porfimer sodium with the trade name Photofrin, which is a hematoporphyrin (HpD) derivative. The hematoporphyrin derivative (HpD; Photofrin®) was the first photosensitizer to be thoroughly investigated (Tetard et al., 2014). It accumulates in cancer cells and is activated by laser light with a wavelength of 630 nm (red light) and contact with tissue oxygen to produce highly reactive excited singlet oxygen, which oxidizes cancer cell components, e.g., mitochondrial enzymes, and leads to their destruction. In addition, this compound is used to enhance the fluorescence of PpIX in the intraoperative treatment of gliomas (Eljamel et al., 2008; Yang et al., 2015). The selectivity of Photofrin in settling in tumor cells may be due to the increased expression of LDL receptors on their surface, which bind lipoproteins circulating in the blood, which are carriers of photosensitizers, including sodium porfimer (Candide et al., 1986; Korbelik, 1992; Korbelik, 1993; Tsukagoshi, 1995; Misawa et al., 2005). This mechanism, as well as the participation of other plasma proteins in the distribution of photosensitizers, creates a field for discussion and further research. Currently, PS molecules are divided into three generations of compounds. The first generation includes naturally occurring porphyrins - including hematoporphyrins and HpD (Photofrin®, a mixture of dimers and oligomers of porphyrins, also known as sodium porfimer). The second generation includes chlorides (sodium talaporfin and temoporfin), benzoporphyrin derivatives, texapyrins, thiopurine derivatives, bacteriochlorin analogs, phthalocyanines and 5-aminolevulinic acid. These compounds are activated at a wavelength >600nm, show greater efficiency in the formation of singlet oxygen and seem to be more effective (Kwiatkowski et al., 2018). Third-generation photosensitizers show greater selectivity for tumor cells and have minimal accumulation in normal tissue. This group includes combinations of photosensitizers of the second generation with molecules targeting the tumor cell receptor, a combination with LDL lipoprotein, a monoclonal antibody targeting a specific antigen or tumor surface markers (such as growth factor receptors, transferrins or some hormones) (Josefsen et al., 2008).
3.4.1 Other photosensitizers in PDT
Turubanova et al. used a standard mixture of PS (di-, tri- and tetrasubstituted fractions of aluminum phthalocyanine) and PD (bis-N-methylglucoamine chloride e6) at a concentra-tion of 10 μg/ml as photosensitizers. Their behavior under the influence of light with wavelengths between 320 and 850 nm for PS and 300–700 nm for PD was studied, re-spectively. In vitro studies on the mouse glioblastoma line GL261 have shown that both PS-PDT and PD-PDT are strong inducers of cancer cell death at a light dose of 20 J/cm2 (λex 615–635 nm) (Turubanova et al., 2019). Similar results to 5-ALA are also achieved using 10–20 times lower concentrations of lipophilic 5-aminolevulinic acid esters, such as benzyl-ALA and hex-yl-ALA. This property may result from increased penetration through the cell membrane (Hirschberg et al., 2002). Another potential photosensitizer in PDT of brain glioma may be TiO(2)/PEG, i.e., a combination of polyethylene glycol with titanium oxide, the therapeutic effect of which was confirmed on the spheroids of the rat C6 glioma line (Yamaguchi et al., 2010). Verteporphyrin (VP) may also be of importance as a potential drug in the treatment of glioblastoma of the brain, which, at marginal concentrations and when treated with a 689 nm laser beam, turns out to be a good photosensitizer. This was proved by the in vitro studies of Jeising et al., in which the effect of VP on the LN229 and HSR-GBM1 glioblastoma cell lines was examined (Jeising et al., 2022). Among others, sodium sinoporphyrin and me-so-tetra[3-(N,N-diethyl)aminomethyl-4-methoxy]phenylchlorone (TMPC) may be of importance as photosensitizers in PDT of brain glioma (An et al., 2020; Wang et al., 2015). Many other potential photosensitizers may prove to be good alternatives to 5-ALA and hematoporphyrin in the future. More research is needed to prove or disprove their effectiveness.
The photosensitizer is usually administered intravenously and the substance accumulates in the target cancer cells (Eljamel, 2008; Eljamel, 2010; Quirk et al., 2015; Mahmoudi et al., 2019; Bartusik-Aebisher et al., 2022). In the case of GBM, there are 2 methods of irradiating the tumor with a laser of a specific wavelength: intracavitary PDT and interstitial PDT (iPDT) (Dupont et al., 2019; Vermandel et al., 2021). Initially, argon-dye and xenon lasers were widely used, then around 2000, diode lasers were introduced, which are still used today. An alternative is light-emitting diodes (LEDs). They have recently been shown to be as effective and less expensive than their traditional counterparts. Optical fiber devices with cylindrical ends of dispersing fibers are also used (Schmidt et al., 2004). In the case of intracavitary PDT, an expandable irradiating balloon filled with a diluted liquid photodistributor is used and introduced into the operating cavity after prior surgical resection of the primary tumor. In turn, iPDT is a minimally invasive method used in the case of inoperable or recurrent GBM tumors. It consists of stereotactic placement of the previously mentioned scattering fibers directly in the brain tissue (Powers and Brown, 1986; Zhan et al., 2018). After irradiation, the photosensitizer is activated - energy is transferred from PS to molecular oxygen to produce ROS. These reactions occur directly at the irradiated site, sparing healthy tissues. The correlation between the width of the emission band of the light source and the width of the absorption band of the dye is important (Dolmans et al., 2003). The photosensitizer can be administered in various ways, intravenously or topically on the skin, which affects its biodistribution. Upon absorption of light (photons), the sensitizer is converted from its ground state (singlet state) to a relatively long-lasting excited state (triplet state) via a short-lived excited singlet state. The excited triplet form can undergo two types of reactions. Type I is a direct reaction of an excited triplet with a substrate: a cell membrane or a molecule forming radicals such as hydroxy radical that are able to damage cellular structured. In Type II reactions, thetriplet transfers its energy directly to the oxygen to form highly reactive singlet oxygen. Both types of reactions can occur simultaneously, however, for tissue-based PS it is assumed that mechanism II is dominant and determines the effectiveness of therapy. The ROS generated is influenced by substrate and oxygen concentration, pH of the environment and dye quantum yield (Gomer and Razum, 1984; Henderson and Dougherty, 1992; Pass, 1993; Luksiene, 2003; Vrouenraets et al., 2003; Allison et al., 2006). Photodynamic therapy uses different wavelengths of visible light depending on the photosensitizer and its absorption range, as well as the desired depth of penetration of the light into the tissue. The general wavelength range is from about 405 to 900 nm (Quirk et al., 2015). When it comes to photosensitizers, they must meet several conditions: they must be systemically non-toxic, concentrate in the cancerous tissue, absorb light of the appropriate wavelength and not cause damage to the adjacent healthy tissues.
So far, the following PS have been used for this purpose: hematoporphyrin and its derivative HpD, photophrin, boron porphyrin, talaporfin sodium, metatetrahydroxyphenylchlorin (mTHPC) and metabolic precursors of protoporphyrin, such as 5-aminolevulinic acid. These substances can be divided into 3 generations - the first includes natural porphyrins, hematoporphyrins and their derivatives, the second includes chlorins - sodium talaporfin and temoporfin, and the third generation consists of nanoparticles carrying a photosensitizer conjugated with molecules facilitating tumor targeting (Josefsen and Boyle, 2008; Kou et al., 2017; Stallivieri et al., 2018). Photodynamic therapy can destroy cancer cells in three ways. Due to the generation of reactive oxygen species, such as singlet molecular oxygen, hydroxyl radicals and/or superoxide anions, tumor cells are directly destroyed by oxidation of the constituent cell organelles. Cell death can occur through necrosis, apoptosis, autophagy, necroptosis and paraptosis (Kessel, 2019). However, the effectiveness of this method depends on several factors: even distribution of the photosensitizer within the tumor, the distance between the tumor and blood vessels in the case of intravenous administration of the photosensitizer, and the availability of oxygen in the tissue (Tromberg et al., 1990; Korbelik and Krosl, 1994; Messmann et al., 1995; Pogue and Hasan, 1997). Another anticancer mechanism of PDT is the destruction of the tumor microcirculation. Documented studies on PDT with the use of such photosensitizers as porfimer sodium, pyrophorbide derivatives and benzoporphyrin derivatives indicated narrowing of tumor microcirculation vessels, as well as thrombus formation leading to reduced blood supply to the diseased tissue, and thus, its death. On the other hand, the use of PDT contributed to the increased expression of angiogenic factors such as vascular endothelial growth factor (VEGF) and cyclooxygenase (COX)-2, but this fact is not often mentioned in the literature and seems to be less important than the positive effect of PDT on the destruction of tumor vascularization (Henderson and Fingar, 1989; Chen et al., 1996; Fingar et al., 1997; Fingar et al., 1999; Ferrario et al., 2000; Busch et al., 2002; Dolmans et al., 2002; Ferrario et al., 2002). The third mode of action of PDT is participation in the activation of the immune system, which leads to the influx of immune cells into the tumor tissue: leukocytes, lymphocytes and macrophages, and triggers an inflammatory reaction. The process also involves vasoactive substances, components of complement and coagulation cascades, acute phase proteins, proteinases, peroxidases, ROS, leukocyte chemo-attractants, cytokines, growth factors, as well as inflammatory interleukins IL-1 and IL-6. The accumulation of neutrophils has also been found to limit the rate of tumor development (Shumaker and Hetzel, 1987; de Vree et al., 1996; Gollnick et al., 1997). In addition, PTD contributes to the development of an immune response directed against cancer cells, by, e.g., release of tumor antigens from damaged cells. This leads to the stimulation of antigen-presenting cells, such as dendritic cells, which in turn enables the next stages of “setting” the body to fight the diseased cells (Etminan et al., 2011; Li et al., 2011). This is related to the recently discovered use of PDT in the temporary opening of the blood-brain barrier (OBBB). This creates new perspectives for the delivery of drugs to the CNS, which is currently difficult due to the BBB. According to new reports, OBBB enables the activation of lymphatic drainage of brain tissues through the lymphatic vessels of the meninges. Cells and macromolecules, including cancer antigen-presenting cells from PDT-damaged GBM cells, are removed via this route from the CNS to the deep cervical lymph nodes. In the lymph nodes, antigens are presented and CD4+/CD8+ lymphocytes are activated, which migrate back to the vicinity of the tumor and stimulate the destruction of its mass (Semyachkina-Glushkovskaya et al., 2018; Hu et al., 2020; Kanamori and Kipnis, 2020; Semyachkina-Glushkovskaya et al., 2020). Currently, due to the immunomodulatory functions of PDT, research is being conducted on the issue of using GBM cells changed during therapy as a vaccine for this type of glioblastoma (Hirschberg et al., 2018; Madsen et al., 2018). Further research is needed to visualize the distribution of photosensitizers in the body, as well as to improve the methods of measuring the amount of oxygen produced necessary to destroy cancer cells (Mahmoudi et al., 2019). Limitations of PDT include the fact that the remaining, undegraded photosensitizer must be removed from the body, and the possibility of photosensitivity of the skin after its administration. In addition, destruction of hypoxic tumors may be hindered by the low availability of oxygen for the necessary reactions. These problems are likely to be solved in the near future (Eljamel, 2010; Quirk et al., 2015; Mahmoudi et al., 2019; Bartusik-Aebisher et al., 2022). Figure 4 shows mechanisms of destroying cancer cells by PDT.
3.5 Standard methods in the treatment of a brain tumor
Treatment methods for glioblastoma aim to remove the tumor completely or at least reduce its size in order to alleviate the symptoms and prolong the patient’s life with as much comfort as possible. The choice of method depends on many factors, including the type, size and location of the tumor, as well as age, medical history, general health, and the intensity and nature of the symptoms observed in the patient. Standard therapy is based on three methods: surgery, radio-(RT) and chemotherapy (Shah and Kochar, 2018). Surgical resection is usually used in the early stages of cancer, especially in the case of benign tumors, because then it can ensure a complete cure. The main limitation of this method is that many tumors are located in places where access is limited, and interference could result in damage to important brain structures with serious health consequences for the patient. Complications may also occur after a properly performed procedure. Moreover, often the patient’s general condition does not allow for surgical intervention with the use of general anesthesia (Shah and Kochar, 2018). Another method is radiotherapy, which is based on the destruction of cancer cells using ionizing radiation - photon, electron or proton. Radiation causes ionization of molecules in the irradiated tissue, which results in DNA damage and cell death. Radiation energy is absorbed directly by cellular structures: DNA, organelles and cell membrane or indirectly by induction of highly reactive free radicals in the cytosol. This process is more effective in an environment rich in molecular oxygen. Due to the method of delivering RT radiation, it can be divided into brachytherapy, where the source of rays is located in the immediate vicinity of the tumor or inside it, which allows for precise delivery of a large dose to the desired structure, and teleradiotherapy, where the irradiation of tissues takes place from a certain distance (with using a linear accelerator as a source of photons). A more precise method, derived from teleradiotherapy, are stereotactic techniques, also referred to as stereotactic radiosurgery using, for example, a gamma knife [GK]. Radiotherapy is used to treat most types of brain tumors, but with varying success - for example, glioblastomas tend to progress despite high doses of radiation. Radiotherapy is also associated with many annoying side effects, such as fatigue, headaches, nausea and hair loss (Laperriere and Bernstein, 1994; Johnson et al., 2008; Minniti et al., 2012). Another standard method of treatment is chemotherapy, based on the administration of cytostatic drugs that inhibit cell division. Its main limitations include the systemic toxic effect of the compounds used and the disruption of the differentiation of healthy cells, e.g., in the bone marrow, which results in a reduction in the number of immune cells and impairment of the body’s immune functions. Standard first-line chemotherapy involves the use of temozolomide (75 mg/m2 daily) during radiotherapy, followed by 6 consecutive cycles of this drug (150–200 mg/m2 on days 1–5 every 28 days). The most common side effects are nausea, thrombocytopenia and neutropenia. Usually, after the first course of treatment, the disease recurs within 6 months. In the second line, alkylating chemotherapy is usually used: lomustine, carmustine, and a second attempt at temozolomide is made. The effectiveness of chemotherapeutics is often reduced by the need to cross the blood-brain barrier and limited accumulation in tumor cells (Tan et al., 2020; Saito, 2021).
3.6 Targeted methods in the treatment of a brain tumor
Targeted therapy aims to increase the precision of cancer therapy by increasing the toxicity of drugs used for diseased cells while sparing healthy tissues. Such selective action can be achieved by using receptors on cancer cells and absent or present in a reduced amount on healthy cells. It is, among others in the case of LDL receptors, which are used to deliver photosensitizers to cancer cells (Gutman et al., 2000). Glioblastoma cells also differ from normal cells by the presence or overexpression of interleukin-13 (IL13) receptors, fibroblast growth factor (FGF) receptor, Neu/ErbB2 receptor, tumor-specific antigens including MAGE, and tumor-associated extracellular matrix proteins, such as chondroitin sulfate proteoglycan. These and other receptors can become a target for appropriately modified antibodies, which enables new directions of therapy (Gutman et al., 2000). Methods that target enzymes or growth factors crucial for tumor division and growth, such as tyrosine kinase (TKI) or vascular endothelial growth factor (VEGF), are also being investigated. Monoclonal antibodies such as Bevacizumab, which binds to vascular endothelial growth factor (VEGF) and thus slows down tumor angiogenesis, are used here. It is often used in combination with chemotherapy (Diaz et al., 2017). It is well known that in cancer cells there are mutations in the p53 protein gene as well as in the genetic material of the MDM2 and MDM4 proteins that regulate it, so that p53 does not perform its functions in controlling the cell cycle and preventing excessive cell proliferation. For this reason, inhibitors of defective proteins are being developed, such as the MDM2-AMG 232 inhibitor, which can be used to slow down tumor progression (Yang et al., 2022). Glioblastoma immunotherapy, on the other hand, focuses on stimulating the immune system in order to “tune” it to fight the diseased cells, which is related to the work on cancer vaccines. In addition, research is being conducted on the tumor microenvironment (TME), consisting of immune cells (mostly macrophages), fibroblasts, endothelial cells, extracellular matrix (ECM), vascular system and chemokines. TAMs is an abbreviation for tumor-associated macrophages and microglial cells that are recruited into the tumor environment to produce factors that promote tumor growth, including by promoting angiogenesis. These macrophages have receptors for the colony-forming factor CSF-1R on their surface, which can be blocked using specific inhibitors, e.g., BLZ945 and PLX3397. Another method of affecting immune cells is tumor antigen vaccines designed to stimulate dendritic cells (e.g., DCVax-L) and other APCs. Antigens can be obtained, for example, from heat shock proteins or purified peptides derived from tumor cells, as in the case of the NCT01814813 and NCT03018288 vaccines (Miyauchi and Tsirka, 2018). After contact with a foreign antigen, dendritic cells present it to T lymphocytes, which mobilizes them to destroy cancer cells. An example of a DC vaccine is ICT-107, which sensitizes APCs to tumor antigens such as HER2, TRP-2, gp100, MAGE-1, IL13Rα2 and AIM-2. DC vaccination may contribute to the prolongation of patients’ lives and prolongation of the disease progression-free period (Yang et al., 2015; Hoang-Minh and Mitchell, 2018; Miyauchi and Tsirka, 2018). An important discovery was also the development of immune checkpoint inhibitors.
So-called checkpoints, i.e., PD-1 and CTLA-4 molecules, are found on the surface of T and B lymphocytes. In the case of chronic inflammation, as well as in the course of cancer, these receptors are upregulated, which by connecting with their ligands (PD-L1) present on cancer cells, macrophages and APCs within the tumor cause the inhibition of proliferation and apoptosis of T lymphocytes (except for Tregs). All this contributes to a decrease in the population of Th and Tc lymphocytes, an increase in the number of Tregs, and thus immunosuppression favoring the development of cancer. For this reason, the discovery of checkpoint inhibitors: anti-CTLA-4 (e.g., ipilimumab) and anti-PD-1/PD-L1 (e.g., nivolumab, pembrolizumab) ICIs seems to be a promising method in the treatment of glioblastoma (Prins et al., 2011; Preus et al., 2015; Berghoff et al., 2016; Butowski et al., 2016; Quail and Joyce, 2017). Another direction of immunotherapy, which is gaining more and more importance, are CAR-T, i.e., modified T lymphocytes, taken from a patient or a healthy donor, to which the CAR receptor is attached, which can recognize cancer antigens, bind to them and activate the T lymphocyte, thus cancer cells are destroyed in different ways. The chimeric CAR itself consists of an extracellular antigen-sensing domain and an intracellular signaling-lymphocyte-activating domain linked by a transmembrane linker. In the case of brain tumors, the following are considered as targets for modified lymphocytes: EGRFvIII - mutated epidermal growth factor receptor (EGFR), which is the most common variant of this receptor in cancer cells, IL13Rα2 - receptor for IL-13, which is expressed in more than 75% of GBM and heralds a high aggressiveness of the tumor and poor prognosis, HER-2, i.e., a receptor for human epidermal growth factor, which is overexpressed in many types of cancer and in about 80% of GBM, as well as such cancer antigens as B7-H3, CD147 or GD2. The limitation of CAR-T therapy are difficulties in the delivery and accumulation of lymphocytes in the tumor tissue due to the need to penetrate the blood-brain barrier. The solution may be to place the modified cells directly in the tumor mass or in the cavity after its resection. CAR-T is often combined with other methods of treatment, such as radio- and chemotherapy, and other forms of immunotherapy in order to achieve a better therapeutic effect (Ch et al., 2020; Lindo et al., 2021; Marei et al., 2021). An interesting method is also gene therapy, which uses various vectors, including viruses, to introduce changes in the genetic material of cancer cells and thus to destroy them. The introduction of modified genes contributes to the damage of cancer cells in various ways. It may be cytotoxic, directly causing cell death by blocking DNA synthesis, as well as restoring mutated tumor suppressor genes to normal function, thereby controlling the cell cycle and preventing excessive cell proliferation, as well as blocking tumor angiogenesis. Modified viruses can also lead to the destruction of cancer cells themselves. Viruses that are being studied for adaptation to therapy include: Delta-24-RGD adenovirus (DNX-2401), measles virus, herpes simplex virus and polio virus PVS-RIPO (NCT01491893), as well as genetically modified HSV, reoviruses and Newcastle disease virus. Glioblastoma cells are characterized by an increased expression of the CD155 receptor, which is recognized by poliovirus. When combined, PVS-RIPO leads to immunogenic cell death with the release of tumor antigens, which are taken up by dendritic cells and presented to T lymphocytes, thereby stimulating the immune system to fight the tumor.
The destruction of tumor cells is also carried out by implanting the genetic material of the virus into their genome, e.g., thymidine kinase gene. Then, antiviral drugs are administered, e.g., ganciclovir, which destroys the cells that produce viral proteins. In turn, the use of modified adenoviruses expressing IL-12 may lead to the transformation of TAMs into anticancer phenotypes (Dunn-Pirio and Vlahovic, 2017; Maxwell et al., 2017). Figure 5A shows mechanism of selective attachment of CAR-T lymphocytes to tumor cell receptors and Figure 5B structure of a modified T lymphocyte with the CAR receptor.
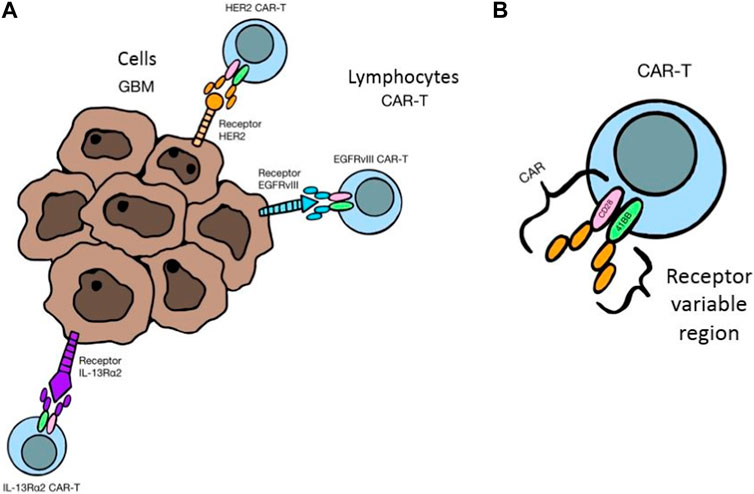
FIGURE 5. (A) Mechanism of selective attachment of CAR-T lymphocytes to tumor cell receptors, 3 (B). Structure of a modified T lymphocyte with the CAR receptor.
3.6.1 Nanoparticles supporting PDT
More and more often, in combination with PDT, nanocomposites and nanoparticles are used to support the effectiveness of therapy (Jin et al., 2021). Research shows that the use of various elements of nanomedicine improves treatment results. Below are some examples of nanocomposites supporting PDT. One example is nanoparticles that reverse tumor hypoxia (Wang et al., 2019). This group of nanoparticles includes quinones and nitroimidazoles. Nanoparticles consisting of quinones are selectively activated in a site with limited oxygen saturation, mainly in the environment of tumor cells. In turn, nitroimidazole is usually one of the elements developed in PDT. May be part of a complex in combination with ROS (Qian et al., 2016). Another example is upconverting nanoparticles (UCNPs). According to Zhang et al., the upconversion of Au-doped nanoparticles has a relatively high light intensity. In particular, Au-doped UCNPs are non-toxic to tissues, making them extremely effective (Zhang et al., 2022). In turn, the Tumor Microenvironment (TME)-Responsive nanoparticles group includes such platforms as: nanogels, hybrid micelles, nanocoatings, and miceplexes. According to Qin et al., nanogels are an effective and safe method of drug delivery in therapy (Qin et al., 2020). Just like hybrid micelles or miceplexes. Chemo-photodynamic therapy and the use of organometallic structures in combination with photosensitizers are gaining in popularity. Designed and applied by Zheng et al., complexes of nanoparticles with organometallic substrates had high anticancer activity and satisfactory biocompatibility (Zeng et al., 2021). Another example is carrier-free nanoplatforms. According to Ji et al., the nanoplatform designed in this way allows the drug to be applied for the application of the photosensitizer without the occurrence of potential side effects. Another example is the study carried out by Wang et al., who constructed a carrier-free nanoplatform in combination with ICG and αPD-L1, which can self-assemble into nanoparticles (Wang et al., 2019). The last mentioned group of nanoparticles are subcellular targeted nanoparticles in PDT. The principle of operation of these consists in targeted targeting of cellular structures, i.e., mitochondria, lysosomes, cell nuclei or the plasma membrane. According to Ji et al., central delivery of photosensitizers to, e.g., mitochondria using a nanocomposite may be a potential strategy to enhance cancer immunotherapy (Ji et al., 2022). Figure 6 shows examples of nanostructures used in PDT.
There is still a need for studies evaluating the correlation of PDT with various nanocomposites or nanostructures in combination with applied photosensitizers. Advanced developed structures in the field of nanomedicine can be a promising and, above all, a common therapeutic tool.
3.6.2 The advantages and the disadvantages of PDT compared to the other therapies
Surgical resection is the most common tool in cancer treatment (Juvekar et al., 2023). Its highest effectiveness occurs when applied in the early stages. Despite its commonness, it has several challenges and problems that PDT can solve. The first is the location of the tumor in the patient’s body. Tumors located deep or in hard-to-reach places are a challenge for surgeons. In addition, organs made of connective tissue are localized and constitute an important structure, such as bronchi or the brain. In such cases, the doctor does not decide to perform resection. In such cases, minimally invasive methods such as PDT are necessary. PDT as a therapeutic method damages cancer cells, leaving the collagen structure intact (Overchuk et al., 2023). Therefore, in cases where resection is impossible, PDT is applied. Another disadvantage of surgical resection are the side effects and complications after the procedure, i.e., nausea, problems with the healing wound, pain (Gan, 2017). In turn, PDT has fewer side effects and the wounds after the procedure are very small or do not occur at all. Another aspect of resection is that when removing cancerous tissues, the doctor also removes a several millimeter margin of healthy tissue. The solution is PDT, which works selectively. The applied photosensitizer selectively accumulates in cancer cells, and not in the surrounding healthy cells without signs of disease. Therefore, PDT is a cancer-selective method (Hong et al., 2016). The last aspect is the whole resection procedure. It requires prior preparation. In contrast, PDT can be used on an outpatient basis. Compared to radiotherapy, PDT can be used many times, so it is reproducible. In addition, like surgery, radiotherapy is associated with side effects, which makes PDT an alternative in this aspect. The most crucial difference between PDT and radiotherapy is that it takes work to assess dosimetry at the time of treatment in PDT accurately. Furthermore PDT compared to radiotherapy uses nonionizing radiation (Zhu and Finlay, 2008). It can be used for a long time, in stages or sequentially. Therefore, it is necessary to establish a method to evaluate the therapeutic effect on tumor cells and side effects on normal brain cells with high reliability. Compared to chemotherapy, PDT does not work systemically, but locally. Thanks to this, the patient is not exposed to adverse side effects. In turn, immunotherapy is a very expensive and more expensive method than other forms. In turn, PDT generates lower costs, which makes it widely applicable. In addition, immunotherapy may correlate with other applied therapies, which reduces the effectiveness of treatment. According to Calixto et al., one of the main disadvantages of PDT is the hypersensitivity of patients to light after treatment. This aspect does not appear with other methods. In addition, at the moment there is no clearly defined effective dose of the photosensitizer and the time of exposure to light. The effectiveness of the therapy depends on the most precise delivery of the light source. In PDT, it is also necessary to properly oxygenate the treated tissue, which is not the case in other therapies. Additionally, PDT is not used to treat metastatic cancer (Calixto et al., 2016).
4 Summary
Malignant tumors of the brain and central nervous system (CNS) are a serious threat to health and cause the death of people. Current standard treatments for brain tumors have some limitations. New therapeutic methods are constantly being sought. One such solution is PDT. The main principle of operation of PDT is based on the effect of combining photosensitizer molecules with cancer cells and their activation by excitation with laser light of the appropriate wavelength. The main advantage of this method is its high precision—cancer tissue or tumor vasculature containing PS can be selectively irradiated sparing healthy tissue. Photodynamic therapy was first approved in 1993 using the photosensitizer Photofrin for the treatment of bladder cancer. Since then, many papers have been published confirming the advantages of PDT in the treatment of gliomas and showing its advantage over standard surgical therapy. Currently, in the PDT treatment of brain tumors, the selection of the photosensitizer and, consequently, the selection of the light source still remains a challenge. Therefore, the solution may be targeted therapies or the use of nanocomposites.
5 Conclusion
Brain tumors, including glioblastoma multiforme, are currently the cause of suffering and death of tens of thousands of people worldwide. Despite the progress of medicine, the average patient survival from the moment of diagnosis of GBM with the use of standard treatment methods, such as surgical resection, radio- and chemotherapy, is less than 4 years. The development of new therapeutic methods targeting brain tumors may extend life and provide greater comfort to patients. Particular attention should be paid to photodynamic therapy, the effectiveness of which in the treatment of GBM, resulting in prolongation of progression-free survival, has been confirmed in many scientific studies (although still leaving the fact of the effectiveness of photosensitizers to be improved). Another important aspect is the ongoing development of immunological therapies for brain tumors, which have already been used in the treatment of other cancers with positive results. Cancer vaccines, monoclonal antibodies, gene therapies or modified CAR-T lymphocytes form a dynamically progressing branch of medicine, which is of interest to many scientists and may revolutionize the future of brain tumor treatment.
Author contributions
All authors listed have made a substantial, direct, and intellectual contribution to the work and approved it for publication.
Conflict of interest
The authors declare that the research was conducted in the absence of any commercial or financial relationships that could be construed as a potential conflict of interest.
Publisher’s note
All claims expressed in this article are solely those of the authors and do not necessarily represent those of their affiliated organizations, or those of the publisher, the editors and the reviewers. Any product that may be evaluated in this article, or claim that may be made by its manufacturer, is not guaranteed or endorsed by the publisher.
References
Alexander, B. M., and Cloughesy, T. F. (2017). Adult glioblastoma. J. Clin. Oncol. 35 (21), 2402–2409. doi:10.1200/JCO.2017.73.0119
Allison, R. R., Bagnato, V. S., Cuenca, R., Downie, G. H., and Sibata, C. H. (2006). The future of photodynamic therapy in oncology. Future Oncol. 2 (1), 53–71. doi:10.2217/14796694.2.1.53
An, Y-W., Liu, H. Q., Zhou, Z. Q., Wang, J. C., Jiang, G. Y., Li, Z. W., et al. (2020). Sinoporphyrin sodium is a promising sensitizer for photodynamic and sonodynamic therapy in glioma. Oncol. Rep. 44, 1596–1604. doi:10.3892/or.2020.7695
Awad, A. J., and Sloan, A. (2014). The use of 5-ALA in glioblastoma resection: Two cases with long-term progression-free survival. Cureus 6, e202. doi:10.7759/cureus.202
Bartusik-Aebisher, D., Żołyniak, A., Barnaś, E., Machorowska-Pieniążek, A., Oleś, P., Kawczyk-Krupka, A., et al. (2022). The use of photodynamic therapy in the treatment of brain tumors-A review of the literature. Molecules 27 (20), 6847. doi:10.3390/molecules27206847
Batash, R., Asna, N., Schaffer, P., Francis, N., and Schaffer, M. (2017). Glioblastoma multiforme, diagnosis and treatment; recent literature review. Curr. Med. Chem. 24 (27), 3002–3009. doi:10.2174/0929867324666170516123206
Beck, T. J., Kreth, F. W., Beyer, W., Mehrkens, J. H., Obermeier, A., Stepp, H., et al. (2007). Interstitial photodynamic therapy of nonresectable malignant glioma recurrences using 5-aminolevulinic acid induced protoporphyrin IX. Lasers Surg. Med. 39 (5), 386–393. doi:10.1002/lsm.20507
Berghoff, A. S., Venur, V. A., Preusser, M., and Ahluwalia, M. S. (2016). Immune checkpoint inhibitors in brain metastases: From biology to treatment. Annu. Meet. 35, e116–e122. doi:10.1200/EDBK_100005
Busch, T. M., Wileyto, E. P., Emanuele, M. J., Del Piero, F., Marconato, L., Glatstein, E., et al. (2002). Photodynamic therapy creates fluence rate-dependent gradients in the intratumoral spatial distribution of oxygen. Cancer Res. 62 (24), 7273–7279.
Bush, N. A., Chang, S. M., and Berger, M. S. (2017). Current and future strategies for treatment of glioma. Neurosurg. Rev. 40 (1), 1–14. doi:10.1007/s10143-016-0709-8
Butowski, N., Colman, H., De Groot, J. F., Omuro, A. M., Nayak, L., Wen, P. Y., et al. (2016). Orally administered colony stimulating factor 1 receptor inhibitor PLX3397 in recurrent glioblastoma: An ivy foundation early phase clinical trials consortium phase II study. Neuro-oncology 18 (4), 557–564. doi:10.1093/neuonc/nov245
Calixto, G. M., Bernegossi, J., de Freitas, L. M., Fontana, C. R., and Chorilli, M. (2016). Nanotechnology-based drug delivery systems for photodynamic therapy of cancer: A review. Molecules 21 (3), 342. doi:10.3390/molecules21030342
Candide, C., Morlière, P., Mazière, J. C., Goldstein, S., Santus, R., Dubertret, L., et al. (1986). In vitro interaction of the photoactive anticancer porphyrin derivative photofrin II with low density lipoprotein, and its delivery to cultured human fibroblasts. FEBS Lett. 207 (1), 133–138. doi:10.1016/0014-5793(86)80026-6
Casas, A. (2020). Clinical uses of 5-aminolaevulinic acid in photodynamic treatment and photodetection of cancer: A review. Cancer Lett. 490, 165–173. doi:10.1016/j.canlet.2020.06.008
Chou, C-J., Lin, C. F., Chen, Y. W., Huang, P. I., Yang, Y. P., Wang, M. L., et al. (2020). The update of chimeric antigen receptor-T cells therapy in glioblastoma. J. Chin. Med. Assoc. JCMA 83 (5), 442–445. doi:10.1097/JCMA.0000000000000302
Chaichana, K. L., Parker, S. L., Olivi, A., and Quiñones-Hinojosa, A. (2009). Long-term seizure outcomes in adult patients undergoing primary resection of malignant brain astrocytomas: Clinical article. J. Neurosurg. 111, 282–292. doi:10.3171/2009.2.JNS081132
Chen, Q., Chen, H., and Hetzel, F. W. (1996). Tumor oxygenation changes post-photodynamic therapy. Photochem Photobiol. 63 (1), 128–131. doi:10.1111/j.1751-1097.1996.tb03003.x
Chen, X., Wang, C., Teng, L., Liu, Y., Yang, G., et al. (2014). Calcitriol enhances 5-aminolevulinic acid-induced fluorescence and the effect of photodynamic therapy in human glioma. Acta Oncol. Stockh. Swed. 53 (3), 405–413. doi:10.3109/0284186X.2013.819993
Corti, C., Antonarelli, G., Criscitiello, C., Lin, N. U., Carey, L. A., Cortés, J., et al. (2022). Targeting brain metastases in breast cancer. Cancer Treat. Rev. 103, 102324. doi:10.1016/j.ctrv.2021.102324
Dajani, S., Hill, V. B., Kalapurakal, J. A., Horbinski, C. M., Nesbit, E. G., Sachdev, S., et al. (2022). Imaging of GBM in the age of molecular markers and MRI guided adaptive radiation therapy. J. Clin. Med. 11 (19), 5961. doi:10.3390/jcm11195961
de Paiva, A. C. M., Ferreira, M. D. C., and da Fonseca, A. S. (2022). Photodynamic therapy for treatment of bacterial keratitis. Photodiagnosis Photodyn. Ther. 37, 102717. doi:10.1016/j.pdpdt.2022.102717
de Paula, L. B., Primo, F. L., and Tedesco, A. C. (2017). Nanomedicine associated with photodynamic therapy for glioblastoma treatment. Biophys. Rev. 9 (5), 761–773. doi:10.1007/s12551-017-0293-3
de Vree, W. J., Essers, M. C., de Bruijn, H. S., Star, W. M., Koster, J. F., and Sluiter, W. (1996). Evidence for an important role of neutrophils in the efficacy of photodynamic therapy in vivo. Cancer Res. 56 (13), 2908–2911.
DeCordova, S., Shastri, A., Tsolaki, A. G., Yasmin, H., Klein, L., Singh, S. K., et al. (2020). Molecular heterogeneity and immunosuppressive microenvironment in glioblastoma. Front. Immunol. 11, 1402. doi:10.3389/fimmu.2020.01402
Diaz, R. J., Ali, S., Qadir, M. G., De La Fuente, M. I., Ivan, M. E., and Komotar, R. J. (2017). The role of bevacizumab in the treatment of glioblastoma. J. neuro-oncology 133 (3), 455–467. doi:10.1007/s11060-017-2477-x
Dolmans, D. E., Fukumura, D., and Jain, R. K. (2003). Photodynamic therapy for cancer. Nat. Rev. Cancer 3 (5), 380–387. doi:10.1038/nrc1071
Dolmans, D. E., Kadambi, A., Hill, J. S., Waters, C. A., Robinson, B. C., Walker, J. P., et al. (2002). Vascular accumulation of a novel photosensitizer, MV6401, causes selective thrombosis in tumor vessels after photodynamic therapy. Cancer Res. 62 (7), 2151–2156.
Dubey, S. K., Pradyuth, S. K., Saha, R. N., Singhvi, G., Alexander, A., Agrawal, M., et al. (2019). Application of photodynamic therapy drugs for management of glioma. J. Porphyr. Phthalocyanines 23, 1216–1228. , Kessel D, Vicente MG and Reiners Jr JJ. Autophagy 2006; 2: 289–290. doi:10.1142/s1088424619300192
Dunn-Pirio, A. M., and Vlahovic, G. (2017). Immunotherapy approaches in the treatment of malignant brain tumors. Cancer 123 (5), 734–750. doi:10.1002/cncr.30371
Dupont, C., Vermandel, M., Leroy, H. A., Quidet, M., Lecomte, F., Delhem, N., et al. (2019). INtraoperative photoDYnamic therapy for GliOblastomas (INDYGO): Study protocol for a phase I clinical trial. Neurosurgery 84 (6), E414–E419. doi:10.1093/neuros/nyy324
Eljamel, M. S., Goodman, C., and Moseley, H. (2008a). ALA and photofrin fluorescence-guided resection and repetitive PDT in glioblastoma multiforme: A single centre phase III randomised controlled trial. Lasers Med. Sci. 23 (4), 361–367. doi:10.1007/s10103-007-0494-2
Eljamel, M. S. (2008). Brain photodiagnosis (PD), fluorescence guided resection (FGR) and photodynamic therapy (PDT): Past, present and future. Photodiagnosis Photodyn. Ther. 5 (1), 29–35. doi:10.1016/j.pdpdt.2008.01.006
Eljamel, M. S., Goodman, C., and Moseley, H. (2008b). ALA and photofrin fluorescence-guided resection and repetitive PDT in glioblastoma multiforme: A single centre phase III randomised controlled trial. Lasers Med. Sci. 23 (4), 361–367. doi:10.1007/s10103-007-0494-2
Eljamel, S. (2010). Photodynamic applications in brain tumors: A comprehensive review of the literature. Photodiagnosis Photodyn. Ther. 7 (2), 76–85. doi:10.1016/j.pdpdt.2010.02.002
Etminan, N., Peters, C., Lakbir, D., Bünemann, E., Börger, V., Sabel, M. C., et al. (2011). Heat-shock protein 70-dependent dendritic cell activation by 5-aminolevulinic acid-mediated photodynamic treatment of human glioblastoma spheroids in vitro. Br. J. Cancer 105 (7), 961–969. doi:10.1038/bjc.2011.327
Fan, Y., Zhang, X., Gao, C., Jiang, S., Wu, H., Liu, Z., et al. (2022). Burden and trends of brain and central nervous system cancer from 1990 to 2019 at the global, regional, and country levels. Arch. Public Health 80 (1), 209. doi:10.1186/s13690-022-00965-5
Ferrario, A., Von Tiehl, K., Wong, S., Luna, M., and Gomer, C. J. (2002). Cyclooxygenase-2 inhibitor treatment enhances photodynamic therapy-mediated tumor response. Cancer Res. 62 (14), 3956–3961.
Ferrario, A., von Tiehl, K. F., Rucker, N., Schwarz, M. A., Gill, P. S., and Gomer, C. J. (2000). Antiangiogenic treatment enhances photodynamic therapy responsiveness in a mouse mammary carcinoma. Cancer Res. 60 (15), 4066–4069.
Fingar, V. H., Kik, P. K., Haydon, P. S., Cerrito, P. B., Tseng, M., Abang, E., et al. (1999). Analysis of acute vascular damage after photodynamic therapy using benzoporphyrin derivative (BPD). Br. J. Cancer 79 (11-12), 1702–1708. doi:10.1038/sj.bjc.6690271
Fingar, V. H., Wieman, T. J., and Haydon, P. S. (1997). The effects of thrombocytopenia on vessel stasis and macromolecular leakage after photodynamic therapy using photofrin. Photochem Photobiol. 66 (4), 513–517. doi:10.1111/j.1751-1097.1997.tb03182.x
Fuentes, R., Osorio, D., Expósito Hernandez, J., Simancas-Racines, D., Martinez-Zapata, M. J., and Bonfill Cosp, X. (2018). Surgery versus stereotactic radiotherapy for people with single or solitary brain metastasis. Cochrane Database Syst. Rev. 8 (8), CD012086. doi:10.1002/14651858.CD012086.pub2
Fujisawa, H., Reis, R. M., Nakamura, M., Colella, S., Yonekawa, Y., Kleihues, P., et al. (2000). Loss of heterozygosity on chromosome 10 is more extensive in primary (de novo) than in secondary glioblastomas. Lab. Invest. 80 (1), 65–72. doi:10.1038/labinvest.3780009
Furnari, F. B., Fenton, T., Bachoo, R. M., Mukasa, A., Stommel, J. M., Stegh, A., et al. (2007). Malignant astrocytic glioma: Genetics, biology, and paths to treatment. Genes Dev. 21 (21), 2683–2710. doi:10.1101/gad.1596707
Gan, T. J. (2017). Poorly controlled postoperative pain: Prevalence, consequences, and prevention. J. Pain Res. 10, 2287–2298. doi:10.2147/JPR.S144066
Gholami, L., Shahabi, S., Jazaeri, M., Hadilou, M., and Fekrazad, R. (2023). Clinical applications of antimicrobial photodynamic therapy in dentistry. Front. Microbiol. 13, 1020995. doi:10.3389/fmicb.2022.1020995
Gollnick, S. O., Liu, X., Owczarczak, B., Musser, D. A., and Henderson, B. W. (1997). Altered expression of interleukin 6 and interleukin 10 as a result of photodynamic therapy in vivo. Cancer Res. 57 (18), 3904–3909.
Gomer, C. J., and Razum, N. J. (1984). Acute skin response in albino mice following porphyrin photosensitization under oxic and anoxic conditions. Photochem Photobiol. 40 (4), 435–439. doi:10.1111/j.1751-1097.1984.tb04614.x
Gutman, R. L., Peacock, G., and Lu, D. R. (2000). Targeted drug delivery for brain cancer treatment. J. Control Release 65 (1-2), 31–41. doi:10.1016/s0168-3659(99)00229-1
Hefti, M., Albert, I., and Luginbuehl, V. (2012). Phenytoin reduces 5-aminolevulinic acid-induced protoporphyrin IX accumulation in malignant glioma cells. J. neuro-oncology 108 (3), 443–450. doi:10.1007/s11060-012-0857-9
Henderson, B. W., and Dougherty, T. J. (1992). How does photodynamic therapy work? Photochem Photobiol. 55 (1), 145–157. doi:10.1111/j.1751-1097.1992.tb04222.x
Henderson, B. W., and Fingar, V. H. (1989). Oxygen limitation of direct tumor cell kill during photodynamic treatment of a murine tumor model. Photochem Photobiol. 49 (3), 299–304. doi:10.1111/j.1751-1097.1989.tb04110.x
Hirschberg, H., Berg, K., and Peng, Q. (2018). Photodynamic therapy mediated immune therapy of brain tumors. Neuroimmunol. Neuroinflamm 5, 27. doi:10.20517/2347-8659.2018.31
Hirschberg, H., Sun, C. H., Tromberg, B. J., and Madsen, S. J. (2002). ALA- and ALA-ester-mediated photodynamic therapy of human glioma spheroids. J. neuro-oncology 57, 1–7. doi:10.1023/a:1015784926550
Hirschberg, H., Sun, C. H., Tromberg, B. J., Yeh, A. T., and Madsen, S. J. (2004). Enhanced cytotoxic effects of 5-aminolevulinic acid-mediated photodynamic therapy by concur-rent hyperthermia in glioma spheroids. J. neuro-oncology 70 (3), 289–299. doi:10.1007/s11060-004-9161-7
Hoang-Minh, L. B., and Mitchell, D. A. (2018). Immunotherapy for brain tumors. Curr. Treat. Options Oncol. 19 (11), 60. doi:10.1007/s11864-018-0576-3
Holland, E. C. (2000). Glioblastoma multiforme: The terminator. Proc. Natl. Acad. Sci. U. S. A. 97 (12), 6242–6244. doi:10.1073/pnas.97.12.6242
Hong, E. J., Choi, D. G., and Shim, M. S. (2016). Targeted and effective photodynamic therapy for cancer using functionalized nanomaterials. Acta Pharm. Sin. B 6 (4), 297–307. doi:10.1016/j.apsb.2016.01.007
Hsia, T., Small, J. L., Yekula, A., Batool, S. M., Escobedo, A. K., Ekanayake, E., et al. (2023). Systematic review of photodynamic therapy in gliomas. Cancers (Basel) 15 (15), 3918. doi:10.3390/cancers15153918
Hu, X., Deng, Q., Ma, L., Li, Q., Chen, Y., Liao, Y., et al. (2020). Meningeal lymphatic vessels regulate brain tumor drainage and immunity. Cell Res. 30, 229–243. doi:10.1038/s41422-020-0287-8
Ibarra, L. E., Vilchez, M. L., Caverzán, M. D., and Milla Sanabria, L. N. (2021). Understanding the glioblastoma tumor biology to optimize photodynamic therapy: From molecular to cellular events. J. Neurosci. Res. 99 (4), 1024–1047. doi:10.1002/jnr.24776
Ihata, T., Nonoguchi, N., Fujishiro, T., Omura, N., Kawabata, S., Kajimoto, Y., et al. (2022). The effect of hypoxia on photodynamic therapy with 5-aminolevulinic acid in malignant gliomas. Photodiagnosis Photodyn. Ther. 40, 103056. doi:10.1016/j.pdpdt.2022.103056
Jamali, Z., Hejazi, S. M., Ebrahimi, S. M., Moradi-Sardareh, H., and Paknejad, M. (2018). Effects of LED-based photodynamic therapy using red and blue lights, with natural hydrophobic photosensitizers on human glioma cell line. Photodiagnosis Photodyn. Ther. 21, 50–54. doi:10.1016/j.pdpdt.2017.11.002
Jeising, S., Geerling, G., Guthoff, R., Hänggi, D., Sabel, M., Rapp, M., et al. (2022). In-vitro use of verteporfin for photodynamic therapy in glioblastoma. Photodiagnosis pho-todynamic Ther. 40, 103049. doi:10.1016/j.pdpdt.2022.103049
Ji, B., Wei, M., and Yang, B. (2022). Recent advances in nanomedicines for photodynamic therapy (PDT)-driven cancer immunotherapy. Theranostics 12 (1), 434–458. doi:10.7150/thno.67300
Jin, F., Liu, D., Xu, X., Ji, J., and Du, Y. (2021). Nanomaterials-based photodynamic therapy with combined treatment improves antitumor efficacy through boosting immunogenic cell death. Int. J. Nanomedicine 16, 4693–4712. doi:10.2147/IJN.S314506
Johnson, W. D., Loredo, L. N., and Slater, J. D. (2008). Surgery and radiotherapy: Complementary tools in the management of benign intracranial tumors. Neurosurg. Focus 24 (5), E2. doi:10.3171/FOC/2008/24/5/E2
Josefsen, L. B., and Boyle, R. W. (2008). Photodynamic therapy: Novel third-generation photosensitizers one step closer? Br. J. Pharmacol. 154, 1–3. doi:10.1038/bjp.2008.98
Josefsen, L. B., and Boyle, R. W. (2008). Photodynamic therapy: Novel third-generation photosensitizers one step closer? Br. J. Pharmacol. 154 (1), 1–3. doi:10.1038/bjp.2008.98
Juvekar, P., Torio, E., Bi, W. L., Bastos, D. C. D. A., Golby, A. J., and Frisken, S. F. (2023). Mapping resection progress by tool-tip tracking during brain tumor surgery for real-time estimation of residual tumor. Cancers 15, 825. doi:10.3390/cancers15030825
Kanamori, M., and Kipnis, J. (2020). Meningeal lymphatics “drain” brain tumors. Cell Res. 30, 191–192. doi:10.1038/s41422-020-0286-9
Kang, J., Liu, J. J., Fang, Y. H., Lin, Y. Y., Gong, W., Wang, H. Y., et al. (2023). Hemoporfin-mediated photodynamic therapy for port-wine stains on extremities. Dermatol Ther. (Heidelb). 13 (8), 1857–1871. doi:10.1007/s13555-023-00970-8
Kanu, O. O., Mehta, A., Di, C., Lin, N., Bortoff, K., Bigner, D. D., et al. (2009). Glioblastoma multiforme: A review of therapeutic targets. Expert Opin. Ther. Targets 13 (6), 701–718. doi:10.1517/14728220902942348
Kawczyk-Krupka, A., Bugaj, A. M., Latos, W., Zaremba, K., and Sieroń, A. (2013). Photodynamic therapy in treatment of cutaneous and choroidal melanoma. Photodiagnosis Photodyn. Ther. 10 (4), 503–509. doi:10.1016/j.pdpdt.2013.05.006
Kessel, D. (2019). Apoptosis, paraptosis and autophagy: Death and survival pathways associated with photodynamic therapy. Photochem Photobiol. 95 (1), 119–125. doi:10.1111/php.12952
Khandwala, K., Mubarak, F., and Minhas, K. (2021). The many faces of glioblastoma: Pictorial review of atypical imaging features. Neuroradiol. J. 34 (1), 33–41. doi:10.1177/1971400920965970
Korbelik, M. (1993). Cellular delivery and retention of photofrin: III. Role of plasma proteins in photosensitizer clearance from cells. Photochem Photobiol. 57 (5), 846–850. doi:10.1111/j.1751-1097.1993.tb09222.x
Korbelik, M., and Krosl, G. (1994). Cellular levels of photosensitisers in tumours: The role of proximity to the blood supply. Br. J. Cancer 70 (4), 604–610. doi:10.1038/bjc.1994.358
Korbelik, M. (1992). Low density lipoprotein receptor pathway in the delivery of photofrin: How much is it relevant for selective accumulation of the photosensitizer in tumors? J. Photochem Photobiol. B 12 (1), 107–109. doi:10.1016/1011-1344(92)85022-m
Kou, J., Dou, D., and Yang, L. (2017). Porphyrin photosensitizers in photodynamic therapy and its applications. Oncotarget 8 (46), 81591–81603. doi:10.18632/oncotarget.20189
Kumar, A., Shankar, S., Singh, A., Mishra, S., Kumar, P., and Arora, A. (2022). Photodynamic therapy in the treatment of circumscribed choroidal hemangioma: Current perspectives. Photodiagnosis Photodyn. Ther. 39, 103000. doi:10.1016/j.pdpdt.2022.103000
Kwiatkowski, S., Knap, B., Przystupski, D., Saczko, J., Kędzierska, E., Knap-Czop, K., et al. (2018). Photodynamic therapy – mechanisms, photosensitizers and combinations. Biomed. Pharmacother. 106, 1098–1107. doi:10.1016/j.biopha.2018.07.049
Laperriere, N. J., and Bernstein, M. (1994). Radiotherapy for brain tumors. CA a cancer J. Clin. 44 (2), 96–108. doi:10.3322/canjclin.44.2.96
Lazaridis, L., Bumes, E., Cäcilia Spille, D., Schulz, T., Heider, S., Agkatsev, S., et al. (2022). First multicentric real-life experience with the combination of CCNU and temozolomide in newly diagnosed MGMT promoter methylated IDH wildtype glioblastoma. Neurooncol Adv. 4 (1), vdac137. doi:10.1093/noajnl/vdac137
Li, A., Fang, R., Mao, X., and Sun, Q. (2022). Photodynamic therapy in the treatment of rosacea: A systematic review. Photodiagnosis Photodyn. Ther. 38, 102875. doi:10.1016/j.pdpdt.2022.102875
Li, F., Cheng, Y., Lu, J., Hu, R., Wan, Q., and Feng, H. (2011). Photodynamic therapy boosts anti-glioma immunity in mice: A dependence on the activities of T cells and complement C3. J. Cell Biochem. 112 (10), 3035–3043. doi:10.1002/jcb.23228
Lietke, S., Schmutzer, M., Schwartz, C., Weller, J., Siller, S., Aumiller, M., et al. (2021). Interstitial photodynamic therapy using 5-ALA for malignant glioma recurrences. Cancers (Basel) 13 (8), 1767. doi:10.3390/cancers13081767
Lindo, L., Wilkinson, L. H., and Hay, K. A. (2021). Befriending the hostile tumor microenvironment in CAR T-cell therapy. Front. Immunol. 11, 618387. doi:10.3389/fimmu.2020.618387
Louis, D. N., Perry, A., Wesseling, P., Brat, D. J., Cree, I. A., Figarella-Branger, D., et al. (2021). The 2021 WHO classification of tumors of the central nervous system: A summary. Neuro Oncol. 23 (8), 1231–1251. doi:10.1093/neuonc/noab106
Luksiene, Z. (2003). Photodynamic therapy: Mechanism of action and ways to improve the efficiency of treatment. Med. Kaunas. 39 (12), 1137–1150.
Lyons, M., Phang, I., and Eljamel, S. (2012). The effects of PDT in primary malignant brain tumours could be improved by intraoperative radiotherapy. Photodiagnosis Photodyn. Ther. 9 (1), 40–45. doi:10.1016/j.pdpdt.2011.12.001
Ma, R., Taphoorn, M. J. B., and Plaha, P. (2021). Advances in the management of glioblastoma. J. Neurol. Neurosurg. Psychiatry 92 (10), 1103–1111. doi:10.1136/jnnp-2020-325334
Madsen, S. J., Christie, C., Huynh, K., Peng, Q., Uzal, F. A., Krasieva, T. B., et al. (2018). Limiting glioma development by photodynamic therapy-generated macrophage vaccine and allo-stimulation: An in vivo histological study in rats. J. Biomed. Opt. 23 (2), 1–7. doi:10.1117/1.JBO.23.2.028001
Madsen, S. J., Mathews, M. S., Angell-Petersen, E., Sun, C. H., Vo, V., Sanchez, R., et al. (2009). Motexafin gadolinium enhances the efficacy of aminolevulinic acid mediated-photodynamic thera-py in human glioma spheroids. J. neuro-oncology 91 (2), 141–149. doi:10.1007/s11060-008-9692-4
Maher, E. A., Brennan, C., Wen, P. Y., Durso, L., Ligon, K. L., Richardson, A., et al. (2006). Marked genomic differences characterize primary and secondary glioblastoma subtypes and identify two distinct molecular and clinical secondary glioblastoma entities. Cancer Res. 66 (23), 11502–11513. doi:10.1158/0008-5472.CAN-06-2072
Mahmoudi, K., Garvey, K. L., Bouras, A., Cramer, G., Stepp, H., Jesu Raj, J. G., et al. (2019). 5-aminolevulinic acid photodynamic therapy for the treatment of high-grade gliomas. J. Neurooncol 141 (3), 595–607. doi:10.1007/s11060-019-03103-4
Mansi, M., Howley, R., Chandratre, S., and Chen, B. (2022). Inhibition of ABCG2 transporter by lapatinib enhances 5-aminolevulinic acid-mediated protopor-phyrin IX fluorescence and photodynamic therapy response in human glioma cell lines. Biochem. Pharmacol. 200, 115031. doi:10.1016/j.bcp.2022.115031
Marei, H. E., Althani, A., Afifi, N., Hasan, A., Caceci, T., Pozzoli, G., et al. (2021). Current progress in chimeric antigen receptor T cell therapy for glioblastoma multiforme. Cancer Med. 10, 5019–5030. doi:10.1002/cam4.4064
Martin, D. H., Bianchi, E., Ben Mustapha, S., and Frères, P. (2021). Le glioblastome [glioblastoma]. Rev. Med. Liege 76 (5-6), 419–424.
Maxwell, R., Luksik, A. S., Garzon-Muvdi, T., and Lim, M. (2017). The potential of cellular- and viral-based immunotherapies for malignant glioma-dendritic cell vaccines, adoptive cell transfer, and oncolytic viruses. Curr. Neurol. Neurosci. Rep. 17 (6), 50. doi:10.1007/s11910-017-0754-x
Mazur, A., Koziorowska, K., Dynarowicz, K., Aebisher, D., and Bartusik-Aebisher, D. (2022). Photodynamic therapy for treatment of disease in children-A review of the literature. Child. (Basel). 9 (5), 695. doi:10.3390/children9050695
Mazur, E., and Reich, A. (2023). Photodynamic therapy is an effective treatment of facial pigmented actinic keratosis. Dermatol Ther. (Heidelb). 13 (6), 1265–1276. doi:10.1007/s13555-023-00924-0
Messmann, H., Mlkvy, P., Buonaccorsi, G., Davies, C. L., MacRobert, A. J., and Bown, S. G. (1995). Enhancement of photodynamic therapy with 5-aminolaevulinic acid-induced porphyrin photosensitisation in normal rat colon by threshold and light fractionation studies. Br. J. Cancer 72 (3), 589–594. doi:10.1038/bjc.1995.378
Minniti, G., Goldsmith, C., and Brada, M. (2012). Radiotherapy. HCN Ser. 104, 215–228. doi:10.1016/B978-0-444-52138-5.00016-5
Misawa, J., Moriwaki, S., Kohno, E., Hirano, T., Tokura, Y., and Takigawa, M. (2005). The role of low-density lipoprotein receptors in sensitivity to killing by Photofrin-mediated photodynamic therapy in cultured human tumor cell lines. J. Dermatol Sci. 40 (1), 59–61. doi:10.1016/j.jdermsci.2005.01.009
Miyauchi, J. T., and Tsirka, S. E. (2018). Advances in immunotherapeutic research for glioma therapy. J. Neurol. 265 (4), 741–756. doi:10.1007/s00415-017-8695-5
Mroz, P., Yaroslavsky, A., Kharkwal, G. B., and Hamblin, M. R. (2004). Cell death pathways in photodynamic therapy of cancer. Photodiagn. Photodyn. Ther. 1, 279–293.
Müller, P., Abdel Gaber, S. A., Zimmermann, W., Wittig, R., and Stepp, H. (2020). ABCG2 influence on the efficiency of photodynamic therapy in glioblastoma cells. J. pho-tochemistry Photobiol. B, Biol. 210, 111963. doi:10.1016/j.jphotobiol.2020.111963
Newton, H. B. (2000). Novel chemotherapeutic agents for the treatment of brain cancer. Expert Opin. Investig. Drugs 9 (12), 2815–2829. doi:10.1517/13543784.9.12.2815
Ostrom, Q. T., Cioffi, G., Waite, K., Kruchko, C., and Barnholtz-Sloan, J. S. (2021). CBTRUS statistical report: Primary brain and other central nervous system tumors diagnosed in the United States in 2014-2018. Neuro Oncol. 23 (12), iii1–iii105. doi:10.1093/neuonc/noab200
Overchuk, M., Weersink, R. A., Wilson, B. C., and Zheng, G. (2023). Photodynamic and photothermal therapies: Synergy opportunities for nanomedicine. ACS Nano 17 (9), 7979–8003. doi:10.1021/acsnano.3c00891
Page, M. J., McKenzie, J. E., Bossuyt, P. M., Boutron, I., Hoffmann, T. C., Mulrow, C. D., et al. (2021). The PRISMA 2020 statement: An updated guideline for reporting systematic reviews. BMJ 372, n71. doi:10.1136/bmj.n71
Parsons, D. W., Jones, S., Zhang, X., Lin, J. C., Leary, R. J., Angenendt, P., et al. (2008). An integrated genomic analysis of human glioblastoma multiforme. Science 321 (5897), 1807–1812. doi:10.1126/science.1164382
Pass, H. I. (1993). Photodynamic therapy in oncology: Mechanisms and clinical use. J. Natl. Cancer Inst. 85 (6), 443–456. doi:10.1093/jnci/85.6.443
Pogue, B. W., and Hasan, T. (1997). A theoretical study of light fractionation and dose-rate effects in photodynamic therapy. Radiat. Res. 147 (5), 551–559. doi:10.2307/3579621
Powers, S. K., and Brown, J. T. (1986). Light dosimetry in brain tissue: An in vivo model applicable to photodynamic therapy. Lasers Surg. Med. 6 (3), 318–322. doi:10.1002/lsm.1900060305
Preusser, M., Lim, M., Hafler, D. A., Reardon, D. A., and Sampson, J. H. (2015). Prospects of immune checkpoint modulators in the treatment of glioblastoma. Nat. Rev. Neurol. 11, 504–514. doi:10.1038/nrneurol.2015.139
Prins, R. M., Soto, H., Konkankit, V., Odesa, S. K., Eskin, A., Yong, W. H., et al. (2011). Gene expression profile correlates with T-cell infiltration and relative survival in glioblastoma patients vaccinated with dendritic cell immunotherapy. Clin. cancer Res. official J. Am. Assoc. Cancer Res. 17, 1603–1615. doi:10.1158/1078-0432.CCR-10-2563
Qian, C., Yu, J., Chen, Y., Hu, Q., Xiao, X., Sun, W., et al. (2016). Light-activated hypoxia-responsive nanocarriers for enhanced anticancer therapy. Adv. Mater 28 (17), 3313–3320. doi:10.1002/adma.201505869
Qin, L., Cao, J., Shao, K., Tong, F., Yang, Z., Lei, T., et al. (2020). A tumor-to-lymph procedure navigated versatile gel system for combinatorial therapy against tumor recurrence and metastasis. Sci. Adv. 6 (36), eabb3116. doi:10.1126/sciadv.abb3116
Quail, D. F., and Joyce, J. A. (2017). The microenvironmental landscape of brain tumors. Cancer Cell 31 (3), 326–341. doi:10.1016/j.ccell.2017.02.009
Quirk, B. J., Brandal, G., Donlon, S., Vera, J. C., Mang, T. S., Foy, A. B., et al. (2015). Photodynamic therapy (PDT) for malignant brain tumors--where do we stand? Photodiagnosis Photodyn. Ther. 12 (3), 530–544. doi:10.1016/j.pdpdt.2015.04.009
Raporty WHO (2023). International Agency for Research on Cancer. Available at: https://gco.iarc.fr/today/online-analysis-multi-bars?v=2020&mode=population&mode_population=continents&population=900&populations=900&key=total&sex=0&cancer=31&type=0&statistic=5&prevalence=0&population_group=0&ages_group%5B%5D=0&ages_group%5B%5D=17&nb_items=10&group_cancer=1&include_nmsc=0&include_nmsc_other=1&type_multiple=%257B%2522inc%2522%253Atrue%252C%2522mort%2522%253Atrue%252C%2522prev%2522%253Afalse%257D&orientation=horizontal&type_sort=0&type_nb_items=%257B%2522top%2522%253Atrue%252C%2522bottom%2522%253Afalse%257D
Rohle, D., Popovici-Muller, J., Palaskas, N., Turcan, S., Grommes, C., Campos, C., et al. (2013). An inhibitor of mutant IDH1 delays growth and promotes differentiation of glioma cells. Science 340 (6132), 626–630. doi:10.1126/science.1236062
Scaringi, C., Agolli, L., and Minniti, G. (2018). Technical advances in radiation therapy for brain tumors. Anticancer Res. 38 (11), 6041–6045. doi:10.21873/anticanres.12954
Schmidt, M. H., Meyer, G. A., Reichert, K. W., Cheng, J., Krouwer, H. G., Ozker, K., et al. (2004). Evaluation of photodynamic therapy near functional brain tissue in patients with recurrent brain tumors. J. Neurooncol 67 (1-2), 201–207. doi:10.1023/b:neon.0000021804.50002.85
Schumacher, T., Bunse, L., Pusch, S., Sahm, F., Wiestler, B., Quandt, J., et al. (2014). A vaccine targeting mutant IDH1 induces antitumour immunity. Nature 512 (7514), 324–327. doi:10.1038/nature13387
Semyachkina-Glushkovskaya, O., Abdurashitov, A., Klimova, M., Dubrovsky, A., Shirokov, A., Fomin, A., et al. (2020). Photostimulation of cerebral and peripheral lymphatic functions. Transl. Biophot. 2, e201900036. doi:10.1002/tbio.201900036
Semyachkina-Glushkovskaya, O., Chehonin, V., Borisova, E., Fedosov, I., Namykin, A., Abdurashitov, A., et al. (2018). Photodynamic opening of the blood-brain barrier and pathways of brain clearing. J. Biophot. 11 (8), e201700287. doi:10.1002/jbio.201700287
Semyachkina-Glushkovskaya, O., Terskov, A., Khorovodov, A., Telnova, V., Blokhina, I., Saranceva, E., et al. (2022a). Photodynamic opening of the blood-brain barrier and the meningeal lymphatic system: The new Niche in immunotherapy for brain tumors. Pharmaceutics 14 (12), 2612. doi:10.3390/pharmaceutics14122612
Semyachkina-Glushkovskaya, O., Terskov, A., Khorovodov, A., Telnova, V., Blokhina, I., Saranceva, E., et al. (2022b). Photodynamic opening of the blood-brain barrier and the meningeal lymphatic system: The new Niche in immunotherapy for brain tumors. Pharmaceutics 14 (12), 2612. doi:10.3390/pharmaceutics14122612
Shah, V., and Kochar, P. (2018). Brain cancer: Implication to disease, therapeutic strategies and tumor targeted drug delivery approaches. Recent Pat. Anticancer Drug Discov. 13 (1), 70–85. doi:10.2174/1574892812666171129142023
Shinoda, Y., Kato, D., Ando, R., Endo, H., Takahashi, T., Tsuneoka, Y., et al. (2021). Systematic review and meta-analysis of in vitro anti-human cancer experiments investigating the use of 5-aminolevulinic acid (5-ALA) for photodynamic therapy. Pharm. (Basel, Switz. 14 (3), 229. doi:10.3390/ph14030229
Shumaker, B. P., and Hetzel, F. W. (1987). Clinical laser photodynamic therapy in the treatment of bladder carcinoma. Photochem Photobiol. 46 (5), 899–901. doi:10.1111/j.1751-1097.1987.tb04866.x
Soucek, P., and Cihelkova, I. (2006). Photodynamic therapy with verteporfin in subfoveal amelanotic choroidal melanoma (A controlled case). Neuro Endocrinol. Lett. 27 (1-2), 145–148.
Stallivieri, A., Colombeau, L., Devy, J., Etique, N., Chaintreuil, C., Myrzakhmetov, B., et al. (2018). New photodynamic molecular beacons (PMB) as potential cancer-targeted agents in PDT. Bioorg Med. Chem. 26 (3), 688–702. doi:10.1016/j.bmc.2017.12.034
Stummer, W., Beck, T., Beyer, W., Mehrkens, J. H., Obermeier, A., Etminan, N., et al. (2008). Long-sustaining response in a patient with non-resectable, distant recurrence of glioblastoma multiforme treated by interstitial photodynamic therapy using 5-ALA: Case report. J. Neurooncol 87 (1), 103–109. doi:10.1007/s11060-007-9497-x
Stummer, W., Pichlmeier, U., Meinel, T., Wiestler, O. D., Zanella, F., Reulen, H. J., et al. (2006). Fluorescence-guided surgery with 5-aminolevulinic acid for resection of malignant glioma: A randomised controlled multicentre phase III trial. Lancet Oncol. 7 (5), 392–401. doi:10.1016/S1470-2045(06)70665-9
Stylli, S. S., Kaye, A. H., MacGregor, L., Howes, M., and Rajendra, P. (2005). Photodynamic therapy of high grade glioma - long term survival. J. Clin. Neurosci. 12 (4), 389–398. doi:10.1016/j.jocn.2005.01.006
Stylli, S. S., and Andrew, H. K. (2006). Photodynamic therapy of cerebral glioma--a review Part I--a biological basis. A Biol. basis” J. Clin. Neurosci. 13 (6), 615–625. doi:10.1016/j.jocn.2005.11.014
Sun, W., Kajimoto, Y., Inoue, H., Miyatake, S. I., Ishikawa, T., and Kuroiwa, T. (2013). Gefitinib enhances the efficacy of photodynamic therapy using 5-aminolevulinic acid in malignant brain tumor cells. Photodiagnosis Photodyn. Ther. 10 (1), 42–50. doi:10.1016/j.pdpdt.2012.06.003
Tan, A. C., Ashley, D. M., López, G. Y., Malinzak, M., Friedman, H. S., and Khasraw, M. (2020). Management of glioblastoma: State of the art and future directions. CA a cancer J. Clin. 70 (4), 299–312. doi:10.3322/caac.21613
Teng, L., Nakada, M., Zhao, S. G., Endo, Y., Furuyama, N., Nambu, E., et al. (2011). Silencing of ferrochelatase enhances 5-aminolevulinic acid-based fluorescence and photodynamic therapy efficacy. Br. J. cancer 104 (5), 798–807. doi:10.1038/bjc.2011.12
Tetard, M-C., Vermandel, M., Mordon, S., Lejeune, J. P., and Reyns, N. (2014). Experimental use of photodynamic therapy in high grade gliomas: A review focused on 5-aminolevulinic acid. Photodiagnosis Photodyn. Ther. 11 (3), 319–330. doi:10.1016/j.pdpdt.2014.04.004
Tromberg, B. J., Orenstein, A., Kimel, S., Barker, S. J., Hyatt, J., Nelson, J. S., et al. (1990). In vivo tumor oxygen tension measurements for the evaluation of the efficiency of photodynamic therapy. Photochem Photobiol. 52 (2), 375–385. doi:10.1111/j.1751-1097.1990.tb04193.x
Tsukagoshi, S. (1995). Porfimer sodium (Photofrin-II). Gan Kagaku Ryoho 22 (9), 1271–1278. Japanese.
Turubanova, V. D., Balalaeva, I. V., Mishchenko, T. A., Catanzaro, E., Alzeibak, R., Peskova, N. N., et al. (2019). Immunogenic cell death induced by a new photodynamic therapy based on photosens and photodithazine. J. Immunother. cancer 7, 350. doi:10.1186/s40425-019-0826-3
Velazquez, O. C. (2007). Angiogenesis and vasculogenesis: Inducing the growth of new blood vessels and wound healing by stimulation of bone marrow-derived progenitor cell mobilization and homing. J. Vasc. Surg. 45, A39–A47. doi:10.1016/j.jvs.2007.02.068
Vermandel, M., Dupont, C., Lecomte, F., Leroy, H. A., Tuleasca, C., Mordon, S., et al. (2021). Standardized intraoperative 5-ALA photodynamic therapy for newly diagnosed glioblastoma patients: A preliminary analysis of the INDYGO clinical trial. J. Neurooncol 152 (3), 501–514. doi:10.1007/s11060-021-03718-6
Vrouenraets, M. B., Visser, G. W., Snow, G. B., and van Dongen, G. A. (2003). Basic principles, applications in oncology and improved selectivity of photodynamic therapy. Anticancer Res. 23 (1B), 505–522.
Wang, D., Wang, T., Yu, H., Feng, B., Zhou, L., Zhou, F., et al. (2019b). Engineering nanoparticles to locally activate T cells in the tumor microenvironment. Sci. Immunol. 4 (37), eaau6584. doi:10.1126/sciimmunol.aau6584
Wang, L-X., Li, J. W., Huang, J. Y., Li, J. H., Zhang, L. J., O'Shea, D., et al. (2015). Antitumor activity of photodynamic therapy with a chlorin derivative in vitro and in vivo. Tumour Biol. J. Int. Soc. Oncodevelopmental Biol. Med. 36, 6839–6847. doi:10.1007/s13277-015-3395-1
Wang, P., Wang, B., Zhang, L., Liu, X., Shi, L., Kang, X., et al. (2023). Clinical practice Guidelines for 5-Aminolevulinic acid photodynamic therapy for acne vulgaris in China. Photodiagnosis Photodyn. Ther. 41, 103261. doi:10.1016/j.pdpdt.2022.103261
Wang, Y., Shang, W., Niu, M., Tian, J., and Xu, K. (2019a). Hypoxia-active nanoparticles used in tumor theranostic. Int. J. Nanomedicine 14, 3705–3722. doi:10.2147/IJN.S196959
Werner, M., Lyu, C., Stadlbauer, B., Schrader, I., Buchner, A., Stepp, H., et al. (2022). The role of Shikonin in improving 5-aminolevulinic acid-based photodynamic therapy and chemo-therapy on glioblastoma stem cells. Photodiagnosis Photodyn. Ther. 39, 102987. doi:10.1016/j.pdpdt.2022.102987
Wirsching, H. G., Galanis, E., and Weller, M. (2016). Glioblastoma. Handb. Clin. Neurol. 134, 381–397. doi:10.1016/B978-0-12-802997-8.00023-2
Xu, H. Z., Li, T. F., Ma, Y., Li, K., Zhang, Q., Xu, Y. H., et al. (2022). Targeted photodynamic therapy of glioblastoma mediated by platelets with photo-controlled release property. Biomaterials 290, 121833. doi:10.1016/j.biomaterials.2022.121833
Yamaguchi, S., Kobayashi, H., Narita, T., Kanehira, K., Sonezaki, S., Kubota, Y., et al. (2010). Novel photodynamic therapy using water-dispersed TiO2-polyethylene glycol compound: Evalu-ation of antitumor effect on glioma cells and spheroids in vitro. Photochem. Photobiol. 86, 964–971. doi:10.1111/j.1751-1097.2010.00742.x
Yang, K., Wu, Z., Zhang, H., Zhang, N., Wu, W., Wang, Z., et al. (2022). Glioma targeted therapy: Insight into future of molecular approaches. Mol. Cancer 21 (1), 39. doi:10.1186/s12943-022-01513-z
Yang, L., Guo, G., Niu, X. y., and Liu, J. (2015b). Dendritic cell-based immunotherapy treatment for glioblastoma multiforme. BioMed Res. Int. 2015, 717530. doi:10.1155/2015/717530
Yang, M., Li, J., Gu, P., and Fan, X. (2020). The application of nanoparticles in cancer immunotherapy: Targeting tumor microenvironment. Bioact. Mater 6 (7), 1973–1987. doi:10.1016/j.bioactmat.2020.12.010
Yang, X., Li, W., Palasuberniam, P., Myers, K. A., Wang, C., and Chen, B. (2015a). Effects of silencing heme biosynthesis enzymes on 5-aminolevulinic acid-mediated protoporphyrin IX fluorescence and photodynamic therapy. Photochem Photobiol. 91 (4), 923–930. doi:10.1111/php.12454
Yu, M. W., and Quail, D. F. (2021). Immunotherapy for glioblastoma: Current progress and challenges. Front. Immunol. 12, 676301. doi:10.3389/fimmu.2021.676301
Zeng, R., He, T., Lu, L., Li, K., Luo, Z., and Cai, K. (2021). Ultra-thin metal-organic framework nanosheets for chemo-photodynamic synergistic therapy. J. Mater Chem. B 9 (20), 4143–4153. doi:10.1039/d1tb00528f
Zhang, J., Jiang, C., Figueiró Longo, J. P., Azevedo, R. B., Zhang, H., and Muehlmann, L. A. (2018). An updated overview on the development of new photosensitizers for anticancer photodynamic therapy. Acta Pharm. Sin. B 8 (2), 137–146. doi:10.1016/j.apsb.2017.09.003
Zhang, W., Lu, Y., Zang, Y., Han, J., Xiong, Q., and Xiong, J. (2022). Photodynamic therapy and multi-modality imaging of up-conversion nanomaterial doped with AuNPs. Int. J. Mol. Sci. 23 (3), 1227. doi:10.3390/ijms23031227
Zhao, S-G. U., Chen, X. F., Wang, L. G., Yang, G., Han, D. Y., Teng, L., et al. (2013). Increased expression of ABCB6 enhances protoporphyrin IX accumulation and photodynamic effect in human glioma. Ann. Surg. Oncol. 20, 4379–4388. doi:10.1245/s10434-011-2201-6
Keywords: PDT, brain cancer, glioblastoma, diagnostics, treatment
Citation: Bartusik-Aebisher D, Serafin I, Dynarowicz K and Aebisher D (2023) Photodynamic therapy and associated targeting methods for treatment of brain cancer. Front. Pharmacol. 14:1250699. doi: 10.3389/fphar.2023.1250699
Received: 30 June 2023; Accepted: 11 September 2023;
Published: 28 September 2023.
Edited by:
Mohamed Soliman, Cairo University, EgyptReviewed by:
Sankha Bhattacharya, SVKM’s Narsee Moonjee Institute of Management and Studies (NMIMS), IndiaNaosuke Nonoguchi, Osaka Medical and Pharmaceutical University, Japan
Annegret Glasow, Leipzig University, Germany
Copyright © 2023 Bartusik-Aebisher, Serafin, Dynarowicz and Aebisher. This is an open-access article distributed under the terms of the Creative Commons Attribution License (CC BY). The use, distribution or reproduction in other forums is permitted, provided the original author(s) and the copyright owner(s) are credited and that the original publication in this journal is cited, in accordance with accepted academic practice. No use, distribution or reproduction is permitted which does not comply with these terms.
*Correspondence: David Aebisher, ZGFlYmlzaGVyQHVyLmVkdS5wbA==