- 1Department of Gastric and Colorectal Surgery, General Surgery Center, The First Hospital of Jilin University, Changchun, Jilin, China
- 2Division of Clinical Epidemiology, The First Hospital of Jilin University, Changchun, China
Hepatocellular carcinoma (HCC), one of the most notorious malignancies globally, has a high fatality and poor prognosis. Though remarkable breakthroughs have been made in the therapeutic strategies recently, the overall survival of HCC remains unsatisfactory. Consequently, the therapy of HCC remains a great challenge. Epigallocatechin gallate (EGCG), a natural polyphenol extracted from the leaves of the tea bush, has been extensively investigated for its antitumor effects. In this review, we summarize the previous literature to elucidate the roles of EGCG in the chemoprophylaxis and therapy of HCC. Accumulating evidence has confirmed EGCG prevents and inhibits the hepatic tumorigenesis and progression through multiple biological mechanisms, mainly involving hepatitis virus infection, oxidative stress, proliferation, invasion, migration, angiogenesis, apoptosis, autophagy, and tumor metabolism. Furthermore, EGCG enhances the efficacy and sensitivity of chemotherapy, radiotherapy, and targeted therapy in HCC. In conclusion, preclinical studies have confirmed the potential of EGCG for chemoprevention and therapy of HCC under multifarious experimental models and conditions. Nevertheless, there is an urgent need to explore the safety and efficacy of EGCG in the clinical practice of HCC.
Introduction
Liver cancer, particularly hepatocellular carcinoma (HCC), is one of the most frequent malignant tumors worldwide and a major threat to human health. Statistics shown that in 2020 alone, nearly 1 million patients were diagnosed with HCC globally (Sung et al., 2021). Currently, HCC is recognized as one of the top five principal causes of cancer-related death worldwide with relative consistency between morbidity and mortality annually, and the 5-year survival for most patients is less than 20% (Chidambaranathan-Reghupaty et al., 2021; Siegel et al., 2022). These statistical data suggest the treatment and prognosis of HCC remain extremely unsatisfactory. Chronic viral hepatitis B and C, cirrhosis, alcohol, fatty liver disease, diabetes, and aflatoxins are major hepatocarcinogenic risk factors that should be focused on prevention (Yang J. D. et al., 2019). Furthermore, several drugs have been confirmed to have some chemoprevention against HCC, such as aspirin, metformin, pioglitazone, statin, and obeticholic acid, but they seem to lack evidence of clinical benefit, instead, multiple side effects (Lange et al., 2021; Goh and Sinn, 2022; Zeng et al., 2023). Up to now, surgical resection, local ablation, liver transplantation, transcatheter interventional treatment, and systemic treatment are still the main therapeutic strategies for HCC with different clinical stages (Forner et al., 2018). Although recent breakthroughs have been made in systemic therapy of HCC, such as the establishment of standardized protocols based on immune checkpoint inhibitors (ICIs), most patients may lack a response to therapy and ultimately succumb to this cancer (Vogel et al., 2022). Consequently, the therapy of HCC remains a huge challenge for global healthcare, which suggests the demand to explore novel antitumor drugs.
Recently, the anti-cancer effects of natural compounds extracted from plants, especially tea trees, have been the fundamental area of concern to researchers. Globally, tea has become one of the oldest and most prevalent beverages. There are various kinds of tea, mainly divided into green tea, black tea, and oolong tea (Abudureheman et al., 2022). Abundant studies have unveiled the natural compounds in green tea have significantly antioxidant properties that are beneficial to health and reduce the risk of multiple diseases, such as inflammation, infection, cardiovascular disease, and cancer (Kochman et al., 2020; Musial et al., 2020). The pivotal reason for this is that green tea is made from the fresh leaves or buds of tea trees via fixation, twisting, and drying processes without the fermentation of black tea and oolong tea, thus retaining many nutrients of the tea, such as catechins, methylxanthines, amino acids and vitamins, among which catechins content is the most abundant, accounting for up to 30% of the dry weight of green tea (Kim et al., 2020; Ferrari et al., 2022; Trisha et al., 2022). On the basis of the different molecular structure, catechins are categorized into epigallocatechin gallate (EGCG), epigallocatechin (EGC), epicatechin gallate (ECG), gallocatechin gallate (GCG), epicatechin (EC), gallocatechin (GC), catechin gallate (CG), and catechin (C) (Li et al., 2018; Zhang et al., 2019b; Ferrari et al., 2022). Among these compounds, EGCG is the most bioactive and abundant, accounting for 50%–80% of all catechins in green tea (Samavat et al., 2019; Almatroodi et al., 2020; Ferrari et al., 2022).
EGCG, a polyphenol compound synthesized by esterification of epigallocatechin and gallic acid, consists of a dihydropyran heterocycle ring and 3 aromatic rings, where a total of 8 phenolic hydroxyl groups are contained (Bimonte et al., 2019). Since the polyphenol structure of EGCG allows electron delocalization to quench free radicals, EGCG is endowed with strong antioxidant capacity (Alam et al., 2022). Evidence has demonstrated that EGCG not only interacts with a variety of proteins, but enters the nucleus to regulate gene expression (Li et al., 2018). Based on these premises, numerous researchers have investigated the antitumor properties of EGCG recently. An expanding body of studies has revealed the potential of EGCG in chemoprophylaxis and therapy of multiple malignancies (Huang Y. J. et al., 2020; Romano and Martel, 2021; Wang et al., 2023).
In this article, we reviewed the potential role of EGCG in HCC. We comprehensively introduce the roles of EGCG in the chemoprevention and therapy of HCC as well as its molecular mechanisms in vivo and in vitro from multiple perspectives, such as hepatitis virus infection, hepatic tumorigenesis, oxidative stress, proliferation, invasion, migration, angiogenesis, apoptosis, autophagy, and metabolism, in order to provide new contributions to its clinical application.
The roles of EGCG in chemoprevention and anti-cancer in vitro
Accumulating preclinical evidence has suggested the chemoprophylactic and anti-cancer properties of EGCG involve multiple molecular targets and tumor-related signaling pathways (Figure 1). We discussed the antiviral and antitumor effects of EGCG in vitro, and devoted ourselves to clarifying its mechanisms in regulating proliferation, invasion, metastasis, angiogenesis, apoptosis, autophagy, and metabolism of hepatoma cells (Table 1).
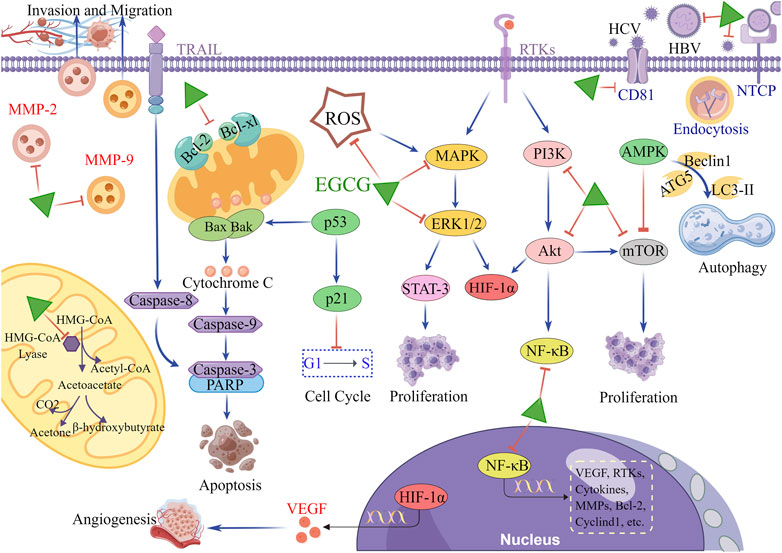
FIGURE 1. The main molecular mechanism of EGCG in HCC. When hepatocytes are exposed to hepatitis virus, EGCG inhibits the viral entry into the cells by reducing the viral adherence and downregulating viral receptors on the surface of hepatocytes. In terms of antitumor, EGCG downregulates multiple tumor-related molecules and signaling pathways, including RTKs, MAPK/ERK, PI3K/Akt, mTOR, NF-κB, STAT-3, HIF-1α and MMPs, to inhibit proliferation, angiogenesis, invasion, and migration. Simultaneously, EGCG upregulates p21 expression to induce cell cycle arrest. Additionally, the antitumor effects of EGCG are manifested in the stimulation of apoptosis through activating mitochondrial cytochrome C and TRAIL/TRAILR pathways. The activation of AMPK pathway is also involved in the regulation of EGCG in autophagy of hepatoma cells. In mitochondria, EGCG blocks the metabolic reprogramming of hepatoma cells by inhibiting the activity of HMG-CoA lyase, thereby reducing the production of ketone body. This figure was drew using Figdraw.
Antiviral activities
Hepatitis B virus (HBV) is an extremely crucial pathogenic factor in cirrhosis and HCC (McGlynn et al., 2021). HBV, a hepatotropic DNA virus, not only causes chronic progressive hepatocyte damage, but integrates viral DNA into the host genome, thus inducing genetic alteration to result in HCC (Yang G. et al., 2022; Fung et al., 2022). According to reports, 10%–25% of HBV carriers undergo the hazard of developing HCC during their lifetime (McGlynn et al., 2015). However, globally, there is still a lack of highly satisfactory antiviral treatments. Evidence has revealed EGCG dose-dependently inhibits HBV-DNA replication and hepatitis B surface antigen (HBsAg) mRNA levels in immortalized human primary hepatocytes infected with HBV (Lai et al., 2018). Similarly, it has been demonstrated that EGCG can significantly block viral DNA replication and antigens expression in HepG2.2.15 cells through downregulating hepatocyte nuclear factor 4α (HNF4α) that are mediated by the extracellular signal-regulated kinase (ERK) signaling pathway (Wang et al., 2020). Even, the inhibitory effects of EGCG on the secretion of HBsAg, hepatitis B e antigen (HBeAg) may be stronger than lamivudine (Pang et al., 2014). As a vital transcriptional regulatory factor, EGCG can also effectively inhibit HBV promoter transcription through antagonizing farnesoid X receptor alpha (FXRα), a member of liver-enriched transcription factors that regulate transcription of liver virus mRNA, thereby reducing HBV replication (Xu et al., 2016). Notably, EGCG may effectively prevent HBV from entering hepatocytes by regulating endocytosis and degradation of sodium taurocholate co-transporting polypeptide (NTCP), the HBV receptors on the cell membrane, thus blocking a substantial proportion of HBV infection (Huang et al., 2014). Moreover, previous study has found EGCG can mediate lysosome acidification in hepatocytes, thus interfering with incomplete autophagosome formation necessary for HBV replication (Zhong et al., 2015).
In addition to HBV, hepatitis C virus (HCV) is another infamous pathogen causing HCC (Shen et al., 2023). In total, it is estimated that there are about 150 million patients who suffer from HCV infection worldwide (Luna-Cuadros et al., 2022). Studies have shown EGCG significantly enhances antiviral innate immune response in hepatocytes by inducing the expression of toll-like receptor 3 (TLR3), retinoic acid-inducible gene I (RIG-I), and interferon lambda 1 (IFN-λ1) (Wang et al., 2016; Wang Y. Z. et al., 2017). Additionally, EGCG can also effectively inhibit HCV entry into hepatocytes. Ample evidence has confirmed the potential mechanism by which EGCG retards HCV infection is that EGCG directly changes the viral particle structure of HCV, ultimately reducing the ability of HCV to adhere to the hepatocyte surface (Ciesek et al., 2011; Calland et al., 2012; Calland et al., 2015). HCV envelope proteins or capsid proteins may be involved in this process (Mathew et al., 2014; Shahid et al., 2021). Different from its direct effect on virus particles, EGCG may also inhibit CD81 receptors that is a critical pathway for HCV to enter hepatocytes through upregulating the expression of certain miRNAs, such as miR-548m and miR-194, thus reducing HCV infectivity in Huh7 cell lines (Mekky et al., 2015; Mekky et al., 2019). Furthermore, EGCG is far superior to HCV embelin, an effective HCV protease inhibitor, in inhibiting the activity of HCV NS3/4A protease that is the necessary enzyme to promote HCV shearing and maturation (Zhong et al., 2012).
Briefly, EGCG, as a valuable natural compound against liver virus, has the effective chemoprophylaxis against HCC. Hence, we consider that EGCG combined with antiviral drugs is promising as a novel therapy strategy for patients with viral hepatitis or liver cancer, especially for their prevention of viral reinfection after liver transplantation, but it remains to be further investigated.
Antioxidative activities
The antioxidant capacity of EGCG depends on its polyphenol groups, which provide the prerequisite for electron delocalization, thus significantly quenching various reactive oxygen species (ROS) (Alam et al., 2022). This antioxidative activity is further elevated by the presence of the trihydroxyl structure in the aromatic ring of EGCG (Singh et al., 2011). As the main cause of cellular oxidative stress, ROS can damage DNA and cause accumulation of carcinogenic alterations, eventually initiating tumor pathogenesis (Srinivas et al., 2019). Concurrently, ROS plays the regulatory roles in tumor microenvironment (TME), cell metabolism, and immune response through a multiple signaling pathways, which are the crucial actions towards cell survival, proliferation, invasion, and metastasis in hepatoma cells (Cheung and Vousden, 2022). Evidence has suggested EGCG inhibits the adhesion and invasion based on its antioxidant capacity in ROS-potentiated AH109A cells induced by hypoxanthine and xanthine oxidase (Zhang et al., 2000). Besides, when the concentration of EGCG ranges from 10 μM to 30μM, EGCG can dose-dependently inhibit ROS generation induced by miR483-3p and attenuate the metastasis potential of HCC (Kang et al., 2021). Briefly, as a strong free radical scavenger, EGCG may inhibit hepatic tumorigenesis and progression by neutralizing intracellular ROS.
Interestingly, it is now realized EGCG also plays a prooxidant role under certain conditions, due to its chemical reactivity making it susceptible to generate ROS (Kang et al., 2008; Chen et al., 2020; Yang L. et al., 2022). Under cell culture conditions, when the medium containing EGCG is exposed directly to air, the phenol hydroxyl groups on the specific aromatic rings of EGCG are converted to o-quinone by autooxidation, yielding superoxide anion radicals and EGCG radicals, which may be beneficial for killing tumor cells (Ouyang et al., 2020). Besides, when the concentration of transition metal ions rises, EGCG can significantly chelate metal ions, including copper ions and iron ions (Bimonte et al., 2019). The chelation of free ions will hamper the antioxidant activity of phenol hydroxyl groups, resulting in EGCG autooxidation and hydrogen peroxide generation to induce cell apoptosis (Alam et al., 2022). Moreover, both EGCG concentration and pH in matrices determine which physicochemical properties EGCG should select (Zhou and Elias, 2013). In general, EGCG might represent an antioxidant at low doses (nanomolar levels), whereas EGCG manifested as a prooxidant at high doses (micromolar levels) in hepatic cells (Kang et al., 2008; Zhou and Elias, 2013; Ouyang et al., 2020). However, the specific critical concentration remains to be determined. Meanwhile, EGCG mainly acted as a prooxidant effect at low pH (pH ≤ 4), while its antioxidant effect was observed at relative high pH (pH = 7) (Zhou and Elias, 2012; 2013). Forementioned findings imply EGCG has dual effects on ROS regulation and may act as a prooxidant to play an antitumor cytotoxic role under certain conditions.
Antiproliferative effects
The receptor tyrosine kinases (RTKs) are the pivotal factors in regulating physiological function of normal cell. Dysregulation of certain RTKs, especially insulin-like growth factor (IGF)-1 receptor (IGF-1R), epidermal growth factor receptor (EGFR), EGFR type 2 (HER2), and vascular endothelial growth factor (VEGF) receptor (VEGFR), are largely related to the acquisition of malignant hallmarks of tumor, as a result of which RTKs have become the most practical molecular targets for HCC therapy (Shimizu et al., 2011a; Park et al., 2022). Increasing evidence has proved EGCG can suppress the abnormal activation of multiple RTKs and their downstream signaling molecules in HCC. The possible mechanism is that it may bind directly or indirectly to RTKs, thereby blocking their tyrosine kinase activities. Specifically, EGCG reduces IGF expression and inhibits IGF-1R and corresponding downstream signaling molecules, such as ERK and phosphatidylinositol-3-kinase (PI3K)/protein kinase B (Akt), which are major signaling targets of RTKs activation, ultimately suppressing the proliferation of HCC through such IGF/IGF-1R axis (Shimizu et al., 2008; Shimizu et al., 2011a). Meanwhile, EGCG can directly inhibit downstream signaling of IGF-1R through acting on the adenosine triphosphate (ATP)-binding domain of 78 kDa glucose regulated protein (GRP78), a multifunctional chaperone protein that aggrandizes the phosphorylation and activation of IGF-1R (Yin et al., 2017). Recently, estrogen receptor alpha 36 (ERα36), a newly discovered estrogen receptor α-subtype in liver, which is prominently upregulated only in hepatoma cells but not in normal hepatocytes, has been recognized as a potential EGCG target in HCC. Evidence suggests EGCG can directly or indirectly target ERα36 in hepatoma cells, thus downregulating EGFR and HER2, as well as blocking PI3K/Akt and mitogen-activated protein kinases (MAPK)/ERK pathways (Chen et al., 2019). Moreover, VEGFR is significantly highly expressed in hepatoma cells, especially VEGFR-2, which is an essential regulator of tumor growth and angiogenesis (Bai et al., 2020; Teng et al., 2020). When researchers treated hepatoma cells with EGCG, VEGFR-2 expression markedly decreased in a time- and dose-dependent manner, implying EGCG may inhibit HCC cell growth by targeting VEGF-VEGFR axis (Shirakami et al., 2009). These findings suggest EGCG can hinder the proliferation of hepatoma cells by acting as an RTKs inhibitor.
Not only that, EGCG can also inhibit HCC growth by affecting cell cycle progression. EGCG significantly reduces the expression of cyclin D1 protein in hepatoma cells, which leads to cell cycle arrest, especially when combined with metformin (Shan et al., 2014; Sabry et al., 2019). Remarkably, the choice of EGCG between cell cycle arrest and apoptosis is largely dependent on p53 status. For p53-positive HepG2 cells, EGCG can induce G1-phase cell cycle arrest through increasing the expression of p53 and p21 successively, whereas EGCG can induce the apoptosis of p53-negative Hep3B cells, in which p21 is not affected by EGCG (Huang et al., 2009). Additionally, EGCG inhibits self-renewal of liver cancer stem cells (CSCs), weakens the stemness characteristics and disrupts the proliferation-related signaling molecules, including never in mitosis A (NIMA)-related kinase 2 (Nek2) and Akt in CSCs (Wubetu et al., 2016). Nevertheless, the regulatory mechanism of EGCG in CSCs remains obscure and needs to be further investigation.
Antiinvasive and antimigratory effects
As the primary malignant hallmarks, invasion and metastasis bring severe challenges to HCC treatment. Several authors have confirmed matrix metalloproteinases (MMPs) participate in various processes of tumor progression, particularly tumor invasion and metastasis (de Almeida et al., 2022). MMPs remove barriers for invasion and migration by breaking the extracellular matrix and basement membrane (Huang et al., 2022). For instance, MMP-2 and MMP-9 can degrade type IV collagen in the basement membrane to help tumor cells invade blood vessels and lymphatic vessels (Cabral-Pacheco et al., 2020). Furthermore, MMPs induce the epithelial mesenchymal transformation of HCC cells to promote tumor invasion and migration (Scheau et al., 2019). Extensive evidence has confirmed EGCG suppresses HCC invasion and migration in a dose-dependent manner by reducing the activities of MMP-2 and MMP-9 (Lee et al., 2007; Roomi et al., 2010). Zhang et al. (2013) also has uncovered the inhibitory roles of EGCG in HCC metastasis relies on the suppression of MMP-2 and MMP-9 activities, but the detailed regulatory mechanism has not been explained yet. We speculate EGCG may directly bind to MMP-2 and MMP-9 to inhibit their activities, which has been supported by several additional studies (Sazuka et al., 1997; Jha et al., 2016; Saeki et al., 2018). It is worth noting that RNA-seq data analysis of hepatoma cells revealed EGCG downregulated the expression of some MMPs, such as MMP-11 and MMP-24 (Agioutantis et al., 2020). Given previous scientific research, we have also proposed some potential signaling pathways on how EGCG inhibits MMPs expression, including nuclear factor-kappaB (NF-κB), MAPK/ERK, and PI3K/Akt (Luo et al., 2017; Won et al., 2021; Tanabe et al., 2023).
EGCG also inhibits HCC invasion and migration through other pathways. Evidence has suggested prostaglandin E2 (PGE2) can bind to hepatocellular prostaglandin E receptors (EP1) to stimulate the progression and migration of hepatoma cells (Chen et al., 2022). EGCG can hinder the proliferation and migration of hepatoma cells though downregulating the expression of EP1 as well as the generation of PGE2 (Jin et al., 2012; Yang H. et al., 2019). Moreover, osteopontin, a turnover protein regulating extracellular matrix, is correlated with aggressiveness and prognosis of HCC (Song et al., 2021). Zapf et al. (2015) has revealed EGCG reduces the half-life of osteopontin mRNA, rather than affecting the transcription of osteopontin. This may be related to the attenuated migration of hepatoma cells. In brief, these results imply EGCG may inhibit malignant hallmarks of HCC by activating multiple molecular mechanisms.
Antiangiogenic effects
Angiogenesis, a critical pathological hallmark in HCC, is the most practical therapeutic target for HCC (Morse et al., 2019). Evidence has suggested EGCG significantly reduces the expression of VEGF that directly acts on VEGFR on vascular endothelial cells and subsequently leads to the angiogenic dysregulation in HCC (Shirakami et al., 2009; Sabry et al., 2019). The relatively hypoxic environment inside the tumor can stimulate the expression of hypoxia-inducible factor-1 (HIF-1) to adapt to the hypoxic state in tumor tissue, thus activating various downstream target genes including VEGF (You et al., 2021). In hepatoma cells, EGCG inhibits hypoxia-induced HIF-1α accumulation through PI3K/Akt and ERK1/2 pathways, and subsequently causes a strong decrease in VEGF expression (Zhang et al., 2006). Additionally, EGCG directly inhibits signal transducer and activator of transcription 3 (STAT3), a complex transcriptional regulator that can activate HIF-1α expression and cooperate with HIF-1α in binding to the VEGF promoter to maximize the activation of transcription and angiogenesis (Wang et al., 2013; Tolomeo and Cascio, 2021). However, EGCG may have some limitations in anti-angiogenesis, such as relatively unstable chemical structure and poor bioavailability. To compensate for the deficiency of EGCG, EGCG derivative Y6 was synthesized through substituting ethoxy groups for six phenolic hydroxyl groups of EGCG (Zhou et al., 2020). Compared with EGCG, optimized EGCG more significantly downregulates HIF-1α and VEGF through inhibiting PI3K/Akt and MAPK/ERK1/2 pathways, thereby inhibiting angiogenesis of HCC (Liao et al., 2020; Zhou et al., 2020). Another derivative, methylated-EGCG, blocks VEGF-induced angiogenesis by significantly repressing the formation of tubular structures in human umbilical vascular endothelial cells, consistent with the validation in xenografted mice with liver cancer (Hashimoto et al., 2014). With the clarification of the abovementioned antiangiogenic mechanism, EGCG may become an auxiliary strategy for targeted therapy of HCC in future clinical practice.
Proapoptotic effects
Abundant evidence has suggested EGCG-induced apoptosis is relevant to the apoptosis-related proteins. More narrowly, EGCG plays the prominent regulatory roles in a series of apoptosis-related signaling molecules in hepatoma cells, including upregulating the expression of p53, Bax, caspase-9, and caspase-3, downregulating the expression of NF-κB, survivin, and BCL2 apoptosis regulator (Bcl-2), and promoting the release of cytochrome c (Zhang et al., 2015; Yang H. et al., 2019; Sabry et al., 2019). Shen et al. (2014) has deciphered EGCG induces cell cycle arrest and apoptosis of hepatoma cells though suppressing PI3K/Akt pathway, in which the phosphorylation at Ser473 of Akt are decreased with EGCG treatment. Notably, some miRNAs can regulate the apoptotic induced by EGCG in hepatoma cells. For instance, miRNA microarray has unveiled EGCG enhances the levels of miR-16, which modulates EGCG-induced apoptosis by targeting Bcl-2 (Tsang and Kwok, 2010). Additionally, EGCG further induces apoptosis of hepatoma cell by coordinating tumor necrosis factor (TNF)-related apoptosis-inducing ligand (TRAIL) (Nishikawa et al., 2006). The reason may be that EGCG elevates the sensitivity of hepatoma cells to TRAIL-induced apoptosis by enhancing the expression of TRAIL receptor (Abou El Naga et al., 2013). Meanwhile, EGCG also directly inhibits the activity of phosphofructokinase (PFK), a glycolytic rate-limiting enzyme, resulting in metabolic stress-related apoptosis with the release of pro-apoptotic protein Bad (Li et al., 2016).
Autophagy regulation
Previous investigation has found EGCG can induce autophagy of hepatoma cells by inhibiting the formation of microtubule-associated protein light chain 3 (LC3)-I dimer and promoting the synthesis of characteristic autophagic protein LC3-II, thus modulating the degradation of α-fetoprotein (AFP) that is relevant to malignant differentiation, metastasis, and poor prognosis of HCC (Zhao et al., 2017). Furthermore, EGCG induces autophagy in hepatoma cells by activating adenosine monophosphate-activated protein kinase (AMPK) pathway, which is mainly manifested as formation of autophagosomes, acidification of lysosomes, and enhancement of autophagic flux (Zhou et al., 2014). Nevertheless, Chen et al. elucidated EGCG could reduce the autophagy activity of Hep3B cells, which was mainly manifested as reduced autophagy vacuoles and downregulated expression of autophagic proteins, including autophagy-related protein 5 (ATG5) and beclin1 (Chen et al., 2014). This may be related to the reversely double effects of autophagy on tumors in distinct contexts and stages (Li et al., 2020). Overall, EGCG are likely to play an anticarcinogenic role through modulating autophagy of hepatoma cells.
Metabolic regulation
To maintain the energy requirements of tumor cell growth and proliferation, tumor cells often initiate metabolic reprogramming to overcome the energy deficiency. As is known to all, ketone bodies are generated by the liver, but the liver of normal adults lacks the enzymes to consume them. However, studies have found that under nutritional deprivation of HCC, hepatoma cells can re-induce the synthesis of ketolytic enzymes to activate the ketone catabolism, which prevents excessive autophagy of hepatoma cells, thus promoting the progression of HCC (Huang D. et al., 2016). Simultaneously, β-hydroxybutyrate can induce the β-hydroxybutyrylation of p53, leading to an obvious decrease in the acetylation levels and activities of p53, which ultimately promote the growth of tumor cells (Liu et al., 2019). These evidence supports the regulatory roles of ketone bodies in HCC progression. EGCG can non-competitively block the activity of 3-hydroxy-3-methylglutaryl-coenzyme A (HMG-CoA) lyase that catalyzes the cleavage of HMG-CoA into ketone bodies in hepatoma cells (Nakagawa et al., 2013). This implies the anti-cancer effects of EGCG in HCC may involve the regulation of ketone metabolism. Furthermore, different from normal cells, tumor cells prefer aerobic glycolysis for energy despite the availability of oxygen, a phenomenon known as the “Warburg effect” (Zhou et al., 2021). EGCG suppresses the phosphofructokinase activity in hepatoma cells by converting its oligomer structure into an inactive state, thus resulting in aerobic glycolysis inhibition and tumor cell death (Li et al., 2016). Briefly, these findings suggest EGCG may inhibit the reprogramming of HCC energy metabolism. In addition to regulating energy metabolism, EGCG can inhibit the mechanistic target of rapamycin (mTOR) signal and the activities of lipogenic enzymes of hepatoma cells by activating AMPK pathway, subsequently obstructing the protein and lipid synthesis in HCC (Huang et al., 2009). EGCG can also induce autophagy stimulated by AMPK pathway to clear lipids, which promotes lipid metabolism in hepatoma cells (Zhou et al., 2014). Overall, EGCG affects the material and energy metabolism of HCC to a large extent.
The roles of EGCG in chemoprevention and anti-cancer in vivo
In vivo animal experiments are the important basis to investigate the potential of EGCG in the prevention and therapy of HCC as they provide references to the pharmacological effects, which can subsequently be extrapolated to humans (Romano and Martel, 2021). We discuss the preventive and anti-cancer roles of EGCG in vivo from following perspectives (Table 2).
Antiviral activities
In a previous study, an animal model of HBV infection was established using C57BL/6 mice in which intraperitoneal administration of EGCG for 5 consecutive days was performed. The results suggested EGCG markedly decreased HBV-DNA, HBsAg and HBeAg levels in serum, and HBV-RNA transcription as well as hepatitis B core antigen (HBcAg) expression in liver tissue (Wang et al., 2020). Similarly, in human liver chimeric mice, serological and immunohistochemical detection of liver also suggested EGCG injected through the tail vein significantly reduced HBV-DNA, HBsAg, and HBcAg levels (Lai et al., 2018). These findings further confirm EGCG can effectively suppress HBV infection, which is beneficial to block the development of HCC. Additionally, a randomized clinical trial compared the efficacy and safety of Catvira, a new formulation consisting of EGCG, sofosbuvir and ribavirin, with sofosbuvir and ribavirin tablets in patients with HCV genotype 4 (Shiha et al., 2021). The results demonstrated the patients treated with Catvira underwent a more rapid decline in viral load, with stable hemoglobin levels. In contrast, the patients treated with sofosbuvir and ribavirin suffered from a significant reduction in hemoglobin levels. This suggests EGCG not only inhibits HCV infection, but may also resist hemolysis from ribavirin treatment. However, this clinical trial reported only the results of pilot study with a small sample size, and did not include patients with pre-existing cirrhosis, HCC, and other HCV genotypes. Therefore, the effectiveness of EGCG combined with antiviral drugs remains to be further clinical trials.
Preventive roles in tumorigenesis
Although EGCG acts a certain chemoprophylactic roles in the etiology of HCC in vitro and in vivo, the roles of EGCG in the actual HCC incidence and the corresponding molecular mechanism still need to be further elaborated. Accumulating evidence confirm EGCG can inhibit the tumorigenesis and development of HCC. A team from Japan was the first to investigate the inhibitory roles of EGCG in spontaneous hepatoma models (Nishida et al., 1994). In that study, after treating 72 mice with different concentrations of EGCG in drinking water, researchers observed EGCG minimized the HCC incidence from 83.3% to 52.2%. Later on, another study from Japan revealed EGCG attenuates obesity-related hepatocarcinogenesis though suppressing the IGF/IGF-1R axis, alleviating hyperinsulinemia, and reducing chronic inflammation (Shimizu et al., 2011b). Darweish et al. found EGCG injected intraperitoneally markedly decreased the average number of liver nodules in thioacetamide-induced rat models of HCC by 70% and elevated the survival of animals from 30% to 80% (Darweish et al., 2014). Also, previous evidence suggested ingestion of EGCG-rich tea infusion markedly diminished the size and number of hepatic neoplastic nodules induced by diethylnitrosamine, and reduced the HCC incidence from 87.50% to a staggering 8.33% (Liao et al., 2019). Further analysis indicated EGCG could regulate the hepatocarcinogenesis process though suppressing the inflammatory response caused by NF-κB and corresponding downstream signals. Simultaneously, Tang et al. uncovered EGCG prevent HCC in diethylnitrosamine-induced rat models through upregulating p21 expression and downregulating cell division cycle 25A (CDC25A) expression (Tang et al., 2020). In addition, Sur et al. deciphered EGCG could restrain the tumorigenesis of HCC through shrinking the proportion of hepatocyte progenitor cells or CSCs, and modulating Wnt and hedgehog signaling pathways, in which the downregulated β-catenin, cyclinD1, c-Myc and EGFR but upregulated E-cadherin were detected (Sur et al., 2016). The forementioned results indicates that EGCG has an obvious chemoprophylaxis effect on the tumorigenesis of HCC.
Antioxidative activities
Unlike ordinary tea drinks, EGCG-rich green tea infusion significantly increases the antioxidant capacity in the diethylnitrosamine-induced HCC models through elevating the activity of superoxide dismutase, glutathione peroxidase, and hepatic catalase in serum, decreasing the content of malondialdehyde in liver, and reducing ROS production caused by DNA damage (Liao et al., 2019). Darweish et al. supports the above conclusion that EGCG attenuates the oxidative stress of HCC and protect liver function in vivo (Darweish et al., 2014). Also, in a study conducted on oolong tea extracts, the combined application of polysaccharide and polyphenols (52.17% content of EGCG) not only synergically improves the antioxidant capacity and immune level of mice, but inhibits the growth and proliferation of hepatoma cells (Wang J. et al., 2017). Subsequently, Kang et al. observed EGCG could effectively inhibit the lung metastasis of hepatoma cells after injecting luciferase-labelled hepatoma cells with overexpressed miR483-3p (a miRNA to facilitate the metastasis of HCC by inducing oxidative stress) into tail veins of mice (Kang et al., 2021). Taken together, EGCG can protect the liver from oxidative stress damage, and may even attenuate HCC progression through antioxidant effects.
Antiangiogenic effects
Consistent with experimental results in vitro, EGCG significantly suppresses the growth of Huh7 xenografts in nude mice by reducing VEGF expression and inhibiting the activation of VEGFR-2 and associated downstream signaling molecules, such as Akt and ERK (Shirakami et al., 2009). Interestingly, Liao et al. (2020) directly observed EGCG and its derivative Y6 can significantly hinder the angiogenesis of chorioallantoic membrane in chicken embryos, and also clearly revealed them can significantly inhibit intratumoral angiogenesis in HepG2 xenograft models. As expected, this inhibitory effects in xenografts were found to be relevant to the inhibition of PI3K/Akt and MAPK/ERK signaling pathways and the downregulation of HIF-1α and VEGF subsequently. Similarly, Hashimoto et al. used CD31 to specifically label the Huh7 xenograft models and found that EGCG’s methylated derivatives could also significantly inhibit angiogenesis and growth of xenograft tumors (Hashimoto et al., 2014). In summary, these findings suggest EGCG has high antitumor activity in vivo by inhibiting the angiogenesis of HCC.
Regulatory roles in apoptosis, autophagy, and necrosis
In addition to chemoprophylactic, antioxidant, and antiangiogenic effects have been proven in vivo, EGCG can also regulate the apoptosis, autophagy, and necrosis to obstruct the growth of HCC. Evidence showed abundant apoptotic cells and downregulated apoptosis suppressors, including Bcl-2 and Bcl-xl, were detected in the xenograft sections after feeding nude mice with EGCG (Nishikawa et al., 2006). Since NF-κB partially modulates the transcription of these apoptosis suppressors, researchers considered EGCG might trigger apoptosis process by inhibiting NF-κB signal. Likewise, green tea extract rich in EGCG significantly increases the expression levels of p53, caspase-3 and Bax, while decrease the expression levels of proliferating cell nuclear antigen (PCNA) in rat liver (Liao et al., 2019). Predictably, such proapoptotic effect of EGCG was further traced to the PI3K/Akt pathway. Furthermore, one research conducted on combination drugs reported that, in the HCC-LM3 xenograft models, EGCG combined with sorafenib prominently increased the percentage of apoptosis compared to monotherapy (Li et al., 2016). Another study involving drug therapy showed EGCG combined with doxorubicin increased apoptotic cells by about 50% and prominently attenuated the autophagy levels compared with doxorubicin alone in Hep3B xenograft models of nude mice (Chen et al., 2014). Moreover, in vivo experiments also found EGCG not only suppressed the growth of Hep3B xenograft in a dose-dependent manner, but markedly induced the necrosis of a large number of tumor cells (Chen et al., 2019). In conclusion, EGCG affects tumor growth and drug susceptibility by regulating apoptosis, autophagy, and necrosis procedures.
Target prediction of EGCG
To further elucidate the molecular mechanism of EGCG in HCC, we supplemented the additional potential targets of EGCG through the Swiss Target Prediction (http://www.swisstargetprediction.ch/) platform, which can predict the most likely macromolecular targets for a small molecule drug (Daina et al., 2019). The results showed more than ten proteins had high probability as EGCG targets (Table 3). For instance, DNA methyltransferase (DNMT) is highly suspected as a molecular target of EGCG among them, which can transfer methyl groups from S-adenosine methionine to the cytosine bases of CpG dinucleotides. This epigenetic alteration results in the dysregulated expression of oncogenes and tumor suppressor genes, which is the pivotal biological processes of hepatic tumorigenesis and metastasis (Nagaraju et al., 2022). Related evidence has demonstrated EGCG induces demethylation of abnormally hypermethylated tumor suppressor genes, which may be attributed to its inhibitory roles in DNMT activity as a direct competitive inhibitor of DNMT (Moreno et al., 2016). In addition, some researchers have argued EGCG induces the downregulation and degradation of DNMT by inhibiting the association of DNMT with Ubiquitin-like with PHD and RING finger domains 1 (UHRF1), a ligase of E3 ubiquitin (Qadir Nanakali et al., 2022). These findings suggest EGCG may reduce the development of tumor by correcting the dysregulated epigenetic mechanisms. However, the effect of EGCG on epigenetic modification of HCC remains to be verified by subsequent experiments. Briefly, these potential targets may provide insights for the investigation and therapy of EGCG in HCC.
Mechanisms of EGCG in the regulation of target molecules
As mentioned above, we summarized the multiple molecular targets and signaling pathways by which EGCG plays the preventive and antitumor roles in HCC. However, how EGCG simultaneously regulates these molecular targets or signaling pathways in HCC remains to be further discussed. Computational docking analysis has demonstrated the mechanism of physical interactions between EGCG and its molecular targets involving MAPK, PI3K, Akt, NF-κB, STAT-3, RTKs, and MMPs. Specifically, Wang et al. (2019) identified extensive hydrogen bonds were formed between the hydroxyl groups of EGCG and critical residues of many vital tumor-related molecules, including MAPK and Akt, and some enzymatic activities were inhibited by EGCG in vitro. Van Aller et al. (2011) found the ATP binding pocket of PI3K accommodated the 3, 4, 5-trihydroxybenzoate portion of EGCG, which implies EGCG may be an ATP competitive inhibitor of PI3K. Ample evidence indicated the inhibitory effect of EGCG on NF-κB-mediated transcriptional activation was attributable to the formation of a covalent bond between EGCG and residue of NF-κB p65 subunit (Lakshmi et al., 2020; Suhail et al., 2022). Simultaneously, STAT-3 binding assay confirmed EGCG interacted with Arg-609, a critical residue in the STAT-3 SH2 domain that contributes to the binding of STAT-3 and phosphorylated peptides (Wang et al., 2013). Hsu et al. (2015) revealed that in addition to the hydroxyl groups of EGCG binding to residues F795 and E804 in EGFR via hydrogen bonds, the gallate moiety of EGCG interacted with specific anchor residues G796, C797, and D800 through van der Waals forces. Likewise, Singh and Bast also fully illustrated lipophilic, hydrophobic, electrostatic hydrogen bond, π-π stacking, and π-cationic interactions were key contributors in protein-ligand interactions between EGCG and other RTKs, including IGF-1R and VEGFR (Singh and Bast, 2015). In addition, evidence suggested EGCG interacted with several amino acid residues in MMP-9 via hydrogen bond, π-π stacking, π-cationic, and π-σ chemical interactions, and galloyl group of EGCG seem to play an important role in the inhibition of MMP-9 (Sarkar et al., 2016; Nakano et al., 2018).
On the other hand, EGCG’s regulatory effect on epigenetic events may be another underlying mechanism. DNA methylation and histone deacetylation act pivotal roles in shutting down gene expression. The former blocks the binding between transcription factors and promoters, while the latter results in a compact and inaccessible chromatin structure (Hogg et al., 2020). Scientific studies found EGCG interacted with DNMT by hydrogen bond to regulate DNA methylation, thereby reactivating a range of methylation-silenced cancer suppressor genes (Kedhari Sundaram et al., 2020; Li et al., 2022). Studies also revealed EGCG modulated histone acetylation status through docking to histone deacetylases (HDAC) and competitively inhibiting enzymatic activity (Khan et al., 2015; Negri et al., 2018). Inhibiting HDAC will lead to upregulation of histone acetylation on p21 and Bax promoters and hyperacetylation of histone H3 (Li et al., 2022). Meanwhile, inhibiting HDAC will upregulate phosphatase and tensin homolog (PTEN), resulting in Akt inactivation and PI3K/Akt pathway inhibition in HCC (Liu et al., 2021). However, the further mechanism about how EGCG regulates multiple target molecules simultaneously remains to be investigated in the future.
The potential of EGCG in HCC therapy
Currently, there are a variety of therapeutic strategies for HCC, mainly including surgical resection, liver transplantation, percutaneous ablation, transarterial chemoembolization, radiotherapy, chemotherapy, targeted therapy, and immunotherapy (Llovet et al., 2021; Vogel et al., 2022). Although remarkable progress has been made in the systematic immunotherapy of HCC in recent years, the long-term survival of HCC patients remains unsatisfactory and the treatment of HCC still faces great challenges. Therefore, it is vital to explore new anti-cancer drugs and combined therapeutic strategies. Based on these premises, we summarize the therapeutic roles of EGCG reported in the literature.
Chemotherapy
Chemotherapy resistance is the most intractable problem in cancer therapy, which is strong relevant to the evolution of CSCs towards drug resistance, eventually causing tumor recurrence and metastasis (Barbato et al., 2019). A study involving CSCs of hepatoma revealed EGCG attenuated the expression of ATP-binding cassette (ABC) transporters, which participate in the multidrug resistance by transporting chemotherapy drugs out of the tumor cells (Wubetu et al., 2016). This means the combination of EGCG with chemotherapeutic agents seems warranted.
5-Fluorouracil, a traditional chemotherapy agent, is still routinely used for advanced HCC. Unfortunately, non-specific cytotoxicity and chemotherapy resistance due to long-term application limit its clinical efficacy (Xu et al., 2020). Accumulating studies have found cyclooxygenase-2 (COX-2), an enzyme that catalyzes prostaglandin synthesis, is highly expressed in multiple cancers, and is involved in tumorigenicity and chemotherapy resistance, which may be related to 5-fluorouracil resistance in HCC (Zhang S. et al., 2019; Hashemi Goradel et al., 2019; Xu et al., 2020). Coincidentally, EGCG enhances the inhibitory roles of 5-fluorouracil in hepatoma growth by eliminating 5-fluorouracil-induced COX-2 overexpression and PGE2 secretion (Yang et al., 2012). This suggests that combinations of EGCG and 5-fluorouracil provide an effective clinical strategy for HCC therapy.
Daunorubicin is an anthracycline chemotherapy agent. The study of Huang et al. demonstrated EGCG overcame the chemotherapy resistance of hepatoma cells to daunorubicin and reduce the cardiotoxicity of daunorubicin through directly targeting carbonyl reductase 1 (CBR1) to inhibit the conversion of daunorubicin to daunorubicinol (Huang et al., 2010). Similarly, Y6, an ethylated derivative of EGCG, was also revealed to significantly inhibit the CBR1 expression in hepatoma cells, thus enhancing the efficacy of daunorubicin against HCC (Zhou et al., 2020).
As for doxorubicin, another anthracycline, EGCG can enhance its antitumor ability on hepatoma cells, and re-sensitize hepatoma cells resistant to it through reversing the multiple resistance mechanisms, such as suppressing multidrug resistance 1 (MDR1) expression, enhancing intracellular doxorubicin accumulation, and decreasing P-glycoprotein efflux pump activity of ABC transporter family members (Liang et al., 2010). Meanwhile, Satonaka et al. (2017) confirmed EGCG reversed the above doxorubicin resistance in hepatoma cells by synergistically inhibiting MAPK/ERK and PI3K/Akt signaling pathways. Wen et al., 2019 also confirmed EGCG derivative Y6 reversed doxorubicin resistance in hepatoma cells through competitively inhibiting efflux pump activity of ABC transporter. Additionally, Chen et al., 2014 found EGCG could kill hepatoma cells synergically with doxorubicin through inhibiting doxorubicin-induced autophagy. In conclusion, EGCG enhances the efficacy of chemotherapy through multiple mechanisms, which provides the important strategies for combination therapy of HCC.
Radiotherapy
Besides improving the efficacy of chemotherapy agents, EGCG is also involved in the regulation of radiotherapy for HCC. It has been confirmed that hepatic stellate cells, as one of the most important components of the TME, induces HCC angiogenesis, inhibits antitumor immune response, and accelerates HCC progression (Ruan et al., 2020). Increasing evidence suggests radiotherapy activates hepatic stellate cells to reshape the TME through multiple molecular mechanisms, such as secreting cytokines, and driving hepatic fibrosis, ultimately resulting in enhanced invasion and metastasis of residual hepatoma cells (Barry et al., 2020; Ruan et al., 2020; Ezhilarasan and Najimi, 2023). This may be the reason why radiotherapy for HCC often fails. Therefore, targeting hepatic stellate cells is a novel strategy for the therapy of HCC. EGCG can suppress the activation of hepatic stellate cells though binding to laminin receptor (67 LR) to block activated toll-like receptor 4 (TLR4) signal transduction (Shen et al., 2022). Once TLR4 signal is blocked, it will be conducive to the inhibition of NF-κB and various inflammatory factors, so as to suppresses the hepatic tumorigenesis and progression. These findings suggest EGCG can be applied as an adjunct to HCC radiotherapy to reduce the invasion and metastasis abilities of residual hepatoma cells after radiotherapy.
Targeted therapy
Sorafenib, the first multi-kinase inhibitor used in the systematic targeted therapy of HCC, which simultaneously inhibits tumor cell proliferation and angiogenesis, is still used as the first-line treatment for HCC (Huang A. et al., 2020; Benson et al., 2021). Sorafenib resistance in HCC has been confirmed to be closely relevant to the process of aerobic glycolysis (Feng et al., 2020b). Sorafenib combined with glycolysis inhibitors can markedly retarded the growth of hepatoma cells resistant to sorafenib (Xia et al., 2020). Hepatoma cells are re-sensitized to sorafenib through blocking the expression of glycolytic enzymes, including PFK and pyruvate kinase M2 (PKM2) (Li et al., 2017; Feng et al., 2020a). As expected, EGCG can increase the inhibitory effects of sorafenib on aerobic glycolytic hepatoma cells and xenograft models of mice through directly inhibiting PFK activity (Li et al., 2016). Furthermore, an interesting study demonstrated EGCG combined with low doses of sorafenib did not differ in inhibiting angiogenesis of HCC rats compared with standard doses of sorafenib, which was beneficial in preventing resistance and reducing toxicity of sorafenib (Irawan et al., 2022). Nevertheless, the application of EGCG as an adjuvant for targeted therapy of HCC remains to be further investigated clinically.
Immunotherapy
In the systematic therapy of HCC, the therapeutic options of some patients are limited due to the resistance to traditional chemotherapy drugs. As a new treatment method, immunotherapy overturns the traditional protocols of HCC therapy, and has achieved encouraging outcomes in terms of safety and efficacy (Kole et al., 2020). So far, several ICIs have been approved for the therapy of HCC, such as atezolizumab, camrelizumab, sintilimab and tislelizumab. Unfortunately, there have been no reports of EGCG affecting the efficacy of ICIs in HCC. Even the effects of EGCG on the TME of HCC remains unclear. But we are hopeful that future research will be carried out.
Challenge of EGCG in the therapy of HCC
Although EGCG can enhance the efficacy of chemotherapy, radiotherapy, and targeted therapy, like other natural compounds, EGCG also has its own relative limitations, such as unstable chemical structure, low oral bioavailability, and potential hepatotoxicity at high doses, which may limit its clinical application in HCC. Encouragingly, these slight deficiencies of EGCG can be improved by chemical modification or nanosystem.
Instability
The stability of EGCG is influenced by many physicochemical factors, such as temperature, pH, oxygen concentration, and ion concentration. Among them, temperature and pH are the main reasons, since they promote the epimerization and autoxidation of EGCG (Mehmood et al., 2022). Specifically, the autooxidation mainly occurs at temperatures below 50°C, producing ROS, while the epimerization of EGCG mainly occurs at higher temperatures, producing GCG. In addition to temperature-induced instability, EGCG is highly unstable at alkaline pH, especially when pH exceeds 8, whereas that is highly stable at the acidic pH ranging from 2.0 to 5.5 (Krupkova et al., 2016b). Considering the temperature and pH factors synthetically, the degradation rate of EGCG is lowest at pH 3 and 25°C, but that is highest at pH 8 and 135°C, which implies EGCG is less stable at high temperature and alkaline pH (Xu et al., 2019). Thus, in addition to improving green tea production processes, the instability of EGCG provides guidance for future EGCG extraction, storage, and solvent selection. Notably, although EGCG remains relatively stable in gastric acid, it is less stable in the alkaline environment of the duodenum, resulting in the possibility of low bioavailability in oral administration. We hope this limitation will be further ameliorated through certain chemical modification of EGCG.
Bioavailability
Due to the hydrophilicity and lack of active transporters of EGCG, EGCG enters intestinal epithelial cells mainly through passive diffusion (Dai et al., 2020; Alam et al., 2022). There are widely distributed efflux transporters on the surface of intestinal epithelial cells, such as P-glycoprotein, multidrug resistance-associated proteins (MRP), which may excrete intracellular EGCG into the intestinal lumen, thus reducing the absorption of EGCG (Krupkova et al., 2016a; Rashidinejad et al., 2021; Mehmood et al., 2022). In the liver, MRP on the surface of hepatocytes also mediates the excretion of polyphenols into bile (Alam et al., 2022). Besides, the metabolism of intestinal microbial flora also affects the absorption of EGCG (Gan et al., 2018). These findings imply that, in addition to the forementioned instability of EGCG in alkaline intestinal fluids, passive absorption, active excretion, and microbial decomposition may limit its oral bioavailability. According to the previous investigation of Nakagawa et al., the maximum plasma concentrations of EGCG in fasting rats and humans were 1047 ng/ml and 156 ng/ml, respectively, after treating them with 56 mg and 97 mg of EGCG orally, which further confirmed the relatively poor bioavailability of EGCG (Nakagawa and Miyazawa, 1997). Therefore, the development of lipophilic EGCG and nano-delivery system will be of substantial significance for the clinical application of EGCG in HCC.
Hepatotoxicity
Although the time-honored tea consumption has demonstrated the safety of green tea and many green tea extracts are commercially available as dietary supplements, a growing number of researchers are concerned about their hepatotoxicity (James et al., 2018). Previously, a host of researchers believed EGCG, as a safe and non-toxic natural compound, has an inhibitory role in tumor cells and a protective effect on healthy cells (Singh et al., 2011; Niedzwiecki et al., 2016; Shiha et al., 2021). Nevertheless, subsequent animal experiments unveiled the risk of hepatotoxicity at high dosages of EGCG. Evidence suggested the intragastric administration of a 750 mg/kg once daily for 3 days caused an 80-fold increase in plasma alanine aminotransferase (ALT) levels and a 59% decrease in hepatic reduced glutathione levels of mice (James et al., 2015). In a clinical study conducted on the safety of EGCG, Siblini et al. reported that 800 mg of EGCG daily was a safe dose for reproductive-aged women, with no drug-induced liver injury in all subjects (Siblini et al., 2023). Paradoxically, in postmenopausal women, Dostal et al. reported 6.7% of them experienced elevated ALT and 1.3% of them suffered from serious adverse events when taking green tea extract containing 843 mg of EGCG daily (Dostal et al., 2015). Although the two clinical trials used similar doses of EGCG, even from the same country, the reasons for the contrary results of hepatotoxicity may be related to age and race of the patients. Overall, even though the hepatotoxicity of EGCG remains controversial, we believe there is sufficient evidence to doubt the hepatotoxic effects at high doses of EGCG. Several researchers suggested 338 mg EGCG ingested as solid doses of tea preparations daily was a safe level for adults based on toxicology and human safety data, while 704 mg EGCG ingested as beverages of tea preparations daily might be an observed safe level based on human adverse event data (Hu et al., 2018). Unfortunately, the safe dose range of EGCG for the patients with HCC remains to be determined based on clinical practice.
Application of EGCG nanosystems in HCC
As an emerging tumor therapy, nanosystems have been widely investigated and applied in recent years (Li et al., 2021). Many natural compounds are coated and delivered by nanosystems to regulate drug release, increase biofilm permeability, alter distribution in the body, which helps to improve the bioavailability and reduce the dosage-induced hepatotoxicity (Gan et al., 2018; Aiello et al., 2021). Therefore, the application of nanosystems is expected to address the challenges of EGCG in the therapy of HCC.
Several EGCG nanosystems have been found to inhibit HCC. Tang et al. designed the drug-delivery nanoparticles loaded with EGCG-functionalized chitin and honokiol, which significantly reduced mitochondrial membrane potential, arrested cell cycle, and inhibited the HCC proliferation (Tang et al., 2018). Mostafa et al. found EGCG-capped gold nanoparticles had better antitumor effects on HCC than EGCG alone (Mostafa et al., 2020). After treatment with this gold nanoparticles, the expressions of caspase-3 and tumor suppressant factors were upregulated, while the expression of c-Myc proteins were decreased in hepatoma cells. Analogously, Gao et al. confirmed EGCG-loaded gold nanocages with photothermal effect significantly upregulated the levels of apoptosis-related proteins in HepG2 cells, including caspase-9, caspase-3 and Bax, whereas inhibited the levels of Bcl-2 (Gao et al., 2022). Moreover, Zhang et al. utilized EGCG and ursolic acid to construct a new “core shell” co-assembly carrier-free nanosystem, which could synergize immunotherapy via activating innate immunity and acquired immunity (Zhang et al., 2021). In conclusion, the development of EGCG nanosystems provides new insights and interventions for the therapy of HCC.
Although the technology of drug-delivery nanosystems in HCC is becoming more sophisticated, the suitable encapsulation methods for EGCG remain limited. In addition, there are still few studies on the safety of EGCG-delivery nanosystems, and no comparison of the efficacy between different nanosystems loaded EGCG in the therapy of HCC. Thus, there is still a host of work and challenges to be done and overcome before EGCG-delivery nanosystems are available clinically.
Clinical studies of EGCG in HCC
Ample preclinical studies have confirmed that EGCG has prominent antitumor effects and comprehensive therapeutic advantages from multiple perspectives. To date, the corresponding clinical trials about the antitumor roles of EGCG have also been performed in several tumors according to ClinicalTrials.gov (www.clinicaltrials.gov) database (Table 4). However, there is still short of relevant intervention trials to unveil the chemoprophylaxis and clinical efficacy of EGCG in HCC. An early clinical trial with limited participants only explored the safety and tolerability of EGCG in patients with hepatic cirrhosis, but did not examine whether EGCG alleviated the pathological changes or clinical symptoms of cirrhosis, nor did it follow up the incidence of HCC (Halegoua-De Marzio et al., 2012). In recent years, a promising clinical trial (NCT03278925) has been registered in the ClinicalTrials.gov database (www.clinicaltrials.gov) to investigate how well EGCG works in prevents HCC in patients with cirrhosis, but no results have been posted or published yet. The results of clinical trials from other oncology fields may provide some references for the therapeutic effect of EGCG in HCC. For example, a clinical randomized controlled trial has confirmed that green tea extract with EGCG as the main active ingredient has the expected safety, which can prevent the colorectal neoplasia to some extent during 3-year follow-up period (Seufferlein et al., 2022). However, the development of colorectal tumors is a relatively long process, and further extension of follow-up period may result in more than expected outcomes. Another phase II preoperative randomized controlled trial involving patients with bladder cancer showed EGCG-rich green tea extract significantly reduced biological markers of bladder cancer tissue, including PCNA and clusterin, which are associated with tumor proliferation, invasion, and migration (Gee et al., 2017). In view of this, we speculate EGCG is promising to show outstanding efficacy in future clinical trials for HCC.
Luckily, numerous observational studies have confirmed that tea consumption is notably relevant to a reduced risk of HCC (Kim et al., 2020). A comprehensive analysis of cohort and case-control studies demonstrated the summary relative risk of HCC among the highest tea drinkers (≥5 cups daily) decreased to 0.62 (95% confidence interval: 0.49–0.79) compared with non-tea drinkers (Ni et al., 2017). Similarly, in Asian, the summary relative risk for the populations with the highest green tea consumption was 0.88 (95% confidence interval: 0.81–0.97) (Huang Y. Q. et al., 2016). To a certain extent, these studies provide evidence for the role of EGCG in the prevention of HCC. Nevertheless, other bioactive ingredients contained in green tea, such as caffeine, polysaccharides, and vitamins, may have a confounding effect on the hepatic tumorigenesis, even if they are not present in the largest amounts. Hence, further specific high-level prospective randomized controlled trials need to be conducted to further confirm the efficacy of EGCG in the future.
Future perspectives
Currently, there is a lack of high-quality clinical trials of EGCG in HCC. Even though EGCG has shown significant antitumor evidence in HCC in animal models, we remain ignorant about whether EGCG can also effectively intervene in the development and improve the prognosis of HCC. Therefore, large sample size and multi-center clinical trials are urgently needed to confirm the safety and efficacy of EGCG in HCC, which may become the future focus of research on natural polyphenol compounds in the field of HCC therapy.
Considering the hepatotoxicity caused by high-dosage EGCG, when investigating the clinical efficacy of EGCG in HCC in the future, we should closely monitor the changes in liver function of patients, especially those who have suffered from cirrhosis or partial hepatectomy. Also, we should focus on finding the optimal dose that can inhibit hepatoma cells without damaging the function of normal hepatocytes. Simultaneously, greater efforts should be made to design the EGCG derivatives and EGCG-delivery systems to help control drug release and increase the stability, bioavailability, and bioactivity in response to the challenges of future clinical applications. Remarkably, preclinical studies have demonstrated that melatonin and EGCG are ideal partners in the therapy of HCC. Melatonin has the dual roles in attenuating the hepatotoxicity and enhancing the anti-cancer potential of EGCG (Wang et al., 2015; Zhang et al., 2019a). Therefore, the combination of EGCG and melatonin is also promising to be a novel therapeutic strategy for HCC in the future.
Although EGCG enhances sensitivity to chemotherapy, radiotherapy and targeted therapy in HCC, the roles of EGCG in HCC immunotherapy have been poorly investigated in vitro and in vivo. Even, the roles and mechanisms of EGCG in the tumor immune microenvironment of HCC remain obscure. Recent research has suggested EGCG inhibits the expression of PD-L1 and PD-L2 in melanoma by targeting the Janus kinase (JAK)/STAT signaling pathway and its downstream transcriptional regulator IRF1, causing the reactivation of the immune response of cytotoxic T lymphocytes (Ravindran Menon et al., 2021). More surprisingly, although EGCG does not work through blocking immune checkpoints, its inhibitory roles in tumor growth are comparable to ICIs. Therefore, we speculate EGCG could increase the therapeutic effects of ICIs, and might even be an alternative therapeutic strategy to block the PD-L1/PD-1 axis. Based on this premise, it is essential to explore the association between EGCG and tumor immune microenvironment of HCC in the future, so as to provide evidence for combined therapy of EGCG and ICIs.
In addition, the antitumor roles of EGCG may involve the mobilization of intracellular copper ions. It was found that with the increase of intracellular copper ion levels, DNA breakage in hepatoma cells induced by EGCG was also increased, which may be correlated with the fact that EGCG produces ROS in a pro-oxidative manner by targeting copper accumulation in hepatoma cells (Farhan et al., 2015). However, recent studies have discovered intracellular accumulation of copper can trigger cuproptosis, a novel copper-induced mode of mitochondrial cell death (Tang et al., 2022; Tsvetkov et al., 2022). Perhaps, there are a partial correlation between the antitumor effects of EGCG and cuproptosis. This still needs to be validated by a host of experimental data in the future.
Conclusion
In summary, sufficient evidence confirms EGCG suppresses the tumorigenesis and progression of HCC by various molecular mechanisms in vitro and in vivo. Furthermore, EGCG significantly enhances the efficacy of chemotherapy, radiotherapy, and targeted therapy, which provides a novel therapeutic strategy for HCC. Nevertheless, we still need to conduct large sample size, multi-center clinical trials to investigate the safety and efficacy of EGCG in HCC therapy to further promote its clinical application in the future.
Author contributions
DL, DC, and XC: conception and design. YC and YS: data collection and visualization. DL: drafting of manuscript. XC and JJ: revision of manuscript. All authors contributed to the article and approved the submitted version.
Funding
This work was supported by grants from National Natural Science Foundation of China (82002932), Health Commission of Jilin Province (2021JC002), Jilin Province Department of Finance (JLSWSRCZX 2021-073), and Scientific and Technological Development program of Jilin Province (20210101333JC).
Acknowledgments
The figure in this review was drew using Figdraw platform. We appreciate the graphic technical support provided by Figdraw.
Conflict of interest
The authors declare that the research was conducted in the absence of any commercial or financial relationships that could be construed as a potential conflict of interest.
Publisher’s note
All claims expressed in this article are solely those of the authors and do not necessarily represent those of their affiliated organizations, or those of the publisher, the editors and the reviewers. Any product that may be evaluated in this article, or claim that may be made by its manufacturer, is not guaranteed or endorsed by the publisher.
References
Abou El Naga, R. N., Azab, S. S., El-Demerdash, E., Shaarawy, S., El-Merzabani, M., and Ammar el, S. M. (2013). Sensitization of TRAIL-induced apoptosis in human hepatocellular carcinoma HepG2 cells by phytochemicals. Life Sci. 92 (10), 555–561. doi:10.1016/j.lfs.2013.01.017
Abudureheman, B., Yu, X., Fang, D., and Zhang, H. (2022). Enzymatic oxidation of tea catechins and its mechanism. Molecules 27 (3), 942. doi:10.3390/molecules27030942
Agioutantis, P. C., Kotsikoris, V., Kolisis, F. N., and Loutrari, H. (2020). RNA-seq data analysis of stimulated hepatocellular carcinoma cells treated with epigallocatechin gallate and fisetin reveals target genes and action mechanisms. Comput. Struct. Biotechnol. J. 18, 686–695. doi:10.1016/j.csbj.2020.03.006
Aiello, P., Consalvi, S., Poce, G., Raguzzini, A., Toti, E., Palmery, M., et al. (2021). Dietary flavonoids: Nano delivery and nanoparticles for cancer therapy. Semin. Cancer Biol. 69, 150–165. doi:10.1016/j.semcancer.2019.08.029
Alam, M., Ali, S., Ashraf, G. M., Bilgrami, A. L., Yadav, D. K., and Hassan, M. I. (2022). Epigallocatechin 3-gallate: From green tea to cancer therapeutics. Food Chem. 379, 132135. doi:10.1016/j.foodchem.2022.132135
Almatroodi, S. A., Almatroudi, A., Khan, A. A., Alhumaydhi, F. A., Alsahli, M. A., and Rahmani, A. H. (2020). Potential therapeutic targets of epigallocatechin gallate (EGCG), the most abundant catechin in green tea, and its role in the therapy of various types of cancer. Molecules 25 (14), 3146. doi:10.3390/molecules25143146
Bai, J., Wu, J., Tang, R., Sun, C., Ji, J., Yin, Z., et al. (2020). Emodin, a natural anthraquinone, suppresses liver cancer in vitro and in vivo by regulating VEGFR(2) and miR-34a. Investig. New Drugs 38 (2), 229–245. doi:10.1007/s10637-019-00777-5
Barbato, L., Bocchetti, M., Di Biase, A., and Regad, T. (2019). Cancer stem cells and targeting strategies. Cells 8 (8), 926. doi:10.3390/cells8080926
Barry, A. E., Baldeosingh, R., Lamm, R., Patel, K., Zhang, K., Dominguez, D. A., et al. (2020). Hepatic stellate cells and hepatocarcinogenesis. Front. Cell Dev. Biol. 8, 709. doi:10.3389/fcell.2020.00709
Benson, A. B., D'Angelica, M. I., Abbott, D. E., Anaya, D. A., Anders, R., Are, C., et al. (2021). Hepatobiliary cancers, version 2.2021, NCCN clinical practice guidelines in oncology. J. Natl. Compr. Canc Netw. 19 (5), 541–565. doi:10.6004/jnccn.2021.0022
Bimonte, S., Albino, V., Piccirillo, M., Nasto, A., Molino, C., Palaia, R., et al. (2019). Epigallocatechin-3-gallate in the prevention and treatment of hepatocellular carcinoma: Experimental findings and translational perspectives. Drug Des. Devel Ther. 13, 611–621. doi:10.2147/dddt.S180079
Cabral-Pacheco, G. A., Garza-Veloz, I., Castruita-De la Rosa, C., Ramirez-Acuña, J. M., Perez-Romero, B. A., Guerrero-Rodriguez, J. F., et al. (2020). The roles of matrix metalloproteinases and their inhibitors in human diseases. Int. J. Mol. Sci. 21 (24), 9739. doi:10.3390/ijms21249739
Calland, N., Albecka, A., Belouzard, S., Wychowski, C., Duverlie, G., Descamps, V., et al. (2012). (-)-Epigallocatechin-3-gallate is a new inhibitor of hepatitis C virus entry. Hepatology 55 (3), 720–729. doi:10.1002/hep.24803
Calland, N., Sahuc, M. E., Belouzard, S., Pène, V., Bonnafous, P., Mesalam, A. A., et al. (2015). Polyphenols inhibit hepatitis C virus entry by a new mechanism of action. J. Virol. 89 (19), 10053–10063. doi:10.1128/jvi.01473-15
Chen, A., Jiang, P., Zeb, F., Wu, X., Xu, C., Chen, L., et al. (2020). EGCG regulates CTR1 expression through its pro-oxidative property in non-small-cell lung cancer cells. J. Cell Physiol. 235 (11), 7970–7981. doi:10.1002/jcp.29451
Chen, C., Guan, J., Gu, X., Chu, Q., and Zhu, H. (2022). Prostaglandin E2 and receptors: Insight into tumorigenesis, tumor progression, and treatment of hepatocellular carcinoma. Front. Cell Dev. Biol. 10, 834859. doi:10.3389/fcell.2022.834859
Chen, J., Chen, L., Lu, T., Xie, Y., Li, C., Jia, Z., et al. (2019). ERα36 is an effective target of epigallocatechin-3-gallate in hepatocellular carcinoma. Int. J. Clin. Exp. Pathol. 12 (9), 3222–3234.
Chen, L., Ye, H. L., Zhang, G., Yao, W. M., Chen, X. Z., Zhang, F. C., et al. (2014). Autophagy inhibition contributes to the synergistic interaction between EGCG and doxorubicin to kill the hepatoma Hep3B cells. PLoS One 9 (1), e85771. doi:10.1371/journal.pone.0085771
Cheung, E. C., and Vousden, K. H. (2022). The role of ROS in tumour development and progression. Nat. Rev. Cancer 22 (5), 280–297. doi:10.1038/s41568-021-00435-0
Chidambaranathan-Reghupaty, S., Fisher, P. B., and Sarkar, D. (2021). Hepatocellular carcinoma (HCC): Epidemiology, etiology and molecular classification. Adv. Cancer Res. 149, 1–61. doi:10.1016/bs.acr.2020.10.001
Ciesek, S., von Hahn, T., Colpitts, C. C., Schang, L. M., Friesland, M., Steinmann, J., et al. (2011). The green tea polyphenol, epigallocatechin-3-gallate, inhibits hepatitis C virus entry. Hepatology 54 (6), 1947–1955. doi:10.1002/hep.24610
Dai, W., Ruan, C., Zhang, Y., Wang, J., Han, J., Shao, Z., et al. (2020). Bioavailability enhancement of EGCG by structural modification and nano-delivery: A review. J. Funct. Foods 65, 103732. doi:10.1016/j.jff.2019.103732
Daina, A., Michielin, O., and Zoete, V. (2019). SwissTargetPrediction: Updated data and new features for efficient prediction of protein targets of small molecules. Nucleic Acids Res. 47 (1), W357–w364. doi:10.1093/nar/gkz382
Darweish, M. M., Abbas, A., Ebrahim, M. A., and Al-Gayyar, M. M. (2014). Chemopreventive and hepatoprotective effects of epigallocatechin-gallate against hepatocellular carcinoma: Role of heparan sulfate proteoglycans pathway. J. Pharm. Pharmacol. 66 (7), 1032–1045. doi:10.1111/jphp.12229
de Almeida, L. G. N., Thode, H., Eslambolchi, Y., Chopra, S., Young, D., Gill, S., et al. (2022). Matrix metalloproteinases: From molecular mechanisms to physiology, pathophysiology, and Pharmacology. Pharmacol. Rev. 74 (3), 712–768. doi:10.1124/pharmrev.121.000349
Dostal, A. M., Samavat, H., Bedell, S., Torkelson, C., Wang, R., Swenson, K., et al. (2015). The safety of green tea extract supplementation in postmenopausal women at risk for breast cancer: Results of the Minnesota green tea trial. Food Chem. Toxicol. 83, 26–35. doi:10.1016/j.fct.2015.05.019
Ezhilarasan, D., and Najimi, M. (2023). Deciphering the possible reciprocal loop between hepatic stellate cells and cancer cells in the tumor microenvironment of the liver. Crit. Rev. Oncol. Hematol. 182, 103902. doi:10.1016/j.critrevonc.2022.103902
Farhan, M., Rizvi, A., Naseem, I., Hadi, S. M., and Ahmad, A. (2015). Targeting increased copper levels in diethylnitrosamine induced hepatocellular carcinoma cells in rats by epigallocatechin-3-gallate. Tumour Biol. 36 (11), 8861–8867. doi:10.1007/s13277-015-3649-y
Feng, J., Dai, W., Mao, Y., Wu, L., Li, J., Chen, K., et al. (2020a). Simvastatin re-sensitizes hepatocellular carcinoma cells to sorafenib by inhibiting HIF-1α/PPAR-γ/PKM2-mediated glycolysis. J. Exp. Clin. Cancer Res. 39 (1), 24. doi:10.1186/s13046-020-1528-x
Feng, J., Li, J., Wu, L., Yu, Q., Ji, J., Wu, J., et al. (2020b). Emerging roles and the regulation of aerobic glycolysis in hepatocellular carcinoma. J. Exp. Clin. Cancer Res. 39 (1), 126. doi:10.1186/s13046-020-01629-4
Ferrari, E., Bettuzzi, S., and Naponelli, V. (2022). The potential of epigallocatechin gallate (EGCG) in targeting autophagy for cancer treatment: A narrative review. Int. J. Mol. Sci. 23 (11), 6075. doi:10.3390/ijms23116075
Forner, A., Reig, M., and Bruix, J. (2018). Hepatocellular carcinoma. Lancet 391 (10127), 1301–1314. doi:10.1016/s0140-6736(18)30010-2
Fung, S., Choi, H. S. J., Gehring, A., and Janssen, H. L. A. (2022). Getting to HBV cure: The promising paths forward. Hepatology 76 (1), 233–250. doi:10.1002/hep.32314
Gan, R. Y., Li, H. B., Sui, Z. Q., and Corke, H. (2018). Absorption, metabolism, anti-cancer effect and molecular targets of epigallocatechin gallate (EGCG): An updated review. Crit. Rev. Food Sci. Nutr. 58 (6), 924–941. doi:10.1080/10408398.2016.1231168
Gao, W., Fan, X., Bi, Y., Zhou, Z., and Yuan, Y. (2022). Preparation of NIR-responsive gold nanocages as efficient carrier for controlling release of EGCG in anticancer application. Front. Chem. 10, 926002. doi:10.3389/fchem.2022.926002
Gee, J. R., Saltzstein, D. R., Kim, K., Kolesar, J., Huang, W., Havighurst, T. C., et al. (2017). A phase II randomized, double-blind, presurgical trial of polyphenon E in bladder cancer patients to evaluate pharmacodynamics and bladder tissue biomarkers. Cancer Prev. Res. (Phila) 10 (5), 298–307. doi:10.1158/1940-6207.Capr-16-0167
Goh, M. J., and Sinn, D. H. (2022). Statin and aspirin for chemoprevention of hepatocellular carcinoma: Time to use or wait further? Clin. Mol. Hepatol. 28 (3), 380–395. doi:10.3350/cmh.2021.0366
Halegoua-De Marzio, D., Kraft, W. K., Daskalakis, C., Ying, X., Hawke, R. L., and Navarro, V. J. (2012). Limited sampling estimates of epigallocatechin gallate exposures in cirrhotic and noncirrhotic patients with hepatitis C after single oral doses of green tea extract. Clin. Ther. 34 (12), 2279–2285. doi:10.1016/j.clinthera.2012.10.009
Hashemi Goradel, N., Najafi, M., Salehi, E., Farhood, B., and Mortezaee, K. (2019). Cyclooxygenase-2 in cancer: A review. J. Cell Physiol. 234 (5), 5683–5699. doi:10.1002/jcp.27411
Hashimoto, O., Nakamura, A., Nakamura, T., Iwamoto, H., Hiroshi, M., Inoue, K., et al. (2014). Methylated-(3'')-epigallocatechin gallate analog suppresses tumor growth in Huh7 hepatoma cells via inhibition of angiogenesis. Nutr. Cancer 66 (4), 728–735. doi:10.1080/01635581.2013.783601
Hogg, S. J., Beavis, P. A., Dawson, M. A., and Johnstone, R. W. (2020). Targeting the epigenetic regulation of antitumour immunity. Nat. Rev. Drug Discov. 19 (11), 776–800. doi:10.1038/s41573-020-0077-5
Hsu, K. C., Sung, T. Y., Lin, C. T., Chiu, Y. Y., Hsu, J. T., Hung, H. C., et al. (2015). Anchor-based classification and type-C inhibitors for tyrosine kinases. Sci. Rep. 5, 10938. doi:10.1038/srep10938
Hu, J., Webster, D., Cao, J., and Shao, A. (2018). The safety of green tea and green tea extract consumption in adults - results of a systematic review. Regul. Toxicol. Pharmacol. 95, 412–433. doi:10.1016/j.yrtph.2018.03.019
Huang, A., Yang, X. R., Chung, W. Y., Dennison, A. R., and Zhou, J. (2020a). Targeted therapy for hepatocellular carcinoma. Signal Transduct. Target Ther. 5 (1), 146. doi:10.1038/s41392-020-00264-x
Huang, C. H., Tsai, S. J., Wang, Y. J., Pan, M. H., Kao, J. Y., and Way, T. D. (2009). EGCG inhibits protein synthesis, lipogenesis, and cell cycle progression through activation of AMPK in p53 positive and negative human hepatoma cells. Mol. Nutr. Food Res. 53 (9), 1156–1165. doi:10.1002/mnfr.200800592
Huang, C., Xu, S., Luo, Z., Li, D., Wang, R., and Wang, T. (2022). Epidemiological evidence between variants in matrix metalloproteinases-2, -7, and -9 and cancer risk. Front. Oncol. 12, 856831. doi:10.3389/fonc.2022.856831
Huang, D., Li, T., Wang, L., Zhang, L., Yan, R., Li, K., et al. (2016a). Hepatocellular carcinoma redirects to ketolysis for progression under nutrition deprivation stress. Cell Res. 26 (10), 1112–1130. doi:10.1038/cr.2016.109
Huang, H. C., Tao, M. H., Hung, T. M., Chen, J. C., Lin, Z. J., and Huang, C. (2014). (-)-Epigallocatechin-3-gallate inhibits entry of Hepatitis B virus into hepatocytes. Antivir. Res. 111, 100–111. doi:10.1016/j.antiviral.2014.09.009
Huang, W., Ding, L., Huang, Q., Hu, H., Liu, S., Yang, X., et al. (2010). Carbonyl reductase 1 as a novel target of (-)-epigallocatechin gallate against hepatocellular carcinoma. Hepatology 52 (2), 703–714. doi:10.1002/hep.23723
Huang, Y. J., Wang, K. L., Chen, H. Y., Chiang, Y. F., and Hsia, S. M. (2020b). Protective effects of epigallocatechin gallate (EGCG) on endometrial, breast, and ovarian cancers. Biomolecules 10 (11), 1481. doi:10.3390/biom10111481
Huang, Y. Q., Lu, X., Min, H., Wu, Q. Q., Shi, X. T., Bian, K. Q., et al. (2016b). Green tea and liver cancer risk: A meta-analysis of prospective cohort studies in asian populations. Nutrition 32 (1), 3–8. doi:10.1016/j.nut.2015.05.021
Irawan, A., Prabowo, E., Riwanto, I., and Atmodjo, W. L. (2022). Anti-angiogenic effect of the combination of low-dose sorafenib and EGCG in HCC-induced Wistar rats. F1000Res 11, 289. doi:10.12688/f1000research.109142.2
James, K. D., Forester, S. C., and Lambert, J. D. (2015). Dietary pretreatment with green tea polyphenol, (-)-epigallocatechin-3-gallate reduces the bioavailability and hepatotoxicity of subsequent oral bolus doses of (-)-epigallocatechin-3-gallate. Food Chem. Toxicol. 76, 103–108. doi:10.1016/j.fct.2014.12.009
James, K. D., Kennett, M. J., and Lambert, J. D. (2018). Potential role of the mitochondria as a target for the hepatotoxic effects of (-)-epigallocatechin-3-gallate in mice. Food Chem. Toxicol. 111, 302–309. doi:10.1016/j.fct.2017.11.029
Jha, S., Kanaujia, S. P., and Limaye, A. M. (2016). Direct inhibition of matrix metalloproteinase-2 (MMP-2) by (-)-epigallocatechin-3-gallate: A possible role for the fibronectin type II repeats. Gene 593 (1), 126–130. doi:10.1016/j.gene.2016.07.061
Jin, J., Chang, Y., Wei, W., He, Y. F., Hu, S. S., Wang, D., et al. (2012). Prostanoid EP1 receptor as the target of (-)-epigallocatechin-3-gallate in suppressing hepatocellular carcinoma cells in vitro. Acta Pharmacol. Sin. 33 (5), 701–709. doi:10.1038/aps.2012.13
Kang, N. J., Lee, K. M., Kim, J. H., Lee, B. K., Kwon, J. Y., Lee, K. W., et al. (2008). Inhibition of gap junctional intercellular communication by the green tea polyphenol (-)-epigallocatechin gallate in normal rat liver epithelial cells. J. Agric. Food Chem. 56 (21), 10422–10427. doi:10.1021/jf801981w
Kang, Q., Tong, Y., Gowd, V., Wang, M., Chen, F., and Cheng, K. W. (2021). Oral administration of EGCG solution equivalent to daily achievable dosages of regular tea drinkers effectively suppresses miR483-3p induced metastasis of hepatocellular carcinoma cells in mice. Food Funct. 12 (8), 3381–3392. doi:10.1039/d1fo00664a
Kedhari Sundaram, M., Haque, S., Somvanshi, P., Bhardwaj, T., and Hussain, A. (2020). Epigallocatechin gallate inhibits HeLa cells by modulation of epigenetics and signaling pathways. 3 Biotech. 10 (11), 484. doi:10.1007/s13205-020-02473-1
Khan, M. A., Hussain, A., Sundaram, M. K., Alalami, U., Gunasekera, D., Ramesh, L., et al. (2015). (-)-Epigallocatechin-3-gallate reverses the expression of various tumor-suppressor genes by inhibiting DNA methyltransferases and histone deacetylases in human cervical cancer cells. Oncol. Rep. 33 (4), 1976–1984. doi:10.3892/or.2015.3802
Kim, T. L., Jeong, G. H., Yang, J. W., Lee, K. H., Kronbichler, A., van der Vliet, H. J., et al. (2020). Tea consumption and risk of cancer: An umbrella review and meta-analysis of observational studies. Adv. Nutr. 11 (6), 1437–1452. doi:10.1093/advances/nmaa077
Kochman, J., Jakubczyk, K., Antoniewicz, J., Mruk, H., and Janda, K. (2020). Health benefits and chemical composition of matcha green tea: A review. Molecules 26 (1), 85. doi:10.3390/molecules26010085
Kole, C., Charalampakis, N., Tsakatikas, S., Vailas, M., Moris, D., Gkotsis, E., et al. (2020). Immunotherapy for hepatocellular carcinoma: A 2021 update. Cancers (Basel) 12 (10), 2859. doi:10.3390/cancers12102859
Krupkova, O., Ferguson, S. J., and Wuertz-Kozak, K. (2016a). Stability of (-)-epigallocatechin gallate and its activity in liquid formulations and delivery systems. J. Nutr. Biochem. 37, 1–12. doi:10.1016/j.jnutbio.2016.01.002
Krupkova, O., Ferguson, S. J., and Wuertz-Kozak, K. (2016b). Stability of (-)-epigallocatechin gallate and its activity in liquid formulations and delivery systems. J. Nutr. Biochem. 37, 1–12. doi:10.1016/j.jnutbio.2016.01.002
Lai, Y. H., Sun, C. P., Huang, H. C., Chen, J. C., Liu, H. K., and Huang, C. (2018). Epigallocatechin gallate inhibits Hepatitis B virus infection in human liver chimeric mice. BMC Complement. Altern. Med. 18 (1), 248. doi:10.1186/s12906-018-2316-4
Lakshmi, S. P., Reddy, A. T., Kodidhela, L. D., and Varadacharyulu, N. C. (2020). The tea catechin epigallocatechin gallate inhibits NF-κB-mediated transcriptional activation by covalent modification. Arch. Biochem. Biophys. 695, 108620. doi:10.1016/j.abb.2020.108620
Lange, N. F., Radu, P., and Dufour, J. F. (2021). Prevention of NAFLD-associated HCC: Role of lifestyle and chemoprevention. J. Hepatol. 75 (5), 1217–1227. doi:10.1016/j.jhep.2021.07.025
Lee, S. J., Lee, K. W., Hur, H. J., Chun, J. Y., Kim, S. Y., and Lee, H. J. (2007). Phenolic phytochemicals derived from red pine (Pinus densiflora) inhibit the invasion and migration of SK-Hep-1 human hepatocellular carcinoma cells. Ann. N. Y. Acad. Sci. 1095, 536–544. doi:10.1196/annals.1397.058
Li, F., Qasim, S., Li, D., and Dou, Q. P. (2022). Updated review on green tea polyphenol epigallocatechin-3-gallate as a cancer epigenetic regulator. Semin. Cancer Biol. 83, 335–352. doi:10.1016/j.semcancer.2020.11.018
Li, F., Wang, Y., Li, D., Chen, Y., Qiao, X., Fardous, R., et al. (2018). Perspectives on the recent developments with green tea polyphenols in drug discovery. Expert Opin. Drug Discov. 13 (7), 643–660. doi:10.1080/17460441.2018.1465923
Li, S., Dai, W., Mo, W., Li, J., Feng, J., Wu, L., et al. (2017). By inhibiting PFKFB3, aspirin overcomes sorafenib resistance in hepatocellular carcinoma. Int. J. Cancer 141 (12), 2571–2584. doi:10.1002/ijc.31022
Li, S., Wu, L., Feng, J., Li, J., Liu, T., Zhang, R., et al. (2016). In vitro and in vivo study of epigallocatechin-3-gallate-induced apoptosis in aerobic glycolytic hepatocellular carcinoma cells involving inhibition of phosphofructokinase activity. Sci. Rep. 6, 28479. doi:10.1038/srep28479
Li, X., He, S., and Ma, B. (2020). Autophagy and autophagy-related proteins in cancer. Mol. Cancer 19 (1), 12. doi:10.1186/s12943-020-1138-4
Li, X., Li, W., Wang, M., and Liao, Z. (2021). Magnetic nanoparticles for cancer theranostics: Advances and prospects. J. Control Release 335, 437–448. doi:10.1016/j.jconrel.2021.05.042
Liang, G., Tang, A., Lin, X., Li, L., Zhang, S., Huang, Z., et al. (2010). Green tea catechins augment the antitumor activity of doxorubicin in an in vivo mouse model for chemoresistant liver cancer. Int. J. Oncol. 37 (1), 111–123.
Liao, S., Lin, J., Liu, J., Chen, T., Xu, M., and Zheng, J. (2019). Chemoprevention of elite tea variety CFT-1 rich in EGCG against chemically induced liver cancer in rats. Food Sci. Nutr. 7 (8), 2647–2665. doi:10.1002/fsn3.1121
Liao, Z. H., Zhu, H. Q., Chen, Y. Y., Chen, R. L., Fu, L. X., Li, L., et al. (2020). The epigallocatechin gallate derivative Y6 inhibits human hepatocellular carcinoma by inhibiting angiogenesis in MAPK/ERK1/2 and PI3K/AKT/HIF-1α/VEGF dependent pathways. J. Ethnopharmacol. 259, 112852. doi:10.1016/j.jep.2020.112852
Liu, K., Li, F., Sun, Q., Lin, N., Han, H., You, K., et al. (2019). p53 β-hydroxybutyrylation attenuates p53 activity. Cell Death Dis. 10 (3), 243. doi:10.1038/s41419-019-1463-y
Liu, Y. R., Wang, J. Q., Huang, Z. G., Chen, R. N., Cao, X., Zhu, D. C., et al. (2021). Histone deacetylase-2: A potential regulator and therapeutic target in liver disease (review). Int. J. Mol. Med. 48 (1), 131. doi:10.3892/ijmm.2021.4964
Llovet, J. M., Kelley, R. K., Villanueva, A., Singal, A. G., Pikarsky, E., Roayaie, S., et al. (2021). Hepatocellular carcinoma. Nat. Rev. Dis. Prim. 7 (1), 6. doi:10.1038/s41572-020-00240-3
Luna-Cuadros, M. A., Chen, H. W., Hanif, H., Ali, M. J., Khan, M. M., and Lau, D. T. (2022). Risk of hepatocellular carcinoma after hepatitis C virus cure. World J. Gastroenterol. 28 (1), 96–107. doi:10.3748/wjg.v28.i1.96
Luo, K. W., Wei, C., Lung, W. Y., Wei, X. Y., Cheng, B. H., Cai, Z. M., et al. (2017). EGCG inhibited bladder cancer SW780 cell proliferation and migration both in vitro and in vivo via down-regulation of NF-κB and MMP-9. J. Nutr. Biochem. 41, 56–64. doi:10.1016/j.jnutbio.2016.12.004
Mathew, S., Faheem, M., Archunan, G., Ilyas, M., Begum, N., Jahangir, S., et al. (2014). In silico studies of medicinal compounds against hepatitis C capsid protein from north India. Bioinform Biol. Insights 8, 159–168. doi:10.4137/bbi.S15211
McGlynn, K. A., Petrick, J. L., and El-Serag, H. B. (2021). Epidemiology of hepatocellular carcinoma. Hepatology 73 (1), 4–13. doi:10.1002/hep.31288
McGlynn, K. A., Petrick, J. L., and London, W. T. (2015). Global epidemiology of hepatocellular carcinoma: An emphasis on demographic and regional variability. Clin. Liver Dis. 19 (2), 223–238. doi:10.1016/j.cld.2015.01.001
Mehmood, S., Maqsood, M., Mahtab, N., Khan, M. I., Sahar, A., Zaib, S., et al. (2022). Epigallocatechin gallate: Phytochemistry, bioavailability, utilization challenges, and strategies. J. Food Biochem. 46 (8), e14189. doi:10.1111/jfbc.14189
Mekky, R. Y., El-Ekiaby, N., El Sobky, S. A., Elemam, N. M., Youness, R. A., El-Sayed, M., et al. (2019). Epigallocatechin gallate (EGCG) and miR-548m reduce HCV entry through repression of CD81 receptor in HCV cell models. Arch. Virol. 164 (6), 1587–1595. doi:10.1007/s00705-019-04232-x
Mekky, R. Y., El-Ekiaby, N. M., Hamza, M. T., Elemam, N. M., El-Sayed, M., Esmat, G., et al. (2015). Mir-194 is a hepatocyte gate keeper hindering HCV entry through targeting CD81 receptor. J. Infect. 70 (1), 78–87. doi:10.1016/j.jinf.2014.08.013
Moreno, F. S., Heidor, R., and Pogribny, I. P. (2016). Nutritional epigenetics and the prevention of hepatocellular carcinoma with bioactive food constituents. Nutr. Cancer 68 (5), 719–733. doi:10.1080/01635581.2016.1180410
Morse, M. A., Sun, W., Kim, R., He, A. R., Abada, P. B., Mynderse, M., et al. (2019). The role of angiogenesis in hepatocellular carcinoma. Clin. Cancer Res. 25 (3), 912–920. doi:10.1158/1078-0432.Ccr-18-1254
Mostafa, S. M., Gamal-Eldeen, A. M., Maksoud, N. A. E., and Fahmi, A. A. (2020). Epigallocatechin gallate-capped gold nanoparticles enhanced the tumor suppressors let-7a and miR-34a in hepatocellular carcinoma cells. Acad Bras Cienc 92 (4), e20200574. doi:10.1590/0001-3765202020200574
Musial, C., Kuban-Jankowska, A., and Gorska-Ponikowska, M. (2020). Beneficial properties of green tea catechins. Int. J. Mol. Sci. 21 (5), 1744. doi:10.3390/ijms21051744
Nagaraju, G. P., Dariya, B., Kasa, P., Peela, S., and El-Rayes, B. F. (2022). Epigenetics in hepatocellular carcinoma. Semin. Cancer Biol. 86 (3), 622–632. doi:10.1016/j.semcancer.2021.07.017
Nakagawa, K., and Miyazawa, T. (1997). Chemiluminescence-high-performance liquid chromatographic determination of tea catechin, (-)-epigallocatechin 3-gallate, at picomole levels in rat and human plasma. Anal. Biochem. 248 (1), 41–49. doi:10.1006/abio.1997.2098
Nakagawa, S., Kojima, Y., Sekino, K., and Yamato, S. (2013). Effect of polyphenols on 3-hydroxy-3-methylglutaryl-coenzyme A lyase activity in human hepatoma HepG2 cell extracts. Biol. Pharm. Bull. 36 (12), 1902–1906. doi:10.1248/bpb.b13-00334
Nakano, S., Megro, S. I., Hase, T., Suzuki, T., Isemura, M., Nakamura, Y., et al. (2018). Computational molecular docking and X-ray crystallographic studies of catechins in new drug design strategies. Molecules 23 (8), 2020. doi:10.3390/molecules23082020
Negri, A., Naponelli, V., Rizzi, F., and Bettuzzi, S. (2018). Molecular targets of epigallocatechin-gallate (EGCG): A special focus on signal transduction and cancer. Nutrients 10 (12), 1936. doi:10.3390/nu10121936
Ni, C. X., Gong, H., Liu, Y., Qi, Y., Jiang, C. L., and Zhang, J. P. (2017). Green tea consumption and the risk of liver cancer: A meta-analysis. Nutr. Cancer 69 (2), 211–220. doi:10.1080/01635581.2017.1263754
Niedzwiecki, A., Roomi, M. W., Kalinovsky, T., and Rath, M. (2016). Anticancer efficacy of polyphenols and their combinations. Nutrients 8 (9), 552. doi:10.3390/nu8090552
Nishida, H., Omori, M., Fukutomi, Y., Ninomiya, M., Nishiwaki, S., Suganuma, M., et al. (1994). Inhibitory effects of (-)-epigallocatechin gallate on spontaneous hepatoma in C3H/HeNCrj mice and human hepatoma-derived PLC/PRF/5 cells. Jpn. J. Cancer Res. 85 (3), 221–225. doi:10.1111/j.1349-7006.1994.tb02085.x
Nishikawa, T., Nakajima, T., Moriguchi, M., Jo, M., Sekoguchi, S., Ishii, M., et al. (2006). A green tea polyphenol, epigalocatechin-3-gallate, induces apoptosis of human hepatocellular carcinoma, possibly through inhibition of Bcl-2 family proteins. J. Hepatol. 44 (6), 1074–1082. doi:10.1016/j.jhep.2005.11.045
Ouyang, J., Zhu, K., Liu, Z., and Huang, J. (2020). Prooxidant effects of epigallocatechin-3-gallate in health benefits and potential adverse effect. Oxid. Med. Cell Longev. 2020, 9723686. doi:10.1155/2020/9723686
Pang, J. Y., Zhao, K. J., Wang, J. B., Ma, Z. J., and Xiao, X. H. (2014). Green tea polyphenol, epigallocatechin-3-gallate, possesses the antiviral activity necessary to fight against the Hepatitis B virus replication in vitro. J. Zhejiang Univ. Sci. B 15 (6), 533–539. doi:10.1631/jzus.B1300307
Park, H., Park, H., Baek, J., Moon, H., and Ro, S. W. (2022). Target therapy for hepatocellular carcinoma: Beyond receptor tyrosine kinase inhibitors and immune checkpoint inhibitors. Biol. (Basel) 11 (4), 585. doi:10.3390/biology11040585
Qadir Nanakali, N. M., Maleki Dana, P., Sadoughi, F., Asemi, Z., Sharifi, M., Asemi, R., et al. (2022). The role of dietary polyphenols in alternating DNA methylation in cancer. Crit. Rev. Food Sci. Nutr., 1–14. doi:10.1080/10408398.2022.2100313
Rashidinejad, A., Boostani, S., Babazadeh, A., Rehman, A., Rezaei, A., Akbari-Alavijeh, S., et al. (2021). Opportunities and challenges for the nanodelivery of green tea catechins in functional foods. Food Res. Int. 142, 110186. doi:10.1016/j.foodres.2021.110186
Ravindran Menon, D., Li, Y., Yamauchi, T., Osborne, D. G., Vaddi, P. K., Wempe, M. F., et al. (2021). EGCG inhibits tumor growth in melanoma by targeting JAK-STAT signaling and its downstream PD-L1/PD-L2-PD1 Axis in tumors and enhancing cytotoxic T-cell responses. Pharm. (Basel) 14 (11), 1081. doi:10.3390/ph14111081
Romano, A., and Martel, F. (2021). The role of EGCG in breast cancer prevention and therapy. Mini Rev. Med. Chem. 21 (7), 883–898. doi:10.2174/1389557520999201211194445
Roomi, M. W., Monterrey, J. C., Kalinovsky, T., Rath, M., and Niedzwiecki, A. (2010). Comparative effects of EGCG, green tea and a nutrient mixture on the patterns of MMP-2 and MMP-9 expression in cancer cell lines. Oncol. Rep. 24 (3), 747–757. doi:10.3892/or_00000917
Ruan, Q., Wang, H., Burke, L. J., Bridle, K. R., Li, X., Zhao, C. X., et al. (2020). Therapeutic modulators of hepatic stellate cells for hepatocellular carcinoma. Int. J. Cancer 147 (6), 1519–1527. doi:10.1002/ijc.32899
Sabry, D., Abdelaleem, O. O., El Amin Ali, A. M., Mohammed, R. A., Abdel-Hameed, N. D., Hassouna, A., et al. (2019). Anti-proliferative and anti-apoptotic potential effects of epigallocatechin-3-gallate and/or metformin on hepatocellular carcinoma cells: In vitro study. Mol. Biol. Rep. 46 (2), 2039–2047. doi:10.1007/s11033-019-04653-6
Saeki, K., Hayakawa, S., Nakano, S., Ito, S., Oishi, Y., Suzuki, Y., et al. (2018). In vitro and in silico studies of the molecular interactions of epigallocatechin-3-O-gallate (EGCG) with proteins that explain the health benefits of green tea. Molecules 23 (6), 1295. doi:10.3390/molecules23061295
Samavat, H., Wu, A. H., Ursin, G., Torkelson, C. J., Wang, R., Yu, M. C., et al. (2019). Green tea catechin extract supplementation does not influence circulating sex hormones and insulin-like growth factor Axis proteins in a randomized controlled trial of postmenopausal women at high risk of breast cancer. J. Nutr. 149 (4), 619–627. doi:10.1093/jn/nxy316
Sarkar, J., Nandy, S. K., Chowdhury, A., Chakraborti, T., and Chakraborti, S. (2016). Inhibition of MMP-9 by green tea catechins and prediction of their interaction by molecular docking analysis. Biomed. Pharmacother. 84, 340–347. doi:10.1016/j.biopha.2016.09.049
Satonaka, H., Ishida, K., Takai, M., Koide, R., Shigemasa, R., Ueyama, J., et al. (2017). (-)-Epigallocatechin-3-gallate down-regulates doxorubicin-induced overexpression of P-glycoprotein through the coordinate inhibition of PI3K/Akt and MEK/ERK signaling pathways. Anticancer Res. 37 (11), 6071–6077. doi:10.21873/anticanres.12055
Sazuka, M., Imazawa, H., Shoji, Y., Mita, T., Hara, Y., and Isemura, M. (1997). Inhibition of collagenases from mouse lung carcinoma cells by green tea catechins and black tea theaflavins. Biosci. Biotechnol. Biochem. 61 (9), 1504–1506. doi:10.1271/bbb.61.1504
Scheau, C., Badarau, I. A., Costache, R., Caruntu, C., Mihai, G. L., Didilescu, A. C., et al. (2019). The role of matrix metalloproteinases in the epithelial-mesenchymal transition of hepatocellular carcinoma. Anal. Cell Pathol. (Amst) 2019, 9423907. doi:10.1155/2019/9423907
Seufferlein, T., Ettrich, T. J., Menzler, S., Messmann, H., Kleber, G., Zipprich, A., et al. (2022). Green tea extract to prevent colorectal adenomas, results of a randomized, placebo-controlled clinical trial. Am. J. Gastroenterol. 117 (6), 884–894. doi:10.14309/ajg.0000000000001706
Shahid, F., NoreenAli, R., Badshah, S. L., Jamal, S. B., Ullah, R., et al. (2021). Identification of potential HCV inhibitors based on the interaction of epigallocatechin-3-gallate with viral envelope proteins. Molecules 26 (5), 1257. doi:10.3390/molecules26051257
Shan, X., Li, Y., Meng, X., Wang, P., Jiang, P., and Feng, Q. (2014). Curcumin and (-)-epigallocatechin-3-gallate attenuate acrylamide-induced proliferation in HepG2 cells. Food Chem. Toxicol. 66, 194–202. doi:10.1016/j.fct.2014.01.046
Shen, C., Jiang, X., Li, M., and Luo, Y. (2023). Hepatitis virus and hepatocellular carcinoma: Recent advances. Cancers (Basel) 15 (2), 533. doi:10.3390/cancers15020533
Shen, X., Zhang, Y., Feng, Y., Zhang, L., Li, J., Xie, Y. A., et al. (2014). Epigallocatechin-3-gallate inhibits cell growth, induces apoptosis and causes S phase arrest in hepatocellular carcinoma by suppressing the AKT pathway. Int. J. Oncol. 44 (3), 791–796. doi:10.3892/ijo.2014.2251
Shen, X., Zhao, J., Wang, Q., Chen, P., Hong, Y., He, X., et al. (2022). The invasive potential of hepatoma cells induced by radiotherapy is related to the activation of hepatic stellate cells and could be inhibited by EGCG through the TLR4 signaling pathway. Radiat. Res. 197 (4), 365–375. doi:10.1667/rade-21-00129.1
Shiha, G., Soliman, R., Elbasiony, M., Darwish, N. H. E., and Mousa, S. A. (2021). Novel combined single dose anti-hepatitis C therapy: A pilot study. Sci. Rep. 11 (1), 4623. doi:10.1038/s41598-021-84066-3
Shimizu, M., Adachi, S., Masuda, M., Kozawa, O., and Moriwaki, H. (2011a). Cancer chemoprevention with green tea catechins by targeting receptor tyrosine kinases. Mol. Nutr. Food Res. 55 (6), 832–843. doi:10.1002/mnfr.201000622
Shimizu, M., Sakai, H., Shirakami, Y., Yasuda, Y., Kubota, M., Terakura, D., et al. (2011b). Preventive effects of (-)-epigallocatechin gallate on diethylnitrosamine-induced liver tumorigenesis in obese and diabetic C57BL/KsJ-db/db Mice. Cancer Prev. Res. (Phila) 4 (3), 396–403. doi:10.1158/1940-6207.Capr-10-0331
Shimizu, M., Shirakami, Y., Sakai, H., Tatebe, H., Nakagawa, T., Hara, Y., et al. (2008). EGCG inhibits activation of the insulin-like growth factor (IGF)/IGF-1 receptor axis in human hepatocellular carcinoma cells. Cancer Lett. 262 (1), 10–18. doi:10.1016/j.canlet.2007.11.026
Shirakami, Y., Shimizu, M., Adachi, S., Sakai, H., Nakagawa, T., Yasuda, Y., et al. (2009). (-) Epigallocatechin gallate suppresses the growth of human hepatocellular carcinoma cells by inhibiting activation of the vascular endothelial growth factor-vascular endothelial growth factor receptor axis. Cancer Sci. 100 (10), 1957–1962. doi:10.1111/j.1349-7006.2009.01241.x
Siblini, H., Al-Hendy, A., Segars, J., González, F., Taylor, H. S., Singh, B., et al. (2023). Assessing the hepatic safety of epigallocatechin gallate (EGCG) in reproductive-aged women. Nutrients 15 (2), 320. doi:10.3390/nu15020320
Siegel, R. L., Miller, K. D., Fuchs, H. E., and Jemal, A. (2022). Cancer statistics, 2022. CA Cancer J. Clin. 72 (1), 7–33. doi:10.3322/caac.21708
Singh, B. N., Shankar, S., and Srivastava, R. K. (2011). Green tea catechin, epigallocatechin-3-gallate (EGCG): Mechanisms, perspectives and clinical applications. Biochem. Pharmacol. 82 (12), 1807–1821. doi:10.1016/j.bcp.2011.07.093
Singh, P., and Bast, F. (2015). Screening of multi-targeted natural compounds for receptor tyrosine kinases inhibitors and biological evaluation on cancer cell lines, in silico and in vitro. Med. Oncol. 32 (9), 233. doi:10.1007/s12032-015-0678-8
Song, Z., Chen, W., Athavale, D., Ge, X., Desert, R., Das, S., et al. (2021). Osteopontin takes center stage in chronic liver disease. Hepatology 73 (4), 1594–1608. doi:10.1002/hep.31582
Srinivas, U. S., Tan, B. W. Q., Vellayappan, B. A., and Jeyasekharan, A. D. (2019). ROS and the DNA damage response in cancer. Redox Biol. 25, 101084. doi:10.1016/j.redox.2018.101084
Suhail, M., Rehan, M., Tarique, M., Tabrez, S., Husain, A., and Zughaibi, T. A. (2022). Targeting a transcription factor NF-κB by green tea catechins using in silico and in vitro studies in pancreatic cancer. Front. Nutr. 9, 1078642. doi:10.3389/fnut.2022.1078642
Sung, H., Ferlay, J., Siegel, R. L., Laversanne, M., Soerjomataram, I., Jemal, A., et al. (2021). Global cancer statistics 2020: GLOBOCAN estimates of incidence and mortality worldwide for 36 cancers in 185 countries. CA Cancer J. Clin. 71 (3), 209–249. doi:10.3322/caac.21660
Sur, S., Pal, D., Mandal, S., Roy, A., and Panda, C. K. (2016). Tea polyphenols epigallocatechin gallete and theaflavin restrict mouse liver carcinogenesis through modulation of self-renewal Wnt and hedgehog pathways. J. Nutr. Biochem. 27, 32–42. doi:10.1016/j.jnutbio.2015.08.016
Tanabe, H., Suzuki, T., Ohishi, T., Isemura, M., Nakamura, Y., and Unno, K. (2023). Effects of epigallocatechin-3-gallate on matrix metalloproteinases in terms of its anticancer activity. Molecules 28 (2), 525. doi:10.3390/molecules28020525
Tang, D., Chen, X., and Kroemer, G. (2022). Cuproptosis: A copper-triggered modality of mitochondrial cell death. Cell Res. 32 (5), 417–418. doi:10.1038/s41422-022-00653-7
Tang, P., Sun, Q., Yang, H., Tang, B., Pu, H., and Li, H. (2018). Honokiol nanoparticles based on epigallocatechin gallate functionalized chitin to enhance therapeutic effects against liver cancer. Int. J. Pharm. 545 (1-2), 74–83. doi:10.1016/j.ijpharm.2018.04.060
Tang, Y., Cao, J., Cai, Z., An, H., Li, Y., Peng, Y., et al. (2020). Epigallocatechin gallate induces chemopreventive effects on rats with diethylnitrosamine-induced liver cancer via inhibition of cell division cycle 25A. Mol. Med. Rep. 22 (5), 3873–3885. doi:10.3892/mmr.2020.11463
Teng, F., Zhang, J. X., Chang, Q. M., Wu, X. B., Tang, W. G., Wang, J. F., et al. (2020). LncRNA MYLK-AS1 facilitates tumor progression and angiogenesis by targeting miR-424-5p/E2F7 axis and activating VEGFR-2 signaling pathway in hepatocellular carcinoma. J. Exp. Clin. Cancer Res. 39 (1), 235. doi:10.1186/s13046-020-01739-z
Tolomeo, M., and Cascio, A. (2021). The multifaced role of STAT3 in cancer and its implication for anticancer therapy. Int. J. Mol. Sci. 22 (2), 603. doi:10.3390/ijms22020603
Trisha, A. T., Shakil, M. H., Talukdar, S., Rovina, K., Huda, N., and Zzaman, W. (2022). Tea polyphenols and their preventive measures against cancer: Current trends and directions. Foods 11 (21), 3349. doi:10.3390/foods11213349
Tsang, W. P., and Kwok, T. T. (2010). Epigallocatechin gallate up-regulation of miR-16 and induction of apoptosis in human cancer cells. J. Nutr. Biochem. 21 (2), 140–146. doi:10.1016/j.jnutbio.2008.12.003
Tsvetkov, P., Coy, S., Petrova, B., Dreishpoon, M., Verma, A., Abdusamad, M., et al. (2022). Copper induces cell death by targeting lipoylated TCA cycle proteins. Science 375 (6586), 1254–1261. doi:10.1126/science.abf0529
Van Aller, G. S., Carson, J. D., Tang, W., Peng, H., Zhao, L., Copeland, R. A., et al. (2011). Epigallocatechin gallate (EGCG), a major component of green tea, is a dual phosphoinositide-3-kinase/mTOR inhibitor. Biochem. Biophys. Res. Commun. 406 (2), 194–199. doi:10.1016/j.bbrc.2011.02.010
Vogel, A., Meyer, T., Sapisochin, G., Salem, R., and Saborowski, A. (2022). Hepatocellular carcinoma. Lancet 400 (10360), 1345–1362. doi:10.1016/s0140-6736(22)01200-4
Wang, D., Wei, Y., Wang, T., Wan, X., Yang, C. S., Reiter, R. J., et al. (2015). Melatonin attenuates (-)-epigallocatehin-3-gallate-triggered hepatotoxicity without compromising its downregulation of hepatic gluconeogenic and lipogenic genes in mice. J. Pineal Res. 59 (4), 497–507. doi:10.1111/jpi.12281
Wang, J., Liu, W., Chen, Z., and Chen, H. (2017a). Physicochemical characterization of the oolong tea polysaccharides with high molecular weight and their synergistic effects in combination with polyphenols on hepatocellular carcinoma. Biomed. Pharmacother. 90, 160–170. doi:10.1016/j.biopha.2017.03.059
Wang, L., Li, P., and Feng, K. (2023). EGCG adjuvant chemotherapy: Current status and future perspectives. Eur. J. Med. Chem. 250, 115197. doi:10.1016/j.ejmech.2023.115197
Wang, W., Xiong, X., Li, X., Zhang, Q., Yang, W., and Du, L. (2019). In silico investigation of the anti-tumor mechanisms of epigallocatechin-3-gallate. Molecules 24 (7), 1445. doi:10.3390/molecules24071445
Wang, Y., Li, J., Wang, X., Peña, J. C., Li, K., Zhang, T., et al. (2016). (-)-Epigallocatechin-3-Gallate enhances hepatitis C virus double-stranded RNA intermediates-triggered innate immune responses in hepatocytes. Sci. Rep. 6, 21595. doi:10.1038/srep21595
Wang, Y., Ren, X., Deng, C., Yang, L., Yan, E., Guo, T., et al. (2013). Mechanism of the inhibition of the STAT3 signaling pathway by EGCG. Oncol. Rep. 30 (6), 2691–2696. doi:10.3892/or.2013.2743
Wang, Y. Z., Li, J. L., Wang, X., Zhang, T., and Ho, W. Z. (2017b). (-)-Epigallocatechin-3-gallate enhances poly I:C-induced interferon-λ1 production and inhibits hepatitis C virus replication in hepatocytes. World J. Gastroenterol. 23 (32), 5895–5903. doi:10.3748/wjg.v23.i32.5895
Wang, Z. Y., Li, Y. Q., Guo, Z. W., Zhou, X. H., Lu, M. D., Xue, T. C., et al. (2020). ERK1/2-HNF4α axis is involved in epigallocatechin-3-gallate inhibition of HBV replication. Acta Pharmacol. Sin. 41 (2), 278–285. doi:10.1038/s41401-019-0302-0
Wen, Y., Zhao, R., Gupta, P., Fan, Y., Zhang, Y., Huang, Z., et al. (2019). The epigallocatechin gallate derivative Y(6) reverses drug resistance mediated by the ABCB1 transporter both in vitro and in vivo. Acta Pharm. Sin. B 9 (2), 316–323. doi:10.1016/j.apsb.2018.10.001
Won, H. R., Lee, P., Oh, S. R., and Kim, Y. M. (2021). Epigallocatechin-3-Gallate suppresses the expression of TNF-α-induced MMP-1 via MAPK/ERK signaling pathways in human dermal fibroblasts. Biol. Pharm. Bull. 44 (1), 18–24. doi:10.1248/bpb.b20-00304
Wubetu, G. Y., Shimada, M., Morine, Y., Ikemoto, T., Ishikawa, D., Iwahashi, S., et al. (2016). Epigallocatechin gallate hinders human hepatoma and colon cancer sphere formation. J. Gastroenterol. Hepatol. 31 (1), 256–264. doi:10.1111/jgh.13069
Xia, S., Pan, Y., Liang, Y., Xu, J., and Cai, X. (2020). The microenvironmental and metabolic aspects of sorafenib resistance in hepatocellular carcinoma. EBioMedicine 51, 102610. doi:10.1016/j.ebiom.2019.102610
Xu, J., Gu, W., Li, C., Li, X., Xing, G., Li, Y., et al. (2016). Epigallocatechin gallate inhibits Hepatitis B virus via farnesoid X receptor alpha. J. Nat. Med. 70 (3), 584–591. doi:10.1007/s11418-016-0980-6
Xu, T., Guo, P., Pi, C., He, Y., Yang, H., Hou, Y., et al. (2020). Synergistic effects of curcumin and 5-fluorouracil on the hepatocellular carcinoma in vivo and vitro through regulating the expression of COX-2 and NF-κB. J. Cancer 11 (13), 3955–3964. doi:10.7150/jca.41783
Xu, Y.-Q., Yu, P., and Zhou, W. (2019). Combined effect of pH and temperature on the stability and antioxidant capacity of epigallocatechin gallate (EGCG) in aqueous system. J. Food Eng. 250, 46–54. doi:10.1016/j.jfoodeng.2019.01.016
Yang, G., Wan, P., Zhang, Y., Tan, Q., Qudus, M. S., Yue, Z., et al. (2022a). Innate immunity, inflammation, and intervention in HBV infection. Viruses 14 (10), 2275. doi:10.3390/v14102275
Yang, H., Wang, M., Sun, H., Zhu, S., and Jin, J. (2019a). Synergetic effect of EP1 receptor antagonist and (-)-Epigallocatechin-3-gallate in hepatocellular carcinoma. Pharmacology 104 (5-6), 267–275. doi:10.1159/000502076
Yang, J. D., Hainaut, P., Gores, G. J., Amadou, A., Plymoth, A., and Roberts, L. R. (2019b). A global view of hepatocellular carcinoma: Trends, risk, prevention and management. Nat. Rev. Gastroenterol. Hepatol. 16 (10), 589–604. doi:10.1038/s41575-019-0186-y
Yang, L., Jia, L., Li, X., Zhang, K., Wang, X., He, Y., et al. (2022b). Prooxidant activity-based guideline for a beneficial combination of (-)-epigallocatechin-3-gallate and chlorogenic acid. Food Chem. 386, 132812. doi:10.1016/j.foodchem.2022.132812
Yang, X. W., Wang, X. L., Cao, L. Q., Jiang, X. F., Peng, H. P., Lin, S. M., et al. (2012). Green tea polyphenol epigallocatechin-3-gallate enhances 5-fluorouracil-induced cell growth inhibition of hepatocellular carcinoma cells. Hepatol. Res. 42 (5), 494–501. doi:10.1111/j.1872-034X.2011.00947.x
Yin, Y., Chen, C., Chen, J., Zhan, R., Zhang, Q., Xu, X., et al. (2017). Cell surface GRP78 facilitates hepatoma cells proliferation and migration by activating IGF-IR. Cell Signal 35, 154–162. doi:10.1016/j.cellsig.2017.04.003
You, L., Wu, W., Wang, X., Fang, L., Adam, V., Nepovimova, E., et al. (2021). The role of hypoxia-inducible factor 1 in tumor immune evasion. Med. Res. Rev. 41 (3), 1622–1643. doi:10.1002/med.21771
Zapf, M. A., Kothari, A. N., Weber, C. E., Arffa, M. L., Wai, P. Y., Driver, J., et al. (2015). Green tea component epigallocatechin-3-gallate decreases expression of osteopontin via a decrease in mRNA half-life in cell lines of metastatic hepatocellular carcinoma. Surgery 158 (4), 1039–1047. discussion 1047-1038. doi:10.1016/j.surg.2015.06.011
Zeng, R. W., Yong, J. N., Tan, D. J. H., Fu, C. E., Lim, W. H., Xiao, J., et al. (2023). Meta-analysis: Chemoprevention of hepatocellular carcinoma with statins, aspirin and metformin. Aliment. Pharmacol. Ther. 57 (6), 600–609. doi:10.1111/apt.17371
Zhang, B., Jiang, J., Wu, P., Zou, J., Le, J., Lin, J., et al. (2021). A smart dual-drug nanosystem based on co-assembly of plant and food-derived natural products for synergistic HCC immunotherapy. Acta Pharm. Sin. B 11 (1), 246–257. doi:10.1016/j.apsb.2020.07.026
Zhang, G., Miura, Y., and Yagasaki, K. (2000). Suppression of adhesion and invasion of hepatoma cells in culture by tea compounds through antioxidative activity. Cancer Lett. 159 (2), 169–173. doi:10.1016/s0304-3835(00)00545-0
Zhang, L., He, Y., Wu, X., Zhao, G., Zhang, K., Yang, C. S., et al. (2019a). Melatonin and (-)-Epigallocatechin-3-Gallate: Partners in fighting cancer. Cells 8 (7), 745. doi:10.3390/cells8070745
Zhang, L., Ho, C. T., Zhou, J., Santos, J. S., Armstrong, L., and Granato, D. (2019b). Chemistry and biological activities of processed camellia sinensis teas: A comprehensive review. Compr. Rev. Food Sci. Food Saf. 18 (5), 1474–1495. doi:10.1111/1541-4337.12479
Zhang, Q., Tang, X., Lu, Q., Zhang, Z., Rao, J., and Le, A. D. (2006). Green tea extract and (-)-epigallocatechin-3-gallate inhibit hypoxia- and serum-induced HIF-1alpha protein accumulation and VEGF expression in human cervical carcinoma and hepatoma cells. Mol. Cancer Ther. 5 (5), 1227–1238. doi:10.1158/1535-7163.Mct-05-0490
Zhang, S., Guo, N., Wan, G., Zhang, T., Li, C., Wang, Y., et al. (2019c). pH and redox dual-responsive nanoparticles based on disulfide-containing poly(β-amino ester) for combining chemotherapy and COX-2 inhibitor to overcome drug resistance in breast cancer. J. Nanobiotechnology 17 (1), 109. doi:10.1186/s12951-019-0540-9
Zhang, Y., Duan, W., Owusu, L., Wu, D., and Xin, Y. (2015). Epigallocatechin-3-gallate induces the apoptosis of hepatocellular carcinoma LM6 cells but not non-cancerous liver cells. Int. J. Mol. Med. 35 (1), 117–124. doi:10.3892/ijmm.2014.1988
Zhang, Y., Owusu, L., Duan, W., Jiang, T., Zang, S., Ahmed, A., et al. (2013). Anti-metastatic and differential effects on protein expression of epigallocatechin-3-gallate in HCCLM6 hepatocellular carcinoma cells. Int. J. Mol. Med. 32 (4), 959–964. doi:10.3892/ijmm.2013.1446
Zhao, L., Liu, S., Xu, J., Li, W., Duan, G., Wang, H., et al. (2017). A new molecular mechanism underlying the EGCG-mediated autophagic modulation of AFP in HepG2 cells. Cell Death Dis. 8 (11), e3160. doi:10.1038/cddis.2017.563
Zhong, L., Hu, J., Shu, W., Gao, B., and Xiong, S. (2015). Epigallocatechin-3-gallate opposes HBV-induced incomplete autophagy by enhancing lysosomal acidification, which is unfavorable for HBV replication. Cell Death Dis. 6 (5), e1770. doi:10.1038/cddis.2015.136
Zhong, Y., Ma, C. M., and Shahidi, F. (2012). Antioxidant and antiviral activities of lipophilic epigallocatechin gallate (EGCG) derivatives. J. Funct. Foods 4 (1), 87–93. doi:10.1016/j.jff.2011.08.003
Zhou, H., Fu, L. X., Li, L., Chen, Y. Y., Zhu, H. Q., Zhou, J. L., et al. (2020). The epigallocatechin gallate derivative Y6 reduces the cardiotoxicity and enhances the efficacy of daunorubicin against human hepatocellular carcinoma by inhibiting carbonyl reductase 1 expression. J. Ethnopharmacol. 261, 113118. doi:10.1016/j.jep.2020.113118
Zhou, J., Farah, B. L., Sinha, R. A., Wu, Y., Singh, B. K., Bay, B. H., et al. (2014). Epigallocatechin-3-gallate (EGCG), a green tea polyphenol, stimulates hepatic autophagy and lipid clearance. PLoS One 9 (1), e87161. doi:10.1371/journal.pone.0087161
Zhou, L., and Elias, R. J. (2013). Antioxidant and pro-oxidant activity of (-)-epigallocatechin-3-gallate in food emulsions: Influence of pH and phenolic concentration. Food Chem. 138 (2-3), 1503–1509. doi:10.1016/j.foodchem.2012.09.132
Zhou, L., and Elias, R. J. (2012). Factors influencing the antioxidant and pro-oxidant activity of polyphenols in oil-in-water emulsions. J. Agric. Food Chem. 60 (11), 2906–2915. doi:10.1021/jf204939h
Zhou, Y., Lin, F., Wan, T., Chen, A., Wang, H., Jiang, B., et al. (2021). ZEB1 enhances Warburg effect to facilitate tumorigenesis and metastasis of HCC by transcriptionally activating PFKM. Theranostics 11 (12), 5926–5938. doi:10.7150/thno.56490
Glossary
Keywords: epigallocatechin gallate, hepatocellular carcinoma, cancer prevention, cancer therapy, anticancer
Citation: Li D, Cao D, Cui Y, Sun Y, Jiang J and Cao X (2023) The potential of epigallocatechin gallate in the chemoprevention and therapy of hepatocellular carcinoma. Front. Pharmacol. 14:1201085. doi: 10.3389/fphar.2023.1201085
Received: 06 April 2023; Accepted: 16 May 2023;
Published: 24 May 2023.
Edited by:
Zhaofeng Liang, Jiangsu University, ChinaReviewed by:
Subhayan Sur, Indian Institute of Science Education and Research Kolkata, IndiaMadan Kumar Perumal, Central Food Technological Research Institute (CSIR), India
Sherin Zakaria, Kafrelsheikh University, Egypt
Xueting Deng, Nanjing Medical University, China
Copyright © 2023 Li, Cao, Cui, Sun, Jiang and Cao. This is an open-access article distributed under the terms of the Creative Commons Attribution License (CC BY). The use, distribution or reproduction in other forums is permitted, provided the original author(s) and the copyright owner(s) are credited and that the original publication in this journal is cited, in accordance with accepted academic practice. No use, distribution or reproduction is permitted which does not comply with these terms.
*Correspondence: Jing Jiang, amlhbmdqaW5nMTk3MDIwMDBAamx1LmVkdS5jbg==; Xueyuan Cao, amQzZDJ1YkBqbHUuZWR1LmNu