- Department of Otolaryngology Head and Neck Surgery, Shengjing Hospital of China Medical University, Shenyang, China
Wnt signaling plays an important role in regulating the biological behavior of cancers, and many drugs targeting this signaling have been developed. Recently, a series of research have revealed that Wnt signaling could regulate DNA damage response (DDR) which is crucial for maintaining the genomic integrity in cells and closely related to cancer genome instability. Many drugs have been developed to target DNA damage response in cancers. Notably, different components of the Wnt and DDR pathways are involved in crosstalk, forming a complex regulatory network and providing new opportunities for cancer therapy. Here, we provide a brief overview of Wnt signaling and DDR in the field of cancer research and review the interactions between these two pathways. Finally, we also discuss the possibility of therapeutic agents targeting Wnt and DDR as potential cancer treatment strategies.
1 Introduction
The Wnt signaling pathway is a complex protein regulatory network cascade, which participates in various biological processes, including embryonic development, tissue development and regeneration, and cancer development and progression. The abnormal activation of Wnt signaling will result in many pathological processes, such as cancer, inflammatory and immune diseases, and metabolic diseases (Clevers and Nusse, 2012; Nusse and Clevers, 2017).
DNA damage response (DDR) is a hierarchical signaling pathway that functions to maintain the genome integrity and stability and is coordinated by a variety of proteins. Numerous studies have demonstrated that DDR deficiency is closely associated with several diseases (Jackson and Bartek, 2009; Ciccia and Elledge, 2010; Lee and Paull, 2021; Stoof et al., 2021; Ye et al., 2021), among which cancer has become a focus of research. DDR has been extensively involved in regulation of cell senescence, carcinogenesis, and cancer progression as well as influencing the efficacy of cancer radiotherapy and chemotherapy. As the majority of anti-tumor therapies primarily target the genomic DNA of cancer cells, enhancement of DDR is closely related to their therapeutic efficacy (Lord and Ashworth, 2012; Su et al., 2023; Traphagen et al., 2023).
Previous studies have reported that Wnt signaling can affect DDR by regulating a variety of factors (Lento et al., 2014; Tao et al., 2015; Liang et al., 2018). In this review, we first introduce the Wnt/β-catenin and DDR signaling pathways and focus on the role of signaling in the regulation of DDR. Wnt/β-catenin pathway and DDR pathway members are potential therapeutic targets for many types of cancer (Lord and Ashworth, 2012; Krishnamurthy and Kurzrock, 2018; Pilié et al., 2019; Wang et al., 2021a). Could this possibly shed new light on clinical decision-making? We describe the molecular mechanisms and associations of the Wnt and DDR pathways with the biological processes involved in cancer and the treatment of cancer and their potential impact on the development of new treatment regimens.
2 Wnt signaling pathway
The Wnt signaling can be activated through the binding of Wnt family member ligand to the membrane receptors like Frizzled (FZD) family receptors, low-density lipoprotein receptor-related protein 5/6 (LRP5/6), and receptor tyrosine kinase-like orphan receptors (ROR1/2). Wnt signaling activation leads to transcriptional activation of multiple downstream effectors (Clevers and Nusse, 2012), and extracellular signals are transmitted through this pathway into the cell via activation of the intracellular segments of receptors on the cell surface, which in turn activates β-catenin-dependent or -independent Wnt signaling cascades. There are several separate Wnt pathways which can engage in crosstalk with one another; these have been summarized as canonical (β-catenin-dependent) and non-canonical signaling (Wnt/Ca2+ (calcium) pathway and Wnt/PCP (planar cell polarity) pathway) pathways (Figure 1). Generally, the canonical Wnt signaling pathway mainly participates in regulating progenitor cell self-renewal, proliferation, or differentiation, while the non-canonical signaling pathway is primarily responsible for the maintenance of cell stemness, cell motility, or antagonism of the canonical pathway (Fodde and Brabletz, 2007; Clevers and Nusse, 2012; Nusse and Clevers, 2017).
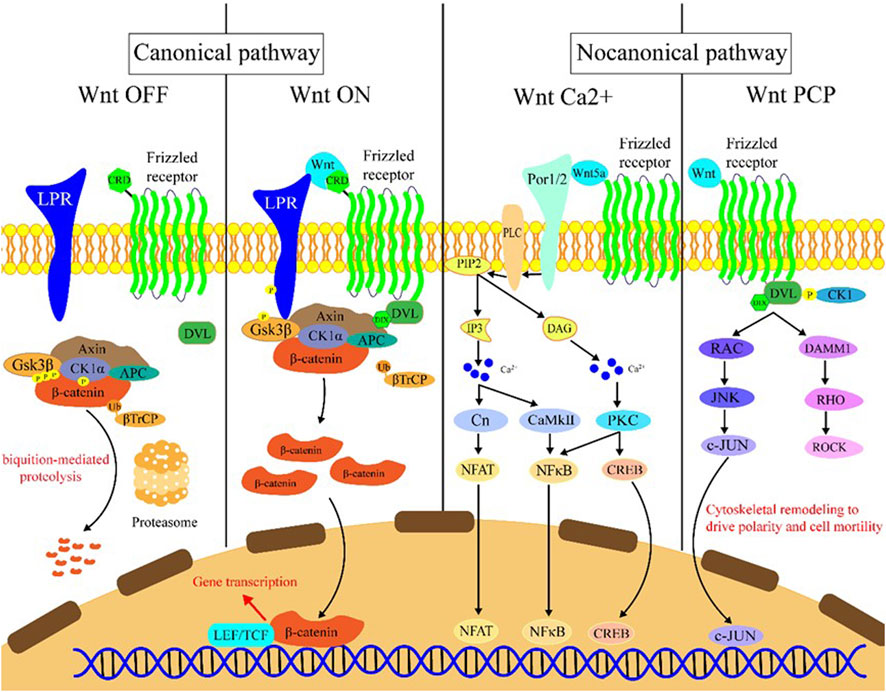
FIGURE 1. Introduction of the Wnt signaling pathway. Wnt OFF: In the absence of Wnt ligand, β-catenin binds to the destruction complex which comprises AXIN, CK1α, GSK-3β, and APC. Subsequently, a series of phosphorylation events occur to create a docking site on β-catenin for β-TrCP, and then β-catenin is ubiquitinated by β-TrCP and subjected to ubiquitin-proteasomal degradation. Wnt ON: In the presence of Wnt ligands, Frizzled receptors and the co-receptors LRP5/6 multimerize at the cell surface. This leads to recruitment of the cytoplasmic protein Dvl to the cell membrane by interacting with cytoplasmic domains of Frizzled receptors. The Frizzled-bound Dvl recruits the destruction complex through Dvl and axin, GSK-3β in the destruction complex initiates phosphorylation of the LRP5/6 and subsequent phosphorylation by CK1s, and more destruction complexes are recruited to the cell membrane that further phosphorylate LRP5/6 as a positive feedback loop. Wnt Ca2+: Wnt/Frizzled ligand receptor interaction with the participating co-receptor ROR1/2 leads to the activation of PLC and then hydrolyzes PIP2 into products IP3 and DAG. IP3 causes the release of Ca2+ from the endoplasmic reticulum, and two kinases CaMKII and Cn are activated, which in turn activate NFAT and NF-κB. DAG is activated by released calcium from the endoplasmic reticulum. Subsequently, PKC is activated which then activates NF-κB and CREB. These factors translocate to the nucleus where downstream gene expression is regulated. Wnt PCP: In the planar cell polarity pathway, after binding to Frizzled receptors and recruiting Dvl, which forms a complex with DAAM1, Wnt then activates the small GTPase Rho, which in turn activates ROCK. Alternatively, Dvl could also form a complex with RAC to activate JNK and then increase JNK-dependent c-JUN transcription activity.
2.1 The canonical Wnt/β-catenin pathway
The key members of the canonical Wnt signaling pathway include the Wnt family proteins, FZD/LRP6, disheveled protein (Dvl), β-catenin, T lymphocytokine/lymphoenhancer factor (TCF), human lymphoid enhancer factor (LEF), and the β-catenin destruction complex which comprises adenomatous polyposis coli (APC), axin, glycogen synthase kinase-3β (GSK-3β), and casein kinase 1α (CK1α). In the absence of a Wnt signal, β-catenin binds to the destruction complex, resulting in its ubiquitination via proteasomal degradation mediated by β-TrCP. In the presence of Wnt signal, Wnt binds to the FZD and LRP5/6 receptors to activate Dvl protein and inhibit the destruction complex, resulting in the accumulation of free unphosphorylated β-catenin in the cytoplasm, which is then transported to the nucleus where it can combine with TCF/LEF to induce transcription of downstream Wnt signaling genes (Clevers and Nusse, 2012; Nusse and Clevers, 2017).
2.2 The non-canonical Wnt pathway
2.2.1 The Wnt/Ca2+ pathway
The Wnt proteins could bind to the FZD receptors on the cell transmembrane and participate in several cellular processes that are involved in stimulating the isotrimer G protein to further activate PLC. PLC activation could result in elevated release of intracellular Ca2+, reduced cyclic guanosine (cGMP) levels, and activation of Ca2+/calmodulin-dependent protein kinase-II (CaMKII) and PKC; these processes can stimulate NFAT and other transcription factors such as CREB1 (De, 2011).
2.2.2 Wnt/PCP signaling
In Wnt/PCP signaling (Wang et al., 2021a), PCP mainly regulates cell movement direction and cell morphology. Wnt ligands bind to the ROR–FZD receptor complex to recruit and activate Dvl which binds to the small GTPase Rho. Then, the small GTPase Rac1 and Rho together trigger ROCK (Rho kinase) and JNK, thereby contributing to the regulation of cytoskeletal and transcriptional responses.
3 DDR regulators and signaling pathways
Under physiological conditions, DNA damage can be caused by various environmental stimuli and intracellular stress. After suffering DNA damage, cells can have three possible fates: recovering to the normal physiological state, initiation of apoptosis, or survival with damage. Through various regulatory pathways, cells can efficiently recognize DNA damage lesions, activate the DDR, and initiate the corresponding repair mechanism. The DDR involves a series of complex and elaborate regulatory events, including the identification of lesions, activation of checkpoints, remodeling of chromatin, DNA damage repair, cell cycle arrest, and apoptosis.
The DNA repair pathways vary in response to different types of DNA damage, which can be classified as follows: base excision repair (BER), nucleotide excision repair (NER), mismatch repair (MMR), homologous recombination (HR; where another nucleotide chain is required as a repair template), and non-homologous end-joining (NHEJ, where the broken ends are directly connected to the DNA strand). HR and NHEJ are the main repair ways for double-strand breaks (DSBs). HR always occurs in G2 and S phases, during which sister chromatids are present and can provide a template strand, while NHEJ is cell cycle phase-independent. For eukaryotes, NHEJ repairs most DSBs, and HR is preferentially used when the lesion occurs at DNA replication forks.
DDR is majorly regulated by two protein families: the phosphatidylinositol 3-kinase-like protein kinase (PIKK) family (including ATM, ATR, and DNA-PK) and the 16-member poly (ADP) ribose polymerase (PARP) family. Generally speaking, ATM, ATR, DNA-PK, and PARP mainly function as sensors for DNA damage. ATM is recruited by the MRN (MRE11/RAD50/NBS1) complex following recognition of DSBs (Rupnik et al., 2010; Blackford and Jackson, 2017), and ATM is the kinase typically responsible for modulation of cellular responses to DSBs, which comprise DNA repair, checkpoint activation, apoptosis, senescence, and alterations in the chromatin structure (Shiloh and Ziv, 2013; Blackford and Jackson, 2017). ATR is recruited by 9-1-1 (RAD9–RAD1–HUS1) complex to extend tracts of ssDNA (Blackford and Jackson, 2017). ATR is the DNA replication stress response kinase which could phosphorylate numerous substrates under the stimulation of genotoxic stresses. Following detection of DSBs, DNA-PK is recruited by Ku to initiate NHEJ (Graham et al., 2016). Among PARP family proteins, the structure and function of PARP-1 have been well elucidated by researchers. PARP plays a multifaceted role in cellular response to DNA damage. For example, PARP acts as a sensor in DDR that catalyzes the addition of poly (ADP-ribose) chains to histones and other nuclear proteins to recruit DDR factors to chromatin at breakpoints (Amé et al., 2004). PARP could also cause chromatin remodeling and recruit DNA-repair-associated proteins (Haince et al., 2008). In Figure 2, we summarize the DDR processes involving these different sensors, namely, ATM, ATR, DNA-PK, and PARP.
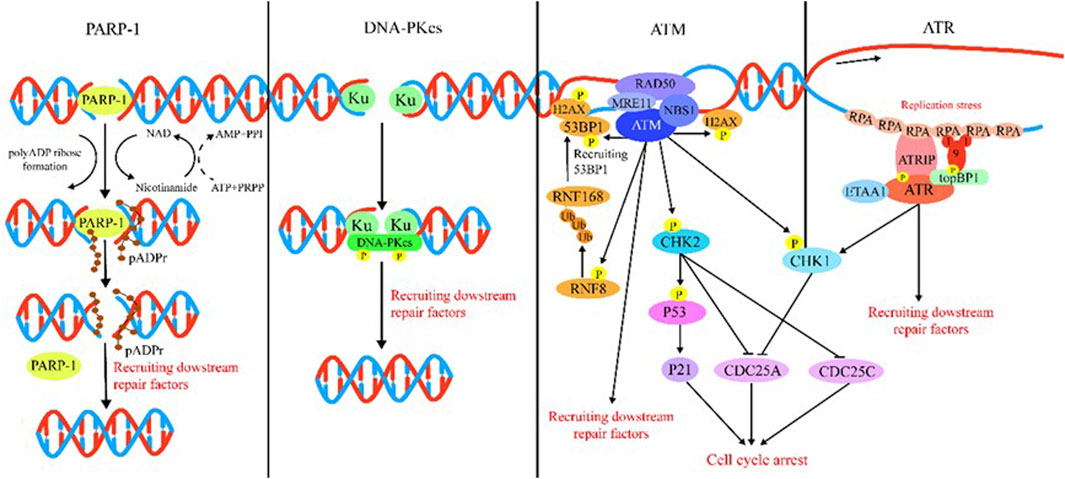
FIGURE 2. Introduction of DDR. PARP-1: PARP-1 detects and binds to damaged DNA and subsequently synthesizes poly (ADP) ribose (pADPr) on acceptor proteins. The high-density negative charge of pADPr gradually accumulates, leading to PARP-1 release from DNA simultaneously with the recruitment of DNA repair protein complex. Lastly, after the DNA break is repaired, the repair complex is dissociated from DNA and poly (ADP-ribose) glycohydrolase (PARG), and ADP-ribose hydrolase 3 (ARH3) hydrolyzes pADPr into ADP-ribose molecules and free pADPr. DNA-PKcs: DSBs are rapidly bound by the Ku heterodimer (Ku70 and Ku80) and loads onto DSB ends. Within seconds, Ku loads and activates DNA-PKcs to initiate NHEJ. Additional NHEJ core factors are subsequently recruited for the ends to be closely aligned and ligated. ATM: The MRN complex recruits ATM to DNA lesions and stimulates ATM kinase activity in response to DSBs. ATM phosphorylates histone H2AX and MDC1 in response to DSBs and lays the foundation for the chromatin-based signaling cascade involving phosphorylation and ubiquitination, which are mediated by RNF8 and RNF168 that results in ubiquitination of H2A to promote recruitment of 53BP1, which recruits its effectors to repair lesion. In addition, ATM phosphorylation activates Chk1/2, which in turn influences downstream factor cell to arrest the cell cycle. ATR: After suffering various forms of damaged DNA or helicase-polymerase uncoupling at stalled replication forks, RPA coats the ssDNA protecting it from degradation. ATR is recruited to RPA-ssDNA by its partner protein ATRIP and activated by TopBP1 or ETAA1; TopBP1 plays a role in ATR activation, which requires interaction with the RAD9–HUS1–RAD1 (9-1-1) clamp complex. ETAA1 is recruited to RPA-ssDNA via direct binding to RPA. In the downstream, ATR signaling activates the CHK1 kinase. CHK1 activation causes CDC25A degradation and slowing of cell cycle progression to arrest the cell cycle.
The downstream effects of these repair processes involve activation of various substrates to elicit appropriate responses involved in cell fate determination. These downstream effectors include H2AX, CHK1, CHK2, and p53, among others. H2AX is phosphorylated to form γH2AX, which is one of the earliest events in DDR. γH2AX not only generates a signaling cascade to amplify the signal of DDR and recruits the DDR proteins but also regulates chromatin relaxation (Rogakou et al., 1998). CHK1 and CHK2, coordinating DDR by arresting the cell cycle (Abraham, 2001), can phosphorylate p53 at Ser20 (Bode and Dong, 2004). P53 plays an important role in activating repair proteins to promote DNA repair, arresting the cell cycle in G1/S to provide ample time to repair damage, and initiating apoptosis to prevent abnormal genetic information from dividing and growing if the damage proves to be irreparable (Shiloh and Ziv, 2013; Li et al., 2016). Several characteristics of p53 are particularly notable. In the normal physiological state, p53 is presented at low levels in cells because of proteasome-mediated degradation, which is closely related to complex formation with various types of E3 ubiquitin ligases, the most important of which is Mdm2 (Brooks and Gu, 2006). HIPK2, a p53 Ser46 kinase, is retained in an inactive status because of the targeted proteolysis by E3 ubiquitin ligases in unstressed cells, making it an important regulator of stress signaling and DDR (Matt and Hofmann, 2016). When DNA damage is encountered, ATM and ATR kinases could phosphorylate Siah1, resulting in the dissociation of the HIPK2–Siah1 complex and increased stability of the HIPK2 protein (Winter et al., 2008).
4 Wnt and its crosstalk with DDR
Numerous studies have shown that Wnt signaling is highly intertwined with DDR (Zhang et al., 2011; Karimaian et al., 2017; Zhao et al., 2018b). In this section, we elaborate on these specific connections by outlining the roles of key factors (Figure 3).
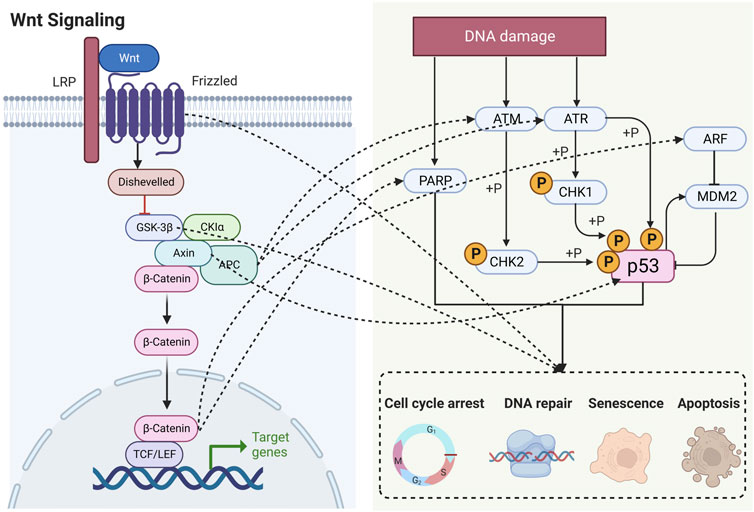
FIGURE 3. Schematic diagram showing that Wnt signaling is highly intertwined with DDR. LRP, lipoprotein receptor-related protein; APC, adenomatous polyposis coli; GSK-3β, glycogen synthase kinase-3β; CK1α, casein kinase 1α; TCF, T lymphocytokine/lymphoenhancer factor; LEF, human lymphoid enhancer factor; PARP, poly (ADP) ribose polymerase; ATM, ataxia telangiectasia mutated proteins; ATR, ataxia telangiectasia and Rad3-related protein; CHK, checkpoint kinase; ARF, ADP-ribosylation factor; MDM2, murine double minute 2.
4.1 The role of β-catenin in DDR
β-catenin is implicated in the regulation of DDR (Xu et al., 2008; Zhang et al., 2011; Priolli et al., 2013; Tavana et al., 2013; Chandra et al., 2015; Serebryannyy et al., 2017). Activation of downstream target genes of β-catenin such as cyclin D1 and c-myc proto-oncogenes leads to cell proliferation, differentiation, and maturation (Tulac et al., 2003). Notably, there is a growing list of Wnt/β-catenin target genes which have been found in various species and have been cataloged at: http://www.stanford.edu/group/nusselab/cgi-bin/wnt/target_genes. Based on these data, we have summarized the target genes closely related to DDR and briefly describe their roles in DDR.
4.2 The role of APC in DDR
APC is a key effector of the canonical Wnt signaling pathway involved in downregulating the β-catenin level. Many previous studies have shown that the loss of APC function can cause chromosomal instability (Fodde et al., 2001; Kaplan et al., 2001; Bienz, 2002). Clarke et al. found that APC loss leads to DNA damage and genomic instability in the live cell in a process closely associated with p53 (Méniel et al., 2015). In addition, there have been reports that APC is involved in the BER (Jaiswal and Narayan, 2008; Jaiswal and Narayan, 2011). Moreover, APC loss decreases cancer cell sensitivity to chemotherapy by reducing phosphorylation of ATM/Chk1/Chk2, which in turn influences DDR (Stefanski et al., 2019). APC loss can also increase STAT3 activation, leading to chemotherapy resistance (VanKlompenberg et al., 2017). STAT3 has been shown to take part in DNA repair, as low STAT3 levels can reduce ATM and ATR signaling through MDC1 (Barry et al., 2010).
4.3 Regulation of Axin on DDR
Axin, a key scaffolding protein, is responsible for the formation of the β-catenin destruction complex. Stability of axin protein is regulated by the ubiquitin-proteasome system, and enhancing axin stabilization is a common method of inhibiting Wnt/β-catenin signaling. In DDR, axin interacts with PML to regulate p53-dependent apoptosis in response to DNA damage (Li et al., 2011). Axin is involved in regulating phosphorylation of p53 Ser46 by forming a p53–axin–HIPK2 complex (Rui et al., 2004). Additionally, Lin et al. found that Tip60 interacts with axin in an ATM/ATR/CHK1-dependent way and abrogates Pirh2–axin binding, forming an axin–Tip60–HIPK2–p53 complex and leading to p53 phosphorylation in response to genotoxic stress during radiochemotherapy (Li et al., 2009).
4.4 Regulation of FZD on DDR
FZD is the seven-pass transmembrane receptor of Wnt. FZD5 is a member of the FZD family. Zhao et al. revealed that FZD5 has been involved in triple-negative breast cancer (TNBC) cell G1/S transition and DNA damage repair partially dependent on Wnt7B (Sun et al., 2020). Moreover, FZD5 modulates the downstream effecter FOXM1 in a Wnt/β-catenin-dependent manner to regulate the cell cycle, DNA replication, and DNA damage repair (Sun et al., 2020). In addition, a study on ovarian cancer found that another FZD family member, FZD7, can protect cells from chemotherapy-induced oxidative stress through the FZD7–β-catenin–Tp63–GPX4 pathway (Wang et al., 2021b). Similarly, another study showed that FZD10 can regulate Wnt signaling in BRCA-mutated epithelial ovarian cancers, ultimately contributing to increased HR activity (Fukumoto et al., 2019).
4.5 The effects of GSK-3β on DDR
GSK-3β is involved in multiple intracellular signaling pathways and is a component of the β-catenin destruction complex in Wnt signaling, which determines β-catenin stabilization. In DDR, GSK-3β can phosphorylate several DNA repair factors and affect their interaction with chromatin. For instance, through phosphorylating cyclin D1, CDC25A, and CRY2, GSK-3β can tune DNA repair and cell cycle (Diehl et al., 1998; Harada et al., 2005; Kang et al., 2008). Furthermore, GSK-3β can also phosphorylate B-Myb, leading to the dissociation of B-Myb from the MRN-mediated response to DNA damage (Henrich et al., 2017). Translin-associated protein X (TRAX) is a DNA/RNA-binding protein that participates in various functions, including DNA repair and physical interaction with GSK-3β and other DNA repair factors, to further influence NHEJ-mediated repair (Weng et al., 2018). In the cytoplasm, GSK-3β could phosphorylate targets to trigger proteasomal degradation and promote activation of NF-κB activity to evade apoptosis (Lin et al., 2020). On radiation exposure, GSK-3β translocates from the cytoplasm to the nucleus and phosphorylates 53BP1, an important regulator for DNA repair (Yang et al., 2018). There is strong evidence that GSK-3β plays an important role in DDR; however, whether those functions of GSK-3β depend on Wnt/β-catenin signaling requires further investigation.
4.6 The role of p53 in DDR
In DDR, p53 can be activated in various ways. For example, ATM and ATR can phosphorylate p53 on Ser15 which is thought to inhibit interaction of p53 with the ubiquitin ligase, MDM2, leading to its dissociation from MDM2, thereby contributing to p53 stabilization (Shiloh and Ziv, 2013). Likewise, the p53 co-factor, hnRNPK, can be phosphorylated by ATM and protect p53 from MDM2-mediated degradation (Moumen et al., 2013). In the Wnt pathway, Wnt/β-catenin can promote the expression of ARF, which binds to MDM2 leading to its inactivation (Kubbutat et al., 1997). In contrast, the activation of p53 could trigger the Siah/SIP/Skp1/Ebi pathway for β-catenin ubiquitination degradation and then reduce activity of TCF/LEF (Matsuzawa and Reed, 2001). Accordingly, p53 is a key factor in the crosstalk between the Wnt/β-catenin signaling pathway and DDR.
4.7 Regulation of PARP on DDR
In general, PARP, a DNA damage sensor, is activated by recognizing damaged DNA fragments. DNA damage would induce auto-polyADP-ribosylation of the PARP-1 protein to inhibit the functional interaction of PARP-1 with TCF-4 and participate in the transcriptional regulation of TCF-4/β-catenin complex target genes, such as cyclin D1 and c-myc (Idogawa et al., 2005). In addition, Ku70 is an inhibitor of the β-catenin/TCF-4 transcriptional complex, and PARP-1 could compete with Ku70 for binding to TCF-4, thereby modifying transcriptional activity of TCF-4 (Idogawa et al., 2007). Moreover, there is considerable evidence that Wnt/β-catenin signaling activation can promote resistance to PARP inhibitors (Fukumoto et al., 2019; Yamamoto et al., 2019; Zhu et al., 2021). Therefore, PARP may provide a novel linkage between Wnt/β-catenin signaling and DDR.
In summary, there are many connections between Wnt and DDR (Table 1); however, whether these factors regulate DDR directly via Wnt signaling or independent of the Wnt signaling pathway warrants further investigation.
5 Potential treatment strategies
At present, initiation and increase of DNA damage in cancer cell is a commonly exploited strategy for treating cancer and plays an effective therapeutic role; however, some cancer cells can develop resistance to DNA damage drugs by activating DDR. Increased DNA repair leads to resistance of cancer cells to targeted local (radiotherapy) or systemic (chemotherapy) treatments. Numerous kinds of drugs used for cancer chemotherapy, such as Adriamycin, etoposide, bleomycin, and cisplatin, are characterized as intense inducers of DNA damage and could effectively activate the intracellular DDR (Jackson and Bartek, 2009). All parts of DDR require proper coordination to maintain genome stability, and they can all influence the effects of cancer treatment. Therefore, DDR components can serve as radiation and chemical sensitization targets in cancer treatment. Knowledge of the DDR defects that are present in cancer can also allow for the selection of optimal treatments that can efficiently kill the cancer cells (Goldstein and Kastan, 2015). Currently, many drugs targeting the DDR have been developed to treat tumors, which are shown in Schedule 1. A summary of clinical trials on Wnt/β-catenin-targeted agents is presented in Table 2.
Theoretically, targeting both DDR and Wnt signaling could serve as potential treatment strategies for cancer. Another common denominator of them is that DDR and Wnt signaling have been associated with therapeutic resistance (Martin-Orozco et al., 2019). Alterations in Wnt signaling are also closely associated with maintenance of cancer stem cell (CSCs) proliferation, as hyperactivation of the Wnt signaling pathway is critical in supporting cancer cell survival in the context of treatment with anti-cancer drugs and ultimately leads to cancer progression. Therefore, the design and development of appropriate anti-cancer strategies based on Wnt targets may have important therapeutic value in treating cancer cells, thereby enhancing the therapeutic efficacy of these strategies (Mukherjee and Panda, 2020; Zhang and Wang, 2020). The formation of drug resistance could be attributed to some different mechanisms such as the acquisition of quiescence, the interaction with the microenvironment, drug efflux capacity, increased resistance to apoptosis, and increased DNA repair. For example, FZD5 enhances DNA damage repair and chemoresistance (Sun et al., 2020). GSK-3β promotes resistance of cancer cells to DNA damage chemotherapeutic agents and radiation through regulating DNA repair and stemness of cancer cells (Lin et al., 2020). YAP/TAZ is a Wnt regulator that can be activated to protect cancer cells from DNA damage (de Lau et al., 2014). Furthermore, the role of Wnt signaling in promoting DNA damage repair and inhibiting apoptosis contributes to cancer resistance to radiation (Jun et al., 2016; Zhao et al., 2018a).
Most current anti-cancer chemotherapy approaches function by killing highly proliferating cells, which in many cancers are mostly non-CSCs. However, small CSC populations present in cancer have a higher repair mechanism and are also highly resistant to chemotherapy. In addition, radiotherapy and chemotherapy may trigger a series of cellular stress response mechanisms that enhance stem cell properties of non-CSCs, thereby improving their adaptation and survival. Therefore, compared with previous therapies, new combination therapies, which can kill both CSCs and non-CSCs while preventing the transition from non-CSCs to CSCs, require consideration. It would be possible to develop a therapeutic strategy which combines DDR-targeting drugs with Wnt-targeting drugs to increase sensitivity of cytotoxic drugs while contributing anti-CSC effects. Importantly, the connection points between the DDR and Wnt warrant further investigation, as drugs targeting these points are likely to inhibit both DDR and CSCs simultaneously.
6 Discussion and conclusion
In the context of cancer, increased Wnt signaling is beneficial and reflected in enhancement of DDR, endowing cancer cells with increased ability to repair themselves during radiation or chemotherapy. The association between Wnt signaling and DDR in the post-carcinogenesis stage have been well studied, but the relationship between these two pathways during the process of carcinogenesis requires further investigation. There is a strong correlation between carcinogenesis and inadequate repair of DNA damage; however, whether Wnt signaling is involved in this process remains unknown. Future studies are required to investigate whether Wnt signaling can influence carcinogenesis via regulation of DDR.
Although Wnt signaling and the DDR are closely related, the effects generated by different specific points of crosstalk are unknown. Identification of an appropriate regulator as a therapeutic target may be beneficial to cancer treatment. Compared with single-drug chemotherapy or radiotherapy, drug combination may achieve double efficacy with half the input. From another perspective, a triple therapy including DDR-targeting drugs and Wnt-targeting drugs has the potential to enhance the efficacy of radiotherapy and chemotherapy. Although our theory may be considered oversimplified, identification of crosstalk between the Wnt signaling pathway and DNA damage recognition has potential to provide novel insights into cancer therapy.
Author contributions
XZ and XY: conceptualization. XZ: data curation and writing–original draft preparation. XY: visualization and investigation. XZ and XY: supervision. XZ and XY: writing–reviewing and editing. All authors contributed to the article and approved the submitted version.
Funding
This work was supported by the 345 Talent Project of Shengjing Hospital of China Medical University.
Acknowledgments
The authors thank the Department of Otolaryngology, Head, and Neck Surgery, Shengjing Hospital of China Medical University. This work was supported by the 345 Talent Project of Shengjing Hospital of China Medical University. The authors also thank biorender.com for creating Figure 3.
Conflict of interest
The authors declare that the research was conducted in the absence of any commercial or financial relationships that could be construed as a potential conflict of interest.
Publisher’s note
All claims expressed in this article are solely those of the authors and do not necessarily represent those of their affiliated organizations, or those of the publisher, the editors, and the reviewers. Any product that may be evaluated in this article, or claim that may be made by its manufacturer, is not guaranteed or endorsed by the publisher.
References
Abraham, R. T. (2001). Cell cycle checkpoint signaling through the ATM and ATR kinases. Genes & Dev. 15 (17), 2177–2196. doi:10.1101/gad.914401
Amé, J.-C., Spenlehauer, C., and de Murcia, G. (2004). The PARP superfamily. BioEssays news Rev. Mol. Cell. Dev. Biol. 26 (8), 882–893. doi:10.1002/bies.20085
Barry, S. P., Townsend, P. A., Knight, R. A., Scarabelli, T. M., Latchman, D. S., and Stephanou, A. (2010). STAT3 modulates the DNA damage response pathway. Int. J. Exp. Pathology 91 (6), 506–514. doi:10.1111/j.1365-2613.2010.00734.x
Bienz, M. (2002). The subcellular destinations of APC proteins. Nat. Rev. Mol. Cell Biol. 3 (5), 328–338. doi:10.1038/nrm806
Blackford, A. N., and Jackson, S. P. (2017). ATM, ATR, and DNA-PK: The trinity at the heart of the DNA damage response. Mol. Cell 66 (6), 801–817. doi:10.1016/j.molcel.2017.05.015
Bode, A. M., and Dong, Z. (2004). Post-translational modification of p53 in tumorigenesis. Nat. Rev. Cancer 4 (10), 793–805. doi:10.1038/nrc1455
Brooks, C. L., and Gu, W. (2006). p53 ubiquitination: Mdm2 and beyond. Mol. Cell 21 (3), 307–315. doi:10.1016/j.molcel.2006.01.020
Canesin, G., Evans-Axelsson, S., Hellsten, R., Krzyzanowska, A., Prasad, C. P., Bjartell, A., et al. (2017). Treatment with the WNT5A-mimicking peptide Foxy-5 effectively reduces the metastatic spread of WNT5A-low prostate cancer cells in an orthotopic mouse model. PLoS One 12 (9), e0184418. doi:10.1371/journal.pone.0184418
Chandra, A., Lin, T., Zhu, J., Tong, W., Huo, Y., Jia, H., et al. (2015). PTH1-34 blocks radiation-induced osteoblast apoptosis by enhancing DNA repair through canonical Wnt pathway. J. Biol. Chem. 290 (1), 157–167. doi:10.1074/jbc.M114.608158
Choi, M. Y., Widhopf, G. F., Ghia, E. M., Kidwell, R. L., Hasan, M. K., Yu, J., et al. (2018). Phase I trial: Cirmtuzumab inhibits ROR1 signaling and stemness signatures in patients with chronic lymphocytic leukemia. Cell Stem Cell 22 (6), 951–959.e3. doi:10.1016/j.stem.2018.05.018
Choi, M. Y., Widhopf, G. F., 2ndWu, C. C., Cui, B., Lao, F., Sadarangani, A., et al. (2015). Pre-clinical specificity and safety of UC-961, a first-in-class monoclonal antibody targeting ROR1. Clin. Lymphoma Myeloma Leuk. 15, S167–S169. doi:10.1016/j.clml.2015.02.010
Ciccia, A., and Elledge, S. J. (2010). The DNA damage response: Making it safe to play with knives. Mol. Cell 40 (2), 179–204. doi:10.1016/j.molcel.2010.09.019
Clevers, H., and Nusse, R. (2012). Wnt/β-catenin signaling and disease. Cell 149 (6), 1192–1205. doi:10.1016/j.cell.2012.05.012
Davis, S. L., Cardin, D. B., Shahda, S., Lenz, H. J., Dotan, E., O'Neil, B. H., et al. (2020). A phase 1b dose escalation study of Wnt pathway inhibitor vantictumab in combination with nab-paclitaxel and gemcitabine in patients with previously untreated metastatic pancreatic cancer. Invest. New Drugs 38 (3), 821–830. doi:10.1007/s10637-019-00824-1
De, A. (2011). Wnt/Ca2+ signaling pathway: A brief overview. Acta biochimica biophysica Sinica 43 (10), 745–756. doi:10.1093/abbs/gmr079
de Lau, W., Peng, W. C., Gros, P., and Clevers, H. (2014). The R-spondin/lgr5/rnf43 module: Regulator of Wnt signal strength. Genes & Dev. 28 (4), 305–316. doi:10.1101/gad.235473.113
Diamond, J. R., Becerra, C., Richards, D., Mita, A., Osborne, C., O'Shaughnessy, J., et al. (2020). Phase Ib clinical trial of the anti-frizzled antibody vantictumab (OMP-18R5) plus paclitaxel in patients with locally advanced or metastatic HER2-negative breast cancer. Breast Cancer Res. Treat. 184 (1), 53–62. doi:10.1007/s10549-020-05817-w
Diehl, J. A., Cheng, M., Roussel, M. F., and Sherr, C. J. (1998). Glycogen synthase kinase-3beta regulates cyclin D1 proteolysis and subcellular localization. Genes & Dev. 12 (22), 3499–3511. doi:10.1101/gad.12.22.3499
Dotan, E., Cardin, D. B., Lenz, H. J., Messersmith, W., O'Neil, B., Cohen, S. J., et al. (2020). Phase ib study of Wnt inhibitor ipafricept with gemcitabine and nab-paclitaxel in patients with previously untreated stage IV pancreatic cancer. Clin. Cancer Res. 26 (20), 5348–5357. doi:10.1158/1078-0432.Ccr-20-0489
Fodde, R., and Brabletz, T. J. C. (2007). Wnt/beta-catenin signaling in cancer stemness and malignant behavior. Wnt/β-catenin Signal. cancer stemness malignant Behav. 19 (2), 150–158. doi:10.1016/j.ceb.2007.02.007
Fodde, R., Kuipers, J., Rosenberg, C., Smits, R., Kielman, M., Gaspar, C., et al. (2001). Mutations in the APC tumour suppressor gene cause chromosomal instability. Nat. Cell Biol. 3 (4), 433–438. doi:10.1038/35070129
Fukumoto, T., Zhu, H., Nacarelli, T., Karakashev, S., Fatkhutdinov, N., Wu, S., et al. (2019). N6-Methylation of adenosine of FZD10 mRNA contributes to PARP inhibitor resistance. Cancer Res. 79 (11), 2812–2820. doi:10.1158/0008-5472.CAN-18-3592
Goldsberry, W. N., Meza-Perez, S., Londoño, A. I., Katre, A. A., Mott, B. T., Roane, B. M., et al. (2020). Inhibiting WNT ligand production for improved immune recognition in the ovarian tumor microenvironment. Cancers (Basel) 12 (3), 766. doi:10.3390/cancers12030766
Goldstein, M., and Kastan, M. B. (2015). The DNA damage response: Implications for tumor responses to radiation and chemotherapy. Annu. Rev. Med. 66, 129–143. doi:10.1146/annurev-med-081313-121208
Goswami, V. G., and Patel, B. D. (2021). Recent updates on Wnt signaling modulators: A patent review (2014-2020). Expert Opin. Ther. Pat. 31 (11), 1009–1043. doi:10.1080/13543776.2021.1940138
Graham, T. G. W., Walter, J. C., and Loparo, J. J. (2016). Two-stage synapsis of DNA ends during non-homologous end joining. Mol. Cell 61 (6), 850–858. doi:10.1016/j.molcel.2016.02.010
Haince, J.-F., McDonald, D., Rodrigue, A., Déry, U., Masson, J.-Y., Hendzel, M. J., et al. (2008). PARP1-dependent kinetics of recruitment of MRE11 and NBS1 proteins to multiple DNA damage sites. J. Biol. Chem. 283 (2), 1197–1208. doi:10.1074/jbc.M706734200
Harada, Y., Sakai, M., Kurabayashi, N., Hirota, T., and Fukada, Y. (2005). Ser-557-phosphorylated mCRY2 is degraded upon synergistic phosphorylation by glycogen synthase kinase-3 beta. J. Biol. Chem. 280 (36), 31714–31721. doi:10.1074/jbc.M506225200
Henrich, S. M., Usadel, C., Werwein, E., Burdova, K., Janscak, P., Ferrari, S., et al. (2017). Interplay with the Mre11-Rad50-Nbs1 complex and phosphorylation by GSK3β implicate human B-Myb in DNA-damage signaling. Sci. Rep. 7, 41663. doi:10.1038/srep41663
Idogawa, M., Masutani, M., Shitashige, M., Honda, K., Tokino, T., Shinomura, Y., et al. (2007). Ku70 and poly(ADP-ribose) polymerase-1 competitively regulate beta-catenin and T-cell factor-4-mediated gene transactivation: Possible linkage of DNA damage recognition and Wnt signaling. Cancer Res. 67 (3), 911–918. doi:10.1158/0008-5472.CAN-06-2360
Idogawa, M., Yamada, T., Honda, K., Sato, S., Imai, K., and Hirohashi, S. (2005). Poly(ADP-ribose) polymerase-1 is a component of the oncogenic T-cell factor-4/beta-catenin complex. Gastroenterology 128 (7), 1919–1936. doi:10.1053/j.gastro.2005.03.007
Jackson, S. P., and Bartek, J. (2009). The DNA-damage response in human biology and disease. Nature 461 (7267), 1071–1078. doi:10.1038/nature08467
Jaiswal, A. S., and Narayan, S. (2008). A novel function of adenomatous polyposis coli (APC) in regulating DNA repair. Cancer Lett. 271 (2), 272–280. doi:10.1016/j.canlet.2008.06.024
Jaiswal, A. S., and Narayan, S. (2011). Assembly of the base excision repair complex on abasic DNA and role of adenomatous polyposis coli on its functional activity. Biochemistry 50 (11), 1901–1909. doi:10.1021/bi102000q
Jimeno, A., Gordon, M., Chugh, R., Messersmith, W., Mendelson, D., Dupont, J., et al. (2017). A first-in-human phase I study of the anticancer stem cell agent ipafricept (OMP-54F28), a decoy receptor for Wnt ligands, in patients with advanced solid tumors. Clin. Cancer Res. 23 (24), 7490–7497. doi:10.1158/1078-0432.Ccr-17-2157
Jun, S., Jung, Y.-S., Suh, H. N., Wang, W., Kim, M. J., Oh, Y. S., et al. (2016). LIG4 mediates Wnt signalling-induced radioresistance. Nat. Commun. 7, 10994. doi:10.1038/ncomms10994
Kang, T., Wei, Y., Honaker, Y., Yamaguchi, H., Appella, E., Hung, M.-C., et al. (2008). GSK-3 beta targets Cdc25A for ubiquitin-mediated proteolysis, and GSK-3 beta inactivation correlates with Cdc25A overproduction in human cancers. Cancer Cell 13 (1), 36–47. doi:10.1016/j.ccr.2007.12.002
Kaplan, K. B., Burds, A. A., Swedlow, J. R., Bekir, S. S., Sorger, P. K., and Näthke, I. S. (2001). A role for the Adenomatous Polyposis Coli protein in chromosome segregation. Nat. Cell Biol. 3 (4), 429–432. doi:10.1038/35070123
Karimaian, A., Majidinia, M., Baghi, H. B., and Yousefi, B. J. D. (2017). The crosstalk between Wnt/β-catenin signaling pathway with DNA damage response and oxidative stress: Implications in cancer therapy. DNA Repair 51, 14–19. doi:10.1016/j.dnarep.2017.01.003
Katoh, M. (2018). Multi-layered prevention and treatment of chronic inflammation, organ fibrosis and cancer associated with canonical WNT/β-catenin signaling activation (Review). Int. J. Mol. Med. 42 (2), 713–725. doi:10.3892/ijmm.2018.3689
Kipps, T. J. (2021). Mining the microenvironment for therapeutic targets in chronic lymphocytic leukemia. Cancer J. 27 (4), 306–313. doi:10.1097/ppo.0000000000000536
Krishnamurthy, N., and Kurzrock, R. (2018). Targeting the Wnt/beta-catenin pathway in cancer: Update on effectors and inhibitors. Cancer Treat. Rev. 62, 50–60. doi:10.1016/j.ctrv.2017.11.002
Kubbutat, M. H., Jones, S. N., and Vousden, K. H. (1997). Regulation of p53 stability by Mdm2. Nature 387 (6630), 299–303. doi:10.1038/387299a0
Lee, J. H., Faderl, S., Pagel, J. M., Jung, C. W., Yoon, S. S., Pardanani, A. D., et al. (2020). Phase 1 study of CWP232291 in patients with relapsed or refractory acute myeloid leukemia and myelodysplastic syndrome. Blood Adv. 4 (9), 2032–2043. doi:10.1182/bloodadvances.2019000757
Lee, J. H., and Paull, T. T. (2021). Cellular functions of the protein kinase ATM and their relevance to human disease. Nat. Rev. Mol. Cell Biol. 22 (12), 796–814. doi:10.1038/s41580-021-00394-2
Lento, W., Ito, T., Zhao, C., Harris, J. R., Huang, W., Jiang, C., et al. (2014). Loss of β-catenin triggers oxidative stress and impairs hematopoietic regeneration. Genes & Dev. 28 (9), 995–1004. doi:10.1101/gad.231944.113
Li, C., Liang, Y., Cao, J., Zhang, N., Wei, X., Tu, M., et al. (2019). The delivery of a Wnt pathway inhibitor toward CSCs requires stable liposome encapsulation and delayed drug release in tumor tissues. Mol. Ther. 27 (9), 1558–1567. doi:10.1016/j.ymthe.2019.06.013
Li, Q., He, Y., Wei, L., Wu, X., Wu, D., Lin, S., et al. (2011). AXIN is an essential co-activator for the promyelocytic leukemia protein in p53 activation. Oncogene 30 (10), 1194–1204. doi:10.1038/onc.2010.499
Li, Q., Lin, S., Wang, X., Lian, G., Lu, Z., Guo, H., et al. (2009). Axin determines cell fate by controlling the p53 activation threshold after DNA damage. Nat. Cell Biol. 11 (9), 1128–1134. doi:10.1038/ncb1927
Li, T., Liu, X., Jiang, L., Manfredi, J., Zha, S., and Gu, W. (2016). Loss of p53-mediated cell-cycle arrest, senescence and apoptosis promotes genomic instability and premature aging. Oncotarget 7 (11), 11838–11849. doi:10.18632/oncotarget.7864
Liang, Y., Feng, Y., Zong, M., Wei, X.-F., Lee, J., Feng, Y., et al. (2018). β-catenin deficiency in hepatocytes aggravates hepatocarcinogenesis driven by oncogenic β-catenin and MET. Hepatol. Baltim. Md 67 (5), 1807–1822. doi:10.1002/hep.29661
Lin, J., Song, T., Li, C., and Mao, W. (2020). GSK-3β in DNA repair, apoptosis, and resistance of chemotherapy, radiotherapy of cancer. Biochimica biophysica acta. Mol. Cell Res. 1867 (5), 118659. doi:10.1016/j.bbamcr.2020.118659
Lord, C. J., and Ashworth, A. (2012). The DNA damage response and cancer therapy. Nature 481 (7381), 287–294. doi:10.1038/nature10760
Martin-Orozco, E., Sanchez-Fernandez, A., Ortiz-Parra, I., and Ayala-San Nicolas, M. (2019). WNT signaling in tumors: The way to evade drugs and immunity. Front. Immunol. 10, 2854. doi:10.3389/fimmu.2019.02854
Matsuzawa, S. I., and Reed, J. C. (2001). Siah-1, SIP, and Ebi collaborate in a novel pathway for beta-catenin degradation linked to p53 responses. Mol. Cell 7 (5), 915–926. doi:10.1016/s1097-2765(01)00242-8
Matt, S., and Hofmann, T. G. (2016). The DNA damage-induced cell death response: A roadmap to kill cancer cells. Cell. Mol. life Sci. CMLS 73 (15), 2829–2850. doi:10.1007/s00018-016-2130-4
Méniel, V., Megges, M., Young, M. A., Cole, A., Sansom, O. J., and Clarke, A. R. (2015). Apc and p53 interaction in DNA damage and genomic instability in hepatocytes. Oncogene 34 (31), 4118–4129. doi:10.1038/onc.2014.342
Moore, K. N., Gunderson, C. C., Sabbatini, P., McMeekin, D. S., Mantia-Smaldone, G., Burger, R. A., et al. (2019). A phase 1b dose escalation study of ipafricept (OMP54F28) in combination with paclitaxel and carboplatin in patients with recurrent platinum-sensitive ovarian cancer. Gynecol. Oncol. 154 (2), 294–301. doi:10.1016/j.ygyno.2019.04.001
Moumen, A., Magill, C., Dry, K. L., and Jackson, S. P. (2013). ATM-dependent phosphorylation of heterogeneous nuclear ribonucleoprotein K promotes p53 transcriptional activation in response to DNA damage. Cell cycleGeorget. Tex.) 12 (4), 698–704. doi:10.4161/cc.23592
Mukherjee, N., and Panda, C. K. (2020). Wnt/β-Catenin signaling pathway as chemotherapeutic target in breast cancer: An update on pros and cons. Clin. Breast Cancer 20 (5), 361–370. doi:10.1016/j.clbc.2020.04.004
Nusse, R., and Clevers, H. (2017). Wnt/β-Catenin signaling, disease, and emerging therapeutic modalities. Cell 169 (6), 985–999. doi:10.1016/j.cell.2017.05.016
Osman, J., Bellamkonda, K., Liu, Q., Andersson, T., and Sjölander, A. (2019). The WNT5A agonist Foxy5 reduces the number of colonic cancer stem cells in a xenograft mouse model of human colonic cancer. Anticancer Res. 39 (4), 1719–1728. doi:10.21873/anticanres.13278
Pak, S., Park, S., Kim, Y., Park, J. H., Park, C. H., Lee, K. J., et al. (2019). The small molecule WNT/β-catenin inhibitor CWP232291 blocks the growth of castration-resistant prostate cancer by activating the endoplasmic reticulum stress pathway. J. Exp. Clin. Cancer Res. 38 (1), 342. doi:10.1186/s13046-019-1342-5
Phillips, C., Bhamra, I., Eagle, C., Flanagan, E., Armer, R., Jones, C. D., et al. (2022). The Wnt pathway inhibitor RXC004 blocks tumor growth and reverses immune evasion in Wnt ligand-dependent cancer models. Cancer Res. Commun. 2 (9), 914–928. doi:10.1158/2767-9764.Crc-21-0095
Pilié, P. G., Tang, C., Mills, G. B., and Yap, T. A. (2019). State-of-the-art strategies for targeting the DNA damage response in cancer. Nat. Rev. Clin. Oncol. 16 (2), 81–104. doi:10.1038/s41571-018-0114-z
Priolli, D. G., Canelloi, T. P., Lopes, C. O., Valdívia, J. C. M., Martinez, N. P., Açari, D. P., et al. (2013). Oxidative DNA damage and β-catenin expression in colorectal cancer evolution. Int. J. colorectal Dis. 28 (5), 713–722. doi:10.1007/s00384-013-1688-7
Rodon, J., Argilés, G., Connolly, R. M., Vaishampayan, U., de Jonge, M., Garralda, E., et al. (2021). Phase 1 study of single-agent WNT974, a first-in-class Porcupine inhibitor, in patients with advanced solid tumours. Br. J. Cancer 125 (1), 28–37. doi:10.1038/s41416-021-01389-8
Rogakou, E. P., Pilch, D. R., Orr, A. H., Ivanova, V. S., and Bonner, W. M. (1998). DNA double-stranded breaks induce histone H2AX phosphorylation on serine 139. J. Biol. Chem. 273 (10), 5858–5868. doi:10.1074/jbc.273.10.5858
Rui, Y., Xu, Z., Lin, S., Li, Q., Rui, H., Luo, W., et al. (2004). Axin stimulates p53 functions by activation of HIPK2 kinase through multimeric complex formation. EMBO J. 23 (23), 4583–4594. doi:10.1038/sj.emboj.7600475
Rupnik, A., Lowndes, N. F., and Grenon, M. (2010). MRN and the race to the break. Chromosoma 119 (2), 115–135. doi:10.1007/s00412-009-0242-4
Säfholm, A., Tuomela, J., Rosenkvist, J., Dejmek, J., Härkönen, P., and Andersson, T. (2008). The Wnt-5a-derived hexapeptide Foxy-5 inhibits breast cancer metastasis in vivo by targeting cell motility. Clin. Cancer Res. 14 (20), 6556–6563. doi:10.1158/1078-0432.Ccr-08-0711
Serebryannyy, L. A., Yemelyanov, A., Gottardi, C. J., and de Lanerolle, P. (2017). Nuclear α-catenin mediates the DNA damage response via β-catenin and nuclear actin. J. Cell Sci. 130 (10), 1717–1729. doi:10.1242/jcs.199893
Shah, K., Panchal, S., and Patel, B. (2021). Porcupine inhibitors: Novel and emerging anti-cancer therapeutics targeting the Wnt signaling pathway. Pharmacol. Res. 167, 105532. doi:10.1016/j.phrs.2021.105532
Shiloh, Y., and Ziv, Y. (2013). The ATM protein kinase: Regulating the cellular response to genotoxic stress, and more. Nat. Rev. Mol. Cell Biol. 14 (4), 197–210. doi:10.1038/nrm3546
Stefanski, C. D., Keffler, K., McClintock, S., Milac, L., and Prosperi, J. R. (2019). APC loss affects DNA damage repair causing doxorubicin resistance in breast cancer cells. Neoplasia (New York, N.Y.) 21 (12), 1143–1150. doi:10.1016/j.neo.2019.09.002
Stoof, J., Harrold, E., Mariottino, S., Lowery, M. A., and Walsh, N. (2021). DNA damage repair deficiency in pancreatic ductal adenocarcinoma: Preclinical models and clinical perspectives. Front. Cell Dev. Biol. 9, 749490. doi:10.3389/fcell.2021.749490
Su, Y. L., Xiao, L. Y., Huang, S. Y., Wu, C. C., Chang, L. C., Chen, Y. H., et al. (2023). Inhibiting WEE1 augments the antitumor efficacy of Cisplatin in urothelial carcinoma by enhancing the DNA damage process. Cells 12 (11), 1471. doi:10.3390/cells12111471
Sun, Y., Wang, Z., Na, L., Dong, D., Wang, W., and Zhao, C. (2020). FZD5 contributes to TNBC proliferation, DNA damage repair and stemness. Cell Death Dis. 11 (12), 1060. doi:10.1038/s41419-020-03282-3
Tabernero, J., Van Cutsem, E., Garralda, E., Tai, D., De Braud, F., Geva, R., et al. (2023). A phase ib/II study of WNT974 + encorafenib + cetuximab in patients with BRAF V600e-mutant KRAS wild-type metastatic colorectal cancer. Oncologist 28 (3), 230–238. doi:10.1093/oncolo/oyad007
Tao, S., Tang, D., Morita, Y., Sperka, T., Omrani, O., Lechel, A., et al. (2015). Wnt activity and basal niche position sensitize intestinal stem and progenitor cells to DNA damage. EMBO J. 34 (5), 624–640. doi:10.15252/embj.201490700
Tavana, O., Puebla-Osorio, N., Kim, J., Sang, M., Jang, S., and Zhu, C. (2013). Ku70 functions in addition to nonhomologous end joining in pancreatic β-cells: A connection to β-catenin regulation. Diabetes 62 (7), 2429–2438. doi:10.2337/db12-1218
Traphagen, N. A., Schwartz, G. N., Tau, S., Roberts, A. M., Jiang, A., Hosford, S. R., et al. (2023). Estrogen therapy induces receptor-dependent DNA damage enhanced by PARP inhibition in ER+ breast cancer. Clin. Cancer Res. 23, 488. doi:10.1158/1078-0432.Ccr-23-0488
Tulac, S., Nayak, N. R., Kao, L. C., Van Waes, M., Huang, J., Lobo, S., et al. (2003). Identification, characterization, and regulation of the canonical Wnt signaling pathway in human endometrium. J. Clin. Endocrinol. metabolism 88 (8), 3860–3866. doi:10.1210/jc.2003-030494
Vaisitti, T., Arruga, F., Vitale, N., Lee, T. T., Ko, M., Chadburn, A., et al. (2021). ROR1 targeting with the antibody-drug conjugate VLS-101 is effective in Richter syndrome patient-derived xenograft mouse models. Blood 137 (24), 3365–3377. doi:10.1182/blood.2020008404
VanKlompenberg, M. K., Leyden, E., Arnason, A. H., Zhang, J.-T., Stefanski, C. D., and Prosperi, J. R. (2017). APC loss in breast cancer leads to doxorubicin resistance via STAT3 activation. Oncotarget 8 (61), 102868–102879. doi:10.18632/oncotarget.22263
Wang, J., Feng, D., and Gao, B. (2021a). An overview of potential therapeutic agents targeting WNT/PCP signaling. Handb. Exp. Pharmacol. 269, 175–213. doi:10.1007/164_2021_533
Wang, J., Zhao, G., Condello, S., Huang, H., Cardenas, H., Tanner, E. J., et al. (2021b). Frizzled-7 identifies platinum-tolerant ovarian cancer cells susceptible to ferroptosis. Cancer Res. 81 (2), 384–399. doi:10.1158/0008-5472.CAN-20-1488
Wang, W., Cho, U., Yoo, A., Jung, C. L., Kim, B., Kim, H., et al. (2022). Wnt/β-Catenin inhibition by CWP232291 as a novel therapeutic strategy in ovarian cancer. Front. Oncol. 12, 852260. doi:10.3389/fonc.2022.852260
Weng, Y.-T., Chien, T., Kuan, I. I., and Chern, Y. (2018). The TRAX, DISC1, and GSK3 complex in mental disorders and therapeutic interventions. J. Biomed. Sci. 25 (1), 71. doi:10.1186/s12929-018-0473-x
Winter, M., Sombroek, D., Dauth, I., Moehlenbrink, J., Scheuermann, K., Crone, J., et al. (2008). Control of HIPK2 stability by ubiquitin ligase Siah-1 and checkpoint kinases ATM and ATR. Nat. Cell Biol. 10 (7), 812–824. doi:10.1038/ncb1743
Xu, M., Yu, Q., Subrahmanyam, R., Difilippantonio, M. J., Ried, T., and Sen, J. M. (2008). Beta-catenin expression results in p53-independent DNA damage and oncogene-induced senescence in prelymphomagenic thymocytes in vivo. Mol. Cell. Biol. 28 (5), 1713–1723. doi:10.1128/MCB.01360-07
Yamamoto, T. M., McMellen, A., Watson, Z. L., Aguilera, J., Ferguson, R., Nurmemmedov, E., et al. (2019). Activation of Wnt signaling promotes olaparib resistant ovarian cancer. Mol. Carcinog. 58 (10), 1770–1782. doi:10.1002/mc.23064
Yang, Y., Lei, T., Du, S., Tong, R., Wang, H., Yang, J., et al. (2018). Nuclear GSK3β induces DNA double-strand break repair by phosphorylating 53BP1 in glioblastoma. Int. J. Oncol. 52 (3), 709–720. doi:10.3892/ijo.2018.4237
Ye, Z., Shi, Y., Lees-Miller, S. P., and Tainer, J. A. (2021). Function and molecular mechanism of the DNA damage response in immunity and cancer immunotherapy. Front. Immunol. 12, 797880. doi:10.3389/fimmu.2021.797880
Zhang, D., Wang, H., and Tan, Y. (2011). Wnt/β-catenin signaling induces the aging of mesenchymal stem cells through the DNA damage response and the p53/p21 pathway. PloS one 6 (6), e21397. doi:10.1371/journal.pone.0021397
Zhang, Y., and Wang, X. (2020). Targeting the Wnt/β-catenin signaling pathway in cancer. J. Hematol. Oncol. 13 (1), 165. doi:10.1186/s13045-020-00990-3
Zhao, Y., Tao, L., Yi, J., Song, H., and Chen, L. (2018a). The role of canonical Wnt signaling in regulating radioresistance. Cell. physiology Biochem. Int. J. Exp. Cell. physiology, Biochem. Pharmacol. 48 (2), 419–432. doi:10.1159/000491774
Zhao, Y., Yi, J., Tao, L., Huang, G., Chu, X., Song, H., et al. (2018b). Wnt signaling induces radioresistance through upregulating HMGB1 in esophageal squamous cell carcinoma. Cell Death Dis. 9 (4), 433. doi:10.1038/s41419-018-0466-4
Keywords: Wnt, DNA damage response, cancer, therapy, drug resistance
Citation: Zhang X and Yu X (2023) Crosstalk between Wnt/β-catenin signaling pathway and DNA damage response in cancer: a new direction for overcoming therapy resistance. Front. Pharmacol. 14:1230822. doi: 10.3389/fphar.2023.1230822
Received: 29 May 2023; Accepted: 20 July 2023;
Published: 02 August 2023.
Edited by:
Ayaz Shahid, Western University of Health Sciences, United StatesReviewed by:
Pengcheng Wang, Capital Medical University, ChinaMohammad Ashhar I. Khan, University of North Carolina at Chapel Hill, United States
Copyright © 2023 Zhang and Yu. This is an open-access article distributed under the terms of the Creative Commons Attribution License (CC BY). The use, distribution or reproduction in other forums is permitted, provided the original author(s) and the copyright owner(s) are credited and that the original publication in this journal is cited, in accordance with accepted academic practice. No use, distribution or reproduction is permitted which does not comply with these terms.
*Correspondence: Xiaofeng Yu, eWFua2UxMTAxMTJAMTYzLmNvbQ==