- 1Department of Neurology, Xinqiao Hospital, Third Military Medical University (Army Medical University), Chongqing, China
- 2Department of Neurosurgery and State Key Laboratory of Trauma, Burn and Combined Injury, Southwest Hospital, Third Military Medical University (Army Medical University), Chongqing, China
- 3Chongqing Key Laboratory of Precision Neuromedicine and Neuroregenaration, Southwest Hospital, Third Military Medical University (Army Medical University), Chongqing, China
- 4Chongqing Clinical Research Center for Neurosurgery, Southwest Hospital, Third Military Medical University (Army Medical University), Chongqing, China
Subarachnoid hemorrhage (SAH) can lead to damage to the myelin sheath in white matter. Through classification and analysis of relevant research results, the discussion in this paper provides a deeper understanding of the spatiotemporal change characteristics, pathophysiological mechanisms and treatment strategies of myelin sheath injury after SAH. The research progress for this condition was also systematically reviewed and compared related to myelin sheath in other fields. Serious deficiencies were identified in the research on myelin sheath injury and treatment after SAH. It is necessary to focus on the overall situation and actively explore different treatment methods based on the spatiotemporal changes in the characteristics of the myelin sheath, as well as the initiation, intersection and common action point of the pathophysiological mechanism, to finally achieve accurate treatment. We hope that this article can help researchers in this field to further clarify the challenges and opportunities in the current research on myelin sheath injury and treatment after SAH.
1 Introduction
Subarachnoid hemorrhage (SAH) is one of the most common diseases and normally causes a high mortality rate, 80% of which is caused by intracranial aneurysm rupture. With the rapid development of treatment methods (especially interventional techniques), the survival rate of patients with aneurysmal SAH has reached approximately 65%. However, studies have noted that in the United States, the in-hospital mortality of patients with ruptured aneurysms has not significantly decreased (from 13.7% in 2006% to 13.1% in 2018) (Wahood et al., 2022). Moreover, many survivors suffer from severe neurological deficits (Neifert et al., 2021), thus causing significant negative impacts on not only themselves but also their families and society.
Research on the mechanism of neurological deficits after SAH has been performed for decades, and these deficits have yet to be fully clarified. A large number of studies have suggested that white matter injury plays an important role. It is well known that white matter is mainly composed of axons of neurons and myelin sheaths of oligodendrocytes (OLs). As an important functional unit of OLs, the myelin sheath deserves special attention. A previous study found that the model animals demonstrated myelin sheath injury 4 h after SAH, and active treatment was able to improve the neurological deficits (Toyota et al., 2019). This scenario indicated that myelin sheath injury occurs earlier after SAH, and active treatment is of great significance.
In this study, the spatiotemporal change characteristics, pathophysiological mechanisms and current treatment strategies of myelin sheath injury after SAH are described in detail. Subsequently, through a systematic review and comparative analysis of the important achievements of myelin sheath research in adjacent fields, the shortcomings of the current research were identified. Finally, new research ideas can be adopted in future research on myelin sheath injury and treatment after SAH.
2 Research status of myelin sheath injury and treatment after SAH
2.1 The spatiotemporal change characteristics of myelin sheath injury after SAH
The myelin sheath was shown to be rapidly damaged and slowly recovered after SAH. Animal studies have shown that myelin basic protein (MBP) content in the corpus callosum can significantly decrease at 3 h after SAH (4). Four hours after SAH, 87.5% (35/40) of experimental animals were found to have T2-hyperintensity in white matter (which was closely related to degradation of myelin protein MBP) via cranial MRI (3). Moreover, at 72 h after SAH, the MBP protein content in the corpus callosum decreased to the lowest value (Peng et al., 2019) and then slowly recovered; however, it was still lower than that in the control group at 1 week (Wang et al., 2022). It is well known that the integrity of the myelin sheath is closely related to neurological function. A previous study found that the mNSS score at 2 weeks of SAH experimental animals (Li et al., 2018) and the spatial learning memory and cognitive function at 5 weeks were considerably lower than those of the control group (Wang et al., 2022).
The location of myelin sheath injury is closely related to the puncture point of SAH but is not limited to the area around the puncture point. When considering the threading method as an example, the total incidence of T2-hyperintensity in the white matter was 87.5% (35/40), of which 57% (20/35) were unilateral [of only 10% (2/20) on the same side as the puncture point, 90% (18/20) were contralateral], and 43% (15/35) were bilateral at 4 h after SAH (3). Nevertheless, the collective white matter of the experimental animals exhibited T2-hyperintensity after 24 h of SAH (7).
In summary, SAH-induced myelin sheath injury had obvious spatiotemporal change characteristics, which provides experimental evidence and a research basis for accurately maintaining the integrity of myelin sheath structure and function.
2.2 Pathophysiological mechanism involved in myelin sheath injury caused by SAH
SAH can cause white matter injury through increased intracranial pressure, the outflow of blood components and their metabolites and microcirculation disorders, among causes. In addition, studies have shown that cerebral ischemia and hypoxia caused by microcirculation disorders can induce oxidative stress, excitotoxicity and neuroinflammatory reactions, which can easily damage the myelin sheath. Therefore, under the influence of the abovementioned pathogenic factors, SAH can affect the structural and functional integrity of the myelin sheath through a variety of pathophysiological mechanisms. Thus, this scenario was analyzed as follows (Figure 1).
2.2.1 Involved brain regions
The first analyzed brain area was the frontal lobe. Frontal lobe injury can easily lead to cognitive dysfunction. Moreover, SAH can have an influence on the expression of microRNAs (such as mir-132, mir-134 and mir-138, etc.) in rat frontal lobe brain tissue and subsequently affect the stability of the myelin sheath structure (Ergen et al., 2021). The second analyzed brain area was the entorhinal cortex. Through a systematic RNA sequencing screen of the hippocampus of mice, it was found that SAH-induced damage to the entorhinal cortex may remotely trigger specific hippocampal responses, thereby significantly reducing the expression of myelin structure-specific genes (such as pllp, mbp, mal, mag, cntnap1, plp1, mog and mobp, among other genes), thus leading to injury (Regnier-Golanov et al., 2021). Eventually, the blood‒brain barrier (BBB) in white matter was identified as being a factor. SAH can damage the integrity of the BBB via secondary microcirculation disorders. Therefore, blood components and their metabolites can enter white matter in large quantities and damage the structure and function of the myelin sheath through direct or indirect effects. For example, matrix metalloproteinases (MMPs), especially MMP-9, can destroy the integrity of the BBB in the white matter and myelin sheath; in addition, the inhibition of its expression can produce a protective effect (Egashira et al., 2015).
2.2.2 Involved brain cells
At present, research has mainly focused on astrocytes and microglia. Astrocytes are widely distributed in white matter and can have a rapid impact on the myelin sheath. SAH may cause the rapid expression of lipocalin 2 (LCN2) in the astrocytes located in white matter (which is believed to be the pathological basis of MRI white matter T2-hyperintensity) and subsequently damage the myelin sheath (Toyota et al., 2019). Obviously, astrocytes play an important role in the occurrence and development of myelin sheath injury after SAH. Microglia are closely related to neuroinflammatory reactions, and the persistent inflammatory microenvironment induced by microglia may be the potential mechanism of persistent injury of the myelin sheath after SAH in mice (Wu et al., 2017). In addition, abnormal polar transformation (M1/M2) of microglia also plays an important role in myelin sheath injury. By modulating M2 microglial polarization, it was shown to be helpful in alleviating the myelin sheath injury caused by SAH (4).
2.2.3 Involved molecules and signal pathways
Previous studies have noted that the molecules that cause myelin sheath injury mainly fall into the following three categories after SAH: inflammatory molecules, lipid molecules and apoptotic molecules. For example, when considering inflammatory molecules, MMP-9, ROS, amyloid precursor protein (APP), TNFα, IL-1β, IL-6 and other molecules can directly affect the structure and function of the myelin sheath (Simard et al., 2012; Egashira et al., 2015; Xu et al., 2020). Moreover, ROS are involved in the TXNIP and GNPAT signaling pathways (Xu et al., 2020). When considering lipid molecules, lipoprotein 2 (LCN 2) plays an essential role in myelin sheath injury after SAH (3, 7, 14). The possible mechanism involves the binding of this molecule to its receptor and participation in iron uptake after intracerebral hemorrhage, thus leading to cell injury (Ni et al., 2015) and impaired oligodendrocyte precursor cell differentiation (Li et al., 2022). Endogenous apolipoprotein E (Apo E) may also affect the polarization of microglia through the Shc1/PI3K/Akt signaling pathway and participate in myelin sheath damage (Peng et al., 2019). For apoptotic molecules, NOD-like receptor thermal protein domain associated protein 3 (NLRP3) can destroy the myelin sheath by promoting oligodendrocyte apoptosis (Liu et al., 2020a).
The abovementioned analysis demonstrated that myelin sheath injury after SAH was the result of the combined action of multiple pathophysiological mechanisms involving different brain tissues, brain cells, molecules and signaling pathways. These factors were combined together and interacted, thus making this scenario extremely complex.
2.3 Treatment strategy for myelin sheath injury caused by SAH
The degree of myelin sheath injury was shown to be positively correlated with the severity of SAH. As proof, a previous study found that a higher SAH score corresponded to a larger volume of T2-hyperintensity in white matter (Egashira et al., 2014). Accordingly, the treatment of myelin sheath injury plays a vital role in improving secondary neurological deficits after SAH and has become a research hotspot in this field. At present, there are three main research directions (Table 1).
First, in a previous study, endogenous proteins or molecules, such as heparin and melatonin, were administered. Intravenous infusion of undivided heparin (10 U/kg/h) into rats could significantly reduce myelin sheath injury, neuroinflammatory reactions and trans-synaptic neuronal apoptosis at 12 h after SAH (12). Afterwards, intraperitoneal injection of melatonin (50 mg/kg) after SAH could reduce the apoptosis of oligodendrocytes, the loss of MBP and the abnormal accumulation of APP by regulating the expression of Bim and Bcl-2 (16).
Second, when considering exogenous proteins or molecules (such as COG1410, RvD2 and TRAP) and after SAH, rats were intraperitoneally injected with the apolipoprotein apoE mimic peptide COG1410 (LPR1 ligand), which could significantly relieve myelin sheath injury, reduce brain water content, increase the M2 phenotype of microglia and improve neural function (Peng et al., 2019). Moreover, the administration of the G protein-coupled receptor 18 (GPR18) agonist RvD2 (resolvin D2) can relieve damage to the myelin sheath, reduce oxidative stress and apoptosis and protect the blood‒brain barrier by increasing the expression of MBP and inhibiting the expression of APP(17). Additionally, thrombin receptor antagonist peptide (TRAP, 25 μg/kg) can promote the regeneration of the damaged myelin sheath and the recovery of neural function after SAH (6).
Furthermore, the expression of proteins or molecules can be altered through genetic engineering. For example, the knockout of the lcn2 gene in experimental animals can significantly reduce myelin sheath loss, axon injury, oligodendrocyte death and BBB damage after SAH (3, 7). When the mmp-9 gene was knocked out, the T2 hyperintensity in the white matter of experimental mice was significantly lower than that of wild-type (WT) mice at 24 h after SAH; in addition, at 8 days after SAH, compared with mmp-9 −/− mice, WT mice still had major myelin sheath injury (Egashira et al., 2015). Moreover, the transcription factor protease-activated receptor 1 (PAR1) is the key molecule in F-actin-dependent migration (Jeon et al., 2007). The downregulation of par1 expression with par1 siRNA can promote myelin regeneration after SAH (6). Recent studies have also found that after SAH, the administration of catalase CRISPR (clustered regularly interspaced short palindromic repeats) to rats can also prominently increase the expression of myelin basic protein (MBP), while also reducing APP, IL-6 and TNF α and subsequently cricially alleviate the neurological deficit (Xu et al., 2020).
Generally, to treat myelin sheath injury after SAH, previous researchers have conducted many informative explorations from different aspects and obtained many important achievements, which have provided experimental conclusions and research experience for future precise treatments.
3 Deficiencies in the study of myelin sheath injury and treatment after SAH
Through the abovementioned analysis and summary, it can be easily deduced that the current research on myelin sheath injury after SAH is relatively superficial, and numerous problems have yet to be clarified. By comparing and analyzing the research results in adjacent fields, it has taken little effort to identify the shortcomings of current research. The shortcomings are detailed as follows.
3.1 Research on changes in individual characteristics of the myelin sheath
The individual characteristics of the myelin sheath (mainly including length, thickness, internode length and constituent proteins, etc.) are closely related to its function. Much research has been done on the individual characteristics of the myelin sheath, and considerable important progress has been obtained. As proof, the length of the myelin sheath is determined by the inherent characteristics of oligodendrocytes (Bechler et al., 2015; Swire et al., 2021); moreover, the main regulatory factor is tubulin polymerization promoting protein (TPPP) (Roll-Mecak, 2019), and the length of the myelin sheath in various areas of the central nervous system can be different (Hildebrand et al., 1993). The increase in myelin sheath thickness is a relatively independent stage in the maturation process of oligodendrocytes (Furusho et al., 2012; Kim et al., 2012), which is regulated by the surrounding axons, microglia and extracellular matrix proteins, among other factors (Friedrich and Mugnaini, 1983; Murcia-Belmonte et al., 2016; Nemes-Baran et al., 2020). The internodal length of the myelin sheath is also closely related to its surrounding microenvironment, which involves the number of OPCs, axon activity and extracellular matrix adhesion molecules, among other factors (Murcia-Belmonte et al., 2016; Hamilton et al., 2017; Osanai et al., 2018); however, it has nothing to do with the diameter of axons and the internal characteristics of oligodendrocytes. In addition, the main myelin sheath proteins are expressed and deposited in chronological order (Monge et al., 1986), and there are significant differences in the subcellular assembly positions of different myelin sheath proteins (Gould et al., 1999). These findings provide a basis for our comprehensive and profound understanding of the functional changes in the myelin sheath.
As mentioned above, SAH can obviously damage the individual characteristics of the myelin sheath. However, most of the relevant studies were limited to the influence on myelin sheath thickness and constituent proteins, and only a preliminary discussion had been made on the temporal and spatial changes of myelin sheath injury. Moreover, its pathogenic factors and pathophysiological mechanisms still need to be further clarified. Therefore, multiangle and multidimensional research on the changes in myelin sheath individual characteristics after SAH is helpful to clarify the relationship between the spatiotemporal changes in myelin sheath injury and its functional changes, as well as providing theoretical support and an experimental basis for the clinical treatment of myelin sheath injury and secondary neurological deficits.
3.2 Research on the pathophysiological mechanism of myelin sheath injury
Myelin sheath injury can occur in many central nervous system diseases, such as multiple sclerosis, nerve trauma, infection, cerebral hemorrhage and ischemic brain injury. Through in-depth analysis of the pathophysiological mechanism that causes myelin sheath injury, it was demonstrated that the mechanism mainly focused on the following aspects (Figure 2).
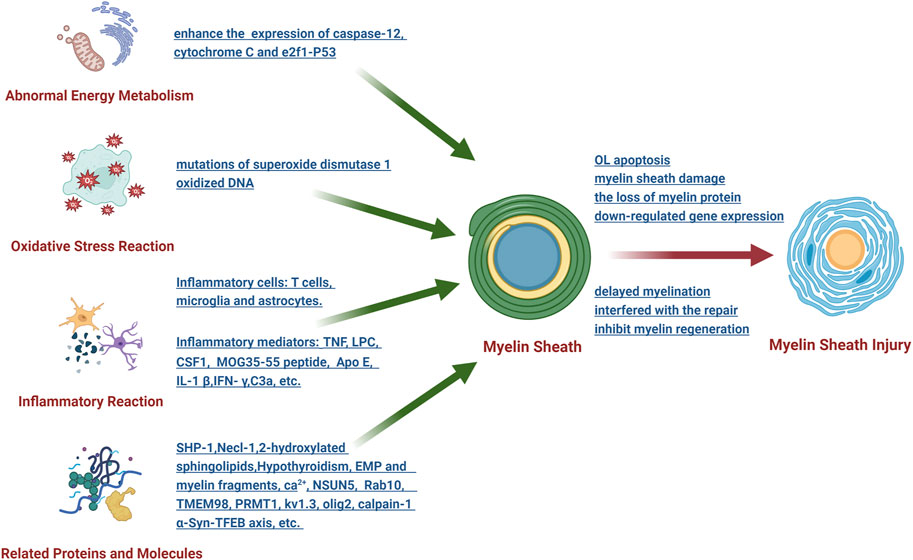
FIGURE 2. Schematic diagram of the research on the pathophysiological mechanism of myelin sheath injury after SAH.
3.2.1 Abnormal energy metabolism
The endoplasmic reticulum and mitochondria are the main participants in oligodendrocyte energy metabolism, which plays an important role in myelin sheath injury. To illustrate this point, after intracerebral hemorrhage, the levels of caspase-12 (a representative protein of endoplasmic reticulum stress) and cytochrome C (an apoptotic factor released by mitochondria) significantly increased, thus leading to oligodendrocyte apoptosis, which correspondingly results in demyelination (Zhuo et al., 2016). Compressive spinal cord injury can enhance the ER-mitochondrial interaction and the expression of e2f1 and initiate demyelination through p53-mediated oligodendrocyte apoptosis (Ma et al., 2017). As mentioned above, SAH can cause abnormal energy metabolism of the white matter, whereas the relationship between abnormal energy metabolism and myelin sheath damage has not been clarified.
3.2.2 Oxidative stress reaction
After the central nervous system is injured, it is simple to produce oxidative stress reactions in its adjacent areas, and the myelin sheath is the most vulnerable structure. It has been demonstrated that mutations of superoxide dismutase 1 in amyotrophic lateral sclerosis can induce myelin sheath damage (Kim et al., 2019). However, the use of nano SIMS technology (nanoscale secondary ion mass spectrometry) demonstrated that oxidative stress can easily downregulate the expression of myelin sheath-related regulator genes through oxidized DNA, thus leading to axon damage and oligodendrocyte death (Giacci et al., 2018). At present, there is only one study on oxidative stress and myelin sheath injury after SAH, which is worthy of further study in the future.
3.2.3 Inflammatory reaction
Inflammatory reactions are the most common pathogenesis, mainly involving inflammatory mediators and inflammatory cells. Research has shown that the inflammatory mediators that can cause myelin sheath damage mainly include TNF, LPC, CSF1, MOG35-55 peptide, Apo E, IL-1 β, IFN- γ and C3a, among others. The inflammatory cells involved in myelin sheath injury include T cells (Batterman et al., 2021), microglia and astrocytes. Inflammatory mediators and inflammatory cells lead to the loss of myelin protein, thus interfering with the repair of myelin sheath and ultimately affecting the structure and function of myelin sheath through their respective downstream pathways. Many studies have been performed on the relationship between inflammatory reactions and myelin sheath injury after SAH, whereas some research conclusions have been reported in other disease models for a long period of time, which deserves our vigilance and attention.
3.2.4 Effects of related proteins and molecules
Previous studies have shown that many proteins and molecules can affect the formation, stability, maturation and regeneration of the myelin sheath. For example, SHP-1 is a key regulator of the myelin sheath development signal, and knockout of its gene can cause a myelin sheath formation disorder in mice (Massa et al., 2004). Moreover, knockout of the necl-1 gene can destroy the initial recognition and adhesion between axons and oligodendrocytes, thus resulting in delayed myelination (Park et al., 2008). In the absence of 2-hydroxylated sphingolipids, a myelin sheath with normal structure and function can be formed, yet it cannot be maintained for a long period of time (Zoller et al., 2008). Additionally, hypothyroidism may cause the wrapping of the myelin sheath to axons to be obstructed. Extracellular matrix proteins and myelin fragments produced by demyelination can inhibit myelin regeneration (Baer et al., 2009; Bauch and Faissner, 2022). Other molecules, such as Ca2+, NSUN5, Rab10, TMEM98, PRMT1, kv1.3, olig2, calpain-1 and α-Syn-TFEB axis, among others, ccan also affect various aspects of the myelin sheath in different ways. As mentioned above, SAH can damage the myelin sheath through different proteins and molecules. Nevertheless, current research has mainly focused on clarifying its role and lacks in-depth discussion regarding its pathophysiological mechanism.
In brief, the pathophysiological mechanism of myelin sheath injury involves many factors and is very complex. At present, research on the mechanism of myelin sheath injury after SAH is associated with problems such as shallowness and lack of systematic methodology, and research has mainly focused only on oligodendrocytes. Whether there is a unique pathophysiological mechanism of myelin sheath injury is worthy of serious discussion.
3.3 Research on the treatment of myelin sheath injury
Myelin sheath injury has a significant impact on the integrity of nerve function; thus, the question of how to treat it is the focus and hotspot of current research. In general, the current research on the treatment of myelin sheath injury in adjacent fields has mainly focused on the following three directions.
3.3.1 Seeking drugs to treat myelin sheath injury
From the perspective of clinical application, it is more convenient and practical to treat myelin sheath injury with drugs. Consequently, most studies have focused on this scenario. Overall, the research in this field can be divided into four facets (Table 2). The first aspect is to identify proteins or molecules that can accurately treat myelin sheath damage. For example, the GPR17 inhibitor pranlukast (Ou et al., 2016), GPR30-specific activator G1 (43), A2bAR selective activator BAY 60-6583 (44), TRPV4 antagonist Rn-1734 (45), LINGO-1 antagonist (Mi et al., 2007), estrogen receptor-β ligand (Crawford et al., 2010), Trk-b agonist Lm22a-4 (48), translocator protein PIGA 1138 (49) and monosialotetrahexosyl ganglioside (GM1) are involved in the regulation of lipid rafts (Zhang et al., 2011), and L-carnitine regulates the PTEN/Akt/mTOR signaling pathway (Ueno et al., 2015). The second facet is to search for proteins or molecules that can treat myelin sheath injury. For example, ethyl pyruvate (EP) (He et al., 2019; He et al., 2021), osteopontin (Selvaraju et al., 2004), structural analog of BDNF(55), nanoparticles containing leukemia inhibitory factor (Rittchen et al., 2015), clemastine (Li et al., 2015), endothelin 2 (58), human hair keratin (Xu et al., 2003), the cycloskeleton adapter protein anilin (ANLN) (Erwig et al., 2019), DL-3-n-butylphthalide (NBP) (Wu et al., 2019) and FMRP (62) can be used to treat this injury. The third aspect is to search for marketed drugs with therapeutic effects on myelin sheath injury, such as clobetasol (Su et al., 2020), trifluoperazine (Khaledi et al., 2021), sildenafil (Nunes et al., 2016), adrenomedullin (Maki et al., 2015), omega-3 polyunsaturated fatty acids (Pu et al., 2013), ascorbic acid (Nam et al., 2019), fluoxetine (Li et al., 2020a), melatonin (Abo Taleb and Alghamdi, 2020) and a mixture containing docosahexaenoic acid, arachidonic acid, vitamin B12, vitamin B9, iron and sphingomyelin (Hauser et al., 2020). The fourth facet is to investigate Chinese herbal medicine and its extract that can treat myelin sheath injury, such as Honokiol (Tan et al., 2022a), Astaxanthin (Lotfi et al., 2021), Shenling oral liquid (Liu et al., 2020b; Zheng et al., 2021), Rosmarinic acid (Li et al., 2020b), Naringenin (Rong et al., 2017), Salvianolic acid B (78), Tanshinone IIA (79), Bushen Yisui capsule (Rabinovich and Kacen, 2012; Zhao et al., 2018), the herbal medicine Ninjin ‘yoeito (Seiwa et al., 2007) and Quercetin (Naeimi et al., 2018; Tan et al., 2022b), among other medicines.
As mentioned above, there have been relatively few studies on the treatment of myelin sheath injury after SAH. The search for effective drugs to treat myelin sheath injury from the abovementioned four aspects has indicated the research direction and broadened the research ideas.
3.3.2 Cell transplantation or genetic engineering to treat myelin sheath injury
It is also an important research direction to treat myelin sheath injury through cell transplantation or genetic engineering. With regard to cell transplantation, umbilical cord mesenchymal stem cells (Zhang et al., 2012; Chen et al., 2013), neural stem cells (Hwang et al., 2009) and f3. olig2 cells (Ahn et al., 2016) can promote myelin sheath repair and improve neural function. Genetic engineering, such as knockout of the G-protein coupled receptor-17 (GPR17) gene, GPR62 gene, hnRNP-K gene, EPAC gene and Lingo-1 gene, as well as overexpression of the Golli myelin basic protein gene, SOX10 gene and NF155 gene and regulation of FGF receptor gene expression (Li and Yao, 2013), can effectively treat myelin sheath injury. Although this research direction has experienced many difficulties in clinical application, it is still worthy of further discussion.
3.3.3 Attempts of physical therapy to treat myelin sheath injury
It has been proven that physical therapy can treat myelin sheath injury in experimental animals. A previous study demonstrated that precisely timed motor learning can promote the recovery of myelin sheath injury by enhancing the remyelination of newborn and surviving oligodendrocytes (Bacmeister et al., 2020). The application of electroacupuncture can promote the proliferation of oligodendrocytes and inhibit their death to mediate the protection of the myelin sheath (Huang et al., 2015). Moreover, electroacupuncture treatment can also reduce myelin sheath fragments, promote microglia and oligodendrocytes to gather to the injured site, upregulate the expression of MBP and AXL in the corpus callosum of demyelinating mice and accelerate myelin sheath regeneration (Kang et al., 2020). These pioneering studies have provided us with new ideas and methods for the clinical treatment of myelin sheath injury after SAH, which is worthy of continuous attention and trial application.
In general, the current research on myelin sheath injury and treatment after SAH has experienced some problems, such as relatively superficial research, ineffective connection between basic research and clinical application and no effective drugs entering clinical application. Knowledge concerning the treatment strategies and achievements in adjacent research fields can provide new ideas for research and new methods for the precise clinical treatment of myelin sheath injury.
4 Perspective
The early diagnosis and treatment of myelin sheath injury after SAH is of great significance. Based on the spatiotemporal change characteristics of myelin sheath injury, the clarification of the pathophysiological mechanism and precise treatment is a practical research approach. In view of this research idea, the following points warrant further discussion.
4.1 Detection of the spatiotemporal change characteristics of myelin sheath injury
The spatiotemporal change characteristics of myelin sheath injury were the basis of treatment research after SAH. Therefore, how to quickly, accurately and comprehensively detect this change is of profound significance. In view of the complexity of the spatiotemporal change characteristics of myelin sheath injury after SAH, the information provided by a single technical method is limited. Hence, multimodal detection combined with neuroimaging, neuroelectrophysiology, electroencephalography, real-time metabonomics and other technologies can allow for the possibility to understand the spatiotemporal change characteristics of myelin sheath injury in a multidimensional, multiangle and systematic manner after SAH. It is possible that neuroimaging can be used to assess the location and extent of myelin sheath injury. As mentioned above, T2-hyperintensity of MRI in white matter is closely related to degradation of MBP after SAH (3). The real-time detection of metabolic biomarkers in the blood, cerebrospinal fluid, tears, saliva, urine and other body fluid samples can accurately reflect the changes in the myelin sheath, which has provided researchers with the possibility to accurately describe the occurrence, development and outcome of myelin sheath injury after SAH. Spatial metabolomics based on high spatial resolution imaging mass spectrometry can visually assess the composition, dynamics and spatial distribution of protein molecules in tissues or cells (Chen et al., 2022a; Chen et al., 2022b). In addition, traditional examination methods such as neuroelectrophysiology and electroencephalography still have value for in-depth research on the continuous monitoring of myelin sheath injury due to their convenience, low cost and high popularity. Consequently, by combining different inspection methods, real-time multimodal detection can be helpful to accurately clarify and monitor the spatiotemporal change characteristics of myelin sheath injury after SAH and can provide experimental data and a theoretical basis for treatment research.
4.2 Screening of research objects for treatment of myelin sheath injury
Nonetheless, various important research achievements have been made in the treatment of myelin sheath injury after SAH in recent years; however precise treatment still has not been achieved. The main reason involves the deviation in the screening of research objects. SAH can cause myelin sheath injury with many pathogenic factors, complex pathophysiological mechanisms and extensive structural and functional damage. Consequently, it is believed that the research object for the treatment of myelin sheath injury should focus on the starting point of SAH, the intersection point of multiple pathophysiological mechanisms and the joint event leading to different myelin sheath structures and functional damage. Via single-cell sequencing, spatial real-time metabonomics, comparative proteomics and other methods, key research objects have been identified, after which drug molecules (preferably with multiple action targets) were screened to finally achieve precise treatment of myelin sheath injury after SAH. Moreover, it is believed that the treatment of myelin sheath injury should not only focus on the myelin sheath but also focus on how to restore the balance of cerebrospinal fluid circulation and the reconstruction of the intracranial barrier structure. Hence, it is necessary to actively explore other treatment methods and study the mechanism of action, such as the abovementioned electroacupuncture treatment.
5 Conclusion
Although research on the diagnosis and treatment of myelin sheath injury after SAH has made many significant achievements, a number of problems remain. Based on the spatiotemporal change characteristics of myelin sheath injury after SAH, a focus on the starting point, intersection point and common event, as well as devoting attention to the overall situation and actively exploring different treatment methods, may result in new breakthroughs for its diagnosis and treatment.
Author contributions
SZ and YC conceptualized and outlined the manuscript. MC and PG drafted the manuscript. XR and HF discussed contents and revised the manuscript. SZ created the figures with Biorender.com. All authors have read and approved the current version of the manuscript.
Funding
This work was supported by the Natural Science Foundation of Chongqing (No. cstc2019jcyj-msxmX0535 to SZ), National Natural Science Foundation of China (82030036 to HF), Chongqing Medical Scientific Research Project (Joint Project of Chongqing Health Commission and Science and Technology Bureau, No. 2023MSXM138 to YC) and State Key Laboratory of Trauma, Burn and Combined Injury (SKLYQ202002 to YC).
Conflict of interest
The authors declare that the research was conducted in the absence of any commercial or financial relationships that could be construed as a potential conflict of interest.
Publisher’s note
All claims expressed in this article are solely those of the authors and do not necessarily represent those of their affiliated organizations, or those of the publisher, the editors and the reviewers. Any product that may be evaluated in this article, or claim that may be made by its manufacturer, is not guaranteed or endorsed by the publisher.
References
Abo Taleb, H. A., and Alghamdi, B. S. (2020). Neuroprotective effects of melatonin during demyelination and remyelination stages in a mouse model of multiple sclerosis. J. Mol. Neurosci. 70 (3), 386–402. doi:10.1007/s12031-019-01425-6
Ahn, J. H., Chen, B. H., Shin, B. N., Cho, J. H., Kim, I. H., Park, J. H., et al. (2016). Intravenously infused F3.Olig2 improves memory deficits via restoring myelination in the aged Hippocampus following experimental ischemic stroke. Cell Transpl. 25 (12), 2129–2144. doi:10.3727/096368916X692230
Bacmeister, C. M., Barr, H. J., McClain, C. R., Thornton, M. A., Nettles, D., Welle, C. G., et al. (2020). Motor learning promotes remyelination via new and surviving oligodendrocytes. Nat. Neurosci. 23 (7), 819–831. doi:10.1038/s41593-020-0637-3
Baer, A. S., Syed, Y. A., Kang, S. U., Mitteregger, D., Vig, R., Ffrench-Constant, C., et al. (2009). Myelin-mediated inhibition of oligodendrocyte precursor differentiation can be overcome by pharmacological modulation of Fyn-RhoA and protein kinase C signalling. Brain 132, 465–481. doi:10.1093/brain/awn334
Batterman, K. V., Cabrera, P. E., Moore, T. L., and Rosene, D. L. (2021). T cells actively infiltrate the white matter of the aging monkey brain in relation to increased microglial reactivity and cognitive decline. Front. Immunol. 12, 607691. doi:10.3389/fimmu.2021.607691
Bauch, J., and Faissner, A. (2022). The extracellular matrix proteins tenascin-C and tenascin-R retard oligodendrocyte precursor maturation and myelin regeneration in a cuprizone-induced long-term demyelination animal model. Cells 11 (11), 1773. doi:10.3390/cells11111773
Bechler, M. E., Byrne, L., and Ffrench-Constant, C. (2015). CNS myelin sheath lengths are an intrinsic property of oligodendrocytes. Curr. Biol. CB 25 (18), 2411–2416. doi:10.1016/j.cub.2015.07.056
Chen, H., Zhang, Y., Yang, Z., and Zhang, H. (2013). Human umbilical cord Wharton's jelly-derived oligodendrocyte precursor-like cells for axon and myelin sheath regeneration. Neural Regen. Res. 8 (10), 890–899. doi:10.3969/j.issn.1673-5374.2013.10.003
Chen, Y., Galea, I., Macdonald, R. L., Wong, G. K. C., and Zhang, J. H. (2022). Rethinking the initial changes in subarachnoid haemorrhage: Focusing on real-time metabolism during early brain injury. EBioMedicine 83, 104223. doi:10.1016/j.ebiom.2022.104223
Chen, Y., Hu, D., Zhao, L., Tang, W., and Li, B. (2022). Unraveling metabolic alterations in transgenic mouse model of Alzheimer's disease using MALDI MS imaging with 4-aminocinnoline-3-carboxamide matrix. Anal. Chim. acta 1192, 339337. doi:10.1016/j.aca.2021.339337
Crawford, D. K., Mangiardi, M., Song, B., Patel, R., Du, S., Sofroniew, M. V., et al. (2010). Oestrogen receptor beta ligand: A novel treatment to enhance endogenous functional remyelination. Brain 133 (10), 2999–3016. doi:10.1093/brain/awq237
Doll, C. A., Yergert, K. M., and Appel, B. H. (2020). The RNA binding protein fragile X mental retardation protein promotes myelin sheath growth. Glia 68 (3), 495–508. doi:10.1002/glia.23731
Dubois, M., Chenivesse, C., Raux, M., Morales-Robles, A., Nierat, M. C., Garcia, G., et al. (2016). Neurophysiological evidence for a cortical contribution to the wakefulness-related drive to breathe explaining hypocapnia-resistant ventilation in humans. J. Neurosci. 36 (41), 10673–10682. doi:10.1523/JNEUROSCI.2376-16.2016
Egashira, Y., Hua, Y., Keep, R. F., and Xi, G. (2014). Acute white matter injury after experimental subarachnoid hemorrhage: Potential role of lipocalin 2. Stroke 45 (7), 2141–2143. doi:10.1161/STROKEAHA.114.005307
Egashira, Y., Zhao, H., Hua, Y., Keep, R. F., and Xi, G. (2015). White matter injury after subarachnoid hemorrhage: Role of blood-brain barrier disruption and matrix metalloproteinase-9. Stroke 46 (10), 2909–2915. doi:10.1161/STROKEAHA.115.010351
Ergen, F. B., Cosan, D. T., Kandemir, T., Dag, I., Mutlu, F., and Cosan, T. E. (2021). An enriched environment leads to increased synaptic plasticity-associated miRNA levels after experimental subarachnoid hemorrhage. J. Stroke Cerebrovasc. Dis. 30 (6), 105766. doi:10.1016/j.jstrokecerebrovasdis.2021.105766
Erwig, M. S., Patzig, J., Steyer, A. M., Dibaj, P., Heilmann, M., Heilmann, I., et al. (2019). Anillin facilitates septin assembly to prevent pathological outfoldings of central nervous system myelin. Elife 8, e43888. doi:10.7554/eLife.43888
Feng, Y., Feng, F., Zheng, C., Zhou, Z., Jiang, M., Liu, Z., et al. (2019). Tanshinone IIA attenuates demyelination and promotes remyelination in A. cantonensis-infected BALB/c mice. Int. J. Biol. Sci. 15 (10), 2211–2223. doi:10.7150/ijbs.35266
Fletcher, J. L., Wood, R. J., Nguyen, J., Norman, E. M. L., Jun, C. M. K., Prawdiuk, A. R., et al. (2018). Targeting TrkB with a brain-derived neurotrophic factor mimetic promotes myelin repair in the brain. J. Neurosci. 38 (32), 7088–7099. doi:10.1523/JNEUROSCI.0487-18.2018
Friedrich, V. L., and Mugnaini, E. (1983). Myelin sheath thickness in the CNS is regulated near the axon. Brain Res. 274 (2), 329–331. doi:10.1016/0006-8993(83)90712-6
Furusho, M., Dupree, J. L., Nave, K. A., and Bansal, R. (2012). Fibroblast growth factor receptor signaling in oligodendrocytes regulates myelin sheath thickness. J. Neurosci. 32 (19), 6631–6641. doi:10.1523/JNEUROSCI.6005-11.2012
Giacci, M. K., Bartlett, C. A., Smith, N. M., Iyer, K. S., Toomey, L. M., Jiang, H., et al. (2018). Oligodendroglia are particularly vulnerable to oxidative damage after neurotrauma in vivo. J. Neurosci. 38 (29), 6491–6504. doi:10.1523/JNEUROSCI.1898-17.2018
Gould, R. M., Freund, C. M., and Barbarese, E. (1999). Myelin-associated oligodendrocytic basic protein mRNAs reside at different subcellular locations. J. Neurochem. 73 (5), 1913–1924.
Hamilton, N. B., Clarke, L. E., Arancibia-Carcamo, I. L., Kougioumtzidou, E., Matthey, M., Karadottir, R., et al. (2017). Endogenous GABA controls oligodendrocyte lineage cell number, myelination, and CNS internode length. Glia 65 (2), 309–321. doi:10.1002/glia.23093
Hauser, J., Sultan, S., Rytz, A., Steiner, P., and Schneider, N. (2020). A blend containing docosahexaenoic acid, arachidonic acid, vitamin B12, vitamin B9, iron and sphingomyelin promotes myelination in an in vitro model. Nutr. Neurosci. 23 (12), 931–945. doi:10.1080/1028415X.2019.1580918
He, Y., An, J., Yin, J. J., Miao, Q., Sui, R. X., Han, Q. X., et al. (2021). Ethyl pyruvate-derived transdifferentiation of astrocytes to oligodendrogenesis in cuprizone-induced demyelinating model. Neurotherapeutics 18 (1), 488–502. doi:10.1007/s13311-020-00947-x
He, Y., An, J., Yin, J. J., Sui, R. X., Miao, Q., Ding, Z. B., et al. (2019). Ethyl pyruvate enhances spontaneous remyelination by targeting microglia phagocytosis. Int. Immunopharmacol. 77, 105929. doi:10.1016/j.intimp.2019.105929
Hildebrand, C., Remahl, S., Persson, H., and Bjartmar, C. (1993). Myelinated nerve fibres in the CNS. Prog. Neurobiol. 40 (3), 319–384. doi:10.1016/0301-0082(93)90015-k
Hirahara, Y., Matsuda, K. I., Yamada, H., Saitou, A., Morisaki, S., Takanami, K., et al. (2013). G protein-coupled receptor 30 contributes to improved remyelination after cuprizone-induced demyelination. Glia 61 (3), 420–431. doi:10.1002/glia.22445
Huang, S., Tang, C., Sun, S., Cao, W., Qi, W., Xu, J., et al. (2015). Protective effect of electroacupuncture on neural myelin sheaths is mediated via promotion of oligodendrocyte proliferation and inhibition of oligodendrocyte death after compressed spinal cord injury. Mol. Neurobiol. 52 (3), 1870–1881. doi:10.1007/s12035-014-9022-0
Hwang, D. H., Kim, B. G., Kim, E. J., Lee, S. I., Joo, I. S., Suh-Kim, H., et al. (2009). Transplantation of human neural stem cells transduced with Olig2 transcription factor improves locomotor recovery and enhances myelination in the white matter of rat spinal cord following contusive injury. BMC Neurosci. 10, 117. doi:10.1186/1471-2202-10-117
Jeon, T. J., Lee, D. J., Lee, S., Weeks, G., and Firtel, R. A. (2007). Regulation of Rap1 activity by RapGAP1 controls cell adhesion at the front of chemotaxing cells. J. Cell Biol. 179 (5), 833–843. doi:10.1083/jcb.200705068
Kang, Z., Zou, Z. F., Sun, J. X., Zhu, K. Y., Jiang, J. W., Wu, G. C., et al. (2020). Electroacupuncture promotes regeneration and repair of myelin sheath of corpus callosum in demyelination mice. Zhen ci yan jiu = Acupunct. Res. 45 (1), 1–7. doi:10.13702/j.1000-0607.1901626
Khaledi, E., Noori, T., Mohammadi-Farani, A., Sureda, A., Dehpour, A. R., Yousefi-Manesh, H., et al. (2021). Trifluoperazine reduces cuprizone-induced demyelination via targeting Nrf2 and IKB in mice. Eur. J. Pharmacol. 909, 174432. doi:10.1016/j.ejphar.2021.174432
Kim, D. H., Morimoto, N., Saburi, W., Mukai, A., Imoto, K., Takehana, T., et al. (2012). Purification and characterization of a liquefying alpha-amylase from alkalophilic thermophilic Bacillus sp. AAH-31. Biosci. Biotechnol. Biochem. 76 (7), 1378–1383. doi:10.1271/bbb.120164
Kim, S., Chung, A. Y., Na, J. E., Lee, S. J., Jeong, S. H., Kim, E., et al. (2019). Myelin degeneration induced by mutant superoxide dismutase 1 accumulation promotes amyotrophic lateral sclerosis. Glia 67 (10), 1910–1921. doi:10.1002/glia.23669
Li, B., Xu, Y., Quan, Y., Cai, Q., Le, Y., Ma, T., et al. (2020). Inhibition of RhoA/ROCK pathway in the early stage of hypoxia ameliorates depression in mice via protecting myelin sheath. ACS Chem. Neurosci. 11 (17), 2705–2716. doi:10.1021/acschemneuro.0c00352
Li, J. S., and Yao, Z. X. (2013). Modulation of FGF receptor signaling as an intervention and potential therapy for myelin breakdown in Alzheimer's disease. Med. Hypotheses 80 (4), 341–344. doi:10.1016/j.mehy.2012.12.008
Li, M., Cui, M. M., Kenechukwu, N. A., Gu, Y. W., Chen, Y. L., Zhong, S. J., et al. (2020). Rosmarinic acid ameliorates hypoxia/ischemia induced cognitive deficits and promotes remyelination. Neural Regen. Res. 15 (5), 894–902. doi:10.4103/1673-5374.268927
Li, Q., Ru, X., Yang, Y., Zhao, H., Qu, J., Chen, W., et al. (2022). Lipocalin-2-Mediated insufficient oligodendrocyte progenitor cell remyelination for white matter injury after subarachnoid hemorrhage via SCL22A17 receptor/early growth response protein 1 signaling. Neurosci. Bull. 38 (12), 1457–1475. doi:10.1007/s12264-022-00906-w
Li, Q., Zhao, H., Pan, P., Ru, X., Zuo, S., Qu, J., et al. (2018). Nexilin regulates oligodendrocyte progenitor cell migration and remyelination and is negatively regulated by protease-activated receptor 1/ras-proximate-1 signaling following subarachnoid hemorrhage. Front. neurology 9, 282. doi:10.3389/fneur.2018.00282
Li, Z., He, Y., Fan, S., and Sun, B. (2015). Clemastine rescues behavioral changes and enhances remyelination in the cuprizone mouse model of demyelination. Neurosci. Bull. 31 (5), 617–625. doi:10.1007/s12264-015-1555-3
Liu, D., Dong, Y., Li, G., Zou, Z., Hao, G., Feng, H., et al. (2020). Melatonin attenuates white matter injury via reducing oligodendrocyte apoptosis after subarachnoid hemorrhage in mice. Turk. Neurosurg. 30 (5), 685–692. doi:10.5137/1019-5149.JTN.27986-19.3
Liu, M., Liu, X., Wang, L., Wang, Y., Dong, F., Wu, J., et al. (2018). TRPV4 inhibition improved myelination and reduced glia reactivity and inflammation in a cuprizone-induced mouse model of demyelination. Front. Cell Neurosci. 12, 392. doi:10.3389/fncel.2018.00392
Liu, Z., Qin, G., Mana, L., Huang, S., Wang, Y., and Wang, P. (2020). Shenzhiling oral liquid protects STZ-injured oligodendrocyte through PI3K/Akt-mTOR pathway. Evid. Based Complement. Altern. Med. 2020, 4527283. doi:10.1155/2020/4527283
Lotfi, A., Soleimani, M., and Ghasemi, N. (2021). Astaxanthin reduces demyelination and oligodendrocytes death in A rat model of multiple sclerosis. Cell J. 22 (4), 565–571. doi:10.22074/cellj.2021.6999
Ma, L., Yu, H. J., Gan, S. W., Gong, R., Mou, K. J., Xue, J., et al. (2017). p53-Mediated oligodendrocyte apoptosis initiates demyelination after compressed spinal cord injury by enhancing ER-mitochondria interaction and E2F1 expression. Neurosci. Lett. 644, 55–61. doi:10.1016/j.neulet.2017.02.038
Ma, Q., Wang, D., Li, Y., Yang, H., Li, Y., Wang, J., et al. (2022). Activation of A(2B) adenosine receptor protects against demyelination in a mouse model of schizophrenia. Exp. Ther. Med. 23 (6), 396. doi:10.3892/etm.2022.11323
Maki, T., Takahashi, Y., Miyamoto, N., Liang, A. C., Ihara, M., Lo, E. H., et al. (2015). Adrenomedullin promotes differentiation of oligodendrocyte precursor cells into myelin-basic-protein expressing oligodendrocytes under pathological conditions in vitro. Stem Cell Res. 15 (1), 68–74. doi:10.1016/j.scr.2015.05.001
Massa, P. T., Wu, C., and Fecenko-Tacka, K. (2004). Dysmyelination and reduced myelin basic protein gene expression by oligodendrocytes of SHP-1-deficient mice. J. Neurosci. Res. 77 (1), 15–25. doi:10.1002/jnr.20155
Mi, S., Hu, B., Hahm, K., Luo, Y., Kam Hui, E. S., Yuan, Q., et al. (2007). LINGO-1 antagonist promotes spinal cord remyelination and axonal integrity in MOG-induced experimental autoimmune encephalomyelitis. Nat. Med. 13 (10), 1228–1233. doi:10.1038/nm1664
Monge, M., Kadiiski, D., Jacque, C. M., and Zalc, B. (1986). Oligodendroglial expression and deposition of four major myelin constituents in the myelin sheath during development. An in vivo study. Dev. Neurosci. 8 (4), 222–235. doi:10.1159/000112255
Murcia-Belmonte, V., Esteban, P. F., Martinez-Hernandez, J., Gruart, A., Lujan, R., Delgado-Garcia, J. M., et al. (2016). Anosmin-1 over-expression regulates oligodendrocyte precursor cell proliferation, migration and myelin sheath thickness. Brain Struct. Funct. 221 (3), 1365–1385. doi:10.1007/s00429-014-0977-4
Naeimi, R., Baradaran, S., Ashrafpour, M., Moghadamnia, A. A., and Ghasemi-Kasman, M. (2018). Querectin improves myelin repair of optic chiasm in lyolecithin-induced focal demyelination model. Biomed. Pharmacother. 101, 485–493. doi:10.1016/j.biopha.2018.02.125
Nam, S. M., Seo, J. S., Nahm, S. S., and Chang, B. J. (2019). Effects of ascorbic acid on osteopontin expression and axonal myelination in the developing cerebellum of lead-exposed rat pups. Int. J. Environ. Res. public health 16 (6), 983. doi:10.3390/ijerph16060983
Neifert, S. N., Chapman, E. K., Martini, M. L., Shuman, W. H., Schupper, A. J., Oermann, E. K., et al. (2021). Aneurysmal subarachnoid hemorrhage: The last decade. Transl. Stroke Res. 12 (3), 428–446. doi:10.1007/s12975-020-00867-0
Nemes-Baran, A. D., White, D. R., and DeSilva, T. M. (2020). Fractalkine-dependent microglial pruning of viable oligodendrocyte progenitor cells regulates myelination. Cell Rep. 32 (7), 108047. doi:10.1016/j.celrep.2020.108047
Nguyen, H. T. H., Wood, R. J., Prawdiuk, A. R., Furness, S. G. B., Xiao, J., Murray, S. S., et al. (2019). TrkB agonist lm22a-4 increases oligodendroglial populations during myelin repair in the corpus callosum. Front. Mol. Neurosci. 12, 205. doi:10.3389/fnmol.2019.00205
Ni, W., Zheng, M., Xi, G., Keep, R. F., and Hua, Y. (2015). Role of lipocalin-2 in brain injury after intracerebral hemorrhage. J. Cereb. Blood Flow. Metab. 35 (9), 1454–1461. doi:10.1038/jcbfm.2015.52
Nunes, A. K., Raposo, C., de Oliveira, W. H., Thome, R., Verinaud, L., Tovar-Moll, F., et al. (2016). Phosphodiesterase-5 inhibition promotes remyelination by MCP-1/CCR-2 and MMP-9 regulation in a cuprizone-induced demyelination model. Exp. Neurol. 275, 143–153. doi:10.1016/j.expneurol.2015.10.013
Osanai, Y., Shimizu, T., Mori, T., Hatanaka, N., Kimori, Y., Kobayashi, K., et al. (2018). Length of myelin internodes of individual oligodendrocytes is controlled by microenvironment influenced by normal and input-deprived axonal activities in sensory deprived mouse models. Glia 66 (11), 2514–2525. doi:10.1002/glia.23502
Ou, Z., Sun, Y., Lin, L., You, N., Liu, X., Li, H., et al. (2016). Olig2-Targeted G-protein-coupled receptor Gpr17 regulates oligodendrocyte survival in response to lysolecithin-induced demyelination. J. Neurosci. official J. Soc. Neurosci. 36 (41), 10560–10573. doi:10.1523/JNEUROSCI.0898-16.2016
Park, J., Liu, B., Chen, T., Li, H., Hu, X., Gao, J., et al. (2008). Disruption of Nectin-like 1 cell adhesion molecule leads to delayed axonal myelination in the CNS. J. Neurosci. 28 (48), 12815–12819. doi:10.1523/JNEUROSCI.2665-08.2008
Peng, J., Pang, J., Huang, L., Enkhjargal, B., Zhang, T., Mo, J., et al. (2019). LRP1 activation attenuates white matter injury by modulating microglial polarization through Shc1/PI3K/Akt pathway after subarachnoid hemorrhage in rats. Redox Biol. 21, 101121. doi:10.1016/j.redox.2019.101121
Pu, H., Guo, Y., Zhang, W., Huang, L., Wang, G., Liou, A. K., et al. (2013). Omega-3 polyunsaturated fatty acid supplementation improves neurologic recovery and attenuates white matter injury after experimental traumatic brain injury. J. Cereb. Blood Flow. Metab. 33 (9), 1474–1484. doi:10.1038/jcbfm.2013.108
Rabinovich, M., and Kacen, L. (2012). Transference in view of a classical conditioning model. Am. J. Psychol. 125 (2), 209–223. doi:10.5406/amerjpsyc.125.2.0209
Regnier-Golanov, A. S., Dundar, F., Zumbo, P., Betel, D., Hernandez, M. S., Peterson, L. E., et al. (2021). Hippocampal transcriptome changes after subarachnoid hemorrhage in mice. Front. Neurol. 12, 691631. doi:10.3389/fneur.2021.691631
Rittchen, S., Boyd, A., Burns, A., Park, J., Fahmy, T. M., Metcalfe, S., et al. (2015). Myelin repair in vivo is increased by targeting oligodendrocyte precursor cells with nanoparticles encapsulating leukaemia inhibitory factor (LIF). Biomaterials 56, 78–85. doi:10.1016/j.biomaterials.2015.03.044
Roll-Mecak, A. (2019). A microtubule-myelination connection. Cell 179 (1), 54–56. doi:10.1016/j.cell.2019.08.046
Rong, W., Pan, Y. W., Cai, X., Song, F., Zhao, Z., Xiao, S. H., et al. (2017). The mechanism of Naringin-enhanced remyelination after spinal cord injury. Neural Regen. Res. 12 (3), 470–477. doi:10.4103/1673-5374.202923
Seiwa, C., Yamamoto, M., Tanaka, K., Fukutake, M., Ueki, T., Takeda, S., et al. (2007). Restoration of FcRgamma/Fyn signaling repairs central nervous system demyelination. J. Neurosci. Res. 85 (5), 954–966. doi:10.1002/jnr.21196
Selvaraju, R., Bernasconi, L., Losberger, C., Graber, P., Kadi, L., Avellana-Adalid, V., et al. (2004). Osteopontin is upregulated during in vivo demyelination and remyelination and enhances myelin formation in vitro. Mol. Cell Neurosci. 25 (4), 707–721. doi:10.1016/j.mcn.2003.12.014
Simard, J. M., Tosun, C., Ivanova, S., Kurland, D. B., Hong, C., Radecki, L., et al. (2012). Heparin reduces neuroinflammation and transsynaptic neuronal apoptosis in a model of subarachnoid hemorrhage. Transl. Stroke Res. 3, 155–165. doi:10.1007/s12975-012-0166-9
Su, X., Yuan, H., Bai, Y., Chen, J., Sui, M., Zhang, X., et al. (2020). Clobetasol attenuates white matter injury by promoting oligodendrocyte precursor cell differentiation. Pediatr. Neurosurg. 55 (4), 188–196. doi:10.1159/000509521
Swire, M., Assinck, P., McNaughton, P. A., Lyons, D. A., Ffrench-Constant, C., and Livesey, M. R. (2021). Oligodendrocyte HCN2 channels regulate myelin sheath length. J. Neurosci. official J. Soc. Neurosci. 41 (38), 7954–7964. doi:10.1523/JNEUROSCI.2463-20.2021
Tan, Y., Yu, H., Sun, S., Gan, S., Gong, R., Mou, K. J., et al. (2022). Honokiol exerts protective effects on neural myelin sheaths after compressed spinal cord injury by inhibiting oligodendrocyte apoptosis through regulation of ER-mitochondrial interactions. J. spinal cord Med. 45 (4), 595–604. doi:10.1080/10790268.2021.1890878
Tan, Z., Yang, G., Qiu, J., Yan, W., Liu, Y., Ma, Z., et al. (2022). Quercetin alleviates demyelination through regulating microglial phenotype transformation to mitigate neuropsychiatric symptoms in mice with vascular dementia. Mol. Neurobiol. 59 (5), 3140–3158. doi:10.1007/s12035-021-02712-3
Toyota, Y., Wei, J., Xi, G., Keep, R. F., and Hua, Y. (2019). White matter T2 hyperintensities and blood-brain barrier disruption in the hyperacute stage of subarachnoid hemorrhage in male mice: The role of lipocalin-2. CNS Neurosci. Ther. 25 (10), 1207–1214. doi:10.1111/cns.13221
Tremolanti, C., Cavallini, C., Meyer, L., Klein, C., Da Pozzo, E., Costa, B., et al. (2022). Translocator protein ligand PIGA1138 reduces disease symptoms and severity in experimental autoimmune encephalomyelitis model of primary progressive multiple sclerosis. Mol. Neurobiol. 59 (3), 1744–1765. doi:10.1007/s12035-022-02737-2
Ueno, Y., Koike, M., Shimada, Y., Shimura, H., Hira, K., Tanaka, R., et al. (2015). L-carnitine enhances axonal plasticity and improves white-matter lesions after chronic hypoperfusion in rat brain. J. Cereb. Blood Flow. Metab. 35 (3), 382–391. doi:10.1038/jcbfm.2014.210
Wahood, W., Rizvi, A. A., Alexander, A. Y., Yolcu, Y. U., Lanzino, G., Brinjikji, W., et al. (2022). Trends in admissions and outcomes for treatment of aneurysmal subarachnoid hemorrhage in the United States. Neurocrit Care 37 (1), 209–218. doi:10.1007/s12028-022-01476-5
Wang, Y., Xu, J., You, W., Shen, H., Li, X., Yu, Z., et al. (2022). Roles of Rufy3 in experimental subarachnoid hemorrhage-induced early brain injury via accelerating neuronal axon repair and synaptic plasticity. Mol. Brain 15 (1), 35. doi:10.1186/s13041-022-00919-6
Wu, Y., Dong, L., Huang, Q., Sun, L., Liao, Y., Tang, Y., et al. (2019). Multiple functional therapeutic effects of DL-3-n-butylphthalide in the cuprizone model of demyelination. Life Sci. 232, 116501. doi:10.1016/j.lfs.2019.05.057
Wu, Y., Peng, J., Pang, J., Sun, X., and Jiang, Y. (2017). Potential mechanisms of white matter injury in the acute phase of experimental subarachnoid haemorrhage. Brain a J. neurology 140 (6), e36. doi:10.1093/brain/awx084
Xu, W., Yan, J., Chen, S., Ocak, U., Shao, A., and Zhang, J. (2020). Peroxisomal dysfunction contributes to white matter injury following subarachnoid hemorrhage in rats via thioredoxin-interacting protein-dependent manner. Front. Cell Dev. Biol. 8, 576482. doi:10.3389/fcell.2020.576482
Xu, X. J., Piao, Y. J., and Huo, X. (2003). [Effect of human hair keratin implant on oligodendrocyte proliferation and differentiation in rats with acute spinal cord injury]. Di 1 jun yi da xue xue bao = Acad. J. first Med. Coll. PLA 23, 542–545.
Yuen, T. J., Johnson, K. R., Miron, V. E., Zhao, C., Quandt, J., Harrisingh, M. C., et al. (2013). Identification of endothelin 2 as an inflammatory factor that promotes central nervous system remyelination. Brain 136, 1035–1047. doi:10.1093/brain/awt024
Zhang, T., Zuo, G., and Zhang, H. (2022). GPR18 agonist resolvin D2 reduces early brain injury in a rat model of subarachnoid hemorrhage by multiple protective mechanisms. Cell Mol. Neurobiol. 42 (7), 2379–2392. doi:10.1007/s10571-021-01114-2
Zhang, Y. J., Zhang, W., Lin, C. G., Ding, Y., Huang, S. F., Wu, J. L., et al. (2012). Neurotrophin-3 gene modified mesenchymal stem cells promote remyelination and functional recovery in the demyelinated spinal cord of rats. J. Neurol. Sci. 313 (1-2), 64–74. doi:10.1016/j.jns.2011.09.027
Zhang, Y. P., Huang, Q. L., Zhao, C. M., Tang, J. L., and Wang, Y. L. (2011). GM1 improves neurofascin155 association with lipid rafts and prevents rat brain myelin injury after hypoxia-ischemia. Braz. J. Med. Biol. Res. = Revista brasileira de pesquisas medicas e Biol. 44 (6), 553–561. doi:10.1590/s0100-879x2011000600009
Zhao, P. Y., Ji, J., Liu, X. H., Zhao, H., Xue, B., Jin, L. Y., et al. (2020). Bu-shen-Yi-sui capsule, an herbal medicine formula, promotes remyelination by modulating the molecular signals via exosomes in mice with experimental autoimmune encephalomyelitis. Oxid. Med. Cell Longev. 2020, 7895293. doi:10.1155/2020/7895293
Zhao, P. Y., Wang, Y. Q., Liu, X. H., Zhu, Y. J., Zhao, H., Zhang, Q. X., et al. (2018). Bu Shen Yi Sui capsule promotes remyelination correlating with Sema3A/NRP-1, LIF/LIFR and Nkx6.2 in mice with experimental autoimmune encephalomyelitis. J. Ethnopharmacol. 217, 36–48. doi:10.1016/j.jep.2018.02.014
Zheng, M., Liu, Z., Mana, L., Qin, G., Huang, S., Gong, Z., et al. (2021). Shenzhiling oral liquid protects the myelin sheath against Alzheimer's disease through the PI3K/Akt-mTOR pathway. J. Ethnopharmacol. 278, 114264. doi:10.1016/j.jep.2021.114264
Zhu, Z., Ding, L., Qiu, W. F., Wu, H. F., and Li, R. (2016). Salvianolic acid B protects the myelin sheath around injured spinal cord axons. Neural Regen. Res. 11 (3), 487–492. doi:10.4103/1673-5374.179068
Zhuo, F., Qiu, G., Xu, J., Yang, M., Wang, K., Liu, H., et al. (2016). Both endoplasmic reticulum and mitochondrial pathways are involved in oligodendrocyte apoptosis induced by capsular hemorrhage. Mol. Cell. Neurosci. 72, 64–71. doi:10.1016/j.mcn.2016.01.009
Zoller, I., Meixner, M., Hartmann, D., Bussow, H., Meyer, R., Gieselmann, V., et al. (2008). Absence of 2-hydroxylated sphingolipids is compatible with normal neural development but causes late-onset axon and myelin sheath degeneration. J. Neurosci. 28 (39), 9741–9754. doi:10.1523/JNEUROSCI.0458-08.2008
Keywords: subarachnoid hemorrhage, white matter, myelin sheath, oligodendrocyte, secondary brain injury
Citation: Chen M, Guo P, Ru X, Chen Y, Zuo S and Feng H (2023) Myelin sheath injury and repairment after subarachnoid hemorrhage. Front. Pharmacol. 14:1145605. doi: 10.3389/fphar.2023.1145605
Received: 16 January 2023; Accepted: 24 March 2023;
Published: 03 April 2023.
Edited by:
Chongjie Cheng, Chongqing Medical University, ChinaReviewed by:
Jiaxin Ren, First Affiliated Hospital of Jilin University, ChinaYue He, Huazhong University of Science and Technology, China
Yue Wu, First Affiliated Hospital of Chongqing Medical University, China
Copyright © 2023 Chen, Guo, Ru, Chen, Zuo and Feng. This is an open-access article distributed under the terms of the Creative Commons Attribution License (CC BY). The use, distribution or reproduction in other forums is permitted, provided the original author(s) and the copyright owner(s) are credited and that the original publication in this journal is cited, in accordance with accepted academic practice. No use, distribution or reproduction is permitted which does not comply with these terms.
*Correspondence: Yujie Chen, Y2hlbnlqQHRtbXUuZWR1LmNu; Shilun Zuo, c2hpbHVuenVvQHRtbXUuZWR1LmNu
†These authors have contributed equally to this work