- 1Department of Nutrition, School of Public Health and Health Sciences, University of Massachusetts, Amherst, MA, United States
- 2Department of Food Science, University of Massachusetts, Amherst, MA, United States
- 3Chobanian and Avedisian School of Medicine, Boston University, Boston, MA, United States
- 4UMass Cancer Center, University of Massachusetts Chan Medical School, Worcester, MA, United States
Introduction: Diet-induced obesity has been shown to decrease the abundance of Turicibacter, a genus known to play a role in the serotonin signaling system, which is associated with colorectal tumorigenesis, making the presence of Turicibacter potentially influential in the protection of intestinal tumorigenesis. Recently, Antrodia camphorata (AC), a medicinal fungus native to Taiwan, has emerged as a promising candidate for complementary and alternative cancer therapy. Small molecules and polysaccharides derived from AC have been reported to possess health-promoting effects, including anti-cancer properties.
Methods: Bacterial culture followed with cell culture were used in this study to determine the role of Turicibacter in colorectal tumorigenesis and to explore the anti-cancer mechanism of AC with Turicibacter fermentation.
Results: Turicibacter fermentation and the addition of AC polysaccharide led to a significant increase in the production of nutrients and metabolites, including α-ketoglutaric acid and lactic acid (p < 0.05). Treatment of Turicibacter fermented AC polysaccharide was more effective in inhibiting serotonin signaling-related genes, including Tph1, Htr1d, Htr2a, Htr2b, and Htr2c (p < 0.05), and Wnt-signaling related protein and downstream gene expressions, such as phospho-GSK-3β, active β-catenin, c-Myc, Ccnd1, and Axin2 (p < 0.05). Additionally, it triggered the highest generation of reactive oxygen species (ROS), which activated PI3K/Akt and MAPK/Erk signaling and resulted in cleaved caspase-3 expression. In comparison, the treatment of AC polysaccharide without Turicibacter fermentation displayed a lesser effect.
Discussion: Our findings suggest that AC polysaccharide effectively suppresses the tumorigenic serotonin and Wnt-signaling pathways, and promotes ROS-mediated apoptosis in Caco-2 cells. These processes are further enhanced by Turicibacter fermentation.
1 Introduction
Colorectal cancer (CRC) is the third most commonly diagnosed cancer in both men and women and the second leading cause of cancer-related death worldwide (Siegel et al., 2022). In the United States, nearly 153,000 new cases are expected to be diagnosed in 2023, which translates to approximately one in 24 people developing this disease during their life. Additionally, there were approximately 52,000 CRC-related deaths per year, making it the second leading cause of cancer fatalities in the country (American Cancer Society, 2023).
The widely recognized risk factors of CRC include age, genetic and environmental factors (Thanikachalam and Khan, 2019). Among these identified risk factors, diet is one of the most controllable factors and has been studied extensively. In a meta-analysis, certain foods that possess pro-inflammatory potentials identified by the Dietary Inflammatory Index (DII), such as energy, total fat, trans fat, cholesterol, and saturated fatty acids, are associated with an increased risk of CRC. A 7% higher risk of CRC was reported for each 1-point increase in the DII score (Shivappa et al., 2014; Shivappa et al., 2017). It is reported that high-fat diets alter the intestinal microbial composition and short-chain fatty acid (SCFA) formation, which can promote intestinal tumorigenesis (Schulz et al., 2014; Chen et al., 2023). The Western-style diet, characterized by high saturated fat content, significantly promotes the obesity epidemic and CRC (Kopp, 2019; O'Neill et al., 2016; Benninghoff et al., 2020; Mehta et al., 2017). Therefore, understanding the mechanisms underlying diet-induced colorectal tumorigenesis can help practitioners develop strategies to reduce the increased risk of CRC associated with the obese population in Western countries, which has reached an epidemic level (Hruby and Hu, 2015).
A healthy gut microbiome, known as eubiosis, can protect the gastrointestinal tract from damage arising from daily digestion. In contrast, an imbalanced microbiome, known as dysbiosis, facilitates systemic and local inflammation and a microenvironment favoring colorectal tumorigenesis (Rebersek, 2021). Limited but fascinating data indicates that high-fat diets attenuate the abundance of genus Turicibacter in the gut microbiome (Everard et al., 2014; Guo et al., 2017), but little is known about the role of Turicibacter in CRC or any other gastrointestinal disease. Some studies suggested that the depletion of Turicibacter might be linked to colitis and may be correlated with increased Tnf and NF-κB1 expression and decreased intestinal butyrate level (Jones-Hall et al., 2015; Zhong et al., 2015; Liu et al., 2016). As evidence has shown that butyrate is capable of relieving metabolic syndrome, maintaining the intestinal barrier, reducing inflammation, and inhibiting the growth of CRC cells. The presence of Turicibacter, therefore, might be associated with anti-inflammatory and anti-cancer properties (Canani et al., 2011). In addition, limited work indicates that Turicibacter may play a role in the serotonin-signaling system, which is intimately affiliated with intestinal inflammation and cancer development (Mawe and Hoffman, 2013; Fung et al., 2019; Kannen et al., 2020; Ala, 2022). Nevertheless, the casual relationship between Turicibacter and CRC remains largely undefined.
Chemotherapy is the primary strategy for treating CRC in addition to surgical therapy and is often accompanied by side effects. Accordingly, the development of complementary and alternative medicine is urgent, especially the use of natural products as potential anti-cancer remedies. Antrodia camphorata (AC) is a medicinal fungus rich in complementary polysaccharides and bioactive components for a Western-style diet. It has been reported to suppress proliferation, metastasis and epithelial-to-mesenchymal transition and induce apoptosis in human CRC cells particularly by suppressing the Wnt/β-catenin signaling pathway (Park et al., 2013; Hseu et al., 2017; Wang et al., 2017; Ding et al., 2021). However, the molecular mechanisms behind the effects remain largely unclear and necessitate further investigation. In the present study, we cultured Caco-2 cells with a conditioned medium of Turicibacter culture to determine the role of Turicibacter in colorectal tumorigenesis and to explore the anti-cancer mechanism of AC with Turicibacter fermentation. This innovative approach aims to investigate whether Turicibacter can enhance the effects of AC through biotransformation by microorganisms (Chávarri et al., 2021). By understanding the molecular interactions between AC and Turicibacter, we hope to shed light on the potential synergistic anti-cancer properties of this combination.
2 Materials and methods
2.1 Reagents and chemicals
AC supplementation powder (freeze-dried powder of concentrated supernatant collected from AC culture media, contains 44% polysaccharide) was obtained from New Bellus Enterprises Co., Ltd. (Tainan, Taiwan). Gibco™ Dulbecco’s modified Eagle medium (DMEM), Gibco™ fetal bovine serum (FBS), Gibco™ phosphate buffered saline, Gibco™ trypsin-EDTA, Gibco™ penicillin-streptomycin, TRIzol® reagent, Applied Biosystems™ cDNA Reverse Transcription Kit, SYBR™ Green Master Mix, Pierce™ BCA Protein Assay Kit, Pierce™ Protease and Phosphatase Inhibitor, and Image-IT™ LIVE Green Reactive Oxygen Species Detection Kit were purchased from Thermo Fisher Scientific Co. (Waltham, MA). 3-(4,5-Dimethyl-2-thiazolyl)-2,5-diphenyl-2H-tetrazolium Bromide (MTT), puromycin dihydrochloride, RIPA lysis buffer, bovine serum albumin (BSA), and N-Acetyl-L-cysteine (NAC) were purchased from MilliporeSigma (Burlington, MA). Luciferase Assay System was purchased from Promega Co. (Madison, WI). Cignal Lenti TCF/LEF Reporter (luc), Cignal Lenti Negative Control (luc), and SureENTRY™ Transduction Reagent were purchased from Qiagen Inc. (Germantown, MD). Antibodies against β-catenin, p-NF-κB p65 (Ser536), p-Akt (Ser473), p-Mek (Ser221), p-Erk1/2 (Thr202/Tyr204), p-Gsk3β (Ser9), Cleaved caspase 3 (Asp175), GAPDH, and Anti-rabbit IgG, Horseradish peroxidase (HRP)-linked antibody were purchased from Cell Signaling Technology (Beverly, MA).
2.2 Bacterial strains and bacterial culture
A strain of Turicibacter, Turicibacter sanguinis DSM 14220 (originally isolated from human blood), was purchased from DSMZ (Braunschweig, Germany) and was used in this study. Frozen bacterial stocks were first retrieved in PYG TWEEN FA/GLC from Anaerobe systems (Morgan Hill, CA), then were grown and maintained in a non-commercial anaerobic bacterial culture medium with peptone, tryptone, yeast extract, casein hydrolysate, soluble starch, L-cysteine hydrochloride, bile salts, ferrous sulfate, hemin, salt solution, vitamin solution, Tween 80, and glucose with or without 1% AC supplementation at 37°C under anaerobic conditions. Supernatants were filter-sterilized and stored at −80°C until use. Metabolite profiling of supernatants was measured by hydrophilic interaction liquid chromatography (HILIC) positive and amide negative methods as previously described (Kimberly et al., 2017; Paynter et al., 2018).
2.3 Hydrophilic interaction liquid chromatography (HILIC)
AC supplementation powder is derived from the submerged fermentation of AC mycelium. Due to its complex composition, measuring all nutrients and bioactive constituents in the powder is challenging. To address this, HILIC method was employed to analyze identifiable components among four groups of cell-free supernatants from bacterial culture media: (1) bacterial culture medium only (CM), (2) medium with AC supplementation (ACCM), (3) Turicibacter cultured medium (TCM), and (4) Turicibacter cultured medium with AC supplementation (TACCM). This analysis can provide valuable information regarding the enrichment of nutrients from the AC supplementation and the differences in secondary metabolites after Turicibacter fermentation. Briefly, the supernatants of bacterial culture media were subjected to HILIC using a 2.1 × 100 mm, 3.5-μm XBridge™ amide column (Waters™, Milford, MA). Mobile phase A was 95:5 (v/v) water/acetonitrile, with 20 mM ammonium acetate and 20 mM ammonium hydroxide (pH 9.5). Mobile phase B was acetonitrile. For amide-negative mode, the chromatography system consisted of a 1260 Infinity autosampler (Agilent Technologies, Santa Clara, CA) connected to a 1290 Infinity HLPC binary pump system (Agilent Technologies, Santa Clara, CA). For the positive mode, the system utilized an HTS PAL autosampler (Leap Technologies, Morrisville, NC) connected to a 1260 HPLC binary system (Agilent Technologies, Santa Clara, CA). Metabolite quantification was determined by integrating peak areas using MassHunter QQQ Quant (Agilent Technologies, Santa Clara, CA) or MultiQuant™ software (Version 2.0, Sciex, Framingham, MA).
2.4 Cell line and cell culture
Human colon cancer cell line Caco-2 was obtained from American Tissue Culture Collection (ATCC, Manassas, VA). Caco-2 cells were maintained in DMEM supplemented with 10% FBS, 100 U/mL penicillin and 100 μg/mL streptomycin, and 1 mM sodium pyruvate at 37°C in a humidified incubator containing 5% CO2. After 48 h of culture, cells were sub-cultured at 80% confluence or were harvested for following cell experiments.
2.5 Cell viability assays
Caco-2 cells were seeded in 96-well plates with 2×104 cells per well and incubated overnight. Subsequently, cells were starved in DMEM containing 0.5% FBS overnight. After starvation, cells were either mock-treated or pretreated with NAC (100 μM) for 1 h, then treated for 24 h with conditioned media of four cell-free supernatants collected from bacterial culture media: bacterial culture medium only (CM), medium with AC supplementation (ACCM), Turicibacter cultured medium (TCM), and Turicibacter cultured medium with AC supplementation (TACCM) or control medium. Conditioned media were prepared based on the percentage of supernatant (10%–90%) in the cell culture medium. Blanks were incubated with cell culture medium without seeding cells. Cell viability was determined by incubation with MTT (0.5 mg/mL) for 2 h. Formazan crystals were dissolved in DMSO, and the absorbance was measured by a BioTek Synergy H1 microplate reader (Agilent Technologies, Santa Clara, CA) at 570 nm wavelength. The average value obtained from the blanks was subtracted from the average values obtained from the treatment and control groups. Cell viability was expressed as a percentage compared to the control.
2.6 Quantitative real-time polymerase chain reaction (RT-PCR) analyses
Total RNAs from Caco-2 cells were extracted by using TRIzol® reagent (Invitrogen™, Carlsbad, CA) according to the manufacturer’s instructions. Total RNA concentration as well as purity of RNA samples were determined by using NanoDrop™ Lite Spectrophotometer (Thermo Scientific™, Waltham, MA). The first-strand cDNAs were synthesized from RNA samples by using high-capacity cDNA reverse transcription kit (Applied Biosystems™, Carlsbad, CA). The relative expression of target genes was measured by using SYBR™ Green Master Mix (Applied Biosystems™, Carlsbad, CA) and ViiA™ 7 Real-Time PCR System (Applied Biosystems®, Carlsbad, CA) with the following conditions: hold stage starting at 50°C for 2 min, 95°C for 10 min, 40 cycles starting at 95°C for 15 s, 60°C for 1 min, melt curve stage starting at 95°C for 15 s, 60°C for 1 min, and 95°C for 15 s. The primers for target genes (Tph1, Htr1d, Htr2a, Htr2b, Htr2c, c-Myc, Ccnd1, Axin2, and GAPDH) were designed using PrimerBank (https://pga.mgh.harvard.edu/primerbank/). A list of the primers used can be found in Supplementary Table S1. The gene expression was normalized to the housekeeping gene Gapdh (
2.7 Immunoblotting
Western blot was performed to analyze protein expression as previously described (Lin et al., 2021). Briefly, 20 μg of protein from each group was loaded into 10% SDS-PAGE gel for protein separation, then was blotted onto Immun-Blot® PVDF Membrane (Bio-Rad, Hercules, CA). After membranes were blocked in 5% BSA (Sigma-Aldrich, St. Louis, MO) in Tris Buffered Saline with Tween 20 (TBST) for 1 h, they were incubated overnight at 4°C with primary antibodies against β-catenin (1:1000), p-NF-κB p65 (1:1000), p-Akt (1:1000), p-Mek (1:1000), p-Erk1/2 (1:1000), p-Gsk3β (1:1000), cleaved caspase 3 (1:1000) and GAPDH (1:10000), followed by incubation with HRP-conjugated secondary antibody (1:5000) for 1 h at room temperature. Antibody binding was detected by incubating membranes with enhanced chemiluminescence substrate (Bio-Rad, Hercules, CA) and images were captured by Odyssey® Fc Imaging System (LI-COR Biosciences, Lincoln, NE). Relative protein expression was quantified using Image J software after normalizing to the corresponding loading control. In general, phosphorylated levels of proteins reflect their functional impact. While total protein expression informs translational regulation, phosphorylation reflects changes at both translation and post-modification levels. Specifically, phosphorylated protein levels were measured using GAPDH as the loading control to gain insights into the total functional mechanisms underlying protein activity.
2.8 ROS generation assays
The presence of H2O2 reactive oxygen species in live cells was detected using the 5(6)-carboxy-2′,7′-dichlorodihydrofluorescein diacetate (carboxy-H2DCFDA) method. Generation of ROS was measured by using Image-IT™ LIVE Green Reactive Oxygen Species Detection Kit (Invitrogen™, Carlsbad, CA) according to the manufacturer’s instructions. Briefly, Caco-2 cells were seeded in a 96-well black plate with a clear bottom at a density of 2×104 cells per well and were starved in DMEM containing 0.5% FBS. Then, the cells were either mock-treated or pretreated with NAC (100 μM) for 1 h. Subsequently, the cells were treated with conditioned media containing 30% supernatants collected from four groups of bacterial culture media or control medium for 6 h. After treatment, 25 μM carboxy-H2DCFDA was applied to cells and incubated for 30 min at 37°C. During the last 5 min of the incubation, 1.0 μM Hoechst 33342 was added to cells. Images were captured immediately after staining using the EVOS™ M5000 Imaging System (Invitrogen™, Carlsbad, CA), with wavelengths of 495 nm (excitation) and 529 nm (emission) for the detection of ROS generation, and wavelengths of 350 nm (excitation) and 461 nm (emission) for the detection of the cell nucleus. Relative fluorescence intensity was quantified using ImageJ software.
2.9 Lentiviral vector transduction
Caco-2 cells were transduced with the Wnt-signaling reporter gene using the Cignal Lenti TCF/LEF Reporter (luc) kit (Qiagen, Germantown, MD), while cells transduced with the Cignal Lenti Negative Control (luc) kit (Qiagen, Germantown, MD) served as the negative control. First, Caco-2 cells were grown in 35 × 10 mm cell culture dishes to 80% confluence, and then transduced with Cignal lentiviral particles diluted 1:10 in growth medium without antibiotics, along with SureENTRY™ Transduction Reagent (Qiagen, Germantown, MD). After 48 h, the viral particle-containing medium was replaced with growth medium containing 2 μg/mL puromycin, and cells were cultured for 2 weeks to select for a stably transduced cell line.
2.10 Firefly luciferase assays
Caco-2 cells transduced with the Cignal Lenti Reporter gene were seeded in 96-well plates at a density of 2×104 cells per well and incubated overnight. The cells were then starved in DMEM containing 0.5% FBS overnight. After starvation, the cells were either mock-treated or pretreated with NAC (100 μM) for 1 h and then treated with conditioned media containing 30% supernatants collected from four groups of bacterial culture media or control medium for 6 h. After treatment, relative luciferase activity was measured using the Luciferase Assay System (Promega, Madison, WI) according to the manufacturer’s instructions, and the luminescence was detected using BioTek Synergy H1 microplate reader (Agilent Technologies, Santa Clara, CA).
2.11 Statistical analyses
Data are presented as means ± SEM. Statistical analyses were performed using SAS program (Version 9.4, SAS Institute, Cary, NC) and GraphPad Prism (Version 9, GraphPad Software, San Diego, CA). Two-tailed, unpaired t-test was applied to test the differences between two groups, one-way ANOVA was applied to test the differences among groups, and the dose-dependent relationship was assessed by the Cochran-Armitage test for trend.
3 Results
3.1 Turicibacter fermentation increased the production of bioactive substances
Table 1 shows the results of the HILIC analysis on supernatants, which demonstrated that the addition of AC supplementation to the bacterial culture medium significantly increased the levels of all the listed substances, particularly the levels of α-ketoglutaric acid (1.000
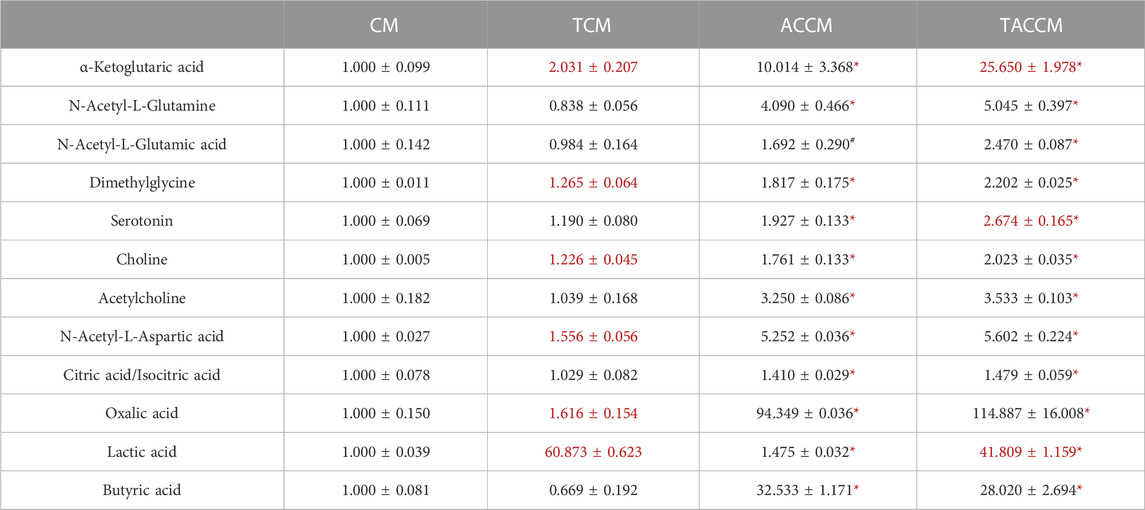
TABLE 1. Bioactive Component analyses among 4 groups of supernatants by HILIC method. CM, fresh bacterial culture medium; TCM, Turicibacter culture medium; ACCM, fresh medium with AC; TACCM, Turicibacter culture medium with AC. Data was presented as relative mean AUC ±SEM compared to CM, n = 3. Red color indicates the statistical significances between CM and TCM or ACCM and TACCM. #p < 0.1, *p < 0.05 indicate the differences between CM and ACCM or TCM and TACCM.
3.2 Turicibacter metabolites of Antrodia camphorata supplementation promoted cytotoxic effect against Caco-2 cells
To determine the appropriate treatment condition, we treated Caco-2 cells with conditioned media containing different percentages of supernatants collected from four groups of bacterial culture media: CM, TCM, ACCM, and TACCM. Our results showed that, in all four groups, cell viability started to decrease as the percentage of supernatant in the conditioned medium increased beyond 20% when compared to untreated cells. When the percentage of supernatants (CM, TCM, ACCM, and TACCM) fell between 10% and 30%, all these conditioned media only resulted in modest cytotoxic responses with <20% inhibition of cell viability (Figure 1A). Therefore, we decided to conduct further experiments by treating cells with conditioned media containing 10%–30% of supernatants.
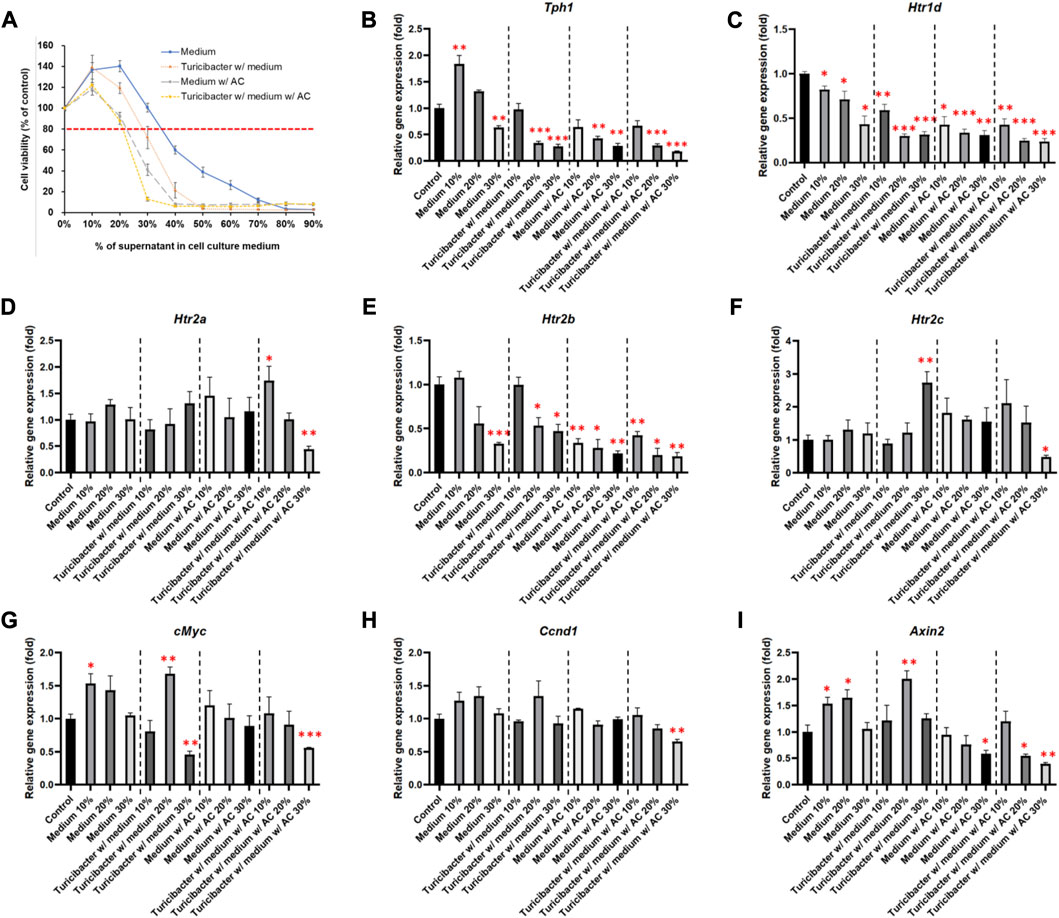
FIGURE 1. Cell viability and gene expression levels of Caco-2 cells cultured in conditioned medium. (A) Cell viability of Caco-2 cells cultured in conditioned medium with the supernatant of fresh bacterial culture medium, Turicibacter culture medium, fresh medium with AC, and Turicibacter culture medium with AC for 24 h (B–I) Gene expression levels of Tph1, Htr1d, Htr2a, Htr2b, Htr2c, c-Myc, Ccnd1, and Axin2 in Caco-2 cells after 6 h of treatment by conditioned medium. Data was presented as mean ± SEM, n = 3. *p < 0.05, **p < 0.01, ***p < 0.001, as compared to the control. Abbreviation: w/, with; w/o, without.
Among the treatments of the four groups of conditioned media, supernatants from TACCM exhibited the most cytotoxicity, followed by supernatants from ACCM, TCM, and CM (Figure 1A).
3.3 Turicibacter metabolism enhanced the inhibitory effects of Antrodia camphorata supplementation on tumorigenic serotonin and Wnt pathways
As shown in Figure 1, treatments of the four groups of conditioned media containing 20% and 30% of supernatant dose-dependently suppressed Tph1 (tryptophan hydroxylase 1) expression, which is the rate-limiting enzyme of serotonin synthesis (p < 0.01, Figure 1B). Moreover, the expression of Htr1d and Htr2b was also decreased dose-dependently (p < 0.05, Figures 1C, E), whereas the expression of Htr2a and Htr2c was significantly inhibited only by the treatment of conditioned media containing 30% of supernatant from TACCM (p < 0.05, Figures 1D, F).
Regarding the expression of Wnt-signaling downstream genes, c-Myc expression was significantly suppressed by treating with conditioned media containing 30% of supernatant from TCM and TACCM (p < 0.01, Figure 1G). Ccnd1 expression was significantly suppressed only by treating with conditioned media containing 30% of supernatant from TACCM (p < 0.01, Figure 1H). Axin2 expression was significantly suppressed by treating with conditioned media containing 30% of supernatant from ACCM and TACCM (p < 0.05, Figure 1I).
The results of immunoblotting showed that expression of protein involved in Wnt-signaling, such as β-catenin and p-Gsk-3β, was significantly inhibited only by the treatment of conditioned media containing 30% of supernatant from TACCM (p < 0.05, Figure 2). In contrast, the protein expressions of p-Akt, p-Mek, and p-Erk1/2 were dose-dependently increased upon treatment with conditioned media containing 10%–30% of supernatant from ACCM and TACCM, with the TACCM groups showing a stronger promotion effect (p < 0.05, Figure 2).
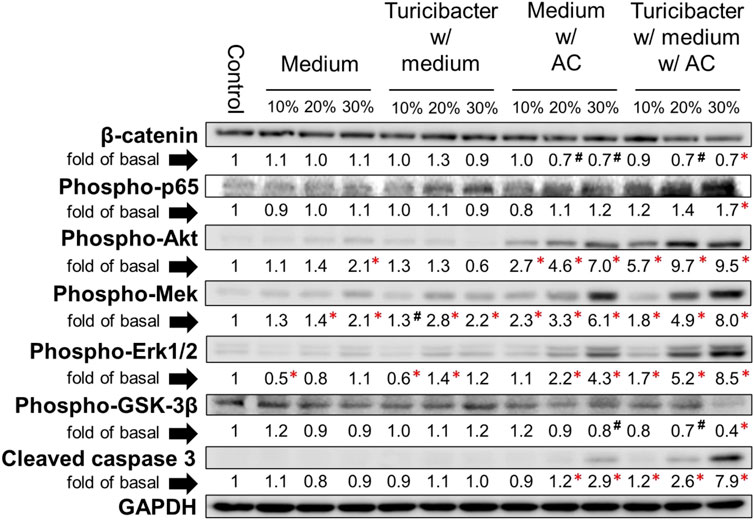
FIGURE 2. Protein expression of Caco-2 cells treated with conditioned medium. Western blots show the effects of conditioned medium treatment on the expression of β-catenin, phospho-p65, phospho-Akt, phospho-Mek, phospho-Erk1/2, phospho-Gsk-3β, and cleaved caspase 3 in Caco-2 cells. Data was presented as mean ± SEM, n = 3. #p < 0.1, *p < 0.05, as compared to the control. Abbreviation: w/, with; w/o, without.
3.4 Turicibacter metabolites of Antrodia camphorata supplementation promoted ROS generation and ROS-induced cell apoptosis
As illustrated in Figures 3A, C, compared to the untreated control group, treatment with conditioned media containing 30% of supernatant from TCM, ACCM, and TACCM stimulated ROS generation. The TACCM group showed the greatest promoting effect, followed by ACCM and TCM groups (p < 0.001). Pretreatment with NAC, a ROS production inhibitor, effectively attenuated the ROS production induced by the treatments (p < 0.05).
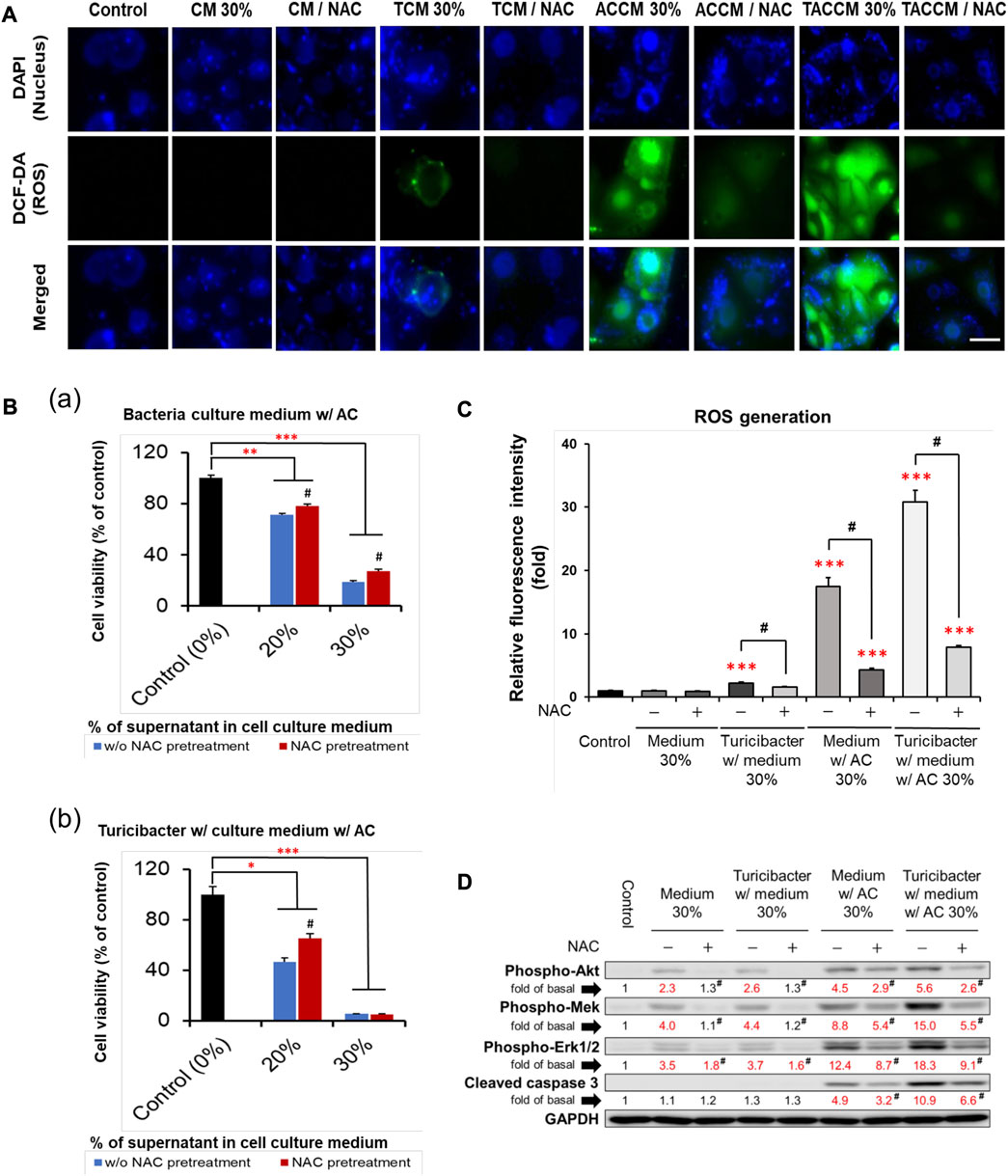
FIGURE 3. ROS generation and apoptosis of Caco-2 cells cultured in conditioned medium with and without pre-treatment of ROS inhibitor NAC. (A) Fluorescence microscopy of live Caco-2 cells untreated (control) or treated with conditioned medium with or without NAC (100 μM) pre-treatment. Carboxy-H2DCFDA was loaded to detect H2O2 in live cells (green color), and Hoechst 33342 was used for nucleic acid stain (blue color). (B) Cell viability of Caco-2 cells cultured in conditioned medium ACCM (a) and TACCM (b) for 24 h with or without NAC (100 μM) pre-treatment. (C) Relative H2O2 generation levels of Caco-2 cells untreated (control) or treated with conditioned media with or without NAC (100 μM) pre-treatment. (D) Western blots show the effects of NAC (100 μM) pre-treatment on the expression of proteins in conditioned media-treated Caco-2 cells. Red color specifies the statistical significance as compared to the control. ROS, Reactive Oxygen Species; NAC, ROS inhibitor N-acetyl-l-cysteine. CM, fresh bacterial culture medium; TCM, Turicibacter culture medium; ACCM, fresh medium with AC; TACCM, Turicibacter culture medium with AC. Data was presented as mean ± SEM, n = 3. *p < 0.05, **p < 0.01, ***p < 0.001, as compared to the control; #p < 0.05 indicates the impact of NAC. Abbreviation: w/, with; w/o, without.
The results of cell viability assays showed that NAC pretreatment partially abolished the cytotoxicity effect of conditioned media containing supernatant from ACCM and TACCM, with the strongest protective effect observed in the treatment of conditioned medium containing 20% of supernatant from TACCM (p < 0.05, Figure 3B). The increased expression of cleaved caspase 3 promoted by the treatment of conditioned media containing supernatant from ACCM and TACCM was significantly attenuated by pre-treatment of the ROS inhibitor NAC (p < 0.05, Figure 3D). This result reiterated the dose-dependent elevation of cleaved caspase 3 upon treatment with conditioned media containing 10%–30% of supernatant from ACCM and TACCM, with the TACCM groups showing a stronger promotion effect (p < 0.05, Figure 2).
3.5 Conditioned medium of Turicibacter cultured with Antrodia camphorata supplementation inhibited tumorigenic serotonin and Wnt pathways independent of ROS production
Although ROS inhibitor NAC inhibited ROS production and attenuated ROS-mediated apoptosis, which was otherwise promoted by the conditioned medium from Turicibacter fermentation of Antrodia camphorata supplementation (Figure 3), NAC pretreatment (100 μM) did not affect the activation of tumorigenic serotonin and Wnt-signaling pathways when exposed to conditioned media (Figure 4). Caco-2 cells treated with the supernatants from CM, TCM, ACCM, and TACCM inhibited the expression of genes related to the serotonin and Wnt pathways with the strongest suppression for TACCM, whereas the NAC pretreatment did not mediate this impact (Figure 4A). Similarly, the supernatants from CM, TCM, ACCM, and TACCM inhibited Wnt/β-catenin signaling, as indicated by TCF/LEF luciferase reporter gene activation, whereas the impact was independent to NAC pre-treatment (Figure 4B).
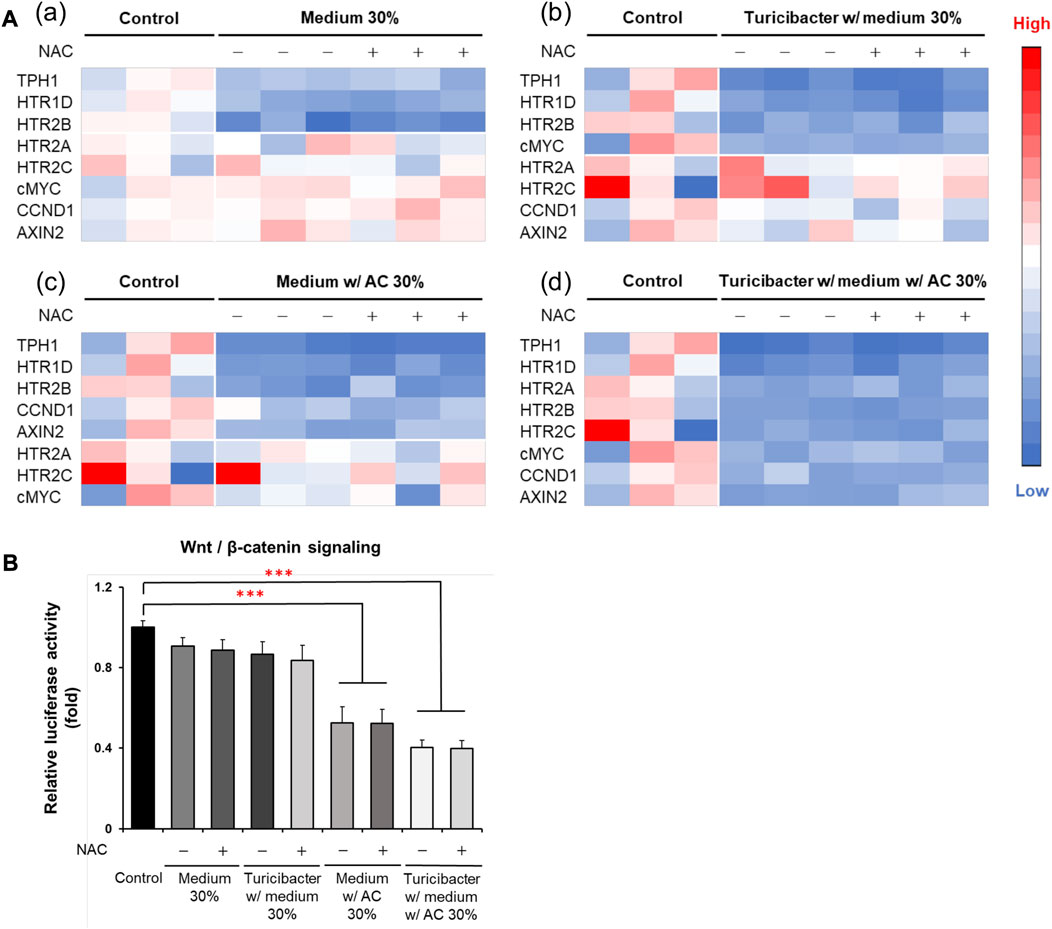
FIGURE 4. Heatmaps for expression of genes related to serotonin and Wnt pathways and Wnt/β-catenin signaling in Caco-2 cells cultured in conditioned media with and without ROS inhibitor NAC pre-treatment. (A) Gene expression heatmaps for the effect of NAC (100 μM) pre-treatment on expression of genes related to serotonin and Wnt pathways in conditioned medium-treated Caco-2 cells. (B) Wnt/β-catenin signaling, as indicated by TCF/LEF luciferase reporter gene activation, in response to NAC (100 μM) pre-treatment in conditioned medium-treated Caco-2 cells. ROS, Reactive Oxygen Species; NAC, ROS inhibitor N-acetyl-l-cysteine. Data was presented as mean ± SEM, n = 3. ***p < 0.001, as compared to the control. Abbreviation: w/, with; w/o, without.
4 Discussion
Studies have shown that CRC patients have a distinct microbiome composition compared to healthy individuals, with alterations in the abundance of specific bacterial species (Vogtmann et al., 2016; Thomas et al., 2019). Accumulating evidence suggests that the composition and activity of the gut microbiome can affect the development and progression of CRC (Nyangale et al., 2012; Schwabe and Jobin, 2013; Irrazábal et al., 2014; Louis et al., 2014; Liu et al., 2021), whereas the mechanism(s) by which the microbiome affects CRC remains insufficiently apprehended. Emerging evidence suggests that microbiome modulation through dietary interventions, pre- and probiotics may be a potential preventive or therapeutic strategy for CRC (Cheng et al., 2020). The present study suggests the role of an understudied genus, Turicibacter, and the potential of a specific mushroom Antrodia camphorata in the inhibition of the tumorigenic serotonin and Wnt pathways and the promotion of ROS-mediated apoptosis in the Caco-2 CRC cell line.
Previously, we discovered that the abundance of the rarely explored Turicibacter was significantly reduced in the gut microbiome of high-fat diet-induced obese C57BL/6 mice and APC+/1638N mice, accompanied by elevated inflammation and Wnt-signaling activation (Liu et al., 2016; Guo et al., 2017). In the present study, based on the metabolite profiling of the medium supernatants by HILIC, it is not surprising that the addition of AC supplementation significantly enriched a great number of bioactive components (Table 1; Supplementary Tables S2, S3) in the cell-free supernatants of bacterial culture media (ACCM vs. CM and TACCM vs. TCM). It is also important to note that Turicibacter fermentation resulted in an increased number of certain metabolites including α-ketoglutaric acid and lactic acid regardless of AC supplementation (TCM vs. CM and TACCM vs. ACCM) and serotonin with the presence of AC supplementation (TACCM vs. ACCM) (Table 1).
α-ketoglutaric acid is a critical intermediate in the tricarboxylic acid (TCA) cycle, which is one of the most important pathways for energy metabolism. It was reported that the depletion of α-ketoglutaric acid and glutamine could activate Wnt-signaling and promote cancer dedifferentiation in CRC with Apc mutation in mouse and human ex vivo organoids, whereas the supplementation of α-ketoglutaric acid antagonized tumorigenesis through epigenetic reprogramming (Tran et al., 2020). α-ketoglutaric acid has also been shown in various cancer cell lines to induce ROS generation, leading to pyroptosis (Zhang et al., 2021). Moreover, α-ketoglutaric acid was shown to alleviate intestinal inflammation in C57BL/6 mice with chemically-induced CRC and was capable of increasing the abundance of anti-inflammatory and SCFA-producing bacteria (Li et al., 2019). In alignment with the previous findings, our results indicated that treatment of supernatants containing higher levels of α-ketoglutaric acid attenuated Wnt-signaling, increased ROS generation, and ROS-mediated apoptosis (Figures 2–4), implying that the role of Turicibacter mediates tumorigenic pathways via the intermediate metabolite α-ketoglutaric acid.
A previous report showed that Turicibacter could stimulate the production of serotonin in the gut of healthy individuals (Yano et al., 2015), and production was likely through short-chain fatty acids (Reigstad et al., 2015). Reciprocally, serotonin has also been reported to promote Turicibacter colonization (Fung et al., 2019). In this study, we did not observe that Turicibacter fermentation increased butyric acid production and only slightly increased serotonin in the medium; this is probably due to differences between the in vitro and in vivo models used. In contrast, this study clearly demonstrated that the conditioned medium of Turicibacter significantly suppressed the expression of Tph1 and serotonin receptors in Caco-2 cells, with the addition of AC supplementation further enhancing this effect (Figures 1B–F). This suggests that Turicibacter and AC supplementation could potentially impact the development of CRC by altering serotonin signaling (Mawe and Hoffman, 2013; Ala, 2022). In fact, the serotonin pathway in CRC development is extremely complicated. Growing evidence suggests that serotonin plays a dual role in CRC development, with its presence promoting DNA damage repair in early stages but contributing to proliferation, angiogenesis, and metastasis in the later stages via receptor signaling or re-uptake transporters (Kannen et al., 2020). Notably, the activation of serotonin receptor (5-HT) 1 and 2 has been linked to the amplification of tumorigenic signaling pathways, such as β-catenin, MAPK, and AKT signaling (Karmakar and Lal, 2021; Ala, 2022). Previous research has also shown that treatment with a 5-HT2A receptor antagonist, Pizotifen, inhibits proliferation and migration of HCT-116 cells through inhibiting the Wnt/β-catenin signaling pathway (Piao and Shang, 2019). Therefore, our results demonstrate that Turicibacter and AC supplementation suppress serotonin signaling, suggesting a potential anti-tumorigenic effect in Caco-2 cells.
Our results showed that treating Caco-2 cells with conditioned medium ACCM and TACCM dramatically increased ROS generation and ROS-mediated apoptosis as indicated by the increased expression of cleaved caspase 3, with the strongest effect observed in the latter treatment (Figure 3). In addition, we observed increased expression of MAPK/Erk and PI3K/Akt signaling by the conditioned media ACCM and TACCM (Figures 2, 3D). Though the activation of MAPK/Erk and PI3K/Akt signals typically promote cell proliferation, survival, and tumorigenesis, emerging evidence suggests that the sustained accumulation of Erk1/2 above a certain threshold in the cytoplasm and nucleus, triggered by stimuli such as ROS, can lead to cell apoptosis (Sugiura et al., 2021). In addition, it has been suggested that there is a positive feedback loop between ROS and abnormally activated PI3K/Akt signaling, whereby hyperactive PI3K/Akt signaling may contribute to the production of excessive ROS and ROS-mediated apoptosis (Koundouros and Poulogiannis, 2018), which is consistent with what is observed in this study. In line with our results, previous research has shown that piperlongumine, a bioactive compound derived from long peppers, induces ROS-mediated apoptosis through the MAPK/Erk pathway in HT-29 cells (Randhawa et al., 2013).
Though previous research has shown that CRC cells exhibit higher ROS levels compared to normal colon cells, which contribute to cancer cell growth, the excessive production of ROS over a threshold can also lead to cancer cell apoptosis (Zeng et al., 2021). The elevated ROS levels in CRC cells are a result of dysregulated redox homeostasis. While normal cells maintain a balance to neutralize ROS damage, cancer cells experience disrupted redox balance, leading to moderate ROS levels that may support cancer cell survival (Liu et al., 2017). Notably, CRC cells display reduced activity of enzymes involved in antioxidant functions compared to normal colon cells (De Giani et al., 2022). This diminished antioxidant capacity in cancer cells makes them more susceptible to damage caused by excessive ROS or oxidative stress compared to normal cells. Therefore, targeting ROS-mediated apoptosis has emerged as a promising anti-cancer strategy due to this vulnerability in CRC cells. Inducing apoptosis through ROS enables selective targeting of cancer cells while sparing normal cells from adverse effects.
The vast majority (>90%) of CRC in humans possess over-activation of the Wnt pathway and there is strong evidence that this activation plays a pivotal role in colorectal carcinogenesis (Taketo, 2004; Klaus and Birchmeier, 2008; Network, 2012). The present study explicitly demonstrated that the Turicibacter fermentation together with AC supplementation (TACCM) diminished the Wnt/β-catenin signaling in Caco-2 cells, indicating an anti-colorectal tumorigenic function (Figures 1G–I and Figure 2). Moreover, this impact on Wnt/β-catenin signaling (serotonin pathway as well) is independent of ROS production and ROS-mediated apoptosis (Figures 3D, Figure 4).
In summary, based on our findings of the impacts on the serotonin pathway, Wnt/β-catenin signaling, and ROS-mediated apoptosis, AC supplementation exhibits an anti-CRC property which is further enhanced by Turicibacter fermentation. As such, this unique AC mushroom and the rarely explored Turicibacter have the potential to be developed into a complementary strategy for the prevention and treatment of CRC. Furthermore, previous safety assessments indicate that AC is safe for oral consumption, making it suitable for further investigation in animal experiments using a mouse model (Lin et al., 2015; EFSA Panel on Nutrition et al., 2022). Conducting an in vivo study will provide valuable insights into the potential therapeutic benefits of AC and Turicibacter fermentation on CRC, helping researchers understand their effects on tumor growth, metastasis, and other relevant parameters, and supporting the development of potential treatments for CRC.
Data availability statement
The original contributions presented in the study are included in the article/Supplementary Material, further inquiries can be directed to the corresponding author.
Author contributions
T-CL, ZL, and MM contributed to conception and design of the study. T-CL performed the experiments and wrote the manuscript. YG and YT assisted in Caco-2 cell culture. AS and MM assisted in Turicibacter culture. All authors contributed to the article and approved the submitted version.
Funding
This work was supported in part by USDA/NIFA AFRI grants (2022-67018-36986, ZL), USDA/NIFA Hatch project (MAS00586, ZL) and Industrial funds. The contents are solely the responsibility of the authors and do not necessarily represent the official views of the USDA or NIFA.
Acknowledgments
We thank Liv Dedon, Asha Rani, and David Sela for their invaluable assistance in determining the anaerobic conditions necessary for growing Turicibacter.
Conflict of interest
The authors declare that the research was conducted in the absence of any commercial or financial relationships that could be construed as a potential conflict of interest.
Publisher’s note
All claims expressed in this article are solely those of the authors and do not necessarily represent those of their affiliated organizations, or those of the publisher, the editors and the reviewers. Any product that may be evaluated in this article, or claim that may be made by its manufacturer, is not guaranteed or endorsed by the publisher.
Supplementary material
The Supplementary Material for this article can be found online at: https://www.frontiersin.org/articles/10.3389/fphar.2023.1203087/full#supplementary-material
References
Ala, M. (2022). Tryptophan metabolites modulate inflammatory bowel disease and colorectal cancer by affecting immune system. Int. Rev. Immunol. 41, 326–345. doi:10.1080/08830185.2021.1954638
Benninghoff, A. D., Hintze, K. J., Monsanto, S. P., Rodriguez, D. M., Hunter, A. H., Phatak, S., et al. (2020). Consumption of the total western diet promotes colitis and inflammation-associated colorectal cancer in mice. Nutrients 12, 544. doi:10.3390/nu12020544
Canani, R. B., Costanzo, M. D., Leone, L., Pedata, M., Meli, R., and Calignano, A. (2011). Potential beneficial effects of butyrate in intestinal and extraintestinal diseases. World J. Gastroenterol. 17, 1519–1528. doi:10.3748/wjg.v17.i12.1519
Chen, J., Xiao, Y., Li, D., Zhang, S., Wu, Y., Zhang, Q., et al. (2023). New insights into the mechanisms of high-fat diet mediated gut microbiota in chronic diseases. Chilworth: iMeta, n/a, e69.
Cheng, Y., Ling, Z., and Li, L. (2020). The intestinal microbiota and colorectal cancer. Front. Immunol. 11, 615056. doi:10.3389/fimmu.2020.615056
M. CHñVARRI, L. DIEZ-GUTI¢RREZ, D. MARAIn. DHANASEKARAN, and A. SANKARANARAYANAN (Editors) (2021). “Microorganisms in food and health,” Advances in probiotics (Massachusetts, United States: Academic Press).
De Giani, A., Oldani, M., Forcella, M., Lasagni, M., Fusi, P., and Di Gennaro, P. (2022). Synergistic antioxidant effect of prebiotic ginseng berries extract and probiotic strains on healthy and tumoral colorectal cell lines. Int. J. Mol. Sci. 24, 373. doi:10.3390/ijms24010373
Ding, R., Ning, X., Ye, M., and Yin, Y. (2021). Antrodia camphorata extract (ACE)-induced apoptosis is associated with BMP4 expression and p53-dependent ROS generation in human colon cancer cells. J. Ethnopharmacol. 268, 113570. doi:10.1016/j.jep.2020.113570
Efsa Panel On Nutrition, N. F., Allergens, F., Turck, D., Bohn, T., Castenmiller, J., De Henauw, S., et al. (2022). Safety of freeze-dried mycelia of Antrodia camphorata as a novel food pursuant to regulation (EU) 2015/2283. EFSA J. 20, e07380. doi:10.2903/j.efsa.2022.7380
Everard, A., Lazarevic, V., GaÿA, N., Johansson, M., StõHLMAN, M., Backhed, F., et al. (2014). Microbiome of prebiotic-treated mice reveals novel targets involved in host response during obesity. Isme J. 8, 2116–2130. doi:10.1038/ismej.2014.45
Fung, T. C., Vuong, H. E., Luna, C. D. G., Pronovost, G. N., Aleksandrova, A. A., Riley, N. G., et al. (2019). Intestinal serotonin and fluoxetine exposure modulate bacterial colonization in the gut. Nat. Microbiol. 4, 2064–2073. doi:10.1038/s41564-019-0540-4
Guo, X., Li, J., Tang, R., Zhang, G., Zeng, H., Wood, R. J., et al. (2017). High fat diet alters gut microbiota and the expression of paneth cell-antimicrobial peptides preceding changes of circulating inflammatory cytokines. Mediat. Inflamm. 2017, 9474896. doi:10.1155/2017/9474896
Hruby, A., and Hu, F. B. (2015). The epidemiology of obesity: a big picture. Pharmacoeconomics 33, 673–689. doi:10.1007/s40273-014-0243-x
Hseu, Y. C., Chao, Y. H., Lin, K. Y., Way, T. D., Lin, H. Y., Thiyagarajan, V., et al. (2017). Antrodia camphorata inhibits metastasis and epithelial-to-mesenchymal transition via the modulation of claudin-1 and Wnt/β-catenin signaling pathways in human colon cancer cells. J. Ethnopharmacol. 208, 72–83. doi:10.1016/j.jep.2017.07.001
IrrazñBAL, T., Belcheva, A., Girardin, S. E., Martin, A., and Philpott, D. J. (2014). The multifaceted role of the intestinal microbiota in colon cancer. Mol. Cell. 54, 309–320. doi:10.1016/j.molcel.2014.03.039
Jones-Hall, Y. L., Kozik, A., and Nakatsu, C. (2015). Ablation of tumor necrosis factor is associated with decreased inflammation and alterations of the microbiota in a mouse model of inflammatory bowel disease. PLoS One 10, e0119441. doi:10.1371/journal.pone.0119441
Kannen, V., Bader, M., Sakita, J. Y., Uyemura, S. A., and Squire, J. A. (2020). The dual role of serotonin in colorectal cancer. Trends Endocrinol. Metab. 31, 611–625. doi:10.1016/j.tem.2020.04.008
Karmakar, S., and Lal, G. (2021). Role of serotonin receptor signaling in cancer cells and anti-tumor immunity. Theranostics 11, 5296–5312. doi:10.7150/thno.55986
Kimberly, W. T., O'Sullivan, J. F., Nath, A. K., Keyes, M., Shi, X., Larson, M. G., et al. (2017). Metabolite profiling identifies anandamide as a biomarker of nonalcoholic steatohepatitis. JCI Insight 2, e92989. doi:10.1172/jci.insight.92989
Klaus, A., and Birchmeier, W. (2008). Wnt signalling and its impact on development and cancer. Nat. Rev. Cancer 8, 387–398. doi:10.1038/nrc2389
Kopp, W. (2019). How western diet and lifestyle drive the pandemic of obesity and civilization diseases. Diabetes Metab. Syndr. Obes. 12, 2221–2236. doi:10.2147/DMSO.S216791
Koundouros, N., and Poulogiannis, G. (2018). Phosphoinositide 3-kinase/akt signaling and redox metabolism in cancer. Front. Oncol. 8, 160. doi:10.3389/fonc.2018.00160
Li, S., Fu, C., Zhao, Y., and He, J. (2019). Intervention with α-ketoglutarate ameliorates colitis-related colorectal carcinoma via modulation of the gut microbiome. Biomed. Res. Int. 2019, 8020785. doi:10.1155/2019/8020785
Lin, C. C., Kumar, K. J. S., Liao, J. W., Kuo, Y. H., and Wang, S. Y. (2015). Genotoxic, teratotoxic and oral toxic assessments of Antrodia cinnamomea health food product (Leader Deluxe Antrodia cinnamomea(®)). Toxicol. Rep. 2, 1409–1417. doi:10.1016/j.toxrep.2015.10.007
Lin, T. C., Germagian, A., and Liu, Z. (2021). The NF-[Formula: see text]B signaling and wnt/[formula: see text]-catenin signaling in MCF-7 breast cancer cells in response to bioactive components from mushroom Antrodia camphorata. Am. J. Chin. Med. 49, 199–215. doi:10.1142/S0192415X21500117
Liu, H., Liu, X., Zhang, C., Zhu, H., Xu, Q., Bu, Y., et al. (2017). Redox imbalance in the development of colorectal cancer. J. Cancer 8, 1586–1597. doi:10.7150/jca.18735
Liu, P., Wang, Y., Yang, G., Zhang, Q., Meng, L., Xin, Y., et al. (2021). The role of short-chain fatty acids in intestinal barrier function, inflammation, oxidative stress, and colonic carcinogenesis. Pharmacol. Res. 165, 105420. doi:10.1016/j.phrs.2021.105420
Liu, W., Crott, J. W., Lyu, L., Pfalzer, A. C., Li, J., Choi, S. W., et al. (2016). Diet- and genetically-induced obesity produces alterations in the microbiome, inflammation and Wnt pathway in the intestine of apc(+/1638N) mice: comparisons and contrasts. J. Cancer 7, 1780–1790. doi:10.7150/jca.15792
Louis, P., Hold, G. L., and Flint, H. J. (2014). The gut microbiota, bacterial metabolites and colorectal cancer. Nat. Rev. Microbiol. 12, 661–672. doi:10.1038/nrmicro3344
Mawe, G. M., and Hoffman, J. M. (2013). Serotonin signalling in the gut--functions, dysfunctions and therapeutic targets. Nat. Rev. Gastroenterol. Hepatol. 10, 473–486. doi:10.1038/nrgastro.2013.105
Mehta, R. S., Song, M., Nishihara, R., Drew, D. A., Wu, K., Qian, Z. R., et al. (2017). Dietary patterns and risk of colorectal cancer: analysis by tumor location and molecular subtypes. Gastroenterology 152, 1944–1953. doi:10.1053/j.gastro.2017.02.015
Network, C. G. A. (2012). Comprehensive molecular characterization of human colon and rectal cancer. Nature 487, 330–337. doi:10.1038/nature11252
Nyangale, E. P., Mottram, D. S., and Gibson, G. R. (2012). Gut microbial activity, implications for health and disease: the potential role of metabolite analysis. J. Proteome Res. 11, 5573–5585. doi:10.1021/pr300637d
O'Neill, A. M., Burrington, C. M., Gillaspie, E. A., Lynch, D. T., Horsman, M. J., and Greene, M. W. (2016). High-fat Western diet-induced obesity contributes to increased tumor growth in mouse models of human colon cancer. Nutr. Res. 36, 1325–1334. doi:10.1016/j.nutres.2016.10.005
Park, D. K., Lim, Y. H., and Park, H. J. (2013). Antrodia camphorata grown on germinated brown rice inhibits HT-29 human colon carcinoma proliferation through inducing G0/G1 phase arrest and apoptosis by targeting the β-catenin signaling. J. Med. Food 16, 681–691. doi:10.1089/jmf.2012.2605
Paynter, N. P., Balasubramanian, R., Giulianini, F., Wang, D. D., Tinker, L. F., Gopal, S., et al. (2018). Metabolic predictors of incident coronary heart disease in women. Circulation 137, 841–853. doi:10.1161/CIRCULATIONAHA.117.029468
Piao, S. S., and Shang, B. (2019). Pizotifen inhibits the proliferation and migration of colon cancer HCT116 cells by down-regulating Wnt signaling pathway. Ann. Clin. Lab. Sci. 49, 183–188.
Randhawa, H., Kibble, K., Zeng, H., Moyer, M. P., and Reindl, K. M. (2013). Activation of ERK signaling and induction of colon cancer cell death by piperlongumine. Toxicol Vitro 27, 1626–1633. doi:10.1016/j.tiv.2013.04.006
Rebersek, M. (2021). Gut microbiome and its role in colorectal cancer. BMC Cancer 21, 1325. doi:10.1186/s12885-021-09054-2
Reigstad, C. S., Salmonson, C. E., Rainey, J. F., 3R. D., Szurszewski, J. H., Linden, D. R., Sonnenburg, J. L., et al. (2015). Gut microbes promote colonic serotonin production through an effect of short-chain fatty acids on enterochromaffin cells. Faseb J. 29, 1395–1403. doi:10.1096/fj.14-259598
Schulz, M. D., Atay, C., Heringer, J., Romrig, F. K., Schwitalla, S., Aydin, B., et al. (2014). High-fat-diet-mediated dysbiosis promotes intestinal carcinogenesis independently of obesity. Nature 514, 508–512. doi:10.1038/nature13398
Schwabe, R. F., and Jobin, C. (2013). The microbiome and cancer. Nat. Rev. Cancer 13, 800–812. doi:10.1038/nrc3610
Shivappa, N., Godos, J., H¢Bert, J. R., Wirth, M. D., Piuri, G., Speciani, A. F., et al. (2017). Dietary inflammatory index and colorectal cancer risk-A meta-analysis. Nutrients 9, 1043. doi:10.3390/nu9091043
Shivappa, N., Steck, S. E., Hurley, T. G., Hussey, J. R., and H¢Bert, J. R. (2014). Designing and developing a literature-derived, population-based dietary inflammatory index. Public Health Nutr. 17, 1689–1696. doi:10.1017/S1368980013002115
Siegel, R. L., Miller, K. D., Fuchs, H. E., and Jemal, A. (2022). Cancer statistics, 2022. CA A Cancer J. Clin. 72, 7–33. doi:10.3322/caac.21708
Sugiura, R., Satoh, R., and Takasaki, T. (2021). Erk: a double-edged sword in cancer. ERK-dependent apoptosis as a potential therapeutic strategy for cancer. Cells 10, 2509. doi:10.3390/cells10102509
Taketo, M. M. (2004). Shutting down Wnt signal-activated cancer. Nat. Genet. 36, 320–322. doi:10.1038/ng0404-320
Thanikachalam, K., and Khan, G. (2019). Colorectal cancer and nutrition. Nutrients 11, 164. doi:10.3390/nu11010164
Thomas, A. M., Manghi, P., Asnicar, F., Pasolli, E., Armanini, F., Zolfo, M., et al. (2019). Metagenomic analysis of colorectal cancer datasets identifies cross-cohort microbial diagnostic signatures and a link with choline degradation. Nat. Med. 25, 667–678. doi:10.1038/s41591-019-0405-7
Tran, T. Q., Hanse, E. A., Habowski, A. N., Li, H., Ishak Gabra, M. B., Yang, Y., et al. (2020). α-Ketoglutarate attenuates Wnt signaling and drives differentiation in colorectal cancer. Nat. Cancer 1, 345–358. doi:10.1038/s43018-020-0035-5
Vogtmann, E., Hua, X., Zeller, G., Sunagawa, S., Voigt, A. Y., Hercog, R., et al. (2016). Colorectal cancer and the human gut microbiome: reproducibility with whole-genome shotgun sequencing. PLoS One 11, e0155362. doi:10.1371/journal.pone.0155362
Wang, G., Wan, Y., Zhao, J., and Hong, Z. (2017). Ethanol extract of Antrodia camphorata inhibits proliferation of HCT-8 human colorectal cancer cells by arresting cell cycle progression and inducing apoptosis. Mol. Med. Rep. 16, 4941–4947. doi:10.3892/mmr.2017.7207
Yano, J. M., Yu, K., Donaldson, G. P., Shastri, G. G., Ann, P., Ma, L., et al. (2015). Indigenous bacteria from the gut microbiota regulate host serotonin biosynthesis. Cell. 161, 264–276. doi:10.1016/j.cell.2015.02.047
Zeng, J., Li, M., Xu, J. Y., Xiao, H., Yang, X., Fan, J. X., et al. (2021). Aberrant ROS mediate cell cycle and motility in colorectal cancer cells through an oncogenic CXCL14 signaling pathway. Front. Pharmacol. 12, 764015. doi:10.3389/fphar.2021.764015
Zhang, J. Y., Zhou, B., Sun, R. Y., Ai, Y. L., Cheng, K., Li, F. N., et al. (2021). The metabolite α-KG induces GSDMC-dependent pyroptosis through death receptor 6-activated caspase-8. Cell. Res. 31, 980–997. doi:10.1038/s41422-021-00506-9
Keywords: mushroom polysaccharide, microbiome, Turicibacter, serotonin, Wnt/βcatenin signaling, reactive oxygen species, colorectal cancer
Citation: Lin T-C, Soorneedi A, Guan Y, Tang Y, Shi E, Moore MD and Liu Z (2023) Turicibacter fermentation enhances the inhibitory effects of Antrodia camphorata supplementation on tumorigenic serotonin and Wnt pathways and promotes ROS-mediated apoptosis of Caco-2 cells. Front. Pharmacol. 14:1203087. doi: 10.3389/fphar.2023.1203087
Received: 10 April 2023; Accepted: 07 August 2023;
Published: 16 August 2023.
Edited by:
Defang Li, Binzhou Medical University, ChinaReviewed by:
K. B. Arun, Christ University, IndiaDingka Song, Shanghai Jiao Tong University, China
Veronica M. Ringel-Scaia, Charles River Laboratories, United States
Copyright © 2023 Lin, Soorneedi, Guan, Tang, Shi, Moore and Liu. This is an open-access article distributed under the terms of the Creative Commons Attribution License (CC BY). The use, distribution or reproduction in other forums is permitted, provided the original author(s) and the copyright owner(s) are credited and that the original publication in this journal is cited, in accordance with accepted academic practice. No use, distribution or reproduction is permitted which does not comply with these terms.
*Correspondence: Zhenhua Liu, emxpdUBudXRyaXRpb24udW1hc3MuZWR1