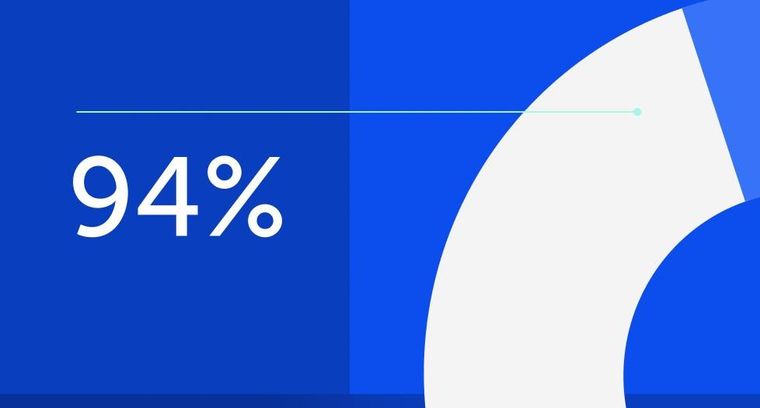
94% of researchers rate our articles as excellent or good
Learn more about the work of our research integrity team to safeguard the quality of each article we publish.
Find out more
ORIGINAL RESEARCH article
Front. Pharmacol., 19 June 2023
Sec. Cardiovascular and Smooth Muscle Pharmacology
Volume 14 - 2023 | https://doi.org/10.3389/fphar.2023.1147360
Objective: This is the first study to explore the mechanism of colchicine in treating coronary artery disease using network pharmacology and molecular docking technology, aiming to predict the key targets and main approaches of colchicine in treating coronary artery disease. It is expected to provide new ideas for research on disease mechanism and drug development.
Methods: Traditional Chinese Medicine Systems Pharmacology Database and Analysis Platform (TCMSP), Swiss Target Prediction and PharmMapper databases were used to obtain drug targets. GeneCards, Online Mendelian Inheritance in Man (OMIM), Therapeutic Target Database (TTD), DrugBank and DisGeNET databases were utilized to gain disease targets. The intersection of the two was taken to access the intersection targets of colchicine for the treatment of coronary artery disease. The Sting database was employed to analyze the protein-protein interaction network. Gene Ontology (GO) functional enrichment analysis was performed using Webgestalt database. Reactom database was applied for Kyoto Encyclopedia of Genes and Genomes (KEGG) enrichment analysis. Molecular docking was simulated using AutoDock 4.2.6 and PyMOL2.4 software.
Results: A total of 70 intersecting targets of colchicine for the treatment of coronary artery disease were obtained, and there were interactions among 50 targets. GO functional enrichment analysis yielded 13 biological processes, 18 cellular components and 16 molecular functions. 549 signaling pathways were obtained by KEGG enrichment analysis. The molecular docking results of key targets were generally good.
Conclusion: Colchicine may treat coronary artery disease through targets such as Cytochrome c (CYCS), Myeloperoxidase (MPO) and Histone deacetylase 1 (HDAC1). The mechanism of action may be related to the cellular response to chemical stimulus and p75NTR-mediated negative regulation of cell cycle by SC1, which is valuable for further research exploration. However, this research still needs to be verified by experiments. Future research will explore new drugs for treating coronary artery disease from these targets.
Coronary artery disease (CAD) is a common heart disease characterized by insufficient blood and oxygen supply to the myocardium (Liang et al., 2021). It is caused by atherosclerosis and atherosclerotic plaque rupture leading to arterial occlusion or obstruction of smaller branches of coronary arteries by material dislodged from the plaque (Prasad and Elefteriades, 2021). Inflammatory activation and dysfunction of the coronary endothelium is a key link in the development of atherosclerosis, as well as being strongly associated with an increased risk of cardiovascular events (Medina-Leyte et al., 2021). Currently, CAD remains the leading cause of death worldwide (Lawton et al., 2022) and is a major source of global cardiovascular disease morbidity, mortality, and economic burden on health (Virani et al., 2021). Clinically, management of diet as well as lifestyle and usage of statins as well as antiplatelet drugs are mainly used as prophylactic treatment for CAD (Jia et al., 2020; Wong et al., 2022). Although antiplatelet aggregation, lipid regulation to stabilize plaque and control of risk factors have reduced the cardiovascular risk of patients to some extent (Imazio et al., 2020), the annual risk of major cardiovascular events is still as high as 3% (Amico et al., 2016; Eikelboom et al., 2017), so CAD is still in urgent need of intervention with novel therapy regimens. Colchicine has been reported to have potential application in cardiovascular disease (Tong et al., 2020), significantly reducing major adverse cardiovascular events (MACE) in patients with CAD (Nidorf et al., 2020; Kurup et al., 2021), and is expected to be a new drug for second-level prevention of CAD (Roubille and Tardif, 2020; Slomski, 2020). Colchicine was found to reduce hs-CRP at 0.36 mg/L and mean leukocytes at 371.75/L in patients with CAD (Sethuramalingam et al., 2021), implying that colchicine can reduce the inflammatory response in patients with CAD. Meta-analysis demonstrated that colchicine reduced MACE by approximately 27%–35%% (Yu et al., 2021; Khan and Lee, 2022a; Casula et al., 2022; Ma et al., 2022), and this benefit was independent of the clinical phenotype of CAD (Xia et al., 2021). In another study, colchicine was also shown to significantly reduce the incidence of stroke by 75% (Wudexi et al., 2021) and the incidence of ACS by 36% (Abrantes et al., 2021). This evidence suggests that colchicine has great potential in the treatment of CAD.
Colchicine, one of the oldest drugs still in use today, is derived from the dried bulb and seeds of the colchicum (Imazio and Nidorf, 2021). It is a low-cost drug with a wide range of anti-inflammatory properties and is currently used in diseases such as gout and familial mediterranean fever (Liuzzo and Patrono, 2020). Several recent meta-analyses have shown that colchicine significantly reduces MACE (Fiolet et al., 2021; Xiang et al., 2021; Andreis et al., 2022) and decreases the level of inflammatory markers in patients with CAD (Sethuramalingam et al., 2021), and the benefit of MACE still holds after percutaneous coronary intervention (Aw et al., 2022). Nevertheless, the mechanism of action of colchicine in the treatment of CAD has not been elucidated, and it is difficult to determine its long-term efficacy and safety.
Network pharmacology is to establish a network model of effective drug targets, involving emerging interdisciplinary subjects such as artificial intelligence and big data (Xia, 2017; Khan and Lee, 2022a). In the development of clinical trials of therapeutic drug candidates, the direction of their research can be guided by visualizing the “drug-targeted disease” network, which is of great significance for exploring the pathways of natural drug molecules related to the treatment of various diseases and other related research (Khan and Lee, 2022b). Therefore, this study aimed to predict the key targets and major pathways of colchicine for the treatment of CAD using network pharmacology and molecular docking techniques, and to explore the potential mechanisms of drug action.
The Traditional Chinese Medicine Systems Pharmacology Database and Analysis Platform (Zhang et al., 2016) (TCMSP, https://tcmsp-e.com/, accessed on 10 February 2022) was used to identify the targets of “Colchicine”. The Swiss Target Prediction database (Gfeller et al., 2013) (http://www.swisstargetprediction.ch/, accessed on 10 February 2022) was also used to predict the target of colchicine, and we use probability >0 as the screening condition to screen target. PharmMapper database (Wang et al., 2017) (http://www.lilab-ecust.cn/pharmmapper/, accessed on 10 February 2022) was utilized to predict the targets of colchicine, where we set up Generate Conformers as “yes”, Maximum Generated Conformations as “300” and Select Targets Set as “Druggable Pharmacophore Models (v2017, 16,159)”. The Uniprot database (Wang et al., 2021) (https://www.uniprot.org/, accessed on 10 February 2022) was used for matching the gene names of each target protein, and then the target genes from the three databases were pooled to obtain the drug targets of colchicine.
The GeneCards (Stelzer et al., 2016) (https://www.genecards.org/, accessed on 10 February 2022), OMIM (Amberger et al., 2015) (https://mirror.omim.org/, accessed on 10 February 2022), DrugBank (Wishart et al., 2018) (https://go.drugbank.com/, accessed on 10 February 2022), DisGeNET (Bauer-Mehren et al., 2010) (https://www.disgenet.org/, accessed on 10 February 2022), and TTD (Zhou et al., 2022) (http://db.idrblab.net/ttd/, accessed on 10 February 2022) databases were searched for “Coronary Artery Disease” to obtain the relevant disease targets. We used the default search function of the database, and included all CAD related targets provided by the database. The disease targets from the five databases were combined to obtain the disease targets for CAD. The drug targets were then intersected with the disease targets to obtain the intersecting targets, and the Venn diagram of the drug-disease intersecting targets was drawn using R-4.0.2-win software.
The intersecting targets of drugs and diseases were imported into the String network platform (Szklarczyk et al., 2021) (https://string-db.org/, accessed on 10 February 2022). The protein type was set to “Homo sapiens”. The confidence level was set to >0.9. The free targets were deleted, and the protein-protein interaction network (PPI) was constructed. After importing the nodal relationship information into Cytoscape 3.8.0 software to calculate the degree, the key targets for colchicine in the treatment of CAD were predicted based on the degree (Long et al., 2020), and the PPI was mapped using Cyroscape3.8.0 software.
The drug-disease intersection targets were imported into the WebGestalt database (Liao et al., 2019) (http://www.webgestalt.org/, accessed on 10 February 2022). The restricted species was “H. sapiens”. The method was over characterization analysis. Using the genomic protein code as the reference set, GO functional enrichment analysis of biological process (BP), molecular function (MF) and cellular component (CC) was performed on the intersection targets.
The drug-disease intersection targets were uploaded into the Reactome database (Griss et al., 2020) (https://reactome.org/, accessed on 10 February 2022). The species was qualified as “H. sapiens”. p < 0.05 and FDR < 0.05 were set. KEGG pathway enrichment analysis was performed on the intersecting targets. GO functional enrichment analysis and KEGG enrichment analysis were mapped using R-4.0.2-win software, and drug-target-pathway mesh maps were mapped using Cytoscape 3.8.0 software.
The protein-data-bank (PDB) IDs of the intersecting targets were searched through the String network platform, and the corresponding protein structures were downloaded from the RCSB PDB database (https://www.rcsb.org/, accessed on 10 February 2022) based on the PDB IDs. If multiple PDB IDs exist for the same target, the best protein crystal structure was selected based on the root mean square deviation of the resolution. The proteins were processed using AutoDock4.2.6 (Goodsell et al., 2021) (https://autodock.scripps.edu/) and PyMOL2.4 (https://pymol.org/2/) (Rigsby and Parker, 2016) to remove small molecule ligands and all water molecules. The location of the docking box was determined based on the small molecule composition provided by the PBD database. If the PDB database did not provide the small molecule composition, the POCASA platform (http://g6altair.sci.hokudai.ac.jp/g6/service/pocasa/, accessed on 10 February 2022) was used to predict the position of the docking box. All parameters were default standard parameters of the software or platform. Molecular docking was carried out after hydrogenation and electron addition to the processed proteins, and the binding activity was judged by the docking score. The docked protein-ligand complexes were imported into the PLIP platform (https://plip-tool.biotec.tu-dresden.de/, accessed on 10 February 2022) for analysis, and molecular docking patterns were drawn in combination with PyMOL2.4 software.
The workflow is demonstrated in Figure 1.
The TCMSP database indicated that colchicine has a Mol ID of MOL013158, a bioavailability of 3.85% and a drug-like property of 0.78. A total of 143 potential targets of colchicine were obtained through TCMSP, Swiss Target Prediction and PharmMapper databases.
A total of 8520 CAD-related targets were obtained through GeneCards, OMIM, DrugBank, DisGeNET and TTD databases. After intersecting them with drug targets, 70 targets of colchicine for the treatment of CAD were obtained (Figure 2).
Seventy intersecting targets were imported into the String database, and the PPI network revealed that there were free targets for ACADS, ACKR1, ACR, BLVRA, CCL24, DAB1, EDC3, FERD3L, FLOT2, FUT8, KLK1, MYNN, PMP2, PRPS1, REPS1, RPA1, SNF8, TCEA3, TOX and TUBB1. And interactions were present for the remaining 50 targets, as shown in Figure 3. The node relationship information was imported into Cytoscape3.8.0 software to calculate the degree values, and the following results were displayed. 1) The degree values of Cytochrome c (CYCS), Myeloperoxidase (MPO), Annexin A5 (ANXA5) and Histone deacetylase 1 (HDAC1) were >10. 2) The degree values of Receptor tyrosine-protein kinase erbB-2 (ERBB2), Prothrombin (F2), Glutathione reductase (GSR), Histone deacetylase 3 (HDAC3), Superoxide Dismutase 2 (SOD2), Hematopoietic prostaglandin D synthase (HPGDS), 72 kDa type IV collagenase (MMP2), Actin, alpha skeletal muscle (ACTA1), Histone deacetylase 6 (HDAC6), Histone deacetylase 2 (HDAC2), Succinate Dehydrogenase Complex Flavoprotein Subunit A (SDHA) and Acetylcholine receptor subunit epsilon (ACHE) were >5. 3) The degree values of the remaining targets were ≤ 5.
The 70 intersecting targets were loaded into the Webgestalt database for GO functional enrichment analysis, and the results shown were as follows (Figure 4). 1) There were 13 BP for colchicine in the treatment of CAD, mainly involving metabolic processes, biological regulation, responses to stimuli, multicellular biological processes and growth processes. 2) There were 18 CC, mainly involving the nucleus, cell membrane, cytoplasm, membrane lumen and vesicles. 3) There were 16 molecular functions (MF), mainly related to protein binding, ion binding, nucleic acid binding, hydrolase activity and nucleotide binding, etc.
70 intersecting targets were imported into the Reactom database for KEGG enrichment analysis. It revealed a total of 549 pathways for colchicine in the treatment of CAD, which involved cellular response to chemical stress, p75NTR-mediated SC1 negative regulation of cell cycle, transcriptional activation of mitochondrial biogenesis, Notch-HLH transcriptional pathway and FOXO-mediated transcription. The top 20 pathways are shown in Figure 5, and the reticulation of drugs, targets, and pathways is shown in Figure 6.
Based on the results of the PPI, key targets with degree values > 5 were molecularly docked to the active ingredient colchicine. The stability between the two can be judged by the binding energy, which can be used to determine how well a protein binds to a ligand compound. When the binding energy of the ligand to the receptor is lower, the more stable the binding conformation of the two and the higher the possibility of interaction, which is generally evaluated at −5 kcal/mol (Liu et al., 2020; Lu and Wang, 2021). The results demonstrated that colchicine had a relatively high binding potential to CYCS, MPO, HDAC1, ERBB2, F2, GSR, HDAC3, HPGDS, MMP2, ACTA1, HDAC6, HDAC2, SDHA and ACHE (Table 1). Among them, the structure of CYCS protein is complex and PLIP cannot obtain the docking state of protein and ligand, so the molecular docking pattern of CYCS was not mapped. Molecular docking mode diagram is shown in Figure 7.
Target prediction yielded a total of 70 targets for colchicine in the treatment of CAD. PPI network further screened 4 key targets with degree values > 10 and 12 key targets with degree values > 5, which may be potential mechanism of colchicine in the treatment of CAD. Both MPO and CYCS are key targets with the highest degree values, and there are numerous studies demonstrating the involvement of MPO in the development and progression of atherosclerosis (Teng et al., 2017). The lack of MPO expression was shown to significantly reduce the risk of cardiovascular disease at the beginning of this century (Kutter et al., 2000), and subsequent studies have pointed out that MPO gene polymorphisms are associated with an increased risk of CAD (Asselbergs et al., 2004). A recent study suggested that elevated plasma MPO in patients with CAD were directly related to the severity of CAD and the risk of developing MACE, and that reducing MPO has important clinical implications for improving prognosis (Cheng et al., 2020). MPO has been reported to contribute to the development of CAD through multiple pathways, including triggering endothelial dysfunction, activation of inflammatory responses and weakening of atherosclerotic plaques (Chaikijurajai and Tang, 2020). Notably, it was reported that MPO can affect nitric oxide (NO) metabolism in the vascular endothelium by reducing NO production and inhibiting Bcl-2 expression, triggering apoptosis of endothelial cells and leading to vascular endothelial dysfunction (Kimak et al., 2018). And colchicine promotes NO production and exerts a protective endothelial function, probably by inhibiting the MPO pathway (Chen et al., 2022). Furthermore, it has been shown that MPO is also involved in the formation of neutrophil extracellular traps (NETs), which can activate the inflammatory response, promote the expression of MPO itself and its associated inflammatory factors, release matrix metalloproteinases and oxidants, and promote the formation, destabilization, as well as rupture of atherosclerotic plaques (Borissoff et al., 2013; Döring et al., 2017). Colchicine has been reported to reduce neutrophil L-selectin expression and regulate E-selectin expression on the cell surface of endothelial cells, thereby impairing neutrophil chemotaxis and adhesion, inhibiting superoxide production and expression of NETs, and attenuating cardiovascular injury from MPO (Tong et al., 2020). Besides, MPO binding to extracellular matrix structural proteins such as type IV collagen and fibronectin, which means that HOCl derived from MPO directly oxidizes the protein, can reduce the adhesion of vascular smooth muscle cells and affect atheromatous plaque stability (Kubala et al., 2013; Bennett et al., 2016). Thus, MPO is an important factor in the development and progression of atherosclerosis (Ndrepepa, 2019).
CYCS is the gene encoding cytochrome C, which is a water-soluble protein located in the inner mitochondrial membrane and is the only peripheral protein in the electron transport chain that plays an important role in mitochondrial energy metabolism (Marenzi et al., 2016). Continuous progressive damage to mitochondria is an important mechanism for the development of myocardial ischemia, which proceeds in a regional subpopulation-dependent manner and at specific sites in the electron transport chain within both groups of mitochondria (Lesnefsky et al., 2017). Because CYCS is located in the inner membrane of mitochondria, it is not detectable from the blood of healthy individuals under physiological conditions (Ow et al., 2008). However, prolonged ischemic injury can cause mitochondrial dysfunction, which in turn leads to CYCS release from mitochondria (Zhu et al., 2018). Colchicine can reduce the expression of NLRP3 inflammatory vesicles in mice with viral myocarditis and inhibit the expression of the apoptotic factor caspase-3, thereby reducing cardiac cell death (Pappritz et al., 2022). Colchicine gel was reported to inhibit myocardial apoptosis and fibrosis in mice with myocardial infarction and improved cardiac function and structure (Chen et al., 2020). Colchicine exerts its anti-inflammatory, anti-apoptotic and anti-fibrotic effects through upregulated hepatic B-cell lymphoma 2 (Bcl-2) and downregulated Bcl-2 associated X protein (BAX) expression and transforming growth factor-β (TGF-β) content, which attenuates rat liver injury induced by renal ischemia-reperfusion injury (Awad et al., 2022). It was shown that when cytochrome C is released from mitochondria, it activates the caspase-3 apoptotic pathway and promotes cardiomyocyte apoptosis, making cardiac function impaired (Radhakrishnan et al., 2007). In summary, colchicine has an inhibitory, anti-apoptotic and anti-fibrotic effect on cardiomyocyte inflammation. Thus, we speculate that colchicine may maintain mitochondrial integrity and reduce the release of cytochrome C after myocardial damage through its inhibitory effect on cardiomyocyte apoptosis. And as an important substance affecting mitochondrial function, CYCS is then likely to be a key target for the treatment of CAD.
ANXA5, also known as membrane-linked protein A5, is a member of the Annexin family (Zhang et al., 2019a). It inhibits the binding and uptake of oxidized LDL by macrophages and plays an important protective role in atherosclerosis formation and plaque rupture (Domeij et al., 2013). Myocardial infarction leads to elevated levels of endogenous ANXA5, which is a self-protective mechanism of the body (Matsuda et al., 2003). Treatment with exogenous ANXA5 reveals that labeled ANXA5 accumulates in a punctate pattern in the infarcted area, which is significantly reduced in the infarcted area of myocardial infarction after 3 weeks (de Jong et al., 2018). The mechanism is the reduced proliferation of macrophages and inhibition of IL-6 production by macrophages derived from bone marrow, attenuating the inflammatory response (de Jong et al., 2018). ANXA5 also significantly inhibits the capture, rolling, adhesion and migration of peripheral blood monocytes on TNF-α-activated endothelial cell layer, which may reduce inflammation of plaques in lesions with advanced disease by interfering with the recruitment and activation of monocytes at the site of inflammatory lesions (Burgmaier et al., 2014). It was noted that colchicine not only binds microtubulin heterodimers to alter the conformation of microtubulin and prevent microtubule growth, thus affecting the assembly of inflammatory vesicles, but also inhibits neutrophil chemotaxis and reduces macrophage formation, thus exerting anti-inflammatory effects (Slobodnick et al., 2015). ANXA5 has also been reported to further stabilize plaques by reducing the content of macrophages and increasing the content of smooth muscle cells (Stöhr et al., 2017). They also suggest that ANXA5 treatment reduces the formation of atherosclerotic plaques, in part due to a reduced rate of apoptosis, thus favoring macrophage infiltration and activation (Stöhr et al., 2017). Colchicine can inhibit lipoprotein uptake by macrophages and is also able to suppress formation of foam cell by promoting lipid efflux from macrophage ABCA1 receptors (Zhang et al., 2022). It has been suggested that ANXA5 enhances ABCA1-mediated cholesterol efflux, thereby improving macrophage lipid metabolism and reducing the risk of CAD (Chen et al., 2021). However, the role of ANXA5 in CAD remains controversial. Burgmaier et al. revealed that in high-risk diabetic patients, the level of circulating ANXA5 correlated with the thickness of the carotid intima, but not with the composition of the coronary plaque (Burgmaier et al., 2017). And Hashemi et al. reported that the frequency of polymorphism in ANXA5 gene was not relevant to the presence of in-stent restenosis (Hashemi et al., 2019). Nevertheless, the anti-atherosclerotic potential of ANXA5 still deserves further attention and research, and ANXA5 may be an important target for the treatment of CAD.
HDAC1, histone deacetylase 1, is an enzyme that removes acetyl groups from lysine residues of histones and non-histones (Pao et al., 2020). HDAC1 has a protective role in models of atherosclerosis and is able to mediate the effects of external and ambient stimuli by modulating key endothelial functions such as angiogenesis, inflammatory signaling, redox homeostasis and nitric oxide signaling (Dunaway and Pollock, 2021). The anti-atherosclerotic effect of HDAC1 may be related to the miR-410/HDAC1/KLF5/IKBα/NF-κB axis, with miR-410 playing a potential role in the pathogenesis of atherosclerosis (Nan et al., 2021). Studies have suggested that blocking miR-410 promotes HDAC1 expression, increases KLF5-mediated IKBα expression, and inhibits the NF-κB pathway, thereby inhibiting the development of atherosclerosis (Nan et al., 2021). It has also been demonstrated that the anti-atherosclerotic effect of HDAC1 is based on the miR-146a-3p/HDAC1/KLF5/IKBα signaling axis, as deletion of miR-146a-3p promotes the expression of HDAC1 and IKBα in atherosclerotic mice, thus promoting the stabilization of pathological plaques (Liu et al., 2021). It has also been reported that the role of HDAC1 is closely related to miR-224-3p and that the over expression of HDAC1 may enhance the anti-atherogenic and endothelial protective effects of miR-224-3p-mediated FOSL2 inhibition by deacetylating HIF1α (Wang et al., 2020). Although there is more evidence that HDAC1 plays a protective role in atherosclerosis and CAD, there is still evidence supporting HDAC1 as an adverse factor in atherosclerosis. It has been noted that HDAC1 can be involved in VCAM-1 expression and promotion of atherosclerosis through inhibition of STAT3 acetylation-dependent methylation of the GATA6 promoter (Hu et al., 2021). It has also been proposed that HDAC1 inhibits miR-182-5p and activates the AKT pathway by improving VAV3, thereby promoting the progression of atherosclerosis (Gao et al., 2021). Furthermore, some reports have demonstrated that high expression of HDAC1 suppresses H3K9ac and promotes the accumulation of total cholesterol, free cholesterol and triglycerides in foam cells (Zhao et al., 2017). All of these factors may contribute to the progression of atherosclerosis and CAD. Although the beneficial and detrimental effects of HDAC1 in atherosclerosis and CAD remain controversial, the important value of HDAC1 in atherosclerosis and CAD cannot be denied. What is certain is that HDAC1 regulates endothelial function through deacetylation of histones and non-histones, which ultimately affects overall physiology and health. The potential mechanism of colchicine in treating CAD is shown in Figure 8.
In molecular docking, colchicine has a high probability of binding to three key targets with degree >10, such as MPO, CYCS and HDAC1, and other 11 key targets with degree >5. MPO is the key target with the highest degree value and lowest binding energy, and the binding energy for docking with colchicine is −8.7 kcal/mol, which is a good fit. Studies have revealed that colchicine can significantly reduce MPO, and this effect has been demonstrated in models of respiratory syncytial virus infection (Lu et al., 2019), ischemia-reperfusion injury in allogeneic lung transplants (Pieróg et al., 2007), and ischemia-reperfusion-induced injury to skeletal muscle (Wang et al., 2016). CYCS is the key target ranked second in terms of degree value, with a binding energy of −6.7 kcal/mol for docking with colchicine and a good fit. Available studies have shown that colchicine promotes the release of CYCS to activate apoptosis in acute lymphoblastic leukemia (Aghvami et al., 2018), as well as the release of CYCS to induce apoptosis in gastric cancer cells and inhibit growth of gastric tumors (Zhang et al., 2019b). It has also been reported that after pretreatment of HPV16-positive and HPV18-positive human cervical cancer cell lines with colchicine for 48 h, there was a significant increase in CYCS expression in the cytoplasm (Yan et al., 2020). This implies that colchicine is a promising candidate for the treatment and prevention of HPV-associated cervical cancer (López-Sánchez and Frade, 2002). ANXA5 is the key target ranked third in degree value, but the binding energy of ANXA5 docked with colchicine is −4.5 kcal/mol, suggesting that the binding of both is less likely. HDAC1 is the last key target with a degree value > 10 and has a good binding energy of −6.9 kcal/mol docked with colchicine, which is a good fit. Yet, the effect of colchicine on HDAC1 has not been reported in the literature and is to be demonstrated by relevant studies.
In the KEGG pathway enrichment analysis, cellular response to chemical stress and p75NTR-mediated SC1 negative regulation of cell cycle may be the main pathways for colchicine to treat CAD. The cellular response to chemical stress is the top-ranked signaling pathway, and CYCS, HDAC3, GSR, SOD2, SIN3B, NCOA2, PPARA, and BLVRA are all intersectional targets enriched in this pathway. Among them, CYCS and HDAC3 are key targets with degree >10, and GSR, SOD2 and SIN3B are the key targets with degree >5. The p75NTR-mediated SC1 negative regulation of cell cycle is the second-ranked signaling pathway, and three key targets, HDAC1, HDAC2, and HDAC3, are enriched in this pathway. They cover the entire range of histone deacetylases in this process and play an integral and important role in mediating the deacetylation of PRDM4 in this pathway (López-Sánchez and Frade, 2002). In GO functional enrichment analysis, the cellular component of colchicine for the treatment of CAD is mainly the nucleus, while the molecular function mainly affects protein binding and the biological process mainly affects metabolic processes. These are consistent with the results of current studies. It has been reported that colchicine inhibits DNA release by inhibiting the formation of neutrophil-spontaneous, foponol-12-myristate-13-acetate-induced and ionomycin-induced reticulation in patients with acute coronary syndrome, which immobilizes the neutrophil microtubule complex around the nucleus (Vaidya et al., 2021).
Although this study predicted potential targets and mechanisms for colchicine in the treatment of CAD, it still has some limitations (shown below). 1) Due to the limitations of the databases, some targets and mechanisms may not have been included in the analysis. Deftereos et al. showed that colchicine can reduce the incidence of in-stent restenosis by inhibiting the in-stent neointima (Deftereos et al., 2013), and Saji et al. found that colchicine can inhibit cardiomyocyte apoptosis by inhibiting the Bax/Bcl-2 pathway (Saji et al., 2007). Manni et al. also found that nerve growth factor (NGF) and brain-derived neurotrophic factor (BDNF) may be related to the pathogenesis of CAD, and the changes in the levels of these two neurotrophins are related to inflammation, so NGF and BDNF may also be potential targets for colchicine in the treatment of CAD (Manni et al., 2005). In addition, the classic anti-inflammatory mechanism of colchicine is to inhibit tubulin polymerization, which means that tubulin-related targets may be the entry point for future research (Chaldakov, 2018). These mechanisms appear to be beneficial for CAD, but this study did not predict them. 2) Network pharmacology and molecular docking can only be used to predict the targets of colchicine for the treatment of CAD, but not for its efficacy and safety assessment. The long-term safety of colchicine in the treatment of CAD is still unclear and this is to be explored in ongoing clinical trials. In fact, the side effects of a drug are closely related to its selectivity, and the broader the range of action of colchicine in the body means that its potential side effects may be stronger. Therefore, some researchers have focused their attention on new colchicine formulations. Eddleston et al. reported that equimolar doses of anti-colchicine Fab given early in life reduced the cardiotoxicity of colchicine (Eddleston et al., 2018). And Chen et al. used an injectable thermosensitive polymer hydrogel as a carrier for colchicine in the treatment of myocardial infarction, which reduced the systemic toxicity of colchicine (Chen et al., 2020). This implies that colchicine preparations acting topically may have a higher safety profile, and this may be a future research direction.
This study predicts the potential targets and mechanisms of action of colchicine in the treatment of CAD. Colchicine may treat CAD through targets such as CYCS, MPO and HDAC1. The mechanism of action may be related to the cellular response to chemical stimulus and p75NTR-mediated negative regulation of cell cycle by SC1, which is valuable for further research exploration. However, our research lacks experimental verification, and we look forward to future experimental studies to confirm our results and continue to explore new drugs for treating CAD on this basis.
The original contributions presented in the study are included in the article/Supplementary Material. Further inquiries can be directed to the corresponding author.
YY participated in the conception and design of this study. YY and MZ wrote the first draft of the manuscript. XL was in charge of software operation and data analysis. MZ adjusted the research protocol. SY was responsible for language revision. GH and XY participated in database retrieval and target sorting. WJ reviewed and revised the manuscript. RY put forward the preliminary conception of the research, participated in the overall design of the research, and guided the revision of the manuscript. All authors contributed to the article and approved the submitted version.
National Natural Science Foundation of China (81973753) and Natural Science Foundation of Hunan Province (2018JJ3405).
The authors declare that the research was conducted in the absence of any commercial or financial relationships that could be construed as a potential conflict of interest.
All claims expressed in this article are solely those of the authors and do not necessarily represent those of their affiliated organizations, or those of the publisher, the editors and the reviewers. Any product that may be evaluated in this article, or claim that may be made by its manufacturer, is not guaranteed or endorsed by the publisher.
The Supplementary Material for this article can be found online at: https://www.frontiersin.org/articles/10.3389/fphar.2023.1147360/full#supplementary-material
Abrantes, A. M., Nogueira-Garcia, B., Alves, M., Teixeira Passos, D., Brito, D., Pinto, F. J., et al. (2021). Low-Dose colchicine in coronary artery disease - systematic review and meta-analysis. Circ. Rep. 3 (8), 457–464. doi:10.1253/circrep.CR-21-0065
Aghvami, M., Eshghi, P., Zarei, M. H., Arefi, H., Sattari, F., Zarghi, A., et al. (2018). Novel colchicine analogues target mitochondrial PT pores using free tubulins and induce ROS-mediated apoptosis in cancerous lymphocytes. Iran. J. Pharm. Res. 17, 1476–1487.
Amberger, J. S., Bocchini, C. A., Schiettecatte, F., Scott, A. F., and Hamosh, A. (2015). OMIM.org: Online Mendelian Inheritance in Man (OMIM®), an online catalog of human genes and genetic disorders. Nucleic Acids Res. 43, D789–D798. doi:10.1093/nar/gku1205
Amico, F., Schlesinger, A., and Mazzoni, J. (2016). Long-term use of ticagrelor in patients with prior heart attack: Ticagrelor plus aspirin versus aspirin monotherapy. Postgrad. Med. 128, 164–169. doi:10.1080/00325481.2016.1134270
Andreis, A., Imazio, M., Piroli, F., Avondo, S., Casula, M., Paneva, E., et al. (2022). Efficacy and safety of colchicine for the prevention of major cardiovascular and cerebrovascular events in patients with coronary artery disease: A systematic review and meta-analysis on 12869 patients. Eur. J. Prev. Cardiol. 28, 1916–1925. doi:10.1093/eurjpc/zwab045
Asselbergs, F. W., Reynolds, W. F., Cohen-Tervaert, J. W., Jessurun, G. A., and Tio, R. A. (2004). Myeloperoxidase polymorphism related to cardiovascular events in coronary artery disease. Am. J. Med. 116, 429–430. doi:10.1016/j.amjmed.2003.10.025
Aw, K. L., Koh, A., Lee, H. L., Kudzinskas, A., and De Palma, R. (2022). Colchicine for symptomatic coronary artery disease after percutaneous coronary intervention. Open Heart 9, e001887. doi:10.1136/openhrt-2021-001887
Awad, A. S., Elariny, H. A., and Sallam, A. S. (2022). Colchicine attenuates renal ischemia-reperfusion-induced liver damage: Implication of TLR4/NF-κB, TGF-β, and BAX and bcl-2 gene expression. Can. J. Physiol. Pharmacol. 100 (1), 12–18. doi:10.1139/cjpp-2021-0007
Bauer-Mehren, A., Rautschka, M., Sanz, F., and Furlong, L. I. (2010). DisGeNET: A Cytoscape plugin to visualize, integrate, search and analyze gene-disease networks. Bioinformatics 26 (22), 2924–2926. doi:10.1093/bioinformatics/btq538
Bennett, M. R., Sinha, S., and Owens, G. K. (2016). Vascular smooth muscle cells in atherosclerosis. Circ. Res. 118 (4), 692–702. doi:10.1161/CIRCRESAHA.115.306361
Borissoff, J. I., Joosen, I. A., Versteylen, M. O., Brill, A., Fuchs, T. A., Savchenko, A. S., et al. (2013). Elevated levels of circulating DNA and chromatin are independently associated with severe coronary atherosclerosis and a prothrombotic state. Arterioscler. Thromb. Vasc. Biol. 33 (8), 2032–2040. doi:10.1161/ATVBAHA.113.301627
Burgmaier, M., Reith, S., Schurgers, L., Kahles, F., Marx, N., and Reutelingsperger, C. (2017). Circulating annexin A5 levels are associated with carotid intima-media thickness but not coronary plaque composition. Diab Vasc. Dis. Res. 14 (5), 415–422. doi:10.1177/1479164117710392
Burgmaier, M., Schutters, K., Willems, B., van der Vorst, E. P., Kusters, D., Chatrou, M., et al. (2014). AnxA5 reduces plaque inflammation of advanced atherosclerotic lesions in apoE(-/-) mice. J. Cell. Mol. Med. 18 (10), 2117–2124. doi:10.1111/jcmm.12374
Casula, M., Andreis, A., Avondo, S., Vaira, M. P., and Imazio, M. (2022). Colchicine for cardiovascular medicine: A systematic review and meta-analysis. Future Cardiol. 18 (8), 647–659. doi:10.2217/fca-2020-0206
Chaikijurajai, T., and Tang, W. H. W. (2020). Myeloperoxidase: A potential therapeutic target for coronary artery disease. Expert Opin. Ther. Targets 24, 695–705. doi:10.1080/14728222.2020.1762177
Chaldakov, G. N. (2018). Colchicine, a microtubule-disassembling drug, in the therapy of cardiovascular diseases. Cell. Biol. Int. 42 (8), 1079–1084. doi:10.1002/cbin.10988
Chen, T., Liu, G., and Yu, B. (2022). Colchicine for coronary artery disease: A review. Front. Cardiovasc Med. 9, 892588. doi:10.3389/fcvm.2022.892588
Chen, W., Li, L., Wang, J., Zhang, R., Zhang, T., Wu, Y., et al. (2021). The ABCA1-efferocytosis axis: A new strategy to protect against atherosclerosis. Clin. Chim. Acta 518, 1–8. doi:10.1016/j.cca.2021.02.025
Chen, Y., Shi, J., Zhang, Y., Miao, J., Zhao, Z., Jin, X., et al. (2020). An injectable thermosensitive hydrogel loaded with an ancient natural drug colchicine for myocardial repair after infarction. J. Mater Chem. B 8 (5), 980–992. doi:10.1039/c9tb02523e
Cheng, M., Cheng, M., and Wei, Q. (2020). Association of myeloperoxidase, homocysteine and high-sensitivity C-reactive protein with the severity of coronary artery disease and their diagnostic and prognostic value. Exp. Ther. Med. 20, 1532–1540. doi:10.3892/etm.2020.8817
de Jong, R. C. M., Pluijmert, N. J., de Vries, M. R., Pettersson, K., Atsma, D. E., Jukema, J. W., et al. (2018). Annexin A5 reduces infarct size and improves cardiac function after myocardial ischemia-reperfusion injury by suppression of the cardiac inflammatory response. Sci. Rep. 8 (1), 6753. doi:10.1038/s41598-018-25143-y
Deftereos, S., Giannopoulos, G., Raisakis, K., Kossyvakis, C., Kaoukis, A., Panagopoulou, V., et al. (2013). Colchicine treatment for the prevention of bare-metal stent restenosis in diabetic patients. J. Am. Coll. Cardiol. 61 (16), 1679–1685. doi:10.1016/j.jacc.2013.01.055
Domeij, H., Hua, X., Su, J., Bäcklund, A., Yan, Z., Frostegård, A. G., et al. (2013). Annexin A5 inhibits atherogenic and pro-inflammatory effects of lysophosphatidylcholine. Prostagl. Other Lipid Mediat 106, 72–78. doi:10.1016/j.prostaglandins.2013.01.004
Döring, Y., Soehnlein, O., and Weber, C. (2017). Neutrophil extracellular traps in atherosclerosis and atherothrombosis. Circ. Res. 120 (4), 736–743. doi:10.1161/CIRCRESAHA.116.309692
Dunaway, L. S., and Pollock, J. S. (2021). HDAC1: An environmental sensor regulating endothelial function. Cardiovasc Res. 118, 1885–1903. doi:10.1093/cvr/cvab198
Eddleston, M., Fabresse, N., Thompson, A., Al Abdulla, I., Gregson, R., King, T., et al. (2018). Anti-colchicine Fab fragments prevent lethal colchicine toxicity in a porcine model: A pharmacokinetic and clinical study. Clin. Toxicol. (Phila) 56 (8), 773–781. doi:10.1080/15563650.2017.1422510
Eikelboom, J. W., Connolly, S. J., Bosch, J., Dagenais, G. R., Hart, R. G., Shestakovska, O., et al. (2017). Rivaroxaban with or without aspirin in stable cardiovascular disease. N. Engl. J. Med. 377, 1319–1330. doi:10.1056/NEJMoa1709118
Fiolet, A. T. L., Opstal, T. S. J., Mosterd, A., Eikelboom, J. W., Jolly, S. S., Keech, A. C., et al. (2021). Efficacy and safety of low-dose colchicine in patients with coronary disease: A systematic review and meta-analysis of randomized trials. Eur. Heart J. 42, 2765–2775. doi:10.1093/eurheartj/ehab115
Gao, Y., Pan, L., Zhao, L., and Dang, X. (2021). HDAC1 promotes artery injury through activation of VAV3 by binding to miR-182-5p in atherosclerotic mice model. Cell. Signal 78, 109840. doi:10.1016/j.cellsig.2020.109840
Gfeller, D., Michielin, O., and Zoete, V. (2013). Shaping the interaction landscape of bioactive molecules. Bioinformatics 29 (23), 3073–3079. doi:10.1093/bioinformatics/btt540
Goodsell, D. S., Sanner, M. F., Olson, A. J., and Forli, S. (2021). The auto docksuite at 30. Protein Sci. 30 (1), 31–43. doi:10.1002/pro.3934
Griss, J., Viteri, G., Sidiropoulos, K., Nguyen, V., Fabregat, A., and Hermjakob, H. (2020). ReactomeGSA - efficient multi-omics comparative pathway analysis. Mol. Cell. Proteomics 19 (12), 2115–2124. doi:10.1074/mcp.TIR120.002155
Hashemi, S. M., Baktashian, M., Moghaddam, K. H., Salehi, M., Soflaei, S. S., Ferns, G., et al. (2019). The association between genetic polymorphisms of the interleukin-10, tumor necrosis factor-alpha, and annexin A5 gene loci and restenosis after percutaneous coronary angioplasty and stenting. J. Res. Med. Sci. 24, 68. doi:10.4103/jrms.JRMS_293_18
Hu, C., Peng, K., Wu, Q., Wang, Y., Fan, X., Zhang, D. M., et al. (2021). HDAC1 and 2 regulate endothelial VCAM-1 expression and atherogenesis by suppressing methylation of the GATA6 promoter. Theranostics 11 (11), 5605–5619. doi:10.7150/thno.55878
Imazio, M., Andreis, A., Brucato, A., Adler, Y., and De Ferrari, G. M. (2020). Colchicine for acute and chronic coronary syndromes. Heart 106, 1555–1560. doi:10.1136/heartjnl-2020-317108
Imazio, M., and Nidorf, M. (2021). Colchicine and the heart. Eur. Heart J. 42, 2745–2760. doi:10.1093/eurheartj/ehab221
Jia, S., Liu, Y., and Yuan, J. (2020). Evidence in guidelines for treatment of coronary artery disease. Adv. Exp. Med. Biol. 1177, 37–73. doi:10.1007/978-981-15-2517-9_2
Khan, S. A., and Lee, T. (2022a). Network pharmacology and molecular docking-based investigations of Kochiae Fructus's active phytomolecules, molecular targets, and pathways in treating COVID-19. Front. Microbiol. 13, 972576. doi:10.3389/fmicb.2022.972576
Khan, S. A., and Lee, T. K. W. (2022b). Investigations of nitazoxanide molecular targets and pathways for the treatment of hepatocellular carcinoma using network pharmacology and molecular docking. Front. Pharmacol. 13, 968148. doi:10.3389/fphar.2022.968148
Kimak, E., Zięba, B., Duma, D., and Solski, J. (2018). Myeloperoxidase level and inflammatory markers and lipid and lipoprotein parameters in stable coronary artery disease. Lipids Health Dis. 17 (1), 71. doi:10.1186/s12944-018-0718-4
Kubala, L., Kolářová, H., Víteček, J., Kremserová, S., Klinke, A., Lau, D., et al. (2013). The potentiation of myeloperoxidase activity by the glycosaminoglycan-dependent binding of myeloperoxidase to proteins of the extracellular matrix. Biochim. Biophys. Acta 1830 (10), 4524–4536. doi:10.1016/j.bbagen.2013.05.024
Kurup, R., Galougahi, K. K., Figtree, G., Misra, A., and Patel, S. (2021). The role of colchicine in atherosclerotic cardiovascular disease. Heart Lung Circ. 30, 795–806. doi:10.1016/j.hlc.2020.11.010
Kutter, D., Devaquet, P., Vanderstocken, G., Paulus, J. M., Marchal, V., and Gothot, A. (2000). Consequences of total and subtotal myeloperoxidase deficiency: Risk or benefit? Acta Haematol. 104, 10–15. doi:10.1159/000041062
Lawton, J. S., Tamis-Holland, J. E., Bangalore, S., Bates, E. R., Beckie, T. M., et al. (2022). ACC/AHA/SCAI guideline for coronary artery revascularization: A report of the American College of cardiology/American heart association joint committee on clinical practice guidelines. J. Am. Coll. Cardiol. 79, e21-e129. doi:10.1016/j.jacc.2021.09.006
Lesnefsky, E. J., Chen, Q., Tandler, B., and Hoppel, C. L. (2017). Mitochondrial dysfunction and myocardial ischemia-reperfusion: Implications for novel therapies. Annu. Rev. Pharmacol. Toxicol. 57, 535–565. doi:10.1146/annurev-pharmtox-010715-103335
Liang, B., Cai, X. Y., and Gu, N. (2021). Marine natural products and coronary artery disease. Front. Cardiovasc Med. 8, 739932. doi:10.3389/fcvm.2021.739932
Liao, Y., Wang, J., Jaehnig, E. J., Shi, Z., and Zhang, B. (2019). WebGestalt 2019: Gene set analysis toolkit with revamped UIs and APIs. Nucleic Acids Res. 47 (1), W199–W205. doi:10.1093/nar/gkz401
Liu, H., Wang, H., Ma, J., Qiao, Z., Zhang, L., and Ge, G. (2021). MicroRNA-146a-3p/HDAC1/KLF5/IKBα signal axis modulates plaque formation of atherosclerosis mice. Life Sci. 284, 119615. doi:10.1016/j.lfs.2021.119615
Liu, Q., He, Z., Yang, H., Liu, X., Zeng, Q., Zhang, M., et al. (2020). Exploration on active compounds of Feiduqing for treatment of COVID-19 based on network pharmacology and molecular docking. Chin. Traditional Herb. Drugs 51, 1713–1722. doi:10.7501/j.issn.0253-2670.2020.07.005
Liuzzo, G., and Patrono, C. (2020). Low-dose colchicine: A new tool in the treatment of chronic coronary disease? Comment on the low-dose colchicine (LoDoCo)2 trial. Eur. Heart J. 41, 3880–3881. doi:10.1093/eurheartj/ehaa782
Long, X., Liu, L., Xu, X., Li, L., and Zhang, G. (2020). A network pharmacology study on the effects of Ma Xing Shi Gan Decoction on influenza. Digit. Chin. Med. 3, 163–179. doi:10.1016/j.dcmed.2020.09.003
López-Sánchez, N., and Frade, J. M. (2002). Control of the cell cycle by neurotrophins: Lessons from the p75 neurotrophin receptor. Histol. Histopathol. 17, 1227–1237. doi:10.14670/HH-17.1227
Lu, N., Yang, Y., Liu, H., Ding, X., Ou, Y., Xia, J., et al. (2019). Inhibition of respiratory syncytial virus replication and suppression of RSV-induced airway inflammation in neonatal rats by colchicine. 3 Biotech. 9, 392. doi:10.1007/s13205-019-1917-z
Lu, Y., and Wang, X. (2021). The network pharmacological mechanism of guishen pills in the treatment of polycystic ovary syndrome. Traditional Chin. Drug Res. Clin. Pharmacol. 32, 825–833. doi:10.19378/j.issn.1003-9783.2021.06.011
Ma, Z., Chen, J., Jin, K., and Chen, X. (2022). Colchicine and coronary heart disease risks: A meta-analysis of randomized controlled clinical trials. Front. Cardiovasc Med. 9, 947959. doi:10.3389/fcvm.2022.947959
Manni, L., Nikolova, V., Vyagova, D., Chaldakov, G. N., and Aloe, L. (2005). Reduced plasma levels of NGF and BDNF in patients with acute coronary syndromes. Int. J. Cardiol. 102 (1), 169–171. doi:10.1016/j.ijcard.2004.10.041
Marenzi, G., Cosentino, N., Boeddinghaus, J., Trinei, M., Giorgio, M., Milazzo, V., et al. (2016). Diagnostic and prognostic utility of circulating cytochrome c in acute myocardial infarction. Circ. Res. 119 (12), 1339–1346. doi:10.1161/CIRCRESAHA.116.309792
Matsuda, R., Kaneko, N., Kikuchi, M., Chiwaki, F., Toda, M., Ieiri, T., et al. (2003). Clinical significance of measurement of plasma annexin V concentration of patients in the emergency room. Resuscitation 57 (2), 171–177. doi:10.1016/s0300-9572(03)00034-0
Medina-Leyte, D. J., Zepeda-García, O., Domínguez-Pérez, M., González-Garrido, A., Villarreal-Molina, T., and Jacobo-Albavera, L. (2021). Endothelial dysfunction, inflammation and coronary artery disease: Potential biomarkers and promising therapeutical approaches. Int. J. Mol. Sci. 22, 3850. doi:10.3390/ijms22083850
Nan, S., Wang, Y., Xu, C., and Wang, H. (2021). Interfering microRNA-410 attenuates atherosclerosis via the HDAC1/KLF5/IKBα/NF-κB axis. Mol. Ther. Nucleic Acids 24, 646–657. doi:10.1016/j.omtn.2021.03.009
Ndrepepa, G. (2019). Myeloperoxidase - a bridge linking inflammation and oxidative stress with cardiovascular disease. Clin. Chim. Acta 493, 36–51. doi:10.1016/j.cca.2019.02.022
Nidorf, S. M., Fiolet, A. T. L., Mosterd, A., Eikelboom, J. W., Schut, A., Opstal, T. S. J., et al. (2020). Colchicine in patients with chronic coronary disease. N. Engl. J. Med. 383, 1838–1847. doi:10.1056/NEJMoa2021372
Ow, Y. P., Green, D. R., Hao, Z., and Mak, T. W. (2008). Cytochrome c: Functions beyond respiration. Nat. Rev. Mol. Cell. Biol. 9 (7), 532–542. doi:10.1038/nrm2434
Pao, P. C., Patnaik, D., Watson, L. A., Gao, F., Pan, L., Wang, J., et al. (2020). HDAC1 modulates OGG1-initiated oxidative DNA damage repair in the aging brain and Alzheimer's disease. Nat. Commun. 11, 2484. doi:10.1038/s41467-020-16361-y
Pappritz, K., Lin, J., El-Shafeey, M., Fechner, H., Kühl, U., Alogna, A., et al. (2022). Colchicine prevents disease progression in viral myocarditis via modulating the NLRP3 inflammasome in the cardiosplenic axis. Esc. Heart Fail 9 (2), 925–941. doi:10.1002/ehf2.13845
Pieróg, J., Kubisa, B., Grodzki, T., Wójcik, J., Pankowski, J., Ostrowska, J., et al. (2007). Colchicine against ischemia-reperfusion injury in experimental lung transplantation. Ann. Transpl. 12, 32–37.
Prasad, K., and Elefteriades, J. A. (2021). Coronary artery disease: Part I-medical. Int. J. Angiol. 30, 1–3. doi:10.1055/s-0041-1723943
Radhakrishnan, J., Wang, S., Ayoub, I. M., Kolarova, J. D., Levine, R. F., and Gazmuri, R. J. (2007). Circulating levels of cytochrome c after resuscitation from cardiac arrest: A marker of mitochondrial injury and predictor of survival. Am. J. Physiol. Heart Circ. Physiol. 292 (2), H767–H775. doi:10.1152/ajpheart.00468.2006
Rigsby, R. E., and Parker, A. B. (2016). Using the PyMOL application to reinforce visual understanding of protein structure. Biochem. Mol. Biol. Educ. 44 (5), 433–437. doi:10.1002/bmb.20966
Roubille, F., and Tardif, J. C. (2020). Colchicine for secondary cardiovascular prevention in coronary disease. Circulation 142, 1901–1904. doi:10.1161/CIRCULATIONAHA.120.051240
Saji, K., Fukumoto, Y., Suzuki, J., Fukui, S., Nawata, J., and Shimokawa, H. (2007). Colchicine, a microtubule depolymerizing agent, inhibits myocardial apoptosis in rats. Tohoku J. Exp. Med. 213 (2), 139–148. doi:10.1620/tjem.213.139
Sethuramalingam, S., Maiti, R., Hota, D., and Srinivasan, A. (2021). Effect of colchicine in reducing inflammatory biomarkers and cardiovascular risk in coronary artery disease: A meta-analysis of clinical trials. Am. J. Ther. 30, e197–e208. doi:10.1097/MJT.0000000000001409
Slobodnick, A., Shah, B., Pillinger, M. H., and Krasnokutsky, S. (2015). Colchicine: Old and new. Am. J. Med. 128 (5), 461–470. doi:10.1016/j.amjmed.2014.12.010
Slomski, A. (2020). Colchicine reduces cardiovascular events in chronic coronary disease. JAMA 324, 1599. doi:10.1001/jama.2020.20646
Stelzer, G., Rosen, N., Plaschkes, I., Zimmerman, S., Twik, M., Fishilevich, S., et al. (2016). The GeneCards suite: From gene data mining to disease genome sequence analyses. Curr. Protoc. Bioinforma. 54, 1. doi:10.1002/cpbi.5
Stöhr, R., Schurgers, L., van Gorp, R., Jaminon, A., Marx, N., and Reutelingsperger, C. (2017). Annexin A5 reduces early plaque formation in ApoE -/- mice. PLoS One 12 (12), e0190229. doi:10.1371/journal.pone.0190229
Szklarczyk, D., Gable, A. L., Nastou, K. C., Lyon, D., Kirsch, R., Pyysalo, S., et al. (2021). The STRING database in 2021: Customizable protein-protein networks, and functional characterization of user-uploaded gene/measurement sets. Nucleic Acids Res. 49 (1), D605–D612. doi:10.1093/nar/gkaa1074
Teng, N., Maghzal, G. J., Talib, J., Rashid, I., Lau, A. K., and Stocker, R. (2017). The roles of myeloperoxidase in coronary artery disease and its potential implication in plaque rupture. Redox Rep. 22, 51–73. doi:10.1080/13510002.2016.1256119
Tong, D. C., Quinn, S., Nasis, A., Hiew, C., Roberts-Thomson, P., Adams, H., et al. (2020). Colchicine in patients with acute coronary syndrome: The Australian COPS randomized clinical trial. Circulation 142, 1890–1900. doi:10.1161/CIRCULATIONAHA.120.050771
Vaidya, K., Tucker, B., Kurup, R., Khandkar, C., Pandzic, E., Barraclough, J., et al. (2021). Colchicine inhibits neutrophil extracellular trap formation in patients with acute coronary syndrome after percutaneous coronary intervention. J. Am. Heart Assoc. 10 (1), e018993. doi:10.1161/JAHA.120.018993
Virani, S. S., Alonso, A., Aparicio, H. J., Benjamin, E. J., Bittencourt, M. S., Callaway, C. W., et al. (2021). Heart disease and stroke statistics-2021 update: A report from the American heart association. Circulation 143, e254–e743. doi:10.1161/CIR.0000000000000950
Wang, H., Sugimoto, K., Lu, H., Yang, W. Y., Liu, J. Y., Yang, H. Y., et al. (2020). HDAC1-mediated deacetylation of HIF1α prevents atherosclerosis progression by promoting miR-224-3p-mediated inhibition of FOSL2. Mol. Ther. Nucleic Acids 23, 577–591. doi:10.1016/j.omtn.2020.10.044
Wang, L., Shan, Y., Chen, L., Lin, B., Xiong, X., Lin, L., et al. (2016). Colchicine protects rat skeletal muscle from ischemia/reperfusion injury by suppressing oxidative stress and inflammation. Iran. J. Basic Med. Sci. 19, 670–675.
Wang, X., Shen, Y., Wang, S., Li, S., Zhang, W., Liu, X., et al. (2017). PharmMapper 2017 update: A web server for potential drug target identification with a comprehensive target pharmacophore database. Nucleic Acids Res. 45 (1), W356–W360. doi:10.1093/nar/gkx374
Wang, Y., Wang, Q., Huang, H., Huang, W., Chen, Y., McGarvey, P. B., et al. (2021). A crowdsourcing open platform for literature curation in UniProt. PLoS Biol. 19 (12), e3001464. doi:10.1371/journal.pbio.3001464
Wishart, D. S., Feunang, Y. D., Guo, A. C., Lo, E. J., Marcu, A., Grant, J. R., et al. (2018). DrugBank 5.0: A major update to the DrugBank database for 2018. Nucleic Acids Res. 46 (1), D1074–D1082. doi:10.1093/nar/gkx1037
Wong, N. D., Budoff, M. J., Ferdinand, K., Graham, I. M., Michos, E. D., Reddy, T., et al. (2022). Atherosclerotic cardiovascular disease risk assessment: An American Society for Preventive Cardiology clinical practice statement. Am. J. Prev. Cardiol. 10, 100335. doi:10.1016/j.ajpc.2022.100335
Wudexi, I., Shokri, E., Abo-Aly, M., Shindo, K., and Abdel-Latif, A. (2021). Comparative effectiveness of anti-inflammatory drug treatments in coronary heart disease patients: A systematic review and network meta-analysis. Mediat. Inflamm. 2021, 5160728. doi:10.1155/2021/5160728
Xia, M., Yang, X., and Qian, C. (2021). Meta-analysis evaluating the utility of colchicine in secondary prevention of coronary artery disease. Am. J. Cardiol. 140, 33–38. doi:10.1016/j.amjcard.2020.10.043
Xia, X. (2017). Bioinformatics and drug discovery. Curr. Top. Med. Chem. 17 (15), 1709–1726. doi:10.2174/1568026617666161116143440
Xiang, Z., Yang, J., Yang, J., Zhang, J., Fan, Z., Yang, C., et al. (2021). Efficacy and safety of colchicine for secondary prevention of coronary heart disease: A systematic review and meta-analysis. Intern Emerg. Med. 16, 487–496. doi:10.1007/s11739-020-02606-7
Yan, L., Huang, H., Zhang, Y., Yuan, X., Yan, Z., Cao, C., et al. (2020). Involvement of p53-dependent apoptosis signal in antitumor effect of Colchicine on human papilloma virus (HPV)-positive human cervical cancer cells. Biosci. Rep. 40, BSR20194065. doi:10.1042/BSR20194065
Yu, Y., Zhou, M., Luo, X., Zhao, Y., Zhou, X., Zhang, Y., et al. (2021). Efficacy of colchicine for patients with coronary artery disease: Meta-analysis and trial sequential analysis. Chin. Circulation J. 36 (07), 659–665. doi:10.3760/cma.j.cn501113-20210705-00317
Zhang, F. S., He, Q. Z., Qin, C. H., Little, P. J., Weng, J. P., and Xu, S. W. (2022). Therapeutic potential of colchicine in cardiovascular medicine: A pharmacological review. Acta Pharmacol. Sin. 43 (9), 2173–2190. doi:10.1038/s41401-021-00835-w
Zhang, L., Qin, Z., Li, R., Wang, S., Wang, W., Tang, M., et al. (2019a). The role of ANXA5 in DBP-induced oxidative stress through ERK/Nrf2 pathway. Environ. Toxicol. Pharmacol. 72, 103236. doi:10.1016/j.etap.2019.103236
Zhang, L., Chen, W., Jiang, X., Liu, L., Wei, K., Du, H., et al. (2019b). Anticancer effects and underlying mechanism of Colchicine on human gastric cancer cell lines in vitro and in vivo. Biosci. Rep. 39, BSR20181802. doi:10.1042/BSR20181802
Zhang, Y., Mao, X., Guo, Q., Lin, N., Li, S., et al. (2016). Network pharmacology-based approaches capture essence of Chinese herbal medicines. Chin. Herb. Med. 8 (2), 107–116. doi:10.1016/s1674-6384(16)60018-7
Zhao, Q., Li, S., Li, N., Yang, X., Ma, S., Yang, A., et al. (2017). miR-34a targets HDAC1-regulated H3K9 acetylation on lipid accumulation induced by homocysteine in foam cells. J. Cell. Biochem. 118, 4617–4627. doi:10.1002/jcb.26126
Zhou, Y., Zhang, Y., Lian, X., Li, F., Wang, C., Zhu, F., et al. (2022). Therapeutic target database update 2022: Facilitating drug discovery with enriched comparative data of targeted agents. Nucleic Acids Res. 50 (1), D1398–D1407. doi:10.1093/nar/gkab953
Keywords: colchicine, coronary artery disease, network pharmacology, molecular docking, mechanism of action
Citation: Yu Y, Zhou M, Long X, Yin S, Hu G, Yang X, Jian W and Yu R (2023) Study on the mechanism of action of colchicine in the treatment of coronary artery disease based on network pharmacology and molecular docking technology. Front. Pharmacol. 14:1147360. doi: 10.3389/fphar.2023.1147360
Received: 18 January 2023; Accepted: 07 June 2023;
Published: 19 June 2023.
Edited by:
Malik Hassan Mehmood, Government College University Faisalabad, PakistanReviewed by:
Genyan Qin, Changde Hospital of Traditional Chinese Medicine, ChinaCopyright © 2023 Yu, Zhou, Long, Yin, Hu, Yang, Jian and Yu. This is an open-access article distributed under the terms of the Creative Commons Attribution License (CC BY). The use, distribution or reproduction in other forums is permitted, provided the original author(s) and the copyright owner(s) are credited and that the original publication in this journal is cited, in accordance with accepted academic practice. No use, distribution or reproduction is permitted which does not comply with these terms.
*Correspondence: Rong Yu, eXVyb25nMTk2OTA1QDE2My5jb20=; Weixiong Jian, ZGF4aW9uZzIwMDAxOTc3QDE2My5jb20=
†These authors have contributed equally to this work and share first authorship
Disclaimer: All claims expressed in this article are solely those of the authors and do not necessarily represent those of their affiliated organizations, or those of the publisher, the editors and the reviewers. Any product that may be evaluated in this article or claim that may be made by its manufacturer is not guaranteed or endorsed by the publisher.
Research integrity at Frontiers
Learn more about the work of our research integrity team to safeguard the quality of each article we publish.