- 1Guangzhou University of Chinese Medicine, Guangzhou, Guangdong, China
- 2Department of Psychiatry, The Third Affiliated Hospital of Guangzhou Medical University, Guangzhou, Guangdong, China
- 3Department of Oncology, The First Affiliated Hospital of Guangzhou University of Chinese Medicine, Guangzhou University of Chinese Medicine, Guangzhou, Guangdong, China
- 4Department of Hepatology, TCM-Integrated Hospital of Southern Medical University, Guangzhou, Guangdong, China
- 5Department of Hepatopancreatobiliary, Cancer Center, Southern Medical University, Guangzhou, Guangdong, China
- 6Science and Technology Innovation Center, Guangzhou University of Chinese Medicine, Guangzhou, Guangdong, China
Tumor-associated macrophages (TAMs) are essential components of the immune cell stroma of hepatocellular carcinoma. TAMs originate from monocytic myeloid-derived suppressor cells, peripheral blood monocytes, and kupffer cells. The recruitment of monocytes to the HCC tumor microenvironment is facilitated by various factors, leading to their differentiation into TAMs with unique phenotypes. TAMs can directly activate or inhibit the nuclear factor-κB, interleukin-6/signal transducer and signal transducer and activator of transcription 3, Wnt/β-catenin, transforming growth factor-β1/bone morphogenetic protein, and extracellular signal-regulated kinase 1/2 signaling pathways in tumor cells and interact with other immune cells via producing cytokines and extracellular vesicles, thus affecting carcinoma cell proliferation, invasive and migratory, angiogenesis, liver fibrosis progression, and other processes to participate in different stages of tumor progression. In recent years, TAMs have received much attention as a prospective treatment target for HCC. This review describes the origin and characteristics of TAMs and their mechanism of action in the occurrence and development of HCC to offer a theoretical foundation for further clinical research of TAMs.
Introduction
Hepatocellular carcinoma (HCC) accounts for roughly 90% of all cases of primary liver carcinoma, making it the most prevalent form. It ranked as the 6th most common carcinoma and the 3rd most common cause of cancer-related fatalities in 2020. It was projected that HCC caused 906,000 new cases and 830,000 deaths worldwide due to HCC (Sung et al., 2021; Ding et al., 2022). HCC is associated with inflammation and can be caused by various factors, including environmental and genetic risk factors (Giraud et al., 2021). Chronic infection caused by hepatitis C virus (HCV), hepatitis B virus (HBV), and non-alcoholic fatty liver disease/non-alcoholic steatohepatitis (NAFLD/NASH) are the leading etiologies of liver cirrhosis, which can significantly increase the likelihood of progression to HCC (Kew, 2014). Chronic liver injury triggers reparative mechanisms aimed at restoring its form and function. However, this persistent inflammation can lead to ongoing regenerative repair of hepatocytes, which may contribute to the emergence and advancement of HCC (Endig et al., 2016; Gao et al., 2019). The malignant level of a tumor is related to the characteristics of cancerous cells and different components in the tumor microenvironment (TME) (Wang et al., 2014; Ge and Ding, 2020). These constituents have a pivotal function in connecting inflammatory mediators and tumors (Chen et al., 2022). The TME is a significant site for the interaction between carcinoma cells and the human immune system. It contains neoplastic cells, immune cells, blood vessel cells, tumor-associated fibroblasts, other non-tumor cells, extracellular matrix, and various cytokines (Li et al., 2018). In recent years, the immune microenvironment has gained an increasing focus, and it is indicated that the TME can facilitate tumor progression. Research has indicated that the high infiltration of tumor-associated macrophages (TAMs) in liver cancer is strongly tied to a negative prognosis for patients (Li et al., 2018b). Compared to other organs, the liver has a greater percentage of macrophages (van der Heide et al., 2019). Kupffer cells (KCs), as a stationary tissue-resident macrophage subset of the liver, are positioned in the blood sinuses, which is pivotal in maintaining homeostasis (Lopez et al., 2011; Scott et al., 2016). Inflammation in the liver or depletion of KCs leads to recruiting monocyte-derived macrophages into the stem tissue (Mossanen et al., 2016; Surewaard and Kubes, 2017). Tumor cells secrete different cytokines that can induce monocyte differentiation into TAMs, thereby altering the functional phenotype of macrophages (Mφ) in the TME (Rabold et al., 2017). These mutual transformations are a common occurrence in cancer and are correlated with tumor occurrence and development. The dysregulation of the two types of TAMs polarization is frequently involved in the development of pathological complications (Qian and Pollard, 2010). Interactions between tumor cells, TAMs, and other immune cells in the immune microenvironment affect the proliferation, invasion, migration, liver fibrosis, and immune killing of tumor cells through the secretion of cytokines and exosomes and changes in the expression of related proteins, ultimately affecting the progression of HCC. The mechanisms and signaling pathways involved are very complex. Therefore, this article provides a review of the definition, source, and polarization of TAMs and the mechanisms of interaction among TAMs, tumors, and other immune cells in HCC.
Definition of TAMs in HCC
Mφ are one of the most significant components of the HCC TME. The liver, as an immune-exempt organ, contains a significant number of Mφ, including resident KCs and recruited Mφ (MacParland et al., 2018; Mattos  et al., 2022). Based on protein expression, secreted cytokines, and function, TAMs are typically divided into two subgroups (Zhou et al., 2022): classical activated TAMs (M1-TAMs) and alternative activated TAMs (M2-TAMs) (Lin et al., 2019). M1-TAMs are mainly present in the tumor adjacent tissue, while M2-TAMs are mainly present in the liver cancer tissue (Luo et al., 2017; Englinger et al., 2019). M1-TAMs promote more inducible nitric oxide synthase (iNOS) and mainly secrete interleukin (IL)-1β, tumor necrosis factor (TNF)-α, highly expressed cluster of differentiation (CD)80, and CD86. M2-TAMs decreased the protein expression of CD80 and CD86, while elevating the expression of CD163, CD206, and arginase (Arg)-1. They also secreted less TNF-α and IL-1β, but more transforming growth factor (TGF)-β and IL-10 (Dong et al., 2016; Minami et al., 2018; Li et al., 2021; Sen et al., 2022). Moreover, CD68 was identified as a reliable marker for pan-Mφ or M1-TAMs (Minami et al., 2018). Additionally, the M2-TAMs could be further classified into four subsets (M2a, M2b, M2c, and M2d) based on the type of stimulus (Yang et al., 2019). When stimulated with interferon-gamma (IFN-γ) or IFN-γ integrated with lipopolysaccharide (LPS), Mφ become classically activated Mφ or M1-TAMs. Unlike M1-TAMs, which are polarized by proinflammatory stimuli, IL-4, IL-13, IL-10, and TGF-β can induce Mφ polarization into M2-TAMs, also known as alternatively activated Mφ. These Mφ highly express mannose receptor (MR) and Arg-1, which are connected to anti-inflammatory Th2 immunoreaction and can promote HCC progression (Yang et al., 2018a). Both M1-TAMs and M2-TAMs can be converted into each other in certain conditions and with specific stimuli (Xiang et al., 2021). M1-TAMs appear to eliminate HCC cells in the primary stages of tumorigenesis, but as tumor progression advances, M1-TAMs are replaced by M2-TAMs (Yao et al., 2018). Specifically, Mφ that infiltrate tumors tend to exhibit M2 phenotypes that promote tumor growth, rather than M1 phenotypes that have antitumor effects (Chen et al., 2018). Currently, many studies have found that the phenotype and function of TAMs are very complex, and it is limited to classify TAMs simply as M1 and M2 phenotypes. Therefore, there are many studies to distinguish different TAM subgroups through the special phenotype expression of TAMs and to investigate the impact of different subgroups on the progression of HCC. Intra-tumoral TAMs in HCC are often characterized by low expression of CD169 and high expression of CD204. Reversing this trend can improve patient prognosis (Li et al., 2017). The pro-inflammatory response induced by a high-fat diet leads to an increase in TNF-α+ Mφ infiltration, promoting the early onset of HCC (de Oliveira et al., 2019). Chemokine (CC motif) receptor (CCR)2+ monocytes and triggering receptor expressed on myeloid cells (TREM) 2+ Mφ in the TME can terminally differentiate to matrix metalloproteinase (MMP)9+ TAMs to promote the progression of HCC by stimulating the peroxisome proliferator-activated receptor (PPAR)γ signal (Lu et al., 2022). Siglec-10hi TAMs have characteristics and functions similar to M2-TAMs and can inhibit the function of CD8+ T cells (Xiao et al., 2021). These phenotypic TAMs play an immunosuppressive role in the TME of HCC. By contrast, CD38hi Mφ in the TME can secrete more IFN-γ, which helps CD8+ T cells to kill tumors (Ng et al., 2020). In addition, forkhead box O1 (FOXO1) expressed in TAMs inhibits the expression of IL-6 through the interferon regulatory factor (IRF)-1/nitric oxide (NO) axis and ultimately suppresses the progression of HCC (Cui et al., 2023). Furthermore, loss of FOXO1 leads to a decrease in major histocompatibility complex (MHC)-II expression, which weakens the antigen-presenting ability of TAMs and hinders the ability of immune cells to kill tumor cells. The hypoxic microenvironment in HCC induces the downregulation of FOXO1 expression in TAMs (Yang et al., 2018b). Typically, the majority of TAMs within the TME display an immunosuppressive type, and these TAMs promote immune suppression and facilitate tumor progression (Allavena et al., 2008). To further elaborate, TAMs refer to immunosuppressive Mφ in the HCC TME in a narrow sense.
Origin of TAMs in HCC
TAMs originate from peripheral blood monocytes, M-MDSCs, and KCs. Under normal conditions, Mφ can be distinguished from peripheral monocytes in tissues. However, during inflammation and cancer, peripheral monocytes derived from the bone marrow (BM) were one of the principal sources of Mφ, particularly TAMs. Under the stimulation of inflammatory and tumor signals, circulating monocytes can mobilize and infiltrate in the TME, where they transform into tissue Mφ (Shi and Pamer, 2011; Nowarski et al., 2013). HCC produces extracellular vesicles (EVs) containing PKM2, which induce phosphorylation of the signal transducer and activator of transcription 3 (STAT3) in monocytes, promoting their differentiation into TAMs (Hou et al., 2020). Chronic liver injury is closely related to the infiltration of M2-TAMs derived from monocytes (Delire et al., 2018). Most TAMs are derived from CCR2 + monocytes in BM (Cheng et al., 2022). Monocytes are attracted to the TME through the (CC motif) ligand 2 (CCL2)/CCR2 axis, transformed into TAMs, polarized toward M2-TAMs, and involved in the HCC progression (Sahin et al., 2010; Li et al., 2017). CCR2+ myeloid cells are necessary for senescence surveillance, and CCR2 ablation leads to HCC outgrowth (Eggert et al., 2016). The TME causes the overexpression of dynamin-related protein 1 (Drp1) in the HCC cells, which stimulates mitochondrial fission and induces cytoplasmic mitochondrial DNA (mtDNA) stress, activating the TLR9-mediated NF-κB signaling pathway and promoting the nuclear translocation of phosphorylated P65, thereby promoting the secretion of chemokine CCL2 (Bao et al., 2019). Additionally, the expression level of APOBEC3B (A3B) is upregulated in HCC cells, and A3B interacts with polycomb repressive complex 2 (PRC2), leading to reduced occupancy of H3K27me3 on the CCL2 promoter to recruit massive TAMs (Wang et al., 2019). Meanwhile, the expression of CCL2 by tumor cells is also regulated by Yes-associated protein (YAP)/TEA domain family member 4 (TEAD4) dependence (Thomann et al., 2021). Blocking CCL2/CCR2 and cysteine-X-cysteine motif chemokine ligand (CXCL)1/CXCR2 can inhibit the infiltration levels of Mφ and neutrophils in the TME (Tian et al., 2022). Meanwhile, the high expression of hsa_circ_0003410 in HCC induces the secretion of CCL5, activating and recruiting M2-TAMs (Cao et al., 2022). The upregulation of MMP-21 expression in tumor cells promotes the secretion of CCL14, which induces the recruitment of monocytes through binding to CCR1 on these cells (Zhou et al., 2022). CSF1 is a chemotactic factor for monocytes and can facilitate recruitment of monocytes and polarization of Mφ to M2-TAMs (Zhu et al., 2019; Jiang et al., 2022). The colony-stimulating factor 1 receptor (CSF-1R) is mainly expressed by TAMs and monocyte-restricted cells (Jiang et al., 2020). TAMs promote Mφ migration by upregulating the expression of M-CSFR and CXCR4 through hypoxia inducible factor (HIF)-2α mediation (Imtiyaz et al., 2010). Prostaglandin E (PGE)2 secreted by TAMs stimulates the expression of UHRF1 in tumor cells, which in turn induces the upregulation of CSF1 expression (Zhang et al., 2022). IL-1β secreted by TAMs induces the upregulation of solute carrier family 7 member 11 (SLC7A11) expression in HCC. SLC7A11 upregulation promotes HIF-1α expression by reducing α-ketoglutarate (αKG) levels, which in turn promotes the expression of programmed death ligand 1 (PD-L1) and CSF1 in tumor cells (He et al., 2021). Circular RNA (circRNA) CircASAP1 competes with tumor suppressor miRNAs (miR-326 and miR-532-5p) that target CSF1, also promoting the expression of CSF1 (Hu et al., 2020). The overexpression of stanniocalcin-1 (STC1) in tumor cells inhibits the expression of monocyte chemokine receptors chemokine CCR2, CCR4, and colony-stimulating factor 1 receptor (CSF1R), thereby suppressing the recruitment of monocytes to the TME (Leung and Wong, 2020).
TAMs can also originate from myeloid-derived suppressor cells (MDSCs), which also come from BM. MDSCs are composed of two major groups: granulocytic or polymorphonuclear (PMN-MDSCs and Ly6C− Ly6G+) and monocytic (M-MDSCs and Ly6C+ Ly6C−) (Tcyganov et al., 2018). PMN-MDSCs share similar characteristics with neutrophils, while M-MDSCs are more akin to monocytes (Gabrilovich et al., 2012). In the context of tumor tissues, M-MDSCs have the ability to quickly differentiate into TAMs and inflammatory dendritic cells (DCs).
Although bone marrow-derived monocytes were previously regarded as the only origin of TAMs, recent research studies indicated that tissue-determined Mφ can infiltrate tumors and transform into TAMs in specific tumors (Zhu et al., 2017; Conte, 2022). Liver-resident Mφ, like KCs (Matsuda and Seki, 2020), can stem from erythro-myeloid progenitors (EMPs) in the embryonic liver or the yolk sac, which express the Mφ CSF1R (Laviron and Boissonnas, 2019; Cheng et al., 2022). These Mφ can self-renew, but recent studies have shown their input from the bone marrow as well (Gomez Perdiguero et al., 2015; Hoeffel et al., 2015; Scott et al., 2016). As one of the subsets of TAMs, KCs can also facilitate the progression of HCC while participating in anti-tumor immunity (Yang et al., 2011). Signals from the local microenvironment stimulate immunogenic KCs and induce their functional differentiation. Danger signals in HCC can activate the inflammatory regulation of KCs and promote drawing immune cells to the liver. The production of CCL2 by tumor cells leads to the absence of embryonic KCs in tissue and promotes the infiltration of monocyte-derived KCs and immature monocytes (M0) (Thomann et al., 2021; Vanderborght et al., 2023). Myeloid precursor cells are recruited to infiltrate HCC, and the TME blocks its maturation process. The loss of Shp2 expression in KCs results in apoptosis of KCs and exacerbate the recruitment and differentiation of CCR2+ monocytes into TAMs, reshaping the immunosuppressive microenvironment (Du et al., 2023). However, some studies suggest that activated KCs also produce TNF-a, and IL-1, inducing the expression of IL-8 in HCC cells and promoting Mφ recruitment (Thornton et al., 1991). Expressing neurotensin (NTS) in tumor cells activates the mitogen-activated protein kinase (MAPK)/nuclear factor (NF)-κB signaling pathway, inducing the production of IL-8 (Xiao et al., 2018). In addition, the upregulation of AlkB homolog 5 (ALKBH5) promotes the upregulation of MAP3K8 expression in tumor cells, which induces the phosphorylation of the c-Jun N-terminal kinase (JNK)/extracellular signal-regulated kinase (ERK) pathway, resulting in the upregulation of IL-8 expression. IL-8 promotes the recruitment of PD-L1+TAMs and polarization of M2-TAMs (You et al., 2022). TAMs originate not only from the recruitment of monocytes or MDSCs but also from the proliferation of TAMs themselves residing in the TME. Tumor cells stimulates TAMs to secrete granulocyte-macrophage colony stimulating factor (GM-CSF), which induces the upregulation of A2A receptor expression in TAMs. This process synergistically stimulates the proliferation of TAMs in coordination with small molecules of adenosine produced by tumor cells, leading to increased infiltration of TAMs (Wang et al., 2021).
Cancer-associated fibroblasts (CAFs) express endosialin (CD248/TEM1) and interact with CD68, inducing the recruitment of Mφ. Meanwhile, this process induces GAS6 expression in CAFs, promoting the polarization of TAMs toward M2-TAMs (Yang et al., 2020). M2-TAMs secrete the hepatocyte growth factor (HGF) to help recruiting Mφ, and promote the infiltration of M2-TAMs (Dong et al., 2019). Infiltrating monocytes in HCC stimulate the production of TNF-α through the NF-κB signaling pathway, inducing the expression of their own c-Met. When interacting with HGF, this enhances their migration ability (Zhao et al., 2015). Autophagy inhibition in Mφ induces self-recruitment through the activation of the CCL20/CCR6 signaling pathway (Gao et al., 2023). However, in HCC cells, interferon regulatory factor (IRF8) inhibits the transcription of activator protein-1(AP1) signal (c-fos), resulting in a decrease in the expression of CCL20 and the inhibition of TAMs recruitment, thereby suppressing tumor progression (Wu et al., 2022). In M1-TAMs, the extracellular matrix protein SPON2 activates RhoA/Rac1-Hippo signaling through α4β1 integrin, leading to F-actin reorganization. The upregulation of F-actin expression inhibits large tumor suppressor kinase 1 (LATS1) phosphorylation and promotes YAP nuclear translocation, promoting the recruitment of M1-TAMs and inhibiting HCC progression. The specific mechanism by which YAP affects Mφ migration remains unclear (Zhang et al., 2018). However, the high expression of YAP in tumor cells induces an increase in IL-6 secretion, which plays an opposite role in tumor progression (Zhou et al., 2018). Hepatic stellate cells activated within tumors exhibit high expression of fatty acid-binding protein 4 (FABP4), inducing NF-κB nuclear translocation and promoting the secretion of IL-6 and IL-1A (Chiyonobu et al., 2018). By activating the IL-6-IL6R/STAT3 signaling pathway in Mφ, IL-6 induces the recruitment of Mφ and polarization toward the M2 phenotype (Guo et al., 2017; Yang and Xing, 2021). Hypoxia leads to the expression of high-mobility group box 1 (HMGB1), which induces the infiltration of TAMs and an increase in IL-6 expression through HIF-1α (Jiang et al., 2018). (Figure 1)
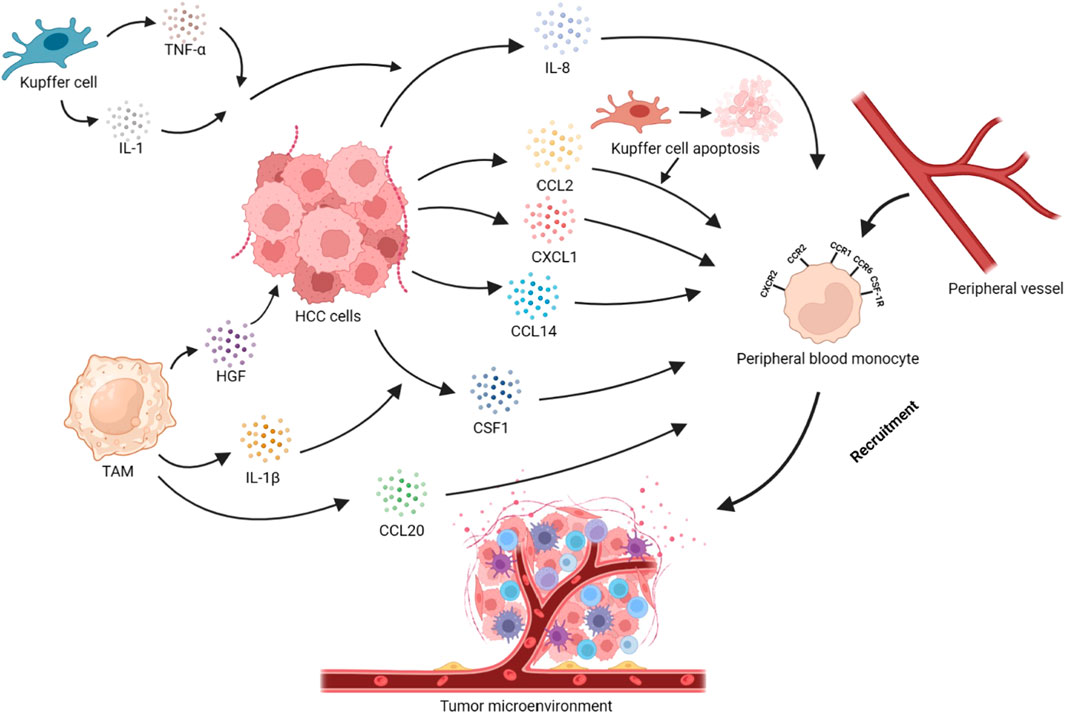
FIGURE 1. Recruitment of peripheral blood monocytes in HCC. Tumor cells secrete cytokines or chemokines such as CCL2, CXCL1, CCL14, CCL20, and CSF1, which recruit monocytes into the TME via the CCL2/CCR2, CXCL1/CXCR2, CCL14/CCR1, and CSF1/CSF-1R axes. The apoptosis of KCs promotes the recruitment of monocytes through the CCL2/CCR2 axis. IL-1, and TNF-α secreted by KCs act on HCC cells, promoting the secretion of IL-8 and, thus, recruiting monocytes. IL-1β secreted by TAMs can promote HCC cells to secrete CSF1. HGF secreted by TAMs is closely related to monocyte recruitment in HCC cells. At the same time, TAMs can also secrete CCL20 to achieve self-recruitment.
In the TME, tumor cells, KCs, TAMs with different phenotypes, hepatic stellate cells, and CAFs can produce multiple cytokines that promote the generation of TAMs. These chemokines play a significant role in recruiting and infiltrating macrophages into tumor tissues. The recruitment and chemotaxis of TAMs play a promoting part not only in liver cancer (Shieh et al., 2009) but also in many malignant tumors. How to inhibit the recruitment and chemotaxis of Mφ has gradually become one of the hotspots in the therapy of HCC.
TME affects the polarization of TAMs
The tumor immune microenvironment contains cytokines and extracellular vesicles secreted by tumor cells, which can induce the polarization of TAMs. The polarization of TAMs is also related to the transport of zinc and ferritin, as well as the metabolism of carbohydrates and lipids. As liver cancer advances, pro-tumorigenic factors stimulate tissue-resident Mφ, leading to a phenotypic shift and the development of TAMs (Cheng et al., 2022). In HCC, IL-37 is highly expressed in M1-TAMs and lowly expressed in M2-TAMs. IL-37 promotes the polarization of M2-TAMs toward M1-TAMs in HCC by inhibiting IL-6/STAT3 signaling in TAMs (Zhang et al., 2020a). The high expression of B7 homolog 3 (B7-H3) in HCC polarizes TAMs toward the M2 direction by the activation of STAT6 signaling (Kang et al., 2015). The Wnt ligand produced by tumor cells activates the Wnt/β-catenin signaling pathway in Mφ, promoting the nuclear translocation of β-catenin and transcriptional upregulation of C-MYC, inducing M2-TAMs polarization (Yang et al., 2018). In HCC cells, the lncRNA LINC00662 can competitively bind to miR-15a, miR-16, and miR-107, upregulating WNT3A secretion (Tian et al., 2020). Tumor cells secrete EVs containing miR4458H, which induces the upregulation of Arg1 expression in TAMs, promoting M2-TAMs polarization (Ye et al., 2023). Receptor-interacting protein 140 (RIP140) is overexpressed in TAMs, which inhibits the alternative activation of Mφ and has an inhibitory effect on the progression of HCC by suppressing the NF-κB/IL-6 axis (Hu et al., 2017). IL-17+ cells induce epithelial cells to secrete CXCL9, CXCL10, and CXCL11, recruiting CXCR3+B cells and promoting their maturation. CXCR3+B cells polarize M2b through IgG signaling (Liu et al., 2015). TREM1 is highly expressed in M2 TAMs. The downregulation of TREM1 expression reverses M2-TAMs into M1-TAMs by inhibiting the PI3K/AKT/mTOR signaling pathway (Chen et al., 2021). HCC produces HMGB1, which activates the Toll-like receptor 2 (TLR2)/NADPH oxidase 2 (NOX2)/autophagic axis in TAMs. NOX2 induces reactive oxygen species (ROS) production, which induces autophagy. The autophagic process degrades NF-κB p65 through the p62/SQSYM1 signaling pathway, inhibiting the secretion of IL-12 (which has anti-tumor effects) and promoting polarization of Mφ toward the M2 phenotype. Meanwhile, TLR2 mediates the phosphorylation and activation of ERK1/2, promoting the degradation process of NF-κB p65 and synergistically promoting the polarization of TAMs toward the M2 direction (Chang et al., 2013; Shiau et al., 2020). The expression loss of liver cell zinc finger protein Miz1 activates the NF-κB signaling pathway, promoting Mφ polarization toward the pro-inflammatory phenotype and promoting the progression of HCC (Zhang et al., 2021). Zinc/iron-regulated transporter-like protein (Zip) 9, which is associated with zinc uptake, is highly expressed in M2-TAMs of HCC and promotes M2 polarization by activating the STAT6 signaling pathway, while inhibiting M1 polarization by inhibiting IκBα/β signaling (Gou et al., 2022). The ferrous iron content in M1-TAMs is higher than in M2-TAMs. The high expression of transferrin in HCC cells leads to insufficient iron uptake by TAMs, which promotes the polarization of M2-TAMs through the upregulation of HIF-1α (Sun et al., 2021). (Figure 2)
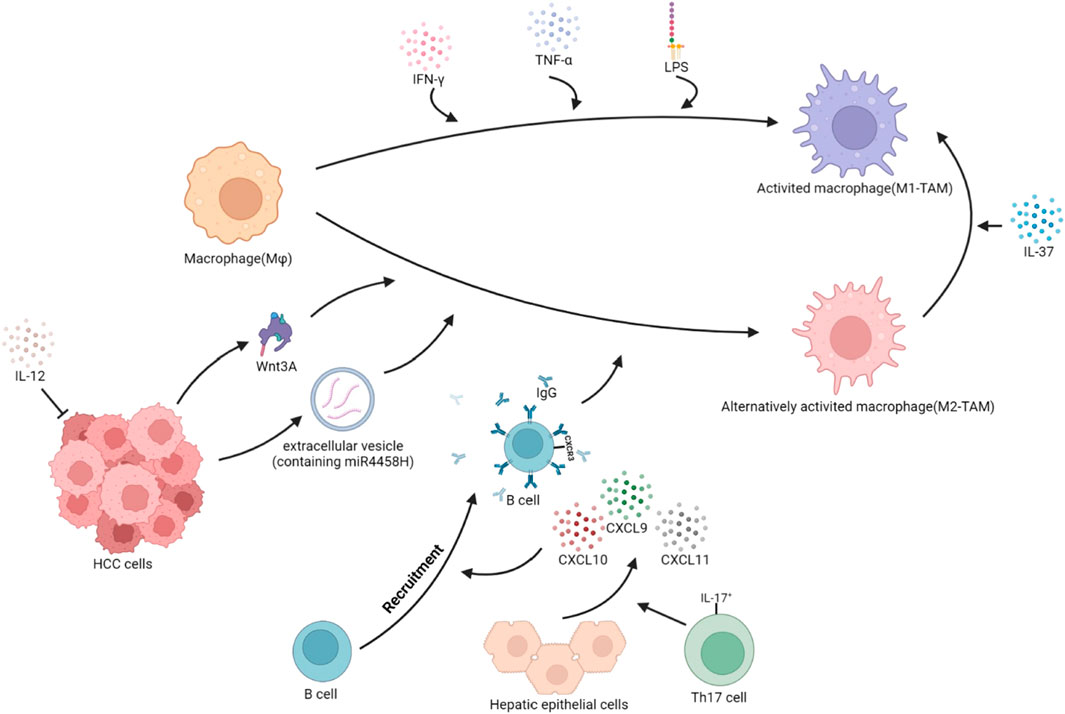
FIGURE 2. Polarization of TAMs in HCC. The local cytokine environment can determine the polarization of macrophages. Based on protein expression, secreted cytokines, and function, TAMs are typically divided into two subgroups: classical activated TAMs (M1-TAMs) and alternative activated TAMs (M2-TAMs). HCC cells secrete Wnt3A ligands and extracellular vesicles containing miR4458H, which can induce the polarization of Mφ toward M2-TAMs. IL-17+ cells stimulate hepatocytes to secrete CXCL9, CXCL10, and CXCL11, promoting the recruitment of CXCR3+B cells and inducing the polarization of Mφ towards M2-TAMs through the IgG pathway. IL-12, which has anti-tumor effects, reduces secretion and indirectly promotes the polarization of Mφ toward M2-TAMs. Conversely, when stimulated with IFN-γ, or IFN-γ integrated with LPS, Mφ become classically activated Mφ, or M1-TAMs. Additionally, in the TME, IL-37 can induce M2-TAMs to reverse into M1-TAMs.
Abnormalities in glucose and lipid metabolism in HCC affect the function and polarization of TAMs, accelerating tumor progression. In HCC, aerobic glycolysis promotes the activation of M1-TAMs, but the hypoxic microenvironment and insufficient glucose in the tumor lead to an increase in fatty acid oxidation (FAO) and a decrease in aerobic glycolysis. This promotes the expression of ROS in TAMs and induces TAMs to transform into an M2 phenotype through nuclear factor (erythroid-derived 2)-like 2 (Nrf2) mediation (Feng et al., 2018). The hypoxic microenvironment of HCC leads to abnormal glucose metabolism in tumor cells, with an increase in anaerobic glycolysis and lactate secretion. However, even in the presence of sufficient oxygen, tumor cells preferentially undergo anaerobic glycolysis, exhibiting the Warburg effect. The overexpression of pH-regulating molecule V-ATPase in M2-TAMs induces hypoxic and acidic metabolism, reshaping the immunosuppressive microenvironment (Kuchuk et al., 2018). The expression of receptor-interacting protein kinase 3 (RIPK3) in HCC is closely related to glucose and lipid metabolism. The downregulation of RIPK3 activates the PPAR signaling pathway to promote fatty acid metabolism and FAO, inducing M2 polarization, which depends on the mediation of ROS-caspase1 (Wu et al., 2020). The downregulation of sirtuin (SIRT)5 expression in liver cancer induces the production of bile acids (BAs) (Sun et al., 2022). Abnormal BAs metabolism induces M2-TAMs polarization, and the excessive production of BAs reshapes the immunosuppressive microenvironment. On the contrary, (SIRT)1 promotes the polarization of Mφ toward the M1 direction, inhibiting the progression of HCC (Zhou et al., 2019). Therefore, targeting these processes and signaling pathways can alter the polarization process of TAMs in the TME and reverse the immune-suppressive microenvironment.
TAMs impact the malignant biological behaviors of HCC
Many studies have indicated that Mφ infiltrating HCC affect the progression of the disease by secreting cytokines, chemokines, and matrix metalloproteases and releasing EVs containing non-coding RNA that have an impact on HCC cells, including proliferation, apoptosis, angiogenesis, cancer stem cell properties, invasion and migration abilities, liver fibrosis progression, and the immune microenvironment. Proteins, lipids, and RNAs are contained in exosomes, which mediate intercellular communication between different cell types, thus affecting the progression of cancer (Colombo et al., 2014). There have been reports that demonstrated that some signaling pathway-mediated crosstalk between Mφ and HCC cells cause M2-TAM polarization, leading to HCC growth, migration and metastasis, such as IL-6/STAT3, Wnt/β-catenin, and NF-κB (Mano et al., 2013; Yan et al., 2015; Yang et al., 2018).
TAMs could affect the level of cancer stem cells in HCC
The transformation of HCC cells into stem-cell like entities is facilitated by alterations in the TME. Consequently, HCC cells can acquire characteristics including self-renewal, differentiative capacity, and rejection of treatment, which are the stemness of cancerous cells (DeLuca and Saleh, 2023). The multiplication of HCC cells is corresponding to the cancer stem cells (CSCs). Patients with high stemness scores presented a higher infiltration level of TAMs, implying the presence of an immunosuppressive microenvironment (Zhang et al., 2022). Furthermore, CSCs high-risk patients with CD133 as a marker may exhibit immune liver homeostasis disorder (Yu et al., 2020). In addition, there is a positive interrelation between the infiltration of CD68+ TAMs and the number of OV6+ CSCs or EpCAM+ CSCs in HCC (Yao et al., 2016). The infiltration of these three types of cells is related to inferior overall survival (OS) and progression-free survival (PFS) of individuals with HCC (Fan et al., 2014; Wang et al., 2022). M2-TAMs could secrete CXCL1 and CXCL2 as potential paracrine factors. In addition to their CSCs properties, CXCL1 and CXCL2 were found to stimulate the transcription of BCL-2 while suppressing the transcription of BAD and BAX. BCL-2 is an optimistic target for eliminating CSCs in HCC (Wang et al., 2022). The targeting of CD90 by miR-125a/b was found to have a central impact on HCC CSCs. TAMs exosomes with reduced ranks of miR-125a and miR-125b facilitate HCC cell multiplication and stemness (Wang et al., 2019). It was observed that CD163+ TAMs expressed arachidonic acid 5-lipoxygenase (5-LOX) and generated LTB4 and LTC/D/E4 in a mouse model of HCC. These molecules were found to facilitate oncogenesis and CSCs properties by activating the phosphorylation of ERK1/2 and CSCs-associated genes (Nosaka et al., 2023). In vitro research studies have confirmed that CD44+ cells derived from both HCC specimens and cell lines exhibit CSCs actions. Furthermore, the secretion of IL-6 by TAMs were found to facilitate the expansion of CSCs in HCC and boost the migration process (Mano et al., 2013; Wan et al., 2014). It is indicated that IL-6 produced by cocultured Mφ activated STAT3 signaling of Hep1-6 cells induced the invasion of HCC cells in the hypoxic environment (Jiang et al., 2018). TAMs can secrete S100 calcium-binding protein A9 (S100A9) to induce the pro-inflammatory milieu in HCC. This upregulation occurs in a Ca2+-dependent manner and activates the NF-κB pathway via the advanced glycosylation end product-specific receptor (AGER). This activation enhances the stemness of HepG2 and MHCC-97H cells (Wei et al., 2021). Therefore, TAMs promote their M2 polarization and proliferation by expressing relevant proteins through paracrine cytokines and EVs.
CSCs existing in HCC are closely bound to enhanced invasion and migration, which contributes to the aggressive nature of this cancer, besides unrestrained cell replication in cancer. The releasement of TNF-α by M2-TAMs has been shown to facilitate EMT and CSCs via the Wnt/β-catenin signaling (Chen et al., 2019). Additionally, M2-TAMs secrete CCL17 to enhance the stemness and epithelial–mesenchymal transition (EMT), as well as TGF-β1 and Wnt/β-catenin signaling transduction of HCC cells (Zhu et al., 2016). In addition to cytokines, EVs from M2-TAMs could also affect the CSCs-like characteristics and invasive migration ability of HCC cells (Liang et al., 2016; Cheng et al., 2022). The miR-17-92 cluster, deriving from EVs of M2-TAMs, was observed to disrupt balance in the TGF-β1/bone morphogenetic protein (BMP)-7 signaling in cancerous cells. This was achieved by inhibiting the post-translational ubiquitylation of activin A receptor type 1 (ACVR1) and inducing the post-transcriptional silencing of TGF-β type II receptor (TGFBR2) through the targeting of Smad ubiquitylation regulatory factor 1 (Smurf1). Creating a disturbance in the TGF-β1/BMP-7 pathways can effectively enhance the incursion and CSCs properties of malignant cells by upregulating the inhibitor of differentiation 1 (ID1) expression (Ning et al., 2021). Specifically, TAMs facilitate the development of CSC-like characteristics through TGF-β1-induced EMT, and they could potentially aid in the study of HCC prognosis (Fan et al., 2014). Targeting CSCs may, therefore, be a promising strategy for preventing HCC metastasis and improving patient outcomes.
TAMs affect the proliferation of HCC cells
Long non-coding RNAs (lncRNAs) are a type of RNA that exceed 200 bases in length (Han et al., 2019). TAMs secrete exosomes containing M2 Mφ polarization associated lncRNA (lncMMPA). In HCC cells, lncMMPA has been found to interact with miR-548s, increasing the mRNA level of ALDH1A3, and induce cell glycolysis and proliferation (Xu et al., 2022). HBeAg, an antigen associated with HBV, resulted in an increased level of lncRNA MAPKAPK5_AS1 (MAAS) of M2-TAMs via boosting the N6-methyladenosine adjustment of MAAS through the action of methyltransferase-like 3. M2 macrophage-derived exosomes containing MAAS facilitated the stimulation of cyclin-dependent kinase 4 (CDK4), CDK6, and S-phase kinase-associated protein 2 transcription that were induced by the MYC proto-oncogene (c-Myc), via sustaining the c-Myc protein, leading to the facilitation of G1/S transition. The multiplication of HBV-positive HCC cells was attributed to this phenomenon (Tao et al., 2022). The levels of exosomes containing microRNA (miR)-375 derived from TAMs subjected to IL-2 (ExoIL2−TAM) were higher than those TAMs not subjected to IL-2 (ExoTAM). These exosomes could decrease HCC cells multiplication and metastasis and facilitate apoptosis both in vivo and in vitro (Chen et al., 2022).
Promoting M1-TAMs polarization increased propensity for cell killing and phagocytosis (Lujambio et al., 2013). Nonetheless, a single investigation revealed that M1-TAMs augmented the NF-κB p-p65/p65 ratio in HCC cells, thereby facilitating the nuclear translocation of p65. This, in turn, led to an elevated amount of malignant liver cells in the phases of the cell cycle known as S and G2/M, as well as the upregulation of CDK1, CDK2, and cyclin D1 expression. These studies indicated that M1-TAMs increased tumor cell proliferation. It is noteworthy that inhibiting the nuclear translocation of p65 brought on the reversal of alterations in the cell cycle, anti-apoptotic capacity, and protein expression induced by M1-TAMs of HCC (Sharen et al., 2022).
The M2-TAMs overexpressed IL-17 in HCC, particularly upon oxaliplatin treatment. Activation of the IL-17 receptor and lysosome-associated membrane protein 2A is crucial for chaperone-mediated autophagy induction by IL-17 in HCC cells. This, in turn, hinders apoptotic processes in response to oxaliplatin treatment (Guo et al., 2017). A significant increase in T cell immunoglobulin and mucin-domain containing protein-3 (Tim-3) expression was observed in TAMs of HCC patients. By activating NF-κB in Mφ, Tim-3 promotes IL-6 releasing, and the multiplication of liver cancer cells is, therefore, enhanced. However, receptor interacting protein 140 (RIP140), which is expressed in TAMs, exerts the opposite effect (Yan et al., 2015; Hu et al., 2017). STAT3 phosphorylation promoted cell proliferation and migration after IL-6 stimulation (Mano et al., 2013). Additionally, the progression of tumors in relation to alcohol consumption has been linked to a crucial mechanism involving IL6-STAT3 signaling (Zhao et al., 2019). The production of CXCL8 by TAMs resulted in an increase in the miR-17 cluster, comprising miR-18a and miR-19a. These two miRNAs were observed to stimulate tumor metastasis and cell proliferation in HCC and were related to elevated metastasis and prolonged survival in HCC patients during clinical investigations (Yin et al., 2017). According to a research study, the creation of insulin-like growth factor-1 (IGF-1) by M2-TAMs could boost the expansion and spread of HCC cells (Sprinzl et al., 2015).
Dectin-3, a C-type lectin receptor (CLR), is responsible for inducing the apoptosis of tumor cells and inhibiting their proliferation by regulating the glycolysis of Mφ. Mφ deficient in Dectin-3 were found to significantly facilitate the proliferation of H22 cells and suppress their apoptosis (Qu et al., 2022). Releasing complement C1q from Mφ present in the inflammatory milieu was regarded as an unorthodox mechanism for activating the β-catenin pathway in periportal hepatic progenitor cells, which results in the enlargement and de-differentiation (Ho et al., 2020). The finding highlights the function of Mφ in triggering the pathway activation in the hepatic progenitor cells. TAMs induce proliferation of HCC cells through these mechanisms. Targeting these pathways or inhibiting the secretion of TAMs cytokines and exosomes is an effective way to suppress the proliferation of HCC cells.
TAMs have the potential to influence invasion, migration, and angiogenesis in HCC
EMT is responsible for inducing a temporary and reversible loss of differentiation in epithelial cells, leading to the development of a mesenchymal-like or mesenchymal phenotype (Giannelli et al., 2016). This allows them to detach from the original tumor and migrate to distant locations, leading to metastasis. Activated Mφ promotes the invasion, migration, and angiogenesis of HCC (Wu and Zheng, 2012). There are multiple signaling pathways that regulate EMT, like TGF-β and Wnt/β-catenin, and entail the activation of transcriptional regulators, namely, Snail, Slug, and Twist. Low expression of CD86+, overexpression of CD206+, and oncoprotein-induced transcript 3 (OIT3) in TAMs are significantly associated with tumor invasion abilities such as multifocal tumors and late-stage tumor lymph node metastasis (TNM) (Jiang et al., 2021; Yang et al., 2022). M2-TAMs can facilitate the process of EMT and proliferation in liver cancer (Li et al., 2018b; Xiao et al., 2018; Jiang et al., 2021), while M1-TAMs act opposite to the action of M2-TAMs (Zhang et al., 2020; Chen et al., 2021). Of note, CD68+ human leukocyte antigen (HLA)-DR+ M1-TAMs promoted carcinoma cell movement through the activation of NF-κB/focal adhesion kinase (FAK) signaling (Wang et al., 2014). During the polarization process of U937 Mφ from the M2-TAM to M1-TAM type, lnc-Ma301 was found to be overexpressed. This overexpression led to an interaction between lnc-Ma301 and caprin-1 and then ultimately inhibited the metastasis of HCC and EMT through the AKT/ERK1 signaling (Luo et al., 2021). M2-TAM leads to a significant increase in expressing the mesenchymal-associated markers N-cadherin and vimentin of malignant liver cells, along with Snail, Twist, and ZEB1. At the same time, it decreases the ratio of E-cadherin/N-cadherin in cancerous cells (Zhu et al., 2015; Li et al., 2019; Jiang et al., 2021). Carbonic anhydrase XII (CA12) expressed in TAMs stimulates the production of significant quantities of CCL8, which promoted cancer cell EMT (Ning et al., 2022). Additionally, M2-TAMs promote the process of EMT through paracrine releasing vascular endothelial growth factor (VEGF), IL-10, and CCL18 (Wang et al., 2017; Li et al., 2018c; Feng et al., 2018). The TGF-β1 secreted by TAMs initiated activate Gli2/IGF-II/ERK1/2 pathway in HCC cells (Liu et al., 2020). In addition, TGF-β1 inhibiting HCC miR-28-5p expression could be a vicious cycle (Zhou et al., 2016). The aforementioned signals were demonstrated to stimulate the multiplication, invasiveness, and migratory potential of HCC cells. M2-TAMs facilitated the attaching Smad2/3 to the miR-362-3p promoter, bringing about an upregulation of miR-362-3p, which was attributed to the release of TGF-β. The maintenance of EMT was regulated by miR-362-3p through the modulation of CD82, a significant member of the tetraspanin family (Zhang et al., 2019). M2-TAMs have been shown to release IL-1β, which stimulates the production of HIF-1α in cancerous cells through cyclooxygenase-2 (COX-2) (Zhang et al., 2018; Zhang et al., 2018; Gao et al., 2023). Additionally, IL-1 was shown to facilitate EMT in HCC cells via activating the IL-1R1/IκB/IKK/NF-κB pathway (Wang et al., 2020; Meng et al., 2022). Inflammatory cytokines secreted by activated macrophages can cause a reduction of E-cadherin expression in HCC. This is achieved through activating NF-κB/Slug signaling and destabilizing the E-cadherin/β-catenin complex. The instability of the E-cadherin/β-catenin complex was caused by phosphorylating β-catenin and E-cadherin with tyrosine kinases c-Src and EGFR (Lin et al., 2006; Wang et al., 2014). TAMs secreted CXCL12 to bind with CXCR4 of HCC cells, activating the ERK and AKT pathways, promoting HCC proliferation and metastasis (Song et al., 2021). Activated macrophages, known as TAMs, can trigger the EMT of HCC cells. This process is mediated by the JAK2/STAT3/Snail pathway, which is activated by the inflammatory cytokine IL-8 (Fu et al., 2015). The diminishment in the G protein-coupled receptor kinase 2 (GRK2) expression of M2-polarized macrophages, which are stimulated by β2-adrenoceptors (β2-ARs), induces the activation of the cyclic adenosine monophosphate (cAMP)/protein kinase A/cAMP response element binding protein and cAMP/IL-6/STAT3 signaling pathways. This decrease in GRK2 expression contributes to the release of associated cytokines, such as VEGF, MMP-9, and IL-6. Ultimately, this leads to the enhancement of malignant biological activity in cancer cells (Wu et al., 2019). MiR-15b expression was upregulated in M2-TAMs and transferred to HCC cells through EVs. This transfer of miR-15b in EVs suppressed the activation of the Hippo pathway through LATS1. LATS1-mediated ubiquitination and degradation of YAP1 were suppressed. The displacement of YAP1 to the nucleus stimulates the upregulation of oncogenes, leading to increased multiplication, invasion, and propagation of neoplastic liver cells (Li et al., 2021). The interplay between TAMs and EMT is intricate and can facilitate the advancement and metastasis of cancer. Targeting these processes can be a hopeful approach for cancer treatment.
In well-differentiated HCC, there exists a correspondence between the number of TAMs and tumor blood vessels (Fujita et al., 2014; Wang et al., 2020). TAMs are linked to the promotion of neovascularization, and the count of tumor micro-vessels (MVs) is related to carcinoma invasion and metastasis (Peng et al., 2005). One mechanism is the production of MMP-9 by TAMs, which contributes to tumor angiogenesis, extracellular matrix (ECM) remodeling, and cancer cell invasion through the ECM, ultimately promoting cancer cell metastasis (Wu et al., 2019; Wang et al., 2020). VEGF is generally regarded as one of the most significant angiogenic factors released by Mφ (Zhou et al., 2017). Abnormal angiogenesis was linked to the activation of platelets by tissue factor (TF) secreted by invading Mφ and endothelial cells. Tumoral angiogenesis is significantly influenced by HIF-1α, VEGF, and TF (Dupuy et al., 2003; Zang et al., 2018). In tumor tissues, the presence of M-CSF, also known as CSF1, promotes carcinoma growth and angiogenesis by a paracrine effect on the CSF1R (Awasthi et al., 2023). The absence of functional polarization, as indicated by the M0 signature and the heightened presence of CCL2 receptors such as CCR2 and CX3CR1, as well as pro-angiogenic factors, were observed. The M0 signature was found to be linked with adverse clinical results, while the expression of CCL2 was linked to YAP and the formation of vascular networks (Thomann et al., 2021). The promotion of TAMs on EMT and angiogenesis are all important processes in carcinoma growth and metastasis, and understanding the interactions between these processes may lead to the emergence of novel cancer treatments.
TAMs possess the capability to impact hepatic fibrosis in HCC
Hepatic stellate cells are responsible for hepatic fibrosis by depositing extracellular matrix proteins. These cells are activated, in part, by TAMs. Exacerbation of liver fibrotic changes, as well as induction of neighboring epithelial cells transformed into HCC, occurs when the aging response in hepatic stellate cells, which relies on p53, is eliminated (Lujambio et al., 2013). Long-term alcohol abuse facilitates the TAMs infiltration in HCC, exacerbating inflammation, fibrosis, and EMT in the disease process of HCC (Yan et al., 2017). In vitro experiments have demonstrated that glucagon-like peptide-1 (GLP-1) can suppress the proinflammatory characteristics of Mφ (Kawakubo et al., 2020). M2-TAMs engage in the procedure of hepatic fibrosis in HCC advancement and are managed via the PI3K-AKT-mTOR signaling (Zhang et al., 2022). Activation of the Notch signaling pathway in Mφ promotes hepatic fibrosis by upregulating NF-κB via cylindromatosis (CYLD) (He et al., 2015). In TAMs with autophagy defects, mitochondrial ROS enhances the NF-κB signaling and increases the releasement of IL-1α/β. NOX enzymes were the principal source of ROS. NOX1 and secretion of IL-1α/β and TNF-α facilitated the advancement of hepatic fibrosis and inflammation (Amano et al., 2017; Sun et al., 2017; Vandierendonck et al., 2021). Targeting the phenotype of TAMs and the cytokines they secrete is the key to blocking the progression of the fibrotic liver into HCC (Table 1).
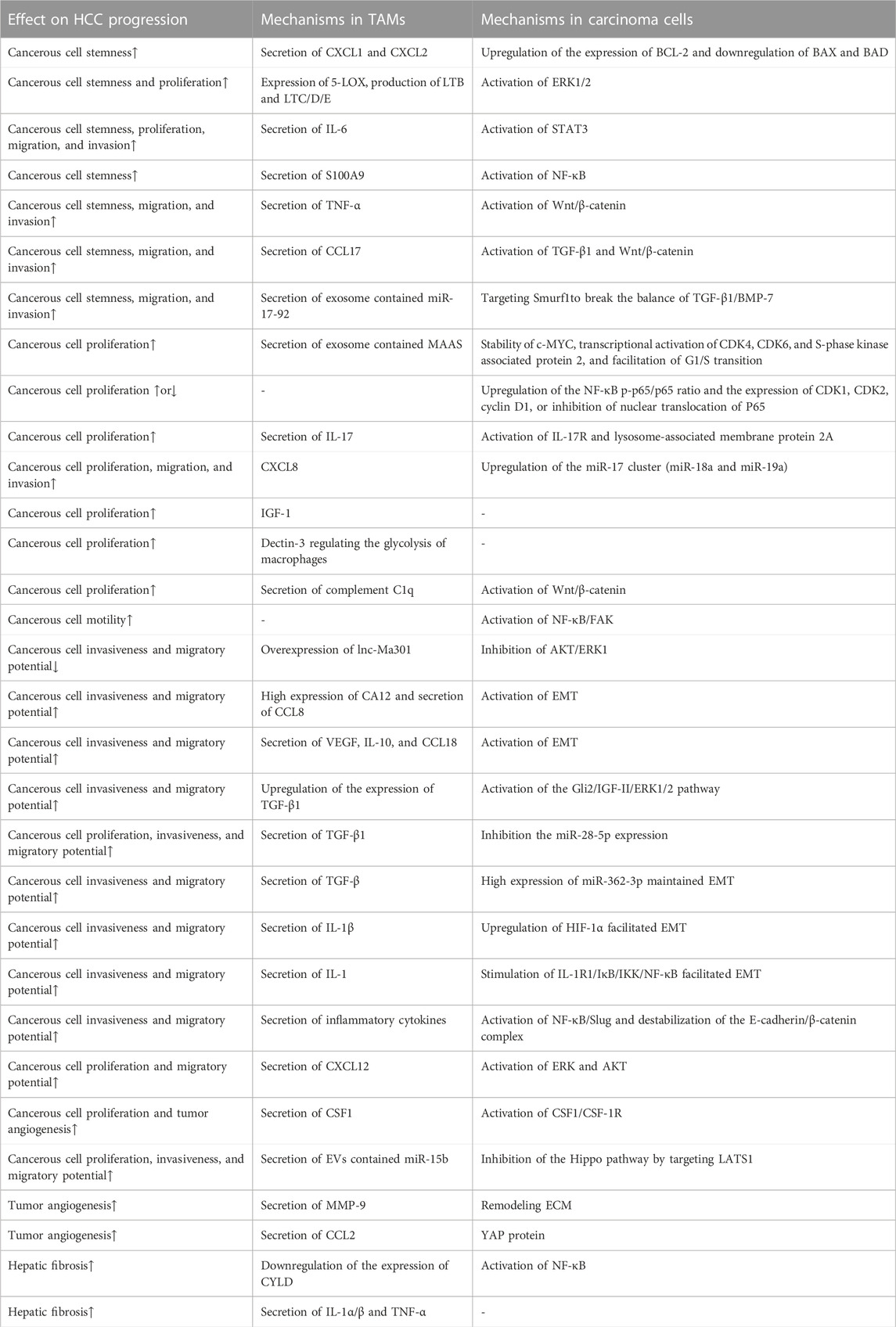
TABLE 1. Mechanisms of TAMs influencing the progression of HCC. (↑ means promoting the process to promote HCC progression, and ↓ means inhibiting HCC progression by inhibiting the process.)
Interplay between TAMs and other immune cells reshapes the immune microenvironment in HCC
The TME contains various immune cells, such as TAMs, T cells, B cells, and NK cells, whose secretion of cytokines leads to cellular interactions and ultimately reshapes the immune microenvironment, affecting the progression of the tumor. The TME often experiences hypoxia, a common phenomenon in various cancer types. This hypoxia can negatively impact cytotoxic T cells’ actions and promote recruiting regulatory T cells (Tregs), ultimately inducing a reduction in the tumor’s immunogenicity (Wu et al., 2022). In hypoxic tumor microenvironments, Tregs can impair the function of cytotoxic T cells. This is due to the induction of TREM1 in TAMs by HIF-1α under hypoxic conditions. The increase in TREM1 activates the ERK/NF-κB pathway as a reaction to oxygen starvation and cancer-specific metabolites, resulting in the increased expression of CCL20. This leads to the accumulation of CCR6+Foxp3+ Tregs (Wu et al., 2019). CD69+ T cells could trigger TAMs to produce indoleamine 2,3-dioxygenase (IDO), while TAMs can secrete IL-12 to activate T cells. Furthermore, IL-12 can also enhance the expression of IDO, which subsequently brings about Tregs expansion and the suppression of T cells’ reactions and proliferation (Zhao et al., 2012; Ye et al., 2016). The presence of CD74+ Mφ in HCC was discovered to have a correlation with more CD8+ cytotoxic T lymphocyte (CTL) infiltration, which displayed improved effector capabilities (Xiao et al., 2022). CD169+ Mφ were observed to significantly augment the multiplication, cytotoxic potential, and cell secretory product generation of CD8+ T cells in a manner relying on CD169. The downregulation of CD169 expression in these cells is linked to autocrine TGF-β secretion. This is opposite to the survival prognosis tendency of the entire amount of CD68+ macrophages (Zhang et al., 2016). In non-responders, the TME is typical to the close proximity of CD8+ T cells and Arg1hi TAMs, rather than CD4+ T cells (Mi et al., 2022). M2-TAMs suppress the activity of NK cells (Xu et al., 2021).
As a type of co-inhibitory molecule, PD-L1 can bind to the programmed death-1 (PD-1) receptor on the surface of T cells to regulate immune responses and inhibit immune cells from killing tumor cells, and is closely related to T-cell exhaustion and tumor immune evasion. PD-L1 can be expressed in tumor cells, TAMs, and vascular endothelial cells, but PD-L1 expression was predominantly witnessed on CD68+ TAMs rather than HCC cells in the TME. Additionally, high expression of PD-L1 was detected on TREM-1+ TAMs (Wu et al., 2019; Park et al., 2021). Abnormal levels of cytokines such as HGF, VEGF, TNF-α, and IL-6 are often detected in the serum of HCC patients and are closely related to liver cancer prognosis. Among them, elevated levels of IL-6 in serum lead to the downregulation of protein tyrosine phosphatase receptor O (PTPRO) expression in monocytes and Mφ. A depletion of PTPRO mediates high expression of PD-L1 in monocytes and Mφ through the JAK2/STAT1 and JAK2/STAT3/C-MYC pathways (Zhang et al., 2020). Changes in the expression of enzymes related to abnormal glycolipid metabolism can also affect the expression of PD-L1 in TAMs. The overexpression of key glycolytic enzyme PFKFB3 in the monocytes of the TME induces high expression of PD-L1 through mediation of the NF-κB signaling pathway (Chen et al., 2019). Monocytes upregulate IL-10 secretion through the expression of lipid-binding protein FABP5 and inhibition of the PPARα pathway, and IL-10 can promote the expression of PD-L1 in Tregs and TAMs (Liu et al., 2022), ultimately resulting in immunosuppression. Abnormal metabolism of tumor cells can lead to increased secretion of IgA. Stimulation of IgA signaling induces M2 polarization and upregulates PD-L1 expression through YAP, leading to reduced CD8+ T-cell infiltration (Sung et al., 2022). Upregulating PD-L1 in TAMs was also found to be mediated by STAT3, which is induced by tumor-derived Sonic hedgehog (Shh) signaling (Petty et al., 2021). Tumor cells activate the hedgehog (Hh) signaling pathway to suppress Mφ to produce CXCL10 and CXCL9 (Petty et al., 2019). CXCL10 secreted by Mφ attaches to CXC motif chemokine receptor (CXCR)3 on B cells, causing them to differentiate into IgG-producing plasma cells. IgG stimulates Fc receptors on Mφ, which in turn release cell secretory product like IL-10, IL-6, and CCL20, causing a diminish in the recruitment and response of antineoplastic CD8+ effector cells (Wei et al., 2019). The secretion of CXCL9 by TREM2+ TAMs was reduced, while Galectin-1 (Gal-1) secretion was increased. This resulted in PD-L1 highly expressing in vascular endothelial cells, which impeded the recruiting and infiltration of CD8+ T cells into the TME (Tan et al., 2023). The high levels of DNA damage in HCC cells activate the cyclic GMP–AMP synthase (cGAS)–stimulator of interferon genes (STING) signaling, leading to PD-L1 expression in M1-TAMs through the STING-IRF3-STAT1 pathway. This promotes immunosuppression, facilitating oncogenesis and malignant development. Additionally, M1-TAMs with a stimulated cGAS–STING signaling can enlist T lymphocytes via the STING-IRF3 signaling (Ma et al., 2023). The IL-27 secreted by Mφ leads to the phosphorylation of STAT1 and reproduces an IFN-γ-like reaction in cancer cells, which is similar to the effect of IFN-γ, leading to the upregulation of TAP2 and MHC-I proteins, thereby increasing the immune clearance rate. Simultaneously, it induces T-cell exhaustion by promoting PD-L1 expression and IDO secretion (Rolvering et al., 2018). Furthermore, as antigen-presenting cells, the infiltration of MHC IIlow TAMs is positively correlated with the progression of HCC (Wang et al., 2011). The overexpression of PD-L1 in HCC is also related to the inhibition of PD-L1 ubiquitination and degradation processes in tumor cells and TAMs. Golgi membrane protein 1 (GOLM1) in HCC cells inhibits the ubiquitination and degradation of PD-L1 by COP9 signalosome 5 and promotes the secretion of EVs containing PD-L1 in HCC cells, upregulating the expression of PD-L1 in TAMs (Chen et al., 2021). The lack of GSK3β in TAMs can also inhibit the ubiquitination and degradation of PD-L1 (Sun et al., 2022). On one hand, high expression of PD-L1 can reshape the immune inhibitory microenvironment. However, on the other hand, increased levels of PD-L1 expression can facilitate the treatment of PD-1 inhibitors (Figure 3).
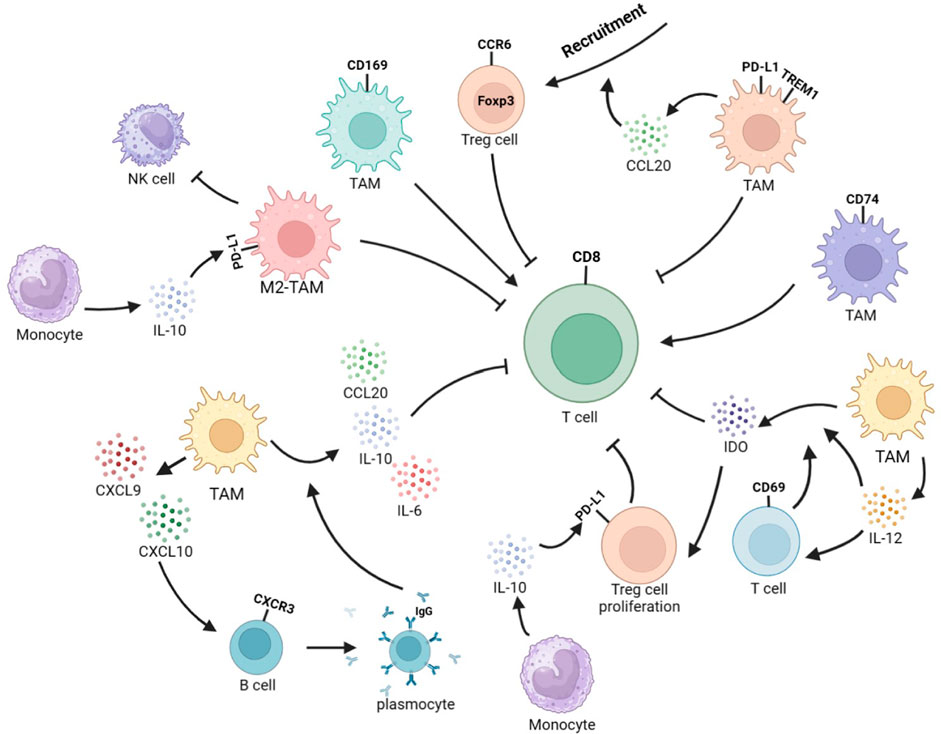
FIGURE 3. Interplay between TAMs and other immune cells reshapes the immune microenvironment in HCC. TREM1+TAMs secrete CCL20 to recruit CCR6+Foxp3+ Tregs and exert immunosuppressive function. CD69+ T cells can stimulate TAMs to produce IDO, and TAMs can secrete IL-12 to activate T cells. In addition, IL-12 can enhance IDO expression, leading to Treg expansion and inhibition of T-cell response and proliferation. CD74+ TAMs induce stronger effector function in CD8+ CTLs. CD169+ TAMs significantly enhance the proliferation, cytotoxic potential, and secretion of CD8+ T cells in a CD169-dependent manner. M2-TAMs can to inhibit NK-cell activity. Monocytes-secreted IL-10 promotes PD-L1 expression in Tregs and TAMs. TAMs could secret CXCL9 and CXCL10. CXCL10 stimulates CXCR3+B cells to differentiate into IgG-producing plasma cells. IgG stimulates Fc receptors on TAMs, leading to the secretion of IL-10, IL-6, and CCL20 to inhibit the recruitment and function of CD8+ T cells.
Conclusion
TAMs originate from peripheral blood monocytes, M-MDSCs, and KCs. The cytokines and EVs secreted by tumor cells, stromal cells including TAMs, and other interstitial cells within the tumor tissue can induce the recruitment of peripheral monocytes. Meanwhile, TAMs in the tumor tissue can also increase their infiltration by proliferation. Altered glucose and lipid metabolism in HCC and cytokines secreted by tumor cells induce Mφ polarization and functional phenotype changes. However, due to the influence of the TME, TAMs often exert immunosuppressive functions, inducing the occurrence of malignant behavior in HCC. TAMs directly activate or inhibit the NF-κB, IL-6/STAT3, Wnt/β-catenin, TGF-β1/BMP, and ERK1/2 signaling pathways in HCC cells by producing cytokines and exosomes and overexpressing related proteins, affecting carcinoma cell proliferation, invasion and migration ability, angiogenesis, and liver fibrosis progression, thus affecting the progression of HCC. Additionally, the coordination between TAMs and immune cells reshapes the immune-suppressive microenvironment within cancer. Among them, elevated PD-L1 expression in TAMs suppresses the stimulation of CD8+ T cells, induces CTL exhaustion, and promotes Treg recruitment, which is one of the key mechanisms for inhibiting CD8+ T-cell killing of HCC cells. The resistance mechanism of the small-molecule targeted drug sorafenib used in HCC treatment is also linked with TAMs. Therefore, focusing on the mechanism of TAMs interacting with tumor and immune cells in HCC helps to target relevant pathways to inhibit the progression of HCC.
Author contributions
YY and DW were the major contributors to the original draft, JL prepared the figures, YZ and DH collected the related papers, and TG and ZZ helped to collect references. YC, D-YZ, and YT revised the manuscript. All authors contributed to the article and approved the submitted version.
Funding
This work was supported by the National Natural Science Foundation of China (Grant No. 82160506 and 82205220) and Science and Technology Department of Xinjiang Uygur Autonomous Region (Grant No. 2021D01C016).
Conflict of interest
The authors declare that the research was conducted in the absence of any commercial or financial relationships that could be construed as a potential conflict of interest.
Publisher’s note
All claims expressed in this article are solely those of the authors and do not necessarily represent those of their affiliated organizations, or those of the publisher, the editors, and the reviewers. Any product that may be evaluated in this article, or claim that may be made by its manufacturer, is not guaranteed or endorsed by the publisher.
References
Allavena, P., Sica, A., Garlanda, C., and Mantovani, A. (2008). The Yin-Yang of tumor-associated macrophages in neoplastic progression and immune surveillance. Immunol. Rev. 222, 155–161. doi:10.1111/j.1600-065X.2008.00607.x
Amano, Y., Shimizu, F., Yasuno, H., Harada, A., Tsuchiya, S., Isono, O., et al. (2017). Non-alcoholic steatohepatitis-associated hepatic fibrosis and hepatocellular carcinoma in a combined mouse model of genetic modification and dietary challenge. Hepatol. Res. 47 (1), 103–115. doi:10.1111/hepr.12709
Awasthi, B. P., Guragain, D., Chaudhary, P., Jee, J. G., Kim, J. A., and Jeong, B. S. (2023). Antitumor activity of a pexidartinib bioisostere inhibiting CSF1 production and CSF1R kinase activity in human hepatocellular carcinoma. Chem. Biol. Interact. 369, 110255. doi:10.1016/j.cbi.2022.110255
Bao, D., Zhao, J., Zhou, X., Yang, Q., Chen, Y., Zhu, J., et al. (2019). Mitochondrial fission-induced mtDNA stress promotes tumor-associated macrophage infiltration and HCC progression. Oncogene 38 (25), 5007–5020. doi:10.1038/s41388-019-0772-z
Cao, P., Ma, B., Sun, D., Zhang, W., Qiu, J., Qin, L., et al. (2022). hsa_circ_0003410 promotes hepatocellular carcinoma progression by increasing the ratio of M2/M1 macrophages through the miR-139-3p/CCL5 axis. Cancer Sci. 113 (2), 634–647. doi:10.1111/cas.15238
Chang, C. P., Su, Y. C., Hu, C. W., and Lei, H. Y. (2013). TLR2-dependent selective autophagy regulates NF-κB lysosomal degradation in hepatoma-derived M2 macrophage differentiation. Cell Death Differ. 20 (3), 515–523. doi:10.1038/cdd.2012.146
Chen, D., Xie, J., Fiskesund, R., Dong, W., Liang, X., Lv, J., et al. (2018). Chloroquine modulates antitumor immune response by resetting tumor-associated macrophages toward M1 phenotype. Nat. Commun. 9 (1), 873. doi:10.1038/s41467-018-03225-9
Chen, D. P., Ning, W. R., Jiang, Z. Z., Peng, Z. P., Zhu, L. Y., Zhuang, S. M., et al. (2019b). Glycolytic activation of peritumoral monocytes fosters immune privilege via the PFKFB3-PD-L1 axis in human hepatocellular carcinoma. J. Hepatol. 71 (2), 333–343. doi:10.1016/j.jhep.2019.04.007
Chen, H., Tang, C., Tan, C., Wu, F., Li, Z., Ji, W., et al. (2022). IL-2 modulates TAMs derived exosomal MiRNAs to ameliorate hepatocellular carcinoma development and progression. J. Oncol. 2022, 3445350. doi:10.1155/2022/3445350
Chen, J., Lin, Z., Liu, L., Zhang, R., Geng, Y., Fan, M., et al. (2021b). GOLM1 exacerbates CD8(+) T cell suppression in hepatocellular carcinoma by promoting exosomal PD-L1 transport into tumor-associated macrophages. Signal Transduct. Target Ther. 6 (1), 397. doi:10.1038/s41392-021-00784-0
Chen, M., Lai, R., Lin, X., Chen, W., Wu, H., and Zheng, Q. (2021a). Downregulation of triggering receptor expressed on myeloid cells 1 inhibits invasion and migration of liver cancer cells by mediating macrophage polarization. Oncol. Rep. 45 (4), 37. doi:10.3892/or.2021.7988
Chen, Y., Wen, H., Zhou, C., Su, Q., Lin, Y., Xie, Y., et al. (2019a). TNF-α derived from M2 tumor-associated macrophages promotes epithelial-mesenchymal transition and cancer stemness through the Wnt/β-catenin pathway in SMMC-7721 hepatocellular carcinoma cells. Exp. Cell Res. 378 (1), 41–50. doi:10.1016/j.yexcr.2019.03.005
Cheng, K., Cai, N., Zhu, J., Yang, X., Liang, H., and Zhang, W. (2022). Tumor-associated macrophages in liver cancer: from mechanisms to therapy. Cancer Commun. Lond. Engl. 42 (11), 1112–1140. doi:10.1002/cac2.12345
Chiyonobu, N., Shimada, S., Akiyama, Y., Mogushi, K., Itoh, M., Akahoshi, K., et al. (2018). Fatty acid binding protein 4 (FABP4) overexpression in intratumoral hepatic stellate cells within hepatocellular carcinoma with metabolic risk factors. Am. J. Pathol. 188 (5), 1213–1224. doi:10.1016/j.ajpath.2018.01.012
Colombo, M., Raposo, G., and Thery, C. (2014). Biogenesis, secretion, and intercellular interactions of exosomes and other extracellular vesicles. Annu. Rev. Cell Dev. Biol. 30, 255–289. doi:10.1146/annurev-cellbio-101512-122326
Conte, E. (2022). Targeting monocytes/macrophages in fibrosis and cancer diseases: therapeutic approaches. Pharmacol. Ther. 234, 108031. doi:10.1016/j.pharmthera.2021.108031
Cui, X., Zhao, H., Wei, S., Du, Q., Dong, K., Yan, Y., et al. (2023). Hepatocellular carcinoma-derived FOXO1 inhibits tumor progression by suppressing IL-6 secretion from macrophages. Neoplasia 40, 100900. doi:10.1016/j.neo.2023.100900
de Oliveira, S., Houseright, R. A., Graves, A. L., Golenberg, N., Korte, B. G., Miskolci, V., et al. (2019). Metformin modulates innate immune-mediated inflammation and early progression of NAFLD-associated hepatocellular carcinoma in zebrafish. J. Hepatol. 70 (4), 710–721. doi:10.1016/j.jhep.2018.11.034
Delire, B., Henriet, P., Lemoine, P., Leclercq, I. A., and Starkel, P. (2018). Chronic liver injury promotes hepatocarcinoma cell seeding and growth, associated with infiltration by macrophages. Cancer Sci. 109 (7), 2141–2152. doi:10.1111/cas.13628
DeLuca, V. J., and Saleh, T. (2023). Insights into the role of senescence in tumor dormancy: mechanisms and applications. Cancer metastasis Rev. 42 (1), 19–35. doi:10.1007/s10555-023-10082-6
Ding, Y., Liu, X., Yuan, Y., Sheng, Y., Li, D., Ojha, S. C., et al. (2022). THRSP identified as a potential hepatocellular carcinoma marker by integrated bioinformatics analysis and experimental validation. Aging 14 (4), 1743–1766. doi:10.18632/aging.203900
Dong, N., Shi, X., Wang, S., Gao, Y., Kuang, Z., Xie, Q., et al. (2019). M2 macrophages mediate sorafenib resistance by secreting HGF in a feed-forward manner in hepatocellular carcinoma. Br. J. Cancer 121 (1), 22–33. doi:10.1038/s41416-019-0482-x
Dong, P., Ma, L., Liu, L., Zhao, G., Zhang, S., Dong, L., et al. (2016). CD86⁺/CD206⁺, diametrically polarized tumor-associated macrophages, predict hepatocellular carcinoma patient prognosis. Int. J. Mol. Sci. 17 (3), 320. doi:10.3390/ijms17030320
Du, L., Ji, Y., Xin, B., Zhang, J., Lu, L. C., Glass, C. K., et al. (2023). Shp2 deficiency in kupffer cells and hepatocytes aggravates hepatocarcinogenesis by recruiting non-kupffer macrophages. Cell Mol. Gastroenterol. Hepatol. 15 (6), 1351–1369. doi:10.1016/j.jcmgh.2023.02.011
Dupuy, E., Hainaud, P., Villemain, A., Bodevin-Phedre, E., Brouland, J. P., Briand, P., et al. (2003). Tumoral angiogenesis and tissue factor expression during hepatocellular carcinoma progression in a transgenic mouse model. J. Hepatol. 38 (6), 793–802. doi:10.1016/s0168-8278(03)00086-2
Eggert, T., Wolter, K., Ji, J., Ma, C., Yevsa, T., Klotz, S., et al. (2016). Distinct functions of senescence-associated immune responses in liver tumor surveillance and tumor progression. Cancer Cell 30 (4), 533–547. doi:10.1016/j.ccell.2016.09.003
Endig, J., Buitrago-Molina, L. E., Marhenke, S., Reisinger, F., Saborowski, A., Schütt, J., et al. (2016). Dual role of the adaptive immune system in liver injury and hepatocellular carcinoma development. Cancer Cell 30 (2), 308–323. doi:10.1016/j.ccell.2016.06.009
Englinger, B., Pirker, C., Heffeter, P., Terenzi, A., Kowol, C. R., Keppler, B. K., et al. (2019). Metal drugs and the anticancer immune response. Chem. Rev. 119 (2), 1519–1624. doi:10.1021/acs.chemrev.8b00396
Fan, Q. M., Jing, Y. Y., Yu, G. F., Kou, X. R., Ye, F., Gao, L., et al. (2014). Tumor-associated macrophages promote cancer stem cell-like properties via transforming growth factor-beta1-induced epithelial-mesenchymal transition in hepatocellular carcinoma. Cancer Lett. 352 (2), 160–168. doi:10.1016/j.canlet.2014.05.008
Feng, R., Morine, Y., Ikemoto, T., Imura, S., Iwahashi, S., Saito, Y., et al. (2018). Nrf2 activation drive macrophages polarization and cancer cell epithelial-mesenchymal transition during interaction. Cell Commun. Signal 16 (1), 54. doi:10.1186/s12964-018-0262-x
Fu, X. T., Dai, Z., Song, K., Zhang, Z. J., Zhou, Z. J., Zhou, S. L., et al. (2015). Macrophage-secreted IL-8 induces epithelial-mesenchymal transition in hepatocellular carcinoma cells by activating the JAK2/STAT3/Snail pathway. Int. J. Oncol. 46 (2), 587–596. doi:10.3892/ijo.2014.2761
Fujita, N., Nishie, A., Aishima, S., Kubo, Y., Asayama, Y., Ishigami, K., et al. (2014). Role of tumor-associated macrophages in the angiogenesis of well-differentiated hepatocellular carcinoma: pathological-radiological correlation. Oncol. Rep. 31 (6), 2499–2505. doi:10.3892/or.2014.3138
Gabrilovich, D. I., Ostrand-Rosenberg, S., and Bronte, V. (2012). Coordinated regulation of myeloid cells by tumours. Nat. Rev. Immunol. 12 (4), 253–268. doi:10.1038/nri3175
Gao, L., Lv, G., Li, R., Liu, W. T., Zong, C., Ye, F., et al. (2019). Glycochenodeoxycholate promotes hepatocellular carcinoma invasion and migration by AMPK/mTOR dependent autophagy activation. Cancer Lett. 454, 215–223. doi:10.1016/j.canlet.2019.04.009
Gao, Z., Feng, S. R., Chen, J. F., Li, X. G., Shi, Y. H., Tang, Z., et al. (2023). Inhibition of autophagy in macrophage promotes IL-1β-mediated hepatocellular carcinoma progression via inflammasome accumulation and self-recruitment. Biomed. Pharmacother. 161, 114560. doi:10.1016/j.biopha.2023.114560
Ge, Z., and Ding, S. (2020). The crosstalk between tumor-associated macrophages (TAMs) and tumor cells and the corresponding targeted therapy. Front. Oncol. 10, 590941. doi:10.3389/fonc.2020.590941
Giannelli, G., Koudelkova, P., Dituri, F., and Mikulits, W. (2016). Role of epithelial to mesenchymal transition in hepatocellular carcinoma. J. Hepatol. 65 (4), 798–808. doi:10.1016/j.jhep.2016.05.007
Giraud, J., Chalopin, D., Blanc, J. F., and Saleh, M. (2021). Hepatocellular carcinoma immune landscape and the potential of immunotherapies. Front. Immunol. 12, 655697. doi:10.3389/fimmu.2021.655697
Gomez Perdiguero, E., Klapproth, K., Schulz, C., Busch, K., Azzoni, E., Crozet, L., et al. (2015). Tissue-resident macrophages originate from yolk-sac-derived erythro-myeloid progenitors. Nature 518 (7540), 547–551. doi:10.1038/nature13989
Gou, Y., Yang, D., Tian, T., Zhu, X., Zhang, R., Ren, J., et al. (2022). The transcription of ZIP9 is associated with the macrophage polarization and the pathogenesis of hepatocellular carcinoma. Front. Immunol. 13, 725595. doi:10.3389/fimmu.2022.725595
Guo, B., Li, L., Guo, J., Liu, A., Wu, J., Wang, H., et al. (2017b). M2 tumor-associated macrophages produce interleukin-17 to suppress oxaliplatin-induced apoptosis in hepatocellular carcinoma. Oncotarget 8 (27), 44465–44476. doi:10.18632/oncotarget.17973
Guo, X., Zhao, Y., Yan, H., Yang, Y., Shen, S., Dai, X., et al. (2017a). Single tumor-initiating cells evade immune clearance by recruiting type II macrophages. Genes & Dev. 31 (3), 247–259. doi:10.1101/gad.294348.116
Han, D., Fang, Y., Guo, Y., Hong, W., Tu, J., and Wei, W. (2019). The emerging role of long non-coding RNAs in tumor-associated macrophages. J. Cancer 10 (26), 6738–6746. doi:10.7150/jca.35770
He, F., Guo, F. C., Li, Z., Yu, H. C., Ma, P. F., Zhao, J. L., et al. (2015). Myeloid-specific disruption of recombination signal binding protein Jκ ameliorates hepatic fibrosis by attenuating inflammation through cylindromatosis in mice. Hepatology 61 (1), 303–314. doi:10.1002/hep.27394
He, Q., Liu, M., Huang, W., Chen, X., Zhang, B., Zhang, T., et al. (2021). IL-1β-Induced elevation of solute carrier family 7 member 11 promotes hepatocellular carcinoma metastasis through up-regulating programmed death ligand 1 and colony-stimulating factor 1. Hepatology 74 (6), 3174–3193. doi:10.1002/hep.32062
Ho, T. C., Wang, E. Y., Yeh, K. H., Jeng, Y. M., Horng, J. H., Wu, L. L., et al. (2020). Complement C1q mediates the expansion of periportal hepatic progenitor cells in senescence-associated inflammatory liver. Proc. Natl. Acad. Sci. U. S. A. 117 (12), 6717–6725. doi:10.1073/pnas.1918028117
Hoeffel, G., Chen, J., Lavin, Y., Low, D., Almeida, F. F., See, P., et al. (2015). C-Myb(+) erythro-myeloid progenitor-derived fetal monocytes give rise to adult tissue-resident macrophages. Immunity 42 (4), 665–678. doi:10.1016/j.immuni.2015.03.011
Hou, P. P., Luo, L. J., Chen, H. Z., Chen, Q. T., Bian, X. L., Wu, S. F., et al. (2020). Ectosomal PKM2 promotes HCC by inducing macrophage differentiation and remodeling the tumor microenvironment. Mol. Cell 78 (6), 1192–1206.e10. doi:10.1016/j.molcel.2020.05.004
Hu, Y. C., Yi, Z. J., Zhou, Y., Li, P. Z., Liu, Z. J., Duan, S. G., et al. (2017). Overexpression of RIP140 suppresses the malignant potential of hepatocellular carcinoma by inhibiting NF‑κB‑mediated alternative polarization of macrophages. Oncol. Rep. 37 (5), 2971–2979. doi:10.3892/or.2017.5551
Hu, Z. Q., Zhou, S. L., Li, J., Zhou, Z. J., Wang, P. C., Xin, H. Y., et al. (2020). Circular RNA sequencing identifies CircASAP1 as a key regulator in hepatocellular carcinoma metastasis. Hepatology 72 (3), 906–922. doi:10.1002/hep.31068
Imtiyaz, H. Z., Williams, E. P., Hickey, M. M., Patel, S. A., Durham, A. C., Yuan, L. J., et al. (2010). Hypoxia-inducible factor 2alpha regulates macrophage function in mouse models of acute and tumor inflammation. J. Clin. Invest. 120 (8), 2699–2714. doi:10.1172/JCI39506
Jiang, J., Wang, G. Z., Wang, Y., Huang, H. Z., Li, W. T., and Qu, X. D. (2018). Hypoxia-induced HMGB1 expression of HCC promotes tumor invasiveness and metastasis via regulating macrophage-derived IL-6. Exp. Cell Res. 367 (1), 81–88. doi:10.1016/j.yexcr.2018.03.025
Jiang, Q., Tan, X. P., Zhang, C. H., Li, Z. Y., Li, D., Xu, Y., et al. (2022). Non-coding RNAs of extracellular vesicles: key players in organ-specific metastasis and clinical implications. Cancers (Basel). 14 (22), 5693. doi:10.3390/cancers14225693
Jiang, Q., Zeng, Y., Xu, Y., Xiao, X., Liu, H., Zhou, B., et al. (2020). Ultrasound molecular imaging as a potential non-invasive diagnosis to detect the margin of hepatocarcinoma via CSF-1R targeting. Front. Bioeng. Biotechnol. 8, 783. doi:10.3389/fbioe.2020.00783
Jiang, Y., Han, Q., Zhao, H., and Zhang, J. (2021). Promotion of epithelial-mesenchymal transformation by hepatocellular carcinoma-educated macrophages through Wnt2b/β-catenin/c-Myc signaling and reprogramming glycolysis. J. Exp. Clin. Cancer Res. 40 (1), 13. doi:10.1186/s13046-020-01808-3
Kang, F. B., Wang, L., Li, D., Zhang, Y. G., and Sun, D. X. (2015). Hepatocellular carcinomas promote tumor-associated macrophage M2-polarization via increased B7-H3 expression. Oncol. Rep. 33 (1), 274–282. doi:10.3892/or.2014.3587
Kawakubo, M., Tanaka, M., Ochi, K., Watanabe, A., Saka-Tanaka, M., Kanamori, Y., et al. (2020). Dipeptidyl peptidase-4 inhibition prevents nonalcoholic steatohepatitis-associated liver fibrosis and tumor development in mice independently of its anti-diabetic effects. Sci. Rep. 10 (1), 983. doi:10.1038/s41598-020-57935-6
Kew, M. C. (2014). Hepatocellular carcinoma: epidemiology and risk factors. J. Hepatocell. Carcinoma 1, 115–125.
Kuchuk, O., Tuccitto, A., Citterio, D., Huber, V., Camisaschi, C., Milione, M., et al. (2018). pH regulators to target the tumor immune microenvironment in human hepatocellular carcinoma. Oncoimmunology 7 (7), e1445452. doi:10.1080/2162402X.2018.1445452
Laviron, M., and Boissonnas, A. (2019). Ontogeny of tumor-associated macrophages. Front. Immunol. 10, 1799. doi:10.3389/fimmu.2019.01799
Leung, C. C. T., and Wong, C. K. C. (2020). Effects of stanniocalcin-1 overexpressing hepatocellular carcinoma cells on macrophage migration. PLoS One 15 (11), e0241932. doi:10.1371/journal.pone.0241932
Li, C., Pan, X. Y., Ma, M., Zhao, J., Zhao, F., and Lv, Y. P. (2021a). Astragalus polysacharin inhibits hepatocellular carcinoma-like phenotypes in a murine HCC model through repression of M2 polarization of tumour-associated macrophages. Pharm. Biol. 59 (1), 1533–1539. doi:10.1080/13880209.2021.1991384
Li, J., Xue, J., Ling, M., Sun, J., Xiao, T., Dai, X., et al. (2021b). MicroRNA-15b in extracellular vesicles from arsenite-treated macrophages promotes the progression of hepatocellular carcinomas by blocking the LATS1-mediated Hippo pathway. Cancer Lett. 497, 137–153. doi:10.1016/j.canlet.2020.10.023
Li, J. Q., Yu, X. J., Wang, Y. C., Huang, L. Y., Liu, C. Q., Zheng, L., et al. (2017a). Distinct patterns and prognostic values of tumor-infiltrating macrophages in hepatocellular carcinoma and gastric cancer. J. Transl. Med. 15 (1), 37. doi:10.1186/s12967-017-1139-2
Li, L., Sun, P., Zhang, C., Li, Z., Cui, K., and Zhou, W. (2018b). MiR-98 modulates macrophage polarization and suppresses the effects of tumor-associated macrophages on promoting invasion and epithelial-mesenchymal transition of hepatocellular carcinoma. Cancer Cell Int. 18, 95. doi:10.1186/s12935-018-0590-3
Li, L., Sun, P., Zhang, C., Li, Z., and Zhou, W. (2018c). MiR-98 suppresses the effects of tumor-associated macrophages on promoting migration and invasion of hepatocellular carcinoma cells by regulating IL-10. Biochimie 150, 23–30. doi:10.1016/j.biochi.2018.04.016
Li, Q., Ma, L., Shen, S., Guo, Y., Cao, Q., Cai, X., et al. (2019). Intestinal dysbacteriosis-induced IL-25 promotes development of HCC via alternative activation of macrophages in tumor microenvironment. J. Exp. Clin. Cancer Res. 38 (1), 303. doi:10.1186/s13046-019-1271-3
Li, W., Ng, J. M., Wong, C. C., Ng, E. K. W., and Yu, J. (2018a). Molecular alterations of cancer cell and tumour microenvironment in metastatic gastric cancer. Oncogene 37 (36), 4903–4920. doi:10.1038/s41388-018-0341-x
Li, X., Yao, W., Yuan, Y., Chen, P., Li, B., Li, J., et al. (2017b). Targeting of tumour-infiltrating macrophages via CCL2/CCR2 signalling as a therapeutic strategy against hepatocellular carcinoma. Gut 66 (1), 157–167. doi:10.1136/gutjnl-2015-310514
Liang, L., Sun, H., Zhang, W., Zhang, M., Yang, X., Kuang, R., et al. (2016). Meta-Analysis of EMT datasets reveals different types of EMT. PLoS One 11 (6), e0156839. doi:10.1371/journal.pone.0156839
Lin, C. Y., Lin, C. J., Chen, K. H., Wu, J. C., Huang, S. H., and Wang, S. M. (2006). Macrophage activation increases the invasive properties of hepatoma cells by destabilization of the adherens junction. FEBS Lett. 580 (13), 3042–3050. doi:10.1016/j.febslet.2006.04.049
Lin, Y., Xu, J., and Lan, H. (2019). Tumor-associated macrophages in tumor metastasis: biological roles and clinical therapeutic applications. J. Hematol. Oncol. 12 (1), 76. doi:10.1186/s13045-019-0760-3
Liu, J., Sun, B., Guo, K., Yang, Z., Zhao, Y., Gao, M., et al. (2022). Lipid-related FABP5 activation of tumor-associated monocytes fosters immune privilege via PD-L1 expression on Treg cells in hepatocellular carcinoma. Cancer Gene Ther. 29 (12), 1951–1960. doi:10.1038/s41417-022-00510-0
Liu, M., Zhong, Y. B., Shao, J., Zhang, C., and Shi, C. (2020). Tumor-associated macrophages promote human hepatoma Huh-7 cell migration and invasion through the Gli2/IGF-II/ERK1/2 axis by secreting TGF-β1. Cancer Biol. Ther. 21 (11), 1041–1050. doi:10.1080/15384047.2020.1824478
Liu, R. X., Wei, Y., Zeng, Q. H., Chan, K. W., Xiao, X., Zhao, X. Y., et al. (2015). Chemokine (C-X-C motif) receptor 3-positive B cells link interleukin-17 inflammation to protumorigenic macrophage polarization in human hepatocellular carcinoma. Hepatology 62 (6), 1779–1790. doi:10.1002/hep.28020
Lopez, B. G., Tsai, M. S., Baratta, J. L., Longmuir, K. J., and Robertson, R. T. (2011). Characterization of Kupffer cells in livers of developing mice. Comp. Hepatol. 10 (1), 2. doi:10.1186/1476-5926-10-2
Lu, Y., Yang, A., Quan, C., Pan, Y., Zhang, H., Li, Y., et al. (2022). A single-cell atlas of the multicellular ecosystem of primary and metastatic hepatocellular carcinoma. Nat. Commun. 13 (1), 4594. doi:10.1038/s41467-022-32283-3
Lujambio, A., Akkari, L., Simon, J., Grace, D., Tschaharganeh, D. F., Bolden, J. E., et al. (2013). Non-cell-autonomous tumor suppression by p53. Cell 153 (2), 449–460. doi:10.1016/j.cell.2013.03.020
Luo, H. L., Chen, J., Luo, T., Wu, F. X., Liu, J. J., Wang, H. F., et al. (2017). Downregulation of macrophage-derived T-UCR uc.306 associates with poor prognosis in hepatocellular carcinoma. Cell Physiol. Biochem. 42 (4), 1526–1539. doi:10.1159/000479269
Luo, H. L., Luo, T., Liu, J. J., Wu, F. X., Bai, T., Ou, C., et al. (2021). Macrophage polarization-associated lnc-Ma301 interacts with caprin-1 to inhibit hepatocellular carcinoma metastasis through the Akt/Erk1 pathway. Cancer Cell Int. 21 (1), 422. doi:10.1186/s12935-021-02133-1
Ma, H., Kang, Z., Foo, T. K., Shen, Z., and Xia, B. (2023). Disrupted BRCA1-PALB2 interaction induces tumor immunosuppression and T-lymphocyte infiltration in HCC through cGAS-STING pathway. Hepatology 77 (1), 33–47. doi:10.1002/hep.32335
MacParland, S. A., Liu, J. C., Ma, X. Z., Innes, B. T., Bartczak, A. M., Gage, B. K., et al. (2018). Single cell RNA sequencing of human liver reveals distinct intrahepatic macrophage populations. Nat. Commun. 9 (1), 4383. doi:10.1038/s41467-018-06318-7
Mano, Y., Aishima, S., Fujita, N., Tanaka, Y., Kubo, Y., Motomura, T., et al. (2013). Tumor-associated macrophage promotes tumor progression via STAT3 signaling in hepatocellular carcinoma. Pathobiology 80 (3), 146–154. doi:10.1159/000346196
Matsuda, M., and Seki, E. (2020). Hepatic stellate cell-macrophage crosstalk in liver fibrosis and carcinogenesis. Semin. Liver Dis. 40 (3), 307–320. doi:10.1055/s-0040-1708876
Mattos Â, Z., Debes, J. D., Vogel, A., Arrese, M., Revelo, X., Pase, T. H. S., et al. (2022). Non-alcoholic fatty liver disease-related hepatocellular carcinoma: is there a role for immunotherapy? World J. gastroenterology 28 (28), 3595–3607. doi:10.3748/wjg.v28.i28.3595
Meng, Q., Duan, X., Yang, Q., Xue, D., Liu, Z., Li, Y., et al. (2022). SLAMF6/Ly108 promotes the development of hepatocellular carcinoma via facilitating macrophage M2 polarization. Oncol. Lett. 23 (3), 83. doi:10.3892/ol.2022.13203
Mi, H., Ho, W. J., Yarchoan, M., and Popel, A. S. (2022). Multi-scale spatial analysis of the tumor microenvironment reveals features of cabozantinib and nivolumab efficacy in hepatocellular carcinoma. Front. Immunol. 13, 892250. doi:10.3389/fimmu.2022.892250
Minami, K., Hiwatashi, K., Ueno, S., Sakoda, M., Iino, S., Okumura, H., et al. (2018). Prognostic significance of CD68, CD163 and Folate receptor-beta positive macrophages in hepatocellular carcinoma. Exp. Ther. Med. 15 (5), 4465–4476. doi:10.3892/etm.2018.5959
Mossanen, J. C., Krenkel, O., Ergen, C., Govaere, O., Liepelt, A., Puengel, T., et al. (2016). Chemokine (C-C motif) receptor 2-positive monocytes aggravate the early phase of acetaminophen-induced acute liver injury. Hepatology 64 (5), 1667–1682. doi:10.1002/hep.28682
Ng, H. H. M., Lee, R. Y., Goh, S., Tay, I. S. Y., Lim, X., Lee, B., et al. (2020). Immunohistochemical scoring of CD38 in the tumor microenvironment predicts responsiveness to anti-PD-1/PD-L1 immunotherapy in hepatocellular carcinoma. J. Immunother. Cancer 8 (2), e000987. doi:10.1136/jitc-2020-000987
Ning, J., Ye, Y., Bu, D., Zhao, G., Song, T., Liu, P., et al. (2021). Imbalance of TGF-β1/BMP-7 pathways induced by M2-polarized macrophages promotes hepatocellular carcinoma aggressiveness. Mol. Ther. 29 (6), 2067–2087. doi:10.1016/j.ymthe.2021.02.016
Ning, W. R., Jiang, D., Liu, X. C., Huang, Y. F., Peng, Z. P., Jiang, Z. Z., et al. (2022). Carbonic anhydrase XII mediates the survival and prometastatic functions of macrophages in human hepatocellular carcinoma. J. Clin. Invest. 132 (7), e153110. doi:10.1172/JCI153110
Nosaka, T., Murata, Y., Takahashi, K., Naito, T., Ofuji, K., Matsuda, H., et al. (2023). Hepatocellular carcinoma progression promoted by 5-lipoxygenase activity in CD163(+) tumor-associated macrophages. Biomed. Pharmacother. 162, 114592. doi:10.1016/j.biopha.2023.114592
Nowarski, R., Gagliani, N., Huber, S., and Flavell, R. A. (2013). Innate immune cells in inflammation and cancer. Cancer Immunol. Res. 1 (2), 77–84. doi:10.1158/2326-6066.CIR-13-0081
Park, D. J., Sung, P. S., Lee, G. W., Cho, S., Kim, S. M., Kang, B. Y., et al. (2021). Preferential expression of programmed death ligand 1 protein in tumor-associated macrophages and its potential role in immunotherapy for hepatocellular carcinoma. Int. J. Mol. Sci. 22 (9), 4710. doi:10.3390/ijms22094710
Peng, S. H., Deng, H., Yang, J. F., Xie, P. P., Li, C., Li, H., et al. (2005). Significance and relationship between infiltrating inflammatory cell and tumor angiogenesis in hepatocellular carcinoma tissues. World J. gastroenterology 11 (41), 6521–6524. doi:10.3748/wjg.v11.i41.6521
Petty, A. J., Dai, R., Lapalombella, R., Baiocchi, R. A., Benson, D. M., Li, Z., et al. (2021). Hedgehog-induced PD-L1 on tumor-associated macrophages is critical for suppression of tumor-infiltrating CD8+ T cell function. JCI Insight 6 (6), e146707. doi:10.1172/jci.insight.146707
Petty, A. J., Li, A., Wang, X., Dai, R., Heyman, B., Hsu, D., et al. (2019). Hedgehog signaling promotes tumor-associated macrophage polarization to suppress intratumoral CD8+ T cell recruitment. J. Clin. Invest. 129 (12), 5151–5162. doi:10.1172/JCI128644
Qian, B-Z., and Pollard, J. W. (2010). Macrophage diversity enhances tumor progression and metastasis. Cell 141 (1), 39–51. doi:10.1016/j.cell.2010.03.014
Qu, W., Qiao, S., Liu, L., Chen, Y., Peng, C., Hou, Y., et al. (2022). Dectin3 protects against hepatocellular carcinoma by regulating glycolysis of macrophages. Int. Immunopharmacol. 113, 109384. doi:10.1016/j.intimp.2022.109384
Rabold, K., Netea, M. G., Adema, G. J., and Netea-Maier, R. T. (2017). Cellular metabolism of tumor-associated macrophages - functional impact and consequences. FEBS Lett. 591 (19), 3022–3041. doi:10.1002/1873-3468.12771
Rolvering, C., Zimmer, A. D., Ginolhac, A., Margue, C., Kirchmeyer, M., Servais, F., et al. (2018). The PD-L1- and IL6-mediated dampening of the IL27/STAT1 anticancer responses are prevented by α-PD-L1 or α-IL6 antibodies. J. Leukoc. Biol. 104 (5), 969–985. doi:10.1002/JLB.MA1217-495R
Sahin, H., Trautwein, C., and Wasmuth, H. E. (2010). Functional role of chemokines in liver disease models. Nat. Rev. Gastroenterol. Hepatol. 7 (12), 682–690. doi:10.1038/nrgastro.2010.168
Scott, C. L., Zheng, F., De Baetselier, P., Martens, L., Saeys, Y., De Prijck, S., et al. (2016). Bone marrow-derived monocytes give rise to self-renewing and fully differentiated Kupffer cells. Nat. Commun. 7, 10321. doi:10.1038/ncomms10321
Sen, B., Aggarwal, S., Nath, R., Sehgal, R., Singh, R., Agrawal, K., et al. (2022). Secretome of senescent hepatoma cells modulate immune cell fate by macrophage polarization and neutrophil extracellular traps formation. Med. Oncol. 39 (9), 134. doi:10.1007/s12032-022-01732-w
Sharen, G., Cheng, H., Hu, X., Miao, J., and Zhao, D. (2022). M1‑like tumor‑associated macrophages enhance proliferation and anti‑apoptotic ability of liver cancer cells via activating the NF‑κB signaling pathway. Mol. Med. Rep. 26 (5), 331. doi:10.3892/mmr.2022.12847
Shi, C., and Pamer, E. G. (2011). Monocyte recruitment during infection and inflammation. Nat. Rev. Immunol. 11 (11), 762–774. doi:10.1038/nri3070
Shiau, D. J., Kuo, W. T., Davuluri, G. V. N., Shieh, C. C., Tsai, P. J., Chen, C. C., et al. (2020). Hepatocellular carcinoma-derived high mobility group box 1 triggers M2 macrophage polarization via a TLR2/NOX2/autophagy axis. Sci. Rep. 10 (1), 13582. doi:10.1038/s41598-020-70137-4
Shieh, Y. S., Hung, Y. J., Hsieh, C. B., Chen, J. S., Chou, K. C., and Liu, S. Y. (2009). Tumor-associated macrophage correlated with angiogenesis and progression of mucoepidermoid carcinoma of salivary glands. Ann. Surg. Oncol. 16 (3), 751–760. doi:10.1245/s10434-008-0259-6
Song, J. S., Chang, C. C., Wu, C. H., Dinh, T. K., Jan, J. J., Huang, K. W., et al. (2021). A highly selective and potent CXCR4 antagonist for hepatocellular carcinoma treatment. Proc. Natl. Acad. Sci. U. S. A. 118 (13), e2015433118. doi:10.1073/pnas.2015433118
Sprinzl, M. F., Puschnik, A., Schlitter, A. M., Schad, A., Ackermann, K., Esposito, I., et al. (2015). Sorafenib inhibits macrophage-induced growth of hepatoma cells by interference with insulin-like growth factor-1 secretion. J. Hepatol. 62 (4), 863–870. doi:10.1016/j.jhep.2014.11.011
Sun, G., Liu, H., Zhao, J., Zhang, J., Huang, T., Sun, G., et al. (2022b). Macrophage GSK3β-deficiency inhibits the progression of hepatocellular carcinoma and enhances the sensitivity of anti-PD1 immunotherapy. J. Immunother. Cancer 10 (12), e005655. doi:10.1136/jitc-2022-005655
Sun, J. L., Zhang, N. P., Xu, R. C., Zhang, G. C., Liu, Z. Y., Abuduwaili, W., et al. (2021). Tumor cell-imposed iron restriction drives immunosuppressive polarization of tumor-associated macrophages. J. Transl. Med. 19 (1), 347. doi:10.1186/s12967-021-03034-7
Sun, K., Xu, L., Jing, Y., Han, Z., Chen, X., Cai, C., et al. (2017). Autophagy-deficient Kupffer cells promote tumorigenesis by enhancing mtROS-NF-κB-IL1α/β-dependent inflammation and fibrosis during the preneoplastic stage of hepatocarcinogenesis. Cancer Lett. 388, 198–207. doi:10.1016/j.canlet.2016.12.004
Sun, R., Zhang, Z., Bao, R., Guo, X., Gu, Y., Yang, W., et al. (2022a). Loss of SIRT5 promotes bile acid-induced immunosuppressive microenvironment and hepatocarcinogenesis. J. Hepatol. 77 (2), 453–466. doi:10.1016/j.jhep.2022.02.030
Sung, H., Ferlay, J., Siegel, R. L., Laversanne, M., Soerjomataram, I., Jemal, A., et al. (2021). Global cancer statistics 2020: GLOBOCAN estimates of incidence and mortality worldwide for 36 cancers in 185 countries. CA Cancer J. Clin. 71 (3), 209–249. doi:10.3322/caac.21660
Sung, P. S., Park, D. J., Roh, P. R., Mun, K. D., Cho, S. W., Lee, G. W., et al. (2022). Intrahepatic inflammatory IgA+PD-L1high monocytes in hepatocellular carcinoma development and immunotherapy. J. Immunother. Cancer 10 (5), e003618. doi:10.1136/jitc-2021-003618
Surewaard, B. G. J., and Kubes, P. (2017). Measurement of bacterial capture and phagosome maturation of Kupffer cells by intravital microscopy. Methods 128, 12–19. doi:10.1016/j.ymeth.2017.05.004
Tan, J., Fan, W., Liu, T., Zhu, B., Liu, Y., Wang, S., et al. (2023). TREM2(+) macrophages suppress CD8(+) T-cell infiltration after transarterial chemoembolisation in hepatocellular carcinoma. J. Hepatol. 79, 126–140. doi:10.1016/j.jhep.2023.02.032
Tao, L., Li, D., Mu, S., Tian, G., and Yan, G. (2022). LncRNA MAPKAPK5_AS1 facilitates cell proliferation in Hepatitis B virus -related hepatocellular carcinoma. Lab. Invest. 102 (5), 494–504. doi:10.1038/s41374-022-00731-9
Tcyganov, E., Mastio, J., Chen, E., and Gabrilovich, D. I. (2018). Plasticity of myeloid-derived suppressor cells in cancer. Curr. Opin. Immunol. 51, 76–82.
Thomann, S., Weiler, S. M. E., Wei, T., Sticht, C., De La Torre, C., Toth, M., et al. (2021). YAP-induced Ccl2 expression is associated with a switch in hepatic macrophage identity and vascular remodelling in liver cancer. Liver Int. 41 (12), 3011–3023. doi:10.1111/liv.15048
Thornton, A. J., Ham, J., and Kunkel, S. L. (1991). Kupffer cell-derived cytokines induce the synthesis of a leukocyte chemotactic peptide, interleukin-8, in human hepatoma and primary hepatocyte cultures. Hepatology 14 (6), 1112–1122. doi:10.1002/hep.1840140627
Tian, X., Wu, Y., Yang, Y., Wang, J., Niu, M., Gao, S., et al. (2020). Long noncoding RNA LINC00662 promotes M2 macrophage polarization and hepatocellular carcinoma progression via activating Wnt/β-catenin signaling. Mol. Oncol. 14 (2), 462–483. doi:10.1002/1878-0261.12606
Tian, Z., Hou, X., Liu, W., Shao, C., Gao, L., Jiang, J., et al. (2022a). Targeted blocking of CCR2 and CXCR2 improves the efficacy of transarterial chemoembolization of hepatocarcinoma. Cancer Cell Int. 22 (1), 362. doi:10.1186/s12935-022-02771-z
Vanderborght, B., De Muynck, K., Gijbels, E., Lefere, S., Scott, C. L., Guilliams, M., et al. (2023). Transient Kupffer cell depletion and subsequent replacement by infiltrating monocyte-derived cells does not alter the induction or progression of hepatocellular carcinoma. Int. J. Cancer 152 (12), 2615–2628. doi:10.1002/ijc.34505
van der Heide, D., Weiskirchen, R., and Bansal, R. (2019). Therapeutic targeting of hepatic macrophages for the treatment of liver diseases. Front. Immunol. 10, 2852.
Vandierendonck, A., Degroote, H., Vanderborght, B., Verhelst, X., Geerts, A., Devisscher, L., et al. (2021). NOX1 inhibition attenuates the development of a pro-tumorigenic environment in experimental hepatocellular carcinoma. J. Exp. Clin. Cancer Res. 40 (1), 40. doi:10.1186/s13046-021-01837-6
Wan, S., Zhao, E., Kryczek, I., Vatan, L., Sadovskaya, A., Ludema, G., et al. (2014). Tumor-associated macrophages produce interleukin 6 and signal via STAT3 to promote expansion of human hepatocellular carcinoma stem cells. Gastroenterology 147 (6), 1393–1404. doi:10.1053/j.gastro.2014.08.039
Wang, B., Li, Q., Qin, L., Zhao, S., Wang, J., and Chen, X. (2011). Transition of tumor-associated macrophages from MHC class II(hi) to MHC class II(low) mediates tumor progression in mice. BMC Immunol. 12, 43. doi:10.1186/1471-2172-12-43
Wang, B., Li, Q., Wang, J., Zhao, S., Nashun, B., Qin, L., et al. (2020b). Plasmodium infection inhibits tumor angiogenesis through effects on tumor-associated macrophages in a murine implanted hepatoma model. Cell Commun. Signal 18 (1), 157. doi:10.1186/s12964-020-00570-5
Wang, D., Li, X., Li, J., Lu, Y., Zhao, S., Tang, X., et al. (2019a). APOBEC3B interaction with PRC2 modulates microenvironment to promote HCC progression. Gut 68 (10), 1846–1857. doi:10.1136/gutjnl-2018-317601
Wang, H., Wang, X., Li, X., Fan, Y., Li, G., Guo, C., et al. (2014b). CD68(+)HLA-DR(+) M1-like macrophages promote motility of HCC cells via NF-κB/FAK pathway. Cancer Lett. 345 (1), 91–99. doi:10.1016/j.canlet.2013.11.013
Wang, H. C., Haung, L. Y., Wang, C. J., Chao, Y. J., Hou, Y. C., Yen, C. J., et al. (2022b). Tumor-associated macrophages promote resistance of hepatocellular carcinoma cells against sorafenib by activating CXCR2 signaling. J. Biomed. Sci. 29 (1), 99. doi:10.1186/s12929-022-00881-4
Wang, J., Wang, Y., Chu, Y., Li, Z., Yu, X., Huang, Z., et al. (2021). Tumor-derived adenosine promotes macrophage proliferation in human hepatocellular carcinoma. J. Hepatol. 74 (3), 627–637. doi:10.1016/j.jhep.2020.10.021
Wang, M. D., Xiang, H., Zhang, L., and Wang, C. (2022a). Integration of OV6 expression and CD68(+) tumor-associated macrophages with clinical features better predicts the prognosis of patients with hepatocellular carcinoma. Transl. Oncol. 25, 101509. doi:10.1016/j.tranon.2022.101509
Wang, T. T., Yuan, J. H., Ma, J. Z., Yang, W. J., Liu, X. N., Yin, Y. P., et al. (2017). CTGF secreted by mesenchymal-like hepatocellular carcinoma cells plays a role in the polarization of macrophages in hepatocellular carcinoma progression. Biomed. Pharmacother. 95, 111–119. doi:10.1016/j.biopha.2017.08.004
Wang, X., Tan, Y., Zhang, Y., Xu, Z., Xu, B., Lei, H., et al. (2020a). The novel glycyrrhetinic acid-tetramethylpyrazine conjugate TOGA induces anti-hepatocarcinogenesis by inhibiting the effects of tumor-associated macrophages on tumor cells. Pharmacol. Res. 161, 105233. doi:10.1016/j.phrs.2020.105233
Wang, X., Wang, H., Li, G., Song, Y., Wang, S., Zhu, F., et al. (2014a). Activated macrophages down-regulate expression of E-cadherin in hepatocellular carcinoma cells via NF-κB/Slug pathway. Tumour Biol. 35 (9), 8893–8901. doi:10.1007/s13277-014-2159-7
Wang, Y., Wang, B., Xiao, S., Li, Y., and Chen, Q. (2019b). miR-125a/b inhibits tumor-associated macrophages mediated in cancer stem cells of hepatocellular carcinoma by targeting CD90. J. Cell Biochem. 120 (3), 3046–3055. doi:10.1002/jcb.27436
Wei, R., Zhu, W. W., Yu, G. Y., Wang, X., Gao, C., Zhou, X., et al. (2021). S100 calcium-binding protein A9 from tumor-associated macrophage enhances cancer stem cell-like properties of hepatocellular carcinoma. Int. J. Cancer 148 (5), 1233–1244. doi:10.1002/ijc.33371
Wei, Y., Lao, X. M., Xiao, X., Wang, X. Y., Wu, Z. J., Zeng, Q. H., et al. (2019). Plasma cell polarization to the immunoglobulin G phenotype in hepatocellular carcinomas involves epigenetic alterations and promotes hepatoma progression in mice. Gastroenterology 156 (6), 1890–1904.e16. doi:10.1053/j.gastro.2019.01.250
Wu, H., Li, Y., Shi, G., Du, S., Wang, X., Ye, W., et al. (2022a). Hepatic interferon regulatory factor 8 expression suppresses hepatocellular carcinoma progression and enhances the response to anti-programmed cell death protein-1 therapy. Hepatology 76 (6), 1602–1616. doi:10.1002/hep.32316
Wu, J. J., Yang, Y., Peng, W. T., Sun, J. C., Sun, W. Y., and Wei, W. (2019a). G protein-coupled receptor kinase 2 regulating β2-adrenergic receptor signaling in M2-polarized macrophages contributes to hepatocellular carcinoma progression. Onco Targets Ther. 12, 5499–5513. doi:10.2147/OTT.S209291
Wu, L., Zhang, X., Zheng, L., Zhao, H., Yan, G., Zhang, Q., et al. (2020). RIPK3 orchestrates fatty acid metabolism in tumor-associated macrophages and hepatocarcinogenesis. Cancer Immunol. Res. 8 (5), 710–721. doi:10.1158/2326-6066.CIR-19-0261
Wu, Q., You, L., Nepovimova, E., Heger, Z., Wu, W., Kuca, K., et al. (2022b). Hypoxia-inducible factors: master regulators of hypoxic tumor immune escape. J. Hematol. Oncol. 15 (1), 77. doi:10.1186/s13045-022-01292-6
Wu, Q., Zhou, W., Yin, S., Zhou, Y., Chen, T., Qian, J., et al. (2019b). Blocking triggering receptor expressed on myeloid cells-1-positive tumor-associated macrophages induced by hypoxia reverses immunosuppression and anti-programmed cell death ligand 1 resistance in liver cancer. Hepatology 70 (1), 198–214. doi:10.1002/hep.30593
Wu, Y., and Zheng, L. (2012). Dynamic education of macrophages in different areas of human tumors. Cancer Microenviron. 5 (3), 195–201. doi:10.1007/s12307-012-0113-z
Xiang, X., Wang, J., Lu, D., and Xu, X. (2021). Targeting tumor-associated macrophages to synergize tumor immunotherap y. Signal Transduct. Target Ther. 6 (1), 75. doi:10.1038/s41392-021-00484-9
Xiao, N., Li, K., Zhu, X., Xu, B., Liu, X., Lei, M., et al. (2022). CD74(+) macrophages are associated with favorable prognosis and immune contexture in hepatocellular carcinoma. Cancer Immunol. Immunother. 71 (1), 57–69. doi:10.1007/s00262-021-02962-z
Xiao, N., Zhu, X., Li, K., Chen, Y., Liu, X., Xu, B., et al. (2021). Blocking siglec-10(hi) tumor-associated macrophages improves anti-tumor immunity and enhances immunotherapy for hepatocellular carcinoma. Exp. Hematol. Oncol. 10 (1), 36. doi:10.1186/s40164-021-00230-5
Xiao, P., Long, X., Zhang, L., Ye, Y., Guo, J., Liu, P., et al. (2018). Neurotensin/IL-8 pathway orchestrates local inflammatory response and tumor invasion by inducing M2 polarization of Tumor-Associated macrophages and epithelial-mesenchymal transition of hepatocellular carcinoma cells. Oncoimmunology 7 (7), e1440166. doi:10.1080/2162402X.2018.1440166
Xu, D., Wang, Y., Wu, J., Zhang, Z., Chen, J., Xie, M., et al. (2021). ECT2 overexpression promotes the polarization of tumor-associated macrophages in hepatocellular carcinoma via the ECT2/PLK1/PTEN pathway. Cell Death Dis. 12 (2), 162. doi:10.1038/s41419-021-03450-z
Xu, M., Zhou, C., Weng, J., Chen, Z., Zhou, Q., Gao, J., et al. (2022). Tumor associated macrophages-derived exosomes facilitate hepatocellular carcinoma malignance by transferring lncMMPA to tumor cells and activating glycolysis pathway. J. Exp. Clin. Cancer Res. 41 (1), 253. doi:10.1186/s13046-022-02458-3
Yan, G., Wang, X., Sun, C., Zheng, X., Wei, H., Tian, Z., et al. (2017). Chronic alcohol consumption promotes diethylnitrosamine-induced hepatocarcinogenesis via immune disturbances. Sci. Rep. 7 (1), 2567. doi:10.1038/s41598-017-02887-7
Yan, W., Liu, X., Ma, H., Zhang, H., Song, X., Gao, L., et al. (2015). Tim-3 fosters HCC development by enhancing TGF-beta-mediated alternative activation of macrophages. Gut 64 (10), 1593–1604. doi:10.1136/gutjnl-2014-307671
Yang, F., Wei, Y., Han, D., Li, Y., Shi, S., Jiao, D., et al. (2020). Interaction with CD68 and regulation of GAS6 expression by endosialin in fibroblasts drives recruitment and polarization of macrophages in hepatocellular carcinoma. Cancer Res. 80 (18), 3892–3905. doi:10.1158/0008-5472.CAN-19-2691
Yang, J., and Xing, Z. (2021). Ligustilide counteracts carcinogenesis and hepatocellular carcinoma cell-evoked macrophage M2 polarization by regulating yes-associated protein-mediated interleukin-6 secretion. Exp. Biol. Med. (Maywood) 246 (17), 1928–1937. doi:10.1177/15353702211010420
Yang, J. B., Zhao, Z. B., Liu, Q. Z., Hu, T. D., Long, J., Yan, K., et al. (2018b). FoxO1 is a regulator of MHC-II expression and anti-tumor effect of tumor-associated macrophages. Oncogene 37 (9), 1192–1204. doi:10.1038/s41388-017-0048-4
Yang, J. D., Nakamura, I., and Roberts, L. R. (2011). The tumor microenvironment in hepatocellular carcinoma: current status and therapeutic targets. Semin. Cancer Biol. 21 (1), 35–43. doi:10.1016/j.semcancer.2010.10.007
Yang, R., Liao, Y., Wang, L., He, P., Hu, Y., Yuan, D., et al. (2019). Exosomes derived from M2b macrophages attenuate DSS-induced colitis. Front. Immunol. 10, 2346.
Yang, S., Zhang, J., Xu, Y., Wang, J., Zhao, H., Lei, J., et al. (2022). OIT3 mediates macrophage polarization and facilitates hepatocellular carcinoma progression. Cancer Immunol. Immunother. 71 (11), 2677–2689. doi:10.1007/s00262-022-03188-3
Yang, Y., Ye, Y. C., Chen, Y., Zhao, J. L., Gao, C. C., Han, H., et al. (2018a). Crosstalk between hepatic tumor cells and macrophages via Wnt/β-catenin signaling promotes M2-like macrophage polarization and reinforces tumor malignant behaviors. Cell Death Dis. 9 (8), 793. doi:10.1038/s41419-018-0818-0
Yao, H., Liu, N., Lin, M. C., and Zheng, J. (2016). Positive feedback loop between cancer stem cells and angiogenesis in hepatocellular carcinoma. Cancer Lett. 379 (2), 213–219. doi:10.1016/j.canlet.2016.03.014
Yao, R. R., Li, J. H., Zhang, R., Chen, R. X., and Wang, Y. H. (2018). M2-polarized tumor-associated macrophages facilitated migration and epithelial-mesenchymal transition of HCC cells via the TLR4/STAT3 signaling pathway. World J. Surg. Oncol. 16 (1), 9. doi:10.1186/s12957-018-1312-y
Ye, L. Y., Chen, W., Bai, X. L., Xu, X. Y., Zhang, Q., Xia, X. F., et al. (2016). Hypoxia-induced epithelial-to-mesenchymal transition in hepatocellular carcinoma induces an immunosuppressive tumor microenvironment to promote metastasis. Cancer Res. 76 (4), 818–830. doi:10.1158/0008-5472.CAN-15-0977
Ye, Y., Wang, M., Wang, G., Mai, Z., Zhou, B., Han, Y., et al. (2023). lncRNA miR4458HG modulates hepatocellular carcinoma progression by activating m6A-dependent glycolysis and promoting the polarization of tumor-associated macrophages. Cell Mol. Life Sci. 80 (4), 99. doi:10.1007/s00018-023-04741-8
Yin, Z., Huang, J., Ma, T., Li, D., Wu, Z., Hou, B., et al. (2017). Macrophages activating chemokine (C-X-C motif) ligand 8/miR-17 cluster modulate hepatocellular carcinoma cell growth and metastasis. Am. J. Transl. Res. 9 (5), 2403–2411.
You, Y., Wen, D., Zeng, L., Lu, J., Xiao, X., Chen, Y., et al. (2022). ALKBH5/MAP3K8 axis regulates PD-L1+ macrophage infiltration and promotes hepatocellular carcinoma progression. Int. J. Biol. Sci. 18 (13), 5001–5018. doi:10.7150/ijbs.70149
Yu, H., Zhu, X., Lin, H., Pan, H., Zhao, F., Zhu, M., et al. (2020). A new risk model comprising genes highly correlated with CD133 identifies different tumor-immune microenvironment subtypes impacting prognosis in hepatocellular carcinoma. Aging 12 (12), 12234–12250. doi:10.18632/aging.103409
Zang, M., Li, Y., He, H., Ding, H., Chen, K., Du, J., et al. (2018). IL-23 production of liver inflammatory macrophages to damaged hepatocytes promotes hepatocellular carcinoma development after chronic Hepatitis B virus infection. Biochim. Biophys. Acta Mol. Basis Dis. 1864 (12), 3759–3770. doi:10.1016/j.bbadis.2018.10.004
Zhang, J., Zhang, H., Ding, X., Hu, J., Li, Y., Zhang, J., et al. (2022a). Crosstalk between macrophage-derived PGE(2) and tumor UHRF1 drives hepatocellular carcinoma progression. Theranostics 12 (8), 3776–3793. doi:10.7150/thno.69494
Zhang, J., Zhang, Q., Lou, Y., Fu, Q., Chen, Q., Wei, T., et al. (2018b). Hypoxia-inducible factor-1α/interleukin-1β signaling enhances hepatoma epithelial-mesenchymal transition through macrophages in a hypoxic-inflammatory microenvironment. Hepatology 67 (5), 1872–1889. doi:10.1002/hep.29681
Zhang, K., Xie, K., Huo, X., Liu, L., Liu, J., Zhang, C., et al. (2022b). Development and optimization of a prognostic model associated with stemness genes in hepatocellular carcinoma. Biomed. Res. Int. 2022, 9168441. doi:10.1155/2022/9168441
Zhang, Q., Huang, F., Yao, Y., Wang, J., Wei, J., Wu, Q., et al. (2019). Interaction of transforming growth factor-beta-Smads/microRNA-362-3p/CD82 mediated by M2 macrophages promotes the process of epithelial-mesenchymal transition in hepatocellular carcinoma cells. Cancer Sci. 110 (8), 2507–2519. doi:10.1111/cas.14101
Zhang, Q., Wang, H., Mao, C., Sun, M., Dominah, G., Chen, L., et al. (2018c). Fatty acid oxidation contributes to IL-1β secretion in M2 macrophages and promotes macrophage-mediated tumor cell migration. Mol. Immunol. 94, 27–35. doi:10.1016/j.molimm.2017.12.011
Zhang, W., Liu, Y., Yan, Z., Yang, H., Sun, W., Yao, Y., et al. (2020b). IL-6 promotes PD-L1 expression in monocytes and macrophages by decreasing protein tyrosine phosphatase receptor type O expression in human hepatocellular carcinoma. J. Immunother. Cancer 8 (1), e000285. doi:10.1136/jitc-2019-000285
Zhang, W., Zhangyuan, G., Wang, F., Jin, K., Shen, H., Zhang, L., et al. (2021). The zinc finger protein Miz1 suppresses liver tumorigenesis by restricting hepatocyte-driven macrophage activation and inflammation. Immunity 54 (6), 1168–1185.e8. doi:10.1016/j.immuni.2021.04.027
Zhang, Y., Li, J. Q., Jiang, Z. Z., Li, L., Wu, Y., and Zheng, L. (2016). CD169 identifies an anti-tumour macrophage subpopulation in human hepatocellular carcinoma. J. Pathol. 239 (2), 231–241. doi:10.1002/path.4720
Zhang, Y. L., Li, Q., Yang, X. M., Fang, F., Li, J., Wang, Y. H., et al. (2018a). SPON2 promotes M1-like macrophage recruitment and inhibits hepatocellular carcinoma metastasis by distinct integrin-rho GTPase-hippo pathways. Cancer Res. 78 (9), 2305–2317. doi:10.1158/0008-5472.CAN-17-2867
Zhang, Y. Z., Zeb, A., and Cheng, L. F. (2022c). Exploring the molecular mechanism of hepatitis virus inducing hepatocellular carcinoma by microarray data and immune infiltrates analysis. Front. Immunol. 13, 1032819. doi:10.3389/fimmu.2022.1032819
Zhang, Z., Zhang, J., He, P., Han, J., and Sun, C. (2020a). Interleukin-37 suppresses hepatocellular carcinoma growth through inhibiting M2 polarization of tumor-associated macrophages. Mol. Immunol. 122, 13–20. doi:10.1016/j.molimm.2020.03.012
Zhao, J., O'Neil, M., Vittal, A., Weinman, S. A., and Tikhanovich, I. (2019). PRMT1-Dependent macrophage IL-6 production is required for alcohol-induced HCC progression. Gene Expr. 19 (2), 137–150. doi:10.3727/105221618X15372014086197
Zhao, L., Wu, Y., Xie, X. D., Chu, Y. F., Li, J. Q., and Zheng, L. (2015). c-Met identifies a population of matrix metalloproteinase 9-producing monocytes in peritumoural stroma of hepatocellular carcinoma. J. Pathol. 237 (3), 319–329. doi:10.1002/path.4578
Zhao, Q., Kuang, D. M., Wu, Y., Xiao, X., Li, X. F., Li, T. J., et al. (2012). Activated CD69+ T cells foster immune privilege by regulating Ido expression in tumor-associated macrophages. J. Immunol. 188 (3), 1117–1124. doi:10.4049/jimmunol.1100164
Zhou, B., Yang, Y., and Li, C. (2019). SIRT1 inhibits hepatocellular carcinoma metastasis by promoting M1 macrophage polarization via NF-κB pathway. Onco Targets Ther. 12, 2519–2529. doi:10.2147/OTT.S195234
Zhou, D. Y., Qin, J., Huang, J., Wang, F., Xu, G. P., Lv, Y. T., et al. (2017). Zoledronic acid inhibits infiltration of tumor-associated macrophages and angiogenesis following transcatheter arterial chemoembolization in rat hepatocellular carcinoma models. Oncol. Lett. 14 (4), 4078–4084. doi:10.3892/ol.2017.6717
Zhou, J., Liu, L., Hu, X., Feng, R., Zhao, N., Zhang, L., et al. (2022b). Matrix metalloproteinase-21 promotes metastasis via increasing the recruitment and M2 polarization of macrophages in HCC. Cancer Sci. 114 (2), 423–435. doi:10.1111/cas.15368
Zhou, S. L., Hu, Z. Q., Zhou, Z. J., Dai, Z., Wang, Z., Cao, Y., et al. (2016). miR-28-5p-IL-34-macrophage feedback loop modulates hepatocellular carcinoma metastasis. Hepatology 63 (5), 1560–1575. doi:10.1002/hep.28445
Zhou, T. Y., Zhou, Y. L., Qian, M. J., Fang, Y. Z., Ye, S., Xin, W. X., et al. (2018). Interleukin-6 induced by YAP in hepatocellular carcinoma cells recruits tumor-associated macrophages. J. Pharmacol. Sci. 138 (2), 89–95. doi:10.1016/j.jphs.2018.07.013
Zhou, Z., Wang, Z., Gao, J., Lin, Z., Wang, Y., Shan, P., et al. (2022a). Noncoding RNA-mediated macrophage and cancer cell crosstalk in hepatocellular carcinoma. Mol. Ther. oncolytics 25, 98–120. doi:10.1016/j.omto.2022.03.002
Zhu, C., Kros, J. M., Cheng, C., and Mustafa, D. (2017). The contribution of tumor-associated macrophages in glioma neo-angiogenesis and implications for anti-angiogenic strategies. Neuro Oncol. 19 (11), 1435–1446. doi:10.1093/neuonc/nox081
Zhu, F., Li, X., Chen, S., Zeng, Q., Zhao, Y., and Luo, F. (2016). Tumor-associated macrophage or chemokine ligand CCL17 positively regulates the tumorigenesis of hepatocellular carcinoma. Med. Oncol. 33 (2), 17. doi:10.1007/s12032-016-0729-9
Zhu, F., Li, X., Jiang, Y., Zhu, H., Zhang, H., Zhang, C., et al. (2015). GdCl3 suppresses the malignant potential of hepatocellular carcinoma by inhibiting the expression of CD206 in tumor-associated macrophages. Oncol. Rep. 34 (5), 2643–2655. doi:10.3892/or.2015.4268
Zhu, Y., Yang, J., Xu, D., Gao, X. M., Zhang, Z., Hsu, J. L., et al. (2019). Disruption of tumour-associated macrophage trafficking by the osteopontin-induced colony-stimulating factor-1 signalling sensitises hepatocellular carcinoma to anti-PD-L1 blockade. Gut 68 (9), 1653–1666. doi:10.1136/gutjnl-2019-318419
Keywords: hepatocellular carcinoma, tumor-associated macrophages, tumor microenvironment, cancer stem cells, carcinoma cell proliferation, invasion and migration, angiogenesis, hepatic fibrosis
Citation: Yuan Y, Wu D, Li J, Huang D, Zhao Y, Gao T, Zhuang Z, Cui Y, Zheng D-Y and Tang Y (2023) Mechanisms of tumor-associated macrophages affecting the progression of hepatocellular carcinoma. Front. Pharmacol. 14:1217400. doi: 10.3389/fphar.2023.1217400
Received: 05 May 2023; Accepted: 23 June 2023;
Published: 17 August 2023.
Edited by:
Aijun Qiao, University of Alabama at Birmingham, United StatesReviewed by:
Xinchen Wang, Zhaoqing University, ChinaTianyi Zhao, Tianjin University of Traditional Chinese Medicine, China
Copyright © 2023 Yuan, Wu, Li, Huang, Zhao, Gao, Zhuang, Cui, Zheng and Tang. This is an open-access article distributed under the terms of the Creative Commons Attribution License (CC BY). The use, distribution or reproduction in other forums is permitted, provided the original author(s) and the copyright owner(s) are credited and that the original publication in this journal is cited, in accordance with accepted academic practice. No use, distribution or reproduction is permitted which does not comply with these terms.
*Correspondence: Ying Cui, NDA5NzY1NzY0QHFxLmNvbQ==; Da-Yong Zheng, emhlbmdkeUBzbXUuZWR1LmNu; Ying Tang, dGFuZ3lpbmdAZ3p1Y20uZWR1LmNu
†These authors share first authorship