- 1Platforme de Recherche et D’analyse en Sciences de L’environnement (EDST-PRASE), Beirut, Lebanon
- 2Department of Biology, American University of Beirut, Beirut, Lebanon
- 3Department of Nutrition, University of Petra, Amman, Jordan
- 4UMRT INRE 1158 BioEcoAgro, Laboratorie BIOPI, University of Picardie Jules Verne, Amiens, France
- 5Aix-Marseille Univ, CNRS, Centrale Marseille, iSM2, Marseille, France
Pancreatic cancer is a highly aggressive malignancy and a leading cause of cancer-related deaths worldwide. Moreover, the incidence and mortality rates for pancreatic cancer are projected to keep increasing. A major challenge in the treatment of pancreatic cancer is the lack of effective screening approaches, which contributes to its poor prognosis, indicating the need for new treatment regimens and alternative therapies, such as herbal medicine. The medicinal plant A. strigosa, which is widely distributed in the Eastern Mediterranean region, is a short prickly plant from the Boraginaceae family that has been widely used in traditional medicine for treating various diseases. Nevertheless, its effect on human pancreatic cancer remains poorly investigated. In the present study, we screened the phytochemical content of Anchusa strigosa aqueous extracts obtained by maceration and ultrasound-assisted methods (ASM and ASU, respectively) and evaluated their antioxidant effects. We also investigated their anticancer effects and possible underlying mechanisms. The results show that both extracts were rich in bioactive molecules, with slight differences in their composition. Both extracts exhibited remarkable antioxidant potential and potent radical-scavenging activity in vitro. Additionally, non-cytotoxic concentrations of both extracts attenuated cell proliferation in a time- and concentration-dependent manner, which was associated with a decrease in the proliferation marker Ki67 and an induction of the intrinsic apoptotic pathway. Furthermore, the extracts increased the aggregation of pancreatic cancer cells and reduced their migratory potential, with a concomitant downregulation of integrin β1. Finally, we showed that the ASM extract caused a significant decrease in the levels of COX-2, an enzyme that has been linked to inflammation, carcinogenesis, tumor progression, and metastasis. Taken together, our findings provide evidence that A. strigosa extracts, particularly the extract obtained using the maceration method, have a potential anticancer effect and may represent a new resource for the design of novel drugs against pancreatic cancer.
1 Introduction
Cancer is the second leading cause of death worldwide, after heart disease. New cases have been growing by 1% each year since the late 1990s, affecting both men and women, and reaching over 495,000 new cases in 2020 (Cancer, Net, 2023; World Cancer Research Fund International, 2023). Numbers are expected to grow, due to the aging and growth of the global population, from 18.1 million new cases in 2018 to around 29.4 million cases in 2040, equivalent to a predicted 60% increase (The Cancer Atlas, 2018). Moreover, experts estimate that it will be the top cause of death worldwide in this century (Saez, 2018). Although our understanding of cancer has evolved throughout the years, cancer continues to wreck lives, bringing sorrow and pain. The burden of cancer on society and health systems is huge. Of particular concern is pancreatic cancer, which is considered one of the most aggressive and lethal malignancies because of its poor prognosis. Pancreatic cancer is asymptomatic and silent for a long time and, by the time it becomes symptomatic, it is often incurable. At the time of diagnosis, only 10%–20% can have surgery, which until now has been the best chance for cure and the only curative option (Lambert et al., 2019). However, local or distant relapse remains a major obstacle. If the tumor is unresectable, treatment consists of chemotherapy, sometimes in combination with radiation, with often inconsistent results, poor outcomes, and controversial benefits (Hajatdoost et al., 2018). In other words, pancreatic cancer accounts for almost as many deaths as cases. Moreover, the incidence of pancreatic cancer is on the rise, prompting an urgent need to improve survival rates (Rahib et al., 2014; Rawla et al., 2019). As such, researchers have been looking for alternative treatments for pancreatic cancer, with herbal medicine increasingly gaining interest and popularity due its lower costs and fewer side effects. So far, natural products and herbal formulas have been shown to be safe and effective options with good therapeutic effects against cancer in general and pancreatic cancer in particular (Saif, 2008; Zhang et al., 2022).
Anchusa strigosa, commonly known as prickly alkanet or “Lisan Al-thawr” in Arabic, is a perennial weed from the family Boraginaceae, that, is widely distributed in the Eastern Mediterranean region and native to Lebanon, Syria, Jordan, Iraq, and Iran. It grows on roadsides and has the ability to adapt to a wide range of habitats, from Mediterranean woods and steppe vegetation to true deserts (Tohme and Tohmé, 2014). The plant consists of a basal rosette of leaves and an inflorescence of pure white or cobalt blue flowers borne on a stem that can reach up to 1 m in length (Figure 1). It is traditionally used in folk medicine for analgesic and sedative treatments, and to relieve pain (Al-douri 2000; Yeşil and Inal, 2021). Extracts from A. strigosa have been shown to be effective in the alleviation or treatment of several diseases (Yarmolinsky et al., 2022). It has been reported to have anti-bacterial (Abutbul et al., 2005; Al-Salihi et al., 2007; Al-Salihi et al., 2009), anti-fungal (Ali Shtayeh and Abu Ghdeib, 1999), anti-ulcer (Disi et al., 1998; Abbas et al., 2009), anti-arthritic (Alallan et al., 2018), and antioxidant (Alali et al., 2007; Dibas et al., 2017; Al-Khateeb et al., 2019) activities. The anticancer activity of A. strigosa against several cancer cell lines from breast and colorectal carcinomas has also been investigated (Dibas et al., 2017; Al-Khatib et al., 2021). This wide range of activities is attributed to the fact that a variety of bioactive compounds and phytochemicals, namely, polyphenols, flavonoids, terpenoids, saponins, alkaloids, glycosides, steroids, and sterols, are bountiful in A. strigosa extracts (Alallan et al., 2018; Ghalib and Kadhim, 2021). These studies suggest that A. strigosa may have beneficial anticancer properties, which promoted us to investigate its effect in the context of pancreatic cancer.
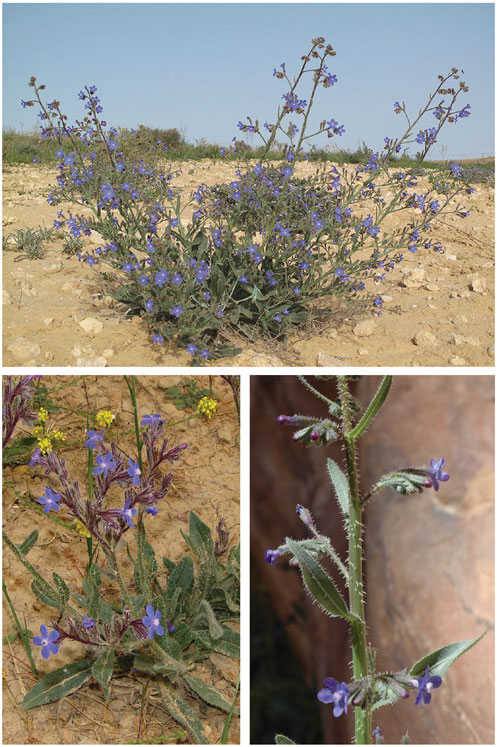
FIGURE 1. Anchusa strigosa plant. Images obtained from https://powo.science.kew.org/taxon/urn:lsid:ipni.org:names:113367-1 (accessed on 25 March 2023).
The extraction of plant material is a crucial first step in the preparation of plant samples rich in active biomolecules (Stéphane et al., 2021). Selection of appropriate extraction methods needs meticulous evaluation in order to ensure that therapeutic phytochemicals are preserved and stable.
In this study, the phytochemical composition and antioxidant activity of aqueous extracts of A. strigosa were evaluated using the traditional maceration technique (ASM) and the more modern ultrasound-assisted method (ASU). Moreover, the types of compounds in the extracts of A. strigosa leaves were also investigated. Finally, their effect on the malignant phenotype of the human pancreatic ductal adenocarcinoma cells (Capan-2) was tested. We examined the effects of the ASM and ASU extracts on the proliferation, migration, adhesion, and aggregation of Capan-2 cells. In addition, the molecular and mechanistic basis of their action was also investigated.
2 Materials and Methods
2.1 Aqueous extracts of A. strigosa
Leaves of A. strigosa were collected from Akkar, Lebanon, at 500 m altitude in April 2022. The leaves were rinsed, air-dried in the dark at room temperature, and then placed in an oven at 40°C for 24 h. The dried leaves were then crushed and ground into fine powder. Aqueous crude extracts of A. strigosa, ASM and ASU, were obtained by maceration and ultrasound-assisted extraction, respectively.
2.1.1 Maceration (ASM)
A. strigosa powder was resuspended in distilled water at a ratio of 1:10 (w/v) in the dark for 48 h, with constant shaking. The aqueous suspension was centrifuged at 2,500 rpm for 10 min and vacuum-filtered. The supernatant was then lyophilized, and the powder obtained was dissolved in sterile ultrapure water at 100 mg/mL and kept in the dark at 4°C.
2.1.2 Ultrasound-assisted extraction (ASU)
A. strigosa powder was immersed in distilled water at a ratio of 1:10 (w/v) and placed in an ultrasound bath (Wiseclean Sonicator) at 40 kHz for 30 min at a temperature below 40°C. The process of separation, filtration, and lyophilization was similar to that described above for ASM.
2.2 Total flavonoid content (TFC)
The total flavonoid content (TFC) of A. strigosa was determined by the aluminum chloride colorimetric assay, as reported by Quettier-Deleu et al. (2000) with slight modification. Each extract was prepared at a concentration of 1 mg/mL of sterile ultrapure water and an aliquot (1 mL) was mixed with 1 mL of 2% methanolic aluminum chloride. The mixture was incubated for 30 min in the dark and at room temperature. The absorbance was measured at 415 nm, using rutin as standard. The TFC was expressed as mg rutin equivalents (RE) per Gram of extract (mg RE/g). Analysis of TFC was repeated three times and data are presented as mean values ±SEM.
2.3 Total phenolic content (TPC)
With slight modification, the total phenolic content (TPC) of A. strigosa extracts was evaluated using the Folin–Ciocalteu method (Phuyal et al., 2020). A stock of each A. strigosa extract was prepared at a concentration of 1 mg/mL. An aliquot (500 μL) of the extract was oxidized with 2.5 mL of 0.2 N Folin–Ciocalteu reagent for 5 min. Then the reaction was neutralized with 2 mL of 75 g/L sodium carbonate and incubated in the dark at 37°C for 1 h. The absorbance was measured at 760 nm, where gallic acid was used as a standard. The TPC was expressed as a percentage of total gallic acid equivalents per Gram of extract (mg GAE/g). Analysis of TPC was repeated three times and data are presented as mean values ±SEM.
2.4 Assay of antioxidant activity
The antioxidant activity of the extracts was determined using the free-radical-scavenging activity of α, α-diphenyl-β-picrylhydrazyl (DPPH), as previously described in Farhan et al. (2012), with some modification. Increasing concentrations of each extract were tested (100, 200, 400, 600, 800 and 1,000 μg/mL). 0.5 mL from each concentration was mixed with 0.5 mL of DPPH solution (0.5 mM in methanol) and 3 mL of methanol. The blank consisted of 0.5 mL of distilled water with 0.5 mL of DPPH, and 3 mL of methanol. Ascorbic acid was used as a standard. The samples were then kept in the dark and at room temperature for 30 min and the absorbance was measured at a wavelength of 517 nm using a Hitachi U-2900 UV-Vis spectrophotometer. The DPPH- scavenging activity of each concentration of the extract was calculated as a percentage inhibition using the following formula:
2.5 Liquid chromatography-mass spectrophotometry
For HPLC-PDA-MS/MS analysis, the mass spectrometer (LC-MS 8050; Shimadzu, Japan) was coupled to a triple quadruple spectrometer with an ESI source. The samples underwent chromatographic separation using a Zorbax Eclipse XDB-C18 column (4.6 mm i.d. × 150 mm long, 3.5 µm particle size; Agilent, Santa Clara, CA, United States). Two mobile phases, water and acetonitrile (ACN), were used and the gradient applied from 5% to 60% ACN over 40 min at a flow rate of 1 mL/min. The extracts were injected via an autosampler (SIL-40C xs) controlled by LC solution software (Shimadzu, Japan). The ions were collected in the negative mode.
2.6 Cell culture
Capan-2 human pancreatic cancer cells [CLS (Cell Line Service), Eppelheim, Germany] were maintained in a humidified (37°C and 5% CO2) chamber in high-glucose DMEM (Dulbecco’s modified Eagle’s medium), supplemented with 10% fetal bovine serum (FBS) (both from Sigma-Aldrich, St. Louis, MO, United States) and 1% penicillin/streptomycin (Lonza, Switzerland).
2.7 Assay of cell viability
Capan-2 cells (5 × 103) were seeded in 96-well plates and allowed to grow until they reached 30%–40% confluency. The cells were then treated with increasing concentrations of extract and incubated for a total period of 72 h. Cell viability was assessed by the reduction of 3-(4,5- dimethylthiazol-2-yl)-2,5-diphenyltetrazolium bromide (MTT; Sigma-Aldrich, St. Louis, MO, United States). Cell growth was determined as the proportional viability of the treated cells in comparison with the vehicle (sterile ultrapure water)-treated cells, the viability of which was assumed to be 100%. The assay was performed in triplicate and repeated three times. Data are presented as mean values ±SEM.
2.8 Analysis of apoptotic morphological changes
Morphological changes characteristic of apoptotic cells were observed using a phase-contrast inverted microscope. For this, cells were grown in 6-well plates in the presence or absence of different concentrations of ASM and ASU. Pictures were taken after 24 h at 4×, ×10, and ×20 magnifications.
Changes in nuclear morphology characteristic of apoptosis were determined by 4′, 6-diamidino-2-phenylindole, dihydrochloride (DAPI) staining. Capan-2 cells were grown in 12-well plates in the presence or absence of the indicated concentrations of ASM and ASU for 24 h. Then the cells were fixed with 4% formaldehyde, stained with DAPI (Cell Signaling #4083) according to the manufacturer’s instructions, and visualized by fluorescence microscopy.
2.9 Wound-healing assay
Capan-2 cells were grown in 12-well plates until confluent. A wound scratch was made through the confluent monolayer using a sterile 200-μL plastic pipette tip. The culture medium was then removed and the cells were washed twice with phosphate-buffered saline (PBS; Sigma-Aldrich, St. Louis, MO, United States) to remove the remaining cellular debris. Fresh medium in the presence or absence of the indicated concentrations of ASM and ASU was added, and cells were further incubated at 37°C. Photomicrographs were taken at 0 (baseline), 8, and 12 h time points using an inverted phase-contrast microscope (objective ×4). The wound width was expressed as the average ±SEM between the measurements taken at time zero and the corresponding time points. The assay was repeated three times and data are presented as mean ± SEM.
2.10 Aggregation assay
Cell aggregation was assessed by harvesting cells from confluent plates using 2 mM EDTA in calcium and magnesium-free (CMF)-PBS and aliquoting them onto fresh empty dishes, with or without treatment. Cells were incubated at 37°C with shaking for 4 h and then fixed with 1% formaldehyde. Pictures were taken using the Olympus IX 71 inverted microscope.
2.11 Analysis of western blots
Cells were washed twice with PBS, scraped, and lysed using 2% SDS, 60 mM Tris lysis buffer (pH 6.8). The cell lysates were centrifuged at 5,000 g for 10 min and the protein concentration of the supernatants was determined using the Bradford protein assay kit (Bio-Rad, Hercules, CA, United States). Aliquots of 25–30 μg were resolved by 10% sodium dodecyl sulfate-polyacrylamide gel electrophoresis and transferred to a polyvinylidene difluoride membrane (Immobilon PVDF; Bio-Rad, Hercules, CA, United States). The membrane was then blocked with 5% nonfat dry milk in TBST (TBS and 0.05% Tween) for 1 h at room temperature. Immunodetection was carried out using the specified primary antibodies. Horseradish peroxidase-conjugated anti-IgG was used as secondary antibody and immunoreactive bands were detected using the Clarity Western ECL substrate kit (Bio-Rad, Hercules, CA, United States), according to the manufacturer’s instructions. All primary and secondary antibodies were obtained from Cell Signaling (Cell Signaling Technology, Inc., Danvers, MA, United States: β-actin #3700, caspase-3 #9662, BCL-2 #15071, integrin β1 #9699, and COX2 #12282) and abcam (Ki67 ab92742). For quantification, experiments were repeated three times. Data are presented as mean values ±SEM.
2.12 Statistical analysis
Data were statistically evaluated using the Student’s t-test with GraphPad Prism version 5.0. For the comparison of more than two means, ANOVA was used: either a one-way ANOVA (with Dunnett’s post-hoc test) or a two-way ANOVA (with Tukey–Kramer’s post-hoc test). Data are presented as mean ± standard error of the mean (SEM). A p-value of less than 0.05 is considered as significant.
3 Results
3.1 Analysis of A. strigosa extracts by LC-MS
Aqueous extracts obtained from A. strigosa leaves using the two extraction methods (maceration and ultrasonic extraction) were subjected to liquid chromatography analysis. The chromatographic peak profiles of ASM and ASU differed in the composition of their bioactive compounds, with the ASM extract showing more and higher peaks (Figure 2). Moreover, the study revealed the presence of 38 phytochemicals in ASM and 39 in ASU based on their MS, MS/MS spectral data, and retention times (Table 1). Rosmarinic acid was particularly abundant in the ASM extract.
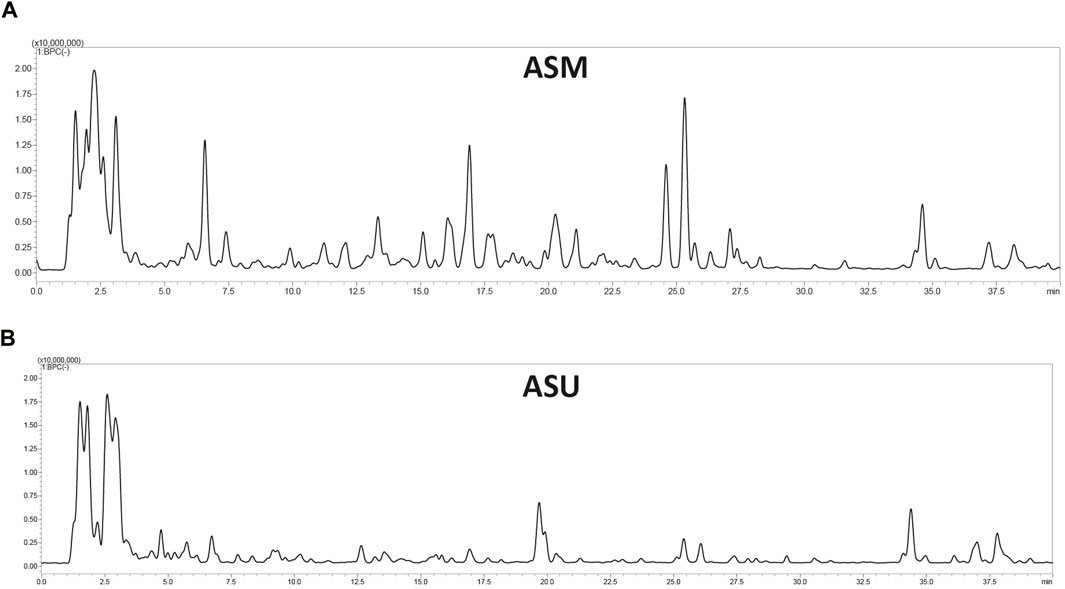
FIGURE 2. LC-MS profile of A. strigosa extracts obtained by maceration (A) and ultrasound-assisted (B) extraction methods.
This indicates that the ASM extract contains more secondary bioactive metabolites. According to our results, the maceration method could be selected as being more effective for the extraction of bioactive compounds from A. strigosa than the ultrasound-assisted method.
3.2 The phenolic and flavonoid contents of A. strigosa extracts
Polyphenolic compounds are a large family of secondary metabolites that account for most of the antioxidant properties of plants. Our results show that the highest TPC and TFC contents were recorded in the ASM extract with 284.79 ± 12.4 mg GAE/g and 27.93 ± 0.39 mg RE/g extract, respectively, compared to 169.06 ± 7.11 mg GAE/g and 25.20 ± 0.21 mg RE/g extract, respectively, for ASU (Table 2). This is in accordance with the LC-MS data, which clearly highlighted the differences in the composition of the extracts and emphasized their differential abundance with regards to polyphenols.
3.3 The antioxidant potential of A. strigosa extracts
Antioxidants are molecules that can prevent and decrease the oxidation of other molecules, reducing their capacity to damage by reacting with free radicals. Referred to as free-radical scavengers, antioxidant molecules can delay or inhibit oxidative stress or cellular damage.
In the present study, both ASM and ASU extracts showed concentration-dependent free-radical scavenging activity (Figure 3). More specifically, ASM exhibited a stronger reducing power than ASU. These results correlate with the TPC, TFC, and LC-MS data, confirming that the greater the concentration of phenolics and flavonoids, the greater the antioxidant ability of the extract.
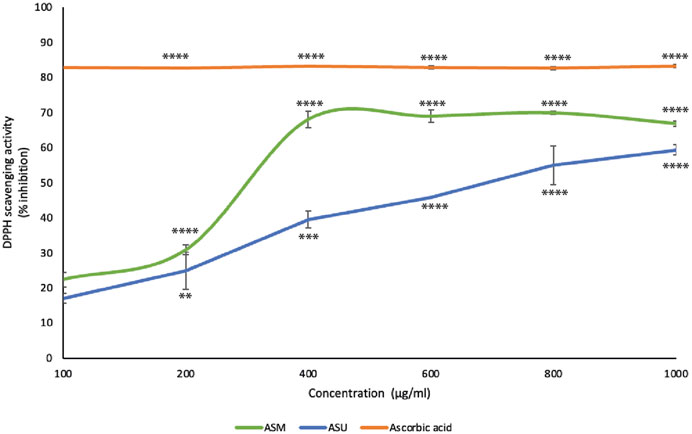
FIGURE 3. A. strigosa extracts have remarkable antioxidant potential. The antioxidant activity of the indicated concentrations of ASM and ASM was measured by the 2,2-di-phenyl-1-picrylhydrazyl (DPPH) assay for radical-scavenging capacity assay as described in Materials and Methods. Data represent the means ± SEM of three independent experiments (**p < 0.005, ***p < 0.001, ****p < 0.0001).
3.4 ASM and ASU inhibit the proliferation of Capan-2 pancreatic cancer cells
To investigate the anti-proliferative effect of ASM and ASU extracts on pancreatic cancer, the effect of various concentrations of the extracts (0, 100, 200, 400, 600, and 1,000 μg/mL) was tested on the human pancreatic ductal adenocarcinoma Capan-2 cell line at 24, 48, and 72 h of treatment. Results show that ASM and ASU treatments both decreased cell viability in a concentration- and time-dependent manner, with ASM exhibiting stronger activity (Figure 4A). For example, at 48 h of treatment, cell viability using 100, 200, 400, 600 μg/mL of ASM to treat Capan-2 cells was 74% ± 1.1%, 62% ± 4.0%, 49% ± 7.2%, and 44% ± 4.4% that of control cells compared to 81% ± 2.2%, 78% ± 1.6%, 61% ± 6.2, and 53% ± 5.7% when ASU was used. The half-maximal inhibitory concentration (IC50) was 2136.6, 404.98, and 370.6 μg/mL at 24, 48, and 72 h, respectively with ASM and 2994.97, 847.92, and 687.46 μg/mL at 24, 48, and 72 h, respectively with ASU. Based on these values, 200 and 400 μg/mL ASM and ASU were used in further experiments.
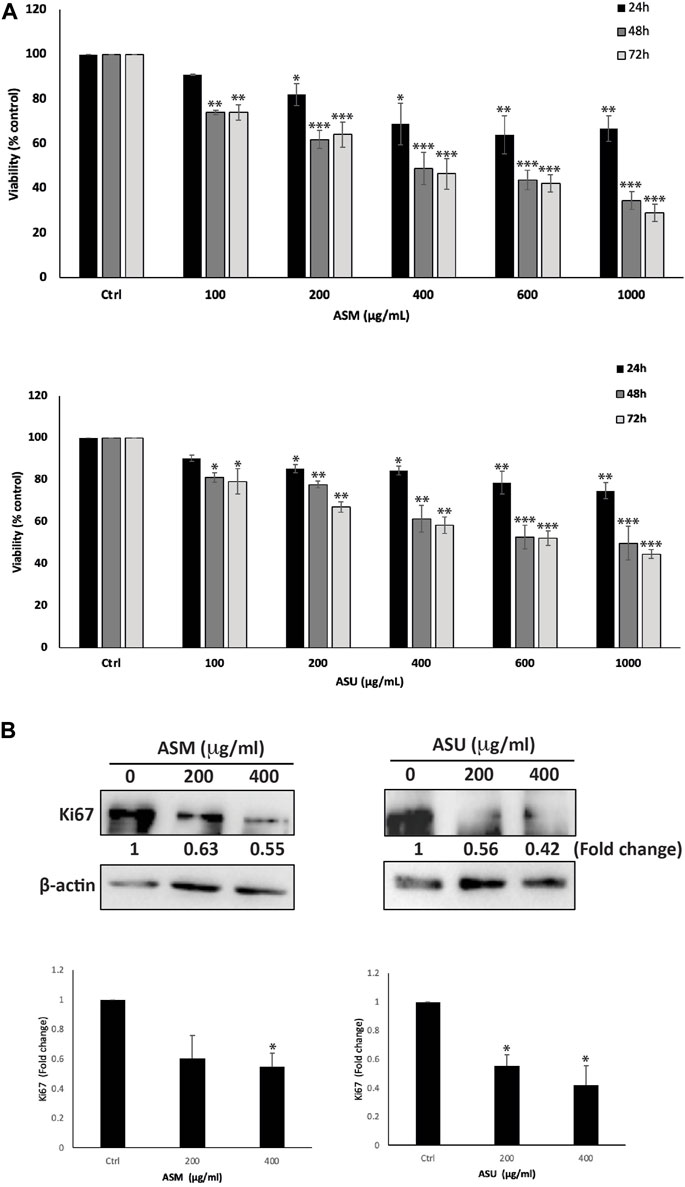
FIGURE 4. A. strigosa extracts inhibit the cellular proliferation of Capan-2 pancreatic cancer cells. (A) Capan-2 cells were treated with and without the indicated concentrations of ASM and ASU for 24, 48, and 72 h. Cell viability was examined using the metabolism-based MTT assay, as described in Materials and Methods. Data represent the mean ± SEM of three independent experiments (n = 3) performed in triplicate and expressed as a percentage of the corresponding control cells. (B) Capan-2 cells were incubated for 24 h with and without the indicated concentrations of ASM and ASU. Cells were then lysed, and protein lysates were subject to Western blotting with a Ki67 antibody, using β-actin as the loading control. Data represent the mean ± SEM of three independent experiments (n = 3) and are expressed as a percentage of the corresponding control cells. Statistical analysis was performed using one-way ANOVA followed by LSD post hoc test (*p < 0.05, **p < 0.005, ***p < 0.001).
To confirm the anti-proliferative effect of ASM and ASU on a molecular level, protein lysates from Capan-2-treated cells were immunoblotted with an anti-Ki67 antibody, which is a widely used biomarker that reflects the cell proliferation state and indicates the prognosis for many cancers, including pancreatic cancer (Lee et al., 2013). Figure 4B shows that treatment of Capan-2 cells with 200 and 400 μg/mL of ASM and ASU caused a significant decrease in the levels of Ki67 in a concentration-dependent manner, 24 h after treatment. These data suggest that ASM and ASU inhibit the growth of Capan-2 cells by interfering with their cell-proliferation process.
3.5 ASM and ASU induce intrinsic apoptosis in Capan-2 cells
At 24 h after treatment with ASM and ASU, Capan-2 cells were examined using an inverted phase-contrast microscope. Results showed a concentration-dependent decrease in the total number of cells per microscopic field. Further analysis of the morphology of the treated and DAPI-stained cells revealed apoptotic characteristics (Figure 5A). Indeed, treated cells clearly showed cytoplasmic shrinkage, rounded morphology, condensation of the nuclear material, the appearance of membrane blebbing, and apoptotic bodies, suggesting the possible induction of apoptosis in Capan-2 cells by ASM and ASU treatment.
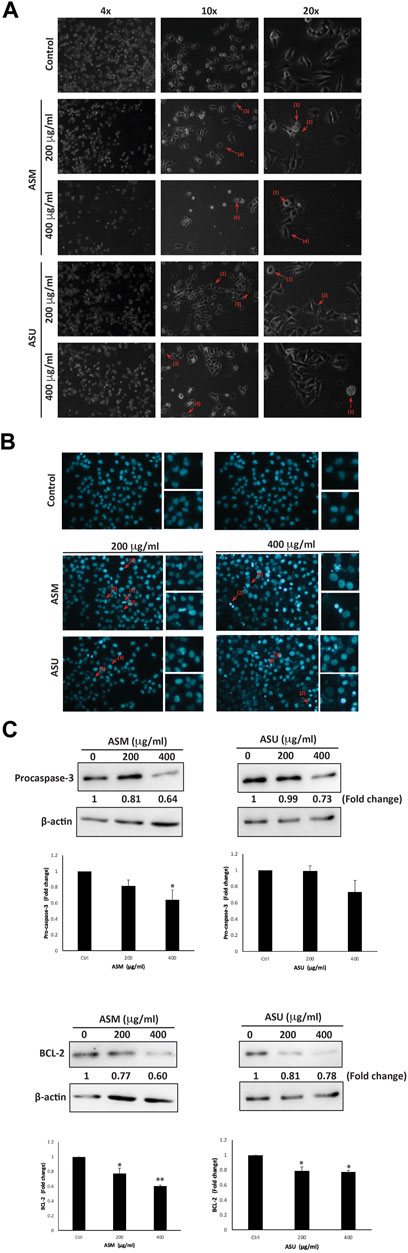
FIGURE 5. A. strigosa induces apoptosis in Capan-2 cells. (A) Capan-2 cells were treated with and without the indicated concentrations of ASM and ASU. Morphological changes were observed by light microscopy. Arrows show (1) apoptotic bodies, (2) echinoid spikes, (3) shrinkage and (4) membrane blebbing. (B) Cells were incubated with the corresponding concentrations of ASM and ASU for 24 h and stained with 4′,6-diamidino-2-phenylindole (DAPI) to visualize nuclear alterations characteristic of apoptotic features such as (1) chromatin lysis, (2) nuclear condensation, and (3) aggregation of apoptotic bodies. (C) Cells were incubated with and without the indicated concentrations of ASM and ASU for 24 h. Protein levels of procaspase-3 and BCL-2 were determined by Western blotting, using β-actin as loading control. Control images are re-used for illustrative purposes. Data represent the mean of ±SEM of three independent experiments (n = 3; *p < 0.05, and **p < 0.01).
We next sought to confirm further that apoptosis is activated in ASM- and ASU-treated cells. To this end, we first evaluated the levels of procaspase-3, which has a crucial role in apoptosis. Procaspase-3 is processed and cleaved into the lower-molecular-weight caspase-3, which is required for the efficient execution of the intrinsic apoptosis pathway. Results showed a significant decrease in the levels of procaspase-3 in cells treated with ASM and ASU in a concentration-dependent manner, achieving a significant decrease from the control at 400 μg/mL (Figure 5C). More specifically, ASM had a stronger effect than ASU, with 0.64 ± 0.13- and 0.73 ± 0.14-fold reductions, respectively.
B-cell lymphoma 2 (BCL-2) is an antiapoptotic protein that also plays an important role in the intrinsic apoptosis pathway, and its dysregulation has been linked with the chemoresistance of many cancers by blocking drug-induced apoptosis (Maji et al., 2018). In our study, both ASM and ASU extracts remarkably decreased the level of BCL-2 protein in Capan-2 cells 24 h after treatment. ASM had a greater effect than ASU in a concentration-dependent manner, showing 0.77 ± 0.05- and 0.60 ± 0.02-fold reductions at 200 and 400 μg/mL, respectively, relative to vehicle-treated cells (Figure 5B). Together, these results strongly suggest that A. strigosa extracts induce cell death by targeting apoptotic mechanisms and that they could have a potential role in the treatment of pancreatic cancer.
3.6 ASM and ASU increase the aggregation of Capan-2 cells
Epithelial-mesenchymal transition (EMT) is a complex developmental program that enables cancer cells to infiltrate and metastasize by suppressing their epithelial features, losing their adherence junctions, and transforming into mesenchymal cells to attain mobility. Pancreatic cancer cells, including Capan-2, exhibit an EMT phenotype (Wang et al., 2017). Therefore, blocking or reversing EMT by allowing cells to regain their epithelial properties, such as cell-cell adhesion, could be an effective strategy to improve cancer treatment (Sinha et al., 2020). Toward this end, an aggregation assay was performed to test the effect of A. strigosa extracts on the cell-cell adhesion properties of Capan-2 cells in suspension. Figure 6 shows that ASM and ASU caused a concentration-dependent increase in cell-cell aggregates compared to the control cells, with significant 75.8% ± 1.9% and 76.4% ± 5.4% increases, respectively, after 4 h of incubation at 400 μg/mL.
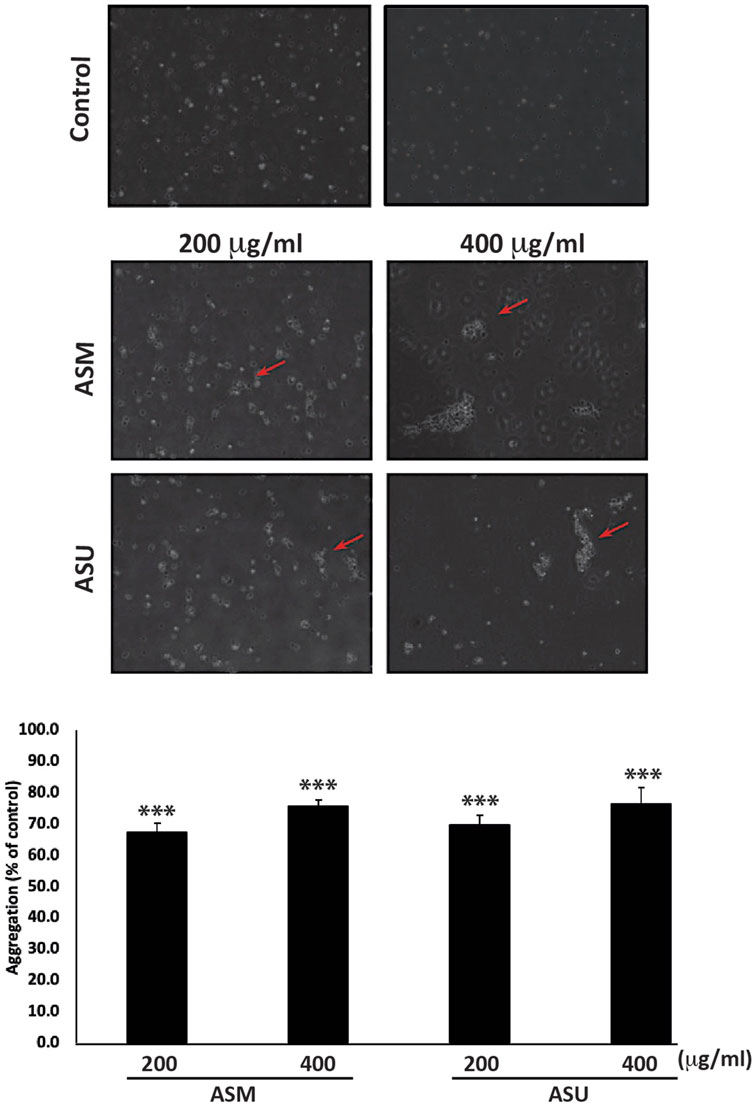
FIGURE 6. A. strigosa extracts increase the cell-cell aggregation of Capan-2 cells. Capan-2 cells were treated with and without the indicated concentrations of ASM and ASU and subjected to an aggregation assay as described in Materials and Methods. Micrographs were taken after 4 h and the percentage of cell-cell aggregates was measured using the equation: % aggregation = (1—Nt/Nc) x 100, where Nt is the number of single cells in the control and Nc is the number of single cells in the treated sample. Data represent the mean ± SEM of three independent experiments (n = 3; *p < 0.05, and **p < 0.005).
3.7 ASM and ASU reduces the migration property of Capan-2 cells
Cell migration is an essential mechanism during physiological processes such as embryonic morphogenesis, immune responses, and wound repair. It is also a pivotal process contributing to the initial steps of cancer metastasis and a main characteristic of a malignant phenotype. The effect of ASM and ASU extracts on the migration of Capan-2 cells was examined using the wound-healing assay. Figure 7A shows that both A. strigosa extracts decreased the Capan-2 cells’ capacity to migrate and fill the wound in a concentration- and time-dependent fashion. For example, 8 h after the scratch was done on the cell monolayer, the distance migrated by Capan-2 cells treated with ASM and ASU at 400 μg/mL was 0.65 ± 0.04- and 0.76 ± 0.08-fold, respectively, that of the control cells.
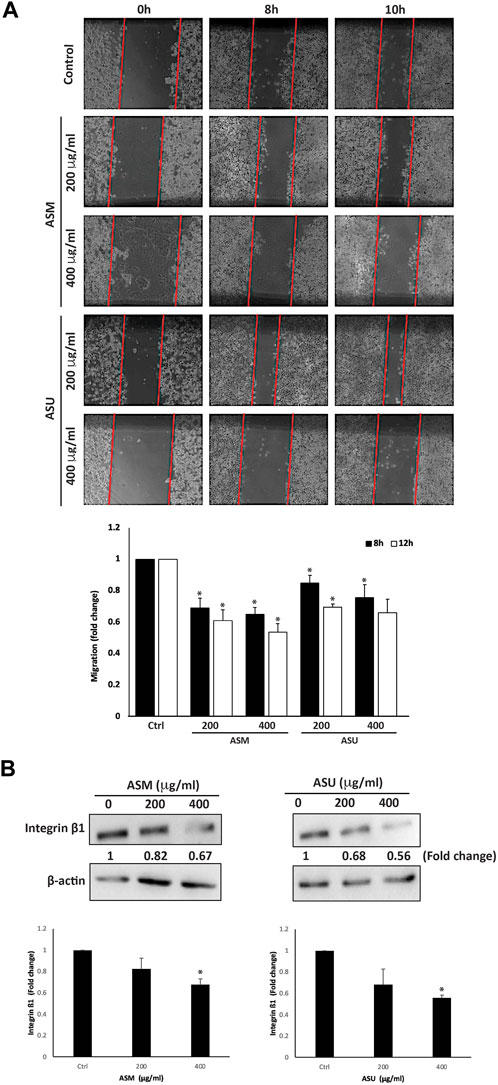
FIGURE 7. A. strigosa extracts inhibit the migration of Capan-2 cells. (A) Confluent cultures of Capan-2 cells were wounded by scratching with a pipette tip. The cells were then incubated with and without the indicated concentrations of ASM and ASU. The wound was photographed after 8 and 10 h using an inverted phase-contrast microscope, measured, and analyzed. Values are plotted as fold change compared with the control cells. (B) Capan-2 cells were incubated for 24 h with or without the indicated concentrations of ASM and ASU, and whole-cell extracts were subjected to Western blotting analysis for integrin β1 expression using β-actin as the loading control. Control images are re-used for illustrative purposes. Data represent the mean ± SEM of three independent experiments (*p < 0.05).
The spread of a cancer from its primary location to other parts of the body is a multi-step process. Each step requires specific molecular interactions between the tumor cells and the extracellular matrix (ECM) that starts with the adhesion of the cells to the ECM. The cells then secrete hydrolytic enzymes that degrade the matrix, thereby enabling cells to migrate through these modified regions. The adhesion of the cells to the ECM is mediated by the interaction between matrix proteins and specific adhesion molecules, such as integrins, expressed on the cell surface. These allow the formation of focal contacts that drive the cell’s migration process. Overall, integrins play a crucial role in the metastasis of cancers by modulating the interaction of the tumor cells with a wide range of matrix proteins in the ECM (Bozzuto et al., 2010). Studies have shown that the upregulation of integrin subunits is associated with an increase in the metastatic potential of tumor cells, including those of pancreatic adenocarcinoma (Grzesiak et al., 2007). Here, we investigated the levels of integrin β1 in Capan-2 cells after treatment with A. strigosa extracts. The results showed that both ASM and ASU at 400 μg/mL significantly decreased the levels of integrin β1 by 0.67 ± 0.05- and 0.56 ± 0.02-fold compared to the control cells, suggesting that A. strigosa inhibits the migration capacity of Capan-2 cells by disrupting the integrin-ECM axis (Figure 7B).
3.8 ASM inhibits the COX-2 signaling pathway
Cyclooxygenase-2 (COX-2), a key enzyme mediating prostaglandin synthesis, has been shown to play a significant role in carcinogenesis. Studies have shown that COX-2 is overexpressed in most cancers, including pancreatic ductal adenocarcinoma, and, that is, associated with poor prognosis (Hill et al., 2012). In fact, it has been linked with tumor development, proliferation, progression, and metastasis, in addition to chemoresistance. Moreover, the inhibition of COX-2 has been shown to reduce the risk of metastasis and sensitize cancer cells to radio- and chemotherapy. Therefore targeting the COX-2 pathway has a promising therapeutic potential, paving the way for the development of new anticancer drugs (Mohsin et al., 2022). Our results showed that ASM treatment at 200 and 400 μg/mL caused a significant decrease in COX-2 levels by 0.73 ± 0.06- and 0.63 ± 0.03-fold, respectively, compared to the control (Figure 8). However, no significant effect on COX-2 levels was observed in cells treated with the ASU extract, suggesting that ASM potentially contains specific biomolecules implicated in targeting the COX-2 pathway.
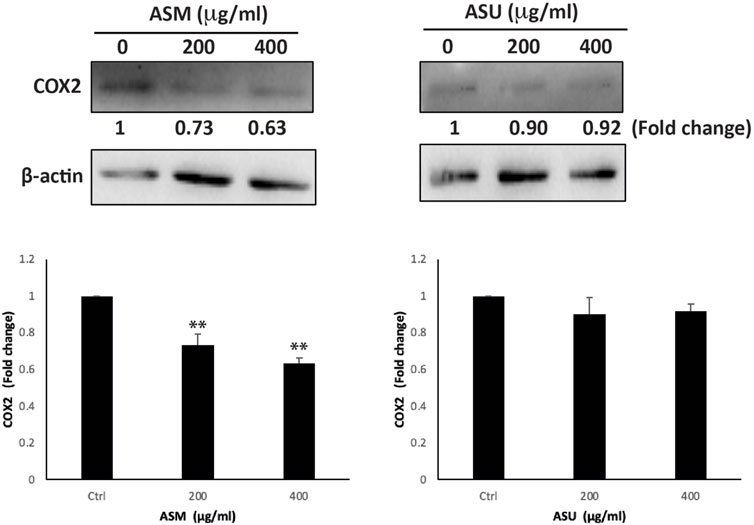
FIGURE 8. ASM downregulates the COX-2 levels in Capan-2 cells. Capan-2 cells were treated with and without the indicated concentrations of ASM and ASU. After 24 h, protein lysates were examined for the level of COX-2, using β-actin as the loading control. Control images are re-used for illustrative purposes. Data represent the mean ± SEM of three independent experiments (**p < 0.005).
4 Discussion
In addition to being a reservoir of phytochemicals that protect the plant against environmental constraints and facilitate adaptation, plants are rich in secondary metabolites with varied biological functions that play key roles in the plant’s growth and development (Siciliano et al., 2005; Bhattacharya, 2019). Many of these secondary metabolites are known to possess therapeutic properties with strong antioxidant, anti-inflammatory, and anticancer effects. Contextually, the use of plants and herbal extracts in the treatment of cancer, either alone or in combination with established chemotherapeutic agents, has been increasingly drawing attention. More specifically, several experimental and clinical studies using herbal extracts have shown beneficial effects in pancreatic cancer with low side-effects, suggesting that natural products will play a pivotal role in the identification of novel treatments for this aggressive cancer (Kim et al., 2021; He et al., 2022; Triantafillidis et al., 2022). In the study of medicinal plants, the extraction technique is crucial as it can greatly influence the extract obtained and further the isolation of bioactive compounds (Abubakar and Haque, 2020). In the present study, we investigated the effect of two green extraction methods, maceration, and ultrasound-assisted extraction (UAE), on the composition and antioxidant activity of A. strigosa-derived aqueous extracts, as well as their antitumor capabilities against pancreatic cancer. The significance of this study arises from the applicability of the model used, accessibility of the plant, and the cost-effectiveness of the process.
Maceration is a conventional technique that was initially used in wine making and has been widely adopted in plant medicinal research (Azwanida, 2015). It is considered one of the simplest techniques for the extraction of bioactive compounds, and consists of soaking coarse or powdered dried plant material in solvents such as water, ethanol, methanol, ethyl acetate, acetone, etc. Although simple and inexpensive, the maceration technique is time-consuming as it often requires large amounts of solvent, which then needs to be concentrated. However, it is convenient for thermolabile plant material. The more-modern UAE method, also called sonication, uses sound energy at high frequency in the extraction in order to disrupt the plant cell wall and facilitate solvent penetration due to cavitation, thereby improving the release of intracellular compounds into the solvent and increasing the extraction yield. UAE has several advantages: it is simple, time-saving, and requires low amounts of solvent. However, it is often difficult to reproduce, and the ultrasonic energy applied may often produce undesirable changes in the phytochemicals or even degrade them, in addition to producing free radicals during the cavitation phenomenon (Vilkhu et al., 2008; Kentish and Feng, 2014; Ameer et al., 2017). This method requires extraction conditions to be optimized as they play a critical role in the biological quality of the extract.
Indeed, several studies have proven the antioxidant capacity of A. strigosa (Al-Khateeb et al., 2019; Ghalib and Kadhim, 2021). This plant was also shown to possess several biological and medicinal properties including antimicrobial (Ali-Shtayeh et al., 1998; Al-Salihi et al., 2007; Al-Salihi et al., 2009; Abdulla and Shihab, 2022), neuroprotective (Tsalkovich et al., 2015), anti-ulcer (Abuereish, 1998; Disi et al., 1998; Abbas et al., 2009), anti-arthritis (Alallan et al., 2018), and anti-hyperglycemic (Bacanlı et al., 2016) properties. Moreover, its tumorigenic activity has been recently investigated in a study using root and leaf extracts, highlighting that the latter had a more potent anti-proliferative effect against human breast and colorectal cancer cell lines (Al-Khatib et al., 2021). However, the effect of A. strigosa on the malignant phenotype of pancreatic cancer has not been studied to date. Overall, our results show that A. strigosa as a crude extract has potent in vitro anti-pancreatic cancer activities and particularly highlight the ASM extract as a potential source of bioactive compounds with anticancer properties. Uncontrolled proliferation is a major hallmark of cancer and is characterized by the faulty regulation of cell division and cell death. Consequently, we first demonstrated that both ASM and ASU extracts exhibited strong anti-proliferative activity against Capan-2 cells, in a dose-dependent manner. This was concomitant with a decrease in the proliferation marker Ki67, which is highly expressed in pancreatic cancer and closely correlated with tumor severity. Additionally, inducing apoptosis is an important strategy to control excessive cancer cell proliferation. As such, in the development of cancer medication, targeting apoptotic pathways has been of increasing interest. To further understand the anti-proliferative mechanism of the A. strigosa extracts, we investigated the induction of apoptosis. This was characterized by an activation of caspase-3 and downregulation of BCL-2, with a more pronounced effect observed by the ASM extract, suggesting that A. strigosa potentially induces intrinsic apoptosis in Capan-2 cells.
We also showed that the extracts affected cell-cell adhesion and the migratory potential of Capan-2 cells, which are crucial elements of the epithelial-mesenchymal transition (EMT) and an indication of the cancer’s progression to metastasis. Indeed, the reduction of cell-cell adhesion increases the migratory and invasive capacities of cancer cells. In our study, treatment of Capan-2 cells with ASM and ASU extracts promoted both the formation of cell-cell aggregates and attenuated their migration, indicating that the EMT phenotype had been reversed and metastasis therefore hindered. Moreover, EMT has been increasingly associated with resistance to targeted therapies and chemotherapies (Song and Faber, 2019). In the case of pancreatic cancer, the poor prognosis and dismal 5-year survival rate of only 9% is attributed to the invasive behavior of the disease as well chemoresistance, with EMT being a major contributor to the development of drug resistance (Gaianigo et al., 2017; Hu and Chen, 2021). EMT is a reversible process as cancer cells can still revert to an epithelial phenotype and therefore anti-EMT compounds are considered an attractive target for cancer treatment, with great therapeutic significance. As such, bioactive compounds in both the ASM and ASU extract may be used to suppress the tumorigenicity of pancreatic cancer and its metastatic potential.
Cyclooxygenase-2 (COX-2) is a key enzyme in the biosynthesis of prostaglandins and plays an important role in inflammation. COX-2 and its downstream effector prostaglandin E2 (PGE2), a major mediator of inflammation and angiogenesis, are involved in shaping the tumor microenvironment through the modulation of inflammatory mediators, thereby promoting tumor growth and immune escape (Bell et al., 2022). Enhanced levels of PGE2 due to the upregulation of COX-2 have been associated with cancer genesis by promoting apoptotic resistance, proliferation, and metastasis of cancer cells. Moreover, elevated COX-2 levels have been associated with resistance of cancer cells to conventional chemotherapy (Pang et al., 2016). Inhibitors of COX-2 could therefore play a unique role in cancer chemotherapy. Indeed, they have been shown to overcome drug resistance in several cancers, including gastric cancer (Xu et al., 2016), head and neck squamous-cell carcinoma (Saito et al., 2021), and breast cancer (Bell et al., 2022). Contextually, COX-2 is upregulated in pancreatic ductal adenocarcinoma and has been shown to play an important role in its proliferation (Hill et al., 2012). Interestingly, ASM treatment caused a significant decrease in COX-2 levels in a dose-dependent manner compared to the ASU-treated pancreatic cancer cells, suggesting that the ASM extract contains bioactive compounds specifically targeting COX-2 signaling and potentially mediating anti-inflammatory responses that confer its enhanced anti-pancreatic cancer activity compared to the ASU extract. In fact, A. strigosa is widely used as a herbal medicine for the treatment of rheumatism and arthritis (Ali-Shtayeh et al., 2000; El Beyrouthy et al., 2008; Abu-Rabia, 2015; Alallan et al., 2018). Its anti-inflammatory activity has been studied in vivo against Complete Freund’s adjuvant (CFA)-induced arthritis in rats (Alallan et al., 2018) and ethanol-induced gastric ulcers in laboratory animals (Disi et al., 1998; Abbas et al., 2009). However, the mechanism of action behind the anti-inflammatory effect of A. strigosa remains unknown.
The LC-MS analysis revealed that there were differences in the composition of the extracts in terms of type and quantity. We have confirmed the presence of secondary metabolites in A. strigosa as previously reported in the literature, namely, rosmarinic acid, caffeic acid, quercetin rutinoside, and kaempferol (Braca et al., 2003; Ghalib and Kadhim, 2021; Yarmolinsky et al., 2022). It has been documented that rosmarinic acid, a major phytoconstituent in ASM, exhibits potent anti-cancer effects against pancreatic cancer (Han et al., 2019; Zhou et al., 2022). Other major secondary metabolites of ASM that are known for their anti-cancer effects include syringic acid (Abaza et al., 2013; Periyannan and Veerasamy, 2018; Gheena and Ezhilarasan, 2019), kaempferol (Lee and Kim, 2016; Kim et al., 2018), and salvianolic acid (Katary et al., 2019; Chuang et al., 2020; Teng et al., 2021). The presence of syringic acid, kaempferol and its derivatives, rosmarinic acid, and salvianolic acids in higher amounts in ASM compared to ASU may be responsible for the superior effects observed in ASM. The differences in the phytoconstituents of ASM and ASU might be due to the degradation of phytochemicals as a result of sonication, suggesting that maceration is a more efficient method for the extraction of bioactive compounds from A. strigosa. This is in agreement with several studies proving that while advanced methods such as ultrasound- and microwave-assisted extractions provide efficient extracts with a high yield in a much shorter period of time, conventional methods such as maceration are less damaging (El Maaiden et al., 2022). Moreover, the high sound energy applied in ultrasonic-assisted extraction requires close monitoring of the temperature to prevent excessive heating, in addition to producing free radicals, therefore raising the issue of reproducibility (Azwanida, 2015; Abubakar and Haque, 2020).
5 Conclusion
The present study provides preliminary data that highlight the potential of A. strigosa aqueous extracts as a source of natural antioxidants with potential health benefits and pharmacological applications. In summary, our results show that A. strigosa aqueous extracts obtained by both maceration and ultrasound-assisted techniques exhibit strong antioxidant potential, with the ASM extract showing a more potent capacity as observed by its higher total phenolic content and stronger radical-scavenging activity. This correlates with their anti-proliferative and anti-metastatic effects on the aggressive phenotype of Capan-2 cells, suggesting that A. strigosa may be a cost-effective and attractive plant for the development of drugs to alleviate pancreatic cancer. Exploring key proteins involved in antioxidant pathways, such as superoxide dismutase (SOD), glutathione peroxidase (GPx), glutathione reductase (GR), and nuclear factor erythroid 2-related factor 2 (Nrf2), is needed to confirm the observed antioxidant profile and to understand the underlying molecular mechanism of action. Moreover, the ASM extract showed a particularly stronger inhibition of the pro-inflammatory mediator COX-2. Overall, our findings provide evidence for the in vitro anticancer effect of A. strigosa and open new opportunities for investigating its anti-inflammatory effect. While the UAE method provides an effective extract, the conventional maceration method offers an extract with more potent biological properties, proving that maceration is more suitable, leading to minimal changes and not affecting the properties of the extract. It is important to mention that the synergy between the various bioactives in a plant crude extract confers its biological activity. Therefore, characterizing and isolating the bioactive molecules in the ASM and ASU extracts and further analyzing the differences in their composition would be worth investigating. As such, bioassay guided fractionation will be carried out to isolate active compounds from the extracts and study their mechanism of action. Moreover, further in vivo studies using animal models of pancreatic cancer are needed to validate the efficacy and safety of the A. strigosa extracts.
Data availability statement
The raw data supporting the conclusion of this article will be made available by the authors, without undue reservation.
Author contributions
JM and EB: conceptualization. ZC, RA, and JM: methodology, validation, and data curation. ZC, JM, RA, KH, AH, MM, AB, and EB: resources, writing—review and editing, and supervision. ZC and JM: writing—original draft preparation. EB, AB, and MM: funding acquisition. All authors contributed to the article and approved the submitted version.
Funding
This work was funded by the University Research Board of the American University of Beirut, Lebanon by a grant to EB and the University of Petra, Jordan by a grant to AB.
Acknowledgments
The authors would like to thank the URB of the American University of Beirut, University of Petra for their funding. The authors also thank Sandra Hillman for editing the manuscript.
Conflict of interest
The authors declare that the research was conducted in the absence of any commercial or financial relationships that could be construed as a potential conflict of interest.
Publisher’s note
All claims expressed in this article are solely those of the authors and do not necessarily represent those of their affiliated organizations, or those of the publisher, the editors and the reviewers. Any product that may be evaluated in this article, or claim that may be made by its manufacturer, is not guaranteed or endorsed by the publisher.
References
Abaza, M.-S., Al-Attiyah, R. a., Bhardwaj, R., Abbadi, G., Koyippally, M., and Afzal, M. (2013). Syringic acid from Tamarix aucheriana possesses antimitogenic and chemo-sensitizing activities in human colorectal cancer cells. Pharm. Biol. 51 (9), 1110–1124. doi:10.3109/13880209.2013.781194
Abbas, M., Disi, A., and Al-Khalil, S. (2009). Isolation and identification of anti-ulcer components from Anchusa strigosa root. Jordan J. Pharm. Sci. 2 (2), 131139.
Abdulla, D. N., and Shihab, B. A. (2022). “The antifungal effect of crude ethanolic extract of Anchusa strigosa on T. pedis,” in International scientific congress of pure (Kochi: Applied and Technological Sciences), 372.
Abu-Rabia, A. (2015). Indigenous medicine among the Bedouin in the Middle East. New York: Berghahn Books.
Abubakar, A. R., and Haque, M. (2020). Preparation of medicinal plants: Basic extraction and fractionation procedures for experimental purposes. J. Pharm. Bioallied Sci. 12 (1), 1–10. doi:10.4103/jpbs.JPBS_175_19
Abuereish, G. M. (1998). Pepsin inhibitor from roots of Anchusa strigosa. Phytochemistry 48 (2), 217–221. doi:10.1016/s0031-9422(97)01131-x
Abutbul, S., Golan-Goldhirsh, A., Barazani, O., Ofir, R., and Zilberg, D. (2005). Screening of desert plants for use against bacterial pathogens in fish. Israeli J. Aquac. - Bamidgeh 57 (2), 71–80. doi:10.46989/001c.20405
Al-douri, N. A. (2000). A survey of medicinal plants and their traditional uses in Iraq. Pharm. Biol. 38 (1), 74–79. doi:10.1076/1388-0209(200001)3811-BFT074
Al-Khateeb, E. H., Al-Assi, G. A., Shakya, A. K., Al-Rawi, N., and Shalan, N. (2019). Antioxidant potential of Pistacia vera L. fruit hull, Anchusa strigosa flowers and Ilex paraguariensis A. St.-Hil. leaves extract. Orient. J. Chem. 35 (3), 982–986. doi:10.13005/ojc/350309
Al-Khatib, A., Al-Khateeb, I., Abu–Dahab, R., and Al-Rawi, N. (2021). The cytotoxic effect of the extract of Anchusa strigosa (Him Him) grown in Jordan against different cancer cell lines. Baghdad Sci. J. 18 (1), 0070. doi:10.21123/bsj.2021.18.1.0070
Al-Salihi, F., Al-Ameri, A., and Al-Juobory, T. (2007). Antimicrobial activity of total lipids extracted from Anchusa strigosa Lab. J. Surra Man Raa 3 (6), 11–20.
Al-Salihi, F., Yasseen, A., and Al-Salihi, S. (2009). Antimicrobial activity of volatile oil and fixed oil extracted from Anchusa strigosa Lab. Tikrit J. Pure Sci. 14 (2), 21–24.
Alali, F. Q., Tawaha, K., El-Elimat, T., Syouf, M., El-Fayad, M., Abulaila, K., et al. (2007). Antioxidant activity and total phenolic content of aqueous and methanolic extracts of Jordanian plants: An ICBG project. Nat. Prod. Res. 21 (12), 1121–1131. doi:10.1080/14786410701590285
Alallan, L., Agha, M. I. H., Omerein, A. N., and Al Balkhi, M. H. (2018). Anti-arthritic effects of Anchusa strigosa extracts on complete Freund’s adjuvant-induced arthritis in rats. J. Pharmacogn. Phytochemistry 7 (6), 679–685.
Ali Shtayeh, M., and Abu Ghdeib, S. I. (1999). Antifungal activity of plant extracts against dermatophytes. Mycoses 42 (11-12), 665–672. doi:10.1046/j.1439-0507.1999.00499.x
Ali-Shtayeh, M. S., Yaniv, Z., and Mahajna, J. (2000). Ethnobotanical survey in the Palestinian area: A classification of the healing potential of medicinal plants. J. Ethnopharmacol. 73 (1-2), 221–232. doi:10.1016/s0378-8741(00)00316-0
Ali-Shtayeh, M., Yaghmour, R. M.-R., Faidi, Y., Salem, K., and Al-Nuri, M. (1998). Antimicrobial activity of 20 plants used in folkloric medicine in the Palestinian area. J. Ethnopharmacol. 60 (3), 265–271. doi:10.1016/s0378-8741(97)00153-0
Ameer, K., Shahbaz, H. M., and Kwon, J. H. (2017). Green extraction methods for polyphenols from plant matrices and their byproducts: A review. Compr. Rev. Food Sci. Food Saf. 16 (2), 295–315. doi:10.1111/1541-4337.12253
Azwanida, N. (2015). A review on the extraction methods use in medicinal plants, principle, strength and limitation. Med. Aromatic Plants 4 (196), 2167–0412. doi:10.4172/2167-0412.1000196
Bacanlı, M., Göktaş, H. G., Başaran, N., Arı, N., and Başaran, A. A. (2016). "Beneficial effects of commonly used phytochemicals in diabetes mellitus."
Bell, C. R., Pelly, V. S., Moeini, A., Chiang, S.-C., Flanagan, E., Bromley, C. P., et al. (2022). Chemotherapy-induced COX-2 upregulation by cancer cells defines their inflammatory properties and limits the efficacy of chemoimmunotherapy combinations. Nat. Commun. 13 (1), 2063. doi:10.1038/s41467-022-29606-9
Bhattacharya, A. (2019). Effect of high temperature on crop productivity and metabolism of macro molecules. London, UK: Elsevier, 391–484.High-temperature stress and metabolism of secondary metabolites in plants
Bozzuto, G., Ruggieri, P., and Molinari, A. (2010). Molecular aspects of tumor cell migration and invasion. Ann. dell'Istituto Super. sanità 46, 66–80. doi:10.4415/ANN_10_01_09
Braca, A., Bader, A., Siciliano, T., Morelli, I., and De Tommasi, N. (2003). New pyrrolizidine alkaloids and glycosides from Anchusa strigosa. Planta medica 69 (09), 835–841. doi:10.1055/s-2003-43202
Chuang, C.-Y., Ho, Y.-C., Lin, C.-W., Yang, W.-E., Yu, Y.-L., Tsai, M.-C., et al. (2020). Salvianolic acid A suppresses MMP-2 expression and restrains cancer cell invasion through ERK signaling in human nasopharyngeal carcinoma. J. Ethnopharmacol. 252, 112601. doi:10.1016/j.jep.2020.112601
Dibas, J. I., Yaghi, B. M., Mansi, I. A., Mhaidat, N. M., and Al-Abrounie, K. F. (2017). Screening for cytotoxic and antioxidant activity of selected wild plants at Shafa Badran, Amman, Jordan. Res. J. Pharm. Biol. Chem. Sci. 8 (2), 489–497.
Disi, A. M., Tamimi, S. O., and Abuereish, G. M. (1998). Effects of Anchusa strigosa root aqueous extract on gastric ethanol-induced ulcer in laboratory animals. J. Ethnopharmacol. 60 (3), 189–198. doi:10.1016/s0378-8741(97)00134-7
El Beyrouthy, M., Arnold, N., Delelis-Dusollier, A., and Dupont, F. (2008). Plants used as remedies antirheumatic and antineuralgic in the traditional medicine of Lebanon. J. Ethnopharmacol. 120 (3), 315–334. doi:10.1016/j.jep.2008.08.024
El Maaiden, E., Bouzroud, S., Nasser, B., Moustaid, K., El Mouttaqi, A., Ibourki, M., et al. (2022). A comparative study between conventional and advanced extraction techniques: Pharmaceutical and cosmetic properties of plant extracts. Molecules 27 (7), 2074. doi:10.3390/molecules27072074
Farhan, H., Rammal, H., Hijazi, A., Hamad, H., and Badran, B. (2012). Phytochemical screening and extraction of polyphenol from stems and leaves of a Lebanese Euphorbia macrolada schyzoceras Boiss. Ann. Biol. Res. 3 (1), 149–156.
Gaianigo, N., Melisi, D., and Carbone, C. (2017). EMT and treatment resistance in pancreatic cancer. Cancers 9 (9), 122. doi:10.3390/cancers9090122
Ghalib, S. A., and Kadhim, E. J. (2021). The investigation of some phytochemical compounds found in Anchusa strigosa L. grown naturally in Iraq. Iraqi J. Pharm. Sci. 30 (1), 179–188. doi:10.31351/vol30iss1pp179-188
Gheena, S., and Ezhilarasan, D. (2019). Syringic acid triggers reactive oxygen species–mediated cytotoxicity in HepG2 cells. Hum. Exp. Toxicol. 38 (6), 694–702. doi:10.1177/0960327119839173
Grzesiak, J. J., Ho, J. C., Moossa, A. R., and Bouvet, M. (2007). The integrin-extracellular matrix axis in pancreatic cancer. Pancreas 35 (4), 293–301. doi:10.1097/mpa.0b013e31811f4526
Hajatdoost, L., Sedaghat, K., Walker, E. J., Thomas, J., and Kosari, S. (2018). Chemotherapy in pancreatic cancer: A systematic review. Medicina 54 (3), 48. doi:10.3390/medicina54030048
Han, Y., Ma, L., Zhao, L., Feng, W., and Zheng, X. (2019). Rosmarinic inhibits cell proliferation, invasion and migration via up-regulating miR-506 and suppressing MMP2/16 expression in pancreatic cancer. Biomed. Pharmacother. 115, 108878. doi:10.1016/j.biopha.2019.108878
He, X., Wang, N., Zhang, Y., Huang, X., and Wang, Y. (2022). The therapeutic potential of natural products for treating pancreatic cancer. Front. Pharmacol. 13, 1051952. doi:10.3389/fphar.2022.1051952
Hill, R., Li, Y., Tran, L. M., Dry, S., Calvopina, J. H., Garcia, A., et al. (2012). Cell intrinsic role of COX-2 in pancreatic cancer development. Mol. Cancer Ther. 11 (10), 2127–2137. doi:10.1158/1535-7163.MCT-12-0342
Hu, X., and Chen, W. (2021). Role of epithelial-mesenchymal transition in chemoresistance in pancreatic ductal adenocarcinoma. World J. Clin. Cases 9 (19), 4998–5006. doi:10.12998/wjcc.v9.i19.4998
Katary, M. A., Abdelsayed, R., Alhashim, A., Abdelhasib, M., and Elmarakby, A. A. (2019). Salvianolic acid B slows the progression of breast cancer cell growth via enhancement of apoptosis and reduction of oxidative stress, inflammation, and angiogenesis. Int. J. Mol. Sci. 20 (22), 5653. doi:10.3390/ijms20225653
Kentish, S., and Feng, H. (2014). Applications of power ultrasound in food processing. Annu. Rev. Food Sci. Technol. 5, 263–284. doi:10.1146/annurev-food-030212-182537
Kim, A., Ha, J., Kim, J., Cho, Y., Ahn, J., Cheon, C., et al. (2021). Natural products for pancreatic cancer treatment: From traditional medicine to modern drug discovery. Nutrients 13 (3801), 3801. doi:10.3390/nu13113801
Kim, T. W., Lee, S. Y., Kim, M., Cheon, C., and Ko, S.-G. (2018). Kaempferol induces autophagic cell death via IRE1-JNK-CHOP pathway and inhibition of G9a in gastric cancer cells. Cell death Dis. 9 (9), 875. doi:10.1038/s41419-018-0930-1
Lambert, A., Schwarz, L., Borbath, I., Henry, A., Van Laethem, J.-L., Malka, D., et al. (2019). An update on treatment options for pancreatic adenocarcinoma. Ther. Adv. Med. Oncol. 11, 1758835919875568. doi:10.1177/1758835919875568
Lee, C., He, H., Jiang, Y., Di, Y., Yang, F., Li, J., et al. (2013). Elevated expression of tumor miR-222 in pancreatic cancer is associated with Ki67 and poor prognosis. Med. Oncol. 30, 700–706. doi:10.1007/s12032-013-0700-y
Lee, J., and Kim, J. H. (2016). Kaempferol inhibits pancreatic cancer cell growth and migration through the blockade of EGFR-related pathway in vitro. PloS one 11 (5), e0155264. doi:10.1371/journal.pone.0155264
Maji, S., Panda, S., Samal, S. K., Shriwas, O., Rath, R., Pellecchia, M., et al. (2018). Bcl-2 antiapoptotic family proteins and chemoresistance in cancer. Adv. Cancer Res. 137, 37–75. doi:10.1016/bs.acr.2017.11.001
Mohsin, N. u. A., Aslam, S., Ahmad, M., Irfan, M., Al-Hussain, S. A., and Zaki, M. E. (2022). Cyclooxygenase-2 (COX-2) as a target of anticancer agents: A review of novel synthesized scaffolds having anticancer and COX-2 inhibitory potentialities. Pharmaceuticals 15 (12), 1471. doi:10.3390/ph15121471
Pang, L. Y., Hurst, E. A., and Argyle, D. J. (2016). Cyclooxygenase-2: A role in cancer stem cell survival and repopulation of cancer cells during therapy. Stem Cells Int. 2016, 2048731. doi:10.1155/2016/2048731
Periyannan, V., and Veerasamy, V. (2018). Syringic acid may attenuate the oral mucosal carcinogenesis via improving cell surface glycoconjugation and modifying cytokeratin expression. Toxicol. Rep. 5, 1098–1106. doi:10.1016/j.toxrep.2018.10.015
Phuyal, N., Jha, P. K., Raturi, P. P., and Rajbhandary, S. (2020). Total phenolic, flavonoid contents, and antioxidant activities of fruit, seed, and bark extracts of Zanthoxylum armatum DC. Sci. World J. 2020, 8780704. doi:10.1155/2020/8780704
Quettier-Deleu, C., Gressier, B., Vasseur, J., Dine, T., Brunet, C., Luyckx, M., et al. (2000). Phenolic compounds and antioxidant activities of buckwheat (Fagopyrum esculentum Moench) hulls and flour. J. Ethnopharmacol. 72 (1-2), 35–42. doi:10.1016/s0378-8741(00)00196-3
Rahib, L., Smith, B. D., Aizenberg, R., Rosenzweig, A. B., Fleshman, J. M., and Matrisian, L. M. (2014). Projecting cancer incidence and deaths to 2030: The unexpected burden of thyroid, liver, and pancreas cancers in the United States. Cancer Res. 74 (11), 2913–2921. doi:10.1158/0008-5472.CAN-14-0155
Rawla, P., Sunkara, T., and Gaduputi, V. (2019). Epidemiology of pancreatic cancer: Global trends, etiology and risk factors. World J. Oncol. 10 (1), 10–27. doi:10.14740/wjon1166
Saez, C. (2018). New statistics show cancer burden rising in the world, lung cancer biggest killer. Geneva: Health Policy Watch.
Saif, M. W. (2008). Is there a role for herbal medicine in the treatment of pancreatic cancer. J. Pancreas 10, 403–407.
Saito, S., Ozawa, H., Imanishi, Y., Sekimizu, M., Watanabe, Y., Ito, F., et al. (2021). Cyclooxygenase-2 expression is associated with chemoresistance through cancer stemness property in hypopharyngeal carcinoma. Oncol. Lett. 22 (1), 533–543. doi:10.3892/ol.2021.12794
Siciliano, T., De Leo, M., Bader, A., De Tommasi, N., Vrieling, K., Braca, A., et al. (2005). Pyrrolizidine alkaloids from Anchusa strigosa and their antifeedant activity. Phytochemistry 66 (13), 1593–1600. doi:10.1016/j.phytochem.2005.05.002
Sinha, D., Saha, P., Samanta, A., and Bishayee, A. (2020). Emerging concepts of hybrid epithelial-to-mesenchymal transition in cancer progression. Biomolecules 10 (11), 1561. doi:10.3390/biom10111561
Song, K.-A., and Faber, A. C. (2019). Epithelial-to-mesenchymal transition and drug resistance: Transitioning away from death. J. Thorac. Dis. 11 (6), E82–E85. doi:10.21037/jtd.2019.06.11
Stéphane, F., Jules, B., Batiha, G., Ali, I., and Bruno, L. N. (2021). Extraction of bioactive compounds from medicinal plants and herbs. Nat. Med. Plants. doi:10.5772/intechopen.98602
Teng, M., Hu, C., Yang, B., Xiao, W., Zhou, Q., Li, Y., et al. (2021). Salvianolic acid B targets mortalin and inhibits the migration and invasion of hepatocellular carcinoma via the RECK/STAT3 pathway. Cancer Cell Int. 21, 264–277. doi:10.1186/s12935-021-02367-z
Tohme, G., and Tohmé, H. (2014). Illustrated flora of Lebanon. Beirut, Lebanon: National Council for Scientific Research.
Triantafillidis, J. K., Triantafyllidi, E., Sideris, M., Pittaras, T., and Papalois, A. E. (2022). Herbals and plants in the treatment of pancreatic cancer: A systematic review of experimental and clinical studies. Nutrients 14 (3), 619. doi:10.3390/nu14030619
Tsalkovich, L., Sallon, S., Paavilainen, H., and Rosenmann, H. (2015). Anti Alzheimer’s disease related activities of Israeli medicinal plants. JSM Alzheimer’s Dis. Relat. Dementia 2, 1015–1022.
Vilkhu, K., Mawson, R., Simons, L., and Bates, D. (2008). Applications and opportunities for ultrasound assisted extraction in the food industry - a review. Innovative Food Sci. Emerg. Technol. 9 (2), 161–169. doi:10.1016/j.ifset.2007.04.014
Wang, S., Huang, S., and Sun, Y. L. (2017). Epithelial-mesenchymal transition in pancreatic cancer: A review. BioMed Res. Int. 2017, 2646148. doi:10.1155/2017/2646148
World Cancer Research Fund International (2023). Pancreatic cancer statistics. Retrieved May 16, 2023).
Xu, H.-B., Shen, F.-M., and Lv, Q.-Z. (2016). Celecoxib enhanced the cytotoxic effect of cisplatin in chemo-resistant gastric cancer xenograft mouse models through a cyclooxygenase-2-dependent manner. Eur. J. Pharmacol. 776, 1–8. doi:10.1016/j.ejphar.2016.02.035
Yarmolinsky, L., Budovsky, A., Khalfin, B., Yarmolinsky, L., and Ben-Shabat, S. (2022). Medicinal properties of Anchusa strigosa and its active compounds. Molecules 27 (23), 8239. doi:10.3390/molecules27238239
Yeşil, Y., and Inal, I. (2021). Ethnomedicinal plants of hasankeyf (batman-Turkey). Front. Pharmacol. 11, 624710. doi:10.3389/fphar.2020.624710
Zhang, Z., Wang, J., Liu, B., Liu, Y., Shi, X., Li, W., et al. (2022). Anticancer effects of herbal medicines in pancreatic ductal adenocarcinoma through modulation of steroid hormone response proteins. Sci. Rep. 12 (1), 9910–9911. doi:10.1038/s41598-022-14174-1
Keywords: Anchusa strigosa, pancreatic cancer, herbal medicine, bioactive compounds, conventional extraction, ultrasound-assisted extraction, apoptosis, COX-2
Citation: Chebaro Z, Abdallah R, Badran A, Hamade K, Hijazi A, Maresca M, Mesmar JE and Baydoun E (2023) Study of the antioxidant and anti-pancreatic cancer activities of Anchusa strigosa aqueous extracts obtained by maceration and ultrasonic extraction techniques. Front. Pharmacol. 14:1201969. doi: 10.3389/fphar.2023.1201969
Received: 07 April 2023; Accepted: 18 July 2023;
Published: 01 August 2023.
Edited by:
Latifa Bouissane, Université Sultan Moulay Slimane, MoroccoReviewed by:
Agustina Dwi Retno Nurcahyanti, Atma Jaya Catholic University of Indonesia, IndonesiaMohammed Sikander, The University of Texas Rio Grande Valley, United States
Copyright © 2023 Chebaro, Abdallah, Badran, Hamade, Hijazi, Maresca, Mesmar and Baydoun. This is an open-access article distributed under the terms of the Creative Commons Attribution License (CC BY). The use, distribution or reproduction in other forums is permitted, provided the original author(s) and the copyright owner(s) are credited and that the original publication in this journal is cited, in accordance with accepted academic practice. No use, distribution or reproduction is permitted which does not comply with these terms.
*Correspondence: Joelle Edward Mesmar, am0xMDRAYXViLmVkdS5sYg==; Elias Baydoun, ZWxpYXNiYXlAYXViLmVkdS5sYg==