- 1Clinical Pathology Department, Faculty of Veterinary Medicine, Mansoura University, Mansoura, Egypt
- 2Department of Haematology and Immunology, Faculty of Medicine, Umm Alqura University, Makkah, Saudi Arabia
- 3Laboratory Medicine Department, Faculty of Applied Medical Sciences, Umm Al-Qura University, Makkah, Saudi Arabia
- 4Pathology Department, Faculty of Veterinary Medicine, Mansoura University, Mansoura, Egypt
Introduction: Nonalcoholic fatty liver disease (NAFLD) is a chronic disease characterized by fat deposits in liver cells, which can lead to hepatitis and fibrosis. This study attempted to explore the protective effect of vitamin D3 (VitD) against NAFLD.
Methods: Adult male albino rats were randomized into four separate groups: the negative control group was fed a standard rat chow; the positive group received a high-fat diet (20%) and 25% fructose water (NAFLD); the VitD control group was intramuscularly treated with VitD (1,000 IU/kg BW) 3 days per week for 10 weeks; and the NAFLD group was treated with VitD therapy. Biochemical and hepatic histological analyses were performed. Hepatic oxidative stress and inflammatory conditions were also studied. Hepatic expression of sterol regulatory element-binding protein 1-c (SREBP-1-c), peroxisome proliferator-activated receptor alpha (PPAR-α), and insulin receptor substrate-2 was analyzed by quantitative real-time polymerase chain reaction.
Results and discussion: The NAFLD rats exhibited elevated terminal body weight, hepatic injury markers, dyslipidemia, glucose intolerance, and insulin resistance. Moreover, the NAFLD rats had increased SREBP-1-c expression and reduced PPAR-α and IRS-2 expressions. Histological analysis showed hepatic steatosis and inflammation in the NAFLD group. In contrast, VitD administration improved the serum biochemical parameters and hepatic redox status in NAFLD rats. Also, VitD treatment ameliorated hepatic inflammation and steatosis in the NAFLD group by decreasing the expression of SREBP-1-c and increasing the expression of PPAR-α. Overall, these results suggest that VitD could have a protective effect against NAFLD and its associated complication.
1 Introduction
Nonalcoholic fatty liver disease (NAFLD) is a common disease characterized by the presence of triglyceride deposits in more than 5% of hepatocytes, in the absence of excessive alcohol consumption or other documented causes of liver damage (Rahimi and Landaverde, 2013; Shojaei Zarghani et al., 2018). It is divided into two main forms: the non-progressive form, which rarely develops into cirrhosis and is known as nonalcoholic fatty liver disease, and the progressive form, which leads to cirrhosis and hepatocellular carcinoma and is known as nonalcoholic steatohepatitis (NASH) (Loomba and Sanyal, 2013). Since NAFLD is related to obesity and insulin resistance, it is the best-known liver disease that chronically affects people of different ages (Kaufmann et al., 2021). Significant evidence suggests that excessive consumption of high-fat diets and sugar-sweetened beverages is related to the development and progression of NAFLD (Rahimi and Landaverde, 2013; Softic et al., 2016). Additionally, excessive carbohydrate and fat consumption increases the levels of blood sugar and free fatty acids, resulting in excessive neutral lipid deposition in the liver (Zivkovic et al., 2007). The severity of NAFLD can be increased by excessive fructose consumption as it promotes insulin resistance, de novo lipogenesis, and the development of an inflammatory, oxidative stress state (Jarukamjorn et al., 2016). Since NAFLD is a critical cause of abnormal liver enzymes, cryptogenic cirrhosis, and liver transplantation, it is important to manage and control NAFLD (Souza et al., 2012; Softic et al., 2016).
Vitamin D is a fat-soluble prohormone that can be formed in the skin after direct exposure to ultraviolet rays or taken with food (Sharifi and Amani, 2019). To achieve its biological activity, a series of sequential biochemical reactions must take place, including 25-hydroxylation in the liver and then 1-hydroxylation in the kidney (Yin et al., 2012). Classically, it has been implicated in calcium and phosphorus hemostasis, but recent studies indicate its role in managing diseases associated with inflammation and oxidative stress in both human and animal models (Sharifi and Amani, 2019). It also plays a role in improving the lipid profile (Faraji and Alizadeh, 2020). Several lines of evidence suggest that VitD can modulate liver inflammation and improve hepatic responsiveness to insulin by binding to its specific receptor in the liver (Barchetta et al., 2017). It has been demonstrated that an active form of VitD can reduce oxidative stress, generation of inflammatory factors, and hepatic fibrosis in NAFLD resulting from a high-fat diet (Liu et al., 2020). It can also alleviate fatty liver disease in an NAFLD rat model by modulating lipid metabolism and/or impeding cell senescence (Yin et al., 2012; Liu et al., 2020). Moreover, VitD intake could reduce NAFLD severity and its risk factors including dyslipidemia and obesity (Sangouni et al., 2019). Therefore, VitD supplementation may be helpful in the prevention of NAFLD by modulating some serum liver function markers, lipid profile, hepatic redox status, selective molecules involved in the inflammatory and antiinflammatory processes, and mRNA expression levels of regulatory molecules, SREBP-1-c, PPAR-α, and IRS-2 involved in lipogenesis, lipolysis, and insulin signaling, respectively.
2 Materials and methods
2.1 Materials
2.1.1 Chemicals
Cholecalciferol (vitamin D3) was brought from Memphis Company for Pharmaceutical and Chemical Industries (Cairo, Egypt) in the form of ampoules (100,000 IU/mL).
2.1.2 Experimental animals
A total of 32 healthy male albino rats (230–250 g) of about 9 weeks of age were procured from the Animal House of Zagazig University. The rats were housed in standard polypropylene cages (four rats per cage) under appropriate conditions of temperature (25°C ± 2°C), humidity (60%–70%), and light (12-h dark/light cycles). They were provided ad libitum with a commercial rodent diet and plain water. The experimental protocol was approved by the Ethical Animal Research Committee of Faculty of Veterinary Medicine, Mansoura University, Egypt (Approval No. 2021; M/35).
2.2 Methods
2.2.1 Induction of NAFLD
To establish NAFLD, the rats received a high-fat diet (HFD) with 20% fat. The HFD was prepared according to the work of Shin et al. (2016) with some modifications (we used 19 g of butter oil and 1 g of soybean oil as a source of fat instead of corn oil and lard). In addition, 25% fructose solution was added to the drinking water for 10 weeks (about 2.5 months) (high-fat, high-fructose diet).
2.2.2 Animal treatment
After 2 weeks of acclimatization, the experimental animals were assigned to four groups (eight each), detailed as follows:
• Control group: Rats were maintained on standard chow and fructose-free water
• NAFLD group: Rats were fed an HFD and 25% fructose water
• VitD group: Rats received standard chow, fructose-free water, and VitD treatment
• NAFLD + VitD group: Rats received an HFD, 25% fructose water, and VitD treatment
Vitamin D3 was diluted in sterile saline and then injected intramuscularly (1,000 IU/kg BW) 3 days per week throughout the experimental period as mentioned by BaSalamah et al. (2018). The body weight of each rat was taken on day 0 and then every week.
2.2.3 Collection and preparation of samples
After the 10th week, experimental rats were injected intraperitoneally with xylazine and ketamine mixture at doses of 10 mg/kg and 50 mg/kg, respectively, and retro-orbital puncture was performed to collect peripheral blood samples. The blood samples were carefully centrifuged at 1,198 × g for 10 min to separate the sera. The obtained sera were then used to study some biochemical parameters. After that, hepatic tissue specimens were collected from the decapitated rats and cut into several parts. One part was used to prepare liver homogenates to assess the hepatic redox status and hepatic pro/antiinflammatory status. The protein content in the homogenates was determined, as reported by Bradford (1976). A hepatic specimen of 1 gm was maintained in RNAlater (Quigen, Germany) for gene expression studies. For histological investigation, hepatic tissue sections were formalin-fixed and then stained with hematoxylin and eosin (H&E). In addition, other sections were frozen, fixed, and stained with Oil Red O.
2.2.4 Serum biochemical analysis
Available commercial kits were utilized to evaluate the biomarkers of liver function: alanine and aspartate aminotransferases (ALT and AST, respectively) (Human Diagnostics Worldwide, Wiesbaden, Germany); alkaline phosphatase (ALP) (ELITech, Paris, France); bilirubin (total and direct) (Diamond, Cairo, Egypt); and total protein and albumin (Stanbio Laboratory, TX, United States). A Spinreact kit was used to analyze the serum glucose level, while serum insulin and 25(OH) VitD levels were quantified by enzyme-linked immunosorbent assay using the appropriate kits (Biospes, Chongqing, China, and DiaMetra SRL, Perugia, Italy, respectively). Homeostasis model assessment of insulin resistance (HOMA-IR) was detected according to the work of Matthews et al. (1985).
Different lipid parameters [triglyceride (TG), total cholesterol (TC), and high-density lipoprotein cholesterol (HDL-C)] were estimated using ready-made assay kits (Spinreact, Sant Esteve de Bas, Spain), and exceptionally very-low- and low-density lipoprotein cholesterol (VLDL-C and LDL-C) values were calculated as recorded by Friedewald et al. (1972). The biochemical serum analyses were performed following the manufacturer’s guidelines and measured using a spectrophotometer (BM, Germany, 5010).
2.2.5 Assessment of hepatic redox status and serum total antioxidant capacity
Malondialdehyde (MDA) concentration and the enzymatic activities of both superoxide dismutase (SOD) and catalase (CAT), together with the reduced glutathione (GSH) level, were determined in the hepatic tissue using standard assay kits from Bio-Diagnostic Co. (Cairo, Egypt), according to the instructions in each corresponding pamphlet. Serum total antioxidant capacity (sTAC) was also estimated by using Bio-Diagnostic kits.
2.2.6 Assessment of hepatic pro- and antiinflammatory status
Specific rat ELISA kits purchased from Cusabio (Wuhan, China) were utilized for the determination of the hepatic concentration of nuclear factor kappa β (NF-κβ), whereas R&D Systems ELISA Kits (Minneapolis, MN, United States) were used to measure hepatic interleukin-10 (IL-10) levels, following the protocol in the assay kits.
2.2.7 Assessment of hepatic gene expression
RNA was first extracted from the hepatic tissue following the RNeasy Mini Kit instructions (Qiagen, Hilden, Germany). Then, cDNA was synthesized using RevertAid Reverse Transcriptase Kits from Thermo Fisher Scientific (Massachusetts, United States), following the manufacturer’s instructions. Quantitative RT-PCR was performed to evaluate the relative expression levels of the following genes: sterol regulatory element-binding protein 1-c (SREBP-1-c), peroxisome proliferator-activated receptor alpha (PPAR-α), and insulin receptor substrate-2 (IRS-2). Primer sequences of SREBP-1-c (Ren et al., 2019), PPAR-α (Ding et al., 2014), and IRS-2 (Kanuri et al., 2016) are shown in Table 1. RT-PCR assay was performed using the MX3005P QPCR system. The preparation of the PCR master mix and cycling conditions was performed using the QuantiTect SYBR Green PCR Kit (Qiagen, Germany), following the manufacturer’s instructions. Rat β-actin (Banni et al., 2010) was used as a housekeeping gene, and a comparative Ct method (2−ΔΔCT) was used to detect mRNA expression levels, according to the work of Livak and Schmittgen (2001).
2.2.8 Histopathological assessment of the liver
Liver sections were preserved in 10% formaldehyde solution and then immersed in paraffin. The obtained tissue was sliced into 5 μm-thick tissue blocks that were stained with hematoxylin and eosin (H&E). The obtained blocks were inspected under a light microscope, as mentioned by Kleiner et al. (2005). To assess hepatic lipid deposition, 10-μm frozen liver sections were prepared using a cryostat (LEICA CM 1800), fixed, and then, stained with ORO (Green and Kehinde, 1974). Using the XSZ-107BN microscope (China) and the Apex Minigrab (UK), the obtained sections were randomly photographed and then automatically analyzed using ImageJ (https://imagej.nih.gov/ij).
2.2.9 Data analysis
The outputs were given as means ± SEM. The Statistical Package of Social Services, version 22 (SPSS 22), was used to perform all statistics using ANOVA, followed by Duncan’s multiple-range test to perform the comparisons between the tested groups. Statistical significance was displayed at p < 0.05. Positive ORO-stained areas were evaluated by ANOVA, followed by Tukey’s test for group comparison (p-value ≤ 0.05), using GraphPad Prism for macOS, version 9.2.0 (283).
3 Results
3.1 Effect of vitamin D treatment on body weight
Figure 1 shows that the initial body weights were similar in all the experimental groups. However, a remarkable increase was noted in the terminal body weights of the NAFLD rats compared to the control rats (p < 0.05). No substantial variation was observed in the body weight of the NAFLD + VitD compared with the NAFLD group.
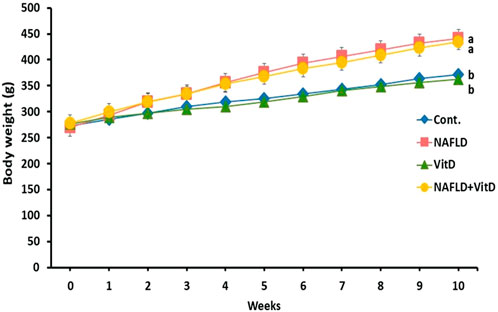
FIGURE 1. Changes in the body weight (g) of experimental groups during the study period. Data are expressed as means ± SEM, in which those with different superscripts differed significantly (p < 0.05). a is used for the high value, while b is used for the lower one. Cont., control; NAFLD, nonalcoholic fatty liver disease; and VitD, vitamin D.
3.2 Vitamin D treatment improved serum biochemical parameters in NAFLD rats
As shown in Table 2, NAFLD rats showed marked (p < 0.05) elevation in the serum ALT, AST, and ALP activities compared to the control rats. Conversely, VitD administration in rats with NAFLD markedly (p < 0.05) normalized ALT and ALP serum activities and caused a substantial (p < 0.05) decrease in serum AST activity but did not return it to its normal value, suggesting that VitD has hepatoprotective properties. Additionally, the total and direct bilirubin concentrations were pronouncedly (p < 0.05) increased in the NAFLD group (Table 2), whereas the total protein and globulin levels were pronouncedly (p < 0.05) decreased as opposed to the control rats (Table 2). VitD had no significant (p > 0.05) effect on either bilirubin or proteinogram serum results in the NAFLD + VitD group versus NAFLD rats.
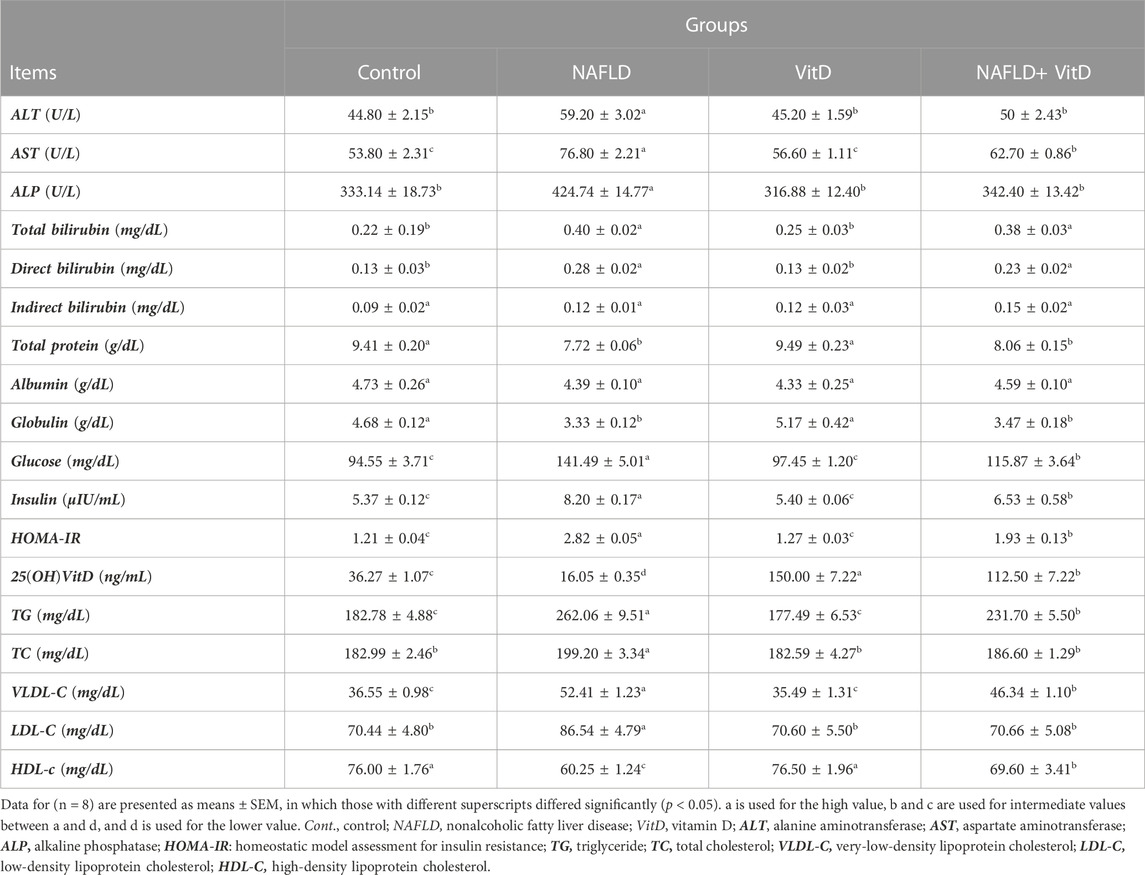
TABLE 2. Impact of VitD on alterations in the serum liver injury indices, proteinogram, glucose hemostasis parameters, 25(OH)VitD, and lipid profile of NAFLD rats.
Serum glucose and insulin levels, together with HOMA-IR index, were sustainably (p < 0.05) increased, while the serum 25 (OH)VitD level was decreased (p < 0.05) significantly in the NAFLD group compared the control group, suggesting disorders of glucose metabolism and a state of insulin resistance. However, VitD treatment markedly (p < 0.05) attenuated the high-fat and fructose diet (HFFD)-mediated increase in glucose, insulin, and HOMA-IR values and significantly (p < 0.05) improved the serum 25(OH)VitD level in the NAFLD+ VitD group compared to the untreated NAFLD rats (Table 2).
3.3 Vitamin D treatment improved the serum lipid profile in NAFLD rats
Table 2 shows that rats in the NAFLD group had significantly (p < 0.05) higher TG, TC, VLDL-C, and LDL-C serum levels, while the HDL-C serum level significantly decreased compared with the control rats, indicating impaired lipid homeostasis. Serum levels of TG and VLDL-C notably (p < 0.05) decreased, while TC and LDL-C levels almost returned to their basal values, and the HDL-C serum concentration was significantly increased in the NAFLD + VitD group by intramuscular VitD treatment as opposed to the NAFLD group.
3.4 Vitamin D improved hepatic antioxidant defense status and serum total antioxidant capacity in NAFLD rats
As shown in Table 3, NAFLD rats exhibited an imbalance in hepatic redox status, as evidenced by a substantial (p < 0.05) increment in the hepatic MDA concentration and marked decreases in sTAC, hepatic SOD, GSH, and CAT concentrations compared to the control group. In contrast, VitD effectivity decreased the MDA hepatic concentration and increased hepatic SOD and GSH concentrations in the NAFLD + VitD group compared to the NAFLD group.
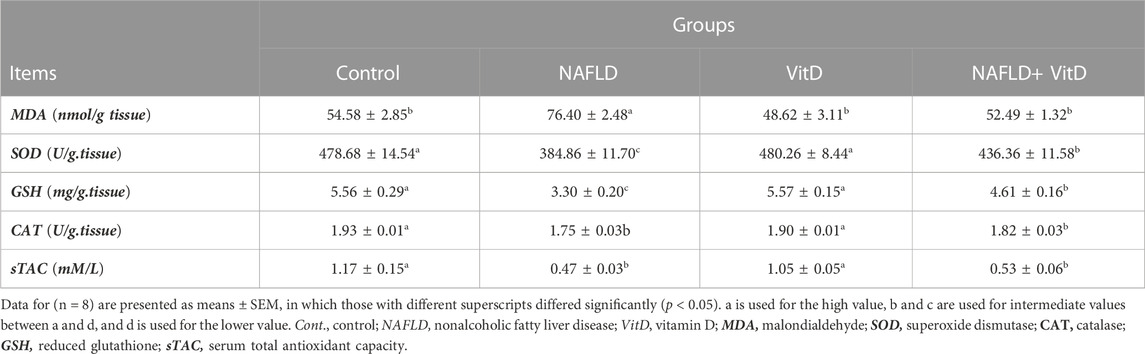
TABLE 3. Impact of VitD on alterations in the hepatic redox system and serum total antioxidant capacity of NAFLD rats.
3.5 Vitamin D treatment modulated the hepatic pro- and antiinflammatory status in NAFLD rats
A significant (p < 0.05) increase in the NF-κB level and a sustainable (p < 0.05) decrease in the IL-10 level were observed in the liver of NAFLD rats with respect to the control group, indicative of hepatic inflammation. As expected, vitamin D treatment reduced hepatic inflammation and ameliorated the antiinflammatory status as indicated in the NAFLD + VitD group versus the untreated NAFLD group (Table 4).
3.6 Vitamin D improved gene expression in the liver of NAFLD rats
Hepatic gene expression analysis shown in Figure 2 revealed that the hepatic mRNA expression of SREBP-1-c was markedly (p < 0.05) upregulated, while hepatic PPAR-α and IRS-2 mRNA expressions levels were markedly (p < 0.05) downregulated in the NAFLD rats relative to the control group. Moreover, VitD treatment significantly (p < 0.05) abolished the HFFD-mediated downregulation of SREBP-1-c and significantly promoted the mRNA expression of PPAR-α and IRS-2 in the liver of the NAFLD + VitD group versus the NAFLD group.
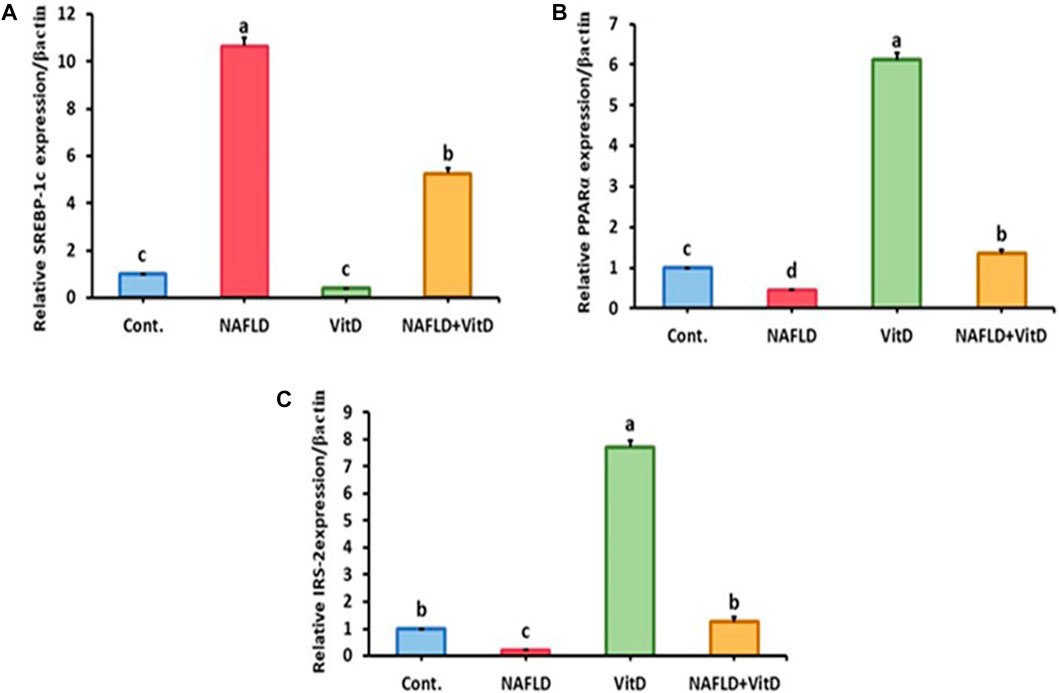
FIGURE 2. Impact of VitD on the hepatic mRNA expression levels of NAFLD rats. (A) SREBP-1-c, (B) PPAR-α, and (C) IRS-2. Data are shown as means ± SEM, in which those with different superscripts differed significantly (p < 0.05). a is used for the high value, b and c are used for intermediate values between a and d, and d is used for the lower value. Cont., control; NAFLD, nonalcoholic fatty liver disease; and VitD, vitamin D. SREBP-1-c, sterol regulatory element-binding protein 1-c; PPAR-α, peroxisome proliferator-activated receptor alpha; and RS-2, insulin receptor substrate-2.
3.7 Vitamin D ameliorated the hepatic histopathological changes observed in NAFLD rats
Histopathological analysis of the hepatic sections revealed that the livers of the control and VitD-treated rats had normal tissue architecture without any detected pathological changes (Figures 3A, E). On the contrary, Table 5 shows that the liver of the NAFLD rats showed parenchyma with intracytoplasmic fat vacuoles and a centrally located nucleus indicating microvesicular fatty changes. The hepatic parenchyma also showed ballooning degeneration and lobular inflammation as opposed to the negative control group (Figures 3B, C). In addition, the liver of the NAFLD rats showed periportal microgranuloma, which can be defined as aggregates of epithelioid cells and other inflammatory cells, including lymphocytes. On the other hand, VitD effectively ameliorated these histopathological abnormalities in the NAFLD + VitD group, as evidenced by a decrease in the observed hepatic microvesicular fatty changes and inflammatory infiltrates when compared to the NAFLD group (Figures 3F, G).
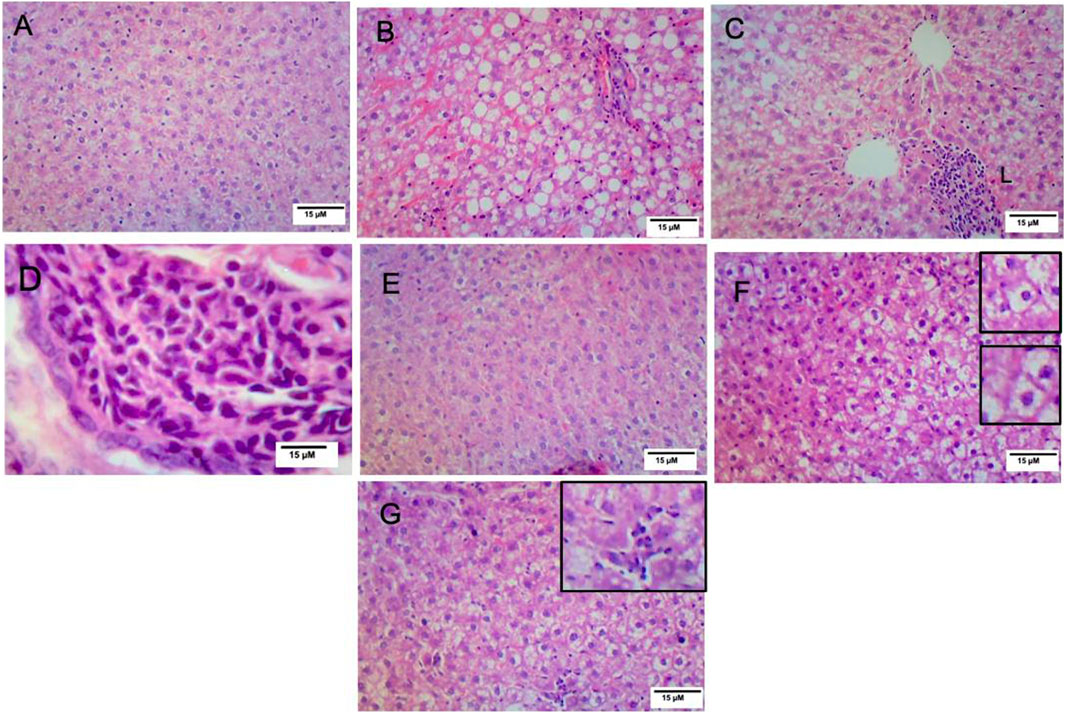
FIGURE 3. Effect of VitD on hepatic histopathological alterations in NAFLD rats (H&E staining). (A,E) Micrographs of the control group and vitamin D-treated group showing normal liver histology (10x). (B) Micrograph of the NAFLD group showing microvesicular steatosis and ballooning degeneration (10x). (C) Micrograph of the NAFLD group showing lobular inflammation consisting mainly of polymorphonuclear cell infiltration (10x). (D) Micrograph of the NAFLD group showing periportal microgranuloma (40x). (F) Micrograph of the NAFLD + VitD group showing decreased microvascular steatosis (bottom inset) and hepatocyte ballooning injury (top inset) (10x) compared to the NAFLD group. (G) Micrograph of the NAFLD + VitD group showing mild lobular inflammation (inset) (10x).
Figure 4 shows photomicrographs of ORO-stained hepatic sections. Histology of the liver was normal in the control and VitD groups (Figures 4A, C), respectively. In the NAFLD group, the liver showed widespread lipid droplets of varied sizes occupying the cytoplasm of hepatocytes (Figure 4B). As expected, the liver of the NAFLD + VitD group had a fewer number of lipid droplets than the NAFLD group (Figures 4D, E).
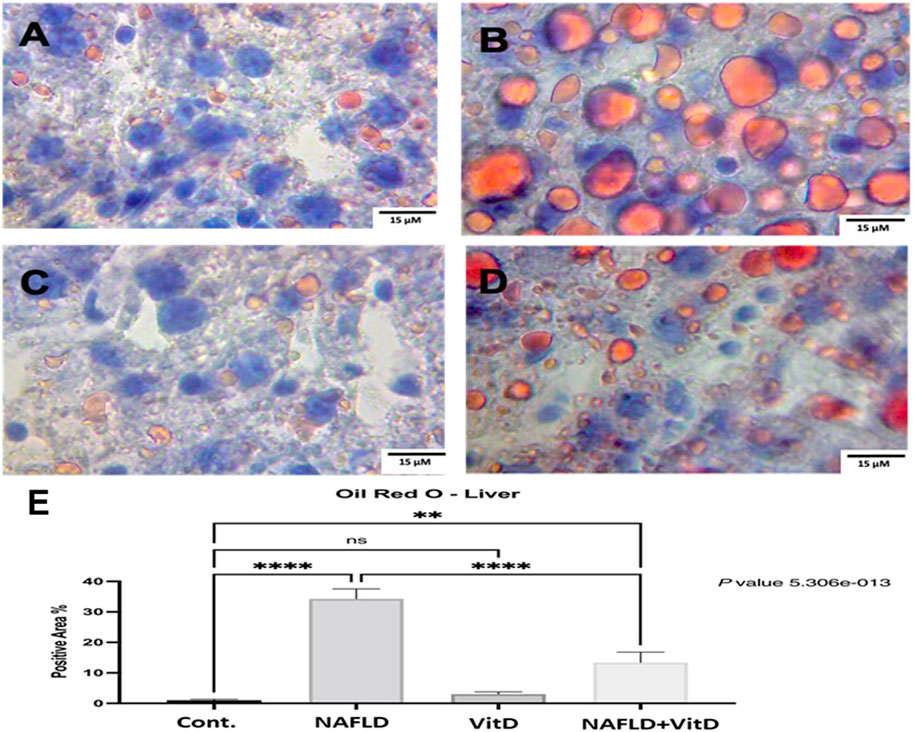
FIGURE 4. Photomicrographs of Oil Red O-stained hepatic sections (40x). (A,C) Cont. group and vitamin D-treated group: showing normal liver histology. (B) NAFLD group: showing widespread lipid droplets of variable size occupying the cytoplasm of hepatocytes. (D) NAFLD + VitD group: showing decreased lipid deposits compared with the NAFLD group. (E) Mean positive area percentage ±SEM in each group. Data were analyzed using one-way ANOVA, followed by Tukey’s test for group comparison. ns = not significant. * = p value ≤ 0.05. ** = p value ≤ 0.01. *** = p value ≤ 0.001. **** = p value ≤ 0.0001.
4 Discussion
Nonalcoholic fatty liver disease is a common liver disorder that can range from asymptomatic steatosis to inflammation and fibrosis, which may lead to hepatic cirrhosis and carcinoma (Katsiki et al., 2016). Routine treatments for NAFLD include lifestyle adjustment and surgical therapy; however, the most effective therapeutic regimens for NAFLD are still under study (Liu et al., 2020). Nowadays, the various functions of VitD are known due to its immunomodulatory, antiinflammatory, and insulin-sensitizing properties (Barchetta et al., 2020). Therefore, VitD could be effectively used as a therapy for NAFLD (Eliades and Spyrou, 2015). From this point of view, this study was conducted to explore the possible protective effects of VitD administration against NAFLD caused by a high-fat and fructose diet (HFFD).
In the current study, the HFFD caused a marked increase in the body weight of rats, which may be attributed to the ability of fructose to promote leptin resistance and serum ghrelin levels (Muriel et al., 2021). Vitamin D could not modulate the increase in body weight induced by the HFFD that was previously recorded in rats fed an obesogenic diet and treated with vitamin D (Mazzone et al., 2018; Shojaei Zarghani et al., 2018; Al-Badarein and Ahmad, 2021).
Evaluation of the serum activity of ALT is considered a valuable tool for the detection of NAFLD. In our study, liver function biomarkers, including ALT, AST, ALP, and total and direct bilirubin, were markedly increased in the NAFLD group, indicating hepatocellular damage. This may be due to the lipogenic potential of fructose as it increases hepatic de novo lipogenesis (DNL) at multiple levels, leading to intrahepatic fat accumulation (Mouzaki and Allard, 2012; Charrez et al., 2015; Jin and Vos, 2015; Softic et al., 2016). This was confirmed histopathologically in our work with H&E and ORO staining. Administration of VitD had a hepatoprotective effect, as detected by lowering the elevated liver enzymes activities (Han et al., 2015; Zhu et al., 2017), which was confirmed histopathologically by a significant reduction in the hepatic lipid burden. The same findings were reported previously by Shojaei Zarghani et al. (2018) in rats fed an HFFD and treated with a vitamin D and calcium combination. The prevention of intrahepatic fat accumulation and the reduction in hepatic cholesterol content may be the cause of the reduction in the hepatic lipid burden (Mazzone et al., 2018; Shojaei Zarghani et al., 2018). Hence, VitD has been reported to downregulate SREBP-1-c and stimulate PPAR-α (Yin et al., 2012).
In the current study, the NAFLD group showed glucose intolerance and impaired insulin sensitivity, which is consistent with the findings obtained by Geetha et al. (2014). This may be a result of high fructose consumption, as it has been reported that fructose-1-phosphate activates mitogen-activated protein kinase, which phosphorylates insulin receptor substrate 1 at the serine residue, leading to hepatic insulin resistance (IR) (Lim et al., 2010; Chang et al., 2014). In addition, fructose itself promotes forkhead box protein O1 synthesis in the liver, leading to an increase in gluconeogenesis, which, together with IR, leads to hyperglycemia and glucose intolerance (Mouzaki and Allard, 2012). In contrast, VitD administration improved glucose hemostasis in the NAFLD group (Sergeev and Song, 2014). This improvement could be attributed to the ability of calcitriol to activate AMPK via the calcium/calmodulin protein kinase beta pathway, leading to attenuating gluconeogenesis and promoting glycolysis (Leung, 2016; Szymczak-Pajor et al., 2020). Additionally, Sung et al. (2012) claimed that VitD can promote insulin action either directly by stimulating insulin receptor expression or indirectly through the regulation of Ca++ levels.
Since NAFLD is associated with lipid disorders, serum lipid profile parameters were investigated. The NAFLD group showed elevations in the serum concentration of TG, TC, VLDL-C, and LDL-C but lower serum HDL-C concentrations. This impairment may be contributed to increase DNL induced by fructose consumption, leading to the formation of fatty acids to produce hepatic TG. The increased hepatic lipid content leads to a decrease in intracellular apo-B degradation and increased production and secretion of VLDL-C (Stanhope and Havel, 2010). On the other hand, the decreased HDL blood level was contributed to the decreased post-heparin plasma LPL/HL ratio and the increased VLDL levels (Mooradian et al., 2008). Concerning the impact of VitD on lipid profile parameters, it ameliorated lipid levels in the serum, which may refer to its ability to promote calcium absorption from the intestine. This calcium forms insoluble soap with fatty acids, especially saturated fatty acids, resulting in increased fecal fat excretion and a decrease in its digestibility (Christensen et al., 2009; Subih et al., 2018). It may also increase lipoprotein lipase enzymes, promote the formation of high-density lipoprotein cholesterol particles, and regulate serum apo-lipoprotein A-1 levels (Hassan et al., 2020; Melguizo-Rodríguez et al., 2021). Our result was previously reported by Farhangi et al. (2017)and El-Sherbiny et al. (2018). In contrast, Shojaei Zarghani et al. (2018) reported insignificant changes in the serum level of TG, TC, and LDL in groups fed with an HFFD and treated with a vitamin D and calcium combination.
Hepatic redox imbalance has been known to be involved in the development and progression of NAFLD from simple steatosis to a more severe form (Spahis et al., 2016). Our data indicated that NAFLD rats had increased hepatic MDA and decreased hepatic antioxidant enzymes and GSH, which was previously reported by Nasri et al. (2015). This may be due to increased superoxide and hydrogen peroxide ions from HFFD feeding (Jarukamjorn et al., 2016). In addition, the antioxidant potential of cells decreases when the excess fructose activates DNL, leading to a reduction in NADPH (Charrez et al., 2015). In agreement with Zhu et al. (2017), our data demonstrated that VitD improved hepatic redox status. The antioxidant effect of VitD may be associated with the inhibition of NADPH oxidase and the enhancement of nuclear factor erythroid 2-related factor 2 nuclear translocation (Nakai et al., 2013; Zhu et al., 2017). Additionally, VitD enhances the synthesis of metal ion protein carriers. These metal ions are critical for the action of various enzymes such as antioxidant enzymes (Hassan et al., 2020).
Our data revealed that NAFLD rats showed an increase in hepatic NF-κB and a decline in the IL-10 levels. There are many potential cellular mechanisms leading to activating inflammatory signaling in NAFLD as a high-caloric diet activates the IKK/NF-κB pathway in adipocytes, hepatocytes, and associated macrophages. IKK activation leads to NF-κB translocation and increased expression of numerous markers and potential mediators of inflammation (Pradhan, 2007). Therefore, the activation of the NF-κB signaling pathway as the most enriched pathway was associated with hepatic inflammation and resulted in an increase in NF-κB expression in NAFLD (Sangouni et al., 2019; Zhao et al., 2022). Meanwhile, the decreased IL-10 levels are linked to T-helper-2 malfunctions (Cano Barquilla et al., 2014). Our findings were previously recorded by Theodoro et al. (2021). On the contrary, VitD administration reduced hepatic inflammation, which was confirmed in our histopathological results by decreasing inflammatory cell infiltration. This could be due to the ability of calcitriol to interfere with NF-κB via increasing the expression of the inhibitory protein (IκB) in peripheral blood mononuclear cells and reducing the nuclear translocation of the NF-κB subunit p65 (Krishnan and Feldman, 2011; Chen et al., 2020). Moreover, the IL-10 hepatic level was significantly enhanced upon VitD treatment, which is in agreement with a prior report (Refaat et al., 2021). This could be attributed to enhancing T-helper-2 cell differentiation and overproduction of antiinflammatory cytokines such as IL-10 after VitD administration (Bishop et al., 2021; Sharma et al., 2021).
To further confirm the hepatic lipid burden in our NAFLD model with a decrease in its oxidation, we performed qualitative real-time PCR for analyzing the hepatic expression of lipid metabolic genes including SREBP-1-c and PPAR-α. The results revealed disturbances in the expression of both genes in the NAFLD group including an increase in SREBP-1-c expression and a decrease in PPAR-α expression, which was previously observed by Wang et al. (2022). The explanation for the observed changes in the hepatic SREBP-1-c and PPAR-α mRNA levels is provided by Nagai et al. (2002), who observed that fructose can stimulate SREBP-1-c but suppress PPAR-α expression levels in rat liver. VitD administration resulted in reverse changes in both genes’ expression in the liver of the NAFLD group. This may be related to the ability of VitD to interfere with the activation of SREBP-1-c by enhancing the ubiquitin-mediated degradation of the SREBP cleavage-activating protein (Asano et al., 2017). Similar results were previously observed in male rats fed with an HFD and treated with calcitriol at doses 5 μg/kg B.W, I/P, and 5 ng/g B.W, I/M, twice per week, respectively (Yin et al., 2012; Kong et al., 2014).
The impairment of the insulin signal and insulin resistance was further confirmed in our NAFLD model by a significant decrease in the hepatic expression of IRS-2. This may refer to the ability of SREBP-1-c to suppress IRS-2 promoter activity by competing with its transactivator (Ide et al., 2004). Several studies confirmed that hepatic IRS-2 mRNA expression was downregulated in HFD-fed rodent models (Xing et al., 2011; Qiu et al., 2015; Yang et al., 2019). Contrariwise, vitamin D administration enhanced hepatic IRS-2 expression in NAFLD rats. Our result is in parallel with that obtained by Szymczak-Pajor et al. (2020), who speculated that the active form of VitD enhances the transcriptional activation of the IR gene, which improves insulin signaling.
5 Conclusion
This study clarified that an HFFD led to a substantial increase in body weight and hepatic injury indices, along with disorders in glucose hemostasis and lipid metabolism. In addition, hepatic inflammation and oxidative damage occurred, which led to the development and progression of NAFLD. We also concluded that VitD may protect against HFFD-induced NAFLD through its antioxidant and antiinflammatory effects. In addition, VitD had ameliorative effects on glucose hemostasis and lipid profile.
Data availability statement
The original contributions presented in the study are included in the article/Supplementary Material; further inquiries can be directed to the corresponding author.
Ethics statement
The animal study was reviewed and approved by the Ethical Animal Research Committee of Faculty of Veterinary Medicine, Mansoura University, Egypt (Approval No. 2021 M/35).
Author contributions
DR: methodology, formal analysis, data curation, and writing—original draft, review, and editing. GE, ER, and FA: conceptualization, validation, visualization, and editing—final draft and supervision. HM, TA, SA, BR, and ME-B: methodology, data curation and analysis, and writing and reviewing. FA: final reviewing and preparing the manuscript for publication. All authors read and approved the final manuscript. All authors listed made a substantial, direct, and intellectual contribution to the work and approved it for publication. All authors contributed to the article and approved the submitted version.
Funding
The present study was a self-funded study.
Acknowledgments
The authors would like to express their sincere gratitude to Professor Abeer E. Aziza, Professor of Nutrition and Nutritional Deficiency Diseases at Mansoura University, Faculty of Veterinary Medicine, for her assistance with the preparation of the experimental diets.
Conflict of interest
The authors declare that the research was conducted in the absence of any commercial or financial relationships that could be construed as a potential conflict of interest.
Publisher’s note
All claims expressed in this article are solely those of the authors and do not necessarily represent those of their affiliated organizations, or those of the publisher, the editors, and the reviewers. Any product that may be evaluated in this article, or claim that may be made by its manufacturer, is not guaranteed or endorsed by the publisher.
References
Al-Badarein, B. O., and Ahmad, M. N. (2021). Body weight, insulin resistance, and inflammatory biomarkers in rats fed normal-fat, high-fat, and ketogenic diets supplemented with vitamin D. J. Agric. Sci. 17 (1), 1–16. doi:10.35516/jjas.v17i1.64
Asano, L., Watanabe, M., Ryoden, Y., Usuda, K., Yamaguchi, T., Khambu, B., et al. (2017). Vitamin D metabolite, 25-hydroxyvitamin D, regulates lipid metabolism by inducing degradation of SREBP/SCAP. Cell Chem. Biol. 24 (2), 207–217. doi:10.1016/j.chembiol.2016.12.017
Banni, M., Messaoudi, I., Said, L., El Heni, J., Kerkeni, A., and Said, K. (2010). Metallothionein gene expression in liver of rats exposed to cadmium and supplemented with zinc and selenium. Archives Environ. Contam. Toxicol. 59 (3), 513–519. doi:10.1007/s00244-010-9494-5
Barchetta, I., Cimini, F. A., and Cavallo, M. G. (2020). Vitamin D and metabolic dysfunction-associated fatty liver disease (MAFLD): An update. Nutrients 12 (11), 3302. doi:10.3390/nu12113302
Barchetta, I., Cimini, F. A., and Cavallo, M. G. (2017). Vitamin D supplementation and non-alcoholic fatty liver disease: Present and future. Nutrients 9 (9), 1015. doi:10.3390/nu9091015
BaSalamah, M. A., Abdelghany, A. H., El-Boshy, M., Ahmad, J., Idris, S., and Refaat, B. (2018). Vitamin D alleviates lead induced renal and testicular injuries by immunomodulatory and antioxidant mechanisms in rats. Sci. Rep. 8 (1), 4853. doi:10.1038/s41598-018-23258-w
Bishop, L. E., Ismailova, A., Dimeloe, S., Hewison, M., and White, J. H. (2021). Vitamin D and immune regulation: Antibacterial, antiviral, anti-inflammatory. JBMR plus 5 (1), e10405. doi:10.1002/jbm4.10405
Bradford, M. M. (1976). A rapid and sensitive method for the quantitation of microgram quantities of protein utilizing the principle of protein-dye binding. Anal. Biochem. 72 (1), 248–254. doi:10.1006/abio.1976.9999
Cano Barquilla, P., Pagano, E. S., Jiménez-Ortega, V., Fernández-Mateos, P., Esquifino, A. I., and Cardinali, D. P. (2014). Melatonin normalizes clinical and biochemical parameters of mild inflammation in diet-induced metabolic syndrome in rats. J. pineal Res. 57 (3), 280–290. doi:10.1111/jpi.12168
Chang, W.-C., Jia, H., Aw, W., Saito, K., Hasegawa, S., and Kato, H. (2014). Beneficial effects of soluble dietary Jerusalem artichoke (Helianthus tuberosus) in the prevention of the onset of type 2 diabetes and non-alcoholic fatty liver disease in high-fructose diet-fed rats. Br. J. Nutr. 112 (5), 709–717. doi:10.1017/S0007114514001421
Charrez, B., Qiao, L., and Hebbard, L. (2015). The role of fructose in metabolism and cancer. Hormone Mol. Biol. Clin. Investigation 22 (2), 79–89. doi:10.1515/hmbci-2015-0009
Chen, J., Tang, Z., Slominski, A. T., Li, W., Żmijewski, M. A., Liu, Y., et al. (2020). Vitamin D and its analogs as anticancer and anti-inflammatory agents. Eur. J. Med. Chem. 207, 112738. doi:10.1016/j.ejmech.2020.112738
Christensen, R., Lorenzen, J. K., Svith, C. R., Bartels, E., Melanson, E., Saris, W., et al. (2009). Effect of calcium from dairy and dietary supplements on faecal fat excretion: A meta-analysis of randomized controlled trials. Obes. Rev. 10 (4), 475–486. doi:10.1111/j.1467-789X.2009.00599.x
Ding, L., Li, J., Song, B., Xiao, X., Huang, W., Zhang, B., et al. (2014). Andrographolide prevents high-fat diet–induced obesity in C57bl/6 mice by suppressing the sterol regulatory element-binding protein pathway. J. Pharmacol. Exp. Ther. 351 (2), 474–483. doi:10.1124/jpet.114.217968
El-Sherbiny, M., Eldosoky, M., El-Shafey, M., Othman, G., Elkattawy, H. A., Bedir, T., et al. (2018). Vitamin D nanoemulsion enhances hepatoprotective effect of conventional vitamin D in rats fed with a high-fat diet. Chemico-Biological Interact. 288, 65–75. doi:10.1016/j.cbi.2018.04.010
Eliades, M., and Spyrou, E. (2015). Vitamin D: A new player in non-alcoholic fatty liver disease? World J. gastroenterology WJG 21 (6), 1718–1727. doi:10.3748/wjg.v21.i6.1718
Faraji, S., and Alizadeh, M. (2020). Mechanistic effects of vitamin D supplementation on metabolic syndrome components in patients with or without vitamin D deficiency. J. Obes. Metab. Syndr. 29 (4), 270–280. doi:10.7570/jomes20003
Farhangi, M. A., Nameni, G., Hajiluian, G., and Mesgari-Abbasi, M. (2017). Cardiac tissue oxidative stress and inflammation after vitamin D administrations in high fat-diet induced obese rats. BMC Cardiovasc. Disord. 17 (1), 161. doi:10.1186/s12872-017-0597-z
Friedewald, W. T., Levy, R. I., and Fredrickson, D. S. (1972). Estimation of the concentration of low-density lipoprotein cholesterol in plasma, without use of the preparative ultracentrifuge. Clin. Chem. 18 (6), 499–502. doi:10.1093/clinchem/18.6.499
Geetha, R., Yogalakshmi, B., Sreeja, S., Bhavani, K., and Anuradha, C. V. (2014). Troxerutin suppresses lipid abnormalities in the heart of high-fat–high-fructose diet-fed mice. Mol. Cell. Biochem. 387 (1), 123–134. doi:10.1007/s11010-013-1877-2
Green, H., and Kehinde, O. (1974). Sublines of mouse 3T3 cells that accumulate lipid. Cell 1 (3), 113–116. doi:10.1016/0092-8674(74)90126-3
Han, H., Cui, M., You, X., Chen, M., Piao, X., and Jin, G. (2015). A role of 1,25(OH)2D3 supplementation in rats with nonalcoholic steatohepatitis induced by choline-deficient diet. Nutr. Metabolism Cardiovasc. Dis. 25 (6), 556–561. doi:10.1016/j.numecd.2015.02.011
Hassan, F., El-Said, E.-S. E.-S., El-sayed, G. R., El-Sayed, S. A. E.-S., and Awadin, W. F. (2020). Nano-particles of trace minerals in poultry nutrition: Potential applications and future prospects. Comp. Clin. Pathol. 29 (3), 591–612. doi:10.1007/s12011-019-01862-9
Ide, T., Shimano, H., Yahagi, N., Matsuzaka, T., Nakakuki, M., Yamamoto, T., et al. (2004). SREBPs suppress IRS-2-mediated insulin signalling in the liver. Nat. Cell Biol. 6 (4), 351–357. doi:10.1038/ncb1111
Jarukamjorn, K., Jearapong, N., Pimson, C., and Chatuphonprasert, W. (2016). A high-fat, high-fructose diet induces antioxidant imbalance and increases the risk and progression of nonalcoholic fatty liver disease in mice. Scientifica 2016, 5029414. doi:10.1155/2016/5029414
Jin, R., and Vos, M. B. (2015). Fructose and liver function – is this behind nonalcoholic liver disease? Curr. Opin. Clin. Nutr. Metabolic Care 18 (5), 490–495. doi:10.1097/MCO.0000000000000203
Kanuri, G., Landmann, M., Priebs, J., Spruss, A., Löscher, M., Ziegenhardt, D., et al. (2016). Moderate alcohol consumption diminishes the development of non-alcoholic fatty liver disease (NAFLD) in ob/ob mice. Eur. J. Nutr. 55 (3), 1153–1164. doi:10.1007/s00394-015-0929-7
Katsiki, N., Mikhailidis, D. P., and Mantzoros, C. S. (2016). Non-alcoholic fatty liver disease and dyslipidemia: An update. Metabolism 65 (8), 1109–1123. doi:10.1016/j.metabol.2016.05.003
Kaufmann, B., Reca, A., Wang, B., Friess, H., Feldstein, A. E., and Hartmann, D. (2021). Mechanisms of nonalcoholic fatty liver disease and implications for surgery. Langenbeck's Archives Surg. 406 (1), 1–17. doi:10.1007/s00423-020-01965-1
Kleiner, D. E., Brunt, E. M., Van Natta, M., Behling, C., Contos, M. J., Cummings, O. W., et al. (2005). Design and validation of a histological scoring system for nonalcoholic fatty liver disease. Hepatology 41 (6), 1313–1321. doi:10.1002/hep.20701
Kong, M., Zhu, L., Bai, L., Zhang, X., Chen, Y., Liu, S., et al. (2014). Vitamin D deficiency promotes nonalcoholic steatohepatitis through impaired enterohepatic circulation in animal model. Am. J. Physiology-Gastrointestinal Liver Physiology 307 (9), G883–G893. doi:10.1152/ajpgi.00427.2013
Krishnan, A. V., and Feldman, D. (2011). Mechanisms of the anti-cancer and anti-inflammatory actions of vitamin D. Annu. Rev. Pharmacol. Toxicol. 51, 311–336. doi:10.1146/annurev-pharmtox-010510-100611
Leung, P. S. (2016). The potential protective action of vitamin D in hepatic insulin resistance and pancreatic islet dysfunction in type 2 diabetes mellitus. Nutrients 8 (3), 147. doi:10.3390/nu8030147
Lim, J. S., Mietus-Snyder, M., Valente, A., Schwarz, J.-M., and Lustig, R. H. (2010). The role of fructose in the pathogenesis of NAFLD and the metabolic syndrome. Nat. Rev. Gastroenterology Hepatology 7 (5), 251–264. doi:10.1038/nrgastro.2010.41
Liu, Y., Wang, M., Xu, W., Zhang, H., Qian, W., Li, X., et al. (2020). Active vitamin D supplementation alleviates initiation and progression of nonalcoholic fatty liver disease by repressing the p53 pathway. Life Sci. 241, 117086. doi:10.1016/j.lfs.2019.117086
Livak, K. J., and Schmittgen, T. D. (2001). Analysis of relative gene expression data using real-time quantitative PCR and the 2(-Delta Delta C(T)) Method. Methods 25 (4), 402–408. doi:10.1006/meth.2001.1262
Loomba, R., and Sanyal, A. J. (2013). The global NAFLD epidemic. Nat. Rev. Gastroenterology Hepatology. 10 (11), 686–690. doi:10.1038/nrgastro.2013.171
Matthews, D. R., Hosker, J. P., Rudenski, A. S., Naylor, B. A., Treacher, D. F., and Turner, R. C. (1985). Homeostasis model assessment: Insulin resistance and β-cell function from fasting plasma glucose and insulin concentrations in man. Diabetologia 28 (7), 412–419. doi:10.1007/BF00280883
Mazzone, G., Morisco, C., Lembo, V., D’Argenio, G., D’Armiento, M., Rossi, A., et al. (2018). Dietary supplementation of vitamin D prevents the development of Western diet-induced metabolic, hepatic and cardiovascular abnormalities in rats. United Eur. Gastroenterology J. 6 (7), 1056–1064. doi:10.1177/2050640618774140
Melguizo-Rodríguez, L., Costela-Ruiz, V. J., García-Recio, E., De Luna-Bertos, E., Ruiz, C., and Illescas-Montes, R. (2021). Role of vitamin D in the metabolic syndrome. Nutrients 13 (3), 830. doi:10.3390/nu13030830
Mooradian, A. D., Haas, M. J., Wehmeier, K. R., and Wong, N. C. W. (2008). Obesity-related changes in high-density lipoprotein metabolism. Obesity 16 (6), 1152–1160. doi:10.1038/oby.2008.202
Mouzaki, M., and Allard, J. P. (2012). The role of nutrients in the development, progression, and treatment of nonalcoholic fatty liver disease. J. Clin. Gastroenterology 46 (6), 457–467. doi:10.1097/MCG.0b013e31824cf51e
Muriel, P., López-Sánchez, P., and Ramos-Tovar, E. (2021). Fructose and the liver. Int. J. Mol. Sci. 22 (13), 6969. doi:10.3390/ijms22136969
Nagai, Y., Nishio, Y., Nakamura, T., Maegawa, H., Kikkawa, R., and Kashiwagi, A. (2002). Amelioration of high fructose-induced metabolic derangements by activation of PPARalpha. Am. J. Physiology-Endocrinology Metabolism 282 (5), E1180–E1190. doi:10.1152/ajpendo.00471.2001
Nakai, K., Fujii, H., Kono, K., Goto, S., Kitazawa, R., Kitazawa, S., et al. (2013). Vitamin D activates the nrf2-keap1 antioxidant pathway and ameliorates nephropathy in diabetic rats. Am. J. Hypertens. 27 (4), 586–595. doi:10.1093/ajh/hpt160
Nasri, R., Abdelhedi, O., Jemil, I., Daoued, I., Hamden, K., Kallel, C., et al. (2015). Ameliorating effects of goby fish protein hydrolysates on high-fat-high-fructose diet-induced hyperglycemia, oxidative stress and deterioration of kidney function in rats. Chemico-Biological Interact. 242, 71–80. doi:10.1016/j.cbi.2015.08.003
Pradhan, A. (2007). Obesity, metabolic syndrome, and type 2 diabetes: Inflammatory basis of glucose metabolic disorders. Nutr. Rev. 65 (3), S152–S156. doi:10.1111/j.1753-4887.2007.tb00354.x
Qiu, X., Gao, D.-H., Xiang, X., Xiong, Y.-F., Zhu, T.-S., Liu, L.-G., et al. (2015). Ameliorative effects of lutein on non-alcoholic fatty liver disease in rats. World J. Gastroenterol. 21 (26), 8061–8072. doi:10.3748/wjg.v21.i26.8061
Rahimi, R. S., and Landaverde, C. (2013). Nonalcoholic fatty liver disease and the metabolic syndrome: Clinical implications and treatment. Nutr. Clin. Pract. 28 (1), 40–51. doi:10.1177/0884533612470464
Refaat, B., Abdelghany, A. H., Ahmad, J., Abdalla, O. M., Elshopakey, G. E., Idris, S., et al. (2021). Vitamin D3 enhances the effects of omega-3 oils against metabolic dysfunction-associated fatty liver disease in rat. BioFactors.
Ren, L., Sun, D., Zhou, X., Yang, Y., Huang, X., Li, Y., et al. (2019). Chronic treatment with the modified Longdan Xiegan Tang attenuates olanzapine-induced fatty liver in rats by regulating hepatic de novo lipogenesis and fatty acid beta-oxidation-associated gene expression mediated by SREBP-1c, PPAR-alpha and AMPK-alpha. J. Ethnopharmacol. 232, 176–187. doi:10.1016/j.jep.2018.12.034
Sangouni, A. A., Ghavamzadeh, S., and Jamalzehi, A. (2019). A narrative review on effects of vitamin D on main risk factors and severity of Non-Alcoholic Fatty Liver Disease. Diabetes & Metabolic Syndrome Clin. Res. Rev. 13 (3), 2260–2265. doi:10.1016/j.dsx.2019.05.013
Sergeev, I. N., and Song, Q. (2014). High vitamin D and calcium intakes reduce diet-induced obesity in mice by increasing adipose tissue apoptosis. Mol. Nutr. Food Res. 58 (6), 1342–1348. doi:10.1002/mnfr.201300503
Sharifi, N., and Amani, R. (2019). Vitamin D supplementation and non-alcoholic fatty liver disease: A critical and systematic review of clinical trials. Crit. Rev. food Sci. Nutr. 59 (4), 693–703. doi:10.1080/10408398.2017.1389693
Sharma, K., Zajc, I., and Žiberna, L. (2021). Dietary vitamin D equilibrium in serum ameliorates direct bilirubin associated diabetes mellitus. Chemico-Biological Interact. 337, 109399. doi:10.1016/j.cbi.2021.109399
Shin, S.-K., Cho, S.-J., Jung, U. J., Ryu, R., and Choi, M.-S. (2016). Phlorizin supplementation attenuates obesity, inflammation, and hyperglycemia in diet-induced obese mice fed a high-fat diet. Nutrients 8 (2), 92. doi:10.3390/nu8020092
Shojaei Zarghani, S., Soraya, H., and Alizadeh, M. (2018). Calcium and vitamin D 3 combinations improve fatty liver disease through AMPK-independent mechanisms. Eur. J. Nutr. 57, 731–740. doi:10.1007/s00394-016-1360-4
Softic, S., Cohen, D. E., and Kahn, C. R. (2016). Role of dietary fructose and hepatic de novo lipogenesis in fatty liver disease. Dig. Dis. Sci. 61 (5), 1282–1293. doi:10.1007/s10620-016-4054-0
Souza, M. RdA., Diniz, M. d. F. F. d. M., Medeiros-Filho, J. E. M. d., and Araújo, M. S. T. d. (2012). Metabolic syndrome and risk factors for non-alcoholic fatty liver disease. Arq. Gastroenterol. 49, 89–96. doi:10.1590/s0004-28032012000100015
Spahis, S., Delvin, E., Borys, J.-M., and Levy, E. (2016). Oxidative stress as a critical factor in nonalcoholic fatty liver disease pathogenesis. Antioxidants Redox Signal. 26 (10), 519–541. doi:10.1089/ars.2016.6776
Stanhope, K. L., and Havel, P. J. (2010). Fructose consumption: Recent results and their potential implications. Ann. N. Y. Acad. Sci. 1190 (1), 15–24. doi:10.1111/j.1749-6632.2009.05266.x
Subih, H., Al-Tamimi, H., Hamdan, H., Bawadi, H., and Janakat, S. (2018). Decreased weight gain and enhanced serum biochemical parameters in rats after vitamin D and Ca supplementation. Malays. J. Nutr. 24 (2).
Sung, C.-C., Liao, M.-T., Lu, K.-C., and Wu, C.-C. (2012). Role of vitamin D in insulin resistance. J. Biomed. Biotechnol. 2012, 634195. doi:10.1155/2012/634195
Szymczak-Pajor, I., Drzewoski, J., and Śliwińska, A. (2020). The molecular mechanisms by which vitamin D prevents insulin resistance and associated disorders. Int. J. Mol. Sci. 21 (18), 6644. doi:10.3390/ijms21186644
Theodoro, J. M. V., Martinez, O. D. M., Grancieri, M., Toledo, R. C. L., Dias Martins, A. M., Dias, D. M., et al. (2021). Germinated millet flour (Pennisetum glaucum (L) R Br) reduces inflammation, oxidative stress, and liver steatosis in rats fed with high-fat high-fructose diet. J. Cereal Sci. 99, 103207. doi:10.1016/j.jcs.2021.103207
Wang, J.-H., Hwang, S.-J., Lim, D.-W., and Son, C.-G. (2022). Cynanchum atratum alleviates non-alcoholic fatty liver by balancing lipogenesis and fatty acid oxidation in a high-fat, high-fructose diet mice model. Cells 11 (1), 23. doi:10.3390/cells11010023
Xing, L.-J., Zhang, L., Liu, T., Hua, Y.-Q., Zheng, P.-Y., and Ji, G. (2011). Berberine reducing insulin resistance by up-regulating IRS-2 mRNA expression in nonalcoholic fatty liver disease (NAFLD) rat liver. Eur. J. Pharmacol. 668 (3), 467–471. doi:10.1016/j.ejphar.2011.07.036
Yang, P., Liang, Y., Luo, Y., Li, Z., Wen, Y., Shen, J., et al. (2019). Liraglutide ameliorates nonalcoholic fatty liver disease in diabetic mice via the IRS2/PI3K/Akt signaling pathway. Diabetes Metab. Syndr. Obes. 12, 1013–1021. doi:10.2147/DMSO.S206867
Yin, Y., Yu, Z., Xia, M., Luo, X., Lu, X., and Ling, W. (2012). Vitamin D attenuates high fat diet–induced hepatic steatosis in rats by modulating lipid metabolism. Eur. J. Clin. Investigation 42 (11), 1189–1196. doi:10.1111/j.1365-2362.2012.02706.x
Zhao, W., Guo, M., Feng, J., Gu, Z., Zhao, J., Zhang, H., et al. (2022). Myristica fragrans extract regulates gut microbes and metabolites to attenuate hepatic inflammation and lipid metabolism disorders via the AhR–FAS and NF-κB signaling pathways in mice with non-alcoholic fatty liver disease. Nutrients 14 (9), 1699. doi:10.3390/nu14091699
Zhu, C.-g., Liu, Y.-x., Wang, H., Wang, B.-p., Qu, H.-q., Wang, B.-l., et al. (2017). Active form of vitamin D ameliorates non-alcoholic fatty liver disease by alleviating oxidative stress in a high-fat diet rat model. Endocr. J. 64 (7), 663–673. doi:10.1507/endocrj.EJ16-0542
Keywords: hepatic steatosis, vitamin D, high-fat diet, insulin resistance, oxidative stress
Citation: Reda D, Elshopakey GE, Albukhari TA, Almehmadi SJ, Refaat B, Risha EF, Mahgoub HA, El-Boshy ME and Abdelhamid FM (2023) Vitamin D3 alleviates nonalcoholic fatty liver disease in rats by inhibiting hepatic oxidative stress and inflammation via the SREBP-1-c/ PPARα-NF-κB/IR-S2 signaling pathway. Front. Pharmacol. 14:1164512. doi: 10.3389/fphar.2023.1164512
Received: 12 February 2023; Accepted: 02 May 2023;
Published: 16 May 2023.
Edited by:
Thomas Brzozowski, Jagiellonian University Medical College, PolandReviewed by:
Fabrizio Dal Piaz, University of Salerno, ItalyWenhuan Feng, Nanjing University, China
Amira Alsemeh, Zagazig University, Egypt
Hamid Soraya, Urmia University of Medical Sciences, Iran
Copyright © 2023 Reda, Elshopakey, Albukhari, Almehmadi, Refaat, Risha, Mahgoub, El-Boshy and Abdelhamid. This is an open-access article distributed under the terms of the Creative Commons Attribution License (CC BY). The use, distribution or reproduction in other forums is permitted, provided the original author(s) and the copyright owner(s) are credited and that the original publication in this journal is cited, in accordance with accepted academic practice. No use, distribution or reproduction is permitted which does not comply with these terms.
*Correspondence: Fatma M. Abdelhamid, bWF5X21va2hAbWFucy5lZHUuZWc=