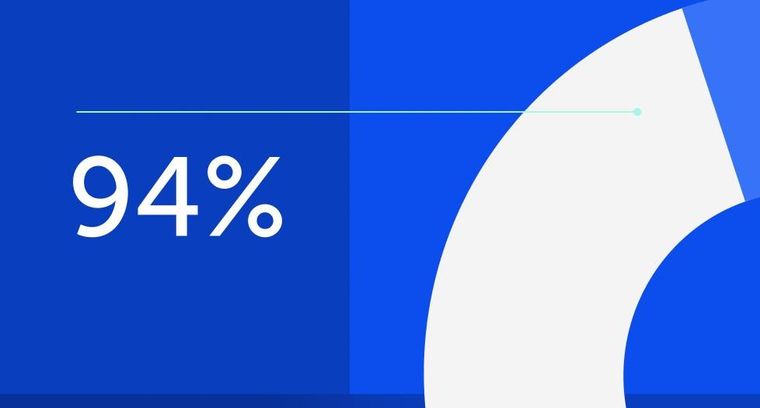
94% of researchers rate our articles as excellent or good
Learn more about the work of our research integrity team to safeguard the quality of each article we publish.
Find out more
REVIEW article
Front. Pharmacol., 26 June 2023
Sec. Renal Pharmacology
Volume 14 - 2023 | https://doi.org/10.3389/fphar.2023.1144918
This article is part of the Research TopicReceptor Biology and Cell Signaling in Diabetes: Volume IIView all 6 articles
Diabetes mellitus is mainly classified into four types according to its pathogenesis, of which type 2 diabetes mellitus (T2DM) has the highest incidence rate and is most relevant to obesity. It is characterized by high blood glucose, which is primarily due to insulin resistance in tissues that are responsible for glucose homeostasis (such as the liver, skeletal muscle, and white adipose tissue (WAT)) combined with insufficiency of insulin secretion from pancreatic β-cells. Treatment of diabetes, especially treatment of diabetic complications (such as diabetic nephropathy), remains problematic. Obesity is one of the main causes of insulin resistance, which, however, could potentially be treated by activating thermogenic adipose tissues, like brown and beige adipose tissues, because they convert energy into heat through non-shivering thermogenesis and contribute to metabolic homeostasis. In this review, we summarize the function of certain anti-diabetic medications with known thermogenic mechanisms and focus on various receptor signaling pathways, such as previously well-known and recently discovered ones that are involved in adipose tissue-mediated thermogenesis and could be potentially targeted to combat obesity and its associated diabetes, for a better understanding of the molecular mechanisms of non-shivering thermogenesis and the development of novel therapeutic interventions for obesity-related diabetes and potentially diabetic complications.
Obesity is one of the main pathogeneses of type 2 diabetes mellitus (T2DM). The incidence of obesity and T2DM is increasing worldwide due to changes in human lifestyle and dietary structure. High glucose levels in blood circulation lead to dysfunction in the cardiovascular system, kidneys, nervous system, etc. Diabetic nephropathy is one of the principal causes of diabetic mortality. Obesity-related diabetes is largely attributed to the development of insulin resistance, which is primarily caused by “unhealthy” white adipose tissue (WAT) expansion and pro-inflammatory macrophage-mediated WAT inflammation (Odegaard et al., 2007). Additionally, insufficient insulin secretion from pancreatic β-cells is another important cause of T2DM. Reasons for pancreatic β-cell dysfunction in T2DM patients may include the accumulation of lipid droplets and dysfunctional mitochondria in these cells (Horii et al., 2022; Aoyagi et al., 2023).
Except for diet control and kinesitherapy, which are difficult to sustain, therapeutic strategies for the treatment of T2DM include increasing the sensitivity of target cells to insulin [sulfonylureas and thiazolidinediones (TZDs)/peroxisome proliferator-activated receptor γ (PPARγ) activators], moderate glucose levels either by increasing glucose uptake and usage in peripheral tissues such as skeletal muscle (metformin) or by inhibiting α-glucosidase in intestinal mucosal epithelial cells to reduce glucose uptake (α-glucosidase inhibitors), inhibit glucose reabsorption through renal tubules [sodium-glucose co-transporter 2 (SGLT2) inhibitors], and facilitate insulin secretion from pancreatic β-cells [dipeptidyl peptidase 4 (DPP4) inhibitors, glucagon-like peptide-1 receptor (GLP-1R) agonists, and amphetamine-acid derivatives] and insulin treatment. Drug safety profiles are certainly a big concern. Early-developed drugs, such as sulfonylureas, show a high risk of hypoglycemia and weight gain and may be associated with a hyperosmolar hyperglycemic state or pancreatic dysfunction (Pfeifer et al., 1984; Stoner et al., 2005). Although used as a first-line therapy in the past and present, the main concern with metformin is its gastrointestinal side effects (Florez et al., 2010). Off-target effects of several drugs, such as TZDs and DPP4 inhibitors, are also reported, and some are even fatal; for example, TZDs could cause hepatotoxicity, myocardial infarction, bladder cancer, and even liver/heart failure (Nesto et al., 2003; Rogue et al., 2010; Lewis et al., 2011), while DPP4 inhibitors often lead to upper respiratory tract infections and nasopharyngitis (Monami et al., 2011; Karagiannis et al., 2012). Due to the renal excretion of most of the drugs, renal impairment during anti-diabetic treatment is always under observation, and most of them, including SGLT2 inhibitors, are even prohibited for use in patients with severe renal dysfunction (Guthrie, 2012). Primarily, weight control is the most fundamental treatment for T2DM. Among the aforementioned therapeutic interventions, GLP-1R agonism alternatively seems to be an anti-obesity treatment, as GLP-1R agonists work as potent extrinsic appetite suppressants. Certain GLP-1R agonists could penetrate through the blood–brain barrier and probably act on the hypothalamus to increase central satiety on the one hand (Seufert and Gallwitz, 2014; Blundell et al., 2017), and on the other hand, they could act on the gastrointestinal tract to delay gastric emptying (Friedrichsen et al., 2021). Probably, GLP-1R agonism could be a competent therapeutic strategy to combat obesity-related diabetes with minimal side effects (such as nausea and vomiting), also because of its beneficial effects on the nervous and cardiovascular systems and metabolic equilibrium (Zhao et al., 2021). Interestingly, the antiatherogenic benefit of GLP-1R agonists is independent of GLP-1Rs in either endothelial cells or hematopoietic cells, whereas the inhibitory effects of GLP-1R agonists on cytokine expression, triglyceride accumulation, and fibrosis in the high-fat, high-cholesterol diet-fed mouse liver require GLP-1R expression in the cells targeted by Tie2-Cre (possibly T cells) (McLean et al., 2021). However, the invasive subcutaneous injection route of administration and high cost have limited the clinical application of GLP-1R agonists. Renal outcomes have been assessed and compared between these common anti-diabetic medications. SGLT2 inhibitors are known for their protective effects on the kidney in both diabetic and non-diabetic patients (Palmer and Clegg, 2023). Furthermore, SGLT2 inhibitors, GLP-1 analogs, and metformin have been shown to be associated with a lower risk of adverse renal outcomes compared with other types of anti-diabetic medications (Xie et al., 2020; Hung et al., 2023).
Of note, two types of thermogenic adipose tissue have been proven to exist in humans: brown adipose tissue (BAT) and beige adipose tissue. Unlike WAT, thermogenic adipose tissue could convert surplus energy into heat by mitochondria through the mechanism of so-called non-shivering thermogenesis, contributing to energy homeostasis. Classic mechanisms of non-shivering thermogenesis include stimulation of β-adrenergic receptors (β-ARs) by norepinephrine (NE), which is released from the sympathetic nerve terminal, and UCP1-mediated heat generation through mitochondria. In particular, beige adipose tissue is heterogeneous with high plasticity and could emerge in the depot of WAT. They could be most potentially targeted for the treatment of obesity, and thus, obesity-related diabetes. Human BAT is mainly located in the cervical, supraclavicular, paravertebral, and periadrenal areas, according to previous human studies (Nedergaard et al., 2007). Notably, the activity of BAT in humans is negatively correlated with body mass index (BMI) and positively correlated with glucose tolerance and insulin sensitivity (Matsushita et al., 2014). It has been subsequently indicated that BAT also exists in the human perirenal adipose tissue depot but with a broad difference among individuals (Svensson et al., 2014). It mainly consists of unilocular adipocytes with gene expression partially overlapping with either periadrenal multilocular adipocytes (UCP1, etc.) or subcutaneous WAT, which differ in their morphology from the cells near the adrenal gland (Jespersen et al., 2019). However, the function of perirenal BAT is poorly described.
In addition to being a thermogenic organ, brown and beige adipose tissue is also recognized as an endocrine organ, involved in the regulation of distant organs through the secretion of factors and microRNAs, which are termed “batokines” (Figure 1). So far, these distant organs include the heart, skeletal muscle, brain, bone, liver, pancreas, and WAT, as summarized by several groups (Thomou et al., 2017; Villarroya et al., 2019; Ahmad et al., 2021). In recent years, a crosstalk between BAT and the kidney has also been described (Figure 1). It has been demonstrated that the browning of renal peritumoral adipose tissue stimulates the expression of epithelial–mesenchymal transition markers in human renal cells (Ferrando et al., 2022), suggesting a detrimental aspect of the perirenal adipose tissue that potentially contributes to tumor development and metastasis. Nevertheless, BAT activation shows metabolic benefits and renoprotective effects in diabetic mice (Cai et al., 2019). Particularly, transplantation of BAT from normal mice to diabetic mice near the interscapular region significantly ameliorates diabetic kidney disease (on the aspects of renal dysfunction, inflammation, oxidative stress, and fibrosis) by downregulating the nephropathy-related genes Runx1 and Snail1 in BAT-derived microRNA-30b in diabetic kidneys, a telecrine effect of BAT (Zhang et al., 2021). Massive investigations are required to further explore the function of perirenal BAT and the regulatory mechanisms of possible BAT–kidney crosstalk.
FIGURE 1. Crosstalk between brown/beige adipose tissue and distant organs and regulation of perirenal BAT (black dots in perirenal adipocytes represent UCP1). The figure was created using BioRender (https://biorender.com/).
Various anti-diabetic medications have thus been reassessed to investigate whether they play a role in the regulation of thermogenic adipose tissue. In this review, we summarize findings on the thermogenic effects of certain anti-diabetic medications commonly used in the clinic, and potential receptors in adipose tissue that could be targeted to combat obesity, providing novel viewpoints in the intervention and treatment of obesity-related diabetes and possibly diabetic complications (such as diabetic kidney disease).
Owing to the potential of brown and beige adipose tissue in combating obesity, which remains the leading cause of T2DM, targeting these thermogenic adipose tissues may be theoretically and practically feasible to benefit and ameliorate obesity-related diabetes. Along with the increasing knowledge of these two types of thermogenic adipose tissue over the decades, it is questionable whether the medications clinically used for T2DM treatment also play a role in the regulation of brown/beige adipose tissue-mediated thermogenesis. Thus, certain anti-diabetic medications have been reassessed for their effects on thermogenesis. Certain types of anti-diabetic medications have been reported to potentially regulate thermogenic adipose tissue. They include sulfonylureas, TZDs, metformin, SGLT2 inhibitors, and GLP-1 analogs, as shown in Figure 2.
FIGURE 2. Classical non-shivering thermogenesis and the mechanisms of certain anti-diabetic medications. AMPK, AMP-activated protein kinase; AR, adrenergic receptor; GLP-1, glucagon-like peptide-1; NE, norepinephrine; PKA, protein kinase A; PPARγ, peroxisome proliferator-activated receptor gamma; PGC-1α, PPARγ coactivator-1α; PRDM16, protein PR domain-containing 16; SGLT2, sodium-glucose co-transporter 2; SIRT1, sirtuin 1; TZDs, thiazolidinediones; UCP1, uncoupling protein 1. The figure was created using BioRender (https://biorender.com/).
Sulfonylureas have been found to lower blood glucose by stimulating the release of insulin from pancreatic β-cells, inhibiting glucagon secretion from pancreatic α-cells, directly acting on the liver to reduce the clearance rate of insulin and glycogenolysis, and enhancing the sensitivity of peripheral tissues to insulin (Lv et al., 2020). Specifically, the actions of sulfonylureas on pancreatic β-cells include binding with their receptors, which are tightly linked to ATP-sensitive potassium (KATP) channels, inhibiting the activity of KATP channels, whose closure is related to calcium ion flow into the cells and thus insulin secretion (Ashcroft, 2000; Mikhailov et al., 2000). Furthermore, sulfonylureas activate cyclic adenosine monophosphate (cAMP), and stimulate the translocation of exchange protein2 activated by cAMP (Epac2A) to the membrane, which is a protein involved in the cAMP–protein kinase A (PKA) pathway, or directly activate Epac2A/Rap1 signaling by binding to Epac2A, which regulates the calcium kinetics to enhance insulin secretion (Shibasaki et al., 2007; Seino et al., 2017; Robichaux and Cheng, 2018). The actions of sulfonylureas on pancreatic α-cells include a direct inhibition of glucagon secretion by sulfonylureas and an indirect inhibition of glucagon secretion by sulfonylurea-induced insulin from adjacent β-cells in a paracrine manner (Landstedt-Hallin et al., 1999; ter Braak et al., 2002). Direct effects of sulfonylureas on adipose tissue may include inhibition of lipolysis and triglyceride lipase and promotion of glucose uptake and oxidation (Sola et al., 2015). One of the sulfonylureas, glibenclamide, has been found to improve high-fat diet (HFD)-induced depressive-like symptoms in mice by restoring KATP channel expression on the surface of brown adipocytes, which is accompanied by improved sympathetic innervation of interscapular BAT (involvement of KATP channel closure, sympathetic nerve depolarization, and promotion of NE release) and activation of β3 AR-PKA signaling (Kuo et al., 2020). However, in this study, they did not show any direct evidence of improved activation of BAT or enhanced thermogenesis and energy expenditure induced by glibenclamide, though an improved sympathetic innervation of BAT may indicate these effects. Further investigations are thus required in this aspect.
PPARγ is a nuclear receptor that is highly expressed in adipose tissue along with other tissues in the colon and placenta (Rosen and Spiegelman, 2000). It plays an important role in the regulation of lipid and glucose metabolism in concert with PPARγ coactivator-1α (PGC-1α), fulfilling the oxidation of fatty acids in mitochondria and maintaining insulin sensitivity in skeletal muscle, adipose tissue, and liver (Hevener et al., 2003; Alaynick, 2008). Thus, PPARγ activation in adipose tissue results in the induction of adipogenesis, lipid metabolism, and promotion of glucose homeostasis (Ahmadian et al., 2013). TZDs are synthetic PPARγ activators designed to improve insulin sensitivity in patients with T2DM. PPARγ, as one of the key factors regulating brown and beige adipogenesis, mediates the effect of TZDs on brown and beige adipose tissue. Indeed, different TZDs, such as lobeglitazone, rosiglitazone, and pioglitazone, show different efficiencies in the regulation of thermogenesis and glycometabolism (Sohn et al., 2018). Rosiglitazone has been found to induce beige fat development in mice probably through the activation of the sirtuin 1 (SIRT1)–PR domain-containing protein 16 (PRDM16) pathway (Ohno et al., 2012; Qiang et al., 2012). Despite inducing beige adipose tissue development, certain TZDs, like pioglitazone, have also been characterized by a weight gain effect. Evidence shows that pioglitazone significantly reduces the activation of BAT in response to cold in humans, which may partially explain the weight gain effect of pioglitazone (Loh et al., 2018). In addition, the full agonism of PPARγ by TZDs is associated with various side effects. Partial or non-TZD PPARγ agonism may reduce side effects while treating metabolic disorders with PPARγ activators. Hence, a novel non-TZD partial PPARγ activator, which is an indole-based chemical and inhibits the phosphorylation of PPARγ, WO95E, has recently been developed with minimal side effects (Wu et al., 2021). It not only ameliorates insulin resistance and glucose tolerance in HFD-induced obese mice but also induces beiging in the inguinal WAT of mice and increases energy expenditure to counter obesity. It also shows beneficial effects on adipose tissue inflammation and obesity-associated liver steatosis. Thus, potential PPARγ activators with minimal side effects are desired to accomplish the treatment of obesity and related diabetes by targeting thermogenic adipose tissue.
Metformin, as a potent AMP-activated protein kinase (AMPK) activator, is a first-line anti-diabetic medication and plays a very important role in glucose metabolism and energy homeostasis. It targets various organs and exerts metabolic effects. In adipose tissue, it promotes glucose uptake and attenuates fat accumulation and inflammation through AMPK activation and modulation of other factors (FGF21, mTOR, etc.) (Ziqubu et al., 2023). In addition, metformin has been found to promote thermogenesis and have an anti-obesity effect. Its treatment in mice led to an increase in BAT mass, an induction of beige adipocytes in inguinal WAT, and a probable promotion in adipocyte proliferation and differentiation, in addition to an enhancement in the expression of various thermogenic markers in BAT, which was probably accompanied by improved mitochondrial biogenesis, lipolysis, and fatty acid uptake in BAT (Karise et al., 2019). Introduction of metformin through dissolvable poly-microneedles to the subcutaneous WAT of obese mice with the assistance of iontophoresis led to a marked beiging in inguinal WAT, an increase in energy expenditure, a decrease in body weight and fat mass, and thus an improvement in energy metabolism (Abbasi et al., 2022). Evidence shows that the AMPK α1 isoform mediates the DNA demethylation in the promoter of Prdm16, which is very essential for brown adipogenesis, through a key metabolite of the Krebs cycle, α-ketoglutarate (Yang et al., 2016). In addition, Zhang E. et al. (2022) revealed that the effects of metformin on BAT may also include intestine–BAT communication through intestinal α1AMPK-mediated regulation of gut microbiota and their metabolites. Thus, the therapeutic effect of metformin on diabetes may be partially dependent on its acting on brown and beige adipose tissue through various mechanisms.
SGLT2 inhibitors work as anti-diabetic medications through the inhibition of glucose reabsorption through the renal tubules, which could reduce blood glucose by increasing glucosuria. A weight loss effect of SGLT2 inhibitors has been observed; however, it is less than expected based on the energy excretion via glucosuria. Evidence shows that certain SGLT2 inhibitors, such as canagliflozin, ipragliflozin, and empagliflozin, increase energy expenditure and thus ameliorate diet-induced metabolic changes. They promote thermogenesis via inducing beiging in subcutaneous WAT, protect mice from HFD-induced fat accumulation in BAT, enhance mitochondrial biogenesis and respiratory activity, and promote lipolysis and fatty acid oxidation in adipose tissue to varying degrees (Lee et al., 2021; Xu et al., 2021; Yang et al., 2021). The mechanisms of the aforementioned regulation may involve the activation of AMPK and therefore its downstream factors, SIRT1 and PGC-1α, and elevation of the sympathetic innervation of adipose tissue, which facilitates fat mobilization via the β3-adrenergic receptor (β3AR)–cAMP–PKA signaling pathway. Thus, the beneficial effects of SGLT2 inhibition in diabetes may also include its various regulatory effects on adipose tissue.
GLP-1 analogs, also known as GLP-1R agonists, have become popular anti-diabetic drugs in recent years because of their additional anti-obesity effect. Administration of one of the GLP-1 analogs, semaglutide, to obese diabetic patients, results in a significant reduction in their weight, BMI, fasting glucose levels, and hemoglobin A1c levels without affecting the skeletal muscle mass (Ozeki et al., 2022). Several studies were performed over the years to clarify whether there are other mechanisms by which GLP-1Rs regulate energy metabolism in addition to suppression of appetite and adipose/hepatic lipogenesis and promotion of insulin secretion and sensitivity. GLP-1R activation led to the induction of interscapular BAT thermogenesis with an improved sympathetic innervation. However, GLP-1R signaling is not required for cold-induced thermogenesis since GLP-1R-knockout mice display a normal thermogenic response to cold exposure, suggesting that induction of BAT thermogenesis may be only one of the metabolic effects of GLP-1R signaling on metabolic regulation (Lockie et al., 2012). Similarly, GLP-1R signaling is not required for NE-stimulated oxygen consumption in BAT in mice fed with a chow diet; however, it impacts NE-induced increases in BAT oxygen consumption in mice fed with HFD (Heppner et al., 2015). In addition, chronic administration of the GLP-1R agonist, liraglutide (even at a higher dose, 30 nmol/kg per day), is unable to activate BAT in the presence of GLP-1R in diet-induced obese mice (Heppner et al., 2015), which further indicates that GLP-1R signaling is not indispensable for the BAT-related weight-lowering effect of liraglutide. Ulteriorly, it was demonstrated that central injection of the GLP-1R agonist, liraglutide, in mice could stimulate thermogenesis in BAT and beiging in WAT by activating AMPK in the hypothalamic ventromedial nucleus (Beiroa et al., 2014). Kooijman et al. (2015) observed similar effects and improved glycolipid metabolism after central administration of another GLP-1 analog, exendin-4, suggesting that the beneficial effects of GLP-1 signaling activation on BAT and WAT are probably mediated by enhancing sympathetic innervation of these adipose tissues. Nevertheless, a protective effect of exendin-4 on diabetic kidneys has been suggested to be independent of BAT activation (Fang et al., 2020). Further investigations are required to clarify the regulatory mechanisms of GLP-1 signaling intervention in the treatment of obesity and diabetes.
Despite the thermogenic effects of the aforementioned anti-diabetic medications, the regulatory mechanisms of these medications on adipose tissue are unclear. In addition, the contribution of these medications to combating obesity and obesity-related diabetes by targeting brown and beige adipose tissue is very limited. Due to the strong energy dissipation capability of these thermogenic adipose tissues, the regulatory mechanisms involved in evoking their activity have recently attracted great interest. Notably, receptors in adipose tissue could be readily targeted by their agonists or antagonists. Here, we also highlight several prominent receptors in adipose tissue that are involved in the regulation of non-shivering thermogenesis and could be potentially targeted to achieve efficient energy dissipation.
Several promising receptor signaling pathways have been previously summarized in one of our reviews, such as β2/β3-AR and A2A/A2B adenosine receptor signaling pathways (Pan et al., 2020; Pan and Chen, 2022). Investigations of these receptor signaling pathways including the effects of some competent receptor agonists on fat metabolism, have been gradually performed in human cells and human bodies. Excitingly, the dual-active adenosine receptor ligand LJ-4378, with A2A receptor agonist and A3 receptor antagonist activity, showed a stronger lipolytic effect than single-substance administration alone in mice, with significant anti-obesity and glucose-improving effects (Kim et al., 2022). Despite great advancements, there remains bemusement in treating obesity and metabolic disorders by targeting these receptors. Reasons include (1) side effects (such as on the cardiovascular system) caused by a high dosage of β3-AR agonist (mirabegron) that is still effective in increasing lipolysis and energy expenditure; and (2) limited investigations of β2-AR agonists and A2B receptor agonists in humans. Thus, the challenges of these receptor agonisms require us to perform further investigations.
PPARs play an important role in maintaining whole-body glucose and lipid homeostasis. PPAR isoforms show different tissue distributions, among which PPARγ and PPARβ/δ show similar expression levels in WAT and BAT, while PPARα shows the highest expression in BAT (Gross et al., 2017). Evidence shows that PPARα is indispensable for BAT growth and thus for non-shivering thermogenesis in response to cold, regulating BAT biology probably through its cooperation with PGC-1a and PRDM16 (Barbera et al., 2001; Hondares et al., 2011). The PPARα agonist fenofibrate (clinically used for the treatment of dyslipidemia), has shown a beiging effect on subcutaneous WAT in obese mice, increasing energy expenditure (Rachid et al., 2015a, b). Another selective PPARα agonist, pirinixic acid, has been shown to counter HFD-induced BAT whitening in mice, probably by activating the thermogenic pathway and enhancing lipolysis (Miranda et al., 2020). PPARβ/δ has been shown to activate fatty acid oxidation so that it enhances thermogenesis and prevents obesity, which may involve the participation of PGC-1α. However, its agonists have not yet been used in the clinic. The importance of PPARγ, the most expressive PPAR isoform in adipose tissue, has been shown in the regulation of fat metabolism. Its agonists, TZDs, as insulin sensitizers, are commonly used for the treatment of T2DM, which also fulfills a beiging effect (Ohno et al., 2012; Qiang et al., 2012; Wu et al., 2021). As for the challenges and advancements of PPARγ agonism for the treatment of obesity and T2DM, they have been discussed in the previous section.
Glucocorticoid stimulation has been found to diminish thermogenic capacity with a decreased uncoupling protein 1 (UCP1) protein level and an increased accumulation of lipids in BAT, which has thus been considered to mediate glucocorticoid-induced obesity (Poggioli et al., 2013; Scotney et al., 2017; Thuzar et al., 2018). Importantly, the effects of glucocorticoids on brown adipocytes are dependent on their binding to their receptors, which are glucocorticoid receptors located in cytosolic fractions. The liganded glucocorticoid receptors then transferred to the nucleus, where they could bind to various binding sites on the ucp1 gene and regulate ucp1 transcription (Luijten et al., 2019). Among them, glucocorticoid receptors binding to the upstream of the transcription start site of ucp1 cause inhibition in ucp1 transcription. Thus, the inhibitory effect of glucocorticoid receptor signaling on thermogenic capacity is probably due to the binding of glucocorticoid receptors to ucp1 that inhibits ucp1 transcription (“masking” of UCP1). Accordingly, administration of glucocorticoid receptor antagonists inhibits glucocorticoid receptor activity and contributes to UCP1 “unmasking,” most of which (e.g., CORT125281 and C108297) prevent mice from HFD-induced lipid accumulation in BAT and/or result in a decrease in fat mass and body weight (Kroon et al., 2018; van den Heuvel et al., 2016). Furthermore, mineralocorticoid receptor antagonism by spironolactone, drospirenone, and finerenone also shows positive effects on HFD-induced lipid accumulation in BAT and fat accumulation (Armani et al., 2014; Marzolla et al., 2020). In addition, spironolactone and drospirenone possess a beiging effect in the WAT of HFD mice (Armani et al., 2014). In addition, spironolactone treatment in healthy humans results in an enhancement in BAT function (activity, volume, and supraclavicular skin temperature) in response to cold and an elevation in supraclavicular skin temperature and energy production rate in response to a mixed meal (Thuzar et al., 2019). The use of these antagonists is still limited in clinical practice because of the simultaneous antagonism of androgen and progesterone receptors, which cause parallel clinical symptoms (Lainscak et al., 2015; Luijten et al., 2019). In addition, abnormal renal function is associated with a higher risk of hyperkalemia in patients treated with spironolactone, which could threaten the patients’ lives (Schepkens et al., 2001). Thus, great efforts are required to achieve more research progress in this field.
Thyroid hormones have long been known to increase energy consumption and reduce fat. Early findings revealed that they bind to various thyroid hormone receptors (including α and β isoforms) in adipocytes to promote adaptive thermogenesis through crosstalk between thyroid hormone receptor signaling and β AR signaling (Ribeiro et al., 2001). Lateral ventricular injection of triiodothyronine (T3) led to an increase in sympathetic output and an enhancement in BAT activity and thermogenesis (Lopez et al., 2010). In addition, T3 has also been demonstrated to induce beiging (Martinez-Sanchez et al., 2017). Due to the probable but unexpected adverse effects on the heart, bones, etc., caused by excess thyroid hormones, thyroid hormone analogs have been developed to obtain the beneficial effects of thyroid hormone receptor activation with minimal side effects. As such, pharmacological stimulation of thyroid hormone receptors by their agonist GC-1 (β-isoform-selective) has been shown to markedly promote beiging in WAT and induce adaptive thermogenesis in mice (Lin et al., 2015). Notably, the chemical hybridization of glucagon and thyroid hormone results in the concentration of thyroid hormone in WAT, where high levels of glucagon are expressed, the subsequent induction of beige fat development, and an improvement in energy expenditure (Finan et al., 2016). Despite the prolonged controversy on the thermogenic mechanisms of thyroid hormones, it has been recently revealed that T3-thyroid hormone receptor signaling plays a crucial role in the regulation of energy metabolism and BAT proliferation (Zekri et al., 2022). Moreover, the regulatory effects of T3 on BAT hyperplasia have been shown to be mediated by thyroid hormone receptor α through the promotion of progenitor cell proliferation (Liu et al., 2022). Thus, the development of selective agonists against the α isoform of thyroid hormone receptors and further evaluation of their potential effects on thermogenesis is also required.
Several other prominent receptors in adipocytes mediate the stimulatory or inhibitory effects of external signals on energy metabolism. They have been summarized in some of our previous reviews, which include the secretin receptor, G protein-coupled receptor 3, neurotensin receptor 2, fibroblast growth factor receptor 3, interleukin-27 receptor α subunit, androgen receptor, estrogen receptors, and follicle-stimulating hormone receptor (Pan et al., 2020; Pan and Chen, 2022). Recently, the specific roles of some other receptors have also been identified. The interleukin (IL)-18 receptor mediates the IL-18 signaling in WAT to promote insulin sensitivity and glucose uptake, whereas the Na-Cl co-transporter mediates the IL-18 signaling in BAT to enhance thermogenesis (Zhang X. et al., 2022). The nuclear bile acid receptor-farnesoid X receptor has been recently found to negatively regulate BAT development and function in mice (Yang et al., 2023). Hydroxycarboxylic acid receptor 1 in BAT plays an important role in maintaining glucose homeostasis (Min et al., 2022).
The basic treatment of diabetes remains complex, inefficient, and sometimes contradictory, even though more and more anti-diabetic medications are being developed. Especially when patients have complications such as diabetic nephropathy, treatment with anti-diabetic drugs becomes problematic due to renal excretion of most of the medications. Obesity is one of the main causes of T2DM, so combating obesity could potentially be the primary treatment for T2DM and its complications. Due to the potent function of BAT and beige adipose tissue on fat burning and energy expenditure, targeting these thermogenic adipose tissues could be a promising strategy to combat obesity and thus obesity-related diabetes, independent of insulin and glucose levels. In addition, as an endocrine organ, activated thermogenic adipose tissue secretes “batokines” that may be involved in the regulation of distant organs. The crosstalk between these adipose tissues and the kidney has been partially described (Figure 1), and there exist special types of BAT in the perirenal region per se (Figure 1). The function of the perirenal BAT and the crosstalk between BAT and the kidney should be further investigated.
Meanwhile, the function of the classic anti-diabetic medications on thermogenic adipose tissue has been studied by many groups. The potential roles of these medications in the regulation of non-shivering thermogenesis are shown in Figure 2. Certain anti-diabetic drugs play well-defined roles in the regulation of thermogenic adipose tissue, such as PPARγ activators, metformin, SGLT2 inhibitors, and GLP-1R agonists, which are also summarized in Table 1. However, investigations of the mechanisms of the effects of these anti-diabetic medications on thermogenic adipose tissue are so far very limited and truly require further research.
TABLE 1. Common anti-diabetic medications that play a role in the regulation of thermogenic adipose tissue.
In addition, numerous receptors in adipose tissue have been found to mediate the action of various substances on thermogenic adipose tissue that could theoretically be targeted to induce BAT and beige adipose tissue activity. Main receptors and their actions on thermogenic adipose tissue (when agonized or antagonized) and the limitations of their use have been summarized in Table 2. Progress in the development of receptor agonists or antagonists has been made to minimize the side effects. However, with the exception of PPARγ inhibitors for the treatment of obesity-related diabetes, most of them have not been approved to treat obesity. In addition, thermogenic mechanisms involving other promising receptors are gradually being discovered, although agonists or antagonists are not yet available. Although challenging, further investigations of receptor signaling are required for a significant transition from basic research to clinical practice and better and more effective treatment of obesity-related diabetes.
RP wrote the manuscript. JL edited the figures. YC revised the manuscript. All authors contributed to the article and approved the submitted version.
This work was supported by a grant from the National Natural Science Foundation of China (Grant numbers 82070859 and 82270910 to YC and RP) and a grant from Tongji Hospital in Huazhong University of Science and Technology (Grant number 2201103295 to YC).
The authors declare that the research was conducted in the absence of any commercial or financial relationships that could be construed as a potential conflict of interest.
All claims expressed in this article are solely those of the authors and do not necessarily represent those of their affiliated organizations, or those of the publisher, the editors, and the reviewers. Any product that may be evaluated in this article, or claim that may be made by its manufacturer, is not guaranteed or endorsed by the publisher.
Abbasi, M., Fan, Z., Dawson, J. A., and Wang, S. (2022). Transdermal delivery of metformin using dissolving microneedles and iontophoresis patches for browning subcutaneous adipose tissue. Pharmaceutics 14, 879. doi:10.3390/pharmaceutics14040879
Ahmad, B., Vohra, M. S., Saleemi, M. A., Serpell, C. J., Fong, I. L., and Wong, E. H. (2021). Brown/Beige adipose tissues and the emerging role of their secretory factors in improving metabolic health: The batokines. Biochimie 184, 26–39. doi:10.1016/j.biochi.2021.01.015
Ahmadian, M., Suh, J. M., Hah, N., Liddle, C., Atkins, A. R., Downes, M., et al. (2013). PPARγ signaling and metabolism: The good, the bad and the future. Nat. Med. 19, 557–566. doi:10.1038/nm.3159
Alaynick, W. A. (2008). Nuclear receptors, mitochondria and lipid metabolism. Mitochondrion 8, 329–337. doi:10.1016/j.mito.2008.02.001
Aoyagi, K., Yamashita, S. I., Akimoto, Y., Nishiwaki, C., Nakamichi, Y., Udagawa, H., et al. (2023). A new beta cell-specific mitophagy reporter mouse shows that metabolic stress leads to accumulation of dysfunctional mitochondria despite increased mitophagy. Diabetologia 66, 147–162. doi:10.1007/s00125-022-05800-8
Armani, A., Cinti, F., Marzolla, V., Morgan, J., Cranston, G. A., Antelmi, A., et al. (2014). Mineralocorticoid receptor antagonism induces browning of white adipose tissue through impairment of autophagy and prevents adipocyte dysfunction in high-fat-diet-fed mice. FASEB J. 28, 3745–3757. doi:10.1096/fj.13-245415
Ashcroft, S. J. (2000). The beta-cell K(ATP) channel. J. Membr. Biol. 176, 187–206. doi:10.1007/s00232001095
Barbera, M. J., Schluter, A., Pedraza, N., Iglesias, R., Villarroya, F., and Giralt, M. (2001). Peroxisome proliferator-activated receptor alpha activates transcription of the Brown fat uncoupling protein-1 gene. A link between regulation of the thermogenic and lipid oxidation pathways in the Brown fat cell. J. Biol. Chem. 276, 1486–1493. doi:10.1074/jbc.M006246200
Beiroa, D., Imbernon, M., Gallego, R., Senra, A., Herranz, D., Villarroya, F., et al. (2014). GLP-1 agonism stimulates Brown adipose tissue thermogenesis and browning through hypothalamic AMPK. Diabetes 63, 3346–3358. doi:10.2337/db14-0302
Blundell, J., Finlayson, G., Axelsen, M., Flint, A., Gibbons, C., Kvist, T., et al. (2017). Effects of once-weekly semaglutide on appetite, energy intake, control of eating, food preference and body weight in subjects with obesity. Diabetes Obes. Metab. 19, 1242–1251. doi:10.1111/dom.12932
Cai, Y. Y., Zhang, H. B., Fan, C. X., Zeng, Y. M., Zou, S. Z., Wu, C. Y., et al. (2019). Renoprotective effects of Brown adipose tissue activation in diabetic mice. J. Diabetes 11, 958–970. doi:10.1111/1753-0407.12938
Fang, S., Cai, Y., Lyu, F., Zhang, H., Wu, C., Zeng, Y., et al. (2020). Exendin-4 improves diabetic kidney disease in C57bl/6 mice independent of Brown adipose tissue activation. J. Diabetes Res. 2020, 9084567. doi:10.1155/2020/9084567
Ferrando, M., Bruna, F. A., Romeo, L. R., Contador, D., Moya-Morales, D. L., Santiano, F., et al. (2022). Renal peritumoral adipose tissue undergoes a browning process and stimulates the expression of epithelial-mesenchymal transition markers in human renal cells. Sci. Rep. 12, 8687. doi:10.1038/s41598-022-12746-9
Finan, B., Clemmensen, C., Zhu, Z., Stemmer, K., Gauthier, K., Muller, L., et al. (2016). Chemical hybridization of glucagon and thyroid hormone optimizes therapeutic impact for metabolic disease. Cell 167, 843–857. doi:10.1016/j.cell.2016.09.014
Florez, H., Luo, J., Castillo-Florez, S., Mitsi, G., Hanna, J., Tamariz, L., et al. (2010). Impact of metformin-induced gastrointestinal symptoms on quality of life and adherence in patients with type 2 diabetes. Postgrad. Med. 122, 112–120. doi:10.3810/pgm.2010.03.2128
Friedrichsen, M., Breitschaft, A., Tadayon, S., Wizert, A., and Skovgaard, D. (2021). The effect of semaglutide 2.4 mg once weekly on energy intake, appetite, control of eating, and gastric emptying in adults with obesity. Diabetes Obes. Metab. 23, 754–762. doi:10.1111/dom.14280
Gross, B., Pawlak, M., Lefebvre, P., and Staels, B. (2017). PPARs in obesity-induced T2DM, dyslipidaemia and NAFLD. Nat. Rev. Endocrinol. 13, 36–49. doi:10.1038/nrendo.2016.135
Guthrie, R. M. (2012). Evolving therapeutic options for type 2 diabetes mellitus: An overview. Postgrad. Med. 124, 82–89. doi:10.3810/pgm.2012.11.2614
Heppner, K. M., Marks, S., Holland, J., Ottaway, N., Smiley, D., Dimarchi, R., et al. (2015). Contribution of Brown adipose tissue activity to the control of energy balance by GLP-1 receptor signalling in mice. Diabetologia 58, 2124–2132. doi:10.1007/s00125-015-3651-3
Hevener, A. L., He, W., Barak, Y., Le, J., Bandyopadhyay, G., Olson, P., et al. (2003). Muscle-specific Pparg deletion causes insulin resistance. Nat. Med. 9, 1491–1497. doi:10.1038/nm956
Hondares, E., Rosell, M., Diaz-Delfin, J., Olmos, Y., Monsalve, M., Iglesias, R., et al. (2011). Peroxisome proliferator-activated receptor α (PPARα) induces PPARγ coactivator 1α (PGC-1α) gene expression and contributes to thermogenic activation of Brown fat: Involvement of PRDM16. J. Biol. Chem. 286, 43112–43122. doi:10.1074/jbc.M111.252775
Horii, T., Kozawa, J., Fujita, Y., Kawata, S., Ozawa, H., Ishibashi, C., et al. (2022). Lipid droplet accumulation in beta cells in patients with type 2 diabetes is associated with insulin resistance, hyperglycemia and beta cell dysfunction involving decreased insulin granules. Front. Endocrinol. (Lausanne) 13, 996716. doi:10.3389/fendo.2022.996716
Hung, A. M., Hackstadt, A. J., Griffin, M. R., Grijalva, C. G., Greevy, R. A., and Roumie, C. L. (2023). Comparative effectiveness of metformin versus sulfonylureas on kidney function decline or death among patients with reduced kidney function: A retrospective cohort study. CMAJ Open 11, E77–E89. doi:10.9778/cmajo.20210207
Jespersen, N. Z., Feizi, A., Andersen, E. S., Heywood, S., Hattel, H. B., Daugaard, S., et al. (2019). Heterogeneity in the perirenal region of humans suggests presence of dormant Brown adipose tissue that contains Brown fat precursor cells. Mol. Metab. 24, 30–43. doi:10.1016/j.molmet.2019.03.005
Karagiannis, T., Paschos, P., Paletas, K., Matthews, D. R., and Tsapas, A. (2012). Dipeptidyl peptidase-4 inhibitors for treatment of type 2 diabetes mellitus in the clinical setting: Systematic review and meta-analysis. BMJ 344, e1369. doi:10.1136/bmj.e1369
Karise, I., Bargut, T. C., Del Sol, M., Aguila, M. B., and Mandarim-de-Lacerda, C. A. (2019). Metformin enhances mitochondrial biogenesis and thermogenesis in Brown adipocytes of mice. Biomed. Pharmacother. 111, 1156–1165. doi:10.1016/j.biopha.2019.01.021
Kim, K., Im, H., Son, Y., Kim, M., Tripathi, S. K., Jeong, L. S., et al. (2022). Anti-obesity effects of the dual-active adenosine A(2A)/A(3) receptor-ligand LJ-4378. Int. J. Obes. (Lond). 46, 2128–2136. doi:10.1038/s41366-022-01224-x
Kooijman, S., Wang, Y., Parlevliet, E. T., Boon, M. R., Edelschaap, D., Snaterse, G., et al. (2015). Central GLP-1 receptor signalling accelerates plasma clearance of triacylglycerol and glucose by activating Brown adipose tissue in mice. Diabetologia 58, 2637–2646. doi:10.1007/s00125-015-3727-0
Kroon, J., Koorneef, L. L., van den Heuvel, J. K., Verzijl, C. R. C., van de Velde, N. M., Mol, I. M., et al. (2018). Selective glucocorticoid receptor antagonist CORT125281 activates Brown adipose tissue and alters lipid distribution in male mice. Endocrinology 159, 535–546. doi:10.1210/en.2017-00512
Kuo, Y. Y., Lin, J. K., Lin, Y. T., Chen, J. C., Kuo, Y. M., Chen, P. S., et al. (2020). Glibenclamide restores dopaminergic reward circuitry in obese mice through interscauplar Brown adipose tissue. Psychoneuroendocrinology 118, 104712. doi:10.1016/j.psyneuen.2020.104712
Lainscak, M., Pelliccia, F., Rosano, G., Vitale, C., Schiariti, M., Greco, C., et al. (2015). Safety profile of mineralocorticoid receptor antagonists: Spironolactone and eplerenone. Int. J. Cardiol. 200, 25–29. doi:10.1016/j.ijcard.2015.05.127
Landstedt-Hallin, L., Adamson, U., and Lins, P. E. (1999). Oral glibenclamide suppresses glucagon secretion during insulin-induced hypoglycemia in patients with type 2 diabetes. J. Clin. Endocrinol. Metab. 84, 3140–3145. doi:10.1210/jcem.84.9.6002
Lee, J. Y., Lee, M., Lee, J. Y., Bae, J., Shin, E., Lee, Y. H., et al. (2021). Ipragliflozin, an SGLT2 inhibitor, ameliorates high-fat diet-induced metabolic changes by upregulating energy expenditure through activation of the AMPK/SIRT1 pathway. Diabetes Metab. J. 45, 921–932. doi:10.4093/dmj.2020.0187
Lewis, J. D., Ferrara, A., Peng, T., Hedderson, M., Bilker, W. B., Quesenberry, C. P., et al. (2011). Risk of bladder cancer among diabetic patients treated with pioglitazone: Interim report of a longitudinal cohort study. Diabetes Care 34, 916–922. doi:10.2337/dc10-1068
Lin, J. Z., Martagon, A. J., Cimini, S. L., Gonzalez, D. D., Tinkey, D. W., Biter, A., et al. (2015). Pharmacological activation of thyroid hormone receptors elicits a functional conversion of white to Brown fat. Cell Rep. 13, 1528–1537. doi:10.1016/j.celrep.2015.10.022
Liu, S., Shen, S., Yan, Y., Sun, C., Lu, Z., Feng, H., et al. (2022). Triiodothyronine (T3) promotes Brown fat hyperplasia via thyroid hormone receptor α mediated adipocyte progenitor cell proliferation. Nat. Commun. 13, 3394. doi:10.1038/s41467-022-31154-1
Lockie, S. H., Heppner, K. M., Chaudhary, N., Chabenne, J. R., Morgan, D. A., Veyrat-Durebex, C., et al. (2012). Direct control of Brown adipose tissue thermogenesis by central nervous system glucagon-like peptide-1 receptor signaling. Diabetes 61, 2753–2762. doi:10.2337/db11-1556
Loh, R. K. C., Formosa, M. F., Eikelis, N., Bertovic, D. A., Anderson, M. J., Barwood, S. A., et al. (2018). Pioglitazone reduces cold-induced Brown fat glucose uptake despite induction of browning in cultured human adipocytes: A randomised, controlled trial in humans. Diabetologia 61, 220–230. doi:10.1007/s00125-017-4479-9
Lopez, M., Varela, L., Vazquez, M. J., Rodriguez-Cuenca, S., Gonzalez, C. R., Velagapudi, V. R., et al. (2010). Hypothalamic AMPK and fatty acid metabolism mediate thyroid regulation of energy balance. Nat. Med. 16, 1001–1008. doi:10.1038/nm.2207
Luijten, I. H. N., Cannon, B., and Nedergaard, J. (2019). Glucocorticoids and Brown Adipose Tissue: Do glucocorticoids really inhibit thermogenesis? Mol. Asp. Med. 68, 42–59. doi:10.1016/j.mam.2019.07.002
Lv, W., Wang, X., Xu, Q., and Lu, W. (2020). Mechanisms and characteristics of sulfonylureas and glinides. Curr. Top. Med. Chem. 20, 37–56. doi:10.2174/1568026620666191224141617
Martinez-Sanchez, N., Moreno-Navarrete, J. M., Contreras, C., Rial-Pensado, E., Ferno, J., Nogueiras, R., et al. (2017). Thyroid hormones induce browning of white fat. J. Endocrinol. 232, 351–362. doi:10.1530/JOE-16-0425
Marzolla, V., Feraco, A., Gorini, S., Mammi, C., Marrese, C., Mularoni, V., et al. (2020). The novel non-steroidal MR antagonist finerenone improves metabolic parameters in high-fat diet-fed mice and activates Brown adipose tissue via AMPK-ATGL pathway. FASEB J. 34, 12450–12465. doi:10.1096/fj.202000164R
Matsushita, M., Yoneshiro, T., Aita, S., Kameya, T., Sugie, H., and Saito, M. (2014). Impact of Brown adipose tissue on body fatness and glucose metabolism in healthy humans. Int. J. Obes. (Lond). 38, 812–817. doi:10.1038/ijo.2013.206
McLean, B. A., Wong, C. K., Kaur, K. D., Seeley, R. J., and Drucker, D. J. (2021). Differential importance of endothelial and hematopoietic cell GLP-1Rs for cardiometabolic versus hepatic actions of semaglutide. JCI Insight 6, e153732. doi:10.1172/jci.insight.153732
Mikhailov, M. V., Mikhailova, E. A., and Ashcroft, S. J. (2000). Investigation of the molecular assembly of beta-cell K(ATP) channels. FEBS Lett. 482, 59–64. doi:10.1016/s0014-5793(00)02035-4
Min, H. Y., Hwang, J., Choi, Y., and Jo, Y. H. (2022). Overexpressing the hydroxycarboxylic acid receptor 1 in mouse Brown adipose tissue restores glucose tolerance and insulin sensitivity in diet-induced obese mice. Am. J. Physiol. Endocrinol. Metab. 323, E231–E241. doi:10.1152/ajpendo.00084.2022
Miranda, C. S., Silva-Veiga, F., Martins, F. F., Rachid, T. L., Mandarim-De-Lacerda, C. A., and Souza-Mello, V. (2020). PPAR-Alpha activation counters Brown adipose tissue whitening: A comparative study between high-fat- and high-fructose-fed mice. Nutrition 78, 110791. doi:10.1016/j.nut.2020.110791
Monami, M., Dicembrini, I., Martelli, D., and Mannucci, E. (2011). Safety of dipeptidyl peptidase-4 inhibitors: A meta-analysis of randomized clinical trials. Curr. Med. Res. Opin. 27 (3), 57–64. doi:10.1185/03007995.2011.602964
Nedergaard, J., Bengtsson, T., and Cannon, B. (2007). Unexpected evidence for active Brown adipose tissue in adult humans. Am. J. Physiol. Endocrinol. Metab. 293, E444–E452. doi:10.1152/ajpendo.00691.2006
Nesto, R. W., Bell, D., Bonow, R. O., Fonseca, V., Grundy, S. M., Horton, E. S., et al. (2003). Thiazolidinedione use, fluid retention, and congestive heart failure: A consensus statement from the American heart association and American diabetes association. October 7, 2003. Circulation. 108, 2941–2948. doi:10.1161/01.CIR.0000103683.99399.7E
Odegaard, J. I., Ricardo-Gonzalez, R. R., Goforth, M. H., Morel, C. R., Subramanian, V., Mukundan, L., et al. (2007). Macrophage-specific PPARgamma controls alternative activation and improves insulin resistance. Nature 447, 1116–1120. doi:10.1038/nature05894
Ohno, H., Shinoda, K., Spiegelman, B. M., and Kajimura, S. (2012). PPARγ agonists induce a white-to-Brown fat conversion through stabilization of PRDM16 protein. Cell Metab. 15, 395–404. doi:10.1016/j.cmet.2012.01.019
Ozeki, Y., Masaki, T., Kamata, A., Miyamoto, S., Yoshida, Y., Okamoto, M., et al. (2022). The effectiveness of GLP-1 receptor agonist semaglutide on body composition in elderly obese diabetic patients: A pilot study. Med. (Basel) 9, 47. doi:10.3390/medicines9090047
Palmer, B. F., and Clegg, D. J. (2023). Kidney-protective effects of SGLT2 inhibitors. Clin. J. Am. Soc. Nephrol. 18, 279–289. doi:10.2215/CJN.09380822
Pan, R., and Chen, Y. (2022). Latest advancements on combating obesity by targeting human Brown/beige adipose tissues. Front. Endocrinol. (Lausanne) 13, 884944. doi:10.3389/fendo.2022.884944
Pan, R., Zhu, X., Maretich, P., and Chen, Y. (2020). Combating obesity with thermogenic fat: Current challenges and advancements. Front. Endocrinol. (Lausanne) 11, 185. doi:10.3389/fendo.2020.00185
Pfeifer, M. A., Halter, J. B., Judzewitsch, R. G., Beard, J. C., Best, J. D., Ward, W. K., et al. (1984). Acute and chronic effects of sulfonylurea drugs on pancreatic islet function in man. Diabetes Care 7 (1), 25–34.
Poggioli, R., Ueta, C. B., Drigo, R. A., Castillo, M., Fonseca, T. L., and Bianco, A. C. (2013). Dexamethasone reduces energy expenditure and increases susceptibility to diet-induced obesity in mice. Obes. (Silver Spring) 21, E415–E420. doi:10.1002/oby.20338
Qiang, L., Wang, L., Kon, N., Zhao, W., Lee, S., Zhang, Y., et al. (2012). Brown remodeling of white adipose tissue by SirT1-dependent deacetylation of Pparγ. Cell 150, 620–632. doi:10.1016/j.cell.2012.06.027
Rachid, T. L., Penna-de-Carvalho, A., Bringhenti, I., Aguila, M. B., Mandarim-de-Lacerda, C. A., and Souza-Mello, V. (2015b). PPAR-alpha agonist elicits metabolically active Brown adipocytes and weight loss in diet-induced obese mice. Cell Biochem. Funct. 33, 249–256. doi:10.1002/cbf.3111
Rachid, T. L., Penna-de-Carvalho, A., Bringhenti, I., Aguila, M. B., Mandarim-de-Lacerda, C. A., and Souza-Mello, V. (2015a). Fenofibrate (PPARalpha agonist) induces beige cell formation in subcutaneous white adipose tissue from diet-induced male obese mice. Mol. Cell Endocrinol. 402, 86–94. doi:10.1016/j.mce.2014.12.027
Ribeiro, M. O., Carvalho, S. D., Schultz, J. J., Chiellini, G., Scanlan, T. S., Bianco, A. C., et al. (2001). Thyroid hormone-sympathetic interaction and adaptive thermogenesis are thyroid hormone receptor isoform-specific. J. Clin. Invest. 108, 97–105. doi:10.1172/JCI12584
Robichaux, W. G., and Cheng, X. (2018). Intracellular cAMP sensor EPAC: Physiology, pathophysiology, and therapeutics development. Physiol. Rev. 98, 919–1053. doi:10.1152/physrev.00025.2017
Rogue, A., Spire, C., Brun, M., Claude, N., and Guillouzo, A. (2010). Gene expression changes induced by PPAR gamma agonists in animal and human liver. PPAR Res. 2010, 325183. doi:10.1155/2010/325183
Rosen, E. D., and Spiegelman, B. M. (2000). Peroxisome proliferator-activated receptor gamma ligands and atherosclerosis: Ending the heartache. J. Clin. Invest. 106, 629–631. doi:10.1172/JCI10909
Schepkens, H., Vanholder, R., Billiouw, J. M., and Lameire, N. (2001). Life-threatening hyperkalemia during combined therapy with angiotensin-converting enzyme inhibitors and spironolactone: An analysis of 25 cases. Am. J. Med. 110, 438–441. doi:10.1016/s0002-9343(01)00642-8
Scotney, H., Symonds, M. E., Law, J., Budge, H., Sharkey, D., and Manolopoulos, K. N. (2017). Glucocorticoids modulate human Brown adipose tissue thermogenesis in vivo. Metabolism 70, 125–132. doi:10.1016/j.metabol.2017.01.024
Seino, S., Sugawara, K., Yokoi, N., and Takahashi, H. (2017). β-Cell signalling and insulin secretagogues: A path for improved diabetes therapy. Diabetes Obes. Metab. 19 (1), 22–29. doi:10.1111/dom.12995
Seufert, J., and Gallwitz, B. (2014). The extra-pancreatic effects of GLP-1 receptor agonists: A focus on the cardiovascular, gastrointestinal and central nervous systems. Diabetes Obes. Metab. 16, 673–688. doi:10.1111/dom.12251
Shibasaki, T., Takahashi, H., Miki, T., Sunaga, Y., Matsumura, K., Yamanaka, M., et al. (2007). Essential role of Epac2/Rap1 signaling in regulation of insulin granule dynamics by cAMP. Proc. Natl. Acad. Sci. U. S. A. 104, 19333–19338. doi:10.1073/pnas.0707054104
Sohn, J. H., Kim, J. I., Jeon, Y. G., Park, J., and Kim, J. B. (2018). Effects of three Thiazolidinediones on metabolic regulation and cold-induced thermogenesis. Mol. Cells 41, 900–908. doi:10.14348/molcells.2018.0294
Sola, D., Rossi, L., Schianca, G. P., Maffioli, P., Bigliocca, M., Mella, R., et al. (2015). Sulfonylureas and their use in clinical practice. Arch. Med. Sci. 11, 840–848. doi:10.5114/aoms.2015.53304
Svensson, P. A., Lindberg, K., Hoffmann, J. M., Taube, M., Pereira, M. J., Mohsen-Kanson, T., et al. (2014). Characterization of Brown adipose tissue in the human perirenal depot. Obes. (Silver Spring) 22, 1830–1837. doi:10.1002/oby.20765
ter Braak, E. W., Appelman, A. M., van der Tweel, I., Erkelens, D. W., and van Haeften, T. W. (2002). The sulfonylurea glyburide induces impairment of glucagon and growth hormone responses during mild insulin-induced hypoglycemia. Diabetes Care 25, 107–112. doi:10.2337/diacare.25.1.107
Thomou, T., Mori, M. A., Dreyfuss, J. M., Konishi, M., Sakaguchi, M., Wolfrum, C., et al. (2017). Adipose-derived circulating miRNAs regulate gene expression in other tissues. Nature 542, 450–455. doi:10.1038/nature21365
Thuzar, M., Law, W. P., Dimeski, G., Stowasser, M., and Ho, K. K. Y. (2019). Mineralocorticoid antagonism enhances Brown adipose tissue function in humans: A randomized placebo-controlled cross-over study. Diabetes Obes. Metab. 21, 509–516. doi:10.1111/dom.13539
Thuzar, M., Law, W. P., Ratnasingam, J., Jang, C., Dimeski, G., and Ho, K. K. Y. (2018). Glucocorticoids suppress Brown adipose tissue function in humans: A double-blind placebo-controlled study. Diabetes Obes. Metab. 20, 840–848. doi:10.1111/dom.13157
van den Heuvel, J. K., Boon, M. R., van Hengel, I., Peschier-van der Put, E., van Beek, L., van Harmelen, V., et al. (2016). Identification of a selective glucocorticoid receptor modulator that prevents both diet-induced obesity and inflammation. Br. J. Pharmacol. 173, 1793–1804. doi:10.1111/bph.13477
Villarroya, J., Cereijo, R., Gavalda-Navarro, A., Peyrou, M., Giralt, M., and Villarroya, F. (2019). New insights into the secretory functions of Brown adipose tissue. J. Endocrinol. 243, R19–R27. doi:10.1530/JOE-19-0295
Wu, D., Eeda, V., Undi, R. B., Mann, S., Stout, M., Lim, H. Y., et al. (2021). A novel peroxisome proliferator-activated receptor gamma ligand improves insulin sensitivity and promotes browning of white adipose tissue in obese mice. Mol. Metab. 54, 101363. doi:10.1016/j.molmet.2021.101363
Xie, Y., Bowe, B., Gibson, A. K., McGill, J. B., Maddukuri, G., Yan, Y., et al. (2020). Comparative effectiveness of SGLT2 inhibitors, GLP-1 receptor agonists, DPP-4 inhibitors, and sulfonylureas on risk of kidney outcomes: Emulation of a target trial using health care databases. Diabetes Care 43, 2859–2869. doi:10.2337/dc20-1890
Xu, L., Xu, C., Liu, X., Li, X., Li, T., Yu, X., et al. (2021). Empagliflozin induces white adipocyte browning and modulates mitochondrial dynamics in KK cg-ay/J mice and mouse adipocytes. Front. Physiol. 12, 745058. doi:10.3389/fphys.2021.745058
Yang, J., de Vries, H. D., Mayeuf-Louchart, A., Stroeve, J. H., Bloks, V. W., Koehorst, M., et al. (2023). Role of bile acid receptor FXR in development and function of Brown adipose tissue. Biochim. Biophys. Acta Mol. Cell Biol. Lipids 1868, 159257. doi:10.1016/j.bbalip.2022.159257
Yang, Q., Liang, X., Sun, X., Zhang, L., Fu, X., Rogers, C. J., et al. (2016). AMPK/α-Ketoglutarate Axis dynamically mediates DNA demethylation in the Prdm16 promoter and Brown adipogenesis. Cell Metab. 24, 542–554. doi:10.1016/j.cmet.2016.08.010
Yang, X., Liu, Q., Li, Y., Ding, Y., Zhao, Y., Tang, Q., et al. (2021). Inhibition of the sodium-glucose co-transporter SGLT2 by canagliflozin ameliorates diet-induced obesity by increasing intra-adipose sympathetic innervation. Br. J. Pharmacol. 178, 1756–1771. doi:10.1111/bph.15381
Zekri, Y., Guyot, R., Suner, I. G., Canaple, L., Stein, A. G., Petit, J. V., et al. (2022). Brown adipocytes local response to thyroid hormone is required for adaptive thermogenesis in adult male mice. Elife 11, e81996. doi:10.7554/eLife.81996
Zhang, E., Jin, L., Wang, Y., Tu, J., Zheng, R., Ding, L., et al. (2022a). Intestinal AMPK modulation of microbiota mediates crosstalk with Brown fat to control thermogenesis. Nat. Commun. 13, 1135. doi:10.1038/s41467-022-28743-5
Zhang, X., Luo, S., Wang, M., Cao, Q., Zhang, Z., Huang, Q., et al. (2022b). Differential IL18 signaling via IL18 receptor and Na-Cl co-transporter discriminating thermogenesis and glucose metabolism regulation. Nat. Commun. 13, 7582. doi:10.1038/s41467-022-35256-8
Zhang, Y., Cai, Y., Zhang, H., Zhang, J., Zeng, Y., Fan, C., et al. (2021). Brown adipose tissue transplantation ameliorates diabetic nephropathy through the miR-30b pathway by targeting Runx1. Metabolism 125, 154916. doi:10.1016/j.metabol.2021.154916
Zhao, X., Wang, M., Wen, Z., Lu, Z., Cui, L., Fu, C., et al. (2021). GLP-1 receptor agonists: Beyond their pancreatic effects. Front. Endocrinol. (Lausanne) 12, 721135. doi:10.3389/fendo.2021.721135
Keywords: diabetes, obesity, brown adipose tissue, beige adipose tissue, receptor
Citation: Pan R, Liu J and Chen Y (2023) Treatment of obesity-related diabetes: significance of thermogenic adipose tissue and targetable receptors. Front. Pharmacol. 14:1144918. doi: 10.3389/fphar.2023.1144918
Received: 15 January 2023; Accepted: 12 June 2023;
Published: 26 June 2023.
Edited by:
Keizo Kanasaki, Shimane University, JapanReviewed by:
Hong-Ping Guan, Rezubio Pharmaceuticals Co. Ltd., ChinaCopyright © 2023 Pan, Liu and Chen. This is an open-access article distributed under the terms of the Creative Commons Attribution License (CC BY). The use, distribution or reproduction in other forums is permitted, provided the original author(s) and the copyright owner(s) are credited and that the original publication in this journal is cited, in accordance with accepted academic practice. No use, distribution or reproduction is permitted which does not comply with these terms.
*Correspondence: Yong Chen, dGoueS5jaGVuQHZpcC4xNjMuY29t
Disclaimer: All claims expressed in this article are solely those of the authors and do not necessarily represent those of their affiliated organizations, or those of the publisher, the editors and the reviewers. Any product that may be evaluated in this article or claim that may be made by its manufacturer is not guaranteed or endorsed by the publisher.
Research integrity at Frontiers
Learn more about the work of our research integrity team to safeguard the quality of each article we publish.