- 1Department of Pharmacy, Sixth People’s Hospital of Chengdu, Chengdu, China
- 2State Key Laboratory of Southwestern Chinese Medicine Resources, School of Pharmacy, Chengdu University of Traditional Chinese Medicine, Chengdu, China
Cantharidin (CTD), a natural compound derived from Mylabris, is widely used in traditional Oriental medicine for its potent anticancer properties. However, its clinical application is restricted due to its high toxicity, particularly towards the liver. This review provides a concise understanding of the hepatotoxic mechanisms of CTD and highlights novel therapeutic strategies to mitigate its toxicity while enhancing its anticancer efficacy. We systematically explore the molecular mechanisms underlying CTD-induced hepatotoxicity, focusing on the involvement of apoptotic and autophagic processes in hepatocyte injury. We further discuss the endogenous and exogenous pathways implicated in CTD-induced liver damage and potential therapeutic targets. This review also summarizes the structural modifications of CTD derivatives and their impact on anticancer activity. Additionally, we delve into the advancements in nanoparticle-based drug delivery systems that hold promise in overcoming the limitations of CTD derivatives. By offering valuable insights into the hepatotoxic mechanisms of CTD and outlining potential avenues for future research, this review contributes to the ongoing efforts to develop safer and more effective CTD-based therapies.
1 Introduction
Cancer continues to be the leading cause of death worldwide, significantly impacting human health, with an estimated 10 million fatalities expected in 2020 (Sung et al., 2021). Current clinical treatments for tumors, such as surgical resection, chemotherapy, and radiotherapy, often inflict considerable harm and pain on patients due to side effects (Nussbaumer et al., 2011). As a result, there is a pressing need for highly effective, targeted therapies with fewer side effects (Saad, 2022; Staudt et al., 2022; Zhang et al., 2022). Natural products have proven to be a valuable source for antitumor drug discovery, with approximately 50% of antitumor drugs in use today being derived directly or indirectly from plants, animals, and microorganisms (Harvey et al., 2015; Sznarkowska et al., 2017; Newman, 2020). Promising compounds for tumor treatment include alkaloids, flavonoids, terpenoids, polyphenols, quinones, and saponins. There has been growing interest in recent years in the use of toxic traditional Oriental medicine to treat malignant tumors. Long-term clinical practice in the Asia has shown that toxic traditional Oriental medicine, such as Mylabris, Aconiti lateralis Radix Praeparata, Strychni semen, and Bufonis venenum, possess significant antitumor effects. Active ingredients in these toxic compounds, including cantharidin (Wang et al., 2021), aconitine (Wang et al., 2020; Luan et al., 2022), and toadstool (Pan et al., 2019), exhibit unique pharmacology, offering promising therapeutic options for cancer treatment despite their toxicity.
Mylabris (Chinese: Banmao), a renowned TCM derived from the dried bodies of Mylabris phalerata Pallas or Mylabris sichorii Linnaeus, used for over 2000 years and is included in Sheng Nong’s Herbal Classic (Commission, 2020). CTD, a mono-terpene phosphoprotein phosphatase inhibitor, is the primary active ingredient of Mylabris, primarily used for the topical treatment of warts (Cotton, 2021; Guenthner et al., 2021). Recent studies have shown that CTD induces apoptosis in tumor cells, positioning it as a promising treatment for various malignancies, particularly hepatocellular carcinoma (Zeng et al., 2020). CTD demonstrates a unique advantage over first-line chemotherapeutic drugs by elevating leukocytes and cytokines, thus improving immune function (Wang, 1989; Florea and Büsselberg, 2011; Shou et al., 2013). CTD also exerts its antitumor effects by blocking the cell cycle, inducing apoptosis, and reversing multidrug resistance through various mechanisms (Zheng et al., 2008). Several Mylabris-/CTD-based drug formulations are available in the Chinese market, including Aidi injection, disodium cantharidinate injection, and compound Mylabris capsules, which have shown effective anti-tumor effects against liver cancer, lung cancer, rectal cancer, and malignant lymphoma (Liu et al., 2021; Wang et al., 2021; Wei et al., 2021; Yang et al., 2022). However, the therapeutic dose of CTD is very close to its toxic dose, with a lethal oral dose ranging from 10–60 mg and a median lethal dose (LD50) of 1.71 mg/kg in mice (Li et al., 2017).
Despite its potential as an antitumor agent (Shaoting et al., 2023), the low bioavailability, intestinal irritation, and significant hepatotoxicity of CTD limit its clinical application (Youyou et al., 2020). The current lack of a comprehensive understanding of CTD toxicity complicates effective clinical prevention and treatment. Consequently, developing an efficient delivery system for CTD or reducing its toxicity through structural modification may provide a solution. This review aims to summarize the hepatotoxicity mechanism of CTD and briefly introduce the progress in developing delivery systems for CTD and its derivatives, providing a reference for researchers and clinicians.
2 Hepatotoxicity of cantharidin
CTD poisoning has been associated with multi-organ damage, with acute circulatory failure and acute renal failure as leading causes of death in affected patients. Following oral administration, Mylabris initially stimulates the stomach, intestines, and other digestive organs, resulting in multi-organ damage with inflammation of the digestive tract, mucosal necrosis, and hepatocyte damage, including turbidity, steatosis, and necrosis (Bagatell et al., 1969; Zhang et al., 2020b; He et al., 2022). Toxic substances such as CTD in Mylabris can cause glomerular degeneration, tubular epithelial edema, and hemorrhage, leading to renal impairment and a significant increase in serum blood urea nitrogen and creatinine levels (Massicot et al., 2005; Cotovio et al., 2013). At the same time, toxic substances excreted from the kidneys can stimulate the urinary tract, ultimately causing symptoms such as urinary urgency, painful urination, and urinary abnormalities like hematuria and proteinuria. Stimulation of the urethra can also cause abnormal penile erection (Peng, 2014). Consequently, Mylabris is used as an aphrodisiac in some parts of the world but is deadly (Diaz et al., 2020). The absorption of toxic substances can directly damage capillary endothelial cells, leading to cell gap dilation and increased vascular permeability, resulting in the extravasation of plasma components. CTD can also cause turbid swelling of cardiomyocytes and myocardial hemorrhage (Knapp et al., 1997; Knapp et al., 1998; Zhang et al., 2020a).
Various degrees of liver injury have been observed in reported cases of CTD poisoning or death (Zhang et al., 2018). As the primary organ involved in drug metabolism and detoxification, the liver is more vulnerable to drug damage than other organs (He et al., 2019). Studies have shown that the liver is the main target organ of CTD-induced toxicity (Wu et al., 2015; Zhang J. Y. et al., 2020; Liu et al., 2020c). Inflammatory cell infiltration, hepatocyte injury, degeneration, and necrosis are the primary pathological manifestations of CTD hepatotoxicity (Xu et al., 2013). Biochemical markers of liver injury, such as bilirubin, aspartate aminotransferase (AST), alanine aminotransferase (ALT), and alkaline phosphatase (ALP), were significantly upregulated in models with varying degrees of liver injury and cases of toxicity. CTD-mediated hepatotoxicity is mainly associated with endoplasmic reticulum stress (ERS), autophagy, activation of the cysteine signaling pathway, mitochondrial dysfunction, and bile acid cycle (Wu et al., 2015; Zhang J. Y. et al., 2020; Liu et al., 2020c; Liu et al., 2020; Yu et al., 2020) (Figure 1, Table 1).
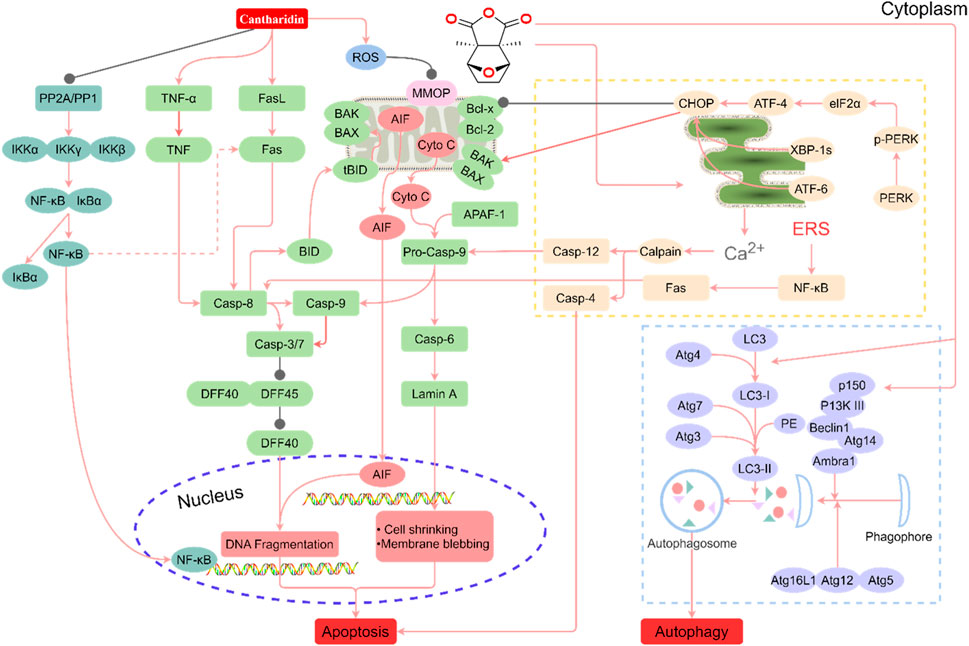
FIGURE 1. Summary of the mechanism of hepatotoxicity of cantharidin. In hepatocytes, cantharidin inhibits proliferation, promotes apoptosis and autophagy, and exacerbates the inflammatory response. These effects are associated with the inhibition of protein phosphatase (PP) 1, PP2A, Toll-like receptor (TIL)-4, nuclear factor-κB (NF-κB), ERK, and DFF45, and the promotion of the tumor necrosis factor (TNF)-α, FASL, ROS, caspase-4, caspase-6, caspase-8, caspase-9, caspase-12, protein kinase R-like ER kinase (PERK), inositol-requiring enzyme 1, transcription factor 6 (ATF6), BID, BAK, BAX, Cyto C, LC3-I, p150, Atg7, P13K Ⅲ, eIF2α, ATF-4, and CCAAT/enhancer-binding proteins-homologous protein (CHOP) pathways.
2.1 Endoplasmic reticulum stress
The endoplasmic reticulum (ER) is an essential organelle in hepatocytes, serving as the primary site of drug metabolism and responsible for proper folding and post-translational modification of membrane and secreted proteins (Wang and Kaufman, 2016). Unfavorable internal and external factors, such as drug-induced toxicity, hypoxia, and nutrient deprivation, can cause ER misfolding, unfolded protein accumulation, and calcium ion imbalance (Tabas and Ron, 2011). To counteract these disruptions, the ER initiates a signaling cascade called the ERS response, which aims to re-establish intracellular homeostasis and promote cell survival (Westrate et al., 2015). The ERS response activates through three transmembrane proteins: Protein kinase R-like ER kinase (PERK), inositol-requiring enzyme 1, and activating transcription factor 6 (ATF6). This response, known as the unfolded protein response, is triggered by the accumulation of misfolded or unfolded proteins and excessive calcium release due to membrane leakage (Di Conza and Ho, 2020). ERS and autophagy-related signaling pathways interact, playing a crucial role in acute liver injury. Under mild stress, hepatocytes activate ERS and autophagy mechanisms to protect cells from stress injury while inhibiting apoptosis. However, when stress levels increase and persist, apoptotic pathways are activated. Under severe stress, hepatocytes undergo complete necrosis, leading to tissue destruction (Liu et al., 2020).
A significant hallmark of ERS pathway-mediated apoptosis is the activation of CHOP, a member of the CCAAT/enhancer-binding proteins family encoded by the DDIT3 gene (Gu et al., 2017). CHOP can also act as a pro-apoptotic factor, promoting the expression of pro-apoptotic proteins such as BAK/BAX. Under normal physiological conditions, CHOP protein expression is extremely low. However, under various pathological conditions, including ERS, CHOP expression increases dramatically, activating apoptosis (Shah and Kumar, 2016; Yang et al., 2020). Liu et al. (2020) established an in vitro study model using LO2 cells and found that CTD mediates CHOP protein expression, leading to cell damage and apoptosis. Continuous treatment of LO2 cells with low concentrations of CTD activates the ATF6 and PERK pathways, initiating downstream signaling pathways and continuous accumulation of CHOP proteins, thereby inducing apoptosis. High concentrations of CTD (50 μM) inhibit ERS, promoting autophagy and apoptosis, and inducing toxicity in LO2 cells. Additionally, ERS causes an imbalance in calcium homeostasis, allowing calcium ions to escape from the ER to the cytoplasm, activating calpain and ultimately pro-apoptotic proteins caspase-4/7/12 (Kurokawa and Kornbluth, 2009; Hsia et al., 2014).
2.2 Autophagy
Autophagy is a conserved catabolic process in eukaryotic cells that maintains intracellular homeostasis and prevents various diseases (White, 2015). Although nutritional deficiencies typically activate autophagy, it is also associated with numerous physiological and pathological processes, including development, differentiation, neurodegenerative diseases, stress, infection, and cancer (Codogno et al., 2011). Induction of autophagy requires a class III PI3K complex containing hVps34, Beclin-1, p150, and Atg14-like proteins (Papinski and Kraft, 2014; Schneider and Cuervo, 2014). Atg genes regulate autophagosome formation through the Atg12-Atg5 and LC3-II complexes. Atg12 binds to Atg5 via a ubiquitin-like reaction requiring Atg7 and Atg10 (corresponding to E1 and E2-like enzymes, respectively). The Atg12-Atg5 complex non-covalently interacts with Atg16, forming a larger complex. Atg4 protease cleaves LC3/Atg8 at the carboxyl terminus, producing cytoplasmic LC3-I. LC3-I then binds to phosphatidylethanolamine through a ubiquitin-like reaction requiring Atg7 and Atg3 (corresponding to E1 and E2-like enzymes, respectively). The lipidated form of LC3, also known as LC3-II, attaches to the autophagosome membrane (Papinski and Kraft, 2014). Autophagy and apoptosis can be positively or negatively linked, with numerous interactions between the two processes. Bcl-2 inhibits Beclin-1-dependent autophagy, functioning as both a pro-survival and anti-autophagy regulatory molecule. Several pro-apoptotic signals, such as TNF, TRAIL, and FADD, can also induce autophagy. Liu et al. (2020) reported that treating LO2 cells with high concentrations of CTD in vitro significantly upregulated the expression of LC3, Beclin-1, Atg3, Atg4A, Atg4B, and Atg7, proteins associated with activated autophagy.
2.3 Caspase
Caspases, a group of cysteine proteases, play a critical and synergistic role in apoptotic signaling cascades. These enzymes can be activated via both exogenous and endogenous apoptotic pathways (Van Opdenbosch and Lamkanfi, 2019; Ketelut-Carneiro and Fitzgerald, 2022). Apoptosis is triggered by the activation of death receptors, such as Fas, TNFαR, DR3, DR4, and DR5, upon binding to their respective ligands. DNA damage, Ca2+ homeostatic imbalance, and ERS can also initiate apoptosis (Chao et al., 2022). Upon activation of pro-apoptotic factor receptors, caspases cleave and activate downstream effector caspases, including caspase-3, -6, and -7 (Swanton et al., 1999). Fas ligand binding results in Fas trimerization, which recruits the initiator caspase-8 through the adaptor protein FADD. Caspase-8 then undergoes oligomerization and autocatalytic activation (Pirnia et al., 2002). Subsequently, caspase-8 cleaves BID into truncated BID (tBID), which disrupts the outer mitochondrial membrane (Kaufmann et al., 2012). This disruption leads to the release of the pro-apoptotic factor cytochrome c (Cyto C), a crucial component for pro-caspase-9 activation (Kantari and Walczak, 2011). Cyto C, released from the membrane gap, binds to APAF1 (apoptosis protease activator-1), which recruits and activates caspase-9, ultimately leading to caspase-3 activation (Srinivasula et al., 1998; Pirnia et al., 2002). The activation of caspase-3 signifies the irreversible phase of apoptosis. During apoptosis, pro-apoptotic factors such as AIF, SMAC (mitochondrial-derived caspase activator), and DIABLO are released from the mitochondria alongside Cyto C. These factors promote caspase activation by inhibiting IAP (inhibitor of apoptosis) family proteins (Kaufmann et al., 2012). ERS induces Ca2+-mediated activation of caspase-12. TNF-α interaction with TNFαR activates the NF-κB pathways through NIK/IKKα/β/γ. The activation of NF-κB triggers the expression of pro-survival genes, including Bcl-2 and FLIP, which directly inhibit caspase-8 activation (Swanton et al., 1999; Pinkoski et al., 2000; Pirnia et al., 2002). According to Yu et al. (2020), male Sprague-Dawley rats exposed to low (1.34 mg/kg) and high (2.67 mg/kg) doses of CTD displayed increased expression levels of TNF-α protein and IKK-α genes as CTD doses increased. Wu et al. (2015) observed that CTD-induced chronic liver injury was associated with inflammatory cell infiltration and abnormal upregulation of TNF-α. Inhibition of the Toll-like receptor 4/NF-κB pathway attenuated CTD-induced hepatotoxicity.
2.4 Mitochondrial dysfunction
Mitochondria function as the center of cellular energy metabolism and play vital roles in cellular processes such as cell proliferation, genetic information transfer, immune regulation, cell cycle control, and apoptosis. Additionally, they are a major site for reactive oxygen species (ROS) production. Damage or dysfunction of mitochondria leads to metabolic abnormalities and functional organ decline in the body (Rustin et al., 1994), making it a potential contributor to liver toxicity. For example, in rats treated with CTD, liver mitochondria exhibited swelling and disappearance of cristae, potentially due to increased production of ROS free radicals causing organ dysfunction (Blajszczak and Bonini, 2017). Furthermore, the activation of BAK and BAX, two crucial apoptosis effectors, results in mitochondrial outer membrane permeabilization and Cyto C release (Bock and Tait, 2020).
2.5 Bile acid cycle
Glutathione plays a critical role in defending against oxidative damage and promoting integrated detoxification by scavenging nitrogen radicals and ROS, as well as reducing hydrogen peroxide. A decrease in glutathione levels is considered a potential early activation signal for apoptosis (Calabrese et al., 2017). Studies have demonstrated that CTD interferes with several biometabolic processes in mouse liver, causing significant disruptions in glutathione metabolism, taurine and hypotaurine metabolism, and the interconversion of pentose and glucuronide (Zhu et al., 2019). Specifically, oxidized glutathione, glutathione, 3-sulfoalanine, and deoxycholic acid 3-glucosylate are involved in three significantly disordered metabolic pathways (Zhu et al., 2019).
The bile acid cycle may play a crucial role in CTD-mediated hepatotoxicity, as disruption of BA homeostasis can lead to the accumulation of toxic BAs, resulting in cholestasis, bile duct infarction, liver fibrosis, and cirrhosis (Yang et al., 2017). Several studies have reported that BAs play an essential role in the hepatotoxicity of various drugs. For example, impaired BA homeostasis has been associated with milliporeline-induced hepatotoxicity and increased intracellular bile acid levels (Xiong et al., 2014). Taurine β-muricholic acid (TβMCA), taurocholic acid, and taurodeoxycholic acid (TDCA) are potential biomarkers of oleanolic acid-induced hepatotoxicity (Feng et al., 2020). Cheng W. et al. (2021) demonstrated that TβMCA levels significantly increased in rat liver following CTD (1.0 mg/kg) intervention. TβMCA is a competitive and reversible antagonist of the ligand-activated farnesoid X receptor, and elevated TβMCA levels can inhibit farnesoid X receptor activation and disrupt BA homeostasis. HCA, TUDCA, and TβMCA can serve as biomarkers for CTD-induced hepatotoxicity in rats (Cheng W. et al., 2021). However, the specific mechanisms underlying the roles of HCA, TUDCA, and TβMCA in CTD-induced hepatotoxicity warrant further investigation.
2.6 Other signaling pathway
In addition to causing severe hepatotoxicity, research on CTD poisoning has revealed that CTD can induce cardiotoxicity and nephrotoxicity (Zhang et al., 2018). CTD has been demonstrated to induce non-endothelium-dependent vasoconstriction in bovine coronary artery rings in a time- and concentration-dependent manner (Knapp et al., 1997; Knapp et al., 1998). Furthermore, exposure of erythrocytes to CTD results in erythrocyte shrinkage and membrane disorders, eventually leading to suicidal erythrocyte death (Alzoubi et al., 2015). In vitro exposure of HK-2 cells to CTD elevates levels of intracellular pro-apoptotic protein caspase-3 expression and the BAX/Bcl-2 ratio (He et al., 2020). CTD also activates the ERS-dependent PERK/CHOP pathway, inducing macroautophagy and apoptosis, which contributes to toxic effects on rat and HK-2 cells (He et al., 2022). Results from in vivo and in vitro experiments have shown that the expression levels of ERS regulatory genes, such as PERK, eIF2α, CHOP, and ATF4, are elevated alongside pro-apoptotic proteins, including GRP78, ATF4, LC3, Beclin-1, Atg3, Atg7, caspase-3, and the BAX/Bcl-2 ratio (He et al., 2022). Similar to CTD-mediated hepatotoxicity, CTD induces autophagy and apoptosis through ERS, leading to cardiotoxicity and nephrotoxicity.
3 Anticancer activity of cantharidin
Numerous clinical and experimental studies have evidenced the potent and wide-ranging antitumor properties of CTD on diverse cancer cell types. A comprehensive overview of these studies is provided in Table 2. These include investigations into cancers such as leukemia (Huh et al., 2004; Zhang et al., 2004; Dorn et al., 2009; Sun et al., 2016), bladder cancer (Huan et al., 2006; Kuo et al., 2010; Huang et al., 2013), rectal cancer (Huang et al., 2011; Han et al., 2014; Sheng et al., 2015), Ehrlich ascites cancer (Verma and Prasad, 2012; 2013), Dalton’s lymphoma (Prasad and Verma, 2013), oral cancer (Xi et al., 2015), pancreatic cancer (Wu et al., 2014; Xie et al., 2015), lung cancer (Hsia et al., 2014; Hsia et al., 2015a; Hsia et al., 2015b; Hsia et al., 2016), gastric cancer (Zhang et al., 2014), breast cancer (Zhang and Yan, 2015; Gu et al., 2017), renal cell carcinoma (Ren et al., 2016), skin cancer (Li et al., 2017), bile duct cancer (Zhou et al., 2018), and notably, liver cancer (Lu et al., 2014; Le et al., 2016; Zhang et al., 2017; Ma et al., 2018).
CTD primarily exerts its antitumor functions via multiple pathways, which include the inhibition of cell growth and proliferation, restriction of migration and invasion, along with the induction of apoptosis and autophagy. Succinctly, CTD obstructs the cell cycle, curbs cell migration, and triggers apoptosis in tumor cells through the regulation of an array of factors. These factors comprise apoptotic proteins (e.g., caspase-3/7/8, BAX, Bcl-2, Bcl-10, Fas/FasL, Beclin-1, Atg3/7), transcription factors (e.g., PPARα, NF-κB, Nrf2, STAT3), enzymes (e.g., AST, COX-2, SOD, eNOS), protein kinases (e.g., ERK, JAK2, p38, p53, P13K/Akt, mTOR), growth factors (e.g., TGF-β1, VEGAF, PDGF), and inflammatory cytokines (e.g., TNF-α, IL-1, IL-6, MCP-1). For a more in-depth exploration of the specific signaling pathways involved, a review of the antitumor effects of norcantharidin (a derivative of CTD) by Zhou et al. (2020) is recommended.
4 Potential solutions to cantharidin-mediated toxicity
Given the potent anticancer properties of CTD, alongside its severe side effects, it is crucial to develop approaches that reduce toxicity while preserving its activity. Although CTD demonstrates potent inhibition of protein phosphatase 2A and cytotoxic activity in cancer cells, its preclinical development might be hindered by its toxicity. To tackle this issue, chemists have synthesized various CTD derivatives (Figure 2) and developed several nano-precision delivery systems, some of which have shown promising antitumor potential.
4.1 Structural modification of cantharidin
Cantharidin is a natural protein phosphatase monoterpene anhydride inhibitor (Ren and Kinghorn, 2021), primarily composed of a six-membered carbon ring with an oxygen bridge and a five-membered anhydride ring. The activity of CTD is highly dependent on retaining the 1-O group (McCluskey et al., 1996; McCluskey et al., 2003b; Baba et al., 2005; Dong et al., 2007; Deng et al., 2013). Although the 1-O of CTD can be replaced by N, S, or -CH2- using the isostere principle, structural modification at the 1-position may significantly reduce or even eliminate the pharmacological activity of CTD derivatives. It is speculated that the 1-O can form a hydrogen bond with the receptor, facilitating proper binding between CTD and the receptor. Consequently, CTD derivatives with other atoms or groups replacing the oxygen atom might not bind effectively to the receptor, resulting in a loss of activity (Dong et al., 2007). Studies suggest that eliminating the bridging ether oxygen on the ring can decrease cytotoxicity (Yeh et al., 2010). Moreover, the presence of 2-C and 5-C substituents eliminates the inhibitory activity of CTD derivatives on all protein phosphatase 2A, even those with minimal steric hindrance (McCluskey et al., 1996; McCluskey et al., 2000b). Substitutions at 3-C and 4-C also significantly impact the toxicity and efficacy of CTD. For instance, norcantharidin, a derivative of CTD with comparable anti-tumor efficacy but fewer side effects than CTD, is primarily used for hepatocellular carcinoma treatment. It retains functions of raising leukocytes, protecting liver cells, and regulating immune function (Zhou et al., 2020). Substitutions at 6-C and 7-C increase the molecule’s spatial resistance, leading to decreased activity or selectivity. The transformation into carbon-carbon double bonds is an effective means of modification (Tatlock et al., 1997; Dong et al., 2007).
Modifying the CTD anhydride site has proven effective in enhancing its anti-hepatocarcinogenic activity. Cantharidic acid, a binary carboxylic acid formed by the ring-opening of CTD’s five-membered carboxylic anhydride ring, exhibits the same inhibitory activity against PPA as CTD (McCluskey et al., 2000b). Carboxylate derivatives of CTD are significant modifications, and sodium cantharidinate, which has been clinically applied, has a substantially superior antitumor effect compared to CTD, with lower toxicity and irritation (McCluskey et al., 2000b; Feng I. C. et al., 2018; Ji and He, 2019). The inhibitory activities of carboxylate derivatives of CTD against PPA1, PPA2A, and PPA2B vary depending on their structures. It has been reported that an amination reaction at the CTD anhydride site or the introduction of basic groups into the structure can effectively improve its anti-hepatocarcinoma activity (Dong et al., 2007; Wang et al., 2018; Zhou et al., 2020). The activity of imide derivatives varies significantly depending on the substituents attached to the nitrogen atom.
4.2 Cantharidin targeted delivery system
In clinical practice, CTD is typically administered at a dose range of 0.5–4 mg/d (Dang and Zhu, 2013). To address issues related to membrane irritation, in vivo release control, and limited bioavailability, it is essential to develop an effective drug delivery system. Nanoparticle-based drug delivery systems show promise in overcoming the limitations of conventional anticancer drug therapy. Sheng et al. developed folic acid-targeted nanoparticles loaded with CTD, which effectively killed colorectal cancer cells via a PP2A-dependent mechanism (Sheng et al., 2015). Chi et al. (2019) reported on norcantharidin-conjugated carboxymethyl chitosan conjugates for hepatocellular carcinoma treatment, which significantly inhibited the proliferation and migration of BEL-7402 cells. Dang and Zhu (2013) developed CTD-loaded solid lipid nanoparticles (CA-SLNs) with an oral bioavailability and sustained release profile after oral administration. Xie et al. (2021) designed a TriPt prodrug, combining cisplatin, artesunate, and CTD in equimolar ratios. TriPt NPs exhibited substantial antitumor effects in 7404DDP tumor-bearing mice and significantly improved drug efficacy compared to free drug combination therapy. Sun et al. (2021) developed a hepatic-targeting hyaluronic acid-mPEG-modified CTD nanostructured liposome (HA-mPEG-CTD-NLC) that inhibited hepatoma carcinoma cell growth and prolonged survival in tumor-bearing mice. Zhu K. et al. (2020) developed a novel CTD-loaded nanoliposome 18-GA-Suc-CTD-Lip, modified with 3-succinyl-30-stearyl glycyrrhetinic acid, which showed high toxicity against hepatocellular carcinoma cells. Cheng X. et al. (2021) developed a CTD-loaded biomimetic metal-organic framework nanoparticle cascade, PPy-CTD@MIL-100@MPCM nanoparticles (PCMM NPs), which accumulated in tumor tissue through encapsulated macrophage cell membranes (MPCMs) targeting inflamed tissue. This study suggested that PCMM NPs could serve as a combined treatment platform to enhance the Fenton reaction-based amplified photothermal therapy. Finally, Guo et al. (2020) developed a cell membrane-coated biomimetic nanoparticle (m-CTD@Te) with strong homologous targeting capabilities that effectively suppressed cancer through synergistic treatment. Encapsulated Te in m-CTD@Te triggered PDT and PPT by NIR laser irradiation, and PTT further triggered the release of CTD. Due to the outer cell membrane coating of m-CTD@Te, these nanoparticles exhibited good biocompatibility with healthy cells.
In brief, the advanced delivery systems discussed above have shown potential to reduce the toxicity of CTD and their derivatives by precise delivery to target cells, avoiding the potential side effects (Table 3). However, these systems do not fully meet the high clinical requirements needed. Although these systems can improve the therapeutic of CTD and its derivatives compared to direct utilization of CTD, their delivery efficiency, cell and tissue selectivity, and the physicochemical properties of the compounds delivered can significantly impact the therapeutic effects of CTD (Li et al., 2017). Hence, further research efforts are necessary to investigate these factors to improve the therapeutic efficacy and safety of CTD.
5 The clinical usage of cantharidin
Although CTD has some toxicity to humans, its anticancer effects should not be overlooked. To reduce these side effects, several CTD derivatives, such as norcantharidin, disodium cantharidinate, and methylcantharidinmide, have been produced. These derivatives retain the antitumor effects of CTD, while reducing its toxic side effects and providing application advantages. Currently, National Medical Products Administration of China has approved several antitumor chemicals based on these CTD derivatives and several antitumor proprietary Chinese medicines containing Mylabris for the treatment of various solid tumors, particularly liver cancer. Table 4 provides a summary of the names, dosage forms, compositions, indications, specifications, and usage of these marketed preparations in China. However, it is vital to acknowledge that while such marketed products, which include CTD as one of the therapeutic components, have shown positive therapeutic outcomes in clinical settings, these beneficial effects may be attributed to other molecules contained within the products. Therefore, further elucidation of the exact pharmacological impacts of individual molecules is crucial to enhance future clinical application and usage guidelines.
6 Conclusion
Despite the outstanding efficacy of CTD and high demand, Chinese patent medicines made of Mylabris or CTD, such as Aidi injection, disodium cantharidinate injection, and compound Mylabris capsules, have excellent efficacy in treating malignant tumors like liver cancer, breast cancer, leukemia, and other difficult-to-treat diseases. However, the direct use of CTD causes strong irritation to the skin and gastrointestinal mucosa, as well as significant damage to major organs like the liver, kidney, and heart, especially significant hepatotoxicity. Notably, drug-related liver injury is a leading cause of drug development interruption or marketed drug withdrawal, making it important to increase studies on the toxicity of CTD and its derivatives beyond hepatotoxicity to further enhance their clinical applications (Kaplowitz, 2005; Navarro and Senior, 2006; Hebels et al., 2014).
In this review, we summarized the mechanism by which CTD induces hepatotoxicity, leading to different degrees of liver injury through the activation of endogenous and exogenous pathways, resulting in apoptosis and autophagy in hepatocytes. Future research should focus on understanding the toxic reactions of CTD (Hong et al., 2022), studying the mechanism of CTD toxicity in-depth, and developing methods to reduce toxicity and improve the efficacy of CTD analogs based on the mechanism of CTD toxic reactions. Additionally, exploiting the unique advantage of CTD to enhance leukocytes among many antitumor drugs and increasing the use of CTD analogs alone or in combination with other antitumor drugs is a promising approach (Swati and Raghuvir, 2022). Furthermore, the development of nano-precision delivery systems to control the side effects of CTDs and enhance their targeting of tumor sites presents an exciting avenue for future research. By offering valuable insights into the hepatotoxic mechanisms of CTD and outlining potential avenues for future research, this review contributes to the ongoing efforts to develop safer and more effective cantharidin-based therapies.
In addition to these advancements, the development of targeted protein degradation technology has revolutionized the study of traditional small molecule compounds (Lin et al., 2022). PROTAC molecules, composed of E3 ubiquitin ligase ligand, protein of interest, and linker, have shown potential to break through existing applications when using natural products as protein of interest (Dhanusha and Craig, 2020). Therefore, the use of CTD and its derivatives, or other toxic compounds from traditional oriental drugs, as potential protein of interest, could enhance the therapeutic potential of CTD (Miaomiao et al., 2022). Although no results have been reported yet, this strategy deserves attention. In addition to targeted nano-delivery systems, antibody-drug conjugate technology offers a promising avenue by combining “specific” targeting and “efficient” killing of cancer cells (Anish et al., 2016). These drugs act like precision-guided “biological missiles” that can destroy cancer cells with precision, increase the therapeutic window, and reduce off-target side effects (Carmen et al., 2021). Research in this area may provide a significant breakthrough in the clinical use of CTDs, but further experimental validation is necessary.
Author contributions
DJ and J-XW contributed to the conception and design of the study. DJ and N-NH organized the database and performed the statistical analysis. DJ and N-NH wrote the first draft of the manuscript. DJ and J-XW contributed to the manuscript revision. All authors contributed to the article and approved the submitted version.
Funding
Financial support was provided by the 2019 Sichuan Medical Association venous embolism embolism Prevention and Treatment (Hengrui) special scientific research project, Grant/Award Number: 2019HR36.
Conflict of interest
The authors declare that the research was conducted in the absence of any commercial or financial relationships that could be construed as a potential conflict of interest.
Publisher’s note
All claims expressed in this article are solely those of the authors and do not necessarily represent those of their affiliated organizations, or those of the publisher, the editors and the reviewers. Any product that may be evaluated in this article, or claim that may be made by its manufacturer, is not guaranteed or endorsed by the publisher.
References
Alzoubi, K., Egler, J., Briglia, M., Fazio, A., Faggio, C., and Lang, F. (2015). Induction of suicidal erythrocyte death by cantharidin. Toxins 7 (8), 2822–2834. doi:10.3390/toxins7082822
An, P., Lu, D., Zhang, L., Lan, H., Yang, H., Ge, G., et al. (2022). Synergistic antitumor effects of compound-composed optimal formula from Aidi injection on hepatocellular carcinoma and colorectal cancer. Phytomedicine 103, 154231. doi:10.1016/j.phymed.2022.154231
Anish, T., Beverly, A. T., and Raffit, H. (2016). Antibody-drug conjugates for cancer therapy. Lancet Oncol. 17 (6), e254–e262. doi:10.1016/S1470-2045(16)30030-4
Baba, Y., Hirukawa, N., and Sodeoka, M. (2005). Optically active cantharidin analogues possessing selective inhibitory activity on Ser/Thr protein phosphatase 2B (calcineurin): Implications for the binding mode. Bioorg. Med. Chem. 13 (17), 5164–5170. doi:10.1016/j.bmc.2005.05.013
Bagatell, F. K., Dugan, K., and Wilgram, G. F. (1969). Structural and biochemical changes in tissues isolated from the cantharidin-poisoned rat with special emphasis upon hepatic subcellular particles. Toxicol. Appl. Pharmacol. 15 (2), 249–261. doi:10.1016/0041-008x(69)90024-6
Blajszczak, C., and Bonini, M. G. (2017). Mitochondria targeting by environmental stressors: Implications for redox cellular signaling. Toxicology 391, 84–89. doi:10.1016/j.tox.2017.07.013
Bock, F. J., and Tait, S. W. G. (2020). Mitochondria as multifaceted regulators of cell death. Nat. Rev. Mol. Cell Biol. 21 (2), 85–100. doi:10.1038/s41580-019-0173-8
Calabrese, G., Morgan, B., and Riemer, J. (2017). Mitochondrial glutathione: Regulation and functions. Antioxid. Redox Signal 27 (15), 1162–1177. doi:10.1089/ars.2017.7121
Carmen, C., Stefania, M., and Giuseppe, C. (2021). Antibody-drug conjugates in solid tumors: A look into novel targets. J. Hematol. Oncol. 14 (1), 20. doi:10.1186/s13045-021-01035-z
Chao, W., Xiangui, D., Shudi, G., Xuemei, W., and Jing, C. (2022). Cantharidin inhibits proliferation of liver cancer by inducing DNA damage via KDM4A-dependent histone H3K36 demethylation. Evid. Based Complement. Altern. Med. 2022, 2197071. doi:10.1155/2022/2197071
Cheng, W., Wang, Y., Liu, J., Li, X., Yu, M., Duan, C., et al. (2021). Hepatotoxicity of cantharidin is associated with the altered bile acid metabolism. J. Appl. Toxicol. 42 (6), 970–980. doi:10.1002/jat.4267
Cheng, X., Liu, Y., Zhou, H., Leng, J., Dai, X., Wang, D., et al. (2021). Cantharidin-loaded biomimetic MOF nanoparticle cascade to enhance the Fenton reaction based on amplified photothermal therapy. Biomater. Sci. 9 (23), 7862–7875. doi:10.1039/d1bm01396c
Chi, J. H., Jiang, Z. W., Chen, X. T., Peng, Y. F., Liu, W. S., Han, B. S., et al. (2019). Studies on anti-hepatocarcinoma effect, pharmacokinetics and tissue distribution of carboxymethyl chitosan based norcantharidin conjugates. Carbohydr. Polym. 226, 115297. doi:10.1016/j.carbpol.2019.115297
Codogno, P., Mehrpour, M., and Proikas-Cezanne, T. (2011). Canonical and non-canonical autophagy: Variations on a common theme of self-eating? Nat. Rev. Mol. Cell Biol. 13 (1), 7–12. doi:10.1038/nrm3249
Cotovio, P., Silva, C., Guedes Marques, M., Ferrer, F., Costa, F., Carreira, A., et al. (2013). Acute kidney injury by cantharidin poisoning following a silly bet on an ugly beetle. Clin. Kidney J. 6 (2), 201–203. doi:10.1093/ckj/sft001
Cotton, C. H. (2021). Pediatric game changers: Cantharidin for treatment of facial molluscum contagiosum: A retrospective review. J. Am. Acad. Dermatol. 84 (6), 1800. doi:10.1016/j.jaad.2021.02.015
Dang, Y. J., and Zhu, C. Y. (2013). Oral bioavailability of cantharidin-loaded solid lipid nanoparticles. Chin. Med. 8 (1), 1. doi:10.1186/1749-8546-8-1
Deng, X., Liang, J., Wu, F. S., Li, Y. B., and Tang, Y. F. (2011). Effects of the Ganning formula on liver fibrosis in patients with chronic Hepatitis B. J. Tradit. Chin. Med. 31 (4), 282–287. doi:10.1016/s0254-6272(12)60005-3
Deng, L. P., Dong, J., Cai, H., and Wang, W. (2013). Cantharidin as an antitumor agent: A retrospective review. Curr. Med. Chem. 20 (2), 159–166. doi:10.2174/092986713804806711
Dhanusha, A. N., and Craig, M. C. (2020). PROTACs: An emerging therapeutic modality in precision medicine. Cell Chem. Biol. 27 (8), 998–1014. doi:10.1016/j.chembiol.2020.07.020
Di Conza, G., and Ho, P. C. (2020). ER stress responses: An emerging modulator for innate immunity. cells 9 (3), 695. doi:10.3390/cells9030695
Diaz, P., Carneiro, A., Montes, V., and Alves, S. (2020). A potentially fatal aphrodisiac: Cantharidin poisoning. Acta Med. Port. 33 (4), 284–287. doi:10.20344/amp.11567
Dong, H. W., Liu, C. M., He, Q. Q., and Zhao, L. H. (2007). Research progress on the antitumor structure-activity relationship of cantharidin and its derivatives. J. Pharm. Pract. (05), 276–280.
Dong, X. L., Gong, Y., Chen, Z. Z., and Wang, Y. J. (2014). Delisheng Injection, a Chinese medicinal compound, enhanced the effect of cis-platinum on lung carcinoma cell line PGCL3. Chin. J. Integr. Med. 20 (4), 286–291. doi:10.1007/s11655-013-1335-0
Dorn, D. C., Kou, C. A., Png, K. J., and Moore, M. A. (2009). The effect of cantharidins on leukemic stem cells. Int. J. Cancer 124 (9), 2186–2199. doi:10.1002/ijc.24157
Efferth, T., Rauh, R., Kahl, S., Tomicic, M., Böchzelt, H., Tome, M. E., et al. (2005). Molecular modes of action of cantharidin in tumor cells. Biochem. Pharmacol. 69 (5), 811–818. doi:10.1016/j.bcp.2004.12.003
Fan, C. X. (2010). The observation of the effects of disodium norcantharidate in the treatment of advanced primary liver cancer. J. Basic Clin. Oncol. 23 (01), 50–51.
Feng, I. C., Hsieh, M. J., Chen, P. N., Hsieh, Y. H., Ho, H. Y., Yang, S. F., et al. (2018). Cantharidic acid induces apoptosis through the p38 MAPK signaling pathway in human hepatocellular carcinoma. Environ. Toxicol. 33 (3), 261–268. doi:10.1002/tox.22513
Feng, S. M., Zhu, J., Xia, K. S., Yu, W., Wang, Y. T., Wang, J. J., et al. (2018). Cantharidin inhibits anti-apoptotic Bcl-2 family proteins and induces apoptosis in human osteosarcoma cell lines MG-63 and MNNG/HOS via mitochondria-dependent pathway. Med. Sci. Monit. 24, 6742–6749. doi:10.12659/Msm.910294
Feng, H., Wu, Y. Q., Xu, Y. S., Wang, K. X., Qin, X. M., and Lu, Y. F. (2020). LC-MS-based metabolomic study of oleanolic acid-induced hepatotoxicity in mice. Front. Pharmacol. 11, 747. doi:10.3389/fphar.2020.00747
Florea, A. M., and Büsselberg, D. (2011). Cisplatin as an anti-tumor drug: Cellular mechanisms of activity, drug resistance and induced side effects. Cancers (Basel) 3 (1), 1351–1371. doi:10.3390/cancers3011351
Fukamachi, T., Chiba, Y., Wang, X., Saito, H., Tagawa, M., and Kobayashi, H. (2010). Tumor specific low pH environments enhance the cytotoxicity of lovastatin and cantharidin. Cancer Lett. 297 (2), 182–189. doi:10.1016/j.canlet.2010.05.010
Graziano, M. J., and Casida, J. E. (1987). Comparison of the acute toxicity of endothal and cantharidic acid on mouse liver in vivo. Toxicol. Lett. 37 (2), 143–148. doi:10.1016/0378-4274(87)90150-0
Graziano, M. J., Waterhouse, A. L., and Casida, J. E. (1987). Cantharidin poisoning associated with specific binding site in liver. Biochem. Biophys. Res. Commun. 149 (1), 79–85. doi:10.1016/0006-291x(87)91607-x
Gu, Y. H., Wang, Y., Bai, Y., Liu, M., and Wang, H. L. (2017). Endoplasmic reticulum stress and apoptosis via PERK-eIF2α-CHOP signaling in the methamphetamine-induced chronic pulmonary injury. Environ. Toxicol. Pharmacol. 49, 194–201. doi:10.1016/j.etap.2017.01.003
Guenthner, S., McFalda, W., Tate, M., Eads, K., Rieger, J., Glover, D. K., et al. (2021). Phase II, double-blind, vehicle-controlled study to determine the cantharidin dose regimen, efficacy, safety, and tolerability of VP-102 in subjects with external genital warts. Am. J. Clin. Dermatol. 22 (6), 867–875. doi:10.1007/s40257-021-00635-2
Guo, Z. M., Liu, Y., Cheng, X., Wang, D., Guo, S. B., Jia, M. L., et al. (2020). Versatile biomimetic cantharidin-tellurium nanoparticles enhance photothermal therapy by inhibiting the heat shock response for combined tumor therapy. Acta Biomater. 110, 208–220. doi:10.1016/j.actbio.2020.03.028
Harvey, A. L., Edrada-Ebel, R., and Quinn, R. J. (2015). The re-emergence of natural products for drug discovery in the genomics era. Nat. Rev. Drug Discov. 14 (2), 111–129. doi:10.1038/nrd4510
Han, L., Sun, Y. J., Pan, Y. F., Ding, H., Chen, X., and Zhang, X. (2014). Cantharidin combined with chemotherapy for Chinese patients with metastatic colorectal cancer. Asian Pac. J. Cancer Prev. 15 (24), 10977–10979. doi:10.7314/apjcp.2014.15.24.10977
He, S., Zhang, C., Zhou, P., Zhang, X., Ye, T., Wang, R., et al. (2019). Herb-induced liver injury: Phylogenetic relationship, structure-toxicity relationship, and herb-ingredient network analysis. Int. J. Mol. Sci. 20 (15), 3633. doi:10.3390/ijms20153633
He, T. M., Liu, J. X., Wang, X. N., Duan, C. C., Li, X. F., and Zhang, J. Y. (2020). Analysis of cantharidin-induced nephrotoxicity in HK-2 cells using untargeted metabolomics and an integrative network pharmacology analysis. Food Chem. Toxicol. 146, 111845. doi:10.1016/j.fct.2020.111845
He, T. M., Liu, J. X., Duan, C. C., Li, X. F., and Zhang, J. Y. (2021). Effective material basis and mechanism analysis of compound Banmao capsule against tumors using integrative network pharmacology and molecular docking. Evid. Based Complement. Altern. Med. 2021, 6653460. doi:10.1155/2021/6653460
He, T., Wang, Q., Ao, J., Chen, K., Li, X., Zhang, J., et al. (2022). Endoplasmic reticulum stress contributes to autophagy and apoptosis in cantharidin-induced nephrotoxicity. Food Chem. Toxicol. 163, 112986. doi:10.1016/j.fct.2022.112986
Hebels, D. G., Jetten, M. J., Aerts, H. J., Herwig, R., Theunissen, D. H., Gaj, S., et al. (2014). Evaluation of database-derived pathway development for enabling biomarker discovery for hepatotoxicity. Biomark. Med. 8 (2), 185–200. doi:10.2217/bmm.13.154
Hong, S., Pei, W., Chunqi, W., Yifan, L., and Yalin, Z. (2022). The detoxification enzymatic responses of plutella xylostella (lepidoptera: Plutellidae) to cantharidin. J. Econ. Entomol. 115 (5), 1551–1556. doi:10.1093/jee/toac139
Hsia, T. C., Yu, C. C., Hsu, S. C., Tang, N. Y., Lu, H. F., Huang, Y. P., et al. (2014). Cantharidin induces apoptosis of H460 human lung cancer cells through mitochondria-dependent pathways. Int. J. Oncol. 45 (1), 245–254. doi:10.3892/ijo.2014.2428
Hsia, T. C., Lin, J. H., Hsu, S. C., Tang, N. Y., Lu, H. F., Wu, S. H., et al. (2015a). Cantharidin induces DNA damage and inhibits DNA repair-associated protein levels in NCI-H460 human lung cancer cells. Environ. Toxicol. 30 (10), 1135–1143. doi:10.1002/tox.21986
Hsia, T. C., Yu, C. C., Hsu, S. C., Tang, N. Y., Lu, H. F., Yu, C. S., et al. (2015b). cDNA microarray analysis of the effect of cantharidin on DNA damage, cell cycle and apoptosis-associated gene expression in NCI-H460 human lung cancer cells in vitro. Mol. Med. Rep. 12 (1), 1030–1042. doi:10.3892/mmr.2015.3538
Hsia, T. C., Yu, C. C., Hsiao, Y. T., Wu, S. H., Bau, D. T., and Lu, H. F. (2016). Cantharidin impairs cell migration and invasion of human lung cancer NCI-H460 cells via UPA and MAPK signaling pathways. Anticancer Res. 36 (11), 5989–5997. doi:10.21873/anticanres.11187
Hsiao, Y. P., Tsai, C. H., Wu, P. P., Hsu, S. C., Liu, H. C., Huang, Y. P., et al. (2014). Cantharidin induces G2/M phase arrest by inhibition of Cdc25c and Cyclin A and triggers apoptosis through reactive oxygen species and the mitochondria-dependent pathways of A375.S2 human melanoma cells. Int. J. Oncol. 45 (6), 2393–2402. doi:10.3892/ijo.2014.2689
Hu, S., Chang, J., Ruan, H., Zhi, W., Wang, X., Zhao, F., et al. (2021). Cantharidin inhibits osteosarcoma proliferation and metastasis by directly targeting miR-214-3p/DKK3 axis to inactivate β-catenin nuclear translocation and LEF1 translation. Int. J. Biol. Sci. 17 (10), 2504–2522. doi:10.7150/ijbs.51638
Huan, S. K., Lee, H. H., Liu, D. Z., Wu, C. C., and Wang, C. C. (2006). Cantharidin-induced cytotoxicity and cyclooxygenase 2 expression in human bladder carcinoma cell line. Toxicology 223 (1-2), 136–143. doi:10.1016/j.tox.2006.03.012
Huang, W. W., Ko, S. W., Tsai, H. Y., Chung, J. G., Chiang, J. H., Chen, K. T., et al. (2011). Cantharidin induces G2/M phase arrest and apoptosis in human colorectal cancer colo 205 cells through inhibition of CDK1 activity and caspase-dependent signaling pathways. Int. J. Oncol. 38 (4), 1067–1073. doi:10.3892/ijo.2011.922
Huang, Y. P., Ni, C. H., Lu, C. C., Chiang, J. H., Yang, J. S., Ko, Y. C., et al. (2013). Suppressions of migration and invasion by cantharidin in TSGH-8301 human bladder carcinoma cells through the inhibitions of matrix metalloproteinase-2/-9 signaling. Evid. Based Complement. Altern. Med. 2013, 190281. doi:10.1155/2013/190281
Huang, X., Tang, W., Lin, C., Sa, Z., Xu, M., Liu, J., et al. (2021). Protective mechanism of astragalus polysaccharides against cantharidin-induced liver injury determined in vivo by liquid chromatography/mass spectrometry metabolomics. Basic Clin. Pharmacol. Toxicol. 129 (1), 61–71. doi:10.1111/bcpt.13585
Huh, J. E., Kang, K. S., Chae, C., Kim, H. M., Ahn, K. S., and Kim, S. H. (2004). Roles of p38 and JNK mitogen-activated protein kinase pathways during cantharidin-induced apoptosis in U937 cells. Biochem. Pharmacol. 67 (10), 1811–1818. doi:10.1016/j.bcp.2003.12.025
Ji, X. L., and He, M. (2019). Sodium cantharidate targets STAT3 and abrogates EGFR inhibitor resistance in osteosarcoma. Aging-Us 11 (15), 5848–5863. doi:10.18632/aging.102193
Ji, B. C., Hsiao, Y. P., Tsai, C. H., Chang, S. J., Hsu, S. C., Liu, H. C., et al. (2015). Cantharidin impairs cell migration and invasion of A375.S2 human melanoma cells by suppressing MMP-2 and -9 through PI3K/NF-κB signaling pathways. Anticancer Res. 35 (2), 729–738.
Kantari, C., and Walczak, H. (2011). Caspase-8 and bid: Caught in the act between death receptors and mitochondria. Biochim. Biophys. Acta 1813 (4), 558–563. doi:10.1016/j.bbamcr.2011.01.026
Kaplowitz, N. (2005). Idiosyncratic drug hepatotoxicity. Nat. Rev. Drug Discov. 4 (6), 489–499. doi:10.1038/nrd1750
Kaufmann, T., Strasser, A., and Jost, P. J. (2012). Fas death receptor signalling: Roles of bid and XIAP. Cell Death Differ. 19 (1), 42–50. doi:10.1038/cdd.2011.121
Ketelut-Carneiro, N., and Fitzgerald, K. A. (2022). Apoptosis, pyroptosis, and necroptosis-Oh my! the many ways a cell can die. J. Mol. Biol. 434 (4), 167378. doi:10.1016/j.jmb.2021.167378
Kim, J. A., Kim, Y., Kwon, B. M., and Han, D. C. (2013). The natural compound cantharidin induces cancer cell death through inhibition of heat shock protein 70 (HSP70) and Bcl-2-associated athanogene domain 3 (BAG3) expression by blocking heat shock factor 1 (HSF1) binding to promoters. J. Biol. Chem. 288 (40), 28713–28726. doi:10.1074/jbc.M113.488346
Knapp, J., Bokník, P., Linck, B., Lüss, H., Müller, F. U., Nacke, P., et al. (1997). The effect of the protein phosphatases inhibitor cantharidin on beta-adrenoceptor-mediated vasorelaxation. Br. J. Pharmacol. 120 (3), 421–428. doi:10.1038/sj.bjp.0700929
Knapp, J., Bokník, P., Huke, S., Lüss, H., Müller, F. U., Müller, T., et al. (1998). The mechanism of action of cantharidin in smooth muscle. Br. J. Pharmacol. 123 (5), 911–919. doi:10.1038/sj.bjp.0701668
Kuo, J. H., Chu, Y. L., Yang, J. S., Lin, J. P., Lai, K. C., Kuo, H. M., et al. (2010). Cantharidin induces apoptosis in human bladder cancer TSGH 8301 cells through mitochondria-dependent signal pathways. Int. J. Oncol. 37 (5), 1243–1250. doi:10.3892/ijo_00000775
Kuo, J. H., Shih, T. Y., Lin, J. P., Lai, K. C., Lin, M. L., Yang, M. D., et al. (2015). Cantharidin induces DNA damage and inhibits DNA repair-associated protein expressions in TSGH8301 human bladder cancer cell. Anticancer Res. 35 (2), 795–804.
Kurokawa, M., and Kornbluth, S. (2009). Caspases and kinases in a death grip. Cell 138 (5), 838–854. doi:10.1016/j.cell.2009.08.021
Le, A. P., Zhang, L. L., Liu, W., and Shi, Y. F. (2016). Cantharidin inhibits cell proliferation and induces apoptosis through G2/M phase cell cycle arrest in hepatocellular carcinoma stem cells. Oncol. Rep. 35 (5), 2970–2976. doi:10.3892/or.2016.4684
Li, W., Xie, L., Chen, Z., Zhu, Y., Sun, Y., Miao, Y., et al. (2010). Cantharidin, a potent and selective PP2A inhibitor, induces an oxidative stress-independent growth inhibition of pancreatic cancer cells through G2/M cell-cycle arrest and apoptosis. Cancer Sci. 101 (5), 1226–1233. doi:10.1111/j.1349-7006.2010.01523.x
Li, W., Chen, Z., Zong, Y., Gong, F., Zhu, Y., Zhu, Y., et al. (2011). PP2A inhibitors induce apoptosis in pancreatic cancer cell line PANC-1 through persistent phosphorylation of IKKα and sustained activation of the NF-κB pathway. Cancer Lett. 304 (2), 117–127. doi:10.1016/j.canlet.2011.02.009
Li, S., Guan, H., Qian, Z., Sun, Y., Gao, C., Li, G., et al. (2017). Taurine inhibits 2,5-hexanedione-induced oxidative stress and mitochondria-dependent apoptosis in PC12 cells. Ind. Health 55 (2), 108–118. doi:10.2486/indhealth.2016-0044
Lin, Z., Jia, Z., Kunhong, Z., Aiping, T., and Da, J. (2022). Targeted protein degradation: Mechanisms, strategies and application. Signal Transduct. Target. Ther. 7 (1), 113. doi:10.1038/s41392-022-00966-4
Liu, F., Duan, C., Zhang, J., and Li, X. (2020). Cantharidin-induced LO2 cell autophagy and apoptosis via endoplasmic reticulum stress pathway in vitro. J. Appl. Toxicol. 40 (12), 1622–1635. doi:10.1002/jat.4022
Liu, F., Wang, X. N., Duan, C. C., Zhang, J. Y., and Li, X. F. (2020c). Hepatoxicity mechanism of cantharidin-induced liver LO2 cells by LC-MS metabolomics combined traditional approaches. Toxicol. Lett. 333, 49–61. doi:10.1016/j.toxlet.2020.07.024
Liu, X., Zhang, L., Thu, P. M., Min, W., Yang, P., Li, J., et al. (2021). Sodium cantharidinate, a novel anti-pancreatic cancer agent that activates functional p53. Sci. China Life Sci. 64 (8), 1295–1310. doi:10.1007/s11427-019-1753-3
Lu, S., Gao, Y., Huang, X. L., and Wang, X. H. (2014). Cantharidin exerts anti-hepatocellular carcinoma by Mir-214 modulating macrophage polarization. Int. J. Biol. Sci. 10 (4), 415–425. doi:10.7150/ijbs.8002
Luan, S. X., Gao, Y. Y., Liang, X. X., Zhang, L., Wu, Q., Hu, Y. K., et al. (2022). Aconitine linoleate, a natural lipo-diterpenoid alkaloid, stimulates anti-proliferative activity reversing doxorubicin resistance in MCF-7/ADR breast cancer cells as a selective topoisomerase II alpha inhibitor. Naunyn Schmiedeb. Arch. Pharmacol. 395 (1), 65–76. doi:10.1007/s00210-021-02172-5
Ma, Q. X., Feng, Y. Y., Deng, K. W., Shao, H. Z., Sui, T. T., Zhang, X., et al. (2018). Unique responses of hepatocellular carcinoma and cholangiocarcinoma cell lines toward cantharidin and norcantharidin. J. Cancer 9 (12), 2183–2190. doi:10.7150/jca.25454
Massicot, F., Dutertre-Catella, H., Pham-Huy, C., Liu, X. H., Duc, H. T., and Warnet, J. M. (2005). In vitro assessment of renal toxicity and inflammatory events of two protein phosphatase inhibitors cantharidin and nor-cantharidin. Basic Clin. Pharmacol. Toxicol. 96 (1), 26–32. doi:10.1111/j.1742-7843.2005.pto960104.x
McCluskey, A., Taylor, C., Quinn, R. J., Suganuma, M., and Fujiki, H. (1996). Inhibition of protein phosphatase 2A by cantharidin analogues. Bioorg. Med. Chem. Lett. 6 (9), 1025–1028. doi:10.1016/0960-894X(96)00166-7
McCluskey, A., Bowyer, M. C., Collins, E., Sim, A. T. R., Sakoff, J. A., and Baldwin, M. L. (2000a). Anhydride modified cantharidin analogues: Synthesis, inhibition of protein phosphatases 1 and 2A and anticancer activity. Bioorg. Med. Chem. Lett. 10 (15), 1687–1690. doi:10.1016/s0960-894x(00)00323-1
McCluskey, A., Keane, M. A., Mudgee, L.-M., Sim, A. T. R., Sakoff, J., and Quinn, R. J. (2000b). Anhydride modified cantharidin analogues. Is ring opening important in the inhibition of protein phosphatase 2A? Eur. J. Med. Chem. 35 (10), 957–964. doi:10.1016/S0223-5234(00)00186-0
McCluskey, A., Ackland, S. P., Bowyer, M. C., Baldwin, M. L., Garner, J., Walkom, C. C., et al. (2003a). Cantharidin analogues: Synthesis and evaluation of growth inhibition in a panel of selected tumour cell lines. Bioorg. Chem. 31 (1), 68–79. doi:10.1016/s0045-2068(02)00524-2
McCluskey, A., Sakoff Jennette, A., Ackland, S., and Sim Alistair, T. R. (2003b). Anhydride modified cantharidin analogues useful in the treatment of cancer. US20030703336.
Miaomiao, L., Alexander, P. M., and Ronald, J. Q. (2022). Natural product-based PROteolysis TArgeting chimeras (PROTACs). Nat. Prod. Rep. 39 (12), 2292–2307. doi:10.1039/d2np00038e
Mu, Z., and Sun, Q. (2018). Cantharidin inhibits melanoma cell proliferation via the miR-21-mediated PTEN pathway. Mol. Med. Rep. 18 (5), 4603–4610. doi:10.3892/mmr.2018.9440
Navarro, V. J., and Senior, J. R. (2006). Drug-related hepatotoxicity. N. Engl. J. Med. 354 (7), 731–739. doi:10.1056/NEJMra052270
Newman, D. J. (2020). Modern traditional Chinese medicine: Identifying, defining and usage of TCM components. Adv. Pharmacol. 87, 113–158. doi:10.1016/bs.apha.2019.07.001
Nussbaumer, S., Bonnabry, P., Veuthey, J. L., and Fleury-Souverain, S. (2011). Analysis of anticancer drugs: A review. Talanta 85 (5), 2265–2289. doi:10.1016/j.talanta.2011.08.034
Pan, Z. H., Qu, C. J., Chen, Y., Chen, X. Y., Liu, X. N., Hao, W. J., et al. (2019). Bufotalin induces cell cycle arrest and cell apoptosis in human malignant melanoma A375 cells. Oncol. Rep. 41 (4), 2409–2417. doi:10.3892/or.2019.7032
Papinski, D., and Kraft, C. (2014). Atg1 kinase organizes autophagosome formation by phosphorylating Atg9. Autophagy 10 (7), 1338–1340. doi:10.4161/auto.28971
Peng, C. (2014). Toxicology of Chinese medicine. Beijing: China Press of Traditional Chinese Medicine.
Pinkoski, M. J., Heibein, J. A., Barry, M., and Bleackley, R. C. (2000). Nuclear translocation of granzyme B in target cell apoptosis. Cell Death Differ. 7 (1), 17–24. doi:10.1038/sj.cdd.4400604
Pirnia, F., Schneider, E., Betticher, D. C., and Borner, M. M. (2002). Mitomycin C induces apoptosis and caspase-8 and -9 processing through a caspase-3 and Fas-independent pathway. Cell Death Differ. 9 (9), 905–914. doi:10.1038/sj.cdd.4401062
Prasad, S. B., and Verma, A. K. (2013). Cantharidin-mediated ultrastructural and biochemical changes in mitochondria lead to apoptosis and necrosis in murine dalton's lymphoma. Microsc. Microanal. 19 (6), 1377–1394. doi:10.1017/S143192761301324x
Qiao-Ling, Z., Yi, Z., Min, G., Di-Jia, Y., Xiao-Feng, Z., Yang, L., et al. (2012). Hepatocyte-targeted delivery using pH-sensitive liposomes loaded with lactosylnorcantharidin phospholipid complex: Preparation, characterization, and therapeutic evaluation in vivo and in vitro. Curr. Med. Chem. 19 (33), 5754–5763. doi:10.2174/092986712803988857
Ren, Y. L., and Kinghorn, A. D. (2021). Antitumor potential of the protein phosphatase inhibitor, cantharidin, and selected derivatives. Bioorg. Med. Chem. 32, 116012. doi:10.1016/j.bmc.2021.116012
Ren, Y., Zhang, S. W., Xie, Z. H., Xu, X. M., Chen, L. L., Lou, Z. G., et al. (2016). Cantharidin induces G2/M arrest and triggers apoptosis in renal cell carcinoma. Mol. Med. Rep. 14 (6), 5614–5618. doi:10.3892/mmr.2016.5963
Rustin, P., Chretien, D., Bourgeron, T., Gérard, B., Rötig, A., Saudubray, J. M., et al. (1994). Biochemical and molecular investigations in respiratory chain deficiencies. Clin. Chim. Acta 228 (1), 35–51. doi:10.1016/0009-8981(94)90055-8
Saad, A. A. (2022). Targeting cancer-associated glycans as a therapeutic strategy in leukemia. All Life 15 (1), 378–433. doi:10.1080/26895293.2022.2049901
Sagawa, M., Nakazato, T., Uchida, H., Ikeda, Y., and Kizaki, M. (2008). Cantharidin induces apoptosis of human multiple myeloma cells via inhibition of the JAK/STAT pathway. Cancer Sci. 99 (9), 1820–1826. doi:10.1111/j.1349-7006.2008.00872.x
Sakoff, J. A., Ackland, S. P., Baldwin, M. L., Keane, M. A., and McCluskey, A. (2002). Anticancer activity and protein phosphatase 1 and 2A inhibition of a new generation of cantharidin analogues. Invest. New Drugs 20 (1), 1–11. doi:10.1023/a:1014460818734
Schneider, J. L., and Cuervo, A. M. (2014). Autophagy and human disease: Emerging themes. Curr. Opin. Genet. Dev. 26, 16–23. doi:10.1016/j.gde.2014.04.003
Shah, A., and Kumar, A. (2016). Methamphetamine-mediated endoplasmic reticulum (ER) stress induces type-1 programmed cell death in astrocytes via ATF6, IRE1α and PERK pathways. Oncotarget 7 (29), 46100–46119. doi:10.18632/oncotarget.10025
Shan, H. B., Cai, Y. C., Liu, Y., Zeng, W. N., Chen, H. X., Fan, B. T., et al. (2006). Cytotoxicity of cantharidin analogues targeting protein phosphatase 2A. Anticancer Drugs 17 (8), 905–911. doi:10.1097/01.cad.0000217428.90325.35
Shaoting, L., Xufeng, W., Gang, F., Kui, D., Liping, D., et al. (2023). Exploring cantharidin and its analogues as anticancer agents: A review. Curr. Med. Chem. 30 (18), 2006–2019. doi:10.2174/0929867330666221103151537
Shen, M., Wu, M. Y., Chen, L. P., Zhi, Q. M., Gong, F. R., Chen, K., et al. (2015). Cantharidin represses invasion of pancreatic cancer cells through accelerated degradation of MMP2 mRNA. Sci. Rep. 5, 11836. doi:10.1038/srep11836
Sheng, S. H., Zhang, T., Li, S. J., Wei, J., Xu, G. J., Sun, T. H., et al. (2015). Targeting vitamin E TPGS-cantharidin conjugate nanoparticles for colorectal cancer therapy. Rsc Adv. 5 (66), 53846–53856. doi:10.1039/c5ra08154h
Shou, L. M., Zhang, Q. Y., Li, W., Xie, X., Chen, K., Lian, L., et al. (2013). Cantharidin and norcantharidin inhibit the ability of MCF-7 cells to adhere to platelets via protein kinase C pathway-dependent downregulation of α2 integrin. Oncol. Rep. 30 (3), 1059–1066. doi:10.3892/or.2013.2601
Srinivasula, S. M., Ahmad, M., Fernandes-Alnemri, T., and Alnemri, E. S. (1998). Autoactivation of procaspase-9 by apaf-1-mediated oligomerization. Mol. Cell. 1 (7), 949–957. doi:10.1016/s1097-2765(00)80095-7
Staudt, R. E., Carlson, R. D., and Snook, A. E. (2022). Targeting gastrointestinal cancers with chimeric antigen receptor (CAR)-T cell therapy. Cancer Biol. Ther. 23 (1), 127–133. doi:10.1080/15384047.2022.2033057
Su, C. C., Liu, S. H., Lee, K. I., Huang, K. T., Lu, T. H., Fang, K. M., et al. (2015). Cantharidin induces apoptosis through the calcium/PKC-regulated endoplasmic reticulum stress pathway in human bladder cancer cells. Am. J. Chin. Med. 43 (3), 581–600. doi:10.1142/s0192415x15500366
Sun, X. Y., Cai, X. T., Yang, J., Chen, J., Guo, C. X., and Cao, P. (2016). Cantharidin overcomes imatinib resistance by depleting BCR-ABL in chronic myeloid leukemia. Mol. Cells 39 (12), 869–876. doi:10.14348/molcells.2016.0023
Sun, S., Shang, E. Y., Ju, A. X., Li, Y. L., Wu, Q., Li, Q. H., et al. (2021). Tumor-targeted hyaluronic acid-mPEG modified nanostructured lipid carriers for cantharidin delivery: An in vivo and in vitro study. Fitoterapia 155, 105033. doi:10.1016/j.fitote.2021.105033
Sung, H., Ferlay, J., Siegel, R. L., Laversanne, M., Soerjomataram, I., Jemal, A., et al. (2021). Global cancer statistics 2020: GLOBOCAN estimates of incidence and mortality worldwide for 36 cancers in 185 countries. CA Cancer J. Clin. 71 (3), 209–249. doi:10.3322/caac.21660
Swanton, E., Savory, P., Cosulich, S., Clarke, P., and Woodman, P. (1999). Bcl-2 regulates a caspase-3/caspase-2 apoptotic cascade in cytosolic extracts. Oncogene 18 (10), 1781–1787. doi:10.1038/sj.onc.1202490
Swati, S., and Raghuvir, S. T. (2022). Cantharidin downregulates PSD1 expression and inhibits autophagic flux in yeast cells. FEBS Open Bio 12 (5), 1017–1035. doi:10.1002/2211-5463.13196
Sznarkowska, A., Kostecka, A., Meller, K., and Bielawski, K. P. (2017). Inhibition of cancer antioxidant defense by natural compounds. Oncotarget 8 (9), 15996–16016. doi:10.18632/oncotarget.13723
Tabas, I., and Ron, D. (2011). Integrating the mechanisms of apoptosis induced by endoplasmic reticulum stress. Nat. Cell Biol. 13 (3), 184–190. doi:10.1038/ncb0311-184
Tatlock, J. H., Angelica Linton, M., Hou, X. J., Kissinger, C. R., Pelletier, L. A., Showalter, R. E., et al. (1997). Structure-based design of novel calcineurin (PP2B) inhibitors. Bioorg. Med. Chem. Lett. 7 (8), 1007–1012. doi:10.1016/S0960-894X(97)00141-8
Tian, X. G., Zeng, G., Li, X., Wu, Z. Z., and Wang, L. (2015). Cantharidin inhibits cell proliferation and promotes apoptosis in tongue squamous cell carcinoma through suppression of miR-214 and regulation of p53 and Bcl-2/Bax. Oncol. Rep. 33 (6), 3061–3068. doi:10.3892/or.2015.3942
Van Opdenbosch, N., and Lamkanfi, M. (2019). Caspases in cell death, inflammation, and disease. Immunity 50 (6), 1352–1364. doi:10.1016/j.immuni.2019.05.020
Verma, A. K., and Prasad, S. B. (2012). Bioactive component, cantharidin from Mylabris cichorii and its antitumor activity against Ehrlich ascites carcinoma. Cell Biol. Toxicol. 28 (3), 133–147. doi:10.1007/s10565-011-9206-6
Verma, A. K., and Prasad, S. B. (2013). Antitumor effect of blister beetles: An ethno-medicinal practice in Karbi community and its experimental evaluation against a murine malignant tumor model. J. Ethnopharmacol. 148 (3), 869–879. doi:10.1016/j.jep.2013.05.032
Wang, M., and Kaufman, R. J. (2016). Protein misfolding in the endoplasmic reticulum as a conduit to human disease. Nature 529 (7586), 326–335. doi:10.1038/nature17041
Wang, C. C., Wu, C. H., Hsieh, K. J., Yen, K. Y., and Yang, L. L. (2000). Cytotoxic effects of cantharidin on the growth of normal and carcinoma cells. Toxicology 147 (2), 77–87. doi:10.1016/s0300-483x(00)00185-2
Wang, Q., Zhang, L., Hu, W., Hu, Z. H., Bei, Y. Y., Xu, J. Y., et al. (2010). Norcantharidin-associated galactosylated chitosan nanoparticles for hepatocyte-targeted delivery. Nanomedicine 6 (2), 371–381. doi:10.1016/j.nano.2009.07.006
Wang, W.-J., Wu, M.-Y., Shen, M., Zhi, Q., Liu, Z.-Y., Gong, F.-R., et al. (2015). Cantharidin and norcantharidin impair stemness of pancreatic cancer cells by repressing the β-catenin pathway and strengthen the cytotoxicity of gemcitabine and erlotinib. Int. J. Oncol. 47 (5), 1912–1922. doi:10.3892/ijo.2015.3156
Wang, P., Wang, X. J., Pan, X. X., Yang, C., and Huang, C. (2018). Syntheses, activities and structure-activity relationship anti-hepatoma of cantharidin derivatives. Chemistry 81 (04), 355–360. doi:10.14159/j.cnki.0441-3776.2018.04.009
Wang, X. Y., Lin, Y. Y., and Zheng, Y. (2020). Antitumor effects of aconitine in A2780 cells via estrogen receptor beta-mediated apoptosis, DNA damage and migration. Mol. Med. Rep. 22 (3), 2318–2328. doi:10.3892/mmr.2020.11322
Wang, C. Q., Zheng, X. T., Chen, X. F., Jiang, H., Huang, J., Jiang, Y., et al. (2021). The optimal adjuvant strategy of aidi injection with gemcitabine and cisplatin in advanced non-small cell lung cancer: A meta-analysis of 70 randomized controlled trials. Front. Pharmacol. 12, 582447. doi:10.3389/fphar.2021.582447
Wang, G.-S. (1989). Medical uses of mylabris in ancient China and recent studies. J. Ethnopharmacol. 26 (2), 147–162. doi:10.1016/0378-8741(89)90062-7
Wei, J., Ma, L., Liu, W., Wang, Y., Shen, C., Zhao, X., et al. (2021). Identification of the molecular targets and mechanisms of compound mylabris capsules for hepatocellular carcinoma treatment through network pharmacology and bioinformatics analysis. J. Ethnopharmacol. 276, 114174. doi:10.1016/j.jep.2021.114174
Westrate, L. M., Lee, J. E., Prinz, W. A., and Voeltz, G. K. (2015). Form follows function: The importance of endoplasmic reticulum shape. Annu. Rev. Biochem. 84, 791–811. doi:10.1146/annurev-biochem-072711-163501
White, E. (2015). The role for autophagy in cancer. J. Clin. Invest. 125 (1), 42–46. doi:10.1172/Jci73941
Wu, M. Y., Xie, X., Xu, Z. K., Xie, L., Chen, Z., Shou, L. M., et al. (2014). PP2A inhibitors suppress migration and growth of PANC-1 pancreatic cancer cells through inhibition on the Wnt/β-catenin pathway by phosphorylation and degradation of β-catenin. Oncol. Rep. 32 (2), 513–522. doi:10.3892/or.2014.3266
Wu, W., Su, M., Li, T., Wu, K., Wu, X., and Tang, Z. (2015). Cantharidin-induced liver injuries in mice and the protective effect of vitamin C supplementation. Int. Immunopharmacol. 28 (1), 182–187. doi:10.1016/j.intimp.2015.06.003
Xi, Y., Garshott, D. M., Brownell, A. L., Yoo, G. H., Lin, H. S., Freeburg, T. L., et al. (2015). Cantharidins induce ER stress and a terminal unfolded protein response in OSCC. J. Dent. Res. 94 (2), 320–329. doi:10.1177/0022034514559376
Xie, P., Jin, Q., Li, Y. F., Zhang, J. B., Kang, X., Zhu, J. L., et al. (2021). Nanoparticle delivery of a triple-action Pt(iv) prodrug to overcome cisplatin resistance via synergistic effect. Biomater. Sci. 10 (1), 153–157. doi:10.1039/d1bm01556g
Xie, X., Wu, M. Y., Shou, L. M., Chen, L. P., Gong, F. R., and Chen, K. (2015). Tamoxifen enhances the anticancer effect of cantharidin and norcantharidin in pancreatic cancer cell lines through inhibition of the protein kinase C signaling pathway. Oncol. Lett. 9 (2), 837–844. doi:10.3892/ol.2014.2711
Xiong, A., Yang, F., Fang, L., Yang, L., He, Y., Wan, Y. J., et al. (2014). Metabolomic and genomic evidence for compromised bile acid homeostasis by senecionine, a hepatotoxic pyrrolizidine alkaloid. Chem. Res. Toxicol. 27 (5), 775–786. doi:10.1021/tx400451q
Xu, Q. M., Jia, D., Gao, H. W., Zhang, M. M., He, W. J., Pan, S., et al. (2013). In vitro and in vivo protective effects of gingenosides on acute renal injury induced by cantharidin. J. Funct. Foods 5 (4), 2012–2018. doi:10.1016/j.jff.2013.08.005
Yang, T., Shu, T., Liu, G., Mei, H., Zhu, X., Huang, X., et al. (2017). Quantitative profiling of 19 bile acids in rat plasma, liver, bile and different intestinal section contents to investigate bile acid homeostasis and the application of temporal variation of endogenous bile acids. J. Steroid. Biochem. Mol. Biol. 172, 69–78. doi:10.1016/j.jsbmb.2017.05.015
Yang, L., Guo, N., Fan, W., Ni, C., Huang, M., Bai, L., et al. (2020). Thioredoxin-1 blocks methamphetamine-induced injury in brain through inhibiting endoplasmic reticulum and mitochondria-mediated apoptosis in mice. Neurotoxicology 78, 163–169. doi:10.1016/j.neuro.2020.03.006
Yang, M., Shen, C., Zhu, S. J., Zhang, Y., Jiang, H. L., Bao, Y. D., et al. (2022). Chinese patent medicine Aidi injection for cancer care: An overview of systematic reviews and meta-analyses. J. Ethnopharmacol. 282, 114656. doi:10.1016/j.jep.2021.114656
Yeh, C. B., Su, C. J., Hwang, J. M., and Chou, M. C. (2010). Therapeutic effects of cantharidin analogues without bridging ether oxygen on human hepatocellular carcinoma cells. Eur. J. Med. Chem. 45 (9), 3981–3985. doi:10.1016/j.ejmech.2010.05.053
Youyou, Z., Yalei, Y., Jie, Z., Chuhuai, G., Liang, L., and Liang, R. (2020). Molecular biomarkers of cantharidin-induced cardiotoxicity in Sprague-Dawley rats: Troponin T, vascular endothelial growth factor and hypoxia inducible factor-1α. J. Appl. Toxicol. 40 (8), 1153–1161. doi:10.1002/jat.3974
Yu, Y., Zhang, Y., Zhang, J., Guan, C., Liu, L., and Ren, L. (2020). Cantharidin-induced acute hepatotoxicity: The role of TNF-α, IKK-α, bcl-2, bax and caspase3. J. Appl. Toxicol. 40 (11), 1526–1533. doi:10.1002/jat.4003
Zeng, Y., Guo, Y., Zhang, Y., Wang, X., Jiang, Y., and Yang, D. (2020). Rapid profiling of cantharidin analogs in Mylabris phalerata Pallas by ultra-performance liquid chromatography-quadrupole time-of-flight-tandem mass spectrometry. Biomed. Chromatogr. 34 (6), e4801. doi:10.1002/bmc.4801
Zhang, H., and Yan, X. L. (2015). Cantharidin modulates the E2F1/MCM7-miR-106b-93/p21-PTEN signaling axis in MCF-7 breast cancer cells. Oncol. Lett. 10 (5), 2849–2855. doi:10.3892/ol.2015.3681
Zhang, J. P., Ying, K., Xiao, Z. Y., Zhou, B., Huang, Q. S., Wu, H. M., et al. (2004). Analysis of gene expression profiles in human HL-60 cell exposed to cantharidin using cDNA microarray. Int. J. Cancer 108 (2), 212–218. doi:10.1002/ijc.11405
Zhang, C. J., Chen, Z. T., Zhou, X. L., Xu, W., Wang, G., Tang, X. X., et al. (2014). Cantharidin induces G(2)/M phase arrest and apoptosis in human gastric cancer SGC-7901 and BGC-823 cells. Oncol. Lett. 8 (6), 2721–2726. doi:10.3892/ol.2014.2611
Zhang, Y. Y., Zhou, X. W., Zhang, J., Guan, C. H., and Liu, L. (2018). Cantharides poisoning: A retrospective analysis from 1996 to 2016 in China. Regul. Toxicol. Pharmacol. 96, 142–145. doi:10.1016/j.yrtph.2018.05.007
Zhang, Y. Y., Liu, L., and Liang, R. (2020a). RNA-sequencing-based transcriptome analysis of cantharidin-induced myocardial injury. J. Appl. Toxicol. 40 (11), 1491–1497. doi:10.1002/jat.4000
Zhang, X., Lin, C. C., Chan, W. K. N., Liu, K. L., Yang, Z. J., and Zhang, H. Q. (2017). Augmented anticancer effects of cantharidin with liposomal encapsulation: in vitro and in vivo evaluation. Molecules 22 (7).
Zhang, Y. Y., Yu, Y. L., Zhang, J., Guan, C. H., Ren, L., and Liu, L. (2020b). Research progress on multiple organ damage and mechanism of cantharidin poisoning. J. Forensic Med. 36 (4), 545–548. doi:10.12116/j.issn.1004-5619.2020.04.021
Zhang, X. Y., Liu, Q. B., Zhang, T. T., Gao, P., Wang, H., Yao, L., et al. (2022). Bone-targeted nanoplatform enables efficient modulation of bone tumor microenvironment for prostate cancer bone metastasis treatment. Drug Deliv. 29 (1), 889–905. doi:10.1080/10717544.2022.2050845
Zhang, J. Y., Chen, Q. H., Wang, L., Chen, K., Mu, W. B., Duan, C. C., et al. (2020a). Study on the mechanism of cantharidin-induced hepatotoxicity in rat using serum and liver metabolomics combined with conventional pathology methods. J. Appl. Toxicol. 40 (9), 1259–1271. doi:10.1002/jat.3983
Zheng, L. H., Bao, Y. L., Wu, Y., Yu, C. L., Meng, X., and Li, Y. X. (2008). Cantharidin reverses multidrug resistance of human hepatoma HepG2/ADM cells via down-regulation of P-glycoprotein expression. Cancer Lett. 272 (1), 102–109. doi:10.1016/j.canlet.2008.06.029
Zhou, C. J., Qiao, L. M., Zhao, L. H., and Li, Z. Y. (2015). Evaluation of the impact of cantharidin on rat CYP enzymes by using a cocktail of probe drugs. Fitoterapia 107, 49–53. doi:10.1016/j.fitote.2015.10.008
Zhou, H., Xu, J., Wang, S., and Peng, J. (2018). Role of cantharidin in the activation of IKKα/IκBα/NF‑κB pathway by inhibiting PP2A activity in cholangiocarcinoma cell lines. Mol. Med. Rep. 17 (6), 7672–7682. doi:10.3892/mmr.2018.8860
Zhou, J. B., Ren, Y. L., Tan, L., Song, X. M. T., Wang, M., Li, Y. Z., et al. (2020). Norcantharidin: Research advances in pharmaceutical activities and derivatives in recent years. Biomed. Pharmacother. 131, 110755. doi:10.1016/j.biopha.2020.110755
Zhu, S. S., Long, R., Song, T., Zhang, L., Dai, Y. L., Liu, S. W., et al. (2019). UPLC-Q-TOF/MS based metabolomics approach to study the hepatotoxicity of cantharidin on mice. Chem. Res. Toxicol. 32 (11), 2204–2213. doi:10.1021/acs.chemrestox.9b00233
Zhu, K., Zhou, L. L., Zou, M. S., Ning, S. C., Liu, S. L., Zhou, Y. L., et al. (2020). 18-GA-Suc modified liposome loading cantharidin for augmenting hepatic specificity: Preparation, characterization, antitumor effects, and liver-targeting efficiency. J. Pharm. Sci. 109 (6), 2038–2047. doi:10.1016/j.xphs.2020.03.001
Keywords: cantharidin, hepatotoxicity, autophagy, apoptosis, natural producct
Citation: Jin D, Huang N-N and Wei J-X (2023) Hepatotoxic mechanism of cantharidin: insights and strategies for therapeutic intervention. Front. Pharmacol. 14:1201404. doi: 10.3389/fphar.2023.1201404
Received: 06 April 2023; Accepted: 01 June 2023;
Published: 13 June 2023.
Edited by:
Irwin Rose Alencar de Menezes, Regional University of Cariri, BrazilReviewed by:
Juan Liu, Tsinghua University, ChinaSeong Lin Teoh, National University of Malaysia, Malaysia
Copyright © 2023 Jin, Huang and Wei. This is an open-access article distributed under the terms of the Creative Commons Attribution License (CC BY). The use, distribution or reproduction in other forums is permitted, provided the original author(s) and the copyright owner(s) are credited and that the original publication in this journal is cited, in accordance with accepted academic practice. No use, distribution or reproduction is permitted which does not comply with these terms.
*Correspondence: Jing-Xia Wei, MTY1NzM1MjUxMEBxcS5jb20=
†These authors have contributed equally to this work and share first authorship