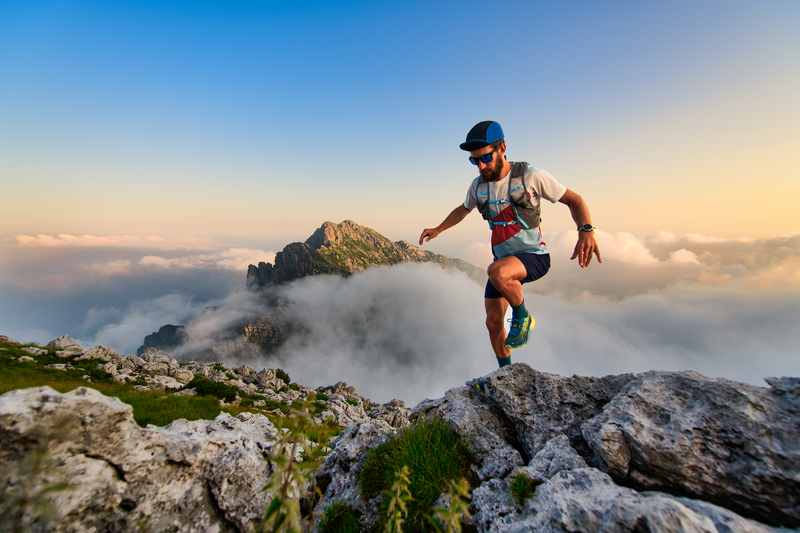
95% of researchers rate our articles as excellent or good
Learn more about the work of our research integrity team to safeguard the quality of each article we publish.
Find out more
ORIGINAL RESEARCH article
Front. Pharmacol. , 11 October 2023
Sec. Respiratory Pharmacology
Volume 14 - 2023 | https://doi.org/10.3389/fphar.2023.1250154
We have provided indirect pharmacological evidence that hypoxia may trigger release of the S-nitrosothiol, S-nitroso-L-cysteine (L-CSNO), from primary carotid body glomus cells (PGCs) of rats that then activates chemosensory afferents of the carotid sinus nerve to elicit the hypoxic ventilatory response (HVR). The objective of this study was to provide direct evidence, using our capacitive S-nitrosothiol sensor, that L-CSNO is stored and released from PGCs extracted from male Sprague Dawley rat carotid bodies, and thus further pharmacological evidence for the role of S-nitrosothiols in mediating the HVR. Key findings of this study were that 1) lysates of PGCs contained an S-nitrosothiol with physico-chemical properties similar to L-CSNO rather than S-nitroso-L-glutathione (L-GSNO), 2) exposure of PGCs to a hypoxic challenge caused a significant increase in S-nitrosothiol concentrations in the perfusate to levels approaching 100 fM via mechanisms that required extracellular Ca2+, 3) the dose-dependent increases in minute ventilation elicited by arterial injections of L-CSNO and L-GSNO were likely due to activation of small diameter unmyelinated C-fiber carotid body chemoafferents, 4) L-CSNO, but not L-GSNO, responses were markedly reduced in rats receiving continuous infusion (10 μmol/kg/min, IV) of both S-methyl-L-cysteine (L-SMC) and S-ethyl-L-cysteine (L-SEC), 5) ventilatory responses to hypoxic gas challenge (10% O2, 90% N2) were also due to the activation of small diameter unmyelinated C-fiber carotid body chemoafferents, and 6) the HVR was markedly diminished in rats receiving L-SMC plus L-SEC. This data provides evidence that rat PGCs synthesize an S-nitrosothiol with similar properties to L-CSNO that is released in an extracellular Ca2+-dependent manner by hypoxia.
Endogenous S-nitrosothiols, such as L-S-nitrosocysteine (L-CSNO) and L-S-nitrosoglutathione (L-GSNO), modulate the activities of numerous neural circuits in the central nervous system (Lei et al., 1992; Lipton et al., 1993; Lipton et al., 1994; Takahashi et al., 2007; Tegeder et al., 2011; Raju et al., 2015; Tarasenko, 2015; Nakamura and Lipton, 2016). Moreover, these S-nitrosothiols modulate numerous peripheral neural systems (Meller et al., 1990; Matsuda et al., 1995; Savidge, 2011; Lee et al., 2013; Tooker and Vigh, 2015; Gaston et al., 2020). The proposed mechanisms by which S-nitrosothiols exert their effects on neuronal systems include breakdown to nitric oxide and creation of iron-nitrosothiol complexes that activate soluble guanylate cyclase and down-stream cGMP-dependent protein kinase signaling events (Mellion et al., 1983; Travis et al., 1996; Severina et al., 2003; Lima et al., 2010; Martínez-Ruiz et al., 2013; Marozkina and Gaston, 2015; Vanin, 2019). In addition, it is clear that S-nitrosothiols exert their effects by transferring NO+ to sulfur atoms (S-nitrosylation) in a large number of functional proteins (Jaffrey et al., 2001; Joksovic et al., 2007; Foster et al., 2009; Lima et al., 2010; Rudkouskaya et al., 2010; Marozkina and Gaston, 2012; 2020; Anand et al., 2014; Pires da Silva et al., 2016; Wynia-Smith and Smith, 2017; Stomberski et al., 2019).
L-CSNO is an endothelium-derived S-nitrosothiol (Myers et al., 1990; Bates et al., 1991; Kukreja, et al., 1993) that is stored in cytoplasmic vesicles (Seckler et al., 2020). L-CSNO may be subject to exocytotic release within peripheral vascular beds (Davisson et al., 1996a; Batenburg et al., 2004a; b, 2009; Hashmi-Hill et al., 2007), and from lumbar sympathetic vasodilator nerves within the hindlimb vascular beds (Davisson et al., 1994; 1996a; b,c; 1997a; Possas and Lewis, 1997; Possas et al., 2006). Many of the pharmacological effects of SNOs, such as L-CSNO and S-nitroso-β,β-dimethyl-L-cysteine, are heavily reliant on their stereoisomeric configuration (Lewis et al., 1996; 2005a; b, 2006; Davisson et al., 1996d; 1997b; Travis et al., 1996; 1997; 2000; Ohta et al., 1997; Hoque et al., 1999; 2000; Lipton et al., 2001; Gaston et al., 2020). For example, the microinjection of L-CSNO into the nucleus tractus solitarius (nTS) decreases mean arterial blood pressure in anesthetized rats (Ohta et al., 1997), and microinjection of L-CSNO into the lateral (Davisson et al., 1997b) or fourth (Lewis et al., 1996) ventricles of freely-moving rats produce a complex array of hemodynamic responses, whereas similar microinjections of the D-isomer (D-CSNO) elicit minor responses. Although the identities of the functional proteins that may represent the stereoselective L-CSNO binding sites have not been fully characterized, we demonstrated that L-CSNO, but not D-CSNO, directly binds to and alters the activities of voltage-gated K+-channels (Kv-channels) that does not require S-nitrosylation of the ion channel (Gaston et al., 2020).
With respect to the control of breathing, it is known that microinjections of L-CSNO into the nTS increase minute ventilation in awake freely-moving rats by stereoselective-dependent mechanisms unrelated to the potential decomposition of L-CSNO to nitric oxide (Lipton et al., 2001). At the peripheral level, the carotid body contains, numerous excitatory and inhibitory neurotransmitters released by primary glomus cells (PGCs) in response to changes in ventilation (Prabhakar, 1994; Iturriaga and Alcayaga, 2004; Rey and Iturriaga, 2004; Bairam and Carroll, 2005; Kumar, 2007; Shirahata et al., 2007; Iturriaga et al., 2009; Nurse and Piskuric, 2013; Nurse, 2014; Gonkowski, 2020; Bardsley et al., 2021; Gold et al., 2022). However, there is no current agreement as to the identity of the primary neurotransmitter released by PGCs to drive the hypoxic ventilatory response (Eyzaguirre and Fidone, 1980; Iturriaga and Alcayaga, 2004; Nurse, 2005; Lahiri et al., 2006; Iturriaga et al., 2009; Gonzalez et al., 2010; Prieto-Lloret and Aaronson, 2017; Aldossary et al., 2020; Gold et al., 2022).
We have reported that arterial injections of L-CSNO (catheter tip positioned close to the carotid body artery) elicit dose-dependent increases in frequency of breathing (Freq), tidal volume (TV) and minute ventilation (MV) in freely-moving rats that are not mimicked by similar injections of D-CSNO (Gaston et al., 2020). We also reported that these L-CSNO-induced responses were reduced in rats with 1) prior bilateral transection of the carotid sinus nerves (CSNX) and 2) those receiving continuous intravenous infusion of S-methyl-L-cysteine (L-SMC), which reduces the ventilatory responses elicited by L-CSNO, but not those elicited by other S-nitrosothiols, such as L-GSNO (Gaston et al., 2020). Previous research has shown that nitric oxide has an inhibitory role in carotid body chemotransduction processes (Prabhakar et al., 1993; Chugh et al., 1994; Prabhakar, 1994; Trzebski, et al., 1995; Wang et al., 1995a; b; Summers et al., 1999; Iturriaga, 2001; Silva and Lewis, 2002; Rey and Iturriaga, 2004; Campanucci et al., 2012; Moya et al., 2012; Prabhakar and Peers, 2014). Consistent with this evidence, we found that arterial injections of the nitric oxide donor, MAHMA NONOate, inhibited breathing via actions in the carotid body (Gaston et al., 2020). This and our findings that inhibition of soluble guanylate cyclase reversed the effects of MAHMA NONOate, but minimally affected the excitatory actions of L-CSNO (Gaston et al., 2020), suggests that the excitatory actions of L-CSNO in the carotid body are not dependent on its decomposition to and activity of nitric oxide.
Our data led us to hypothesize that hypoxic challenges induce the release of preformed pools (perhaps vesicular stores subject to exocytosis) of L-CSNO from rat carotid body PGCs that activate small diameter C-fiber carotid body chemoafferents to initiate the hypoxic ventilatory response. The first set of objectives of this study was to provide direct evidence for our hypothesis, using an ultra-sensitive capacitive S-nitrosothiol sensor (Seckler et al., 2017), by determining whether 1) rat carotid body PGCs contain an S-nitrosothiol with physico-chemical properties similar to those of L-CSNO, 2) whether hypoxic challenges can stimulate isolated rat carotid body PGCs to release S-nitrosothiols, in particular L-CSNO, and 3) entry of extracellular Ca2+ via depolarization-induced opening of voltage-sensitive Ca2+-channels (Ca2+VS-channels) during hypoxic challenge (Donnelly and Kholwadwala, 1992; Summers et al., 2000; Kumar, 2007; Pandit and Buckler, 2009) is essential to trigger the release of S-nitrosothiols from PGCs. The second set of objectives was to provide further in vivo evidence to support our hypothesis by examining the ventilatory responses to intra-arterial injections of L-CSNO and L-GSNO during hypoxic gas (10% O2, 90% N2) challenge in 1) adult SHAM and CSNX rats, 2) adult rats treated as neonates with a bolus subcutaneous injection of vehicle or capsaicin in order to eliminate unmyelinated small-diameter C-fibers afferents (Meller et al., 1991), and 3) in rats receiving continuous intravenous infusions of L-SMC (10 μmol/kg/min) plus another inhibitor of L-CSNO activity, S-ethyl-L-cysteine (L-SEC, 10 μmol/kg/min) (Gaston et al., 2020).
All animal studies were carried out in accordance with the National Institutes of Health Guide for the Care and Use of Laboratory Animals (NIH Publication No. 80.23) revised in 1996, and in strict compliance with the latest ARRIVE (Animal Research: Reporting of In Vivo Experiments) guidelines (http://www.nc3rs.org.uk/). The protocols were approved by the Institutional Animal Care and Use Committees of the University of Virginia, Case Western Reserve University, and Galleon Pharmaceuticals, Inc.
Adult male Sprague Dawley rats were obtained from Harlan Laboratories, Inc (Indianapolis, IN). The rats were caged in standard housing conditions with free access to food and water. Room temperature (22.2–22.4°C), humidity (48%–50%) and light-dark cycle (12:12 h) were maintained consistently in rooms where the rats were housed and where the studies were performed. All protocols involved the use of rats that had been implanted with an arterial catheter and/or a jugular vein catheter under isoflurane (2.5%–3.5%) anesthesia 5 days previously. To allow arterial injections of vehicle or S-nitrosothiols, the left common carotid artery was exposed and separated carefully from the sympathetic trunk and vagus nerve. Two 3.0 silk ligatures (1.5 cm apart) were placed around the common carotid artery and pulled tight to temporarily occlude blood flow (less than 60 s in total). A small hole was placed in the artery between the ligatures with a 23-gauge needle, and a non-occlusive catheter (PE-10) was inserted with the extruded tip positioned at the trifurcation of the common carotid artery where the external and internal carotid arteries split and the occipital artery branches off the external carotid artery to feed the carotid body, such that the tip of the catheter lay within 1–2 mm of the carotid body artery (Lacolley et al., 2006a; Lacolley et al., 2006b). The volumes of each intra-arterial catheter (overall mean ± SEM of 112 ± 3 μL) were measured before implantation. Following implantation, the catheter was glued in place (Super Glue, Elmer’s Products Inc.), and the ligatures were gradually released until full blood flow was restored. To allow for continuous infusions of L-SEC plus L-SMC, a second catheter (PE-50 connected to PE-10, the latter inserted into the vein) was inserted into the right jugular vein. All catheters were exteriorized at the back of the neck, and the wounds coated with triple antibiotic (neomycin, polymyxin B, bacitracin) ointment (Fougera Pharmaceuticals, Inc.) and then sutured.
Sprague Dawley dams with litters containing 10–12 cross-fostered male pups were purchased from ENVIGO (Indianapolis, IN, United States) and delivered at least 48 h prior to experimentation. The carotid body primary glomus cell isolation procedure was adapted from Burlon et al. (2009). Briefly, three Sprague Dawley male rats (P11–16) were anesthetized with sevoflurane and decapitated. The head and neck were placed in ice cold, oxygenated (95% O2-5% CO2) Dulbecco’s phosphate buffered saline (DPBS) without Ca2+ or Mg2+. These two ions were absent from the DPBS to suppress activity of the cells. The carotid bodies were removed, cleaned of surrounding connective tissue, and transferred to a Petri dish containing digestive enzyme solution consisting of 0.4 mg/ml collagenase type I, 220 u/mg (Worthington Biochemical Corporation, Lakewood, NJ), and 0.2 mg/ml trypsin type I, 10,100 BAEE u/mg (Sigma Aldrich, St. Louis, MO) in DPBS with low CaCl2 (86 μM) and MgCl2 (350 μM) for 20 min incubation at 37 °C in a humidified, 5% CO2/air incubator. The carotid bodies were then mechanically teased apart and incubated for another 7 min. The tissue was then centrifuged, supernatant removed, and the cells triturated to mechanically dissociate the primary glomus cells from the remaining tissue (e.g., nerve endings, vascular endothelial cells, and type II sustentacular cells). The cells were then plated on poly-D- lysine coated glass coverslips and maintained at 37OC in a humidified 5% CO2/air incubator. The primary glomus cells were allowed to adhere for 2–3 h before use in the experiments.
Briefly coverslips were placed in a perfusion/recording chamber (RC-26GLP, Warner Instruments a division of Harvard Biosciences, Inc., Hamden, CT) and mounted on the stage of an upright microscope (BX51WI, Olympus, Shinjuku, Tokyo, Japan). The primary glomus cells were superfused with oxygenated (21% O2-5% CO2-balanced N2) Tyrode solution ((in mM): KCl, 4.5; NaCl, 117; CaCl2•2H2O, 2.5; MgCl2•6H2O, 1; glucose, 11; NaHCO3, 23, adjusted to pH 7.4 when bubbled with 5% CO2/air) controlled at 37 °C by an inline heater (TC-324C, Warner Instruments a division of Harvard Biosciences, Inc., Hamden, CT). For a subset of experiments the primary glomus cells were superfused with oxygenated (21% O2-5% CO2-balanced N2) Ca2+-free Tyrode solution ((in mM): KCl, 4.5; NaCl, 117; MgCl2•6H2O, 1; glucose, 11; NaHCO3, 23, adjusted to pH 7.4 when bubbled with 5% CO2/air) to assess whether L-CSNO release from carotid body primary glomus cells is via calcium-dependent vesicular fusion and exocytosis. After primary glomus cells have been superfused with oxygenated Tyrode solution, with or without calcium for 10 min a micropipette collected 200 μL of solution directly from the recording chamber (this is the normoxia group) and put it in a 500 μL Eppendorf tube for analysis by means of our capacitive biosensor (Seckler et al., 2017). Next the glomus cell perfusion was switched to a hypoxic (0%–8% O2-5% CO2-balanced N2) Tyrode solution, either with or without Ca2+, and allowed to equilibrate for 10 min before 200 μL of solution was collected directly from the recording chamber (this is the hypoxia group) and put in a 500 μL Eppendorf tube for analysis by means of our capacitive biosensor. Finally, the glomus cell perfusion was switched back to the oxygenated Tyrode solution, either with or without Ca2+, and allowed to equilibrate for 10 min before 200 μL of solution was collected directly from the recording chamber (this is the return group) and put it in a 500 μL Eppendorf tube for analysis by means of our capacitive biosensor.
Carotid body primary glomus cells were derived from Sprague Dawley male rats (P11–16) as described above and then incubated for 10 min in DPBS with 1% (v/v) Triton X-100% and 0.5% (v/v) formaldehyde with either: nothing, 100 μM HgCl2, or 100 μM HgCl2, while being constantly exposed to UV light. This mixture was then diluted 1 to 1,000 in DPBS with 0.5% (v/v) formaldehyde and incubated for a further 5 min. The Triton X-100 served to lyse the cells, the formaldehyde served to block all free thiols and amines in the cells, and the HgCl2 and UV light served to degrade extant S-nitrosothiols serving as a negative control. We also measured the response generated by our system to either L-CSNO, or L-GSNO to serve as positive controls. The resulting mixture was filtered through a 10 kDa spin filter to remove membrane aggregates and large proteins, and the flow through was measured by means of our capacitive biosensor.
The levels of S-nitrosothiols in solution were measured by means of our capacitive biosensor as described previously (Seckler et al., 2017). Briefly, 3 functionalized electrodes were attached to 3 separate pre-amplifiers (SR560, Standford Research), and connected to three separate channels of an ITC-1600 (HEKA Corporation). These electrodes were houses inside of a well-ventilated Faraday cage to block out all ambient electrical noise and to ensure no exposure to formaldehyde fumes. A small Ag-AgCl ground pellet (E205, Warner Instruments) connected to a DC channel of the same ITC-1600 provided the current injection. The three electrodes were continuously perfused with DPBS with 0.5% (v/v) formaldehyde during measurements. Data was taken in 5 min intervals with a 5 min baseline, followed by sample injection and 5 min of sample wash-in, and finally 5 min of sample washout. The current response of each electrode was integrated over the duration of the current injection and that number was recorded. The difference in response between the baseline (b) and washout (w) was calculated to form a normalized ratio (r) where:
This produces a number between 0 and 1, where 0 represents all cases where the signal from the washout reading was less than or equal to the signal from the baseline reading and 1 represents the washout signal being infinitely larger than the baseline signal. In practice, any ration value greater than 0.4 represents the presence of S-nitrosothiols.
Ventilatory parameters, frequency of breathing (Freq), tidal volume (TV) and minute ventilation (MV) were recorded in freely-moving rats by whole body plethysmography (PLY3223; Data Sciences International, St. Paul, MN) as detailed previously (May et al., 2013a; May et al., 2013b; Young et al., 2013; Getsy et al., 2014; 2020; Henderson et al., 2014; Baby et al., 2019; Gaston et al., 2020; 2021; Baby S. M. et al., 2021; Baby M. S. et al., 2021; Jenkins et al., 2021; Seckler et al., 2022). The rats were given 60 min in order to acclimatize to the chambers and to allow true resting ventilatory parameters to be established before commencing the studies.
These rats consisted of sham-operated (SHAM) rats and those in which both carotid sinus nerves (CSNX) were transected 7 days prior as described previously (Getsy et al., 2020; 2021a). A single arterial catheter was inserted after the transections were completed (see above for detailed description regarding arterial catheter implantation). After a 60 min acclimatization period in the plethysmography chambers, to allow the rats to settle, each carotid artery catheter was loaded with vehicle (saline), L-CSNO (300 pmol/μL) or L-GSNO (300 pmol/μL) to completely fill the catheter as per the recorded volume. A small volume (10 μL) was injected to ensure that the first test injection delivered the responses elicited by L-CSNO and L-GSNO, whereas vehicle elicited minor responses only. The doses (given as a slow bolus over 3 s) for L-CSNO were 2.5, 5, 10, 25, and 50 nmol/kg and those for L-GSNO were 5, 10, 25, 50 and 75 nmol/kg. For example, to deliver 2.5, 5, 10, 25, and 50 nmol/kg doses of L-CSNO, the volumes to be delivered would be 2.5, 5, 10, 25, and 50 μL, respectively. Each injection was given 5–10 min apart when baseline breathing values had returned to pre-injection levels for at least 90 s. The volumes given to each rat to achieve the required dose were adjusted by body weight. Equal volumes of vehicle (saline) were given to determine control (injection) responses. Other groups of adult SHAM and CSNX rats (no catheters inserted) received a 10 min hypoxic gas (10% O2, 90% N2) challenge. The dose response curves to L-CSNO and L-GSNO were designed to move through a minimal effective dose for elevating MV to one that elicits a robust response while minimally affecting mean arterial blood pressure (Gaston et al., 2020).
These rats had received a subcutaneous injection of vehicle (saline, 200 μL) or capsaicin (50 mg/kg) on day 3 post-birth as described previously (Meller et al., 1991). These rats received an arterial catheter as adults to give injections of vehicle or L-CSNO (2.5–50 nmol/kg, IA) or in separate rats, vehicle or L-GSNO (5–75 nmol/kg, IA). Other groups of adult vehicle- or capsaicin-treated rats (with no catheters) received a 10 min hypoxic gas (10% O2, 90% N2) challenge.
These rats received a jugular vein catheter and arterial catheter as described above. The arterial catheter allowed for bolus injections of vehicle or L-CSNO (2.5–50 nmol/kg) and in other rats, vehicle or L-GSNO (5–75 nmol/kg). Groups of rats received continuous infusions of vehicle (20 μL/min) or L-SMC (10 μmol/kg/min) plus L-SEC (10 μmol/kg/min). Other groups of rats receiving vehicle or L-SMC (10 μmol/kg/min) plus L-SEC (10 μmol/kg/min) were exposed to a 10 min hypoxic gas (10% O2, 90% N2) challenge beginning 30–35 min after starting the infusions (30 min was a sufficient amount of time for ventilatory parameters to stabilize).
All data are presented as mean ± SEM and were evaluated using one-way and two-way ANOVA followed by Bonferroni corrections for multiple comparisons between means using the error mean square terms from each ANOVA analysis (Winer, 1971; Wallenstein et al., 1980; Ludbrook, 1998; McHugh, 2011) as detailed previously (Getsy et al., 2021a; Jenkins et al., 2021). A value of p < 0.05 was taken as the initial level of statistical significance (Wallenstein et al., 1980; Ludbrook, 1998; McHugh, 2011). The statistical analyses were performed using GraphPad Prism software (GraphPad Software, Inc., La Jolla, CA). A detailed description of these statistical procedures is provided in the Supplementary Material (see Detailed description of Statistical Approaches).
As can be seen in Figure 1, primary glomus cell lysates contained S-nitrosothiol concentrations equivalent to 100 fM concentrations of L-CSNO and 100 fM concentrations of L-GSNO. The addition of HgCl2 (100 μM) abolished the S-nitrosothiol signal from the primary glomus cell lysate and authentic L-CSNO (100 fM) but not that of L-GSNO. The S-nitrosothiol signals from the primary glomus cell lysate, L-CSNO (100 fM) and GSNO (100 fM) were effectively abolished by co-application of HgCl2 (100 μM) and UV light. Additionally, DPBS was used as a negative control (blanks). As can be seen in Figure 2, no significant S-nitrosothiol signal above blank (DPBS) was observed in the extracellular media under normoxic conditions (21% O2-5% CO2-balanced N2). After 10 min exposure to hypoxia ((0%–8% O2-5% CO2-balanced N2), the concentrations of S-nitrosothiols in the extracellular media containing Ca2+ reached peak levels similar to those produced by a 100 fM concentration of authentic L-CSNO, and no significant level of S-nitrosothiols was detected in the Ca2+-free media. After 10 min return to normoxia the S-nitrosothiol levels returned to the pre-hypoxic levels in the extracellular media containing Ca2+. These results show that S-nitrosothiols release from carotid body glomus cells is calcium-dependent and most likely involves calcium-dependent vesicular fusion and release.
FIGURE 1. The response coefficients of our S-nitrosothiol biosensor to a 1,000x dilution of primary glomus cell lysates (analyte), 100 fM S-nitroso-L-cysteine (L-CSNO) or 100 fM S-nitrosoglutathione (L-GSNO). Definitions: Columns designated as “Blank” refer to the electrode reaction to Dulbecco’s phosphate buffered saline (DPBS) alone for each study. Columns designated as “Analyte” refer to the electrode reaction to primary glomus cell lysates (Glomus cells), L-CSNO (100 fM) or L-GSNO (100 fM). The columns designated as “Analyte + HgCl2” refer to the electrode reactions to primary glomus cell lysates (Glomus cells), L-CSNO (100 fM) or L-GSNO (100 fM) that were exposed to HgCl2 (100 μM). The columns designated as “Analyte + HgCl2 + UVL” refer to the electrode reactions to primary glomus cell lysates (Glomus cells), L-CSNO (100 fM) or L-GSNO (100 fM) that were exposed to HgCl2 (100 μM) and ultraviolet light (UVL). The data are shown as mean ± SEM from 6-7 samples. *p < 0.05, significant change from control conditions (Analyte columns).
FIGURE 2. The response coefficient of the S-nitrosothiol biosensors to perfusion media collected from the chamber of plated PGCs exposed to normoxia, hypoxia, or return to normoxia after hypoxic challenge. All of the electrodes were exposed to DPBS before the experiment (blank) to serve as a negative control. After each experiment, electrodes were exposed to a solution of 100 fM S-nitroso-L-cysteine (L-CSNO) to ensure that the electrodes were properly detecting S-nitrosothiols and specifically, L-CSNO. The data are presented as mean ± SEM. There were 6 samples in each of the Ca2+-present studies and 5 samples in each of the Ca2+-absent studies. *p < 0.05, significant difference from blank and normoxia values. †p < 0.05, Ca2+-free versus Ca2+-values resulting from hypoxic challenge. ‡p < 0.05, Ca2+-free versus Ca2+-values upon return to room-air.
The body weights and number of rats in each group are summarized in Supplementary Table S1 (there were no between-group differences in body weights for any study (p > 0.05, for all comparisons). The actual Freq, TV and MV data are summarized in Supplementary Table S2-S5. The data show that i) resting ventilatory parameters in the CSNX groups were generally lower than those in the SHAM groups, ii) resting parameters were similar in the VEH and CAP groups and iii) resting parameters in the groups of rats receiving infusion of L-SMC + L-SEC were generally lower than those receiving infusions of vehicle. In addition, Supplementary Table S6 shows that the infusion of L-SMC + L-SEC caused sustained decreases in Freq, TV and MV from initial control values, whereas ventilatory values remained at pre-infusion values in rats receiving vehicle (saline).
As can be seen in Panel A of Figure 3, exposure to a hypoxic gas (10% O2, 90% N2) challenge for 10 min elicited a robust increase in MV in sham-operated (SHAM rats) and markedly smaller responses in bilateral CSN-transected (CSNX) rats. Similar patterns of responses were observed for Freq and TV (Supplementary Figure S1, Panels A and D). As seen in Panel B of Figure 3, exposure to a hypoxic gas (10% O2, 90% N2) challenge for 10 min elicited a robust increase in MV in neonatal vehicle-injected (VEH) rats and markedly smaller responses in neonatal capsaicin-injected (CAP) rats. Similar patterns of responses were observed for Freq and TV (Supplementary Figure S1, Panels B and E). Moreover, as seen in Panel C of Figure 3, exposure to a hypoxic gas (10% O2, 90% N2) challenge for 10 min elicited a robust increase in MV in rats receiving an intravenous infusion of vehicle (VEH) rats and markedly smaller responses in those receiving an infusion of L-SMC + L-SEC. Similar patterns of responses were observed for Freq and TV (Supplementary Figure S1, Panels C and F).
FIGURE 3. Arithmetic changes in minute ventilation during a hypoxic gas challenge (10% O2, 90% N2) for 10 min. (A) Adult sham-operated (SHAM) rats (n = 6) and those with bilateral carotid sinus nerve transection (CSNX) (n = 6). (B) Adult rats treated as neonates with vehicle (VEH; n = 9) or capsaicin (CAP; 50 mg/kg, SC; n = 9). (C) Adult rats receiving a continuous intravenous infusion of vehicle (VEH; 20 μL/min, IV; n = 9) or S-methyl-L-cysteine (L-SMC; 10 μmol/min, IV; n = 9) plus S-ethyl-L-cysteine (L-SEC; 10 μmol/min, IV; n = 9). The data are shown as mean ± SEM. *p < 0.05, significant response. †p < 0.05, CSNX versus SHAM, CAP versus VEH, L-SMC + L-SEC versus VEH.
As can be seen in panels A and B of Figure 4, respectively, intra-arterial injections of L-CSNO (2.5–50 nmol/kg) or L-GSNO (5–75 nmol/kg) elicited dose-dependent increases in MV in SHAM rats and markedly smaller responses in CSNX rats. As seen in panels C and D of Figure 4, respectively, these injections of L-CSNO or L-GSNO elicited dose-dependent increases in MV in VEH rats and markedly smaller responses in CAP rats. As seen in panel E of Figure 4, the injections of L-CSNO elicited dose-dependent increases in MV in rats receiving VEH infusion and markedly smaller responses in rats receiving an infusion of L-SMC + L-SEC. In contrast, the dose-dependent increases in MV elicited by L-GSNO in the L-SMC + L-SEC rats were similar to those in VEH rats (Figure 4F,). Similar patterns of responses were observed for Freq and TV for L-CSNO (Supplementary Figure S2) and for L-GSNO (Supplementary Figure S3) in all three groups.
FIGURE 4. Arithmetic changes in minute ventilation elicited by injections of L-CSNO (left-hand panels) and L-GSNO (right-hand panels). (A) and (B) Adult sham-operated (SHAM) rats (n = 6) and those with bilateral carotid sinus nerve transection (CSNX) (n = 6). (C) and (D) Adult rats treated as neonates with vehicle (VEH; n = 9) or capsaicin (CAP; 50 mg/kg, SC; n = 9). (E) and (F) Adult rats receiving a continuous intravenous infusion of vehicle (VEH; 20 μL/min, IV; n = 9) or S-methyl-L-cysteine (L-SMC; 10 μmol/min, IV; n = 9) plus S-ethyl-L-cysteine (L-SEC; 10 μmol/min, IV; n = 9). The data are shown as mean ± SEM. *p < 0.05, significant response. †p < 0.05, CSNX versus SHAM, CAP versus VEH, L-SMC + L-SEC versus VEH.
Capacitive sensors when coupled to a semiconducting material represent a method to detect trace quantities of a chemical in complex solutions. We have taken advantage of the semiconducting and chemical properties of polydopamine to construct a capacitive sensor, which specifically senses S-nitrosothiols in complex biological solutions (Seckler et al., 2020). Our capacitive biosensor employs a thin layer of polydopamine, which will crosslink to all free amines, free thiols, and S-nitrosothiols within the body. To make our sensor selective for small molecule S-nitrosothiols we used the following process: 1) We ran our sample through a 10 kDa spin filter to remove all large proteins and other molecules, 2) We incubated our sample in 0.4% formaldehyde to block all free thiols and free amines, but not S-nitrosothiols, 3) We divided this sample into three aliquots and performed 2 separate negative controls 4) We pre-incubated one negative control to HgCl2, and 5) We exposed the other negative control to UV light with an emission of 350 nm. These steps ensure that we selectively sense S-nitrosothiols. If our signal, for example, instead came from a small molecule which can bind to polydopamine, but cannot be fully blocked by formaldehyde, such as urea, then steps 4 and 5 would fail as HgCl2 and/or UV light would not be capable of abolishing a signal. Additionally, if our signal was produced by a small molecule, such as cysteine sulfinic acid, it would show a signal when exposed to UV light (as our UV does not emit in the 300 nm absorption band of sulfinic acid), but not in HgCl2 (which is capable of degrading sulfinic acid). Therefore, the method by which the capacitive biosensor studies were performed left no alternative targets for the biosensor apart from small molecule S-nitrosothiols.
The first major finding of the present study was that rat PGCs contained an S-nitrosothiol with physico-chemical properties more closely resembling those of L-CSNO than L-GSNO. The presence of pre-formed S-nitrosothiols in intracellular vesicles of PGCs is not shocking as there is published evidence showing the presence of S-nitrosothiols in cytoplasmic vesicles of endothelial cells (Seckler et al., 2020). There is also direct evidence that rat PGCs contain vesicles that store neurotransmitters, such as catecholamines (e.g., dopamine) and ATP that are subject to depolarization-induced (Weiss and Donnelly, 1996), Ca2+-dependent exocytosis in response to stimuli, including hypoxia (Echeverría et al., 1977; 1978; Hansen, 1977; Grönblad and Eränkö, 1978; Krammer, 1978; Grönblad et al., 1979; 1980; Grönblad, 1983; Conde et al., 2009; Yan et al., 2012; Ortega-Sáenz et al., 2016; Tse et al., 2018) via the presence of VGCa-channels (Silva and Lewis, 1995; Peers and Buckler, 1995; Overholt and Prabhakar, 1997; Carpenter et al., 2000; Peers, 2004; Makarenko et al., 2015; Conde et al., 2006) and Ca2+-binding sites (Hansen and Smith, 1979) in the plasma membranes of PGCs. These observations are clinically relevant since human PGCs contain large numbers of cytoplasmic vesicles (Abrahám, 1970; 1981; Kobayashi, 1971a; Kobayashi, 1971b; Hervonen and Korkala, 1972) that contain gap-junctions (Kondo, 2002), enkephalin (Khan, et al., 1990) and numerous other peptides/polypeptides (Smith et al., 1990; Scraggs et al., 1992), such as calcitonin, calcitonin gene-related peptide, cholecystokinin and Substance P (Kummer and Habeck, 1991; Wang, et al., 1993). The question arises as to how S-nitrosothiols, such as L-CSNO, may be stored in vesicles subject to exocytosis. Neuronal and endothelial forms of nitric oxide synthase (nNOS and eNOS, respectively) are expressed in several structures in the carotid bodies, including sensory nerve terminals, ganglion cells and vascular endothelium (Prabhakar et al., 1993; Wang et al., 1993; Grimes et al., 1994; 1995; Höhler et al., 1994; Atanasova et al., 2016; 2020). Importantly, while several studies have not found eNOS or nNOS to be expressed in carotid body primary or secondary (sustenacular) glomus cells (Prabhakar et al., 1993; Höhler et al., 1994; Grimes et al., 1995; Moya et al., 2012; Atanasova et al., 2016; 2020), others have demonstrated the presence of eNOS in rat (Yamamoto et al., 2006) and cat (Valdés et al., 2003) PGCs and nNOS in rabbit PGCs (Li et al., 2010). Moreover, Grimes et al. (1994) observed weak NADPH diaphorase staining in rat PGCs and Tanaka and Chiba (1994) demonstrated intense NADPH diaphorase staining in sub-populations of guinea-pig PGCs. Although Atanasova et al. (2020) reported that PGCs were largely negative for NADPH diaphorase, close analysis of their figures shows that it is present in fine granules in the glomus cells, but not as robust as in the encircling fine neural positive varicosities. These findings are relevant because of our evidence that NADPH diaphorase is a histochemical marker for S-nitrosylated proteins and small molecular weight S-nitrosothiols (Seckler et al., 2020). With respect to how S-nitrosothiols may be generated in PGCs, there is substantial evidence that plasma membranes of intracellular vesicles in other structures, such as nerve terminals and endothelial cells, contain NOS (Loesch et al., 1993; 1994; Dikranian et al., 1994; Loesch and Burnstock, 1995; Sosunov et al., 1995; Loesch and Burnstock, 1996; Sosunov et al., 1996; Loesch and Burnstock, 1998), whereas NADPH diaphorase is localized strictly to the lumen of these vesicles (Loesch et al., 1993). Our evidence that NADPH diaphorase detects S-nitrosothiols/S-nitrosylated proteins (e.g., NOS) led us to determine that cytoplasmic vesicles extracted from rat femoral artery endothelium are indeed rich in S-nitrosothiols (Seckler et al., 2020). Moreover, the presence of cysteine transporters, such as excitatory amino acid transporters 1 and 3 on PGC membranes of rats and humans (Li et al., 2020), would allow for entry of L-cysteine into PGCs for potential transformation to L-CSNO.
The second major finding of this study was that hypoxic challenge caused the release of S-nitrosothiols (presumably L-CSNO) from these PGCs that was strictly dependent on the presence of extracellular Ca2+. The question arises as to whether the hypoxia-stimulated Ca2+-dependent release of L-CSNO from the carotid body PGCs is from de novo synthesized L-CSNO or potentially from pre-formed vesicular pools that are subject to exocytosis. L-CSNO is poorly lipophilic (Clancy et al., 2001) and its entry into cells occurs predominantly/exclusively via L-amino acid transporter systems (Li et al., 2020; Nemoto et al., 2003; Li and Whorton, 2007). Although to our knowledge there is no evidence that L-CSNO can be transported out of cells via these L-amino acid transporter systems, it may be possible that hypoxia elicits a Ca2+-dependent increase in L-CSNO synthesis within PGCs that then undergo transport to the extracellular environment. It would also seem plausible that hypoxia elicits Ca2+-dependent exocytosis of vesicular pools of L-CSNO. To our knowledge there is no evidence that the plasma membranes of PGCs or vesicles within PGCs contain fusion proteins that are known to subserve exocytosis on other cell-types (Sudhof, 2004; Südhof and Rothman, 2009; Wang et al., 2019). Nonetheless, such a process would allow for dynamic regulation of carotid body chemoafferent activity in response to graded levels of hypoxia and presumably hypercapnia and acidosis. It would also seem plausible that these putative stores of L-CSNO may be depleted upon repetitive hypoxic gas challenges when NOS in PGC is inhibited, thereby preventing the replenishing of these L-CSNO stores resulting in progressively smaller ventilatory responses. Such use-dependent loss of vasodilation in the presence of NOS inhibitors has been observed in response to repetitive stimulation of the vascular endothelium (Davisson et al., Batenburg et al., 2004a; b, 2009) and lumbar sympathetic fibers (Davisson et al., 1996b; Davisson et al., 1996c; Davisson et al., 1997a). Indeed, Gozal et al. (1985) reported the hypoxic ventilatory response in freely-moving rats rapidly declines in the presence of a non-specific inhibitor of eNOS and nNOS or a specific inhibitor of nNOS.
The third major finding was that the ventilatory responses elicited by L-CSNO and a hypoxic gas challenge in freely-moving adult Sprague Dawley rats were markedly diminished in rats receiving a continuous infusion of L-SMC + L-SEC. The observation that the ventilatory responses to L-GSNO were not diminished by the L-SMC + L-SEC infusion provides support for the above biochemical findings that rat PGCs store L-CSNO and release it response to hypoxic challenge. We have previously reported that the responses to hypoxia and L-CSNO were diminished in rats receiving L-SMC alone, but we wanted to further our studies to include L-SEC, since our preliminary cardiovascular studies are showing that L-SEC is a better inhibitor than L-SMC in some vascular beds (Lewis, unpublished observations). Taken with the other in vivo studies presented here (CSNX and CAP studies) it seems that hypoxia may release L-CSNO from PGCs that in turn activate small diameter unmyelinated C-fiber chemoafferents to mediate the HVR. The hypoxic ventilatory responses recorded in this study are consistent with evidence that the ventilatory responses to hypoxic gas challenges are largely abolished in adult rats with CSNX (Getsy et al., 2020; 2021a) and adult rats treated with capsaicin as neonates (McQueen and Mir, 1984; De Sanctis et al., 1991). Our data that the ventilatory responses elicited by L-CSNO and L-GSNO are markedly diminished in CSNX and CAP rats greatly expands our understanding of the pharmacology of these endogenous S-nitrosothiols. It should be noted that the infusion of L-SEC + L-SEC caused a reduction in resting frequency and tidal volume, and therefore minute ventilation in naïve rats. The decreases in these parameters could be due to numerous effects, such as sedation (not observed to be the case) or a decrease in resting carotid body chemoafferent activity via inhibition of the possible tonic effects of PGC-released L-CSNO that may occur under normoxic conditions. The decreases in ventilatory parameters appear to be consistent with those that occur during application of the Dejours’ test (100% oxygen), which is known to silence carotid body chemoafferents (Haouzi et al., 2014).
A study limitation is that although the signals from the PGC lysates exposed to HgCl2 and UV light showed a degradation pattern consistent with the signals being due to the presence of L-CSNO, but not L-GSNO, it remains possible that the S-nitrosothiol present in PGCs is another small molecule S-nitrosothiol with a free primary amine, such as S-nitroso-cysteamine, S-nitroso-coenzyme A, or S-nitroso-glutamylcysteine (for which there is no evidence currently for their existence in PGCs). The development of a selective antibody to L-CSNO would allow immunohistochemical studies to confirm the presence of L-CSNO in PGCs. Another study limitation is that the S-nitrosothiol detection experiments were performed in PGCs from P11-P16 rat pups and not in cells from adult rats that were used for the whole body plethysmography studies. PGCs from rat pups were used 1) because it is extremely difficult to isolate PGCs from adult carotid bodies due to extensive connective tissue and other technical matters, and 2) to match on-going electrophysiological whole cell patch-clamp recordings that are examining the effects of L-CSNO on carotid body PGC responses to hypoxia that are historically only performed successfully in young rat pups (P10-P25). Another limitation pertains to the lack of S-nitrosothiol detection studies in PGCs from female rats and plethsmography studies in freely-moving female rats since it is well-established that sex differences occur in terms of specific patterns of changes in breathing parameters and cell-signaling events in response to hypoxic and hypercapnic gas challenges (Getsy et al., 2021b; Getsy et al., 2021c). Additionally, our study is limited in that only Sprague Dawley rats were used and it would be important to extend our studies to include other strains of rats and also larger species to get a more complete picture of the potential role of PGC-derived S-nitrosothiols in the carotid body control of ventilation. Finally, dose-response studies with L-SMC + L-SEC would also further the pharmacological analyses regarding the role of PGC-derived L-CSNO in ventilatory control processes. The intra-arterial doses of L-CSNO used in these studies (2.5–50 nmol/kg) elicited increases in MV that were substantially less in magnitude than those elicited by the hypoxic gas challenge. These doses were chosen because they elicit minimal falls in arterial blood pressure that may confound the interpretation of the MV responses (Gaston et al., 2020). Although the present findings raise the distinct possibility that primary glomus cell-derived L-CSNO has a possible role in the expression of the hypoxic ventilatory response (HVR), we have preliminary evidence that high doses of L-CSNO (e.g., 100 nmol/kg, IA) elicit a rise in MV of magnitude closely matching that of the hypoxic gas challenge. Although the combination of L-SMC + L-SEC markedly diminished the responses to L-CSNO and hypoxic gas challenge, there were certainly residual responses in the order of 50 ml/min in both the hypoxic gas challenge and the 50 nmol/kg dose of L-CSNO. At present we do not know whether higher doses of L-SMC + L-SEC would elicit a more complete blockade of the L-CSNO responses, or whether L-S-phenyl-cysteine (L-SPC), which we used in combination with L-SMC to identify L-CSNO interacting proteins (Gaston et al., 2020), would in combination with L-SMC and L-SEC provide a more complete blockade of the effects of L-CSNO and hypoxia on MV. The tentative idea driving the use of various combinations of L-SMC, L-SEC and L-SPC is that they may differentially block the numerous functional proteins (e.g., Kv-channels) activated by L-CSNO (Gaston et al., 2020).
As presented in Figure 5, our major conclusions are that carotid body PGCs from male Sprague Dawley rats contain an S-nitrosothiol with physico-chemical properties resembling those of L-CSNO, and that a hypoxic gas challenge releases L-CSNO in a manner dependent upon Ca2+-entry into PGCs. Our studies with L-SMC + L-SEC support the concept that the PGC-derived S-nitrosothiol is L-CSNO, since the ventilatory actions of L-CSNO and hypoxic gas challenge were markedly attenuated by L-SMC + L-SEC, whereas the responses elicited by L-GSNO were not. Accordingly, our data support the concept that the release of L-CSNO from PGCs plays a primary role in the expression of the HVR (Figure 5). Whether PGC-derived L-CSNO directly excites carotid body afferents and/or releases excitatory neurotransmitters from PGCs is undetermined. Whether L-CSNO contributes to the ventilatory responses that occur post-hypoxic (Getsy et al., 2021b) and post-hypercapnic (Getsy et al., 2021c) challenges, and which post-response depends upon carotid body chemoafferents (Getsy et al., 2020; Getsy et al., 2021a), is also undetermined. Other possible mechanisms of action for L-CSNO may include a) L-CSNO-stimulated release of neurotransmitters from primary glomus cells, which activate chemoafferents, and/or b) L-CSNO-mediated S-nitrosylation of functional proteins on the chemoafferent terminals thereby allowing other neurotransmitters to directly activate the afferents. While the ventilatory responses elicited by IA injections of L-CSNO appear to involve the direct/indirect activation of carotid body chemoafferents, the residual responses observed for the higher doses of L-CSNO in the CSNX and CAP rats raises the possibility that L-CSNO may exert its effects via actions on other afferent fibers or at brain sites that are devoid of a blood-brain barrier, such as the area postrema (Johnson and Gross, 1993). The ability of glomus cell-derived L-CSNO to activate the carotid body chemoreflex may be part of a coordinated role of this S-nitrosothiol in regulating cardio-respiratory function at the brainstem level. More specifically, we reported that the NADPH diaphorase technique visualizes S-nitrosothiols/S-nitrosylated proteins in the brain (Seckler et al., 2020), and it is evident that afferent fibers entering the nucleus tractus solitarius (NTS) and numerous intrinsic cells within the NTS stain for NADPH diaphorase (Ruggiero et al., 1996). This and the ability of microinjections of L-CSNO into the NTS of rats to lower mean arterial blood pressure (Ohta et al., 1997) provide tentative support for the concept that primary afferent terminals or intrinsic neurons release L-CSNO or related S-nitrosothiols as neurotransmitters/neuromulators to drive cardiovascular and potentially ventilatory response processes within the NTS. The possibility that the loss of afferent input to the NTS in CSNX or CAP rats alters the responsiveness of NTS neurons to intrinsic L-CSNO is an intriguing question.
FIGURE 5. Proposed mechanism by which S-nitroso-L-cysteine (L-CSNO) is stored in primary glomus cells (PGCs) and released in response to hypoxia to act as a neurotransmitter mediating the hypoxic ventilatory response. Vesicular stores of L-CSNO within PGCs are generated by L-cysteine uptake into vesicles via membrane-bound L-amino acid transporter (L-AT) and then S-nitrosylation of L-cysteine to L-CSNO by membrane-bound nitric oxide synthase (NOS). Hypoxia causes PGCs to depolarize thereby activating voltage-gated Ca2+-channels (VGCa-channels) leading to extracellular Ca2+-dependent release of the vesicular stores of L-CSNO. The released L-CSNO then binds stereoselectively to extracellular domains of voltage-gated K+-channels (Kv-channels), such as Kvβ2 subunits, on carotid body chemoafferent nerve terminals to actively close the Kv-channels thereby preventing K+ release from the terminals. Diminished K+ release depolarizes the terminals thereby generating action potentials that activate neurons in the commissural nucleus tractus solitarius (cNTS) leading to neurotransmitter release that elicits the hypoxic ventilatory response (HVR). In addition, L-CSNO released by the PGCs is actively transported into chemoafferent terminals in the carotid body via plasma membrane-bound L-AT.
The raw data supporting the conclusion of this article will be made available by the authors, without undue reservation.
The animal study was approved by Case Western Reserve University. The study was conducted in accordance with the local legislation and institutional requirements.
The study was conceived and planned by JS, PG, BG, JB, and SL. All of the experiments were performed by JS, PG, WM, TL, SB and SL. The data were collated and statistically analyzed by JS, PG, and SL. The figures and tables were prepared by JS, TL, PG, and SL. All authors contributed to the article and approved the submitted version.
This research was funded in part by grants to National Institutes of Health grants to SL (NIH NIDA U01DA051373 and BG (NIH 1R61HL154136-01).
The authors wish to thank the staff at the animal care facilities at Case Western Reserve University, University of Virginia and Galleon Pharmaceuticals, Inc., for their expert assistance with the care of the rats.
SB was employed by Galleon Pharmaceuticals, Inc.
The remaining authors declare that the research was conducted in the absence of any commercial or financial relationships that could be construed as a potential conflict of interest.
All claims expressed in this article are solely those of the authors and do not necessarily represent those of their affiliated organizations, or those of the publisher, the editors and the reviewers. Any product that may be evaluated in this article, or claim that may be made by its manufacturer, is not guaranteed or endorsed by the publisher.
The Supplementary Material for this article can be found online at: https://www.frontiersin.org/articles/10.3389/fphar.2023.1250154/full#supplementary-material
PGCs, primary glomus cells; L-CSNO, S-nitroso-L-cysteine; L-GSNO, L-S-nitrosoglutathione; L-SMC, S-methyl-L-cysteine; L-SEC, S-ethyl-L-cysteine; Freq, frequency of breathing; TV, tidal volume; MV, minute ventilation; Kv-channels, voltage-gated K + -channels; HgCl2, mercurous chloride; UV light, ultra-violet light; CSNX, carotid sinus nerve transection; CAP, capsaicin; VEH, vehicle.
Abrahám, A. (1970). Electron microscopic studies on human carotid bodies. Z. Mikrosk. Anat. Forsch. 81, 413–453.
Abrahám, A. (1981). Light- and electron-microscopic investigations on human carotid bodies. Z. Mikrosk. Anat. Forsch. 95, 33–71.
Aldossary, H. S., Alzahrani, A. A., Nathanael, D., Alhuthail, E. A., Ray, C. J., Batis, N., et al. (2020). G-Protein-Coupled receptor (GPCR) signaling in the carotid body: Roles in hypoxia and cardiovascular and respiratory disease. Int. J. Mol. Sci. 21, 6012. doi:10.3390/ijms21176012
Anand, P., Hausladen, A., Wang, Y. J., Zhang, G. F., Stomberski, C., Brunengraber, H., et al. (2014). Identification of S-nitroso-CoA reductases that regulate protein S-nitrosylation. Proc. Natl. Acad. Sci. U. S. A. 111, 18572–18577. doi:10.1073/pnas.1417816112
Atanasova, D. Y., Dandov, A. D., Dimitrov, N. D., and Lazarov, N. E. (2020). Histochemical and immunohistochemical localization of nitrergic structures in the carotid body of spontaneously hypertensive rats. Acta histochem. 122, 151500. doi:10.1016/j.acthis.2019.151500
Atanasova, D. Y., Dimitrov, N. D., and Lazarov, N. E. (2016). Expression of nitric oxide-containing structures in the rat carotid body. Acta histochem. 118, 770–775. doi:10.1016/j.acthis.2016.09.007
Baby, M. S., Discala, F. G., Gruber, R., Getsy, M. P., Cheng, F., Damron, S. D., et al. (2021b). Tempol reverses the negative effects of morphine on arterial blood-gas chemistry and tissue oxygen saturation in freely-moving rats. Front. Pharmacol. 12, 749084. doi:10.3389/fphar.2021.749084
Baby, S. M., Gruber, R. B., Young, A. P., MacFarlane, P. M., Teppema, L. J., and Lewis, S. J. (2019). Bilateral carotid sinus nerve transection exacerbates morphine-induced respiratory depression. Eur. J. Pharmacol. 834, 17–29. doi:10.1016/j.ejphar.2018.07.018
Baby, S. M., Gruber, R., Discala, J., Puskovic, V., Jose, N., Cheng, F., et al. (2021a). Systemic administration of tempol attenuates the cardiorespiratory depressant effects of fentanyl. Front. Pharmacol. 12, 690407. doi:10.3389/fphar.2021.690407
Bairam, A., and Carroll, J. L. (2005). Neurotransmitters in carotid body development. Respir. Physiol. Neurobiol. 149, 217–232. doi:10.1016/j.resp.2005.04.017
Bardsley, E. N., Pen, D. K., McBryde, F. D., Ford, A. P., and Paton, J. F. R. (2021). The inevitability of ATP as a transmitter in the carotid body. Auton. Neurosci. 234, 102815. doi:10.1016/j.autneu.2021.102815
Batenburg, W. W., de Vries, R., Saxena, P. R., and Danser, A. H. (2004a). L-S-nitrosothiols: endothelium-derived hyperpolarizing factors in porcine coronary arteries? J. Hypertens. 22, 1927–1936. doi:10.1097/00004872-200410000-00015
Batenburg, W. W., Kappers, M. H., Eikmann, M. J., Ramzan, S. N., de Vries, R., and Danser, A. H. (2009). Light-induced vs. bradykinin-induced relaxation of coronary arteries: do S-nitrosothiols act as endothelium-derived hyperpolarizing factors? J. Hypertens. 27, 1631–1640. doi:10.1097/HJH.0b013e32832bff54
Batenburg, W. W., Popp, R., Fleming, I., de Vries, R., Garrelds, I. M., Saxena, P. R., et al. (2004b). Bradykinin-induced relaxation of coronary microarteries: S-nitrosothiols as EDHF? Br. J. Pharmacol. 142, 125–135. doi:10.1038/sj.bjp.0705747
Bates, J. N., Harrison, D. G., Myers, P. R., and Minor, R. L. (1991). EDRF: nitrosylated compound or authentic nitric oxide. Basic Res. Cardiol. 86 (2), 17–26. doi:10.1007/978-3-642-72461-9_3
Burlon, D. C., Jordan, H. L., and Wyatt, C. N. (2009). Presynaptic regulation of isolated neonatal rat carotid body type I cells by histamine. Respir. Physiol. Neurobiol. 168, 218–223. doi:10.1016/j.resp.2009.07.002
Campanucci, V. A., Dookhoo, L., Vollmer, C., and Nurse, C. A. (2012). Modulation of the carotid body sensory discharge by NO: an up-dated hypothesis. Respir. Physiol. Neurobiol. 184, 149–157. doi:10.1016/j.resp.2012.04.005
Carpenter, E., Hatton, C. J., and Peers, C. (2000). Effects of hypoxia and dithionite on catecholamine release from isolated type I cells of the rat carotid body. J. Physiol. 523, 719–729. doi:10.1111/j.1469-7793.2000.00719.x
Chugh, D. K., Katayama, M., Mokashi, A., Bebout, D. E., Ray, D. K., and Lahiri, S. (1994). Nitric oxide-related inhibition of carotid chemosensory nerve activity in the cat. Respir. Physiol. 97, 147–156. doi:10.1016/0034-5687(94)90022-1
Clancy, R., Cederbaum, A. I., and Stoyanovsky, D. A. (2001). Preparation and properties of S-nitroso-L-cysteine ethyl ester, an intracellular nitrosating agent. J. Med. Chem. 44, 2035–2038. doi:10.1021/jm000463f
Conde, S. V., Caceres, A. I., Vicario, I., Rocher, A., Obeso, A., and Gonzalez, C. (2006). An overview on the homeostasis of Ca2+ in chemoreceptor cells of the rabbit and rat carotid bodies. Adv. Exp. Med. Biol. 580, 215–222. discussion 351-359. doi:10.1007/0-387-31311-7_33
Conde, S. V., Obeso, A., Monteiro, E. C., and Gonzalez, C. (2009). The A2B-D2 receptor interaction that controls carotid body catecholamines release locates between the last two steps of hypoxic transduction cascade. Adv. Exp. Med. Biol. 648, 161–168. doi:10.1007/978-90-481-2259-2_18
Davisson, R. L., Bates, J. N., Johnson, A. K., and Lewis, S. J. (1996a). Use-dependent loss of acetylcholine- and bradykinin-mediated vasodilation after nitric oxide synthase inhibition. Evidence for preformed stores of nitric oxide-containing factors in vascular endothelial cells. Hypertension 28, 354–360. doi:10.1161/01.hyp.28.3.354
Davisson, R. L., Johnson, A. K., and Lewis, S. J. (1994). Nitrosyl factors mediate active neurogenic hindquarter vasodilation in the conscious rat. Hypertension 23, 962–966. doi:10.1161/01.hyp.23.6.962
Davisson, R. L., Possas, O. S., Murphy, S. P., and Lewis, S. J. (1997a). Neurogenically derived nitrosyl factors mediate sympathetic vasodilation in the hindlimb of the rat. Am. J. Physiol. 272, H2369–H2376. doi:10.1152/ajpheart.1997.272.5.H2369
Davisson, R. L., Shaffer, R. A., Johnson, A. K., and Lewis, S. J. (1996c). Stimulation of lumbar sympathetic nerves may produce hindlimb vasodilation via the release of pre-formed stores of nitrosyl factors. Neuroscience 72, 881–887. doi:10.1016/0306-4522(96)00090-5
Davisson, R. L., Shaffer, R. A., Johnson, A. K., and Lewis, S. J. (1996b). Use-dependent loss of active sympathetic neurogenic vasodilation after nitric oxide synthase inhibition in conscious rats. Evidence for the presence of preformed stores of nitric oxide-containing factors. Hypertension 28, 347–353. doi:10.1161/01.hyp.28.3.347
Davisson, R. L., Travis, M. D., Bates, J. N., Johnson, A. K., and Lewis, S. J. (1997b). Stereoselective actions of S-nitrosocysteine in central nervous system of conscious rats. Am. J. Physiol. 272, H2361–H2368. doi:10.1152/ajpheart.1997.272.5.H2361
Davisson, R. L., Travis, M. D., Bates, J. N., and Lewis, S. J. (1996d). Hemodynamic effects of L- and D-S-nitrosocysteine in the rat. Stereoselective S-nitrosothiol recognition sites. Circ. Res. 79, 256–262. doi:10.1161/01.res.79.2.256
De Sanctis, G. T., Green, F. H., and Remmers, J. E. (1991). Ventilatory responses to hypoxia and hypercapnia in awake rats pretreated with capsaicin. J. Appl. Physiol. (1985) 70 (3), 1168–1174. doi:10.1152/jappl.1991.70.3.1168
Dikranian, K., Loesch, A., and Burnstock, G. (1994). Localisation of nitric oxide synthase and its colocalisation with vasoactive peptides in coronary and femoral arteries. An electron microscope study. J. Anat. 184, 583–590.
Donnelly, D. F., and Kholwadwala, D. (1992). Hypoxia decreases intracellular calcium in adult rat carotid body glomus cells. J. Neurophysiol. 67, 1543–1551. doi:10.1152/jn.1992.67.6.1543
Echeverría, O. M., Vázquez-Nin, G. H., and Aguilar, R. (1978). Cytochemical study on the mechanism of secretion of catecholamines. Acta Anat. (Basel). 100, 51–60. doi:10.1159/000144881
Echeverría, O. M., Vázquez-Nin, G. H., and Chávez, B. (1977). Correlated ultrastructural and biochemical studies on the mechanisms of secretion of catecholamines. Acta Anat. (Basel). 98, 313–324. doi:10.1159/000144807
Eyzaguirre, C., and Fidone, S. J. (1980). Transduction mechanisms in carotid body: glomus cells, putative neurotransmitters, and nerve endings. Am. J. Physiol. 239, C135–C152. doi:10.1152/ajpcell.1980.239.5.C135
Foster, M. W., Hess, D. T., and Stamler, J. S. (2009). Protein S-nitrosylation in health and disease: a current perspective. Trends Mol. Med. 15, 391–404. doi:10.1016/j.molmed.2009.06.007
Gonzalez, C., Agapito, M. T., Rocher, A., Gomez-Niño, A., Rigual, R., Castañeda, J., et al. (2010). A revisit to O2 sensing and transduction in the carotid body chemoreceptors in the context of reactive oxygen species biology. Respir. Physiol. Neurobiol. 174, 317–330. doi:10.1016/j.resp.2010.09.002
Grimes, P. A., Lahiri, S., Stone, R., Mokashi, A., and Chug, D. (1994). Nitric oxide synthase occurs in neurons and nerve fibers of the carotid body. Adv. Exp. Med. Biol. 360, 221–224. doi:10.1007/978-1-4615-2572-1_33
Gaston, B., Baby, S. M., May, W. J., Young, A. P., Grossfield, A., Bates, J. N., et al. (2021). D-Cystine di(m)ethyl ester reverses the deleterious effects of morphine on ventilation and arterial blood gas chemistry while promoting antinociception. Sci. Rep. 11, 10038. doi:10.1038/s41598-021-89455-2
Gaston, B., Smith, L., Bosch, J., Seckler, J., Kunze, D., Kiselar, J., et al. (2020). Voltage-gated potassium channel proteins and stereoselective S-nitroso-l-cysteine signaling. JCI Insight 5, e134174. doi:10.1172/jci.insight.134174
Getsy, P. M., Sundararajan, S., May, W. J., von Schill, G. C., McLaughlin, D. K., Palmer, L. A., et al. (2021b). Short-term facilitation of breathing upon cessation of hypoxic challenge is impaired in male but not female endothelial NOS knock-out mice. Sci. Rep. 11, 18346. doi:10.1038/s41598-021-97322-3
Getsy, P. M., Coffee, G. A., and Lewis, S. J. (2020). The role of carotid sinus nerve Input in the hypoxic-hypercapnic ventilatory response in juvenile rats. Front. Physiol. 11, 613786. doi:10.3389/fphys.2020.613786
Getsy, P. M., Davis, J., Coffee, G. A., May, W. J., Palmer, L. A., Strohl, K. P., et al. (2014). Enhanced non-eupneic breathing following hypoxic, hypercapnic or hypoxic-hypercapnic gas challenges in conscious mice. Respir. Physiol. Neurobiol. 204, 147–159. doi:10.1016/j.resp.2014.09.006
Getsy, P. M., Sundararajan, S., and Lewis, S. J. (2021a). Carotid sinus nerve transection abolishes the facilitation of breathing that occurs upon cessation of a hypercapnic gas challenge in male mice. J. Appl. Physiol. (1985) 131, 821–835. doi:10.1152/japplphysiol.01031.2020
Getsy, P. M., Sundararajan, S., May, W. J., von Schill, G. C., McLaughlin, D. K., Palmer, L. A., et al. (2021c). Ventilatory responses during and following hypercapnic gas challenge are impaired in male but not female endothelial NOS knock-out mice. Sci. Rep. 11, 20557. doi:10.1038/s41598-021-99922-5
Gold, O. M. S., Bardsley, E. N., Ponnampalam, A. P., Pauza, A. G., and Paton, J. F. R. (2022). Cellular basis of learning and memory in the carotid body. Front. Synaptic. Neurosci. 14, 902319. doi:10.3389/fnsyn.2022.902319
Gonkowski, S. (2020). Vasoactive intestinal polypeptide in the carotid body-A history of forty years of research. A mini review. Int. J. Mol. Sci. 21, 4692. doi:10.3390/ijms21134692
Gozal, D., Torres, J. E., Gozal, Y. M., and Littwin, S. M. (1996). Effect of nitric oxide synthase inhibition on cardiorespiratory responses in the conscious rat. J. Appl. Physiol. (1985) 81 (5), 2068–2077. doi:10.1152/jappl.1996.81.5.2068
Grimes, P. A., Mokashi, A., Stone, R. A., and Lahiri, S. (1995). Nitric oxide synthase in autonomic innervation of the cat carotid body. J. Auton. Nerv. Syst. 54, 80–86. doi:10.1016/0165-1838(95)00006-j
Grönblad, M., Akerman, K. E., and Eränkö, O. (1979). Induction of exocytosis from glomus cells by incubation of the carotid body of the rat with calcium and ionophore A23187. Anat. Rec. 195, 387–395. doi:10.1002/ar.1091950211
Grönblad, M., Akerman, K. E., and Eränkö, O. (1980). Ultrastructural evidence of exocytosis from glomus cells after incubation of adult rat carotid bodies in potassium-rich calcium-containing media. Brain Res. 189, 576–581. doi:10.1016/0006-8993(80)90372-8
Grönblad, M., and Eränkö, O. (1978). Fine structure of dense-cored vesicles in glomus cells of rat carotid body after fixation with permanganate or glutaraldehyde. Histochemistry 57, 305–312. doi:10.1007/BF00492666
Grönblad, M. (1983). Improved demonstration of exocytotic profiles in glomus cells of rat carotid body after perfusion with glutaraldehyde fixative containing a high concentration of potassium. Cell Tissue Res. 229, 627–637. doi:10.1007/BF00207702
Hansen, J. T. (1977). Freeze-fracture study of the carotid body. Am. J. Anat. 148, 295–300. doi:10.1002/aja.1001480207
Hansen, J. T., and Smith, N. K. (1979). Calcium binding sites in the vesicles of the carotid and aortic body chief cells. Cell Tissue Res. 199, 145–151. doi:10.1007/BF00237734
Haouzi, P., Sonobe, T., and Chenuel, B. (2014). Oxygen-related chemoreceptor drive to breathe during H₂S infusion. Respir. Physiol. Neurobiol. 201, 24–30. doi:10.1016/j.resp.2014.05.012
Hashmi-Hill, M. P., Sandock, K., Bates, J. N., Robertson, T. P., and Lewis, S. J. (2007). Flavin adenine dinucleotide may release preformed stores of nitrosyl factors from the vascular endothelium of conscious rats. J. Cardiovasc. Pharmacol. 50, 142–154. doi:10.1097/FJC.0b013e31805c1646
Henderson, F., May, W. J., Gruber, R. B., Discala, J. F., Puskovic, V., Young, A. P., et al. (2014). Role of central and peripheral opiate receptors in the effects of fentanyl on analgesia, ventilation and arterial blood-gas chemistry in conscious rats. Respir. Physiol. Neurobiol. 191, 95–105. doi:10.1016/j.resp.2013.11.005
Hervonen, A., and Korkala, O. (1972). Fine structure of the carotid body of the midterm human fetus. Z. Anat. Entwicklungsgesch. 138, 135–144. doi:10.1007/BF00519376
Höhler, B., Mayer, B., and Kummer, W. (1994). Nitric oxide synthase in the rat carotid body and carotid sinus. Cell Tissue Res. 276, 559–564. doi:10.1007/BF00343953
Hoque, A., Bates, J. N., and Lewis, S. J. (1999). In vivo evidence that L-S-nitrosocysteine may exert its vasodilator effects by interaction with thiol residues in the vasculature. Eur. J. Pharmacol. 384, 169–172. doi:10.1016/s0014-2999(99)00686-x
Hoque, A., Bates, J. N., and Lewis, S. J. (2000). Redox regulation of S-nitrosocysteine-mediated vasodilation in vivo. Eur. J. Pharmacol. 408, 195–198. doi:10.1016/s0014-2999(00)00779-2
Iturriaga, R., Alcayaga, J., and Gonzalez, C. (2009). Neurotransmitters in carotid body function: the case of dopamine - invited article. Adv. Exp. Med. Biol. 648, 137–143. doi:10.1007/978-90-481-2259-2_16
Iturriaga, R., and Alcayaga, J. (2004). Neurotransmission in the carotid body: transmitters and modulators between glomus cells and petrosal ganglion nerve terminals. Brain. Res. Brain. Res. Rev. 47, 46–53. doi:10.1016/j.brainresrev.2004.05.007
Iturriaga, R. (2001). Nitric oxide and carotid body chemoreception. Biol. Res. 34, 135–139. doi:10.4067/s0716-97602001000200019
Jaffrey, S. R., Erdjument-Bromage, H., Ferris, C. D., Tempst, P., and Snyder, S. H. (2001). Protein S-nitrosylation: a physiological signal for neuronal nitric oxide. Nat. Cell Biol. 3, 193–197. doi:10.1038/35055104
Jenkins, M. W., Khalid, F., Baby, S. M., May, W. J., Young, A. P., Bates, J. N., et al. (2021). Glutathione ethyl ester reverses the deleterious effects of fentanyl on ventilation and arterial blood-gas chemistry while prolonging fentanyl-induced analgesia. Sci. Rep. 11, 6985. doi:10.1038/s41598-021-86458-x
Johnson, A. K., and Gross, P. M. (1993). Sensory circumventricular organs and brain homeostatic pathways. FASEB J. 7, 678–686. doi:10.1096/fasebj.7.8.8500693
Joksovic, P. M., Doctor, A., Gaston, B., and Todorovic, S. M. (2007). Functional regulation of T-type calcium channels by s-nitrosothiols in the rat thalamus. J. Neurophysiol. 97, 2712–2721. doi:10.1152/jn.00926.2006
Khan, Q., Smith, P., and Heath, D. (1990). The distribution of enkephalins in human carotid bodies showing cellular proliferation and chronic glomitis. Arch. Pathol. Lab. Med. 114, 1232–1235.
Kobayashi, S. (1971a). Comparative cytological studies of the carotid body. 1. Demonstration of monoamine-storing cells by correlated chromaffin reaction and fluorescence histochemistry. Arch. Histol. Jpn. 33, 319–339. doi:10.1679/aohc1950.33.319
Kobayashi, S. (1971b). Comparative cytological studies of the carotid body. 2. Ultrastructure of the synapses on the chief cell. Arch. Histol. Jpn. 33, 397–420. doi:10.1679/aohc1950.33.397
Kondo, H. (2002). Are there gap junctions between chief (glomus, type I) cells in the carotid body chemoreceptor? A review. Microsc. Res. Tech. 59, 227–233. doi:10.1002/jemt.10196
Krammer, E. B. (1978). Carotid body chemoreceptor function: hypothesis based on a new circuit model. Proc. Natl. Acad. Sci. USA. 75, 2507–2511. doi:10.1073/pnas.75.5.2507
Kukreja, R. C., Wei, E. P., Kontos, H. A., and Bates, J. N. (1993). Nitric oxide and S-nitroso-L-cysteine as endothelium-derived relaxing factors from acetylcholine in cerebral vessels in cats. Stroke 24, 2010–2014. discussion 2014-2015. doi:10.1161/01.str.24.12.2010
Kumar, P. (2007). Sensing hypoxia in the carotid body: from stimulus to response. Essays Biochem. 43, 43–60. doi:10.1042/BSE0430043
Kummer, W., and Habeck, J. O. (1991). Substance P- and calcitonin gene-related peptide-like immunoreactivities in the human carotid body studied at light and electron microscopical level. Brain Res. 554, 286–292. doi:10.1016/0006-8993(91)90202-7
Lacolley, P., Owen, J. R., Sandock, K., Lewis, T. H., Bates, J. N., Robertson, T. P., et al. (2006b). 5-HT activates vagal afferent cell bodies in vivo: role of 5-HT2 and 5-HT3 receptors. Neuroscience 143, 273–287. doi:10.1016/j.neuroscience.2006.07.032
Lacolley, P., Owen, J. R., Sandock, K., Lewis, T. H., Bates, J. N., Robertson, T. P., et al. (2006a). Occipital artery injections of 5-HT may directly activate the cell bodies of vagal and glossopharyngeal afferent cell bodies in the rat. Neuroscience 143, 289–308. doi:10.1016/j.neuroscience.2006.08.047
Lahiri, S., Roy, A., Baby, S. M., Hoshi, T., Semenza, G. L., and Prabhakar, N. R. (2006). Oxygen sensing in the body. Prog. Biophys. Mol. Biol. 91, 249–286. doi:10.1016/j.pbiomolbio.2005.07.001
Lee, J., Nelson, M. T., Rose, K. E., and Todorovic, S. M. (2013). Redox mechanism of S-nitrosothiol modulation of neuronal CaV3.2 T-type calcium channels. Mol. Neurobiol. 48, 274–280. doi:10.1007/s12035-013-8493-8
Lei, S. Z., Pan, Z. H., Aggarwal, S. K., Chen, H. S., Hartman, J., Sucher, N. J., et al. (1992). Effect of nitric oxide production on the redox modulatory site of the NMDA receptor-channel complex. Neuron 8, 1087–1099. doi:10.1016/0896-6273(92)90130-6
Lewis, S. J., Graves, J. E., Bates, J. N., and Kooy, N. W. (2005b). Peroxynitrite elicits dysfunction of stereoselective S-nitrosocysteine recognition sites. J. Cardiovasc. Pharmacol. 46, 637–645. doi:10.1097/01.fjc.0000181717.87204.2f
Lewis, S. J., Hoque, A., and Bates, J. N. (2005a). Differentiation of L- and D-S-nitrosothiol recognition sites in vivo. J. Cardiovasc. Pharmacol. 46, 660–671. doi:10.1097/01.fjc.0000181714.94827.5d
Lewis, S. J., Travis, M. D., and Bates, J. N. (1996). Stereoselective S-nitrosocysteine recognition sites in rat brain. Eur. J. Pharmacol. 312, R3–R5. doi:10.1016/0014-2999(96)00607-3
LewisOwen, S. J. J. R., and Bates, J. N. (2006). S-nitrosocysteine elicits hemodynamic responses similar to those of the Bezold-Jarisch reflex via activation of stereoselective recognition sites. Eur. J. Pharmacol. 531, 254–258. doi:10.1016/j.ejphar.2005.11.027
Li, C., Huang, L., Jia, X., Zhao, B., Chen, L., and Liu, Y. (2020). Functional glutamate transporters are expressed in the carotid chemoreceptor. Respir. Res. 21, 208. doi:10.1186/s12931-020-01468-z
Li, S., and Whorton, A. R. (2007). Functional characterization of two S-nitroso-L-cysteine transporters, which mediate movement of NO equivalents into vascular cells. Am. J. Physiol. Cell Physiol. 292, C1263–C1271. doi:10.1152/ajpcell.00382.2006
Li, Y. L., Zheng, H., Ding, Y., and Schultz, H. D. (2010). Expression of neuronal nitric oxide synthase in rabbit carotid body glomus cells regulates large-conductance Ca2+-activated potassium currents. J. Neurophysiol. 103, 3027–3033. doi:10.1152/jn.01138.2009
Lima, B., Forrester, M. T., Hess, D. T., and Stamler, J. S. (2010). S-nitrosylation in cardiovascular signaling. Circ. Res. 106, 633–646. doi:10.1161/CIRCRESAHA.109.207381
Lipton, A. J., Johnson, M. A., Macdonald, T., Lieberman, M. W., Gozal, D., and Gaston, B. (2001). S-nitrosothiols signal the ventilatory response to hypoxia. Nature 413, 171–174. doi:10.1038/35093117
Lipton, S. A., Choi, Y. B., Pan, Z. H., Lei, S. Z., Chen, H. S., Sucher, N. J., et al. (1993). A redox-based mechanism for the neuroprotective and neurodestructive effects of nitric oxide and related nitroso-compounds. Nature 364, 626–632. doi:10.1038/364626a0
Lipton, S. A., Singel, D. J., and Stamler, J. S. (1994). Nitric oxide in the central nervous system. Prog. Brain Res. 103, 359–364. doi:10.1016/s0079-6123(08)61149-8
Loesch, A., and Burnstock, G. (1996). Ultrastructural study of perivascular nerve fibres and endothelial cells of the rat basilar artery immunolabelled with monoclonal antibodies to neuronal and endothelial nitric oxide synthase. J. Neurocytol. 25, 525–534. doi:10.1007/BF02284820
Loesch, A., Belai, A., and Burnstock, G. (1994). An ultrastructural study of NADPH-diaphorase and nitric oxide synthase in the perivascular nerves and vascular endothelium of the rat basilar artery. J. Neurocytol. 23, 49–59. doi:10.1007/BF01189816
Loesch, A., Belai, A., and Burnstock, G. (1993). Ultrastructural localization of NADPH-diaphorase and colocalization of nitric oxide synthase in endothelial cells of the rabbit aorta. Cell Tissue Res. 274, 539–545. doi:10.1007/BF00314551
Loesch, A., and Burnstock, G. (1998). Perivascular nerve fibres and endothelial cells of the rat basilar artery: immuno-gold labelling of antigenic sites for type I and type III nitric oxide synthase. J. Neurocytol. 27, 197–204. doi:10.1023/a:1026493425977
Loesch, A., and Burnstock, G. (1995). Ultrastructural localization of nitric oxide synthase and endothelin in coronary and pulmonary arteries of newborn rats. Cell Tissue Res. 279, 475–483. doi:10.1007/BF00318161
Ludbrook, J. (1998). Multiple comparison procedures updated. Clin. Exp. Pharmacol. Physiol. 25, 1032–1037. doi:10.1111/j.1440-1681.1998.tb02179.x
Makarenko, V. V., Peng, Y. J., Yuan, G., Fox, A. P., Kumar, G. K., Nanduri, J., et al. (2015). CaV3.2 T-type Ca2⁺ channels in H₂S-mediated hypoxic response of the carotid body. Am. J. Physiol. Cell Physiol. 308, C146–C154. doi:10.1152/ajpcell.00141.2014
Marozkina, N., and Gaston, B. (2020). An update on thiol signaling: S-nitrosothiols, hydrogen sulfide and a putative role for thionitrous acid. Antioxidants (Basel). 9, 225. doi:10.3390/antiox9030225
Marozkina, N. V., and Gaston, B. (2015). Nitrogen chemistry and lung physiology. Annu. Rev. Physiol. 77, 431–452. doi:10.1146/annurev-physiol-021113-170352
Marozkina, N. V., and Gaston, B. (2012). S-Nitrosylation signaling regulates cellular protein interactions. Biochim. Biophys. Acta 1820, 722–729. doi:10.1016/j.bbagen.2011.06.017
Martínez-Ruiz, A., Araújo, I. M., Izquierdo-Álvarez, A., Hernansanz-Agustín, P., Lamas, S., and Serrador, J. M. (2013). Specificity in S-nitrosylation: a short-range mechanism for NO signaling? Antioxid. Redox Signal 19, 1220–1235. doi:10.1089/ars.2012.5066
Matsuda, T., Bates, J. N., Lewis, S. J., Abboud, F. M., and Chapleau, M. W. (1995). Modulation of baroreceptor activity by nitric oxide and S-nitrosocysteine. Circ. Res. 76, 426–433. doi:10.1161/01.res.76.3.426
May, W. J., Gruber, R. B., Discala, J. F., Puskovic, V., Henderson, F., Palmer, L. A., et al. (2013a). Morphine has latent deleterious effects on the ventilatory responses to a hypoxic challenge. Open J. Mol. Integr. Physiol. 3, 166–180. doi:10.4236/ojmip.2013.34022
May, W. J., Henderson, F., Gruber, R. B., Discala, J. F., Young, A. P., Bates, J. N., et al. (2013b). Morphine has latent deleterious effects on the ventilatory responses to a hypoxic-hypercapnic challenge. Open. J. Mol. Integr. Physiol. 3, 134–145. doi:10.4236/ojmip.2013.33019
McHugh, M. L. (2011). Multiple comparison analysis testing in ANOVA. Biochem. Med. Zagreb. 21, 203–209. doi:10.11613/bm.2011.029
McQueen, D. S., and Mir, A. K. (1984). Changes in carotid body amine levels and effects of dopamine on respiration in rats treated neonatally with capsaicin. Br. J. Pharmacol. 83 (4), 909–918. doi:10.1111/j.1476-5381.1984.tb16531.x
Meller, S. T., Lewis, S. J., Bates, J. N., Brody, M. J., and Gebhart, G. F. (1990). Is there a role for an endothelium-derived relaxing factor in nociception? Brain Res. 531, 342–345. doi:10.1016/0006-8993(90)90798-g
Meller, S. T., Lewis, S. J., Ness, T. J., Brody, M. J., and Gebhart, G. F. (1991). Neonatal capsaicin treatment abolishes the nociceptive responses to intravenous 5-HT in the rat. Brain Res. 542, 212–218. doi:10.1016/0006-8993(91)91569-m
Mellion, B. T., Ignarro, L. J., Myers, C. B., Ohlstein, E. H., Ballot, B. A., Hyman, A. L., et al. (1983). Inhibition of human platelet aggregation by S-nitrosothiols. Heme-dependent activation of soluble guanylate cyclase and stimulation of cyclic GMP accumulation. Mol. Pharmacol. 23, 653–664.
Moya, E. A., Alcayaga, J., and Iturriaga, R. (2012). NO modulation of carotid body chemoreception in health and disease. Respir. Physiol. Neurobiol. 184, 158–164. doi:10.1016/j.resp.2012.03.019
Myers, P. R., Minor, R. L., Guerra, R., Bates, J. N., and Harrison, D. G. (1990). Vasorelaxant properties of the endothelium-derived relaxing factor more closely resemble S-nitrosocysteine than nitric oxide. Nature 345, 161–163. doi:10.1038/345161a0
Nakamura, T., and Lipton, S. A. (2016). Protein S-nitrosylation as a therapeutic target for neurodegenerative diseases. Trends Pharmacol. Sci. 37, 73–84. doi:10.1016/j.tips.2015.10.002
Nemoto, T., Shimma, N., Horie, S., Saito, T., Okuma, Y., Nomura, Y., et al. (2003). Involvement of the system L amino acid transporter on uptake of S-nitroso-L-cysteine, an endogenous S-nitrosothiol, in PC12 cells. Eur. J. Pharmacol. 458, 17–24. doi:10.1016/s0014-2999(02)02699-7
Nurse, C. A. (2005). Neurotransmission and neuromodulation in the chemosensory carotid body. Auton. Neurosci. 120, 1–9. doi:10.1016/j.autneu.2005.04.008
Nurse, C. A., and Piskuric, N. A. (2013). Signal processing at mammalian carotid body chemoreceptors. Semin. Cell Dev. Biol. 24, 22–30. doi:10.1016/j.semcdb.2012.09.006
Nurse, C. A. (2014). Synaptic and paracrine mechanisms at carotid body arterial chemoreceptors. J. Physiol. 592, 3419–3426. doi:10.1113/jphysiol.2013.269829
Ohta, H., Bates, J. N., Lewis, S. J., and Talman, W. T. (1997). Actions of S-nitrosocysteine in the nucleus tractus solitarii are unrelated to release of nitric oxide. Brain Res. 746, 98–104. doi:10.1016/s0006-8993(96)01188-2
Ortega-Sáenz, P., Macías, D., Levitsky, K. L., Rodríguez-Gómez, J. A., González-Rodríguez, P., Bonilla-Henao, V., et al. (2016). Selective accumulation of biotin in arterial chemoreceptors: requirement for carotid body exocytotic dopamine secretion. J. Physiol. 594, 7229–7248. doi:10.1113/JP272961
Overholt, J. L., and Prabhakar, N. R. (1997). Ca2+ current in rabbit carotid body glomus cells is conducted by multiple types of high-voltage-activated Ca2+ channels. J. Neurophysiol. 78, 2467–2474. doi:10.1152/jn.1997.78.5.2467
Pandit, J. J., and Buckler, K. J. (2009). Differential effects of halothane and sevoflurane on hypoxia-induced intracellular calcium transients of neonatal rat carotid body type I cells. Br. J. Anaesth. 103, 701–710. doi:10.1093/bja/aep223
Peers, C., and Buckler, K. J. (1995). Transduction of chemostimuli by the type I carotid body cell. J. Membr. Biol. 144, 1–9. doi:10.1007/BF00238411
Peers, C. (2004). Interactions of chemostimuli at the single cell level: studies in a model system. Exp. Physiol. 89, 60–65. doi:10.1113/expphysiol.2003.002657
Pires da Silva, M., de Almeida Moraes, D. J., Mecawi, A. S., Rodrigues, J. A., and Varanda, W. A. (2016). Nitric oxide modulates HCN channels in magnocellular neurons of the supraoptic nucleus of rats by an S-Nitrosylation-Dependent mechanism. J. Neurosci. 36, 11320–11330. doi:10.1523/JNEUROSCI.1588-16.2016
Possas, O. S., Johnson, A. K., and Lewis, S. J. (2006). Role of nitrosyl factors in the hindlimb vasodilation elicited by baroreceptor afferent nerve stimulation. Am. J. Physiol. Regul. Integr. Comp. Physiol. 290, R741–R748. doi:10.1152/ajpregu.00660.2005
Possas, O. S., and Lewis, S. J. (1997). NO-containing factors mediate hindlimb vasodilation produced by superior laryngeal nerve stimulation. Am. J. Physiol. 273, H234–H243. doi:10.1152/ajpheart.1997.273.1.H234
Prabhakar, N. R., Kumar, G. K., Chang, C. H., Agani, F. H., and Haxhiu, M. A. (1993). Nitric oxide in the sensory function of the carotid body. Brain Res. 625, 16–22. doi:10.1016/0006-8993(93)90132-7
Prabhakar, N. R. (1994). Neurotransmitters in the carotid body. Adv. Exp. Med. Biol. 360, 57–69. doi:10.1007/978-1-4615-2572-1_6
Prabhakar, N. R., and Peers, C. (2014). Gasotransmitter regulation of ion channels: a key step in O2 sensing by the carotid body. Physiol. (Bethesda) 29, 49–57. doi:10.1152/physiol.00034.2013
Prieto-Lloret, J., and Aaronson, P. I. (2017). Hydrogen sulfide as an O2 sensor: A critical analysis. Adv. Exp. Med. Biol. 967, 261–276. doi:10.1007/978-3-319-63245-2_15
Raju, K., Doulias, P. T., Evans, P., Krizman, E. N., Jackson, J. G., Horyn, O., et al. (2015). Regulation of brain glutamate metabolism by nitric oxide and S-nitrosylation. Sci. Signal 8, ra68. doi:10.1126/scisignal.aaa4312
Rey, S., and Iturriaga, R. (2004). Endothelins and nitric oxide: vasoactive modulators of carotid body chemoreception. Curr. Neurovasc. Res. 1, 465–473. doi:10.2174/1567202043361857
Rudkouskaya, A., Sim, V., Shah, A. A., Feustel, P. J., Jourd'heuil, D., and Mongin, A. A. (2010). Long-lasting inhibition of presynaptic metabolism and neurotransmitter release by protein S-nitrosylation. Free Radic. Biol. Med. 49, 757–769. doi:10.1016/j.freeradbiomed.2010.05.032
Ruggiero, D. A., Mtui, E. P., Otake, K., and Anwar, M. (1996). Central and primary visceral afferents to nucleus tractus solitarii may generate nitric oxide as a membrane-permeant neuronal messenger. J. Comp. Neurol. 364, 51–67. doi:10.1002/(SICI)1096-9861(19960101)364:1<51:AID-CNE5>3.0.CO;2-R
Savidge, T. C. (2011). S-nitrosothiol signals in the enteric nervous system: lessons learnt from big brother. Front. Neurosci. 5, 31. doi:10.3389/fnins.2011.00031
Scraggs, M., Smith, P., and Heath, D. (1992). Glomic cells and their peptides in the carotid body of the human fetus. Pediatr. Pathol. 12, 823–834. doi:10.3109/15513819209024239
Seckler, J. M., Grossfield, A., May, W. J., Getsy, P. M., and Lewis, S. J. (2022). Nitrosyl factors play a vital role in the ventilatory depressant effects of fentanyl in unanesthetized rats. Biomed. Pharmacother. 146, 112571. doi:10.1016/j.biopha.2021.112571
Seckler, J. M., Meyer, N. M., Burton, S. T., Bates, J. N., Gaston, B., and Lewis, S. J. (2017). Detection of trace concentrations of S-nitrosothiols by means of a capacitive sensor. PLoS One 12, e0187149. doi:10.1371/journal.pone.0187149
Seckler, J. M., Shen, J., Lewis, T. H. J., Abdulameer, M. A., Zaman, K., Palmer, L. A., et al. (2020). NADPH diaphorase detects S-nitrosylated proteins in aldehyde-treated biological tissues. Sci. Rep. 10, 21088. doi:10.1038/s41598-020-78107-6
Severina, I. S., Bussygina, O. G., Pyatakova, N. V., Malenkova, I. V., and Vanin, A. F. (2003). Activation of soluble guanylate cyclase by NO donors-S-nitrosothiols, and dinitrosyl-iron complexes with thiol-containing ligands. Nitric Oxide 8, 155–163. doi:10.1016/s1089-8603(03)00002-8
Shirahata, M., Balbir, A., Otsubo, T., and Fitzgerald, R. S. (2007). Role of acetylcholine in neurotransmission of the carotid body. Respir. Physiol. Neurobiol. 157, 93–105. doi:10.1016/j.resp.2006.12.010
Silva, J. M., and Lewis, D. L. (2002). Nitric oxide enhances Ca2+-dependent K+ channel activity in rat carotid body cells. Pflugers Arch. 443, 671–675. doi:10.1007/s00424-001-0745-1
Silva, M. J., and Lewis, D. L. (1995). L- and N-type Ca2+ channels in adult rat carotid body chemoreceptor type I cells. J. Physiol. 489, 689–699. doi:10.1113/jphysiol.1995.sp021083
Smith, P., Gosney, J., Heath, D., and Burnett, H. (1990). The occurrence and distribution of certain polypeptides within the human carotid body. Cell Tissue Res. 261, 565–571. doi:10.1007/BF00313536
Sosunov, A. A., Hassall, C. J., Loesch, A., Turmaine, M., and Burnstock, G. (1996). Nitric oxide synthase-containing neurones and nerve fibres within cardiac ganglia of rat and guinea-pig: an electron-microscopic immunocytochemical study. Cell Tissue Res. 284, 19–28. doi:10.1007/s004410050563
Sosunov, A. A., Hassall, C. J., Loesch, A., Turmaine, M., and Burnstock, G. (1995). Ultrastructural investigation of nitric oxide synthase-immunoreactive nerves associated with coronary blood vessels of rat and guinea-pig. Cell Tissue Res. 280, 575–582. doi:10.1007/BF00318361
Stomberski, C. T., Hess, D. T., and Stamler, J. S. (2019). Protein S-nitrosylation: Determinants of specificity and enzymatic regulation of S-Nitrosothiol-Based signaling. Antioxid. Redox Signal 30, 1331–1351. doi:10.1089/ars.2017.7403
Südhof, T. C., and Rothman, J. E. (2009). Membrane fusion: grappling with SNARE and SM proteins. Science 323, 474–477. doi:10.1126/science.1161748
Sudhof, T. C. (2004). The synaptic vesicle cycle. Annu. Rev. Neurosci. 27, 509–547. doi:10.1146/annurev.neuro.26.041002.131412
Summers, B. A., Overholt, J. L., and Prabhakar, N. R. (2000). Augmentation of calcium current by hypoxia in carotid body glomus cells. Adv. Exp. Med. Biol. 475, 589–599. doi:10.1007/0-306-46825-5_57
Summers, B. A., Overholt, J. L., and Prabhakar, N. R. (1999). Nitric oxide inhibits L-type Ca2+ current in glomus cells of the rabbit carotid body via a cGMP-independent mechanism. J. Neurophysiol. 81, 1449–1457. doi:10.1152/jn.1999.81.4.1449
Takahashi, H., Shin, Y., Cho, S. J., Zago, W. M., Nakamura, T., Gu, Z., et al. (2007). Hypoxia enhances S-nitrosylation-mediated NMDA receptor inhibition via a thiol oxygen sensor motif. Neuron 53, 53–64. doi:10.1016/j.neuron.2006.11.023
Tanaka, K., and Chiba, T. (1994). Nitric oxide synthase containing neurons in the carotid body and sinus of the guinea pig. Microsc. Res. Tech. 29, 90–93. doi:10.1002/jemt.1070290205
Tarasenko, A. S. (2015). The effect of nitric oxide on synaptic vesicle proton gradient and mitochondrial potential of brain nerve terminals. Ukr. Biochem. J. 87, 64–75. doi:10.15407/ubj87.06.064
Tegeder, I., Scheving, R., Wittig, I., and Geisslinger, G. (2011). SNO-ing at the nociceptive synapse? Pharmacol. Rev. 63, 366–389. doi:10.1124/pr.110.004200
Tooker, R. E., and Vigh, J. (2015). Light-evoked S-nitrosylation in the retina. J. Comp. Neurol. 523, 2082–2110. doi:10.1002/cne.23780
Travis, M. D., Davisson, R. L., Bates, J. N., and Lewis, S. J. (1997). Hemodynamic effects of L- and D-S-nitroso-beta,beta-dimethylcysteine in rats. Am. J. Physiol. 273, H1493–H1501. doi:10.1152/ajpheart.1997.273.3.H1493
Travis, M. D., Hoque, A., Bates, J. N., and Lewis, S. J. (2000). Blockade of voltage-sensitive Ca2+-channels markedly diminishes nitric oxide- but not L-S-nitrosocysteine- or endothelium-dependent vasodilation in vivo. Eur. J. Pharmacol. 408, 289–298. doi:10.1016/s0014-2999(00)00792-5
Travis, M. D., Stoll, L. L., Bates, J. N., and Lewis, S. J. (1996). L- and D-S-nitroso-beta,beta-dimethylcysteine differentially increase cGMP in cultured vascular smooth muscle cells. Eur. J. Pharmacol. 318, 47–53. doi:10.1016/s0014-2999(96)00719-4
Trzebski, A., Sato, Y., Suzuki, A., and Sato, A. (1995). Inhibition of nitric oxide synthesis potentiates the responsiveness of carotid chemoreceptors to systemic hypoxia in the rat. Neurosci. Lett. 190, 29–32. doi:10.1016/0304-3940(95)11492-f
Tse, A., Lee, A. K., Takahashi, N., Gong, A., Kasai, H., and Tse, F. W. (2018). Strong stimulation triggers full fusion exocytosis and very slow endocytosis of the small dense core granules in carotid glomus cells. J. Neurogenet. 32, 267–278. doi:10.1080/01677063.2018.1497629
Valdés, V., Mosqueira, M., Rey, S., Del Rio, R., and Iturriaga, R. (2003). Inhibitory effects of NO on carotid body: contribution of neural and endothelial nitric oxide synthase isoforms. Am. J. Physiol. Lung Cell Mol. Physiol. 284, L57–L68. doi:10.1152/ajplung.00494.2001
Vanin, A. F. (2019). What is the mechanism of nitric oxide conversion into nitrosonium Ions ensuring S-nitrosating processes in living organisms. Cell biochem. Biophys. 77, 279–292. doi:10.1007/s12013-019-00886-1
Wallenstein, S., Zucker, C. L., and Fleiss, J. L. (1980). Some statistical methods useful in circulation research. Circ. Res. 47, 1–9. doi:10.1161/01.res.47.1.1
Wang, H., Zhang, C., and Xiao, H. (2019). Mechanism of membrane fusion: protein-protein interaction and beyond. Int. J. Physiol. Pathophysiol. Pharmacol. 11, 250–257.
Wang, Y. Y., Perrin, D. G., and Cutz, E. (1993). Localization of cholecystokinin-like and calcitonin-like peptides in infant carotid bodies: a light- and electron-microscopic immunohistochemical study. Cell Tissue Res. 272, 169–174. doi:10.1007/BF00323583
Wang, Z. Z., Dinger, B. G., Stensaas, L. J., and Fidone, S. J. (1995b). The role of nitric oxide in carotid chemoreception. Biol. Signals. 4, 109–116. doi:10.1159/000109430
Wang, Z. Z., Stensaas, L. J., Dinger, B. G., and Fidone, S. J. (1995a). Nitric oxide mediates chemoreceptor inhibition in the cat carotid body. Neuroscience 65, 217–229. doi:10.1016/0306-4522(94)00437-a
Weiss, N., and Donnelly, D. F. (1996). Depolarization is a critical event in hypoxia-induced glomus cell secretion. Adv. Exp. Med. Biol. 410, 181–187. doi:10.1007/978-1-4615-5891-0_26
Wynia-Smith, S. L., and Smith, B. C. (2017). Nitrosothiol formation and S-nitrosation signaling through nitric oxide synthases. Nitric Oxide 63, 52–60. doi:10.1016/j.niox.2016.10.001
Yamamoto, Y., König, P., Henrich, M., Dedio, J., and Kummer, W. (2006). Hypoxia induces production of nitric oxide and reactive oxygen species in glomus cells of rat carotid body. Cell Tissue Res. 325, 3–11. doi:10.1007/s00441-006-0178-4
Yan, L., Lee, A. K., Tse, F. W., and Tse, A. (2012). Ca2+ homeostasis and exocytosis in carotid glomus cells: role of mitochondria. Cell Calcium 51, 155–163. doi:10.1016/j.ceca.2011.12.003
Young, A. P., Gruber, R. B., Discala, J. F., May, W. J., McLaughlin, D., Palmer, L. A., et al. (2013). Co-activation of μ- and δ-opioid receptors elicits tolerance to morphine-induced ventilatory depression via generation of peroxynitrite. Respir. Physiol. Neurobiol. 186, 255–264. doi:10.1016/j.resp.2013.02.028
Keywords: primary glomus cells, S-nitroso-L-cysteine, L-S-nitrosoglutathione, Smethyl-L-cysteine, S-ethyl-L-cysteine, minute ventilation
Citation: Seckler JM, Getsy PM, May WJ, Gaston B, Baby SM, Lewis THJ, Bates JN and Lewis SJ (2023) Hypoxia releases S-nitrosocysteine from carotid body glomus cells—relevance to expression of the hypoxic ventilatory response. Front. Pharmacol. 14:1250154. doi: 10.3389/fphar.2023.1250154
Received: 29 June 2023; Accepted: 13 September 2023;
Published: 11 October 2023.
Edited by:
Ying-Jie Peng, The University of Chicago, United StatesReviewed by:
Mohamed Ahmed, University of Arizona, United StatesCopyright © 2023 Seckler, Getsy, May, Gaston, Baby, Lewis, Bates and Lewis. This is an open-access article distributed under the terms of the Creative Commons Attribution License (CC BY). The use, distribution or reproduction in other forums is permitted, provided the original author(s) and the copyright owner(s) are credited and that the original publication in this journal is cited, in accordance with accepted academic practice. No use, distribution or reproduction is permitted which does not comply with these terms.
*Correspondence: Stephen J. Lewis, c2psNzhAY2FzZS5lZHU=
†Present addresses: Santhosh M. Baby, Translational Sciences Treatment Discovery, Galvani Bioelectronics, Inc., Pennsylvania
James N. Bates, Chief Medical Officer, Atelerix Life Sciences Inc., Virginia
Disclaimer: All claims expressed in this article are solely those of the authors and do not necessarily represent those of their affiliated organizations, or those of the publisher, the editors and the reviewers. Any product that may be evaluated in this article or claim that may be made by its manufacturer is not guaranteed or endorsed by the publisher.
Research integrity at Frontiers
Learn more about the work of our research integrity team to safeguard the quality of each article we publish.