- 1Programa de Pós-graduação em Produtos Naturais e Sintéticos Bioativos, Centro de Ciências da Saúde, Universidade Federal da Paraíba, João Pessoa, Paraíba, Brazil
- 2Graduação em Farmácia, Departamento de Ciências Farmacêuticas, Centro de Ciências da Saúde, Universidade Federal da Paraíba, João Pessoa, Paraíba, Brazil
- 3Departamento de Ciências Farmacêuticas, Centro de Ciências da Saúde, Universidade Federal da Paraíba, João Pessoa, Paraíba, Brazil
- 4Departamento de Fisiologia e Patologia, Centro de Ciências da Saúde, Universidade Federal da Paraíba, João Pessoa, Paraíba, Brazil
Asthma is one of the main non-communicable chronic diseases and affects a huge portion of the population. It is a multifactorial disease, classified into several phenotypes, being the allergic the most frequent. The pathophysiological mechanism of asthma involves a Th2-type immune response, with high concentrations of allergen-specific immunoglobulin E, eosinophilia, hyperreactivity and airway remodeling. These mechanisms are orchestrated by intracellular signaling from effector cells, such as lymphocytes and eosinophils. Ion channels play a fundamental role in maintaining the inflammatory response on asthma. In particular, transient receptor potential (TRP), stock-operated Ca2+ channels (SOCs), Ca2+-activated K+ channels (IKCa and BKCa), calcium-activated chloride channel (TMEM16A), cystic fibrosis transmembrane conductance regulator (CFTR), piezo-type mechanosensitive ion channel component 1 (PIEZO1) and purinergic P2X receptor (P2X). The recognition of the participation of these channels in the pathological process of asthma is important, as they become pharmacological targets for the discovery of new drugs and/or pharmacological tools that effectively help the pharmacotherapeutic follow-up of this disease, as well as the more specific mechanisms involved in worsening asthma.
1 Introduction
Characterized by variable respiratory symptoms, asthma can be defined as a chronic inflammatory disease, and the subject might present wheezing, increased chest pressure, cough, shortness of breath and limited expiratory flow. Asthma also can be triggered by several factors, including the exposure to allergens or irritants, respiratory infections, climate change or physical exercise. In the last decade approximately 260 million individuals were reported worldwide, causing 455,000 deaths and observing an increase in the prevalence of bronchial asthma (Who, 2023; Gina, 2022).
This condition involves a collaboration between various cells of the innate and adaptive immune system, alongside epithelial cells, to enhance bronchial hyperreactivity. It is characterized by an hyperresponsiveness of smooth muscle cells in the bronchial lumen to non-specific triggers, like cold air and physical exertion. Additionally, it leads to excessive mucus production, remodeling of the airway walls, and constriction of the passageways through which air flows (Hammad and Lambrecht, 2021).
At present, the primary approach to treating asthma primarily revolves around the utilization of bronchodilators. These include cholinergic antagonists, β-adrenergic agonists, antileukotrienes, and non-specific phosphodiesterase inhibitors. Alternatively, treatment may also involve the suppression of inflammation, wherein glucocorticoids serve as the primary option (Gina, 2022). These strategies help to control asthma symptoms, however they have several side effects, such as glaucoma, metabolic imbalance, tachycardia, dry mouth, mydriasis, constipation and immunosuppression (Walsh, 2005; Donohue et al., 2019). Therefore, studies to better elucidate possible pharmacological targets, such as ion channels, open the possibility of looking forward to new anti-asthmatic therapies. Therefore, this review will provide scientific support about ion channels that play an important role in the pathophysiology of asthma. These specialized membrane proteins regulate the flow of ions across cell membranes, thereby influencing various cellular processes. In asthma, specific ion channels participate in the dysregulation of bronchoconstriction, airway inflammation, and mucus production (Valverde et al., 2011; Bartoszewski et al., 2017; Pelletier and Savignac, 2018).
2 Epidemiological data on asthma
In 2019, approximately 262 million individuals worldwide were affected by asthma. The prevalence of this condition continues to rise, with projections indicating an expected increase of over 100 million people by the year 2025 (Global Asthma Network, 2018; Tohidinik et al., 2019; Who, 2023). Epidemiological data show that over the decades there has been almost double the number of cases (Antó, 2012).
Asthma stands as the most prevalent chronic disease among children, impacting approximately 24% of this population. Moreover, it affects around 19% of teenagers (Who, 2023). From a global perspective, the financial burden of asthma surpasses the combined costs associated with tuberculosis and HIV/AIDS (ASBAI, 2023). Despite the concerning statistics, asthma remains underdiagnosed. The World Health Survey (WHS) estimated that merely 4.3% of asthma cases were diagnosed globally, showcasing substantial variations of up to 21-fold between countries (To et al., 2012). These discrepancies in asthma prevalence emphasize the importance of conducting further studies to enable comparisons and facilitate the planning of appropriate interventions.
Brazil holds the 8th position globally in terms of asthma prevalence. In 2015, there were 383,000 reported deaths and according to the Sistema Único de Saúde (SUS), among the cases of hospitalization, asthma attacks ranked as the fourth type of cause (Cardoso et al., 2017).
3 Etiological factors
Asthma is a chronic inflammatory disease and its development involves environmental and genetic factors (Hammad and Lambrecht, 2021). This disease is characterized by its different phenotypes, which have distinct pathophysiological mechanisms and clinical manifestations, making diagnosis difficult (Moore et al., 2010). The possibility of developing and progressing asthma in genetically susceptible individuals is determined by various factors, including aspects related to immunological maturation and the timing of exposure to local agents, particularly during the childhood. These factors play an essential role in the likelihood of developing asthma in susceptible individuals (Busse and Lemanske, 2001).
Genetic factors, several comorbidities and risk factors are involved on the development of asthma, such as in individuals with severe or uncontrolled asthma. Exposure to dust mites, pet dander, cockroaches and Aspergillus genus fungi are notable risk factors. Other risk factors include smoking, physical exercise, and comorbidities such as obesity, respiratory infections, psychiatric illnesses, stress, rhinosinusitis, and non-steroidal anti-inflammatory drugs (Figure 1). These factors can collaborate to the worsening of asthma symptoms and should be carefully considered in the management and treatment of individuals with asthma.
Recent studies have placed increasing emphasis on the heterogeneity of asthma, leading to the recognition that asthma comprises multiple phenotypes. These phenotypes represent distinct groups with specific characteristics and varied expressions of the disease (Wenzel, 2012). Numerous phenotypes of asthma were identified, nevertheless the most common is allergic asthma. This particular phenotype typically emerges during childhood and is frequently linked to a family genetic conditions of allergy like rhinitis, eczema or allergies to food or medications. Identifying the allergic asthma phenotype is crucial for implementing tailored management strategies that involve allergen avoidance, immunotherapy, and targeted medication interventions to address the specific allergic triggers involved (Bel, 2004). Other phenotypes include the non-allergic asthma, aggravated by obesity and exercise (Moore et al., 2010; Wenzel, 2012).
3.1 Pathophysiology of asthma
Asthma is a chronic inflammatory disease of the airways, which causes bronchial hyperresponsiveness, tissue remodeling, lumen narrowing and excessive mucus production, especially by the action of immune system cells in association with epithelial cells (Hammad and Lambrecht, 2021). Since the immunity can be classified into type 1 and 2 T helper, asthma was regarded as a pathological process Th2 profile which is strongly linked to hypersensitivity reactions of type I (allergy). In fact, data from several studies suggest that most asthma cases fit this traditional view (Moore et al., 2010; Siroux et al., 2011).
In the lungs of several patients with allergic asthma, mainly Th2 profile cells are found (Robinson et al., 1992), which are responsible for the generation of cytokines that stimulate the synthesis of immunoglobulin E (IgE) antibodies by modulating IL-4. Additionally, IL-13, IL-5 and IL-9 are also important in this process, aiding in the recruitment of eosinophils and mast cells, culminating in increased bronchial hyperresponsiveness - an essential feature of asthma (Holgate and Polosa, 2008).
Activation of adaptive immunity depends on antigen-presenting cells (APCs), such as dendritic cells (DCs), whose role is crucial in triggering the Th2 response in asthma. DCs perform this action by presenting antigen through the major histocompatibility complex type II (MHC II) molecule. In the lung, DCs are distributed throughout the airways, vasculature, pleura, lung interstitial space, and bronchial lymph nodes. Their strategic positioning allows them to efficiently capture and process antigens, initiating immune responses (Lambrecht et al., 2019).
In allergic patients, whose immune response is directed towards the Th2 profile, activation of the T cell-specific trans-action transcription factor (GATA-3) occurs and characteristic cytokines are synthesized and secreted, such as IL-4, IL-5 and IL-13. The cytokines responsible for the isotype switch from IgG to IgE by plasma cells are IL-4 and IL-13, especially (Romagnani, 2000), in addition to the structural remodeling observed in chronic asthma. These structural changes include metaplasia of epithelial cells into Goblet cells and subepithelial fibrosis, mucus hypersecretion, fibroblasts differentiation to myofibroblasts and smooth muscle hyperplasia (Doucet et al., 1998).
Recruitment of eosinophils to lung tissue is fundamentally carried out by IL-5. The development of eosinophils in the bone marrow and their subsequent migration to the pulmonary mucosa and interstitium are made possible by the action of eotaxins 1, 2 and 3 (CCL11, CCL24 and CCL26, respectively). The action of these chemokines occurs by activation of the chemokine receptor CCR3, whose location occurs numerously in eosinophils, leading to their targeted recruitment to inflamed lung tissue (Ponath et al., 1996). Once stimulated, eosinophils assume a crucial pro-inflammatory role by producing cysteinyl-leukotrienes (Cys-LTs), along with additional Th2 cytokines. These inflammatory mediators contribute to the multiplication of the inflammatory response and exacerbate the allergic cascade in asthma (Akuthota et al., 2008). Products derived from eosinophils, such as eosinophilic peroxidase (EPO), major basic protein (MBP) and eosinophilic cationic protein (ECP), directly cause tissue damage and bronchial hyperresponsiveness, in addition to activating adaptive immunity through effects on DCs (Coyle et al., 1995; Chu et al., 2014).
Other important cells in allergic asthma are mast cells, which express type I Fcε receptors (FcεRI) and reside near the mucosal surface and in blood vessels. Local factors influence the maturation of these cells, mainly stem cell factor (SCF) and IL-3 (Gurish and Austen, 2012). Immediate hypersensitivity reactions can be initiated by mast cells upon subsequent allergen challenges. They degranulate in response to signals from both the adaptive immune system, through antigen cross-linking to IgEs bound to FcεRI receptors, and the innate immune system, triggered by Toll-like receptor agonists or cytokines like IL-33 (Stone et al., 2006; Silver et al., 2010).
This degranulation process stimulates the release of several inflammatory mediators, including histamine, prostaglandins, leukotrienes, and cytokines. Once activated, these cells release histamine, Cys-LTs, prostaglandin D2 (PGD2), platelet activating factor (PAF), in addition to cytokines and chemokines (Galli et al., 2005; Barrett and Austen, 2009). Basophils, which are circulating granulocytes, also express FcεRI and amplifying immediate hypersensitivity responses. The contribution to the allergic cascade also occurs by IL-4 production (Yoshimoto et al., 2009). Mast cell granules store previously synthesized cytokines, including IL-5, IL-6 (Bradding et al., 1993), IL-4 (Reed et al., 1995), tumor necrosis factor (TNF) (Gordon and Galli, 1990), as well as transforming growth factor β (TGF-β) (Lindstedt et al., 2001) and vascular endothelial growth factor (VEGF) (Boesiger et al., 1998; Grutzkau et al., 1998).
After the challenge with the allergen, cytokines such as IL-33, derived from epithelial and inflammatory cells, have the ability to activate innate immune cells such as eosinophils, mast cells, basophils, innate lymphoid cells type 2 (ILC2), acting in combination with the stem cell factor (SCF) and the IgE receptor (Cherry et al., 2008; Schneider et al., 2009; Saenz et al., 2010; Silver et al., 2010; Ramadas et al., 2011). The activity of Th2 cytokines maintains the epithelial responses triggered by allergens, reacting to the cytokines IL-4 and IL-13, producing chemokines such as CCL11, CCL17 and IL-8, which, in turn, attract eosinophils, neutrophils, among other cells of the Th2 profile lingering asthma symptoms. This feedback loop of immune cell recruitment and inflammatory response contributes to the worsening of this disease (Matsukura et al., 2001; Lordan et al., 2002). Figure 2 represents the immune mechanism of allergic asthma. The complete cascade of inflammation and abnormal processes of attempts at repair generate structural changes in the walls of the airways of asthmatic individuals, contributing to tissue remodeling throughout the bronchial structure, which are more pronounced in the small airways (Saetta et al., 1991; Elias et al., 1999).
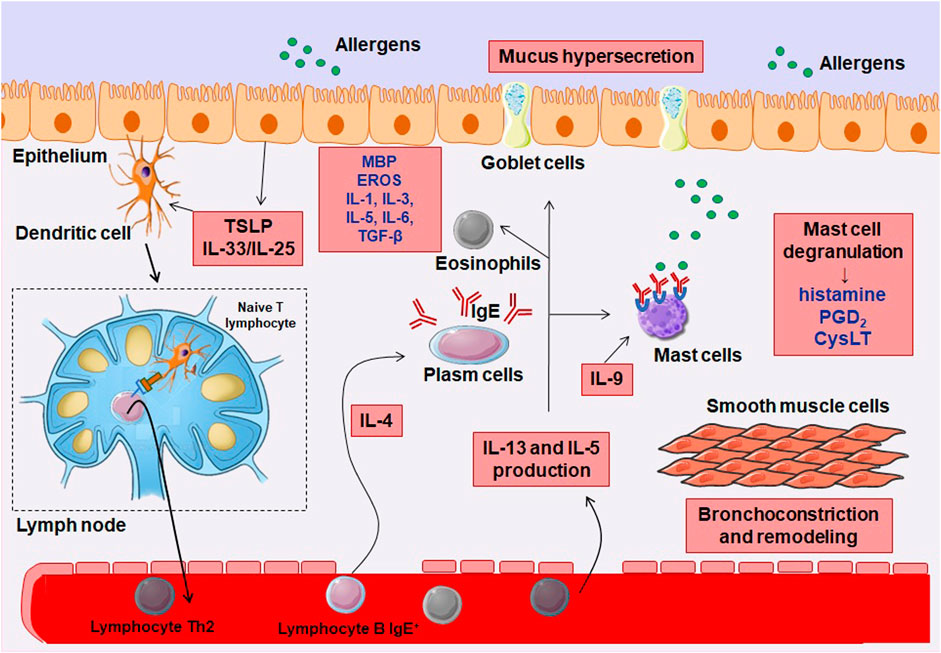
FIGURE 2. Inflammatory pathophysiology of allergic asthma. In the presence of an allergen, there is the release of cytokines from the epithelium, particularly IL-25 and IL-33, which induces co-stimulatory molecule of T lymphocytes in dendritic cells (DCs), which act to promote T cell survival and expansion. DCs are mobilized to local lymph nodes, where they present the antigenic peptide to naive CD4+ T cells, activating and converting them to a competent IL-4-producing state. These T cells migrate to B cell zones where they differentiate into follicular helper T cells (TFH) and move into the circulation to complete their maturation as helper T cells 2 (Th2). IL-4 secreting TFH cells in areas of B cells mediate IgE class switching in B lymphocytes, which attach to the surface of mast cells, basophils and eosinophils (not shown in figure), mediating the degranulation of these cells. IL-9 also stimulates mast cells. Th2 cells migrate to the airway epithelium and to the submucosa layer, where they secrete IL-5 and IL-13 to mediate the inflammatory response, with the accumulation of mast cells and eosinophils, airway remodeling and bronchial hyperreactivity.
In asthmatic patients, the airway epithelium is fragile and, in some areas, absent in ciliated cells (Lackie et al., 1997). The maintenance of the integrity of the bronchial epithelial membrane depends on the presence of adherent junctions, which are responsible for binding and maintaining the cohesion of bronchial epithelial cells. Airway epithelial alterations involve desquamation, loss of hair cells and goblet cell hyperplasia (Aikawa et al., 1992; Carroll et al., 1993; Xiao Q et al., 2011). Due to the abnormal repair, epithelial desquamation leads to increased proliferation, contributing to thickening and fibrosis of the epithelium (Cohen et al., 2007), accompanied by an increase in the area of the subepithelial mucous glands and hyperplasia of Goblet cells (Ordoñez et al., 2001; Jenkins et al., 2003).
Another cytokine that plays an important role in the development of effects associated with bronchial remodeling is transforming growth factor β (TGF-β), produced by several cell types in the lungs, such as lymphocytes, fibroblasts, macrophages, eosinophils, and epithelial cells (Tagaya and Tamaoki, 2007). It has the ability to induce fibroblasts to express α-actin, which leads to their transformation into myofibroblasts (Batra et al., 2004). As part of abnormal repair, TGF-β induces goblet cell proliferation and the trans differentiation of ciliated cells and Clara cells into Goblet cells, increasing mucus production in the remodeling process (Le Cras et al., 2011). These mucous cells produce the mucin glycoprotein (MUC), the predominant form in the airways being MUC5AC; and the submucosal glands mainly produce MUC5B, with MUC5B being predominant under normal conditions and MUC5AC over-regulated in asthma (Evans et al., 1999; Kirkham et al., 2002; Rose and Voynow, 2006).
One of the most notable alterations associated with airway remodeling is the hypertrophy and hyperplasia of smooth muscle cell (Carroll et al., 1993). Additionally, mesenchymal cells undergo differentiation and migration to the smooth muscle cell layer, contributing to the overall increase. This increase in smooth muscle cell size and proliferation is a major contributor to the increased contractility seen in asthma, which is a primary underlying factor for bronchial hyperresponsiveness (Martin et al., 2000; Schmidt et al., 2003; Bergeron et al., 2009).
3.2 Asthma treatment
Current asthma pharmacotherapy is based on two fronts: bronchodilation or broncho protection, by direct inhibition of contraction or facilitation of relaxation of airway smooth muscle; and minimize inflammatory mediators that can induce bronchoconstriction and thus improve asthma symptoms (Pera and Penn, 2016). For the rapid control of asthma attacks and relief of symptoms, bronchodilators are used as first choice drugs, which act mainly by activating G protein-coupled receptors (GPCR)-Gs or antagonism of GPCR-Gq/11. These agents are formed by β-adrenergic agonists, that activate GPCR-Gs, such as salbutamol, salmeterol, and formoterol, and antagonists of muscarinic M3 receptors, such as ipratropium and tiotropium, or Cys-LT receptors, such as montelukast and zafirlukast, that inhibits GPCR-Gq/11. Besides these, another class used in the treatment of asthma, but which does not act directly on the GPCRs, are phosphodiesterase inhibitors, enzymes that degrade cyclic nucleotides, the example of theophylline (Prado et al., 2014).
The airway inflammation is controlled through the use of steroidal anti-inflammatory drugs, corticosteroids, orally (OCS) or inhaled (ICS), which act in the long term by inhibiting the inflammatory process, such as fluticasone and budesonide (Pera and Penn, 2016). Since their emergence and introduction in the treatment of asthma, corticosteroids have presented problems related, especially, to their side effects, so that new drugs in the class have been and are being developed with the aim of improving their potency and, mainly, their systemic effects (Colice, 2006). Common side effects of corticosteroids include oral candidiasis, glaucoma, cataracts, skin bruising and osteoporosis. However, it is important to note that the likelihood of experiencing these complications is significantly lower with inhaled versus oral glucocorticoids (Gina, 2022). Other anti-inflammatory drugs are cromoglycates that act by stabilizing mast cells, such as cromolyn sodium, preventing mast cell degranulation. Both corticosteroids and cromolyn are used to treat mild persistent asthma (Lane and Lee, 1996).
New classes of drugs have been developed for treating severe asthma, including anti-IgE antibodies such as omalizumab, especially for corticosteroid-resistant patients (Humbert et al., 2014). Furthermore, new drugs studied include anti-IL-13 antibodies, with promising results in clinical studies (Hodsman et al., 2013; De Boever et al., 2014; Powell et al., 2015). So far, no therapy for asthma has been used to prevent the airway remodeling. As the first-line choice, anti-inflammatory drugs continue to be used, being crucial in the management of the disease. Although the emergence of new pharmacological therapies for asthma has been strengthened, there is still a need for the development of additional effective treatments (Berair and Brightling, 2014), in this sense, knowledge of the ion channels involved in the pathophysiology of asthma is of fundamental importance for a possible more effective therapeutic strategy.
4 Ion channels involved in asthma
4.1 Transient receptor potential (TRP)
4.1.1 Molecular structure
TRP cationic channel superfamily, composed of 28 members, is divided into seven subfamilies in mammalians. Of these, six are found in humans: ankyrin (TRPA, 1 member), canonical (TRPC, 6 members), melastatin (TRPM, 8 members), mucolipin (TRPML, 3 members), polycystine (TRPP, 3 members), and vanilloid (TRPV, 6 members) (Zhao et al., 2021).
Structurally, TRP channels share a common feature, consisting of six transmembrane domains (S1-S6) and a pore-forming loop between the fifth and sixth segments (Benemei et al., 2015). These channels have four pore-forming TRP protein subunits, and can be expressed as homo- and heterotetramers (Moran et al., 2011). Both the intracellular C- and N-terminal regions regulate channel assembly and function through the present domains. In addition to these structures shared by all TRPs, others are also present among some subfamilies. TRPM, TRPA and TRPC channels have a coiled domain in the C-terminal region that stabilizes the tetrameric assembly. TRPA and TRPV channels have ankyrin repeats in the N-terminal region that are capable of transducing conformational changes to the pore. Thus, one of the structural characteristics that differentiate the subfamilies of this channel are the coiled-coil regions, TRP motifs and the number of ankyrin repeats (Hilton et al., 2019).
4.1.2 Molecular physiological and biophysical properties
TRP channels function as detectors that react to alterations in the cellular surroundings, encompassing variations in temperature, mechanical stretch, oxidative processes, osmolarity, and pH levels. They are also stimulated by the activation of GPCR and the action of several intracellular molecules, including phospholipase C (PLC), phosphatidyl inositol 4,5-bisphosphate (PIP2), diacylglycerol (DAG), protein kinase A (PKA) and C (PKC), adenosine triphosphate (ATP), calmodulin and arachidonic acid metabolites, in addition to agonists such as capsaicin, phorbol esters, menthol, eucalyptol, mustard oil and cinnamaldehyde (Castillo et al., 2018; Michalick and Kuebler, 2020).
Most TRP channels, with the exception of TRPM4 and TRPM5, exhibit permeability to both Ca2+ and monovalent cations. However, the channels permeable to Ca2+ display limited selectivity for this ion, as their conductance rate in relation to Na+ (PCa/PNa) typically ranges from 0.3 to 10. Notwithstanding, TRPV5 and TRPV6 stand out as selective channels for Ca2+ with PCa/PNa > 100. TRP activation, therefore, also serves as an important Ca2+ influx pathway, contributing to Ca2+ signaling (Guibert et al., 2011; Hasan and Zhang, 2018).
Due to their wide expression and distribution, TRP channels present significant roles in physiological processes. These processes include crucial sensory functions like pheromone signaling, nociception and thermoregulation (Wang and Siemens, 2015; Laing and Dhaka, 2016); homeostatic functions such as reabsorption of Ca2+ and Mg2+, regulation of osmolarity, metabolism and adipogenesis (Uchida et al., 2017; Dhakal and Lee, 2019; Startek et al., 2019); besides to muscle contraction and vasomotor control (Lonso-Carbajo et al., 2017).
4.1.3 Expression profile and contributions to asthma
Within the respiratory system, in different cell types, several TRP channels are present and expressed at varying levels. However, among them, TRPA1, TRPV1, and TRPV4 are particularly noteworthy due to their strategic localization, greater quantity, and role in maintaining airway function (Figure 3) (Logu et al., 2016). The expression of TRPA1 has been observed in sensory neurons that innervate the airways, immune cells implicated in the inflammatory response associated with chronic obstructive pulmonary disease (COPD) and asthma. These immune cells include mast cells, B cells, and CD4+ and CD8+ T cells (Banner et al., 2011).
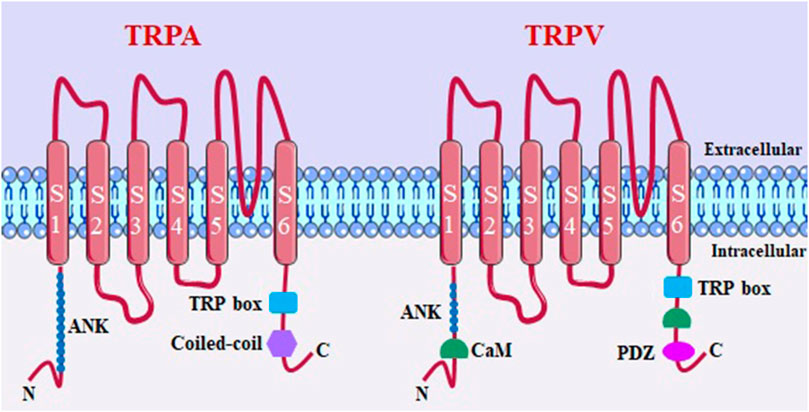
FIGURE 3. TRPA and TRPV channel structure. ANK, ankyrin repeats (varies in number among members of subfamilies); coiled-coil domains; TRP box; CaM, calmodulin binding site; PDZ specific reason for binding to PDZ.
Additionally, TRPA1 was evidenced to be present in human lung bronchial epithelial cell lines (Mukhopadhyay et al., 2011; Büch et al., 2013; Wortley et al., 2016), and it is present in sensory neurons, bronchial epithelial cells, lung dendritic and smooth muscle cells, and CD4+ T cells (Bertin et al., 2014; Samivel et al., 2016). TRPV4, on the other hand, can be found in airway smooth muscle, vascular endothelial cells, macrophages, alveolar, bronchial, and tracheal epithelium (Rosenbaum et al., 2020).
In recent years, there has been evidence highlighting the significant role of TRP channels in respiratory diseases, including asthma (Kytikova et al., 2020). The activation of these channels contributes to inflammatory processes and structural alterations in the respiratory tract, leading to bronchoconstriction (Taylor-Clark, 2016). Since activators of TRP channels, such as reactive oxygen species or cigarette smoke, can exacerbate asthma symptoms, the involvement of these channels in the pathophysiology of this disease becomes evident (Preti et al., 2012).
TRPA1 appears to have involvement in multiple aspects of asthma, as the activation of this channel leads to increase of airway hyperreactivity, a characteristic feature of this disease (Tränkner et al., 2014). Additionally, studies have shown a correlation between variants of the TRPA1 gene and childhood asthma, further suggesting the participation of this channel in the pathogenesis of asthma. Other studies also showed that knockout mice for this channel (TRPA1−/−) showed reduced infiltration of immune cells into the bronchoalveolar fluid in the ovalbumin-induced asthma model, highlighting the contribution of this channel to airway inflammation in mice model asthma (Gallo et al., 2017). Moreover, TRPA1 expressed in sensory neurons that innervate the airways can be activated by inhaled irritants, resulting in influx of calcium ions and release of inflammatory substances. These substances may influence the recruitment of neutrophils and eosinophils in the lungs, contributing to the inflammatory response associated with respiratory conditions such as asthma (Reese et al., 2020).
Of these released substances, it can be mentioned pro-inflammatory neuropeptides (neurokinin A, substance P and the peptides related to the calcitonin gene) responsible for the development of neurogenic inflammation in the airways. These, in turn, mediate the initial inflammatory response, such as vasodilation, leukocyte extravasation, mucus hypersecretion, and airway constriction (Yang and Li, 2016). This response was also observed through the positive modulation of TRPV1 channels (Moran et al., 2011; McMahon et al., 2015), taking into account that the neurogenic inflammation caused by the release of these neuropeptides contributed to the worsening of asthma. This condition also occurred due to the production of cytokines such as tumor necrosis factor-α, IL-1, IL-6 and IL-8 by human bronchial epithelial cells, considering that these factors can cause bronchoconstriction (Lee and Yang, 2012; Song et al., 2017; Kytikova et al., 2020).
Human studies reveal that patients with severe asthma have been reported to exhibit an increase in TRPV1 expression in the airway epithelium (McGarvey et al., 2014). Other data showed that compared to healthy children, asthmatic children presented an increase in TRPV1 gene expression (Ren et al., 2015). In addition, exposure to inflammatory stimuli, such as capsaicin and high temperatures, has been reported to increase TRPV1 expression (Sadofsky et al., 2012). Moreover, it has been observed that the loss of this channel-specific gene is related to reduced susceptibility to active childhood asthma. This suggests that variations in TRPV1 function may play a role in modulating asthma risk and severity in affected individuals. This suggests that variations in TRPV1 function may play a role in modulating the risk and severity of asthma in affected individuals (Cantero-Recasens et al., 2010).
Baker et al. (2016) showed that in TRPV1 knockout mice (TRPV4−/−) in a house dust mite-induced asthma model, a reduction in IgE levels, inflammation and airway hyperresponsiveness was observed. In addition, they showed the role of a blocker of this channel, XEN-D0501, and although IgE levels were not altered, the action of this inhibitor resulted in a reduction in the airway inflammation profile, suggesting that TRPV1 blockade attenuates the allergic asthma phenotype.
Studies have provided evidence that TRPV4 plays a regulatory role in allergic asthma. In an mice model of allergic asthma, it has been observed that TRPV4 can modulate airway wall thickness, collagen synthesis, recruitment of goblet cells, expression of TGF-β and fibrosis remodeling in the airways (Scheraga et al., 2017). Additionally, another study using a mouse model of allergic asthma induced by ovalbumin found that exposure to high relative humidity and formaldehyde intensified the activation of TRPV4 in the lungs. This, in turn, resulted in the inflammation and mucus hypersecretion in the airways, leading to asthma exacerbation (Duan et al., 2020). In a mite-induced asthma model, knockout mice for this channel (TRPV4−/−) was protected from airway remodeling. Likewise, isolated lung fibroblasts from asthmatic individuals showed increased TRPV4 activity (Gombedza et al., 2017).
Therefore, based on these studies, it is suggested that reduced activation of TRP family channels, especially TRPA1, TRPV1 and TRPV4, may be potential targets for new therapies for asthma (Dietrich, 2019; Reese et al., 2020).
4.2 Storage-operated Ca2+ channels (SOCs)
4.2.1 Molecular structure
Ca2+ release-activated Ca2+ channels (CRAC) are widely distributed category of store-operated Ca2+ channels (SOCs). These channels are triggered due to the depletion of Ca2+ within the endoplasmic reticulum (ER) lumen. The function of CRACs depends on two classes of membrane proteins: the Ca2+-sensing stromal interacting molecules (STIM1 and STIM2), located in the ER membrane; and the plasma membrane channel pore-forming proteins (Orai1, Orai2 and Orai3). All types of STIM and Orai proteins are present in mammals, however, STIM1 and Orai1 are the most studied (Niu et al., 2020; Zhang et al., 2020).
The STIM protein has in its structure (Figure 4A) facing the ER lumen, EF-hand domains (a helix–loop–helix structural domain or motif found), a canonical that binds to Ca2+, and a non-canonical structure, in addition to a sterile alpha motif (EF-SAM). The cytoplasmic region has a putative coiled-coil (CC1) domain; an STIM-Orai activation domain, called CAD/SOAR, which also comprises the coiled-coil CC2 and CC3; as well as a polybasic domain (PDB), which interacts with molecules in the plasma membrane, including PIP2 (Bakowski et al., 2020; Lewis, 2020). Considered dimeric at rest, STIM1 is stabilized by hydrophobic interactions and hydrogen bonds between CAD/SOAR monomers. To avoid spontaneous activation of STIM1, the EF-SAM luminal regions are kept separate when bound to Ca2+, allowing the CC1 cytosolic domain to interact with CAD/SOAR, keeping it close to the ER membrane (Korzeniowski et al., 2017).
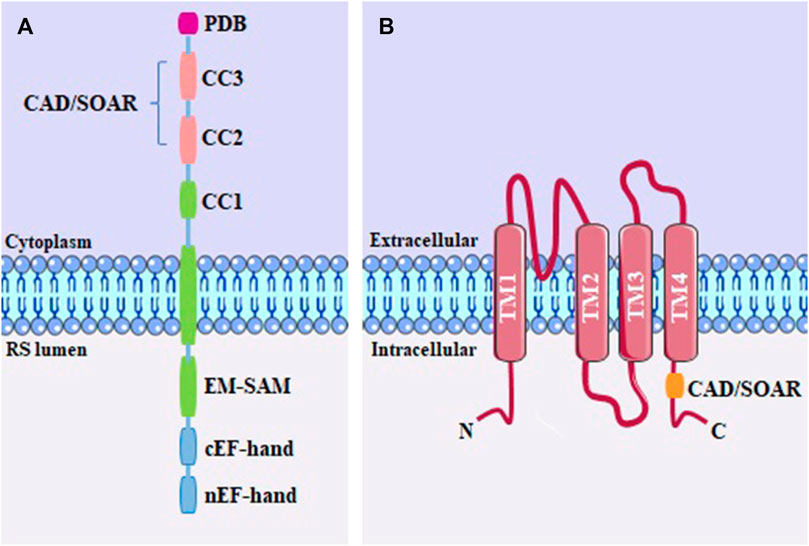
FIGURE 4. (A) STIM monomeric structure, including the PDB domain; the coiled-coil domains (CC1, CC2 and CC3); CAD/SOAR domain; sterile alpha motif (SAM) and EF-hand; (B) Structure of the Orai channel, containing four transmembrane domains (TM1-TM4) and the CAD/SOAR binding domain.
The Orai1 channel (Figure 4B) was revealed to be a hexameric oligomer, where each subunit is a protein spanning four transmembrane expansion domains (TM1-4) (Hou et al., 2012). The pore of the Orai1 channel is formed by the N-terminal transmembrane helix (TM1). The second and third transmembrane helix (TM2 and TM3) are packed around the pore in each subunit (Cai et al., 2016). The C-terminal region of the protein contains the fourth transmembrane helix (TM4) with an extended cytoplasmic segment. This cytoplasmic extension is recognized as the primary site for interaction with STIM proteins (Nwokonko et al., 2017; Zhou et al., 2017).
4.2.2 Molecular physiological and biophysical properties
When the concentration of Ca2+ in the ER decreases, Ca2+ dissociates from the EF-hand luminal domain. This dissociation leads to the unfolding of the EF-SAM domain, resulting in a conformational change in the cytosolic region, that promotes the formation of STIM1 oligomers and facilitates the dissociation of the CC1 domain from CAD/SOAR. After Ca2+ depletion, the STIM1 oligomers migrate to the cortical ER associate with the plasma membrane, at a distance short enough for the direct interaction between STIM1 and Orai1 through the exposure of the CAD/SOAR domains, which in turn interact with Orai1 to activate this channel, resulting in influx of Ca2+ (Bakowski et al., 2020; Baraniak et al., 2020; Fahrner et al., 2020).
Activation of CRAC channels occurs proportionally to the reduction in Ca2+ levels, allowing for the entry of extracellular Ca2+ to replenish the depleted stores (Qiu and Lewis, 2019). These channels play important roles in regulating various cellular functions, including cell proliferation, gene expression, cell migration, and apoptosis (Kappel et al., 2019). SOC signaling is particularly essential for several aspects of T cell function, such as T cell development and differentiation, stimulation of CD8+ T cells followed by release of cytotoxic granules, cytokine production, and control of mast cell and neutrophil function (Vaeth and Feske, 2018; Vaeth et al., 2020).
Under physiological conditions, ER Ca2+ stores are depleted by the action of second messenger inositol triphosphate (IP3). IP3 is generated from the hydrolysis of the membrane phospholipid, PIP2, by the action of PLC enzymes. Several types of PLC are stimulated by different G proteins or receptor tyrosine kinases, depending on the tissue and the ligand (Lopez et al., 2020). IP3 can then bind to its receptors (IP3R) on the ER membrane, favoring the Ca2+ release to the cytoplasm. In excitable cells, ryanodine receptors (RyR) are reported to play a greater role in luminal ER Ca2+ depletion than IP3R. That said, after the decrease of Ca2+ stored in the ER, CRACs are activated, providing a sustained influx of Ca2+ (Zhu et al., 2017).
Interestingly, the stoichiometry of the STIM-Orai coupling is variable (Yen and Lewis, 2019). The optimal activation of the Orai channel occurs with a coupling ratio of 2:1, suggesting that a single hexameric Orai1 channel interact with six STIM1 dimers (Hoover and Lewi, 2011). However, structural studies present an alternative perspective, proposing a bimolecular interaction model. According to this model, two adjacent Orai1 subunits interact with an STIM1 dimer (Maus et al., 2015).
4.2.3 Expression profile and contributions to asthma
CRAC channels represent potential targets for the treatment of respiratory disorders, since they are involved in several diseases such as those related to the immune system. In airway immune cells, CRAC inhibition modulates the chronic inflammation seen in asthma. Furthermore, CRAC channels are expressed in airway smooth muscle and epithelial cells, making them attractive targets for therapeutic interventions in asthma (Johnson and Trebak, 2019; Bakowski et al., 2020). Studies have shown that the expression of STIM1 and Orai1 proteins was positively modulated in smooth muscle cells of tracheal and bronchial tissue isolated from ovalbumin-challenged mice with asthma. The reduced gene expression of these proteins significantly inhibited chemotactic migration and smooth muscle cell proliferation, highlighting the role of STIM1 and Orai1 as targets for smooth muscle remodeling during asthma (Spinelli et al., 2012).
Previously, experimental studies have also shown the significant involvement of SOC in a model of allergic asthma in guinea pigs. These results showed decreased airway hyperreactivity at single doses of a STIM-Orai coupling blocker, 3-fluoropyridine-4-carboxylic acid (FPCA), as well as decreased basal airway reactivity and presented anti-inflammatory response in long-term administration of this blocker (Šutovská et al., 2013).
Šutovská et al. (2015) reported the effect of long-term administration of FPCA blocker on respiratory epithelial cells in conditions of allergic asthma induced by ovalbumin in guinea pigs. The reduction in cytokine levels confirmed an anti-inflammatory effect of this blocker (Šutovská et al., 2015). Furthermore, according to Šutovská et al. (2021), the effects of the STIM-Orai antagonist SKF 96365 were investigated through inhalation in guinea pigs with ovalbumin-induced airway remodeling. Three increasing doses of the antagonist were administered, and its impact on hyperresponsiveness was evaluated. Histology and immunohistochemical analyzes confirmed the prevention of airway remodeling alterations induced by repetitive exposure to ovalbumin in inhibition of the STIM-Orai pathway. The researchers observed that the inhibition of tissue remodeling promoted by SKF 96365 occurred through suppression of IL-4, IL-5, IL-12, IL-13, interferon γ (INF-γ) and TNF-ɑ pathway.
The inhibition or reversal of airway tissue remodeling in asthma is a therapeutically important target. Thus, the STIM-ORAI pathway stands out as a possible mechanism responsible for this effect, making store-operated Ca2+ channels as promising therapeutic targets for an effective treatment for asthma (Johnson and Trebak, 2019; Šutovská et al., 2021).
4.3 Intermediate and big conductance Ca2+-activated K+ channels (IKCa and BKCa)
4.3.1 Molecular structure
Ca2+-activated K+ channels (KCa) comprise several ion channels with different characteristics. Based on their conductance levels, Ca2+-activated K+ channels can be categorized into three subfamilies: small conductance (SKCa), intermediate conductance (IKCa), and large conductance (BKCa). Among these subfamilies, IKCa and BKCa channels are particularly involved in the pathogenesis of asthma. These channels play significant roles in regulating airway smooth muscle tone and bronchial hyperresponsiveness, key factors contributing to asthma symptoms and airway constriction (Kshatri et al., 2018).
IKCa (or KCa3.1) channels have intracellular C- and N-terminal regions and four α subunits, each with six transmembrane helices (S1-S6). The pore-forming region comprises the S5 and S6 helices, being K+ selective (Figure 5A) (Adelman, 2016; Kshatri et al., 2018). BKCa (or KCa1.1) channels have a multimeric structure, comprising four identical pore-forming α subunits and up to four β and γ regulatory subunits (Figure 5B). These channels have seven transmembrane segments (S0-S6), with S0 being a domain that leads the N-terminal portion to the extracellular side. Furthermore, within segments S2, S3, and S4 are charged amino acid residues that play roles in voltage detection (Al-Karagholi et al., 2020).
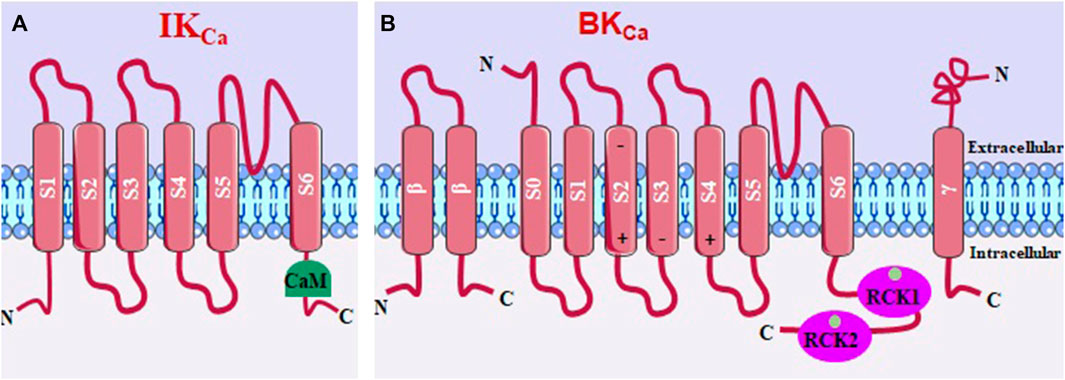
FIGURE 5. (A) IKCa structure, with 6 transmembrane segments and the calmodulin binding domain (CaM). (B) BKCa, with seven transmembrane segments and two Ca2+-sensitive domains that regulate K+ conductance.
4.3.2 Molecular physiological and biophysical properties
IKCa channels are independent of membrane voltage and are activated by a small increase in [Ca2+]c through a mechanism that consists of binding this ion to calmodulin (CaM), forming a complex that activates the calmodulin binding domain (CaMBD) in the C-terminal region of the IKCa (Adelman, 2016; Kshatri et al., 2018).
The β1 and β4 subunits stabilize the voltage detection domain of BKCa channels in an active conformation, leading to increased channel activity and promoting membrane potential hyperpolarization. This results in more negative values of the membrane potential. In contrast, the β2 and β3 subunits favor an inactive conformation of BKCa channels, limiting K+ efflux and membrane hyperpolarization (Hite et al., 2017). The cytosolic C-terminal region of BKCa channels is relatively large and consists of two K+ conductance regulator domains (RCK1 and RCK2) that contain a high-affinity Ca2+ binding site. When Ca2+ binds to these sites, it directly activates BKCa channels without involving the calcium-binding protein calmodulin (CaM). This direct interaction with Ca2+ leads to the opening of the channel pore and the efflux of K+ ions (Lee and Cui, 2010; Yuan et al., 2012; Hoshi et al., 2013).
4.3.3 Expression profile and contributions to asthma
IKCa channel is expressed in mast cells, macrophages, fibroblasts, T lymphocytes, epithelial cells and smooth muscle cells in the airways of normal and asthmatic individuals (Girodet et al., 2013; Pelletier and Savignac, 2018), and can control several cellular processes, such as proliferation, chemotaxis, cell activation and smooth muscle responsiveness (Van der Velden et al., 2013). In addition, IKCa participates in the pathophysiology of rheumatoid arthritis, multiple sclerosis, atherosclerosis, fibrosis, rhinitis, and asthma (Ohya and Kito, 2018).
In humans, BKCa channels have a wide expression profile, being found in various tissues, including respiratory systems (Latorre et al., 2017; Gonzalez-Perez and Lingle, 2019). The opening of BKCa regulates several physiological processes, such as smooth muscle contraction, hormonal secretion, neuronal excitation and gene expression (Yang et al., 2015). Studies have reported that mutations in the KCNMA1 gene encoding the BKCa channel, known as KCNMA1-linked channelopathy in humans, are associated with neurological conditions such as movement disorders, seizures and developmental delay. In addition, there is evidence of the association of other polymorphisms in this channel with diseases such as asthma (Bailey et al., 2019).
Yu et al. (2013) demonstrated the role of IKCa in the pathogenesis of inflammation and airway remodeling in ovalbumin-induced allergic asthma in mice. Thus, it was reported that in this disease model, IKCa expression was elevated, especially in bronchial smooth muscle cells. In addition, blocking this channel by triarylmethane (TRAM-34) resulted in the inhibition of inflammation, remodeling and airway hyperresponsiveness, as well as in the interruption of bronchial smooth muscle cell proliferation in asthmatic humans (Yu et al., 2013).
In a mouse model of chronic asthma, studies have demonstrated that blocking IKCa channels using TRAM-34 has beneficial effects. Treatment with TRAM-34 has been shown to reduce airway remodeling, decrease airway eosinophilia and collagen deposition. Additionally, when TRAM-34 was used in combination with preventive and therapeutic treatments, it was found to reduce bronchial hyperresponsiveness to methacholine. These findings suggest that targeting IKCa channels with blockers like TRAM-34 may hold potential as a therapeutic strategy for managing asthma and its associated symptoms (Girodet et al., 2013). Another study has shown that the administration of senicapoc, an IKCa blocker, was effective in reducing bronchoconstriction, pulmonary resistance, airway hyperresponsiveness to carbachol and eosinophilia in bronchoalveolar fluid in an ovine model of mite-induced experimental asthma (Van der Velden et al., 2013).
Inhibition of inflammation, remodeling, and airway hyperresponsiveness were also observed through deletion of the IKCa gene. In this study, it was also shown, for the first time, that the suppression of the proliferation of bronchial smooth muscle cells in asthmatic humans occurred because of the inhibition of calcium influx via TRPV4 due to the deletion of the IKCa gene, given that these channels are colocalized and functionally interact to regulate [Ca2+]c in these cells (Yu et al., 2017). This is because in airway smooth muscle cells, the IKCa channels act as Ca2+ detectors and amplifiers, since the membrane hyperpolarization caused by the activation of this channel positively regulates Ca2+ influx (Guéguinou et al., 2014).
BKCa polymorphisms have been associated with susceptibility to asthma in an African population (Mantese et al., 2011). Furthermore, a BKCa activator, NS1619, decreased in vivo and in vitro airway hyperreactivity, ciliary beat frequency, inflammatory cell infiltration, pro-inflammatory cytokines and exhaled nitric oxide in a guinea pig model of ovalbumin-induced allergic asthma (Kocmalova et al., 2015).
Studies have also shown that rottlerin, a BKCa activator, attenuated both airway hyperreactivity in vivo and in vitro, and infiltration of inflammatory cells in the lung, by reducing the production of TH2 profile cytokines in ovalbumin and mite-induced asthma models (Goldklang et al., 2013). In addition, andolast, another BKCa activator, is in clinical phase III for a potential treatment of allergic rhinitis, COPD, and bronchial asthma (Al-Karagholi et al., 2020).
Based on the evidence implicating IKCa and BKCa channels in asthma, there is growing support for considering these channels as promising targets for the treatment of the disease. The involvement of these channels in regulating airway smooth muscle tone, bronchial hyperresponsiveness, and inflammation highlights their potential as therapeutic targets. By modulating the activity of IKCa and BKCa channels, it may be possible to mitigate the pathological features of asthma and improve symptoms. Further research and development of specific modulators or blockers for these channels could lead to novel therapeutic interventions for the treatment of asthma.
4.4 Calcium-activated chloride channel (TMEM16A)
4.4.1 Molecular structure
Ca2+−activated chloride channels (CaCCs) are channels that allow the passage of chloride ions (Cl−) and can be activated by the presence of intracellular calcium and also by voltage. A specific type of CaCCs, called anoctamine-1 (ANO1) or transmembrane protein 16A (TMEM16A), was initially described in Xenopus oocytes. The ANO1/TMEM16A gene encodes a protein that belongs to a family known as anoctamins, which is composed of 10 members (ANO1–ANO10) in mammals (Schroeder et al., 2008; Yang et al., 2008; Kamaleddin, 2018).
TMEM16A channels (Figure 6) are formed by transmembrane homodimers, in which each subunit is regulated independently (Jeng et al., 2016). They have N- and C-terminal domains and the transmembrane domain starts with two short α-helices (α0a and α0b), followed by ten membrane-spanning segments (α1–α10). Between helices, there are calcium-binding regions, and the intracellular loop between α6–α8 contains six amino acids (N650, E654, E702, E705, E734 and D738) that participate in the formation of principal calcium-binding pocket, thus regulating the opening of this channel. The α3–α7 helices form the pore of the channel (Brunner et al., 2014; Paulino et al., 2017).
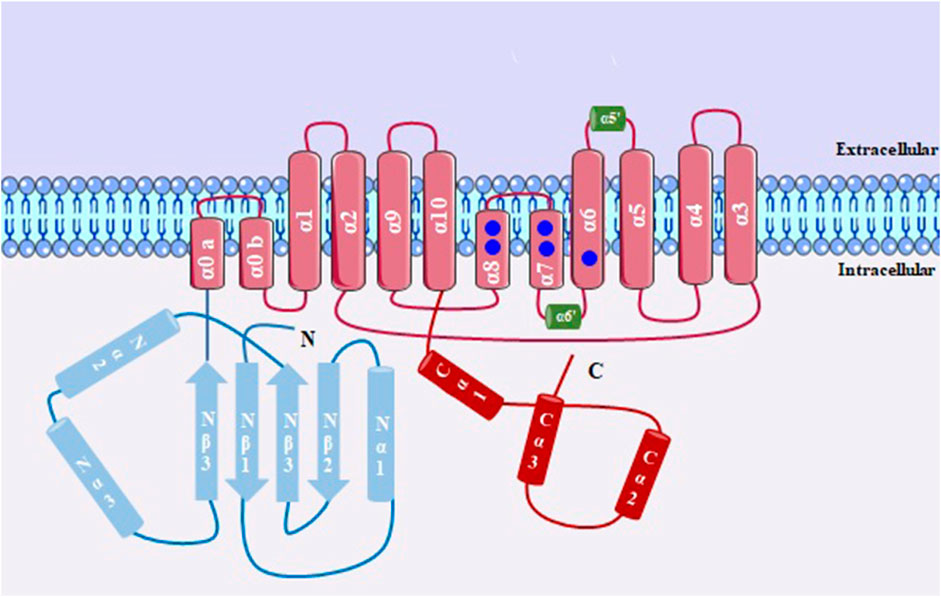
FIGURE 6. Structure of a subunit of TMEM16A, with 10 transmembrane domains. Between α3-α7 there is the formation of the channel pore; Ca2+ binding sites is shown in blue circles.
4.4.2 Molecular physiological and biophysical properties
Most naturally expressed CaCCs are sensitive to low [Ca2+]c (100 nM to 1–2 μM), so that a considerable increase in [Ca2+]c leads to inactivation of this channel (Schreiber et al., 2010; Yang et al., 2008). Associated with this, changing the membrane potential to fewer negative values can also cause activation of these channels (Xiao C et al., 2011). This occurs because the calcium binding site is located in the transmembrane region of the channel, which is also sensitive to changes in membrane potential. Furthermore, the sensitivity of the channel to activation by cytosolic calcium decreases when the membrane potential is hyperpolarized (Yang et al., 2008; Pedemonte and Galietta, 2014).
When activated, the chloride ion conductance in a single channel is 0.5–5 pS. This channel also showed relative permeability for the following monovalent anions, in increasing order of permeability: NO3−, I−, Br−, Cl− and F− (Yang et al., 2008; Alexander et al., 2021). Studies have also shown that TMEM16A can be activated in the presence of other divalent ions such as Ba2+, Sr2+ and Ni2+ (Yuan et al., 2013). Previous research has established that activation of phospholipase C leads to the hydrolysis of PIP2, producing IP3, which in turn releases calcium from intracellular compartments, potentially activating TMEM16A (Cruz-Rangel et al., 2015; Jesús-Pérez et al., 2018).
4.4.3 Expression profile and contributions to asthma
These channels are found in a variety of organisms and tissues, suggesting their functional diversity. TMEM16A has already been reported to be present in neuronal, vascular, myocardial, epithelial and smooth muscle cells (Large and Wang, 1996; Pedemonte and Galietta, 2014; Rottgen et al., 2018). In humans, TMEM16A is widely expressed in the respiratory tract, from the bronchi to the alveoli (Kunzelmann et al., 2012). In addition, it is largely related to the regulation of transepithelial transport and smooth muscle contraction (Pedemonte and Galietta, 2014). TMEM16A hyperactivity plays a role in neuropathic pain, cell migration and proliferation in different types of cancer, as well as in asthma (Zhang et al., 2013; Crottès and Jan, 2019; Kulkarni et al., 2023).
Studies suggest that the increase in TMEM16A activity is related to the pathogenesis of asthma, so that the inhibition of this protein characterizes a therapeutic alternative for this disease, especially related to the control of mucus hypersecretion, a potentially serious characteristic that culminates in airway obstruction. Thus, regulation of the expression of secretory cells and mucus secretion are shown to be important targets (Liu et al., 2021).
The upregulation of TMEM16A in airway smooth muscle cells was also confirmed by Zhang et al. (2013). It has been highlighted that activation of TMEM16A contributes to agonist-induced contraction, which activate these chloride channels to depolarize the membrane. Thus, deletion of TMEM16A rendered Ca2+ sparks incapable of activating chloride efflux and weakened caffeine and methacholine-induced muscle fiber shortening in knockout TMEM16A−/− airway smooth muscle cells. Furthermore, TMEM16A blockers niflumic acid and benzbromarone, prevented hyperresponsiveness in vivo and in vitro in an ovalbumin-induced asthma model in C57BL/6 mice (Zhang et al., 2013).
Increased expression of TMEM16A on the surface of airway epithelial secretory cells has been reported both from C57BL/6 mice sensitized and challenged with ovalbumin and from asthmatic patients. They also showed that the inhibition of this protein by blockers such as benzbromarone and dichlorophen negatively modulated mucus secretion in human epithelial cells, in addition to reducing contraction in response to methacholine in smooth muscle cells of the airways of mice and humans (Huang et al., 2012).
Kondo et al. (2017) demonstrated overexpression of TMEM16A in the apical portion of the epithelium with goblet cell metaplasia in the trachea of guinea pigs sensitized with ovalbumin, associated with an increase in chloride ion conductance through this protein. Furthermore, it was shown that the presence of the blockers T16Ainh-A01, benzbromarone and niflumic acid in the culture of tracheal epithelial cells, the increase in chloride ion transport induced by the calcium-dependent agonist UTP was inhibited. T16Ainh-A01 also inhibited the mucus secretion that was shown in the trachea of sensitized animals, suggesting that TMEM16A inhibitors may be viable in inhibiting hypersecretion in asthma (Kondo et al., 2017).
Based on these data, there are indications that TMEM16A may be a unique therapeutic target for the treatment of asthma. TMEM16A-CaCCs channel blockers have the potential to treat both mucus hypersecretion and airway hyperresponsiveness in animal models, and may positively impact asthma symptoms.
4.5 Cystic fibrosis transmembrane conductance regulator (CFTR)
4.5.1 Molecular structure
CFTR belongs to ATP-binding cassette (ABC) superfamily, a family with 48 members, grouped into 7 subfamilies (ABCA-G). CFTR belongs to ABCC subfamily, subtype 7 being the only one that functions as an ion channel, transporting mostly chloride ions, but also bicarbonate and other ions. ABC transporters use the chemical energy of ATP hydrolysis to transport substrates against the electrochemical gradient, with the exception of CFTR that conduct anions in favor of the electrochemical gradient (Dean and Annilo, 2005; Gadsby et al., 2006).
The CFTR protein is structurally (Figure 7) characterized by having two canonical transmembrane domains (TMDs) and each domain has 6 transmembrane segments, being S1-S6 in TMD1 and S7-S12 in TMD2, these being the structures responsible for the formation of the channel pore. Each TMD is followed by a cytosolic nucleotide-binding domain (NBD1 and NBD2). This channel also has a unique regulatory domain (R), located between TMD-NBD complexes that contain multiple sites for phosphorylation (Sohma and Hwang, 2015).
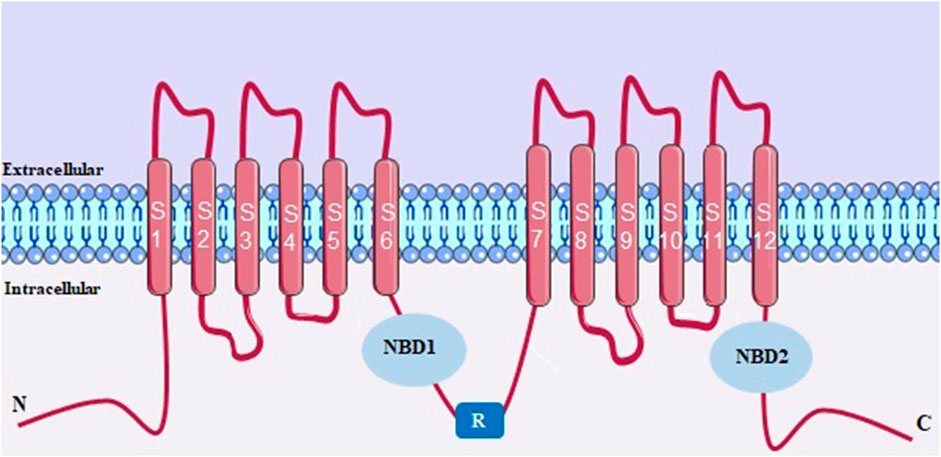
FIGURE 7. CFTR structure with two canonical transmembrane domains (TMDs). NBD, cytosolic nucleotide-binding domain. R, regulatory domain.
4.5.2 Molecular physiological and biophysical properties
The literature describes that CFTR channel can present in 2 conformations, the open one, requires phosphorylation of R domain by protein kinase A (PKA) and the ATP binding site induces the opening of the channel and passage of ions. This channel has 2 distinct sites for ATP, “consensus” which is catalytically active and responsible for ATP hydrolysis and “degenerate” which has no catalytic activity. The ATP hydrolysis that occurs in the “consensus” induces pore closure. Pore opening in absence of ATP or its closure without ATP hydrolysis may also occur, but this is rare (Aleksandrov et al., 2002; Csanady et al., 2010; Wang et al., 2010).
The other conformation is the closed one, which occurs after phosphorylation of the R domain, inducing the folding of nucleotide-binding domains that interact with the transmembrane domains, leading to ATP hydrolysis and altering the channel conformation from an open state to closed one. In this conformation, the NBDs are separated by approximately 20 Å (Liu et al., 2017).
When analyzing the CFTR conductance in isolation, a concentration-dependent saturation of chloride was observed, suggesting a general affinity for the pore of approximately 55 mM (Linsdell, 2017).
4.5.3 Expression profile and contributions to asthma
CFTR is located in the apical membrane of epithelial cells, especially in pancreatic duct and nasal polyps, and to a lesser extent in the intestine, sweat glands, placenta, liver, and predominantly in the male reproductive system, particularly in vas deferens and lungs. Among these, the pulmonary secretory cells are the most crucial in expressing CFTR in this organ. This channel function is to regulate and transport ions essential for the homeostasis of bodily fluids (Harris et al., 1991; Okuda et al., 2021).
In open state, CFTR allows chloride ions to pass through the epithelial tissues at the apical surface, facilitating the cotransport of bicarbonate (HCO3−). This function directly influences pH of surfaces of epithelial cells and mucus. CFTR has also been reported to have indirect effects on other channels, such as a strong inhibitory effect on ENaCs, as well as modulating other chloride channels like calcium-activated chloride channels (CaCC) and outward rectifying chloride channels (ORCC) (Gentzsch et al., 2010; Gustafsson et al., 2012; Cant et al., 2014).
Mutations or polymorphisms in gene encoding CFTR can lead to disruptions in the homeostatic functioning of various organs, including the lungs. While cystic fibrosis is the most well-known disease associated with mutations in this gene, studies have also linked these mutations to asthma. Individuals who are heterozygous for CFTR mutations but do not develop cystic fibrosis symptoms, known as carriers, have been found to have an increased risk of developing asthma (Miller et al., 2020; Deletang and Taulan-Cadars, 2022).
Non-clinical studies have characterized the involvement of CFTR in asthma, citing data obtained from human airway tissues. These studies demonstrated that chronic exposure to IL-4 and IL-13 increases CFTR activity (Danahay et al., 2002; Galietta et al., 2002). Moreover, this was confirmed in the trachea of mice subjected to allergic inflammation model induced by Aspergillus fumigatus extract (Anagnostopoulou et al., 2010).
These findings were supported by Crespo-Lessmann et al. (2021), who conducted a comparative multicenter cross-sectional descriptive study with 100 asthmatic patients, including those with the classic phenotype of asthma, without hypersecretion, and those with severe asthma (with hypersecretion). They observed that a significant number of these patients had polymorphisms in the CFTR gene, and these mutations coincided with more severe asthma and poorer clinical control. This suggests a potential positive correlation between severe asthma and genetic alterations in CFTR.
Despite on these evidences, there are still aspects of the CFTR-asthma correlation that remain not fully elucidated, necessitating further extensive research to validate the evidence in this field. These efforts are aimed at gaining a better understanding of asthma and opening up new perspectives for treatment.
4.6 Piezo-type mechanosensitive ion channel component 1 (PIEZO1)
4.6.1 Molecular structure
Discovered a few years ago, PIEZO1 is a non-selective and mechanosensitive cation channel, which operates as a mechanical transducer, being controlled by the tension in the cell membrane. This channel has a unique and differentiated structure in relation to other ion channels, being characterized by a trimeric complex, with a structure similar to a helix around the central ionic pore that detects mechanical forces. PIEZO1 is mainly present in the lipid bilayer, and can also be found in the cytoplasm, endoplasmic reticulum and nuclear envelope (Coste et al., 2010; Coste et al., 2012).
PIEZO1 is a large protein (900 kDa) containing around 2547 amino acids, with each helix having 38 transmembrane segments, which are folded into nine transmembrane helical subunits (THUs), each of which has four segments (Figure 8). The last two transmembrane helices that form the channel pore, the outer (OH) and inner (IH) connected by a C-terminal domain (CED), are fundamental for channel activity, regulating conductance and selectivity (Douguet and Honoré, 2019; Fang et al., 2021; Jiang et al., 2021).
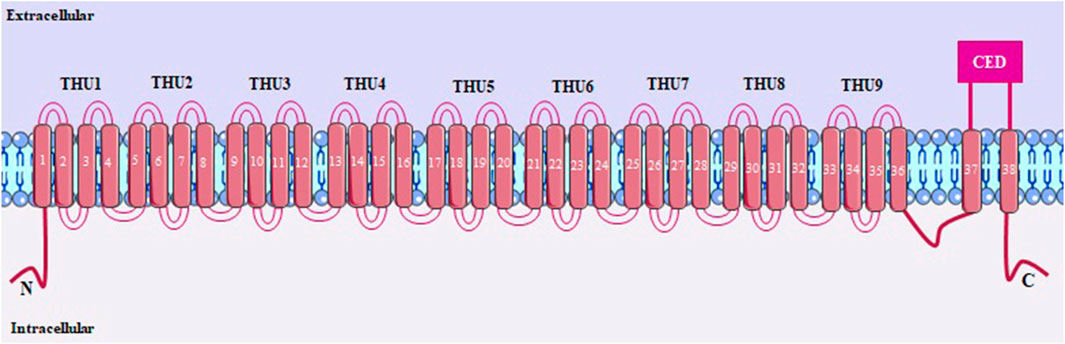
FIGURE 8. PIEZO1 structure, with 38 transmembrane segments. THUs, transmembrane helical subunits; OH and IH, outer and inner transmembrane helices, respectively; CED, C-terminal domain.
4.6.2 Molecular physiological and biophysical properties
Changes in membrane tension around 1.4 pN nm−1, including membrane stretching or compression, shear stress, ultrasound or osmotic pressure can activate PIEZO1 (Bagriantsev et al., 2014), inducing increased conductance to the cations K+, Na+, Mg2+ and, preferably, Ca2+ (Volkers et al., 2015). The single-channel conductance for PIEZO1 is 29 pS and the currents turn on in a microsecond scale (<5 msec) with rapid subsequent inactivation (16 msec) (Wu et al., 2016).
4.6.3 Expression profile and contributions to asthma
PIEZO1 is predominant in many types of mechanosensitive cells, such as endothelial, epithelial or immune cells, and is found in organs such as the bladder, skin, kidney, colon and lung (Coste et al., 2010). Several pathophysiological processes involve the participation of PIEZO1, such as blood pressure regulation (Wang et al., 2016), vascular remodeling (Li et al., 2014), red blood cell volume regulation (Cahalan et al., 2015), iron metabolism (Ma et al., 2021), renal fibrosis (He et al., 2022), cancer (Yu and Liao, 2021), diabetes (Zhu et al., 2022), immune cell diapedesis (Offermanns et al., 2021), epithelial homeostasis (Eisenhoffer et al., 2012), regulation of surfactant production (Diem et al., 2020) and lung injury induced by mechanical ventilation (Zhang et al., 2021).
Activation of PIEZO1 through its specific agonist, Yoda1, was found to cause changes in the biomechanics and contractile machinery of airway smooth muscle cells, resulting in relaxation of these cells. These biomechanical behaviors, such as cellular stiffness and traction force, play a key role in cellular functions related to contraction and relaxation. Consequently, the assessment of these parameters in airway smooth muscle is essential to understand the development of hyperresponsiveness in asthma and to identify new therapeutic targets and strategies for the treatment of this disease (Luo et al., 2023).
Another study was performed to determine the role of PIEZO1 in the regulation of adherent junctions in the airway epithelium under positive end-expiratory alveolar pressure (Zhou et al., 2021), which is the result of overinflation due to bronchoconstriction, edema and airflow obstruction that occurs in an asthma attack. The proper functioning of the protective barrier of the airway epithelium depends on the integrity of the adherent junctions (Godfrey, 1997), and damage to these proteins represents damage to the epithelium, which occurs in most respiratory diseases such as asthma (Gon and Hashimoto, 2018).
According to Zhou et al. (2021), the expression of PIEZO1 in bronchial epithelial cells of asthmatic mice in a model induced by ovalbumin was higher compared to healthy animals. Furthermore, treatment with an inhibitor of this channel, GsMTx4, reduced the degradation of adhesion junction proteins, such as occludin, zonula occludens-1 and claudin-18, in primary epithelial cells of human airways subjected to pressure, thus simulating the airways of asthmatic patients in vitro. Thus, it was suggested that PIEZO1 plays a crucial role in the degradation of these proteins and compromises the airway epithelial barrier under pressure conditions (Zhou et al., 2021).
4.7 Purinergic receptor (P2X)
4.7.1 Molecular structure
P2X receptors are channels that allow the passage of a diversity of ions, such as sodium (Na+), calcium (Ca2+) and potassium (K+), through activation by the presence of extracellular adenosine 5′-triphosphate (ATP). In 1994 the P2X gene was first cloned, thereafter, in 2012, the ATP binding site was discovered, providing information that upon channel activation the ATP-bound state conformation changes (Hattori and Gouaux, 2012; North, 2016; Sheng and Hattori, 2022).
There are seven types of P2X receptors, from P2X1 to P2X7, they exist as either homotrimers or heterotrimers, and when ATP binds to their subunits, it induces a coordinated flexing motion within their extracellular domain and a separation in the transmembrane domain, leading to the opening of a central channel. Each protomer of P2X receptors has a dolphin-like shape, resembling the body, head, dorsal fin, left flipper (LF), and right flipper (RF). The extracellular domain is hydrophilic and contains multiple β-strands. The RF domain has a glycosylation site stabilized by five conserved disulfide bonds. The LF domain, along with the DF domain, plays a crucial role in channel gating. The transmembrane domain consists of two α-helices (TM1 and TM2), with TM2 forming the ion-conducting pore within the inner tunnel (Figure 9) (Burnstock, 2016; Sheng and Hattori, 2022).
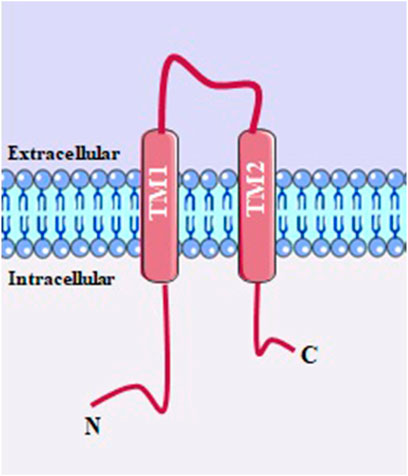
FIGURE 9. P2X structure with two transmembrane domain α-helices (TM1 and TM2), with TM2 forming the ion-conducting pore within the inner tunnel.
4.7.2 Molecular physiological and biophysical properties
P2X receptors exhibit permeability to sodium, potassium, and calcium ions. Some of them additionally demonstrate notable permeability to chloride ions. Quantifying the channel currents of P2X receptors presents challenges due to their rapid flickering nature. Single homomeric P2X2 channels display a unitary conductance of approximately 30 pS and exhibit transient open states that likely correspond to alterations in protein conformation. The permeation properties of the 30-pS state have been extensively studied. Furthermore, during bursts of activity, certain P2X2 channels undergo transitions into a 14-pS state, with some channels exclusively opening to this state (Khakh, 2001; Egan et al., 2006).
4.7.3 Expression profile and contributions to asthma
P2X receptors are widely expressed throughout the body and can be found in both excitatory and non-excitatory cells. They play significant roles in various physiological and pathological processes in mammals, such as pain, inflammation, taste perception, and smooth muscle contraction (Suprernant and North, 2009). In neuroinflammatory conditions observed in both in vitro and in vivo models, there are reported changes in the expression of P2X receptor subtypes. P2X4 receptors are linked to the early inflammatory mediator, PGE2. Similar to P2X7, P2X4 receptors form large conductance pores on the cell membrane, enabling ion efflux and subsequent inflammasome activation. The P2X4 receptor might serve as an initial trigger, while the P2X7 receptor, along with pannexin 1, appears to amplify the signal. In mice, the contribution of the P2X4 receptor to PGE2 release is relatively minor compared to that of P2X7 receptors (Burnstock, 2016).
In asthma, it is already established that P2X receptors play crucial roles in the pathophysiology. Among these, findings suggest that P2X7 receptors could have a significant role in contributing to the undesirable activation of mast cells during chronic inflammatory conditions when there is an increase in extracellular ATP levels (Oguma et al., 2007; Wareham and Seward, 2016). Also, although the molecular mechanism through which P2X4R influences airway remodeling in allergic asthma remains largely unidentified, recent studies suggest that in mice with allergic asthma, the P2X4 receptor (P2X4R) plays a role in airway inflammation and airway remodeling by directly acting on the phenotype switching of BSMCs (Wang, et al., 2018). Another study showed both healthy and asthmatic human eosinophils expressed transcripts for P2X1, P2X4, and P2X5 receptors (Wright et al., 2016).
5 Conclusion
Based on the studies covered in this review, the importance of ion channels such as transient receptor potential (TRP), stock-operated Ca2+ channels (SOCs), Ca2+-activated K+ channels (IKCa and BKCa), calcium-activated chloride channel (TMEM16A), cystic fibrosis transmembrane conductance regulator (CFTR), piezo-type mechanosensitive ion channel component 1 (PIEZO1) and purinergic P2X receptor were highlighted in asthma, and these are identified as key elements in the pathogenesis of this disorder (Figure 10). Hence, these channels emerge as potential targets for drug discovery and the development of novel pharmacological tools. Their significance lies in their involvement not only in immune cell function but also in other cell types implicated in asthma, including epithelial cells and airway smooth muscle cells (Figure 11). Nonetheless, additional research is necessary to elucidate the full extent of their promising role in asthma pathophysiology and treatment.
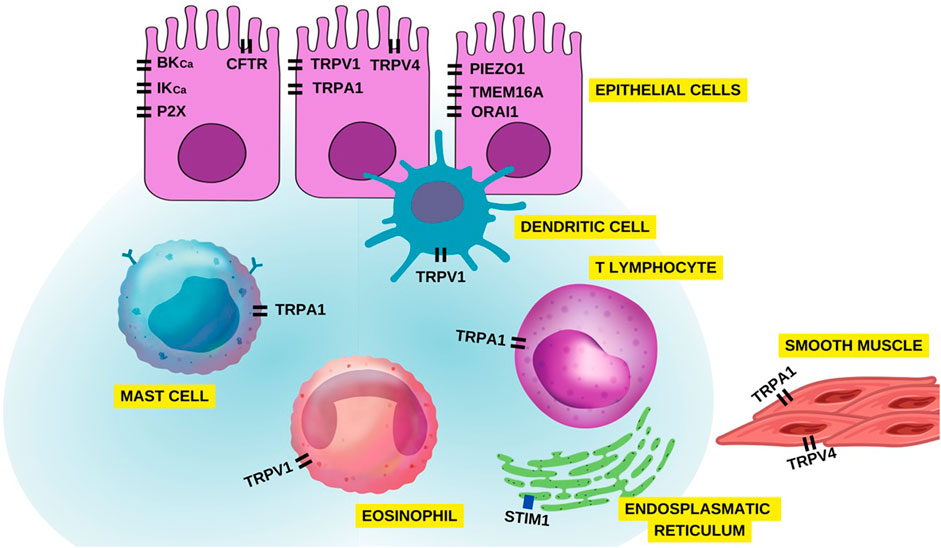
FIGURE 11. Overview of ion channels in different airway cells associated with asthma pathophysiology.
Author contributions
IF and SF carried out the research and wrote the manuscript. LV, FC, and BS guided the preparation of the manuscript. All authors contributed to the article and approved the submitted version.
Funding
The Coordenação de Aperfeiçoamento de Pessoal de Nível Superior (Capes) and Conselho Nacional de Desenvolvimento Científico e Tecnológico (CNPq) for providing financial support.
Acknowledgments
The authors thank Coordenação de Aperfeiçoamento de Pessoal de Nível Superior (Capes), Conselho Nacional de Desenvolvimento Científico e Tecnológico (CNPq) and Pró-Reitoria de Pesquisa (Propesq) for providing financial support, and Universidade Federal da Paraíba (UFPB) for institutional support. IF, SF, and JF carried out the research and wrote the manuscript. LV, FC, and BS guided the preparation of the manuscript.
Conflict of interest
The authors declare that the research was conducted in the absence of any commercial or financial relationships that could be construed as a potential conflict of interest.
Publisher’s note
All claims expressed in this article are solely those of the authors and do not necessarily represent those of their affiliated organizations, or those of the publisher, the editors and the reviewers. Any product that may be evaluated in this article, or claim that may be made by its manufacturer, is not guaranteed or endorsed by the publisher.
References
Adelman, J. P. (2016). SK channels and calmodulin. Channels 10, 1–6. doi:10.1080/19336950.2015.1029688
Aikawa, T., Shimura, S., Sasaki, H., Ebina, M., and Takishima, T. (1992). Marked goblet cell hyperplasia with mucus accumulation in the airways of patients who died of severe acute asthma attack. Chest 101, 916–921. doi:10.1378/chest.101.4.916
Akuthota, P., Wang, H. B., Spencer, L. A., and Weller, P. F. (2008). Immunoregulatory roles of eosinophils: A new look at a familiar cell. Clin. Exp. Allergy 38, 1254–1263. doi:10.1111/j.1365-2222.2008.03037.x
Al-Karagholi, M. A. M., Gram, C., Nielsen, C. A. W., and Ashina, M. (2020). Targeting BKCa channels in migraine: Rationale and perspectives. CNS Drugs 34, 325–335. doi:10.1007/s40263-020-00706-8
Aleksandrov, L., Aleksandrov, A. A., Chang, X. B., and Riordan, J. R. (2002). The first nucleotide binding domain of cystic fibrosis transmembrane conductance regulator is a site of stable nucleotide interaction, whereas the second is a site of rapid turnover. J. Biol. Chem. 277, 15419–15425. doi:10.1074/jbc.M111713200
Alexander, S. P., Mathie, A., Peters, J. A., Veale, E. L., Striessnig, J., Kelly, E., et al. (2021). The concise guide to pharmacology 2021/22: Ion channels. Br. J. Pharmacol. 178, S157–S245. doi:10.1111/bph.15539
Anagnostopoulou, P., Dai, L., Schatterny, J., Hirtz, S., Duerr, J., and Mall, M. A. (2010). Allergic airway inflammation induces a pro-secretory epithelial ion transport phenotype in mice. Eur. Respir. J. 36 (6), 1436–1447. doi:10.1183/09031936.00181209
Antó, J. M. (2012). Recent advances in the epidemiologic investigation of risk factors for asthma: A review of the 2011 literature. Curr. Allergy Asthma Rep. 12, 192–200. doi:10.1007/s11882-012-0254-7
ASBAI (2023). Associação Brasileira de Alergia e Imunologia - asbai. Available at: http://www.asbai.org.br/secao.asp?s=81&id=940 (Accessed January 12, 2023).
Bagriantsev, S. N., Gracheva, E. O., and Gallagher, P. G. (2014). Piezo proteins: Regulators of mechanosensation and other cellular processes. J. Biol. Chem. 289 (46), 31673–31681. doi:10.1074/jbc.R114.612697
Bailey, C. S., Moldenhauer, H. J., Park, S. M., Keros, S., and Meredith, A. L. (2019). KCNMA1-linked channelopathy. J. Gen. Physiol. 151, 1173–1189. doi:10.1085/jgp.201912457
Baker, K., Raemdonck, K., Dekkak, B., Snelgrove, R. J., Ford, J., Shala, F., et al. (2016). Role of the ion channel, transient receptor potential cation channel subfamily V member 1 (TRPV1), in allergic asthma. Respir. Res. 17, 67–10. doi:10.1186/s12931-016-0384-x
Bakowski, D., Murray, F., and Parekh, A. B. (2020). Store-operated Ca2+ channels: Mechanism, function, pharmacology and therapeutic targets. Annu. Rev. Pharmacol. Toxicol. 61, 629–654. doi:10.1146/annurev-pharmtox-031620-105135
Banner, K. H., Igney, F., and Poll, C. (2011). TRP channels: Emerging targets for respiratory disease. Pharmacol. Ther. 130, 371–384. doi:10.1016/j.pharmthera.2011.03.005
Baraniak, J. H., Zhou, Y., Nwokonko, R. M., and Gill, D. L. (2020). The intricate coupling between STIM proteins and Orai channels. Curr. Opin. Psychol. 17, 106–114. doi:10.1016/j.cophys.2020.07.018
Barrett, N. A., and Austen, K. F. (2009). Innate cells and T helper 2 cell immunity in airway inflammation. Immunity 31, 425–437. doi:10.1016/j.immuni.2009.08.014
Bartoszewski, R., Matalon, S., and Collawn, J. F. (2017). Ion channels of the lung and their role in disease pathogenesis. Am. J. Physiol. Lung Cell. Mol. Physiol. 313 (5), L859–L872. doi:10.1152/ajplung.00285.2017
Batra, V., Musani, A. I., Hastie, A. T., Khurana, S., Carpenter, K. A., Zangrilli, J. G., et al. (2004). Bronchoalveolar lavage fluid concentrations of transforming growth factor (TGF)-beta1, TGF-beta2, interleukin (IL)-4 and IL-13 after segmental allergen challenge and their effects on alpha-smooth muscle actin and collagen III synthesis by primary human lung fibroblasts. Clin. Exp. Allergy 34, 437–444. doi:10.1111/j.1365-2222.2004.01885.x
Bel, E. H. (2004). Clinical phenotypes of asthma. Curr. Opin. Pulm. Med. 10, 44–50. doi:10.1097/00063198-200401000-00008
Benemei, S., Patacchini, R., Trevisani, M., and Geppetti, P. (2015). TRP channels. Curr. Opin. Pharmacol. 22, 18–23. doi:10.1016/j.coph.2015.02.006
Berair, R., and Brightling, C. E. (2014). Asthma therapy and its effect on airway remodelling. Drugs 74, 1345–1369. doi:10.1007/s40265-014-0250-4
Bergeron, C., Al-Ramli, W., and Hamid, Q. (2009). Remodeling in asthma. Ann. Am. Thorac. Soc. 6, 301–305. doi:10.1513/pats.200808-089RM
Bertin, S., Aoki-Nonaka, Y., De Jong, P. R., Nohara, L. L., Xu, H., Stanwood, S. R., et al. (2014). The ion channel TRPV1 regulates the activation and proinflammatory properties of CD4+ T cells. Nat. Immunol. 15, 1055–1063. doi:10.1038/ni.3009
Boesiger, J., Tsai, M., Maurer, M., Yamaguchi, M., Brown, L. F., Claffey, K. P., et al. (1998). Mast cells can secrete vascular permeability factor/vascular endothelial cell growth factor and exhibit enhanced release after immunoglobulin E-dependent upregulation of Fcε receptor I expression. J. Exp. Clin. Med. 188, 1135–1145. doi:10.1084/jem.188.6.1135
Bradding, P., Feather, I. H., Wilson, S., Bardin, P. G., Heusser, C. H., Holgate, S. T., et al. (1993). Immunolocalization of cytokines in the nasal mucosa of normal and perennial rhinitic subjects. The mast cell as a source of IL-4, IL-5, and IL-6 in human allergic mucosal inflammation. J. Immunol. 151, 3853–3865. doi:10.4049/jimmunol.151.7.3853
Brunner, J. D., Lim, N. K., Schenck, S., Duerst, A., and Dutzler, R. (2014). X-ray structure of a calcium-activated TMEM16 lipid scramblase. Nature 516, 207–212. doi:10.1038/nature13984
Büch, T. R. H., Schäfer, E. A. M., Demmel, M. T., Boekhoff, I., Thiermann, H., Gudermann, T., et al. (2013). Functional expression of the transient receptor potential channel TRPA1, a sensor for toxic lung inhalants, in pulmonary epithelial cells. Chem. Biol. Interact. 206, 462–471. doi:10.1016/j.cbi.2013.08.012
Burnstock, G. (2016). P2X ion channel receptors and inflammation. Purinergic Signal 12 (1), 59–67. doi:10.1007/s11302-015-9493-0
Busse, W. W., and Lemanske, R. F. (2001). Asthma. N. Engl. J. Med. 344, 350–362. doi:10.1056/NEJM200102013440507
Cahalan, S. M., Lukacs, V., Ranade, S. S., Chien, S., Bandell, M., and Patapoutian, A. (2015). PIEZO1 links mechanical forces to red blood cell volume. Elife 4, e07370. doi:10.7554/eLife.07370
Cai, X., Zhou, Y., Nwokonko, R. M., Loktionova, N. A., Wang, X., Xin, P., et al. (2016). The Orai1 store-operated calcium channel functions as a hexamer. J. Biol. Chem. 291, 25764–25775. doi:10.1074/jbc.M116.758813
Cant, N., Pollock, N., and Ford, R. C. (2014). CFTR structure and cystic fibrosis. Int. J. Biochem. Cell. Biol. 52, 15–25. doi:10.1016/j.biocel.2014.02.004
Cantero-Recasens, G., Gonzalez, J. R., Fandos, C., Duran-Tauleria, E., Smit, L. A., Kauffmann, F., et al. (2010). Loss of function of transient receptor potential vanilloid 1 (TRPV1) genetic variant is associated with lower risk of active childhood asthma. J. Biol. Chem. 285, 27532–27535. doi:10.1074/jbc.C110.159491
Cardoso, T. A., Roncada, C., Silva, E. R., Pinto, L. A., Jones, M. H., Stein, R. T., et al. (2017). The impact of asthma in Brazil: A longitudinal analysis of data from a Brazilian national database system. J. Bras. Pneumol. 43, 163–168. doi:10.1590/S1806-37562016000000352
Carroll, N., Elliot, J., Morton, A., and James, A. (1993). The structure of large and small airways in nonfatal and fatal asthma. Am. Rev. Respir. Dis. 147, 405–410. doi:10.1164/ajrccm/147.2.405
Castillo, K., Diaz-Franulic, I., Canan, J., Gonzalez-Nilo, F., and Latorre, R. (2018). Thermally activated TRP channels: Molecular sensors for temperature detection. Phys. Biol. 15, 021001. doi:10.1088/1478-3975/aa9a6f
Cherry, W. B., Yoon, J., Bartemes, K. R., Iijima, K., and Kita, H. (2008). A novel IL-1 family cytokine, IL-33, potently activates human eosinophils. J. Allergy Clin. Immunol. 121, 1484–1490. doi:10.1016/j.jaci.2008.04.005
Chu, D. K., Jimenez-Saiz, R., Verschoor, C. P., Walker, T. D., Goncharova, S., Llop-Guevara, A., et al. (2014). Indigenous enteric eosinophils control DCs to initiate a primary TH2 immune response in vivo. J. Exp. Clin. Med. 211, 1657–1672. doi:10.1084/jem.20131800
Cohen, L., Xueping, E., Tarsi, J., Ramkumar, T., Horiuchi, T. K., Cochran, R., et al. (2007). Epithelial cell proliferation contributes to airway remodeling in severe asthma. Am. J. Respir. Crit. Care Med. 176, 138–145. doi:10.1164/rccm.200607-1062OC
Colice, G. L. (2006). New developments in inhaled corticosteroids. Allergy Asthma Proc. 27, 332–340. doi:10.2500/aap.2006.27.2929
Coste, B., Mathur, J., Schmidt, M., Earley, T. J., Ranade, S., Petrus, M. J., et al. (2010). PIEZO1 and Piezo2 are essential components of distinct mechanically activated cation channels. Science 330, 55–60. doi:10.1126/science.1193270
Coste, B., Xiao, B., Santos, J. S., Syeda, R., Grandl, J., Spencer, K. S., et al. (2012). Piezo proteins are pore-forming subunits of mechanically activated channels. Nature 483 (7388), 176–181. doi:10.1038/nature10812
Coyle, A. J., Ackerman, S. J., Burch, R., Proud, D., and Irvin, C. G. (1995). Human eosinophil-granule major basic protein and synthetic polycations induce airway hyperresponsiveness in vivo dependent on bradykinin generation. J. Clin. Investig. 95, 1735–1740. doi:10.1172/JCI117850
Crespo-Lessmann, A., Bernal, S., Del Río, E., Rojas, E., Martínez-Rivera, C., Marina, N., et al. (2021). Association of the CFTR gene with asthma and airway mucus hypersecretion. Plos one 16 (6), e0251881. doi:10.1371/journal.pone.0251881
Crottès, D., and Jan, L. Y. (2019). The multifaceted role of TMEM16A in cancer. Cell. Calcium 82, 102050. doi:10.1016/j.ceca.2019.06.004
Cruz-Rangel, S., De Jesús-Pérez, J. J., Contreras-Vite, J. A., Pérez-Cornejo, P., Hartzell, H. C., and Arreola, J. (2015). Gating modes of calcium-activated chloride channels TMEM16A and TMEM16B. J. Physiol. 593 (24), 5283–5298. doi:10.1113/JP271256
Csanády, L., Vergani, P., and Gadsby, D. C. (2010). Strict coupling between CFTR’s catalytic cycle and gating of its Cl-ion pore revealed by distributions of open channel burst durations. Proc. Natl. Acad. Sci. U. S. A. 107, 1241–1246. doi:10.1073/pnas.0911061107
Danahay, H., Atherton, H., Jones, G., Bridges, R. J., and Poll, C. T. (2002). Interleukin-13 induces a hypersecretory ion transport phenotype in human bronchial epithelial cells. Am. J. Physiol. Lung Cell. Mol. Physiol. 282 (2), L226–L236. doi:10.1152/ajplung.00311.2001
De Boever, E. H., Ashman, C., Cahn, A. P., Locantore, N. W., Overend, P., Pouliquen, I. J., et al. (2014). Efficacy and safety of an anti–IL-13 mAb in patients with severe asthma: A randomized trial. J. Allergy Clin. Immunol. 133, 989–996. doi:10.1016/j.jaci.2014.01.002
Dean, M., and Annilo, T. (2005). Evolution of the ATP-binding cassette (ABC) transporter superfamily in vertebrates. Annu. Rev. Genomics Hum. Genet. 6, 123–142. doi:10.1146/annurev.genom.6.080604.162122
Deletang, K., and Taulan-Cadars, M. (2022). Splicing mutations in the CFTR gene as therapeutic targets. Gene Ther. 29, 399–406. doi:10.1038/s41434-022-00347-0
Dhakal, S., and Lee, Y. (2019). Transient receptor potential channels and metabolism. Mol. Cells 42, 569–578. doi:10.14348/molcells.2019.0007
Diem, K., Fauler, M., Fois, G., Hellmann, A., Winokurow, N., Schumacher, S., et al. (2020). Mechanical stretch activates PIEZO1 in caveolae of alveolar type I cells to trigger ATP release and paracrine stimulation of surfactant secretion from alveolar type II cells. FASEB J. 34 (9), 12785–12804. doi:10.1096/fj.202000613RRR
Dietrich, A. (2019). Transient receptor potential (TRP) channels in health and disease. Cells 8, 413. doi:10.3390/cells8050413
Donohue, J. F., Kerwin, E., Sethi, S., Haumann, B., Pendyala, S., Dean, L., et al. (2019). Revefenacin, a once-daily, lung-selective, long-acting muscarinic antagonist for nebulized therapy: Safety and tolerability results of a 52-week phase 3 trial in moderate to very severe chronic obstructive pulmonary disease. Respir. Med. 153, 38–43. doi:10.1016/j.rmed.2019.05.010
Doucet, C., Brouty-Boyé, D., Pottin-Clémenceau, C., Canonica, G. W., Jasmin, C., and Azzarone, B. (1998). Interleukin (IL) 4 and IL-13 act on human lung fibroblasts. Implication in asthma. J. Clin. Investig. 101, 2129–2139. doi:10.1172/JCI741
Douguet, D., and Honoré, E. (2019). Mammalian mechanoelectrical transduction: Structure and function of force-gated ion channels. Cell. 179 (2), 340–354. doi:10.1016/j.cell.2019.08.049
Duan, J., Xie, J., Deng, T., Xie, X., Liu, H., Li, B., et al. (2020). Exposure to both formaldehyde and high relative humidity exacerbates allergic asthma by activating the TRPV4-p38 MAPK pathway in Balb/c mice. Environ. Pollut. 256, 113375. doi:10.1016/j.envpol.2019.113375
Egan, T. M., Samways, D. S. K., and Li, Z. (2006). Biophysics of P2X receptors. Pflügers Archiv Eur. J. Physiology 452 (5), 501–512. doi:10.1007/s00424-006-0078-1
Eisenhoffer, G. T., Loftus, P. D., Yoshigi, M., Otsuna, H., Chien, C. B., Morcos, P. A., et al. (2012). Crowding induces live cell extrusion to maintain homeostatic cell numbers in epithelia. Nature 484 (7395), 546–549. doi:10.1038/nature10999
Elias, J. A., Zhu, Z., Chupp, G., and Homer, R. J. (1999). Airway remodeling in asthma. J. Clin. Invest. 104, 1001–1006. doi:10.1172/JCI8124
Evans, P., Lyras, L., and Halliwell, B. (1999). Measurement of protein carbonyls in human brain tissue. Methods Enzym. 300, 145–156. doi:10.1016/s0076-6879(99)00122-6
Fahrner, M., Grabmayr, H., and Romanin, C. (2020). Mechanism of STIM activation. Curr. Opin. Psychol. 17, 74–79. doi:10.1016/j.cophys.2020.07.006
Fang, X. Z., Zhou, T., Xu, J. Q., Wang, Y. X., Sun, M. M., He, Y. J., et al. (2021). Structure, kinetic properties and biological function of mechanosensitive Piezo channels. Cell. Biosc 11, 13–20. doi:10.1186/s13578-020-00522-z
Gadsby, D. C., Vergani, P., and Csanády, L. (2006). The ABC protein turned chloride channel whose failure causes cystic fibrosis. Nature 440, 477–483. doi:10.1038/nature04712
Galietta, L. J., Pagesy, P., Folli, C., Caci, E., Romio, L., Costes, B., et al. (2002). IL-4 is a potent modulator of ion transport in the human bronchial epithelium in vitro. J. Immunol. 168 (2), 839–845. doi:10.4049/jimmunol.168.2.839
Galli, S. J., Nakae, S., and Tsai, M. (2005). Mast cells in the development of adaptive immune responses. Nat. Immunol. 6, 135–142. doi:10.1038/ni1158
Gallo, V., Dijk, F. N., Holloway, J. W., Ring, S. M., Koppelman, G. H., Postma, D. S., et al. (2017). TRPA 1 gene polymorphisms and childhood asthma. Pediatr. Allergy Immunol. 28, 191–198. doi:10.1111/pai.12673
Gentzsch, M., Dang, H., Dang, Y., Garcia-Caballero, A., Suchindran, H., Boucher, R. C., et al. (2010). The cystic fibrosis transmembrane conductance regulator impedes proteolytic stimulation of the epithelial Na+ channel. J. Biol. Chem. 285 (42), 32227–32232. doi:10.1074/jbc.M110.155259
Girodet, P. O., Ozier, A., Carvalho, G., Ilina, O., Ousova, O., Gadeau, A. P., et al. (2013). Ca2+-activated K+ channel-3.1 blocker TRAM-34 attenuates airway remodeling and eosinophilia in a murine asthma model. Am. J. Respir. Cell. Mol. Biol. 48, 212–219. doi:10.1165/rcmb.2012-0103OC
Global Asthma Network (2018). The global asthma report 2018. Available at: http://www.globalasthmareport.org (Accessed October 12, 2022).
GINA - Global Initiative for Asthma (2022). Global strategy for asthma management and prevention. Available at: http://www.ginathma.org (Accessed December 12, 2022).
Godfrey, R. W. A. (1997). Human airway epithelial tight junctions. Micros. Res. Tech. 38 (5), 488–499. doi:10.1002/(SICI)1097-0029(19970901)38:5<488::AID-JEMT5>3.0.CO;2-E
Goldklang, M. P., Perez-Zoghbi, J. F., Trischler, J., Nkyimbeng, T., Zakharov, S. I., Shiomi, T., et al. (2013). Treatment of experimental asthma using a single small molecule with anti-inflammatory and BK channel activating properties. FASEB J. 27, 4975–4986. doi:10.1096/fj.13-235176
Gombedza, F. C., Kondeti, V., Al-Azzam, N., Thodeti, C., and Paruchuri, S. (2017). Transient receptor potential vanilloid 4 (TRPV4) regulates fibroblast differentiation in vitro and D. farinae-induced airway remodeling in asthma in vivo via modulation of matrix synthesis and matrix degradation mechanisms. FASEB J. 31, 772.2. doi:10.1096/fasebj.31.1_supplement.772.2
Gon, Y., and Hashimoto, S. (2018). Role of airway epithelial barrier dysfunction in pathogenesis of asthma. Allergol. Int. 67 (1), 12–17. doi:10.1016/j.alit.2017.08.011
Gonzalez-Perez, V., and Lingle, C. J. (2019). Regulation of BK channels by beta and gamma subunits. Annu. Rev. Physiol. 81, 113–137. doi:10.1146/annurev-physiol-022516-034038
Gordon, J. R., and Galli, S. J. (1990). Mast cells as a source of both preformed and immunologically inducible TNF-alpha/cachectin. Nature 346, 274–276. doi:10.1038/346274a0
Grutzkau, A., Kruger-Krasagakes, S., Baumeister, H., Schwarz, C., Kogel, H., Welker, P., et al. (1998). Synthesis, storage, and release of vascular endothelial growth factor/vascular permeability factor (VEGF/VPF) by human mast cells: Implications for the biological significance of VEGF206. Mol. Biol. Cell. 9, 875–884. doi:10.1091/mbc.9.4.875
Guéguinou, M., ChantÔme, A., Fromont, G., Bougnoux, P., Vandier, C., and Potier-Cartereau, M. (2014). KCa and Ca2+ channels: The complex thought. Biochim. Biophys. Acta – Mol. Cell. Res. 1843, 2322–2333. doi:10.1016/j.bbamcr.2014.02.019
Guibert, C., Ducret, T., and Savineau, J. P. (2011). “Expression and physiological roles of TRP channels in smooth muscle cells,” in Transient receptor potential channels. Editor M. Islam (Dordrecht: Springer), 687–706.
Gurish, M. F., and Austen, K. F. (2012). Developmental origin and functional specialization of mast cell subsets. Immunity 37, 25–33. doi:10.1016/j.immuni.2012.07.003
Gustafsson, J. K., Ermund, A., Ambort, D., Johansson, M. E., Nilsson, H. E., Thorell, K., et al. (2012). Bicarbonate and functional CFTR channel are required for proper mucin secretion and link cystic fibrosis with its mucus phenotype. J. Exp. Med. 209 (7), 1263–1272. doi:10.1084/jem.20120562
Hammad, H., and Lambrecht, B. N. (2021). The basic immunology of asthma. Cell. 184 (6), 1469–1485. doi:10.1016/j.cell.2021.02.016
Harris, A., Chalkley, G., Goodman, S., and Coleman, L. (1991). Expression of the cystic fibrosis gene in human development. Development 113, 305–310. doi:10.1242/dev.113.1.305
Hattori, M., and Gouaux, E. (2012). Molecular mechanism of ATP binding and ion channel activation in P2X receptors. Nature 485 (7397), 207–212. doi:10.1038/nature11010
Hasan, R., and Zhang, X. (2018). Ca2+ regulation of TRP ion channels. Int. J. Mol. Sci. 19, 1256. doi:10.3390/ijms19041256
He, Y., Deng, B., Liu, S., Luo, S., Ning, Y., Pan, X., et al. (2022). Myeloid PIEZO1 deletion protects renal fibrosis by restraining macrophage infiltration and activation. Hypertension 79 (5), 918–931. doi:10.1161/HYPERTENSIONAHA.121.18750
Hilton, J. K., Kim, M., and Van Horn, W. D. (2019). Structural and evolutionary insights point to allosteric regulation of TRP ion channels. Acc. Chem. Res. 52, 1643–1652. doi:10.1021/acs.accounts.9b00075
Hite, R., Tao, X., and MacKinnon, R. (2017). Structural basis for gating the high-conductance Ca2+-activated K+ channel. Nature 541, 52–57. doi:10.1038/nature20775
Hodsman, P., Ashman, C., Cahn, A., Boever, E., Locantore, N., Serone, A., et al. (2013). A phase 1, randomized, placebo-controlled, dose-escalation study of an anti- IL-13 monoclonal antibody in healthy subjects and mild asthmatics. Br. J. Clin. Pharmacol. 75, 118–128. doi:10.1111/j.1365-2125.2012.04334.x
Holgate, S. T., and Polosa, R. (2008). Treatment strategies for allergy and asthma. Nat. Rev. Immunol. 8, 218–230. doi:10.1038/nri2262
Hoover, P. J., and Lewi, R. S. (2011). Stoichiometric requirements for trapping and gating of Ca2+ release-activated Ca2+ (CRAC) channels by stromal interaction molecule 1 (STIM1). Pro. Natl. Acad. Sci. 108, 13299–13304. doi:10.1073/pnas.1101664108
Hoshi, T., Pantazis, A., and Olcese, R. (2013). Transduction of voltage and Ca2+ signals by Slo1 BK channels. Physiology 28, 172–189. doi:10.1152/physiol.00055.2012
Hou, X., Pedi, L., Diver, M. M., and Long, S. B. (2012). Crystal structure of the calcium release–activated calcium channel Orai. Science 338, 1308–1313. doi:10.1126/science.1228757
Huang, F., Zhang, H., Wu, M., Yang, H., Kudo, M., Peters, C. J., et al. (2012). Calcium-activated chloride channel TMEM16A modulates mucin secretion and airway smooth muscle contraction. Proc. Natl. Acad. Sci. U.S.A. 109 (40), 16354–16359. doi:10.1073/pnas.1214596109
Humbert, M., Busse, W., Hanania, N. A., Lowe, P. J., Canvin, J., Erpenbeck, V. J., et al. (2014). Omalizumab in asthma: An update on recent developments. J. Allergy Clin. Immunol. 2, 525–536. doi:10.1016/j.jaip.2014.03.010
Jeng, G., Aggarwal, M., Yu, W. P., and Chen, T. Y. (2016). Independent activation of distinct pores in dimeric TMEM16A channels. J. Gen. Physiol. 148, 393–404. doi:10.1085/jgp.201611651
Jenkins, H. A., Cool, C., Szefler, S. J., Covar, R., Brugman, S., Gelfand, E. W., et al. (2003). Histopathology of severe childhood asthma: A case series. Chest 124, 32–41. doi:10.1378/chest.124.1.32
Jesús-Pérez, J. J., Cruz-Rangel, S., Espino-Saldaña, Á. E., Martínez-Torres, A., Qu, Z., Hartzell, H. C., et al. (2018). Phosphatidylinositol 4, 5-bisphosphate, cholesterol, and fatty acids modulate the calcium-activated chloride channel TMEM16A (ANO1). Biochim. Biophys. Acta Mol. Cell. Biol. Lipids 3, 299–312. 1863. doi:10.1016/j.bbalip.2017.12.009
Jiang, Y., Yang, X., Jiang, J., and Xiao, B. (2021). Structural designs and mechanogating mechanisms of the mechanosensitive piezo channels. Trends biochem. Sci. 46, 472–488. doi:10.1016/j.tibs.2021.01.008
Johnson, M., and Trebak, M. (2019). ORAI channels in cellular remodeling of cardiorespiratory disease. Cell. Calcium 79, 1–10. doi:10.1016/j.ceca.2019.01.005
Kamaleddin, M. A. (2018). Molecular, biophysical, and pharmacological properties of calcium-activated chloride channels. J. Cell. Physiol. 233 (2), 787–798. doi:10.1002/jcp.25823
Kappel, S., Borgström, A., Stok, P., Dörr, K., and Peinelt, C. (2019). Store-operated calcium entry in disease: Beyond STIM/Orai expression levels. Semin. Cell. Dev. Biol. 94, 66–73. doi:10.1016/j.semcdb.2019.01.003
Khakh, S. B. (2001). Molecular physiology of p2x receptors and atp signalling at synapses. Nat. Rev. 2, 165–174. doi:10.1038/35058521
Kirkham, S., Sheehan, J. K., Knight, D., Richardson, P. S., and Thornton, D. J. (2002). Heterogeneity of airways mucus: Variations in the amounts and glycoforms of the major oligomeric mucins MUC5AC and MUC5B. Biochem. J. 361, 537–546. doi:10.1042/0264-6021:3610537
Kocmalova, M., Oravec, M., Adamkov, M., Sadlonova, V., Kazimierova, I., Medvedova, I., et al. (2015). Potassium ion channels and allergic asthma. Ad. Exp. Med. Biol. 838, 35–45. doi:10.1007/5584_2014_76
Kondo, M., Tsuji, M., Hara, K., Arimura, K., Yagi, O., Tagaya, E., et al. (2017). Chloride ion transport and overexpression of TMEM 16A in a Guinea-pig asthma model. Clin. Exp. Allergy 47 (6), 795–804. doi:10.1111/cea.12887
Korzeniowski, M. K., Wisniewski, E., Baird, B., Holowka, D. A., and Balla, T. (2017). Molecular anatomy of the early events in STIM1 activation – oligomerization or conformational change? J. Cell. Sci. 130, 2821–2832. doi:10.1242/jcs.205583
Kshatri, A. S., Gonzalez-Hernandez, A., and Giraldez, T. (2018). Physiological roles and therapeutic potential of Ca2+ activated potassium channels in the nervous system. Front. Mol. Neurosci. 11, 258. doi:10.3389/fnmol.2018.00258
Kulkarni, S., Li, Q., Singhi, A. D., Liu, S., Monga, S. P., and Feranchak, A. P. (2023). TMEM16A partners with mTOR to influence pathways of cell survival, proliferation and migration in cholangiocarcinoma. Am. J. Physiol. Gastrointest. Liver Physiol. 325, G122–G134. doi:10.1152/ajpgi.00270.2022
Kunzelmann, K., Tian, Y., Martins, J. R., Faria, D., Kongsuphol, P., Ousingsawat, J., et al. (2012). Airway epithelial cells--functional links between CFTR and anoctamin dependent Cl- secretion. Int. J. Biochem. Cell. Biol., 44, 1897–1900. doi:10.1016/j.biocel.2012.06.011
Kytikova, O. Y., Novgorodtseva, T. P., Denisenko, Y. K., Antonyuk, M. V., and Gvozdenko, T. A. (2020). Dysfunction of transient receptor potential ion channels as an important pathophysiological mechanism in asthma. Open Med. J. 9, 102. doi:10.15275/rusomj.2020.0102
Lackie, P. M., Baker, J. E., Gunthert, U., and Holgate, S. T. (1997). Expression of CD44 isoforms is increased in the airway epithelium of asthmatic subjects. Am. J. Respir. Cell. Mol. Biol. 16, 14–22. doi:10.1165/ajrcmb.16.1.8998074
Laing, R. J., and Dhaka, A. (2016). Thermo TRPs and pain. Neurosci. 22, 171–187. doi:10.1177/1073858414567884
Lambrecht, B. N., Hammad, H., and Fahy, J. V. (2019). The cytokines of asthma. Immunity 50 (4), 975–991. doi:10.1016/j.immuni.2019.03.018
Lane, S. J., and Lee, T. H. (1996). Mast cell effector mechanisms. J. Allergy Clin. Immunol. 98, S67–S71. doi:10.1016/s0091-6749(96)80131-x
Large, W. A., and Wang, Q. (1996). Characteristics and physiological role of the Ca(2+)-activated Cl- conductance in smooth muscle. Am. J. Physiol. 271, C435–C454. doi:10.1152/ajpcell.1996.271.2.C435
Latorre, R., Castillo, K., Carrasquel-Ursulaez, W., Sepulveda, R. V., Gonzalez-Nilo, F., Gonzalez, C., et al. (2017). Molecular determinants of BK channel functional diversity and functioning. Physiol. Rev. 97, 39–87. doi:10.1152/physrev.00001.2016
Le Cras, T. D., Acciani, T. H., Mushaben, E. M., Kramer, E. L., Pastura, P. A., Hardie, W. D., et al. (2011). Epithelial EGF receptor signaling mediates airway hyperreactivity and remodeling in a mouse model of chronic asthma. Am. J. Physiol. Lung Cell. Mol. Physiol. 300, 414–421. doi:10.1152/ajplung.00346.2010
Lee, I. T., and Yang, C. M. (2012). Role of NADPH oxidase/ROS in pro-inflammatory mediators-induced airway and pulmonary diseases. Biochem. Pharmacol. 84, 581–590. doi:10.1016/j.bcp.2012.05.005
Lee, U. S., and Cui, J. (2010). BK channel activation: Structural and functional insights. Trends Neurosci. 33, 415–423. doi:10.1016/j.tins.2010.06.004
Lewis, R. S. (2020). Store-operated calcium channels: From function to structure and back again. Cold Spring Harb. Perspect. Biol. 12, a035055. doi:10.1101/cshperspect.a035055
Li, J., Hou, B., Tumova, S., Muraki, K., Bruns, A., Ludlow, M. J., et al. (2014). PIEZO1 integration of vascular architecture with physiological force. Nature 515 (7526), 279–282. doi:10.1038/nature13701
Lindstedt, K. A., Wang, Y., Shiota, N., Saarinen, J., Hyytiäinen, M., Kokkonen, J. O., et al. (2001). Activation of paracrine TGF-beta1 signaling upon stimulation and degranulation of rat serosal mast cells: A novel function for chymase. FASEB J. 15, 1377–1388. doi:10.1096/fj.00-0273com
Linsdell, P. (2017). Architecture and functional properties of the CFTR channel pore. Cell. Mol. Life Sci. 74, 67–83. doi:10.1007/s00018-016-2389-5
Liu, F., Zhang, Z., Csanády, L., Gadsby, D. C., and Chen, J. (2017). Molecular structure of the human CFTR ion channel. Cell. 169, 85–95. doi:10.1016/j.cell.2017.02.024
Liu, Y., Liu, Z., and Wang, K. (2021). The Ca2+-activated chloride channel ANO1/TMEM16A: An emerging therapeutic target for epithelium-originated diseases? Acta Pharm. Sin. B 11 (6), 1412–1433. doi:10.1016/j.apsb.2020.12.003
Logu, F., Patacchini, R., Fontana, G., and Geppetti, P. (2016). “TRP functions in the broncho-pulmonary system,” in Seminars in immunopathology (Springer Berlin Heidelberg), 321–329.
Lonso-Carbajo, L., Kecskes, M., Jacobs, G., Pironet, A., Syam, N., Talavera, K., et al. (2017). Muscling in on TRP channels in vascular smooth muscle cells and cardiomyocytes. Cell. Calcium 66, 48–61. doi:10.1016/j.ceca.2017.06.004
Lopez, J. J., Jardin, I., Albarrán, L., Sanchez-Collado, J., Cantoner, C., Salido, G. M., et al. (2020). “Molecular basis and regulation of store-operated calcium entry,” in Calcium signaling. Editor M. Islam (Cham: Springer), 445–469.
Lordan, J., Bucchieri, F., Richter, A., Konstantinidis, A., Holloway, J. W., Thornber, M., et al. (2002). Cooperative effects of TH2 cytokines and allergen on normal and asthmatic bronchial epithelial cells. J. Immunol. 169, 407–414. doi:10.4049/jimmunol.169.1.407
Luo, M., Ni, K., Gu, R., Qin, Y., Guo, J., Che, B., et al. (2023). Chemical activation of PIEZO1 alters biomechanical behaviors toward relaxation of cultured airway smooth muscle cells. Biol. Pharm. Bull. 46 (1), 1–11. doi:10.1248/bpb.b22-00209
Ma, S., Dubin, A. E., Zhang, Y., Mousavi, S. A. R., Wang, Y., Coombs, A. M., et al. (2021). A role of PIEZO1 in iron metabolism in mice and humans. Cell. 184 (4), 969–982.e13. doi:10.1016/j.cell.2021.01.024
Mantese, V. J., Rafaels, N., Murray, T., Vergara, C., Gao, L., Araujo, M. I., et al. (2011). Replication of a genetic association between polymorphisms in KCNMA1 and asthma in six populations of african ancestry. J. Allergy Clin. Immunol. 127, AB213. doi:10.1016/j.jaci.2010.12.846
Martin, J. G., Duguet, A., and Eidelman, D. H. (2000). The contribution of airway smooth muscle to airway narrowing and airway hyperresponsiveness in disease. Eur. Respir. J. 16, 349–354. doi:10.1034/j.1399-3003.2000.16b25.x
Matsukura, S., Stellato, C., Georas, S. N., Casolaro, V., Plitt, J. R., Miura, K., et al. (2001). Interleukin-13 upregulates eotaxin expression in airway epithelial cells by a STAT6-dependent mechanism. Am. J. Respir. Cell. Mol. Biol. 24, 755–761. doi:10.1165/ajrcmb.24.6.4351
Maus, M., Jairaman, A., Stathopulos, P. B., Muik, M., Fahrner, M., Weidinger, C., et al. (2015). Missense mutation in immunodeficient patients shows the multifunctional roles of coiled-coil domain 3 (CC3) in STIM1 activation. Proc. Natl. Acad. Sci. 112, 6206–6211. doi:10.1073/pnas.1418852112
McGarvey, L. P., Butler, C. A., Stokesberry, S., Polley, L., McQuaid, S., Ashraf, S., et al. (2014). Increased expression of bronchial epithelial transient receptor potential vanilloid 1 channels in patients with severe asthma. J. Allergy Clin. Immunol. 133, 704–712. doi:10.1016/j.jaci.2013.09.016
McMahon, S. B., La Russa, F., and Bennett, D. L. (2015). Crosstalk between the nociceptive and immune systems in host defence and disease. Nat. Rev. Neurosci. 16, 389–402. doi:10.1038/nrn3946
Michalick, L., and Kuebler, W. M. (2020). TRPV4—A missing link between mechanosensation and immunity. Front. Immunol. 11, 413. doi:10.3389/fimmu.2020.00413
Miller, A. C., Comellas, A. P., Hornick, D. B., Stoltz, D. A., Cavanaugh, J. E., Gerke, A. K., et al. (2020). Cystic fibrosis carriers are at increased risk for a wide range of cystic fibrosis-related conditions. Proc. Natl. Acad. Sci. U. S. A. 117, 1621–1627. doi:10.1073/pnas.1914912117
Moore, W. C., Meyers, D. A., Wenzel, S. E., Teague, W. G., Li, H., Li, X., et al. (2010). Identification of asthma phenotypes using cluster analysis in the Severe Asthma Research Program. Am. J. Respir. Crit. Care Med. 181, 315–323. doi:10.1164/rccm.200906-0896OC
Moran, M., McAlexander, M. A., Biro, T., and Szallasi, A. (2011). Transient receptor potential channels as therapeutic targets. Nat. Rev. Drug Discov. 10, 601–620. doi:10.1038/nrd3456
Mukhopadhyay, I., Gomes, P., Aranake, S., Shetty, M., Karnik, P., Damle, M., et al. (2011). Expression of functional TRPA1 receptor on human lung fibroblast and epithelial cells. J. Recept Signal Transduct. Res. 31, 350–358. doi:10.3109/10799893.2011.602413
Niu, L., Wu, F., Li, K., Li, J., Zhang, S. L., Hu, J., et al. (2020). STIM1 interacts with termini of Orai channels in a sequential manner. J. Cell. Sci. 133, jcs239491. doi:10.1242/jcs.239491
North, R. A. (2016). P2X receptors. Philosophical Trans. R. Soc. B Biol. Sci. 371, 20150427. doi:10.1098/rstb.2015.0427
Nwokonko, R. M., Cai, X., Loktionova, N. A., Wang, Y., Zhou, Y., and Gill, D. L. (2017). “The STIM-orai pathway: Conformational coupling between STIM and Orai in the activation of store-operated Ca2+ entry,” in Store-operated Ca2⁺ entry (SOCE) pathways. Advances in experimental Medicine and biology. Editors K. Groschner, W. Graier, and C. Romanin (Springer), 83–98.
Offermanns, S., Wang, S., Shi, Y., Moeller, T., Stegmeyer, R., Strilic, B., et al. (2021). Mechanosensation by endothelial PIEZO1 is required for leukocyte diapedesis. Blood. 140. doi:10.21203/rs.3.rs-208666/v1
Oguma, T., Ito, S., Kondo, M., Makino, Y., Shimokata, K., Honjo, H., et al. (2007). Roles of P2X receptors and Ca2+sensitization in extracellular adenosine triphosphate-induced hyperresponsiveness in airway smooth muscle. Clin. Exp. Allergy 37 (6), 893–900. doi:10.1111/j.1365-2222.2007.02719.x
Ohya, S., and Kito, H. (2018). Ca2+-activated K+ channel KCa3.1 as a therapeutic target for immune disorders. Biol. Pharm. Bull. 41, 1158–1163. doi:10.1248/bpb.b18-00078
Okuda, K., Dang, H., Kobayashi, Y., Carraro, G., Nakano, S., Chen, G., et al. (2021). Secretory cells dominate airway CFTR expression and function in human airway superficial epithelia. Am. J. Respir. Crit. Care Med. 203, 1275–1289. doi:10.1164/rccm.202008-3198OC
Ordoñez, C. L., Khashayar, R., Wong, H. H., Ferrando, R., Wu, R., Hyde, D. M., et al. (2001). Mild and moderate asthma is associated with airway goblet cell hyperplasia and abnormalities in mucin gene expression. Am. J. Respir. Crit. Care Med. 163, 517–523. doi:10.1164/ajrccm.163.2.2004039
Paulino, C., Kalienkova, V., Lam, A. K. M., Neldner, Y., and Dutzler, R. (2017). Activation mechanism of the calcium-activated chloride channel TMEM16A revealed by cryo-EM. Nature 552, 421–425. doi:10.1038/nature24652
Pedemonte, N., and Galietta, L. J. V. (2014). Structure and function of TMEM16 proteins (anoctamins). Physiol. Rev. 94 (2), 419–459. doi:10.1152/physrev.00039.2011
Pelletier, L., and Savignac, M. (2018). Involvement of ion channels in allergy. Curr. Opin. Immunol. 52, 60–67. doi:10.1016/j.coi.2018.04.006
Pera, T., and Penn, R. B. (2016). Bronchoprotection and bronchorelaxation in asthma: New targets, and new ways to target the old ones. Pharmacol. Ther. 164, 82–96. doi:10.1016/j.pharmthera.2016.04.002
Ponath, P. D., Qin, S., Post, T. W., Wang, J., Wu, L., Gerard, N. P., et al. (1996). Molecular cloning and characterization of a human eotaxin receptor expressed selectively on eosinophils. J. Exp. Med. 183, 2437–2448. doi:10.1084/jem.183.6.2437
Powell, C., Milan, S. J., Dwan, K., Bax, L., and Walters, N. (2015). Mepolizumab versus placebo for asthma. Cochrane Database Syst. Rev. 7, CD010834. doi:10.1002/14651858.CD010834.pub2
Prado, C. M., Righetti, R. F., Silva Pigati, P. A., Possa, S. S., and Santos, A. S. A. (2014). New pharmacological targets for asthma drug development. J. Allergy Ther. 5, 170. doi:10.4172/2155-6121.1000170
Preti, D., Szallasi, A., and Patacchini, R. (2012). TRP channels as therapeutic targets in airway disorders: A patent review. Expert Opin. Ther. Pat. 22, 663–695. doi:10.1517/13543776.2012.696099
Qiu, R., and Lewis, R. S. (2019). Structural features of STIM and Orai underlying store-operated calcium entry. Curr. Opin. Cell. Biol. 57, 90–98. doi:10.1016/j.ceb.2018.12.012
Ramadas, R. A., Ewart, S. L., Medoff, B. D., and Levine, A. M. (2011). Interleukin-1–family member 9 stimulates chemokine production and neutrophil influx in mouse lungs. Am. J. Respir. Cell. Mol. Biol. 44, 134–145. doi:10.1165/rcmb.2009-0315OC
Reed, J. A., Albino, A. P., and Mcnutt, N. S. (1995). Human cutaneous mast cells express basic fibroblast growth factor. Lab. Invest. 72, 215–222.
Reese, R. M., Dourado, M., Anderson, K., Warming, S., Stark, K. L., Balestrini, A., et al. (2020). Behavioral characterization of a CRISPR-generated TRPA1 knockout rat in models of pain, itch, and asthma. Sci. Rep. 10, 979. doi:10.1038/s41598-020-57936-5
Ren, Y. F., Li, H., Xing, X. H., Guan, H. S., Zhang, B. A., Chen, C. L., et al. (2015). Preliminary study on pathogenesis of bronchial asthma in children. Pediatr. Res. 77, 506–510. doi:10.1038/pr.2015.11
Robinson, D. S., Hamid, Q., Ying, S., Tsicopoulos, A., Barkans, J., Bentley, A. M., et al. (1992). Predominant TH2-like bronchoalveolar T-lymphocyte population in atopic asthma. N. Engl. J. Med. 326, 298–304. doi:10.1056/NEJM199201303260504
Romagnani, S. (2000). The role of lymphocytes in allergic disease. J. Allergy Clin. Immunol. 105, 399–408. doi:10.1067/mai.2000.104575
Rose, M. C., and Voynow, J. A. (2006). Respiratory tract mucin genes and mucin glycoproteins in health and disease. Physiol. Ver. 86, 245–278. doi:10.1152/physrev.00010.2005
Rosenbaum, T., Benítez-Angeles, M., Sánchez-Hernández, R., Morales-Lázaro, S. L., Hiriart, M., Morales-Buenrostro, L. E., et al. (2020). TRPV4: A physio and pathophysiologically significant ion channel. Int. J. Mol. Sci. 21, 3837. doi:10.3390/ijms21113837
Rottgen, T., Nickerson, A., and Rajendran, V. (2018). Calcium-activated Cl− channel: Insights on the molecular identity in epithelial tissues. Int. J. Mol. Sci. 19 (5), 1432. doi:10.3390/ijms19051432
Sadofsky, L. R., Ramachandran, R., Crow, C., Cowen, M., Compton, S. J., and Morice, A. H. (2012). Inflammatory stimuli up-regulate transient receptor potential vanilloid-1 expression in human bronchial fibroblasts. Exp. Lung Res. 38, 75–81. doi:10.3109/01902148.2011.644027
Saenz, S. A., Siracusa, M. C., Perrigoue, J. G., Spencer, S. P., Urban, J. F., Tocker, J. E., et al. (2010). IL25 elicits a multipotent progenitor cell population that promotes TH2 cytokine responses. Nature 464, 1362–1366. doi:10.1038/nature08901
Saetta, M., Di Stefano, A., Rosina, C., Thiene, G., and Fabbri, L. M. (1991). Quantitative structural analysis of peripheral airways and arteries in sudden fatal asthma. Am. Ver. Respir. Dis. 143, 138–143. doi:10.1164/ajrccm/143.1.138
Samivel, R., Kim, D. W., Son, H. R., Rhee, Y. H., Kim, E. H., Kim, J. H., et al. (2016). The role of TRPV1 in the CD4+ T cell-mediated inflammatory response of allergic rhinitis. Oncotarget 7, 148–160. doi:10.18632/oncotarget.6653
Scheraga, R. G., Southern, B. D., Grove, L. M., and Olman, M. A. (2017). The role of transient receptor potential vanilloid 4 in pulmonary inflammatory diseases. Front. Immunol. 8, 503. doi:10.3389/fimmu.2017.00503
Schmidt, M., Sun, G., Stacey, M. A., Mori, L., and Mattoli, S. (2003). Identification of circulating fibrocytes as precursors of bronchial myofibroblasts in asthma. J. Immunol. 171, 380–389. doi:10.4049/jimmunol.171.1.380
Schneider, E., Petit-Bertron, A. F., Bricard, R., Levasseur, M., Ramadan, A., Girard, J. P., et al. (2009). IL-33 activates unprimed murine basophils directly in vitro and induces their in vivo expansion indirectly by promoting hematopoietic growth factor production. J. Immunol. 183, 3591–3597. doi:10.4049/jimmunol.0900328
Schroeder, B. C., Cheng, T., Jan, Y. N., and Jan, L. Y. (2008). Expression cloning of TMEM16A as a calcium-activated chloride channel subunit. Cell. 134 (6), 1019–1029. doi:10.1016/j.cell.2008.09.003
Schreiber, R., Uliyakina, I., Kongsuphol, P., Warth, R., and Mirza, M. (2010). Expression and function of epithelial anoctamins. J. Biol. Chem. 285 (10), 7838–7845. doi:10.1074/jbc.M109.065367
Sheng, D., and Hattori, M. (2022). Recent progress in the structural biology of P2X receptors. Proteins 90 (10), 1779–1785. doi:10.1002/prot.26302
Silver, M. R., Margulis, A., Wood, N., Goldman, S. J., Kasaian, M., and Chaudhary, D. (2010). IL-33 synergizes with IgE-dependent and IgE-independent agents to promote mast cell and basophil activation. Inflamm. Res. 59, 207–218. doi:10.1007/s00011-009-0088-5
Siroux, V., Basagaña, X., Boudier, A., Pin, I., Garcia-Aymerich, J., Vesin, A., et al. (2011). Identifying adult asthma phenotypes using a clustering approach. Eur. Respir. J. 38, 310–317. doi:10.1183/09031936.00120810
Sohma, Y., and Hwang, T. C. (2015). “Cystic fibrosis and the CFTR anion channel,” in Handbook of ion channels. Editors J. Zheng, and M. C. Trudeau (Boca Raton, FL: Taylor & Francis Group, LLC), 627–648. doi:10.1201/b18027-48
Song, J., Kang, J., Lin, B., Li, J., Zhu, Y., Du, J., et al. (2017). Mediating role of TRPV1 Ion channels in the co-exposure to PM2.5 and formaldehyde of Balb/c mice asthma model. Sci. Rep. 7, 11926. doi:10.1038/s41598-017-11833-6
Spinelli, A. M., González-Cobos, J. C., Zhang, X., Motiani, R. K., Rowan, S., Zhang, W., et al. (2012). Airway smooth muscle STIM1 and Orai1 are upregulated in asthmatic mice and mediate PDGF-activated SOCE, CRAC currents, proliferation and migration. Pflugers Arch. Eur. J. Physiol. 464, 481–492. doi:10.1007/s00424-012-1160-5
Startek, J. B., Boonen, B., Talavera, K., and Meseguer, V. (2019). TRP channels as sensors of chemically-induced changes in cell membrane mechanical properties. Int. J. Mol. Sci. 20, 371. doi:10.3390/ijms20020371
Stone, K. D., Prussin, C., and Metcalfe, D. D. (2006). IgE, mast cells, basophils, and eosinophils. J. Allergy Clin. Immunol. 117, S73–S80. doi:10.1016/j.jaci.2009.11.017
Surprenant, A., and North, R. A. (2009). Signaling at purinergic P2X receptors. Annu. Rev. Physiology 71 (1), 333–359. doi:10.1146/annurev.physiol.70.113006.100630
Šutovská, M., Kocmálová, M., Adamkov, M., Výbohová, D., Mikolka, P., Mokrá, D., et al. (2013). The long-term administration of Orai1 antagonist possesses antitussive, bronchodilatory and anti-inflammatory effects in experimental asthma model. Gen. Physiol. Biophys. 32, 251–259. doi:10.4149/gpb_2013018
Šutovská, M., Kocmálová, M., Jošková, M., Adamkov, M., and Fraňová, S. (2015). The effect of long-term administered CRAC channels blocker on the functions of respiratory epithelium in Guinea pig allergic asthma model. Gen. Physiol. Biophys. 34, 167–176. doi:10.4149/gpb_2014031
Šutovská, M., Kocmálová, M., Kazimierová, I., Forsberg, C. I. N., Jošková, M., Adamkov, M., et al. (2021). “Effects of inhalation of STIM-orai antagonist SKF 96365 on ovalbumin-induced airway remodeling in Guinea pigs,” in Advances in experimental medicine and biology (New York, NY: Springer), 1–15.
Tagaya, E., and Tamaoki, J. (2007). Mechanisms of airway remodeling in asthma. Allergol. Int. 56, 331–340. doi:10.2332/allergolint.R-07-152
Taylor-Clark, T. E. (2016). Role of reactive oxygen species and TRP channels in the cough reflex. Cell. Calcium 60, 155–162. doi:10.1016/j.ceca.2016.03.007
To, T., Stanojevic, S., Moores, G., Gershon, A. S., Bateman, E. D., Cruz, A. A., et al. (2012). Global asthma prevalence in adults: Findings from the cross-sectional world health survey. BMC Public Health 12, 204. doi:10.1186/1471-2458-12-204
Tohidinik, H. R., Mallah, N., and Takkouche, B. (2019). History of allergic rhinitis and risk of asthma; a systematic review and meta-analysis. World Allergy Organ. J. 12, 100069. doi:10.1016/j.waojou.2019.100069
Tränkner, D., Hahne, N., Sugino, K., Hoon, M. A., and Zuker, C. (2014). Population of sensory neurons essential for asthmatic hyperreactivity of inflamed airways. Proc. Natl. Acad. Sci. U. S. A. 111, 11515–11520. doi:10.1073/pnas.1411032111
Uchida, K., Dezaki, K., Yoneshiro, T., Watanabe, T., Yamazaki, J., Saito, M., et al. (2017). Involvement of thermosensitive TRP channels in energy metabolism. J. Physiol. Sci. 67, 549–560. doi:10.1007/s12576-017-0552-x
Vaeth, M., and Feske, S. (2018). Ion channelopathies of the immune system. Curr. Opin. Immunol. 52, 39–50. doi:10.1016/j.coi.2018.03.021
Vaeth, M., Kahlfuss, S., and Feske, S. (2020). CRAC channels and calcium signaling in T cell-mediated immunity. Trends Immunol. 41, 878–901. doi:10.1016/j.it.2020.06.012
Valverde, M. A., Cantero-Recasens, G., Garcia-Elias, A., Jung, C., Carreras-Sureda, A., and Vicente, R. (2011). Ion channels in asthma. J. Biol.Chem. 286 (38), 32877–32882. doi:10.1074/jbc.R110.215491
Van Der Velden, J., Sum, G., Barker, D., Koumoundouros, E., Barcham, G., Wulff, H., et al. (2013). KCa3.1 channel-blockade attenuates airway pathophysiology in a sheep model of chronic asthma. PLoS One 8, e66886–e66887. doi:10.1371/journal.pone.0066886
Volkers, L., Mechioukhi, Y., and Coste, B. (2015). Piezo channels: From structure to function. Pflug. Arch. Eur. J. Physiol. 467, 95–99. doi:10.1007/s00424-014-1578-z
Walsh, G. M. (2005). Novel therapies for asthma-advances and problems. Curr. Pharm. 11, 3027–3038. doi:10.2174/1381612054864984
Wang, H., and Siemens, J. (2015). TRP ion channels in thermosensation, thermoregulation and metabolism. Temperature 2, 178–187. doi:10.1080/23328940.2015.1040604
Wang, L., Feng, X., Hu, B., Xia, Q., Ni, X., and Song, W. (2018). P2X4R promotes airway remodeling by acting on the phenotype switching of bronchial smooth muscle cells in rats. Purinergic Signal. 14 (4), 433–442. doi:10.1007/s11302-018-9625-4
Wang, S., Chennupati, R., Kaur, H., Iring, A., Wettschureck, N., and Offermanns, S. (2016). Endothelial cation channel PIEZO1 controls blood pressure by mediating flow-induced ATP release. J. Clin. Investig. 126 (12), 4527–4536. doi:10.1172/JCI87343
Wang, W., Wu, J., Bernard, K., Li, G., Wang, G., Bevensee, M. O., et al. (2010). ATP-independent CFTR channel gating and allosteric modulation by phosphorylation. Proc. Natl. Acad. Sci. U. S. A. 107 (8), 3888–3893. doi:10.1073/pnas.0913001107
Wareham, K. J., and Seward, E. P. (2016). P2X7 receptors induce degranulation in human mast cells. Purinergic Signal. 12 (2), 235–246. doi:10.1007/s11302-016-9497-4
Wenzel, S. E. (2012). Asthma phenotypes: The evolution from clinical to molecular approaches. Nat. Med. 18, 716–725. doi:10.1038/nm.2678
World Health Organization (WHO) (2023). Asthma. Available at: https://www.who.int/en/news-room/fact-sheets/detail/asthma (Accessed May 04, 2023).
Wortley, M. A., Birrell, M. A., and Belvisi, M. G. (2016). “Drugs affecting TRP channels,” in Pharmacology and therapeutics of asthma and COPD. Editors C. Page, and P. Barnes (Cham: Springer), 213–241.
Wright, A., Mahaut-Smith, M., Symon, F., Sylvius, N., Ran, S., Bafadhel, M., et al. (2016). Impaired P2X1 receptor–mediated adhesion in eosinophils from asthmatic patients. J. Immunol. 196 (12), 4877–4884. doi:10.4049/jimmunol.1501585
Wu, J., Goyal, R., and Grandl, J. (2016). Localized force application reveals mechanically sensitive domains of PIEZO1. Nat. Commun. 7 (1), 12939. doi:10.1038/ncomms12939
Xiao C, C., Puddicombe, S. M., Field, S., Haywood, J., Broughton-Head, V., Puxeddu, I., et al. (2011). Defective epithelial barrier function in asthma. J. Allergy Clin. Immunol. 128, 549–556. doi:10.1016/j.jaci.2011.05.038
Xiao Q, Q., Yu, K., Perez-Cornejo, P., Cui, Y., Arreola, J., and Hartzell, H. C. (2011). Voltage- and calcium-dependent gating of TMEM16A/Ano1 chloride channels are physically coupled by the first intracellular loop. Proc. Natl. Acad. Sci. U.S.A. 108 (21), 8891–8896. doi:10.1073/pnas.1102147108
Yang, H., and Li, S. (2016). Transient receptor potential ankyrin 1 (TRPA1) channel and neurogenic inflammation in pathogenesis of asthma. Med. Sci. Mon. Int. Med. J. Exp. Clin. Res. 22, 2917–2923. doi:10.12659/MSM.896557
Yang, H., Zhang, G., and Cui, J. (2015). BK channels: Multiple sensors, one activation gate. Front. Physiol. 6, 29–16. doi:10.3389/fphys.2015.00029
Yang, Y. D., Cho, H., Koo, J. Y., Tak, M. H., Cho, Y., Shim, W. S., et al. (2008). TMEM16A confers receptor-activated calcium-dependent chloride conductance. Nature 455, 1210–1215. doi:10.1038/nature07313
Yen, M., and Lewis, R. S. (2019). Numbers count: How STIM and Orai stoichiometry affect store-operated calcium entry. Cell. Calcium 79, 35–43. doi:10.1016/j.ceca.2019.02.002
Yoshimoto, T., Yasuda, K., Tanaka, H., Nakahira, M., Imai, Y., Fujimori, Y., et al. (2009). Basophils contribute to TH2-IgE responses in vivo via IL-4 production and presentation of peptide-MHC class II complexes to CD4+ T cells. Nat. Immunol. 10, 706–712. doi:10.1038/ni.1737
Yu, J. L., and Liao, H. Y. (2021). Piezo-type mechanosensitive ion channel component 1 (PIEZO1) in human cancer. Biomed. Pharmacother., 140, 111692. doi:10.1016/j.biopha.2021.111692
Yu, Z. H., Xu, J. R., Wang, Y. X., Xu, G. N., Xu, Z. P., Yang, K., et al. (2013). Targeted inhibition of KCa3.1 channel attenuates airway inflammation and remodeling in allergic asthma. Am. J. Respir. Cell. Mol. Biol. 48, 685–693. doi:10.1165/rcmb.2012-0236OC
Yu, Z., Wang, Y., Qin, L., and Chen, H. (2017). Functional cooperation between KCa3.1 and TRPV4 channels in bronchial smooth muscle cell proliferation associated with chronic asthma. Front. Pharmacol. 8, 559. doi:10.3389/fphar.2017.00559
Yuan, H., Gao, C., Chen, Y., Jia, M., Geng, J., Zhang, H., et al. (2013). Divalent cations modulate TMEM16A calcium-activated chloride channels by a common mechanism. J. Membr. Biol. 246, 893–902. doi:10.1007/s00232-013-9589-9
Yuan, P., Leonetti, M. D., Hsiung, Y., and MacKinnon, R. (2012). Open structure of the Ca2+ gating ring in the high-conductance Ca2+-activated K+ channel. Nature 481, 94–97. doi:10.1038/nature10670
Zhang, C. H., Li, Y., Zhao, W., Lifshitz, L. M., Li, H., Harfe, B. D., et al. (2013). The transmembrane protein 16A Ca2+-activated Cl− channel in airway smooth muscle contributes to airway hyperresponsiveness. Am. J. Respir. Crit. Care Med. 187 (4), 374–381. doi:10.1164/rccm.201207-1303OC
Zhang, X., Xin, P., Yoast, R. E., Emrich, S. M., Johnson, M. T., Pathak, T., et al. (2020). Distinct pharmacological profiles of ORAI1, ORAI2 and ORAI3 channels. Cell. Calcium 91, 102281. doi:10.1016/j.ceca.2020.102281
Zhang, Y., Jiang, L., Huang, T., Lu, D., Song, Y., Wang, L., et al. (2021). Mechanosensitive cation channel PIEZO1 contributes to ventilator-induced lung injury by activating RhoA/ROCK1 in rats. Resp. Res. 22 (1), 1–14. doi:10.1186/s12931-021-01844-3
Zhao, Y., McVeigh, B. M., and Moiseenkova-Bell, V. Y. (2021). Structural pharmacology of TRP channels. J. Mol. Biol. 433 (17), 166914. doi:10.1016/j.jmb.2021.166914
Zhou, J., Zhou, X. D., Xu, R., Du, X. Z., Li, Q., Li, B., et al. (2021). The degradation of airway epithelial tight junctions in asthma under high airway pressure is probably mediated by Piezo-1. Front. Phys. 12, 637790. doi:10.3389/fphys.2021.637790
Zhou, Y., Cai, X., Nwokonko, R. M., Loktionova, N. A., Wang, Y., and Gill, D. L. (2017). The STIM-Orai coupling interface and gating of the Orai1 channel. Cell. Calcium 63, 8–13. doi:10.1016/j.ceca.2017.01.001
Zhu, J., Feng, Q., and Stathopulos, P. B. (2017). “The STIM-orai pathway: STIM-orai structures: Isolated and in complex,” in Store-operated Ca2⁺ entry (SOCE) pathways. Advances in experimental medicine and biology. Editors K. Groschner, W. Graier, and C. Romanin (Cham: Springer), 15–38.
Zhu, W., Guo, S., Homilius, M., Nsubuga, C., Wright, S. H., Quan, D., et al. (2022). PIEZO1 mediates a mechanothrombotic pathway in diabetes. Sci. Transl. Med. 14, 626. doi:10.1126/scitranslmed.abk1707
Glossary
Keywords: asthma, TRP channels, ORAI channels, KCa channels, TMEM16A channel, CFTR, Piezo1 channel, P2X receptor
Citation: Figueiredo IAD, Ferreira SRD, Fernandes JM, Silva BA, Vasconcelos LHC and Cavalcante FA (2023) A review of the pathophysiology and the role of ion channels on bronchial asthma. Front. Pharmacol. 14:1236550. doi: 10.3389/fphar.2023.1236550
Received: 07 June 2023; Accepted: 08 September 2023;
Published: 28 September 2023.
Edited by:
Frederic Becq, University of Poitiers, FranceReviewed by:
Alexander V. Zholos, Taras Shevchenko National University of Kyiv, UkraineHuanghe Yang, Duke University, United States
Copyright © 2023 Figueiredo, Ferreira, Fernandes, Silva, Vasconcelos and Cavalcante. This is an open-access article distributed under the terms of the Creative Commons Attribution License (CC BY). The use, distribution or reproduction in other forums is permitted, provided the original author(s) and the copyright owner(s) are credited and that the original publication in this journal is cited, in accordance with accepted academic practice. No use, distribution or reproduction is permitted which does not comply with these terms.
*Correspondence: Bagnólia Araújo da Silva, YmFnbm9saWFAbHRmLnVmcGIuYnI=; Luiz Henrique César Vasconcelos, bGhjdkBhY2FkZW1pY28udWZwYi5icg==