- Nantes Université, Univ Angers, INSERM, CNRS, CRCI2NA, Nantes, France
Part of the broader glycosphingolipid family, gangliosides are composed of a ceramide bound to a sialic acid-containing glycan chain, and locate at the plasma membrane. Gangliosides are produced through sequential steps of glycosylation and sialylation. This diversity of composition is reflected in differences in expression patterns and functions of the various gangliosides. Ganglioside GD2 designates different subspecies following a basic structure containing three carbohydrate residues and two sialic acids. GD2 expression, usually restrained to limited tissues, is frequently altered in various neuroectoderm-derived cancers. While GD2 is of evident interest, its glycolipid nature has rendered research challenging. Physiological GD2 expression has been linked to developmental processes. Passing this stage, varying levels of GD2, physiologically expressed mainly in the central nervous system, affect composition and formation of membrane microdomains involved in surface receptor signaling. Overexpressed in cancer, GD2 has been shown to enhance cell survival and invasion. Furthermore, binding of antibodies leads to immune-independent cell death mechanisms. In addition, GD2 contributes to T-cell dysfunction, and functions as an immune checkpoint. Given the cancer-associated functions, GD2 has been a source of interest for immunotherapy. As a potential biomarker, methods are being developed to quantify GD2 from patients’ samples. In addition, various therapeutic strategies are tested. Based on initial success with antibodies, derivates such as bispecific antibodies and immunocytokines have been developed, engaging patient immune system. Cytotoxic effectors or payloads may be redirected based on anti-GD2 antibodies. Finally, vaccines can be used to mount an immune response in patients. We review here the pertinent biological information on GD2 which may be of use for optimizing current immunotherapeutic strategies.
1 Introduction
Although GD2-specific immunotherapies have shown clinical successes, our understanding of the biology of GD2, both in normal development and tumorigenesis, remains insufficient. Improving insufficient knowledge would make it easier to optimize anti-GD2 immunotherapies in a more informed and logical manner. GD2 ganglioside is a sialic acid-containing glycosphingolipid, whose structure is characterized by two distinct portions with different physicochemical properties. It is composed of a hydrophobic ceramide and a hydrophilic oligosaccharide containing one or more negatively charged sialic acids. Thus, gangliosides are amphiphilic molecules that have the ability to establish both hydrophilic and hydrophobic interactions. The ceramide, a sphingoid base linked to a fatty acid, interacts with other membrane lipids and allows GD2 to be tightly anchored to the cell surface. This ceramide tail is generally shared by all other gangliosides species. The oligosaccharide head is oriented towards the extracellular environment and interacts, via mild hydrophilic bonds, with neighboring membrane molecules or extracellular ligands. The term ganglioside GD2 is based on Svennerholm’s nomenclature (Svennerholm, 1963), where G stands for ganglioside, D for the number of sialic acid residues, and 2 corresponds to the order of its migration on thin-layer chromatograph. According to the IUPAC-IUBMB nomenclature, GD2 is named II3Neu5Ac2-Gg3-Cer, where the Roman number indicates the position of the sugar residue to which the sialic acid is linked considering the glucose in first position, the exponent Arabic number stands for the linkage position, the index Arabic number is for the number of sialic acids, and Gg3 indicates the following specific ganglio trisaccharide sequence: β-GalNAc-(1-4)β-Gal-(1-4)β-Glc-(1-1) (Chester, 1998). Thus, the chemical structure of human GD2 is β-GalNAc-(1–4)[α-Neu5Ac-(2-8)-α-Neu5Ac-(2–3)]β-Gal-(1-4)β-Glc-(1-1)Ceramide (Figure 1). Interestingly, GD2 has a universal base structure in all known species in contrast to proteins with variable homology between human and nonhuman species. However, the term ganglioside GD2 actually identifies a mixture of different GD2 subspecies with either different ceramides or sialic acids. These structural modifications impact both the biological and immunological properties of GD2 molecules as we will review here.
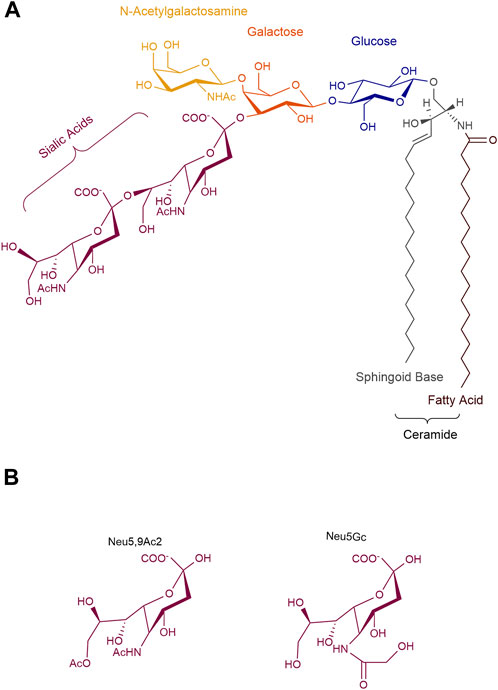
FIGURE 1. Structure of ganglioside GD2. (A) GD2 is an amphiphilic molecule that combines a hydrophobic ceramide to a hydrophilic oligosaccharide, containing two N-acetyl neuraminic acids. (B) Natural substitutions of sialic acids in GD2. Neu5Ac, N-acetyl neuraminic; Neu5Gc, N-glycolyl neuraminic acid; Neu5,9Ac2, 9-O-acetyl-5-N-acetyl neuraminic acid.
2 Biological significance of GD2 structural diversity
As described above, GD2 ganglioside is an amphiphilic molecule that combines a hydrophobic ceramide to a hydrophilic oligosaccharide, containing two negatively charged sialic acids at most physiological pH values (Figure 1). Variations in GD2’s oligosaccharide moiety mostly occur on the sialic acids, and are detected either by classical chromatographic techniques combined with antibody staining (Diaz et al., 2009), or, by combination of chromatography coupled with mass spectrometry analysis with higher sensitivity (Zarei et al., 2010). For example, 9-O-acetylated sialic acids on GD2 have been detected (Fleurence et al., 2017). This modification occurs during GD2 biosynthesis. The O-acetyl group is added by an O-acetyltransferase, possibly CASD1 (Baumann et al., 2015), to the carbon 7 of the terminal α2-8 linked sialic acid residue. This acetyl group further migrates to carbon 9 when exposed to higher pH (Fleurence et al., 2017). While the addition of the O-acetyl group on the terminal sialic acid of GD2 decreases polarity and hydrophobicity of the gangliosides, it does not affect general conformation: O-acetyl GD2 (OAcGD2) can be detected by most anti-GD2 monoclonal antibodies (Ye and Cheung, 1992; Fleurence et al., 2017). O-Acetylated gangliosides are often found in developping tissues and are regarded as onco-fetal antigens present on different tumors (Kohla et al., 2002), representing amounts up to 50% that of GD2 (Fleurence et al., 2017). As such, this GD2 subspecies, with more restricted normal expression than GD2 (see below) is of the most therapeutical significance.
The main sialic acid residue found in human ganglioside is N-acetylneuraminic acid (Neu5Ac), as an exon of the CMAH gene, encoding for the cytidine monophosphate-N-acetyl-neuraminic acid hydroxylase, the enzyme responsible for the synthesis of Neu5Gc is deleted in humans (Bashir et al., 2020). Another sialic acid variant is uses N-glycolylneuramic acid (Neu5Gc), usually observed in non-human mammals such as mice, and occasionally incorporated in human gangliosides from the diet through the consumption of red meat (Varki, 2009; Bashir et al., 2020). The exogenous incorporation is particularly seen in cancer cells due to their increased metabolism and induction of the sialin sialic acid transporter by hypoxia (Labrada et al., 2018). The presence of Neu5Gc leads to the development of varying levels of antibodies, which can lead to chronic inflammation and exacerbate cancer in mice models (Hedlund et al., 2008; Bashir et al., 2020). Noteworthy, Neu5Gc-containing GD2 gangliosides have been described (Nishio and Furukawa, 2004), which could affect the affinity of anti-GD2 antibodies which binds in part to the terminal sialic acid (Ahmed et al., 2013), to limited effect due to ganglioside recycling and the minimal proportion these modified gangliosides represent (Nishio and Furukawa, 2004). This might represent, however, an opportunity to increase immunogenicity of GD2 gangliosides in vaccination efforts.
Disialogangliosides are also prone to form lactones at low pH. Lactones are cyclic esters of hydroxycarboxylic acids, formed through intramolecular esterification. GD3-lactone was identified in mouse brain (Gross et al., 1980) and human melanoma cells (Kawashima et al., 1994). However, no such report exists for GD2-lactone. Nonetheless, synthetic lactonization of GD2 was described, and demonstrated to possess increased immunogenicity. GD2-lactones induced an active humoral response in vaccination efforts, producing superior results compared to native GD2 (Ragupathi et al., 2003).
Structural heterogeneity is also found in the ceramide moiety. This can consist of different fatty acids and/or sphingoid bases. The latter consist of sphinganine, sphingosine, and phytosphingosine of different chain lengths, which can be further O-acetylated (Pruett et al., 2008; Hama, 2010). The most common sphingoid base found in human gangliosides is sphingosine (Ando and Yu, 1984; Sarbu et al., 2021), but GD2 ceramide can also include eicosphingosine, sphinganine, or phytosphingosine (Ando and Yu, 1984; Colsch et al., 2011; Merrill, 2011; Sibille et al., 2016). In addition, fatty acids can be unsaturated, saturated, oxygenated (Ladisch et al., 1989), and of different lengths, ranging from C16 to C24 (Ando and Yu, 1984; Sjoberg et al., 1992; Serb et al., 2009; Balis et al., 2020; Fabris et al., 2021). The functional consequences of the heterogeneities of the ceramide tail remain largely unknown. There are some indications that the lipid anchor composition determines ganglioside insertion into glycolipid-enriched microdomains through hydrophobic bonds with phospholipids, cholesterol, and glycosylphosphatidylinositol-anchored proteins (Furukawa et al., 2012). Within these microdomains, gangliosides interact with signaling molecules including receptor tyrosine kinases (Furukawa et al., 2012). Changes in the composition of the ceramide tail can thus result in the modification of plasma membrane fluidity and consequently in the deregulation of cellular signals. Furthermore, GD2 with shorter fatty acid chain (16 or 18 carbons) are more likely to be shed in the extracellular environment than longer chain GD2, in which they will be able to produce effects on bystander cells, including immunosuppression (Li and Ladisch, 1991; Ladisch et al., 1994). As another example, both fatty acid chain length and sphingoid structure influence the immunogenicity of glycosphingolipids, and thus, are of particular relevance to GD2 vaccination efforts (Okuda et al., 2019; Okuda, 2021). The ceramide moiety can also mask the receptor function of the oligosaccharide head through interaction with membrane cholesterol (Kolter, 2012). This observation raises inquiries about the asymmetrical distribution of glycosphingolipids in cellular membranes and tissue as GD2 detection and targeting is mediated by the oligosaccharide head. Indeed, ganglioside profiling should take into account both the oligosaccharide and the ceramide moieties.
3 GD2 biosynthesis
While the heterogeneity of the lipid tail results from the ceramide biosynthesis at the endoplasmic reticulum (Mandon et al., 1992; Sandhoff et al., 2018), the structural diversity of GD2 ganglioside oligosaccharide is generated within the Golgi apparatus as thoroughly reviewed elsewhere (Sandhoff et al., 2018; Sandhoff and Sandhoff, 2018). Intracellular synthesis of GD2 ganglioside begins with formation of the ceramide core. The synthesis of the sphingoid base is initiated by the condensation of a coenzyme A-activated fatty acid with L-serine, catalyzed by the serine palmitoyltransferase (SPT), to form 3-keto sphinganine (Ikushiro and Hayashi, 2011). Although palmitoyl CoA (16C) is the main substrate, SPT can also metabolize other acyl-CoAs, from C14 to C18, forming a variety of long-chain bases that differ by structure and function. In fact, SPT consists of three different core subunits (SPTLC1, SPTLC2, and SPTLC3) that condition the substrate specificity (Sandhoff et al., 2018). As an example, SPLTC3 induces a shift towards myristoyl-CoA (14C) and with the addition of serine, it produces sphingoid bases 16, 18 or 20 carbon long (Sandhoff et al., 2018).
The next step is the reduction of 3-keto sphinganine by 3-keto sphinganine reductase followed by acylation of sphinganine to hydroceramides of different chain lengths (Ikushiro and Hayashi, 2011). Six isoforms of ceramide synthase have been described and determine preferences for fatty acids of different chain length (Cingolani et al., 2016). At this stage, dihydroceramides can be dehydrogenated to ceramide by the dihydroceramide desaturases des1 (Fabrias et al., 2012), or hydroxylated to phytoceramides by des2 (Sandhoff et al., 2018).
Ceramides are then transported to the early Golgi apparatus vesicular transport or protein-bound transfer (Hanada et al., 2009; Sandhoff et al., 2018).
On the early Golgi’s cytosolic leaflet, addition of glucose to ceramides, mediated by UDP-glucose-ceramide-glucosyltransferase, produces glucosylceramide β-Glc-(1-1) Ceramide (GlcCer). GlcCer is then translocated to the luminal leaflet and the biosynthesis of the oligosaccharide moiety is synthesized in the Golgi apparatus by the sequential action of different glycosyltransferases (GT) and sialyltransferases (ST) (Figure 2). The sugar donor groups used in ganglioside synthesis are nucleotide sugars: UDP-saccharides for the sugar backbone, and CMP-sialic acid for the sialylations. Starting from GlcCer, the addition of a sialic acid by GM3 synthase (ST3 GAL 5, ST3 β-galactoside α-2,3-sialyltransferase 5) forms GM3 (Figure 2) (Ishii et al., 1998). Thereafter, GM3 can be converted by GD3 synthase (ST8SIA1, ST8 α-N-acetylneuraminate α-2,8-sialytransferase 1) to form GD3, the key precursor to GD2 (Nagata et al., 1992). A final sialic acid added to GD3 by ST8Sia3 or ST8sia5 will form GT3. Downstream of these three precursors, gangliosides are synthesized by multienzyme hetero or homodimers, capable of using any of the precursor gangliosides as substrate. Using GD3, β4GalNT1 will link N-acetylgalactosamine (GalNAc) to the terminal galactose to form GD2. GD2 may in turn be used as substrate by β3GalT4, producing GD1b. This highlights GD2’s role as a metabolic intermediate (Figure 2).
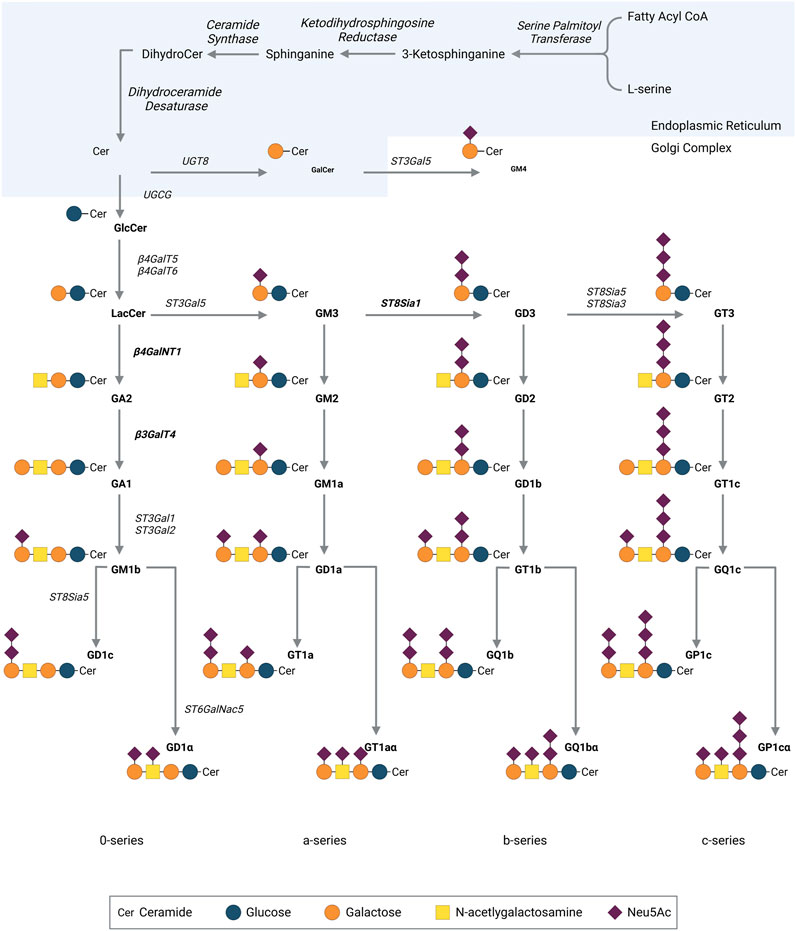
FIGURE 2. Pathway for ganglioside biosynthesis. Cer, ceramide, N-acylsphingosine; Lac-Cer, lactosyl ceramide; Gal, galactose; GalNAc, N-acetylgalactosamine; Glc, glucose; Sia, sialic acid; ST, sialyltransferase; GalT, galactosyltransferase; GalNT, N-acetyl-galactosaminyltransferase; GlcT, glucosyltransferase. Neu5Ac, N-acetyl neuraminic acid. Created with QmlvUmVuZGVyLmNvbQ==.
GD2 ganglioside can be further modified by the addition of an O-acetyl group to the external sialic acid (Sjoberg et al., 1992). The O-acetylation reaction is catalyzed by Sialyl O-Acetyl Transferases (SOAT) and localized on membrane fractions and in the Golgi (Higa et al., 1989; Vandamme-Feldhaus and Schauer, 1998). With the help of recent screening methods, a single enzyme fitting all the necessary requirements was identified, encoded by the CASD1 (capsule structure1 domain containing 1) gene, whose overexpression in cellular models led to an increased expression of O-acetylated gangliosides (Arming et al., 2011). Additional work demonstrated that CASD1 transfers O-acetyl group on free sialic acids, and knock-out of CASD1 impedes cellular expression of O-acetylated gangliosides, underlining its essential role in this process (Baumann et al., 2015; Cavdarli et al., 2021).
While the acetyl donor group is universally identified as acetyl-CoA, there are divergent models regarding the formation process of O-acetyl-GD2 (OAcGD2). Conflicting studies suggest that the O-acetylation can either occur on C7 or C9 (Kamerling et al., 1987; Sjoberg et al., 1992). It is thought that the transfer primarily occurs on C7, with subsequent spontaneous migration to C9 possible under physiological pH (Varki and Diaz, 1984). Other reports show that OAcGD2 can be either obtained by the O-acetylation of GD2 by the SAOT or by the conversion of OAcGD3 by the β3GalT4 (Baumann et al., 2015; Cavdarli et al., 2020). Finally, according to initial models, the O-acetyl group is added on the terminal sialic acid, yet, recent data highlight the possibility of inner sialic acids’ acetylation (Cavdarli et al., 2020). Noteworthy, none of these mechanisms are exclusive, and may take place simultaneously with different importance. Interestingly, O-acetylation of sialic acids diminishes affinity of neuraminidases, which might consequently hamper the first step of ganglioside degradation (Hunter et al., 2018).
Surprisingly, OAcGD2 is concomitantly expressed with GD2 at the tumor cell surface (Alvarez-Rueda et al., 2011). This observation suggests another point of control in OAcGD2 biosynthesis. There are some indications that the synthesis of OAcGD2 can be regulated by the quantity of acetyl-CoA concentrations within the Golgi apparatus (Higa et al., 1989). This mechanism does not further exclude a possible turnover of O-acetyl esters bound to sialic acids of gangliosides controlled by sialate-O-acetylesterases (SIAE) (Butor et al., 1993). Thus, the expression of OAcGD2 in a cell type may be the result of the conjunction of, at least, three parameters: the balance between two enzymatic systems, SAOT and SIAE; the activity of the GM2/GD2 synthase that synthesizes OAcGD2 from OAcGD3; and the activity of GM1/GD1b synthase that forms GD1b from GD2 (Figure 2). Given the complexity of this biosynthetic model, the clarification of the mechanisms that regulate the expression of O-acetyl-GD2 remains challenging. At the end of the biosynthetic process, gangliosides are transported through vesicular transport on the surface of secretory vesicles to be expressed on the membrane.
Once at the cell surface membrane, GD2 ganglioside can be internalized. The kinetics of GD2 internalization have been reported in several studies after binding to specific antibodies (Wargalla and Reisfeld, 1989; Buhtoiarov et al., 2011; Tibbetts et al., 2022). The phenomena of GD2 internalization at the cell surface has several practical implications for designing anti-GD2 immunotherapies. For example, anti-GD2 immunotoxins have been designed and demonstrated some efficacy against GD2-expressing tumor cells in vitro and in vivo (Wargalla and Reisfeld, 1989; Mujoo et al., 1991). On the other hand, anti-GD2 internalization can also represent a mechanism for immunotherapy escape (Tibbetts et al., 2022). Yet, the mechanisms by which this occurs are not well understood. Studies performed with other ganglioside species suggest the existence of different endocytic processes such as clathrin- or caveolin-dependent mechanisms and autophagy (Sandhoff et al., 2018). Once in the early endosome, gangliosides can follow different pathways. Of note, the saturation of the ceramide acyl chain was reported to influence trafficking fate of gangliosides, with unsaturated acyl chain promoting recycling (Chinnapen et al., 2012). Ganglioside catabolism starts with the removal of sialic acids. This process is carried out by membrane-bound sialidases mainly located in the late endosomes. Once GD2 ganglioside is converted to its monosialylated GM2 equivalent, the terminal N-acetylgalactosamine is then removed by hexosaminidase, forming GM3. The final sialic acid is detached by sialidases and SAP-B to produce LacCer. β-galactosidases with their cofactors will produce GlcCer, and then ceramides under the influence of β-glucosidases with SAP-C. Finally, ceramidases and SAP-D split ceramides into sphingoid bases and fatty acids. Starting from GlcCer, catabolic fragments such as LacCer, ceramides or sphingosine can leave the lysosome to be used in new metabolic reactions, constituting salvage pathways (Tettamanti et al., 2003). To avoid catabolism, some gangliosides can be directly recycled back to the plasma membrane, and others can go through “direct glycosylation”, in which they are reintegrated in the Golgi and follow later stages of the biosynthesis pathway (Yu et al., 2008).
While glycosidases play a significant role in the breakdown of gangliosides, it has long been believed that the expression of cellular gangliosides primarily depends on the biosynthetic rather than catabolic processes. Essentially, in order to enhance the presence of a specific ganglioside, the cell needs to increase the enzyme responsible for its synthesis and/or reduce the activity of the enzyme that utilizes this ganglioside as its substrate (Bieberich and Yu, 1999; Mabe et al., 2022). As a consequence, it is difficult to modulate the expression of a single ganglioside species at the enzymatic level.
4 Regulation of GD2 expression
As will be seen in further details later in this work, there is a tissue- and age-dependent restriction of GD2 expression (Svennerholm et al., 1991; Lammie et al., 1993; Huang et al., 2022). GD2 biosynthesis is mostly regulated by the activity of different glycosyltransferases, with emphasis on ST8SIA1, B4GALNT1 & B3GALT4 (Sorokin et al., 2020; Sha et al., 2022), and is controlled epigenetically, transcriptionally, and post-transcriptionally.
Common to the transcriptional regulation of all aforementioned genes, and ganglioside glycosyltransferase in general, is their lack of TATA- and CCAAT-boxes, or any known core promoter elements (Kasprowicz et al., 2022). GC-rich boxes were however observed in the promoters (Yu et al., 2004). These features correspond to housekeeping genes, genes under little regulation. This apparent lack of regulation underlines the importance of another level of gene expression regulation: epigenetic modifications (Itokazu et al., 2017). Epigenetic regulation of ganglioside patterns is seen during development in physiological conditions (Tsai and Yu, 2014; Itokazu et al., 2017). In cancers expressing low levels of GD2 such as Ewing sarcoma and some neuroblastomas (NB), inhibition of silencing epigenetic modifications (detailed later in this work) increases significantly GD2 expression (Kroesen et al., 2016; Kailayangiri et al., 2019; van den Bijgaart et al., 2019; Mabe et al., 2022). However, the same treatment on healthy counterpart cells did not lead to increased GD2 (Mabe et al., 2022). This suggests additional levels of regulation differentiating normal from aberrant cells. This distinction can be a result of varying transcriptional regulations in the three genes mainly responsible for GD2 expression (Sorokin et al., 2020; Sha et al., 2022). This additionally means that GD2 can be a useful stage-specific marker molecule of developing cells.
ST8SIA1, the gene coding for the GD3 synthase is essential to GD2 expression through the production of GD3, precursor and bottleneck to all b-series ganglioside synthesis (Freischütz et al., 1995; Tsai and Yu, 2014; Mabe et al., 2022). The gene possesses multiple initiation sites in the human promoter region. However, alternative transcripts have rarely been observed, with weak expression in some cancers with no particular effect described (Kasprowicz et al., 2022). Of interest, the promoter region contains binding sites for transcription factors such as c-Ets-1, CREB, AP-1, and NF-κB (Kasprowicz et al., 2022), associated with cancers and inflammation. In addition, work on rat PC12 cells showed that alternative use of transcription factors could differentiate between high- and low-ST8SIA expressing cells (Yu et al., 2008). This implies a potential modulation of GD2 expression by microenvironmental cues. A negative control region has similarly been described, but data is lacking regarding its relevance (Furukawa et al., 2003).
B4GALNT1, the gene coding for GD2/GM2 synthase, is under complex regulation. There are three promoters for this gene, corresponding to three transcription start sites and three different exons. In the different promoters, distinct transcription factor binding sites are present, implying differential regulation. Furthermore, an enhancer for two promoters, as well as a silencer for the third have been described (Yu et al., 2004). Alternative use of promoters, enhancers and silencers may regulate cell-specific expression.
B3GALT4, the gene coding for the GD1b/GM1 synthase similarly possesses multiple promoters with associated transcription factor binding sites, and transcription start sites (Al-Obaide et al., 2015). Tissue or cell specificity was noted for promoter use and transcription factor binding sites. Alternative promoter or transcription factor use may be a mechanism leading to higher GD2 expression (Yang et al., 2022). In addition, the promoter regions contain cancer-associated transcription factors binding sites, linking tumorigenesis to ganglioside expression (Al-Obaide et al., 2015).
In a final regulation layer, glycosyltransferases form complexes in their respective compartments. Multiple enzymes can form heterodimers in addition to homodimers: ST8Sia1/ST3Gal5, ST8Sia1/B4GalNT1, B4GalNT1/B3GalT4 (Yu et al., 2004). Dimer formation is influenced by enzyme composition: overexpression of either ST8Sia1 or B4GalNT1 in normal cells lead to increased ST8Sia1/B4GalNT1 with concomitant decrease of dimers using other partners (Bieberich et al., 2002). While dimerized, ST8Sia1 & B4GalNT1 transactivate each other to produce GD2 from GD3 (Bieberich et al., 2002). These mechanisms potentiate simple enzyme up- or downregulation and lead to increased surface GD2. In this model, additional regulation of GD2 synthesis may be mediated by post-translational modifications of glycosyltransferases, such as N-glycosylation that has been described to affect enzyme cellular localization (Bieberich et al., 2000). However, limited details concerning the specifics of these modifications, potentially druggable, are available in the literature (Yu et al., 2004; Yang et al., 2022).
5 GD2 expression in normal tissues
GD2 expression has been reported in the brain to varying degrees according to developmental stages (Svennerholm et al., 1991; van den Bijgaart et al., 2019). While GD2 represents 5%–7% of brain gangliosides during gestation, the proportion progressively declines until it reaches 2% in adult brains (Svennerholm et al., 1991; van den Bijgaart et al., 2019). In comparison, its precursor, GD3, maintains a stable proportion of 5% of brain gangliosides through development (Svennerholm et al., 1989). This underlies development-dependent restriction of GD2 expression, most likely in favor of more complex gangliosides highly expressed in adult brains such as downstream GD1 (Svennerholm et al., 1989; Sipione et al., 2020). After birth, GD2 expression has been reported in various brain zones such as the hypophysis, the hypothalamus, the hippocampus, and the midbrain (Svennerholm et al., 1994; Yuki et al., 1997; Colsch et al., 2011). Concerning nervous tissues outside the brain, GD2 expression has been detected on the spinal cord, cauda equina, and the peripheral nerves (Svennerholm et al., 1994; Yuki et al., 1997; Colsch et al., 2011). GD2 expression was additionally detected in the skin (Hersey et al., 1988), and in human prostate cells (Shiraishi et al., 1988).
Regarding cellular expression, surface GD2 has been detected in various populations. Melanocytes, the pigmental cells of the skin, have also been shown to express GD2, in line with their neuroectodermic origin (Svennerholm et al., 1994). Noteworthy, expression of GD2 has been detected on T cells activated artificially or in disease (Hersey and Jamal, 1989; Villanueva-Cabello et al., 2015; Villanueva-Cabello and Martinez-Duncker, 2016). This may have an impact on GD2-targeting therapies that aim to engage the immune system, as T cells may be targeted as well, with particular relevance for GD2 CAR-T cells. Among immune populations, one report noted GD2 expression on B cells and reticular dendritic cells in lymph nodes of melanoma patients (Hersey and Jamal, 1989). More recently, GD2 was identified as a marker of mesenchymal stromal cells (MSC), be they from the bone marrow or umbilical cord (Martinez et al., 2007; Santilli et al., 2022). This point is of particular interest in the field of oncology, as MSC are often found in tumors with various pro-tumorigenic roles such as immune-inhibition and angiogenesis (Kidd et al., 2008; Lee and Hong, 2017). Targeting of tumors expressing GD2 may as such yield additional benefits in microenvironment regulation, although further studies are needed for validation on cancer-associated MSCs.
Of note, the O-acetylated form of GD2, OAcGD2, displays more restricted expression than GD2. Among 32 tissues tested by immunohistochemistry, OAcGD2 was only weakly detected in specific zones of few tissues. OAcGD2 was detected in the zona reticularis of the adrenal medulla, some macrophages of the bone marrow, the germinal centers of lymphoid follicles, as well as the Purkinje neurons and the gray matter of the dorsal horns (Alvarez-Rueda et al., 2011).
6 GD2 expression in cancer
Overexpression of GD2—significant increase in GD2 compared to healthy tissue–has been reported in various cancers (Tab.1) (Nazha et al., 2020). Interestingly, most of these tumors are pediatric cancers. As such, increased GD2 may reflect aberrant developmental processes, with cells less differentiated compared to healthy counterparts and not having completed the transition to expression of more complex gangliosides such as GD1 (Filbin and Monje, 2019). In this sense, it is intriguing to note that GD2 is increased specifically on breast cancer stem cells. Additionally, in several GD2-positive cancers, OAcGD2 is correspondingly overexpressed (Table 1). Combined with its more restricted normal expression and considering that anti-OAcGD2 antibodies have no cross-reactivity with GD2, targeting OAcGD2 may decrease on-target off-tumor anti-GD2 immunotherapy adverse effects in patients (Alvarez-Rueda et al., 2011; Terme et al., 2014).
GD2 overexpression can be further correlated with the upregulation of ST8SIA1 and B4GALNT1, or the downregulation of B3GALT4 (Sorokin et al., 2020; Yoshida et al., 2020; Kasprowicz et al., 2022; Mabe et al., 2022; Sha et al., 2022). So far, no mutation has been reported for these genes, and the specific causes of GD2 overexpression remain unclear.
At the plasma membrane level, GD2 has been identified as a lipid raft component (Sha et al., 2022). Lipid rafts are specialized, sphingolipid-enriched, dynamic microdomains within the membrane that can compartmentalize cellular processes. Within lipid rafts, signaling proteins such as tyrosine kinases can either be recruited or excluded, to regulate signal transduction (Mollinedo and Gajate, 2020). Several reports have demonstrated the role of GD2 in modulation of cell signaling. In NB cells, enrichment of GD2 has been observed in lipid rafts, and inhibition of GD2 synthesis through B3GALT4 silencing, leads to decreased rafts within cells (Sha et al., 2022).
While GD2 and OAcGD2 represent interesting targets for these multiple cancers, recent works suggest that GD2 expression is rather heterogeneous in tumors, including neuroblastoma (Schumacher-Kuckelkorn et al., 2017; Terzic et al., 2018; Dondero et al., 2021). Several reports further suggest that GD2 expression level may predict anti-GD2 antibody therapeutic responses (Terzic et al., 2018; Hirabayashi et al., 2021; Theruvath et al., 2022). As such, studies on the mechanisms influencing GD2 tumor cell expression are required to optimize anti-GD2 therapies. Several parameters associated with the tumor microenvironment have been identified so far. For example, it has been noted that hypoxic conditions led to overexpression of the key enzyme to GD2 production, ST8SIA1, in colon tumor cell lines (Yin et al., 2010). Similarly, osteosarcoma spheroids had gradually increased GD2 expression over time, concurrent with cell growth and densification of the sphere causing a decrease in oxygen availability (Wiebel et al., 2021). Further studies are required to confirm the link between hypoxia and GD2 expression. Immunostimulatory cytokines were also tested on diverse cancer models for their capacity to enhance GD2 expression. The cytokines, IL-4, TNF-α and IFN-γ, all increased GD2 expression as a single agent or in combination with each other (Hoon et al., 1991a; Hoon et al., 1991b). TNF-α and IFN-γ are cytokines produced either by innate or adaptive immune cells (Castro et al., 2018; Josephs et al., 2018). Therefore, it is of particular relevance to immunotherapies as immune cell activation may lead to increased target expression by bystander cells.
GD2 expression in tumors has also been studied in respect to the cellular intra-tumoral heterogeneity and tumor cell plasticity. A small subpopulation of cells within tumors with self-renewal capacities are named cancer stem cells (CSC). CSCs have been linked with resistance to conventional chemotherapy and radiotherapy, and thus, are believed to drive tumor progression and disease recurrence (Phi et al., 2018). Targeting CSCs is therefore important for limiting tumor spread and recurrence. Interestingly, the expression of GD2 has been evidenced in breast carcinoma CSCs (Battula et al., 2012; Liang et al., 2013). In parallel, factors modulating the expression of GD2 in breast CSCs have been identified. In various models of triple negative cancer cell lines, oxidative stress, caused by disturbances in the normal redox state of cells, induced increased stemness in cells and as such increased percentages of GD2-positive cells (Jaggupilli et al., 2022). In addition, OAcGD2 expression was also confirmed in breast CSCs (Cheng et al., 2021).
Another source of intra-tumoral heterogeneity with regards to GD2 expression stems from plasticity. Cell plasticity allows tumor cells to change their phenotypic characteristics without necessitating further genetic mutations in response to environmental cues. For instance, in NB there is a more differentiated “noradrenergic” state, and a less-differentiated “mesenchymal” state (Boeva et al., 2017; van Groningen et al., 2017). When diagnosing human primary NBs, the noradrenergic state is found to be the predominant state in the tumor (Boeva et al., 2017; van Groningen et al., 2017), while the MES state may be more prevalent in relapse and metastatic disease according to studies on human tumors and mouse models (Boeva et al., 2017; van Groningen et al., 2019; McNerney et al., 2022). Interestingly, “mesenchymal” NB cells demonstrate lower GD2 expression level than “noradrenergic” NB cell lines, with greater resistance to anti-GD2 immunotherapy (Mabe et al., 2022; McNerney et al., 2022).
Given the emerging role of cancer plasticity in anti-GD2 immunotherapy, the development of strategies targeting the underlying mechanisms of plasticity may lead to durable responses. At the cellular level, epigenetic modification is a promising approach that allows regulation of glycosyltransferase gene expression (Kroesen et al., 2016; Kailayangiri et al., 2019; Mabe et al., 2022). Histones, the DNA packaging proteins, can be acetylated or methylated leading to changes in chromatin architecture that can increase or decrease gene expression through DNA accessibility. EZH2 is part of the PRC2 complex and is involved in histone methylation, such as the silencing modification of histone 3, H3K27me3. Use of EZH2 inhibitors in various potentially GD2-positive cancers such as neuroblastoma and Ewing sarcoma led to increased GD2 expression through upregulation of ST8SIA1, and in some cases B4GALNT1 (Kailayangiri et al., 2019; Mabe et al., 2022). Similarly, Tazemetostat, an inhibitor of EZH2, successfully altered the cellular state in “mesenchymal” NB. This reprogramming led to the activation of “noradrenergic” gene expression, including ST8SIA1, resulting in an increase in GD2 expression (Mabe et al., 2022).
In the same line, histone deacetylase (HDAC) catalyzes, amongst others, deacetylation of the activating modification H3K27ac. Use of HDAC inhibitors enhanced GD2 expression on neuroblastoma cells through upregulation of ST8SIA1 (Kroesen et al., 2016; van den Bijgaart et al., 2019). This effect could be further augmented through exogenous addition of sialic acids (van den Bijgaart et al., 2019).
Although both HDAC inhibitors and EZH2 inhibitors, have FDA-approval in different cancers (Mann et al., 2007; Straining and Eighmy, 2022), these treatments are nonspecific and lead to a general decrease of silencing marks or increase of activating marks. This causes up- and downregulation of numerous other genes and as such precautions are necessary in their use.
In a more technical approach, but particularly relevant to the use of 2D and 3D in vitro models, it has been demonstrated that cell confluency modulates GD2 expression, whether in monolayer or spheroid culture (Wiebel et al., 2021). If unchecked, this parameter may negatively impact data reproducibility. The mechanisms linking cell confluency and GD2 expression have not been described, but hypoxia and oxidative stress due to reduced nutrient may play a role. In addition, these microenvironmental stress factors may induce epigenetic rewiring, of particular importance for GD2 expression as mentioned above.
7 Functional aspects of GD2 ganglioside in cancer progression
As described above, GD2 is overexpressed in several cancer types. Nevertheless, comprehension of the biological consequences stemming from its overexpression have yet to be unequivocally determined. Several roles of GD2 have been suggested using different experimental approaches. For example, exogenous GD2 can be added in the culture media to be incorporated in cells’ plasma membranes (Li et al., 1996). Anti-GD2 blocking antibodies can also be used to disrupt GD2 interaction with other cell membrane components. Pharmacological depletion of the cellular gangliosides can be achieved using glucosylceramide synthase inhibitors such as PDMP (Inokuchi et al., 1990) or PPMP (Lee et al., 1999). Alternative methods consist in gene transfer (Yoshida et al., 2001) or gene editing (Bhat et al., 2023).
However, it should be noted that these experimental approaches have technical limitations. Because of the nature of GD2 as a metabolic intermediate in a sequential process, targeting the enzymes responsible for GD2 biosynthesis may result in a complex pattern of interference making it difficult to identify the molecular ganglioside species involved. For example, knocking out GD3 synthase will result in the accumulation of GD3 precursors and the disappearance of its downstream products. While GD2-blocking antibodies have the advantage of precisely identifying GD2, their different modes of actions such as antigen blocking, masking, clustering or internalization hinder understanding of the normal roles in cancer cells. Nonetheless, these different approaches have evidenced several biological effects of GD2 particularly relevant to tumor progression and immunosuppression as discussed below.
Among first studies were conflicting reports pointing to a link between GD2 expression and either adhesive or anti-adhesive properties. Regarding anti-adhesive properties, one work has shown that GD2 is a cellular receptor for tenascin-C, an extracellular matrix protein found in embryonic, inflamed, or cancerous tissues, leading to cell detachment from fibronectin through inhibition of focal adhesion (Probstmeier and Pesheva, 1999). A study using osteosarcoma cells similarly showed that GD2 overexpression led to increased cell motility (Shibuya et al., 2012). In that context, GD2 expression has been linked with the EMT process in bladder cancer which could be reversed upon inhibition of GD2 synthesis (Vantaku et al., 2017). Contrariwise, multiple work based on cancerous models such as melanoma and neuroblastoma indicate a role in adhesion. Acting as a co-receptor for integrins in binding to various extracellular matrix proteins such as fibronectin, vitronectin and collagen, blocking of GD2 can inhibit adhesion (Cheresh et al., 1986a; 1987; Yesmin et al., 2021). Altogether, these observations suggest that effects of GD2 on adhesion are context dependent, with a possible dependence on integrin type (Furukawa et al., 2012).
It is interesting to note in either case, GD2 is associated with integrins. In this line, different works have demonstrated localization of GD2 in adhesion plaques and presence of trimeric complexes of GD2, Focal Adhesion Kinase (FAK) and integrins, (Cheresh et al., 1984; Aixinjueluo et al., 2005). In SCLC cells, GD2-specific antibodies disrupted the GD2/integrin complexes at the tumor cell surface resulting in the dephosphorylation of FAK and subsequent anoikis. Inversely, overexpression of GM2/GD2 synthase reduced anoikis in melanoma models. The association of GD2 with FAK/AKT signaling pathway were later reported by others in high-expressing GD2 melanoma cells (Yesmin et al., 2021), glioma cells (Iwasawa et al., 2018), prostate cancer cells (Nguyen et al., 2018; Xing et al., 2021), triple negative breast cancer cells (Nguyen et al., 2018), and osteosarcoma cells (Liu et al., 2014). FAK signal through the AKT/mTOR pathway to promote processes such as cell survival, cell motility, angiogenesis (Chuang et al., 2022). In the context of cell attachment, FAK activation is a necessary step in the outside-in signaling mediated by integrins when bound to matrix proteins, and leads to pro-survival signaling (Mitra and Schlaepfer, 2006). In the context of cell detachment and motility, FAK activation promotes anchorage independent growth and consequently tumor cell survival during dissemination (Duxbury et al., 2004; Liu et al., 2008; Deng et al., 2021). As such, activation of FAK, possibly facilitated by its localization in the increased GD2-dependent lipid rafts, may be a link between the opposite adhesive/anti-adhesive roles of GD2 in different contexts. In this sense, another example of modulation of the tumor signaling pathways by GD2 was identified in breast cancer cells. In GD3 synthase transfected breast cancer cells, GD2 colocalized with the receptor tyrosine kinase cMET and was responsible for its constitutive activation, leading to increased proliferation through downstream MAPK signaling (Cazet et al., 2012). This effect was blocked either by silencing GM2/GD2 synthase, or by anti-GD2 antibodies treatment (Cazet et al., 2012).
Both adhesive/anti-adhesive mechanisms link back to a positive correlation between GD2 expression and invasiveness in different context such as melanomas, osteosarcoma (Shibuya et al., 2012; Yesmin et al., 2021), gliomas (Iwasawa et al., 2018), triple negative breast cancer (TNBC) and prostate cancer (Nguyen et al., 2018; Xing et al., 2021). In this sense, anti-GD2 incubation decreased matrix-metalloproteinase 2 production in osteosarcoma (Liu et al., 2014).
Regarding other processes of cancer dissemination, overexpression of GD2/GM2 synthase in a melanoma model increased angiogenesis, potentially through a drastic increase in periostin production, a protein with implications in angiogenesis and migration (Huizer et al., 2020; Yoshida et al., 2020).
Other molecular alterations induced by anti-GD2 may be implicated in antigen-positive cells’ apoptosis. In different cancer context such as neuroblastoma and small cell lung cancer, incubation of anti-GD2 antibodies led to cell death without any immune mediator, through activation of the caspase-3 pathway inducing apoptosis (Yoshida et al., 2001; Kowalczyk et al., 2009; Tsao et al., 2015; Iwasawa et al., 2018). Interestingly, use of antibodies directed against O-acetyl GD2 similarly induced apoptosis in OAcGD2-expressing neuroblastoma cells through caspase-3 activation (Cochonneau et al., 2013).
Along the same lines, in reports based on neuroblastoma cell lines, incubation with anti-GD2 diminished Aurora kinase A and MYCN expression, while PHDLA1, P53 and c-jun’s were strengthened (Horwacik et al., 2015; Horwacik et al., 2013). Aurora kinase A is involved in cell proliferation and is crucial to complete mitosis. Aurora kinase A additionally protects from degradation MYCN, a major driver of oncogenesis in neuroblastoma. Inversely, aurora kinase A promotes tumor-suppressor p53 degradation. On the opposite, PHDLA1 is a tumor suppressor inhibiting AKT and Aurora kinase A, and is regulated by p53 (Chen et al., 2018). As such, incubation with anti-GD2 induces a shift from a tumor-promoting function characterized by increased Aurora A kinase expression, to a tumor-suppressing function characterized by increased PHDLA1, in line with the observed increased apoptosis. However, c-jun expression–regarded as a proto-oncogene–was similarly increased following anti-GD2 treatment. Interestingly, c-jun may rather favor apoptosis, as it mediates expression of pro-apoptotic factor following Jun kinase activation, as described after anti-GD2 incubation (Yoshida et al., 2002).
In addition, GD2 may be implicated with regulation other hallmark of cancer, such as cancer stem cells (CSC) and treatment resistance. Expressed on breast CSCs in TNBC, it has been reported that the genes associated with CSC properties such as SOX2, BCL11A, FOXC1, are in fact tightly regulated by ST8SIA1 (Nguyen et al., 2018). Still in TNBC, upregulation of ST8SIA1 was associated in patients with chemoresistance. Inhibition of ST8SIA1 enhanced the efficacy of the chemotherapy, concomitant with a suppression of the FAK/Akt/mTOR and Wnt/β-catenin pathways (Wan et al., 2021). Both of these reports may be linked by the fact that cancer stem cells generally display increased chemoresistance (Abdullah and Chow, 2013; Zhao, 2016).
Although unrelated to cancer functions, injection of anti-GD2 is often followed by allodynia, due to GD2 expression on peripheral nerves (Anghelescu et al., 2015). This was explained by antibody mediated activation of Src kinases cascading to activation of the NMDA-Receptor, involved in peripheral nerve sensitization. It is interesting to note that FAK kinase activation mediates among others Src kinase activation.
Apart from tumor-centric roles, GD2 might be involved in regulation of the tumor immune microenvironment. In gliomas, knock-out of ST8SIA1 increased the number, and activation status, of microglia/macrophages inside the tumor via reduced IL-6 and TGF-β1 (Zhang et al., 2021). Going further, the general changes in chemokine and cytokine production observed following the knock-out favored polarization towards the M1 phenotype rather than the M2-tumor promoting phenotype enriched in wild-type gliomas (Zhang et al., 2021). In neuroblastomas, based on overexpression of B3GALT4, forming GD1b from GD2, one report described enhanced recruitment of CD8+ T cells. The enzyme overexpression decreased GD2 quantity and consequently the formation of lipid rafts. In turn, this reduced c-Met signaling and downstream AKT/mTOR/IRF-1 pathway, with one finality being the increased expression of CXCL9 and CXCL10, responsible for cytotoxic T cell recruitment (Sha et al., 2022). It can thus be hypothesized that GD2 has a role in immune microenvironment suppression, both at the innate and adaptive level.
Like other glycosphingolipids, GD2 is in part shed from the plasma membrane, mostly as micelles or membrane vesicles (Ladisch et al., 1987; Li and Ladisch, 1991; Kong et al., 1998). Circulating GD2 can be measured in the serum of patients with GD2-expressing tumors, such as NB and retinoblastomas (Valentino et al., 1990; Portoukalian et al., 1993). Thus, it can be proposed as a companion diagnostics to anti-GD2 immunotherapies.
Shed gangliosides can be also incorporated in the plasma membrane of the neighboring cells (Chang et al., 1997), thus it is possible that GD2 can modulate tumor-bystander cell interactions. In this line, GD2-positive melanoma cells with increased cell growth, invasion, and adhesion secreted extracellular vesicles that could enhance properties of neighboring GD2 negative cells to similar levels (Yesmin et al., 2023). There are also several studies evidencing a role of GD2 in inhibition of T cell reactivity both in vitro and in vivo (Floutsis et al., 1989; Ladisch et al., 1992; Li et al., 1996). This diminished activity, evaluated through lymphoproliferative responses was valid for both non-specific mitogens such as PHA and soluble antigens such as toxoids (Ladisch et al., 1992). The effects on T cells, while significantly lower, were comparable to potent doses of cyclosporine A, an immunosuppressive agent (Li et al., 1996). Interestingly, shed GD2 was detected incorporated into T cells, and these T cells were significantly more apoptotic than GD2 negative ones. In addition, variations in immunosuppressive potential were observed depending on the ganglioside subspecies. Shorter fatty acid chains (C18 or less) were associated with increased immunosuppression for GD2 (Ladisch et al., 1994). Surprisingly, GD2 extracted from tumors were more immunosuppressive compared to healthy human brains’, which might imply enrichment of different GD2 subspecies in cancer than in physiological conditions (Ladisch et al., 1994). Other modifications modulating this property are modifications to sialic acids, with lactones less immunosuppressive than their classical counterparts, and hydroxylation of the fatty acid, displaying a similar trend (Ladisch et al., 1992; Ladisch et al., 1995). Similarly, using purified GD2, and inhibition of dendropoiesis, and dendritic cell activation by four-fold was shown (Shurin et al., 2001). This implies dysfunctions in the generation of possible adaptive immune responses to GD2 expressing cancers. On the contrary, incubation of NK cells with shed GD2 did not hinder cell cytotoxicity, nor their maturation (Ando et al., 1987; Grayson and Ladisch, 1992; Liu et al., 2022).
Finally, GD2 can also regulate direct tumor-immune cell interactions. In a recent study, GD2 blocking antibodies allowed the identification of GD2 as a ligand for the immune checkpoint receptor siglec-7, expressed on monocytes and NK cells (Ito et al., 2001; Theruvath et al., 2022). This finding arises on the observation that a Fc-dead anti-GD2 antibody enhances ADCP of GD2-positive tumor cells by macrophages in the absence of Fc binding or complement activation (Theruvath et al., 2022). The final demonstration was brought by evidencing the binding of recombinant siglec-7 onto GD2 gangliosides (Theruvath et al., 2022). Given these observations, similar work conducted on NK cells might yield interesting results.
8 GD2 as a target antigen for immunotherapies
8.1 First successes: monoclonal antibodies
The most representative therapeutic targeting of GD2, paving the way for all other forms immunotherapies, are monoclonal antibodies. The murine IgG3 monoclonal antibody (mAb) 3F8, mediates tumor cell killing directly through GD2 binding, as mentioned earlier, and through antibody dependent cell cytotoxicity (ADCC), complement dependent cytotoxicity (CDC), as well as phagocytosis of intact GD2 positive cells (Munn and Cheung, 1989; Munn and Cheung, 1990; Kushner and Cheung, 1991). Phase II studies on patients with stage 4 neuroblastoma, 40% responded with in some cases nearly 50% of patients progression-free (Cheung et al., 1998a; Cheung et al., 1998b). These clinical studies confirmed the implication of ADCC, with both granulocytes and NK cells’ activation potential correlating positively with treatment outcome (Delgado et al., 2010; Cheung et al., 2012; Tarek et al., 2012).
Another murine antibody, 14G2a, IgG2a class-switched from the IgG3 14.18, was shown to enable ADCC and CDC (Mujoo et al., 1987; Mujoo et al., 1989). In Phase I studies in various contexts such as melanoma, neuroblastoma, and osteosarcoma clinical utility of 14G2a varied depending on the context, going from 25% of patients respondent, up to 66% in neuroblastoma, with two complete remissions and two partial remissions (Handgretinger et al., 1992; Saleh et al., 1992; Murray et al., 1994).
A similarity of 3F8 and 14G2a is that both of them are cross-reactive with NCAM, a neural adhesion molecule (Patel et al., 1989; Agrawal and Frankel, 2010). Affinity for GD2 however is a differentiating factor as 3F8 had higher affinity than 14G2a (Cheung et al., 2012).
In order to reduce production of human anti-mouse antibodies, efforts were carried out to reduce murine components of these antibodies (Handgretinger et al., 1992; Saleh et al., 1992; Murray et al., 1994; Cheung et al., 1998a; Cheung et al., 1998b).
Chimeric antigen ch14.18 was produced by fusing heavy and light chains of murine 14.18 (Gillies et al., 1989). Chimeric ch14.18 increased efficiency of ADCC (Mueller et al., 1990) and prolonged half-life (Uttenreuther-Fischer et al., 1995). Analysis on 11 years follow-up of the 1st large scale study demonstrated benefit on overall survival when compared to chemotherapy for maintenance in neuroblastoma (Simon et al., 2004; 2011; Perez Horta et al., 2016). Building up on the role of ADCC in treatment outcome, a phase III trial combining Ch14.18 with IL-2, GM-CSF and retinoic acid for neuroblastoma showed superiority to the standard of care chemotherapy considering both overall survival (73.2%) and progression free survival (56.6%) at 5 years (Yu et al., 2010; Perez Horta et al., 2016; Yu et al., 2021). These results led to its marketing authorization by the FDA in the United States of America in 2015. In 2017, the European Commission granted marketing authorization of dinutuximab ß, a ch14.18 mAb (expressed in CHO cells instead of SP2O cells) after a phase III clinical trial conducted by the SIOPEN (Ladenstein et al., 2018).
The murine 3F8 was similarly humanized by fusing complementarity-determining regions to a human IgG1 framework, thus forming hu3F8. The hu3F8 maintained affinity of the murine antibody, but increased ADCC capacity and drastically lowered CDC capacities (Cheung et al., 2012). In a pilot Phase I trial of hu3F8 on resistant neuroblastoma, 79% of patient treated with highest acceptable dosage had partial or complete response (Kushner et al., 2018). This led in 2020 to accelerated approval of hu3F8 in relapsed/refractory neuroblastoma patients (Markham, 2021), increasing the panel of approved anti-GD2 therapies available to patients. Acute pain during antibody infusion and occasional neuropathy were observed but with no late toxicities reported in long-term follow-up of patients treated with anti-GD2 mAbs (Ozkaynak et al., 2000; Yu et al., 2010; Kushner et al., 2011; Ladenstein et al., 2018).
Clinical success of anti-GD2 antibodies demonstrated the potential of anti-GD2 immunotherapies. However, their clinical use is hindered by neurologic adverse effects of on-target off-tumor nature. Moreover, a number of patients remain refractory or relapse, even in GD2-positive cancers. In this context, several strategies to optimize success of therapies will be discussed here. Avenues to optimize anti-GD2 treatment rely either on increasing the administrable dose of antibodies, limited by the side effects, or exploring other immunotherapeutic mechanisms (Figure 3).
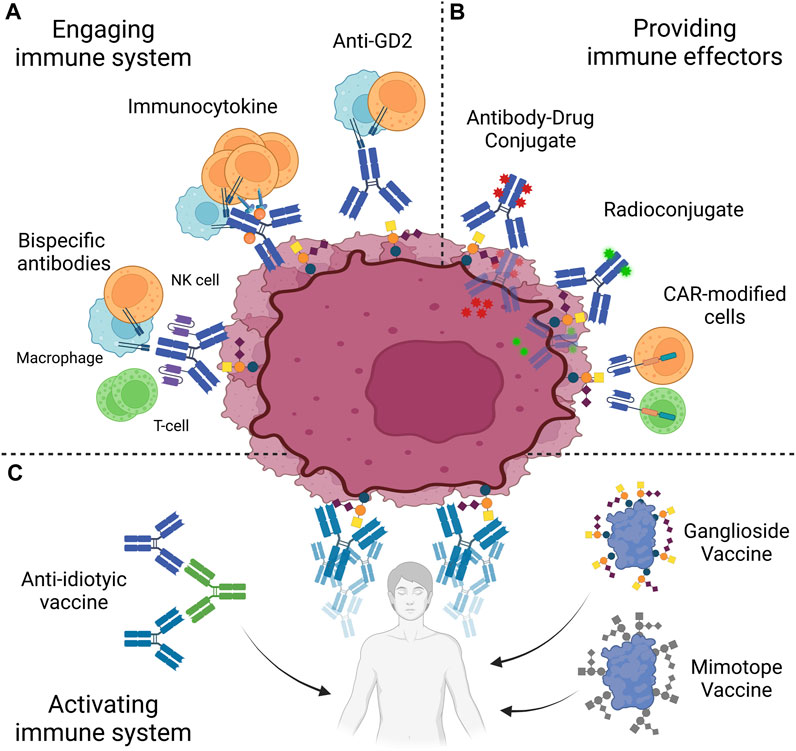
FIGURE 3. Anti-GD2 immunotherapies. Strategies to target GD2 are based on three approaches. (A) Patient immune system is engaged against GD2-positive cells through antibodies, immunoctykines or bispecific antibodies. (B) Immune effectors, such as antibody-drug conjugates, radioconjugates, or CAR-modified cells, are directly provided to the patient. (C) Patient immune system may be activated to target GD2 through the use of vaccines, based on purified GD2, peptide mimotopes or anti-idiotypes. NK, Natural Killer; CAR, Chimeric Antigen Receptor. Created with QmlvUmVuZGVyLmNvbQ==.
8.2 Necessity of companion diagnostics
As stated earlier, GD2 expression is heterogeneous both between and within tumors, which could pose an obstacle for effective tumor targeting. GD2 is heterogeneously expressed in various cancers, and may as such hold diagnostic and prognostic value, whether expressed on tissues, or shed as was mentioned in the context of cancer previously. Conflicting reports undermine GD2-expression’s prognostic relevance (Valentino et al., 1990; Czaplicki et al., 2009; Balis et al., 2020; Erber et al., 2021; Galan et al., 2023). However, shed or tumor-expressed GD2 may be used as a companion diagnostic for anti-GD2 immunotherapies, particularly in cancers where heterogeneous expression has been identified (See Table 1) (Portoukalian et al., 1993; Wiebel et al., 2021). In addition, GD2 may be used as a diagnostic tool. Studies have shown that serum levels of GD2 separate between patients with NB and healthy controls (Schulz et al., 1984). Serum level of GD2 of patients with ovarian cancers was reported to hold higher diagnostic power than standard CA125 detection for the screening of ovarian cancer of all types (Galan et al., 2023). In this context, the challenge lies in developing clinically applicable routine assays, due to GD2 biochemical nature and the absence of reference controls. Initially, detection of circulating/shed GD2 was conducted after ganglioside extraction, followed by chromatographic separation using high-performance thin layer chromatography and densitometric scanning (Ladisch et al., 1987; Valentino et al., 1990; Portoukalian et al., 1993). Since then, different methods have emerged to detect circulating GD2. A more recent standard for GD2 detection and quantification is the use of high-performance liquid chromatography coupled to mass spectrometry (Busch et al., 2018; Balis et al., 2020). This technique enables quantification of gangliosides with precision as low as 4–6 ng/mL, and separation of GD2 subspecies based on long chain bases. In a simplified technique, ganglioside stripped of their ceramide and fluorescently labeled were quantified using HPLC only through the differential retention time of their glycan moiety, producing results comparable to HP-TLC (Czaplicki et al., 2009). In a recent report, a quantitative ELISA was performed on serum samples and displayed similar sensitivity as HPLC-MS for GD2 when using a purified standard (Galan et al., 2023). Circulating GD2 may additionally be detected indirectly. A surprising report suggests that the epitope detection in monocytes (EDIM) blood test utilizing GD2 could function as a sensitive and non-invasive diagnostic tool for detecting tumor cells in patients with NB (Stagno et al., 2022). EDIM is based on antigen expression by macrophages which phagocytose parts of neoplastic cells, extracellular vesicles, or circulating tumor cells, and subsequent identification by flow cytometry in blood of patients (Stagno et al., 2022). Further studies on this method are, however, required before it can be widely adopted as a standard diagnostic approach. Apart from circulation, GD2 expression may also be detected on tumor cells. This aspect has particular relevance in therapeutic decisions.
The current gold standard for tissue expression of GD2 is immunohistochemistry, which can be conducted on primary tumors of bone marrow aspirates (Schulz et al., 1984; Berthold et al., 1989; Sariola et al., 1991; Chang et al., 1992; Corrias et al., 2008).
In the context of residual disease such as bone marrow infiltration, quantification of B4GALNT1 (GM2/GD2S) via RT-qPCR similarly showed high sensitivity (Hoon et al., 2001; Lo Piccolo et al., 2001; Cheung et al., 2003; Laurent et al., 2010). One report comparing both methods in this context revealed higher sensitivity of RT-qPCR quantification of GD2S than immunohistochemistry (Lo Piccolo et al., 2001). RT-qPCR however possesses the caveat of not quantifying strictly GD2 levels, as GM2 may instead be upregulated by this same enzyme. These two techniques may then be used for different purposes, with immunohistochemistry revealing pertinence of anti-GD2 therapy in a patient, and B4GALNT1 quantification used for precise monitoring of remission.
8.3 Engaging the patient’s immune system
As mentioned earlier, a common acute side effect upon anti-GD2 mAb infusions is severe pain that limits the administrable dose to patients (Ozkaynak et al., 2000; Yu et al., 2010; Kushner et al., 2011; Tong et al., 2015; Ladenstein et al., 2018). This neuropathic pain has been attributed to complement activation on anti-GD2 bound peripheral nerves (Sorkin et al., 2010). To reduce this side effect, a single point mutation (K322A) was introduced in the Fc receptor of the humanized 14.18 anti-GD2 mAb to decrease C1q binding while maintaining ADCC capabilities (Sorkin et al., 2010). The resulting antibody hu14.18K322A elicited less allodynia in rats than dinutuximab (Sorkin et al., 2010). At the clinical level, this enabled higher antibody dosage (Anghelescu et al., 2015), and the result of a phase II clinical trial showed that usage of hu14.18K322A displays similar efficacy with reduced pain in patients with NB (Furman et al., 2022). An alternative approach to mitigate severe pain associated with anti-GD2 treatment involves targeting OAcGD2, expressed on GD2-positive tumors but not on peripheral nerves (Alvarez-Rueda et al., 2011). The mAb 8B6, specifically targeting OAcGD2, demonstrated comparable immune-mediated tumor-killing effects in vitro and inhibition of tumor growth in vivo to anti-GD2 antibody, without inducing allodynia in rats ((Alvarez-Rueda et al., 2011; Terme et al., 2014). Clinical trials are anticipated to explore optimal dosing of anti-OAcGD2 mAb.
To potentiate antibody-based treatment, strategies to increase patient immune cells’ engagement may be devised. Based on the anti-GD2 antibodies partial reliance on immune effectors such as NK cells and macrophages, immunocytokines were developed. Immunocytokines are conjugate between an antibody and cytokines, thus limiting their systemic effect, while bolstering activity and proliferation of tumor microenvironment immune cells (Neri and Sondel, 2016). The anti-GD2 hu14.18 antibody expressed as a fusion protein with interleukin-2 (hu14.18-IL2) has shown promise in treating NB during preclinical and clinical testing. In a Phase II study of this therapeutic on relapsed or refractory neuroblastoma, a fifth of 23 patients responded to treatments, all displaying complete response from 9 to 35 months (Shusterman et al., 2010). However, doubts remain regarding the overall therapeutic benefits of IL-2 due to the severe toxicities associated with higher doses and the unestablished effectiveness at lower doses (Shusterman et al., 2010). Another potentially useful cytokine to bolster the patient’s immune system is IL-15 (Vincent et al., 2013). Interestingly, in a preclinical study, hu14.18-IL-15 & IL-21 outperformed hu14.18-IL2 in immunocompetent mice with syngeneic NB with increased survival (Nguyen et al., 2022). The increase in survival was concurrent with increased tumor CD8+ T cells and M1 macrophages, and decreased Treg. Interestingly this increased recruitment was mediated by the CXCL9/10 axis, seen to be activated during decreased lipid raft formation caused by overexpression of GD1S, which could similarly apply to antibody incubation, thus producing a synergistic effect (Nguyen et al., 2022).
Anti-GD2 monoclonal antibodies have also paved the way for the development of bi- and tri-specific antibodies. These novel antibody constructs redirect T-cell cytotoxicity towards GD2-positive cells by linking tumoral antigens and T-cell receptors via anti-CD3 (Cheng et al., 2015; Cheng et al., 2016). Bispecific antibodies, such as huOKT3-hu3F8, have demonstrated potent tumor control (Cheng et al., 2016). Trispecific antibodies, formed by linking ScFv to anti-GD2 IgG’s light chains, not only redirect T-cells but also engage Fc-receptor bearing immune cells. In murine models, trispecific antibodies based on ch14.18 or hu3F8 exhibited remarkable control over metastatic neuroblastoma, melanoma, and osteosarcoma tumors (Xu et al., 2015; Park and Cheung, 2020; Nakajima et al., 2021; Park et al., 2021; Zirngibl et al., 2021). Encouraged by preclinical success, phase I/II clinical trials employing trispecific antibodies (hu3F8) have been initiated for neuroblastoma, osteosarcoma, and small cell lung cancer (Hattinger et al., 2019; Yankelevich et al., 2019; Ordóñez-Reyes et al., 2022). Initial results from the neuroblastoma and osteosarcoma trials indicate a 33% clinical response rate among patients with relapsed/refractory disease who completed Phase I (Yankelevich et al., 2019). Phase II results will provide further insights into the effectiveness of this therapeutic approach.
8.4 Providing immune effectors
Another strategy is to directly provide cytotoxic effectors redirected via antigen specific whole or fragment antibody. Radiolabeled antibodies, enables targeted therapy combining diagnostic and therapeutic capabilities (aka theranostics). A pilot study using 131I-GD2 ch14.18 in patients with NB demonstrated tumor uptake in 65% of cases with acceptable organ doses (Zhang et al., 2022). A Phase II study on medulloblastoma using 131I-3F8 resulted in 15 long-term survivors out of 42 patients, showing promising outcomes (Kramer et al., 2018). The Sloan Kettering Cancer Center (New York City, NY, United States) adopted 131I-labeled anti-GD2 3F8 as a standard protocol for patients with high-risk NB older than 1 year, starting from reference protocol N7 (Cheung et al., 2001). Of note, imaging with ch14.18-labeled 99mTc improved NB recurrence detection (Reuland et al., 2001). In small animal bearing NB tumors, gold particles conjugated to anti-GD2 hu14.18K322A showed enhanced CT contrast imaging, while exhibiting an increased NK cell cytotoxicity against GD2-positive tumors compared to that elicited by unlabeled hu14.18K322A. Conjugation of anti-GD2 to gold particles may additionally enable photo-thermolysis of GD2-positive cells following near-infrared laser light exposure (Peng and Wang, 2011).
Building up on data showing internalization of the 14G2a antibody, various payloads were conjugated to anti-GD2 (Kalinovsky et al., 2022; Tibbetts et al., 2022). Surprisingly, there is a scarcity of studies on classical antibody-drug conjugates based on anti-GD2. Recently, conjugation of ch14.18 with the microtubular poisons monomethyl auristatin E & F was achieved via a cleavable linker to release the payload inside target cells after endocytosis. Both antibody drug conjugates achieved superior tumor control compared to the parent ch14.18 antibody, with significant differences in mice GD2-positive melanoma and lymphoma tumor volume. This opens the way for further work based on this model, exploiting the full diversity of drug-conjugates already in clinical trials (Fu et al., 2022). Of note, hydrophobic drugs may be used in anti-GD2-linked liposomes and delivered effectively (Di Paolo et al., 2011; Jose et al., 2020).
The anti-GD2 14G2a and 3F8 mAbs have also been used to develop chimeric antigen receptors expressed particularly on αβ T cells. CARs consist of a cell surface antigen-specific single-chain variable fragment (ScFv) linked to signaling domains. Co-stimulatory domains, such as CD28, CD137, and/or OX40, are utilized in CAR-T cells, linked to the CD3ζ signaling domain. Clinical trials using GD2 CAR-T cells have been conducted, primarily in relapsed/refractory neuroblastoma (Louis et al., 2011; Heczey et al., 2017). Third-generation CAR-T cells using a 14G2a ScFv showed promising results, with complete remission achieved in a subset of patients (Del Bufalo et al., 2023). A Phase I/II trial using a hu3F8-based ScFv on a fourth-generation CAR demonstrated a 1-year overall survival rate of 74% and partial responses in a portion of patients (Yang et al., 2017; Yu et al., 2022). A comparison of these trials highlighted that increased affinity and the use of a fourth-generation construct did not necessarily result in superior effects. GD2 CAR-T cells have also been tested in glioblastoma and H3K27M-mutated diffuse intrinsic midline gliomas, showing clinical improvements in some patients (Majzner et al., 2022).
CAR-T cells are not the only cell types modified with CARs for GD2-positive cancers. Expression of GD2-specific CAR on macrophages and mesenchymal cells led to GD2-positive tumor cell cytotoxicity, proving potential utility, although their naturally pro-tumorigenic role in the tumor microenvironment remains to be addressed (Golinelli et al., 2022; Golinelli et al., 2020; Zhang et al., 2023). NK cells and γδ T cells, which play crucial roles in antitumor immunity and are found infiltrated in tumors, have also been modified with CARs, resulting in GD2-positive cancer cell lysis (Capsomidis et al., 2018; Chabab et al., 2020; Cózar et al., 2021; Zuo et al., 2023). However, these cell types have a shorter lifespan and memory cell formation remains uncertain compared to αβ T cells (Sun et al., 2011; Comeau et al., 2020). NKT cells, semi-innate lymphoid cells sharing properties of both NK cells and T cells, have shown efficacy in preclinical studies, and a Phase I trial using CAR GD2 iNKT cells derived from the 14G2a antibody was initiated for neuroblastoma patients, showing potential with complete and partial remissions observed (Heczey et al., 2014; Xu et al., 2019). Overall, CAR-based immunotherapies targeting GD2 have demonstrated promising results in various cancers, including neuroblastoma and glioblastoma, with manageable safety profiles. The utilization of different cell types and CAR designs provides a range of options for personalized treatment approaches.
8.5 Activating the patient’s immune system
A final strategy is complete reliance on the patient’s immune system activation, named active immunotherapy. In the case of GD2, a glycolipid with poor immunogenicity, vaccine development has been the primary focus. GD2’s glycan portion, which constitutes its hydrophilic extracellular membrane, poses a challenge for vaccine development since carbohydrate antigens are not strongly immunogenic. To enhance immunogenicity, GD2 has been conjugated to carrier proteins like keyhole limpet hemocyanin (KLH) and combined with adjuvants. Initial attempts using GD2-KLH and Monophosphoryl-Lipid A (MPLA) adjuvant failed to induce anti-GD2 antibodies in glioma patients. However, GD2-lactones (GD2L) conjugated to KLH with QS-21 adjuvant successfully elicited anti-GD2 antibodies in over 80% of melanoma patients, with responses lasting more than 6 months (Nores et al., 1987; Ragupathi et al., 2003). Vaccine trials involving mixtures of ganglioside conjugates such as GD2L and GD3L-KLH with OPT-821 and ß-glucan demonstrated encouraging results in neuroblastoma, leading to high anti-GD2 titers and more than 90% long-term survival rates (Kushner et al., 2014; Cheung et al., 2021). A bivalent vaccine targeting GM2 and GD2-KLH induced both anti-GM2 and anti-GD2 responses in nearly half of melanoma patients (Chapman et al., 2000). However, a trivalent vaccine combining GM2, GD3L, and GD2L-KLH with OPT-821 showed no advantage in overall or progression-free survival compared to adjuvant alone in a phase II study on relapsed sarcoma patients (Rosenbaum et al., 2022). This suggests that the addition of ß-glucan adjuvant may have been beneficial. The context and selection of ganglioside vaccines are critical factors to consider for the success of this approach.
In order to circumvent the difficulties linked to the use of purified GD2 gangliosides, approaches were developed to produce mimics. Mimotopes are peptide sequences developed to imitate molecules. In the case of GD2, phage display technology was used to raise peptides against the ch14.18 antibody, aiming to produce structural copies of GD2 (Förster-Waldl et al., 2005; Fest et al., 2006; Riemer et al., 2006). Mimotopes could be delivered in vivo directly, conjugated to KLH to increase immunogenicity, or through DNA vaccines with adjuvant (Fest et al., 2006; Riemer et al., 2006). In both cases, this induced a humoral response, capable of clearing liver metastases with NK activation with the DNA vaccine. Interestingly, DNA vaccines expressing mimotopes based on the 14G2a antibody led to production of antibodies reactive against GD2 and NCAM, just as 14G2a (Bolesta et al., 2005). One mimotope vaccine mimicking both GD2 and Lewis Y antigen, conjugated to a pan T-cell epitope and with adjuvant, was used in a Phase I trial in stage IV breast cancer patients (Hutchins et al., 2017). Encouragingly, this led to production of antigens to both antigens in all patients, and may pave the way for further studies.
Another technique mimicking GD2 is the development of anti-idiotype vaccines. An anti-idiotype is an antibody raised against the variable region of another antibody, thus adopting a similar conformation to the epitope of this second antibody. This theory was applied to GD2 after the observation of anti-idiotype (anti-Id) antibodies in melanoma patients following treatment with the murine 14G2a (Saleh et al., 1993). By immunizing rodents with either 3F8 or 14G2a, anti-Id antibodies were generated, capable of inhibiting anti-GD2 binding to GD2-positive targets. Furthermore, immunization of allogenic mice with these anti-Id led to in vivo production of anti-GD2 (Cheung et al., 1993; Sen et al., 1998). Similar results were observed in primates using 1A7, the anti-Id raised against 14G2a, with QS-21 adjuvant, leading to clinical evaluation of 1A7 (Sen et al., 1997). Phase I clinical trials were conducted on patients with advanced melanoma. In these trials, 85% of patients developed an anti-GD2 response with 1 complete response and 12 stable diseases on 47 patients going for 1 + year (Foon et al., 1998; Foon et al., 2000). In contrast, in high-risk neuroblastoma following complete remission, all patients developed anti-GD2, and 85% of patients in first remission remained without disease progression, compared to 10% for patients in second remission (Batova et al., 2004). More recently, another anti-Id, named ganglidiomab was raised in mice against 14G2a, and further humanized to produce the chimeric ganglidiximab (Lode et al., 2013; Eger et al., 2016). Ganglidiximab was capable of raising anti-GD2 responses, and may increase the specificity of this response, enhancing effect of this therapeutic against tumoral challenges.
Interestingly, GD2 carbohydrate head-groups displayed on a multivalent polyamidoamine scaffold used as vaccines induced rapid expansion of γδ T cells in mice, followed by a second-wave CD8+ T cells expansion (Bartish et al., 2020).
Intriguingly, a role for GD2 has a target for un-engineered cytotoxic T cells was described in mice. Immunization of mice with a GD2 expressing cell line induced a cytotoxic T cell response, carried out by CD8+ T cell (Zhao and Cheung, 1995). The cytotoxicity was dependent on the TCR, independent of NK cells, and restricted to GD2 and H-2b bearing targets. Functionally, it was suggested that GD2 residues could be linked to peptides, working as haptens, that bind to the MHC class I pocket. Pending confirmation, this could pave the way for alternative targeting of GD2-positive cancers, such as vaccine therapy and TCR engineering.
9 Conclusion and perspectives
While encouraging results have been observed using different therapeutic modalities, cases of refractory or relapsed disease are still frequent. Thus, the different treatment may benefit from potentiation based on biological properties of GD2. Based on monoclonal antibodies’ inhibition of the FAK/AKT/mTOR pathway in GD2-positive tumors, inhibition of components of the pathway or others involved in cell survival may achieve targeted and synergistic effect, thus enhancing therapeutic effect. Building up on GD2’s role as an immune checkpoint for macrophages, synergistic use of anti-GD2 and anti-CD47—a phagocytosis suppressor–has been used to attain synergy and eradicate in vivo osteosarcoma and small cell lung cancer tumors (Ito et al., 2001; Theruvath et al., 2022).
The potential expression of GD2 on T-cell warrants particular attention in T-cell based immunotherapies, as it could potentially lead to fratricidal killing and drop in therapeutic efficacy, as seen in other contexts (Kozani et al., 2021). The same may apply with CAR-macrophages since after tumor cell phagocytosis they may recycle the tumor cell GD2 on their cellular membrane (Stagno et al., 2022). Immune effector cell candidates for CAR approaches should be tested respectively against the immunosuppressive properties of tumor cell shed GD2. A different approach, based on GD2’s propension to be shed from tumor cells, is the inhibition of extravesicular vesicles’ secretion has proven effective in a preclinical model (Liu et al., 2022). While this might inhibit transfer of malignant properties from GD2-positive to antigen-negative cells, it especially potentiated anti-GD2’s therapeutic effect through reduction of decoy targets and immunosuppressive properties of circulating GD2. Another strategy will be the use of anti-GD2 immunotherapies at the time of minimal residual disease.
Regarding the development of vaccines, different alternatives can be suggested to further improve efficacy. As mentioned, the choice of GD2 subspecies may matter, with varying immunogenicity among the different structures, as seen with increased efficacy using lactonized forms of GD2 (Nores et al., 1987). In addition, the potential of GD2 stabilizing peptides to induce cytotoxic T-cell response remains to be explored.
Finally, a commonality between all treatments is the superior results obtained in the treatment of high-risk neuroblastoma compared to other indications. One specificity of neuroblastoma is high expression of GD2, observable in nearly all cells, with very rare cases of antigen loss (Wu et al., 1986; Kramer et al., 2001). As such, other GD2-positive cancer may benefit from strategies to increase antigen expression to levels similar to neuroblastoma. Different compounds may achieve this effect, such as epigenetic modulators (HDAC or EZH2 inhibitors), cytokines (IFNγ, TNFα, IL-4) which may additionally potentiate immune effectors, retinoids (Fenretinide), or polyamine (nanospermidine) as more recently described (Hoon et al., 1991a; Hoon et al., 1991b; Shibina et al., 2013; Kroesen et al., 2016; van den Bijgaart et al., 2019; Kailayangiri et al., 2019; Mabe et al., 2022; Galassi et al., 2023). Identifying the most suitable patients and the optimal timing for these approaches should also be taken into consideration to improve the patients’ outcome. In this regard, the quantification of tumor shed GD2 can provide a diagnostic companion for anti-GD2 immunotherapies.
Author contributions
PM retrieved the literature and completed the first draft, so he is the first author. PM and SB examined the literature-PM and SB contributed in the scientific writing of the manuscript. EM and SB revised the manuscript. All authors contributed to the article and approved the submitted version.
Funding
This work is supported by La Fondation Bristol Myers Squibb pour la Recherche en Oncoimmunologie and by la Ligue contre le Cancer comité 44 et la Ligue contre le Cancer comité 59.
Conflict of interest
SB is inventor of patents covering the therapeutic use of anti-OAcGD2 immunotherapies.
The remaining authors declare that the research was conducted in the absence of any commercial or financial relationships that could be construed as a potential conflict of interest.
Publisher’s note
All claims expressed in this article are solely those of the authors and do not necessarily represent those of their affiliated organizations, or those of the publisher, the editors and the reviewers. Any product that may be evaluated in this article, or claim that may be made by its manufacturer, is not guaranteed or endorsed by the publisher.
References
Abdullah, L. N., and Chow, E. K.-H. (2013). Mechanisms of chemoresistance in cancer stem cells. Clin. Transl. Med. 2, 3. doi:10.1186/2001-1326-2-3
Agrawal, V., and Frankel, A. E. (2010). 14G2a anti-GD2 crossreactivity with the CD166 antigen. J. Immunother. Hagerst. Md 33, 1014–1015. doi:10.1097/CJI.0b013e3181f3bf36
Ahmed, M., Goldgur, Y., Hu, J., Guo, H.-F., and Cheung, N.-K. V. (2013). In silico driven redesign of a clinically relevant antibody for the treatment of GD2 positive tumors. PloS One 8, e63359. doi:10.1371/journal.pone.0063359
Aixinjueluo, W., Furukawa, K., Zhang, Q., Hamamura, K., Tokuda, N., Yoshida, S., et al. (2005). Mechanisms for the apoptosis of small cell lung cancer cells induced by anti-GD2 monoclonal antibodies: roles of anoikis. J. Biol. Chem. 280, 29828–29836. doi:10.1074/jbc.M414041200
Al-Obaide, M. A. I., Alobydi, H., Abdelsalam, A. G., Zhang, R., and Srivenugopal, K. S. (2015). Multifaceted roles of 5’-regulatory region of the cancer associated gene B4GALT1 and its comparison with the gene family. Int. J. Oncol. 47, 1393–1404. doi:10.3892/ijo.2015.3136
Alvarez-Rueda, N., Desselle, A., Cochonneau, D., Chaumette, T., Clemenceau, B., Leprieur, S., et al. (2011). A monoclonal antibody to O-acetyl-GD2 ganglioside and not to GD2 shows potent anti-tumor activity without peripheral nervous system cross-reactivity. PloS One 6, e25220. doi:10.1371/journal.pone.0025220
Ando, I., Hoon, D. S., Suzuki, Y., Saxton, R. E., Golub, S. H., and Irie, R. F. (1987). Ganglioside GM2 on the K562 cell line is recognized as a target structure by human natural killer cells. Int. J. Cancer 40, 12–17. doi:10.1002/ijc.2910400104
Ando, S., and Yu, R. K. (1984). Fatty acid and long-chain base composition of gangliosides isolated from adult human brain. J. Neurosci. Res. 12, 205–211. doi:10.1002/jnr.490120208
Anghelescu, D. L., Goldberg, J. L., Faughnan, L. G., Wu, J., Mao, S., Furman, W. L., et al. (2015). Comparison of pain outcomes between two anti-GD2 antibodies in patients with neuroblastoma. Pediatr. Blood Cancer 62, 224–228. doi:10.1002/pbc.25280
Arming, S., Wipfler, D., Mayr, J., Merling, A., Vilas, U., Schauer, R., et al. (2011). The human Cas1 protein: a sialic acid-specific O-acetyltransferase? Glycobiology 21, 553–564. doi:10.1093/glycob/cwq153
Balis, F. M., Busch, C. M., Desai, A. V., Hibbitts, E., Naranjo, A., Bagatell, R., et al. (2020). The ganglioside GD2 as a circulating tumor biomarker for neuroblastoma. Pediatr. Blood Cancer 67, e28031. doi:10.1002/pbc.28031
Bartish, M., Del Rincón, S. V., Rudd, C. E., and Saragovi, H. U. (2020). Aiming for the sweet spot: glyco-immune checkpoints and γδ T cells in targeted immunotherapy. Front. Immunol. 11, 564499. doi:10.3389/fimmu.2020.564499
Bashir, S., Fezeu, L. K., Leviatan Ben-Arye, S., Yehuda, S., Reuven, E. M., Szabo de Edelenyi, F., et al. (2020). Association between Neu5Gc carbohydrate and serum antibodies against it provides the molecular link to cancer: french NutriNet-santé study. BMC Med. 18, 262. doi:10.1186/s12916-020-01721-8
Batova, A., Yu, A. L., Strother, D., Angelini, P., and Eskenazi, A. (2004). Promising results of a pilot trial of a GD2 directed anti-idiotypic antibody as a vaccine for high risk neuroblastoma. J. Clin. Oncol. 22, 8511. doi:10.1200/jco.2004.22.90140.8511
Battula, V. L., Shi, Y., Evans, K. W., Wang, R.-Y., Spaeth, E. L., Jacamo, R. O., et al. (2012). Ganglioside GD2 identifies breast cancer stem cells and promotes tumorigenesis. J. Clin. Invest. 122, 2066–2078. doi:10.1172/JCI59735
Baumann, A.-M. T., Bakkers, M. J. G., Buettner, F. F. R., Hartmann, M., Grove, M., Langereis, M. A., et al. (2015). 9-O-Acetylation of sialic acids is catalysed by CASD1 via a covalent acetyl-enzyme intermediate. Nat. Commun. 6, 7673. doi:10.1038/ncomms8673
Berthold, F., Schneider, A., Schumacher, R., and Bosslet, K. (1989). Detection of minimal disease in bone marrow of neuroblastoma patients by immunofluorescence. Pediatr. Hematol. Oncol. 6, 73–83. doi:10.3109/08880018909034273
Bhat, A. M., Mohapatra, B. C., Luan, H., Mushtaq, I., Chakraborty, S., Dutta, S., et al. (2023). Role of GD2 and its biosynthetic enzyme GD3 synthase in prostate cancer tumorigenesis. BioRxiv Prepr. Serv. Biol. 2023. doi:10.1101/2023.03.18.533299
Bieberich, E., MacKinnon, S., Silva, J., Li, D. D., Tencomnao, T., Irwin, L., et al. (2002). Regulation of ganglioside biosynthesis by enzyme complex formation of glycosyltransferases. Biochemistry 41, 11479–11487. doi:10.1021/bi0259958
Bieberich, E., Tencomnao, T., Kapitonov, D., and Yu, R. K. (2000). Effect of N-glycosylation on turnover and subcellular distribution of N-acetylgalactosaminyltransferase I and sialyltransferase II in neuroblastoma cells. J. Neurochem. 74, 2359–2364. doi:10.1046/j.1471-4159.2000.0742359.x
Bieberich, E., and Yu, R. K. (1999). Multi-enzyme kinetic analysis of glycolipid biosynthesis. Biochim. Biophys. Acta 1432, 113–124. doi:10.1016/s0167-4838(99)00085-0
Boeva, V., Louis-Brennetot, C., Peltier, A., Durand, S., Pierre-Eugène, C., Raynal, V., et al. (2017). Heterogeneity of neuroblastoma cell identity defined by transcriptional circuitries. Nat. Genet. 49, 1408–1413. doi:10.1038/ng.3921
Bolesta, E., Kowalczyk, A., Wierzbicki, A., Rotkiewicz, P., Bambach, B., Tsao, C.-Y., et al. (2005). DNA vaccine expressing the mimotope of GD2 ganglioside induces protective GD2 cross-reactive antibody responses. Cancer Res. 65, 3410–3418. doi:10.1158/0008-5472.CAN-04-2164
Buhtoiarov, I. N., Neal, Z. C., Gan, J., Buhtoiarova, T. N., Patankar, M. S., Gubbels, J. A. A., et al. (2011). Differential internalization of hu14.18-IL2 immunocytokine by NK and tumor cell: impact on conjugation, cytotoxicity, and targeting. J. Leukoc. Biol. 89, 625–638. doi:10.1189/jlb.0710422
Busch, C. M., Desai, A. V., Moorthy, G. S., Fox, E., and Balis, F. M. (2018). A validated HPLC-MS/MS method for estimating the concentration of the ganglioside, GD2, in human plasma or serum. J. Chromatogr. B Anal. Technol. Biomed. Life. Sci. 1103, 60–65. doi:10.1016/j.jchromb.2018.10.010
Butor, C., Diaz, S., and Varki, A. (1993). High level O-acetylation of sialic acids on N-linked oligosaccharides of rat liver membranes. Differential subcellular distribution of 7- and 9-O-acetyl groups and of enzymes involved in their regulation. J. Biol. Chem. 268, 10197–10206. doi:10.1016/s0021-9258(18)82190-1
Capsomidis, A., Benthall, G., Van Acker, H. H., Fisher, J., Kramer, A. M., Abeln, Z., et al. (2018). Chimeric antigen receptor-engineered human gamma delta T cells: enhanced cytotoxicity with retention of cross presentation. Mol. Ther. J. Am. Soc. Gene Ther. 26, 354–365. doi:10.1016/j.ymthe.2017.12.001
Castro, F., Cardoso, A. P., Gonçalves, R. M., Serre, K., and Oliveira, M. J. (2018). Interferon-Gamma at the crossroads of tumor immune surveillance or evasion. Front. Immunol. 9, 847. doi:10.3389/fimmu.2018.00847
Cavdarli, S., Schröter, L., Albers, M., Baumann, A.-M., Vicogne, D., Le Doussal, J.-M., et al. (2021). Role of Sialyl-O-acetyltransferase CASD1 on GD2 ganglioside O-acetylation in breast cancer cells. Cells 10, 1468. doi:10.3390/cells10061468
Cavdarli, S., Yamakawa, N., Clarisse, C., Aoki, K., Brysbaert, G., Le Doussal, J.-M., et al. (2020). Profiling of O-acetylated gangliosides expressed in neuroectoderm derived cells. Int. J. Mol. Sci. 21, 370. doi:10.3390/ijms21010370
Cazet, A., Bobowski, M., Rombouts, Y., Lefebvre, J., Steenackers, A., Popa, I., et al. (2012). The ganglioside G(D2) induces the constitutive activation of c-Met in MDA-MB-231 breast cancer cells expressing the G(D3) synthase. Glycobiology 22, 806–816. doi:10.1093/glycob/cws049
Chabab, G., Boissière-Michot, F., Mollevi, C., Ramos, J., Lopez-Crapez, E., Colombo, P.-E., et al. (2020). Diversity of tumor-infiltrating, γδ T-cell abundance in solid cancers. Cells 9, 1537. doi:10.3390/cells9061537
Chang, F., Li, R., and Ladisch, S. (1997). Shedding of gangliosides by human medulloblastoma cells. Exp. Cell Res. 234, 341–346. doi:10.1006/excr.1997.3619
Chang, H. R., Cordon-Cardo, C., Houghton, A. N., Cheung, N. K., and Brennan, M. F. (1992). Expression of disialogangliosides GD2 and GD3 on human soft tissue sarcomas. Cancer 70, 633–638. doi:10.1002/1097-0142(19920801)70:3<633::aid-cncr2820700315>3.0.co;2-f
Chapman, P. B., Morrisey, D., Panageas, K. S., Williams, L., Lewis, J. J., Israel, R. J., et al. (2000). Vaccination with a bivalent G(M2) and G(D2) ganglioside conjugate vaccine: a trial comparing doses of G(D2)-keyhole limpet hemocyanin. Clin. Cancer Res. Off. J. Am. Assoc. Cancer Res. 6, 4658–4662.
Chen, Y., Takikawa, M., Tsutsumi, S., Yamaguchi, Y., Okabe, A., Shimada, M., et al. (2018). PHLDA1, another PHLDA family protein that inhibits Akt. Cancer Sci. 109, 3532–3542. doi:10.1111/cas.13796
Cheng, J.-Y., Hung, J.-T., Lin, J., Lo, F.-Y., Huang, J.-R., Chiou, S.-P., et al. (2021). O-Acetyl-GD2 as a therapeutic target for breast cancer stem cells. Front. Immunol. 12, 791551. doi:10.3389/fimmu.2021.791551
Cheng, M., Ahmed, M., Xu, H., and Cheung, N.-K. V. (2015). Structural design of disialoganglioside GD2 and CD3-bispecific antibodies to redirect T cells for tumor therapy. Int. J. Cancer 136, 476–486. doi:10.1002/ijc.29007
Cheng, M., Santich, B. H., Xu, H., Ahmed, M., Huse, M., and Cheung, N.-K. V. (2016). Successful engineering of a highly potent single-chain variable-fragment (scFv) bispecific antibody to target disialoganglioside (GD2) positive tumors. Oncoimmunology 5, e1168557. doi:10.1080/2162402X.2016.1168557
Cheresh, D. A., Harper, J. R., Schulz, G., and Reisfeld, R. A. (1984). Localization of the gangliosides GD2 and GD3 in adhesion plaques and on the surface of human melanoma cells. Proc. Natl. Acad. Sci. U. S. A. 81, 5767–5771. doi:10.1073/pnas.81.18.5767
Cheresh, D. A., Pierschbacher, M. D., Herzig, M. A., and Mujoo, K. (1986a). Disialogangliosides GD2 and GD3 are involved in the attachment of human melanoma and neuroblastoma cells to extracellular matrix proteins. J. Cell Biol. 102, 688–696. doi:10.1083/jcb.102.3.688
Cheresh, D. A., Pytela, R., Pierschbacher, M. D., Klier, F. G., Ruoslahti, E., and Reisfeld, R. A. (1987). An Arg-Gly-Asp-directed receptor on the surface of human melanoma cells exists in an divalent cation-dependent functional complex with the disialoganglioside GD2. J. Cell Biol. 105, 1163–1173. doi:10.1083/jcb.105.3.1163
Cheresh, D. A., Rosenberg, J., Mujoo, K., Hirschowitz, L., and Reisfeld, R. A. (1986b). Biosynthesis and expression of the disialoganglioside GD2, a relevant target antigen on small cell lung carcinoma for monoclonal antibody-mediated cytolysis. Cancer Res. 46, 5112–5118.
Chester, M. A. (1998). IUPAC-IUB joint commission on biochemical nomenclature (JCBN). Nomenclature of glycolipids--recommendations 1997. Eur. J. Biochem. 257, 293–298. doi:10.1046/j.1432-1327.1998.2570293.x
Cheung, I. Y., Cheung, N.-K. V., Modak, S., Mauguen, A., Feng, Y., Basu, E., et al. (2021). Survival impact of anti-GD2 antibody response in a phase II ganglioside vaccine trial among patients with high-risk neuroblastoma with prior disease progression. J. Clin. Oncol. Off. J. Am. Soc. Clin. Oncol. 39, 215–226. doi:10.1200/JCO.20.01892
Cheung, I. Y., Hsu, K., and Cheung, N.-K. V. (2012). Activation of peripheral-blood granulocytes is strongly correlated with patient outcome after immunotherapy with anti-GD2 monoclonal antibody and granulocyte-macrophage colony-stimulating factor. J. Clin. Oncol. Off. J. Am. Soc. Clin. Oncol. 30, 426–432. doi:10.1200/JCO.2011.37.6236
Cheung, I. Y., Lo Piccolo, M. S., Kushner, B. H., Kramer, K., and Cheung, N.-K. V. (2003). Quantitation of GD2 synthase mRNA by real-time reverse transcriptase polymerase chain reaction: clinical utility in evaluating adjuvant therapy in neuroblastoma. J. Clin. Oncol. Off. J. Am. Soc. Clin. Oncol. 21, 1087–1093. doi:10.1200/JCO.2003.02.055
Cheung, N.-K. V., Guo, H., Hu, J., Tassev, D. V., and Cheung, I. Y. (2012). Humanizing murine IgG3 anti-GD2 antibody m3F8 substantially improves antibody-dependent cell-mediated cytotoxicity while retaining targeting in vivo. Oncoimmunology 1, 477–486. doi:10.4161/onci.19864
Cheung, N. K., Canete, A., Cheung, I. Y., Ye, J. N., and Liu, C. (1993). Disialoganglioside GD2 anti-idiotypic monoclonal antibodies. Int. J. Cancer 54, 499–505. doi:10.1002/ijc.2910540324
Cheung, N. K., Kushner, B. H., Cheung, I. Y., Kramer, K., Canete, A., Gerald, W., et al. (1998a). Anti-G(D2) antibody treatment of minimal residual stage 4 neuroblastoma diagnosed at more than 1 year of age. J. Clin. Oncol. Off. J. Am. Soc. Clin. Oncol. 16, 3053–3060. doi:10.1200/JCO.1998.16.9.3053
Cheung, N. K., Kushner, B. H., LaQuaglia, M., Kramer, K., Gollamudi, S., Heller, G., et al. (2001). N7: A novel multi-modality therapy of high risk neuroblastoma (NB) in children diagnosed over 1 year of age. Med. Pediatr. Oncol. 36, 227–230. doi:10.1002/1096-911X(20010101)36:1<227::AID-MPO1055>3.0.CO;2-U
Cheung, N. K., Kushner, B. H., Yeh, S. D., and Larson, S. M. (1998b). 3F8 monoclonal antibody treatment of patients with stage 4 neuroblastoma: a phase II study. Int. J. Oncol. 12, 1299–1306. doi:10.3892/ijo.12.6.1299
Chinnapen, D. J.-F., Hsieh, W.-T., te Welscher, Y. M., Saslowsky, D. E., Kaoutzani, L., Brandsma, E., et al. (2012). Lipid sorting by ceramide structure from plasma membrane to ER for the cholera toxin receptor ganglioside GM1. Dev. Cell 23, 573–586. doi:10.1016/j.devcel.2012.08.002
Chuang, H.-H., Zhen, Y.-Y., Tsai, Y.-C., Chuang, C.-H., Hsiao, M., Huang, M.-S., et al. (2022). FAK in cancer: from mechanisms to therapeutic strategies. Int. J. Mol. Sci. 23, 1726. doi:10.3390/ijms23031726
Cingolani, F., Futerman, A. H., and Casas, J. (2016). Ceramide synthases in biomedical research. Chem. Phys. Lipids 197, 25–32. doi:10.1016/j.chemphyslip.2015.07.026
Cochonneau, D., Terme, M., Michaud, A., Dorvillius, M., Gautier, N., Frikeche, J., et al. (2013). Cell cycle arrest and apoptosis induced by O-acetyl-GD2-specific monoclonal antibody 8B6 inhibits tumor growth in vitro and in vivo. Cancer Lett. 333, 194–204. doi:10.1016/j.canlet.2013.01.032
Colsch, B., Jackson, S. N., Dutta, S., and Woods, A. S. (2011). Molecular microscopy of brain gangliosides: illustrating their distribution in hippocampal cell layers. ACS Chem. Neurosci. 2, 213–222. doi:10.1021/cn100096h
Comeau, K., Paradis, P., and Schiffrin, E. L. (2020). Human and murine memory γδ T cells: evidence for acquired immune memory in bacterial and viral infections and autoimmunity. Cell. Immunol. 357, 104217. doi:10.1016/j.cellimm.2020.104217
Corrias, M. V., Parodi, S., Haupt, R., Lacitignola, L., Negri, F., Sementa, A. R., et al. (2008). Detection of GD2-positive cells in bone marrow samples and survival of patients with localised neuroblastoma. Br. J. Cancer 98, 263–269. doi:10.1038/sj.bjc.6604179
Cózar, B., Greppi, M., Carpentier, S., Narni-Mancinelli, E., Chiossone, L., and Vivier, E. (2021). Tumor-infiltrating natural killer cells. Cancer Discov. 11, 34–44. doi:10.1158/2159-8290.CD-20-0655
Czaplicki, D., Horwacik, I., Kowalczyk, A., Wieczorek, A., Bolek-Marzec, K., Balwierz, W., et al. (2009). New method for quantitative analysis of GD2 ganglioside in plasma of neuroblastoma patients. Acta Biochim. Pol. 56, 423–431. doi:10.18388/abp.2009_2476
Del Bufalo, F., De Angelis, B., Caruana, I., Del Baldo, G., De Ioris, M. A., Serra, A., et al. (2023). GD2-CART01 for relapsed or refractory high-risk neuroblastoma. N. Engl. J. Med. 388, 1284–1295. doi:10.1056/NEJMoa2210859
Delgado, D. C., Hank, J. A., Kolesar, J., Lorentzen, D., Gan, J., Seo, S., et al. (2010). Genotypes of NK cell KIR receptors, their ligands, and Fcγ receptors in the response of neuroblastoma patients to Hu14.18-IL2 immunotherapy. Cancer Res. 70, 9554–9561. doi:10.1158/0008-5472.CAN-10-2211
Deng, Z., Wang, H., Liu, J., Deng, Y., and Zhang, N. (2021). Comprehensive understanding of anchorage-independent survival and its implication in cancer metastasis. Cell Death Dis. 12, 629. doi:10.1038/s41419-021-03890-7
Di Paolo, D., Ambrogio, C., Pastorino, F., Brignole, C., Martinengo, C., Carosio, R., et al. (2011). Selective therapeutic targeting of the anaplastic lymphoma kinase with liposomal siRNA induces apoptosis and inhibits angiogenesis in neuroblastoma. Mol. Ther. J. Am. Soc. Gene Ther. 19, 2201–2212. doi:10.1038/mt.2011.142
Diaz, S. L., Padler-Karavani, V., Ghaderi, D., Hurtado-Ziola, N., Yu, H., Chen, X., et al. (2009). Sensitive and specific detection of the non-human sialic Acid N-glycolylneuraminic acid in human tissues and biotherapeutic products. PloS One 4, e4241. doi:10.1371/journal.pone.0004241
Dobrenkov, K., Ostrovnaya, I., Gu, J., Cheung, I. Y., and Cheung, N.-K. V. (2016). Oncotargets GD2 and GD3 are highly expressed in sarcomas of children, adolescents, and young adults. Pediatr. Blood Cancer 63, 1780–1785. doi:10.1002/pbc.26097
Dondero, A., Morini, M., Cangelosi, D., Mazzocco, K., Serra, M., Spaggiari, G. M., et al. (2021). Multiparametric flow cytometry highlights B7-H3 as a novel diagnostic/therapeutic target in GD2neg/low neuroblastoma variants. J. Immunother. Cancer 9, e002293. doi:10.1136/jitc-2020-002293
Duxbury, M. S., Ito, H., Zinner, M. J., Ashley, S. W., and Whang, E. E. (2004). Focal adhesion kinase gene silencing promotes anoikis and suppresses metastasis of human pancreatic adenocarcinoma cells. Surgery 135, 555–562. doi:10.1016/j.surg.2003.10.017
Eger, C., Siebert, N., Seidel, D., Zumpe, M., Jüttner, M., Brandt, S., et al. (2016). Generation and characterization of a human/mouse chimeric GD2-mimicking anti-idiotype antibody ganglidiximab for active immunotherapy against neuroblastoma. PloS One 11, e0150479. doi:10.1371/journal.pone.0150479
Erber, R., Kailayangiri, S., Huebner, H., Ruebner, M., Hartmann, A., Häberle, L., et al. (2021). Variable expression of the disialoganglioside GD2 in breast cancer molecular subtypes. Cancers 13, 5577. doi:10.3390/cancers13215577
Fabrias, G., Muñoz-Olaya, J., Cingolani, F., Signorelli, P., Casas, J., Gagliostro, V., et al. (2012). Dihydroceramide desaturase and dihydrosphingolipids: debutant players in the sphingolipid arena. Prog. Lipid Res. 51, 82–94. doi:10.1016/j.plipres.2011.12.002
Fabris, D., Karmelić, I., Muharemović, H., Sajko, T., Jurilj, M., Potočki, S., et al. (2021). Ganglioside composition distinguishes anaplastic ganglioglioma tumor tissue from peritumoral brain tissue: complementary mass spectrometry and thin-layer chromatography evidence. Int. J. Mol. Sci. 22, 8844. doi:10.3390/ijms22168844
Fest, S., Huebener, N., Weixler, S., Bleeke, M., Zeng, Y., Strandsby, A., et al. (2006). Characterization of GD2 peptide mimotope DNA vaccines effective against spontaneous neuroblastoma metastases. Cancer Res. 66, 10567–10575. doi:10.1158/0008-5472.CAN-06-1158
Filbin, M., and Monje, M. (2019). Developmental origins and emerging therapeutic opportunities for childhood cancer. Nat. Med. 25, 367–376. doi:10.1038/s41591-019-0383-9
Fleurence, J., Fougeray, S., Bahri, M., Cochonneau, D., Clémenceau, B., Paris, F., et al. (2017). Targeting O-Acetyl-GD2 ganglioside for cancer immunotherapy. J. Immunol. Res. 2017, 5604891. doi:10.1155/2017/5604891
Floutsis, G., Ulsh, L., and Ladisch, S. (1989). Immunosuppressive activity of human neuroblastoma tumor gangliosides. Int. J. Cancer 43, 6–9. doi:10.1002/ijc.2910430103
Foon, K. A., Lutzky, J., Baral, R. N., Yannelli, J. R., Hutchins, L., Teitelbaum, A., et al. (2000). Clinical and immune responses in advanced melanoma patients immunized with an anti-idiotype antibody mimicking disialoganglioside GD2. J. Clin. Oncol. Off. J. Am. Soc. Clin. Oncol. 18, 376–384. doi:10.1200/JCO.2000.18.2.376
Foon, K. A., Sen, G., Hutchins, L., Kashala, O. L., Baral, R., Banerjee, M., et al. (1998). Antibody responses in melanoma patients immunized with an anti-idiotype antibody mimicking disialoganglioside GD2. Clin. Cancer Res. Off. J. Am. Assoc. Cancer Res. 4, 1117–1124.
Förster-Waldl, E., Riemer, A. B., Dehof, A. K., Neumann, D., Brämswig, K., Boltz-Nitulescu, G., et al. (2005). Isolation and structural analysis of peptide mimotopes for the disialoganglioside GD2, a neuroblastoma tumor antigen. Mol. Immunol. 42, 319–325. doi:10.1016/j.molimm.2004.07.011
Freischütz, B., Saito, M., Rahmann, H., and Yu, R. K. (1995). Characterization of sialyltransferase-IV activity and its involvement in the c-pathway of brain ganglioside metabolism. J. Neurochem. 64, 385–393. doi:10.1046/j.1471-4159.1995.64010385.x
Fu, Z., Li, S., Han, S., Shi, C., and Zhang, Y. (2022). Antibody drug conjugate: the “biological missile” for targeted cancer therapy. Signal Transduct. Target. Ther. 7, 93. doi:10.1038/s41392-022-00947-7
Furman, W. L., McCarville, B., Shulkin, B. L., Davidoff, A., Krasin, M., Hsu, C.-W., et al. (2022). Improved outcome in children with newly diagnosed high-risk neuroblastoma treated with chemoimmunotherapy: updated results of a phase II study using hu14.18K322A. J. Clin. Oncol. Off. J. Am. Soc. Clin. Oncol. 40, 335–344. doi:10.1200/JCO.21.01375
Furukawa, K., Hamamura, K., Ohkawa, Y., Ohmi, Y., and Furukawa, K. (2012). Disialyl gangliosides enhance tumor phenotypes with differential modalities. Glycoconj. J. 29, 579–584. doi:10.1007/s10719-012-9423-0
Furukawa, K., Horie, M., Okutomi, K., Sugano, S., and Furukawa, K. (2003). Isolation and functional analysis of the melanoma specific promoter region of human GD3 synthase gene. Biochim. Biophys. Acta 1627, 71–78. doi:10.1016/s0167-4781(03)00076-9
Galan, A., Papaluca, A., Nejatie, A., Matanes, E., Brahimi, F., Tong, W., et al. (2023). GD2 and GD3 gangliosides as diagnostic biomarkers for all stages and subtypes of epithelial ovarian cancer. Front. Oncol. 13, 1134763. doi:10.3389/fonc.2023.1134763
Galassi, L., Rossi, M., Lodeserto, P., Lenzi, M., Borsetti, F., Voltattorni, M., et al. (2023). Naxitamab activity in neuroblastoma cells is enhanced by nanofenretinide and nanospermidine. Pharmaceutics 15, 648. doi:10.3390/pharmaceutics15020648
Gillies, S. D., Lo, K. M., and Wesolowski, J. (1989). High-level expression of chimeric antibodies using adapted cDNA variable region cassettes. J. Immunol. Methods 125, 191–202. doi:10.1016/0022-1759(89)90093-8
Golinelli, G., Grisendi, G., Dall’Ora, M., Casari, G., Spano, C., Talami, R., et al. (2022). Anti-GD2 CAR MSCs against metastatic Ewing’s sarcoma. Transl. Oncol. 15, 101240. doi:10.1016/j.tranon.2021.101240
Golinelli, G., Grisendi, G., Prapa, M., Bestagno, M., Spano, C., Rossignoli, F., et al. (2020). Targeting GD2-positive glioblastoma by chimeric antigen receptor empowered mesenchymal progenitors. Cancer Gene Ther. 27, 558–570. doi:10.1038/s41417-018-0062-x
Grant, S. C., Kostakoglu, L., Kris, M. G., Yeh, S. D., Larson, S. M., Finn, R. D., et al. (1996). Targeting of small-cell lung cancer using the anti-GD2 ganglioside monoclonal antibody 3F8: a pilot trial. Eur. J. Nucl. Med. 23, 145–149. doi:10.1007/BF01731837
Grayson, G., and Ladisch, S. (1992). Immunosuppression by human gangliosides. II. Carbohydrate structure and inhibition of human NK activity. Cell. Immunol. 139, 18–29. doi:10.1016/0008-8749(92)90096-8
Gross, S. K., Williams, M. A., and McCluer, R. H. (1980). Alkali-labile, sodium borohydride-reducible ganglioside sialic acid residues in brain. J. Neurochem. 34, 1351–1361. doi:10.1111/j.1471-4159.1980.tb11215.x
Hama, H. (2010). Fatty acid 2-Hydroxylation in mammalian sphingolipid biology. Biochim. Biophys. Acta 1801, 405–414. doi:10.1016/j.bbalip.2009.12.004
Hanada, K., Kumagai, K., Tomishige, N., and Yamaji, T. (2009). CERT-mediated trafficking of ceramide. Biochim. Biophys. Acta 1791, 684–691. doi:10.1016/j.bbalip.2009.01.006
Handgretinger, R., Baader, P., Dopfer, R., Klingebiel, T., Reuland, P., Treuner, J., et al. (1992). A phase I study of neuroblastoma with the anti-ganglioside GD2 antibody 14.G2a. G2a. Cancer Immunol. Immunother. CII 35, 199–204. doi:10.1007/BF01756188
Hattinger, C. M., Patrizio, M. P., Magagnoli, F., Luppi, S., and Serra, M. (2019). An update on emerging drugs in osteosarcoma: towards tailored therapies? Expert Opin. Emerg. Drugs 24, 153–171. doi:10.1080/14728214.2019.1654455
Heczey, A., Liu, D., Tian, G., Courtney, A. N., Wei, J., Marinova, E., et al. (2014). Invariant NKT cells with chimeric antigen receptor provide a novel platform for safe and effective cancer immunotherapy. Blood 124, 2824–2833. doi:10.1182/blood-2013-11-541235
Heczey, A., Louis, C. U., Savoldo, B., Dakhova, O., Durett, A., Grilley, B., et al. (2017). CAR T cells administered in combination with lymphodepletion and PD-1 inhibition to patients with neuroblastoma. Mol. Ther. J. Am. Soc. Gene Ther. 25, 2214–2224. doi:10.1016/j.ymthe.2017.05.012
Hedlund, M., Padler-Karavani, V., Varki, N. M., and Varki, A. (2008). Evidence for a human-specific mechanism for diet and antibody-mediated inflammation in carcinoma progression. Proc. Natl. Acad. Sci. U. S. A. 105, 18936–18941. doi:10.1073/pnas.0803943105
Hersey, P., and Jamal, O. (1989). Expression of the gangliosides GD3 and GD2 on lymphocytes in tissue sections of melanoma. Pathol. (Phila.) 21, 51–58. doi:10.3109/00313028909059531
Hersey, P., Jamal, O., Henderson, C., Zardawi, I., and D’Alessandro, G. (1988). Expression of the gangliosides GM3, GD3 and GD2 in tissue sections of normal skin, naevi, primary and metastatic melanoma. Int. J. Cancer 41, 336–343. doi:10.1002/ijc.2910410303
Higa, H. H., Butor, C., Diaz, S., and Varki, A. (1989). O-acetylation and de-O-acetylation of sialic acids. J. Biol. Chem. 264, 19427–19434. doi:10.1016/s0021-9258(19)47318-3
Hirabayashi, K., Du, H., Xu, Y., Shou, P., Zhou, X., Fucá, G., et al. (2021). Dual targeting CAR-T cells with optimal costimulation and metabolic fitness enhance antitumor activity and prevent escape in solid tumors. Nat. Cancer 2, 904–918. doi:10.1038/s43018-021-00244-2
Hoon, D. S., Banez, M., Okun, E., Morton, D. L., and Irie, R. F. (1991a). Modulation of human melanoma cells by interleukin-4 and in combination with gamma-interferon or alpha-tumor necrosis factor. Cancer Res. 51, 2002–2008.
Hoon, D. S., Kuo, C. T., Wen, S., Wang, H., Metelitsa, L., Reynolds, C. P., et al. (2001). Ganglioside GM2/GD2 synthetase mRNA is a marker for detection of infrequent neuroblastoma cells in bone marrow. Am. J. Pathol. 159, 493–500. doi:10.1016/S0002-9440(10)61721-X
Hoon, D. S., Okun, E., Banez, M., Irie, R. F., and Morton, D. L. (1991b). Interleukin 4 alone and with gamma-interferon or alpha-tumor necrosis factor inhibits cell growth and modulates cell surface antigens on human renal cell carcinomas. Cancer Res. 51, 5687–5693.
Horwacik, I., Durbas, M., Boratyn, E., Sawicka, A., Węgrzyn, P., Krzanik, S., et al. (2015). Analysis of genes involved in response to doxorubicin and a GD2 ganglioside-specific 14G2a monoclonal antibody in IMR-32 human neuroblastoma cells. Acta Biochim. Pol. 62, 423–433. doi:10.18388/abp.2015_1035
Horwacik, I., Durbas, M., Boratyn, E., Węgrzyn, P., and Rokita, H. (2013). Targeting GD2 ganglioside and aurora A kinase as a dual strategy leading to cell death in cultures of human neuroblastoma cells. Cancer Lett. 341, 248–264. doi:10.1016/j.canlet.2013.08.018
Huang, F., Bailey, L. S., Gao, T., Jiang, W., Yu, L., Bennett, D. A., et al. (2022). Analysis and comparison of mouse and human brain gangliosides via two-stage matching of MS/MS spectra. ACS Omega 7, 6403–6411. doi:10.1021/acsomega.1c07070
Huizer, K., Zhu, C., Chirifi, I., Krist, B., Zorgman, D., van der Weiden, M., et al. (2020). Periostin is expressed by pericytes and is crucial for angiogenesis in glioma. J. Neuropathol. Exp. Neurol. 79, 863–872. doi:10.1093/jnen/nlaa067
Hunter, C. D., Khanna, N., Richards, M. R., Rezaei Darestani, R., Zou, C., Klassen, J. S., et al. (2018). Human neuraminidase isoenzymes show variable activities for 9- O-Acetyl-sialoside substrates. ACS Chem. Biol. 13, 922–932. doi:10.1021/acschembio.7b00952
Hutchins, L. F., Makhoul, I., Emanuel, P. D., Pennisi, A., Siegel, E. R., Jousheghany, F., et al. (2017). Targeting tumor-associated carbohydrate antigens: a phase I study of a carbohydrate mimetic-peptide vaccine in stage IV breast cancer subjects. Oncotarget 8, 99161–99178. doi:10.18632/oncotarget.21959
Ikushiro, H., and Hayashi, H. (2011). Mechanistic enzymology of serine palmitoyltransferase. Biochim. Biophys. Acta 1814, 1474–1480. doi:10.1016/j.bbapap.2011.02.005
Inokuchi, J., Jimbo, M., Momosaki, K., Shimeno, H., Nagamatsu, A., and Radin, N. S. (1990). Inhibition of experimental metastasis of murine Lewis lung carcinoma by an inhibitor of glucosylceramide synthase and its possible mechanism of action. Cancer Res. 50, 6731–6737.
Ishii, A., Ohta, M., Watanabe, Y., Matsuda, K., Ishiyama, K., Sakoe, K., et al. (1998). Expression cloning and functional characterization of human cDNA for ganglioside GM3 synthase. J. Biol. Chem. 273, 31652–31655. doi:10.1074/jbc.273.48.31652
Ito, A., Handa, K., Withers, D. A., Satoh, M., and Hakomori, S. (2001). Binding specificity of siglec7 to disialogangliosides of renal cell carcinoma: possible role of disialogangliosides in tumor progression. FEBS Lett. 504, 82–86. doi:10.1016/s0014-5793(01)02734-x
Itokazu, Y., Tsai, Y.-T., and Yu, R. K. (2017). Epigenetic regulation of ganglioside expression in neural stem cells and neuronal cells. Glycoconj. J. 34, 749–756. doi:10.1007/s10719-016-9719-6
Iwasawa, T., Zhang, P., Ohkawa, Y., Momota, H., Wakabayashi, T., Ohmi, Y., et al. (2018). Enhancement of malignant properties of human glioma cells by ganglioside GD3/GD2. Int. J. Oncol. 52, 1255–1266. doi:10.3892/ijo.2018.4266
Jaggupilli, A., Ly, S., Nguyen, K., Anand, V., Yuan, B., El-Dana, F., et al. (2022). Metabolic stress induces GD2+ cancer stem cell-like phenotype in triple-negative breast cancer. Br. J. Cancer 126, 615–627. doi:10.1038/s41416-021-01636-y
Jose, G., Lu, Y.-J., Hung, J.-T., Yu, A. L., and Chen, J.-P. (2020). Co-delivery of CPT-11 and panobinostat with anti-GD2 antibody conjugated immunoliposomes for targeted combination chemotherapy. Cancers 12, 3211. doi:10.3390/cancers12113211
Josephs, S. F., Ichim, T. E., Prince, S. M., Kesari, S., Marincola, F. M., Escobedo, A. R., et al. (2018). Unleashing endogenous TNF-alpha as a cancer immunotherapeutic. J. Transl. Med. 16, 242. doi:10.1186/s12967-018-1611-7
Kailayangiri, S., Altvater, B., Lesch, S., Balbach, S., Göttlich, C., Kühnemundt, J., et al. (2019). EZH2 inhibition in ewing sarcoma upregulates GD2 expression for targeting with gene-modified T cells. Mol. Ther. J. Am. Soc. Gene Ther. 27, 933–946. doi:10.1016/j.ymthe.2019.02.014
Kalinovsky, D. V., Kibardin, A. V., Kholodenko, I. V., Svirshchevskaya, E. V., Doronin, I. I., Konovalova, M. V., et al. (2022). Therapeutic efficacy of antibody-drug conjugates targeting GD2-positive tumors. J. Immunother. Cancer 10, e004646. doi:10.1136/jitc-2022-004646
Kamerling, J. P., Schauer, R., Shukla, A. K., Stoll, S., Van Halbeek, H., and Vliegenthart, J. F. (1987). Migration of O-acetyl groups in N,O-acetylneuraminic acids. Eur. J. Biochem. 162, 601–607. doi:10.1111/j.1432-1033.1987.tb10681.x
Kasprowicz, A., Sophie, G.-D., Lagadec, C., and Delannoy, P. (2022). Role of GD3 synthase ST8Sia I in cancers. Cancers 14, 1299. doi:10.3390/cancers14051299
Kawashima, I., Kotani, M., Ozawa, H., Suzuki, M., and Tai, T. (1994). Generation of monoclonal antibodies specific for ganglioside lactones: evidence of the expression of lactone on human melanoma cells. Int. J. Cancer 58, 263–268. doi:10.1002/ijc.2910580220
Kidd, S., Spaeth, E., Klopp, A., Andreeff, M., Hall, B., and Marini, F. C. (2008). The (in) auspicious role of mesenchymal stromal cells in cancer: be it friend or foe. Cytotherapy 10, 657–667. doi:10.1080/14653240802486517
Kohla, G., Stockfleth, E., and Schauer, R. (2002). Gangliosides with O-acetylated sialic acids in tumors of neuroectodermal origin. Neurochem. Res. 27, 583–592. doi:10.1023/a:1020211714104
Kong, Y., Li, R., and Ladisch, S. (1998). Natural forms of shed tumor gangliosides. Biochim. Biophys. Acta 1394, 43–56. doi:10.1016/s0005-2760(98)00096-4
Kowalczyk, A., Gil, M., Horwacik, I., Odrowaz, Z., Kozbor, D., and Rokita, H. (2009). The GD2-specific 14G2a monoclonal antibody induces apoptosis and enhances cytotoxicity of chemotherapeutic drugs in IMR-32 human neuroblastoma cells. Cancer Lett. 281, 171–182. doi:10.1016/j.canlet.2009.02.040
Kozani, P. S., Kozani, P. S., and Rahbarizadeh, F. (2021). CAR-T cell therapy in T-cell malignancies: is success a low-hanging fruit? Stem Cell Res. Ther. 12, 527. doi:10.1186/s13287-021-02595-0
Kramer, K., Gerald, W. L., Kushner, B. H., Larson, S. M., Hameed, M., and Cheung, N. K. (2001). Disaloganglioside GD2 loss following monoclonal antibody therapy is rare in neuroblastoma. Med. Pediatr. Oncol. 36, 194–196. doi:10.1002/1096-911X(20010101)36:1<194::AID-MPO1046>3.0.CO;2-B
Kramer, K., Pandit-Taskar, N., Humm, J. L., Zanzonico, P. B., Haque, S., Dunkel, I. J., et al. (2018). A phase II study of radioimmunotherapy with intraventricular 131 I-3F8 for medulloblastoma. Pediatr. Blood Cancer 65, e26754. doi:10.1002/pbc.26754
Kroesen, M., Büll, C., Gielen, P. R., Brok, I. C., Armandari, I., Wassink, M., et al. (2016). Anti-GD2 mAb and Vorinostat synergize in the treatment of neuroblastoma. Oncoimmunology 5, e1164919. doi:10.1080/2162402X.2016.1164919
Kushner, B. H., Cheung, I. Y., Modak, S., Basu, E. M., Roberts, S. S., and Cheung, N.-K. (2018). Humanized 3F8 anti-GD2 monoclonal antibody dosing with granulocyte-macrophage colony-stimulating factor in patients with resistant neuroblastoma: a phase 1 clinical trial. JAMA Oncol. 4, 1729–1735. doi:10.1001/jamaoncol.2018.4005
Kushner, B. H., Cheung, I. Y., Modak, S., Kramer, K., Ragupathi, G., and Cheung, N.-K. V. (2014). Phase I trial of a bivalent gangliosides vaccine in combination with β-glucan for high-risk neuroblastoma in second or later remission. Clin. Cancer Res. 20, 1375–1382. doi:10.1158/1078-0432.CCR-13-1012
Kushner, B. H., and Cheung, N. K. (1991). Clinically effective monoclonal antibody 3F8 mediates nonoxidative lysis of human neuroectodermal tumor cells by polymorphonuclear leukocytes. Cancer Res. 51, 4865–4870.
Kushner, B. H., Kramer, K., Modak, S., and Cheung, N.-K. V. (2011). Successful multifold dose escalation of anti-GD2 monoclonal antibody 3F8 in patients with neuroblastoma: a phase I study. J. Clin. Oncol. 29, 1168–1174. doi:10.1200/JCO.2010.28.3317
Labrada, M., Dorvignit, D., Hevia, G., Rodríguez-Zhurbenko, N., Hernández, A. M., Vázquez, A. M., et al. (2018). GM3(Neu5Gc) ganglioside: an evolution fixed neoantigen for cancer immunotherapy. Semin. Oncol. 45, 41–51. doi:10.1053/j.seminoncol.2018.04.003
Ladenstein, R., Pötschger, U., Valteau-Couanet, D., Luksch, R., Castel, V., Yaniv, I., et al. (2018). Interleukin 2 with anti-GD2 antibody ch14.18/CHO (dinutuximab beta) in patients with high-risk neuroblastoma (HR-NBL1/SIOPEN): A multicentre, randomised, phase 3 trial. Lancet Oncol. 19, 1617–1629. doi:10.1016/S1470-2045(18)30578-3
Ladisch, S., Becker, H., and Ulsh, L. (1992). Immunosuppression by human gangliosides: I. Relationship of carbohydrate structure to the inhibition of T cell responses. Biochim. Biophys. Acta 1125, 180–188. doi:10.1016/0005-2760(92)90043-u
Ladisch, S., Hasegawa, A., Li, R., and Kiso, M. (1995). Immunosuppressive activity of chemically synthesized gangliosides. Biochemistry 34, 1197–1202. doi:10.1021/bi00004a012
Ladisch, S., Li, R., and Olson, E. (1994). Ceramide structure predicts tumor ganglioside immunosuppressive activity. Proc. Natl. Acad. Sci. U. S. A. 91, 1974–1978. doi:10.1073/pnas.91.5.1974
Ladisch, S., Sweeley, C. C., Becker, H., and Gage, D. (1989). Aberrant fatty acyl alpha-hydroxylation in human neuroblastoma tumor gangliosides. J. Biol. Chem. 264, 12097–12105. doi:10.1016/s0021-9258(18)80178-8
Ladisch, S., Wu, Z. L., Feig, S., Ulsh, L., Schwartz, E., Floutsis, G., et al. (1987). Shedding of GD2 ganglioside by human neuroblastoma. Int. J. Cancer 39, 73–76. doi:10.1002/ijc.2910390113
Lammie, G., Cheung, N., Gerald, W., Rosenblum, M., and Cordoncardo, C. (1993). Ganglioside gd(2) expression in the human nervous-system and in neuroblastomas - an immunohistochemical study. Int. J. Oncol. 3, 909–915. doi:10.3892/ijo.3.5.909
Laurent, V. E., Otero, L. L., Vazquez, V., Camarero, S., Gabri, M. R., Labraga, M., et al. (2010). Optimization of molecular detection of GD2 synthase mRNA in retinoblastoma. Mol. Med. Rep. 3, 253–259. doi:10.3892/mmr_00000248
Lee, H.-Y., and Hong, I.-S. (2017). Double-edged sword of mesenchymal stem cells: cancer-promoting versus therapeutic potential. Cancer Sci. 108, 1939–1946. doi:10.1111/cas.13334
Lee, L., Abe, A., and Shayman, J. A. (1999). Improved inhibitors of glucosylceramide synthase. J. Biol. Chem. 274, 14662–14669. doi:10.1074/jbc.274.21.14662
Li, R., Gage, D., McKallip, R., and Ladisch, S. (1996). Structural characterization and in vivo immunosuppressive activity of neuroblastoma GD2. Glycoconj. J. 13, 385–389. doi:10.1007/BF00731471
Li, R. X., and Ladisch, S. (1991). Shedding of human neuroblastoma gangliosides. Biochim. Biophys. Acta 1083, 57–64. doi:10.1016/0005-2760(91)90124-z
Liang, Y.-J., Ding, Y., Levery, S. B., Lobaton, M., Handa, K., and Hakomori, S. (2013). Differential expression profiles of glycosphingolipids in human breast cancer stem cells vs. cancer non-stem cells. Proc. Natl. Acad. Sci. U. S. A. 110, 4968–4973. doi:10.1073/pnas.1302825110
Liu, B., Wu, Y., Zhou, Y., and Peng, D. (2014). Endothelin A receptor antagonism enhances inhibitory effects of anti-ganglioside GD2 monoclonal antibody on invasiveness and viability of human osteosarcoma cells. PloS One 9, e93576. doi:10.1371/journal.pone.0093576
Liu, G., Meng, X., Jin, Y., Bai, J., Zhao, Y., Cui, X., et al. (2008). Inhibitory role of focal adhesion kinase on anoikis in the lung cancer cell A549. Cell Biol. Int. 32, 663–670. doi:10.1016/j.cellbi.2008.01.292
Liu, X., Wills, C. A., Chen, L., Zhang, J., Zhao, Y., Zhou, M., et al. (2022). Small extracellular vesicles induce resistance to anti-GD2 immunotherapy unveiling tipifarnib as an adjunct to neuroblastoma immunotherapy. J. Immunother. Cancer 10, e004399. doi:10.1136/jitc-2021-004399
Lo Piccolo, M. S., Cheung, N. K., and Cheung, I. Y. (2001). GD2 synthase: a new molecular marker for detecting neuroblastoma. Cancer 92, 924–931. doi:10.1002/1097-0142(20010815)92:4<924::aid-cncr1402>3.0.co;2-o
Lode, H. N., Schmidt, M., Seidel, D., Huebener, N., Brackrock, D., Bleeke, M., et al. (2013). Vaccination with anti-idiotype antibody ganglidiomab mediates a GD(2)-specific anti-neuroblastoma immune response. Cancer Immunol. Immunother. CII 62, 999–1010. doi:10.1007/s00262-013-1413-y
Longee, D. C., Wikstrand, C. J., Månsson, J. E., He, X., Fuller, G. N., Bigner, S. H., et al. (1991). Disialoganglioside GD2 in human neuroectodermal tumor cell lines and gliomas. Acta Neuropathol. (Berl.) 82, 45–54. doi:10.1007/BF00310922
Louis, C. U., Savoldo, B., Dotti, G., Pule, M., Yvon, E., Myers, G. D., et al. (2011). Antitumor activity and long-term fate of chimeric antigen receptor-positive T cells in patients with neuroblastoma. Blood 118, 6050–6056. doi:10.1182/blood-2011-05-354449
Mabe, N. W., Huang, M., Dalton, G. N., Alexe, G., Schaefer, D. A., Geraghty, A. C., et al. (2022). Transition to a mesenchymal state in neuroblastoma confers resistance to anti-GD2 antibody via reduced expression of ST8SIA1. Nat. Cancer 3, 976–993. doi:10.1038/s43018-022-00405-x
Majzner, R. G., Ramakrishna, S., Yeom, K. W., Patel, S., Chinnasamy, H., Schultz, L. M., et al. (2022). GD2-CAR T cell therapy for H3K27M-mutated diffuse midline gliomas. Nature 603, 934–941. doi:10.1038/s41586-022-04489-4
Mandon, E. C., Ehses, I., Rother, J., van Echten, G., and Sandhoff, K. (1992). Subcellular localization and membrane topology of serine palmitoyltransferase, 3-dehydrosphinganine reductase, and sphinganine N-acyltransferase in mouse liver. J. Biol. Chem. 267, 11144–11148. doi:10.1016/s0021-9258(19)49887-6
Mann, B. S., Johnson, J. R., Cohen, M. H., Justice, R., and Pazdur, R. (2007). FDA approval summary: vorinostat for treatment of advanced primary cutaneous T-cell lymphoma. Oncol. 12, 1247–1252. doi:10.1634/theoncologist.12-10-1247
Martinez, C., Hofmann, T. J., Marino, R., Dominici, M., and Horwitz, E. M. (2007). Human bone marrow mesenchymal stromal cells express the neural ganglioside GD2: a novel surface marker for the identification of MSCs. Blood 109, 4245–4248. doi:10.1182/blood-2006-08-039347
McNerney, K. O., Karageorgos, S., Ferry, G. M., Wolpaw, A. J., Burudpakdee, C., Khurana, P., et al. (2022). TH-MYCN tumors, but not tumor-derived cell lines, are adrenergic lineage, GD2+, and responsive to anti-GD2 antibody therapy. Oncoimmunology 11, 2075204. doi:10.1080/2162402X.2022.2075204
Merrill, A. H. (2011). Sphingolipid and glycosphingolipid metabolic pathways in the era of sphingolipidomics. Chem. Rev. 111, 6387–6422. doi:10.1021/cr2002917
Mitra, S. K., and Schlaepfer, D. D. (2006). Integrin-regulated FAK-Src signaling in normal and cancer cells. Curr. Opin. Cell Biol. 18, 516–523. doi:10.1016/j.ceb.2006.08.011
Mollinedo, F., and Gajate, C. (2020). Lipid rafts as signaling hubs in cancer cell survival/death and invasion: implications in tumor progression and therapy. J. Lipid Res. 61, 611–635. doi:10.1194/jlr.TR119000439
Mueller, B. M., Romerdahl, C. A., Gillies, S. D., and Reisfeld, R. A. (1990). Enhancement of antibody-dependent cytotoxicity with a chimeric anti-GD2 antibody. J. Immunol. Balt. Md 1950 144, 1382–1386. doi:10.4049/jimmunol.144.4.1382
Mujoo, K., Cheresh, D. A., Yang, H. M., and Reisfeld, R. A. (1987). Disialoganglioside GD2 on human neuroblastoma cells: target antigen for monoclonal antibody-mediated cytolysis and suppression of tumor growth. Cancer Res. 47, 1098–1104.
Mujoo, K., Kipps, T. J., Yang, H. M., Cheresh, D. A., Wargalla, U., Sander, D. J., et al. (1989). Functional properties and effect on growth suppression of human neuroblastoma tumors by isotype switch variants of monoclonal antiganglioside GD2 antibody 14.18. Cancer Res. 49, 2857–2861.
Mujoo, K., Reisfeld, R. A., Cheung, L., and Rosenblum, M. G. (1991). A potent and specific immunotoxin for tumor cells expressing disialoganglioside GD2. Cancer Immunol. Immunother. CII 34, 198–204. doi:10.1007/BF01742313
Munn, D. H., and Cheung, N. K. (1989). Antibody-dependent antitumor cytotoxicity by human monocytes cultured with recombinant macrophage colony-stimulating factor. Induction of efficient antibody-mediated antitumor cytotoxicity not detected by isotope release assays. J. Exp. Med. 170, 511–526. doi:10.1084/jem.170.2.511
Munn, D. H., and Cheung, N. K. (1990). Phagocytosis of tumor cells by human monocytes cultured in recombinant macrophage colony-stimulating factor. J. Exp. Med. 172, 231–237. doi:10.1084/jem.172.1.231
Murray, J. L., Cunningham, J. E., Brewer, H., Mujoo, K., Zukiwski, A. A., Podoloff, D. A., et al. (1994). Phase I trial of murine monoclonal antibody 14G2a administered by prolonged intravenous infusion in patients with neuroectodermal tumors. J. Clin. Oncol. Off. J. Am. Soc. Clin. Oncol. 12, 184–193. doi:10.1200/JCO.1994.12.1.184
Nagata, Y., Yamashiro, S., Yodoi, J., Lloyd, K. O., Shiku, H., and Furukawa, K. (1992). Expression cloning of beta 1,4 N-acetylgalactosaminyltransferase cDNAs that determine the expression of GM2 and GD2 gangliosides. J. Biol. Chem. 267, 12082–12089. doi:10.1016/s0021-9258(19)49809-8
Nakajima, M., Guo, H.-F., Hoseini, S. S., Suzuki, M., Xu, H., and Cheung, N.-K. V. (2021). Potent antitumor effect of T cells armed with anti-GD2 bispecific antibody. Blood Cancer 68, e28971. doi:10.1002/pbc.28971
Nazha, B., Inal, C., and Owonikoko, T. K. (2020). Disialoganglioside GD2 expression in solid tumors and role as a target for cancer therapy. Front. Oncol. 10, 1000. doi:10.3389/fonc.2020.01000
Neri, D., and Sondel, P. M. (2016). Immunocytokines for cancer treatment: past, present and future. Curr. Opin. Immunol. 40, 96–102. doi:10.1016/j.coi.2016.03.006
Nguyen, K., Yan, Y., Yuan, B., Dasgupta, A., Sun, J., Mu, H., et al. (2018). ST8SIA1 regulates tumor growth and metastasis in TNBC by activating the FAK-AKT-mTOR signaling pathway. Mol. Cancer Ther. 17, 2689–2701. doi:10.1158/1535-7163.MCT-18-0399
Nguyen, R., Zhang, X., Sun, M., Abbas, S., Seibert, C., Kelly, M. C., et al. (2022). Anti-GD2 antibodies conjugated to IL15 and IL21 mediate potent antitumor cytotoxicity against neuroblastoma. Clin. Cancer Res. Off. J. Am. Assoc. Cancer Res. 28, 3785–3796. doi:10.1158/1078-0432.CCR-22-0717
Nishio, M., and Furukawa, K. (2004). Incorporation, remodeling and re-expression of exogenous gangliosides in human cancer cell lines in vitro and in vivo. Nagoya J. Med. Sci. 67, 35–44.
Nores, G. A., Dohi, T., Taniguchi, M., and Hakomori, S. (1987). Density-dependent recognition of cell surface GM3 by a certain anti-melanoma antibody, and GM3 lactone as a possible immunogen: requirements for tumor-associated antigen and immunogen. J. Immunol. Balt. Md 1950 139, 3171–3176. doi:10.4049/jimmunol.139.9.3171
Okuda, T. (2021). Application of the antibody-inducing activity of glycosphingolipids to human diseases. Int. J. Mol. Sci. 22, 3776. doi:10.3390/ijms22073776
Okuda, T., Shimizu, K., Hasaba, S., and Date, M. (2019). Induction of specific adaptive immune responses by immunization with newly designed artificial glycosphingolipids. Sci. Rep. 9, 18803. doi:10.1038/s41598-019-55088-9
Ordóñez-Reyes, C., Garcia-Robledo, J. E., Chamorro, D. F., Mosquera, A., Sussmann, L., Ruiz-Patiño, A., et al. (2022). Bispecific antibodies in cancer immunotherapy: a novel response to an old question. Pharmaceutics 14, 1243. doi:10.3390/pharmaceutics14061243
Ozkaynak, M. F., Sondel, P. M., Krailo, M. D., Gan, J., Javorsky, B., Reisfeld, R. A., et al. (2000). Phase I study of chimeric human/murine anti-ganglioside G(D2) monoclonal antibody (ch14.18) with granulocyte-macrophage colony-stimulating factor in children with neuroblastoma immediately after hematopoietic stem-cell transplantation: a children’s cancer group study. J. Clin. Oncol. 18, 4077–4085. doi:10.1200/JCO.2000.18.24.4077
Park, J. A., and Cheung, N.-K. V. (2020). GD2 or HER2 targeting T cell engaging bispecific antibodies to treat osteosarcoma. J. Hematol. Oncol.J Hematol. Oncol. 13, 172. doi:10.1186/s13045-020-01012-y
Park, J. A., Santich, B. H., Xu, H., Lum, L. G., and Cheung, N.-K. V. (2021). Potent ex vivo armed T cells using recombinant bispecific antibodies for adoptive immunotherapy with reduced cytokine release. J. Immunother. Cancer 9, e002222. doi:10.1136/jitc-2020-002222
Patel, K., Rossell, R. J., Pemberton, L. F., Cheung, N. K., Walsh, F. S., Moore, S. E., et al. (1989). Monoclonal antibody 3F8 recognises the neural cell adhesion molecule (NCAM) in addition to the ganglioside GD2. Br. J. Cancer 60, 861–866. doi:10.1038/bjc.1989.380
Peng, C.-A., and Wang, C.-H. (2011). Anti-neuroblastoma activity of gold nanorods bound with GD2 monoclonal antibody under near-infrared laser irradiation. Cancers 3, 227–240. doi:10.3390/cancers3010227
Perez Horta, Z., Goldberg, J. L., and Sondel, P. M. (2016). Anti-GD2 mAbs and next-generation mAb-based agents for cancer therapy. Immunotherapy 8, 1097–1117. doi:10.2217/imt-2016-0021
Phi, L. T. H., Sari, I. N., Yang, Y.-G., Lee, S.-H., Jun, N., Kim, K. S., et al. (2018). Cancer stem cells (CSCs) in drug resistance and their therapeutic implications in cancer treatment. Stem Cells Int. 2018, 5416923. doi:10.1155/2018/5416923
Poon, V. I., Roth, M., Piperdi, S., Geller, D., Gill, J., Rudzinski, E. R., et al. (2015). Ganglioside GD2 expression is maintained upon recurrence in patients with osteosarcoma. Clin. Sarcoma Res. 5, 4. doi:10.1186/s13569-014-0020-9
Portoukalian, J., David, M. J., Gain, P., and Richard, M. (1993). Shedding of GD2 ganglioside in patients with retinoblastoma. Int. J. Cancer 53, 948–951. doi:10.1002/ijc.2910530614
Probstmeier, R., and Pesheva, P. (1999). Tenascin-C inhibits beta1 integrin-dependent cell adhesion and neurite outgrowth on fibronectin by a disialoganglioside-mediated signaling mechanism. Glycobiology 9, 101–114. doi:10.1093/glycob/9.2.101
Pruett, S. T., Bushnev, A., Hagedorn, K., Adiga, M., Haynes, C. A., Sullards, M. C., et al. (2008). Biodiversity of sphingoid bases (“sphingosines”) and related amino alcohols. J. Lipid Res. 49, 1621–1639. doi:10.1194/jlr.R800012-JLR200
Ragupathi, G., Livingston, P. O., Hood, C., Gathuru, J., Krown, S. E., Chapman, P. B., et al. (2003). Consistent antibody response against ganglioside GD2 induced in patients with melanoma by a GD2 lactone-keyhole limpet hemocyanin conjugate vaccine plus immunological adjuvant QS-21. Clin. Cancer Res. Off. J. Am. Assoc. Cancer Res. 9, 5214–5220.
Reuland, P., Geiger, L., Thelen, M. H., Handgretinger, R., Haase, B., Müller-Schauenburg, W., et al. (2001). Follow-up in neuroblastoma: comparison of metaiodobenzylguanidine and a chimeric anti-GD2 antibody for detection of tumor relapse and therapy response. J. Pediatr. Hematol. Oncol. 23, 437–442. doi:10.1097/00043426-200110000-00009
Riemer, A. B., Förster-Waldl, E., Brämswig, K. H., Pollak, A., Zielinski, C. C., Pehamberger, H., et al. (2006). Induction of IgG antibodies against the GD2 carbohydrate tumor antigen by vaccination with peptide mimotopes. Eur. J. Immunol. 36, 1267–1274. doi:10.1002/eji.200535279
Rosenbaum, E., Chugh, R., Ryan, C. W., Agulnik, M., Milhem, M. M., George, S., et al. (2022). A randomised phase II trial of a trivalent ganglioside vaccine targeting GM2, GD2 and GD3 combined with immunological adjuvant OPT-821 versus OPT-821 alone in metastatic sarcoma patients rendered disease-free by surgery. Eur. J. Cancer Oxf. Engl. 176, 155–163. doi:10.1016/j.ejca.2022.09.003
Roth, M., Linkowski, M., Tarim, J., Piperdi, S., Sowers, R., Geller, D., et al. (2014). Ganglioside GD2 as a therapeutic target for antibody-mediated therapy in patients with osteosarcoma. Cancer 120, 548–554. doi:10.1002/cncr.28461
Saleh, M. N., Khazaeli, M. B., Wheeler, R. H., Dropcho, E., Liu, T., Urist, M., et al. (1992). Phase I trial of the murine monoclonal anti-GD2 antibody 14G2a in metastatic melanoma. Cancer Res. 52, 4342–4347.
Saleh, M. N., Stapleton, J. D., Khazaeli, M. B., and LoBuglio, A. F. (1993). Generation of a human anti-idiotypic antibody that mimics the GD2 antigen. J. Immunol. Balt. 151, 3390–3398. doi:10.4049/jimmunol.151.6.3390
Sandhoff, R., and Sandhoff, K. (2018). Emerging concepts of ganglioside metabolism. FEBS Lett. 592, 3835–3864. doi:10.1002/1873-3468.13114
Sandhoff, R., Schulze, H., and Sandhoff, K. (2018). Ganglioside metabolism in health and disease. Prog. Mol. Biol. Transl. Sci. 156, 1–62. doi:10.1016/bs.pmbts.2018.01.002
Santilli, F., Fabrizi, J., Pulcini, F., Santacroce, C., Sorice, M., Delle Monache, S., et al. (2022). Gangliosides and their role in multilineage differentiation of mesenchymal stem cells. Biomedicines 10, 3112. doi:10.3390/biomedicines10123112
Sarbu, M., Petrica, L., Clemmer, D. E., Vukelić, Ž., and Zamfir, A. D. (2021). Gangliosides of human glioblastoma multiforme: a comprehensive mapping and structural analysis by ion mobility tandem mass spectrometry. J. Am. Soc. Mass Spectrom. 32, 1249–1257. doi:10.1021/jasms.1c00088
Sariola, H., Terävä, H., Rapola, J., and Saarinen, U. M. (1991). Cell-surface ganglioside GD2 in the immunohistochemical detection and differential diagnosis of neuroblastoma. Am. J. Clin. Pathol. 96, 248–252. doi:10.1093/ajcp/96.2.248
Schulz, G., Cheresh, D. A., Varki, N. M., Yu, A., Staffileno, L. K., and Reisfeld, R. A. (1984). Detection of ganglioside GD2 in tumor tissues and sera of neuroblastoma patients. Cancer Res. 44, 5914–5920.
Schumacher-Kuckelkorn, R., Volland, R., Gradehandt, A., Hero, B., Simon, T., and Berthold, F. (2017). Lack of immunocytological GD2 expression on neuroblastoma cells in bone marrow at diagnosis, during treatment, and at recurrence. Pediatr. Blood Cancer 64, 46–56. doi:10.1002/pbc.26184
Sen, G., Chakraborty, M., Foon, K. A., Reisfeld, R. A., and Bhattacharya-Chatterjee, M. B. (1998). Induction of IgG antibodies by an anti-idiotype antibody mimicking disialoganglioside GD2. J. Immunother. Hagerst. Md 1997 21, 75–83. doi:10.1097/00002371-199801000-00010
Sen, G., Chakraborty, M., Foon, K. A., Reisfeld, R. A., and Bhattacharya-Chatterjee, M. (1997). Preclinical evaluation in nonhuman primates of murine monoclonal anti-idiotype antibody that mimics the disialoganglioside GD2. Clin. Cancer Res. 3, 1969–1976.
Serb, A., Schiopu, C., Flangea, C., Vukelić, Z., Sisu, E., Zagrean, L., et al. (2009). High-throughput analysis of gangliosides in defined regions of fetal brain by fully automated chip-based nanoelectrospray ionization multi-stage mass spectrometry. Eur. J. Mass Spectrom. Chichester Engl. 15, 541–553. doi:10.1255/ejms.1009
Sha, Y.-L., Liu, Y., Yang, J.-X., Wang, Y.-Y., Gong, B.-C., Jin, Y., et al. (2022). B3GALT4 remodels the tumor microenvironment through GD2-mediated lipid raft formation and the c-met/AKT/mTOR/IRF-1 axis in neuroblastoma. J. Exp. Clin. Cancer Res. CR 41, 314. doi:10.1186/s13046-022-02523-x
Shibina, A., Seidel, D., Somanchi, S. S., Lee, D. A., Stermann, A., Maurer, B. J., et al. (2013). Fenretinide sensitizes multidrug-resistant human neuroblastoma cells to antibody-independent and ch14.18-mediated NK cell cytotoxicity. J. Mol. Med. Berl. Ger. 91, 459–472. doi:10.1007/s00109-012-0958-0
Shibuya, H., Hamamura, K., Hotta, H., Matsumoto, Y., Nishida, Y., Hattori, H., et al. (2012). Enhancement of malignant properties of human osteosarcoma cells with disialyl gangliosides GD2/GD3. Cancer Sci. 103, 1656–1664. doi:10.1111/j.1349-7006.2012.02344.x
Shiraishi, T., Kinter, M. T., Mills, S. E., Lippert, M. C., Bova, G. S., and Young, W. W. (1988). The glycosphingolipids of human prostate tissue. Biochim. Biophys. Acta 961, 160–169. doi:10.1016/0005-2760(88)90109-9
Shurin, G. V., Shurin, M. R., Bykovskaia, S., Shogan, J., Lotze, M. T., and Barksdale, E. M. (2001). Neuroblastoma-derived gangliosides inhibit dendritic cell generation and function. Cancer Res. 61, 363–369.
Shusterman, S., London, W. B., Gillies, S. D., Hank, J. A., Voss, S. D., Seeger, R. C., et al. (2010). Antitumor activity of hu14.18-IL2 in patients with relapsed/refractory neuroblastoma: a children’s oncology group (COG) phase II study. J. Clin. 28, 4969–4975. doi:10.1200/JCO.2009.27.8861
Sibille, E., Berdeaux, O., Martine, L., Bron, A. M., Creuzot-Garcher, C. P., He, Z., et al. (2016). Ganglioside profiling of the human retina: comparison with other ocular structures, brain and plasma reveals tissue specificities. PloS One 11, e0168794. doi:10.1371/journal.pone.0168794
Simon, T., Hero, B., Faldum, A., Handgretinger, R., Schrappe, M., Klingebiel, T., et al. (2011). Long term outcome of high-risk neuroblastoma patients after immunotherapy with antibody ch14.18 or oral metronomic chemotherapy. BMC Cancer 11, 21. doi:10.1186/1471-2407-11-21
Simon, T., Hero, B., Faldum, A., Handgretinger, R., Schrappe, M., Niethammer, D., et al. (2004). Consolidation treatment with chimeric anti-GD2-antibody ch14.18 in children older than 1 year with metastatic neuroblastoma. J. Clin. Oncol. 22, 3549–3557. doi:10.1200/JCO.2004.08.143
Sipione, S., Monyror, J., Galleguillos, D., Steinberg, N., and Kadam, V. (2020). Gangliosides in the brain: physiology, pathophysiology and therapeutic applications. Front. Neurosci. 14, 572965. doi:10.3389/fnins.2020.572965
Sjoberg, E. R., Manzi, A. E., Khoo, K. H., Dell, A., and Varki, A. (1992). Structural and immunological characterization of O-acetylated GD2. Evidence that GD2 is an acceptor for ganglioside O-acetyltransferase in human melanoma cells. J. Biol. Chem. 267, 16200–16211. doi:10.1016/s0021-9258(18)41986-2
Sorkin, L. S., Otto, M., Baldwin, W. M., Vail, E., Gillies, S. D., Handgretinger, R., et al. (2010). Anti-GD(2) with an FC point mutation reduces complement fixation and decreases antibody-induced allodynia. Pain 149, 135–142. doi:10.1016/j.pain.2010.01.024
Sorokin, M., Kholodenko, I., Kalinovsky, D., Shamanskaya, T., Doronin, I., Konovalov, D., et al. (2020). RNA sequencing-based identification of ganglioside GD2-positive cancer phenotype. Biomedicines 8, 142. doi:10.3390/biomedicines8060142
Stagno, M. J., Schmidt, A., Bochem, J., Urla, C., Handgretinger, R., Cabanillas Stanchi, K. M., et al. (2022). Epitope detection in monocytes (EDIM) for liquid biopsy including identification of GD2 in childhood neuroblastoma-a pilot study. Br. J. Cancer 127, 1324–1331. doi:10.1038/s41416-022-01855-x
Straining, R., and Eighmy, W. (2022). Tazemetostat: EZH2 inhibitor. J. Adv. Pract. Oncol. 13, 158–163. doi:10.6004/jadpro.2022.13.2.7
Sujjitjoon, J., Sayour, E., Tsao, S.-T., Uiprasertkul, M., Sanpakit, K., Buaboonnam, J., et al. (2021). GD2-specific chimeric antigen receptor-modified T cells targeting retinoblastoma - assessing tumor and T cell interaction. Transl. Oncol. 14, 100971. doi:10.1016/j.tranon.2020.100971
Sun, J. C., Lopez-Verges, S., Kim, C. C., DeRisi, J. L., and Lanier, L. L. (2011). NK cells and immune “memory”. J. Immunol. Balt. 186, 1891–1897. doi:10.4049/jimmunol.1003035
Svennerholm, L., Boström, K., Fredman, P., Jungbjer, B., Lekman, A., Månsson, J. E., et al. (1994). Gangliosides and allied glycosphingolipids in human peripheral nerve and spinal cord. Biochim. Biophys. Acta 1214, 115–123. doi:10.1016/0005-2760(94)90034-5
Svennerholm, L., Boström, K., Fredman, P., Månsson, J. E., Rosengren, B., and Rynmark, B. M. (1989). Human brain gangliosides: developmental changes from early fetal stage to advanced age. Biochim. Biophys. Acta 1005, 109–117. doi:10.1016/0005-2760(89)90175-6
Svennerholm, L. (1963). Chromatographic separation of human brain gangliosides. J. Neurochem. 10, 613–623. doi:10.1111/j.1471-4159.1963.tb08933.x
Svennerholm, L., Rynmark, B. M., Vilbergsson, G., Fredman, P., Gottfries, J., Månsson, J. E., et al. (1991). Gangliosides in human fetal brain. J. Neurochem. 56, 1763–1768. doi:10.1111/j.1471-4159.1991.tb02078.x
Tarek, N., Le Luduec, J.-B., Gallagher, M. M., Zheng, J., Venstrom, J. M., Chamberlain, E., et al. (2012). Unlicensed NK cells target neuroblastoma following anti-GD2 antibody treatment. J. Clin. Invest. 122, 3260–3270. doi:10.1172/JCI62749
Terme, M., Dorvillius, M., Cochonneau, D., Chaumette, T., Xiao, W., Diccianni, M. B., et al. (2014). Chimeric antibody c.8B6 to O-acetyl-GD2 mediates the same efficient anti-neuroblastoma effects as therapeutic ch14.18 antibody to GD2 without antibody induced allodynia. PloS One 9, e87210. doi:10.1371/journal.pone.0087210
Terzic, T., Cordeau, M., Herblot, S., Teira, P., Cournoyer, S., Beaunoyer, M., et al. (2018). Expression of disialoganglioside (GD2) in neuroblastic tumors: A prognostic value for patients treated with anti-GD2 immunotherapy. Pediatr. Dev. Pathol. 21, 355–362. doi:10.1177/1093526617723972
Tettamanti, G., Bassi, R., Viani, P., and Riboni, L. (2003). Salvage pathways in glycosphingolipid metabolism. Biochimie 85, 423–437. doi:10.1016/s0300-9084(03)00047-6
Theruvath, J., Menard, M., Smith, B. A. H., Linde, M. H., Coles, G. L., Dalton, G. N., et al. (2022). Anti-GD2 synergizes with CD47 blockade to mediate tumor eradication. Nat. Med. 28, 333–344. doi:10.1038/s41591-021-01625-x
Tibbetts, R., Yeo, K. K., Muthugounder, S., Lee, M.-H., Jung, C., Porras-Corredor, T., et al. (2022). Anti-disialoganglioside antibody internalization by neuroblastoma cells as a mechanism of immunotherapy resistance. Cancer Immunol. Immunother. CII 71, 153–164. doi:10.1007/s00262-021-02963-y
Tong, W., Maira, M., Gagnon, M., and Saragovi, H. U. (2015). Ligands binding to cell surface ganglioside GD2 cause src-dependent activation of N-Methyl-D-Aspartate receptor signaling and changes in cellular morphology. PloS One 10, e0134255. doi:10.1371/journal.pone.0134255
Tsai, Y.-T., and Yu, R. K. (2014). Epigenetic activation of mouse ganglioside synthase genes: implications for neurogenesis. J. Neurochem. 128, 101–110. doi:10.1111/jnc.12456
Tsao, C.-Y., Sabbatino, F., Cheung, N.-K. V., Hsu, J. C.-F., Villani, V., Wang, X., et al. (2015). Anti-proliferative and pro-apoptotic activity of GD2 ganglioside-specific monoclonal antibody 3F8 in human melanoma cells. Oncoimmunology 4, e1023975. doi:10.1080/2162402X.2015.1023975
Uttenreuther-Fischer, M. M., Huang, C. S., and Yu, A. L. (1995). Pharmacokinetics of human-mouse chimeric anti-GD2 mAb ch14.18 in a phase I trial in neuroblastoma patients. Cancer Immunol. Immunother. CII 41, 331–338. doi:10.1007/BF01526552
Valentino, L., Moss, T., Olson, E., Wang, H. J., Elashoff, R., and Ladisch, S. (1990). Shed tumor gangliosides and progression of human neuroblastoma. Blood 75, 1564–1567. doi:10.1182/blood.v75.7.1564.bloodjournal7571564
van den Bijgaart, R. J. E., Kroesen, M., Wassink, M., Brok, I. C., Kers-Rebel, E. D., Boon, L., et al. (2019). Combined sialic acid and histone deacetylase (HDAC) inhibitor treatment up-regulates the neuroblastoma antigen GD2. J. Biol. Chem. 294, 4437–4449. doi:10.1074/jbc.RA118.002763
van Groningen, T., Akogul, N., Westerhout, E. M., Chan, A., Hasselt, N. E., Zwijnenburg, D. A., et al. (2019). A NOTCH feed-forward loop drives reprogramming from adrenergic to mesenchymal state in neuroblastoma. Nat. Commun. 10, 1530. doi:10.1038/s41467-019-09470-w
van Groningen, T., Koster, J., Valentijn, L. J., Zwijnenburg, D. A., Akogul, N., Hasselt, N. E., et al. (2017). Neuroblastoma is composed of two super-enhancer-associated differentiation states. Nat. Genet. 49, 1261–1266. doi:10.1038/ng.3899
Vandamme-Feldhaus, V., and Schauer, R. (1998). Characterization of the enzymatic 7-O-acetylation of sialic acids and evidence for enzymatic O-acetyl migration from C-7 to C-9 in bovine submandibular gland. J. Biochem. (Tokyo) 124, 111–121. doi:10.1093/oxfordjournals.jbchem.a022069
Vantaku, V., Donepudi, S. R., Ambati, C. R., Jin, F., Putluri, V., Nguyen, K., et al. (2017). Expression of ganglioside GD2, reprogram the lipid metabolism and EMT phenotype in bladder cancer. Oncotarget 8, 95620–95631. doi:10.18632/oncotarget.21038
Varki, A., and Diaz, S. (1984). The release and purification of sialic acids from glycoconjugates: methods to minimize the loss and migration of O-acetyl groups. Anal. Biochem. 137, 236–247. doi:10.1016/0003-2697(84)90377-4
Varki, A. (2009). Multiple changes in sialic acid biology during human evolution. Glycoconj. J. 26, 231–245. doi:10.1007/s10719-008-9183-z
Villanueva-Cabello, T. M., and Martinez-Duncker, I. (2016). Preparation of CD4<sup>+</sup> T cells for analysis of GD3 and GD2 ganglioside membrane expression by microscopy. J. Vis. Exp. JoVE 54569, 54569. doi:10.3791/54569
Villanueva-Cabello, T. M., Mollicone, R., Cruz-Muñoz, M. E., López-Guerrero, D. V., and Martínez-Duncker, I. (2015). Activation of human naïve Th cells increases surface expression of GD3 and induces neoexpression of GD2 that colocalize with TCR clusters. Glycobiology 25, 1454–1464. doi:10.1093/glycob/cwv062
Vincent, M., Bessard, A., Cochonneau, D., Teppaz, G., Solé, V., Maillasson, M., et al. (2013). Tumor targeting of the IL-15 superagonist RLI by an anti-GD2 antibody strongly enhances its antitumor potency. Int. J. Cancer 133, 757–765. doi:10.1002/ijc.28059
Wan, H., Li, Z., Wang, H., Cai, F., and Wang, L. (2021). ST8SIA1 inhibition sensitizes triple negative breast cancer to chemotherapy via suppressing Wnt/β-catenin and FAK/Akt/mTOR. Clin. Transl. Oncol. 23, 902–910. doi:10.1007/s12094-020-02484-7
Wang, L., Li, S., Mei, J., and Ye, L. (2022). Immunotherapies of retinoblastoma: effective methods for preserving vision in the future. Front. Oncol. 12, 949193. doi:10.3389/fonc.2022.949193
Wargalla, U. C., and Reisfeld, R. A. (1989). Rate of internalization of an immunotoxin correlates with cytotoxic activity against human tumor cells. Proc. Natl. Acad. Sci. U. S. A. 86, 5146–5150. doi:10.1073/pnas.86.13.5146
Wiebel, M., Kailayangiri, S., Altvater, B., Meltzer, J., Grobe, K., Kupich, S., et al. (2021). Surface expression of the immunotherapeutic target GD2 in osteosarcoma depends on cell confluency. Cancer Rep. Hob. N. J. 4, e1394. doi:10.1002/cnr2.1394
Wingerter, A., El Malki, K., Sandhoff, R., Seidmann, L., Wagner, D.-C., Lehmann, N., et al. (2021). Exploiting gangliosides for the therapy of ewing’s sarcoma and H3K27m-mutant diffuse midline glioma. Cancers 13, 520. doi:10.3390/cancers13030520
Wu, Z. L., Schwartz, E., Seeger, R., and Ladisch, S. (1986). Expression of GD2 ganglioside by untreated primary human neuroblastomas. Cancer Res. 46, 440–443.
Xing, P., Wang, Y., Zhang, L., Ma, C., and Lu, J. (2021). Knockdown of lncRNA MIR4435-2HG and ST8SIA1 expression inhibits the proliferation, invasion and migration of prostate cancer cells in vitro and in vivo by blocking the activation of the FAK/AKT/β-catenin signaling pathway. Int. J. Mol. Med. 47, 93. doi:10.3892/ijmm.2021.4926
Xu, H., Cheng, M., Guo, H., Chen, Y., Huse, M., and Cheung, N.-K. V. (2015). Retargeting T cells to GD2 pentasaccharide on human tumors using Bispecific humanized antibody. Cancer Immunol. Res. 3, 266–277. doi:10.1158/2326-6066.CIR-14-0230-T
Xu, X., Huang, W., Heczey, A., Liu, D., Guo, L., Wood, M., et al. (2019). NKT cells coexpressing a GD2-specific chimeric antigen receptor and IL15 show enhanced in vivo persistence and antitumor activity against neuroblastoma. Clin. Cancer Res. Off. J. Am. Assoc. Cancer Res. 25, 7126–7138. doi:10.1158/1078-0432.CCR-19-0421
Yang, J., Han, L., Sha, Y., Jin, Y., Li, Z., Gong, B., et al. (2022). A novel ganglioside-related risk signature can reveal the distinct immune landscape of neuroblastoma and predict the immunotherapeutic response. Front. Immunol. 13, 1061814. doi:10.3389/fimmu.2022.1061814
Yang, L., Ma, X., Liu, Y.-C., Zhao, W., Yu, L., Qin, M., et al. (2017). Chimeric antigen receptor 4SCAR-GD2-modified T cells targeting high-risk and recurrent neuroblastoma: a phase II multi-center trial in China. Blood 130, 3335. doi:10.1182/blood.V130.Suppl_1.3335.3335
Yankelevich, M., Modak, S., Chu, R., Lee, D. W., Thakur, A., Cheung, N.-K. V., et al. (2019). Phase I study of OKT3 x hu3F8 bispecific antibody (GD2Bi) armed T cells (GD2BATs) in GD2-positive tumors. J. Clin. Oncol. 37, 2533. doi:10.1200/JCO.2019.37.15_suppl.2533
Ye, J. N., and Cheung, N. K. (1992). A novel O-acetylated ganglioside detected by anti-GD2 monoclonal antibodies. Int. J. Cancer 50, 197–201. doi:10.1002/ijc.2910500207
Yesmin, F., Bhuiyan, R. H., Ohmi, Y., Yamamoto, S., Kaneko, K., Ohkawa, Y., et al. (2021). Ganglioside GD2 enhances the malignant phenotypes of melanoma cells by cooperating with integrins. Int. J. Mol. Sci. 23, 423. doi:10.3390/ijms23010423
Yesmin, F., Furukawa, K., Kambe, M., Ohmi, Y., Bhuiyan, R. H., Hasnat, M. A., et al. (2023). Extracellular vesicles released from ganglioside GD2-expressing melanoma cells enhance the malignant properties of GD2-negative melanomas. Sci. Rep. 13, 4987. doi:10.1038/s41598-023-31216-4
Yin, J., Miyazaki, K., Shaner, R. L., Merrill, A. H., and Kannagi, R. (2010). Altered sphingolipid metabolism induced by tumor hypoxia - new vistas in glycolipid tumor markers. FEBS Lett. 584, 1872–1878. doi:10.1016/j.febslet.2009.11.019
Yoshida, H., Koodie, L., Jacobsen, K., Hanzawa, K., Miyamoto, Y., and Yamamoto, M. (2020). B4GALNT1 induces angiogenesis, anchorage independence growth and motility, and promotes tumorigenesis in melanoma by induction of ganglioside GM2/GD2. Sci. Rep. 10, 1199. doi:10.1038/s41598-019-57130-2
Yoshida, S., Fukumoto, S., Kawaguchi, H., Sato, S., Ueda, R., and Furukawa, K. (2001). Ganglioside G(D2) in small cell lung cancer cell lines: enhancement of cell proliferation and mediation of apoptosis. Cancer Res. 61, 4244–4252.
Yoshida, S., Kawaguchi, H., Sato, S., Ueda, R., and Furukawa, K. (2002). An anti-GD2 monoclonal antibody enhances apoptotic effects of anti-cancer drugs against small cell lung cancer cells via JNK (c-Jun terminal kinase) activation. Jpn. J. Cancer Res. Gann 93, 816–824. doi:10.1111/j.1349-7006.2002.tb01324.x
Yu, A. L., Gilman, A. L., Ozkaynak, M. F., London, W. B., Kreissman, S. G., Chen, H. X., et al. (2010). Anti-GD2 antibody with GM-CSF, interleukin-2, and isotretinoin for neuroblastoma. N. Engl. J. Med. 363, 1324–1334. doi:10.1056/NEJMoa0911123
Yu, A. L., Gilman, A. L., Ozkaynak, M. F., Naranjo, A., Diccianni, M. B., Gan, J., et al. (2021). Long-term follow-up of a phase III study of ch14.18 (dinutuximab) + cytokine immunotherapy in children with high-risk neuroblastoma: COG study ANBL0032. Clin. Cancer Res. 27, 2179–2189. doi:10.1158/1078-0432.CCR-20-3909
Yu, L., Huang, L., Lin, D., Lai, X., Wu, L., Liao, X., et al. (2022). GD2-specific chimeric antigen receptor-modified T cells for the treatment of refractory and/or recurrent neuroblastoma in pediatric patients. J. Cancer Res. Clin. Oncol. 148, 2643–2652. doi:10.1007/s00432-021-03839-5
Yu, R. K., Ariga, T., Yanagisawa, M., and Zeng, G. (2008). “Gangliosides in the nervous system: biosynthesis and degradation,” in Glycoscience: Chemistry and chemical biology Editors B. O. Fraser-Reid, K. Tatsuta, and J. Thiem (Berlin, Heidelberg: Springer), 1671–1695. doi:10.1007/978-3-540-30429-6_41
Yu, R. K., Bieberich, E., Xia, T., and Zeng, G. (2004). Regulation of ganglioside biosynthesis in the nervous system. J. Lipid Res. 45, 783–793. doi:10.1194/jlr.R300020-JLR200
Yuki, N., Yamada, M., Tagawa, Y., Takahashi, H., and Handa, S. (1997). Pathogenesis of the neurotoxicity caused by anti-GD2 antibody therapy. J. Neurol. Sci. 149, 127–130. doi:10.1016/s0022-510x(97)05390-2
Zarei, M., Müthing, J., Peter-Katalinić, J., and Bindila, L. (2010). Separation and identification of GM1b pathway Neu5Ac- and Neu5Gc gangliosides by on-line nanoHPLC-QToF MS and tandem MS: toward glycolipidomics screening of animal cell lines. Glycobiology 20, 118–126. doi:10.1093/glycob/cwp154
Zhang, J., Webster, S., Duffin, B., Bernstein, M. N., Steill, J., Swanson, S., et al. (2023). Generation of anti-GD2 CAR macrophages from human pluripotent stem cells for cancer immunotherapies. Stem Cell Rep. 18, 585–596. doi:10.1016/j.stemcr.2022.12.012
Zhang, P., Ohkawa, Y., Yamamoto, S., Momota, H., Kato, A., Kaneko, K., et al. (2021). St8sia1-deficiency in mice alters tumor environments of gliomas, leading to reduced disease severity. Nagoya J. Med. Sci. 83, 535–549. doi:10.18999/nagjms.83.3.535
Zhang, Y., Kupferschlaeger, J., Lang, P., Reischl, G., Handgretinger, R. J., Fougère, C., et al. (2022). 131I-GD2-ch14.18 scintigraphy to evaluate option for radioimmunotherapy in patients with advanced tumors. J. Nucl. Med. Off. Publ. Soc. Nucl. Med. 63, 205–211. doi:10.2967/jnumed.120.261854
Zhao, J. (2016). Cancer stem cells and chemoresistance: the smartest survives the raid. Pharmacol. Ther. 160, 145–158. doi:10.1016/j.pharmthera.2016.02.008
Zhao, X. J., and Cheung, N. K. (1995). GD2 oligosaccharide: target for cytotoxic T lymphocytes. J. Exp. Med. 182, 67–74. doi:10.1084/jem.182.1.67
Zirngibl, F., Ivasko, S. M., Grunewald, L., Klaus, A., Schwiebert, S., Ruf, P., et al. (2021). GD2-directed bispecific trifunctional antibody outperforms dinutuximab beta in a murine model for aggressive metastasized neuroblastoma. J. Immunother. Cancer 9, e002923. doi:10.1136/jitc-2021-002923
Keywords: ganglioside, GD2, cancer, immunotherapy, antibody, CAR, vaccine
Citation: Machy P, Mortier E and Birklé S (2023) Biology of GD2 ganglioside: implications for cancer immunotherapy. Front. Pharmacol. 14:1249929. doi: 10.3389/fphar.2023.1249929
Received: 29 June 2023; Accepted: 31 July 2023;
Published: 21 August 2023.
Edited by:
Horacio Uri Saragovi, Lady Davis Institute (LDI), CanadaReviewed by:
Aldo Alejandro Vilcaes, Universidad Nacional de Córdoba, ArgentinaAli Nejatie, McGill University, Canada
Copyright © 2023 Machy, Mortier and Birklé. This is an open-access article distributed under the terms of the Creative Commons Attribution License (CC BY). The use, distribution or reproduction in other forums is permitted, provided the original author(s) and the copyright owner(s) are credited and that the original publication in this journal is cited, in accordance with accepted academic practice. No use, distribution or reproduction is permitted which does not comply with these terms.
*Correspondence: Stéphane Birklé, c3RlcGhhbmUuYmlya2xlQHVuaXYtbmFudGVzLmZy